Isolation and Culturing of Primary Murine Chondroprogenitor Cells: A Mammalian Model of Chondrogenesis
Roland Takács, Roland Takács, Csaba Matta, Csaba Matta, Csilla Somogyi, Csilla Somogyi, Judit Vágó, Judit Vágó, Krisztina Bíróné Barna, Krisztina Bíróné Barna, Róza Zákány, Róza Zákány, Eun-Jung Jin, Eun-Jung Jin
Abstract
Embryonic limb bud-derived micromass cultures are valuable tools for investigating cartilage development, tissue engineering, and therapeutic strategies for cartilage-related disorders. This collection of fine-tuned protocols used in our laboratories outlines step-by-step procedures for the isolation, expansion, and differentiation of primary mouse limb bud cells into chondrogenic micromass cultures. Key aspects covered in these protocols include synchronized fertilization of mice (Basic Protocol 1), tissue dissection, cell isolation, micromass formation, and culture optimization parameters, such as cell density and medium composition (Basic Protocol 2). We describe techniques for characterizing the chondrogenic differentiation process by histological analysis (Basic Protocol 3). The protocols also address common challenges encountered during the process and provide troubleshooting strategies. This fine-tuned comprehensive protocol serves as a valuable resource for scientists working in the fields of developmental biology, cartilage tissue engineering, and regenerative medicine, offering an updated methodology for the study of efficient chondrogenic differentiation and cartilage tissue regeneration. © 2024 The Authors. Current Protocols published by Wiley Periodicals LLC.
Basic Protocol 1 : Synchronized fertilization of mice
Basic Protocol 2 : Micromass culture of murine embryonic limb bud-derived cells
Basic Protocol 3 : Qualitative assessment of cartilage matrix production using Alcian blue staining
INTRODUCTION
Hyaline articular cartilage is a highly specialized connective tissue found in most diarthrodial joints. It covers the ends of bones in these joints, providing a smooth surface that allows for frictionless movement. Cartilage is made up of sparsely distributed chondrocytes (∼5% by volume), which maintain the abundant cartilage extracellular matrix (ECM) in which they are embedded. This ECM is composed of collagen fibers (mainly type II collagen), proteoglycans, and structural water. Collagen provides the cartilage with tensile strength, while proteoglycans, particularly aggrecan, trap water molecules, imparting compressive resistance to the tissue (Sophia Fox et al., 2009). The unique composition of hyaline articular cartilage enables it to absorb and distribute mechanical forces during locomotion, protecting the underlying bones from damage (Jahr et al., 2015). Unlike the other connective tissue types, cartilage is avascular, and chondrocytes receive oxygen and nutrients primarily by diffusion. Hyaline articular cartilage is lubricated by synovial fluid, which further reduces friction and ensures smooth movement in joints and provides chondrocytes with nutrients and oxygen.
Mature chondrocytes become practically postmitotic, hence the probability of cell renewal is minimal in adult articular cartilage (Chen et al., 1995). Chondrocytes maintain the cartilage tissue by continuously producing and maintaining the ECM at a very low rate (Richardson et al., 2016). Due to its unique cellular and matrix constitution, hyaline articular cartilage has limited regenerative abilities in adults. Injuries or damage to the cartilage are difficult to heal naturally because of the lack of blood vessels, as well as the low metabolic activity and postmitotic nature of chondrocytes.
Articular cartilage forms during early fetal life (Cancedda et al., 2000), which makes it particularly challenging to study in vivo. During embryonic development, hyaline cartilage is formed from mesenchymal cells through a process called chondrogenesis. During this process, mesenchymal cells condense and differentiate into chondroblasts, which secrete the cartilage ECM (Fig. 1). As chondroblasts become enclosed in lacunae within the developing ECM, they mature into chondrocytes. Chondrogenesis is a tightly regulated process involving various signaling molecules and transcription factors that orchestrate the differentiation and maturation of chondrocytes, ensuring the proper formation and growth of cartilage during embryonic development (Richardson et al., 2016). A better understanding of the molecular mechanisms that control the differentiation of hyaline cartilage in the limb mesenchyme during embryonic life may help us develop more efficient regenerative approaches and enhance the healing response of articular cartilage.
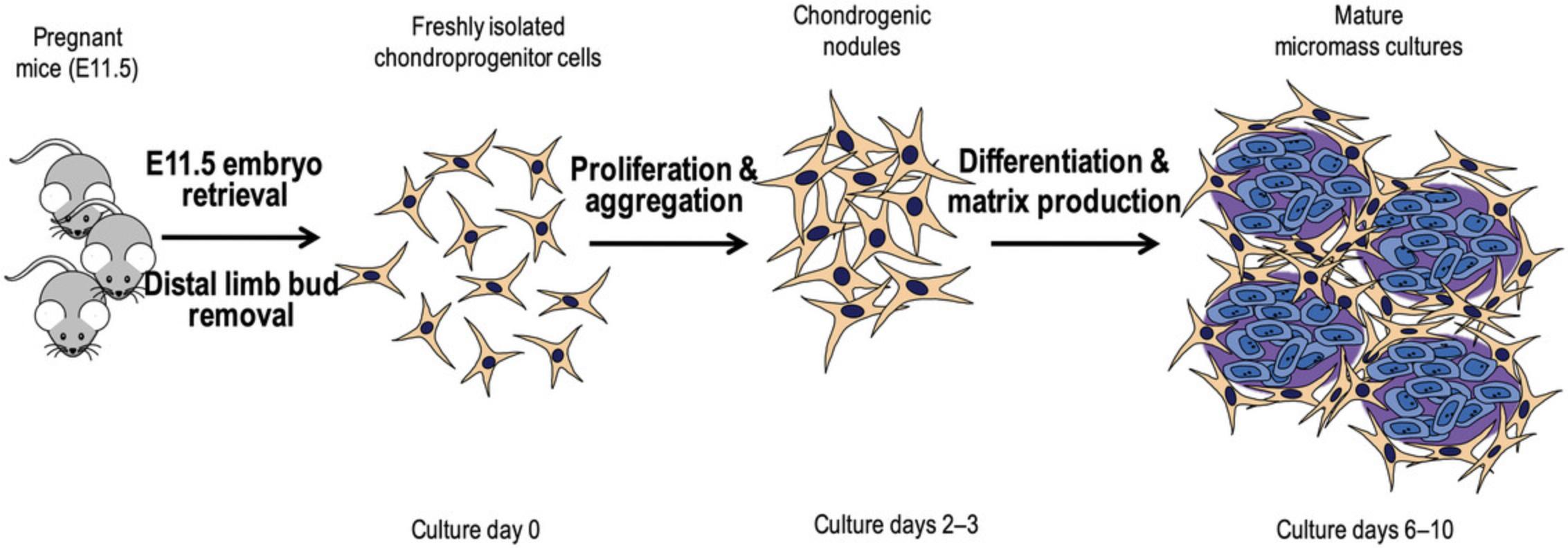
The micromass culture model is a widely used in vitro technique in chondrogenesis research, allowing the study of the formation and differentiation of cartilage under controlled laboratory conditions. This model provides a three-dimensional environment that mimics the natural conditions of chondrogenesis more closely than traditional two-dimensional cell culture. The high density micromass cell culture model established from limb buds of chicken or mouse embryos is widely used to recapitulate embryonic chondrogenesis (Ahrens et al., 1977; Takacs, Vago, et al., 2023; Takacs et al., 2013). The isolated chondroprogenitor cells are mixed with a small volume of culture medium, forming droplets (micromasses) typically ranging from 10 to 100 μl in size. These droplets are placed in the center of laboratory plasticware (Petri dishes or culture wells), allowing the cells to adhere and form a three-dimensional structure. The cells within the micromasses proliferate and differentiate into chondroblasts, which start producing cartilage-specific ECM, including collagen type II and proteoglycans. The cells organize themselves into multiple nodules within the micromass, forming a structure that resembles early cartilage (Takacs, Juhasz, et al., 2023; Takacs, Vago, et al., 2023). The chondrogenic micromasses can be analyzed at various time points to assess the progression of chondrogenesis and to evaluate the quality and quantity of cartilage-specific matrix components using techniques, e.g., histological staining, immunohistochemistry, gene expression analysis, and biochemical assays (Takacs, Juhasz, et al., 2023).
The micromass culture model provides a controlled and physiologically relevant platform for investigating the molecular background of chondrogenesis, making it an essential tool in both basic research and regenerative medicine applications related to cartilage tissue engineering and repair. We have previously published a detailed protocol for culturing limb bud mesenchymal cells from chick embryos (Takacs, Juhasz, et al., 2023). Here, we describe a method for the synchronized fertilization of mice (Basic Protocol 1). Then, we outline a protocol for establishing micromass cultures of limb bud-derived chondrogenic progenitor cells obtained from early-stage mouse embryos refined in our laboratories (Basic Protocol 2). Lastly, we provide a refined approach on the qualitative and quantitative assessment of chondrogenic differentiation and cartilage matrix production using Alcian blue staining (Basic Protocol 3).
STRATEGIC PLANNING
The following should be considered:
Αn Animal Research License is required before implementing the experiments described below. Depending on your country/region, you may need to obtain personal license(s) and a project license. Please make sure that the relevant authorities issue the required licenses before carrying out the experiments. Consider the full timeline of the experiment (Fig. 2). Note that a single experiment from start (i.e., conception) to finish (i.e., 10-day-old micromass cultures) takes 23 days to complete. Carefully plan the time of setting up mating pairs of mice so that none of the “critical” or laborious days falls on a weekend. A typical experiment requires at least 20 embryos, which should be at the same developmental stage (E11.5). We therefore recommend choosing mouse strains with a reputation of good mating and high fertility rates (e.g., NMRI or Black-6) unless it is necessary to work with special strains (in case of knock-out, recombinant, or transgenic models). Instead of experimenting with setting up timed fertilization of mice in your laboratory setting, it is recommended that pregnant mice are ordered from certain vendors (e.g., the Jackson Laboratory, or Charles River Laboratories). They provide properly staged pregnant mice with precise timing requested by the customer. If you are keen on setting up the synchronized fertilization protocol (Basic Protocol 1) in your laboratory, an appropriate number of young female (6 to 9 weeks) and male mice are needed (see below). Please note that young mice should be at least two months of age before they are suitable for the experiment. It may be useful to synchronize the estrus cycle of female mice, which provides higher fertility rates and thus higher yield of embryos. This can be achieved by exposing females to male urine to initiate the estrus cycle, which is favorable for timed mating (Hurst et al., 2005). In addition to exposing female mice to male urine or soiled bedding to synchronize the estrus cycle and gaining enhanced fertilization rate, other methods include the use of pregnant mare serum gonadotropin (PMSG) or human chorionic gonadotropin (hCG). This is known as superovulation; a process that induces female mice to release more eggs than normal, thereby increasing the chances of obtaining a higher number of embryos. Do not be surprised if the first two synchronization attempts do not work out as expected and provide low fertility rates. It takes time to harmonize the cycles of the female mice. Synchronization is more efficient if the temperature, humidity, and light-dark cycle are properly set in the breeding room. To achieve a high number of pregnant mice, a stress-free environment is needed after mating. Avoid unnecessary cage changing and keep room temperature and feeding habits constant. Otherwise, the implantation sites might become compromised/absorbed. We recommend spatially separating the experimental procedure. (1) Sacrifice the mice and dissect the uteri in a prep room, and (2) continue the procedure by retrieving the embryos and dissecting the limb buds in a laminar flow cabinet in the cell culture facility. To save time, before retrieving the embryos from the uteri, we recommend preparing the equipment and solutions. Depending on the experimental plan (and the number of embryos to be processed), at least two members of staff are required for the experiment (Basic Protocol 2). One person is tasked with sacrificing the mice and retrieving the embryos while the other person dissects the limb buds under a stereomicroscope. In case a high number of embryos need to be processed (e.g., over ∼30), involvement of three members of staff is recommended. The appropriate method for sacrificing the mice depends on local guidelines. As described, we use cervical dislocation. After the animal has been sacrificed, make sure to retrieve the embryos quickly because blood accumulates within the uterine horns as time passes, making it difficult to get rid of blood during limb bud harvesting. It is recommended to sacrifice the mice sequentially rather than all at once.
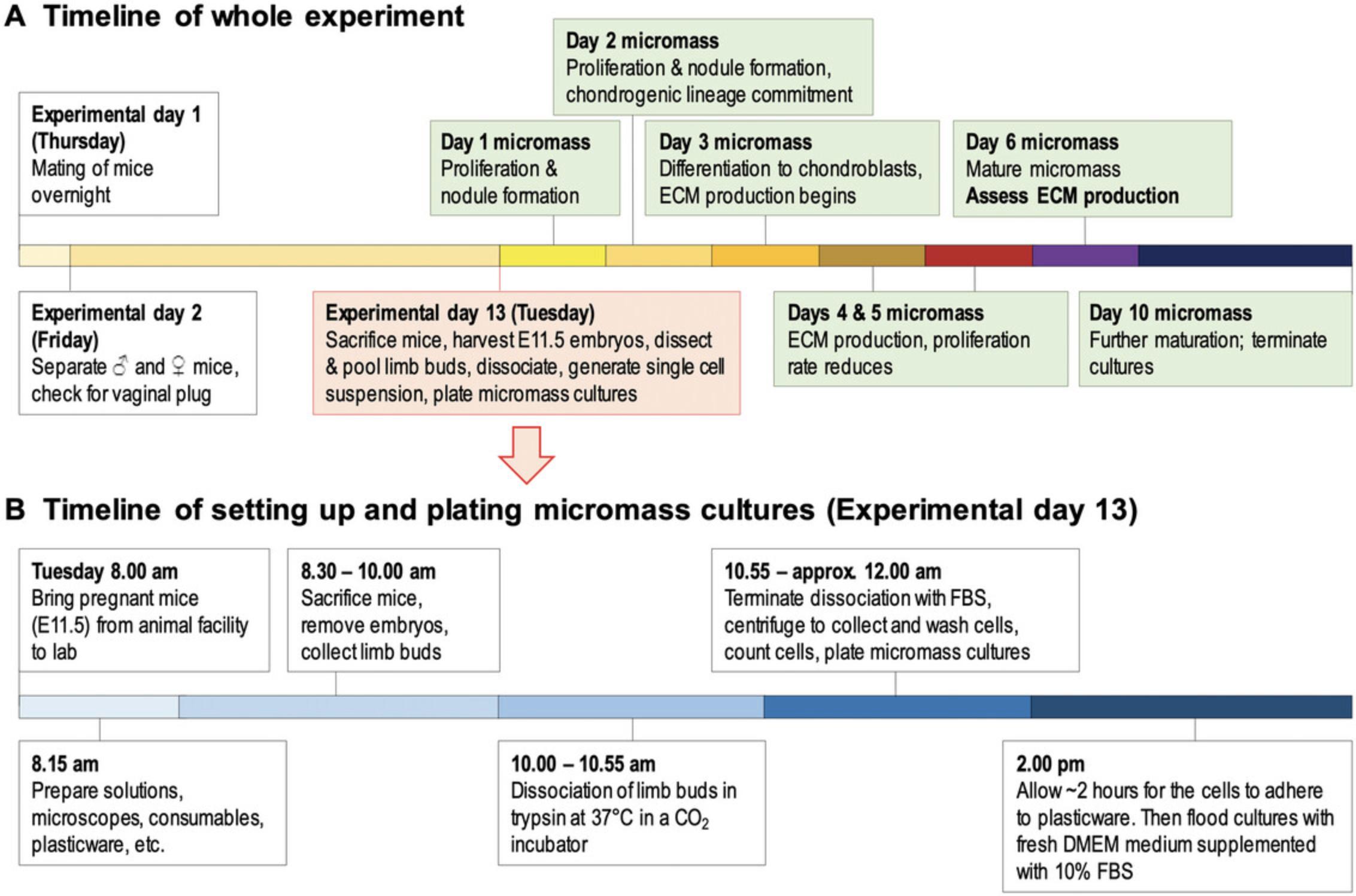
NOTE : This protocol relies on sacrificing adult mice. Please proceed using this protocol only if alternative protocols, such as cell line-based or chicken embryo-based methods (Takacs, Juhasz, et al., 2023), are unsuitable. When using this protocol, please make sure that the experimental plans are robust and will provide meaningful results that are reported in line with the ARRIVE guidelines (see Internet Resources). Please make sure that measures are taken to minimize any suffering of the animals where appropriate (see Internet Resources). All protocols involving animals must be reviewed and approved by the appropriate Animal Care and Use Committee and must follow regulations for the care and use of laboratory animals.
Basic Protocol 1: SYNCHRONIZED FERTILIZATION OF MICE
Certain research projects require mouse embryos at a given differentiation stage. For these experiments, it is important to know the exact time of the conception, which can be accomplished by the synchronized fertilization of female mice. This means that one male mouse is placed with female mice for one night in a cage, and the next morning females are checked for the presence of the vaginal plug, which is a sign of sexual interaction between the male and the female. The day when male mice are separated from the females is considered as gestational day E0.5 (DeLise et al., 2000). This is sometimes referred to as 0.5 days post coitum (0.5 dpc). However, mouse embryos can be staged according to a variety of criteria, the most general of which are those described by Karl Theiler (1989). It may be worthwhile to familiarize oneself with the e-Mouse Atlas Project (EMA) (see Internet Resources). EMA is a detailed model of the developing mouse. It provides information regarding the shape, gross anatomy, and histology of mouse embryos (Armit et al., 2015).
Materials
-
Mice (see Strategic Planning)
-
Personal protective equipment (PPE), including nitrile gloves
-
Permanent marker pen (Edding 751 paint marker, or similar)
1.Thirteen days before the planned date of micromass cell culturing, divide female mice into groups: each cage should contain up to 4 females.
2.On the same day, at ∼3 to 5 pm, place one male mouse into each cage containing up to 4 female mice. This is considered experimental day 1.Mating of the mice happens overnight.
3.On the next day, between ∼7 and 9 am, separate the male mice from the female mice and replace them into their own cages. After removing the male mice, check female mice for the presence of the copulatory or vaginal plug one by one. If the plug is visible, mark those animals for plug positivity (Fig. 3).
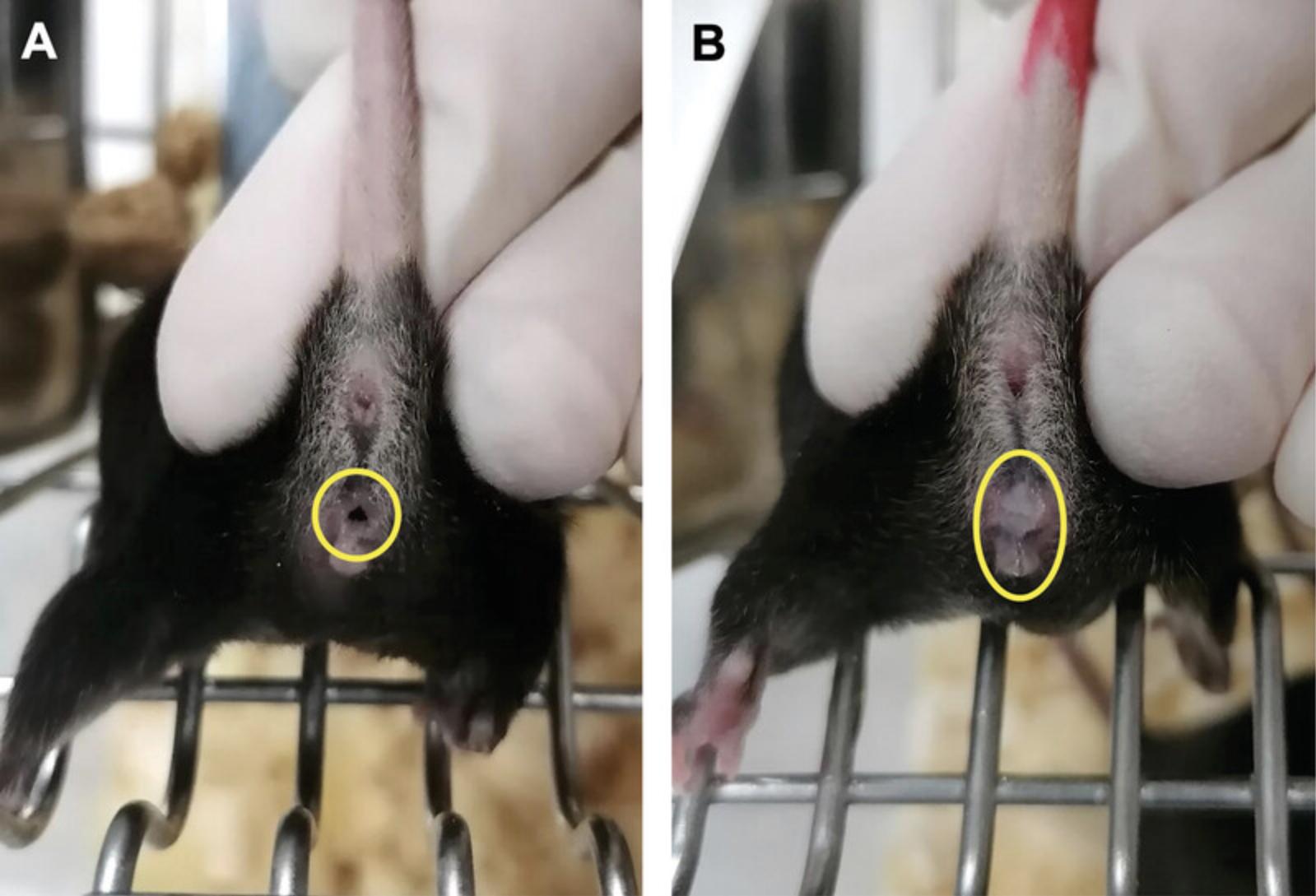
4.On gestational day 11.5 (experimental day 13), check marked females for signs of pregnancy (e.g., increased volume of the abdominal region, swollen breasts) before starting the cell culturing procedure (Basic Protocol 2). According to our suggested timeline, this is a Tuesday.
Basic Protocol 2: MICROMASS CULTURE OF MURINE EMBRYONIC LIMB BUD-DERIVED CELLS
In vitro hyaline cartilage formation is often studied using chondrifying micromass cultures established from chondroprogenitor cells derived from embryonic limb buds. Ahrens et al. (1977) were the first to describe this widely used and reliable experimental method that relies on limb buds of early-stage chicken embryos. Chondroprogenitor cells can also be harvested from the distal parts of the anterior and posterior limb buds of early-stage (E11.5) mouse embryos (Umansky, 1966). After dissociating the limb buds in trypsin, the density of the cell suspension is diluted to 1.0 × 107 to establish the chondrifying micromass cultures. These cell cultures go through a specific sequence of chondrogenic differentiation: on the first 3 days, cells proliferate intensively, then differentiate into chondroblasts; by the sixth day in culture, a well-detectable hyaline cartilage-specific ECM is formed (DeLise et al., 2000).
Materials
-
96% ethanol, diluted to 70% (v/v) (VWR, cat. no. EM1.59010.2500)
-
Calcium and magnesium-free phosphate-buffered saline (CMF-PBS) (see recipe)
-
Gentamycin solution (Sigma-Aldrich, cat. no. G1397), or equivalent
-
Trypsin-EDTA solution (see recipe)
-
Fetal bovine serum (FBS), qualified (Thermo Fisher Scientific, cat. no. 26140079)
-
Dulbecco's Modified Eagle Medium (DMEM) (Capricorn Scientific, cat. no. DMEM-HA), or equivalent
-
0.4% trypan blue solution (Sigma-Aldrich, cat. no. 93595)
-
L-glutamine (Euroclone, cat. no. ECB3000D)
-
5000 U/ml penicillin, 5 mg/ml streptomycin solution (Sigma-Aldrich, cat. no. P4458), or equivalent
-
Personal protective equipment (PPE) including nitrile gloves
-
0.2-mm/115-mm micro forceps, curved (SUBAN Instruments, cat. no. PC-855-11)
-
0.2-mm/110-mm micro forceps, straight (SUBAN Instruments, cat. no. PC-858-11)
-
105-mm fine surgical scissors, straight (SUBAN instruments, cat. no. PB-088-10)
-
130-mm operating scissors, straight (SUBAN instruments, cat. no. PB-020-13)
-
130-mm dressing forceps, standard (SUBAN instruments, cat. no. PC-010-13)
-
10-mm soda lime glass Petri culture dishes, Pyrex Vista (Thermo Fisher Scientific, cat. no. 07-770-214)
-
Digital water bath, Daihan Scientific, LWB-111D, or equivalent
-
Stereomicroscope, Leica S6 E LED 2500 (Leica Microsystems), or equivalent
-
Laminar flow cabinet, FlowFAST H (Faster S.R.L), or equivalent
-
Plastic bag
-
Crystallizing dishes, Pyrex (Merck, cat. no. SLW1470/02D)
-
Pipettes and appropriate pipette tips (5-ml, 1000-µl, 200-µl, and 10-µl)
-
30-ml weighing bottle, Duran (Thermo Fisher Scientific, cat. no. 11740944)
-
Incubator, BINDER model BD 23, or equivalent
-
50-ml centrifuge tubes (Corning, cat. no. 430290)
-
Benchtop centrifuge, Eppendorf 5804/5804 R, or equivalent
-
Steriflip, 20-µm nylon net filter (Merck, cat. no. SCNY00020)
-
50-ml Steriflip centrifuge tube (Millipore, cat. no. SCNY00020)
-
Luna automated cell counter (Logos Biosystems), or equivalent
-
35-mm cell culture Petri dishes (Eppendorf, cat. no. 0030700112) and/or 24-well cell culture plate (Eppendorf, cat. no. 0030722116)
1.Twelve days following the overnight mating of mice, on gestational day 11.5, bring female mice indicated to be pregnant to the laboratory (Fig. 2).
2.Sacrifice female mice by cervical dislocation, in accordance with the local ethical regulations and guidelines.
3.Place the sacrificed mouse on its back (dorsal side).
4.Clean the abdominal (ventral) area by spraying 70% (v/v) ethanol on the intact mouse.
5.Perform a Caesarean section to open the lower part of the abdomen and abdominal wall, using sterile operating scissors and dressing forceps (Fig. 4A).
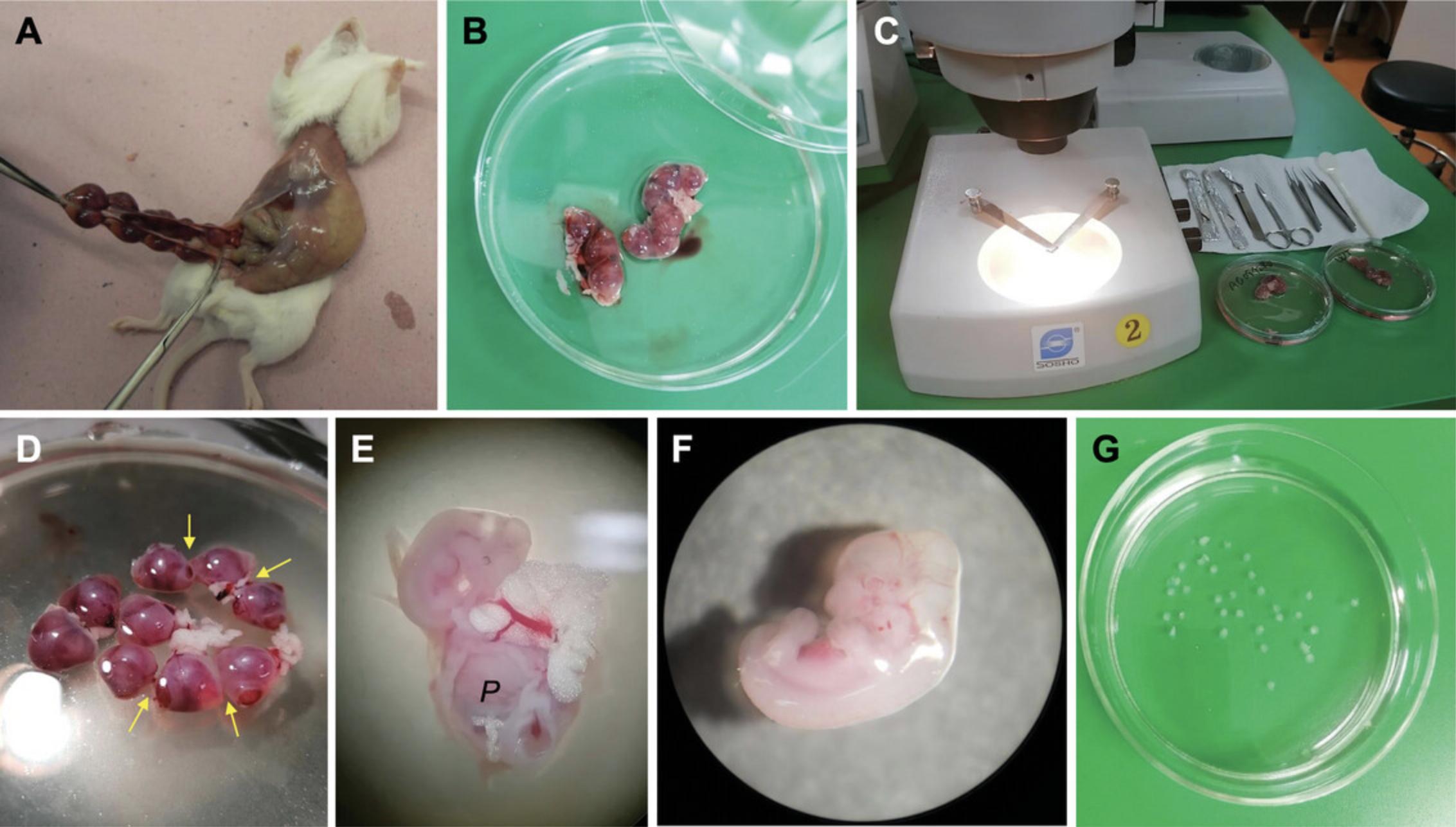
6.Remove the bicornuate uterus containing the embryos (Fig. 4A and 4B). Free the uterus by cutting through the mesentery and other membranes attaching it to the abdominal cavity and place the uterine horns, resembling beads on strings, into a sterile glass Petri dish filled with ∼10 ml prewarmed (37°C) gentamycin-enriched CMF-PBS (0.2 mg/ml).
7.Place the Petri dishes containing the isolated uteri under stereomicroscopes placed in a laminar hood (Fig. 4B and 4C).
8.Cut open the uteri in the following, specific way: between the bead-like individual implantation sites (containing the embryo), there is a visibly narrower area that will indicate the sites for the cuts (Fig. 4D, arrows).
9.After cutting the uterus into individual pieces, gently retrieve the embryos from the placental membranes and remove the yolk sac.
10.Transfer the retrieved embryos into a new sterile glass Petri dish filled with ∼10 ml gentamycin-supplemented CMF-PBS (Fig. 4E).
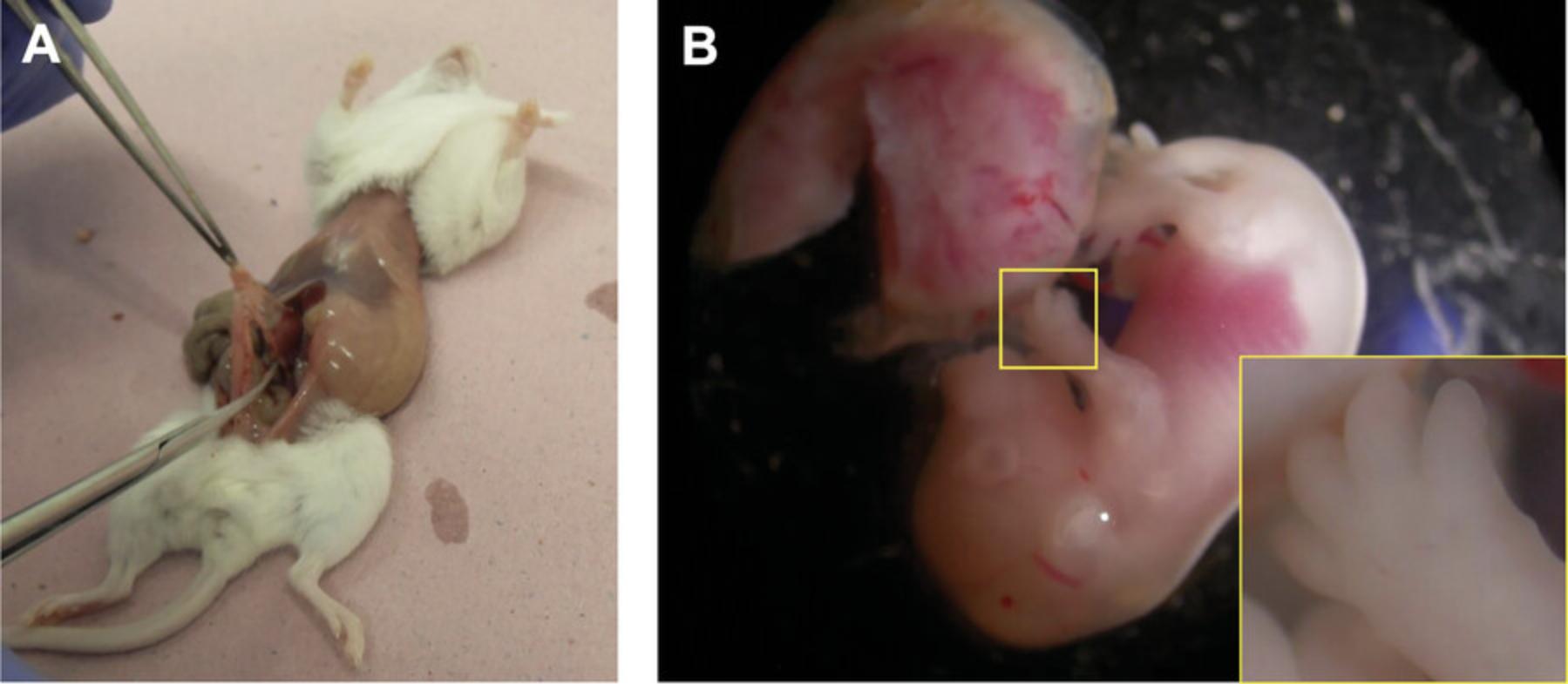
11.Remove the distal parts of all four limb buds from each embryo using a stereomicroscope, and a pair of straight and a pair of curved microdissection forceps. Pool isolated limb buds in a crystallizing dish containing ∼5 ml prewarmed (37°C) gentamycin-supplemented CMF-PBS (Fig. 4G).
12.After removing and pooling the limb buds from every isolated embryo, undesired tissue pieces and debris (e.g., epithelial membranes, improper portions of the embryo) should be removed from the crystallizing dish.
13.Carefully aspirate the CMF-PBS from the dish and pipette the limb buds to 2 to 8 ml trypsin-EDTA solution preincubated at 37°C in a 30-ml weighing bottle with a glass lid. Incubate for 30 min at 37°C in a CO2 incubator.
14.At the end of the digestion, gently pipette the trypsin a few times using a 5-ml pipette to dissociate the limb buds. The enzymatic dissociation is terminated by adding an equal volume of fetal bovine serum (FBS). Mix the solution well, then transfer it to a new 50-ml centrifuge tube.
15.Centrifuge the cell suspension 5 min at 150 × g , room temperature. Cells should form a pellet at the bottom of the centrifuge tube.
16.Remove the supernatant carefully with a pipette or a vacuum aspirator. Resuspend the cell pellet in high glucose (HG; 4.5 g/L) DMEM culture medium supplemented with 10% FBS. For cells liberated from ∼80 limb buds (the expected yield of limb buds from 20 embryos), add 2 ml culture medium.
17.Filter the cell suspension through a 20-µm nylon net filter.
18.Centrifuge the solution 5 min at 150 × g , room temperature. Resuspend the cell pellet in an appropriate volume of HG-DMEM culture medium supplemented with 10% FBS.
19.Determine cell density with the help of an automated cell counter. Set the final volume by adding the calculated volume of DMEM medium to the cell suspension to reach the final cell concentration of 1.0 × 107 cells/ml.
20.Plate the cells by inoculating droplets of the diluted cell suspension of appropriate volume into 35-mm cell culturing dishes or 24-well plates. The volume of the droplet varies from 30 to 100 µl, depending on the purpose of the experiment. Cells are allowed to attach to the surface for 2 hr in a CO2 incubator (37°C, 5% CO2, 90% humidity).
21.After the 2-hr incubation time (when the cells have adhered to the surface of the culture dish), flood the cultures with medium [HG-DMEM supplemented with 10% FBS, 0.5 mM L-glutamine (if needed), and 1% penicillin/streptomycin solution]. Replace the culture medium on every second day during the full length of the experiments.
Basic Protocol 3: QUALITATIVE ASSESSMENT OF CARTILAGE MATRIX PRODUCTION USING ALCIAN BLUE STAINING
Cartilage-specific extracellular matrix (ECM), produced by chondroblasts and mature chondrocytes, can be detected and visualized by qualitative staining procedures, such as Alcian blue staining. This cationic dye selectively binds to the negatively charged sulfated components of the ECM, e.g., glycosaminoglycans (GAGs) and proteoglycans (PGs), when applied low pH. The stained tissue components can be observed with a light blue color (Green & Pastewka, 1974; Scott & Dorling, 1965).
Materials
- PBS tablets (Thermo Fisher Scientific Inc., cat. no. 003002), or equivalent
- Kahle's fixative (see recipe)
- 1% (w/v) Alcian blue 8GX solution (see recipe)
NOTE : Each step is carried out in a 24-well plate at room temperature. Add ∼500 µl of solution per well at each step with the help of plastic Pasteur pipettes. When removing the solvents, using a vacuum aspirator is optional.
1.Wash micromass cultures from Basic Protocol 2, step 20 (established from 30-µl droplets and cultured on 12-mm cover glasses in a 24-well plate for various days) two times in 1× PBS.
2.Fix samples in Kahle's fixative for 10 min.
3.Wash samples 3 times with 1× PBS (5 min each).
4.Add 500 µl of 1% (w/v) Alcian blue 8GX solution per well. Stain the samples by keeping them in a dark place overnight at room temperature.
5.On the next day, remove the excess dye from the wells and rinse samples 3 times in 1× PBS.
6.Do not remove the last round of PBS from the samples. Take pictures of the stained cultures while they soak in PBS (see Figs. 6 and 7).
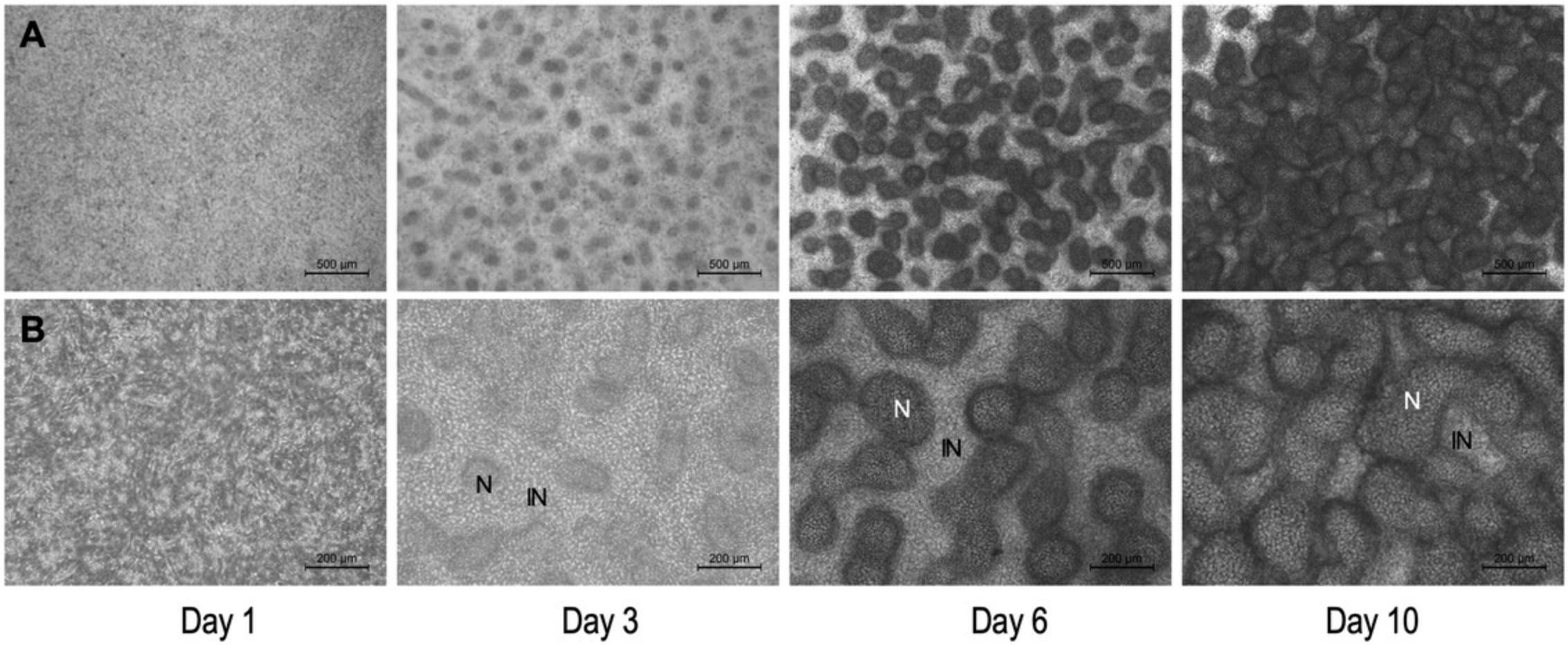
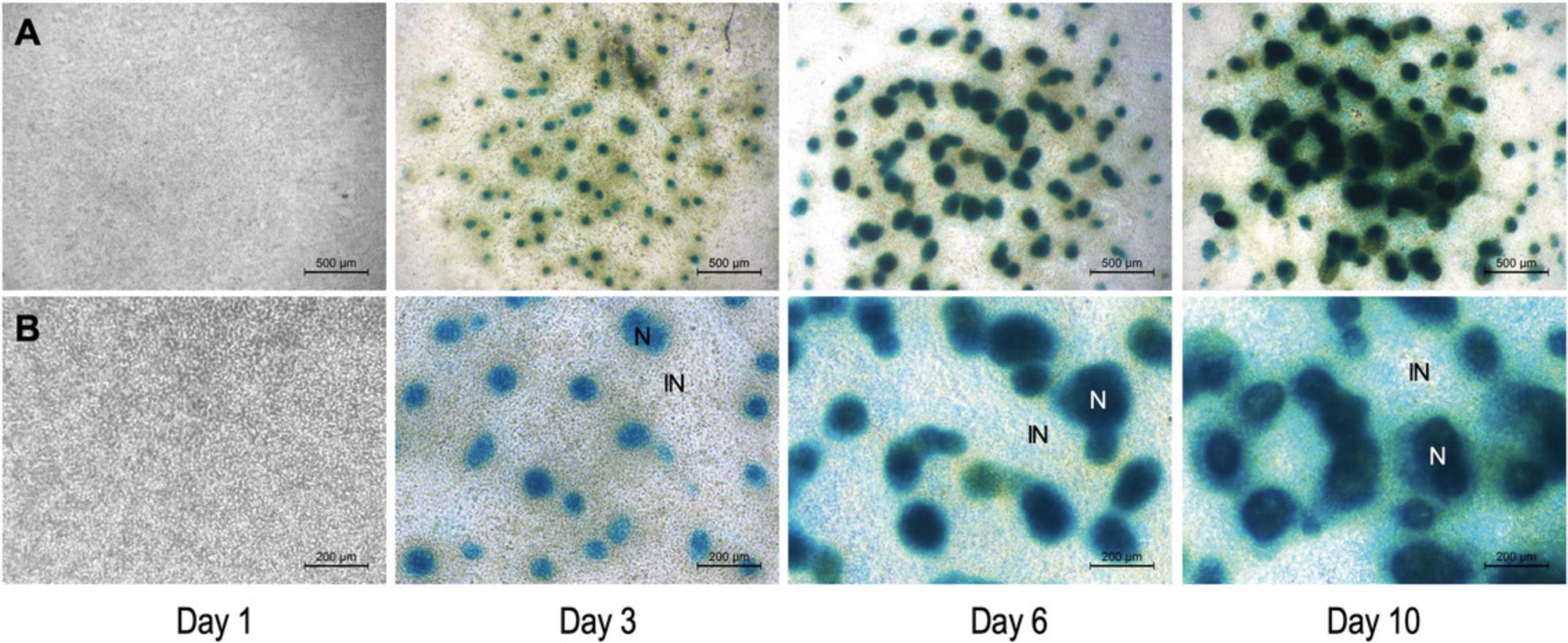
REAGENTS AND SOLUTIONS
Alcian blue 8GX solution, 1% (w/v)
- 100 ml of 0.1 N HCl (Current Protocols, 2006)
- 1 g Alcian blue 8GX (Sigma-Aldrich, cat no. A5268-25MG)
- Store in dark bottles up to 1 year at room temperature
CMF-PBS, 10×
- 80 g NaCl
- 3 g KCl
- 1.84 g Na2HPO4·12H2O
- 0.2 g KH2PO4
- 20 g D(+)-glucose monohydrate (VWR Chemicals, cat. no. 24369.290)
- Bring volume to 1000 ml with deionized H2O
- Adjust pH to 7.6 using HCl or NaOH
- Sterilize using a 0.2-micron filter in a laminar flow hood
- Store up to 3 months at 4°C
Kahle's fixative
- 28.9% (v/v) ethanol (Sigma-Aldrich, cat. no. 1.00983)
- 3.9% (v/v) acetic acid (Merck, cat. no. 1.00063)
- 0.37% (v/v) formaldehyde (Sigma-Aldrich, cat. no. F1635-500ML)
- Store up to 1 year at room temperature
Trypsin-EDTA solution
- 0.75 g trypsin from porcine pancreas (Sigma-Aldrich, cat. no. T6567), or equivalent
- 0.3 g EDTA (VWR, cat. no. 20301.290)
- 300 ml of 1× CMF-PBS (see recipe)
- Sterilize using a 0.2-micron filter before use
- Store in 30 ml aliquots up to 3 months at −20°C
COMMENTARY
Background Information
The micromass culture technique, particularly using mouse limb bud cells, is an extensively applied in vitro model in developmental biology, providing significant insights into the processes of chondrogenesis and cartilage development. Several studies have contributed to our understanding of this complex process, each exploring different aspects of cell differentiation and the factors influencing it. The fact that murine mesenchymal cells from 11-day embryonic limb buds are intrinsically capable of differentiating into connective tissue cell types including chondrocytes has been known for half a century (Umansky, 1966). More recently, Takashi et al. demonstrated the induction of cartilage from mouse mesenchymal stem cells in high-density micromass culture (Iezaki et al., 2019). This method involves isolating mesenchymal cells from mouse limb buds, followed by culturing at high densities, which is crucial for chondrogenesis initiation. Limb buds from embryonic mice are routinely dissected and digested with enzymes to generate a single-cell suspension, and the cells are then seeded at high density. This protocol underlines the simplicity and effectiveness of the micromass culture method in inducing cartilage formation without the need for specialized equipment or complex reagents. Further advancing this technique, a study on modeling appendicular skeletal cartilage development employed a modified, miniaturized micromass culture model using human bone marrow-derived mesenchymal progenitor cells (hBM-MPCs) (Pirosa et al., 2019). This approach incorporated a methacrylated gelatin overlay, enhancing the system's capacity for moderate throughput and high-content analysis of chondrogenesis. The study revealed robust chondrogenesis, as indicated by increased Alcian blue staining and the production of collagen type II and aggrecan, along with stage-specific chondrogenic gene expression. Stott and Chuong (1997) and others have shown that both chondro-induction and cell proliferation are responsible for the growth of cartilage nodules in mouse limb bud cultures and understanding these mechanisms is crucial for comprehending the intricacies of cartilage development and growth. Primary murine limb bud mesenchymal cells in long-term culture can complete chondrocyte differentiation. Studies have shown that factors like TGF-β can delay hypertrophy and PGE2 can inhibit terminal differentiation, indicating the complex interplay of biochemical factors in chondrogenesis (Clark et al., 2009; Wang et al., 2014).
Mouse micromass cultures offer a range of advantages in various research fields. One of the primary benefits is their ability to closely mimic in vivo developmental processes, especially chondrogenesis, making them an ideal model for studying cell differentiation and development (Akiyama et al., 2002). This model provides a highly controlled environment where researchers can manipulate and precisely monitor experimental conditions, including cell density and the biochemical milieu (Voskamp et al., 2020; Yamashita et al., 2009). Such control is crucial for dissecting the specific factors and pathways influencing cell development. The versatility of mouse micromass cultures extends across several research fields, including developmental biology, toxicology, and pharmacology (Genschow et al., 2002). They are particularly valuable for assessing the impact of substances on embryonic development and for evaluating the safety and efficacy of new pharmaceutical compounds (Parsons et al., 1990). An added benefit of these cultures is the capacity for real-time observation of cellular processes, allowing researchers to track the progression from stem cells to fully differentiated chondrocytes (Takacs, Vago, et al., 2023). Genetic studies also benefit greatly from mouse micromass cultures. They provide a platform for manipulating genes to understand their roles in developmental processes. This manipulation can reveal the impact of specific genes on the development and differentiation of cells, particularly in the context of chondrogenesis (Liang et al., 2022; Takacs, Vago, et al., 2023). The application of mouse micromass cultures extends to tissue engineering and regenerative medicine. They offer a testing ground for new tissue regeneration methods, including those aimed at cartilage repair, which remains a significant challenge in the field of orthopedics.
Critical Parameters and Troubleshooting
There are several critical factors that influence the protocol and to which special attention should be paid during the procedures. These are listed below. We also provide a troubleshooting guide for the mating and fertilization of mice, micromass culturing, and subsequent analysis of ECM production (Table 1).
Problem | Possible cause | Solution |
---|---|---|
Low number of plug-positive female mice | Plug is missing | Plugs are absorbed, translucent or positioned deeper in the vaginal canal |
Females are not in the same estrous cycle | Continue synchronization; generally, it takes 2 consecutive mating dates to enhance the synchronization of the female estrous cycle (Mader et al., 2009) | |
Males are exhausted, because of consecutive, multiple previous matings; alternatively, male mice are not young enough, with reduced sexual activity | The same strained males were used for mating with other females belonging to other experimental groups; give them at least 3-5 days of break for regeneration; refresh the male mouse population | |
Lack of certain vitamins (vitamin E or B6) | Revise the environmental conditions and diet, and supplement food with vitamins if necessary (Saito et al., 2020; Thiessen et al., 1975) | |
Disturbance during maintenance and increased stress | Optimize environmental conditions; provide stability during maintenance (stable room temperature, silence in the rooms, minimize handling) | |
Low embryo yield | The fertility of the mice is low | Refresh the mouse population |
Females lack certain vitamins | Supplement the females’ diet with additional vitamins (see above) | |
Stressful environment | Optimize environmental conditions | |
Out-of-stage embryos | Incorrect calculation for the experimental settings | Check the planned experiment |
Consecutive mating without keeping proper intervals | Because of the lack of plug positivity, females were housed with males within a short period of time (3 days) again | |
Poor chondrogenic differentiation | Embryos are out of developmental stage | Adjust mating time and duration of pregnancy so that embryos are in the correct stage (E11.5) |
Inappropriate part(s) of embryos collected (e.g., tail, heart, etc.), or contamination with embryonic membranes | Make sure to remove inappropriate embryo parts or pieces of membranes before trypsin dissociation | |
Inappropriate size of limb buds collected (contains proximal part as well) | Make sure to only remove the distal part of the developing limb bud | |
Limb bud removal and collection step took >60 min | Make sure that the process of limb bud removal and collection does not take >60 min | |
Dissociation in trypsin took >30 min | Make sure that the dissociation step does not take >30 min; to facilitate dissociation, resuspend the solution once or twice during enzymatic dissociation | |
Partially adherent cells were washed away during feeding | Let the culture medium run down the sides of the well so as not to disturb the cells | |
Not all 4 limb buds have been removed (low yield of chondrogenic cells) | Make sure to remove all 4 limb buds of the embryos | |
Inappropriate culture medium is used (there might be minor but critical differences between culture media made by different suppliers) | Check and compare the contents of the culture medium with the one applied in this protocol. | |
Cultures detach from the surface of plasticware/glassware, or do not form stable droplets | Glass coverslips were heat-sterilized more than once | Make sure not to heat-sterilize the glass coverslips more than once |
Inappropriate plasticware (non-cell culture treated) | Make sure to use cell culture-treated plasticware | |
Improper culture conditions | Check the incubator settings (temperature, humidity, CO2 levels, etc.) and the composition of solutions (e.g., medium, etc.) | |
EDTA carry-over to the culture medium from the dissociation step | Change the culture medium to fresh one | |
Bacterial/fungal infection | Signs of bacterial infection of cultures (yellow, turbid medium) | Sterilize the incubators and all the equipment needed for the culturing |
Abdomen of female mice not properly decontaminated | Decontaminate the abdomen more thoroughly by wiping them with ample 70% ethanol | |
Non-sterile equipment/solutions used | Sterilize the equipment and filter sterilize the solution(s) | |
Inappropriate use of PPE | Wear appropriate PPE | |
Poor metachromatic ECM production | Insufficient seeding density | Make sure to adjust the cell density correctly (to 1 × 107 cells/ml); apply trypan blue during cell counting to check cell viability |
Low or no L-ascorbic acid in medium | Add 50 mg/L of L-ascorbic acid to the culture medium | |
Improper/poor staining | Cultures were kept in the fixative or in wash solution for too long | Follow the protocol carefully |
Incorrect pH of the staining solutions | Adjust the pH of the solutions as recommended | |
Staining solution expired | Prepare a fresh Alcian blue solution | |
Dark spots on cultures after staining | Imperfectly dissolved clumps of dye solution | Filter the stain solutions using a filter paper |
Carefully consider the number of fertilized female mice necessary for the experiment. An abundance of factors has an influence on the pregnancy rate [including the amount and rhythmicity of light (Nakamura et al., 2023), room temperature (Kolbe et al., 2022), the number of mice in not just their cage, but also in the entire room (Svenson & Paigen, 2019), etc.]. With our recommendations above, this rate can be maximized; however, a small proportion of female mice will usually be pseudo-pregnant. Furthermore, another low proportion of embryos will likely not be in the appropriate developmental stage.
For more information on the successful breeding of laboratory mice, please see “Handbook on Genetically Standardized Mice – A Jackson Laboratory Resource Manual” (see Internet Resources).
As described in the Strategic Planning section, we recommend synchronizing the estrous cycle of female mice either by applying male urine or inducing superovulation with PMSG. If the number of embryos is still low, it might be worthwhile to switch to another strain (NMRI or Black-6) or to refresh the current mouse population.
It may happen that there are embryos in different developmental stages within the uterine horns because mouse mating was repeated 2 or 3 days after the previous attempt based on the lack of plug positive females. During embryo retrieval, the out-of-stage (too small or too large, see Fig. 5) embryos must be rejected because these are unsuitable for generating chondrifying micromass cultures.
When sacrificing mice, for instance during cervical dislocation, great care must be taken to be quick in order to reduce suffering (see Internet Resources), and to not injure the gravid uterus as intrauterine bleeding decreases the chances of obtaining a high number of viable cells, which is absolutely necessary for chondrogenesis in the plated cultures.
Limb buds must be harvested from the embryos within a 60 min time frame, as the cells within the isolated limb buds begin to lose their vitality.
Inexperienced individuals should exclusively undertake the removal and collection of limb buds while being supervised. It is crucial to focus solely on extracting the distal portions of the developing limb buds, as extracting sections of the body wall along with the limb bud mesenchyme can negatively impact trypsin dissociation and potentially introduce unsuitable cell populations into the micromass cultures. Verifying the pooled limb buds for any lingering membranes and undesirable “contaminants” (e.g., portions of the tail, heart, or unidentifiable body parts, etc.) is advisable.
Limit the trypsin digestion of the limb buds to a maximum of 30 min, even if the limb buds appear to be intact. Prolonged digestion beyond this time frame will threaten the integrity of the cells, leading to cell death. If chondrogenesis and cell viability are poor, it may be worthwhile to decrease the digestion time from 30 min to 20 min.
Achieving a “critical” cell mass is imperative for advancing chondrogenic differentiation and for the formation of the ECM. Hence, it is crucial to carefully adjust the seeding density to the appropriate level, specifically at 1.0 × 107 cells/ml.
During cell plating, prevent the presence of air bubbles or irregularly shaped drops, as they can disrupt the morphology of micromass cultures and impede chondrogenesis.
Allow the cells to adhere to the surface of plasticware/glassware for a duration of 2 hr. When feeding the cells, gently introduce the medium to the newly formed micromass cultures. Allow the medium to flow down the sides of the wells or Petri dishes to avoid disturbing the cells. At this stage, cell attachments are delicate, and introducing the medium too rapidly may result in cell detachment from the plate, leading to cell death and negatively impacting chondrogenesis.
It is essential to use cell culture-treated plasticware to ensure proper adhesion of the micromasses. Based on our observations, tissue culture plasticware from brands, e.g., Nunc, Eppendorf, Thermo, and Greiner, including 6- and 24-well tissue culture plates, as well as 35-mm Petri dishes, have shown the most favorable results for optimal adhesion of these micromass cultures.
Understanding Results
Cartilage formation (chondrogenesis) spontaneously occurs in mouse embryonic limb bud-derived micromass cultures in a manner similar to that seen in the limbs of an intact embryo (Jiang et al., 1995). Chondrogenesis in the model described above is characterized by the following stages: day of seeding (culture day 0), proliferation and nodule formation (culture days 1 and 2), chondrogenic differentiation (culture days 3 and 4), ECM production (starting from culture day 3). After approximately culture day 10, hypertrophic chondrocytes appear within the cultures. Mature micromass cultures accumulate an abundant, hyaline cartilage-like ECM rich in proteoglycans and glycosaminoglycans, which can be visualized by Alcian blue staining (Fig. 6 and 7).
The method described in this protocol (Basic Protocol 2) relies on pooling chondroprogenitor cells derived from forelimbs and hindlimbs of mouse embryos. However, it is possible to set up micromass cultures from cells obtained from forelimbs and hindlimbs separately. Please note that the forelimb and hindlimb micromass cultures display differences in patterning and size of chondrogenic nodules (Butterfield et al., 2017).
It is worth highlighting that mature (6-day-old) micromass cultures are three-dimensional “organoids”, with a heterogeneous structure. They are made up of compact clusters of aggregated cells forming chondrogenic nodules, and monolayers of cells between the nodules (Figs. 6 and 7). There is also a considerable amount of cartilage ECM present in the cultures (Fig. 7). The multi-layered nature of the micromass cultures, and the abundant ECM in mature cultures may interfere with downstream analytical applications including, but not limited to, single-cell or live-cell imaging techniques, patch clamp experiments, and immunohistochemistry.
Although typical limb histogenetic capacities tend to be preserved and recapitulated in micromass cultures, it is also clear that in vitro morphogenesis is different from what is happening in vivo (Moscona & Moscona, 1952). While the micromass model is not a typical two-dimensional cell culture, the distribution of cells on laboratory plasticware or glass surfaces in which a high proportion shares an interface with the culture medium and in which limb bud ectoderm is lacking, results in nodules, rods, and fused masses of cartilage (Figs. 6 and 7), rather than the characteristic shapes of limb skeletal primordia (Moscona & Moscona, 1952). Nevertheless, while this model is unsuitable for studying the morphogenesis of limb cartilage anlagen, it provides a simple and efficient model to study the molecular processes that regulate cartilage histodifferentiation.
Time Considerations
Planning in advance is highly recommended, given the long timeline of the experiment, including preparation for the mating of mice, in utero development of mouse embryos (gestational days 11.5 to 12.5), embryo retrieval, and micromass culturing.
Preparing for the mating of mice takes ∼30 min. This should be done overnight, 12 days in advance of the experiment. Checking for vaginal plugs takes ∼30 min (Basic Protocol 1). In utero development of mouse embryos takes 11.5 days. Preparation time for the experiment (prepare solutions, i.e., CMF–PBS, trypsin, cell culture medium; disinfect laboratory surfaces, glassware, and dissection tools) takes ∼2 hr. Collecting pregnant mice from animal house facility, euthanizing mice, embryo retrieval & harvesting takes ∼1 hr. Dissection and collection of limb buds takes ∼1 hr (maximum). Dissociation of limb buds in trypsin-EDTA takes up to 30 min. Washing & centrifuging cells, cell counting, plating of micromass cultures takes ∼ 1 hr. Allow ∼2 hr for the cells to adhere. Feeding of plated micromass cultures with fresh complete cell culture medium takes ∼30 min (depending on actual experimental setting) (Basic Protocol 2). Micromass culturing takes 6 to 10 days, but cultures can be maintained for longer periods of time; however, in older cultures, cell viability may decrease, and cultures often detach from the surface. Detection of chondrogenesis by fixing and staining the cultures with Alcian blue solution, including preparation time, takes ∼2 hr (Basic Protocol 3).
For more details and a visual representation of the experimental timeline, see Figure 2.
Acknowledgments
The authors are thankful to Ms. Rana Abdelsattar Mansour Ebeid for proofreading this article. The important role of Dr. Zoltan Meszar (Department of Anatomy, Histology and Embryology, University of Debrecen) in helping us with animal handling at the beginning of our experiments is gratefully acknowledged. Zsofia Foldvari, Barbara Furstal and Tamas Juhasz have also participated in fine-tuning the model in our laboratory, for which we are grateful. C.M. and J.V. were supported by the Young Researcher Excellence Program (grant number: FK-134304) of the National Research, Development and Innovation Office, Hungary. C.M. was also supported by the Bolyai János Research Fellowship of the Hungarian Academy of Sciences. This research was supported by the UNKP-23-5 and UNKP-23-4 New National Excellence Program of the Ministry for Culture and Innovation from the source of the National Research, Development and Innovation Fund, Hungary, awarded to C.M. (UNKP-23-5-DE-487) and R.T. (UNKP-23-4-II-DE-378). C.M. and R.T. acknowledge financial support from the European Cooperation in Science and Technology (COST) Association, Action CA21110 – Building an open European Network on OsteoArthritis research (NetwOArk; https://www.cost.eu/actions/CA21110/).
Author Contributions
Judit Vágó : Formal analysis; investigation; methodology; writing original draft. Csilla Somogyi : Conceptualization; data curation; formal analysis; methodology; writing original draft. Roland Takács : Formal analysis; methodology; writing original draft. Krisztina Bíróné Barna : Data curation; formal analysis; investigation; methodology; writing original draft. Eun-Jung Jin : Formal analysis; methodology; writing original draft; writing review and editing. Róza Zákány : Conceptualization; formal analysis; methodology; writing original draft; writing review and editing. Csaba Matta : Conceptualization; funding acquisition; methodology; supervision; writing original draft; writing review and editing.
Conflict of Interest
The authors declare no conflicts of interest. This paper was written by the authors within the scope of their academic and research positions. None of the authors have any relationships that could be construed as biased or inappropriate. The funding bodies were not involved in the study design, data collection, analysis, and interpretation. The decision to submit the paper for publication was not influenced by any funding bodies.
Open Research
Data Availability Statement
Data sharing is not applicable to this article as no datasets were generated or analyzed during the current study.
Literature Cited
- Ahrens, P. B., Solursh, M., & Reiter, R. S. (1977). Stage-related capacity for limb chondrogenesis in cell culture. Developmental Biology , 60(1), 69–82. https://doi.org/10.1016/0012-1606(77)90110-5
- Akiyama, H., Chaboissier, M. C., Martin, J. F., Schedl, A., & de Crombrugghe, B. (2002). The transcription factor Sox9 has essential roles in successive steps of the chondrocyte differentiation pathway and is required for expression of Sox5 and Sox6. Genes & Development, 16(21), 2813–2828. https://doi.org/10.1101/gad.1017802
- Armit, C., Richardson, L., Hill, B., Yang, Y., & Baldock, R. A. (2015). eMouseAtlas informatics: Embryo atlas and gene expression database. Mammalian Genome , 26(9-10), 431–440. https://doi.org/10.1007/s00335-015-9596-5
- Butterfield, N. C., Qian, C., & Logan, M. P. O. (2017). Pitx1 determines characteristic hindlimb morphologies in cartilage micromass culture. PLoS ONE , 12(7), e0180453. https://doi.org/10.1371/journal.pone.0180453
- Cancedda, R., Castagnola, P., Cancedda, F. D., Dozin, B., & Quarto, R. (2000). Developmental control of chondrogenesis and osteogenesis. International Journal of Developmental Biology , 44(6), 707–714.
- Chen, Q., Johnson, D. M., Haudenschild, D. R., & Goetinck, P. F. (1995). Progression and recapitulation of the chondrocyte differentiation program: Cartilage matrix protein is a marker for cartilage maturation. Developmental Biology , 172(1), 293–306. https://doi.org/10.1006/dbio.1995.0024
- Clark, C. A., Li, T. F., Kim, K. O., Drissi, H., Zuscik, M. J., Zhang, X., & O'Keefe, R. J. (2009). Prostaglandin E2 inhibits BMP signaling and delays chondrocyte maturation. Journal of Orthopaedic Research , 27(6), 785–792. https://doi.org/10.1002/jor.20805
- Current Protocols (2006). Commonly Used Reagents. Current Protocols in Microbiology , 00, A.2A.1–A.2A.15. https://doi.org/10.1002/9780471729259.mca02as00
- DeLise, A. M., Stringa, E., Woodward, W. A., Mello, M. A., & Tuan, R. S. (2000). Embryonic limb mesenchyme micromass culture as an in vitro model for chondrogenesis and cartilage maturation. Methods in Molecular Biology , 137, 359–375. https://doi.org/10.1385/1-59259-066-7:359
- Genschow, E., Spielmann, H., Scholz, G., Seiler, A., Brown, N., Piersma, A., Brady, M., Clemann, N., Huuskonen, H., Paillard, F., Bremer, S., & Becker, K. (2002). The ECVAM international validation study on in vitro embryotoxicity tests: Results of the definitive phase and evaluation of prediction models. European Centre for the Validation of Alternative Methods. Alternatives to Laboratory Animals , 30(2), 151–176. https://doi.org/10.1177/026119290203000204
- Green, M. R., & Pastewka, J. V. (1974). Simultaneous differential staining by a cationic carbocyanine dye of nucleic acids, proteins and conjugated proteins. II. Carbohydrate and sulfated carbohydrate-containing proteins. Journal of Histochemistry and Cytochemistry , 22(8), 774–781. https://doi.org/10.1177/22.8.774
- Hurst, J. L., Thom, M. D., Nevison, C. M., Humphries, R. E., & Beynon, R. J. (2005). MHC odours are not required or sufficient for recognition of individual scent owners. Proceedings. Biological Sciences , 272(1564), 715–724. https://doi.org/10.1098/rspb.2004.3004
- Iezaki, T., Fukasawa, K., Yamada, T., Hiraiwa, M., Kaneda, K., & Hinoi, E. (2019). Cartilage induction from mouse mesenchymal stem cells in high-density micromass culture. Bio-Protocol , 9(1), e3133. https://doi.org/10.21769/BioProtoc.3133
- Jahr, H., Matta, C., & Mobasheri, A. (2015). Physicochemical and biomechanical stimuli in cell-based articular cartilage repair. Current Rheumatology Reports , 17(3), 22. https://doi.org/10.1007/s11926-014-0493-9
- Jiang, H., Soprano, D. R., Li, S. W., Soprano, K. J., Penner, J. D., Gyda, M. 3rd., & Kochhar, D. M. (1995). Modulation of limb bud chondrogenesis by retinoic acid and retinoic acid receptors. International Journal of Developmental Biology , 39(4), 617–627.
- Kolbe, T., Lassnig, C., Poelzl, A., Palme, R., Auer, K. E., & Rulicke, T. (2022). Effect of different ambient temperatures on reproductive outcome and stress level of lactating females in two mouse strains. Animals , 12(16), 2141. https://doi.org/10.3390/ani12162141
- Liang, T., Li, P., Liang, A., Zhu, Y., Qiu, X., Qiu, J., Peng, Y., Huang, D., Gao, W., & Gao, B. (2022). Identifying the key genes regulating mesenchymal stem cells chondrogenic differentiation: An in vitro study. BMC Musculoskeletal Disorders [Electronic Resource] , 23(1), 985. https://doi.org/10.1186/s12891-022-05958-7
- Mader, S. L., Libal, N. L., Pritchett-Corning, K., Yang, R., & Murphy, S. J. (2009). Refining timed pregnancies in two strains of genetically engineered mice. Lab Animal , 38(9), 305–310. https://doi.org/10.1038/laban0909-305
- Moscona, A., & Moscona, H. (1952). The dissociation and aggregation of cells from organ rudiments of the early chick embryo. Journal of Anatomy , 86(3), 287–301.
- Nakamura, T. J., Takasu, N. N., Sakazume, S., Matsumoto, Y., Kawano, N., Pendergast, J. S., Yamazaki, S., & Nakamura, W. (2023). Long days restore regular estrous cyclicity in mice lacking circadian rhythms. Heliyon , 9(6), e16970. https://doi.org/10.1016/j.heliyon.2023.e16970
- Parsons, J. F., Rockley, J., & Richold, M. (1990). In vitro micromass teratogen test: Interpretation of results from a blind trial of 25 compounds using three separate criteria. Toxicology in Vitro , 4(4-5), 609–611. https://doi.org/10.1016/0887-2333(90)90125-d
- Pirosa, A., Clark, K. L., Tan, J., Yu, S., Yang, Y., Tuan, R. S., & Alexander, P. G. (2019). Modeling appendicular skeletal cartilage development with modified high-density micromass cultures of adult human bone marrow-derived mesenchymal progenitor cells. Stem Cell Research & Therapy, 10(1), 388. https://doi.org/10.1186/s13287-019-1505-5
- Richardson, S. M., Kalamegam, G., Pushparaj, P. N., Matta, C., Memic, A., Khademhosseini, A., Mobasheri, R., Poletti, F. L., Hoyland, J. A., & Mobasheri, A. (2016). Mesenchymal stem cells in regenerative medicine: Focus on articular cartilage and intervertebral disc regeneration. Methods , 99, 69–80. https://doi.org/10.1016/j.ymeth.2015.09.015
- Saito, H., Hara, K., Kitajima, S., & Tanemura, K. (2020). Effect of vitamin E deficiency on spermatogenesis in mice and its similarity to aging. Reproductive Toxicology , 98, 225–232. https://doi.org/10.1016/j.reprotox.2020.10.003
- Scott, J. E., & Dorling, J. (1965). Differential staining of acid glycosaminoglycans (mucopolysaccharides) by alcian blue in salt solutions. Histochemie. Histochemistry Histochimie , 5(3), 221–233. https://doi.org/10.1007/BF00306130
- Sophia Fox, A. J., Bedi, A., & Rodeo, S. A. (2009). The basic science of articular cartilage: Structure, composition, and function. Sports Health , 1(6), 461–468. https://doi.org/10.1177/1941738109350438
- Stott, N. S., & Chuong, C. M. (1997). Dual action of sonic hedgehog on chondrocyte hypertrophy: Retrovirus mediated ectopic sonic hedgehog expression in limb bud micromass culture induces novel cartilage nodules that are positive for alkaline phosphatase and type X collagen. Journal of Cell Science , 110(Pt 21), 2691–2701. https://doi.org/10.1242/jcs.110.21.2691
- Svenson, K. L., & Paigen, B. (2019). Recommended housing densities for research mice: Filling the gap in data-driven alternatives. Faseb Journal , 33(3), 3097–3111. https://doi.org/10.1096/fj.201801972R
- Takács, R., Juhász, T., Katona, É., Somogyi, C., Vágó, J., Hajdú, T., Barna, K. B., Nagy, P., Zákány, R., & Matta, C. (2023). Isolation and micromass culturing of primary chicken chondroprogenitor cells for cartilage regeneration. Current Protocols , 3(7), e835. https://doi.org/10.1002/cpz1.835
- Takacs, R., Matta, C., Somogyi, C., Juhasz, T., & Zakany, R. (2013). Comparative analysis of osteogenic/chondrogenic differentiation potential in primary limb bud-derived and C3H10T1/2 cell line-based mouse micromass cultures. International Journal of Molecular Sciences , 14(8), 16141–16167. https://doi.org/10.3390/ijms140816141
- Takács, R., Vágó, J., Póliska, S., Pushparaj, P. N., Ducza, L., Kovács, P., Jin, E. J., Barrett-Jolley, R., Zákány, R., & Matta, C. (2023). The temporal transcriptomic signature of cartilage formation. Nucleic Acids Research , 51(8), 3590–3617. https://doi.org/10.1093/nar/gkad210
- Teiler, K. (1989). The House Mouse - Atlas of Emryonic Development ( 2nd ed.). Springer-Verlag.
- Thiessen, D. D., Ondrusek, G., & Coleman, R. V. (1975). Vitamin E and sex behavior in mice. Nutrition and Metabolism , 18(3), 116–119. https://doi.org/10.1159/000175585
- Umansky, R. (1966). The effect of cell population density on the developmental fate of reaggregating mouse limb bud mesenchyme. Developmental Biology , 13(1), 31–56. https://doi.org/10.1016/0012-1606(66)90048-0
- Voskamp, C., Koevoet, W., Somoza, R. A., Caplan, A. I., Lefebvre, V., van Osch, G., & Narcisi, R. (2020). Enhanced Chondrogenic Capacity of Mesenchymal Stem Cells After TNFalpha Pre-treatment. Frontiers in Bioengineering and Biotechnology , 8, 658. https://doi.org/10.3389/fbioe.2020.00658
- Wang, W., Rigueur, D., & Lyons, K. M. (2014). TGFbeta signaling in cartilage development and maintenance. Birth Defects Research. Part C, Embryo Today , 102(1), 37–51. https://doi.org/10.1002/bdrc.21058
- Yamashita, A., Krawetz, R., & Rancourt, D. E. (2009). Loss of discordant cells during micro-mass differentiation of embryonic stem cells into the chondrocyte lineage. Cell Death and Differentiation , 16(2), 278–286. https://doi.org/10.1038/cdd.2008.149
Key References
- Moscona and Moscona (1952). See above.
- This is the first paper that describes the concept of self-aggregation of dissociated cells isolated from early embryos.
- Umansky (1966). See above.
- This is the first original research article on the developmental fate of reaggregating mouse limb bud mesenchymal cells in micromass cultures.
- Ahrens et al. (1977). See above.
- This is the original paper describing the embryonic chicken limb bud-derived micromass cultures.
Internet Resources
- https://arriveguidelines.org
- The ARRIVE guidelines (Animal Research: Reporting of In Vivo Experiments) are a checklist of recommendations for the full and transparent reporting of research involving animals. Last accessed February 3, 2024.
- https://nc3rs.org.uk/who-we-are/our-mission
- The NC3Rs primary mission is to accelerate the replacement, reduction, and refinement of the use of animals in research. Last accessed February 3, 2024.
- www.emouseatlas.org
- eMouseAtlas is a comprehensive online resource to visualize mouse development and investigate gene expression in the mouse embryo. Last accessed February 3, 2024.
- https://resources.jax.org/misc/jax-handbook-genetically-standardized-mice
- The 6th edition of The Jackson Laboratory Handbook on Genetically Standardized Mice. Last accessed February 3, 2024.