Intravital Microscopy for the Study of Hepatic Glucose Uptake
Megan L. Stefkovich, Megan L. Stefkovich, Sun Woo Sophie Kang, Sun Woo Sophie Kang, Natalie Porat-Shliom, Natalie Porat-Shliom
Abstract
The liver is central in maintaining glucose homeostasis. Indeed, impaired hepatic glucose uptake has been implicated in the development of hyperglycemia in type II diabetes (T2D) and non-alcoholic fatty liver disease (NAFLD). However, current approaches to evaluate glucose mobilization rely on indirect measurements that do not provide spatial and temporal information. Here, we describe confocal-based intravital microscopy (IVM) of the liver that allows the identification of hepatocyte spatial organization and glucose transport. Specifically, we describe a method to fluorescently label hepatic landmarks to identify different compartments within the liver. In addition, we outline an in vivo fluorescent glucose uptake assay to quantitatively measure glucose mobilization in space and time. These protocols allow direct investigation of hepatic glycemic control and can be further applied to murine models of liver disease. © Published 2021. This article is a U.S. Government work and is in the public domain in the USA.
Basic Protocol 1 : Mouse surgical procedure and positioning for liver intravital imaging
Basic Protocol 2 : Fluorescent labeling and intravital imaging of mouse hepatic compartments
Basic Protocol 3 : Mouse hepatic glucose uptake assay and intravital imaging analysis
INTRODUCTION
The liver plays a vital role in regulating glucose homeostasis by coordinating various glucose metabolic pathways, including glycogenesis, glycogenolysis, glycolysis, and gluconeogenesis. Dysregulation of glucose homeostasis is a hallmark of metabolic diseases such as T2D and NAFLD. Despite its importance in disease progression, hepatic glucose uptake cannot be measured directly with spatial and temporal precision using current methods.
Hepatocytes are organized into a repetitive hexagonal unit called the hepatic lobule (Fig. 1A). Blood flows directionally from the six corners of the lobule toward a single central vein, generating a gradient of nutrients, oxygen, and hormones across the porto-central axis. Consequently, hepatocytes are exposed to different microenvironments, which affects their gene expression patterns and metabolic activities—a phenomenon known as liver zonation (Ben-Moshe & Itzkovitz, 2019). Specifically, periportal (PV) hepatocytes are known to engage in gluconeogenesis, whereas pericentral (CV) hepatocytes are involved in glycolysis. As such, liver zonation is an important consideration for hepatic glucose homeostasis.
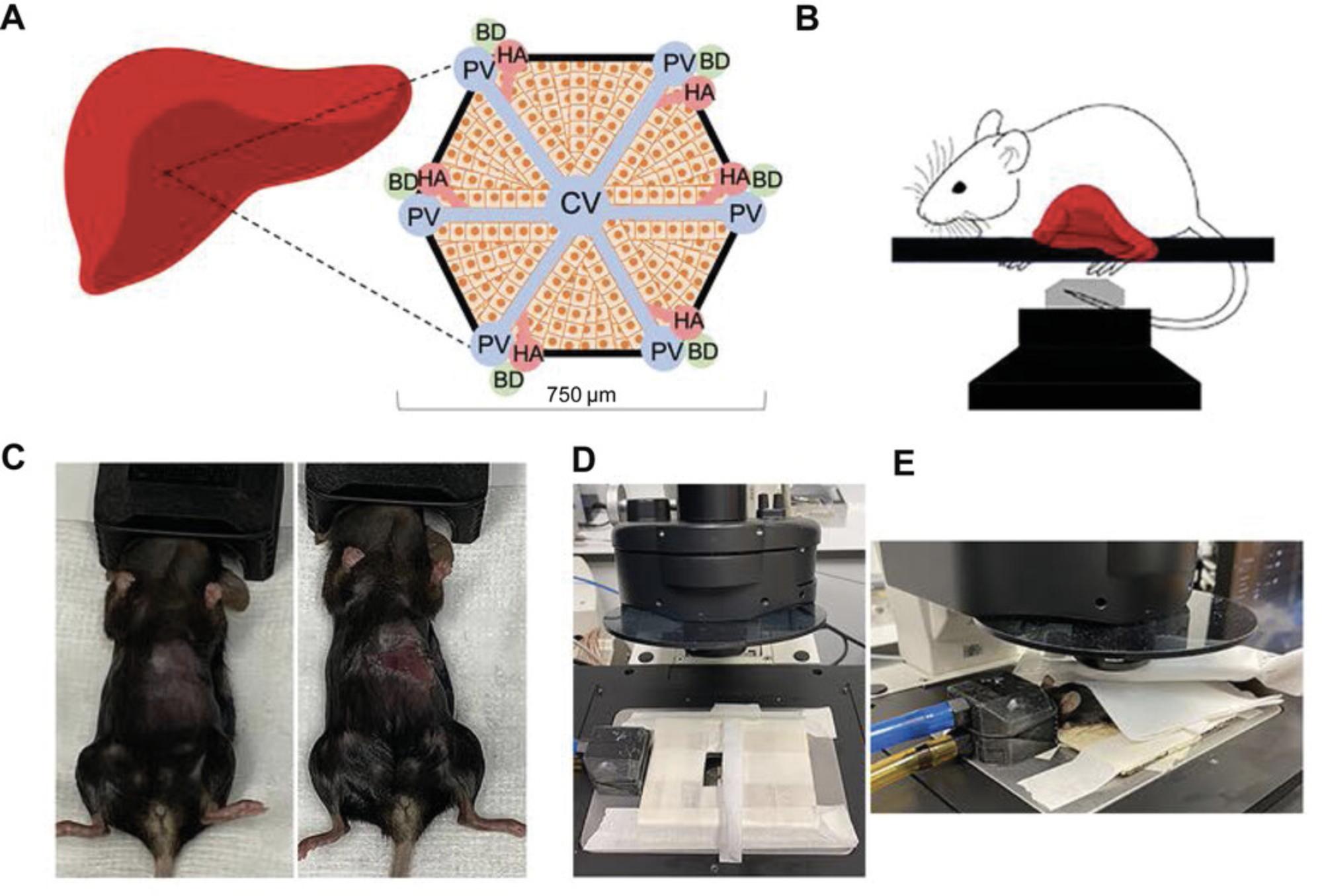
Fluorescent light microscopy has revolutionized the ability to visualize cellular processes in living systems. In the past two decades, the development of fluorescent protein variants, along with advancements in optics and image analysis, have made it possible to track processes in space and time in cultured cells (Lippincott-Schwartz, Snapp, & Kenworthy, 2001). More recently, new technologies to manipulate the genomes of various organisms, as well as new in vivo imaging modalities, have allowed the extension of these light-based approaches to intact tissues (Entenberg et al., 2018; Weigert, Porat-Shliom, & Amornphimoltham, 2013). This collection of light microscopy approaches applied to live, intact tissues is collectively referred to as intravital microscopy (IVM). IVM methods now offer great opportunities to understand liver structure and function (Gola et al., 2021; Meyer et al., 2017; Porat-Shliom et al., 2016; Ritsma et al., 2013; Tavakoli, Tsekouras, Day, Dunn, & Presse, 2019). Furthermore, these approaches will be powerful in resolving the functional heterogeneity of hepatocytes, which is impossible to recreate in vitro.
Here, we present a comprehensive protocol for performing intravital microscopy of the murine liver. In Basic Protocol 1, we outline the steps for surgical exposure of the liver and its positioning on the microscope stage. We also discuss considerations taken to avoid tissue damage/ischemia and to monitor mouse body temperature during the procedure. Next, in Basic Protocol 2, we delineate the steps taken to fluorescently label hepatic compartments and to identify PV and CV hepatocytes. Finally, in Basic Protocol 3, we describe a functional assay to evaluate glucose uptake using 2-(N -(7-nitrobenz-2-oxa-1,3-diazol-4-yl)amino)-2-deoxyglucose (2-NBDG). Basic Protocol 3 also includes an image analysis strategy to quantify the rate of glucose uptake and its retention within hepatocytes in different parts of the lobule. Together, IVM of hepatocyte spatial organization in combination with dynamic transport of fluorescent glucose provides physiological insight into hepatic glycemic control in real time. These assays can be further applied to different mouse models of liver disease.
Basic Protocol 1: MOUSE SURGICAL PROCEDURE AND POSITIONING FOR LIVER INTRAVITAL IMAGING
Here, we describe in detail microscope setup, mouse preparation, mouse anesthesia, and a surgical procedure for liver exposure. Next, we provide guidelines on how to position the mouse on the microscope stage to minimalize motion artifacts due to heartbeat and respiration while maintaining proper perfusion.
IMPORTANT NOTE : All experiments involving animals must be performed under an animal protocol approved by your animal care and ethics committee. Personnel should be properly trained before proceeding.
Materials
-
Clidox disinfectant (VWR, MSPP-96118F)
-
Mus musculus
-
70% (v/v) ethanol
-
FORANE (isoflurane, USP) liquid for inhalation, 250 ml (Baxter, 10019-360-60)
-
Heparin sodium, 1000 USP U/ml (Hikma, 0641-0391-12)
-
Normal saline (0.9% sodium chlorine; Fisher Scientific, cat. no. 7210-16)
-
Leica SP8 (or other commercial) confocal microscope
-
Custom-made stage cardboard of appropriate dimensions (see Table 1)
-
Custom-made aluminum stage insert (3 mm thick) with 35-mm depression
-
40-mm coverslips (Bioptech, 40-1313-0319)
-
Surgical gauze
-
½-inch. masking tape
-
Anesthesia machine: AMD-3 plus (Somni, AMD-17106)
-
Reusable chemical heat packs
-
Mouse heat pad (ALA Scientific Instruments, HEATINGPAD-1/2)
-
Space heat pad (AIMSTM, AIMS™ GGP1)
-
Ultra Fine U-100 Insulin Syringes, 31-G (BD, BD328438)
-
Hair clipper
-
Surgical scissors
-
Cautery System (Gemini, GEM 5917)
-
Surgical forceps, curved tip
-
Cotton-tip applicators
-
Heat pad with rectal probe (ALA scientific instruments, HEATINGPAD-1/2)
-
Aquasonic 100 ultrasound transmission gel (Parker lab Inc)
Mouse body weight (g) | Board (cm) | Board thickness (mm) | Imaging window (cm) | |
---|---|---|---|---|
Small (S) | 17-20 | 12 × 8 | 1.5 | 2.5 × 2.3 |
Medium (M) | 22-25 | 15 × 8 | 2.5 | 2.0 × 2.0 |
Large (L) | >30 | 15.5 × 8 | 4.0 | 3.5 × 1.8 |
Microscope preparation
1.IVM of the liver can be performed on any commercial inverted confocal microscope (Fig. 1B). Standard microscope stages have various inserts to accommodate slides or tissue culture plates. For IVM, a flat surface with a 35-mm circular opening covered with a 40-mm coverglass is required. In some cases, 35-mm-plate inserts can be modified to hold a mouse. Alternatively, a custom-made metal insert, 3 mm in thickness, with a central 35-mm circular opening can be fabricated. The coverglass should be cleaned thoroughly on both sides using 70% ethanol before use.
2.Select the objective based on area size, spatial resolution, and the length of the imaging session. In general, lower magnification is useful for imaging larger areas at lower resolution, whereas higher magnification increases the resolution of smaller structures. One should consider that motion artifact (see below) will be more noticeable when using higher magnifications.
3.Select stage cardboard dimensions based on mouse weight (see Table 1) and tape the stage insert with the imaging window (in the cardboard) directly over the coverslip (Fig. 1D). Minimizing motion artifacts due to respiration and/or heartbeat is central to IVM; the cardboard is used to absorb such motion while also creating a barrier between the body and the microscope stage. Place a small strip of gauze (1 × 8 cm) across the cardboard imaging window and fix it with masking tape on one side (Fig. 1D).
4.Warm the stage area using chemical heat pads at least 30 min prior to imaging. This will help maintain the mouse body temperature during the imaging experiment.
Mouse anesthesia and surgical procedure
5.Clean anesthesia induction chamber with Clidox disinfectant; this will remove odor of the previous mouse, which reduces stress.
6.Place the mouse in the induction chamber, and turn on the oxygen condenser and 3% isoflurane for 5 min. When the mouse is anesthetized, transfer it onto the heating pad and maintain anesthesia using 1% isoflurane delivered via a nose cone (Fig. 1C).
7.Inject 20 U heparin intraperitoneally (i.p.; dilute 20 μl heparin from 1000 U/ml stock into 30 μl saline). The liver is prone to injury and ischemia; heparin ensures proper perfusion during the imaging study.
8.Shave the mouse abdomen using a hair clipper and remove hair using gauze soaked with 70% ethanol (Fig. 1C, left).
9.Expose the liver by making a 2- to 3-cm incision along the ribcage starting from the xiphoid and ending at the left lateral side of the abdomen. Initially, the outer skin is cut with scissors, and then the muscle layer is cut, using a cauterizer as needed to avoid bleeding. To avoid burning the liver with the cauterizer, carefully open the abdominal cavity on the lateral side and insert wet gauze (soaked in normal saline) between the liver and muscle layer before starting with the major incision (Fig. 1C, right).
Positioning of the mouse
10.Position mouse on the microscope stage with the exposed left lateral lobe of the liver laid against the coverslip. Use gauze to separate the liver lobe from the intestines and mouse by placing it between the liver and abdomen (Fig. 1D). Stretching the mouse flat on the microscope stage may help reduce motion (Fig. 1E).
11.Reposition the mouse if breathing causes the liver position to shift on the coverslip. Adjust the liver position very gently using a cotton-tipped applicator soaked in normal saline.
12.Secure the mouse's neck to the stage using tape to prevent motion artifacts upon retro-orbital injection of fluorescent tracers during imaging. Adjust the nose cone so that the mouse remains under the plane of anesthesia while allowing access to the eye. Maintain mouse anesthesia through delivery of 1% isoflurane via a nose cone on the microscope stage.
13.Monitor and maintain the mouse body temperature using a heat pad with a rectal probe. Avoid placing the heat pad directly against the skin to avoid overheating. Additionally, avoid tissue dehydration by (1) using a microscope with an enclosed chamber with humidity control or (2) applying Aquasonic 100 ultrasound transmission gel at the edges of the exposed cavity.
Basic Protocol 2: FLUORESCENT LABELING AND INTRAVITAL IMAGING OF MOUSE HEPATIC COMPARTMENTS
This protocol describes how to (1) fluorescently label different compartments within the hepatic lobule, (2) identify PV regions, and (3) select the ideal imaging area for time-lapse IVM.
Materials
- Appropriate fluorescent dyes (see Table 2)
- 31-G insulin syringe
Fluorescent dyes | Compartment | Concentration | Source (cat. no.) |
---|---|---|---|
Alexa Fluor® 647–conjugated anti-mouse/human CD324 (E-cadherin) antibody | PV hepatocytes | 0.5 µg/g | Biolegend (147308) |
2 M tetramethylrhodamine-dextran | Vasculature | 18 µg/g | Thermo Fisher (D7139) |
Hoechst 33342 | Nuclei | 2 μg/g | Thermo Fisher (H357 |
- Additional reagents and equipment for mouse anesthesia, surgery, and intravital imaging (Basic Protocol 1)
1.Anesthetize the mouse and maintain anesthesia using nose cone (Basic Protocol 1).
2.Inject 0.5 µg/g Alexa Fluor® 647–conjugated anti-mouse/human CD324 (E-cadherin) antibody diluted in 1× heparin (total volume of 50 μl) via retro-orbital injection using a 31-G insulin syringe.
3.Inject 2 µg/g Hoechst 33342 diluted in 1× heparin (total volume 50 μl), via retro-orbital injection using a 31-G insulin syringe. Use the other eye for Hoechst 33342 injection, as the probe tend to precipitate if combined with other fluorescent probes.
4.Incubate 30-45 min to allow labeling of PV hepatocytes and nuclei, while controlling the body temperature with a heating pad.
5.Prepare microscope and mouse for imaging (Basic Protocol 1).
6.Secure the mouse's neck to the stage with tape.
7.Minimize motion, then retro-orbitally inject 18 µg/g of fluorescent dextran diluted in 10 USP units/ml heparin (total volume 50 μl) using a 31-G insulin syringe.
8.Assess blood flow by observing the fluorescent dextran signal via the eyepiece. Strong labeling of the vasculature should occur within 1 s of injection (Fig. 2).
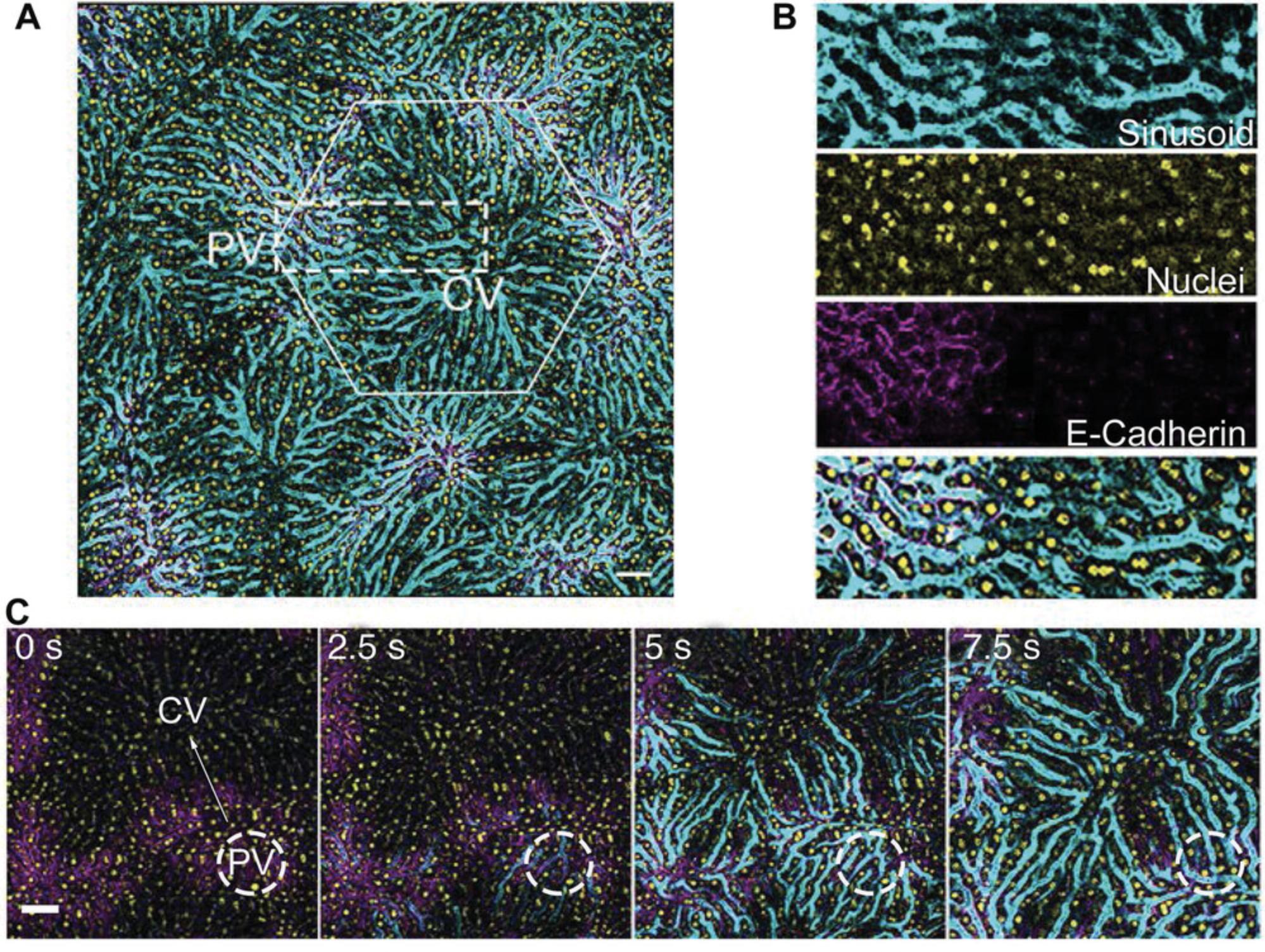
9.Identify PV from CV areas of the lobule using E-cadherin labeling and the direction of the blood flow. Blood flows from the PV towards the CV regions (Fig. 2C).
10.Select an imaging area that is stable and has a good blood flow. This can be assessed by looking through the eyepiece and acquiring a short IVM time-lapse to evaluate stability over time.
11.Set up time-lapse IVM to acquire 512 × 512 pixels every 2.5 s for 10 min using the 20× objective (Fig. 2 and Video 1).
Basic Protocol 3: MOUSE HEPATIC GLUCOSE UPTAKE ASSAY AND INTRAVITAL IMAGE ANALYSIS
This protocol describes how to use the fluorescent glucose analog 2-(N -(7-nitrobenz-2-oxa-1,3-diazol-4-yl)amino)-2-deoxyglucose (2-NBDG) to measure glucose mobilization between different compartments in the hepatic lobule of a live, anesthetized mouse. A step-by-step guideline for image analysis follows. This procedure provides an alternative to other methods of measuring hepatic glucose uptake by providing spatiotemporal information. The protocol can be completed in 45-60 min per mouse.
Materials
-
Dextrose 50% (Hospira, 000622)
-
2-NBDG (Thermo Fisher, N13195)
-
31-G insulin syringe
-
ImageJ software or similar
-
Excel spreadsheet software
-
Additional reagents and equipment for mouse anesthesia, surgery, and intravital imaging (Basic Protocol 1)
Image acquisition
1.Fast the mice for 4-6 hr prior to imaging.
2.Prepare the microscope and surgically expose the liver as described in Basic Protocol 1.
3.Secure the mouse's neck to the stage with tape.
4.Minimize motion, then retro-orbitally inject 18 µg of dextran per gram of mouse body weight (diluted to a total volume of 50 μl in 10 USP U/ml heparin) using a 31-G insulin syringe.
5.Set up time-lapse IVM to acquire 512 × 512 pixels every 2.5 s for 30 min. Use the 20× objective and select the field of view as described in Basic Protocol 2 (Video 2).
COMMENTARY
Background Information
IVM was first described in 1839 (Wagner, 1839), when it was used to observe the movement of leukocytes through blood vessels in the translucent, webbed feet of frogs. The development of novel fluorescent probes since 1994 has made its application to a wide range of intact tissues possible. IVM is particularly suited for studying liver physiology because the liver can easily be slipped out of the body cavity onto a coverslip and is highly amenable to administration of fluorescent probes, eliminating the need to create transgenic animals expressing fluorescently tagged proteins. In these protocols, we have described a novel method for measuring hepatic glucose uptake in real time in live mice using intravital microscopy and the fluorescent glucose analog 2-NBDG. Previous methods have measured hepatic glucose uptake in rats (Tsumura, Tsushima, Tamura, Hasebe, & Kobayashi, 2019) and humans (Mari, Wahren, DeFronzo, & Ferrannini, 1994) by perfusing the livers with stable-isotope-labeled glucose and quantifying the ratio of the carbon isotopes to determine net uptake. Positron emission tomography (PET) has also been used to measure glucose uptake in the liver (Honka et al., 2018). Although these methods allow quantification of hepatic glucose uptake in vivo, they lack spatial and temporal resolution.
Here we describe protocols for IVM of the liver, labeling of liver compartments, and evaluation of glucose mobilization using the fluorescent tracer 2-NBDG. Collectively, these protocols allow the investigation of zone-specific differences in hepatic glucose transport. Applying these assays to liver disease models will provide invaluable information on hepatocyte regulation of glucose homeostasis at the cellular level. The increased spatial resolution also permits examination into the roles that non-parenchymal liver cells, the microenvironment, or the vasculature may have in the regulation of glucose transport. For example, hepatic glucose uptake might be impaired in the liver due to inadequate vascularization rather than dysfunctional glucose transporters, a distinction that is impossible to test in isolated hepatocytes. This assay is not without limitations, however, as it cannot be used to determine downstream fates of glucose, such as glycogen deposition. Once taken up by hepatocytes, the NBD group is cleaved from the glucose before the glucose is phosphorylated in the first reaction of glycolysis (Yoshioka et al. 1996).
Critical Parameters
Basic Protocol 1: Liver exposure and positioning on the microscope stage are key to obtaining robust results. While surgically exposing the liver, special attention should be paid to avoid excessive bleeding and damage to the liver parenchyma. In addition, positioning of the mouse on the microscope stage is equally critical for two main reasons. First, stable preparation for imaging is a prerequisite for extracting quantitative information from the images. Second, tissue damage may occur during the positioning of the mouse on the microscope stage. Therefore, these steps are particularly sensitive to user manipulation and require precision and practice.
Basic Protocol 2: Labeling of the different hepatic compartments is critically dependent on the fluorescent probe concentrations and the effective delivery of the probes. The concentrations for the fluorescent probes described here were determined empirically in wild-type (C57Bl/6J) mice. Applying this protocol to other lines and/or disease models may require adjusting these concentrations. In addition, retro-orbital injection, like any other procedure, requires precision and practice. It is therefore advisable to master the technique before performing experiments.
Basic Protocol 3: The ability to quantify the rate of glucose uptake is dependent on the stability of the region imaged. Minimizing motion artifacts is extremely important but should be done with caution. Quantification of glucose retention in the liver, however, can still be done even if significant movement persists because individual frames can be analyzed independently. Another key aspect of this assay is ensuring that probes are sufficiently bright once their signal appears in the liver, because a dim signal will impede accurate thresholding.
Troubleshooting
Table 3 lists problems that may arise with these procedure along with their possible causes and solutions.
Problem | Possible cause | Solution |
---|---|---|
Mouse not becoming anesthetized | Isoflurane tank needs to be refilled or output is too low. | Fill tank or increase isoflurane output to 2%. |
If administering anesthesia via i.p. injection, an insufficient volume may have been injected. | Inject an additional half dose, especially if the mouse weighs >20 g. | |
Excessive bleeding during surgical prep | Damage to muscle layer or liver parenchyma occurred. | Cauterize to stop/prevent bleeding. Be mindful of avoiding heat damage to the liver while cauterizing. If direct damage to the liver occurs or bleeding persists, euthanize the mouse. |
Inability to find stable field of view | Isoflurane levels are too high, causing the mouse to gasp. | Lower isoflurane levels to 1-1.5% |
Liver is too close to the body cavity. | Use a saline-soaked cotton-tipped applicator to gently separate the liver from the other internal organs. Liver damage can be minimized by gently squeezing the sides of the mouse's abdomen without use of the applicator to coax the liver away from the rest of the body. Soak a strip of gauze in saline and tape it to the insert such that the liver is positioned below it and the rest of the internal organs rest on top. | |
Liver-coverslip interface is poor. | Ensure that the liver is moist but not submerged in liquid on the coverslip. Use Kimteck tissue to absorb any excess fluids. Allow 10 min for the tissue to settle and for the formation of stable interface between the tissue and the coverslip. | |
Mouse is a heavy breather. | Adjust the mouse's body to minimize breathing motion. This may involve shifting the mouse further up the stage insert so that the ribcage is elevated away from the liver. Choose areas of the liver close to the edges of the lobe, as these are usually less prone to motion artifacts. | |
Poor signal from the dextran or 2-NBDG probe | Insufficient probe was injected. | Increase the probe concentration to be injected. |
The probe was not correctly injected into the capillary bed behind the mouse's eye. | Ensure that the syringe is inserted at the corner of the eye closest to the nose and behind the eyeball to get to the back of the eye socket. If the first retro-orbital injection fails, try injecting through the other eye. | |
Laser power is too low. | Increase laser power while minimizing photobleaching. | |
Blood flow in the selected area is poor. | Evaluate the fluorescence signal in other areas of the tissue. If the signal is poor throughout the tissue, this may indicate extensive disruption to blood flow and may require euthanasia of the mouse. It is not uncommon to have local disruptions in blood flow; therefore, if fluorescent signal is good in other regions, they can be imaged. | |
Liver damage | Poor blood flow resulted in ischemia. | Identify an area with normal perfusion. Blood flow can be seen indirectly, without any labeling through the eyepiece. |
The liver was subjected to excessive handling. | Handle the liver with cotton-tipped applicators as little as possible. Use gravity to coax the liver out of the body cavity and onto the coverslip. Gently squeeze the sides of the mouse to further extrude the liver. If using an applicator, soak the cotton tip in saline. | |
Poor labeling of PV hepatocytes with E-cadherin or Hoechst 33342 | Retro-orbital injection did not work. | Reinject the probe. |
Probe concentration is too low. | Increase the probe concentration 10×. | |
Blood flow is poor due to damage. | Try the procedure again while minimizing tissue damage. |
Statistical Analysis
A Student's t -test should be used in Basic Protocol 3.
Understanding Results
Basic Protocol 1
The goal is to surgically expose the liver and position the mouse on the microscope (Fig. 1B and E) to allow visualization of the hepatic lobule (Fig. 1A). To achieve this, the abdomen should be shaved, and the incision should expose the largest liver lobe. Bleeding is to be expected once the muscle layer is cut and can be controlled using a cauterizer (Fig. 1C). The microscope stage should be heated, and a cardboard insert taped to elevate the mouse's body away from the exposed liver. A narrow strip of gauze soaked in saline attached to the insert can keep the liver hydrated and separated from the other internal organs (Fig. 1D). The mouse should be positioned on the stage such that its abdomen is flat against the insert and its nose just inside the nose cone to maintain anesthesia while allowing access to an eye for retro-orbital injection. Gauze placed over the mouse and heating pad connected to a rectal probe help control body temperature. In addition, ultrasound gel around the edges of the incision prevents the liver from drying out (Fig. 1E).
Basic Protocol 2
Retro-orbital injection is a convenient mode to administer fluorescent probes to label hepatic compartments. The sinusoids are labeled with high-molecular-weight dextran (2 M K d), a probe that is retained for the duration of the imaging experiment (Fig. 2A-C). Similarly, Hoechst 33342 and Alexa Fluor® 647 anti-mouse/human CD324 are used to label the nuclei and E-cadherin, respectively. Expect the nuclei of all hepatocytes to be evenly labeled with Hoechst, whereas the E-cadherin antibody should selectively label PV hepatocytes (Fig. 2B and C and Video 1). The hepatic lobule receives blood from six portal veins that drain into a single central vein (Fig. 1A). When using a 20× objective, multiple lobules can be visible within the field of view (Fig. 2A and Video 1). Portal veins can be identified using the E-cadherin label and/or the site where dextran appears first when acquiring IVM time-lapse (Fig. 2C and Video 1).
Basic Protocol 3
After analyzing the rate of 2-NBDG uptake, the slope of the linear increase in 2-NBDG mean gray value can be compared between mice and shown as a bar graph, as in Figure 3C. 2-NBDG retention is best shown as a line graph, as in Figure 3D, to allow comparison of 2-NBDG retention at different time points and between mice. The retention is calculated in Basic Protocol 3 as the ratio of 2-NBDG mean gray value in the hepatocytes compared to the sinusoids. A ratio <1 indicates that more 2-NBDG is present in the sinusoids than in the hepatocytes, a ratio of 1 indicates that the 2-NBDG levels are about the same in sinusoids and hepatocytes, and a ratio >1 indicates that 2-NBDG levels are higher in hepatocytes than in sinusoids.
Interestingly, although CV hepatocytes are known to rely on glycolysis more than PV hepatocytes (Rui, 2014), we have found that the rate of glucose uptake and retention is comparable between the three zones (Fig. 3C and Video 2). This unexpected result may be due to the strategic distribution of GLUT family transporters along the hepatic lobule. The glucose concentration is higher in PV than CV hepatocytes after a meal (Ricken et al., 2015), and GLUT2, which has a low affinity for glucose, is primarily found in PV hepatocytes (Ogawa et al., 1996), whereas GLUT1, with a high affinity for glucose, is primarily found in CV hepatocytes (Bilir et al., 1993). The low affinity of GLUT2 for glucose around the PV leaves some glucose in the blood for use by the CV hepatocytes, which can rapidly take up glucose even at low concentrations due to the high affinity of the GLUT1 transporter. The organization of glucose transporters in this fashion may be crucial for maintaining glucose homeostasis, as the liver appears to clear 30%-40% of the glucose entering the portal vein (Pagliassotti & Cherrington, 1992). Inadequate glucose uptake due to loss of glucose transporter zonation or expression may contribute to high circulating blood glucose levels and lead to insulin resistance, which could be investigated by using this protocol in disease models.
Time Considerations
Basic Protocol 1 can be completed in 45-60 min.
Basic Protocol 2 can be completed in 30-45 min.
Basic Protocol 3 can be completed in 45-60 min.
Acknowledgments
This work was supported by the Intramural Research Program at the US National Institutes of Health, National Cancer Institute. We would like to thank members of the Porat-Shliom lab, Drs. Constance Cultraro and Rory Cunningham, for critically reading the protocols.
Author Contributions
Megan Stefkovich: Conceptualization, Data curation, Formal analysis, Methodology, Writing-original draft, Writing-review & editing, Sun Woo Sophie Kang: Data curation, Methodology, Writing-original draft, Natalie Porat-Shliom: Conceptualization, Formal analysis, Methodology, Visualization, Writing-original draft, Writing-review & editing
Conflict of Interest
The authors declare no conflict of interest.
Open Research
Data Availability Statement
The data that supports the findings of this study are available in the supplementary material of this article.
Literature Cited
- Ben-Moshe, S., & Itzkovitz, S. (2019). Spatial heterogeneity in the mammalian liver. Nature Reviews Gastroenterology and Hepatology , 16(7), 395–410. doi: 10.1038/s41575-019-0134-x.
- Bevan, R. K., Rose, M. A., & Duggan, K. A. (1997). Evidence for direct interaction of ketamine with alpha 1- and beta 2-adrenoceptors. Clinical and Experimental Pharmacology and Physiology , 24(12), 923–926. doi: 10.1111/j.1440-1681.1997.tb02720.x.
- Bilir, B. M., Gong, T. W., Kwasiborski, V., Shen, C. S., Fillmore, C. S., Berkowitz, C. M., & Gumucio, J. J. (1993). Novel control of the position-dependent expression of genes in hepatocytes. The GLUT-1 transporter. Journal of Biological Chemistry , 268(26), 19776–19784. Retrieved from https://www.ncbi.nlm.nih.gov/pubmed/7690040.
- Entenberg, D., Voiculescu, S., Guo, P., Borriello, L., Wang, Y., Karagiannis, G. S., … Condeelis, J. (2018). A permanent window for the murine lung enables high-resolution imaging of cancer metastasis. Nature Methods , 15(1), 73–80. doi: 10.1038/nmeth.4511.
- Gola, A., Dorrington, M. G., Speranza, E., Sala, C., Shih, R. M., Radtke, A. J., … Germain, R. N. (2021). Commensal-driven immune zonation of the liver promotes host defence. Nature , 589(7840), 131–136. doi: 10.1038/s41586-020-2977-2.
- Honka, M. J., Latva-Rasku, A., Bucci, M., Virtanen, K. A., Hannukainen, J. C., Kalliokoski, K. K., & Nuutila, P. (2018). Insulin-stimulated glucose uptake in skeletal muscle, adipose tissue and liver: a positron emission tomography study. European Journal of Endocrinology , 178(5), 523–531. doi: 10.1530/EJE-17-0882.
- Lippincott-Schwartz, J., Snapp, E., & Kenworthy, A. (2001). Studying protein dynamics in living cells. Nature Reviews Molecular Cell Biology , 2(6), 444–456. doi: 10.1038/35073068.
- Mari, A., Wahren, J., DeFronzo, R. A., & Ferrannini, E. (1994). Glucose absorption and production following oral glucose: comparison of compartmental and arteriovenous-difference methods. Metabolism , 43(11), 1419–1425. doi: 10.1016/0026-0495(94)90038-8.
- Meyer, K., Ostrenko, O., Bourantas, G., Morales-Navarrete, H., Porat-Shliom, N., Segovia-Miranda, F., … Zerial, M. (2017). A Predictive 3D multi-scale model of biliary fluid dynamics in the liver lobule. Cell Systems , 4(3), 277–290.e279. doi: 10.1016/j.cels.2017.02.008.
- Ogawa, A., Kurita, K., Ikezawa, Y., Igarashi, M., Kuzumaki, T., Daimon, M., … Sasaki, H. (1996). Functional localization of glucose transporter 2 in rat liver. Journal of Histochemistry and Cytochemistry , 44(11), 1231–1236. doi: 10.1177/44.11.8918897.
- Pagliassotti, M. J., & Cherrington, A. D. (1992). Regulation of net hepatic glucose uptake in vivo. Annual Review of Physiology , 54, 847–860. doi: 10.1146/annurev.ph.54.030192.004215.
- Porat-Shliom, N., Tietgens, A. J., Van Itallie, C. M., Vitale-Cross, L., Jarnik, M., Harding, O. J., … Arias, I. M. (2016). Liver kinase B1 regulates hepatocellular tight junction distribution and function in vivo. Hepatology , 64(4), 1317–1329. doi: 10.1002/hep.28724.
- Ricken, T., Werner, D., Holzhutter, H. G., Konig, M., Dahmen, U., & Dirsch, O. (2015). Modeling function-perfusion behavior in liver lobules including tissue, blood, glucose, lactate and glycogen by use of a coupled two-scale PDE-ODE approach. Biomechanics and Modeling in Mechanobiology , 14(3), 515–536. doi: 10.1007/s10237-014-0619-z.
- Ritsma, L., Steller, E. J., Ellenbroek, S. I., Kranenburg, O., Borel Rinkes, I. H., & van Rheenen, J. (2013). Surgical implantation of an abdominal imaging window for intravital microscopy. Nature Protocols , 8(3), 583–594. doi: 10.1038/nprot.2013.026.
- Rui, L. (2014). Energy metabolism in the liver. Comprehensive Physiology , 4(1), 177–197. doi: 10.1002/cphy.c130024.
- Tavakoli, M., Tsekouras, K., Day, R., Dunn, K. W., & Presse, S. (2019). Quantitative kinetic models from intravital microscopy: a case study using hepatic transport. Journal of Physical Chemistry B , 123(34), 7302–7312. doi: 10.1021/acs.jpcb.9b04729.
- Tsumura, Y., Tsushima, Y., Tamura, A., Hasebe, M., & Kobayashi, T. (2019). Ex vivo method to simultaneously evaluate glucose utilization, uptake, and production in rat liver. Analytical Sciences , 35(4), 455–460. doi: 10.2116/analsci.18P427.
- Wagner, R. (1839). Erlauterungstaflen zur Physiologie und Entwicklungsgeschichte. Leipzig, German: Leopold Voss.
- Weigert, R., Porat-Shliom, N., & Amornphimoltham, P. (2013). Imaging cell biology in live animals: ready for prime time. Journal of Cell Biology , 201(7), 969–979. doi: 10.1083/jcb.201212130.
- Yoshioka, K., Saito, M., Oh, K. B., Nemoto, Y., Matsuoka, H., Natsume, M., & Abe, H. (1996). Intracellular fate of 2-NBDG, a fluorescent probe for glucose uptake activity, in Escherichia coli cells. Bioscience, Biotechnolology, and Biochemistry , 60(11), 1899.–1901. doi: 10.1271/bbb.60.1899.
Citing Literature
Number of times cited according to CrossRef: 3
- Stan F. J. Graaf, Coen C. Paulusma, Wietse In het Panhuis, Getting in the zone: Metabolite transport across liver zones, Acta Physiologica, 10.1111/apha.14239, 240 , 11, (2024).
- Suehelay Acevedo‐Acevedo, Megan L. Stefkovich, Sun Woo Sophie Kang, Rory P. Cunningham, Constance M. Cultraro, Natalie Porat‐Shliom, LKB1 acts as a critical brake for the glucagon‐mediated fasting response, Hepatology Communications, 10.1002/hep4.1942, 6 , 8, (1949-1961), (2022).
- Rory P. Cunningham, Natalie Porat-Shliom, Liver Zonation – Revisiting Old Questions With New Technologies, Frontiers in Physiology, 10.3389/fphys.2021.732929, 12 , (2021).