Establishment of Humanized Mice from Peripheral Blood Mononuclear Cells or Cord Blood CD34+ Hematopoietic Stem Cells for Immune-Oncology Studies Evaluating New Therapeutic Agents
Bhavna Verma, Bhavna Verma, Amy Wesa, Amy Wesa
humanized mouse model
immune oncology
patient-derived xenograft (PDX) models
tumor-infiltrating lymphocytes
Abstract
The clinical success of immune checkpoint modulators and the development of next-generation immune-oncology (IO) agents underscore the need for robust preclinical models to evaluate novel IO therapeutics. Human immune system (HIS) mouse models enable in vivo studies in the context of the HIS via a human tumor. The immunodeficient mouse strains NOG (Prkdcscid Il2rgtm1Sug) and triple-transgenic NOG-EXL [Prkdcscid Il2rgtm1Sug Tg (SV40/HTLV-IL3, CSF2)], which expresses human IL-3 and GM-CSF, allow for human CD34+ hematopoietic stem cell (huCD34+ HSC) engraftment and multilineage immune cell development by 12 to 16 weeks post-transplant and facilitate studies of immunomodulatory agents. A more rapid model of human immune engraftment utilizes peripheral blood mononuclear cells (PBMCs) transplanted into immunodeficient murine hosts, permitting T-cell engraftment within 2 to 3 weeks without outgrowth of other human immune cells. The PBMC-HIS model can be limited due to onset of xenogeneic graft-versus-host disease (xGVHD) within 3 to 5 weeks post-implantation. Host deficiency in MHC class I, as occurs in beta-2 microglobulin knockout in either NOG or NSG mice, results in resistance to xGVHD, which permits a longer therapeutic window. In this article, detailed processes for generating humanized mice by transplantation of HSCs from cord blood–derived huCD34+ HSCs or PBMCs into immunodeficient mouse strains to respectively generate HSC-HIS and PBMC-HIS mouse models are provided. In addition, the co-engraftment and growth kinetics of patient-derived and cell line–derived xenograft tumors in humanized mice and recovery of tumor-infiltrating lymphocytes from growing tumors to evaluate immune cell subsets by flow cytometry are described. © 2020 The Authors.
Basic Protocol 1 : Establishment of patient-derived xenograft tumors in CD34+ hematopoietic stem cell–humanized mice
Basic Protocol 2 : Establishment of patient-derived xenograft tumors in peripheral blood mononuclear cell–humanized mice
Support Protocol 1 : Flow cytometry assessment of humanization in mice
Support Protocol 2 : Flow cytometry assessment of tumor-infiltrating lymphocytes in tumor-bearing humanized mouse models
INTRODUCTION
Human immune system (HIS)–reconstituted mice are a valuable tool to recapitulate the interactions between immune components and tumors of human origin, allowing evaluation of immune-oncology (IO) therapeutic modalities. Advances in developing murine strains with either truncation or complete loss of the IL-2 receptor gene has resulted in immunodeficient mice that lack mature T, B, and NK cells but retain dysfunctional macrophages and dendritic cells (DCs). Use of these mice permits the efficient engraftment of human CD34+ hematopoietic stem cells (huCD34+ HSCs). After transplantation of huCD34+ HSCs, hematopoiesis occurs in situ, leading to immune reconstitution. Lymphoid cells undergo negative selection during differentiation into T and B cells, allowing tolerance to the mouse host, with limited myeloid development (Katano, Ito, Eto, Aiso, & Ito, 2011). Transgenic expression of the human cytokines IL-3 and GM-CSF in NOG (Prkdcscid Il2rgtm1Sug) mice can improve engraftment of human progenitor cells, supporting enhanced myeloid lineage development while preserving T-cell development (Ito et al., 2013).
Basic Protocol 1, as described in this article, outlines a protocol for generating humanized mice by transplanting cord blood huCD34+ HSCs. This protocol describes co-implantation of a non-small-cell lung carcinoma (NSCLC) patient-derived xenograft (PDX) into humanized mice, along with evaluation of growth kinetics. Humanization is assessed in Support Protocol 1, and tumor-infiltrating lymphocytes (TILs) are assessed in Support Protocol 2. Basic Protocol 2 describes the humanization of mice using peripheral blood mononuclear cells (PBMCs). This model provides the advantage of faster engraftment (2 to 3 weeks) in comparison to the huCD34+ HSC-HIS model (10 to 12 weeks). Although PBMCs comprise mature T, B, NK, and myeloid cells, only T cells engraft in this model. The mature T cells are not thymically selected in the mouse, ensuring human MHC class I recognition. Further, mice lacking MHC class I have been demonstrated to have a more favorable profile for avoiding the development of xenogeneic graft-versus-host disease (xGVHD) in these models. These models are ideal for short-term studies using cell line–derived xenograft (CDX) tumors or moderate- to fast-growing PDX tumors. In this article, we also provide details on tumor growth kinetics in PBMC-HIS mice as well as infiltration of immune subsets in tumors using the breast cancer cell line MDA-MB-231 and an NSCLC PDX.
STRATEGIC PLANNING
Successful generation of humanized mouse models with human tumors (CDX or PDX) requires careful planning of variables of interest (please refer to Table 1). Additional details are discussed in the Critical Parameters section.
Mouse strain | Humanization cell source | Tumor model |
---|---|---|
NOG, NSG, NSG-SGM3, NOG-EXL, NOG-IL-6 | CD34+ HSCs | CDX or PDX |
NOG, NSG, NSG-B2M, NOG-B2M | PBMCs | CDX or PDX |
NOTE : A barrier facility is required to house immunodeficient mice in a pathogen-free environment, using sterile techniques, microisolator caging, and appropriate husbandry practices (National Research Council Committee on Immunologically Compromised Rodents, 1989). All studies described here were conducted in an AALAC-certified facility with full IACUC approval, under the review of a laboratory veterinarian.
NOTE : Humanized mice, tumor-bearing humanized mice, and mouse bedding and cages should be considered potential biological biohazards and handled with appropriate personal protective equipment at animal biosafety level 2 in accordance with institutional biosafety guidelines.
Basic Protocol 1: ESTABLISHMENT OF PATIENT-DERIVED XENOGRAFT TUMORS IN CD34+ HEMATOPOIETIC STEM CELL–HUMANIZED MICE
HIS reconstitution using cord blood–derived huCD34+ HSCs in sublethally irradiated immunodeficient mouse strains is described in detail in this protocol. After huCD34+ HSC engraftment into NOG or NOG-EXL mice, subcutaneous implantation of PDX tumors into these mice generates a tumor-bearing humanized mouse model (please refer to Fig. 1 for a schema).
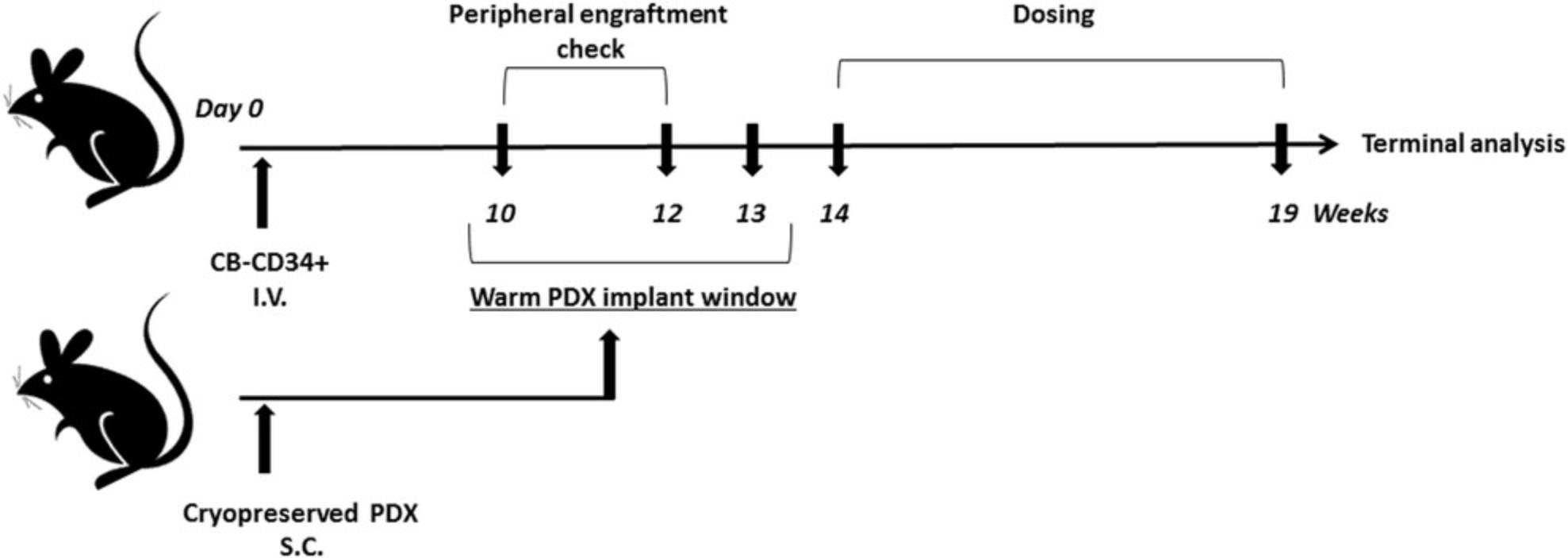
Materials
-
4- to 7-week-old female NOG (NOD.Cg-Prkdcscid Il2rgtm1Sug /JicTac) or NOG-EXL [NOD.Cg-Prkdcscid Il2rgtm1Sug Tg (SV40/HTLV-IL3, CSF2)10-7Jic/JicTac] mice (Taconic), housed in individually ventilated microisolator cages
-
Cryopreserved cord blood huCD34+ HSCs, 1 × 106/vial (Lonza or equivalent)
-
1× phosphate-buffered saline (PBS; Invitrogen)
-
RPMI-1640 medium (ATCC, cat. no. 30-2001)
-
Diet gel or other suitable feed (Tekland)
-
Cryopreserved PDX tumors
-
Nude mice: Hsd: Athymic Nude-Foxn1 nu (Envigo; optional)
-
Irradiation cages
-
Irradiation source (e.g., RS 2000 Biological Research Irradiator, Rad Source Technologies)
-
37°C water bath
-
15-ml polypropylene tubes
-
Class II biosafety cabinet (BSC)
-
Standard tabletop centrifuge
-
Hematocytometer, trypan blue, and light microscope or automated cell counter (e.g., Cellometer Auto 2000, Nexcelom Bioscience)
-
1-cc tuberculin syringes with 25-G × ⅝-in. needle
-
Scale
-
Scalpel
-
Forceps
-
Isoflurane anesthesia machine
-
Vernier or digital calipers
-
K2EDTA tubes
-
Scissors
-
Digital calipers
-
Additional reagents and equipment for flow cytometry and phenotyping (see Support Protocol 1), subcutaneous tumor implantation, and mouse euthanasia
1.Approximately 4 hr prior to inoculation, sublethally irradiate 4- to 7-week-old female NOG or NOG-EXL mice in irradiation cages with 175 cGy whole-body irradiation from an irradiation source.
2.Thaw cryopreserved cord blood huCD34+ HSCs (1 × 106 CB-CD34+ cells per vial for 8 to 10 mice) in a 37°C water bath until a sliver of ice remains. Then, transfer cells to a 15-ml polypropylene tube in a class II BSC and wash twice in 10 ml of 1× PBS at room temperature, with centrifugation for 5 min at 300 × g. Resuspend cells in 0.5 to 1 ml RPMI-1640 medium and count viable cells either manually, using a hematocytometer, trypan blue, and a light microscope, or with an automated cell counter.
3.Adjust concentration of huCD34+ HSCs by resuspension to 6 × 105 cells/ml in RPMI-1460 and store cells briefly on ice prior to injection. Using a 1-cc tuberculin syringe with a 25-G × ⅝-in. needle, inject 0.2 ml (1.2 × 105 HSCs) into lateral tail vein of each irradiated mouse by intravenous (IV) injection.
4.Monitor animals via clinical observation and record body weight at days 0, 3, 6, and 8 of the humanization period and once weekly thereafter for 3 months.
5.Provide mice with diet gel or other suitable feed for 2 weeks during recovery after irradiation. Provide water ad libitum.
6.After 10 to 12 weeks, evaluate HIS reconstitution by flow cytometry every 2 weeks (see Support Protocol 1).
7.At the time of humanization, thaw cryopreserved PDX tumors in a 37°C water bath, implant subcutaneously (SC) into a parallel cohort of immunocompromised mice (nude or NOG) bilaterally while under isoflurane anesthesia, and monitor for growth using Vernier or digital calipers (see Current Protocols article; Verma, Ritchie, & Mancini, 2017).
8.At 10 and 12 weeks post-transplant of huCD34+ HSCs, assess human chimerism in circulating cells by collecting ∼100 µl peripheral blood from mice via the facial vein into K2EDTA tubes and prepare cells for flow cytometry phenotyping (refer to Figs. 2 and 3 for data and to Support Protocol 1 for phenotyping details).
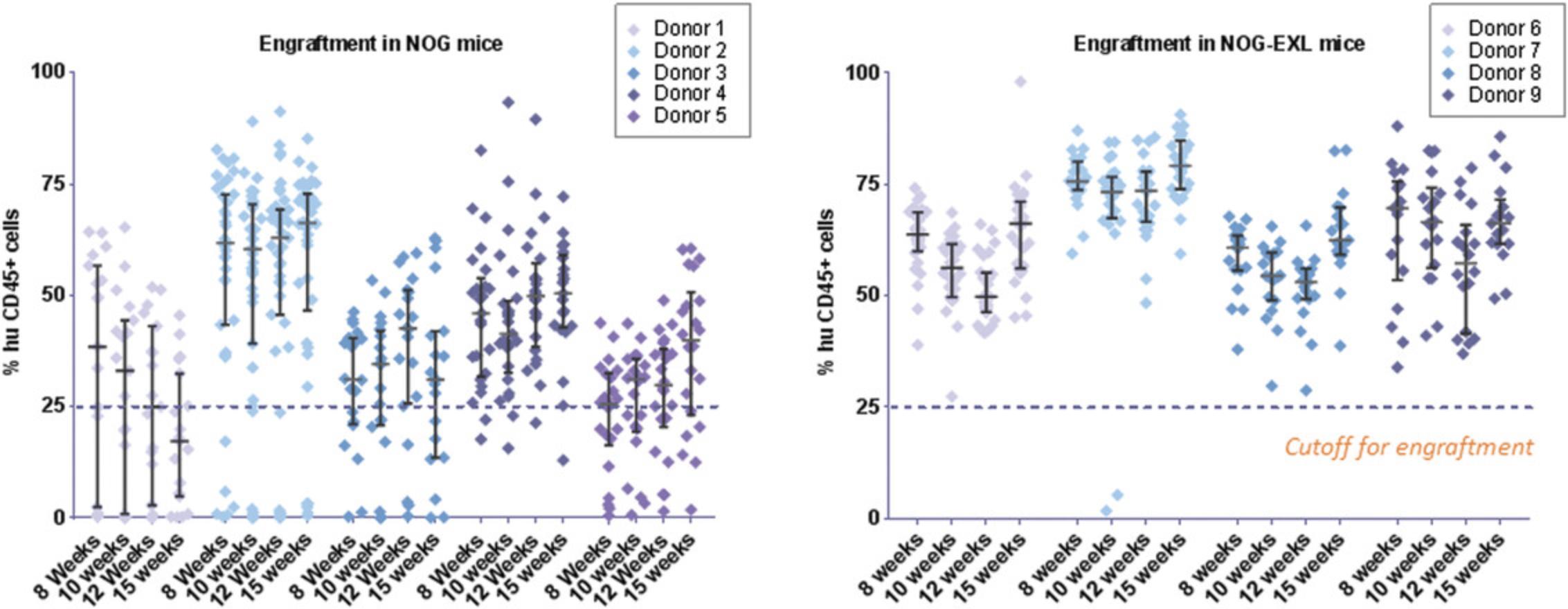
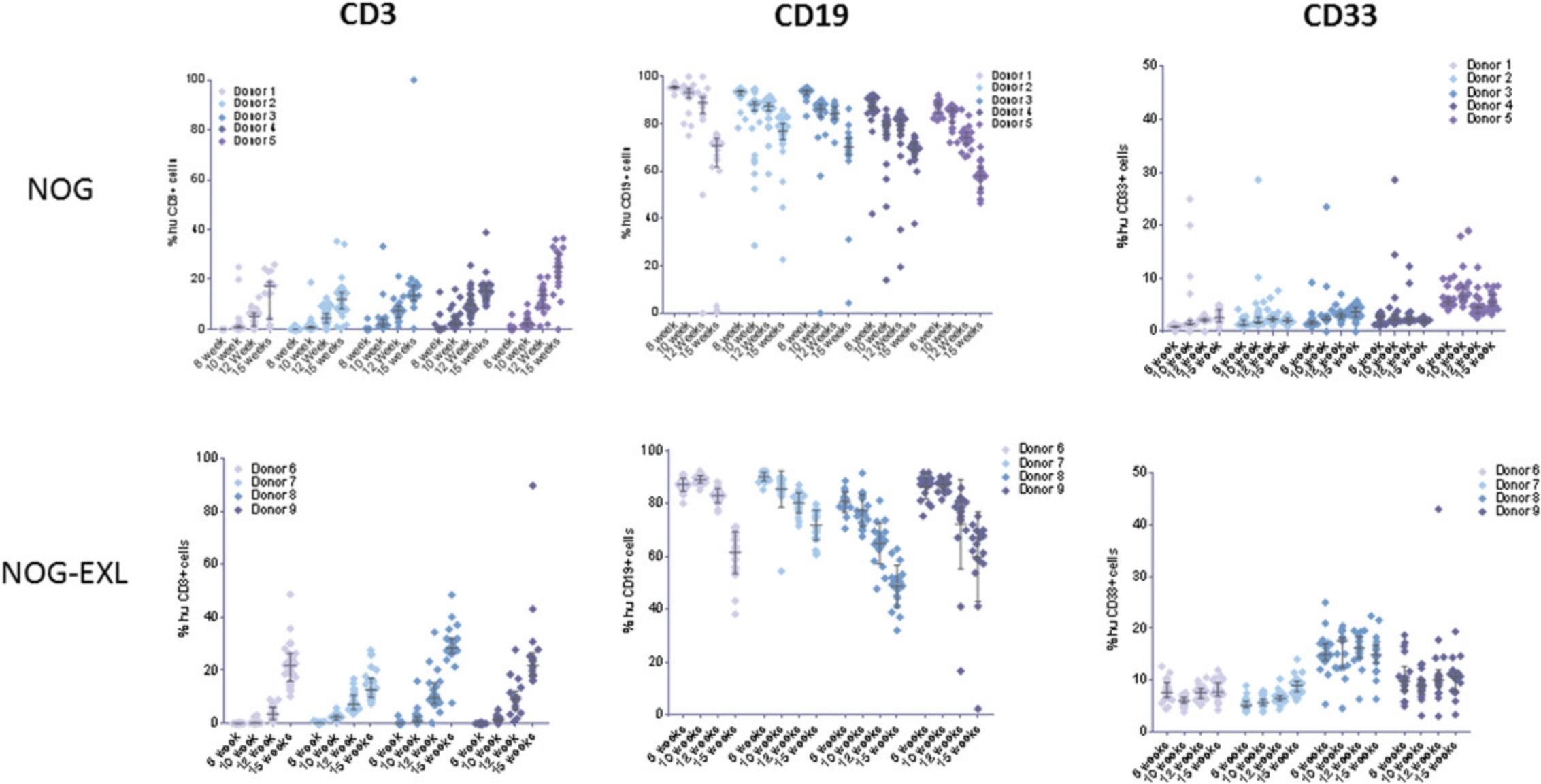
9.To generate humanized PDX mice, harvest PDX tumors from stock mice (see step 7) using a scalpel. Generate 5 × 5 × 5–mm fragments using scissors and implant SC into flank of the humanized mice under isoflurane anesthesia.
10.Following subcutaneous tumor implantation, monitor tumor growth kinetics by digital caliper measurements of length and width to calculate volume, with the first measurements taken on the seventh day of tumor implantation. Record tumor volume measurements (TVMs) at two timepoints to ensure that tumors are growing prior to randomization into study groups, performed when tumor volumes range from 80 to 150 mm3, as shown in Figure 4.Continue TVMs twice weekly throughout duration of the study (see Fig. 5 for example data). Calculate tumor volume using the following formula:

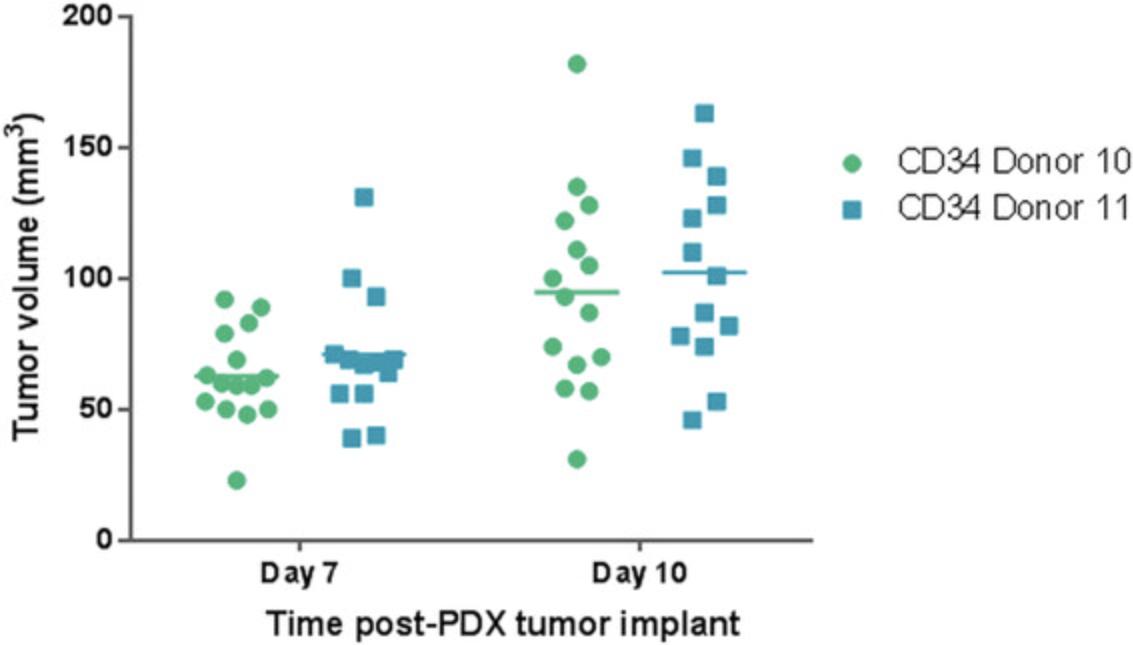
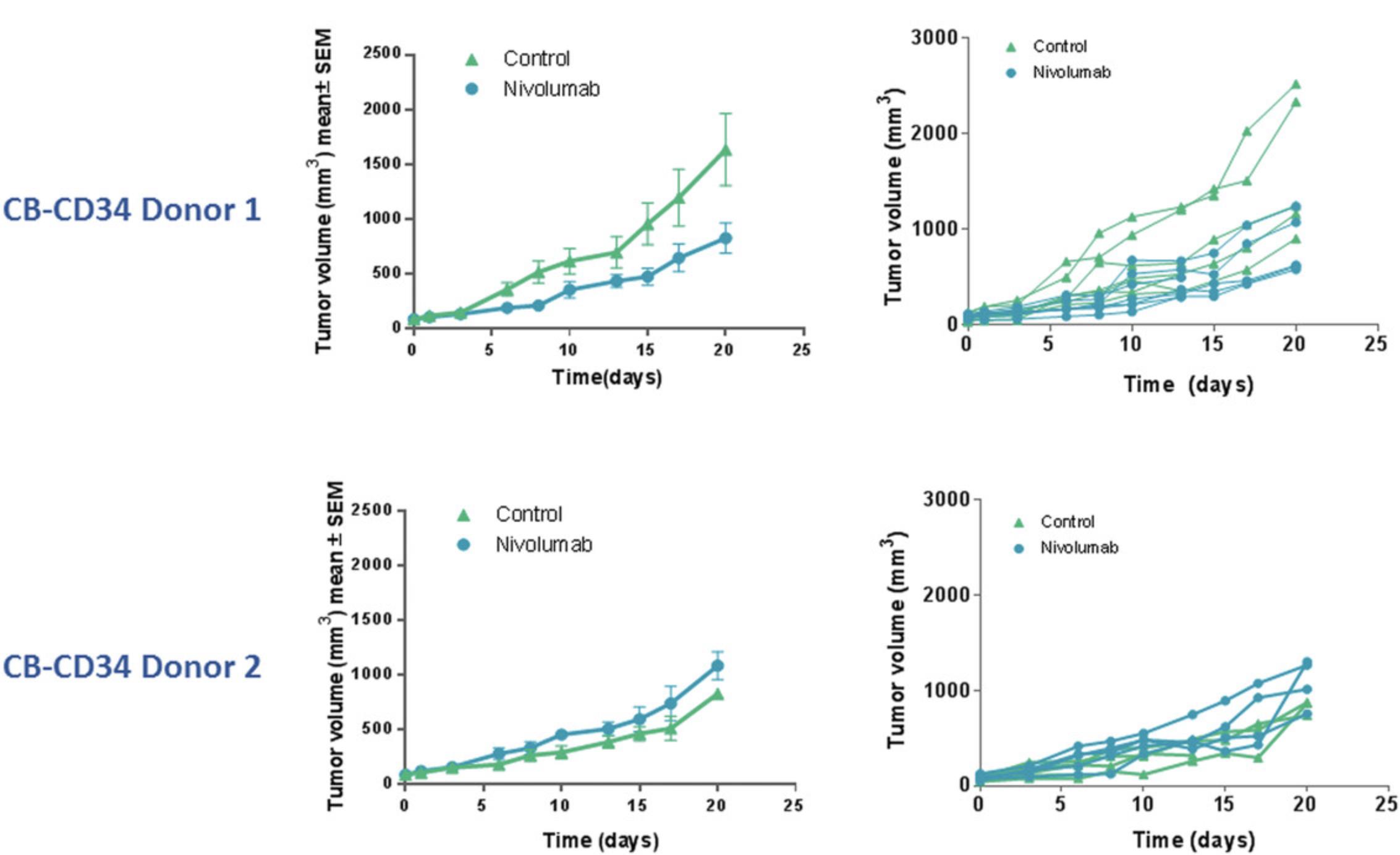
11.At the endpoint of the study, euthanize mice as per IACUC-approved protocol and collect tumors for downstream analysis.
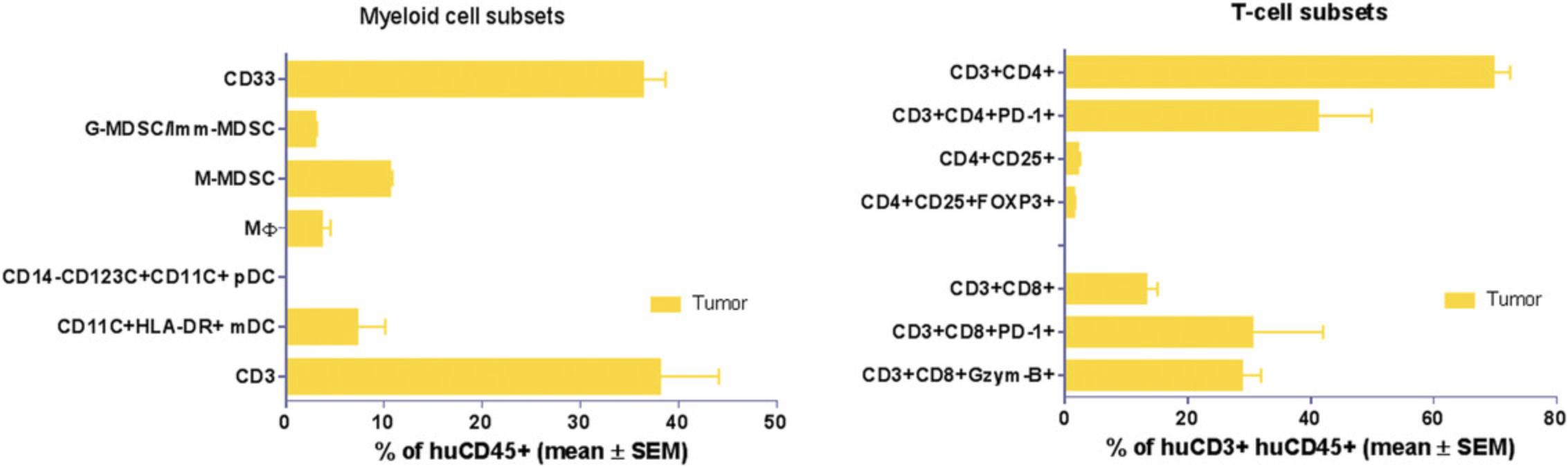
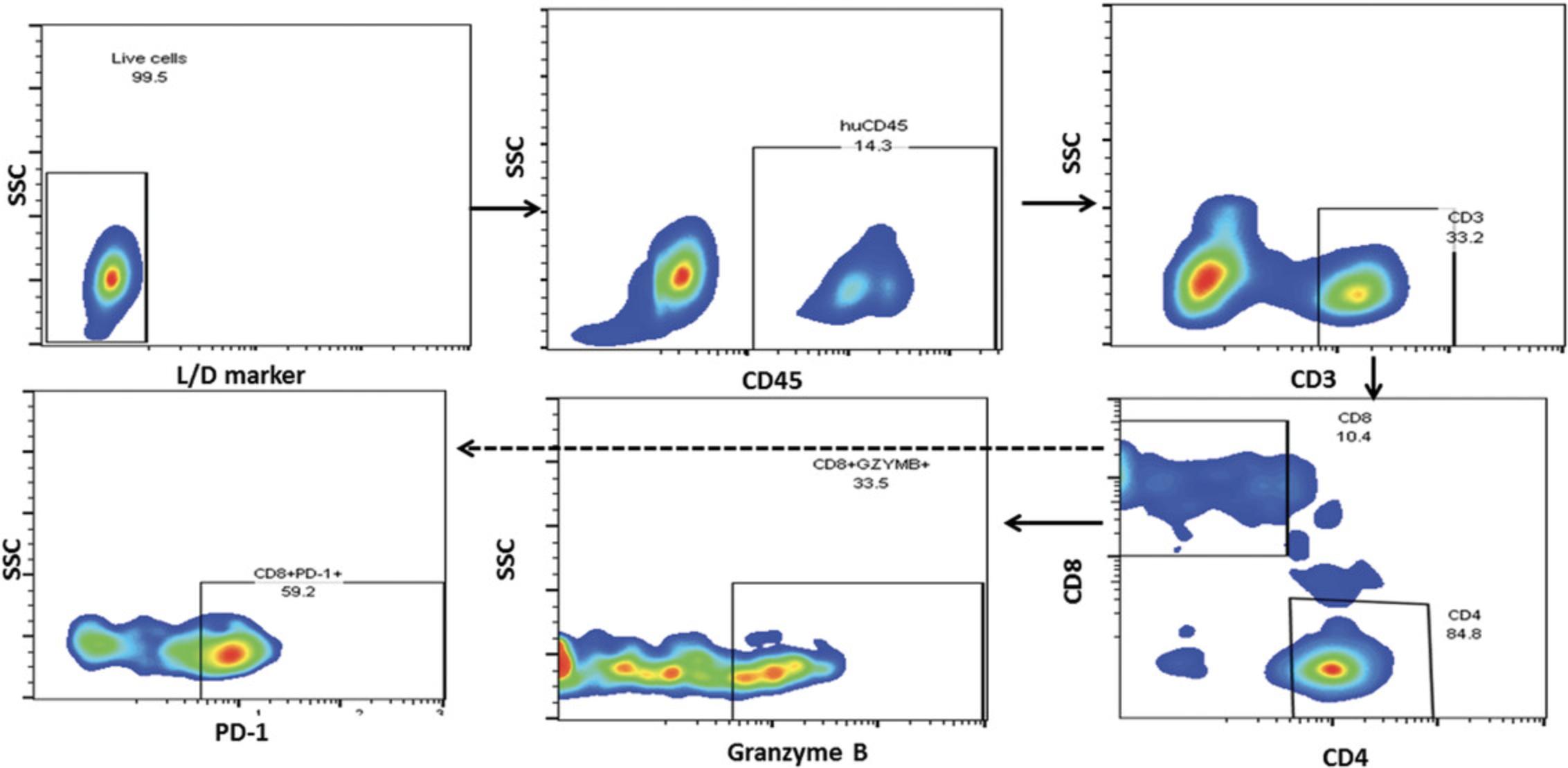
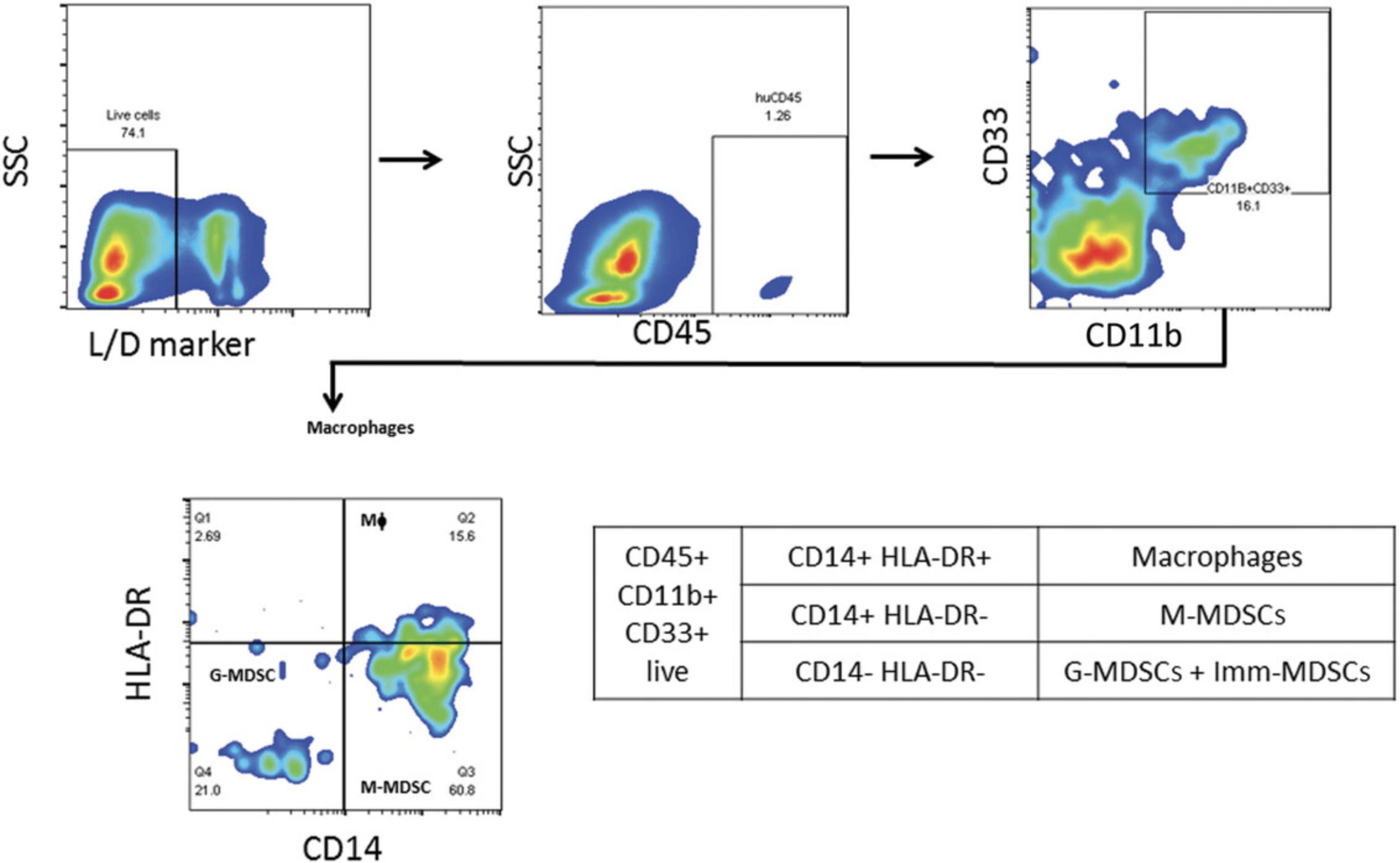
Basic Protocol 2: ESTABLISHMENT OF PATIENT-DERIVED XENOGRAFT TUMORS IN PERIPHERAL BLOOD MONONUCLEAR CELL–HUMANIZED MICE
This protocol describes generation of humanized mice by injecting PBMCs isolated from healthy adult donors. After a PDX or CDX is implanted SC, PBMCs are transplanted within several days via IV injection. Tumor growth kinetics are recorded, and PBMC engraftment is monitored as described in Support Protocol 1.For PBMC-humanized mice, no irradiation is used, as this would enhance the onset and severity of xGVHD.
Additional Materials (also see Basic Protocol 1)
- Cryopreserved PDX or CDX tumors
- 4- to 7-week-old female NOG (NOD.Cg-Prkdcscid Il2rgtm1Sug /JicTac) or NOG-B2M (Taconic) mice or NSG (NOD.Cg-Prkdcscid Il2rgtm1Wjl /SzJ) or NSG-B2M (NOD.Cg-B2mtm1Unc Prkdcscid Il2rgtm1Wjl /SzJ) mice (The Jackson Laboratory), housed in individually ventilated microisolator cages
- Cryopreserved PBMCs, isolated from normal human donor leukopaks by density gradient centrifugation with Ficoll-Paque Premium (Thomas Scientific) as per manufacturer's recommendation
1.Implant cryopreserved PDX or CDX tumors SC into 4- to 7-week-old female NOG, NOG-B2M, NSG, or NSG-B2M mice prior to humanization with PBMCs.
2.Thaw cryopreserved PBMCs in a 37°C water bath and transfer cells to a 15-ml polypropylene tube in a class II BSC. Wash twice in 10 ml of 1× PBS by centrifugation for 5 min at 350 × g , room temperature. Resuspend cells in 0.5 to 1 ml RPMI-1640 medium and enumerate viable cells either manually, using a hematocytometer, trypan blue, and a light microscope, or with an automated cell counter.
3.Adjust PBMC concentration by resuspension to 5 × 107 cells/ml in RPMI-1640 and store on ice briefly prior to injection. Using a 1-cc tuberculin syringe with a 25-G × ⅝-in. needle, inject 0.2 ml (1 × 107 PBMCs) into lateral tail vein of each mouse by IV injection.
4.Monitor animals via clinical observation and record body weight twice weekly for signs of xGVHD throughout duration of the study.
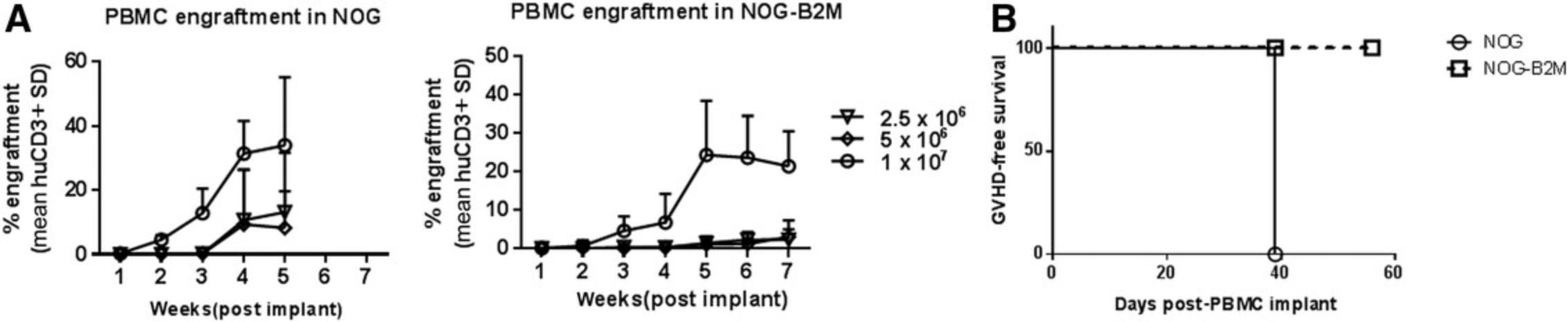
5.Collect 100 µl peripheral blood from mice via the facial vein into K2EDTA tubes weekly or at least every 2 weeks post–PBMC implant for assessment of humanization by flow cytometry phenotyping (see Figs. 9 to 11 and Support Protocol 1).
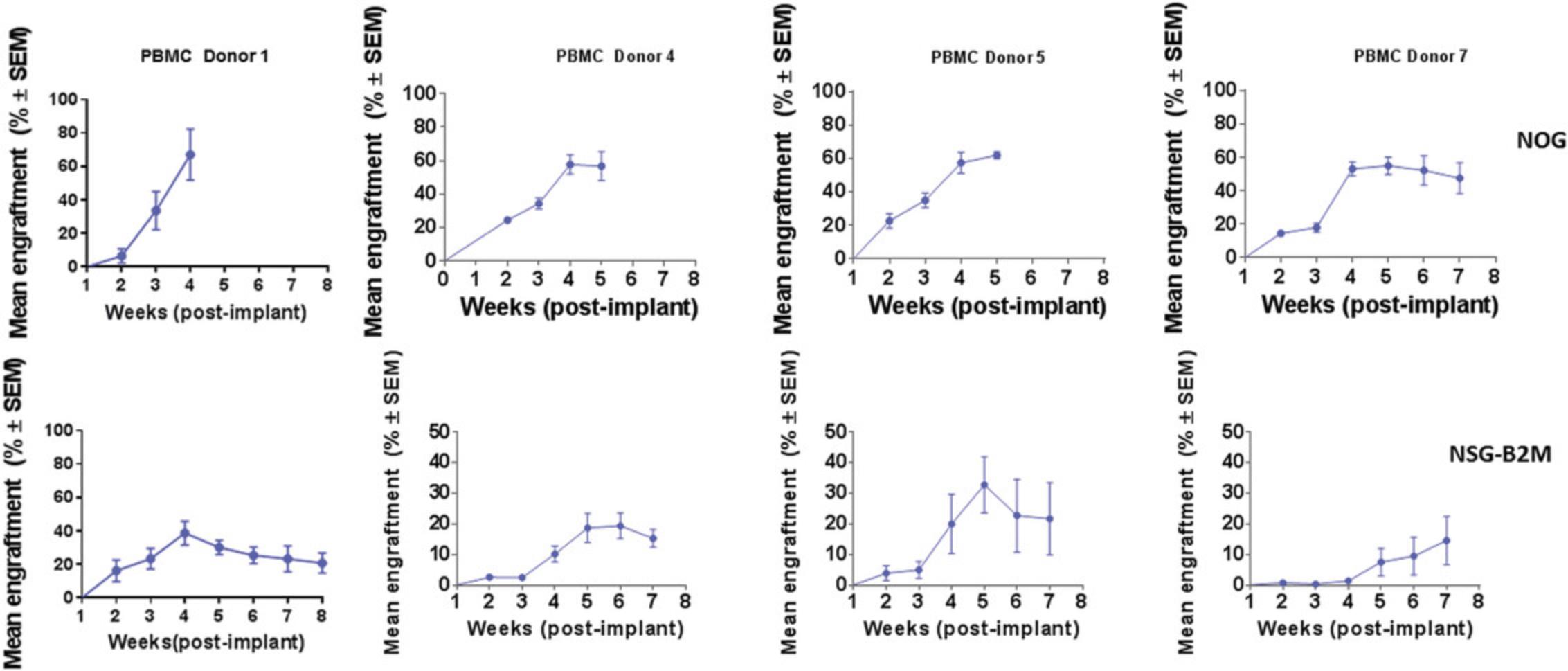
6.Following subcutaneous tumor implantation, monitor tumor growth kinetics by digital caliper measurements of length and width to calculate tumor volume (typical results are shown in Fig. 12). Randomize mice into study groups within 7 days of CDX implantation or when PDX tumor volumes are in the range of 80 to 150 mm3. Continue TVMs twice weekly throughout duration of the study. Calculate tumor volume using the following formula:

7.At the study endpoint or another predetermined time point, euthanize tumor-bearing humanized mice as per IACUC-approved protocol and collect tumors for downstream analysis.
Support Protocol 1: FLOW CYTOMETRY ASSESSMENT OF HUMANIZATION IN MICE
This protocol provides a detailed procedure for assessment of engraftment levels of chimerism in immunodeficient mice implanted with huCD34+ HSCs (Basic Protocol 1) or PBMCs (Basic Protocol 2). The percent chimerism is calculated by flow cytometric assessment of levels of human pan-leukocyte marker (CD45) in the peripheral blood in comparison to total live cells, as follows:
Materials
-
Mouse whole blood, freshly collected via facial vein into K2EDTA tubes (see Basic Protocols 1 and 2)
-
Streck CD-Chex™ Plus Immunophenotyping Control (Fisher Scientific, cat. no. 23-046-500D)
-
Human BD Fc Block (BD Biosciences, cat. no. 564220)
-
FACS buffer: MACSQuant Running Buffer (Miltenyi Biotec, cat. no. 130-092-747) + 5% (v/v) fetal bovine serum (FBS)
-
Fluorochrome-labeled antibodies: antibodies to murine CD45 and human CD45 (clone HI30), CD3 (clone UCHT1), CD4 (clone RM4-5), CD8 (clone SK1), CD33 (clone p67.6), and CD19 (clone HIB19; all Biolegend; see Table 2)
-
1× red blood cell–lysing buffer (diluted from 10×; BD Pharm Lyse, BD Biosciences, cat. no. 555899)
-
7-Aminoactinomycin D (7-AAD; Biolegend, cat. no. 420403)
-
96-deep-well plates (Analytical Sales and Services, cat. no. 59623-23)
-
5-ml Falcon round-bottom tubes (VWR, cat. no. 60819-820)
-
Tube rocker
-
Aluminum foil
-
5°C centrifuge with plate rotor (Eppendorf 5810 or equivalent)
-
Flow cytometer [MACSQuant® Analyzer 10 (Miltenyi Biotec) or equivalent flow analyzer with three-laser (405/488/635 nm) configuration]
-
Flow cytometry analysis software (FlowJo V8 or equivalent)
NOTE : A maximum of 150 µl blood can be drawn weekly from each mouse, as per IACUC guidelines.
Humanization cell source | Engraftment panel | Time post-implant for engraftment checks to begin |
---|---|---|
CD34+ HSCs | huCD45, muCD45, huCD3, huCD4, huCD8, huCD33, huCD19, live/dead marker | 10 weeks |
PBMCs | muCD45, huCD3, huCD4, huCD8, live/dead marker | 2 weeks |
1.Aliquot 60 μl mouse whole blood per sample into each well of a 96-deep-well plate. Include 50 μl Streck CD-Chex™ Plus Immunophenotyping Control as a positive control in first well and pool small aliquots of blood for a 60-μl unstained negative control.
2.Incubate samples with 0.6 μl Human BD Fc Block + 0.14 μl FACS buffer (2 μl total; 2.5 μg/million cells) for 10 min.
3.Prepare a flow cytometry antibody staining cocktail in a 5-ml Falcon round-bottom tube using fluorochrome-labeled antibodies at the manufacturer's recommended concentration in FACS buffer as per the flow panel being tested. Add antibody staining cocktail to all whole-blood samples, followed by incubation for 30 min at room temperature in the dark.
4.Add 1.25 ml of 1× red blood cell–lysing buffer per sample. Incubate samples at room temperature on a tube rocker for 20 min, covered with aluminum foil.
5.Centrifuge samples for 5 min at 388 × g , 5°C, and discard supernatant.
6.Wash off excess antibody and lysing buffer by adding 1.25 ml FACS buffer per sample, centrifuging for 5 min at 388 × g , 5°C, and discarding supernatant. Repeat this step for a total of two washes. After the last wash, discard supernatant and resuspend each cell pellet in a final total volume of 150 μl FACS buffer.
7.Add 1 μl viability stain (7-AAD) per sample. Incubate for 5 min at room temperature in dark.
8.Acquire samples using a flow cytometer, ensuring collection of 20,000 gated live-cell events.
9.Perform flow cytometry data analysis with flow cytometry analysis software (Fig. 3).
Support Protocol 2: FLOW CYTOMETRY ASSESSMENT OF TUMOR-INFILTRATING LYMPHOCYTES IN TUMOR-BEARING HUMANIZED MOUSE MODELS
Flow cytometric assessment of TILs in tumor-bearing humanized mice (Basic Protocols 1 and 2) is described in detail in this support protocol. At the study endpoint, tumors are harvested from the humanized mice and dissociated to obtain a single-cell suspension for staining and marker assessment.
Additional Materials (also see Support Protocol 1)
-
Surgically excised PDX or CDX tumors (see Basic Protocols 1 and 2), collected from mice in tubes with MACS Tissue Storage Solution (Miltenyi Biotec, cat. no. 130-100-008) and kept on ice until processing
-
Tumor Dissociation Kit, human (Miltenyi Biotec, cat. no. 130-095-929), including Enzymes A, H, and R (see recipes)
-
RPMI-1640 medium (ATCC, cat. no. 30-2001)
-
1× red blood cell–lysing buffer (diluted from 10×; Miltenyi Biotec, cat. no. 130-094-183)
-
Class II BSC
-
Small petri dishes
-
Forceps
-
Scalpel
-
gentleMACS C Tubes (Miltenyi Biotec, cat. no. 130-096-334)
-
gentleMACS Dissociator (Miltenyi Biotec, cat. no. 130-093-235) or gentleMACS Octo Dissociator with Heaters (Miltenyi Biotec, cat. no. 130-096-427)
-
15- and 50-ml conical tubes (Thermo Fisher, cat. no. 339650 and cat. no. 339652, respectively)
-
MACS SmartStrainers (30 µm; Miltenyi Biotec, cat. no. 130-098-458)
-
Room-temperature centrifuge (Eppendorf 5810 or equivalent)
-
Hematocytometer, trypan blue, and light microscope or automated cell counter (e.g., Cellometer Auto 2000, Nexcelom Bioscience)
1.In a class II BSC, transfer each surgically excised PDX or CDX tumor to a small petri dish. Using a forceps and scalpel, dissect each tumor into pieces of 2 to 4 mm3 in the dish.
2.Transfer tumor fragments into a gentleMACS C Tube and add Enzymes A, H, and R from Tumor Dissociation Kit according to the manufacturer's instructions. Place tube in a gentleMACS Dissociator or gentleMACS Octo Dissociator with Heaters and use appropriate setting based on tumor toughness according to the manufacturer's instructions.
3.After completion of tumor digestion, empty contents of the C Tube into a 15-ml conical tube, strain into a 50-ml conical tube using a MACS SmartStrainer, and add 10 ml RPMI-1640 medium.
4.When filtration has stopped, cap tube and centrifuge 10 min at 300 × g. Remove supernatant and add 2 ml of 1× 1× red blood cell–lysing buffer per sample to dissociated tumor cells.
5.Incubate cells for 20 min on a tube rocker at room temperature. Centrifuge 5 min at 350 × g , 5°C, and discard supernatant.
6.Add 2 ml FACS buffer, centrifuge as in step 5, discard supernatant, and resuspend cells in 0.5 to 1 ml FACS buffer. Count cells with a hematocytometer, trypan blue, and light microscope or an automated cell counter and aliquot 500,000 live cells in 60 μl per sample per well of a 96-deep-well plate.
7.Incubate cells with 0.3 μl Human BD Fc Block + 0.7 μl FACS buffer (1 μl total; 2.5 μg/million cells) for 10 min.
8.Prepare a flow cytometry antibody staining cocktail in a 5-ml Falcon round-bottom tube using fluorochrome-labeled antibodies at the manufacturer's recommended concentration in FACS buffer as per the flow panel being tested. Add antibody staining cocktail to cells, followed by incubation for 30 min at 4°C in the dark.
9.Wash cells twice with 1.25 ml FACS buffer by centrifugation for 5 min at 350 × g , 5°C. After the last wash, discard supernatant and resuspend each cell pellet in a final total volume of 150 μl FACS buffer.
10.Add 1.5 μl viability stain (7-AAD) and incubate at room temperature in dark for 5 min.
11.Acquire samples using a flow cytometer, ensuring collection of a minimum of 200,000 total events.
12.Perform flow cytometry data analysis with flow cytometry analysis software as per defined gating strategy (Figs. 7 and 8).
REAGENTS AND SOLUTIONS
Enzyme A
Reconstitute Enzyme A (lyophilized powder from Tumor Dissociation Kit, human, Miltenyi Biotec, cat. no. 130-095-929) by adding 1 ml Buffer A (provided with kit) to vial and mixing gently (do not vortex). Prepare 0.5-ml aliquots of Enzyme A solution in 1.5-ml tubes to avoid multiple freeze-thaw cycles. Store aliquots ≤6 months at −20°C, until ready for use.
Enzyme H
Reconstitute Enzyme H (lyophilized powder from Tumor Dissociation Kit, human, Miltenyi Biotec, cat. no. 130-095-929) by adding 3 ml RPMI-1640 medium (ATCC, cat. no. 30-2001) to vial and mixing gently (do not vortex). Prepare 1-ml aliquots of Enzyme H solution in 1.5-ml tubes to avoid too many freeze-thaw cycles. Store aliquots ≤6 months at −20°C, until ready for use.
Enzyme R
Reconstitute Enzyme R (lyophilized powder from Tumor Dissociation Kit, human, Miltenyi Biotec, cat. no. 130-095-929) by adding 2.7 ml RPMI-1640 medium (ATCC, cat. no. 30-2001) to vial and mixing gently (do not vortex). Prepare 1-ml aliquots of Enzyme R solution in 1.5-ml tubes to avoid multiple freeze-thaw cycles. Store aliquots ≤6 months at −20°C, until ready for use. Mix Enzyme R very well by pipetting up and down immediately before use in a reaction.
COMMENTARY
Background Information
There is an increasing demand for improved preclinical animal models to support mechanistic and therapeutic evaluation of novel agents targeting activation of the immune system against cancer. Data obtained from immunocompetent mice do not always support translation into human therapies due to species-specific differences (Mestas & Hughes, 2004), underscoring the need for humanized mouse models comprising both human immune cells and a human tumor compartment. Generation of immunodeficient mouse strains carrying either mutation or truncation of IL-2rγ [e.g., NOD/Shi-scid-IL2rγnull (NOG) or NOD/LtSz-scid IL2rγnull (NSG)] results in mice deficient in T, B, and NK cells and allows for efficient immune reconstitution (Ito et al., 2002; see Current Protocols article; Pearson, Greiner, & Shultz, 2008). Next-generation strains include immunodeficient transgenic mice expressing human cytokines, namely GM-CSF and IL-3 [NOG-EXL: Prkdcscid Il2rgtm1Sug Tg (SV40/HTLV-IL3, CSF2)] or cKIT-L, GM-CSF, and IL-3 (NSG-SGM3), which further support engraftment of CD34 cells, and particularly the development of myeloid cells (Bryce et al., 2016; Ito et al., 2013). Such models have also been used as an improved platform for preclinical efficacy studies of checkpoint inhibitors (Wang et al., 2018). Adult human PBMCs engraft readily and efficiently in NOG and NSG mice. However, due to human T-cell recognition of MHC class I and MHC class II on murine host cells, xGVHD has somewhat limited human PBMCs’ application in preclinical oncology studies with PDX tumors that require longer timelines. Development of mice that lack MHC antigens has led to reduced xGVHD (Brehm, Wiles, Greiner, & Shultz, 2014). An MHC class I and MHC class II double-knockout mouse strain developed on the NOG background was evaluated for anti-PD-1 efficacy in a PBMC-CDX model system. TIL profiling showed that more exhausted (PD-1+TIM3+LAG3+) T cells were maintained in anti-PD-1-antibody-treated tumors. A greater number of CD8+ and granzyme-producing T cells infiltrated the tumors in mice treated with the anti-PD-1 antibody (Ashizawa et al., 2017).
PDX models, which preserve the histology of patient tumors and exhibit similar gene expression patterns, represent an asset for testing therapeutic agents in development (Izumchenko et al., 2017). A comprehensive review of PDX model generation and use in humanized mouse models was published earlier by our group (see Current Protocols article; Verma et al., 2017).
Critical Parameters and Troubleshooting
The HIS-PDX platform is a valuable translational tool to study and modulate the interactions between immune components and tumors of human origin, allowing for evaluation of IO therapeutics. However, its success is dependent on several critical parameters, understanding of the limitations, and careful planning of studies.
Humanized model system
The basic protocols described in the article (Basic Protocols 1 and 2) offer two different methods for reconstitution of the HIS in immunodeficient mice. Selection of either one is dependent on the experimental design and the hypothesis being tested (please refer to Fig. 14). The huCD34+ HSC engraftment model (Basic Protocol 1) provides the advantage of stable and long-term humanization with minimal risk of xGVHD, allowing for complex biological evaluations of humanized tumor-bearing models in which long-term analysis is required (Wang et al., 2017). HuCD34+ HSC engraftment leads to development of various immune lineages, such as T cells, B cells, DCs, and myeloid cells (Audigé et al., 2017). One major caveat is that human T-cell education occurs in the mouse thymus, and hence, tumor recognition is mediated by alloreactive or xenoreactive T cells (Walsh et al., 2017). PBMC engraftment (Basic Protocol 2) facilitates adult human T-cell investigation and is well suited for examining the function of the mature immune system and even antigen-specific responses if the donor and tumor are HLA matched. The study timeline is considerably shorter for PBMC-HIS than for huCD34+ HSC-HIS models. However, the PBMC-HIS platform supports investigation of immune checkpoint drugs and their combinations (Sanmamed et al., 2015; Spranger, Frankenberger, & Schendel, 2012) and therapeutic assessment of bispecific antibodies. In particular, Bacac et al. (2016) showed in vivo therapeutic efficacy using carcinoembryonic antigen (CEA) T-cell bispecific antibody against CEA-expressing xenograft tumors with variable amounts of immune infiltrate.
Mouse strains for humanization
Currently, there are many immunodeficient mouse strains available on the market that can support reconstitution of the HIS using both huCD34+ HSC (Basic Protocol 1) and PBMC (Basic Protocol 2) platforms. This article focuses on the utility of NOG mice [and transgenic NOG-EXL mice: Prkdcscid Il2rgtm1Sug Tg (SV40/HTLV-IL3, CSF2] expressing human IL-3 and GM-CSF for humanization using huCD34+ HSCs (Basic Protocol 1). NOG mice engrafted with huCD34+ HSCs reach the acceptable threshold of 25% circulating huCD45+ cells by 10 to 12 weeks, with reconstitution of B, T, and myeloid cells. The frequency of T cells circulating in the periphery varies between 10% and 30%, depending on donor engraftment efficiency (as in Fig. 2). This model is suitable for evaluation of T cell–based therapeutics. NOG-EXL mice expressing huIL-3 and huGM-CSF have a greater ability to undergo myeloid reconstitution and are suitable for testing therapeutic agents that require or may modulate the myeloid compartment (Ito et al., 2013). Engraftment of mice using PBMCs (Basic Protocol 2) can be successfully achieved using NOG mice; however, these mice will succumb to xGVHD by ∼4 weeks, providing a very narrow therapeutic window for assessment, or may also require PBMC dose optimization for optimal engraftment levels. Alternatively, MHC class I–null B2M knockout mice on the NOG or NSG background provide a longer therapeutic window, namely up to 8 weeks (Fig. 10). The PBMC-HIS model is exclusively a T cell–based model, with both CD4+ and CD8+ T-cell subsets represented (Fig. 11).
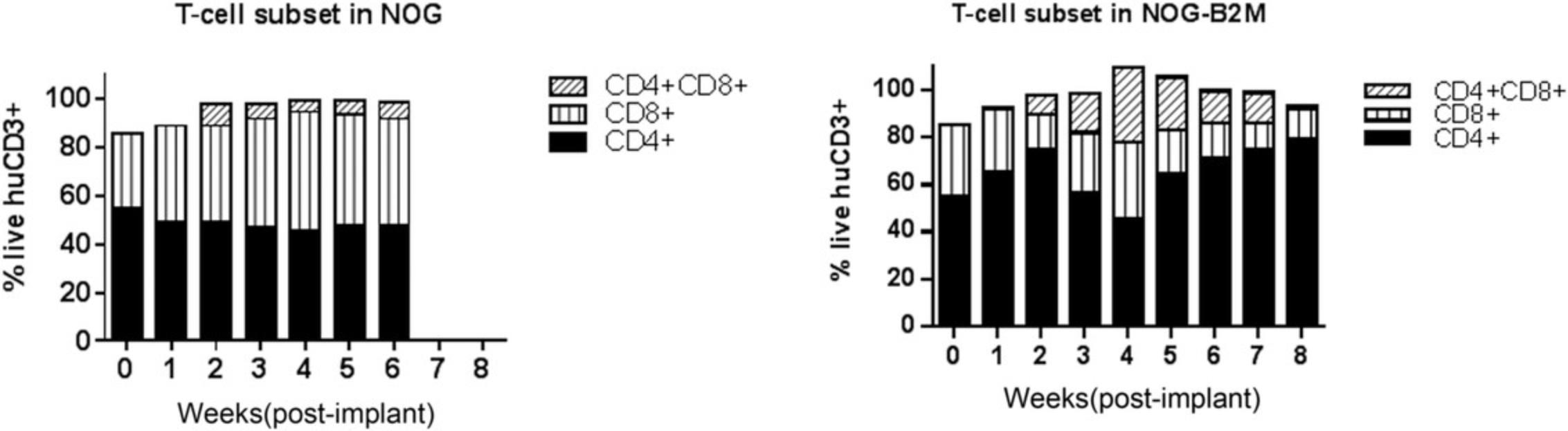
Human donor cells
HuCD34+ HSCs
The major sources of huCD34+ HSCs (Basic Protocol 1) include umbilical cord blood, fetal liver tissue, bone marrow, and mobilized peripheral blood. Umbilical cord blood–derived huCD34+ HSCs have several advantages, including that they a) are readily available and b) demonstrate high pluripotency and reconstitution potential. Fetal liver–derived huCD34+ HSCs result in similarly robust engraftment. However, ethical issues associated with their use necessitate IRB approval and oversight (Drake, Chen, & Chen, 2012). Substituting different sources of huCD34+ HSCs may impact the overall engraftment rates and kinetics, and hence, cell doses should be titrated when the source of cells differs. Donor-to-donor variability in engraftment and response to therapeutic efficacy is an important factor to be considered, and thus, it is recommended to have mice engrafted separately with cells from at least three or four donors in parallel cohorts (mixed donor populations should be avoided to prevent graft-versus-graft allogeneic interactions). We have also evaluated tumor responses to the checkpoint inhibitor nivolumab (10 mg/kg) utilizing two CB-CD34 donors. Dosing was initiated at a tumor volume of 80 to 150 mm3. Donor-dependent antitumor efficacy was observed: CB-CD34 donor 1's cells inhibited tumor growth in the nivolumab group in comparison to the control group, whereas for the CB-CD34 donor 2's cells, no efficacy was observed in the nivolumab group (data shown in Fig. 5).
Cell purity and huCD34+ HSC viability (>70%) are critical parameters to be monitored for optimal study performance.
PBMCs
Leukopaks are the ideal source for preparation of adult PBMCs (Basic Protocol 2), ensuring that large enough numbers of humanized mice with cells from a single donor can be generated for a study. As for HSCs, post-thaw viabilities of >70% are required. In some instances, the possibility of recalling healthy donors for repeat blood draws can lend more consistency across studies. Intrinsic donor-to-donor variability is expected in the model system, and hence, two or four donors should be used (see example data in Fig. 10).
Tumor model system
Both humanization protocols (Basic Protocols 1 and 2) support the evaluation of immunotherapeutic agents using CDX or PDX. The huCD34+ HSC-HIS platform (Basic Protocol 1) allows for evaluation of slow-growing tumors, whereas the PBMC-HIS platform (Basic Protocol 2) is applicable to moderate- to fast-growing tumors due to the limited therapeutic window. These parameters should be considered while designing a study for implantation of tumors in humanized mice.
Understanding Results
HuCD34+ HSC-HIS model
Both NOG and NOG-EXL mice successfully undergo engraftment of CD34+ cells, resulting in circulating levels of huCD45+ cells that are detectable 8 to 10 weeks after transfer. Doses of 1–1.2 × 105 cord blood CD34+ cells are enough to achieve a 25% level of engraftment in mice 10 weeks post-implant. Head-to-head comparison of NOG and NOG-EXL strains revealed that NOG-EXL mice are superior in engraftment efficiency. In particular, 100% engraftment was observed across four huCD34+ HSC donors, in comparison to ∼80% engraftment efficiency in NOG mice across five donors, with some variation by donor in terms of the overall level of engraftment (Fig. 2). Overall high levels of circulating huCD45+ cells were observed in NOG-EXL mice (50% to 75%) and were stable for up to 15 weeks. Typically, engraftment can be expected to be maintained for up to 25 weeks or more (data not shown). T-cell lineage development was equivalent in the two strains at 12 weeks post-HSC transplantation. Improved myeloid lineage (CD33+) development was found in NOG-EXL animals (Fig. 3). Although minimal impact on tumor growth rate was observed with both humanization techniques (data not shown), in some cases, donor-mediated effects can impede tumor growth rates. HuCD45+ lymphocytes were detected in tumors collected from humanized mice at 19 to 20 weeks post-humanization. Further analysis of TILs revealed ∼35% CD3+ T cells, with greater frequencies of CD4+ T cells detected in comparison to CD8+ cells for this model and these donors. CD8+ T cells appeared to be functional, as evidenced by production of granzyme B. High PD-1 expression was observed in T cells (> 60%); however, few regulatory T cells (CD4+CD25+FOXP3+) were present. Among human cell infiltrates in the tumor, ∼35% of these were myeloid cells. Myeloid subpopulations were characterized by marker expression through gating on CD45+CD11b+CD33+ cells. Flow cytometric analysis revealed the presence of CD14+HLA-DR+ macrophages (10%), CD14+HLA-DR- monocytic myeloid-derived suppressor cells (M-MDSCs; <20%), and CD14-HLA-DR- granulocytic MDSCs (G-MDSCs)/immature MDSCs (<10%) in humanized NOG-EXL tumors (Fig. 6). The NSCLC PDX model (CTG-1932) was infiltrated with various myeloid populations in this humanized model using NOG-EXL mice; however, the exact subsets present in a tumor model (CDX or PDX) may vary based on factors produced by each tumor.
PBMC-HIS model
A single IV dose of PBMCs in NOG or NSG mice leads to 20% to 60% human chimerism for up to 5 weeks (as shown in Fig. 9). The majority (>95%) of huCD45+ cells in circulation are CD3+ T cells, with a phenotype ratio of CD4/CD8 (40:60), which is stable throughout the 5 weeks. NOG mice succumb to xGVHD (characterized by body weight loss, dehydration, and worsening body condition) within ∼40 days post-implant. Transplant of the same donor PBMCs in NOG-B2M mice results in slower engraftment, with 10% circulating huCD45+ cell levels at 4 weeks and stable increased levels of huCD45+ cells (30%) at up to 8 weeks. An increased CD4/CD8 ratio is observed in B2M-deficient mice (data shown in Fig. 11). Similar trends were observed when multiple donors were evaluated using cell engraftment into NOG or NSG-B2M mice, as shown in Figure 10, with some donor-dependent effects on the magnitude and kinetics of engraftment. A donor-dependent effect on tumor growth kinetics was observed after 5 weeks with the CDX MDA-MB-231 tumor cell line using three different donors (please refer to Fig. 12 for data).
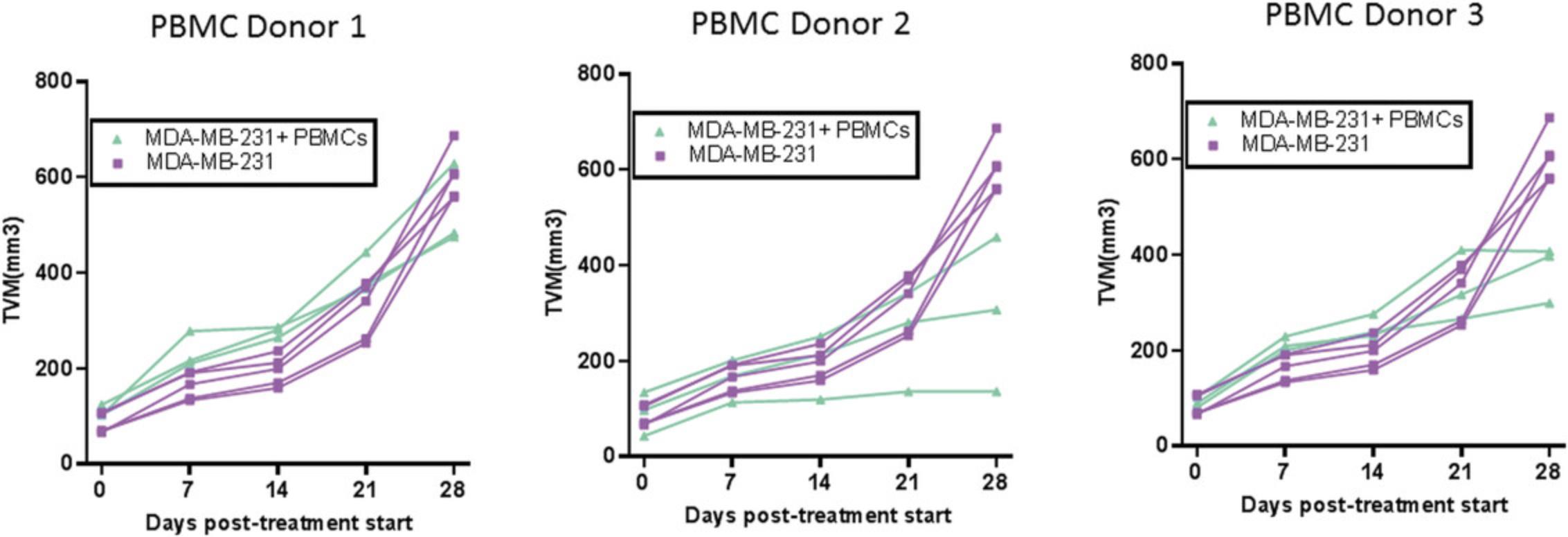
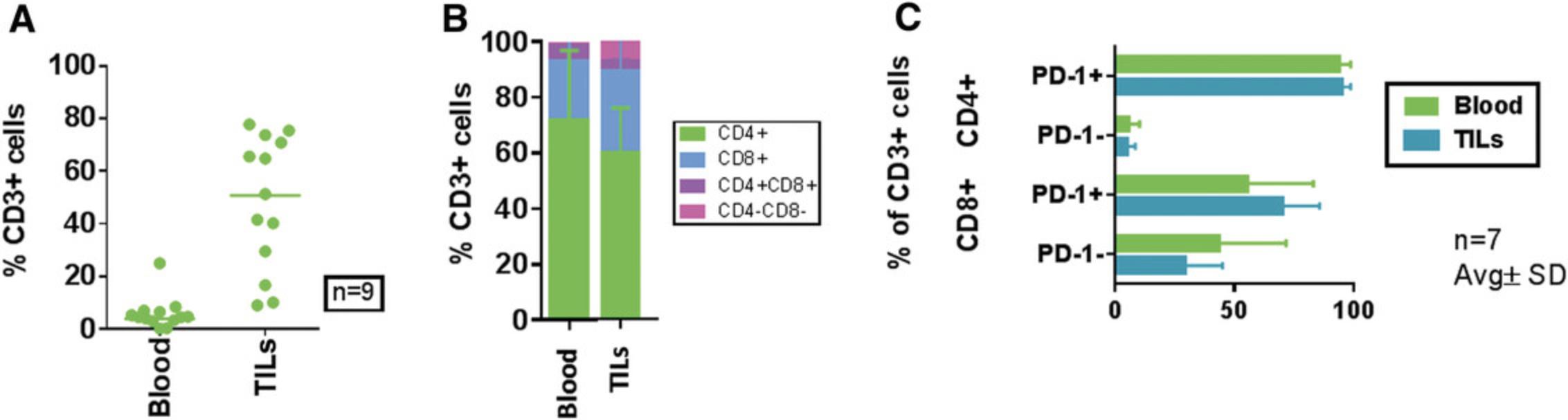
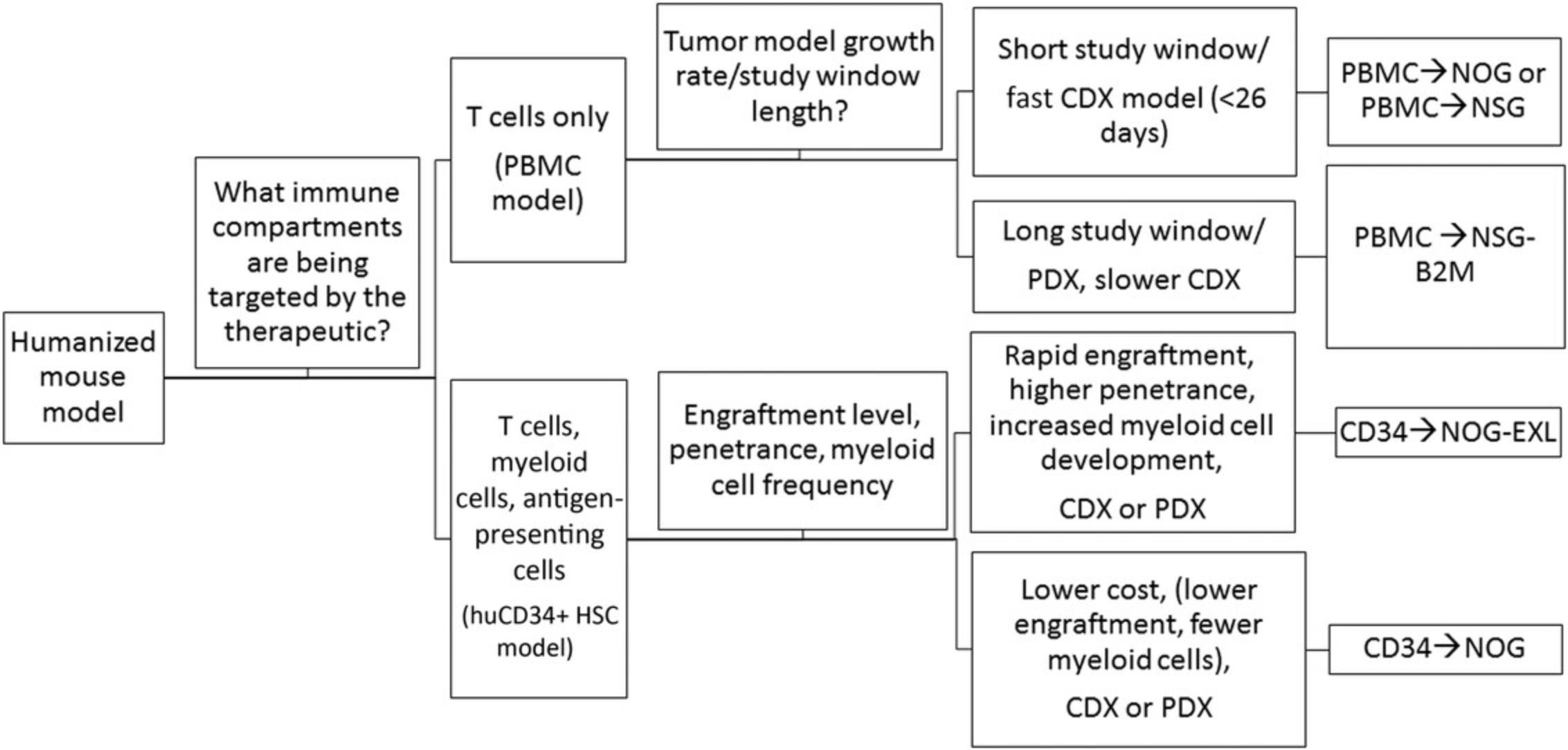
Time Considerations
Establishment of humanized PDX models using huCD34+ HSCs, as described in Basic Protocol 1, requires up to 12 weeks for engraftment post–-HSC implant. Tumors are then SC implanted into humanized mice, requiring an additional 2 to 3 weeks to establish the tumor prior to initiation of dosing for a therapeutic model. Dosing schedule and study endpoints define the length of the study post-randomization of the tumor-bearing humanized mice. A total of 19 to 20 weeks is thus required for completion of Basic Protocol 1.
The PBMC-HIS model (Basic Protocol 2) has an abbreviated timeline as compared to the huCD34+ HSC-HIS model (Basic Protocol 1). The timing of PBMC implantation is dictated by the study design and the tumor model selected (see Current Protocols article; Verma et al., 2017). A PDX tumor study requires subcutaneous PDX implantation prior to PBMC implantation, followed by randomization and dosing, whereas in a CDX study, PBMCs can be implanted before or after tumor cell engraftment. A total of 6 to 9 weeks is required for completion of Basic Protocol 2, depending on the duration of dosing and the tumor growth kinetics.
For Support Protocols 1 and 2, a total of 2 to 3 days are required for processing and acquisition followed by data analysis.
Acknowledgments
Special thanks to Linda St. John for excellent technical support and Dr. Geoffery Cole for review of the manuscript.
Literature Cited
- Ashizawa, T., Iizuka, A., Nonomura, C., Kondou, R., Maeda, C., Miyata, H., … Akiyama, Y. (2017). Antitumor effect of programmed death-1 (PD-1) blockade in humanized the NOG-MHC double knockout mouse. Clinical Cancer Research , 23(1), 149–158. doi: 10.1158/1078-0432.CCR-16-0122.
- Audigé, A., Rochat, M. A., Li, D., Ivic, S., Fahrny, A., Muller, C. K. S., … Speck, R. F. (2017). Long-term leukocyte reconstitution in NSG mice transplanted with human cord blood hematopoietic stem and progenitor cells. BMC Immunology , 18(1), 28. doi: 10.1186/s12865-017-0209-9.
- Bacac, M., Fauti, T., Sam, J., Colombetti, S., Weinzierl, T., Ouaret, D., … Umaña, P. (2016). A novel carcinoembryonic antigen T-cell bispecific antibody (CEA TCB) for the treatment of solid tumors. Clinical Cancer Research , 22, 3286–3297. doi: 10.1158/1078-0432.CCR-15-1696.
- Brehm, M. A., Wiles, M. V., Greiner, D. L., & Shultz, L. D. (2014). Generation of improved humanized mouse models for human infectious diseases. Journal of Immunological Methods , 410, 3–17. doi: 10.1016/j.jim.2014.02.011.
- Bryce, P. J., Falahati, R., Kenney, L. L., Leung, J., Bebbington, C., Tomasevic, N., … Brehm, M. A. (2016). Humanized mouse model of mast cell-mediated passive cutaneous anaphylaxis and passive systemic anaphylaxis. Journal of Allergy and Clinical Immunology , 138, 769–779. doi: 10.1016/j.jaci.2016.01.049.
- Drake, A. C., Chen, Q., & Chen, J. (2012). Engineering humanized mice for improved hematopoietic reconstitution. Cellular and Molecular Immunology , 9, 215–224. doi: 10.1038/cmi.2012.6.
- Ito, M., Hiramatsu, H., Kobayashi, K., Suzue, K., Kawahata, M., Hioki, K., … Nakahata, T. (2002). NOD/SCID/γcnull mouse: An excellent recipient mouse model for engraftment of human cells. Blood , 100, 3175–3182. doi: 10.1182/blood-2001-12-0207.
- Ito, R., Takahashi, T., Katano, I., Kawai, K., Kamisako, T., Ogura, T., … Ito, M. (2013). Establishment of a human allergy model using human IL-3/GM-CSF-transgenic NOG mice. The Journal of Immunology , 191, 2890–2899. doi: 10.4049/jimmunol.1203543.
- Izumchenko, E., Paz, K., Ciznadija, D., Sloma, I., Katz, A., Vasquez-Dunddel, D., … Sidransky, D. (2017). Patient-derived xenografts effectively capture responses to oncology therapy in a heterogeneous cohort of patients with solid tumors. Annals of Oncology , 28, 2595–2605. doi: 10.1093/annonc/mdx416.
- Katano, I., Ito, R., Eto, T., Aiso, S., & Ito, M. (2011). Immunodeficient NOD-scid IL-2Rγnull mice do not display T and B cell leakiness. Experimental Animals , 60, 181–186. doi: 10.1538/expanim.60.181.
- Mestas, J., & Hughes, C. C. W. (2004). Of mice and not men: Differences between mouse and human immunology. The Journal of Immunology , 172, 2731–2738. doi: 10.4049/jimmunol.172.5.2731.
- National Research Council (US) Committee on Immunologically Compromised Rodents. (1989). Immunodeficient rodents: A guide to their immunobiology, husbandry, and use. Washington (DC): National Academies Press (US).
- Pearson, T., Greiner, D. L., & Shultz, L. D. (2008). Creation of “humanized” mice to study human immunity. Current Protocols in Immunology , 81, 15.21.1–15.21.21. doi: 10.1002/0471142735.im1521s81.
- Sanmamed, M. F., Rodriguez, I., Schalper, K. A., Oñate, C., Azpilikueta, A., Rodriguez-Ruiz, M. E., … Melero, I. (2015). Nivolumab and urelumab enhance antitumor activity of human T lymphocytes engrafted in Rag2-/-IL2Rγnull immunodeficient mice. Cancer Research , 75, 3466–3478. doi: 10.1158/0008-5472.CAN-14-3510.
- Spranger, S., Frankenberger, B., & Schendel, D. J. (2012). NOD/scid IL-2Rg null mice: A preclinical model system to evaluate human dendritic cell-based vaccine strategies in vivo. Journal of Translational Medicine , 10, 30. doi: 10.1186/1479-5876-10-30.
- Verma, B., Ritchie, M., & Mancini, M. (2017). Development and applications of patient-derived xenograft models in humanized mice for oncology and immune-oncology drug discovery. Current Protocols in Pharmacology , 78, 14.41.1–14.41.12. doi: 10.1002/cpph.26.
- Walsh, N. C., Kenney, L. L., Jangalwe, S., Aryee, K.-E., Greiner, D. L., Brehm, M. A., & Shultz, L. D. (2017). Humanized mouse models of clinical disease. Annual Review of Pathology: Mechanisms of Disease , 12, 187–215. doi: 10.1146/annurev-pathol-052016-100332.
- Wang, M., Yao, L. C., Cheng, M., Cai, D., Martinek, J., Pan, C. X., … Keck, J. G. (2018). Humanized mice in studying efficacy and mechanisms of PD-1-targeted cancer immunotherapy. FASEB Journal , 32, 1537–1549. doi: 10.1096/fj.201700740R.
- Wang, W., Fujii, H., Kim, H. J., Hermans, K., Usenko, T., Xie, S., … Zandstra, P. W. (2017). Enhanced human hematopoietic stem and progenitor cell engraftment by blocking donor T cell-mediated TNF signaling. Science Translational Medicine , 9, eaag3214. doi: 10.1126/scitranslmed.aag3214.
Citing Literature
Number of times cited according to CrossRef: 41
- Kang-Hyun Kim, Sang-wook Lee, In-Jeoung Baek, Hye-Young Song, Seon-Ju Jo, Je-Won Ryu, Seung-Hee Ryu, Jin-Hee Seo, Jong-Choon Kim, Seung-Ho Heo, CD47;Rag2;IL-2rγ triple knock-out mice pre-conditioning with busulfan could be a novel platform for generating hematopoietic stem cells engrafted humanized mice, Frontiers in Immunology, 10.3389/fimmu.2024.1365946, 15 , (2024).
- Xueyuan Zhou, Felix Klaus Geyer, Dominic Happel, Jeffrey Takimoto, Harald Kolmar, Brian Rabinovich, Using protein geometry to optimize cytotoxicity and the cytokine window of a ROR1 specific T cell engager, Frontiers in Immunology, 10.3389/fimmu.2024.1323049, 15 , (2024).
- Tingting Huang, Bernice Leung, Yuyang Huang, Laura Price, Jiang Gui, Bonnie W. Lau, A murine model to evaluate immunotherapy effectiveness for human Fanconi anemia-mutated acute myeloid leukemia, PLOS ONE, 10.1371/journal.pone.0292375, 19 , 1, (e0292375), (2024).
- Teja Celhar, Xinyi Li, Yunqian Zhao, Hui Chien Tay, Andrea Lee, Hui Hua Liew, Edwin Kunxiang Shepherdson, Ravisankar Rajarethinam, Yiping Fan, Anselm Mak, Jerry Kok Yen Chan, Amit Singhal, Takeshi Takahashi, Fetal liver CD34+ contain human immune and endothelial progenitors and mediate solid tumor rejection in NOG mice, Stem Cell Research & Therapy, 10.1186/s13287-024-03756-7, 15 , 1, (2024).
- Elinor Willis, Jillian Verrelle, Esha Banerjee, Charles-Antoine Assenmacher, James C. Tarrant, Nicholas Skuli, Moriah L. Jacobson, Donald M. O’Rouke, Zev A. Binder, Enrico Radaelli, Humanization with CD34-positive hematopoietic stem cells in NOG-EXL mice results in improved long-term survival and less severe myeloid cell hyperactivation phenotype relative to NSG-SGM3 mice, Veterinary Pathology, 10.1177/03009858231222216, 61 , 4, (664-674), (2024).
- Jane Tian, Amir M. Ashique, Sabrina Weeks, Tian Lan, Hong Yang, Hung-I Harry Chen, Christina Song, Kikuye Koyano, Kalyani Mondal, Daniel Tsai, Isla Cheung, Mehrdad Moshrefi, Avantika Kekatpure, Bin Fan, Betty Li, Samir Qurashi, Lauren Rocha, Jonathan Aguayo, Col Rodgers, Marchelle Meza, Darren Heeke, Sara M. Medfisch, Chun Chu, Shelley Starck, Nandini Pal Basak, Satish Sankaran, Mohit Malhotra, Suzanne Crawley, Thomas-Toan Tran, Dana Y. Duey, Carmence Ho, Igor Mikaelian, Wenhui Liu, Lee B. Rivera, Jiawei Huang, Kevin J. Paavola, Kyle O'Hollaren, Lisa K. Blum, Vicky Y. Lin, Peirong Chen, Anjushree Iyer, Sisi He, Julie M. Roda, Yan Wang, James Sissons, Alan K. Kutach, Daniel D. Kaplan, Geoffrey W. Stone, ILT2 and ILT4 Drive Myeloid Suppression via Both Overlapping and Distinct Mechanisms, Cancer Immunology Research, 10.1158/2326-6066.CIR-23-0568, 12 , 5, (592-613), (2024).
- Funda Meric-Bernstam, Michael W. Lloyd, Soner Koc, Yvonne A. Evrard, Lisa M. McShane, Michael T. Lewis, Kurt W. Evans, Dali Li, Lawrence Rubinstein, Alana Welm, Dennis A. Dean, Anuj Srivastava, Jeffrey W. Grover, Min J. Ha, Huiqin Chen, Xuelin Huang, Kaushik Varadarajan, Jing Wang, Jack A. Roth, Bryan Welm, Ramaswamy Govinden, Li Ding, Salma Kaochar, Nicholas Mitsiades, Luis Carvajal-Carmona, Meenhard Herylyn, Michael A. Davies, Geoffrey I. Shapiro, Ryan Fields, Jose G. Trevino, Joshua C. Harrell, James H. Doroshow, Jeffrey H. Chuang, Jeffrey A. Moscow, Assessment of Patient-Derived Xenograft Growth and Antitumor Activity: The NCI PDXNet Consensus Recommendations, Molecular Cancer Therapeutics, 10.1158/1535-7163.MCT-23-0471, 23 , 7, (924-938), (2024).
- Carla Panisello, Rosario Aschero, Alba Martinez-Moreno, Heleia Roca Ho, Aida Falgas, Europa Azucena González-Navarro, Julieta Carabelli, Edwards Pradenas, María Lázaro-Díez, Julia G Prado, Julià Blanco, Jorge Carrillo, Manel Juan, Ángel M Carcaboso, Clara Bueno, Pablo Menendez, NSGS mice humanized with cord blood mononuclear cells show sustained and functional myeloid–lymphoid representation with limited graft-versus-host disease, Journal for ImmunoTherapy of Cancer, 10.1136/jitc-2024-009198, 12 , 10, (e009198), (2024).
- Lin Chen, Yinyu Chen, Liangyu Ge, Qian Zhang, Jian Meng, Recent advances in patient‐derived tumor organoids for reconstructing TME of head and neck cancer, Journal of Oral Pathology & MedicineJournal of Oral Pathology & MedicineJournal of Oral Pathology & Medicine, 10.1111/jop.13532, 53 , 4, (238-245), (2024).
- Séverine Ménoret, Florence Renart-Depontieu, Gaelle Martin, Kader Thiam, Ignacio Anegon, Efficient generation of human immune system rats using human CD34+ cells, Stem Cell Reports, 10.1016/j.stemcr.2024.07.005, (2024).
- Wenjing Li, Chunlei Xia, Kun Wang, Liting Xue, Yan Wang, Janine Y. Yang, Mingkun Zhang, Ming Yin, Cunxiang Ju, Zhenchuan Miao, Ying Li, Xiaofeng Zhao, Zhijian Yang, Renhong Tang, WenQing Yang, Technical considerations and strategies for generating and optimizing humanized mouse tumor models in immuno-oncology research, International Immunopharmacology, 10.1016/j.intimp.2024.112722, 139 , (112722), (2024).
- Roxane Crouigneau, Yan-Fang Li, Jamie Auxillos, Eliana Goncalves-Alves, Rodolphe Marie, Albin Sandelin, Stine Falsig Pedersen, Mimicking and analyzing the tumor microenvironment, Cell Reports Methods, 10.1016/j.crmeth.2024.100866, 4 , 10, (100866), (2024).
- Maria Francesca Baietti, Eleonora Leucci, Humanized mouse models for anti-cancer therapy, Cell-based Cancer Immunotherapy, 10.1016/bs.mcb.2023.06.002, (317-333), (2024).
- Yulin Xu, Wei Shan, Qian Luo, Meng Zhang, Dawei Huo, Yijin Chen, Honghu Li, Yishan Ye, Xiaohong Yu, Yi Luo, He Huang, Establishment of a humanized mouse model using steady‐state peripheral blood‐derived hematopoietic stem and progenitor cells facilitates screening of cancer‐targeted T‐cell repertoires, Cancer Innovation, 10.1002/cai2.118, 3 , 3, (2024).
- Lourdes Rodríguez-Fragoso, Anahí Rodríguez-López, Janet Sánchez-Quevedo, Models of Hepatotoxicity for the Study of Chronic Liver Disease, Animal Models and Experimental Research in Medicine, 10.5772/intechopen.106219, (2023).
- Bei Jia, Chenchen Zhao, Kentaro Minagawa, Hiroko Shike, David F. Claxton, W. Christopher Ehmann, Witold B. Rybka, Shin Mineishi, Ming Wang, Todd D. Schell, K. Sandeep Prabhu, Robert F. Paulson, Yi Zhang, Leonard D. Shultz, Hong Zheng, Acute Myeloid Leukemia Causes T Cell Exhaustion and Depletion in a Humanized Graft-versus-Leukemia Model, The Journal of Immunology, 10.4049/jimmunol.2300111, 211 , 9, (1426-1437), (2023).
- Chi Yan, Caroline A. Nebhan, Nabil Saleh, Rebecca Shattuck-Brandt, Sheau-Chiann Chen, Gregory D. Ayers, Vivian Weiss, Ann Richmond, Anna E. Vilgelm, Generation of Orthotopic Patient-Derived Xenografts in Humanized Mice for Evaluation of Emerging Targeted Therapies and Immunotherapy Combinations for Melanoma, Cancers, 10.3390/cancers15143695, 15 , 14, (3695), (2023).
- Wing Keung Chan, Jessica Williams, Kinnari Sorathia, Betsy Pray, Kaled Abusaleh, Zehua Bian, Archisha Sharma, Ian Hout, Shamama Nishat, Walter Hanel, Shelby L. Sloan, Aneeq Yasin, Nathan Denlinger, Xiaoli Zhang, Natarajan Muthusamy, Sumithira Vasu, Marcos de Lima, Yiping Yang, Robert Baiocchi, Lapo Alinari, A novel CAR-T cell product targeting CD74 is an effective therapeutic approach in preclinical mantle cell lymphoma models, Experimental Hematology & Oncology, 10.1186/s40164-023-00437-8, 12 , 1, (2023).
- Amelia Foss, Manav Pathania, Pediatric Glioma Models Provide Insights into Tumor Development and Future Therapeutic Strategies, Developmental Neuroscience, 10.1159/000531040, 46 , 1, (22-43), (2023).
- Jane Chuprin, Hannah Buettner, Mina O. Seedhom, Dale L. Greiner, James G. Keck, Fumihiko Ishikawa, Leonard D. Shultz, Michael A. Brehm, Humanized mouse models for immuno-oncology research, Nature Reviews Clinical Oncology, 10.1038/s41571-022-00721-2, 20 , 3, (192-206), (2023).
- Kanve N. Suvilesh, Yariswamy Manjunath, Klaus Pantel, Jussuf T. Kaifi, Preclinical models to study patient-derived circulating tumor cells and metastasis, Trends in Cancer, 10.1016/j.trecan.2023.01.004, 9 , 4, (355-371), (2023).
- Yicun Han, Xiuqi Fan, Liyan Fan, Yaosong Wu, Zhexu Zhou, Ge Wang, Lanwei Guo, Wendong Gao, Yulong Chen, Qilong Gao, Liujunzi decoction exerts potent antitumor activity in oesophageal squamous cell carcinoma by inhibiting miR-34a/STAT3/IL-6R feedback loop, and modifies antitumor immunity, Phytomedicine, 10.1016/j.phymed.2023.154672, 111 , (154672), (2023).
- Hong Kyu Lee, Min-Woo Nam, Ryeo-Eun Go, Jihye Koo, Tae Hun Kim, Jun-Eui Park, Kyung-Chul Choi, TGF-β2 antisense oligonucleotide enhances T-cell mediated anti-tumor activities by IL-2 via attenuation of fibrotic reaction in a humanized mouse model of pancreatic ductal adenocarcinoma, Biomedicine & Pharmacotherapy, 10.1016/j.biopha.2022.114212, 159 , (114212), (2023).
- Selen Kum Özşengezer, Zekiye S. Altun, Animal Models for Cancer Immunology, Current Molecular Biology Reports, 10.1007/s40610-023-00154-5, 9 , 4, (33-43), (2023).
- Jui-Ling Wang, Wen-Hui Ma, Tak-Wah Wong, Chun-Keung Yu, Personalized Immuno-Oncology with Immunodeficiency Mouse Models, 10.1007/16833_2023_133, (2023).
- Preeti Kanikarla Marie, Alexey V. Sorokin, Lea A. Bitner, Rebecca Aden, Michael Lam, Ganiraju Manyam, Melanie N. Woods, Amanda Anderson, Anna Capasso, Natalie Fowlkes, Michael J. Overman, David G. Menter, Scott Kopetz, Autologous humanized mouse models to study combination and single-agent immunotherapy for colorectal cancer patient-derived xenografts, Frontiers in Oncology, 10.3389/fonc.2022.994333, 12 , (2022).
- Shihong Peng, Pan Hu, Yu-Tian Xiao, Weiqiang Lu, Dandan Guo, Shixiu Hu, Jiayi Xie, Minna Wang, Weiwei Yu, Junjie Yang, Huang Chen, Xiaomin Zhang, Yasheng Zhu, Ye Wang, Yue Yang, Guanghui Zhu, Sujun Chen, Jian Wang, Bo Zhang, Weidong Chen, Huangan Wu, Zhenliang Sun, Tao Ding, Hankun Zhang, Zhengfang Yi, Mingyao Liu, Shancheng Ren, Single-Cell Analysis Reveals EP4 as a Target for Restoring T-Cell Infiltration and Sensitizing Prostate Cancer to Immunotherapy, Clinical Cancer Research, 10.1158/1078-0432.CCR-21-0299, 28 , 3, (552-567), (2022).
- Kelli A. Connolly, Brittany Fitzgerald, Martina Damo, Nikhil S. Joshi, Novel Mouse Models for Cancer Immunology, Annual Review of Cancer Biology, 10.1146/annurev-cancerbio-070620-105523, 6 , 1, (269-291), (2022).
- Chen Chen, Weiqiang Jing, Yu Chen, Ganyu Wang, Mohnad Abdalla, Lin Gao, Maosen Han, Chongdeng Shi, Anning Li, Peng Sun, Xin Jiang, Zhenmei Yang, Shengchang Zhang, Jing Zhang, Chunwei Tang, Ying Liu, Rui Zhang, Fengbo Xu, Baixiang Dong, Xueen Li, Minglu Liu, Bangming Qiang, Yanhua Sun, Xia Wei, Jun Li, Quanyin Hu, Xinyi Jiang, Intracavity generation of glioma stem cell–specific CAR macrophages primes locoregional immunity for postoperative glioblastoma therapy, Science Translational Medicine, 10.1126/scitranslmed.abn1128, 14 , 656, (2022).
- Masoud H. Manjili, The adaptation model of immunity: Is the goal of central tolerance to eliminate defective T cells or self‐reactive T cells?, Scandinavian Journal of Immunology, 10.1111/sji.13209, 96 , 4, (2022).
- Garima Kaushik, Shivaprasad Venkatesha, Bhavna Verma, Bandana Vishwakarma, Ai-Hong Zhang, Amy Wesa, Preclinical In Vitro and In Vivo Models for Adoptive Cell Therapy of Cancer, The Cancer Journal, 10.1097/PPO.0000000000000609, 28 , 4, (257-262), (2022).
- Ryoji Ito, Ikumi Katano, Immanuel W.H. Kwok, Lai Guan Ng, Miyuki Ida-Tanaka, Yusuke Ohno, Yunmei Mu, Hanako Morita, Eiko Nishinaka, Chiyoko Nishime, Misa Mochizuki, Kenji Kawai, Tay Hui Chien, Zhao Yunqian, Fan Yiping, Liew Hui Hua, Teja Celhar, Jerry Kok Yen Chan, Takeshi Takahashi, Motohito Goto, Tomoyuki Ogura, Riichi Takahashi, Mamoru Ito, Efficient differentiation of human neutrophils with recapitulation of emergency granulopoiesis in human G-CSF knockin humanized mice, Cell Reports, 10.1016/j.celrep.2022.111841, 41 , 12, (111841), (2022).
- Parmida Sadat Pezeshki, Nima Rezaei, Preclinical Cancer Models for the Evaluation of Immunotherapies: From Cell Lines to Animal Models, Handbook of Cancer and Immunology, 10.1007/978-3-030-80962-1_206-1, (1-21), (2022).
- Guilherme Ribeiro Romualdo, Kaat Leroy, Cícero Júlio Silva Costa, Gabriel Bacil Prata, Bart Vanderborght, Tereza Cristina da Silva, Luís Fernando Barbisan, Wellington Andraus, Lindsey Devisscher, Niels Olsen Saraiva Câmara, Mathieu Vinken, Bruno Cogliati, In Vivo and In Vitro Models of Hepatocellular Carcinoma: Current Strategies for Translational Modeling, Cancers, 10.3390/cancers13215583, 13 , 21, (5583), (2021).
- Morgane M. Cogels, Redouane Rouas, Ghanem E. Ghanem, Philippe Martinive, Ahmad Awada, Dirk Van Gestel, Mohammad Krayem, Humanized Mice as a Valuable Pre-Clinical Model for Cancer Immunotherapy Research, Frontiers in Oncology, 10.3389/fonc.2021.784947, 11 , (2021).
- Wenwen Guo, Caiqin Zhang, Tianyun Qiao, Jumei Zhao, Changhong Shi, Strategies for the Construction of Mouse Models With Humanized Immune System and Evaluation of Tumor Immune Checkpoint Inhibitor Therapy, Frontiers in Oncology, 10.3389/fonc.2021.673199, 11 , (2021).
- Darel Martínez Bedoya, Valérie Dutoit, Denis Migliorini, Allogeneic CAR T Cells: An Alternative to Overcome Challenges of CAR T Cell Therapy in Glioblastoma, Frontiers in Immunology, 10.3389/fimmu.2021.640082, 12 , (2021).
- Sandra D. Scherer, Alessandra I. Riggio, Fadi Haroun, Yoko S. DeRose, H. Atakan Ekiz, Maihi Fujita, Jennifer Toner, Ling Zhao, Zheqi Li, Steffi Oesterreich, Ahmed A. Samatar, Alana L. Welm, An immune-humanized patient-derived xenograft model of estrogen-independent, hormone receptor positive metastatic breast cancer, Breast Cancer Research, 10.1186/s13058-021-01476-x, 23 , 1, (2021).
- Peeyush K. Lala, Pinki Nandi, Ali Hadi, Chidambra Halari, A crossroad between placental and tumor biology: What have we learnt?, Placenta, 10.1016/j.placenta.2021.03.003, 116 , (12-30), (2021).
- Aitziber Buqué, Lorenzo Galluzzi, Preface: Chemical carcinogenesis in mice as a model of human cancer: Pros and cons, Carcinogen-driven mouse models of oncogenesis, 10.1016/S0091-679X(21)00040-6, (xvii-xxv), (2021).
- Nahee Park, Kamal Pandey, Sei Kyung Chang, Ah-Young Kwon, Young Bin Cho, Jin Hur, Nar Bahadur Katwal, Seung Ki Kim, Seung Ah Lee, Gun Woo Son, Jong Min Jo, Hee Jung Ahn, Yong Wha Moon, Preclinical platform for long-term evaluation of immuno-oncology drugs using hCD34+ humanized mouse model, Journal for ImmunoTherapy of Cancer, 10.1136/jitc-2020-001513, 8 , 2, (e001513), (2020).