Assays to Study Enzymatic and Non-Enzymatic Protein Lysine Acetylation In Vitro
Leonie G. Graf, Leonie G. Graf, Robert Vogt, Robert Vogt, Anna-Theresa Blasl, Anna-Theresa Blasl, Chuan Qin, Chuan Qin, Sabrina Schulze, Sabrina Schulze, Daniela Zühlke, Daniela Zühlke, Susanne Sievers, Susanne Sievers, Michael Lammers, Michael Lammers
Abstract
Proteins can be lysine-acetylated both enzymatically, by lysine acetyltransferases (KATs), and non-enzymatically, by acetyl-CoA and/or acetyl-phosphate. Such modification can be reversed by lysine deacetylases classified as NAD+-dependent sirtuins or by classical Zn2+-dependent deacetylases (KDACs). The regulation of protein lysine acetylation events by KATs and sirtuins/KDACs, or by non-enzymatic processes, is often assessed only indirectly by mass spectrometry or by mutational studies in cells. Mutational approaches to study lysine acetylation are limited, as these often poorly mimic lysine acetylation. Here, we describe protocols to assess the direct regulation of protein lysine acetylation by both sirtuins/KDACs and KATs, as well as non-enzymatically. We first describe a protocol for the production of site-specific lysine-acetylated proteins using a synthetic biological approach, the genetic code expansion concept (GCEC). These natively folded, lysine-acetylated proteins can then be used as direct substrates for sirtuins and KDACs. This approach addresses various limitations encountered with other methods. First, results from sirtuin/KDAC-catalyzed deacetylation assays obtained using acetylated peptides as substrates can vary considerably compared to experiments using natively folded substrate proteins. In addition, producing lysine-acetylated proteins for deacetylation assays by using recombinantly expressed KATs is difficult, as these often do not yield proteins that are homogeneously and quantitatively lysine acetylated. Moreover, KATs are often huge multi-domain proteins, which are difficult to recombinantly express and purify in soluble form. We also describe protocols to study the direct regulation of protein lysine acetylation, both enzymatically, by sirtuins/KDACs and KATs, and non-enzymatically, by acetyl-CoA and/or acetyl-phosphate. The latter protocol also includes a section that explains how specific lysine acetylation sites can be detected by liquid chromatography coupled to tandem mass spectrometry (LC-MS/MS). The protocols described here can be useful for providing a more detailed understanding of the enzymatic and non-enzymatic regulation of lysine acetylation sites, an important aspect to judge their physiological significance. © 2021 The Authors. Current Protocols published by Wiley Periodicals LLC.
Basic Protocol 1 : Preparation of N-(ε)-lysine-acetylated proteins using the genetic code expansion concept (GCEC)
Basic Protocol 2 : In vitro sirtuin (SIRT)-catalyzed deacetylation of lysine-acetylated proteins prepared by the GCEC
Basic Protocol 3 : In vitro KDAC/HDAC-catalyzed deacetylation of lysine-acetylated proteins
Basic Protocol 4 : In vitro lysine acetylation of recombinantly expressed proteins by lysine acetyltransferases (KATs)
Basic Protocol 5 : In vitro non-enzymatic lysine acetylation of proteins by acetyl-CoA and/or acetyl-phosphate
INTRODUCTION
Lysine acetylation is a post-translational modification that was originally found in the 1960s in histones, affecting RNA synthesis (Allfrey, Faulkner, & Mirsky, 1964; Phillips, 1963). Today, it is known that lysine acetylation is present in thousands of proteins in organisms of all domains of life, regulating fundamental cellular processes (Choudhary et al., 2009; Lundby et al., 2012; Weinert et al., 2011). A dysfunction in the post-translational machinery responsible for this modification is associated with the development of severe diseases in humans, ranging from neurodegenerative disorders and cancer to metabolic diseases. Moreover, it is directly involved in the aging process of organisms (Imai & Guarente, 2016; Zullo, Simone, Grimaldi, Musto, & Mancini, 2018). Identifying proteins subjected to this modification and understanding its regulation, is, thus, of significant relevance.
Lysine acetylation is tightly connected to the cellular metabolic state, as lysine acetyltransferases, enzymes that catalyze protein lysine acetylation, use the central metabolic molecule acetyl-CoA as donor molecule for the acetylation of the ε-amino group of lysine side chains, and sirtuins (SIRT: silent information regulator), the corresponding deacetylases, use NAD+ as a stoichiometric co-substrate for catalysis (Fig. 1A; Albaugh, Arnold, & Denu, 2011; Dittenhafer-Reed, Feldman, & Denu, 2011; Feldman, Dittenhafer-Reed, & Denu, 2012; Schmidt, Smith, Jackson, & Denu, 2004). In addition to enzymatic acetylation, non-enzymatic acetylation has been described in eukaryotes and prokaryotes (Dieterich et al., 2019; Weinert et al., 2013). The level of non-enzymatic acetylation depends on the concentration of the high-energy molecules acetyl-CoA and acetyl-phosphate, the major drivers of non-enzymatic acetylation in eukaryotes and in bacteria, respectively, (Kuhn et al., 2014; Weinert et al., 2013; Fig. 1A). Notably, recent reports suggest that non-enzymatic, chemical acetylation can even occur in a site-specific manner, and protein sequence and structure might affect its efficiency (Baeza, Smallegan, & Denu, 2015; Chen & Li, 2019; Lindahl, Lawton, Baeza, Dowell, & Denu, 2019; Wang, You, & Ye, 2017; Fig. 1A).
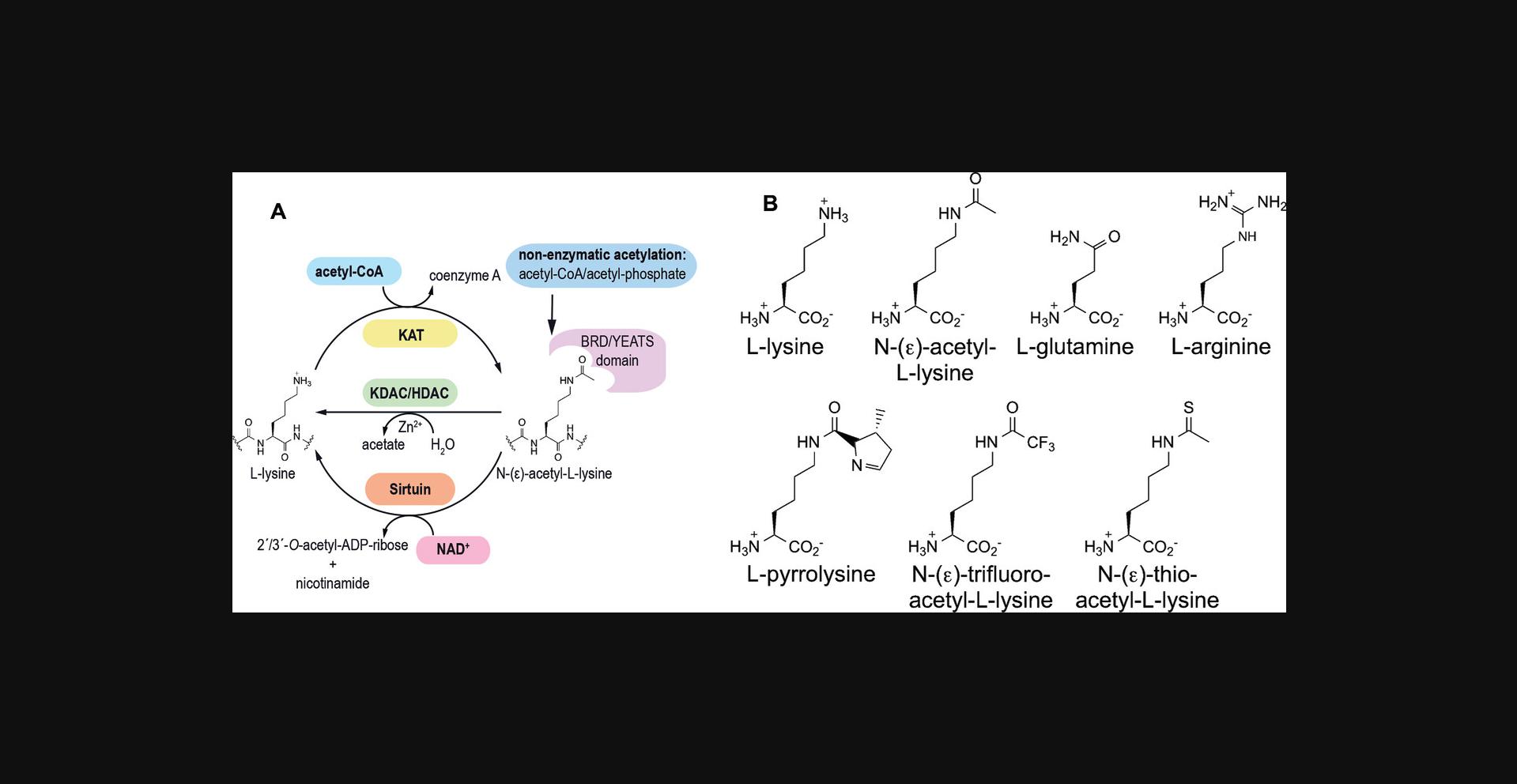
The massive progress in mass spectrometry (MS) in the last two decades has enabled the identification of thousands of lysine acetylation sites in proteins from organisms of all domains of life (Baeza et al., 2014; Baeza et al., 2020; Choudhary et al., 2009; Choudhary, Weinert, Nishida, Verdin, & Mann, 2014; Thomas, Haws, Borth, & Denu, 2020; Weinert et al., 2015; Weinert et al., 2018; Weinert et al., 2011). Importantly, however, these experiments have limitations in assessing whether acetyl transfer events are direct or indirect with regard to an enzyme-substrate connection. Follow-up experiments with purified materials are needed to complement such genetic studies.
Other reported methods to identify KAT/KDAC/sirtuin substrates employ acetylated peptides or microarrays consisting of small acetylated/non-acetylated peptides spotted on a surface (Rauh et al., 2013; Schutkowski, Fischer, Roessler, & Steegborn, 2014). These experiments assume that the primary sequence is the major molecular determinant of substrate specificity, and do not take into account that the three-dimensional structure of the protein can have a profound effect on the recognition of an individual acetylation site by lysine deacetylases. We have shown, by using natively folded proteins that have been site-specifically lysine acetylated as substrates, that the protein structure is a major factor determining substrate specificity for sirtuins and acetyltransferases (Knyphausen et al., 2016). Again, it is important to then validate sites identified by these microarray methods in follow-up experiments and to evaluate the contribution of protein structure and, ultimately, the physiological importance of these sites.
Several laboratories have performed mutational studies not only in vivo , in mammalian cells, but also in vitro , using recombinantly expressed and purified proteins, to derive the role of lysine acetylation and its consequences on protein function. Mutation of lysine to glutamine (Lys to Gln) is often used in these studies as a mimic for acetyl lysine, and mutation of lysine to arginine (Lys to Arg) is typically used to conserve the non-acetylated state (Fig. 1B). However, from a chemical perspective, the structural mimicry of acetyl-lysine (AcK) by Gln and that of Lys by Arg is rather crude in terms of sterics and physicochemical properties (Fig. 1B; de Boor et al., 2015; Knyphausen, Lang, Baldus, Extra, & Lammers, 2016). In fact, it has been shown that mutation of Lys to Gln is often a poor mimic of lysine acetylation at the molecular level. Apart from these points, recruitment of a lysine-acetylated protein to a reader domain [bromodomain (BRD), YEATS domain] is not captured by these mutations (Fig. 1B). Moreover, it is not clear if these mutations can mimic crosstalk of lysine acetylation with other post-translational modifications. These examples demonstrate that it is important to show the reliability of using these mutations to study lysine acetylation, particularly in cellular studies, in order to draw valid conclusions from the experiments and not misinterpret the results and the physiological consequences of a lysine-acetylation event.
A major step in the field was the development of the genetic code expansion concept (GCEC), which enables the study of the real consequences of a site-specific lysine acetylation in a protein, in its natively folded state (Kuhlmann et al., 2016; Lammers, 2018; Lammers, Neumann, Chin, & James, 2010; Neumann, Peak-Chew, & Chin, 2008). This system is based on a synthetically evolved orthogonal acetyl-lysyl-tRNA-synthetase (AcKRS3)/tRNACUA (PylT)-pair originating from pyrrolysyl-tRNA-synthetase (PylRS) from methanogenic archaea of the genus Methanosarcina. We have used the acetyl-lysyl-tRNA-synthetase/tRNACUA pair from M. barkeri to prepare proteins that are homogenously, site-specifically, and quantitatively lysine acetylated. Moreover, the proteins used in this system are natively folded, which is a major advantage in studying the role of lysine acetylation in regulating protein function compared to acetylated peptides, which often do not represent the correct three-dimensional structural context. The AcKRS3/PylT pair from M. barkeri has been shown to be orthogonal in all model organisms, i.e., it does not interfere with the endogenous translation system. AcKRS3 charges the cognate tRNA PylT with acetyl-L-lysine, and this can thereby be co-translationally incorporated into proteins in response to an amber stop codon, using the endogenous cellular translational machinery.
Here, we describe protocols that help address some of the limitations explained above to directly study the regulation of protein lysine acetylation, both enzymatically, by sirtuins/KDACs as well as KATs, and non-enzymatically, by acetyl-CoA and acetyl-phosphate. These include a protocol for producing a protein that is natively folded and site-specifically and homogenously lysine-acetylated via the GCEC, in yields and quality compatible with biophysical studies, including X-ray crystallography (Basic Protocol 1; Fig. 2A). The precise lysine acetylation afforded by this approach permits a more accurate determination of its potential regulatory roles. Moreover, genetic incorporation of acetyl lysine allows the investigator to assess the real consequences of a protein lysine-acetylation event, rather than using Lys to Gln or Lys to Arg mutants as it is often done in cell culture experiments.
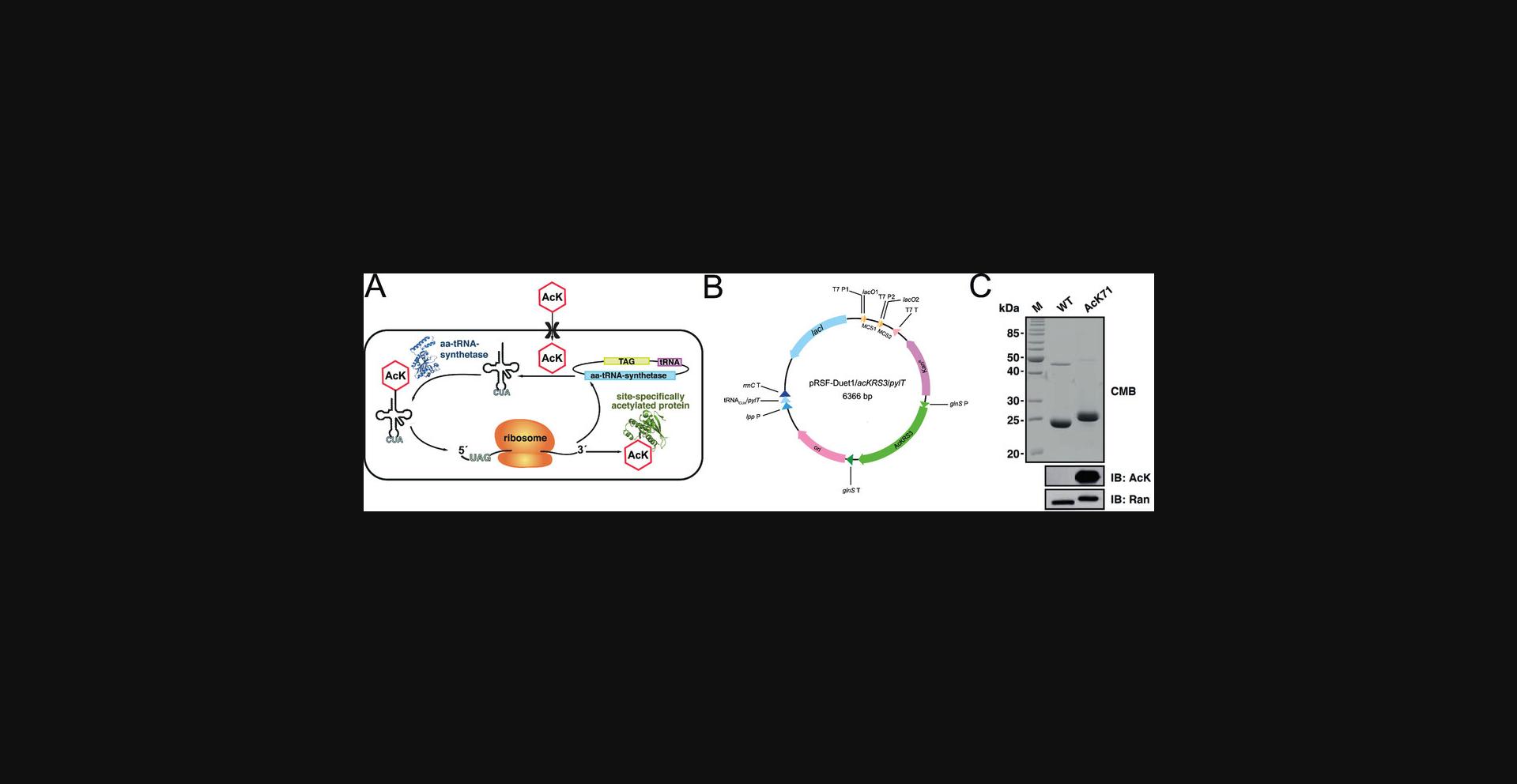
While most mass spectrometry−based data on KDACs/sirtuins, KATs, and non-enzymatic acetylation driven by acetyl-phosphate/acetyl-CoA do not reveal direct substrates, recombinantly expressed and purified acetylated and non-acetylated proteins are often used to identify direct targets of these enzymatic and non-enzymatic reactions. Knowledge about the regulation of an individual acetylation event is important to judge its cellular spatial and temporal coordination, as well as its physiological significance. We thus describe protocols for using site-specifically acetylated proteins as substrates for sirtuins, allowing the characterization of deacetylation reactions catalyzed by these enzymes (Basic Protocol 2). Moreover, this allows studying enzyme activities in their natural sequence and structural context rather than using reporter peptides, such as Fluor-de-Lys peptides, which are mostly histone-derived or p53-derived peptides. We also describe a protocol that includes preparation of acetylated proteins to then study the deacetylation catalyzed by classical deacetylases (Basic Protocol 3). To complete the assessment of the lysine acetylation cycle, we also describe the preparation of non-acetylated proteins to study their acetylation, both enzymatically, by lysine acetyltransferases (Basic Protocol 4), and non-enzymatically, by acetyl-CoA and/or acetyl-phosphate (Basic Protocol 5). In combination with LC-MS/MS analyses, this facilitates the direct identification of individual lysine substrate sites in proteins. An overview of the protocols described in this article is shown in Figure 3.
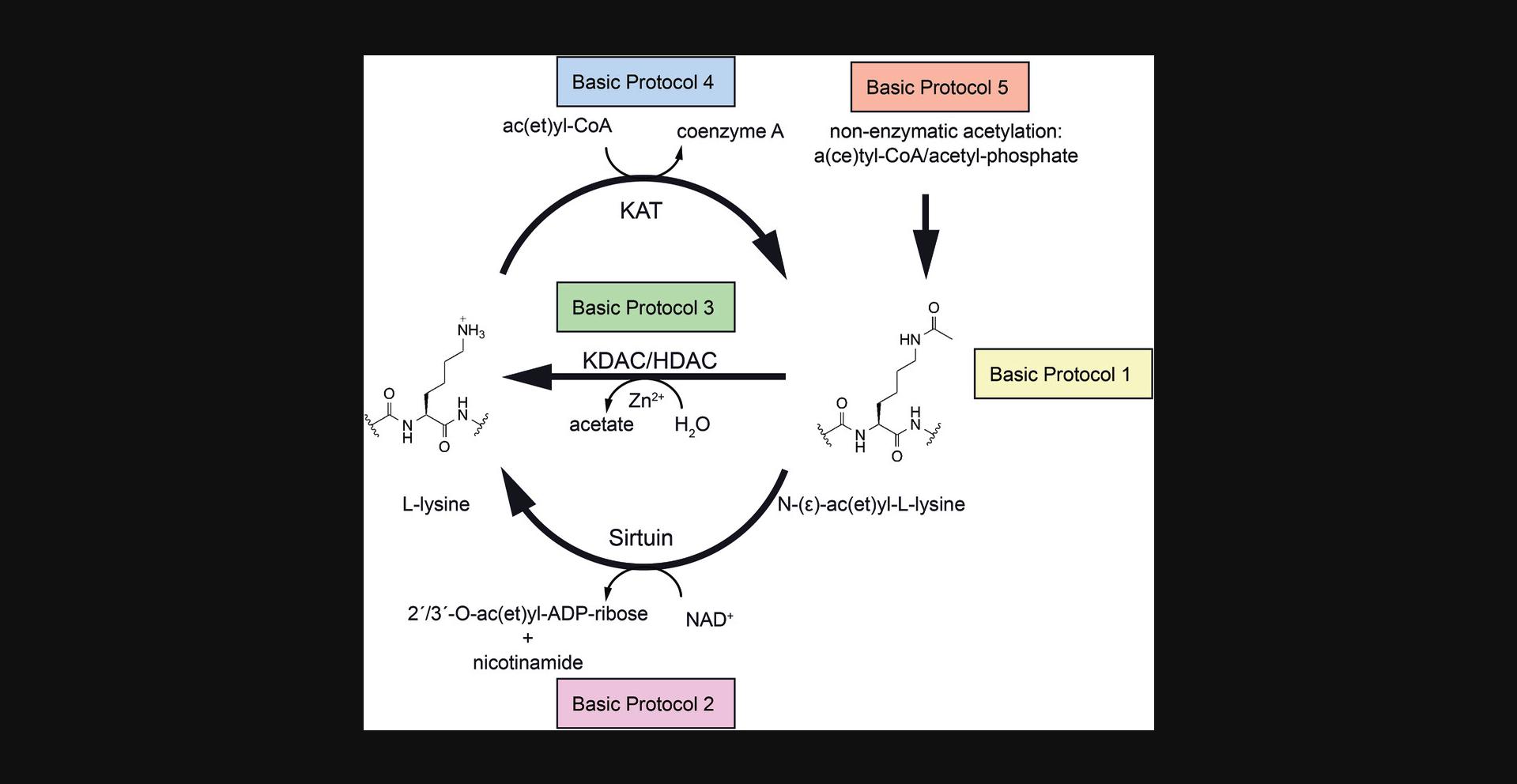
Overall, these protocols aim to allow users to gain a comprehensive understanding of the regulation of lysine acetylation of a protein, both enzymatically and non-enzymatically, to judge the physiological significance of an individual lysine acetylation site.
Strategic Planning
For the preparation of site-specifically lysine-acetylated proteins using the GCEC, the gene encoding the desired protein of interest (POI) carrying an amber stop codon (POIUAG) must first be cloned into an expression plasmid. Either the expression is performed using a one-plasmid system—encoding for the protein of interest, the Methanosarcina barkeri acetyl-lysyl-tRNA synthetase (AcKRS3), and the Mb tRNACUA (Fig. 2B)—or a two-plasmid one. In the latter case, the coding region of the protein of interest can be cloned together with the Mb tRNACUA, including the promoter and terminator regions, in one plasmid, and the coding region for acyl-lysyl-tRNA synthetase, including promoter and terminator regions, can be cloned in another plasmid. We constructed a vector based on pRSF-Duet1 that encodes for the synthetically evolved acetyl-lysyl-tRNA-synthetase(AcKRS3)/Mb tRNACUA (PylT)-pair from Methanosarcina barkeri next to the protein of interest (Fig. 2B). Here, the expression of acKRS3 is under the control of a constitutive glutaminyl-tRNA-synthetase promotor (glnS P) and terminator (glnS T), and pylT expression is under control of a constitutive lipoprotein (lpp)-promotor and rrnC terminator (Fig. 2B; Lammers, 2018). In pRSF-Duet1, the gene of interest is expressed under the control of a T7 promotor (T7 P) and T7 terminator (T7 T). This allows inducing expression by adding IPTG. Importantly, the origins of replication of both plasmids should belong to different incompatibility groups, and they should contain genes encoding for different antibiotic resistances, to allow propagation and maintenance in E. coli.
For the other protein purification protocols described here, appropriate expression constructs must be prepared beforehand. The choice of the expression plasmid depends on several factors such as solubility of the target protein and compatibility with buffer components needed for the purification, such as imidazole, which is needed for elution of a His6-tagged protein. Small-scale tests can be performed in advance to define the optimal expression and purification conditions before applying the GCEC to initiate expression and purification of the acetylated protein. Optimization parameters should include: expression host, expression temperature and duration, IPTG concentration, method used for cell lysis (sonication, French press, microfluidics, lysozyme, etc.), the buffer system, and further buffer components used for resuspension and for purification and optimization of the purification strategy. The purification of proteins should be conducted as quickly as possible in a continuous process to ensure that unstable proteins do not precipitate during the process. Proteins should be shock-frozen in liquid nitrogen in small aliquots to avoid repeated freeze-thaw cycles, and then stored long-term at −80°C. The optimal purification conditions, including purification affinity tags, buffer components, and buffer pH, should be analyzed carefully to obtain properly folded proteins in high yield. In that context, it is also recommended to analyze if codon optimization for E. coli expression results in an increase in the yield of soluble protein. In our experience, this can increase the amount of soluble target protein. Several companies offer the synthesis of codon-optimized genes for expression in various expression hosts.
For the purification of the classical Zn2+-dependent KDACs (Basic Protocol 3), prepare a Zn2+-charged Zn2+-NTA-column in advance. To this end, connect an Ni2+-NTA column to an automated FPLC system. Strip the column with 2 column volumes (CVs) of 0.1 M EDTA, wash with 10 CVs of water, recharge the column with 3 CVs of 0.1 M ZnCl2 solution, and wash again with 5 CVs of water before equilibrating it with KDAC lysis buffer.
To select the correct size-exclusion chromatography (SEC) column type for the purification of the target protein on a preparative scale, perform analytical SEC runs beforehand. These analytical SEC analyses can be performed on different columns (S200 Increase 10/300 GL, S75 Increase 10/300 GL, Superose 6 Increase 10/300 GL) to determine the elution volume and the oligomeric state of the target protein.
Basic Protocol 1: PREPARATION OF N-(ε)-LYSINE-ACETYLATED PROTEINS USING THE GENETIC CODE EXPANSION CONCEPT (GCEC)
This protocol describes the generation of site-specifically acetylated proteins that can be used as substrates for deacetylases to analyze deacetylation by sirtuin deacetylases and/or classical deacetylases (KDACs). To generate site-specifically lysine-acetylated proteins, the genetic code expansion concept (GCEC) represents a powerful tool. The GCEC is based on a synthetically evolved orthogonal acetyl-lysyl-tRNA-synthetase (AcKRS3)/tRNACUA pair derived from the pyrrolysyl-tRNA-synthetase (PylRS)/tRNACUA (PylT) pair from an archaea of the genus Methanosarcina. Here, as an example, we describe the site-specific lysine acetylation of the small GTP-binding protein Ran at Lys71 (Ran AcK71), applying the GCEC (Fig. 2A), using a one-plasmid system. The application of the GCEC allows the production of site-specifically and quantitatively lysine-acetylated proteins, which are natively folded (Knyphausen et al., 2016; Lammers, 2018). These natively folded proteins can then be used to study sirtuin- (Basic Protocol 2) and KDAC-catalyzed (Basic Protocol 3) deacetylation.
Materials
-
Competent E. coli BL21 (DE3) cells (Novagen®; Sigma-Aldrich, cat. no: 69450)
-
Plasmid pRSF-Duet1 (Sigma-Aldrich, cat. no.: 71341) expressing ackRS3 /MbpylT/ranUAG71 (see Strategic Planning)
-
Lysogeny broth (LB) agar plates (see recipe) containing 50 µg ml-1 kanamycin
-
Lysogeny broth (LB) medium (sterilize by autoclaving) (see recipe)
-
Kanamycin sulfate (50 mg ml-1 stock in H2O; Carl Roth, CAS no. 25389-94-0)
-
1 M N-(ε)-acetyl-L-lysine (Chem-Impex Int.; CAS no. 692-04-6, cat. no. 05364) in sterile-filtered (0.22-µm filter) deionized water (prepare fresh)
-
2 M nicotinamide (Sigma-Aldrich; CAS no. 98-92-0, cat. no. N3376) in sterile-filtered (0.22-µm filter) deionized water (prepare fresh)
-
Isopropyl β-D-1-thiogalactopyranoside (IPTG; 1 M stock in H2O; Carl Roth, CAS no. 367-93-1)
-
Buffers 1.1, 1.2, 1.3, 1.4, and 1.5 (see recipes)
-
Liquid nitrogen (CAUTION : handle with care; use protective equipment for extremely low temperatures and provide a well-ventilated environment)
-
5× SDS-PAGE sample buffer (see recipe)
-
12% v/v SDS-PAGE gel (see recipe)
-
SDS-PAGE running buffer (see recipe)
-
SDS-PAGE staining solution (see recipe)
-
SDS-PAGE destaining solution (see recipe)
-
100-ml and 5-L baffled Erlenmeyer flasks (VWR, cat. no. 214-1131 and 214-1137)
-
Incubator (e.g., Function Line B20; Heraeus Holding GmbH)
-
Shaking incubator (e.g., Infors HT Multitron Standard; Infors AG)
-
UV/Vis spectrophotometer (e.g., Varian Cary 50 UV-Vis spectrophotometer or NanoDrop ND 1000, VWR International GmbH)
-
Heat block (e.g., Mixing Block MB-102, Bioer Technology Co.; and Digital Heatblock, VWR International GmbH)
-
Refrigerated microcentrifuge
-
Centrifuge (e.g., SORVALL RC 6 with Rotor SLA-1500 and Rotor SS-34, Thermo Fisher GmbH)
-
250-ml glass beaker (VWR, cat. no. 213-1121)
-
Sonicator [e.g., Sonopulse HD 4100 with UW 100, TS133V, SH 100G, and tip TT213 (ᴓ12.7 mm); BANDELIN Electronic GmbH & Co. KG, Berlin, Germany]
-
5-ml HisTrapTM FF crude column (Cytiva, cat. no. 17-5286-01)
-
Automated FPLC system and fraction collector (Cytiva, Bio-Rad)
-
Sterile bottle-top filters, 0.45-μm pore size
-
Electrophoresis chamber and power supply (e.g., Bio-Rad Laboratories GmbH)
-
Amicon® Ultra-15 centrifugal filter unit (10 kDa MW cut off; Merck, cat. no. UFC901024)
-
Black Corning® 96-well plate with flat bottom
-
Preparative size-exclusion column: HiLoad® 26/60 Superdex®75 pg column (Cytiva, cat. no. 28-9893-34)
-
Medium-pressure chromatography system (low MPA range) with UV detection (280 nm) (e.g., Äkta Purifier, GE Healthcare) and a fraction collector
-
Nitrocellulose/PVDF membrane (Carl Roth GmbH & Co. KG)
-
Semi-dry Blotter Trans-Blot® TurboTM Transfer System (e.g., Bio-Rad Laboratories GmbH)
-
Chemiluminescent detection system (e.g., Octoplus QUPLEX, Intas Science Imaging Instruments GmbH)
-
1.5-ml microtubes (BRAND, cat. no. 780500)
-
Additional reagents and equipment for SDS-PAGE (see Current Protocols article: Gallagher, 2012) and immunoblotting (Western blotting; Ni, Xu, & Gallagher, 2016)
Expression of His6-Ran AcK71
1.Transform E. coli (BL21) DE3 cells with pRSFDuet-1/ackRS3 /MbpylT/ranUAG71
2.Plate cells on selective LB agar plates containing 50 µg ml-1 kanamycin and incubate the plates overnight at 37°C.
3.Add 25 ml of LB medium containing 50 µg ml-1 kanamycin to a 100-ml baffled Erlenmeyer flask. Inoculate this pre-culture with four to five single colonies from the LB agar plate from step 2.Incubate the pre-culture overnight (∼16 hr, 37°C, and 160 rpm)
4.Prepare eight 5-L baffled Erlenmeyer flasks, each with 1.25 L of LB medium supplemented with 50 µg ml-1 kanamycin. Inoculate each of these (main culture) with 12.5 ml of the pre-culture (i.e., prepare a 1:100 dilution) and grow to an OD600 of 0.6 (37°C, 140 rpm).
5.Supplement each flask with 12.5 ml of 1 M N-(ε)-acetyl-L-lysine (final concentration in culture: 10 mM) and 12.5 ml of 2 M nicotinamide (final concentration in culture: 20 mM). Reduce temperature to 18°-20°C.
6.After 30 min, add IPTG to a final concentration of 100 µM to induce the expression of the POI (here: His6-Ran AcK71) and incubate for 12-24 hr (at 18°-20°C, 140 rpm).
7.Harvest the cells by centrifugation (10,000 × g , 4°C, 20 min).
8.Resuspend the cell pellet in resuspension buffer (buffer 1.1) and store the pellet at −80°C. Use ∼10-15 ml of resuspension buffer per pellet from 1 L of culture.
Purification of Ran AcK71 (see Current Protocols article: Wingfield, 2015)
9.Thaw the cell pellet on ice. Transfer the suspension into a glass beaker and lyse cells by sonication on ice (five cycles of 2 min, intensity 60%, pulse 0.5 s/2.0 s).
10.Centrifuge the lysate at 39,000 × g , 4°C, for 45-60 min to remove the insoluble fraction. While waiting for the centrifugation, prepare the Ni2+-NTA metal affinity column (5-ml HisTrapTM FF crude column) by equilibrating it with 5 column volumes (CVs) of buffer 1.1.
11.Load the cleared supernatant (which contains the POI; here: His6-Ran AcK71) onto the Ni2+-NTA metal affinity column (5 ml HisTrapTM FF crude column) using an automated FPLC system (Cytiva, Bio-Rad) at 4°C.
12.Wash the column with >10 CVs washing buffer (buffer 1.2) to remove unspecifically bound proteins and contaminants. Wash with 2 CVs of buffer 1.3.
13.Elute the POI on an FPLC system, applying a 10-500 mM imidazole gradient using buffer 1.3 and buffer 1.4.Follow the absorption at 280 nm (A 280) to monitor elution of the POI and the conductivity to monitor the imidazole gradient over time. Collect the flow-through using automated FPLC system and fraction collector.
14.Analyze the protein-containing fractions by SDS-PAGE (see Current Protocols article: Gallagher, 2012).
15.Pool the fractions containing the POI and concentrate via ultrafiltration (4°C, 4000 × g) using an Amicon® Ultra centrifugal filter device with a suitable MWCO, following the manufacturer's instructions.
16.Equilibrate the SEC column with >2 CVs of SEC equilibration buffer (buffer 1.5).
17.Load the concentrated POI (from step 15) onto the equilibrated SEC column and elute on medium-pressure chromatography system using buffer 1.5.Monitor the elution by following the A 280. Collect the eluate using a fraction collector.
18.Analyze the protein-containing fractions by SDS-PAGE (Gallagher, 2012) to identify the POI-containing fractions (see Gallagher, 2012). Then, pool the POI-containing fractions, concentrate the POI using an Amicon Ultrafiltration device, and determine the concentration of the POI (by measuring A 280). Aliquot the POI-containing solution, snap freeze the solution in liquid nitrogen, and store the POI at −80°C until further use.
19.Evaluate the successful incorporation of acetyl-L-lysine by immunoblotting using a specific anti-acetyl-lysine antibody (Fig. 2C) (see Basic Protocol 2).
Basic Protocol 2: IN VITRO SIRTUIN (SIRT)-CATALYZED DEACETYLATION OF LYSINE-ACETYLATED PROTEINS PREPARED BY THE GCEC
This protocol describes how natively folded and site-specifically lysine-acetylated proteins prepared by genetic code expansion (GCEC; Basic Protocol 1) can be used as substrates for sirtuin-catalyzed deacetylation assays. Sirtuin deacetylases (short: sirtuins; SIRT: silent information regulator) are NAD+-dependent enzymes, which are important regulators of fundamental cellular processes (Fig. 2A). Of the seven mammalian sirtuins known (SIRT1-7), SIRT 1-3 are well-documented to possess robust lysine deacetylase activity, while the others show more complex enzymatic activities. Here, we describe the expression and purification of SIRT2 (Fig. 4B and 4C), as well as a deacetylation assay using the site-specifically lysine-acetylated protein His6-Ran AcK71 (Basic Protocol 1) as a substrate (Fig. 4D). This assay is designed to directly identify deacetylases for individual acetylation sites in proteins. Following the initial expression of the gene encoding for the sirtuin of interest in E. coli (here: His6-SIRT250-356), a two-step purification strategy consisting of Ni2+-NTA affinity chromatography (Fig. 4B) and size-exclusion chromatography (SEC) (Fig. 4C) is used to obtain a protein sample of sufficient purity for the deacetylation assay, which uses immunoblotting with a specific anti-acetyl-lysine antibody as a readout (Fig. 4D).
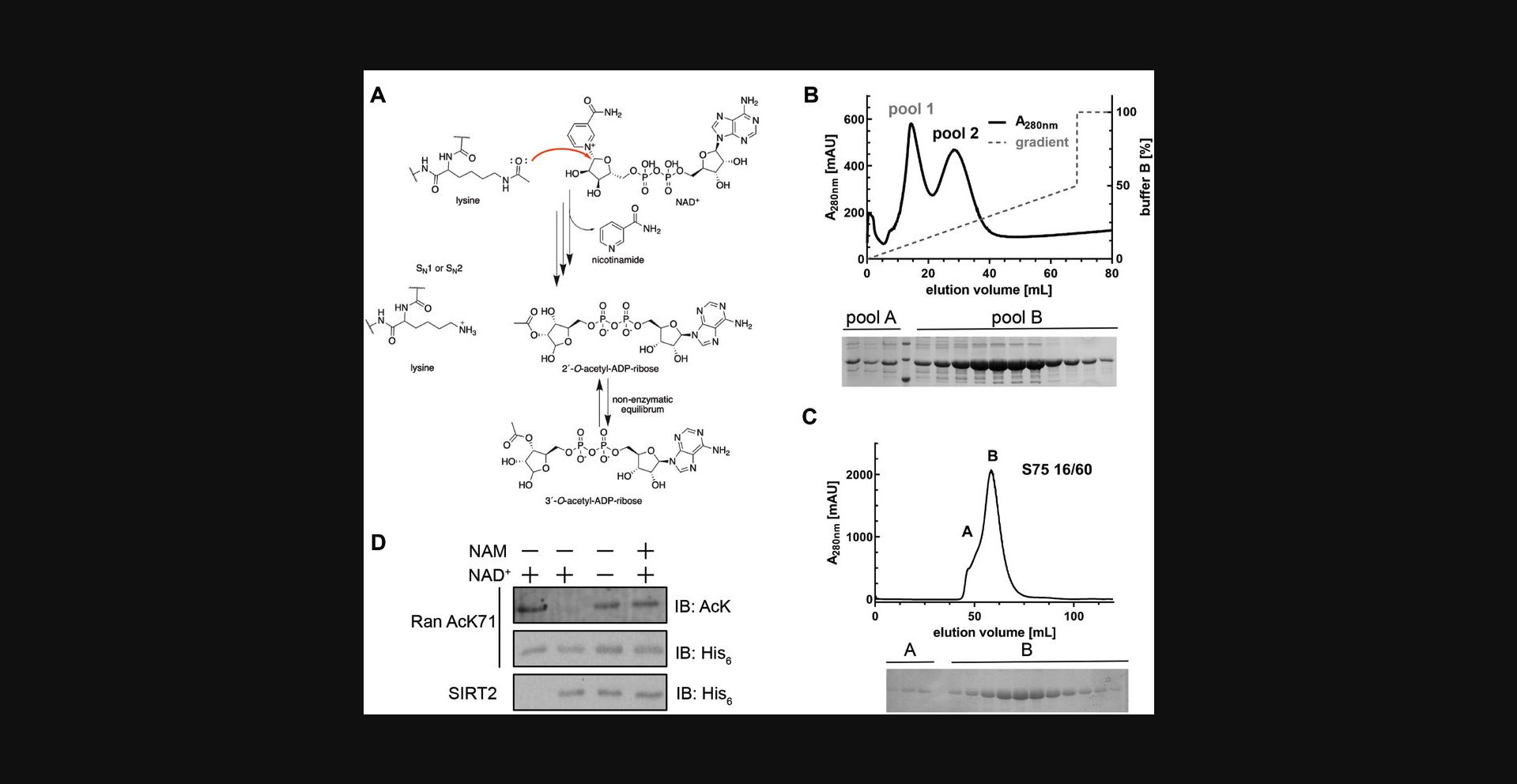
Materials
-
Competent E. coli (BL21) DE3 cells (Novagen®; Sigma-Aldrich, cat. no: 69450)
-
Plasmid pRSF-Duet1 (Sigma-Aldrich, cat. no. 71341) encoding the sirtuin of interest (here, His6-SIRT250-356)
-
LB agar plates (see recipe) containing 50 µg L-1 kanamycin (Carl Roth, cat. no: T832.2)
-
Lysogeny broth (LB) medium (see recipe)
-
Kanamycin sulfate (50 mg ml-1 stock in H2O; Carl Roth, CAS no. 25389-94-0)
-
Isopropyl β-D-1-thiogalactopyranoside (IPTG), 1 M stock, in water (Carl Roth, CAS no. 367-93-1)
-
Buffers 2.1 (resuspension buffer), 2.2, 2.3, 2.4, 2.5 (assay buffer), 2.6 (stripping buffer) (see recipes)
-
5× SDS-PAGE sample buffer (see recipe)
-
my-Budget Pre-stained Protein Ladder (Bio-Budget, cat. no. 86-1000-0500)
-
4%-12% (v/v) SDS-PAGE gels
-
SDS-PAGE running buffer (see recipe)
-
SDS-PAGE staining solution (see recipe)
-
Liquid nitrogen (CAUTION : handle with care; use protective equipment for extremely low temperatures and provide a well-ventilated environment)
-
Acetylated POIAck (see Basic Protocol 1 for His6-Ran AcK71)
-
200 mM nicotinamide (Sigma-Aldrich, cat. no. N3376) in sterile filtered (0.22 µm filter) deionized water. Prepare fresh
-
5 mM NAD+ stock solution (β-nicotinamide adenine dinucleotide sodium salt; Sigma-Aldrich, cat. no.: N0632)
-
Ponceau S red (VWR, Lot No.: 0447C180) in 10% acetic acid
-
PBS-T buffer (see recipe)
-
5% skimmed milk powder in PBS-T (see recipe)
-
Immunoblot transfer buffer (see recipe)
-
Methanol (Carl Roth, CAS no. 67-56-1)
-
Anti-acetyl-L-lysine antibody (abcam 21623, anti-rabbit; dilute 1:1000 in 5% w/v skimmed milk/PBS-T)
-
Goat HRP-coupled anti-rabbit IgG (ab6271, dilute 1: 10,000 in 5% w/v skimmed milk/PBS-T)
-
ECL solution (ROTI®Lumin; Carl Roth GmbH & Co- KG, cat. no. P078.2)
-
Phosphate-buffered saline (PBS; see recipe)
-
Anti-His6-tag antibody (ab18184, 1:1000 in 5% w/v skimmed milk/PBS-T)
-
Rabbit HRP-coupled anti-mouse IgG (ab6728, 1: 10.000 in 5% w/v skimmed milk/PBS-T)
-
5-L baffled Erlenmeyer flasks (VWR, cat. no. 214-1137)
-
Incubator (e.g., Function Line B20; Heraeus Holding GmbH)
-
Shaking incubator (e.g., Infors HT Multitron Standard; Infors AG)
-
UV/Vis spectrophotometer (e.g., Varian Cary 50 UV-Vis spectrophotometer or NanoDrop ND 1000, VWR International GmbH)
-
Heat block at 30°C and 95°C (Mixing Block MB-102, Bioer Technology Co.)
-
Centrifuge (SORVALL RC 6, ThermoFisher GmbH)
-
Refrigerated microcentrifuge
-
Sonicator (Sonopulse HD 4100, BANDELIN Electronic GmbH & Co. KG, Berlin, Germany)
-
1.5-ml microtubes (SARSTEDT AG & Co. KG, REF 72.690.001)
-
Sterile bottle-top filters, 0.45-μm pore size
-
5-ml His-TrapTM High Performance column (Cytiva, cat. no. 17524801, with Ni Sepharose® High Performance)
-
Automated FPLC system and fraction collector (Cytiva, Bio-Rad)
-
Electrophoresis chamber and power supply (e.g., Bio-Rad Laboratories GmbH)
-
Amicon® Ultra centrifugal filter unit (10 kDa; Merck, cat. no. UFC901024)
-
NanoDrop microspectrophotometer (NanoDrop ND 1000, VWR International GmbH)
-
Preparative size-exclusion column: HiLoad® 16/600 Superdex®75 pg column (Cytiva, cat. no. 2898933)
-
Medium-pressure chromatography system (low MPA range) with UV detection (280 nm) (e.g., Äkta Purifier, GE Healthcare)
-
0.2-ml microtubes
-
Nitrocellulose/PVDF membrane (Carl Roth GmbH & Co. KG)
-
Semi-dry Blotter Trans-Blot® TurboTM Transfer System (e.g., Bio-Rad Laboratories GmbH)
-
Chemiluminescent detection system (e.g., Octoplus QUPLEX, Intas Science Imaging Instruments GmbH)
-
Additional reagents and equipment for SDS-PAGE (see Current Protocols article: Gallagher, 2012) and immunoblotting (Western blotting; Ni, Xu, & Gallagher, 2016)
Expression of His6-SIRT250-356
1.Transform competent E. coli BL21 (DE3) cells with pRSF-Duet1/his6-sirt250-356.
2.Plate cells on LB agar plates containing 50 µg L-1 kanamycin.
3.Incubate overnight at 37°C (16-18 hr).
4.Prepare eight 5-L baffled flasks, each containing 1 L of LB medium with 50 µg L-1 kanamycin.
5.Inoculate each flask with 12.5 ml (i.e., 1:100 dilution) of the overnight preculture.
6.Grow cells under constant shaking (160 rpm) at 37°C.
7.Measure the optical density OD600 every 30 min until it reaches 0.6.
8.Adjust the temperature to 18°-20°C (this takes approximately 30 min).
9.Induce expression of the POI (here: His6-SIRT250-356) by adding IPTG to a final concentration of 100-400 µM.
10.Allow POI expression by incubating the cells at 18-20°C at 160 rpm overnight (for 12-16 hr).
11.Harvest the cells by centrifugation (4500 × g , 20 min, 4°C).
12.Discard the supernatant.
13.Resuspend the cells in ∼20 ml of resuspension buffer (buffer 2.1) and freeze the suspension at −80°C or proceed with protein purification (step 14).
Purification of His6-SIRT250-356
14.If frozen, thaw the cell suspension from step 13 on ice.
15.Lyse cells by sonication (two cycles with 5 min, intensity 55%, pulse 0.5 s/1.5 s).
16.Centrifuge the lysate at 50,000 × g for 45 min.
17.Transfer the supernatant containing the POI into a fresh 1.5-ml tube and discard the pellet.
18.Filter the supernatant using a bottle-top filter with a 0.45-µm pore size to remove any remaining cell debris.
19.Equilibrate the Ni2+-NTA affinity column (5 ml His-TrapTM High Performance column) with ≥3 CVs of buffer 2.2.
20.Load the supernatant onto the equilibrated Ni2+-NTA column using an automated FPLC system (4°C, flow rate ≤2 ml min-1).
21.Wash the column with 10 CVs of buffer 2.3 (flow rate 1-2 ml min-1 dependent on the column used). Follow the absorption at 280 nm (A 280) and wash the column until A 280 reaches a stable minimum.
22.Wash the column with buffer 2.2 (>2 CVs) to exchange the high-salt buffer 2.3.
23.Elute the SIRT2 by applying a 10-500 mM imidazole gradient from 100% buffer 2.2 to 100% buffer 2.4 over 10 CVs (flow rate 1 ml min-1). Collect the eluate in fractions of 1 ml using an automated FPLC system and a fraction collector (Fig. 4B). Monitor A 280 and the conductivity to follow protein elution and the formation of the imidazole gradient, respectively.
24.Select possible His6-SIRT250-356-containing fractions based on A 280 and prepare SDS-PAGE samples of each fraction (10 μl sample + 2.5 μl 5× sample buffer). Microcentrifuge briefly at room temperature. Heat the mix for 5 min at 95°C. Microcentrifuge briefly at room temperature.
25.Load 10 μl of each mix and 5 μl of pre-stained ladder on a 4% v/v-12% v/v SDS-PAGE gel. Run the gel in SDS-PAGE running buffer at 180 V until the dye front reaches the bottom of the gel (approximately 50 min).
26.Pool the fractions containing the POI and concentrate using an Amicon® Ultra centrifugal filter device with suitable molecular weight cutoff (MWCO).
27.Measure the concentration based on the extinction coefficient (EC) of the POI by assessing A 280 using a NanoDrop microspectrophotometer and applying the Lambert-Beer law.
28.Equilibrate a suitable size-exclusion chromatography (SEC) column (depending on the molecular weight, oligomeric state, and total amount of POI) with ≥1.5 CVs of equilibration buffer 2.5.
29.Perform size-exclusion chromatography (SEC) with buffer 2.5 using a medium-pressure chromatography system (low MPA range) with UV detection (280 nm) and running specifications according to the selected column.
30.Select possible POI-containing fractions by monitoring the A 280 and subsequent analysis of the protein-containing fractions by SDS-PAGE (Fig. 4B).
31.Pool the POI-containing fractions (separated by oligomeric state if necessary).
32.Concentrate the POI-containing fractions using an Amicon® Ultra centrifugal filter device with a suitable molecular weight cutoff (MWCO).
33.Determine the concentration of the POI by measuring A 280 using a NanoDrop microspectrophotometer and applying the Lambert-Beer law using the POI's specific extinction coefficient.
34.Flash-freeze the concentrated POI in liquid nitrogen and store in suitable aliquots at −80°C.
Sirtuin deacetylation assay
35.Thaw one aliquot of the acetylated POIAck (see Basic Protocol 1 for His6-Ran AcK71) and one aliquot of the prepared SIRT (here: His6-SIRT250-356; step 34) on ice.
36.Microcentrifuge (5 min at 13,500 rpm, 4°C) both tubes to remove precipitated proteins and other precipitants.
37.Transfer the supernatants to separate, fresh, pre-cooled 1.5-ml tubes and store on ice.
38.Measure the concentration of the POIAck and SIRT by applying the Lambert-Beer law using each protein's specific extinction coefficient and A 280 determined using a NanoDrop microspectrophotometer.
39.Calculate the volume of protein needed for preparing a 20-μl solution containing 5 µM POIAck (volume needed in assay: V A) and 1 µM SIRT (volume needed in assay: V B).
40.Prepare four tubes, e.g., 0.2-ml tubes, and label them with 1 through 4.
41.Use the pipetting scheme described in Table 1 for conducting the SIRT deacetylase assay.
Final concentration | Sample 1 -SIRT/+NAD+ | Sample 2 +SIRT/+NAD+ | Sample 3 +SIRT/-NAD+ | Sample 4 +SIRT/+NAD+/+NAM |
---|---|---|---|---|
5 µM POIAck | VA | VA | VA | VA |
1 µM sirtuin | - | VB | VB | VB |
0.5 mM NAD+ (5 mM stock) | 2 µl | 2 µl | - | 2 µl |
10 mM nicotinamide (200 mM stock) | - | - | - | 1 µl |
Assay buffer (buffer 2.5) to 20 µl | X µl | X µl | X µl | X µl |
- a
Add the SIRT as last component and immediately start the incubation.
- b
Sample 1 corresponds to the non-enzyme control, sample 2 contains the SIRT co-substrate NAD+, sample 3 lacks the co-substrate NAD+ and nicotinamide (NAM), and sample 4 contains NAD+ and NAM in addition to SIRT and POIAcK.
- c
Applying this pipetting scheme allows one to confirm an NAD+-dependent SIRT-catalyzed deacetylation of POIAcK. VA, VB: volume of acetylated substrate protein (POIAcK) or sirtuin deacetylase, respectively.
42.Incubate samples in a heat block, incubator, or PCR cycler at 30°C for 2 hr 30 min.
43.Add 10 μl of H2O and 12 μl of 5× SDS-PAGE sample buffer to each reaction. Microcentrifuge briefly at room temperature. Heat the samples for 5 min at 95°C. Microcentrifuge briefly at room temperature.
44.Store samples at −20°C until further use, or proceed to SDS-PAGE and immunoblotting (detection of deacetylation by immunoblotting, step 45).
Detection of deacetylation by immunoblotting
45.If frozen, thaw samples from step 44.Load 10 μl of each sample for analysis by SDS-PAGE (step 48) and 5 μl of pre-stained ladder on a 4% v/v-12% v/v SDS-PAGE gel. Run the SDS-PAGE gel in SDS-PAGE running buffer at 180 V until the dye front reaches the bottom of the gel (∼50 min); also see step 24.
46.Blot on a PVDF or nitrocellulose membrane by semi-dry transfer in immunoblot transfer buffer at 150 mA for 30 min (for additional details, see Niet al., 2016).
47.To analyze the efficiency of the transfer of the proteins from the SDS-PAGE gel to the membrane, stain the membrane for 5 min with Ponceau S followed by washing with distilled, deionized H2O (for details, see Ni et al., 2016).
48.Wash the membrane in PBS-T at least three times, each time for 5 min at room temperature, until Ponceau S red−stained protein bands are no longer visible.
49.Block the membrane with 5% (w/v) skimmed milk in PBS-T for 1 hr at room temperature.
50.Incubate the membrane with primary anti-acetyl-L-lysine antibody overnight at 4°C with mild agitation.
51.Wash the membrane at least three times with PBS-T buffer, each time for 5 min.
52.Incubate with secondary goat HRP-coupled anti-rabbit IgG for 1 hr at room temperature with mild agitation.
53.Wash the membrane at least three times with PBS-T buffer, each time for 5 min.
54.Detect lysine-acetylated proteins using an enhanced chemiluminescence substrate for HRP (ECL solution) (Fig. 4C) using a suitable documentation system.
55.Incubate two times for 10 min with fresh stripping buffer (buffer 2.6).
The following steps are designed as an additional loading control by detecting the His6-tag of the SIRT and the POIAck
56.Wash two times for 5 min with PBS-T buffer followed by two times for 5 min with PBS buffer.
57.Continue with step 49 of this protocol but use anti-His6 antibody (ab18184, diluted 1:1000 in 5% w/v skimmed milk/PBS-T) as the primary antibody and HRP-coupled anti mouse IgG (ab6728, 1: 10,000 in 5% w/v skimmed milk/PBST) as the secondary antibody.
Basic Protocol 3: IN VITRO KDAC/HDAC-CATALYZED DEACETYLATION ON LYSINE-ACETYLATED PROTEINS
In this protocol, we describe the use of acetylated proteins to assess deacetylation catalyzed by classical KDACs. The enzymatic activity of purified KDAC can be determined in vitro with acetylated proteins produced via the GCEC method (Basic Protocol 1), using immunoblotting (Basic Protocol 2) and mass spectrometry (Basic Protocol 5) as readouts. As classical deacetylases produce acetate as a result of deacetylation, enzyme-coupled assays can be used to determine the amount of released acetate (Fig. 5A). Alternatively, fluorescently labeled peptides can be used to determine the activity of classical KDACs (and of sirtuins). This Fluor-de-Lys® assay uses histone- or p53-derived peptides that carry an acetyl-L-lysine and a coumarin fluorophore at the C-terminus. Upon deacetylation of the lysine in these peptides, the peptide becomes cleavable by a trypsin protease, which then liberates and unquenches the fluorogenic coumarin group, which allows detection by measuring fluorescence intensity. As such, this end-point assay allows one to quantify deacetylase activity and to screen for potential KDAC inhibitors (Wegener, Wirsching, Riester, & Schwienhorst, 2003). In this protocol, we use the Bacillus subtilis classical deacetylase AcuC (acu : acetoin utilization) as an example to describe this deacetylation assay. We describe the steps for expression of the test deacetylase in E. coli BL21 (DE3) and its purification, and the procedures for activity determination of classical KDACs (Fig. 5B). For determination of AcuC activity, we describe two assays. We report how the Fluor-de-Lys assay can be used to determine the activity of classical KDACs (Fig. 5C). In addition, we describe the assessment of deacetylation of the AcuC substrate AcsA (acetyl-CoA synthetase) using immunoblotting with a specific anti-acetyl-lysine antibody (anti-AcK antibody) as a readout, as described in Basic Protocol 2 (Fig. 5D).
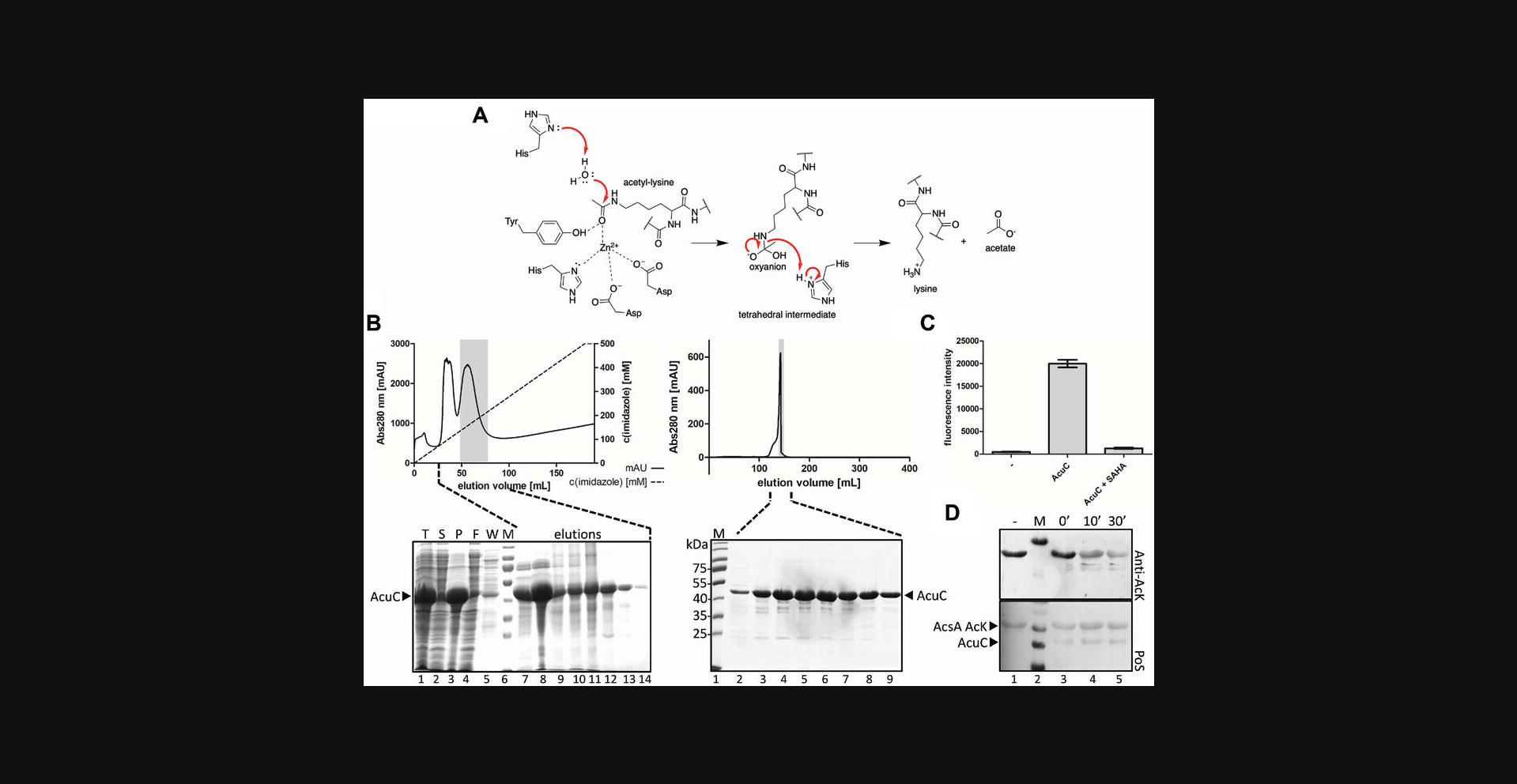
Materials
-
Competent E. coli BL21 (DE3) cells (Novagen®; Sigma-Aldrich, cat. no. 69450)
-
pET-45b(+) vector (Novagen, Darmstadt, Germany) with (a) the acuC (N-terminal His6-Tag, UniProtKB entry: P39067), (b) acsA (N-terminal His6-Tag, UniProtKB entry: P39062), and (c) acuA sequence (N-terminal His6-tag, UniProtKB entry: P39065)
-
We used codon-optimized, synthetic genes prepared by BioCat (https://www1.biocat.com/).
-
LB agar plates (see recipe) containing 100 µg ml-1 ampicillin
-
Ampicillin sodium salt (100 mg ml-1 stock in H2O; Sigma-Aldrich, CAS no. 69-52-3)
-
Lysogeny broth (LB) medium (sterilize by autoclaving; see recipe)
-
Terrific broth (TB; sterilize by autoclaving; see recipe)
-
200 mM ZnCl2 (Carl Roth, CAS no. 7646-85-7) in water
-
Isopropyl β-D-1-thiogalactopyranoside (IPTG) (1 M stock in H2O; Carl Roth, CAS no. 367-93-1)
-
Buffer 3.1 (KDAC lysis buffer), 3.2 (KDAC washing buffer), 3.3 (KDAC standard buffer), and 3.4 (KDAC elution buffer) (see recipes)
-
5× SDS-PAGE sample buffer (see recipe)
-
Ladder: my -Budget Pre-stained Protein Ladder (Bio-Budget, cat. no. 86-1000-0500)
-
12% v/v SDS-PAGE gel and SDS-PAGE running buffer (see recipe)
-
SDS-PAGE staining solution (see recipe)
-
SDS-PAGE destaining solution (see recipe)
-
Ponceau S red (VWR, Lot No.: 0447C180) in 10% acetic acid
-
Bradford assay (Carl Roth, cat. no.: K015.1)
-
Liquid nitrogen (CAUTION : handle with care; use protective equipment for extremely low temperatures and provide a well-ventilated environment)
-
Fluorogenic KDAC substrate (Boc-Lys(Ac)-AMC; BACHEM, cat. no. I-1875)
-
DMSO (Cayman Chemical, CAS no. 149647-78-9)
-
Trypsin (Sigma Aldrich, CAS no. 9002-07-7)
-
10 mM stock solution of SAHA (suberoylanilide hydroxamic acid) in DMSO (Cayman Chemical, CAS no. 149647-78-9)
-
10 mM stock of Trichostatin A (TSA) in DMSO (Hycultec, cat. no. HY-15144)
-
25% (v/v) HCl
-
2× Fluor-de-Lys developer solution (see recipe)
-
50 mM Tris⋅HCl, pH 8.0
-
50 mM KCl
-
Acetylated POIAck (see Basic Protocol 4 for His6-AcsA)
-
PBS-T (see recipe)
-
Immunoblotting transfer buffer (see recipe)
-
5% (w/v) skimmed milk powder in PBS-T (see recipe)
-
Antibodies (5% w/v skimmed milk/PBS-T):
- Primary antibody: 1:1000; anti-acetyl-L-lysine antibody (abcam, cat. no. ab21623)
- Secondary antibody: 1:10,000; goat Anti-Rabbit IgG H&L (HPR) (abcam, cat. no. ab6721)
-
ECL solution A and B (see recipes)
-
100-ml and 5-L baffled Erlenmeyer flasks (VWR cat. no. 214-1131 and 214-1137)
-
Incubator (e.g., Function Line B20, Heraeus Holding GmbH)
-
Shaking incubator (e.g., Infors HT Multitron Standard, Infors AG)
-
Heat block (e.g., Mixing Block MB-102, Bioer Technology Co. and Digital Heatblock, VWR International GmbH)
-
UV/Vis spectrophotometer (e.g., Varian Cary 50 UV-Vis spectrophotometer or NanoDrop ND 1000, VWR International GmbH)
-
Refrigerated microcentrifuge
-
Centrifuge (e.g., SORVALL RC 6 with Rotor SLA-1500 and Rotor SS-34, Thermo Fisher GmbH)
-
Sonicator [e.g., Sonopulse HD 4100 with UW 100, TS133V, SH 100G, and tip TT213 (ᴓ12.7 mm); BANDELIN Electronic GmbH & Co. KG, Berlin, Germany]
-
5-ml HisTrapTM High Performance column (Cytiva, cat. no. 17524801, packed with Ni Sepharose® High Performance)
-
Amicon® Ultracentrifugal filter unit (10 kDa MW cut off; Merck, cat. no. UFC901024)
-
Electrophoresis chamber and power Supply (e.g., Bio-Rad Laboratories GmbH)
-
Preparative size-exclusion chromatography column: HiLoad® 16/600 Superdex®75 pg column and HiLoad® 16/600 Superdex®200 pg (Cytiva, cat. no. 2898933 and 28989335) or Superose 6 Increase 10/300 GL (Cytiva, cat. no. 29091596)
-
Medium pressure chromatography system (low MPA range) with UV detection (280 nm) (e.g., Äkta Purifier, GE Healthcare) and a fraction collector
-
Black Corning 96-well plates with flat bottom
-
Sealing tape for 96-well plates (Greiner Bio-One International GmbH, cat. no. 676001)
-
Plate reader Infinite 200 PRO (Tecan), or similar
-
1.5-ml microtubes (SARSTEDT AG & Co. KG, REF 72.690.001)
-
0.45-µm syringe filter
-
Polyvinylidene fluoride (PVDF) membrane and filter paper (Bio-Rad, cat. no 1620263)
-
Semi-dry Blotter Trans-Blot® TurboTM Transfer System (e.g., Bio-Rad Laboratories GmbH)
-
Shaker/Nutator
-
Chemiluminescent detection system (e.g., Octoplus QUPLEX, Intas Science Imaging Instruments GmbH)
-
Additional reagents and equipment for SDS-PAGE (see Current Protocols article: Gallagher, 2012) and immunoblotting (Western blotting; Ni, Xu, & Gallagher, 2016)
Expression of acuC
1.Transform E. coli BL21 (DE3) cells with the expression plasmid [here: pET-45b(+)] encoding for B. subtilis AcuC. Plate the cells on a LB agar plates containing 100 µg ml-1 ampicillin.
2.Pick 4-5 single colonies to inoculate a 50-ml preculture in LB medium containing 100 µg ml-1 ampicillin. Grow overnight at 150-160 rpm, 37°C.
3.The next day, transfer 20 ml of the preculture to 2 L of TB (i.e., do a 1:100 dilution) in a 5-L Erlenmeyer flask and grow the culture to an optical density at 600 nm (OD600) of 0.3 (37°C, 140 rpm).
4.Lower the temperature to 18°C and add ZnCl2 to the medium to a final concentration of 200 µM.
5.Grow the cells for an additional 30 min and induce the expression of acuC overnight by adding 400 μl of a 1 M IPTG stock solution (final concentration: 200 µM of IPTG) to the flask containing the 2 L of culture.
6.The next day, harvest the cells by centrifugation (4000 × g , 10 min, 4°C).
7.Resuspend the cell pellet in 40 ml of KDAC lysis buffer (buffer 3.1).
8.Lyse the cells by sonication on ice (cycle 5 min, intensity 55%, pulse 0.5/1.5 s).
9.Centrifuge the lysate (45 min, 40,000 × g , 4°C) and use the supernatant for protein purification. Discard pellet.
Protein purification of AcuC from B. subtilis (AcuC)
Have a Zn2+-charged Zn2+-NTA column prepared, as described in Strategic Planning.
10.Load the supernatant containing all soluble components in KDAC lysis buffer (buffer 3.1) onto an equilibrated zinc-charged NTA column (Zn2+-NTA; HisTrapTM High Performance column).
11.Wash the column with 10 CVs of KDAC washing buffer (buffer 3.2).
12.Elute the protein by applying a 0-500 mM imidazole gradient from 100% KDAC standard buffer (buffer 3.3) to 100% KDAC elution buffer 3.4 over 5 CVs.
13.Analyze the elution fractions by SDS-PAGE (see Current Protocols article: Gallagher, 2016). Collect the fractions containing the POI and concentrate the solution using an Amicon® Ultra centrifugal filter device. Microcentrifuge (4000 × g , 5 min, 4˚C) the concentrated protein solution to remove precipitants. Determine the protein concentration by Bradford assay.
14.Equilibrate the size-exclusion chromatography (SEC) column (HiLoad® 26/600 Superdex®200 pg) with the KDAC standard buffer (buffer 3.3). Load the protein and elute it using the same buffer (buffer 3.3).
15.Analyze the elution fractions by SDS-PAGE (see Current Protocols article: Gallagher, 2012). Collect the POI-containing fractions and concentrate the target protein using an Amicon ultrafiltration device.
16.Flash-freeze the concentrated protein aliquots in liquid nitrogen and store them at –80°C.
Fluor-de-Lys assay
17.Prepare a 10 mM stock solution of the fluorogenic KDAC substrate in DMSO. Boc-Lys(Ac)-AMC is highly soluble in DMSO. Protect the fluorogenic substrate solution from prolonged exposure to light, and store at −20°C.
18.Prepare 2× Fluor-de-Lys developer solution as described in Reagents and Solutions.
19.Prepare two Fluor-de-Lys reaction mixes, one with and one without KDAC inhibitor (SAHA or TSA). Prepare a mixture containing:
- 50 mM Tris·HCl (pH 8.0)
- 50 mM KCl
- 5 µM KDAC (AcuC from step 16)
- 20 µM fluorogenic KDAC substrate
- 1% v/v DMSO.
- To one of the mixes, add 50 µM KDAC inhibitor (SAHA or TSA). To the other, add the corresponding volume of DMSO.
For the reactions with KDAC inhibitor, incubate the mixture with KDAC and inhibitor on ice for 15 min before adding the KDAC substrate to the mixture, and mix in the last step.
20.Add 100 μl of the Fluor-de-Lys reaction mixtures, containing 5 µM KDAC and the KDAC substrate (here: (Boc-Lys(Ac)-AMC), to wells of a black Corning® 96-well plate with flat bottom.
21.Cover the plate with sealing tape and incubate at room temperature or 37˚C in the dark for 30 min.
22.Quench the reaction and initiate the cleavage of the deacetylated substrate by trypsin protease by adding 100 μl of the Fluor-de-Lys developer solution from step 18.Incubate at room temperature in the dark for 30 min.
23.Using a 96-well microtiter plate reader, determine the fluorescence at 460 nm by exciting the fluorophore at the wavelength of 390 nm.
Deacetylation assay with acetylated protein
24.Prepare 200 μl of a reaction mixture in 1.5-ml tubes containing:
- 10 nM-5 µM KDAC (AcuC from step 16)
- 10 µM of acetylated protein (Basic Protocol 4)
- 1% v/v DMSO
- 50 mM KCl
- 50 mM Tris·HCl (pH 8).
- After adding the acetylated protein, incubate the mixture at room temperature or 37°C.
The KDAC concentration and substrate concentration to use are enzyme dependent. For a qualitative assay to compare different enzymes or different mutant versions of one enzyme, it is important to ensure which assay settings (enzyme/substrate concentration, incubation time, incubation temperature, buffer conditions, etc.) are selected which ensure that the reaction is still within the dynamic range, i.e., the substrate is not consumed. If that were to happen, it would not be possible to relatively compare the enzymes/mutants. For quantitative analyses, such as for Michaelis-Menten kinetics, the enzyme concentration should be less than 10-fold below the substrate concentration of the reaction, and the substrate must not be fully consumed during the reaction. Ideally, less than 10%-20% turnover of substrate should occur under the experimental settings used.
25.At defined time points (0, 5, 10, 20, 30, 45, 60, 90, and 120 min), take 20 μl of the sample and stop the reaction by adding 5 μl of 5× SDS-PAGE sample buffer supplemented with 2.5-50 µM SAHA.
26.Denature the samples at 95°C for 5 min and analyze by immunoblotting as described below (see Current Protocols article: Ni et al., 2016).
Immunoblotting
27.Run the denatured samples on an SDS-PAGE (Gallagher, 2012).
28.Transfer the proteins from the SDS-PAGE gel to a PVDF membrane in a blotting device using immunoblot transfer buffer (Ni et al., 2016). After transfer, rinse the membrane with PBS-T.
29.Incubate the membrane in Ponceau S red solution (0.25% Ponceau S, 10% acetic acid) for 5 min on a shaker, carefully rinse with distilled water five times, and document the result as loading control.
30.Incubate the membrane with blocking solution (PBS-T supplemented with 5% w/v skimmed milk powder) for half an hour on a shaker.
31.Incubate the membrane with an appropriate dilution of an anti-acetyl-lysine antibody (here: abcam, cat. no. ab21623, 1:1000 in blocking buffer) overnight at 4°C on a nutator.
32.Wash the membrane three times with PBS-T, each with shaking on a nutator for 5 min. Incubate the membrane with a secondary antibody solution (use an appropriate dilution of a horseradish peroxidase coupled secondary antibody in the blocking buffer; here: 1:10,000 of abcam, cat. no. ab6721) for 1 hr at room temperature on a nutator.
33.Wash the membrane three times with PBS-T, each time for 5 min.
34.Mix 1 ml of ECL solution A (ECL A) and 1 ml of ECL solution B (ECL B), and rinse the membrane with the development solution mix. Document the result using a blot imaging system.
Basic Protocol 4: IN VITRO LYSINE ACETYLATION OF RECOMBINANTLY EXPRESSED PROTEINS BY LYSINE ACETYLTRANSFERASES (KATs)
In addition to the site-specific incorporation of acetyl-L-lysine into proteins by the genetic code expansion concept (GCEC; Basic Protocol 1), proteins can also be enzymatically acetylated at lysines. This protocol describes the steps to do this, using the enzymatic acetylation of K549 of acetyl-coenzyme A synthetase (AcsA) by a lysine acetyltransferase (AcuA) from Bacillus subtilis as an example (Fig. 6). Enzymatic lysine acetylation first requires the recombinant expression and purification of the substrate enzyme (in this case, AcsA) and the lysine acetyltransferase (in this example, AcuA; Fig. 6B). Expression is performed in Escherichia coli BL21 (DE3) cells, and purification takes place in two steps: firstly via an Ni-NTA affinity chromatography (IMAC: immobilized metal ion affinity chromatography) by the N-terminal His6-tag and, secondly, via a size-exclusion chromatography (SEC). The respective purification steps are monitored by recording the absorption at 280 nm (A 280) and additionally analyzed by SDS-PAGE. After purification, the proteins are concentrated using an Amicon® Ultra ultrafiltration device with a suitable molecular weight cutoff (MWCO) and flash-frozen in small aliquots (∼20 μl) in liquid nitrogen, which are stored at –80°C until use. Subsequently, the substrate protein (AcsA) can be lysine-acetylated by the test lysine acetyltransferase (AcuA) in the presence of acetyl-coenzyme A (acetyl-CoA). The acetylation is then detected by immunoblotting (Fig. 6C).
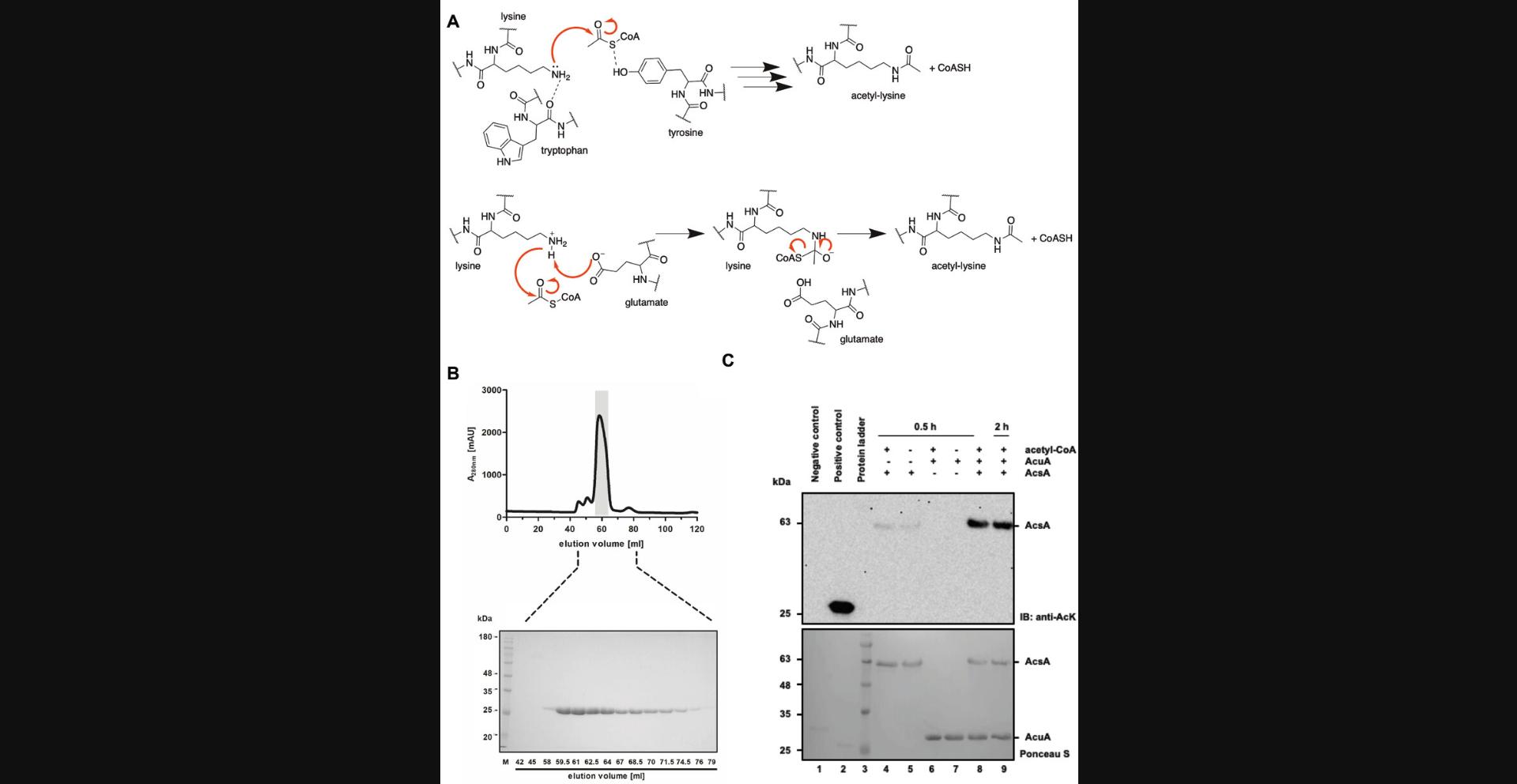
Materials
-
Competent E. coli BL21 (DE3) cells (Novagen®; Sigma-Aldrich, cat. no: 69450)
-
pET-45b(+) vector with the acsA sequence (N-terminal His6-Tag, UniProtKB entry: P39062) and pET-45b(+) vector with acuA sequence (N-terminal His6-Tag, UniProtKB entry: P39065) ordered as codon-optimized, synthetic DNA construct, from BioCat (https://www1.biocat.com/)
-
LB agar plates (see recipe) containing 100 µg ml-1 ampicillin
-
Ampicillin sodium salt (100 mg ml-1 stock in H2O; Sigma-Aldrich, CAS no. 69-52-3)
-
LB medium (sterilize by autoclaving; see recipe)
-
Terrific broth medium (TB; sterilize by autoclaving; see recipe)
-
Isopropyl β-D-1-thiogalactopyranoside (IPTG; 1 M stock in H2O; Carl Roth, CAS no. 367-93-1)
-
Resuspension buffer: Buffer 4.1 (see recipe) plus Pefabloc®SC Protease Inhibitor (Carl Roth, CAS no. 30827-99-7)
-
Buffer 4.1 (assay buffer), buffer 4.2 (Ni-II buffer), and buffer 4.3 (Ni-III buffer) (see recipe)
-
5× SDS-PAGE sample buffer (see recipe)
-
Ladder: my -Budget Prestained Protein Ladder (Bio-Budget, cat. no. 86-1000-0500)
-
12% v/v SDS-PAGE gel and SDS-PAGE running buffer (see recipe)
-
SDS-PAGE staining solution (see recipe)
-
SDS-PAGE destaining solution (see recipe)
-
Bradford assay (Carl Roth, cat. no.: K015.1)
-
Liquid nitrogen (CAUTION : handle with care; use protective equipment for extremely low temperatures and provide a well-ventilated environment)
-
Acetyl-coenzyme A sodium salt (e.g., Sigma Aldrich, CAS no. 102029-73-2)
-
Ponceau S red Solution (abcam, cat. no. ab270042)
-
Blocking buffer: 5% w/v skimmed milk powder in PBS-T (see recipe)
-
Antibodies (1: 10,000 in 5% w/v skimmed milk/PBS-T):
- Primary antibody: anti-acetyl-L-lysine antibody (abcam, cat. no. ab21623)
- Secondary antibody: goat Anti-Rabbit IgG H&L (HPR) (abcam, cat. no. ab6721)
-
PBS-T solution (see recipe)
-
Immunoblot transfer buffer (see recipe)
-
Phosphate-buffered saline (PBS; see recipe)
-
ECL solutions A and B (see recipes)
-
100-ml and 5-L baffled Erlenmeyer flasks (VWR, cat. no. 214-1131 and 214-1137)
-
Incubator (e.g., Function Line B20, Heraeus Holding GmbH)
-
Shaking incubator (e.g., Infors HT Multitron Standard, Infors AG)
-
Heat block (e.g., Mixing Block MB-102, Bioer Technology Co. and Digital Heatblock, VWR International GmbH)
-
UV/Vis spectrophotometer (e.g., Varian Cary 50 UV-Vis spectrophotometer or NanoDrop ND 1000, VWR International GmbH)
-
Refrigerated microcentrifuge
-
Centrifuge (e.g., SORVALL RC 6 with Rotor SLA-1500 and Rotor SS-34, Thermo Fisher GmbH)
-
100-ml glass beaker
-
Sonicator (e.g., Sonopulse HD 4100 with UW 100, TS133V, SH 100G, and tip TT213 (ᴓ12.7 mm) (BANDELIN Electronic GmbH & Co. KG, Berlin, Germany)
-
5-ml HisTrapTM High Performance column (Cytiva, cat. no. 17524801, packed with Ni Sepharose® High Performance) for AcuA
-
5-ml HiTrapTM TALON® crude column (Cytiva, cat. no. 28-9537-67)
-
Amicon® Ultra Centrifugal filter unit (10 kDa MW cut off; Merck, cat. no. UFC901024)
-
Preparative size-exclusion chromatography column: HiLoad® 16/600 Superdex®75 pg column and HiLoad® 16/600 Superdex®200 pg (Cytiva, cat. no. 2898933 and 28989335)
-
Medium pressure chromatography system (low MPA range) with UV detection (280 nm) (e.g., Äkta Purifier, GE Healthcare) and a fraction collector
-
1.5-ml microtubes (BRAND, cat. no. 780500)
-
Electrophoresis chamber and power supply (e.g., Bio-Rad Laboratories GmbH)
-
0.45 µm Polyvinylidene fluoride (PVDF) membrane and filter paper (Bio-Rad, cat. no 1620263)
-
Semi-dry Blotter Trans-Blot® TurboTM Transfer System (e.g., Bio-Rad Laboratories GmbH)
-
Chemiluminescent detection system (e.g., Octoplus QUPLEX, Intas Science Imaging Instruments GmbH)
-
Additional reagents and equipment for SDS-PAGE (see Current Protocols article: Gallagher, 2012) and immunoblotting (Western blotting; Ni, Xu, & Gallagher, 2016)
Expression of AcsA and AcuA
1.Transform two sets of E. coli BL21 (DE3) cells with the pET-45b(+) expression vectors containing the genes acsA and acuA , encoding for the enzymes AcsA or AcuA, respectively.
2.Plate cells after transformation on separate selective LB agar plates containing 100 µg ml-1 ampicillin and incubate the plates overnight at 37°C.
3.For each transformation, add 25 ml of LB medium containing 100 µg ml-1 ampicillin to a 100-ml baffled Erlenmeyer flask. Take 4-5 single colonies from each of the plates on step 2 and inoculate the corresponding pre-cultures. Incubate the pre-cultures overnight (37°C, 160 rpm).
4.Prepare two 5-L baffled Erlenmeyer flasks with 2 L TB medium supplemented with 100 µg ml-1 ampicillin. One will be for inducing expression of acsA and the other, for acuA. Inoculate the corresponding flasks with 20 ml of the appropriate pre-culture, E. coli BL21 (DE3)/pET45b(+)/acsA or E. coli BL21 (DE3)/pET45b(+)/acuA.
5.Incubate the cultures (37°C, 130 rpm) until the OD600 reaches 0.4.Induce protein expression by adding 400 μl of a 1 M IPTG stock (final IPTG concentration 200 µM). Lower the temperature to 18°C and continue the incubation with shaking for an additional 18 hr.
6.Harvest the cells by centrifugation (10,000 × g , 4°C, 20 min). Discard the supernatant and either immediately use the pellet and continue with cell lysis, or store the pellet at –20°C or –80°C until use.
Purification of AcsA and AcuA
The purification process of AcsA is almost identical to that of AcuA. Perform each step at 4°C.
7.Resuspend the cell pellet on ice in 40 ml of resuspension buffer (20 g wet cell pellet in 40 ml of resuspension buffer) and transfer the suspension into a 100-ml glass beaker. Disrupt the cells by sonication on ice (six cycles with 2 min, intensity 55%, pulse 0.5 s/1.5 s).
8.Centrifuge the cell lysate (39,000 × g , 4°C, 60 min) to remove insoluble cell debris.
9.Load the supernatant on a 5-ml metal affinity column equilibrated with assay buffer (buffer 4.1) (use for AcsA a HiTrap. TALON® crude metal affinity column and for AcuA a Ni Sepharose® High Performance metal affinity column) using a medium pressure chromatography system (low MPa range) with UV detection (280 nm) at 4°C. Wash the column with 15 column volumes (CVs) of buffer 4.2 to remove unbound proteins. Elute the target protein using an imidazole gradient from 20 mM imidazole (buffer 4.2) to 500 mM imidazole (buffer 4.3).
10.Pool the fractions containing the POI (here: AcsA and AcuA) based on monitoring the absorption at 280 nm (A 280) and the subsequent analysis of the protein containing fractions by SDS-PAGE; determine the concentration by Bradford assay and concentrate to 10-20 g/L protein by ultrafiltration at 4°C with a 10 kDa MW cut off Amicon® Ultra Centrifugal filter unit.
11.To obtain a high-purity POI sample, perform size-exclusion chromatography (SEC). Equilibrate the size-exclusion column (HiLoad® 26/600 Superdex®200 pg for AcsA; HiLoad® 26/600 Superdex®75 pg for AcuA) at 4°C with 1.5-2 CVs of assay buffer (buffer 4.1).
12.Load the concentrated target protein on the equilibrated size-exclusion column at 4°C and elute with 340 ml of assay buffer (buffer 4.1) using a medium pressure chromatography system (low MPa range). Set the flow rate to an appropriate flow rate to keep the pressure limit of the column (0.3 MPa) and collect the eluate in 3-ml fractions.
13.Pool the pure protein fractions representing a single oligomeric species from the SEC elution following analyses by SDS-PAGE (Gallagher, 2012; an example of SEC results and the subsequent identification of pure fractions by SDS-PAGE for AcuA is shown in Fig. 6B) and concentrate at 4°C to approximately 10 g L-1 using Amicon Ultra Centrifugal filter unit (10 kDa MW cut off). Afterwards, flash-freeze small aliquots (∼20 μl) in liquid nitrogen and store at −80°C until use.
In vitro lysine acetylation of AcsAK549 by lysine acetyltransferase AcuA
14.Prepare the following mix in a 1.5-ml microcentrifuge tube and mix gently by pipetting up and down:
- 34.4 μl of assay buffer (buffer 4.1)
- 1 μl of 10 mg ml−1 AcuA (final concentration 10 µM)
- 2.6 μl of 10 mg ml−1 AcsA (final concentration 10 µM)
- 2 μl of 1 mM acetyl-coenzyme A (final concentration 50 µM).
Acetyl-CoA is a thioester that is quite unstable in solution. Ideally, prepare a fresh solution right before starting the experiment. Add acetyl-CoA as final component to start the reaction.
15.Incubate at 37°C (e.g., Mixing Block MB-102) for 30 min.
16.After incubation, stop the reaction with 10 μl of 5× SDS-PAGE sample buffer and incubate the sample at 95°C in a heat block for 8 min.
17.To detect the acetylation signal, load 5 μl of the stopped sample in a 12% (v/v) SDS-PAGE gel (Gallagher, 2012). In addition, load a negative (a non-acetylated protein; here: Ran) and positive control (a lysine-acetylated protein; here: His6-Ran AcK71; see BP1) and a pre-stained protein ladder onto the gel. The controls are important to judge the immunoblotting procedure. After the electrophoresis, transfer the proteins from the SDS-PAGE gel onto a PVDF membrane.
18.As a loading control, and to assess the efficiency of protein transfer, stain the membrane with Ponceau S red solution for 5 min at room temperature and wash the membrane with Milli-Q water until signals are visible (Fig. 6C).
19.Block unspecific antibody binding sites on the membrane with 5% (w/v) skimmed milk powder (blocking buffer) for 2 hr at room temperature.
20.Incubate the membrane with the primary anti-acetyl-L-lysine antibody (here: abcam, cat. no. ab21623) for 2 hr at room temperature (prepare 10 ml at a dilution 1:5000 in blocking buffer).
21.Wash the membrane three times with PBS-T for 10 min and incubate with the secondary antibody (here: abcam, cat. no. ab6721) for 2 hr at room temperature (prepare 10 ml at a dilution of 1:5000 in blocking buffer)
22.Wash the membrane three times with PBS and incubate the membrane with 2 ml of ECL A and 2 ml of ECL B solution for 1 min at room temperature. Detect the ECL signal with a chemiluminescent imager (Fig. 6C).
Basic Protocol 5: IN VITRO NON-ENZYMATIC LYSINE ACETYLATION OF PROTEINS BY ACETYL-CoA AND/OR ACETYL-PHOSPHATE
This protocol describes the analysis of non-enzymatic, chemical acetylation of proteins and its detection using immunoblotting and LC-MS/MS. Determining the stoichiometry of a lysine acetylation event is important in order to judge its physiological significance. Under physiological conditions, N-(ε)-amino groups of lysines can be acetylated either enzymatically or non-enzymatically. As discussed above, non-enzymatic acetylation in the cell occurs at high concentrations of either acetyl-coenzyme A (acetyl-CoA; e.g., in the millimolar range in mitochondria of eukaryotic cells) or acetyl-phosphate (AcP; e.g., in the low millimolar range in Escherichia coli) and alkaline pH. This protocol describes the steps to perform non-enzymatic acetylation of N-(ε)-amino groups of lysine side chains of natively folded proteins. The concentration of acetyl-coenzyme A (20-1000 μM) or acetyl-phosphate (1-20 mM), the incubation temperature (25˚C-37˚C) and/or the incubation time (3-18 hr), and the accessibility of the N-(ε)-amino groups of lysine side chains of a protein determine the degree of lysine acetylation. The acetylation of a protein can be qualitatively detected by immunoblotting using an anti-acetyl-L-lysine antibody (anti-AcK antibody) in combination with a secondary antibody that allows detection of the signal (e.g., HRP-coupled or fluorescence-labeled compound). To unravel the specific site of lysine acetylation, liquid chromatography−tandem mass spectrometry (LC-MS/MS) is performed, as described below. We exemplify these protocols by describing non-enzymatic acetylation of a recombinantly expressed and purified protein by treatment with acetyl-CoA or acetyl-phosphate, and the subsequent identification of the acetylation sites applying LC–MS/MS.
Materials
-
Recombinantly expressed and purified protein of interest (for protein purification protocols, see Basic Protocols 2-4)
-
Bradford assay (Carl Roth, cat. no.: K015.1)
-
100 mM lithium potassium acetyl-phosphate (see recipe)
-
10 mM acetyl-coenzyme A trilithium (see recipe)
-
5× reaction buffer (see recipe)
-
5× SDS-PAGE sample buffer (see recipe)
-
Ladder: my -Budget Prestained Protein Ladder (Bio-Budget, cat. no. 86-1000-0500)
-
12% v/v SDS-PAGE gel and SDS-PAGE running buffer (see recipe)
-
SDS-PAGE staining solution (see recipe)
-
SDS-PAGE destaining solution (see recipe)
-
12% v/v mini-PROTEAN SDS-PAGE gel (Bio-Rad)
-
Pre-stained marker (Thermofisher scientific, cat. no. 26617)
-
Positive control (an lysine-acetylated protein; here: His6-Ran AcK71; see Basic Protocol 1)
-
Negative control (a non-acetylated protein; here: Ran; see Basic Protocol 1)
-
ECL solution A and B (see recipe)
-
Blocking buffers: 1%, 3%, and 5% (w/v) skimmed milk powder in PBS-T (see recipe)
-
Rabbit anti-acetyl-L-lysine antibody (abcam, cat. no. ab21623)
-
Goat anti-rabbit-HRP antibody (abcam, cat. no. ab6217)
-
Immunoblot transfer buffer (see recipe)
-
PBS-T solution (see recipe)
-
Methanol (Carl Roth, CAS no. 67-56-1)
-
50 mM triethylammonium bicarbonate (TEAB) buffer, pH 8.5 (Sigma Aldrich, cat. no. T7408)
-
RapiGestTM SF (Waters, cat. no. 186001861)
-
500 mM TCEP (tris-(2-carboxyethyl) phosphine hydrochloride) buffer (Sigma Aldrich, cat. no. C4706)
-
100 mM iodoacetamide (Sigma Aldrich, cat. no. I6125)
-
Endopeptidase trypsin solution (see recipe)
-
Trifluoroacetic acid (Sigma Aldrich, cat. no. 74564)
-
Wetting solution: 0.1% v/v acetic acid (3738.4, Carl Roth) in 70% v/v acetonitrile (7330.1, Carl Roth)
-
Equilibration solution (0.1% v/v acetic acid in 3% v/v acetonitrile)
-
Washing solution (0.1% v/v acetic acid in water)
-
Elution solution (0.1% v/v acetic acid in 60% v/v acetonitrile)
-
Buffer B (0.1% v/v acetic acid in acetonitrile)
-
ReproSil-Pur 120 C18-AQ (R13.aq.0003, Dr. Maisch GmbH, Ammerbuch-Entringen, Germany)
-
Tabletop microcentrifuge (Micro Star 17R, VWR, or equivalent)
-
1.5-ml microtubes (BRAND, cat. no. 780500)
-
NanoDrop spectrophotometer (DS-11 Series Spectrophotometer, DeNovix, or equivalent) or UV-Vis spectrophotometer (Cary 60 UV-Vis spectrophotometer, or equivalent)
-
Heating block (PCR machine) or water bath
-
SDS-PAGE system (Mini-PROTEAN Tetra handcast system, Bio-Rad, or equivalent)
-
Semi-dry blotting system (Trans-blot turbo transfer system Bio-Rad, or equivalent)
-
Immobilon-P membrane, PVDF, 0.45 μm (MERCK, Millipore cat. no. IVPH00010)
-
Shaker/Nutator
-
Gel documentation system (Octoplus OPLEX, NHDyeAGNOSTICS, INTAS Science Imaging, or equivalent)
-
Pierce C18 Tips (Thermo Fisher Scientific, cat. no. 87782)
-
Concentrator plus vacuum centrifuge (Eppendorf, cat. no. 5305000703)
-
Micro-flow UPLC system (EASY nLC, Thermo Fisher Scientific, or equivalent)
-
20-cm length, 100-µm inner diameter column
-
Mass spectrometer (Q Exactive Plus Orbitrap, Thermo Fisher Scientific, or equivalent)
-
MaxQuant, Version 1.6.17.0 (https://maxquant.org/)
-
Additional reagents and equipment for SDS-PAGE (see Current Protocols article: Gallagher, 2012) and immunoblotting (Western blotting; Ni, Xu, & Gallagher, 2016)
Non-enzymatic acetylation of proteins using Ac-CoA or AcP
Here, we describe the protocol for any protein. Start with one aliquot of purified protein (50-100 μl, 1-10 g/L).
1.Microcentrifuge the purified protein solution in a 1.5-ml microcentrifuge tube (10 min, 11,000 rpm, 4˚C).
2.Pipette the supernatant into a fresh 1.5-ml microcentrifuge tube. Avoid transferring any protein precipitation or protein pellet into the tube.
3.Determine the concentration of the pure protein solution by measuring the absorption at 280 nm (A 280) or by performing a Bradford assay [measuring the absorption of the reaction product at 595 nm (A 595)] or a similar procedure (e.g., BCA or amido black protein assay) to determine protein concentration with a UV-Vis spectrophotometer.
4.Dilute the protein sample with a suitable buffer to a concentration of 20-200 μM.
5.Set up one of the reactions shown in Table 2 or 3 for the non-enzymatic acetylation. Use either acetyl-phosphate or acetyl-coenzyme A. The tables describe a setup for testing acetylation at three different incubation temperatures (25, 30, and 37°C) and four concentrations of acetyl-phosphate or acetyl-CoA (0, 1, 5, and 20 mM) resulting in 12 conditions.
X(μl)=cproteininassaycproteinstock×totalvolume(μl)=10μM200μM×20μl=1.0μl
1 | 2 | 3 | 4 | 5 | 6 | 7 | 8 | 9 | 10 | 11 | 12 | |
---|---|---|---|---|---|---|---|---|---|---|---|---|
Sample | Incubation temperature 25°C | Incubation temperature 30°C | Incubation temperature 37°C | |||||||||
Volume of acetyl-phosphate [µl] | 0 | 2 (10 mM stock) | 10 (10 mM stock) | 4 (100 mM stock) | 0 | 2 (10 mM stock) | 10 (10 mM stock) | 4 (100 mM stock) | 0 | 2 (10 mM stock) | 10 (10 mM stock) | 4 (100 mM stock) |
Protein concentration [µM] | 10 | 10 | 10 | 10 | 10 | 10 | 10 | 10 | 10 | 10 | 10 | 10 |
Reaction buffer (5×) [µl] | 4 | 4 | 4 | 4 | 4 | 4 | 4 | 4 | 4 | 4 | 4 | 4 |
Filtered ddH2O [µl] | Y | Y | Y | Y | Y | Y | Y | Y | Y | Y | Y | Y |
Total assay volume [µl] | 20 | 20 | 20 | 20 | 20 | 20 | 20 | 20 | 20 | 20 | 20 | 20 |
6.Incubate the reactions in a heat block, e.g., PCR machine or water bath (25˚C, 30˚C/37˚C, 3-18 hr; also see Tables 2 and 3, 4).
1 | 2 | 3 | 4 | 5 | 6 | 7 | 8 | 9 | 10 | 11 | 12 | |
---|---|---|---|---|---|---|---|---|---|---|---|---|
Sample | Incubation temperature 25°C | Incubation temperature 30°C | Incubation temperature 37°C | |||||||||
Volume of acetyl-coenzyme A [µl] | 0 | 5 (100 μM stock) | 5 (1 mM stock) | 2 (10 mM stock) | 0 | 5 (100 μM stock) | 5 (1 mM stock) | 2 (10 mM stock) | 0 | 5 (100 μM stock) | 5 (1 mM stock) | 2 (10 mM stock) |
Protein concentration [µM] | 10 | 10 | 10 | 10 | 10 | 10 | 10 | 10 | 10 | 10 | 10 | 10 |
Reaction buffer (5×) [µl] | 4 | 4 | 4 | 4 | 4 | 4 | 4 | 4 | 4 | 4 | 4 | 4 |
Filtered ddH2O [µl] | Y | Y | Y | Y | Y | Y | Y | Y | Y | Y | Y | Y |
Total assay volume [µl[ | 20 | 20 | 20 | 20 | 20 | 20 | 20 | 20 | 20 | 20 | 20 | 20 |
Problem | Possible Cause | Solution |
---|---|---|
Basic Protocol Basic Protocol 1 | ||
High amounts of translational termination product | Translational stop at amber stop codon by RF1 | Check whether AcK was added and probably increase concentration; use E. coli ΔRF1 for expression. |
ESI-MS does not show correct molecular weight of POI and/or immunoblotting for acetyl lysine does not show any signal |
CobB might have deacetylated POI during expression. Anti-acetyl-lysine antibody does not recognize the site |
Check whether nicotinamide was added to the culture and/or increase the concentration; use E. coli ΔCobB for expression Check dilution of the antibody used and amount of protein loaded on the SDS-PAGE gel, and increase accordingly. Prepare a fresh antibody dilution. Check transfer of proteins from the SDS- PAGE to the membrane. |
High amounts of translational termination product but also of full-length protein | Low acetyl-L-lysine incorporation efficiency | Use C-terminal affinity tag to remove translational termination product; add additional copies of AckRS3 and/or MbPylTCUA encoding genes on compatible vector. |
Basic Protocol Basic Protocol 2 | ||
No acetylation signal | POIAck is not acetylated or antibody does not detect POIAck | Analyze POIAck by ESI-MS for acetyl-L-lysine incorporation and evaluate using antibody at different concentrations. |
No deacetylation | POIAck is no substrate | Repeat the assay with different SIRT. |
Sirtuin is not active | Test activity with known SIRT substrate. | |
Slow substrate turnover | Extend assay incubation time or use a higher SIRT concentration. | |
Weak deacetylation | Access of SIRT to acetylation site is limited, substrate is not a physiological substrate, or other factors are missing to enhance SIRT-catalyzed deacetylation | Extend incubation time or use different SIRT constructs. |
Deacetylation with NAM | NAM concentration is too low | Repeat with new solution. |
NAM was added following addition of the SIRT | Always add the SIRT last, particularly if the substrate is efficiently deacetylated by the SIRT. | |
Basic Protocol Basic Protocol 3 | ||
Strong aggregation of KDAC | Protein is denatured/partially unfolded in the KDAC elution buffer |
Reduce the time for Zn2+-NTA affinity chromatography and SEC. Include an initial purification step via Superose 6 SEC following Zn2+-NTA chromatography. |
Protein is insoluble following expression | Optimize expression conditions (expression medium, expression time, modify IPTG concentration, expression host, etc.). | |
Green/blue protein solution | KDAC binds Ni2+ ions and of Zn2+ ions | Strip the Ni2+-NTA resin with EDTA-containing stripping buffer and charge it with ZnCl2. |
No deacetylation activity in FdL assay | Acidification affects the fluorescence and enzyme activity | Check pH value of assay. If necessary, increase the concentration of buffer agent. |
Deactivation of KDAC in the Zn2+-ion-rich environment | Avoid adding additional Zn2+ to the assay buffer. | |
No cleavage by trypsin | Trypsin is inactive | Prepare fresh developer solution, increase trypsin concentration. |
No acetylation signal in immunoblotting | H2O2 is consumed in ECL solution B | Prepare fresh ECL solution B. |
Anti-acetyl-lysine antibody does not recognize the acetylation site on the substrate | Use alternative approaches (mass spectrometry, acetate detection by coupled-enzymatic assay, etc.) to detect the deacetylation of the substrate. | |
Basic Protocol Basic Protocol 4 | ||
Substrate protein was not acetylated | Acetyl-coenzyme A is degraded | Use fresh solutions. |
Acetyltransferase is inactive | Optimize the buffer. | |
Weak signal in immunoblot | Weak chemiluminescent signal | Extend exposure time. |
Insufficient amount of protein was transferred from the SDS-PAGE gel to the membrane |
Replace ECL solution B, the H2O2 might be consumed. Increase the blotting transfer time. |
|
Basic Protocol Basic Protocol 5 | ||
Protein was not lysine acetylated | The LiK-acetyl-phosphate/acetyl-coenzyme A was degraded | Use fresh solutions. |
No/poor immunoblot signal | Transfer of protein to the PVDF membrane was insufficient | The PVDF membrane was not activated by methanol. |
Increase the transfer time to 30-60 min. | ||
Increase the transfer voltage to 20 V. | ||
The PVDF membrane was blocked too much | Reduce the concentration of the milk solution to 1%-3% w/v. | |
Reduce blocking incubation time. | ||
The anti-acetyl-L-lysine antibody did not bind | The anti-acetyl-L-lysine antibody concentration was too low. Increase the concentration to 1:1000. | |
Increase the incubation time. | ||
Decrease the milk concentration to 1%-3% w/v. | ||
The antibody was degraded. Use a fresh antibody solution. | ||
The secondary antibody did not bind | The antibody concentration was too low. Increase the antibody concentration to 1:5000. | |
Increase incubation time. | ||
Decrease milk concentration to 0-0.5% w/v. | ||
The antibody was degraded. Prepare a fresh antibody solution. | ||
The ECL solution was degraded | Use fresh solutions. | |
No peptides detected in the LC-MS/MS | The protein was not denatured properly before digestion | Ensure the correct concentrations of iodoacetamide and TCEP. |
The endopeptidase trypsin was degraded and/or inactive | Use fresh enzymes. | |
The incubation time for trypsin digestion was too short | Increase the incubation time to overnight. | |
The pH for the trypsin digestion was incorrect | Check the pH of the reaction. It should be between 8.3 and 8.5. | |
The Pierce C18 Tip dried out | Always keep the Pierce C18 Tip moist in LC-MS/MS buffer. |
Immunoblotting
7.Add 5 μl of 5× SDS sample buffer to each sample. Mix by carefully pipetting up and down.
8.Incubate the samples for 10 min at 95˚C.
9.Microcentrifuge the samples (1 min, 11,000 rpm, room temperature).
10.Load the samples (as much volume as possible, depending on the loading capacity in the lanes of the SDS-PAGE gel used), including a pre-stained protein marker (3 μl), a positive control (an lysine-acetylated protein; here: His6-Ran AcK71), and a negative control (a non-acetylated protein; here: Ran) on an SDS-PAGE gel and run the SDS-PAGE (constant volt at 160 V, 1 hr, room temperature for a 12% v/v mini-PROTEAN SDS-PAGE gel) in SDS running buffer until the dye front is just off the bottom of the gel (see Gallagher, 2012).
11.Transfer the proteins from the SDS-PAGE gel onto a methanol-activated PVDF membrane in immunoblot transfer buffer for 25 min at 15 V and at room temperature (also see Ni et al., 2016).
12.Rinse the PVDF membrane briefly in PBS-T buffer.
13.Block unspecific binding sites on the PVDF membrane with 3%-5% w/v skimmed milk powder in PBS-T for 1 hr at room temperature on a nutator.
14.Wash the membrane three times by incubating it for 15 min each time in PBS-T at room temperature on the nutator.
15.Incubate the membrane with the primary antibody (e.g., rabbit anti-acetyl-L-lysine, 1:2000) in 3% (w/v) skimmed milk/PBS-T overnight in a cold room at 4˚C on a nutator.
16.Wash the membrane three times by incubating it for 15 min, each time in PBS-T at room temperature on the nutator.
17.Incubate the membrane with the secondary antibody (e.g., goat anti-rabbit-HRP, 1:10,000) in 1% (w/v) skimmed milk/PBS-T for 1 hr at room temperature on a nutator.
18.Wash the membrane three times by incubating it for 15 min each time in PBS-T at room temperature on the nutator.
19.Incubate the membrane with 2 ml of ECL developing solution (1 ml ECL A plus 1 ml ECL B) in the dark for 1 min at room temperature.
20.Expose the membrane to a digital chemiluminescence reader (e.g., INTAS Science Imaging documentation system).
Liquid chromatography-tandem mass spectrometry (LC-MS/MS)
21.Dilute proteins (1-5 g/L) in 50 mM TEAB/ 0.1% RapiGestTM SF
22.Reduce proteins using TCEP at a final concentration of 5 mM for 45 min at 60°C (stock concentration of TCEP: 500 mM) in a final reaction volume of 50 μl.
23.Add 5 μl of a 100 mM iodoacetamide stock solution to reach a final concentration of 10 mM. Vortex the samples and incubate in the dark at room temperature for 15 min.
24.Reconstitute trypsin in the provided trypsin resuspension buffer according to the manufacturer's instructions. Add trypsin at a protease:protein ratio of 1:200 and incubate for 5 hr at 37°C at 900 rpm.
25.Precipitate RapiGestTM SF by adding 1 μl of trifluoroacetic acid, incubate for 45 min at 37°C, and microcentrifuge three times for 10 min at 15,000 rpm at 4°C to remove RapiGestTM SF.
26.Purify peptide mixture using Pierce C18 Tips (100 μl bed volume)
27.Wet the C18 material by pipetting 100 μl of wetting solution twice.
28.Equilibrate the C18 material by pipetting 100 μl of the equilibration solution twice.
29.Bind peptides by pipetting the peptide solution 10 times up and down; pipet the supernatant back into reaction tube.
30.Wash bound peptides on C18 material by pipetting 100 μl of washing solution. Repeat this step four times.
31.Elute peptides from C18 material by pipetting 100 μl of elution solution. Transfer the eluted peptide mixture into glass vial.
32.Dry down in a vacuum centrifuge and resolve prior to MS analysis in 10 μl of 0.1% v/v acetic acid in water.
LC-MS/MS analysis and confirmation of site-specific N-(ε)-L-lysine acetylation
33.Separate peptides by liquid chromatography (e.g., EASY nLC 1200) running a binary linear gradient of 4% (v/v) to 26% (v/v) buffer B and a flow rate of 0.3 μl/min for 20 min on an analytical column (e.g., self-packed of 20 cm length and 100 µm inner diameter; filled with 3 µm ReproSil-Pur 120 C18-AQ. Couple to an Orbitrap mass spectrometer (e.g., QExactive HF).
34.Acquire mass spectra with an automatic gain control (AGC) target of 3 × 106 at a resolution of 60,000 in a mass range of 300-1650 m/z.
35.Set the maximum injection time to 100 ms for ion accumulation.
36.Measure the MS/MS events for the 15 most abundant peaks (Top 15 method) in the data-dependent mode of high mass accuracy Orbitrap after HCD (Higher energy C-trap Dissociation) fragmentation in a 200-2000 m/z mass range.
37.Set the resolution to 15,000, the injection time to 50 ms, and an AGC target of 1 × 105.
38.Process raw files with a suitable analysis program (e.g., MaxQuant). Perform searches with trypsin specificity allowing two missed cleavages and set carbamidomethyl at cysteine residues as a fixed modification. Set methionine oxidation and acetylation on lysine as variable modifications.
39.Set the minimal peptide length to 5 and the false discovery rate (FDR) on proteins and peptides to 1% for analysis.
40.Analyze the obtained MS/MS spectra for peptides containing acetylated N-(ε)-amino groups of lysines (m/z for peptide, peptide score, quality of obtained b- and y-ion series) and identify the exact L-lysine acceptor sites.
REAGENTS AND SOLUTIONS
Acetyl-coenzyme A trilithium, 10 mM
- 8.27 mg acetyl-coenzyme A trilithium (AppliChem, BioChemica, cat. no. A3753,0050; MW: 827.37 g/mol)
- 1000 μl filtered distilled deionized H2O
- Pipette 50-µl solution aliquots
- Store at –80˚C for 1 month
Buffer 1.1
- 25 mM Tris·HCl, pH 8
- 500 mM NaCl
- 5 mM MgCl2
- 2 mM 2-mercaptoethanol
- 10 mM imidazole
- 200 µM Pefabloc (Carl Roth, CAS no. 30827-99-7)
- Filter and degas buffer before addition of 2-mercaptoethanol. Store up to 2 months at 4°C without 2-mercaptoethanol; otherwise do not store. If used for equilibration of affinity column or SEC prepare buffer without Pefabloc.
Buffer 1.2
- 25 mM Tris·HCl, pH 8
- 1 M NaCl
- 5 mM MgCl2
- 2 mM 2-mercaptoethanol
- 10 mM imidazole
- Filter and degas buffer before addition of 2-mercaptoethanol. Store up to 2 months at 4°C without 2-mercaptoethanol, otherwise do not store.
Buffer 1.3
- 25 mM Tris·HCl, pH 8
- 300 mM NaCl
- 5 mM MgCl2
- 2 mM 2-mercaptoethanol
- 10 mM imidazole
- Filter and degas buffer before addition of 2-mercaptoethanol. Store up to 2 months at 4°C without 2-mercaptoethanol, otherwise do not store.
Buffer 1.4
- 25 mM Tris·HCl, pH 8
- 300 mM NaCl
- 5 mM MgCl2
- 2 mM 2-mercaptoethanol
- 500 mM imidazole
- Filter and degas buffer before addition of 2-mercaptoethanol. Store up to 2 months at 4°C without 2-mercaptoethanol; otherwise do not store.
Buffer 1.5
- 50 mM Tris·HCl, pH 8
- 100 mM NaCl
- 5 mM MgCl2
- 2 mM 2-mercaptoethanol
- Filter and degas buffer before addition of 2-mercaptoethanol. Store up to 2 months at 4°C without 2-mercaptoethanol, otherwise do not store.
Buffer 2.1 (resuspension buffer)
- 50 mM Tris⋅HCl, pH 7.4
- 100 mM NaCl
- 5 mM MgCl2
- 2 mM 2-mercaptoethanol
- 200 µM Pefabloc (Carl Roth, CAS no. 30827-99-7)
- Filter and degas buffer before addition of 2-mercaptoethanol. Store up to 2 months at 4°C without 2-mercaptoethanol, otherwise do not store.
Buffer 2.2
- 50 mM Tris⋅HCl, pH 7.4
- 100 mM NaCl
- 5 mM MgCl2
- 2 mM 2-mercaptoethanol
- 10 mM imidazole
- Filter and degas buffer before addition of 2-mercaptoethanol. Store up to 2 months at 4°C without 2-mercaptoethanol, otherwise do not store.
Buffer 2.3
- 50 mM Tris⋅HCl, pH 7.4
- 500 mM NaCl
- 5 mM MgCl2
- 2 mM 2-mercaptoethanol
- 10 mM imidazole
- Filter and degas buffer before addition of 2-mercaptoethanol. Store up to 2 months at 4°C without 2-mercaptoethanol, otherwise do not store.
Buffer 2.4
- 50 mM Tris⋅HCl, pH 7.4
- 100 mM NaCl
- 5 mM MgCl2
- 2 mM 2-mercaptoethanol
- 500 mM imidazole
- Filter and degas buffer before addition of 2-mercaptoethanol. Store up to 2 months at 4°C without 2-mercaptoethanol, otherwise do not store.
Buffer 2.5 (assay buffer)
- 50 mM Tris⋅HCl, pH 7.4
- 100 mM NaCl
- 5 mM MgCl2
- 2 mM 2-mercaptoethanol
- Filter and degas buffer before addition of 2-mercaptoethanol. Store up to 2 months at 4°C without 2-mercaptoethanol, otherwise do not store.
Buffer 2.6 (stripping buffer)
- 15 g glycine
- 1 g SDS
- 10 ml Tween-20
- H2O to 1 L
- pH 2.2
Buffer 3.1 (KDAC lysis buffer)
- 50 mM Tris·HCl, pH 8.0
- 50 mM KCl
- 5% (v/v) glycerol
- 2 mM 2-mercaptoethanol
- 200 µM Pefabloc® (Carl Roth, cat. no. 2931.3)
- Filter and degas buffer before addition of 2-mercaptoethanol. Store up to 2 months at 4°C without 2-mercaptoethanol, otherwise do not store.
Buffer 3.2 (KDAC washing buffer)
- 50 mM Tris·HCl, pH 8.0
- 50 mM KCl
- 350 mM NaCl
- 5% (v/v) glycerol
- 10 mM imidazole
- 2 mM 2-mercaptoethanol
- Filter and degas buffer before addition of 2-mercaptoethanol. Store up to 2 months at 4°C without 2-mercaptoethanol, otherwise do not store.
Buffer 3.3 (KDAC standard buffer)
- 50 mM Tris·HCl, pH 8.0
- 50 mM KCl
- 5% (v/v) glycerol
- 2 mM 2-mercaptoethanol
- Filter and degas buffer before addition of 2-mercaptoethanol. Store up to 2 months at 4°C without 2-mercaptoethanol, otherwise do not store.
Buffer 3.4 (KDAC elution buffer)
- 50 mM Tris·HCl, pH 8.0
- 50 mM KCl
- 5% (v/v) glycerol
- 500 mM imidazole
- 2 mM 2-mercaptoethanol
- Filter and degas buffer before addition of 2-mercaptoethanol. Store up to 2 months at 4°C without 2-mercaptoethanol, otherwise do not store.
Buffer 4.1 (assay buffer)
- 50 mM Tris·HCl, pH 7.4 (at 4°C)
- 100 mM sodium chloride
- 5 mM magnesium chloride
- 2 mM 2-mercaptoethanol
- Add 200 µM Pefabloc (Carl Roth, CAS no. 30827-99-7) if used as resuspension buffer
- Filter and degas buffer before addition of 2-mercaptoethanol. Store up to 2 months at 4°C without 2-mercaptoethanol, otherwise do not store.
Buffer 4.2 (Ni-II buffer)
- 50 mM Tris·HCl, pH 7.4 (at 4°C)
- 500 mM sodium chloride
- 5 mM magnesium chloride
- 20 mM imidazole
- 2 mM 2-mercaptoethanol
- Filter and degas buffer before addition of 2-mercaptoethanol. Store up to 2 months at 4°C without 2-mercaptoethanol, otherwise do not store.
Buffer 4.3 (Ni-III buffer)
- 50 mM Tris·HCl, pH 7.4 (at 4°C)
- 100 mM sodium chloride
- 5 mM magnesium chloride
- 500 mM imidazole
- 2 mM 2-mercaptoethanol
- Filter and degas buffer before addition of 2-mercaptoethanol. Store up to 2 months at 4°C without 2-mercaptoethanol, otherwise do not store.
ECL A solution
- 5 ml of 1 M Tris·HCl, pH 8.5
- 500 µl of 250 mM luminol (Sigma-Aldrich, cat. no. A4685)
- 220 µl of 90 mM p -coumaric acid (Sigma-Aldrich, cat. no. 55823)
- Add distilled deionized H2O to a final volume of 50 ml
- Store at 4°C for up to 1 month
ECL B solution
- 5 ml of 1 M Tris·HCl, pH 8.5
- 30 µl of 30% (v/v) H2O2
- Add distilled deionized H2O to a final volume of 50 ml
- Store at 4°C for up to 1 month
Endopeptidase trypsin solution
- Reconstitute 1 mg lyophilized trypsin (Promega, cat. no. V511A) in 2 ml of 1 mM HCl solution
- Pipette 50-μl aliquots
- Store at −80˚C for 3 months
Fluor-de-Lys reaction mixture
- 50 mM Tris·HCl, pH 8.0
- 50 mM KCl
- 5 µM KDAC
- 20 µM fluorogenic KDAC substrate
- with/without 50 µM KDAC inhibitor
- 1% (v/v) DMSO
Fluor-de-Lys developer solution, 2×
- 200 mg/L trypsin
- 100 µM SAHA
- 100 µM TSA
Immunoblot transfer buffer
- 150 mM glycine
- 25 mM Tris base
- 10% (v/v) methanol
- Store at room temperature for up to 1 month
LC-MS/MS buffer
- 80% (v/v) acetonitrile (ACN)
- 0.1% (v/v) formic acid (AC)
Lithium potassium acetyl-phosphate, 100 mM
- 18.4 mg LiK acetyl-phosphate (MERCK, Sigma-Aldrich, cat. no. 94249-01-1; MW: 184.06 g/mol)
- 1000 μl filtered distilled deionized H2O
- Pipette 50-μl aliquots
- Store at −80˚C for up to 12 months
Lysogeny broth (LB) medium
- 10 g NaCl
- 10 g tryptone
- 5 g yeast extract
- H2O to 1 L
- Sterilize by autoclaving
- Store up to 1 month at 4°C-8°C
Lysogeny broth (LB) agar plates
- 10 g NaCl
- 10 g tryptone
- 5 g yeast extract
- 15 g agar
- H2O to 1 L
- Sterilize by autoclaving
- Pour 15-20 ml per 92-mm petri dish (diameter: 92 mm, height: 16 mm; Sarstedt, cat. no.: 82.1472.001). Store plates at 4°C-8°C.
- For antibiotic-containing plates, add the antibiotic after autoclaving, once the medium has cooled down to ∼55°C. Then, mix well and pour the plates.
Phosphate-buffered saline (PBS)
- 10.1 mM Na2HPO4
- 1.8 mM KH2PO4
- 137 mM NaCl
- 2.7 mM KCl
- Adjust pH with HCl to 7.4
- Store at room temperature up to 1 month
PBS-T
- 10.1 mM Na2HPO4
- 1.8 mM KH2PO4
- 137 mM NaCl
- 2.7 mM KCl
- 0.1% v/v Tween-20
- Adjust pH with HCl to 7.4
- Store at room temperature up to 1 month
Reaction buffer (5×)
- Dissolve 8.15 g K2HPO4 and 0.444 g KH2PO4 in 80 ml distilled deionized H2O
- Check the pH with a pH electrode or a pH test strip (it should be pH 8.0)
- Add distilled deionized H2O to a final volume of 100 ml
- Store at room temperature up to 1 month
SDS-PAGE gel, 12% (w/v)
- Stacking gel :
- 1.65 ml 30% acrylamide/bis -acrylamide solution (Carl Roth, cat. no. 3029.1)
- 100 μl 12% SDS
- 8 μl TEMED (Thermo Fisher Scientific, cat. no. 17919)
- 80 μl 10% (w/v) ammonium persulfate (APS; Thermo Fisher Scientific, cat. no. 17873)
- 1.4 ml 2 M Tris⋅HCl, pH 6.8
- 7 ml distilled deionized H2O .
- Separating gel:
- 8 ml 30% acrylamide/bis -acrylamide solution (Carl Roth, cat. no. 3029.1)
- 200 μl 12% w/v SDS
- 12 μl TEMED
- 120 μl 10% w/v APS
- 3.7 ml 2 M Tris⋅HCl, pH 8.8
- 8 ml distilled deionized H2O
SDS-PAGE destaining solution
- 10% (v/v) ethanol
- 20% (v/v) acetic acid
- Store up to 3 months at room temperature
SDS-PAGE running buffer
- Dissolve 14.4 g glycine, 3 g Tris base, and 1 g SDS in 800 ml. Add distilled deionized H2O to a final volume of 1 L. Store at 4°C up to 1 month.
SDS-PAGE sample buffer, 5×
- 50 mM Tris·HCl (pH 6.8)
- 10 mM 2-mercaptoethanol
- 50% (v/v) glycerol
- 10% (w/v) SDS
- 0.5% (w/v) bromophenol blue
- Store up to 6 months at −20°C
SDS-PAGE staining solution
- 40% (v/v) methanol
- 60% (v/v) distilled deionized H2O
- 3% (w/v) Coomassie blue G250
- 0.3% (w/v) Coomassie blue R250
- Store up to 3 months at room temperature
Skimmed milk powder in PBS-T, 5% (w/v)
- 0.75 g skimmed milk powder
- 15 ml PBS-T (see recipe)
- Store at –20°C up to 1 month
Terrific broth (TB) medium
- 5 ml glycerol
- 12 g peptone
- 24 g yeast extract
- 2.31 g KH2PO4
- 12.54 g K2HPO4
- H2O to 1 L
- Sterilize by autoclaving
- Store at 4°C-8°C
COMMENTARY
Background Information
The protocols described here allow a comprehensive characterization of enzymatic and non-enzymatic regulation of protein lysine acetylation on proteins of interest. We report the steps to perform protein lysine acetylation enzymatically, by lysine acetyltransferases (KATs), and non-enzymatically, by acetyl-CoA (Ac-CoA) and acetyl-phosphate (AcP). Moreover, we also describe an assay for the deacetylation of lysine-acetylated proteins by classical Zn2+-dependent deacetylases (KDACs) and NAD+-dependent sirtuin deacetylases (short: sirtuins). As substrates for these deacetylation reactions, lysine-acetylated proteins are generated using the genetic code expansion concept (GCEC), as described in the introduction (Kuhlmann et al., 2016; Lammers, 2018; Lammers et al., 2010; Neumann et al., 2008). PylRS has been used in the past for the incorporation of more than 100 unnatural amino acids (UNAA) in response to an amber stop codon into proteins, including aromatic side chains, aliphatic side chains, azides, and alkynes for click chemistry (Guo et al., 2014; Wan, Tharp, & Liu, 2014). PylRS has various characteristics that make it a powerful tool in synthetic biology: it lacks an editing domain, which is needed in other aminoacyl-tRNA synthetases for amino acid substrate specificity to ensure that the cognate tRNA is only charged with the cognate amino acid. This accounts for the high side-chain promiscuity of PylRS. The hydrophobic substrate binding site in PylRS is capable of binding to structurally diverse side chains with low degree of selectivity. Additionally, PylRS shows a low degree of selectivity towards the tRNA anticodon, making it possible to reassign the tRNA anticodon to recognize other mRNA codons, such as ochre (5′-UAA-3′), opal (5′-UGA-3′), and even quadruplet codons (5′AGGAG-3′) (Neumann, Wang, Davis, Garcia-Alai, & Chin, 2010). Moreover, PylRS is quite unselective towards the PylRS α-amine, also allowing the incorporation of α-hydroxy acids into proteins (Wan et al., 2014). Structurally, PylRS is composed of an N-terminal domain of varying length and a C-terminal catalytic domain that can be classified into class II aminoacyl-tRNA-synthetases (AARS), showing similarity to eukaryotic phenylalanyl-tRNA-synthetase (PheRS) (Kavran et al., 2007). Another parameter accounting for the success of PylRS as an important synthetic biological tool is that it is orthogonal in major model organisms: Drosophila melanogaster , Mus musculus , Saccharomyces cerevisiae , Caenorhabditis elegans , and Escherichia coli (Bianco, Townsley, Greiss, Lang, & Chin, 2012; Chin, 2014; Gautier, Deiters, & Chin, 2011; Gautier et al., 2010; Greiss & Chin, 2011; Hancock, Uprety, Deiters, & Chin, 2010). This orthogonality allows the use of PylRS/PylT even together with other engineered AARS/tRNA-pairs such as the Methanocaldococcus jannaschii tyrosyl-tRNA-synthetase (Mj TyrRS)/Mj tRNACUA-pair, making it possible to simultaneously incorporate two UNAA into proteins by decoding different codons. Mj TyrRS/Mj tRNACUA was the first aminoacyl-tRNA-synthetase/tRNA-pair that was engineered by the Schultz laboratory to incorporate UNAA such as p -benzoyl-L-phenylalanine (pBP) or p -azido-L-phenylalanine (pAP) into proteins (Chin, Martin, King, Wang, & Schultz, 2002; Chin et al., 2002). Other orthogonal aminoacyl-tRNA-synthetase/tRNACUA pairs based on Methanosarcina barkeri and/or Methanosarcina mazei PylRS/tRNACUA were developed that allow the incorporation of methyl-lysine, phosphoserine, phosphothreonine, and ubiquitin (Nguyen, Garcia Alai, Virdee, & Chin, 2010; Rogerson et al., 2015; Virdee et al., 2011; Zhang et al., 2017). These were obtained by powerful screening strategies of complex libraries encoding the variants. It was shown that novel deep-sequencing technologies can be efficiently used to screen complex libraries, simplifying and complementing negative-positive-negative selection strategies. These systems have had a great impact on studies of post-translational modifications (Zhang et al., 2017).
Methanosarcina barkeri Mb PylRS/Mb tRNACUA was synthetically evolved by the laboratory of Jason Chin to incorporate acetyl-L-lysine into proteins in response to an amber stop codon. The first studies applying this genetic code expansion concept (GCEC) showed successful incorporation of acetyl-L-lysine into myoglobin, histones, and the peptidyl-prolyl-cis /trans isomerase cyclophilin A (Lammers et al., 2010; Neumann et al., 2009; Neumann et al., 2008). Next to PylRS, it was shown that evolving its cognate tRNACUA resulted in a significant increase of incorporation efficiency of UNAAs (Fan, Xiong, Reynolds, & Soll, 2015). The amber stop codon is the least-used stop codon in E. coli (∼7%). However, the amber stop codon is recognized by release factor 1 (RF1), resulting in premature termination of translation. Moreover, the UNAA is also incorporated at endogenous amber codons, resulting in unwanted off-target effects. To this end, further improvements of the system include the genomic deletion of the gene encoding for RF1 and removing all amber stop codons in the E. coli genome and replacing them by ochre codons, to abolish the genetic dependence on RF1 (Isaacs et al., 2011; Johnson et al., 2011; Mukai et al., 2010; Wals & Ovaa, 2014). Moreover, PylRS/tRNACUA of other archaea was also reported as efficient orthogonal synthetic biological tools for site-specific incorporation of UNAAs in proteins (Beranek, Willis, & Chin, 2019). Systems for the rapid discovery of novel orthogonal aminoacyl-tRNA-synthetase/tRNACUA-pairs for incorporation of unnatural amino acids in proteins have been reported (Cervettini et al., 2020).
The GCEC makes it possible to produce quantitatively and homogenously lysine-acetylated proteins that are suitable for structural studies by X-ray crystallography. Following expression, the lysine-acetylated protein can be purified by standard purification strategies (Lammers, 2018). Previous attempts to produce site-specifically lysine-acetylated proteins involved using purified KATs. However, KATs are often multidomain proteins that are difficult to purify. Moreover, KATs often result in non-quantitative acetylation, and they also might acetylate multiple lysine side chains, particularly when working with the isolated catalytic domains. Other experimental approaches involve synthetic or semi-synthetic methods, including native chemical ligation. However, these approaches are often limited to the protein termini (Lammers, 2018; Lammers et al., 2010; Li et al., 2011; Spicer & Davis, 2014). By applying the GCEC, we were able to solve the first crystal structure of a protein carrying a post-translationally relevant lysine acetylation. We obtained high resolution structures of K125-acetylated Cyclophilin A, and discovered its influence on enzymatic activity and protein function (Lammers et al., 2010). The system has even been used to structurally characterize a double lysine-acetylated protein, namely RhoGDIα (Kuhlmann et al., 2016; Kuhlmann, Wroblowski, Scislowski, & Lammers, 2016). Notably, the simultaneous incorporation of several acetyl lysines in proteins in response to several amber stop codons is possible. However, the incorporation efficiency is strongly reduced in these cases (about 50% for RhoGDIα, but this depends on the individual protein). The efficiency can be increased in these cases by using the E. coli strain carrying genomic deletion of the gene encoding RF1, which would otherwise compete for binding to the amber stop codon, resulting in termination of translation, as stated above.
The recombinantly expressed and purified site-specifically lysine-acetylated proteins obtained by the GCEC (Basic Protocol 1) can be directly used as substrates for classical deacetylases and/or sirtuins (Knyphausen et al., 2016; Lammers, 2018). The advantage of this approach is that it allows the investigator to directly follow the deacetylation at an individual site in the context of a natively folded protein. Our laboratory showed that the protein structure is, in addition to the primary sequence, important for determination of deacetylase substrate specificity (Knyphausen et al., 2016). This could not be assessed, for instance, using lysine-acetylated peptides. The described approach allows one to perform enzyme kinetics on lysine-acetylated and natively folded proteins, which is not possible with any other method. This allows the characterization of the deacetylase activity of classical deacetylases and sirtuins on natively folded substrate proteins (de Boor et al., 2015; Knyphausen et al., 2016; Kuhlmann et al., 2016; Kuhlmann et al., 2016; Lammers, 2018). For the characterization of the acetylation of proteins by KATs, and non-enzymatically with acetyl-CoA and/or acetyl-phosphate, we use recombinantly expressed and purified proteins as substrates (de Boor et al., 2015; Knyphausen et al., 2016; Kuhlmann et al., 2016; Kuhlmann et al., 2016; Lammers, 2018). Several studies have employed cells in which genes encoding for KDACs/sirtuins/KATs were deleted, in combination with transient overexpression of the protein of interest, or they performed immunoprecipitation of the endogenous proteins combined with LC-MS/MS to identify lysine substrate side chains. However, these approaches are indirect, as they only show that the genomic deletion of the gene affects the acetylation state of the protein of interest. These results have to be validated with further experiments, such as mutational analyses, to confirm the substrate site.
The detection of the acetylation state of the substrate proteins can be achieved by various methods. Immunoblotting using an anti-acetyl-L-lysine antibody can be used to detect the acetylated protein and to follow its acetylation or deacetylation. Electrospray ionization mass-spectrometry (ESI-MS) allows the investigator to determine the mass of the substrate protein (acetyl: 42 Da), and LC-MS/MS makes it possible to determine the site of lysine acetylation (de Boor et al., 2015; Kuhlmann et al., 2016; Lammers, 2018). For the deacetylation by KDACs/sirtuins, fluorescence-based assays were developed that allow detection of deacetylase-catalyzed deacetylation in an end-point assay (Fluor-de-Lys assay). Recently, a continuous assay format was developed for KDAC/sirtuin deacetylase activity based on deacetylation of firefly luciferase, dependent on the incorporation of acetyl-lysine into the enzyme by the GCEC affecting enzyme activity (Spinck, Ecke, Sievers, & Neumann, 2018). For the classical deacetylases, the production of acetate during substrate deacetylation can also be followed in an enzyme-coupled assay format including acetate-kinase, phosphotransacetylase, and lactate-dehydrogenase (Megazyme Ltd.).
In summary, using recombinantly expressed proteins to follow the acetylation of substrate proteins and site-specifically lysine-acetylated proteins prepared by GCEC to follow KDAC/sirtuin catalyzed deacetylation, combined with sensitive detection methods, is a powerful strategy to characterize substrate lysine acetylation and deacetylation.
Critical Parameters
Basic Protocol 1
It is often observed that the expression yield of the lysine-acetylated protein is lower compared to the non-acetylated protein. This is due to the fact that although the decoded amber stop codon is the least used stop codon in E. coli (∼7%), it competes with the release factor 1 (RF1) for translational termination. Moreover, endogenous amber stop codons are also decoded, and acetyl-lysine is incorporated at these sites, which might interfere with cellular fitness, which could affect the expression yield. To this end, E. coli strains have been constructed in which all endogenous amber stop codons have been replaced by the ochre stop codon, and a strain was constructed that carries a genomic deletion of the gene encoding RF1 (E. coli ΔRF1) (Isaacs et al., 2011; Johnson et al., 2011; Mukai et al., 2010). If problems should arise with solubility of the proteins, consider using different expression vectors (low copy vs. high copy; different promotors) or using different fusion tags (His6-tag, MBP-tag, GST-tag, etc.). Notably, lysine acetylation might also affect the solubility and stability of the POI, and the expression and purification conditions used for the non-acetylated protein might not always be directly transferable to the lysine-acetylated protein.
Analyzing the soluble and insoluble fractions of an expression using the GCEC to incorporate acetyl-L-lysine into a POI by SDS-PAGE gel reveals if a high amount of translational termination product is generated. A strategy to improve the expression of full-length protein of UNAA-incorporated POI is to position the affinity tag at the C-terminus and to use the E. coli ΔRF1 strain for expression. Moreover, E. coli encodes for a single sirtuin deacetylase, CobB, which might deacetylate the acetylated POI during expression. To this end, 2 mM nicotinamide is added during bacterial cultivation. Nicotinamide is a non-competitive inhibitor for CobB. If this is not sufficient to avoid translational truncation, an E. coli strain with a genomic deletion of CobB, E. coli ΔCobB, might be used for expression. Finally, the amount of acetyl-L-lysine supplemented into the culture can be varied to ensure an efficient charging of the Mb tRNACUA. Nicotinamide (final concentration: 2 mM) and acetyl-L-lysine (final concentration: 10 mM) are added 30 min before induction of expression of the gene of interest to ensure that the cells are adjusted for an efficient incorporation of the UNAA into the POI.
Basic Protocol 2
Protein purity and activity
Obtaining protein in high quantity and purity is critical for the success of this procedure. Since relatively low amounts of SIRT are needed for a single deacetylase assay, the focus should be on obtaining a high-purity protein preparation. Freezing the SIRT preparation directly following purification in suitable aliquot volumes to avoid subsequent repeated freeze-thaw cycles ensures high enzyme activity and reproducible enzyme activity of different enzyme preparations. After long storage periods at –80°C (>6 months), the activity of the SIRT should be analyzed by assessing deacetylation of a known substrate before testing new substrates.
Detecting strong acetylation signals
A second critical parameter for this protocol is the detection of the acetylated substrate used. Our data on immunoblotting of site-specifically lysine-acetylated proteins showed that the commercially available anti-acetyl lysine antibodies exhibit a sequence bias in detection (de Boor et al., 2015). Therefore, it is important to analyze the anticipated acetylation signal using an anti-acetyl-lysine antibody before the assay is performed. If necessary, the substrate and SIRT concentrations or the incubation time of the assay can be adjusted accordingly.
Basic Protocol 3
Protein solubility, aggregation, and purity
The solubility of the protein is essential for the success of the described expression and purification strategy. Several parameters can be modified and optimized for improving the solubility of the POI (cultivation medium, expression duration, expression temperature, IPTG concentration, expression host, etc.). If these parameters do not improve the solubility of the POI, a different expression system apart from E. coli might be considered, such eukaryotic expression systems like insect or yeast cells. For mammalian classical KDACs, only HDAC1, HDAC2, and HDAC8 provide good soluble expression in E. coli. The other mammalian KDACS have been expressed in eukaryotic expression systems. For AcuC, we observed that it forms higher oligomers following Zn2+-NTA purification. These oligomeric state(s) are in equilibrium with the AcuC dimeric state. To this end, a purification strategy consisting of an initial SEC using a Superose 6 Increase column, which is suited for high-molecular-weight proteins and protein complexes, followed by an SEC on a Superdex 200 10/300 pg column, was used for purification of dimeric B. subtilis AcuC. Moreover, we observed aggregation to higher oligomers if AcuC is stored in KDAC elution buffer overnight. To this end, the purification by SEC should be conducted without any delay directly following Zn2+-NTA elution. To improve the purity of the POI, the washing step of the Zn2+-NTA affinity chromatography can be extended dependent on the development of A 280, and/or additional purification steps might be included in the purification strategy such as ion-exchange chromatography.
Assessment of KDAC deacetylase activity
Different KDACs exhibit diverse activities towards the used Boc-acetyl-L-lysine-AMC (Boc-AcK-AMC) substrate in the Fluor-de-Lys assay. When implementing an activity assay for an uncharacterized deacetylase, a high deacetylase:substrate ratio (up to 2:1) and extended incubation times (up to overnight) can be considered. Furthermore, the buffer components might also interfere with KDAC activity. We have observed that low pH values and the addition of Zn2+ to the assay buffer have an inhibitory effect on AcuC activity in the Fluor-de-Lys assay. As the deacetylase activity of AcuC diminishes over time, even if the enzyme preparation is stored on ice, it is important to complete the expression, purification, and activity assay as quickly as possible. The protein should be flash-frozen in liquid nitrogen and stored in appropriate small aliquots at –80°C to avoid repeated thawing and freezing.
Specificity and activity to protein substrate
Boc-AcK-AMC is a widely accepted KDAC substrate. However, KDACs exhibit substrate specificity dependent on both the substrates’ primary and three-dimensional structure. To this end, even if no activity can be observed for the commercially available Fluor-de-Lys substrates such as Boc-AcK-AMC, or p53- and histone-derived peptides, it is possible that the individual KDAC has a very restricted, narrow substrate range and shows activity only towards those substrates. The GCEC can be applied to produce site-specifically acetylated KDAC substrate proteins to understand the molecular determinants of KDAC substrate specificity. As an example, acetylated Bacillus subtilis acetyl-CoA synthetase A (AcsA), a known substrate of AcuC, might not be a substrate for other KDACs or sirtuins. Although other bacterial HDAC homologs such as Bordetella/Alcaligenes FB188 HDAH have been shown to be able to deacetylate fluorogenic Boc-AcK-AMC substrate or histone-derived substrates (Nielsen, Hildmann, Dickmanns, Schwienhorst, & Ficner, 2005), the identification of endogenous protein substrates is important to classify those enzymes as protein lysine deacetylases. The Boc-AcK-AMC contains only a single amino acid, i.e., the acetyl-L-lysine, and is not part of a peptide or protein with additional neighboring residues.
Basic Protocol 4
For the in vitro acetylation assay, rapid purification of the enzymes is essential, as we have observed that enzyme activity decreases over time. To ensure a high level of activity, it is important to perform the purification at 4°C and to store the protein preparation on wet ice. Storage (2-4 days) at 4°C leads to a strong decrease in acetyltransferase activity. Furthermore, it is important to use pure protein for the in vitro assay, so that negative or positive influences on the acetyltransferase activity in the assay due to impurities are excluded. Another important parameter is the buffer. Depending on the lysine acetyltransferase, a different pH, a different ionic strength, or additional divalent ions are preferred. This protocol provides a buffer that was optimized for studies on AcuA. It might be necessary to modify it for other lysine acetyltransferases. Importantly, the concentration of both the acetyl-group donor molecule acetyl-CoA and of the substrate used in the assay must also be optimized for the KAT of interest. Here, we use an acetyl-CoA concentration of 50 µM for AcuA. However, lower (20 µM) or higher concentrations (100 µM) were also reported in KAT-catalyzed acetylation reactions (Wang et al., 2017). To determine a suitable acetyl-CoA concentration, various samples with different acetyl-CoA concentrations could be tested. Note that higher acetyl-CoA concentrations and longer incubation times (1-6 hr) may lead to non-enzymatic acetylation (Kuhn et al., 2014; Vo et al., 2020). If a basic pH is used in the assay, non-enzymatic acetylation is additionally favored because the lysine side chain ε-amino groups are more deprotonated compared to the state at physiological pH (pH 7.4). To this end, lysine side chains are more reactive, i.e., the nucleophilicity is increased to attack the acetyl-CoA/acetyl-phosphate carbonyl carbon, resulting in more prevalent non-enzymatic acetylation (Kuhn et al., 2014).
Basic Protocol 5
The non-enzymatic protocol includes several chemicals and enzymes that are prone to degradation. Acetyl-coenzyme A and lithium potassium LiK-acetyl-phosphate need to be prepared and used fresh, as these chemicals are unstable in solution. Acetyl-coenzyme A degrades within 2 weeks, even when frozen at −20˚C. Further, antibodies degrade quickly when not maintained in an appropriate solution and at low temperatures. For LC-MS/MS analysis, proteins are fragmented to peptides, which is essential to evaluate acetylated L-lysines in the protein structure. To achieve fragmentation, the endopeptidase trypsin is used. This protein is stable for 12 months under optimal conditions. Temperature differences during transfer from −20˚C to 4˚C for pipetting the enzymes to the assay are not optimal. This should be kept in mind and may mean that the actual stability and activity of the enzymes might be reduced and, therefore, a slightly higher concentration or/and a longer incubation time might be needed.
For LC-MS/MS analysis, it is especially important to work in a clean environment. Keratin, which originates from skin and hair, is one of the most common contaminants detected in mass spectrometry. It is, therefore, recommended to always wear gloves and a laboratory coat, and to work in a dust-reduced environment when preparing samples for MS.
Troubleshooting
Table 4 lists common problems with the protocols, their causes, and potential solutions, organized by basic protocol.
Understanding Results
Basic Protocol 1
Using the GCEC to incorporate acetyl-L-lysine site-specifically into proteins is a powerful approach to study the impact of this post-translational modification on protein function. The general expression and purification strategies that are applied to non-acetylated proteins also apply for the POI with incorporated UNAA. However, the conditions and parameters might need to be adjusted, and are sometimes not transferable from the expression of the non-acetylated protein to the acetylated protein counterpart. The system for genetically encoding acetyl-L-lysine relies on the expression of genes encoding for the orthogonal acetyl-L-lysyl-tRNA-synthetase/tRNACUA-pair from archaea of genus Methanosarcina (Fig. 1B). To this end, the cultures for incorporation of acetyl-L-lysine are supplemented with nicotinamide and acetyl-lysine to inhibit CobB deacetylase and to allow charging of Mb tRNACUA with acetyl-L-lysine. If the incorporation of the UNAA is not quantitative, formation of premature termination products might be observed on SDS-PAGE and/or by ESI-MS. To confirm the site-specific incorporation of acetyl-L-lysine into the POI, the protein should be analyzed by immunoblotting using a specific anti-acetyl-lysine antibody, by ESI-MS to confirm the correct molecular mass, and by LC-MS/MS to confirm the correct site of acetyl-lysine incorporation (Lammers, 2018).
Basic Protocol 2
This protocol should yield high amounts (10-30 g L-1 from a 10 L culture) of sirtuin with just minimal amounts of impurities, which will vary depending on the sirtuin isoform and the fragment expressed. As for the purification shown in Figure 4B and 4C, the final yield of His6-SIRT250-356 was approximately 10 mg L-1 culture. The sirtuin assay includes the essential controls to yield a robust answer for whether POIAck deacetylation is catalyzed by the SIRT analyzed. Figure 4D shows the result of the deacetylation of the acetylated His6-SIRT250-356 substrate Ran Ack71 (see Basic Protocol 1). A His6-Ran AcK71 acetylation signal is not detectable only in sample 2, in the presence of the stoichiometric co-substrate NAD+. On the other hand, no significant reduction in the His6-Ran AcK71 acetylation signal can be seen in the absence of NAD+ (sample 3, lane 3), nor upon addition of NAD+ and nicotinamide (NAM) (sample 4, lane 4), compared to the non-enzyme control (sample 1, lane 1).
Basic Protocol 3
This protocol is used to express and purify a non-characterized KDAC, which can display different characteristics regarding solubility and oligomeric state. As shown on the SDS-PAGE for Bc AcuC (Fig. 5), a small portion of the total expressed AcuC (lane 1) is present in the soluble fraction (lane 2) and binds to the Zn2+-NTA affinity chromatography resin (lane 3) (Fig. 5B). This soluble AcuC was subjected to further purification by Zn2+-NTA affinity chromatography (lanes 10-13) (Fig. 5B). Finally, dimeric AcuC was obtained by Superose 6 Increase SEC and S200 SEC performed in KDAC standard buffer. From a 5-L E. coli Bl21 (DE3) culture grown in TB medium, approximately 20 mg of AcuC protein could be purified with a high degree of purity, sufficient to perform enzyme kinetics assays and for further biophysical characterization. The AcuC enzyme was active against the Boc-AcK-AMC substrate, and the enzyme activity could be inhibited with suberoylanilide hydroxamic acid (SAHA). AcuC was capable of deacetylating recombinantly expressed, purified, and enzymatically acetylated (using purified Bs AcuA KAT) AcsA, as shown by immunoblotting using an anti-acetyl-L-lysine antibody (Fig. 5D). These data show that the protocol described can be applied to express and purify active KDACs.
Basic Protocol 4
In vitro acetylation assay
If successful acetylation is detected, the potential KAT can acetylate the tested substrate in vitro. However, this is no proof of the physiological significance of such an acetylation. The limited number of lysine acetyltransferases encoded in the human genome and also in bacteria suggests that KAT substrate specificity is determined by their presence in multi-protein complexes. However, our data using isolated KATs in in vitro studies suggest that these enzymes are not unspecific, as we observe KAT-catalyzed acetylation only for a limited number of target proteins and not for all proteins tested. The latter case would be an indication of an unspecific reaction. However, their degree of specificity and potency in vivo might be modulated by additional proteins, interaction partners, and other regulatory mechanisms, such as post-translational modifications, that are not represented in these in vitro studies. To this end, these studies have to be complemented by in vivo studies in cells or organisms to confirm the role of the KAT-catalyzed post-translational modification of the target protein. It can also occur that some KATs are active in vivo but not in vitro because the assay format in vitro lacks stimulatory factors that are present in cells. Importantly, in vitro KAT assays must include appropriate controls to show that the reactions are not non-enzymatic and to confirm that they are enzyme catalyzed. Due to the high reactivity of acetyl-CoA, which acts as a mediator for non-enzymatic acetylation in mammals, and the high reactivity of lysine side chains dependent on the sequence and structural context, these are prone to non-enzymatic acetylation. Controls that assess the degree of acetylation in a no-enzyme-containing control are, therefore, essential to rule out non-enzymatic acetylation. Often, KAT enzymes show auto-acetyltransferase activity, which can be used as a control to show activity of the enzymes and to confirm the technical validity of the conducted experiment (Fig. 6B).
Basic Protocol 5
N-(ε)-lysine acetylation is revealed in the immunoblot when a reaction (light) signal is detected. The signal can vary in intensity depending on the acetyl-coenzyme A/acetyl-phosphate concentrations used, or on the incubation time. A negative control sample is of significance here, as it should indicate that the antibodies (primary as well as secondary) bind specifically, and no false-positive signal is detected. The immunoblot signals are faint if only a small percentage of lysine side chains are acetylated. This might occur at low acetyl-coenzyme A/acetyl-phosphate concentrations, at short incubation times, or if only very few lysines are present in the protein. If several lysines are present in the protein, it might indicate that only accessible, surface-exposed lysines side chains are acetylated. Moreover, not all proteins at every lysine position might be acetylated. Strong signals, on the other hand, might occur at high acetyl-coenzyme A/acetyl-phosphate concentrations and with long incubation times, and reflect full acetylation of almost or all lysines of the protein. With immunoblot analysis, it is not possible to detect at which L-lysine position the acetylation occurred.
With LC-MS/MS, peptide fragments of the protein should be detected. The peptides and peptide lengths can be theoretically determined from the protein sequence. Since trypsin cleaves C-terminal of a L-lysine or L-arginine residue, all peptides of the protein can be specified. Thus, the resulting peptides can be predicted beforehand. LC-MS/MS analysis allows detecting the molecular masses of the peptides, and an acetylated L-lysine within a peptide can be determined by a molecular mass shift of 42 g mol-1. The peptide of interest can then be aligned with the complete protein sequence, and the exact position of the acetyl-L-lysine can be specified. The physiological significance of the determined acetylation site has to be evaluated with further experiments, such as enzymatic acetylation/deacetylation assays (Basic Protocols 4 and 2/3). A subsequent experiment could show if certain deacetylases are able to remove the acetylation at specific L-lysines. In this way, a physiologically relevant acetylation site might be identified. An enzymatic acetylation experiment could further validate a physiologically relevant acetylation site if a protein-specific acetyltransferase is known.
Time Considerations
Basic Protocol 1
The expression of the POI, including transformation, preparation, and cultivation of the pre-culture (overnight), the cultivation of the main culture (12-16 hr), and cell harvest takes 3 days, but depends on the optimal time for expression. Protein purification by a two-step purification strategy, including an affinity chromatography such as Ni2+-NTA chromatography and SEC, takes about 1-2 days. Depending on the level of purity obtained using this two-step purification protocol, additional purification steps might be included in the strategy, such as ion-exchange chromatography. If this is the case, the time for purification will be extended accordingly. The immunoblotting can be performed in 1-2 days, depending on the incubation times used for the individual steps (i.e., incubation with primary antibody can be executed overnight or only for 1-2 hr, depending on the antibody used). Moreover, the time taken for this assay depends on the duration of all individual steps and on the type of loading control performed—i.e., Ponceau S/amido black staining of the membrane can be done directly following the transfer, but staining with an anti-His6 antibody needs further steps (i.e., stripping of first antibody, washing, blocking, incubation with anti-His6 antibody, washing, detection). The determination of the molecular mass by ESI-MS (not shown here, but explained in Lammers (2018) and the position of acetyl-L-lysine incorporation by tryptic digest and LC-MS/MS (see Basic Protocol 5) analyses can be done in 2-3 days.
Basic Protocol 2
Expression and purification His6-SIRT250-356
The expression His6-SIRT250-356 requires 3-4 days [day 1: transformation of E. coli BL21 (DE3) cells; day 2: inoculation of pre-culture; day 3: inoculation and cultivation of main culture/expression of POI; day 4: harvesting of cells]. The purification of His6-SIRT250-356 takes 2-3 days (day 1: cell lysis, separation of insoluble and soluble fractions, Ni2+-NTA chromatography; day 2: elution from Ni2+-NTA chromatography, SEC; day 3: concentration of POI and freezing of POI).
The SIRT deacetylation assay takes 3-4 hr, including the preparation and thawing of the enzymes, determination of protein concentrations, pipetting, and incubation of the samples. The detection of the deacetylase reactions by immunoblotting requires 2-3 days, depending on the incubation times and on the type of loading control performed. If antibody staining is performed as loading control, such as anti-His6 staining, the immunoblotting takes 3 days.
Basic Protocol 3
Expression and purification of KDAC
The expression and purification of acuC is conducted in 5 days. The E. coli BL21 (DE3) cells are transformed with pET45b(+)/His6-acuC on the first day. On the second day, the pre-culture is inoculated with 4-5 single colonies; on the third day, the main culture is inoculated with the pre-culture, and acuC expression is conducted overnight. On the fourth day, the cells are harvested and prepared for purification. As stated above, it is recommended to perform Zn2+-NTA affinity purification without any delay following expression and do cell harvest on day 4. The subsequent SEC runs (Superose 6 SEC followed by S200 SEC) are conducted on day 5 to avoid degradation or aggregation of the protein. Check the elution fractions of the SEC runs by SDS-PAGE, pool the pure AcuC-containing fractions, flash-freeze the protein in small aliquots in liquid nitrogen, and store at –80°C.
Fluor-de-Lys assay
For determination of protein concentrations, pipetting of the reactions, performing the reaction, development, and the final measurement of the fluorescence intensities, approximately 4 hr are needed. The total time needed depends on the number of samples to be prepared and the incubation time used.
Protein deacetylation
Preparation of reaction mixes takes 2-3 hr, and includes thawing the proteins, spinning to remove precipitants, and determination of protein concentration by measurement of the A 280 and applying the Lambert-Beer law using the protein-specific extinction coefficient (EC, ε). The EC can be calculated using the ProtParam tool within the Expasy bioinformatics resource portal of the SIB Swiss Institute of Bioinformatics (https://web.expasy.org/protparam/). The time needed for deacetylation of a substrate depends on the enzyme activity and efficiency of the substrate. This can vary dependent on the KDAC and the substrate analyzed. The AcuC is a highly active KDAC. Protein substrates can be prepared by applying the GCEC, by chemical acetylation using acetyl-CoA or acetyl-phosphate, or enzymatically using purified lysine acetyltransferases (KATs) such as AcuA (Basic Protocol 4). Following the enzymatic reaction, the samples are prepared for SDS-PAGE and immunoblotting by addition of SDS sample buffer and boiling (5 min, 95°C). These samples can be stored at −20°C until immunoblotting.
Immunoblotting
Running the SDS-PAGE, transferring of the proteins from the SDS-PAGE gel to the membrane, incubating with blocking solution and antibodies, and documenting the immunoblotting takes 1-2 days. The blocking takes 2 hr, and the membrane is incubated with the primary anti-acetyl-lysine antibody overnight. Depending on the loading control, stripping and staining with secondary antibody and development take 3-4 hr, including all washing steps and another blocking step.
Basic Protocol 4
In vitro lysine acetylation assay
The expression of acuA and acsA requires 3 days. The purification of each enzyme can take 1-2 days. The acetylation assay takes approximately 4 hr. The immunoblotting, including SDS-PAGE, protein transfer, antibody staining, and detection, takes 1-2 days depending on the length of incubation times.
Basic Protocol 5
To complete the non-enzymatic acetylation protocol, including immunoblot analysis and/or LC-MS/MS, 5 working days should be considered.
Acknowledgments
This study was funded by the German Research Foundation (DFG; Deutsche Forschungsgemeinschaft) Grant LA2984-5/1 (Project: 389564084) and LA2984-6/1 (Project: 449703098). The authors thank Susanne de Boor for generating figures.
Open access funding enabled and organized by Projekt DEAL.
Author Contributions
Leonie G. Graf : writing original draft, writing review and editing; Robert Vogt : writing original draft, writing review and editing; Anna-Theresa Blasl : writing original draft, writing review and editing; Chuan Qin : writing original draft, writing review and editing; Sabrina Schulze : writing original draft, writing review and editing; Daniela Zühlke : writing original draft, writing review and editing; Susanne Sievers : supervision; Michael Lammers : conceptualization, funding acquisition, supervision, writing original draft, writing review and editing.
Conflict of Interest
The authors declare no conflict of interest.
Open Research
Data Availability Statement
Data sharing is not applicable to this article as no new data were created or analyzed in this study.
Literature Cited
- Albaugh, B. N., Arnold, K. M., & Denu, J. M. (2011). KAT(ching) metabolism by the tail: Insight into the links between lysine acetyltransferases and metabolism. Chembiochem , 12(2), 290–298. doi: 10.1002/cbic.201000438.
- Allfrey, V. G., Faulkner, R., & Mirsky, A. E. (1964). Acetylation and methylation of histones and their possible role in the regulation of RNA synthesis. Proceedings of the National Academy of Sciences of the United States of America , 51, 786–794. doi: 10.1073/pnas.51.5.786.
- Baeza, J., Dowell, J. A., Smallegan, M. J., Fan, J., Amador-Noguez, D., Khan, Z., & Denu, J. M. (2014). Stoichiometry of site-specific lysine acetylation in an entire proteome. Journal of Biological Chemistry , 289(31), 21326–21338. doi: 10.1074/jbc.M114.581843.
- Baeza, J., Lawton, A. J., Fan, J., Smallegan, M. J., Lienert, I., Gandhi, T., … Denu, J. M. (2020). Revealing dynamic protein acetylation across subcellular compartments. Journal of Proteome Research , 19(6), 2404–2418. doi: 10.1021/acs.jproteome.0c00088.
- Baeza, J., Smallegan, M. J., & Denu, J. M. (2015). Site-specific reactivity of nonenzymatic lysine acetylation. ACS Chemical Biology , 10(1), 122–128. doi: 10.1021/cb500848p.
- Beranek, V., Willis, J. C. W., & Chin, J. W. (2019). An evolved Methanomethylophilus alvus pyrrolysyl-tRNA synthetase/tRNA pair is highly active and orthogonal in mammalian cells. Biochemistry , 58(5), 387–390. doi: 10.1021/acs.biochem.8b00808.
- Bianco, A., Townsley, F. M., Greiss, S., Lang, K., & Chin, J. W. (2012). Expanding the genetic code of Drosophila melanogaster. Nature Chemical Biology , 8(9), 748–750. doi: 10.1038/nchembio.1043.
- Blasl, A. T., Schulze, S., Qin, C., Graf, L. G., Vogt, R., & Lammers, M. (2021). Post-translational lysine ac(et)ylation in health, ageing and disease. Biological Chemistry. Online ahead of print. doi: 10.1515/hsz-2021-0139.
- Cervettini, D., Tang, S., Fried, S. D., Willis, J. C. W., Funke, L. F. H., Colwell, L. J., & Chin, J. W. (2020). Rapid discovery and evolution of orthogonal aminoacyl-tRNA synthetase-tRNA pairs. Nature Biotechnology , 38(8), 989–999. doi: 10.1038/s41587-020-0479-2.
- Chen, Y., & Li, Y. (2019). Site-specific determination of lysine acetylation stoichiometries on the proteome-scale. Methods in Enzymology , 626, 115–132. doi: 10.1016/bs.mie.2019.06.021.
- Chin, J. W. (2014). Expanding and reprogramming the genetic code of cells and animals. Annual Review of Biochemistry , 83, 379–408. doi: 10.1146/annurev-biochem-060713-035737.
- Chin, J. W., Martin, A. B., King, D. S., Wang, L., & Schultz, P. G. (2002). Addition of a photocrosslinking amino acid to the genetic code of Escherichia coli. Proceedings of the National Academy of Sciences of the United States of America , 99(17), 11020–11024. doi: 10.1073/pnas.172226299.
- Chin, J. W., Santoro, S. W., Martin, A. B., King, D. S., Wang, L., & Schultz, P. G. (2002). Addition of p-azido-L-phenylalanine to the genetic code of Escherichia coli. Journal of the American Chemical Society , 124(31), 9026–9027. doi: 10.1021/ja027007w.
- Choudhary, C., Kumar, C., Gnad, F., Nielsen, M. L., Rehman, M., Walther, T. C., … Mann, M. (2009). Lysine acetylation targets protein complexes and co-regulates major cellular functions. Science , 325(5942), 834–840. doi: 10.1126/science.1175371.
- Choudhary, C., Weinert, B. T., Nishida, Y., Verdin, E., & Mann, M. (2014). The growing landscape of lysine acetylation links metabolism and cell signalling. Nature Reviews Molecular Cell Biology , 15(8), 536–550. doi: 10.1038/nrm3841.
- de Boor, S., Knyphausen, P., Kuhlmann, N., Wroblowski, S., Brenig, J., Scislowski, L., … Lammers, M. (2015). Small GTP-binding protein Ran is regulated by posttranslational lysine acetylation. Proceedings of the National Academy of Sciences of the United States of America , 112(28), E3679–3688. doi: 10.1073/pnas.1505995112.
- Dieterich, I. A., Lawton, A. J., Peng, Y., Yu, Q., Rhoads, T. W., Overmyer, K. A., … Puglielli, L. (2019). Acetyl-CoA flux regulates the proteome and acetyl-proteome to maintain intracellular metabolic crosstalk. Nature Communications , 10(1), 3929. doi: 10.1038/s41467-019-11945-9.
- Dittenhafer-Reed, K. E., Feldman, J. L., & Denu, J. M. (2011). Catalysis and mechanistic insights into sirtuin activation. Chembiochem , 12(2), 281–289. doi: 10.1002/cbic.201000434.
- Edelhoch, H. (1967). Spectroscopic determination of tryptophan and tyrosine in proteins. Biochemistry , 6(7), 1948–1954. doi: 10.1021/bi00859a010.
- Fan, C., Xiong, H., Reynolds, N. M., & Soll, D. (2015). Rationally evolving tRNAPyl for efficient incorporation of noncanonical amino acids. Nucleic Acids Research , 43(22), e156. doi: 10.1093/nar/gkv800.
- Feldman, J. L., Dittenhafer-Reed, K. E., & Denu, J. M. (2012). Sirtuin catalysis and regulation. Journal of Biological Chemistry , 287(51), 42419–42427. doi: 10.1074/jbc.R112.378877.
- Gallagher, S. R. (2012). SDS-Polyacrylamide Gel Electrophoresis (SDS-PAGE). Current Protocols Essential Laboratory Techniques , 6(1), 7.3.1−7.3.28. doi: 10.1002/9780470089941.et0703s06.
- Gasteiger, E., Gattiker, A., Hoogland, C., Ivanyi, I., Appel, R. D., & Bairoch, A. (2003). ExPASy: The proteomics server for in-depth protein knowledge and analysis. Nucleic Acids Research , 31(13), 3784–3788. doi: 10.1093/nar/gkg563.
- Gautier, A., Deiters, A., & Chin, J. W. (2011). Light-activated kinases enable temporal dissection of signaling networks in living cells. Journal of the American Chemical Society , 133(7), 2124–2127. doi: 10.1021/ja1109979.
- Gautier, A., Nguyen, D. P., Lusic, H., An, W., Deiters, A., & Chin, J. W. (2010). Genetically encoded photocontrol of protein localization in mammalian cells. Journal of the American Chemical Society , 132(12), 4086–4088. doi: 10.1021/ja910688s.
- Greiss, S., & Chin, J. W. (2011). Expanding the genetic code of an animal. Journal of the American Chemical Society , 133(36), 14196–14199. doi: 10.1021/ja2054034.
- Guo, L. T., Wang, Y. S., Nakamura, A., Eiler, D., Kavran, J. M., Wong, M., … Soll, D. (2014). Polyspecific pyrrolysyl-tRNA synthetases from directed evolution. Proceedings of the National Academy of Sciences of the United States of America , 111(47), 16724–16729. doi: 10.1073/pnas.1419737111.
- Hancock, S. M., Uprety, R., Deiters, A., & Chin, J. W. (2010). Expanding the genetic code of yeast for incorporation of diverse unnatural amino acids via a pyrrolysyl-tRNA synthetase/tRNA pair. Journal of the American Chemical Society , 132(42), 14819–14824. doi: 10.1021/ja104609m.
- Imai, S. I., & Guarente, L. (2016). It takes two to tango: NAD(+) and sirtuins in aging/longevity control. NPJ Aging and Mechanisms of Disease , 2, 16017. doi: 10.1038/npjamd.2016.17.
- Isaacs, F. J., Carr, P. A., Wang, H. H., Lajoie, M. J., Sterling, B., Kraal, L., … Church, G. M. (2011). Precise manipulation of chromosomes in vivo enables genome-wide codon replacement. Science , 333(6040), 348–353. doi: 10.1126/science.1205822.
- Johnson, D. B., Xu, J., Shen, Z., Takimoto, J. K., Schultz, M. D., Schmitz, R. J., … Wang, L. (2011). RF1 knockout allows ribosomal incorporation of unnatural amino acids at multiple sites. Nature Chemical Biology , 7(11), 779–786. doi: 10.1038/nchembio.657.
- Kavran, J. M., Gundllapalli, S., O'Donoghue, P., Englert, M., Soll, D., & Steitz, T. A. (2007). Structure of pyrrolysyl-tRNA synthetase, an archaeal enzyme for genetic code innovation. Proceedings of the National Academy of Sciences of the United States of America , 104(27), 11268–11273. doi: 10.1073/pnas.0704769104.
- Knyphausen, P., de Boor, S., Kuhlmann, N., Scislowski, L., Extra, A., Baldus, L., … Lammers, M. (2016). Insights into lysine deacetylation of natively folded substrate proteins by sirtuins. Journal of Biological Chemistry , 291(28), 14677–14694. doi: 10.1074/jbc.M116.726307.
- Knyphausen, P., Lang, F., Baldus, L., Extra, A., & Lammers, M. (2016). Insights into K-Ras 4B regulation by post-translational lysine acetylation. Biological Chemistry , 397(10), 1071–1085. doi: 10.1515/hsz-2016-0118.
- Kuhlmann, N., Wroblowski, S., Knyphausen, P., de Boor, S., Brenig, J., Zienert, A. Y., … Lammers, M. (2016). Structural and mechanistic insights into the regulation of the fundamental rho regulator RhoGDIalpha by lysine acetylation. Journal of Biological Chemistry , 291(11), 5484–5499. doi: 10.1074/jbc.M115.707091.
- Kuhlmann, N., Wroblowski, S., Scislowski, L., & Lammers, M. (2016). RhoGDIalpha acetylation at K127 and K141 affects binding toward nonprenylated RhoA. Biochemistry , 55(2), 304–312. doi: 10.1021/acs.biochem.5b01242.
- Kuhn, M. L., Zemaitaitis, B., Hu, L. I., Sahu, A., Sorensen, D., Minasov, G., … Wolfe, A. J. (2014). Structural, kinetic and proteomic characterization of acetyl phosphate-dependent bacterial protein acetylation. PloS One , 9(4), e94816. doi: 10.1371/journal.pone.0094816.
- Ladner, C. L., Yang, J., Turner, R. J., & Edwards, R. A. (2004). Visible fluorescent detection of proteins in polyacrylamide gels without staining. Analytical Biochemistry , 326(1), 13–20. doi: 10.1016/j.ab.2003.10.047.
- Lammers, M. (2018). Expression and purification of site-specifically lysine-acetylated and natively-folded proteins for biophysical investigations. Methods in Molecular Biology , 1728, 169–190. doi: 10.1007/978-1-4939-7574-7_11.
- Lammers, M., Neumann, H., Chin, J. W., & James, L. C. (2010). Acetylation regulates cyclophilin A catalysis, immunosuppression and HIV isomerization. Nature Chemical Biology , 6(5), 331–337. doi: 10.1038/nchembio.342.
- Li, F., Allahverdi, A., Yang, R., Lua, G. B., Zhang, X., Cao, Y., … Liu, C. F. (2011). A direct method for site-specific protein acetylation. Angewandte Chemie (International Ed. in English) , 50(41), 9611–9614. doi: 10.1002/anie.201103754.
- Lindahl, A. J., Lawton, A. J., Baeza, J., Dowell, J. A., & Denu, J. M. (2019). Site-specific lysine acetylation stoichiometry across subcellular compartments. Methods in Molecular Biology , 1983, 79–106. doi: 10.1007/978-1-4939-9434-2_6.
- Lundby, A., Lage, K., Weinert, B. T., Bekker-Jensen, D. B., Secher, A., Skovgaard, T., … Olsen, J. V. (2012). Proteomic analysis of lysine acetylation sites in rat tissues reveals organ specificity and subcellular patterns. Cell Reports , 2(2), 419–431. doi: 10.1016/j.celrep.2012.07.006.
- Mukai, T., Hayashi, A., Iraha, F., Sato, A., Ohtake, K., Yokoyama, S., & Sakamoto, K. (2010). Codon reassignment in the Escherichia coli genetic code. Nucleic Acids Research , 38(22), 8188–8195. doi: 10.1093/nar/gkq707.
- Neumann, H., Hancock, S. M., Buning, R., Routh, A., Chapman, L., Somers, J., … Chin, J. W. (2009). A method for genetically installing site-specific acetylation in recombinant histones defines the effects of H3 K56 acetylation. Molecular Cell , 36(1), 153–163. doi: 10.1016/j.molcel.2009.07.027.
- Neumann, H., Peak-Chew, S. Y., & Chin, J. W. (2008). Genetically encoding N(epsilon)-acetyllysine in recombinant proteins. Nature Chemical Biology , 4(4), 232–234. doi: 10.1038/nchembio.73.
- Neumann, H., Wang, K., Davis, L., Garcia-Alai, M., & Chin, J. W. (2010). Encoding multiple unnatural amino acids via evolution of a quadruplet-decoding ribosome. Nature , 464(7287), 441–444. doi: 10.1038/nature08817.
- Nguyen, D. P., Garcia Alai, M. M., Virdee, S., & Chin, J. W. (2010). Genetically directing varepsilon-N, N -dimethyl-l-lysine in recombinant histones. Chemistry & Biology, 17(10), 1072–1076. doi: 10.1016/j.chembiol.2010.07.013.
- Ni, D., Xu, P., & Gallagher, S. (2016). Immunoblotting and immunodetection. Current Protocols in Immunology , 114, 8.10.11–18.10.36. doi: 10.1002/cpim.10.
- Nielsen, T. K., Hildmann, C., Dickmanns, A., Schwienhorst, A., & Ficner, R. (2005). Crystal structure of a bacterial class 2 histone deacetylase homologue. Journal of Molecular Biology , 354(1), 107–120. doi: 10.1016/j.jmb.2005.09.065.
- Olson, B. (2016). Assays for determination of protein concentration. Current Protocols in Pharmacology , 73, A.3A.1–A.3A.32. doi: 10.1002/cpph.3.
- Pace, C. N., Vajdos, F., Fee, L., Grimsley, G., & Gray, T. (1995). How to measure and predict the molar absorption coefficient of a protein. Protein Science , 4(11), 2411–2423. doi: 10.1002/pro.5560041120.
- Phillips, D. M. (1963). The presence of acetyl groups of histones. Biochemical Journal , 87, 258–263. doi: 10.1042/bj0870258.
- Rauh, D., Fischer, F., Gertz, M., Lakshminarasimhan, M., Bergbrede, T., Aladini, F., … Steegborn, C. (2013). An acetylome peptide microarray reveals specificities and deacetylation substrates for all human sirtuin isoforms. Nature Communications , 4, 2327. doi: 10.1038/ncomms3327.
- Riggs, P. D. (2018). Overview of protein expression vectors for E. coli. Current Protocols Essential Laboratory Techniques , 17(1), e23. doi: 10.1002/cpet.23.
- Rogerson, D. T., Sachdeva, A., Wang, K., Haq, T., Kazlauskaite, A., Hancock, S. M., … Chin, J. W. (2015). Efficient genetic encoding of phosphoserine and its nonhydrolyzable analog. Nature Chemical Biology , 11(7), 496–503. doi: 10.1038/nchembio.1823.
- Schmidt, M. T., Smith, B. C., Jackson, M. D., & Denu, J. M. (2004). Coenzyme specificity of Sir2 protein deacetylases: Implications for physiological regulation. Journal of Biological Chemistry , 279(38), 40122–40129. doi: 10.1074/jbc.M407484200.
- Schutkowski, M., Fischer, F., Roessler, C., & Steegborn, C. (2014). New assays and approaches for discovery and design of Sirtuin modulators. Expert Opinion on Drug Discovery , 9(2), 183–199. doi: 10.1517/17460441.2014.875526.
- Spicer, C. D., & Davis, B. G. (2014). Selective chemical protein modification. Nature Communications , 5, 4740. doi: 10.1038/ncomms5740.
- Spinck, M., Ecke, M., Sievers, S., & Neumann, H. (2018). Highly sensitive lysine deacetylase assay based on acetylated firefly luciferase. Biochemistry , 57(26), 3552–3555. doi: 10.1021/acs.biochem.8b00483.
- Terpe, K. (2006). Overview of bacterial expression systems for heterologous protein production: From molecular and biochemical fundamentals to commercial systems. Applied Microbiology and Biotechnology , 72(2), 211–222. doi: 10.1007/s00253-006-0465-8.
- Thomas, S. P., Haws, S. A., Borth, L. E., & Denu, J. M. (2020). A practical guide for analysis of histone post-translational modifications by mass spectrometry: Best practices and pitfalls. Methods , 184, 53–60. doi: 10.1016/j.ymeth.2019.12.001.
- Virdee, S., Kapadnis, P. B., Elliott, T., Lang, K., Madrzak, J., Nguyen, D. P., … Chin, J. W. (2011). Traceless and site-specific ubiquitination of recombinant proteins. Journal of the American Chemical Society , 133(28), 10708–10711. doi: 10.1021/ja202799r.
- Vo, T. T. L., Park, J. H., Lee, E. J., Nguyen, Y. T. K., Han, B. W., Nguyen, H. T. T., … Seo, J. H. (2020). Characterization of lysine acetyltransferase activity of recombinant human ARD1/NAA10. Molecules , 25(3), 588. doi: 10.3390/molecules25030588.
- Wals, K., & Ovaa, H. (2014). Unnatural amino acid incorporation in E. coli : Current and future applications in the design of therapeutic proteins. Frontiers in Chemistry , 2, 15. doi: 10.3389/fchem.2014.00015.
- Wan, W., Tharp, J. M., & Liu, W. R. (2014). Pyrrolysyl-tRNA synthetase: An ordinary enzyme but an outstanding genetic code expansion tool. Biochimica et Biophysica Acta , 1844(6), 1059–1070. doi: 10.1016/j.bbapap.2014.03.002.
- Wang, M. M., You, D., & Ye, B. C. (2017). Site-specific and kinetic characterization of enzymatic and nonenzymatic protein acetylation in bacteria. Scientific Reports , 7(1), 14790. doi: 10.1038/s41598-017-13897-w.
- Wegener, D., Wirsching, F., Riester, D., & Schwienhorst, A. (2003). A fluorogenic histone deacetylase assay well suited for high-throughput activity screening. Chemistry & Biology, 10(1), 61–68. doi: 10.1016/s1074-5521(02)00305-8.
- Weinert, B. T., Iesmantavicius, V., Moustafa, T., Scholz, C., Wagner, S. A., Magnes, C., … Choudhary, C. (2015). Acetylation dynamics and stoichiometry in Saccharomyces cerevisiae. Molecular Systems Biology , 11(10), 833. doi: 10.15252/msb.156513.
- Weinert, B. T., Iesmantavicius, V., Wagner, S. A., Scholz, C., Gummesson, B., Beli, P., … Choudhary, C. (2013). Acetyl-phosphate is a critical determinant of lysine acetylation in E. coli. Molecular Cell , 51(2), 265–272. doi: 10.1016/j.molcel.2013.06.003.
- Weinert, B. T., Narita, T., Satpathy, S., Srinivasan, B., Hansen, B. K., Scholz, C., … Choudhary, C. (2018). Time-resolved analysis reveals rapid dynamics and broad scope of the CBP/p300 acetylome. Cell , 174(1), 231–244.e212. doi: 10.1016/j.cell.2018.04.033.
- Weinert, B. T., Wagner, S. A., Horn, H., Henriksen, P., Liu, W. R., Olsen, J. V., … Choudhary, C. (2011). Proteome-wide mapping of the Drosophila acetylome demonstrates a high degree of conservation of lysine acetylation. Science Signaling , 4(183), ra48. doi: 10.1126/scisignal.2001902.
- Wingfield, P. T. (2015). Overview of the purification of recombinant proteins. Current Protocols in Protein Science , 80, 6.1.1–6.1.35. doi: 10.1002/0471140864.ps0601s80.
- Zhang, M. S., Brunner, S. F., Huguenin-Dezot, N., Liang, A. D., Schmied, W. H., Rogerson, D. T., & Chin, J. W. (2017). Biosynthesis and genetic encoding of phosphothreonine through parallel selection and deep sequencing. Nature Methods , 14(7), 729–736. doi: 10.1038/nmeth.4302.
- Zullo, A., Simone, E., Grimaldi, M., Musto, V., & Mancini, F. P. (2018). Sirtuins as Mediator of the anti-ageing effects of calorie restriction in skeletal and cardiac muscle. International Journal of Molecular Sciences , 19(4), 928. doi: 10.3390/ijms19040928.
Citing Literature
Number of times cited according to CrossRef: 1
- Vincenza Capone, Laura Della Torre, Daniela Carannante, Mehrad Babaei, Lucia Altucci, Rosaria Benedetti, Vincenzo Carafa, HAT1: Landscape of Biological Function and Role in Cancer, Cells, 10.3390/cells12071075, 12 , 7, (1075), (2023).