Methods to Identify Immune Cells in Tissues With a Focus on Skin as a Model
Peter R. Murphy, Peter R. Murphy, Divyaa Narayanan, Divyaa Narayanan, Snehlata Kumari, Snehlata Kumari
flow cytometry
inflammation
immune cells
immunofluorescence
immunohistochemistry
multiplex
skin
skin inflammation
skin immune cells
Abstract
The skin protects our body from external challenges, insults, and pathogens and consists of two layers, epidermis and dermis. The immune cells of the skin are an integral part of protecting the body and essential for mediating skin immune homeostasis. They are distributed in the epidermal and dermal layers of the skin. Under homeostatic conditions, the mouse and human skin epidermis harbors immune cells such as Langerhans cells and CD8+ T cells, whereas the dermis contains dendritic cells (DCs), mast cells, macrophages, T cells, and neutrophils. Skin immune homeostasis is maintained through communication between epidermal and dermal cells and soluble factors. This communication is important for proper recruitment of immune cells in the skin to mount immune responses during infection/injury or in response to external/internal insults that alter the local cellular milieu. Imbalance in this crosstalk that occurs in association with inflammatory skin disorders such as psoriasis and atopic dermatitis can lead to alterations in the number and type of immune cells contributing to pathological manifestation in these disorders. Profiling changes in the immune cell type, localization, and number can provide important information about disease mechanisms and help design interventional therapeutic strategies. Toward this end, skin cells can be detected and characterized using basic techniques like immunofluorescence, immunohistochemistry, and flow cytometry, and recently developed methods of multiplexing. This article provides an overview on the basic techniques that are widely accessible to researchers to characterize immune cells of the skin. © 2022 The Authors. Current Protocols published by Wiley Periodicals LLC.
INTRODUCTION
The epidermis and underlying dermis of mouse and human skin harbor a variety of immune and non-immune cells. The epidermis in mice and humans mainly consists of keratinocytes, melanocytes, and immune cells, such as Langerhans cells (LCs) and CD8+ T cells. Mouse skin also hosts immune cells called dendritic epidermal T cells (DETCs), which are absent in human skin. Below the epidermis is the dermis, which is populated with diverse groups of immune cells such as dendritic cells (DCs), mast cells, macrophages, and T cells, as well as stromal cells like fibroblasts, all of which are embedded in a network of extracellular matrices and blood vessels (Nestle, Di Meglio, Qin, & Nickoloff, 2009; Pasparakis, Haase, & Nestle, 2014). The following publications will help researchers understand the immune cell diversity in mouse and human skin (Chu, Di Meglio, & Nestle, 2011; Conrad, Meller, & Gilliet, 2009; Kortekaas Krohn et al., 2021; Lakschevitz et al., 2016; Merad, Ginhoux, & Collin, 2008; Nestle & Nickoloff, 2007; Nestle et al., 2009; Nguyen & Soulika, 2019; Pasparakis et al., 2014; Reynolds et al., 2021; Xu et al., 2021).
Proper communication among cells making up the network of immune cells in the skin is crucial to maintain skin homeostasis (Nestle et al., 2009; Pasparakis et al., 2014) and prevent the development of skin pathologies, such as skin inflammation and skin cancer. Understanding the biology and landscape of the immune cells in normal and pathological skin conditions is crucial to identify prognostic biomarkers and drug targets for therapeutic interventions. The most used state-of-the-art methodologies to characterize immune cells in the skin are flow cytometry, immunohistochemistry (IHC), and immunofluorescence (IF). These techniques are widely accessible and have been constantly evolving with enhanced efficiency and resolutions to delineate the immune diversity in the skin.
Historically, the 20th century ushered in techniques for labeling antibodies with dyes, enabling their visualization via light microscopy (Heidelberger, Kendall, & Soo Hoo, 1933). Later, the successful conjugation of a fluorophore to an antibody resulted in the immunofluorescence revolution, enabling localization of antigens in cells and tissues through fluorescence microscopy (Coons, 1951; Coons & Kaplan, 1950). However, it was not until the 1960s that this technique was applied to dermatology. In 1964, Beutner and Jordon (1964) demonstrated that antibodies within the skin could be detected via indirect IF staining in patients with pemphigus vulgaris. By the 1970s, methods to detect antigens on formalin-fixed paraffin embedded (FFPE) tissues were also developed (Taylor & Burns, 1974). During this period, inefficient epitope retrieval limited the use of FFPE tissues when compared to cryosectioned tissues. However, in the 1990s, demasking techniques using heat-induced epitope retrieval on paraffin-embedded tissues were developed, which enhanced the capacity of antigen-antibody detection and opened up the possibility of using archival FFPE-processed tissue (Shi, Key, & Kalra, 1991).
Flow cytometry was available throughout the 20th and 21st centuries for the detection of immune cells within the skin. Flow cytometers were developed in the 1960s and their refinement led to the development of one of the first fluorescence-activated cell sorters (FACSs), manufactured in the 1970s (Hulett, Bonner, Barrett, & Herzenberg, 1969; Hulett, Bonner, Sweet, & Herzenberg, 1973; Melamed, 2001). Since then, the technology has expanded, with many more antibodies and fluorophores becoming available to detect a wider range of markers on a given cell (McKinnon, 2018). The advancements in flow cytometry range from the development of the automated high-throughput screening system that incorporates a fast sample-loading technology and powerful data analysis capabilities (Black, Duensing, Trinkle, & Dunlay, 2011; Edwards, Kuckuck, Prossnitz, Ransom, & Sklar, 2001) to multicolor flow cytometry that can analyze more than 20 markers (Liechti et al., 2021). Selection of the right combination of fluorophores and antibody conjugates, and availability of the respective flow cytometry machines are the crucial considerations when planning to use multicolor flow cytometry (Baumgarth & Roederer, 2000).
The possibility of combining multiple fluorophore-conjugated markers is advantageous when using flow cytometry; however, it cannot determine the spatial distribution of immune cells. Therefore, if the experimental aim is to address localization, niche, and distribution, IF/IHC or in situ hybridization should be the method of choice, or these must be used in combination. If the experimental aim is to quantitatively characterize the immune cells in the skin, flow cytometry is recommended. The capabilities of each method (IF, IHC, and flow cytometry) are compared in Table 1.
Flow cytometry | Immunofluorescence | Immunohistochemistry | |
---|---|---|---|
Detection | Fluorescence | Fluorescence | Chromogenic |
Origin tissue | Fresh | Fixed or cryopreserved | Fixed or cryopreserved |
Processed tissue | Single-cell suspension | Sectioned tissue | Sectioned tissue |
Antigen detection | 20+ | 1-6 | 1-2 |
Time to analyze cells | (>10,000 cells/sec) | Slower | Slower |
Spatial context | No | Yes | Yes |
Identify marker co-localization | No | Yes | Yes, limited to two or three antigens |
Identify cell-cell interactions | No | Yes | Yes |
Rare cell types | Yes | Comparatively less sensitive | Comparatively less sensitive |
Multiplexing (Table 3) | Yes | Yes (up to 50-60) | Yes (up to 25-30) |
Instrumentation | Flow cytometer | Fluorescence microscope | Light microscope |
The research field has evolved further with the development of multiplexing imaging techniques that enable the quantification of numerous proteins in tissues in the spatial and morphological contexts. Multiplexing refers to the ability to detect multiple markers on one tissue section or simultaneously, often via the use of multiple fluorophore-conjugated antibodies or probes. Originally, multiplexing involved two or three different markers; however, the field has evolved, and now there are several different multiplexing technologies available for detecting more than 50 markers simultaneously (Phillips et al., 2021). It is important to note that due to possible repetitive cycles of enzymatic labeling, image scanning, and stripping of the chromogenic substrate on the tissue, it is now possible to detect more markers using multiplex IHC staining. However, compared to IF, fewer markers are detected in IHC-based multiplexing (Eng et al., 2022).
In multiplex imaging, the phenotypic composition of immune cells can be combined with the identification of cellular networks, such as cell-cell and cell-soluble factor interactions. Multiplexing is increasingly being used for biomarker discoveries as well as in precision medicine, particularly in the cancer research field such as in cutaneous melanoma (Zhang, Song, Sheng, Bai, & Liang, 2021). It is crucial to understand the methodologies, platforms, and the analytic systems of these multiplexing strategies, which are still in the development phase; hence, they are not yet accessible to or cost-effective for every researcher. Multiplexing is a topic in itself and is not covered thoroughly in this overview. We have summarized some of these multiplexing imaging systems in Table 2, and refer two reviews that cover multiplexing in more detail (Eng et al., 2022; Tan et al., 2020).
Imaging system | Imaging modality |
---|---|
Codex | DNA-barcoding |
Chip cytometry | Fluorescence system |
CyTOF imaging | Metal-based system |
DISCOVERY ULTRA | Fluorescence/chromogenic system |
Digital Spatial Profiling (DSP) | DNA-barcoding system |
InSituPlex | DNA-barcoding system |
Multiplexed Ion Beam Imaging (MIBI) | Metal system |
Vectra | Fluorescence system |
The aim of this overview is to review widely used state-of-the-art technologies, flow cytometry, IHC, and IF, which are widely accessible to researchers to detect and characterize immune cells in the skin.
MAJOR TOPICS
IF and IHC Staining
IF and IHC are the most commonly used techniques to detect in situ immune cells in the skin via microscopy. Figure 1 summarizes the IF and IHC processes.
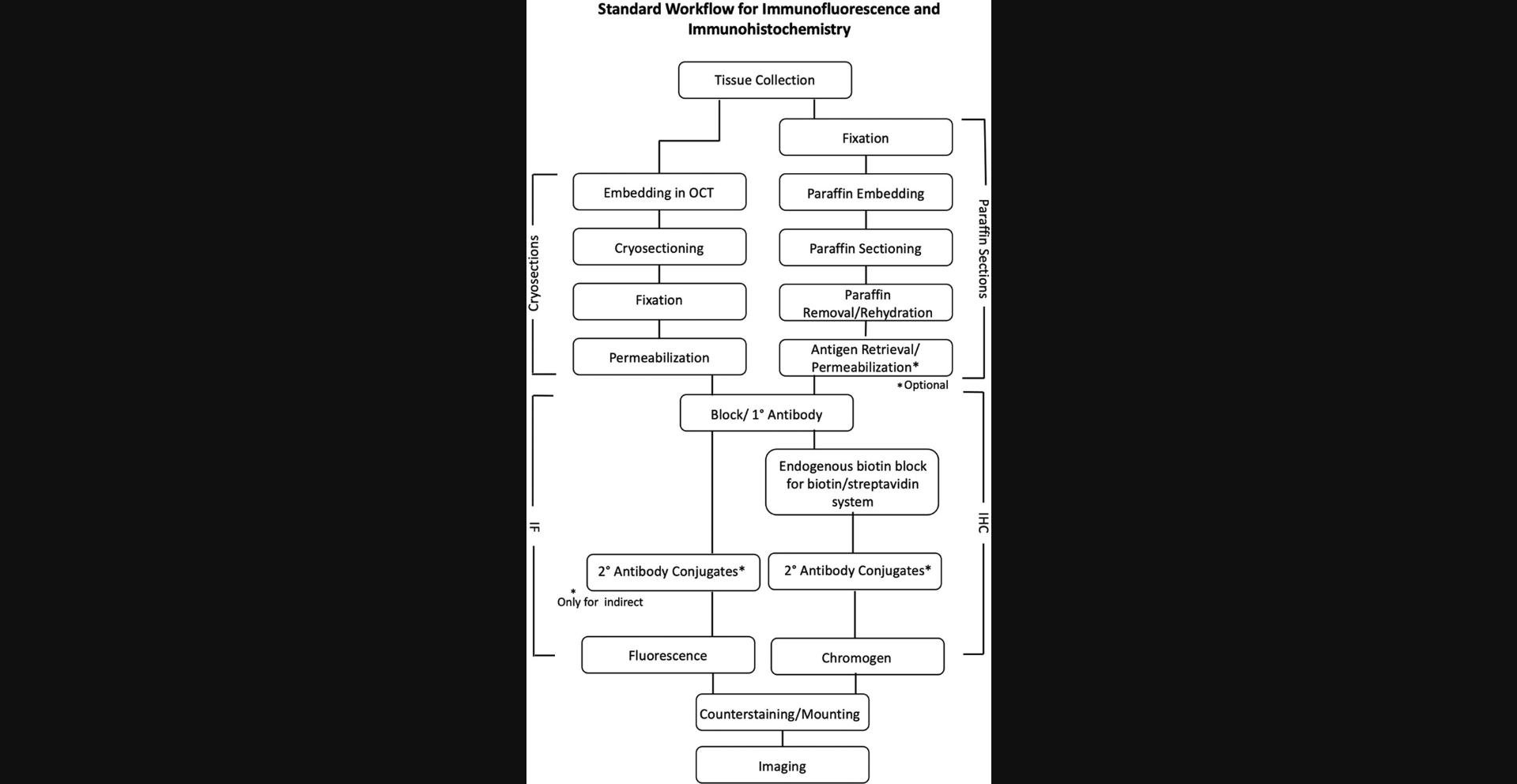
IHC relies upon antibody-enzyme conjugates and a chromogen for the detection method, whereas IF is based on antibody-fluorophore conjugates with fluorescence detection to identify antigens within the tissues. One of the key differences between IF and IHC is that defining an immune cell in the spatial context on the same tissue is easier in IF compared to IHC. Basic IF can detect more antigens than IHC due to availability of the fluorophore-conjugated antibodies, DNA-binding dyes, and ion indicator dyes. The method chosen for detecting the cell type depends on the experimental aims and accessibility of the materials. For example, if the experimental aim is to perform co-localization of multiple immune cell markers on the same skin section, IF should be the method of choice. The differences between IHC and IF are briefly explained in Table 1.
The skin samples collected for IF or IHC should either be cryopreserved in optimal cutting temperature (OCT) compound or as formalin-fixed paraffin-embedded (FFPE) sections. When collecting tissue, it is important to know the differences between cryosectioning and paraffin embedding methods, which we have highlighted in Table 3.
Cryopreserved | FFPE | |
---|---|---|
Antigen demasking | No | Yes |
DNA/RNA/lipid analysis | Yes | No |
Tissue morphology | Comparatively less preserved | Preserved |
Section thickness/resolution | Thicker section (less resolution) | Thinner section (higher resolution) |
Stability of sample | Short term | Long term |
Sectioning instrumentation | Cryostat | Microtome |
Storage temperature | −80°C | Room temperature |
Optimization for specific antibody | Yes | Yes |
The method of choice depends on the experimental aims. For example, if skin morphology is to be investigated, FFPE is the better option as it retains tissue morphology better than cryopreservation. FFPE also allows for cutting thinner sections (∼3 µm), thus enabling, higher resolution in detecting antigens compared to cryosections (>4 µm) (Hira et al., 2019). However, it is difficult to analyze post-translational modification of proteins as well as DNA or RNA in FFPE tissues, whereas cryopreserved tissues can be used for this purpose (Groelz, Viertler, Pabst, Dettmann, & Zatloukal, 2018). Cryopreserved tissues are less stable for long-term storage but superior in the preservation of antigenicity; in contrast, FFPE tissues are good for long-term storage, but antigens are altered due to tissue fixation. Therefore, FFPE sections require an additional antigen-retrieval step to unmask antigens before staining (Shi et al., 1991). For FFPE, common fixatives are formalin or methanol. Each of these fixation methods can impact staining of certain antigens, so care should be taken to choose the correct fixative. Collection of both cryopreserved and FFPE tissues and optimization of each antibody are recommended prior to the detection of immune cell markers. This review does not aim to provide information on the fixative methods to be chosen; these are discussed elsewhere (Howat & Wilson, 2014; Srinivasan, Sedmak, & Jewell, 2002). Once fixed, the tissues are dehydrated and embedded in paraffin for long-term storage or for proceeding with the sectioning. The sectioning instruments are different for cryosectioning and FFPE. Cryosectioning requires the tissue to be sectioned in a cryostat at subzero temperatures, whereas FFPE tissues can be sectioned at room temperature and are therefore easier to handle. After sectioning, the cryosections are often fixed using a mild fixative such as methanol, ethanol, or acetone. Due to this being a mild form of fixation, there is no need for antigen retrieval as with FFPE.
The key differences between cryopreserved and FFPE tissues are highlighted in Table 3 to help researchers decide the suitability of the methods accordingly.
Deparaffinization is a process whereby paraffin is removed from the tissues prior to staining. This generally involves the incubation of skin sections in xylene solution, followed by incubations in a gradient of decreasing ethanol concentration to gradually rehydrate the tissues. Post deparaffinization, antigen unmasking or retrieval is performed. There are two commonly used methods for antigen retrieval, heat-induced epitope retrieval (HIER) and protease-induced epitope retrieval (PIER; Krenacs, Krenacs, Stelkovics, & Raffeld, 2010). PIER relies on enzymes such as proteinase K, trypsin, or pepsin to unmask antigens by degrading the protein crosslinks, whereas HIER unfolds protein crosslinks to expose epitopes to be detected by antibodies. HIER is commonly performed using a microwave, pressure cooker, or water bath in citrate buffer (pH 2, 6, or 9). The tissue type, fixation method, method availability, and antibody are the key determinants of method selection, and therefore it is recommended to optimize them accordingly based on the manufacturer's instructions. An example of tissue type–dependent selection is that the pressure cooker could be harsh for a thin section but suitable for a thick tissue section of the skin.
After antigen retrieval and fixation, the skin sections are blocked with blocking reagents to prevent nonspecific binding of primary antibodies. Normal goat serum, bovine serum albumin, fish skin gelatin, or a mixture of different components are commonly used in the blocking solutions. Optionally, the use of Triton-X or Tween-20 detergent in the blocking solution or antibody diluent may be required for better penetration of antibodies into the tissues if staining for intracellular markers (Bachman, 2013; D'Amico, Skarmoutsou, & Stivala, 2009; Im, Mareninov, Diaz, & Yong, 2019; Pileri et al., 1997).
Before proceeding to the next step of antigen detection using primary and secondary antibodies, IHC requires an additional step to block endogenous biotin if a biotin-streptavidin conjugation system is used for staining. Endogenous enzymes such as peroxidase can interfere with peroxidase-based detection systems, and endogenous biotin can interfere with biotin-avidin–based systems (Chen, Cho, & Yang, 2010).
Two forms of antigen detection methods are used in IF and IHC, direct and indirect. The direct approach requires a fluorophore-/enzyme-conjugated primary antibody, whereas the indirect method initially labels antigens with an unlabeled antibody followed by labeling of the primary antibody with a secondary antibody that is conjugated to a fluorophore/enzyme. The visualization method for IHC-stained sections is different from that in IF-stained sections. The IF-stained sections are examined with a fluorescence microscope to visualize the fluorophores; however, in IHCs, IHC antibodies are usually conjugated with an enzyme, such as horseradish peroxidase (HRP) or alkaline phosphatase (Hofman & Taylor, 2013) and visualized using a light microscope. Chromogens such as aminoethyl carbazole (AEC) and 3,3′- diaminobenzidine (DAB) are the most commonly used substrates for IHC visualization methods (Hira et al., 2019). It is important to note that if the signal of an antibody is weak, an additional amplification step or staining step can be performed to amplify the signal intensity (Magaki, Hojat, Wei, So, & Yong, 2019). At the end, counterstaining with DAPI (4′,6- diamidino-2-phenylindole) or propidium iodide (PI) in IF and with hematoxylin in IHC is a common practice to visualize the cell nucleus and tissue morphology.
Flow Cytometry
Flow cytometric detection of the immune cells found within human or mouse skin requires fresh skin biopsies. It is often a method of choice for the quantitative characterization of the immune cell populations of the skin. Flow cytometry is comparatively more sensitive than IHC/IF and can therefore easily detect rare immune cell types.
The process of flow cytometry starts with collection of the skin, digestion, and processing to prepare a single-cell suspension, followed by blocking and staining with antibodies and data acquisition via a flow cytometer. A general workflow for flow cytometry is summarized in Figure 2. Prior to the collection of human or mouse skin, the hair must be removed, as this can interfere with tissue processing. The amount of skin needed for flow cytometry will depend on the experimental requirements. For example, if researchers want to characterize rare cell populations, the starting material should be more than what would be required for immune cells in abundance. Unlike IF/IHC, the detection and characterization of immune cells via flow cytometry require tissue digestion and preparation of a single-cell suspension.
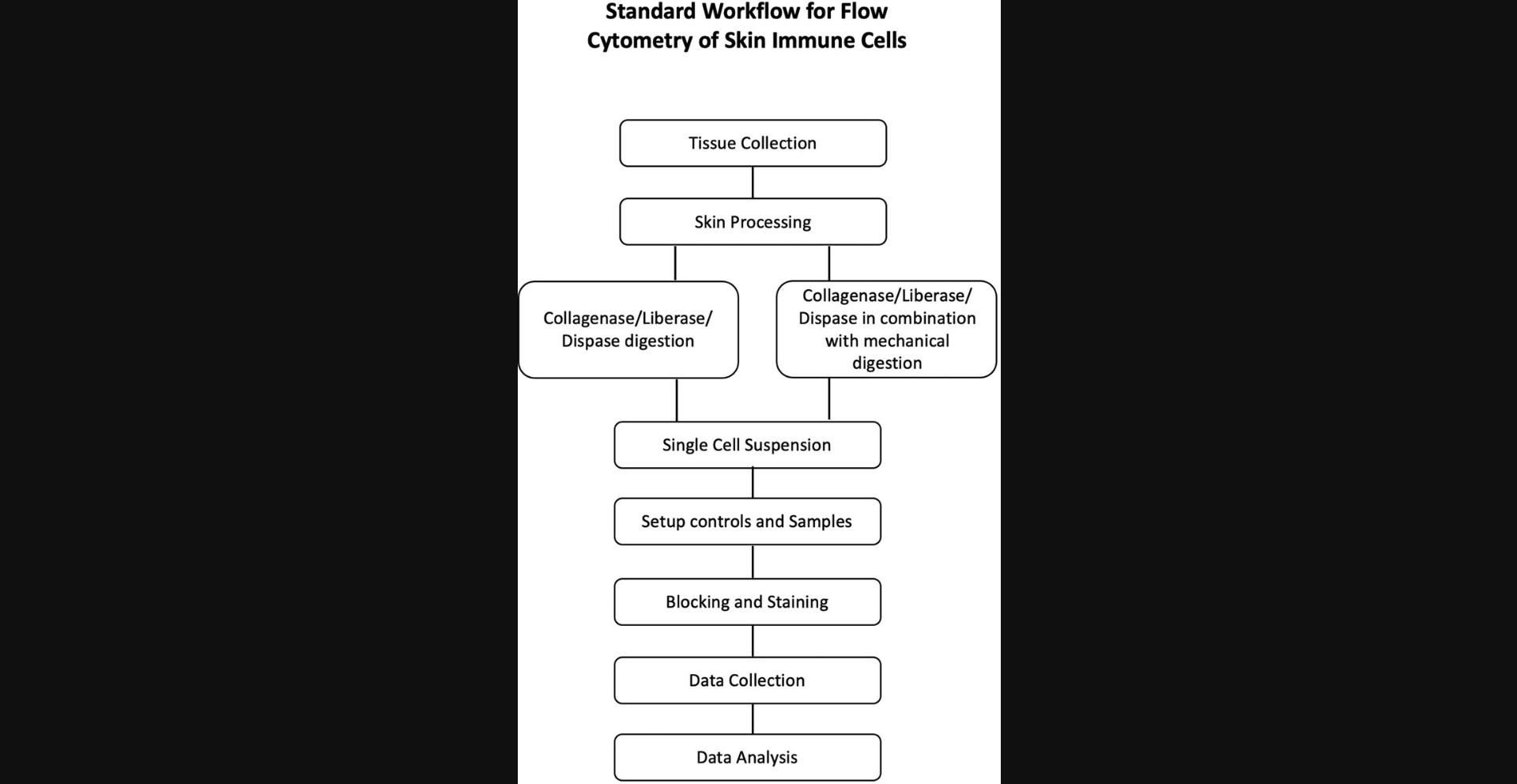
Before starting a flow cytometry experiment, care needs to be taken to choose the markers, fluorophores, titer of antibody, and number of fluorophores detectable by the machine. Depending on the flow cytometer, it is possible to detect more than 20 markers, providing a robust platform to delineate lineages of immune cells in the skin. In this review, we aim to provide a comprehensive overview on the basic principles and markers commonly used in flow cytometry; however, investigators can find detailed information in the comprehensive reviews by Ashhurst, Smith, and King (2017) and Cossarizza et al. (2019).
The locations of immune cells in the epidermis and dermis of human and mouse skin are different; therefore, if the aim is to detect epidermal cells, the dermis can be separated, or if the experimental aim is complete immune profiling, the skin can be processed and analyzed as a whole tissue. For detection of immune cells and their diversity, a combination of lineage-specific markers, cell surface markers, and gating strategies can be used. Figure 3 outlines the similarities and differences among these methods. Moreover, the gender and strain of the mouse can also affect the detection of immune cells (Hensel, Khattar, Ashton, & Ponnazhagan, 2019). Figure 3 summarizes some of the commonly used markers for immune cells in IF, IHC, and flow cytometry of human and mouse skin. For example, a population of cells existing in only mice but not humans is a subset of γδ T cells called dendritic epidermal T cells (DETCs; Castillo-Gonzalez, Cibrian, & Sanchez-Madrid, 2021). Further information on the markers of specific immune cells in humans and mice can be obtained from several resources including the following references (Hey, Tan, & O'Neill, 2015; Maecker, McCoy, & Nussenblatt, 2012; Meindl, Schmidt, Vaculik, & Elbe-Burger, 2006; Mousset et al., 2019; Nakayama, 2014; Riedl, Tada, & Udey, 2004; Stankovic et al., 2018; Szabo, Miron, & Farber, 2019).
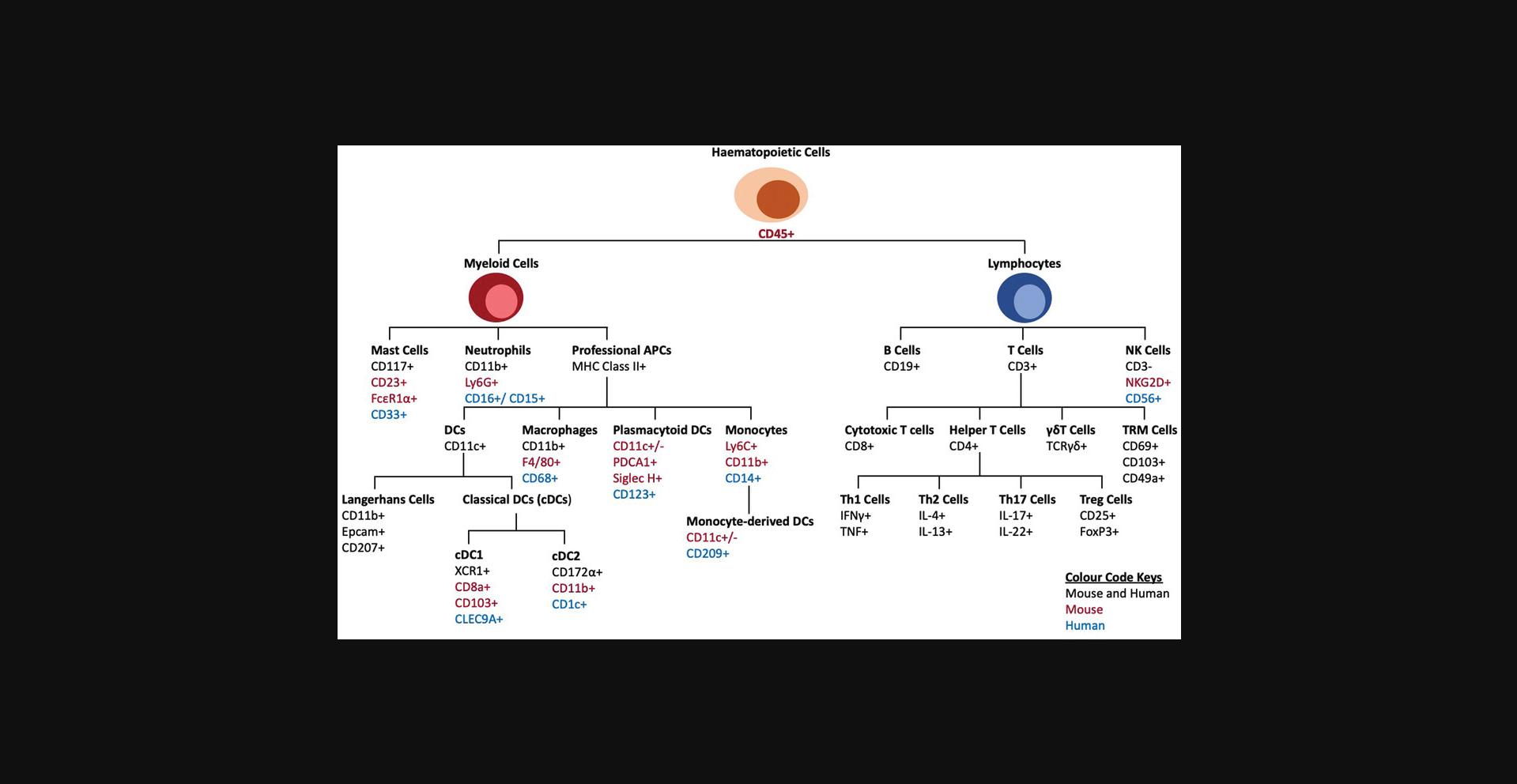
Optimal digestion of skin tissue is crucial to obtain a single-cell suspension and can be achieved using enzymes, mechanical dissociation, or a combination of both. Dispase, collagenase, liberase, and trypsin are commonly used either alone or in combination to digest human or mouse skin (Adam et al., 2020; Benck, Martinov, Fife, & Chatterjea, 2016; Kumari et al., 2013; Li, Gothard, Coles, & Ambler, 2018; Lou, Sun, & Wang, 2020). As discussed above, if the aim is to analyze the immune cells of the epidermis and dermis separately, they can be separated using dispase (Lou et al., 2020). Variations in the yield of the immune cell populations have been observed using different enzymatic methods. Here, we have provided multiple references for general and skin-specific protocols for tissue digestion and their limitations to help investigators decide which enzymatic method must be used (Autengruber, Gereke, Hansen, Hennig, & Bruder, 2012; Kashem & Kaplan, 2018; Kumari et al., 2013; Li et al., 2018; Liu, Gu, Shin, Zhang, & Ginhoux, 2020; Lou et al., 2020; Singh, Zhang, Hwang, & Farber, 2019; Stoitzner, Romani, McLellan, Tripp, & Ebner, 2010; Sumaria et al., 2011; Willenborg et al., 2021). Li et al. (2018) compared dispase with liberase/collagenase D and found that although similar numbers of CD45+ cells were isolated, dispase II gave a 10-fold higher number of total cells with a 20-fold higher number of DETCs. This suggests that dispase II is more effective for digesting the epidermal layers of the skin in comparison to liberase/collagenase D (Li et al., 2018). However, multiple studies including that of Li et al. detected a reduction in staining of some markers, such as CD4 in dispase compared to collagenase (Autengruber et al., 2012; Li et al., 2018). A similar finding from spleen immune cells has been described by Autengruber et al. (2012) in which dispase digestion of spleen resulted in the reduction of cell-surface expression of CD4, CD8, and CD25 compared to collagenase. Therefore, using an enzymatic method requires careful consideration and optimization.
The duration of enzymatic digestion is another consideration, and optimization of the duration and medium used is recommended prior to working with experimental samples. For the skin, incubation in cell culture medium, PBS, or HBSS for 30 min to 2 hr at 37°C is sufficient for most of the digestive enzymes, but optimization of the duration is recommended. DNase I is often added to the solution to digest nucleic acids and prevent cell aggregates that could potentially block the flow cytometer during sample acquisition. To obtain clean flow cytometry data, there are some considerations. Failure to exclude dead cells, cell aggregates, and unblocked Fc receptors contribute to phenotypic artifacts in the flow cytometry data. Filtration of cell suspensions through 70-µm and 40-µm strainers prior to measurement also helps in obtaining a good single-cell suspension and preventing blockage of the flow cytometer. The use of anticoagulants such as EDTA in FACS buffers helps prevent cell aggregation during processing.
Prior to incubation with fluorophore-conjugated primary antibodies, the single-cell suspension must be blocked to prevent nonspecific binding of antibodies to Fc receptors. For mice, this is typically done with an anti-CD16/32 antibody or any other suitable Fc receptor blocker. Similarly, a number of commercially available Fc blockers can be used for humans (Benck et al., 2016; Li et al., 2018). After blocking, the single-cell suspension is incubated with fluorophore-conjugated primary antibodies. Titration of antibodies is highly recommended to obtain optimal staining. Before the final step of analyzing the cells in the machine, dead cell exclusion is recommended using different types of live/dead cell stains. Dead cells generated during tissue processing can lead to false positive results, (Perfetto, Chattopadhyay, & Roederer, 2004). The selection of live/dead cell stains depends on post-staining processes. Researchers can proceed with the flow cytometric measurement and use nucleic acid–binding dyes such as PI and 7-AAD as live/dead stains (O'Brien & Bolton, 1995). However, if the cells have been fixed/permeabilized for intracellular staining or after staining to preserve and extend the time between staining and measurement (Lanier & Warner, 1981), nucleic acid–binding dyes cannot be used; protein-binding dyes prior to fixation are recommended (Perfetto et al., 2004). Once stained, the cells are washed and resuspended in FACS buffer. One of the important considerations during sample preparation is to prepare control cells for each color to set channel voltages and calculate compensation values. All the steps are performed on ice, and stained cells must not be exposed to light.
During the acquisition of flow cytometry data, compensation and gating strategies should be considered. The selected strategy will depend on the FACS machine used by the investigator. Selection of an appropriate FACS machine is discussed elsewhere (Cossarizza et al., 2019; Liechti et al., 2021; Wang & Hoffman, 2017).
Fluorescence In situ Hybridization
Fluorescence in situ hybridization (FISH) is a method to detect spatiotemporal expression of DNA and RNA sequences in cells (Cui, Shu, & Li, 2016). DNA or RNA strands conjugated with fluorophores are used as probes to visualize localization of the gene of interest by hybridizing through complementary base pairing (Wang et al., 2021). A fluorescence microscope is then used to visualize the genetic maps created by the hybridization of the probes (Pickup & Ahmed, 2020). Although not widely used for detecting immune cells, FISH is used in conjunction with other immunological methods such as IF and IHC to link genotypes to phenotypes in unknown diseased states (Muraleedharan, Nardini, Waclaw, & Dasgupta, 2021). Additionally, with multiple probe sets to detect immune cells and genes of interest, for example, cytokine/chemokine expression, this method can be used to depict spatial expression of genes in specific immune cells (Fe Lanfranco, Loane, Mocchetti, Burns, & Villapol, 2017; Wang et al., 2021), and therefore can identify specific subsets of the immune cells. This method needs to be performed in an RNase-free environment to avoid the degradation of RNA.
PERSPECTIVE AND FUTURE DIRECTIONS
In this review, we have provided an overview of the methodologies that are commonly used and accessible to most researchers for the characterization of immune cells, with a focus on skin as a model. We have also reviewed the advantages and disadvantages, basic steps, considerations, and limitations of these methods throughout the review to help researchers decide on the method of choice.
Although not discussed here, through advancements in multiplexing technologies such as multicolor flow cytometry and multiplex imaging, which utilize multicolor fluorophores, we have started to understand the depth of the profound immune diversity. We have briefly discussed these multiplexing methodologies and provided a summary and resources in Table 2.
In the coming decades, these multiplexing strategies will revolutionize how we define and visualize immune cells within the tissues spatially and temporally. It will be possible to characterize immune cells in the spatial context of the tissue and measure their functionalities in the tissue. Multiplexing can be applied to study biomarkers in precision medicine as well as to define drug resistance in individual patients across inflammatory diseases, infections, and cancer of the skin. Although these systems are currently not accessible to every researcher, we envision that in future, they will become more available, and along with flow cytometry, IHC, and IF, they will become part of the technical armamentarium that we use in basic and translational cutaneous biology research.
AUTHOR CONTRIBUTIONS
Peter Murphy : methodology, writing review & editing; Divyaa Narayanan : methodology, writing review & editing; Snehlata Kumari : conceptualization, funding acquisition, methodology, resources, supervision, writing original draft, writing review & editing.
CONFLICT OF INTEREST
Authors declare no conflict of interest.
Open Research
DATA AVAILABILITY STATEMENT
Data sharing is not applicable to this article.
LITERATURE CITED
- Adam, R. C., Yang, H., Ge, Y., Infarinato, N. R., Gur-Cohen, S., Miao, Y., … Fuchs, E. (2020). NFI transcription factors provide chromatin access to maintain stem cell identity while preventing unintended lineage fate choices. Nature Cell Biology , 22(6), 640–650. doi: 10.1038/s41556-020-0513-0
- Ashhurst, T. M., Smith, A. L., & King, N. J. C. (2017). High-dimensional fluorescence cytometry. Current Protocols in Immunology , 119, 5.8.1–5.8.38. doi: 10.1002/cpim.37
- Autengruber, A., Gereke, M., Hansen, G., Hennig, C., & Bruder, D. (2012). Impact of enzymatic tissue disintegration on the level of surface molecule expression and immune cell function. European Journal of Microbiology and Immunology (Bp) , 2(2), 112–120. doi: 10.1556/EuJMI.2.2012.2.3
- Bachman, J. (2013). Immunohistochemistry on freely floating fixed tissue sections. Methods in Enzymology , 533, 207–215. doi: 10.1016/B978-0-12-420067-8.00013-1
- Baumgarth, N., & Roederer, M. (2000). A practical approach to multicolor flow cytometry for immunophenotyping. Journal of Immunology Methods , 243(1-2), 77–97. doi: 10.1016/s0022-1759(00)00229-5
- Benck, C. J., Martinov, T., Fife, B. T., & Chatterjea, D. (2016). Isolation of infiltrating leukocytes from mouse skin using enzymatic digest and gradient separation. Journal of Visualized Experiments , 107, e53638. doi: 10.3791/53638
- Beutner, E. H., & Jordon, R. E. (1964). Demonstration of skin antibodies in sera of pemphigus vulgaris patients by indirect immunofluorescent staining. Proceedings of the Society for Experimental Biology , 117, 505–510. doi: 10.3181/00379727-117-29622
- Black, C. B., Duensing, T. D., Trinkle, L. S., & Dunlay, R. T. (2011). Cell-based screening using high-throughput flow cytometry. ASSAY and Drug Development Technologies , 9(1), 13–20. doi: 10.1089/adt.2010.0308
- Castillo-Gonzalez, R., Cibrian, D., & Sanchez-Madrid, F. (2021). Dissecting the complexity of gammadelta T-cell subsets in skin homeostasis, inflammation, and malignancy. Journal of Allergy and Clinical Immunology , 147(6), 2030–2042. doi: 10.1016/j.jaci.2020.11.023
- Chen, X., Cho, D. B., & Yang, P. C. (2010). Double staining immunohistochemistry. North American Journal of Medicine and Science , 2(5), 241–245. doi: 10.4297/najms.2010.2241
- Chu, C. C., Di Meglio, P., & Nestle, F. O. (2011). Harnessing dendritic cells in inflammatory skin diseases. Seminars in Immunology , 23(1), 28–41. doi: 10.1016/j.smim.2011.01.006
- Conrad, C., Meller, S., & Gilliet, M. (2009). Plasmacytoid dendritic cells in the skin: To sense or not to sense nucleic acids. Seminars in Immunology , 21(3), 101–109. doi: 10.1016/j.smim.2009.01.004
- Coons, A. H. (1951). Fluorescent antibodies as histochemical tools. Federation Proceedings , 10(2), 558–559. Retrieved from https://www.ncbi.nlm.nih.gov/pubmed/14849613
- Coons, A. H., Creech, H. J., & Jones, R. N. (1941). Immunological properties of an antibody containing a fluorescent group. Proceedings of the Society for Experimental Biology and Medicine , 47(2), 200–202. doi: 10.3181/00379727-47-13084P
- Coons, A. H., & Kaplan, M. H. (1950). Localization of antigen in tissue cells; improvements in a method for the detection of antigen by means of fluorescent antibody. Journal of Experimental Medicine , 91(1), 1–13. doi: 10.1084/jem.91.1.1
- Cossarizza, A., Chang, H. D., Radbruch, A., Acs, A., Adam, D., Adam-Klages, S., … Zychlinsky, A. (2019). Guidelines for the use of flow cytometry and cell sorting in immunological studies (second edition). European Journal of Immunology , 49(10), 1457–1973. doi: 10.1002/eji.201970107
- Cui, C., Shu, W., & Li, P. (2016). Fluorescence in situ hybridization: Cell-based genetic diagnostic and research applications. Frontiers in Cell and Developmental Biology , 4, 89. doi: 10.3389/fcell.2016.00089
- D'Amico, F., Skarmoutsou, E., & Stivala, F. (2009). State of the art in antigen retrieval for immunohistochemistry. Journal of Immunological Methods , 341(1-2), 1–18. doi: 10.1016/j.jim.2008.11.007
- Edwards, B. S., Kuckuck, F. W., Prossnitz, E. R., Ransom, J. T., & Sklar, L. A. (2001). HTPS flow cytometry: A novel platform for automated high throughput drug discovery and characterization. Journal of Biomolecular Screening , 6(2), 83–90. doi: 10.1177/108705710100600204
- Eng, J., Bucher, E., Hu, Z., Zheng, T., Gibbs, S. L., Chin, K., & Gray, J. W. (2022). A framework for multiplex imaging optimization and reproducible analysis. Communications Biology , 5(1), 438. doi: 10.1038/s42003-022-03368-y
- Fe Lanfranco, M., Loane, D. J., Mocchetti, I., Burns, M. P., & Villapol, S. (2017). Combination of fluorescent in situ hybridization (FISH) and immunofluorescence imaging for detection of cytokine expression in microglia/macrophage cells. Bio-Protocol , 7(22), e2608. doi: 10.21769/BioProtoc.2608
- Groelz, D., Viertler, C., Pabst, D., Dettmann, N., & Zatloukal, K. (2018). Impact of storage conditions on the quality of nucleic acids in paraffin embedded tissues. PLoS One , 13(9), e0203608. doi: 10.1371/journal.pone.0203608
- Heidelberger, M., Kendall, F. E., & Soo Hoo, C. M. (1933). Quantitative studies on the precipitin reaction: Antibody production in rabbits injected with an Azo protein. Journal of Experimental Medicine , 58(2), 137–152. doi: 10.1084/jem.58.2.137
- Hensel, J. A., Khattar, V., Ashton, R., & Ponnazhagan, S. (2019). Characterization of immune cell subtypes in three commonly used mouse strains reveals gender and strain-specific variations. Laboratory Investigation , 99(1), 93–106. doi: 10.1038/s41374-018-0137-1
- Hey, Y. Y., Tan, J. K., & O'Neill, H. C. (2015). Redefining myeloid cell subsets in murine spleen. Frontiers in Immunology , 6, 652. doi: 10.3389/fimmu.2015.00652
- Hira, V. V. V., de Jong, A. L., Ferro, K., Khurshed, M., Molenaar, R. J., & Van Noorden, C. J. F. (2019). Comparison of different methodologies and cryostat versus paraffin sections for chromogenic immunohistochemistry. Acta Histochemica , 121(2), 125–134. doi: 10.1016/j.acthis.2018.10.011
- Hofman, F. M., & Taylor, C. R. (2013). Immunohistochemistry. Current Protocols in Immunology , 103, 21.24.21–21.24.26. doi: 10.1002/0471142735.im2104s103
- Howat, W. J., & Wilson, B. A. (2014). Tissue fixation and the effect of molecular fixatives on downstream staining procedures. Methods , 70(1), 12–19. doi: 10.1016/j.ymeth.2014.01.022
- Hulett, H. R., Bonner, W. A., Barrett, J., & Herzenberg, L. A. (1969). Cell sorting: Automated separation of mammalian cells as a function of intracellular fluorescence. Science , 166(3906), 747–749. doi: 10.1126/science.166.3906.747
- Hulett, H. R., Bonner, W. A., Sweet, R. G., & Herzenberg, L. A. (1973). Development and application of a rapid cell sorter. Clinical Chemistry , 19(8), 813–816. Retrieved from https://www.ncbi.nlm.nih.gov/pubmed/4200173
- Im, K., Mareninov, S., Diaz, M. F. P., & Yong, W. H. (2019). An introduction to performing immunofluorescence staining. Methods in Molecular Biology , 1897, 299–311. doi: 10.1007/978-1-4939-8935-5_26
- Kashem, S. W., & Kaplan, D. H. (2018). Isolation of murine skin resident and migratory dendritic cells via enzymatic digestion. Current Protocols in Immunology , 121(1), e45. doi: 10.1002/cpim.45
- Kortekaas Krohn, I., Aerts, J. L., Breckpot, K., Goyvaerts, C., Knol, E., Van Wijk, F., & Gutermuth, J. (2021). T-cell subsets in the skin and their role in inflammatory skin disorders. Allergy , 77(3), 827–842. doi: 10.1111/all.15104
- Krenacs, L., Krenacs, T., Stelkovics, E., & Raffeld, M. (2010). Heat-induced antigen retrieval for immunohistochemical reactions in routinely processed paraffin sections. Methods in Molecular Biology , 588, 103–119. doi: 10.1007/978-1-59745-324-0_14
- Kumari, S., Bonnet, M. C., Ulvmar, M. H., Wolk, K., Karagianni, N., Witte, E., … Haase, I. (2013). Tumor necrosis factor receptor signaling in keratinocytes triggers interleukin- 24-dependent psoriasis-like skin inflammation in mice. Immunity , 39(5), 899–911. doi: 10.1016/j.immuni.2013.10.009
- Lakschevitz, F. S., Hassanpour, S., Rubin, A., Fine, N., Sun, C., & Glogauer, M. (2016). Identification of neutrophil surface marker changes in health and inflammation using high-throughput screening flow cytometry. Experimental Cell Research , 342(2), 200–209. doi: 10.1016/j.yexcr.2016.03.007
- Lanier, L. L., & Warner, N. L. (1981). Paraformaldehyde fixation of hematopoietic cells for quantitative flow cytometry (FACS) analysis. Journal of Immunological Methods , 47(1), 25–30. doi: 10.1016/0022-1759(81)90253-2
- Li, Z., Gothard, E., Coles, M. C., & Ambler, C. A. (2018). Quantitative methods for measuring repair rates and innate-immune cell responses in wounded mouse skin. Frontiers in Immunology , 9, 347. doi: 10.3389/fimmu.2018.00347
- Liechti, T., Weber, L. M., Ashhurst, T. M., Stanley, N., Prlic, M., Van Gassen, S., & Mair, F. (2021). An updated guide for the perplexed: Cytometry in the high-dimensional era. Nature Immunology , 22(10), 1190–1197. doi: 10.1038/s41590-021-01006-z
- Liu, Z., Gu, Y., Shin, A., Zhang, S., & Ginhoux, F. (2020). Analysis of myeloid cells in mouse tissues with flow cytometry. STAR Protocols , 1(1), 100029. doi: 10.1016/j.xpro.2020.100029
- Lou, F., Sun, Y., & Wang, H. (2020). Protocol for flow cytometric detection of immune cell infiltration in the epidermis and dermis of a psoriasis mouse model. STAR Protocols , 1(3), 100115. doi: 10.1016/j.xpro.2020.100115
- Maecker, H. T., McCoy, J. P., & Nussenblatt, R. (2012). Standardizing immunophenotyping for the human immunology project. Nature Reviews Immunology , 12(3), 191–200. doi: 10.1038/nri3158
- Magaki, S., Hojat, S. A., Wei, B., So, A., & Yong, W. H. (2019). An introduction to the performance of immunohistochemistry. Methods in Molecular Biology , 1897, 289–298. doi: 10.1007/978-1-4939-8935-5_25
- McKinnon, K. M. (2018). Flow cytometry: An overview. Current Protocols in Immunology , 120, 5.1.1–5.1.11. doi: 10.1002/cpim.40
- Meindl, S., Schmidt, U., Vaculik, C., & Elbe-Burger, A. (2006). Characterization, isolation, and differentiation of murine skin cells expressing hematopoietic stem cell markers. Journal of Leukocyte Biology , 80(4), 816–826. doi: 10.1189/jlb.0106015
- Melamed, M. R. (2001). A brief history of flow cytometry and sorting. Methods in Cell Biology , 63, 3–17. doi: 10.1016/s0091-679x(01)63005-x
- Merad, M., Ginhoux, F., & Collin, M. (2008). Origin, homeostasis and function of langerhans cells and other langerin-expressing dendritic cells. Nature Reviews Immunology , 8(12), 935–947. doi: 10.1038/nri2455
- Mousset, C. M., Hobo, W., Woestenenk, R., Preijers, F., Dolstra, H., & van der Waart, A. B. (2019). Comprehensive phenotyping of T cells using flow cytometry. Cytometry A , 95(6), 647–654. doi: 10.1002/cyto.a.23724
- Muraleedharan, R., Nardini, D., Waclaw, R. R., & Dasgupta, B. (2021). Analysis of reactive astrogliosis in mouse brain using in situ hybridization combined with immunohistochemistry. STAR Protocols , 2(1), 100375. doi: 10.1016/j.xpro.2021.100375
- Nakayama, M. (2014). Antigen presentation by MHC-dressed cells. Frontiers in Immunology , 5, 672. doi: 10.3389/fimmu.2014.00672
- Nestle, F. O., Di Meglio, P., Qin, J. Z., & Nickoloff, B. J. (2009). Skin immune sentinels in health and disease. Nature Reviews Immunology , 9(10), 679–691. doi: 10.1038/nri2622
- Nestle, F. O., & Nickoloff, B. J. (2007). Deepening our understanding of immune sentinels in the skin. Journal of Clinical Investigation , 117(9), 2382–2385. doi: 10.1172/JCI33349
- Nguyen, A. V., & Soulika, A. M. (2019). The dynamics of the skin's immune system. International Journal of Molecular Sciences , 20(8), 1811. doi: 10.3390/ijms20081811
- O'Brien, M. C., & Bolton, W. E. (1995). Comparison of cell viability probes compatible with fixation and permeabilization for combined surface and intracellular staining in flow cytometry. Cytometry , 19(3), 243–255. doi: 10.1002/cyto.990190308
- Pasparakis, M., Haase, I., & Nestle, F. O. (2014). Mechanisms regulating skin immunity and inflammation. Nature Reviews Immunology , 14(5), 289–301. doi: 10.1038/nri3646
- Perfetto, S. P., Chattopadhyay, P. K., & Roederer, M. (2004). Seventeen-colour flow cytometry: Unravelling the immune system. Nature Reviews Immunology , 4(8), 648–655. doi: 10.1038/nri1416
- Phillips, D., Schurch, C. M., Khodadoust, M. S., Kim, Y. H., Nolan, G. P., & Jiang, S. (2021). Highly multiplexed phenotyping of immunoregulatory proteins in the tumor microenvironment by CODEX tissue imaging. Frontiers in Immunology , 12, 687673. doi: 10.3389/fimmu.2021.687673
- Pickup, M. E., & Ahmed, M. I. (2020). Detection of MicroRNAs by in situ hybridization in skin. Methods in Molecular Biology , 2154, 187–196. doi: 10.1007/978-1-0716-0648-3_16
- Pileri, S. A., Roncador, G., Ceccarelli, C., Piccioli, M., Briskomatis, A., Sabattini, E., … Falini, B. (1997). Antigen retrieval techniques in immunohistochemistry: Comparison of different methods. Journal of Pathology , 183(1), 116–123. doi: 10.1002/(SICI)1096- 9896(199709)183:1<116::AID-PATH1087>3.0.CO;2-2.
- Reynolds, G., Vegh, P., Fletcher, J., Poyner, E. F. M., Stephenson, E., Goh, I., … Haniffa, M. (2021). Developmental cell programs are co-opted in inflammatory skin disease. Science , 371(6527), eaba6500. doi: 10.1126/science.aba6500
- Riedl, E., Tada, Y., & Udey, M. C. (2004). Identification and characterization of an alternatively spliced isoform of mouse langerin/CD207. Journal of Investigative Dermatology , 123(1), 78–86. doi: 10.1111/j.0022-202X.2004.22718.x
- Shi, S. R., Key, M. E., & Kalra, K. L. (1991). Antigen retrieval in formalin-fixed, paraffin-embedded tissues: An enhancement method for immunohistochemical staining based on microwave oven heating of tissue sections. Journal of Histochemistry & Cytochemistry, 39(6), 741–748. doi: 10.1177/39.6.1709656
- Singh, T. P., Zhang, H. H., Hwang, S. T., & Farber, J. M. (2019). IL-23- and imiquimod-induced models of experimental psoriasis in mice. Current Protocols in Immunology , 125(1), e71. doi: 10.1002/cpim.71
- Srinivasan, M., Sedmak, D., & Jewell, S. (2002). Effect of fixatives and tissue processing on the content and integrity of nucleic acids. American Journal of Pathology , 161(6), 1961–1971. doi: 10.1016/S0002-9440(10)64472-0
- Stankovic, B., Bjorhovde, H. A. K., Skarshaug, R., Aamodt, H., Frafjord, A., Muller, E., … Corthay, A. (2018). Immune cell composition in human non-small cell lung cancer. Frontiers in Immunology , 9, 3101. doi: 10.3389/fimmu.2018.03101
- Stoitzner, P., Romani, N., McLellan, A. D., Tripp, C. H., & Ebner, S. (2010). Isolation of skin dendritic cells from mouse and man. Methods in Molecular Biology , 595, 235–248. doi: 10.1007/978-1-60761-421-0_16
- Sumaria, N., Roediger, B., Ng, L. G., Qin, J., Pinto, R., Cavanagh, L. L., … Weninger, W. (2011). Cutaneous immunosurveillance by self-renewing dermal gammadelta T cells. Journal of Experimental Medicine , 208(3), 505–518. doi: 10.1084/jem.20101824
- Szabo, P. A., Miron, M., & Farber, D. L. (2019). Location, location, location: Tissue resident memory T cells in mice and humans. Science Immunology , 4(34), eaas9673. doi: 10.1126/sciimmunol.aas9673
- Tan, W. C. C., Nerurkar, S. N., Cai, H. Y., Ng, H. H. M., Wu, D., Wee, Y. T. F., … Lim, T. K. H. (2020). Overview of multiplex immunohistochemistry/immunofluorescence techniques in the era of cancer immunotherapy. Cancer Communication (Lond) , 40(4), 135–153. doi: 10.1002/cac2.12023
- Taylor, C. R., & Burns, J. (1974). The demonstration of plasma cells and other immunoglobulin-containing cells in formalin-fixed, paraffin-embedded tissues using peroxidase-labelled antibody. Journal of Clinical Pathology , 27(1), 14–20. doi: 10.1136/jcp.27.1.14
- Wang, A., Fogel, A. L., Murphy, M. J., Panse, G., McGeary, M. K., McNiff, J. M., … Damsky, W. (2021). Cytokine RNA in situ hybridization permits individualized molecular phenotyping in biopsies of psoriasis and atopic dermatitis. JID Innovations , 1(2), 100021. doi: 10.1016/j.xjidi.2021.100021
- Wang, L., & Hoffman, R. A. (2017). Standardization, calibration, and control in flow cytometry. Current Protocols in Cytometry , 79, 1.3.1–1.3.27. doi: 10.1002/cpcy.14
- Willenborg, S., Sanin, D. E., Jais, A., Ding, X., Ulas, T., Nuchel, J., … Eming, S. A. (2021). Mitochondrial metabolism coordinates stage-specific repair processes in macrophages during wound healing. Cell Metabolism , 33(12), 2398–2414.e2399. doi: 10.1016/j.cmet.2021.10.004
- Xu, Y., Zhang, J., Hu, Y., Li, X., Sun, L., Peng, Y., … Rong, Z. (2021). Single-cell transcriptome analysis reveals the dynamics of human immune cells during early fetal skin development. Cell Reports , 36(6), 109524. doi: 10.1016/j.celrep.2021.109524
- Zhang, J., Song, J., Sheng, J., Bai, X., & Liang, T. (2021). Multiplex imaging reveals the architecture of the tumor immune microenvironment. Cancer Biology & Medicine, 18(4), 949–954. doi: 10.20892/j.issn.2095-3941.2021.0494