Tunable Synthetic Hydrogels to Study Angiogenic Sprouting
Giuseppe Trapani, Giuseppe Trapani, Martin Sebastian Weiß, Martin Sebastian Weiß, Britta Trappmann, Britta Trappmann
angiogenic sprouting
cell-matrix interactions
extracellular matrix
endothelial cell migration
synthetic hydrogels
Abstract
Angiogenic sprouting, the formation of new blood vessels from pre-existing vasculature, is tightly regulated by the properties of the surrounding tissue microenvironment. Although the extracellular matrix has been shown to be a major regulator of this process, it is not clear how individual biochemical and mechanical properties influence endothelial cell sprouting. This information gap is largely due to the lack of suitable in vitro models that recapitulate angiogenic sprouting in a 3D environment with independent control over matrix properties. Here, we present protocols for the preparation of endothelial cell spheroid-laden synthetic, dextran-based hydrogels, which serve as a highly tunable 3D scaffold. The adjustment of the hydrogels’ adhesiveness, stiffness, and degradability is demonstrated in detail. Finally, we describe assays to elucidate how individual matrix properties regulate angiogenic sprouting, including their analysis by immunofluorescence staining and imaging. © 2023 The Authors. Current Protocols published by Wiley Periodicals LLC.
Basic Protocol 1 : Synthesis of methacrylated dextran (DexMA)
Basic Protocol 2 : Generation of endothelial cell spheroids in microwells
Basic Protocol 3 : Endothelial cell sprouting in hydrogels of tunable stiffness
Basic Protocol 4 : Endothelial cell sprouting in hydrogels of tunable adhesiveness
Basic Protocol 5 : Endothelial cell sprouting in hydrogels of tunable degradability
Basic Protocol 6 : Imaging of endothelial cell spheroid-laden hydrogels
Support Protocol 1 : Preparation of pro-angiogenic cocktail for endothelial cell sprouting
INTRODUCTION
During angiogenic sprouting, endothelial cells are triggered to exit pre-existing blood vessels and form new vascular structures. This complex, multi-step process is regulated by many cues from the surrounding tissue microenvironment (Grainger & Putnam, 2013). One critical regulator is the extracellular matrix (ECM), the three-dimensional network of proteins and polysaccharides, which not only provides structural support but also presents important biochemical and mechanical signals to residing cells. Here, matrix adhesiveness, stiffness, and degradability have emerged as important parameters regulating cell and tissue function (Madl & Heilshorn, 2018). However, how individual matrix properties affect angiogenic sprouting in 3D tissues remains largely unknown. Answering this question requires 3D hydrogel models, whose hydrated, porous architecture mimics the natural tissue microenvironment while offering full and independent control over individual matrix properties of interest.
In principle, hydrogels for in vitro models of angiogenic sprouting are based on either naturally occurring or synthetic materials. Natural hydrogels are composed of ECM proteins (such as type I collagen or fibrin) that self-assemble into hydrated fibrous 3D networks with micrometer-sized pores, and are characterized by inherent biocompatibility. Although such hydrogels are used in angiogenesis studies (Kim et al., 2013; Nguyen et al., 2013), it is difficult to tune one ECM parameter (e.g., stiffness) without concurrently affecting another one (e.g., adhesiveness) (Ingber, 1990). This limitation can be overcome through the use of synthetic hydrogels, which are based on an initially cell- and protein-inert polymer backbone that is subsequently functionalized with bioactive molecules of interest, thereby offering full control over ECM properties. Synthetic hydrogels are linear elastic (spring-like behavior) and mechanically isotropic (uniform stiffness in all directions) materials that are characterized by pores in the size range of tens to hundreds of nanometers (Trappmann & Chen, 2013; Trappmann et al., 2012). Additionally, their mechanical properties can be tuned to span the stiffness range of in vivo tissues (Discher et al., 2005; Engler et al., 2006; Yeung et al., 2005). Examples of commonly used fully synthetic or naturally occurring backbone polymers are poly(ethylene glycol), dextran, and hyaluronic acid.
In this article, we describe the preparation of a synthetic, dextran-based hydrogel with independent control over stiffness, adhesiveness, and matrix metalloproteinase (MMP)-mediated degradability, and its use in angiogenic sprouting assays of embedded endothelial cell spheroids (ECSs). In this system, the invasion of endothelial cells into the surrounding hydrogel is initiated by a cocktail of pro-angiogenic factors, in a process that resembles in vivo angiogenic sprouting. The formation and extension of endothelial cell sprouts can be studied in real time, and matrix-dependent changes in sprout phenotypes can be directly correlated with a hydrogel property of interest.
In Basic Protocol 1, we report the synthesis and purification of methacrylated dextran (DexMA), the main constituent of our synthetic hydrogels. In Basic Protocol 2, we describe the preparation of ECSs as a model to examine angiogenic sprouting. ECSs are then embedded within DexMA hydrogels of varying stiffness in Basic Protocol 3, of different adhesive ligand densities in Basic Protocol 4, and of varying degradability in Basic Protocol 5. As a means to analyze endothelial cell sprouts within synthetic hydrogels, procedures for immunofluorescence staining and imaging of the 3D samples are outlined in Basic Protocol 6. Finally, Support Protocol 1 details the preparation of the pro-angiogenic cocktail used to drive endothelial cell sprouting.
NOTE : Although we have focused here on human umbilical cord vein endothelial cells (HUVECs) as a model cell type in this protocol, the techniques described are also directly applicable to other endothelial cell types, such as human microvascular cells (Liu et al., 2021).
Basic Protocol 1: SYNTHESIS OF METHACRYLATED DEXTRAN (DexMA)
DexMA serves as an ideal backbone for a tunable 3D cell culture matrix, the most important building block for an in vitro model of angiogenic sprouting. First, DexMA chains are cytocompatible, enabling live cell encapsulation during gelation; second, DexMA is intrinsically resistant to protein adsorption and cell adhesion, allowing the controlled introduction of any biofunctionality of choice; and third, the polysaccharide backbone displays abundant functional groups, thereby providing complete and orthogonal control over bioactive moieties and crosslinking components (Liu et al., 2021; Trappmann et al., 2017). In particular, hydrogel stiffness can be adjusted solely by tuning the degree of network crosslinking, without changes in polymer content, which in turn affect pore size and solute diffusion—an advantage over other backbones with limited functionalities, such as poly(ethylene glycol) diacrylate (PEGDA; Trappmann et al., 2017). Additionally, functionalization with balanced concentrations of hydrophobic pendant groups can eliminate hydrogel swelling, thereby enabling 3D molding applications needed for more complex models of angiogenic sprouting that also take the structural features of native blood vessels into account (Trappmann et al., 2017).
Here, we describe a method for the methacrylation of dextran through its base-catalyzed reaction with glycidyl methacrylate (Fig. 1). The crude product is purified by precipitation and dialysis to remove unreacted compounds and then is recovered as a pale orange product after lyophilization (Fig. 2). Dextran methacrylation levels can be analyzed through 1H nuclear magnetic resonance (1H-NMR) spectroscopy.
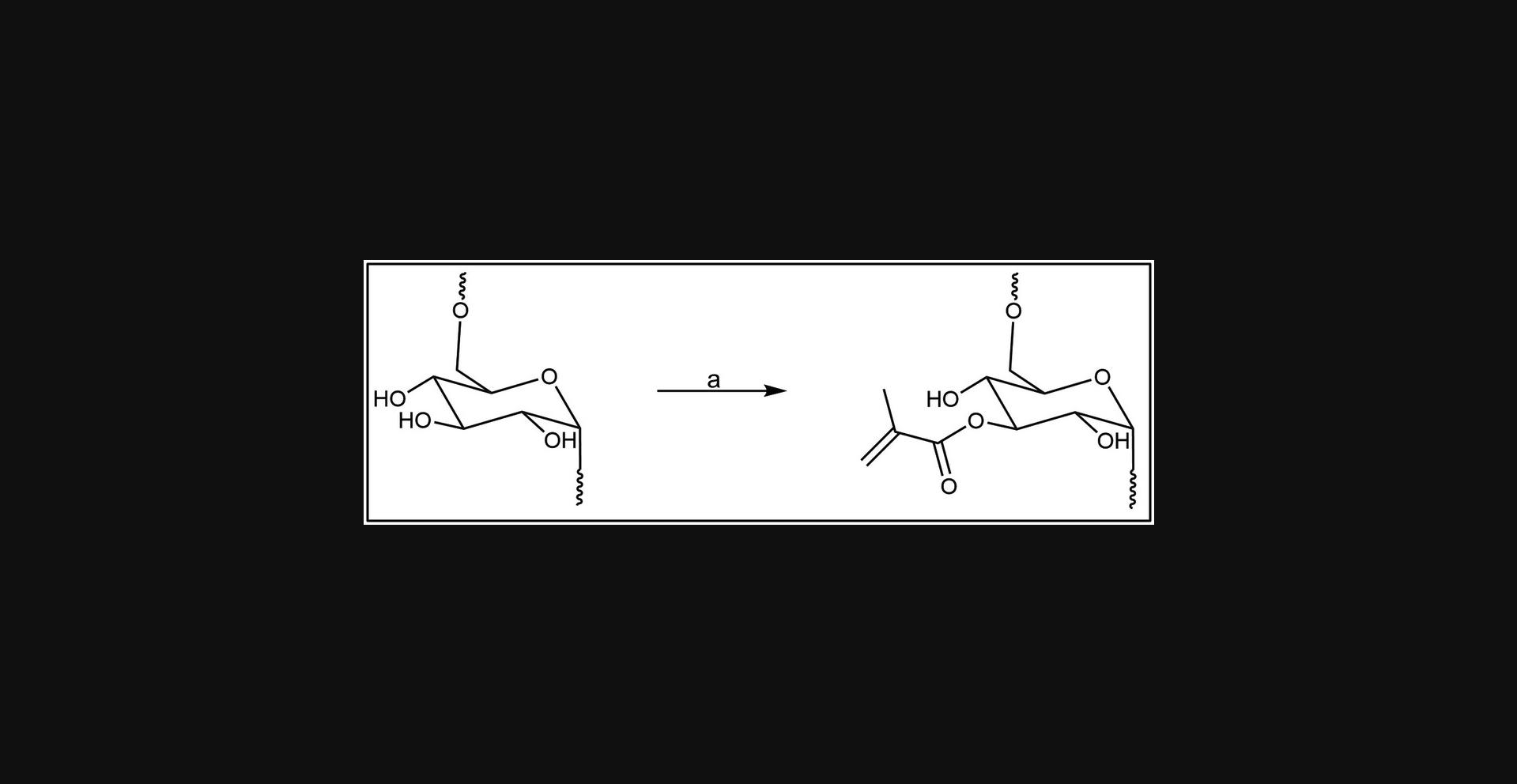
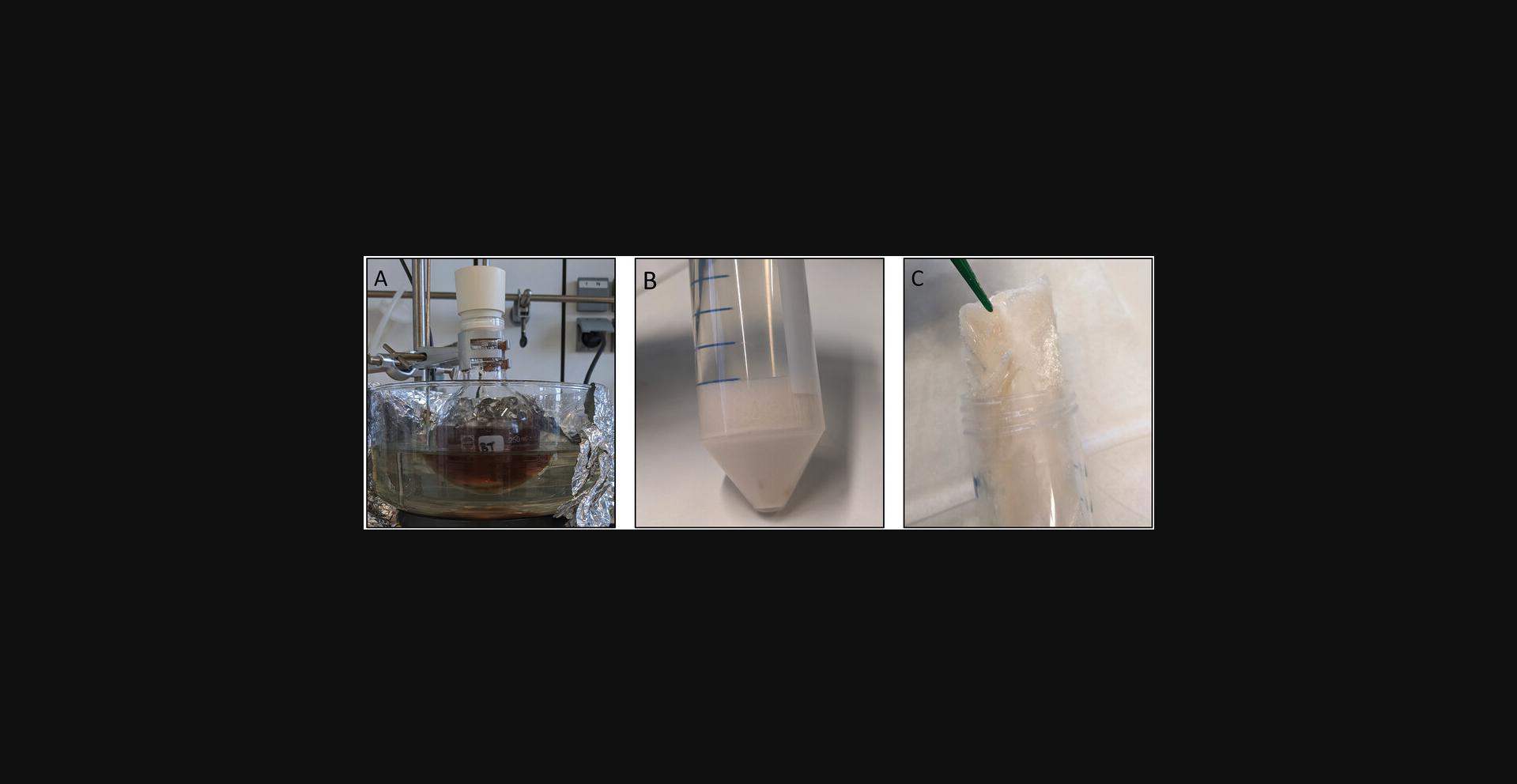
Materials
- Dextran (MP Biomedicals; MW 60,000-90,000 Da, cat. no. 20519580)
- Anhydrous dimethyl sulfoxide (DMSO; Sigma-Aldrich, cat. no. 276855)
- 4-Dimethylaminopyridine (DMAP; Fluka Analytical, cat. no. 39405)
- Glycidyl methacrylate (GMA; Sigma-Aldrich, cat. no. 779342)
- 2-Propanol (IPA; VWR chemicals, cat. no. 20842.330), 4°C
- Milli-Q water
- Deuterium oxide (D2O; Sigma-Aldrich, cat. no. 151882)
- Scale
- 250-ml round-bottom flask and septum
- Stir bar
- Magnetic hotplate
- Syringe
- Serological glass pipet
- Rubber bulb
- Oil bath
- Temperature sensor
- Tin foil
- Parafilm
- 2- and 4-L beakers
- 50-ml conical centrifuge tubes (Corning® Falcon®, cat. no. 352070)
- Centrifuge
- Dialysis tubing (SnakeSkin Dialysis Tubing, 3500 MWCO, 35 mm dry I.D., 35 feet; ThermoScientific, cat. no. 88244)
- Clamps
- −20°C freezer
- Freeze-dryer (lyophilizer), canisters, and adaptors
CAUTION : The methacrylation of dextran involves the use of hazardous chemicals and must be performed in a chemical fume hood.
DexMA synthesis
1.Weigh 20 g dextran into a 250-ml round-bottom flask and add a stir bar.
2.Using a syringe, add 100 ml anhydrous DMSO while mixing on a magnetic hotplate. Immediately close the flask with a septum.
3.After the dextran has completely dissolved, add 2 g DMAP.
4.After DMAP has dissolved, add 24.6 ml (1.5 equivalents relative to dextran repeating units) of GMA under vigorous stirring using a serological glass pipet with a rubber bulb. Ensure that the flask is well sealed with the septum, as the reaction is moisture sensitive.
5.Place the round-bottom flask into an oil bath and heat to 45°C, using a temperature sensor for most precise heating. Stir the mixture vigorously for 24 hr and cover the round-bottom flask with tin foil to minimize exposure of the solution to light (Fig. 2A).
DexMA purification and lyophilization
6.Cool down the solution to room temperature and precipitate DexMA by slowly pouring the reaction mixture into a beaker containing 1 L of cold (4°C) IPA under vigorous stirring.
7.Transfer the precipitate containing solution to 50-ml conical centrifuge tubes and centrifuge 2 min at 1600 rcf, 4°C.
8.Discard the supernatant and repeat centrifugation of the pellets for 2 min at 1600 rcf, 4°C, to expel most of the solvent.
9.Resuspend the pellets in Milli-Q water.
10.Cut dialysis tubing into the required length and soak in water for a few minutes to rehydrate the membrane.
11.Close one end of each dialysis tube with a clamp and transfer the DexMA solution, being careful not to fill the tubes completely. Close the other end of the tube with a clamp and transfer everything to a 4-L beaker filled with Milli-Q water and a stir bar. Perform dialysis with stirring at 4°C in the dark.
12.Replace dialysis water with fresh, ice-cold Milli-Q water twice per day for three consecutive days.
13.Transfer dialyzed dextran solution to 50-ml conical centrifuge tubes using a serological pipet. Place conical centrifuge tubes horizontally inside a −20°C freezer and freeze the solution overnight.
14.Lyophilize the frozen solution for ∼3 days to completely dry the polymer.
15.Store the lyophilized polymer (Fig. 2C) at −20°C.
16.Analyze the degree of methacrylate functionalization of the obtained product by 1H-NMR spectroscopy using deuterium oxide as solvent.
Basic Protocol 2: GENERATION OF ENDOTHELIAL CELL SPHEROIDS IN MICROWELLS
The basis for all in vitro models of angiogenic sprouting are endothelial cells, which in vivo line the inner wall of blood vessels and are triggered to migrate into the surrounding matrix by chemokine gradients (Heiss et al., 2015). More specifically, HUVECs have evolved as a major primary model cell type as they can be easily obtained in large numbers (Jiménez et al., 2013). Historically, HUVECs were generally cultured on surfaces; however, this configuration fails to recapitulate the natural sprouting phenotype in a 3D tissue microenvironment and frequently results in the loss of their differentiated phenotype (Fina et al., 1990). The maintenance of a more natural phenotype, in particular, can be supported by a more physiological 3D culture setup, e.g., in the form of spheroids (Heiss et al., 2015).
Over the years, many different methods have been developed to generate ECSs reproducibly and efficiently. All techniques drive cells to self-aggregate by preventing their adhesion to an underlying culture substrate (Fang & Eglen, 2017). One common approach is the culture of endothelial cell suspensions as hanging drops, leading to cell aggregation and spheroid formation (Foty, 2011). Although this method does not require special equipment and is therefore easily accessible to many labs, it often results in a comparably low spheroid yield and a low degree of homogeneity. Instead, low-adhesion wells with U- or V-shaped geometries can be optimized for high spheroid shape reproducibility, yet here, again, the number of generated spheroids is generally low. To improve yield, spheroids can be generated in low-adhesion microwell plates. Here, a standardized array of microwells (e.g., 400 µm × 400 µm in size) permits the formation of many, highly uniform spheroids from cell suspensions.
This protocol aims to generate spheroids of HUVECs in a 24-well low-adhesion AggreWellTM microwell plate. Before the cell suspension is added, an anti-adherence rinsing solution must be applied to the well to prevent cell adhesion to the microwells and to initiate spheroid formation (Fang & Eglen, 2017). The input cell density can be adjusted to control the individual sizes of each of the ∼1200 ECSs that can be yielded per well. The cell density applied in this protocol will result in spheroids of ∼140 µm in diameter after an incubation period of 24 hr (Fig. 3). At this time, they can be harvested from the microwells and used for downstream applications.
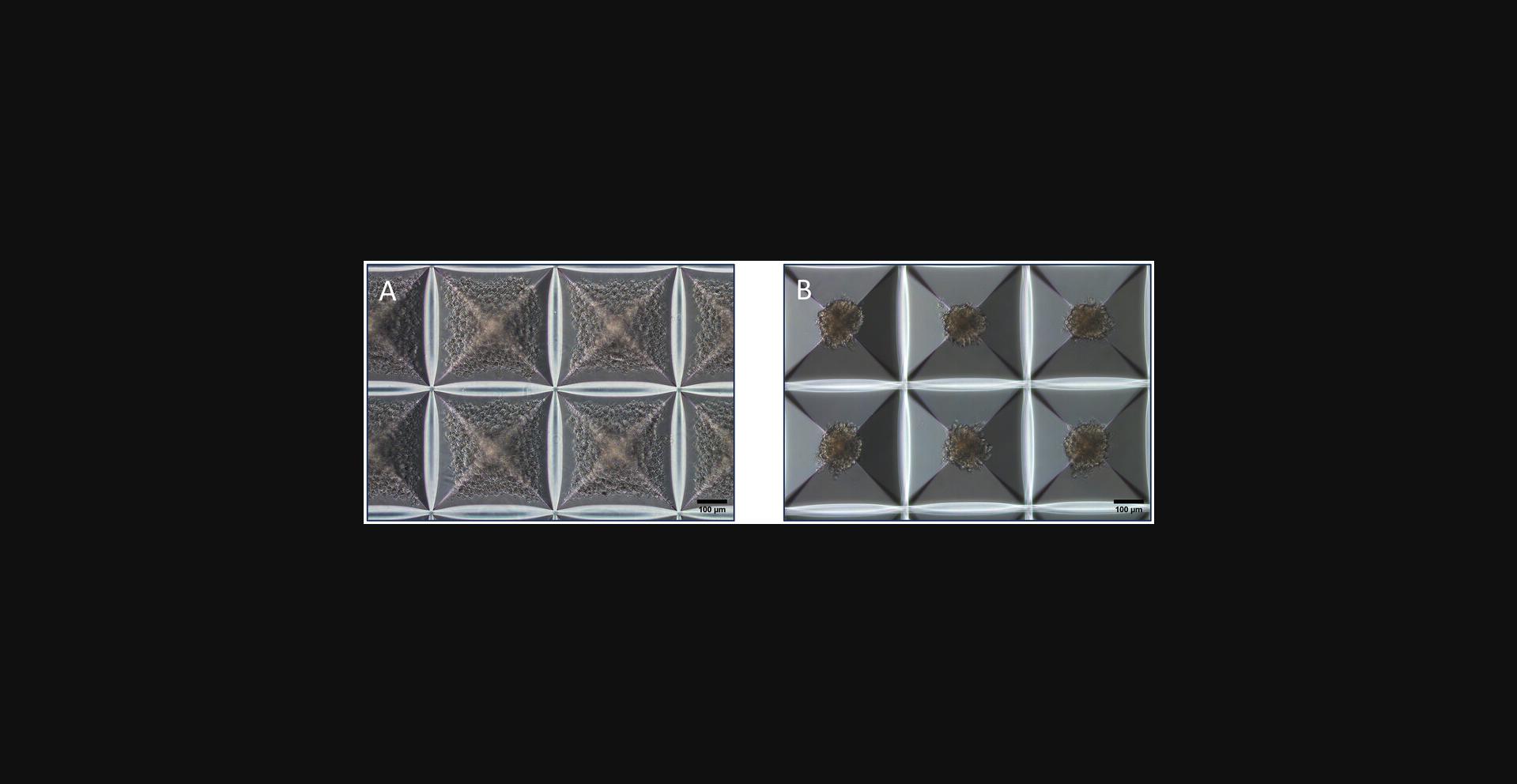
Materials
-
Anti-Adherence Rinsing Solution (StemCell Technologies, cat. no. 07010)
-
Dulbecco's Phosphate-Buffered Saline (PBS; Corning, cat. no. 21-031-CV)
-
Suspension of HUVECs (from pooled donors; Lonza, cat. no. C2519A; we used lot. no. 0000611160), cultivated following the vendor's instructions (advised cell passage ≤6)
-
Trypsin-EDTA (0.05%), with phenol red (ThermoFisher, Gibco, cat. no. 25300-054)
-
Endothelial Cell Growth Medium 2 (EGM-2) Kit (PromoCell, cat. no. C-22111) including Basal Medium 2 (PromoCell, cat. no. C-22211) and Supplement Pack (PromoCell, cat. no. C-39211)
-
Biological safety cabinet
-
10-cm tissue culture dishes
-
Set of single-channel pipets with respective tips
-
AggreWell™400 24-well Plates (StemCell Technologies, cat. no. 34411)
-
24-well plate (Greiner Bio-One CELLSTAR®, cat. no. 662160)
-
Centrifuge 5804/5804 R (Eppendorf, cat. no. 5805.000.010) or equivalent
-
Neubauer cell counting chamber (Marienfeld, cat. no. 0640110, or equivalent)
-
Tissue culture microscope
-
Water bath
-
Serological pipets
-
50-ml conical centrifuge tubes (Corning Falcon®, cat. no. 352070)
-
Rotor for Deepwell plates (Kisker-Biotech, cat. no. 033258, or equivalent)
-
Humidified cell culture incubator (37°C, 5% CO2)
NOTE : All steps of ECS preparation need to be performed in a biological safety cabinet.
Prepare AggreWell™400 plates with anti-adherence solution to prevent cell adhesion
1.Follow the manufacturer's instructions by adding the 0.5 ml of sterile anti-adherence solution to one well of the AggreWell™400 24-well plate. Prepare a balance plate using a standard 24-well plate filled with water to match the weight and filled positions of the AggreWell™400 24-well plate. Centrifuge both plates for 5 min at 1300 rcf, room temperature.
2.Aspirate the anti-adherence solution and wash once with 2 ml PBS. Aspirate PBS and add 1 ml EGM-2 to the well.
Prepare a cell suspension for spheroid generation
3.Prepare a cell suspension from a confluent 10-cm dish of HUVECs (∼4.5 million cells): Wash cells twice with PBS and spread 1 ml of trypsin-EDTA solution across the cell layer. Aspirate the solution and detach the cells by tapping the dish from the side. Check the degree of cell detachment under a cell culture microscope.
4.Take up cells with 10 ml EGM-2 medium using a serological pipet and transfer the suspension to a conical centrifuge tube. Count cells in a counting chamber or equivalent. Prepare a cell suspension of 1.2 × 106 cells/ml or as desired (see AggreWell™400 datasheet) in a fresh conical centrifuge tube.
Generate spheroids
5.Add 1 ml of cell suspension to the EGM-2-containing well of the AggreWell™400 24-well plate prepared in step 2, to achieve a total volume of 2 ml. Mix gently by pipetting up and down to ensure an even distribution of cells throughout the well.
6.Centrifuge the plate for 3 min at 100 rcf, room temperature, to capture cells in the microwells along with the balance plate.
7.Incubate the plate at 37°C with 5% CO2 and 95% humidity for 24 hr. Observe the cells under a microscope. Cells will assemble into spheroids after 24 hr (Fig. 3B).
Basic Protocol 3: ENDOTHELIAL CELL SPROUTING IN HYDROGELS OF TUNABLE STIFFNESS
Matrix stiffness is known to regulate endothelial cell migration patterns during angiogenic sprouting. In particular, we have shown that in both soft (∼0.8 kPa) and stiff (∼6 kPa) matrices, endothelial cells migrate as single cells, whereas cells acquire a collective migratory phenotype in matrices of intermediate stiffness (∼1.5 kPa) (Trappmann et al., 2017). Multicellular migration is required for subsequent vascular lumen formation, a critical step toward the formation of functional lab-grown blood vessels with in vivo -like properties (Liu et al., 2021; see Background Information for more details).
In Basic Protocol 3, we describe the preparation of DexMA hydrogels (Fig. 4) with tunable stiffness, which are optimally designed to study the stiffness-dependent sprouting phenotype of ECSs. The protocol is divided into four sections: First, glass-coverslip-supported PDMS wells are prepared as holders for the spheroid-laden hydrogels; next, DexMA is functionalized with the integrin-binding peptide RGD to enable cell-matrix engagement; subsequently, the spheroid-laden precursor solution is crosslinked into MMP-cleavable hydrogels; and finally, cells are initiated to sprout by a pro-angiogenic cocktail (Fig. 5). DexMA functionalization with cell-adhesive peptides as well as hydrogel formation through coupling of MMP-cleavable peptide crosslinkers occur through the methacrylate groups introduced on the sugar backbone in Basic Protocol 1.Methacrylates undergo Michael-type addition with thiols present in cysteine residues of the peptides (mono-cysteine adhesive ligands, bis-cysteine crosslinkers; Fig. 4). Specifically, the presence of a base catalyzes the formation of nucleophilic thiolate anions, which react with methacrylates (Nair et al., 2014). DexMA hydrogel stiffness can be tuned by changing the concentration of MMP-cleavable peptides (Table 1; refer also to Trappmann et al., 2017 for stiffness values as a function of different crosslinker concentration). Changing hydrogel stiffness while keeping the content of DexMA polymer chains constant is a crucial design criterion of our system, because it ensures a comparable pore size and hence biomolecule diffusivity across the entire stiffness range (Trappmann et al., 2017). DexMA hydrogel stiffness can be measured through nanoindentation, a widely applied technique to probe the mechanical properties of materials, described in detail elsewhere (Ebenstein & Pruitt, 2006; Xu et al., 2022). An example of stiffness-dependent changes in endothelial cell migration mode is presented in Figure 6.
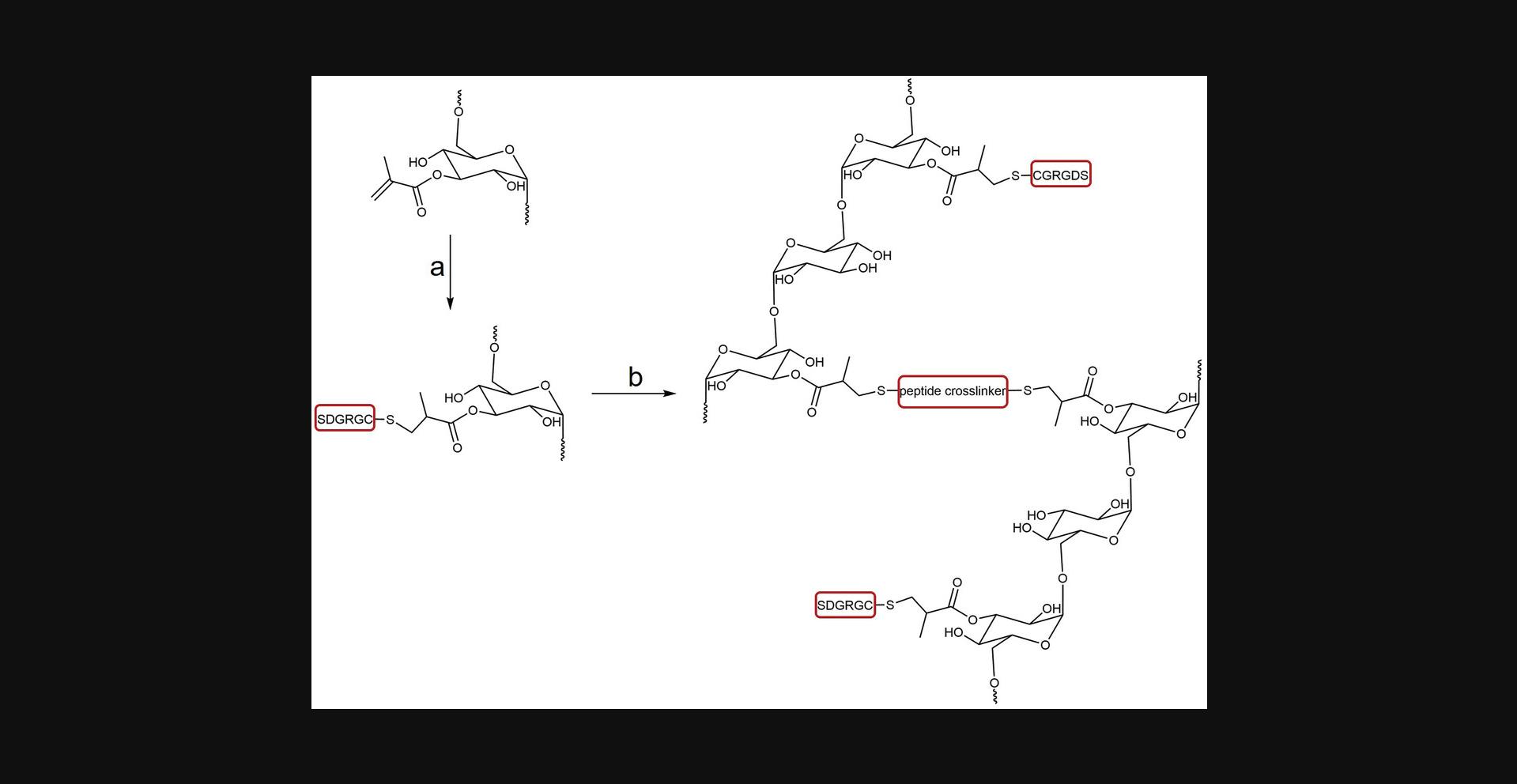
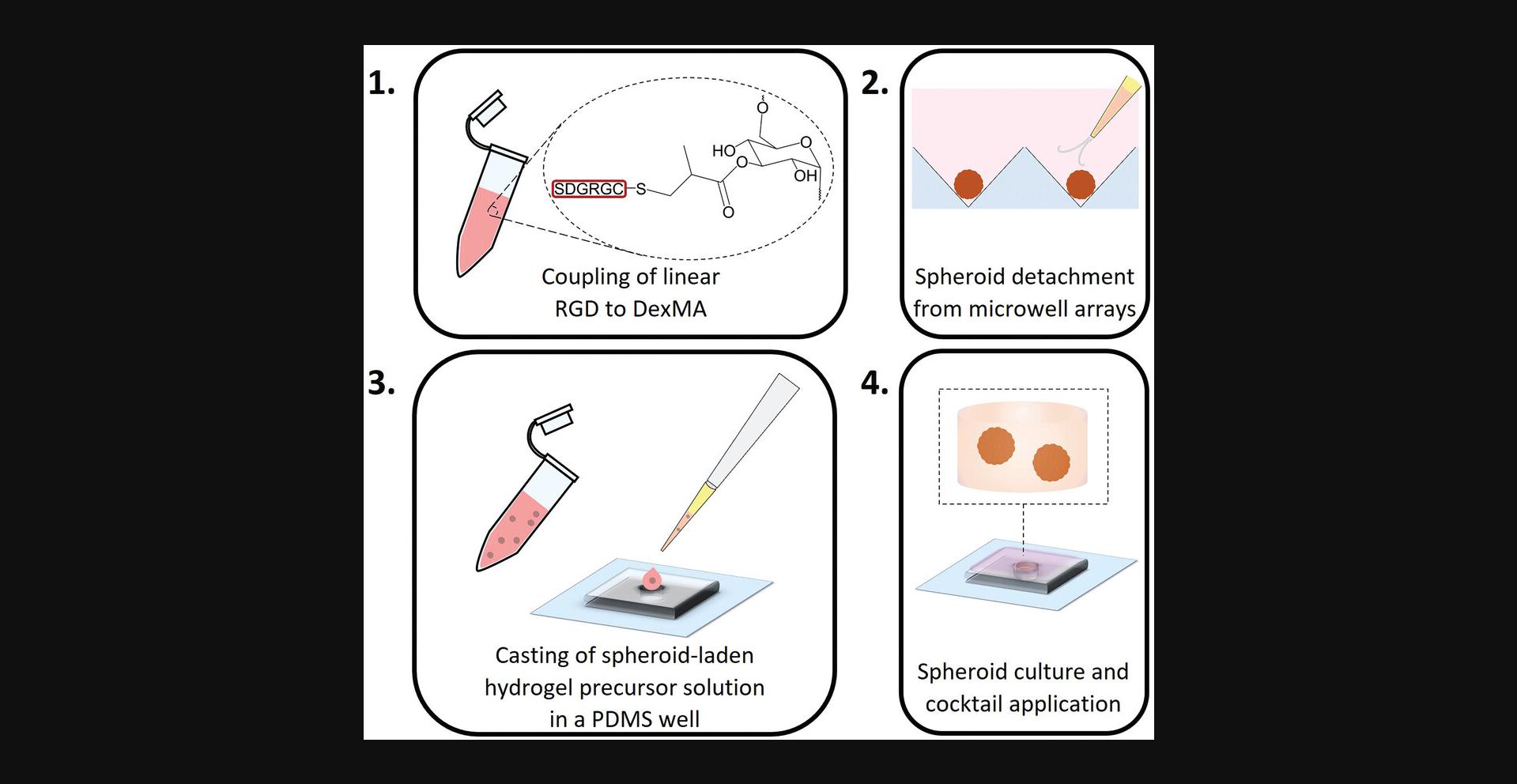
1st step (reaction time: 30 min) | 2nd step (reaction time: 30 min) | Final concentrations | |||||||||
---|---|---|---|---|---|---|---|---|---|---|---|
Expected stiffness values (kPa) | DMEM, pH 7.0 (µl) | RGD, 40 mM (µl) | DexMA, 250 mg/ml (µl) | NaOH, 1 M (µl) | DMEM, pH 7.0 (µl) | NCD, 200 mg/ml (µl) | NaOH, 1 M (µl) | ECS susp. (µl) | RGD (mM) | DexMA (% w/v) | NCD (mM) |
0.8 | 14.1 | 13.5 | 16 | 1.40 | 25.2 | 9.00 | 2.50 | 8.30 | 6 | 4.4 | 17.4 |
1 | 14.1 | 13.5 | 16 | 1.40 | 19.6 | 13.5 | 3.60 | 8.30 | 6 | 4.4 | 26.2 |
1.5 | 14.1 | 13.5 | 16 | 1.40 | 16.6 | 15.8 | 4.30 | 8.30 | 6 | 4.4 | 30.6 |
5 | 14.1 | 13.5 | 16 | 1.40 | 2.50 | 27 | 7.20 | 8.30 | 6 | 4.4 | 52.3 |
-
Final hydrogel volume: 90 µl. Linear RGD sequence: CGRGDS, free N- and C-termini (molecular weight 593.61 Da), used as HCl salt. Native collagen degradability (NCD) crosslinker sequence: CGPQGIAGQGCR, free N- and C-termini (molecular weight 1146.30 Da), used as HCl salt.
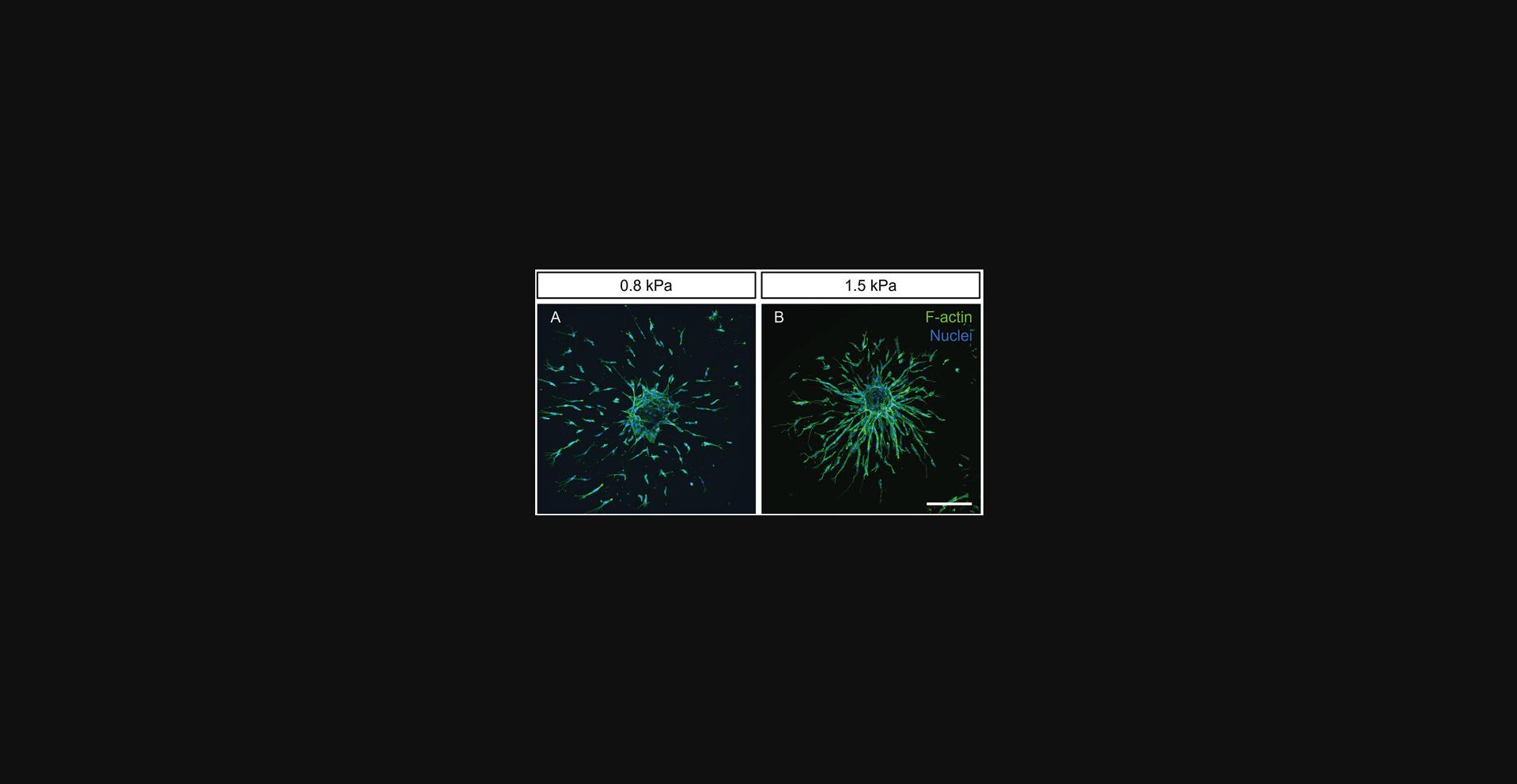
All recipes listed in Table 1 report the use of a bis-cysteine peptide crosslinker of native collagen degradability (NCD; sequence: CGPQGIAGQGCR, free N- and C-termini) and 6 mM linear RGD (sequence: CGRGDS, free N- and C-termini) as integrin-binding epitope. To prepare hydrogels of different degradability or adhesive epitope concentrations, refer to Basic Protocols 4 and 5, which describe the design criteria to adapt the recipes accordingly.
Materials
-
SYLGARD™ 184 PDMS Silicone Elastomer 2-Component Kit (polymer and curing agent; Biesterfeld, cat. no. 5498840000)
-
Linear RGD aliquots (see recipe)
-
DexMA aliquots (see recipe)
-
DMEM, pH 7.0 and 7.4, without NaHCO3 (Gibco, cat. no. 12800017)
-
Sterile 1 M NaOH (Sigma-Aldrich, cat. no. S5881)
-
Antibiotic-Antimycotic (Gibco, cat. no. 15240062) diluted 1:100 in PBS (Corning, cat. no. 21-031-CV)
-
NCD peptide crosslinker solution (see recipe)
-
Endothelial cell spheroids (ECSs; see Basic Protocol 2)
-
Endothelial Cell Growth Medium 2 (EGM-2) Kit (PromoCell, cat. no. C-22111) including Basal Medium 2 (PromoCell, cat. no. C-22211) and Supplement Pack (PromoCell, cat. no. C-39211)
-
Pro-angiogenic cocktail (see Support Protocol 1)
-
UV safety goggles
-
Biological safety cabinet
-
0.2-L plastic cup (polypropylene; Papstar, cat. no. 12149)
-
Non-sterile plastic transfer pipets (Fisherbrand, cat. no. 13459108)
-
Desiccator
-
10-cm cell culture dishes (Sarstedt, cat. no. 83.3902)
-
Precision tweezers
-
Cutting mat
-
10-mm (SMI, cat. no. ZBP10)
-
5-mm biopsy punches (Stiefel, cat. no. 05.SF002.07/97)
-
Razor blade (Carl Roth, cat. no. CK07.7)
-
Sticky tape
-
24 × 24-mm square or 15-mm circular cover glasses (VWR, cat. nos. 631-1579 and 631-1571)
-
Omnicure® Series 1500 UV Spot Curing System (Excelitas Technologies Ltd.)
-
Centrifuge
-
Water bath
-
Set of single-channel pipets with respective tips
-
Sterile 0.5- and 1.5-ml reaction tubes
-
Kimwipes® (Kimtech Science, cat. no. 7552)
-
Scale
-
Spatulas
-
Humidified cell culture incubator (37°C, 5% CO2)
NOTE : UV safety goggles need to be worn during UV sterilization of glass-coverslip-supported PDMS wells. All steps of hydrogel preparation need to be performed in a biological safety cabinet.
Preparation of glass-coverslip-supported PDMS wells
1.Follow the manufacturer's instructions and pour the desired volume of liquid PDMS pre-polymer solution into a plastic cup. Add the dedicated curing agent at a 1:10 ratio using a plastic pipet and mix thoroughly for several minutes.
2.To cast a flat PDMS sheet, degas the mixture in a vacuum chamber or desiccator until all bubbles disappear. Pour 7 g of PDMS into a 10-cm culture dish, allow it to spread out evenly, and solidify on a level surface at 60°C for ∼4 hr. Alternatively, keep it at room temperature for 24 hr.
3.To prepare PDMS wells, extract the solid PDMS layer from the dish with tweezers. Cut out circular pieces of PDMS using a 10-mm-diameter biopsy punch or, alternatively, 2 cm × 2 cm squares using a razor blade on a cutting mat.
4.Cut out holes of 5 mm diameter in each piece of PDMS. These cavities will function as hydrogel chambers.
5.Remove dust and particles from the PDMS pieces using sticky tape and attach each PDMS piece to a glass coverslip, ensuring conformal contact (24 × 24-mm square coverslip for square pieces or 15-mm circular coverslips for circular pieces).
6.Sterilize the glass-coverslip-supported PDMS wells using a UV light source (e.g., Omnicure® Series 1500 UV Spot Curing System, 10 mW/cm2 for 60 s; intensity measured at 365 nm).
Linear RGD coupling to DexMA: First step of DexMA hydrogel preparation
7.Follow Table 1 to select the stiffness of interest.
8.Thaw aliquots of linear RGD and DexMA and equilibrate to room temperature.
9.Centrifuge the aliquots to spin down any liquid left on the walls of the vessels.
10.Add the required volume of DMEM, pH 7.0, to a sterile 0.5-ml reaction tube.
11.Homogenize the RGD solution by pipetting up and down several times.
12.Add the required volume of linear RGD solution to DMEM, pH 7.0.
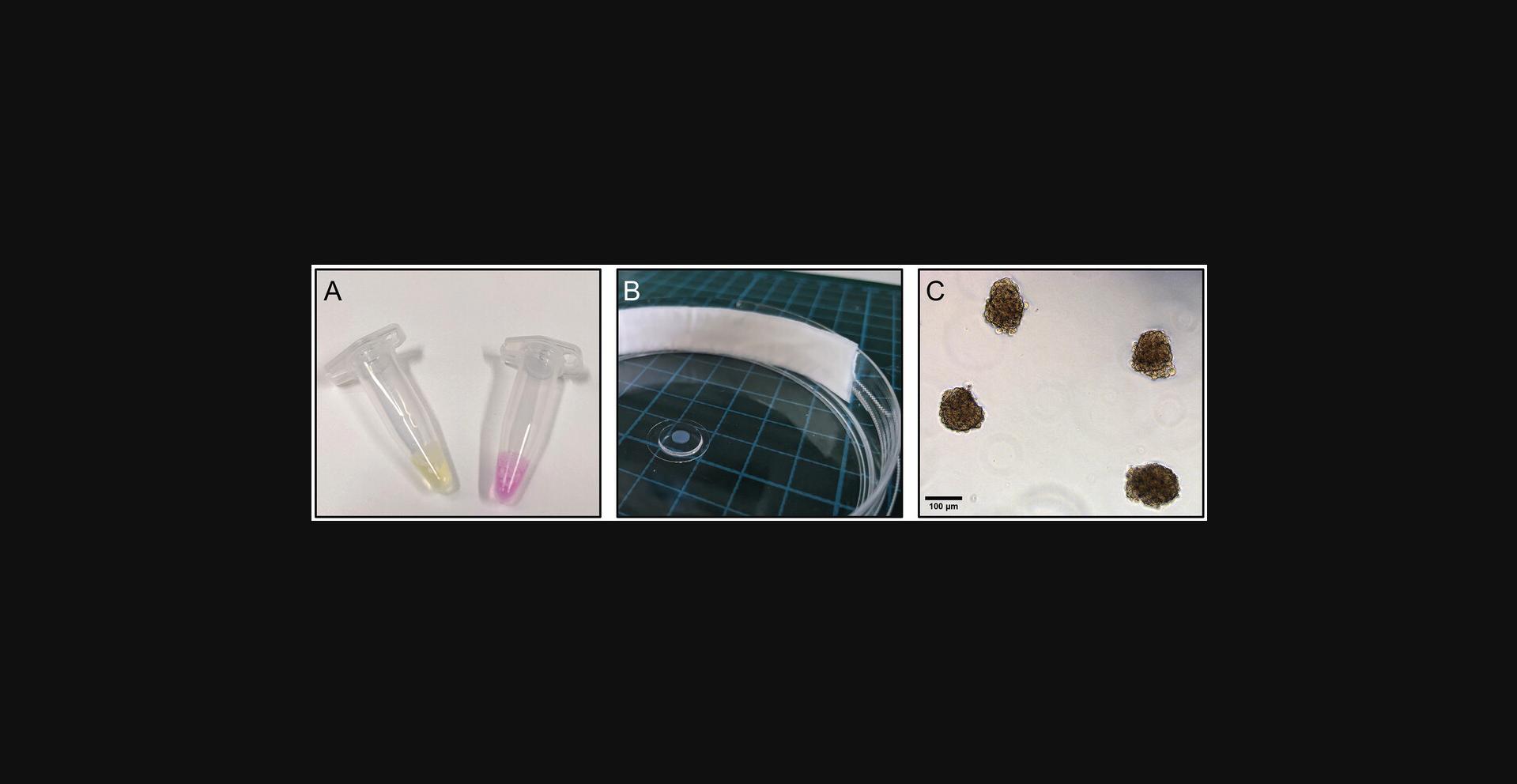
13.Homogenize the DexMA solution by stirring with a pipet tip several times.
14.Dispense the required volume of DexMA solution into the reaction tube containing the mixture of DMEM and RGD and pipet up and down several times to wash the inner side of the tip.
15.Dispense the required volume of 1 M NaOH solution while quickly stirring.
16.Allow the reaction to proceed for 30 min at room temperature.
Formation of ECS-laden hydrogels: Second step of DexMA hydrogel preparation
17.Transfer a glass-coverslip-supported PDMS well to a 10-cm tissue culture dish with an adhesive surface.
18.Wet a Kimwipe with 2 ml antibiotic/antimycotic solution in PBS, fold it to obtain a long strip, and fit it inside the PDMS-coated dish by pressing it against the walls of the dish (Fig. 7B).
19.In a biological safety cabinet, cut off the end of a 200-µl pipet tip using a razor blade.
20.During the 30-min incubation period after the first step of DexMA hydrogel preparation, detach ECSs from microwells by pipetting up and down against the walls of each well.
21.Check ECS detachment under a cell culture microscope and repeat step 20 in case ECSs are still present in the microwells.
22.Transfer ECS suspension to a 1.5-ml reaction tube using a cut tip and centrifuge for 4 min at 16 rcf, room temperature.
23.Carefully aspirate the supernatant with a P100 pipet and resuspend spheroids in the desired volume of DMEM, pH 7.4 (prewarmed to 37°C in a water bath).
24.Add the required volume of DMEM, pH 7.0, to the reaction mixture prepared in the first step of DexMA hydrogel preparation (steps 7-16).
25.Add the NCD peptide solution and pipet up and down several times.
26.Add 1 M NaOH solution under rigorous stirring.
27.Add the required volume of ECS suspension (from step 23) using a cut tip and pipet up and down gently to homogenize the solution.
28.Quickly cast the hydrogel precursor solution, ∼20 µl, within the glass-coverslip-supported PDMS well using a cut 200-µl tip, and flip the dish repeatedly to ensure an appropriate positioning of the spheroid until the hydrogel has formed (Fig. 7C). Let the hydrogel polymerize fully for 30 min outside the biological safety cabinet. Cover the outside of the dish with wet tissues to create a moisturized environment for polymerization.
Induction of ECS sprouting by pro-angiogenic cocktail
29.After 30 min of polymerization, carefully add 200 µl EGM-2 on top of the samples. Keep the samples in a cell culture incubator (37°C, 5% CO2) for ∼4 hr.
30.Replace EGM-2 with 200 µl of pro-angiogenic cocktail on top of the samples. Transfer the samples to a cell culture incubator (37°C, 5% CO2) to induce sprouting.
31.Culture ECSs while refreshing pro-angiogenic cocktail every 24 hr.
Basic Protocol 4: ENDOTHELIAL CELL SPROUTING IN HYDROGELS OF TUNABLE ADHESIVENESS
In blood vessels, the endothelium is supported by a surrounding basement membrane consisting mainly of ECM proteins, such as laminin, type IV collagen, and heparan sulfate proteoglycans (HSPGs). Importantly, not only does this basement membrane serve as a support structure for endothelial cells, but its biochemical properties directly affect endothelial cell function, e.g., by regulating integrin activation through the presentation of adhesive ligands (Leclech et al., 2020). To study the regulatory role of individual adhesive ligands in vitro , synthetic hydrogels can be functionalized with peptides recapitulating the adhesive sites presented to endothelial cells in natural basement membranes. This approach offers an advantage over commonly used hydrogels of natural origin, such as type I collagen and fibrin, which do not provide control over the selection and concentration of adhesive ligands.
In our standard DexMA hydrogels (Basic Protocol 3), the engagement of endothelial cells with their surrounding ECM is mediated through an RGD peptide (extended sequence: CGRGDS, free N- and C-termini). This peptide activates both αvβ3 and α5β1 integrins (Kapp et al., 2017), which are known regulators of endothelial cell function during angiogenic sprouting in vivo (Brooks et al., 1994; Francis et al., 2002). By using DexMA hydrogels functionalized with different concentrations of RGD, our lab was able to elucidate the role of ECM adhesiveness in the regulation of multicellular sprouting. In particular, endothelial cells were only able to sprout multicellularly through matrices functionalized with high concentrations of linear RGD, whereas they adopted a single-cell migratory phenotype at low peptide concentrations (Liu et al., 2021).
In Basic Protocol 4, we report the recipes for the preparation of DexMA hydrogels of a constant stiffness (∼1.5 kPa) (see Basic Protocol 3) and varying concentrations of RGD ligand, ranging from 0.15 to 6 mM (Table 2)—a range of concentrations shown to support HUVEC adhesion in 2D (Liu et al., 2021). These recipes will allow users to test the effect of matrix adhesiveness on ECS angiogenic sprouting independently from stiffness (Fig. 8). To keep chemical properties of hydrogels constant across the entire RGD concentration range, the adhesiveness is tuned by varying the ratio of RGD to RGE ligands, the latter not eliciting integrin activation (Takahashi et al., 2007).
1st step (reaction time: 30 min) | 2nd step (reaction time: 30 min) | Final concentrations | |||||||||||
---|---|---|---|---|---|---|---|---|---|---|---|---|---|
Expected stiffness values (kPa) | DMEM, pH 7.0 (µl) | RGD, 40 mM (µl) | RGE, 40 mM (µl) | DexMA, 250 mg/ml (µl) | NaOH, 1 M (µl) | DMEM, pH 7.0 (µl) | NCD, 200 mg/ml (µl) | NaOH, 1 M (µl) | ECS susp. (µl) | RGD (mM) | RGE (mM) | DexMA (% w/v) | NCD (mM) |
1.5 | 14.1 | 0.34 | 13.2 | 16 | 1.40 | 16.6 | 15.8 | 4.30 | 8.30 | 0.15 | 5.85 | 4.4 | 30.6 |
1.5 | 14 | 2.30 | 11.3 | 16 | 1.40 | 16.6 | 15.8 | 4.30 | 8.30 | 1 | 5 | 4.4 | 30.6 |
1.5 | 14 | 6.80 | 6.80 | 16 | 1.40 | 16.6 | 15.8 | 4.30 | 8.30 | 3 | 3 | 4.4 | 30.6 |
1.5 | 14.1 | 13.5 | – | 16 | 1.40 | 16.6 | 15.8 | 4.30 | 8.30 | 6 | 0 | 4.4 | 30.6 |
-
Final hydrogel volume: 90 µl. Linear RGD sequence: CGRGDS, free N- and C-termini (molecular weight 593.61 Da), used as HCl salt. Linear RGE sequence: CGRGES, free N- and C-termini (molecular weight 607.64 Da), used as HCl salt. Native collagen degradability (NCD) crosslinker sequence: CGPQGIAGQGCR, free N- and C-termini (molecular weight 1146.30 Da), used as HCl salt.
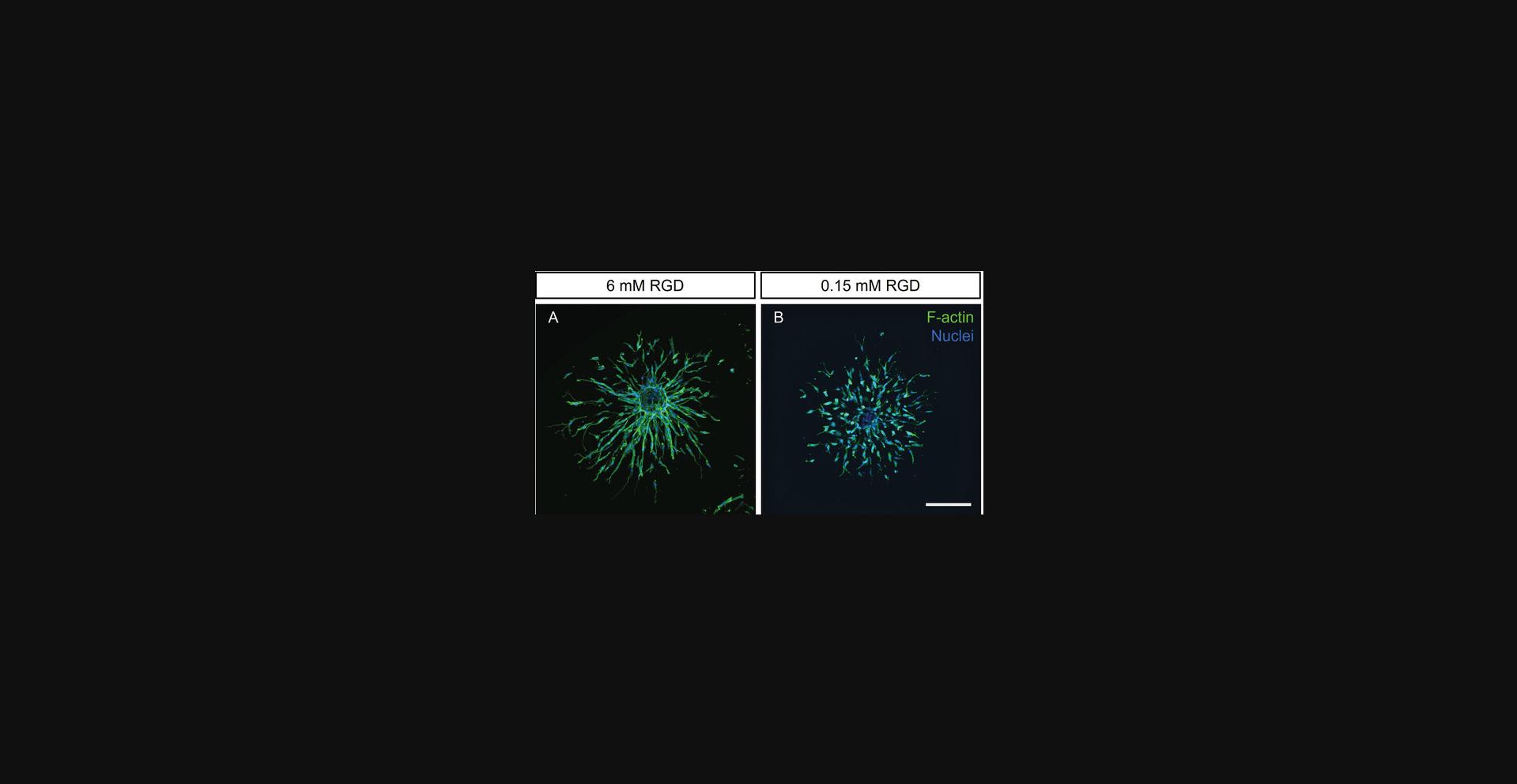
The strategy described in this protocol can be applied to any other adhesive ligand of interest, such as selective ligands for α5β1 integrins (Liu et al., 2021) or αvβ3 integrins (cyclic RGD, extended sequence: c[RGDfK(C)]; Aumailley et al., 1991; Francis et al., 2002; Haubner et al., 1996).
Materials
-
SYLGARD™ 184 PDMS Silicone Elastomer 2-Component Kit (polymer and curing agent; Biesterfeld, cat. no. 5498840000)
-
Linear RGD aliquots (see recipe)
-
Linear RGE aliquots (see recipe)
-
DexMA aliquots (see recipe)
-
DMEM, pH 7.0 and 7.4, without NaHCO3 (Gibco, cat. no. 12800017)
-
Sterile 1 M NaOH (Sigma-Aldrich, cat. no. S5881)
-
Antibiotic-Antimycotic (Gibco, cat. no. 15240062) diluted 1:100 in PBS (Corning, cat. no. 21-031-CV)
-
NCD peptide crosslinker solution (see recipe)
-
Endothelial cell spheroids (ECSs; see Basic Protocol 2)
-
Endothelial Cell Growth Medium 2 (EGM-2) Kit (PromoCell, cat. no. C-22111) including Basal Medium 2 (PromoCell, cat. no. C-22211) and Supplement Pack (PromoCell, cat. no. C-39211)
-
Pro-angiogenic cocktail (see Support Protocol 1)
-
UV safety goggles
-
Biological safety cabinet
-
0.2-L plastic cup (polypropylene; Papstar, cat. no. 12149)
-
Non-sterile plastic transfer pipets (Fisherbrand, cat. no. 13459108)
-
Desiccator
-
10-cm cell culture dishes (Sarstedt, cat. no. 83.3902)
-
Precision tweezers
-
Cutting mat
-
10-mm (SMI, cat. no. ZBP10)
-
5-mm biopsy punches (Stiefel, cat. no. 05.SF002.07/97)
-
Razor blade (Carl Roth, cat. no. CK07.7)
-
Sticky tape
-
24 × 24-mm square or 15-mm circular cover glasses (VWR, cat. nos. 631-1571 and 631-1579)
-
Omnicure® Series 1500 UV Spot Curing System (Excelitas Technologies Ltd.)
-
Sterile 0.5- and 1.5-ml reaction tubes
-
Centrifuge
-
Water bath
-
Set of single-channel pipets with respective tips
-
Kimwipes® (Kimtech Science, cat. no. 7552)
-
Scale
-
Spatulas
-
Humidified 37°C, 5% CO2 cell culture incubator
NOTE : UV safety goggles need to be worn during UV sterilization of glass-coverslip-supported PDMS wells. All steps of hydrogel preparation need to be performed in a biological safety cabinet.
1.Prepare glass-coverslip-supported PDMS wells as described in Basic Protocol 3, steps 1-6.
2.Follow Table 2 and select the linear RGD concentrations of interest.
3.Thaw aliquots of linear RGD, RGE, and DexMA and equilibrate them to room temperature.
4.Centrifuge the aliquots to spin down any drops left on the walls of the vessels.
5.Add the required volume of DMEM, pH 7.0 (see Table 2), to a sterile 0.5-ml reaction tube.
6.Homogenize linear RGD and RGE aliquots by pipetting the solution up and down several times.
7.Add the required volume of linear RGD solution to DMEM, pH 7.0.
8.Add the required volume of linear RGE solution to DMEM, pH 7.0. Then continue with steps 13-31 of Basic Protocol 3.
Basic Protocol 5: ENDOTHELIAL CELL SPROUTING IN HYDROGELS OF TUNABLE DEGRADABILITY
Synthetic hydrogels are nanoporous polymer networks that require active cleavage of crosslinker moieties to allow cell spreading and migration (West & Hubbell, 1999). Our DexMA hydrogels are crosslinked through peptide sequences that can be cleaved by specific sets of cell-secreted MMPs. The speed at which a given volume of covalently crosslinked hydrogel is cleaved by MMPs defines the degradability of the hydrogel. This degradability is affected by the concentration of the crosslinker as well as its susceptibility to MMP activity. Thus, in covalently crosslinked hydrogels, changes in stiffness through crosslinker concentration are always accompanied by differences in hydrogel degradability (Trappmann et al., 2017). In order to dissect the individual roles of these two parameters in endothelial cell sprouting, crosslinker peptides with different MMP susceptibility can be used to generate hydrogels of varying degradability but constant stiffness. Using this approach, our lab recently demonstrated that endothelial cells switch their migration pattern between single-cell and multicellular modes depending on the degradability of the matrix (Trappmann et al., 2017).
In Basic Protocol 5, we describe recipes for preparing DexMA hydrogels crosslinked with peptide sequences of different MMP susceptibility while keeping the stiffness (∼0.8 kPa) and adhesiveness (6 mM linear RGD) constant (Table 3). Specifically, a crosslinker sequence derived from the cleavage site of native collagen, called a “native collagen degradability” (NCD) sequence (CGPQGIAGQGCR, free N- and C-termini), introduced in Basic Protocols 3 and 4, is used as our standard sequence. Introduction of an amino acid mismatch to this sequence lowers its susceptibility to MMPs and therefore generates crosslinks of low degradability (LD, sequence: CGPQGPAGQGCR, free N- and C-termini) (Nagase & Fields, 1996). The applicability of the resulting hydrogels is demonstrated by the degradability-dependent changes in endothelial cell migration modes in Figure 9.
1st step (reaction time: 30 min) | 2nd step (reaction time: 30 min) | Final concentrations | |||||||||||
---|---|---|---|---|---|---|---|---|---|---|---|---|---|
Expected stiffness values (kPa) | DMEM, pH 7.0 (µl) | RGD, 40 mM (µl) | DexMA, 250 mg/ml (µl) | NaOH, 1 M (µl) | DMEM, pH 7.0 (µl) | NCD, 200 mg/ml (µl) | LD, 200 mg/ml (µl) | NaOH, 1 M (µl) | ECS susp. (µl) | RGD (mM) | DexMA (% w/v) | NCD (mM) | LD (mM) |
0.8 | 14.1 | 13.5 | 16 | 1.40 | 25.2 | 9.00 | – | 2.50 | 8.30 | 6 | 4.4 | 17.4 | – |
0.8 | 14.1 | 13.5 | 16 | 1.40 | 25.4 | – | 8.85 | 2.50 | 8.30 | 6 | 4.4 | – | 17.4 |
-
Final hydrogel volume: 90 µl. Linear RGD sequence: CGRGDS, free N- and C-termini (molecular weight 593.61 Da), used as HCl salt. Native collagen degradability (NCD) crosslinker sequence: CGPQGIAGQGCR, free N- and C-termini (molecular weight 1146.30 Da), used as HCl salt. Low-degradability (LD) crosslinker sequence: CGPQGPAGQGCR, free N- and C-termini (molecular weight 1130.26 Da), used as HCl salt.
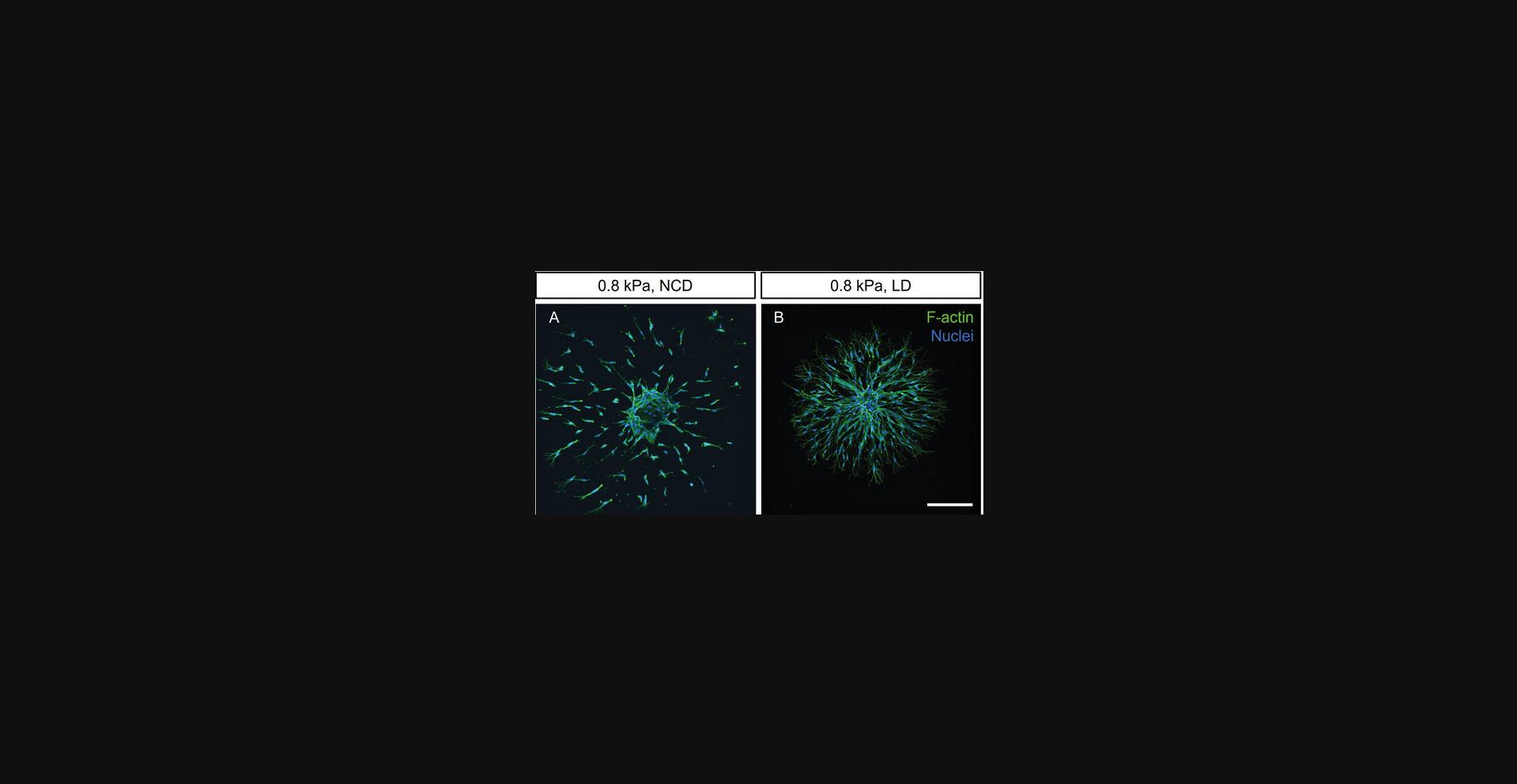
The recipes reported in Basic Protocol 5 can be adapted to any other MMP-cleavable crosslinker processable by cell-secreted MMPs. A reference for MMP-cleavable peptide sequences is Turk et al., 2001.For further information on additional crosslinker peptides used in similar experimental conditions, refer to Liu et al., 2021.
Materials
-
SYLGARD™ 184 PDMS Silicone Elastomer 2-Component Kit (polymer and curing agent; Biesterfeld, cat. no. 5498840000)
-
Linear RGD aliquots (see recipe)
-
DexMA aliquots (see recipe)
-
DMEM, pH 7.0 and 7.4, without NaHCO3 (Gibco, cat. no. 12800017)
-
Sterile 1 M NaOH (Sigma-Aldrich, cat. no. S5881)
-
Antibiotic-Antimycotic (Gibco, cat. no. 15240062) diluted 1:100 in PBS (Corning, cat. no. 21-031-CV)
-
NCD peptide crosslinker solution (see recipe) or LD peptide crosslinker solution (see recipe)
-
Endothelial cell spheroids (ECSs; see Basic Protocol 2)
-
Endothelial Cell Growth Medium 2 (EGM-2) Kit (PromoCell, cat. no. C-22111) including Basal Medium 2 (PromoCell, cat. no. C-22211) and Supplement Pack (PromoCell, cat. no. C-39211)
-
Pro-angiogenic cocktail (see Support Protocol 1)
-
UV safety goggles
-
Biological safety cabinet
-
0.2-L plastic cup (polypropylene; Papstar, cat. no. 12149)
-
Non-sterile plastic transfer pipets (Fisherbrand, cat. no. 13459108)
-
Desiccator
-
10-cm cell culture dishes (Sarstedt, cat. no. 83.3902)
-
Precision tweezers
-
Cutting mat
-
10-mm (SMI, cat. no. ZBP10)
-
5-mm biopsy punches (Stiefel, cat. no. 05.SF002.07/97)
-
Razor blade (Carl Roth, cat. no. CK07.7)
-
Sticky tape
-
24 × 24-mm square or 15-mm circular cover glasses (VWR, cat. nos. 631-1571 and 631-1579)
-
Omnicure® Series 1500 UV Spot Curing System (Excelitas Technologies Ltd)
-
Water bath
-
Set of single-channel pipets with respective tips
-
Sterile 0.5- and 1.5-ml reaction tubes
-
Kimwipes® (Kimtech Science, cat. no. 7552)
-
Centrifuge
-
Scale
-
Spatulas
-
Humidified 37°C, 5% CO2 cell culture incubator
NOTE : UV safety goggles need to be worn during UV sterilization of glass-coverslip-supported PDMS wells. All hydrogel preparation steps need to be performed in a biological safety cabinet.
1.Follow Table 3 and prepare hydrogels of desired degradability following the steps described in Basic Protocol 3. For hydrogels of low degradability, simply use the required volume of LD crosslinker solution instead of the NCD crosslinker.
Basic Protocol 6: IMAGING OF ENDOTHELIAL CELL SPHEROID-LADEN HYDROGELS
To gain insights into matrix-dependent signaling events, cultured ECSs within 3D DexMA hydrogel scaffolds can be assessed via immunofluorescence assays, including standard fixation methods, antibody staining, and fluorescence microscopy. Yet the surrounding nanoporous hydrogel hinders diffusion-based access to the cells. To achieve sufficient tissue preservation and antibody labeling, the application of all fixatives, antibodies, and fluorophores requires extended incubation times.
The imaging of embedded spheroids with a confocal fluorescence microscope requires the consideration of a set of additional parameters that may limit the acquisition results: Scattering of light by the cells and the hydrogel matrix, as well as mismatches in refractive indices between the hydrogel and the sample buffer (Riss & Trask, 2021). To reduce background noise from out-of-plane fluorescence, samples are best imaged with a confocal microscope with a uniform laser illumination. Due to the thickness of 3D samples, fast image acquisition with a spinning-disc microscope is advised. The objectives of choice must provide extensive working distances of up to several hundred micrometers to reach and image the spheroids embedded at distant z -layers within the hydrogel.
When assessing cells and spheroids in 3D, acquisition of structures close to the glass surface should be avoided. Cells can sense the stiffness of an underlying glass support surface up to a few tens of micrometers and thus must be excluded from the analysis in matrix-stiffness-related studies (Tusan et al., 2018). The imaging of ECSs close to the top of the hydrogel is advised, as these have better access to nutrients.
Materials
-
Dulbecco's Phosphate-Buffered Saline (PBS; Corning, cat. no. 21-031-CV)
-
Fixation solution: 4% paraformaldehyde (PFA), prepared by diluting 16% paraformaldehyde in PBS (ThermoFisher, cat. no. 28906)
-
Permeabilization solution: 0.5% Triton X-100 prepared by diluting Triton X-100 (Sigma-Aldrich, cat. no. T8787) in PBS
-
Invitrogen Hoechst 33342 (Fisher Scientific, cat. no. H3570), reconstituted following manufacturer's instructions
-
Alexa Fluor 488 Phalloidin (Invitrogen, cat. no. A12379), reconstituted following manufacturer's instructions
-
Blocking solution: 3% bovine serum albumin (BSA; Sigma-Aldrich, cat. no. A8412) in PBS
-
Primary antibody (CD144 [VE-cadherin], mouse anti-human, ThermoFisher, cat. no. 14-1449-82)
-
Washing solution: 0.1% Tween 20 (Sigma-Aldrich, cat. no. P1379) in PBS
-
Secondary antibody: Goat anti-mouse conjugated to Alexa Fluor 647 (ThermoFisher, cat. no. A-21235)
-
Chemical fume hood
-
Serological pipets
-
Set of single-channel pipets with respective tips
-
50-ml conical centrifuge tubes (Corning Falcon, cat. no. 352070)
-
0.5- and 1.5-ml reaction tubes
-
Platform rocker
-
6-well plates
-
Confocal (spinning disc) microscope (e.g., Dragonfly by Andor or equivalent)
NOTE : PFA is carcinogenic—handle it in a fume hood.
Fixation and permeabilization
1.Wash the sample twice with PBS. To fix cells, add a 4% PFA solution in PBS on top. Incubate at room temperature for 1 hr.
2.Wash three times with PBS, and add permeabilization solution (0.5% Triton-X in PBS) to permeabilize cells at room temperature for 1 hr.
Staining of ECSs
Staining of cell nuclei and F-actin
3.Wash the sample twice with PBS and then prepare a staining solution: Dilute Hoechst 1:200 and phalloidin 1:500 in PBS. Add 200 µl of the solution to the sample. Keep at room temperature in a moist atmosphere overnight.
Antibody staining for proteins of interest
4.To prevent nonspecific antibody binding, add a blocking solution of 3% BSA in PBS on top. Keep at room temperature for 1 hr.
5.Prepare a primary antibody solution by diluting a defined volume of desired antibody according to its datasheet with 3% BSA blocking solution. Place the solution on top of the sample and keep at 4°C for 24 hr.
6.To wash out excess antibody, soak the sample in washing solution (PBS containing 0.1% Tween 20). Keep it in a 6-well plate on a rocker at 4°C for 2 days, replacing the solution twice per day.
7.Prepare a secondary antibody solution by diluting a defined volume of desired antibody according to its datasheet with 3% BSA blocking solution. Place the solution on top of the sample and keep at 4°C overnight.
8.Wash out excess antibody by soaking the sample in PBS with 0.1% Tween 20. Place on a rocker at 4°C for 24 hr. Replace the solution twice.
9.Stain nuclei with Hoechst (1:200) and F-actin with phalloidin (1:500) in PBS. Place the solution on top of the sample and keep at room temperature overnight.
10.Image the samples with a spinning-disc confocal microscope.
Support Protocol 1: PREPARATION OF PRO-ANGIOGENIC COCKTAIL FOR ENDOTHELIAL CELL SPROUTING
Angiogenic sprouting in vivo is promoted by pro-angiogenic cues that activate endothelial cells. Three factors commonly associated with angiogenesis have been identified and confirmed to induce robust and reproducible sprouting in vitro : Vascular endothelial growth factor (VEGF), sphingosine-1-phosphate (S1P), and phorbol 12-myristate 13-acetate (PMA) (Nguyen et al., 2013). VEGF and S1P both bind to their specific receptors on endothelial cells and activate downstream signaling cascades to promote cell migration and tube formation (Carmeliet & Jain, 2011; Takuwa, 2010). PMA passes through the plasma membrane and activates protein kinase C (PKC), which leads to the activation of important pro-angiogenic signaling pathways (e.g., ERK), resulting, for example, in elevated cell proliferation (Mebratu & Tesfaigzi, 2009; Verin et al., 2000; Wang et al., 1999).
To recapitulate the in vivo initiation of sprouting in the presented in vitro spheroid assay, a defined pro-angiogenic cocktail containing VEGF, S1P, and PMA at titrated concentrations is administered to the sample (Wang et al., 2020). A diffusion-based, chemoattractive gradient establishes within the synthetic hydrogel, triggering the endothelial cells to exit the spheroid body and invade the surrounding matrix.
Materials
- Recombinant Human VEGF165 Protein (R&D Systems, cat. no. 293-VE)
- Sphingosine-1-phosphate (S1P; Cayman Chemicals, cat. no. 62570)
- Phorbol 12-myristate 13-acetate (PMA; Sigma-Aldrich, cat. no. 1585)
- Bovine serum albumin (BSA; Sigma-Aldrich, cat. no. A8412)
- Dulbecco's Phosphate-Buffered Saline (PBS; Corning, cat. no. 21-031-CV)
- Sterile 0.3 M NaOH (Sigma-Aldrich, cat. no. S5881)
- Sterile dimethyl sulfoxide (DMSO; Sigma-Aldrich, cat. no. D2650-100ML)
- Endothelial Cell Growth Medium 2 (EGM-2) Kit (PromoCell, cat. no. C-22111) including Basal Medium 2 (PromoCell, cat. no. C-22211) and Supplement Pack (PromoCell, cat. no. C-39211)
- Biological safety cabinet
- 0.5- and 1.5-ml reaction tubes
- Set of single-channel pipets with respective tips
NOTE : All steps of pro-angiogenic cocktail preparation need to be performed in a biological safety cabinet.
Prepare aliquots of pro-angiogenic cocktail components
1.Follow the manufacturer's instructions to reconstitute pro-angiogenic cocktail components as detailed in Table 4.Prepare stock solution aliquots in 0.5-ml reaction tubes. Store the tubes at −20°C; avoid freeze-thaw cycles.
1st dilution | 2nd dilution | |||||||
---|---|---|---|---|---|---|---|---|
Pro-angiogenic cocktail components | Concentration of stock solutions | Solvent | Dilution factor | Solvent | Concentration of intermediate solutions | Dilution factor | Solvent | Component concentrations in pro-angiogenic cocktail |
VEGF | 10 µg/ml | 0.1% BSA in PBS | – | – | – | 1:267 | EGM-2 | 37.5 ng/ml |
S1P | 0.1 mM | 0.3 M NaOH | 1:10 | EGM-2 | 10 µM | 1:80 | EGM-2 | 125 nM |
PMA | 1 mg/ml | DMSO | 1:100 | EGM-2 | 10 µg/ml | 1:133 | EGM-2 | 75 ng/ml |
Preparation of pro-angiogenic cocktail
2.Thaw required aliquots of VEGF, S1P, and PMA stock solutions. Equilibrate to room temperature.
3.Dilute stock solutions of S1P and PMA in warm EGM-2 as described in Table 4 to prepare intermediate solutions.
4.Follow Table 4 to prepare the pro-angiogenic cocktail by combining stock solution of VEGF with intermediate solutions of PMA and S1P in warm EGM-2.
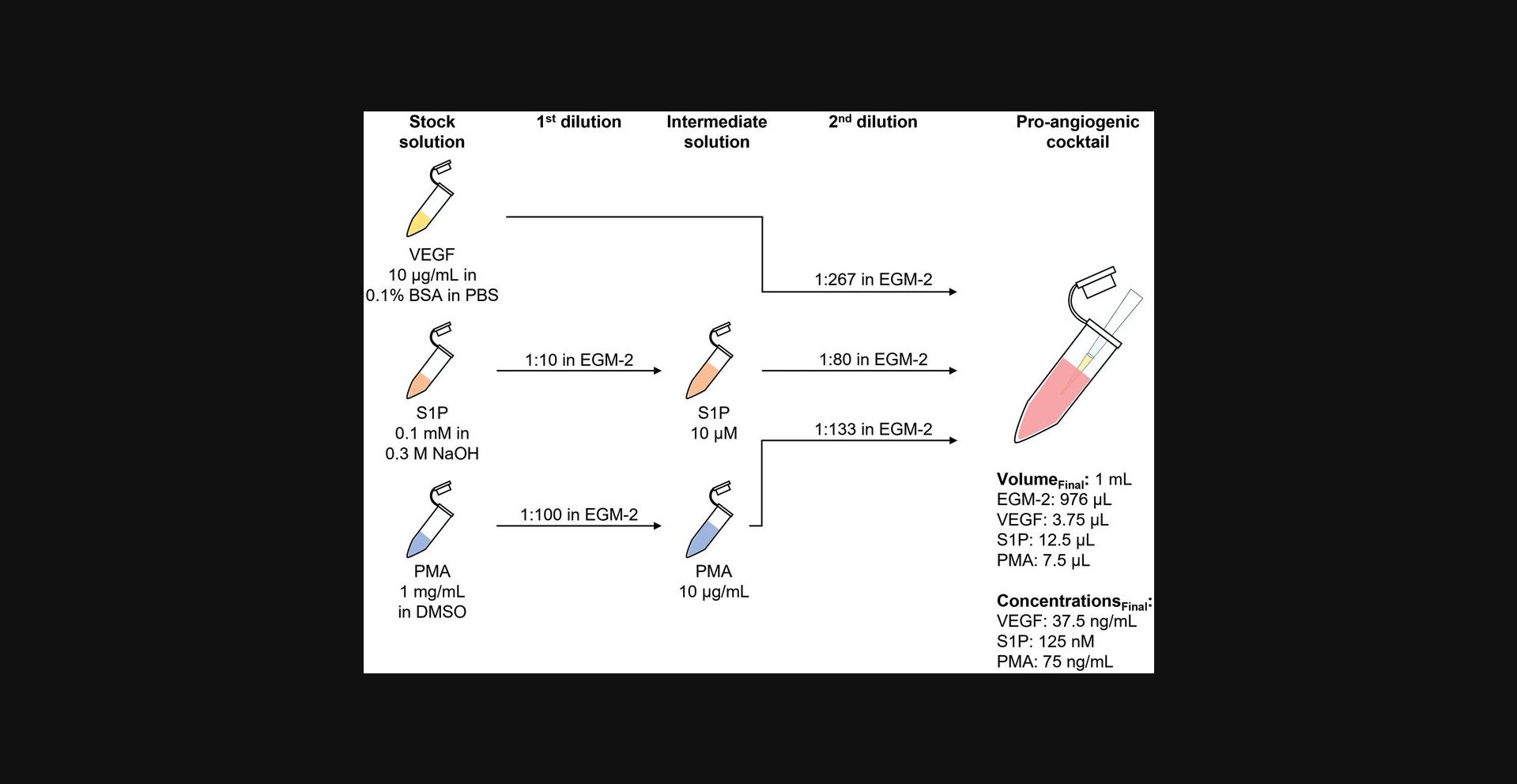
REAGENTS AND SOLUTIONS
DexMA aliquots (250 mg/ml in DMEM, pH 7.0)
Allow lyophilized DexMA to equilibrate from −20°C to room temperature. Weigh DexMA in a glass vial and dissolve in DMEM, pH 7.0 (Gibco, cat. no. 12800017), to a final concentration of 250 mg/ml. Dissolve at room temperature in the dark on a tube roller (a few hours will be required). Then, centrifuge the vial for 5 min at 1600 rcf, 4°C, to collect DexMA from the walls of the vessel. For sterile filtration, transfer the solution to Spin-X tube filters (0.22-µm-pore CA membrane; Costar, cat. no. 8160) in a biological safety cabinet and centrifuge 10 min at 20,000 rcf. Store aliquots in 0.5-ml reaction tubes under sterile conditions for up to 1 year at −80°C.
LD peptide crosslinker solution (200 mg/ml in DMEM, pH 7.0)
Sequence: CGPQGPAGQGCR, free N- and C-termini (molecular weight: 1130.26 Da), used as HCl salt
Allow LD crosslinker powder (custom synthesized by Genscript at >95% purity) to equilibrate from −80°C to room temperature before opening the vial. Transfer LD crosslinker to a 2-ml reaction tube and dissolve in DMEM, pH 7.0 (Gibco, cat. no. 12800017), to a final concentration of 200 mg/ml. Dissolve LD crosslinker at room temperature for few minutes with the help of a vortex mixer. For sterile filtration, transfer the solution to Spin-X tube filters (0.22-µm-pore CA membrane; Costar, cat. no. 8160) in a biological safety cabinet and centrifuge 5 min at 20,000 rcf. Homogenize the solution by pipetting up and down several times before usage. Prepare fresh each time.
Linear RGD aliquots (40 mM in DMEM, pH 7.0)
Sequence: CGRGDS, as HCl salt (free N- and C-termini; molecular weight: 593.61 Da)
Allow RGD powder (custom synthesized by Genscript at >95% purity) to equilibrate from −80°C to room temperature before opening the vial. Transfer RGD to a 2-ml reaction tube and dissolve in DMEM, pH 7.0 (Gibco, cat. no. 12800017), to a final concentration of 40 mM. For sterile filtration, transfer the solution to Spin-X tube filters (0.22-µm-pore CA membrane; Costar, cat. no. 8160) in a biological safety cabinet and centrifuge 5 min at 20,000 rcf. Store aliquots in 0.5-ml reaction tubes under sterile conditions for up to 1 year at −80°C.
Linear RGE aliquots (40 mM in DMEM, pH 7.0)
Sequence: CGRGES, as HCl salt (free N- and C-termini; molecular weight: 607.64 Da)
Equilibrate RGE powder (custom synthesized by Genscript at >95% purity) from −80°C to room temperature before opening the vial. Transfer RGE to a 2-ml reaction tube and dissolve in DMEM, pH 7.0 (Gibco, cat. no. 12800017), to a final concentration of 40 mM. For sterile filtration, transfer the solution to Spin-X tube filters (0.22-µm-pore CA membrane; Costar, cat. no. 8160) in a biological safety cabinet and centrifuge 5 min at 20,000 rcf. Store aliquots in 0.5-ml reaction tubes under sterile conditions for up to 1 year at −80°C.
NCD crosslinker solution (200 mg/ml in DMEM, pH 7.0)
Sequence: CGPQGIAGQGCR, as HCl salt (free N- and C-termini; molecular weight: 1146.30 Da)
Allow NCD crosslinker powder (custom synthesized by Genscript at >95% purity) to equilibrate from −80°C to room temperature before opening the vial. Add NCD crosslinker in a 2 ml reaction tube and dissolve in DMEM pH 7.0 (Gibco™, cat. no. 12800017) for a final concentration of 200 mg/ml. Dissolve NCD crosslinker at room temperature for few minutes with the help of a vortex mixer. For sterile filtration, transfer the solution to Spin-X tube filters (0.22 µm pore CA membrane, Costar, cat. no. 8160) in a biological safety cabinet and centrifuge at 20,000 rcf for 5 min. Homogenize the solution by pipetting up and down several times before usage. Prepare fresh each time.
COMMENTARY
Background Information
To examine the complex, multi-step process of angiogenic sprouting, a variety of experimental systems to observe and manipulate newly formed capillary vessels have arisen. Although in vivo models (e.g., mouse dorsal window chamber) capture the full complexity of blood vessel formation, it is very difficult to systematically study the role of individual signaling events or tissue properties of interest. Instead, in vitro cell culture models, such as the spheroid assay described here, have been developed to reduce this complexity by systematically mimicking and visualizing the most important steps of angiogenic sprouting in a well-controlled environment. These techniques have gradually evolved to include more and more relevant aspects, like a 3D scaffold with defined matrix properties and anatomical resemblance to the in vivo situation. Although the spheroid assay is a proven and reliable tool to recapitulate angiogenic sprouting, however, it does not capture other important steps of the in vivo process, such as directed sprouting towards a source of pro-angiogenic factors, continuous flow (Nguyen et al., 2013), and lumen formation. Biomimetic, microfluidic systems have recently emerged to manage these additional complexities (Nguyen et al., 2013). For example, Nguyen et al. developed a microfluidic platform consisting of a perfusable, cylindrical, endothelium-lined channel that is embedded within a surrounding type I collagen hydrogel. Application of a pro-angiogenic cocktail to a second, parallel channel generates a chemokine gradient through the hydrogel that triggers endothelial cells to sprout out into the matrix (Nguyen et al., 2013). Whereas historically such models were based on non-tunable, natural hydrogels, we have recently integrated synthetic DexMA hydrogels to study the impact of matrix properties on angiogenic sprouting (Trappmann et al., 2017). To further widen the scope of the methodology and also capture aspects of angiogenic sprouting that require longer culture periods, we replaced the hydrolytically labile methacrylates with more stable vinyl sulfone moieties. Using this modified hydrogel system, we were able to demonstrate the formation of perfusable, lumenized neo-vessels emanating from the parent channel (Liu et al., 2021). The vascular structures not only were functional in transporting fluid across the hydrogel, but also showed characteristic hallmarks of in vivo blood vessels, such as apical-basal polarity and deposition of basement-membrane-specific proteins, such as laminin and type IV collagen.
Critical Parameters
During the preparation of ECS-laden hydrogels, special attention needs to be paid to the pH of the precursor solutions upon addition of NaOH (in both the first and second steps), which can be monitored based on the color of an added pH indicator. Users should always aim for the same pH (8.0) to ensure a high degree of reproducibility. Indeed, differences in the pH of the precursor solutions may profoundly alter the kinetics of the Michael-type addition reaction, ultimately affecting the final hydrogel stiffness and degree of adhesive ligand functionalization. Additionally, we recommend not exceeding pH 8 to avoid fast hydrolysis of the ester bonds present on the polymer chains, leading to the formation of more compliant hydrogels. Another important parameter to observe is the speed at which the NaOH solution is added to initiate the Michael addition. The solution needs to be mixed quickly to avoid a steep local increase in pH, which ultimately may lead to inhomogeneities within the hydrogel. Finally, attention needs to be paid to the dissolution of the bis-cysteine MMP-cleavable crosslinker peptides. The thiol groups of cysteine residues are prone to oxidation, leading to the formation of disulfide bonds that are no longer reactive towards methacrylates and thereby reducing the final stiffness of hydrogels. As oxidation of cysteine residues is likely to occur after dissolution of the MMP-cleavable crosslinker peptide powder, we highly recommend preparing the solution of this compound directly before it is required. Attention should be paid to the complete dissolution of the peptide. Moreover, appropriate storage and handling of the peptide in its powder form are also crucial: after thawing, always allow the powder to equilibrate to room temperature before opening the vial, and store the powder at −80°C again soon after use. Storage under inert gas (e.g., Ar or N2) is also recommended.
Troubleshooting
Table 5 lists common problems which may be encountered with our reported protocols. Each problem is listed together with its possible cause and solutions.
Problem | Possible cause | Solution |
---|---|---|
Low DexMA functionalization | Presence of water in the reaction mixture | Repeat the reaction using anhydrous solvent and keep the reaction flask tightly closed throughout the entire reaction. |
Formation of an agglomerate of DexMA precipitate | Overly fast pouring of reaction solution in DMSO into cold IPA | Manually transfer the precipitate into a weighing dish and dissolve in ultrapure water, and then dialyze. |
Precursor solution not turning pink after addition of 1 M NaOH solution (pH ∼8) | Different peptide batch with a different acid content | Repeat the preparation of the sample and increase the volume of 1 M NaOH. Subtract the additional volume from DMEM, pH 7.0, to keep a constant final volume of 90 µl. |
Unusual morphology of angiogenic sprouts | Cells that are not confluent | Trypsinize a confluent dish of HUVECs with cobblestone pattern for the preparation of ECSs (no less than ∼4 million cells should be harvested from a 10-cm tissue culture dish). |
Incorrect pro-angiogenic cocktail | Prepare fresh aliquots of pro-angiogenic cocktail components paying attention to the dilution factors and avoid freeze-thaw cycles. | |
Low fluorescence signal | Too low antibody concentration | Titrate the antibody concentration. |
Insufficient incubation time with antibody staining solutions | Prolong incubation to allow for good diffusion of antibodies into the hydrogel sample. | |
High background signal | Insufficient washing steps | Replace washing buffer (PBS + 0.1% Tween 20) multiple times per day (at least three times). |
Non-reproducible ECS-laden hydrogels | Inaccurate pipetting | Pay careful attention when pipetting 1 M NaOH solutions and viscous DexMA. |
Contamination | Inappropriate handling or sterilization of glass-coverslip-supported PDMS wells, tissue culture dishes, and/or solutions | Sterilize solutions using Spin-X tube filters. Sterilize tissue culture dishes and glass-coverslip-supported PDMS wells with UV light. |
Understanding Results
Following this protocol, the researcher should be able to generate reproducible synthetic hydrogels with consistent mechanical properties. Mechanical testing of samples can be performed by nanoindentation, as shown by others (Ebenstein & Pruitt, 2006; Xu et al., 2022).
When performing the ECS sprouting assay, the researcher should observe mainly two migration phenotypes, depending on the hydrogel properties: single and collective cell migration. The expected experimental outcomes are summarized as follows:
Effect of matrix stiffness at constant adhesiveness (6 mM RGD) and degradability (NCD crosslinker) :
- Single-cell migration is expected in a 0.8-kPa hydrogel;
- Collective cell migration is expected in a 1.5-kPa hydrogel.
- Effect of matrix degradability at constant adhesiveness (6 mM RGD) and stiffness (0.8 kPa) :
- Single-cell migration is expected in a hydrogel with NCD crosslinker peptide;
- Collective cell migration is expected in a hydrogel with LD crosslinker peptide.
- Effect of matrix adhesiveness at constant stiffness (1.5 kPa) and degradability (NCD crosslinker) :
- Single-cell migration is expected in a hydrogel containing 0.15 mM RGD;
- Collective cell migration is expected in a hydrogel containing 6 mM RGD.
The migration mode can be evaluated by 3D confocal imaging of samples with fluorescently stained nuclei and F-actin using 3D analysis software (e.g., IMARIS x64, version 9.1.1, https://imaris.oxinst.com). First, the total cell number can best be determined by an (automatic) detection of all nuclei within the cohort of migrated cells (IMARIS Spots assistant). Then, the number of cells (nuclei) in sprouts has to be manually counted and saved as subsets. An empirically determined threshold of six or more nuclei in a collective strand connected to the main body is advised as a measure for the interpretation of collectivity (Trappmann et al., 2017). If the connection of the sprout to the main body is lost, the respective cells are counted as single cells. The degree of collectivity is the ratio of the collectively migrating cells to the total number of migrating cells. If a high density of cells in close proximity complicates the judgement of collectivity, additional staining of the cell membrane through R18 membrane dye or cell junctions via antibodies against VE-cadherin or catenins may improve the evaluation.
Time Considerations
Synthesis and purification of DexMA (Basic Protocol 1) require 8 days in total: 1 day for the coupling reaction, 1 day for DexMA precipitation in IPA and dissolution in water, 3 days of dialysis against water, and 3 days of lyophilization.
ECS formation (Basic Protocol 2) takes ∼1 day: 1 hr for microwell coating and HUVEC trypsinization, counting, and seeding into microwells of a AggreWell™400 plate plus an additional 24 hr of culture in the cell culture incubator to allow cell aggregation.
Generation of ECS-laden hydrogel samples and angiogenic sprouting (Basic Protocols 3, 4, or 5) require ∼2.5 days total: 3 hr for hydrogel preparation, 4 hr of sample incubation with EGM-2 after DexMA gelation, and 48 hr of culture in presence of pro-angiogenic cocktail to induce sprouting. An additional 5 hr should be considered for the preparation of glass-coverslip-supported PDMS wells: ∼30 min for mixing of PDMS pre-polymer solution with curing agent, degassing, and distribution within tissue culture dishes, 4 hr for curing in the oven, and ∼30 min for fabrication of glass-coverslip-supported PDMS wells.
Staining of ECS-laden hydrogel (Basic Protocol 6) for visualization of nuclei and F-actin requires ∼1 day: 1 hr for sample fixation, 1 hr for sample permeabilization, plus overnight incubation with Hoechst and phalloidin staining solution. If antibody staining needs to be performed, the staining procedure takes up to 6 days: 3 hr in total for fixation, permeabilization, and blocking (1 hr for each step), 1 day for primary antibody incubation followed by 2 days of washing, overnight incubation with secondary antibody followed by 1 day of washing, and finally overnight incubation with Hoechst and phalloidin solution.
Preparation of pro-angiogenic cocktail (Support Protocol 1) takes a few hours for the preparation of aliquots of VEGF, S1P, and PMA stock and intermediate solutions (∼1 hr for each component). The final dilution of VEGF, S1P, and PMA in EGM-2 for the pro-angiogenic cocktail preparation requires ∼10 min.
Acknowledgments
We thank N. Mote, P. Günther, I. Schröter, and M. Schütterle for helpful discussions. This work was financially supported by the Max Planck Society (MPG; B.T.) and the German Research Foundation (DFG, SFB1348 A07; B.T.). G.T. is supported by the International Max Planck Research School–Molecular Biomedicine, Münster, Germany.
Open access funding enabled and organized by Projekt DEAL.
Author Contributions
Giuseppe Trapani : Data curation, methodology, visualization, writing—original draft, writing—review and editing. Martin Weiß : Data curation, methodology, visualization, writing—original draft, writing—review and editing. Britta Trappmann : Funding acquisition, resources, supervision, writing—original draft, writing—review and editing.
Conflict of Interest
The authors declare no competing interests.
Open Research
Data Availability Statement
All data reported are available within this article or from the corresponding author upon reasonable request.
Literature Cited
- Aumailley, M., Gurrath, M., Müller, G., Calvete, J., Timpl, R., & Kessler, H. (1991). Arg-Gly-Asp constrained within cyclic pentapeptides Strong and selective inhibitors of cell adhesion to vitronectin and laminin fragment P1. FEBS Letters , 291(1), 50–54. https://doi.org/10.1016/0014-5793(91)81101-D
- Brooks, P. C., Clark, R. A., & Cheresh, D. A. (1994). Requirement of vascular integrin alpha v beta 3 for angiogenesis. Science , 264(5158), 569–571. https://doi.org/10.1126/science.7512751
- Carmeliet, P., & Jain, R. K. (2011). Molecular mechanisms and clinical applications of angiogenesis. Nature , 473, 298–307. https://doi.org/10.1038/nature10144
- Discher, D. E., Janmey, P., & Wang, Y. L. (2005). Tissue cells feel and respond to the stiffness of their substrate. Science , 310, 1139–1143. https://doi.org/10.1126/science.1116995
- Ebenstein, D. M., & Pruitt, L. A. (2006). Nanoindentation of biological materials. Nano Today , 1(3), 26–33. https://doi.org/10.1016/S1748-0132(06)70077-9
- Engler, A. J., Sen, S., Sweeney, H. L., & Discher, D. E. (2006). Matrix elasticity directs stem cell lineage specification. Cell , 126(4), 677–689. https://doi.org/10.1016/j.cell.2006.06.044
- Fang, Y., & Eglen, R. M. (2017). Three-dimensional cell cultures in drug discovery and development. SLAS Discovery: Advancing Life Sciences R &D, 22(5), 247255521769679–247255521769679. https://doi.org/10.1177/2472555217696795
- Fina, L., Molgaard, H. V., Robertson, D., Bradley, N. J., Monaghan, P., Delia, D., Sutherland, D. R., Baker, M. A., & Greaves, M. F. (1990). Expression of the CD34 gene in vascular endothelial cells. Blood , 75(12), 2417–2426. https://static.yanyin.tech/literature/current_protocol/10.1002/cpz1.859/attachments/2417.pdf
- Foty, R. (2011). A simple hanging drop cell culture protocol for generation of 3D spheroids. Journal of Visualized Experiments , May 6(51), 2720. https://doi.org/10.3791/2720
- Francis, S. E., Goh, K. L., Hodivala-Dilke, K., Bader, B. L., Stark, M., Davidson, D., & Hynes, R. O. (2002). Central roles of α5β1 integrin and fibronectin in vascular development in mouse embryos and embryoid bodies. Arteriosclerosis, Thrombosis, and Vascular Biology , 22(6), 927–933. https://doi.org/10.1161/01.ATV.0000016045.93313.F2
- Grainger, S. J., & Putnam, A. J. (2013). ECM Remodeling in angiogenesis. In C. A. Reinhart-King (Ed.), Mechanical and Chemical Signaling in Angiogenesis (pp. 185–209). Springer. https://doi.org/10.1007/978-3-642-30856-7_9
- Haubner, R., Gratias, R., Diefenbach, B., Goodman, S. L., Jonczyk, A., & Kessler, H. (1996). Structural and functional aspects of RGD-containing cyclic pentapeptides as highly potent and selective integrin α(v)β3 antagonists. Journal of the American Chemical Society , 118(32), 7461–7472. https://doi.org/10.1021/ja9603721
- Heiss, M., Hellström, M., Kalén, M., May, T., Weber, H., Hecker, M., Augustin, H. G., & Korff, T. (2015). Endothelial cell spheroids as a versatile tool to study angiogenesis in vitro. FASEB Journal , 29(7), 3076–3084. https://doi.org/10.1096/fj.14-267633
- Ingber, D. E. (1990). Fibronectin controls capillary endothelial cell growth by modulating cell shape. Proceedings of the National Academy of Sciences of the United States of America , 87(9), 3579–3583. https://doi.org/10.1073/pnas.87.9.3579
- Jiménez, N., Krouwer, V. J. D., & Post, J. A. (2013). A new, rapid and reproducible method to obtain high quality endothelium in vitro. Cytotechnology , 65(1), 1–14. https://doi.org/10.1007/s10616-012-9459-9
- Kapp, T. G., Rechenmacher, F., Neubauer, S., Maltsev, O. V., Cavalcanti-Adam, E. A., Zarka, R., Reuning, U., Notni, J., Wester, H. J., Mas-Moruno, C., Spatz, J., Geiger, B., & Kessler, H. (2017). A comprehensive evaluation of the activity and selectivity profile of ligands for RGD-binding Integrins. Scientific Reports , 7, 39805. https://doi.org/10.1038/srep39805
- Kim, S., Lee, H., Chung, M., & Jeon, N. L. (2013). Engineering of functional, perfusable 3D microvascular networks on a chip. Lab on a Chip , 13(8), 1489–1500. https://doi.org/10.1039/c3lc41320a
- Leclech, C., Natale, C. F., & Barakat, A. I. (2020). The basement membrane as a structured surface – role in vascular health and disease. Journal of Cell Science , 133(18), jcs239889–. https://doi.org/10.1242/jcs.239889
- Liu, J., Long, H., Zeuschner, D., Räder, A. F. B., Polacheck, W. J., Kessler, H., Sorokin, L., & Trappmann, B. (2021). Synthetic extracellular matrices with tailored adhesiveness and degradability support lumen formation during angiogenic sprouting. Nature Communications , 12(1), 3402. https://doi.org/10.1038/s41467-021-23644-5
- Madl, C. M., & Heilshorn, S. C. (2018). Engineering hydrogel microenvironments to recapitulate the stem cell niche. Annual Review of Biomedical Engineering , 20, 21–47. https://doi.org/10.1146/annurev-bioeng-062117-120954
- Mebratu, Y., & Tesfaigzi, Y. (2009). How ERK1/2 activation controls cell proliferation and cell death: Is subcellular localization the answer? Cell Cycle , 8(8), 1168–1175. https://doi.org/10.4161/cc.8.8.8147
- Nagase, H., & Fields, G. B. (1996). Human matrix metalloproteinase specificity studies using collagen sequence-based synthetic peptides. Biopolymers , 40(4), 399–416. https://doi.org/10.1002/(sici)1097-0282(1996)40:4<399::aid-bip5>3.0.co;2-r
- Nair, D. P., Podgórski, M., Chatani, S., Gong, T., Xi, W., Fenoli, C. R., & Bowman, C. N. (2014). The thiol-Michael addition click reaction: A powerful and widely used tool in materials chemistry. Chemistry of Materials , 26(1), 724–744. https://doi.org/10.1021/cm402180t
- Nguyen, D. H. T., Stapleton, S. C., Yang, M. T., Cha, S. S., Choi, C. K., Galie, P. A., & Chen, C. S. (2013). Biomimetic model to reconstitute angiogenic sprouting morphogenesis in vitro. Proceedings of the National Academy of Sciences , 110(17), 6712–6717. https://doi.org/10.1073/pnas.1221526110
- Raj, M. K., & Chakraborty, S. (2020). PDMS microfluidics: A mini review. Journal of Applied Polymer Science , 137, 48958. https://doi.org/10.1002/app.48958
- Riss, T., & Trask, O. J. (2021). Factors to consider when interrogating 3D culture models with plate readers or automated microscopes. In Vitro Cellular and Developmental Biology -Animal , 57, 238–256.
- Takahashi, S., Leiss, M., Moser, M., Ohashi, T., Kitao, T., Heckmann, D., Pfeifer, A., Kessler, H., Takagi, J., Erickson, H. P., & Fässler, R. (2007). The RGD motif in fibronectin is essential for development but dispensable for fibril assembly. Journal of Cell Biology , 178(1), 167–178. https://doi.org/10.1083/jcb.200703021
- Takuwa, Y. (2010). Roles of sphingosine-1-phosphate signaling in angiogenesis. World Journal of Biological Chemistry , 1(10), 298–298. https://doi.org/10.4331/wjbc.v1.i10.298
- Trappmann, B., Baker, B. M., Polacheck, W. J., Choi, C. K., Burdick, J. A., & Chen, C. S. (2017). Matrix degradability controls multicellularity of 3D cell migration. Nature Communications , 8(1), 371. https://doi.org/10.1038/s41467-017-00418-6
- Trappmann, B., & Chen, C. S. (2013). How cells sense extracellular matrix stiffness: A material's perspective. Current Opinion in Biotechnology , 24(5), 948–953.
- Trappmann, B., Gautrot, J. E., Connelly, J. T., Strange, D. G., Li, Y., Oyen, M. L., Cohen Stuart, M. A., Boehm, H., Li, B., Vogel, V., Spatz, J. P., Watt, F. M., & Huck, W. T. (2012). Extracellular-matrix tethering regulates stem-cell fate. Nature Materials , 11(7), 642–649. https://doi.org/10.1038/nmat3339
- Turk, B. E., Huang, L. L., Piro, E. T., & Cantley, L. C. (2001). Determination of protease cleavage site motifs using mixture-based oriented peptide libraries. Nature Biotechnology , 19(7), 661–667. https://doi.org/10.1038/90273
- Tusan, C. G., Man, Y. H., Zarkoob, H., Johnston, D. A., Andriotis, O. G., Thurner, P. J., Yang, S., Sander, E. A., Gentleman, E., Sengers, B. G., & Evans, N. D. (2018). Collective cell behavior in mechanosensing of substrate thickness. Biophysical Journal , 114(11), 2743–2755. https://doi.org/10.1016/j.bpj.2018.03.037
- van Dijk-Wotthuis, W. N. E., Franssen, O., Talsma, H., van Steenbergen, M. J., Kettenes-van den Bosch, J. J., & Hennink, W. E. (1995). Synthesis, characterization, and polymerization of glycidyl methacrylate derivatized dextran. Macromolecules , 28(18), 6317–6322. https://doi.org/10.1021/ma00122a044
- Verin, A. D., Liu, F., Bogatcheva, N., Borbiev, T., Hershenson, M. B., Wang, P., & Garcia, J. G. (2000). Role of ras-dependent ERK activation in phorbol ester-induced endothelial cell barrier dysfunction. American Journal of Physiology-Lung Cellular and Molecular Physiology , 279(2), L360–370. https://doi.org/10.1152/ajplung.2000.279.2.L360
- Wang, Q. J., Bhattacharyya, D., Garfield, S., Nacro, K., Marquez, V. E., & Blumberg, P. M. (1999). Differential localization of protein kinase C δ by phorbol esters and related compounds using a fusion protein with green fluorescent protein. Journal of Biological Chemistry , 274(52), 37233–37239. https://doi.org/10.1074/jbc.274.52.37233
- Wang, W. Y., Lin, D., Jarman, E. H., Polacheck, W. J., & Baker, B. M. (2020). Functional angiogenesis requires microenvironmental cues balancing endothelial cell migration and proliferation. Lab on a Chip , 20(6), 1153–1166. https://doi.org/10.1039/C9LC01170F
- West, J. L., & Hubbell, J. A. (1999). Polymeric biomaterials with degradation sites for proteases involved in cell migration. Macromolecules , 32(1), 241–244. https://doi.org/10.1021/ma981296k
- Xu, D., Harvey, T., Begiristain, E., Domínguez, C., Sánchez-Abella, L., Browne, M., & Cook, R. B. (2022). Measuring the elastic modulus of soft biomaterials using nanoindentation. Journal of the Mechanical Behavior of Biomedical Materials , 133, 105329. https://doi.org/10.1016/j.jmbbm.2022.105329
- Yeung, T., Georges, P. C., Flanagan, L. A., Marg, B., Ortiz, M., Funaki, M., Zahir, N., Ming, W., Weaver, V., & Janmey, P. A. (2005). Effects of substrate stiffness on cell morphology, cytoskeletal structure, and adhesion. Cell Motility and the Cytoskeleton , 60(1), 24–34. https://doi.org/10.1002/cm.20041
Citing Literature
Number of times cited according to CrossRef: 1
- Martin S. Weiß, Giuseppe Trapani, Hongyan Long, Britta Trappmann, Matrix Resistance Toward Proteolytic Cleavage Controls Contractility‐Dependent Migration Modes During Angiogenic Sprouting, Advanced Science, 10.1002/advs.202305947, 11 , 19, (2024).