Theoretical Considerations for Optimizing the Use of Optogenetics with Complex Behavior
Bess Glickman, Bess Glickman, Ryan T. LaLumiere, Ryan T. LaLumiere
activity-controlled optogenetics
cell-type specificity
frequency specificity
stimulation pattern
temporal precision
Abstract
Optogenetic approaches have allowed researchers to address complex questions about behavior that were previously unanswerable. However, as optogenetic procedures involve a large parameter space across multiple dimensions, it is crucial to consider such parameters in conjunction with the behaviors under study. Here, we discuss strategies to optimize optogenetic approaches with complex behavior by identifying critical experimental design considerations, including frequency specificity, temporal precision, activity-controlled optogenetics, stimulation pattern, and cell-type specificity. We highlight potential limitations or theoretical considerations to be made when manipulating each of these factors of optogenetic experiments. This overview emphasizes the importance of optimizing optogenetic study design to enhance the conclusions that can be drawn about the neuroscience of behavior. © 2023 The Authors. Current Protocols published by Wiley Periodicals LLC.
INTRODUCTION
Over the past two decades, the development of optogenetics has opened major new avenues for understanding the relationship between the brain and behavior, with the ability to control the activity of genetically targeted cells with a high degree of temporal and spatial precision and to do so in awake, behaving animals. In previous decades, the ability to influence neural activity in conjunction with behavior was more limited, with significant restrictions across multiple domains. For example, pharmacological approaches, even via intracranial microinjections, are considerably more temporally imprecise and do not allow targeting of specific pathways. Thus, determining the role of specific neural pathways in controlling behavior was difficult, if not impossible, and often required more inference than the data directly indicated. Similarly, identifying specific epochs of neural activity necessary for a particular behavioral phenomenon has also become easier with the temporal control afforded by optogenetic approaches. Although optogenetic approaches have enhanced scientific findings, as with all techniques, they have limitations and, in conjunction with behavior, must be thoughtfully used and optimized to draw the most rigorous conclusions. This overview will highlight different topics—frequency specificity, temporal precision, activity-controlled optogenetics, stimulation pattern, and cell-type specificity—that must be considered when investigating complex behavior using optogenetics.
FREQUENCY SPECIFICITY
A major advantage of optogenetic approaches is the ability to apply neuronal stimulation in a frequency-specific manner. Early work with channelrhodopsin-2 (ChR2) observed excellent fidelity of spiking in transduced neurons in response to pulses of light up to 20-30 Hz (Berndt et al., 2011; Boyden et al., 2005). Later mutated versions of ChR2 led to fidelity at even higher frequencies, though the upper limit likely depends on the neurons’ intrinsic firing capabilities (Berndt et al., 2011; Gunaydin et al., 2010; Yizhar et al., 2011). Consequently, using ChR2 and mutated derivatives enables the investigation of different stimulation frequencies and patterns in conjunction with behavior. Although determining optimal stimulation parameters for influencing behavior does not necessarily reveal how neurons normally fire, such findings provide critical insight into questions regarding neuronal functioning that were not possible previously.
Work from our laboratory found that stimulation of basolateral amygdala (BLA) cell bodies and BLA projections to other brain regions influenced memory consolidation in a frequency-dependent manner—i.e., stimulation at a specific frequency produced memory enhancement or impairment not observed with other frequencies (Huff et al., 2016; Huff et al., 2013; Wahlstrom et al., 2018). Moreover, the stimulation frequency determined whether there were downstream alterations in synaptic plasticity-associated proteins (Wahlstrom et al., 2021). A substantial body of literature supports the idea that specific neural rhythms or oscillations play a role in facilitating neural activity across structures important for memory and suggests the importance of these oscillations in memory consolidation (Bauer et al., 2007; Buzsaki, 2005; Buzsaki & Moser, 2013; Kanta et al., 2019; Popescu et al., 2009). Although optogenetic stimulation at specific frequencies is not necessarily identical to producing endogenous oscillations, specific frequencies might relate to oscillations that are believed to be critical for communication between brain structures.
Additional evidence suggests that stimulating the same neuronal population at different frequencies could influence different types of behavior. Recent research examined whether rats would press a lever to optogenetically self-stimulate dopamine neurons in the ventral tegmental area (Millard et al., 2022). The findings indicate that rats pressed a lever for 50 Hz neuronal stimulation but not for 20 Hz stimulation, even though 50 Hz stimulation exceeds the physiological limits of the spiking capabilities of the neurons. However, 20 Hz stimulation of these neurons increased time spent reward seeking, suggesting that stimulation at this frequency promoted learning. Such findings raise interesting and important questions about how the results of optogenetic studies should be interpreted when only a single frequency is tested, as different results may be obtained under other stimulation parameters, even if such parameters are outside the bounds of physiological relevance.
Other research has utilized stabilized step function opsins (SSFOs) to optogenetically stimulate certain brain regions and pathways. Using SSFOs eliminates frequency specificity parameter issues in stimulation experiments, as SSFO activation in cell bodies puts the cells into a more depolarized state. Similarly, SSFO activation at axonal terminals enhances synaptic release without altering endogenous vesicle release patterns (Liu et al., 2016). Slice recordings in mice indicate that illumination given to the terminals of SSFO-expressing neurons of the medial prefrontal cortex (mPFC) or BLA increased presynaptic neurotransmitter release probability and spontaneous excitatory postsynaptic current (EPSC) frequency in the nucleus accumbens (Liu et al., 2016; Wang et al., 2020). Laser stimulation of such opsins likely indirectly evokes neurotransmitter release by increasing the probability of action potentials without directly evoking neurotransmitter release. This suggests that neural pathways can be stimulated without frequency-specific stimulation parameters. SSFOs have been used to manipulate cell bodies and axonal terminals in sucrose-seeking and cocaine-seeking tasks (Liu et al., 2016; Muller Ewald et al., 2019; Wang et al., 2020). Although the use of SSFOs likely capitalizes on endogenous firing patterns, perhaps allowing for more physiologically relevant data, there are still many unknowns about the mechanisms underlying terminal stimulation utilizing such opsins. For example, Liu and colleagues (2016) discussed the possibility of a secondary mechanism, such as Ca2+ accumulation driving neurotransmitter release. Therefore, more research is needed to discover the mechanism by which SSFOs allow for optogenetic neural stimulation, especially at terminals, and to determine whether this optogenetic tool could be used to stimulate cell bodies and terminals without accounting for stimulation frequency.
Many behavioral neuroscience studies do not report results for different stimulation frequencies. Undoubtedly, the parameter space is virtually limitless, so it is not surprising that when researchers identify an effective set of parameters, they rarely deviate from them due to the effort involved in conducting such work. In the past, pharmacological microinjections were one of the most frequently used methods for altering neural activity to influence behavior, and the use of dose-response curves was common. With optogenetic approaches, even if many studies find 20 Hz stimulation, for example, to be most effective across a variety of neural systems, it would be beneficial to determine why and under which circumstances such stimulation produces optimal results. Nonetheless, it should be noted that, under some circumstances, the particular frequency used for stimulation does not appear to be critical. For example, Warlow and colleagues observed similar levels of attraction to an aversive stimulus when optogenetically stimulating the central amygdala (CeA) regardless of the stimulation frequency (10, 25, or 40 Hz) (Warlow et al., 2020). Understanding why some neural systems are sensitive to different stimulation frequencies while others are not is yet another line of unexplored research. Such work would provide better insight into how the brain normally functions and is capable of functioning and would provide important complementary data to electrophysiological recordings.
TEMPORAL PRECISION
One of the most significant advances in investigating brain-behavior relationships provided by optogenetics was the temporal precision for when neuronal activity could be altered. With fast kinetics for opsin channels to open and close or pumps to turn on and off, optogenetic manipulations permit tight temporal control of neuronal firing (Yizhar et al., 2011). In contrast, pharmacological manipulations typically involve alterations in neural activity on the scale of minutes to hours, while optogenetic approaches allow for control on the order of milliseconds to seconds, strengthening the conclusions that can be made about the relationship between neuronal activity and changes in behavior. Taking full advantage of this temporal precision requires obtaining a positive finding with a manipulation within a relevant epoch and obtaining a comparison negative finding outside that epoch. For example, our laboratory examined the extinction of cocaine-seeking behavior in rats and found that optogenetically inhibiting the infralimbic cortex for 20 s immediately following an unreinforced lever press impaired extinction encoding (Gutman et al., 2017). Critically, we also found that giving the same total amount of inhibition but in a pseudo-random manner (i.e., not temporally linked to the lever press) during the extinction session had no effect, providing evidence for a specific temporal window of infralimbic cortex activity, rather than general activity, being important for such encoding. In a recent follow-up study, we found that inhibiting infralimbic terminals in the nucleus accumbens shell and the amygdala for 20 s after an unreinforced lever press also impaired extinction encoding (Nett et al., 2023). In this case, a control experiment found that giving such inhibition during the period 20 to 40 s after the lever press had no effect, indicating that the activity in these pathways involved in extinction encoding does not extend beyond the first 20 s after the unreinforced lever press. These studies illustrate the value of temporally precise optogenetic control over neural activity in identifying specific epochs when such activity is important and the value of key control experiments that further strengthen what can be concluded.
Evidence also indicates that the duration of stimulation influences behavior. For example, optogenetic stimulation of medium spiny neurons (MSNs) expressing D1 or D2 dopamine receptors in the nucleus accumbens differentially drove behavior in conditioned place preference depending on the duration of stimulation (Soares-Cunha et al., 2020). Providing brief (1 s, 40 Hz pulses) optogenetic stimulation to both D1 and D2 MSNs increased preference for the laser stimulation chamber in a conditioned place preference task both with optogenetic stimulation alone and when paired with cocaine conditioning, pointing to the idea that D1 and D2 MSNs induce positive reinforcement. However, prolonged optogenetic stimulation (60 s, 40 Hz pulses) to D1 or D2 MSNs produced aversion for the chamber paired with optogenetic stimulation. Furthermore, prolonged D2 MSN stimulation paired with cocaine conditioning decreased cocaine-conditioning effects, but D1 MSN stimulation had no effect on chamber preference. These behavioral differences are likely a result of downstream regions, such as the ventral tegmental area and ventral pallidum, where nucleus accumbens D1 and D2 MSNs project, respectively, ultimately disinhibiting dopamine activity in the ventral tegmental area.
Further work by Soares-Cunha and colleagues supports the idea that changing the timing of optogenetic manipulations during a behavioral task produces important behavioral differences. In their recent work, rats were trained on a progressive ratio task to increase lever pressing on one of two levers to receive a single food pellet reward (Soares-Cunha et al., 2022). A cue light above one lever indicated that the lever yielded a reward. They observed that stimulating or inhibiting terminals of nucleus accumbens D2 MSNs in the ventral pallidum during cue light presentation increased or decreased, respectively, total lever pressing and the maximum number of lever presses performed to earn the reward (breakpoint). However, stimulating or inhibiting terminals of the same neuronal population during reward delivery decreased or increased, respectively, lever pressing and breakpoint. Optogenetic manipulations applied during cue presentation versus reward delivery of this progressive ratio task produced opposite results, shedding light on discrepant findings related to the role of accumbens D2 MSNs in motivated behaviors. This research illustrates ways in which optogenetics can be used to parse the specific temporal relationship between neural activity and behavior. Thus, providing optogenetic manipulations offset by small timeframes or experimental phases may affect whether differences in behavior are observed and shed more insight into the neural mechanisms regulating specific behaviors.
ACTIVITY-CONTROLLED OPTOGENETICS
Optogenetic approaches not only allow for neural inhibition or stimulation in a temporally precise manner, but also the ability to pair the delivery of optogenetic illumination with specific behavior. In a series of studies, Berridge and colleagues explored the role of the CeA in incentive motivation and addiction-like behaviors using an activity-controlled approach. In these studies, rats were trained on a two-choice sucrose-reward task (Robinson et al., 2014) or an instrumental cocaine self-administration task (Warlow et al., 2017). In each experiment, a lever press or nosepoke either led to a sucrose pellet or cocaine infusion paired with CeA stimulation or resulted in a sucrose pellet or cocaine infusion alone. In each task, rats preferred the option paired with optogenetic stimulation of the CeA, suggesting a role for the CeA in incentive motivation. Further work found that CeA stimulation given when a rat engaged with an aversive stimulus (a shock rod) increased attraction to touching the shock rod and increased consummatory behaviors such as sniffing, nibbling, and biting the shock rod, even though rats found the shock aversive and touched it less when not paired with stimulation (Warlow et al., 2020). Additionally, these studies examined whether rats would self-administer CeA stimulation. In these experiments, rats received optogenetic stimulation of the CeA when they entered a certain corner in a place self-administration test or when they touched a spout in a spout-touch self-administration test (Robinson et al., 2014; Warlow et al., 2020; Warlow et al., 2017). Interestingly, rats did not self-administer CeA stimulation. This research suggests that stimulation of the CeA alone is not reinforcing but that CeA stimulation paired with seeking and receiving a certain reward or aversive stimulus altered incentive motivation. Thus, the use of an activity-controlled design in these studies enabled conclusions to be drawn about the role of the CeA in behavior that otherwise would be difficult, if not impossible, to parse and enhanced behavioral observations related to optogenetic manipulations that are linked to certain behaviors with strong temporal precision.
Other work has focused on using real-time electrophysiological activity to control optogenetic stimulation. Work by Kanta and colleagues took advantage of online monitoring of gamma rhythms to apply optogenetic stimulation to the BLA during different phases (trough, peak, or random) of locally generated gamma rhythms (Kanta et al., 2019). Stimulation was given when a certain amplitude and phase of a mid-gamma cycle was detected. Notably, although the stimulation was provided by way of blue light activation of BLA neurons, this stimulation ultimately had inhibitory effects on the principal neurons and excited fast-spiking interneurons, which are known to play a critical role in generating gamma rhythms. Their findings suggest that blue light pulses delivered during the trough phase of the gamma rhythm enhanced gamma rhythms and retrieval on a spatial memory task. Identical stimulation delivered during the peak gamma phase yielded the opposite results for both gamma rhythms and spatial memory performance. Similarly, optogenetic stimulation of the hippocampus during different phases of theta rhythms differentially affected performance on encoding and retrieval of a spatial navigation task (Siegle & Wilson, 2014). Together, these findings suggest that altering brain rhythm activity alters behavior. Indeed, such approaches have also been used to increase the duration of specific sharp-wave ripples during a learning and memory task and indicate that behavioral differences depend on whether stimulation prolonged endogenous oscillations or was applied randomly (Fernandez-Ruiz et al., 2019). These studies have been some of the first to provide strong evidence indicating a functional role for brain rhythms and sharp-wave ripples in behavior. The importance of these findings in their respective fields underscores how the ability to manipulate neural activity with respect to real-time behavior or brain rhythms strengthens the conclusions from optogenetic studies of behavior.
STIMULATION PATTERN
Evidence suggests that the pattern of optogenetic stimulation—e.g., giving small bursts of light pulses versus continuous stimulation or variable pulse widths—also influences behavior. Early studies examining optogenetic stimulation in neural systems indicated that delivering stimulation as discrete pulses versus continuous illumination differentially drove neuronal firing in cultured hippocampal neurons (Boyden et al., 2005). Whereas pulsed illumination reliably drove temporally precise action potentials, continuous illumination was less precise after the initial spike. Indeed, other research suggests that different populations of neurons optimally fire under differing pulse-width stimulation parameters (Herman et al., 2014). In contrast to these electrophysiological differences related to stimulation pattern, several studies indicate that a pulsed versus continuous stimulation pattern may not impact behavior (Adamantidis et al., 2007; Johansen et al., 2010; Warlow et al., 2020). For example, Johansen and colleagues trained rats on a fear conditioning procedure where lateral amygdala stimulation acted as the unconditioned stimulus and was paired with a tone (conditioned stimulus) (Johansen et al., 2010). Although 20 Hz pulsed stimulation increased action potential firing compared to constant illumination, fear learning was similar in both groups, suggesting both stimulation patterns evoked fear conditioning when acting as the unconditioned stimulus. It is unclear why different stimulation patterns cause different spiking patterns while maintaining similar behaviors, and this is an area that deserves further examination in optogenetic studies of complex behavior.
Furthermore, stimulation delivery via a continuous sinusoidal or square pulsatile pattern is another element that can drive behavioral differences in experiments using optogenetic manipulations. Padilla-Coreano and colleagues optogenetically stimulated ventral hippocampus terminals in the mPFC in mice as a continuous oscillatory sinusoidal pattern or square pulsatile pattern (Padilla-Coreano et al., 2019). Their findings suggest that 8 Hz stimulation delivered in an oscillatory pattern decreased both percentage of time spent in and entries into the open arms of an elevated plus maze. However, when 8 Hz stimulation was delivered as square pulses, there was no effect on either of these measures. Furthermore, stimulation of ventral hippocampus terminals in the mPFC with either sinusoidal or pulsatile patterns increased EPSC frequency, yet pulsatile, but not sinusoidal, stimulation led to time-locked EPSCs. These findings suggest a functional link between behavior and EPSCs as both were differentially affected by stimulation design choices and highlight the importance of stimulation pattern parameters when using optogenetics to study behavior, an issue that we have rarely seen addressed in other studies.
CELL-TYPE SPECIFICITY
Optogenetic approaches enable genetic targeting that enhances the spatial precision of manipulations by pinpointing specific cell types within a brain region responsible for certain behaviors. One of the first examples demonstrating cell-type specificity using optogenetics indicated that distinct cell types differentially drove barrel cortex oscillations as stimulating fast-spiking interneurons, but not pyramidal neurons, amplified gamma oscillations (Cardin et al., 2009). Building on this finding, further research suggested that optogenetic stimulation of these fast-spiking interneurons in the gamma range enhanced sensory processing (Siegle et al., 2014). Optogenetically targeting different cell types within a single brain region allows researchers to identify which cells are important for influencing certain behaviors. Such specificity allows stronger conclusions to be drawn about the neurobiology that underlies behavior.
This spatially targeted approach has been used to identify populations of neurons underlying behavioral responses related to conditioned fear. One such example indicates that distinct subpopulations of GABAergic neurons in the CeA differentially mediated Pavlovian conditioned fear responses (Fadok et al., 2017). Whereas neurons expressing corticotropin-releasing factor drove a flight response categorized by increased speed and number of jumps in response to conditioning, somatostatin-positive neurons drove freezing behavior. Furthermore, Fadok and colleagues (2017) suggest these neuronal subpopulations compete as optogenetically stimulating either subpopulation increases inhibitory postsynaptic currents in the other. Similarly, such competition was observed in behavior. Stimulating neurons expressing corticotropin-releasing factor during exposure to the conditioned stimulus that normally produced freezing (tone exposure) decreased freezing and increased flight behavior. In contrast, stimulating somatostatin-positive neurons during exposure to the conditioned stimulus that normally produced flight (white noise exposure) increased freezing and decreased flight. Targeting specific cell types within a larger brain region or circuit, such as different subpopulations of GABAergic neurons, is a crucial tool to reveal why studies observe contrasting behavioral findings. For example, if neurons expressing corticotropin-releasing factor and somatostatin-positive neurons are manipulated simultaneously but have contrasting roles in regulating the behavior under scrutiny, this could lead to discrepant and unreliable findings. Using optogenetic approaches to target specific cell types enhances our understanding of how different neural populations contribute to behavior.
CONCLUSIONS
The development of optogenetic approaches has enabled neuroscientists to address many research questions in ways that were challenging, if not impossible, previously. The examples discussed here contain a subset of such work that illustrates the use of optogenetics with complex behavior. Figure 1 summarizes the critical elements discussed in each section of this overview. These studies not only promote interesting uses for optogenetics but also raise important theoretical considerations for the ongoing use of this technique. Thus, researchers must not only consider whether optogenetics is the best approach for addressing a question, but also which parameters to use and how behavioral results may differ with various parameters. Choices of methodological design, such as using the appropriate opsin based on desired physiological properties, must be at the forefront of researchers’ minds when conducting this type of research. Whereas this overview focused on theoretical considerations of using optogenetics with complex behavior, other reviews have addressed the selection of appropriate optogenetic tools as well as practical issues such as those surrounding light properties (Emiliani et al., 2022; Yizhar et al., 2011). Figure 2 summarizes the practical issues and decision points in designing an optogenetic experiment. Nonetheless, the ideas addressed in this overview raise important points to consider when combining optogenetic approaches with behavior. Such considerations are crucial in drawing conclusions about complex behavior, as using one stimulation frequency over another or a square pulse instead of sinusoidal oscillation may drastically change the findings of an experiment. Furthermore, it is important to consider the meaning behind behavioral differences when optogenetic parameters vary. For example, although it is interesting that supraphysiological stimulation of a population of neurons produces different behavioral results than stimulating at a physiologically relevant frequency, it is not always clear how such findings should be interpreted. Moreover, if appropriate control experiments are not performed to test alternative optogenetic parameters, incorrect conclusions may be drawn about the findings. Taking these theoretical considerations of using optogenetics with complex behavior into account will undoubtedly strengthen scientific findings and provide far greater insight into the functioning of the brain in controlling behavior.
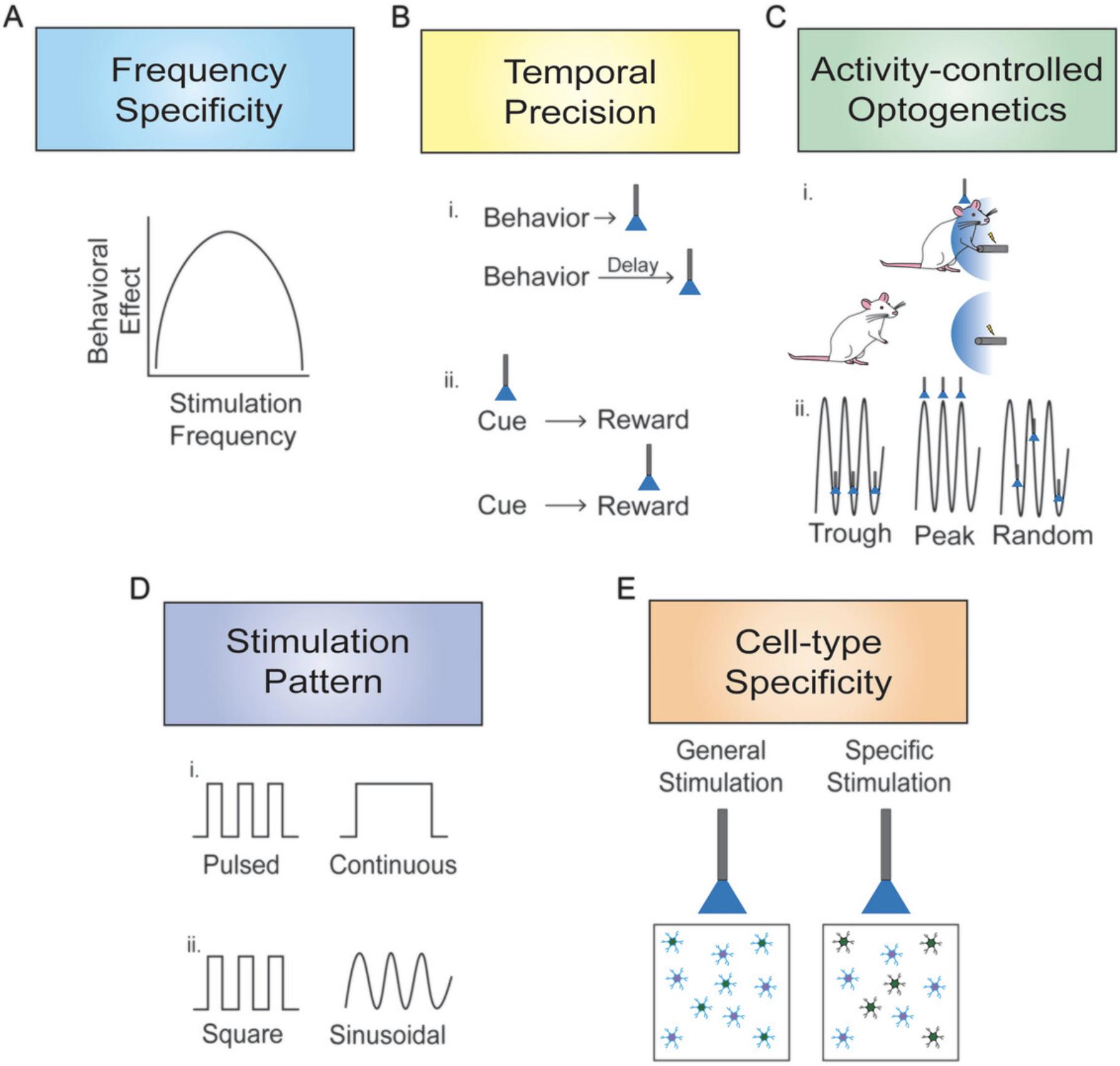
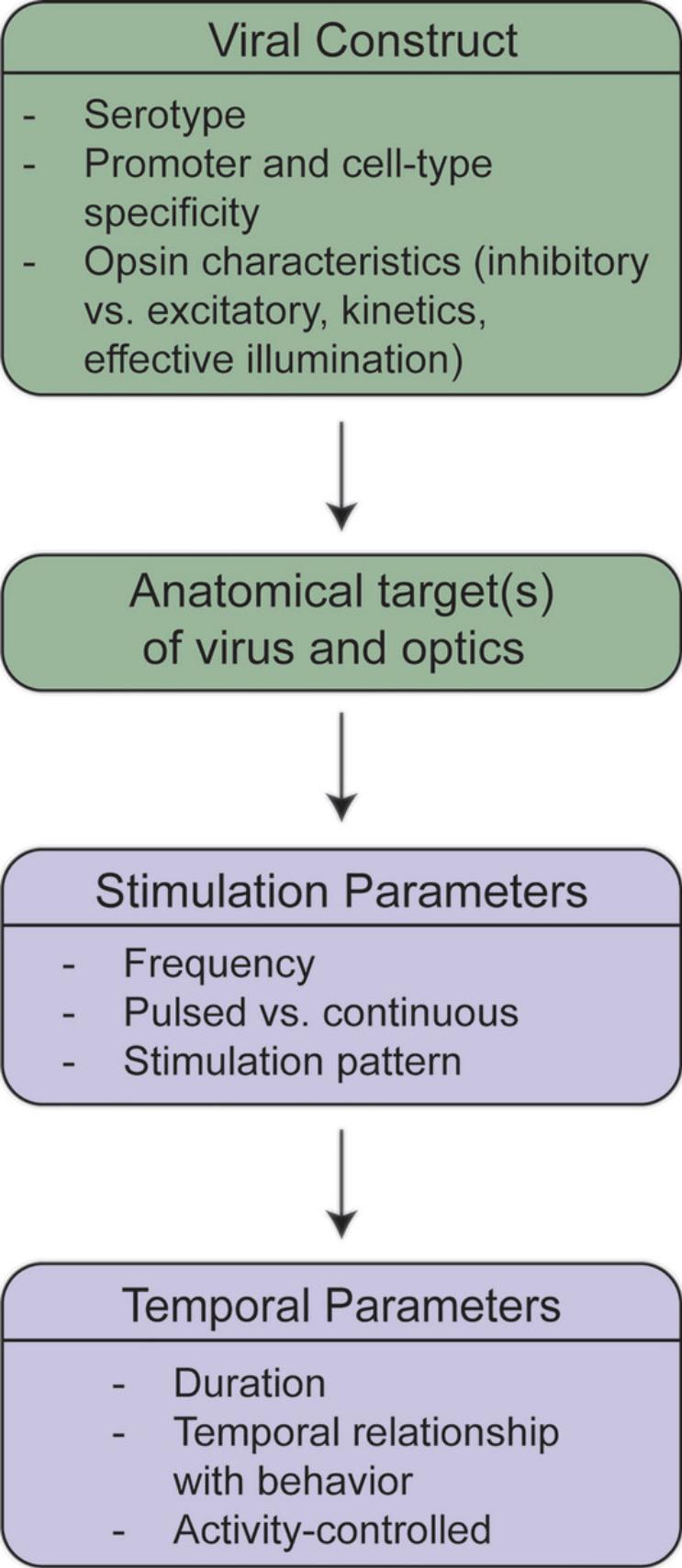
AUTHOR CONTRIBUTIONS
Bess Glickman : Visualization, writing original draft, writing review and editing; Ryan LaLumiere : Funding acquisition, visualization, writing original draft, writing review, and editing.
CONFLICT OF INTEREST
The authors declare no conflict of interest.
Open Research
DATA AVAILABILITY STATEMENT
Data sharing not applicable to this article as no datasets were generated or analyzed during the current study.
LITERATURE CITED
- Adamantidis, A. R., Zhang, F., Aravanis, A. M., Deisseroth, K., & de Lecea, L. (2007). Neural substrates of awakening probed with optogenetic control of hypocretin neurons. Nature , 450(7168), 420–424. https://doi.org/10.1038/nature06310
- Bauer, E. P., Paz, R., & Pare, D. (2007). Gamma oscillations coordinate amygdalo-rhinal interactions during learning. Journal of Neuroscience , 27(35), 9369–9379. https://doi.org/10.1523/JNEUROSCI.2153-07.2007
- Berndt, A., Schoenenberger, P., Mattis, J., Tye, K. M., Deisseroth, K., Hegemann, P., & Oertner, T. G. (2011). High-efficiency channelrhodopsins for fast neuronal stimulation at low light levels. Proceedings of the National Academy of Science USA , 108(18), 7595–7600. https://doi.org/10.1073/pnas.1017210108
- Boyden, E. S., Zhang, F., Bamberg, E., Nagel, G., & Deisseroth, K. (2005). Millisecond-timescale, genetically targeted optical control of neural activity. Nature Neuroscience , 8(9), 1263–1268. https://doi.org/10.1038/nn1525
- Buzsaki, G. (2005). Theta rhythm of navigation: Link between path integration and landmark navigation, episodic and semantic memory. Hippocampus , 15(7), 827–840. https://doi.org/10.1002/hipo.20113
- Buzsaki, G., & Moser, E. I. (2013). Memory, navigation and theta rhythm in the hippocampal-entorhinal system. Nature Neuroscience , 16(2), 130–138. https://doi.org/10.1038/nn.3304
- Cardin, J. A., Carlen, M., Meletis, K., Knoblich, U., Zhang, F., Deisseroth, K., Tsai, H., & Moore, C. I. (2009). Driving fast-spiking cells induces gamma rhythm and controls sensory responses. Nature , 459(7247), 663–667. https://doi.org/10.1038/nature08002
- Emiliani, V., Entcheva, E., Hedrich, R., Hegemann, P., Konrad, K. R., Luscher, C., Mahn, M., Pan, Z. H., Sims, R. R., Vierock, J., & Yizhar, O. (2022). Optogenetics for light control of biological systems. Nature Reviews , 2(56), 55. https://doi.org/10.1038/s43586-022-00149-z
- Fadok, J. P., Krabbe, S., Markovic, M., Courtin, J., Xu, C., Massi, L., Botta, P., Bylund, K., Müller, C., Kovacevic, A., Tovote, P., & Luthi, A. (2017). A competitive inhibitory circuit for selection of active and passive fear responses. Nature , 542(7639), 96–100. https://doi.org/10.1038/nature21047
- Fernandez-Ruiz, A., Oliva, A., Fermino de Oliveira, E., Rocha-Almeida, F., Tingley, D., & Buzsaki, G. (2019). Long-duration hippocampal sharp wave ripples improve memory. Science , 364(6445), 1082–1086. https://doi.org/10.1126/science.aax0758
- Gunaydin, L. A., Yizhar, O., Berndt, A., Sohal, V. S., Deisseroth, K., & Hegemann, P. (2010). Ultrafast optogenetic control. Nature Neuroscience , 13(3), 387–392. https://doi.org/10.1038/nn.2495
- Gutman, A. L., Nett, K. E., Cosme, C. V., Worth, W. R., Gupta, S. C., Wemmie, J. A., & LaLumiere, R. T. (2017). Extinction of cocaine seeking requires a window of infralimbic pyramidal neuron activity after unreinforced lever presses. Journal of Neuroscience , 37(25), 6075–6086. https://doi.org/10.1523/JNEUROSCI.3821-16.2017
- Herman, A. M., Huang, L., Murphey, D. K., Garcia, I., & Arenkiel, B. R. (2014). Cell type-specific and time-dependent light exposure contribute to silencing in neurons expressing Channelrhodopsin-2. Elife , 3, e01481. https://doi.org/10.7554/eLife.01481
- Huff, M. L., Emmons, E. B., Narayanan, N. S., & LaLumiere, R. T. (2016). Basolateral amygdala projections to ventral hippocampus modulate the consolidation of footshock, but not contextual, learning in rats. Learning & Memory, 23(2), 51–60. https://doi.org/10.1101/lm.039909.115
- Huff, M. L., Miller, R. L., Deisseroth, K., Moorman, D. E., & LaLumiere, R. T. (2013). Posttraining optogenetic manipulations of basolateral amygdala activity modulate consolidation of inhibitory avoidance memory in rats. Proceedings of the National Academy of Science USA , 110(9), 3597–3602. https://doi.org/10.1073/pnas.1219593110
- Johansen, J. P., Hamanaka, H., Monfils, M. H., Behnia, R., Deisseroth, K., Blair, H. T., & LeDoux, J. E. (2010). Optical activation of lateral amygdala pyramidal cells instructs associative fear learning. Proceedings of the National Academy of Science USA , 107(28), 12692–12697. https://doi.org/10.1073/pnas.1002418107
- Kanta, V., Pare, D., & Headley, D. B. (2019). Closed-loop control of gamma oscillations in the amygdala demonstrates their role in spatial memory consolidation. Nature Communications , 10(1), 1–14. https://doi.org/10.1038/s41467-019-11938-8
- Liu, Z., Wang, Y., Cai, L., Li, Y., Chen, B., Dong, Y., & Huang, Y. H. (2016). Prefrontal cortex to accumbens projections in sleep regulation of reward. Journal of Neuroscience , 36(30), 7897–7910. https://doi.org/10.1523/JNEUROSCI.0347-16.2016
- Millard, S. J., Hoang, I. B., Greer, Z., O'Connor, S. L., Wassum, K. M., James, M. H., Barker, D. J., & Sharpe, M. J. (2022). The cognitive basis of intracranial self-stimulation of midbrain dopamine neurons. BioRxiv.
- Muller Ewald, V. A., De Corte, B. J., Gupta, S. C., Lillis, K. V., Narayanan, N. S., Wemmie, J. A., & LaLumiere, R. T. (2019). Attenuation of cocaine seeking in rats via enhancement of infralimbic cortical activity using stable step-function opsins. Psychopharmacology , 236(1), 479–490. https://doi.org/10.1007/s00213-018-4964-y
- Nett, K. E., Zimbelman, A. R., McGregor, M. S., Alizo Vera, V., Harris, M., & LaLumiere, R. T. (2023). Infralimbic projections to the nucleus accumbens shell and amygdala regulate the encoding of cocaine extinction learning. Journal of Neuroscience , 43(8), 1348–1359. https://doi.org/10.1523/JNEUROSCI.2023-22.2022
- Padilla-Coreano, N., Canetta, S., Mikofsky, R. M., Alway, E., Passecker, J., Myroshnychenko, M. V., Garcia-Garcia, A. L., Warren, R., Teboul, E., Blackman, D. R., Morton, M. P., Hupalo, S., Tye, K. M., Kellendonk, C., Kupferschmidt, D. A., & Gordon, J. A. (2019). Hippocampal-prefrontal theta transmission regulates avoidance behavior. Neuron , 104(3), 601–610.e604. https://doi.org/10.1016/j.neuron.2019.08.006
- Popescu, A. T., Popa, D., & Pare, D. (2009). Coherent gamma oscillations couple the amygdala and striatum during learning. Nature Neuroscience , 12(6), 801–807. https://doi.org/10.1038/nn.2305
- Robinson, M. J., Warlow, S. M., & Berridge, K. C. (2014). Optogenetic excitation of central amygdala amplifies and narrows incentive motivation to pursue one reward above another. Journal of Neuroscience , 34(50), 16567–16580. https://doi.org/10.1523/JNEUROSCI.2013-14.2014
- Siegle, J. H., Pritchett, D. L., & Moore, C. I. (2014). Gamma-range synchronization of fast-spiking interneurons can enhance detection of tactile stimuli. Nature Neuroscience , 17(10), 1371–1379. https://doi.org/10.1038/nn.3797
- Siegle, J. H., & Wilson, M. A. (2014). Enhancement of encoding and retrieval functions through theta phase-specific manipulation of hippocampus. Elife , 3, e03061. https://doi.org/10.7554/eLife.03061
- Soares-Cunha, C., de Vasconcelos, N. A. P., Coimbra, B., Domingues, A. V., Silva, J. M., Loureiro-Campos, E., Gaspar, R., Sotiropoulos, I., Sousa, N., & Rodrigues, A. J. (2020). Nucleus accumbens medium spiny neurons subtypes signal both reward and aversion. Molecular Psychiatry , 25(12), 3241–3255. https://doi.org/10.1038/s41380-019-0484-3
- Soares-Cunha, C., Domingues, A. V., Correia, R., Coimbra, B., Vieitas-Gaspar, N., de Vasconcelos, N. A. P., Pinto, L., Sousa, N., & Rodrigues, A. J. (2022). Distinct role of nucleus accumbens D2-MSN projections to ventral pallidum in different phases of motivated behavior. Cell Reports , 38(7), 110380. https://doi.org/10.1016/j.celrep.2022.110380
- Wahlstrom, K. L., Alvarez-Dieppa, A. C., McIntyre, C. K., & LaLumiere, R. T. (2021). The medial entorhinal cortex mediates basolateral amygdala effects on spatial memory and downstream activity-regulated cytoskeletal-associated protein expression. Neuropsychopharmacology , 46(6), 1172–1182. https://doi.org/10.1038/s41386-020-00875-6
- Wahlstrom, K. L., Huff, M. L., Emmons, E. B., Freeman, J. H., Narayanan, N. S., McIntyre, C. K., & LaLumiere, R. T. (2018). Basolateral amygdala inputs to the medial entorhinal cortex selectively modulate the consolidation of spatial and contextual learning. Journal of Neuroscience , 38(11), 2698–2712. https://doi.org/10.1523/JNEUROSCI.2848-17.2018
- Wang, Y., Liu, Z., Cai, L., Guo, R., Dong, Y., & Huang, Y. H. (2020). A critical role of basolateral amygdala-to-nucleus accumbens projection in sleep regulation of reward seeking. Biological Psychiatry , 87(11), 954–966. https://doi.org/10.1016/j.biopsych.2019.10.027
- Warlow, S. M., Naffziger, E. E., & Berridge, K. C. (2020). The central amygdala recruits mesocorticolimbic circuitry for pursuit of reward or pain. Nature Communications , 11(1), 2716. https://doi.org/10.1038/s41467-020-16407-1
- Warlow, S. M., Robinson, M. J. F., & Berridge, K. C. (2017). Optogenetic central amygdala stimulation intensifies and narrows motivation for cocaine. Journal of Neuroscience , 37(35), 8330–8348. https://doi.org/10.1523/JNEUROSCI.3141-16.2017
- Yizhar, O., Fenno, L. E., Davidson, T. J., Mogri, M., & Deisseroth, K. (2011). Optogenetics in neural systems. Neuron , 71(1), 9–34. https://doi.org/10.1016/j.neuron.2011.06.004