mRNA Synthesis and Encapsulation in Ionizable Lipid Nanoparticles
Rebecca Elizabeth McKenzie, Rebecca Elizabeth McKenzie, Jordan James Minnell, Jordan James Minnell, Mitch Ganley, Mitch Ganley, Gavin Frank Painter, Gavin Frank Painter, Sarah Louise Draper, Sarah Louise Draper
Abstract
mRNA vaccines have recently generated significant interest due to their success during the COVID-19 pandemic. Their success is due to advances in mRNA design and encapsulation into ionizable lipid nanoparticles (iLNPs). This has highlighted the potential for the use of mRNA-iLNPs in other settings such as cancer, gene therapy, or vaccines for different infectious diseases. Here, we describe the production of mRNA-iLNPs using commercially available reagents that are suitable for use as vaccines and therapeutics. This article contains detailed protocols for the synthesis of mRNA by in vitro transcription with enzymatic capping and tailing and the encapsulation of the mRNA into iLNPs using the ionizable lipid DLin-MC3-DMA. DLin-MC3-DMA is often used as a benchmark for new formulations and provides an efficient delivery vehicle for screening mRNA design. The protocol also describes how the formulation can be adapted to other lipids. Finally, a stepwise methodology is presented for the characterization and quality control of mRNA-iLNPs, including measuring mRNA concentration and encapsulation efficiency, particle size, and zeta potential. © 2023 The Authors. Current Protocols published by Wiley Periodicals LLC.
Basic Protocol 1 : Synthesis of mRNA by in vitro transcription and enzymatic capping and tailing
Basic Protocol 2 : Encapsulation of mRNA into ionizable lipid nanoparticles
Alternate Protocol : Small-scale encapsulation of mRNA using preformed vesicles
Basic Protocol 3 : Characterization and quality control of mRNA ionizable lipid nanoparticles
INTRODUCTION
Therapeutics and vaccines based on nucleic acids have received considerable attention over the last 30 years; however, only recently have mRNA design and delivery options provided these products with safety and efficacy profiles acceptable for market approval. The COVID-19 mRNA-based vaccines developed by Pfizer-BioNTech and Moderna have validated the mRNA vaccine platform and generated significant interest in the application of mRNA in vaccines for various indications such as infectious diseases and cancer (Chaudhary et al., 2021; Patel et al., 2022). These vaccines are based on mRNA encapsulated in ionizable lipid nanoparticles (iLNPs) which are ∼100 nm in diameter, have a neutral surface charge and a dense electron-rich oily core. iLNPs are currently the most clinically advanced delivery vehicle for mRNA (Chaudhary et al., 2021). Further optimization and development of the iLNPs and mRNA is likely required for application of mRNA to these other areas; therefore, an accessible method for the manufacture of mRNA-iLNPs allows more researchers to evaluate and advance them. Here, we describe the production of mRNA-iLNPs, from the synthesis of mRNA to the formulation and characterization of the iLNP.
There are currently no clear protocols for scientists new to the field to produce an encapsulated mRNA product (i.e., mRNA-iLNPs) using commercially available reagents from planning stages to a final product. While some reagent suppliers provide detailed procedures for using their products, the overall process to synthesize mRNA is comprised of many steps and is carried out over several days. Consideration of the whole process from the DNA template design stage to suitable storage conditions in between steps is critical. The formulation of iLNPs is described widely in the literature; however, the use of proprietary lipids or formulations often means that sufficient details to reproduce the experiments are not published. This protocol aims to describe the formulation and characterization of iLNPs in detail using commercially available reagents, including steps typically not included or detailed in published literature.
Basic Protocol 1 (Fig. 1A) describes a 3-step method using components widely available through commercial suppliers to synthesize mRNA via in vitro transcription (IVT) from a DNA template, followed by enzymatic capping and tailing. This method, which allows each component of the mRNA molecule [coding region, non-coding cis-regulatory regions, 5′ cap and 3′ poly(A) tail] to be investigated independently, is the most efficient capping method (100% capped mRNA after enzymatic treatment) (Linares-Fernández et al., 2020) and avoids difficulties with poly(A) tract instability during DNA template production (Grier et al., 2016). Purification of the mRNA by lithium chloride precipitation and analysis by gel electrophoresis allows adjustment of the mRNA concentration required for easy flow on to iLNP encapsulation in Basic Protocol 2.
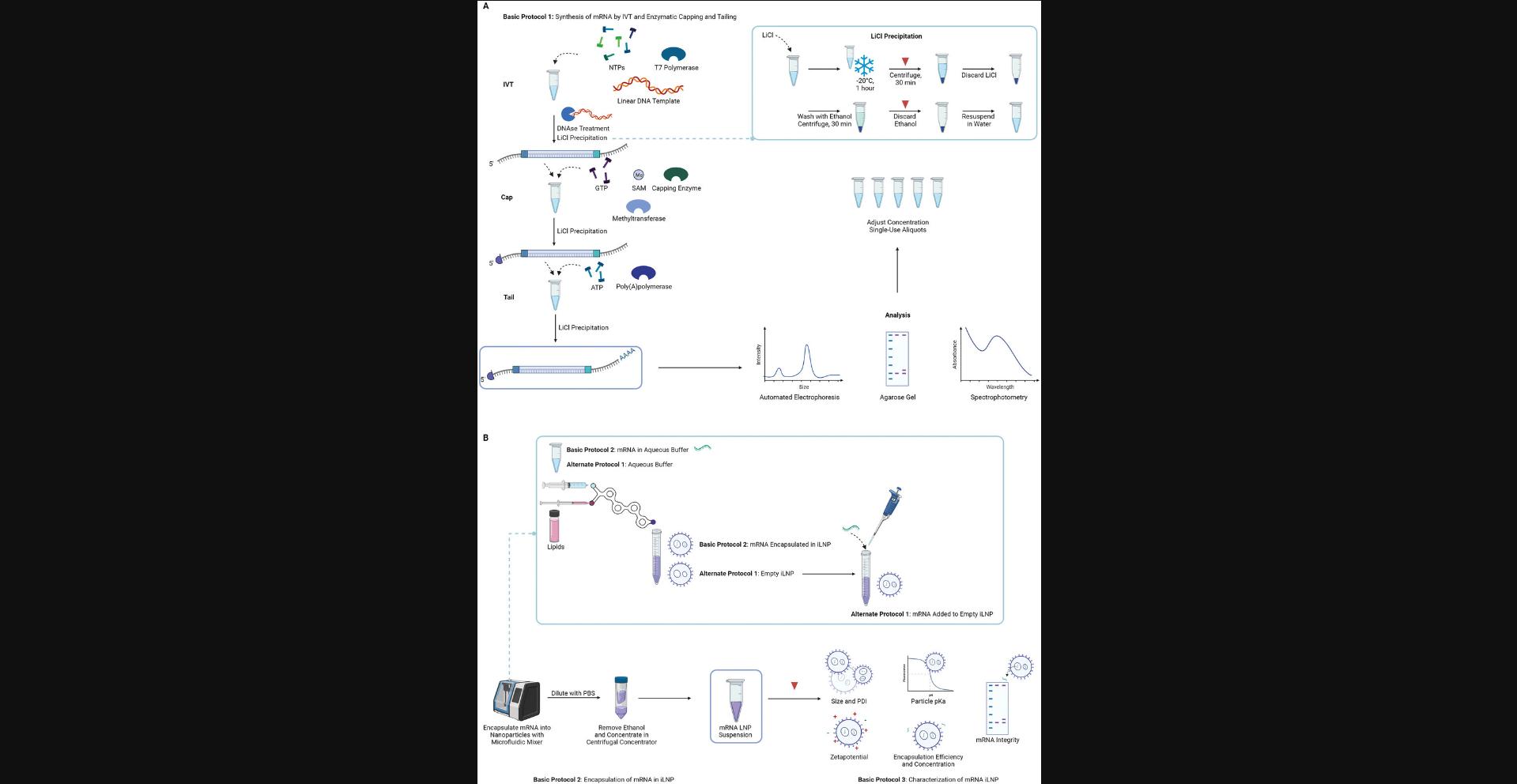
Basic Protocol 2 describes the formulation of mRNA in iLNPs using a microfluidics mixer; Alternate Protocol outlines the process for the formulation of small amounts of mRNA by complexing the mRNA to preformed vesicles and downstream processing. Basic Protocol 3 covers the characterization of iLNPs including routine analysis of the size, polydispersity, charge, and encapsulation efficiency, and the less routine analysis of the apparent particle pKa and quality of mRNA after encapsulation (Fig. 1B). The encapsulation is demonstrated using the ionizable lipid DLin-MC3-DMA (MC3) which is often used to benchmark new formulations for vaccines and therapeutics and was the first iLNP to be used in the clinic for delivery of siRNA (Akinc et al., 2019). The protocol can be adapted to different lipids, payloads, and formulation parameters, as required. Examples of this are included in the Understanding Results section. iLNPs created with MC3 are versatile and can be used to evaluate mRNA design in a vaccination or therapeutic setting.
STRATEGIC PLANNING
DNA Template
The DNA template should be linear and can be produced by linearization of a purified plasmid or generated via PCR. The template requires the T7 promoter sequence 5′ TAATACGACTCACTATA 3′ to allow initiation of in vitro transcription (IVT) by the T7 RNA polymerase and should encode the antigen of interest in an open reading frame (ORF) flanked by a 5′ and 3′ untranslated region (UTR), if desired. For further details on template design and preparation see Henderson et al. (2021).
Prior to mRNA production, calculating how much DNA template is needed for IVT is required. For example, in Basic Protocol 1, ∼1 µg of linearized template is required for the preparation of 150 µg of mRNA, and this amount should be scaled up accordingly. It is important to prepare the plasmid DNA from bacteria using a low endotoxin plasmid preparation method. Commercially available plasmid chromatography kits such as the PureLink HiPure Plasmid Midiprep Kit (Thermo Fisher Scientific) should provide at least 100 µg of low-endotoxin plasmid DNA, which will be sufficient for downstream linearization and multiple IVT reactions. Following linearization with restriction enzyme, DNA can be purified by phenol/chloroform extraction and/or alcohol precipitation (Green & Sambrook, 2017).
Modified Nucleoside Triphosphates
Modified nucleoside triphosphates (NTPs) such as pseudouridine (Ѱ), N1-methyl-pseudouridine (m1Ѱ) and 5-methoxyuridine (5moU) reduce the innate immune stimulating properties of mRNA as they have less specificity to RNA-sensing receptors (Vaidyanathan et al., 2018). This reduces the production of type I IFN, increasing transfection, translation efficiency, and reduces toxicity (Alexopoulou et al., 2001; Diebold et al., 2006). Full or partial substitution can be made with the immune suppression increasing proportionally with the proportion of modified NTPs. Full substitution will likely be a highly desirable approach for therapeutic applications, such as protein replacement and gene editing therapies, mRNA-based monoclonal antibodies, and cell transfection (Karikó et al., 2005; Qin et al., 2022). For vaccines, the ratio of modified/unmodified NTPs will require optimization by the user for the specific application, route of administration, and iLNP formulation. It is worth noting that the use of modified nucleotides will reduce the yield of mRNA, and this will need to be taken into account when considering the scale of the reaction.
mRNA Storage
Unnecessary freeze-thawing of mRNA should be avoided (Davis et al., 2000; Kellman et al., 2021). The final product should be frozen in small single-use aliquots and defrosted only as needed.
mRNA can be stored long term at –80°C in water or a low pH solution suitable for encapsulation, such as sodium acetate. The downstream application should be considered when choosing a storage solution. For mRNA that will be encapsulated, we recommend storage of these aliquots in 100 mM sodium acetate to reduce handling. For other applications, such as in vitro expression screening using commercial transfection reagents or analysis, storage in nuclease-free water is most versatile.
Appropriate storage of mRNA between steps is important to consider reducing degradation and handling. The mRNA is recovered from each step by precipitation with lithium chloride followed by a wash with ethanol. Storage of the pellet in ethanol is recommended as a safe stopping point (Fig. 1A). The pellet in ethanol can be stored at −20°C for several months and resuspended in RNase-free water prior to the next step as required (Dowhan, 2012; Walker & Lorsch, 2013). This prevents additional freeze-thaw cycles of dissolved mRNA from aqueous solutions (Pogocki & Schöneich, 2000; Mikkola et al., 2001).
Encapsulation of mRNA in Ionizable Lipid Nanoparticles
Encapsulation of the mRNA should be carried out within 1 day. The RiboGreen assay (Basic Protocol 3) and dynamic light scattering (DLS) analysis may be carried out at a later stage but before the particles are used for biological applications. The iLNP is ideally used fresh so formulation close to the date of use should be planned.
Scale
The desired amount of encapsulated mRNA should be considered when planning the scale of mRNA synthesis. About 300 µg of mRNA is required to return 100 µg encapsulated mRNA using Basic Protocol 2 and 150 µg is required for the same yield using Alternate Protocol.
mRNA Purification Methods
After each of the three steps in mRNA synthesis outlined in Basic Protocol 1 (IVT, capping and tailing) a purification step is required to isolate the mRNA product of interest and remove reaction components, such as the buffer, enzymes and NTPs (Fig. 1A). In this protocol we describe precipitation of mRNA using lithium chloride, an inexpensive, quick method that typically results in quantitative yield using common laboratory reagents. For RNA products ≤100 nt ethanol precipitation should be used (Walker & Lorsch, 2013).
For less experienced users, precipitation can be exchanged for the use of a column-based RNA clean up kit such as Monarch RNA Cleanup Kit (NEB cat. no. T2050L) or a bead-based clean up step such as RNAClean XP (Beckman Coulter cat. no. A63987). These alternative clean up steps may also be preferred when time is limited and overnight precipitation following the capping and tailing steps is inconvenient.
Lithium Chloride Precipitation
Typically, the IVT reaction results in a high concentration of mRNA which should immediately precipitate out of solution upon the addition of cold lithium chloride (if no precipitate is observed, leave it to precipitate overnight before proceeding to the next step). This quick precipitation step allows IVT and capping to be carried out in 1 day if desired, reducing the amount of time required to complete the protocol. As the capping and tailing reactions are carried out in a larger volume the concentration of mRNA is reduced; therefore, the precipitation step greatly benefits from an overnight incubation at −20°C (Barman et al., 2017).
CAUTION : RNA is extremely sensitive to degradation by RNases prior to encapsulation. Before beginning, check reagents and consumables are certified RNase free. Gloves should be worn and changed regularly, and surfaces and equipment should also be wiped down with an RNase decontaminant agent before use.
NOTE : We recommend using commercially available high-grade phenol/chloroform/isoamyl alcohol reagents, as in-house preparation of phenol requires distillation to remove oxidized contaminants. The commercially available reagent will be at the appropriate pH and include stabilizers, which saves time and reduces the handling of phenol, a toxic compound and severe skin irritant.
Basic Protocol 1: SYNTHESIS OF mRNA BY IN VITRO TRANSCRIPTION AND ENZYMATIC CAPPING AND TAILING
This protocol begins with an IVT reaction to enable the production of an mRNA transcript from a linearized DNA template (see Strategic Planning and Fig. 1A). This protocol is based on the kit protocol provided by New England Biolabs (see Internet Resources) and has been further optimized as described here. The mRNA yield (mass recovery) of this reaction can vary; a reduction in yield can be expected when modified NTPs such as m1Ѱ are used. Reactions can be scaled as necessary. After IVT the mRNA is capped enzymatically by combining the vaccinia capping system and cap 2′-O-methyltransferase to result in the installation of a cap1 structure to the 5′ end of the construct. The capped mRNA is then tailed enzymatically using the Escherichia coli poly(A) polymerase resulting in a poly(A) tail of >100 bases (Fig. 1A). For the IVT, capping, and tailing reactions, reagents are combined, mixed thoroughly, and incubated for the appropriate amount of time at 37°C. After each reaction the mRNA is purified away from DNA, salts, free nucleotides, and proteins using lithium chloride precipitation (Fig. 1A). Lithium neutralizes the negative charge of the mRNA phosphate backbone allowing the neutralized molecules to precipitate. Although lithium chloride precipitation of mRNA is an efficient method of purifying mRNA from solutions containing DNA, some DNA can co-precipitate. Therefore, we recommend carrying out a DNase treatment following the IVT step to ensure that no DNA is carried through to the successive reactions. Once purified, the mRNA can be visualized using gel electrophoresis which separates mRNA molecules based on size (exploiting the negatively charged backbone) (Fig. 2). This allows assessment of the mRNA integrity, size, presence of the poly(A) tail and, in some cases, the approximate length of the tail. Here, we describe visualization of the mRNA with both a 1% agarose gel and the Agilent TapeStation automated electrophoresis system which is commonly used to determine sample integrity (amount of intact vs degraded RNA). mRNA should be adjusted to a final concentration of 1 mg/ml in 100 mM sodium acetate at pH 3 to 5 to allow the user to continue immediately to the encapsulation procedure (see Basic Protocol 2).
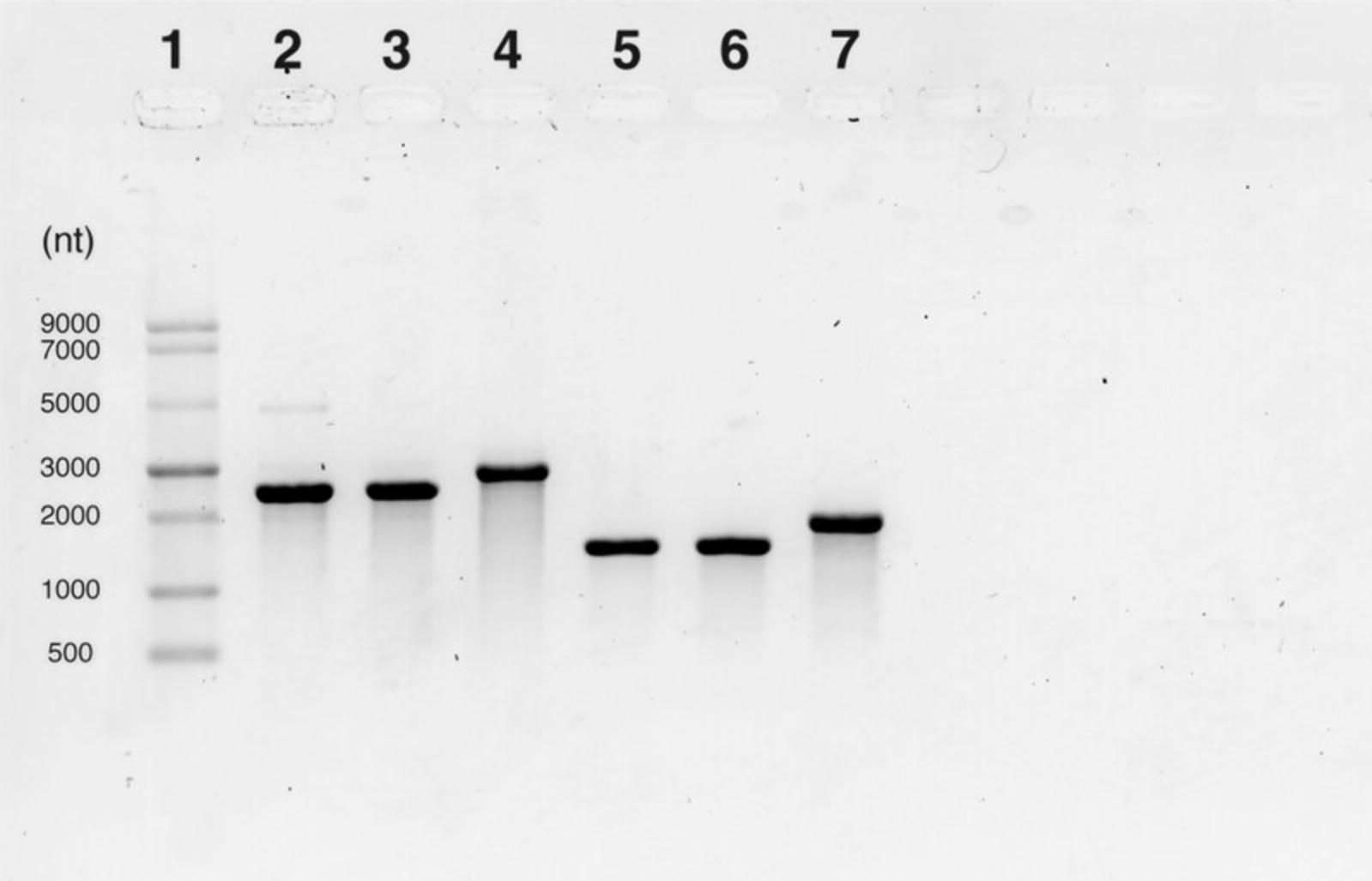
Materials
-
10% bleach solution
-
RNaseZAP (Ambion, cat. no. AM9782), RNase AWAY (Thermo Fisher Scientific, cat. no. 7002PK) or similar RNase-decontaminant solution
-
70% (v/v) ethanol solution (RNase free)
-
RNase- and DNase-free H2O (e.g., Thermo Fisher Scientific, cat. no. AM9937 or Thomas Scientific, cat. no. C788W45)
-
HiScribe kit (NEB, cat. no. E2040S)
- 10× T7 reaction buffer
- T7 polymerase mix
- 100 mM ATP
- 100 mM CTP
- 100 mM GTP
- 100 mM UTP
- FLuc control template (DNA)
-
N1-methylpseudouridine (BOC sciences, cat. no. 1428903-59-6), optional
-
∼500 ng/µl purified, linearized DNA transcription template
-
Dithiothreitol (DTT) (Thermo Fisher Scientific, cat. no. P2325), optional
-
10× DNase I reaction buffer (NEB, cat. no. B0303, or similar)
-
DNase I (RNase free) (NEB, cat. no. M0303)
-
6 M lithium chloride (Sigma, cat. no. 203637-50G)
-
3 M sodium acetate (Sigma, cat. no. S7899-100 ml)
-
70% (v/v) ethanol solution (RNase free), pre-chilled
-
Vaccinia Capping System (NEB, cat. no. M2080S)
- Vaccinia capping enzyme
- 10× capping buffer
- S-adenosylmethionine (SAM)
- 10 mM GTP
-
RNase inhibitor (NEB, cat. no. M0314, or similar), optional
-
Cap 2′-O-methyltransferase (NEB, cat. no. M0366S)
-
E. coli poly (A) polymerase (NEB, cat. no. M0276L)
- 10 mM ATP
- 10× poly(A) polymerase reaction buffer
-
Agarose (Thermo Fisher Scientific, cat. no.16500500)
-
50× TAE buffer (BioRad, cat. no. 1610743)
-
SYBR Safe (Thermo Fisher Scientific, cat. no. S33102)
-
NEB ssRNA ladder (NEB, cat. no. N0362)
-
2× RNA loading dye (NEB, cat. no. B0363S)
-
RNA ScreenTape (Agilent, cat. no. 5067-5576)
-
RNA ScreenTape ladder (Agilent, cat. no. 5067-5578)
-
RNA ScreenTape sample buffer (Agilent, cat. no. 5067-5577)
-
Laminar flow hood (Labconco Purifier BSC Class II, or equivalent) or RNase-free work bench
-
Cold block, to store enzymes while working
-
Vortex mixer (e.g., VELP F202A0280)
-
Mini-centrifuge (e.g., DLAB D1008 or similar)
-
Benchtop centrifuge (e.g., Eppendorf 5810R)
-
Micropipette set and 20-µl, 200-µl, and 1000-µl filter pipette tips (Corning, cat. no. TF-400/200/1000-L-R-S)
-
PCR tubes (Mediray, cat. no. EP0030124359)
-
37°C PCR thermocycler
-
Heat block, water bath, or incubator that can reach 65°C
-
1.7-ml Eppendorf tubes, nuclease-free (Mediray, cat. no. EP0030108418)
-
Nanodrop spectrophotometer
-
Microwave
-
Gel electrophoresis cell, tray, and combs
-
Gel imager
-
TapeStation 4150 (Agilent, or similar automated gel electrophoresis)
-
Optical tube, 8× strip (Agilent cat. no. 401428)
-
Optical cap, 8× strip (Agilent cat. no. 401425)
-
Orbital shaker (e.g., IKA MS3, or similar)
Production of mRNA by IVT
1.Clean the laminar flow hood or lab bench space to remove dust and RNase contamination as follows:
- Wipe down surfaces with 10% bleach, followed by RNase-free water or an RNase-decontaminant solution.
- Wipe pipettes and tip boxes with RNase-decontaminant solution.
- Wear clean gloves at all times that have been sterilized with RNase-decontaminant solution.
2.Remove a single tube of each of the reagents required for IVT listed in Table 1 from the freezer and transfer to the clean laminar flow hood or lab bench. Allow the 10× T7 reaction buffer and NTPs to equilibrate to room temperature.
Reagent | Volume (µl) | Final concentration |
---|---|---|
Nuclease-free water | 6 | |
10× T7 reaction buffer | 2 | 1× |
NTP mix | 8 | 40 mM |
3.Mix, spin in a mini-centrifuge 10 s at maximum speed, room temperature, and use micropipettes to combine NTPs equally into a 100 mM final concentration (i.e., 25 mM of each NTP).
4.Set up the reaction in a 200-µl PCR tube, the final volume will be 20 µl. Add the required amounts of each reagent in the order listed in Table 1 to reach a 16 µl total. Mix the reaction thoroughly by vortexing and spin in a mini-centrifuge 10 s at maximum speed, room temperature, to get the liquid to the bottom of the tube.
5.To reach 20 µl final volume add the DNA template and RNA polymerase as listed in Table 2 to the tube. Mix them gently with a pipette and spin in a mini-centrifuge 10 s at maximum speed, room temperature, to get all liquid to the bottom.
Reagent | Volume (µl) | Final concentration |
---|---|---|
500 ng/µl DNA template | 2 | 50 ng/µl |
RNA polymerase mix | 2 | 2 µl/20 µl |
6.Place tubes in a thermocycler or heat block and incubate for 2 hr once 37°C is reached.
DNase I treatment
7.Thaw and mix the tube of the DNase I buffer supplied with the DNase I enzyme (NEB). After incubation is complete (step 6) add the reagents to the reaction in the order listed in Table 3.Mix the reaction gently with a pipette and incubate the reaction for 15 min at 37°C in the heat block or thermocycler.
Reagent | Volume (µl) | Final Concentration |
---|---|---|
Nuclease-free water | 68 | |
10× DNase I buffer | 10 | 1× |
DNase I | 2 | 4 U/µg DNA |
Precipitation of mRNA
8.To precipitate the mRNA the reaction should be transferred to a tube able to fit the centrifuge that will be used for precipitation e.g., a 1.7 ml Eppendorf tube. Half the sample volume of 6 M lithium chloride should be added to the DNase I-treated IVT reaction. In this case add 50 µl of 6 M lithium chloride.
9.Mix the reaction and lithium chloride mixture by gently inverting the tube and spin in a mini-centrifuge 10 s at maximum speed, room temperature, before storing at −20°C for at least 30 min.
10.Cool the centrifuge to 4°C. Spin the sample for at least 30 min at >14,000 × g , 4°C.
11.Discard the lithium chloride and retain the pellet. Wash the sample by adding 1 ml pre-chilled 70% ethanol. Gently flick the tube until the pellet comes loose and can be rinsed by the ethanol.
12.Spin the pellet down 5 min, >14,000 × g , 4°C.
13.Discard the ethanol and retain the pellet. Remove as much ethanol as possible with a pipette and allow the pellet to dry.
14.Resuspend the pellet in 150 µl nuclease-free water by pipetting gently up and down. The expected yield is 150 µg of mRNA.
15.Take an aliquot of at least 5 µl of mRNA for quality control. Measure and record the concentration of the mRNA by measuring the absorbance at 260 nm using a nanodrop spectrophotometer.
Enzymatic capping of mRNA
16.Heat one heat block or thermocycler, water bath or incubator to 65°C and another to 37°C.
17.Remove a single tube of 10× capping buffer, GTP and SAM (see Table 4) from the freezer and allow to equilibrate to room temperature. Mix and spin in a mini-centrifuge 10 s at maximum speed, room temperature, to ensure the liquid is at the bottom before opening.
Reagent | Volume (µl) | Final concentration |
---|---|---|
Uncapped RNA | 150 | 1 µg/µl |
Nuclease-free water | 65.5 | |
RNase inhibitor | 7.5 | 1 U/µl |
10× capping buffer | 30 | 1× |
10 mM GTP | 15 | 0.5 mM |
32 mM SAM | 2 | 0.2 mM |
18.Denature the precipitated mRNA from the IVT reaction (from Basic Protocol 1, step 14) at 65°C for 5 min in the preheated device selected above (step 16).
19.During the denaturation step of the uncapped mRNA, add all other reagents in Table 4 to an Eppendorf tube in the order listed, mix reagents thoroughly and spin in a mini-centrifuge 10 s at maximum speed, room temperature, to ensure the mixture is in the bottom of the tube.
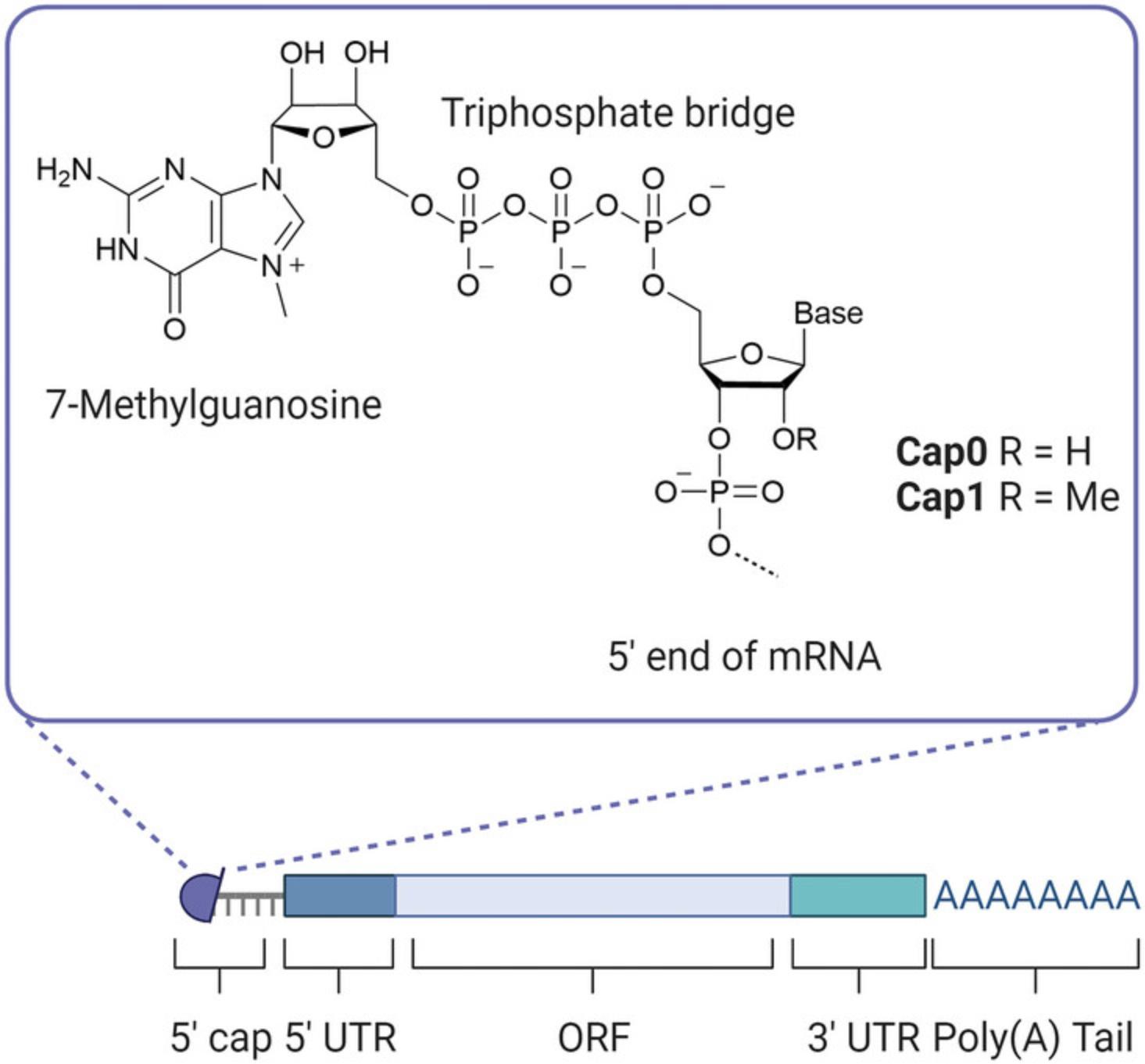
20.Add the denatured and cooled mRNA to this reaction.
21.Add the enzymes listed in Table 5 to the reaction and mix by gently pipetting. Spin in a mini-centrifuge 10 s at maximum speed, room temperature, the tube and incubate at 37°C for 1 hr.
Reagent | Volume (µl) | Final concentration |
---|---|---|
10 U/µl vaccinia capping enzyme | 15 | 1 U/µg mRNA |
50 U/µl cap-2′-O-methyltransferase | 15 | 5 U/µg mRNA |
22.After incubation, proceed with precipitation of the mRNA and resuspension in nuclease-free water as described above in steps 8 to 14.
23.Take an aliquot of at least 5 µl of the capped mRNA for quality control. Measure and record the concentration of the mRNA using a nanodrop spectrophotometer as described in step 15.
Enzymatic tailing of mRNA
24.Heat the heat block, thermocycler, or water bath or incubator to 37°C.
25.To set up the enzymatic tailing reaction add the reagents in Table 6 in the order listed. The ‘capped mRNA’ component is the mRNA resuspended in water during ‘Enzymatic capping of mRNA’ (Basic Protocol 1, step 22). Mix gently, spin in a mini-centrifuge 10 s at maximum speed, room temperature and incubate at 37°C for 1 hr.
Reagent | Volume (µl) | Final concentration |
---|---|---|
Capped mRNA | 150 | 1 µg/µl |
Nuclease-free water | 67.5 | |
RNase inhibitor | 7.5 | 1 U/µl |
10× E. coli poly(A) polymerase reaction buffer | 30 | 1× |
10 mM ATP | 30 | 1 mM |
5 U/µl poly(A) polymerase | 15 | 0.5 U/µg |
26.After incubation, proceed directly to precipitate the capped and tailed mRNA as described in steps 8 to 13.This will both stop the reaction and separate the mRNA product from reagents. Resuspend the washed pellet in slightly less nuclease-free water than above aiming for a concentration above 1 mg/ml.
27.Take an aliquot of at least 5 µl of the capped and tailed mRNA for quality control. Measure and record the concentration of the mRNA using a nanodrop spectrophotometer as described in step 15.
28.Adjust the mRNA to a final concentration of 1 mg/ml in 100 mM sodium acetate buffer, pH 3 to 5, using equations 1 to 3 to calculate the volume of nuclease-free water (VWater) and 3 M sodium acetate (VNaOAc) to be added.
29.Aliquot the mRNA into 100-µl single-use aliquots and freeze at −80°C for long-term storage until required for encapsulation as described in Basic Protocol 2.
Assessment of poly(A)tail presence by gel electrophoresis
30.Measure agarose and add to appropriate volume of 1× TAE buffer to make a 1 % gel (e.g., 1 g agarose in 100 ml TAE buffer). Mix agarose and buffer and melt in microwave. Once melted add 1 µl SYBR Safe (10,000× stock) for every 10 ml. Mix well and pour into gel tray with comb to set.
31.Prepare the ssRNA ladder by adding 3 µl ladder to 9 µl 2× RNA loading dye. Prepare the samples by adding 2× RNA loading dye and the mRNA samples 1:1.Denature ladder and sample for 5 min at 72°C in a pre-heated heat block, thermocycler or a PCR thermocycler programmed to reach 72°C.
32.Once set, submerge gel in running cell filled with 1× TAE buffer. Remove comb and load ssRNA ladder followed by samples. A control sample of mRNA from the IVT reaction (e.g., not yet enzymatically tailed) should be included as a reference to screen for a size increase. Run gel at 120 V for 45 min.
33.Image gel and assess the presence of the poly(A) tail after the enzymatic tailing reaction through an increase in size on the gel (see Understanding Results, Fig. 2).
Assessment of mRNA integrity with automated gel electrophoresis
34.Dilute samples to ∼100 ng/µl in nuclease-free water for best results.
35.Remove a 1 µl aliquot of RNA ScreenTape ladder from the −80°C freezer and place on ice.
36.Using the optical tubes 8× strip, pipette 5 µl sample buffer into each tube that will contain a sample plus one extra tube for the ladder.
37.Pipette 1 µl of each sample into a tube containing sample buffer. Cover the tubes using the optical caps, mix the samples by shaking the tubes in the orbital shaker at 2000 rpm for ∼1 min, and spin in a mini-centrifuge 10 s at maximum speed, room temperature.
38.Heat the samples in a PCR thermocycler for 3 min at 72°C, followed by cooling the tubes immediately on ice for 2 min.
39.Centrifuge the samples in a mini-centrifuge 10 s at maximum speed, room temperature, so all the liquid is at the bottom before placing the tubes in the TapeStation 4150 machine and removing the caps.
40.Place an RNA ScreenTape in the TapeStation and label samples using the TapeStation controller software and click start. Follow all prompts to start the run.
41.View the results in the TapeStation analysis software and define the sample peak by deleting all peaks (18S and 28S) and then reassigning a sample peak by right clicking > add peak (Fig. 4).
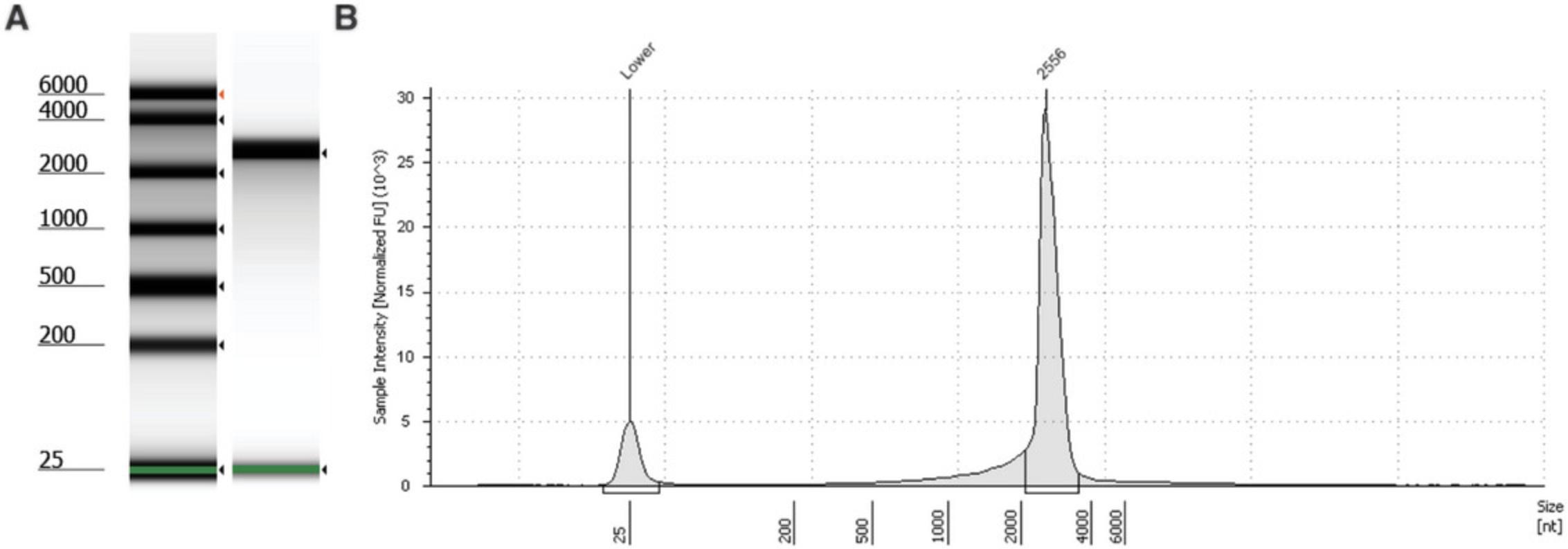
42.Press the region button to define the peak region of the mRNA by adding an upper and lower size value. The region table will then give the percentage of mRNA calculated to be within the region you have defined (see Understanding Results, Fig. 5, Table 7).
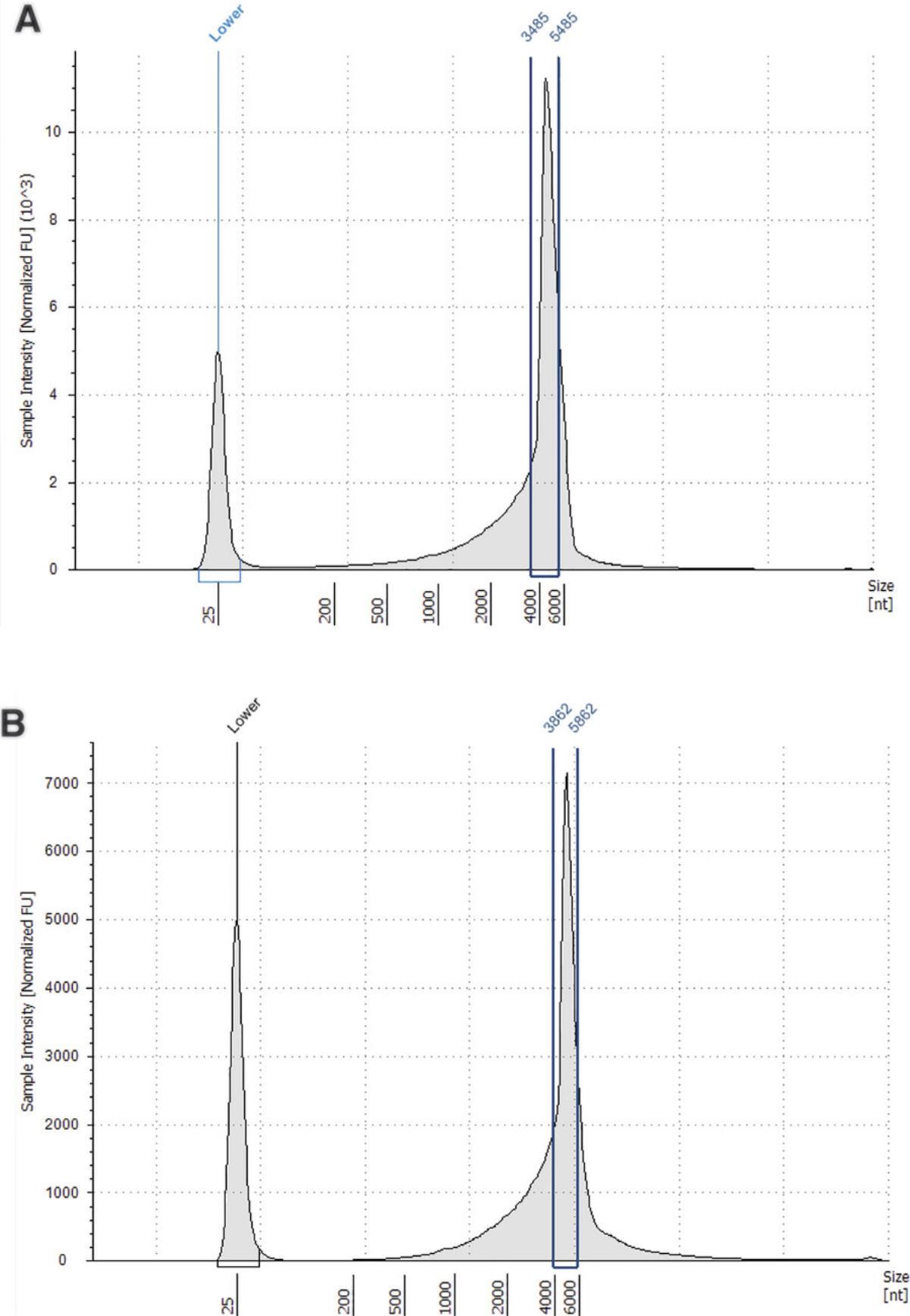
Sample description | Lower limit (nt) | Upper limit (nt) | Peak size (nt) | % total mRNA within defined region |
---|---|---|---|---|
mRNA | 3485 | 5485 | 3485 | 53.67 |
mRNA post-encapsulation | 3862 | 5862 | 4862 | 47.54 |
Basic Protocol 2: ENCAPSULATION OF mRNA IN IONIZABLE LIPID NANOPARTICLES
This protocol describes the encapsulation of mRNA into ionizable lipid nanoparticles (iLNPs) (Fig. 6). The protocol uses a microfluidic mixer to control nanoprecipitation of the lipids and mRNA in an acidic buffer, followed by buffer exchange to DPBS resulting in a rearrangement and stabilization of the particles (Fig. 1B). Neutral nanoparticles with >80% encapsulation efficiency that are 80 to 150 nm in diameter can be expected (see Understanding Results section). It is expected that following Basic Protocol 2 will produce 100 µg of encapsulated mRNA in iLNPs using the Dlin-MC3-DMA (MC3) ionizable lipid (Jayaraman et al., 2012). Generally, this should be sufficient for routine characterization and subsequent murine experiments (for example, two 5 µg doses for five mice). Users can easily scale up the protocol to encapsulate up to 1 mg of mRNA.
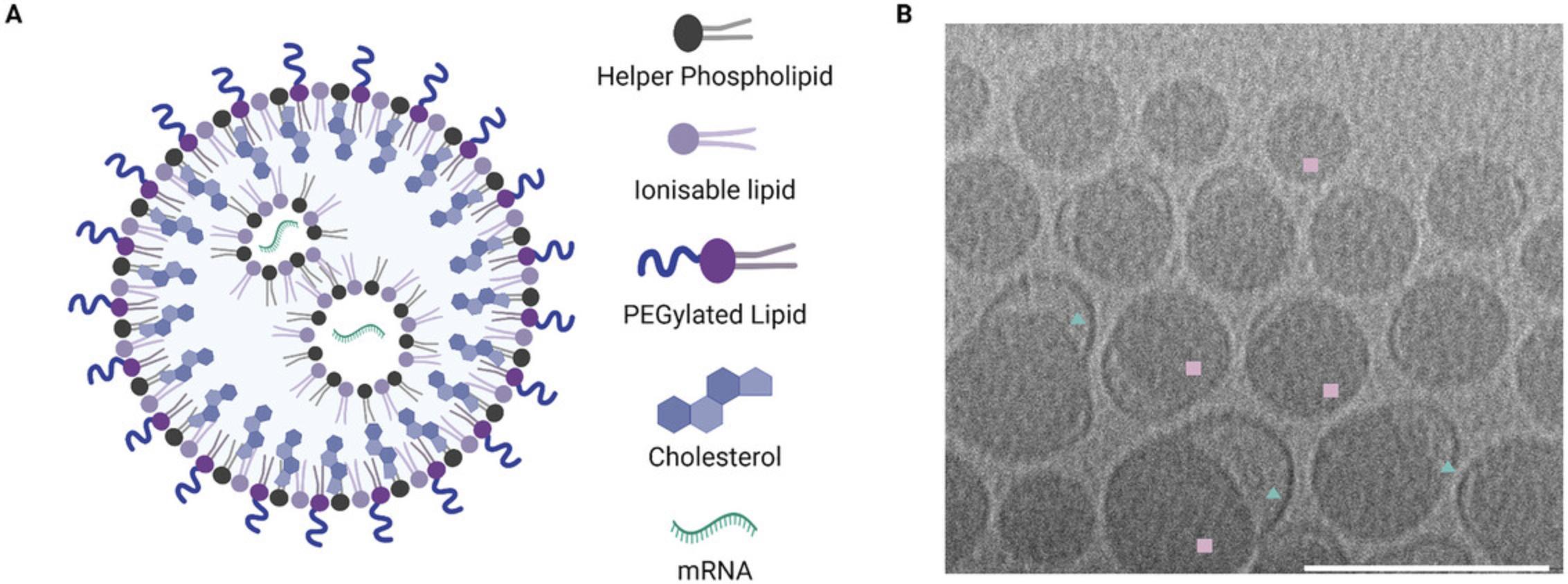
The protocol can be adapted to produce particles with different cargo (for example, siRNA or saRNA) or different lipids; however, optimization may be required. A spreadsheet is provided in the supplementary information to alter the recipe as required for different outputs. The formulation described here has a standard lipid molar ratio of 50 ionizable/10 DSPC/38.5 cholesterol/1.5 DMG-PEG2000 and an N/P ratio of 4 (N being the ionizable amine and P the phosphates in the mRNA backbone). For mRNA it is generally recommended that an N/P of 3 to 8 is used. The PEGylated lipid component should consist of 1% to 2% of the formulation (Belliveau et al., 2012; Kulkarni et al., 2018; Ly et al., 2022). The helper phospholipid should be 8% to 12% of the formulation, with DSPC (1,2-distearoyl-sn -glycero-3-phosphocholine) and DOPE (dioleoylphosphatidylethanolamine) being the most commonly used. DSPC is used in all of the currently approved iLNP products, likely because it is known to improve stability of other lipid nanoparticles such as liposomes. DOPE, which forms a cone structure as opposed to the cylindrical structure of DSPC, has been reported to improve the packing properties of iLNPs resulting in a more uniformly spherical particle. The conical shape of DOPE may lead to increased intracellular delivery of mRNA but the particles may be less stable (Albertson et al., 2022; Cheng & Lee, 2016; Kauffman et al., 2015; Leung et al., 2015; Ly et al., 2022). The ionizable lipid can be reduced to 40% and still produce the expected type of iLNP (see Understanding Results section for examples of different iLNPs generated using this protocol), and the cholesterol should be adjusted to make up the remaining percentage of lipids. The structure of the ionizable lipid is likely to have the largest effect on the properties of the lipid nanoparticle and should be selected carefully. Some ionizable lipids and lipidoids contain more than one ionizable head group and this should be factored into the N/P calculation.
This protocol makes use of a NanoAssemblr Ignite microfluidic mixer but may be adapted to suit other microfluidic mixers employing a syringe pump and a T-mixer or staggered herringbone mixer. Centrifugal spin filters are used here to exchange buffers and concentrate the iLNP solution as they provide a convenient and high recovery solution when working at this small scale. Alternatively, dialysis or tangential flow filtration may be used when reduced recovery is not an issue or larger scales are used, respectively.
Basic Protocol 2 encapsulates the mRNA in the iLNP during the microfluidics nanoprecipitation step; however, this results in a significant amount of wasted mRNA due to dead volumes and instrument waste. Where the mRNA is expensive or in limited supply, the use of preformed vesicles outlined in Alternate Protocol may be preferable.
NOTE : It is important to work in an environment and with reagents that are nuclease-free to prevent degradation of the mRNA, as described in Basic Protocol 1.If the particles are to be used in biological experiments (such as animals or cells) it is important to work in a sterile environment and use sterile reagents and consumables.
Additional Materials (also see Basic Protocol 1)
-
Dlin-MC3-DMA (MC3) (NanoSoft Polymers, cat. no. 25325)
-
Cholesterol (Sigma-Aldrich, cat. no. C3045)
-
DSPC (1,2-distearoyl-sn -glycero-3-phosphocholine) (Avanti, cat. no. 850365P)
-
DMG-PEG2000 (1,2-dimyristoyl-rac –glycero-3-methoxypolyethylene glycol-2000) (NanoSoft Polymers, cat. no. 25404)
-
100% ethanol
-
Dulbecco's phosphate-buffered saline (DPBS), sterile (e.g., Thermo Fisher Scientific, cat. no. 14190144)
-
mRNA in aqueous solution, 1 mg/ml in sodium acetate buffer pH 5 stored at −80°C
-
100 mM sodium acetate buffer, pH 5
-
Glass vials (e.g., Agilent, cat. no. 5182-0866 or Merck, cat. no. M1901)
-
Balance (e.g., Mettler Toledo, cat. no. XPR205S)
-
Amber glass vial (e.g., Agilent, cat. no. 5182-0558)
-
Amicon Ultra-15 centrifugal concentrators, 30,000 MWCO (Merck, cat. no. UFC903024)
-
Centrifuge tube (e.g., Falcon 15-ml conical centrifuge tube, cat. no. 352096)
-
NanoAssemblr Ignite (Precision Nanosystems)
-
1- and 3-ml plastic syringes (BD, 309659 and 309658)
-
NxGen cartridge (Precision Nanosystems, cat. no. NIN0061)
-
Blunt needle (e.g., TERUMO drawing up needle 18G × 1.5 in.)
-
2-ml cryovial (Mediray, cat. no. GR126263) or 1.7-ml Eppendorf tubes, nuclease-free (Mediray, cat. no. EP0030108418)
-
200-µl long pipette tips (Watson BioLabs, cat. no. 1252-801CS)
1.To prepare lipid stock solutions (MC3, Cholesterol, DSPC, DMG-PEG2000), allow the lipids to equilibrate to room temperature before opening the bottle to prevent moisture getting into the bottle. Weigh at least 3 mg of each lipid individually into clean glass 4 ml vials on a 4 decimal place balance.
2.Add the appropriate volume of ethanol to the lipids with an autopipette such that the final concentration is 10 mg/ml. The lipid stock solutions can be stored at −20°C for later use.
3.Prepare the lipid mixture solution as described in Table 8 in a clean glass 2 ml vial. Vortex briefly to mix.
Lipids | Volume (µl) |
---|---|
MC3 | 274 |
Cholesterol | 127 |
DSPC | 67 |
DMG-PEG2000 | 32 |
Entry | Lipids Mixture | Encapsulation method | N/Pa | Size (nm) | PDIb | Zeta (mV) | EE (%) |
---|---|---|---|---|---|---|---|
1 | 50 MC3/10 DSPC/38.5 cholesterol/1.5 DMG-PEG2000 | Basic Protocol 2 | 4 | 91 | 0.069 | 4.9 | 91 |
2 | 50 MC3/10 DSPC/38.5 cholesterol/1.5 DMG-PEG2000 | Alternate Protocol | 4 | 117 | 0.13 | 6.5 | 90 |
3 | 50 ALC-0315/10 DSPC/38.5 cholesterol/1.5 DMG-PEG2000 | Basic Protocol 2 | 4 | 111 | 0.10 | -4.6 | 85 |
4 | 50 ALC-0315/10 DSPC/38.5 cholesterol/1.5 DMG-PEG2000 | Basic Protocol 2 | 6 | 104 | 0.10 | 3.7 | 84 |
5 | 46.3 ALC-0315/9.4 DSPC/42.7 cholesterol/1.6 DMG-PEG2000 | Basic Protocol 2 | 6 | 88 | 0.066 | -0.4 | 89 |
6 | 46.3 ALC-0315/9.4 DSPC/42.7 cholesterol/1.6 ALC-0159 | Basic Protocol 2 | 6 | 85 | 0.070 | 2.8 | 85 |
- a
N/P ratio where N is the ionizable amine and P is the phosphates in the RNA backbone.
- b
Polydispersity Index.
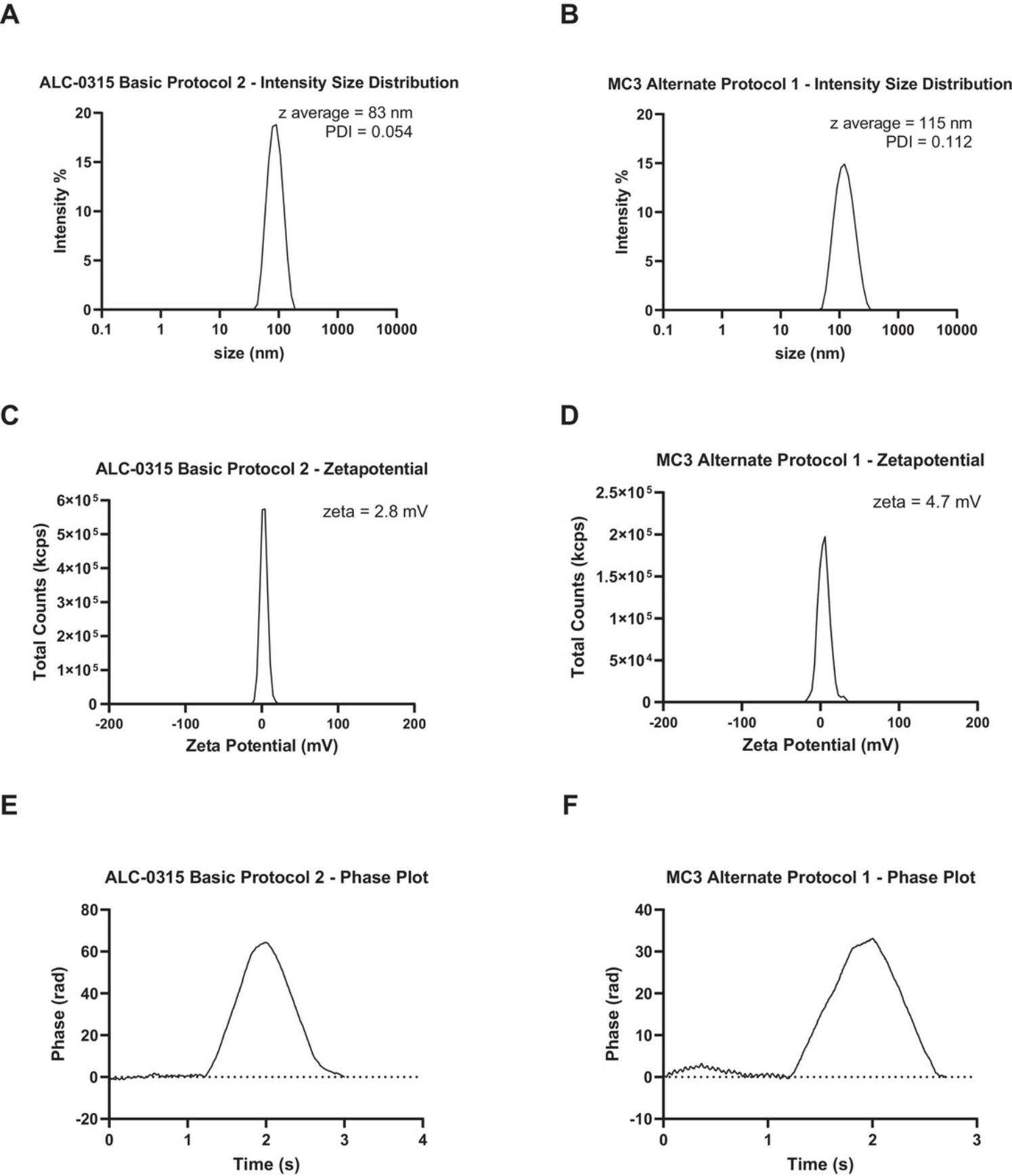
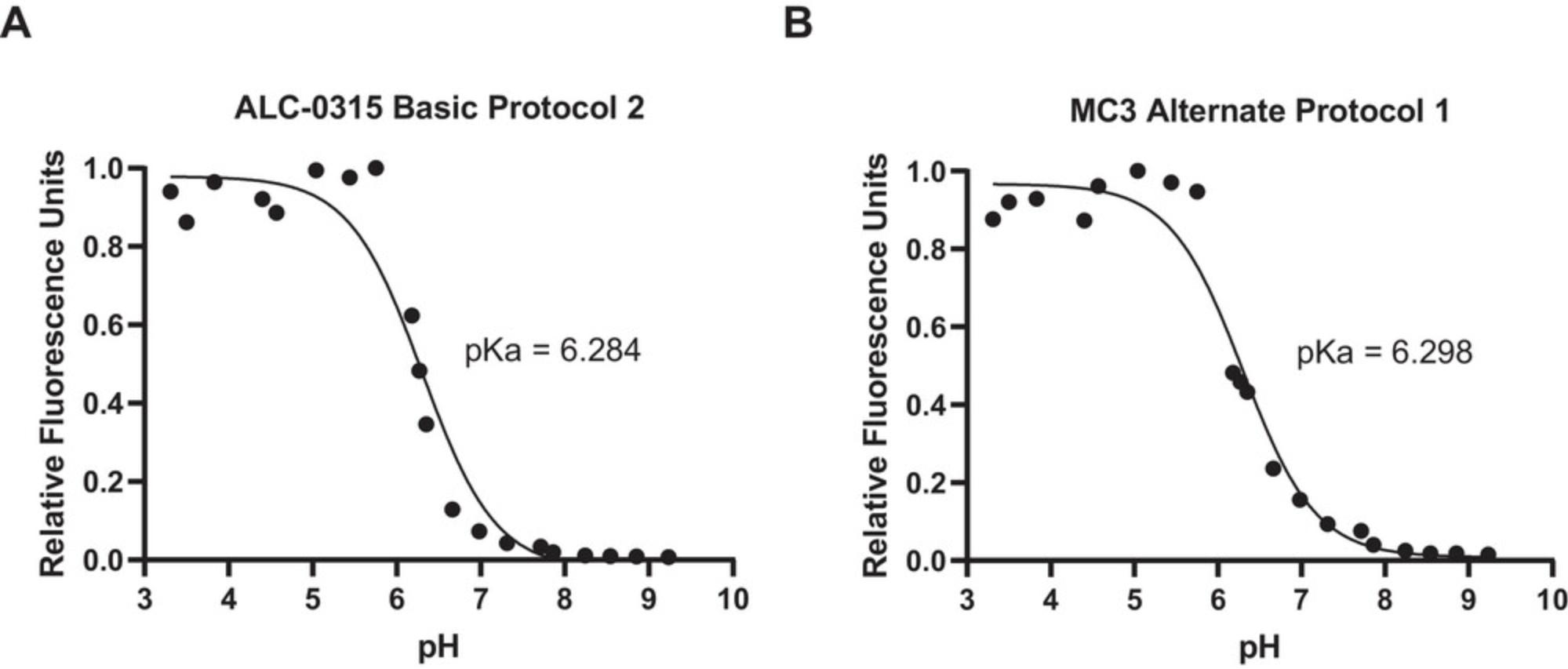
4.Wipe down the laminar flow hood and pipettes with 70% ethanol and RNase-decontaminant solution. Spray gloves and anything entering the cabinet (e.g., vials of lipids) with RNase-decontaminant solution before entering.
5.Prepare two Amicon Ultra-15 centrifugal concentrators. Add 10 ml DPBS and centrifuge 20 min at 4000 × g , room temperature, until ∼5 ml of the DPBS has passed through to rinse the filter. Discard filtrate and retentate and immediately add 13 ml DPBS to each tube.
6.Thaw 300 µg of the mRNA aliquot(s) in the fridge (4°C) or on ice. Once thawed and just before use, allow to warm to room temperature.
7.Prepare 100 mM pH 5 sodium acetate buffer by diluting 1 ml of 3 M stock into 30 ml using RNase-free, sterile water. Vortex briefly to mix. Measure the pH of the buffer to ensure it is still ∼5.
8.Adjust the concentration of the mRNA solution to 241.5 µg/ml by diluting the aliquot with room temperature 100 mM sodium acetate buffer and combine aliquots if necessary.
9.Enter the formulation parameters into the NanoAssemblr Ignite according to Table 10.
Parameter | Value |
---|---|
Total volume | 1.6 ml |
TFRa | 12 ml/min |
FRRb | 3:1 |
Start waste | 0.25 ml |
End waste | 0.05 ml |
C syringe volume | 3 ml |
R syringe volume | 1 ml |
- a
Total Flow Rate.
- b
Flow Rate Ratio.
10.Insert a new NxGen cartridge into the instrument and check that it has been recognized. Insert the waste and 15-ml sample centrifuge tubes.
11.Draw the aqueous mRNA solution into the 3-ml syringe using a blunt needle. Draw in some extra air and invert the syringe so the needle is pointing up and tap the syringe to dislodge any air bubbles. Remove the needle and plunge the syringe to remove any remaining air. Place the syringe firmly in the C position of the cartridge.
12.Draw the lipids solution into the 1-ml syringe using a blunt needle. Remove the needle and plunge the syringe so that there is no air remaining. Place the syringe firmly in the R position of the cartridge.
13.Ensure the instrument is in the ready state and start the formulation.
14.When the formulation is complete, immediately transfer the lipid nanoparticle suspension to the DPBS in the pre-rinsed spin filters.
15.Concentrate the iLNP solution in DPBS to the initial volume of iLNP added by centrifugation for 30 min at ≤4000 × g , 4°C (if the centrifuge is equipped with cooling). Repeat the centrifugation step if needed (see annotation below).
16.Transfer the concentrated iLNP solution to an appropriate sterile, RNase-free vessel (cryovial, Eppendorf tube, centrifuge tube) and note the final volume.
17.Characterize the iLNP as desired according to Basic Protocol 3.
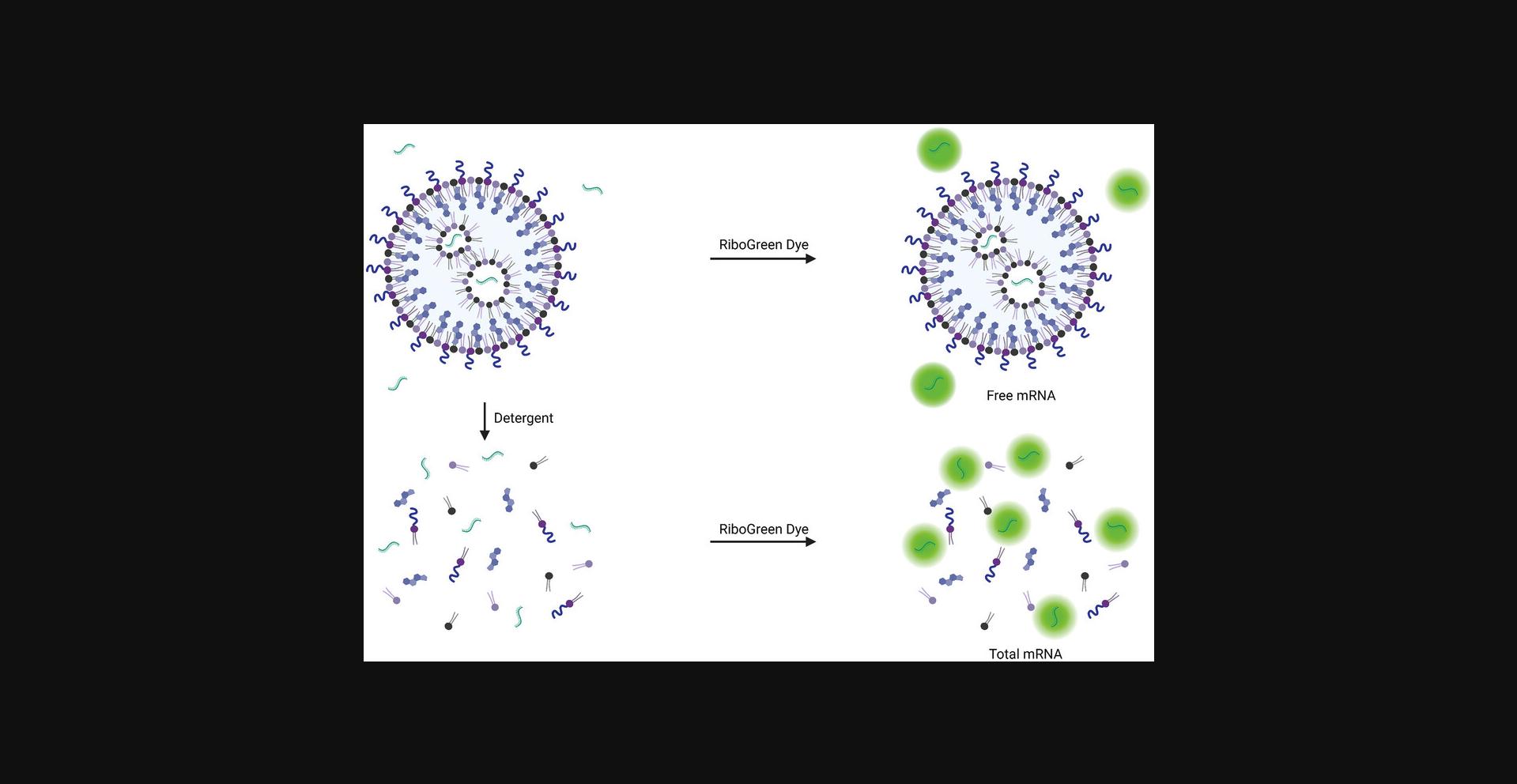
18.Store the iLNP solution at 4°C (do not freeze). It is preferable to use the product immediately, but it can be stored for up to 1 month in the fridge.
Alternate Protocol: SMALL-SCALE ENCAPSULATION OF mRNA USING PREFORMED VESICLES
This alternate protocol is conceptually similar to Basic Protocol 2 and will follow many of the same steps: generating a lipid nanoparticle using microfluidics, complexing mRNA to a cationic iLNP at low pH, fusion of the lipid nanoparticles at neutral pH, concentration, and analysis. However, the mRNA is complexed to the iLNP after it is formulated rather than when the iLNP is formed using microfluidics (Fig. 1B). This means it can be carried out on a smaller scale and with less waste.
The Alternate Protocol may be required when mRNA is made in small quantities due to expensive reagents or special modifications. In this case 300 µg of mRNA as described in Basic Protocol 2 may be too much to produce or the amount of waste (∼80 µg) is too significant. A preformed vesicle (PFV) approach involves forming the lipid nanoparticle by microfluidics without the mRNA present during the nanoprecipitation and adding this preformed vesicle to the mRNA at low pH so the electrostatic interactions can still occur (Fig. 1B). The resulting particles are then neutralized at pH 7.4 and processed as normal. This process results in minimal waste compared to encapsulating during the microfluidics process; however, it can lead to a higher PDI and lower encapsulation efficiency (see Understanding Results). For payload optimization or screening, this may not be a hindrance.
As in Basic Protocol 2, the steps described are expected to return 100 µg mRNA encapsulated in iLNPs. In contrast to Basic Protocol 2, there is no minimum scale, and it is much easier to scale down. If required, 4-ml instead of 15-ml spin filters may be used if more appropriate to the scale.
Materials
- See Basic Protocol 2
1.Follow steps 1 to 5 of Basic Protocol 2 (prepare lipids and spin filters).
2.Thaw at least 150 µg of the mRNA aliquot(s) in the fridge (4°C) or on ice. Once thawed and just before use, allow to warm to room temperature.
3.Enter the formulation parameters into the NanoAssemblr Ignite (Table 11).
Parameter | Value |
---|---|
Total volume | 1.5 ml |
TFRa | 12 ml/min |
FRRb | 3:1 |
Start waste | 0.25 ml |
End waste | 0.05 ml |
C syringe volume | 3 ml |
R syringe volume | 1 ml |
- a
Total Flow Rate.
- b
Flow Rate Ratio.
4.Insert a new NxGen cartridge into the instrument and check that it has been recognized. Insert the waste and 15-ml sample centrifuge tubes.
5.Draw a little more than the required volume of 100 mM acetate buffer into the 3-ml syringe using a blunt needle. Draw in some extra air and invert the syringe so the needle is pointing up and tap the syringe to dislodge any air bubbles. Remove the needle and plunge the syringe to remove any remaining air. Place the syringe firmly in the C position of the cartridge.
6.Draw the lipids solution into the 1-ml syringe using a blunt needle. Remove the needle and plunge the syringe so that there is no air remaining. Place the syringe firmly in the R position of the cartridge.
7.Ensure the instrument is in the ready state and start the formulation.
8.When the formulation of the preformed vesicles is complete, immediately add the appropriate volume of preformed vesicle to the mRNA solution and pipette up and down to mix thoroughly.
9.Immediately transfer the lipid nanoparticle suspension to the DPBS in the pre-rinsed spin filters from Basic Protocol 2, step 5.
10.Follow steps 15-18 of Basic Protocol 2 to complete the formulation.
Basic Protocol 3: CHARACTERIZATION AND QUALITY CONTROL OF mRNA LIPID NANOPARTICLES
Basic Protocol 3 describes the routine analysis and quality control of iLNPs including the mRNA concentration and encapsulation efficiency (RiboGreen), particle size, and zeta potential (DLS). The protocol also describes extraction of mRNA from the nanoparticle to assess the integrity of the mRNA. An assay to determine the apparent pKa of the particles is also described in Basic Protocol 3 but is not required for routine analysis. In depth analysis of the morphology and structure of iLNPs using techniques such as cryo-TEM is not described here. Detailed studies on the structure of iLNPs have been described by Brader et al. (2021), Kulkarni et al. (2018) and Li et al. (2022). Examples of results obtained and how to interpret them using the methods described in Basic Protocol 3 are in the Understanding Results section.
The RiboGreen experiment should typically be carried out first to determine the encapsulation efficiency and concentration of encapsulated mRNA. The concentration should be adjusted to 100 µg/ml according to Basic Protocol 2, step 17.The remaining analyses (DLS, apparent pKa, mRNA extraction) should be carried out with the diluted solution.
The RiboGreen assay uses a dye that is non-fluorescent when free in solution but becomes fluorescent in the presence of RNA. The detergent Triton X-100 is added to the iLNP sample to disrupt the particle so that the total mRNA present in the well can be detected (Fig. 9). The difference between the total mRNA sample (with Triton X-100) and the free, non-encapsulated mRNA is used to determine the concentration of encapsulated mRNA and the encapsulation efficiency. The encapsulation efficiency should typically be greater than 80%.
The particle size and polydispersity index is measured using dynamic light scattering (DLS). DLS measures the fluctuations of scattered light by particles as they move in solution according to Brownian motion and uses algorithms to determine hydrodynamic diameter of the particles and the polydispersity index (PDI). The DLS instrument can also be used to measure the zeta potential of particles. Zeta potential is deduced from the electrophoretic mobility of charged particles under an applied electric field and is therefore a measure of the surface charge of the particles. iLNPs should typically be a uniform distribution of particles (i.e., one peak) with a diameter of 50 to 200 nm and a polydispersity index below 0.2.As iLNPs were designed to have a neutral surface charge, the zeta potential of particles generated using Basic Protocol 2 should be -10 to +10 mV. The size and charge of iLNPs have an effect on the activity and stability of the particles, therefore it is important to characterize the particles and adjust the formulation in Basic Protocol 2 as required (Hassett et al., 2021; Nakamura et al., 2020).
The apparent pKa of particles is determined by measuring the fluorescence of 6-(p -toluidino)-2-napthalenesulfonic acid sodium salt (TNS) in buffers with pH 3 to 9.TNS is a negatively charged dye which becomes fluorescent as it partitions into the lipid membrane of the iLNP, attracted by the positive charge of the particle at low pH (Fig. 10). The pH-dependent behavior of the ionizable lipid generates a pH titration curve for the iLNP used to describe the apparent pKa of the particle. This assay can be used to evaluate the properties of novel ionizable lipids. The optimal apparent pKa for iLNPs is 6 to 7 as this allows the particles to have a neutral charge in neutral pH, which reduces toxicity and improves stability. A pKa in this range also means that under the reduced pH conditions in the endosome the ionizable lipid becomes protonated, facilitating the interaction with negatively charged endosomal phospholipids and eventual endosomal escape of the RNA cargo (Carrasco et al., 2021; Patel et al., 2021).
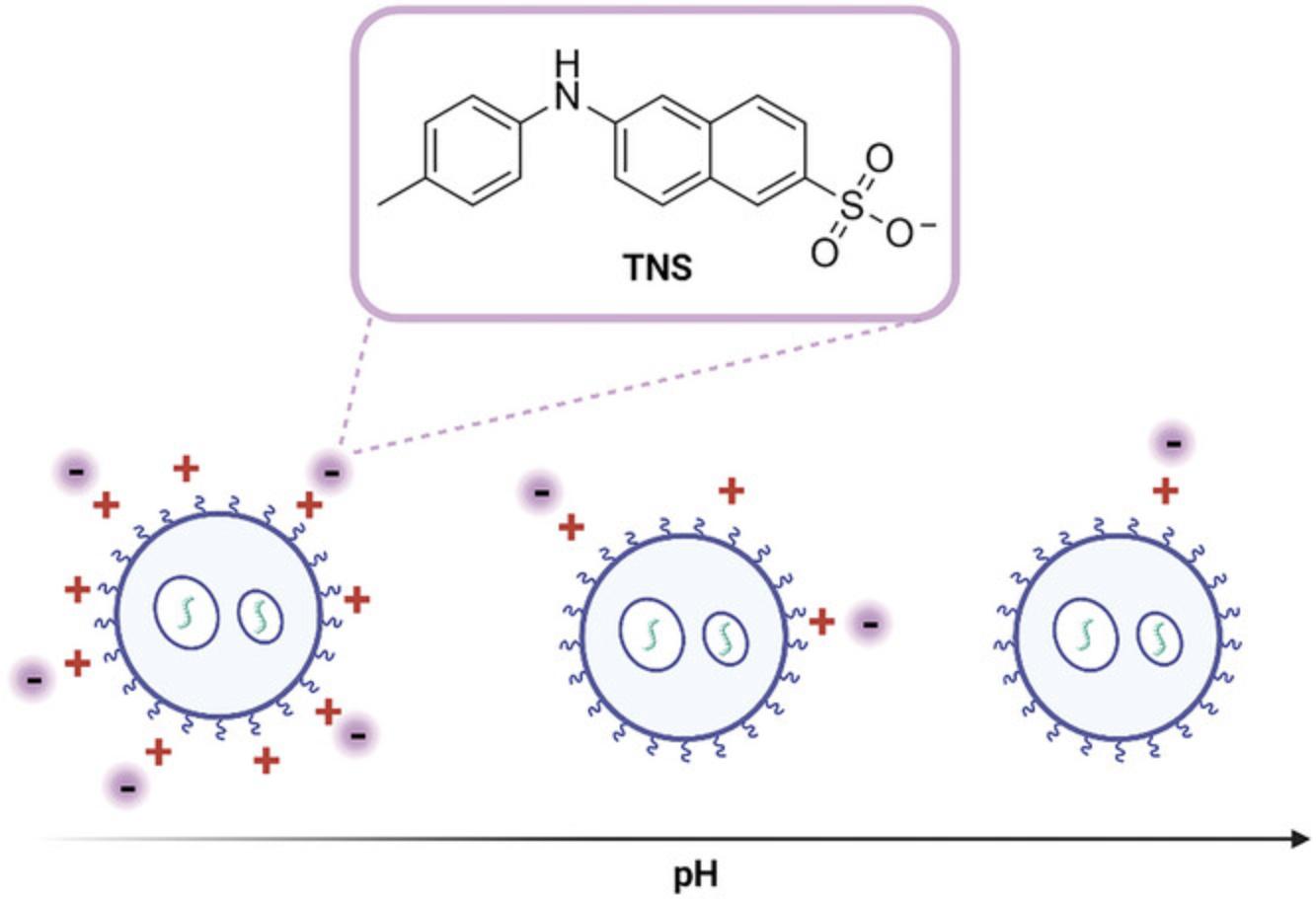
Extraction of the mRNA from the iLNP is achieved using detergent and/or ethanol to break open the particle followed by adsorption and subsequent release of the mRNA from a solid phase. This provides an alternative to the phenol/chloroform/isoamyl alcohol extraction with safer reagents and a simple protocol. This analysis may not be required routinely if the nanoparticles are being used within a few days; however, it may be useful if the iLNP has been stored for a few weeks. It may also be adapted for nuclease-protection assays [not described here, see Geall et al. (2012)].
Additional Materials (also see Basic Protocols 1 and 2)
- QuantIT RiboGreen RNA assay kit (Thermo Fisher Scientific, cat. no. R11490)
- Triton X-100 (Sigma-Aldrich, cat. no. T8787)
- Zymo Quick-RNA Miniprep kit (Zymo Research, cat. no. R1054)
- Phosphate-citrate-borate buffer with NaCl (see recipe)
- 1 M NaOH (Current Protocols, 2006)
- 1 M NaCl (Current Protocols, 2006)
- 6-(p -toluidino)-2-napthalenesulfonic acid sodium salt (TNS) (Sigma-Aldrich, cat. no. T9792)
- Dimethyl sulfoxide (DMSO) (Sigma-Aldrich, cat. no. D8418)
- Pipette basin (e.g., Fisherbrand, cat. no. 13-681-509)
- Multichannel pipette (e.g., Eppendorf Research plus, cat. no. 3125000044)
- 96-well plate for fluorescence (e.g., Corning, cat. no. CORN3904 or OptiPlate, cat. no. 6005270)
- Incubator (e.g., Cole-Palmer, cat. no. EW-51705)
- Microplate reader (e.g., Molecular Devices SpectraMax M3 Multi-Mode Microplate Reader)
- DLS instrument (e.g., Malvern Panalytical Zetasizer Pro)
- DLS cuvette (e.g., Malvern Panalytical, cat. no. ZEN0040)
- Folded capillary zeta cell (e.g., Malvern Panalytical, cat. no. DTS1070)
- 3-ml disposable syringe (e.g., B. Braun inject Luer Lock Solo)
- 60-ml Nalgene bottle (Sigma-Aldrich, cat. no. B9657)
- Benchtop pH meter (e.g., Mettler Toledo Seven Direct SD20)
RiboGreen assay
1.Remove QuantIT RiboGreen RNA reagent kit from the fridge and warm to room temperature.
2.Prepare 1× TE buffer and 1× TE buffer with 2% (v/v) Triton X-100 (2% Triton buffer) from the 20× TE buffer supplied with the kit and RNase-free water. Pour into separate pipette basins if using a multichannel pipette.
3.In the top row (A) of a 96-well plate (opaque or black walled for fluorescence measurements) add 7.5 µl of each iLNP solution and a DPBS blank. See Figure 11A for plate layout.
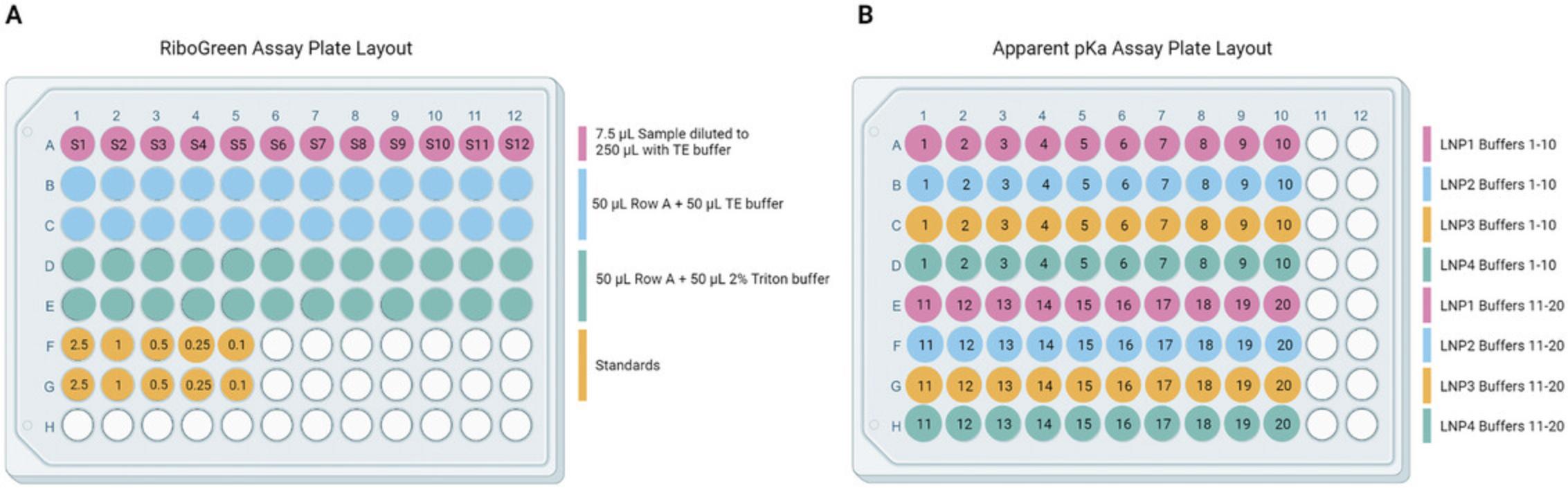
4.Use a pipette (multichannel if available) to add 1× TE buffer to each well to a final volume of 250 µl. Pipette up and down to mix.
5.Add 50 µl 1× TE buffer to the two wells below the samples (rows B and C).
6.Add 50 µl 2% Triton buffer to the two wells below the samples (rows D and E).
7.Add 50 µl of the diluted sample from row A to each of the four wells below (rows B, C, D and E).
8.Set up a standard curve in duplicate according to Table 12.The RNA standard provided with the kit, or the mRNA used in the formulation can be used to generate the standard curve. Dilute the RNA standard or mRNA from the formulation to 20 µg/ml with 1× TE buffer.
Concentration of RNA in well (µg/ml) | RNA stock (µl) | 1×TE buffer (µl) | 2% Triton buffer (µl) | Total volume per well (µl) |
---|---|---|---|---|
2.5 | 25 | 25 | 50 | 100 |
1 | 10 | 40 | 50 | 100 |
0.5 | 5 | 45 | 50 | 100 |
0.25 | 2.5 | 47.5 | 50 | 100 |
0.1 | 1 | 49 | 50 | 100 |
9.With the samples and standards plated, incubate the plate at 37°C for 10 min.
10.Meanwhile, prepare the RiboGreen dye solution by diluting the RiboGreen dye reagent 1:100 in 1×TE buffer in a centrifuge tube and vortex to mix.
11.Add 100 µl of the diluted RiboGreen dye solution to each sample and standard well. If bubbles have formed in the well, pop them with a needle.
12.Read the fluorescence with a plate reader using λex 485 nm and λem 528 nm.
13.Plot the standard curve and calculate the concentration of mRNA in the sample wells (Fig. 12).
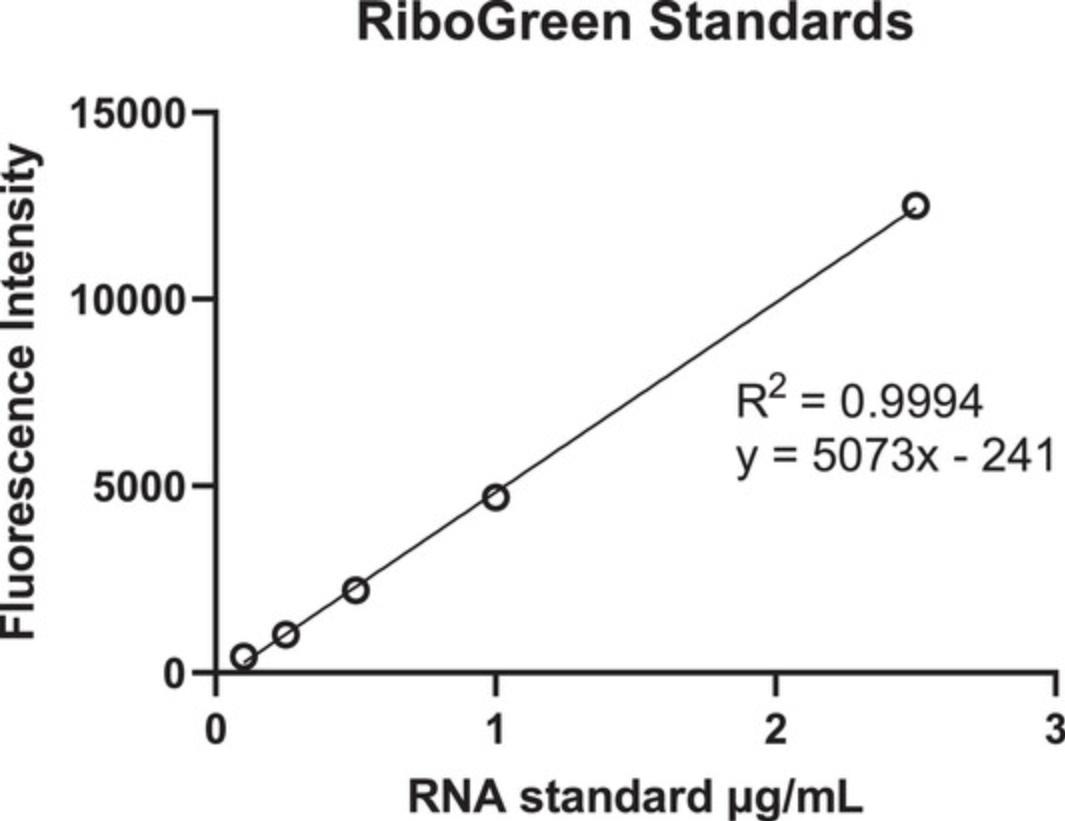
14.Calculate the concentration of mRNA in the bulk solution (µg/ml) according to Equation 4.
15.To determine the concentration of encapsulated mRNA, subtract the concentration of the samples without Triton X-100 from the samples with Triton X-100 according to Equation 5.Average the duplicate samples. Use this value and the known volume of the sample to dilute the sample to 100 µg/ml encapsulated mRNA as described in Basic Protocol 2, step 17.
16.To determine the encapsulation efficiency, divide the concentration of encapsulated mRNA by the concentration of the well with Triton X-100 (total mRNA) and multiply by 100. See Equation 6 below.
Particle size
17.Turn on the DLS instrument at least 30 min prior to use and set the temperature to 25°C.
18.Prepare the sample for DLS measurement by diluting 10 µl of the 100 µg/ml iLNP sample with 90 µl DPBS in the disposable low volume zetasizer cuvette.
19.Insert the cuvette into the instrument and collect DLS data. Example parameters for a Malvern Panalytical Zetasizer:
- Dispersant: water
- Dispersant refractive index: 1.330
- Viscosity (cP): 0.8872
- Material refractive index: 1.45
- Material absorption: 0
- Temperature: 25°C
- Equilibration time: 120 s
- Measurement duration: automatic (instrument will determine this based on count rate)
- Number of measurements: 3
20.In the DLS software, average the results of the three acquisitions and report the z-average as the particle size and polydispersity index. See the Understanding Results section for examples (Fig. 7A and 14B).
21.Remove the sample from the instrument.
Zeta potential
22.Turn on the DLS instrument at least 30 min prior to use and set the temperature to 25°C.
23.Prepare the sample for zeta potential measurement by diluting 20 µl of the 100 µg/ml iLNP solution with 980 µl water in an appropriate container such as an Eppendorf tube.
24.Prepare the folded capillary zeta potential cuvette as follows:
-
To facilitate wetting, flush the cell with ethanol by taking up 1-2 ml ethanol in a 3-ml syringe.
-
Attach the ethanol syringe and an empty syringe to each port.
-
Flush the ethanol through the capillary into the empty syringe then flush back. Repeat 2 to 3 times.
-
Repeat this process with water.
-
Ensure all the liquid is in one of the syringes (the capillary is empty) and remove the syringes.
-
Discard solution.
25.Load the sample into the zeta potential cuvette as follows:
-
Use a fresh syringe to draw up the sample and attach to one of the ports of the cuvette.
-
Invert the cuvette and inject the sample until it has filled about half of the U tube.
-
Carefully return the cuvette upright and inject the rest of the sample to just below the ‘fill’ line.
-
Ensure there are no air bubbles.
-
Remove the syringe and fit the stoppers.
26.Insert the cuvette into the instrument and collect zeta potential data. Example parameters for a Malvern Panalytical Zetasizer:
- Dispersant: water
- Dispersant refractive index: 1.330
- Viscosity (cP): 0.8872
- Dispersant dielectric constant: 78.5
- Temperature: 25°C
- Equilibration time: 120 s
- Measurement duration: automatic (instrument will determine this based on count rate)
- Number of measurements: 3
27.In the DLS software average the results of the three acquisitions and report the zeta potential in millivolts (mV) (Fig. 7C to F). See Understanding Results for examples of how the data should look and how to report results.
28.Remove the sample from the instrument then remove the sample from the cuvette by attaching a syringe to one port, draw up the solution until the liquid has been removed from half of the U-bend. Invert the cell and remove the remaining contents. Repeat the wash step with water as described in step 24.
29.If the cuvette will be used for another sample, load as described in step 25.If the cuvette is not being used for any more samples, flush the cell with ethanol as described in step 24 and leave to dry.
Extract mRNA for quality assessment
30.Prepare RNA wash buffer by diluting the RNA wash buffer concentrate (provided in the Zymo Quick-RNA Microprep kit) five-fold with ethanol.
31.In an RNase-free Eppendorf tube add 20 µl of the iLNP sample at 100 µg/ml, followed by 10 µl RNA lysis buffer then 10 µl ethanol. Pipette up and down to mix.
32.Transfer the solution to a Zymo-Spin IC column in a collection tube, provided with the kit. Centrifuge 30 s at 10,000 to 16,000 × g , room temperature. Discard flow through.
33.Add 400 µl RNA prep buffer provided with the kit to the column and centrifuge as in step 32, discard flow through.
34.Add 700 µl RNA wash buffer prepared in step 30 to the column and centrifuge as in step 32, discard flow through.
35.Add 400 µl RNA wash buffer to the column and centrifuge 60 s at 10,000 to 16,000 × g , room temperature.
36.Transfer the column to a new RNase-free Eppendorf tube. Add 15 µl RNase-free water to the column and centrifuge as in step 32.
37.The flow through contains the extracted mRNA. Analyze by automated gel electrophoresis as in Basic Protocol 1 step 34 to 41.See the Understanding Results section for examples and interpretation (Fig. 5, Fig. 13, and Table 7).
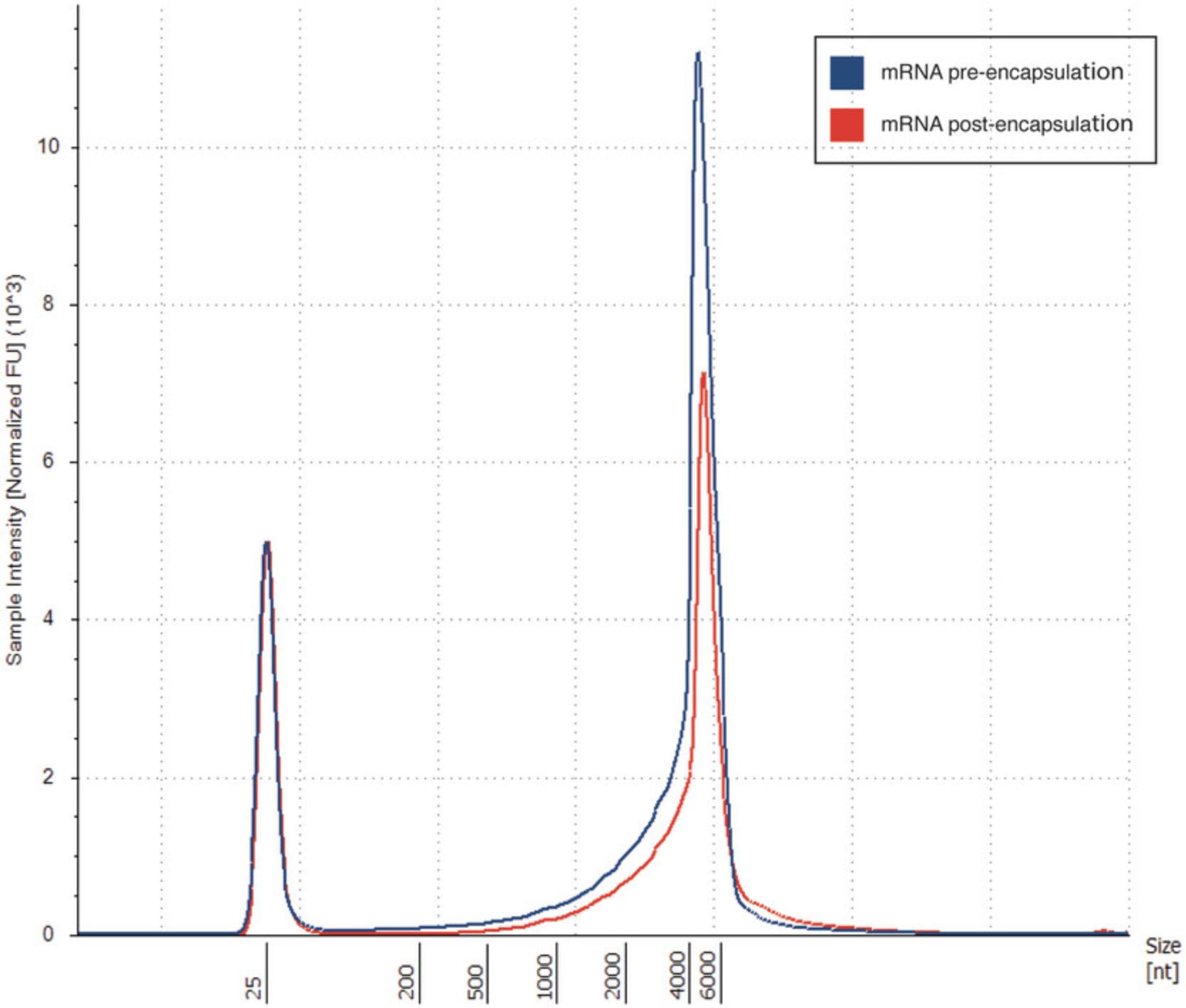
Apparent pKa assay
38.Portion the phosphate-citrate-borate buffer evenly across 20 containers (50 ml each). Adjust the pH of each aliquot using 1 M NaOH and 1 M HCl to achieve roughly even increments between pH 3 and 9 (∼0.3 units).
39.Dissolve the TNS reagent in DMSO to 0.1 mg/ml.
40.Aliquot 1 ml of each of the 20 buffers into an appropriate container such as an Eppendorf tube or cryovial. Add 22 µl of 0.1 mg/ml TNS in DMSO solution to each buffer and vortex.
41.Add 2.5 µl of each iLNP sample into 20 wells of an opaque or black walled 96-well plate. Layout samples according to Figure 11B.
42.Add 92.5 µl of each buffer containing TNS into the wells with iLNP sample so that each iLNP well has a different pH buffer added to it.
43.Read the fluorescence of the TNS reagent with a plate reader using λex 325 nm and λem 435 nm.
44.Convert the fluorescence readings to relative fluorescence (divide all values by the highest reading) and fit the relative fluorescence data to the Henderson-Hasselbalch equation (see Equation 7 below). Report the apparent pKa for each lipid (pH at which half of the maximum TNS fluorescence is reached). Examples of fluorescence curves and fitting data is described in the Understanding Results section (Fig. 8).
REAGENTS AND SOLUTIONS
Phosphate-citrate-borate buffer with sodium chloride
- 1.42 g sodium phosphate dibasic (10 mM)
- 1.27 g sodium borate (10 mM)
- 2.94 g trisodium citrate tribasic dihydrate (10 mM)
- 8.77 g NaCl (150 mM)
- Bring volume up to 1 L with MiliQ water or equivalent
- Store at −20°C for up to 12 months
- Adjust pH as required with 1 M HCl or 1 M NaOH
COMMENTARY
Background Information
The interest in the use of nucleic acids for vaccine development stems from their ability to induce antigen expression and adaptive immune responses from encoded antigens. Compared to traditional vaccine platforms such as attenuated or inactivated pathogens that require the growth and containment of highly infective pathogens on scale, mRNA can be produced by means of an enzymatic manufacturing process. This process is relatively inexpensive, scalable, and can be modified easily, making the mRNA an attractive platform for vaccine development. This was highlighted during the COVID-19 pandemic where mRNA vaccines were developed at unprecedented speed, entering clinical trials within 66 days and approval within 11 months of development (Chaudhary et al., 2021; Corbett et al., 2020).
mRNA vaccines comprise mRNA molecules prepared via in vitro transcription that represent the minimal genetic information required to produce defined target antigens (normally proteins or protein subunits), that are only transiently expressed until the mRNA has been degraded. One of the main challenges associated with mRNA vaccines is the sensitivity towards ribonucleases that hydrolyze the polyribophosphate backbone, and the highly anionic and hydrophilic nature that impairs its cellular uptake. For translation to occur, mRNA needs to gain entry to the cytoplasm where ribosomes are located. Several strategies have been developed for RNA delivery among which non-viral vectors have become the preferred delivery vehicle. The recent development of mRNA vaccines has been facilitated by breakthroughs in the packaging of mRNA into iLNPs that is made possible with advances in microfluidics mixing suitable for large scale production, and the technical progress in the mRNA construct using modified nucleotides for improved stability and translation (Buschmann et al., 2021; Hou et al., 2021; Schoenmaker et al., 2021).
Synthesis of mRNA
Production of mRNA via in vitro transcription is commonly carried out by the T7 RNA polymerase due to its rapid transcription rate and ability to incorporate modified NTPs (Borkotoky & Murali, 2018). Transcription is initiated at the T7 promoter which is required in the DNA template added to the reaction. While not discussed in depth in this protocol, DNA template elements, design and quality are important influencers of resulting antigen longevity and IVT reaction yields (Kim et al., 2022; New England Biolabs, see Internet Resources). To avoid triggering innate immune responses, and to prolong the stability and translation of the transcripts in vivo, a 5′ cap and 3′ poly(A) tail (Fig. 3) are added to IVT-produced mRNAs to closely mimic host mRNAs (Decroly et al., 2012; Gallie, 1991; Passmore & Coller, 2022). The cap and tail can both be added post-transcriptionally (as described here, Fig. 1A) and co-transcriptionally. While the latter requires much less handling of the mRNA reducing the likelihood of degradation, the former enzymatic capping and tailing systems are widely available through commercial suppliers at research and GMP grades. This means they are less affected by the current IP landscape, thus avoiding the requirement of expensive licensing fees.
The vaccinia capping enzyme used in this protocol adds cap0 consisting of a 7-methylguanylate structure to the 5′ end of the mRNA, the addition of a 2′-O-methyltransferase to the reaction then facilitates the conversion of cap0 to cap1 (Fig. 3). Cap1, defined by an extra methyl group at the 2′-O position of the first nucleotide adjacent to the cap, has been shown to enhance gene expression and is essential for the evasion of host defense (Decroly et al., 2012; Furuichi, 2015; Vaidyanathan et al., 2018). Co-transcriptional capping methods have undergone significant advancements from the initial anti-reverse cap analog (ARCA) (Stepinski et al., 2001) to the current CleanCap technology (Henderson et al., 2021), enabling high yields of mRNA in a one-step reaction where transcription and capping occur synergistically. However, the CleanCap reagent has been highlighted as the major cost component for developing vaccines making traditional methods like the vaccinia capping system more accessible to all (Kis et al., 2021). While post-transcriptional tailing using the commercially available enzyme E. coli poly(A) polymerase consistently results in tails >100 bases, it introduces variation in tail lengths between molecules. Alternatively, the poly(A) tail could be DNA template vector-encoded, reducing handling time and variation in tail length. However, the poly(A) tract can be lost during the production of the DNA template and requires stringent quality control to check the tail length before proceeding with IVT (Grier et al., 2016). While technology for the synthesis of mRNA is rapidly advancing and being adopted, post-transcriptional capping and tailing reactions allow small-scale, cost efficient and flexible production of mRNA for the pre-clinical research phase when optimizing quality control procedures and the set-up of specific assays do not require both capping and tailing.
Ionizable lipid nanoparticles
iLNPs are composed of a cationic lipid with an ionizable head group, PEGylated lipid, helper phospholipid and cholesterol (Figs. 4A and 5), generally in a ratio of 50/1.5/10/38.5, respectively. The headgroup of the ionizable lipid contains a tertiary amine which is neutral at physiological pH but becomes protonated at low pH such as that encountered in the endosome. Under these conditions the positively charged ionizable lipid fuses with the endosomal membrane and releases the mRNA into the cytosol for translation (Hou et al., 2021; Reichmuth et al., 2016). The apparent pKa of the resulting particle is one of the most important factors in determining the stability and cellular delivery of iLNPs, with the ideal pKa reported as 6.2 to 6.9, and is mostly driven by the properties of the ionizable lipid (Hassett et al., 2019; Jayaraman et al., 2012). Some ionizable lipids are commercially available, including MC3, ALC-0315 utilized in the COVID-19 Comirnaty vaccine by Pfizer/BioNTech, and SM-102 utilized in Spikevax by Moderna (Schoenmaker et al., 2021). ALC-0315 and SM-102 contain esters in place of the double bonds in MC3 that make the lipids more biodegradable resulting in improved lipid clearance (Hassett et al., 2019; Sabnis et al., 2018) (Fig. 14). Typically for mRNA, the N/P ratio (ionizable lipid amine ‘N’ to phosphate backbone on mRNA ‘P’) of 3 to 8 provides sufficient encapsulation efficiency and delivery. iLNPs containing ionizable lipids adopt a range of different morphologies, typically including an electron dense lipid-filled core (squares, Fig. 6B) and sometimes containing an electron-lucent bleb structure attached (triangles, Fig. 6B) or completely detached (liposome, not shown here).
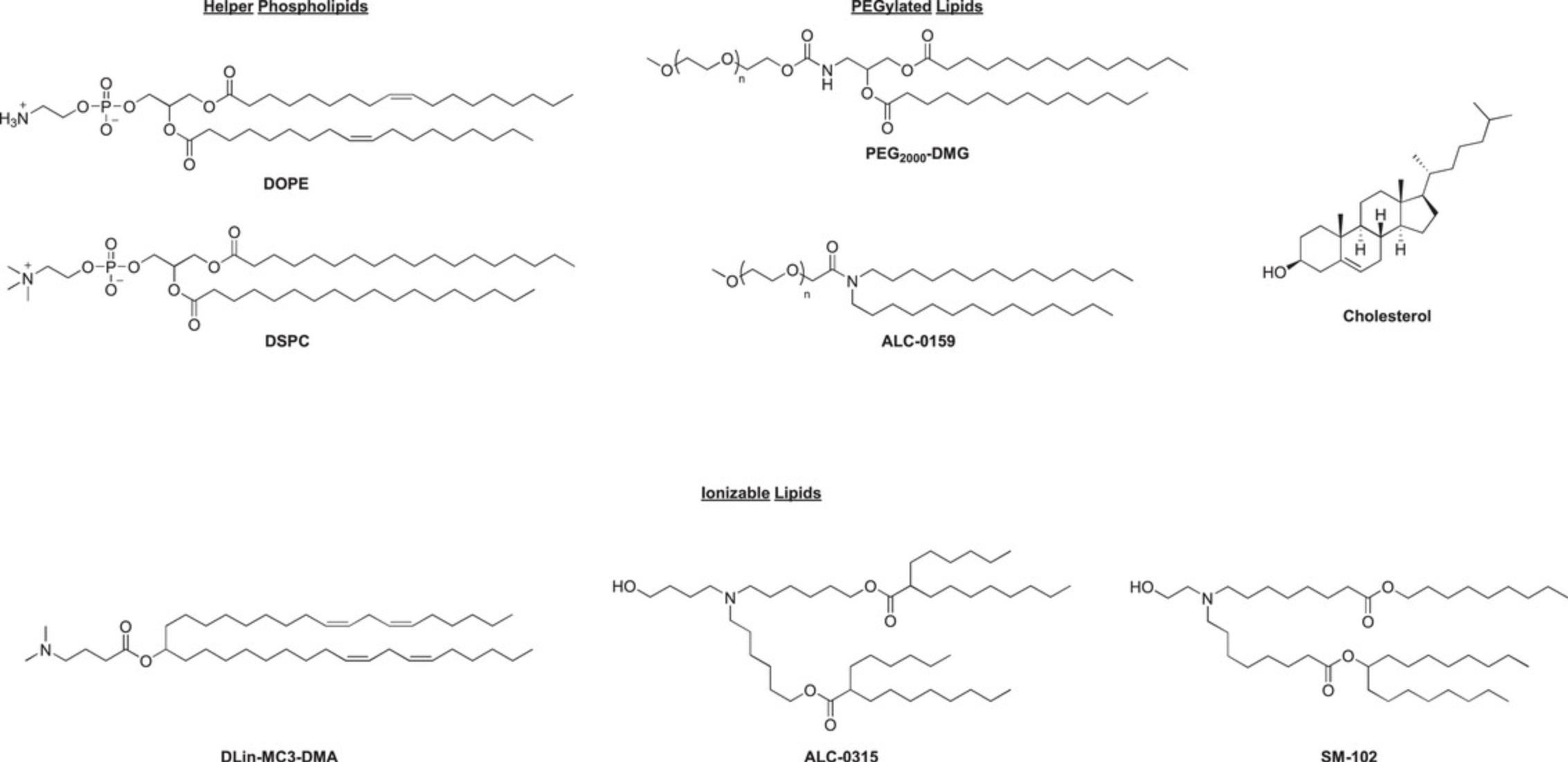
iLNPs result from spontaneous aggregation of amphiphilic lipids in an aqueous solution. This can be achieved practically using a top-down approach where large particles are made smaller or a bottom-up approach building the particle from monomers (Tenchov et al., 2021). The top-down approach (typically thin film hydration followed by extrusion or sonication) is widely used in research laboratories to make liposomes. However, this method suffers from poor reproducibility and is challenging to scale-up for manufacture. Nanoprecipitation of lipids dissolved in a water-miscible solvent in an aqueous solution is more suited to scaling up and has better reproducibility. Early examples include ethanol precipitation, where the lipids are dropped into a stirring container of aqueous solution, however this method suffers from poor particle size polydispersity due to the turbulent mixing being inhomogeneous. To overcome this, microfluididic mixers based on laminar flow have been developed. This technique uses fast flow and narrow tubing to prevent premature mixing of the two solutions until they reach a controlled mixing chamber containing a T-junction, staggered herringbone mixer or ring mixer (Fig. 15) (Ripoll et al., 2022). Precise control of the temperature, solvents and flow rates can produce low polydispersity particles with desired size and is essentially scale-independent through controlled nanoprecipitation (Reichmuth et al., 2016; Webb et al., 2019).
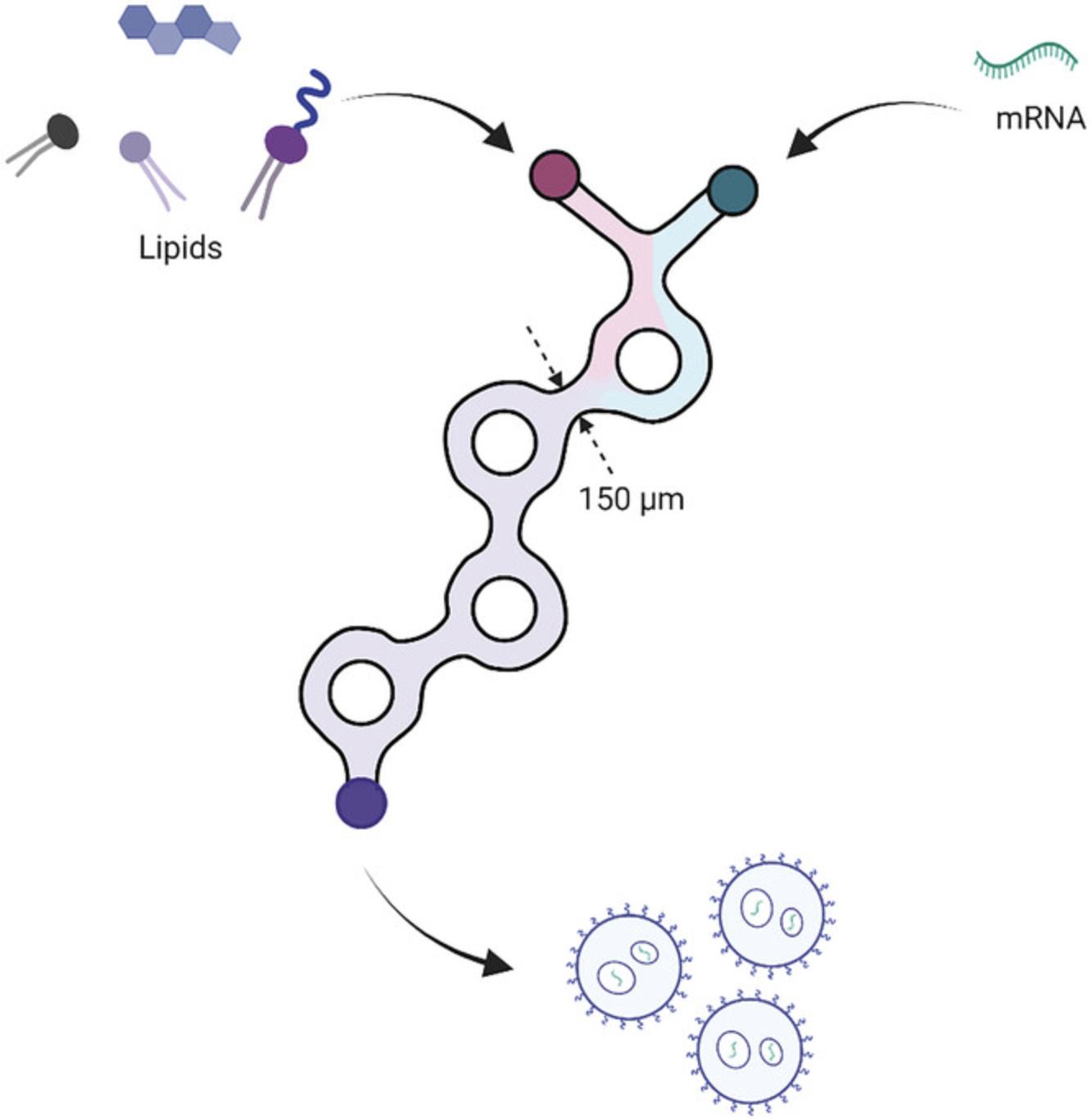
Formulation of iLNPs using microfluidic mixing, such as that described in Basic Protocol 2, is carried out using lipids dissolved in ethanol and mRNA dissolved in an acidic aqueous buffer such as acetate or citrate buffer, pH 3 to 5 (Fig. 15). At low pH, the ionizable lipid is positively charged and electrostatically interacts with the negatively charged mRNA. The pH of the solution is then increased to 7.4 by dialysis or dilution with DPBS resulting in a rearrangement of the particles as the ionizable lipid is neutralized. The rearrangement is largely determined by the steric shielding of the PEGylated lipid preventing further fusion of vesicles (Kulkarni et al., 2018). It is possible to encapsulate the mRNA without it being present in the initial aqueous solution. This may be desirable if the start/end waste, and the amount required in the syringe, results in too much waste for an expensive construct or there is a very small amount of mRNA to work with. This method, termed preformed vesicle encapsulation and described in Alternate Protocol, introduces the mRNA to the positively charged lipid nanoparticle after it has been formulated while it is still in acidic buffer (Fig. 1B). This allows the electrostatic interaction between the iLNP and mRNA to occur before adjusting the pH as carried out in the standard procedure. For larger nucleic acid molecules such as mRNA and saRNA (Blakney et al., 2019), it is important that the ethanol content is sufficiently high at the time the RNA is added, such that the nanoparticles are deformable allowing the mRNA to have maximum contact with the cationic particles and to be encapsulated (Maurer et al., 2001). Smaller RNA molecules such as siRNA can be encapsulated in the absence of ethanol (Kulkarni et al., 2020). The preformed vesicle method does have limitations; the particles tend to be less uniform and encapsulation efficiency slightly lower than those produced with the mRNA in the aqueous phase of the microfluidic mixing. However, for research purposes these limitations may not be critical.
Characterization and quality control of ionizable lipid nanoparticles
Size and polydispersity index
There are several parameters to describe the properties of the iLNPs generated from Basic Protocol 2. Some of these include routine analysis required to ensure the particles are of a suitable quality for administration while others are less routine and may be useful for characterizing new formulations, lipids or assessing stability upon storage. Routine analysis includes the use of dynamic light scattering (DLS) to determine the particle size and the polydispersity index (PDI) of the particles. DLS measures the fluctuations of scattered light by particles as they move in solution according to Brownian motion (Stetefeld et al., 2016). The hydrodynamic diameter of the particle is calculated as a function of the diffusion coefficient which is inversely proportional to the particle size according to the Stokes-Einstein equation. Algorithms are used to generate a secondary readout parameter, the most relevant of which are the ‘z-average diameter’ which is an intensity-weighted mean diameter based on a cumulant analysis, and the PDI. The PDI is a measure of the heterogeneity of the sample with lower values suggesting the particle distribution is less disperse. Samples with a low PDI are considered monodisperse, which means the sample is predominantly made up of particles with the same diameter. This is in contrast to a polydisperse sample that has two or more distinct populations of peaks. DLS is a simple technique to use, samples can generally be analyzed in relevant solvents such as PBS, water, or other aqueous buffers, and uses relatively little material when small volume cuvettes are used. DLS can, however, suffer from poor data quality if the sample is colored, too concentrated, aggregating or contains large particles. The Malvern software usefully interprets the quality of the data and suggests if any of these phenomena are occurring. One limitation of DLS is that it is not good at resolving populations of particles close in size, because it measures the bulk solution, and the algorithm makes assumptions about all the particles based on the average movement. Other techniques that detect one particle at a time such as nanoparticle tracking analysis (NTA) or resistive pulse sensing (RPS) are much more accurate. However, these techniques are more challenging to run and optimize, and may require altering the analyte buffer, for example to include a detergent. Therefore, DLS is the most convenient technique and provides sufficient detail for routine characterization of particle size.
Zeta potential
DLS can not only measure the hydrodynamic radius, but it can also be used to measure the zeta potential of particles. Zeta potential is deduced from the electrophoretic mobility of charged particles under an applied electric field (Bhattacharjee, 2016). When a charged particle is dispersed, ions tend to cluster nearby. There is an immobile layer of ions of the opposite charge which adhere tightly to the surface of the particle, called the Stern layer. A second diffuse outer layer is comprised of loosely associated ions. Together these make up the electrical double layer (Clogston & Patri, 2011). The barrier between the electric double layer and the bulk solution is called the slipping plane and the electric potential across this plane is called the zeta potential. In practice, the electric double layer moves with the particle during electrophoresis, creating a hypothetical plane which acts as a barrier between moving particles and its associated ions and the bulk solution. The potential of the particle itself cannot be measured directly so the zeta potential must be measured instead. Zeta potential measurements are affected by pH, temperature, viscosity, and ionic strength of the solvent which must all be kept as constant as possible. In most uses of zeta potential only the magnitude of the measurement is robust, as the positive/negative finding can be influenced by a variety of factors not related to the surface charge. A neutral surface charge of −10 to +10 mV is typical for iLNPs, whereas less than −30 and more than +30 mV indicates strongly charged surfaces (Arab et al., 2021; Bhattacharjee, 2016; Clogston & Patri, 2011).
RiboGreen assay
The RiboGreen assay is used routinely to determine the mRNA encapsulation efficiency and the concentration of encapsulated mRNA which is required to administer the correct dose. Unbound RiboGreen dye reagent is essentially nonfluorescent. Upon binding to nucleic acid, the dye exhibits a significant fluorescence enhancement with a high quantum yield making the detection of mRNA very sensitive (Jones et al., 1998). Quantification of mRNA using the dye is linear across four orders of magnitude in nucleic acid concentration and is a very simple and rapid experiment to run. In order to determine the amount of mRNA inside particles, the particle must be destroyed for the dye to bind to the mRNA. This is achieved by incubating the iLNP suspension with a detergent before adding the dye. Destroying the particle means the dye detects the total mRNA in the sample, including the unencapsulated mRNA (Fig. 9). To calculate the encapsulation efficiency and the concentration of the mRNA inside the particle, the unencapsulated mRNA is quantified by adding the dye to an iLNP sample that does not contain the detergent. The unencapsulated mRNA is subtracted from the total mRNA to give the concentration of encapsulated mRNA, and this is divided by the total mRNA to give the encapsulation efficiency (see Equation 6). The RiboGreen assay is robust and simple to run and there are no other high-throughput, routine alternatives.
mRNA extraction for quality assessment
Extraction of the mRNA from iLNPs is a useful technique to establish the ability of the particle to protect the mRNA from degradation by RNases or to evaluate storage conditions but is not required for routine characterization. The method outlined in Basic Protocol 3 utilizes a kit designed to extract mRNA from cells. The lysis buffer contains a detergent and is diluted with ethanol which dissolves the iLNP, resulting in the mRNA being in solution. The mRNA is loaded onto a silica-based spin column and the lipid components are washed away. Finally, the mRNa is eluted from the column in water for analysis of the mRNA integrity and concentration. The mRNA recovery described can be applied to other commercial kits designed to extract or purify mRNA. If the kit does not come with a lysis buffer (i.e., it is designed to purify synthetic mRNA), a suitable detergent such as 2% Triton X-100 may be used to disrupt the particle as described by Ly et al. (2022). The spin column methods are compatible with RNase protection assays (treatment with RNase followed by inactivation with proteinase K). Phenol/chloroform/isoamyl alcohol is commonly used to remove protein from nucleic acids and has been used in RNase protection assays for iLNPs (Blakney et al., 2019). However, this mixture contains toxic, corrosive, and odorous chemicals that can be avoided by using detergent and spin columns. Analysis of the extracted mRNA should use an appropriate technique to determine the quality of the mRNA. In Basic Protocol 3 we have described analysis using automated gel electrophoresis, however, other electrophoretic instruments can be used with a suitable ladder and control sample.
Apparent pKa assay
Determining the apparent pKa of the particle is important for understanding the activity of new formulations or lipids but is not required for routine analysis. The apparent pKa of the particle is closely linked to the activity and properties of the iLNP and stems from the ionization of the tertiary amine in the ionizable lipid. The apparent pKa of iLNPs is determined by the TNS assay. TNS is a negatively charged fluorescent dye (Fig. 10) whose fluorescence is quenched in the presence of water. The partition of the dye into the positively charged lipid membrane via electrostatic interactions increases the fluorescence due to the absence of water. The increase in fluorescence of TNS in the presence of increasing cationic ionizable lipids provides an estimate of the surface charge of the iLNP. This can be used to report an apparent pKa of the particle as the lipids change their ionization state according to the pH of the buffer (Fig. 10). Relative fluorescence intensity plotted against buffer pH results in a Henderson-Hasselbalch curve that can be described by a sigmoidal curve (see Equation 7). Several variations of the TNS assay have been reported in the literature using slightly different buffer conditions and dye concentrations but when compared there was not a significant difference between the methods (Carrasco et al., 2021; Jayaraman et al., 2012; Sabnis et al., 2018; Zhang et al., 2011). The buffer used by Sabnis et al. (2018) is described in Basic Protocol 3 as it uses buffer components readily available in most laboratories. The alternative buffers described in the literature will give similar results if those reagents are more readily available. Zeta potential has been used to estimate pKa however the nature of the technique as a measure of the electric potential of the whole particle means the iLNP acts as a polyelectrolyte (Carrasco et al., 2021). This causes a broadening of the titration curve whereas the TNS dye only interacts with the surface of the particle.
Critical Parameters
RNase-free practices
It is crucial that Basic Protocols 1 and 2 and Alternate Protocol be carried out using RNase-free practices to reduce the likelihood of mRNA degradation by RNase contamination. This can be achieved by regularly changing gloves, spraying gloves and equipment with an RNase-decontamination solution, and working with RNase-free reagents and consumables where possible.
mRNA quality control procedures
Gel electrophoresis is a well described and easily accessible method for assessing the integrity of mRNA. However, there are many more aspects of the product that may need to be screened depending on the next steps in the experimental or production pipeline. Quality control and keeping a record of the integrity of each mRNA batch is an extremely important step in the production and study of iLNP-encapsulated mRNA. mRNA must be of high integrity and RNase-free before encapsulation to ensure the mRNA delivered via the iLNP for further in vitro or in vivo studies is intact, functional, and able to elicit a response. It is also possible to screen the quality of mRNA post-encapsulation (see Basic Protocol 3). Screening of mRNA quality includes confirming the size, sequence, absence of contaminants and RNases, the presence of the 5′ cap and 3′ poly(A) tail. Quality control assays are constantly being developed and optimized to make the process more efficient, affordable and user friendly. In Table 13 we summarize some of the currently available methods to assess various properties of the mRNA produced via this protocol.
Quality control for | QC method | Reference |
---|---|---|
mRNA integrity | Agarose gel | Henderson et al., 2021; Pregeljc et al., 2023 |
mRNA integrity | Stability assay | Korenč et al., 2021 |
mRNA integrity | Nanodrop | Henderson et al., 2021 |
mRNA integrity |
Agilent TapeStation Bioanalyzer Fragment analyzer |
See Basic Protocol 1 and Understanding Results; Malmanger et al., 2010 |
mRNA integrity | CImMultus PrimaS | Korenč et al., 2021 |
mRNA integrity | RT-qPCR | Brisco & Morley, 2012 |
Presence of the poly(A) tail | Agarose gel | See Basic Protocol 1 and Understanding Results |
Presence of the poly(A) tail | OligodT purification | Korenč et al., 2021 |
dsRNA | Dot blot | Baiersdörfer et al., 2019 |
dsRNA | ELISA | Schonborn et al., 1991 |
Presence of the cap | Ribozyme assay | Vlatkovic et al., 2022 |
Presence of the cap | Enzymatic assay | Chiron & Jais, 2017 |
Presence of the cap | LC-MS | Beverly et al., 2016 |
mRNA integrity post-encapsulation |
Agilent TapeStation Gel electrophoresis |
See Basic Protocol 1 and Understanding Results |
iLNP encapsulation
The temperature of reagents for formulation should be controlled at room temperature as it effects the nanoprecipitation process. All reagents must also be fully dissolved before loading syringes. The lipids may require gentle heating in order to dissolve but should only be used once they cool to room temperature again. The pH of the aqueous buffer used in the microfluidics nanoprecipitation of the lipids must be between 3 and 5 for the ionizable lipid to be positively charged, therefore the pH of this solution at the dilution used should be measured and adjusted, if necessary, before use. The formulation recipe may be adjusted to suit the application. Generally, for mRNA the N/P ratio 4 to 6 gives consistently good encapsulation efficiency and delivery. The lipids ratio 50 ionizable/38.5 cholesterol/10 helper phospholipid/1.5 PEGylated lipid is commonly used and does not tend to deviate from this more than ∼10%. Formulation within this range is robust for most ionizable lipids.
It is important not to leave the freshly formulated iLNP exposed to ethanol for too long as this can result in aggregation and leakage of the mRNA from the iLNP. Do not freeze the solution of iLNPs without optimizing freezing conditions for each iLNP. There are a limited number of published studies on freezing iLNPs. Sugar-based cryoprotectants, such as sucrose, mannitol and trehalose have been reported, and the selection of buffers and freezing conditions have been investigated (Ball et al., 2017; Henderson et al., 2022; Kafetzis et al., 2023; Kim et al., 2023; Zhao et al., 2020). The optimal conditions reported for freezing mRNA-iLNPs appears to be variable, depending on the ionizable lipid or lipidoid and RNA cargo. Until more research is carried out on the storage conditions of a wide range of iLNPs, this must be optimized for each formulation. The iLNPs generated using Basic Protocol 2 and Alternate Protocol are stable in the fridge for at least a few weeks, but the integrity of the mRNA should be checked using the mRNA extraction and analysis described in Basic Protocol 3 if the use is beyond that time frame.
Formulation should ideally be carried out in a sterile environment to not only avoid RNase contamination and maintain sterility, but also reduce the likelihood of introducing other contaminants such as endotoxin. This is especially important if the iLNPs are going to be tested in a vaccination setting.
Troubleshooting
Table 14 lists possible causes and solutions to problems encountered in mRNA synthesis, encapsulation, and analysis.
Problem | Possible cause | Solution |
---|---|---|
Low yield after IVT | Low quality template | Consider running IVT with control DNA template to confirm reagents are as expected; add 5 mM DTT (final concentration) to the reaction; confirm the correct concentration of the DNA template added to the reaction; increase IVT reaction time, this is construct dependent, e.g., for short constructs extending the reaction to 16 hr may help; run an additional purification of the DNA template before use |
Low yield after IVT | Reaction components not mixed thoroughly |
Ensure each reaction component is thawed and mixed thoroughly before adding DNA template and RNA polymerase; mix gently after the addition of these more sensitive reagents |
Low yield after IVT | Reaction buffer not fresh (oxidized) | Open new tube of reaction buffer |
Low yield after IVT | DNA template concentration too low for shorter transcripts | Add up to 2 µg DNA template |
Presence of large mRNA transcripts after IVT | DNA template not completely linearized | Repeat linearization of DNA template; purify linearized DNA using FPLC methods |
Loss of mRNA after precipitation | RNA too dilute |
Add glycogen (1 µg/µl) during LiCl precipitation |
Loss of mRNA after precipitation | RNase contamination | Clean entire working space, change gloves, open new reagents, and repeat synthesis protocol |
RiboGreen assay shows no mRNA is encapsulated | Triton X-100 did not break open the iLNP to release mRNA | Incubate the plate longer (extra 5 min) or increase the amount of Triton X-100 to 5% (v/v) |
RiboGreen assay shows no mRNA is encapsulated | RNase contamination | Repeat the encapsulation with new acetate buffer, clean workspace, change gloves |
RiboGreen standards not linear | Gain on instrument too high | Adjust gain on instrument until the standards are linear |
RiboGreen standards not linear | Clear 96-well plate used | Use an opaque plate or black walled plate |
RiboGreen assay unexpected reading | Bubbles present in Triton X-100 wells | Pop bubbles with a needle and run again |
Instrument indicated poor data quality during zetapotential measurement | Particle has a neutral charge resulting in poor phase quality data | This is common for neutral particles and is not a problem for this type of particle |
Zeta potential cuvette electrodes turn black | Too many runs with one cuvette | Get a new cuvette every 10 runs |
Zeta potential cuvette electrodes turn black | Sample contains too much salt | Dilute the sample with water, use a new cuvette |
DLS size distribution poor quality | Sample too concentrated (turbid) | Sample should be slightly turbid but still transparent; dilute until only slight haziness is visible |
DLS size distribution poor quality | Sample contains large particles | Filter DPBS before using it to dilute sample for measurement; DLS software is good at reporting the cause of poor-quality data, follow the instructions |
Poor encapsulation efficiency | pH of acidic aqueous buffer used in formulation is too high | Check the pH is between 3-5 at the dilution used for formulation; adjust with HCl if necessary |
Poor encapsulation efficiency | Freshly formulated iLNP exposed too ethanol for too long | Dilute the iLNP in DPBS and remove ethanol promptly |
Lipids will not dissolve in ethanol | High melting temperature of lipid | Gently warm the lipid solution in warm water bath with swirling to dissolve |
Particles too large | Low formulation flow rate | Increase the total flow rate (e.g., 20 ml/min) |
Particles too large | Salt present in aqueous buffer for formulation | Ensure the acidic buffer used for formulation does not contain salt |
Particles too large | Prolonged exposure to ethanol | Dilute the iLNP in DPBS and remove ethanol promptly |
Understanding Results
Interpretation of agarose gels
Gel electrophoresis is a technique commonly used to separate molecules based on their charge and size (Lee et al., 2012). Larger molecules will move through the agarose matrix at a slower rate allowing researchers to screen for the presence of the poly(A) tail after the tailing reaction due to the increase in size of the molecule when the extra bases (A) are added. The size can be assessed more accurately if the sample is denatured, for example with formamide (Masek et al., 2005). The denatured mRNA can then be run on native agarose gels and compared to a known RNA size marker.
After the tailing reaction an increase in size is observed for tailed molecules (Fig. 2, lanes 4 and 7) when compared to the untailed samples in Figure 2 (lanes 2, 3, 5 and 6). Analysis of the gel gives a rough estimation of tail size as being greater than 100 bases. Smearing underneath the expected product size (lower molecular weight) can indicate degradation of the mRNA. Due to handling of mRNA during the capping and tailing procedures more smearing is often present in the final product post-tailing (Fig. 2, lane 5 compared to lane 7). Note that smearing can also be seen when an excessive amount of mRNA (e.g., ∼1 µg) is loaded in the gel (Fig. 2, lane 3). We recommend keeping the loaded RNA between 200 and 400 ng as overloading can lead to inaccurate assessment of degradation.
Due to the exposure to heat we recommend shorter running times (30 to 40 min) for accurate assessment of mRNA using a known RNA marker (Fig. 2, lane 1). When samples are not denatured prior to loading, a high molecular weight band double the size of the expected construct can often be observed (Fig. 16, lane 2). This band can also sometimes be seen in gels where samples have been denatured (Fig. 2, lane 2) indicating that denaturing may not have been successful or that a higher molecular weight product such as dsRNA is present in the sample. When crude lysate from an enzymatic reaction, in this case tailing, is run on a gel higher molecular weight smearing may be seen due to salts present in the reaction (Fig. 16, lane 4).
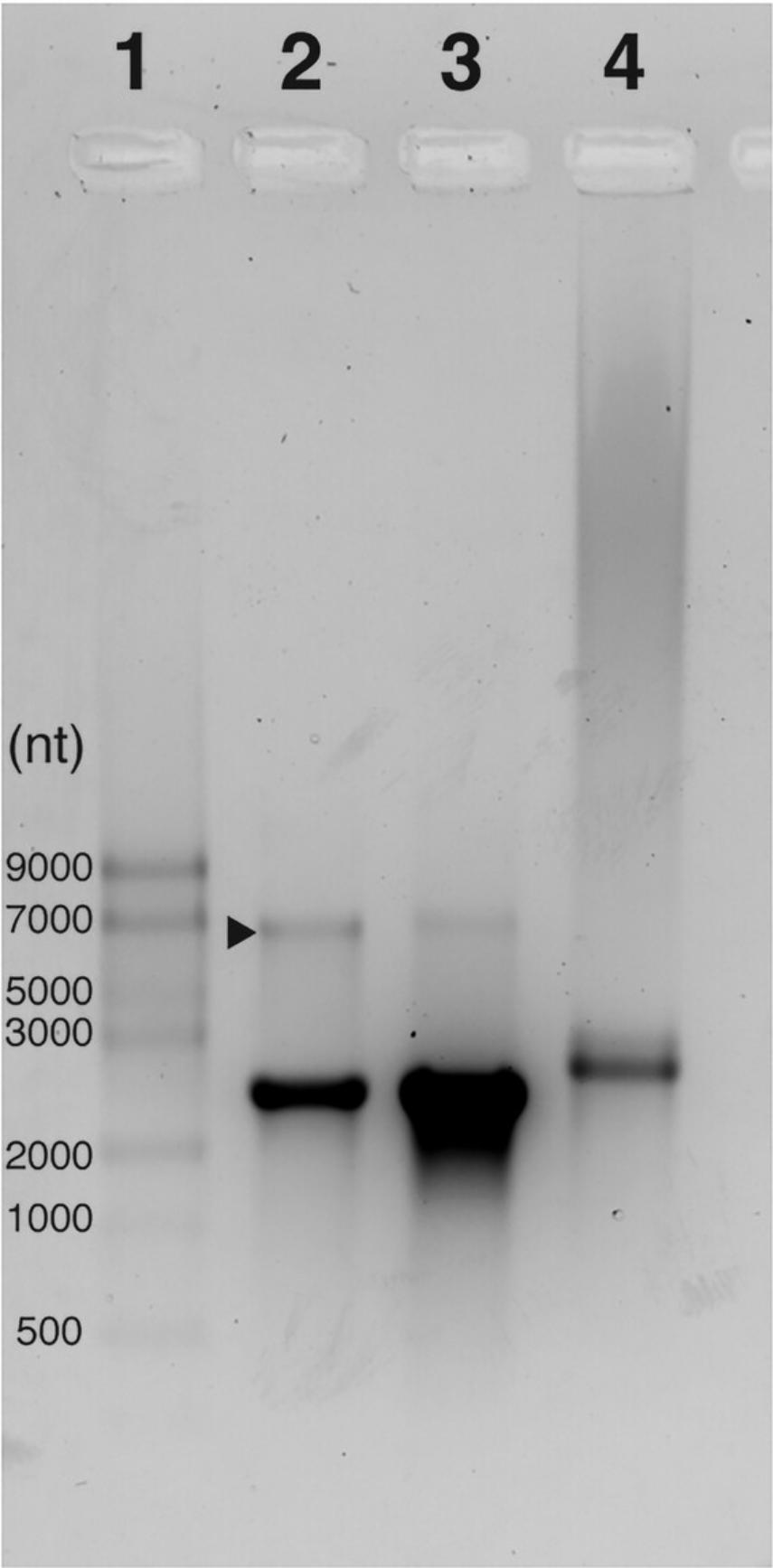
Interpretation of TapeStation results
While gel electrophoresis is common and accessible to most researchers there are alternative techniques for analyzing mRNA integrity and size that utilize more specialized equipment. These include but are not limited to HPLC (Azarani & Hecker, 2001), in some cases FPLC (Korenč et al., 2021), Bioanalyzer, Fragment Analyzer or TapeStation. The advantages of the TapeStation analysis outlined in Basic Protocol 1 are the requirement for very small amounts of mRNA, faster running times and simultaneous concentration measurements. The results are presented in a gel-like image (Fig. 4A) or in an electropherogram a plot of fluorescence against migration time which enables more in-depth analysis (Fig. 4B). Note that the TapeStation reports the RNA Integrity Number (RIN) for each sample. When applied to RNA extracted from biological samples, this is an estimate of how degraded versus intact the RNA sample is based on the ratio of 18S and 28S ribosomal RNA that it detects (Schroeder et al., 2006). However, a sample produced by IVT does not contain these same features. Therefore, the reported RIN is meaningless and should be ignored.
The TapeStation analysis software has region analysis capabilities which allow the user to define a region of interest. In this protocol the region of interest is defined as 1000 bases above and below the sample peak (Fig. 5 and Table 7). Once defined, the software will calculate the percentage of the peak with the selected region giving an estimate of mRNA integrity or how much of the sample is within the expected size range (Table 7). A small percentage change in the mRNA integrity suggests that electropherogram traces can also be overlaid for visual comparison of samples (Fig. 13). This function is particularly useful for comparison of mRNA samples for example before and after encapsulation by Basic Protocol 2, extracting the mRNA using Basic Protocol 3 ‘Extract mRNA for Quality Assessment’ after 2 months of storage at 4°C as depicted in Fig. 13.
Encapsulation of mRNA into iLNPs
Following nanoprecipitation using Basic Protocol 2 the solution will appear transparent or very slightly cloudy. Once concentrated at pH 7.4, the sample can appear very turbid, and in some instances opaque, depending on the particle size and concentration. The solution should appear homogeneous (no sedimentation or flotation of particles). When the preformed vesicles are added to the mRNA in Alternate Protocol, the solution can become more turbid than the preformed vesicles on their own.
The size, PDI, zeta potential and encapsulation efficiency should be reported for each iLNP, as shown in Table 9. The expected properties for particles produced using Basic Protocol 2 and Alternate Protocol with different commercially available lipid and reagent ratios is summarized in Table 9.
Interpretation of DLS data (Size, PDI, Zeta Potential)
DLS data for two representative formulations–Entry 2 and 6 in Table 9 (henceforth referred to as ‘MC3 Alternate Protocol’ and ‘ALC-0315 Basic Protocol 2’, respectively), is depicted in Figure 7. Z-average size and PDI should be reported for the size and zeta potential from zeta distribution should be reported. Size distribution by intensity as in Figure 13A and 13B should be investigated to ensure there are no peaks at large particle size that would indicate the presence of dust or aggregation and the peak should be a smooth distribution. To generate a zeta potential distribution with a single peak as in Fig. 7C and 14D the phase plot for zeta potential measurements should look as depicted in Fig. 7E and 14F).
RiboGreen assay
The RiboGreen standards analyzed in duplicate should generate a straight line. Exact values will depend on spectrometer settings, but the relationship should be linear, with a linear correlation of at least R2 of 0.99. An example of the fluorescence readings and the resulting standard curve are shown in Table 15 and Figure 12.
Concentration of RNA in well (µg/ml) | Fluorescence intensity replicate 1 (a.u.) | Fluorescence intensity replicate 2 (a.u.) |
---|---|---|
2.5 | 13006 | 12011 |
1 | 4715 | 4674 |
0.5 | 2211 | 2213 |
0.25 | 1064 | 971 |
0.1 | 420 | 443 |
Use the equation generated from the standards to interpolate the concentration of mRNA in each well, then calculate the bulk concentration using equation 8. An example of how to work through the calculation is shown in Table 16.
Sample | Fluorescence intensity (a.u.) | Concentration mRNA in well (µg/ml) | Aliquot volume (µl) | Bulk mRNA concentration (µg/ml) |
---|---|---|---|---|
iLNP replicate 1 | 138 | 0.07 | 7.5 | 10 |
iLNP replicate 2 | 138 | 0.07 | 7.5 | 10 |
iLNP + Triton replicate 1 | 11018 | 2.22 | 7.5 | 296 |
iLNP + Triton replicate 2 | 10413 | 2.10 | 7.5 | 280 |
Calculate the concentration of encapsulated mRNA by subtracting the mean unencapsulated mRNA concentration (mean of iLNP Replicates 1 and 2 in Table 16) from the total mRNA concentration (mean of iLNP + Triton Replicates 1 and 2 in Table 16). Report this as the concentration and dilute as required.
Mean iLNP = 10 µg/ml
Mean + Triton = 288 µg/ml
288 − 10 = 278 µg/ml encapsulated mRNA
Calculate the encapsulation efficiency according to the calculations. Report this value as the encapsulation efficiency, this should be over 80%.
Apparent pKa assay
Results for the apparent pKa assay of two representative formulations, Entry 2 and 6 (‘MC3 Alternate Protocol’ and ‘ALC-0315 Basic Protocol 2’, respectively) in Table 9, are depicted in Figure 8.
Plot the relative fluorescence intensity, RFU (all intensity values divided by the highest fluorescence reading) against the pH of the solution. Apply a model with the sigmoidal Equation 7 and report the pH where the fluorescence is halfway between maximum and minimum RFU.
Time Considerations
Refer to Figure 1 for safe stopping points indicated by red arrows.
Basic Protocol 1: Synthesis of mRNA by in vitro transcription and enzymatic capping and tailing
Allow 2 to 4 days to complete the entire protocol including the production and assessment of capped and tailed mRNA. Four days will be required if precipitations are planned overnight. The IVT, capping and tailing reactions all take approximately half a day. All reactions can be set up in ∼1 hr followed by the required incubation time of two hours for IVT and one hour for capping/tailing. Precipitation of the mRNA requires a 30 min centrifugation step and resuspension of the RNA takes ∼10 min. Quality assessment of the mRNA by Nanodrop, agarose gel and automated gel electrophoresis takes 1 hr.
Note when scaling up, more time is required particularly when multiple or larger mRNA pellets need to be resuspended in nuclease-free water.
Basic Protocol 2: Encapsulation of mRNA into iLNPs
Preparation of lipid solutions may be carried out in advance of the formulation step. Otherwise, the entire encapsulation protocol must be carried out on the same day. Allow an hour for all the reagents to come to room temperature before use and an hour to carry out the formulation and dilution into DPBS step. The centrifugal concentration step is dependent on the particle size, total sample volume and desired end volume. Typically allow 1 to 4 hr. Once concentrated, the iLNP solution can be stored in the fridge until the dilution requirements are determined by the RiboGreen assay.
Alternate Protocol: Small-scale encapsulation of mRNA using preformed vesicles
Same as for Basic Protocol 2.
Basic Protocol 3: Characterization and quality control of mRNA iLNPs
Allow 1 to 2 hr for the RiboGreen assay including allowing the kit to warm to room temperature from the fridge. DLS (size, PDI, zeta potential) should be carried out on the final, diluted sample before it is used for biological evaluation. Preparation of samples takes a few minutes and analysis time varies across different instruments, but it is typically 5 to 10 min per sample.
The buffers used in the TNS assay must be at room temperature before use. Depending on the size of the aliquot this may take several hours. Buffers can be moved to the fridge the night before the assay is to be run to reduce the time required to warm the buffers. The assay requires 40 aliquots in a 96-well plate per iLNP sample therefore allow 10 to 15 min per iLNP sample to prepare the plate and 10 min to read the plate.
Allow 30 min for the mRNA extraction protocol. See above (Basic Protocol 1) for analysis of extracted mRNA by automated gel electrophoresis.
Acknowledgments
Figures created with BioRender.com.
This work was supported by funding from New Zealand Ministry of Business Innovation and Employment (RTVU1603 and Vac 19008) and Research Trust of Victoria University of Wellington (project number 400662).
We thank the Otago Micro Nano Imaging Electron Microscopy Unit at the University of Otago for assistance with cyro-TEM imaging and the Hugh Green Cytometry Centre for technical assistance.
Open access publishing facilitated by Victoria University of Wellington, as part of the Wiley - Victoria University of Wellington agreement via the Council of Australian University Librarians.
Author Contributions
Rebecca Elizabeth McKenzie : Conceptualization; data curation; formal analysis; methodology; project administration; writing original draft; writing review and editing. Jordan James Minnell : Conceptualization; investigation; methodology; writing review and editing. Mitch Ganley : Conceptualization; investigation; methodology; writing review and editing. Gavin Frank Painter : Conceptualization; funding acquisition; project administration; resources; supervision; writing review and editing. Sarah Louise Draper : Conceptualization; data curation; formal analysis; investigation; methodology; project administration; writing original draft; writing review and editing.
Conflict of Interest
The authors declare no conflict of interest.
Open Research
Data Availability Statement
The data that support the findings of this study are available from the corresponding author upon reasonable request.
Supporting Information
Filename | Description |
---|---|
cpz1898-sup-0001-SuppMat.xlsx16.1 KB | Spreadsheet that can be used to alter the formulation parameters in Basic Protocol 2. |
Please note: The publisher is not responsible for the content or functionality of any supporting information supplied by the authors. Any queries (other than missing content) should be directed to the corresponding author for the article.
Literature Cited
- Albertson, A. H., Kulkarni, J. A., Witzigmann, D., Lind, M., Petersson, K., & Simonsen, J. B. (2022). The role of lipid components in lipid nanoparticles for vaccines and gene therapy. Advanced Drug Delivery Reviews , 188, 114416. https://doi.org/10.1016/j.addr.2022.114416
- Alexopoulou, L., Holt, A., Medzhitov, R., & Flavell, R. A. (2001). Recognition of double-stranded RNA and activation of NF-κB by Toll-like receptor 3. Nature , 413, 732–738. https://doi.org/10.1038/35099560
- Akinc, A., Maier, M. A., Manoharan, M., Fitzgerald, K., Jayaraman, M., Barros, S., Ansell, S., Du, X., Hope, M. J., Madden, T. D., Mui, B. L., Semple, S. C., Tam, Y. K., Ciufolini, M., Witzigmann, D., Kulkarni, J. A., van der Meel, R., & Cullis, P. R. (2019). The Onpattro story and the clinical translation of nanomedicines containing nucleic acid-based drugs. Nature Nanotechnology , 14(12), 1084–1087. https://doi.org/10.1038/s41565-019-0591-y
- Arab, T., Mallick, E. R., Huang, Y., Dong, L., Liao, Z., Zhao, Z., Gololobova, O., Smith, B., Haughey, N. J., Pienta, K. J., Slusher, B. S., Tarwater, P. M., Tosar, J. P., Zivkovic, A. M., Vreeland, W. N., Paulaitis, M. E., & Witwer, K. W. (2021). Characterization of extracellular vesicles and synthetic nanoparticles with four orthogonal single-particle analysis platforms. Journal of Extracellular Vesicles , 10(6), e12079. https://doi.org/10.1002/jev2.12079
- Azarani, A., & Hecker, K. H. (2001). RNA analysis by ion-pair reversed-phase high performance liquid chromatography. Nucleic Acids Research , 29(2), E7. https://doi.org/10.1093/nar/29.2.e7
- Baiersdörfer, M., Boros, G., Muramatsu, H., Mahiny, A., Vlatkovic, I., Sahin, U., & Karikó, K. (2019). A Facile Method for the Removal of dsRNA Contaminant from InVitro -Transcribed mRNA. Molecular Therapy. Nucleic Acids , 15, 26–35. https://doi.org/10.1016/j.omtn.2019.02.018
- Ball, R. L., Bajaj, P., & Whitehead, K. A. (2017). Achieving long-term stability of lipid nanoparticles: Examining the effect of pH, temperature, and lyophilization. International Journal of Nanomedicine , 12, 305–315. https://doi.org/10.2147/IJN.S123062
- Barman, P., Choudhary, A. K., & Geeta, R. (2017). A modified protocol yields high-quality RNA from highly mucilaginous Dioscorea tubers. Biotech , 7(2), 150. https://doi.org/10.1007/s13205-017-0775-9
- Belliveau, N. M., Huft, J., Lin, P. J., Chen, S., Leung, A. K., Leaver, T. J., Wild, A. W., Lee, J. B., Taylor, R. J., Tam, Y. K., Hansen, C. L., & Cullis, P. R. (2012). Microfluidic synthesis of highly potent limit-size lipid nanoparticles for in vivo delivery of siRNA. Molecular Therapy. Nucleic Acids , 1(8), e37. https://doi.org/10.1038/mtna.2012.28
- Beverly, M., Dell, A., Parmar, P., & Houghton, L. (2016). Label-free analysis of mRNA capping efficiency using RNase H probes and LC-MS. Analytical and Bioanalytical Chemistry , 408(18), 5021–5030. https://doi.org/10.1007/s00216-016-9605-x
- Bhattacharjee, S. (2016). DLS and zeta potential – What they are and what they are not? Journal of Controlled Release , 235, 337–351. https://doi.org/10.1016/j.jconrel.2016.06.017
- Blakney, A. K., McKay, P. F., Yus, B. I., Aldon, Y., & Shattock, R. J. (2019). Inside out: Optimization of lipid nanoparticle formulations for exterior complexation and in vivo delivery of saRNA. Gene Therapy , 26(9), 363–372. https://doi.org/10.1038/s41434-019-0095-2
- Borkotoky, S., & Murali, A. (2018). The highly efficient T7 RNA polymerase: A wonder macromolecule in biological realm. International Journal of Biological Macromolecules , 118, 49–56. https://doi.org/10.1016/j.ijbiomac.2018.05.198
- Brader, M. L., Williams, S. J., Banks, J. M., Hui, W. H., Zhou, Z. H., & Jin, L. (2021). Encapsulation state of messenger RNA inside lipid nanoparticles. Biophysical Journal , 120(14), 2766–2770. https://doi.org/10.1016/j.bpj.2021.03.012
- Brisco, M. J., & Morley, A. A. (2012). Quantification of RNA integrity and its use for measurement of transcript number. Nucleic Acids Research , 40(18), e144–e144. https://doi.org/10.1093/nar/gks588
- Buschmann, M. D., Carrasco, M. J., Alishetty, S., Paige, M., Alameh, M. G., & Weissman, D. (2021). Nanomaterial Delivery Systems for mRNA Vaccines. Vaccines , 9(1), 65. https://doi.org/10.3390/vaccines9010065
- Carrasco, M. J., Alishetty, S., Alameh, M. G., Said, H., Wright, L., Paige, M., Soliman, O., Weissman, D., Cleveland, T. E. 4th., Grishaev, A., & Buschmann, M. D. (2021). Ionization and structural properties of mRNA lipid nanoparticles influence expression in intramuscular and intravascular administration. Communications Biology , 4(1), 956. https://doi.org/10.1038/s42003-021-02441-2
- Chaudhary, N., Weissman, D., & Whitehead, K. A. (2021). mRNA vaccines for infectious diseases: Principles, delivery and clinical translation. Nature Reviews Drug Discovery , 20, 817–838. https://doi.org/10.1038/s41573-021-00283-5
- Cheng, X., & Lee, R. J. (2016). The role of helper lipids in lipid nanoparticles (LNPs) designed for oligonucleotide delivery. Advanced Drug Delivery Reviews , 99, 129–137. https://doi.org/10.1016/j.addr.2016.01.022
- Chiron, S., & Jais, P. H. (2017). Non-radioactive monitoring assay for capping of messenger RNA. Journal of Translational Genetics and Genomics , 1, 46–49.
- Clogston, J. D., & Patri, A. K. (2011). Zeta Potential Measurement. In S. E. McNeil (Ed.), Characterization of nanoparticles intended for drug delivery (pp. 63–70). Totowa, NJ: Humana Press.
- Corbett, K. S., Edwards, D. K., Leist, S. R., Abiona, O. M., Boyoglu-Barnum, S., Gillespie, R. A., Himansu, S., Schäfer, A., Ziwawo, C. T., DiPiazza, A. T., Dinnon, K. H., Elbashir, S. M., Shaw, C. A., Woods, A., Fritch, E. J., Martinez, D. R., Bock, K. W., Minai, M., Nagata, B. M., … Graham, B. S. (2020). SARS-CoV-2 mRNA vaccine design enabled by prototype pathogen preparedness. Nature , 586(7830), 567–571. https://doi.org/10.1038/s41586-020-2622-0
- Current Protocols. (2006). Commonly Used Reagents. Current Protocols in Microbiology , 00, A.2A.1–A.2A.15. https://doi.org/10.1002/9780471729259.mca02as00
- Davis, D. L., O'Brie, E. P., & Bentzley, C. M. (2000). Analysis of the degradation of oligonucleotide strands during the freezing/thawing processes using MALDI-MS. Analytical Chemistry , 72(20), 5092–5096. https://doi.org/10.1021/ac000225s
- Decroly, E., Ferron, F., Lescar, J., & Canard, B. (2012). Conventional and unconventional mechanisms for capping viral mRNA. Nature Reviews Microbiology , 10(1), 51–65. https://doi.org/10.1038/nrmicro2675
- Diebold, S., Massacrier, C., Akira, S., Paturel, C., Morel, Y., & Reis e Sousa, C. (2006). Nucleic acid agonists for toll-like receptor 7 are defined by the presence of uridine ribonucleotides. European Journal of Immunology , 36, 3256–3267. https://doi.org/10.1002/eji.200636617
- Dowhan, D. H. (2012). Purification and concentration of nucleic acids. Current Protocols Essential Laboratory Techniques , 6, 5.2.1–5.2.21. https://doi.org/10.1002/9780470089941.et0502s06
- Furuichi, Y. (2015). Discovery of m(7)G-cap in eukaryotic mRNAs. Proceedings of the Japan Academy, Series B , 91(8), 394–409. https://doi.org/10.2183/pjab.91.394
- Gallie, D. R. (1991). The cap and poly(A) tail function synergistically to regulate mRNA translational efficiency. Genes & Development, 5(11), 2108–2116. https://doi.org/10.1101/gad.5.11.2108
- Geall, A. J., Verma, A., Otten, G. R., Shaw, C. A., Hekele, A., Banerjee, K., Cu, Y., Beard, C. W., Brito, L. A., Krucker, T., ’'Hagan, D. T., Singh, M., Mason, P. W., Valiante, N. M., Dormitzer, P. R., Barnett, S. W., Rappuoli, R., Ulmer, J. B., & Mandl, C. W. (2012). Nonviral delivery of self-amplifying RNA vaccines. Proceedings of the National Academy of Sciences , 109(36), 14604–14609. https://doi.org/10.1073/pnas.1209367109
- Green, M. R., & Sambrook, J. (2017). Isolation of high-molecular-weight DNA using organic solvents. Cold Spring Harbour Protocols , 2017(4), pdb–prot093450. https://doi.org/10.1101/pdb.prot093450
- Grier, A. E., Burleigh, S., Sahni, J., Clough, C. A., Cardot, V., Choe, D. C., Krutein, M. C., Rawlings, D. J., Jensen, M. C., Scharenberg, A. M., & Jacoby, K. (2016). pEVL: A linear plasmid for generating mRNA IVT templates with extended encoded poly(A) sequences. Molecular Therapy. Nucleic Acids , 5, e306. https://doi.org/10.1038/mtna.2016.21
- Hassett, K. J., Benenato, K. E., Jacquinet, E., Lee, A., Woods, A., Yuzhakov, O., Himansu, S., Deterling, J., Geilich, B. M., Ketova, T., Mihai, C., Lynn, A., McFadyen, I., Moore, M. J., Senn, J. J., Stanton, M. G., Almarsson, Ö., Ciaramella, G., & Brito, L. A. (2019). Optimization of lipid nanoparticles for intramuscular administration of mRNA vaccines. Molecualr Therapy Nucleic Acids , 15, 1–11. https://doi.org/10.1016/j.omtn.2019.01.013
- Hassett, K. J., Higgins, J., Woods, A., Levy, B., Xia, Y., Hsiao, C. J., Acosta, E., Almarsson, Ö., Moore, M. J., & Brito, L. A. (2021). Impact of lipid nanoparticle size on mRNA vaccine immunogenicity. Journal of Controlled Release , 335(10), 237–246. https://doi.org/10.1016/j.jconrel.2021.05.021
- Henderson, M. I., Eygeris, Y., Jozic, A., Herrera, M., & Sahay, G. (2022). Leveraging biological buffers for efficient messenger RNA delivery via lipid nanoparticles. Molecular Pharmaceutics , 19(11), 4275–4285. https://doi.org/10.1021/acs.molpharmaceut.2c00587
- Henderson, J. M., Ujita, A., Hill, E., Yousif-Rosales, S., Smith, C., Ko, N., McReynolds, T., Cabral, C. R., Escamilla-Powers, J. R., & Houston, M. E. (2021). Cap 1 messenger RNA synthesis with Co-transcriptional CleanCap® analog by in vitro transcription. Current Protocols , 1(2), e39. https://doi.org/10.1002/cpz1.39
- Hou, X., Zaks, T., Langer, R., & Dong, Y. (2021). Lipid nanoparticles for mRNA delivery. Nature Review Materials , 6(12), 1078–1094. https://doi.org/10.1038/s41578-021-00358-0
- Jayaraman, M., Ansell, S. M., Mui, B. L., Tam, Y. K., Chen, J., Du, X., Butler, D., Eltepu, L., Matsuda, S., Narayanannair, J. K., Rajeev, K. G., Hafez, I. M., Akinc, A., Maier, M. A., Tracy, M. A., Cullis, P. R., Madden, T. D., Manoharan, M., & Hope, M. J. (2012). Maximizing the potency of siRNA lipid nanoparticles for hepatic gene silencing in vivo. Angewandte Chemie International Edition in English , 51(34), 8529–8533. https://doi.org/10.1002/anie.201203263
- Jones, L. J., Yue, S. T., Cheung, C.-Y., & Singer, V. L. (1998). RNA quantitation by fluorescence-based solution assay: RiboGreen reagent characterization. Analytical Biochemistry , 265(2), 368–374. https://doi.org/10.1006/abio.1998.2914
- Kafetzis, K. N., Papalamprou, N., McNulty, E., Thong, K. X., Sato, Y., Mironov, A., Purohit, A., Welsby, P. J., Harashima, H., Yu-Wai-Man, C., & Tagalakis, A. D. (2023). The effect of cryoprotectants and storage conditions on the transfection efficiency, stability, and safety of lipid-based nanoparticles for mRNA and DNA delivery. Advanced Healthcare Materials , 12, 2203022. https://doi.org/10.1002/adhm.202203022
- Karikó, K., Buckstein, M., Ni, H., & Weissman, D. (2005). Suppression of RNA recognition by toll-like receptors: The impact of nucleoside modification and the evolutionary origin of RNA immunity. Immunity , 23(2), 165–175. https://doi.org/10.1016/j.immuni.2005.06.008
- Kauffman, K. J., Dorkin, J. R., Yang, J. H., Heartlein, M. W., DeRosa, F., Mir, F. F., Fenton, O. S., & Anderson, D. G. (2015). Optimization of lipid nanoparticle formulations for mRNA delivery in vivo with fractional factorial and definitive screening designs. Nano Letters , 15(11), 7300–7306. https://doi.org/10.1021/acs.nanolett.5b02497
- Kellman, B. P., Baghdassarian, H. M., Pramparo, T., Shamie, I., Gazestani, V., Begzati, A., Li, S., Nalabolu, S., Murray, S., Lopez, L., Pierce, K., Courchesne, E., & Lewis, N. E. (2021). Multiple freeze-thaw cycles lead to a loss of consistency in poly(A)-enriched RNA sequencing. BMC Genomics , 22(1), 69. https://doi.org/10.1186/s12864-021-07381-z
- Kim, S. C., Sekhon, S. S., Shin, W.-R., Ahn, G., Cho, B.-K., Ahn, J.-Y., & Kim, Y.-H. (2022). Modifications of mRNA vaccine structural elements for improving mRNA stability and translation efficiency. Molecular & Cellular Toxicology, 18(1), 1–8. https://doi.org/10.1007/s13273-021-00171-4
- Kim, B., Hosn, R. R., Remba, T., Yun, D., Li, N., Abraham, W., Melo, M. B., Cortes, M., Li, B., Zhang, Y., Dong, Y., & Irvine, D. J. (2023). Optimization of storage conditions for lipid nanoparticle-formulated self-replicating RNA vaccines. Journal of Controlled Release , 353, 241–253. https://doi.org/10.1016/j.jconrel.2022.11.022
- Kis, Z., Kontoravdi, C., Shattock, R., & Shah, N. (2021). Resources, production scales and time required for producing RNA vaccines for the global pandemic demand. Vaccines , 9(1), 3. Retrieved from https://www.mdpi.com/2076-393X/9/1/3
- Koontz, L. (2013). Chapter four – agarose gel electrophoresis. In J. Lorsch (Ed.), Methods in Enzymology (Vol. 529, pp. 35–45). Academic Press. https://doi.org/10.1016/B978-0-12-418687-3.00004-5
- Korenč, M., Mencin, N., Puc, J., Skok, J., Nemec, K. Š., Celjar, A. M., Gagnon, P., Štrancar, A., & Sekirnik, R. (2021). Chromatographic purification with CIMmultus™ Oligo dT increases mRNA stability. Cell and Gene Therapy Insights , 7(9), 1207. https://doi.org/10.18609/cgti.2021.161
- Kulkarni, J. A., Darjuan, M. M., Mercer, J. E., Chen, S., van der Meel, R., Thewalt, J. L., Tam, Y. Y. C., & Cullis, P. R. (2018). On the formation and morphology of lipid nanoparticles containing ionizable cationic lipids and siRNA. ACS Nano , 12(5), 4787–4795. https://doi.org/10.1021/acsnano.8b01516
- Kulkarni, J. A., Thomson, S. B., Zaifman, J., Leung, J., Wagner, P. K., Hill, A., Tam, Y. Y. C., Cullis, P. R., Petkau, T. L., & Leavitt, B. R. (2020). Spontaneous, solvent-free entrapment of siRNA within lipid nanoparticles. Nanoscale , 12(47), 23959–23966. https://doi.org/10.1039/d0nr06816k
- Lee, P. Y., Costumbrado, J., Hsu, C. Y., & Kim, Y. H. (2012). Agarose gel electrophoresis for the separation of DNA fragments. Journal of Visualized Experiments: JoVE , (62), 3923. https://doi.org/10.3791/3923
- Leung, A. K. K., Leung, Y. C., Tam, S. C., Ismail, M. H., & Cullis, P. R. (2015). Microfluidic mixing: A general method for encapsulating macromolecules in lipid nanoparticle systems. The Journal of Physical Chemistry B , 119(28), 8698–8706. https://doi.org/10.1021/acs.jpcb.5b02891
- Li, S., Hu, Y., Li, A., Lin, J., Hsieh, K., Schneiderman, Z., Zhang, P., Zhu, Y., Qiu, C., Kokkoli, E., Wang, T. H., & Mao, H. Q. (2022). Payload distribution and capacity of mRNA lipid nanoparticles. Nature Communications , 13, 5561. https://doi.org/10.1038/s41467-022-33157-4
- Linares-Fernández, S., Lacroix, C., Exposito, J.-Y., & Verrier, B. (2020). Tailoring mRNA vaccine to balance innate/adaptive immune response. Trends in Molecular Medicine , 26(3), 311–323. https://doi.org/10.1016/j.molmed.2019.10.002
- Ly, H. H., Daniel, S., Soriano, S. K. V., Kis, Z., & Blakney, A. K. (2022). Optimization of lipid nanoparticles for saRNA expression and cellular activation using a design-of-experiment approach. Molecular Pharmaceutics , 19(6), 1892–1905. https://doi.org/10.1021/acs.molpharmaceut.2c00032
- Malmanger, B., Harrington, C., & Savage, S. (2010). Comparison of agilent 2100 bioanalyzer and caliper life sciences GX II in functionality, total RNA scoring algorithms and reproducibility to evaluate total RNA integrity. Journal of Biomolecular Techniques , 23, (Suppl), S49–S50.
- Masek, T., Vopalensky, V., Suchomelova, P., & Pospisek, M. (2005). Denaturing RNA electrophoresis in TAE agarose gels. Analytical Biochemistry , 336(1), 46–50. https://doi.org/10.1016/j.ab.2004.09.010
- Maurer, N., Wong, K. F., Stark, H., Louie, L., McIntosh, D., Wong, T., Scherrer, P., Semple, S. C., & Cullis, P. R. (2001). Spontaneous entrapment of polynucleotides upon electrostatic interaction with ethanol-destabilized cationic liposomes. Biophysical Journal , 80(5), 2310–2326. https://doi.org/10.1016/S0006-3495(01)76202-9
- Mikkola, S., Kaukinen, U., & Lönnberg, H. (2001). The effect of secondary structure on cleavage of the phosphodiester bonds of RNA. Cell biochemistry and Biophysics , 34(1), 95–119. https://doi.org/10.1385/CBB:34:1:95
- Nakamura, T., Kawai, M., Sato, Y., Maeki, M., Tokeshi, M., & Harashima, H. (2020). The effect of size and charge of lipid nanoparticles prepared by microfluidic mixing on their lymph node transitivity and distribution. Molecular Therapeutics , 17(3), 944–953. https://doi.org/10.1021/acs.molpharmaceut.9b01182
- Passmore, L. A., & Coller, J. (2022). Roles of mRNA poly(A) tails in regulation of eukaryotic gene expression. Nature Reviews Molecular Cell Biology , 23(2), 93–106. https://doi.org/10.1038/s41580-021-00417-y
- Patel, P., Ibraham, N. M., & Cheng, K. (2021). The importance of apparent pKa in the development of nanoparticles encapsulating siRNA and mRNA. Trends in Pharmacological Sciences , 42(6), 448–460. https://doi.org/10.1016/j.tips.2021.03.002
- Patel, R., Kaki, M., Potluri, V. S., Kahar, P., & Khanna, D. (2022). A comprehensive review of SARS-CoV-2 vaccines: Pfizer, Moderna & Johnson & Johnson. Human Vaccines & Immunotherapeutics, 18(1), 2002083. https://doi.org/10.1080/21645515.2021.2002083
- Pogocki, D., & Schöneich, C. (2000). Chemical stability of nucleic acid-derived drugs. Journal of Pharmaceutical Sciences , 89(4), 443–456. https://doi.org/10.1002/(SICI)1520-6017(200004)89:4<443::AID-JPS2>3.0.CO;2-W
- Pregeljc, D., Skok, J., Vodopivec, T., Mencin, N., Krušič, A., Ličen, J., Nemec, K. Š., Štrancar, A., & Sekirnik, R. (2023). Increasing yield of in vitro transcription reaction with at-line high pressure liquid chromatography monitoring. Biotechnology and Bioengineering , 120(3), 737–747. https://doi.org/10.1002/bit.28299
- Qin, S., Tang, X., Chen, Y., Chen, K., Fan, N., Xiao, W., Zheng, Q., Li, G., Teng, Y., Wu, M., & Song, X. (2022). mRNA-based therapeutics: Powerful and versatile tools to combat diseases. Signal Transduction and Targeted Therapy , 7(1), 166. https://doi.org/10.1038/s41392-022-01007-w
- Reichmuth, A. M., Oberli, M. A., Jaklenec, A., Langer, R., & Blankschtein, D. (2016). mRNA vaccine delivery using lipid nanoparticles. Therapeutic Delivery , 7(5), 319–334. https://doi.org/10.4155/tde-2016-0006
- Rio, D. C., Ares, M., Hannon, G. J., & Nilson, T. W. (2010). Polyacrylamide Gel Electrophoresis of RNA. Cold Spring Harbour Protocols , 6, pdb–prot5444. https://doi.org/10.1101/pdb.prot5444
- Ripoll, M., Martin, E., Enot, M., Robbe, O., Rapisarda, C., Nicolai, M. C., Deliot, A., Tabeling, P., Authelin, J. R., Nakach, M., & Wils, P. (2022). Optimal self-assembly of lipid nanoparticles (LNP) in a ring micromixer. Scientific Reports , 12(1), 9483. https://doi.org/10.1038/s41598-022-13112-5
- Sabnis, S., Kumarasinghe, E. S., Salerno, T., Mihai, C., Ketova, T., Senn, J. J., Lynn, A., Bulychev, A., McFadyen, I., Chan, J., Almarsson, Ö., Stanton, M. G., & Benenato, K. E. (2018). A novel amino lipid series for mRNA delivery: Improved endosomal escape and sustained pharmacology and safety in non-human primates. Molecular Therapy , 26(6), 1509–1519. https://doi.org/10.1016/j.ymthe.2018.03.010
- Schindelin, J., Arganda-Carreras, I., Frise, E., Kaynig, V., Longair, M., Pietzsch, T., Preibisch, S., Rueden, C., Saalfeld, S., Schmid, B., Tinevez, J. Y., White, D. J., Hartenstein, V., Eliceiri, K., Tomancak, P., & Cardona, A. (2012). Fiji: An open-source platform for biological-image analysis. Nature Methods , 9(7), 676–682. https://doi.org/10.1038/nmeth.2019
- Schoenmaker, L., Witzigmann, D., Kulkarni, J. A., Verbeke, R., Kersten, G., Jiskoot, W., & Crommelin, D. J. A. (2021). mRNA-lipid nanoparticle COVID-19 vaccines: Structure and stability. International Journal of Pharmaceutics , 601, 120586. https://doi.org/10.1016/j.ijpharm.2021.120586
- Schonborn, J., Oberstraβ, J., Breyel, E., Tittgen, J., Schumacher, J., & Lukacs, N. (1991). Monoclonal antibodies to double-stranded RNA as probes of RNA structure in crude nucleic acid extracts. Nucleic Acids Research , 19(11), 2993–3000. https://doi.org/10.1093/nar/19.11.2993
- Schroeder, A., Mueller, O., Stocker, S., Salowsky, R., Leiber, M., Gassmann, M., Lightfoot, S., Menzel, W., Granzow, M., & Ragg, T. (2006). The RIN: An RNA integrity number for assigning integrity values to RNA measurements. BMC Molecular Biology , 7, 3. https://doi.org/10.1186/1471-2199-7-3
- Stepinski, J., Waddell, C., Stolarski, R., Darzynkiewicz, E., & Rhoads, R. E. (2001). Synthesis and properties of mRNAs containing the novel “anti-reverse” cap analogs 7-methyl(3′-O-methyl)GpppG and 7-methyl(3′-deoxy)GpppG. RNA , 7(10), 1486–1495. https://doi.org/10.1017/S1355838201014078
- Stetefeld, J., McKenna, S. A., & Patel, T. R. (2016). Dynamic light scattering: A practical guide and applications in biomedical sciences. Biophysical Reviews , 8(4), 409–427. https://doi.org/10.1007/s12551-016-0218-6
- Tenchov, R., Bird, R., Curtze, A. E., & Zhou, Q. (2021). Lipid Nanoparticles─From Liposomes to mRNA Vaccine Delivery, a Landscape of Research Diversity and Advancement. ACS nano , 15(11), 16982–17015. https://doi.org/10.1021/acsnano.1c04996
- Vaidyanathan, S., Azizian, K. T., Haque, A. K. M. A., Henderson, J. M., Hendel, A., Shore, S., Antony, J. S., Hogrefe, R. I., Kormann, M. S. D., Porteus, M. H., & McCaffrey, A. P. (2018). Uridine depletion and chemical modification increase Cas9 mRNA activity and reduce immunogenicity without HPLC purification. Molecular Therapy. Nucleic Acids , 12, 530–542. https://doi.org/10.1016/j.omtn.2018.06.010
- Vlatkovic, I., Ludwig, J., Boros, G., Szabó, G. T., Reichert, J., Buff, M., Baiersdörfer, M., Reinholz, J., Mahiny, A. J., Şahin, U., & Karikó, K. (2022). Ribozyme assays to quantify the capping efficiency of in vitro-transcribed mRNA. Pharmaceutics , 14(2), 328. https://doi.org/10.3390/pharmaceutics14020328
- Walker, S. E., & Lorsch, J. (2013). Chapter Nineteen – RNA Purification – Precipitation Methods. In J. Lorsch (Ed.), Methods in Enzymology (Vol. 530, pp. 337–343): Academic Press.
- Webb, C., Khadke, S., Schmidt, S. T., Roces, C. B., Forbes, N., Berrie, G., & Perrie, Y. (2019). The impact of solvent selection: Strategies to guide the manufacturing of liposomes using microfluidics. Pharmaceutics , 11(12), 653. https://doi.org/10.3390/pharmaceutics11120653
- Zhang, J., Fan, H., Levorse, D. A., & Crocker, L. S. (2011). Ionization behavior of amino lipids for siRNA delivery: Determination of ionization constants, SAR, and the impact of lipid pKa on cationic lipid-biomembrane interactions. Langmuir , 27(5), 1907–1914. https://doi.org/10.1021/la104590k
- Zhao, P., Hou, X., Yan, J., Du, S., Xue, Y., Li, W., Xiang, G., & Dong, Y. (2020). Long-term storage of lipid-like nanoparticles for mRNA delivery, Bioactive Materials , 5(2), 358–363. https://doi.org/10.1016/j.bioactmat.2020.03.001
Internet Resources
- https://www.nebiolabs.co.nz/protocols/0001/01/01/dna-template-preparation-e2040
- New England Biolabs: DNA Template Preparation (E2040).
- https://www.nebiolabs.co.nz/protocols/0001/01/01/standard-rna-synthesis-e2040
- New England Biolabs: Standard RNA Synthesis (E2040).