In Vitro Assessment of Cardiac Fibroblast Activation at Physiologic Stiffness
Robert S. Goldsmith, Robert S. Goldsmith, Yao-Chang Tsan, Yao-Chang Tsan, Rachel E. Scissors, Rachel E. Scissors, Adam S. Helms, Adam S. Helms, Matthew J. Brody, Matthew J. Brody
Abstract
Cardiac fibroblasts (CF) are an essential cell type in cardiac physiology, playing diverse roles in maintaining structural integrity, extracellular matrix (ECM) synthesis, and tissue repair. Under normal conditions, these cells reside in the interstitium in a quiescent state poised to sense and respond to injury by synthesizing and secreting collagen, vimentin, hyaluronan, and other ECM components. In response to mechanical and chemical stimuli, these “resident” fibroblasts can undergo a transformation through a continuum of activation states into what is commonly known as a “myofibroblast,” in a process critical for injury response. Despite progress in understanding the contribution of fibroblasts to cardiac health and disease, much remains unknown about the signaling mediating this activation, in part owing to technical challenges in evaluating CF function and activation status in vitro. Given their role in monitoring the ECM, CFs are acutely sensitive to stiffness and pressure. High basal activation of isolated CFs is common due to the super-physiologic stiffness of traditional cell culture substrates, making assays dependent on quiescent cells challenging. To overcome this problem, cell culture parameters must be tightly controlled, and the use of dishes coated with biocompatible reduced-stiffness substrates, such as 8-kPa polydimethylsiloxane (PDMS), has shown promise in reducing basal activation of fibroblasts. Here, we describe cell culture protocol for maintaining CF quiescence in vitro to enable a dynamic range for the assessment of activation status in response to fibrogenic stimuli using PDMS-coated coverslips. Our protocol provides a cost-effective tool to study fibroblast signaling and activity, allowing researchers to better understand the underlying mechanisms involved in cardiac fibrosis. © 2024 The Authors. Current Protocols published by Wiley Periodicals LLC.
Basic Protocol 1 : Generation of 8-kPa polydimethylsiloxane (PDMS)/gelatin-coated coverslips for cardiac fibroblast cell culture
Basic Protocol 2 : Isolation of adult cardiac fibroblasts and plating onto PDMS coverslips
Basic Protocol 3 : Assessment of cardiac fibroblast activation by α smooth muscle actin (αSMA) immunocytochemistry
INTRODUCTION
Cardiac fibroblasts have gained recognition as crucial cellular regulators that contribute to both normal cardiac function and disease progression (Fu et al., 2018; Ivey & Tallquist, 2016; Kanisicak et al., 2016; Tallquist & Molkentin, 2017). Although they were traditionally considered support cells involved in the maintenance of the extracellular matrix (ECM), it is now well established that cardiac fibroblasts play multifaceted roles in the heart (Tallquist, 2020). From regulating ECM homeostasis and providing structural integrity, CFs also actively participate in tissue repair, remodeling, and the inflammatory response (Tallquist, 2020). However, the complex interactions and signaling cascades responsible for modulating these cellular behaviors remain incompletely understood.
Recently, significant efforts have been made to elucidate the molecular mechanisms that underlie the different phenotypic states of cardiac fibroblasts (Fu et al., 2020; Kanisicak et al., 2016; Umbarkar et al., 2021). Though normally in a quiescent state, CFs undergo a transformation known as activation during pathological conditions, for example in response to myocardial injury or chronic pressure overload (Bretherton et al., 2020). Cardiac fibroblast activation is a complex process that involves the transition from a quiescent state to the canonical “myofibroblast” phenotype, characterized by increased proliferation, migration, release of inflammatory cytokines, and synthesis of ECM proteins such as collagens (Alter et al., 2023; Gibb et al., 2020; Khalil et al., 2019). This phenotypic and morphological transformation is triggered by a variety of stimuli, including pro-inflammatory cytokines and peptide hormones released from surrounding cells, as well as physical stimuli such as mechanical stress (Bertaud et al., 2023; Herum et al., 2017). The dysregulated activation of myofibroblasts underlies the development and progression of maladaptive scar formation in the heart known as cardiac fibrosis, which is a hallmark feature of ischemic heart disease, as well as many inherited and acquired cardiomyopathies and heart failure (Kanisicak et al., 2016; Teuber et al., 2022). Elucidating the intricate signaling networks involved in cardiac fibroblast activation could potentially reveal novel therapeutic targets for preventing or reversing cardiac fibrosis. Therefore, there is an urgent need for comprehensive investigations aimed at unraveling mechanisms that drive cardiac fibroblast activation.
In vitro assays are indispensable tools for studying cardiac fibroblast activation under controlled conditions; however, researchers working with these cells have encountered a number of technical challenges associated with traditional cell culture. Due to their role in sensing and modifying the properties of the cardiac microenvironment, cardiac fibroblasts are highly responsive to the stiffness of surrounding tissue (Childers et al., 2021; Meagher et al., 2021). The use of common two-dimensional (2D) cell culture methods involving glass or plastic substrates thus poses limitations arising from their non-physiologic stiffness that can trigger a spontaneous increase in fibroblast activation (Herum et al., 2017). As a result, the relative magnitude of induction of fibroblast activation observed when using pro-fibrotic agonists such as transforming growth factor β1 (TGFβ1) becomes less significant, as the activation relative to the “unstimulated” control condition is blunted. This confounding factor compromises the ability to study the specific mechanisms underlying the transition from quiescent to activated cardiac fibroblasts, as well as their responsiveness to external stimuli.
To overcome these challenges, researchers have begun to optimize 2D cell culture conditions for maintaining quiescent populations of cardiac fibroblasts. Previous work in the field has established highly variable fibroblast responses as a result of differences in the composition of medium and serum, time in culture, and plate stiffness (Landry et al., 2019; Santiago et al., 2010). However, even with consistent cell density and culture conditions and when all other variables are tightly controlled, high plate stiffness is still likely to result in a highly activated fibroblast population in vitro. Efforts to address this have focused on generating substrates with physiologic stiffness ratings to be used as alternatives to traditional cell culture plastic. Polydimethylsiloxane (PDMS) substrates have become widely utilized for cell culture due to their tunable mechanical properties and compatibility with a wide range of assays (Palchesko et al., 2012; Yeh et al., 2017). By adjusting the formulation and curing process of PDMS, researchers can tailor the substrate stiffness to better mimic the mechanical properties of native tissue, including more appropriately mimicking the physiologic stiffness of the myocardium. This enables the creation of a more physiologically relevant microenvironment for studying cardiac fibroblast behavior and activation.
The use of “soft” substrates has become increasingly common in fibroblast in vitro experimentation because of the attenuation of the mechanosensitive activation observed with traditional cell culture, as soft substrates allow a better representation of the physiological behavior of these cells (Cheng et al., 2021; Landry et al., 2019; Morningstar et al., 2021; Shiraishi et al., 2023; Wang et al., 2021). Further, using soft substrates has been shown to reduce cardiac fibroblast activation and promote a more quiescent phenotype (Landry et al., 2019). Consequently, the use of soft substrates at physiological stiffness provides researchers with a valuable tool for studying the mechanisms underlying cardiac fibroblast activation and their responses to various stimuli. However, commercially available options for cell culture plates with physiological stiffness ratings can be prohibitively expensive, underlining the need for a more cost-effective alternative.
Here, we developed a cost-effective protocol for culturing cardiac fibroblasts at physiologic stiffness on PDMS-coated coverslips to address the limitations associated with traditional cardiac fibroblast activation assays. These PDMS-coated coverslips, generated in house, provide an affordable and accessible alternative that yield a comparable level of baseline cardiac fibroblast quiescence to that obtained with commercially available cell culture soft substrates (Landry et al., 2019). Additionally, we present a comprehensive cell culture experimental timeline for assessing cardiac fibroblast activation from quiescent cells cultured on PDMS-coated coverslips. This protocol will enable investigation of the cellular and molecular mechanisms governing cardiac fibroblast activation using an in vitro system that more accurately models the physiologic stiffness of the myocardium.
NOTE : All protocols involving animals must be reviewed and approved by the appropriate Animal Care and Use Committee and must follow regulations for the care and use of laboratory animals.
Basic Protocol 1: GENERATION OF 8-kPa POLYDIMETHYLSILOXANE (PDMS)/GELATIN-COATED COVERSLIPS FOR CARDIAC FIBROBLAST CELL CULTURE
This protocol describes a detailed procedure for the generation of 8-kPa PDMS (Palchesko et al., 2012) and the steps for applying this PDMS solution to coat cell culture coverslips for use in in vitro fibroblast assays. First, commercially available PDMS solutions of various stiffness ratings are mixed to generate an 8-kPa polymer that more closely replicates the endogenous pressure of the cardiac fibroblast environment. A thin layer of this polymer is then applied to coverslips and cured. After sterilization, the PDMS-coated coverslips can then be additionally coated with gelatin to increase cell adherence before being used in downstream applications.
Materials
-
Sylgard 184 and curing agent (Krayden Dow, cat. no. NC9285739)
-
Sylgard 527 A and B (Krayden Dow, cat. no. NC1208196)
-
70% (v/v) ethanol
-
Phosphate-buffered saline (PBS; Corning, cat. no. 21-040-CV)
-
Porcine skin gelatin aliquots (see recipe)
-
24-well plates (Fisherbrand, FB012929)
-
12-mm glass coverslips (Electron Microscopy Sciences, cat. no. 72230-01)
-
Precision balance
-
Disposable plastic cups (Dixie cups; Krayden Dow, cat. no. NC9285739)
-
1-, 5-, and 10-ml serological pipets (Falcon, cat. nos. 357506, 357543, and 357551)
-
Serological pipet controller
-
50-ml conical tubes (Fisherbrand, cat. no. 05-539-7) and tube holder
-
Disposable plastic cell scrapers (Santa Cruz, cat. no. sc-395251)
-
10-, 20-, and 200-μl (P10, P20, and P200) pipets
-
10- and 200-μl (P10 and P200) pipet tips (TipOne, cat. nos. 1110-3700 and 1111-1706)
-
Vortex mixer
-
Tabletop centrifuge
-
20-μl (P20) filter pipet tips (SureOne, cat. no. 02-707-432)
-
Vacuum desiccator
-
50°C oven
-
Fine-tip straight forceps (WPI, cat. no. 14099) or equivalent
-
Bent-needle lifting tool made from a 1-ml syringe (Becton Dickinson, cat. no. 309659) and a 1.5-inch, 21-G PrecisionGlide needle (Becton Dickinson, cat. no. 305167) (see Fig. 1)
-
Class II biological safety cabinet with UV light
-
37°C water bath
-
Vacuum aspirator
-
37°C, 5% CO2 incubator
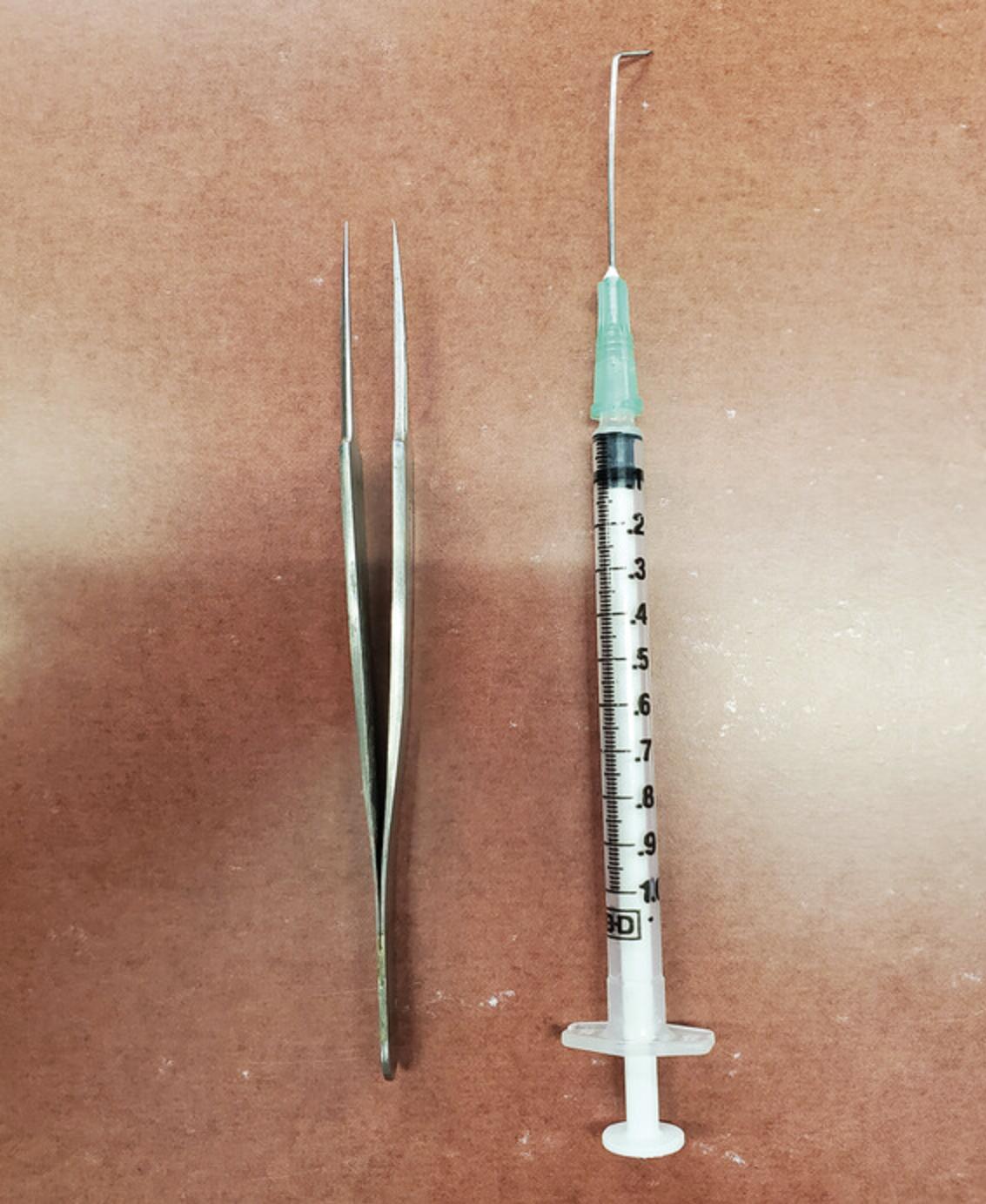
NOTE : PDMS is difficult to remove from surfaces with normal solvents. Cover any workspaces during the creation of the PDMS mixture to ensure ease of clean-up in the event of a spill.
PDMS creation and coverslip coating
For this protocol, 10 g of 8-kPa PDMS solution is made by mixing two different Sylgard solutions together at the appropriate ratio.
1.Carefully place one 12-mm coverslip into each well of a 24-well plate with forceps.
2.Separately, prepare two different Sylgard mixtures:
- Sylgard 184: Place an empty disposable plastic cup onto a precision balance. To it, add exactly 10 g Sylgard 184 base using a 10-ml serological pipet (tip can be cut off to improve pipetting efficiency), followed by exactly 1 g Sylgard 184 curing agent with a 5-ml serological pipet. Remove the cup from the balance, and using a plastic cell scraper, mix vigorously for 5 min. Place the cup with mixed components into the vacuum desiccator to remove bubbles.
Because PDMS is highly viscous and does not readily dissolve in common solvents, disposable containers such as the cups described in this protocol are recommended for ease of handling and cleaning.
-
Sylgard 527: Again, place an empty disposable plastic cup onto a precision balance. With a 10-ml serological pipet, add exactly 6 g Sylgard 527 Part A to the disposable cup. Then, with a new 10-ml serological pipet, add exactly 6 g Sylgard 527 Part B to the cup for a total mass of 12 g. Mix vigorously in the cup with a plastic cell scraper for 5 min. Bubbles will likely not be present in this solution (does not require vacuum desiccation).
3.Combine the Sylgard 184 and 527 at the precise ratio of 0.102:9.90 (v/v) to obtain an 8-kPa PDMS solution:
-
Place an empty 50-ml conical tube into a small tube holder that fits on the precision balance. Place tube and holder onto the balance and tare the balance. Using a P10 pipet and pipet tip, without depressing the pipet, dip the tip into the Sylgard 184 mixture. Once the solution has adhered to the tip, move the tip over to the 50-ml conical tube on the balance and allow it to drip off without depressing the pipet. Repeat untilexactly102 mg has been added to the conical tube. Be careful to achieve this mass as closely as possible. When 102 mg is reached, tare the balance.
-
Using a 5-ml serological pipet, add 9.90 g Sylgard 527 to the tube containing the 102 mg Sylgard 184. We recommend using the serological pipet for the majority of the mass being added, and then, at the end, using a P200 tip to add the Sylgard 527 dropwise, in a fashion similar to that in step 3a, to reach exactly 9.90 g.
The specific gravity of PDMS is .918. The main reason we use the weighing method is because, due to the high viscosity of PDMS, it is very difficult to accurately and reproducibly measure volumes.
4.Mix the two PDMS solutions very thoroughly:
-
Secure the lid on the conical tube and vortex the tube on high for ∼10 s, before orbitally tilting the tube and rolling the Sylgard mixture around the interior surface for another ∼10 s. Repeat vortexing and mixing five times to ensure an extremely well-mixed, homogenous solution.
-
Centrifuge the mixture for 3 min at 3200 ×g, room temperature, to get the PDMS to the bottom of the conical tube.
-
Vortex again for several seconds while tilting the tube in different directions.
5.Pipet the PDMS solution onto coverslips:
- Load a P20 pipet with a 20-µl filter tip, and then cut off the tip of the pipet (∼2-3 mm). Set the volume on the pipet to 14 µl and pipet the thoroughly mixed PDMS solution up and down into the tip slowly three times to coat the inside of the pipet and reduce the formation of bubbles.
The repeated pipetting is only necessary upon first introduction of the solution to the tip, before the first coverslip loading.
- Eject all of the 14 µl of mixed PDMS onto the direct center of each coverslip within each well of the 24-well plate. To achieve consistent volumes, after pulling the PDMS into the pipet, drag the tip along the inside of the tube to “brush off” extra PDMS that coats the outside of the pipet tip (it is highly viscous) before pipetting onto the coverslip.
Once the PDMS has been applied, it will slowly begin to spread out to the edges of the coverslip. It is normal for the PDMS to not be completely spread out prior to curing in the oven, and it will likely be uniformly distributed following the overnight incubation in the 50°C oven.
Any unused PDMS solution remaining after coverslip coating should be discarded, as it will solidify. A fresh PDMS solution should be prepared for each subsequent batch of coverslips at later dates.
6.Place the lids on the 24-well plates and place plates with coated coverslips into the vacuum desiccator for 30-45 min.
7.Transfer the plates to the 50°C oven and cure PDMS overnight.
8.Remove plastic plates containing the cured coverslips from the oven. Coverslips can be stored at room temperature indefinitely in the plastic plates that they were cured in until needed for experiments. Only proceed to gelatin coating (step 9-13) when ready to perform experiments, as once gelatin coating is performed, it is recommended that the coverslips be used immediately.
Preparation, sterilization, and gelatin coating of coverslips
9.Load new 24-well experimental plates with unattached PDMS-coated coverslips from the plastic plates in which they were cured:
-
Using the forceps and a bent-needle/syringe lifting tool (see Fig.1), lift the unattached, freely moving PDMS-coated coverslips from the preparation plate, and transfer the desired number of coverslips to a new experimental 24-well plate.
When transferring coverslips, use the forceps, pointed down toward the bottom of the well, to push each coverslip sideways gently against the plastic well with one hand and gingerly lift up, just enough to break the surface tension between the coverslip and the plastic. Simultaneously, with the other hand, using the bent-needle lifting tool, position the needle under the coverslip that is being barely lifted with the forceps. Once the needle is under the coverslip, the forceps can be released. While elevating the coverslip with the lifting tool, grip the edge of the coverslip with the forceps as minimally as possible so as not to disturb the PDMS coating and lift, and then transfer the coverslip to the desired location. As you release the coverslip from the forceps, the PDMS-coated side may stick to the forceps. When this happens, the bent-needle tool can be used to gently scrape the attachment point of the forceps to the PDMS to release it.
10.Place the plate in the BSC, remove the lid, and flip it so that the outer surface of the lid rests on the cabinet surface. Liberally spray the PDMS-coated coverslips and rest of the plastic plate, including the overturned lid, with 70% ethanol, effectively soaking them. Ethanol should cover the coverslip entirely. Close the cabinet sash, turn on the UV light, and allow coverslips to sit in ethanol under UV for 20-30 min. In the meantime, place a bottle of sterile PBS and an aliquot of porcine skin gelatin stock (see recipe) in a 37°C water bath.
11.Thoroughly aspirate the ethanol covering each coverslip by pointing the 1-ml serological pipet toward the side of the well to avoid direct contact with the coverslips. Additionally, aspirate the lid of the plastic dish. Once the majority of the ethanol has been removed, allow the remainder to air dry in the cabinet for ∼20-30 min until dry. Coverslips are now ready for downstream applications.
12.Dilute porcine skin gelatin stock in sterile, warm (37°C) PBS to make a sufficient volume of porcine skin gelatin working solution (10-100 µg/ml; concentration can be adjusted depending on cell adherence; adapted from Landry et al., 2021). Each coverslip requires 500 µl of working solution. To make enough 100 µg/ml solution to coat one 24-well dish (12 ml), use a serological pipet to transfer 15 ml prewarmed PBS into a 50-ml conical tube. Then add 150 µl porcine skin gelatin stock solution from the prewarmed aliquot to the PBS. Mix by pipetting up and down with the serological pipet. Pipet 500 µl of the porcine skin gelatin working solution onto each PDMS-coated coverslip, ensuring that each coverslip is completely submerged in the solution. Cover the plate with the lid and place the plate in the 37°C, 5% CO2 incubator overnight.
13.The next day, when ready for cell plating, move the plates from the 37°C, 5% CO2 incubator back into the BSC. Remove plate lids as before, aspirate using a 1-ml serological pipet to remove the gelatin solution from each well, and allow the coverslips to air dry in the BSC for ∼10 min. Coverslips are now ready for cell plating (see Basic Protocol 2, step 22).
Basic Protocol 2: ADULT CARDIAC FIBROBLAST ISOLATION AND PLATING ONTO PDMS-COATED COVERSLIPS
This protocol describes the isolation of primary murine adult cardiac fibroblasts for use in in vitro fibroblast assays adapted from Kanisicak et al. (2016), along with their direct plating onto PDMS-coated coverslips. First, mouse hearts are enzymatically digested to separate myocardial tissue into its cellular components. The cell suspension is then plated onto PDMS/gelatin-coated coverslips to allow preferential adherence of fibroblasts. This process maintains the fibroblasts on a physiologically relevant substrate for the duration of the experiment and avoids any alterations to the fibroblasts associated with passaging primary cardiac fibroblasts prior to experimental assays.
NOTE : All experiments involving animals should abide by institutional protocols governing animal use and care (IACUC) and governmental agency guidelines, and protocols should be reviewed and approved prior to conducting any experimental procedures.
NOTE : ACFs are most commonly isolated and cultured from 8- to 12-week-old mice of the C57/Bl6 strain but can be isolated from adult mice of any strain or genetic background.
NOTE : For an alternative mouse cardiac fibroblast isolation protocol, see article on isolation of adult mouse cardiac fibroblasts by Almazloum & Khalil (2023).
Materials
-
Dulbecco's Modified Eagle's Medium (DMEM; Corning, cat. no. 10-013-CV)
-
FBS aliquot(s) (see recipe)
-
Pen/Strep (P/S; Corning, cat. no. 30-002-CV)
-
Dispase buffer (see recipe)
-
Enzymatic digestion buffer (see recipe)
-
C57BL/6 mice, aged 8-12 weeks
-
DMEM/10% FBS with 1% P/S (see recipe)
-
PDMS/gelatin coated coverslips (see Basic Protocol 1)
-
Ice bucket
-
Gyromini Nutator (Labnet, cat. no. S0500) or equivalent
-
37°C, 5% CO2 incubator
-
37°C water bath
-
15-ml conical tubes (Basix, cat. no. 14-955-237)
-
50-ml conical tubes (Fisherbrand, cat. no. 05-539-12)
-
10-cm culture dishes (Fisherbrand, cat. no. FB012924)
-
10-ml serological pipets (Falcon, 357551)
-
Serological pipet controller
-
1-ml serological pipets (Falcon, cat. no. 357506)
-
Fine-tip straight forceps (WPI, cat. no. 14099) or equivalent (2)
-
Surgical scissors
-
Class II biological safety cabinet (BSC)
-
P1000 pipet
-
P1000 pipet tips (TipOne, cat. no. 1112-1720)
-
Razor blades
-
40-µm mesh strainer (Fisherbrand, cat. no. 22-363-547)
-
Tabletop centrifuge
-
Vacuum aspirator
-
Inverted bright-field tissue culture microscope
Initial preparations
1.Before beginning, place DMEM and one (or more, depending on number of hearts being isolated) FBS aliquot into the 37°C water bath. Warm P/S and dispase buffer (see recipe) to room temperature, prepare an ice bucket, and place nutator in the 37°C, 5% CO2 incubator.
2.Determine the number of hearts to be isolated and prepare enzymatic digestion buffer (see recipe) accordingly. Store in water bath at 37°C until ready to use.
3.Prelabel three conical tubes per sample.
4.When DMEM is warm and enzymatic digestion buffer is ready, pipet 5-10 ml of serum-free DMEM into first set of prelabeled 15-ml conical tubes using a 10-ml serological pipet. Then, pipet 10 ml serum-free DMEM into one or multiple 10-cm culture dishes using a 10-ml serological pipet, one for each sample condition. Bring the 10-cm dish(es) and 15-ml conical(s) with medium to the location of animal sacrifice and heart isolation. Prepare the benchtop ahead of time to contain relevant euthanasia and sterile surgical tools (forceps, scissors) necessary to remove and dissect the heart after sacrifice.
Isolation of heart(s)
5.Sacrifice the animal in accordance with respective approved animal protocols, and then use surgical scissors to open the thoracic cavity and expose the heart. Using two sets of forceps, with one set grip the aorta and extracardiac tissue superior to the ventricles, and with the other set gently grip and pull the ventricles away from chest cavity to isolate the heart.
6.Place the isolated heart into the 10-cm dish containing prewarmed DMEM (from step 4) and squeeze the heart repeatedly with forceps in prewarmed DMEM to remove remaining blood cells. Move each heart with the forceps to the respective prelabeled 15-ml conical tube containing 5-10 ml serum-free DMEM and bring the tubes containing the heart(s) to the BSC.
7.Using the P1000 pipet with filter tips, pipet 2 ml per heart of fresh enzymatic digestion buffer into an empty prelabeled 15- or 50-ml conical tube, as applicable (see annotation to step 3).
8.Remove the lid from a sterile 10-cm dish and then place the internal side of the lid facing up. Pour out the 15-ml conical tube containing the medium and isolated hearts into the base of the 10-cm dish, and then transfer (using forceps or lifting up with razor blades) each heart from the medium in the 10-cm dish to the dry lid that was set facing up. Pinch the heart with the forceps, and use a razor blade to make a transverse cut of roughly the “top” 1 mm of the heart to remove atria and vasculature above the ventricles, while maintaining as much of the ventricles as possible (see Fig. 2). Then, mince ventricles using a razor blade and/or surgical scissors, forming pieces approximately 1- to 2-mm3 pieces (∼8-10 pieces per heart), and transfer minced ventricular tissue from one or more pooled heart(s) into the 15- or 50-ml conical tube containing the enzymatic digestion buffer for that sample.
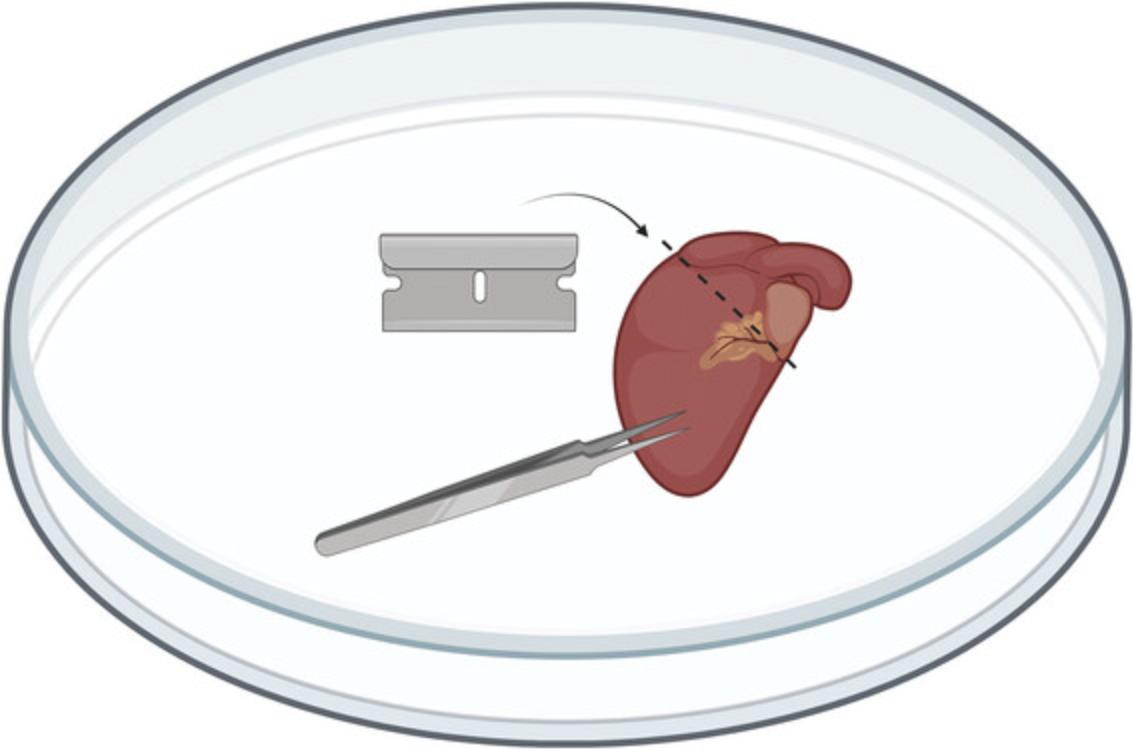
Dissociation of cardiac tissue
9.When all samples are in their corresponding tubes, cap tubes and then transfer and secure the tubes in a nutator inside the 37°C, 5% CO2 incubator to provide gentle agitation. Incubate tissues in the incubator for 20 min.
10.Remove the tubes from the nutator, transfer the tubes back to the BSC, and for each tube, perform manual trituration 12-15 times with a 5-ml serological pipet until all tissue pieces are able to travel through the pipet without resistance.
11.Allow the tissue to settle by sedimentation (∼30 s to 1 min), and carefully collect the supernatant using a P1000 filter pipet tip without disturbing the tissue at the bottom of the tube.
12.Using the P1000 pipet, pass the supernatant through a 40-μm-mesh strainer into its corresponding prelabeled 50-ml conical tube and immediately store on ice.
13.Using the P1000, add 2 ml fresh enzymatic digestion buffer per heart to the previously sedimented ventricular tissue (not the digested supernatant on ice) in its existing tube, and place the tube back on the nutator in the 37°C, 5% CO2 incubator for 20 min.
14.Remove the tubes from the nutator, transfer the tubes back to the BSC, and for each tube, perform manual trituration 12-15 times with a P1000 pipet tip until there is no resistance.
15.Allow the tissue to settle by sedimentation (∼30 s to 1 min), and carefully collect the supernatant using a P1000 pipet without disturbing the tissue at the bottom of the tube.
16.Using the P1000 pipet, pipet the supernatant through a 40-μm-mesh strainer (either reused from the same sample or a new filter if doing bulk preps and the previous filter is clogged) into the same corresponding 50-ml collection tube that was on ice, and return the tube(s) to the ice bucket.
Final enzymatic digestion and centrifugation
17.Using the P1000 pipet, add the final 2 ml of fresh enzymatic digestion buffer per heart to the previously sedimented tissue in its existing tube and place on the nutator in the 37°C, 5% CO2 incubator for a final 20-min digestion. Place premade DMEM/10% FBS with 1% P/S in the 37°C water bath, and precool the tabletop centrifuge to 4°C.
18.Remove the tubes from the 37°C, 5% CO2 incubator, transfer to the BSC, and perform manual trituration 12-15 times through a P1000 pipet tip.
19.Allow the tissue to settle by sedimentation (∼30 s to 1 min), and carefully collect the supernatant using a P1000 pipet tip without disturbing the tissue at the bottom of the tube.
20.Using the P1000 pipet, pipet the supernatant through a 40-μm-mesh strainer (either reused from the same sample or a new filter if doing bulk preps and the previous filter is clogged) into the corresponding 50-ml collection tube.
21.Centrifuge the pooled sample supernatant from the three digestions (6 ml total per heart per tube) in the 50-ml collection tube(s) for 20 min at 350 × g , 4°C, using a fixed-angle rotor.
Incubation of cells on PDMS/gelatin-coated coverslips
22.During the spin, remove PDMS/gelatin-coated coverslips from the 37°C, 5% CO2 incubator, and aspirate gelatin solution to prepare for cell plating (see step 13, Basic Protocol 1).
23.Carefully remove tube(s) from centrifuge so as not to disturb the pelleted cells. Gently pour off supernatant from each tube and discard, with the pellet facing “up” in such a way that the supernatant is not flowing over the cell pellet as it leaves the tube.
24.Resuspend the cell pellet in 6.5 ml (per heart) of fresh DMEM/10% FBS with 1% P/S (prewarmed in a 37°C water bath) using a 10-ml serological pipet.
25.Pipet 500 µl of the cell suspension/medium mixture onto each PDMS/gelatin-coated coverslip, so that the coverslip is submerged in the solution. Place lid on 24-well plate. Transfer the plates to the 37°C, 5% CO2 incubator for 2 hr to allow the fibroblasts to adhere to the coverslips (see Fig. 3).
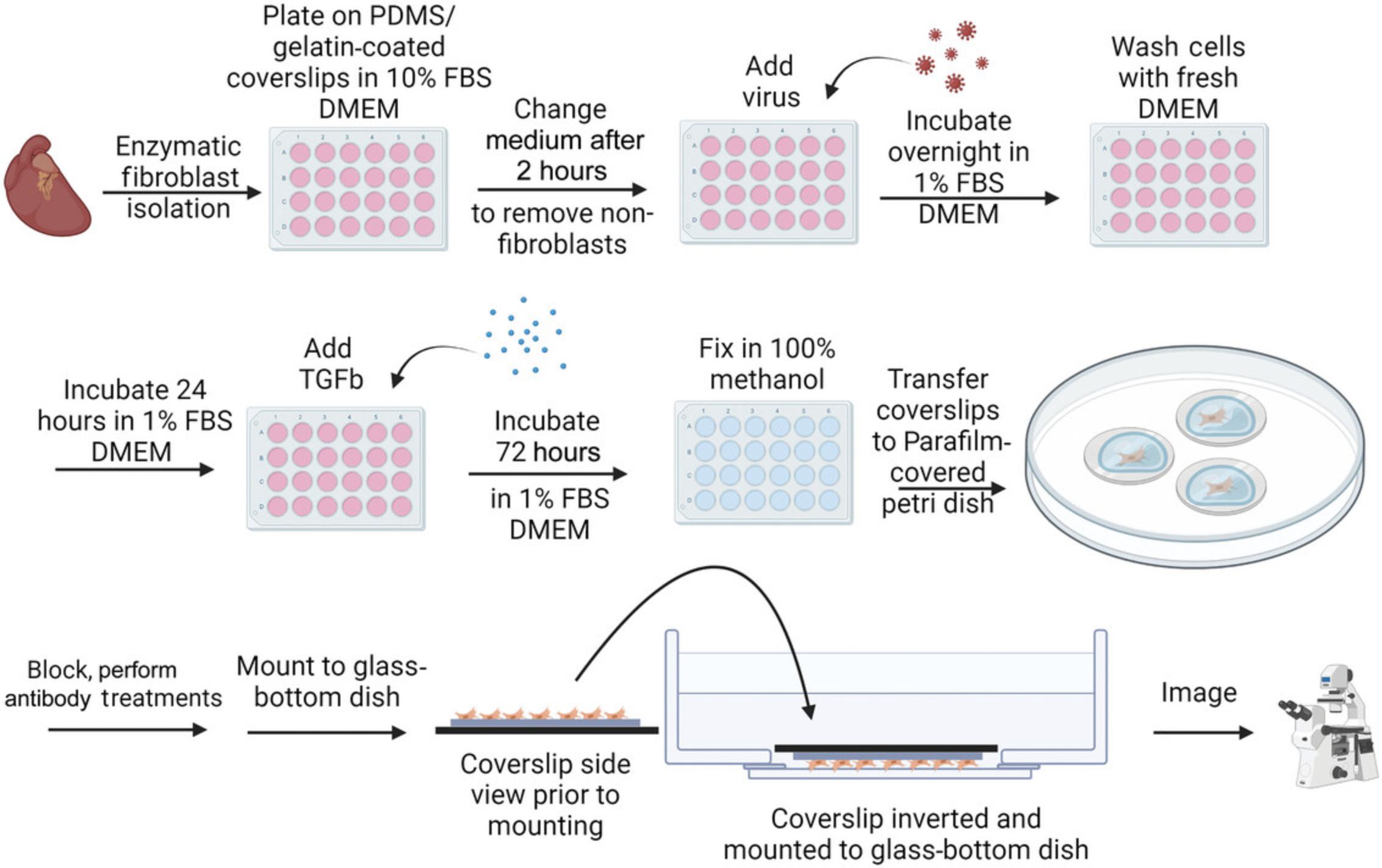
26.Warm an FBS aliquot and P/S to room temperature, and warm serum-free DMEM in the 37°C water bath. After the 2-hr incubation is finished, transfer the plates to the BSC and gently wash the cell solution (that is, non-fibroblast cells, as fibroblasts will have attached to coverslips) from the coverslips. Aspirate medium/cell suspension from the plate ∼4 wells at a time using a 1-ml serological pipet. Then, using the P1000 pipet, immediately cover each coverslip again in fresh medium by pipetting 500 µl warm serum-free DMEM onto the side of each well gently to avoid disturbing attached cells. Repeat until all wells in the dish have been washed once, and then repeat the washing process until the majority of the free floating/dead cells have been removed (∼3 washes per coverslip total).
27.After the final wash, the incubation medium composition and volume will depend on downstream assay requirements. If no transduction is required, use a P1000 pipet to replace the wash medium with 500 µl of freshly made DMEM/1% FBS with 1% P/S using the prewarmed components from step 26.Incubate for 48 hr before proceeding to Basic Protocol 3, step 3.If transduction is required, immediately following the isolation washing step, use the P1000 pipet to pipet 250 µl DMEM/1% FBS (without P/S) into each well and proceed to Basic Protocol 3, step 1.
Basic Protocol 3: ASSESSMENT OF CARDIAC FIBROBLAST ACTIVATION BY α SMOOTH MUSCLE ACTIN (αSMA) IMMUNOCYTOCHEMISTRY
This protocol describes the process of performing an immunocytochemical assay using primary adult cardiac fibroblasts to assess their transformation into activated myofibroblasts. Parameters have been optimized for reduced background activation, efficient transduction in vitro (if applicable), and successful induction of activation using a profibrotic agonist. After cell culture, fibroblasts on PDMS-coated coverslips are then fixed and prepared for downstream immunocytochemistry to detect α-smooth muscle actin (αSMA).
Materials
-
Adenovirus (if applicable)
-
Adult mouse cardiac fibroblast cultures in 24-well plates (Basic Protocol 2)
-
Dulbecco's Modified Eagle's Medium (DMEM; Corning, cat. no. 10-013-CV)
-
Fetal bovine serum (FBS; Corning, cat. no. 35-010-CV)
-
Pen/Strep (P/S, Corning, cat. no. 30-002-CV)
-
TGFβ1 aliquots (see recipe)
-
Phosphate-buffered saline (PBS; Corning, cat. no. 21-040-CV)
-
Methanol (Sigma, cat. no. 179337), ice cold
-
Blocking solution (see recipe)
-
Anti-αSMA primary antibody (Sigma, cat. no. A5228)
-
Goat anti-mouse Alexa Fluor 488 sary antibody (Invitrogen, cat. no. A-11029)
-
Prolong Gold with DAPI Mounting Solution (Cell Signaling, cat. no. 8961)
-
10-, 20-, 200-, and 1000-μl (P10, P20, P200, and P1000) pipets
-
10- and 20-μl (P10 and P20) filter pipet tips (SureOne, cat. nos. 02-707-001 and 02-707-432)
-
Class II biosafety cabinet (BSC) with UV light
-
37°C water bath
-
1000-μl (P1000) filter tips (VWR, cat. no. 76322-156)
-
37°C, 5% CO2 incubator
-
Gyromini Nutator (Labnet, cat. no. S0500) or equivalent
-
15-cm plastic petri dishes (Fisherbrand, cat. no. FB012925)
-
Parafilm (Fisher Scientific, cat. no. S37440)
-
P200 pipet tips (TipOne, cat. no. 1111-1706)
-
Fine-tip straight forceps (WPI, cat. no. 14099) or equivalent
-
Bent-needle lifting tool made from a 1-ml syringe (Becton Dickinson, cat. no. 309659) and 1.5-inch, 21-G PrecisionGlide needle (Becton Dickinson, cat. no. 305167) (see Fig. 1)
-
Vacuum aspirator
-
1-ml serological pipet
-
Paper towels (Scott, cat. no. 01510)
-
Cold room or refrigerator, 4°C
-
35-mm glass-bottom dishes (Cellvis, D35-28-1.5-N)
-
Confocal or epifluorescence microscope compatible with imaging of 35-mm dishes
-
ImageJ software (optional)
Fibroblast activation with TGFβ1
1.If the assay requires a transduction step, thaw adenovirus and, using a P10 pipet with filter tips, pipet the appropriate volume of virus into each experimental well of the 24-well plate of adult murine cardiac fibroblasts in a BSC (see Fig. 3). Incubate overnight.
2.Gently aspirate virus-containing medium from the coverslips. Remove medium from the plate ∼4 wells at a time using a P1000 pipet with filter tips, and immediately cover each coverslip again in fresh warm (37°C) serum-free DMEM by using the P1000 to gently pipet 500 µl medium into each well. Repeat until all the wells have been washed once, and then repeat twice more, adding warm 500 µl freshly made DMEM/1% FBS with 1% P/S after the final wash. Incubate for 24 hr in the 37°C, 5% CO2 incubator.
3.Following the post-isolation 48-hr incubation with or without virus, prepare treatment solutions (see Fig. 3). Calculate for 500 µl per well to determine appropriate volumes of medium and saline (PBS)/TGFβ1 treatments. Briefly thaw frozen TGFβ1 aliquot(s) (see recipe) at room temperature. To make treatment solutions, prepare prewarmed DMEM/1% FBS with P/S, and into separate 15-ml conical tubes, pipet 1 µl of TGFβ1 or PBS per milliliter of medium for a 1:1000 dilution (to achieve a final concentration of 10 ng/ml TGFβ1). Mix the treatment solutions by placing them on the nutator inside the 37°C, 5% CO2 incubator for 5 min.
4.To begin treatments, remove medium from the wells ∼4 wells at a time using P1000 pipet with filter tips, and then immediately cover each coverslip with either PBS or one of the 10 ng/ml treatment solutions prepared in step 3, as appropriate. Incubate in the 37°C, 5% CO2 incubator for 72 hr.
αSMA immunocytochemistry
5.Remove medium from the wells ∼4 wells at a time using P1000 pipet with filter tips and then, using the P1000, gently pipet 500 µl PBS onto each coverslip. Repeat so that all coverslips have been washed twice with PBS.
6.After the second wash, remove PBS with P1000 filter tips. Then, with the P1000 pipet, add 500 µl ice-cold methanol onto each coverslip. Incubate for 15 min at 4°C.
7.Remove methanol from each coverslip with P1000 filter tips, and gently wash twice with PBS with P1000 filter tips.
Immunocytochemistry is performed as described (Brody et al., 2012; Essandoh et al., 2023) and adapted for detection and imaging of αSMA stress fibers in adult cardiac fibroblasts cultured on PDMS-coated coverslips.
8.Coat a 15-cm non-sterile petri dish with one layer of Parafilm to create an incubation chamber for immunostaining. Using the forceps and bent-needle lifting tool (see Fig. 1), remove each coverslip from its well and place onto the Parafilm layer with the cells facing up. Immediately cover each coverslip with ∼70 µl PBS using the P200 pipet. The PBS should form a meniscus on the coverslip.
9.When all coverslips have been placed on the Parafilm, aspirate PBS with 1-ml serological pipet, and use a P200 pipet to add ∼70 µl blocking solution to each coverslip, enough to sufficiently cover the whole surface. Incubate 1 hr at room temperature.
10.Prepare 1:1000 anti-αSMA primary antibody dilution in blocking solution (e.g., 1 µl of primary antibody in 1 ml blocking solution). Aspirate the blocking solution from each coverslip using a 1-ml serological pipet, and using P200 pipet, add ∼70 µl of this diluted primary antibody onto each coverslip.
11.Wet small pieces of paper towels, fold, and place on the sides of the 15-cm dish away from the coverslips to create a humidity chamber; then replace the lid to prevent coverslips from drying out. Move to a cold room or refrigerator and incubate overnight at 4°C.
12.The following day, wash each coverslip three times with 50-100 µl PBS. Then, add blocking solution containing goat anti-mouse Alexa Fluor 488 sary antibody diluted 1:1000 in PBS to the coverslips and incubate for 1 hr at room temperature.
13.Wash each coverslip three times with PBS in a similar fashion as in previous washing steps.
14.Mount coverslips onto 35-mm glass-bottom dishes for imaging. Three coverslips can be mounted on each 35-mm dish. Label dishes to appropriately identify coverslips before mounting. Using a P20 filter tip, apply three small drops of Prolong Gold with DAPI mounting solution to each glass-bottom dish in a triangular pattern (see Fig. 4).
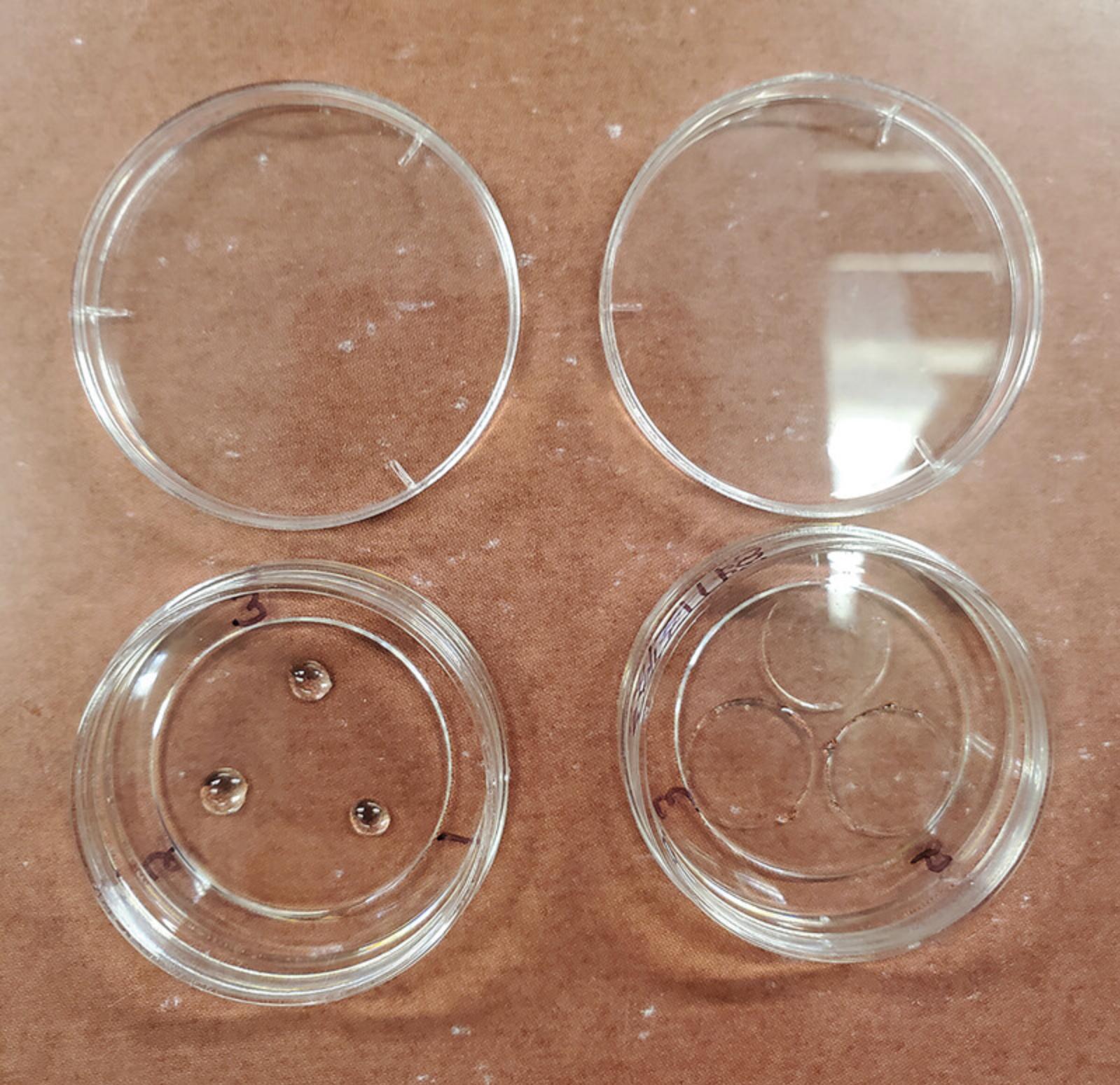
15.Lift each coverslip from the Parafilm, flip it with the forceps so that the cell side makes contact with the drop of mounting solution, and gently lay it over the drop of mounting solution in the 35-mm glass-bottom dish (see Fig. 4). Coverslips can be left to dry at room temperature and are then ready for imaging.
16.Coverslips can now be imaged using fluorescence microscopy (see Fig. 5). Images can be taken with lower magnification (10×) to achieve fields of view (FOV) with an ample number of cells (50+ ACFs per FOV) to be representative during quantification steps.
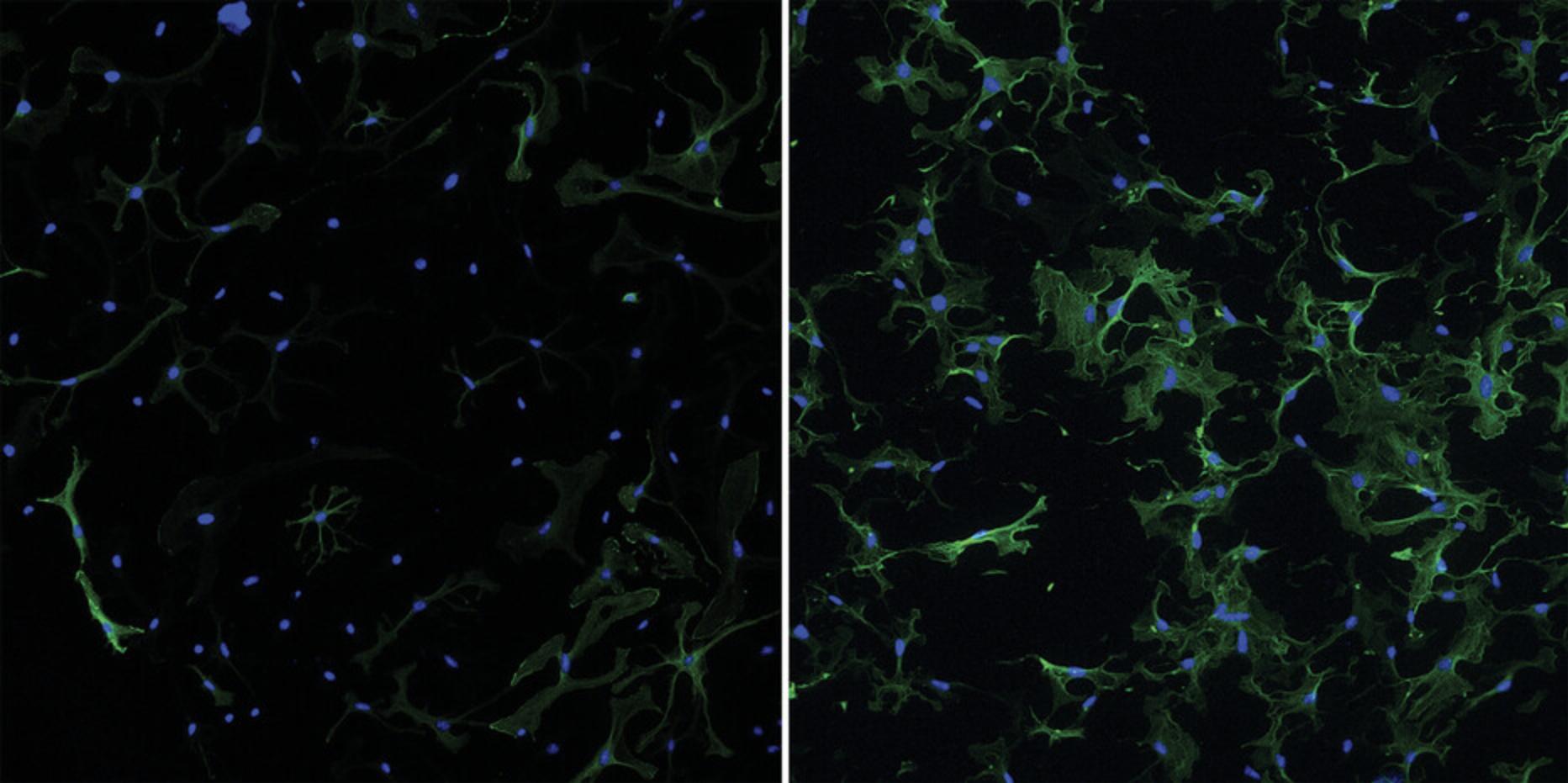
17.Quantify differences in αSMA response using ImageJ, if desired.
REAGENTS AND SOLUTIONS
Blocking solution
- 1.Prepare 1 liter of 10× phosphate-buffered saline (PBS).
NaCl | 80 g | (Fisher BioReagents, cat. no. BP358) |
KCl | 2 g | (Sigma, cat. no. P9541) |
Na2HPO4 | 14.4 g | (Sigma, cat. no. S9763) |
KH2PO4 | 2.4 g | (Sigma, cat. no. P5655) |
pH to 7.4 |
- 2.Dilute 10× PBS 1:10 in ddH2O to generate 1× PBS.
- 3.Generate blocking solution starting with 1× PBS:
- 1× PBS
- 5% (v/v) goat serum (Sigma Aldrich, cat. no. G6767)
- 1% (w/v) bovine serum albumin (BSA; Sigma Aldrich, cat. no. A9647)
- 1% (w/v) glycine (Thermo Scientific, cat. no. AAA138160E)
- 0.2% (v/v) Triton X-100 (Fisher BioReagents, cat. no. BP151-500)
Dispase buffer
For 300 ml stock solution:
- 1.On a precision balance, weigh:
- 3.57 g HEPES (Fisher BioReagents, cat. no. BP310)
- 2.63 g NaCl (Fisher BioReagents, cat. no. BP358)
Transfer weighed solids to a 500-ml glass beaker containing ∼280 ml double-distilled water (ddH2O).
- 2.Calibrate pH meter. Stir solution. Add KOH dropwise until the solution reaches pH 7.4.
- 3.Transfer solution to a graduated cylinder and bring volume to 300 ml with ddH2O. Sterilize solution with 0.2-µm-mesh filter flasks (Fisherbrand, cat. no. FB12566500) and create six 50-ml conical aliquots to store at 4°C until use. Aliquots should be used within 6 months.
DMEM/10% FBS with 1% penicillin/streptomycin (P/S)
- 1.Open a fresh 500 ml bottle of DMEM. Using a serological pipet, remove 55 ml of medium and discard.
- 2.Using a new serological pipet, add 50 ml FBS (Corning, cat. no. 35-010-CV).
- 3.Using a new serological pipet, add 5 ml Pen/Strep (Corning, cat. no. 30-002-CV).
- 4.Cap bottle and store at 4°C. Use within ∼2 months.
Enzymatic digestion buffer
- 1.Prewarm serum-free DMEM in the 37°C water bath. Incubate dispase buffer (see recipe), an FBS aliquot (see recipe), and P/S at room temperature.
- 2.Using the precision balance, weigh out 14 mg of dispase II (Sigma, cat. no. D4693) and 11 mg of collagenase IV (Worthington, cat. no. LS004188). Bring weighed solids to the biosafety cabinet.
- 3.Using a serological pipet, add 1.5 ml room-temperature dispase buffer to a 50-ml conical tube. Carefully pour in the 14 mg dispase II. Invert the tube manually and gently swirl to dissolve the solid.
- 4.Using a serological pipet, add 4.1 ml of prewarmed serum-free DMEM to the 50-ml conical tube.
- 5.Using a pipet, add 300 µl P/S and 120 µl FBS to the tube.
- 6.Carefully pour in the 11 mg collagenase IV to the tube, cap, and again manually invert the tube to ensure a mixed solution. Place the tube in the 37°C water bath until ready to use.
The steps listed here will yield 6 ml enzymatic digestion buffer, enough for one heart. To generate larger amounts, just multiply the listed amounts by the number of hearts being isolated.
FBS aliquots
- 1.Thaw frozen FBS (Corning, cat. no. 35-010-CV) and pipet up and down with serological pipet until solution is mixed.
- 2.Aliquot small volumes of FBS into 1.5-ml microcentrifuge tubes, in amounts sufficient for isolating cells from a few hearts with some extra.
Example: For an aliquot for a five-heart isolation, 5 × 120 µl FBS per heart is 600 µl, plus 20 µl extra for a total of 620 µl per aliquot.
- 3.Store at −20°C until ready for use. Always use a fresh aliquot for each isolation—do not use FBS that has been freeze/thawed.
Porcine skin gelatin aliquots, 1% (w/v)
- 1.In a glass autoclave bottle, add 100 ml double-distilled water, cap the bottle, and incubate in 37°C water bath.
- 2.When warm, add 1 g Porcine Gelatin Type A (Sigma-Aldrich, cat. no. G1980). Allow solution to dissolve.
- 3.Once dissolved, autoclave the solution at 121°C, 15 psi, for 30 min to ensure sterility.
- 4.Carefully remove the solution from the autoclave, and aliquot, working in the biological safety cabinet, while the solution is still warm (∼50°C). Aliquots can be stored indefinitely at 4°C.
This procedure for making 1% (w/v) porcine skin gelatin aliquots to coat was previously described by Landry et al. (2021).
TGFβ1 aliquots
- 1.Reconstitute lyophilized TGFβ1 (Peprotech, cat. no. 100-21) according to manufacturer's instructions. For Peprotech recombinant human TGFβ1, reconstitute in sterile filtered 10 mM citric acid (Sigma, cat. no. 251275) in double-distilled water, pH 3.0, at a concentration of 20 µg/ml. Incubate 10 min at 4°C.
- 2.Further dilute 1:1 by adding an equal volume of 0.2% bovine serum albumin (BSA; Sigma Aldrich, cat. no. A9647) in PBS to the TGFβ1/citric acid solution to achieve a final stock concentration of 10 µg/ml TGFβ1/0.1% BSA.
- 3.Aliquot the final TGFβ1 solution into 0.2-ml PCR tubes (VWR, cat. no. 93001-118) by pipetting 10 µl into each tube. Aliquots can be stored up to 1 year at −20°C.
COMMENTARY
Background Information
Recent exploration of the mechanisms underlying cardiac fibroblast activity and phenotypic transformation to elicit ECM remodeling and cardiac fibrosis in many forms of heart disease is an exciting frontier for cardiac biology. Given the sensitivity of cardiac fibroblasts to their environment, 2D cell culture experimentation can represent a significant technical challenge. Toward solving this problem, researchers have adopted new strategies including the use of substrates such as polydimethylsiloxane (PDMS), a silicon-based organic polymer that is widely used in cell biology and bioengineering for this application due to its unique biocompatibility, transparency, and tunable elasticity (Palchesko et al., 2012). Coverslips coated with PDMS provide a simple yet robust means to model a variety of in vivo environments in vitro , with a range of practical applications spanning cell and tissue culture, biomechanical studies, and high-resolution imaging applications. In the context of fibroblast research, it has been demonstrated that fibroblasts plated and maintained on 8-kPa soft-substrate-coated plates exhibit reduced baseline activation compared to those on standard plastic plates used for in vitro studies (Landry et al., 2019). Here we provide a straightforward and cost-effective protocol for the assessment of cardiac fibroblast activation.
Critical Parameters
Cardiac fibroblasts are particularly sensitive to a variety of cell culture conditions that can influence experimental results, and attention to small details throughout the course of preparation, from coverslip generation to activation treatments, is necessary for these assays. Carefully mixing the PDMS reagents at the proper ratios when generating batches of coverslips is critical to obtaining consistent results between experiments. Coverslips must be cured at the same temperature and length of time in order to achieve the proper stiffness rating; curing for too much or too little time may result in variation in substrate performance. The gelatin coating step is additionally important to ensure that the fibroblasts properly attach to the PDMS substrate during the cell plating step. Ensure that the filtered supernatants are kept on ice for the duration of the isolation process, including precooling the centrifuge. After the cells are spun down, the cell pellet should appear red at the center of the pellet (red blood cells), with the larger white/pale portion of the cell pellet containing the fibroblasts. When pouring off the medium to resuspend the pellet, hold the tube in such a way that the medium does not travel over the pellet as it is being poured, to reduce loss of fibroblasts in this step. During plating, achieving the proper confluency of fibroblasts is critical for the success of the 5-day activation experiment and may need to be adjusted to accommodate different experimental time courses. Overly confluent (>∼40%-50%) cells on the coverslips in the beginning of the experiment will lead to high baseline activation, as after 5 days of growth the monolayer will be overly confluent. Meanwhile, fibroblast populations that are under confluent (<∼15%) at the beginning of the experiment will not grow properly and there will not be enough cells to analyze at the conclusion of the assay. Ideally, the confluency should be kept between 20% and 80% confluent throughout the 5-day time course. In all washing steps following plating, the amount of time that the cells are not covered in medium should be minimized as much as possible. The hydrophobicity of the PDMS can lead to the cells drying out much faster than on standard coverslips, and thus it is critical to swiftly reintroduce fresh medium. When pipetting medium into the dishes, it should be pipetted gently into the side of the well in order to reduce cells being washed away. Fibroblasts generally attach well to their plates; however, on the PDMS they are more easily dislodged. If using a virus in the experiment, the transduction process will need to take place as early as possible so as to reduce the total amount of time in culture for the experimental time course. Generally, 48 hr after transduction provides sufficient time for robust expression of the desired constructs before the activation assay begins. Additionally, the amount of virus needed to successfully transduce cardiac fibroblasts will likely be far greater than that needed for other cell types. As fibroblasts exhibit increased activation with higher concentrations of FBS (10%-20%), the TGFβ induction of activation will be less effective at these FBS concentrations. Thus, 1% FBS DMEM should be used for all culture steps aside from the initial isolation and plating steps.
Troubleshooting
For a list of possible problems, causes, and solutions, see Table 1.
Problem | Possible cause | Solution |
---|---|---|
PDMS-coated coverslips consistently stuck to bottom of loading plate | Overloading coverslips with PDMS; pipetting not centered | Reduce loading volume: optimal range is between 12 and 14 µl per coverslip |
Low fibroblast isolation yield | Not completing overnight gelatin coating | Make sure to coat wells overnight in gelatin solution |
Losing cells in washing step | Add medium very gently during washing steps, and make sure the amount of time without medium during each wash is as short as possible (aspirate only ∼4 wells at a time) | |
Non-fibroblasts present in plates | Extended plating duration | Reduce plating time before wash |
Fibroblasts are overly confluent | Insufficient dilution when plating | Increase dilution prior to plating |
Cells show high baseline activation without stimulation | Errors during PDMS generation | Remake solution/coverslips |
Cells become over-confluent by time of fixing | Increase dilution to reduce endpoint confluency (ideally <80% at day 5) | |
Low transduction efficiency | Insufficient viral load | Increase viral load (300-500 MOI) |
Insufficient transduction time | Transduce with viruses overnight | |
Cells are not activated with agonist | Insufficient treatment time course | Treat with TGFβ for full 72 hr |
FBS concentration too high | Use 1% FBS DMEM | |
Cells appear damaged when imaging | Pushing down on coverslips during mounting | Allow the mounting solution to fully seal the coverslip without disturbing it manually |
Cells are contaminated | Insufficient ethanol coverage or UV treatment in sterilization step | Make sure that ethanol covers coverslip and time course in ethanol and UV is carried out |
Medium/aliquot sterility not carefully maintained | Maintain strict adherence to sterile technique; use sterile filter flasks when applicable |
Understanding Results
Carrying out this protocol will allow the maintenance of a quiescent population of primary murine cardiac fibroblasts for a 5-day time course. One of the most common ways in which cardiac fibroblasts are classified as activated is through their expression of αSMA. Successful completion of this protocol is simply interpreted through evaluation of immunocytochemical detection of αSMA expression in the cytoskeleton of the fibroblasts. Quiescent cells will predominantly lack this activated fibroblast marker (∼20% positive for αSMA), as seen in Figure 5, left panel. Induction of activation by TGFβ1 treatment leads to a significant upregulation of αSMA expression, as seen in Figure 5, right panel (∼50%-60% positive for αSMA). Due to the extreme sensitivity of these cells to substrate stiffness, false negatives of αSMA expression are not to be expected, and thus a population of cells that predominantly lacks αSMA for 3-5 days in culture would be considered quiescent.
Time Considerations
Coverslip preparation: PDMS coating
Making the PDMS-coated coverslips will take ∼2 hr of work followed by an overnight incubation. This step can produce between 150 and 200 coverslips at a time, which can be used at the researcher's discretion over the course of many experiments. PDMS-coated coverslips (without gelatin coating) can be stored at room temperature indefinitely.
Coverslip preparation: Gelatin coating
Coating the coverslips with gelatin (beginning the day before fibroblast isolation) takes ∼15 min of work followed by an overnight incubation. Once the gelatin coating is performed on the PDMS-coated coverslips, it is recommended that they be used immediately.
Cardiac fibroblast isolation
The cardiac fibroblast isolation takes ∼2 hr of work, followed by a 2-hr incubation period while the fibroblasts attach to the coverslip and then a ∼15 min washing process. The process in its entirety will span ∼4.5 hr, though only ∼2.5 hr of this process involves active work.
Cell culture/activation assay
The activation assay takes 5 full days, beginning at the washing step following fibroblast isolation and plating. The first 24-48 hr are used for desired experimental genetic manipulations (viral transduction and expression of engineered constructs). 72-hrs is needed for TGFβ1 treatment to induce sufficient activation in the fibroblasts.
Acknowledgments
This work was supported by grants from the American Heart Association (825996) to RSG and the U.S. National Institute of Health (R00HL136695 and R01HL166274) to MJB.
Author Contributions
R.S. Goldsmith : Conceptualization; data curation; formal analysis; investigation; methodology; validation; visualization; writing—original draft; writing—review and editing. Y.-C. Tsan : Conceptualization; investigation; methodology. R.E. Scissors : Investigation; methodology. A.S. Helms : Project administration; supervision; writing—review and editing. M.J. Brody : Conceptualization; funding acquisition; project administration; supervision; writing—review and editing.
Conflict of Interest
The authors declare no conflict of interest.
Open Research
Data Availability Statement
No new data generated.
Literature Cited
- Almazloum, A., & Khalil, H. (2023). Isolation of adult mouse cardiac fibroblasts. Current Protocols , 3(7), e840. https://doi.org/10.1002/cpz1.840
- Alter, C., Henseler, A. S., Owenier, C., Hesse, J., Ding, Z., Lautwein, T., Bahr, J., Hayat, S., Kramann, R., Kostenis, E., Scheller, J., & Schrader, J. (2023). IL-6 in the infarcted heart is preferentially formed by fibroblasts and modulated by purinergic signaling. Journal of Clinical Investigation , 133(11), e163799. https://doi.org/10.1172/JCI163799
- Bertaud, A., Joshkon, A., Heim, X., Bachelier, R., Bardin, N., Leroyer, A. S., & Blot-Chabaud, M. (2023). Signaling pathways and potential therapeutic strategies in cardiac fibrosis. International Journal of Molecular Sciences , 24(2), 1756. https://doi.org/10.3390/ijms24021756
- Bretherton, R., Bugg, D., Olszewski, E., & Davis, J. (2020). Regulators of cardiac fibroblast cell state. Matrix Biology , 91-92, 117–135. https://doi.org/10.1016/j.matbio.2020.04.002
- Brody, M. J., Hacker, T. A., Patel, J. R., Feng, L., Sadoshima, J., Tevosian, S. G., Balijepalli, R. C., Moss, R. L., & Lee, Y. (2012). Ablation of the cardiac-specific gene leucine-rich repeat containing 10 (Lrrc10) results in dilated cardiomyopathy. PLoS ONE , 7(12), e51621. https://doi.org/10.1371/journal.pone.0051621
- Cheng, X., Wang, L., Wen, X., Gao, L., Li, G., Chang, G., Qin, S., & Zhang, D. (2021). TNAP is a novel regulator of cardiac fibrosis after myocardial infarction by mediating TGF-β/Smads and ERK1/2 signaling pathways. EBioMedicine , 67, 103370. https://doi.org/10.1016/j.ebiom.2021.103370
- Childers, R. C., Lucchesi, P. A., & Gooch, K. J. (2021). Decreased substrate stiffness promotes a hypofibrotic phenotype in cardiac fibroblasts. International Journal of Molecular Sciences , 22(12), 6231. https://doi.org/10.3390/ijms22126231
- Davis, J., Salomonis, N., Ghearing, N., Lin, S. C., Kwong, J. Q., Mohan, A., Swanson, M. S., & Molkentin, J. D. (2015). MBNL1-mediated regulation of differentiation RNAs promotes myofibroblast transformation and the fibrotic response. Nature Communications , 6, 10084. https://doi.org/10.1038/ncomms10084
- Essandoh, K., Subramani, A., Ferro, O. A., Teuber, J. P., Koripella, S., & Brody, M. J. (2023). zDHHC9 regulates cardiomyocyte Rab3a Activity and atrial natriuretic peptide secretion through palmitoylation of Rab3gap1. JACC: Basic to Translational Science , 8(5), 518–542. https://doi.org/10.1016/j.jacbts.2022.11.003
- Fu, X., Khalil, H., Kanisicak, O., Boyer, J. G., Vagnozzi, R. J., Maliken, B. D., Sargent, M. A., Prasad, V., Valiente-Alandi, I., Blaxall, B. C., & Molkentin, J. D. (2018). Specialized fibroblast differentiated states underlie scar formation in the infarcted mouse heart. Journal of Clinical Investigation , 128(5), 2127–2143. https://doi.org/10.1172/jci98215
- Fu, X., Liu, Q., Li, C., Li, Y., & Wang, L. (2020). Cardiac fibrosis and cardiac fibroblast lineage-tracing: Recent advances. Frontiers in Physiology , 11, 416. https://doi.org/10.3389/fphys.2020.00416
- Gibb, A. A., Lazaropoulos, M. P., & Elrod, J. W. (2020). Myofibroblasts and fibrosis: Mitochondrial and metabolic control of cellular differentiation. Circulation Research , 127(3), 427–447. https://doi.org/10.1161/circresaha.120.316958
- Herum, K. M., Choppe, J., Kumar, A., Engler, A. J., & McCulloch, A. D. (2017). Mechanical regulation of cardiac fibroblast profibrotic phenotypes. Molecular Biology of the Cell , 28(14), 1871–1882. https://doi.org/10.1091/mbc.E17-01-0014
- Herum, K. M., Lunde, I. G., McCulloch, A. D., & Christensen, G. (2017). The soft- and hard-heartedness of cardiac fibroblasts: Mechanotransduction signaling pathways in fibrosis of the heart. Journal of Clinical Medicine , 6(5), 53. https://doi.org/10.3390/jcm6050053
- Ivey, M. J., & Tallquist, M. D. (2016). Defining the cardiac fibroblast. Circulation Journal , 80(11), 2269–2276. https://doi.org/10.1253/circj.CJ-16-1003
- Kanisicak, O., Khalil, H., Ivey, M. J., Karch, J., Maliken, B. D., Correll, R. N., Brody, M. J., SC, J. L., Aronow, B. J., Tallquist, M. D., & Molkentin, J. D. (2016). Genetic lineage tracing defines myofibroblast origin and function in the injured heart. Nature Communications , 7, 12260. https://doi.org/10.1038/ncomms12260
- Khalil, H., Kanisicak, O., Vagnozzi, R. J., Johansen, A. K., Maliken, B. D., Prasad, V., Boyer, J. G., Brody, M. J., Schips, T., Kilian, K. K., Correll, R. N., Kawasaki, K., Nagata, K., & Molkentin, J. D. (2019). Cell-specific ablation of Hsp47 defines the collagen-producing cells in the injured heart. JCI Insight , 4(15), e128722. https://doi.org/10.1172/jci.insight.128722
- Landry, N. M., Rattan, S. G., & Dixon, I. M. C. (2019). An improved method of maintaining primary murine cardiac fibroblasts in two-dimensional cell culture. Scientific Reports , 9(1), 12889. https://doi.org/10.1038/s41598-019-49285-9
- Landry, N. M., Rattan, S. G., & Dixon, I. M. C. (2021). Soft substrate culture to mechanically control cardiac myofibroblast activation. Methods in Molecular Biology , 2299, 171–179. https://doi.org/10.1007/978-1-0716-1382-5_13
- Meagher, P. B., Lee, X. A., Lee, J., Visram, A., Friedberg, M. K., & Connelly, K. A. (2021). Cardiac fibrosis: Key role of integrins in cardiac homeostasis and remodeling. Cells , 10(4), 770. https://doi.org/10.3390/cells10040770
- Morningstar, J. E., Gensemer, C., Moore, R., Fulmer, D., Beck, T. C., Wang, C., Moore, K., Guo, L., Sieg, F., Nagata, Y., Bertrand, P., Spampinato, R. A., Glover, J., Poelzing, S., Gourdie, R. G., Watts, K., Richardson, W. J., Levine, R. A., Borger, M. A., & Norris, R. A. (2021). Mitral valve prolapse induces regionalized myocardial fibrosis. Journal of the American Heart Association , 10(24), e022332. https://doi.org/10.1161/jaha.121.022332
- Palchesko, R. N., Zhang, L., Sun, Y., & Feinberg, A. W. (2012). Development of polydimethylsiloxane substrates with tunable elastic modulus to study cell mechanobiology in muscle and nerve. PLoS ONE , 7(12), e51499. https://doi.org/10.1371/journal.pone.0051499
- Santiago, J. J., Dangerfield, A. L., Rattan, S. G., Bathe, K. L., Cunnington, R. H., Raizman, J. E., Bedosky, K. M., Freed, D. H., Kardami, E., & Dixon, I. M. (2010). Cardiac fibroblast to myofibroblast differentiation in vivo and in vitro: Expression of focal adhesion components in neonatal and adult rat ventricular myofibroblasts. Developmental Dynamics , 239(6), 1573–1584. https://doi.org/10.1002/dvdy.22280
- Shiraishi, M., Suzuki, K., & Yamaguchi, A. (2023). Effect of mechanical tension on fibroblast transcriptome profile and regulatory mechanisms of myocardial collagen turnover. FASEB Journal , 37(4), e22841. https://doi.org/10.1096/fj.202201899R
- Tallquist, M. D. (2020). Cardiac fibroblast diversity. Annual Review of Physiology , 82, 63–78. https://doi.org/10.1146/annurev-physiol-021119-034527
- Tallquist, M. D., & Molkentin, J. D. (2017). Redefining the identity of cardiac fibroblasts. Nature Reviews Cardiology , 14(8), 484–491. https://doi.org/10.1038/nrcardio.2017.57
- Teuber, J. P., Essandoh, K., Hummel, S. L., Madamanchi, N. R., & Brody, M. J. (2022). NADPH oxidases in diastolic dysfunction and heart failure with preserved ejection fraction. Antioxidants , 11(9), 1822. https://doi.org/10.3390/antiox11091822
- Umbarkar, P., Ejantkar, S., Tousif, S., & Lal, H. (2021). Mechanisms of fibroblast activation and myocardial fibrosis: lessons learned from FB-Specific conditional mouse models. Cells , 10(9), 2412. https://doi.org/10.3390/cells10092412
- Wang, A., Cao, S., Stowe, J. C., & Valdez-Jasso, D. (2021). Substrate stiffness and stretch regulate profibrotic mechanosignaling in pulmonary arterial adventitial fibroblasts. Cells , 10(5), 1000. https://doi.org/10.3390/cells10051000
- Yeh, Y. C., Corbin, E. A., Caliari, S. R., Ouyang, L., Vega, S. L., Truitt, R., Han, L., Margulies, K. B., & Burdick, J. A. (2017). Mechanically dynamic PDMS substrates to investigate changing cell environments. Biomaterials , 145, 23–32. https://doi.org/10.1016/j.biomaterials.2017.08.033