Cap 1 Messenger RNA Synthesis with Co-transcriptional CleanCap® Analog by In Vitro Transcription
Jordana M. Henderson, Jordana M. Henderson, Andrew Ujita, Andrew Ujita, Elizabeth Hill, Elizabeth Hill, Sally Yousif-Rosales, Sally Yousif-Rosales, Cory Smith, Cory Smith, Nicholas Ko, Nicholas Ko, Taylor McReynolds, Taylor McReynolds, Charles R. Cabral, Charles R. Cabral, Julienne R. Escamilla-Powers, Julienne R. Escamilla-Powers, Michael E. Houston, Michael E. Houston
Abstract
Synthetic messenger RNA (mRNA)−based therapeutics are an increasingly popular approach to gene and cell therapies, genome engineering, enzyme replacement therapy, and now, during the global SARS-CoV-2 pandemic, vaccine development. mRNA for such purposes can be synthesized through an enzymatic in vitro transcription (IVT) reaction and formulated for in vivo delivery. Mature mRNA requires a 5′-cap for gene expression and mRNA stability. There are two methods to add a cap in vitro: via a two-step multi-enzymatic reaction or co-transcriptionally. Co-transcriptional methods minimize reaction steps and enzymes needed to make mRNA when compared to enzymatic capping. CleanCap® AG co-transcriptional capping results in 5 mg/ml of IVT with 94% 5′-cap 1 structure. This is highly efficient compared to first-generation cap analogs, such as mCap and ARCA, that incorporate cap 0 structures at lower efficiency and reaction yield. This article describes co-transcriptional capping using TriLink Biotechnology's CleanCap® AG in IVT. © 2021 The Authors.
This article was corrected on 22 November 2021 and 26 July 2022 . See the end of the full text for details.
Basic Protocol 1 : IVT with CleanCap
Basic Protocol 2 : mRNA purification and analysis
INTRODUCTION
Synthetic messenger RNA (mRNA) has shown great potential as a therapeutic in various clinical applications such as vaccines and genetic disease treatments, i.e., protein replacement therapies, stem cell reprograming, and gene editing (Sahin, Karikó, & Türeci, 2014; Warren et al., 2010). Transient expression of synthetic mRNA is desirable in such applications because it avoids the risk of genomic insertion events presented by viral systems. Advances in mRNA manufacturing, scalability, and delivery have expanded mRNA's utility as a therapeutic (Kowalski, Rudra, Miao, & Anderson, 2019). Currently, multiple mRNA vaccines against SARS-CoV-2 are in clinical trials, resulting in some of the fastest drug development programs in modern biotechnology history (Kis et al., 2020; Krammer, 2020). Two mRNA vaccines are now authorized by U.S. FDA for emergency use (Haynes, 2020), one of which uses CleanCap technology (Sahin et al., 2020).
mRNA is transcribed from DNA by RNA polymerase enzymes via a process called transcription. RNA polymerase binds to the promoter region of DNA and forms a transcription initiation bubble. Nucleotides complementary to the DNA are added in the 5′-to-3′ direction by the formation of phosphodiester bonds. In vitro, the same process is achieved by combining a DNA template, RNA polymerase, and nucleotide triphosphates (NTPs) with magnesium-containing buffer, RNase inhibitor, and inorganic pyrophosphatase (Chamberlin & Ring, 1973).
Most mature eukaryotic mRNA consists of a 5′-cap, 5′-untranslated region (UTR), coding sequence or open reading frame (ORF), 3′-UTR, and poly(A) tail, as shown in Figure 1. The 5′-cap is vital for mRNA stability, translation, and self- versus non-self identification, but several variations of this structure exist in nature (Furuichi, 2015; Wang et al., 2019). A cap 1 structure is critical for successful expression in cells (Ramanathan, Robb, & Chan, 2016). Therefore, creating this structure is a vital part of the synthetic process.

Traditional T7 RNA polymerase reactions will initiate transcription with guanosine triphosphate, forming a 5′-triphosphate RNA. Generating a cap structure then requires a second enzymatic treatment (Ensinger, Martin, Paoletti, & Moss, 1975; Moss, Gershowitz, Wei, & Boone, 1976). Alternatively, capped transcripts can be produced by using a cap analog during the in vitro transcription (IVT) reaction (Hadas et al., 2019; Ishikawa et al., 2009; Sikorski et al., 2020; Stepinski et al., 2001). Termed co-transcriptional capping, the cap analog is introduced into the IVT reaction along with NTPs, DNA plasmid, and RNA polymerase in a single reaction vessel, resulting in mature mRNA. This “one-pot” reaction decreases the number of manufacturing steps, which reduces overall handling time, purification steps, and number of enzymes required. Such reductions are critical for reducing the cost and complexity of mRNA manufacturing. First-generation cap analogs resulted in cap 0 structures with low reaction yields and capping efficiency. Therefore, a new cap analog resulting in cap 1 structure incorporated by the RNA polymerase was developed. Co-transcriptional capping with CleanCap AG trimer as shown in Figure 2A produces 94% (or higher) cap 1 mRNA in a one-pot synthesis, resulting in mRNA yields of approximately 5 mg mRNA per ml of IVT reaction. Transcription initiates downstream of the T7 promoter sequence when the CleanCap trimer binds to the +1 and +2 nucleotides of the template through complementary base pairing followed by incorporation of the complementary NTP at the +3 position, as shown in Figure 2B.
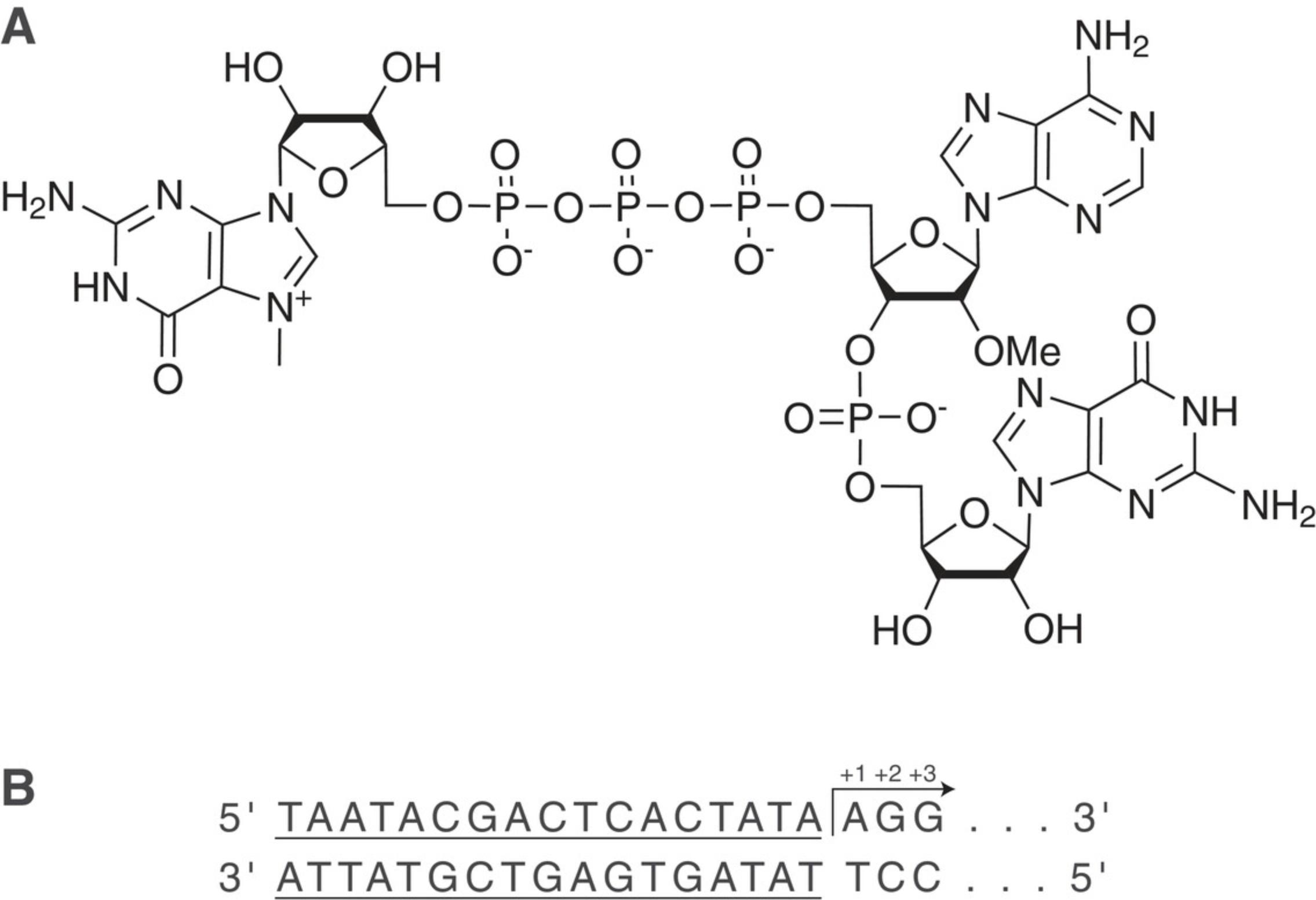
This method describes co-transcriptional capping using CleanCap AG reagent with optional DNase I treatment for synthesis of 5 mg/ml cap 1 mRNA through IVT reaction (Basic Protocol 1). Following IVT, the mRNA is purified and analyzed by UV-vis spectrophotometry and denaturing gel electrophoresis, as described in Basic Protocol 2.
STRATEGIC PLANNING
DNA Template Design
DNA template design is an integral part of successful transcription. IVT of an mRNA requires a DNA template coding the desired message with an upstream RNA polymerase promoter site. Templates can be in the form of a linearized plasmid or a PCR product. Templates must contain the T7 RNA polymerase promoter sequence, a 5′-UTR, ORF, and 3′-UTR. Sequence optimization of the ORF using bioinformatic software may improve therapeutic applications (Vaidyanathan et al., 2018). A poly(A) tail is required for functional translation of an mRNA. The poly(A) tail can be included in the plasmid, added via PCR, or added post-transcriptionally by enzymatic polyadenylation. Of note, enzymatic polyadenylation results in heterogenous tail lengths. Optimal poly(A) tail length may vary by sequence and application (Jalkanen, Coleman, & Wilusz, 2014). Plasmid designs must include a unique restriction enzyme cut site for linearization downstream of the desired 3′-end, as shown in Figure 3A. A PCR template may be preferred if you require a longer poly(A) than can be maintained within the plasmid and/or if your current plasmid does not contain the T7 promoter region needed for transcription (Fig. 3B).
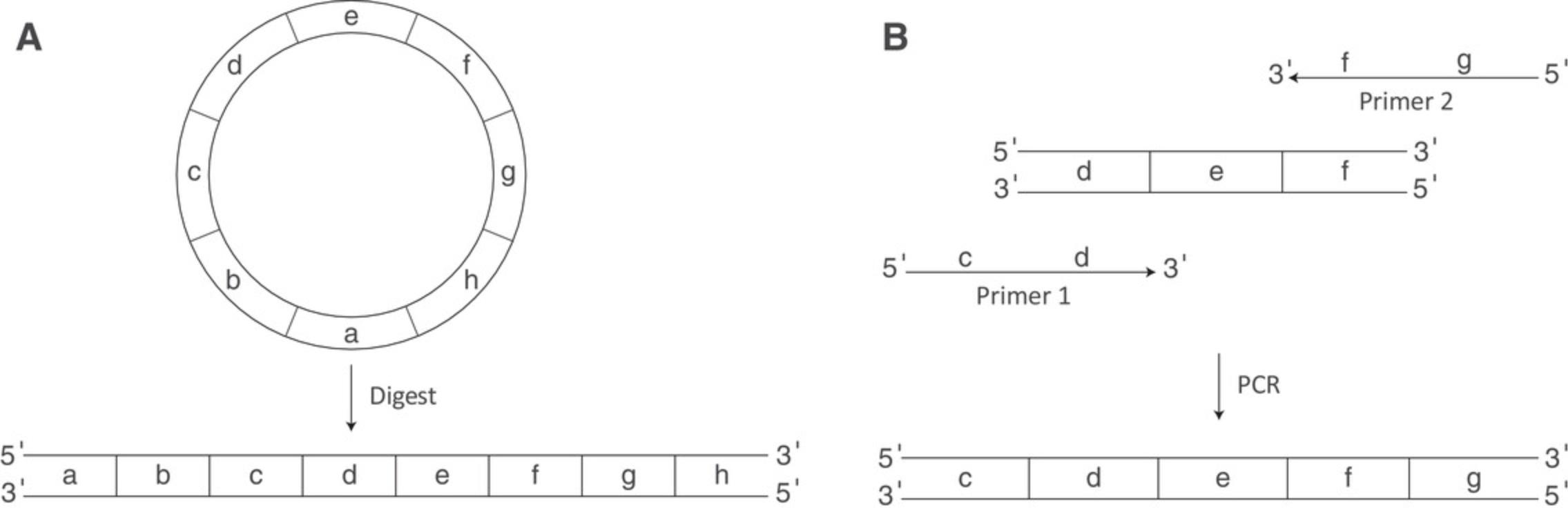
While it is likely that CleanCap would function with SP6 or T3 RNA polymerases, to the best of our knowledge CleanCap AG has only been tested with T7 RNA polymerase. Figure 2B shows the correct T7 promoter sequence (underlined) and initiator sequence for CleanCap AG.
Following restriction digest or PCR, the DNA template must be purified to remove enzymes and reaction components. Commercially available column purification such as QIAGEN's Plasmid Plus kits (cat no. 12941, or similar), or phenol/chloroform extraction followed by ethanol precipitation (see Current Protocols article: Dowhan, 2012), are common methods for DNA cleanup. If the template is prepared by phenol/chloroform extraction, all residual phenol must be removed from DNA template prior to IVT, as enzymes may be denatured.
RNase-free environment
RNases in an IVT reaction will degrade an mRNA product. It is essential that all reagents be rigorously RNase free. When possible, use dedicated RNase-free pipettes with filter tips.
Post IVT
Determine if any additional enzymatic reactions will be required after transcription. For example, DNase I treatment is employed to degrade the template, and is essential when using a purification method that does not readily separate RNA from DNA. Purification and analysis methods should be determined prior to beginning IVT. The quality of IVT products is negatively impacted during long periods of being kept in the crude reaction mixture. Make sure all equipment and reagents for downstream steps are available. Refer to Figure 4 for workflow options.
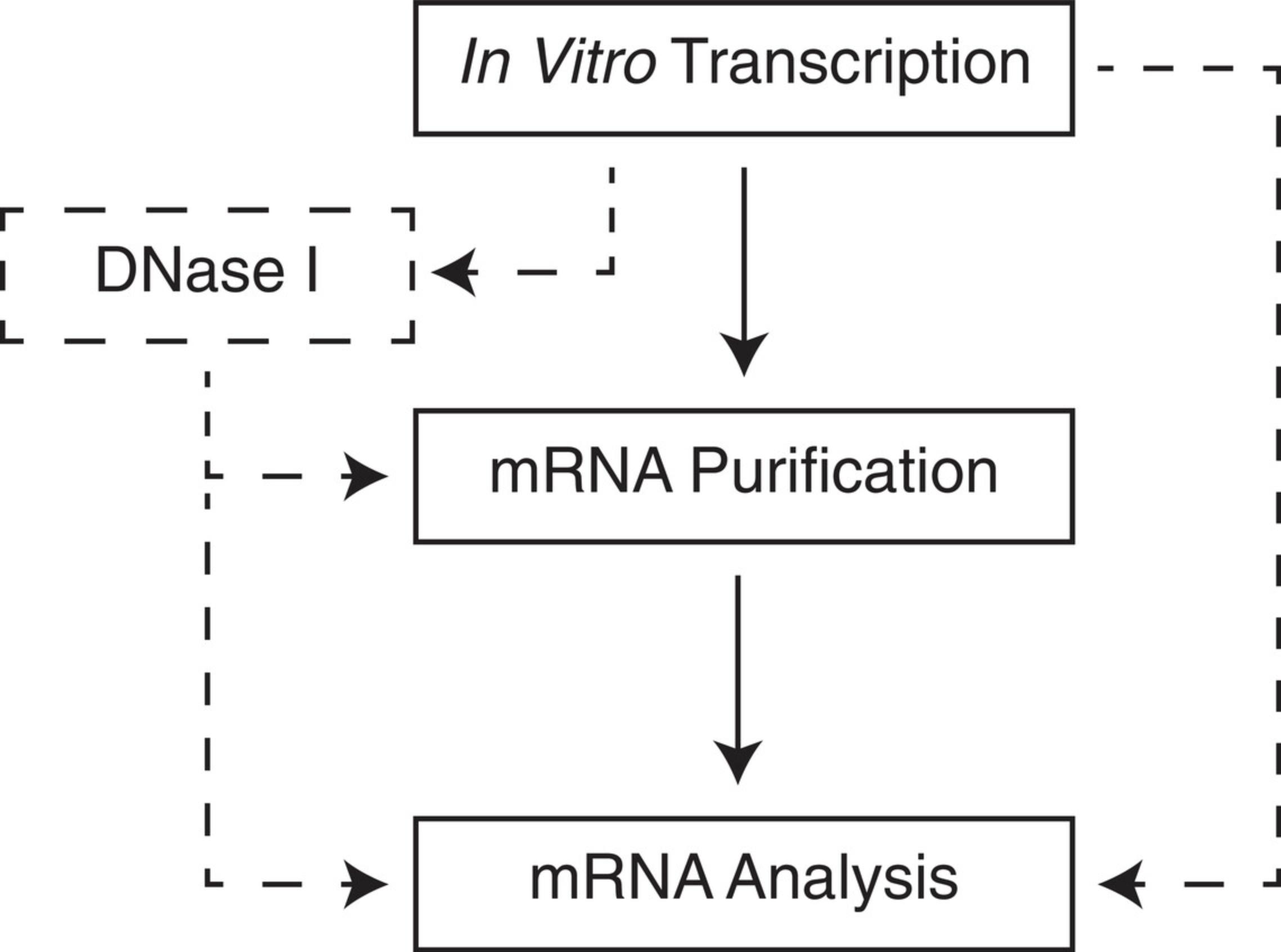
Basic Protocol 1: IVT WITH CleanCap
This procedure describes IVT of a DNA template containing a T7 promoter site and poly(A) track, with a co-transcriptional CleanCap AG analog, to produce a cap 1 mRNA. An optional DNase I treatment step is described. Reaction components will be mixed and incubated at 37°C for an extended time to allow RNA polymerization to occur. Reaction size may be scaled to achieve desired amounts of capped mRNA as necessary. The basic protocol below yields 5 mg of capped RNA per ml of reaction on average; however, template length and quality may affect actual results. Higher reaction yields may be possible with optimization of the synthesis protocol. If desired, an aliquot of crude mRNA can be analyzed by gel electrophoresis prior to purification, to verify reaction success (step 12). Purification of IVT product should immediately follow (see Basic Protocol 2).
Materials
-
10% bleach solution
-
RNase- and DNase-free water
-
RNaseZAP™ (Ambion, cat. no. AM9782) or similar decontaminant solution
-
70% ethanol solution
-
ATP, 100 mM (Thermo Fisher, cat no. R0481 or similar)
-
CTP, 100 mM (Thermo Fisher, cat no. R0481 or similar)
-
GTP, 100 mM (Thermo Fisher, cat no. R0481 or similar)
-
UTP, 100 mM (Thermo Fisher, cat no. R0481 or similar
-
CleanCap AG®, 100 mM (TriLink Biotechnologies, cat. no. N-7113)
-
10× in vitro transcription (IVT) buffer (see recipe)
-
Purified, linearized DNA transcription template (500-1000 μg/ml)
-
10× DNase I reaction buffer (optional; NEB, cat no. B0303, or similar)
-
DNase I, RNase-free (optional, NEB, cat no. M0303, or similar)
-
RNase inhibitor, murine, 40 U/µl (NEB, cat no. M0314, or similar)
-
Inorganic pyrophosphatase, yeast, 0.1 U/µl (NEB, cat. no. M2403, or similar)
-
T7 RNA polymerase, 50 U/µl (NEB, cat. no. M0251 or similar)
-
37°C water bath or heat block
-
Microcentrifuge tubes, nuclease-free (VWR, cat. no. 87003-294, or similar)
-
Benchtop vortex
-
Microcentrifuge
-
Micropipettes
-
Cryosafe enzyme cooler or ice bucket
-
Parafilm
Prepare reagents
1.Clean the work area prior to starting the reaction to avoid common lab contaminants. Treat surfaces and pipettes with 10% bleach to ensure no nucleic acid cross contamination. Allow bleach to rest on surfaces for 10 min. Wipe all bleached surfaces and pipettes with RNase-free water followed by RNaseZap and 70% ethanol.
2.Equilibrate water bath to 37°C.
3.Thaw NTPs, 10× IVT buffer, and linearized plasmid transcription template at room temperature. Vortex NTPs and 10× IVT buffer until homogenous. Spin tubes briefly to collect any liquid from caps.
Prepare reaction
4.Combine the components in an RNase-free tube. For a 1-ml reaction, use volumes listed in Table 1.Reactions may be scaled up or down using these ratios depending on desired final mRNA yield, based on approximately 5 mg mRNA per 1 ml reaction. Add transcription components in the order listed in the table.
Component | Final concentration | Amount |
---|---|---|
DNase/RNase-free water | — | Up to 1000 µl |
ATP | 5 mM | 50 µl |
CTP | 5 mM | 50 µl |
GTP | 5 mM | 50 µl |
UTP | 5 mM | 50 µl |
CleanCap AG | 4 mM | 40 µl |
10× transcription buffer | 1× | 100 µl |
DNA template | 25-50 μg/ml | 25-50 µg |
Murine RNase inhibitor | 1 U/μl | 25 µl |
Yeast inorganic pyrophosphatase | 0.002 U/μl | 20 µl |
T7 RNA polymerase | 8 U/μl | 160 µl |
Total volume | — | 1000 µl |
5.Mix total reaction well by gentle pipetting until homogenous. Seal tube with parafilm.
Incubate reaction
6.Incubate the reaction at 37°C in a water bath or heat block.
DNase I treatment (optional)
7.Thaw 10× DNase I reaction buffer and vortex to mix.
8.Add the 10× buffer to the finished IVT reaction mixture. Volumes in Table 2 may be scaled up or down to match IVT volume used.
Component | Final concentration | Volume |
---|---|---|
IVT reaction | — | 1000 µl |
10× DNase buffer | 1× | 133 µl |
DNase I | 0.3 U/µl | 200 µl |
Total volume | — | 1333 µl |
9.Add DNase I enzyme to the finished IVT reaction mixture.
10.Gently mix new reaction until homogenous.
11.Incubate DNase reaction at 37°C for at least 15 min.
Retain crude sample for analysis
12.Save 20 µl of crude IVT reaction at 4°C for troubleshooting purposes.
Purify IVT product
13.Take remaining IVT reaction forward to purification (Basic Protocol 2, step 3).
Basic Protocol 2: mRNA PURIFICATION AND ANALYSIS
Several options for purification of capped mRNA are available. Methods utilized for uncapped, enzymatically capped, or ARCA-capped mRNA purification are appropriate for use with CleanCap AG mRNA. This protocol describes lithium chloride precipitation of IVT material, similar to Dowhan (2012) or Walker and Lorsch (2013), with helpful tips for successful mRNA recovery. Lithium chloride precipitation employs elevated concentrations of lithium cations to selectively precipitate RNA. The precipitated mRNA is pelleted and the supernatant containing IVT salts, free NTPs, proteins, and DNA is discarded. DNase I treatment post-transcription is optional with this RNA purification method, but will ensure complete template DNA removal.
Following purification, the mRNA product should be measured and visualized to assess reaction yield and quality. The most common visualization technique is gel electrophoresis. Electrophoretic techniques achieve size-based separation of RNA molecules by exploiting their constant mass-to-charge ratios. Electrophoresis is commonly performed by means of agarose gel electrophoresis, which uses the porosity of an agarose slab cast at a sample-specific concentration to separate nucleic acids by size alongside known size markers. Voltage is applied to a sample solution, and the negatively charged RNA molecules are forced to migrate toward an anode through a size-selective matrix. Under denaturing conditions RNA migration rates through the matrix are determined nearly exclusively by their length (Armstrong & Shultz, 2008; Goda & Minton, 1995). This protocol provides steps to denature the RNA using a glyoxal loading dye (Rio, 2015), which improves sizing accuracy by eliminating the effects of secondary structure on gel migration.
Materials
-
70% (v/v) ethanol
-
Crude mRNA (Basic Protocol 1, step 13)
-
7.5 M lithium chloride solution (Thermo Fisher, cat. no. AM9480 or similar)
-
Resuspension buffer of choice: e.g., RNase-free water, 1× TE buffer, pH 8.0, 1 mM sodium citrate pH 6.4
-
Agarose
-
NorthernMax™-Gly Gel Prep/Running Buffer, 10× (Thermo Fisher, cat. no. AM8678)
-
NorthernMax™-Gly Sample Loading Dye (Thermo Fisher, cat. no. AM8551, or similar)
-
Ethidium bromide
-
Millennium™ RNA Marker (Thermo Fisher, cat. no. AM7150, or similar )
-
Refrigerated centrifuge and rotor appropriate for centrifugation at 18,500 × g
-
Centrifuge tubes of appropriate volume and strength
-
NanoDrop (Thermo Fisher) or UV-vis spectrophotometer with cuvette
-
50°C water bath or heat block
-
Gel rig with power supply
-
Gel imager
-
Additional reagents and equipment for agarose gel electrophoresis (see Current Protocols article: Armstrong & Schulz, 2008)
Prepare reagents
1.Prepare 70% ethanol and chill at −20°C for at least 1 hr before use.
2.Begin chilling centrifuge to 4°C.
Precipitate sample
3.Transfer crude mRNA from Basic Protocol 1, step 13, to a centrifuge tube that can tolerate at least 18,500 × g with a capacity of 1.5× its current volume.
4.Add LiCl to 2.5 M final concentration.
5.Mix by gentle inversion or swirling. Do not vortex or shake.
6.Chill at −20°C for at least 30 min.
7.Centrifuge 30 min at 18,500 × g , 4°C.
8.Discard the supernatant, taking care to retain the pellet and, potentially, any smaller pellet fragments.
9.Wash the pellet with the cold 70% ethanol prepared in step 1.Use maximum volume allowed by the precipitation tube. Pipet the ethanol over the pellet until it dislodges from the wall of the tube, and allow the pellet to move gently through the wash.
10.Centrifuge the pellet in 70% ethanol 5 min at 12,000 × g , 4°C.
11.Discard the ethanol supernatant. Use a pipet to remove as much of the residual wash as possible without disturbing the pellet.
12.Repeat steps 9-11 to perform a second wash.
13.Dry the pellet.
Resuspend pelleted mRNA sample
14.Resuspend the pellet in a buffer of choice.
Measure mRNA concentration
15.Use NanoDrop per manufacturer's instructions to read the absorbance of the purified mRNA at 260 nm.
Visualize mRNA by gel electrophoresis
Refer to Current Protocols article Armstrong and Schulz (2008) for general guidelines.
16.Melt agarose in 1× NothernMax™-Gly Running Buffer.
17.Allow agarose to cool 10 min. Add 1 µl of ethidium bromide per 100 ml of melted agarose. Swirl to mix. Pour agarose into the rig and allow to set for 30 min. Once gel is set, fill chambers with 1× running buffer.
18.Prepare RNA sample(s) with NorthernMax™-Gly Sample Loading Dye. Incubate samples for 30 min at 50°C.
19.Load size marker and denatured RNA samples in gel wells and apply voltage.
20.Image gel.
Aliquot sample
21.After purification, aliquot the capped mRNA into single-use vials and store long term at −80°C.
REAGENTS AND SOLUTIONS
In vitro transcription (IVT) buffer, 10×
- 400 mM Tris·HCl pH 8.0
- 165 mM magnesium acetate
- 100 mM dithiothreitol (DTT)
- 20 mM spermidine
- 0.02% (v/v) Triton X-100
Fresh transcription buffer is crucial for optimal polymerase activity, as the DTT may oxidize over time. Store at −20°C in single-use aliquots or mix 10× buffer fresh before use.
COMMENTARY
Background Information
As the intermediate code of gene expression, messenger RNA can be harnessed for several research and therapeutic purposes. By directly delivering a synthetic mRNA to a cell, almost any protein of interest can be transiently expressed. In vitro mRNA production should be optimized for high purity and intact molecular structure. This can become time consuming and costly if the appropriate tools are missing. Purity and structure of the IVT product become especially critical during delivery to the cell. Pattern recognition receptors (PRR) detect self- from non-self RNA and trigger the downstream release of interferons, interleukins, and pro-inflammatory cytokines upon binding double-stranded RNA (dsRNA), uncapped RNA, or partially capped RNA (Decroly, Ferron, Lescar, & Canard, 2011). To address dsRNA-induced immunogenicity, modified NTPs such as pseudouridine (Ѱ), N1-methyl-Ѱ, 5-methoxyuridine, and others have been substituted for uridine bases to evade PRR activation (Karikó, Buckstein, Ni, & Weissman, 2005; Vaidyanathan et al., 2018). Alternatively, ion-pairing reversed-phase (IP-RP) HPLC methods (Karikó, Muramatsu, Ludwig, & Weissman, 2011) and/or cellulose chromatography (Baiersdörfer et al., 2019) can be used to clear dsRNA contaminants post IVT. To address the 5′-cap, the only structural element not directly derived from template DNA, two main approaches have been developed: multi-step enzymatic capping or co-transcriptional incorporation of cap analogs.
The 5′-cap structure consists of an N7-methylated guanosine (m7G) connected by a 5′- to 5′-O -triphosphate bridge to the first nucleotide (known as cap 0). An optional 2′-O -methylation of the +1 nucleotide's ribose sugar results in a cap 1 structure (m7GpppN2′OmeN), and 2′-O -methylation of the +1 and +2 ribose sugars results in a cap 2 structure (Topisirovic, Svitkin, Sonenberg, & Shatkin, 2011). Enzymatic reactions using the vaccinia capping enzyme can efficiently produce cap 0, which can be converted to cap 1 with an additional enzyme, 2′-O -methyltransferase. These reactions require the temperature-sensitive methyl donor S -adenosylmethionine, and occur after the T7 RNA polymerase IVT reaction (Ensinger et al., 1975). This second enzymatic reaction requires more product handling and longer incubation time at elevated temperature to denature any structure at the 5′-end of the molecule for enzyme access, which can lead to mRNA degradation.
Co-transcriptional capping with ARCA dimer produces a cap 0 structure and requires a limited GTP concentration in IVT to allow for the cap analog to outcompete the single nucleotide for initiation of the transcript (Stepinski, Waddell, Stolarski, Darzynkiewicz, & Rhoads, 2001). This results in lower reaction yield (approximately 3 mg/ml of reaction volume), lower mRNA quality (truncation of full-length product as limited NTP runs out), and inefficient 5′-capping that requires additional purifications to eliminate immunogenic 5′-triphosphate species from the final mRNA product (Hadas et al., 2019). In mammalian in vivo use, cap 1 typically results in higher gene expression (Furiuchi, 2015), while cap 2 mRNAs are still widely unstudied, without appropriate research tools (Werner et al., 2011). Thus, there is a need for scalable co-transcriptional cap 1 mRNA production in a single step.
Extending chemically synthesized cap analogs from dimers to trimers allows for direct cap 1 production with more flexibility for the +1 nucleotide base composition (U.S. Patent No. 20180273576; Hogrefe, Lebedev, McCaffrey, & Shin, 2018). Similarly, extending the cap analog from trimer to tetramer allows for the possibility of cap 2−initiated 5′-ends during IVT. CleanCap AG trimer allows for 94% or higher cap 1 mRNA synthesis in a one-pot reaction, with increased RNA yield from 3 to 5 mg/ml of IVT. With no limitation in NTP concentrations during transcription, the quality for full-length mRNA also increases compared to other methods, which often show truncated products as GTP substrates run out (Hadas et al., 2019).
Recent work from Sikorski et al. (2020) supports the concept of co-transcriptional trimeric cap analogs, with results showing increased gene expression by cap 1 versus cap 0 in certain cell types. However, template sequences were not appropriately adjusted for trimer hybridization and cap analogs incorporated at −1 and/or +2 positions relative to the “TATA” box. We find that cap efficiency improves when the DNA template is designed to hybridize with the cap analog at both the +1 and +2 positions (Fig. 2B).
Critical Parameters and Troubleshooting
Low product yield or quality
Component mixing and setup are critical for all IVTs to ensure reaction completion. Low mRNA yields and/or quality may occur if the reaction is not homogenized, a component is not homogenized before use, or a component is omitted. Of note, the reaction buffer requires fresh DTT for optimal enzyme activity. Oxidized buffer may contribute to low yields and/or low mRNA quality with increased production of truncated transcripts. Incomplete heat transfer may also contribute to low reaction yields; water baths with mixing are optimal. Avoid reaction inhibitors such as RNase and EDTA. Limit the amount of NaCl in IVT to less than 100 mM. Maintain pH at or below 8 for the duration of the reaction as well as any downstream processes to avoid alkaline hydrolysis of mRNA. Addition of DNase enzyme will immediately degrade the DNA template substrate for T7 RNA polymerase and should only be done after the allotted IVT reaction time.
The ratio of CleanCap reagent to NTPs during IVT is critical for complete capping as well as reaction yield of mRNA. Incomplete capping may occur if the reaction setup includes each NTP at a high molar excess over CleanCap, allowing NTPs to outcompete the CleanCap trimer for initiation (hybridization to template at the +1 and +2 positions). The appropriate ratio in Basic Protocol 1 is 5 mM each NTP to 4 mM cap analog.
DNA template sequence and quality will also influence mRNA quality. The template DNA sequence must include the full T7 promoter consensus sequence (underlined), followed by 5′-AG (TAATACGACTCACTATAAG). The 3′-A in the “TATA” box does not serve as the initiation site. Legacy transcription templates designed for enzymatic or ARCA capping must be mutagenized to initiate with a 5′-AG. Should a highly structured sequence such as a T7 termination site or thermodynamically favored hairpin exist in the template, creating a block in polymerization, truncation of the mRNA may occur. Optimal template design may need to be determined empirically. Confirm that the A 260/A 280 purity ratio of DNA is close to 1.8. A lower ratio may indicate residual phenol, which absorbs maximally at 280 nm. If a longer- or shorter-than-expected RNA molecule is observed after IVT, ensure that the DNA template is of the appropriate size. Incomplete linearization may contribute to longer RNA molecules, as the T7 RNA polymerase will continue to read through any undigested DNA template available. Shorter-than-expected molecules may arise from truncated DNA templates inadvertently formed by multiple cut sites in a plasmid digest, or multiple primer-binding sites in PCR.
RNA integrity
RNA can be difficult to work with, as it is less stable than DNA and readily degraded by ribonucleases, commonly referred to as RNases. RNases can be introduced through reagents, tips, tubes, bottles, surfaces, and human contact. As discussed in Strategic Planning, only use RNase-free consumables. Always wear gloves during setup, transcription, and handling of mRNA product. Change gloves often. Surfaces and pipettes can be wiped down with RNaseZap or equivalent RNase cleaning solution to destroy RNases.
Planning for necessary purifications and aliquotting is critical to maintaining integrity of the molecule. It is critical to limit handling and hold times throughout this process. Reduce the number of processing steps and handling time of the mRNA following IVT. Avoid freeze-thaws and prepare single-use aliquots for storage of final product.
mRNA purification
It is essential that the transcription reaction be quenched and purified to obtain mRNA free of proteins, DNA, NTPs, and buffer components such as magnesium, which may cause phosphodiester cleavage at elevated temperatures. Residual DNA and proteins may interfere with downstream applications of the mRNA, while residual free NTPs will result in inflated quantity measurements by UV spectrometry. Removal of dsRNA contaminants is essential for in vivo use, to prevent immunostimulatory reactions (Baiersdörfer et al., 2019; Karikó et al., 2011).
Precipitations are a manual purification method that may require a practiced technique to avoid loss of material. Alternative purification methods include silica membrane columns, preparative HPLC, or oligo(dT) capture of poly(A) tails. Silica membrane purification kits such as RNeasy (Qiagen, cat no. 75162) treat RNA solutions with ethanol and chaotropic salt to selectively bind nucleic acids, allowing salts, free NTPs, and proteins to fall through in a shorter protocol than lithium chloride precipitation (see Current Protocols article: Dowhan, 2012). Such membranes will retain intact template DNA; removal requires DNase I treatment post-transcription. Immobilized oligo(dT) will bind the poly(A) tail of mRNA. This method results in high purity of full-length samples, with most truncated mRNA molecules washed away. Typically, immobilized oligo(dT) resin and mRNA samples are equilibrated in high-salt buffer (∼0.5 M NaCl) to prevent repulsion between negatively charged phosphate backbones. mRNA is applied to the resin, and its poly(A) tails form hydrogen bonds to the oligo(dT) for immobilization via canonical base pairing. An intermediate salt wash (∼0.1 M NaCl) removes unbound impurities, and purified mRNA is eluted with low-salt buffer or water (Aviv & Leder, 1972; Green & Sambrook, 2019). Commercial kits suitable for small-scale oligo(dT) purifications using spin columns or magnetic beads are widely available (for example, Qiagen, cat no. 70022, NEB, cat no. E7490, or Thermo Fisher, cat no. AM1922.)
mRNA analysis
Depending on the application, additional or more sensitive analysis of IVT-produced mRNA beyond what is presented in this article may be necessary. These may include cap efficiency, sequence verification, residual solvents, residual DNA, dsRNA content, and/or poly(A) tail length confirmation. Capping efficiency may be assayed in various ways. However, the most sensitive methods employ mass spectrometry (Efimov et al., 2001; Galloway et al., 2020; Hengesbach, Meusburger, Lyko, & Helm, 2008; Sikorski et al., 2020; Wang et al., 2019).
Alternative techniques for interrogating mRNA length and quality with improved sensitivity over gels include capillary electrophoresis and analytical HPLC. Capillary gel electrophoresis requires more elaborate instrumentation such as a Bioanalyzer or Fragment Analyzer (Agilent part number G2939BA or M5310AA, respectively). The RNA sample is injected into fused silica capillaries filled with a sieving gel, generally polymer solutions such as linear polyacrylamide or hydroxyethylcellulose. Voltage is applied along the length of the capillaries, and the RNA migrates to the anode through the medium. Entanglement between polymers impedes RNA migration, effecting size-based separation. Intercalating fluorophores present in the gel allow quantitative detection when RNA molecules reach a detector positioned near the capillary ends. The result is a plot of fluorescence signal against time, termed an electropherogram. RNA ladders are run alongside samples to establish a relationship between migration time and RNA size, enabling quantification of test sample fragment sizes. mRNA quality can be quantified by comparing fluorescence signal from full-length transcripts to that of degradation products or other contaminants (Todorov, de Carmejane, Walter, & Morris, 2001).
In principle, analytical HPLC is achievable by any chromatographic technique capable of resolving mRNAs by size. The two most established techniques are IP-RP HPLC and size-exclusion chromatography (SEC). IP-RP binds mRNA samples to a hydrophobic resin, including polymers such as polystyrene-divinylbenzene, C18 or other hydrophobic ligands on silica supports, or a combination of the two. The mobile phase contains an ion-pairing reagent, usually an alkylamine such as triethylamine or n -hexylamine, which mediates interaction between the RNA phosphate backbone and the hydrophobic stationary phase. A gradient of organic solvent such as acetonitrile will then elute the RNA in order of increasing size. SEC applies RNA samples to a column packed with inert porous beads. Smaller RNA exhibits slower linear flow down the length of the column because it diffuses into the beads more readily. SEC and IP-RP generally employ UV-vis detectors measuring absorbance at 260 nm (Azarani & Hecker, 2001; Fujii et al., 2014).
Understanding Results
UV-vis measurement of RNA
The expected reaction yield (mass of RNA per volume of IVT) of purified CleanCap AG mRNA using this protocol is 5 mg/ml, with an absorbance maximum at 260 nm and a value of 2.0-2.2 for A 260/A 280 ratio. Figure 5 shows a typical IVT sample post purification with peak absorbance at 260 nm and purity ratio of 2.15 (A 260/A 280). If no absorbance peak is observed at 260 nm, there is little or no RNA in the sample. If traditional cuvette measurements are done in lieu of a NanoDrop, the optimal dilution factor will vary by sample concentration and cuvette pathlength. Refer to the instrumentation manual to ensure an accurate reading within the instrument's quantitative range. If a large dilution factor is used for cuvette reading, RNA concentration may be below the instrument's limit of detection. Try measuring more samples to validate the original reading.
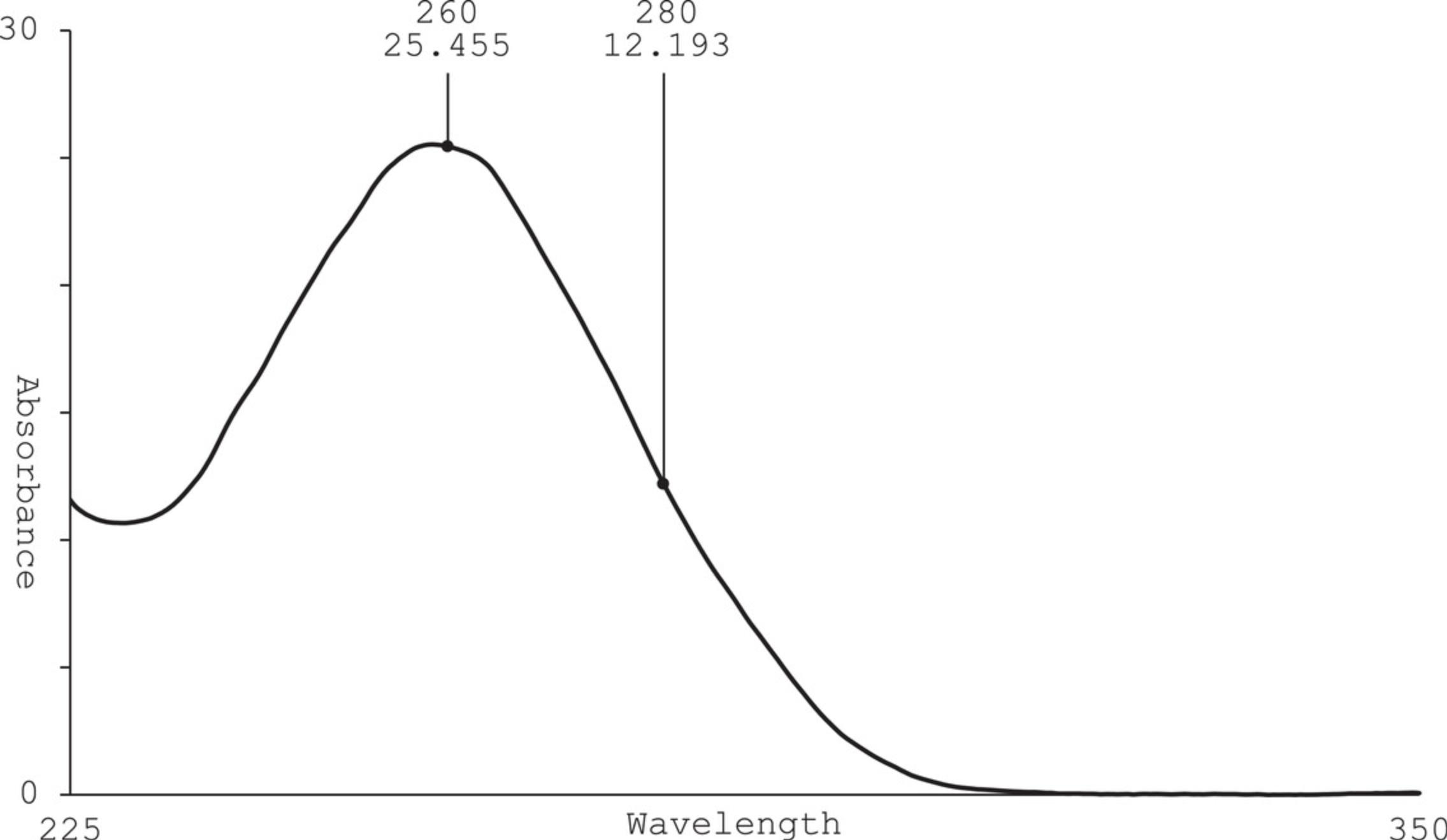
If reaction yield is truly lower than expected, compare crude IVT (sample retained in Basic Protocol 1, step 12) to purified mRNA by gel electrophoresis. This comparison can be used to determine if the RNA was lost during purification or if the IVT reaction was unsuccessful. For gel-loading purposes, assume a crude IVT yield of 5 mg/ml and load equal masses for crude and purified samples. In the gel image following electrophoresis, the band intensity correlates with the mass load. If a dark band is observed in crude sample but not in purified sample, then the RNA may have been lost during precipitation. If a band of lower intensity is observed in crude IVT, then a suboptimal reaction likely occurred. See Troubleshooting guidelines above to improve IVT reaction.
An A 260/A 280 ratio of 1.8 or lower may indicate residual DNA or protein. The mRNA may not be pure enough for downstream applications. Another round of purification or an alternative purification method is recommended.
Interpretation of gel image
Relative to an appropriate size marker, purified RNA in a glyoxal gel will migrate close to true size (length in nucleotides). A gel band at unexpected size can indicate a variety of issues, such as contamination, incomplete template digestion, or incomplete IVT reaction. A typical glyoxal gel of CleanCap mRNA is shown in Figure 6.
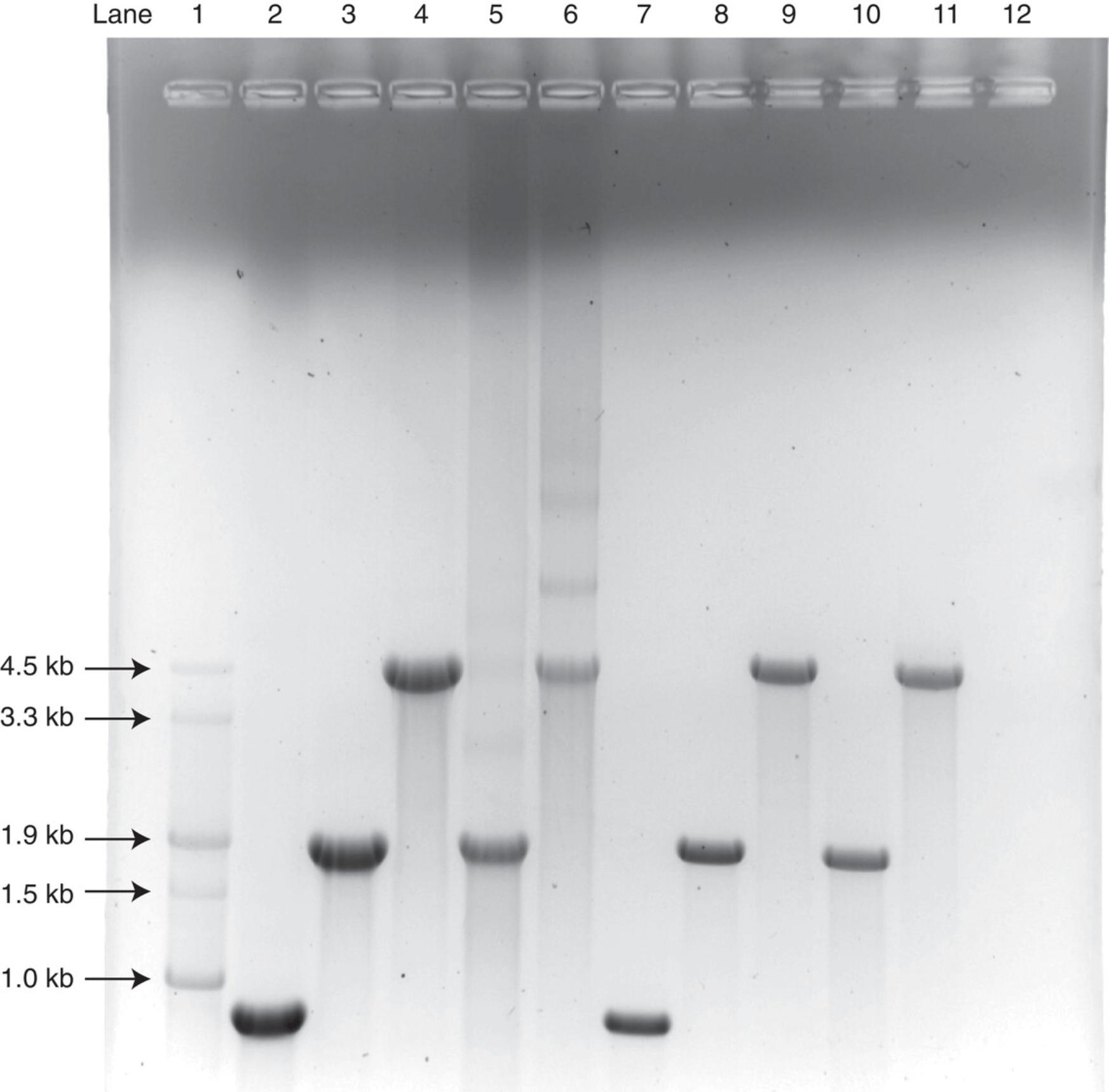
A gel band at expected size with lower-molecular-weight smears indicates degradation of the RNA sample, while higher-molecular-weight smearing can occur in unpurified samples due to residual salts. Lower-molecular-weight smearing is also observed when band intensity is increased by gel overloading. Longer RNA molecules are generally less stable, because the probability of at least one phosphodiester bond break increases with the number of bonds, i.e., RNA length. Thus, lower-molecular-weight smearing is more prevalent for longer RNAs. Significant lower- and higher-molecular-weight smearing is observed in the crude samples shown in Figure 6, lanes 4-6. Following sample purification, these smears are typically reduced, and sample analysis shows a clean single band as shown in Figure 6, lanes 10 (purified sample shown in lane 5) and 11 (purified sample shown in lane 6).
A lack of denaturation, i.e., analysis of RNA by native gels, will result in relatively higher mobility, producing bands that run at lower-than-expected size and/or multiple bands.
Time Considerations
IVT, purification, and analysis can be done in 1-2 days. IVT setup will take approximately 1 hr, including reagent thaw time. The IVT reaction will take 2-3 hr, and optional DNase I treatment will take approximately 30 min. Purification by LiCl may be split over 2 days, with samples incubating overnight at −20°C. UV measurements are completed in 10 min or less, and gel analysis may range from 30 to 90 min depending on gel size and precast gel options.
Acknowledgments
We would like to thank and acknowledge Drs. Richard Hogrefe, Alexandre Lebedev, Anton McCaffrey, and Dongwon Shin for their contributions to the invention of CleanCap®. We thank Dr. Krist Azizian and Sabrina Shore for their previous contributions toward the development of this procedure.
Author Contributions
Jordana M. Henderson : Investigation; methodology; visualization; writing-original draft; writing-review & editing. Andrew Ujita : Investigation; validation; writing-original draft; writing-review & editing. Elizabeth Hill : Visualization; writing-original draft. Sally Yousif-Rosales : writing-original draft. Cory Smith : writing-original draft. Nicholas Ko : writing-original draft. Taylor McReynolds : writing-original draft. Charles R. Cabral : Supervision. Julienne R. Escamilla-Powers : Data curation; methodology; resources; supervision; validation; writing-review & editing. Michael E. Houston : Resources; supervision; writing-review & editing.
Conflict of Interest
The authors are employees of TriLink Biotechnologies, LLC.
Open Research
Data Availability Statement
Data sharing not applicable–no new data generated.
Literature Cited
- Armstrong, J. A., & Schulz, J. R. (2008). Agarose gel electrophoresis. Current Protocols Essential Laboratory Techniques , 00, 7.2.1–7.2.20. doi: 10.1002/9780470089941.et0702s00.
- Aviv, H., & Leder, P. (1972). Purification of biologically active globin messenger RNA by chromatography on oligothymidylic acid−cellulose. Proceedings of the National Academy of Sciences of the United States of America , 69(6), 1408–1412.
- Azarani, A., & Hecker, K. H. (2001). RNA analysis by ion-pair reversed-phase high performance liquid chromatography. Nucleic Acids Research , 29(2), e7. doi: 10.1093/nar/29.2.e7.
- Baiersdörfer, M., Boros, G., Muramatsu, H., Mahiny, A., Vlatkovic, I., Sahin, U., & Karikó, K. (2019). A facile method for the removal of dsRNA contaminant from in vitro-transcribed mRNA. Molecular Therapy Nucleic Acids , 15, 26–35. doi: 10.1016/j.omtn.2019.02.018.
- Chamberlin, M., & Ring, J. (1973). Characterization of T7-specific ribonucleic acid polymerase. 1. General properties of the enzymatic reaction and the template specificity of the enzyme. The Journal of Biological Chemistry , 248(6), 2235–2244. doi: 10.1016/S0021-9258(19)44211-7.
- Decroly, E., Ferron, F., Lescar, J., & Canard, B. (2011). Conventional and unconventional mechanisms for capping viral mRNA. Nature Reviews: Microbiology , 10(1), 51–65. doi: 10.1038/nrmicro2675.
- Dowhan, D. H. (2012). Purification and concentration of nucleic acids. Current Protocols Essential Laboratory Techniques , 5, 2.1–5.2.21. doi: 10.1002/9780470089941.et0502s06.
- Efimov, V. A., Chakhmakhcheva, O. G., Archdeacon, J., Fernandez, J. M., Fedorkin, O. N., Dorokhov, Y. L., & Atabekov, J. G. (2001). Detection of the 5′-cap structure of messenger RNAs with the use of the cap-jumping approach. Nucleic Acids Research , 29(22), 4751–4759. doi: 10.1093/nar/29.22.4751.
- Ensinger, M. J., Martin, S. A., Paoletti, E., & Moss, B. (1975). Modification of the 5′-terminus of mRNA by soluble guanylyl and methyl transferases from vaccinia virus. Proceedings of the National Academy of Sciences of the United States of America , 72(7), 2525–2529. doi: 10.1073/pnas.72.7.2525.
- Fujii, S., Inagaki, K., Miyashita, S., Groombridge, A. S., Nagasawa, K., Chiba, K., & Takatsu, A. (2014). Separation and quantification of RNA molecules using size-exclusion chromatography hyphenated with inductively coupled plasma-mass spectrometry. Electrophoresis , 35, 1315–1318. doi: 10.1002/elps.201300477
- Furuichi, Y. (2015). Discovery of m(7)G-cap in eukaryotic mRNAs. Proceedings of the Japan Academy. Series B, Physical and Biological Sciences , 91(8), 394–409. doi: 10.2183/pjab.91.394.
- Gallagher, S. R., & Desjardins, P. (2011). Quantitation of nucleic acids and proteins. Current Protocols Essential Laboratory Techniques , 5, 2.2.1–2.2.36. doi: 10.1002/9780470089941.et0202s5.
- Galloway, A., Atrih, A., Grzela, R., Darzynkiewicz, E., Ferguson, M., & Cowling, V. H. (2020). CAP-MAP: Cap analysis protocol with minimal analyte processing, a rapid and sensitive approach to analysing mRNA cap structures. Open Biology , 10(2), 190306. doi: 10.1098/rsob.190306.
- Goda, S. K., & Minton, N. P. (1995). A simple procedure for gel electrophoresis and northern blotting of RNA. Nucleic Acids Research , 23(16), 3357–3358. doi: 10.1093/nar/23.16.3357.
- Green, M. R., & Sambrook, J. (2019). Isolation of poly(A)+ messenger RNA using magnetic oligo(dT) beads. Cold Spring Harbor Protocols , 2019(10).
- Hadas, Y., Sultana, N., Youssef, E., Sharkar, M., Kaur, K., Chepurko, E., & Zangi, L. (2019). Optimizing modified mRNA in vitro synthesis protocol for heart gene therapy. Molecular Therapy Methods & Clinical Development, 14, 300–305.
- Haynes, B. F. (2020). A New vaccine to battle Covid-19. The New England Journal of Medicine , NEJMe2035557. Advance online publication. doi: 10.1056/NEJMe2035557.
- Hengesbach, M., Meusburger, M., Lyko, F., & Helm, M. (2008). Use of DNAzymes for site-specific analysis of ribonucleotide modifications. RNA (New York, N.Y.) , 14(1), 180–187. doi: 10.1261/rna.742708.
- Hogrefe, R. I., Lebedev, A., McCaffrey, A. P., & Shin, D. (2018). U.S. Patent No. 20180273576. Washington, DC: U.S. Patent and Trademark Office.
- Ishikawa, M., Murai, R., Hagiwara, H., Hoshino, T., & Suyama, K. (2009). Preparation of eukaryotic mRNA having differently methylated adenosine at the 5′-terminus and the effect of the methyl group in translation. Nucleic Acids Symposium Series , 53, 129–131. doi: 10.1093/nass/nrp065.
- Jalkanen, A. L., Coleman, S. J., & Wilusz, J. (2014). Determinants and implications of mRNA poly(A) tail size–does this protein make my tail look big? Seminars in Cell & Developmental Biology, 34, 24–32. doi: 10.1016/j.semcdb.2014.05.018.
- Karikó, K., Buckstein, M., Ni, H., & Weissman, D. (2005). Suppression of RNA recognition by toll-like receptors: The impact of nucleoside modification and the evolutionary origin of RNA. Immunity , 23(2), 165–175. doi: 10.1016/j.immuni.2005.06.008.
- Karikó, K., Muramatsu, H., Ludwig, J., & Weissman, D. (2011). Generating the optimal mRNA for therapy: HPLC purification eliminates immune activation and improves translation of nucleoside-modified, protein-encoding mRNA. Nucleic Acids Research , 39(21), e142. doi: 10.1093/nar/gkr695.
- Kis, Z., Kontoravdi, C., Dey, A. K., Shattock, R., & Shah, N. (2020). Rapid development and deployment of high-volume vaccines for pandemic response. Journal of Advanced Manufacturing Processes , 2, e10060. doi: 10.1002/amp2.10060.
- Kowalski, P. S., Rudra, A., Miao, L., & Anderson, D. G. (2019). Delivering the messenger: Advances in technologies for therapeutic mRNA delivery. Molecular Therapy: The Journal of the American Society of Gene Therapy , 27(4), 710–728. doi: 10.1016/j.ymthe.2019.02.012.
- Krammer, F. (2020). SARS-CoV-2 vaccines in development. Nature , 586(7830), 516–527. doi: 10.1038/s41586-020-2798-3.
- Moss, B., Gershowitz, A., Wei, C. M., & Boone, R. (1976). Formation of the guanylylated and methylated 5′-terminus of vaccinia virus mRNA. Virology , 72(2), 341–351. doi: 10.1016/0042-6822(76)90163-X.
- Ramanathan, A., Robb, G. B., & Chan, S. H. (2016). mRNA capping: Biological functions and applications. Nucleic Acids Research , 44(16), 7511–7526. doi: 10.1093/nar/gkw551.
- Rio, D. C. (2015). Denaturation and electrophoresis of RNA with glyoxal. Cold Spring Harbor Protocols , 2015(2), 223–226.
- Sahin, U., Karikó, K., & Türeci, Ö (2014). mRNA-based therapeutics–developing a new class of drugs. Nature Reviews Drug Discovery , 13(10), 759–780. doi: 10.1038/nrd4278.
- Sahin, U., Muik, A., Derhovanessian, E., Vogler, I., Kranz, L. M., Vormehr, M., … Türeci, Ö. (2020). COVID-19 vaccine BNT162b1 elicits human antibody and TH1 T cell responses. Nature , 586, 594–599. doi: 10.1038/s41586-020-2814-7.
- Sikorski, P. J., Warminski, M., Kubacka, D., Ratajczak, T., Nowis, D., Kowalska, J., & Jemielity, J. (2020). The identity and methylation status of the first transcribed nucleotide in eukaryotic mRNA 5′ cap modulates protein expression in living cells. Nucleic Acids Research , 48(4), 1607–1626. doi: 10.1093/nar/gkaa032.
- Stepinski, J., Waddell, C., Stolarski, R., Darzynkiewicz, E., & Rhoads, R. E. (2001). Synthesis and properties of mRNAs containing the novel “anti-reverse” cap analogs 7-methyl(3′-O -methyl)GpppG and 7-methyl (3′-deoxy)GpppG. RNA (New York, N.Y.) , 7(10), 1486–1495.
- Todorov, T. I., de Carmejane, O., Walter, N. G., & Morris, M. D. (2001). Capillary electrophoresis of RNA in dilute and semidilute polymer solutions. Electrophoresis , 22(12), 2442–2447. doi: 10.1002/1522-2683(200107)22:12%3c2442::AID-ELPS2442%3e3.0.CO;2-9.
- Topisirovic, I., Svitkin, Y. V., Sonenberg, N., & Shatkin, A. J. (2011). Cap and cap-binding proteins in the control of gene expression. Wiley Interdisciplinary Reviews RNA , 2(2), 277–298. doi: 10.1002/wrna.52.
- Vaidyanathan, S., Azizian, K. T., Haque, A., Henderson, J. M., Hendel, A., Shore, S., … McCaffrey, A. P. (2018). Uridine depletion and chemical modification increase Cas9 mRNA activity and reduce immunogenicity without HPLC purification. Molecular Therapy Nucleic Acids , 12, 530–542. doi: 10.1016/j.omtn.2018.06.010.
- Walker, S. E., & Lorsch, J. (2013). RNA purification-precipitation methods. Methods in Enzymology , 530, 337–343. doi: 10.1016/B978-0-12-420037-1.00019-1.
- Wang, J., Alvin Chew, B. L., Lai, Y., Dong, H., Xu, L., Balamkundu, S., … Dedon, P. C. (2019). Quantifying the RNA cap epitranscriptome reveals novel caps in cellular and viral RNA. Nucleic Acids Research , 47(20), e130. doi: 10.1093/nar/gkz751.
- Warren, L., Manos, P. D., Ahfeldt, T., Loh, Y. H., Li, H., Lau, F., … Rossi, D. J. (2010). Highly efficient reprogramming to pluripotency and directed differentiation of human cells with synthetic modified mRNA. Cell Stem Cell , 7(5), 618–630. doi: 10.1016/j.stem.2010.08.012.
- Werner, M., Purta, E., Kaminska, K. H., Cymerman, I. A., Campbell, D. A., Mittra, B., … Bujnicki, J. M. (2011). 2′-O-ribose methylation of cap2 in human: Function and evolution in a horizontally mobile family. Nucleic Acids Research , 39(11), 4756–4768. doi: 10.1093/nar/gkr038.
Corrections
In this publication, the following corrections have been made:
An error was corrected for the concentration of Triton X–100 in the recipe for 10× in vitro transcription (IVT) buffer in the Reagents and Solutions section, which now reads 0.02% (not 0.2%).
Author's conflict of interest and data availability statement have been added.
The current version online now includes this correction and may be considered the authoritative version of record.
Citing Literature
Number of times cited according to CrossRef: 112
- Anya M Hillery, The science of mRNA, mRNA Therapeutics, 10.1016/B978-0-443-28934-7.00003-0, (67-94), (2025).
- Bangjie Chen, Yipin Yang, Xinyi Wang, Wenzhi Yang, You Lu, Daoyue Wang, Enba Zhuo, Yanchao Tang, Junhong Su, Guozheng Tang, Song Shao, Kangsheng Gu, mRNA vaccine development and applications: A special focus on tumors (Review), International Journal of Oncology, 10.3892/ijo.2024.5669, 65 , 2, (2024).
- Zhongyan Wu, Weilu Sun, Hailong Qi, Recent Advancements in mRNA Vaccines: From Target Selection to Delivery Systems, Vaccines, 10.3390/vaccines12080873, 12 , 8, (873), (2024).
- Maciej Lukaszewicz, Application of Mammalian Nudix Enzymes to Capped RNA Analysis, Pharmaceuticals, 10.3390/ph17091195, 17 , 9, (1195), (2024).
- Wei He, Xinya Zhang, Yangxiaoyu Zou, Ji Li, Chong Wang, Yucai He, Qiuheng Jin, Jianren Ye, Effective Synthesis of High-Integrity mRNA Using In Vitro Transcription, Molecules, 10.3390/molecules29112461, 29 , 11, (2461), (2024).
- Robin Lenk, Werner Kleindienst, Gábor Tamás Szabó, Markus Baiersdörfer, Gábor Boros, Jason M. Keller, Azita J. Mahiny, Irena Vlatkovic, Understanding the impact of in vitro transcription byproducts and contaminants, Frontiers in Molecular Biosciences, 10.3389/fmolb.2024.1426129, 11 , (2024).
- Karol Kurpiejewski, Anna Stankiewicz-Drogon, Karolina Piecyk, Eliza Rajkowska, Paulina Skrzypczyk, Jingping Geng, Edward Darzynkiewicz, Renata Grzela, Marzena Jankowska-Anyszka, The potential of N2-modified cap analogues for precise genetic manipulation through mRNA engineering, Frontiers in Molecular Biosciences, 10.3389/fmolb.2023.1269028, 10 , (2024).
- Yuxuan Hu, Chengtao Pu, Boxiang Liu, Liang Zhang, The rational design of mRNA vaccine: From empirical method to artificial intelligence-based design, Chinese Science Bulletin, 10.1360/TB-2024-0478, (2024).
- Ruei-Min Lu, Hsiang-En Hsu, Ser John Lynon P. Perez, Monika Kumari, Guan-Hong Chen, Ming-Hsiang Hong, Yin-Shiou Lin, Ching-Hang Liu, Shih-Han Ko, Christian Angelo P. Concio, Yi-Jen Su, Yi-Han Chang, Wen-Shan Li, Han-Chung Wu, Current landscape of mRNA technologies and delivery systems for new modality therapeutics, Journal of Biomedical Science, 10.1186/s12929-024-01080-z, 31 , 1, (2024).
- Hiva Azizi, Tyler M Renner, Gerard Agbayani, Bryan Simard, Renu Dudani, Blair A Harrison, Umar Iqbal, Yimei Jia, Michael J McCluskie, Bassel Akache, Self-amplifying RNAs generated with the modified nucleotides 5-methylcytidine and 5-methyluridine mediate strong expression and immunogenicity in vivo , NAR Molecular Medicine, 10.1093/narmme/ugae004, 1 , 2, (2024).
- Chaobin Zhang, Yuhang Wang, Jianding Peng, Xiaotian Wen, Youwen Zhang, Kejun Li, Hanjian Du, Xiaofei Hu, Decoding trends in mRNA vaccine research: A comprehensive bibliometric study, Human Vaccines & Immunotherapeutics, 10.1080/21645515.2024.2355037, 20 , 1, (2024).
- Ruhui Yao, Chunyuan Xie, Xiaojun Xia, Recent progress in mRNA cancer vaccines, Human Vaccines & Immunotherapeutics, 10.1080/21645515.2024.2307187, 20 , 1, (2024).
- Combes Francis, Pettersson Frida J, Bui Thanh-Huong, Molska Alicja, Komissarov Artem, Parot Jérémie, Borgos Sven Even, Urea supplementation improves mRNA in vitro transcription by decreasing both shorter and longer RNA byproducts, RNA Biology, 10.1080/15476286.2024.2321764, 21 , 1, (1-6), (2024).
- Mathew Miller, Oscar Alvizo, Scott Baskerville, Avinash Chintala, Chinping Chng, Justin Dassie, Jonathan Dorigatti, Gjalt Huisman, Stephan Jenne, Supriya Kadam, Neil Leatherbury, Stefan Lutz, Melissa Mayo, Arpan Mukherjee, Antoinette Sero, Stuart Sundseth, Jonathan Penfield, James Riggins, Xiyun Zhang, An engineered T7 RNA polymerase for efficient co-transcriptional capping with reduced dsRNA byproducts in mRNA synthesis, Faraday Discussions, 10.1039/D4FD00023D, (2024).
- Sara Sousa Rosa, Shuran Zhang, Yustika Sari, Marco P. C. Marques, A (RP)UHPLC/UV analytical method to quantify dsRNA during the mRNA vaccine manufacturing process, Analytical Methods, 10.1039/D4AY00560K, 16 , 30, (5146-5153), (2024).
- Anna Slezak, Kevin Chang, Samir Hossainy, Aslan Mansurov, Stuart J. Rowan, Jeffrey A. Hubbell, Mustafa O. Guler, Therapeutic synthetic and natural materials for immunoengineering, Chemical Society Reviews, 10.1039/D3CS00805C, 53 , 4, (1789-1822), (2024).
- U. Sandy Tretbar, Joel G. Rurik, Even H. Rustad, Duran Sürün, Ulrike Köhl, Johanna Olweus, Frank Buchholz, Zoltán Ivics, Stephan Fricke, Ulrich Blache, Non-viral vectors for chimeric antigen receptor immunotherapy, Nature Reviews Methods Primers, 10.1038/s43586-024-00348-w, 4 , 1, (2024).
- Yustika Sari, Sara Sousa Rosa, Jack Jeffries, Marco P. C. Marques, Comprehensive evaluation of T7 promoter for enhanced yield and quality in mRNA production, Scientific Reports, 10.1038/s41598-024-59978-5, 14 , 1, (2024).
- Xiaozhu Zhang, Briana Van Treeck, Connor A. Horton, Jeremy J. R. McIntyre, Sarah M. Palm, Justin L. Shumate, Kathleen Collins, Harnessing eukaryotic retroelement proteins for transgene insertion into human safe-harbor loci, Nature Biotechnology, 10.1038/s41587-024-02137-y, (2024).
- Norbert Pardi, Florian Krammer, mRNA vaccines for infectious diseases — advances, challenges and opportunities, Nature Reviews Drug Discovery, 10.1038/s41573-024-01042-y, (2024).
- Marcin Warminski, Edyta Trepkowska, Miroslaw Smietanski, Pawel J. Sikorski, Marek R. Baranowski, Marcelina Bednarczyk, Hanna Kedzierska, Bartosz Majewski, Adam Mamot, Diana Papiernik, Agnieszka Popielec, Remigiusz A. Serwa, Brittany A. Shimanski, Piotr Sklepkiewicz, Marta Sklucka, Olga Sokolowska, Tomasz Spiewla, Diana Toczydlowska-Socha, Zofia Warminska, Karol Wolosewicz, Joanna Zuberek, Jeffrey S. Mugridge, Dominika Nowis, Jakub Golab, Jacek Jemielity, Joanna Kowalska, Trinucleotide mRNA Cap Analogue N 6-Benzylated at the Site of Posttranscriptional m6 A m Mark Facilitates mRNA Purification and Confers Superior Translational Properties In Vitro and In Vivo , Journal of the American Chemical Society, 10.1021/jacs.3c12629, 146 , 12, (8149-8163), (2024).
- Aritra Sarkar, Guogang Dong, Jennifer Quaglia-Motta, Kelly Sackett, Flow-NMR as a Process-Monitoring Tool for mRNA IVT Reaction, Journal of Pharmaceutical Sciences, 10.1016/j.xphs.2023.11.021, 113 , 4, (900-905), (2024).
- Xue Feng, Zhengjun Li, Zhiguo Su, Shiyi Che, Baiqian Dai, Yuan Cheng, Songping Zhang, Rapid and high recovery isolation of mRNA from in vitro transcription system by ammonium sulphate precipitation at room temperature, Separation and Purification Technology, 10.1016/j.seppur.2024.126331, 336 , (126331), (2024).
- Zohre Eftekhari, Horieh Zohrabi, Akbar Oghalaie, Tahereh Ebrahimi, Fatemeh Sadat Shariati, Mahdi Behdani, Fatemeh Kazemi-Lomedasht, Advancements and challenges in mRNA and ribonucleoprotein-based therapies: From delivery systems to clinical applications, Molecular Therapy - Nucleic Acids, 10.1016/j.omtn.2024.102313, 35 , 3, (102313), (2024).
- Xijun Piao, Yujie Tang, Xiuzhi Li, Weicheng Zhang, Wei Yang, Xining Xu, Wenjing Wang, Jiajia Jiang, Jun Xu, Kunkun Hu, Meiling Xu, Mengjie Liu, Mengfei Sun, Lin Jin, Supercoiled DNA percentage: A key in-process control of linear DNA template for mRNA drug substance manufacturing, Molecular Therapy - Nucleic Acids, 10.1016/j.omtn.2024.102223, 35 , 2, (102223), (2024).
- Alberto Rubio-Casillas, David Cowley, Mikolaj Raszek, Vladimir N. Uversky, Elrashdy M. Redwan, Review: N1-methyl-pseudouridine (m1Ψ): Friend or foe of cancer?, International Journal of Biological Macromolecules, 10.1016/j.ijbiomac.2024.131427, 267 , (131427), (2024).
- Daniel M. Dayeh, Jaclyn Cika, Youmi Moon, Steven Henderson, Deanna Di Grandi, Yue Fu, Kathir Muthusamy, Nisha Palackal, Peter M. Ihnat, Erica A. Pyles, Comprehensive chromatographic assessment of forced degraded in vitro transcribed mRNA, Journal of Chromatography A, 10.1016/j.chroma.2024.464885, 1722 , (464885), (2024).
- Emily A. Dewar, Peter Guterstam, David Holland, Susanna Lindman, Peter Lundbäck, Susana Brito dos Santos, Sheng-ching Wang, Andrew R. Swartz, Improved mRNA affinity chromatography binding capacity and throughput using an oligo-dT immobilized electrospun polymer nanofiber adsorbent, Journal of Chromatography A, 10.1016/j.chroma.2024.464670, 1717 , (464670), (2024).
- Letao Guo, Zhikai Liu, Shirong Song, Wang Yao, Mei Yang, Guangwen Chen, Maximizing the mRNA productivity for in vitro transcription by optimization of fed-batch strategy, Biochemical Engineering Journal, 10.1016/j.bej.2024.109412, 210 , (109412), (2024).
- Mariona Estapé Senti, Lucía García del Valle, Raymond M. Schiffelers, mRNA delivery systems for cancer immunotherapy: Lipid nanoparticles and beyond, Advanced Drug Delivery Reviews, 10.1016/j.addr.2024.115190, 206 , (115190), (2024).
- Wajid Hussain, Sadia Chaman, Hafiza Nazia Koser, Syed Muhammad Aun, Zainab Bibi, Ayesha Nasir Pirzadi, Jawad Hussain, Zubaria Zubaria, Ghulam Nabi, Muhammad Wajid Ullah, Shenqi Wang, Ishrat Perveen, Nanoparticle-Mediated Mucosal Vaccination: Harnessing Nucleic Acids for Immune Enhancement, Current Microbiology, 10.1007/s00284-024-03803-9, 81 , 9, (2024).
- Qianying Liang, Bowen Tu, Lun Cui, Recombinant T7 RNA polymerase production using ClearColi BL21(DE3) and animal-free media for in vitro transcription, Applied Microbiology and Biotechnology, 10.1007/s00253-023-12939-w, 108 , 1, (2024).
- Máté Vadovics, Hiromi Muramatsu, András Sárközy, Norbert Pardi, Production and Evaluation of Nucleoside-Modified mRNA Vaccines for Infectious Diseases, RNA Vaccines, 10.1007/978-1-0716-3770-8_7, (167-181), (2024).
- Mengzhu Chai, Xinyue Ma, Shuaifa Chang, Xiaofei Cheng, Isolation of Double-Stranded RNAs by Lithium Chloride Fractionation, Double-Stranded RNA, 10.1007/978-1-0716-3702-9_1, (1-5), (2024).
- Boran Li, A Possible Blue Print of Addressing mRNA Vaccine Efficacy, Highlights in Science, Engineering and Technology, 10.54097/hset.v36i.6265, 36 , (1433-1438), (2023).
- Alexey D. Perenkov, Alena D. Sergeeva, Maria V. Vedunova, Dmitri V. Krysko, In Vitro Transcribed RNA-Based Platform Vaccines: Past, Present, and Future, Vaccines, 10.3390/vaccines11101600, 11 , 10, (1600), (2023).
- Jingjing Zhang, Yuheng Liu, Chao Li, Qin Xiao, Dandan Zhang, Yang Chen, Joseph Rosenecker, Xiaoyan Ding, Shan Guan, Recent Advances and Innovations in the Preparation and Purification of In Vitro-Transcribed-mRNA-Based Molecules, Pharmaceutics, 10.3390/pharmaceutics15092182, 15 , 9, (2182), (2023).
- Han Sun, Yu Zhang, Ge Wang, Wen Yang, Yingjie Xu, mRNA-Based Therapeutics in Cancer Treatment, Pharmaceutics, 10.3390/pharmaceutics15020622, 15 , 2, (622), (2023).
- Theofanis Vavilis, Eleni Stamoula, Alexandra Ainatzoglou, Athanasios Sachinidis, Malamatenia Lamprinou, Ioannis Dardalas, Ioannis S. Vizirianakis, mRNA in the Context of Protein Replacement Therapy, Pharmaceutics, 10.3390/pharmaceutics15010166, 15 , 1, (166), (2023).
- Masahito Inagaki, Cell Reprogramming and Differentiation Utilizing Messenger RNA for Regenerative Medicine, Journal of Developmental Biology, 10.3390/jdb12010001, 12 , 1, (1), (2023).
- Sarfaraz K. Niazi, RNA Therapeutics: A Healthcare Paradigm Shift, Biomedicines, 10.3390/biomedicines11051275, 11 , 5, (1275), (2023).
- Maryam Youssef, Cynthia Hitti, Julia Puppin Chaves Fulber, Amine A. Kamen, Enabling mRNA Therapeutics: Current Landscape and Challenges in Manufacturing, Biomolecules, 10.3390/biom13101497, 13 , 10, (1497), (2023).
- Nikoletta Y. Papaioannou, Petros Patsali, Basma Naiisseh, Panayiota L. Papasavva, Lola Koniali, Ryo Kurita, Yukio Nakamura, Soteroula Christou, Maria Sitarou, Claudio Mussolino, Toni Cathomen, Marina Kleanthous, Carsten W. Lederer, High-efficiency editing in hematopoietic stem cells and the HUDEP-2 cell line based on in vitro mRNA synthesis, Frontiers in Genome Editing, 10.3389/fgeed.2023.1141618, 5 , (2023).
- Cao Minh Nguyen, Bac An Luong, Thu Thuy Thi Tran, Hoai Nghia Nguyen, Le Son Tran, Design and Generation of MRNAS Encoding Conserved Regions of SARS-Cov-2 ORf1Ab for T Cell-Mediated Immune Activation, Future Virology, 10.2217/fvl-2023-0066, 18 , 8, (501-516), (2023).
- Binhe Du, Jinzhong Lin, Purification of IVT mRNA by 5′ cap affinity chromatography, Chinese Science Bulletin, 10.1360/TB-2023-1013, (2023).
- Yu-Shiuan Wang, Monika Kumari, Guan-Hong Chen, Ming-Hsiang Hong, Joyce Pei-Yi Yuan, Jui-Ling Tsai, Han-Chung Wu, mRNA-based vaccines and therapeutics: an in-depth survey of current and upcoming clinical applications, Journal of Biomedical Science, 10.1186/s12929-023-00977-5, 30 , 1, (2023).
- Jiacheng Sun, Lu Wang, Rachel C. Matthews, Gregory P. Walcott, Yu-An Lu, Yuhua Wei, Yang Zhou, Lior Zangi, Jianyi Zhang, CCND2 Modified mRNA Activates Cell Cycle of Cardiomyocytes in Hearts With Myocardial Infarction in Mice and Pigs, Circulation Research, 10.1161/CIRCRESAHA.123.322929, 133 , 6, (484-504), (2023).
- V. R. Litvinova, A. P. Rudometov, L. I. Karpenko, A. A. Ilyichev, mRNA Vaccine Platform: mRNA Production and Delivery, Russian Journal of Bioorganic Chemistry, 10.1134/S1068162023020152, 49 , 2, (220-235), (2023).
- Londiwe Simphiwe Mbatha, Jude Akinyelu, Fiona Maiyo, Tukayi Kudanga, Future prospects in mRNA vaccine development, Biomedical Materials, 10.1088/1748-605X/aceceb, 18 , 5, (052006), (2023).
- David Sáez Moreno, Udi Qimron, Joana Azeredo, Lucília Domingues, Towards T7 RNA polymerase (T7RNAP)-based expression system in yeast: challenges and opportunities, Bioengineered, 10.1080/21655979.2023.2180579, 13 , 7-12, (14947-14959), (2023).
- Vivian Weiwen Xue, Sze Chuen Cesar Wong, Bo Li, William Chi Shing Cho, The discovery and development of mRNA vaccines for the prevention of SARS-CoV-2 infection, Expert Opinion on Drug Discovery, 10.1080/17460441.2023.2218083, 18 , 7, (769-780), (2023).
- Lizhou Zhang, Kunal R. More, Amrita Ojha, Cody B. Jackson, Brian D. Quinlan, Hao Li, Wenhui He, Michael Farzan, Norbert Pardi, Hyeryun Choe, Effect of mRNA-LNP components of two globally-marketed COVID-19 vaccines on efficacy and stability, npj Vaccines, 10.1038/s41541-023-00751-6, 8 , 1, (2023).
- Helen M. Gunter, Senel Idrisoglu, Swati Singh, Dae Jong Han, Emily Ariens, Jonathan R. Peters, Ted Wong, Seth W. Cheetham, Jun Xu, Subash Kumar Rai, Robert Feldman, Andy Herbert, Esteban Marcellin, Romain Tropee, Trent Munro, Tim R. Mercer, mRNA vaccine quality analysis using RNA sequencing, Nature Communications, 10.1038/s41467-023-41354-y, 14 , 1, (2023).
- Masahito Inagaki, Naoko Abe, Zhenmin Li, Yuko Nakashima, Susit Acharyya, Kazuya Ogawa, Daisuke Kawaguchi, Haruka Hiraoka, Ayaka Banno, Zheyu Meng, Mizuki Tada, Tatsuma Ishida, Pingxue Lyu, Kengo Kokubo, Hirotaka Murase, Fumitaka Hashiya, Yasuaki Kimura, Satoshi Uchida, Hiroshi Abe, Cap analogs with a hydrophobic photocleavable tag enable facile purification of fully capped mRNA with various cap structures, Nature Communications, 10.1038/s41467-023-38244-8, 14 , 1, (2023).
- Ahmet Hınçer, Recep Erdem Ahan, Ebru Aras, Urartu Özgür Şafak Şeker, Making the Next Generation of Therapeutics: mRNA Meets Synthetic Biology, ACS Synthetic Biology, 10.1021/acssynbio.3c00253, 12 , 9, (2505-2515), (2023).
- Xiaoqing Liu, Pei Huang, Rusen Yang, Hongzhang Deng, mRNA Cancer Vaccines: Construction and Boosting Strategies, ACS Nano, 10.1021/acsnano.3c05635, 17 , 20, (19550-19580), (2023).
- Zhongfeng Ye, Joseph Harmon, Wei Ni, Yamin Li, Douglas Wich, Qiaobing Xu, The mRNA Vaccine Revolution: COVID-19 Has Launched the Future of Vaccinology, ACS Nano, 10.1021/acsnano.2c12584, 17 , 16, (15231-15253), (2023).
- Marvin Möhler, Andres Jäschke, Future Perspectives for the Identification and Sequencing of Nicotinamide Adenine Dinucleotide-Capped RNAs, Accounts of Chemical Research, 10.1021/acs.accounts.3c00446, 56 , 21, (3000-3009), (2023).
- Marcin Warminski, Adam Mamot, Anaïs Depaix, Joanna Kowalska, Jacek Jemielity, Chemical Modifications of mRNA Ends for Therapeutic Applications, Accounts of Chemical Research, 10.1021/acs.accounts.3c00442, 56 , 20, (2814-2826), (2023).
- Chiara F. Magnani, Renier Myburgh, Silvan Brunn, Morgane Chambovey, Marianna Ponzo, Laura Volta, Francesco Manfredi, Christian Pellegrino, Steve Pascolo, Csaba Miskey, Nicolás Sandoval-Villegas, Zoltán Ivics, Judith A. Shizuru, Dario Neri, Markus G. Manz, Anti-CD117 CAR T cells incorporating a safety switch eradicate human acute myeloid leukemia and hematopoietic stem cells, Molecular Therapy - Oncolytics, 10.1016/j.omto.2023.07.003, 30 , (56-71), (2023).
- Anna Andrzejewska, Renata Grzela, Anna Stankiewicz-Drogon, Piotr Rogujski, Siranjeevi Nagaraj, Edward Darzynkiewicz, Barbara Lukomska, Miroslaw Janowski, Mesenchymal stem cell engineering by ARCA analog-capped mRNA, Molecular Therapy - Nucleic Acids, 10.1016/j.omtn.2023.07.006, 33 , (454-468), (2023).
- Tomokazu Amano, Hong Yu, Misa Amano, Erica Leyder, Maria Badiola, Priyanka Ray, Jiyoung Kim, Akihiro C. Ko, Achouak Achour, Nan-ping Weng, Efrat Kochba, Yotam Levin, Minoru S.H. Ko, Controllable self-replicating RNA vaccine delivered intradermally elicits predominantly cellular immunity, iScience, 10.1016/j.isci.2023.106335, 26 , 4, (106335), (2023).
- Diana D. Kang, Haoyuan Li, Yizhou Dong, Advancements of in vitro transcribed mRNA (IVT mRNA) to enable translation into the clinics, Advanced Drug Delivery Reviews, 10.1016/j.addr.2023.114961, 199 , (114961), (2023).
- Anamika Sengupta, mRNA vaccines for COVID-19, Viral Infections and Antiviral Therapies, 10.1016/B978-0-323-91814-5.00007-6, (611-624), (2023).
- Satyendra Prakash, mRNA-Based Nanomedicine: A New Strategy for Treating Infectious Diseases and Beyond, European Journal of Drug Metabolism and Pharmacokinetics, 10.1007/s13318-023-00849-1, 48 , 5, (515-529), (2023).
- Gamaleldin I. Harisa, Tarek M. Faris, Abdelrahman Y. Sherif, Riyad F. Alzhrani, Saleh A. Alanazi, Neveen A. Kohaf, Fars K. Alanazi, Coding Therapeutic Nucleic Acids from Recombinant Proteins to Next-Generation Vaccines: Current Uses, Limitations, and Future Horizons, Molecular Biotechnology, 10.1007/s12033-023-00821-z, 66 , 8, (1853-1871), (2023).
- Hamed Tabasi, Samaneh Mollazadeh, Elham Fazeli, Khalil Abnus, Seyed Mohammad Taghdisi, Mohammad Ramezani, Mona Alibolandi, Transitional Insight into the RNA-Based Oligonucleotides in Cancer Treatment, Applied Biochemistry and Biotechnology, 10.1007/s12010-023-04597-5, 196 , 3, (1685-1711), (2023).
- Ivan R. Corrêa, Eric J. Wolf, Erbay Yigit, S. Hong Chan, Ribonucleases for Sequencing and Characterization of RNA by LC–MS, RNA Structure and Function, 10.1007/978-3-031-36390-0_27, (613-628), (2023).
- Guilherme J. Guimaraes, Jaeah Kim, Michael G. Bartlett, Characterization of mRNA therapeutics, Mass Spectrometry Reviews, 10.1002/mas.21856, 43 , 5, (1066-1090), (2023).
- Steve Pascolo, Nonreplicating synthetic mRNA vaccines: A journey through the European (Journal of Immunology) history, European Journal of Immunology, 10.1002/eji.202249941, 53 , 7, (2023).
- Rebecca Elizabeth McKenzie, Jordan James Minnell, Mitch Ganley, Gavin Frank Painter, Sarah Louise Draper, mRNA Synthesis and Encapsulation in Ionizable Lipid Nanoparticles, Current Protocols, 10.1002/cpz1.898, 3 , 9, (2023).
- Shiyi Che, Xue Feng, Zhengjun Li, Zhiguo Su, Guanghui Ma, Zhikao Li, Aibing Yu, Minsu Liu, Songping Zhang, On‐column capping of poly dT media‐tethered mRNA accomplishes high capping efficiency, enhanced mRNA recovery, and improved stability against RNase, Biotechnology and Bioengineering, 10.1002/bit.28560, 121 , 1, (206-218), (2023).
- Jerry Pelletier, Nahum Sonenberg, The Eukaryotic mRNA Cap Structure, Encyclopedia of Life Sciences, 10.1002/9780470015902.a0029559, (1-10), (2023).
- Andrei A. Deviatkin, Ruslan A. Simonov, Kseniya A. Trutneva, Anna A. Maznina, Elena M. Khavina, Pavel Y. Volchkov, Universal Flu mRNA Vaccine: Promises, Prospects, and Problems, Vaccines, 10.3390/vaccines10050709, 10 , 5, (709), (2022).
- Zoltán Kis, Stability Modelling of mRNA Vaccine Quality Based on Temperature Monitoring throughout the Distribution Chain, Pharmaceutics, 10.3390/pharmaceutics14020430, 14 , 2, (430), (2022).
- Jie Tang, Larry Cai, Chuanfei Xu, Si Sun, Yuheng Liu, Joseph Rosenecker, Shan Guan, Nanotechnologies in Delivery of DNA and mRNA Vaccines to the Nasal and Pulmonary Mucosa, Nanomaterials, 10.3390/nano12020226, 12 , 2, (226), (2022).
- Ajit Magadum, Modified mRNA Therapeutics for Heart Diseases, International Journal of Molecular Sciences, 10.3390/ijms232415514, 23 , 24, (15514), (2022).
- Marcin Ryczek, Martyna Pluta, Leszek Błaszczyk, Agnieszka Kiliszek, Overview of Methods for Large-Scale RNA Synthesis, Applied Sciences, 10.3390/app12031543, 12 , 3, (1543), (2022).
- Reese Jalal Ladak, Alexander J. He, Yu-Hsun Huang, Yu Ding, The Current Landscape of mRNA Vaccines Against Viruses and Cancer–A Mini Review, Frontiers in Immunology, 10.3389/fimmu.2022.885371, 13 , (2022).
- Dylan Kairuz, Nazia Samudh, Abdullah Ely, Patrick Arbuthnot, Kristie Bloom, Advancing mRNA technologies for therapies and vaccines: An African context, Frontiers in Immunology, 10.3389/fimmu.2022.1018961, 13 , (2022).
- S. Hong Chan, Joseph M. Whipple, Nan Dai, Theresa M. Kelley, Kathryn Withers, George Tzertzinis, Ivan R. Corrêa, G. Brett Robb, RNase H-based analysis of synthetic mRNA 5′ cap incorporation, RNA, 10.1261/rna.079173.122, 28 , 8, (1144-1155), (2022).
- Wout De Mey, Arthur Esprit, Kris Thielemans, Karine Breckpot, Lorenzo Franceschini, RNA in Cancer Immunotherapy: Unlocking the Potential of the Immune System, Clinical Cancer Research, 10.1158/1078-0432.CCR-21-3304, 28 , 18, (3929-3939), (2022).
- Eric J Wolf, Sebastian Grünberg, Nan Dai, Tien-Hao Chen, Bijoyita Roy, Erbay Yigit, Ivan R Corrêa, Human RNase 4 improves mRNA sequence characterization by LC–MS/MS, Nucleic Acids Research, 10.1093/nar/gkac632, 50 , 18, (e106-e106), (2022).
- Akon Higuchi, Tzu-Cheng Sung, Ting Wang, Qing-Dong Ling, S. Suresh Kumar, Shih-Tien Hsu, Akihiro Umezawa, Material Design for Next-Generation mRNA Vaccines Using Lipid Nanoparticles, Polymer Reviews, 10.1080/15583724.2022.2106490, 63 , 2, (394-436), (2022).
- Jonathan K. Watts, Michael J. Gait, Synthesis of Nucleic Acids, Nucleic Acids in Chemistry and Biology, 10.1039/9781837671328-00279, (279-323), (2022).
- Eduarde Rohner, Ran Yang, Kylie S. Foo, Alexander Goedel, Kenneth R. Chien, Unlocking the promise of mRNA therapeutics, Nature Biotechnology, 10.1038/s41587-022-01491-z, 40 , 11, (1586-1600), (2022).
- Enyue Fang, Xiaohui Liu, Miao Li, Zelun Zhang, Lifang Song, Baiyu Zhu, Xiaohong Wu, Jingjing Liu, Danhua Zhao, Yuhua Li, Advances in COVID-19 mRNA vaccine development, Signal Transduction and Targeted Therapy, 10.1038/s41392-022-00950-y, 7 , 1, (2022).
- Alison Obinna Nwokeoji, Tachung Chou, Eleojo Ahuva Nwokeoji, Low Resource Integrated Platform for Production and Analysis of Capped mRNA, ACS Synthetic Biology, 10.1021/acssynbio.2c00609, 12 , 1, (329-339), (2022).
- Naoko Abe, Akihiro Imaeda, Masahito Inagaki, Zhenmin Li, Daisuke Kawaguchi, Kaoru Onda, Yuko Nakashima, Satoshi Uchida, Fumitaka Hashiya, Yasuaki Kimura, Hiroshi Abe, Complete Chemical Synthesis of Minimal Messenger RNA by Efficient Chemical Capping Reaction, ACS Chemical Biology, 10.1021/acschembio.1c00996, 17 , 6, (1308-1314), (2022).
- Steffen Fahr, Samantha Ayde Peña-Benavides, Lukas Thiel, Carl Sengoba, Kaan Karacasulu, Nina Ihling, Juan Eduardo Sosa-Hernández, Gary Gilleskie, John M. Woodley, Roberto Parra-Saldivar, Seyed Soheil Mansouri, Kosan Roh, Mobile On Demand COVID-19 Vaccine Production Units for Developing Countries, Industrial & Engineering Chemistry Research, 10.1021/acs.iecr.2c01217, 61 , 35, (13191-13204), (2022).
- Friederike Knipping, Gregory A. Newby, Cindy R. Eide, Amber N. McElroy, Sarah C. Nielsen, Kyle Smith, Yongxing Fang, Tatjana I. Cornu, Caroline Costa, Alejandra Gutierrez-Guerrero, Samuel P. Bingea, Colby J. Feser, Benjamin Steinbeck, Keli L. Hippen, Bruce R. Blazar, Anton McCaffrey, Claudio Mussolino, Els Verhoeyen, Jakub Tolar, David R. Liu, Mark J. Osborn, Disruption of HIV-1 co-receptors CCR5 and CXCR4 in primary human T cells and hematopoietic stem and progenitor cells using base editing, Molecular Therapy, 10.1016/j.ymthe.2021.10.026, 30 , 1, (130-144), (2022).
- Jiali Yang, Jiafeng Zhu, Jiaojiao Sun, Yiyun Chen, Yaran Du, Yiling Tan, Linpeng Wu, Mengting Zhai, Lixiang Wei, Na Li, Ke Huang, Qiangbo Hou, Zhenbo Tong, Andreas Bechthold, Hao Tian, Zhenhua Sun, Chijian Zuo, Intratumoral delivered novel circular mRNA encoding cytokines for immune modulation and cancer therapy, Molecular Therapy - Nucleic Acids, 10.1016/j.omtn.2022.09.010, 30 , (184-197), (2022).
- Wout de Mey, Phaedra De Schrijver, Dorien Autaers, Lena Pfitzer, Bruno Fant, Hanne Locy, Arthur Esprit, Lien Lybaert, Cedric Bogaert, Magali Verdonck, Kris Thielemans, Karine Breckpot, Lorenzo Franceschini, A synthetic DNA template for fast manufacturing of versatile single epitope mRNA, Molecular Therapy - Nucleic Acids, 10.1016/j.omtn.2022.08.021, 29 , (943-954), (2022).
- Miroslaw Janowski, Anna Andrzejewska, The legacy of mRNA engineering: A lineup of pioneers for the Nobel Prize, Molecular Therapy - Nucleic Acids, 10.1016/j.omtn.2022.07.003, 29 , (272-284), (2022).
- Rein Verbeke, Michael J. Hogan, Karin Loré, Norbert Pardi, Innate immune mechanisms of mRNA vaccines, Immunity, 10.1016/j.immuni.2022.10.014, 55 , 11, (1993-2005), (2022).
- Mohammad M. Pourseif, Yosef Masoudi-Sobhanzadeh, Erfan Azari, Sepideh Parvizpour, Jaleh Barar, Rais Ansari, Yadollah Omidi, Self-amplifying mRNA vaccines: Mode of action, design, development and optimization, Drug Discovery Today, 10.1016/j.drudis.2022.103341, 27 , 11, (103341), (2022).
- Itziar Gómez-Aguado, Julen Rodríguez-Castejón, Marina Beraza-Millor, Alicia Rodríguez-Gascón, Ana del Pozo-Rodríguez, María Ángeles Solinís, mRNA delivery technologies: Toward clinical translation, mRNA-Based Therapeutics, 10.1016/bs.ircmb.2022.04.010, (207-293), (2022).
- Suji Kwon, Minseon Kwon, Seongeun Im, Kyuri Lee, Hyukjin Lee, mRNA vaccines: the most recent clinical applications of synthetic mRNA, Archives of Pharmacal Research, 10.1007/s12272-022-01381-7, 45 , 4, (245-262), (2022).
- Dylan Kairuz, Prashika Singh, Tiffany Smith, Patrick Arbuthnot, Abdullah Ely, Kristie Bloom, Synthetic mRNA Gene Therapies and Hepatotropic Non-viral Vectors for the Treatment of Chronic HBV Infections, Messenger RNA Therapeutics, 10.1007/978-3-031-08415-7_8, (157-179), (2022).
- Günther Schmalzing, Fritz Markwardt, Established Protocols for cRNA Expression and Voltage-Clamp Characterization of the P2X7 Receptor in Xenopus laevis Oocytes, The P2X7 Receptor, 10.1007/978-1-0716-2384-8_9, (157-192), (2022).
- See more