Optimized Recombinant Expression and Purification of the SARS-CoV-2 Polymerase Complex
Ana Podadera, Ana Podadera, Lucas Campo, Lucas Campo, Fasih Rehman, Fasih Rehman, Nikola Kolobaric, Nikola Kolobaric, Adriana Zutic, Adriana Zutic, Kenneth K-S. Ng, Kenneth K-S. Ng
Abstract
An optimized protocol has been developed to express and purify the core RNA-dependent RNA polymerase (RdRP) complex from the severe acute respiratory syndrome coronavirus-2 (SARS-CoV-2). The expression and purification of active core SARS-CoV-2 RdRp complex is challenging due to the complex multidomain fold of nsp12, and the assembly of the multimeric complex involving nsp7, nsp8, and nsp12. Our approach adapts a previously published method to express the core SARS-CoV-2 RdRP complex in Escherichia coli and combines it with a procedure to express the nsp12 fusion with maltose-binding protein in insect cells to promote the efficient assembly and purification of an enzymatically active core polymerase complex. The resulting method provides a reliable platform to produce milligram amounts of the polymerase complex with the expected 1:2:1 stoichiometry for nsp7, nsp8, and nsp12, respectively, following the removal of all affinity tags. This approach addresses some of the limitations of previously reported methods to provide a reliable source of the active polymerase complex for structure, function, and inhibition studies of the SARS-CoV-2 RdRP complex using recombinant plasmid constructs that have been deposited in the widely accessible Addgene repository. © 2024 The Authors. Current Protocols published by Wiley Periodicals LLC.
Basic Protocol 1 : Expression and production of SARS-CoV-2 nsp7, nsp8, and nsp12 in E. coli cells
Support Protocol : Establishment and maintenance of insect cell cultures
Basic Protocol 2 : Generation of recombinant baculovirus in Sf9 cells and production of nsp12 fusion protein in T. ni cells
Basic Protocol 3 : Purification of the SARS-CoV-2 core polymerase complex
INTRODUCTION
After the emergence of SARS-CoV-2, the etiological agent of COVID-19, intensive research efforts have focused on the structural and enzymatic characterization of the SARS-CoV-2 RNA-dependent RNA polymerase (RdRP) complex (Dwivedy et al., 2021; Gao et al., 2020; Hillen et al., 2020; Malone et al., 2021; Peng et al., 2020; B. Wang et al., 2021). Three virally encoded non-structural proteins (nsp7, nsp8, and nsp12) spontaneously assemble both in vitro and in vivo to form the core of the coronavirus replication-transcription complex (RTC) (Hillen, 2021; Lou & Rao, 2022; Malone et al., 2022). Many different expression and purification systems have been developed to express the core RTC, especially following the onset of the COVID-19 pandemic in 2019 (Bertolin et al., 2021; Biswal et al., 2021; Bravo et al., 2021; Campagnola et al., 2022; Chen et al., 2020; Chien et al., 2020; Conti et al., 2021; Dangerfield et al., 2020; Dangerfield et al., 2021; Dejmek et al., 2021; Dwivedy et al., 2021; Eydoux et al., 2021; Gao et al., 2020; Gordon et al., 2020; Hillen et al., 2020; Kirchdoerfer & Ward, 2019; Li et al., 2021; Lu et al., 2020; Madru et al., 2021; Naydenova et al., 2021; Peng et al., 2020; Shannon et al., 2020; Shannon et al., 2022; Shi et al., 2020; Slanina et al., 2021; Walker et al., 2021; B. Wang et al., 2021; B. Wang, Svetlov, Wolf, et al., 2021; Q. Wang et al., 2020; Wilamowski et al., 2021; Yan et al., 2020; Yan et al., 2021; Yin et al., 2021). However, the production of large amounts of an enzymatically active form of the complex suitable for detailed biochemical and biophysical studies remains challenging. Two central challenges appear to be the folding of the large, multidomain nsp12 subunit and the assembly of the heterotetrameric complex involving nsp7, nsp8, and nsp12. To address these challenges, we report in detail a novel protocol to efficiently purify milligram quantities of the enzymatically active polymerase complex for enzymatic characterization, inhibitor discovery, and structure-function studies. Although the co-expression of nsp7, nsp8, and nsp12 in Escherichia coli generates a modest amount of the core RdRP complex, the amount of nsp12 is limiting, resulting in a large stoichiometric excess of nsp7 and nsp8. By adding nsp12, which is expressed at much higher levels in baculovirus-infected insect cells, it is possible to purify a much larger amount of the core RdRP complex through this newly described method.
Basic Protocol 1 describes the expression of recombinant forms of SARS-CoV-2 nsp7, His-tagged nsp8, and nsp12 in E. coli. Basic Protocol 2 describes the expression of a recombinant form of SARS-CoV-2 nsp12 bearing a His-tag and MBP-tag at the N-terminus in baculovirus-infected Trichoplusia ni cells. Basic Protocol 3 describes the purification of the core RdRP complex formed by nsp7, nsp8, and nsp12, and the removal of all artificial affinity tags by proteolytic digestion. Support Protocol describes the establishment and maintenance of the insect cell cultures used in Basic Protocol 2 (Fig. 1).
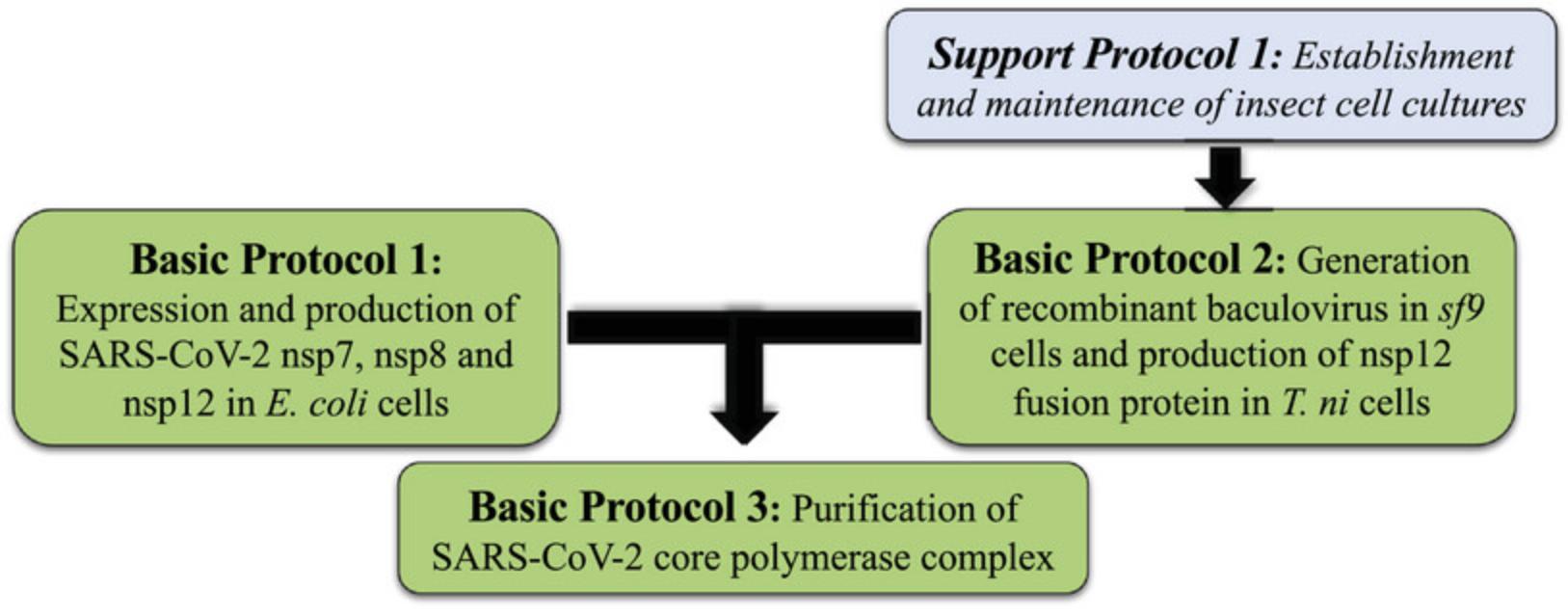
Basic Protocol 1: EXPRESSION AND PRODUCTION OF SARS-CoV-2 nsp7, nsp8, nsp12 IN E. coli CELLS
This protocol was adapted from a previously published method to express the SARS-CoV-2 core polymerase complex in E. coli (Madru et al., 2021) and describes the production of untagged forms of nsp7 and nsp12, and nsp8 bearing a His14-tag at the N-terminus.
Materials
-
pRSFDuet-1(nsp8-nsp7)(nsp12) plasmid (Addgene, plasmid #165451)
-
H2O, sterile, molecular grade (Multicell, #809115LL)
-
Rosetta(DE3) competent cells (Millipore, #70954-3)
-
S.O.C. medium (Thermo Fisher, #15544034)
-
LB broth medium and LB agar plates (see recipe)
-
Kanamycin (Wisent, #400-145-IG)
-
IPTG (Wisent, #800-050-IG)
-
L1 buffer (see recipe)
-
Ice
-
Water bath, 42°C
-
Bacteria incubator, 37°C
-
1-L glass Erlenmeyer flask
-
2.8-L glass Fernbach flask
-
Cuvettes for Biophotometer
-
Biophotometer or another spectrophotometer
-
Incubator-cooler with shaking, 18°C
-
Refrigerated centrifuge, 4°C
-
50-ml centrifuge tubes (Sarstedt, #62.547.205)
Plasmid transformation in E. coli Rosetta(DE3) cells
Expression and purification of the nsp8/nsp7/nsp12 complex is carried out employing the pRSFDuet-1(nsp8-nsp7)(nsp12) plasmid described by Madru et al. (2021) (Fig. 2). The plasmid is transformed into Rosetta(DE3) cells, a strain of E. coli derived from BL21(DE3) that carries the pRARE plasmid containing the native genes and promoters for six tRNAs that are normally expressed at lower levels.
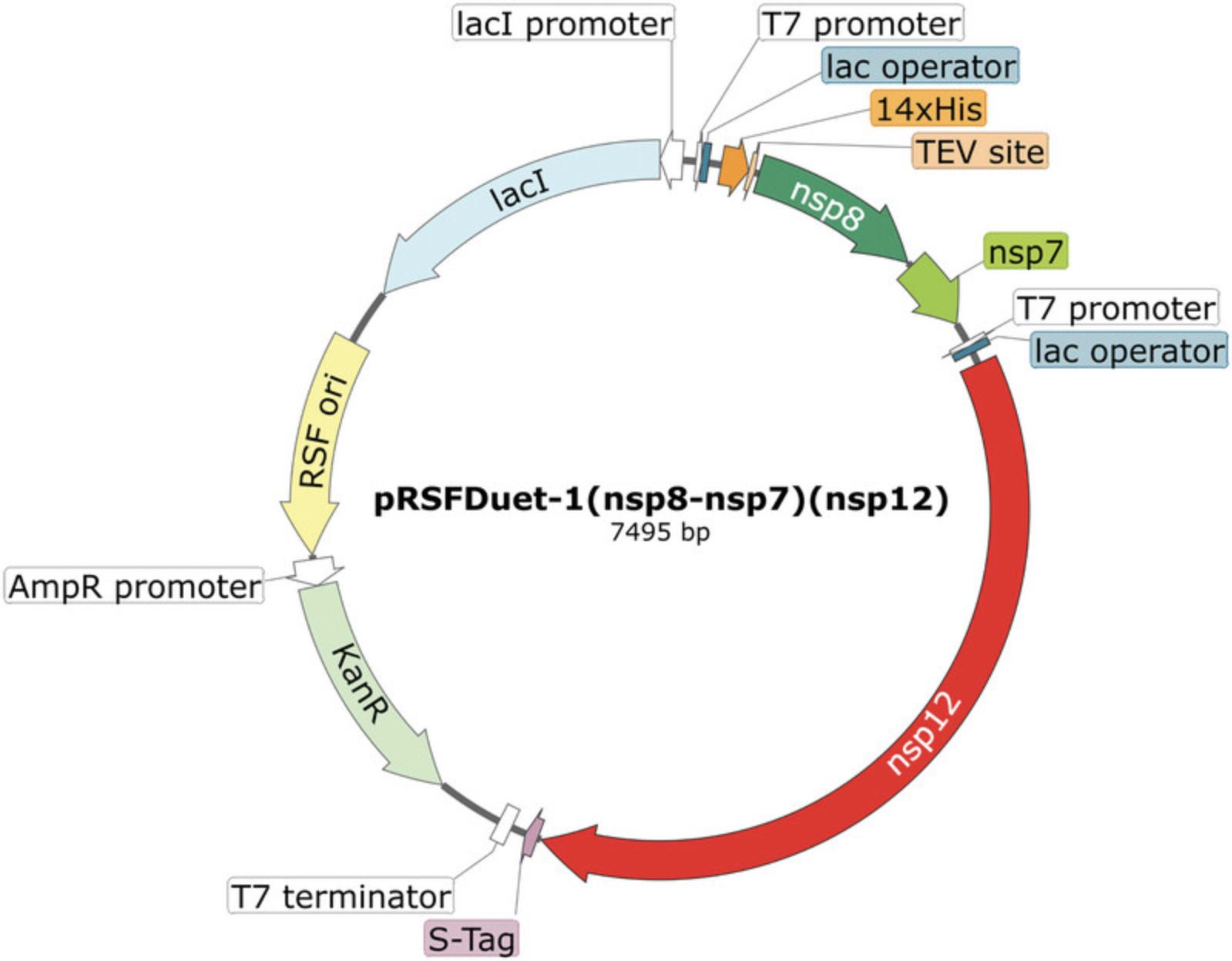
1.Thaw the plasmid solution and dilute with sterile water (molecular grade) to a final concentration of 50 ng/μl. Meanwhile, thaw an aliquot of chemically competent Rosetta(DE3) cells and place on ice.
2.Add 1 μl plasmid solution into 20 μl Rosetta(DE3) cell suspension. Avoid pipetting up and down but stir the pipette tip to gently mix the cell suspension.
3.After incubating for 30 min on ice, place the tube in a 42°C water bath for 30 s. Avoid disturbing or agitating the tube, and place the tube on ice immediately afterwards.
4.Add 450 μl S.O.C. medium preheated at 37°C, and incubate the tube with the transformed bacterial cells at 37°C with shaking (250 rpm) for 1 hr.
5.Streak 100 μl of the transformed cell suspension onto pre-heated LB agar plates containing 30 μg/ml kanamycin and incubate the plates upside down in a 37°C incubator overnight. If needed, prepare 1:10 or 1:100 dilution of the cell suspension to obtain isolated colonies.
Scaling up the co-expression and production of nsp7, nsp8, and nsp12
6.Inoculate 200 ml LB broth medium supplemented with 30 μg/ml kanamycin in a 1 L Erlenmeyer flask with one colony of the Rosetta(DE3) cells transformed with pRSFDuet-1(nsp8-nsp7)(nsp12) plasmid. Incubate at 37°C for 16 hr with shaking (250 rpm).
7.Inoculate 1 L LB broth medium supplemented with 30 μg/ml kanamycin in a 2.8 L Fernbach flask with 25 ml starter culture from step 6 and incubate for ∼2 to 3 hr at 37°C (250 rpm) until the optical density (OD) of the culture is ∼0.4, as determined by measuring OD600 using a Biophotometer and cuvettes.
8.Pre-cool the culture by shaking at 18°C for 1 hr prior to starting induction. Add IPTG to achieve a final concentration of 0.5 mM and incubate the culture for 20 hr at 18°C with shaking at 250 rpm.
9.Harvest cells by centrifuging 15 to 20 min at 3060 × g , 4°C.
10.Resuspend cell pellet with 35 ml L1 buffer per L culture, and store in a 50-ml tube at −80°C until use.
11.Proceed with Basic Protocol 3 for protein purification.
Support Protocol: ESTABLISHMENT AND MAINTENANCE OF INSECT CELL CULTURES
Reliable insect cell cultures are needed for generating and supporting the growth and replication of recombinant baculovirus (Lynn, 2002). We describe how the Sf9 cells (derived from the pupal ovarian tissue of Spodoptera frugiperda) are maintained in adherent culture conditions to generate, maintain, and titer recombinant baculoviral stocks. Meanwhile, T. ni cells (derived from the ovarian tissue of Trichoplusia ni) are grown in suspension to carry out baculoviral infections and to scale up the production of nsp12.Frozen insect cells are commonly purchased or stored in complete medium containing 10% DMSO under liquid nitrogen. If performed correctly, the user should expect cultures with cell viability equal ≥90%.
Materials
-
Gibco Sf9 cells in Sf-900 II SFM (Fisher Scientific, #11496015)
-
T. ni cells in ESF-921 (Expression Systems, #94-002F)
-
I-MAX complete medium (see recipe)
-
ESF-921 growth medium (see recipe)
-
Water bath, 37°C
-
Class II biosafety cabinet (Nuaire #NV-540-600)
-
5- and 10-ml serological pipettes (Sarstedt, #86.1253.001 and #86.1254.001)
-
15-ml conical tube
-
Centrifuge compatible with 15- and 50-ml conical tubes
-
75-cm² (T-75) Nunc EasYFlask cell culture flasks (Fisher Scientific #12-565-349)
-
Gravity convection incubator, non-CO2, 28°C (VWR #89511-420)
-
250-ml Erlenmeyer flasks (VWR #10536-914)
-
Genie Temp-Shaker 300, flask clamps (Scientific Industries, #SI-G1600)
Establishment and maintenance of S. frugiperda (Sf9) cells in adherent culture conditions
1.Thaw the frozen vial with Sf9 cells in a 37°C water bath for 1 min, or until only a small piece of ice remains.
2.Inside the biosafety cabinet, using a 5-mL serological pipette transfer the thawed cell suspension into a 15-ml tube containing 9 ml complete I-MAX complete medium prewarmed to room temperature.
3.Pellet the viable cells by centrifuging 10 min at 47 × g , room temperature.
4.Discard supernatant and resuspend cells with 10 ml pre-warmed I-MAX complete medium employing a 10-mL serological pipette.
5.Place the cell suspension into a 75-cm2 T-75 cell culture flask.
6.Incubate cell flasks with loose caps at 28°C in a non-CO2 incubator.
7.Split cells when 90% confluency is reached (every 2 to 3 days), seeding ∼2.5–3 × 105 viable cells/ml.
8.Once the cell line is established and doubling regularly, freeze viable cells at a low passage number (<10) for optimal long-term use.
Establishment and maintenance of T. ni cells in suspension conditions culture
9.Thaw the frozen vial with T. ni cells in a water bath at 37°C for 1 min, or until only a small piece of ice remains.
10.Inside the biosafety cabinet, using a 5-mL pipette transfer the thawed cell suspension into a 15-ml tube containing 9 ml ESF-921 growth medium prewarmed to room temperature.
11.Pellet the viable cells by centrifuging 10 min at 47 × g , room temperature.
12.Discard supernatant and resuspend cells with 10 ml pre-warmed ESF-921 growth medium, employing a 10-mL serological pipette.
13.Place the cell suspension into a sterile 250-ml Erlenmeyer flask with 40 ml ESF-921 growth medium.
14.Incubate cell-containing flasks at 28°C with shaking at 110 to 120 rpm and without CO2 atmosphere.
15.Subculture cells when 3.5–4 × 106 cells/ml confluency is reached (every 2 to 3 days), splitting at a concentration of 5 × 105 viable cells/ml.
Basic Protocol 2: GENERATION OF RECOMBINANT BACULOVIRUS IN Sf9 CELLS AND PRODUCTION OF nsp12 FUSION PROTEIN IN T. ni CELLS
The baculovirus expression vector system (BEVS) has become a well-known commercial manufacturing platform enabling fast, flexible, and scalable protein production (Felberbaum, 2015; Irons et al., 2018). In this protocol, standard methods for recombinant baculovirus generation in adherent cultures of insect cells (Sf9) will be employed to produce a highly infectious recombinant baculoviral stock expressing SARS-CoV-2 nsp12, whereas T. ni cells will be used as the host for establishing a cost-efficient system to produce nsp12.The production of histidine-MBP tagged nsp12 expressed by recombinant baculovirus will allow to produce high quantity of soluble active nsp12, overcoming insolubility and misfolding issues observed when expressing SARS-CoV-2 nsp12 in bacteria (Dangerfield et al., 2021; Madru et al., 2021; B. Wang et al., 2021). If performed correctly, the user should expect yields of ∼5.6 ± 1.6 mg of soluble protein per 500 ml of cell culture (this yield was calculated from three individual purifications of nsp12).
Materials
-
pFastBac-His-MBP-SCoV2-nsp12 plasmid (Addgene, plasmid #154759)
-
MAX Efficiency DH10Bac competent cells (Gibco, #10361012)
-
LB broth medium (see recipe)
-
Kanamycin (Wisent, #400-145-IG)
-
Gentamycin solution (Wisent, #450-135-XL)
-
Tetracycline hydrochloride (Wisent, #400-180-XG)
-
GeneJET plasmid miniprep kit (Thermo Scientific, #K0503)
-
Gibco Sf9 cells in Sf-900 II SFM (Fisher Scientific, #11496015)
-
I-MAX serum-free medium with L-glutamine (Wisent, #301-045-CL)
-
I-MAX complete medium (see recipe)
-
Cellfectin II reagent (Gibco, #10362-100)
-
I-MAX 5% FBS medium (see recipe)
-
Autoclaved agarose, low-melting point (BioBasic, #D0012)
-
Neutral Red solution, 0.33% (Sigma, #N2889)
-
Phosphate-buffered saline (PBS), 1× (Wisent, # 311-010-CL)
-
T. ni cells in ESF-921 (Expression Systems, #94-002F)
-
ESF-921 growth medium (see recipe)
-
Production Boost Additive (PBA) (Expression Systems, #95-006-100)
-
L1 buffer (see recipe)
-
Bacteria incubator with shaking, 37°C
-
6-well clear flat bottom, TC-treated multiwell cell culture plate (Corning, #353046) or similar
-
Incubator, 28°C
-
15-ml conical tubes
-
Centrifuge compatible with 15- and 50-ml conical tubes
-
75-cm² (T-75) Nunc EasYFlask cell culture flasks (Fisher Scientific, #12-565-349) or similar
-
Class II biosafety cabinet (Nuaire #NV-540-600)
-
1-L sterile glass Erlenmeyer flasks
-
50-ml centrifuge tubes
Bacmid generation
1.Transform the pFastBac-His-MBP-SCoV2-nsp12 plasmid (Fig. 3) into DH10Bac cells according to the manufacturer's instructions for pFastBac products.
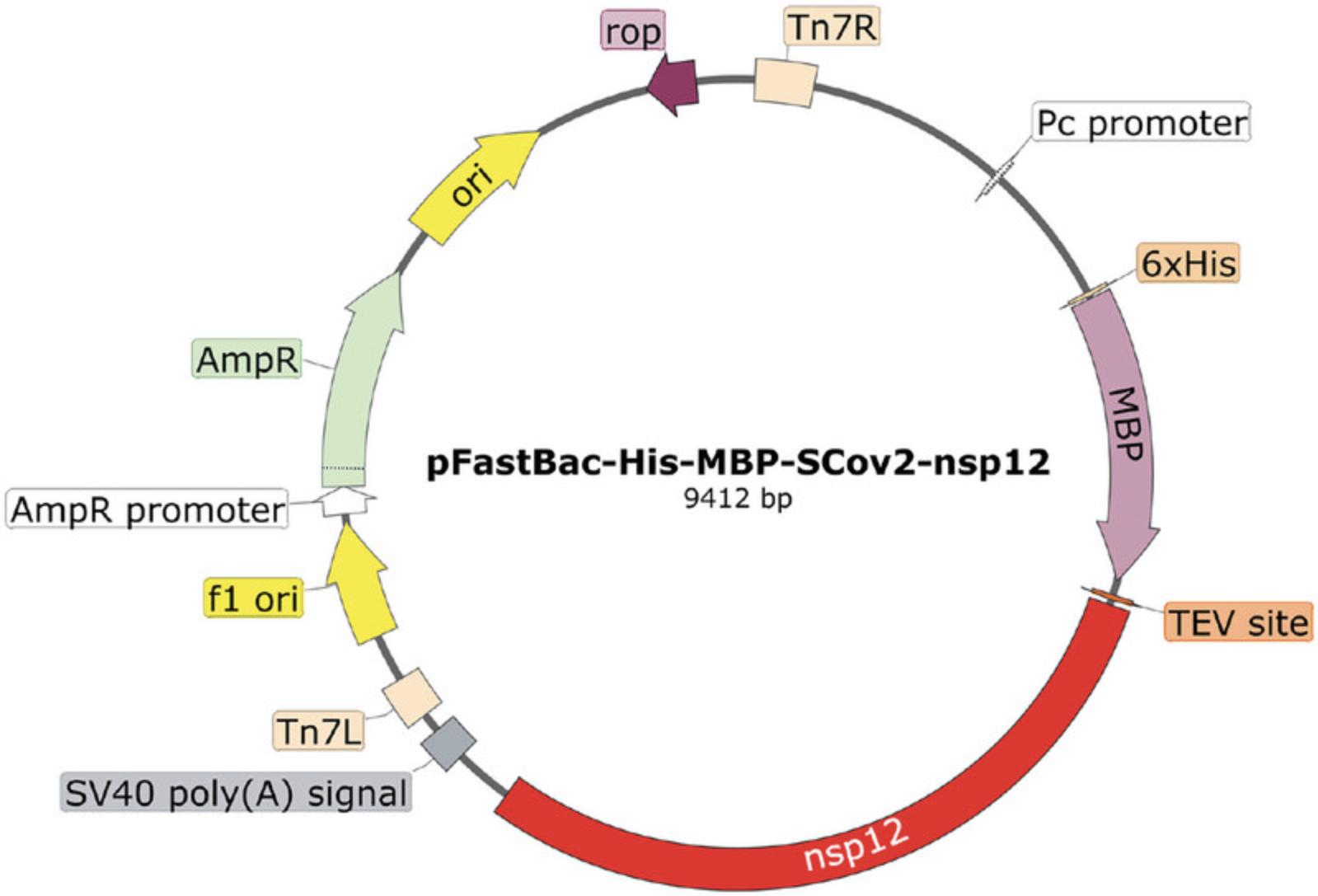
2.Select 3 to 4 of the most isolated white colonies to avoid cross-contamination and grow them separately in 5 ml LB broth supplemented with 50 μg/ml of kanamycin, 7 μg/ml gentamycin and 10 μg/ml tetracycline overnight at 37°C with shaking (250 rpm).
3.Extract and purify the His6-MBP-nsp12 bacmid from 1.5 ml bacterial culture by employing a plasmid purification kit.
Transfection of bacmid into Sf9 cells for baculovirus generation
4.Prepare a 6-well plate with Sf9 cells at a cell density of 8 × 105 to 1 × 106 cells per well using 2 ml I-MAX serum-free medium with L-glutamine. Incubate the plate at 28°C for 30 to 60 min until the cells attach to the plate surface.
5.While waiting for the cell incubation, prepare the Cellfectin II master mix consisting of 8 μl Cellfectin II reagent with 100 μl serum-free and antibiotic-free medium, and keep it at room temperature.
6.In a new tube, dilute the purified 6His-MBP-nsp12 bacmid in serum-free and antibiotic-free medium to a final quantity of 0.5 to 1 μg in a final volume of 112 μl. Add 108 μl Cellfectin II master mix to each tube containing a bacmid solution and incubate for 20 to 30 min at room temperature.
7.Remove the medium from the wells of the 6-well plate and add the desired transfection mixture (220 μl) spreading it all over the bottom well surface dropwise and avoiding disruption of the cell monolayer. Complete up to 1 ml/well with serum-free and antibiotic-free medium making sure that all the cell monolayer is covered. Incubate the plate for 4 to 5 hr at 28°C.
8.Remove the transfection solution and add 2 ml/well I-MAX complete medium and allow the recombinant baculovirus generation by incubating the cells for 3 to 4 days at 28°C, checking for cytopathic effect (CPE) every day (Fig. 4).
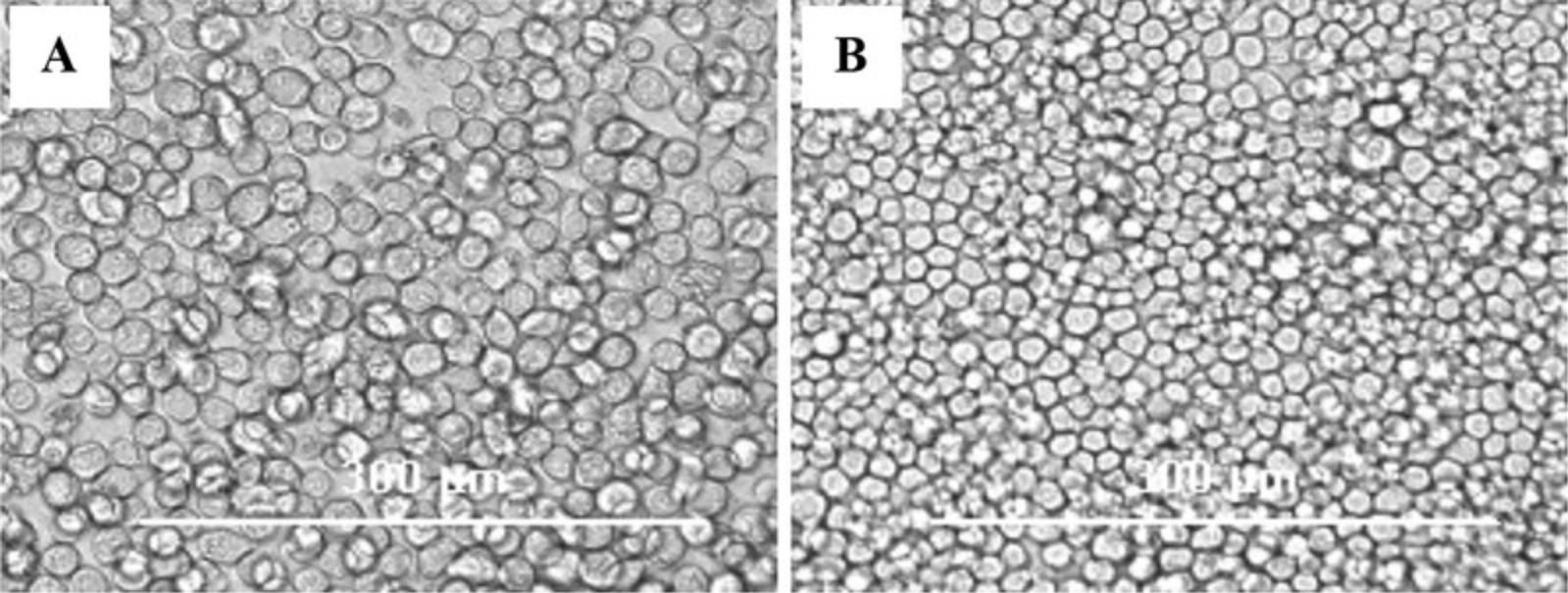
9.Once CPEs appear, harvest cells and supernatant from each well in a 15-ml conical tube and centrifuge them 5 min at 750 × g , 4°C. Transfer the supernatant to a new 15-ml conical tube and store at −80°C for long-term storage or at 4°C for use in 3 to 7 days.
Propagation of recombinant baculovirus expressing nsp12 of SARS-CoV-2
10.Prepare a 6-well plate with Sf9 cells at a cell density of ∼1 × 106 cells per well. Incubate for 1 hr to let the cells settle and attach to the bottom plate surface.
11.Discard the supernatant and avoid disrupting the cell monolayer. Per well, add 0.5 ml of the supernatant (rBAC p0) obtained after transfection from step 9.Bring up to 2 ml with I-MAX 5% FBS medium.
12.Incubate for 72 to 96 hr at 28°C until the cell monolayer is clearly infected showing CPEs (Fig. 4). Harvest the supernatant from all the infected wells and clear it by centrifugation at 5 min at 750 × g , room temperature.
13.The second passage of the virus is produced on 75-cm2 T-75 cell culture flasks. Seed Sf9 cells in 4 to 5 T-75 flasks at 60% confluence (∼7–8 × 106 cells/flask). Allow the cells to attach to the bottom surface of the flasks by incubating for 1 hr at 28°C.
14.Inoculate each of the flasks with 1 ml of rBAC p1 supernatant and bring up to 10 ml with I-MAX 5% FBS medium. Incubate for 72 to 96 hr (depending on the CPEs) at 28°C.
15.Collect the supernatant from all the infected cell culture T-75 flasks and centrifuge 5 min at 750 × g , 4°C, to obtain a cleared rBAC p2 solution.
Titration of the generated recombinant baculovirus stock
16.Prepare a 6-well plate with Sf9 cells at a cell density of 8 × 105 to 1 × 106 cells/well. Allow the cells to attach to the plate surface by incubating for 1 hr at 28°C.
17.Change the medium to serum-free and antibiotic-free medium (2 ml/well) and incubate the plate at 28°C while preparing the baculovirus dilutions.
18.Prepare serial dilutions from 10-2 to 10-7 of the rBAC p2 solution with a final volume of 1.2 ml per dilution in medium without serum or antibiotics.
19.Remove the medium from the cells and add 1 ml of the corresponding baculoviral dilution per well and incubate the plate for 60 to 90 min at 28°C.
20.Following the incubation, mix 7 ml melted agarose with 7 ml I-MAX complete medium.
21.Discard the baculoviral inoculate from each well and add 1 ml of the agarose-medium mixture. Incubate at room temperature inside the hood until the agarose polymerizes and add 1 ml of I-MAX complete medium over the agarose layer.
22.Incubate the plate for 4 to 5 days at 28°C.
23.Add 0.6 ml Neutral Red solution into 10 ml of 1× PBS. Discard the remaining medium over the agarose layers from each well and add 1 ml of the Neutral Red dilution.
24.Incubate for at least 4 hr at 28°C. Discard the staining solution from the top of the agarose layer. Place the plate upsidedown overnight in the dark at room temperature.
25.The following day, observe and count the lysis plaques for each tested dilution of baculovirus (Fig. 5). Calculate the viral titer as for any other virus plaque-assay titration employing the formula below:
Plaque-forming units (pfu)/ml = # plaques lysis × dilution factor ×
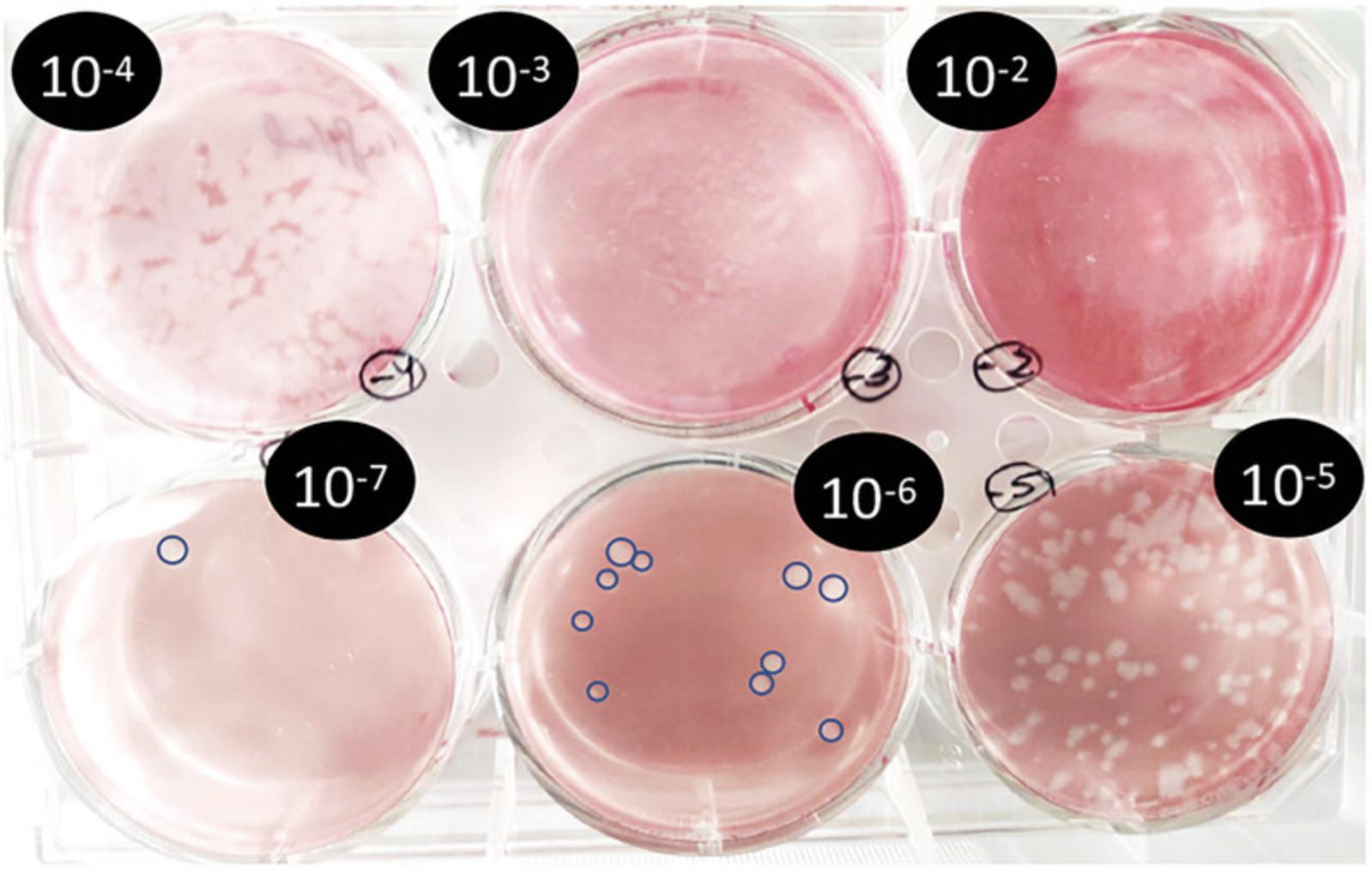
Infection of T. ni cells for SARS-CoV-2 nsp12 fusion protein production
26.Seed four 1 L Erlenmeyer flasks at a cell density of 1 × 106 cells/ml in a volume of 250 ml each of ESF-921 growth medium.
27.Infect each flask with a sufficient baculoviral solution for infecting at a multiplicity of infection (m.o.i.) of 1.
28.Incubate for 22 to 24 hr at 28°C with shaking (120 rpm).
29.Add 0.1% Production Boost Additive to each flask.
30.Incubate for 48 hr at 28°C with shaking (120 rpm).
31.Collect all infected cultures and centrifuge 15 min at 1200 × g , 4°C.
32.Discard the supernatant and resuspend the pellet from 1 L of culture in 100 ml L1 buffer.
33.Split the resuspended cell pellet into four 50-ml centrifuge tubes and store at −80°C until use.
Basic Protocol 3: PURIFICATION OF THE SARS-CoV-2 CORE POLYMERASE COMPLEX
The combined purification of proteins expressed in both systems increases the final yield of the purified complex. In addition, with this procedure, the ratio between proteins in the final purified complex is similar to the expected 1:2:1 stoichiometry for nsp7, nsp8, and nsp12, whereas the complex expressed and purified from E. coli expression alone shows accessory subunits (nsp7 and nsp8) in large stoichiometric excess (Madru et al., 2021).
Materials
-
Lysozyme (BioBasic, #LDB0308)
-
DNase Type I (BioBasic, #DD0099)
-
cOmplete protease inhibitor cocktail (Roche, #05892791001)
-
L1 buffer (see recipe)
-
20% ethanol
-
Milli-Q H2O
-
A1 buffer (see recipe)
-
B1 buffer (see recipe)
-
Purified His-tagged TEV protease or equivalent
-
Sodium carbonate (Na2CO3)
-
Disodium ethylenediaminetetraacetic acid (Na2-EDTA)
-
D1 buffer (see recipe)
-
B2 buffer (see recipe)
-
D2 buffer (see recipe)
-
QA-50 buffer (see recipe)
-
QA-0 buffer (see recipe)
-
QB buffer (see recipe)
-
Water bath, 35° to 37°C
-
Ice
-
Small foam box
-
100-ml beakers
-
Magnetic stir bar and stirrer
-
Q500 sonicator with standard 0.5-in diameter probe (Q SONICA, #Q500E)
-
Avanti JXN-26 centrifuge (Beckman Coulter) or equivalent high-speed centrifuge, 4°C
-
Polyethersulfone (PES) syringe filters:
- 0.8 μm × 25 mm (Sterlitech, #PES082550S)
- 0.45 μm × 25 mm (Sterlitech, #PES452550S)
- 0.22 μm × 25 mm (Sterlitech, #PES022550S)
-
1-ml PureCube compact cartridge Ni-NTA (Cube Biotech, #31302)
-
Amylose resin (New England BioLabs, #E8021L)
-
Regenerated cellulose dialysis membrane, MWCO 6000 to 8000 (VWR, #S632650) or equivalent
-
Peristaltic pump (New Era Pumps Systems, #NE-9000G)
-
UV detector (Aurora, #MR-A-280)
-
Nanodrop
-
1-ml G-Trap Q Agarose Fast Flow column (GBiosciences, #786-1014)
-
Syringe pumps (New Era Pumps Systems, #DUAL-1000)
-
Conductivity meter (VWR, #21800-012)
-
10,000 MWCO Amicon Ultra-4 and Amicon Ultra-15 centrifugal filters (Millipore #UFC801024 and #UFC901024, respectively)
-
Refrigerated centrifuge compatible with 15-ml conical tubes, 4°C
-
0.2-ml PCR tubes
-
Liquid nitrogen
-
Additional reagents and equipment for SDS-PAGE (Gallagher, 2008)
Cell lysis of combined cell pellets
1.Thaw one 50 ml aliquot of cell pellet from (nsp8-nsp7)(nsp12) complex expression in E. coli (Basic Protocol 1, steps 6 to 11), as well as 2 tubes of T .ni cell pellets expressing the nsp12 fusion protein (Basic Protocol 2, steps 26 to 33).
2.Gently swirl the tubes in a water bath set at 35° to 37°C. Put tubes into ice when only a small piece of ice remains inside.
3.Combine cell pellets into a 100-ml glass beaker pre-chilled in a water-ice slush bath (inside a small foam box). Place a stir bar inside the beaker.
4.Add a small scoop each of lysozyme powder and DNase Type I powder directly into the cell suspension.
5.Stir employing a magnetic stirrer at a low speed to not generate foam or bubbles.
6.Add 2 tablets of cOmplete protease inhibitor cocktail previously diluted in 2 ml L1 buffer.
7.Sonicate the cell suspension at 60% amplitude in 10 to 40 s ON-OFF duty cycle for 1 min of pulse time.
8.Leave the sample stirring on ice for 1 min to cool down before proceeding with the next sonication cycle.
9.Repeat for a total of 5 cycles of sonication.
10.Centrifuge sonicated cell lysate in the Avanti JXN-26 centrifuge 2 hr at 18,500 × g , 4°C.
11.Carefully pour off supernatant into a clean 100-ml beaker without disturbing the pellet.
12.Filter supernatant through a 0.8 μm × 25 mm PES filter and then through 0.45 μm × 25 mm PES filter.
Combined IMAC/amylose-affinity chromatography
13.Sequentially connect a 1-ml Ni-NTA PureCube column and 5-ml amylose resin pre-packed in a column. Columns are typically stored at 4°C in 20% ethanol (Fig. 6).
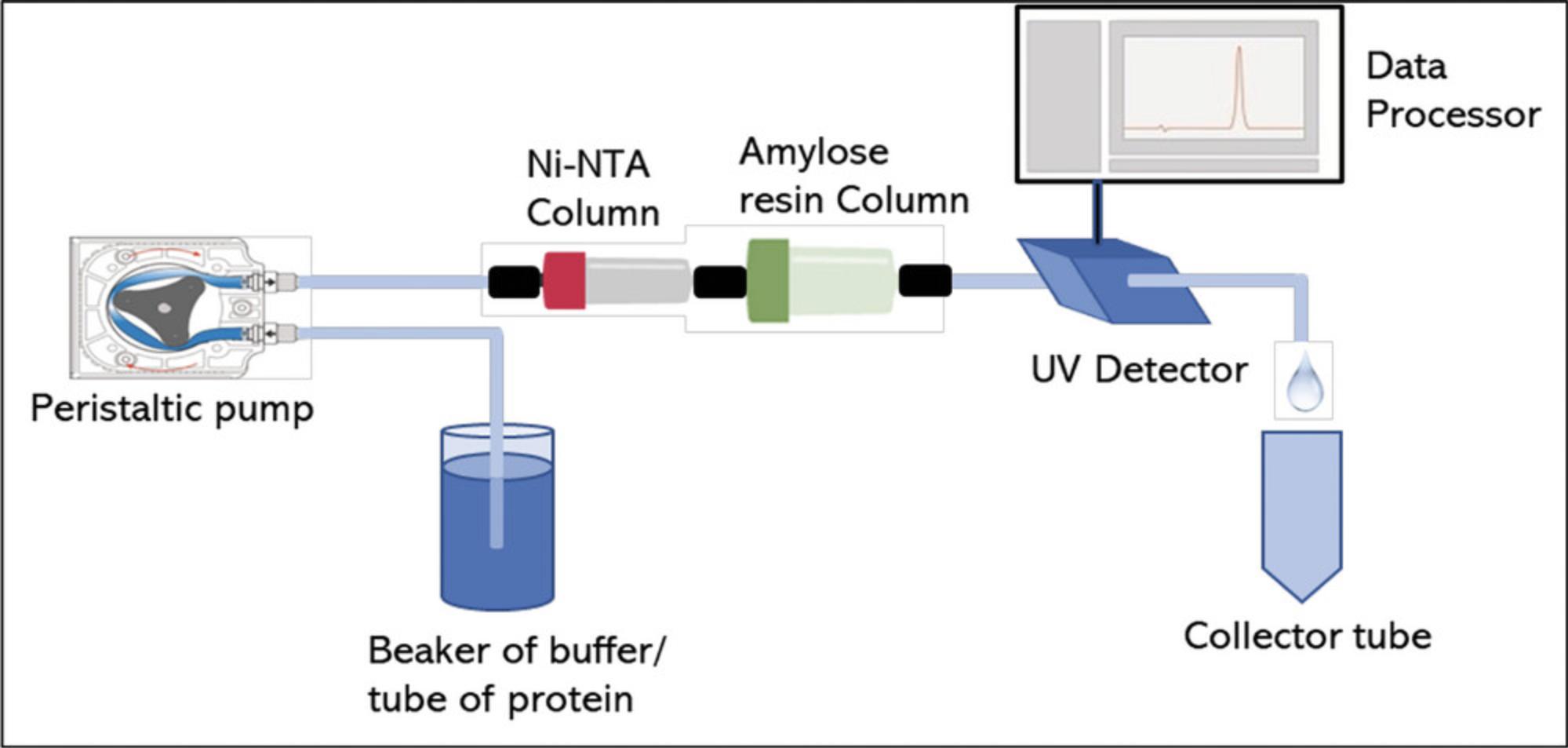
14.Wash both columns with 10 ml Milli-Q H2O at 1 ml/min.
15.Equilibrate with 25 ml A1 buffer at 1 ml/min.
16.Load the filtered supernatant from step 12 at 0.5 ml/min.
17.Wash the unbound proteins with A1 buffer at 1.5 ml/min with a total of volume of 60 ml.
18.Elute proteins with a total volume of 25 ml B1 buffer at 1 ml/min flow rate.
19.Wash columns with additional 25 ml Milli-Q water at 1 ml/min.
20.Store columns at 4°C in 20% ethanol.
21.Perform an SDS-PAGE analysis of collected samples (Fig. 7).
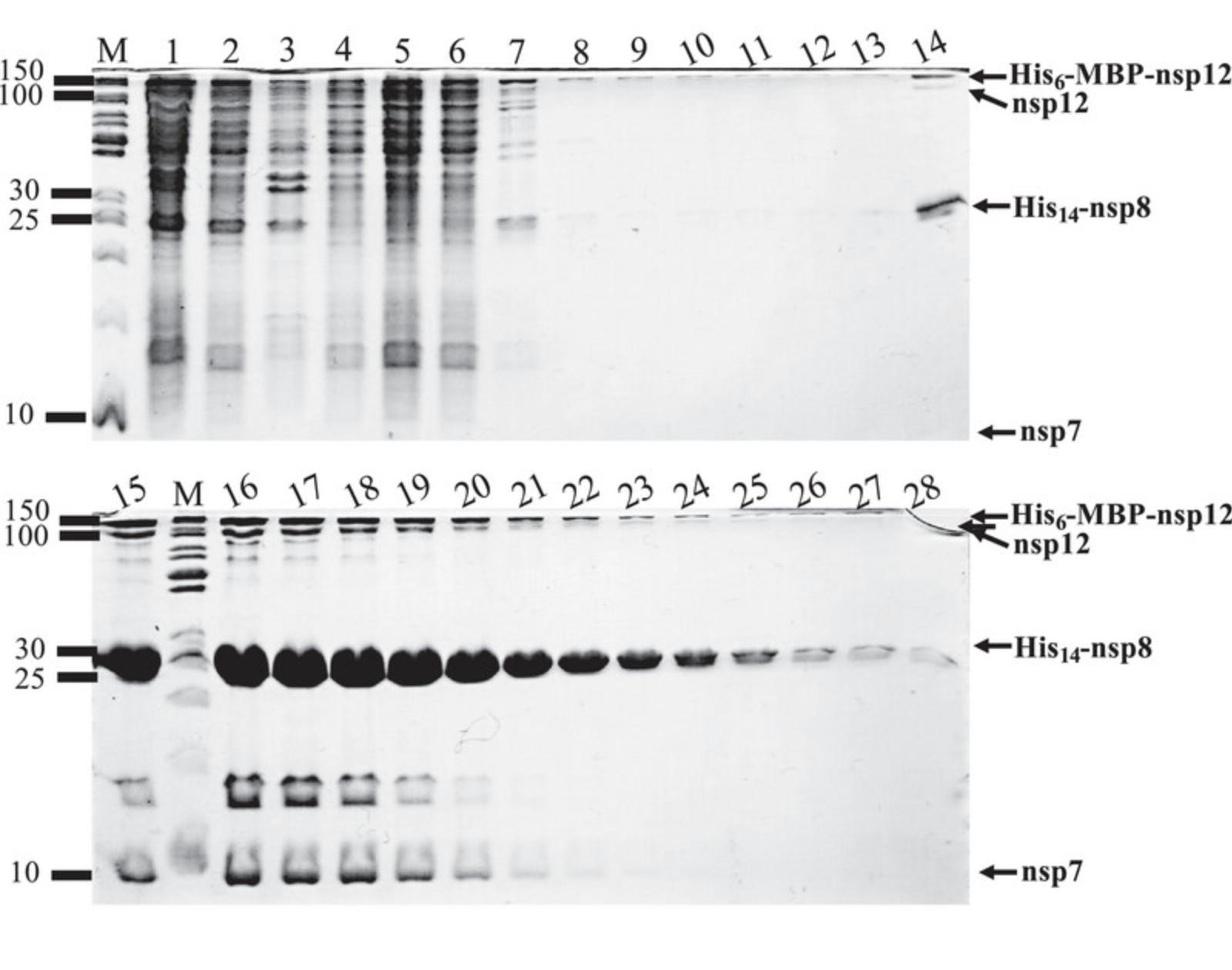
Cleavage of N-terminal fusion tags and dialysis
22.Combine eluted fractions with the higher concentration of desired proteins.
23.Estimate the total quantity of protein present in that sample.
24.Add TEV-protease in final mass proportion of 4% with respect to the complex.
25.Place the sample in a pre-washed and activated regenerated cellulose dialysis membrane tubing.
26.Cap the termini of each membrane tightly and place them into a beaker with 1.4 L pre-chilled D1 buffer.
27.Incubate overnight at 4°C with gentle stirring.
Removal of His-tagged fragments using Ni-NTA chromatography
28.The following day, wash out the peristaltic pump tubing with 10 ml milli-Q water at 2 ml/min.
29.Connect peristaltic pump to a 1-ml Ni-NTA PureCube column.
30.Wash with 10 ml milli-Q water at 1 ml/min.
31.Carefully transfer the tubing to a beaker with pre-chilled A1 buffer placed inside a foam box with ice.
32.Equilibrate the column with 10 ml A1 buffer at 1 ml/min.
33.Zero the UV detector.
34.Recover all the dialyzed sample from step 27.
35.Filter the dialyzed sample through a 0.22 μm × 25 mm PES syringe filter and place on ice for immediate use.
36.Load the sample into the 1-ml Ni-NTA PureCube column at 0.5 ml/min flow rate.
37.When UV signal increases above the baseline, start collecting the flowthrough in a single tube placed in a water-ice slush bath to always keep the sample cool.
38.Once the loading has finished, transfer the tubing to a beaker with A1 buffer.
39.Wash at 1 ml/min with 10 to 15 ml of A1 buffer or until UV signal reduces to zero.
40.Collect separated fractions of the flowthrough during the wash once the digested protein collection has finished.
41.Transfer the peristaltic pump inlet tubing into a beaker or 50-ml tube with B2 buffer.
42.Elute the bound proteins with a total of 20 ml at 1 ml/min flow rate.
43.Start fraction collector to collect 1 ml fractions of eluted proteins.
44.Remove fractions from the collector, cap, mix by inversion and put on ice immediately.
45.Wash column with additional 15 ml Milli-Q water at 1 ml/min.
46.Store column at 4°C in 20% ethanol.
47.Measure OD280 from flowthrough, wash, and elution fractions employing a nanodrop.
48.Analyze by SDS-PAGE the presence of digested proteins, tagged-fragments, His-TEV protease, or undigested proteins from various fractions (see Fig. 8 as example).
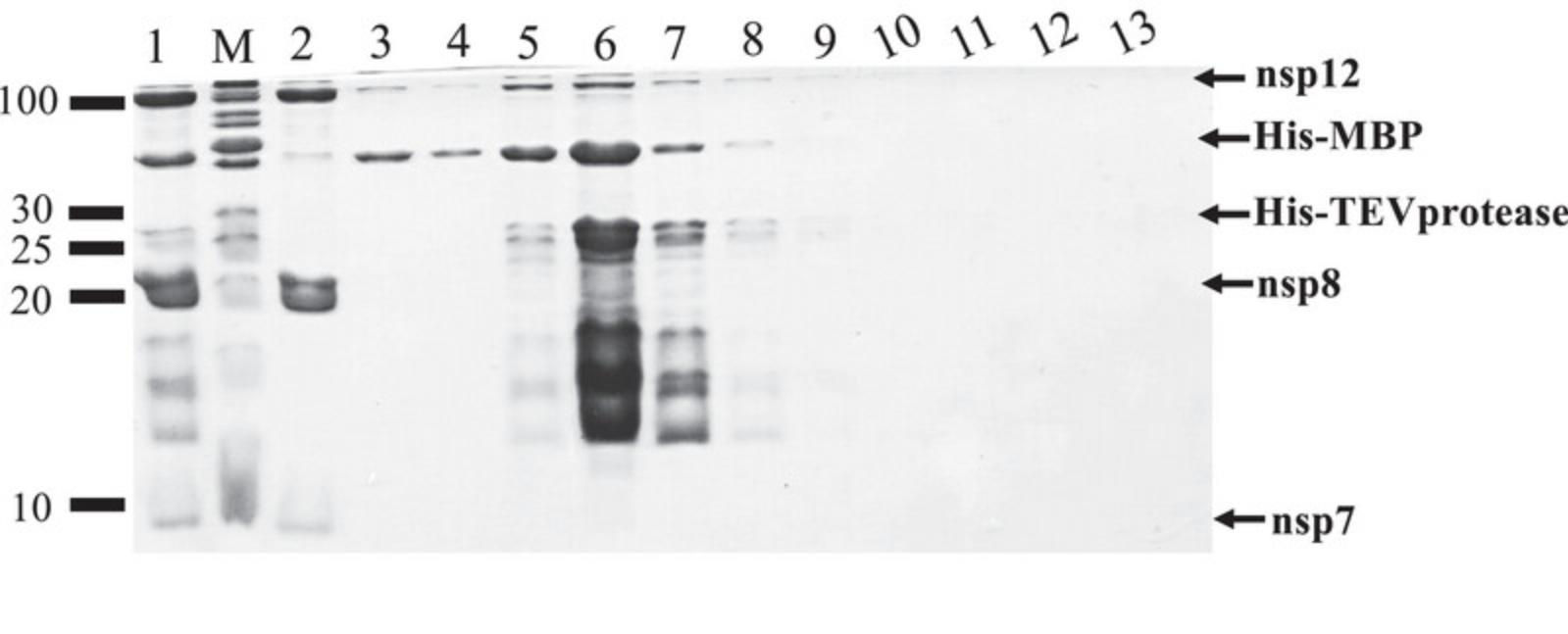
Dialysis and sample preparation for ion-exchange chromatography
49.Transfer the flow-through fraction containing the digested nsp12, nsp8, and nsp7 into dialysis bags prepared from pre-washed dialysis membranes.
50.Carefully close the ends of each dialysis bag and place them into a beaker with 1.4 L pre-chilled D2 buffer.
51.Incubate overnight at 4°C with gentle stirring.
Ion-exchange chromatography
52.The following day, wash out the peristaltic pump tubing with 10 ml Milli-Q water at 2 ml/min.
53.Connect the peristaltic pump tubing to the 1-ml G-Trap Q agarose column (Fig. 9).
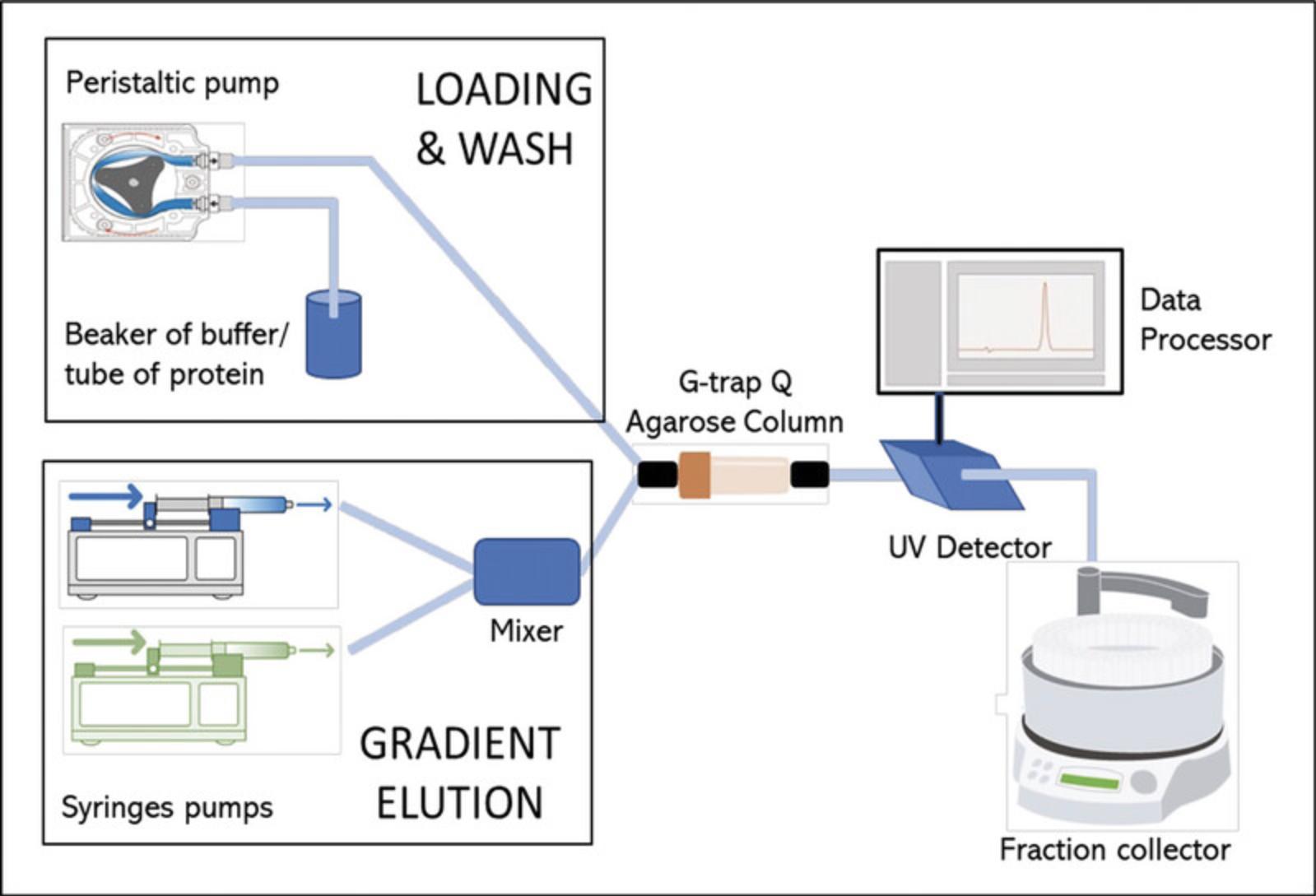
54.Wash with 10 ml Milli-Q water at 2 ml/min.
55.Connect the column to the UV detector.
56.Wash with an additional 10 ml Milli-Q water at 2 ml/min.
57.Equilibrate the column with 10 ml QA-50 buffer at 1 ml/min.
58.Zero the UV detector.
59.Collect the dialyzed sample and always keep it on ice.
60.Measure the conductivity of the sample.
61.Dilute the sample by adding QA-0 buffer until the conductivity of the sample is the same or slightly lower than for the QA-50 buffer.
62.Filter the diluted sample through a 0.22 μm × 25 mm PES syringe filter.
63.Load into the 1-ml G-Trap Q agarose column at 0.5 ml/min.
64.Collect flowthrough fractions.
65.Wash the column with 10 ml QA-50 buffer at 1 ml/min.
66.Collect two 5 ml fractions of flowthrough.
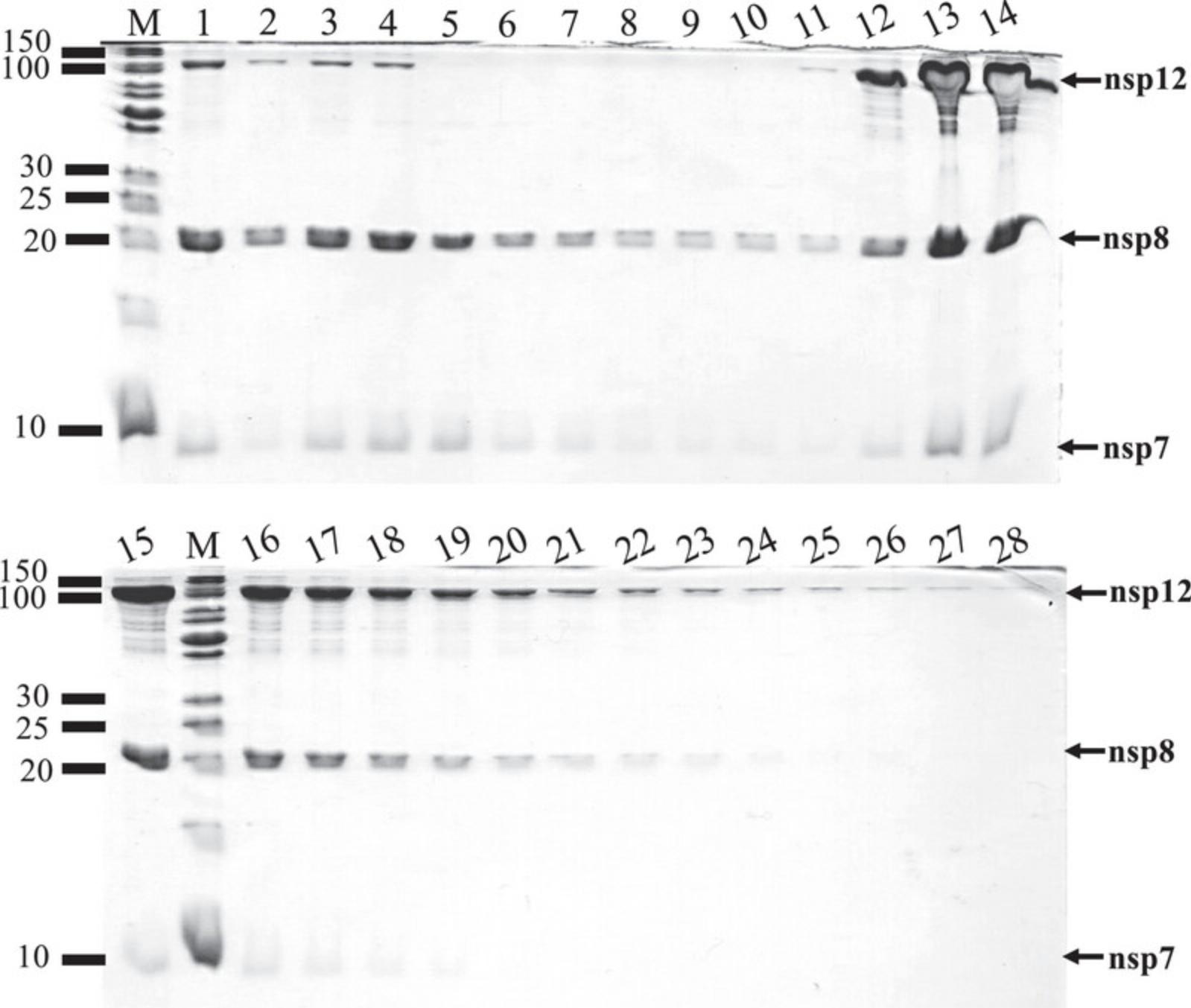
67.Elute with 30 ml, employing a gradient from 0% to 100% of QB buffer at 1 ml/min generated by syringe pumps (Fig. 9).
68.Collect 1 ml fractions, mix by inversion, and place them on ice immediately.
69.Wash the 1-ml G-Trap Q agarose column with 10 ml Milli-Q water.
70.Store column and tubing in 20% ethanol. Measure the OD280 and analyze by SDS-PAGE samples of flowthrough, wash, and eluted fractions (Fig. 10).
Protein concentration & freezing
71.Based on results from the previous step, combine the desired eluted fractions containing the three proteins, nsp12, nsp8, and nsp7.
72.Concentrate the protein employing an Amicon Ultra-4 centrifugal filter (cutoff ∼10,000 MW).
73.Pre-wet the centrifugal filter membrane with 2 ml QA-0 buffer and centrifuge 10 min at 3000 × g , 4°C.
74.Load the sample into the filter (register initial volume of the sample as well as OD280 signal).
75.Centrifuge 5 min at 3000 × g , 4°C, as many times as needed until the desired absorbance is achieved at OD280 (final recommended protein concentrations are OD280 = 2.0 to 2.5).
76.Aliquot the concentrated sample into 0.2-ml PCR tubes in 25 to 50 μl aliquots, and flash-freeze them employing liquid nitrogen.
77.Store at −80°C until use.
REAGENTS AND SOLUTIONS
A1 buffer, 500 ml
- 50 mM NaH2PO4, pH 8.0
- 20 mM imidazole-HCl, pH 8.0
- 300 mM NaCl
- 15% (w/v) glycerol (Wisent, #800-040-LL)
- Store up to 2 weeks at 4°C
B1 buffer, 100 ml
- 50 mM NaH2PO4, pH 8.0
- 200 mM imidazole-HCl, pH 8.0
- 300 mM NaCl
- 116.9 mM D-maltose
- 15% (w/v) glycerol (Wisent, #800-040-LL)
- Prepare fresh
B2 buffer, 100 ml
- 50 mM NaH2PO4, pH 8.0
- 200 mM imidazole-HCl, pH 8.0
- 300 mM NaCl
- 15% (w/v) glycerol (Wisent, #800-040-LL)
- Prepare fresh
D1 buffer, 1.5 L
- 50 mM NaH2PO4, pH 8.0
- 300 mM NaCl
- 15% (w/v) glycerol (Wisent, #800-040-LL)
- Prepare fresh
D2 buffer, 1.5 L
- 20 mM Tris·HCl, pH 8.0
- 100 mM NaCl
- 2 mM MgCl2
- 20% (w/v) glycerol (Wisent, #800-040-LL)
- 2 mM DTT
- Prepare fresh
ESF-921 growth medium
- 1 L ESF-921 medium (Expression Systems, #96-001-01)
- 100 U/ml penicillin and 100 μg/ml streptomycin (added from pen/strep solution; Wisent, #450-200-E)
- 10 μg/ml gentamycin (Wisent, #450-134-XL)
- 0.25 μg/ml amphotericin B (Wisent, #450-105-QL)
- Store up to 1 month at 4°C
I-MAX complete medium
- I-MAX serum-free medium with L-glutamine (Wisent, #301-045-CL)
- 10% heat-inactivated fetal bovine serum (FBS) (Wisent, #080-150)
- 10 μg/ml gentamycin (Wisent, #450-134-XL)
- 0.25 μg/ml amphotericin B (Wisent, #450-105-QL)
- 100 U/ml penicillin and 100 μg/ml streptomycin (added from pen/strep solution; Wisent, #450-200-E)
- Filter through a 500-ml vacuum filter storage bottle system, 0.22-µm pore, 33.2-cm², PES membrane (Corning, #431097)
- Store up to 1 month at 4°C
I-MAX 5% FBS medium
- I-MAX serum-free medium with L-glutamine (Wisent, #301-045-CL)
- 5% heat-inactivated FBS (Wisent, #080-150)
- 10 μg/ml gentamycin (Wisent, #450-134-XL)
- 0.25 μg/ml amphotericin B (Wisent, #450-105-QL)
- 100 U/ml penicillin and 100 μg/ml streptomycin (added from pen/strep solution; Wisent, #450-200-E)
- Filter through a 500-ml vacuum filter storage bottle system, 0.22-µm pore, 33.2-cm², PES membrane (Corning, #431097)
- Store up to 1 month at 4°C
L1 buffer, 500 ml
- 50 mM NaH2PO4, pH 8.0
- 10 mM imidazole-HCl, pH 8.0
- 300 mM NaCl
- 15% (w/v) glycerol (Wisent, #800-040-LL)
- Store up to 2 weeks at 4°C
LB broth medium and LB agar plates
- For LB broth medium:
- 25 g Miller LB broth (Wisent, #800-061-LG)
- Bring up to 1 L with H2O
- Autoclave for 20 min at 121°C
- Store up to 1 month at room temperature
- For LB agar plates:
- Add 3 g bacteriological agar (Wisent, #800-010-LG) per 200 ml LB broth medium
- If applicable, add 30 μg/ml kanamycin (Wisent, #400-145-IG) immediately before use
- Store up to 1 month at room temperature
QA-0 buffer, 100 ml
- 20 mM Tris·HCl, pH 8.0
- 2 mM MgCl2
- 20% (w/v) glycerol (Wisent, #800-040-LL)
- 2 mM DTT
- Prepare fresh
QA-50 buffer, 100 ml
- 20 mM Tris·HCl, pH 8.0
- 50 mM NaCl
- 2 mM MgCl2
- 20% (w/v) glycerol (Wisent, #800-040-LL)
- 2 mM DTT
- Prepare fresh
QB buffer, 50 ml
- 20 mM Tris·HCl, pH 8.0
- 1 M NaCl
- 2 mM MgCl2
- 20% (w/v) glycerol (Wisent, #800-040-LL)
- 2 mM DTT
- Prepare fresh
COMMENTARY
Critical Parameters
Basic Protocol 3
It is important to perform the whole purification process at 4°C. During cell lysis, the amplitude of sonication should be as low as possible to avoid overheating and nsp12 precipitation (it depends on sonicator probes features and volumes of samples).
Troubleshooting
Small deviations at several critical steps can lead to some commonly encountered problems when expressing and purifying the core SARS-CoV-2 polymerase complex (Table 1).
Problem | Possible cause | Solution |
---|---|---|
Poor overexpression of proteins expressed in E. coli | Suboptimal control of temperature in incubators | Check the temperature of bacterial cultures located in different locations in the incubator with a calibrated IR thermometer before and after induction |
Bacterial culture density was too high prior to induction | The bacterial culture density (OD600) after cooling down to 18°C should not exceed 1.0 before inducing protein expression with IPTG | |
Loss of plasmid from bacterial cultures stored in glycerol stocks | Transform bacteria with plasmid and avoid overgrowing starter cultures where antibiotics have been depleted | |
Infected T. ni cells showed reduced protein production | The number of infectious recombinant baculovirus particles is too low | Produce new high titer recombinant baculovirus stocks by either generating new recombinant baculovirus or propagating previous recombinant baculoviral stocks (Basic Protocol 2) |
Low yield of purified complex from ion exchange chromatography | Excess salt in the loaded sample will weaken attractive electrostatic interactions between the protein complex and the G-Trap Q agarose column | Dilute samples with salt-free buffer to lower conductivity to a value equal to or lower than that of the equilibration buffer |
Inconsistencies in protein concentration measurements | Aggregation of proteins may be occurring especially after thawing previously frozen protein complex | Flash-freeze small aliquots of protein in thin-walled PCR tubes using liquid nitrogen to minimize ice formation; centrifuge protein solutions (2 min at 6153 × g, 4°C) prior to measuring absorbance at 280 nm |
Understanding Results
The diversity of recombinant expression systems and purification procedures that have been reported to produce the SARS-CoV-2 core polymerase complex helps to highlight some of the challenges with producing this multisubunit protein complex. Although the accessory subunits nsp7 and nsp8 are usually expressed efficiently in bacterial cells, soluble and active nsp12 is typically expressed at much higher levels in insect cells when compared to bacteria. The main advantage of our method is to combine the efficiency of producing nsp12 at high levels in insect cells with the convenience of producing large amounts of nsp7 and nsp8 in bacterial cells. Our optimized protocol can routinely produce milligram quantities of enzymatically active complex with typical yields of 4.25 ± 1.86 mg of complex per L of bacterial culture + 0.5 L of insect cell culture. Although the yield of purified complex is lower than in a previously reported protocol where yields and activities are carefully described (Dangerfield et al., 2021), the lower number of purification steps in our protocol makes it cost- and time-efficient (Table 2). In addition, our method takes advantage of the excess amounts of nsp7 and nsp8 proteins, which are separated during the final ion exchange chromatography step. As shown previously (Bertolin et al., 2021; Rehman, 2022) and in the work presented here, the addition of nsp7 and nsp8 subunits in stoichiometric excess can also increase the enzymatic activity of the SARS-CoV-2 core polymerase complex (Fig. 11). An advantage of our method is that the final ion exchange chromatography step yields an active complex with the expected 1:2:1 stoichiometry for nsp7, nsp8, and nsp12 (Fig. 12) that we can supplement with amounts of nsp7 and nsp8 to enhance polymerase activity in a controlled manner.
Protocol | Steps | Total time | Details |
---|---|---|---|
Basic Protocol 1: Expression and production of SARS-CoV-2 nsp7, nsp8 and nsp12 in E. coli cells | Bacteria transformation | 2 days |
Day 1: 2 hr Day 2: 30 min |
Scaling-up bacteria cultures and induction of SARS-CoV-2 polymerase expression in bacteria | 2 days |
Day 1: 2 hr Day 2: 1 hr |
|
Basic Protocol 2: Generation of recombinant baculovirus in Sf9 cells and production of nsp12 fusion protein in T. ni cells | Bacmid preparation | 3 days |
Day 1: 2 hr Day 2: 30 min Day 3: 1 hr |
Recombinant baculovirus generation | 5 days |
Day 1: 4 hr Day 5: 30 min |
|
Amplification and titration of baculoviral stock expressing nsp12 fusion protein | 15 days |
Day 1: 2 hr Day 5: 1.5 hr Day 9: 3 hr Day 14: 30 min Day 15: 15 min |
|
T. ni cells infection and nsp12 fusion protein production | 4 days |
Day 1: 1.5 hr Day 2: 15 min Day 4: 1 hr |
|
Basic Protocol 3: Purification of SARS-CoV-2 core polymerase complex | First purification and TEV proteolysis | 1 day | Day 1: 8 hr |
Second purification | 1 day | Day 1: 5 hr | |
Third purification and protein concentration | 2 days |
Day 1: 8 hr Day 2: 2 hr |
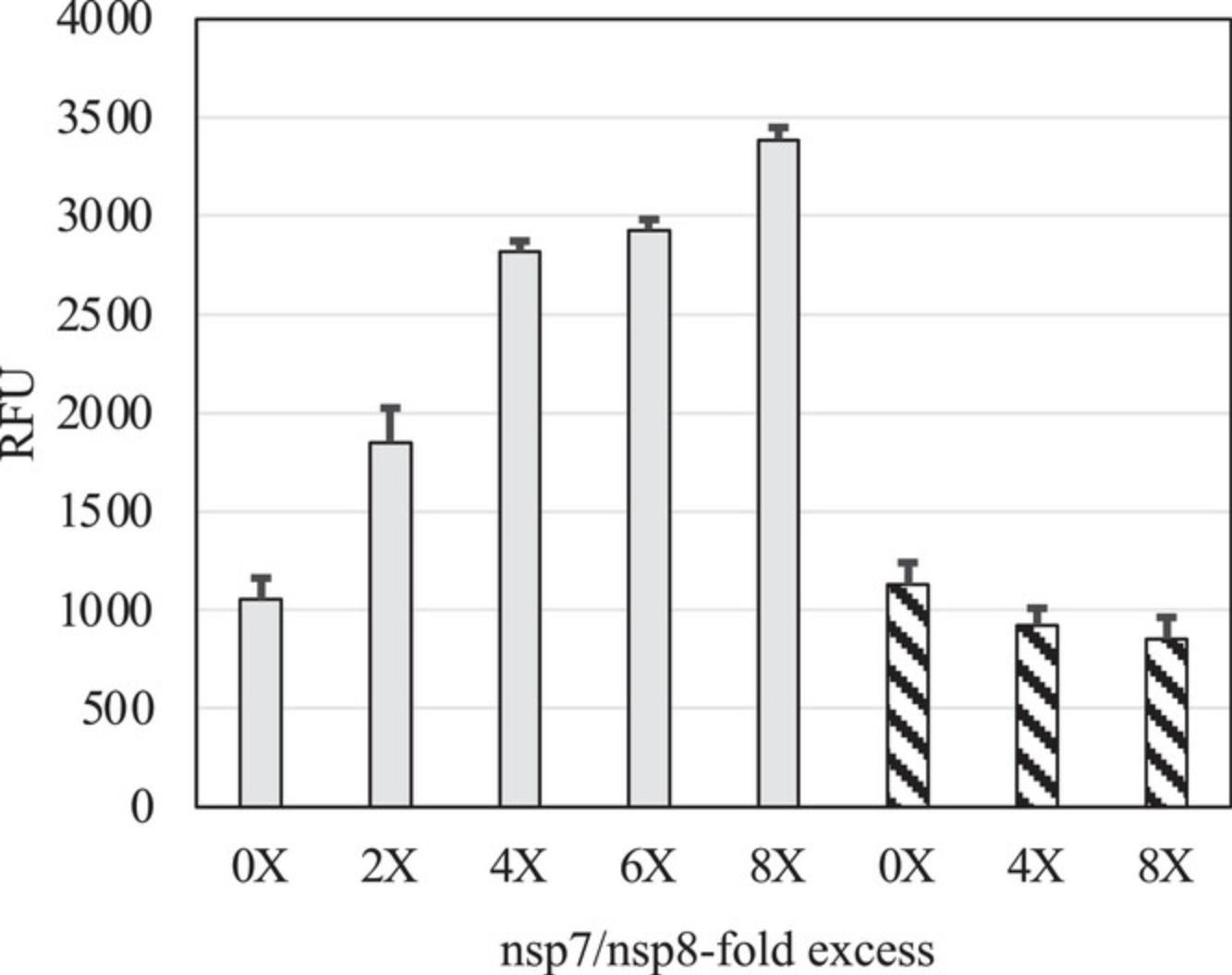
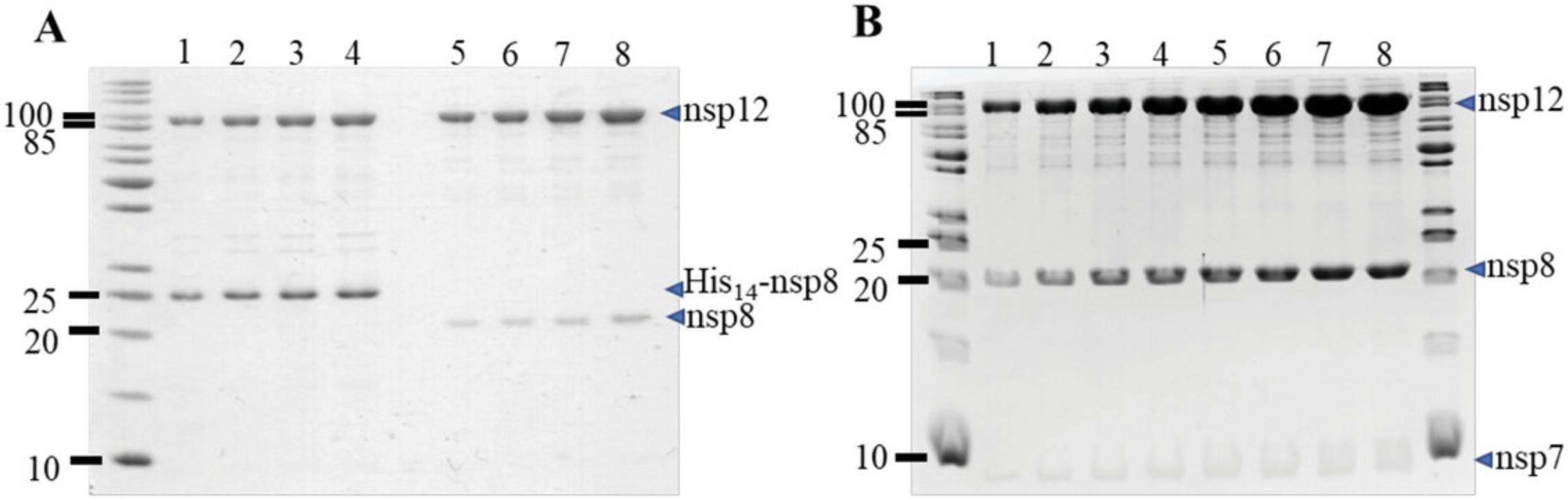
The apparent insolubility and misfolding of bacterially expressed nsp12 from SARS-CoV-2 and SARS-CoV-1 has been previously reported, with some beneficial effects observed through the co-expression of chaperones and the careful optimization of growth conditions prior to inducing protein expression (Dangerfield et al., 2021; Madru et al., 2021; Shannon et al., 2020). Although the co-expression of chaperones with nsp12 in E. coli appears to increase the solubility and presumably the efficiency of folding of nsp12, the specific effects of chaperones are not yet clearly documented (Shannon et al., 2020), and significant amounts of the nsp12 expressed in recombinant E. coli expression systems appear to be insoluble and presumably misfolded even with the overexpression of chaperone proteins (Dangerfield et al., 2020, 2021). A careful study also reported negative effects on the efficiency of protein folding and activity from the use of codon-optimized sequences of nsp12 for expression in bacterial cells (B. Wang et al., 2021). Conformational differences that correlated with RNA-binding and polymerization activity differences were documented for samples of nsp12 expressed from genes containing differences in the selection of codons at positions normally found to have rare codons in natural isolates of SARS-CoV-2. This suggests that pausing at specific steps during the translation of nsp12 may affect cotranslational folding, especially affecting interactions between the polymerase and NiRAN domains of nsp12 (B. Wang et al., 2021).
Time Considerations
See Table 2 for typical scheduling of steps for the purification of the SARS-CoV-2 polymerase complex.
Acknowledgments
This work was supported by an operating grant from the Canadian Institutes for Health Research (170708) and a Discovery Grant from the Natural Sciences and Engineering Research Council of Canada (05287). We gratefully acknowledge the deposition of expression plasmids by P. Cramer and M. Delarue into the Addgene repository to facilitate our research.
Author Contributions
Ana Podadera : Conceptualization; data curation; formal analysis; investigation; methodology; project administration; writing original draft; writing review and editing. Lucas Campo : Conceptualization; formal analysis; investigation; methodology; writing review and editing. Fasih Rehman : Conceptualization; formal analysis; investigation; methodology; writing review and editing. Nikola Kolobaric : Formal analysis; investigation; methodology; writing review and editing. Adriana Zutic : Formal analysis; investigation; methodology; writing review and editing. Kenneth Ng : Conceptualization; data curation; funding acquisition; project administration; supervision; writing review and editing.
Conflict of Interest
The authors declare no conflict of interest.
Open Research
Data Availability Statement
The data and materials that support the protocol are openly available in Addgene at http://addgene.org, reference numbers 154759 and 165451.
Literature Cited
- Bertolin, A. P., Weissmann, F., Zeng, J., Posse, V., Milligan, J. C., Canal, B., Ulferts, R., Wu, M., Drury, L. S., Howell, M., Beale, R., & Diffley, J. F. X. (2021). Identifying SARS-CoV-2 antiviral compounds by screening for small molecule inhibitors of nsp12/7/8 RNA-dependent RNA polymerase. Biochemical Journal , 478(13), 2425–2443. https://doi.org/10.1042/BCJ20210200
- Biswal, M., Diggs, S., Xu, D., Khudaverdyan, N., Lu, J., Fang, J., Blaha, G., Hai, R., & Song, J. (2021). Two conserved oligomer interfaces of NSP7 and NSP8 underpin the dynamic assembly of SARS-CoV-2 RdRP. Nucleic Acids Research , 49(10), 5956–5966. https://doi.org/10.1093/nar/gkab370
- Bravo, J. P. K., Dangerfield, T. L., Taylor, D. W., & Johnson, K. A. (2021). Remdesivir is a delayed translocation inhibitor of SARS-CoV-2 replication. Molecular Cell , 81(7), 1548–1552. e4. https://doi.org/10.1016/j.molcel.2021.01.035
- Campagnola, G., Govindarajan, V., Pelletier, A., Canard, B., & Peersen, O. B. (2022). The SARS-CoV nsp12 polymerase active site is tuned for large-genome replication. Journal of Virology , 96(16), e0067122. https://doi.org/10.1128/jvi.00671-22
- Chen, J., Malone, B., Llewellyn, E., Grasso, M., Shelton, P. M. M., Olinares, P. D. B., Maruthi, K., Eng, E. T., Vatandaslar, H., Chait, B. T., Kapoor, T. M., Darst, S. A., & Campbell, E. A. (2020). Structural basis for helicase-polymerase coupling in the SARS-CoV-2 replication-transcription complex. Cell , 182(6), 1560–1573. e13. https://doi.org/10.1016/j.cell.2020.07.033
- Chien, M., Anderson, T. K., Jockusch, S., Tao, C., Li, X., Kumar, S., Russo, J. J., Kirchdoerfer, R. N., & Ju, J. (2020). Nucleotide analogues as inhibitors of SARS-CoV-2 polymerase, a key drug target for COVID-19. Journal of Proteome Research , 19(11), 4690–4697. https://doi.org/10.1021/acs.jproteome.0c00392
- Conti, B. J., Leicht, A. S., Kirchdoerfer, R. N., & Sussman, M. R. (2021). Mass spectrometric based detection of protein nucleotidylation in the RNA polymerase of SARS-CoV-2. Communications Chemistry , 4(1), 41. https://doi.org/10.1038/s42004-021-00476-4
- Dangerfield, T. L., Huang, N. Z., & Johnson, K. A. (2020). Remdesivir is effective in combating COVID-19 because it is a better substrate than ATP for the viral RNA-dependent RNA polymerase. iScience , 23(12), 101849. https://doi.org/10.1016/j.isci.2020.101849
- Dangerfield, T. L., Huang, N. Z., & Johnson, K. A. (2021). Expression and purification of tag-free SARS-CoV-2 RNA-dependent RNA polymerase in Escherichia coli. STAR Protocols , 2(1), 100357. https://doi.org/10.1016/j.xpro.2021.100357
- Dejmek, M., Konkoľová, E., Eyer, L., Straková, P., Svoboda, P., Šála, M., Krejčová, K., Růžek, D., Boura, E., & Nencka, R. (2021). Non-nucleotide RNA-dependent RNA polymerase inhibitor that blocks SARS-CoV-2 replication. Viruses , 13(8), 1585. https://doi.org/10.3390/v13081585
- Dwivedy, A., Mariadasse, R., Ahmad, M., Chakraborty, S., Kar, D., Tiwari, S., Bhattacharyya, S., Sonar, S., Mani, S., Tailor, P., Majumdar, T., Jeyakanthan, J., & Biswal, B. K. (2021). Characterization of the NiRAN domain from RNA-dependent RNA polymerase provides insights into a potential therapeutic target against SARS-CoV-2. PLOS Computational Biology , 17(9), e1009384. https://doi.org/10.1371/journal.pcbi.1009384
- Eydoux, C., Fattorini, V., Shannon, A., Le, T.-T.-N., Didier, B., Canard, B., & Guillemot, J.-C. (2021). A fluorescence-based high throughput-screening assay for the SARS-CoV RNA synthesis complex. Journal of Virological Methods , 288, 114013. https://doi.org/10.1016/j.jviromet.2020.114013
- Felberbaum, R. S. (2015). The baculovirus expression vector system: A commercial manufacturing platform for viral vaccines and gene therapy vectors. Biotechnology Journal , 10(5), 702–714. https://doi.org/10.1002/biot.201400438
- Gallagher, S. R. (2008). SDS-Polyacrylamide Gel Electrophoresis (SDS-PAGE). Current Protocols Essential Laboratory Techniques , 00(1), 7.3.1–7.3.25. https://doi.org/10.1002/9780470089941.et0703s00
- Gao, Y., Yan, L., Huang, Y., Liu, F., Zhao, Y., Cao, L., Wang, T., Sun, Q., Ming, Z., Zhang, L., Ge, J., Zheng, L., Zhang, Y., Wang, H., Zhu, Y., Zhu, C., Hu, T., Hua, T., Zhang, B., … Rao, Z. (2020). Structure of the RNA-dependent RNA polymerase from COVID-19 virus. Science , 368(6492), 779–782. https://doi.org/10.1126/science.abb7498
- Gordon, C. J., Tchesnokov, E. P., Woolner, E., Perry, J. K., Feng, J. Y., Porter, D. P., & Götte, M. (2020). Remdesivir is a direct-acting antiviral that inhibits RNA-dependent RNA polymerase from severe acute respiratory syndrome coronavirus 2 with high potency. Journal of Biological Chemistry , 295(20), 6785–6797. https://doi.org/10.1074/jbc.RA120.013679
- Hillen, H. S. (2021). Structure and function of SARS-CoV-2 polymerase. Current Opinion in Virology , 48, 82–90. https://doi.org/10.1016/j.coviro.2021.03.010
- Hillen, H. S., Kokic, G., Farnung, L., Dienemann, C., Tegunov, D., & Cramer, P. (2020). Structure of replicating SARS-CoV-2 polymerase. Nature , 584(7819), 154–156. https://doi.org/10.1038/s41586-020-2368-8
- Irons, S. L., Chambers, A. C., Lissina, O., King, L. A., & Possee, R. D. (2018). Protein production using the baculovirus expression system. Current Protocols in Protein Science , 91(1), 5.5.1–5.5.22. https://doi.org/10.1002/cpps.45
- Kapust, R. B., Tözsér, J., Fox, J. D., Anderson, D. E., Cherry, S., Copeland, T. D., & Waugh, D. S. (2001). Tobacco etch virus protease: Mechanism of autolysis and rational design of stable mutants with wild-type catalytic proficiency. Protein Engineering, Design and Selection , 14(12), 993–1000. https://doi.org/10.1093/protein/14.12.993
- Kirchdoerfer, R. N., & Ward, A. B. (2019). Structure of the SARS-CoV nsp12 polymerase bound to nsp7 and nsp8 co-factors. Nature Communications , 10(1), 2342. https://doi.org/10.1038/s41467-019-10280-3
- Li, Q., Yi, D., Lei, X., Zhao, J., Zhang, Y., Cui, X., Xiao, X., Jiao, T., Dong, X., Zhao, X., Zeng, H., Liang, C., Ren, L., Guo, F., Li, X., Wang, J., & Cen, S. (2021). Corilagin inhibits SARS-CoV-2 replication by targeting viral RNA-dependent RNA polymerase. Acta Pharmaceutica Sinica B , 11(6), 2342. https://doi.org/10.1016/j.apsb.2021.02.011
- Lou, Z., & Rao, Z. (2022). The life of SARS-CoV-2 inside cells: Replication–transcription complex assembly and function. Annual Review of Biochemistry , 91(1), 381–401. https://doi.org/10.1146/annurev-biochem-052521-115653
- Lu, G., Zhang, X., Zheng, W., Sun, J., Hua, L., Xu, L., Chu, X., Ding, S., & Xiong, W. (2020). Development of a simple in vitro assay to identify and evaluate nucleotide analogs against SARS-CoV-2 RNA-dependent RNA polymerase. Antimicrobial Agents and Chemotherapy , 65(1), e01508-20. https://doi.org/10.1128/AAC.01508-20
- Lynn, D. E. (2002). Methods for maintaining insect cell cultures. Journal of Insect Science , 2(1), 9. https://doi.org/10.1093/jis/2.1.9
- Madru, C., Tekpinar, A. D., Rosario, S., Czernecki, D., Brûlé, S., Sauguet, L., & Delarue, M. (2021). Fast and efficient purification of SARS-CoV-2 RNA dependent RNA polymerase complex expressed in Escherichia coli. PLoS ONE , 16(4), e0250610. https://doi.org/10.1371/journal.pone.0250610
- Malone, B., Chen, J., Wang, Q., Llewellyn, E., Choi, Y. J., Olinares, P. D. B., Cao, X., Hernandez, C., Eng, E. T., Chait, B. T., Shaw, D. E., Landick, R., Darst, S. A., & Campbell, E. A. (2021). Structural basis for backtracking by the SARS-CoV-2 replication–transcription complex. Proceedings of the National Academy of Sciences , 118(19), e2102516118. https://doi.org/10.1073/pnas.2102516118
- Malone, B., Urakova, N., Snijder, E. J., & Campbell, E. A. (2022). Structures and functions of coronavirus replication–transcription complexes and their relevance for SARS-CoV-2 drug design. Nature Reviews Molecular Cell Biology , 23(1), 21–39. https://doi.org/10.1038/s41580-021-00432-z
- Naydenova, K., Muir, K. W., Wu, L.-F., Zhang, Z., Coscia, F., Peet, M. J., Castro-Hartmann, P., Qian, P., Sader, K., Dent, K., Kimanius, D., Sutherland, J. D., Löwe, J., Barford, D., & Russo, C. J. (2021). Structure of the SARS-CoV-2 RNA-dependent RNA polymerase in the presence of favipiravir-RTP. Proceedings of the National Academy of Sciences , 118(7), e2021946118. https://doi.org/10.1073/pnas.2021946118
- Peng, Q., Peng, R., Yuan, B., Zhao, J., Wang, M., Wang, X., Wang, Q., Sun, Y., Fan, Z., Qi, J., Gao, G. F., & Shi, Y. (2020). Structural and Biochemical Characterization of the nsp12-nsp7-nsp8 core polymerase complex from SARS-CoV-2. Cell Reports , 31(11), 107774. https://doi.org/10.1016/j.celrep.2020.107774
- Rehman, F. (2022). Development and Characterization of a Platform to Characterize Putative Inhibitors Targeting the SARS CoV-2 RNA-Dependent RNA Polymerase [Master's Thesis]. University of Windsor.
- Shannon, A., Fattorini, V., Sama, B., Selisko, B., Feracci, M., Falcou, C., Gauffre, P., El Kazzi, P., Delpal, A., Decroly, E., Alvarez, K., Eydoux, C., Guillemot, J.-C., Moussa, A., Good, S. S., la Colla, P., Lin, K., Sommadossi, J.-P., Zhu, Y., … Canard, B. (2022). A dual mechanism of action of AT-527 against SARS-CoV-2 polymerase. Nature Communications , 13(1), 621. https://doi.org/10.1038/s41467-022-28113-1
- Shannon, A., Selisko, B., Le, N.-T.-T., Huchting, J., Touret, F., Piorkowski, G., Fattorini, V., Ferron, F., Decroly, E., Meier, C., Coutard, B., Peersen, O., & Canard, B. (2020). Rapid incorporation of Favipiravir by the fast and permissive viral RNA polymerase complex results in SARS-CoV-2 lethal mutagenesis. Nature Communications , 11(1), 4682. https://doi.org/10.1038/s41467-020-18463-z
- Shi, W., Chen, M., Yang, Y., Zhou, W., Chen, S., Yang, Y., Hu, Y., & Liu, B. (2020). A dynamic regulatory interface on SARS-CoV-2 RNA polymerase. bioRxiv , 2020.07.30.229187. https://doi.org/10.1101/2020.07.30.229187
- Slanina, H., Madhugiri, R., Bylapudi, G., Schultheiß, K., Karl, N., Gulyaeva, A., Gorbalenya, A. E., Linne, U., & Ziebuhr, J. (2021). Coronavirus replication–transcription complex: Vital and selective NMPylation of a conserved site in nsp9 by the NiRAN-RdRp subunit. Proceedings of the National Academy of Sciences , 118(6), e2022310118. https://doi.org/10.1073/pnas.2022310118
- Walker, A. P., Fan, H., Keown, J. R., Knight, M. L., Grimes, J. M., & Fodor, E. (2021). The SARS-CoV-2 RNA polymerase is a viral RNA capping enzyme. Nucleic Acids Research , 49(22), 13019–13030. https://doi.org/10.1093/nar/gkab1160
- Wang, B., Svetlov, D., & Artsimovitch, I. (2021). NMPylation and de-NMPylation of SARS-CoV-2 nsp9 by the NiRAN domain. Nucleic Acids Research , 49(15), 8822–8835. https://doi.org/10.1093/nar/gkab677
- Wang, B., Svetlov, V., Wolf, Y. I., Koonin, E. V., Nudler, E., & Artsimovitch, I. (2021). Allosteric activation of SARS-CoV-2 RNA-dependent RNA polymerase by remdesivir triphosphate and other phosphorylated nucleotides. mBio , 12(3), e0142321. https://doi.org/10.1128/mBio.01423-21
- Wang, Q., Wu, J., Wang, H., Gao, Y., Liu, Q., Mu, A., Ji, W., Yan, L., Zhu, Y., Zhu, C., Fang, X., Yang, X., Huang, Y., Gao, H., Liu, F., Ge, J., Sun, Q., Yang, X., Xu, W., … Rao, Z. (2020). Structural basis for RNA replication by the SARS-CoV-2 polymerase. Cell , 182(2), 417–428. e13. https://doi.org/10.1016/j.cell.2020.05.034
- Wilamowski, M., Hammel, M., Leite, W., Zhang, Q., Kim, Y., Weiss, K. L., Jedrzejczak, R., Rosenberg, D. J., Fan, Y., Wower, J., Bierma, J. C., Sarker, A. H., Tsutakawa, S. E., Pingali, S. V., O'Neill, H. M., Joachimiak, A., & Hura, G. L. (2021). Transient and stabilized complexes of Nsp7, Nsp8, and Nsp12 in SARS-CoV-2 replication. Biophysical Journal , 120(15), 3152–3165. https://doi.org/10.1016/j.bpj.2021.06.006
- Yan, L., Ge, J., Zheng, L., Zhang, Y., Gao, Y., Wang, T., Huang, Y., Yang, Y., Gao, S., Li, M., Liu, Z., Wang, H., Li, Y., Chen, Y., Guddat, L. W., Wang, Q., Rao, Z., & Lou, Z. (2021). Cryo-EM structure of an extended SARS-CoV-2 replication and transcription complex reveals an intermediate state in cap synthesis. Cell , 184(1), 184–193. e10. https://doi.org/10.1016/j.cell.2020.11.016
- Yan, L., Zhang, Y., Ge, J., Zheng, L., Gao, Y., Wang, T., Jia, Z., Wang, H., Huang, Y., Li, M., Wang, Q., Rao, Z., & Lou, Z. (2020). Architecture of a SARS-CoV-2 mini replication and transcription complex. Nature Communications , 11(1), 5874. https://doi.org/10.1038/s41467-020-19770-1
- Yin, W., Luan, X., Li, Z., Zhou, Z., Wang, Q., Gao, M., Wang, X., Zhou, F., Shi, J., You, E., Liu, M., Wang, Q., Jiang, Y., Jiang, H., Xiao, G., Zhang, L., Yu, X., Zhang, S., & Eric Xu, H. (2021). Structural basis for inhibition of the SARS-CoV-2 RNA polymerase by suramin. Nature Structural & Molecular Biology, 28(3), 319–325. https://doi.org/10.1038/s41594-021-00570-0