Single-Molecule Localization Microscopy Using Time-Lapse Imaging of Single-Antibody Labeling
Thilini Perera, Thilini Perera, Hirushi Gunasekara, Hirushi Gunasekara, Ying S. Hu, Ying S. Hu
antibodies
multiplexed imaging
single-antibody labeling
single-molecule localization microscopy
superresolution imaging
time-lapse imaging
Abstract
In single-molecule localization microscopy (SMLM), immunofluorescence (IF) staining affects the quality of the reconstructed superresolution images. However, optimizing IF staining remains challenging because IF staining is a one-step, irreversible process. Sample labeling through reversible binding presents an alternative strategy, but such techniques require significant technological advancements to enhance the dissociation of labels without sacrificing their binding specificity. In this article, we introduce time-lapse imaging of single-antibody labeling. Our versatile technique utilizes commercially available dye-conjugated antibodies. The method controls the antibody concentrations to capture single-antibody labeling of subcellular targets, thereby achieving SMLM through the labeling process. We further demonstrate dual-color single-antibody labeling to enhance the sample labeling density. The new approach allows the evaluation of antibody binding at the single-antibody level and within the cellular environment. This comprehensive guide offers step-by-step instructions for time-lapse imaging of single-antibody labeling experiments and enables the application of the single-antibody labeling technique to a wide range of targets. © 2023 The Authors. Current Protocols published by Wiley Periodicals LLC.
Basic Protocol 1 : Sample preparation for single-antibody labeling
Basic Protocol 2 : Data acquisition for single-molecule localization microscopy
Alternate Protocol : Dual-color single-antibody labeling using OptoSplit II equation
Basic Protocol 3 : Image analysis
INTRODUCTION
In single-molecule localization microscopy (SMLM), immunofluorescence (IF) staining remains one of the most widely used techniques for sample labeling. IF staining immobilizes the molecular target dye-conjugated primary or secondary antibodies. To this end, the antibody quality determines the quality of the superresolution images. Most common SMLM techniques utilize IF staining, including direct stochastic optical reconstruction microscopy (d STORM) and DNA-based points accumulation for imaging in nanoscale topography (DNA-PAINT) (Heilemann et al., 2008; Jungmann et al., 2010, 2014; Rust et al., 2006; Schnitzbauer et al., 2017). The optimization of sample labeling often involves empirical adjustments to the antibody concentration and other IF staining conditions. However, evaluating antibody labeling is nearly impossible until after the superresolution image reconstruction is complete.
An alternative labeling strategy is through fluorescently labeled probes that transiently bind to the target. This approach offers several advantages, including stoichiometric labeling, reduced linkage error, flexibility in the choice of the fluorophore, enhanced labeling densities, and multiplexing imaging. Universal PAINT (u-PAINT) utilizes fluorescently labeled ligands and antibodies to label tagged and endogenous membrane proteins (Giannone et al., 2010). Protein-PAINT (pPAINT) employs signaling proteins to investigate T-cell signaling with multiplexing capability (Farrell et al., 2022). Another related approach is image reconstruction by integrating exchangeable single-molecule localization (IRIS). IRIS utilizes rapidly exchanging probes to achieve high-density labeling and multiplexed imaging (Kiuchi et al., 2015). Recently, the IRIS technology has been extended to using fast-dissociating antibodies produced through hybridoma technology and antibody fragments (Miyoshi et al., 2021) and engineered fast-dissociating antibody fragments against molecular epitope tags generated through protein engineering (Zhang et al., 2022). Reversible binding has also been chemically enhanced in the superresolution census of molecular epitope tags (SR-COMET) technique (Gunasekara et al., 2022). Although transient labeling represents significant technological advancements, there is still a need for a more versatile approach that uses readily available antibodies. To this end, we introduced an SMLM technique using time-lapse imaging of single-antibody labeling (Fig. 1; Gunasekara et al., 2023).
![Details are in the caption following the image Overview of the concept of time-lapse imaging of single-antibody labeling. (A) Schematic illustration of single-molecule localization microscopy (SMLM) using single-antibody labeling of a fixed cell. (B) Time-lapse imaging with single-antibody labeling [optimization of antibody concentration, non-illuminating interval (NII) selection, and imaging]. (C) Reconstructed superresolution image from single-antibody labeling.](https://static.yanyin.tech/literature_test/cpz1908-fig-0001-m.jpg)
This article presents a comprehensive step-by-step guide for single-antibody labeling, consisting of three basic protocols and an alternate protocol. Basic Protocol 1 focuses on the sample preparation steps for imaging microtubules and mitochondria in U2OS cells using dye-conjugated primary or secondary antibodies. The protocol can be modified and adapted for imaging other subcellular targets in different cells. Basic Protocol 2 covers time-lapse imaging with single-antibody labeling, multiplexed imaging with single-antibody labeling, and dual-color single-target imaging with single-antibody labeling. Basic Protocol 2 details optimizing antibody concentrations and non-illuminating intervals (NIIs), and the Alternate Protocol details dual-color imaging using OptoSplit II. Basic Protocol 3 provides steps for single-molecule analysis and superresolution image reconstruction using the ThunderSTORM ImageJ plug-in. Additionally, we provide critical tips for sample preparation and imaging procedures, a troubleshooting guide, and timing information for planning the experiments.
Basic Protocol 1: SAMPLE PREPARATION FOR SINGLE-ANTIBODY LABELING
The sample preparation follows a standard IF staining protocol. This protocol provides a guide for preserving subcellular structures (microtubules and mitochondria) in U2OS cells and describes sample preparation for two distinct single-antibody labeling approaches: 1) single-antibody labeling using dye-conjugated primary antibodies and 2) single-antibody labeling using dye-conjugated secondary antibodies on samples stained with unconjugated primary antibodies.
Materials
- U2OS cell line (ATCC, HTB-96)
- Complete DMEM (see recipe)
- 0.25% (w/v) trypsin-EDTA (Fisher Scientific, 25200-056)
- DPBS (Thermo Fisher Scientific, 14190-144, 500 ml)
- Fixation buffer (see recipe; make fresh)
- Blocking buffer (containing permeabilization reagent; see recipe; make fresh)
- Post-fixation buffer (see recipe; make fresh; optional)
- Unconjugated primary antibodies (see Table 1)
Antibody | Target | Antibody concentration (nM) |
---|---|---|
ReadyTag anti-HA (12CA5) (Bio X Cell, RT0268, 25 mg) | 3×HA tag on α-tubulin (microtubule) | 67 |
Rabbit polyclonal anti-Tom20 (Thermo Fisher Scientific, MA5-32148) | Tom20 (mitochondria) | 27 |
-
100 × 20–mm tissue culture dishes (Falcon, 353003)
-
Tube of choice
-
Standard tabletop centrifuge
-
8-well chambered cover glasses (Thermo Fisher Scientific, 55411, or Cellvis, C8-1-N)
-
Parafilm (Amcor, PM996)
-
Additional reagents and equipment for counting cells (see Current Protocols article: Phelan & May, 2015)
CAUTION : Paraformaldehyde is a carcinogenic, toxic chemical. Wear protective gloves, protective clothing, and eye protection. Avoid breathing vapors. Wash skin thoroughly after handling.
CAUTION : Glutaraldehyde is a toxic chemical that is harmful if swallowed or inhaled and can cause skin burns and eye damage. Wear protective gloves, protective clothing, and eye protection. Avoid ingestion and inhalation. Wash skin thoroughly after handling.
CAUTION : Sodium azide is a hazardous substance that can be fatal if ingested, comes into contact with skin, or inhaled. Wear protective gloves, protective clothing, and eye protection. Wash skin thoroughly after handling.
NOTE : All the steps are conducted at room temperature unless otherwise specified.
NOTE : All solutions and equipment coming into contact with cells must be sterile, and proper sterile technique should be used accordingly, including use of a biological safety cabinet (Thermo Fisher Scientific, 1300 Series A2).
NOTE : All culture incubations are performed in a humidified 37°C, 5% CO2 incubator (Thermo Fisher Scientific, Series 8000 WJ) unless otherwise specified.
Cell seeding and fixation
1.Maintain the U2OS cell line in 10 ml complete DMEM (initial cell density of 2 × 104 cells/cm2) in 100 × 20–mm tissue culture dishes in a humidified atmosphere with 5% CO2 at 37°C. Subculture when the cells reach ∼70% confluency.
2.Trypsinize cells with 1 ml of 0.25% trypsin-EDTA, incubate for 3 min, neutralize with 9 ml complete DMEM, place in tube of choice, and centrifuge 3 min at 250 × g. Then, seed ∼5000 cells in 400 µl complete DMEM per well in an 8-well chambered cover glass. Let the cells grow at 37°C and 5% CO2 for 36 hr before fixing.
3.Wash cells with 400 µl DPBS once.
4.Add 200 µl fixation buffer and incubate the sample for 15 to 20 min at room temperature.
5.Remove the fixation buffer and wash the sample two times with DPBS.
6.Add 400 µl fresh DPBS, cover sample chambers with parafilm to prevent the cells from drying out during storage, and store fixed samples for ≤1 month at 4°C.
Sample preparation for single-antibody labeling using dye-conjugated primary antibodies
7a. Add 200 µl blocking buffer (containing permeabilization reagent) to the sample. Incubate for 30 min at room temperature.
8a. Optional: Add 200 µl post-fixation buffer. Incubate the sample for 10 min at room temperature.
9a. Optional: Wash the sample two times with DPBS.
10a. Add 200 µl fresh DPBS. Proceed for Basic Protocol 2 for time-lapse imaging with single-antibody labeling using dye-conjugated primary antibodies.
Sample preparation for single-antibody labeling using dye-conjugated secondary antibodies
7b. Add 200 µl blocking buffer (containing permeabilization reagent) to the sample. Incubate for 30 min at room temperature.
8b. Dilute the unconjugated primary antibody in 200 µl blocking buffer to a final concentration within the manufacturer-recommended range.
9b. Replace the blocking buffer with the diluted primary antibody. Incubate the sample for 2 hr at room temperature.
10b. Wash the sample three times with DPBS.
11b. Add 200 µl post-fixation buffer. Incubate the sample for 10 min at room temperature.
12b. Wash the sample three times with DPBS. Add 200 µl fresh DPBS after washing. Proceed to time-lapse imaging with single-antibody labeling using dye-conjugated secondary antibodies, as described in Basic Protocol 2.
Basic Protocol 2: DATA ACQUISITION FOR SINGLE-MOLECULE LOCALIZATION MICROSCOPY
The following protocol provides the steps for three implementations of single-antibody labeling: 1) time-lapse imaging with single-antibody labeling, 2) multiplexed imaging with single-antibody labeling, and 3) dual-color single-target imaging with single-antibody labeling.
The first section of the protocol after data acquisition initiation outlines the steps for a basic single-antibody labeling experiment. This section includes the steps for initiating the imaging process, identifying the optimal antibody concentration, selecting the optimal NII, and setting up the imaging parameters for a subcellular target. Fiducial markers need to be added to the sample for drift correction. A short trial run must be performed to determine the optimal antibody concentration and NII before proceeding with extended acquisitions. This protocol is written for using an inverted microscope (Nikon Instruments, Eclipse Ti2E) with a 100×/1.49 oil objective and a Prime 95B sCMOS camera. The laser power densities indicated in the steps are measured after the objective. Imaging is performed using a total internal reflection fluorescence (TIRF) microscopy setup. Data acquisition is performed using ND acquisition in Nikon NIS-Elements software (version 5.21.03).
The second section of the protocol outlines the extended steps for multiplexed single-antibody labeling using different antibodies. The third section of the protocol focuses on dual-color single-antibody labeling of a single target. The rationale for developing this assay is that single-antibody labeling can yield lower sample labeling densities than other SMLM techniques. Dual-color single-antibody labeling increases the labeling density while minimizing the spatial overlap between molecules. This strategy uses a mixture of the same antibody conjugated with two different dyes. Additionally, the Alternate Protocol describes the use of OptoSplit II for dual-color imaging.
Materials
- Chamber slide with target of interest prepared according to Basic Protocol 1
- Index-matching immersion oil (Cargille Laboratories, 16245; refractive index 1.5150)
- Fiducial markers (TetraSpeck microspheres, Thermo Fisher Scientific, T7280, 0.2 μm, or gold colloids, Ted Pella, 15711−20, 100 nm)
- DPBS (Thermo Fisher Scientific,14190-144, 500 ml) or MilliQ water
- Dye-conjugated antibodies (see Table 2)
Antibody | Target | Antibody concentration (nM) |
---|---|---|
ReadyTag anti-HA (12CA5) (Bio X Cell, RT0268, 25 mg)-Alexa Fluor 647a | 3×HA tag on α-tubulin (microtubule) | 0.5 |
F(ab′)2-Goat anti-Mouse IgG Alexa Fluor 647 (Thermo Fisher Scientific, A21237) | Anti-HA (12CA5) | 0.063 |
α-tubulin monoclonal antibody (DMIA) Alexa Fluor 488 (Thermo Fisher Scientific, 53− 4502−82) | α-tubulin (microtubule) | 0.003 |
Goat anti-rabbit Alexa Fluor 647 IgG (Thermo Fisher Scientific, Superclonal Recombinant, A27040, 1 mg) | Rabbit polyclonal anti-Tom20 | 0.125 |
- a
In-house dye conjugation with AF 647 (Gunasekara et al., 2022). U2OS cells expressing 3×HA-α-tubulin were used with the ReadyTag anti-HA antibody.
- Inverted fluorescence microscope (Nikon Instruments, Eclipse Ti2E with Perfect Focus System)
- Prime 95B sCMOS camera (serial no. A18B203004)
- Acquisition computer with image acquisition software (Nikon, NIS Elements version 5.21.03)
- Laser unit (Nikon, LUN-F, serial no. 3-476)
- 100×/1.49 oil-immersion objective (100× Apo TIRF objective, numerical aperture 1.49, working distance 0.12)
- Quad-band set (Chroma Technology, TRF89901-ET-405/488/561/640 nm)
- Emission filters (Chroma Technology, ET450/50m, ET525/50m, ET600/50m, ET700/75m)
- Vortex or bath sonicator
- Parafilm (Amcor, PM996)
Initiating data acquisition
1.Allow the sample (chamber slide with a target of interest prepared according to Basic Protocol 1) to reach room temperature before proceeding if stored at 4°C.
2.Turn on the inverted fluorescence microscope, Prime 95B sCMOS camera, acquisition computer with image acquisition software, and laser unit.
3.Select the objective (i.e., 100×/1.49 oil-immersion objective) and apply a layer of index-matching immersion oil.
4.Position the sample on the microscope stage and raise the objective (e.g., 100×/1.49 oil objective) until the immersion oil makes contact with the sample.
5.Open the image acquisition software that controls the data acquisition.
6.Choose the optical configuration (excitation laser, quad-band set, and emission filter) for the fluorophore selected for single-antibody labeling.
7.Select the laser power intensity level and set the camera parameters.
8.Prepare fiducial markers by vortexing TetraSpeck microspheres for 30 s or sonicating gold colloids for 3 min before use. Dilute TetraSpeck microspheres (size: 200 nm) 1:1000 in DPBS. Dilute gold colloids (size: 100 nm) 1:5 in Milli-Q water.
9.Add fiducial markers (i.e., TetraSpeck microspheres or gold colloids) to the sample. Incubate sample with TetraSpeck microspheres for 5 min and gold colloids for 10 to 20 min.
10.Using brightfield illumination, locate a cell and focus on it.
11.Identify fiducial markers near the cell that can be used for drift correction (Fig. 2).
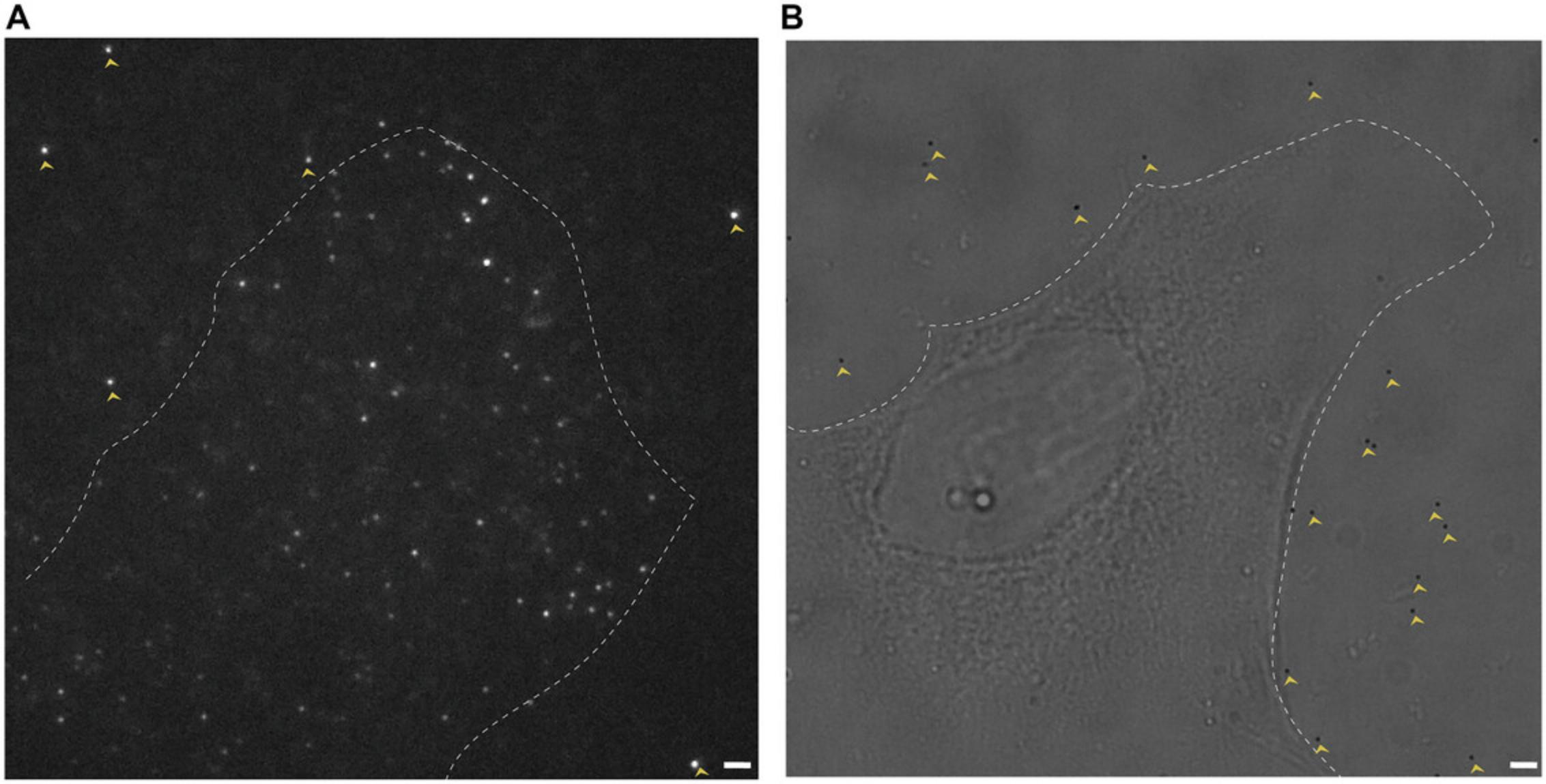
12.Replace the fiducial marker solution with 200 µl DPBS.
Time-lapse imaging with single-antibody labeling
13a. Starting with an antibody concentration of 0.003 to 0.5 nM, prepare the imaging buffer by diluting the dye-conjugated antibody in DPBS.
![Details are in the caption following the image Single-molecule event density for single-antibody labeling. Representative single-molecule events detected (A) before and (B) after concentration optimization in three antibody-target systems from Table 2. The higher number of overlapping events in (A) renders single-molecule localization difficult. The antibody concentrations used are as follows: [12CA5-AF 647] =1 nM (A) and 0.5 nM (B); [DMIA-AF 488] = 1 nM (A) and 0.003 nM (B); [F(ab′)<sub>2</sub>-AF 647] = 0.5 nM (A) and 0.063 nM (B). NII= 20 s. Scale bars: 2 μm.](https://static.yanyin.tech/literature_test/cpz1908-fig-0003-m.jpg)
14a. Add 300 µl imaging buffer to the mounted sample well.
15a. Increase the laser power density (100 to 400 W/cm2) and set the exposure time (50 ms).
16a. Adjust the TIRF angle to achieve the maximum SNR.
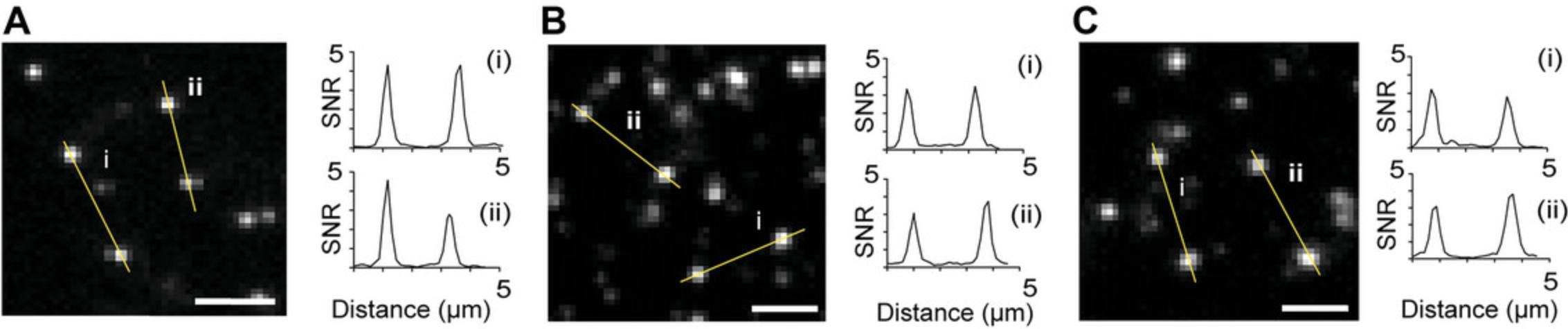
17a. Open the image acquisition software (NIS Elements) and right click to find acquisition controls. Go to “ND Acquisition.”
18a. In the “Time” tab, input the NII in the “interval” column. Input the frames in the “loops” column.
19a. Select “Time” tab and click “Run now” to record a movie consisting of at least 50 frames.
20a. Replay the recorded movie and evaluate the sparsity of the binding events.
21a. To determine the average number of single-molecule events/μm², select three representative regions (e.g., 15 × 15 μm) of the movie captured in step 20a.
22a. Count the number of single-molecule events in each frame and calculate the average number of events/μm².
23a. Adjust the antibody concentration of the imaging buffer to generate the optimal single-molecule event density for single-molecule localization microscopy (see step 13a).
24a. Cover the sample well with parafilm to prevent the imaging buffer from evaporating.
25a. Select the number of frames in the “loops” column of the ND acquisition.
26a. Capture sufficient single-molecule localizations to resolve the underlying structure (refer to Figs. 1I and 2C in Gunasekara et al., 2023).
![Details are in the caption following the image Superresolution images of microtubules in U2OS cells using single-antibody labeling. A comparison of reconstructed images after (A) 500, (B) 1000, (C) 2000, and (D) 3000 frames is shown for microtubules. DMIA-AF 488 was used to label endogenous α-tubulin. [DMIA-AF 488] = 0.003 nM, NII = 20 s, and exposure time = 50 ms. Scale bars: 1 μm.](https://static.yanyin.tech/literature_test/cpz1908-fig-0005-m.jpg)
Multiplexed imaging with single-antibody labeling
13b. Optimize the antibody concentrations for each target as described in steps 13a to 23a.
14b. Prepare the imaging buffer by diluting all antibodies. Add the imaging buffer to the sample well and set the NII.
15b. Choose the optical configurations (excitation lasers, quad-band set, and emission filters) based on the fluorophores selected for multiplexed imaging. Set the laser power density (100 to 400 W/cm2) and exposure time (50 ms).
16b. Set the number of frames and start the image acquisition.
Dual-color single-target imaging with single-antibody labeling
13c. Prepare the imaging buffer by diluting the antibodies. Add the imaging buffer to the sample well and set the NII.
14c. Select the optical configurations (excitation lasers, quad-band set, and emission filters) based on the fluorophores selected for multiplexed imaging. Set the laser power density (100 to 400 W/cm2) and exposure time (50 ms).
15c. Set the number of frames and start the image acquisition.
Alternate Protocol: DUAL-COLOR SINGLE-ANTIBODY LABELING USING OptoSplit II
In this protocol, we utilize OptoSplit II to perform dual-color single-target imaging. OptoSplit II allows a single camera to simultaneously capture images from two distinct optical wavelengths. Identify the set of filters and beam splitter for your specific application. For detailed information on the installation and usage, refer to the manual provided by the manufacturer.
Additional Materials (also see Basic Protocol 2)
- Acquisition software (Nikon, NIS-AR 5.42)
- Software add-on for OptoSplit II (Nikon, MQS41410: NIS-D SPLITTER Dual View)
- Filter cube to split 488/647 nm (Cairn Research):
- T565LPXR-UF2 Dichroic
- AT540/30M emission filter 25 mm
- ET655LP long pass emission filter 25 mm
- Filter cube to split 561/647 nm (Cairn Research):
- T640LPXR-UF2 LP Dichroic 26 × 38 × 2 mm
- ET615/40M emission filter 25 mm
- ET655LP long pass emission filter 25 mm
- OptoSplit II (OptoSplit II LS 1.0× Cairn Research)
1.Turn on the microscope, camera for OptoSplit II, acquisition computer with acquisition software and the software add-on for OptoSplit II, and lasers.
2.Open the acquisition software. Select the optical configuration (excitation lasers, quad-band set, and filter cube to split 488/647 nm or 561/647 nm).
3.Prepare the imaging buffer by diluting the antibodies. Add the imaging buffer to the sample well and set the NII.
4.Set the laser power density (100 to 400 W/cm2) and the number of frames (500 to 3000).
5.Go to “Live” mode.
6.Use split adjusters and aperture adjusters in OptoSplit II to visualize and adjust the position of the region of interest (ROI).
7.Cover the sample well with parafilm to prevent the imaging buffer from evaporation.
8.Select “Time” in the ND acquisition tab and input the NII in the “interval” column. Input the frames in the “loops” column.
9.Start image acquisition.
Basic Protocol 3: IMAGE ANALYSIS
ThunderSTORM is an ImageJ plug-in designed for the analysis of fluorescence single-molecule data and the reconstruction of superresolution images using SMLM.
Materials
- ImageJ software: https://imagej.nih.gov/ij/download.html
- Thunder_STORM.jar file: https://github.com/zitmen/thunderstorm
- Raw data file (acquired according to Basic Protocol 2)
Initializing image reconstructions using ThunderSTORM plug-in
1.Install ImageJ software and download the Thunder_STORM.jar file. Copy it into the plug-in subfolder of the ImageJ installation. Locate the ThunderSTORM plug-in in the “Plugins” menu of ImageJ to confirm successful installation.
2.Load the raw data file (acquired according to Basic Protocol 2) in ImageJ. Open the ThunderSTORM plug-in and select “Run analysis”.
3.Select “camera setup” and set the parameters such as effective pixel size, photoelectrons per A/D count, and base level.
4.Set the other parameters such as image filtering, approximate localization of molecules, sub-pixel localization of molecules, and visualization of the results. Use the selected parameters and click “Preview”.
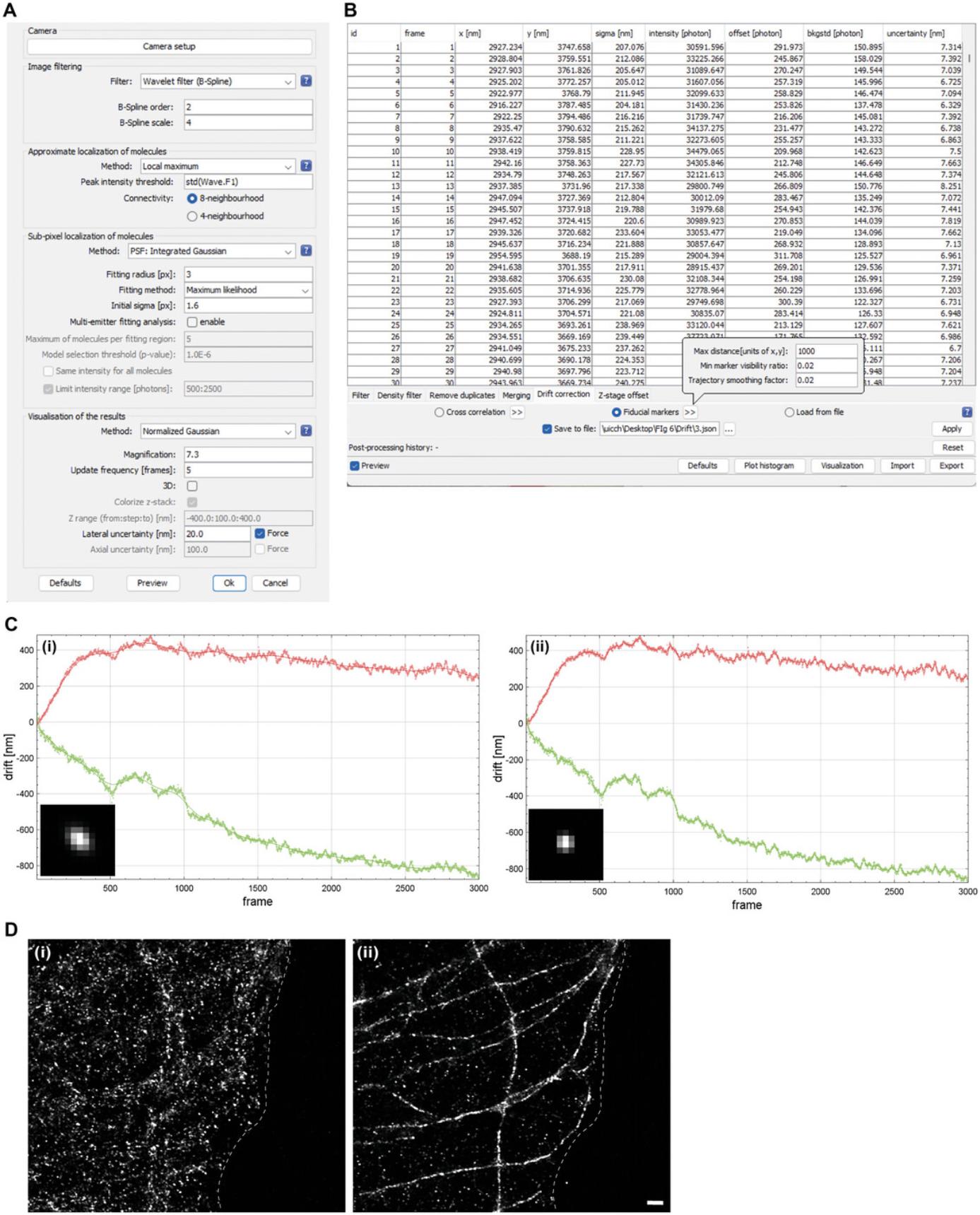
5.Visualize the detected molecules in the “Detections in frame 1” result window. If the visualization indicates a lower or higher count of detected molecules, adjust the parameters in step 4 and redo the “Preview”.
Drift correction using a fiducial marker
6.While the file is open in ImageJ, use the square selection tool to identify a fiducial marker present during the entire acquisition.
7.Open the ThunderSTORM plug-in and select “Run analysis” using the parameters set for image reconstruction.
8.In the result window, click the “Drift correction” tab.
9.Check the “Fiducial marker” and set parameters for drift correction.
10.Check “Save to file” and define a path to save the drift-corrected file. Click “Apply”.
Analyzing raw single-molecule data and applying drift correction
11.Unselect the square selection from step 6.Select “Run analysis” in ThunderSTORM and click “Ok”.
12.Apply the drift correction by clicking the “Drift correction” tab in the results table. Check “Load from file”.
13.In the “Visualization” tab, set the visualization method and magnification to render the final superresolution image.
Overlaying channels in multiplexed and dual-color single-antibody labeling
14.Open the reconstructed images of each channel separately in ImageJ.
15.Select “Image” and go to “Color”. Click “Merge Channels” from the drop-down menu. Assign colors for each channel using the “Merge Channels” dialog box.
16.Select “Create Composite” and click “OK” to create the overlay. Use “Save As” to save the overlay image as a new file.
17.Superimpose the images by aligning the fiducial markers from both channels. Select “Image” and go to “Transform”. Click “Translate” from the drop-down menu. Adjust X and Y offset.
REAGENTS AND SOLUTIONS
Blocking buffer
- For blocking buffer with 5% (w/v) bovine serum albumin (BSA ) :
- 100 μl 10% (w/v) BSA (MilliporeSigma, A7906-100G; 5% final)
- 20 μl 3% (v/v) Triton X-100 (MilliporeSigma, X100-1 litre; 0.3% final)
- 80 μl DPBS (Thermo Fisher Scientific, 14190-144, 500 ml)
- Prepare fresh immediately before use
- For blocking buffer with 10% (v/v) goat serum :
- 20 μl goat serum (Thermo Fisher Scientific, New Zealand origin, 16210072, 500 ml; 10% final)
- 20 μl 3% (v/v) Triton X-100 (MilliporeSigma, X100-1 litre; 0.3% final)
- 160 μl DPBS (Thermo Fisher Scientific, 14190-144, 500 ml)
- Prepare fresh immediately before use
Complete DMEM
- DMEM (Fisher Scientific, 11960069, 500 ml)
- 10% (v/v) fetal bovine serum (FBS; MilliporeSigma, F0926, 500 ml)
- 2 mM L-glutamine (Fisher Scientific, 25030− 081, 100 ml)
- 100 U/ml penicillin and 100 μg/ml streptomycin (diluted from 10,000 U/ml penicillin and 10,000 μg/ml streptomycin; Fisher Scientific, 15140−122, 100 ml)
- Store ≤4 months at 4°C
Fixation buffer
- 46 μl 16% (v/v) paraformaldehyde (Electron Microscopy Sciences, 15710; 3.7% final)
- 2 μl 10% (v/v) glutaraldehyde (Electron Microscopy Sciences, 16120; 0.1% final)
- 152 μl DPBS (Thermo Fisher Scientific, 14190-144, 500 ml)
- Prepare fresh immediately before use
The components of the extraction buffer used in our prior study (Gunasekara et al., 2023) are as follows: paraformaldehyde [3.7%(v/v)], glutaraldehyde [0.1% (v/v)], and Triton X-100 [0.5% (v/v)] in cytoskeleton buffer. In that study, the permeabilization step was combined with the fixation step to preserve the morphology of the microtubules during chemical fixation. The components and the final concentrations of the cytoskeleton buffer are as follows: MES (10 mM, pH 6.1; (MilliporeSigma, M3671-50G, MW 195.24), potassium chloride (90 mM; Fisher Scientific, P217−500, MW 74.55), magnesium chloride hexahydrate (3 mM; ACROS Organics, 413415000, MW 203.31), and EGTA (2 mM; MilliporeSigma, E3889-25G, MW 380.35) (Kiuchi et al., 2015). This solution can be stored ≤1 month at room temperature.
Post-fixation buffer
- 46 μl 16% (v/v) paraformaldehyde (Electron Microscopy Sciences, 15710; 3.7% final)
- 154 μl DPBS (Thermo Fisher Scientific, 14190-144, 500 ml)
- Prepare fresh immediately before use
COMMENTARY
Background Information
IF staining is a critical step in sample preparation for SMLM, typically accomplished through immunolabeling using dye-conjugated primary or secondary antibodies. The quality of the resulting superresolution image relies on the quality and specificity of the antibodies utilized. Currently, the selection of antibodies for IF staining for SMLM largely relies on empirical methods, emphasizing the need for careful antibody validation and optimization.
The interactions between antibodies and epitope targets are often characterized by the equilibrium dissociation constant (KD), typically falling within the nanomolar range. In conventional IF staining, primary antibodies are commonly used at concentrations ranging from 2 to 10 µg/ml or 13.3 to 66.7 nM (for full-length IgG, the molecular weight is ∼150 kDa). These concentrations exceed the KD values by 1 to 2 orders of magnitude. The large excess of antibodies labels the majority of the target at equilibrium. In our study, we employed low antibody concentrations, below the nanomolar range. This process scales down the on-rate, enabling the capture of antibody labeling through single-molecule imaging. Thus, single-antibody labeling captures the progression of the immunolabeling process one antibody at a time.
Furthermore, we implemented a stroboscopic imaging scheme by incorporating an NII between consecutive frames to ensure that each frame captures an adequate number of single-molecule events without significant spatial overlap. As manifested by the extended acquisition time, the off-rate of the antibody is unmodulated and remains slow. This is a distinct feature compared to existing PAINT techniques that promote the off-rate of the labeling probes. However, a noted advantage of our technique is the higher SNR due to multiple dyes per antibody. Signal amplification may also be achieved by multiple dye–conjugated secondary antibodies interacting with a single primary antibody. The extended image acquisition time represents a major limitation of our technique. Among approaches to accelerate the dissociation are antibody fragmentation and protein engineering methods (Gunasekara et al., 2022; Miyoshi et al., 2021; Zhang et al., 2022). However, promoting antibody dissociation without compromising binding specificity is a challenging task that highly relies on the specific interactions between the antibody and antigen. To that end, our dual-color single-target antibody labeling strategy provides an alternative approach to increasing labeling density within a given duration of acquisition. Our imaging method also enables the screening and evaluation of antibody labeling within the native cellular environment. Our approach provides valuable insights into the behavior and performance of antibodies in their cellular environment, augmenting existing assays to enhance the understanding of their performance for superresolution imaging applications.
Critical Parameters
A critical task in image acquisition is optimizing the dye-conjugated antibody concentration and NII to capture sufficient single-molecule events without significant spatial overlap (steps 13a to 23a, Basic Protocol 2). The optimal concentration and NII vary for different antibody-target systems. A single-molecule event density of 0.09 to 0.3 events/µm2 serves as a starting point for optimization. We maintain the concentration within the range of 0.003 to 0.5 nM (Table 2) while maintaining an NII of 20 s.
When preparing the samples for imaging using dye-conjugated secondary antibodies, selecting the appropriate concentration of unconjugated primary antibody is important (step 8b, Basic Protocol 1). As a starting point, use the manufacturer-suggested concentration. If the resulting superresolution image does not demonstrate the anticipated labeling density, consider increasing the primary antibody concentration to enhance the substrate abundance. For instance, in our study, we used a primary antibody (12CA5) concentration of 10 µg/ml (67 nM) for the labeling of HA tag on α-tubulin to image with secondary antibody F(ab′)2-AF 647.
Troubleshooting
During each of the procedures outlined in the protocols, it is anticipated that several challenges may arise. To address these issues, Table 3 presents a summary of the possible problems, potential causes, and solutions.
Problem | Possible cause | Solution |
---|---|---|
Single-molecule events outside the cell (equal to or significantly greater than within the cell) | Nonspecific binding is high | Extend the blocking time (up to 2 hr) if using bovine serum albumin in the blocking buffer or use serum from the species that corresponds to the secondary antibody in the blocking buffer |
Increase washing steps (to five times) after primary antibody staining (step 10b, Basic Protocol 1) | ||
Uneven illumination observed following sample mounting | Bubbles in immersion oil | Wipe off the immersion oil completely and then reapply it to the objective |
Let the chamber slide reach room temperature before mounting | ||
Low signal-to-noise ratio (SNR, <3) | High background signal | Decrease the antibody concentration (by 10- to 100-fold) in the imaging buffer to reduce the background signal |
Adjust the TIRF angle to restrict out-of-focus excitation to maximize the SNR (step 16a, Basic Protocol 2) | ||
Overlapping single-molecule events | High antibody concentration in imaging buffer | Decrease the antibody concentration (by 10- to 100-fold) in the imaging buffer (step 23a, Basic Protocol 2) |
Introduce a photobleaching step (with a maximum laser power density) every 20-50 frames in the image acquisition | ||
Insufficient number of fiducial markers for drift correction | Cannot identify fiducial markers near the cell to be imaged | Sonicate (gold colloids) or vortex (TetraSpeck microspheres) the stock solutions to uniformly suspend the fiducials (step 8, Basic Protocol 2) |
Increase the concentration of fiducial markers (5-fold) or incubate the fiducial markers longer (until ≥3 settle down) | ||
Diffusive appearance of the morphology (e.g., microtubules) after image reconstruction | Overlapping single-molecule events | Decrease the antibody concentration (by 10- to 100-fold) in the imaging buffer (step 23a, Basic Protocol 2) |
Apply post-processing by selecting the parameter of choice (e.g., sigma) in ThunderSTORM analysis (refer to ThunderSTORM user's guide, version 1.3, post-processing modules) |
Understanding the Results
Our study used microtubules and mitochondria to demonstrate the imaging method. Basic Protocol 1 outlines the key steps for preserving subcellular structures in U2OS cells. Basic Protocol 2 outlines the steps for using NIS Elements software to perform single-antibody labeling. A comparable software package with time-lapse imaging capabilities can be used for this.
Tables 1 and 2 provide the antibody concentrations used in our study for sample preparation and time-lapse imaging with single-antibody labeling (at NII = 20 s), respectively. We optimized the antibody concentration and NII to achieve a single-molecule event density ranging from 0.09 to 0.3 events/μm². Fig. 3 shows the single-molecule event density before and after optimization for three antibody-target systems. Fig. 4 illustrates cross-sectional intensity profiles showing the SNR for different antibody-target systems. This was obtained using a laser power density of 100 to 400 W/cm2 and an exposure time of 50 ms.
We use the ThunderSTORM plug-in, available in ImageJ (Ovesný et al., 2014), for image processing. Open the raw data file (acquired according to Basic Protocol 2) in ImageJ and run ThunderSTORM analysis to obtain a superresolution image. To process the data, set the camera parameters: effective pixel size, photoelectrons per A/D count, and base level. In our study, we used an effective pixel size of 147 nm, a photoelectrons per A/D count of 0.98, and a base level of 100. Select the image filtering parameters and methods to identify the localization of molecules. In our study, we used Wavelet filter, Local maximum, and PSF: Integrated Gaussian (Fig. 6A). For visualization of the superresolution image during data analysis, select the visualization methods. In our study, we used Averaged shifted histograms or Normalized Gaussian. Preview the molecular localizations with given parameters. If the visualization indicates a lower or higher count of detected molecules, consider adjusting the image filtering parameters (e.g., B-Spline order and scale if using Wavelet filter). After the analysis, the reconstructed superresolution images and the table of localized molecules (results table) are considered the final results. Then, apply the drift correction (Fig. 6D). The post-processing methods can be applied to remove any poor-quality data points, further refining the obtained results and the ThunderSTORM user's guide. In our study, events with a sigma value exceeding 170 exhibited a diffusive appearance and were removed in the post-processing. The superresolution images of microtubules obtained by single-antibody labeling are shown in Fig. 5.
To process the data from multiple channel acquisitions (e.g., multiplexed and dual-color imaging), begin by opening each channel individually in ImageJ. Perform image analysis according to the steps outlined in Basic Protocol 3. Once the reconstructed superresolution images are generated, proceed to creating overlaid images using ImageJ (steps 14 to 17, Basic Protocol 3). Use the fiducials to align images generated from different channels. Representative superresolution images from multiplexed imaging of microtubules and mitochondria are shown in Fig. 7. Representative dual-color images of microtubules are shown in Fig. 8. We achieved 61- to 83-nm resolution for adjacent microtubules by single-antibody labeling (Gunasekara et al., 2023).
![Details are in the caption following the image Multiplexed superresolution imaging with single-antibody labeling. Superresolution images of (A) microtubules and (B) mitochondria. (C) Dual-color overlay of the superresolution images from (A) and (B). Microtubules are shown in magenta, and mitochondria are shown in green. For microtubule imaging, DMIA-AF 488 was used to label endogenous α-tubulin. [DMIA-AF 488] = 0.003 nM. For mitochondria imaging, Tom20 was labeled using the primary antibody anti-Tom20 and imaged with the secondary antibody goat anti-rabbit-AF 647. [Goat anti-rabbit-AF 647] = 0.125 nM. NII = 20 s. The reconstructed image is from 3000 frames. Scale bars: 1 μm.](https://static.yanyin.tech/literature_test/cpz1908-fig-0007-m.jpg)
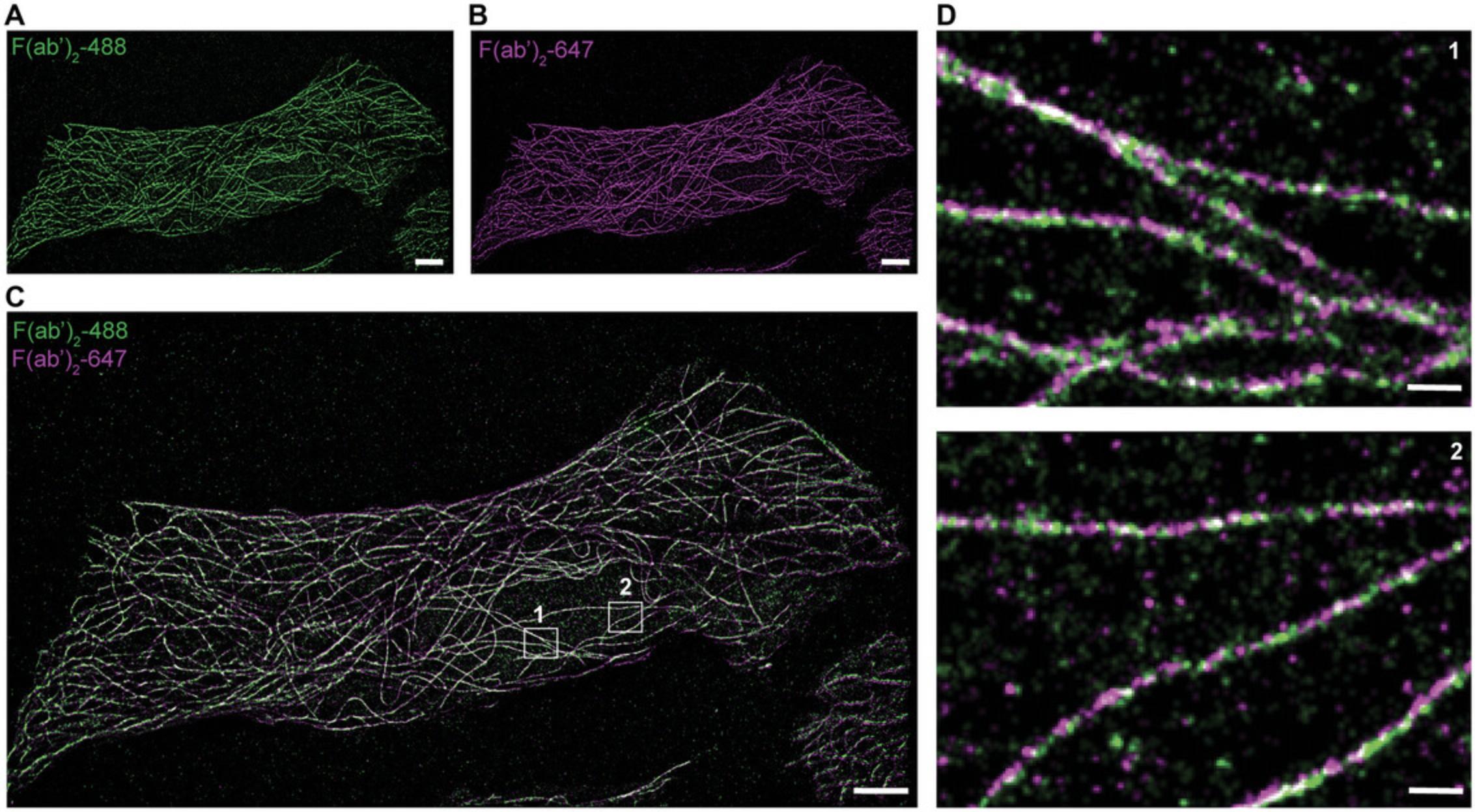
Time Considerations
The complete set of protocols outlined here requires ∼5 days for completion. Table 4 summarizes the time considerations for each basic protocol.
Protocol | Time considerations |
---|---|
Basic Protocol 1 |
Day 1: Cell seeding (10-20 min) Day 3 (after 36 hr of seeding): Fixation (15-20 min) Day 3: Sample preparation for single-antibody labeling using dye-conjugated primary antibodies (1-2.5 hr)a Day 3: Sample preparation for single-antibody labeling using dye-conjugated secondary antibodies (3-7 hr)a |
Basic Protocol 2 |
Day 4: Initiating data acquisition (30-40 min) Day 4: Determination of optimal antibody concentration and NII (steps 13a to 23a) (1-3 hr) Day 4/5: Time-lapse imaging with single-antibody labeling (1-17 hr)b Day 4/5: Multiplexed imaging with single-antibody labeling (1-17 hr)b |
Alternate Protocol | Day 4/5: Dual-color single-target imaging with single-antibody labeling (1-17 hr)b |
Basic Protocol 3 |
Day 5/6: Initializing image reconstructions (30 min) Day 5/6: Analyzing raw single-molecule data and applying drift correction (1 hr) |
- a The timing depends on the antibody-target system.
- b The timing depends on the NII and the number of frames selected for the acquisition (for instance, with a 1-s NII, capturing 3000 frames takes 1 hr, whereas with a 20-s NII, capturing 3000 frames extends to 17 hr).
Acknowledgments
The work was financially supported by the NIH (R35GM146786, YSH) and the College of Liberal Arts and Sciences at the University of Illinois Chicago. The authors thank J. Anderson, B. Saed, N. Ramseier, and N. Kesta for their work on developing this set of protocols. The authors thank B. Saed and A. Burgess for proofreading the manuscript.
Author Contributions
Thilini Perera : Conceptualization; data curation; formal analysis; investigation; methodology; validation; visualization; writing–original draft; writing–review and editing. Hirushi Gunasekara : Conceptualization; data curation; formal analysis; investigation; methodology; validation; visualization; writing–original draft; writing–review and editing. Ying S. Hu : Conceptualization; funding acquisition; project administration; resources; software; supervision; writing–review and editing.
Conflict of Interest
The authors declare no conflict of interest.
Open Research
Data Availability Statement
Images and movies relevant to this article have been published in our earlier manuscript (Gunasekara et al., 2023). New images have been incorporated as figures in this work. The data that support this article are available from the corresponding author upon reasonable request.
Literature Cited
- Farrell, M. V., Nunez, A. C., Yang, Z., Pérez-Ferreros, P., Gaus, K., & Goyette, J. (2022). Protein-PAINT: Superresolution microscopy with signaling proteins. Science Signaling , 15(719), eabg9782. https://doi.org/10.1126/scisignal.abg9782
- Giannone, G., Hosy, E., Levet, F., Constals, A., Schulze, K., Sobolevsky, A. I., Rosconi, M. P., Gouaux, E., Tampé, R., Choquet, D., & Cognet, L. (2010). Dynamic superresolution imaging of endogenous proteins on living cells at ultra-high density. Biophysical Journal , 99(4), 1303–1310. https://doi.org/10.1016/j.bpj.2010.06.005
- Gunasekara, H., Munaweera, R., Novotná, L., Lillemeier, B. F., & Hu, Y. S. (2022). Chaotropic perturbation of noncovalent interactions of the hemagglutinin tag monoclonal antibody fragment enables superresolution molecular census. ACS Nano , 16(12), 21645. https://doi.org/10.1021/acsnano.2c10526
- Gunasekara, H., Perera, T., Anderson, J., Saed, B., Ramseier, N., Keshta, N., & Hu, Y. S. (2023). Superresolution imaging with single-antibody labeling. Bioconjugate Chemistry , 34(5), 825–833. https://doi.org/10.1021/acs.bioconjchem.3c00178
- Heilemann, M., van de Linde, S., Schüttpelz, M., Kasper, R., Seefeldt, B., Mukherjee, A., Tinnefeld, P., & Sauer, M. (2008). Subdiffraction-resolution fluorescence imaging with conventional fluorescent probes. Angewandte Chemie , 47(33), 6172–6176. https://doi.org/10.1002/anie.200802376
- Jungmann, R., Avendaño, M. S., Woehrstein, J. B., Dai, M., Shih, W. M., & Yin, P. (2014). Multiplexed 3D cellular super-resolution imaging with DNA-PAINT and Exchange-PAINT. Nature Methods , 11(3), 313–318. https://doi.org/10.1038/nmeth.2835
- Jungmann, R., Steinhauer, C., Scheible, M., Kuzyk, A., Tinnefeld, P., & Simmel, F. C. (2010). Single-molecule kinetics and super-resolution microscopy by fluorescence imaging of transient binding on DNA origami. Nano Letters , 10(11), 4756–4761. https://doi.org/10.1021/nl103427w
- Kiuchi, T., Higuchi, M., Takamura, A., Maruoka, M., & Watanabe, N. (2015). Multitarget super-resolution microscopy with high-density labeling by exchangeable probes. Nature Methods , 12(8), 743–746. https://doi.org/10.1038/nmeth.3466
- Miyoshi, T., Zhang, Q., Miyake, T., Watanabe, S., Ohnishi, H., Chen, J., Vishwasrao, H. D., Chakraborty, O., Belyantseva, I. A., Perrin, B. J., Shroff, H., Friedman, T. B., Omori, K., & Watanabe, N. (2021). Semi-automated single-molecule microscopy screening of fast-dissociating specific antibodies directly from hybridoma cultures. Cell Reports , 34(5), 108708. https://doi.org/10.1016/j.celrep.2021.108708
- Ovesný, M., Křížek, P., Borkovec, J., Svindrych, Z., & Hagen, G. M. (2014). ThunderSTORM: A comprehensive ImageJ plug-in for PALM and STORM data analysis and super-resolution imaging. Bioinformatics , 30(16), 2389–2390. https://doi.org/10.1093/bioinformatics/btu202
- Phelan, K., & May, K. M. (2015). Basic techniques in mammalian cell tissue culture. Current Protocols in Cell Biology , 66, 1.1.1–1.1.22. https://doi.org/10.1002/0471143030.cb0101s66
- Rust, M. J., Bates, M., & Zhuang, X. (2006). Sub-diffraction-limit imaging by stochastic optical reconstruction microscopy (STORM). Nature Methods , 3(10), 793–795. https://doi.org/10.1038/nmeth929
- Schnitzbauer, J., Strauss, M. T., Schlichthaerle, T., Schueder, F., & Jungmann, R. (2017). Super-resolution microscopy with DNA-PAINT. Nature Protocols , 12(6), 1198–1228. https://doi.org/10.1038/nprot.2017.024
- Zhang, Q., Miyamoto, A., Watanabe, S., Arimori, T., Sakai, M., Tomisaki, M., Kiuchi, T., Takagi, J., & Watanabe, N. (2022). Engineered fast-dissociating antibody fragments for multiplexed super-resolution microscopy. Cell Reports Methods , 2(10), 100301. https://doi.org/10.1016/j.crmeth.2022.100301
Internet Resources
- https://imagej.nih.gov/ij/download.html
- ImageJ software.
- https://github.com/zitmen/thunderstorm
- Thunder_STORM.jar file.