Super-Resolution Fluorescence Microscopy Methods for Assessing Mouse Biology
Jeremy Sanderson, Jeremy Sanderson, Jessica Valli, Jessica Valli
pixel reassignment
single molecule localization microscopy
stimulated emission depletion microscopy
structured illumination microscopy
super resolution microscopy
super resolution radial fluctuations
Abstract
Super-resolution (diffraction unlimited) microscopy was developed 15 years ago; the developers were awarded the Nobel Prize in Chemistry in recognition of their work in 2014. Super-resolution microscopy is increasingly being applied to diverse scientific fields, from single molecules to cell organelles, viruses, bacteria, plants, and animals, especially the mammalian model organism Mus musculus. In this review, we explain how super-resolution microscopy, along with fluorescence microscopy from which it grew, has aided the renaissance of the light microscope. We cover experiment planning and specimen preparation and explain structured illumination microscopy, super-resolution radial fluctuations, stimulated emission depletion microscopy, single-molecule localization microscopy, and super-resolution imaging by pixel reassignment. The final section of this review discusses the strengths and weaknesses of each super-resolution technique and how to choose the best approach for your research. © 2021 The Authors. Current Protocols published by Wiley Periodicals LLC.
INTRODUCTION
The mechanism of development cannot usually be reduced to a single gene, rather occurring through nonlinear interactions of many genes in a dynamic network. Development also occurs at all scales (Megason, Srinivas, Dickinson, & Hadjantonakis, 2011; Xavier da Silveira Dos Santos & Liberali, P 2019; Zinner, Lukonin, & Liberali, 2020), and so super-resolution microscopy is crucial as a foundation for imaging at other developmental scales (Maiques, Georgouli, & Sanz-Moreno, 2019; Tröger et al., 2020). Fluorescence microscopy is a powerful and versatile tool that has proved essential for biological discoveries (e.g., Dunn & Sutton, 2008; Hecksher-Sørensen & Sharpe, 2001; Mertz, 2019; Nowotschin, Ferrer-Vaquer, & Hadjantonakis, 2010; Renz, 2013; Ricci et al., 2020; Turney & Lichtman 2008; Udan & Dickinson, 2010), and even before the widespread adoption of fluorescence contrast, microscopy has been instrumental for research on the mouse (Auerbach, 1954; Lyon, 1955; Serbedzija, Bronner-Fraser, & Fraser, 1992; Zucker, Hunter, & Rogers, 1998) as a model organism (Choi, Kwok, & Yun, 2015; Phifer-Rixey & Nachman, 2015). Classical fluorescence microscopes are, however, limited in resolution because of the wave nature of light and the inability of optical systems to focus these waves precisely owing to diffraction and unavoidable aberrations inherent in the optical system.
Why, therefore, not give up on the optical microscope and pursue electron microscopy (EM)? Indeed, with the introduction of EM to support research scientists from the late 1940s, EM was regarded as the ultimate in microscopy. However, light microscopy persisted for the following simple and compelling reasons. Apart from size, cost, and maintenance issues, electron microscopes generally have to be operated under vacuum, which precludes observing and analyzing living organisms. The beam energy is extremely high, and samples can be very easily damaged—particularly in the hands of novice operators. Moreover, the electron beam can only reveal surface features (scanning EM) or penetrate a shallow depth into cells, necessitating very thin sections (transmission EM). Electron micrographs are more prone to artifacts (not all of which are easily recognized and dealt with) than light microscope images. They are also monochrome and can only be pseudocolored. With a light microscope it is possible to observe a wide range of biological activity, in color, and to easily label multiple targets with fluorescent markers. This is why optical nanoscopy or super-resolution microscopy has been developed and is now a powerful research tool of the 21st century. This review does not consider light microscopy‒EM correlative methods. The reader is directed to Verkade & Collinson (2019) for a thorough treatment of this rapidly developing technology. For a concise, readable, and well-referenced historical review of light microscopy from the 17th century to the development of super-resolution techniques, see Wollman, Nudd, Hedlund, & Leake (2015).
Diffraction at the specimen and interference of the diffracted rays of light are the sine qua non of image formation. The German physicist Ernst Abbe (1873) realized that image formation by a lens occurs by the recombination of light separated by scattering or diffraction at the object and that the finer the detail, the greater the scattering angle. In so doing, he defined the minimum distance (dmin) that must separate two points in order for them to be resolved by a given optical system. For a simple explanation of the phenomenon of diffraction see Bradbury (1984), and for an in-depth treatment see Hammond (2015). How diffraction and interference underlie the formation of microscopical images is explained in a Current Protocols article by Sanderson (2020).
The resolved detail, dmin, is determined by the angular aperture of the lens, expressed as sinα where α is the angle between the direct and most widely scattered or diffracted beams accepted by the aperture of the objective. dmin is also determined by the wavelength of the light, which for an oil immersion lens is λ/n where λ is the wavelength of light in air and n is the refractive index of the immersion oil. Finally, the angular range of convergence of the incident light on the object (maximum value sinα) is accounted for by the factor 2. Abbe's equation, carved on his memorial at Jena, is d = λ / 2n.sinα. The number n.sinα Abbe called the numerical aperture (NA)—a number that is engraved on all objectives. Abbe did not include any formulae in his paper, and his theory was misunderstood for some time afterward (Köhler, 1981; Lauterbach, 2012). For an excellent detailed discussion and review of resolution, see Sheppard (2017).
Resolution just occurs (the Rayleigh criterion) when the central maximum of the diffraction pattern from the first feature coincides with the first minimum of the diffraction pattern of the second, closely apposing, feature in an object (Demmerle, Wegel, Schermelleh, & Dobbie, 2015; Fig. 2 from a Current Protocols article by Sanderson, 2020). At this point, the dip in intensity between the two diffraction patterns is about 26%. Sparrow suggested that two points can just be resolved when the central minimum dip in intensity between them is zero (Sparrow, 1916). With Sparrow's criterion dmin = 0.47 λ/NA, a closer approximation to the Abbe limit of 0.50 λ/NA is achieved. This criterion is better suited to digitally recorded images but is very dependent on signal-to-noise ratio (SNR) and is not easily compared to the full width at half maximum (FWHM) as an estimator of the Rayleigh criterion.
The resolving power of the objective is therefore approximately equal to the wavelength of light divided by twice the numerical aperture of the objective. This means that for a standard fluorescence system, such as a confocal microscope, the lateral resolving power is at best limited to around 200 to 250 nm and axial resolution to around 600 nm. A practical measure of resolving power of the overall microscope system (i.e., objective, intermediate optics, and camera or photomultiplier tube [PMT]) using a subresolution fluorescent bead is to measure the FWHM of the intensity profile of a z -stack of the point spread function (PSF) of the bead (e.g., Cole, Jinadasa, & Brown, 2011). The advantage of this artificial system is that if one knows a priori that two point sources exist in the sample, measuring their separation and deriving a resolving power limit is limited in practice only by SNR.
The limits of resolution (about 0.2 μm laterally and 0.6 μm axially) proposed by the criteria of Abbe, Rayleigh, and Sparrow are based on the following assumptions and so cannot be absolute: a single objective lens, single-photon absorption and emission in a time-independent linear process at the same frequencies, and uniform illumination across the specimen with a wavelength in the visible range. If these assumptions are negated, then enhanced resolution is feasible. So often Abbe's limit d min = λ/2.NA has been regarded as inviolable, largely because the equation is simple to understand and is powerful through being cited so often without question that it is regarded as absolute. Yet Abbe was aware that by altering these conditions (e.g., nonlinear two-photon microscopy; stimulated emission depletion [STED]; structural illumination), enhanced resolution is made possible (Abbe, 1873; Lauterbach, 2012, p. 5): “I must regard the actual capability of the microscope in the rigorous sense as completely exhausted with the above described limit—as long as no entities are claimed that are out of the reach of the derived theory.”
Beside the simplicity of the oft-cited equation above, Stefan Hell, the 2014 Nobel Laureate, also suggests that the “diffraction barrier” persisted for so long because Abbe and Rayleigh's equations refer to propagating waves and the limited passband of spatial frequencies carried by waves through the microscope, which is fundamentally a spatial filter (Hell, 2009). Hell (2009, p. 29) comments, “The only effective pathway of optical microscopy to the nanoscale seemed to be [given] by the physical confinement of light, that is, by near-field optics.” In the far field, light diffracts and is refracted by dioptric lenses to form images in the classical manner, propagating in an unconfined manner, with the distances involved being greater than the wavelength of light; radiative power decreases according to the square of the distance travelled. In the near field, light is focused through an aperture with a diameter smaller than the excitation wavelength, resulting in a nonpropagating evanescent field (or near field) on the far side of the aperture that decomposes rapidly (see Lereu, Passian, & Dumas, 2012; Huszka & Gijs, 2019). Near-field techniques are diffraction unlimited but are constrained to surface inspection techniques, such as total internal reflection fluorescence (TIRF) microscopy (Mattheyses, Simon, & Rappoport, 2010; Oheim, Salomon, Weissman, Brunstein, & Becherer, 2019) or scanning probe techniques (Gerber & Lang, 2006; Kalinin et al., 2016).
Since the turn of the millennium, an increasing number of microscopy techniques have emerged that are able to overcome the resolution limit of traditional far-field light microscopy (Betzig et al., 2006; Heilemann et al., 2008; Hess, Girirajan, & Mason, 2006; Klar, Jakobs, Dyba, Egner, & Hell, 2000; Rust, Bates, & Zhuang, 2006), and these are collectively known as super-resolution microscopy techniques. In fact, 2006 has been described as “an annus mirabilis for the field” (Hell, 2014). Super-resolution techniques can be performed on a range of microscope configurations, including widefield, confocal, TIRF, and light sheet systems, and this will have implications for both the resolution and the imaging depth achievable (reviewed in Thorn, 2016; Haage, 2018). The term “super-resolution microscopy” is here to stay but is disliked by many, including Stefan Hell (Marx, 2013) one of the Nobel Laureates in the field, as being imprecise.
Super-resolution imaging can be performed on various types of microscope setups, and it is important to understand the relative benefits of each (Fig. 1). In widefield setups, excitation light evenly illuminates the entire field of view. This allows for rapid imaging of larger areas but also suffers from blurring due to out-of-focus light reaching the camera detector (Fig. 1A; reviewed in Verdaasdonk, Stephens, Haase, & Bloom, 2014). Confocal imaging overcomes this issue by providing optical sectioning, so that only light from the plane of focus reaches the detector. This is achieved through the use of a pinhole, which blocks out-of-focus light (Fig. 1B; reviewed in Conchello & Lichtman, 2005). In short, this provides point illumination and point detection (Sanderson, 2019). However, using this approach, samples are excited point by point rather than the entire field of view being illuminated. In traditional confocal systems, the excitation point is scanned across the field of view, meaning that acquisition is quite slow. Spinning disk confocal setups increase imaging speed by parallelizing acquisition using a spinning metal disk with multiple holes that act as pinholes, but these systems have slightly lower resolution and are not compatible with certain confocal-based super-resolution techniques (Hosny et al., 2013; Qin, Isbaner, Gregor, & Enderlein, 2021; Shimozawa et al., 2013). Other setups that allow for optical sectioning include TIRF and light sheet microscopes. TIRF uses a very steep laser angle and a mismatch in refractive index of the immersion and sample medium to cause the laser light to reflect off the coverslip so that the sample is only illuminated by an evanescent field coming off the laser light (Fig. 1C; reviewed in Martin-Fernandez, Tynan, & Webb, 2013). The evanescent wave only propagates a few hundred nanometers into the sample, so while this provides optical sectioning, it only allows for imaging of a very thin layer right next to the coverslip. Light sheet microscopy, meanwhile, uses multiple objectives to produce a thin sheet of excitation light perpendicular to the imaging objective (Fig. 1D; reviewed in Girkin & Carvalho, 2018; Power & Huisken, 2017; Watkins & St Croix, 2018). As in widefield, the entire field of view is illuminated allowing for rapid imaging, but the optical sectioning prevents blurring from out-of-focus light (Strobl, Schmitz, & Stelzer, 2017; Wan, McDole, & Keller, 2019).
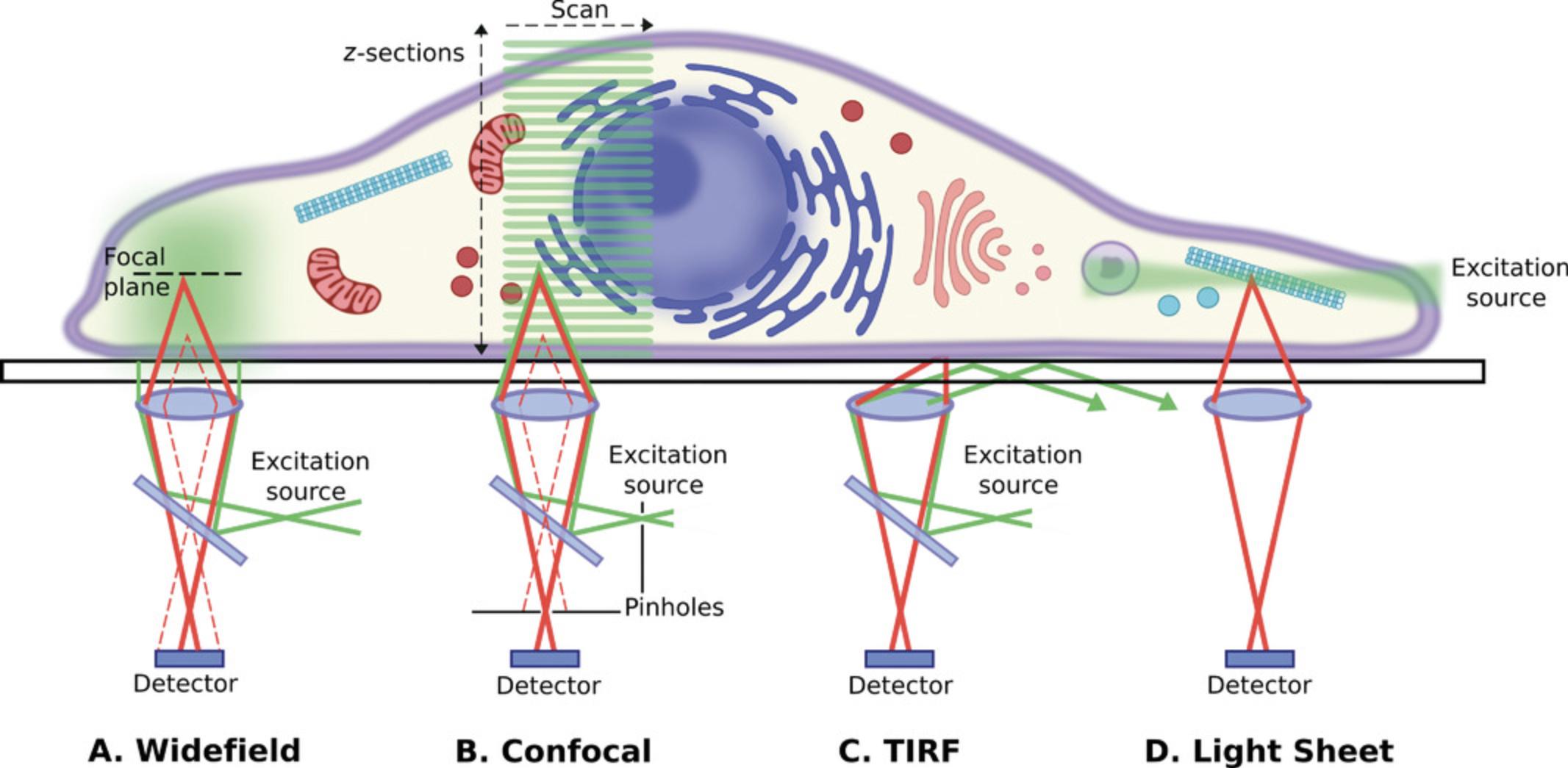
Super-resolution techniques can take many forms, either requiring specialized configurations to optically overcome the diffraction limit or combining traditional setups with sample preparation or image processing techniques. The most widely available super-resolution techniques will be discussed below with a focus on sample preparation requirements and suitability to different questions in mouse biology. Zimmermann (2017) distinguishes between (1) methods that shift the resolving power of the microscope beyond the Abbe limit but that are still constrained by it and (2) methods that increase resolution beyond the diffraction limit and that are not limited by it.
In the first group (1) are 4Pi microscopy (Hell & Stelzer, 1992), the widefield equivalent image interference microscopy (InM; Gustafsson, 1999), and structured illumination microscopy (SIM; Ingerman, London, Heintzmann, & Gustafsson, 2019; Prakash, Diederich, Reichelt, Heintzmann, & Schermelleh, 2021; Vangindertael et al., 2018). 4Pi and InM (usually I5M; Bewersdorf, Schmidt, & Hell, 2006; Gustafsson, Agard, & Sedat, 1999) techniques use orthogonally oriented dual objectives—necessarily with high Strehl ratios—to increase the collection angle of image-forming diffracted rays, also known as aperture enlargement (Sahl, Schönle, & Hell, 2019). Both techniques demand precise alignment and ideally refractive index homogeneity of the sample. The widefield method demands near-perfect optical conditions and is particularly susceptible to misalignment from thermal drift and mechanical shock. The 4Pi commercial turnkey microscope is no longer available, but a recently published, very comprehensive protocol allows researchers to self-build a 4Pi single-molecule localization microscope (Wang et al., 2021). For all these reasons given above, and since self-building a 4Pi microscope is a specialist endeavor, these interferometric methods are not considered further, and only SIM is discussed in this article.
The second group (2) of super-resolution techniques that are discussed here include PSF engineering methods (STED, ground state depletion [GSD]) and stochastic methods. These are software-based super-resolution radial fluctuations (SRRF) and single-molecule localization methods (direct stochastic optical reconstruction microscopy [dSTORM], fluorescence photoactivated localization microscopy [fPALM], PALM, and DNA-based point accumulation for imaging in nanoscale topography [DNA-PAINT]) whereby organic fluorophores, quantum dots, or fluorescent proteins are switched stochastically between dark and fluorescent states. STED, GSD, and saturated SIM (SSIM) fall under the umbrella of reversible saturable optical fluorescence transitions (RESOLFT) microscopy, which is based on the use of reversible saturable optical transitions between fluorescent and nonfluorescent states to inhibit fluorescence in the sample in a controlled way (Tam & Merino, 2015). The actual resolution improvement below the Abbe limit depends on the photon yield, camera detector sensitivity, and resolving power (Früh & Schoen, 2017; Mund & Ries, 2020; Thompson, Larson, & Webb, 2002).
Strategic Planning: Selection of Imaging Technique
No imaging system is perfect, and there will always be a trade-off between spatial resolution, imaging speed, and SNR. SNR is the intensity of the signal divided by the variance in that signal due to noise. Image contrast is the difference between the signal and the background and may also be referred to as the signal-to-background ratio (Vizcay-Barrena, Webb, Martin-Fernandez, & Wilson, 2011). The inter-relationship of these factors is explained in Stelzer (1998). The occurrence of SNR and the fact that the image is only a representation of the object means that it is crucial to consider the biological question being asked and which factors will be most powerful in elucidating this before deciding on the appropriate microscopy technique. The additional restrictions imposed by the so-called “photon budget” and the very low tolerance of living cells to light means that it is better to acquire noisy data and clean this up by postprocessing image analysis than to rely on data of dubious scientific quality taken from dead or dying cells. Data can be analyzed either by commercial software supplied with a turnkey system or open source freeware. ImageJ/Fiji (Laine et al., 2019; Schmied et al., 2021; Schroeder et al., 2021) is both well known and widely used, and at the end of each section, ImageJ plugins are suggested for each super-resolution technique.
This leads to experimental planning. Scientific images are not merely pretty pictures: they are datasets of spatial and/or temporal photo intensity. For that reason, experiments should be properly planned, and the statistical analysis of data should also be considered and not just be the “bit tacked onto the end” of an experiment once all the image data have been collected. We suggest you refer to Deagle, Wee, & Brown (2017); Jonkman, Brown, Wright, Anderson, & North (2020); Jost & Waters (2019); Lee & Kitaoka (2018); Sanderson (2019, p. 646); and Wait, Reiche, & Chew (2020) for further advice and guidance on planning an imaging experiment. You should also test your samples on a classic diffraction-limited fluorescence microscope first to ensure they have been prepared satisfactorily and give good results before moving on to use a super-resolution system.
In their excellent review paper, Jonkman et al. (2020) describe a pentagon, rather than the triangle illustrated in Figure 2. They suggest five trade-offs: speed, resolution, and contrast (i.e., SNR) as above, as well as the two extra parameters depth penetration and sample viability. Whichever aide-memoire you adopt, it is crucial that you consider these parameters when planning your imaging experiment and (if you have the choice) selecting which microscope to use. Figure 8 in a Current Protocols article by Sanderson (2020) gives a flow chart to help you decide which microscope to use for each imaging task. Figure 3 here also shows the inter-relationships between different techniques and how these techniques can be combined. In short, consider whether the sample is living or fixed and then how thick it is. In classic fluorescence microscopy, thin samples should preferably be imaged using widefield microscopy, while thicker sections should be imaged using a confocal microscope; for very thick sections or tissue slices, a multiphoton microscope is the instrument of choice. When considering super-resolution microscopy, most samples will be very thin individual cells or monolayers on coverslips of measured thickness. If the cells are thick or tissue sections are being studied, then either a confocal-based, TIRF-compatible, or light sheet‒compatible method needs to be used.
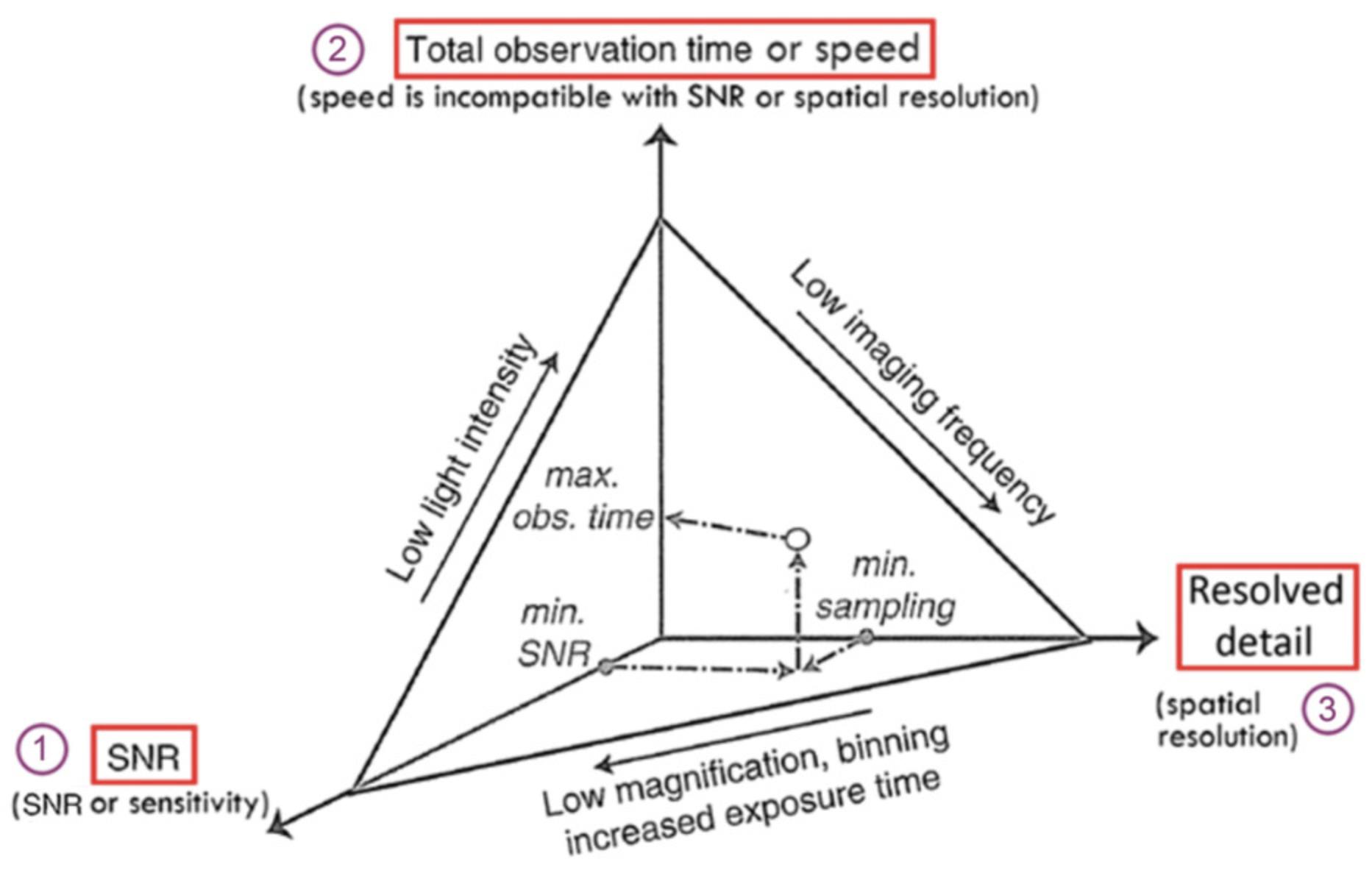
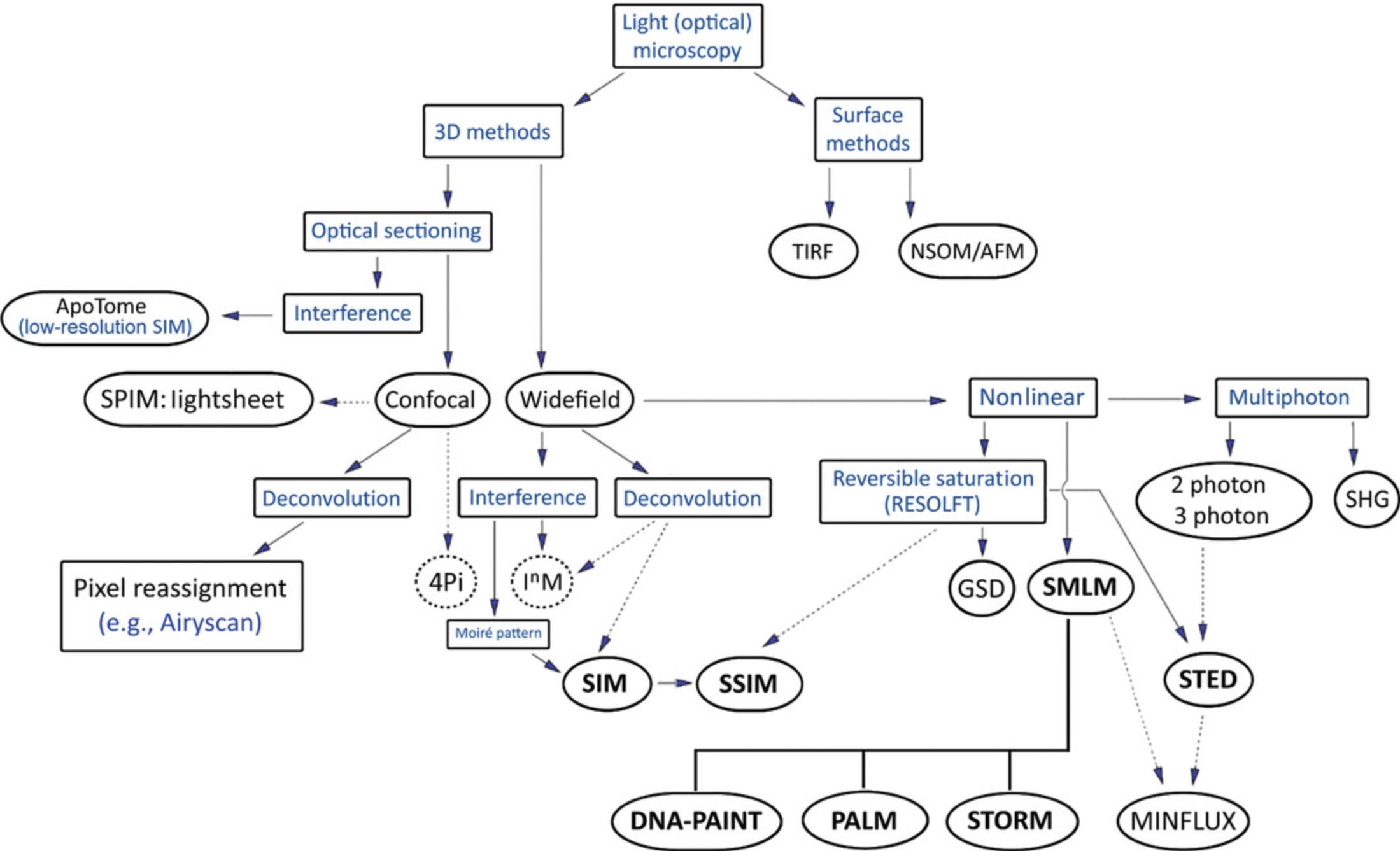
One of the most fundamental factors in deciding which super-resolution technique to use is the size of the object of interest or the resolution required to identify features of interest. This applies to classic microscopy also, but it is even more important when considering using super-resolution techniques. While super resolution microscopy is trendy, Lambert & Waters (2017) rightly question whether it is needed in all cases, and their review paper ought to be essential reading for anyone considering using super-resolution techniques. As they put it, “super resolution requires super optimization.” Lambert and Waters also make the often-overlooked point that the figures reported for the ultimate resolving power achievable with super-resolution techniques by developers and commercial manufacturers are based on the best-possible results acquired under limited conditions with an optimized sample. We cite similar figures drawn from several sources, but these caveats hold true regardless. As stated above with the iron triangle of imaging—the practical limitations of which are speed, resolution, and contrast—SNR, sampling rate, fluorophore labeling density, optical aberrations, and the potential for artifacts (Culley et al., 2018; Endesfelder & Heilemann, 2014) are also important factors to consider and build into any super-resolution imaging protocol. Super-resolution microscopes are particularly sensitive to optical aberrations. The issue of spherical aberration is dealt with in the section on specimen preparation below, but the other major aberrative effect is chromatic aberration (Lambert & Waters, 2017; Möckl & Moerner, 2020). This must be addressed in order to align excitation and depletion lasers in STED and where multicolor imaging is being performed (Bates, Dempsey, Chen, & Zhuang, 2012), particularly with respect to image registration in SIM and single-molecule localization microscopy (SMLM).
Essentially, be very clear in your own mind whether you require (1) sensitivity over resolving power or (2) image analysis over resolving power. For example, increasing the sensitivity and SNR may allow you to determine the presence of subresolution structures smaller than the diffraction limit, and this may be sufficient to answer the hypothesis that the imaging experiment is designed to answer. Equally, computer-assisted image analysis can reveal information that cannot necessarily be seen by eye (Verdaasdonk et al., 2014; commentary by Swedlow, 2010, on an article by Carlton et al., 2010). Further examples are given in Lambert & Waters (2017).
If you are examining dead fixed tissue, the issue of photon flux or “photon budget” does not arise. However, when studying living cells this becomes very important, and the light flux directed onto the specimen must be considered within the constraints of the iron triangle above. The exact reasons and mechanisms for light-induced cell toxicity or the toxic effect of fluorophore labeling is still not understood.
Phototoxicity, with references, has been covered in a Current Protocols article by Sanderson (2020). In addition Wäldchen, Lehmann, Klein, van de Linde, & Sauer (2015) have shown that transfection reduces photoresistance and increases the likelihood of cell death with high irradiance. The influence of wavelength has long been known. The summary information on phototoxicity given in Box 1 of Stelzer (2015) is particularly drawn to readers’ attention. Cells can withstand ∼1 kW/cm2 at 640 nm for several minutes but only ∼5 mW/cm2 at 405 nm. When fluorophores are excited to reactive states, such as long-lived triplet states, they form reactive oxygen species (ROS), a major cause of phototoxicity. Tosheva, Yuan, Pereira, Culley, & Henriques (2020) and Cordes, Maiser, Steinhauer, Schermelleh, & Tinnefeld (2011) have studied the effect of phototoxicity in super-resolution microscopy and suggest several stratagems to reduce this, such as low phototoxicity fluorescent probes, using longer wavelength illumination with long exposures rather than short bursts, and including oxygen scavengers or bubbling nitrogen through the medium to reduce the formation of ROS.
HeLa cells are reportedly more robust than other cell lines. Stockley et al. (2017) have developed a modified Eagle's medium from which they removed photoactive components to assist cell growth and division during imaging. However, Morawski, Motley, & Campbell (2019) report that antibody-conjugated fluorophores lose signal more rapidly in serum-free medium, so it is a question of working out by trial and practice whether your fluorescent probe and cell model will work.
Data processing is a vital step in super-resolution microscopy. A great deal of processing power, RAM, and storage are required. The reasons for gigabytes or even terabytes of data storage space are because of the very large datasets that are required. It can help to arrange for data to be streamed to a separate processing computer and for GPU computing to assist the core processing unit. See Pietzsch, Saalfeld, Preibisch, & Tomancak (2015) and Bria, Iannello, Onofri, & Peng (2016) for further advice.
Fixation and Labeling for Super-Resolution Imaging
Good preservation of subcellular structure is critical for successful super-resolution imaging (e.g., Jimenez, Friedl, & Leterrier, 2020). Fixation with formaldehyde has long represented the standard in fluorescence microscopy, but the advent of super-resolution techniques has shown formaldehyde fixation to be slow and incomplete. Super-resolution imaging has highlighted how the use of formaldehyde as fixative agent can result in loss of morphologic integrity and even mislocalization of proteins (Schnell, Dijk, Sjollema, & Giepmans, 2012; Tanaka et al., 2010). Methanol, methanol-containing formalin, and other precipitating or coagulating fixatives (Eltoum, Fredenburgh, Myers, & Grizzle, 2001) should be avoided. In EM, where ultrastructural preservation is paramount, the standard for chemical fixation is glutaraldehyde, which acts faster and results in more complete fixation than formaldehyde (Huebinger, Spindler, Holl, & Koos, 2018). However, glutaraldehyde fixation cannot so easily be applied to fluorescence imaging, as the extensive crosslinking can block access of antibodies to epitopes and structures of interest (Hayat, 2002).
The small dialdehyde glyoxal represents a good compromise between these two options, providing faster, stronger fixation than formaldehyde but nonetheless leaves epitopes accessible for antibody detection (Bussolati et al., 2017; Dapson, 2007; Richter et al., 2018). Glyoxal is able to fix both cells and tissue without issues of penetration, producing strong fixation of proteins and nucleic acids and preserving overall cell morphology (Richter et al., 2018). An optimal glyoxal fixative solution should contain 3% (w/v) glyoxal, 20% (v/v) ethanol, and 0.75% (v/v) acetic acid, adjusted to pH of 4 with 1 M NaOH (Richter et al., 2018). The solution should be used within a few days to avoid precipitation of glyoxal (Richter et al., 2018). Fixation should be performed for at least 1 hr.
Glyoxal fixation may not be suitable in all cases, as certain cell types such as oocytes, embryos, and stem cells may show superior immunofluorescent labeling in formaldehyde-fixed cells (Celikkan et al., 2020). There may also be differences in structural preservation and immunolabeling of certain subcellular targets. Glyoxal appears to provide vastly improved preservation of membrane and soluble proteins, while results are mixed for detection of mitochondrial targets (Richter et al., 2018).
Choice of labeling strategy is also crucial in super-resolution imaging. Organic dyes have excellent photophysical properties (Heilemann, van de Linde, Mukherjee, & Sauer, 2009) but suffer high background fluorescence from nonspecific binding. A recent review (Choi, Lee, Park, Lee, & Lee, 2021) lists various suitable probes for subcellular organelles, and Hu, Nguyen, Rabasco, Oomen, & Ewing (2021) and Dankovich & Rizzoli (2021) both present in-depth reviews of recent super-resolution labeling strategies. A particular challenge lies in identifying suitable probes for live-cell single-molecule studies (Wang, Frei, Salim, & Johnsson, 2019; Zhang & Raymo, 2020). Working with live cells avoids the artifacts that fixation may introduce, but other factors such as permeability, steric size, toxicity, and photoswitching efficiency become important.
SNAP-tag and HaloTag technology (Banaz, Mäkelä, & Uphoff, 2019; Erdmann et al., 2019; Hoelzel & Zhang, 2020) are efficient methods for conjugating fluorophore markers to proteins for labeling both living and fixed cells. Nevertheless, these labeling schemes often suffer from high background arising from nonspecific binding of organic dyes to the substrate and cellular membranes. Fluorogenic probes (Beliu et al., 2019; Kozma & Kele, 2019; Wang et al., 2020) have the advantage that they do not fluoresce unless bound to their specific small-molecule fluorogens. These probes potentially allow rapid, specific labeling with a high SNR in multicolor single-molecule studies. Photoswitchable and photoconvertible fluorescent probes (e.g., Dunsing et al., 2018; Henriques & Mhlanga, 2009; Uno et al., 2015) have from the start been used for localization microscopy. GMars-Q, a fluorescence protein specifically developed for single-molecule localization experiments from mMaple and rsFusionReds from mCherry (Duwé & Dedecker, 2019), is a brighter, more photostable fluorescent protein. Another fluorogenic photoswitching fluorescent protein that shows promise is holoUnaG (Kwon et al., 2020). FPBase (https://www.fpbase.org) is a useful resource for selecting a suitable fluorescent protein from the many available, while Vaidyanathan et al. (2021) have developed an open-source freeware to help select multiple fluorescent proteins.
In diffraction-limited microscopy, the image is greater by two orders of magnitude than the size of any fluorophore, so the signal appears continuous. Fluorophore brightness, potential degradation, and labeling density are far more critical issues with super-resolution microscopy, particularly as the resolving power approaches the size of the fluorescent label used. Inadequate labeling can lead to confusing or erroneous images (see the explanation and Fig. 2 in Lambert & Waters, 2017). Effectively, the fluorophore labeling density must satisfy Nyquist's criterion (Shroff, Galbraith, Galbraith, & Betzig, 2008), otherwise ultimate resolving power may be compromised. For example, to achieve a resolution of 20 nm, the structure of interest (e.g., a microtubule) must be labeled at least every 10 nm, and so small-sized fluorophores (nanobodies), which reduce steric hindrance, and directly labeled primary antibodies are to be preferred (Carrington, Tomlinson, & Peckham, 2019; Dong et al., 2019; Gomes de Castro, Rammner, & Opazo, 2016). However, oversampling does not achieve extra resolving power beyond the diffraction limit, merely leading to smaller-sized sampling pixels, decreased sensitivity, and higher SNR values.
General Sample Preparation Considerations for Super-Resolution Imaging
While each technique will have its own specific requirements, there are several common considerations in sample preparation that apply in general to super-resolution imaging. Major considerations for achieving super resolution include high precision of coverslip (coverglass) thickness, refractive indices of immersion liquids and mounting media, and choice of labeling strategy. Sample preparation for super-resolution microscopy demands attention to detail—in a very similar manner to EM.
Most current microscope objectives are designed for use with 170-μm thick coverslips (Jonkman et al., 2020; Sanderson, 2019). While no. 1.5 coverslips are designed to be 170 μm thick, inconsistencies in the manufacturing process mean that they actually vary in thickness between 160 and 190 μm (Sanderson, 1994). No. 1.5H coverslips, on the other hand, are precise to within ±5 μm (Cromey, 2020) and can be bought from various suppliers (e.g., CellPath, Marienfeld, Ibidi, Thorlabs, Zeiss). This is particularly relevant for super-resolution imaging, as error in coverslip thickness (Uhlig, 1964) causes spherical aberration, with the deleterious effect increasing with the NA of the objective. As super-resolution techniques typically require very high NA objectives, this means that even small variations in coverslip thickness can lead to significant losses in detected intensity due to spherical aberration (for a very useful tutorial review, see Diel, Lichtman, & Richardson, 2020) and an increase in SNR. All super-resolution imaging should therefore be performed using no. 1.5H high-precision coverslips for the best results, with the cell monolayer grown onto or the specimen mounted against the underside of the coverslip.
Another fundamental consideration for sample preparation for super-resolution imaging is selection of the correct objective for imaging and ensuring that the refractive index of the mounting medium matches that of the immersion liquid as closely as possible. Typical objectives used for super-resolution imaging are designed for use with immersion liquids with refractive indices of 1.518 for oil objectives, 1.466 for glycerol objectives, 1.404 for silicone oil objectives (Olympus), and 1.33 for water objectives. (Note that you should only use immersion liquids specifically recommended by the objective manufacturer.) Zeiss covers most of these options by producing multi-immersion objectives. Oil immersion objectives generally allow for the highest NA and have the added advantage of matching the refractive index of glass coverslips, producing an optically homogenous system (Sanderson, 2019; Uhlig, 1964). Oil immersion objectives with a refractive index‒matched mounting medium can therefore produce the highest-resolution results and can be particularly beneficial for samples with weak signal due to the greater collection of light afforded by a higher NA. There are, however, situations in which glycerol or water immersion objectives are better suited to the samples being imaged. For example, live-cell imaging will usually involve samples in aqueous medium and should therefore be performed using a water immersion objective (Arimoto & Murray, 2004; Heine et al., 2018; Wan, Rajadhyaksha, & Webb, 2000).
Both water and glycerol objectives often have a long working distance and are therefore better suited to imaging thick samples than oil objectives (Fig. 9 on p. 1408 of Murray, 2011). It may help to use 2,2′-thiodiethanol, which is miscible with water and compatible with fluorescence imaging (Staudt, Lang, Medda, Engelhardt, & Hell, 2007). It can then be used to adjust the average refractive index of the sample to be as homogeneous as possible. Szczurek, Contu, Hoang, Dobrucki, & Mai (2018) suggest using sucrose- and iohexol-based medium mixed with the commercial mounting medium Vectashield. Regardless of choice of objective, it is crucial to match the refractive index of the mounting medium as closely as possible with that of the relevant immersion liquid. A mismatch in refractive index causes spherical aberration that significantly deteriorates the PSF, resulting in loss of resolution, particularly in the axial dimension (Diaspro, Federici, & Robello, 2002). Moreover, this effect is not only exacerbated with increasing focus depth but also causes a decrease in signal intensity and a shift in the objective focus producing image distortion along the z -axis (Besseling, Jose, & Van Blaaderen, 2015; Diaspro et al., 2002; Egner & Hell 2006). An important exception to this rule is in the use of TIRF-based super-resolution techniques, where a mismatch in the refractive index of the sample medium and immersion liquid is in fact required for achieving reflection of the excitation laser off of the coverslip and illumination of the sample via an evanescent wave (Martin-Fernandez et al., 2013).
Table 1 lists commercially available mounting media that most closely match the refractive indices of typical immersion objectives. It is best to use liquid or soft-setting mountants, since hard-setting mounting medium can compress tissues and cell samples, particularly in the axial direction.
Medium | Product | Supplier, cat. no. | Notes |
---|---|---|---|
Mounting mediuma | Prolong Diamond | Thermo Fisher Scientific, P36965 | Glycerol based; RI ∼1.47; stays fluid; good for fluorescent proteins |
Prolong Glass | Thermo Fisher Scientific, P36980 | Hardens; RI ∼1.52 after 48 hr curing | |
Prolong Gold | Thermo Fisher Scientific, P10144 | Hardens; RI ∼1.42 for Alexa dyes; not recommended for organic dyes or fluorescent proteins | |
Immersion oil | Immersol | Carl Zeiss | 518F 1.518 at 23°C; free of fluorescence and halogen; certified according to ISO 8036-1/2 |
Immersol | Carl Zeiss | W 1.334 at 23°C | |
Cargille water-RI immersion oils | Cargille Labs | For both 23°C 1.330 and 37°C 1.335; “AAA” series of immersion oils | |
Olympus Silicone immersion oil | Olympus, SIL300CS | 1.406 at 23°C | |
Leica Type F | Leica Microsystems | 1.518 at 23°C |
- RI, refractive index.
- a Leica and Abberior say: “Please do not use Vectashield, Vectashield Hardset, or (other) embedding media containing p-phenylenediamine (PPD) as antifading reagent.”
- References: Boothe et al. (2017); Fouquet et al. (2015); Musielak, Slane, Liebig, & Bayer (2016); Peterson, Mermelstein, & Meisel (2015); Tejedo et al. (2019).
Super-Resolution Imaging by Structured Illumination Microscopy
SIM uses patterned illumination to help detect high-frequency information in the sample that would be outside of the usual sampling range of the microscope (Fig. 4; Gustafsson, 2000). This relies on interference of the different high-frequency patterns of the illumination and sample, producing lower-frequency information, which can be used to back-calculate the subresolution features of the sample (Fig. 4A; Heintzmann & Huser, 2017). A reconstructed SIM image requires several acquisitions with the illumination patterns in different positions and orientations (Förster et al., 2014; Gustafsson, 2000). Traditional SIM implementations use sinusoidal illumination patterns with either two-beam illumination for 2D SIM or three-beam illumination for 3D SIM (Shao, Kner, Rego, & Gustafsson, 2011). Numerous adaptations of SIM have been developed with different patterns of illumination, including multispot (York et al., 2012; York et al., 2013), parallel Bessel beam (Gao et al., 2012), or hexagonal lattice (Chen et al., 2014) illumination.
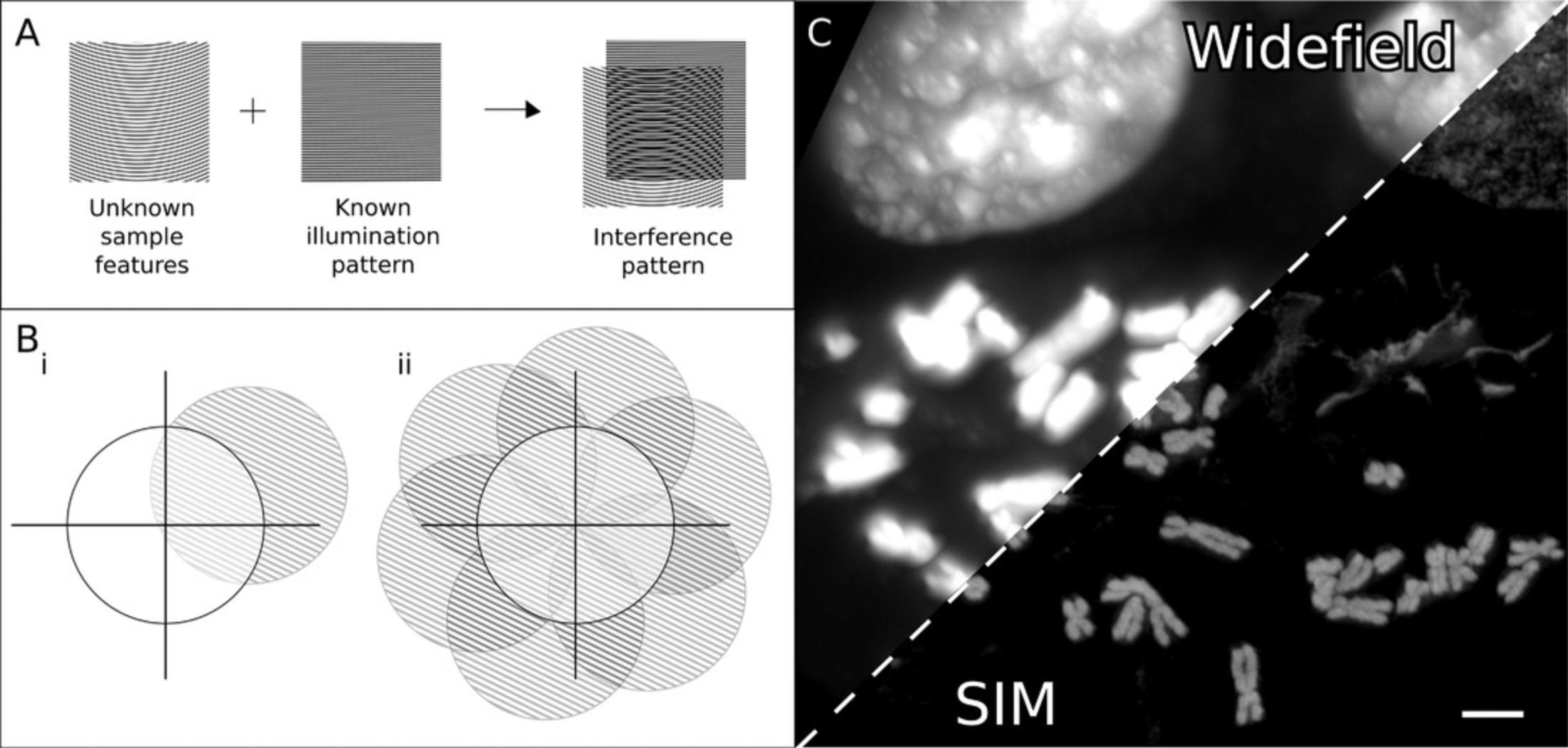
Most implementations of SIM can achieve a two-fold improvement in resolution (in x and y on 2D SIM systems and all three dimensions on 3D SIM systems) over diffraction-limited systems (Gustafsson, 2000; Gustafsson et al., 2008). The improvement in resolution is limited to two-fold because the highest frequency pattern that can be used for illumination is equivalent to the frequency limit of the system optics (Gustafsson, 2000). However, SSIM (Gustafsson, 2005) and nonlinear SIM (NL-SIM; Rego et al., 2012) are able to overcome this limitation through nonlinear responses of the emission rate to the illumination by saturating the fluorophore-excited state with very high illumination intensities in the case of SSIM or through reversible fluorophore photoswitching in the case of NL-SIM (Zheng et al., 2021). These nonlinear responses allow for theoretically unlimited resolution.
While SIM is perhaps one of the more complex super-resolution techniques when it comes to understanding the physical principles behind it (e.g., Wicker & Heintzmann, 2014), it is in fact one of the easier techniques to use in terms of sample preparation, as linear SIM techniques are compatible with standard fluorescence microscopy samples and can easily be used for multicolor imaging (Bates et al., 2012; Gustafsson, 2000; Prakash et al., 2021). SIM can, however, be somewhat limited in imaging thicker samples. 2D SIM particularly struggles with out-of-focus fluorescence in samples thicker than 5 µm, as this background fluorescence will degrade the illumination pattern, decreasing resolution (Ingerman et al., 2019; Mandula et al., 2012). A simple solution for overcoming this issue for 2D SIM imaging is implementing this technique in a TIRF setup (Fiolka, Beck, & Stemmer, 2008; Roth, Mehl, & Rohrbach, 2020). Traditional 3D SIM with sinusoidal illumination provides some computational optical sectioning capabilities but cannot remove shot noise, making it unsuitable for thicker samples (York et al., 2012). However, this has been overcome in newer adaptions of SIM, such as multifocal SIM (which combines structured illumination with optical sectioning via the use of pinholes; York et al., 2012) and lattice light sheet (which applies a 2D lattice illumination pattern as ultrathin light sheets; Sancataldo et al., 2019), and as the illumination is perpendicular to the imaging plane, there is no out-of-focus emission (Chen et al., 2014).
As SIM systems are generally based on widefield setups with sensitive cameras, this technique has advantages in terms of high-speed imaging capabilities and lesser requirements in illumination intensity compared with techniques such as STED microscopy (discussed below), making it perhaps the best super-resolution technique for live-cell imaging (Shao et al., 2011). While SIM requires multiple raw images for reconstruction, the number is far fewer than in single-molecule localization techniques (9 to 15 for SIM vs thousands for SMLM). Even so, capturing these few multiple images can still usefully reduce temporal resolution by performing image processing operations optically instead of digitally (York et al., 2013), making it possible to view super-resolved SIM data in real time. SIM instrumentation has also improved to allow for rapid imaging at 100 frames per second (fps) with minimal photobleaching (Markwirth et al., 2019). There are exceptions, notably SSIM, where the saturation conditions cause higher rates of photobleaching and phototoxicity, making it less suitable for live or biological samples in general (Gustafsson, 2005). While home building a SIM instrument is not trivial, a detailed protocol has been published with a parts list and fully assembly instructions (Curd et al., 2015).
There are some ImageJ plugins specifically written for handling and analyzing SIM data. FairSIM (Müller, Mönkemöller, Hennig, Hübner, & Huser, 2016), QSIM (Gao, 2015), and SIMcheck (Ball et al., 2015) are three of the most widely used. SIMcheck is a suite of ImageJ plugins; these plugins assist troubleshooting, avoid common problems with 3D SIM data, and include calibration tools and utilities (Demmerle et al., 2017) for common image processing tasks. Two particularly useful reviews (Sage et al., 2015, 2019) compare the various software analysis packages.
Software-Based Super-Resolution Imaging (Super-Resolution Radial Fluctuations)
Perhaps the most accessible super-resolution techniques are those that require no specialized microscope setups but instead rely on software processing of images captured on traditional microscopes. One of the most commonly used techniques in this category is SRRF, which uses natural fluctuations of fluorophores, captured over hundreds of frames, to predict the location of each fluorophore at improved resolution (Gustafsson et al., 2016). It can therefore be applied to, and is particularly suited to, live-cell microscopy because SRRF operates over a wide range of fluorophore labeling densities and laser excitation powers, whereas with PALM or STORM it is challenging to maintain sufficient density of fluorophores emitting in each acquired frame. Short video clips (e.g., 40 to 80 fps) can be processed postimage acquisition to achieve super resolution without the need for specialized optics and fluorescent markers or very high laser power illumination.
The SRRF algorithm is available as the NanoJ-SRRF ImageJ/Fiji plugin (Gustafsson et al., 2016; https://www.ucl.ac.uk/~ucbpngu/SRRF.html; https://blogs.dal.ca/dellairelab/soms/). The authors say that SRRF outperforms both the similar multi-emitter fitting (e.g., Munck et al., 2012) and super-resolution optical fluctuation imaging (Dertinger et al., 2013; Geissbuehler, Dellagiacoma, & Lasser, 2011; Girsault et al., 2016; Van den Eynde et al., 2019) approaches and provides a significant reduction in reconstruction artifacts. Cooper et al. (2019) (https://micro-manager.org/wiki/Andor_SRRF-Stream) describe the algorithm used, called “SRRF-Stream,” and image resolution and quality with EMCCD and sCMOS cameras and various fluorophores including fluorescent proteins and organic dyes.
As Culley et al. (2018) point out, the simplest internal control for super-resolution images, and way to identify processing defects, is to directly compare diffraction-limited and super-resolved images of the same sample (e.g., Moeyaert & Dedecker, 2020). To avoid human interpretation bias, the Henriques group has developed the ImageJ/Fiji plugin NanoJ-SQUIRREL (super-resolution quantitative image rating and reporting of error locations). The algorithm can, under certain circumstances, identify common super-resolution artifacts, and it provides complimentary information to SIMcheck, ThunderSTORM, and QUICKPalm.
Super Resolution Imaging by Stimulated Emission Depletion Microscopy
STED microscopy is performed on a confocal laser scanning setup modified with high-powered torus-shaped depletion lasers (Fig. 5). The STED laser is aligned with the excitation beam and depletes emission of the molecules in the region of overlap, producing a subdiffraction region of emission (Fig. 5B; Hell & Wichmann, 1994; Klar et al., 2000). In classic fluorescence techniques, the excitation of a fluorescent molecule causes an electron to jump from the ground state to a higher energy state, and the loss of remaining energy after internal conversion processes results in the emission of a fluorescent photon (Fig. 5Ci; Jablonski, 1933). In STED, this process is interrupted, and the high-energy depletion laser forces the excited fluorescent molecule to immediately return to ground state. This results in the release of a photon with a shorter lifetime and a red-shifted wavelength (Fig. 5Cii; Hell & Wichmann 1994; Klar et al., 2000). The depleted emission can then be excluded from detection by setting specific windows of detection wavelength and through time gating (Klar et al., 2000; Klauss, König, & Hille, 2015; Moffitt, Osseforth, & Michaelis, 2011; Vicidomini et al., 2013).
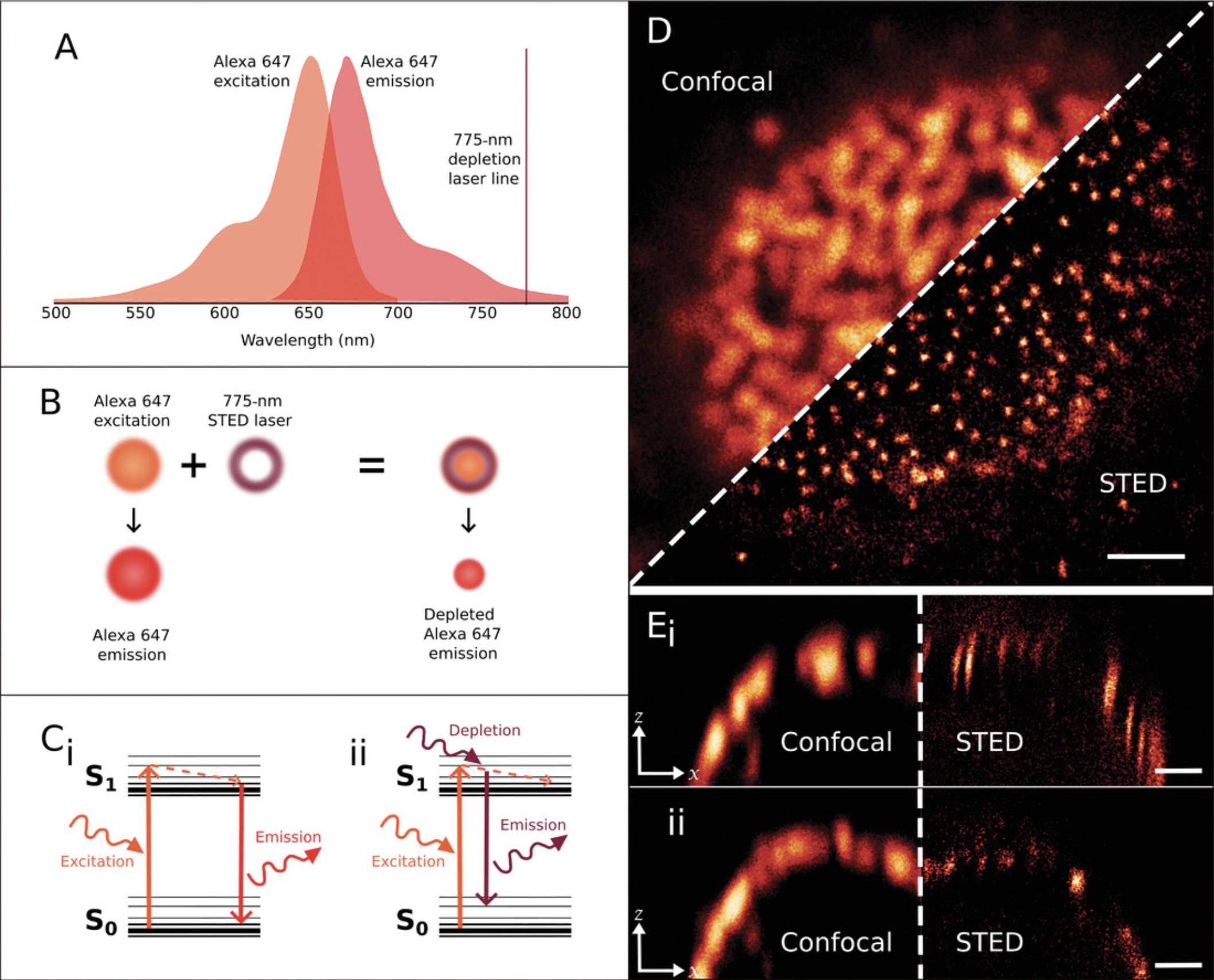
STED and GSD (Hell & Kroug, 1995) microscopy are variants of the RESOLFT concept. Both STED and GSD use a “doughnut” torus-shaped laser to improve resolution, but STED uses this laser to deplete fluorophores after they have been excited, whereas GSD uses this laser to switch molecules into a long-lived metastable dark triplet state in which they cannot be excited (Fölling et al., 2008). The advantage of GSD is that a much lower intensity depletion laser can be used. The potential disadvantage is that fluorophore choice is limited to those with a high triplet state yield.
The resolving power and signal intensity are limited by the de-excitation efficiency and photobleaching of the fluorophore label. Unwanted background noise easily degrades STED image quality and can preclude quantitative analysis. Successful STED imaging is therefore highly dependent on dye or fluorescent protein selection, and this will vary depending on available depletion laser lines (Jahr, Velicky, & Danzl, 2020). Commercial STED systems are currently available from Leica and Abberior, and these are equipped with 592-, 660-, and 775-nm depletion lasers on Leica setups and 595- and 775-nm depletion lasers on Abberior setups (Abberior Instruments, 2020; Leica Microsystems, 2019). In order for a fluorescent molecule to be depleted, the STED laser line must fall within the tail of the emission spectrum for that fluorophore (Fig. 5A; Klar et al., 2000). This means that different fluorophores will be compatible with the different available depletion lasers. Multicolor STED can therefore be challenging because of the undesirable overlap of the wavelengths of excitation and quenching depletion lasers. It is, however, possible and even beneficial to deplete multiple fluorophores with a single STED laser, as long as these fluorophores can be sufficiently separated by their excitation and emission properties. For example, Török et al. (2021) report using large Stokes shift tetrazine-functionalized bio-orthogonal probes for multicolor STED; also see Leica Microsystems and Abberior Instruments guides for appropriate dye selection. As the area of fluorescence emission is determined by the STED torus, imaging multiple fluorophores with a single depletion laser ensures intrinsic alignment of the different channels (Leica Microsystems, 2019).
Commercial systems from Leica include continuous wave 592- and 660-nm STED lasers and a pulsed 775-nm STED laser (Leica Microsystems, 2019), while on Abberior systems, both the 595- and 775-nm STED lasers are pulsed (Abberior Instruments, 2020). Pulsed lasers provide superior results as they reduce unnecessary laser exposure of the sample and thus minimize bleaching (Wildanger, Rittweger, Kastrup, & Hell, 2008). Leica states that typical lateral resolutions on their systems are 50 nm for 592- and 660-nm depletion and 30 nm for 775-nm depletion (though 20 nm has been achieved; Leica Microsystems, 2019), while Abberior claims a typical resolution of 40 nm (but possible down to 25 nm) using their 595-nm depletion laser and a typical resolution of 30 nm (but possible down to 20 nm) with their 775-nm laser (Abberior Instruments, 2020). For directions on building STED systems, see Wu, Wu, Toro, & Stefani (2015) and Klauss et al. (2015).
STED can also be performed in 3D, with isotropic resolutions of down to 40 to 45 nm having been demonstrated on a system with separate STED xy and STED z depletion lasers combined with a 4Pi unit consisting of two juxtaposed objective lenses (Schmidt et al., 2008). This implementation is, however, complex, requiring alignment of multiple beams, meaning that 3D STED is more commonly achieved through the use of a tunable splitting device, which splits the STED beam between the xy plane and the z axis at a user-specified ratio (Osseforth, Moffitt, Schermelleh, & Michaelis, 2014). While this implementation is far simpler, it does result in a tradeoff between lateral and axial resolution. Typical isotropic resolution achieved on these systems are generally around 70 to 75 nm (Leica Microsystems, 2019).
Because of the high laser intensities, live-cell STED is challenging (Lemon & McDole, 2020; Steffens, Wegner, & Willig, 2020). Fluorophore photobleaching can be minimized using fluorophores with a low-absorption cross-section to reduce triplet state excitation (Vicidomini, Bianchini, & Diaspro, 2018), and Abberior has brought out a series of LIVE permeant live-cell dyes for STED and confocal imaging. As with classic confocal microscopy, temporal resolution in STED is limited by scanning speed and the SNR; see Figure 4 in Wu, Xu, & Xi (2021) for a comparison of how different STED configurations compare in 3D axial and temporal resolution. One of the latest approaches to live-cell RESOLFT microscopy is to use multifocal illumination via a “honeycomb” arrangement to switch fluorescent proteins (Bodén et al., 2021; Dreier et al., 2019) and to parallelize the operation in 3D in order to improve temporal resolution and rapidly acquire 3D datasets. Two studies (Kilian et al., 2018; Oracz, Westphal, Radzewicz, Sahl, & Hell, 2017) assess sources of photodamage in STED microscopy and demonstrate that limiting transfection and expression of fluorescent protein markers using antioxidants and ROS-scavenging buffers, employing far-red markers, limiting laser peak intensities, and increasing the pulse duration of the de-excitation STED laser all mitigate the effects of phototoxicity (for a review of fast live-cell 3D imaging, see Vicidomini et al., 2018).
Super-Resolution Imaging by Single-Molecule Localization Microscopy (dSTORM, PALM, DNA-PAINT)
SMLM is a collective term for a plethora of techniques that share the basic principle of differentiating individual fluorophores by causing them to switch on and off stochastically (Fig. 6; Sauer & Heilemann, 2017). While fluorophores within subdiffraction distances of one another cannot be differentiated when fluorescing simultaneously, causing them to fluoresce at different times allows for their localizations to be pinpointed with high precision (Fig. 6A). The main difference between SMLM techniques is the switching mechanism they employ (van de Linde & Sauer, 2014; Zhang & Raymo, 2020). The most commonly used SMLM techniques include dSTORM, PALM, and DNA-PAINT; each of these is briefly described below.
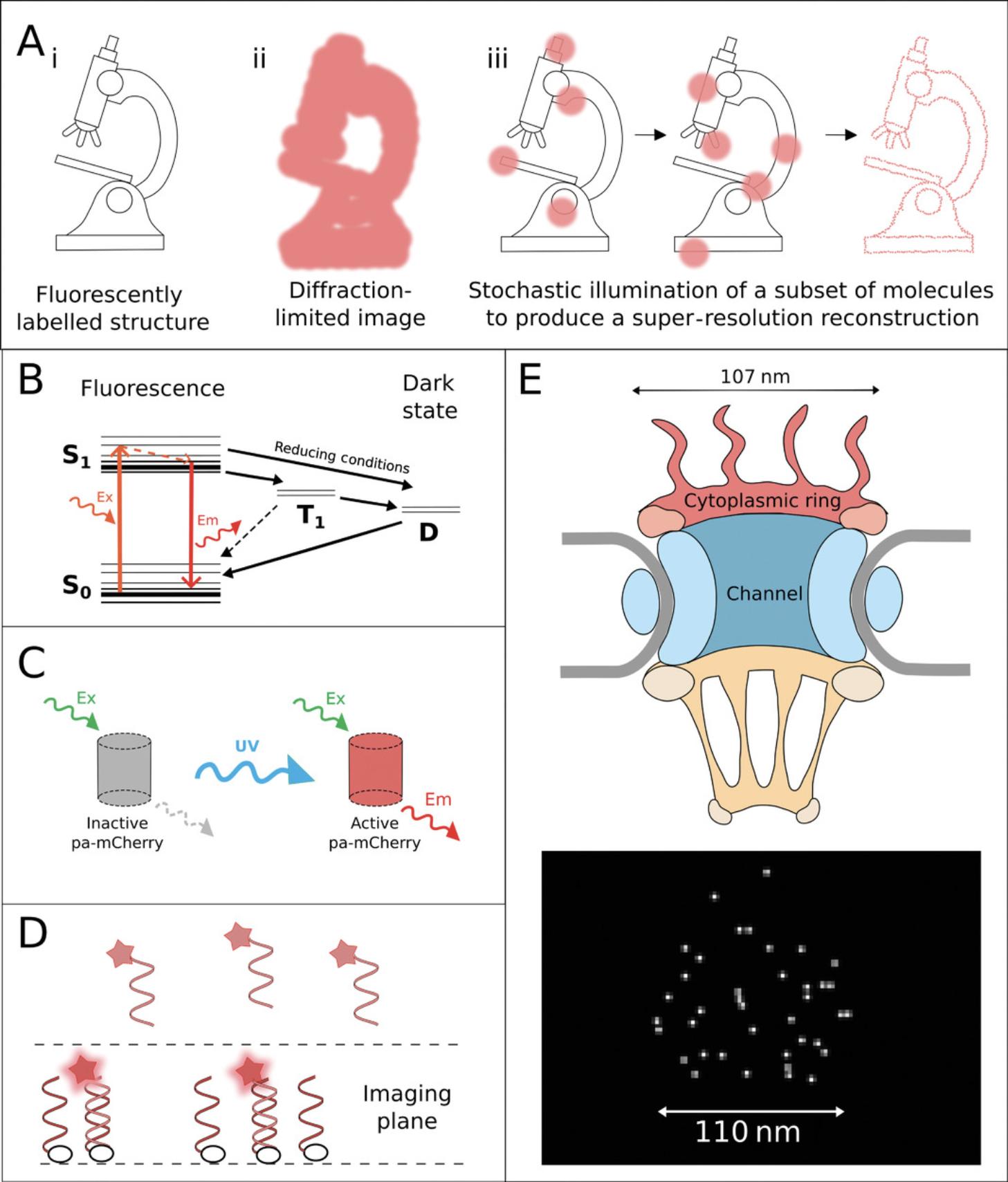
dSTORM takes advantage of natural long-lived dark states of organic dyes, from which the fluorescent molecules cannot be excited without first returning to the ground state (Heilemann et al., 2008). In this technique, organic dyes are driven into the dark state through the use of specialized buffers with reducing and oxygen-scavenging properties (Fig. 6B; Heilemann et al., 2008; Henriques, Griffiths, Hesper Rego, & Mhlanga, 2011; Lehmann, Lichtner, Klenz, & Schmoranzer, 2016). Cycling of dyes into the dark state and stochastically back to ground state causes blinking of individual fluorescent molecules within the sample (Heilemann et al., 2008), which can be influenced by the nature of the fluorophore environment, antifade reagents, and in particular the antioxidant Trolox (Saur & Heilemann, 2017).
PALM, meanwhile, uses specific photoactivatable or photoswitchable fluorophores. Photoactivatable fluorophores are generally found in a native off state, in which they cannot be excited until a conformational change is induced by an activation wavelength, commonly UV (Fig. 6C; Betzig et al., 2006). Nanographenes (Liu et al., 2020) have been proposed as ultrastable, very bright markers for SMLM. Photoswitchable fluorophores, on the other hand, natively fluoresce at a particular wavelength, but an activation wavelength induces a conformational change producing fluorescence at a longer wavelength (Betzig et al., 2006). Single fluorophore localizations are achieved in PALM through cycling of low levels of activation light to turn on or switch a small subset of the fluorophores in a sample (Betzig et al., 2006).
DNA-PAINT is a newer SMLM technique that relies on DNA binding dynamics to achieve blinking. In this technique, target molecules are labeled with antibodies conjugated to single DNA strands (Jungmann et al., 2014; Oi et al., 2020). Fluorophore-bound complementary strands are then introduced into the solution, and these transiently bind to the strands on the sample. This transient binding temporarily immobilizes fluorophores in the imaging plane, resulting in a blinking effect (Fig. 6D; Cnossen et al., 2020). For further explanation and stepwise protocol, see Schnitzbauer, Strauss, Schlichthaerle, Schueder, & Jungmann (2017). A very similar technology is DNA-linked “super beacon” dyes (Pereira, Gustafsson, Marsh, Mhlanga, & Henriques, 2020), which eliminate the need for photodamaging levels of illumination or photoswitching-inducing buffers.
All of these techniques have similar capabilities in terms of resolution, with typical lateral resolutions of 10 to 20 nm (von Diezmann, Shechtman, & Moerner, 2017). SMLM techniques are commonly performed in a TIRF setup, with a very narrow imaging plane close to the coverslip, and are thus commonly performed in 2D. 3D SMLM is, however, possible in widefield setups (von Diezmann et al., 2017) using approaches such as engineering of the PSF to assess z -position or through the use of more specialized setups, such as highly inclined laminated optical sheet (Tokunaga, Imamoto, & Sakata-Sogawa, 2008), selective plane illumination microscopy (Galland et al., 2015), or probe-refresh STORM (Lin, Gagnon, Howard, Halpern, & Vaughan, 2018), a similar method to DNA-PAINT. Given the similarity in resolution, choice of appropriate single-molecule technique will be based on other requirements of the experiment. For example, experiments requiring multicolor imaging can be most easily achieved using DNA-PAINT (or similar PAINT techniques); multicolor imaging can be difficult by dSTORM or PALM due to limitations in compatible dyes and fluorophores (Arsić, Stajković, Spiegel, & Nikić-Spiegel, 2020; Chen, Bestvater, Schaufler, Heintzmann, & Cremer, 2018; Ji, Shroff, Zhong, & Betzig, 2008; Lampe, Haucke, Sigrist, Heilemann, & Schmoranzer, 2012; van de Linde et al., 2009). DNA-PAINT, meanwhile, does not suffer from these limitations and can be extended to labeling of theoretically infinite structures of interest through sequential labeling with washing out of labels in between (Jungmann et al., 2014). Single-particle tracking experiments require the use of live cell‒compatible techniques and are therefore limited to PALM or adapted PAINT techniques such as Tag-PAINT, LIVE-PAINT, or L-H DNA-PAINT (Geertsema et al., 2021; Nieves et al., 2019; Oi et al., 2020). SOMAmers, a class of DNA aptamers that are much smaller than antibodies, can be used to label proteins (Moore & Legant, 2018; Strauss et al., 2018). Finally, experiments requiring quantitation of clustering should take into consideration the multiple localizations that arise from blinking of dyes in techniques such as dSTORM (Puchner, Huang, & Gaub, 2014) and will be better suited to the use of PALM or qPAINT, in which quantitation is based on label-binding kinetics that are more predictable that complex dye kinetics (Jungmann et al., 2016).
The potential errors from SMLM techniques are two-fold: those that degrade resolving power from localization imprecision and position bias (Cohen, Abraham, Ramakrishnan, & Ober, 2019; You, Chao, Cohen, Ward, & Ober, 2021). Poor photoswitching rates and very high local concentrations of fluorophore labeling can also lead to artifacts (Burgert, Letschert, Doose, & Sauer, 2015; Shivanandan, Deschout, Scarselli, & Radenovic, 2014), as can “linkage error” (the distance between the protein target and its fluorescent label). Background signal and camera pixel size directly influence SNR in the image, and—just as with live-cell imaging—stage or sample drift can negate attempts to collect high-quality data (Costello & Cox, 2021; Mund & Ries, 2020). In a recently published paper, Patel, Williamson, Owen, & Cohen (2021) discuss statistical methods to improve stochastic localization precision, mitigating for missed blinks and false positives, while Diekmann et al. (2020) advise on improving speed and sensitivity of image acquisition. See Möckl & Moerner (2020) and Khater, Nabi, & Hamarneh (2020) for good reviews of SMLM techniques, Jimenez et al. (2020) for practical advice regarding sample preparation and probes, and Glushonkov, Réal, Boutant, Mély, & Didier (2018) for a combined PALM-dSTORM stepwise protocol.
The ImageJ plugins ThunderSTORM (Ovesný, Křížek, Borkovec, Svindrych, & Hagen, 2014), rapidSTORM (Wolter et al., 2012), QUICKPalm (Henriques et al., 2010), and SMLocalizer (Bernhem & Brismar, 2018) are all available as open-source software to analyze SMLM data. A 2019 review (van de Linde, 2019) compares these plugins and other tools, such as the Python-based VividSTORM (Barna et al., 2016), for localization microscopy.
Localization microscopy is a widefield technique, so preparing a microscope does not present the same challenges as for SIM or STED microscopy, where additional optical components are required. Almada, Culley, & Henriques (2015) describe how to adapt a system for large fields of view with high throughput using sCMOS detectors. MINFLUX, a recent development (Gwosch et al., 2020), combines SMLM with a STED-like, doughnut-shaped excitation beam to further improve resolution. Molecules directly under the center of this doughnut-shaped beam will not be excited, and MINFLUX thus uses this to probe multiple positions to localize individual switchable fluorophores. Crucially, MINFLUX thus determines the position of the fluorophore not by where fluorescence is present but by where it is absent (Strack, 2017). MINFLUX nanoscopy provides 1- to 3-nm resolution in fixed and living cells. This is at the level of resolution of the size of the conjugated fluorophore probe. The much lower light fluxes permit concomitant increases in temporal resolution and allows imaging of living cells.
Super-Resolution Imaging by Pixel Reassignment
With improved PMT detectors, a great deal of effort has gone into improving the SNR of confocal microscopes. Improving SNR is usually achieved at the expense of image acquisition speed and resolving power—the so-called “iron triangle” (Fig. 2). The underlying concept is the mutual dependency between these three unavoidable constraints: resolving power, acquisition speed, and SNR. These constraints must be considered as tradeoffs when planning an imaging experiment, as discussed in Strategic Planning.
The resolving power of a confocal microscope is marginally improved over that of a widefield microscope because the overall image PSF is the product of both illumination and detection PSFs, but this advantage is achieved only (Cox & Sheppard, 2004) by closing down the pinhole, ultimately to zero, at the expense of rejecting 95% of signal intensity at a setting of 0.2 AU to subsample the PSF. With this smaller pinhole, besides resolving power, contrast and SNR are also increased. Many years ago, Colin Sheppard (1988) realized that collecting this rejected light could be used as a form of structural illumination to improve resolving power. This approach works because a displaced pinhole produces an image about equal to the resolving power of a pinhole aligned to the optical axis but of lower intensity. Since the PSF is the product of illumination and detection, this is true also of the PSF arising from a displaced detector.
A high-sensitivity, multichannel gallium arsenide phosphide PMT detector is able to detect these offset signals with improved SNR. The problem is that, with respect to the detector axis, each individual image is recorded from a slightly different viewing angle and suffers from parallax. Combining these without further processing would lead to a blurred final image, so each individual image must first be shifted onto a common axis (for an excellent illustrated explanation, see York et al., 2013). Software then sums the signal and applies a deconvolution filter to enhance image features near the cutoff frequency. This processing is usually performed in Fourier space (Demmerle et al., 2015) to avoid artifacts before converting back to images. (Fourier transforms describe images in terms of their sine and cosine components.)
Zeiss markets this technology as the Airyscan (Fig. 7; Weisshart, 2014), a detector like an insect's compound eye consisting of 32 elements, each equivalent to a point detector sampled with a 0.2-AU pinhole (Fig. 7A). These elements are arranged in a circular geometry, such that the total detector area is equivalent to a 1.25-AU pinhole setting. The spatial resolution of the final reconstructed image is defined by the sampling of the central pixel, comparable to images acquired with a conventional confocal at a 0.2-AU pinhole setting, while the total sensitivity of the system is equivalent to 1.25 AU (Fig. 7A). The Airyscan is a PMT detector, rather than a CCD/CMOS camera, which enables fast readout. It scans the entire Airy disc, hence the name.
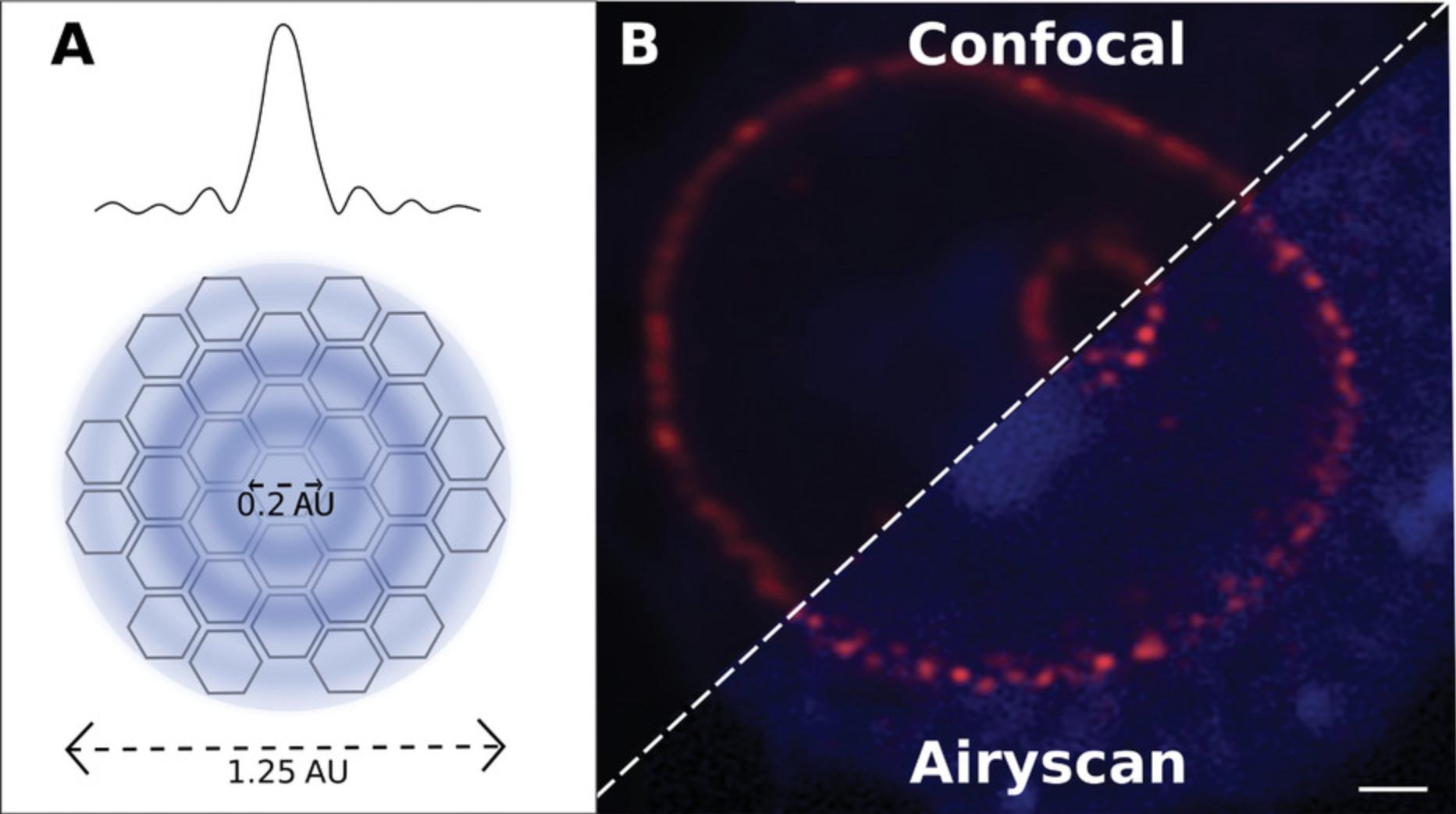
Simple pixel reassignment is not the only method; slightly different image scanning approaches may be taken (Gregor & Enderlein, 2019; Ward & Pal, 2017), such as using digital micromirrors or rescanning the image and shifting the phase of the Fourier image rather than shifting pixels directly in order to improve raster speed and axial resolution. Expanding the beam containing the emitted signal before descanning it (Lam, Cladière, Guillaume, Wassmann, & Bolte, 2017) is the approach adopted by Olympus and Leica, each with patented deconvolution software (Lopez, Fujii, Doi, and Utsunomiya, 2019) since the Airyscan is patented by Zeiss. Overall a 1.7× resolving power improvement is achieved to give a lateral resolving power of 140 nm and an axial resolving power of 400 nm. Since the information still comes from a diffraction-limited pattern, it is limited, as with other structured illumination methods, to a two-fold increase in resolving power.
Assessing Different Super-Resolution Methods: Which One Is Best for You?
First and foremost, which method is best may be determined for you by whether your institution has a particular super-resolution system installed. If you are fortunate to have access to an open-access, dedicated super-resolution facility (e.g., ESRIC run by J.V.) then the choice of which system to use depends on the nature of your sample, how it withstands irradiation, and what information and resolution you require in the final image. Figure 8 shows a comparison of the spatial and temporal resolutions of different techniques. For a guide to selecting the right super-resolution technique based on your research question, see Valli et al. (2021).
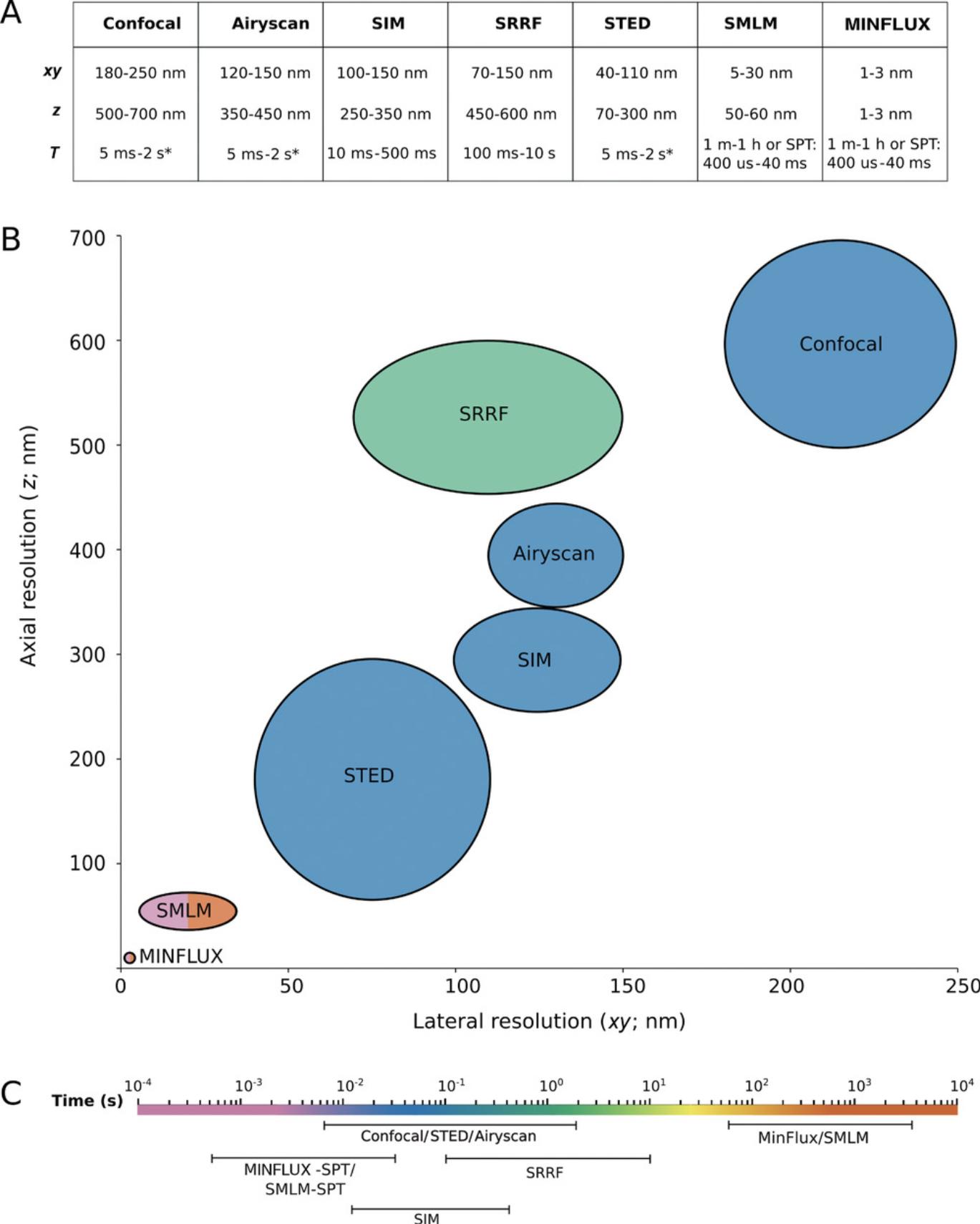
STED imaging involves very high irradiant fluxes, yet its advantage is its similarity to confocal microscopy and that it is the only direct super-resolution technique. It is therefore relatively fast and involves little or no postprocessing of images. SIM will not surpass the Abbe limit by more than two-fold, and localization microscopy (SMLM) is very dependent on labeling density, SNR, and photon count since it never detects all the fluorescent label in a particular structure. For that reason, it is the most difficult technique of the three in which to truly assess resolution.
SIM is not much better than a well-tuned confocal microscope. Since the images are postprocessed, they are particularly prone to reconstruction artifacts, depending on the assumptions made about the PSF and optical transfer function (Wu & Shroff, 2018). SIM is well suited to improving resolution and visibility of widely spaced structures and those that extend axially along the z -axis. SIM uses the same dyes as widefield and confocal microscopy, so multicolor z -stacks are easy to acquire. It is possible to acquire up to four color channels in SIM, as opposed to a maximum of two channels for STED or SMLM. SIM images can be acquired in minutes, its temporal resolving power lying between STED and SMLM.
It is important to remember that with switching fluorophores on and off, super-resolution microscopy records fluorophore distribution rather than structure per se. When once we taught students that the objective is the microscope and the ultimate limit on resolving power and image quality, in super-resolution microscopy that limit is now due to the nature, size, conjugation quality, brightness, photostability, and distribution of the fluorophore(s). If two fluorophores simultaneously fluoresce during a single camera exposure, it is possible for software to assign two locations as one between the two molecules. Thus it is important to use bright fluorophores. Overexpression of fluorescent proteins can significantly perturb the sample and alter the useable image. It is much easier to perform multicolor labeling with SIM than with STED or SMLM, and using multiple wavelengths to give multicolor images is particularly challenging with STED. Despite the high light fluxes, one group (Steffens et al., 2020) has used STED microscopy on account of its 3D sectioning capability to perform intravital live-cell imaging of neurons in mice via an adjustable cranial window. For a recent review of imaging through cranial windows, see Cramer et al. (2021).
STED and localization microscopy make huge demands on fluorophores, so it is crucial to use the latest, most robust dyes (e.g., see Current Protocols article by Kasuboski, Sigal, Joens, Lillemeier, & Fitzpatrick, 2012; Török et al., 2021). You can get away with a lower labeling density with diffraction-limited microscopy, where the diffraction limit “fills the gap” in the structure, but this is not possible with super-resolution microscopy. STED also potentially suffers from high signal to background (Wegel et al., 2016), and when this is the case, SIM and SMLM are superior, particularly for densely packed structures, even though STED images do not require computational postprocessing. Keep in mind the control you design into the experiment so as not to be fooled by potential artifacts (Endesfelder & Heilemann, 2014). One reality check (Marx, 2013) is to computationally blur a super-resolution image to check that it resembles the confocal image that you are familiar with. Another useful check is to process your images through NanoJ-SQUIRREL (Culley et al., 2018). It is also possible to use software (Reinhard, Aufmkolk, Sauer, & Doose, 2019) to automatically register images collected by SIM and dSTORM for comparison with each other to review the real nature of the datasets collected by super-resolution microscopy.
Localization is particularly suited to fixed cells, more so than STED according to Betzig. Localization microscopy can deliver single-molecule spatial precision without the high photon flux of STED (between 10 kW/cm2 and 1 GW/cm2). Hell disagrees and counters that STED and RESLOFT are computationally simpler and so less likely to introduce artifacts into the image. Live-cell super-resolution microscopy is all the more challenging because you need to achieve temporal as well as spatial resolution. Diekmann et al. (2020) offer useful practical insights into optimizing temporal resolution and sensitivity for SMLM. SIM offers the best compromise for live-cell imaging, but any live-cell work with localization microscopy will only collect a few frames (about 1 s worth of video) before the cell is literally fried. Combining SIM systems with light sheet illumination systems (Chatterjee, Wijaya Pratiwi, Wu, Chen, & Bi-Chang, 2018; Wu & Shroff, 2018) or splitting the output of a pulsed laser normally used for multiphoton microscopy (Ji, Magee, & Betzig, 2008) holds promise for the future of live-cell imaging, but combining two technologies like this calls for specialist or home-built imaging platforms and all the complexity that entails. Jacquemet, Carisey, Hamidi, Henriques, & Leterrier (2020) have published a guide to super-resolution microscopy that grades the various techniques according to versatility, availability, resolving power, sample friendliness, image fidelity, and multicolor imaging. Their results are summarized in Table 2.
Characteristic | SIM | STED | Localization | Pixel reassignment | Expansion |
---|---|---|---|---|---|
Versatility, ease of use | Easy | Moderate | Difficult | Easy | Moderate |
Availability, costs | £100,000-£200,000 | £100,000-£300,000 | £50,000-£200,000 | £100,000 | Lab based, low cost |
Sample preparation | Simple | Complex | Complex | Simple | Moderate |
Best lateral xy resolution (nm) | 150 | 20 | 10 | 150 | 30 |
Best axial z resolution (nm) | 300 | 40 | 20 | 600 | 30 |
Image fidelityb | 4 | 5 | 2 | 4 | 3 |
SNRb | 3 | 2 | 2 | 1 | 4 |
Multicolor | Good | Restricted | Limited | Unlimited | Unlimited |
Thick sample friendlinessb | 3 | 2 | 2 | 4 | 5 |
Illumination intensity (kW/cm2)c | 0.01-0.02 | 1000-2000d | 1-20 | 0.01-0.02 | N/A |
Live cell friendlinessb | 4 | 3 | 2 | 5 | 0 |
Temporal resolution (frame rate, Hz)b | 4 (10) | 3 (1-10) | 1 (<1) | 5 (1-10) | 0 (N/A) |
Image acquisition time | Seconds to minutes | Minutes | Minutes to hours | Seconds to minutes | Seconds to minutes |
Maximum penetration depth (μm) | 10 | 50 | 0.2 | 50 | N/Ae |
Comments | Larger fields of view than STED/localization; image requires postprocessing | Good image fidelity; no image postprocessing required | Image requires postprocessing | Requires confocal upgrade; deconvolution software can use confocal samples | For thick samples and whole mouse organs with standard dyes and antibodies |
Merits | Can image thicker sections than localization methods | Confocal speed acquisition | Very high resolution | Improved SNR handles thick mouse tissues | Preserves structural integrity |
Disadvantages | Resolution only up to twice the Abbe limit | Intense depletion laser‒specialized fluorophores | Computational artifacts very slow | Not super-resolution; commercial solution expensive | Potentially artifactual; dependent on fixation and clearing |
Suggested reference | Demmerle et al. (2017) | Vicidomini et al. (2018) | Khater et al. (2020) | Jacquemet et al. (2020) | Truckenbrodt et al. (2019) |
- N/A, not applicable; RESOLFT, reversible saturable optical fluorescence transitions; SIM, structured illumination microscopy; STED, stimulated emission depletion microscopy; SNR, signal-to-noise ratio.
- a Data compiled from Jacquemet et al. (2020), Schermelleh et al. (2019), and a Current Protocols article by Combs & Shroff (2017).
- b Numbers indicate ranking where 5 = best and 1 = poor.
- c The illumination intensity values are approximate and are collated from various sources (e.g., Chen et al., 2018; Ward & Pal, 2017).
- d Photoswitchable RESOLFT is lower kW/cm2.
- e Objective with distance.
The newest approach to resolving structures below the resolving power of classic light microscopes is expansion microscopy. This involves isotropic specimen expansion, physically expanding preserved tissue embedded in a swellable hydrogel to allow subdiffraction-sized structures and cell organelles to be resolved (70-nm lateral and 200-nm axial resolution) and recorded. There are reports that fluorophores are generally quenched to about 50% initial brightness. Fluorophores for STED microscopy, such as Alexa 488 and Atto 565, are recommended. Other dyes, such as Alexa 647 or Cy5, are degraded during the polymerization step. Space precludes a full treatment of this subject here; the reader is referred to Faulkner, Thomas, & Neely (2020); Gao, Asano, & Boyden (2017); a Current Protocols article by Klimas, Gallagher, & Zhao (2019); Truckenbrodt, Sommer, Rizzoli, & Danzl (2019); Wassie, Zhao, & Boyden (2019); and Zhang, Kang, Asano, Gao, & Boyden (2020).
Lambert & Waters (2017, p. 35) profoundly remark, “super-resolution microscopy can greatly reward the rigorous user but has the potential to punish the casual user … requiring careful planning of specimen preparation, rigorous attention to instrument optimization and image acquisition, and a thorough understanding of the practical limitations, sources of error, and artifacts.” The introduction in their paper is well worth reading. In concluding their paper, Lambert and Waters point out that diffraction-limited widefield microscopes are perfectly capable of detecting single molecules and, in most cases, are more sensitive than super-resolution setups. Equally, well-executed image analysis, planning of which we said earlier should precede any experimental work, can be used to make precise measurements of fluorescing structures and features well below the diffraction limit to reveal information that cannot be seen by eye.
CONCLUSIONS
As a powerful emergent technique in the last 15 years, much has been written about nanoscopy—so-called super-resolution microscopy. In 2014, Eric Betzig, Stefan W. Hell, and William E. Moerner equally shared the Nobel Prize for Chemistry for “the development of super-resolved fluorescence microscopy” and how the optical microscope became a nanoscope. We recommend you read their Nobel lectures provided in the Key References, the brief report in Nature (Van Noorden, 2014) that describes how the work of the three Nobel laureates inter-relates, and the superbly readable narrative by Sahl & Hell (2019). There have also been some excellent reviews published by experienced practitioners, and the following are recommended: Follain, Mercier, Osmani, Harlepp, & Goetz (2017); Sahl et al. (2019); Sahl, Hell, & Jakobs (2017); Schermelleh et al. (2019); Turkowyd, Virant, & Endesfelder (2016); Valli et al. (2021); and Vangindertael et al. (2018).
AUTHOR CONTRIBUTIONS
Jessica Valli : writing‒original draft, writing‒review and editing. Jeremy Sanderson : conceptualization, writing‒original draft, writing‒review and editing.
CONFLICT OF INTEREST
The authors declare no conflict of interest.
Open Research
DATA AVAILABILITY STATEMENT
Data sharing is not applicable to this article as no new data were created or analyzed in this study.
LITERATURE CITED
- Abbe, E. (1873). Beiträge zur Theorie des Mikroskops und der mikroskopischen Wahrnehmung. Archiv für Mikroskopische Anatomie , 9, 413–468 doi: 10.1007/BF02956173.
- Almada, P., Culley, S., & Henriques, R. (2015). PALM and STORM: Into large fields and high-throughput microscopy with sCMOS detectors. Methods , 88, 109–121. doi: 10.1016/j.ymeth.2015.06.004.
- Abberior Instruments (2020). Labeling for STED microscopy, Abberior Instruments Guides, Rev. 1.1 20200521. Retrieved from https://static.yanyin.tech/literature/current_protocol/10.1002/cpz1.224/attachments/0240_labeling_for_sted.pdf.
- Arimoto, R., & Murray, J. M. (2004). A common aberration with water-immersion objective lenses. Journal of Microscopy , 216, 49–51. doi: 10.1111/j.0022-2720.2004.01383.x.
- Arsić, A., Stajković, N., Spiegel, R., & Nikić-Spiegel, I. (2020). Effect of Vectashield-induced fluorescence quenching on conventional and super-resolution microscopy. Scientific Reports , 10, 6441. doi: 10.1038/s41598-020-63418-5.
- Auerbach, R. (1954). Analysis of the developmental effects of a lethal mutation in the house mouse. Journal of Experimental Zoology , 127, 305–329. doi: 10.1002/jez.1401270206.
- Ball, G., Demmerle, J., Kaufmann, R., Davis, I., Dobbie, I. M., & Schermelleh, L. (2015). SIMcheck: A toolbox for successful super-resolution structured illumination microscopy. Scientific Reports , 5, 15915. doi: 10.1038/srep15915.
- Banaz, N., Mäkelä, J., & Uphoff, S. (2019). Choosing the right label for single-molecule tracking in live bacteria: Side-by-side comparison of photoactivatable fluorescent protein and Halo tag dyes. Journal of Physics D: Applied Physics , 52, 064002. doi: 10.1088/1361-6463/aaf255.
- Barna, L., Dudok, B., Miczán, V., Horváth, A., László, Z. I., & Katona, I. (2016). Correlated confocal and super-resolution imaging by VividSTORM. Nature Protocols , 11, 163–183. doi: 10.1038/nprot.2016.002.
- Bates, M., Dempsey, G. T., Chen, K. H., & Zhuang, X. (2012). Multicolor super-resolution fluorescence imaging via multi-parameter fluorophore detection. ChemPhysChem , 13, 99–107. doi: 10.1002/cphc.201100735.
- Beliu, G., Kurz, A. J., Kuhlemann, A. C., Behringer-Pliess, L., Meub, M., Wolf, N., … Sauer, M. (2019). Bioorthogonal labeling with tetrazine-dyes for super-resolution microscopy. Communications Biology , 2, 261. doi: 10.1038/s42003-019-0518-z.
- Bernhem, K., & Brismar, H. (2018). SMLocalizer, a GPU accelerated ImageJ plugin for single molecule localization microscopy. Bioinformatics , 34, 137–138. doi: 10.1093/bioinformatics/btx553.
- Besseling, T. H., Jose, J., & Van Blaaderen, A. (2015). Methods to calibrate and scale axial distances in confocal microscopy as a function of refractive index. Journal of Microscopy , 257, 142–150. doi: 10.1111/jmi.12194.
- Betzig, E., Patterson, G. H., Sougrat, R., Lindwasser, O. W., Olenych, S., Bonifacino, J. S., … Hess, H. F. (2006). Imaging intracellular fluorescent proteins at nanometer resolution. Science , 313, 1642–1645. doi: 10.1126/science.1127344.
- Bewersdorf, J., Schmidt, R., & Hell, S. W. (2006). Comparison of I5M and 4Pi-microscopy. Journal of Microscopy , 222, 105–117. doi: 10.1111/j.1365-2818.2006.01578.x.
- Bodén, A., Pennacchietti, F., Coceano, G., Damenti, M., Ratz, M., & Testa, I. (2021). Volumetric live cell imaging with three-dimensional parallelized RESOLFT microscopy. Nature Biotechnology , 39, 609‒618. doi: 10.1038/s41587-020-00779-2.
- Boothe, T., Hilbert, L., Heide, M., Berninger, L., Huttner, W. B., Zaburdaev, V. … Rink, J. C. (2017). A tunable refractive index matching medium for live imaging cells, tissues and model organisms. eLife , 6, e27240. doi: 10.7554/eLife.27240.
- Bradbury, S. (1984). An Introduction to the Optical Microscope. Oxford, UK: Oxford University Press.
- Bria, A., Iannello, G., Onofri, L., & Peng, H. (2016). TeraFly: Real-time three-dimensional visualization and annotation of terabytes of multidimensional volumetric images. Nature Methods , 13, 192–194. doi: 10.1038/nmeth.3767.
- Burgert, A., Letschert, S., Doose, S., & Sauer, M. (2015). Artifacts in single-molecule localization microscopy. Histochemistry and Cell Biology , 144, 123–131. doi: 10.1007/s00418-015-1340-4.
- Bussolati, G., Annaratone, L., Berrino, E., Miglio, U., Panero, M., Cupo, M., … Marchiò, C. (2017). Acid-free glyoxal as a substitute of formalin for structural and molecular preservation in tissue samples. PLoS One , 12, e0182965. doi: 10.1371/journal.pone.0182965.
- Carlton, P. M., Boulanger, J., Kervrann, C., Sibarita, J. B., Salamero, J., Gordon-Messer, S., … Sedat, J. W. (2010). Fast live simultaneous multiwavelength four-dimensional optical microscopy. Proceedings of the National Academy of Sciences of the United States of America , 107, 16016–16022. doi: 10.1073/pnas.1004037107.
- Carrington, G., Tomlinson, D., & Peckham, M. (2019). Exploiting nanobodies and Affimers for superresolution imaging in light microscopy. Molecular Biology of the Cell , 30, 2737–2740. doi: 10.1091/mbc.E18-11-0694.
- Celikkan, F. T., Mungan, C., Sucu, M., Uysal, F., Kahveci Hayme, S., Hayme, S., … Can, A. (2020). PFA is superior to glyoxal in preserving oocyte, embryo, and stem cell proteins evidenced by super-resolution microscopical surveys of epitopes. Journal of Assisted Reproduction and Genetics , 37, 369–384. doi: 10.1007/s10815-019-01666-9.
- Chatterjee, K., Wijaya Pratiwi, F., Wu, F. C. M., Chen, P., & Bi-Chang, C. (2018). Recent progress in light sheet microscopy for biological applications. Applied Spectroscopy , 72, 1137–1169. doi: 10.1177/0003702818778851.
- Chen, B. C., Legant, W. R., Wang, K., Shao, L., Milkie, D. E., Davidson, M. W., … Betzig, E. (2014). Lattice light-sheet microscopy: Imaging molecules to embryos at high spatiotemporal resolution. Science , 346, 1257998. doi: 10.1126/science.1257998.
- Chen, S-Y., Bestvater, F., Schaufler, W., Heintzmann, R., & Cremer, C. (2018). Patterned illumination single molecule localization microscopy (piSMLM): User defined blinking regions of interest. Optics Express , 26, 30009–30020. doi: 10.1364/OE.26.030009.
- Choi, M., Kwok, S. J., & Yun, S. H. (2015). In vivo fluorescence microscopy: Lessons from observing cell behavior in their native environment. Physiology , 30, 40–49. doi: 10.1152/physiol.00019.2014.
- Choi, N. E., Lee, J. Y., Park, E. C., Lee, J. H., & Lee, J. (2021). Recent advances in organelle-targeted fluorescent probes. Molecules , 26, 217. doi: 10.3390/molecules26010217.
- Cnossen, J., Hinsdale, T., Thorsen, R. Ø., Siemons, M., Schueder, F., Jungmann, R., … Stallinga, S. (2020). Localization microscopy at doubled precision with patterned illumination. Nature Methods , 17, 59–63. doi: 10.1038/s41592-019-0657-7.
- Cohen, E. A. K., Abraham, A. V., Ramakrishnan, S., & Ober, R. J. (2019). Resolution limit of image analysis algorithms. Nature Communications , 10, 793. doi: 10.1038/s41467-019-08689-x.
- Cole, R. W., Jinadasa, T., & Brown, C. M. (2011). Measuring and interpreting point spread functions to determine confocal microscope resolution and ensure quality control. Nature Protocols , 6, 1929–1941. doi: 10.1038/nprot.2011.407.
- Combs, C. A., & Shroff, H. (2017). Fluorescence microscopy: A concise guide to current imaging methods. Current Protocols in Neuroscience , 79, 2.1.1–2.1.25. doi: 10.1002/cpns.29.
- Conchello, J. A., & Lichtman, J. (2005). Optical sectioning microscopy. Nature Methods , 2, 920–931. doi: 10.1038/nmeth815.
- Cooper, J., Browne, M., Gribben, H., Catney, M., Coates, C., Mullan, A., & Wilde, G. (2019). Real time multi-modal super-resolution microscopy through Super-Resolution Radial Fluctuations (SRRF-Stream). Proceedings of the Single Molecule Spectroscopy and Superresolution Imaging XII , 10884, 1088418. doi: 10.1117/12.2510761.
- Cordes, T., Maiser, A., Steinhauer, C., Schermelleh, L., & Tinnefeld, P. (2011). Mechanisms and advancement of antifading agents for fluorescence microscopy and single-molecule spectroscopy. Physical Chemistry Chemical Physics , 13, 6699–6709. doi: 10.1039/c0cp01919d.
- Costello, I., & Cox, S. (2021). Analysing errors in single-molecule localisation microscopy. The International Journal of Biochemistry & Cell Biology, 134, 105931. doi: 10.1016/j.biocel.2021.105931.
- Cox, G., & Sheppard, C. J. R. (2004). Practical limits of resolution in confocal and non-linear microscopy. Microscopy Research and Technique , 63, 18–22. doi: 10.1002/jemt.10423.
- Cramer, S. W., Carter, R. E., Aronson, J. D., Kodandaramaiah, S. B., Ebner, T. J., & Chen, C. C. (2021). Through the looking glass: A review of cranial window technology for optical access to the brain. Journal of Neuroscience Methods , 354, 109100. doi: 10.1016/j.jneumeth.2021.109100.
- Cromey, D. (2020). The importance of #1.5 thickness coverslips for microscopy. Retrieved from http://microscopy.arizona.edu/sites/default/files/sites/default/files/upload/coverslips_for_microscopy.pdf.
- Culley, S., Albrecht, D., Jacobs, C., Pereira, P. M., Leterrier, C., Mercer, J., & Henriques, R. (2018). Quantitative mapping and minimization of super-resolution optical imaging artifacts. Nature Methods , 15, 263–266. doi: 10.1038/nmeth.4605.
- Curd, A., Cleasby, A., Makowska, K., York, A., Shroff, H., & Peckham, M. (2015). Construction of an instant structured illumination microscope. Methods , 88, 37–47. doi: 10.1016/j.ymeth.2015.07.012.
- Dankovich, T. M., & Rizzoli, S. O. (2021). Challenges facing quantitative large-scale optical super-resolution, and some simple solutions. iScience, 24 , 102134. doi: 10.1016/j.isci.2021.102134.
- Dapson, R. W. (2007). Glyoxal fixation: How it works and why it only occasionally needs antigen retrieval. Biotechnic & Histochemistry, 82, 161–166. doi: 10.1080/10520290701488113.
- Deagle, R. C., Wee, T. E., & Brown, C. M. (2017). Reproducibility in light microscopy: Maintenance, standards and SOPs. The International Journal of Biochemistry & Cell Biology, 89, 120–124. doi: 10.1016/j.biocel.2017.06.008.
- Demmerle, J., Innocent, C., North, A. J., Ball, G., Müller, M., Miron, E., … Schermelleh, L. (2017). Strategic and practical guidelines for successful structured illumination microscopy. Nature Protocols , 12, 988–1010. doi: 10.1038/nprot.2017.019.
- Demmerle, J., Wegel, E., Schermelleh, L., & Dobbie, I. M. (2015). Assessing resolution in super-resolution imaging. Methods , 88, 3–10. doi: 10.1016/j.ymeth.2015.07.001.
- Dertinger, T., Pallaoro, A., Braun, G., Ly, S., Laurence, T. A., & Weiss, S. (2013). Advances in superresolution optical fluctuation imaging (SOFI). Quarterly Reviews of Biophysics , 46, 210–221. doi: 10.1017/S0033583513000036.
- Diaspro, A., Federici, F., & Robello, M. (2002). Influence of refractive-index mismatch in high-resolution three-dimensional confocal microscopy. Applied Optics , 41, 685–690. doi: 10.1364/AO.41.000685.
- Diekmann, R., Kahnwald, M., Schoenit, A., Deschamps, J., Matti, U., & Ries, J. (2020). Optimizing imaging speed and excitation intensity for single-molecule localization microscopy. Nature Methods , 17, 909–912. doi: 10.1038/s41592-020-0918-5.
- Diel, E. E., Lichtman, J. W., & Richardson, D. S. (2020). Tutorial: Avoiding and correcting sample-induced spherical aberration artifacts in 3D fluorescence microscopy. Nature Protocols , 15, 2773–2784. doi: 10.1038/s41596-020-0360-2.
- Dong, J-X., Lee, Y., Kirmiz, M., Palacio, S., Dumitras, C., Moreno, C. M., … Trimmer, J. S. (2019). A toolbox of nanobodies developed and validated for use as intrabodies and nanoscale immunolabels in mammalian brain neurons. eLife , 8, e48750. doi: 10.7554/eLife.48750.
- Dreier, J., Castello, M., Coceano, G., Cáceres, R., Plastino, J., Vicidomini, G., & Testa, I. (2019). Smart scanning for low-illumination and fast RESOLFT nanoscopy in vivo. Nature Communications , 10, 556. doi: 10.1038/s41467-019-08442-4.
- Dunn, K. W., & Sutton, T. A. (2008). Functional studies in living animals using multiphoton microscopy. ILAR Journal , 49, 66–77. doi: 10.1093/ilar.49.1.66.
- Dunsing, V., Luckner, M., Zühlke, B., Petazzi, R. A., Herrmann, A., & Chiantia, S. (2018). Optimal fluorescent protein tags for quantifying protein oligomerization in living cells. Scientific Reports , 8, 10634. doi: 10.1038/s41598-018-28858-0.
- Duwé, S., & Dedecker, P. (2019). Optimizing the fluorescent protein toolbox and its use. Current Opinion in Biotechnology , 58, 183–191. doi: 10.1016/j.copbio.2019.04.006.
- Egner, A., & Hell, S. W. (2006). Aberrations in confocal and multi-photon fluorescence microscopy induced by refractive index mismatch. In J. Pawley (Ed.), Handbook of biological confocal microscopy ( 3rd ed.). Boston, MA: Springer. doi: 10.1007/978-0-387-45524-2_20.
- Eltoum, I., Fredenburgh, J., Myers, R. B., & Grizzle, W. E. (2001). Introduction to the theory and practice of the fixation of tissues. Journal of Histotechnology , 24, 173–190. doi: 10.1179/his.2001.24.3.173.
- Endesfelder, U., & Heilemann, M. (2014). Art and artifacts in single-molecule localization microscopy: Beyond attractive images. Nature Methods , 11, 235–238. doi: 10.1038/nmeth.2852.
- Erdmann, R. S., Baguley, S. W., Richens, J. H., Wissner, R. F., Xi, Z., Allgeyer, E. S., … Toomre, D. (2019). Labeling strategies matter for super-resolution microscopy: A comparison between HaloTags and SNAP-tags. Cell Chemical Biology , 26, 584–592.e6. doi: 10.1016/j.chembiol.2019.01.003.
- Faulkner, E. L., Thomas, S. G., & Neely, R. K. (2020). An introduction to the methodology of expansion microscopy. The International Journal of Biochemistry & Cell Biology, 124, 105764. doi: 10.1016/j.biocel.2020.105764.
- Fiolka, R., Beck, M., & Stemmer, A. (2008). Structured illumination in total internal reflection fluorescence microscopy using a spatial light modulator. Optics Letters , 33, 1629–1631. doi: 10.1364/OL.33.001629.
- Follain, G., Mercier, L., Osmani, N., Harlepp, S., & Goetz, J. G. (2017). Seeing is believing – multi-scale spatio-temporal imaging towards in vivo cell biology. Journal of Cell Science , 130, 23–38. doi: 10.1242/jcs.189001.
- Fölling, J., Bossi, M., Bock, H., Medda, R., Wurm, C. A., Hein, B., … Hell, S. W. (2008). Fluorescence nanoscopy by ground-state depletion and single-molecule return. Nature Methods , 5, 943–945. doi: 10.1038/nmeth.1257.
- Förster, R., Lu-Walther, H. W., Jost, A., Kielhorn, M., Wicker, K., & Heintzmann, R. (2014). Simple structured illumination microscope setup with high acquisition speed by using a spatial light modulator. Optics Express , 22, 20663–20677. doi: 10.1364/OE.22.020663.
- Fouquet, C., Gilles, J. F., Heck, N., Dos Santos, M., Schwartzmann, R., Cannaya, V., … Bolte, S. (2015). Improving axial resolution in confocal microscopy with new high refractive index mounting media. PLoS One , 10, e0121096. doi: 10.1371/journal.pone.0121096.
- Früh, S. M., & Schoen, I. (2017). Measuring nanometer distances between fluorescent labels step-by-step. Methods in Molecular Biology , 1663, 189–203. doi: 10.1007/978-1-4939-7265-4_16.
- Galland, R., Grenci, G., Aravind, A., Viasnoff, V., Studer, V., & Sibarita, J. B. (2015). 3D high- and super-resolution imaging using single-objective SPIM. Nature Methods , 12, 641–644. doi: 10.1038/nmeth.3402.
- Gao, L. (2015). QSIM: Quantitative structured illumination microscopy image processing in ImageJ. Biomedical Engineering Online , 14, 4. doi: 10.1186/1475-925X-14-4.
- Gao, L., Shao, L., Higgins, C. D., Poulton, J. S., Peifer, M., Davidson, M. W., … Betzig, E. (2012). Noninvasive imaging beyond the diffraction limit of 3D dynamics in thickly fluorescent specimens. Cell , 151, 1370–1385. doi: 10.1016/j.cell.2012.10.008.
- Gao, R., Asano, S. M., & Boyden, E. S. (2017). Q&A: Expansion microscopy. BMC Biology , 15, 50. doi: 10.1186/s12915-017-0393-3.
- Geertsema, H. J., Aimola, G., Fabricius, V., Fuerste, J. P., Kaufer, B. B., & Ewers, H. (2021). Left-handed DNA-PAINT for improved super-resolution imaging in the nucleus. Nature Biotechnology , 39, 551‒554. doi: 10.1038/s41587-020-00753-y.
- Geissbuehler, S., Dellagiacoma, C., & Lasser, T. (2011). Comparison between SOFI and STORM. Biomed Optics Express , 2, 408–420. doi: 10.1364/BOE.2.000408.
- Gerber, C., & Lang, H. P. (2006). How the doors to the nanoworld were opened. Nature Nanotechnology , 1, 3–5. doi: 10.1038/nnano.2006.70.
- Girkin, J. M., & Carvalho, M. T. (2018). The light-sheet microscopy revolution. Journal of Optics , 20, 053002. doi: 10.1088/2040-8986/aab58a.
- Girsault, A., Lukes, T., Sharipov, A., Geissbuehler, S., Leutenegger, M., Vandenberg, W., … Lasser, T. (2016). SOFI simulation tool: A software package for simulating and testing super-resolution optical fluctuation imaging. PLoS One , 11, e0161602. doi: 10.1371/journal.pone.0161602.
- Glushonkov, O., Réal, E., Boutant, E., Mély, Y., & Didier, P. (2018). Optimized protocol for combined PALM-dSTORM imaging. Scientific Reports , 8, 8749. doi: 10.1038/s41598-018-27059-z.
- Gomes de Castro, M. A., Rammner, B., & Opazo, F. (2016). Aptamer stainings for super-resolution microscopy. Methods in Molecular Biology , 1380, 197–210. doi: 10.1007/978-1-4939-3197-2_17.
- Gregor, I., & Enderlein, J. (2019). Image scanning microscopy. Current Opinion in Chemical Biology , 51, 74–83. doi: 10.1016/j.cbpa.2019.05.011.
- Gustafsson, M. G. (1999). Extended resolution fluorescence microscopy. Current Opinion in Structural Biology , 9, 627–634. doi: 10.1016/S0959-440X(99)00016-0.
- Gustafsson, M. G. (2000). Surpassing the lateral resolution limit by a factor of two using structured illumination microscopy. Journal of Microscopy , 198, 82–87. doi: 10.1046/j.1365-2818.2000.00710.x.
- Gustafsson, M. G. (2005). Nonlinear structured-illumination microscopy: Wide-field fluorescence imaging with theoretically unlimited resolution. Proceedings of the National Academy of Sciences of the United States of America , 102, 13081–13086. doi: 10.1073/pnas.0406877102.
- Gustafsson, M. G., Agard, D. A., & Sedat, J. W. (1999). I5M: 3D widefield light microscopy with better than 100 nm axial resolution. Journal of Microscopy , 195, 10–16. doi: 10.1046/j.1365-2818.1999.00576.x.
- Gustafsson, M. G., Shao, L., Carlton, P. M., Wang, C. J., Golubovskaya, I. N., Cande, W. Z., … Sedat, J. W. (2008). Three-dimensional resolution doubling in wide-field fluorescence microscopy by structured illumination. Biophysical Journal , 94, 4957–4970. doi: 10.1529/biophysj.107.120345.
- Gustafsson, N., Culley, S., Ashdown, G., Owen, D. M., Pereira, P. M., & Henriques, R. (2016). Fast live-cell conventional fluorophore nanoscopy with ImageJ through super-resolution radial fluctuations. Nature Communications , 7, 12471. doi: 10.1038/ncomms12471.
- Gwosch, K. C., Pape, J. K., Balzarotti, F., Hoess, P., Ellenberg, J., Ries, J., & Hell, S. W. (2020). MINFLUX nanoscopy delivers 3D multicolor nanometer resolution in cells. Nature Methods , 17, 217–224. doi: 10.1038/s41592-019-0688-0.
- Haage, A. (2018, February 9). What's it all about? Super resolution microscopy. ASCB. Retrieved from https://www.ascb.org/science-news/whats-super-resolution-microscopy.
- Hammond, C. (2015). The basics of crystallography and diffraction ( 4th ed.). Oxford, UK: Oxford Science Publications.
- Hayat, M. A. (2002). Microscopy, immunohistochemistry, and antigen retrieval methods: For light and electron microscopy. Boston, MA: Springer.
- Hecksher-Sørensen, J., & Sharpe, J. (2001). 3D confocal reconstruction of gene expression in mouse. Mechanisms of Development , 100, 59–63. doi: 10.1016/S0925-4773(00)00508-6.
- Heilemann, M., van de Linde, S., Mukherjee, A., & Sauer, M. (2009). Super-resolution imaging with small organic fluorophores. Angewandte Chemie (International Edition in English) , 48, 6903–6908. doi: 10.1002/anie.200902073.
- Heilemann, M., van de Linde, S., Schüttpelz, M., Kasper, R., Seefeldt, B., Mukherjee, A., … Sauer, M. (2008). Subdiffraction-resolution fluorescence imaging with conventional fluorescent probes. Angewandte Chemie (International Edition in English) , 47, 6172–6176. doi: 10.1002/anie.200802376.
- Heine, J., Wurm, C. A., Keller-Findeisen, J., Schönle, A., Harke, B., Reuss, M., … Donnert, G. (2018). Three dimensional live-cell STED microscopy at increased depth using a water immersion objective. Review of Scientific Instruments , 89, 053701. doi: 10.1063/1.5020249.
- Heintzmann, R., & Huser, T. (2017). Super-resolution structured illumination microscopy. Chemical Reviews , 117, 13890–13908. doi: 10.1021/acs.chemrev.7b00218.
- Hell, S. W. (2009). Microscopy and its focal switch. Nature Methods , 6, 24–32. doi: 10.1038/nmeth.1291.
- Hell, S. W. (2014). Biographical, The Nobel Prize in Chemistry 2014. The Nobel Foundation. Retrieved from https://www.nobelprize.org/prizes/chemistry/2014/hell/biographical/.
- Hell, S. W., & Kroug, M. (1995). Ground-state-depletion fluorscence microscopy: A concept for breaking the diffraction resolution limit. Applied Physics B: Photophysics and Laser Chemistry , 60, 495–497. doi: 10.1007/BF01081333.
- Hell, S. W., & Stelzer, E. H. K. (1992). Properties of a 4Pi confocal fluorescence microscope. Journal of the Optical Society of America A , 9, 2159–2166. doi: 10.1364/JOSAA.9.002159.
- Hell, S. W., & Wichmann, J. (1994). Breaking the diffraction resolution limit by stimulated emission: Stimulated-emission-depletion fluorescence microscopy. Optics Letters , 19, 780–782. doi: 10.1364/OL.19.000780.
- Henriques, R., Griffiths, C., Hesper Rego, E., & Mhlanga, M. M. (2011). PALM and STORM: Unlocking live-cell super-resolution. Biopolymers , 95, 322–331. doi: 10.1002/bi21586.
- Henriques, R., Lelek, M., Fornasiero, E. F., Valtorta, F., Zimmer, C., & Mhlanga, M. M. (2010). QuickPALM: 3D real-time photoactivation nanoscopy image processing in ImageJ. Nature Methods , 7, 339–340. doi: 10.1038/nmeth0510-339.
- Henriques, R., & Mhlanga, M. M. (2009). PALM and STORM: What hides beyond the Rayleigh limit? Biotechnology Journal , 4, 846–857. doi: 10.1002/biot.200900024.
- Hess, S. T., Girirajan, T. P., & Mason, M. D. (2006). Ultra-high resolution imaging by fluorescence photoactivation localization microscopy. Biophysical Journal , 91, 4258–4272. doi: 10.1529/biophysj.106.091116.
- Hoelzel, C. A., & Zhang, X. (2020). Visualizing and manipulating biological processes by using HaloTag and SNAP-Tag technologies. ChemBioChem , 21, 1935–1946. doi: 10.1002/cbic.202000037.
- Hosny, N. A., Song, M., Connelly, J. T., Ameer-Beg, S., Knight, M. M., & Wheeler, A. P. (2013). Super-resolution imaging strategies for cell biologists using a spinning disk microscope. PLoS One , 8, e74604. doi: 10.1371/journal.pone.0074604.
- Hu, K., Nguyen, T. D. K., Rabasco, S., Oomen, P. E., & Ewing, A. G. (2021). Chemical analysis of single cells and organelles. Analytical Chemistry , 93, 41–71. doi: 10.1021/acs.analchem.0c04361.
- Huebinger, J., Spindler, J., Holl, K. J., & Koos, B. (2018). Quantification of protein mobility and associated reshuffling of cytoplasm during chemical fixation. Scientific Reports , 8, 17756. doi: 10.1038/s41598-018-36112-w.
- Huszka, G., & Gijs, M. A. M. (2019). Super-resolution optical imaging: A comparison. Micro and Nano Engineering , 2, 7–28. doi: 10.1016/j.mne.2018.11.005.
- Ingerman, E. A., London, R. A., Heintzmann, R., & Gustafsson, M. G. L. (2019). Signal, noise and resolution in linear and nonlinear structured-illumination microscopy. Journal of Microscopy , 273, 3–25. doi: 10.1111/jmi.12753.
- Jabłoński, A. (1933). Efficiency of anti-stokes fluorescence in dyes. Nature , 131, 839–840. doi: 10.1038/131839b0.
- Jacquemet, G., Carisey, A. F., Hamidi, H., Henriques, R., & Leterrier, C. (2020). The cell biologist's guide to super-resolution microscopy. Journal of Cell Science , 133, jcs240713. doi: 10.1242/jcs.240713.
- Jahr, W., Velicky, P., & Danzl, J. G. (2020). Strategies to maximize performance in STimulated Emission Depletion (STED) nanoscopy of biological specimens. Methods , 174, 27–41. doi: 10.1016/j.ymeth.2019.07.019.
- Ji, N., Magee, J. C., & Betzig, E. (2008). High-speed, low-photodamage nonlinear imaging using passive pulse splitters Nature Methods , 5, 197–202. doi: 10.1038/nmeth.1175.
- Ji, N., Shroff, H., Zhong, H., & Betzig, E. (2008). Advances in the speed and resolution of light microscopy. Current Opinion in Neurobiology , 18, 605–616. doi: 10.1016/j.conb.2009.03.009.
- Jimenez, A., Friedl, K., & Leterrier, C. (2020). About samples, giving examples: Optimized single molecule localization microscopy. Methods , 174, 100–114. doi: 10.1016/j.ymeth.2019.05.008.
- Jonkman, J., Brown, C. M., Wright, G. D., Anderson, K. I., & North, A. J. (2020). Tutorial: Guidance for quantitative confocal microscopy. Nature Protocols , 15, 1585–1611. doi: 10.1038/s41596-020-0313-9.
- Jost, A. P., & Waters, J. C. (2019). Designing a rigorous microscopy experiment: Validating methods and avoiding bias. Journal of Cell Biology , 218, 1452–1466. doi: 10.1083/jcb.201812109.
- Jungmann, R., Avendaño, M. S., Dai, M., Woehrstein, J. B., Agasti, S. S., Feiger, Z., … Yin, P. (2016). Quantitative super-resolution imaging with qPAINT. Nature Methods , 13, 439–442. doi: 10.1038/nmeth.3804.
- Jungmann, R., Avendaño, M. S., Woehrstein, J. B., Dai, M., Shih, W. M., & Yin, P. (2014). Multiplexed 3D cellular super-resolution imaging with DNA-PAINT and Exchange-PAINT. Nature Methods , 11, 313–318. doi: 10.1038/nmeth.2835.
- Kalinin, S. V., Strelcov, E., Belianinov, A., Somnath, S., Vasudevan, R. K., Lingerfelt, E. J., … Jesse, S. (2016). Big, deep, and smart data in scanning probe microscopy. ACS Nano , 10, 9068−9086. doi: 10.1021/acsnano.6b04212.
- Kasuboski, J. M., Sigal, Y. J., Joens, M. S., Lillemeier, B. F., & Fitzpatrick, J. A. (2012). Super-resolution microscopy: A comparative treatment. Current Protocols in Cytometry , 62, 2.17.1‒2.17.24. doi: 10.1002/0471142956.cy0217s62.
- Khater, I. M., Nabi, I. R., & Hamarneh, G. (2020). A review of super-resolution single-molecule localization microscopy cluster analysis and quantification methods. Patterns , 1, 100038. doi: 10.1016/j.patter.2020.100038.
- Kilian, N., Goryaynov, A., Lessard, M. D., Hooker, G., Toomre, D., Rothman, J. E., & Bewersdorf, J. (2018). Assessing photodamage in live-cell STED microscopy. Nature Methods , 15, 755–756. doi: 10.1038/s41592-018-0145-5.
- Klar, T. A., Jakobs, S., Dyba, M., Egner, A., & Hell, S. W. (2000). Fluorescence microscopy with diffraction resolution barrier broken by stimulated emission. Proceedings of the National Academy of Sciences of the United States of America , 97, 8206–8210. doi: 10.1073/pnas.97.15.8206.
- Klauss, A., König, M., & Hille, C. (2015). Upgrade of a scanning confocal microscope to a single-beam path STED microscope. PLoS One , 10, e0130717. doi: 10.1371/journal.pone.0130717.
- Klimas, A., Gallagher, B., & Zhao, Y. (2019). Basics of expansion microscopy. Current Protocols in Cytometry , 91, e67. doi: 10.1002/cpcy.67.
- Köhler, H. (1981). On Abbe's theory of image formation in the microscope. Optica Acta: International Journal of Optics , 28, 1691–1701. doi: 10.1080/713820514.
- Kozma, E., & Kele, P. (2019). Fluorogenic probes for super-resolution microscopy. Organic & Biomolecular Chemistry, 17, 215–233. doi: 10.1039/c8ob02711k.
- Kwon, J., Park, J. S., Kang, M., Choi, S., Park, J., Kim, G. T., … Shim, S. H. (2020). Bright ligand-activatable fluorescent protein for high-quality multicolor live-cell super-resolution microscopy. Nature Communications , 11, 273. doi: 10.1038/s41467-019-14067-4.
- Laine, R. F., Tosheva, K. L., Gustafsson, N., Gray, R. D. M., Almada, P., Albrecht, D., … Henriques, R. (2019). NanoJ: A high-performance open-source super-resolution microscopy toolbox. Journal of Physics D: Applied Physics , 52, 163001. doi: 10.1088/1361-6463/ab0261.
- Lam, F., Cladière, D., Guillaume, C., Wassmann, K., & Bolte, S. (2017). Super-resolution for every-body: An image processing workflow to obtain high-resolution images with a standard confocal microscope. Methods , 115, 17–27. doi: 10.1016/j.ymeth.2016.11.003.
- Lambert, T. J., & Waters, J. C. (2017). Navigating challenges in the application of superresolution microscopy. The Journal of Cell Biology , 216, 53–63. doi: 10.1083/jcb.201610011.
- Lampe, A., Haucke, V., Sigrist, S. J., Heilemann, M., & Schmoranzer, J. (2012). Multi-colour direct STORM with red emitting carbocyanines. Biologie Cellulaire , 104, 229–237. doi: 10.1111/boc.201100011.
- Lauterbach, M. A. (2012). Finding, defining and breaking the diffraction barrier in microscopy – a historical perspective. Optical Nanoscopy , 1, 8. doi: 10.1186/2192-2853-1-8.
- Lee, J. Y., & Kitaoka, M. (2018). A beginner's guide to rigor and reproducibility in fluorescence imaging experiments. Molecular Biology of the Cell , 29, 1519–1525. doi: 10.1091/mbc.E17-05-0276.
- Lehmann, M., Lichtner, G., Klenz, H., & Schmoranzer, J. (2016). Novel organic dyes for multicolor localization-based super-resolution microscopy. Journal of Biophotonics , 9, 161–170. doi: 10.1002/jbio.201500119.
- Leica Microsystems (2019). Quick Guide to STED Preparation. Retrieved from https://webcdn.leica-microsystems.com/fileadmin/academy/2019/Quick_Guide_STED_Sample_Preparation/STED_Sample_Preparation_Guide_Online_20190705.pdf.
- Lemon, W. C., & McDole, K. (2020). Live-cell imaging in the era of too many microscopes. Current Opinion in Cell Biology , 66, 34–42. doi: 10.1016/j.ceb.2020.04.008.
- Lereu, A. L., Passian, A., & Dumas, Ph (2012). Near field optical microscopy: A brief review. International Journal of Nanotechnology , 9, 488–501. doi: 10.1504/IJNT.2012.045353.
- Lin, D., Gagnon, L. A., Howard, M. D., Halpern, A. R., & Vaughan, J. C. (2018). Extended-depth 3D super-resolution imaging using probe-refresh STORM. Biophysical Journal , 114, 1980–1987. doi: 10.1016/j.bpj.2018.03.023.
- Liu, X., Chen, S. Y., Chen, Q., Yao, X., Gelléri, M., Ritz, S., … Bonn, M. (2020). Nanographenes: Ultrastable, switchable, and bright probes for super-resolution microscopy. Angewandte Chemie (International Edition in English) , 59, 496–502. doi: 10.1002/anie.201909220.
- Lopez, J., Fujii, S., Doi, A., & Utsunomiya, H. (2019, January 1). Microscopy/image processing: A deconvolution revolution for confocal image enhancement. LaserFocusWorld , 55, 85–88.
- Lyon, M. F. (1955). The development of the otoliths of the mouse. Journal of Embryology and Experimental Morphology , 3, 213–229. doi: 10.1242/dev.3.3.213.
- Maiques, O., Georgouli, M., & Sanz-Moreno, V. (2019). Recent advances in tissue imaging for cancer research. F1000 Research , 8, F1000 Faculty Rev-1980. doi: 10.12688/f1000research.19037.1.
- Mandula, O., Kielhorn, M., Wicker, K., Krampert, G., Kleppe, I., & Heintzmann, R. (2012). Line scan‒structured illumination microscopy super-resolution imaging in thick fluorescent samples. Optics Express , 20, 24167–24174. doi: 10.1364/OE.20.024167.
- Markwirth, A., Lachetta, M., Mönkemöller, V., Heintzmann, R., Hübner, W., Huser, T., & Müller, M. (2019). Video-rate multi-color structured illumination microscopy with simultaneous real-time reconstruction. Nature Communications , 10, 4315. doi: 10.1038/s41467-019-12165-x.
- Martin-Fernandez, M. L., Tynan, C. J., & Webb, S. E. (2013). A 'pocket guide' to total internal reflection fluorescence. Journal of Microscopy , 252, 16–22. doi: 10.1111/jmi.12070.
- Marx, V. (2013). Is super-resolution microscopy right for you? Nature Methods , 10, 1157–1163. doi: 10.1038/nmeth.2756.
- Mattheyses, A. L., Simon, S. M., & Rappoport, J. Z. (2010). Imaging with total internal reflection fluorescence microscopy for the cell biologist. Journal of Cell Science , 123, 3621–3628. doi: 10.1242/jcs.056218.
- Megason, S. G., Srinivas, S., Dickinson, M. E., & Hadjantonakis, A. K. (2011). Microscopy to mechanism across the scales of development. Current Opinion in Genetics & Development, 21, 519–522. doi: 10.1016/j.gde.2011.09.012.
- Mertz, J. (2019). Strategies for volumetric imaging with a fluorescence microscope. Optica , 6, 1261–1268. doi: 10.1364/OPTICA.6.001261.
- Möckl, L., & Moerner, W. E. (2020). Super-resolution microscopy with single molecules in biology and beyond-essentials, current trends, and future challenges. Journal of the American Chemical Society , 142, 17828–17844. doi: 10.1021/jacs.0c08178.
- Moeyaert, B., & Dedecker, P. (2020). A comprehensive dataset of image sequences covering 20 fluorescent protein labels and 12 imaging conditions for use in super-resolution imaging. Data in Brief , 29, 105273. doi: 10.1016/j.dib.2020.105273.
- Moffitt, J. R., Osseforth, C., & Michaelis, J. (2011). Time-gating improves the spatial resolution of STED microscopy. Optics Express , 19, 4242–4254. doi: 10.1364/OE.19.004242.
- Moore, R. P., & Legant, W. R. (2018). Improving probes for super-resolution. Nature Methods , 15, 659–660. doi: 10.1038/s41592-018-0120-1.
- Morawski, P. A., Motley, S. J., & Campbell, D. J. (2019). Rapid light-dependent degradation of fluorescent dyes in formulated serum-free media. ImmunoHorizons , 3, 585–592. doi: 10.4049/immunohorizons.1900080.
- Müller, M., Mönkemöller, V., Hennig, S., Hübner, W., & Huser, T. (2016). Open-source image reconstruction of super-resolution structured illumination microscopy data in ImageJ. Nature Communications , 7, 10980. doi: 10.1038/ncomms10980.
- Munck, S., Miskiewicz, K., Sannerud, R., Menchon, S. A., Jose, L. Heintzmann, R., &Annaert, W. (2012) Sub-diffraction imaging on standard microscopes through photobleaching microscopy with non-linear processing. Journal of Cell Science , 125, 2257–2266. doi: 10.1242/jcs.098939.
- Mund, M., & Ries, J. (2020). How good are my data? Reference standards in superresolution microscopy. Molecular Biology of the Cell , 31, 2093–2096. doi: 10.1091/mbc.E19-04-0189.
- Murray, J. M. (2011). Methods for imaging thick specimens: Confocal microscopy, deconvolution, and structured illumination. Cold Spring Harbor Protocols , 12, 1399–1437. doi: 10.1101/pdb.top066936.
- Musielak, T. J., Slane, D., Liebig, C., Bayer, M. (2016). A versatile optical clearing protocol for deep tissue imaging of fluorescent proteins in Arabidopsis thaliana. PLoS One , 11, e0161107. doi: 10.1371/journal.pone.0161107.
- Nieves, D. J., Hilzenrat, G., Tran, J., Yang, Z., MacRae, H. H., Baker, M. A. B., & Gaus, K. (2019). tagPAINT: Covalent labelling of genetically encoded protein tags for DNA-PAINT imaging. Royal Society Open Science , 6, 191268. doi: 10.1098/rsos.191268.
- Nowotschin, S., Ferrer-Vaquer, A., & Hadjantonakis, A.-K. (2010). Imaging mouse development with confocal time-lapse microscopy. Methods in Enzymology , 476, 351–377. doi: 10.1016/S0076-6879(10)76020-1.
- Oheim, M., Salomon, A., Weissman, A., Brunstein, M., & Becherer, U. (2019). Calibrating evanescent-wave penetration depths for biological TIRF microscopy. Biophysical Journal , 117, 795–809. doi: 10.1016/j.bpj.2019.07.048.
- Oi, C., Gidden, Z., Holyoake, L., Kantelberg, O., Mochrie, S., Horrocks, M. H., & Regan, L. (2020). LIVE-PAINT allows super-resolution microscopy inside living cells using reversible peptide-protein interactions. Communications Biology , 3, 458. doi: 10.1038/s42003-020-01188-6.
- Oracz, J., Westphal, V., Radzewicz, C., Sahl, S. J., & Hell, S. W. (2017). Photobleaching in STED nanoscopy and its dependence on the photon flux applied for reversible silencing of the fluorophore. Scientific Reports , 7, 11354. doi: 10.1038/s41598-017-09902-x.
- Osseforth, C., Moffitt, J. R., Schermelleh, L., & Michaelis, J. (2014). Simultaneous dual-color 3D STED microscopy. Optics Express , 22, 7028–7039. doi: 10.1364/OE.22.007028.
- Ovesný, M., Křížek, P., Borkovec, J., Svindrych, Z., & Hagen, G. M. (2014). ThunderSTORM: A comprehensive ImageJ plug-in for PALM and STORM data analysis and super-resolution imaging. Bioinformatics , 30, 2389–2390. doi: 10.1093/bioinformatics/btu202.
- Patel, L., Williamson, D., Owen, D. M., & Cohen, E. A. K. (2021). Blinking statistics and molecular counting in direct stochastic reconstruction microscopy (dSTORM). Bioinformatics , 27, btab136. doi: 10.1093/bioinformatics/btab136.
- Pereira, P. M., Gustafsson, N., Marsh, M., Mhlanga, M. M., & Henriques, R. (2020). Super-beacons: Open-source probes with spontaneous tuneable blinking compatible with live-cell super-resolution microscopy. Traffic , 21, 375–385. doi: 10.1111/tra.12728.
- Peterson, B. M., Mermelstein, P. G., Meisel, R. L. (2015). Impact of immersion oils and mounting media on the confocal imaging of dendritic spines. Journal of Neuroscience Methods , 242, 106‒111. doi: 10.1016/j.jneumeth.2015.01.014.
- Phifer-Rixey, M., & Nachman, M. W. (2015). Insights into mammalian biology from the wild house mouse Mus musculus. eLife , 4, e05959. doi: 10.7554/eLife.05959.
- Pietzsch, T., Saalfeld, S., Preibisch, S., & Tomancak, P. (2015). BigDataViewer: Visualization and processing for large image data sets. Nature Methods , 12, 481–483. doi: 10.1038/nmeth.3392.
- Power, R. M., & Huisken, J. (2017). A guide to light-sheet fluorescence microscopy for multiscale imaging. Nature Methods , 14, 360–373. doi: 10.1038/nmeth.4224.
- Prakash, K., Diederich, B., Reichelt, S., Heintzmann, R., & Schermelleh, L. (2021). Super-resolution structured illumination microscopy: Past, present and future. Philosophical Transactions of The Royal Society A. Mathematical Physical and Engineering Sciences , 379, 20200143. doi: 10.1098/rsta.2020.0143.
- Puchner, E. M., Huang, B., & Gaub, H. E. (2014). Single molecule techniques–applications in biology. FEBS Letters , 588, 3519. doi: 10.1016/j.febslet.2014.08.020.
- Qin, S., Isbaner, S., Gregor, I., & Enderlein, J. (2021). Doubling the resolution of a confocal spinning-disk microscope using image scanning microscopy. Nature Protocols , 16, 164–181. doi: 10.1038/s41596-020-00408-x.
- Rego, E. H., Shao, L., Macklin, J. J., Winoto, L., Johansson, G. A., Kamps-Hughes, N., … Gustafsson, M. G. (2012). Nonlinear structured-illumination microscopy with a photoswitchable protein reveals cellular structures at 50-nm resolution. Proceedings of the National Academy of Sciences of the United States of America , 109, E135–E143. doi: 10.1073/pnas.1107547108.
- Reinhard, S., Aufmkolk, S., Sauer, M., & Doose, S. (2019). Registration and visualization of correlative super-resolution microscopy data. Biophysical Journal , 116, 2073–2078. doi: 10.1016/j.bpj.2019.04.029.
- Renz, M. (2013). Fluorescence microscopy-a historical and technical perspective. Cytometry. Part A , 83, 767–779. doi: 10.1002/cyto.a.22295.
- Ricci, P., Sancataldo, G., Gavryusev, V., Franceschini, A., Müllenbroich, M. C., Silvestri, L., & Pavone, F. S. (2020). Fast multi-directional DSLM for confocal detection without striping artefacts. Biomed Optics Express , 11, 3111–3124. doi: 10.1364/BOE.390916.
- Richter, K. N., Revelo, N. H., Seitz, K. J., Helm, M. S., Sarkar, D., Saleeb, R. S., … Rizzoli, S. O. (2018). Glyoxal as an alternative fixative to formaldehyde in immunostaining and super-resolution microscopy. EMBO Journal , 37, 139–159. doi: 10.15252/embj.201695709.
- Roth, J., Mehl, J., & Rohrbach, A. (2020). Fast TIRF-SIM imaging of dynamic, low-fluorescent biological samples. Biomed Optics Express , 11, 4008–4026. doi: 10.1364/BOE.391561.
- Rust, M. J., Bates, M., & Zhuang, X. (2006). Sub-diffraction-limit imaging by stochastic optical reconstruction microscopy (STORM). Nature Methods , 3, 793–795. doi: 10.1038/nmeth929.
- Sage, D., Kirshner, H., Pengo, T., Stuurman, N., Min, J., Manley, S., & Unser, M. (2015). Quantitative evaluation of software packages for single-molecule localization microscopy. Nature Methods , 12, 717–724. doi: 10.1038/nmeth.3442.
- Sage, D., Pham, T. A., Babcock, H., Lukes, T., Pengo, T., Chao, J., … Holden, S. (2019). Super-resolution fight club: Assessment of 2D and 3D single-molecule localization microscopy software. Nature Methods , 16, 387–395. doi: 10.1038/s41592-019-0364-4.
- Sahl, S. J., & Hell, S. W. (2019). High-resolution 3D light microscopy with STED and RESOLFT. In J. F. Bille (Ed.), High resolution imaging in microscopy and ophthalmology: New frontiers in biomedical optics (pp. 3–32). Springer.
- Sahl, S. J., Hell, S. W., & Jakobs, S. (2017). Fluorescence nanoscopy in cell biology. Nature Reviews Molecular Cell Biology , 8, 685–701. doi: 10.1038/nrm.2017.71.
- Sahl, S. J., & Moerner, W. E. (2013). Super-resolution fluorescence imaging with single molecules. Current Opinion in Structural Biology , 23, 778–787. doi: 10.1016/j.sbi.2013.07.010.
- Sahl, S. J., Schönle, A., & Hell, S. W. (2019). Fluorescence microscopy with nanometer resolution. In P. W. Hawkes & J. C. H. Spence (Eds.), Springer handbook of microscopy (pp. 1089–1143). Cham, Switzerland: Springer Nature.
- Sancataldo, G., Gavryusev, V., de Vito, G., Turrini, L., Locatelli, M., Fornetto, C., … Pavone, F. S. (2019). Flexible multi-beam light-sheet fluorescence microscope for live imaging without striping artifacts. Frontiers in Neuroanatomy , 13, 7. doi: 10.3389/fnana.2019.00007.
- Sanderson, J. B. (1994). Biological microtechnique (Royal Microscopical Society Microscopy Handbooks No.28). Oxford, UK: BIOS Scientific Publishers.
- Sanderson, J. (2019). Understanding light microscopy. Hoboken, NJ: John Wiley & Sons.
- Sanderson, J. (2020). Fundamentals of microscopy. Current Protocols in Mouse Biology , 10, e76. doi: 10.1002/cpmo.76.
- Sauer, M., & Heilemann, M. (2017). Single-molecule localization microscopy in eukaryotes. Chemical Reviews , 117, 7478–7509. doi: 10.1021/acs.chemrev.6b00667.
- Schermelleh, L., Ferrand, A., Huser, T., Eggeling, C., Sauer, M., Biehlmaier, O., & Drummen, G. P. C. (2019). Super-resolution microscopy demystified. Nature Cell Biology , 21, 72–84. doi: 10.1038/s41556-018-0251-8.
- Schmidt, R., Wurm, C. A., Jakobs, S., Engelhardt, J., Egner, A., & Hell, S. W. (2008). Spherical nanosized focal spot unravels the interior of cells. Nature Methods , 5, 539–544. doi: 10.1038/nmeth.1214.
- Schmied, C., & Jambor, H. K. (2021). Effective image visualization for publications – a workflow using open access tools and concepts. F1000Research , 9, 1373. doi: 10.12688/f1000research.27140.2.
- Schnell, U., Dijk, F., Sjollema, K. A., & Giepmans, B. N. (2012). Immunolabeling artifacts and the need for live-cell imaging. Nature Methods , 9, 152–158. doi: 10.1038/nmeth.1855.
- Schnitzbauer, J., Strauss, M. T., Schlichthaerle, T., Schueder, F., & Jungmann, R. (2017). Super-resolution microscopy with DNA-PAINT. Nature Protocols , 12, 1198–1228. doi: 10.1038/nprot.2017.024.
- Schroeder, A. B., Dobson, E. T. A., Rueden, C. T., Tomancak, P., Jug, F., & Eliceiri, K. W. (2021). The ImageJ ecosystem: Open-source software for image visualization, processing, and analysis. Protein Science , 30, 234–249. doi: 10.1002/pro.3993.
- Serbedzija, G. N., Bronner-Fraser, M., & Fraser, S. E. (1992). Vital dye analysis of cranial neural crest cell migration in the mouse embryo. Development , 116, 297–307. doi: 10.1242/dev.116.2.297.
- Shao, L., Kner, P., Rego, E. H., & Gustafsson, M. G. (2011). Super-resolution 3D microscopy of live whole cells using structured illumination. Nature Methods , 8, 1044–1046. doi: 10.1038/nmeth.1734.
- Sheppard, C. J. R. (1988). Super-resolution in confocal imaging. Optik , 80, 53–54.
- Sheppard, C. J. R. (2017). Resolution and super-resolution. Microscopy Research and Technique , 80, 590–598. doi: 10.1002/jemt.22834.
- Shimozawa, T., Yamagata, K., Kondo, T., Hayashi, S., Shitamukai, A., Konno, D., … Mimori-Kiyosue, Y. (2013). Improving spinning disk confocal microscopy by preventing pinhole cross-talk for intravital imaging. Proceedings of the National Academy of Sciences of the United States of America , 110, 3399–3404. doi: 10.1073/pnas.1216696110.
- Shivanandan, A., Deschout, H., Scarselli, M., & Radenovic, A. (2014). Challenges in quantitative single molecule localization microscopy. FEBS Letters , 588, 3595–3602. doi: 10.1016/j.febslet.2014.06.014.
- Shroff, H., Galbraith, C. G., Galbraith, J. A., & Betzig, E. (2008). Live-cell photoactivated localization microscopy of nanoscale adhesion dynamics. Nature Methods , 5, 417–423. doi: 10.1038/nmeth.1202.
- Sparrow, C. M. (1916). On spectroscopic resolving power. Astrophysical Journal , 44, 76–86.
- Staudt, T., Lang, M. C., Medda, R., Engelhardt, J., & Hell, S. W. (2007). 2,2'-thiodiethanol: A new water soluble mounting medium for high resolution optical microscopy. Microscopy Research and Technique , 70, 1–9. doi: 10.1002/jemt.20396.
- Steffens, H., Wegner, W., & Willig, K. I. (2020). In vivo STED microscopy: A roadmap to nanoscale imaging in the living mouse. Methods , 174, 42–48. doi: 10.1016/j.ymeth.2019.05.020.
- Stelzer, E. H. (2015). Light-sheet fluorescence microscopy for quantitative biology. Nature Methods , 12, 23–26. doi: 10.1038/nmeth.3219.
- Stelzer, E. (1998). Contrast, resolution, pixelation, dynamic range and signal-to-noise ratio: Fundamental limits to resolution in fluorescence light microscopy. Journal of Microscopy , 189, 15–24. doi: 10.1046/j.1365-2818.1998.00290.x.
- Stockley, J. H., Evans, K., Matthey, M., Volbracht, K., Agathou, S., Mukanowa, J., … Káradóttir, R. T. (2017). Surpassing light-induced cell damage in vitro with novel cell culture media. Scientific Reports , 7, 849. doi: 10.1038/s41598-017-00829-x.
- Strack, R. (2017). Taking nanoscopy to the limit. Nature Methods , 14, 221. doi: 10.1038/nmeth.4211.
- Strauss, S., Nickels, P. C., Strauss, M. T., Jimenez Sabinina, V., Ellenberg, J., Carter, J. D., … Jungmann, R. (2018). Modified aptamers enable quantitative sub-10-nm cellular DNA-PAINT imaging. Nature Methods , 15, 685–688. doi: 10.1038/s41592-018-0105-0.
- Strobl, F., Schmitz, A., & Stelzer, E. H. K. (2017). Improving your four-dimensional image: Traveling through a decade of light-sheet-based fluorescence microscopy research. Nature Protocols , 12, 1103–1109. doi: 10.1038/nprot.2017.028.
- Swedlow, J. R. (2010). Advanced hardware and software tools for fast multidimensional imaging of living cells. Proceedings of the National Academy of Sciences of the United States of America , 107, 16005–16006. doi: 10.1073/pnas.1010043107.
- Szczurek, A., Contu, F., Hoang, A., Dobrucki, J., & Mai, S. (2018). Aqueous mounting media increasing tissue translucence improve image quality in structured illumination microscopy of thick biological specimen. Scientific Reports , 8, 13971. doi: 10.1038/s41598-018-32191-x.
- Tam, J., & Merino, D. (2015). Stochastic optical reconstruction microscopy (STORM) in comparison with stimulated emission depletion (STED) and other imaging methods. Journal of Neurochemistry , 135, 643–58. doi: 10.1111/jnc.13257.
- Tanaka, K. A., Suzuki, K. G., Shirai, Y. M., Shibutani, S. T., Miyahara, M. S., Tsuboi, H., … Kusumi, A. (2010). Membrane molecules mobile even after chemical fixation. Nature Methods , 7, 865–866. doi: 10.1038/nmeth.f.314.
- Tejedo, M. I. A., Cervantes, J. C. M., Roldán, A. S. J., Rodriguez, M., Vega, A. G., Piazza, V. (2019). 3,3'-thiodipropanol as a versatile refractive index-matching mounting medium for fluorescence microscopy. Biomedical Optics Express , 10, 1136-1150. doi: 10.1364/BOE.10.001136.
- Thompson, R. E., Larson, D. R., & Webb, W. W. (2002). Precise nanometer localization analysis for individual fluorescent probes. Biophysical Journal , 82, 2775–2783. doi: 10.1016/S0006-3495(02)75618-X.
- Thorn, K. (2016). A quick guide to light microscopy in cell biology. Molecular Biology of the Cell , 27, 219–222. doi: 10.1091/mbc.e15-02-0088.
- Tokunaga, M., Imamoto, N., & Sakata-Sogawa, K. (2008). Highly inclined thin illumination enables clear single-molecule imaging in cells. Nature Methods , 5, 159–161. doi: 10.1038/nmeth1171.
- Török, G., Cserép, G. B., Telek, A., Arany, D., Váradi, M., Homolya, L., … Németh, K. (2021). Large Stokes-shift bioorthogonal probes for STED, 2P-STED and multi-color STED nanoscopy. Methods and Applications in Fluorescence , 9, 015006. doi: 10.1088/2050-6120/abb363.
- Tosheva, K. L., Yuan, Y., Pereira, P. M., Culley, S., & Henriques, R. (2020). Between life and death: Reducing phototoxicity in super-resolution microscopy. Journal of Physics D: Applied Physics , 53, 163001. doi: 10.1088/1361-6463/ab6b95.
- Tröger, J., Hoischen, C., Perner, B., Monajembashi, S., Barbotin, A., Löschberger, A., … Hemmerich, P. (2020). Comparison of multiscale imaging methods for brain research. Cells , 9, 1377. doi: 10.3390/cells9061377.
- Truckenbrodt, S., Sommer, C., Rizzoli, S. O., & Danzl, J. G. (2019). A practical guide to optimization in X10 expansion microscopy. Nature Protocols , 14, 832–863. doi: 10.1038/s41596-018-0117-3.
- Turkowyd, B., Virant, D., & Endesfelder, U. (2016). From single molecules to life: Microscopy at the nanoscale. Analytical and Bioanalytical Chemistry , 408, 6885–6911. doi: 10.1007/s00216-016-9781-8.
- Turney, S. G., & Lichtman, J. W. (2008). Chapter 11: Imaging fluorescent mice in vivo using confocal microscopy. Methods in Cell Biology , 89, 309–327. doi: 10.1016/S0091-679X(08)00611-0.
- Udan, R. S., & Dickinson, M. E. (2010). Imaging mouse embryonic development. Methods in Enzymology , 476, 329–349. doi: 10.1016/S0076-6879(10)76019-5.
- Uhlig, M. (1964). [Effect of the cover glass thickness and the ND value of cover glasses on the spherical aberration in microscope objectives]. Mikroskopie , 19, 161–164.
- Uno, S. N., Tiwari, D. K., Kamiya, M., Arai, Y., Nagai, T., & Urano, Y. (2015). A guide to use photocontrollable fluorescent proteins and synthetic smart fluorophores for nanoscopy. Microscopy , 64, 263–277. doi: 10.1093/jmicro/dfv037.
- Vaidyanathan, P., Appleton, E., Tran, D., Vahid, A., Church, G., & Densmore, D. (2021). Algorithms for the selection of fluorescent reporters. Communications Biology , 4, 118. doi: 10.1038/s42003-020-01599-5.
- Valli, J., Garcia-Burgos, A., Rooney, L. M., Vale de Melo e Oliveira, B., Duncan, R. R., & Rickman, C. (2021). Seeing beyond the limit, a guide to choosing the right super resolution microscopy technique. Journal of Biological Chemistry , 297, 100791. doi: 10.1016/j.jbc.2021.100791.
- van de Linde, S. (2019). Single-molecule localization microscopy analysis with ImageJ. Journal of Physics D: Applied Physics , 52, 203002. doi: 10.1088/1361-6463/ab092f.
- van de Linde, S., Endesfelder, U., Mukherjee, A., Schüttpelz, M., Wiebusch, G., Wolter, S., … Sauer, M. (2009). Multicolor photoswitching microscopy for subdiffraction-resolution fluorescence imaging. Photochemical & Photobiological Sciences, 8, 465–469. doi: 10.1039/b822533h.
- van de Linde, S., & Sauer, M. (2014). How to switch a fluorophore: From undesired blinking to controlled photoswitching. Chemical Society Reviews , 43, 1076–1087. doi: 10.1039/C3CS60195A.
- Van den Eynde, R., Sandmeyer, A., Vandenberg, W., Duw, S., Hübner, W., Huser, T., … Müller, M. (2019). Quantitative comparison of camera technologies for cost-effective super-resolution optical fluctuation imaging (SOFI). Journal of Physics: Photonics , 1, 044001. doi: 10.1088/2515-7647/ab36ae.
- Van Noorden, R. (2014). Insider view of cells scoops Nobel. Nature , 514, 286. doi: 10.1038/nature.2014.16097.
- Vangindertael, J., Camacho, R., Sempels, W., Mizuno, H., Dedecker, P., & Janssen, K. P. F. (2018). An introduction to optical super-resolution microscopy for the adventurous biologist. Methods and Applications in Fluorescence , 6, 022003. doi: 10.1088/2050-6120/aaae0c.
- Verdaasdonk, J. S., Stephens, A. D., Haase, J., & Bloom, K. (2014). Bending the rules: Widefield microscopy and the Abbe limit of resolution. Journal of Cellular Physiology , 229, 132–138. doi: 10.1002/jc24439.
- Verkade, P., & Collinson, L. (2019). Correlative imaging: Focusing on the future. Hoboken, NJ: Wiley.
- Vicidomini, G., Bianchini, P., & Diaspro, A. (2018). STED super-resolved microscopy. Nature Methods , 15, 173–182. doi: 10.1038/nmeth.4593.
- Vicidomini, G., Schönle, A., Ta, H., Han, K. Y., Moneron, G., Eggeling, C., & Hell, S. W. (2013). STED nanoscopy with time-gated detection: Theoretical and experimental aspects. PLoS One , 8, e54421. doi: 10.1371/journal.pone.0054421.
- Vizcay-Barrena, G., Webb, S. E., Martin-Fernandez, M. L., & Wilson, Z. A. (2011). Subcellular and single-molecule imaging of plant fluorescent proteins using total internal reflection fluorescence microscopy (TIRFM). Journal of Experimental Botany , 62, 5419–5428. doi: 10.1093/jxb/err212.
- von Diezmann, A., Shechtman, Y., & Moerner, W. E. (2017). Three-dimensional localization of single molecules for super-resolution imaging and single-particle tracking. Chemical Reviews , 117, 7244–7275. doi: 10.1021/acs.chemrev.6b00629.
- Wait, E. C., Reiche, M. A., & Chew, T-L. (2020). Hypothesis-driven quantitative fluorescence microscopy‒the importance of reverse-thinking in experimental design. Journal of Cell Science , 133, jcs250027. doi: 10.1242/jcs.250027.
- Wäldchen, S., Lehmann, J., Klein, T., van de Linde, S., & Sauer, M. (2015). Light-induced cell damage in live-cell super-resolution microscopy. Scientific Reports , 5, 15348. doi: 10.1038/srep15348.
- Wan, D-S., Rajadhyaksha, M., & Webb, R. H. (2000). Analysis of spherical aberration of a water immersion objective: Application to specimens with refractive indices 1.33–1.40. Journal of Microscopy , 197, 274–284. doi: 10.1046/j.1365-2818.2000.00635.x.
- Wan, Y., McDole, K., & Keller, P. J. (2019). Light-sheet microscopy and its potential for understanding developmental processes. Annual Review of Cell and Developmental Biology , 35, 655–681. doi: 10.1146/annurev-cellbio-100818-125311.
- Wang, J., Allgeyer, E. S., Sirinakis, G., Zhang, Y., Hu, K., Lessard, M. D., … Bewersdorf, J. (2021). Implementation of a 4Pi-SMS super-resolution microscope. Nature Protocols , 16, 677–727. doi: 10.1038/s41596-020-00428-7.
- Wang, L., Frei, M. S., Salim, A., & Johnsson, K. (2019). Small-molecule fluorescent probes for live-cell super-resolution microscopy. Journal of the American Chemical Society , 141, 2770–2781. doi: 10.1021/jacs.8b11134.
- Wang, L., Tran, M., D'Este, E., Roberti, J., Koch, B., Xue, L., & Johnsson, K. (2020). A general strategy to develop cell permeable and fluorogenic probes for multicolour nanoscopy. Nature Chemistry , 12, 165–172. doi: 10.1038/s41557-019-0371-1.
- Ward, E. N., & Pal, R. (2017). Image scanning microscopy: An overview. Journal of Microscopy , 266, 221–228. doi: 10.1111/jmi.12534.
- Wassie, A. T., Zhao, Y., & Boyden, E. S. (2019). Expansion microscopy: Principles and uses in biological research. Nature Methods , 16, 33–41. doi: 10.1038/s41592-018-0219-4.
- Watkins, S. C., & St Croix, C. M. (2018). Light sheet imaging comes of age. Journal of Cell Biology , 217, 1567–1569. doi: 10.1083/jcb.201804016.
- Wegel, E., Göhler, A., Lagerholm, B. C., Wainman, A., Uphoff, S., Kaufmann, R., & Dobbie, I. M. (2016). Imaging cellular structures in super-resolution with SIM, STED and localisation microscopy: A practical comparison. Scientific Reports , 6, 27290. doi: 10.1038/srep27290.
- Weisshart, K. (2014). Technology note: The basic principle of Airyscanning. Carl Zeiss Microscopy.
- Wicker, K., & Heintzmann, R. (2014). Resolving a misconception about structured illumination. Nature Photon , 8, 342–344. doi: 10.1038/nphoton.2014.88.
- Wildanger, D., Rittweger, E., Kastrup, L., & Hell, S. W. (2008). STED microscopy with a supercontinuum laser source. Optics Express , 16, 9614–9621. doi: 10.1364/OE.16.009614.
- Wollman, A. J. M., Nudd, R., Hedlund, E. G., & Leake, M. C. (2015). From Animaculum to single molecules: 300 years of the light microscope. Open Biology , 5, 150019. doi: 10.1098/rsob.150019.
- Wolter, S., Löschberger, A., Holm, T., Aufmkolk, S., Dabauvalle, M. C., van de Linde, S., & Sauer, M. (2012). rapidSTORM: Accurate, fast open-source software for localization microscopy. Nature Methods , 9, 1040–1041. doi: 10.1038/nmeth.2224.
- Wu, Y., & Shroff, H. (2018). Faster, sharper, and deeper: Structured illumination microscopy for biological imaging. Nature Methods , 15, 1011–1019. doi: 10.1038/s41592-018-0211-z.
- Wu, Y., Wu, X., Toro, L., & Stefani, E. (2015). Resonant-scanning dual-color STED microscopy with ultrafast photon counting: A concise guide. Methods , 88, 48–56. doi: 10.1016/j.ymeth.2015.06.019.
- Wu, Z., Xu, X., & Xi, P. (2021). Stimulated emission depletion microscopy for biological imaging in four dimensions: A review. Microscopy Research and Technique , [Epub ahead of print]. doi: 10.1002/jemt.23750.
- Xavier da Silveira Dos Santos, A., & Liberali, P. (2019). From single cells to tissue self-organization. The FEBS Journal , 286, 1495–1513. doi: 10.1111/febs.14694.
- York, A. G., Chandris, P., Nogare, D. D., Head, J., Wawrzusin, P., Fischer, R. S., … Shroff, H. (2013). Instant super-resolution imaging in live cells and embryos via analog image processing. Nature Methods , 10, 1122–1126, doi: 10.1038/nmeth.2687.
- York, A., Parekh, S. H., Dalle Nogare, D., Fischer, R. S., Temprine, K., Mione, M., … Shroff, H. (2012). Resolution doubling in live, multicellular organisms via multifocal structured illumination microscopy. Nature Methods , 9, 749–754. doi: 10.1038/nmeth.2025.
- You, S., Chao, J., Cohen, E. A. K., Ward, E. S., & Ober, R. J. (2021). Microscope calibration protocol for single-molecule microscopy. Optics Express , 29, 182–207. doi: 10.1364/OE.408361.
- Zhang, C., Kang, J. S., Asano, S. M., Gao, R., & Boyden, E. S. (2020). Expansion microscopy for beginners: Visualizing microtubules in expanded cultured HeLa cells. Current Protocols in Neuroscience , 92, e96. doi: 10.1002/cpns.96.
- Zhang, Y., & Raymo, F. M. (2020). Photoactivatable fluorophores for single-molecule localization microscopy of live cells. Methods and Applications in Fluorescence , 8, 032002. doi: 10.1088/2050-6120/ab8c5c.
- Zheng, X., Zhou, J., Wang, L., Wang, M., Wu, W., Chen, J., … Shao, Y. (2021). Current challenges and solutions of super-resolution structured illumination microscopy. APL Photonics , 6, 020901. doi: 10.1063/5.0038065.
- Zimmermann, T. (2017). Superresolution microscopy. In eLS. Hoboken, NJ: John Wiley & Sons.
- Zinner, M., Lukonin, I., & Liberali, P. (2020). Design principles of tissue organisation: How single cells coordinate across scales. Current Opinion in Cell Biology , 67, 37–45. doi: 10.1016/j.ceb.2020.07.004.
- Zucker, R. M., Hunter, S., & Rogers, J. M. (1998). Confocal laser scanning microscopy of apoptosis in organogenesis-stage mouse embryos. Cytometry , 33, 348–354. doi: 10.1002/(SICI)1097-0320(19981101)33:33.0.CO;2-C.
KEY REFERENCES
- Betzig, E. (2014, December 8). Single molecules, cells, and super-resolution optics (Nobel lecture). Retrieved from https://static.yanyin.tech/literature/current_protocol/10.1002/cpz1.224/attachments/betzig-lecture.pdf
Nobel lecture by Eric Betzig following awarding of the Nobel Prize in Chemistry.
- Hell, S. W. (2014, December 8). Nanoscopy with focused light (Nobel lecture). Retrieved from https://static.yanyin.tech/literature/current_protocol/10.1002/cpz1.224/attachments/hell-lecture.pdf
Nobel lecture by Stefan W. Hell following awarding of the Nobel Prize in Chemistry.
- Moerner, W. E. (2014, December 8). Single-molecule spectroscopy, imaging, and photocontrol: Foundations for super-resolution microscopy (Nobel lecture). Retrieved from https://static.yanyin.tech/literature/current_protocol/10.1002/cpz1.224/attachments/moerner-lecture.pdf
Nobel lecture by William E. Moerner following awarding of the Nobel Prize in Chemistry.
Citing Literature
Number of times cited according to CrossRef: 4
- Shima Ghasemi, Monika Shamsabadi, Axel Olesund, Francisco Najera, Andreas Erbs Hillers‐Bendtsen, Fredrik Edhborg, Adil S. Aslam, Wera Larsson, Zhihang Wang, Francoise M. Amombo Noa, Rebecca Jane Salthouse, Lars Öhrström, Helen Hölzel, E. Perez‐Inestrosa, Kurt V. Mikkelsen, Jörg Hanrieder, Bo Albinsson, Ambra Dreos, Kasper Moth‐Poulsen, Pyrene Functionalized Norbornadiene‐Quadricyclane Fluorescent Photoswitches: Characterization of their Spectral Properties and Application in Imaging of Amyloid Beta Plaques, Chemistry – A European Journal, 10.1002/chem.202400322, 30 , 34, (2024).
- Jihong Gong, Ziqi Jin, Huidan Chen, Juan He, Yiran Zhang, Xiaofei Yang, Super-resolution fluorescence microscopic imaging in pathogenesis and drug treatment of neurological disease, Advanced Drug Delivery Reviews, 10.1016/j.addr.2023.114791, 196 , (114791), (2023).
- Jeremy Sanderson, Multi‐Photon Microscopy, Current Protocols, 10.1002/cpz1.634, 3 , 1, (2023).
- Su Jin Kim, Ho-Young Lee, In vivo molecular imaging in preclinical research, Laboratory Animal Research, 10.1186/s42826-022-00142-3, 38 , 1, (2022).