Assessing Protein Synthesis and Degradation Rates in Arabidopsis thaliana Using Amino Acid Analysis
Mark Stitt, Mark Stitt, Hirofumi Ishihara, Hirofumi Ishihara, Thiago A. Moraes, Thiago A. Moraes, Stéphanie Arrivault, Stéphanie Arrivault
Abstract
Plants continually synthesize and degrade proteins, for example, to adjust protein content during development or during adaptation to new environments. In order to estimate global protein synthesis and degradation rates in plants, we developed a relatively simple and inexpensive method using a combination of 13CO2 labeling and mass spectrometry–based analyses. Arabidopsis thaliana plants are subjected to a 24-hr 13CO2 pulse followed by a 4-day 12CO2 chase. Soluble alanine and serine from total protein and glucose from cell wall material are analyzed by gas chromatography time-of-flight mass spectrometry (GC-TOF-MS) and their 13C enrichment (%) is estimated. The rate of protein synthesis during the 13CO2 pulse experiment is defined as the rate of incorporation of labeled amino acids into proteins normalized by a correction factor for incomplete enrichment in free amino acid pools. The rate of protein degradation is estimated as the difference between the rate of protein synthesis and the relative growth rate calculated using the 13C enrichment of glucose from cell wall material. Degradation rates are also estimated from the 12CO2 pulse experiment. The following method description includes setting up and performing labeling experiments, preparation and measurement of samples, and calculation steps. In addition, an R script is provided for the calculations. © 2021 The Authors. Current Protocols published by Wiley Periodicals LLC.
Basic Protocol 1 : Setting up the 13CO2 labeling system and stable isotope labeling of Arabidopsis thaliana rosette leaves
Basic Protocol 2 : Extraction of soluble amino acids for GC-TOF-MS analysis
Basic Protocol 3 : Preparation of amino acids from total protein for GC-TOF-MS analysis
Basic Protocol 4 : Preparation of sugars from cell wall material for GC-TOF-MS analysis
Basis Protocol 5 : GC-TOF-MS analysis of 13C-labeled samples and estimation of 13C enrichment (%)
Basis Protocol 6 : Estimation of protein synthesis and degradation rates
INTRODUCTION
Synthesis and degradation of proteins represent fundamental processes during development, growth, and adjustment to the environment (Nelson & Millar, 2015). Due to the high energy cost of amino acid synthesis (∼5 ATP per amino acid when synthesized for nitrate) and formation of peptide bonds (also ∼5 ATP per amino acid), protein turnover represents a large part of the total energy budget (Amthor et al., 2000; Penning de Vries, 1975). These costs are increased by the need to invest heavily in ribosomes; these large machines have a relatively slow rate of peptide bond formation and can represent 40% or more of total protein content in rapidly growing cells (Warner, 1999; see Pal et al., 2013, for further literature).
Although plant research has made significant advances into understanding the complex networks that regulate protein synthesis and degradation, measurement of protein synthesis and degradation rates remains challenging. In microbial and animal systems, this is done by supplying exogenous label, often as amino acids. In plants, this was done in the last century using 14C-, 35S-, or 15N-labeled amino acids or amino acid precursors, and more recently using 13C-labeled amino acids or amino acid precursors. The use of radioisotopes has the advantage that very small amounts can be supplied with a high specific activity, but assignment of label to specific metabolites can be challenging. This is much easier using stable isotopes linked with mass spectrometry, but with the potential disadvantage that large amounts of exogenous compounds must be applied to obtain a clear signal. This is feasible in microbial or animal systems in which cells are supplied with exogenous medium that can be easily switched between unlabeled and labeled metabolites. It is much more challenging with most plant tissues, as the internal transport pathways are far more complicated with the exception of studies in isolated cells or organelles, or in certain tissues such as developing seeds, which receive nutrients from over the apoplast (Allen, Laclair, Ohlrogge, & Shachar-Hill, 2012). It should be noted that a large supply of external amino acids or precursors like sugars can result in a perturbation of metabolism and, in all likelihood, the rates of protein synthesis and degradation. Further problems are raised by mixing of the labeled metabolite with the internal pool, which leads to dilution of the label and underestimation of flux to protein (see below).
In plants, all carbon is derived directly or indirectly from photosynthetically fixed CO2. We have developed a relatively simple approach to quantify the rates of protein synthesis (K S) and degradation (K D) in Arabidopsis thaliana using 13CO2 labeling experiments (Ishihara, Obata, Sulpice, Fernie, & Stitt, 2015; Ishihara et al., 2017). This approach has three potential advantages over other methods using different stable isotopes or radioisotopes. First, 13CO2 is incorporated via photosynthetic CO2 fixation, allowing introduction of the isotope without perturbation of metabolism or growth. Second, 12CO2 and 13CO2 can be rapidly interchanged in the gas mixture supplied to the plant or leaf, allowing rapid start of the pulse and rapid and complete removal of external label at the start of a chase. Third, 13CO2 should label all amino acids and the extent of labeling can be measured using gas chromatography time-of-flight mass spectrometry (GC-TOF-MS).
We define K S as the rate of 13C-labeled amino acid incorporation into proteins. Note that the enrichment of 13C differs among amino acids. This could be due to differences in the various amino acid synthesis pathways. For example, in A. thaliana , amino acids that are directly synthesized from central carbon metabolism (by amino transferase) show higher 13C enrichment values than those whose synthesis requires multiple steps (Ishihara et al., 2015; Szecowka et al., 2013). It can also reflect compartmentation, for example, the sequestration of part of the amino acid pool in the vacuole that is not immediately involved in metabolism and is labeled only slowly. Furthermore, slow exchange between compartmented pools and the cytoplasmic pool used for protein synthesis can lead to decreased labeling in the latter in a manner that is difficult to predict. Compartmentation is much more prevalent in plants than microbes and animals, posing an especially large challenge to determination of fluxes to protein in plant systems. Therefore, information regarding 13C enrichment values from amino acids in soluble fractions are used to correct for incomplete 13C enrichment of free amino acid pools for accurate estimation of K S.
It has been shown that protein abundance does not vary much within a single diel cycle (Sulpice et al., 2009). Thus, K D can be assessed based on enrichment and decay of 13C-labeled amino acids in the protein fraction in a pulse and chase, respectively, and is estimated with the assumption that proteins that are not used for growth are degraded (Ishihara et al., 2015, 2017). Depletion of 13C enrichment in the protein fraction is due not only to protein degradation but also to dilution of labeled amino acids by tissue growth. Therefore, determination of K D requires information about the relative growth rate (RGR), which can be estimated in various ways, including measuring fresh weigh, dry weight, and size of rosette leaves for at least 3 days and estimating the slope of a log plot over this time (Poorter & Nagel, 2000). In our protocol, the 13C enrichment of glucose in the cell wall fraction is used to estimate structural RGR (RGRSTR) (Ishihara et al., 2015, 2017). We describe here the procedures for estimating K D based on the difference between K S and RGRSTR.
The work flow for estimating the rates of protein synthesis and degradation is divided into six protocols (Fig. 1). Basic Protocol 1 focuses on setting up of the 13CO2 labeling system and the labeling experiment. Basic Protocols 2-4 describe how to obtain soluble (free) amino acids, amino acids from total protein, and sugars from cell wall for GC-TOF-MS analysis. Basic Protocol 5 briefly explains how to obtain, organize, and analyze GC-TOF-MS data to calculate 13C enrichment (%) in compounds obtained using Basic Protocols 2-4. Basic Protocol 6 presents stepwise calculations of K S and K D. Stepwise calculations using R language are also available on the open-source GitHub repository (https://github.com/thiagomaf/Kd_Ks_RGRstr).
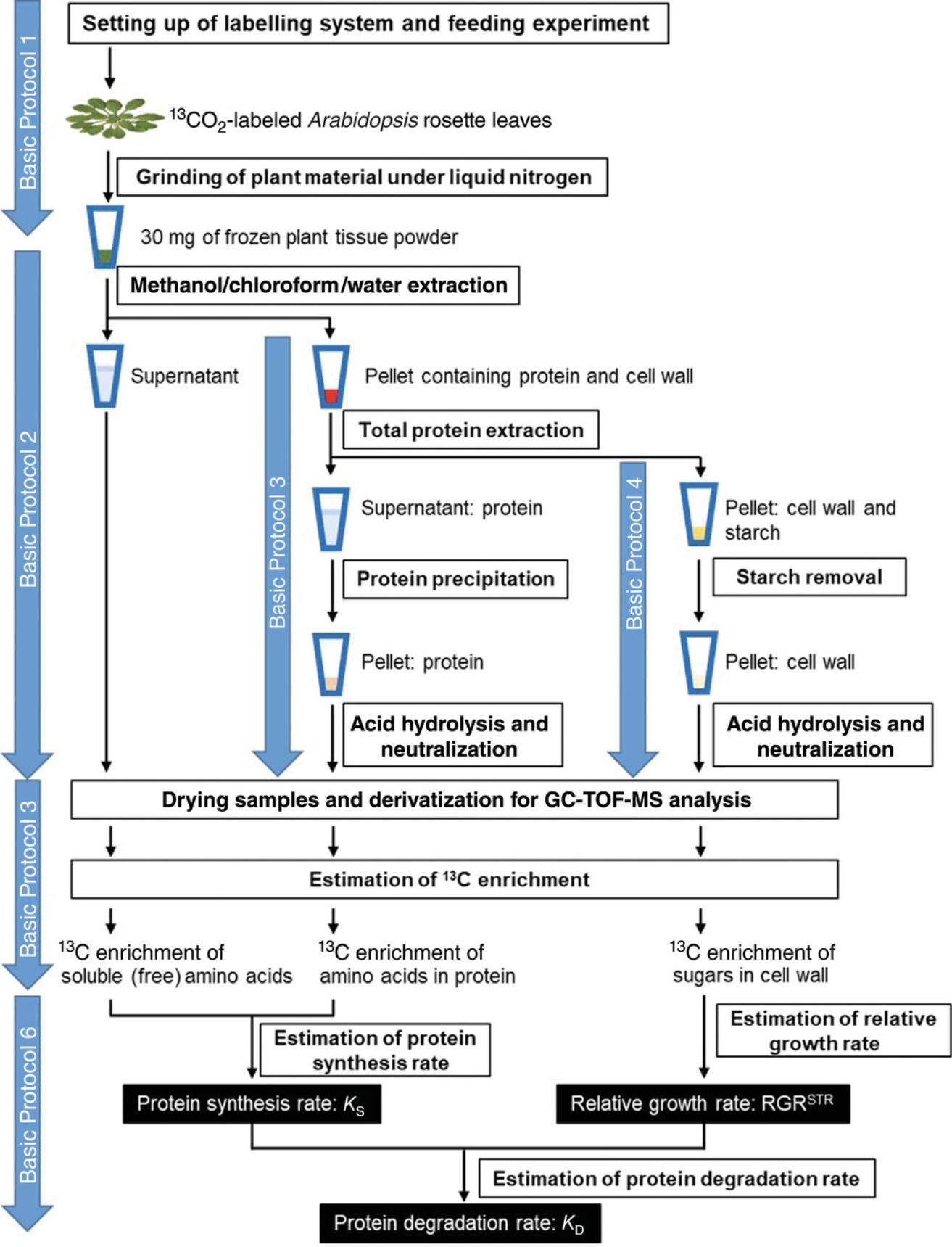
STRATEGIC PLANNING
It should be decided whether to perform a 13CO2 pulse experiment alone or a 13CO2 pulse followed by a 12CO2 chase experiment. In general, a 13CO2 pulse experiment is sufficient to obtain K S, K D, and RGRSTR. The advantage of performing a 13CO2 pulse/12CO2 chase experiment is the estimation of these parameters from two different datasets instead of one.
The temporal distribution of harvest time points and the number of samples per time point needs to be carefully planned in order to obtain robust results. It is recommended to have at least three biological replicates per time point. We use five A. thaliana plants per replicate to reduce biological noise. When using younger and/or smaller plants, it is recommended to grow more plants per pot in order to increase the amount of material harvested. If only a 13CO2 pulse experiment is performed, the number of replicates and time points can be increased, leading to increased statistical power and increased understanding of labeling kinetics in a timely manner.
During a 13CO2 pulse experiment, the first time point is typically at the beginning of the light period (ZT0) and is used to estimate the natural abundance of 13C. A second time point can be taken at the end of a 24-hr 13CO2 pulse (ZT24). If possible, however, it is encouraged to take a few time points during the light period (including at the end of the light period) and a few during the dark period to obtain better temporal information about 13C incorporation into protein and increased precision in the correction for enrichment in free amino acids. It should be noted that 13C enrichment of soluble free amino acids rises in the first 30-60 min after illumination and decreases slightly between the end of the light period and the end of the dark period (Ishihara et al., 2015). Thus, for example, if free amino acids were only measured at ZT24 (corresponding in our case to the end of the dark period), their 13C enrichment values at ZT24 need to be adjusted by considering the decay during the night based on changes previously reported (Ishihara et al., 2017). Generally, we perform a 12CO2 chase for 96 hr and harvest samples at the end of the chase. At this time point, 13C enrichment of alanine, for example, is reduced from 25% to 10%.
In order to estimate K D, it is necessary to have an accurate RGR. We have shown that RGR values estimated from rosette size, fresh weight, and 13C labeling are very similar (Ishihara et al., 2015). If RGR will be determined based on fresh weight, dry weight, or rosette size, extra plants should be grown in the same conditions and at the same time as the plants used for the labeling experiment and harvested 1 day before the start of the experiment (ZT–24), at the beginning of the experiment (ZT0), and 1 day after the start of the experiment (ZT24).
Basic Protocol 1: SETTING UP THE 13CO2 LABELING SYSTEM AND STABLE ISOTOPE LABELING OF ARABIDOPSIS THALIANA ROSETTE LEAVES
A schematic representation of the labeling system is presented in Figure 2A. Stable isotope labeling of A. thaliana rosette leaves is typically done using transparent Plexiglass labeling chambers that can contain up to eighteen 10-cm pots (Fig. 2B). To reduce biological noise, we recommend growing five plants per pot. The labeling chambers are placed inside a plant growth chamber with a controlled environment (Fig. 2B). 13CO2 gas is mixed with a N2/O2 gas mixture using a custom-made tee gas mixer (Fig. 2C). The 13CO2 flow (i.e., concentration) is adjusted and controlled using a mass flow controller. The labeled gas mixture is directed to each labeling chamber at a flow rate of 5 L/min, which is sufficient to completely replace the air in each labeling chamber in 15 min.
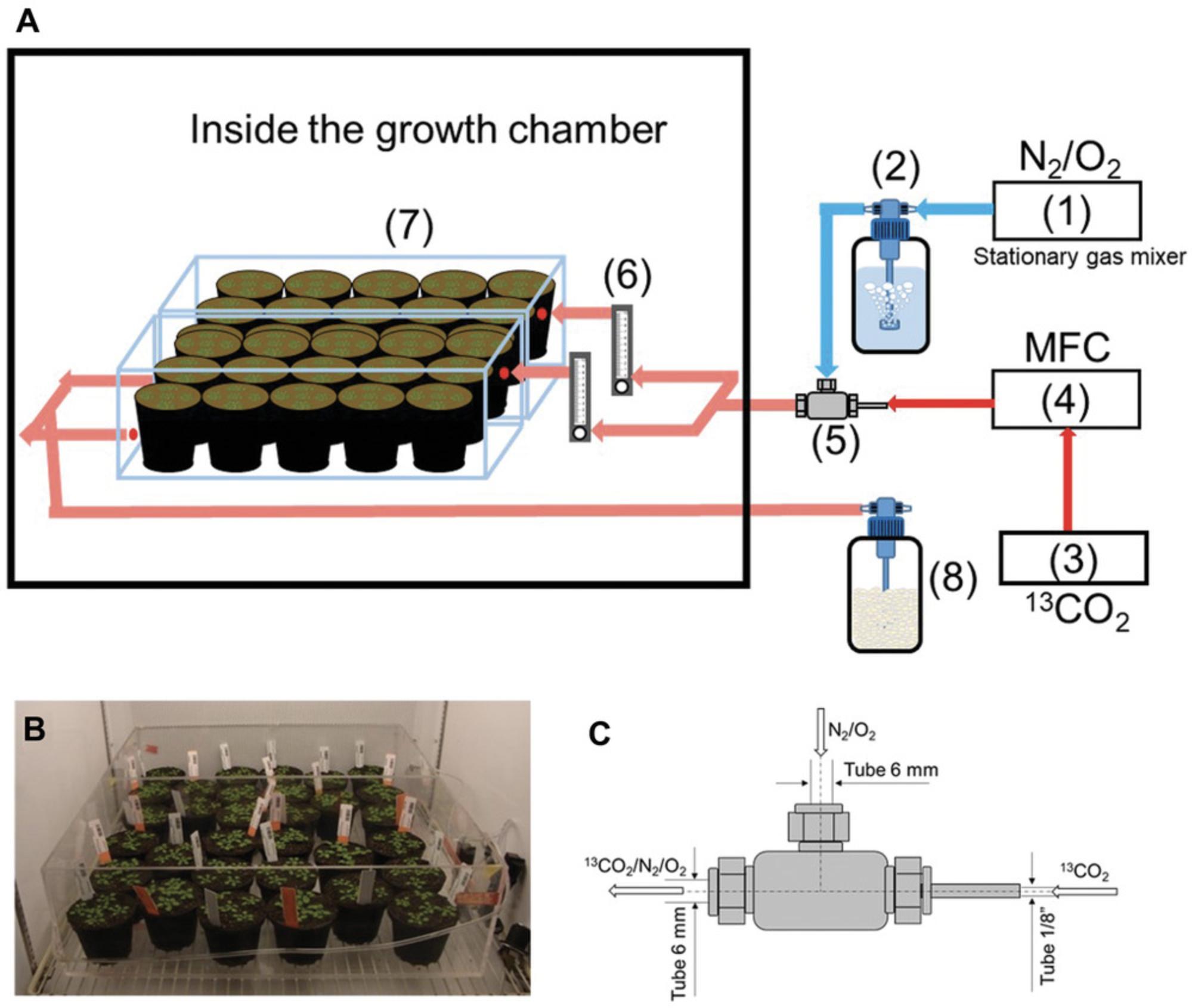
It has been reported that metabolite and transcript levels change when plants are transferred to different growth conditions (Moraes et al., 2019). In order for plants to be adapted to the experimental conditions, they should be transferred to labeling chambers 3 days prior to the labeling experiment. The labeling chambers are not closed with a lid until the start of the experiment.
Materials
-
N2, O2, and 12CO2 gas (e.g., Westfalen, cat. nos. A0034, A0018, A0710)
-
13CO2 gas (e.g., Sigma-Aldrich, cat. no. 364592)
-
Arabidopsis thaliana plants in 10-cm-diameter pots
-
Liquid nitrogen
-
Labeling setup (Fig. 2):
- Plant growth chamber (e.g., Percival Scientific, model E36L)
- Stationary gas mixer (e.g., WMR 4008 Westphal, Brooks Instrument)
- 500-ml gas washing bottles with filter discs (e.g., DURAN, DWK Life Sciences, cat. no. 257040101)
- Cylinder pressure regulator for 13CO2 bottle (e.g., GCE druva, cat. no. FMD 502-14 BCF1,5 CGA180CL6)
- Mass flow controller (e.g., Brooks Portable GC 40 with 0260 series power supply, Brooks Instrument)
- Custom tee gas mixer (Fig. 2C; e.g., Bronkhost High-Tech B.V.)
- 1.1-11 L/min glass tube rotameters (e.g., SHo-RATE, Brooks Instrument, cat. no. 1350GBL3BACV1AAA)
- Plexiglass labeling chambers (internal dimensions, 60 × 31 × 17.4 cm) with holes for gas inlet/outlet (Fig. 2A)
- 6-mm and ⅛-in. (inner diameter) PVC tubes
- Y-shaped hose connectors (e.g., Carl Roth, cat. no. E772.1)
-
CO2 gas analyzer (e.g., LICOR LI-850 CO2/H2O analyzer)
-
Liquid nitrogen–safe scintillation vials (e.g., 20-ml Polyvials, Zinsser Analytic, cat. no. 3071400)
-
Mixer mill (e.g., Retsch, MM400)
-
5- to 7-mm steel balls (e.g., Th. Geyer)
Set up labeling system
1.Three days prior to the labeling experiment, place labeling chambers in the growth chamber.
2.Connect each component of the labeling system as illustrated (Fig. 2A) using 6-mm PVC tubes except between the MFC (4) and tee gas mixer (5). For this connection, use a ⅛-in. PVC tube to increase the gas pressure (Fig. 2C).
3.Inside the growth chamber, divide the tube exiting the tee gas mixer using a Y-shaped hose connector and connect each outlet to a glass tube rotameter (6).
4.Connect the rotameters to the gas inlets of the labeling chambers (7)
5.Connect the PVC tubes exiting the labeling chambers using a Y-shaped hose connector. Connect the resulting single PVC tube to a LICOR LI850 CO2 analyzer connected to a CO2 trap (8).
Set up 13CO2 gas mixture
6.Mix 79% N2 and 21% O2 at a flow rate of 10 L/min using a stationary gas mixer (1).
7.Set the 12CO2 flow rate at 4.5 ml/min using the MFC.
8.Adjust the rotameters to a flow rate of 5 L/min.
9.Close the lids of the labeling chambers.
10.Check the 12CO2 concentration in the gas mixture using a CO2 analyzer. If necessary, adjust the concentration using the MFC.
11.Replace the 12CO2 cylinder with the 13CO2 cylinder.
12.Stop the gas flow, remove the CO2 analyzer from the system, and place the plants in the labeling chambers without lids.
Label rosette leaves
13.Remove unlabeled control plants from the growth chamber 15 min before the start of the light period.
14.As soon as the control plants have been removed, place the lids on the labeling chambers and start the gas flow with the 13CO2 mix.
15.Immediately harvest rosette leaves from the unlabeled control plants and immediately freeze in liquid nitrogen. Transfer frozen material to pre-cooled scintillation vials in liquid nitrogen.
16.For each harvesting time point, slightly open the lids of the labeling chambers, quickly remove the pots, close the lids, and immediately harvest rosette leaves.
17.After performing the 13CO2 pulse for 24 hr, stop the gas flow, replace the 13CO2 cylinder with the 12CO2 cylinder, and restart the gas flow.
18.Harvest samples during the 12CO2 chase.
19.Grind frozen leaves to a fine powder using a mixer mill with steel balls. Store ground frozen material at −80°C until use.
Basic Protocol 2: EXTRACTION OF SOLUBLE AMINO ACIDS FOR GC-TOF-MS ANALYSIS
GC-TOF-MS can identify and quantify a few hundred metabolites in a single plant extract, including sugars, sugar alcohols, phosphorylated intermediates, lipophilic compounds, and organic and amino acids (Lisec, Schauer, Kopka, Willmitzer, & Fernie, 2006). The following sample preparation protocol is relatively simple, established for different plant species such as A. thaliana , cassava, maize, and tomato (Lisec et al., 2006; Obata et al., 2015; Rosado-Souza et al., 2019; Schauer et al., 2006), and adapted for a lower amount of starting material (Ishihara et al., 2015, 2017).
Materials
-
Frozen, ground plant tissue (see Basic Protocol 1)
-
0.2 mg/ml ribitol (Sigma-Aldrich, CAS 488-81-3) in ultrapure water
-
100% methanol (gradient grade for liquid chromatography; Merck, CAS 67-56-1)
-
100% chloroform (gradient grade for liquid chromatography; Merck, CAS 67-66-3)
-
Ultrapure water (∼0.055 μS/cm; e.g., Milli-Q)
-
Argon gas (optional)
-
2-ml screw-cap tubes (e.g., Sarstedt, cat. no. 72.693)
-
Thermomixer (e.g., Eppendorf Thermomixer comfort)
-
6-ml screw-cap glass vials (e.g., DURAN, cat. no. 231751159)
-
2-ml Safe-Lock microcentrifuge tubes (e.g., Eppendorf, cat. no. EP0030123620)
-
Vacuum concentrator (e.g., SpeedVac, Thermo Fischer Scientific)
1.Weigh 30 mg (±10%) frozen, ground plant material in 2-ml screw-cap tubes. Record the weight of each aliquot for data normalization.
2.Mix 280 µl of 100% methanol and 12 µl of 0.2 mg/ml ribitol per sample and cool the mixture on ice.
3.Add 292 µl cold methanol/ribitol to each sample and shake at 100 rpm for 15 min at 70°C using a Thermomixer.
4.Centrifuge at 20,000 × g for 10 min at 4°C.
5.Transfer the supernatant to a 6-ml glass vial. Keep the pellet at −20°C for protein and cell wall preparations.
6.Add 150 µl of 100% chloroform to the supernatant and vortex.
7.Add 300 µl ultrapure water and vortex for precisely 15 s.
8.Centrifuge at 4000 × g for 15 min at 4°C
9.Transfer 150 µl of the upper polar phase to a 2-ml Safe-Lock tube. As a backup, transfer 300 µl to another 2-ml Safe-Lock tube.
10.Dry overnight in a vacuum concentrator without heat.
11.Optional : Fill tubes with argon gas for long-term storage at −80°C.
Basic Protocol 3: PREPARATION OF AMINO ACIDS FROM TOTAL PROTEIN FOR GC-TOF-MS ANALYSIS
Protein solubilization is considered the most problematic step during sample preparation for proteomic studies. A protein extraction buffer including a combination of urea and thiourea was shown to solubilize proteins efficiently (Chan, Lo, & Hodgkiss, 2002). Once the proteins are extracted, amino acids are released from peptides using classical acid hydrolysis with 6 M HCl at 110°C for 24 hr. Neutralization and cleaning of the samples for GC-TOF-MS analysis are carried out by liquid-phase extraction using dioctylamine/chloroform and chloroform.
Materials
-
Pellet containing protein and cell wall (see Basic Protocol 2)
-
100% methanol (gradient grade for liquid chromatography; Merck, CAS 67-56-1)
-
Protein extraction buffer (see recipe)
-
Bovine serum albumin (BSA; Sigma-Aldrich, CAS 9048-46-8)
-
Bio-Rad Protein Assay Dye Reagent Concentrate (Bio-Rad, cat. no. 5000006)
-
Ultrapure water (∼0.055 μS/cm; e.g., Milli-Q)
-
20% (w/v) trichloroacetic acid (TCA; Sigma-Aldrich, CAS 76-03-9) in ultrapure water, ice cold
-
100% acetone (Sigma-Aldrich, CAS 67-64-1)
-
6 M hydrochloric acid (HCl; Sigma-Aldrich, CAS 7647-01-0)
-
Dioctylamine (98% [v/v], Sigma-Aldrich, D201146)
-
100% chloroform (for liquid chromatography; Merck, CAS 67-66-3)
-
Argon gas
-
1.5-ml screw-cap tubes (e.g., Sarstedt, cat. no. 72.692)
-
96-well flat-bottom microtiter plate (e.g., Thermo Fisher Scientific, cat. no, 2205)
-
Open microtiter plate reader (e.g., BioTek 800TS)
-
Thermomixer (e.g., Eppendorf Thermomixer comfort)
-
pH indicator paper (e.g., Macherey-Nagel, cat. no. N361.1)
-
2-ml Safe-Lock microcentrifuge tubes (e.g., Eppendorf, cat. no. 0030123611)
-
Vacuum concentrator (e.g., SpeedVac, Thermo Fischer Scientific)
Extract and quantify protein
1.Carefully wash the pellet containing protein and cell wall material three times with 500 µl of 100% methanol to remove potential amino acid contamination from leftover supernatant.
2.Add 200 µl protein extraction buffer and vortex vigorously at room temperature.
3.Centrifuge at 20,000 × g for 10 min at room temperature. Transfer supernatant to a 1.5-ml screw-cap tube. Keep the pellet at −20°C for cell wall preparation.
4.Prepare BSA standards at 1, 0.5, 0.25, and 0 mg/ml in protein extraction buffer.
5.Dilute 3 µl supernatant in 9 µl protein extraction buffer.
6.Prepare 160 µl of Bio-Rad Protein Assay solution per sample by diluting 1:8 with ultrapure water.
7.Mix 160 µl diluted assay solution with 3 µl of each standard and sample in a 96-well flat-bottom microtiter plate.
8.Measure absorbance at 595 nm (A 595) using an open microtiter plate reader.
9.Calculate protein concentration (µg/µl) using the standard curve generated with the BSA standards.
Precipitate protein
10.Transfer 50 µg sample to a 1.5-ml screw-cap tube.
11.Precipitate protein by adding 2 volumes of 20% (w/v) ice-cold TCA and centrifuging at 20,000 × g for 10 min at 4°C.
12.Discard supernatant and wash pellet three times with 300 µl ice-cold 100% acetone.
13.Air-dry pellet for 15 min.
Hydrolyze protein and neutralize amino acids
14.Resuspend pellet in 300 µl of 6 M HCl and vortex.
15.Hydrolyze proteins by heating at 110°C for 24 hr at atmospheric pressure using a Thermomixer.
16.Neutralize hydrolysate by adding 1 ml of 20% (v/v) dioctylamine in chloroform, vortexing thoroughly, and centrifuging at 20,000 × g for 1 min at room temperature. Discard the organic phase.
17.Repeat neutralization two more times and then check that the pH of the aqueous phase is neutral using pH indicator paper.
18.Remove excess dioctylamine by repeating step 16 four times using 1 ml of 100% chloroform.
19.Transfer the upper polar phase to a 2-ml Safe-Lock tube without taking any chloroform.
20.Dry samples overnight in a vacuum concentrator without heat.
21.Fill tubes with argon gas and store at −80°C to avoid oxidation of metabolites.
Basic Protocol 4: PREPARATION OF SUGARS FROM CELL WALL MATERIAL FOR GC-TOF-MS ANALYSIS
The A. thaliana cell wall is composed of polysaccharides, proteins, and lignin, with polysaccharides accounting for 80% (Zablackis, Huang, Muller, Darvill, & Albersheim, 1995). The polysaccharides are cellulose, hemicellulose, and pectin. Cellulose and the hemicellulose backbone are linear β(1-4) linked d-glucose polymers synthesized from UDP-glucose. On the assumption that there is little or no turnover of these polymers, the 13C enrichment of glucose in the cell wall can be used to estimate how fast plants are growing (RGR). However, the pellet obtained in Basic Protocol 3 contains starch as well as cell wall material. Starch is a polysaccharide composed of d-glucose joined by α1,4-glycoside linkages. In the growth and labeling conditions of our protocol, starch is labeled rapidly and to high enrichment by 13CO2. Starch must therefore be completely removed by repeated incubations with α-amylase and amyloglucosidase before cell wall samples can be used to prepare sugars. Hydrolysis of cell wall material (polysaccharides) is carried out by pre-hydrolysis with 72% (v/v) H2SO4 followed by hydrolysis with 1 M H2SO4 (Saeman, Moore, Mitchell, & Millett, 1954). Neutralization and cleaning of samples for GC-TOF-MS analysis are carried out by liquid-phase extraction using dioctylamine/chloroform and chloroform.
Materials
-
Pellet containing cell wall material (see Basic Protocol 3)
-
Ultrapure water (∼0.055 μS/cm; e.g., Milli-Q)
-
0.1 M NaOH: freshly diluted from 1 M stock (Merck, CAS 1310-73-2) with ultrapure water
-
Neutralization buffer (see recipe)
-
Starch digestion buffer (see recipe)
-
Glucose determination assay buffer (see recipe)
-
2.5 U/ml hexokinase (from Saccharomyces cerevisiae , 1500 U/ml, Roche, 11426362001) in 100 mM HEPES-KOH with 3 mM MgCl2, pH 7.5
-
72% (v/v) sulfuric acid: diluted from 98% stock (Sigma, CAS 7664-93-9) ultrapure water at a ratio of 2.57:1
-
Dioctylamine (98% [v/v], Sigma-Aldrich, D201146)
-
100% chloroform (for liquid chromatography; Merck, CAS 67-66-3)
-
Thermomixer (e.g., Eppendorf Thermomixer comfort)
-
pH indicator paper (e.g., Macherey-Nagel, cat. no. N361.1)
-
Open microtiter plate reader (e.g., BioTek 800TS)
-
96-deep-well plate (e.g., Sigma-Aldrich, cat. no. P8116)
-
96-well flat-bottom microtiter plate (e.g., Thermo Fisher Scientific, cat. no, 2205)
-
2-ml Safe-Lock microcentrifuge tubes (e.g., Eppendorf, cat. no. 0030120094)
-
Vacuum concentrator (e.g., SpeedVac, Thermo Fischer Scientific)
Remove starch
1.Add 700 µl ultrapure water to the pellet containing cell wall material and vortex. Centrifuge at 20,000 × g for 10 min at room temperature and discard supernatant. Repeat at least three more times.
2.Resuspend pellet in 400 µl of 0.1 M NaOH, shake vigorously, and heat at 100°C for 30 min using a Thermomixer.
3.Neutralize by adding 80 µl neutralization buffer and vortex. Check that the pH is neutral using pH indicator paper.
4.Add 100 µl starch digestion buffer and incubate for 16 hr at 37°C in a Thermomixer.
5.Centrifuge at 20,000 × g for 10 min at room temperature. Transfer the supernatant (containing liberated starch) to a 96-deep-well plate. Keep the pellet (containing the desired cell wall material).
6.Add 170 µl glucose determination assay buffer and 30 µl digested starch solution to a 96-well flat-bottom microtiter plate.
7.Using an open microtiter plate reader, begin measuring absorbance at 340 nm (A 340) continuously at 1-min intervals for 2 hr.
8.When the optical density (OD) is stable (15-20 min), remove the plate from the reader, add 1 µl hexokinase to each well, vortex lightly, and return the plate to the plate reader.
9.When the OD is stable again (20-30 min), stop the plate reader and record the OD.
10.Determine the amount of glucose released from starch using the equation: glucose (µmol) = NADPH (µmol) = ΔOD ÷ (2.85 × 6.22).
11.Repeat steps 1-10 with the pellet in step 5 two more times or until no starch remains.
12.Add 700 µl ultrapure water to the pellet, vortex, and centrifuge at 20,000 × g for 10 min at room temperature. Discard the supernatant and air-dry the pellet for 2 hr.
Hydrolyze polysaccharides and neutralize sugars
13.To disrupt the crystallinity of the cellulose, add 63 μl of 72% (v/v) H2SO4 to the pellet, vortex vigorously, and incubate at room temperature for 1 hr.
14.Add 690 μl ultrapure water to dilute the H2SO4 to 1 M and incubate 3 hr at 100°C using a Thermomixer.
15.Neutralize hydrolysate by adding 1 ml of 20% (v/v) dioctylamine in chloroform, vortexing thoroughly, and centrifuging at 20,000 × g for 1 min at room temperature. Discard the organic phase.
16.Repeat neutralization two more times and then check that the pH of the aqueous phase is neutral using pH indicator paper.
17.Remove excess dioctylamine by adding 1 ml of 100% chloroform, vortexing thoroughly, and centrifuging at 20,000 × for 2 min at room temperature. Transfer the upper polar phase to a 2-ml Safe-Lock tube and repeat two more times.
18.Transfer 70-, 140-, and 280-µl aliquots of supernatant to 2-ml Safe-Lock tubes without taking any chloroform.
19.Dry samples overnight in a vacuum concentrator without heat.
20.Fill tubes with argon gas and store at −80°C to avoid oxidation of metabolites.
Basic Protocol 5: GC-TOF-MS ANALYSIS OF 13C-LABELED SAMPLES AND ESTIMATION OF 13C ENRICHMENT (%)
Lisec et al. (2006) published a detailed protocol on how to profile and quantify metabolites from A. thaliana using GC-TOF-MS. This protocol describes in great detail the processing of samples, including derivatization, and the settings for GC-TOF-MS. Heise et al. (2014) provide a protocol describing how to process GC-TOF-MS data to estimate 13C enrichment (%) using CORRECTOR software.
Materials
- Samples containing soluble amino acids, amino acids from total protein, and sugars from cell wall (see Basic Protocols 2-4)
- GC-TOF-MS chromatogram file
- GC-TOF-MS chromatogram analysis software (e.g., TagFinder, Xcalibur)
- CORRECTOR software (https://www.mpimp-golm.mpg.de/54630/Bioinformatik-Tools)
- Intel-compatible platform running Windows 10 /8.1/8 /7 /Vista /XP /2000 Windows Server 2019 /2016 /2012 /2008 /2003
1.Process samples and perform GC-TOF-MS analysis as described (Lisec et al., 2006).
2.Select peak areas of the fragment mass of amino acids and sugars from the GC-TOF-MS chromatograph .cdf file, based on Supplemental Data S1 (Lisec et al., 2006).
3.Generate an input.txt file (see the exemplary file in Supplemental Data S2; also see Heise et al., 2014)
4.Estimate 13C enrichment (%) of amino acids and glucose using CORRECTOR software as described in Heise et al. (2014).
Basic Protocol 6: ESTIMATION OF PROTEIN SYNTHESIS AND DEGRADATION RATES
The absolute rate of protein synthesis in a pulse, K S(pulse), is calculated from the labeling kinetics of amino acids in total protein after correcting for incomplete enrichment in soluble (free) amino acid pools. Many amino acid pools show very low enrichment in the light and decrease rapidly in the dark, and cannot be used to estimate K S(pulse) (Ishihara et al., 2015). Instead, K S(pulse) is calculated using the labeling kinetics of alanine and serine, both of which are rapidly labeled to high enrichment in the day and whose enrichment stays high during the night (Ishihara et al., 2015). The 13C enrichment in the amino acids from protein in a given time interval is corrected by the average enrichment in free amino acids over the time internal. Without 13C enrichment data from the light period, K S(pulse) will be overestimated due to the slight decrease in enrichment between the end of day (ED) and the end of night (EN) (Ishihara et al., 2015). Therefore, we recommend that ED and EN values be obtained. In addition, K S can be estimated from the decay of the enrichment of serine and alanine in a protein during the chase period, K S(chase). The rate of protein degradation during pulse (K D(pulse)) and chase (K D(chase)) periods can be estimated as the difference between the rate of protein synthesis and the relative growth rate (K D = K S − RGR). Since K S, K D, RGR are estimated from descriptive statistics on replicate sample data, we describe here how to estimate rates using Gaussian error propagation with the widely used R language.
Materials
- Spreadsheet editor (e.g.; excel)
- R (https://www.r-project.org/), optional
- R studio (https://rstudio.com/), optional
- 13C enrichment (%) of soluble amino acids, amino acids from total protein, and glucose from cell wall material
- 13C enrichment exemplary data (Supplemental Data S3): 13C enrichment (%) of alanine, serine, and glucose from three independent replicate experiments containing data from thirteen A. thaliana accessions at ZT0, ZT24, and ZT120 (Ishihara et al., 2017)
Estimate protein synthesis rate
The rate of protein synthesis in a 13 CO2 pulse experiment, K S(pulse), is defined as the rate of incorporation of labeled amino acids into proteins normalized by a correction factor for incomplete enrichment in the free amino acid pool (Pocrnjic, Mathews, Rappaport, & Haschemeyer, 1983). It is calculated over the time interval from t 1 and t 2 using Equation 1:
Ks(pulse)=(SB(t2)−SB(t1))/SA(t2−t1)(t2−t1)(1)
where S B(t ) represents the average labeled amino acids in protein at time t , and S A(t 2-t 1) represents the average labeled free amino acids in the interval between t 1 and t 2. Results are expressed as mg protein/mg protein/day. Exemplary results can be found in Supplemental Data S3 and S4.In step form:
1.Calculate the mean and standard error of the 13C enrichment (%) of free amino acids (S A) and of amino acids in total protein (S B) from samples harvested at time points t 1 and t 2 (Supplemental Data S3).
2.Subtract the mean 13C enrichment of amino acids in proteins at time t 1 from time t 2 (SB(t2) – SB(t1)).
3.Calculate the average 13C enrichment of free amino acids in the time interval between t 1 and t 2 (SA(t2−t1)).
4.Divide the result from step 2 by the result from step 3.
5.Divide the result by the time interval between t 1 and t 2 expressed as days (in our case, 1 day).
Estimate relative structural biomass growth rate (RGRSTR)
We estimate RGRSTR based on the labeling kinetics of glucose in cell walls (see Ishihara et al., 2015, 2017 for more details) during a 13CO2 pulse experiment RGRSTR(pulse). The first time point in a 13CO2 pulse experiment (t 1 = 0) corresponds to unlabeled material with no glucose labeling (Glc(t1) = 0). In this case, RGRSTR(pulse) is linearly estimated as the total 13C enrichment (%) in glucose at the end of the pulse period. This is done by subtracting the mean 13C enrichment at t 1 from that at t 2 (Glc(t2) – Glc(t1)) and dividing by the time interval between t 1 and t 2 (in our case, 1 day). Exemplary results can be found in Supplemental Data S3 and S5.
Alternatively, estimation of RGRSTR can be done during a 12CO2 chase, assuming that there is negligible dilution of glucose in cell wall polysaccharides during the analyzed period. RGRSTR(chase) can be estimated considering exponential growth using Equation 2:
RGRSTR(chase)=1−Glc(t2)Glc(t1)t(2)
where t 1 and t 2 are the start and end of the chase, respectively; t is the time interval from t 1 to t 2 (in our case, 4 days); and Glc(t ) represents the average 13C enrichment of glucose in the cell wall material. Exemplary results can be found in Supplemental Data S3 and S8.The unit of RGRSTR (chase) is mg 13C glucose per mg total glucose in cell wall per day.
6.Calculate the mean and standard error of the mean using measurements of glucose 13C enrichment in cell wall fractions collected at the start and end of the chase (t 1 and t 2).
7.Divide mean 13C enrichment at t 2 by mean 13C enrichment at t 1 and calculate the t th root of the result.
8.Subtract the result from 1.
Estimate protein degradation rate
The rate of protein degradation during a 13CO2 pulse experiment, K D(pulse), can be estimated as the difference between the rate of protein synthesis (K S(pulse)) and the relative growth rate (RGRSTR(pulse)) using Equation 3:
KD(pulse)=KS(pulse)−RGRSTR(pulse)(3)
The unit for K D(pulse) is mg protein/mg protein/day. Exemplary results can be found in Supplemental Data S3 and S6.
The rate of protein degradation during a 12CO2 chase experiment, K D(chase), can be estimated as the difference between the rate of decay in 13C enrichment of amino acids in proteins (K S(chase)) and the rate of amino acid enrichment dilution due to tissue growth (RGRSTR(chase)) using Equations 4 and 5:
KS(chase)=−[ln(SB(t2))−ln(SB(t1))]t2−t1(4)
KD(chase)=KS(chase)−RGRSTR(chase)(5)
where S B(t ) represents the average labeled amino acids in protein at time t. The unit for K S(chase) and K D(chase) is mg protein/mg protein/day. Exemplary results can be found in Supplemental Data S3, S7, and S9.
9.Calculate the mean and standard error of the 13C enrichment (%) of amino acids in total protein from samples harvested at time points t 1 and t 2.
10.Calculate the natural logarithm of the mean 13C enrichments at t 1 and t 2 and subtract the latter from the former.
11.Divide the result by the time interval (in our case, 4 days).
12.Subtract RGRSTR(chase) from K S(chase).
Prepare data and estimate RGRSTR, KS, and KD using R language
As previously mentioned, Supplemental Data S3 from Ishihara et al. (2017) is used to illustrate the analysis of a 13CO2 pulse/12CO2 chase experiment. Data analyses were done manually (for Supplemental Data S3) or using R language (https://www.r-project.org/; Supplemental Data S4-S9). Step-by-step instructions are available on the open-source GitHub repository (https://github.com/thiagomaf/Kd_Ks_RGRstr).
Calculations for RGRSTR(pulse) in the pulse experiment involved comparison between ZT0 and ZT24. For the chase experiment, ZT24 and ZT120 were used.
In this example from Ishihara et al. (2017), K S(pulse) was estimated using alanine and serine from a pulse experiment (ZT0 to ZT24; t 2 – t 1 = 1 day) and is expressed as mg protein/mg protein/day.
In this experiment, at ZT0 (t 1 = 0), enrichment of labeled free amino acids is taken as zero (SA(t1) = 0). The correction factor SA(t2−t1) was then defined as the enrichment of free labeled alanine or serine at ZT24. The latter assumes that labeling of free amino acids is roughly constant during the diel cycle, which might not always be true in plants and could lead to under- or overestimation of K S (for more details, see Ishihara et al., 2015). Therefore, Equation 1 is simplified as:
Ks(pulse)=(SB(t2)−SB(t1))/SA(t2)
REAGENTS AND SOLUTIONS
Glucose determination assay buffer
- 100 mM HEPES-KOH with 3 mM magnesium chloride (MgCl2, 1 mM final), pH 7.0
- 1 mM ATP (Roche, cat. no. 10127523001, CAS 34369-07-8)
- 1 mM nicotinamide adenine dinucleotide (NAD+, free acid, grade II, Roche, cat. no. 10127981001, CAS 53-84-9)
- 0.5 U/ml glucose 6-phosphate dehydrogenase (G6PDH, grade II, from Leuconostoc mesenteroides , 100 U/ml, Roche, cat. no. 10165875001)
- 2.25 U/ml hexokinase (from S. cerevisiae , stock 1500 U/ml, Roche, cat. no. 11426362001)
- Store at −80°C
Neutralization buffer
- 0.1 M acetic acid, pH 4.9 (adjust with NaOH)
- 0.5 M HCl
- Store at 4°C
Protein extraction buffer
- 6 M urea (Sigma-Aldrich, cat. no. CAS 57-13-6)
- 2 M thiourea (Sigma-Aldrich, 62-56-6)
- 10 mM HEPES (Sigma-Aldrich, CAS 7365-45-9, adjust to pH 8.0 with KOH)
- Store at room temperature
Starch digestion buffer
- 6.3 U/ml amyloglucosidase (from Aspergillus niger , Roche/Merck, cat. no. 10102857001)
- 50 U/ml α-amylase (Sigma-Aldrich, cat. no. 10070)
- 200 mM sodium acetate (Sigma-Aldrich, 32319-1KG-R, CAS127-09-3), pH 4.8 (adjust with NaOH)
- Always prepare fresh on the day of experiment
COMMENTARY
Background Information
Plants constantly synthesize and degrade proteins to replace damaged proteins, remobilize resources, and change protein content during development or adaptation to new environments (Nelson & Millar, 2015). The rate of protein turnover varies between A. thaliana accessions, and this has a negative impact on growth rate in standard lab conditions. It has been hypothesized that faster protein turnover may aid adjustment to changes in environmental conditions (Ishihara et al., 2017).
Protein synthesis and degradation rates can be measured using stable or radioisotopes. Although the use of radioisotopes has the advantage that very small amounts can be supplied with a high specific activity, it also requires dedicated analytical instruments for accurate determination of isotope incorporation in proteins and the precursor pool (Huffaker & Peterson, 1974). Alternatively, proteins can be labeled by supplying organisms with labeled amino acids or labeled basic elements present in proteins (S, P, C, N, O, H). Cell cultures supplied with labeled amino acids such as [2H4]-lysine and [13C6]-arginine displayed lower enrichment of proteins in the light than in the dark (Gruhler, Schulze, Matthiesen, Mann, & Jensen, 2005; Schütz, Hausmann, Krug, Hampp, & Macek, 2011). However, this method presents several disadvantages, especially when performing experiments in plants. Plants produce amino acids using carbon skeletons that derive from photosynthesis, which in the light will dilute the pool of supplied labeled amino acids (Schütz et al., 2011). Another drawback is that biosynthesis of most amino acids is subject to feedback regulation, meaning that supplying plants with external amino acids in excess will inhibit amino acid biosynthesis (Galili, 1995). Altogether, feeding experiments with labeled amino acids are not suitable for plants (Schütz et al., 2011).
Several studies have used labeled basic elements present in proteins to estimate protein turnover rates. For example, labeling of plant proteins using two different water isotopes (2H2O or H218O) was shown to have a low efficiency due to inhibition of growth (Yang et al., 2010) and a slower incorporation of 18O in proteins (Zhou et al., 2012). N can be incorporated in proteins through inorganic 15N salts such as ammonia and nitrate. 15N is the most common stable isotope used to measure protein synthesis and degradation rates in plant tissues, such as A. thaliana leaf, root, and cell culture (Fan et al., 2016; Li et al., 2017; Nelson, Li, Jacoby, & Millar, 2013), barley (Nelson, Li, & Millar, 2014), and Medicago truncatula (Lyon et al., 2016). Unlike labeled water, 15N salts do not inhibit plant growth. Another advantage of 15N salts is that they are inexpensive compared to labeled amino acids and can be fed to plants grown in liquid medium, solid medium, or black sand (Masclaux-Daubresse & Chardon, 2011; Nelson & Millar, 2015). The major disadvantage of using 15N salts is that they are taken up and moved through the plant quite slowly, with the consequences that the start of the pulse is rather undefined, the isotope is significantly diluted by internal pools, and the isotope is difficult to remove completely from the growth medium and therefore is not suitable for chase experiments.
Since plants are photoautotrophs, 13C can be supplied as 13CO2 and is rapidly incorporated into the plant's metabolism through photosynthesis. To perform a chase experiment, 13CO2 can be easily replaced with 12CO2. As a result, 13CO2 is widely used in plants to measure flux of plant metabolites (Huege et al., 2007; Szecowka et al., 2013; Williams et al., 2010), turnover of specific proteins (Chen et al., 2011), and synthesis of total protein (Ishihara et al., 2015, 2017; Pal et al., 2013). The bottleneck of using 13CO2 is the varying incorporation rates of 13C in different amino acids (Ishihara et al., 2015; Szecowka et al., 2013). This is partially due to the compartmentation of metabolites in plants. GC-TOF-MS analysis, for example, can be used to measure all possible amino acids in order to find the most suitable ones for estimation of protein synthesis and degradation rates. In general, amino acids such as alanine and serine, which are synthesized in the central metabolism by amino transferase, show higher 13C enrichment compared to amino acids requiring multiple biosynthesis steps (Ishihara et al., 2015). Since estimation of protein degradation rates requires the plant growth rate, another advantage of using 13CO2 is that the growth rate can be measured using the same labeled material by quantifying 13C enrichment of glucose in the cell wall (Ishihara et al., 2015, 2017). Here, we present a relatively simple and inexpensive method to determine the rates of protein synthesis, degradation, and plant growth using 13CO2 labeling experiments in A. thaliana.
Critical Parameters and Troubleshooting
Planning of the experiment. It should be decided whether to perform a 13CO2 pulse experiment or a 13CO2 pulse/12CO2 chase experiment. In addition, the temporal distribution of harvest time points and the number of samples per time point need to be carefully planned in order to obtain robust results. If RGR will be calculated by measuring rosette size or fresh weight, extra plants must be grown in parallel to those used in the labeling experiment and in the same conditions. For a more detailed discussion of these points, see Strategic Planning.
Difficulty in mixing CO2. The flow rate (pressure) of the CO2 gas is 200 times lower than that of the N2/O2 gas mixture, which can make it difficult to obtain the desired CO2 concentration in the final gas mixture. The CO2 pressure can be improved by reducing the length and diameter of the tube connecting the MFC to the tee gas mixer. It is important this tube not be bent, as this could cause a backflow.
Amount of starting material. This protocol requires 30 mg frozen plant powder, but the amount of material used could vary. For example, young actively growing leaves contain substantially higher metabolites and protein compared to older slow-growing leaves (Sulpice et al., 2014). Therefore, the amount of starting material can be adjusted depending on the plant material.
Estimation of RGR from cell wall polysaccharide. This estimation is based on 13C enrichment of glucose and is heavily influenced by contamination of cell wall material by starch. Therefore, we recommend digesting starch at least four times and checking that there is no glucose detected before hydrolysis of the cell wall pellets. Plants grown in different growth conditions may contain different amounts of starch, and may need more or less starch hydrolysis.
Unexpectedly high or low 13C enrichment of glucose from cell wall material. Unusually high 13C enrichment levels, especially in samples harvested during the light period, could be due to contamination of cell wall material by starch. As mentioned above, it is important check that starch is completely removed before hydrolyzing cell wall pellets. Unusually high 13C enrichment of glucose could also result from a peak saturation of glucose during GC-TOF-MS analysis. This can lead to over- or even underestimation of 13C enrichment. In this case, we recommend analyzing a dilution series of the sample to determine the best dilution and/or using different split ratios of sample during GC-TOF-MS analysis.
Loss of GC-TOF-MS sensitivity. If sensitivity decreases over the course of the measurement run of amino acids obtained from total protein, it is most likely due to contamination by urea/thiourea from the protein extraction buffer, which will overload the GC column. In this case, the samples should be washed more thoroughly.
Understanding Results
This method provides a quantification of the rates of protein synthesis and degradation. The estimation of protein degradation makes two important assumptions: (1) that plant growth has a dilution effect on the amount of proteins synthesized per day, and (2) that proteins that are not used for growth are degraded.
Time Considerations
The time needed to complete the experiment and analyses varies depending on many factors, such as personal experience, number of samples, and availability of equipment.
Basic Protocol Basic Protocol 1. We grow A. thaliana plants (five plants per pot) under an 8-hr photoperiod for 3 weeks in order to obtain enough plant material. If lower light intensities are used, more time will be needed for plant growth; if higher light intensities or longer photoperiods are used, less time is needed for growth. When growing plants in long photoperiods to study vegetative growth, experiments should be performed before the initiation of flowering. Three days before the start of the labeling experiment, the system is set up and the plants placed in the labeling chambers to adapt to the experimental conditions. The 13CO2 pulse/12CO2 chase experiment is done in 5 days. Grinding and aliquoting the plant material takes 1-2 days, depending on experience.
Basic Protocol Basic Protocol 2. A set of 48 samples can be prepared in 1 day and be ready on day 2 following an overnight drying step.
Basic Protocol Basic Protocol 3. A set of 48 samples can be processed in 2 days and be ready on day 3. Protein extraction and precipitation are done on day 1, followed by acid hydrolysis over 24 hr. On day 2, the hydrolyzed samples are neutralized, cleaned, and dried overnight.
Basic Protocol Basic Protocol 4. A set of 48 samples can be processed in 5 days. It takes 3 days to completely remove starch from the samples. It takes another 2 days for hydrolysis and neutralization.
Basic Protocol Basic Protocol 5. It is recommended to derivatize a maximum of 30 samples per day, including standards, for GC-TOF-MS analysis (Rosado-Souza et al., 2019). The number of samples that can be processed and the measurement time frame are dependent on the availability of the GC-TOF-MS (Rosado-Souza et al., 2019). Analysis of chromatograms is done in a few hours.
Basic Protocol Basic Protocol 6. With the R script provided, it is possible to analyze many data sets at once within a few hours.
Acknowledgments
This research was supported by the Max Planck Society. The authors are grateful to Roosa Laitinen for her critical reading of the manuscript.
Author Contributions
Hirofumi Ishihara: Formal analysis, Investigation, Resources, Writing-original draft, Writing-review & editing, Thiago A. Moraes: Formal analysis, Writing-review & editing, Stéphanie Arrivault: Resources, Writing-review & editing, Mark Stitt: Conceptualization, Funding acquisition, Project administration, Resources, Supervision, Writing-review & editing.
Conflict of Interest
The authors declare no conflict of interest.
Open Research
Data Availability Statement
The data that supports the findings of this study are available in the supplementary material of this article.
Supporting Information
Filename | Description |
---|---|
cpz1114-sup-0001-SuppMat.xlsx51.7 KB | Supplementary Information |
Please note: The publisher is not responsible for the content or functionality of any supporting information supplied by the authors. Any queries (other than missing content) should be directed to the corresponding author for the article.
Literature Cited
- Allen, D. K., Laclair, R. W., Ohlrogge, J. B., & Shachar-Hill, Y. (2012). Isotope labelling of Rubisco subunits provides in vivo information on subcellular biosynthesis and exchange of amino acids between compartments. Plant, Cell & Environment, 35(7), 1232–1244. doi: 10.1111/j.1365-3040.2012.02485.x.
- Amthor, J. S. (2000). The McCree–de Wit–Penning de Vries–Thornley respiration paradigms: 30 years later. Annals of Botany , 86(1), 1–20. doi: 10.1006/anbo.2000.1175.
- Chan, L. L., Lo, S. C.-L., & Hodgkiss, I. J. (2002). Proteomic study of a model causative agent of harmful red tide, Prorocentrum triestinum I: Optimization of sample preparation methodologies for analyzing with two-dimensional electrophoresis. Proteomics , 29, 1169–1186. doi: 10.1002/1615-9861(200209)2:9<1169:26911299:Aid-prot1169>3.0.Co;2-l.
- Chen, W.-P., Yang, X.-Y., Harms, G. L., Gray, W. M., Hegeman, A. D., & Cohen, J. D. (2011). An automated growth enclosure for metabolic labeling of Arabidopsis thaliana with 13C-carbon dioxide—An in vivo labeling system for proteomics and metabolomics research. Proteome Science , 9(1), 9. doi: 10.1186/1477-5956-9-9.
- De Vries, F. W. T. P. (1975). The cost of maintenance processes in plant cells. Annals of Botany , 39(159), 77–92. Retrieved from http://www.jstor.org/stable/42752230.
- Fan, K. T., Rendahl, A. K., Chen, W. P., Freund, D. M., Gray, W. M., Cohen, J. D., & Hegeman, A. D. (2016). Proteome scale-protein turnover analysis using high resolution mass spectrometric data from stable-isotope labeled plants. Journal of Proteome Research , 15(3), 851–867. doi: 10.1021/acs.jproteome.5b00772.
- Galili, G. (1995). Regulation of lysine and threonine synthesis. Plant Cell , 7(7), 899–906. doi: 10.1105/tpc.7.7.899.
- Gruhler, A., Schulze, W. X., Matthiesen, R., Mann, M., & Jensen, O. N. (2005). Stable isotope labeling of Arabidopsis thaliana cells and quantitative proteomics by mass spectrometry. Molecular & Cellular Proteomics, 4(11), 1697–1709. doi: 10.1074/mcp.M500190-MCP200.
- Heise, R., Arrivault, S., Szecowka, M., Tohge, T., Nunes-Nesi, A., Stitt, M., … Fernie, A. R. (2014). Flux profiling of photosynthetic carbon metabolism in intact plants. Nature Protocols , 9(8), 1803–1824. doi: 10.1038/nprot.2014.115.
- Huege, J., Sulpice, R., Gibon, Y., Lisec, J., Koehl, K., & Kopka, J. (2007). GC-EI-TOF-MS analysis of in vivo carbon-partitioning into soluble metabolite pools of higher plants by monitoring isotope dilution after 13CO2 labelling. Phytochemistry , 68(16), 2258–2272. doi: 10.1016/j.phytochem.2007.03.026.
- Huffaker, R. C., & Peterson, L. W. (1974). Protein turnover in plants and possible means of its regulation. Annual Review of Plant Physiology , 25(1), 363–392. doi: 10.1146/annurev.pp.25.060174.002051.
- Ishihara, H., Moraes, T. A., Pyl, E.-T., Schulze, W. X., Obata, T., Scheffel, A., … Stitt, M. (2017). Growth rate correlates negatively with protein turnover in Arabidopsis accessions. Plant Journal , 91(3), 416–429. doi: 10.1111/tpj.13576.
- Ishihara, H., Obata, T., Sulpice, R., Fernie, A. R., & Stitt, M. (2015). Quantifying protein synthesis and degradation in Arabidopsis by dynamic 13CO2 labeling and analysis of enrichment in individual amino acids in their free pools and in protein. Plant Physiology , 168(1), 74–93. doi: 10.1104/pp.15.00209.
- Li, L., Nelson, C. J., Trösch, J., Castleden, I., Huang, S., & Millar, A. H. (2017). Protein degradation rate in Arabidopsis thaliana leaf growth and development. Plant Cell , 29(2), 207–228. doi: 10.1105/tpc.16.00768.
- Lisec, J., Schauer, N., Kopka, J., Willmitzer, L., & Fernie, A. R. (2006). Gas chromatography mass spectrometry–based metabolite profiling in plants. Nature Protocols , 1(1), 387–396. doi: 10.1038/nprot.2006.59.
- Lyon, D., Castillejo, M. A., Mehmeti-Tershani, V., Staudinger, C., Kleemaier, C., & Wienkoop, S. (2016). Drought and recovery: Independently regulated processes highlighting the importance of protein turnover dynamics and translational regulation in Medicago truncatula. Molecular & Cellular Proteomics, 15(6), 1921–1937. doi: 10.1074/mcp.M115.049205.
- Masclaux-Daubresse, C., & Chardon, F. (2011). Exploring nitrogen remobilization for seed filling using natural variation in Arabidopsis thaliana. Journal of Experimental Botany , 62(6), 2131–2142. doi: 10.1093/jxb/erq405.
- Moraes, T. A., Mengin, V., Annunziata, M. G., Encke, B., Krohn, N., Höhne, M., & Stitt, M. (2019). Response of the circadian clock and diel starch turnover to one day of low light or low CO2. Plant Physiology , 179(4), 1457–1478. doi: 10.1104/pp.18.01418.
- Nelson, C. J., Li, L., Jacoby, R. P., & Millar, A. H. (2013). Degradation rate of mitochondrial proteins in Arabidopsis thaliana cells. Journal of Proteome Research , 12(7), 3449–3459. doi: 10.1021/pr400304r.
- Nelson, C. J., Li, L., & Millar, A. H. (2014). Quantitative analysis of protein turnover in plants. Proteomics , 14(4-5), 579–592. doi: 10.1002/pmic.201300240.
- Nelson, C. J., & Millar, A. H. (2015). Protein turnover in plant biology. Nature Plants , 1(3), 15017. doi: 10.1038/nplants.2015.17.
- Obata, T., Witt, S., Lisec, J., Palacios-Rojas, N., Florez-Sarasa, I., Yousfi, S., … Fernie, A. R. (2015). Metabolite profiles of maize leaves in drought, heat, and combined stress field trials reveal the relationship between metabolism and grain yield. Plant Physiology , 169(4), 2665–2683. doi: 10.1104/pp.15.01164.
- Pal, S. K., Liput, M., Piques, M., Ishihara, H., Obata, T., Martins, M. C. M., … Stitt, M. (2013). Diurnal changes of polysome loading track sucrose content in the rosette of wild-type Arabidopsis and the starchless pgm mutant. Plant Physiology , 162(3), 1246–1265. doi: 10.1104/pp.112.212258.
- Pocrnjic, Z., Mathews, R. W., Rappaport, S., & Haschemeyer, A. E. (1983). Quantitative protein synthetic rates in various tissues of a temperate fish in vivo by the method of phenylalanine swamping. Comparative Biochemistry and Physiology. B, Comparative Biochemistry , 74(4), 735–738. doi: 10.1016/0305-0491(83)90135-9.
- Poorter, H., & Nagel, O. (2000). The role of biomass allocation in the growth response of plants to different levels of light, CO2, nutrients and water: A quantitative review. Functional Plant Biology , 27(12), 1191–1191. doi: 10.1071/PP99173_CO.
- Rosado-Souza, L., David, L. C., Drapal, M., Fraser, P. D., Hofmann, J., Klemens, P. A. W., … Fernie, A. R. (2019). Cassava metabolomics and starch quality. Current Protocols in Plant Biology , 4(4), e20102. doi: 10.1002/cppb.20102.
- Saeman, J. F., Moore, W., Mitchell, R. L., & Millett, M. A. J. T. J. (1954). Techniques for the determination of pulp constituents by quantitiative paper chromatography. TAPPI Journal , 37, 336–343.
- Schauer, N., Semel, Y., Roessner, U., Gur, A., Balbo, I., Carrari, F., … Fernie, A. R. (2006). Comprehensive metabolic profiling and phenotyping of interspecific introgression lines for tomato improvement. Nature Biotechnology , 24(4), 447–454. doi: 10.1038/nbt1192.
- Schütz, W., Hausmann, N., Krug, K., Hampp, R., & Macek, B. (2011). Extending SILAC to proteomics of plant cell lines. Plant Cell , 23(5), 1701–1705. doi: 10.1105/tpc.110.082016.
- Sulpice, R., Ishihara, H., Schlereth, A., Cawthray, G. R., Enck, B., Giavalisco, P., … Lambers, H. (2014). Low levels of ribosomal RNA partly account for the very high photosynthetic phosphorus-use efficiency of Proteaceae species. Plant, Cell & Environment, 37(6), 1276–1298. doi: 10.1111/pce.12240.
- Sulpice, R., Pyl, E.-T., Ishihara, H., Trenkamp, S., Steinfath, M., Witucka-Wall, H., … Stitt, M. (2009). Starch as a major integrator in the regulation of plant growth. Proceedings of the National Academy of Sciences , 106(25), 10348–10353. doi: 10.1073/pnas.0903478106.
- Szecowka, M., Heise, R., Tohge, T., Nunes-Nesi, A., Vosloh, D., Huege, J., … Arrivault, S. (2013). Metabolic fluxes in an illuminated Arabidopsis rosette. Plant Cell , 25(2), 694–714. doi: 10.1105/tpc.112.106989.
- Warner, J. R. (1999). The economics of ribosome biosynthesis in yeast. Trends in Biochemical Sciences , 24(11), 437–440. doi: 10.1016/S0968-0004(99)01460-7.
- Williams, T. C. R., Poolman, M. G., Howden, A. J. M., Schwarzlander, M., Fell, D. A., Ratcliffe, R. G., & Sweetlove, L. J. (2010). A genome-scale metabolic model accurately predicts fluxes in central carbon metabolism under stress conditions. Plant Physiology , 154(1), 311–323. doi: 10.1104/pp.110.158535.
- Yang, X.-Y., Chen, W.-P., Rendahl, A. K., Hegeman, A. D., Gray, W. M., & Cohen, J. D. (2010). Measuring the turnover rates of Arabidopsis proteins using deuterium oxide: An auxin signaling case study. Plant Journal , 634, 680–695. doi: 10.1111/j.1365-313X.2010.04266.x.
- Zablackis, E., Huang, J., Muller, B., Darvill, A. G., & Albersheim, P. (1995). Characterization of the cell-wall polysaccharides of Arabidopsis thaliana leaves. Plant Physiology , 107(4), 1129–1138. doi: 10.1104/pp.107.4.1129.
- Zhou, H., Li, W., Wang, S.-P., Mendoza, V., Rosa, R., Hubert, J., … Roddy, T. P. (2012). Quantifying apoprotein synthesis in rodents: Coupling LC-MS/MS analyses with the administration of labeled water. Journal of Lipid Research , 53(6), 1223–1231. doi: 10.1194/jlr.D021295.
Internet Resources
CORRECTOR software.
R language software.
Step-by-step instruction is available on the open-source GitHub repository.
Citing Literature
Number of times cited according to CrossRef: 1
- Anh Thi-Mai Banh, Björn Thiele, Antonia Chlubek, Thomas Hombach, Einhard Kleist, Shizue Matsubara, Combination of long-term 13CO2 labeling and isotopolog profiling allows turnover analysis of photosynthetic pigments in Arabidopsis leaves, Plant Methods, 10.1186/s13007-022-00946-3, 18 , 1, (2022).