Transcriptional Profiling of Skeletal Muscle Stem Cells After In Vivo Engraftment into a Heterologous Niche Environment
Felicia Lazure, Felicia Lazure, Darren M. Blackburn, Darren M. Blackburn, Vahab D. Soleimani, Vahab D. Soleimani
Abstract
Adult stem cells play a critical role in the maintenance and repair of the organs in which they reside. However, their function is highly dependent on the crosstalk with their niche environment that changes during development and in disease states. The niche provides signals to stem cells to activate, proliferate, self-renew, or remain in quiescence. In skeletal muscle, the niche is perturbed in disease contexts such as aging, muscular dystrophies, and cachexia. Therefore, it is important to develop methods that permit the decoupling of niche-mediated from cell-intrinsic changes that occur in muscle stem cells (MuSCs) in development and disease contexts. With the purpose of determining the effect of the niche environment on the MuSC transcriptome, function, or health, we have coupled an allogeneic stem cell transplantation system, meaning the transplantation of MuSCs from a donor mouse into a recipient host mouse, with Switching Mechanism at 5’ End of RNA Template (SMART-Seq) to quantify the effects of the niche on the MuSC transcriptome in vivo. Briefly, MuSCs are isolated from a GFP reporter donor mouse (Pax7-nGFP) and transplanted into the irradiated muscles of immunocompromised allogeneic hosts. The MuSCs are re-isolated by fluorescence-activated cell sorting (FACS) after three weeks of inhabiting the heterologous niche, defined as a niche that is different from their originating niche, and sequencing-ready libraries are created. This method allows for the direct comparison of the transcriptome of stem cells before and after transplantation into a host of a different age, disease status, or genetic background. This method can be used to accurately quantify the direct effect of the niche environment on the stem cell gene expression profile and to decouple cell-intrinsic versus niche-mediated alterations in the stem cell transcriptome. © 2023 The Authors. Current Protocols published by Wiley Periodicals LLC.
Basic Protocol : Allogeneic muscle stem cell transplantation
INTRODUCTION
Muscle stem cells (MuSCs) are imperative for muscle tissue regeneration. When given certain cues, such as in the case of injury, they can activate, differentiate, and repair damaged skeletal muscles (Nguyen et al., 2019; Relaix et al., 2021). However, it is equally important for MuSC activation to be suppressed under homeostatic conditions to maintain quiescence and prevent exhaustion of the stem cell pool (Feige et al., 2018; Relaix et al., 2021). Both processes of MuSC activation and maintenance of quiescence are tightly dependent on communication with their niche environment (Nguyen et al., 2019; Yin et al., 2013). The niche environment includes neighboring cell types such as fibro-adipogenic progenitor cells (FAPs), immune cells, the myofibers upon which the MuSCs reside, and the extracellular matrix (ECM) that surrounds the MuSCs among others (Andrea J. de Micheli et al., 2020; Yin et al., 2013). Understanding how the niche regulates MuSC function is a current topic of great interest, and as such, has led to the generation of multiple in vitro niche models (Laternser et al., 2018; Lee et al., 2020; Maffioletti et al., 2018; Moyle et al., 2020; Quarta et al., 2016), sometimes employing bioengineering methods (Laternser et al., 2018; Lee et al., 2020; Moyle et al., 2020).
Here we present a protocol that can help determine the direct effect of the niche environment on MuSCs under various conditions in vivo. More specifically, this technique involves the transplantation of donor MuSCs into an allogeneic aged host followed by re-isolation of the donor cells after a defined period of exposure to heterologous niche and subsequent transcriptional profiling using SMART-Seq (Switching Mechanism at 5’ End of RNA Template) (Picelli et al., 2014). Thus, this method allows for the direct comparison of the MuSC transcriptome before and after transplantation into an allogeneic host. With proper controls, any observed changes in the transcriptome can therefore be attributed to the host niche environment.
Previous methods have utilized MuSC transplantation for lineage tracing or regeneration studies (Feige & Rudnicki, 2020) and the encapsulation of MuSCs to determine the effect of systemic factors on stem cells (Mashinchian et al., 2022). MuSC transplantation has been used to verify the capacity of MuSCs to lead to the regeneration of new fibers in injured TA muscles under different experimental conditions (Liu et al., 2018; Price et al., 2014). This has been done through histological staining, quantification of fibers of the same color as donor-engrafted cells, or quantification of newly formed dystrophin-positive fibers in the case of the MDX model (Feige & Rudnicki, 2020; Liu et al., 2018; Price et al., 2014; Wang et al., 2019). While MuSC transplantation is routinely used, the novelty of our method is the ability to couple stem cell transplantation with SMART-seq to directly quantify the effect of the entirety of the niche environment, allowing direct comparison of transcriptomic changes before and after transplantation. The use of SMART-Seq technology to generate sequencing-ready libraries for RNA-Seq has the advantage of being capable of using a much lower amount of starting material—in our hands, we have used as few as 38 cells—while simultaneously offering similar depth and coverage as traditional bulk RNA-Seq. However, a disadvantage is that re-isolated MuSCs are pooled in a single reaction, precluding the separation of different subpopulations. Therefore, this technique does not allow the study of how the niche may affect cell population heterogeneity. However, we anticipate that this method can be adapted for use with single-cell RNA sequencing, along with other epigenetic sequencing techniques, while maintaining the same method of transplantation. As a whole, this method allows for the study of the effect of the niche in its entirety on the function of MuSCs. This is in contrast to other techniques, such as parabiosis, which account for only a single aspect of the niche environment. The application of this technique can answer the question of whether the transcriptional changes in MuSC observed in various disease contexts are niche-induced and reversible after transplantation into a healthy host or cell-intrinsic, and thus irreversible after transplantation. The data generated from such studies will have important implications for determining targetable genetic networks and pathways for the development of new therapies.
In this article, we will detail the steps and techniques necessary for the transplantation and re-isolation of MuSCs, along with the generation of RNA-Seq libraries. This method will allow for MuSCs from a specific niche environment to be transplanted into a new one, enabling analysis of the effect of the niche in its entirety on the MuSC transcriptome.
NOTE: All protocols involving animals must be reviewed and approved by the appropriate Animal Care and Use Committee and must follow regulations for the care and use of laboratory animals. Appropriate informed consent is necessary for obtaining and use of human study material.
STRATEGIC PLANNING
The process of allogeneic MuSC transplantation involves isolation of a GFP-reporter cell type of interest, injection of fluorescent donor cells into host mice followed by re-isolation of these cells by FACS, and comparison of their transcriptomes before and after engraftment using SMART-seq (Fig. 1). In order to promote donor cell engraftment into the host niche, the first step involves the irradiation of host hindlimbs (Gross et al., 1999; Morgan et al., 1993; Wernig et al., 2000) (Fig. 2A-B). Without irradiation or injury, donor cell engraftment is very inefficient (Boldrin et al., 2012; Meng et al., 2015). While it is unknown by which precise mechanism irradiation induces MuSC engraftment, studies have shown that reduced competition by host cells and altered niche cell properties are likely to play a role (Boldrin et al., 2012; Doreste et al., 2020). To irradiate, mice are anesthetized and positioned in a MultiRad255 irradiation machine, or equivalent setup, such that only one hindlimb is exposed to the irradiation (Fig. 2A). Two days post-irradiation, counting of donor cells by FACS using counting beads shows a depletion in the host MuSC pool (Fig. 2B).
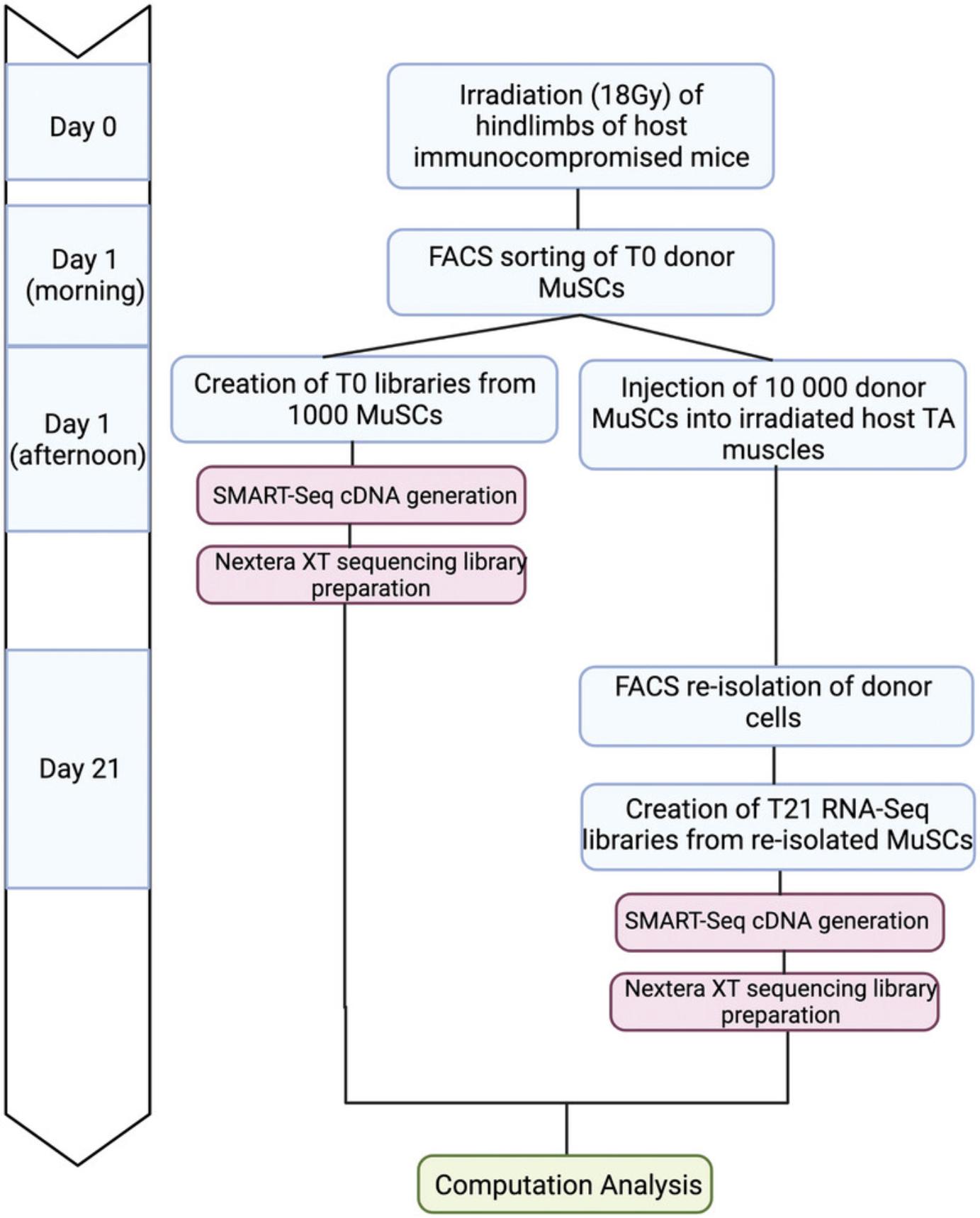
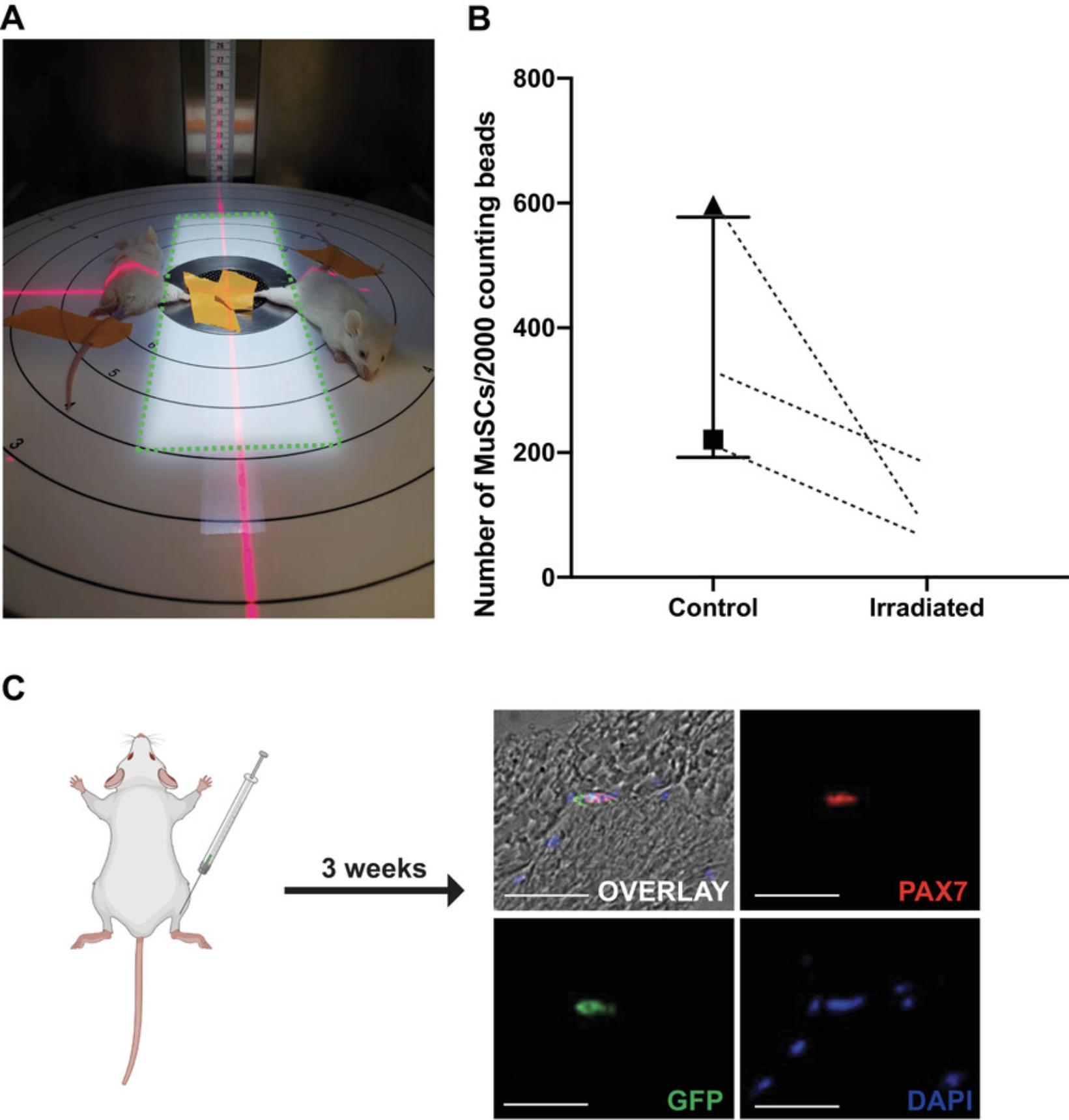
The next step is to use FACS to sort T0 donor MuSCs from a fluorescent reporter mouse, such as Pax7-nGFP (Fig. 3). The fluorescent reporter is necessary to re-isolate only donor cells after transplantation. Other available fluorescent reporter mice, such as Myf5-Cre/ROSA-YFP (Kuang et al., 2007) or Pax7-tdTomato (Keefe et al., 2015; Pawlikowski et al., 2015), may also be employed, depending on the goals and the hypothesis in question. A traditional muscle digestion method using a collagenase/dispase buffer can be used to dissociate muscle cells for sorting, followed by the addition of a live-dead marker (Pasut et al., 2012). To sort for live MuSCs, the DAPI-low, GFP-high population must be gated to isolate viable cells (Fig. 3A). It is important to work quickly after the FACS sorting to create T0 cDNA libraries using SMART-Seq, as well as to inject the rest of the cells (10,000 to 20,000 per host) into the irradiated tibialis anterior (TA) muscles of the host mice. Waiting too long after the sort can cause the mRNA from the T0 SMART-Seq sample to degrade and reduces the viability of the cells to be transplanted. Therefore, it is best to sort the initial 1000 cells for T0 library generation and immediately process these samples using SMART-Seq while waiting for the rest of the cells to be sorted for the engraftment step.
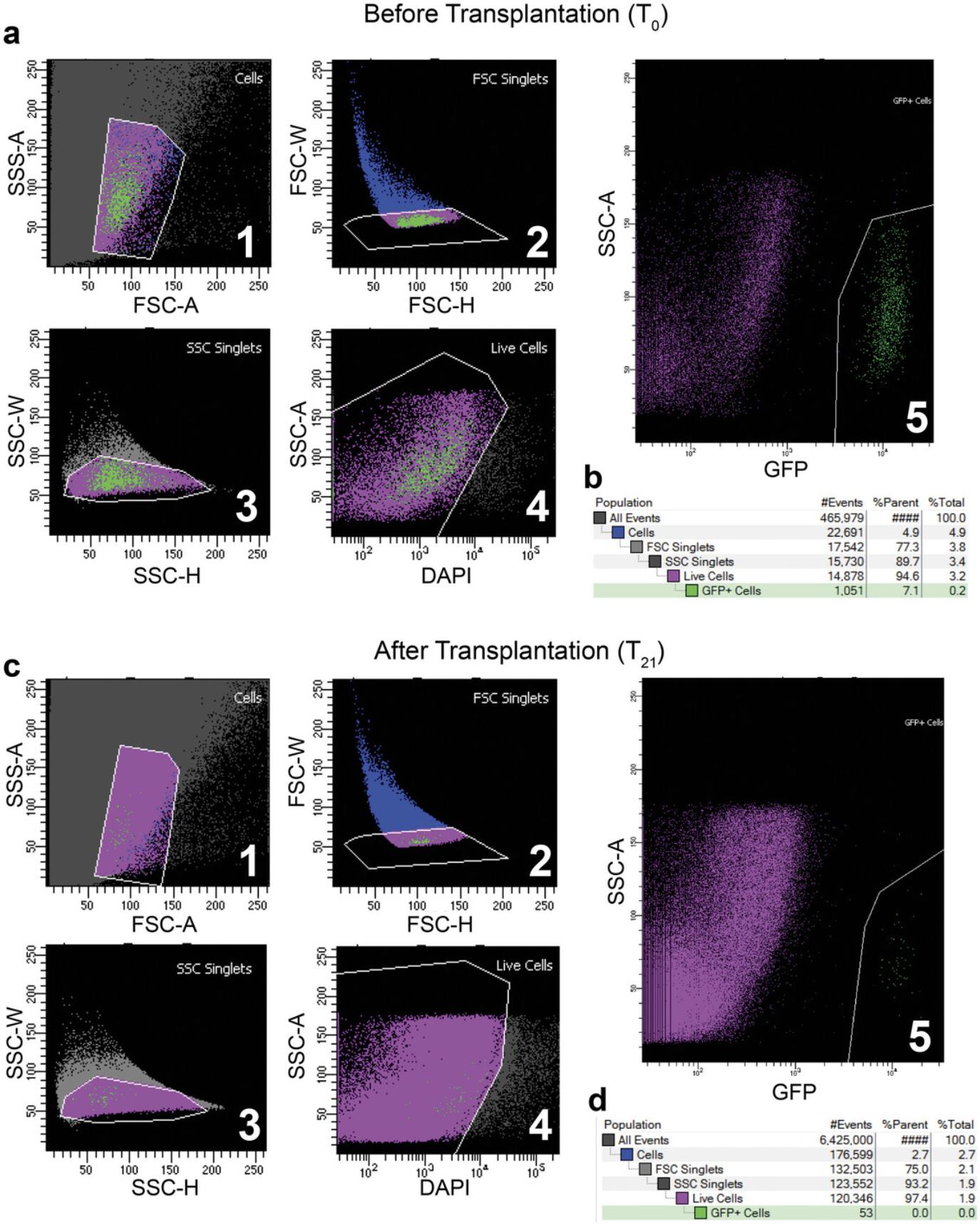
Next, to inject the remaining donor cells into the hosts, donor cells suspended in 2% FBS/PBS are aspirated into a Hamilton syringe, and host mice are anesthetized by isoflurane. Ideally, donor cells are injected into two separate injection sites within the TA muscle, each in a volume of 10 µl. The two injection sites increase the probability of successful engraftment. Throughout these steps, it is important to keep the immune-compromised host mice under sterile conditions.
To ensure sufficient time to home to the niche, the MuSC re-isolation procedure occurs 21 days post-engraftment (T21). Depending on the purpose of the experiment, this time can be extended. To re-isolate the transplanted donor cells, the lower hindlimb muscles of host mice are digested and undergo the same FACS strategy as described above. Given the fluorescence of the donor MuSCs, they can be clearly separated from host cells. The number of GFP+/DAPI- donor cells within the muscles of the host will be greatly reduced due to low engraftment efficiency (Fig. 3). However, the application of SMART-Seq circumvents the limitation on cell number and we were able to generate sequencing-ready libraries from as few as 38 engrafted cells (Table 1).
Samples | Number of cells used | Number of SMART-Seq PCR cycles | Yield after SMART-Seq (ng/µl) | Concentration after Nextera XT library prep starting with 0.15 ng (ng/µl) | Average library size | Number of reads | Number of aligned reads | Number of unique reads | Percent aligned reads | Percent unique reads |
---|---|---|---|---|---|---|---|---|---|---|
Aged T0 | 1000 | 11 | 0.253 | 2.286 | 380 | 58870695 | 32673727 | 11446097 | 55.5 | 19.4 |
1000 | 11 | 0.321 | 1.378 | 400 | 49688879 | 28086575 | 9897017 | 56.5 | 19.9 | |
Young T0 | 1000 | 11 | 1.33 | 1.22 | 467 | 42920426 | 26667590 | 9298834 | 62.1 | 21.7 |
1000 | 11 | 1.36 | 2.9 | 428 | 51092371 | 33301402 | 11165875 | 65.2 | 21.9 | |
1000 | 11 | 4.11 | 1.083 | 504 | 58770931 | 39423797 | 13844857 | 67.1 | 23.6 | |
Aged T21 | 38 | 16 | 0.533 | 1.076 | 478 | 47596086 | 31201999 | 10665092 | 65.6 | 22.4 |
171 | 14 | 0.539 | 2.163 | 472 | 52524544 | 35119392 | 11935463 | 66.9 | 22.7 | |
Young T21 | 187 | 14 | 2.194 | 2.502 | 396 | 48128644 | 21102721 | 8144666 | 43.8 | 16.9 |
205 | 14 | 1.038 | 2.198 | 509 | 46738177 | 27925087 | 10317629 | 59.7 | 22.1 | |
Average | N/A | N/A | N/A | 1.641 | 448.2 | 50703417 | 30611365.6 | 10746170 | 60.3 | 21.2 |
Isolated cells are sorted directly into SMART-Seq 1× reaction buffer, which will immediately lyse the cells upon sorting. This sample must be directly processed using SMART-Seq to generate cDNA from freshly sorted cells. SMART-Seq allows the reverse transcription of minute quantities of cDNA due to its template-switching technology (Picelli et al., 2014). Briefly, mRNA is selectively captured for amplification from the lysed cells using an Oligo(dT) primer, avoiding the need for sample purification and ribosomal RNA depletion. An MMLV reverse transcriptase then reverse transcribes these mRNA templates. Importantly, its template-switching properties allow for the reverse transcription of a hybridized SMART-Seq oligo onto the template strand once it has reached the 5′ end. The SMART-Seq oligo allows for subsequent PCR amplification using complementary primers, all within the same tube (Picelli et al., 2014). After removal of primer dimers by AMPure XP purification, cDNA can then be used for Illumina sequencing library preparation using Nextera XT (Adey et al., 2010), which employs Tn5 transposase technology (Adey et al., 2010; Gertz et al., 2012; Head et al., 2014). The Tn5 transposase allows for simultaneous cleavage of cDNA fragments while adding indexed Illumina adaptors. This procedure is highly efficient in generating RNA-seq libraries from MuSCs after engraftment into a heterologous niche in vivo.
In order to determine which genes are regulated by the niche compared to which remain unchanged after transplantation, it is important to control for the process of engraftment itself. It is possible that the entire procedure, from irradiation to injection of donor cells, may alter the donor cell transcriptome due to stress. Therefore, it is important to have a control where the donor and host are of the same experimental condition (for example, same age if age is the experimental variable) to determine which changes are caused specifically by the transplantation. These “background” changes can then be accounted for when comparing the transcriptome of the donor cells before and after transplantation in the experimental group.
While this protocol is specifically designed for transcriptome analysis before and after transplantation, this method has the potential to be adapted to study a wide range of genomic or functional outputs of cells pre- and post-transplantation. For example, low-input ATAC-Seq of donor cells before and after transplantation can be employed to study how the epigenome of MuSCs changes due to an allogeneic niche. Additionally, donor cells can be analyzed by immunofluorescence staining by using cytospin to fix cells directly after isolation. As a more functional assay, MuSCs before and after transplantation can also be assessed in vitro for their proliferative, cell cycle, or differentiation properties in culture.
Basic Protocol: ALLOGENEIC MUSCLE STEM CELL TRANSPLANTATION
The MuSC niche environment is an important player in the regulation of MuSC function. However, it can be challenging to determine the effect the niche has on the cells outside of the intrinsic changes that may be occurring due to age or a disease state. The method of allogeneic stem cell transplantation relies on a reporter mouse, where the donor cells are labeled with a fluorescent protein such as GFP. These cells will be isolated from the donor mouse using FACS and then injected into a host mouse, where they will be exposed to the new niche for a defined period. Afterward, the donor cells will be reisolated, with the fluorescent protein allowing for the donor cells to be distinguished from the host cells, and RNA-Seq libraries will be generated using SMART-Seq.
NOTE: All protocols involving animals must be reviewed and approved by the appropriate Animal Care and Use Committee and must follow regulations for the care and use of laboratory animals. Appropriate informed consent is necessary for obtaining and use of human study material.
Materials
- Host mice: NOD-Prkdcem26Cd52Il2rgem26Cd22/NjuCrl (NCG) (Charles River)
- Rodent anesthesia cocktail (see recipe)
- Normal saline, 0.9% NaCl
- Donor mice: Tg(Pax7-EGFP)#Tajb (Pax7-nGFP) (Sambasivan et al., 2009)
- 1× PBS
- FACS Digestion Buffer (see recipe)
- Ham's F10 medium (Gibco, 11550043)
- FBS
- 0.5 M EDTA
- DAPI
- Collagenase D (Sigma Aldrich, 11088882001)
- Dispase II (Roche, 4942078001)
- SMART-Seq HT kit (Takara bio, 634456)
- SMART-Seq Reaction buffer (see recipe)
- Nextera XT DNA library preparation kit (Illumina, FC-131-1096)
- TD buffer
- NT buffer
- NPM buffer
- ATM buffer
- Ampure XP beads (Beckman Coulter, A63880)
- Quant-iT PicoGreen dsDNA Assay kit (Invitrogen, P11496)
- Nextera XT Index Kit v2 Set A (96 indexes, 384 samples) (Illumina, FC-131-2001)
- GelGreen dye (41005, Biotium)
- MultiRad225 Irradiation machine (Faxitron)
- 6-cm plates
- 15-ml polypropylene round-bottom Falcon tubes
- Miniature Tilt Platform (Thermo Fisher, T400110)
- 40-µm cell strainers (Falcon, C352340)
- FACSAria Fusion cytometer (BD Biosciences)
- 0.2-ml PCR tubes 10-μl syringe (Hamilton, 80030)
- C1000 Touch Thermal Cycler (Bio-Rad)
- Next Generation Sequencing Platform (Genome Analyzer)
Irradiation of host mice
1.Anesthetize host mice with rodent cocktail (see recipe) by intraperitoneal (IP) injection.
NOTE: All protocols involving animals must be reviewed and approved by the appropriate Animal Care and Use Committee and must follow regulations for the care and use of laboratory animals.
2.Once unconscious, place mice into MultiRad 225 irradiation machine such that their hindlimb is within the X-ray field on the turntable. Make sure that the rest of the body is outside the X-ray field, and secure the leg in place with adhesive tape.
3.On the Multirad225 irradiation machine, set the filter to Cu 0.50 mm, and set the dose to 18 Gy. Press “begin” to administer 18 Gy irradiation to the hindlimb of the host mice.
4.When irradiation is complete, remove the mice from the machine and perform a subcutaneous injection of 1 ml of saline per mouse for hydration. Keep mice in a 37°C incubator until they regain consciousness post-anesthesia. This procedure should take between 15 and 45 min.
Isolation of donor cells by fluorescence-activated cell sorting (FACS)
5.16-20 hr after the irradiation of host mice, sacrifice donor reporter mice (ex: Pax7-nGFP) by CO2 inhalation in preparation for FACS (Fluorescence-Activated cell sorting).
6.Dissect the hindlimb muscles of the upper and lower leg, and place in a 6-cm plate containing cold PBS until the dissection is complete. The plate can be kept at room temperature until the next step.
7.Using forceps, remove visible pieces of fat, tendons, and hair from dissected muscle pieces.
8.Remove PBS and replace with 1 ml fresh PBS. Use scissors to mince the muscle into a slurry.
9.Transfer muscle slurry to a 15-ml falcon tube and add 6 ml of FACS digestion buffer.
10.Place tube of muscle slurry in a 37°C/5% CO2 incubator on a miniature rocking platform at 20 rpm for 30 min.
11.After 30 min, spin down muscle slurry at 600 × g for 30 s to pellet undigested tissue. Released mononuclear cells will remain in the supernatant.
12.Transfer supernatant to a tube containing 9 ml FBS and keep on ice, then add 4 ml of digestion buffer to remaining undigested tissue.
13.Perform a second digestion (steps 9-10) for 25 min in a 37°C/5% CO2 incubator.
14.Combine both digestions and filter through a 40-µm filter into a new tube.
15.Spin down the samples in a centrifuge for 18 min at 600 × g and 4°C and remove supernatant
16.Resuspend sample in 1 ml of 2%FBS in PBS (v:v) with 0.5 mM EDTA and 1 µl of 5 mg/ml DAPI
17.Proceed with flow cytometry, and gate for the GFP+/DAPI- MuSC population (Fig. 3). For troubleshooting see Table 2.
Problem | Possible reason | Solution |
---|---|---|
Low number of donor cells isolated | Insufficient digestion | Increase muscle mincing time by 5 min to ensure complete mincing. Increase digestion time by 5-10 min to ensure no visible chunks remain. |
FACS machine clogging/sorting slowly | Final cell suspension too thick |
Ensure that the original dissection and cleaning steps are done properly i.e., remove as much blood, fat, and tendon as possible. Increase the cell resuspension volume and filter an additional time with a 40 µm cell strainer. Do not over-digest the sample (over 1.5 hr) which can lead to excess debris. Increase the concentration of EDTA in the 2% FBP/PBS (v:v) FACS buffer from 0.5 mM to 1 mM. |
Insufficient/No engraftment | Missed injection | Alter the angle of injection. Make sure to inject into at least 2 different injection sites within the TA muscle in case one fails. Ensure the needle is not being pushed too deep or too far towards the extremities of the TA muscle. |
Low cell viability | Make sure to inject isolated MuSCs into host mice immediately after sorting with as little waiting time as possible to prevent MuSC activation and cell death. | |
Death of NCG host mice after transplantation | Injection site infection | Make sure to use sterile tools when handling NCG mice. Treat injection site with antibiotic cream after injection. |
Mice were not positioned properly in the irradiation machine | Make sure to position only one lower hindlimb of the mice within the field of irradiation, with the contralateral leg, tail, head, and body outside the field of irradiation. | |
Low cDNA yield after SMART-Seq reverse transcription | Cells were not sorted into 0.2-ml tube | Make sure flow cytometry machine is calibrated to sort directly into a 0.2-ml tube. If necessary, perform a test whereby sheath liquid is sorted onto the closed cap of the 0.2-ml tube to visualize sorting accuracy |
Insufficient number of PCR cycles | Different numbers of T21 cells will require differing amounts of PCR cycles. Perform a troubleshooting experiment by varying PCR cycles for a known number of cells, followed by quantification, if necessary. | |
Insufficient elution from Ampure XP Beads | Do not over-dry Ampure beads after washing, since this renders elution more difficult. If Ampure beads clump after the addition of elution buffer instead of becoming a homogenous solution, this is a sign of over-dried beads. If beads are suspected of being overdried, apply the elution buffer and place tubes at 42°C for 20 min with intermittent shaking to promote better elution. | |
Lengthy processing time | Process the MuSC RNA into cDNA immediately after the FACS sort. Reducing the wait time as much as possible prevents mRNA degradation. | |
Low library yield after Nextera XT | Inaccurate timing for the incubation with Tn5 transposase | Do not leave samples incubating with Tn5 transposase for longer than 5 min due to the possibility of over-digesting the cDNA. Make sure to add the neutralizing NT buffer as quickly as possible after the tagmentation step is complete. |
Insufficient elution from Ampure XP Beads | Do not over-dry Ampure beads after washing, since this renders elution more difficult. If Ampure beads clump after the addition of elution buffer instead of becoming a homogenous solution, this is a sign of over-dried beads. If beads are suspected of being overdried, apply the elution buffer and place tubes at 42°C for 20 min with intermittent shaking to promote better elution. |
Injection of donor MuSCs into the host mice
18.Sort at least 10,000 cells per mouse into a 1.5-ml tube containing 200 µl of 2% FBS/PBS (v:v).
19.Spin down cells for 10 min at 600 × g and 4°C and resuspend in the appropriate volume (20 µl per mouse) of 2% FBS/PBS (v:v) for injections
20.Retrieve irradiated recipient mice from steps 1-4 and anesthetize them with isoflurane by placing them in an isoflurane induction chamber.
21.Clean the irradiated hindlimb with ethanol and use a Hamilton syringe to inject the donor MuSCs into the tibialis anterior (TA) muscle of the recipient mouse. Transplantation can be done with two injections of 10 µl each.
22.After injection, cover the site with a dollop of antibiotic cream. For troubleshooting see Table 2.
RNA-Seq library preparation from low cell numbers using SMART-Seq
23.This step should be done in tandem with the FACS in step 18.Sort MuSCs (1000 at T0 or between 30 and 1000 engrafted cells at T21) into a 0.2-ml PCR tube containing 1× SMART-Seq buffer
24.Proceed immediately with primer annealing and cDNA synthesis as described in the SMART-Seq HT user manual.
25.Amplify the cDNA with the appropriate number of PCR cycles.
PAUSE POINT: Once converted into cDNA, samples can be left at 4°C overnight and purified the following day.
26.Purify cDNA libraries using Ampure XP at a 1:1 ratio as described in the SMART-Seq HT Kit user manual.
PAUSE POINT: Purified cDNA can be stored at 4°C for up to 3 days, or at −20°C for longer periods until ready for Nextera library preparation.
Nextera library preparation
27.Quantify cDNA using the Quant-iT PicoGreen dsDNA Assay Kit following the manufacturer's instructions.
28.Transfer 0.15 ng cDNA to a new tube for processing with Nextera XT.
29.Fill tube to 1.25 µl with nuclease-free water.
30.Add 2.5 µl TD buffer and 1.25 µl ATM to each sample.
31.Incubate in thermocycler for 5 min at 56°C to perform tagmentation.
32.Add 1.25 µl NT buffer and incubate for 5 min at room temperature to neutralize the tagmentation reaction.
33.Add 1.25 µl of each Nextera adapter (i5 and i7) and 3.25 µl NPM buffer, and proceed with 12 rounds of PCR as described in the Nextera XT DNA Library Prep Kit user guide.
PAUSE POINT: After PCR, samples can remain at 4°C for up to 2 days, or at −20°C for a longer period until purification.
34.Purify sequencing-ready libraries with Ampure XP beads at a 1:0.85 (sample v: bead v) ratio.
35.Before sending samples out for Bioanalyzer analysis and sequencing, we recommend quantifying libraries in-house with Quant-iT PicoGreen and running 8-10 ng of sequencing-ready libraries on a 1.25% agarose gel prepared with the GelGreen highly sensitive DNA staining solution, as a quality control step for library size. Libraries should be between 200 and 600 bp, concentrated around 450 bp. For troubleshooting see Table 2.
Re-isolation of engrafted cells from the host mice (21 days post-transplantation)
36.Repeat steps 6-17 to isolate donor cells from the host mice. However, dissect only the tibialis anterior (TA) and nearby lower hindlimb muscles surrounding the injection site because the injection was made directly into the TA muscle. Given that MuSCs do not migrate far from the injection site, there are unlikely to be any GFP+ cells in more distal muscles.
37.Sort GFP+ re-isolated donor cells into a 0.2-ml tube containing 1× SMART-Seq reaction buffer
38.Immediately process lysed cells into cDNA using SMART-Seq and Nextera XT as described in step 23 onward. Use the following as a guide for the quantity of SMART-Seq PCR cycles required depending on the number of cells isolated:
-
1000 cells: 11 cycles
-
500-1000 cells: 12 cycles
-
150-500 cells: 14 cycles
-
100-150 cells: 15 cycles
-
50-100 cells: 16 cycles
-
30-50 cells: 17 cycles
REAGENTS AND SOLUTIONS
Use nuclease-free water in all recipes and protocol steps.
FACS digestion buffer
- 0.5 mM CaCl2
- 12 U/ml Dispase II
- 2.4 U/ml Collagenase D
- Prepare the day of the experiment and use immediately.
Rodent anesthesia cocktail
- ketamine (100 mg/kg)
- xylazine (10 mg/kg)
- acepromazine (3 mg/kg)
- Store at 4°C for up to 3 months.
SMART-Seq reaction buffer
- 90% nuclease-free water
- 10% 10× Reaction buffer (1 µl RNase inhibitors, 19 µl 10× lysis buffer)
- Prepare the day of the experiment and use immediately.
COMMENTARY
Background Information
Muscle stem cells (MuSCs) are a rare population that exist in a well-defined niche. They were first identified in 1961 (Mauro, 1961). Since that time, the identity of these cells as the progenitors of muscle fibers and their role in maintaining the tissue have been firmly established (Snow, 1977; Wang & Rudnicki, 2011). While the MuSCs have a potent regenerative capacity, this ability is compromised as we age and during disease (Shcherbina et al., 2020).
The MuSC niche is complex. The MuSCs themselves are located between the basal lamina and the sarcolemma of the myofiber (Wang & Rudnicki, 2011). However, numerous other cell types populate the muscle and these cells have been implicated in the proper functioning of MuSCs. For example, FAPs (Joe et al., 2010; Lukjanenko et al., 2019) and macrophages (Du et al., 2017; Varga et al., 2016) communicate directly with the MuSCs to regulate them. MuSCs are also affected by the myofibers with which they are associated (Lazure et al., 2020). Furthermore, fibroblasts (Mathew et al., 2011), T cells (Dumke & Lees, 2011), endothelial cells (Latroche et al., 2017), and pericytes (Kostallari et al., 2015) also populate the skeletal muscle, and they, along with the stiffness of the ECM (Schuler et al., 2022), also regulate MuSCs.
Not only is the MuSC niche cellularly complex, but it is highly dynamic. In the case of injury, the niche is quickly and continuously remodeled throughout the process of regeneration (de Micheli et al., 2020). For example, shortly after an acute injury the niche will be flooded with pro-inflammatory macrophages, only to have the macrophage type switch afterwards to anti-inflammatory to return the muscle to homeostasis (de Micheli et al., 2020). FAPs will display an altered transcriptome, initially expressing chemokines to attract immune cells, and will also express increased levels of ECM proteins, indicating that they are remodeling the muscle niche (de Micheli et al., 2020). Aging is another condition in which the niche will be heavily altered, we see a change in the macrophage population and the number of FAPs is increased (Lazure et al., 2023). This provides a major challenge in the efforts to uncouple the effects of aging on MuSC function from the effect of the changing niche as the two are inextricably linked.
Previous studies have shown the crucial role the niche plays in MuSC dysfunction in aging. The classic study using heterochronic parabiosis, where the circulatory system of a young mouse is connected to that of an aged mouse, clearly demonstrated that the young circulatory factors lead to an improvement in the function of the aged MuSCs (Conboy & Rando, 2012). However, this study only captured the effect of circulatory factors in the blood and cannot fully decouple the niche from the effect of age. As previously mentioned, the niche is comprised of much more than simply circulatory factors, and to fully capture the effect of the niche, the aged MuSCs must be fully integrated into a new niche. This is the purpose of our development of the heterochronic MuSC transplantation, which is the transplantation of MuSCs from an old donor into a young host.
When a MuSC is successfully transplanted to another mouse, it will be enveloped by the new niche, with no remnant of its previous niche. By analyzing the changes in the transcriptome before and after transplantation we can now fully decouple the effect of the age altered niche on MuSC function from the intrinsic cellular changes caused by age itself.
In this protocol we describe a robust method for transcriptional profiling of muscle stem cells after engraftment into a heterologous niche. In this protocol we used Pax7 GFP mice as the donor and NCG mice as the immune compromised host. This method was applied for the purpose of determining the niche effect in the context of aging on the gene expression profile of MuSCs.
Critical Parameters
Sterility
Because we perform this method on immunocompromised mice, it is essential that every procedure performed on the mice is completely sterile. The syringes used for injections should be autoclaved the day before. Every surface should be wiped down with ethanol before use. After injection, the sites should be covered with an antibiotic cream to reduce the chance of infection.
FACS isolation
A major element of this protocol is the FACS isolation of MuSCs from donor, and subsequently from recipient mice (Fig. 2). From our experience, the initial isolation of donor cells should yield approximately 80,000 to 200,000 MuSCs from young hindlimb muscles, and 30,000 to 60,000 MuSCs from aged hindlimbs. At T21, FACS re-isolation of donor cells has yielded between 30 and 400 MuSCs, in our hands (Table 1). However, a successful T21 re-isolation experiment most typically yields around 100 engrafted cells. Importantly, donor cells at T21 should present as a small, compact population on FACS visualization software, similar to T0 MuSCs (Fig. 2). A sparse or scattered population of donor cells is indicative of MuSC activation or cell death.
Time to transplantation
It is crucial that the cells are injected into the host mouse as quickly as possible. If multiple mice are being sorted for their MuSCs, perform the transplantations as soon as the sort is completed per mouse.
SMART and Nextera technologies
Due to the low number of recoverable donor cells after transplantation, the use of SMART-Seq and Nextera XT technologies is critical to the success of these experiments. As shown in Table 1, RNA-Sequencing library preparation of low numbers of transplanted cells yields sufficient quantities for sequencing. A successful cDNA library preparation with SMART-Seq should yield at least 0.1 ng/µl before Nextera (Table 1). After Nextera library prep, sequencing-ready libraries should average 1.6 ng/µl (Table 1). A 5-min incubation with the Tn5 transposase consistently yields libraries of around 450 bp in size, which is appropriate for sequencing on Illumina sequencers (Table 1). After sequencing and computational processing, we expect similar read density for housekeeping genes, regardless of the time point (T0 or T21) or number of cells used for each sample (Fig. 4).
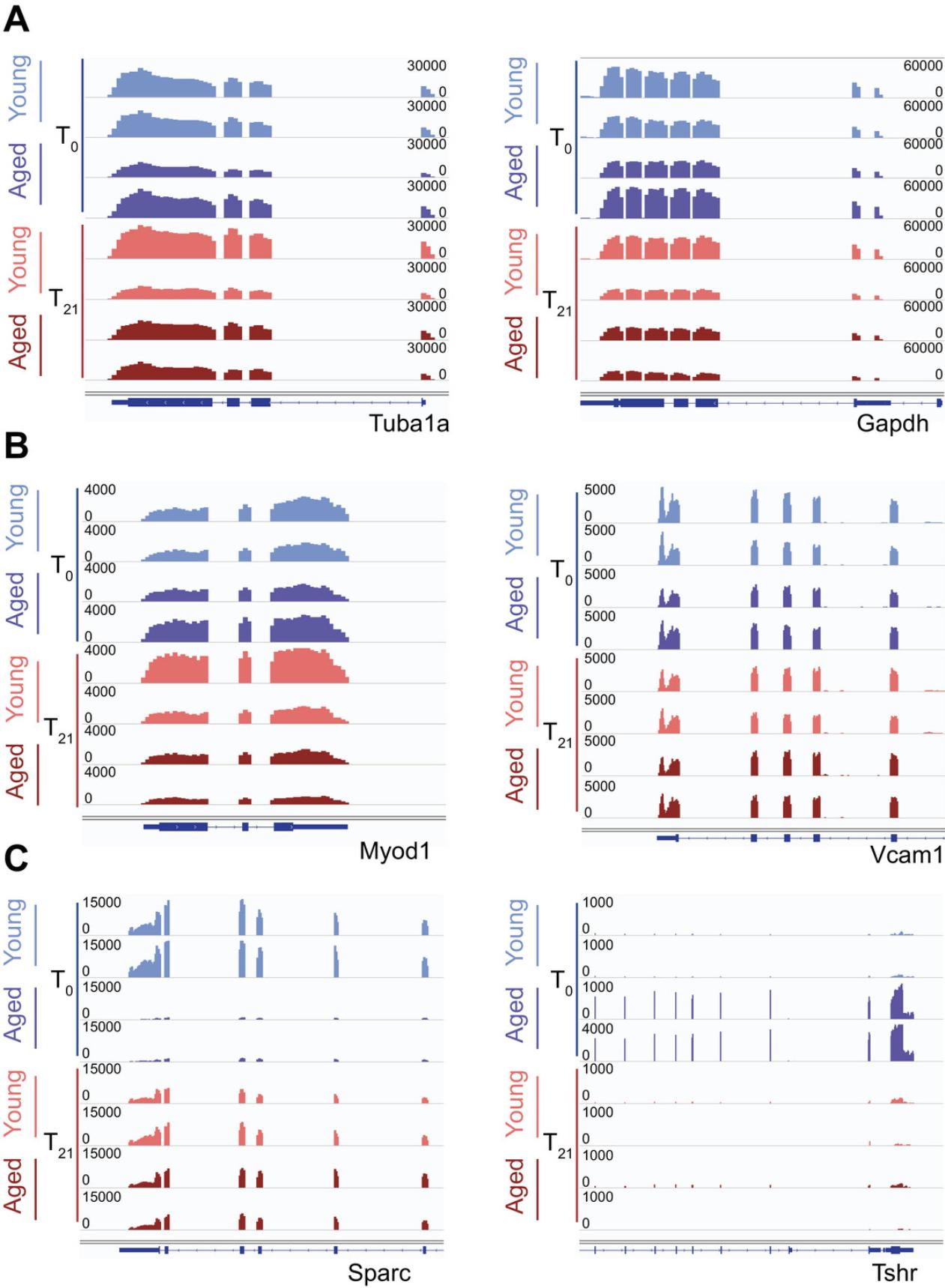
Troubleshooting
Table 2 shows the potential problems, their likely causes, and solutions.
Understanding Results
In our method, we used irradiation to prepare the target muscle, in this case the TA, for MuSC transplantation. However, there is no visual confirmation that the irradiation has successfully depleted the host muscle of MuSCs, nor that the donor MuSCs engrafted, unless the host mouse is euthanized and the muscle harvested. Before beginning FACS re-isolation experiments, we suggest that researchers perform an immunofluorescence assay on host mouse hindlimbs to ensure proper engraftment, or perform a FACS isolation of the donor cells (Fig. 2B-C). Using a standard TA muscle staining protocol with anti-PAX7 and anti-GFP antibodies, researchers should expect to visualize Pax7+/GFP+ donor MuSCs situated at the periphery of the myofibers. Due to the low migration capacity of MuSCs, donor cells will be sparsely spread out, with most cross-sections being void of any visible cells, and only 1-2 GFP+ MuSCs in cross-sections that do contain donor cells (Fig. 2C).
When the fluorescent MuSCs are isolated from the host, they will form a relatively dense cluster of GFP+ cells (Fig. 3A-B). When MuSCs activate they will become larger and on a FACS plot will tend to form a more dispersed population. Once the cells are transplanted into the donor mouse, they will be allowed to home to the niche for 21 days and will return to quiescence. Therefore, when the GFP+ cells are reisolated by FACS, these cells will appear similar to those of the freshly sorted quiescent MuSCs in the FACS plots (Fig. 3B). Notably, there will be much fewer GFP+ cells in the T21 isolation, so the population will appear smaller (Fig. 3C-D).
The FACS-sorted cells, in both T0 and T21 conditions, will be sorted directly into SMART lysis buffer. The preparation of cDNA will result in approximately 0.1 µg/ul cDNA prior to Nextera index incorporation (Table 1). This can be verified using picogreen. Once the indices have been incorporated, the libraries will typically have a yield of 1.6 ng/µl (Table 1). The average library fragment size should be around 450 bp, which is appropriate for sequencing. The size can be verified with a bioanalyzer or by gel electrophoresis.
Once the libraries have been sequenced and the reads mapped, the depth of sequencing and the read counts of housekeeping genes should be similar between conditions (Fig. 4A). It is also important to verify the expression of some marker genes as a proxy for purity of FACS. Here we used MyoD1 and Vcam1 (Fig. 4B). We used the MuSC transplantation method to determine the effect of the aged niche on MuSC function. Here, we highlight two genes that we identified as niche-responsive, meaning they are transcriptionally restored by exposure to a young niche. Sparc is a highly expressed gene in young MuSCs, but its expression is nearly completely lost in aged MuSCs. However, when the aged MuSCs are transplanted into a young host, the Sparc transcript levels are restored (Fig. 4C). In contrast, Tshr is not expressed in young MuSCs but gains in expression in aged mice. In a similar manner, when the aged MuSCs are exposed to a young niche, the Tshr gene loses expression (Fig. 4C).
Limitations
A limitation of this protocol is that the re-isolation of MuSCs from host mice leads to very low yield (30 to 400 cells), due to the low efficiency of engraftment. This low engraftment efficiency could be the result of cell stress due to FACS and the injection procedure, cell loss in the interstitial space due to failure to home to the niche, or cell death post-injection (Bouchentouf et al., 2007; Briggs & Morgan, 2013; Mueller & Bloch, 2019). Using SMART-Seq technology is a way to circumvent low yield, due to the ability to create libraries from 1 to 1000 cells. An additional limitation is the fact that the niche of immunocompromised mice has a defective immune system. Immune cells are an important part of the niche. A consequence of this is that the communication of immune cells with the MuSCs is missing in the host mouse and the new niche is not able to fully encompass a healthy niche. However, proper controls can be used to account for the use of immunocompromised/irradiated mice as hosts such as, for example, the injection of both young and aged cells into host mice to model for the effect of engraftment itself. Finally, a limitation of this protocol is the possibility of failed MuSC engraftment due to technical challenges.
Time Considerations
The protocol will take several days to complete. The host mice must be irradiated 24 hr prior to transplantation, and this process may take 2-3 hr. The isolation and transplantation of the donor MuSCs, along with the collection of the T0 RNA-Seq samples, will then take the entirety of the following day to complete. Once the MuSCs are injected, they will be allowed 21 days to home to the niche. During this period, the T0 RNA-Seq libraries can be generated, a method that will take two days to complete. After the 21-day incubation, the donor MuSCs will be reisolated by FACS into SMART lysis buffer, an experiment that will take the majority of a day. These T21 samples can then be used to generate RNA-Seq libraries, which will take another two days. Further experiments to validate the quality of the RNA-Seq libraries, such as DNA gels or qPCR, may take extra hours to complete.
Acknowledgments
We would like to thank Christian Young from the flow cytometry core at the Lady Davis Institute for Medical Research for their help with FACS sorting experiments. Funding for this research was provided by the Stem Cell Network (SCN) disease team grant and the Canadian Institute for Health Research (CIHR) to VDS.
Authors Contributions
Felicia Lazure : visualization; original draft writing, review, and editing. Darren Blackburn : investigation; methodology. Vahab Soleimani : conceptualization; formal analysis; funding acquisition; supervision; draft review and editing.
Conflict of Interest
The authors declare no conflict of interest.
Open Research
Data Availability Statement
The RNA-seq data utilized in this protocol was retrieved from GSE171998 (Lazure et al., 2023). Other tools and materials (or their source) that support the protocol are available from the corresponding author upon request.
Literature Cited
- Adey, A., Morrison, H. G., Asan, Xun, X., Kitzman, J. O., Turner, E. H., Stackhouse, B., Mackenzie, A. P., Caruccio, N. C., Zhang, X., & Shendure, J. (2010). Rapid, low-input, low-bias construction of shotgun fragment libraries by high-density in vitro transposition. Genome Biology , 11(12), R119. https://doi.org/10.1186/gb-2010-11-12-r119
- Boldrin, L., Neal, A., Zammit, P. S., Muntoni, F., & Morgan, J. E. (2012). Donor satellite cell engraftment is significantly augmented when the host niche is preserved and endogenous satellite cells are incapacitated. Stem Cells , 30(9), 1971–1984. https://doi.org/10.1002/stem.1158
- Bouchentouf, M., Skuk, D., & Tremblay, J. P. (2007). Early and massive death of myoblasts transplanted into skeletal muscle: Responsible factors and potential solutions. Current Opinion in Organ Transplantation , 12(6), 897–898. https://doi.org/10.1097/MOT.0b013e3282f19f20
- Briggs, D., & Morgan, J. E. (2013). Recent progress in satellite cell/myoblast engraftment – relevance for therapy. Febs Journal , 280(17), 4281–4293. https://doi.org/10.1111/febs.12273
- Conboy, I. M., & Rando, T. A. (2012). Heterochronic parabiosis for the study of the effects of aging on stem cells and their niches. Cell Cycle , 11(12), 2260–2267. https://doi.org/10.4161/cc.20437
- de Micheli, A. J., Laurilliard, E. J., Heinke, C. L., Ravichandran, H., Fraczek, P., Soueid-Baumgarten, S., de Vlaminck, I., Elemento, O., & Cosgrove, B. D. (2020). Single-cell analysis of the muscle stem cell hierarchy identifies heterotypic communication signals involved in skeletal muscle regeneration. Cell Reports , 30(10), 3583–3595.e5. https://doi.org/10.1016/j.celrep.2020.02.067
- Doreste, B., Torelli, S., & Morgan, J. (2020). Irradiation dependent inflammatory response may enhance satellite cell engraftment. Scientific Reports , 10(1), 11119. https://doi.org/10.1038/s41598-020-68098-9
- Du, H., Shih, C.-H., Wosczyna, M. N., Mueller, A. A., Cho, J., Aggarwal, A., Rando, T. A., & Feldman, B. J. (2017). Macrophage-released ADAMTS1 promotes muscle stem cell activation. Nature Communications , 8(1), 669. https://doi.org/10.1038/s41467-017-00522-7
- Dumke, B. R., & Lees, S. J. (2011). Age-related impairment of T cell-induced skeletal muscle precursor cell function. American Journal of Physiology. Cell Physiology , 300(6), C1226–1233. https://doi.org/10.1152/ajpcell.00354.2010
- Feige, P., Brun, C. E., Ritso, M., & Rudnicki, M. A. (2018). Orienting muscle stem cells for regeneration in homeostasis, aging, and disease. Cell Stem Cell , 23(5), 653–664. https://doi.org/10.1016/j.stem.2018.10.006
- Feige, P., & Rudnicki, M. A. (2020). Isolation of satellite cells and transplantation into mice for lineage tracing in muscle. Nature Protocols , 15(3), 1082–1097. https://doi.org/10.1038/s41596-019-0278-8
- Gertz, J., Varley, K. E., Davis, N. S., Baas, B. J., Goryshin, I. Y., Vaidyanathan, R., Kuersten, S., & Myers, R. M. (2012). Transposase mediated construction of RNA-seq libraries. Genome Research , 22(1), 134–141. https://doi.org/10.1101/gr.127373.111
- Gross, J. G., Bou-Gharios, G., & Morgan, J. E. (1999). Potentiation of myoblast transplantation by host muscle irradiation is dependent on the rate of radiation delivery. Cell and Tissue Research , 298(2), 371–375. https://doi.org/10.1007/s004419900062
- Head, S. R., Komori, H. K., LaMere, S. A., Whisenant, T., van Nieuwerburgh, F., Salomon, D. R., & Ordoukhanian, P. (2014). Library construction for next-generation sequencing: Overviews and challenges. Biotechniques , 56(2), 61–passim. https://doi.org/10.2144/000114133
- Joe, A. W. B., Yi, L., Natarajan, A., le Grand, F., So, L., Wang, J., Rudnicki, M. A., & Rossi, F. M. V. (2010). Muscle injury activates resident fibro/adipogenic progenitors that facilitate myogenesis. Nature Cell Biology , 12(2), 153–163. https://doi.org/10.1038/ncb2015
- Keefe, A. C., Lawson, J. A., Flygare, S. D., Fox, Z. D., Colasanto, M. P., Mathew, S. J., Yandell, M., & Kardon, G. (2015). Muscle stem cells contribute to myofibres in sedentary adult mice. Nature Communications , 6, 7087. https://doi.org/10.1038/ncomms8087
- Kostallari, E., Baba-Amer, Y., Alonso-Martin, S., Ngoh, P., Relaix, F., Lafuste, P., & Gherardi, R. K. (2015). Pericytes in the myovascular niche promote post-natal myofiber growth and satellite cell quiescence. Development , 142(7), 1242–1253. https://doi.org/10.1242/dev.115386
- Kuang, S., Kuroda, K., le Grand, F., & Rudnicki, M. A. (2007). Asymmetric self-renewal and commitment of satellite stem cells in muscle. Cell , 129(5), 999–1010. https://doi.org/10.1016/j.cell.2007.03.044
- Laternser, S., Keller, H., Leupin, O., Rausch, M., Graf-Hausner, U., & Rimann, M. (2018). A novel microplate 3D bioprinting platform for the engineering of muscle and tendon tissues. SLAS TECHNOLOGY: Translating Life Sciences Innovation , 23(6), 599–613. https://doi.org/10.1177/2472630318776594
- Latroche, C., Weiss-Gayet, M., Muller, L., Gitiaux, C., Leblanc, P., Liot, S., Ben-Larbi, S., Abou-Khalil, R., Verger, N., Bardot, P., Magnan, M., Chrétien, F., Mounier, R., Germain, S., & Chazaud, B. (2017). Coupling between myogenesis and angiogenesis during skeletal muscle regeneration is stimulated by restorative macrophages. Stem Cell Reports , 9(6), 2018–2033. https://doi.org/10.1016/j.stemcr.2017.10.027
- Lazure, F., Blackburn, D. M., Corchado, A. H., Sahinyan, K., Karam, N., Sharanek, A., Nguyen, D., Lepper, C., Najafabadi, H. S., Perkins, T. J., Jahani-Asl, A., & Soleimani, V. D. (2020). Myf6/MRF4 is a myogenic niche regulator required for the maintenance of the muscle stem cell pool. Embo Reports , 21(12), e49499. https://doi.org/10.15252/embr.201949499
- Lazure, F., Farouni, R., Sahinyan, K., Blackburn, D. M., Hernández-Corchado, A., Perron, G., Lu, T., Osakwe, A., Ragoussis, J., Crist, C., Perkins, T. J., Jahani-Asl, A., Najafabadi, H. S., & Soleimani, V. D. (2023). Transcriptional reprogramming of skeletal muscle stem cells by the niche environment. Nature Communications , 14(1), 535. https://doi.org/10.1038/s41467-023-36265-x
- Lee, Y., Choi, J. J., Ahn, S. I., Lee, N. H., Han, W. M., Mohiuddin, M., Shin, E. J., Wood, L., Park, K. D., Kim, Y., & Jang, Y. C. (2020). Engineered heterochronic parabiosis in 3D microphysiological system for identification of muscle rejuvenating factors. Advanced Functional Materials , 30(46), 2002924. https://doi.org/10.1002/adfm.202002924
- Liu, L., Charville, G. W., Cheung, T. H., Yoo, B., Santos, P. J., Schroeder, M., & Rando, T. A. (2018). Impaired notch signaling leads to a decrease in p53 activity and mitotic catastrophe in aged muscle stem cells. Cell Stem Cell , 23(4), 544–556.e544. https://doi.org/10.1016/j.stem.2018.08.019
- Lukjanenko, L., Karaz, S., Stuelsatz, P., Gurriaran-Rodriguez, U., Michaud, J., Dammone, G., Sizzano, F., Mashinchian, O., Ancel, S., Migliavacca, E., Liot, S., Jacot, G., Metairon, S., Raymond, F., Descombes, P., Palini, A., Chazaud, B., Rudnicki, M. A., Bentzinger, C. F., & Feige, J. N. (2019). Aging disrupts muscle stem cell function by impairing matricellular WISP1 secretion from fibro-adipogenic progenitors. Cell Stem Cell , 24(3), 433–446.e7. https://doi.org/10.1016/j.stem.2018.12.014
- Maffioletti, S. M., Sarcar, S., Henderson, A. B. H., Mannhardt, I., Pinton, L., Moyle, L. A., Steele-Stallard, H., Cappellari, O., Wells, K. E., Ferrari, G., Mitchell, J. S., Tyzack, G. E., Kotiadis, V. N., Khedr, M., Ragazzi, M., Wang, W., Duchen, M. R., Patani, R., Zammit, P. S., … Tedesco, F. S. (2018). Three-dimensional human iPSC-derived artificial skeletal muscles model muscular dystrophies and enable multilineage tissue engineering. Cell Reports , 23(3), 899–908. https://doi.org/10.1016/j.celrep.2018.03.091
- Mashinchian, O., Hong, X., Michaud, J., Migliavacca, E., Lefebvre, G., Boss, C., de Franceschi, F., le Moal, E., Collerette-Tremblay, J., Isern, J., Metairon, S., Raymond, F., Descombes, P., Bouche, N., Muñoz-Cánoves, P., Feige, J. N., & Bentzinger, C. F. (2022). In vivo transcriptomic profiling using cell encapsulation identifies effector pathways of systemic aging. Elife , 11, e57393. https://doi.org/10.7554/eLife.57393
- Mathew, S. J., Hansen, J. M., Merrell, A. J., Murphy, M. M., Lawson, J. A., Hutcheson, D. A., Hansen, M. S., Angus-Hill, M., & Kardon, G. (2011). Connective tissue fibroblasts and Tcf4 regulate myogenesis. Development , 138(2), 371–384. https://doi.org/10.1242/dev.057463
- Mauro, A. (1961). Satellite cell of skeletal muscle fibers. Journal of Biophysical and Biochemical Cytology , 9, 493–495. https://doi.org/10.1083/jcb.9.2.493
- Meng, J., Bencze, M., Asfahani, R., Muntoni, F., & Morgan, J. E. (2015). The effect of the muscle environment on the regenerative capacity of human skeletal muscle stem cells. Skeletal Muscle , 5(1), 11. https://doi.org/10.1186/s13395-015-0036-8
- Morgan, J. E., Pagel, C. N., Sherratt, T., & Partridge, T. A. (1993). Long-term persistence and migration of myogenic cells injected into pre-irradiated muscles of mdx mice. Journal of the Neurological Sciences , 115(2), 191–200. https://doi.org/10.1016/0022-510x(93)90224-m
- Moyle, L. A., Jacques, E., & Gilbert, P. M. (2020). Engineering the next generation of human skeletal muscle models: From cellular complexity to disease modeling. Current Opinion in Biomedical Engineering , 16, 9–18. https://doi.org/10.1016/j.cobme.2020.05.006
- Mueller, A. L., & Bloch, R. J. (2019). Skeletal muscle cell transplantation: Models and methods. Journal of Muscle Research and Cell Motility , 41(4), 297–311. https://doi.org/10.1007/s10974-019-09550-w
- Nguyen, J. H., Chung, J. D., Lynch, G. S., & Ryall, J. G. (2019). The microenvironment is a critical regulator of muscle stem cell activation and proliferation. Frontiers in Cell and Developmental Biology , 7, 254. https://doi.org/10.3389/fcell.2019.00254
- Pasut, A., Oleynik, P., & Rudnicki, M. A. (2012). Isolation of muscle stem cells by fluorescence activated cell sorting cytometry. Methods in Molecular Biology , 798, 53–64. https://doi.org/10.1007/978-1-61779-343-1_3
- Pawlikowski, B., Pulliam, C., Betta, N. D., Kardon, G., & Olwin, B. B. (2015). Pervasive satellite cell contribution to uninjured adult muscle fibers. Skeletal Muscle , 5, 42–42. https://doi.org/10.1186/s13395-015-0067-1
- Picelli, S., Faridani, O. R., Bjorklund, A. K., Winberg, G., Sagasser, S., & Sandberg, R. (2014). Full-length RNA-seq from single cells using Smart-seq2. Nature Protocols , 9(1), 171–181. https://doi.org/10.1038/nprot.2014.006
- Price, F. D., von Maltzahn, J., Bentzinger, C. F., Dumont, N. A., Yin, H., Chang, N. C., Wilson, D. H., Frenette, J., & Rudnicki, M. A. (2014). Inhibition of JAK-STAT signaling stimulates adult satellite cell function. Nature Medicine , 20(10), 1174–1181. https://doi.org/10.1038/nm.3655
- Quarta, M., Brett, J. O., Dimarco, R., de Morree, A., Boutet, S. C., Chacon, R., Gibbons, M. C., Garcia, V. A., Su, J., Shrager, J. B., Heilshorn, S., & Rando, T. A. (2016). An artificial niche preserves the quiescence of muscle stem cells and enhances their therapeutic efficacy. Nature Biotechnology , 34(7), 752–759. https://doi.org/10.1038/nbt.3576
- Relaix, F., Bencze, M., Borok, M. J., der Vartanian, A., Gattazzo, F., Mademtzoglou, D., Perez-Diaz, S., Prola, A., Reyes-Fernandez, P. C., Rotini, A., & Taglietti. (2021). Perspectives on skeletal muscle stem cells. Nature Communications , 12(1), 692. https://doi.org/10.1038/s41467-020-20760-6
- Sambasivan, R., Gayraud-Morel, B., Dumas, G., Cimper, C., Paisant, S., Kelly, R. G., & Tajbakhsh, S. (2009). Distinct regulatory cascades govern extraocular and pharyngeal arch muscle progenitor cell fates. Developmental Cell , 16(6), 810–821. https://doi.org/10.1016/j.devcel.2009.05.008
- Schuler, S. C., Liu, Y., Dumontier, S., Grandbois, M., le Moal, E., Cornelison, D., & Bentzinger, C. F. (2022). Extracellular matrix: Brick and mortar in the skeletal muscle stem cell niche. Frontiers in Cell and Developmental Biology , 10, 1056523. https://doi.org/10.3389/fcell.2022.1056523
- Shcherbina, A., Larouche, J., Fraczek, P., Yang, B. A., Brown, L. A., Markworth, J. F., Chung, C. H., Khaliq, M., de Silva, K., Choi, J. J., Fallahi-Sichani, M., Chandrasekaran, S., Jang, Y. C., Brooks, S. V., & Aguilar, C. A. (2020). Dissecting murine muscle stem cell aging through regeneration using integrative genomic analysis. Cell Reports , 32(4), 107964. https://doi.org/10.1016/j.celrep.2020.107964
- Snow, M. H. (1977). Myogenic cell formation in regenerating rat skeletal muscle injured by mincing. II. An autoradiographic study. Anatomical Record , 188(2), 201–217. https://doi.org/10.1002/ar.1091880206
- Varga, T., Mounier, R., Patsalos, A., Gogolák, P., Peloquin, M., Horvath, A., Pap, A., Daniel, B., Nagy, G., Pintye, E., Poliska, S., Cuvellier, S., Ben Larbi, S., Sansbury, B. E., Spite, M., Brown, C. W., Chazaud, B., & Nagy, L. (2016). Macrophage PPARgamma, a lipid activated transcription factor controls the growth factor GDF3 and skeletal muscle regeneration. Immunity , 45(5), 1038–1051. https://doi.org/10.1016/j.immuni.2016.10.016
- Wang, Y. X., Feige, P., Brun, C. E., Hekmatnejad, B., Dumont, N. A., Renaud, J.-M., Faulkes, S., Guindon, D. E., & Rudnicki, M. A. (2019). EGFR-Aurka signaling rescues polarity and regeneration defects in dystrophin-deficient muscle stem cells by increasing asymmetric divisions. Cell Stem Cell , 24(3), 419–432 e416. https://doi.org/10.1016/j.stem.2019.01.002
- Wang, Y. X., & Rudnicki, M. A. (2011). Satellite cells, the engines of muscle repair. Nature Reviews Molecular Cell Biology , 13(2), 127–133. https://doi.org/10.1038/nrm3265
- Wernig, A., Zweyer, M., & Irintchev, A. (2000). Function of skeletal muscle tissue formed after myoblast transplantation into irradiated mouse muscles. The Journal of Physiology , 522 Pt 2(Pt 2), 333–345. https://doi.org/10.1111/j.1469-7793.2000.t01-2-00333.x
- Yin, H., Price, F., & Rudnicki, M. A. (2013). Satellite cells and the muscle stem cell niche. Physiological Reviews , 93(1), 23–67. https://doi.org/10.1152/physrev.00043.2011