Autologous Induced Pluripotent Stem Cell–Based Cell Therapies: Promise, Progress, and Challenges
Marinna Madrid, Marinna Madrid, Cenk Sumen, Cenk Sumen, Suvi Aivio, Suvi Aivio, Nabiha Saklayen, Nabiha Saklayen
Abstract
The promise of human induced pluripotent stem cells (iPSCs) lies in their ability to serve as a starting material for autologous, or patient-specific, stem cell–based therapies. Since the first publications describing the generation of iPSCs from human tissue in 2007, a Phase I/IIa clinical trial testing an autologous iPSC-derived cell therapy has been initiated in the U.S., and several other autologous iPSC-based therapies have advanced through various stages of development. Three single-patient in-human transplants of autologous iPSC-derived cells have taken place worldwide. None of the patients suffered serious adverse events, despite not undergoing immunosuppression. These promising outcomes support the proposed advantage of an autologous approach: a cell therapy product that can engraft without the risk of immune rejection, eliminating the need for immunosuppression and the associated side effects. Despite this advantage, there are currently more allogeneic than autologous iPSC-based cell therapy products in development due to the cost and complexity of scaling out manufacturing for each patient. In this review, we highlight recent progress toward clinical translation of autologous iPSC-based cell therapies. We also highlight technological advancements that would reduce the cost and complexity of autologous iPSC-based cell therapy production, enabling autologous iPSC-based therapies to become a more commonplace treatment modality for patients. © 2021 The Authors.
I INTRODUCTION
Pluripotent stem cells (PSCs) can multiply indefinitely and differentiate into all cell types in the body, making them the ideal starting material for developing a range of cell therapies (Yamanaka, 2020). The ability to generate induced PSCs (iPSCs) from easily harvested human tissue was first reported in 2007 (Takahashi et al., 2007; Yu et al., 2007). Since then, iPSCs have been generated from a range of readily sourced tissues, including blood, urine, and hair follicles (Drozd et al., 2015; Ohmine et al., 2011; Petit et al., 2012; Raab, Klingenstein, Liebau, & Linta, 2014). IPSCs are an attractive alternative to human embryonic stem cells (ESCs), which are sourced from human embryos and thus controversial (Ilic & Ogilvie, 2017). However, the real promise of iPSCs lies in their ability to enable the development of autologous, or patient-specific, stem cell–based therapies. Autologous cell therapies may facilitate long-term engraftment without the need for immunosuppression, providing safer treatments for patients (Hatzimichael & Tuthill, 2010; Majhail, Mau, Denzen, & Arneson, 2013; Osborn, Hallett, Schumacher, & Isacson, 2020). Since the discovery of iPSCs, three in-human transplants of autologous iPSC-derived cells have occurred, with the first transplant taking place in 2014 (Mandai et al., 2017; Schweitzer et al., 2020; Takagi et al., 2019) (Clinical Trial Identification number: jRCTa050190117). None of the patients in these trials has suffered serious adverse events, despite not undergoing immunosuppression, an encouraging outcome for the field of personalized regenerative medicine.
In contrast to autologous cell therapies, which are patient specific, allogeneic iPSC-based cell therapies use donor-derived tissues as a starting material. A greater number of allogeneic iPSC-derived cell therapies are being developed than autologous therapies (Martín-Ibáñez & Sareen, 2020). The advantage of allogeneic treatments is that many doses can be manufactured simultaneously from a single batch of iPSCs and banked. The production process can therefore be “scaled up” and achieve economies of scale that reduce the cost of each dose (Simaria et al., 2014). This also allows for “off-the-shelf” distribution, simplifying logistics and minimizing the time to patient (Sullivan et al., 2018; Yamanaka, 2020). Minimizing the time to patient is critical for certain acute indications where the patient requires immediate care. The disadvantage of an allogeneic approach is the potential for immune rejection. Patients must undergo immunosuppression, which is associated with a higher risk of infection and cardiovascular defects (Miller, 2002). One strategy to reduce immune rejection is to create human leukocyte antigen (HLA)-matched haplobanks, in which donor iPSC lines are generated from individuals with the most common HLA haplotypes to match a range of patients (Hanatani & Takasu, 2020; Sullivan et al., 2018). A 100-line HLA-matched bank would cover 78% of European-Americans. However, it would only cover 52% of Hispanics and <50% of African-Americans (Gourraud, Gilson, Girard, & Peschanski, 2011), making it harder for patients from racial and ethnic minority groups to receive treatment. Although HLA matching reduces the risk of an immune reaction, it does not eliminate the need for immunosuppression. Even “immune-privileged” sites, such as the brain or eye, would require short-term immunosuppression for transplantations of HLA-matched cells (Carson, Doose, Melchior, Schmid, & Ploix, 2006; Osborn et al., 2020; Taylor, 2016).
Another approach to circumventing immune rejection in iPSC-derived transplants is creating universal, or immunocloaked, cells. Generation of these cell lines requires multiple rounds of gene editing; critical genes are deleted to avoid T-cell invasion, whereas others are left intact or knocked in to avoid NK-cell invasion (Gornalusse et al., 2017; Meissner, Strominger, & Cowan, 2015; Xu et al., 2019; Yamanaka, 2020). These approaches could enable coverage of the entire human population with significantly fewer iPSC lines than an HLA-matched haplobank, with the off-the-shelf convenience of a standard allogeneic approach. However, this approach is earlier in its development and therefore farther away from clinical use. The multiplexed gene editing required for this approach comes with safety risks, and any cell products will need to be carefully assessed for off-target effects (Zhang, Tee, Wang, Huang, & Yang, 2015). At this point, the safety of autologous iPSC-derived cell transplants has been validated to a greater extent, both in animal models and in humans (Schweitzer et al., 2020; Sharma et al., 2019; Takagi et al., 2019).
Autologous therapies are considered safer than allogeneic from an immune rejection standpoint (Eliopoulos, Stagg, Lejeune, Pommey, & Galipeau, 2005; Kitala et al., 2016; Sohn et al., 2015; Song et al., 2020). Still, the immunogenicity of autologous iPSCs is not without controversy. At least one study described the rejection of autologous iPSCs transplanted into mice (Zhao, Zhang, Rong, & Xu, 2011). Deuse et al. (2009) proposed that this immunogenicity could occur due to de novo mutations in mitochondrial DNA (mtDNA), potentially warranting screening of mtDNA in the development of autologous cell therapy products. However, most studies have not found rejection of autologous iPSCs or iPSC-derived cells in animal models (Araki et al., 2013; Guha, Morgan, Mostoslavsky, Rodrigues, & Boyd, 2013; Osborn et al., 2020; Schweitzer et al., 2020). Human trials involving transplantation of autologous iPSC-derived cells, although limited in number, have resulted in no serious adverse effects, with the most extended follow-up being 4 years post-transplant (Mandai et al., 2017; Schweitzer et al., 2020; Takagi et al., 2019).
An autologous iPSC-based approach may be the safest path toward developing PSC-based cell therapies for specific indications and patient populations (Miller, 2002; Tepperman et al., 2010). These include patients with chronic progressive diseases, patients who are not covered by major HLA-matched haplobanks, and patients who would do poorly under immunosuppression (Gourraud et al., 2011; Miller, 2002; Papapetrou, 2016; Tepperman et al., 2010). With advancements in the enabling technology landscape and relevant regulatory frameworks, autologous iPSC-derived cell therapies will have the potential to benefit a larger fraction of regenerative medicine patients (Jha, Farnoodian, & Bharti, 2020; Martín-Ibáñez & Sareen, 2020). This review covers the breadth of autologous iPSC-derived cell therapies in development (Section II) and describes logistical complexities and other factors to be considered when moving forward (Section III). Although there are still obstacles to overcome, bringing autologous iPSC-derived cell therapies to patients presents a promising path toward treating a wide range of diseases.
II PROGRESS IN AUTOLOGOUS IPSC-BASED CELL THERAPY DEVELOPMENT
Since the first in-human transplant of autologous iPSC-derived cells in 2014, there has been a growth in recent efforts to translate autologous iPSC-based therapies to the clinic (Fig. 1). This section and Table 1 provide a sampling of the autologous iPSC-based cell therapies currently in development and is not an exhaustive list (Table 1).
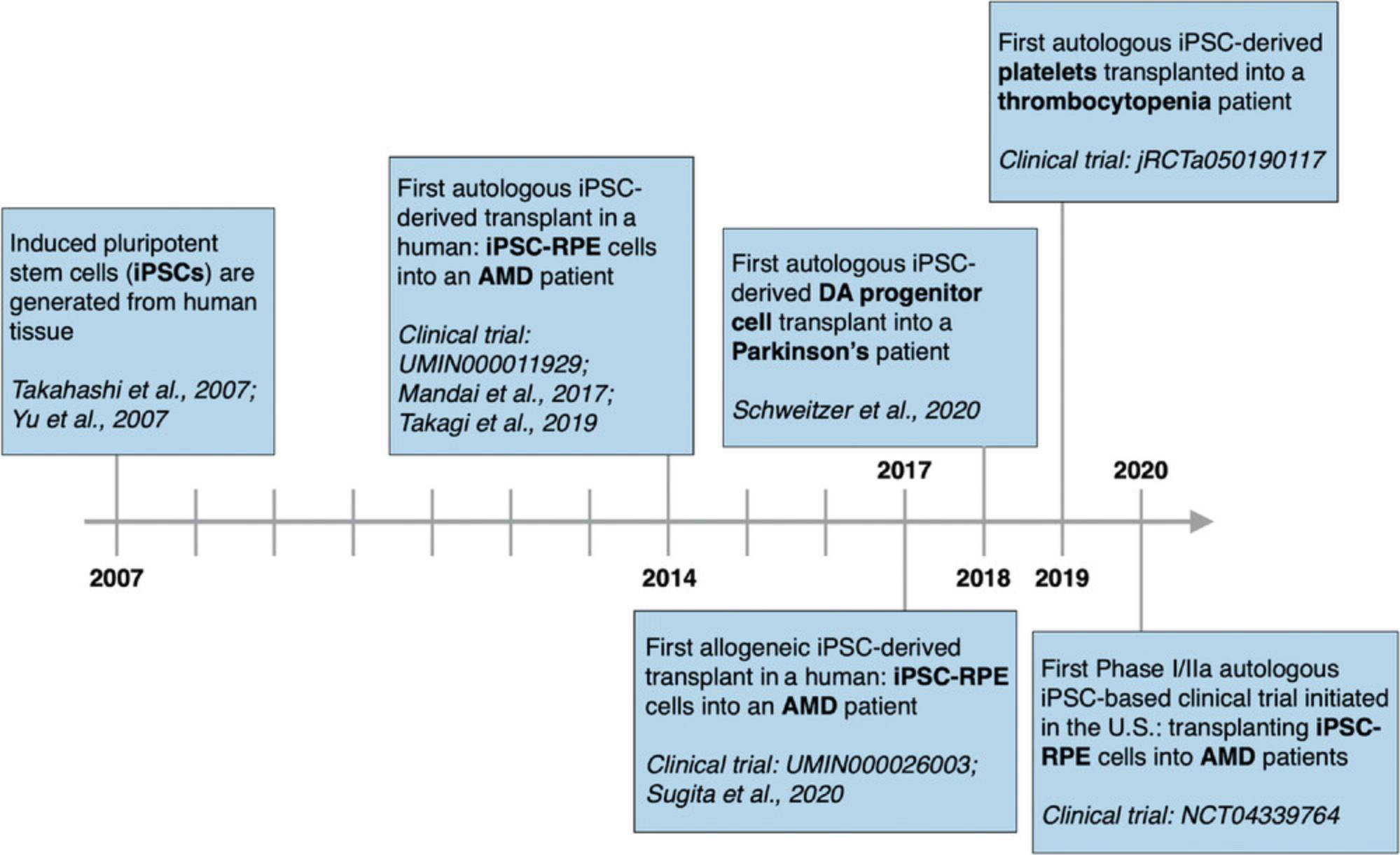
Organ | Indication | Cell product | Reprogramming starting material and chosen technique | Stage | Investigator, institution | Country |
---|---|---|---|---|---|---|
Eye | Dry age-related macular degeneration | Retinal pigment epithelial cell sheet on PLGA scaffold | CD34+ cells, episomal plasmids | Phase I/IIa | Kapil Bharti, NIH/NEI | USA |
Eye | Wet age-related macular degeneration | Retinal pigment epithelial cell sheet | Fibroblasts, episomal plasmids | 1 patient dosed (2014) | Masayo Takahashi, RIKEN | Japan |
Brain | Parkinson's disease | Dopaminergic progenitor cells | Fibroblasts, episomal plasmids | 1 patient dosed (2018) | Kwang-Soo Kim, McLean Hospital/Harvard Medical School | USA |
Brain | Parkinson's disease | Dopaminergic neurons | Fibroblasts, chosen technique not specified in publication | Preparing for IND | Jeanne Loring, Aspen Neuroscience | USA |
Brain | Parkinson's disease | Dopaminergic neurons | Chosen technique for in-human studies not specified in publication | Non-human primate studies published, preparing for IND | Ole Isacson and Penny Hallett, Neuroregeneration Research Institute at McLean Hospital/Harvard Medical School | USA |
Blood | Thrombocytopenia | Platelets | Peripheral blood mononuclear cells, episomal plasmids | 1 patient received multiple doses (2019-2020) | Koji Eto, Kyoto University | Japan |
Skin | Recessive dystrophic epidermolysis bullosa | Gene-edited keratinocytes and fibroblasts | Fibroblasts, RNA | Mouse studies in progress, preparing for IND | Dennis Roop, Gates Center for Regenerative Medicine; Ganna Bilousova and Igor Kogut, University of Colorado School of Medicine | USA |
Skin | Recessive dystrophic epidermolysis bullosa | Gene-edited keratinocytes and fibroblasts | Fibroblasts, episomal plasmids | Mouse studies published, preparing for IND | Angela Christiano, Columbia University | USA |
Skin | Recessive dystrophic epidermolysis bullosa | Gene-edited keratinocytes and fibroblasts | Fibroblasts and keratinocytes, excisable lentiviral approach for reprogramming, excisable AAV approach for gene editing | Mouse studies published, preparing for IND | Anthony Oro, Stanford University | USA |
Skin | Hair loss | Neural crest–derived dermal papillae | Peripheral blood mononuclear cells, Sendai virus | Mouse studies published, preparing for IND | Alexey Terskikh, Sanford Burnham Prebys Medical Discovery Institute/Stemson Therapeutics | USA |
Muscle | Muscular dystrophies | Myogenic stem cells | Peripheral blood mononuclear cells | Received orphan drug designation from FDA, preparing for IND | Douglas Falk, Vita Therapeutics | USA |
Eye | Retinal degenerative disorders (e.g., retinitis pigmentosa) | Photoreceptor precursors | Fibroblasts, Sendai virus | Mouse studies published | Budd Tucker, University of Iowa | USA |
- aTable 1 includes a sample of the autologous iPSC-based cell therapies in development. The reprogramming starting materials and chosen reprogramming techniques shown here are based on available publications and may change as programs advance toward the clinic.
A Indications for In-Human Autologous iPSC-Derived Cell Transplants
i iPSC-derived retinal pigment epithelial cells for age-related macular degeneration
iPSC-derived retinal pigment epithelial cells (RPEs) were the first autologous iPSC-derived cell type to be transplanted into a human, accomplished in 2014 by Masayo Takahashi's team at the RIKEN Center for Developmental Biology in Japan (Mandai et al., 2017; Schweitzer et al., 2020). This transplant was performed to treat age-related macular degeneration (AMD), a chronic disease characterized by the degeneration of RPE cells and the leading cause of vision loss among individuals over age 60 (Sharma, Bose, Maminishkis, & Bharti, 2020). There are three main reasons why RPEs were the first iPSC-derived transplant: (1) the eye is relatively easy to image and access surgically; (2) RPEs were the first ocular cell type to be differentiated from iPSCs, in 2009, just 2 years after the landmark publication demonstrating iPSC generation (Buchholz et al., 2009); and (3) previous surgical experiments had shown proof of concept supporting an autologous cell therapy for AMD. In these experiments, RPEs from the healthy region of an eye of an AMD patient were removed and transplanted into the degenerated region of the same eye (Van Meurs et al., 2004; Van Zeeburg, Maaijwee, Missotten, Heimann, & Van Meurs, 2012). These were complex surgeries requiring significant skill and precision that ultimately resulted in the partial recovery of vision.
In their groundbreaking study, Takahashi's team transplanted an iPSC-RPE sheet measuring 1.3 × 3 mm under the fovea of the right eye of a 77-year-old female patient with neovascular (“wet”) AMD (Mandai et al., 2017). The iPSCs were reprogrammed from fibroblasts using non-integrating episomal vectors, differentiated to RPEs with a 6-week process using a combination of directed and spontaneous differentiation methods, and then purified and expanded over a period of 6 weeks (Kamao et al., 2014; Mandai et al., 2017; Osakada, Ikeda, Sasai, & Takahashi, 2009; Osakada et al., 2009). Over a period of 8 weeks, selected RPE colonies were seeded onto a collagen-coated transwell to form the RPE sheet, which was lifted from the transwell by treatment with collagen IV. From skin tissue collection to shipment of RPE cell sheets, the process took ∼10 months (Mandai et al., 2017). A 4-year follow-up demonstrated that the iPSC-derived RPE sheet had survived and displayed normal morphology (Takagi et al., 2019). The patient experienced no serious adverse events, demonstrating a promising safety profile for iPSC-RPE transplants. Although there was no improvement in vision, the patient's vision remained stable since the surgery, despite continually decreasing in the years before surgery.
Efforts to bring an autologous iPSC-RPE cell therapy to patients have been continued by Kapil Bharti's team at the National Eye Institute of the NIH. Bharti's team developed clinical-grade iPSC-derived RPE patches on a biodegradable poly-(lactic-co-glycolic) acid (PLGA) scaffold. The PLGA scaffold—unique to Bharti's approach—serves several purposes, including enabling the RPEs to form a confluent, correctly polarized monolayer and providing structural integrity for easier transplantation. The patches were successfully transplanted into the Royal College of Surgeons (RCS) rat and laser-induced RPE injury pig models (Sharma et al., 2019). The iPSCs were generated from CD34+ cells isolated from patients’ peripheral blood using a clinical-grade episomal reprogramming protocol (Mack, Kroboth, Rajesh, & Wang, 2011). They were then differentiated to mature RPEs via an 11-week triphasic monolayer directed differentiation protocol. The RPE progenitors were enriched and seeded onto a PLGA scaffold to mature for the final 35 days. Imaging 10 weeks post-transplantation confirmed that the PLGA scaffold had efficiently degraded. The iPSC-RPE patch integrated into both the rat and the pig models, even demonstrating functionality by phagocytosing photoreceptor outer-rod segments and preventing degeneration of the overlying photoreceptors in the pig models (Sharma et al., 2019). Having established the feasibility and efficacy of the iPSC-RPE on a PLGA scaffold in a large animal model, in 2020, the NIH team initiated Phase I/IIa clinical trials to test the safety of autologous transplantation of the iPSC-RPE in 20 dry-AMD patients (National Clinical Trial number: NCT04339764). This clinical trial marks the first and, at the time of this publication, the only Phase I/IIa clinical trial in the U.S. to test autologous iPSC-derived cells in patients and simultaneously opens the door to other scaffold-supported transplant approaches using iPSC-derived cells.
ii iPSC-derived dopaminergic neurons for Parkinson's disease
The second autologous iPSC-derived cell therapy transplanted into a human consisted of iPSC-derived midbrain dopaminergic (mDA) progenitor cells (mDAPs), transplanted into the putamen of a 69-year-old man with a 10-year history of Parkinson's disease (PD) (Schweitzer et al., 2020). PD is a progressive neurodegenerative disease affecting an estimated 7 to 10 million patients worldwide (Dorsey, Sherer, Okun, & Bloemd, 2018). Degeneration of dopaminergic neurons leads to motor symptoms such as tremors and bradykinesia. By the time symptoms have become prominent enough to lead to a diagnosis, ∼60% of the mDA neurons have degenerated (Engelender & Isacson, 2017; Osborn et al., 2020). Today's cell therapy development for PD builds on a 30+ year history of experimentation, in which mDA neurons from fetuses were transplanted into PD patients (Freed et al., 1992; Hagell et al., 2000; Hallett et al., 2014; Kefalopoulou et al., 2014; Kordower et al., 1998; Li et al., 2016; Lindvall et al., 1990; Mendez et al., 2005; Piccini et al., 1999; Redmond, Vinuela, Kordower, & Isacson, 2008). Long-term follow-ups and data collection are essential for assessing the safety and efficacy of such transplants, as the cells may take months or years to integrate into the patient's neural pathways (Barker, Barrett, Mason, & Björklund, 2013; Politis & Piccini, 2012; Politis et al., 2010). Hence, the value of studies such as those conducted by Politis et al. assessing safety and efficacy in patients 15+ years post-transplantation (Politis & Piccini, 2012; Politis et al., 2010). Although the patients followed still suffered from some of the non-motor symptoms of PD related to regulation of sleep and emotion, the relief from motor symptoms was long lasting. Positive improvements were measured by PET neuroimaging of dopamine uptake and reduced scores on the Unified Parkinson's Disease Rating Scale (UPDRS). Moreover, postmortem imaging of brain tissues of patients who had received fetal tissue transplants 4 to 14 years earlier was consistent with these clinical results, showing that the transplanted tissue was healthy and non-atrophied and expressed important dopamine transporters (DATs) (Hallett et al., 2014). Although these findings are promising, fetal transplants are limited by ethical and practical concerns and require patients to undergo immunosuppression. An autologous iPSC-based approach to mDA cell therapy development would address these challenges.
In 2018, autologous iPSC-derived mDAPs were transplanted first into the left hemisphere of a 69-year-old PD patient and then into the right hemisphere 6 months later (Schweitzer et al., 2020). This study was led by Kwang-Soo Kim at McLean Hospital (Harvard Medical School) in collaboration with Bob Carter's and Jeff Schweitzer's teams at Massachusetts General Hospital. The iPSCs were generated from fibroblasts harvested from a skin biopsy and differentiated to mDAPs using a good manufacturing practice (GMP)-grade 28-day protocol (Song et al., 2020). On day 9, the cells were treated with quercetin to remove any undifferentiated iPSCs. The patient-specific iPSC-mDAPs and allogeneic human ESC-derived mDAPs were transplanted into allogeneic humanized mice and patient-humanized mice, both groups without immunosuppression. Imaging of mouse brain sections 2 weeks post-transplantation showed rejection of both graft types in the allogeneic humanized mice and survival of only the patient-specific iPSC-mDAPs in the patient-humanized mice. The PD patient's clinical measurements were taken at 1, 3, 6, 9, and 12 months post-transplantation and then at 6-month intervals. The patient's self-reported UPDRS score decreased from 60 at the time of implantation to 2 in the 24-month follow-up, indicating an improvement of symptoms. The authors responsibly caution against drawing wide-ranging conclusions from the results of a single patient. Still, with no serious adverse events to date, this study is an important step forward in developing autologous iPSC-derived cell therapies for PD.
Autologous iPSC-mDA cell therapies for PD are also being developed by Ole Isacson and Penelope Hallett, co-directors of the Neuroregeneration Research Institute at McLean Hospital (Harvard Medical School), as well as Aspen Neuroscience. In 2015, Isacson and Hallett's team published the first demonstration of functional autologous iPSC-derived dopamine neurons following transplantation into a non-human primate model of PD (Hallett et al., 2015). Autologous iPSCs were derived from three parkinsonian cynomolgus monkeys (CMs). To test the efficacy of the two differentiation protocols, one CM's cell samples were differentiated using the 49-day protocol of Cooper et al. (2010), and the remaining two CMs’ cell samples were differentiated using the 30-day protocol of Sundberg et al. (2013). Cells were transplanted into the putamen of the parkinsonian CMs without immunosuppression. Improvement in PD-like motor symptoms was observed as early as 6 months post-transplantation and for up to 2 years. PET neuroimaging showed an increase in DAT binding sites (Hallett et al., 2015). Postmortem analysis of the brain at 2 years post-surgery showed survival of the engrafted dopaminergic neurons and expression of important markers. Isacson and Hallett's team also demonstrated functional improvement post-transplantation in non-human primate models with 8 years of chronic PD as part of preclinical studies toward developing an autologous iPSC-based cell therapy for PD (Osborn et al., 2020). They were recently awarded a $6M grant from the NIH to support their in-human studies, which will use autologous iPSCs as a starting material for the cell therapy product.
Jeanne Loring of The Scripps Research Institute co-founded Aspen Neuroscience in 2018, which raised a $70M Series A to develop an autologous iPSC-derived neuron replacement therapy for PD (Loring, 2018). Aspen Neuroscience generates iPSCs from dermal fibroblasts using a proprietary artificial intelligence (AI)-based model for assessing pluripotency (Müller et al., 2011). Aspen has two iPSC-derived cell types in their therapeutic pipeline: an autologous iPSC-DA neuron therapy for idiopathic PD, which affects 85% to 90% of PD patients, and an autologous gene-edited iPSC-DA neuron therapy for genetic PD, which affects 10% to 15% of the PD population and qualifies for U.S. Food and Drug Administration (FDA) orphan drug designation (Federoff, 2020). Loring's team is using dopamine neuron differentiation protocols and genomics-based quality control (QC) analyses to assess the safety of the patient-specific iPSC lines and iPSC-derived cells (Bhutani et al., 2016; Loring, 2018). In preparation for an Investigational New Drug (IND) filing to begin clinical trials, Loring's team generated iPSCs from 10 patients and developed QC release criteria for the final cell product (Loring, 2018).
iii iPSC-derived platelets for thrombocytopenia
The third in-human transplant of autologous iPSC-derived cells was a single-patient study led by Koji Eto's team at CiRA, in collaboration with Kyoto University Hospital, in 2019 (Clinical Trial Identification number: jRCTa050190117). The patient suffered from aplastic anemia, a rare disease characterized in part by thrombocytopenia (low platelet count), and complicated by platelet transfusion refractoriness (PTR) (Hod & Schwartz, 2008; Stasi, 2012). Allotransfusion of platelets from blood donors is the standard treatment for thrombocytopenia (Estcourt et al., 2018). However, between 5% and 15% of patients receiving platelet transfusions develop refractoriness, in which an alloimmune response results in the rejection of the immune-incompatible transfused platelets (Pavenski et al., 2013; Saito et al., 2002; Stanworth, Navarrete, Estcourt, & Marsh, 2015).
The patient received three doses of autologous iPSC-derived platelets: 1 × 1010 platelets, 3 × 1010 platelets 3 months later, and then 1 × 1011 platelets 5 months after the second dose. The iPSCs were generated using peripheral blood mononuclear cells (PBMCs) and episomal plasmids. The iPSCs were then differentiated into megakaryocytes, which were expanded and cryopreserved as a master cell bank (MCB). The megakaryocytes could then be thawed, proliferated to 109 to 1010 cells, and differentiated into platelets in a bioreactor shortly before transfusion. In preparation for the transfusion, the iPSC-derived platelets were purified, concentrated, washed, and irradiated. A 1-year follow-up assessing the single-patient trial's safety and efficacy is planned for early 2021 (Clinical Trial Identification number: jRCTa050190117; Clinical research for the transfer of autologous iPS cell-derived platelets to a thrombocytopenia patient, 2020).
B Indications in Earlier (Preclinical) Stages of Development
i iPSC-derived keratinocytes and fibroblasts for epidermolysis bullosa
Inherited epidermolysis bullosa (EB) is a group of rare, incurable, life-threatening skin diseases characterized by severe blistering and scarring (Fine, 2010). The chronic skin wounds are similar to thermal or chemical burns. The severity of symptoms varies across and within subtypes, with some resulting in death in early infancy. Much of the available treatment focuses on pain management, but several in vitro and in vivo studies using mouse models support a cell-based therapeutic approach (Bilousova & Roop, 2014; Jacków et al., 2019; Sebastiano et al., 2014; Shinkuma, 2015; Tolar et al., 2014; Torkelson et al., 2019; Umegaki-Arao et al., 2014; Vanden Oever, Twaroski, Osborn, Wagner, & Tolar, 2018; Wenzel et al., 2014). The skin is an ideal organ for developing cell-based therapies: similar to the eye, it is easy to access and easy to monitor. Each subtype of EB is associated with various genetic mutations, which would need to be corrected in an autologous cell therapy (Fine, 2010). Several research groups are developing autologous gene-edited iPSC-derived cell therapies for EB (Jacków et al., 2019; Roop, Bilousova, & Kogut, 2019; Sebastiano et al., 2014).
Angela Christiano's team at Columbia University used episomal vectors to derive iPSCs from the fibroblasts of two patients with recessive dystrophic EB (RDEB). RDEB is a severe subtype characterized by mutations affecting type VII collagen production (Jacków et al., 2019). Jacków and colleagues tested both plasmid and ribonucleoprotein-based CRISPR-Cas9 approaches for gene editing and differentiated the gene-corrected iPSCs to both keratinocytes and fibroblasts using 60-day and ∼30-day protocols, respectively. The iPSC-derived keratinocytes and fibroblasts were used to generate 3D human skin equivalents (HSEs). When grafted onto immunodeficient mice, the HSEs showed functional restoration of collagen expression and normal dermal morphology. No tumors were seen 9 months after grafting, a promising result toward developing a safe cell-based therapy.
Anthony Oro's team at Stanford University was awarded a $5M grant from the California Institute for Regenerative Medicine (CIRM) to translate an autologous gene-edited iPSC-based cell therapy for RDEB to the clinic (Oro, n.d.). In their 2014 publication, Oro's team used an excisable lentiviral reprogramming method to generate GMP-grade iPSCs from patient-specific skin biopsies (Sebastiano et al., 2014). The mutated gene was corrected using an excisable adeno-associated viral (AAV)-based approach. An ∼110-day protocol was used to differentiate the gene-corrected iPSCs into keratinocytes, which successfully formed sheets of skin in vitro. The iPSC-derived keratinocytes were also able to rebuild mature skin when grafted onto immunocompromised mice, and the grafts survived for 3 weeks post-transplantation. Funding from the CIRM grant will be used to perform additional in vivo studies in preparation for a pre-IND meeting with the FDA.
Ganna Bilousova's and Igor Kogut's teams at the University of Colorado School of Medicine, in collaboration with Dennis Roop, director of the Gates Center for Regenerative Medicine, are also translating an autologous iPSC-based therapy into the clinic for RDEB. Kogut and colleagues developed a clinically relevant RNA-based technology capable of reprogramming ∼90% of individually plated human primary fibroblasts, unprecedentedly high considering that reprogramming efficiencies are typically fractions of a percent or in the single digits (Kogut et al., 2018). Bilousova and colleagues were the first to publish a demonstration of the differentiation of mouse iPSCs into keratinocytes, a critical step in advancing iPSC-derived cell therapies for EB into the clinic. The University of Colorado team is currently developing composite skin grafts from patient-specific iPSC-derived keratinocytes and fibroblasts for transplantation (Roop et al., 2019). Reprogramming and gene correction are performed simultaneously using the proprietary RNA technology. The resulting gene-edited iPSCs are differentiated into keratinocytes and fibroblasts (Kogut et al., 2018; Roop et al., 2019). The University of Colorado team is currently exploring the feasibility of a spray-based applicator from AVITA Medical for delivering iPSC-derived keratinocytes and fibroblasts in mouse models of RDEB (Roop et al., 2019; Sood et al., 2015).
ii iPSC/neural crest–derived dermal papillae for alopecia
Hair loss, or alopecia, is a significant problem that affects nearly 20% of the population. Studies measuring the impact of hair loss on quality of life reveal long-lasting negative effects, including low self-confidence, heightened self-consciousness, and an increased prevalence of depression (Marks et al., 2019; Schmitt, Ribeiro, Souza, Siqueira, & Bebber, 2012; Williamson, Gonzalez, & Finlay, 2001). Follicular unit extraction (FUE), in which individual follicular units are harvested from one region of a patient's scalp and transplanted into a different section of the same patient's scalp, provides a minimally invasive solution for hair transplantation (Rassman et al., 2002). This procedure offers validation of an autologous cell therapy–based approach to hair loss. However, patients with severe hair loss may not have sufficient follicles for FUE transplantation. An autologous iPSC-based approach solves this problem, making it possible to generate large volumes of patient-specific hair follicles for treating patients suffering from severe hair loss. Stemson Therapeutics, based on technology developed in Alexey Terskikh's lab at Sanford Burnham Prebys Medical Discovery Institute, is pursuing such an approach (Gnedeva et al., 2015). Gnedeva and colleagues developed a two-phase 28-day protocol for differentiating ESCs and iPSCs to adherent hair-inducing dermal papillae (DP) cells via a neural crest (NC) cell intermediate stage (Gnedeva et al., 2015). Suspensions of 5 × 105 ESC-DP cells were combined with suspensions of 5 × 105 epidermal cells and successfully transplanted into immunodeficient mice, demonstrating an ability to induce hair follicle formation in vivo, an exciting step toward bringing iPSC-derived hair transplants to patients.
iii iPSC-derived myogenic stem cells for muscular dystrophies
Muscular dystrophies are a group of genetic disorders characterized by muscle wasting: weakening and shrinking of the muscles due to loss of muscle mass (Emery, 2002). Muscle cell transplants into mouse models of muscular dystrophy date back to 1989, and the positive results support a cell therapy approach (Partridge, Morgan, Coulton, Hoffman, & Kunkel, 1989; Tedesco et al., 2012). However, heterologous myoblast transplantation into humans has not resulted in functional improvements; these results are partially due to immune rejection, indicating that an autologous approach may be more promising (Skuk & Tremblay, 2003; Sun, Serra, Lee, & Wagner, 2020). The various dystrophies are characterized by specific genetic mutations, meaning that an autologous approach would require gene editing (Emery, 2002; Ghaoui et al., 2015). Vita Therapeutics, founded out of Johns Hopkins University and based on technology developed by Gabsang Lee at Johns Hopkins School of Medicine and Kathryn Wagner at the Kennedy Krieger Institute, is developing gene-edited cell therapies to regenerate diseased and lost muscle tissue (U.S. Patent No. 15/781,709, 2016). Using a combination of iPSC technology and genome editing, patient-specific cells are differentiated into myogenic stem cells capable of dividing asymmetrically to generate additional satellite cells and myoblasts (Choi et al., 2016; Sun et al., 2020). The myoblasts can then fuse, effectively regenerating muscle fibers (Sun et al., 2020). Vita Therapeutics aims to target specific types of muscular dystrophies, including limb-girdle muscular dystrophies (LGMDs), the most heterogeneous of the muscular dystrophies, and recently received orphan drug designation from the FDA to initiate a clinical study evaluating the use of iPSC-derived myogenic stem cells for Duchenne muscular dystrophy (DMD), which is the most common form of muscular dystrophy and typically affects young boys (Emery, 2002; Sun et al., 2020).
iv iPSC-derived photoreceptor precursors for retinal degenerative blindness
Retinal degenerative disorders, such as retinitis pigmentosa, Stargardt disease, and Leber congenital amaurosis, are characterized by incurable blindness caused by the dysfunction or loss of light-sensing photoreceptors (den Hollander, Roepman, Koenekoop, & Cremers, 2008; Hartong, Berson, & Dryja, 2006; Tsang & Sharma, 2018). In many cases, the neurons of the inner layer of the retina—whose job is to connect the photoreceptors to the brain—remain intact after photoreceptor degeneration, encouraging the pursuit of a photoreceptor transplantation–based cell therapy approach (Cideciyan et al., 2007; Mullins et al., 2012). At least two studies have demonstrated technical feasibility and safety, if not efficacy, of cadaveric photoreceptor transplantation into human patients with retinitis pigmentosa (Berger, Tezel, Del Priore, & Kaplan, 2003; Kaplan, Tezel, Berger, Wolf, & Del Priore, 1997). Several studies have demonstrated transplanted PSC-derived photoreceptor precursors’ ability to restore retinal function and improve vision in animal models of retinal degeneration (Lamba, Gust, & Reh, 2009; Pearson et al., 2012; Tucker et al., 2011). An autologous approach could avoid some of the problems leading to poor long-term survival post-transplantation, which may be due in part to immune responses (Santos-Ferreira, Borsch, & Ade, 2017; West et al., 2010). Budd Tucker's lab at the University of Iowa developed a current GMP (cGMP)-grade protocol for generating patient-specific iPSCs and photoreceptor precursors to support the development of an autologous approach. Fibroblast samples were obtained from 35 patients with inherited retinal degeneration and expanded under cGMP-compliant conditions (Wiley et al., 2016). Sendai virus was used to generate iPSC clones from the patient samples. The iPSCs were differentiated using an ∼16-week 3D suspension–based protocol, resulting in retinal organoids composed primarily of post-mitotic photoreceptor precursor cells. iPSC-derived photoreceptor precursors from eight different patients were transplanted into immunocompromised mice. The transplants resulted in no tumor formation and demonstrated no pluripotent cells in the final cell population, an important concern for cell therapy products (Wiley et al., 2016).
III CHALLENGES AND CONSIDERATIONS IN BRINGING AUTOLOGOUS iPSC-DERIVED CELL THERAPIES TO PATIENTS
With several clinical trials planned or underway, the past 10 years have seen significant progress in developing autologous iPSC-based cell therapies (see Section II). However, an autologous iPSC-derived cell therapy has yet to reach the market, and the majority of iPSC-based cell therapy development efforts are utilizing an allogeneic approach (Martin-Ibanez & Sareen, 2020). For autologous iPSC-based therapies to become a commonplace treatment modality, safety and scalability are crucial. Section III discusses areas where progress would enable autologous iPSC-derived cell therapies to become more widely used. The topics covered are shortening the manufacturing timeline (A), ensuring safety and quality of the final cell product (B), and using scalable manufacturing processes (C).
A Manufacturing Timeline
It takes several months for patient-extracted somatic cells to be reprogrammed and differentiated to produce a cell therapy for patients. This review focuses on accelerating the timelines for reprogramming somatic cells, as the timeline for differentiation is highly cell-type and application dependent and as rapid differentiation techniques have been discussed at length elsewhere (Buchholz et al., 2013; Busskamp et al., 2014; Ehrlich et al., 2017; see Current Protocols article; Fernandopulle et al., 2018; Frega et al., 2017; Ng et al., 2020; Yang et al., 2017). Optimizing the manufacturing timeline will ensure that patients receive their treatments in a timely fashion. This subsection highlights areas of innovation that could compress the autologous iPSC manufacturing timeline.
3.1.1 Timeline for reprogramming somatic cells to iPSCs
The first step in developing an autologous iPSC-based therapy is to create an iPSC MCB for each patient. Automated manufacturing technologies and automation-compatible QC assays hold promise for a faster and more streamlined manufacturing process. However, the biology and reprogramming method limit how rapidly primary tissues can be reprogrammed to produce a validated autologous iPSC MCB. The three most used reprogramming methods use viral vectors (retroviral and Sendai virus), episomal vectors, and mRNA. The field is moving away from retroviral methods due to the potential for permanent, undesired integration of the reprogramming material into the genome (Okita, Ichisaka, & Yamanaka, 2007). Non-integrating, “footprint-free” approaches, such as those using Sendai virus, episomal vectors, and mRNA, are an attractive alternative (Schlaeger et al., 2015).
Figure 2 shows the manufacturing process from somatic cell extraction to reprogramming, differentiation, and transplantation. The current process of reprogramming takes ∼4 months; the exact time depends on the chosen somatic cell source and reprogramming technique. The six steps of reprogramming are as follows: i) patient sample collection, ii) patient sample QC, iii) expansion of patient cells, iv) reprogramming, v) colony expansion and reprogramming vector clearance, and vi) iPSC QC.
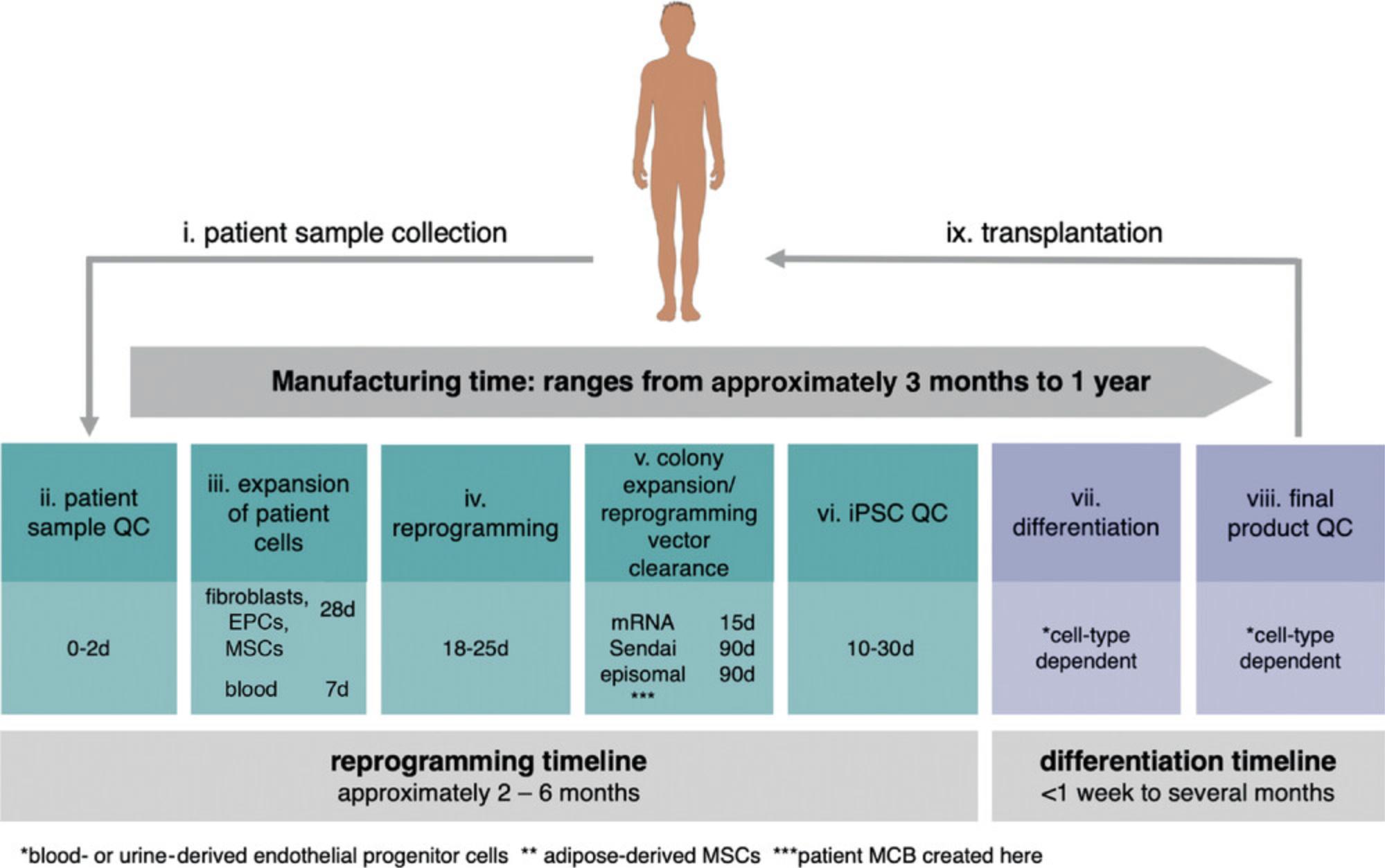
Within this process, shortening steps iii and v will have the biggest impact on the manufacturing timeline.
The sourced patient cells must be expanded to get sufficient starting material for reprogramming. The expansion of patient cells (step iii) can take ∼7 days for peripheral blood CD34+ cells or >28 days for adherent cells. This difference in required expansion time is reflective of differences in the number of extracted cells and the proliferation rate for the two cell types (Loh et al., 2009; Takahashi et al., 2007). Peripheral blood CD34+ cells have advantages as a starting material: shorter expansion timelines and higher reprogramming efficiencies (Eminli et al., 2009). At this time, regulatory agencies do not have specific recommendations regarding patient cell type; the choice of starting material is at the discretion of cell therapy developers (Jha et al., 2020).
The process of reprogramming (step iv) takes ∼28 days, independent of the starting cell type or the chosen reprogramming vector (Ban et al., 2011; Fusaki, Ban, Nishiyama, Saeki, & Hasegawa, 2009; Loh et al., 2009; Okita et al., 2011; Takahashi et al., 2007; Warren et al., 2010). Reprogrammed colonies emerge during this time. Colonies are then expanded, and the reprogramming vector is cleared from the cells over time (step v). Each reprogramming vector has a different clearance rate. For replication-competent episomal and viral methods, the expansion and vector clearance stage can take up to 110 days due to the exogenous reprogramming material's slow elimination rate (Fusaki et al., 2009; Nanbo, Sugden, & Sugden, 2007). On average, each cell division dilutes the episomal plasmids with Epstein-Barr virus (EBV) replicon by 5%, with significant differences between clones (Nanbo et al., 2007). Sendai virus can take up to 20 passages to be diluted out (Fusaki et al., 2009), making it challenging to predict the manufacturing time. It may be possible to streamline the cell division–dependent elimination rate of episomal plasmids and viral vectors by using automation to optimize passaging ratios, media changes, and cell density management (Schorl & Sedivy, 2007).
An mRNA-based approach to reprogramming may have the greatest impact in shortening reprogramming timelines. The mRNA is cleared from the cells within 48 to 72 hr, producing footprint-free iPSCs 60 days faster than episomal or viral vector-based techniques (Warren et al., 2010). However, the transient nature of the mRNA-induced transcription factor expression requires 4 to 8 rounds of mRNA delivery to somatic cells every 48 hr (Kogut et al., 2018; Warren et al., 2010). The mRNA is delivered to somatic cells by electroporation or lipofection-based methods, causing significant stress to the cells and negatively affecting the viability and reprogramming efficiency. To date, only adherent cells, most commonly fibroblasts, have been reprogrammed with mRNAs (Warren & Lin, 2019). New intracellular delivery methods are emerging that might allow for repeated mRNA delivery into blood cells, such as non-viral gene delivery systems from Avectas (O'Dea et al., 2017), SQZ Bio (Ding et al., 2017), Kytopen (Garcia, Ge, Moran, & Buie, 2016), and Cellino (Saklayen et al., 2017). There is a growing interest in developing approaches for mRNA-based reprogramming of blood cells to provide greater options for cell therapy developers.
B Safety and Quality Control
Autologous therapies are considered safer than allogeneic from an immunogenicity perspective (see Section I). Autologous therapies do not suffer from the immensely high QC barrier of allogeneic therapies. There is no risk of introducing infectious agents from one patient into another, as long as sterility and identity chain are maintained during manufacturing. Autologous cell therapies do not have to go through the expensive screening processes that allogeneic donor tissues must undergo for disease-transmitting agents such as viruses (Zika, West Nile, SARS, HIV, HBV, HCV, vaccinia, HTLV, and others), parasites (Treponema pallidum), and transmissible spongiform encephalopathies. Certain safety risks relevant to iPSCs, such as genomic instability, need to be assessed regardless of whether the approach is autologous or allogeneic. Developing standardized regulatory guidelines and validating each autologous iPSC MCB using standardized QC assays can mitigate these safety risks. Standardized QC measurements are also useful for assessing the reproducibility of a manufacturing process, which is an essential consideration for autologous iPSC-based cell therapies, given that the manufacturing process needs to be repeated for each patient. Automated systems for scalable manufacturing (Section IIIC) can play a role in enhancing the reproducibility of a process, as can thoughtful selection of the iPSC culture parameters (this subsection). This subsection describes the currently used QC standards for iPSC manufacturing, relevant tools for assessing key iPSC characteristics, and the status of regulatory guidelines for iPSC-derived products.
i Developing a reproducible iPSC-based process
Achieving reproducibility has been identified as a critical goal in the iPSC field, and a lack thereof has been a barrier to broader adoption of iPSCs, keeping innovators from realizing iPSCs’ full therapeutic potential. The culture factors affecting iPSC outcomes are growth factors, extracellular matrix (ECM) proteins, steroids, hormones, small molecules (agonist/antagonist), shear flow, mechanical strain/stiffness of substrates, ions (such as Ca2+, Mg+, and Cl−), electromagnetic fields, and a host of other parameters (Murphy, McDevitt, & Engler, 2014). In this regard, small molecules can induce efficient and rapid differentiation and enable cells to handle the stress of single-cell seeding strategies such as use of Rho-associated protein kinase (ROCK) inhibitors (Maldonado, Luu, Ramos, & Nam, 2016).
One of the key elements of the cellular microenvironment that shapes differentiation choices is the ECM, typically used to coat the tissue culture plate (substrate) during cell culture. Recent studies have implicated various biomaterial properties as critical elements of the stem cell environment; substrate mechanical stiffness (Engler, Sen, Sweeney, & Discher, 2006; Iwashita et al., 2019; Lee, Abdeen, Zhang, & Kilian, 2013), nanometer-scale topography (Dalby et al., 2007; McMurray et al., 2011; Yim, Darling, Kulangara, Guilak, & Leong, 2010), and chemical functionality (Benoit, Schwartz, Durney, & Anseth, 2008; Saha et al., 2011) each impact stem cell expansion and function. Although many of these earlier studies used inconsistent and poorly reproducible mesenchymal stromal cell (MSC) models, they demonstrate that biomaterials, in principle, can provide an environment that may help control iPSC behavior. Many current iPSC protocols use Matrigel or Geltrex to deliver a physiological ECM substrate for cell adhesion. Both products are related basement membrane matrices made up of secreted ECM proteins, including laminin, collagen IV, heparin sulfate proteoglycans, and entactin/nidogen, purified from murine Engelbreth-Holm-Swarm (EHS) tumor cells. These products are biochemically complex, with over 2000 different protein components. They have an unfortunate but well-documented track record of high variability and lack of reproducibility from batch to batch (Nguyen et al., 2017). Therefore, the selection of custom ECM materials to promote the optimal survival, potency, and differentiation efficiency of iPSC-derived target cells is a product development goal worthy of pursuit on the long, hard road from the bench to the clinic.
ii Quality control
For both autologous and allogeneic approaches, enabling safe delivery of iPSC-derived therapies will require streamlined, rapid QC testing for safety, identity, potency, and purity. Although most of the QC tests developed for a diverse set of cell therapies may translate to the iPSC-derived workflow, there are special considerations to be factored into the QC plan for generating therapeutic cells from iPSCs.
The key to developing a robust and reproducible process for the generation of multipotent cells from iPSCs will be to generate and validate suitable analytics to accurately track and measure the critical quality attributes (CQAs) that will govern the efficiency and consistency of the differentiation method. However, before this, the developer often needs to address the key variables of cell counts and viability. Counting viable cells seems to be one of the simplest and most important steps in developing any cell therapy; it is one of the most fundamental measures for cell-based therapeutics yet is often overlooked or underestimated. The National Institute of Standards and Technology (NIST) and the FDA held a workshop on cell counting in April 2017 with over 50 small and medium-sized enterprises (SMEs) from industry, with the conclusion that for each counting purpose (process and cell type), an appropriate cell counting method needs to be designed, validated, and implemented (Arcidiacono et al., 2018). The ideal instrument needs to robustly determine accurate, reproducible, and validated (21 CFR Part 11 compliant) cell counts for iPSCs and other intermediate cell types in the process with similar size and despite varied clumping characteristics, which can skew results. Instruments such as the ChemoMetec NucleoCounter NC-200 use image cytometry and specialized assays for aggregated cells to overcome these constraints. There is no subjective evaluation of what constitutes a live cell visually, as with traditional counting. Typical errors introduced during pipetting and staining are avoided by a built-in pipet, which is part of the counting cassette. This system uses the permeable dyes acridine orange and DAPI to respectively stain the total and dead cell populations. Our initial but thorough evaluation of the NC-200 compared to a typical lab cell counter using trypan blue (Bio-Rad TC20) has demonstrated the NC-200 to be superior and highly accurate for our purposes. Using these types of novel instruments well suited to GMP manufacturing QC will allow the autologous iPSC developer with limited cell numbers to standardize cell counts for the entire process, from iPSCs to final cell type, and enable a solid foundation for all the cell-based QC assays, such as flow cytometry and enzyme-linked immunosorbent assays (ELISAs) for secreted factors, to demonstrate multipotency and specific differentiation potential.
Genomic (chromosomal) instability is an endemic source of concern for iPSC lines (Henry, Hawkins, Boyle, & Bridger, 2019). Many iPSC lines have been found to contain aneuploidies and pose a serious risk to patients if they are used as input material to generate medically useful cells via controlled differentiation. In fact, the first autologous iPSC clinical trial at RIKEN to restore vision ran into safety concerns when chromosomal abnormalities were detected in iPSCs generated for the second patient (Garber, 2015). There were genetic copy-number alterations at the chromosomal level that were not detected in the patient's original fibroblast sample. The trial was halted until the safety concerns could be addressed. Experiences such as this have taught developers that iPSC chromosomal health needs to be tested and validated at multiple steps along the process workflow. This can be seen as a “digital karyotyping” revolution to replace the standard visual karyotyping that produces beautiful images of striped chromosomes but is otherwise slow and difficult to integrate into future-proof QC plans. In contrast, the recent development of rapid handheld next-generation sequencing tools such as the Oxford Nanopore MinION device has allowed QC for genomic instability to be brought in-line during manufacturing, in between cell line splits, and for the identity of the cells to be validated at important steps along the workflow. Combined with cloud-based systems to store, compile, and analyze digital karyotyping data, such as the FIND Genomics system, with a structural variant detection resolution of 5 Mb or greater, such low-cost, high-data QC tests can be standardized and integrated into regular QC testing (Lu & Sanjana, 2019).
iii Moving toward standardized regulatory guidelines
Autologous iPSC-derived cells have undergone extensive QC validation before being transplanted into patients (Mandai et al., 2017; Schweitzer et al., 2020). However, there is currently no pre-defined regulatory path for autologous iPSC-based cell therapies; for instance, there is no regulatory guidance regarding the choice of starting tissue for iPSC generation (Jha et al., 2020). Development of a standardized regulatory roadmap will require collaboration between subject matter experts and regulatory agencies. Several international bodies, such as the Global Alliance for iPSC Therapies (GAiT) and Alliance for Regenerative Medicine (ARM) are working together with regulatory agencies to reach consensus on CQAs for iPSC-derived cell therapy products (Sullivan et al., 2018). Table 2 summarizes the current QC standards for iPSC manufacturing but, considering the fast pace of progress in the field, these standards are bound to evolve quickly. These guidelines will require frequent reassessment as the field's scientific understanding grows and the enabling technology landscape evolves. Though a well-defined regulatory path for autologous iPSC-derived cells still needs to be developed, several regulatory agencies have introduced new pathways to expedite the review process for regenerative medicine products. Examples of these are the SAKIGAKE in Japan, PRIME in Europe, and RMAT in the U.S., aiming to improve the regulatory review process for regenerative medicines (Nagai, 2019).
Attribute | Assay | Method | Timing | Notes |
---|---|---|---|---|
Safety | Mycoplasma | USP<63>, Ph.Eur.2.6.7 and JP17<G3>, qualified qPCR or culture (broth/agar or Vero cell inoculation/DNA stain method) | Release | Rapid mycoplasma assays such as real-time qPCR (e.g., MycoSEQ) can deliver results in <4 hr versus 28 days for compendial culture-based methods |
Endotoxin | USP<85>, Ph.Eur.2.6.14, JP17<4.01> | Release | Availability of rapid systems such as Endosafe nexgen-PTS allows testing to be performed in-line (at the source) versus at a core QC lab | |
Bacteriology (bioburden/sterility) | USP<71> and <61>, Ph.Eur.2.6.27 and 2.6.1, JP17<4.05> and <4.06> | In-process, release | Rapid sterility testing methods are now available (e.g., Milliflex), which reduce the time from the compendial from 14 to ≤5 days | |
Viral testing | USP<1237>, Ph.Eur.2.6.16, JP17<G3> | Release | Based on risk assessment of starting and raw materials; iPSCs for autologous use have a different viral risk profile than those slated for allogeneic clinical applications | |
Residual vector testing | Appropriate specific assay such as RT-PCR or immunochemistry with vector-specific antibodies | Release | To ensure a detectable absence of vectors (such as Sendai virus) used in iPSC generation | |
Identity | Karyotyping | Chromosome counting, G-banding, array analysis, NanoString (digital karyotyping), fluorescence in situ hybridization (FISH) | In-process, release | Can use compliant cloud-based tracking systems to ensure a chain of identity, such as FIND Genomics |
Short tandem repeat (STR) | Simple and automated process using capillary electrophoresis (CE) of fragments amplified from microsatellite loci with variable numbers of repeats and analysis by PCR | Release | Used to match donor to recipient, existing familiarity, and user base with instruments due to use in forensic analysis | |
Single nucleotide polymorphism (SNP) | SNP chip-based copy number variation (CNV) analysis | Release | Can be queried against database of known CNVs (dgv.tcag.ca/) | |
Genomic stability | RNA-seq-based intensive genetic quality test, whole genome sequencing (WGS), whole exome sequencing (WES), e.g., Illumina transcriptome human gene expression panel | Release | Targeted panels can measure expression levels of >20,000 human RefSeq genes | |
Potency | Pluripotency by differentiation | Confirm differentiation into progeny cells of the three germ layers, e.g., neuron (ectoderm), heart (mesoderm), and liver (endoderm) | Release | May include embryoid body and/or organoid formation and assessment. Teratoma formation is not currently required as a potency assay. |
Pluripotency by gene expression | Expression of pluripotency markers SSEA4, TRA1-60, OCT4, Nanog, and other genes, USP<1027>, Ph.Eur.2.7.24 | Release | Real-time qPCR assays such as TaqMan hPSC Scorecard and genome-wide assays such as PluriTest-compatible PrimeView can be used to assess many markers in parallel | |
Viability, apoptosis, and cell count | Manual (USP<1046>, Ph.Eur.2.7.29 using dye exclusion, e.g., trypan blue), or automated cell counter/flow cytometry, e.g., MACSQuant or NC-200 | Typical release criterion is a viability of >70% post-thaw |
- a Table 2 lists the current typical QC assays for GMP-grade autologous iPSCs (Huang et al., 2019; Jo et al., 2020; Sullivan et al., 2018; Yaffe, Noggle, & Solomon, 2016).
C Scalable Manufacturing
Autologous iPSC-derived cell therapies require repeating the manufacturing process for each patient. Enabling cost-effective autologous iPSC-derived cell therapy manufacturing will require some form of a “process-in-a-box” approach, which will allow scaling up via multiple units each housing one patient's cells. Even though the “expand-the-bioreactor-volume” approach to scaling up allogeneic cell therapy processes may seem more familiar and comparable to biologics manufacturing, autologous iPSC-derived cell therapy processes are well on their way to being scaled out. The process of generating iPSC MCBs is gradually becoming more standardized. Optimization of media components, replacement of recombinant proteins in the media with small molecules that target the same pathways, and generally streamlining the process to produce more target cells of the desired CQAs with shorter process times will all work together to reduce the cost of goods and the complexity of iPSC manufacturing. The automation, closed systems, and data-centric processes described in this subsection are also expected to play a key role in making autologous iPSC-based cell therapy manufacturing more scalable, cost effective, and efficient.
3.3.1 Automation, closed systems, and big data
A typical iPSC-based process will undergo an evolution from a manual, academic protocol to a robust process in a closed, automated system customized to meet the previously determined CQAs and lead to desired clinical outcomes with the final output cell types. Automation and closed systems are considered to be essential for realizing the long-term commercial success of iPSC-derived therapies because one of the primary bottlenecks is the inability to migrate the manufacturing process from a manual, high-risk academic bench protocol to an industrial-scale, GMP-compliant manufacturing system operating in a “factory of the future” to be able to handle patient demand and confidently manage risks to continuity and sustainability. As commonly stated by manufacturing experts, the two main risks for a typical cell therapy process are human error and lack of process understanding (including understanding of CQAs), causing unexpected outcomes; both risks can be effectively managed by housing an evolved process in the appropriate closed, automated system (Leong, Nankervis, & Beltzer, 2019).
The Miltenyi CliniMACS Prodigy instrument is a fully integrated GMP-compliant closed system for the medium-scale manufacturing of cell therapies. This instrument has successfully been used at hundreds of sites globally for clinical manufacturing of suspension cells such as T cells and CD34+ stem cells but initially was not configured to handle adherent cells such as iPSCs and NC cells. Recently, a proof-of-concept study highlighted the upgraded ability of the Prodigy instrument to successfully handle the GMP-compliant differentiation of iPSC-derived mesencephalic dopaminergic (mesDA) progenitor cells, which can be identified based on the integrin-associated protein (IAP) marker CD47 (Lehnen et al., 2017). Another suitable instrument is the Quantum Cell Expansion System (QES) from Terumo Blood and Cell Technologies, based on a large-surface-area hollow-fiber bioreactor membrane. The QES system manufactured iPSCs at large scale using a laminin matrix, with the in-line measurement of lactate providing a consistent readout of cell metabolism and final yield (Paccola Mesquita, Hochman-Mendez, Morrissey, Sampaio, & Taylor, 2019).
Success in this field will be enabled by putting together expert teams of diverse and highly technical stakeholders from industry sponsors, contract development and manufacturing organizations (CDMOs), and academic labs, as well as clinicians and process engineers. As a recent example, in September 2019, the iPSC-derived Cortical neurons (iCone) Consortium, comprising MaSTherCell SA (a CDMO dedicated to the industrialization and production of cell and gene therapies), Ncardia (an industry sponsor), the Pierre Vanderhaeghen Lab at Université Libre de Bruxelles (an academic collaborator), and the “Laboratoire de Chimie Analytique Pharmaceutique” led by Philippe Hubert at Université de Liège (an analytical collaborator), reported their initial success with a new 3D mid- to large-scale process for the production of human iPSC-derived cortical neurons in stirred-tank bioreactors using the Eppendorf DASbox® bioreactor platform (Sumen, 2019).
Lastly, the potential of big data solutions to improve the iPSC manufacturing process cannot be underestimated. AI and machine learning (ML) approaches have leveraged new algorithms, less expensive cloud computing power, and the expansion of various sources of genomic, proteomic, and imaging data to bring new insights to those of us working at the cutting edge to develop CQAs and streamline our processes. Going forward, big data solutions coupled to efficient laboratory information management system/electronic lab notebook (LIMS/ELN) systems will provide new insights while integrating different streams of data using promising computing approaches such as convolutional neural networks (CNNs), recurrent neural networks (RNNs), and generative adversarial networks (GANs) coupled with unsupervised deep learning to extract and relate features to outcomes. These systems are being used to develop new therapies and uncover novel ways for iPSC-derived autologous therapies to address unmet medical needs and provide succor to those suffering from debilitating diseases.
IV CONCLUSION
The promise of iPSCs lies in their ability to serve as a standardizable starting material for the development of autologous, or patient-derived, cell therapies. In contrast to the allogeneic approach, autologous therapies would treat a range of diseases without the need for immunosuppression. An autologous process could be used to treat all patients, not just those who are not covered by HLA haplobanks. Since the first groundbreaking in-human study in 2014, in which autologous iPSC-derived RPE cells were transplanted into the eye of a patient suffering from AMD, two additional patients have received autologous iPSC-derived transplants for other disease indications. None of the patients received immunosuppression, and no serious adverse events were reported for any of these studies, further confirming the promise of autologous iPSC-derived cell therapies to treat patients without subjecting them to the side effects of immunosuppression. The autologous iPSC-derived cell therapy landscape has grown significantly in the past decade, with therapies currently in development for a range of disease indications, including AMD, PD, thrombocytopenia, EB, heart failure, LGMD, and retinitis pigmentosa. With advancements in the enabling technology landscape and the development of well-defined QC standards, autologous iPSC-derived therapies can provide relief to the many individuals suffering from incurable degenerative diseases.
AUTHOR CONTRIBUTIONS
Marinna Madrid: Conceptualization, Data curation, Writing-original draft, Writing-review & editing, Cenk Sumen: Conceptualization, Data curation, Writing-original draft, Writing-review & editing, Suvi Aivio: Data curation, Writing-original draft, Writing-review & editing, Nabiha Saklayen: Conceptualization, Writing-review & editing.
CONFLICT OF INTEREST
The authors M.M., S.A., and N.S. are employees at Cellino. The author C.S. is an employee at Stemson Therapeutics.
Open Research
DATA AVAILABILITY STATEMENT
Data sharing not applicable to this article as no datasets were generated or analyzed during the current study.
LITERATURE CITED
- Araki, R., Uda, M., Hoki, Y., Sunayama, M., Nakamura, M., Ando, S., … Abe, M. (2013). Negligible immunogenicity of terminally differentiated cells derived from induced pluripotent or embryonic stem cells. Nature , 494, 100–104. doi: 10.1038/nature11807.
- Arcidiacono, J. A., Bauer, S. R., Kaplan, D. S., Allocca, C. M., Sarkar, S., & Lin-Gibson, S. (2018). FDA and NIST collaboration on standards development activities supporting innovation and translation of regenerative medicine products. Cytotherapy , 20, 779–784. doi: 10.1016/j.jcyt.2018.03.039.
- Ban, H., Nishishita, N., Fusaki, N., Tabata, T., Saeki, K., Shikamura, M., … Nishikawa, S. I. (2011). Efficient generation of transgene-free human induced pluripotent stem cells (iPSCs) by temperature-sensitive Sendai virus vectors. Proceedings of the National Academy of Sciences of the United States of America , 108(34), 14234–14239. doi: 10.1073/pnas.1103509108.
- Barker, R. A., Barrett, J., Mason, S. L., & Björklund, A. (2013). Fetal dopaminergic transplantation trials and the future of neural grafting in Parkinson's disease. The Lancet Neurology , 12, 84–91. doi: 10.1016/S1474-4422(12)70295-8.
- Benoit, D. S. W., Schwartz, M. P., Durney, A. R., & Anseth, K. S. (2008). Small functional groups for controlled differentiation of hydrogel-encapsulated human mesenchymal stem cells. Nature Materials , 7, 816–823. doi: 10.1038/nmat2269.
- Berger, A. S., Tezel, T. H., Del Priore, L. V., & Kaplan, H. J. (2003). Photoreceptor transplantation in retinitis pigmentosa: Short-term follow-up. Ophthalmology , 110, 383–391. doi: 10.1016/S0161-6420(02)01738-4.
- Bhutani, K., Nazor, K. L., Williams, R., Tran, H., Dai, H., Dzakula, Z., … Loring, J. F. (2016). Whole-genome mutational burden analysis of three pluripotency induction methods. Nature Communications , 7, 10536. doi: 10.1038/ncomms10536.
- Bilousova, G., & Roop, D. R. (2014). Induced pluripotent stem cells in dermatology: Potentials, advances, and limitations. Cold Spring Harbor Perspectives in Medicine , 4(11), a015164. doi: 10.1101/cshperspect.a015164
- Buchholz, D. E., Hikita, S. T., Rowland, T. J., Friedrich, A. M., Hinman, C. R., Johnson, L. V., & Clegg, D. O. (2009). Derivation of functional retinal pigmented epithelium from induced pluripotent stem cells. Stem Cells , 27(10), 2427–2434. doi: 10.1002/stem.189.
- Buchholz, D. E., Pennington, B. O., Croze, R. H., Hinman, C. R., Coffey, P. J., & Clegg, D. O. (2013). Rapid and efficient directed differentiation of human pluripotent stem cells into retinal pigmented epithelium. Stem Cells Translational Medicine , 2(5), 384–393. doi: 10.5966/sctm.2012-0163.
- Busskamp, V., Lewis, N. E., Guye, P., Ng, A. H., Shipman, S. L., Byrne, S. M., … Church, G. M. (2014). Rapid neurogenesis through transcriptional activation in human stem cells. Molecular Systems Biology , 10(11), 760. doi: 10.15252/msb.20145508.
- Carson, M. J., Doose, J. M., Melchior, B., Schmid, C. D., & Ploix, C. C. (2006). CNS immune privilege: Hiding in plain sight. Immunological Reviews , 213, 48–65. doi: 10.1111/j.1600-065X.2006.00441.x.
- Choi, I. Y., Lim, H. T., Estrellas, K., Mula, J., Cohen, T. V., Zhang, Y., … Lee, G. (2016). Concordant but varied phenotypes among Duchenne muscular dystrophy patient-specific myoblasts derived using a human iPSC-based model. Cell Reports , 15, 2301–2312. doi: 10.1016/j.celrep.2016.05.016.
- Cideciyan, A. V., Aleman, T. S., Jacobson, S. G., Khanna, H., Sumaroka, A., Aguirre, G. K., … Swaroop, A. (2007). Centrosomal-ciliary gene CEP290/NPHP6 mutations result in blindness with unexpected sparing of photoreceptors and visual brain: implications for therapy of Leber congenital amaurosis. Human Mutation , 28(11), 1074–1083. doi: 10.1002/humu.20565.
- Clinical research for the transfer of autologous iPS cell-derived platelets to a thrombocytopenia patient (2020). Center for iPS Cell Research and Application, Kyoto University. Retrieved from https://www.cira.kyoto-u.ac.jp/e/pressrelease/news/200325-140000.html.
- Cooper, O., Hargus, G., Deleidi, M., Blak, A., Osborn, T., Marlow, E., … Isacson, O. (2010). Differentiation of human ES and Parkinson's disease iPS cells into ventral midbrain dopaminergic neurons requires a high activity form of SHH, FGF8a and specific regionalization by retinoic acid. Molecular and Cellular Neuroscience , 45, 258–266. doi: 10.1016/j.mcn.2010.06.017.
- Dalby, M. J., Gadegaard, N., Tare, R., Andar, A., Riehle, M. O., Herzyk, P., … Oreffo, R. O. C. (2007). The control of human mesenchymal cell differentiation using nanoscale symmetry and disorder. Nature Materials , 6, 997–1003. doi: 10.1038/nmat2013.
- den Hollander, A. I., Roepman, R., Koenekoop, R. K., & Cremers, F. P. M. (2008). Leber congenital amaurosis: Genes, proteins and disease mechanisms. Progress in Retinal and Eye Research , 27, 391–419. doi: 10.1016/j.preteyeres.2008.05.003.
- Deuse, T., Hu, X., Agbor-Enoh, S., Koch, M., Spitzer, M. H., Gravina, A., … Schrepfer, S. (2019). De novo mutations in mitochondrial DNA of iPSCs produce immunogenic neoepitopes in mice and humans. Nature Biotechnology , 37, 1137–1144. doi: 10.1038/s41587-019-0227-7.
- Ding, X., Stewart, M. P., Sharei, A., Weaver, J. C., Langer, R. S., & Jensen, K. F. (2017). High-throughput nuclear delivery and rapid expression of DNA via mechanical and electrical cell-membrane disruption. Nature Biomedical Engineering , 1, 0039. doi: 10.1038/s41551-017-0039.
- Dorsey, E. R., Sherer, T., Okun, M. S., & Bloemd, B. R. (2018). The emerging evidence of the Parkinson pandemic. Journal of Parkinson's Disease , 8, S3–S8. doi: 10.3233/JPD-181474.
- Drozd, A. M., Walczak, M. P., Piaskowski, S., Stoczynska-Fidelus, E., Rieske, P., & Grzela, D. P. (2015). Generation of human iPSCs from cells of fibroblastic and epithelial origin by means of the oriP/EBNA-1 episomal reprogramming system. Stem Cell Research & Therapy, 6(1), 122. doi: 10.1186/s13287-015-0112-3.
- Ehrlich, M., Mozafari, S., Glatza, M., Starost, L., Velychko, S., Hallmann, A. L., … Kuhlmann, T. (2017). Rapid and efficient generation of oligodendrocytes from human induced pluripotent stem cells using transcription factors. Proceedings of the National Academy of Sciences of the United States of America , 114, E2243–E2252. doi: 10.1073/pnas.1614412114.
- Eliopoulos, N., Stagg, J., Lejeune, L., Pommey, S., & Galipeau, J. (2005). Allogeneic marrow stromal cells are immune rejected by MHC class I- and class II-mismatched recipient mice. Blood , 106(13), 4057–4065. doi: 10.1182/blood-2005-03-1004.
- Emery, A. E. H. (2002). The muscular dystrophies. Lancet , 359, 687–695. doi: 10.1016/S0140-6736(02)07815-7.
- Eminli, S., Foudi, A., Stadtfeld, M., Maherali, N., Ahfeldt, T., Mostoslavsky, G., … Hochedlinger, K. (2009). Differentiation stage determines potential of hematopoietic cells for reprogramming into induced pluripotent stem cells. Nature Genetics , 41, 968–976. doi: 10.1038/ng.428.
- Engelender, S., & Isacson, O. (2017). The threshold theory for Parkinson's disease. Trends in Neurosciences , 40, 4–14. doi: 10.1016/j.tins.2016.10.008.
- Engler, A. J., Sen, S., Sweeney, H. L., & Discher, D. E. (2006). Matrix elasticity directs stem cell lineage specification. Cell , 126, 677–689. doi: 10.1016/j.cell.2006.06.044.
- Estcourt, L. J., Malouf, R., Doree, C., Trivella, M., Hopewell, S., & Birchall, J. (2018). Prophylactic platelet transfusions prior to surgery for people with a low platelet count. Cochrane Database of Systematic Reviews , 9, CD012779. doi: 10.1002/14651858.CD012779.pub2.
- Federoff, H. (2020). No title. In Highlighting the technical and clinical accomplishments of Aspen Neuroscience for 2020. Virtual Conference: Cell & Gene Meeting on the Mesa.
- Fernandopulle, M. S., Prestil, R., Grunseich, C., Wang, C., Gan, L., & Ward, M. E. (2018). Transcription factor-mediated differentiation of human iPSCs into neurons. Current Protocols in Cell Biology , 79, e51. doi: 10.1002/cpcb.51.
- Fine, J. D. (2010). Inherited epidermolysis bullosa. Orphanet Journal of Rare Diseases , 5, 12. doi: 10.1186/1750-1172-5-12.
- Freed, C. R., Breeze, R. E., Rosenberg, N. L., Schneck, S. A., Kriek, E., Qi, J., … Ansari, A. A. (1992). Survival of implanted fetal dopamine cells and neurologic improvement 12 to 46 months after transplantation for Parkinson's disease. New England Journal of Medicine , 327, 1549–1555. doi: 10.1056/nejm199211263272202.
- Frega, M., Van Gestel, S. H. C., Linda, K., Van Der Raadt, J., Keller, J., Van Rhijn, J. R., … Kasri, N. N. (2017). Rapid neuronal differentiation of induced pluripotent stem cells for measuring network activity on micro-electrode arrays. Journal of Visualized Experiments , 54900. doi: 10.3791/54900.
- Fusaki, N., Ban, H., Nishiyama, A., Saeki, K., & Hasegawa, M. (2009). Efficient induction of transgene-free human pluripotent stem cells using a vector based on Sendai virus, an RNA virus that does not integrate into the host genome. Proceedings of the Japan Academy Series B: Physical and Biological Sciences , 85(8), 348–362. doi: 10.2183/pjab.85.348.
- Garber, K. (2015). RIKEN suspends first clinical trial involving induced pluripotent stem cells. Nature Biotechnology , 33, 890–891. doi: 10.1038/nbt0915-890.
- Garcia, P. A., Ge, Z., Moran, J. L., & Buie, C. R. (2016). Microfluidic screening of electric fields for electroporation. Scientific Reports , 6, 21238. doi: 10.1038/srep21238.
- Ghaoui, R., Cooper, S. T., Lek, M., Jones, K., Corbett, A., Reddel, S. W., … Clarke, N. F. (2015). Use of whole-exome sequencing for diagnosis of limb-girdle muscular dystrophy: Outcomes and lessons learned. JAMA Neurology , 72(12), 1424–1432. doi: 10.1001/jamaneurol.2015.2274.
- Gnedeva, K., Vorotelyak, E., Cimadamore, F., Cattarossi, G., Giusto, E., Terskikh, V. V., & Terskikh, A. V. (2015). Derivation of hair-inducing cell from human pluripotent stem cells. PLOS One , 10, e0116892. doi: 10.1371/journal.pone.0116892.
- Gornalusse, G. G., Hirata, R. K., Funk, S. E., Riolobos, L., Lopes, V. S., Manske, G., … Russell, D. W. (2017). HLA-E-expressing pluripotent stem cells escape allogeneic responses and lysis by NK cells. Nature Biotechnology , 35, 765–772. doi: 10.1038/nbt.3860.
- Gourraud, P.-A., Gilson, L., Girard, M., & Peschanski, M. (2011). The role of human leukocyte antigen matching in the development of multiethnic “haplobank” of induced pluripotent stem cell lines. Stem Cells , 30, 180–186. doi: 10.1002/stem.772.
- Guha, P., Morgan, J. W., Mostoslavsky, G., Rodrigues, N. P., & Boyd, A. S. (2013). Lack of immune response to differentiated cells derived from syngeneic induced pluripotent stem cells. Cell Stem Cell , 12, 407–412. doi: 10.1016/j.stem.2013.01.006.
- Hagell, P., Crabb, L., Pogarell, O., Schrag, A., Widner, H., Brooks, D. J., … Lindvall, O. (2000). Health-related quality of life following bilateral intrastriatal transplantation in Parkinson's disease. Movement Disorders , 15, 224–229. doi: 10.1002/1531-8257(200003)15:2<224::AID-MDS1004>3.0.CO;2-W.
- Hallett, P. J., Cooper, O., Sadi, D., Robertson, H., Mendez, I., & Isacson, O. (2014). Long-term health of dopaminergic neuron transplants in Parkinson's disease patients. Cell Reports , 7, 1755–1761. doi: 10.1016/j.celrep.2014.05.027.
- Hallett, P. J., Deleidi, M., Astradsson, A., Smith, G. A., Cooper, O., Osborn, T. M., … Isacson, O. (2015). Successful function of autologous iPSC-derived dopamine neurons following transplantation in a non-human primate model of Parkinson's disease. Cell Stem Cell , 16, 269–274. doi: 10.1016/j.stem.2015.01.018.
- Hanatani, T., & Takasu, N. (2020). CiRA iPSC seed stocks (CiRA's iPSC Stock Project). Stem Cell Research , 50, 102033. doi: 10.1016/j.scr.2020.102033.
- Hartong, D. T., Berson, E. L., & Dryja, T. P. (2006). Retinitis pigmentosa. Lancet , 368, 1795–1809. doi: 10.1016/S0140-6736(06)69740-7.
- Hatzimichael, E., & Tuthill, M. (2010). Hematopoietic stem cell transplantation. Stem Cells and Cloning: Advances and Applications , 3, 105–117. doi: 10.2147/SCCAA.S6815.
- Henry, M. P., Hawkins, J. R., Boyle, J., & Bridger, J. M. (2019). The genomic health of human pluripotent stem cells: Genomic instability and the consequences on nuclear organization. Frontiers in Genetics , 9, 623. doi: 10.3389/fgene.2018.00623.
- Hod, E., & Schwartz, J. (2008). Platelet transfusion refractoriness. British Journal of Haematology , 142, 348–360. doi: 10.1111/j.1365-2141.2008.07189.x.
- Huang, C. Y., Liu, C. L., Ting, C. Y., Chiu, Y. T., Cheng, Y. C., Nicholson, M. W., & Hsieh, P. C. H. (2019). Human iPSC banking: Barriers and opportunities. Journal of Biomedical Science , 26, 87. doi: 10.1186/s12929-019-0578-x.
- Ilic, D., & Ogilvie, C. (2017). Concise review: Human embryonic stem cells-what have we done? What are we doing? Where are we going? Stem Cells , 35(1), 17–25. doi: 10.1002/stem.2450.
- Iwashita, M., Ohta, H., Fujisawa, T., Cho, M., Ikeya, M., Kidoaki, S., & Kosodo, Y. (2019). Brain-stiffness-mimicking tilapia collagen gel promotes the induction of dorsal cortical neurons from human pluripotent stem cells. Scientific Reports , 9, 3068. doi: 10.1038/s41598-018-38395-5.
- Jacków, J., Guo, Z., Hansen, C., Abaci, H. E., Doucet, Y. S., Shin, J. U., … Christiano, A. M. (2019). CRISPR/Cas9-based targeted genome editing for correction of recessive dystrophic epidermolysis bullosa using iPS cells. Proceedings of the National Academy of Sciences of the United States of America , 116(52), 26846–26852. doi: 10.1073/pnas.1907081116.
- Jha, B. S., Farnoodian, M., & Bharti, K. (2020). Regulatory considerations for developing a phase I investigational new drug application for autologous induced pluripotent stem cells-based therapy product. Stem Cells Translational Medicine , 10(2), 198–208. doi: 10.1002/sctm.20-0242.
- Jo, H. Y., Han, H. W., Jung, I., Ju, J. H., Park, S. J., Moon, S., … Kim, J. H. (2020). Development of genetic quality tests for good manufacturing practice-compliant induced pluripotent stem cells and their derivatives. Scientific Reports , 10(1), 3939. doi: 10.1038/s41598-020-60466-9.
- Kamao, H., Mandai, M., Okamoto, S., Sakai, N., Suga, A., Sugita, S., … Takahashi, M. (2014). Characterization of human induced pluripotent stem cell-derived retinal pigment epithelium cell sheets aiming for clinical application. Stem Cell Reports , 2(2), 205–218. doi: 10.1016/j.stemcr.2013.12.007.
- Kaplan, H. J., Tezel, T. H., Berger, A. S., Wolf, M. L., & Del Priore, L. V. (1997). Human photoreceptor transplantation in retinitis pigmentosa: A safety study. Archives of Ophthalmology , 115(9), 1168–1172. doi: 10.1001/archopht.1997.01100160338012.
- Kefalopoulou, Z., Politis, M., Piccini, P., Mencacci, N., Bhatia, K., Jahanshahi, M., … Foltynie, T. (2014). Long-term clinical outcome of fetal cell transplantation for Parkinson disease: Two case reports. JAMA Neurology , 71(1), 83–87. doi: 10.1001/jamaneurol.2013.4749.
- Kitala, D., Kawecki, M., Klama-Baryła, A., łabuś, W., Kraut, M., Glik, J., … Nowak, M. (2016). Allogeneic vs. autologous skin grafts in the therapy of patients with burn injuries: A retrospective, open-label clinical study with pair matching. Advances in Clinical and Experimental Medicine , 25(5), 923–929. doi: 10.17219/acem/61961.
- Kogut, I., McCarthy, S. M., Pavlova, M., Astling, D. P., Chen, X., Jakimenko, A., … Bilousova, G. (2018). High-efficiency RNA-based reprogramming of human primary fibroblasts. Nature Communications , 9(1), 745. doi: 10.1038/s41467-018-03190-3.
- Kordower, J. H., Freeman, T. B., Chen, E. Y., Mufson, E. J., Sanberg, P. R., Hauser, R. A., … Olanow, C. W. (1998). Fetal nigral grafts survive and mediate clinical benefit in a patient with Parkinson's disease. Movement Disorders , 13(3), 383–393. doi: 10.1002/mds.870130303.
- Lamba, D. A., Gust, J., & Reh, T. A. (2009). Transplantation of human embryonic stem cell-derived photoreceptors restores some visual function in Crx-deficient mice. Cell Stem Cell , 4(1), 73–79. doi: 10.1016/j.stem.2008.10.015.
- Lee, J., Abdeen, A. A., Zhang, D., & Kilian, K. A. (2013). Directing stem cell fate on hydrogel substrates by controlling cell geometry, matrix mechanics and adhesion ligand composition. Biomaterials , 34(33), 8140–8148. doi: 10.1016/j.biomaterials.2013.07.074.
- Lehnen, D., Barral, S., Cardoso, T., Grealish, S., Heuer, A., Smiyakin, A., … Knöbel, S. (2017). IAP-based cell sorting results in homogeneous transplantable dopaminergic precursor cells derived from human pluripotent stem cells. Stem Cell Reports , 9(4), 1207–1220. doi: 10.1016/j.stemcr.2017.08.016.
- Leong, W., Nankervis, B., & Beltzer, J. (2019). Automation: What will the cell therapy laboratory of the future look like? Cell & Gene Therapy Insights, 4, 679–694. doi: 10.18609/cgti.2018.067.
- Li, W., Englund, E., Widner, H., Mattsson, B., Van Westen, D., Lätt, J., … Li, J. Y. (2016). Extensive graft-derived dopaminergic innervation is maintained 24 years after transplantation in the degenerating parkinsonian brain. Proceedings of the National Academy of Sciences of the United States of America , 113(23), 6544–6549. doi: 10.1073/pnas.1605245113.
- Lindvall, O., Brundin, P., Widner, H., Rehncrona, S., Gustavii, B., Frackowiak, R., … Björklund, A. (1990). Grafts of fetal dopamine neurons survive and improve motor function in Parkinson's disease. Science , 247(4942), 574–577. doi: 10.1126/science.2105529.
- Loh, Y. H., Agarwal, S., Park, I. H., Urbach, A., Huo, H., Heffner, G. C., … Daley, G. Q. (2009). Generation of induced pluripotent stem cells from human blood. Blood , 113(22), 5476–5479. doi: 10.1182/blood-2009-02-204800.
- Loring, J. F. (2018). Autologous induced pluripotent stem cell-derived neurons to treat Parkinson's disease. Stem Cells and Development , 27(14), 958–959. doi: 10.1089/scd.2018.0107.
- Lu, C., & Sanjana, N. E. (2019). Generation of a knock-in MAP2-tdTomato reporter human embryonic stem cell line with inducible expression of NEUROG2/1 (NYGCe001-A). Stem Cell Research , 41, 101643. doi: 10.1016/j.scr.2019.101643.
- Mack, A. A., Kroboth, S., Rajesh, D., & Wang, W. B. (2011). Generation of induced pluripotent stem cells from CD34+ cells across blood drawn from multiple donors with non-integrating episomal vectors. PLOS One , 6(11), e27956. doi: 10.1371/journal.pone.0027956.
- Majhail, N. S., Mau, L. W., Denzen, E. M., & Arneson, T. J. (2013). Costs of autologous and allogeneic hematopoietic cell transplantation in the United States: A study using a large National Private Claims Database. Bone Marrow Transplantation , 48(2), 294–300. doi: 10.1038/bmt.2012.133.
- Maldonado, M., Luu, R. J., Ramos, M. E. P., & Nam, J. (2016). ROCK inhibitor primes human induced pluripotent stem cells to selectively differentiate towards mesendodermal lineage via epithelial-mesenchymal transition-like modulation. Stem Cell Research , 17(2), 222–227. doi: 10.1016/j.scr.2016.07.009.
- Mandai, M., Watanabe, A., Kurimoto, Y., Hirami, Y., Morinaga, C., Daimon, T., … Takahashi, M. (2017). Autologous induced stem-cell–derived retinal cells for macular degeneration. New England Journal of Medicine , 376(11), 1038–1046. doi: 10.1056/nejmoa1608368.
- Marks, D. H., Penzi, L. R., Ibler, E., Manatis-Lornell, A., Hagigeorges, D., Yasuda, M., … Senna, M. M. (2019). The Medical and Psychosocial Associations of Alopecia: Recognizing hair loss as more than a cosmetic concern. American Journal of Clinical Dermatology , 20(2), 195–200. doi: 10.1007/s40257-018-0405-2.
- Martín-Ibáñez, R. &, & Sareen, D. (2020). Manufacturing of human iPSC-derived cell therapies: Road to the clinic. Cell and Gene Therapy Insights , 6, 177–191. doi: 10.18609/cgti.2020.023.
- McMurray, R. J., Gadegaard, N., Tsimbouri, P. M., Burgess, K. V., McNamara, L. E., Tare, R., … Dalby, M. J. (2011). Nanoscale surfaces for the long-term maintenance of mesenchymal stem cell phenotype and multipotency. Nature Materials , 10(8), 637–644. doi: 10.1038/nmat3058.
- Meissner, T., Strominger, J., & Cowan, C. (2015). The universal donor stem cell: Removing the immune barrier to transplantation using CRISPR/Cas9 (TRAN1P.946). The Journal of Immunology , 194(1 Supplement), 140.28.
- Mendez, I., Sanchez-Pernaute, R., Cooper, O., Viñuela, A., Ferrari, D., Björklund, L., … Isacson, O. (2005). Cell type analysis of functional fetal dopamine cell suspension transplants in the striatum and substantia nigra of patients with Parkinson's disease. Brain , 128(Pt 7), 1498–1510. doi: 10.1093/brain/awh510.
- Miller, L. W. (2002). Cardiovascular toxicities of immunosuppressive agents. American Journal of Transplantation , 2(9), 807–818. doi: 10.1034/j.1600-6143.2002.20902.x.
- Müller, F. J., Schuldt, B. M., Williams, R., Mason, D., Altun, G., Papapetrou, E. P., … Loring, J. F. (2011). A bioinformatic assay for pluripotency in human cells. Nature Methods , 8(4), 315–317. doi: 10.1038/nmeth.1580.
- Mullins, R. F., Kuehn, M. H., Radu, R. A., Enriquez, G. S., East, J. S., Schindler, E. I., … Stone, E. M. (2012). Autosomal recessive retinitis pigmentosa due to ABCA4 mutations: Clinical, pathologic, and molecular characterization. Investigative Ophthalmology & Visual Science, 53(4), 1883–1894. doi: 10.1167/iovs.12-9477.
- Murphy, W. L., McDevitt, T. C., & Engler, A. J. (2014). Materials as stem cell regulators. Nature Materials , 13(6), 547–557. doi: 10.1038/nmat3937.
- Nagai, S. (2019). Flexible and expedited regulatory review processes for innovative medicines and regenerative medical products in the US, the EU, and Japan. International Journal of Molecular Sciences , 20(15), 3801. doi: 10.3390/ijms20153801.
- Nanbo, A., Sugden, A., & Sugden, B. (2007). The coupling of synthesis and partitioning of EBV's plasmid replicon is revealed in live cells. EMBO Journal , 26(19), 4252–4262. doi: 10.1038/sj.emboj.7601853.
- Ng, A. H. M., Khoshakhlagh, P., Rojo Arias, J. E., Pasquini, G., Wang, K., … Church, G. M. (2020). A comprehensive library of human transcription factors for cell fate engineering. Nature Biotechnology , 1–10. doi: 10.1038/s41587-020-0742-6.
- Nguyen, E. H., Daly, W. T., Le, N. N. T., Farnoodian, M., Belair, D. G., Schwartz, M. P., … Murphy, W. L. (2017). Versatile synthetic alternatives to Matrigel for vascular toxicity screening and stem cell expansion. Nature Biomedical Engineering , 1, 0096. doi: 10.1038/s41551-017-0096.
- O'Dea, S., Annibaldi, V., Gallagher, L., Mulholland, J., Molloy, E. L., Breen, C. J., … Curry, F. R. (2017). Vector-free intracellular delivery by reversible permeabilization. PLOS One , 12(3), e0174779. doi: 10.1371/journal.pone.0174779.
- Ohmine, S., Dietz, A. B., Deeds, M. C., Hartjes, K. A., Miller, D. R., Thatava, T., … Ikeda, Y. (2011). Induced pluripotent stem cells from GMP-grade hematopoietic progenitor cells and mononuclear myeloid cells. Stem Cell Research and Therapy , 2(6), 46. doi: 10.1186/scrt87.
- Okita, K., Ichisaka, T., & Yamanaka, S. (2007). Generation of germline-competent induced pluripotent stem cells. Nature , 448(7151), 313–317. doi: 10.1038/nature05934.
- Okita, K., Matsumura, Y., Sato, Y., Okada, A., Morizane, A., Okamoto, S., … Yamanaka, S. (2011). A more efficient method to generate integration-free human iPS cells. Nature Methods , 8(5), 409–412. doi: 10.1038/nmeth.1591.
- Oro's, A. (n.d.). DEBCT: Genetically Corrected, Induced Pluripotent Cell-Derived Epithelial Sheets for Definitive Treatment of Dystrophic Epidermolysis Bullosa. California Institute for Regenerative Medicine. Retrieved from https://www.cirm.ca.gov/our-progress/awards/debct-genetically-corrected-induced-pluripotent-cell-derived-epithelial-sheets.
- Osakada, F., Ikeda, H., Sasai, Y., & Takahashi, M. (2009). Stepwise differentiation of pluripotent stem cells into retinal cells. Nature Protocols , 4(6), 811–824. doi: 10.1038/nprot.2009.51.
- Osakada, F., Jin, Z. B., Hirami, Y., Ikeda, H., Danjyo, T., Watanabe, K., … Takahashi, M. (2009). In vitro differentiation of retinal cells from human pluripotent stem cells by small-molecule induction. Journal of Cell Science , 122(Pt 17), 3169–3179. doi: 10.1242/jcs.050393.
- Osborn, T. M., Hallett, P. J., Schumacher, J. M., & Isacson, O. (2020). Advantages and recent developments of autologous cell therapy for Parkinson's disease patients. Frontiers in Cellular Neuroscience , 14, 58. doi: 10.3389/fncel.2020.00058.
- Paccola Mesquita, F. C., Hochman-Mendez, C., Morrissey, J., Sampaio, L. C., & Taylor, D. A. (2019). Laminin as a potent substrate for large-scale expansion of human induced pluripotent stem cells in a closed cell expansion system. Stem Cells International , 2019, 9704945. doi: 10.1155/2019/9704945.
- Papapetrou, E. P. (2016). Patient-derived induced pluripotent stem cells in cancer research and precision oncology. Nature Medicine , 22(12), 1392–1401. doi: 10.1038/nm.4238.
- Partridge, T. A., Morgan, J. E., Coulton, G. R., Hoffman, E. P., & Kunkel, L. M. (1989). Conversion of mdx myofibres from dystrophin-negative to-positive by injection of normal myoblasts. Nature , 337(6203), 176–179. doi: 10.1038/337176a0.
- Pavenski, K., Rebulla, P., Duquesnoy, R., Saw, C. L., Slichter, S. J., Tanael, S., & Shehata, N. (2013). Efficacy of HLA-matched platelet transfusions for patients with hypoproliferative thrombocytopenia: A systematic review. Transfusion , 53(10), 2230–2242. doi: 10.1111/trf.12175.
- Pearson, R. A., Barber, A. C., Rizzi, M., Hippert, C., Xue, T., West, E. L., … Ali, R. R. (2012). Restoration of vision after transplantation of photoreceptors. Nature , 485(7396), 99–103. doi: 10.1038/nature10997.
- Petit, I., Salman Kesner, N., Karry, R., Robicsek, O., Aberdam, E., Müller, F. J., … Ben-Shachar, D. (2012). Induced pluripotent stem cells from hair follicles as a cellular model for neurodevelopmental disorders. Stem Cell Research , 8(1), 134–140. doi: 10.1016/j.scr.2011.09.003.
- Piccini, P., Brooks, D. J., Björklund, A., Gunn, R. N., Grasby, P. M., Rimoldi, O., … Lindvall, O. (1999). Dopamine release from nigral transplants visualized in vivo in a Parkinson's patient. Nature Neuroscience , 2(12), 1137–1140. doi: 10.1038/16060.
- Politis, M., & Piccini, P. (2012). In vivo imaging of the integration and function of nigral grafts in clinical trials. Progress in Brain Research , 200, 199–220. doi: 10.1016/B978-0-444-59575-1.00009-0.
- Politis, M., Wu, K., Loane, C., Quinn, N. P., Brooks, D. J., Rehncrona, S., … Piccini, P. (2010). Serotonergic neurons mediate dyskinesia side effects in Parkinson's patients with neural transplants. Science Translational Medicine , 2(38), 38ra46. doi: 10.1126/scitranslmed.3000976.
- Raab, S., Klingenstein, M., Liebau, S., & Linta, L. (2014). A comparative view on human somatic cell sources for iPSC generation. Stem Cells International , 2014, 768391. doi: 10.1155/2014/768391.
- Rassman, W. R., Bernstein, R. M., McClellan, R., Jones, R., Worton, E., & Uyttendaele, H. (2002). Follicular unit extraction: Minimally invasive surgery for hair transplantation. Dermatologic Surgery , 28(8), 720–728. doi: 10.1046/j.1524-4725.2002.01320.x.
- Redmond, D. E., Vinuela, A., Kordower, J. H., & Isacson, O. (2008). Influence of cell preparation and target location on the behavioral recovery after striatal transplantation of fetal dopaminergic neurons in a primate model of Parkinson's disease. Neurobiology of Disease , 29(1), 103–116. doi: 10.1016/j.nbd.2007.08.008.
- Roop, D. R., Bilousova, G., & Kogut, I. (2019). Translating a stem cell-based therapy for epidermolysis bullosa into the clinic. Denver: University of Colorado.
- Saha, K., Mei, Y., Reisterer, C. M., Pyzocha, N. K., Yang, J., Muffat, J., … Jaenisch, R. (2011). Surface-engineered substrates for improved human pluripotent stem cell culture under fully defined conditions. Proceedings of the National Academy of Sciences of the United States of America , 108(46), 18714–18719. doi: 10.1073/pnas.1114854108.
- Saito, S., Ota, S., Seshimo, H., Yamazaki, Y., Nomura, S., Ito, T., … Maeda, H. (2002). Platelet transfusion refractoriness caused by a mismatch in HLA-C antigens. Transfusion , 42(3), 302–308. doi: 10.1046/j.1537-2995.2002.00051.x.
- Saklayen, N., Huber, M., Madrid, M., Nuzzo, V., Vulis, D. I., Shen, W., … Mazur, E. (2017). Intracellular delivery using nanosecond-laser excitation of large-area plasmonic substrates. ACS Nano , 11(4), 3671–3680. doi: 10.1021/acsnano.6b08162.
- Santos-Ferreira, T. F., Borsch, O., & Ade, M. (2017). Rebuilding the missing Part—A review on photoreceptor transplantation. Frontiers in Systems Neuroscience , 10, 105. doi: 10.3389/fnsys.2016.00105.
- Schlaeger, T. M., Daheron, L., Brickler, T. R., Entwisle, S., Chan, K., Cianci, A., … Daley, G. Q. (2015). A comparison of non-integrating reprogramming methods. Nature Biotechnology , 33(1), 58–63. doi: 10.1038/nbt.3070.
- Schmitt, J. V., Ribeiro, C. F., Souza, F. H. M., Siqueira, E. B. D., & Bebber, F. R. L. (2012). Hair loss perception and symptoms of depression in female outpatients attending a general dermatology clinic. Anais Brasileiros de Dermatologia , 87, 412–417. doi: 10.1590/s0365-05962012000300010.
- Schorl, C., & Sedivy, J. M. (2007). Analysis of cell cycle phases and progression in cultured mammalian cells. Methods , 41(2), 143–150. doi: 10.1016/j.ymeth.2006.07.022.
- Schweitzer, J. S., Song, B., Herrington, T. M., Park, T.-Y., Lee, N., Ko, S., … Kim, K.-S. (2020). Personalized iPSC-derived dopamine progenitor cells for Parkinson's disease. New England Journal of Medicine , 382(20), 1926–1932. doi: 10.1056/nejmoa1915872.
- Sebastiano, V., Zhen, H. H., Derafshi, B. H., Bashkirova, E., Melo, S. P., Wang, P., … Oro, A. E. (2014). Human COL7A1-corrected induced pluripotent stem cells for the treatment of recessive dystrophic epidermolysis bullosa. Science Translational Medicine , 6(264), 264ra163. doi: 10.1126/scitranslmed.3009540.
- Sharma, R., Bose, D., Maminishkis, A., & Bharti, K. (2020). Retinal pigment epithelium replacement therapy for age-related macular degeneration: Are we there yet? Annual Review of Pharmacology and Toxicology , 60(1), 553–572. doi: 10.1146/annurev-pharmtox-010919-023245.
- Sharma, R., Khristov, V., Rising, A., Jha, B. S., Dejene, R., Hotaling, N., … Bharti, K. (2019). Clinical-grade stem cell-derived retinal pigment epithelium patch rescues retinal degeneration in rodents and pigs. Science Translational Medicine , 11(475), eaat5580. doi: 10.1126/scitranslmed.aat5580.
- Shinkuma, S. (2015). Dystrophic epidermolysis bullosa: A review. Clinical, Cosmetic and Investigational Dermatology , 8, 275–284. doi: 10.2147/CCID.S54681.
- Simaria, A. S., Hassan, S., Varadaraju, H., Rowley, J., Warren, K., Vanek, P., & Farid, S. S. (2014). Allogeneic cell therapy bioprocess economics and optimization: Single-use cell expansion technologies. Biotechnology and Bioengineering , 111(1), 69–83. doi: 10.1002/bit.25008.
- Skuk, D., & Tremblay, J. P. (2003). Myoblast transplantation: The current status of a potential therapeutic tool for myopathies. Journal of Muscle Research and Cell Motility , 24(4-6), 285–300. doi: 10.1023/A:1025425823322.
- Sohn, E. H., Jiao, C., Kaalberg, E., Cranston, C., Mullins, R. F., Stone, E. M., & Tucker, B. A. (2015). Allogenic iPSC-derived RPE cell transplants induce immune response in pigs: A pilot study. Scientific Reports , 5(1), 1–10. doi: 10.1038/srep11791.
- Song, B., Cha, Y., Ko, S., Jeon, J., Lee, N., Seo, H., … Kim, K. S. (2020). Human autologous iPSC-derived dopaminergic progenitors restore motor function in Parkinson's disease models. Journal of Clinical Investigation , 130(2), 904–920. doi: 10.1172/JCI130767.
- Sood, R., Roggy, D. E., Zieger, M. J., Nazim, M., Hartman, B. C., & Gibbs, J. T. (2015). A comparative study of spray keratinocytes and autologous meshed split-thickness skin graft in the treatment of acute burn injuries. Wounds , 27(2), 31–40.
- Stanworth, S. J., Navarrete, C., Estcourt, L., & Marsh, J. (2015). Platelet refractoriness - practical approaches and ongoing dilemmas in patient management. British Journal of Haematology , 171(3), 297–305. doi: 10.1111/bjh.13597.
- Stasi, R. (2012). How to approach thrombocytopenia. Hematology/the Education Program of the American Society of Hematology , 2012, 191–197. doi: 10.1182/asheducation.v2012.1.191.3798260.
- Sullivan, S., Stacey, G. N., Akazawa, C., Aoyama, N., Baptista, R., Bedford, P., … Song, J. (2018). Quality control guidelines for clinical-grade human induced pluripotent stem cell lines. Regenerative Medicine , 13(7), 859–866. doi: 10.2217/rme-2018-0095.
- Sumen, C. (2019). iPSCs: Moving from drug testing to novel cell therapies. Pharma's Almanac. Retrieved from https://www.pharmasalmanac.com/articles/ipscs-moving-from-drug-testing-to-novel-cell-therapies.
- Sun, C., Serra, C., Lee, G., & Wagner, K. R. (2020). Stem cell-based therapies for Duchenne muscular dystrophy. Experimental Neurology , 323, 113086. doi: 10.1016/j.expneurol.2019.113086.
- Sundberg, M., Bogetofte, H., Lawson, T., Jansson, J., Smith, G., Astradsson, A., … Isacson, O. (2013). Improved cell therapy protocols for Parkinson's disease based on differentiation efficiency and safety of hESC-, hiPSC-, and non-human primate iPSC-derived dopaminergic neurons. Stem Cells , 31(8), 154 8–1562. doi: 10.1002/stem.1415.
- Takagi, S., Mandai, M., Gocho, K., Hirami, Y., Yamamoto, M., Fujihara, M., … Takahashi, M. (2019). Evaluation of transplanted autologous induced pluripotent stem cell-derived retinal pigment epithelium in exudative age-related macular degeneration. Ophthalmology Retina , 3(10), 850–859. doi: 10.1016/j.oret.2019.04.021.
- Takahashi, K., Tanabe, K., Ohnuki, M., Narita, M., Ichisaka, T., Tomoda, K., & Yamanaka, S. (2007). Induction of pluripotent stem cells from adult human fibroblasts by defined factors. Cell , 131(5), 861–872. doi: 10.1016/j.cell.2007.11.019.
- Taylor, A. W. (2016). Ocular immune privilege and transplantation. Frontiers in Immunology , 7, 37. doi: 10.3389/fimmu.2016.00037.
- Tedesco, F. S., Gerli, M. F. M., Perani, L., Benedetti, S., Ungaro, F., Cassano, M., … Cossu, G. (2012). Transplantation of genetically corrected human iPSC-derived progenitors in mice with limb-girdle muscular dystrophy. Science Translational Medicine , 4(140), 140ra89. doi: 10.1126/scitranslmed.3003541.
- Tepperman, E., Ramzy, D., Prodaer, J., Sheshgiri, R., Badiwala, M., Ross, H., & Rao, V. (2010). Surgical biology for the clinician: Vascular effects of immunosuppression. Canadian Journal of Surgery , 53(1), 57–63.
- Tolar, J., McGrath, J. A., Xia, L., Riddle, M. J., Lees, C. J., Eide, C., … Wagner, J. E. (2014). Patient-specific naturally gene-reverted induced pluripotent stem cells in recessive dystrophic epidermolysis bullosa. Journal of Investigative Dermatology , 134(5), 1246–1254. doi: 10.1038/jid.2013.523.
- Torkelson, J. L., Hansen, C., Jackow, J., Guo, Z., Hui-Zhen, H., Hayashi, R., … Oro, A. (2019). 1036 Scalable production of CRISPR-corrected autologous iPSC derived skin grafts to treat epidermolysis bullosa. Journal of Investigative Dermatology , 139(5), S179. doi: 10.1016/j.jid.2019.03.1112.
- Tsang, S. H., & Sharma, T. (2018). Stargardt disease. Advances in Experimental Medicine and Biology , 1085, 139–151. doi: 10.1007/978-3-319-95046-4_27.
- Tucker, B. A., Park, I. H., Qi, S. D., Klassen, H. J., Jiang, C., Yao, J., … Young, M. J. (2011). Transplantation of adult mouse iPS cell-derived photoreceptor precursors restores retinal structure and function in degenerative mice. PLOS One , 6(4), e18992. doi: 10.1371/journal.pone.0018992.
- Umegaki-Arao, N., Pasmooij, A. M. G., Itoh, M., Cerise, J. E., Guo, Z., Levy, B., … Christiano, A. M. (2014). Induced pluripotent stem cells from human revertant keratinocytes for the treatment of epidermolysis bullosa. Science Translational Medicine , 6(264), 264ra164. doi: 10.1126/scitranslmed.3009342.
- Lee, G., Choi, I., Lim, H. T., & Wagner, K. R. (2016). U.S. Patent No. 15/781,709. Washington, DC: U.S. Patent and Trademark Office.
- Van Meurs, J. C., Ter Averst, E., Hofland, L. J., Van Hagen, P. M., Mooy, M., Baarsma, G. S., … Van Meurs, J. C. (2004). Autologous peripheral retinal pigment epithelium translocation in patients with subfoveal neovascular membranes. The British Journal of Ophthalmology , 88, 110–113. doi: 10.1136/bjo.88.1.110.
- Van Zeeburg, E. J. T., Maaijwee, K. J. M., Missotten, T. O. A. R., Heimann, H., & Van Meurs, J. C. (2012). A free retinal pigment epithelium-choroid graft in patients with exudative age-related macular degeneration: Results up to 7 years. AJOPHT , 153, 120–127.e2. doi: 10.1016/j.ajo.2011.06.007.
- Vanden Oever, M., Twaroski, K., Osborn, M. J., Wagner, J. E., & Tolar, J. (2018). Inside out: Regenerative medicine for recessive dystrophic epidermolysis bullosa. Pediatric Research , 83(1-2), 318–324. doi: 10.1038/pr.2017.244.
- Warren, L., & Lin, C. (2019). mRNA-based genetic reprogramming. Molecular Therapy , 27(4), 729–734. doi: 10.1016/j.ymthe.2018.12.009.
- Warren, L., Manos, P. D., Ahfeldt, T., Loh, Y. H., Li, H., Lau, F., … Rossi, D. J. (2010). Highly efficient reprogramming to pluripotency and directed differentiation of human cells with synthetic modified mRNA. Cell Stem Cell , 7(5), 618–630. doi: 10.1016/j.stem.2010.08.012.
- Wenzel, D., Bayerl, J., Nyström, A., Bruckner-Tuderman, L., Meixner, A., & Penninger, J. M. (2014). Genetically corrected iPSCs as cell therapy for recessive dystrophic epidermolysis bullosa. Science Translational Medicine , 6, 264ra165. doi: 10.1126/scitranslmed.3010083.
- West, E. L., Pearson, R. A., Barker, S. E., Luhmann, U. F. O., Maclaren, R. E., Barber, A. C., … Ali, R. R. (2010). Long-term survival of photoreceptors transplanted into the adult murine neural retina requires immune modulation. Stem Cells , 28(11), 1997–2007. doi: 10.1002/stem.520.
- Wiley, L. A., Burnight, E. R., Deluca, A. P., Anfinson, K. R., Cranston, C. M., Kaalberg, E. E., … Tucker, B. A. (2016). CGMP production of patient-specific iPSCs and photoreceptor precursor cells to treat retinal degenerative blindness. Scientific Reports , 6(1), 1–16. doi: 10.1038/srep30742.
- Williamson, D., Gonzalez, M., & Finlay, A. Y. (2001). The effect of hair loss in quality of life. Journal of the European Academy of Dermatology and Venereology , 15(2), 137–139. doi: 10.1046/j.1468-3083.2001.00229.x.
- Xu, H., Wang, B., Ono, M., Kagita, A., Fujii, K., Sasakawa, N., … Hotta, A. (2019). Targeted disruption of HLA genes via CRISPR-Cas9 generates iPSCs with enhanced immune compatibility. Cell Stem Cell , 24(4), 566–578.e7. doi: 10.1016/j.stem.2019.02.005.
- Yaffe, M. P., Noggle, S. A., & Solomon, S. L. (2016). Raising the standards of stem cell line quality. Nature Cell Biology , 18(3), 236–237. doi: 10.1038/ncb3313.
- Yamanaka, S. (2020). Pluripotent stem cell-based cell therapy—Promise and challenges. Cell Stem Cell , 27(4), 523–531. doi: 10.1016/j.stem.2020.09.014.
- Yang, N., Chanda, S., Marro, S., Ng, Y. H., Janas, J. A., Haag, D., … Wernig, M. (2017). Generation of pure GABAergic neurons by transcription factor programming. Nature Methods , 14(6), 621–628. doi: 10.1038/nmeth.4291.
- Yim, E. K. F., Darling, E. M., Kulangara, K., Guilak, F., & Leong, K. W. (2010). Nanotopography-induced changes in focal adhesions, cytoskeletal organization, and mechanical properties of human mesenchymal stem cells. Biomaterials , 31(6), 1299–1306. doi: 10.1016/j.biomaterials.2009.10.037.
- Yu, J., Vodyanik, M. A., Smuga-Otto, K., Antosiewicz-Bourget, J., Frane, J. L., Tian, S., … Thomson, J. A. (2007). Induced pluripotent stem cell lines derived from human somatic cells. Science , 318, 1917–1920. doi: 10.1126/science.1151526.
- Zhang, X. H., Tee, L. Y., Wang, X. G., Huang, Q. S., & Yang, S. H. (2015). Off-target effects in CRISPR/Cas9-mediated genome engineering. Molecular Therapy - Nucleic Acids , 4(11), e264. doi: 10.1038/mtna.2015.37.
- Zhao, T., Zhang, Z. N., Rong, Z., & Xu, Y. (2011). Immunogenicity of induced pluripotent stem cells. Nature , 474(7350), 212–215. doi: 10.1038/nature10135.
Citing Literature
Number of times cited according to CrossRef: 56
- Priti Karadbhajne, Akash More, Hellen Y Dzoagbe, Enhancing Endometrial Health in Assisted Reproductive Technology (ART): Evaluating Autologous Endometrial Cells and Platelets-Rich Plasma (PRP) via Hysteroscopic Injections, Cureus, 10.7759/cureus.64068, (2024).
- Gustavo Caldeira Cotta, Rachel Castro Teixeira dos Santos, Guilherme Mattos Jardim Costa, Samyra Maria dos Santos Nassif Lacerda, Reporter Alleles in hiPSCs: Visual Cues on Development and Disease, International Journal of Molecular Sciences, 10.3390/ijms252011009, 25 , 20, (11009), (2024).
- Amatullah Fatehi, Marwa Sadat, Muneera Fayyad, Jean Tang, Duhyun Han, Ian M. Rogers, Drew Taylor, Efficient Generation of Pancreatic Progenitor Cells from Induced Pluripotent Stem Cells Derived from a Non-Invasive and Accessible Tissue Source—The Plucked Hair Follicle, Cells, 10.3390/cells13121010, 13 , 12, (1010), (2024).
- Douglas Pazzin, Thales Previato, João Budelon Gonçalves, Gabriele Zanirati, Fernando Xavier, Jaderson da Costa, Daniel Marinowic, Induced Pluripotent Stem Cells and Organoids in Advancing Neuropathology Research and Therapies, Cells, 10.3390/cells13090745, 13 , 9, (745), (2024).
- Yongkang Wu, Xiangtian Meng, Wai-Yin Cheng, Zhichao Yan, Keqin Li, Jian Wang, Tianfang Jiang, Fei Zhou, Ka-Hing Wong, Chunlong Zhong, Yi Dong, Shane Gao, Can pluripotent/multipotent stem cells reverse Parkinson’s disease progression?, Frontiers in Neuroscience, 10.3389/fnins.2024.1210447, 18 , (2024).
- Katrina Albert, Gundars Goldsteins, Sara Kälvälä, Jukka Jolkkonen, Šárka Lehtonen, Glial cell transplant for brain diseases: the supportive saviours?, Translational Medicine Communications, 10.1186/s41231-024-00182-y, 9 , 1, (2024).
- Su Feng, Ting Zhang, Zhengxiao He, Wenchang Zhang, Yingying Chen, Chunmei Yue, Naihe Jing, Continuous immunosuppression is required for suppressing immune responses to xenografts in non-human primate brains, Cell Regeneration, 10.1186/s13619-024-00191-0, 13 , 1, (2024).
- Paeng Sunwoong, Yeri Alice Rim, Yeowon Sohn, Yoojun Nam, Ji Hyeon Ju, Exploration of Efficient HLA Haplotypes by Comparing the Proportion of Applicable Populations, Cell Transplantation, 10.1177/09636897241283539, 33 , (2024).
- Popat Mohite, Abhijeet Puri, Roshan Dave, Aarati Budar, Shubham Munde, Shruti Bagchi Ghosh, Taha Alqahtani, Humood Al Shmrany, Ajoy Kumer, Bikram Dhara, Unlocking the therapeutic potential: odyssey of induced pluripotent stem cells in precision cell therapies, International Journal of Surgery, 10.1097/JS9.0000000000001892, 110 , 10, (6432-6455), (2024).
- Jonas Cerneckis, Hongxia Cai, Yanhong Shi, Induced pluripotent stem cells (iPSCs): molecular mechanisms of induction and applications, Signal Transduction and Targeted Therapy, 10.1038/s41392-024-01809-0, 9 , 1, (2024).
- Yongshun Lin, Noriko Sato, Sogun Hong, Kenta Nakamura, Elisa A. Ferrante, Zu Xi Yu, Marcus Y. Chen, Daisy S. Nakamura, Xiulan Yang, Randall R. Clevenger, Timothy J. Hunt, Joni L. Taylor, Kenneth R. Jeffries, Karen J. Keeran, Lauren E. Neidig, Atul Mehta, Robin Schwartzbeck, Shiqin Judy Yu, Conor Kelly, Keron Navarengom, Kazuyo Takeda, Stephen S. Adler, Peter L. Choyke, Jizhong Zou, Charles E. Murry, Manfred Boehm, Cynthia E. Dunbar, Long-term engraftment and maturation of autologous iPSC-derived cardiomyocytes in two rhesus macaques, Cell Stem Cell, 10.1016/j.stem.2024.05.005, 31 , 7, (974-988.e5), (2024).
- Hannah W. Song, Jennifer N. Solomon, Fernanda Masri, Amanda Mack, Nisha Durand, Emmanuelle Cameau, Noushin Dianat, Arwen Hunter, Steve Oh, Brianna Schoen, Matthew Marsh, Christopher Bravery, Cenk Sumen, Dominic Clarke, Kapil Bharti, Julie G. Allickson, Uma Lakshmipathy, Bioprocessing considerations for generation of iPSCs intended for clinical application: perspectives from the ISCT Emerging Regenerative Medicine Technology working group, Cytotherapy, 10.1016/j.jcyt.2024.05.024, 26 , 11, (1275-1284), (2024).
- Marinna Madrid, Uma Lakshmipathy, Xiaokui Zhang, Kapil Bharti, Dominic M. Wall, Yoji Sato, George Muschler, Anthony Ting, Nathan Smith, Shuhei Deguchi, Shin Kawamata, Jennifer C. Moore, Bar Makovoz, Stephen Sullivan, Veronica Falco, Arwa Z. Al-Riyami, Considerations for the development of iPSC-derived cell therapies: a review of key challenges by the JSRM-ISCT iPSC Committee, Cytotherapy, 10.1016/j.jcyt.2024.05.022, 26 , 11, (1382-1399), (2024).
- Robin Augustine, Mert Gezek, Vasilios K. Nikolopoulos, Paige Lauren Buck, Nazli Seray Bostanci, Gulden Camci-Unal, Stem Cells in Bone Tissue Engineering: Progress, Promises and Challenges, Stem Cell Reviews and Reports, 10.1007/s12015-024-10738-y, 20 , 7, (1692-1731), (2024).
- Linwei He, Jerome Tan, Shi Yan Ng, King Ho Holden Li, Jongyoon Han, Sing Yian Chew, Han Wei Hou, Label‐Free Impedance Analysis of Induced Pluripotent Stem Cell‐Derived Spinal Cord Progenitor Cells for Rapid Safety and Efficacy Profiling, Advanced Materials Technologies, 10.1002/admt.202400589, 9 , 20, (2024).
- Igor Kizub, Andrii Rozhok, Ganna Bilousova, Induced Pluripotent Stem Cells: Advances and Applications in Regenerative Medicine, Possibilities and Limitations in Current Translational Stem Cell Research, 10.5772/intechopen.109274, (2023).
- Yeonmi Lee, Jongsuk Han, Sae-Byeok Hwang, Soon-Suk Kang, Hyeoung-Bin Son, Chaeyeon Jin, Jae Eun Kim, Beom Hee Lee, Eunju Kang, Selection of iPSCs without mtDNA deletion for autologous cell therapy in a patient with Pearson syndrome, BMB Reports, 10.5483/BMBRep.2022-0204, 56 , 8, (463-468), (2023).
- Kexin Li, Qingji Huo, Bai-Yan Li, Hiroki Yokota, The Double-Edged Proteins in Cancer Proteomes and the Generation of Induced Tumor-Suppressing Cells (iTSCs), Proteomes, 10.3390/proteomes11010005, 11 , 1, (5), (2023).
- Laura Herbst, Ferdinand Groten, Mary Murphy, Georgina Shaw, Bastian Nießing, Robert H. Schmitt, Automated Production at Scale of Induced Pluripotent Stem Cell-Derived Mesenchymal Stromal Cells, Chondrocytes and Extracellular Vehicles: Towards Real-Time Release, Processes, 10.3390/pr11102938, 11 , 10, (2938), (2023).
- Reem Alhejailan, Gloria Garoffolo, Vineesh Raveendran, Maurizio Pesce, Cells and Materials for Cardiac Repair and Regeneration, Journal of Clinical Medicine, 10.3390/jcm12103398, 12 , 10, (3398), (2023).
- Artem Eremeev, Arina Pikina, Yevgeny Ruchko, Alexandra Bogomazova, Clinical Potential of Cellular Material Sources in the Generation of iPSC-Based Products for the Regeneration of Articular Cartilage, International Journal of Molecular Sciences, 10.3390/ijms241914408, 24 , 19, (14408), (2023).
- Oksana I. Sutyagina, Arkadii K. Beilin, Ekaterina A. Vorotelyak, Andrey V. Vasiliev, Immortalization Reversibility in the Context of Cell Therapy Biosafety, International Journal of Molecular Sciences, 10.3390/ijms24097738, 24 , 9, (7738), (2023).
- Manoubia Saidani, Annabelle Darle, Margot Jarrige, Hélène Polveche, Lina El Kassar, Séverine Julié, Sandrine Bessou-Touya, Nathalie Holic, Gilles Lemaitre, Cécile Martinat, Christine Baldeschi, Jennifer Allouche, Generating Functional and Highly Proliferative Melanocytes Derived from Human Pluripotent Stem Cells: A Promising Tool for Biotherapeutic Approaches to Treat Skin Pigmentation Disorders, International Journal of Molecular Sciences, 10.3390/ijms24076398, 24 , 7, (6398), (2023).
- Zahraa I. Khamis, Drishty B. Sarker, Yu Xue, Nancy Al-Akkary, Viviana D. James, Changchun Zeng, Yan Li, Qing-Xiang Amy Sang, Modeling Human Brain Tumors and the Microenvironment Using Induced Pluripotent Stem Cells, Cancers, 10.3390/cancers15041253, 15 , 4, (1253), (2023).
- Eirini Filidou, Leonidas Kandilogiannakis, Gesthimani Tarapatzi, Michail Spathakis, Colin Su, Alin Rai, David W. Greening, Konstantinos Arvanitidis, Vasilis Paspaliaris, George Kolios, A Simplified and Effective Approach for the Isolation of Small Pluripotent Stem Cells Derived from Human Peripheral Blood, Biomedicines, 10.3390/biomedicines11030787, 11 , 3, (787), (2023).
- Laura Barrachina, Tarlan Eslami Arshaghi, Aisling O'Brien, Ana Ivanovska, Frank Barry, Induced pluripotent stem cells in companion animals: how can we move the field forward?, Frontiers in Veterinary Science, 10.3389/fvets.2023.1176772, 10 , (2023).
- Giacomo Roman, Benedicte Stavik, Knut H. Lauritzen, Per Morten Sandset, Sean P. Harrison, Gareth J. Sullivan, Maria Eugenia Chollet, “iPSC-derived liver organoids and inherited bleeding disorders: Potential and future perspectives”, Frontiers in Physiology, 10.3389/fphys.2023.1094249, 14 , (2023).
- Yesuf Siraj, Umberto Galderisi, Nicola Alessio, Senescence induces fundamental changes in the secretome of mesenchymal stromal cells (MSCs): implications for the therapeutic use of MSCs and their derivates, Frontiers in Bioengineering and Biotechnology, 10.3389/fbioe.2023.1148761, 11 , (2023).
- Xiao Xue Zeng, Jie Bangzhe Zeng, Systems Medicine as a Strategy to Deal with Alzheimer’s Disease, Journal of Alzheimer's Disease, 10.3233/JAD-230739, 96 , 4, (1411-1426), (2023).
- AI Stepanov, LV Putlyaeva, DA Didych, AA Galiakberova, NG Gurskaya, KA Lukyanov, ATOH1 factor expression induces rapid differentiation of iPSCs into neurons, Bulletin of Russian State Medical University, 10.24075/brsmu.2023.036, 2023(5), (2023).
- Sangbae Park, Yonghyun Gwon, Shahidul Ahmed Khan, Kyoung-Je Jang, Jangho Kim, Engineering considerations of iPSC-based personalized medicine, Biomaterials Research, 10.1186/s40824-023-00382-x, 27 , 1, (2023).
- Alexander Blümke, Erica Ijeoma, Jessica Simon, Rachel Wellington, Medania Purwaningrum, Sergei Doulatov, Elizabeth Leber, Marta Scatena, Cecilia M. Giachelli, Comparison of osteoclast differentiation protocols from human induced pluripotent stem cells of different tissue origins, Stem Cell Research & Therapy, 10.1186/s13287-023-03547-6, 14 , 1, (2023).
- Margarita E. Bogomiakova, Elizaveta K. Sekretova, Ksenia S. Anufrieva, Polina O. Khabarova, Anastasia N. Kazakova, Pavel A. Bobrovsky, Tatiana V. Grigoryeva, Artem V. Eremeev, Olga S. Lebedeva, Alexandra N. Bogomazova, Maria A. Lagarkova, iPSC-derived cells lack immune tolerance to autologous NK-cells due to imbalance in ligands for activating and inhibitory NK-cell receptors, Stem Cell Research & Therapy, 10.1186/s13287-023-03308-5, 14 , 1, (2023).
- Jonathan R. Soucy, Erika A. Aguzzi, Julie Cho, Michael James Gilhooley, Casey Keuthan, Ziming Luo, Aboozar Monavarfeshani, Meher A. Saleem, Xue-Wei Wang, Juilette Wohlschlegel, Abdelrahman Y. Fouda, Ajay Ashok, Ala Moshiri, Alain Chedotal, Amberlynn A. Reed, Amjad Askary, An-Jey A. Su, Anna La Torre, Archana Jalligampala, Ariadna Silva-Lepe, Arupratan Das, Barbara Wirostko, Benjamin J. Frankfort, Benjamin Sivyer, Bhagwat Alapure, Brent Young, Brian Clark, Bryan William Jones, Chase Hellmer, Claire Mitchell, Claire Ufongene, Dan Goldman, David Feldheim, David H. Gutmann, David J. Calkins, David Krizaj, David M. Gamm, Diana C. Lozano, Diane E. Bovenkamp, Dong Feng Chen, Elena Vecino Cordero, Ephraim F. Trakhtenberg, Feng Tian, Fengquan Zhou, Gillian J. McLellan, Harry A. Quigley, Hashem Abu Serhan, James R. Tribble, Jason Meyer, Jeff Gross, Jeff S. Mumm, Jeremy M. Sivak, Jingliang Simon Zhang, Jiun L. Do, Jonathan Crowston, Julie Chen, Juliette McGregor, Kalyan C. Vinnakota, Kang-Chieh Huang, Karen Peynshaert, Katherine E. Uyhazi, Keith Martin, Ken Muller, Kevin K. Park, Kin-Sang Cho, Kun-Che Chang, Larry Benowitz, Leonard A. Levin, Levi Todd, Lies De Groef, Lieve Moons, Luis Alarcon-Martinez, Mandeep S. Singh, Manuel Vidal-Sanz, Mariana S. Silveira, Marina Pavlou, Matthew B. Veldman, Matthew Van Hook, Melanie Samuel, Mengming Hu, Micalla Peng, Michael Young, Michel Cayouette, Mohammad H. Geranmayeh, Mollie Woodworth, Monica Vetter, Nicholas R. Marsh-Armstrong, Pete A. Williams, Pratheepa Kumari Rasiah, Preeti Subramanian, Qi N. Cui, Rebecca M. Sappington, Reem Amine, Richard Eva, Robert J. Johnston, Roman J. Giger, Ross Ethier, Sadaf Abed, Sehrish Nizar Ali Momin, Seth Blackshaw, Shane A. Liddelow, Stella Mary, Stephen Atolagbe, Supraja Varadarajan, Tareq I. Nabhan, Tasneem Khatib, Tasneem Putliwala Sharma, Thomas Brunner, Tom Greenwell, Tonia S. Rex, Trent Watkins, Tudor C. Badea, V. Vrathasha, Venkata Ramana Murthy Chavali, Viviane M. Oliveira-Valença, Wai Lydia Tai, Wyndham M. Batchelor, Xian-Jie Yang, Yong Park, Yuan Pan, Petr Baranov, Adriana Di Polo, Brad Fortune, Kimberly K. Gokoffski, Jeffrey L. Goldberg, William Guido, Alex L. Kolodkin, Carol A. Mason, Yvonne Ou, Thomas A. Reh, Ahmara G. Ross, Brian C. Samuels, Derek Welsbie, Donald J. Zack, Thomas V. Johnson, Retinal ganglion cell repopulation for vision restoration in optic neuropathy: a roadmap from the RReSTORe Consortium, Molecular Neurodegeneration, 10.1186/s13024-023-00655-y, 18 , 1, (2023).
- Myriam Hemberger, Wendy Dean, The placenta: epigenetic insights into trophoblast developmental models of a generation-bridging organ with long-lasting impact on lifelong health, Physiological Reviews, 10.1152/physrev.00001.2023, 103 , 4, (2523-2560), (2023).
- Devika Bose, Davide Ortolan, Mitra Farnoodian, Ruchi Sharma, Kapil Bharti, Considerations for Developing an Autologous Induced Pluripotent Stem Cell (iPSC)-Derived Retinal Pigment Epithelium (RPE) Replacement Therapy, Cold Spring Harbor Perspectives in Medicine, 10.1101/cshperspect.a041295, 14 , 3, (a041295), (2023).
- Junyi Ji, Hongju Xu, Chen Li, Jiesi Luo, Small-Caliber Tissue-Engineered Vascular Grafts Based on Human-Induced Pluripotent Stem Cells: Progress and Challenges, Tissue Engineering Part B: Reviews, 10.1089/ten.teb.2023.0005, 29 , 4, (441-455), (2023).
- Yuki Fujimura, Itsuki Sakai, Itsuki Shioka, Nozomu Takata, Atsushi Hashimoto, Takuya Funatomi, Satoru Okuda, Machine learning-based estimation of spatial gene expression pattern during ESC-derived retinal organoid development, Scientific Reports, 10.1038/s41598-023-49758-y, 13 , 1, (2023).
- Hsiu-Hui Tsai, Hsiao-Jung Kao, Ming-Wei Kuo, Chin-Hsien Lin, Chun-Min Chang, Yi-Yin Chen, Hsiao-Huei Chen, Pui-Yan Kwok, Alice L. Yu, John Yu, Whole genomic analysis reveals atypical non-homologous off-target large structural variants induced by CRISPR-Cas9-mediated genome editing, Nature Communications, 10.1038/s41467-023-40901-x, 14 , 1, (2023).
- Sabine Kobold, Nils Bultjer, Glyn Stacey, Sabine C. Mueller, Andreas Kurtz, Nancy Mah, History and current status of clinical studies using human pluripotent stem cells, Stem Cell Reports, 10.1016/j.stemcr.2023.03.005, 18 , 8, (1592-1598), (2023).
- Sally Temple, Advancing cell therapy for neurodegenerative diseases, Cell Stem Cell, 10.1016/j.stem.2023.03.017, 30 , 5, (512-529), (2023).
- Aaron Gabriel W. Sandoval, Kelly Y. Gim, Jennifer T. Huang, Karl R. Koehler, Applications of Human Pluripotent Stem Cell–Derived Skin Organoids in Dermatology, Journal of Investigative Dermatology, 10.1016/j.jid.2023.07.017, 143 , 10, (1872-1876), (2023).
- Mario F. Muñoz, Francesco Marotta, Amir Moghadam Ahmadi, Azam Yazdani, Fang He, Roberto Catanzaro, Cristina Garzón-Rodriguez, Antonio Ayala, Anti-aging and Rejuvenation Based on Stem Cell Therapy, Emerging Anti-Aging Strategies, 10.1007/978-981-19-7443-4_5, (79-98), (2023).
- Shashank Pandey, Michal Jirásko, Jan Lochman, Alexandr Chvátal, Magdalena Chottova Dvorakova, Radek Kučera, iPSCs in Neurodegenerative Disorders: A Unique Platform for Clinical Research and Personalized Medicine, Journal of Personalized Medicine, 10.3390/jpm12091485, 12 , 9, (1485), (2022).
- Ana Aragón-González, Pamela J. Shaw, Laura Ferraiuolo, Blood–Brain Barrier Disruption and Its Involvement in Neurodevelopmental and Neurodegenerative Disorders, International Journal of Molecular Sciences, 10.3390/ijms232315271, 23 , 23, (15271), (2022).
- Eleni Damianidou, Lidia Mouratidou, Christina Kyrousi, Research models of neurodevelopmental disorders: The right model in the right place, Frontiers in Neuroscience, 10.3389/fnins.2022.1031075, 16 , (2022).
- Ryusuke Yoshimi, Hideaki Nakajima, Current State and Issues of Regenerative Medicine for Rheumatic Diseases, Frontiers in Medicine, 10.3389/fmed.2022.813952, 9 , (2022).
- Fabian Gather, Irmgard Ihrig-Biedert, Paul Kohlhas, Tamara Krutenko, Michael Peitz, Oliver Brüstle, Andrea Pautz, Hartmut Kleinert, A specific, non-immune system-related isoform of the human inducible nitric oxide synthase is expressed during differentiation of human stem cells into various cell types, Cell Communication and Signaling, 10.1186/s12964-022-00855-x, 20 , 1, (2022).
- Kaivalya Molugu, Giovanni A. Battistini, Tiffany M. Heaster, Jacob Rouw, Emmanuel C. Guzman, Melissa C. Skala, Krishanu Saha, Label-Free Imaging to Track Reprogramming of Human Somatic Cells, GEN Biotechnology, 10.1089/genbio.2022.0001, 1 , 2, (176-191), (2022).
- Igor E. Konstantinov, Gregory King, Enzo R. Porrello, From genome editing to blastocyst complementation: A new horizon in heart transplantation?, JTCVS Techniques, 10.1016/j.xjtc.2022.01.012, 12 , (177-184), (2022).
- Xing Zhen, Sang-Je Park, Se-Hee Choe, Dong-Seok Lee, Jong-Hee Lee, Generation of induced pluripotent stem cells (cmESF-iPS-C5) derived from cynomolgus monkey ear skin fibroblasts (cmESF), Stem Cell Research, 10.1016/j.scr.2022.102977, 65 , (102977), (2022).
- Nidheesh Dadheech, Nerea Cuesta-Gomez, Ila Tewari Jasra, Kevin Verhoeff, Braulio Marfil Garza, Omar Mouhammed, A.M. James Shapiro, Opportunities and impediments of human pluripotent stem cell-derived islets in the treatment of diabetes, Journal of Immunology and Regenerative Medicine, 10.1016/j.regen.2022.100064, 17 , (100064), (2022).
- Masanobu Horie, Noriko Yamano-Adachi, Yoshinori Kawabe, Hidenori Kaneoka, Hideaki Fujita, Eiji Nagamori, Ryosuke Iwai, Yasushi Sato, Kei Kanie, Seiichi Ohta, Masaharu Somiya, Kosuke Ino, Recent advances in animal cell technologies for industrial and medical applications, Journal of Bioscience and Bioengineering, 10.1016/j.jbiosc.2022.03.005, 133 , 6, (509-514), (2022).
- Akira Kunitomi, Ryoko Hirohata, Vanessa Arreola, Mitsujiro Osawa, Tomoaki M. Kato, Masaki Nomura, Jitsutaro Kawaguchi, Hiroto Hara, Kohji Kusano, Yasuhiro Takashima, Kazutoshi Takahashi, Keiichi Fukuda, Naoko Takasu, Shinya Yamanaka, Improved Sendai viral system for reprogramming to naive pluripotency, Cell Reports Methods, 10.1016/j.crmeth.2022.100317, 2 , 11, (100317), (2022).
- Elaheh Izady, Zohreh Saltanatpour, Li-Ping Liu, Akram Alizadeh, Amir Ali Hamidieh, Toward in Vitro Production of Platelet from Induced Pluripotent Stem Cells, Stem Cell Reviews and Reports, 10.1007/s12015-022-10366-4, 18 , 7, (2376-2387), (2022).
- Hyeon Yu, Clayton W. Commander, Joseph M. Stavas, Stem Cell–Based Therapies: What Interventional Radiologists Need to Know, Seminars in Interventional Radiology, 10.1055/s-0041-1736657, 38 , 05, (523-534), (2021).