Two-Tailed RT-qPCR for the Quantification of A-to-I-Edited microRNA Isoforms
Gjendine Voss, Gjendine Voss, Yvonne Ceder, Yvonne Ceder
Abstract
MicroRNAs are short non-coding RNAs with important functions in the regulation of gene expression in healthy and diseased tissues. To optimally utilize the biological and clinical information that is contained in microRNA expression levels, tools for their accurate and cost-effective quantification are needed. While the standard method, qPCR, allows for quick and cheap microRNA quantification, specificity is limited due to the short lengths of microRNAs and the high similarity between closely related microRNA family members. A-to-I editing can further diversify the microRNA pool by altering individual nucleotides. There is currently a lack of protocols for the accurate quantification of A-to-I-edited microRNA isoforms using qPCR. Here, we describe a protocol to quantify microRNA editing isoforms using two-tailed RT-qPCR, with either SYBR Green or hydrolysis probes. The user will perform reverse transcription of RNA samples, generate standard curves, and quantify the resulting cDNA in the following qPCR step. We also give guidelines for primer design and for the evaluation of assays using synthetic oligonucleotides. These tools are expected to be transferable to any A-to-I-edited microRNA and its isoforms. © 2023 The Authors. Current Protocols published by Wiley Periodicals LLC.
Basic Protocol 1 : Two-tailed reverse transcription of A-to-I-edited microRNAs
Basic Protocol 2 : SYBR Green-based qPCR for A-to-I-edited microRNAs
Alternate Protocol : Hydrolysis probe-based qPCR for A-to-I-edited microRNAs
Support Protocol : Preparation of standard curves using synthetic RNA oligonucleotides
INTRODUCTION
MicroRNAs are a class of small RNAs found in animals and plants. Thousands of putative microRNAs have been described and linked to many biological functions. Each one of these short RNA molecules regulates the stability and translation rate of hundreds of mRNAs in the cell and can therefore fine-tune a multitude of biological pathways (Ameres & Zamore, 2013). The deregulation of microRNAs contributes to disease and conversely, the dysregulation of disease-associated biological mechanisms can lead to the de-regulation of microRNAs (Condrat et al., 2020). This association between microRNAs and disease, together with the remarkable stability of microRNAs in biological fluids and tissues (Hui et al., 2009; Mitchell et al., 2008), has contributed to substantial interest in microRNAs throughout the biomarker research community (Condrat et al., 2020). Indeed, with thousands of new publications every year, microRNAs are one of the most widely researched groups of biomarker candidates.
In order for a biomarker to be truly useful in the clinic, there is a need for reliable methods to quantify its abundance. Cost-effective methods based on reverse transcription (RT) and quantitative PCR (qPCR) for the quantification of microRNAs have been widely available for a number of years now. These methods, however, cannot properly distinguish between different isoforms of microRNAs, which often differ by only one nucleotide (Magee, Telonis, Cherlin, Rigoutsos, & Londin, 2017; Nejad et al., 2018; Schamberger & Orbán, 2014; Voss, Edsjo, Bjartell, & Ceder, 2021; Wu et al., 2007). For example, adenosine deaminase acting on RNA (ADAR) enzymes convert one or several adenosines to inosines, a process that happens in virtually all human tissues, and can be associated with disease (Fritzell, Xu, Lagergren, & Öhman, 2018; Nishikura, 2016; Tan et al., 2017). Over 1000 putative editing sites in mature microRNAs have been described, and a further 1800 in pri-miRNAs and pre-miRNAs (Marceca et al., 2021). Although the majority of these editing sites have not yet been functionally characterized, A-to-I editing of microRNAs has been shown to affect their biological functions both by altering their maturation rates and their target preferences (Kawahara et al., 2007, 2008), making it key to develop methods that allow to properly detect and quantify these isoforms. With this in mind, we have recently developed a new method for qPCR quantification of A-to-I-edited microRNAs based on two-tailed RT-qPCR (Voss et al., 2021). Two-tailed RT-qPCR is a method that was recently developed by Androvic and colleagues, and makes use of a microRNA-specific RT primer containing two shorter hemiprobes, rather than one longer primer (Androvic, Valihrach, Elling, Sjoback, & Kubista, 2017). Even a single mismatch would considerably perturb the relatively short helix formed by each hemiprobe, compared to the more stable structure formed by a longer continuous primer, which can otherwise compensate for individual mismatches in the primer binding site. Two-tailed RT-qPCR has been shown to be superior to other qPCR methods in distinguishing microRNAs with highly similar sequences. Compared to approaches that use a universal primer or adapter in the RT step, rather than a microRNA-specific primer, two-tailed RT-qPCR performed much better in a direct comparison (Androvic et al., 2017).
Here, we describe a collection of protocols for using two-tailed RT-qPCR for the detection and quantification of A-to-I-edited microRNAs (Fig. 1). In the Strategic Planning section, we also discuss considerations for primer design. Primers can be designed to be either specific to A-to-I-edited isoforms, or to be purposefully isoform-blind to perform unbiased quantification of a microRNA in all its isoforms. Basic Protocol 1 describes the two-tailed RT step that produces a cDNA product. In this protocol, a microRNA-specific RT primer is used to prompt the RT of the target microRNA. The cDNA product can be quantified in two different ways, namely, using SYBR Green as a fluorescent marker for amplification (Basic Protocol 2), or using hydrolysis probes (Alternate Protocol). The advantages and disadvantages of each approach are discussed in the Commentary section, so that the user can decide which protocol best suits their specific needs. For applications in which absolute numbers of molecules are needed, e.g. for the calculation of the editing frequency of a microRNA, a standard curve needs to be run in parallel. The Support Protocol describes the preparation of such a standard curve using synthetic RNA oligonucleotides. Overall, this collection of protocols supports users with access to basic molecular biology equipment in quantifying any microRNA in a manner that is sensitive to the A-to-I editing status of the microRNA.
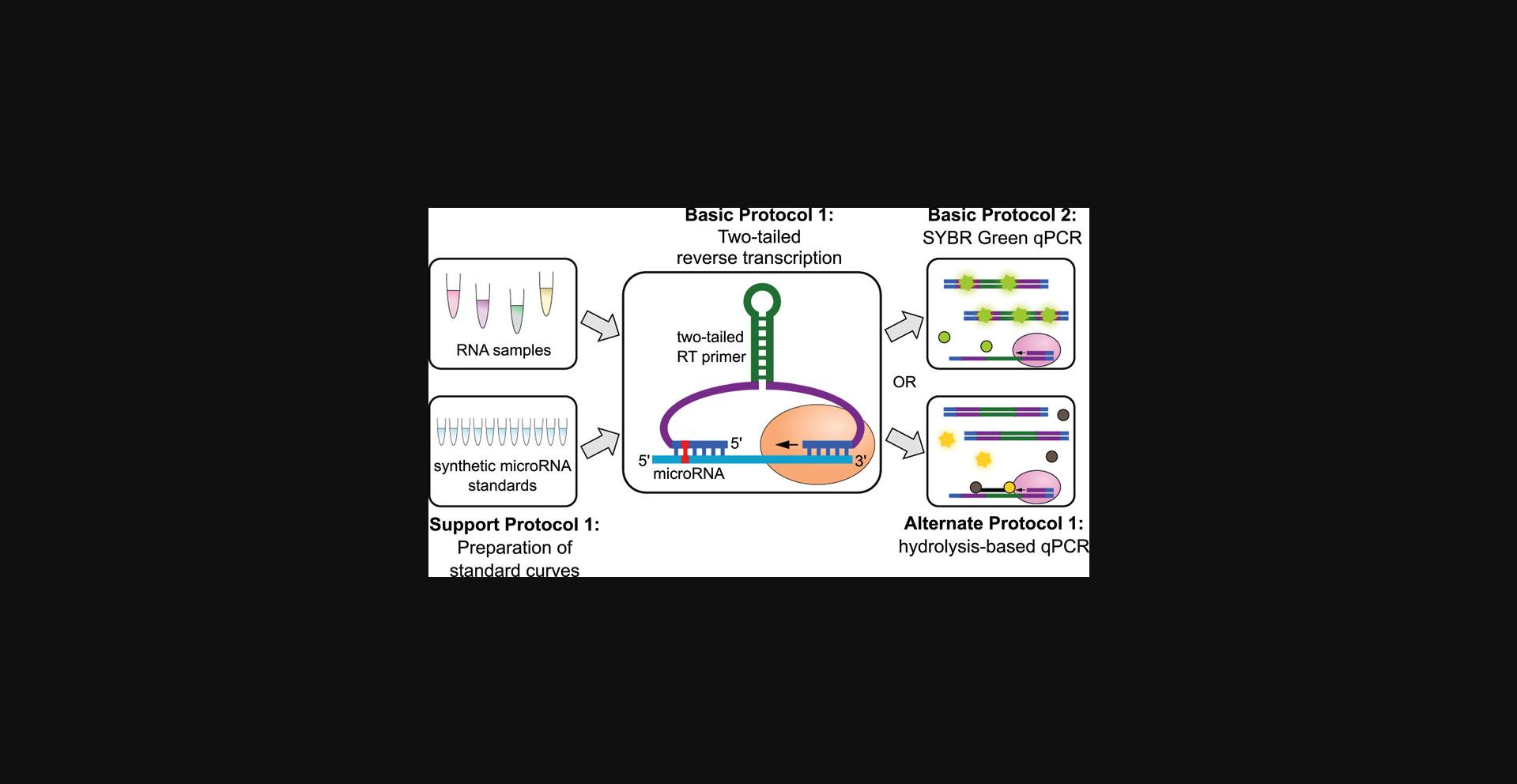
STRATEGIC PLANNING
The protocols described here assume that the user already has RNA samples of interest prepared. We recommend isolating the RNA with a method that will efficiently disrupt RNA-protein complexes, such as guanidinium thiocyanate-phenol-chloroform extraction (Chomczynski & Sacchi, 1987). This is to ensure that interactions between microRNAs and protein complexes will be abolished as much as possible, as sequestering by AGO and other proteins can mask the microRNA and prevent accurate quantification (Bryzgunova, Konoshenko, Zaporozhchenko, Yakovlev, & Laktionov, 2021). Depending on the user's needs and sample availability, both total RNA samples and samples enriched for small RNAs are suitable. When using total RNA, 100 to 500 ng (depending on the microRNA of interest) will be needed, whereas as little as 10 to 20 ng of the small RNA fraction can be sufficient. Importantly, if the original sample from which small RNAs were enriched was of poor quality and already contained high amounts of degraded RNA, the small RNA fraction will contain these fragments of degraded RNA, effectively diluting the microRNAs (Ku et al., 2021). It is therefore important to limit RNA degradation at every step of the way from sample acquisition to microRNA quantification (Armstrong et al., 2018), even though the microRNAs themselves are typically protected by interactions with proteins and therefore relatively stable in biological fluids until RNA extraction (Bryzgunova et al., 2021; Hui et al., 2009; Mitchell et al., 2008). If RNA degradation of longer RNAs has occurred, higher amounts of RNA input will be needed even when using samples that underwent short RNA enrichment. After RNA extraction, even small RNA samples will need to be handled with care to prevent degradation, as the microRNAs will be more sensitive to RNA degradation by RNases or alkaline hydrolysis after they are removed from their protective protein binding partners.
Pre-designed two-tailed probes for edited microRNAs are not currently commercially available. Therefore, users will likely have to design their own probes and primers. As depicted in Figure 2A, the two-tailed RT-qPCR primer consists of a stem-loop structure in the middle, flanked by two primer arms, with the hemiprobes that bind to the 5’ and 3’ end of the microRNA on the 5ʹ and 3ʹ end of the RT primer, respectively. For the design of a two-tailed RT-qPCR primer, we suggest starting with an already published sequence for the stem-loop and primer arms (Androvic et al., 2017; Smieszek et al., 2019; Voss et al., 2021) (purple and green in Fig. 2A). After this, the user should design hemiprobes that match their microRNA of interest (blue in Fig. 2A). In our hands, a 5ʹ hemiprobe with a length of 6 nt and a 3ʹ hemiprobe with a length of 5 nt has proved to be optimal (Voss et al., 2021). In case of a particularly high or low GC content, shorter or longer hemiprobes may be appropriate.
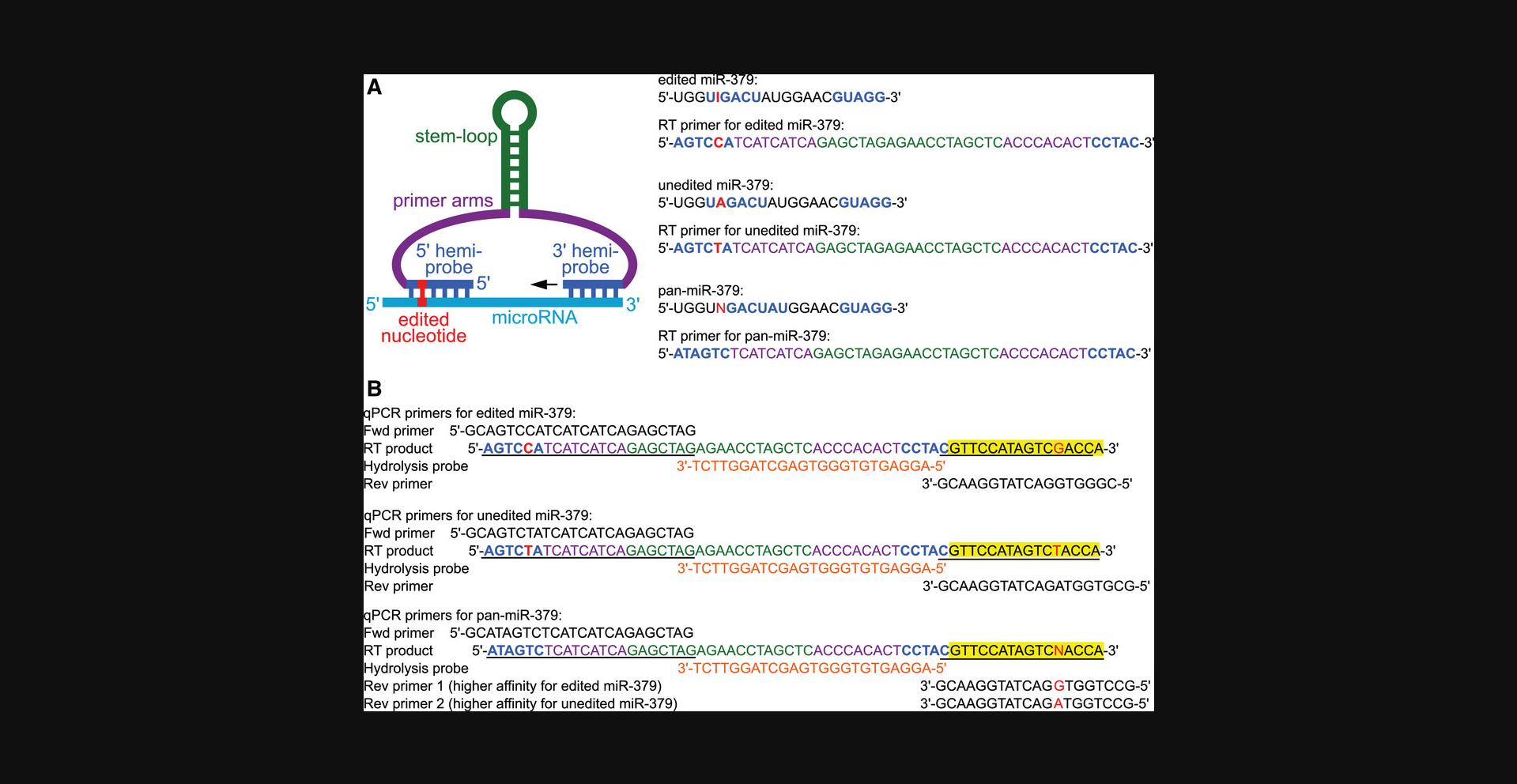
To design an editing-blind assay, neither hemiprobe should bind in an area that contains an editable nucleotide (example: pan-miR-379 in Fig. 2A). In contrast, when designing an assay that should be specific for one editing isoform, it is crucial that one of the hemiprobes covers the edited nucleotide(s) (examples: edited and unedited miR-379 in Fig. 2A). In our hands, the position of the edited nucleotide within the hemiprobe binding site did not have a significant impact on the amplification efficiency or specificity (Voss et al., 2021), but this may vary for each microRNA. For microRNAs that are part of a larger microRNA family, one should compare sequences of closely related microRNAs, which can differ by as little as one or two nucleotides (Androvic et al., 2017). It should be ensured that one or both hemiprobes cover a nucleotide that is unique for the microRNA of interest.
After designing hemiprobes to add to the ends of the primer arms, it is important to ascertain that no secondary structures other than the central stem-loop can form with melting temperatures above room temperature, at which RT will be carried out. Different online tools are available for this (e.g., OligoAnalyzer Tool, https://www.idtdna.com/pages/tools/oligoanalyzer). If undesired secondary structures are predicted, the simplest solution will be to change the nucleotides in the primer arms.
Primers for qPCR are then designed for the predicted RT product (which can be attained by extending the 3ʹ end of the RT primer with the reverse complement of the microRNA of interest), as shown in Figure 2B. Forward and reverse primers should have a similar melting temperature (approximately 55 to 65°C) and should not form significant secondary structures or homo- or heterodimers with high melting temperatures. When designing assays for isoform-blind pan-microRNA analysis, multiple reverse primers will be needed for the different isoforms, and a mixture should be used for qPCR. Due to slight differences in affinity, the ratio of the reverse primers to one another should be optimized to ensure that all isoforms are amplified equally well (Voss et al., 2021).
For users wanting to use hydrolysis-based qPCR (Alternate Protocol), a custom hydrolysis probe is needed as well. The hydrolysis probe is placed in between the two qPCR primers, leaving a gap of at least 1 nt between the hydrolysis probe and the primer, so that primer extension is not blocked by the probe (Fig. 2B). The melting temperature of the hydrolysis probe should be approximately 6 to 8°C higher than that of the primers. If the length of the hemiprobe cannot be extended to reach a high enough melting temperature due to either a low GC content of the sequence or space limitations between the primer binding sites, the qPCR primers can be shortened in order to reach a sufficiently high difference between the melting temperatures. Online tools can aid in the design of the hydrolysis probe (e.g., PrimerQuest Tool, https://www.idtdna.com/pages/tools/primerquest), and most manufacturers of custom hydrolysis probes also offer customer support to assist with the design. It should be ensured that the hydrolysis probe does not form secondary structures or homodimers with melting temperatures higher than the annealing temperature in the qPCR (usually 60°C), or significant heterodimers with the qPCR primers.
Whether the user prefers to design one set of primers or several sets to test different placements of the hemiprobes along the microRNA, we recommend a sequence of pilot experiments using only synthetic RNA oligonucleotides at first (Table 1). These RNA oligonucleotides should be single-stranded unmodified short RNAs, dissolved in a nuclease-free buffer such as IDTE (Integrated DNA Technologies). When testing assays for edited microRNAs, it is important to include inosine, and not replace it with guanosine, which has slightly different base pairing properties (Wright, Force, & Znosko, 2018). Manufacturers such as Integrated DNA Technologies can supply users with RNA oligonucleotides that fulfill these requirements. The optimization experiments will ensure that the primers can efficiently amplify the microRNA of interest and that they are specific for the desired editing isoform(s). If any of the validation steps fail, new primer sets should be designed or qPCR parameters (such as primer concentrations or annealing temperatures) optimized, and the entire validation procedure should be repeated with the new primers and parameters.
Validation phase & aim | Experiment |
---|---|
1. Can the primers amplify the microRNA of interest? | Perform two-tailed RT-qPCR using one or two concentrations of synthetic RNA oligonucleotides of the target microRNA isoform(s) and evaluate the Cq values. For guidance, any primer sets that amplify 106 molecules of microRNA with a Cq < 30 could be considered useful. |
2a. For editing isoform-specific assays: Is the primer set specific for the isoform of interest? | Perform two-tailed RT-qPCR using one or two concentrations of synthetic RNA oligonucleotides of all isoforms and compare Cq values. For guidance, if the amplification of the non-target isoform(s) has a Cq value that is at least 7 units higher than that of the target isoform at the same concentration (this corresponds to a relative detection of less than 0.8%), the primer set could be considered useful. |
2b. For editing isoform-blind pan-assays: Does the primer set amplify all isoforms equally well? | Perform two-tailed RT-qPCR using three or four different concentrations (e.g., 103, 106, 109) of synthetic RNA oligonucleotides of all target microRNA isoforms in separate reactions and compare Cq values. The Cq values of the different isoforms at the same input concentration should be as close to each other as possible. The average difference of Cq values should be 0, which means that there is no bias toward one or the other isoform. It may be useful to optimize the ratios of the different reverse primers to one another at this step. For guidance, if the Cq values at the same concentration differ by no more than 0.5 units, the primer set could be considered useful. |
3. Is there cross-detection of closely related microRNAs from the same microRNA family? | Perform two-tailed RT-qPCR using one or two concentrations of synthetic RNA oligonucleotides of all closely related microRNAs and their isoforms. Ideally, there should be no amplification of the non-target microRNAs. If there is amplification of other family members, the Cq values and the Cq difference compared to that of the target microRNA should be as high as possible. |
4. Does the primer set reach sufficient sensitivity and dynamic range? Is the PCR efficiency near 100%? |
Perform two-tailed RT-qPCR using an entire standard curve of synthetic oligonucleotides of the target microRNA isoform(s). The dynamic range (in which the relationship between the log10 concentration and the Cq value is linear) should be as wide as possible. For guidance, a sensitivity of 102 or 103 molecules and a dynamic range up to 109 or 1010 is very good. Depending on typical microRNA isoform expression levels, a narrower range may be acceptable. Calculate the PCR efficiency from the slope. PCR efficiencies around 85–115% are acceptable. |
5. Does the primer set perform well in a mixture of RNAs? | Perform the same experiment as in validation phase 4, but add 100 ng of yeast total RNA (which does not contain endogenous microRNAs). The resulting standard curve should meet the same requirements as in step 4. |
Depending on the desired outcome, users should consider which and how many microRNAs to quantify. Some possibilities include absolute numbers of a microRNA, editing ratios of a microRNA, and relative expression of a microRNA. To obtain absolute numbers, a standard curve with known concentrations must be created. The Support Protocol describes how to prepare the standard samples; they should be run in parallel with the samples of interest throughout Basic Protocol 1 and Basic Protocol 2 (SYBR Green quantification) or Alternate Protocol (hydrolysis-based quantification). For calculating editing ratios of a microRNA (the fraction of a given microRNA that is edited or unedited), separate reactions will need to be prepared with primers specific for each of the microRNA's editing isoforms. Absolute numbers of each microRNA are needed for this as well, so that standard curves will need to be run for each isoform. In contrast, if the user wants to obtain the relative expression of samples, reference genes should be run on the same samples to normalize the expression. For microRNAs, other small RNAs will typically be used, such as short nucleolar RNAs (snoRNAs) or other microRNAs. Which reference RNAs (or so-called “housekeeping genes”) should be used will depend on the sample type, the cell or tissue type, and the organism in question. We generally recommend using a panel of several reference genes, and to use the geometric mean of them for normalization; and to validate the set of references that will be used on that specific sample set. We refer the users to helpful resources that can assist in the choice of reference genes (Mestdagh et al., 2009; Peltier & Latham, 2008; Taylor et al., 2019; Vandesompele et al., 2002).
Running a large number of samples and using multiple targets and/or reference genes with multiple replicates may quickly exceed the number of wells available on the qPCR plate. In such cases, running all samples and replicates of a given target should be given priority (Taylor et al., 2019), whereas the separate targets can be run on different days. In principle, the different targets can even be run with a different RT-qPCR method, as long as all the different samples that will be compared to one another are run at the same time and with the same method for any given target. For example, it is acceptable to quantify the microRNA of interest (which may have many similar microRNA family members or editing isoforms and therefore needs to be quantified with two-tailed RT-qPCR) using SYBR Green, and to use snoRNAs quantified with commercially available TaqMan probes as housekeeping genes (Voss et al., 2021). Taking these considerations into account, users should determine beforehand how many and which targets and references should be run to enable the required analysis.
Basic Protocol 1: TWO-TAILED REVERSE TRANSCRIPTION OF A-TO-I-EDITED microRNAs
In this Basic Protocol, the procedures for the specific reverse transcription (RT) of microRNAs with a two-tailed primer are described. The user will dilute the previously extracted RNA samples to the required concentration and prepare a master mix containing all reagents needed for RT, including a microRNA-specific primer. Design guidelines for this are provided in the Strategic Planning section. Consider which primers and samples to use; if absolute numbers are desired, a standard curve (Support Protocol) of the target microRNA will be required. If editing isoform-specificity is important, a standard curve of the non-target microRNA should also be run in order to later calculate the relative detection of the non-target isoform. If editing frequencies of a microRNA (i.e., the proportion of a given microRNA pool that is edited) are to be calculated, assays for all editing isoforms will need to be run on the same sample in separate reactions. If the user is interested in relative expression of the microRNA, reference genes will need to be selected (see Strategic Planning) and run in parallel in separate reactions using the same biological sample. Multiplexing of two-tailed assays for different microRNAs in the RT step is possible in principle (Androvic et al., 2017), but we recommend carefully testing this in each particular case. Reactions for different isoforms of one microRNA should be performed separately, since the sequences are too similar to multiplex the RT step.
The reaction mix and RNA samples will be combined in PCR strips and incubated according to the described reaction program (duration: 65 min) to obtain cDNA.
The RT products should not be frozen and thawed before continuing with qPCR. We recommend that users keep the reactions at 4°C or on ice after completion and proceed to qPCR within hours. At most, the cDNA samples can be kept at 4°C overnight.
Materials
-
RNA samples
-
Nuclease-free water (Invitrogen, cat. no. 10977-035 or equivalent)
-
Synthetic RNA oligonucleotides standards (optional; for preparation of standard curves according to the Support Protocol)
-
qScript flex cDNA synthesis kit (QuantaBio, cat. no. 95049-100)
-
1 µM two-tailed reverse transcription primer for each target and reference of interest (custom DNA oligonucleotide, e.g., from Invitrogen), see Strategic Planning and example in Figure 2A
-
IDTE pH 7.5, 1× TE Solution (Integrated DNA Technologies, cat. no. 11-05-01-15)
-
1.5 ml microcentrifuge tubes (Sarstedt, cat. no. 72.690.001 or equivalent)
-
0.2 ml PCR tubes, strips or individual (Sarstedt, cat. no. 72.991.102 or equivalent)
-
Pipettes and filter tips (nuclease/DNA/RNA-free)
-
Ice bucket
-
Vortexer
-
Benchtop mini centrifuge
-
Thermocycler
1.Thaw RNA samples and reagents on ice. The following steps should be performed on ice as well.
2.Dilute all samples to the same desired concentration with nuclease-free water.
3.(Optional) Prepare standard dilutions for each target microRNA (Support Protocol) that will be assayed.
4.Using reagents from the qScript flex cDNA synthesis kit, prepare a RT reaction master mix by combining 5 µl water, 2 µl 5× reaction mix, 1 µl GSP enhancer, 0.5 µl two-tailed RT primer, and 0.5 µl RT enzyme per sample in a 1.5 ml microcentrifuge tube. Vortex thoroughly.
5.Distribute the master mix into 0.2 ml PCR tubes, adding 9 µl of master mix to each tube.
6.Add 1 µl of sample or standard to the prepared reaction mix. Include a “no template” negative control to which only IDTE or water is added.
7.Mix the reaction mixtures by flicking the closed tubes. Spin down in a benchtop mini centrifuge.
8.Place the RT reactions in a thermocycler and incubate for 1 hr at 25°C, then for 5 min at 85°C.
9.Cool the samples at 4°C or place them on ice after the reaction has finished.
10.Proceed immediately to either Basic Protocol 2 for SYBR-Green-based qPCR, or to the Alternate Protocol for hydrolysis probe-based qPCR.
Basic Protocol 2: SYBR GREEN-BASED qPCR FOR A-TO-I-EDITED microRNAs
This basic protocol describes qPCR of the microRNA target reverse-transcribed in Basic Protocol 1, using SYBR Green as a fluorescent marker. The cDNA products from Basic Protocol 1 are diluted and a reaction master mix is prepared. The diluted cDNA products and the master mix are combined in multiple replicates in 384-well plates and qPCR is performed. We recommend performing qPCR reactions for each RT product in triplicate. For microRNAs with extremely low abundance, quadruplicates are advisable, as the stochastic nature of the amplification process in early cycles will have a stronger impact in samples with few target molecules, resulting in a larger variability of the end result, the so-called Monte Carlo effect (Bustin & Nolan, 2004; Karrer et al., 1995; Taylor et al., 2019). The data generated in this protocol is then used to quantify the absolute numbers and/or relative expression of the target microRNA(s).
Materials
-
cDNA sample from Basic Protocol 1
-
Nuclease-free water (Invitrogen, cat. no. 10977-035 or equivalent)
-
PowerUp SYBR Green Master Mix (Applied Biosystems, cat. no. A25742)
-
10 µM forward and reverse qPCR primers for each target and reference molecule of interest (custom DNA oligonucleotides e.g., from Invitrogen), see Strategic Planning and example in Figure 2B
-
Agarose
-
TBE buffer (Appendix 2 ; Common Buffers, Media, and Stock Solutions; Current Protocols in Human Genetics, 2000)
-
DNA loading dye (Thermo Scientific, cat. no. R0621 or equivalent)
-
Low-range DNA ladder (Thermo Scientific, cat. no. SM0383 or equivalent)
-
Nucleic acid gel stain (Biotium, cat. no. 41003 or equivalent)
-
1.5 ml microcentrifuge tubes (Sarstedt, cat. no. 72.690.001 or equivalent)
-
Optical PCR-grade 384-well plates (Applied Biosystems, cat. no. 4309849 or equivalent)
-
Optical adhesive film (Applied Biosystems, cat. no. 4311971 or equivalent)
-
Pipettes and filter tips (nuclease/DNA/RNA-free)
-
Ice bucket
-
Benchtop mini centrifuge
-
Vortexer
-
Adhesive film applicator
-
Benchtop plate spinner
-
qPCR machine (Applied Biosystems QuantStudio 7 Flex or equivalent)
-
Gel electrophoresis apparatus
-
UV transilluminator
1.Thaw the reagents on ice. The following steps, until loading the qPCR plate, should be performed on ice.
2.Dilute the cDNA 1/2 by adding 10 µl of nuclease-free water to each product. Mix by flicking the tubes and spin down in a benchtop mini centrifuge.
3.Prepare a qPCR reaction master mix by combining 5 µl of PowerUp SYBR Green Master Mix, 0.4 µl of 10 µM forward primer, 0.4 µl of 10 µM reverse primer, and 2.2 µl of nuclease-free water per replicate in a 1.5 ml microcentrifuge tube. Vortex thoroughly.
4.Add 8 µl of reaction master mix to the wells of an optical PCR-grade 384-well plate.
5.Add 2 µl of the diluted cDNA to the reaction mixes. Include a “no template” negative control to which water is added instead.
6.Seal the plate with optical adhesive film and spin it down in a benchtop plate spinner.
7.Perform qPCR using a suitable qPCR machine that can quantify SYBR Green fluorescence using the following program:
Step | Temperature | Duration | Number of cycles |
Initial denaturation | 95°C | 30 sec | 1 |
Denaturation Annealing & extension |
95°C 60°C |
$ \left. \def\eqcellsep{&}\begin{array} {l}5 \, \rm{sec}\\ 20 \, \rm{sec} \end{array} \right\} $ | 45 |
8.Run the qPCR products on a 3% agarose/TBE gel and look for a single band of the correct size (roughly 70 to 80 bp). Ensure that the negative controls show no band (see Fig. 3).
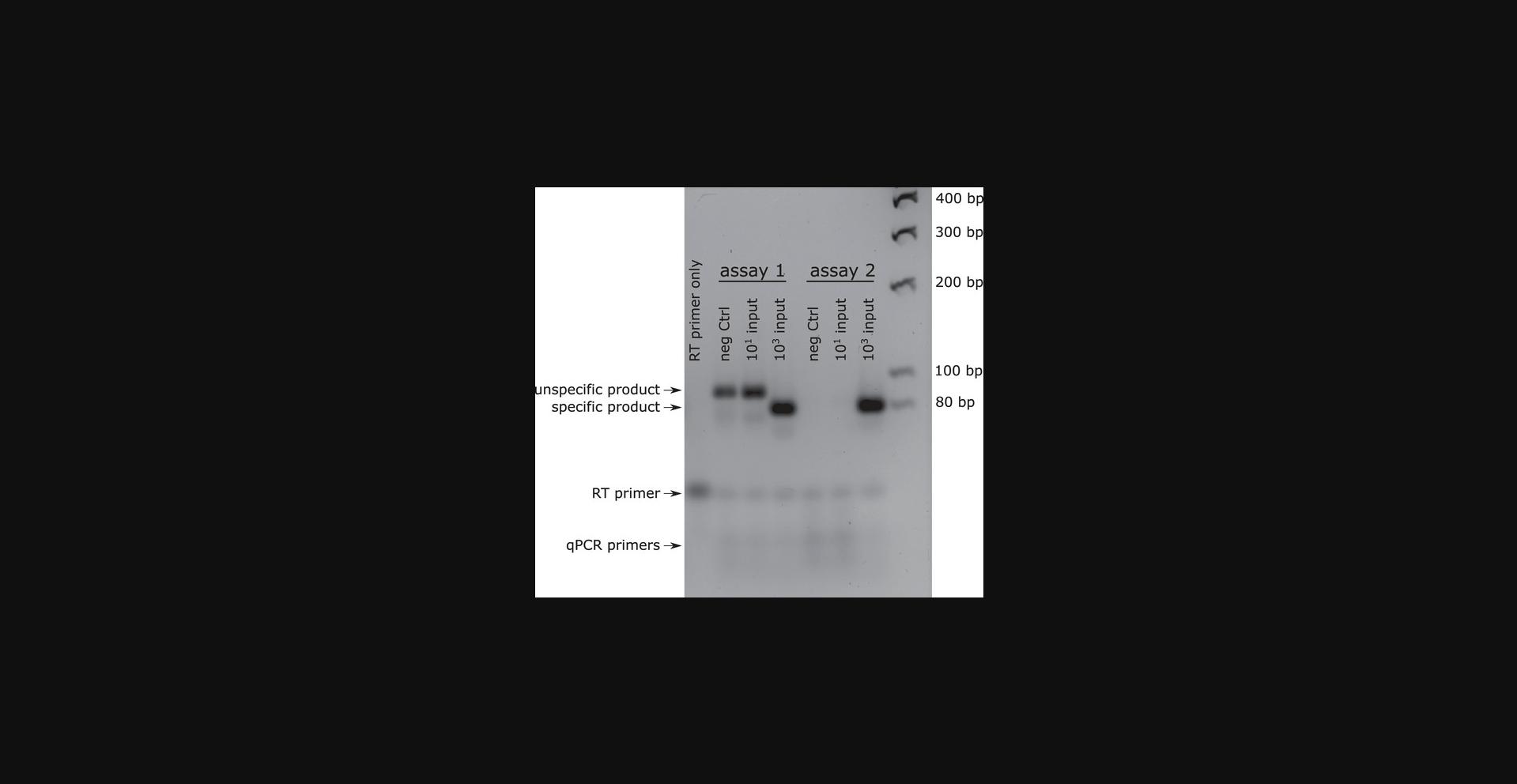
Data Analysis Option 1: Absolute Quantification Using a Standard Curve
9.Construct a standard curve by plotting the Cq values observed for each concentration of synthetic RNA oligonucleotides against the log10 of the input concentration. Perform linear regression to obtain a standard curve.
10.Using the slope of the standard curve, the PCR efficiency can be calculated using the following formula:
For example, the standard curve for the assay for edited miR-379 has a slope of −3.69 (Fig. 4A). This indicates a PCR efficiency of 87%:
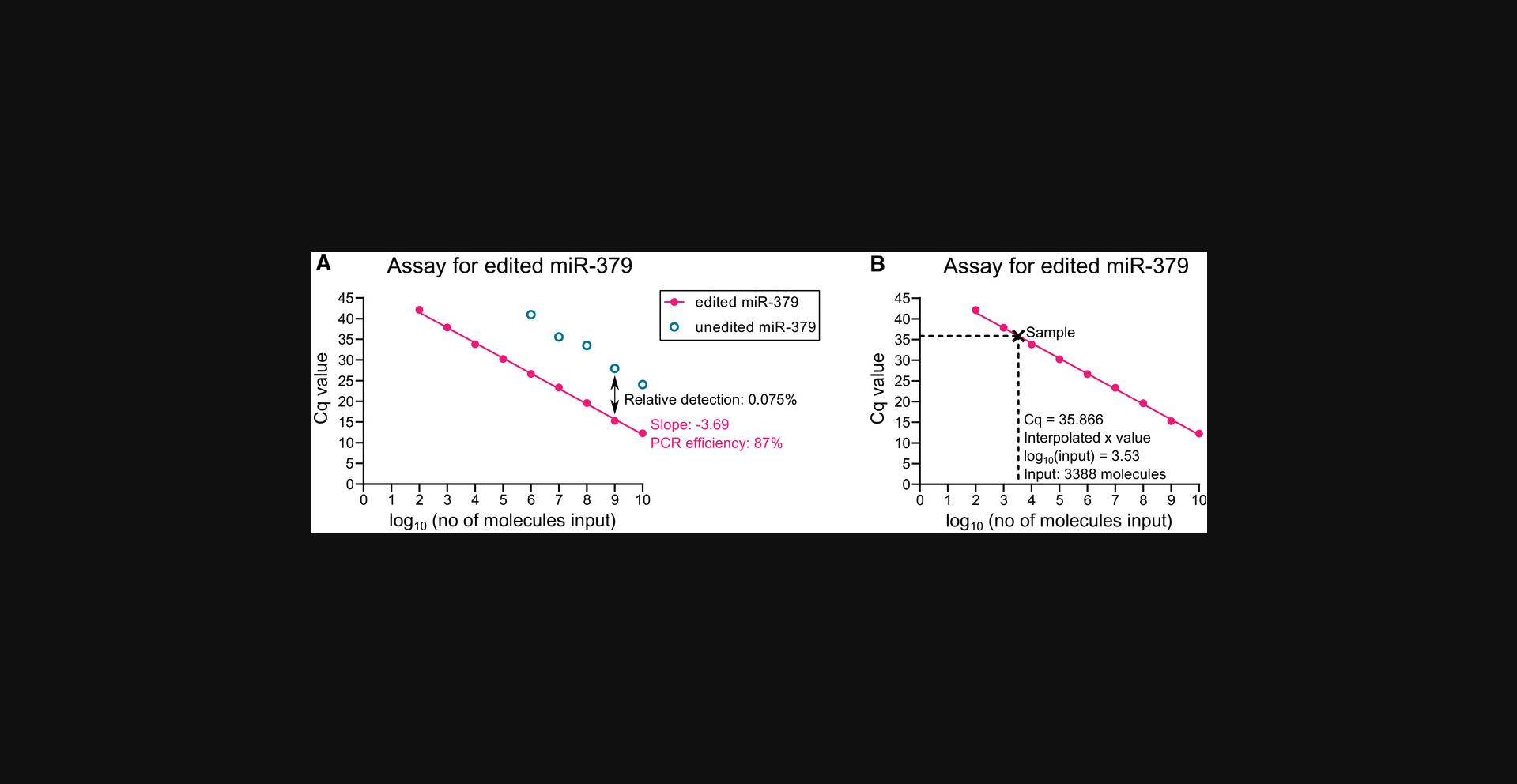
Problem | Possible cause | Solution |
---|---|---|
No amplification | Too little input RNA | Repeat the experiment with higher input. If the RNA concentration is limiting, use less water in the master mix for RT, or concentrate the RNA samples by ethanol precipitation, potentially using a carrier like glycogen if the total RNA amount is low. |
Poor quality of RNA or reagents |
Running the RNA sample on a denaturing agarose gel can identify RNA degradation, whereas spectrophotometry can identify the presence of contaminating chemicals that may inhibit the reaction. Replace reagents with new kits if problems persist. |
|
Poor primer design | To exclude problems with input, RNA quality, and reagents, run experiments using a previously designed and validated set of primers (Androvic et al., 2017; Smieszek et al., 2019; Voss et al., 2021). If these reactions work well, the problem lies in the primer design. Design new primer sets. | |
Amplification in negative controls | Contamination of reagents | Replace reagents with new kits. You may also replace one component at a time to identify the source of the contamination. Clean pipettes and bench surfaces to remove contaminating RNA and cDNA. Make sure to use filter tips for all pipetting steps. |
Poor primer design | Check for potential primer interactions using online tools, especially 3ʹ dimers. Purify the PCR product and send it for Sanger sequencing to identify which product arises and how it is formed. Design new primer sets. | |
Standard curve is not linear | Poor mixing during standard curve preparation | Prepare new standard curves. Make sure to mix each tube well before transferring part of the solution to the next tube. Use a fresh filter tip for each pipetting step. |
Degradation | Prepare the standard curve immediately before RT and perform all steps on ice. | |
Contamination | Replace IDTE and potentially RT reagents. Clean pipettes and bench surfaces to remove contaminating RNA and cDNA. Make sure to use filter tips for all pipetting steps. | |
PCR efficiency is too low (<85%) | Mistakes during standard curve preparation | (see above) |
Poor primer design | Design new primer sets with higher melting temperatures and compare PCR efficiencies. | |
Sub-optimal primer concentration | Optimize the primer concentrations by varying the concentrations of each primer (Fraga, Meulia & Fenster, 2008). | |
Sub-optimal annealing temperature | Lower and optimize the annealing temperature. | |
PCR efficiency is too high (>115%) | Mistakes during standard curve preparation | (see above) |
Presence of PCR inhibitors | Spectrophotometry of the RNA samples can identify the presence of contaminating chemicals that may inhibit the reaction. Diluting the RT products (cDNA) further before qPCR may help to dilute PCR inhibitors. | |
Melt curve with multiple peaks or non-specific product on agarose gel | Poor primer design | Check for potential primer interactions using online tools, especially 3ʹ dimers. Gel-purify the different PCR products and send them for Sanger sequencing to identify which non-specific product arises and how it is formed. Design new primer sets. |
Annealing temperature is too low |
Increase and optimize the annealing temperature to prevent the formation of non-specific products. Alternatively, design new primer sets with lower melting temperatures. |
11.(Optional) If working with editing isoform-specific assays, calculate the relative detection of the non-target isoform at each concentration. Use the following formula:
For example, the Cq value for RT-qPCR of 109 molecules edited miR-379 with the assay for edited miR-379 was 15.271 (Fig. 4A). The Cq value with the same number of molecules of unedited miR-379 using the assay for edited miR-379 was 27.942.This results in a relative detection of 0.015% at this concentration:
12.Interpolate the absolute number of target microRNA molecules in each sample from the average Cq of the triplicates based on the linear regression in Step 9 (Fig. 4B).
13.(Optional) If separate reactions to quantify both the edited and the unedited microRNA isoform from the same sample were performed, the editing frequency of the microRNA can be calculated as:
For example, in prostate cancer cells overexpressing ADAR2 (Voss et al., 2021), the enzyme responsible for miR-379 editing (Kawahara et al., 2008), interpolation of the absolute numbers as described in Step 12 indicated that the sample contained 96,840 molecules of edited miR-379, and 158,171 molecules of unedited miR-379.This results in an editing frequency of 38%:
This was considerably higher than the editing frequency of a sample from control cells with 2222 molecules of edited miR-379, and 292,907 molecules of unedited miR-379, giving an editing frequency of 0.8%:
Data Analysis Option 2: Relative Expression Using Reference Genes
14.If absolute numbers or editing frequencies are not needed, and the user only wishes to calculate the relative expression of a specific microRNA or its isoform, the ΔCq method can be used for relative quantification (Livak & Schmittgen, 2001) with the help of one or multiple housekeeping small RNAs quantified in parallel. Several useful resources are available describing in detail how to perform such a normalization (Bookout, Cummins, Mangelsdorf, Pesola, & Kramer, 2006; Livak & Schmittgen, 2001; Taylor et al., 2019).
Alternate Protocol: HYDROLYSIS PROBE-BASED qPCR FOR A-TO-I-EDITED microRNAs
This protocol is an alternative to Basic Protocol 2 and describes qPCR of the microRNAs reverse-transcribed in Basic Protocol 1 using hydrolysis probes, instead of SYBR Green, to quantify amplification. Hydrolysis probes may be preferred by some users, as they can be combined with any fluorophore. Potentially, this can also be used to multiplex the quantification of several microRNAs in the same qPCR reaction, but users should test this for each application. The cDNA products from Basic Protocol 1 are diluted and a reaction master mix is prepared. The diluted cDNA products and the master mix are combined in multiple replicates in 384-well plates and qPCR is performed. The qPCR reactions for each RT product should be performed in triplicate. Quadruplicates may be more appropriate for microRNAs with extremely low abundance. The qPCR data will then be used to quantify the target microRNA(s) in absolute numbers and/or in relation to housekeeping genes.
Materials
-
cDNA samples from Basic Protocol 1
-
Nuclease-free water (Invitrogen, cat. no. 10977-035 or equivalent)
-
PrimeTime gene expression master mix (Integrated DNA Technologies, cat. no. 1055772)
-
10 µM forward and reverse qPCR primers for each target and reference of interest (custom DNA oligonucleotides e.g., from Invitrogen), see Strategic Planning and example in Figure 2B
-
5 µM hydrolysis probe for each target and reference of interest (e.g., ZEN/Iowa Black FW double-quenched FAM hydrolysis probes; custom order from Integrated DNA Technologies), see Strategic Planning and example in Figure 2B
-
Agarose
-
TBE buffer (Appendix 2 ; Common Buffers, Media, and Stock Solutions; Current Protocols in Human Genetics, 2000)
-
DNA loading dye (Thermo Scientific, cat. no. R0621 or equivalent)
-
Low-range DNA ladder (Thermo Scientific, cat. no. SM0383 or equivalent)
-
Nucleic acid gel stain (Biotium, cat. no. 41003 or equivalent)
-
1.5 ml microcentrifuge tubes (Sarstedt, cat. no. 72.690.001 or equivalent)
-
Optical PCR-grade 384-well plates (Applied Biosystems, cat. no. 4309849 or equivalent)
-
Optical adhesive film (Applied Biosystems, cat. no. 4311971 or equivalent)
-
Pipettes and filter tips (nuclease/DNA/RNA-free)
-
Ice bucket
-
Benchtop mini centrifuge
-
Vortexer
-
Adhesive film applicator
-
Benchtop plate spinner
-
qPCR machine (Applied Biosystems QuantStudio 7 Flex or equivalent)
-
Gel electrophoresis apparatus
-
UV transilluminator
1.Thaw the reagents on ice. The following steps, until loading the qPCR plate, should be performed on ice.
2.Dilute the cDNA samples 1/2 by adding 10 µl nuclease-free water to each product. Mix by flicking the tubes and spin down in a benchtop mini centrifuge.
3.Prepare a qPCR reaction master mix by combining 5 µl of PrimeTime Gene Expression Master Mix, 0.4 µl of 10 µM forward primer, 0.4 µl of 10 µM reverse primer, 0.5 µl of 5 µM hydrolysis probe, and 1.7 µl of nuclease-free water per replicate in a 1.5 ml microcentrifuge tube. Vortex thoroughly.
4.Add 8 µl of the reaction master mix to the wells of an optical PCR-grade 384-well plate.
5.Add 2 µl of the diluted cDNA to the appropriate wells containing the reaction mix. Include a “no template” negative control to which water is added instead.
6.Seal the plate with optical adhesive film and spin it down in a benchtop plate spinner.
7.Perform qPCR following the program below using a suitable qPCR machine that can quantify FAM fluorescence, or the fluorophore of your choice.
Step | Temperature | Duration | Number of cycles |
Initial denaturation | 95°C | 3 min | 1 |
Denaturation Annealing & extension |
95°C 60°C |
$ \left. \def\eqcellsep{&}\begin{array} {l}5 \, \rm{sec}\\ 30 \, \rm{sec} \end{array} \right\} $ | 45 |
8.Run the qPCR products on a 3% agarose/TBE gel and look for a single band of the correct size (roughly 70 to 80 bp). Ensure that the negative controls show no band (see Fig. 3).
9.Detailed instructions and examples for data analysis using either absolute numbers or relative expression are given in Steps 9 to 14 of Basic Protocol 2 and illustrated in Figure 4.
Support Protocol: PREPARATION OF STANDARD CURVES USING SYNTHETIC RNA OLIGONUCLEOTIDES
Here, the user will dilute RNA oligonucleotide standards of the target microRNA to defined concentrations in TE buffer, generating a series of 10-fold dilutions. This will produce dilutions containing 1010, 109, 108, 107, 106, 105, 104, 103, 102, 101, and 100 molecules per µl (tubes 4 to 14 in Fig. 5). These standard dilutions will then be run in parallel with the RNA samples of interest in Basic Protocols 1 and 2 or the Alternate Protocol. The standard curve will aid in the absolute quantification of samples. The standard samples should be used on the same day they are prepared due to the low stability of pure RNA in solution.
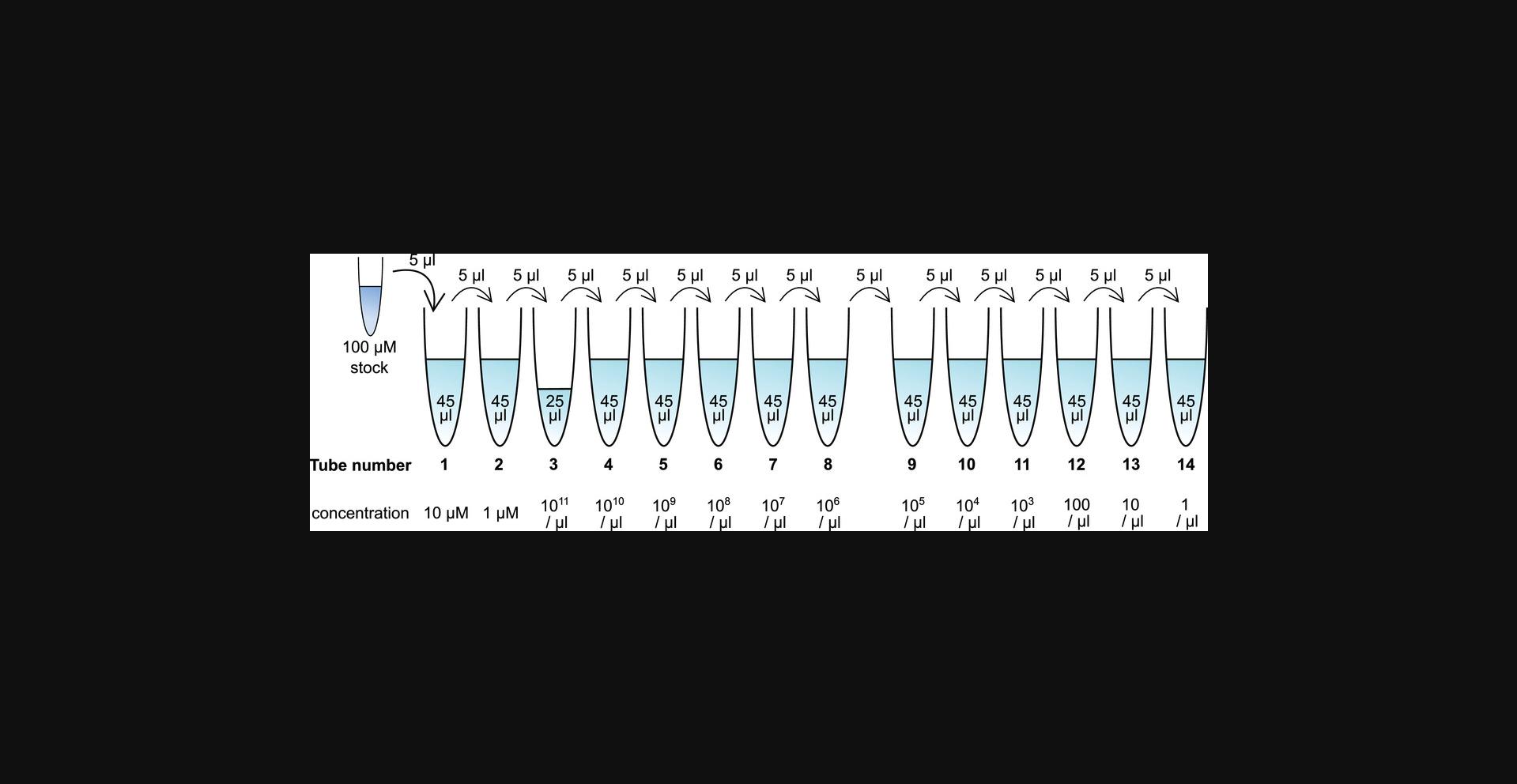
Materials
-
IDTE pH 7.5, 1× TE Solution (Integrated DNA Technologies, cat. no. 11-05-01-15)
-
100 µM synthetic RNA oligonucleotide of the desired microRNA (custom order from Integrated DNA Technologies), see Strategic Planning
-
Pipettes and filter tips (nuclease/DNA/RNA-free)
-
0.2 ml PCR tubes, strips or individual (Sarstedt, cat. no. 72.991.102 or equivalent)
-
Ice bucket
1.Thaw the synthetic RNA oligonucleotide on ice. The following steps should be performed on ice as well. Pipette up and down or flick the tube to ensure a homogenous solution.
2.Prepare IDTE buffer in 0.2 ml PCR strips or tubes as detailed in Figure 5.Add the buffer (depicted in light blue) to 14 tubes in the volumes listed inside the tubes in Figure 5.
3.Add 5 µl of the RNA oligonucleotide stock to tube 1.
4.Mix thoroughly.
5.Using a filter tip, transfer 5 µl from tube 1 to tube 2.
6.Repeat steps 4 and 5 as indicated in Figure 5, until 5 µl from tube 13 has been added to tube 14.Mix this tube thoroughly as well.
7.Keep the tubes on ice until ready for use. RNA standards should be used on the same day that they were prepared. The RNA concentrations are substantially diminished upon freezing and thawing.
8.Proceed to Basic protocol 1 and then either Basic Protocol 2 or the Alternate Protocol.
9.Sample data demonstrating how to plot and interpret the standard curve is shown in Figure 4 and discussed in Steps 9 to 14 of Basic Protocol 2.
COMMENTARY
Background Information
MicroRNAs are a class of short non-coding RNAs that regulate the stability and translation of mRNAs in animal and plant cells (Ameres & Zamore, 2013). This regulation is crucial for development and homeostasis in these organisms, and deregulation and mutation of these non-coding RNAs is associated with disease states (Condrat et al., 2020; Diederichs et al., 2016). As the biological role of microRNAs is becoming increasingly clear, a large diagnostic and therapeutic potential has been ascribed to these highly abundant regulatory molecules (Catela Ivkovic, Voss, Cornella, & Ceder, 2017; Condrat et al., 2020; Mitchell et al., 2008). For microRNAs to fulfill this potential, reliable and affordable methods for their accurate quantification are required.
Quantitative PCR methods have evolved rapidly over the last several years. The common denominator is the quantification of cDNA by observing the amplification of the template using fluorescent reporters. The underlying molecular tools being used for cDNA synthesis, PCR amplification, and fluorescent signals, however, differ vastly across different qPCR methods (Fraga, Meulia, & Fenster, 2008).
RT-qPCR methods for mRNA expression studies typically rely on using oligo(dT) and/or random hexamer primers. Due to the lack of a poly(A) tail on mature microRNAs, it is obvious that oligo(dT) primers are of little use on unmodified microRNAs. However, this is not the only challenge that microRNA RT-qPCR poses compared to qPCR approaches for protein-coding and other longer RNAs. Due to the short length of microRNAs, it is difficult to design a primer that is both efficient at priming RT and results in sufficient specificity, while also generating a large-enough amplicon for subsequent PCR amplification. In order to create a longer amplicon, additional sequence must be added either by the RT primer or through tailing or adapter ligation. Simply using random hexamer primers for RT, as is common practice for gene expression studies, would not be able to add such an additional sequence to the microRNA in a consistent and specific manner, making this approach unsuitable for microRNA expression studies. Several different alternative methods for RT-qPCR of microRNAs have been developed, including both microRNA-specific and universal approaches, both of which will be discussed further below.
One microRNA-specific approach that remains popular to this day is stem-loop RT-qPCR (Chen et al., 2005). This method uses a short microRNA-specific primer that is attached to a stem-loop structure that will increase binding affinity through base-stacking effects, as well as add a primer binding site for subsequent qPCR. The resulting RT product is then amplified in the qPCR step using one universal primer targeting the melted stem-loop, and one microRNA-specific primer (Chen et al., 2005). Stem-loop RT-qPCR of microRNAs is usually performed using TaqMan hydrolysis probes which are sold by Applied Biosystems in their popular line of readily available TaqMan microRNA assays. Quantification of microRNAs using these probes is user-friendly, relatively sensitive, and can be multiplexed (Androvic et al., 2017; Chen et al., 2005; Fraga et al., 2008; Tang, Hajkova, Barton, Lao, & Surani, 2006). On the other hand, the probes are fairly expensive and their specificity is limited. Multiple studies have found that these assays struggle to distinguish closely related microRNAs and microRNA isoforms (Androvic et al., 2017; Magee et al., 2017; Schamberger & Orbán, 2014; Wu et al., 2007).
Stem-loop RT-qPCR requires the use of a microRNA-specific RT primer, which can increase costs and limit high-throughput analysis. Some methods aim to overcome this by adding a poly(N) tail to the microRNA, ligating a linker, or both (Balcells, Cirera, & Busk, 2011; Benes et al., 2015; Mei et al., 2012; Shi & Chiang, 2005). The microRNAs are then reverse-transcribed using a universal primer. This should allow RT of all microRNAs in the sample in a single RT reaction, but in practice these reactions can be less sensitive and the enzymes used for adapter ligation and poly(N) tailing can introduce bias (Yehudai-Resheff & Schuster, 2000; Zhuang, Fuchs, Sun, Zheng, & Robb, 2012). Ligation and tailing-dependent approaches have also been found to be largely unable to differentiate between similar microRNAs and microRNA isoforms (Androvic et al., 2017; Nejad et al., 2018; Voss et al., 2021).
Two-tailed RT-qPCR for microRNA can overcome this lack of specificity. The method is able to distinguish microRNAs that differ by as little as one nucleotide and is, therefore, useful for accurate quantification of microRNAs in closely related microRNA families and for microRNAs that are subject to A-to-I RNA editing (Androvic et al., 2017; Voss et al., 2021). Two-tailed RT-qPCR uses a microRNA-specific RT primer that consists of two short hemiprobes that bind cooperatively to prime RT (Fig. 2). They are connected by a loop that adds enough sequence to the RT product to facilitate qPCR. The separation of the two hemiprobes from each other increases specificity and allows them to target the regions of the microRNA that bear the highest potential to distinguish it from other microRNAs or isoforms. The subsequent qPCR step also includes a microRNA-specific primer so that both the RT step and the qPCR step contribute to the specificity of the amplification (Androvic et al., 2017). The two protocols that are provided in this article allow users to choose between the advantages of either SYBR Green or hydrolysis probes, depending on their individual needs. SYBR Green qPCR is cost-effective, and in our hands, it was more specific and more sensitive than using hydrolysis probes (Voss et al., 2021). The use of hydrolysis probes, on the other hand, can allow for multiplexing of probes with different fluorophores for different microRNAs in the qPCR steps (Fraga et al., 2008).
The primers can be designed either to perform editing isoform-specific or editing-blind quantification. Which is more appropriate will depend on the application; in many cases, isoform-specific analysis can give additional information. This is the case, for example, in biomarker studies (Voss et al., 2021), since RNA editing is often deregulated in disease (Fritzell et al., 2018; Nishikura, 2016); for experiments in which microRNAs are used as a proxy for RNA editing levels or ADAR enzyme activity in a laboratory setting (Voss et al., 2021), or when asking biological questions about the role of different A-to-I editing isoforms. In other cases, for example when wanting to quantify the total expression from a microRNA locus, or when isoforms have the same biological function, an editing isoform-blind pan-microRNA assay can be desirable. These assays will then quantify all present editing isoforms of a microRNA (Voss et al., 2021). Another application for editing-sensitive two-tailed microRNA assays is digital PCR. Hydrolysis-based digital PCR performed especially well with the two-tailed assays in our hands (Voss et al., 2021), and can save the user the need to prepare a standard curve (Quan, Sauzade, & Brouzes, 2018). However, the specificity and sensitivity were lower in digital PCR compared to qPCR, suggesting that optimal implementation of two-tailed assay-based digital PCR will benefit from additional optimization (Voss et al., 2021).
Critical Parameters
When first setting up the experiment, it is important that the RT and qPCR primers are designed with care and tested sufficiently for specificity and efficiency, as described in Strategic Planning. The selection of reliable reference genes is important when the user wants to perform relative expression analysis, which is briefly discussed in Strategic Planning, and more in-depth in other useful resources (Mestdagh et al., 2009; Peltier & Latham, 2008; Taylor et al., 2019; Vandesompele et al., 2002). This section also gives guidelines regarding the type and quality of RNA samples that should be used. Using high quality RNA that contains sufficient amounts of intact short RNAs is critical for the success of the experiment.
Even when starting with high quality RNA, it is crucial to limit RNA degradation during both the RT step of the procedure as well as during the preparation of the standard curve. To prevent RNase contamination, users should work with gloves and use RNase-free materials. We recommend cleaning the bench and the pipettes with a product like RNase AWAY (Molecular BioProducts) or RNaseZap (Invitrogen). In addition, the use of filter tips can prevent contamination with RNases. By performing all steps on ice, the activity of any contaminating RNase and any spontaneous hydrolysis of RNA can be minimized. Keeping reagents and preparing reactions on ice also helps to preserve primer integrity and the activity of the enzymes used.
In the qPCR steps, DNA contamination is the biggest concern. Here, too, filter tips can help in limiting contamination. We recommend preparing qPCR reactions in a space that is separate from the benches on which already amplified PCR products are handled and with pipettes that are dedicated to pre-PCR use only. We also recommend cleaning the workspace and the pipettes with a product like DNA AWAY (Molecular BioProducts).
Troubleshooting
Common problems include lack of amplification, amplification in negative controls, insufficient PCR efficiencies, and unspecific products. Most of these can usually be traced back to poor primer design or contaminations of reagents. Frequently observed issues, their potential causes, and advice for solving these problems are listed in Table 2. For more general troubleshooting of RT-qPCR experiments, we refer the readers to previously published helpful guides (Bustin & Nolan, 2004; Taylor et al., 2019).
Understanding Results
Example data for an adequately functioning editing-specific RT-qPCR assay is shown in Figure 4. A well-designed two-tailed RT-qPCR assay typically has a PCR efficiency in the range of 85 to 110%. If the PCR efficiency based on the standard curve falls outside of this range, primer design and PCR conditions should be reconsidered (see Troubleshooting). Even within this range, if relative expression is to be calculated, the PCR efficiency should be factored into the calculations (Bookout et al., 2006; Livak & Schmittgen, 2001; Taylor et al., 2019).
For an editing-sensitive assay to be truly accurate, the relative detection of the non-target editing isoform(s) of the microRNA should be as low as possible. We recommend that the relative detection of non-target isoforms should be <1%, ideally <0.1%, for rare microRNAs or isoforms. If this is not achievable, it is important to keep these limitations in mind for data interpretation. A calculated editing frequency for a microRNA in a biological sample that lies below the relative detection limit of the assay that was used for quantification is not reliable.
If the designed assay passes these quality checks, the Cq values of biological samples can be used to calculate absolute numbers, relative expression, and editing frequencies of the microRNA of interest as described in the protocols. These will reflect the abundance of a microRNA or its isoforms, which may give useful information in the context of the research question the user wants to answer.
Time Considerations
The entire procedure can be performed in a single workday. If absolute numbers and, therefore, standard curves, are needed, approximately 30 min per microRNA should be calculated for the preparation of standard curves (by an experienced user) as described in the Support Protocol. A new user should expect to spend more time on the preparation of standard curves. It is crucial that standard curves are prepared the same day as the RT in order to ensure that the numbers are accurate. We also recommend that the RT and qPCR (either using Basic Protocol 1 or the Alternate Protocol) are performed on the same day, due to degradation of the RT products upon freezing and thawing. If it is not possible to perform everything on the same day, we suggest running the RT reaction overnight, holding the reaction at 4°C, and performing qPCR immediately the following morning. Avoid freeze-thaw cycles.
Acknowledgements
The development of the method described here was supported by a grant from Kungliga Fysiografiska Sällskapet i Lund (grant no. 40526) awarded to GV, and grants from Cancerfonden (grant nos. CAN 2017/559 and CAN 2019/331), Vetenskapsrådet (grant no. VR-MH 2018-03125) and Prostatacancerfonden awarded to YC.
Author Contributions
Gjendine Voss : Conceptualization, Funding acquisition, Investigation, Methodology, Writing original draft; Yvonne Ceder : Conceptualization, Funding acquisition, Supervision, Writing review & editing.
Conflict of Interest
The authors declare no conflicts of interest.
Open Research
Data Availability Statement
Data sharing not applicable – no new data has been generated.
Literature Cited
- Ameres, S. L., & Zamore, P. D. (2013). Diversifying microRNA sequence and function. Nature Reviews Molecular Cell Biology , 14(8), 475–488. doi: 10.1038/nrm3611
- Androvic, P., Valihrach, L., Elling, J., Sjoback, R., & Kubista, M. (2017). Two-tailed RT-qPCR: A novel method for highly accurate miRNA quantification. Nucleic Acids Research , 45(15), e144. doi: 10.1093/nar/gkx588
- Armstrong, D. A., Dessaint, J. A., Ringelberg, C. S., Hazlett, H. F., Howard, L., Abdalla, M. A. K., … Ashare, A. (2018). Pre-analytical handling conditions and small RNA recovery from urine for miRNA profiling. The Journal of Molecular Diagnostics , 20(5), 565–571. doi: 10.1016/j.jmoldx.2018.04.003
- Balcells, I., Cirera, S., & Busk, P. K. (2011). Specific and sensitive quantitative RT-PCR of miRNAs with DNA primers. BMC Biotechnology , 11, 70. doi: 10.1186/1472-6750-11-70
- Benes, V., Collier, P., Kordes, C., Stolte, J., Rausch, T., Muckentaler, M. U., … Castoldi, M. (2015). Identification of cytokine-induced modulation of microRNA expression and secretion as measured by a novel microRNA specific qPCR assay. Scientific Reports , 5, 11590. doi: 10.1038/srep11590
- Bookout, A. L., Cummins, C. L., Mangelsdorf, D. J., Pesola, J. M., & Kramer, M. F. (2006). High-throughput real-time quantitative reverse transcription PCR. Current Protocols in Molecular Biology , Chapter 15 , doi: 10.1002/0471142727.mb1508s73
- Bryzgunova, O., Konoshenko, M., Zaporozhchenko, I., Yakovlev, A., & Laktionov, P. (2021). Isolation of cell-free miRNA from biological fluids: Influencing factors and methods. Diagnostics (Basel, Switzerland) , 11(5), 865. doi: 10.3390/diagnostics11050865
- Bustin, S. A., & Nolan, T. (2004). Pitfalls of quantitative real-time reverse-transcription polymerase chain reaction. Journal of Biomolecular Techniques , 15(3), 155–166.
- Catela Ivkovic, T., Voss, G., Cornella, H., & Ceder, Y. (2017). microRNAs as cancer therapeutics: A step closer to clinical application. Cancer Letters , 407, 113–122. doi: 10.1016/j.canlet.2017.04.007
- Chen, C., Ridzon, D. A., Broomer, A. J., Zhou, Z., Lee, D. H., Nguyen, J. T., … Guegler, K. J. (2005). Real-time quantification of microRNAs by stem–loop RT–PCR. Nucleic Acids Research , 33(20), e179. doi: 10.1093/nar/gni178
- Chomczynski, P., & Sacchi, N. (1987). Single-step method of RNA isolation by acid guanidinium thiocyanate-phenol-chloroform extraction. Analytical Biochemistry , 162(1), 156–159. doi: 10.1006/abio.1987.9999
- Common Buffers, Media, and Stock Solutions. (2000). Current Protocols in Human Genetics , Appendix 2. doi: 10.1002/0471142905.hga02ds2
- Condrat, C. E., Thompson, D. C., Barbu, M. G., Bugnar, O. L., Boboc, A., Cretoiu, D., … Voinea, S. C. (2020). miRNAs as biomarkers in disease: Latest findings regarding their role in diagnosis and prognosis. Cells , 9(2), 276. doi: 10.3390/cells9020276
- Diederichs, S., Bartsch, L., Berkmann, J. C., Fröse, K., Heitmann, J., Hoppe, C., … Wullenkord, R. (2016). The dark matter of the cancer genome: aberrations in regulatory elements, untranslated regions, splice sites, non-coding RNA and synonymous mutations. EMBO Molecular Medicine , 8(5), 442–457. doi: 10.15252/emmm.201506055
- Fraga, D., Meulia, T., & Fenster, S. (2008). Real-Time PCR. Current Protocols Essential Laboratory Techniques , 00(1), 10.13.11–10.13.34. doi: 10.1002/9780470089941.et1003s00
- Fritzell, K., Xu, L.-D., Lagergren, J., & Öhman, M. (2018). ADARs and editing: The role of A-to-I RNA modification in cancer progression. Seminars in Cell & Developmental Biology, 79, 123–130. doi: 10.1016/j.semcdb.2017.11.018
- Hui, A. B. Y., Shi, W., Boutros, P. C., Miller, N., Pintilie, M., Fyles, T., … Liu, F.-F. (2009). Robust global micro-RNA profiling with formalin-fixed paraffin-embedded breast cancer tissues. Laboratory Investigation , 89(5), 597–606. doi: 10.1038/labinvest.2009.12
- Karrer, E. E., Lincoln, J. E., Hogenhout, S., Bennett, A. B., Bostock, R. M., Martineau, B., … Alexander, D. (1995). In situ isolation of mRNA from individual plant cells: creation of cell-specific cDNA libraries. Proceedings of the National Academy of Sciences of the United States of America , 92(9), 3814–3818. doi: 10.1073/pnas.92.9.3814
- Kawahara, Y., Zinshteyn, B., Sethupathy, P., Iizasa, H., Hatzigeorgiou, A. G., & Nishikura, K. (2007). Redirection of silencing targets by adenosine-to-inosine editing of miRNAs. Science , 315(5815), 1137–1140. doi: 10.1126/science.1138050
- Kawahara, Y., Megraw, M., Kreider, E., Iizasa, H., Valente, L., Hatzigeorgiou, A. G., & Nishikura, K. (2008). Frequency and fate of microRNA editing in human brain. Nucleic Acids Research , 36(16), 5270–5280. doi: 10.1093/nar/gkn479
- Ku, A., Fredsøe, J., Sørensen, K. D., Borre, M., Evander, M., Laurell, T., … Ceder, Y. (2021). High-throughput and automated acoustic trapping of extracellular vesicles to identify microRNAs with diagnostic potential for prostate cancer. Frontiers in Oncology , 11, 631021. doi: 10.3389/fonc.2021.631021
- Livak, K. J., & Schmittgen, T. D. (2001). Analysis of relative gene expression data using real-time quantitative PCR and the 2−ΔΔCT method. Methods (San Diego, Calif.) , 25(4), 402–408. doi: 10.1006/meth.2001.1262
- Magee, R., Telonis, A. G., Cherlin, T., Rigoutsos, I., & Londin, E. (2017). Assessment of isomiR discrimination using commercial qPCR methods. Non-Coding RNA , 3(2), 18. doi: 10.3390/ncrna3020018
- Marceca, G. P., Distefano, R., Tomasello, L., Lagana, A., Russo, F., Calore, F., … Nigita, G. (2021). MiREDiBase, a manually curated database of validated and putative editing events in microRNAs. Scientific Data , 8(1), 199. doi: 10.1038/s41597-021-00979-8
- Mei, Q., Li, X., Meng, Y., Wu, Z., Guo, M., Zhao, Y., … Han, W. (2012). A facile and specific assay for quantifying microRNA by an optimized RT-qPCR approach. PLoS ONE , 7(10), e46890. doi: 10.1371/journal.pone.0046890
- Mestdagh, P., Van Vlierberghe, P., De Weer, A., Muth, D., Westermann, F., Speleman, F., & Vandesompele, J. (2009). A novel and universal method for microRNA RT-qPCR data normalization. Genome Biology , 10(6), R64. doi: 10.1186/gb-2009-10-6-r64
- Mitchell, P. S., Parkin, R. K., Kroh, E. M., Fritz, B. R., Wyman, S. K., Pogosova-Agadjanyan, E. L., … Tewari, M. (2008). Circulating microRNAs as stable blood-based markers for cancer detection. Proceedings of the National Academy of Sciences , 105(30), 10513–10518. doi: 10.1073/pnas.0804549105
- Nejad, C., Pillman, K. A., Siddle, K. J., Pépin, G., Änkö, M.-L., McCoy, C. E., … Gantier, M. P. (2018). miR-222 isoforms are differentially regulated by type-I interferon. RNA , 24(3), 332–341. doi: 10.1261/rna.064550.117
- Nishikura, K. (2016). A-to-I editing of coding and non-coding RNAs by ADARs. Nature Reviews Molecular Cell Biology , 17(2), 83–96. doi: 10.1038/nrm.2015.4
- Peltier, H. J., & Latham, G. J. (2008). Normalization of microRNA expression levels in quantitative RT-PCR assays: Identification of suitable reference RNA targets in normal and cancerous human solid tissues. RNA , 14(5), 844–852. doi: 10.1261/rna.939908
- Quan, P.-L., Sauzade, M., & Brouzes, E. (2018). dPCR: A technology review. Sensors (Basel, Switzerland) , 18(4), 1271. doi: 10.3390/s18041271
- Schamberger, A., & Orbán, T. I. (2014). 3′ IsomiR species and DNA contamination influence reliable quantification of microRNAs by stem-loop quantitative PCR. PLoS ONE , 9(8), e106315. doi: 10.1371/journal.pone.0106315
- Shi, R., & Chiang, V. L. (2005). Facile means for quantifying microRNA expression by real-time PCR. Biotechniques , 39(4), 519–525. doi: 10.2144/000112010
- Smieszek, A., Kornicka, K., Szłapka-Kosarzewska, J., Androvic, P., Valihrach, L., Langerova, L., … Marycz, K. (2019). Metformin increases proliferative activity and viability of multipotent stromal stem cells isolated from adipose tissue derived from horses with equine metabolic syndrome. Cells , 8(2), 80. doi: 10.3390/cells8020080
- Tan, M. H., Li, Q., Shanmugam, R., Piskol, R., Kohler, J., Young, A. N., … Visualization—Ucsc Genomics Institute, U. o. C. S. C. (2017). Dynamic landscape and regulation of RNA editing in mammals. Nature , 550(7675), 249–254. doi: 10.1038/nature24041
- Tang, F., Hajkova, P., Barton, S. C., Lao, K., & Surani, M. A. (2006). MicroRNA expression profiling of single whole embryonic stem cells. Nucleic Acids Research , 34(2), e9. doi: 10.1093/nar/gnj009
- Taylor, S. C., Nadeau, K., Abbasi, M., Lachance, C., Nguyen, M., & Fenrich, J. (2019). The ultimate qPCR experiment: producing publication quality, reproducible data the first time. Trends in Biotechnology , 37(7), 761–774. doi: 10.1016/j.tibtech.2018.12.002
- Vandesompele, J., De Preter, K., Pattyn, F., Poppe, B., Van Roy, N., De Paepe, A., & Speleman, F. (2002). Accurate normalization of real-time quantitative RT-PCR data by geometric averaging of multiple internal control genes. Genome Biology , 3(7), research0034.0031. doi: 10.1186/gb-2002-3-7-research0034
- Voss, G., Edsjo, A., Bjartell, A., & Ceder, Y. (2021). Quantification of microRNA editing using two-tailed RT-qPCR for improved biomarker discovery. RNA , 27(11), 1412–1424. doi: 10.1261/rna.078867.121
- Wright, D. J., Force, C. R., & Znosko, B. M. (2018). Stability of RNA duplexes containing inosine·cytosine pairs. Nucleic Acids Research , 46(22), 12099–12108. doi: 10.1093/nar/gky907
- Wu, H., Neilson, J. R., Kumar, P., Manocha, M., Shankar, P., Sharp, P. A., & Manjunath, N. (2007). miRNA profiling of naïve, effector and memory CD8 T cells. PLoS ONE , 2(10), e1020. doi: 10.1371/journal.pone.0001020
- Yehudai-Resheff, S., & Schuster, G. (2000). Characterization of the E.coli poly(A) polymerase: nucleotide specificity, RNA-binding affinities and RNA structure dependence. Nucleic Acids Research , 28(5), 1139–1144. doi: 10.1093/nar/28.5.1139
- Zhuang, F., Fuchs, R. T., Sun, Z., Zheng, Y., & Robb, G. B. (2012). Structural bias in T4 RNA ligase-mediated 3′-adapter ligation. Nucleic Acids Research , 40(7), e54. doi: 10.1093/nar/gkr1263
Internet Resources
OligoAnalyzerTM Tool (Integrated DNA Technologies) This tool is useful to predict the formation of secondary structures and dimers of newly designed primers.
PrimerQuestTM Tool (Integrated DNA Technologies) This tool can assist with the design of hydrolysis probes for the Alternate Protocol.