Protocol Considerations for In Vitro Metabolic Glycoengineering of Non-Natural Glycans
Kris Dammen-Brower, Kris Dammen-Brower, Elaine Tan, Elaine Tan, Ruben T. Almaraz, Ruben T. Almaraz, Jian Du, Jian Du, Kevin J. Yarema, Kevin J. Yarema
Abstract
Metabolic glycoengineering (MGE) refers to a technique where non-natural monosaccharide analogs are introduced into living biological systems. Once inside a cell, these compounds intercept a targeted biosynthetic glycosylation pathway and in turn are metabolically incorporated into cell-surface-displayed oligosaccharides, where they can modulate a host of biological activities or be exploited as tags for bioorthogonal and chemoselective ligation reactions. Over the past decade, azido-modified monosaccharides have become the go-to analogs for MGE; at the same time, analogs with novel chemical functionalities continue to be developed. Therefore, one emphasis of this article is to describe a general approach for analog selection and then provide protocols to ensure safe and efficacious analog usage by cells. Once cell-surface glycans have been successfully remodeled by MGE methodology, the stage is set for probing changes to the myriad cellular responses modulated by these versatile molecules. This manuscript concludes by detailing how one of these detection methods—flow cytometry—can be successfully utilized to quantify MGE analog incorporation and set the stage for numerous follow-up applications. © 2023 The Authors. Current Protocols published by Wiley Periodicals LLC.
Basic Protocol 1 : Incubation of cells with sugar analogs
Support Protocol : Routine growth and maintenance of Jurkat cells
Basic Protocol 2 : Cell viability assays
Basic Protocol 3 : Periodate-resorcinol assay to measure analog uptake and incorporation into metabolic pathways
Basic Protocol 4 : Quantitation of cell-surface glycoconjugates
INTRODUCTION
Often referred to as metabolic oligosaccharide engineering (MOE) (Almaraz, Mathew et al., 2012; Dube & Bertozzi 2003), metabolic glycoengineering (MGE) has become an integral part of the overall field of glycoengineering (Du et al., 2009; Keppler et al., 2001; Wratil et al., 2016). MGE technology was pioneered in living cells and animals in the early 1990s by Werner Reutter's group, who discovered that certain structural variants of mammalian hexosamines can be taken up by biosynthetic pathways and utilized for oligosaccharide biosynthesis (Figs. 1 and 2) (Kayser, Geilen et al., 1992; Kayser, Zeitler et al., 1992). In particular, ManNAc analogs proved adept at installing the corresponding non-natural sialic acids into glycans, thereby endowing the cell surface with novel biochemical features. Over time, MGE expanded beyond sialic acid to include pathways that process GalNAc (Hang & Bertozzi, 2001), fucose (Sawa et al., 2006), and glucose (Darabedian et al., 2018). As a result, an increased number of glycans found within the glycocalyx can be endowed with novel structural and chemical features (Fig. 1). Additionally, MGE can introduce metabolic labels into the intracellular O -GlcNAc modification (Boyce et al., 2011; Khidekel et al., 2003, 2004), altering metabolic flux and quantitatively modulating this post-translational protein modification (Saeui et al., 2023). In this article, we focus on ManNAc analogs that target the sialic acid pathway in human cells, but the optimization parameters we describe can be readily adapted for other analogs, glycosylation pathways, and cell types.
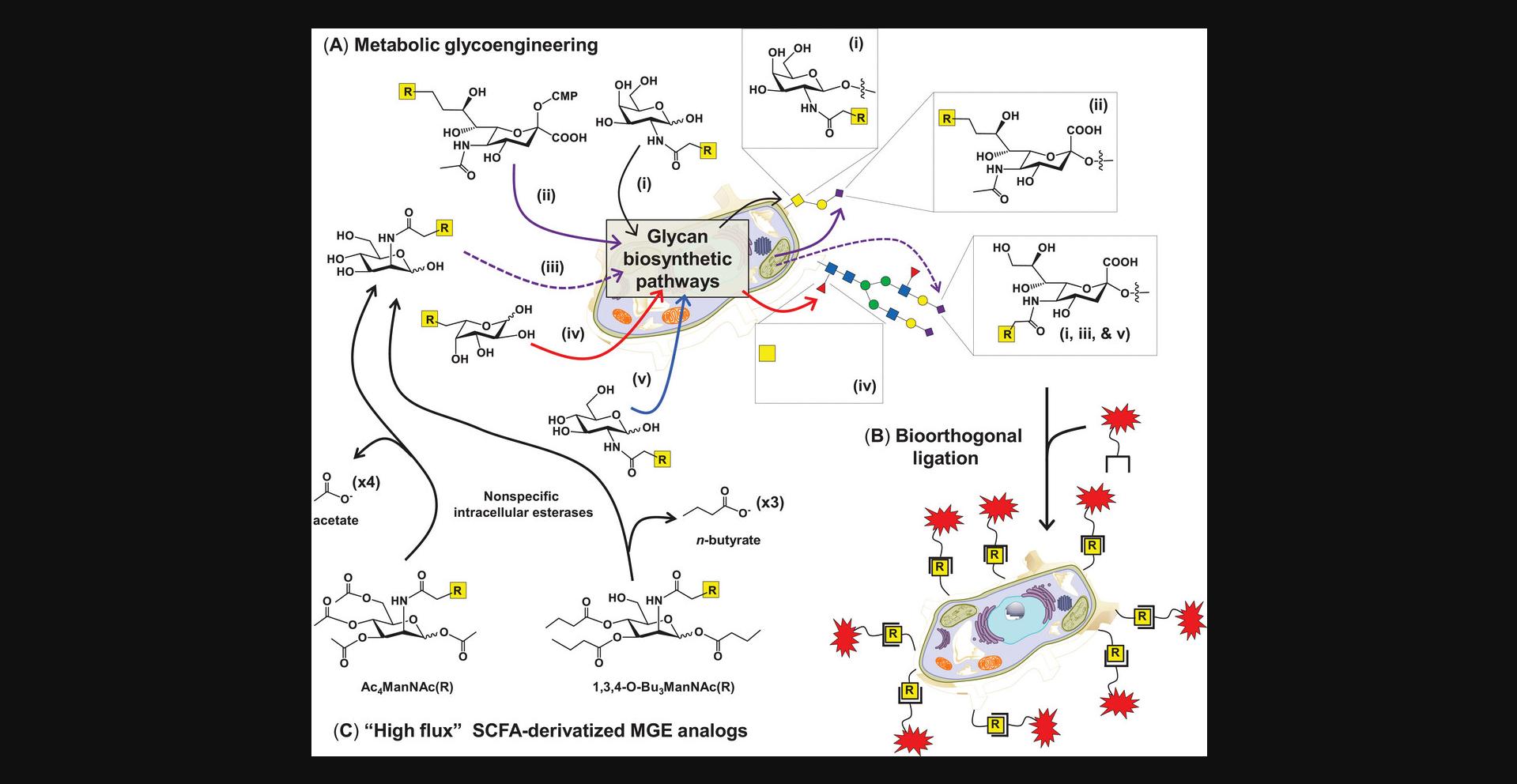
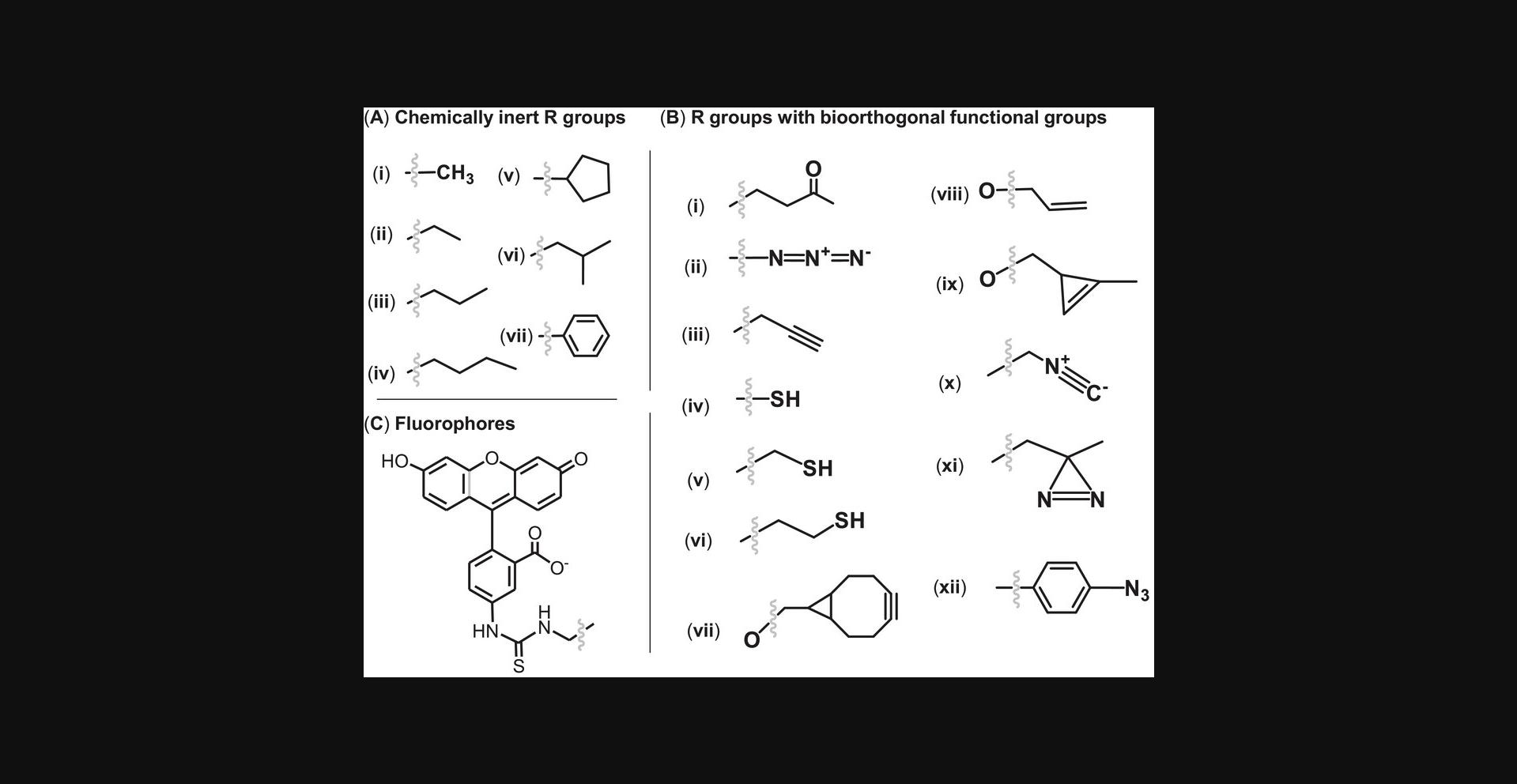
To build on the Reutter group's initial MGE experiments that utilized hexosamine analogs with extended alkane chains at the N -acyl positions (Fig. 2A(i-iv)), incorporation of bioorthogonal chemical functionalities into analog design began with ketone groups (Fig. 2B(i); Mahal et al., 1997). This opened the door to bioorthogonal conjugation of the glyocalyx with complementary probes for applications such as diagnostic imaging as well as drug and gene delivery (Lee et al., 1999; Mahal et al., 1999). From this inception, introduction of new bioorthogonal moieties in MGE workflows rapidly followed, including azides (Fig. 2B(ii); Saxon & Bertozzi, 2000), alkynes (Fig. 2B(iii); Hsu et al., 2007), and thiols (Fig. 2B(iv-vi); Hua et al., 2011; Sampathkumar, Li et al., 2006). These first-generation MGE bioorthogonal ligation reactions often suffered from relatively slow reaction rates, requiring concentrations of labeling reagents and reaction times that are difficult to achieve in vivo (e.g., 1 mM for aminooxy labeling of ketones over 1 hr; Yarema et al., 1998). Efforts to increase conjugation rates, for example, by labeling MGE-installed azido groups using the copper-catalyzed azide-alkyne [3 + 2] cycloaddition reaction, resulted in unacceptable toxicity when used in living systems. A major advance was made with the development of copper-free cyclooctyne strain-promoted labeling agents for azidoglycans (Baskin et al., 2007; Codelli et al., 2008; Ning et al., 2008). In the past decade, additional options for improving conjugation rates have been reported using many variants of ring-strain-promoted reactions for azide-alkyne cycloaddition as well as inverse-electron-demand Diels-Alder reactions (Agatemor et al., 2020).
Another approach, beyond efforts to develop labeling reagents with fast reaction rates for bioorthogonal conjugation to glycan-presented cell-surface azido groups, has been to develop a new generation of monosaccharide analogs with chemical functional groups capable of faster reaction rates themselves (Patterson et al., 2014). One example is the metabolic incorporation of the cyclooctyne moiety into cell-surface glycans (Fig. 2B(vii); Li et al., 2019) instead of using it as the labeling reagent. In a second strategy, tetrazole-based MGE ligation reactions with fast reactions rates have been enabled through ManNAc and sialic acid analogs with terminal alkenes (Fig. 2B(viii); Schart et al., 2019; Späte et al., 2014), cyclopropenes (Fig. 2B(ix); Cole et al., 2013; Hassenrück & Wittmann, 2019; Patterson et al., 2012; Xiong et al., 2015), and isonitriles (Fig. 2B(x); Wainman et al., 2013).
In many cases, monosaccharide analogs used in MGE experiments are intended to modify the cell surface without directly or explicitly altering the biology of the host cell. Instead, responses are intended to be elicited indirectly, e.g., by changing viral binding (Keppler et al., 2001; Wratil et al., 2016) or by directing drugs to the modified glycans (Nauman & Bertozzi, 2001). Over the past two decades, these efforts have largely focused on developing two-step diagnostic, therapeutic, and theragnostic strategies for cancer (Agatemor et al., 2019; Badr et al., 2017; Wang & Mooney, 2020). As it has become evident that non-natural monosaccharides can modulate biological responses directly in a single step (i.e., administration of an MGE analog alone without follow-up administration of a probe; Büttner et al., 2002; Horstkorte et al., 2004; Mahal et al., 2001; Schmidt et al., 1998), efforts aimed at using MGE technology to control cell behavior have intensified. For example, the interrelated abilities of MGE to control cell-substrate binding (Villavicencio-Lorini et al., 2002) and alter gene expression (Elmouelhi et al., 2009; Kontou et al., 2008) opened new horizons for MGE, including the use of ManNAc analogs ranging from promoting sialyl Lewis X expression (Natunen et al., 2013) to enhancing neuronal stem cell differentiation (Du et al., 2021, 2022).
In addition to manipulation of living cells to study and modulate their biology, the use of MGE to glycoengineer therapeutic proteins is gaining momentum. Early efforts included creating protein-drug linkages using the non-natural chemical handles to create antibody-drug conjugates in which the drug is selectively linked to an antibody's Fc glycans (Li et al., 2014; Okeley et al., 2013). MGE can also be taken in a complementary direction by employing it for therapeutic protein production by improving the sialylation of erythropoietin (Wang et al., 2019), monoclonal antibodies (Ludwig et al., 2022; Yin et al., 2017), and the therapeutic enzyme ENPP-1 (Stabach et al., 2021). With the MGE strategies now available, many cellular functions can be studied and manipulated using this versatile technology. Considering the broad scope of this field, the protocols described here can be easily adapted to include the analysis of multiple non-natural glycan-installed chemical moieties (thiol, ketone, azide, and alkyne) across different protein and cellular formulations. Henceforth, this set of protocols is intended to serve as a foundation to broadly adapt MGE for any biological application, which theoretically includes any of the vast array of biological processes modulated by cell-surface carbohydrates.
STRATEGIC PLANNING
This article begins by describing the planning process and general procedures required for characterization of cellular metabolism and measurement of biosynthetic incorporation of novel sugar analogs used in MGE experiments. Because of the many analogs now available (Agatemor et al., 2020; Aich & Yarema, 2008; Almaraz, Mathew et al., 2012; Wratil et al., 2016), numerous options exist for MGE experiments, making it necessary to tailor published protocols for previously untested analogs or cell lines. Accordingly, a perspective of various steps involved in setting up an MGE experiment is provided with a brief discussion of alternative approaches and common pitfalls to avoid. Overall, as outlined in Figure 3, the process begins with the strategic planning required for cell line and analog selection. Experimental work begins with the incubation of cells with non-natural analogs (see Basic Protocol 1), followed by monitoring of cell viability, growth inhibition, and cytotoxicity (see Basic Protocol 2). Next, the periodate-resorcinol assay (see Basic Protocol 3) provides a readout of successful incorporation of analog into intracellular sialic acid metabolism. This is an optional, but in some cases important, part of MGE workflows (see discussion below). Finally, flow cytometry–based methods are described to monitor a critical endpoint in most MGE experiments: cell surface display of non-natural glycans (see Basic Protocol 4). Importantly, instead of labeling cells with fluorophores for quantification as described in this protocol, labeling protocols can be readily adapted to investigate numerous other biological and biomedical endpoints; several of these are indicated in Figure 3 (where they are marked with stars) and throughout this report with relevant literature references.
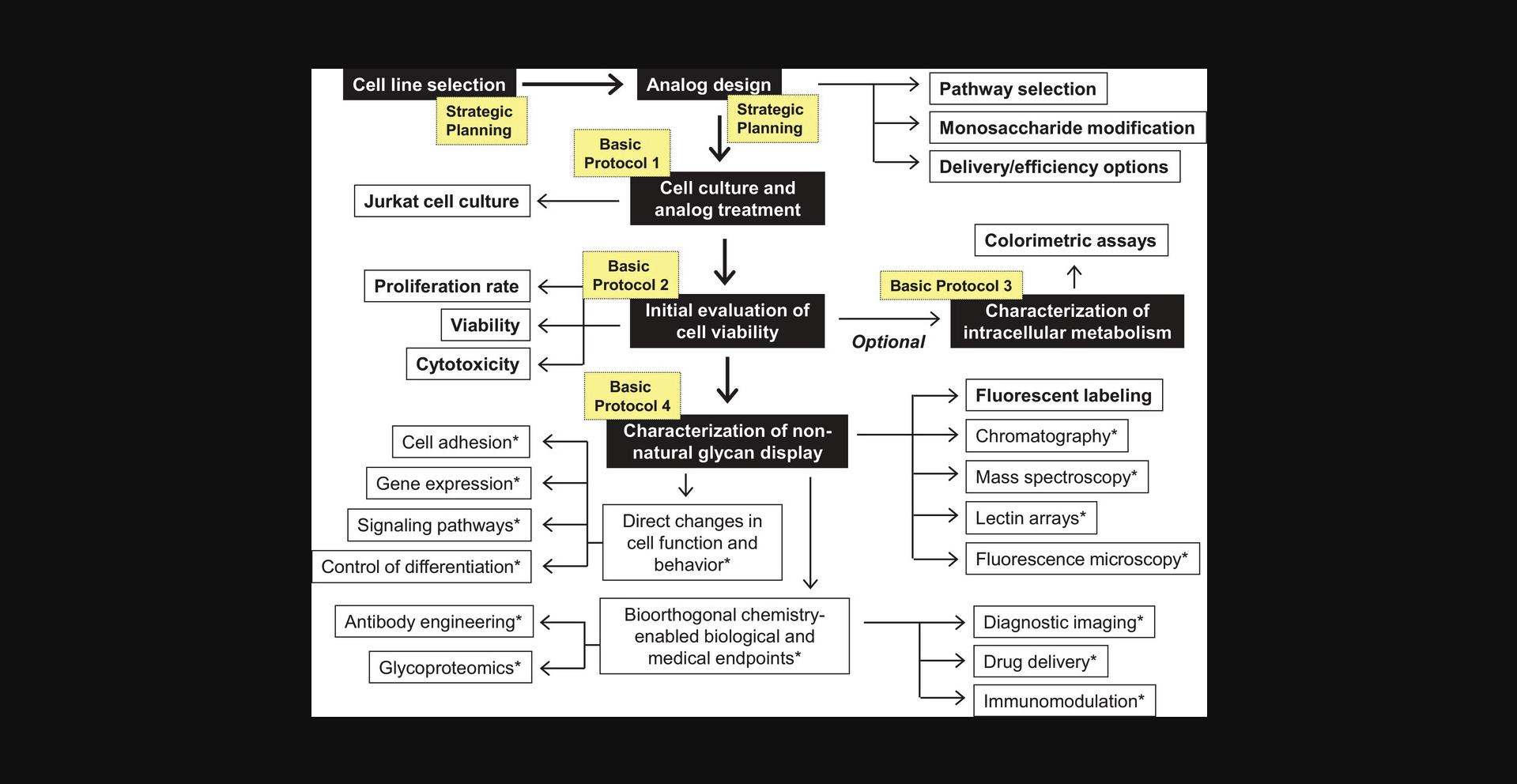
Cell Line Selection
In the early years of MGE, most experiments were performed with human cancer or rodent cell lines, which readily accommodate sugar analog incorporation (Du et al., 2009; Keppler et al., 2001). Although a simplistic explanation, we observed that facile MGE labeling of cells was correlated with rapid cell proliferation (Jones et al., 2004; Yarema, 2002), which requires constant production of membrane components that host metabolically labeled glycans in both glycoproteins and glycolipids (Kayser, Geilen et al., 1992; Kranaster et al., 2022). Over time, MGE has expanded on many fronts to augment cell behavior, target intracellular and even extracellular components such as extracellular vesicles, serve as imaging agents, and improve the production of therapeutic proteins (Agatemor et al., 2019; Buettner et al., 2018; Dammen-Brower et al., 2022). This growing slate of applications includes slowly growing cells, where MGE labeling is less efficient than in rapidly dividing cancer or rodent cells. In one example, the study of MGE in cancer lines benefits from the inclusion of “near-normal” control lines in the experimental workflow (Saeui et al., 2018); the control cells often experience lower analog incorporation. In a second example, the production of therapeutic proteins in the modern biomanufacturing workhorse Chinese hamster ovary (CHO) cell line benefits from slowly growing cells that devote their metabolic resources to recombinant protein production rather than proliferation (Dammen-Brower et al., 2022; Donini et al., 2021).
These examples illustrate the need for a positive control cell line for troubleshooting purposes if lower-than-expected labeling is observed in test cells. The Jurkat cell line, used in seminal MGE experiments from the Bertozzi group (Mahal et al., 1997; Yarema et al., 2001), remains an excellent control to run in parallel with cell lines of unknown MGE capabilities to ensure the fidelity of reagents and experimental procedures. Jurkat cells are attractive positive controls for several reasons. First, they have a modestly sized glycocalyx (∼8 nm thick; Freitas, 1999), which ensures that analog uptake will not be inhibited by the thick glycocalyx found in some cell types (up to microns; Cohen et al., 2004). Instead, the installed glycans are easy to conjugate to labeling agents (Fig. 1B) because they are not buried under a thick layer of hyaluronic acid or other polysaccharides that comprise a steric barrier (Weinbaum et al., 2007) to bioorthogonal probes in some cell types. Second, Jurkat cells grow in suspension under normal culture conditions, making them trivial to harvest and amenable to time-course evaluations. Suspension culture also avoids potential problems with damage to surface glycoconjugates during the detachment steps required for adherent cell lines. Finally, Jurkat cells maintain N-glycosylation profiles common in human cell lines that often are not faithfully reproduced in other species. All in all, when experimenting with metabolic incorporation on a cellular level, the Jurkat cell line is an attractive candidate for use as a positive control. An important caveat for Jurkat cells is that they express truncated O -glycans (Piller et al., 1990), making this line best suited for analyzing analog incorporation into N -glycans. To study mucin type O-glycosylation, one should consider HEK293 cells, which have been used successfully in O-glycosylation modification studies (Sun et al., 2022) and are available in both O -glycan-competent and -deficient varieties (Termini et al., 2017).
Analog Selection
Analog selection requires several decisions. The first is which glycan biosynthetic pathway to target. There are two generic glycosylation pathways: extracellular, which includes N- and O-glycosylation, and intracellular, which is comprised of O-GlcNAcylation. Extracellular N-glycosylation is primarily targeted using two main monosaccharide targets, sialic acid and fucose. O-Glycosylation can be studied using galactosamine analogs, which are incorporated into mucin-type O -glycoproteins (Choi et al., 2010; Du et al., 2009). Interestingly, glycosyltransferases such as GALE can epimerize and interconvert GalNAc and GlcNAc analogs, allowing both sugars to metabolically replace the intracellular O -GlcNAc protein modification (Boyce et al., 2011; Li et al., 2016; Liu et al., 2019). Hence, multiple options are available to experimentally use core ManNAc, GalNAc, fucose, and GlcNAc structures (Fig. 1A) to install non-natural monosaccharides into cell-surface glycans. Sialic acid has been the preferred pathway for cell-surface MGE experiments because there are multiple points to intercept the pathway (e.g., by using ManNAc, Neu5Ac, and CMP-Neu5Ac analogs; Fig. 1A) and because the location of this sugar at the outer termini of mammalian glycoconjugates makes it readily accessible for interactions with the microenvironment or exogenously delivered labeling reagents. In practice, ManNAc analogs are preferred over Neu5Ac or CMP-Neu5Ac analogs for MGE experiments because of their ease of synthesis and the poor cellular uptake properties of CMP-Neu5Ac. A detailed discussion of the relative benefits of ManNAc versus Neu5Ac analogs is provided in our previous publications (Aich & Yarema, 2008; Du et al., 2009).
The next design decision in an MGE experiment involves selecting a chemical modification to install into cell-surface glycans. The options fall into three main categories: chemically inert chemical structures (Fig. 2A), bioorthogonal chemical functional groups (Fig. 2B), and directly installed fluorescent tags (Fig. 2C). The first category is exemplified by the initial analogs bearing elongated alkane chains (Fig. 2A(i-iv) utilized in the Reutter group's landmark MGE experiments; Kayser, Zeitler et al., 1992). This group of compounds now includes branched and ring structures (Fig. 2A(v-vii); Almaraz, Mathew et al., 2012) and even chemical structures ideal for high-flux applications (Kim et al., 2004). However, the difficulty in quantifying or directly modifying these chemically inert sugars after installation into glycans has reduced their popularity for MGE experiments in favor of an increasingly large number of analogs with bioorthogonal chemical functional groups. This second category of analogs (Fig. 2B), most with chemical functional groups not found naturally in sugars, opened the door to a highly versatile and ever-expanding set of chemoselective ligation options for glycan labeling (Agatemor et al., 2020; Lemieux & Bertozzi, 1998; Sletten & Bertozzi, 2009). Finally, the third group of fluorophore-bearing analogs (Fig. 2C) is ideal for easy, one-step labeling of surface glycans; however, because fluorophores tend to be unwieldy and bulky, they are only incorporated into glycans when introduced as CMP-Neu5Ac analogs (Brossmer & Gross, 1994; Gross & Brossmer, 1988). That said, this class of analogs remains in use and can effectively demonstrate sugar turnover when installed onto cell-surface glycans with recombinant glycosyltransferases (Hong et al., 2019; Kondor et al., 2022).
A final design consideration involves the use of short-chain fatty acid (SCFA) protecting groups that increase the lipophilicity and cellular uptake of sugar analogs used in MGE (Fig. 1C). Upon cellular uptake, nonspecific esterases remove the SCFA moieties, regenerating the core sugar (Mathew et al., 2012, 2017; Wang et al., 2009). For context, the original hexosamine analogs used by the Reutter and Bertozzi groups had unmodified hydroxyl groups that rendered these compounds largely membrane impermeable, requiring analog concentrations that were impractically high (e.g., 75 mM for ManNLev; Yarema et al., 1998). A benefit of appending ester-linked SCFAs to mask the hydrophilic hydroxyl groups of a monosaccharide is that the concentration of the resulting analog required to evoke a biological response is dramatically reduced, ranging from an ∼600-fold reduction for acetyl groups (Jones et al., 2004) to more than 2000-fold reduction for n -butyrate (Aich et al., 2008; Kim et al., 2004). In general, the MGE field uses peracetylated analogs, exemplified by Ac4ManNAz, which is widely used to install azido-modified sialic acids into glycans.
As a caveat, SCFA-derivatized analogs have several potential pitfalls. The first is that they can have dose-limiting cytotoxicity, which depends on the type of SCFA used and their position on the core sugar (Aich et al., 2008; Elmouelhi et al., 2009). Notably, SCFA-mediated cytotoxicity is also influenced by N -acyl modification of the analog (Kim et al., 2004).
A second potential pitfall, which can conversely be beneficial, is that the inclusion of SCFA protecting groups in analog design can substantially alter biological activity. For example, both dietary and endogenously produced acetate have metabolic, epigenetic, and oncogenic effects (Moffett et al., 2020); consequently, it is reasonable that acetate liberated intracellularly from MGE analogs such as Ac4ManNAz also affects cellular physiology. Similarly, n -butyrate released from butanoylated MGE analogs (Fig. 1C) can elicit epigenetic effects through HDAC inhibition (Elmouelhi et al., 2009; Sampathkumar, Jones, Meledeo et al., 2006). Our research team has shown that these SCFA-mediated biological responses are not always harmful; indeed, they can be exploited to elicit beneficial responses. For example 1,3,4-tributanoylated ManNAc analogs with the three SCFAs on the C1, C3, and C4 OH groups of the core sugar (Fig. 1C) are minimally toxic and promote the production of recombinant proteins in CHO cells (Aich et al., 2008; Wang et al., 2019). By comparison, if the three SCFA groups are arranged on the C3, C4, and C6 OH groups of a hexosamine analog, the compound has anti-inflammatory properties that can be exploited to attenuate physiological endpoints associated with osteoarthritis (Kim et al., 2016). In our experience, off-target biological activities of SCFA-modified MGE analogs are usually relatively mild and manageable; nonetheless, it is worthwhile for investigators to be aware of their non-glycan-related activities in the event that unexpected cellular responses are observed.
A third potential pitfall of SCFA-modified MGE analogs arises from a phenomenon known as artificial S-glycosylation, which occurs between protein cysteines and per-O -acetylated monosaccharides (Qin et al., 2018, 2020). This non-enzymatic, non-glycan labeling of proteins can confound certain MGE experiments, such as proteomics analyses of azido-modified sialosides when using Ac4ManNAz. This problem can be mitigated using appropriate controls, for example, by performing glycosite analysis (Zhang et al., 2003) to verify that the peptides identified had legitimate N -glycan sites (Tian et al., 2015). In other cases, for example, MGE labeling of cancer cells as a first step in delivery of a diagnostic or therapeutic agent, artificial S-glycosylation is of less concern as long as the cancer cells and tumors are preferentially targeted compared to healthy cells, tissues, or organs. In general, as discussed in a recent article by Darabedian and colleagues, MGE labeling remains a “generally reliable tool” despite artificial S-glycosylation because it can be mitigated with the use of appropriate control experiments (Darabedian et al., 2020). In situations where artificial S-glycosylation must be completely avoided, one suggestion is to forgo the use of peracetylated analogs and instead use the original perhydroxyl sugars (Fig. 1A; Qin et al., 2018) despite their low efficiency. A second suggestion is to use MGE analogs modified with ester-linked SCFAs such as acetate or propionate groups at only the C1 and C3 OH positions; the presence of the SCFAs at these positions increases analog efficiency while their absence from the anomeric position avoids artificial S-glycosylation (Hao et al., 2019).
Analog Sources and Preparation
The number of commercially available, bioorthogonal MGE analogs has dramatically increased within the past decade. Many suppliers (New Zealand Pharmaceuticals, Click Chemistry Tools, Invitrogen, Biosynth, Jena Bioscience, etc.) provide ManNAc core oligosaccharide formulations including Ac4ManNAc, Ac4ManNLev, Ac4ManNAl, and Ac4ManNAz. Additionally, glucosamine and galactosamine analogs such as Ac4GalNAc, Ac4GalNAz, Ac4GlcNAc, and Ac4GlcNAz are available from various vendors including Invitrogen and Sigma-Aldrich. Other analogs such as alkynyl- and azido-modified fucose can often be found through vendors on the internet, although availability can be sporadic. In some cases, analogs must be custom synthesized; protocols for such syntheses are generally available and are not repeated here.
Monosaccharide analogs stored in dried powder form at –20°C are stable for extended time periods (at least several months up to a few years). Stock solutions are commonly prepared by dissolving the SCFA-derivatized analogs (e.g., 1-4 ; Fig. 4) in ethanol or dimethyl sulfoxide at 10 mM and storing at 4°C. Although sugar analogs are stable in this form for several months, it is recommended to prepare modest-sized stock solutions that can be used within 6-8 weeks. Analogs are typically stored in ethanol for two reasons: (1) SCFA-derivatized monosaccharides have limited solubility in aqueous media (often 1 mM or less, which does not pose a problem for biological assays usually performed at <500 µM) and (2) the use of ethanol helps maintain sterility over extended storage periods. DMSO is an alternative solvent vehicle that is used more often for in vivo studies than cell culture, but can be used the ethanol is cytotoxic to the cells being studied. Typically, when the solvent level is kept below 1% (v/v), neither ethanol nor DMSO has measurable effects on endpoints commonly probed in MGE experiments. Nevertheless, the preferred protocol is to avoid any solvent exposure by allowing evaporation before cells are added. When this is not possible, it is important to ensure that the same volume of solvent is added to each sample. If a free hydroxyl monosaccharide (e.g., ManNAc, 5) is used in any experiment, a 500 mM stock solution should be prepared in PBS or serum-free medium and filter sterilized. Stock solutions prepared in this manner should be stored at 4°C. Due to the lack of long-term sterility in PBS or medium (particularly with potential addition of nutrients), these solutions would be used within 6-8 weeks of filter-sterilization.
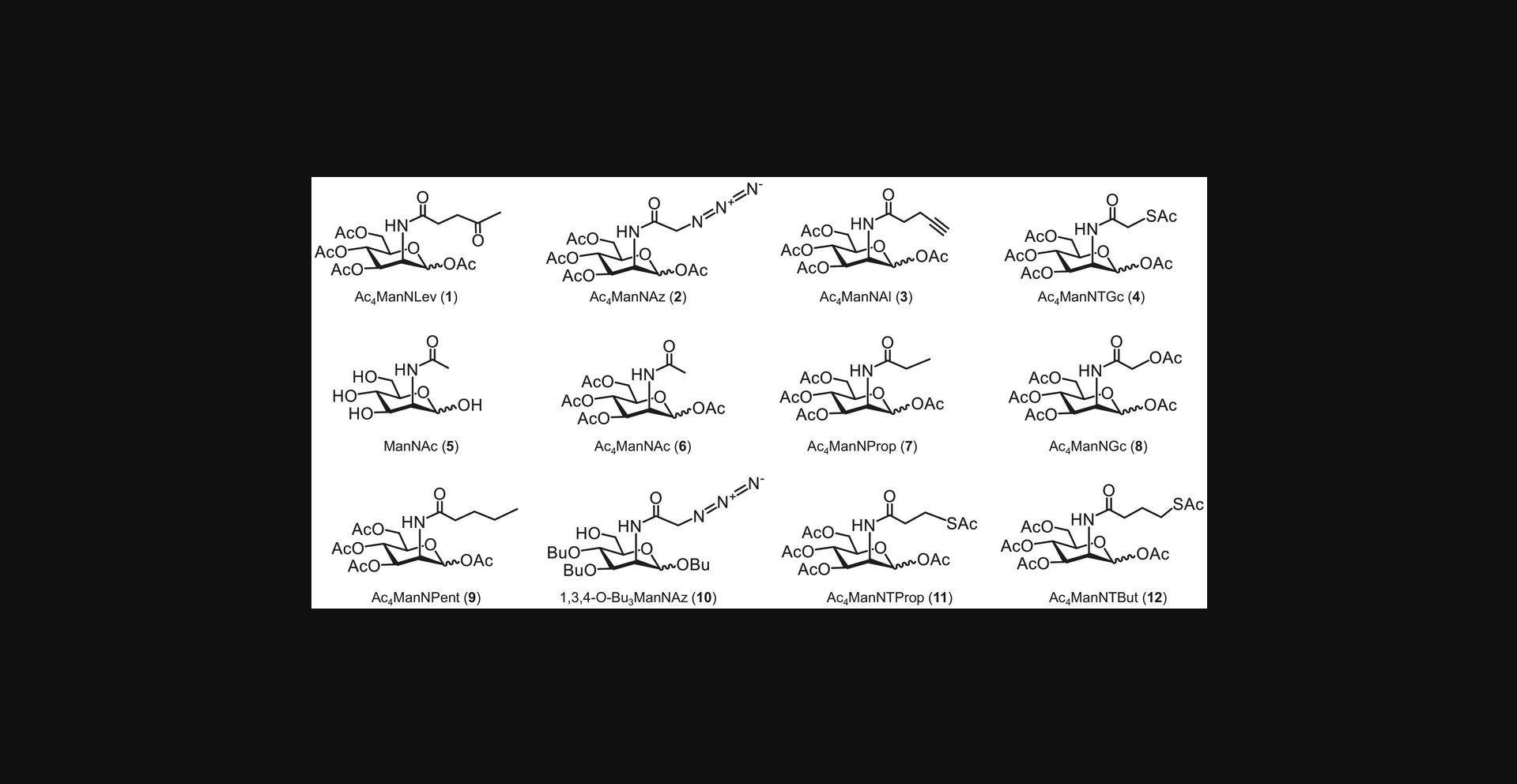
Initial Evaluation of Analog Effects on Cell Viability
Depending on the cell line and the analog under evaluation, biological responses can be realized at concentrations as low as 5 µM for SCFA-derivatized MGE analogs (Elmouelhi et al., 2009; Wen et al., 2019; Zhang et al., 2016), with the dynamic range reaching 75 mM or higher for free-hydroxyl compounds (Yarema et al., 1998). The upper limit for SCFA-derivatized analogs is usually determined by the dose-limiting toxicity (DLT) and can vary significantly (from 25 to >700 µM) depending on cell line (Yarema et al., 1998), cell density (Jones et al., 2004), rate of delivery, and N -acyl substituent (Hao et al., 2019; Kim et al., 2004). For more information on analog cytotoxicity, we recommend a review Takayama et al. (2019). In general, the effects of novel analogs on cell viability should be evaluated over a range of concentrations (typically 0 to 250 or 500 µM for SCFA-derivatized analogs) as a first step in any MGE experiment before undertaking more highly complex procedures, such as the flow cytometry–based quantification of surface glycan display outlined in Basic Protocol 4 (Fig. 3). As described below, two rapid screening assays are recommended to measure cell viability (Basic Protocol 2) and/or metabolic flux (Basic Protocol 3).
Detailed Characterization of Analog Metabolism and Glycan Modification
Confirmation of metabolic flux by colorimetric assays
Analogs sometimes have no deleterious effects during pilot in vitro testing and do not noticeably affect cell viability as tested in Basic Protocol 2. This may mean that the analog legitimately lacks cytotoxicity, or may mean that the analog was not successfully taken up by the test cells. Basic Protocol 3 is designed to discern between these two possibilities and is dispensable for some types of MGE experiments. For example, if the primary endpoint of an experiment is cell-surface display of a non-natural glycans, intracellular flux as measured in Basic Protocol 3 may be a secondary concern and an investigator may wish to bypass these experiments and go directly to Basic Protocol 4. If poor analog incorporation into cell-surface glycans is observed in Basic Protocol 4, we recommend conducting Basic Protocol 3 as a troubleshooting exercise to ensure intracellular metabolism. In other cases, the investigator may want to monitor intracellular metabolism as part of a thorough characterization of the impact of an analog on cellular physiology; we particularly recommend doing so for MGE experiments using previously unreported cell lines or monosaccharide analogs.
One way to monitor monosaccharide analog metabolism by cells is to monitor its depletion from the culture medium. However, because these compounds have chemical properties similar to many components of sugar-rich culture media used for mammalian cells, efforts to probe cellular uptake by measuring residual analog levels in the medium often are cumbersome (Jones et al., 2004). Therefore, in the absence of radiolabeled metabolic precursors (which are useful but prohibitively expensive; Jacobs et al., 2001), colorimetric techniques can provide a ready means for quantitative estimation of analog flux into intracellular glycosylation pathways. Colorimetric methods exist for quantifying flux into several glycosylation pathways, e.g., specifically for glucose (King & Garner, 1947) or for various monosaccharides (Walborg & Christensson, 1965). In our view, the periodate-resorcinol assay is particularly valuable because it selectively detects sialic acids, which are the predominant monosaccharide targeted by MGE, while avoiding competing signal from other monosaccharides (Jourdian et al., 1971). Our laboratory typically uses a modified version of the periodate-resorcinol method (described in Basic Protocol 3) that involves scaling the reaction from the initial 4-ml sample volumes to 100-µl volumes amenable to quantification in 96-well plates (Yarema et al., 2001). Although we continue to employ the periodate-resorcinol assay, we note that alternative sialic acid detection assays have been reported. For example, Matsuno and Suzuki describe a modified version in which sialoglycoproteins are oxidized with periodate and formaldehyde generated from the carbon 9 position of sialic acid is converted to easily detected fluorophores (Matsuno & Suzuki, 2008). In a divergent strategy, Sankoh and co-authors employed a colorimetric sensor for sialic acid detection based on the aggregation of mercaptophenylboronic acid–functionalized gold nanoparticles (Sankoh et al., 2016).
The periodate-resorcinol assay involves two major steps: (1) periodate oxidation, in which a periodate ion reacts with two hydroxyl groups of vicinal diols, breaking the C-C bond to form two aldehyde groups (Tiziani & Cesàro 2003), and (2) heating the sample with resorcinol to improve sensitivity, allowing the detection of a few micrograms of sialic acid (Sussich & Cesàro 2000). This assay is particularly valuable to gauge flux of analogs that do not have bioorthogonal functional groups that can be exploited to readily label the surface display of non-natural glycans as described for ketones, thiols, azide, or alkynes in Basic Protocol 4. Certain analogs (e.g., Ac4ManNHex or Ac4ManNLev; Kim et al., 2004) do not measurably increase intracellular metabolite levels, although they do successfully transit the sialic acid biosynthetic pathway and modify surface glycans (Yarema et al., 1998). Colorimetric assays are not informative for these “low-flux” analogs. Despite these limitations, colorimetric flux assays combined with cell viability assays reliably facilitate optimization of the analog concentration range (e.g., 75-150 µM instead of 5-1500 µM) to use in subsequent MGE experiments.
Quantitative estimation of non-natural analog incorporation into cell-surface glycans
The primary objective of most MGE experiments is to install non-natural monosaccharides into cell surface–displayed glycans. In many cases, these experiments utilize analogs that install unique chemical functional groups (Fig. 2B) into glycans (Kufleitner, Halbert & Wittmann, 2023). In Basic Protocol 4, we present a flow cytometry–based quantitation technique that uses chemoselective ligation reactions to fluorescently label ketone- (Yarema et al., 1998), azido- (Saxon & Bertozzi, 2001), alkynyl- (Chang et al., 2009; Sawa et al., 2006), and thiol-bearing (Du et al., 2022; Sampathkumar, Jones, & Yarema, 2006) metabolically glycoengineered cells. We emphasize that the general methodology described for these four functional groups can be readily adapted to additional functional groups, including photoactivatable groups such as diazirines (Fig. 2B(xi); Bond et al., 2009; Tanaka & Kohler, 2008) and aryl azides (Fig. 2B(xii); Han et al., 2005) in addition to those discussed above.
The Ac4ManNLev (ketone groups), Ac4ManNAz (azido groups), Ac4ManNAl (alkyne groups), and Ac5ManNTGc (thiol groups) analogs were selected for emphasis in this article for several reasons. First, although azido analogs (e.g., Ac4ManNAz) are the workhorse reagents used in MGE, our group and others have noted that analog- and cell line–specific differences exist (Saeui et al., 2018; Scache et al., 2022); consequently, ManNAz analogs are not ideal for all cell lines and alternatives should be considered. Second, these analogs all transit the sialic acid biosynthetic pathway with sufficient flux to install easily detected levels of modified glycans on the cell surface; we note that GlcNAc (Pan et al., 2019), GalNAc (Laughlin et al., 2008), and fucose (Okeley et al., 2013; Soares da Costa et al., 2020) analogs that target monosaccharides other than sialic acids (Fig. 1A) can be easily substituted in Basic Protocol 4. Also, the selected functional groups, when displayed as part of a modified glycan, can all be targeted using chemoselective ligands conjugated to biotin, which in turn can be conjugated to fluorescently conjugated avidin or streptavidin for quantification using flow cytometry or other methods. Specifically, cells displaying ketone groups can be chemoselectively ligated to hydrazides to form acyl hydrazine conjugates (Yarema et al., 1998); cells bearing azido groups can be labeled using strain-promoted azide-alkyne cycloaddition (SPAAC) reactions (Agard et al., 2004; Ning et al., 2008) employing, among several options, dibenzocyclooctyne (DBCO) probes (Agard et al., 2006; Ning et al., 2010); alkynes can be conjugated to azido-probes (Sawa et al., 2006; Vanbeselaere et al., 2013); and cell surface thiols can be ligated to maleimide probes (Sampathkumar, Jones, & Yarema, 2006).
Additional options for characterization of surface glycans in MGE cells
Lectin and antibody staining provides an alternative strategy for characterizing non-natural glycan display on the cell surface. Such an approach is necessary in cases where analog incorporation does not result in cell-surface display of novel bioorthogonal chemical functional groups that can be labeled and quantified as described in Basic Protocol 4 (e.g., when using chemical structures shown in Fig. 2A instead of Fig. 2B). In theory, an attractive option for direct detection of non-natural glycan display is the use of complementary antibodies or lectins (Chefalo et al., 2004; Lemieux & Bertozzi, 2001). In practice, this approach is hindered by a general lack of antibodies and lectins with specificity for non-natural glycans. Accordingly, in cases where analog incorporation does not result in display of a bioorthogonal functional group on the cell surface and there is no appropriate antibody or lectin for detection of the non-natural glycan, high-performance liquid chromatography (HPLC) can be used to estimate metabolic incorporation into glycans (Kamerling & Gerwig, 2006; Stanton et al., 1995); one example is quantification of Sia5Prop after ManNProp treatment (Gagiannis et al., 2007). We note that HPLC-based analysis can also be performed for glycans with bioorthogonal chemical functional groups such as alkynes (Vanbeselaere et al., 2013). For more highly rigorous and definitive characterization, mass spectrometry can be used to identify metabolically glycoengineered glycans (Kamerling & Gerwig, 2006; Schilling et al., 2001; Shajahan et al., 2020). Finally, MGE has the potential to change cell surface glycans in unanticipated ways, which can be probed using lectin array technologies (Gupta et al., 2010; Pilobello et al., 2005; Xia & Gildersleeve, 2015).
Basic Protocol 1: INCUBATION OF CELLS WITH SUGAR ANALOGS
The first step in MGE is the incubation of cells with sugar analogs Ac4ManNAz, Ac4ManNAl, Ac4ManLev, and Ac5ManNTGc (1-4 in Fig. 4). Although an exhaustive bevy of analog controls is not always required, it is recommended to compare a test analog with appropriate control analogs when performing initial experiments with a previously untested cell type. To illustrate this process, we briefly describe appropriate controls for the thiol-modified analog Ac5ManNTGc (4). First, ManNAc (5) serves as a control for increased flux through the sialic acid pathway and Ac4ManNAc (6) controls for increased flux plus any off-target responses engendered by the SCFA protecting groups, either from their scaffold-dependent effects (Elmouelhi et al., 2009) or from SCFA-specific responses (e.g., multifaceted responses from acetate [Moffett et al., 2020] or HDACi activity from butyrate [Sampathkumar, Jones, Meledeo et al., 2006]) after removal by intracellular esterases (Fig. 1C). Taking a step towards increased complexity, Ac4ManNProp (7) and/or Ac5ManNGc (8) are appropriate size-matched controls for an elongated N -acyl side chain reported to enhance signaling propensities (Kontou et al., 2008) or receptor-binding properties (Collins et al., 2000) of these molecules, but without the unique chemical properties of the thiol installed by Ac5ManNTGc at this position (the NProp analog has a methyl and the NGc analog has a hydroxyl instead of a thiol group). Comparably, Ac4ManNAz, Ac4ManNAl, and Ac4ManLev all have an N -acyl group five atoms long, making Ac4ManNPent (9 ; Kim et al., 2004) an appropriate, sized-matched, chemically inert control analog. Note that, although this report provides suggested analogs to use in basic MGE experiments, we acknowledge that analog options continue to expand, providing new MGE opportunities. For example, 1,3,4-tributanoylated analogs (Fig. 1C) such as 1,3,4-O -Bu3ManNAz (10) are used more efficiently by cells than Ac4ManNAz (Almaraz, Aich et al., 2012), and two recently reported thiol analogs (Ac5ManNTProp, 11 , and Ac5ManNTBut, 12) have improved neuromodulatory activity compared to Ac5ManNTGc (Du et al., 2021, 2022).
Materials
- Analog stock solutions (for additional information, see Strategic Planning):
- 10 mM peracetylated analogs (e.g., 1-4 and any control other than 5 ; Fig. 4) in ethanol or DMSO
- 500 mM free hydroxyl monosaccharides (e.g., ManNAc, 5) in PBS or serum-free culture medium, filter sterilized
- Jurkat cells (ATCC, cat. no. TIB-152; see Support Protocol )
- Complete RPMI-1640 (see recipe) or appropriate medium for cells of interest
- Ethanol (200 proof; Pharmco)
- 24-well tissue culture plates
- Cell counter (e.g., Z2 Coulter Particle Counter and Size Analyzer, Beckman Coulter) or hemacytometer
1.Label sterile 24-well tissue culture plates, e.g., with the desired concentrations of SCFA-derivatized analogs (see Table 1) or higher millimolar concentrations of free hydroxyl analogs (e.g., up to 75 mM; Yarema et al., 1998). Set up triplicate wells for each concentration.
Desired concentration of analog (1-4; µM) | 0 | 5 | 10 | 20 | 40 | 80 | 160 | 320 |
Volume of 10 mM analog (1-4; µl) | 0 | 0.25 | 0.5 | 1 | 2 | 4 | 8 | 16 |
Volume of ethanol (µl) (optional, see text) | 16 | 15.75 | 15.5 | 15 | 14 | 12 | 8 | 0 |
2.Add the appropriate volume of 10 mM analog stock solution to give the desired final concentration after 0.5 ml cells are added below.
3.Leave plates in a sterile tissue culture hood with the lids open for ∼15 min to allow the ethanol to evaporate.
4.While ethanol is evaporating, harvest Jurkat cells as described (see Support Protocol) or alternate cell lines by the usual methods.
5.Adjust Jurkat cell density to 5 × 105 cells/ml in complete RPMI-1640 for a 48-hr experiment or 2.5 × 105 cells/ml for a 72-hr experiment. Mix thoroughly to obtain a homogeneous single-cell suspension.
6.Add 0.5 ml cell suspension to each well and incubate cells at 37°C for 48 or 72 hr.
7.After the appropriate time, mix cell culture thoroughly by gently pipetting up and down several times with a 1-ml pipet to obtain a single-cell suspension and then count an aliquot to determine cell density.
8.Study analog incorporation as a function of time.
Support Protocol: ROUTINE GROWTH AND MAINTENANCE OF JURKAT CELLS
This protocol describes routine methods to maintain Jurkat cells, a lymphocyte line derived from human acute T cell leukemia (clone E6-1, often referred to as wildtype Jurkat cells). In general, no special procedures are needed for cell preparation for MGE experiments, so the usual methods used to culture any mammalian cell line of interest can be followed.
Materials
- Jurkat clone E6-1 (ATCC, cat. no. TIB-152)
- Complete RPMI-1640 (see recipe)
- Cell counter (e.g., Z2 Coulter Particle Counter and Size Analyzer, Beckman Coulter) or hemacytometer
- 75-cm2 tissue culture flask (Sarstedt, cat. no. 83.1813)
- 37°C, 5% CO2 humidified incubator
- 15- or 50-ml polypropylene centrifuge tubes
1.Add Jurkat cells to complete RPMI-1640 to attain a cell density of ∼1–2.5 × 105 cells/ml and add to a tissue culture flask or dish (e.g., 1.5 × 106 cells in 15 ml in a 75-cm2 flask).
2.Place in a 37°C, 5% CO2 humidified incubator for up to 4 days to allow cells to reach a density of ∼2 × 106 cells/ml.
3.Transfer cell suspension to a 15- or 50-ml centrifuge tube and centrifuge 3.5 min at 1300 × g , room temperature.
4.Carefully discard supernatant and resuspend cells in 10 ml prewarmed (37°C) complete RPMI-1640.
5.Disperse cell clumps by gently pipetting up and down several times and then count cells using an electronic cell counter or hemacytometer.
6.Replate an aliquot of cells as described (see step 1) and place the stock flask in the incubator. Use the remaining harvested cells (typically 85% to 95% of the total number of cells initially present) in MGE protocols.
Basic Protocol 2: CELL VIABILITY ASSAYS
SCFA-monosaccharide analogs often reduce cell viability, but these deleterious effects vary greatly, ranging from transient growth inhibition to rapid onset of apoptosis at sufficiently high concentrations. Cytotoxicity depends on analog structure. For example, Jurkat cells incubated with peracetylated ManNAc analogs (e.g., 1-4 in Fig. 4) experience cytotoxicity not observed with free hydroxyl monosaccharides (Fig. 1A), and the degree of toxicity is influenced by the N -acyl modification (Kim et al., 2004; Wang et al., 2009), so both hydroxyl and N -acyl modifications affect cell viability. Cell viability can be evaluated with various levels of sophistication and thoroughness. At the simplest, cell counts obtained in Basic Protocol 1 identify analogs that inhibit cell growth, which can be quantified as an IC50 value (the inhibitory concentration that reduces cell counts to 50% of control values). IC50 values are typically a useful endpoint after short-term analog exposure (e.g., 2, 3, or 5 days) because actual cell death from analog exposure is usually slow over concentration ranges used in optimized MGE experiments. Accordingly, additional analyses often are needed to understand the source of reduced viability. To explain briefly, reduced cell numbers could be due to transient growth inhibition from which the cells can recover and resume robust growth; alternatively, the test cells could be fated to die over the next several days (Sampathkumar, Jones, Meledeo et al., 2006; Sampathkumar, Jones, & Yarema, 2006). If this issue needs resolution—in particular in samples that contain fewer cells than the negative controls (i.e., samples without analog), indicating that actual cell death has occurred—trypan blue (or other live/dead assays) can be used to detect if dead cells are already present. In addition, standard apoptosis assays can be run to determine if programmed cell death has been set in motion, or the MTT assay can be used to evaluate the metabolic state of the cells (Kim et al., 2004). A straightforward and reliable way to determine lethality is to continue to monitor cell counts over a 2-week period (Sampathkumar, Jones, Meledeo et al., 2006); in general, IC50 data obtained from 2-, 3-, or 5-day cell counts or LD50 data from 15-day experiments are adequate to determine safe analog levels to use in subsequent metabolic uptake (e.g., Basic Protocol 3) and labeling (Basic Protocol 4) experiments. Below, IC50 and LD50 assays are described.
Additional Materials (also see Basic Protocol 1)
- Phosphate-buffered saline (PBS), pH 7.4 (Invitrogen, cat. no. 10010-049)
- 10 mM analog stock solutions (e.g., Ac4ManNAc (6) plus any additional analogs required for user-specific applications)
1.Incubate Jurkat cells (1 × 105 cells in 0.5 ml) with analog (e.g., 2 at 0-320 µM) in a 24-well plate for 3 days in a 37°C, 5% CO2 incubator (see Basic Protocol 1).
2.On days 3 and 5:
-
Gently pipet Jurkat cells up and down using a 1-ml pipet to dislodge cell clumps.
-
Transfer two 100-µl aliquots to 10 ml PBS in cuvettes.
-
Measure and record the cell density using a cell counter or hemacytometer.
-
Add 1 ml medium to each well and return cells to the incubator.
3.On days 7, 9, 11 and 13:
-
Gently pipet cells to dislodge clumps.
-
Remove 1 ml suspension and add 1 ml fresh medium.
4.On day 15:
-
Gently pipet cells to dislodge clumps.
-
Take a 1-ml aliquot and add it to 10 ml PBS in a cuvette.
-
Measure and record the cell density.
5.Calculate the relative cell number for each analog concentration with respect to the ethanol control for days 3, 5, and 15.Plot relative cell number with respect to analog concentration for days 2 or 3, 5, and 15 to determine the IC50 or LD50 value (concentrations where relative cell numbers are 50% of untreated controls).
Basic Protocol 3: PERIODATE-RESORCINOL ASSAY TO MEASURE ANALOG UPTAKE AND INCORPORATION INTO METABOLIC PATHWAYS
Once the maximum concentration of glycan analog that can be used without deleteriously affecting cell viability has been determined, the next step is to quantify the extent of analog incorporation. The ideal way to measure uptake and metabolism is to quantify non-natural glycan display on the cell surface as described in Basic Protocol 4; this approach is preferred to the periodate-resorcinol assay described here because it measures flux all the way through a metabolic pathway to the cell surface. However, the detection of non-natural glycans on the cell surface is not trivial and requires more highly sophisticated instrumentation than the periodate-resorcinol assay. In brief, the periodate-resorcinol assay is a colorimetric technique that quantifies total and glycoconjugate-bound sialic acids and can provide a rapid quantitative estimation of ManNAc analog incorporation into the sialic acid biosynthetic pathway. It is attractive because it provides a low-cost and technically simple option to monitor cellular uptake and subsequent successful incorporation of high-flux analogs into the sialic acid biosynthetic pathway.
Additional Materials (also see Basic Protocol 1)
-
10 mM Ac4ManNAc (6) stock solution (as positive control) plus additional analogs of choice
-
Phosphate-buffered saline (PBS), pH 7.4 (Invitrogen, cat. no. 10010-049)
-
0.4 M periodic acid (see recipe)
-
6% (w/v) resorcinol (Sigma, cat. no. 108-46-3; store at –20°C)
-
t -Butyl alcohol (2-methyl-propan-2-ol; Sigma, cat. no. 3972-25-6)
-
2.5 mM CuSO4 (see recipe)
-
10 mM sialic acid (see recipe)
-
Concentrated HCl
-
25-cm2 tissue culture flasks
-
15-ml centrifuge tubes
-
1.5-ml microcentrifuge tubes
-
–80° C freezer or pulverized dry ice
-
37°C water bath
-
100°C heating block
-
96-well microtiter plates
-
Microplate reader (µQuant, Bio-Tek Instruments)
1.Incubate Jurkat cells (or other line being tested) with sugar analog(s) as described (see Basic Protocol 1), but use 25-cm2 tissue culture flasks instead of 24-well plates and seed ∼1 × 107 cells in 10 ml.
2.After 2-3 days, harvest Jurkat cells by gently pipetting up and down using a 1-ml pipet to dislodge clumps and transferring to a 15-ml centrifuge tube.
3.Centrifuge cells 5 min at 1000 × g , room temperature. Discard supernatant and resuspend cells in 10 ml PBS by gentle pipetting.
4.Centrifuge 3.5 min at 1300 × g , room temperature. Discard the supernatant and resuspend cells in 5 ml PBS by gentle pipetting. Repeat two more times to wash the cells thoroughly.
5.Remove two 100-µl aliquots and determine density using a cell counter or hemacytometer. Add PBS to each sample to obtain a density of 1 × 106 cells/ml, pipetting gently to obtain a homogenous cell suspension.
6.Aliquot 1 ml suspension to three 1.5-ml microcentrifuge tubes (1 × 106 cells/tube) to yield three replicates per condition.
7.Pellet cells by centrifuging 3.5 min at 1300 × g , room temperature, and resuspend in 300 µl PBS.
8.Freeze tubes in a –80° C freezer for ∼1 hr or on pulverized dry ice for 5-10 min, and then thaw in a 37°C water bath. Repeat for a total of three freeze/thaw cycles.
9.During the freeze-thaw cycles, prepare the assay reagents:
-
Remove 0.4 M periodic acid and 6% (w/v) resorcinol from freezer and thaw at room temperature.
-
Warmt-butyl alcohol to 25°C (just above room temperature).
-
Prewarm heating block to 100°C.
-
Prepare a fresh 1 mM sialic acid solution by diluting the 10 mM stock in PBS. Then, in 1.5-ml microcentrifuge tubes, use the 1 mM solution to prepare 300-µl standards of 0, 10, 20, 30, 40, and 50 μM as follows:
- 300 μl PBS
- 3 μl sialic acid in 297 μl PBS
- 6 μl sialic acid in 294 μl PBS
- 9 μl sialic acid in 291 μl PBS
- 12 μl sialic acid in 288 μl PBS
- 15 μl sialic acid in 285 μl PBS
10.Add 5 µl of 0.4 M periodic acid to each sample and standard. Vortex and place on ice for 15 min.
11.To measure glycoconjugate-bound measurement sialic acids, incubate samples 80 min at 37°C.
12.Prepare resorcinol working reagent:
- 1.5 ml 6% resorcinol
- 1.5 ml 2.5 mM CuSO4
- 6.6 ml deionized H2O
- 5.4 ml conc. HCl
13.Add 500 µl working reagent into each tube, vortex, and incubate 10 min in the 100°C heating block.
14.Remove tubes cool rapidly in room temperature water.
15.Add 500 µl t -butyl alcohol to each tube and mix by vortexing.
16.Centrifuge 2 min at maximum speed in a benchtop microcentrifuge.
17.Aliquot samples into 96-well plates (two wells per sample) and measure absorbance at 630 nm.
Basic Protocol 4: QUANTITATION OF CELL-SURFACE GLYCOCONJUGATES
Cell surface display of non-natural glycans is an inherently inefficient process including entry into a cell, which necessitates the use of SCFA-derivatized analogs (Wang et al., 2009), as well as bottlenecks within intracellular glycosylation pathways (Viswanathan et al., 2003). As a result, analog incorporation into glycans is not a linear function of medium concentration and does not necessarily reflect intracellular metabolite levels as determined by the periodate-resorcinol assay. Consequently, surface display needs to be determined empirically. Non-natural analogs with chemical functional groups such as ketones, azides, alkynes, and thiols that do not occur naturally in the glycocalyx allow quantitative detection and quantification of surface-displayed analogs after conjugation with fluorophores using chemoselective ligation reactions. Commonly, the cell-surface functional groups are reacted with biotin-conjugated chemoselective ligands, then stained using fluorescein-conjugated avidin or streptavidin, and finally quantitated using flow cytometry (Mahal et al., 1997). A brief history of this method is described by Kufleitner, Haiber & Wittmann (2023). Alternatively, protein-specific aptamers can be designed for subcellular measurements of non-natural glycans into specific proteins such as PD-L1 (Zhu et al., 2021).
There are two variations on the basic workflow outlined above and described in detail below that are worth noting. One variation is direct versus indirect labeling. Below, we describe and recommend a two-step labeling process where cell-surface functional groups are first conjugated to biotin linked to an appropriate chemical probe and then the appended biotin is stained with fluorescently tagged streptavidin. As an alternative, glycan-displayed chemical functional groups (fluorophores capable of direct conjugation to the cell surface) are now widely available, enabling direct one-step labeling of the cell surface with fluorescent probes (Liao et al., 2021; Soares da Costa et al., 2020; Vanbeselaere et al., 2013). In our experience, these fluorescent probes have a propensity for nonspecific staining. The two-step approach avoids this pitfall, most likely because fluorescently labeled streptavidin, unlike small-molecule fluorophores, is membrane impermeable, thereby reducing nonspecific background signal.
The second variation is in the quantification approach. Below, we describe quantification via flow cytometry. Alternative approaches that are well-suited for adherent cells involve labeling and staining without cell detachment from the tissue culture plastic. Examples are described elsewhere and include three basic approaches. In one, cells are solubilized after labeling, releasing fluorophores into suspension where they can be quantitatively measured using a fluorescence microplate reader (Smirnov et al., 2020; Wen et al., 2019; Wu et al., 2022). In the second, metabolic incorporation of MGE analogs can be visualized by fluorescence microscopy, which provides rich detail on the subcellular distribution of the non-natural monosaccharide. As an aside, cells can be fixed, stained, and permeabilized before labeling, allowing visualization not only of cell-surface display, but of analog incorporation in glycoprotein secretory pathways (Almaraz, Aich et al., 2012; Scache et al., 2022; Tian et al., 2015; Wang et al., 2017). In the third, a “far western” approach is based on the separation of proteins in a cell lysate using gel electrophoresis. Instead of staining the blots with antibodies as in conventional western blotting, the blots are reacted with biotin-conjugated bioorthogonal probes followed by avidin-based fluorescent probes (Scache et al., 2022). This approach quantifies the incorporation of an unnatural analog in purified protein or cell lysate as an alternative to flow cytometry or plate reader assays. In theory, the same principle can be applied to ELISA assays, creating a sandwich of capturing antibodies and fluorescing molecules (Srinivasan et al., 2015).
The detection and quantification of ketone-, azido-, and alkyne-groups, as described below, follows the same basic set of steps with the main difference being the use of a ketone-, azido-, or alkyne-specific probe. Due to their chemical properties and the slightly oxidizing conditions in the extracellular milieu in normal tissue culture conditions, an additional nuance exists for glycan-displayed thiols; specifically, the majority of glycocalyx-displayed thiols form cis -disulfides (Sampathkumar, Jones, & Yarema, 2006). These disulfides are refractory to labeling probes such as maleimide-conjugated biotin, resulting in significant underestimation of incorporation of thiol-modified analogs such as Ac5ManNTGc into cell-surface glycans. Therefore, to better estimate the total level of surface sugar analog display, this protocol introduces the mild reducing agent tris(2-carboxyethyl)phosphine (TCEP), which reduces disulfide bonds before chemoselective ligation of thiols is performed, thereby providing a more accurate estimate of metabolic incorporation.
Additional Materials (also see Basic Protocol 1)
-
10 mM stock solutions of desired sugar analogs: Ac4ManNLev, Ac4ManNAz, Ac4ManNAl, or Ac5ManNTGc (1-4) for ketone, azide, alkyne, or thiol labeling, respectively, plus any needed controls (e.g., 5 -9)
-
Phosphate-buffered saline (PBS), pH 7.4 (Invitrogen, cat. no. 10010-049)
-
Biotin buffer (see recipe), freshly prepared
-
5 mM biotin hydrazide (see recipe)
-
CuAAC buffer (see recipe)
-
1 mM biotin alkyne (see recipe)
-
4 mM biotin-PEG4-DBCO (see recipe)
-
1 mM biotin azide (see recipe)
-
Tris(2-carboxyethyl)phosphine hydrochloride (TCEP; Sigma, cat. no. C4706)
-
EZ-Link maleimide PEG2-biotin (MB; Pierce Biotechnology, cat. no. 21901)
-
Avidin staining buffer (ASB; see recipe)
-
2.8 mg/ml FITC-avidin (see recipe)
-
6-well tissue culture plates or 35-mm dishes
-
15-ml centrifuge tubes
-
5-ml round-bottom polystyrene tubes, 12 × 75 mm (BD Falcon, BD Biosciences, cat. no. 352054)
-
Flow cytometer with 488-nm argon laser (Becton Dickinson FACSCalibur, BD Biosciences)
Incubate cells with sugar analogs
1.Incubate Jurkat cells with the appropriate test analog (e.g., 1 -4 , Fig. 4) and control (e.g., 5 -9) as described (see Basic Protocol 1), but use 6-well tissue culture plates (or 35-mm dishes) instead of 24-well plates and seed ∼1 × 106 cells in 2 ml for each sample.
Prepare cells for labeling
2.Gently pipet cell suspension up and down using a 5-ml pipet to dislodge cell clumps. Count an aliquot to determine cell density.
3.Centrifuge cells in 15-ml tubes for 3.5 min at 1300 × g , room temperature, and discard supernatant.
4.Add 3 ml PBS and resuspend cells by gentle pipetting.
5.Aliquot 1 ml resuspended cells to three 1.5-ml microcentrifuge tubes (maximum of 2 × 106 cells/tube).
Perform labeling
IMPORTANT : For all labeling reactions, it is crucial that the cells be properly resuspended before and immediately after adding the labeling reagent to avoid cell aggregation and cross-linking. During incubation, tubes should be laid flat on the benchtop with intermittent mixing by gently flipping the tubes end-to-end to ensure uniform labeling. We recommend not vortexing the cells during labeling.
For ketone expression:
6a. Wash cells twice with freshly prepared biotin buffer, then twice with PBS. For each wash, centrifuge cells 2.5 min at 3000 × g , room temperature, discard the supernatant, and gently resuspend in a volume of 1 ml.
7a. Centrifuge cells 3.5 min at 3000 × g , room temperature, and resuspend gently in 250 µl biotin buffer.
8a. Add 250 µl of 5 mM biotin hydrazide and mix immediately by gentle pipetting.
9a. Lay tubes flat on the benchtop at room temperature for 2 hr, mixing by gentle inversion every 15 min. Proceed to step 12.
For azide expression using the copper-catalyzed azide-alkyne [3 + 2] conjugation reaction:
6b. Wash cells as in step 6a.
7b. Centrifuge cells 3.5 min at 3000 × g , room temperature, and resuspend gently in 250 µl CuAAC buffer.
8b. Add 250 µl of 1 mM biotin alkyne and mix immediately by gentle pipetting.
9b. Lay tubes flat on the benchtop at room temperature for 2 hr, mixing by gentle inversion every 15 min. Proceed to step 12.
For azide expression using the copper-free strain-promoted azide-alkyne conjugation reaction:
6c. Wash cells as in step 6a.
7c. Dilute 5 µl of 4 mM biotin-PEG4-DBCO in 495 µl PBS.
8c. Centrifuge cells 3.5 min at 3000 × g , room temperature, and resuspend gently in 500 µl diluted biotin-PEG4-DBCO.
9c. Lay tubes flat on the benchtop at room temperature for 1 hr, mixing by gentle inversion every 15 min. Proceed to step 12.
For alkyne expression:
6d. Wash cells as in step 6a.
7d. Centrifuge cells 3.5 min at 3000 × g , room temperature, and resuspend gently in 250 µl CuAAC buffer.
8d. Add 250 µl of 1 mM biotin azide and mix immediately by gentle pipetting.
9d. Lay tubes flat on the benchtop at room temperature for 2 hr, mixing by gentle inversion every 15 min. Proceed to step 12.
For thiol expression:
6e. Wash cells twice with PBS only. For each wash, centrifuge cells 3.5 min at 3000 × g , room temperature, discard the supernatant, and gently resuspend cells in 1 ml PBS.
7e. Prepare a 10 mM solution of TCEP by adding 2.87 mg TCEP to 1 ml PBS.
8e. Centrifuge cells 3.5 min at 3000 × g , room temperature and discard the supernatant. Resuspend cells gently in 360 µl PBS and then add 40 µl of 10 mM TCEP (final 1 mM).
9e. Lay tubes flat on the benchtop at room temperature for 1 hr, mixing by gentle inversion every 15 min.
10e. Prepare a fresh 5 mM MB solution by adding 3.9 mg EZ-Link maleimide PEG2-biotin to 1.5 ml PBS. Add 100 µl to each sample and mix immediately by gentle pipetting.
11e. Lay tubes flat on the benchtop at room temperature for 1 hr, mixing by gentle inversion every 15 min. Proceed to step 12.
Stain with FITC-avidin
12.While the cells are labeling, cool 50 ml ASB and 50 ml PBS in an ice bath.
13.Centrifuge labeled samples 3.5 min at 3000 × g , room temperature. Discard supernatant and resuspend cells in 1 ml ice-cold ASB.
14.Repeat wash two more times, but resuspend the third pellet in 100 µl ASB.
15.Dilute 2.8 mg/ml FITC-avidin 1:250 in PBS. Mix well and keep in the dark on ice for no more than 12 hr.
16.Add 100 µl diluted FITC-avidin to each tube (final 5.6 µg/ml), mix immediately by gentle pipetting, and incubate on ice out of direct light for 15 min.
17.Centrifuge FITC-labeled cells 3.5 min at 3000 × g , room temperature. Discard supernatant and resuspend cells gently in 100 µl ASB.
18.Repeat FITC-avidin incubation as in step 16.
19.During the second incubation, collect untreated Jurkat cells and add ∼1 × 106 cells to each of two 1.5-ml microcentrifuge tubes. Wash twice, resuspend in 500 µl PBS, and transfer to 5-ml polystyrene tubes. Keep on ice.
20.Centrifuge labeled samples 3.5 min at 3000 × g , room temperature. Discard supernatant and resuspend cells gently in 1 ml ice-cold ASB.
21.Repeat wash two more times, but resuspend the third pellet in 500 µl PBS and transfer to 5-ml polystyrene tubes. Keep on ice out of direct light.
Analyze by flow cytometry
22.Analyze samples by flow cytometry, collecting at least 10,000 events for each run for more accurate results. Run analysis twice for each sample.
23.Measure the relative fluorescence content (and hence relative surface display) of cells using the geometric mean in arbitrary units.
REAGENTS AND SOLUTIONS
Use deionized, distilled water in all recipes and protocol steps.
Avidin staining buffer (ASB)
- 25 ml FBS (HyClone, cat. no. SH30071.03)
- 5 ml 10% (w/v) sodium azide (Pierce Biotechnology, cat. no. AC190380050)
- 500 ml sterile PBS (Invitrogen, cat. no. 10010-049)
- Store up to 6-8 weeks at 4°C
Biotin alkyne, 1 mM
- 10.565 mg biotin alkyne (Click Chemistry Tools, cat. no. 1266-25)
- 20 ml PBS (Invitrogen, cat. no. 10010-049) adjusted to pH 6.5 with conc. HCl
- Store up to 6-8 weeks at 4°C
Biotin alkyne may take up to 1 hr to dissolve. Do not use if precipitate forms during storage.
Biotin azide, 1 mM
- 12.316 mg biotin azide (Click Chemistry Tools, cat. no. 1265-25)
- 20 ml PBS (Invitrogen, cat. no. 10010-049) adjusted to pH 6.5 with conc. HCl
- Store up to 6-8 weeks at 4°C
Biotin azide may take up to 1 hr to dissolve. Do not use if precipitate forms during storage.
Biotin buffer
- 95 ml PBS (Invitrogen, cat. no. 10010-049) adjusted to pH 6.5 with conc. HCl
- 5 ml FBS (HyClone, cat. no. SH30071.03)
- Filter sterilize
- Prepare fresh
Biotin hydrazide, 5 mM
- 25.834 mg biotin hydrazide (Sigma, cat. no. B-7639)
- 20 ml PBS (Invitrogen, cat. no. 10010-049) adjusted to pH 6.5 with conc. HCl
- Store up to 6-8 weeks at 4°C
Biotin hydrazide may take up to 1 hr to dissolve. Do not use if precipitate forms during storage.
Biotin-PEG4-DBCO, 4 mM
- 3 mg biotin-PEG4-DBCO (Click Chemistry Tools, cat. no. A105)
- 1 ml DMSO
- Store up to 6-8 weeks at 4°C
Complete RPMI-1640
- 470 ml RPMI-1640 (Invitrogen, cat. no. 11875-119)
- 25 ml fetal bovine serum (FBS; HyClone, cat. no. SH30071.03)
- 5 ml 100× penicillin-streptomycin (P/S; Sigma, cat. no. P-0781)
- Store up to one 1 month at 4°C
Copper-catalyzed azide-alkyne cycloaddition (CuAAC) buffer
- 348.360 mg dipotassium hydrogen phosphate (Sigma-Aldrich, cat. no. P3786)
- 1.20 ml 2.5 mM CuSO4 (see recipe)
- 2.583 mg BTTAA (Click Chemistry Tools, cat. no. 1236)
- 10.057 mg sodium ascorbate (Sigma-Aldrich, cat. no. A4034)
- 18 ml deionized water
- Store up to 6-8 weeks at 4°C
Copper sulfate (CuSO4), 2.5 mM
- 1 ml 40 mM copper sulfate (Sigma, cat. no. 7758-98-7)
- 15 ml deionized water
- Store up to 6-8 weeks at 4°C
FITC-avidin, 2.8 mg/ml
- 2 mg fluorescein isothiocyanate (FITC)–labeled avidin (Sigma, cat. no. A-2901)
- 714 µl PBS (Invitrogen, cat. no. 10010-049)
- Store up to 6 months in the dark at 2°C
Dissolve FITC-labeled avidin completely in PBS.
Periodic acid, 0.4 M
- 910 mg periodic acid (Sigma, cat. no. 10450-60-9)
- 10 ml deionized water
- Dispense 1-ml aliquots into 1.5-ml microcentrifuge tubes
- Store up to 6 months in the dark at –20°C
Sialic acid, 10 mM
- 10 mg N -acetylneuraminic acid (Sigma, cat. no. A-0812)
- 3.233 ml PBS (Invitrogen, cat. no. 10010-049)
- Store up to 2 weeks at 4°C
COMMENTARY
Background Information
Oligosaccharides on mammalian cell surfaces play critical biological functions, as they contribute to most interactions between the host cell and its environment. These functional roles include numerous biological recognition processes such as viral and bacterial infection, innate and adaptive immunity, and cancer metastasis. Additionally, oligosaccharides, which are commonly conjugated to proteins or lipids to form glycoconjugates, play an essential role in determining glycoconjugate biological activities (Varki, 1993). Accordingly, MGE technology holds great potential for manipulating basic cellular functions for medical applications such as tissue engineering, cancer therapy, and carbohydrate-based medicine. Here (as well as in other publications, e.g., Piller et al., 2015; Wratil & Horstkorte, 2017), the general steps and protocols for characterization of novel sugar analogs used for MGE are described. Critical parameters for consideration and common problems related to these experiments are discussed below.
Critical Parameters and Troubleshooting
Incubation of cells with sugar analogs
For consistent results and optimal surface labeling, experiments should be performed using healthy and robustly growing cells harvested at subconfluency. Of course, if the goal of the experiment is to probe effects of anomalous metabolic states such as hypoxia, toxic waste products from overgrowth of cells (such as ammonia, which affects sialic acid metabolism; Zanghi et al., 1998), or pharmacological agents on glycan production, this caveat does not apply.
The current set of protocols can be adapted to other sugar analogs and cell lines with the conditions described herein (e.g., analog concentrations and incubation time) serving as a reasonable starting point. Variations in the optimal conditions for different cell types and analogs should be expected, but can usually be covered by evaluating cellular responses in the range of 0 to 250 to 500 μM for SCFA-derivatized analogs. The current protocols are also based on 0.5-ml cultures suited for culture in 24-well plates, which typically provide an ample number of cells for flow cytometry characterization, toxicity and viability assays, or functional characterization. If multiple endpoints are to be measured from a single batch of cells, the protocol can readily be scaled to 1-ml cultures in 12-well plates or 2-ml cultures in 6-well plates.
Another potential pitfall is that certain analogs (e.g., Ac4ManNProp or Ac4ManNAz) experience similar rates of elevated flux in human and rodent cells, whereas others (e.g., ManNLev) exhibit substantially lower incorporation (e.g., ∼20-fold) in rodent cells (Yarema et al., 1998). This observation stresses that species-specific MGE labeling differences exist and we recommend conducting a full slate of control experiments if exploring MGE in previously untested species.
Periodate-resorcinol colorimetric assay
The periodate-resorcinol assay measures glycoconjugate-bound sialic acid when the periodate-oxidation step is performed at 37°C and total sialic acid when the oxidation step is performed on ice (see Basic Protocol 3, step 11). Because the oxidation step is highly dependent on temperature, all temperatures stated in the protocol should be carefully controlled to avoid inaccurate results. Another important consideration is that, although conducting the oxidation step at 37°C is generally described as a way to measure “glycoconjugate-bound” sialic acids (Jourdian et al., 1971), we have found that CMP-sialic acid(s) also provides colorimetric signal under these conditions. As a consequence, an increase in “glycoconjugate-bound” signal does not guarantee that cell-surface sialylation has increased, merely that the free monosaccharide form of sialic acid has been converted to the nucleotide sugar form within the cell. Lectin-binding analysis can be used to address this issue in more depth.
Accurate cell enumeration is another critical parameter. Conducting measurements in triplicate minimizes errors and yields results accurate to ±2% (generally expressed as molecules of sialic acid per cell) when cell counting is performed with an electronic cell counter. Moreover, a cell counter can provide cell size information that allows molecules per cell data to be easily converted to molar concentrations within a cell. If an electronic cell counter is not available and cell counts are performed with a hemacytometer, we recommend reserving a portion of the cell sample for standard protein assays and expressing the amount of sialic acid as a function of protein abundance.
Quantification of cell-surface glycoconjugates
Accurate quantitative labeling of surface thiol, ketone, azide, and alkyne groups (see Basic Protocol 4) depends on several variables, including optimal reagent concentrations, incubation times, and reaction temperatures. It is recommended that reagents—e.g., working solutions of TCEP, MB, biotin hydrazide, and FITC-avidin—be prepared in small amounts on the day of the assay and stored at 4°C or on ice until immediately prior to use. Further optimization may be required when a previously untested cell line is used. For example, certain cell types may be less tolerant to the reaction conditions, thereby necessitating shorter labeling periods to maintain cell viability. Similarly, while the basic methodology described can be readily adapted for additional functional groups now available in MGE analogs, customization of reagent concentrations and reaction times is required for each analog, chemoselective ligation partner (and other chemical reagents used), and cell type being tested.
When analog cytotoxicity reduces cell growth, the resulting low number of cells at the conclusion of the incubation period can be compensated by adjusting culture conditions appropriately. In general, increased starting densities of cells ameliorate growth inhibition and help maintain cell viability (Jones et al., 2004). When adherent cells are used, trypsinization and replating often result in a growth lag and increased sensitivity to sugar analog toxicity, which can significantly reduce the final cell count. One solution is to plate the cells and allow them to resume growth for 12-24 hr before introducing the sugar analog into the medium. This approach may reduce analog uptake efficiency, requiring higher analog concentrations to achieve targeted levels of glycan display on the cell surface (Sampathkumar, Jones, & Yarema, 2006).
A common problem, which can be easily detected when samples are run in triplicate, is inconsistent measurement of cell-surface glycan levels. As discussed here (see Basic Protocol 4, step 18) and in detail elsewhere (Yarema, 2002), erratic and lower-than-expected signal may be due to incomplete removal of unreacted biotin reagents from the cell suspension before staining with FITC-avidin. To avoid this problem, samples should be washed at least three times to remove unreacted biotin reagents before FITC-avidin staining is performed. Additionally, as described in the protocol, staining can be performed twice to aid in the removal of excess biotin reagents.
We have encountered situations when surface labeling is much higher than expected when adherent cells were used. This outcome can result from the cell detachment reagents (e.g., trypsin) used for harvesting, which damage the membrane and allow nonspecific uptake of labeling reagents. It is therefore recommended that alternate detachment reagents (e.g., nonenzymatic buffers instead of trypsin) or a cell scraper be used to detach cells. It should be noted that cell scraping causes mechanical damage to some cells, so procedures must be optimized for each cell line tested.
Anticipated Results
Cell viability assays
Typically, monosaccharide analogs used in metabolic labeling have either negligible or relatively minor cytotoxicity that does not deleteriously impact cell viability at concentrations required to label cellular glycans. In cases when analogs such as peracetylated ManNLev do exhibit noticeable toxicity (Kim et al., 2004), these effects can be ameliorated by straightforward procedures such as controlling the rate of analog delivery (Aich et al., 2010) or avoiding very low cell densities (Jones et al., 2004).
Periodate-resorcinol colorimetric assays
The periodate-resorcinol assay provides a quantitative measure of the total sialic acid and glycoconjugate-bound sialic acid content of cells. When compared to untreated cells, it is common that the total sialic acid content of cells treated with high-flux analogs when a natural core sugar is used (such as Ac4ManNAc), or when one with a modest structural alteration (e.g., Ac4ManNProp) is used, can increase by several fold, often 10-fold or more (Kim et al., 2004). Although the assay does not explicitly provide levels of the free monosaccharide form of sialic acid, this endpoint can easily be estimated by subtracting glycoconjugate-bound sialic acids from total levels. Generally, the level of free sialic acid cannot be measured accurately in untreated cells by this assay because it is below the detection limit (roughly 5 µM intracellular level). Upon supplementation with high-flux analogs, intracellular sialic acid levels can increase dramatically to as high as the millimolar level. In other cases, however, flux does not noticeably increase (e.g., with Ac4ManNHex) or actually decreases (e.g., with Ac4ManNLev or Ac4ManNHomoLev) (Kim et al., 2004).
A large increase in intracellular levels of free sialic acid usually does not translate to a corresponding increase in surface display. For example, a 100-fold increase may only result in a 10% increase in sialoglycoconjugate display because of bottlenecks in converting the free sugar to the nucleotide sugar donor, transport into the Golgi lumen, and “empty/available” sialylation sites (i.e., terminal galactose resides on N -glycan termini) on nascent glycans. By contrast, as noted earlier, certain analogs do not measurably change total levels of sialic acid in a cell but nevertheless do successfully transit the pathway, as evidenced by the appearance of their corresponding sialoside on the cell surface when assayed as described in Basic Protocol 4. Conversion of Ac4ManNLev to Sia5Lev provides an example of this phenomenon (Kim et al., 2004). Overall, we reiterate that it is important to be aware that there is not a linear correspondence between analog uptake and incorporation into a metabolic pathway (measured in Basic Protocol 3) and subsequent surface display (measured in Basic Protocol 4).
Quantitation of cell-surface glycoconjugates
Multiple factors can affect the amount of sugar analogs incorporated into glycans and displayed on the cell surface, including cell type, cell density, incubation time, sugar analog, and metabolic status of the cell (Jones et al., 2004). It should also be noted that, although the procedure described is highly reproducible, the exact fluorescence intensity value (geometric mean value) obtained from a flow cytometer will vary based on instrument settings. For standard conditions described here, where actively growing Jurkat cells are incubated with 40 µM of compound 1 for 48 hr, an increase in fluorescence intensity of up to about 10-fold is typically observed compared to no-analog control samples although the absolute intensities of control and test cells are likely to vary from experiment to experiment.
Time Considerations
Incubation of cells with sugar analogs
The time required to set up the incubations should be <2 hr once the sugar analog stock solution has been obtained. Cells are typically incubated with analogs for 2-5 days, depending on the subsequent experiments performed. If adherent cells are used, it is advisable to plate them and allow them to recover and grow for 12-24 hr before adding sugar analogs.
Cell viability assays
After incubation with sugar analogs, cell viability assays typically take an additional 2-5 days, although up to 15 days may be required if long-term toxicity is being evaluated. Cell counting for suspension cells is rather straightforward and should not take longer than 1 hr for each time point (days 3, 5, and 15). Dispersion of cell clumps and changing of medium on days 7, 9, 11, and 13 is also straightforward and can be completed in 30 min per time point. If adherent cells are used, growth inhibition assays typically take 5 days to complete, with cell counting being performed on days 3 and 5. Although the cells will require trypsinization and resuspension before counting, this procedure can be completed within 1 hr per time point.
Periodate-resorcinol assay
In general, after incubation with sugar analogs, the complete assay can be performed in 6-7 hr for 36-48 samples. Cell resuspension, counting, initial washes, and three freeze-thaw cycles should take ∼3.5 hr. Reagent preparation can be done concurrently with the freeze-thaw cycles and therefore does not require additional time. After freeze-thaw cycles are completed, periodic acid oxidation requires ∼30 min on ice (to determine total sialic acid content) or ∼2 hr at 37°C (to determine conjugate-bound sialic acid). Finally, incubation with the resorcinol working reagent, addition of t -butyl alcohol, and measurement of absorbance should take 1 hr. The total time for a set of periodate-resorcinol assays is ∼6-12 hr, depending on the number of samples.
Quantitation of cell-surface glycoconjugates
After initial incubation with sugar analogs, the resuspension of cells and conjugation with biotin reagents (biotin hydrazide, biotin alkyne, biotin azide, or TCEP treatment followed by biotin maleimide) should take ∼3 hr for 24-36 samples. After these reactions are complete, washing of cells to remove unreacted biotinylation reagents, staining with FITC-avidin, and additional washes to remove unbound reagent take another 90 min. Flow cytometry will take up to 2 hr depending on the number of samples. Overall, the entire process can be completed in ∼6-8 hr.
Acknowledgments
We would like to thank the National Institutes of Health (CA112314, CA249381, and NS125232) for financial support.
Author Contributions
Kris Dammen-Brower : Conceptualization, investigation, methodology, supervision, validation, visualization, writing (original draft, review and editing); Elaine Tan : Methodology, data curation, writing (original draft, review and editing); Ruben T. Almaraz : Methodology, data curation, writing (original draft, review and editing); Jian Du : Writing (original draft, review and editing); Kevin J. Yarema : Formal analysis, funding acquisition, project administration, supervision, validation, visualization, writing (original draft, review and editing).
Conflict of Interest
The authors declare no conflict of interest.
Open Research
Data Availability Statement
Data sharing is not applicable to this article as no new data were created or analyzed in this study.
Literature Cited
- Agard, N. J., Baskin, J. M., Prescher, J. A., Lo, A., & Bertozzi, C. R. (2006). A comparative study of bioorthogonal reactions with azides. ACS Chemical Biology , 1, 644–648. https://doi.org/10.1021/cb6003228
- Agard, N. J., Prescher, J. A., & Bertozzi, C. R. (2004). A strain-promoted [3 + 2] azide-alkyne for covalent modification of biomolecules in living systems. Journal of the American Chemical Society , 126, 15046–15047. https://doi.org/10.1021/ja044996f
- Agatemor, C., Buettner, M. J., Ariss, R. S., Muthiah, K., Saeui, C. T., & Yarema, K. J. (2019). Exploiting metabolic glycoengineering to advance healthcare. Nature Reviews Chemistry , 3, 605–620. https://doi.org/10.1038/s41570-019-0126-y
- Agatemor, C., Muthiah, K., Ha, L., Chai, J., Osman, A., Robertson, B. M., & Yarema, K. J. (2020). Imaging glycans with metabolic glycoengineering. In J. Barchi. (Ed.), Comprehensive glycoscience (Vol. 3, Cellular glycobiology) (pp. 253–274). Elsevier. https://doi.org/10.1016/B978-0-12-409547-2.14962-5
- Aich, U., Campbell, C. T., Elmouelhi, N., Weier, C. A., Sampathkumar, S. G., Choi, S. S., & Yarema, K. J. (2008). Regioisomeric SCFA attachment to hexosamines separates metabolic flux from cytotoxicity and MUC1 suppression. ACS Chemical Biology , 3, 230–240. https://doi.org/10.1021/cb7002708
- Aich, U., Meledeo, M. A., Sampathkumar, S.-G., Fu, J., Jones, M. B., Weier, C. A., Chung, S. Y., Tang, B. C., Yang, M., Hanes, J., & Yarema, K. J. (2010). Development of delivery methods for carbohydrate-based drugs: Controlled release of biologically-active short chain fatty acid-hexosamine analogs. Glycoconjugate Journal , 27, 445–459. https://doi.org/10.1007/s10719-010-9292-3
- Aich, U., & Yarema, K. J. (2008). Non-natural sugar analogues: Chemical probes for metabolic oligosaccharide engineering. In B. O. Fraser-Reid, K. Tatsuta, & J. Thiem (Eds.), Glycoscience ( 2nd ed.) (pp. 2133–2190). Springer-Verlag.
- Almaraz, R. T., Aich, U., Khanna, H. S., Tan, E., Bhattacharya, R., Shah, S., & Yarema, K. J. (2012). Metabolic oligosaccharide engineering with N -acyl functionalized ManNAc analogues: Cytotoxicity, metabolic flux, and glycan-display considerations. Biotechnology and Bioengineering , 109, 992–1006. https://doi.org/10.1002/bit.24363
- Almaraz, R. T., Mathew, M. P., Tan, E., & Yarema, K. J. (2012). Metabolic oligosaccharide engineering: Implications for selectin-mediated adhesion and leukocyte extravasation. Annals of Biomedical Engineering , 40, 806–815. https://doi.org/10.1007/s10439-011-0450-y
- Badr, H. A., AlSadek, D. M., El-Houseini, M. E., Saeui, C. T., Mathew, M. P., Yarema, K. J., & Ahmed, H. (2017). Harnessing cancer cell metabolism for theranostic applications using metabolic glycoengineering of sialic acid in breast cancer as a pioneering example. Biomaterials , 116, 158–173. https://doi.org/10.1016/j.biomaterials.2016.11.044
- Baskin, J. M., Prescher, J. A., Laughlin, S. T., Agard, N. J., Chang, P. V., Miller, I. A., Lo, A., Codelli, J. A., & Bertozzi, C. R. (2007). Copper-free click chemistry for dynamic in vivo imaging. Proceedings of the National Academy of Sciences of the United States of America , 104, 16793–16797. https://doi.org/10.1073/pnas.0707090104
- Bond, M. R., Zhang, H., Vu, P. D., & Kohler, J. J. (2009). Photocrosslinking of glycoconjugates using metabolically incorporated diazirine-containing sugars. Nature Protocols , 4, 1044–1063. https://doi.org/10.1038/nprot.2009.85
- Boyce, M., Carrico, I. S., Ganguli, A. S., Yu, S.-H., Hangauer, M. J., Hubbard, S. C., Kohler, J. J., & Bertozzi, C. R. (2011). Metabolic cross-talk allows labeling of O -linked β-N -acetylglucosamine-modified proteins via the N -acetylgalactosamine salvage pathway. Proceedings of the National Academy of Sciences of the United States of America , 108, 3141–3146. https://doi.org/10.1073/pnas.1010045108
- Brossmer, R., & Gross, H. J. (1994). Fluorescent and photoactivatable sialic acids. Methods in Enzymology , 247, 177–193. https://doi.org/10.1016/s0076-6879(94)47014-6
- Buettner, M. J., Shah, S. R., Saeui, C. T., Ariss, R. S., & Yarema, K. J. (2018). Improving immunotherapy through glycodesign. Frontiers in Immunology , 9, 2485. https://doi.org/10.3389/fimmu.2018.02485
- Büttner, B., Kannicht, C., Schmidt, C., Löster, K., Reutter, W., Lee, H.-Y., Nöhring, S., & Horstkorte, R. (2002). Biochemical engineering of cell surface sialic acids stimulates axonal growth. Journal of Neuroscience , 22, 8869–8875. https://doi.org/10.1523/JNEUROSCI.22-20-08869.2002
- Chang, P. V., Chen, X., Smyrniotis, C., Xenakis, A., Hu, T., Bertozzi, C. R., & Wu, P. (2009). Metabolic labeling of sialic acids in living animals with alkynyl sugars. Angewandte Chemie , 48, 4030–4033. https://doi.org/10.1002/anie.200806319
- Chefalo, P., Pan, Y.-B., Nagy, N., Harding, C., & Guo, Z.-W. (2004). Preparation and immunological studies of protein conjugates of N -acylneuraminic acids. Glycoconjugate Journal , 20, 407–414. https://doi.org/10.1023/B:GLYC.0000033997.01760.b9
- Choi, J., Wagner, L. J. S., Timmermans, S. B. P. E., Malaker, S. A., Schumann, B., Gray, M. A., Debets, M. F., Takashima, M., Gehring, J., & Bertozzi, C. R. (2010). Engineering orthogonal polypeptide GalNAc-transferase and UDP-sugar pairs. Journal of the American Chemical Society , 141, 13442–13453. https://doi.org/10.1021/jacs.9b04695
- Codelli, J. A., Baskin, J. M., Agard, N. J., & Bertozzi, C. R. (2008). Second-generation difluorinated cyclooctynes for copper-free click chemistry. Journal of the American Chemical Society , 130, 11486–11493. https://doi.org/10/1021/ja803086r
- Cohen, M., Joester, D., Geiger, B., & Addadi, L. (2004). Spatial and temporal sequence of events in cell adhesion: From molecular assembly to focal adhesion assembly. Chembiochem , 4, 1393–1399. https://doi.org/10.1002/cbic.200400162
- Cole, C. M., Yang, J., Šečkutė, J., & Devaraj, N. K. (2013). Fluorescent live-cell imaging of metabolically incorporated unnatural cyclopropene-mannosamine derivatives. ChemBioChem , 14, 205–208. https://doi.org/10.1002/cbic.201200719
- Collins, B. E., Fralich, T. J., Itonori, S., Ichikawa, Y., & Schnaar, R. L. (2000). Conversion of cellular sialic acid expression from N -acetyl- to N -glycolylneuraminic acid using a synthetic precursor, N -glycolylmannosamine pentaacetate: Inhibition of myelin-associated glycoprotein binding to neural cells. Glycobiology , 10, 11–20. https://doi.org/10.1093/glycob/10.1.11
- Dammen-Brower, K., Epler, P., Zhu, S., Bernstein, Z. J., Stabach, P. R., Braddock, D. T., Spangler, J. B., & Yarema, K. J. (2022). Strategies for glycoengineering therapeutic proteins. Frontiers in Chemistry , 10, 863118. https://doi.org/10.3389/fchem.2022.863118
- Darabedian, N., Gao, J., Chuh, K. N., Woo, C. M., & Pratt, M. R. (2018). The metabolic chemical reporter 6-azido-6-deoxy-glucose further reveals the substrate promiscuity of O -GlcNAc transferase and catalyzes the discovery of intracellular protein modification by O -glucose. Journal of the American Chemical Society , 140, 7092–7100. https://doi.org/10.1021/jacs.7b13488
- Darabedian, N., Yang, B., Ding, R., Cutolo, G., Zaro, B. W., Woo, C. M., & Pratt, M. R. (2020). O -Acetylated chemical reporters of glycosylation can display metabolism-dependent background labeling of proteins but are generally reliable tools for the identification of glycoproteins. Frontiers in Chemistry , 8, 00318. https://doi.org/10.3389/fchem.2020.00318
- Donini, R., Haslam, S. M., & Kontoravdi, C. (2021). Glycoengineering Chinese hamster ovary cells: A short history. Biochemistry Society Transactions , 49, 915–931. https://doi.org/10.1041/BST20200840
- Du, J., Agatemor, C., Saeui, C. T., Bhattacharya, R., Jia, X., & Yarema, K. J. (2021). Glycoengineering human neural and adipose stem cells with novel thiol-modified N -acetylmannosamine (ManNAc) analogs. Cells , 10, 377. https://doi.org/10.3390/cells10020377
- Du, J., Liu, X., Yarema, K. J., & Jia, X. (2022). Glycoengineering human neural stem cells (hNSCs) for adhesion improvement using a novel thiol-modified N -acetylmannosamine (ManNAc) analog. Materials Science and Engineering: C , 134, 112675. https://doi.org/10.1016/j.msec.2022.112675
- Du, J., Meledeo, M. A., Wang, Z., Khanna, H. S., Paruchuri, V. D. P., & Yarema, K. J. (2009). Metabolic glycoengineering: Sialic acid and beyond. Glycobiology , 19, 1382–1401. https://doi.org/10.1093/glycob/cwp115
- Dube, D. H., & Bertozzi, C. R. (2003). Metabolic oligosaccharide engineering as a tool for glycobiology. Current Opinion in Chemical Biology , 7, 616–625. https://doi.org/10.1016/j.cbpa.2003.08.006
- Elmouelhi, N., Aich, U., Paruchuri, V. D. P., Meledeo, M. A., Campbell, C. T., Wang, J. J., Srinivas, R., Khanna, H. S., & Yarema, K. J. (2009). Hexosamine template. A platform for modulating gene expression and for sugar-based drug discovery. Journal of Medicinal Chemistry , 52, 2515–2530. https://doi.org/10.1021/jm801661m
- Freitas, R. A. (1999). Nanomedicine (Vol. 1, Basic capabilities). Landes Bioscience.
- Gagiannis, D., Gossrau, R., Reutter, W., Zimmermann-Kordmann, M., & Horstkorte, R. (2007). Engineering the sialic acid in organs of mice using N -propanoylmannosamine. Biochimica et Biophysica Acta , 1770, 297–306. https://doi.org/10.1016/j.bbagen.2006.09.023
- Gross, H. J., & Brossmer, R. (1988). Enzymatic introduction of a fluorescent sialic acid into oligosaccharide chains of glycoproteins. European Journal of Biochemistry , 177, 583–589. https://doi.org/10.1111/j.1432-1033.1988.tb14410.x
- Gupta, G., Surolia, A., & Sampathkumar, S.-G. (2010). Lectin microarrays for glycomic analysis. OMICS: A Journal of Integrative Biology , 14, 419–436. https://doi.org/10.1089/omi.2009.0150
- Han, S., Collins, B. E., Bengtson, P., & Paulson, J. C. (2005). Homo-multimeric complexes of CD22 revealed by in situ photoaffinity protein-glycan crosslinking. Nature Chemical Biology , 1, 93–97. https://doi.org/10.1038/nchembio713
- Hang, H. C., & Bertozzi, C. R. (2001). Ketone isoteres of 2-N -acetamidosugars as substrates for metabolic cell surface engineering. Journal of the American Chemical Society , 123, 1242–1243. https://doi.org/10.1021/ja002962b
- Hao, Y., Fan, X., Shi, Y., Zhang, C., Sun, D.-E., Qin, K., Qin, W., Zhou, W., & Chen, X. (2019). Next-generation unnatural monosaccharides reveal that ESRRB O -GlcNAcylation regulates pluripotency of mouse embryonic stem cells. Nature Communications , 10, 4065. https://doi.org/10.1038/s41467-019-11942-y
- Hassenrück, J., & Wittmann, V. (2019). Cyclopropene derivatives of aminosugars for metabolic glycoengineering. Beilstein Journal of Organic Chemistry , 15, 584–601. https://doi.org/10.3762/bjoc.15.54
- Hong, S., Sahai-Hernandez, P., Chapla, D. G., Moremen, K. W., Traver, D., & Wu, P. (2019). Direct visualization of live zebrafish glycans via single-step metabolic labeling with fluorophore-tagged nucleotide sugars. Angewandte Chemie , 58, 14327–14333. https://doi.org/10.1002/anie.201907410
- Horstkorte, R., Mühlenhoff, M., Reutter, W., Nöhring, S., Zimmermann-Kordmann, M., & Gerardy-Schahn, R. (2004). Selective inhibition of polysialyltransferase ST8SiaII by unnatural sialic acids. Experimental Cell Research , 298, 268–274. https://doi.org/10.1016/j.yexcr.2004.04.014
- Hsu, T.-L., Hanson, S. R., Kishikawa, K., Wang, S.-K., Sawa, M., & Wong, C.-H. (2007). Alkynyl sugar analogs for the labeling and visualization of glycoconjugates in cells. Proceedings of the National Academy of Sciences of the United States of America , 104, 2614–2619. https://doi.org/10.1073/pnas.0611307104
- Hua, Z., Lvov, A., Morin, T. J., & Kobertz, W. R. (2011). Chemical control of metabolically-engineered voltage-gated K+ channels. Bioorganic & Medicinal Chemistry Letters, 21, 5021–5024. https://doi.org/10.1016/j.bmcl.2011.04.099
- Jacobs, C. L., Goon, S., Yarema, K. J., Hinderlich, S., Hang, H. C., Chai, D. H., & Bertozzi, C. R. (2001). Substrate specificity of the sialic acid biosynthetic pathway. Biochemistry , 40, 12864–12874. https://doi.org/10.1021/bi010862s
- Jones, M. B., Teng, H., Rhee, J. K., Baskaran, G., Lahar, N., & Yarema, K. J. (2004). Characterization of the cellular uptake and metabolic conversion of acetylated N -acetylmannosamine (ManNAc) analogues to sialic acids. Biotechnology and Bioengineering , 85, 394–405. https://doi.org/10.1002/bit.10901
- Jourdian, G. W., Dean, L., & Roseman, S. (1971). The sialic acids. XI. A periodate-resorcinol method for the quantitative estimation of free sialic acids and their glycosides. Journal of Biological Chemistry , 246, 430–435. https://doi.org/10.1016/S0021-9258(18)62508-6
- Kamerling, J. P., & Gerwig, G. J. (2006). Structural analysis of naturally occurring sialic acids. Methods in Molecular Biology , 347, 69–91. https://doi.org/10.1385/1-59745-167-3:69
- Kayser, H., Geilen, C. C., Paul, C., Zeitler, R., & Reutter, W. (1992). Incorporation of N -acyl-2-amino-2-deoxy-hexoses into glycosphingolipids of the pheochromocytoma cell line PC 12. FEBS Letters , 301, 137–140. https://doi.org/10.1016/0014-5793(92)81233-c
- Kayser, H., Zeitler, R., Kannicht, C., Grunow, D., Nuck, R., & Reutter, W. (1992). Biosynthesis of a nonphysiological sialic acid in different rat organs, using N -propanoyl-d-hexosamines as precursors. Journal of Biological Chemistry , 267, 16934–16938. https://doi.org/10.1016/S0021-9258(18)41874-1
- Keppler, O. T., Horstkorte, R., Pawlita, M., Schmidt, C., & Reutter, W. (2001). Biochemical engineering of the N -acyl side chain of sialic acid: Biological implications. Glycobiology , 11, 11R–18R. https://doi.org/10.1093/glycob/11.2.11R
- Khidekel, N., Arndt, S., Lamarre-Vincent, N., Lippert, A., Poulin-Kerstien, K. G., Ramakrishnan, B., Qasba, P. K., & Hsieh-Wilson, L. C. (2003). A chemoenzymatic approach toward the rapid and sensitive detection of O -GlcNAc posttranslational modifications. Journal of the American Chemical Society , 125, 16162–16163. https://doi.org/10.1021/ja038545r
- Khidekel, N., Ficarro, S. B., Peters, E. C., & Hsieh-Wilson, L. C. (2004). Exploring the O -GlcNAc proteome: Direct identification of O -GlcNAc-modified proteins from the brain. Proceedings of the National Academy of Sciences of the United States of America , 101, 13132–13137. https://doi.org/10.1073/pnas.0403471101
- Kim, C., Jeon, O. H., Kim, D. H., Chae, J. J., Shores, L., Bernstein, N., Bhattacharya, R., Coburn, J. M., Yarema, K. J., & Elisseeff, J. H. (2016). Local delivery of a carbohydrate analog for reducing arthritic inflammation and rebuilding cartilage. Biomaterials , 83, 93–101. https://doi.org/10.1016/j.biomaterials.2015.12.029
- Kim, E. J., Sampathkumar, S.-G., Jones, M. B., Rhee, J. K., Baskaran, G., & Yarema, K. J. (2004). Characterization of the metabolic flux and apoptotic effects of O -hydroxyl- and N -acetylmannosamine (ManNAc) analogs in Jurkat (human T-lymphoma-derived) cells. Journal of Biological Chemistry , 279, 18342–18352. https://doi.org/10.1074/jbc.M400205200
- King, E. J., & Garner, R. J. (1947). The colorimetric detection of glucose. Journal of Clinical Pathology , 1, 30–33. https://doi.org/10.1136/jcp.1.1.30
- Kondor, C. A., Gorantla, J. N., Leonard, G. D., & Fehl, C. (2022). Synthesis and mammalian cell compatibility of light-released glycan precursors for controlled metabolic engineering. Bioorganic & Medicinal Chemistry, 70, 116918. https://doi.org/10.1016/j.bmc.2022.116918
- Kontou, M., Bauer, C., Reutter, W., & Horstkorte, R. (2008). Sialic acid metabolism is involved in the regulation of gene expression during neuronal differentiation of PC12 cells. Glycoconjugate Journal , 25, 237–244. https://doi.org/10.1007/s10719-008-9104-1
- Kranaster, P., Blum, J., Dold, J. E. G. A., Wittmann, V., & Leist, M. (2022). Use of metabolic glycoengineering and pharmacological inhibitors to assess lipid and protein sialylation on cells. Journal of Neurochemistry , 164(4), 481–498. https://doi.org/10.1111/jnc.15737
- Kufleitner, M., Halbert, L. M., & Wittmann, V. (2023). Metabolic glycoengineering—Exploring glycosylation with bioorthogonal chemistry. Chemical Society Reviews , 52, 510–535. https://doi.org/10.1039/d2cs00764a
- Laughlin, S. T., Baskin, J. M., Amacher, S. L., & Bertozzi, C. R. (2008). In vivo imaging of membrane-associated glycans in developing zebrafish. Science , 320, 664–667. https://doi.org/10.1126/science.1155106
- Lee, J. H., Baker, T. J., Mahal, L. K., Zabner, J., Bertozzi, C. R., Wiemar, D. F., & Welsh, M. J. (1999). Engineering novel cell surface receptors for virus-mediated gene transfer. Journal of Biological Chemistry , 274, 21878–21884. https://doi.org/10.1074/jbc.274.31.21878
- Lemieux, G. A., & Bertozzi, C. R. (1998). Chemoselective ligation reactions with proteins, oligosaccharides and cells. Trends in Biotechnology , 16, 506–513. https://doi.org/10.1016/s0167-7799(98)01230-x
- Lemieux, G. A., & Bertozzi, C. R. (2001). Modulating cell surface immunoreactivity by metabolic induction of unnatural carbohydrate antigens. Chemistry & Biology, 8, 265–275. https://doi.org/10.1016/s1074-5521(01)00008-4
- Li, J., Wang, J., Wen, L., Zhu, H., Li, S., Huang, K., Jiang, K., Li, X., Ma, C., Qu, J., Parameswaran, A., Song, J., Zhao, W., & Wang, P. G. (2016). An OGA-resistant probe allows specific visualization and accurate identification of O -GlcNAc-modified proteins in cells. ACS Chemical Biology , 11, 3002–3006. https://doi.org/10.1021/acschembio.6b00678
- Li, W., Pan, H., He, H., Meng, X., Ren, Q., Gong, P., Jiang, X., Liang, Z., Liu, L., Zheng, M., Shao, X., Ma, Y., & Cai, L. (2019). Bio-orthogonal T cell targeting strategy for robustly enhancing cytotoxicity against tumor cells. Small , 15, 1804383. https://doi.org/10.1002/small.201804383
- Li, X., Fang, T., & Boons, G.-J. (2014). Preparation of well-defined antibody-drug conjugates through glycan remodeling and strain-promoted azide–alkyne cycloadditions. Angewandte Chemie , 53, 7179–7182. https://doi.org/10.1002/anie.201402606
- Liao, N., Zhang, D., Wu, M., Yang, H., Liu, X., & Song, J. (2021). Enhancing therapeutic effects and in vivo tracking of adipose tissue-derived mesenchymal stem cells for liver injury using bioorthogonal click chemistry. Nanoscale , 13, 1813. https://doi.org/10.1039/d0nr0727a
- Liu, T.-W., Myschyshyn, M., Sinclair, D. A., & Vocadlo, D. J. (2019). A chemical genetic method for monitoring genome-wide dynamics of O -GlcNAc turnover on chromatin-associated proteins. ACS Central Science , 5, 663–670. https://doi.org/10.1021/acscentsci.9b00044
- Ludwig, S. D., Bernstein, Z. J., Agatemor, C., Dammen-Brower, K., Ruffolo, J., Rosas, J. M., Post, J. D., Cole, R. N., Yarema, K. J., & Spangler, J. B. (2022). A versatile design platform for glycoengineering therapeutic antibodies. mAbs , 14, 2095704. https://doi.org/10.1080/19420862.2022.2095704
- Mahal, L. K., Charter, N. W., Angata, K., Fukuda, M., Koshland, D. E. Jr., & Bertozzi, C. R. (2001). A small-molecule modulator of poly-α2,8-sialic acid expression on cultured neurons and tumor cells. Science , 294, 380–381. https://doi.org/10.1126/science.1062192
- Mahal, L. K., Yarema, K. J., & Bertozzi, C. R. (1997). Engineering chemical reactivity on cell surfaces through oligosaccharide biosynthesis. Science , 276, 1125–1128. https://doi.org/10.1126/science.276.5315.1125
- Mahal, L. K., Yarema, K. J., Lemieux, G. A., & Bertozzi, C. R. (1999). Chemical approaches to glycobiology: Engineering cell surface sialic acids for tumor targeting. In Y. Inoue, Y. C. Lee, & F. A. Troy. (Eds.), Sialobiology and other novel forms of glycosylation (pp. 273–280). Gakushin Publishing Company.
- Mathew, M. P., Tan, E., Labonte, J. W., Shah, S., Saeui, C. T., Liu, L., Bhattacharya, R., Bovonratwet, P., Gray, J. J., & Yarema, K. (2017). Glycoengineering of esterase activity through metabolic flux-based modulation of sialic acid. Chembiochem , 18, 1204–1215. https://doi.org/10.1002/cbic.201600698
- Mathew, M. P., Tan, E., Shah, S., Bhattacharya, R., Meledeo, M. A., Huang, J., Espinoza, F. A., & Yarema, K. J. (2012). Extracellular and intracellular esterase processing of SCFA-hexosamine analogs: Implications for metabolic glycoengineering and drug delivery. Bioorganic & Medicinal Chemistry Letters, 22, 6929–6933. https://doi.org/10.1016/j.bmcl.2012.09.017
- Matsuno, K., & Suzuki, S. (2008). Simple fluorimetric method for quantification of sialic acids in glycoproteins. Analytical Biochemistry , 375, 53–59. https://doi.org/10.1016/j.ab.2008.01.002
- Moffett, J. R., Puthillathu, N., Vengilote, R., Jaworks, D. M., & Namboodiri, A. M. (2020). Acetate revisited: A key biomolecule at the nexus of metabolism, epigenetics, and oncogenesis — Part 2: Acetate and ACSS2 in health and disease. Frontiers in Physiology , 11, 580171. https://doi.org/10.3389/fphys.2020.580171
- Natunen, S., Lampinen, M., Suila, H., Ritamo, I., Pitkänen, V., Nairn, A. V., Räbinä, J., Laitinen, S., Moremen, K. W., Reutter, W., & Valmu, L. (2013). Metabolic glycoengineering of mesenchymal stromal cells with N -propanoylmannosamine. Glycobiology , 23, 1004–1012. https://doi.org/10.1093/glycob/cwt039
- Nauman, D. A., & Bertozzi, C. R. (2001). Kinetic parameters for small-molecule drug delivery by covalent cell surface targeting. Biochimica et Biophysica Acta , 1568, 147–154. https://doi.org/10.1016/s0304-4165(01)00211-2
- Ning, X., Guo, J., Wolfert, M. A., & Boons, G.-J. (2008). Visualizing metabolically labeled glycoconjugates of living cells by copper-free and fast Huisgen cycloadditions. Journal of Antimicrobial Chemotherapy , 47, 2253–2255. https://doi.org/10.1002/anie.200705456
- Ning, X., Temming, R. P., Dommerholt, J., Guo, J., Ania, D. B., Debets, M. F., Wolfert, M. A., Boons, G.-J., & van Delft, F. L. (2010). Protein modification by strain-promoted alkyne-nitrone cycloaddition. Angewandte Chemie , 49, 3065–3068. https://doi.org/10.1002/anie.201000408
- Okeley, N. M., Toki, B. E., Zhang, X., Jeffrey, S. C., Burke, P. J., Alley, S. C., & Senter, P. D. (2013). Metabolic engineering of monoclonal antibody carbohydrates for antibody-drug conjugation. Bioconjugate Chemistry , 24, 1650–1655. https://doi.org/10.1021/bc4002694
- Pan, H., Li, P., Li, G., Li, W., Hu, B., He, H., Chen, Z., Wang, F., Liu, L., Gong, Y., Han, Y., Luo, Y., Zheng, M., Ma, Y., Cai, L., & Jin, Y. (2019). Glycometabolic bioorthogonal chemistry-guided viral transduction for robust human T cell engineering. Advanced Functional Materials , 29, 1807528. https://doi.org/10.1002/adfm.201807528
- Patterson, D. M., Nazarova, L. A., & Prescher, J. A. (2014). Finding the right (bioorthogonal) chemistry. ACS Chemical Biology , 9, 592–605. https://doi.org/10.1021/cb400828a
- Patterson, D. M., Nazarova, L. A., Xie, B., Kamber, D. N., & Prescher, J. A. (2012). Functionalized cyclopropenes as bioorthogononal chemical reporters. Journal of the American Chemical Society , 134, 18638–18643. https://doi.org/10.1021/ja3060436
- Piller, F., Mongis, A., & Piller, V. (2015). Metabolic glyco-engineering in eukaryotic cells and selected applications. In A. Castilho (Eds.), Glyco-engineering: Methods and protocols (pp. 335–359). Springer Science+Business Media.
- Piller, V., Piller, F., & Fukuda, M. (1990). Biosynthesis of truncated O -glycans in the T cell line Jurkat. Localization of O -glycan initiation. Journal of Biological Chemistry , 265, 9264–9271.
- Pilobello, K. T., Krishnamoorthy, L., Slawek, D., & Mahal, L. K. (2005). Development of a lectin microarray for the rapid analysis of protein glycopatterns. Chembiochem , 6, 985–989. https://doi.org/10.1002/cbic.200400403
- Qin, K., Zhang, H., Zhao, Z., & Chen, X. (2020). Protein S -glyco-modification through an elimination-addition mechanism. Journal of the American Chemical Society , 140, 9382–9388. https://doi.org/10.1021/jacs.0c02110
- Qin, W., Qin, K., Fan, X., Peng, L., Hong, W., Zhu, Y., Lv, P., Du, Y., Huang, R., Han, M., Cheng, B., Liu, Y., Zhou, W., Wang, C., & Chen, X. (2018). Artificial cysteine S-glycosylation induced by per-O -acetylated unnatural monosaccharides during metabolic glycan labeling. Angewandte Chemie , 57, 1817–1820. https://doi.org/10.1002/anie.201711710
- Saeui, C. T., Nairn, A. V., Galizzi, M., Douville, C., Gowda, P., Park, M., Dharmarha, V., Shah, S. R., Clarke, A., Austin, M., Moremen, K. W., & Yarema, K. J. (2018). Integration of genetic and metabolic features related to sialic acid metabolism distinguishes human breast cell subtypes. PLoS ONE , 13, e0195812. https://doi.org/10.1371/journal.pone.0195812
- Saeui, C. T., Shah, S. R., Fernadez-Gil, B. I., Zhang, C., Agatemor, C., Dammen-Brower, K., Mathew, M. P., Buettner, M., Gowda, P., Khare, P., Otamendi-Lopez, A., Yang, S., Zhang, H., Le, A., Quinones-Hinojosa, A., & Yarema, K. J. (2023). Anticancer properties of hexosamine analogs designed to attenuate flux through the hexosamine biosynthetic pathway. ACS Chemical Biology , 18(1), 151–165. https://doi.org/10.1021/acschembio.2c00784
- Sampathkumar, S.-G., Jones, M. B., Meledeo, M. A., Campbell, C. T., Choi, S. S., Hida, K., Gomutputra, P., Sheh, A., Gilmartin, T., Head, S. R., & Yarema, K. J. (2006). Targeting glycosylation pathways and the cell cycle: Sugar-dependent activity of butyrate-carbohydrate cancer prodrugs. Chemistry & Biology, 13, 1265–1275. https://doi.org/10.1016/j.chembiol.2006.09.016
- Sampathkumar, S.-G., Jones, M. B., & Yarema, K. J. (2006). Metabolic expression of thiol-derivatized sialic acids on the cell surface and their quantitative estimation by flow cytometry. Nature Protocols , 1, 1840–1851. https://doi.org/10.1038/nprot.2006.252
- Sampathkumar, S.-G., Li, A. V., Jones, M. B., Sun, Z., & Yarema, K. J. (2006). Metabolic installation of thiols into sialic acid modulates adhesion and stem cell biology. Nature Chemical Biology , 2, 149–152. https://doi.org/10.1038/nchembio770
- Sankoh, S., Thammakhet, C., Numnuam, A., Limbut, W., Kanatharana, P., & Thavarungkul, P. (2016). 4-Mercaptophenylboronic acid functionalized gold nanoparticles for colorimetric sialic acid detection. Biosensors and Bioelectronics , 85, 743–750. https://doi.org/10.1016/j.bios.2016.05.083
- Sawa, M., Hsu, T.-L., Itoh, T., Sugiyama, M., Hanson, S. R., Vogt, P. K., & Wong, C.-H. (2006). Glycoproteomic probes for fluorescent imaging of fucosylated glycans in vivo. Proceedings of the National Academy of Sciences of the United States of America , 103, 12371–12376. https://doi.org/10.1073/pnas.0605418103
- Saxon, E., & Bertozzi, C. R. (2000). Cell surface engineering by a modified Staudinger reaction. Science , 287, 2007–2010. https://doi.org/10.1126/science.287.5460.2007
- Saxon, E., & Bertozzi, C. R. (2001). Chemical and biological strategies for engineering cell surface glycosylation. Annual Review of Cell and Developmental Biology , 17, 1–23. https://doi.org/10.1146/annurev.cellbio.17.1.1
- Scache, J., Rigolot, V., Lion, C., Mortuaire, M., Lefebvre, T., Biot, C., & Vercoutter-Edouart, A.-S. (2022). Switching azide and alkyne tags on bioorthogonal reporters in metabolic labeling of sialylated glycoconjugates: A comparative study. Scientific Reports , 12, 22129. https://doi.org/10.1038/s41598-022-26521-3
- Schart, V. F., Hassenrück, J., Späte, A.-K., Dold, J. E. G. A., Fahrner, R., & Wittmann, V. (2019). Triple orthogonal labeling of glycans by applying photoclick chemistry. Chembiochem , 20, 166–171. https://doi.org/10.1002/cbic.201800740
- Schilling, B., Goon, S., Samuels, N. M., Gaucher, S. P., Leary, J. A., Bertozzi, C. R., & Gibson, B. W. (2001). Biosynthesis of sialylated lipooligosaccharides in Haemophilus ducreyi is dependent on exogenous sialic acid and not mannosamine. Incorporation studies using N -acylmannosamine analogues, N -glycolylneuraminic acid, and 13C-labeled N -acetylneuraminic acid. Biochemistry , 40, 12666–12677. https://doi.org/10.1021/bi0107849
- Schmidt, C., Stehling, P., Schnitzer, J., Reutter, W., & Horstkorte, R. (1998). Biochemical engineering of neural cell surfaces by the synthetic N -propanoyl-substituted neuraminic acid precursor. Journal of Biological Chemistry , 273, 19146–19152. https://doi.org/10.1074/jbc.273.30.19146
- Shajahan, A., Supekar, N. T., Wu, H., Wands, A. M., Bhat, G., Kalimurthy, A., Matsubara, M., Ranzinger, R., Kohler, J. J., & Azadi, P. (2020). Mass spectrometric method for the unambiguous profiling of cellular dynamic glycosylation. ACS Chemical Biology , 15, 2692–2701. https://doi.org/10.1021/acschembio.0c00453
- Sletten, E. M., & Bertozzi, C. R. (2009). Bioorthogonal chemistry: Fishing for selectivity in a sea of functionality. Angewandte Chemie , 48, 6974–6998. https://doi.org/10.1002/anie.200900942
- Smirnov, I., Sibgatullina, R., Urano, S., Tahara, T., Ahmadi, P., Watanabe, Y., Pradipta, A. R., Kurbangalieva, A., & Tanaka, K. (2020). A strategy for tumor targeting by higher-order glycan pattern recognition: Synthesis and in vitro and in vivo properties of glycoalbumins conjugated with four different N -glycan molecules. Small , 16, 2004831. https://doi.org/10.1002/smll.202004831
- Soares da Costa, D., Sousa, J. C., Dá Mesquita, S., Petkova-Yankova, N. I., Marques, F., Reis, R. L., Sousa, N., & Pashkuleva, I. (2020). Bioorthogonal labeling reveals different expression of glycans in mouse hippocampal neuron cultures during their development. Molecules , 25, 795. https://doi.org/10.3390/molecules25040795
- Späte, A.-K., Schart, V. F., Schöllkpf, S., Niederwieser, A., & Wittmann, V. (2014). Terminal alkenes as versatile chemical reporter groups for metabolic oligosaccharide engineering. Chemistry: A European Journal , 20, 16502–16508. https://doi.org/10.1002/chem.201404716
- Srinivasan, K., Roy, S., Washburn, N., Sipsey, S. F., Meccariella, R., Meador, J. W. III, Ling, L. E., Manning, A. M., & Kaundinya, G. V. (2015). A quantitative microtiter assay for sialylated glycoform analyses using lectin complexes. Journal of Biomolecular Screening , 20, 768–778. https://doi.org/10.1177/1087057115577597
- Stabach, P. R., Zimmerman, K., Adame, A., Kavanagh, D., Saeui, C. T., Agatemor, C., Gray, S., Cao, W., de La Cruz, E., Yarema, K. J., & Braddock, D. T. (2021). Improving the pharmacodynamics and in vivo activity of ENPP1-Fc through protein and glycosylation engineering. Clinical and Translational Science , 14, 362–372. https://doi.org/10.1111/cts.12887
- Stanton, P. C., Shen, Z., Kecorius, E. A., Burgon, P. G., Robertson, D. M., & Hearn, M. T. W. (1995). Application of a sensitive HPLC-based fluorometric assay to determine the sialic acid content of human gonadotropin isoforms. Journal of Biochemical and Biophysical Methods , 30, 37–48. https://doi.org/10.1016/0165-022X(94)00063-J
- Sun, L., Konstantinidi, A., Ye, Z., Nason, R., Zhang, Y., Büll, C., Kahl-Knutson, B., Hansen, L., Leffler, H., Vakhrushev, S. Y., Yang, Z., Clausen, H., & Narimatsu, Y. (2022). Installation of O -glycan sulfation capacities in human HEK293 cells for display of sulfated mucins. Journal of Biological Chemistry , 298, 101382. https://doi.org/10.1016/j.jbc.2021.101382
- Sussich, F., & Cesàro, A. (2000). The kinetics of periodate oxidation of carbohydrates: A calorimetric approach. Carbohydrate Research , 329, 87–95. https://doi.org/10.1016/S0008-6215(00)00158-0
- Takayama, Y., Kusamori, K., & Nishikawa, M. (2019). Click chemistry as a tool for cell engineering and drug delivery. Molecules , 24, 172. https://doi.org/10.3390/molecules24010172
- Tanaka, Y., & Kohler, J. J. (2008). Photoactivatable crosslinking sugars for capturing glycoprotein interactions. Journal of the American Chemical Society , 130, 3278–3279. https://doi.org/10.1021/ja7109772
- Termini, J. M., Silver, Z. A., Connor, B., Antonopoulos, A., Haslam, S. M., Dell, A., & Desrosiers, R. C. (2017). HEK298T cell lines defective for O -linked glycosylation. PLoS ONE , 12, e0179949. https://doi.org/10.1371/journal.pone.0179949
- Tian, Y., Almaraz, R. T., Choi, C. H., Li, Q. K., Saeui, C., Li, D., Shah, P., Bhattacharya, R., Yarema, K. J., & Zhang, H. (2015). Identification of sialylated glycoproteins from metabolically oligosaccharide engineered pancreatic cells. Clinical Proteomics , 12, 11. https://doi.org/10.1186/s12014-015-9083-8
- Tiziani, S., & Cesàro, A. (2003). The kinetics of periodate oxidation of carbohydrates. 2. Polymeric substrates. Carbohydrate Research , 338, 1083–1095. https://doi.org/10.1016/S0008-6215(03)00082-X
- Vanbeselaere, J., Vicogne, D., Matthijs, G., Biot, C., Foulquier, F., & Guerardel, Y. (2013). Alkynyl monosaccharide analogues as a tool for evaluating Golgi glycosylation efficiency: Application to congenital disorders of glycosylation (CDG). Chemical Communications , 49, 11293. https://doi.org/10.1039/c3cc45914d
- Varki, A. (1993). Biological roles of oligosaccharides: All of the theories are correct. Glycobiology , 3, 97–130. https://doi.org/10.1093/glycob/3.2.97
- Villavicencio-Lorini, P., Laabs, S., Danker, K., Reutter, W., & Horstkorte, R. (2002). Biochemical engineering of the acyl side chain of sialic acids stimulates integrin-dependent adhesion of HL60 cells to fibronectin. Journal of Molecular Medicine , 80, 671–677. https://doi.org/10.1007/s00109-002-0382-y
- Viswanathan, K., Lawrence, S., Hinderlich, S., Yarema, K. J., Lee, Y. C., & Betenbaugh, M. (2003). Engineering sialic acid synthetic ability into insect cells: Identifying metabolic bottlenecks and devising strategies to overcome them. Biochemistry , 42, 15215–15225. https://doi.org/10.1021/bi034994s
- Wainman, Y. A., Neves, A. A., Stairs, S., Stöckmann, H., Ireland-Zecchini, H., Brindle, K. M., & Leeper, F. J. (2013). Dual-sugar imaging using isonitrile and azido-based click chemistries. Organic & Biomolecular Chemistry, 11, 7297–7300. https://doi.org/10.1039/c3ob41805g
- Walborg, E. F. Jr., & Christensson, L. (1965). A colorimetric method for the quantitative determination of monosaccharides. Analytical Biochemistry , 13, 186–193. https://doi.org/10.1016/0003-2697(65)90188-0
- Wang, H., & Mooney, D. J. (2020). Metabolic glycan labelling for cancer-targeted therapy. Nature Chemistry , 12, 1102–1114. https://doi.org/10.1038/s41557-020-00587-w
- Wang, H., Wang, R., Cai, K., He, H., Liu, Y., Yen, J., Wang, Z., Xu, M., Sun, Y., Zhou, X., Yin, Q., Tang, L., Dobrucki, I. T., Dobrucki, L. W., Chaney, E. J., Boppart, S. A., Fan, T. M., Lezmi, S., Chen, X., … Cheng, J. (2017). Selective in vivo metabolic cell-labeling-mediated cancer targeting. Nature Chemical Biology , 13, 415–424. https://doi.org/10.1038/nchembio.2297
- Wang, Q., Chung, C.-Y., Yang, W., Yang, G., Chough, S., Chen, Y., Yin, B., Bhattacharya, R., Hu, Y., Saeui, C. T., Yarema, K. J., Betenbaugh, M. J., & Zhang, H. (2019). Combining butyrated ManNAc with glycoengineered CHO cells improves EPO glycan quality and production. Biotechnology Journal , 14, e1800186. https://doi.org/10.1002/biot.201800186
- Wang, Z., Du, J., Che, P.-L., Meledeo, M. A., & Yarema, K. J. (2009). Hexosamine analogs: From metabolic glycoengineering to drug discovery. Current Opinion in Chemical Biology , 13, 565–572. https://doi.org/10.1016/j.cbpa.2009.08.001
- Weinbaum, S., Tarbell, J. M., & Damiano, E. R. (2007). The structure and function of the endothelial glycocalyx layer. Annual Review of Biomedical Engineering , 9, 121–167. https://doi.org/10.1146/annurev.bioeng.9.060906.151959
- Wen, X., Yuan, B., Zhang, J., Meng, X., Guo, Q., Li, L., LI, Z., Jiang, H., & Wang, K. (2019). Enhanced visualization of cell surface glycans via a hybridization chain reaction. Chemical Communications , 55, 6114. https://doi.org/10.1039/c9cc02069a
- Wratil, P. R., & Horstkorte, R. (2017). Metabolic glycoengineering of sialic acid using N -acyl-modified mannosamines. Journal of Visualized Experiments , 129, e55746. https://doi.org/10.3791/55746
- Wratil, P. R., Horstkorte, R., & Reutter, W. (2016). Metabolic glycoengineering with N -acyl side chain modified mannosamines. Angewandte Chemie , 55, 9482–9512. https://doi.org/10.1002/anie.201601123
- Wu, Q., Ye, J., Chao, Y., Dong, S., Niu, M., Wang, Y., Liu, Z., Chen, W., Ge, N., Lu, S., Wang, P. G., & Chen, M. (2022). Chemoenzymatic labeling pathogens containing N -acetylneuraminic acid-α(2,3)-galactose glycans. ACS Infectious Diseases , 8, 657–664. https://doi.org/10.1021/acsinfecdis.2c00011
- Xia, L., & Gildersleeve, J. C. (2015). The glycan array platform as a tool to identify carbohydrate antigens. Methods in Molecular Biology , 2015, 1331, 27–40. https://doi.org/10.1007/978-1-4939-2874-3_3
- Xiong, D.-C., Zhu, J., Han, M.-J., Luo, H.-X., Wang, C., Yu, Y., Ye, Y., Tai, G., & Ye, X.-S. (2015). Rapid probing of sialylated glycoproteins in vitro and in vivo via metabolic oligosaccharide engineering of a minimal cyclopropene reporter. Organic & Biomolecular Chemistry, 13, 3911–3917. https://doi.org/10.1039/c5ob00069f
- Yarema, K. J. (2002). A metabolic substrate-based approach to engineering new chemical reactivity into cellular sialoglycoconjugates. In M. Al-Rubeai (Ed.), Cell engineering (Vol. 3, Glycosylation) (pp. 171–196). Kluwer Academic Publishers.
- Yarema, K. J., Goon, S., & Bertozzi, C. R. (2001). Metabolic selection of glycosylation defects in human cells. Nature Biotechnology , 19, 553–558. https://doi.org/10.1038/89305
- Yarema, K. J., Mahal, L. K., Bruehl, R. E., Rodriguez, E. C., & Bertozzi, C. R. (1998). Metabolic delivery of ketone groups to sialic acid residues. Application to cell surface glycoform engineering. Journal of Biological Chemistry , 273, 31168–31179. https://doi.org/10.1074/jbc.273.47.31168
- Yin, B., Wang, Q., Chung, C.-Y., Bhattacharya, R., Ren, X., Tang, J., Yarema, K. J., & Betenbaugh, M. J. (2017). A novel sugar analog enhances sialic acid production and biotherapeutic sialylation in CHO cells. Biotechnology and Bioengineering , 114, 1899–1902. https://doi.org/10.1002/bit.26291
- Zanghi, J. A., Mendoza, T. P., Schmelzer, A. E., Knop, R. H., & Miller, W. M. (1998). Role of nucleotide sugar pools in the inhibition of NCAM polysialylation by ammonia. Biotechnology Progress , 14, 834–844. https://doi.org/10.1021/bp9800945
- Zhang, H., Li, X.-J., Martin, D. B., & Aebersold, R. (2003). Identification and quantification of N-linked glycoproteins using hydrazide chemistry, stable isotope labeling and mass spectrometry. Nature Biotechnology , 21, 660–666. https://doi.org/10.1038/nbt827
- Zhang, X., LI, R., Chen, Y., Zhang, S., Wang, W., & Li, F. (2016). Applying DNA rolling circle amplification in fluorescence imaging of cell surface glycans labeled by a metabolic method. Chemical Science , 7, 6182. https://doi.org/10.1039/c6sc02089E
- Zhu, L., Xu, Y., Wei, X., Lin, H., Huang, M., Lin, B., Song, Y., & Yang, C. (2021). Coupling aptamer-based protein tagging with metabolic glycan labeling for in situ visualization and biological function study of exosomal protein-specific glycosylation. Angewandte Chemie , 60, 18111–18115. https://doi.org/10.1002/anie.202103696
Citing Literature
Number of times cited according to CrossRef: 1
- Jian Du, Xiao Liu, Subash Marasini, Zhuoran Wang, Kris Dammen‐Brower, Kevin J. Yarema, Xiaofeng Jia, Metabolically Glycoengineered Neural Stem Cells Boost Neural Repair After Cardiac Arrest, Advanced Functional Materials, 10.1002/adfm.202309866, 34 , 17, (2023).