A Rapid and Universal Workflow for Label-Free-Quantitation-Based Proteomic and Phosphoproteomic Studies in Cereals
Mingjie He, Mingjie He, Jiahui Wang, Jiahui Wang, Sandra Herold, Sandra Herold, Lin Xi, Lin Xi, Waltraud X. Schulze, Waltraud X. Schulze
Abstract
Proteomics and phosphoproteomics are robust tools to analyze dynamics of post-transcriptional processes during growth and development. A variety of experimental methods and workflows have been published, but most of them were developed for model plants and have not been adapted to high-throughput platforms. Here, we describe an experimental workflow for proteome and phosphoproteome studies tailored to cereal crop tissues. The workflow consists of two parallel parts that are suitable for analyzing protein/phosphoprotein from total proteins and the microsomal membrane fraction. We present phosphoproteomic data regarding quantification coverage and analytical reproducibility for example preparations from maize root and shoot, wheat leaf, and a microsomal protein preparation from maize leaf. To enable users to adjust for tissue specific requirements, we provide two different methods of protein clean-up: traditional ethanol precipitation (PC) and a recently developed technology termed single-pot, solid-phase-enhanced sample preparation (SP3). Both the PC and SP3 methods are effective in the removal of unwanted substances in total protein crude extracts. In addition, two different methods of phosphopeptide enrichment are presented: a TiO2-based method and Fe(III)-NTA cartridges on a robotized platform. Although the overall number of phosphopeptides is stable across protein clean-up and phosphopeptide enrichment methods, there are differences in the preferred phosphopeptides in each enrichment method. The preferred protocol depends on laboratory capabilities and research objective. © 2022 The Authors. Current Protocols published by Wiley Periodicals LLC.
Basic Protocol 1 : Total protein crude extraction
Basic Protocol 2 : Total protein clean-up with ethanol precipitation
Alternate Protocol 1 : Total protein clean-up with SP3 method
Basic Protocol 3 : Microsomal fraction protein extraction
Basic Protocol 4 : Protein concentration determination by Bradford assay
Basic Protocol 5 : In-solution digestion with trypsin
Basic Protocol 6 : Phosphopeptide enrichment with TiO2
Alternate Protocol 2 : Phosphopeptide enrichment with Fe(III)-NTA cartridges
Basic Protocol 7 : Peptide desalting with C18 material
Basic Protocol 8 : LC-MS/MS analysis of (phospho)peptides and spectrum matching
INTRODUCTION
Maize (Zea mays), wheat (Triticum aestivum), and rice (Oryza sativa) are among the most important cereals globally (Erenstein, Chamberlin, & Sonder, 2021). Productivity and sustainability are constantly challenged by various abiotic and biotic stresses (Grote, Fasse, Nguyen, & Erenstein, 2021), and the variability of the environment is becoming increasingly challenging in the context of climate change. Proteins are known to perceive stress stimuli as sensor proteins, are involved in signaling transduction, and participate in the formation of the plant phenotype by regulating metabolism and development (Lamers, Van Der Meer, & Testerink, 2020). Protein phosphorylation is the most widespread and reversible modification in plants (Millar et al., 2019). It can be stimulated by different stresses, and it modulates protein activity, integrates information from different signaling pathways, and conducts signal transduction at the cellular level, resulting in plant physiology changes (Millar et al., 2019; Schwessinger et al., 2011).
Nowadays, omics platforms provide an opportunity to quantify the stress responses of proteins and their phosphorylation dynamics on a large scale, allowing one to generate a systemic view of stress regulation in plants with new discoveries in a highly efficient manner. For example, proteome profiling revealed common and specific biological responses to different mineral nutrient deficiencies in maize root hairs (Li, Phillip, Neuhäuser, Schulze, & Ludewig, 2015) and suggested important processes involved in drought-tolerant responses and their memory effects (Schulze, Altenbuchinger, He, Kränzlein, & Zörb, 2021). Integration of the transcriptome and proteome created a complementary profile of molecular responses to phosphate deficiency in maize (He et al., 2021; Nie et al., 2021). Phosphoproteomic studies in maize discovered several proteins with differential changes in phosphorylation state that may relate to salt-stress tolerance (Zhao, Bai, Jiang, & Li, 2019) and provided insights into early phosphorylation events triggered by water status (Bonhomme, Valot, Tardieu, & Zivy, 2012). Phosphoproteomic studies also provided insights into phosphorylation dynamics induced by temperature stress in maize and wheat (Hu et al., 2015; Vu et al., 2018) and identified phosphoproteins involved in plant-pathogen iterations (Ding et al., 2016).
Analytical platforms for the proteome and phosphoproteome have been developed and greatly improved over the last decades. The dominant platforms shifted from a 2D gel–based system to liquid chromatography (LC) with tandem mass spectrometry (LC-MS/MS) (Cao et al., 2019; Luís, Alexandre, Oliveira, & Abreu, 2016). The basic workflow of such a system involves protein extraction, protein digestion, phosphopeptide enrichment, and analysis on large-scale mass spectrometry (MS) platforms. Sample processing in each step can be quite diverse depending on the plant materials, the biochemical properties of the materials, and laboratory capabilities. However, specific challenges arise when processing tissues from crop plants: the tissues are commonly rich in fiber and contain a high number of secondary metabolite compounds that significantly interfere with protein extraction and phosphopeptide enrichment (Wu, Gong, & Wang, 2014). After protein digestion, the phosphopeptides can be enriched by charged metal ions (Fe3+, Ga3+, Zr3+) or by complexing with titanium dioxide (TiO2) (Millar et al., 2019). Nowadays, the enrichment procedure has become simpler by using commercial beads or cartridges that are covered or filled with the phosphopeptide-selective materials (Beltran & Cutillas, 2012). Robotized automation of the sample handling process permits multiple-sample processing at high throughput. Here, we compile a simple, rapid, and efficient experimental workflow that is universal for bottom-up proteomic and phosphoproteomic studies in plants, with a special focus on the properties of tissues from cereal crops. To meet the challenge of removing secondary metabolite compounds, we provide two alternative protein clean-up methods, including an ethanol (EtOH) precipitation (PC) method and the single-pot, solid-phase-enhanced sample preparation (SP3) method (Hughes et al., 2019). Because each phosphopeptide enrichment material has different affinities for phosphopeptide subsets, we offer two alternative phosphopeptide enrichment methods, one with a TiO2-based material and one using Fe(III)-NTA cartridges. We have successfully applied this workflow for proteome and phosphoproteome analyses, and the provided protocols also work well with low amounts of tissue input (He et al., 2021; Li et al., 2015; Schulze et al., 2021).
Membranes, especially proteins located in the plasma membrane (PM), are involved in stress perception and play a key role in signal transduction to regulate different biological processes and cellular events. However, these proteins are low in abundance and difficult to detect in total protein extracts. Therefore, microsomal fraction (MF) protein isolation is included to offer a membrane-focused phosphoproteome. Therefore, we adapted a highly efficient single-step method for enrichment of low-abundance phosphopeptides from plant membranes (Wu et al., 2017) to the tissue properties of cereals.
STRATEGIC PLANNING
A typical experimental workflow for phosphoproteome analysis consists of tissue disruption, protein extraction, protein clean-up, trypsin digestion, phosphopeptide enrichment, desalination, and LC-MS/MS runs (Fig. 1). When omitting the phosphopeptide enrichment step, the protocol is a typical workflow for whole proteome analysis, which is usually followed by protein abundance profiling studies. Phosphorylated peptides usually make up a small percentage of all digested peptides in whole proteomes. Therefore, for research questions focusing on phosphorylation events, phosphopeptide enrichment is required to increase the depth and reproducibility of phosphopeptide quantification. By including phosphopeptide enrichment, the focus is put on the proteome subset that is potentially regulated by external treatment and/or active in signaling processes (Millar et al., 2019).
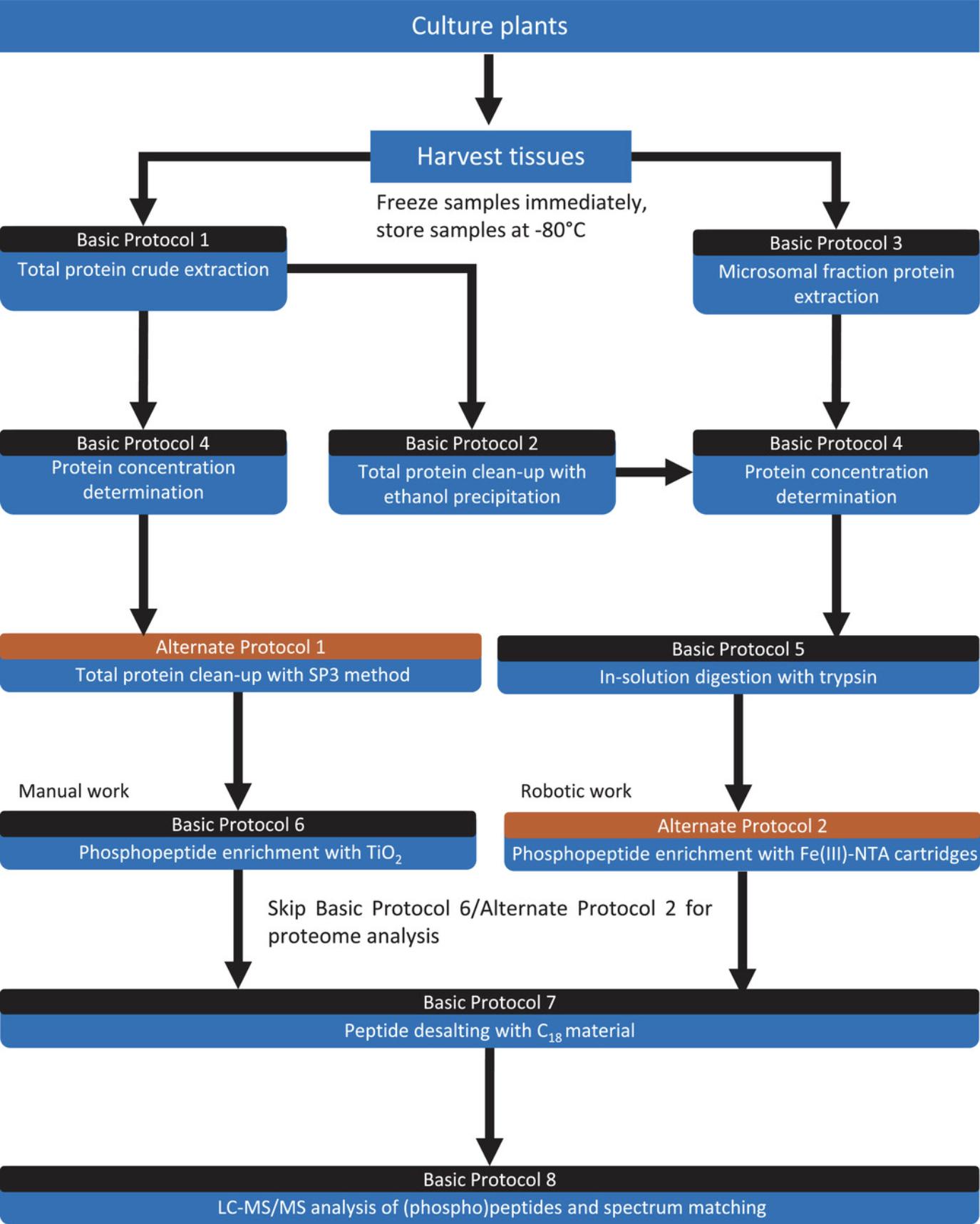
Basic Protocol 1 describes total protein extraction, and Basic Protocol 2 is designed for subsequent clean-up of the protein from Basic Protocol 1 by precipitation (PC method). Alternate Protocol 1 describes protein clean-up using the SP3 method. Basic Protocol 3 describes MF protein isolation, which is independent from Basic Protocols 1 and 2 and suitable for research questions focusing on membrane-located proteins.
Basic Protocol 4 is necessary for label-free-quantification MS analysis; we provide a common protocol using Bradford reagent to determine protein concentration. Basic Protocol 5 provides a protocol for in-solution trypsin digestion. Basic Protocol 6 describes phosphopeptide enrichment with a TiO2-based enrichment method to be performed manually, and in Alternate Protocol 2, we provide an enrichment method using Fe(III)-NTA cartridges with robotics manipulation. Thus, readers can select either method based on accessibility and depending on their research aims.
NOTE : All solutions are in ultrapure water (∼0.055 μS/cm; e.g., Sartorius) unless otherwise indicated.
Basic Protocol 1: TOTAL PROTEIN CRUDE EXTRACTION
This protocol describes total protein crude extraction and requires total protein extraction buffer, which contains the following. The compounds 6 M urea and 2 M thiourea are high-concentration chaotropic compounds that disrupt hydrogen bonds and hydrophobic interactions in proteins, thereby bringing insoluble proteins into solution in an unfolded form. Phenylmethanesulfonyl fluoride (PMSF) is a protease inhibitor that prevents protein degradation during sample preparation. DL-Dithiothreitol (DTT) reduces disulfide bonds to unfold protein complexes to improve protein solubility. Polyvinylpolypyrrolidone (PVPP) has a high affinity toward polyphenols and is used to remove them from crude extracts. For phosphoproteomics applications, it is recommended to add protease and phosphatase inhibitors to prevent phosphorylated proteins from undergoing dephosphorylation during sample preparation.
Materials
-
Liquid nitrogen
-
Frozen plant sample: maize root and shoot or wheat leaf (about 200 mg to 1 g; freshly harvested or frozen at −80°C)
-
Total protein extraction buffer (see recipe; make fresh)
-
Mortar and pestle of proper size
-
2-ml tubes (e.g., Eppendorf)
-
Vortex shaker (e.g., Scientific Industries Vortex-Genie 2 with microtube insert)
-
Refrigerated microcentrifuge (e.g., Eppendorf 5430R), 4°C
-
5-ml tubes (e.g., Eppendorf; optional)
1.Pre-cool a mortar and pestle of proper size with liquid nitrogen, place frozen plant sample into the cold mortar, add liquid nitrogen to cool down sample, and then grind tissue into fine powder with the cold pestle.
2.Pre-cool a 2-ml tube with liquid nitrogen and weigh 50 to 200 mg ground powder into pre-cooled tube.
3.Add 0.5 to 1 ml total protein extraction buffer and mix on a vortex shaker for 1 hr at 4°C.
4.Centrifuge mixture for 10 to 20 min at 21,000 × g , 4°C. Transfer supernatant to a fresh 2- or 5-ml tube.
Basic Protocol 2: TOTAL PROTEIN CLEAN-UP WITH ETHANOL PRECIPITATION
The total protein crude extracts from Basic Protocol 1 contain large amounts of interfering substances, such as phenolics, lipids, organic acids, carbohydrates, terpenes, and pigments that are necessary to remove. A high concentration of EtOH can reduce protein solubility, resulting in protein precipitation. Sodium acetate provides positively charged Na+ to neutralize negatively charged residues on the surface of proteins and acidify the solution environment to assist in reducing protein solubility.
Materials
-
EtOH, absolute (Sigma-Aldrich, cat. no. 34852-M)
-
Total protein extract supernatant (see Basic Protocol 1)
-
2.5 M sodium acetate, pH 5.0 (see recipe)
-
8 M UTU (see recipe; make fresh)
-
Microcentrifuge (e.g., Eppendorf 5430R)
-
Cleaning wipes (e.g., KIMTECH SCIENCE)
1.Add 5 volumes of EtOH to the total protein extract supernatant from Basic Protocol 1.
2.Add 100 to 300 µl (∼1/20 of the total volume) of 2.5 M NaOAc (pH 5.0). Mix gently by inverting several times and incubate at room temperature (24°C) for 3 hr.
3.Centrifuge 20 min at 21,000 × g.
4.Discard supernatant, carefully removing the residual liquid from the tube wall with a cleaning wipe.
5.Gently resuspend pellet with 100 to 300 µl of 8 M UTU as total protein.
6.Proceed to Basic Protocol 4 to determine protein concentration and make protein aliquots.
Alternate Protocol 1: TOTAL PROTEIN CLEAN-UP WITH SP3 METHOD
SP3 technology (Hughes et al., 2019) applies paramagnetic beads coated with carboxyl to bind proteins by hydrophilic interaction. Unbound substrates in the solution will be easily removed with a magnetic rack and pipet. This technique supports on-bead tryptic digestion, so clean-up of proteins and protein digestion will be conducted in the same tube. This protocol is a combined alternative to Basic Protocols 2 and 5.
Materials
-
SpeedBeads magnetic carboxylate-modified particles (GE Healthcare, cat. no. 45152105050250/65152105050250)
-
Ultrapure water (∼0.055 μS/cm; e.g., Sartorius)
-
Total protein extract supernatant (see Basic Protocol 1)
-
8 M UTU (see recipe; make fresh)
-
6.5 mM DTT (e.g., Sigma-Aldrich, cat. no. D0632)
-
27 mM iodoacetamide (IAA; e.g., Sigma-Aldrich, cat. no. I1149)
-
EtOH (e.g., Sigma-Aldrich, cat. no. 34852-M)
-
80% (v/v) EtOH (e.g., Sigma-Aldrich, cat. no. 34852-M)
-
100 mM ammonium bicarbonate (ABC; e.g., Sigma-Aldrich, cat. no. A6141; store ≤1 month in cool place)
-
Lys-C (e.g., Promega, cat. no. VA1170; optional)
-
0.4 μg/μl trypsin, modified sequencing grade (e.g., Promega, cat. no. V5113)
-
10% (v/v) trifluoroacetic acid (TFA; e.g., Thermo, cat. no. 85183)
-
1.5- and 2-ml tubes (e.g., Eppendorf)
-
Magnetic rack
-
Tube shaker (e.g., Thermo-Shaker, BioSan TS-100C), room temperature and 37°C
-
Microcentrifuge (e.g., Eppendorf 5430R)
-
Additional reagents and equipment for determining protein concentration and making protein aliquots (see Basic Protocol 4)
-
CAUTION : Prepare the TFA solution in a fume hood.
Ready-to-use bead preparation
1.Calculate required amount of SpeedBeads magnetic carboxylate-modified particles (abbreviated as “beads” below).
2.Resuspend beads well in the original bottle, immediately transfer required amount of beads (according to the calculation in step 1) to a new 2-ml tube, place tube on a magnetic rack for 2 min to make the beads settle to the bottom of the tube, and then discard supernatant using a pipet.
3.Remove tube from the magnetic rack, add 400 µl ultrapure water and gently mix beads and water by inversion, put tube back on the magnetic rack for 2 min, and then discard supernatant using a pipet.
4.Repeat step 3 two more times.
5.Resuspend beads with 40 µl ultrapure water per 2 mg beads.
Protein aliquots and pre-digestion
6.According to Basic Protocol 4, determine protein concentration and make protein aliquots (e.g., 200 µg protein per aliquot) of the total protein extract supernatant from Basic Protocol 1.
7.Bring up volume of each aliquot to 452 µl with 8 M UTU.
8.Add 4 µl of 6.5 mM DTT per 200 µg protein and incubate at room temperature for 30 min.
9.Add 4 µl of 27 mM IAA per 200 µg protein and incubate in the dark at room temperature for 20 min.
Protein clean-up with ready-to-use beads
10.Add 40 µl ready-to-use beads from step 5 for every 200 µg protein.
11.Add 500 µl EtOH to induce protein-bead binding.
12.Briefly shake to homogenize and incubate at room temperature (24°C) for 15 min with shaking at 1000 rpm.
13.Place tube on the magnetic rack for 2 min and then discard supernatant using a pipet.
14.Remove tube from the rack, add 1.5 ml of 80% EtOH, and mix by pipetting to wash beads.
15.Place tube in the magnetic rack for 2 min and then discard supernatant using a pipet.
16.Repeat steps 14 and 15 two more times.
17.Remove tube from the rack and add 200 µl of 100 mM ABC to tube.
Protein-on-beads digestion
18.Optional: Add 4 μl Lys-C per 200 μg protein, gently shake tube to mix the solution and beads, and incubate at room temperature for 3 hr.
19.Add 4 µl of 0.4 µg/µl trypsin per 200 µg protein, gently shake tube to mix the solution and beads, incubate at 37°C overnight with shaking at 1000 rpm.
20.Centrifuge tube for 1 min at 21,000 × g , at room temperature (24°C).
21.Place tube in the magnetic rack for 2 min.
22.Transfer supernatant to a fresh tube and add 20 µl of 10% TFA solution to acidify sample.
23.Continue to Basic Protocol 6 for phosphoproteome analysis or go directly to Basic Protocol 7 for proteome analysis.
Basic Protocol 3: MICROSOMAL FRACTION PROTEIN EXTRACTION
MF extraction is a common procedure in studies involving membrane proteins. Here, we provide an MF extraction protocol using a glass tissue homogenizer for disruption of cereal plant tissue based on previous work (Pertl-Obermeyer & Obermeyer, 2014; Wu et al., 2017).
Materials
-
Frozen plant sample: maize root and shoot or wheat leaf (about 200 mg to 1 g; freshly harvested or frozen at −80°C)
-
MF extraction buffer (see recipe; make fresh), 4°C
-
8 M UTU (see recipe; make fresh)
-
Aluminum foil
-
Hammer or pestle
-
10-ml glass Potter-Elvehjem homogenizer with Teflon pestle (e.g., 10 ml)
-
Miracloth (e.g., EMD Millipore, cat. no. 475855)
-
Glass funnel (e.g., VWR)
-
50-ml Falcon tubes
-
Refrigerated high-speed benchtop centrifuge (e.g., Sigma-Aldrich 3-30KS), 4°C
-
Ultracentrifuge tubes (30-ml round-bottom tubes, screw cap, for rotor 12158; Sigma-Aldrich 3-30KS)
-
Cleaning wipes (e.g., KIMTECH SCIENCE, cat. no. 05511)
1.Weigh 1 to 3 g frozen plant sample, wrap sample with aluminum foil, and smash wrapped sample into smaller pieces with a hammer or a pestle.
2.Transfer frozen plant material into a 10-ml glass Potter-Elvehjem homogenizer with a Teflon pestle and add 10 ml cold MF extraction buffer, and place homogenizer into an ice bath. Slowly push and pull pestle while twisting it over the sample to generate a homogenate as thoroughly as possible.
3.Place four layers of miracloth on a glass funnel, hold glass funnel atop a 50-ml Falcon tube on ice, and filter homogenate through the miracloth and into the tube.
4.Centrifuge filtered homogenate for 15 min at 7500 × g , 4°C, in a refrigerated high-speed benchtop centrifuge.
5.Transfer supernatant to an ultracentrifuge tube and centrifuge 80 min at 48,000 × g , 4°C.
6.Discard supernatant and carefully remove residual liquid from the tube wall with a cleaning wipe.
7.Gently resuspend pellet with 100 to 500 µl of 8 M UTU as MF protein.
8.Proceed to determining protein concentration and making protein aliquots according to Basic Protocol 4.
Basic Protocol 4: PROTEIN CONCENTRATION DETERMINATION BY BRADFORD ASSAY
The Bradford assay (Bradford, 1976) is a colorimetric method to determine protein concentration based on the unspecific interaction of a dye (Coomassie brilliant blue G-250, or CBB) with proteins. Upon binding to proteins, a shift in the absorption maximum of CBB (from 465 to 595 nm) occurs, which can be detected via photometric measurements. As there is a linear relationship between protein concentration and the shift in the absorption maximum, it is an easy and precise tool for reliable protein quantification. Please note that the Bradford assay is not tolerant to high detergent content in the sample.
Materials
-
Ultrapure water (∼0.055 μS/cm; e.g., Sartorius)
-
1 µg/µl bovine serum albumin (BSA; Sigma-Aldrich, cat. no. A9647)
-
8 M UTU (see recipe; make fresh)
-
Bradford reagent (Sigma-Aldrich, cat. no. B6916)
-
Total protein extract supernatant (Basic Protocol 1) or resuspended proteins (see Basic Protocol 2 or 3)
-
Flat-bottom, transparent 96-well plate (e.g., microplate 96-well flat-bottom clear, Greiner, cat. no. 655101)
-
Spectrophotometer (e.g., Tecan, Infinite M200 PRO)
-
1.5-ml Eppendorf tubes
Standard curve
1.Add reagents to wells of a flat-bottom, transparent 96-well plate as follows:
Reagent | Example well position | |||||
A1 | A2 | A3 | A4 | A5 | A6 | |
Ultrapure water (μl) | 24 | 23 | 22 | 21 | 20 | 19 |
1 μg/μl BSA (μl) | 0 | 1 | 2 | 3 | 4 | 5 |
8 M UTU (μl) | 1 | 1 | 1 | 1 | 1 | 1 |
Bradford reagent (μl) | 200 | 200 | 200 | 200 | 200 | 200 |
2.Incubate at room temperature in the dark for 15 min and then determine absorbance at 595 nm using a spectrophotometer.
3.Generate a standard curve with linear regression (absorbance read A595 = a × protein concentration + b) and display equation and R2 value on the chart.
Resuspended protein concentration
4.To determine the unknown protein concentration, add the following reagents to another well:
Reagent | Example well position |
A7 | |
Ultrapure water (μl) | 24 |
Total protein extract supernatant or resuspended proteins (μl) | 1 |
Bradford reagent (μl) | 200 |
5.Incubate at room temperature in the dark for 15 min and then determine absorbance at 595 nm using a spectrophotometer.
6.Calculate unknown protein concentration.
Protein aliquots
7.Make protein aliquots into fresh 1.5-ml Eppendorf tubes.
Basic Protocol 5: IN-SOLUTION DIGESTION WITH TRYPSIN
Before LC-MS/MS analysis (Basic Protocol 8), the protein should be digested into peptides ideally ranging between 7 and 20 amino acids in length and that likely produce a positive charge during electrospray ionization. The 8 M UTU provides a good buffering environment for protein resuspension and unfolding, making proteins accessible to enzymatic digestion. The DTT and IAA prevent the formation of disulfide bonds from cysteine residues and aid in the complete denaturation of proteins for enzymatic digestion. Endoproteinase Lys-C cleaves peptide chains at the C-terminal side of lysine (K), and trypsin cuts at the C-terminal side of lysine (K) or arginine (R). It is optional to add Lys-C, which reduces the number of proteins identified with miscleavages by trypsin, especially in the high-urea environment. Pre-digestion with Lys-C can increase the number of identified peptides by up to 10%, but on the whole-protein level, it rarely affects the downstream analysis. For phosphopeptide analysis, pre-digestion is recommended, as trypsin often misses cleavage near phosphorylated amino acids. The TFA acidifies the buffer environment to stop the trypsin digestion reaction and adds positive charge to the peptides.
Materials
-
Aliquots of protein from Basic Protocol 2 or 3 (see Basic Protocol 4)
-
8 M UTU (see recipe)
-
6.5 mM DTT (e.g., Sigma-Aldrich, cat. no. D0632)
-
27 mM IAA (e.g., Sigma-Aldrich, cat. no. I1149)
-
Lys-C (e.g., Promega, cat. no. VA1170; optional)
-
10 mM Tris/HCl, pH 8.0 (see recipe)
-
0.4 μg/μl trypsin, modified sequencing grade (e.g., Promega, cat. no. V5113)
-
10% (v/v) TFA (e.g., Thermo, cat. no. 85183)
-
Tube shaker (e.g., Thermo-Shaker, BioSan TS-100C), 37°C
-
pH strips (e.g., Roth, cat. no. 0549.2)
-
Microcentrifuge (e.g., Eppendorf 5430R)
-
1.5-ml Eppendorf tubes
-
Rotary vacuum concentrator (e.g., Christ, RVC 2-25 CD plus; optional)
-
CAUTION : Prepare the TFA solution in a fume hood.
1.Bring up volume of the aliquots of protein from Basic Protocol 2 or 3 to the same level using 8 M UTU.
2.Add 4 µl of 6.5 mM DTT per 200 µg protein and incubate at room temperature for 30 min.
3.Add 4 µl of 27 mM IAA per 200 µg protein and incubate in the dark at room temperature for 20 min.
4.Optional: Add 4 μl Lys-C per 200 μg protein and incubate at room temperature for 3 hr.
5.Dilute solution with 4 volumes of 10 mM Tris/HCl (pH 8.0).
6.Add 4 μl of 0.4 μg/μl trypsin per 200 μg protein and incubate at 37°C overnight with shaking at 1000 rpm.
7.Acidify solution with 0.02 volumes of 10% TFA. Test solution pH with pH strips to verify it has been acidified to around pH 2.
8.Centrifuge acidified solution for 10 min at 21,000 × g and then transfer supernatant to a fresh 1.5-ml Eppendorf tube.
9.Dry supernatant in a rotary vacuum concentrator and then proceed to Alternate Protocol 2 for phosphoproteome analysis or go directly to Basic Protocol 7 for whole proteome analysis of the supernatant.
Basic Protocol 6: PHOSPHOPEPTIDE ENRICHMENT WITH TiO2
TiO2 has a high affinity toward phosphopeptides (and other rather acidic peptides) and is water insoluble. Phosphopeptides can be loaded onto TiO2 beads in an acidic environment (loading buffer) and eluted from TiO2 beads into alkaline buffer (elution buffer). When using TiO2 bulk material to enrich the phosphopeptides, the buffer exchanges and removal of non-phosphopeptides are easily achieved by spinning and pipetting. The formic acid in the final step is used to create positively charged phosphopeptides that can bind to the C18 material in Basic Protocol 7.
Materials
-
TiO2 beads (e.g., GL Science, cat. no. 5020-75000)
-
Methanol (e.g., Sigma-Aldrich, cat. no. 34860)
-
Elution buffer: 1% (v/v) ammonia (NH4OH) solution (Sigma-Aldrich, cat. no. 5438300250)
-
Dried peptides (see Alternate Protocol 1)
-
Loading buffer (see recipe)
-
Wash buffer: 80% (v/v) acetonitrile (ACN; Sigma-Aldrich, cat. no. 34851) and 1% (v/v) TFA (Thermo, cat. no. 85183)
-
10% (v/v) formic acid (FA; e.g., Roth, cat. no. 4724.1)
-
2-ml round-bottom Eppendorf tubes
-
Vortex shaker (e.g., Scientific Industries Vortex-Genie 2 with microtube insert)
-
Microcentrifuge (e.g., VWR MiniStar silverline)
-
1.5-ml Eppendorf tubes
-
CAUTION : Combine the ACN and TFA in the wash buffer in a fume hood and prepare the FA solution in a fume hood.
Ready-to-use TiO2 preparation
1.Weigh 2 mg TiO2 beads per 200 μg protein into a 2-ml round-bottom Eppendorf tube.
2.Add 200 μl methanol and suspend TiO2 beads by shaking on a vortex shaker for 60 s. Then, centrifuge 2 min at 2000 × g and carefully remove methanol with a pipet.
3.Add 200 μl elution buffer to TiO2 beads and shake on vortex shaker for 10 min. Then, centrifuge 2 min at 2000 × g and carefully remove buffer with a pipet.
4.Add 100 μl wash buffer to TiO2 beads and shake on vortex shaker for 2 min. Then, centrifuge 2 min at 2000 × g and carefully remove buffer with a pipet.
5.Repeat step 4 two more times.
Phosphopeptide enrichment with ready-to-use TiO2
6.Resuspend dried peptides from Alternate Protocol 1 with 200 μl loading buffer.
7.Transfer resuspended peptides to the ready-to-use TiO2 beads and incubate for 30 min with continuous shaking on a vortex shaker.
8.Centrifuge 2 min at 2000 × g and carefully transfer supernatant to a fresh tube with a pipet or discard.
9.Add 100 μl loading buffer to TiO2 beads, briefly shake on vortex shaker for 60 s, centrifuge 2 min at 2000 × g , and carefully transfer supernatant to a fresh tube with a pipet or discard.
10.Add 100 μl wash buffer to TiO2 beads, shake on vortex shaker for 2 min, centrifuge 2 min at 2000 × g , and carefully remove supernatant with a pipet.
11.Repeat steps 9 and 10 two more times.
12.Add 80 μl elution buffer to TiO2 beads and incubate for 15 min with continuous shaking on vortex shaker.
13.Centrifuge 2 min at 2000 × g and carefully transfer supernatant to a fresh 1.5-ml Eppendorf tube with a pipet.
14.Repeat steps 12 and 13 for two more cycles.
15.Add 60 μl of 10% FA to 240 μl eluate.
16.Centrifuge acidified solution for 10 min at 21,000 × g , transfer supernatant to a fresh tube, and directly proceed to Basic Protocol 7.
Alternate Protocol 2: PHOSPHOPEPTIDE ENRICHMENT WITH Fe(III)-NTA CARTRIDGES
Robotized automation of sample preparation significantly reduces hands-on time and improves sample handling consistency. This protocol (an alternative to Basic Protocol 6) describes phosphopeptide enrichment using the Agilent Bravo Automated Liquid Handling Platform. The Fe3+ can interact with negatively charged phosphate groups on the peptides, and it has been widely applied as an immobilized metal-ion affinity-based enrichment method. The Fe(III)-NTA cartridge is a standard product for phosphopeptide enrichment using the Agilent Bravo platform (Russell & Murphy, 2016). Of course, other cartridge materials as offered by other manufacturers can also be used following this protocol.
Materials
-
Robot buffers:
- Reversed Phase S Priming buffer and Syringe Wash buffer (70% ACN, 0.1% TFA)
- Reversed Phase S Elution buffer (70% ACN, 0.1% TFA)
- Reversed Phase S Equilibration and Cartridge Wash buffer (5% ACN, 0.1% TFA)
- FE(III)-NTA cartridges Priming buffer and Syringe Wash buffer (99.9% ACN, 0.1% TFA)
- FE(III)-NTA cartridges Elution buffer (99% H2O, 1% NH3)
- FE(III)-NTA cartridges Equilibration and Cartridge Wash buffer (80% ACN, 1% TFA)
-
Dried peptides (see Basic Protocol 5)
-
Loading buffer (see recipe)
-
10% (v/v) FA (Roth, cat. no. 4724.1)
-
Bravo Automated Liquid Handling Platform (Agilent)
-
Labware (all Agilent):
- 96 AssayMAP Head (Agilent, cat. no. G5058A)
- 96 V11 LT 250 Tip Box (cat. no. 19477.002)
- 96AM Cartridge Seating Station (cat. no. G5409-20025)
- 96 AbGene 1127, 1 ml Deep Well, Square Well, Round Bottom
- 96 Greiner 650201, U-Bottom Standard, PolyPro
- 96 Eppendorf 30129300, PCR, Full Skirt, PolyPro
- 12 Column, Low Profile Reservoir, Natural PP (cat. no. 201280-100)
-
AssayMAP Reversed Phase S 5-µl cartridges (Agilent, cat. no. G5496-60033)
-
AssayMAP Fe(III)-NTA cartridges (Agilent, cat. no. G5496-60085)
-
CAUTION : Combine the ACN and TFA in the robot buffers in a fume hood and prepare the FA solution in a fume hood.
Robot system initialization
1.Turn on water pump, robot, thermo controller, and computer for the Bravo Automated Liquid Handling Platform (Agilent).
2.Open “Protein Sample Prep Workbench” application on the computer to connect the robot and access the operation interface. Click on “Utility Library,” go to “System Startup/Shut down v2.2,” and start program “Run Startup.”
Peptide clean-up
3.When the “Run Startup” program has finished, click “APP Library” button and go to program “Peptide Cleanup v3.0.”
4.In the “Select method” area, click “…” button to browse the methods folder. View ready-to-use methods located at “VWorks Workspace” > “Methods” > “AM Peptide Cleanup v3.0.” Select method file with the default setting and click on “Load.”
5.Change parameters according to the experiment requirements. Select labware according to the experiment in the “Labware Table” area on the interface.
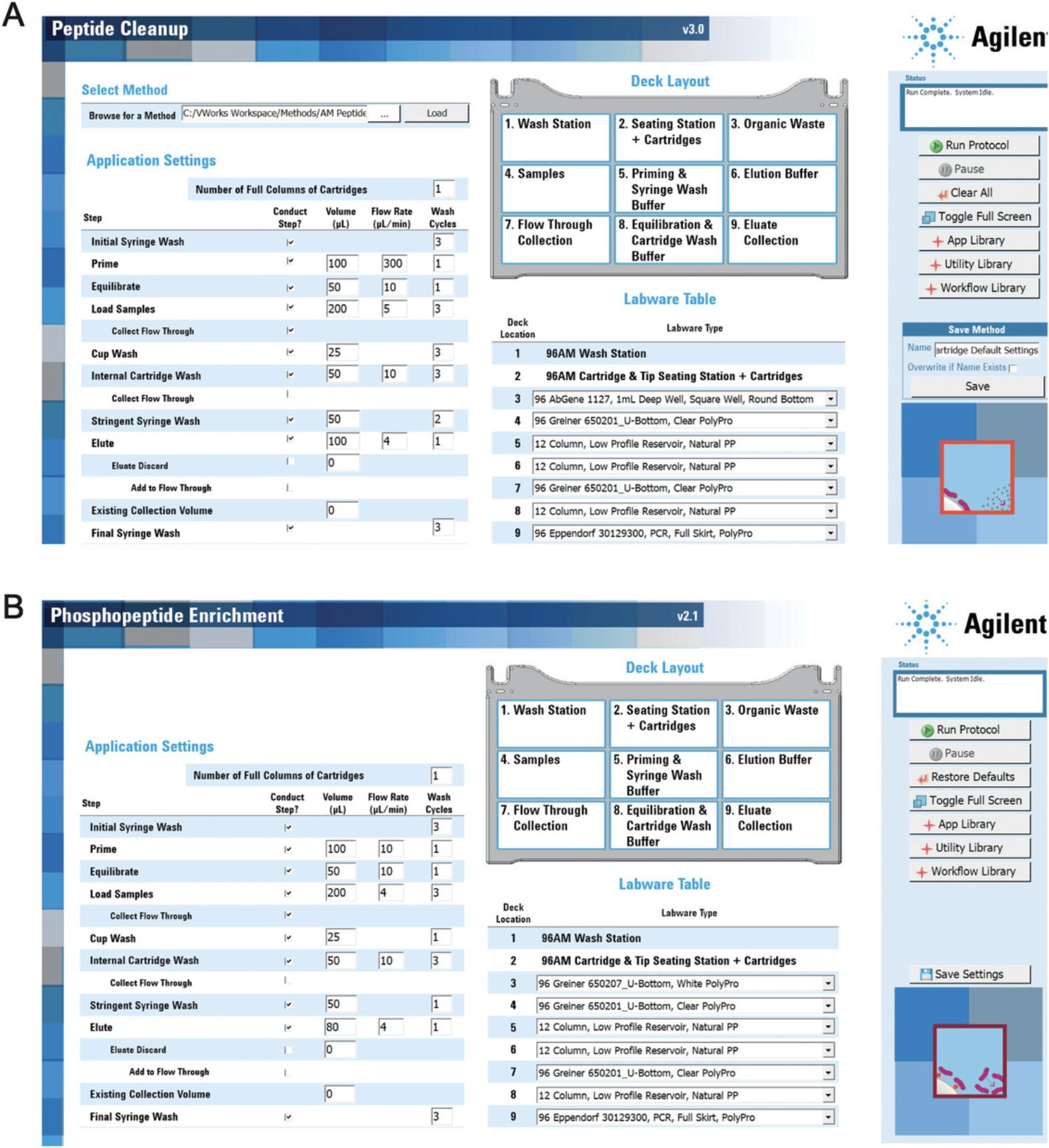
6.According to the “Deck Layout” and “Labware Table,” place corresponding labware and AssayMAP Reversed Phase S 5-µl cartridges at the required deck position on the robot stations.
7.Fill corresponding robot buffers in deck numbers 5, 6, and 8.Resuspend dried peptides from Basic Protocol 5 in 200 ml loading buffer and transfer into appropriate Labware (96 Greiner 650201, U-Bottom Standard, PolyPro) at deck position 4 (see Fig. 2).
8.Click on “Run Protocol.”
Phosphopeptide enrichment with Fe(III)-NTA cartridges
9.When the protocol program finishes, click “App Library” button and go to program “Phosphopeptide Enrichment v2.1.”
10.Change parameters according to the experiment requirements. Select labware in the “Labware Table” area.
11.Prepare corresponding labware plate and AssayMAP Fe(III)-NTA cartridges at the right deck position on the robot stations.
12.Fill corresponding robot buffers into the labware at deck numbers 5, 6, and 8.
13.Click on “Run Protocol.”
14.When the protocol program finishes, take final eluate plate off deck 9 and immediately add 20 µl of 10% FA to acidify samples.
Finalization and robot system shut-down
15.Save leftover buffers or discard into a special waste station and tidy up robot stations.
16.Click “Utility Library” button and go to “System Startup/Shut down v2.2.” Run program “Run Shutdown.” As soon as the program finishes, shut down system according to the user manual from Agilent.
Basic Protocol 7: PEPTIDE DESALTING WITH C18 MATERIAL
Salts can interfere with the column resolution, and peptide solutions containing high salt are usually not suitable to run in LC-MS/MS (Basic Protocol 8) due to highly dominant salt ions. This protocol describes using the Empore Disk C18 material for reversibly binding peptides for desalination and concentration (Rappsilber, Ishihama, & Mann, 2003).
Materials
-
Solution B: 80% (v/v) ACN (Sigma-Aldrich, cat. no. 34851) and 0.5% (v/v) acetic acid (Thermo, cat. no. 85183; store at room temperature or 4°C)
-
Solution A: 0.5% (v/v) acetic acid (Thermo, cat. no. 85183; store at room temperature or 4°C)
-
Supernatant (see Alternate Protocol 1 or 2 or Basic Protocol 5 or 6)
-
Empore Disk C18 (e.g., Varian, cat. no. 12145004)
-
Blunt-tipped hypodermic needle
-
Suitable-diameter stick (to allow passage through needle)
-
200-µl pipet tip (e.g., VWR, cat. no. 89041-378)
-
Centrifuge adapter (for 200-µl pipet tip; e.g., GL Sciences, 5010-21341)
-
2-ml round-bottom Eppendorf tube
-
Microcentrifuge (e.g., Eppendorf 5430R)
-
1.5-ml Eppendorf tube
-
Rotary vacuum concentrator (e.g., Christ, RVC 2-25 CD plus)
-
CAUTION : Prepare Solutions A and B in a fume hood.
Immobilization of C18 material in pipet tip
1.Place an Empore Disk C18 on a flat, clean surface (e.g., disposable plastic petri dish).
2.Press and twist a blunt-tipped hypodermic needle into Empore Disk C18 to punch out two small pieces.
3.Push small pieces of disk out of the needle with a suitable-diameter stick and immobilize it in taper of a 200-µl pipet tip.
Ready-to-use C18 tip preparation
4.Hold C18 tip with a centrifuge adapter and place it into a 2-ml round-bottom Eppendorf tube.
5.Add 50 µl Solution B to C18 tip and centrifuge ∼5 min at 3000 × g , until solution has completely passed through the C18 tip.
6.Discard waste solution in the tube.
7.Add 100 μl Solution A to C18 tip and centrifuge about 5 to 8 min at 3000 × g , until solution has completely passed through the C18 tip.
8.Discard waste solution in the tube.
9.Repeat steps 7 and 8 once more.
Peptide desalting
10.Transfer supernatant from Alternate Protocol 1 or 2 or Basic Protocol 5 or 6 to the ready-to-use C18 tip and centrifuge about 5 to 10 min at 3000 to 6000 × g , until solution has completely passed through the C18 tip.
11.Discard waste solution in the tube.
12.Add 100 μl Solution A to wash peptides bound to the C18 tip and centrifuge about 5 to 10 min at 3000 to 6000 × g , until solution has completely passed through the C18 tip.
13.Discard waste solution in the tube.
14.Repeat steps 12 and 13 once more.
15.Centrifuge C18 tip with bound peptides for 2 min at 3000 × g to remove residual solution on the tip.
16.Place C18 tip in a fresh 1.5-ml Eppendorf tube.
17.Add 20 μl Solution B to elute peptides from the C18 tip and centrifuge 5 min at 3000 × g until solution has completely passed through the C18 tip.
18.Repeat step 17 once more.
19.Concentrate eluted peptides in a rotary vacuum concentrator until completely dry.
Basic Protocol 8: LC-MS/MS ANALYSIS OF (PHOSPHO)PEPTIDES AND SPECTRUM MATCHING
The complex peptide mixture obtained at the end of Basic Protocol 7 is separated by reversed-phase LC and is then directly detected and quantified by tandem MS (MS/MS). We apply the Thermo EASY-nLC 1200 system as a nanoflow liquid ultra-high pressure chromatography system (UHPLC). We use a C18 stationary phase packed into an EASY-Spray analytical column (25 cm × 75 μm inner diameter). Eluting peptides are directly sprayed into the mass spectrometer after ion spray ionization. The MS platform that we use here is the Thermo Q-Exactive HF Hybrid Quadrupole-Orbitrap Mass Spectrometer. The raw spectrum scans from MS are subjected to matching against proteome sequences from the respective organism using the application MaxQuant (Version 2.0.3.0) (Cox & Mann, 2008; Tyanova, Temu, & Cox, 2016). The reference protein sequence database is downloaded from EnsemblPlants (https://plants.ensembl.org/index.html). We describe the parameter settings for LC-MS/MS analysis and MaxQuant data processing for phosphoproteome analysis in Table 1.The settings for non-modified proteins can be found in previous studies (He et al., 2021; Li et al., 2015; Schulze et al., 2021).
Instrument | Parameter | Setting |
---|---|---|
LC system |
Sample loading Gradient length (min) Gradient flow rate (nl/min) Linear gradient (% LC solvent B in LC solvent A) |
At max. pressure 900 bar 90 250 2%-55% for 60 min 55%-95% for 10 min 95% for 5 min 95%-2% for 5 min 2% for 10 min |
Ion source |
Column heater temperature (°C) Spray voltage (kV) Capillary temperature (°C) |
35 2.0 250 |
Mass spectrometer: full MS |
Resolution (at m/z 200) Automatic gain control target (ions) Maximum injection time (ms) Scan range (m/z) |
60,000 500,000 60 300-1800 |
Mass spectrometer: dd-MS2 |
Resolution (at m/z 200) Automatic gain control target (ions) Maximum injection time (ms) Loop count Isolation window (m/z) TopN Scan range (m/z) Normalized collision energy ((N)CE) |
15,000 100,000 60 20 1.6 20 200-2000 27 |
Mass spectrometer: general |
Polarity Intensity threshold Charge exclusion Peptide match Dynamic exclusion (s) |
Positive 210,000 Unassigned, 1, 6-8, >8 Preferred 20 |
Materials
-
Dried (phospho)peptides (see Basic Protocol 7)
-
Resuspension buffer: 5% (v/v) ACN (Sigma-Aldrich, cat. no. 34851) and 0.1% (v/v) TFA (Thermo, cat. no. 85183) (store ≤2 months at room temperature)
-
Microtiter plate (e.g., Thermo, cat. no. AB-0800)
-
Nanoflow UPLC system (e.g., Thermo, EASY-nLC 1200)
-
Packed column (C18 stationary phase packed into EASY-Spray analytical column, 25 cm × 75 μm inner diameter)
-
Mass spectrometer (e.g., Thermo, Q-Exactive HF)
-
MaxQuant application (e.g., Version 2.0.3.0)
-
CAUTION : Combine the ACN and TFA in the resuspension buffer in a fume hood.
1.Resuspend dried (phospho)peptides from Basic Protocol 7 with 5 µl resuspension buffer and transfer them to a microtiter plate. Place microtiter plate into the nanoflow UPLC system for LC detection.
2.Run LC with a packed column using standard settings (see parameters in Table 1).
3.Set parameters for mass spectrometer data acquisition according to Table 1 and run MS analysis.
4.Load LC-MS/MS output raw file into the MaxQuant application and set parameters for fixed and variable modification quantitation and protein identification as proposed in Table 2.
Parameter type | Parameter | Setting |
---|---|---|
Group-specific parameters |
Version Type Multiplicity Enzyme Maximum missed cleavages Variable modifications Fixed modifications Label-free quantification First search p.p.m. Main search p.p.m. |
2.0.3.0 (or newer) Standard 1 Trypsin/P 2 Oxidation (M) Acetylation (protein N-term) Phosphorylation (STY) Carbamidomethyl (C) Fast LFQ 20 4.5 |
Global parameter |
Include contaminants Minimum peptide length (residues) Maximum peptide mass (Da) PSM FDR Protein FDR Site decoy fraction Match between runs Match time window (min) Alignment time window (min) MS/MS tolerance (Fourier transform MS, p.p.m.) Top MS/MS peaks per Da interval |
TRUE 7 4600 0.01 0.01 0.01 TRUE 0.7 20 20 12 |
- a
In-depth information about MaxQuant settings is provided in Tyanova, Temu, & Cox (2016).
5.Begin loading reference sequence into MaxQuant in the tab “Global parameters” > “Sequence” > “Fastafiles.” Click on “Add” button to load the reference sequence from a local folder where the reference sequence was downloaded from the web.
6.Obtain output files and perform statistical analysis.
REAGENTS AND SOLUTIONS
Homogenization buffer
- 330 mM sucrose (Roth, cat. no. 4621)
- 100 mM potassium chloride (Roth, cat. no. 6781)
- 1 mM ethylenediaminetetraacetic acid disodium salt dihydrate (EDTA; Sigma-Aldrich, cat. no. E5134)
- 50 mM Tris/MES, pH 7.5 (see recipe for 1 M)
- Store ≤1 week at 4°C
For experimental results that will be affected by sucrose in the buffer, e.g., analyzing samples with sucrose treatment, 330 mM mannitol can be applied instead of sucrose.
Loading buffer
- 1 M glycolic acid (Sigma-Aldrich, cat. no. 124737)
- 80% (v/v) ACN (Sigma-Aldrich, cat. no. 34851)
- 5% (v/v) TFA (Thermo, cat. no. 85183)
- Store ≤3 months at 4°C
CAUTION: Add the ACN and TFA in a fume hood.
MF extraction buffer
- Homogenization buffer (see recipe) with freshly added inhibitors as follows:
- 0.5% (v/v) protease inhibitor cocktail (PIC; Sigma-Aldrich, cat. no. P9599; store at –20°C)
- 5 mM DTT (Sigma-Aldrich, cat. no. D0632)
- 25 mM sodium fluoride (NaF; Roth, cat. no. P756)
- 1 mM sodium orthovanadate (Na3VO4; Sigma-Aldrich, cat. no. 450243)
- 1 mM benzamidine hydrochloride (Sigma-Aldrich, cat. no. 450243)
- 3 μM leupeptin (Sigma-Aldrich, cat. no. L2884)
- 1 mM PMSF (Sigma-Aldrich, cat. no. 78830) in EtOH
- Prepare fresh immediately before use
Sodium acetate (pH 5.0), 2.5 M
- 30 ml ultrapure H2O
- 10.254 g sodium acetate (NaOAc; Sigma-Aldrich, cat. no. S2889)
- Adjust pH to 5.0 with glacial acetic acid (Roth, cat. no. 3738.4)
- Place solution at 4°C overnight
- Adjust pH once more to 5.0 with glacial acetic acid (Roth, cat. no. 3738.4)
- Ultrapure H2O to 50 ml
- Store ≤3 months at 4°C
CAUTION: Adjust the pH in a fume hood.
Total protein extraction buffer
- 8 M UTU (see recipe) with freshly added inhibitors as follows:
- 5 mM DTT (Sigma-Aldrich, cat. no. D0632)
- 1 mM PMSF (Sigma-Aldrich, cat. no. 78830) in EtOH
- 2% (w/v) PVPP (Sigma-Aldrich, cat. no. 77627)
- Addition for phosphoproteome analysis: 0.5% (v/v) protease inhibitor cocktail (PIC; Sigma-Aldrich, cat. no. P9599; store at –20°C)
- Prepare fresh immediately before use
Tris/HCl (pH 8.0), 10 mM
- 80 ml ultrapure H2O
- 0.121 g Tris base (Sigma-Aldrich, cat. no. T1503)
- Adjust pH to 8.0 with 1 M HCl (Sigma-Aldrich, cat. no. H1758)
- Ultrapure H2O to 100 ml
- Store ≤1 year at room temperature or 4°C
Tris/MES (pH 7.5), 1 M
- 80 ml ultrapure H2O
- 12.114 g Tris base (Sigma-Aldrich, cat. no. T1503)
- Adjust pH to 7.5 with MES monohydrate (Roth, cat. no. 6066)
- Ultrapure H2O to 100 ml
- Store ≤1 year at room temperature or 4°C
UTU, 8 M
- Use 10 mM Tris/HCl, pH 8.0 (see recipe), as solvent to dissolve the following reagents:
- 6 M urea (Sigma-Aldrich, cat. no. U5378)
- 2 M thiourea (Sigma-Aldrich, cat. no. T8656)
- Prepare fresh immediately before use
COMMENTARY
Background Information
Protein extraction
There are three major strategies of total protein extraction for proteome or phosphoproteome analysis. The first one involves applying organic solvents to isolate proteins, for example, TCA/acetone and phenol (Bonhomme et al., 2012; Chao et al., 2016; Ding et al., 2016; Wang, Tai, & Chen, 2008; Wu et al., 2015; Wu, Xiong, Wang, Scali, & Cresti, 2014). The second method involves solubilizing proteins with detergents. This approach is frequently followed by 2D gel–based analysis. The third method uses strong chaotropic reagents to dissolve proteins, such as urea, thiourea, and guanidine chloride. For example, protein extraction with 6 M guanidine chloride was previously used in phosphoproteome analysis in tomato (Hsu et al., 2018), and protein extraction with 8 M urea or 8 M UTU for proteomic studies was applied in Arabidopsis and maize (He et al., 2021; Li et al., 2015; Schulze et al., 2021; Thakur et al., 2011). Strong chaotropic reagents perform better than organic solvents (Luís et al., 2016), and because 8 M UTU is widely used to resuspend purified protein and is compatible with tryptic digestion, we recommend 8 M UTU as a basic lysis buffer (Basic Protocol 1). By already using the urea environment for protein extraction, protein digestion can be performed in the same buffer, thereby reducing sample handling steps and losses, especially when working with very low amounts of tissue (Li et al., 2015).
Protein clean-up
Total protein extracts, especially from leaf samples, contain large amounts of chlorophyll and secondary metabolites such as phenolics, lipids, organic acids, carbohydrates, terpenes, and pigments, which decrease the accuracy of protein concentration determination and reduce the efficiency of tryptic digestion and phosphopeptide enrichment (Niu, Yuan, Gong, Wu, & Wang, 2018; Wu, Gong, & Wang, 2014). Thus, removal of these substances is essential for good protein yield and for high-quality protein to be subjected to mass spectrometric analysis. Including the protein clean-up steps (Basic Protocol 2 and Alternate Protocol 1) results in an 18% increase in quantified proteins compared to when these steps are omitted. A previous study reports a similar result for tomato tissue (Hsu et al., 2018). Currently, the major strategies for protein clean-up include protein precipitation and spin-filter enrichment. The most widely used precipitation solutions are salt (e.g., ammonium sulfate) or organic solvents (e.g., acetone, TCA/acetone, methanol-chloroform, or EtOH). Filter-aided sample preparation (FASP) can be used for removal of detergent from protein solutions and for buffer exchange (Wiśniewski, Zougman, Nagaraj, & Mann, 2009). Because we present a detergent-free protein extraction based on chaotropic reagents and because FASP can cause loss of protein with sizes <30 kDa, we recommend the PC method (Basic Protocol 2) as a suitable clean-up protocol. EtOH is one the most easily accessible reagents with good efficiency for protein precipitation (Yoshikawa, Hirano, Arakawa, & Shiraki, 2012a, 2012b). Besides, SP3 technology (Alternate Protocol 1) is available as a novel method to clean up the protein/peptide sample independent of solubilization agents and quantity of input material. It provides nonselective protein binding and allows on-bead trypsin digestion (Hughes et al., 2019). According to our results, both the PC and SP3 methods effectively remove interfering substances and provide good protein yield for quantification by MS.
Protein concentration determination
Having the same protein content is fundamental for comparing different LC-MS/MS runs with label-free quantitation. Therefore, protein concentration determination is necessary and important in this workflow. The commonly used methods include the Bradford assay (Bradford, 1976), Lowry assay (see Current Protocols article: Olson & Markwell, 2007), and BCA assay (Smith et al., 1985). Different methods have specific advantages and limitations, and compatibility with the applied extraction buffers is more crucial than accuracy. We recommend the Bradford assay (Basic Protocol 4), which is compatible with high concentrations of urea. In cases when protein extracts contain detergents, the Lowry or BCA assay can be chosen instead of the Bradford assay (see Current Protocols article: Olson & Markwell, 2007).
Protein digestion with trypsin
Trypsin is widely used and has become the gold standard for protein digestion (Alternate Protocol 1 and Basic Protocol 5), which is indispensable for bottom-up proteomics. Trypsin is particularly suitable due to the frequency of arginines and lysines in typical proteomes, leading to the creation of positively charged amino acids at the C-terminus of every peptide due to its cleavage preference for C-terminal arginines and lysines (Laskay, Lobas, Srzentić, Gorshkov, & Tsybin, 2013). However, trypsin digestion has some limitations, for example, trypsin activity is decreased when acidic residues (e.g., phosphorylated amino acids) are present on either side of a susceptible bond, resulting in missed cleavages. Furthermore, trypsin has lower activity in higher-salt environments. Pre-digestion of protein with addition of the enzyme Lys-C decreases missed cleavages and thus increases the number of identified peptides, and it improves analytical reproducibility (Glatter et al., 2012; Saveliev et al., 2013).
Phosphopeptide enrichment
Ion suppression by highly abundant non-modified peptides will overwhelm the signals of lower-abundance phosphopeptides, resulting in poor detection of the phosphopeptides (Silva-Sanchez, Li, Chen, & Chen, 2015). Using our protocol workflow, we show that phosphopeptide enrichment in maize root results in more than three times higher phosphopeptide quantification compared to preparations in which this step was eliminated. The most common phosphopeptide enrichment methods are immobilized metal affinity chromatography (IMAC), metal oxide affinity chromatography (MOAC) and TiO2-based enrichment (Beltran & Cutillas, 2012; Gates, Tomer, & Deterding, 2010). These methods all take advantage of the negatively charged phosphate groups on the phosphorylated peptides interacting with positively charged metal ions and compounds. For example, IMAC, which consists of a metal ion such as Fe3+ chelated with nitriloacetic acid (NTA) and immobilized onto resin, has a high efficiency of phosphopeptide selection. These materials are commercially available and immobilized on diverse supporting matrices for different ways of handling, such as in an agarose resin spin-down column from Thermo Scientific™ Pierce™, the TiO2 resin column from Titansphere®, or in the form of magnetic beads from Cytiva. These different phosphopeptide enrichment approaches result in different phosphopeptides identified (Arribas Diez et al., 2021; Gates et al., 2010; Liang et al., 2007), suggesting that different matrices enrich slightly different types of phosphopeptides. New supporting matrices are continuously being developed (Kupcik et al., 2019). The TiO2-based method for phosphopeptide enrichment that we present in Basic Protocol 6 is efficient, simple, and fast and requires smaller amounts of starting proteins compared to the IMAC or MOAC method (Wu et al., 2017). The development of robot systems provides the potential of high-throughput and labor-saving sample processing, for example, with the Agilent Bravo Automated Liquid Handling Platform we use here (Russell & Murphy, 2016), which offers phosphopeptide enrichment via Fe(III)–NTA cartridges (Alternate Protocol 2). Both enrichment strategies result in abundant phosphopeptide detection.
Peptide desalting
The peptides or phosphopeptides obtained after digestion are in a salt-containing buffer, and this salt concentration may not be compatible with MS detection. Salts can interfere with the action of reversed-phase materials, decreasing the column resolution in LC-MS/MS. Particles can easily become irreversibly locked in analytical columns, resulting in complete sample loss. Furthermore, salt ions result in ion suppression of peptide ions, which will then not be detected. The Empore Disk C18 is a material for reversibly binding peptides; therefore, fixing the C18 membrane into a pipet tip allows for desalination and concentration of peptides (Basic Protocol 7) (Rappsilber et al., 2003). Commercial C18 tips or cartridges for desalting also are available, in the form of tips or filter plates, and they can be used as alternatives to Basic Protocol 7.
LC-MS/MS quantification and data processing
LC-MS/MS (Basic Protocol 8) nowadays is a commonly used analytical technique for the analysis of biomolecules, such as peptides and phosphopeptides. Stable-isotope labeling and label-free quantitation are frequently used strategies for comparative proteomics. The stable-isotope approaches offer greater quantitative precision, whereas label-free quantitation performs better when comparing large numbers of samples or conditions at rather low cost (Schulze & Usadel, 2010). Label-free quantification analysis for (phospho)proteomics can be performed by either data-dependent acquisition (DDA) or data-independent acquisition (DIA) (Bekker-Jensen et al., 2020; Kelstrup et al., 2018; Li, Zan, Zhu, Lu, & Krall, 2021; Lou et al., 2021). Various types of software for raw data processing are available (Almeida et al., 2020; Li et al., 2021; Pang et al., 2020). Here, we propose label-free quantitation based on the instrument Q-Exactive HF (Thermo Fisher) and a data analysis workflow in MaxQuant (Version 2.0.3.0).
Critical Parameters
LC-MS/MS platform
Quantification coverage and reproducibility are affected by the capability of the LC-MS/MS platform (Basic Protocol 8) and the quality of sample preparation (Bittremieux et al., 2018; Tuli & Ressom, 2009). Generally, the higher the scan rate and resolution of the LC-MS/MS platform, the better the quantification of peptides.
Tissue disruption
In terms of experimental steps, it is important to thoroughly disrupt tissues (Basic Protocol 1) because plants have rigid cell walls formed by cellulose and lignification, making mechanical disruption difficult. Previous studies have shown that protein extraction yield greatly depends on the fineness of disruption: a finer ground powder will yield a higher amount of total protein (Wang et al., 2008). However, for MF protein extraction (Basic Protocol 3), a gentler cell disruption method using a tissue homogenizer is preferred, which preserves larger membrane fragments in a membrane-friendly buffer environment.
Sample handling steps before mass spectrometry
To prevent proteins from undergoing degradation and modification during sample preparation (Basic Protocol 1), the major critical points include collecting samples fresh, keeping samples frozen before adding extraction buffer, adding appropriate protease and phosphatase inhibitors, and completing all steps as quickly and continuously as possible, ideally in a cooled environment or on ice. Some chemical side reactions might occur during sample preparation, which may affect spectra matching efficiency: urea can cause carbamylation on protein during prolonged incubation time and heating, and overalkylation by IAA can cause N-terminal carbamidomethyl modification at near-neutral pH and prolong incubation time (Bittremieux et al., 2018; Luebker & Koepsell, 2016; Sun, Zhou, Yang, & Zhang, 2014). Tryptic peptides can be lost with prolonged digestion time (Alternate Protocol 1 and Basic Protocol 5), leading to lower coverage of quantitative peptides (Hildonen, Halvorsen, & Reubsaet, 2014). The pH environment is also critical in many steps. For example, UTU at pH 8 is optimal for protein solubility and tryptic digestion. Additionally, binding of peptides to TiO2 and reversed-phase C18 material (Basic Protocols 6 and 7) depends on correct acidification. There is a good linear relationship between quantification coverage and the input amount of phosphopeptides in LC-MS/MS runs. A previous dose-dependent experiment in Arabidopsis demonstrated that more protein input yields higher quantification coverage (Wu et al., 2017). The 200-µg protein aliquot that we propose to be used in Basic Protocol 6 represents a generous protein amount expected to yield over 1000 phosphopeptide identifications. However, users can adjust the input amount of protein according to the accessibility of protein extracts and quantification ability of LC-MS/MS (Basic Protocol 8).
Troubleshooting
Please see Table 3 for a troubleshooting guide.
Potential problem | Possible cause | Solution |
---|---|---|
Difficult or incomplete tissue disruption | Strong cell walls |
Increase disruption time Add grinding beads or quartz sand Cut tissue into small pieces prior to homogenization |
Low yield of protein extraction | Low amount of tissue, inefficient extraction | More tissue input, lower extraction buffer volume, finer grinding |
Protein clean-up failed | Redo protein clean-up | |
Low binding efficiency of TiO2 to phosphopeptides | Decreased affinity of TiO2 toward phosphopeptides | Baking TiO2 at 200°C for 5 hr prior to using it |
Low binding efficiency of Fe (III)-NTA cartridges to phosphopeptides | Wrong buffer used | Double-check layout |
Peptide clean-up failed | Use new reversed-phase cartridges and redo peptide clean-up | |
C18-tip block | Supernatant contains invisible and insoluble particles that block C18-tip | Increase centrifugation time and speed before peptide clean-up |
Statistical Analysis
Statistical analysis will depend on the research question and usually involves respective software packages, as recommended in Basic Protocol 8, step 6. Here, we provide a few simple measures that may be used to describe the dataset and that can be used for quality control.
Sum intensity of quantitative phosphopeptides for each replicate
The summed intensity values in each injection (i.e., each sample) indicate the amount of phosphopeptide obtained from each sample. A view of these phosphopeptide ion intensities typically shows variation across samples from different tissues, clean-up methods, and enrichment methods and also among technical replicates (Fig. 3). A significant difference in these values, as, for example, found between samples from maize root and wheat leaf, indicates different efficiencies of sample preparation steps. Such views are therefore useful when optimizing a protocol or when comparing different methods.
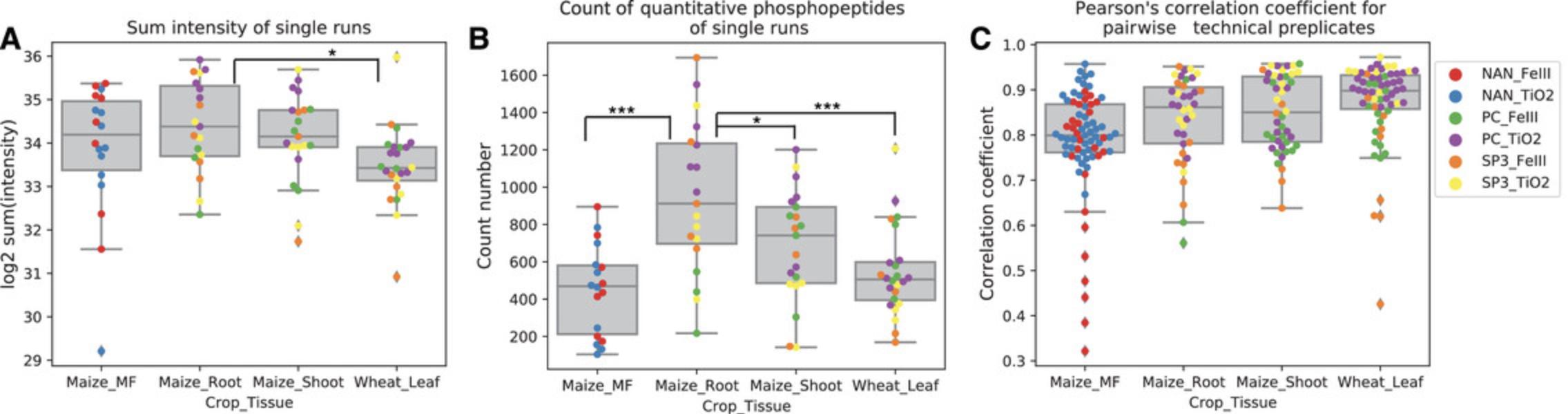
Considering that different methods of protein clean-up and phosphopeptide enrichment have different efficiencies in protein or phosphopeptide recovery, we can now compare combinations of the above basic protocols and alternate protocols regarding the total amount of phosphopeptides identified (Fig. 3). Regarding our basic protocols and alternate protocols described above, neither the clean-up procedure nor the phosphopeptide enrichment method affects the total amount of phosphopeptides obtained from the preparation (Fig. 3A). However, the SP3 method (Alternate Protocol 1) potentially results in higher protein recovery efficiency compared with the recovery efficiency for the PC method (Basic Protocol 2), which is ∼60%, as calculated from protein concentration determination.
Count number of quantitative phosphopeptides per replicate
The count number of quantitative phosphopeptides indicates the quantification capability of LC-MS/MS and the quantification yield of phosphopeptides in each injection (Basic Protocol 8). For example, we observe that the quantification yield in each run is largely determined by the type of tissue because significantly higher yields are achieved in maize root compared to the other tissues. This may relate not only to different protein compositions in different tissues but also to higher content of fibers or interfering substances in shoots (Fig. 3B).
Reproducibility of technical replicates
The reproducibility of technical replicates is analyzed by pairwise correlation between phosphopeptide intensities of different replicates. The example results show that the median value of correlation coefficients in each tissue group, including all values from different methods of protein clean-up and phosphopeptide enrichment, generally range from 0.8 to 0.9 (Fig. 3C). This is indicative of a high reproducibility of technical replicates.
Protein clean-up methods and phosphopeptide enrichment methods yield different results for quantification consistency among technical replicates for different tissues. In maize root, the TiO2-based method (Basic Protocol 6) seems to perform better than the Fe(III)-NTA-based method (Alternate Protocol 2). The averaged correlation coefficient of technical replicates from the TiO2 enrichment method was 0.87, and the averaged correlation coefficient among technical replicates from the Fe(III)-NTA-based enrichment method was 0.78. In protein preparations of maize shoots, the combination of SP3/TiO2 shows the best reproducibility, with a correlation value at 0.92.
Understanding Results
The protocols described here are suitable for proteins extracted from maize shoot, maize root, wheat leaf, and likely tissues from other cereals, such as rice. We present two alternatives for clean-up: either the PC method (Basic Protocol 2) or the SP3 method (Alternate Protocol 1). In parallel, a protocol is presented for MF proteins to allow for membrane-focused studies (Basic Protocol 3). In general, we propose aliquots of 200 µg protein for protein digestion (Basic Protocol 5) and phosphopeptide enrichment. For phosphopeptide enrichment, we present two alternative protocols: either with TiO2 by manual work (Basic Protocol 6) or with Fe(III)-NTA cartridges on a robotic platform (Alternate Protocol 2). For each combination of protein clean-up method and phosphopeptide enrichment method, 3 to 11 technical replicates should be used.
Among all the phosphorylated peptides in each tissue, the phosphorylated amino acids mainly include serine (S), threonine (T), and tyrosine (Y). The distribution of phosphorylated amino acids in samples is expected to consist of 82.9% to 85.6% serines, 13.8% to 16.2% threonines, and a very small percentage of tyrosines (Fig. 4A) in accordance with many previously published studies. The number of phosphorylated sites within an identified phosphopeptide ranges from 1 to 4. Most of them contain single or double phosphorylated sites. The double phosphorylated site count is slightly higher in quantitative phosphopeptides from MF proteins than from the total protein (Fig. 4B).
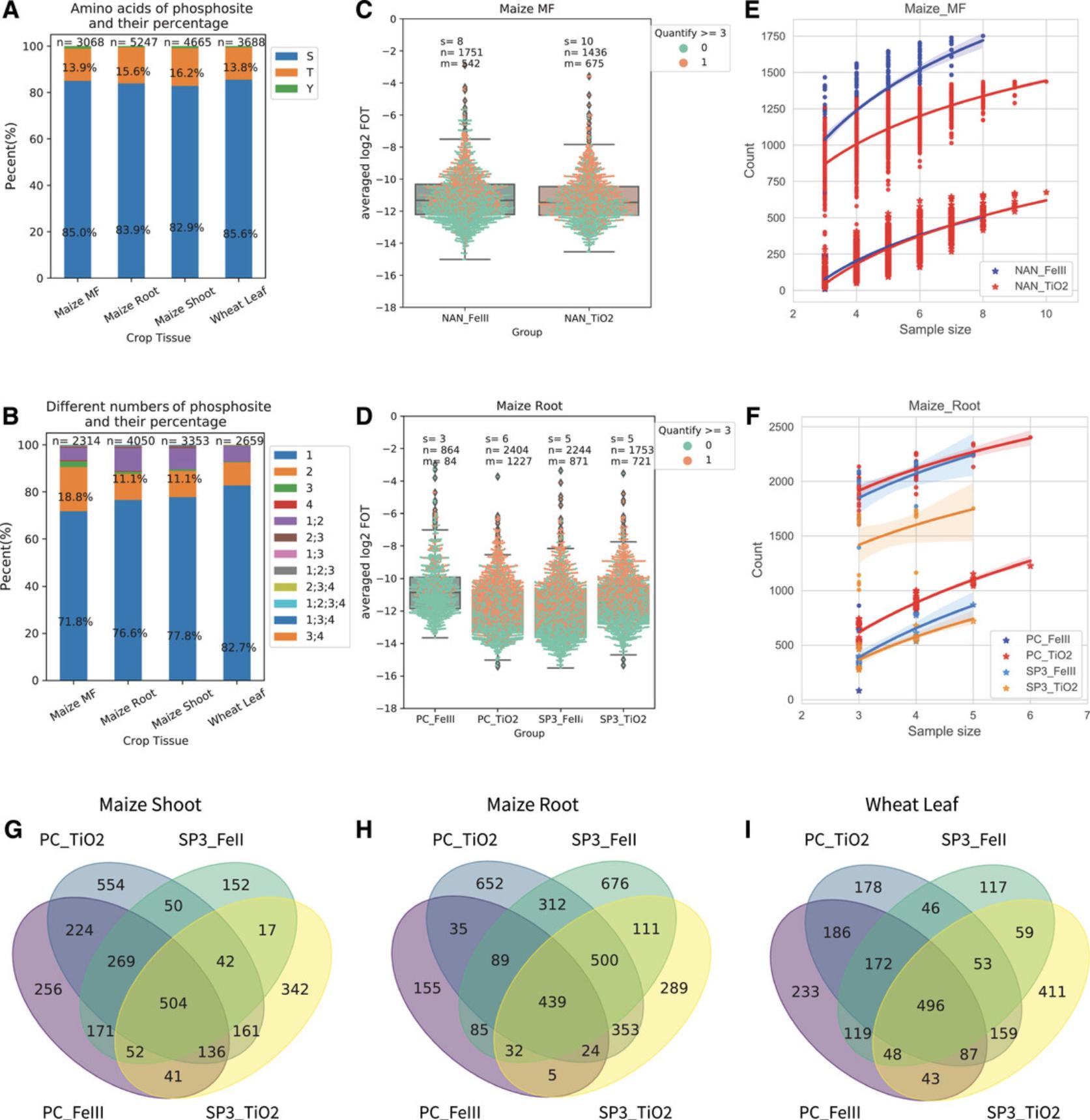
To analyze the quantification values of phosphopeptides between different runs, the original quantitative intensity values of each phosphopeptide should be normalized. We propose a rough and easy normalization on total protein intensity sum called “fraction of total” (FOT). It is calculated by dividing each phosphopeptide intensity value by the total intensity sum of the respective sample. These ratios are then transformed by calculating the logarithm to the basis 2 (log2) to obtain values termed “log2-FOT.” The lower the value of log2-FOT, the lower the abundance of the respective phosphorylated peptides is. Thus, the log2-FOT values can well be used to compare phosphopeptide abundances between different samples.
Typically, in phosphoproteomic datasets, not all phosphopeptides can be quantified in all replicates. The phosphopeptides with at least one quantification among replicates are available only as identity and simple quantitative information, whereas in other cases, phosphopeptides with at least three quantifications are available for full statistical data analysis, pairwise t-testing, and ANOVA, which are frequently used. Therefore, we term phosphopeptides with at least one quantification among technical replicates as “quantitative coverage” and phosphopeptides with at least three quantifications as “statistics-available coverage.”
To obtain an impression of the quantification coverage in a phosphoproteomics experiment, the averaged abundance of all quantitative phosphopeptides across all replicates is calculated and is visualized as dots in a box plot, as exemplified for maize MF and maize root total protein (Fig. 4C and 4D). In principle, lower-abundance proteins/phosphoproteins have a lower probability of being among the statistics-available quantifications. Thus, the higher the abundance of a phosphopeptide, the better the reproducibility of its quantification is. For example, phosphopeptides with log2-FOT greater than –12 are usually found among the statistics-available coverage, whereas phosphopeptides with log2-FOT lower than –14 are usually difficult to quantify as statistics-available. This indicates that when focusing on certain low-abundance phosphopeptides, further optimization of sample preparation, for example by additional enrichment of the target protein or target organelle, is necessary.
Quantification coverage refers to how many phosphopeptides can be quantified in a set of multiple replicates. It can be used to estimate the extent of phosphopeptides available for downstream statistical analysis. Quantitative coverage results in 1751 phosphopeptides using the Fe(III)-based method (Alternate Protocol 2) with 8 technical replicates and in 1436 phosphopeptides using the TiO2-based method (Basic Protocol 6) with 10 technical replicates, for example from maize MF proteins. Statistics-available coverage usually is much smaller; in our example, it covers 542 and 675 phosphopeptides using the Fe(III)-based method and TiO2-based method, respectively (Fig. 4C). Based on such considerations, we can now estimate that with 4 to 6 technical replicates from total protein extracts in all tissues, the quantitative coverage will range from 1110 to 2244 phosphopeptides, and the statistics-available coverage will range from 301 to 1227 phosphopeptides. The highest quantitative coverage is likely found in preparations from root tissue combining the SP3 and Fe(III) methods (Alternate Protocols 1 and 2). This high yield of quantifiable phosphopeptides from roots is likely attributable to better protein extraction efficiency (less fibers) and due to lower content of interfering secondary metabolites (Fig. 4D).
Based on the above considerations, we can now investigate how quantitative and statistics-available coverage increases with technical replicates, as exemplified for phosphopeptides from MF protein (Basic Protocol 3; Fig. 4E) and from root total protein (Basic Protocol 1; Fig. 4F). In general, the quantification coverage increases with sample size (i.e., number of replicates), and the saturation point appears at around eight replicates (Fig. 4E and 4F). These relationships can now be used in decisions on the numbers of necessary technical replicates. Here, we propose up to six replicates with PC/TiO2-based (Basic Protocols 2 and 6) methods to allow statistics-available coverage of around 375 phosphopeptides from MF protein and 1250 phosphopeptides from maize root total protein. In turn, aiming for about 1000 phosphopeptides for statistics-available coverage would require more than six replicates.
In principle, different protein clean-up and phosphopeptide enrichment methods obtain slightly different sets of phosphopeptides, as they work by different mechanisms and sensitivities, leading to slightly selective enrichments. Despite the slight bias of each enrichment method, the results show that most of the phosphopeptides can be quantified irrespective of the enrichment method used (Fig. 4G, 4H, and 4I). Existing datasets can work as a reference to indicate which phosphopeptides may prefer which methods.
Time Considerations
It usually takes ∼1 week to complete all the experimental steps in Basic Protocols 1 to 7, depending on sample numbers. Loading of samples for the LC-MS/MS analysis (Basic Protocol 8) can occur independently, up to several weeks later. In principle, to prevent protein degradation and sample handling variance, it is better to carry out the steps as continuously as possible and to avoid repeated freeze-thawing of samples in a liquid environment. Interruptions at steps that obtain dry samples (e.g., after Basic Protocol 2 or 7) are better than interruptions at steps resulting in liquid samples (e.g., after Basic Protocol 5).
A typical work plan may look like this: On day 1, prepare all required reagents and solutions, except those that should be prepared fresh. For total protein extraction (Basic Protocol 1), spend day 2 or more grinding all samples into fine powder and storing aliquots (50 to 200 mg) at –80°C. On day 3, extract proteins for all samples and perform protein clean-up (Basic Protocol 2 or Alternate Protocol 1). During Basic Protocol 2, one can pause at steps 4 to 6 by storing the samples at –20°C and continue to protein digestion with Basic Protocol 5 the next day (i.e., day 3). If using Alternate Protocol 1, it is recommended to proceed to step 22. Basic Protocol 4 normally takes 30 min to 2 hr, depending on the sample size. If a pause is needed, one can interrupt only at step 6; the samples can be stored at –20°C during the break. For MF protein extraction in Basic Protocol 3, we recommend performing steps 1 to 6 in batches of six samples per day, and these can be stored at –20°C after step 7 until all batches of six samples are at this step.
After protein digestion overnight (Basic Protocol 5), we recommend to directly proceed to phosphopeptide enrichment in Basic Protocol 6 or Alternate Protocol 2 (2 to 3 hr) and to continue to peptide desalting with Basic Protocol 7 (1 to 2 hr) until obtaining dried peptides at step 19. Given that Basic Protocol 6 has many pipetting steps, the number of samples one can process per day depends on the operator's capacity and usually ranges between 10 and 40. Therefore, one should consider how many samples can be processed per day in this step to determine the number of samples to digest. In contrast, using the robotic system, Alternate Protocol 2 can process up to 96 samples per batch. On the robotic platform, peptide clean-up and phosphopeptide enrichment procedures take 4 to 5 hr altogether. The LC-MS/MS run (Basic Protocol 8) takes around 1.5 to 3 hr per sample (depending on the LC-MS/MS setup in use). In Basic Protocol 8, we recommend running all samples from an experiment as a continuous batch using the same column setup.
Acknowledgments
The authors thank M. Sc. Zeeshan for assistance with sample preparation. This work was funded by the Deutsche Forschungsgemeinschaft (DFG), 328017493/GRK 2366 (International Research Training Group “Adaptation of maize-based food-feed-energy systems to limited phosphate resources”).
Open access funding enabled and organized by Projekt DEAL.
Author Contributions
Mingjie He : Data curation, Formal analysis, Investigation, Methodology, Writing — original draft, Writing — review & editing; Jiahui Wang : Data curation, Methodology; Sandra Herold : Methodology, Software; Lin Xi : Conceptualization, Data curation, Investigation, Methodology, Writing — original draft, Writing — review & editing; Waltraud X. Schulze : Conceptualization, Writing — review & editing.
Conflict of Interest
The authors declare no conflict of interest.
Open Research
Data Availability Statement
The data, tools, and materials (or their source) that support the protocols are available from the corresponding author upon reasonable request.
Literature Cited
- Almeida, F. A., Passamani, L. Z., Santa-Catarina, C., Mooney, B. P., Thelen, J. J., & Silveira, V. (2020). Label-free quantitative phosphoproteomics reveals signaling dynamics involved in embryogenic competence acquisition in sugarcane. Journal of Proteome Research , 19, 4145–4157. doi: 10.1021/acs.jproteome.0c00652.
- Arribas Diez, I., Govender, I., Naicker, P., Stoychev, S., Jordaan, J., & Jensen, O. N. (2021). Zirconium(IV)-IMAC revisited: Improved performance and phosphoproteome coverage by magnetic microparticles for phosphopeptide affinity enrichment. Journal of Proteome Research , 20, 453–462. doi: 10.1021/acs.jproteome.0c00508.
- Bekker-Jensen, D. B., Bernhardt, O. M., Hogrebe, A., Martinez-Val, A., Verbeke, L., Gandhi, T., … Olsen, J. V. (2020). Rapid and site-specific deep phosphoproteome profiling by data-independent acquisition without the need for spectral libraries. Nature Communications , 11, 1–12. doi: 10.1038/s41467-020-14609-1.
- Beltran, L., & Cutillas, P. R. (2012). Advances in phosphopeptide enrichment techniques for phosphoproteomics. Amino Acids , 43, 1009–1024. doi: 10.1007/s00726-012-1288-9.
- Bittremieux, W., Tabb, D. L., Impens, F., Staes, A., Timmerman, E., Martens, L., & Laukens, K. (2018). Quality control in mass spectrometry-based proteomics. Mass Spectrometry Reviews , 37, 697–711. doi: 10.1002/mas.21544.
- Bonhomme, L., Valot, B., Tardieu, F., & Zivy, M. (2012). Phosphoproteome dynamics upon changes in plant water status reveal early events associated with rapid growth adjustment in maize leaves. Molecular and Cellular Proteomics , 11, 957–972. doi: 10.1074/mcp.M111.015867.
- Bradford, M. (1976). A rapid and sensitive method for the quantitation of microgram quantities of protein utilizing the principle of protein-dye binding. Analytical Chemistry , 72, 248–254.
- Cao, H., Zhou, Y., Chang, Y., Zhang, X., Li, C., & Ren, D. (2019). Comparative phosphoproteomic analysis of developing maize seeds suggests a pivotal role for enolase in promoting starch synthesis. Plant Science , 289, 110243. doi: 10.1016/j.plantsci.2019.110243.
- Chao, Q., Gao, Z.-F., Wang, Y.-F., Li, Z., Huang, X.-H., Wang, Y.-C., … Wang, B.-C. (2016). The proteome and phosphoproteome of maize pollen uncovers fertility candidate proteins. Plant Molecular Biology , 91, 287–304. doi: 10.1007/s11103-016-0466-7.
- Cox, J., & Mann, M. (2008). MaxQuant enables high peptide identification rates, individualized p.p.b.-range mass accuracies and proteome-wide protein quantification. Nature Biotechnology , 26, 1367–1372. doi: 10.1038/nbt.1511.
- Ding, L., Yang, R., Yang, G., Cao, J., Li, P., & Zhou, Y. (2016). Identification of putative phosphoproteins in wheat spikes induced by Fusarium graminearum. Planta , 243, 719–731. doi: 10.1007/s00425-015-2441-y.
- Erenstein, O., Chamberlin, J., & Sonder, K. (2021). Estimating the global number and distribution of maize and wheat farms. Global Food Security , 30, 100558. doi: 10.1016/j.gfs.2021.100558.
- Gates, M. B., Tomer, K. B., & Deterding, L. J. (2010). Comparison of metal and metal oxide media for phosphopeptide enrichment prior to mass spectrometric analyses. Journal of the American Society for Mass Spectrometry , 21, 1649–1659. doi: 10.1016/j.jasms.2010.06.005.
- Glatter, T., Ludwig, C., Ahrné, E., Aebersold, R., Heck, A. J. R., & Schmidt, A. (2012). Large-scale quantitative assessment of different in-solution protein digestion protocols reveals superior cleavage efficiency of tandem Lys-C/trypsin proteolysis over trypsin digestion. Journal of Proteome Research , 11, 5145–5156. doi: 10.1021/pr300273g.
- Grote, U., Fasse, A., Nguyen, T. T., & Erenstein, O. (2021). Food security and the dynamics of wheat and maize value chains in Africa and Asia. Frontiers in Sustainable Food Systems , 4, 1–17. doi: 10.3389/fsufs.2020.617009.
- He, M., Li, X., Mang, M., Li, Z., Ludewig, U., & Schulze, W. X. (2022). A systems-biology approach identifies co-expression modules in response to low phosphate supply in maize lines of different breeding history. The Plant Journal , 109(5), 1249–1270. doi: 10.1111/tpj.15630.
- Hildonen, S., Halvorsen, T. G., & Reubsaet, L. (2014). Why less is more when generating tryptic peptides in bottom-up proteomics. Proteomics , 14, 2031–2041. doi: 10.1002/pmic.201300479.
- Hong, Y., Flinkman, D., Suomi, T., Pietilä, S., James, P., Coffey, E., & Elo, L. L. (2021). PhosPiR: An automated phospho-proteomic pipeline in R. bioRxiv , 1–11. doi: 10.1101/2021.09.14.460225.
- Hsu, C. C., Zhu, Y., Arrington, J. V., Paez, J. S., Wang, P., Zhu, P., … Tao, W. A. (2018). Universal plant phosphoproteomics workflow and its application to tomato signaling in response to cold stress. Molecular and Cellular Proteomics , 17, 2068–2080. doi: 10.1074/mcp.TIR118.000702.
- Hu, X., Wu, L., Zhao, F., Zhang, D., Li, N., Zhu, G., … Wang, W. (2015). Phosphoproteomic analysis of the response of maize leaves to drought, heat and their combination stress. Frontiers in Plant Science , 6, 298. doi: 10.3389/fpls.2015.00298.
- Hughes, C. S., Moggridge, S., Müller, T., Sorensen, P. H., Morin, G. B., & Krijgsveld, J. (2019). Single-pot, solid-phase-enhanced sample preparation for proteomics experiments. Nature Protocols , 14, 68–85. doi: 10.1038/s41596-018-0082-x.
- Kelstrup, C. D., Bekker-Jensen, D. B., Arrey, T. N., Hogrebe, A., Harder, A., & Olsen, J. V. (2018). Performance evaluation of the Q exactive HF-X for shotgun proteomics. Journal of Proteome Research , 17, 727–738. doi: 10.1021/acs.jproteome.7b00602.
- Kim, H. J., Kim, T., Hoffman, N. J., Xiao, D., James, D. E., Humphrey, S. J., & Yang, P. (2021). PhosR enables processing and functional analysis of phosphoproteomic data. Cell Reports , 34, (8), 108771. doi: 10.1016/j.celrep.2021.108771.
- Kim, H. J., Kim, T., Xiao, D., & Yang, P. (2021). Protocol for the processing and downstream analysis of phosphoproteomic data with PhosR. STAR Protocols , 2(2), 100585. doi: 10.1016/j.xpro.2021.100585.
- Kupcik, R., MacAk, J. M., Rehulkova, H., Sopha, H., Fabrik, I., Anitha, V. C., … Rehulka, P. (2019). Amorphous TiO2 nanotubes as a platform for highly selective phosphopeptide enrichment. ACS Omega , 4, 12156–12166. doi: 10.1021/acsomega.9b00571.
- Lamers, J., Van Der Meer, T., & Testerink, C. (2020). How plants sense and respond to stressful environments. Plant Physiology , 182, 1624–1635. doi: 10.1104/pp.19.01464.
- Laskay, Ü. A., Lobas, A. A., Srzentić, K., Gorshkov, M. V., & Tsybin, Y. O. (2013). Proteome digestion specificity analysis for rational design of extended bottom-up and middle-down proteomics experiments. Journal of Proteome Research , 12, 5558–5569. doi: 10.1021/pr400522h.
- Li, S., Zan, H., Zhu, Z., Lu, D., & Krall, L. (2021). Plant phosphopeptide identification and label-free quantification by MaxQuant and Proteome Discoverer software. In X. N. Wu (Ed.), Plant phosphoproteomics (pp. 179–187). Humana.
- Li, Z., Phillip, D., Neuhäuser, B., Schulze, W. X., & Ludewig, U. (2015). Protein dynamics in young maize root hairs in response to macro- and micronutrient deprivation. Journal of Proteome Research , 14, 3362–3371. doi: 10.1021/acs.jproteome.5b00399.
- Liang, X., Fonnum, G., Hajivandi, M., Stene, T., Kjus, N. H., Ragnhildstveit, E., … Pope, R. M. (2007). Quantitative comparison of IMAC and TiO2 surfaces used in the study of regulated, dynamic protein phosphorylation. Journal of the American Society for Mass Spectrometry , 18, 1932–1944. doi: 10.1016/j.jasms.2007.08.001.
- Lou, R., Liu, W., Li, R., Li, S., He, X., & Shui, W. (2021). DeepPhospho accelerates DIA phosphoproteome profiling through in silico library generation. Nature Communications , 12, 1–15. doi: 10.1038/s41467-021-26979-1.
- Luebker, S. A., & Koepsell, S. A. (2016). Optimization of urea based protein extraction from formalin-fixed paraffin-embedded tissue for shotgun proteomics. International Journal of Proteomics , 2016, 4324987. doi: 10.1155/2016/4324987.
- Luís, I. M., Alexandre, B. M., Oliveira, M. M., & Abreu, I. A. (2016). Selection of an appropriate protein extraction method to study the phosphoproteome of maize photosynthetic tissue. PLOS ONE , 11, 1–16. doi: 10.1371/journal.pone.0164387.
- Millar, A. H., Heazlewood, J. L., Giglione, C., Holdsworth, M. J., Bachmair, A., & Schulze, W. X. (2019). The scope, functions, and dynamics of posttranslational protein modifications. Annual Review of Plant Biology , 70, 119–151. doi: 10.1146/annurev-arplant-050718-100211.
- Nie, Z., Luo, B., Zhang, X., Wu, L., Liu, D., Guo, J., … Gao, S. (2021). Combined transcriptome and proteome analysis of maize (Zea mays l.) reveals a complementary profile in response to phosphate deficiency. Current Issues in Molecular Biology , 43, 1142–1155. doi: 10.3390/cimb43020081.
- Niu, L., Yuan, H., Gong, F., Wu, X., & Wang, W. (2018). Protein extraction methods shape much of the extracted proteomes. Frontiers in Plant Science , 9, 802. doi: 10.3389/fpls.2018.00802.
- Olson, B. J. S. C., & Markwell, J. (2007). Assays for determination of protein concentration. Current Protocols in Protein Science , 48, 3.4.1–3.4.29. doi: 10.1002/0471140864.ps0304s48.
- Pang, Q., Zhang, T., Zhang, A., Lin, C., Kong, W., & Chen, S. (2020). Proteomics and phosphoproteomics revealed molecular networks of stomatal immune responses. Planta , 252, 1–17. doi: 10.1007/s00425-020-03474-3.
- Pertl-Obermeyer, H., & Obermeyer, G. (2014). Pollen cultivation and preparation for proteomic studies. Methods in Molecular Biology , 1072, 435–449. doi: 10.1007/978-1-62703-631-3_30.
- Rappsilber, J., Ishihama, Y., & Mann, M. (2003). STop And Go Extraction tips for matrix-assisted laser desorption/ionization, nanoelectrospray, and LC/MS sample pretreatment in proteomics. Analytical Chemistry , 75, 663–670. doi: 10.1021/ac026117i.
- Ressa, A., Fitzpatrick, M., Van Den Toorn, H., Heck, A. J. R., & Altelaar, M. (2019). PaDuA: A python library for high-throughput (phospho)proteomics data analysis. Journal of Proteome Research , 18, 576–584. doi: 10.1021/acs.jproteome.8b00576.
- Russell, J. D., & Murphy, S. (2016). Agilent AssayMAP bravo technology enables reproducible automated phosphopeptide enrichment from complex mixtures using high‑capacity Fe(III)‑NTA cartridges. 1–15. Retrieved from https://www.agilent.com/cs/library/applications/5991-6073EN.pdf%0Ahttps://www.agilent.com/en/products/automation-solutions/protein-sample-preparation/assaymap-bravo-platform#literature.
- Saveliev, S., Bratz, M., Zubarev, R., Szapacs, M., Budamgunta, H., & Urh, M. (2013). Trypsin/Lys-C protease mix for enhanced protein mass spectrometry analysis. Nature Methods , 10, i–ii. doi: 10.1038/nmeth.f.371.
- Schulze, W. X., Altenbuchinger, M., He, M., Kränzlein, M., & Zörb, C. (2021). Proteome profiling of repeated drought stress reveals genotype-specific responses and memory effects in maize. Plant Physiology and Biochemistry , 159, 67–79. doi: 10.1016/j.plaphy.2020.12.009.
- Schulze, W. X., & Usadel, B. (2010). Quantitation in mass-spectrometry-based proteomics. Annual Review of Plant Biology , 61, 491–516. doi: 10.1146/annurev-arplant-042809-112132.
- Schwessinger, B., Roux, M., Kadota, Y., Ntoukakis, V., Sklenar, J., Jones, A., & Zipfel, C. (2011). Phosphorylation-dependent differential regulation of plant growth, cell death, and innate immunity by the regulatory receptor-like kinase BAK1. PLOS Genetics , 7, e1002046. doi: 10.1371/journal.pgen.1002046.
- Silva-Sanchez, C., Li, H., Chen, S., & Chen, S. (2015). Recent advances and challenges in plant phosphoproteomics. Proteomics , 1, 1–33. doi: 10.1002/pmic.201400410.
- Smith, P. K., Krohn, R. I., Hermanson, G. T., Mallia, A. K., Gartner, F. H., Provenzano, M. D., … Klenk, D. C. (1985). Measurement of protein using bicinchoninic acid. Analytical Biochemistry , 150 ( 1 ) , 76–85. doi: 10.1016/0003-2697(85)90442-7.
- Sun, S., Zhou, J. Y., Yang, W., & Zhang, H. (2014). Inhibition of protein carbamylation in urea solution using ammonium-containing buffers. Analytical Biochemistry , 446, 76–81. doi: 10.1016/j.ab.2013.10.024.
- Thakur, S. S., Geiger, T., Chatterjee, B., Bandilla, P., Fröhlich, F., Cox, J., & Mann, M. (2011). Deep and highly sensitive proteome coverage by LC-MS/MS without prefractionation. Molecular & Cellular Proteomics: MCP, 10, M110.003699. doi: 10.1074/mcp.M110.003699.
- Tuli, L., & Ressom, H. W. (2009). LC-MS based detection of differential protein expression. Journal of Proteomics and Bioinformatics , 2, 416–438. doi: 10.4172/jpb.1000102.
- Tyanova, S., Temu, T., & Cox, J. (2016). The MaxQuant computational platform for mass spectrometry-based shotgun proteomics. Nature Protocols , 11, 2301–2319. doi: 10.1038/nprot.2016.136.
- Tyanova, S., Temu, T., Sinitcyn, P., Carlson, A., Hein, M. Y., Geiger, T., … Cox, J. (2016). The Perseus computational platform for comprehensive analysis of (prote)omics data. Nature Methods , 13, 731–740. doi: 10.1038/nmeth.3901.
- Vu, L. D., Zhu, T., Verstraeten, I., Van De Cotte, B., Gevaert, K., & De Smet, I. (2018). Temperature-induced changes in the wheat phosphoproteome reveal temperature-regulated interconversion of phosphoforms. Journal of Experimental Botany , 69, 4609–4624. doi: 10.1093/jxb/ery204.
- Wang, W., Tai, F., & Chen, S. (2008). Optimizing protein extraction from plant tissues for enhanced proteomics analysis. Journal of Separation Science , 31, 2032–2039. doi: 10.1002/jssc.200800087.
- Wiśniewski, J. R., Zougman, A., Nagaraj, N., & Mann, M. (2009). Universal sample preparation method for proteome analysis. Nature Methods , 6, 359–362. doi: 10.1038/nmeth.1322.
- Wu, L., Hu, X., Wang, S., Tian, L., Pang, Y., Han, Z., … Chen, Y. (2015). Quantitative analysis of changes in the phosphoproteome of maize induced by the plant hormone salicylic acid. Scientific Reports , 5, 1–16. doi: 10.1038/srep18155.
- Wu, X., Gong, F., & Wang, W. (2014). Protein extraction from plant tissues for 2DE and its application in proteomic analysis. Proteomics , 14, 645–658. doi: 10.1002/pmic.201300239.
- Wu, X. N., Xi, L., Pertl-Obermeyer, H., Li, Z., Chu, L.-C., & Schulze, W. X. (2017). Highly efficient single-step enrichment of low abundance phosphopeptides from plant membrane preparations. Frontiers in Plant Science , 8, 1–14. doi: 10.3389/fpls.2017.01673.
- Wu, X., Xiong, E., Wang, W., Scali, M., & Cresti, M. (2014). Universal sample preparation method integrating trichloroacetic acid/acetone precipitation with phenol extraction for crop proteomic analysis. Nature Protocols , 9, 362–374. doi: 10.1038/nprot.2014.022.
- Yoshikawa, H., Hirano, A., Arakawa, T., & Shiraki, K. (2012a). Effects of alcohol on the solubility and structure of native and disulfide-modified bovine serum albumin. International Journal of Biological Macromolecules , 50, 1286–1291. doi: 10.1016/j.ijbiomac.2012.03.014.
- Yoshikawa, H., Hirano, A., Arakawa, T., & Shiraki, K. (2012b). Mechanistic insights into protein precipitation by alcohol. International Journal of Biological Macromolecules , 50, 865–871. doi: 10.1016/j.ijbiomac.2011.11.005.
- Yu, S. H., Ferretti, D., Schessner, J. P., Rudolph, J. D., Borner, G. H. H., & Cox, J. (2020). Expanding the perseus software for omics data analysis with custom plugins. Current Protocols in Bioinformatics , 71, 1–29. doi: 10.1002/cpbi.105.
- Zauber, H., & Schulze, W. X. (2012). Proteomics wants cRacker: Automated standardized data analysis of LC-MS derived proteomic data. Journal of Proteome Research , 11, 5548–5555. doi: 10.1021/pr300413v.
- Zhao, X., Bai, X., Jiang, C., & Li, Z. (2019). Phosphoproteomic analysis of two contrasting maize inbred lines provides insights into the mechanism of salt-stress tolerance. International Journal of Molecular Sciences , 20 ( 8 ) , 1886. doi: 10.3390/ijms20081886.