Flow Virometry for Characterizing the Size, Concentration, and Surface Antigens of Viruses
Mariam Maltseva, Mariam Maltseva, Marc-André Langlois, Marc-André Langlois
enveloped viruses
extracellular vesicles
flow virometry
murine leukemia virus
retroviruses
small-particle flow cytometry
Abstract
Application of flow cytometry principles for the analysis of viruses has been referred to as flow virometry (FVM). FVM is a multiparametric, high-throughput, and sensitive technique that allows viral particles to be detected, quantified, and characterized based on the biophysical properties of the virus and the expression of proteins on their surface. More specifically, by calibrating the flow cytometer with reference materials, it is possible to measure the concentration of intact viral particles in a sample, the abundance of a target antigen on the surface of the virus, and the relative diameter of the virus. Here, we describe a comprehensive overview of procedures used to stain, detect, and quantify viral and host-derived proteins located on the surface of retroviruses. These outlined techniques can be applied for the rapid phenotypic characterization of retroviruses, other enveloped viruses, and generally most viruses at the single-particle level through the direct staining of viruses collected from the supernatant of infected cells, without the need for enrichment or purification. © 2022 The Authors. Current Protocols published by Wiley Periodicals LLC.
Basic Protocol 1 : Virus production
Basic Protocol 2 : Instrument setup, standardization, and quality control for fluorescence quantification
Basic Protocol 3 : Flow virometry analysis
Basic Protocol 4 : Viral surface antigen staining and fluorescence quantification
Support Protocol : Determination of the optimal antibody concentration for virus staining
Basic Protocol 5 : Gain configuration optimization
INTRODUCTION
Viral phenotypic analyses are predominantly performed on a virus population using methodologies such as western blot, ELISA, PCR, and mass spectrometry, which are not designed to characterize viruses at the single-particle level and are incapable of assessing inter-particle variabilities. Although electron microscopy can provide extremely useful structural information on single particles, the time-intensive and low-throughput nature of this technology limits simultaneous virus characterization to only a small portion of the total viral population in a sample. Additionally, these technical approaches are not well suited to characterizing the heterogeneity of viral subpopulations, discern features within these subpopulations, or characterize the antigen composition on the surface of individual viruses on a large scale. However, increasing interest in extracellular vesicles (EVs) has spurred the development of flow cytometry principles for the analysis of submicron particles and renewed the pursuit of analyzing viruses using flow cytometry approaches. In particular, the analysis of viruses by flow cytometry has been referred to as flow virometry (FVM) (Arakelyan, Fitzgerald, Margolis, & Grivel, 2013).
FVM is a rapid, sensitive, and high-throughput technique that can also provide valuable statistical information on the various analyzed features. FVM can measure the concentration of intact viral particles in a sample and characterize some of the physical properties of viruses by the way they scatter light and emit fluorescence when stained with dyes and antibodies (Maltseva & Langlois, 2021; Tang, Renner, Fritzsche, Burger, & Langlois, 2017). When using flow cytometers capable of small-particle analysis, the light scattering properties of the viruses can be used to derive refractive indices (RIs) and viral particle sizes (Brittain et al., 2019; Tang et al., 2019). Additionally, antibodies can be used to stain proteins on the surface of the viruses in a similar manner to cell staining in classical flow cytometry, where fluorescence intensity can provide a measurement of target antigen abundance if adequate calibration is performed on the instrument.
Most enveloped viruses bud at the cell surface or through the endosomal pathway and, in this process, acquire part of the lipid bilayer membrane of the infected cell, which contains cellular and viral proteins (Cantin, Methot, & Tremblay, 2005; Maltseva & Langlois, 2021). This fragment of the membrane with embedded proteins will then constitute the envelope of the released viruses. Characterizing viral envelope proteins is an appealing research venture due to the insight these antigens can provide on virus cell tropism, the cellular identity of infected cell/tissue reservoirs in a host, and potential accessible antigenic targets for vaccine development. FVM is rapidly evolving into a more common method in virology as technological advancements are being made on various fronts, including the development of more sensitive analysis hardware, better reagents, and data and methods standardizations (Thery et al., 2018; Tian et al., 2020; Welsh et al., 2020). Although FVM has shown increasing popularity in recent years, it still faces several technical hurdles that hinder the full deployment of its potential. Analysis of viruses by FVM presents several inherent challenges, primarily due to their very small size compared to cells, which places them at the limit of detection of current instruments (Lippe, 2018). A viral particle's surface area can be 1000- to 10,000-fold smaller than that of a cell, and the magnitude of this difference is reflected in surface antigen density and abundance. Therefore, weakly expressed antigens on viruses may fall at or even below the detection limits of an instrument. Careful consideration should be applied to the experimental setup, assay procedures, and data analysis methods to ensure the most robust and reproducible results from these types of experiments.
This article describes three different methods for the production of viruses: a) by cell transfection, b) by direct infection of a cell population, and c) by generating a chronically infected cell clone (Basic Protocol 1). To ensure the detection of viruses and their resolution at the single-particle level, we outline the workflow for instrument setup, calibration, and fluorescence quantification to optimize FVM measurement sensitivity and standardization (Basic Protocols 2 and 5). We then describe the FVM techniques used to measure the concentration and relative diameter of intact viral particles in a sample (Basic Protocol 3) and how to characterize viral and host-derived proteins on the surface of the viral envelope (Basic Protocol 4 and Support Protocol). Furthermore, these protocols detail the multiple steps required for a comprehensive experimental design and highlight important practical considerations for FVM, virus staining procedures, and best practices for data reporting and instrument calibration.
CAUTION : Viral production and handling should be reviewed by the institution's biosafety officer before beginning experiments.
Basic Protocol 1: VIRUS PRODUCTION
Virus production can be achieved through different means depending on the desired experimental goal. These methods will yield viral particles of varying levels of homogeneity and purity depending on the virus, the type of producer cells, and the medium used. Here, we produce viruses by three common methods and analyze them by light scatter and fluorescence (Basic Protocol 3). In particular, we produce virus by transfecting cells with a virus-expressing plasmid, by harvesting the supernatant of an infected cell population, or by harvesting virus in the supernatant of infected cell clones (Fig. 1). Detailed methods to produce virus and the virus expression plasmids used here have been described elsewhere (Maltseva & Langlois, 2021, Renner, Tang, Burger, & Langlois, 2020; Tang et al., 2017). In our experiments, we used a replicative murine leukemia virus (MLV) that expresses an envelope-superfolder GFP fusion protein (Env-sfGFP) on its surface (Maltseva & Langlois, 2021, Renner et al., 2020; Tang et al., 2019; Tang et al., 2017). Fusing the envelope glycoprotein with a GFP fluorescent reporter makes these viruses intrinsically fluorescent and easily discernible from background noise by FVM. Additionally, these viruses can also be labeled by fluorophore-conjugated antibodies directed against host-derived surface proteins or against the Env-sfGFP fusion protein found on the viral envelope, as we have demonstrated in Basic Protocol 4 (Maltseva & Langlois, 2021, Renner et al., 2020; Tang et al., 2019). As shown in Figure 1A, viruses produced by all three methods are resolved with different efficiencies by both light scatter and fluorescence (Basic Protocol 3). Although viruses produced by all three methods exhibit a homogenous light scatter and fluorescence intensity distribution, virus produced from a chronically infected clonal cell line displayed the most monodisperse population for both properties and had lower background fluorescence compared to virus from the other methods of virus production (Fig. 1B and 1C). Various factors can contribute to the increase in background fluorescence levels, such as the type of medium used, the type of transfection reagent, and EVs that are concomitantly released by the cells (Tang et al., 2017).
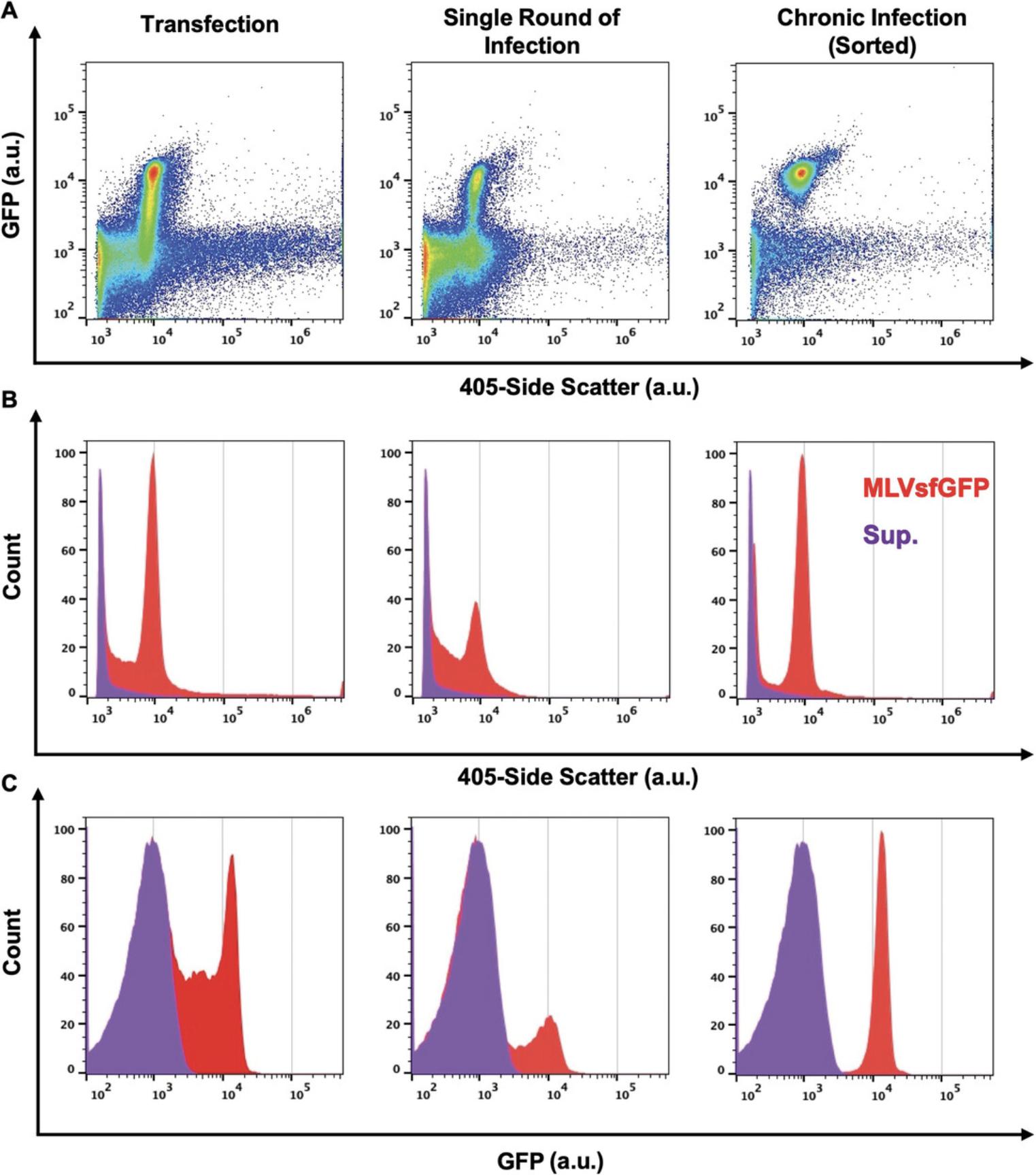
Materials
-
HEK 293T cells (ATCC, CRL-3216)
-
Complete DMEM (see recipe), 37°C
-
Serum-free DMEM (see recipe), 37°C
-
GeneJuice Transfection Reagent (Sigma-Aldrich, cat. no. 70967)
-
Viral plasmid of interest [e.g., virus expression plasmid MLV (Tang et al., 2017) or MLVsfGFP (Renner et al., 2020)]
-
Dulbecco's phosphate-buffered saline (DPBS; Wisent Bioproducts, cat. no. 311-430-CL)
-
Phenol red–free complete DMEM (see recipe), 200-nm-filtered, 37°C
-
NIH 3T3 mouse fibroblast cells (ATCC, CCL-92)
-
6-, 12-, and 96-well plates
-
37°C, 5% CO2 tissue culture incubator (Thermo Fisher Scientific, cat. no. 3110)
-
1.5-ml Eppendorf tubes
-
Vortex
-
Rocker
-
Inverted fluorescence microscope (with 488-nm LED lamp to detect GFP; optional)
-
Flow cytometer
-
100-, 200-, and 450-nm Acrodisc sterile syringe filters (Pall Corporation, cat. no. 4611, 4602, and 4615)
-
10-ml syringes (BD, cat. no. 302995)
-
15-ml conical tubes (Thermo Fisher Scientific, cat. no. 339651)
-
10-cm tissue culture dishes
-
Fluorescence-activated cell sorting (FACS) instrument (MoFlo AstriosEQ FACS cytometer, Beckman Coulter)
-
FACS tubes (5 ml, polystyrene, round bottom; Falcon, cat. no. 352054)
NOTE : All solutions and equipment coming into contact with cells must be sterile, and proper sterile technique should be used accordingly.
Production of virus by transfection
1a. Seed 1.0 × 105 HEK 293T cells in 1 ml complete DMEM per well in a 12-well plate.
2a. Incubate overnight at 37°C, 5% CO2.
3a. Prepare transfection mix by adding 100 µl serum-free DMEM and 3 µl GeneJuice Transfection Reagent to a 1.5-ml Eppendorf tube for each well to be transfected.
4a. Vortex mixture thoroughly and let sit for 5 min at room temperature.
5a. Add viral plasmid of interest and mix by gentle pipetting.
6a. Incubate tubes for 10 min at room temperature.
7a. Add mixture dropwise and evenly across the entire surface of each well.
8a. Gently rock plate biaxially on a flat surface for 1 min.
9a. Incubate cells for 8 hr at 37°C, 5% CO2.
10a. Wash each well with 1 ml DPBS for 30 s, discard wash, and add 1 ml of 200-nm-filtered phenol red–free complete DMEM.
11a. Incubate cells for 64 hr at 37°C, 5% CO2. Validate transfection efficiency by evaluating GFP expression under an inverted fluorescence microscope or by flow cytometry.
12a. Harvest supernatant from the cells and pass through a 450-nm Acrodisc sterile syringe filter attached to a 10-ml syringe and into a 15-ml conical tube.
Production of virus from an infected cell population (single-round infection)
1b. Seed 6.0 × 105 NIH 3T3 mouse fibroblast cells in 2 ml complete DMEM per well in a 6-well plate.
2b. Incubate overnight at 37°C, 5% CO2.
3b. Remove existing medium from the wells carefully, without disturbing the cells, and infect cells using supernatant from 293T cells transfected with the virus expression vector as in steps 1a to 12a.
4b. Incubate for 24 hr at 37°C, 5% CO2 and then wash cells twice with 2 ml DPBS for 30 s each to remove input virus.
5b. Add 2 ml of 200-nm-filtered phenol red–free complete DMEM.
6b. Incubate cells for an additional 48 hr at 37°C, 5% CO2. Validate infection efficiency by evaluating GFP expression under an inverted fluorescence microscope or by flow cytometry.
7b. Harvest supernatant from the cells and pass through a 450-nm Acrodisc sterile syringe filter and into a 15-ml conical tube.
Production of virus by chronically infected cell clones
1c. Carry out steps 1b to 6b, with the modification that 2.5 × 106 NIH 3T3 mouse fibroblast cells are seeded in 10 ml complete DMEM in a 10-cm dish and are infected at various MOIs (0.5, 1, and 2, yielding ∼90% infection).
2c. Harvest cells from each plate and identify and isolate infected cells expressing GFP with a FACS instrument, dispensing one cell in each well of a 96-well plate (one plate per MOI condition) pre-filled with 200 µl complete DMEM.
3c. Incubate cells at 37°C at 5% CO2, and allow cells to expand.
4c. When confluent, transfer all cells from each positive well (i.e., expressing GFP) to a well of a 24-well plate containing 0.5 ml complete DMEM. Incubate cells for 3 days or until confluent.
5c. When confluent, transfer all cells from each positive well to a well of a 6-well plate containing 3 ml complete DMEM per well for further expansion.
6c. Dispense some cells from each clonal expansion into a FACS tube (0.4 × 106 cells per 0.4 ml DPBS) and analyze GFP expression by flow cytometry. Identify clones with the highest GFP fluorescence intensity for further expansion and for downstream analysis of released viruses by FVM (see Basic Protocol 3).
7c. Harvest cell supernatants from GFP-positive clones and pass through a 450-nm Acrodisc sterile syringe filter into a 15-ml conical tube.
8c. Store cell supernatants at 4°C until ready for FVM analysis (see Basic Protocol 3).
Basic Protocol 2: INSTRUMENT SETUP, STANDARDIZATION, AND QUALITY CONTROL FOR FLUORESCENCE QUANTIFICATION
Because the viral particles used here (Basic Protocol 1) are very close to the limits of detection for both light scatter and fluorescence for most commercial flow cytometers, an appropriate experimental design and instrument setup are critical to ensure viral detection and resolution at the single-particle level (Basic Protocol 3). This protocol outlines the necessary steps for fluorescence quantification, relying on the principles for light scatter and fluorescence calibration described in a Current Protocols article by Welsh & Jones (2020). For accurate, reproducible, and standardized data reporting, it is crucial to determine the limit of the assay's and instrument's sensitivity for light scatter and fluorescence measurements and to calibrate these values using standardized reference materials. For this purpose, light scatter calibration is performed using National Institute of Standards and Technology (NIST)-certified standard reference beads. Fluorescence calibration and data reporting in quantitative units (or standard units) such as molecules of equivalent soluble fluorophore (MESF) or equivalent reference fluorophore (ERF) are appropriate for fluorescence intensity conversion from arbitrary units (a.u.) (Welsh et al., 2020). Reporting of experimental data in standard units can be achieved by using FCMPASS software, which calibrates light scatter and fluorescence measurements using commercially available reference standards and enables data reporting to conform with the MIFlowCyt-EV framework (Welsh et al., 2020). As such, this calibration enables an estimation of particle diameter, using the known RIs of the particles, and relative antigen quantitation, which facilitates the comparison of data between experiments and research groups. The protocol below outlines how to prepare fluorescence calibration materials for FCS file calibration and how to evaluate instrument sensitivity and performance. To report data in standard units, calibrated files are generated using FCMPASS software, which plots the acquired signal intensity in a.u. using the manufacturer's predicted values for each bead population and performs a least-squares regression. Figures 2A and 2B respectively display the light scattering and fluorescence features of BD Quantibrite Phycoerythrin (PE) fluorescence and polystyrene NIST-traceable size reference beads used for calibration. Figure 2C shows that the data points for the beads fall within the “good fit” range for scatter modeling using FCMPASS software. Both calibration curves display a high correlation between acquired and predicted values (Fig. 2D). This close correlation is essential to be able to convert fluorescence intensity values into molecule equivalents and report fluorescence measurements in standard units such as PE MESF, as used in this protocol. To ensure consistent detection of individual virus particles, this protocol additionally outlines how to validate that viral particles are interrogated one at a time in the flow cytometer. Simultaneous detection of several particles is a phenomenon called “swarm detection or coincidence,” where the combined interrogation of multiple particles can lead to a decrease in total events but a concurrent increase in fluorescence intensity (van der Pol, van Gemert, Sturk, Nieuwland, & van Leeuwen, 2012). In order to minimize coincidence of viral particles and determine an optimal sample concentration for acquisition, a range of serially diluted virus-containing samples is analyzed and quantified.
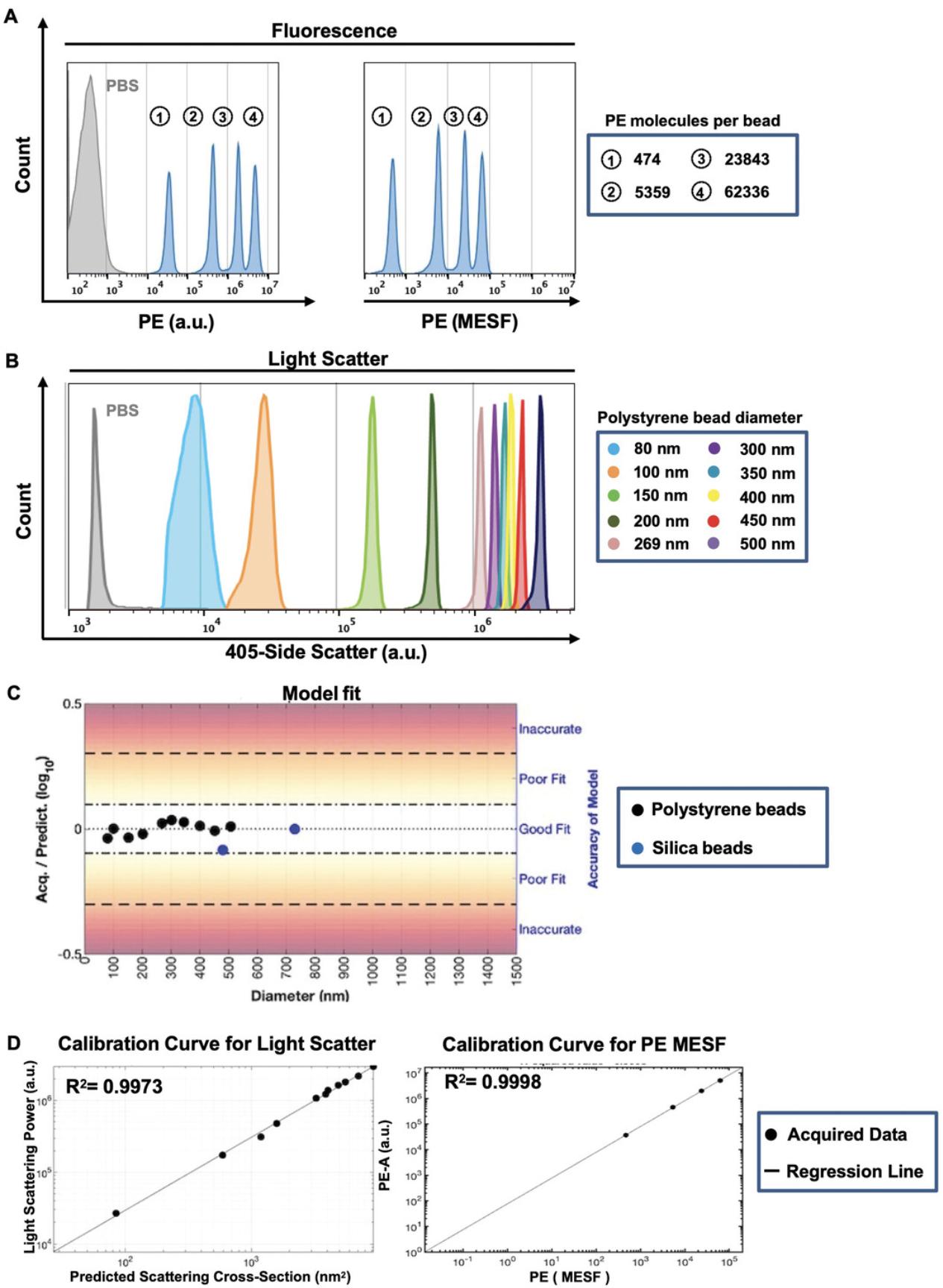
Materials
-
DPBS (Wisent Bioproducts, cat. no. 311-430-CL)
-
BD Quantibrite PE beads (BD Biosciences, cat. no. 340495, lot. no. 47973; Davis, Abrams, Iyer, Hoffman, & Bishop, 1998)
-
Virus-containing supernatant from chronically infected NIH 3T3 cell clone (#6) cells (see Basic Protocol 1, steps 1c to 8c)
-
Flow cytometer (CytoFLEX S with four lasers, Beckman Coulter)
-
100- and 450-nm Acrodisc sterile syringe filters (Pall Corporation, cat. no. 4611 and 4615)
-
10-ml syringes (BD, cat. no. 302995)
-
15- and 50-ml conical tubes (Thermo Fisher Scientific, cat. no. 339651 and 339652)
-
FACS tubes (5 ml, polystyrene, round bottom; Falcon, cat. no. 352054)
-
Vortex
-
FCMPASS software (v3.1, http://nanopass.ccr.cancer.gov; see Current Protocols article; Welsh & Jones, 2020)
1.Set up flow cytometer settings according to the manufacturer's recommendations and the assay being calibrated.
2.Filter DPBS through a 100-nm Acrodisc sterile syringe filter attached to a 10-ml syringe and into a 50-ml conical tube.
3.Run a DPBS-only control in a FACS tube on the flow cytometer.
4.Add 500 µl of 100-nm-filtered DPBS from step 2 to a tube of BD Quantibrite PE beads.
5.Let sit for ∼3 min until beads at the bottom of tube are fully dissolved and then vortex tube thoroughly.
6.Run sample until the desired number of beads is recorded.
7.Once reference beads have been acquired on the flow cytometer, convert raw FCS files into calibrated FCS files using FCMPASS software, which allows reporting of data in standard units following the steps described in the protocol by Welsh & Jones (2020) for fluorescence and light scatter calibration and applying calibration to FCS files.
8.Filter 3 to 5 ml virus-containing supernatant from chronically infected NIH 3T3 cell clone (#6) cells (see Basic Protocol 1, steps 1c to 8c) through a 450-nm Acrodisc sterile syringe filter and into a 15-ml conical tube.
9.Perform serial dilutions of virus-containing supernatant in 100-nm-filtered DPBS (see step 2) directly in FACS tubes and vortex.
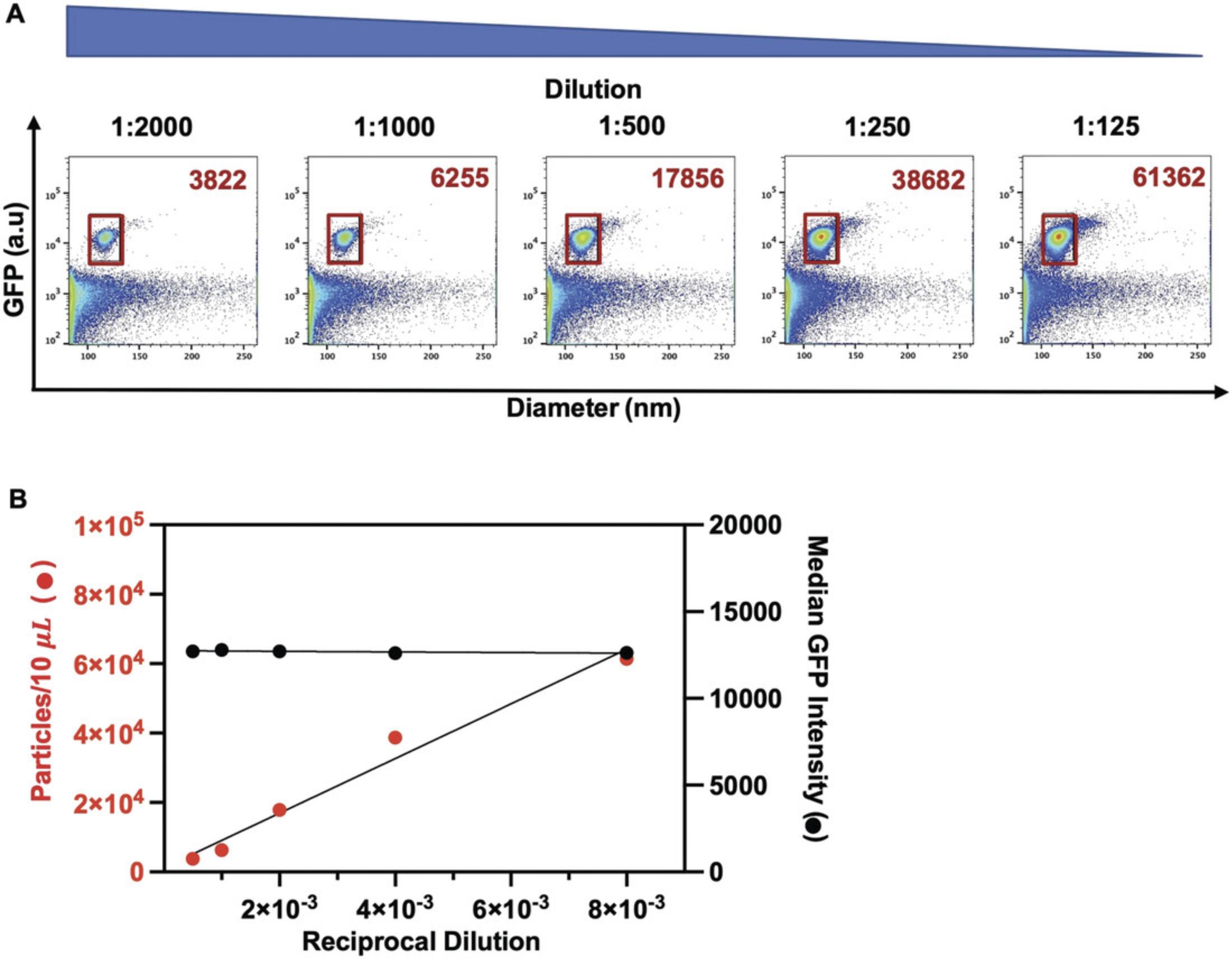
10.Run samples and gate on viral population, which should be distinguishable from the background noise.
Basic Protocol 3: FLOW VIROMETRY ANALYSIS
Analyzing virus particles (Basic Protocol 1) by flow cytometry is challenging due to the fact that they are often near the limit of detection for both light scatter and fluorescence for most commercial flow cytometers. Consequently, an appropriate experimental design and instrument setup are critical to enable virus detection and resolution from background noise before pursuing downstream analyses. This protocol covers the key issues that should be considered to ensure consistent detection and resolution of the virus population at the single-particle level. Similar to the controls outlined in the MIFlowCyt-EV framework for reporting in EV studies, key experimental controls are required for virus detection and quantification studies (Welsh et al., 2020). These controls include buffer only (to evaluate instrument background noise) and supernatant collected from uninfected cells (to evaluate the possible presence of EVs). To evaluate changes in light scatter or fluorescence signals, these experimental controls should all be run at the same acquisition settings (flow rate, trigger threshold, and gain) and during the same session for all samples. Given that several factors influence virus production between experiments, serial dilutions are recommended prior to each experiment in order to determine the optimal particle concentration for acquisition, as illustrated in step 9 of Basic Protocol 2.
Additional Materials (also see Basic Protocol 2)
- Buffer control (100-nm-filtered DPBS; see Basic Protocol 2, step 2)
- Supernatant from uninfected control NIH 3T3 mouse fibroblast cells treated under same conditions as chronically infected NIH 3T3 cell clone (#6) cells (see Basic Protocol 1, steps 1c to 8c)
1.Filter 50 ml of the buffer control (100-nm-filtered DPBS) through a 100-nm Acrodisc sterile syringe filter attached to a 10-ml syringe and into a 50-ml conical tube.
2.Filter 5 ml supernatant from uninfected control NIH 3T3 mouse fibroblast cells treated under the same conditions as the chronically infected NIH 3T3 cell clone (#6) cells (see Basic Protocol 1, steps 1c to 8c) through a 450-nm Acrodisc sterile syringe filter and into a 15-ml conical tube.
3.Filter 5 ml virus-containing supernatant from chronically infected NIH 3T3 cell clone (#6) cells (see Basic Protocol 1, steps 1c to 8c) through a 450-nm Acrodisc sterile syringe filter and into a 15-ml conical tube.
4.Dilute all filtered control and virus samples from steps 2 and 3 at the chosen dilution factor (see Basic Protocol 2, step 9) or at 1:500 in 100-nm-filtered DPBS in FACS tubes and vortex.
5.Acquire assay-control and virus-containing samples diluted in DPBS on the cytometer.
6.Gate on viral population, which should be distinguishable from the background noise.
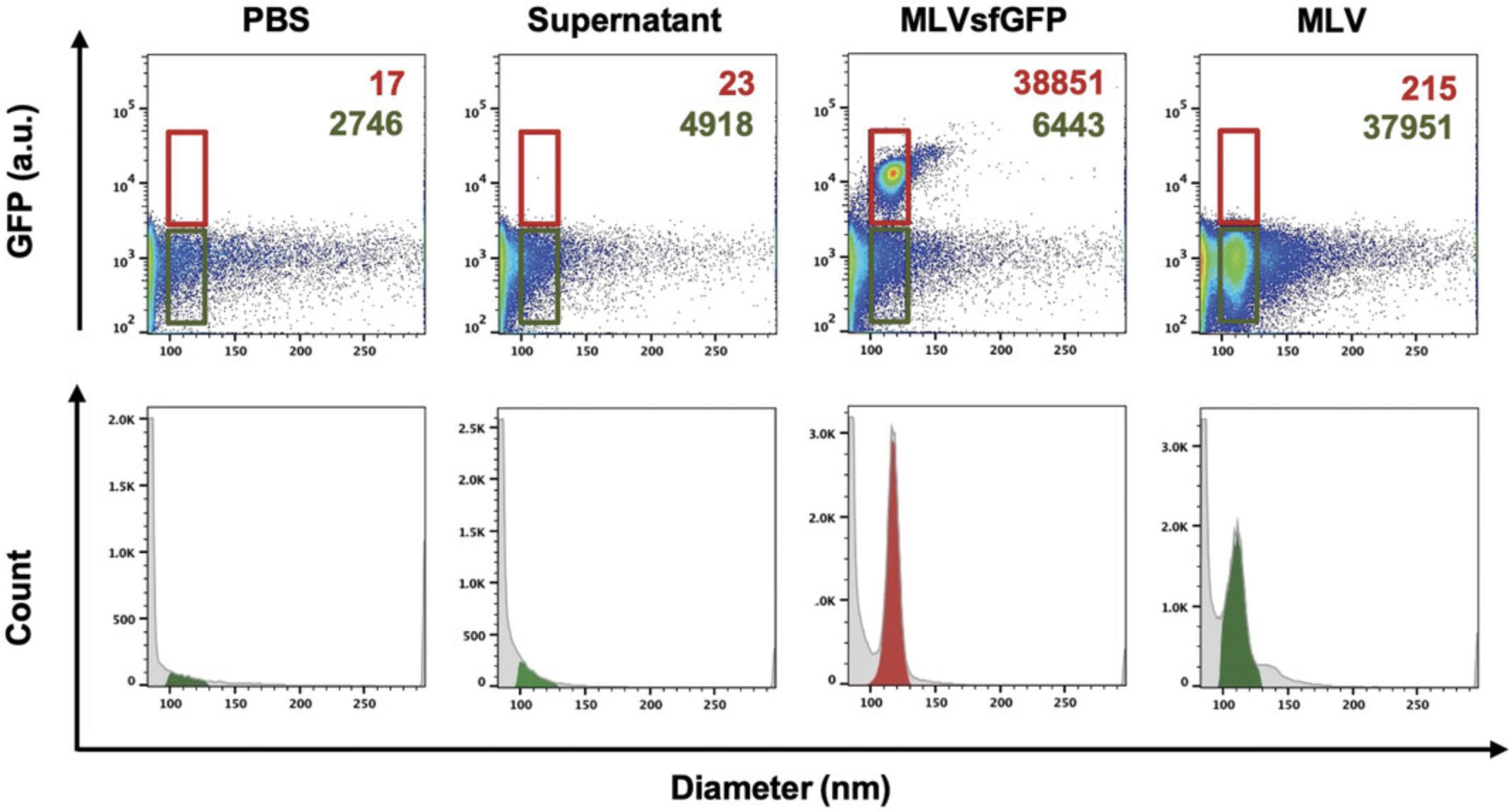
Virusa | Concentration (particles/ml) | Median diameter (nm ± SD) |
---|---|---|
MLV | 1.91 × 109 | 109.8 ± 6.8 |
MLVsfGFP | 1.89 × 109 | 120.3 ± 4.5 |
- a
All samples were passed through a 450-nm filter prior to analysis.
Basic Protocol 4: VIRAL SURFACE ANTIGEN STAINING AND FLUORESCENCE QUANTIFICATION
This protocol outlines the experimental setup for single-color virus staining. Characterization of the antigenic composition and abundance is predominantly achieved by fluorescence quantification. However, the smaller surface area and number of proteins available for staining on the viral surface necessitates a careful consideration of the choice of fluorophore(s) used and the optimal antibody concentration (see Support Protocol). Determining the optimal staining antibody concentration using a titration is especially crucial to resolve dimly stained virus particles from unstained populations and background noise, as illustrated in Figure 5.In this protocol, we optimized the antibody staining conditions using a PE-conjugated antibody that targets the highly expressed Env-sfGFP fusion protein. However, viruses can be labeled by fluorophore-conjugated antibodies directed against host-derived surface proteins or viral glycoproteins found on the viral envelope (Maltseva & Langlois, 2021; Renner et al., 2020; Tang et al., 2019). Similarly to cell staining procedures, attention should be given to the initial virus concentration, antibody incubation time, and final antibody concentration (Cossarizza et al., 2019). As previously mentioned, a set of experimental controls stained under the same conditions as the virus samples should be run during the same data acquisition session in order to evaluate changes in light scatter and fluorescence profiles (Fig. 5A).
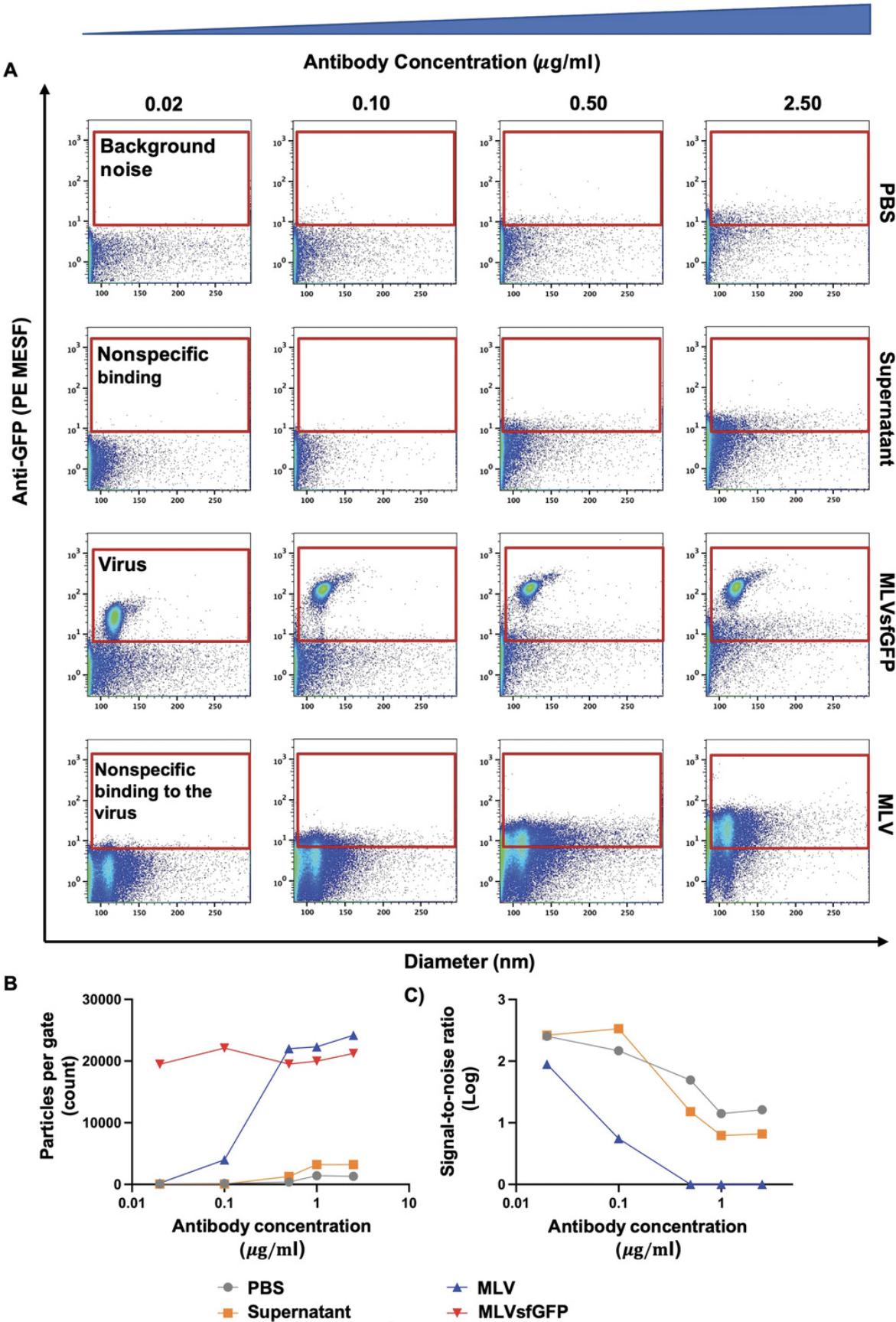
Additional Materials (also see Basic Protocols 2 and 3)
-
Anti-GFP PE monoclonal antibody (BioLegend, clone FM264G)
-
100-nm-filtered DPBS (see Basic Protocol 2, step 2)
-
16% (w/v) paraformaldehyde (PFA; Thermo Scientific, cat. no. 28906)
-
Refrigerated tabletop centrifuge (SorvallTM ST 40, Thermo Fisher Scientific, cat. no. 75004524)
-
37°C, 5% CO2 tissue culture incubator (Thermo Fisher Scientific, cat. no. 3110)
1.Determine concentration of virus in the virus-containing supernatant as described in Basic Protocol 2, step 10.
2.Centrifuge tube of anti-GFP PE monoclonal antibody for 10 min at 17,000 × g , 4°C, in a refrigerated tabletop centrifuge.
3.Prepare a master mixture of antibody diluted in 100-nm-filtered DPBS at twice the predetermined final concentration (see Support Protocol).
4.Add 50 µl virus-containing supernatant or assay controls (DPBS and supernatant collected from uninfected cells) to 50 µl antibody mixture.
5.Pipet mixture thoroughly to mix.
6.Incubate at 37°C for 60 min protected from light.
7.Fix stained virus samples with 16% PFA to obtain a final mixture at 2% PFA and let incubate for 2 hr at 4°C.
8.Dilute sample at the chosen dilution factor (from Basic Protocol 2, step 9) or at 1:500 with 100-nm-filtered DPBS in a FACS tube and vortex.
9.Run sample on the cytometer.
10.Gate on viral population and obtain MFI of the fluorophore during the sample acquisition on the cytometer.
11.Using the procedures described in Basic Protocol 2, step 7, generate calibrated FCS files using NIST-traceable size calibration reference polystyrene and silica beads acquired during the same session and FCMPASS software.
12.Gate on viral population of interest based on the light scatter and/or fluorescence profile, as described in Basic Protocol 3, step 6.
13.Quantify light scatter and MFI of the viral population in standard units.
14.Repeat steps 1 to 13 for supernatant containing control virus that does not express the target protein.
15.Repeat steps 4 to 13 for assay controls (buffer control of 100-nm-filtered DPBS and filtered supernatant collected from uninfected cells).
Virusa | Median PE fluorescence (PE MESF ± SD) | 25th percentile (PE MESF) | 75th percentile (PE MESF) | 99th percentile (PE MESF) |
---|---|---|---|---|
MLV | 3.1 ± 2.8 | 1.6 | 5.3 | 13.0 |
MLVsfGFP | 124.9 ± 26.3 | 107.2 | 141.7 | 236.6 |
- a
All samples were passed through a 450-nm filter prior to analysis. The detection gate for PE fluorescence was applied between 0 and 1000 PE MESF units. Samples were stained at the optimal staining concentration of 0.1 µg/ml. Staining of MLV is nonspecific given that the antibody targets GFP, which is absent in this virus.
Support Protocol: DETERMINATION OF THE OPTIMAL ANTIBODY CONCENTRATION FOR VIRUS STAINING
This protocol outlines the experimental setup for determination of the optimal antibody concentration for single-color virus staining (Basic Protocol 4). Determining the optimal staining antibody concentration using a titration is crucial to resolve dimly stained virus particles from unstained populations and background noise. An antibody titration is especially important in FVM (Basic Protocol 3), as high antibody concentrations can lead to increased background fluorescence and antibody aggregates that can be misinterpreted as positively stained virions.
Materials
- See Basic Protocols 2 and 4.
1.Determine virus particle concentration in the virus-containing supernatant as described in Basic Protocol 2, step 10.
2.Centrifuge tube of anti-GFP PE monoclonal antibody for 10 min at 17,000 × g , 4°C, in a refrigerated tabletop centrifuge.
3.Perform a five-fold titration of the fluorophore-conjugated antibody in 100-nm-filtered DPBS.
4.Mix equal volumes of supernatant containing virus that expresses target protein of interest with supernatant containing control virus that does not express the target protein.
5.Add 50 µl virus-containing supernatant from step 4 to 50 µl antibody mixture from step 3.
6.Perform steps 5 to 15 of Basic Protocol 4.
7.Gate on positively stained and unstained populations to obtain the MFI.
8.Determine highest separation between the positively and negatively labeled populations (stain index).
9.Once a five-fold titration has been evaluated, repeat steps 4 to 8 with a more narrow incremental titration in the range of the optimal concentration determined in step 8.
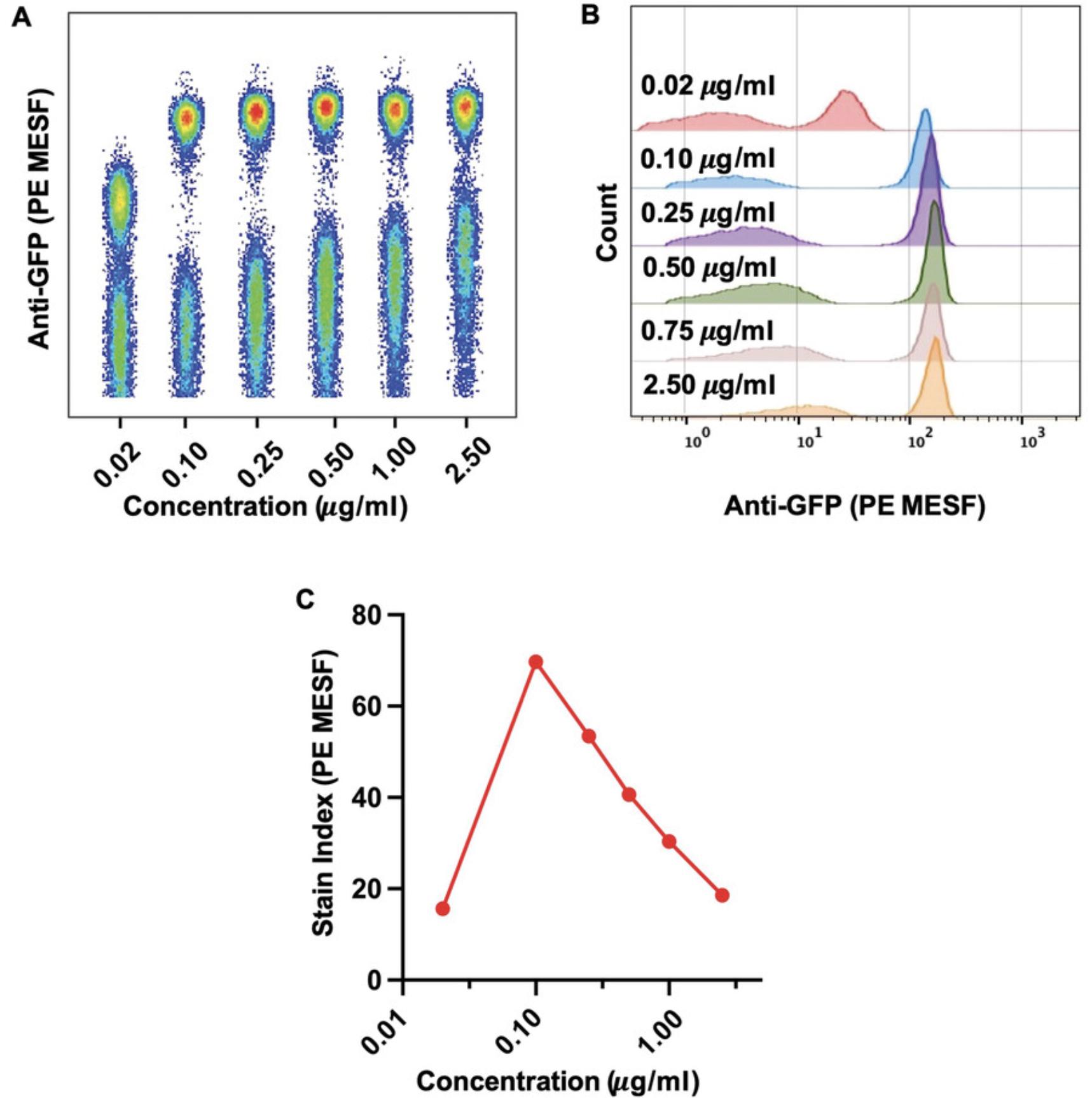
Basic Protocol 5: GAIN CONFIGURATION OPTIMIZATION
To further increase the resolution of the dimly labeled virus population from background noise (Basic Protocol 3), the gain of the parameter being evaluated can be optimized directly on the instrument during acquisition. Similar to the adjustment of acquisition settings in classical flow cytometry for cell analysis, the gain can be optimized to increase the signal and the separation between the positively labeled population and background noise. As shown in Figure 7, we gradually increased the PE gain while keeping the threshold constant and evaluated where the stained viral population or reference beads fell in relation to the gain adjustments. By applying the formula for the calculation of stain index, we determined the optimal separation between our positive and negative populations (Fig. 7B). Furthermore, by acquiring BD Quantibrite PE beads at the same gain setting, we were able to evaluate where the dimmest and brightest bead populations fell in relation to the gain adjustments. This allowed us to evaluate the dynamic range of detection with respect to our labeled viral population of interest (Fig. 7D). Although adjustments in PE gain increase the separation between background noise and the bead population, these can push the brightest bead population off scale and consequently affect the detection range in the PE channel. Once the gain is optimized, the fluorescence signal of the labeled virus can be compared to that of the reference bead population. In our case, this signal is closest to that of the dimmest bead population, as illustrated in Figure 7D. Once voltage has been optimized during the initial setup, reproducibility can be checked using the reference beads prior to each experiment to maintain optimal resolution.
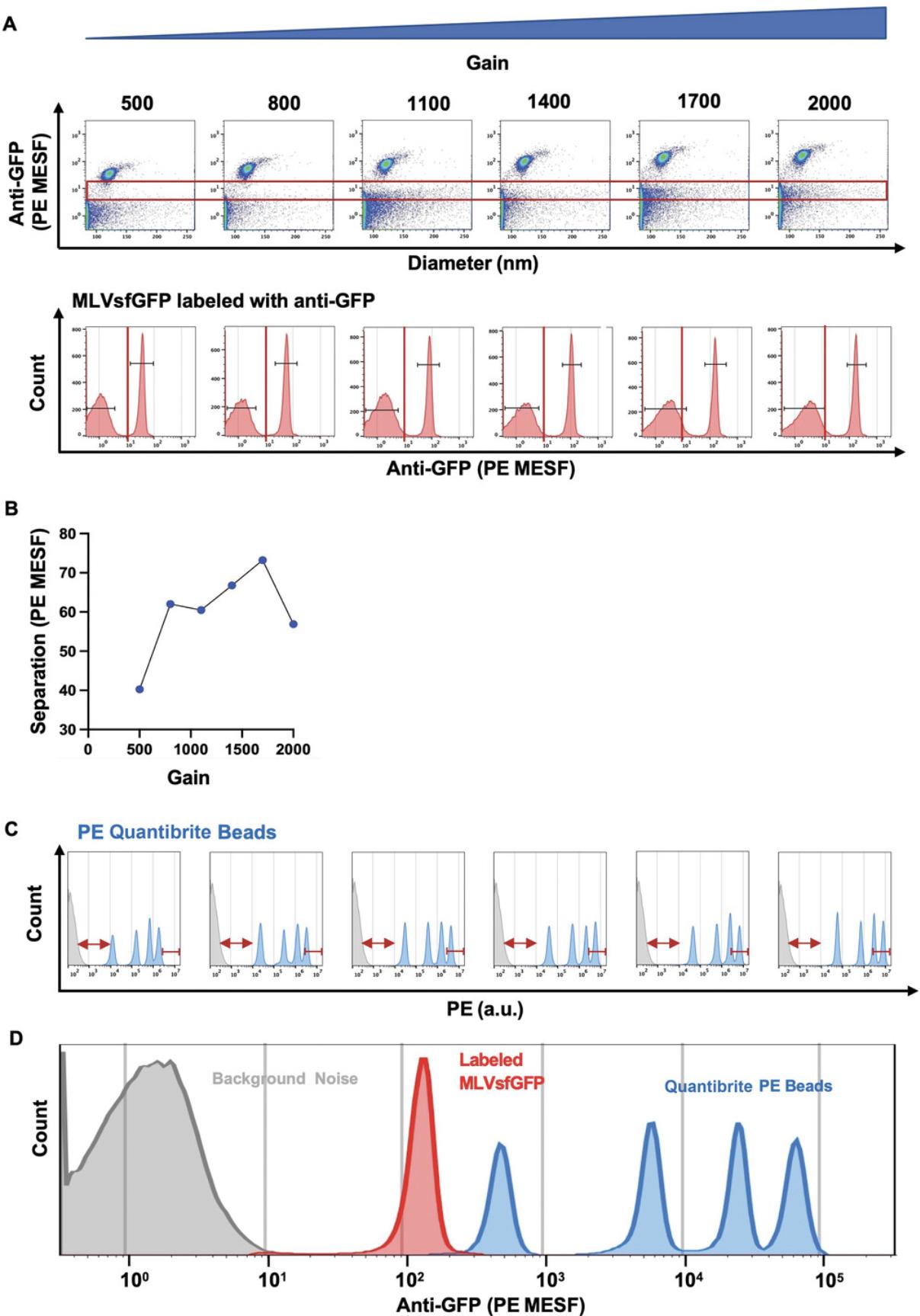
Additional Materials (also see Basic Protocols 2 and 4)
- Buffer control (100-nm-filtered DPBS)
- Stained viral sample of interest
1.Perform steps 1 to 6 of Basic Protocol 2.
2.Run buffer control (100-nm-filtered DBPS) on flow cytometer.
3.While acquiring the buffer control, adjust instrument's gain configuration in order to increase or decrease the signal value.
4.Systematically increase gain configuration.
5.Acquire buffer control (100-nm-filtered DBPS), stained viral sample of interest, and reference beads (BD Quantibrite PE beads) at each gain increment.
6.Gate on positively stained MLVsfGFP population and internal negative population (background noise or MLV in the case of a pre-mixed virus population, as used for Basic Protocol 4, step 4) to obtain the MFI for each.
7.Determine highest separation between the positive and negative populations using the formula for stain index determination (see Support Protocol, step 8).
REAGENTS AND SOLUTIONS
Complete DMEM
- Dulbecco's modified Eagle's medium (DMEM) with 4.5 g/L glucose, with L-glutamine, sodium pyruvate, and phenol red (Wisent Bioproducts, cat. no. 319-005-CL)
- 10% (v/v) FBS (Thermo Fisher Scientific, cat. no. 12483020)
- 1% (v/v) penicillin-streptomycin (GE Healthcare, cat. no. SV30010)
- Store ≤2 months at 4°C
EV-depleted FBS
Ultracentrifuge FBS (Thermo Fisher Scientific, cat. no. 12483020) for 12 hr at 73,000 × g and collect supernatant carefully to avoid disruption of the EV pellet located on the bottom of the ultracentrifuge tube. Store ≤6 months at –20°C.
Phenol red–free complete DMEM
- DMEM with 4.5 g/L glucose, with L-glutamine, sodium pyruvate, and no phenol red (Wisent Bioproducts, cat. no. 319-051-CL)
- 10% (v/v) EV-depleted FBS (see recipe)
- 1% (v/v) penicillin-streptomycin (GE Healthcare, cat. no. SV30010)
- Store ≤2 months at 4°C
Serum-free DMEM
- DMEM with 4.5 g/L glucose, with L-glutamine, sodium pyruvate, and phenol red (Wisent Bioproducts, cat. no. 319-005-CL)
- 1% (v/v) penicillin-streptomycin (GE Healthcare, cat. no. SV30010)
- Store ≤2 months at 4°C
COMMENTARY
Background Information
Here, we described detailed FVM methodology for staining surface proteins on the viral envelope. We used Env-sfGFP as an example, but the procedure would be similar for any desired target, such as tetraspanins CD9, CD63, and CD81 (Maltseva & Langlois, 2021). These protocols highlight a comprehensive experimental design overview and important considerations and practical aspects for FVM analysis and reproducible data reporting as a result of calibration with reference beads. Given the evolution of the field of small-particle flow cytometry and ongoing developments in instrument optics and reference particles, the protocols’ workflow provides a recommended structure for the experimental design that will evolve concurrently to reflect technological advancements. The technique was initially developed by Hercher et al., who were the first to design a custom cytometer that enabled the detection and analysis of bacteriophages by their light scattering properties; direct analysis, quantification, and characterization of viruses followed suit (Hercher, Mueller, & Shapiro, 1979). The first viral staining targeted the genomes of larger DNA viruses (Brussaard, Marie, & Bratbak, 2000; Chen, Lu, Binder, Liu, & Hodson, 2001). Although initially utilized for the purpose of enumerating viruses, flow cytometry principles were later applied for viral characterization and were instrumental in highlighting the extent of viral heterogeneity within a viral population. This was first illustrated in a study by Arakelyan et al., where differential incorporation of host-derived proteins on HIV-1 virions was observed by FVM, revealing heterogeneous antigen expression profiles in the viral envelope (Arakelyan et al., 2013). From a therapeutic standpoint, FVM can also be applied as an analytical tool for the evaluation of the quality of virus-like particle (VLP) vaccine preparations (Tang et al., 2016; Vlasak et al., 2016). Here, we show that virus in the supernatants of infected cells can be directly stained and identified by light scatter and fluorescence without the need for additional concentration or manipulations. Importantly, as highlighted by Renner et al., FVM enables detection, enumeration, and characterization of intact viral particles (Renner et al., 2020). As such, one of the advantages of FVM over other high-throughput techniques is the tailored detection of particles whose signal exceeds the designated light scatter threshold, discerning intact virions from ruptured and irregular virus particles and free protein, which may skew the measurements. However, the many overlapping characteristics between EVs and viruses (e.g., egress pathways, size, biophysical properties) make them considerably difficult to discriminate from one another (Nolte-'t Hoen, Cremer, Gallo, & Margolis, 2016). As such, EV contamination is an important confounding factor in most viral analyses. Furthermore, EVs have also been shown to incorporate genomic as well as viral components when released from infected cells (Nolte-'t Hoen et al., 2016). Currently, there is no consensus on a definitive way to distinguish EVs from non-infectious viral particles, termed VLPs. To address this, we and other groups have fluorescently tagged surface viral glycoproteins or nucleic acid content of viruses to improve viral resolution from EVs (Arakelyan et al., 2013; Brittain et al., 2019; Gaudin & Barteneva, 2015; Landowski, Dabundo, Liu, Nicola, & Aguilar, 2014; Lippe, 2018; Renner et al., 2020; Tang et al., 2017; Maltseva & Langlois, 2021).
Most commercial flow cytometers were designed to analyze cells or particles >500 nm in diameter. Given that viruses are considerably smaller in size than cells, these submicron particles often fall at the threshold of instrument detection, which is around 65 to 80 nm in diameter for our instrument (Brittain et al., 2019). As recommended by the MIFlowCyt-EV framework, data reporting in standard units by way of instrument calibration using reference beads is critical for reproducible and accurate measurements. Despite the current paucity of commercial reference particles with fluorescence intensities biologically comparable to those of viruses or EVs, brighter reference beads remain useful tools for fluorescence calibration and extrapolation of target molecule counts (Tang et al., 2019). Thus, care should be taken during the staining optimization stage and cytometer setup to evaluate the dynamic range between the positively stained viral populations and the background fluorescence of a sample, as weakly expressed proteins could be masked by high levels of background noise. Pre-analytical variables such as virus production, collection, storage, and manipulation should also be carefully considered as variables affecting experimental reproducibility. Consequently, all aspects of the experimental design should be carefully monitored given that FVM measurements are obtained near the limit of detection, where small variations in labeling between experiments can lead to confounding results or inaccurate quantification of epitope abundance. The protocols presented here provide an overview of the instrument setup; methods for virus production; and the staining, detection, and quantification of particle concentration and size and surface antigens on the viral envelope. These protocols should be modified to reflect the goals of the experiment, the flow cytometer used, and the type of virus studied.
Critical Parameters
Cellular expression of viral and host-derived antigens of interest should be phenotypically characterized prior to their characterization on the surface of viruses. Due to the fundamental process of viral egress, proteins incorporated into cellular membranes will form the viral envelope of nascent viruses released from the cell. Thus, the presence of a protein of interest and the degree to which it is expressed should first be confirmed on the viral producer cells prior to its characterization on the surface of viruses. If the abundance is very low on the cell, it may be undetectable on the virus.
Antibody selection (Basic Protocol 4 and Support Protocol) will depend on the research question. In any case, 1) the density of proteins analyzed; 2) the brightness of the antibody-fluorophore conjugate; 3) spectral overlap with other fluorophores; 4) steric hindrance between the antibody and epitopes, which can prevent proper labeling of all available antigens; and 5) the fluorophore-to-protein ratio should be evaluated, among other factors. In our work, a PE-conjugated fluorophore was used; due its large size, the fluorophore-to-antibody and the antibody-to-epitope ratios are both estimated to be 1:1 (Davis et al., 1998). This ratio should be evaluated in order to accurately estimate epitope abundance.
Selection of the trigger parameter (Basic Protocol 2) is dependent on the aim of the experiment and the type of flow cytometer used. As discussed earlier, either light scatter or fluorescence can be chosen as the trigger parameter. The choice reflects whether the goal of a study is to characterize a heterogeneous viral population based on virion size, RI, and physical features or to enumerate fluorescently labeled virions and evaluate surface epitope abundance.
Instrument calibration and careful setting optimizations (Basic Protocols 2 to 5) are critical to efficiently resolve particles of interest and report data in a standardized manner. In the case of homogenous or non-parametric viral populations, data can be reported using percentiles (i.e., 25th, 50th, 75th, and 90th) in order to provide an accurate representation of protein incorporation distribution on individual viruses within a viral population. Positively labeled events can be identified based on detection above a certain gate or threshold, such as two SDs or the 99th percentile above the negative population (Welsh et al., 2020). When reporting measured virus concentrations, light scattering, or fluorescence parameters, the range and limit of detection of the cytometer for each parameter should be included as an appendix to ensure the transparency and reproducibility of the data.
Troubleshooting
Detection of false positive events in buffer and antibody controls
It is critical to evaluate background fluorescence levels in order to assess whether antibody staining (Basic Protocol 4) results in unbound antibody aggregates that are misconstrued as small particles of interest. Thus, the optimal staining concentration (Support Protocol) should be carefully evaluated, as wash steps used for removing unbound antibody in classical cell-based cytometry are primarily omitted in FVM. Furthermore, the optimal signal-to-noise ratio should be identified to ensure maximal separation between positively and negatively stained populations while ensuring minimal levels of nonspecific binding to facilitate resolution of dim signals, as illustrated in Figure 5B.
Detection of false positive events in the supernatant and antibody control
The possible presence and staining of EVs should be carefully evaluated due their concomitant release with viruses from infected cells. As such, supernatant collected from uninfected cells is a critical control for identifying confounding effects related to bystander EV labeling. This control should be compared to a buffer and reagent control and a stained virus control sample to assess differences, if any, in background events and fluorescence signal. Treatment with a detergent (such a Triton X-100) will lyse lipid membrane vesicles, whereas other complexes (e.g., protein complexes, antibody aggregates, buffer salt crystals) will be unaffected. This is therefore an easy way of ensuring that the particles of interest do indeed contain a lipid membrane.
Understanding Results
For accurate, reproducible, and standardized data reporting, it is crucial to determine the instrument's sensitivity for light scatter and fluorescence measurements and to calibrate these values using standardized reference materials. Figure 2 displays the light scattering and fluorescence features of polystyrene NIST-traceable size reference beads and BD Quantibrite PE fluorescence used for calibration. It is essential to have a high correlation between acquired and theoretical values in order to convert fluorescence intensity values into molecule equivalents and report fluorescence measurements in standard units.
Data analysis can be done in most software, such as R, FlowJo, or Kaluza, that can process FSC files and generate visual graphs such as dot plots, histograms, and density plots, among others. To ensure single-particle acquisition, the measured viral particle count should linearly reflect the dilution factor while light scatter intensity or fluorescence signal should be maintained constant, as shown in Basic Protocol 2.
Key experimental controls are required for robust virus detection and to set gates as shown in Basic Protocol 3. Once the gates have been defined, light scatter properties of the viruses can be used to derive viral particle sizes, the concentration of intact viral particles in a sample, and the relative abundance of a target antigen on the surface of the virus, as explained in Basic Protocols 3 and 4. Different metrics of reporting other than MFI can be used in cases of non-parametric distribution of protein expression or particle size within the viral population (such as a percentile measurement), as shown in Tables 1 and 2.
Time Considerations
See Table 3 for time considerations associated with the protocols.
Protocol | Time | Details |
---|---|---|
Basic Protocol 1: Virus production | ||
1. Production of virus by transfection | 4 days |
Day 1: 15 min Day 2: 45 min Day 4: 5 min |
2. Production of virus from an infected cell population | 8 days |
Day 1: 15 min Day 2: 45 min Day 4: 5 min Day 5: 15 min Day 6: 5 min Day 8: 5 min |
3. Production of virus by chronically infected cell clones | 30 days |
Day 1: 15 min Day 2: 45 min Day 4: 5 min Day 5: 15 min Day 6: 5 min Day 8: 120 min Day 12: 10 min Day 15: 10 min Day 18: 10 min Day 21: 10 min Day 24: 10 min Day 27: 80 min Day 28: 10 min Day 30: 5 min |
Basic Protocol 2: Instrument setup, standardization, and quality control for fluorescence quantification | 1 day | Day 1: 100 min |
Basic Protocol 3: Flow virometry analysis | 1 day | Day 1: 30 min |
Basic Protocol 4: Viral surface antigen staining and fluorescence quantification | 1 day | Day 1: 210 min |
Support Protocol for Basic Protocol 4: Determination of the optimal antibody concentration for virus staining |
2 days |
Day 1: 210 min Day 2: 210 min |
Basic Protocol 5: Gain configuration optimization | 1 day | Day 1: 40 min |
Acknowledgments
The authors would like to thank the University of Ottawa Flow Cytometry Core Facility for assistance with some of the flow cytometry analyses. M.M. holds a Queen Elizabeth II Graduate Scholarship in Science and Technology (QEII-GSST). M.-A.L. holds a Canada Research Chair in Molecular Virology and Intrinsic Immunity. This study was supported by a Discovery grant to M.-A.L. by the Natural Sciences and Engineering Research Council (NSERC) of Canada.
Author Contributions
Mariam Maltseva : Performed the experiments; carried out the data analysis; drafted and edited the manuscript; Marc-André Langlois : Conceptualization; funding acquisition; methodology; supervision; writing-review and editing.
Conflict of Interest
The authors declare no conflict of interest.
Open Research
Data Availability Statement
Data files are available from the authors on request.
Supporting Information
Filename | Description |
---|---|
cpz1368-sup-0001-SuppMat.xlsx18.7 KB | Supporting Information, File 1: FCMPASS software output report based on the MIFlowCyt-EV report guidelines and summary of the instrument's parameters, the trigger threshold of the particles analyzed, light scatter, and fluorescence calibration. All samples were acquired at identical acquisition settings as the reference beads and were calibrated using FCMPASS software version 3.1. |
Please note: The publisher is not responsible for the content or functionality of any supporting information supplied by the authors. Any queries (other than missing content) should be directed to the corresponding author for the article.
Literature Cited
- Arakelyan, A., Fitzgerald, W., Margolis, L., & Grivel, J. C. (2013). Nanoparticle-based flow virometry for the analysis of individual virions. Journal of Clinical Investigation , 123(9), 3716–3727. doi: 10.1172/JCI67042.
- Brittain, G. C. t., Chen, Y. Q., Martinez, E., Tang, V. A., Renner, T. M., Langlois, M. A., & Gulnik, S. (2019). A novel semiconductor-based flow cytometer with enhanced light-scatter sensitivity for the analysis of biological nanoparticles. Science Reports , 9(1), 16039. doi: 10.1038/s41598-019-52366-4.
- Brussaard, C. P., Marie, D., & Bratbak, G. (2000). Flow cytometric detection of viruses. Journal of Virological Methods , 85(1-2), 175–182. doi: 10.1016/s0166-0934(99)00167-6.
- Cantin, R., Methot, S., & Tremblay, M. J. (2005). Plunder and stowaways: Incorporation of cellular proteins by enveloped viruses. Journal of Virology , 79(11), 6577–6587. doi: 10.1128/JVI.79.11.6577-6587.2005.
- Chen, F., Lu, J. R., Binder, B. J., Liu, Y. C., & Hodson, R. E. (2001). Application of digital image analysis and flow cytometry to enumerate marine viruses stained with SYBR gold. Applied and Environmental Microbiology , 67(2), 539–545. doi: 10.1128/AEM.67.2.539-545.2001.
- Cossarizza, A., Chang, H. D., Radbruch, A., Acs, A., Adam, D., Adam-Klages, S., … Zychlinsky, A. (2019). Guidelines for the use of flow cytometry and cell sorting in immunological studies (second edition). European Journal of Immunology , 49(10), 1457–1973. doi: 10.1002/eji.201970107.
- Davis, K. A., Abrams, B., Iyer, S. B., Hoffman, R. A., & Bishop, J. E. (1998). Determination of CD4 antigen density on cells: Role of antibody valency, avidity, clones, and conjugation. Cytometry , 33(2), 197–205. doi: 10.1002/(sici)1097-0320(19981001)33:2<197::Aid-cyto14>3.0.co;2-p.
- Gaudin, R., & Barteneva, N. S. (2015). Sorting of small infectious virus particles by flow virometry reveals distinct infectivity profiles. Nature Communication , 6, 6022. doi: 10.1038/ncomms7022.
- Groot Kormelink, T., Arkesteijn, G. J., Nauwelaers, F. A., van den Engh, G., Nolte-'t Hoen, E. N., & Wauben, M. H. (2016). Prerequisites for the analysis and sorting of extracellular vesicle subpopulations by high-resolution flow cytometry. Cytometry Part A: The Journal of the International Society for Analytical Cytology , 89(2), 135–147. doi: 10.1002/cyto.a.22644.
- Hercher, M., Mueller, W., & Shapiro, H. M. (1979). Detection and discrimination of individual viruses by flow cytometry. Journal of Histochemistry and Cytochemistry , 27(1), 350–352. doi: 10.1177/27.1.374599.
- Kornilov, R., Puhka, M., Mannerstrom, B., Hiidenmaa, H., Peltoniemi, H., Siljander, P., … Kaur, S. (2018). Efficient ultrafiltration-based protocol to deplete extracellular vesicles from fetal bovine serum. Journal of Extracellular Vesicles , 7(1), 1422674. doi: 10.1080/20013078.2017.1422674.
- Landowski, M., Dabundo, J., Liu, Q., Nicola, A. V., & Aguilar, H. C. (2014). Nipah virion entry kinetics, composition, and conformational changes determined by enzymatic virus-like particles and new flow virometry tools. Journal of Virology , 88(24), 14197–14206. doi: 10.1128/JVI.01632-14.
- Lippe, R. (2018). Flow virometry: A powerful tool to functionally characterize viruses. Journal of Virology , 92(3), e01765-17. doi: 10.1128/JVI.01765-17.
- Ma, L., Zhu, S., Tian, Y., Zhang, W., Wang, S., Chen, C., … Yan, X. (2016). Label-free analysis of single viruses with a resolution comparable to that of electron microscopy and the throughput of flow cytometry. Angewandte Chemie (International ed in English) , 55(35), 10239–10243. doi: 10.1002/anie.201603007.
- Maltseva, M., & Langlois, M.-A. (2021). Influence of GlycoGag on the incorporation of host membrane proteins into the envelope of the Moloney murine leukemia virus. Frontiers in Virology , 1, 747253. doi: 10.3389/fviro.2021.747253.
- Nolte-'t Hoen, E., Cremer, T., Gallo, R. C., & Margolis, L. B. (2016). Extracellular vesicles and viruses: Are they close relatives? Proceedings of the National Academy of Sciences of the United States of America , 113(33), 9155–9161. doi: 10.1073/pnas.1605146113.
- Renner, T. M., Tang, V. A., Burger, D., & Langlois, M. A. (2020). Intact viral particle counts measured by flow virometry provide insight into the infectivity and genome packaging efficiency of Moloney murine leukemia virus. Journal of Virology , 94(2), e01600-19. doi: 10.1128/JVI.01600-19.
- Tang, V. A., Fritzsche, A. K., Renner, T. M., Burger, D., Lannigan, J. A., Brittain, G. C., … Langlois, M.-A. (2019). Engineered retroviruses as fluorescent biological reference particles for nanoscale flow cytometry. bioRxiv , 614461. doi: 10.1101/614461.
- Tang, V. A., Renner, T. M., Fritzsche, A. K., Burger, D., & Langlois, M. A. (2017). Single-particle discrimination of retroviruses from extracellular vesicles by nanoscale flow cytometry. Science Reports , 7(1), 17769. doi: 10.1038/s41598-017-18227-8.
- Tang, V. A., Renner, T. M., Varette, O., Le Boeuf, F., Wang, J., Diallo, J. S., … Langlois, M. A. (2016). Single-particle characterization of oncolytic vaccinia virus by flow virometry. Vaccine , 34(42), 5082–5089. doi: 10.1016/j.vaccine.2016.08.074.
- Thery, C., Witwer, K. W., Aikawa, E., Alcaraz, M. J., Anderson, J. D., Andriantsitohaina, R., … Zuba-Surma, E. K. (2018). Minimal information for studies of extracellular vesicles 2018 (MISEV2018): A position statement of the International Society for Extracellular Vesicles and update of the MISEV2014 guidelines. Journal of Extracellular Vesicles , 7(1), 1535750. doi: 10.1080/20013078.2018.1535750.
- Tian, Y., Gong, M., Hu, Y., Liu, H., Zhang, W., Zhang, M., … Yan, X. (2020). Quality and efficiency assessment of six extracellular vesicle isolation methods by nano-flow cytometry. Journal of Extracellular Vesicles , 9(1), 1697028. doi: 10.1080/20013078.2019.1697028.
- van der Pol, E., van Gemert, M. J., Sturk, A., Nieuwland, R., & van Leeuwen, T. G. (2012). Single vs. swarm detection of microparticles and exosomes by flow cytometry. Journal of Thrombosis and Haemostasis , 10(5), 919–930. doi: 10.1111/j.1538-7836.2012.04683.x.
- Vlasak, J., Hoang, V. M., Christanti, S., Peluso, R., Li, F., & Culp, T. D. (2016). Use of flow cytometry for characterization of human cytomegalovirus vaccine particles. Vaccine , 34(20), 2321–2328. doi: 10.1016/j.vaccine.2016.03.067.
- Welsh, J. A., & Jones, J. C. (2020). Small particle fluorescence and light scatter calibration using FCMPASS software. Current Protocols in Cytometry , 94(1), e79. doi: 10.1002/cpcy.79.
- Welsh, J. A., Van Der Pol, E., Arkesteijn, G. J. A., Bremer, M., Brisson, A., Coumans, F., … Jones, J. C. (2020). MIFlowCyt-EV: A framework for standardized reporting of extracellular vesicle flow cytometry experiments. Journal of Extracellular Vesicles , 9(1), 1713526. doi: 10.1080/20013078.2020.1713526.
Citing Literature
Number of times cited according to CrossRef: 4
- Jonathan Burnie, Claire Fernandes, Ayushi Patel, Arvin Tejnarine Persaud, Deepa Chaphekar, Danlan Wei, Timothy Kit Hin Lee, Vera A. Tang, Claudia Cicala, James Arthos, Christina Guzzo, Applying Flow Virometry to Study the HIV Envelope Glycoprotein and Differences Across HIV Model Systems, Viruses, 10.3390/v16060935, 16 , 6, (935), (2024).
- Pilar García-Peñarrubia, A novel hypothesis on mechanisms and potential role of host cell membrane proteins incorporated into the viral envelope in alloreactivity, Medical Hypotheses, 10.1016/j.mehy.2024.111412, 189 , (111412), (2024).
- Jonathan Burnie, Claire Fernandes, Deepa Chaphekar, Danlan Wei, Shubeen Ahmed, Arvin Tejnarine Persaud, Nawrah Khader, Claudia Cicala, James Arthos, Vera A. Tang, Christina Guzzo, Identification of CD38, CD97, and CD278 on the HIV surface using a novel flow virometry screening assay, Scientific Reports, 10.1038/s41598-023-50365-0, 13 , 1, (2023).
- Raquel Marcos-Fernández, Borja Sánchez, Lorena Ruiz, Abelardo Margolles, Convergence of flow cytometry and bacteriology. Current and future applications: a focus on food and clinical microbiology, Critical Reviews in Microbiology, 10.1080/1040841X.2022.2086035, 49 , 5, (556-577), (2022).