Animal Models of Osteoarthritis Part 1–Preclinical Small Animal Models: Challenges and Opportunities for Drug Development
Jaqueline Lourdes Rios, Jaqueline Lourdes Rios, Dora Sapède, Dora Sapède, Farida Djouad, Farida Djouad, Anna E. Rapp, Anna E. Rapp, Annemarie Lang, Annemarie Lang, Jonathan Larkin, Jonathan Larkin, Christoph Ladel, Christoph Ladel, Ali Mobasheri, Ali Mobasheri
Abstract
Osteoarthritis (OA) is the most common form of arthritis and a major source of pain and disability in the adult population. There is a significant unmet medical need for the development of effective pharmacological therapies for the treatment of OA. In addition to spontaneously occurring animal models of OA, many experimental animal models have been developed to provide insights into mechanisms of pathogenesis and progression. Many of these animal models are also being used in the drug development pipeline. Here, we provide an overview of commonly used and emerging preclinical small animal models of OA and highlight the strengths and limitations of small animal models in the context of translational drug development. There is limited information in the published literature regarding the technical reliability of these small animal models and their ability to accurately predict clinical drug development outcomes. The cost and complexity of the available models however is an important consideration for pharmaceutical companies, biotechnology startups, and contract research organizations wishing to incorporate preclinical models in target validation, discovery, and development pipelines. Further considerations relevant to industry include timelines, methods of induction, the key issue of reproducibility, and appropriate outcome measures needed to objectively assess outcomes of experimental therapeutics. Preclinical small animal models are indispensable tools that will shine some light on the pathogenesis of OA and its molecular endotypes in the context of drug development. This paper will focus on small animal models used in preclinical OA research. © 2022 The Authors. Current Protocols published by Wiley Periodicals LLC.
INTRODUCTION
Osteoarthritis (OA) is the most common form of arthritis, affecting more than 500 million people globally (Hunter, March, & Chew, 2020). After lower back pain, OA accounts for more pain and functional disability than any other musculoskeletal disease (Luong, Cleveland, Nyrop, & Callahan, 2012) and is a significant contributor to health and social care costs across the world (Conaghan et al., 2015; O'Neill, McCabe, & McBeth, 2018). OA reduces mobility and quality of life in patients, impacting their professional and personal lives. The burden of OA has been steadily rising according to recent estimates from the Global Burden of Disease (GBD) study (Cross et al., 2014; Safiri et al., 2020). OA is increasingly recognized as a serious disease (Hawker, 2019), being described as one of the most debilitating and chronic forms of joint disease (Vos et al., 2012).
Although the pathophysiology of OA is poorly understood (Chan, Ngai, Ip, Lam, & Lai, 2009; Iannone & Lapadula, 2003), the risk factors associated with disease development are well established. They include age (Hügle, Geurts, Nüesch, Müller-Gerbl, & Valderrabano, 2012), obesity (Bliddal, Leeds, & Christensen, 2014), sex (O'Connor, 2006), previous incidence of joint injuries (Andriacchi & Mündermann, 2006; Buckwalter & Brown, 2004), meniscal damage (Englund, 2008), joint instability (Blalock, Miller, Tilley, & Wang, 2015), malalignment (Felson, 2004), genetics (Valdes & Spector, 2011), bone anomalies (including anatomical deformities) (Baker‑LePain & Lane, 2010), sarcopenia (De Ceuninck, Fradin, & Pastoureau, 2014), and metabolic disease (Courties, Sellam, & Berenbaum, 2017; Martel-Pelletier et al., 2016; Mobasheri et al., 2017). Figure 1 illustrates some of the structural changes that are known to occur in OA.
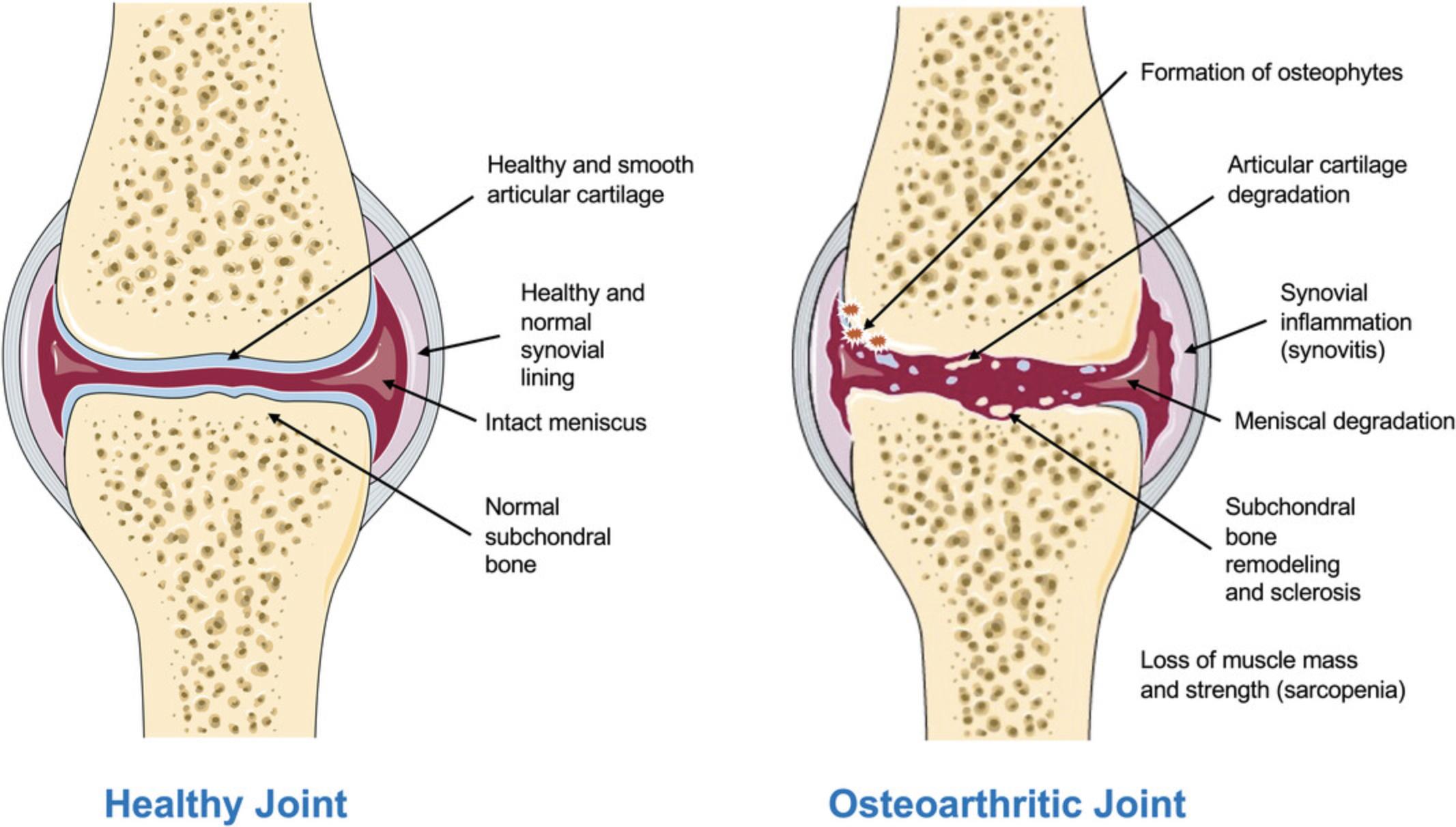
Genetic, genomic, and epigenetic studies have advanced our understanding of the molecular processes underlying OA (Reynard & Barter, 2020). These advances in the understanding of OA pathophysiology have enabled the identification of a variety of novel therapeutic targets involved in the progression of OA, some of which have produced promising results in randomized controlled trials (Eckstein et al., 2021; Oo, Little, Duong, & Hunter, 2021; Sabha, Siaton, & Hochberg, 2020). However, there are still no approved disease-modifying treatments for OA (Malfait & Tortorella, 2019; Oo et al., 2021; Sabha et al., 2020; Van Spil, Kubassova, Boesen, Bay-Jensen, & Mobasheri, 2019). OA drug development, like that in other therapeutic areas, is time-consuming, costly, and plagued by a high attrition rate (Kraus et al., 2011; Oo, Yu, Daniel, & Hunter, 2018; Tonge, Pearson, & Jones, 2014). The OA therapeutic pipeline requires more candidate drugs, higher levels of efficiency, and enhanced/improved translation from preclinical models to humans. Achieving this requires a more comprehensive understanding of the pathophysiological processes involved in the initiation and progression of OA as well as improvements in identification and characterization of phenotype- or endotype-based patient subgroups to enable efficient and effective therapeutic development. Progress in these areas would lead to much needed advances in the field of pharmaceutical and biological therapies for OA (Latourte, Kloppenburg, & Richette, 2020).
Although current OA research is increasingly focused on understanding the underlying pathophysiology and identifying novel therapeutic targets for OA, most of the clinical studies on humans have been conducted in the later stages of the disease. Indeed, the disease is often well advanced before the patient becomes symptomatic (i.e., pain, reduced function, stiffness, joint instability), making it difficult to accurately study and understand the early events involved in the pathogenesis of the disease in humans, missing a vital window of opportunity needed for earlier intervention and the development of personalized therapy (Karsdal et al., 2014). Additionally, the chronic nature of the disease in combination with the significant variability in the rate of disease progression in humans presents further challenges (Karsdal et al., 2014; Matthews, 2013). Due to these challenges and the ethical limitations associated with human medical research, in vitro models (not discussed in this review) and in vivo preclinical animal models of OA have become well established and represent valuable tools for the OA research and development field.
In vivo preclinical animal models have been primarily used to investigate two main areas of research in OA: (1) to study the pathogenesis of the disease with special emphasis on molecular genetic changes leading to cellular and tissue alterations; and (2) to study the therapeutic efficacy of treatment modalities (Kuyinu, Narayanan, Nair, & Laurencin, 2016; Lampropoulou-Adamidou et al., 2014). Some of the small animal models have specifically been developed and used for studying post-traumatic OA (PTOA) (Blaker, Clarke, & Little, 2017; Little & Hunter, 2013). Small animals are particularly popular because of their ease of use and low cost. However, their predictive utility has frequently (if not persistently) been questioned, mainly because the preclinical efficacy of novel therapeutics has translated poorly into human clinical trials (Malfait & Little, 2015). Larger animals are advantageous because of their anatomical similarity to humans and hence their translational potential (Gregory et al., 2012). However, large animal models of OA such as pigs [i.e., Yucatan minipigs (Goetz et al., 2015)], dogs, sheep, goats, horses, and non-human primates are more costly to establish and maintain; they require (for therapeutic interventions) substantial amounts of the investigational drug and some raise considerable ethical challenges (McCoy, 2015) (we refer the reader to the second article in this series for a detailed discussion on translational and large animal models). However, there is no single animal model that can sufficiently recapitulate the complexities of OA in humans (Kuyinu et al., 2016; Little & Zaki, 2012) and the different OA phenotypes. Therefore, many animal models have been developed and reported extensively in the literature to focus on the many features of the disease. Therefore, animal models have played a key role in our understanding of OA pathogenesis and progression. Figure 2 shows the most commonly used in vivo and in vitro models in OA research and clinical development.
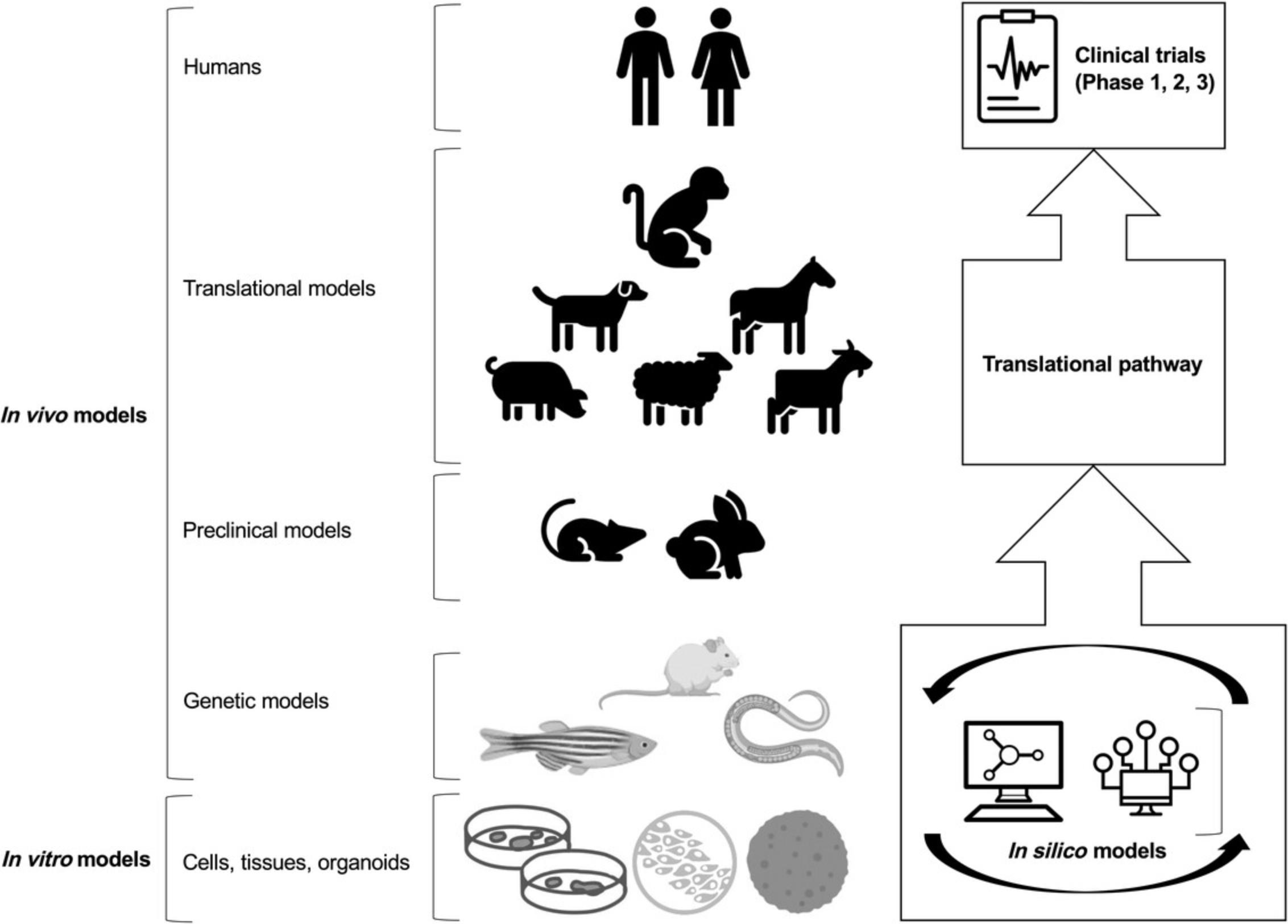
In this review, we discuss the strengths and weaknesses of the most widely used and accepted small animal models of OA, including the zebrafish, mouse, rat, rabbit, and guinea pig. We focus on model validation with respect to key aspects of the human disease state, the technical reliability, and translational predictiveness of each model for drug development and preclinical testing. We also comment on the cost and complexity of the available models, the relevance of each species investigated, timelines, methods of induction, reproducibility, and outcome measures that may also provide insight into the different clinical phenotypes and molecular endotypes of OA in the context of drug development.
THE ZEBRAFISH AS AN EMERGING MODEL FOR DRUG DISCOVERY AND GENETIC MODELING OF OSTEOARTHRITIS
Zebrafish are small tropical freshwater fish that have recently become a popular model for studying vertebrate gene function and skeletal development, and they are also increasingly used for screening of chemical libraries, preclinical drug discovery, and drug development (Chakraborty, Hsu, Wen, Lin, & Agoramoorthy, 2009; Das, McCormick, Thapa, Karki, & Evans, 2013; Driever, Stemple, Schier, & Solnica-Krezel, 1994; Gibert, Trengove, & Ward, 2013; Lessman, 2011). Their high genetic tractability, transparency, large clutch size, rapid and external development, easy handling, and inexpensive maintenance when compared to mammalian models have favored the use of zebrafish for large-scale genetic screening (Lawson & Wolfe, 2011). The high degree of conservation between human and zebrafish disease genes (Howe et al., 2013), the ability to perform transitory knockdown (Nasevicius & Ekker, 2000), and the emergence of efficient genome editing technologies to generate stable mutants in this species (Liu, Petree, Requena, Varshney, & Varshney, 2019; Talbot & Amacher, 2014) have allowed the modeling of a wide range of human pathologies (Lieschke & Currie, 2007) including OA (Modeling osteoarthritis genetics in zebrafish, 2013) in zebrafish. Furthermore, zebrafish are permeable to active molecules dissolved in their environment. This advantage offers the opportunity to design high-throughput in vivo drug-screening tests for preclinical studies (Patton, Zon, & Langenau, 2021).
Although the zebrafish skeleton may appear very different from that of mammals at first glance, the zebrafish bone and cartilage tissues contain all the main cell types found in mammalian species, including osteoblasts, osteocytes, and chondrocytes (Apschner, Schulte-Merker, & Witten, 2011). The skeletal cells of zebrafish express common extracellular matrix (ECM) markers and share similar developmental programs with their mammalian counterparts (Li, Felber, Elks, Croucher, & Roehl, 2009). Therefore, zebrafish have become relevant model organisms for investigating skeletal development and disease (Tonelli et al., 2020) and for identifying novel therapies for skeletal disorders (Bergen, Kague, & Hammond, 2019).
In the field of bone and joint research, an important advantage of using the zebrafish is the possibility to follow dynamic OA-related processes in vivo , which is technically not feasible in mammalian models. For instance, differentiation of cartilage can be monitored in intact zebrafish larvae or adult zebrafish using transgenic reporters for col2a1 and col10a1 , either in physiological (Mitchell et al., 2013) or pathophysiological contexts (Lawrence et al., 2018).
The identification of several genes associated with OA susceptibility in humans has further elevated the status of the zebrafish as a genetic model organism for OA research (Askary et al., 2016; Mitchell et al., 2013). Expression analysis has revealed that these genes (such as col9a2, gdf5, pthlhb, and mcf2l) are expressed in the early development stages of the zebrafish skeleton, suggesting a causal link between OA and early gene dysfunction during cartilage maturation and/or maintenance (Mitchell et al., 2013). Additionally, in silico models on the development of zebrafish jaw for wild-type (Brunt, Roddy, Rayfield, & Hammond, 2016), mutant (Lawrence et al., 2018), and larvae zebrafish exposed to different gravitational fields (Lawrence et al., 2021) are helping understand the effect of load on the joints during development. Taken together, these studies have demonstrated the power and versatility of the zebrafish as a model to study joint morphogenesis and physiopathology in vivo and in silico to investigate the molecular and mechanical basis of naturally occurring OA at various developmental stages. The complete toolbox available in this model might further help design drug-screening strategies to identify and test new drugs for cartilage research or to select the best candidates to be investigated in mammalian models for translation to the clinic.
SMALL ANIMAL MODELS OF OSTEOARTHRITIS
As already outlined in the introduction, animal models of OA are extensively used for investigating disease pathways and preclinical testing of novel disease-modifying therapies (Malfait & Little, 2015). Preclinical models of OA include models with naturally occurring OA or cartilage/joint degeneration, genetically modified models (Tables 1 and 2), and experimentally induced models using chemical, biological, and biomechanical stimuli (Culley et al., 2015, 2021). For example, mouse models of OA have an increasingly important impact on OA research due to advances in microsurgical techniques and the use of genetically modified mice, as well as the development of novel imaging outcomes and functional assessment tools (Fang & Beier, 2014). Nevertheless, some mouse strains develop spontaneous cartilage defects but not a generalized disease pathophysiological phenotype (Stoop et al., 1999).
Animal | Advantages | Disadvantages | Models used | Pharmacological research considerations | Translational potential |
---|---|---|---|---|---|
Zebrafish |
Ease of use Low cost |
Not a mammalian model | Numerous genetic knock-in and knockout models | Targets must be further validated in mammalian models | Valuable genetic discovery and validation tool, important for translation |
Mouse |
Low cost Ease of use Genome-sequenced Can view whole knee on slides |
Thin cartilage, with different cellular tissue architecture Post-operative management difficult |
Genetic DMM a Chemically induced Diet-induced obesity |
Volume of injection (intravenous and intra-articular) needs to be considered Oral administration feasible, limited by volume, i.e., concentration/amount of investigational drug applied Topical administration challenging because mice try to lick the compound away Sampling and sampling timepoints of body fluids very limited; longitudinal analysis in one animal difficult to achieve |
Limited due to different tissue architecture and limits in sampling |
Rat |
Low cost Ease of use Thicker cartilage than mouse Can view whole knee on slides X-ray possible (microCTc) Gait analysis possible (e.g., catwalk) |
Small joints Post-operative management difficult |
Medial meniscus tear Partial medial meniscectomy ACLb transection with partial medial meniscectomy (ACLT) MIAd Chemically induced Diet-induced obesity |
Volume of injection (intravenous and intra-articular) needs to be considered Oral administration feasible, limited by volume, i.e., concentration/amount of investigational drug applied Topical administration challenging because rats try to lick the compound away Pain behavior different (group animal) Direct link to preclinical safety investigations allows for determination of safety margin in one species |
Depending on mode of action of the respective compound: high |
Rabbit |
Ease of use Gait analysis possible |
Knee biomechanics: Different gait/weight bearing properties of the joint Cartilage capable of spontaneous regeneration Post-operative management difficult Different histology from humans Limited number of biomarkers available |
ACL transection Meniscectomy Chemically induced Groove model Drilling model |
All administration routes are feasible Self-healing potential makes longer studies unreliable Handling of animals to be considered for e.g., repeated administration |
Low |
Guinea pig |
Similar histopathology to humans Prone to spontaneous OAe |
Sedentary lifestyle Arthroscopy not possible Difficult to obtain |
Spontaneous Menisectomy Chemically induced |
Formation of antibodies against biologics | High |
- a DMM: destabilization of the medial meniscus.
- b ACL: anterior cruciate ligament.
- c CT: computed tomography.
- d MIA: monoiodoacetate
- e OA: osteoarthritis.
Target | Gene symbol | Functional consequence | Phenotype | Reference |
---|---|---|---|---|
Collagen Type 2 | COL2A1 | Heterozygous knockout | Earlier onset of OAa, higher incidence at 15 months of age; 60%-90% in heterozygous versus 25%-40% in wild type | (Lapveteläinen et al., 2001) |
GDF5 | GDF5 | Homozygous knockout | Underdeveloped femoral condyles and tibial plateaus, no cruciate ligaments, and poorly developed menisci; delayed secondary ossification in the distal femur and proximal tibia; narrower femoral condyles, femoral notches, and tibial plateaus; and curvier medial femoral condyles, shallower trochlea, steeper lateral tibial slopes, and smaller tibial spines | (Pregizer et al., 2018) |
Arginase 2 | ARG | Overexpression | Loss of GAGsb, cartilage erosion, development of osteophytes, SBPc thickening | (Choi et al., 2019) |
Collagen Type 9 | COL9A | Homozygous knockout | Onset of pathological changes at 3 months, loss of cartilage at 9 months of age | (Hu et al., 2006) |
Matrilin3 | MATN3 | Functional knockout, null allele | 100% OA incidence at 1 year of age versus 45% in wild type | (van der Weyden et al., 2006) |
AMPK | PRKAA1 | Double knock out of AMPKalpha1alpha2 | More severe OA phenotype than wild type in spontaneous and induced models (DMMd) | (Zhou et al., 2017) |
- a OA: osteoarthritis.
- b GAGs: glycosaminoglycans.
- c subchondral bone plate.
- d DMM: destabilization of the medial meniscus.
Mechanical instability–induced joint disease in animal models has been used in most preclinical evaluations to model PTOA, a condition similar to OA that develops in humans after a traumatic joint injury. These models comprise destabilization of the medial meniscus (DMM), medial meniscal tear (MMT), and anterior cruciate ligament transection (ACLT) (Lorenz & Grässel, 2014). To induce OA chemically or biologically, chemicals (monoiodoacetate) or ECM-degrading enzymes (papain and collagenase) can be injected into the joint to damage articular cartilage and other synovial joint tissues, leading to a loss of structural integrity, inflammation, and pain (Adães et al., 2014; Grenier, Bhargava, & Torzilli, 2014; Kikuchi, Sakuta, & Yamaguchi, 1998).
Mice, rats, and guinea pigs are predominantly employed to establish these models, which more closely resemble the acute events initiating destabilization and inflammation believed to be precursors of the disease rather than the chronic and slowly progressive condition most frequently associated with human OA. These models are widely used in rheumatoid arthritis (RA) research because of the very progressive destruction and the induced immune response (Billiau & Matthys, 2011). Chemically induced models are less invasive than surgically induced models. However, their pathophysiology is not reflective of the mechanisms underlying PTOA. For this reason, chemically induced models are mainly used to study the mechanisms of pain and as target studies for drug therapies (Kuyinu et al., 2016; Lampropoulou-Adamidou et al., 2014).
POST-TRAUMATIC SMALL ANIMAL MODELS OF OSTEOARTHRITIS
PTOA has a distinct molecular pathophysiology compared to that of spontaneous OA (i.e., without surgical intervention to induce the disease), which may account for the poor translation from preclinical to clinical OA therapeutic trials (Little & Hunter, 2013). There are several ways to surgically induce OA and create a post-traumatic model. Anterior cruciate ligament (ACL) injury results in joint destabilization, which subsequently leads to OA. The anterior cruciate ligament transection (ACLT) model is the most widely used model (Adams, 1983; Johnson, 1986; Lampropoulou-Adamidou et al., 2014; McCoy, 2015; Myers, Brandt, O'Connor, Visco, & Albrecht, 1990) and closely imitates the degradation of articular cartilage associated with the rupture of the ACL in humans. Although it has been used extensively, and in several different animal species, the best species for this model are sheep and goats, as their stifle joints are sufficiently large for easy replication of the procedure, facilitating reproducibility of experimental studies (Frisbie, Cross, & McIlwraith, 2006; Little et al., 2010; Proffen, McElfresh, Fleming, & Murray, 2012) (please refer to the second paper in this series for further details).
Medial meniscal tears in humans result in joint instability and cartilage degradation (Edd, Giori, & Andriacchi, 2015). To mimic this in an animal model, a transection of the medial meniscotibial ligament is performed (medial meniscus destabilization, DMM), followed (or not) by meniscal tear and/or partial meniscectomy, with progressive degenerative changes occurring 3 to 6 weeks post-surgery. (Bendele, 2001; Lorenz & Grässel, 2014; McCoy, 2015). Mice, rats, and guinea pigs are the most studied examples of animals using these models (Bendele, 2001). In rats, there is a rapid progression of cartilage degeneration and substantial change in the subchondral bone, making the meniscal tear model an extremely difficult model for detecting protective effects. The knee joint lesions are morphologically similar to those in human disease, but they occur much more rapidly (Bendele, 2001).
During the preparation of this paper, the authors had numerous discussions reflecting on their experience and interactions with peers at research conferences and the consensus was that there is not such a thing as “the” DMM or meniscal tear procedure [also applicable to other (surgical) procedures], as every laboratory has its own methods to perform the surgery and conduct histopathological grading. These differences are openly disclosed during scientific conferences, but details are often omitted in publications, thereby hampering data interpretation, comparability, and reproducibility. Other examples include the instruments used during surgery; some labs use (micro-) scissors for dissection of the skin, capsule, and meniscotibial ligament, whereas others use scalpels and ophthalmic blades. Another example is resection of the fat pad. In some studies, resection is mentioned in the materials and methods section, whereas others do not comment on it, although it is performed. As the infrapatellar fat pad, an intracapsular, extrasynovial tissue, is highly biologically active and responds to injury by releasing pro-inflammatory and anti-inflammatory cytokines into the synovial fluid, it is important to know whether it was resected to interpret data, especially when considering that inflammation is recognized as an important contributor to the OA pathology.
Another challenge associated with surgeries inducing OA is related to pre-, peri-, and/or post-surgical analgesics as well as anesthetics, as all these affect biological functions. Generally, adequate pain relief is mandatory (1) for ethical reasons and animal welfare and (2) because pain itself generates stress that negatively influences the immunological response and other biological processes (Peterson, Nunamaker, & Turner, 2017). All these small differences in performing the surgery add up to issues regarding reproducibility, faced by the scientific community in general; therefore, adequate reporting is needed. Of note, there is also intralab variability, a fact that is hardly addressed even though everyone is aware of it.
Other factors hampering comparability are different histopathology scoring schemes despite the recommendations of the Osteoarthritis Research International (OARSI) for grading of joint pathologies in mice, rats, and guinea pigs (Gerwin, Bendele, Glasson, & Carlson, 2010; Glasson, Chambers, Van Den Berg, & Little, 2010; Kraus, Huebner, DeGroot, & Bendele, 2010) that are all referred to the OARSI score, without disclosing whether the originally proposed scheme was applied or whether it was a modified score, if it is a summed score or an average, how many sections per animal in which area of the joint were assessed, and at which distance to each other these sections were obtained. In summary, more detailed reporting of procedures including instruments and analgesic medications as well as outcome analyses are highly recommended to foster comparability and reproducibility of data and therefore increase the validity of findings when screening for pharmacological treatments.
Although small/rodent animal models are great models for studying OA, one major limitation is regarding translatability. Usually, the studies are not sufficiently powered to (1) enable detection of effects with small effect sizes and (2) to detect statistically significant differences with less errors. Usually, sample sizes are calculated using an alpha of 0.05 and a power of 0.8. Furthermore, in the light of 3Rs, researchers are asked to keep the sample size as low as possible by the approval authority, making studies with a power of 0.9 or 0.95 extremely difficult to be conducted.
NATURALLY OCCURRING MODELS OF OSTEOARTHRITIS
Naturally occurring models of OA include those that occur spontaneously without the need for any intervention. These include mice (usually inbred strains) (Stoop et al., 1999), certain strains of guinea pigs (Jimenez, Glasson, Trubetskoy, & Haimes, 1997), rabbits (Arzi et al., 2011), dogs (Meeson, Todhunter, Blunn, Nuki, & Pitsillides, 2019), pigs (Macfadyen et al., 2019), sheep, goats, and horses (McIlwraith, Frisbie, & Kawcak, 2012).
SPONTANEOUS AGE-RELATED MOUSE MODELS OF OSTEOARTHRITIS
Mouse models of OA have been in use for several decades and were initially described as spontaneous models in males of certain inbred mouse strains. Early studies by Walton used anatomical and radiological techniques to evaluate macroscopic and microscopic structural changes in the mouse OA joint (Walton, 1977a, 1977b). The disease was radiographically defined in this model as a thickening of the subchondral bone in the medial tibial and femoral condyles, but this is predominantly seen in males rather than females. Microscopic evaluation of advanced lesions revealed patellar dislocation, meniscal ossification, calcification of lateral ligaments accompanying cartilage loss, and subchondral bone eburnation (Walton, 1977a). Later studies showed that stabilization of the knee joint in mice can prevent the development of OA in the medial compartment of the femorotibial joint, highlighting the role of genetics in the anatomical abnormalities in the patella in this model (Walton, 1979).
Comparative histological and radiographic examination of knee joints in the C57BL/6 mouse strain has confirmed all the classical hallmarks of OA, including joint space narrowing, subchondral bone sclerosis, and deformation of the joint epiphyses (Pataki, Fries, Ochsner, & Witzemann, 1987). These joint alterations are accompanied by changes in the menisci, frequently seen as enlargement and deformation. The meniscal alterations in the C57BL mouse model of spontaneous OA was radiologically and histopathologically graded using joint space width as a key quantitative parameter (Pataki et al., 1987). These classic studies have highlighted the importance of inbreeding, genetics, and sex in OA development in mouse models.
Aging is a major factor within the pathogenesis of OA. The thinning of the articular cartilage and chondrocyte death are known to be major contributors to the development of age-related OA. Although several studies have shown that these features can also be observed in surgically induced models (e.g., DMM), the timeline of the occurrence of these features (12 weeks vs. up to 18 months) indicates differences in the disturbed cellular and molecular processes, which are much more biomechanically driven in the DMM. The spontaneous development of OA in the knee joint of commonly used C57Bl/6 mice aged above 17 months has been reported (Fang & Beier, 2014; McNulty et al., 2011). Further studies indicated the presence of differences between strains. Therefore, it has been shown that CD1 mice already exhibit early OA-like changes at an age of 1 year, which increases in severity at 20 and 24 months, affecting the whole joint. The onset of OA has been shown to be comparable between male C57Bl/6 and Balb/c mice at 1 to 2 years, although differences were seen in the location of the cartilage damage, especially in the C57Bl/6 (more pronounced in the lateral compartment), which is contrary to the current understanding (van der Kraan, Stoop, Meijers, Poole, & van den Berg, 2001). Independent of the strain, most studies report changes within the medial tibial plateau, which nicely parallels the human situation. In addition, Moriyama and coworkers observed severe degenerative cartilage loss in Wistar rats at 2 years (Moriyama et al., 2012).
The use of genetically modified mice has exponentially grown during the last decade to investigate the influence of genetic factors and/or genetic defects in the development of OA, enforced by the development of new breeding techniques and evolving knowledge about the genetic risk factors associated with OA in humans. In OA, several genetically modified mice were developed to examine the molecular pathways of the disease progression. Specifically, modifications of matrix components were investigated either to uncover molecular mechanisms or to induce an OA phenotype during aging. A heterozygous knockout of type 2 collagen, the major structural component of cartilage, resulted in an earlier onset of spontaneous OA as well as higher OA incidence at 15 months of age (Lapveteläinen et al., 2001). Homozygous knockout of type 9 collagen led to OA-like changes in the articular cartilage starting at an age of 3 months with loss of proteoglycans that progressed further. At 9 months of age, complete loss of the articular cartilage was evident. The changes went along with increased MMP13 expression and degraded type 2 collagen (Hu et al., 2006). Moreover, knockout of non-collagenous cartilage proteins such as Matrilin-3 led to a higher OA incidence in aged mice (van der Weyden et al., 2006).
Besides ECM proteins, molecular components and regulators of chondrocyte energy metabolism have gained attention in connection to their role in OA pathogenesis (Mobasheri et al., 2017; Zheng, Zhang, Sheng, & Mobasheri, 2020). OA is increasingly recognized as metabolically driven and its symptoms are known to be exacerbated in the presence of comorbidities, especially those of a metabolic nature (Chan et al., 2009; Courties, Berenbaum, & Sellam, 2019; Li, George, Jaarsma, & Mao, 2016; Sellam & Berenbaum, 2013). Examples include overexpression of arginase 2 (Choi et al., 2019) as well as inactivation of AMPK, promoting OA development (Zhou et al., 2017).
In addition to investigating the roles of specific genes in the development of spontaneous OA, genetically modified animals are helpful for analyzing the relevance of genes/proteins in post-traumatic OA and for biomarker research (Kraus & Karsdal, 2021). However, their translational value is questionable, as OA is a multifactorial disease (Glyn-Jones et al., 2015). Poor translatability to the human situation might also be due to the inbred background of mice used in research, whereas the genetic diversity of the human population might be better reflected by outbred strains. In addition, use of knockout strains in the absence of the respective gene(s) during development and growth can lead to false conclusions on the role of a gene or factor. Examples are GDF-5 and FGF-18. KO mice have severe phenotypes, up to premature death, but the genes are involved in OA pathogenesis manifested at later ages of OA patients only. The studies using KO mice should take the influence of genes during development into account and put this in context with human disease in elderly patients. Moreover, traditional breeding is cost-intensive and requires high time commitment. However, due to CRISPR-Cas9, generation of genetically modified mice is quicker than ever. Together with new data from a genome-wide association meta-analysis in humans, gene variants that increase the odds ratio for developing OA can be now investigated on a mid-throughput scale.
Nevertheless, there are significant differences between humans and mice, resulting in a slower translation process (Little & Hunter, 2013). To overcome such hurdles, researchers started to develop so-called “humanized animal models,” meaning the breeding of mice including a human hematopoietic system or genetic components from humans that are of interest (Kollias et al., 2011). This technique prompts the question of functionality and similarity because of the different environments and the far more active metabolism of mice.
Finally, the Dunkin Hartley guinea pig model provides the closest representation of primary human OA and as such has been one of the most widely used animal models to study naturally occurring OA (Jimenez et al., 1997; Kuyinu et al., 2016; Lampropoulou-Adamidou et al., 2014; Yan et al., 2014). Guinea pigs grow rapidly to skeletal maturity, which is a major advantage over larger animal models in terms of timelines and costs of animal maintenance (Poole et al., 2010). At the same time, guinea pigs develop OA lesions with age that are similar to human patients, further strengthening their use as an animal model in therapeutic and pathogenic studies (Jimenez et al., 1997). Additionally, the guinea pig is also a natural model for studying inflammation and biomarkers of low-grade inflammation in the joints (Huebner & Kraus, 2006).
DIET-INDUCED OBESITY IN RODENT MODELS OF OSTEOARTHRITIS
There is accumulating evidence suggesting that metabolic disturbance (i.e., obesity) is associated with a significant increase in the risk of developing knee, hip, and hand OA in humans (Bijlsma, Berenbaum, & Lafeber, 2011; Felson & Chaisson, 1997; Felson, Anderson, Naimark, Walker, & Meenan, 1988; Hart & Spector, 1993; Manninen, Riihimaki, Heliövaara, & Suomalainen, 2004). Increased mechanical loading on weight-bearing joints cannot completely explain OA damage observed in the knee, and systemic inflammatory mediators have a strong relationship with the damage observed, suggesting a new metabolic OA phenotype (Bijlsma et al., 2011; Zhuo, Yang, Chen, & Wang, 2012) that may need further expansion and refinement as an immunometabolic phenotype (Mobasheri et al., 2017). To demonstrate the increased incidence of this new phenotype and understand the underlying mechanism behind OA in people with metabolic disturbance, diet-induced animal models of OA (i.e., metabolic models of OA) have been developed.
One of the first studies on knee OA caused by a high-fat diet (Griffin et al., 2010) exposed mice to either a low- or high-fat diet (10% and 45% kcal from fat, respectively) from 9 to 54 weeks of age. Interestingly, it was possible to delineate mice on the high-fat diet into “resistant” (low body mass and fat) and “prone” (high body mass and fat) populations depending on the extent of their weight gain. The prone mice showed an increased incidence of knee OA, mainly indicated by loss of cartilage proteoglycans. The authors suggested that high susceptibility to obesity was associated with OA-like changes in the knee joint. The same study reported that prone mice were associated with impaired musculoskeletal force generation and motor function compared to controls. Furthermore, the high-fat diet also induced symptoms characteristically displayed by sufferers of OA, including hyperalgesia and anxiety-like behaviors. Additionally, body fat percentage was significantly associated with knee OA severity and serum levels of leptin, adiponectin, and IL-1α. The same model was also used to study short-term wheel-running exercise effects on obesity-induced knee OA in mice (Griffin, Huebner, Kraus, Yan, & Guilak, 2012). Exercise in this cohort improved glucose tolerance and disrupted the co-expression of pro-inflammatory cytokines, suggesting that increased aerobic exercise may act independently of weight loss in promoting joint health as the mice did not lose body mass or body fat when compared to the sedentary group.
A high-fat/high-sucrose model has been successfully used to induce OA in CD Sprague-Dawley rats (Collins, Hart, Reimer, Seerattan, & Herzog, 2015a, 2015b, 2016; Rios et al., 2019). This model was also associated with obesity-prone and obesity-resistant phenotypes, suggesting that individual animals tend to respond differently to the same diet challenge. Furthermore, a high-fat diet resulted in altered chondrocyte function in rabbits, independent of animal weight, and was associated with changes in rabbit cartilage in vivo (Brunner et al., 2012). These preclinical models are essential for understanding the mechanisms through which diabetes mellitus may contribute to OA onset (King & Rosenthal, 2015).
Intestinal permeability may play a role in metabolic OA. It has been suggested that low-grade chronic inflammation in metabolic OA can result from the absorption of endotoxin across the intestinal tract (Brun et al., 2007; Collins et al., 2015a; Creely et al., 2007; Harte et al., 2010; Metcalfe et al., 2012). Additionally, endotoxin absorption has been positively correlated with obesity (Cani et al., 2007; Cluny, Eller, Keenan, Reimer, & Sharkey, 2015; Ley et al., 2005). Other studies (Griffin et al., 2012) have described the OA-inducing effects of a high-fat diet in mouse models, with concomitantly increased levels of inflammatory cytokines. Additionally, rodents fed an obesogenic diet combined with prebiotic supplements did not present high systemic endotoxin levels, and the prebiotics prevented OA development (Rios et al., 2019; Schott et al., 2018). Therefore, endotoxins may be contributing factors to the onset and progression of OA in patients with obesity.
DISCUSSION AND CONCLUSIONS
In this review, we introduced the most widely used small animal models of OA and outlined their strengths and weaknesses. We also discussed the challenges associated with the validation of animal models with respect to key aspects of the human disease state. We also discussed the technical reliability of these animal models and commented on their translational predictiveness, especially in the context of new drug development and preclinical testing. Figure 3 provides an overview of the preclinical models of OA and associated outcomes and assessments, and Table 1 provides a summary of the key advantages and disadvantages of the most commonly used animal models of OA, including consideration of the pharmacological aspects and translational potential of the models.
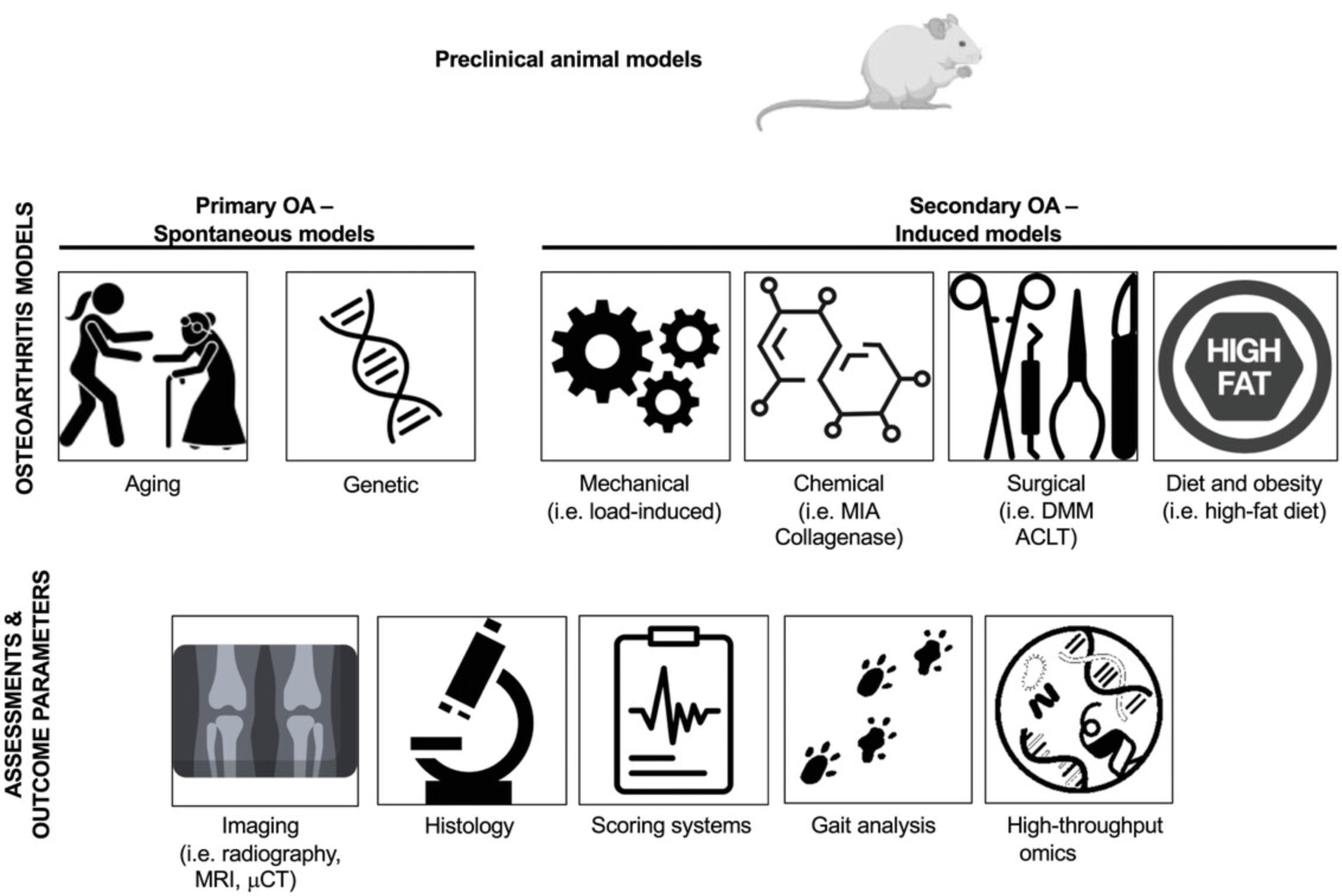
One of the main limitations of preclinical small animal models is the inability to directly translate the findings to humans. However, animal models remain a powerful tool to study disease processes and disease pathophysiologies similar to those observed in humans as long as limitations are acknowledged, and animals are not viewed as representing the human species (Quinn, 2005). Differences in anatomy, gait (i.e., 4 legs vs. 2 legs in humans), physiology, and developmental biology must be considered when analyzing results across animal species, including humans. We must also consider the significant differences in the longevity, environment, diet, and microbiome.
There is no one small animal model that mimics all the facets of human OA, and some models may be better suited to study a specific disease pathway or therapeutic intervention than others. Therefore, a fundamental understanding of each model and how it relates to the research question is critically important in the prioritization and selection process to minimize resource usage and increase the chances of obtaining a clear answer. However, as described in this review, the OA field is still evolving and gaining expert consensus is always a challenge. Although a combination of different animal models to address a question is not the most efficient path, it may be necessary to provide insights into a complex human OA phenotype or therapeutic intervention of interest. For example, a preclinical PTOA model may contain facets of the post-traumatic human OA phenotype caused by acute or repetitive injury to the joint. A diet-induced metabolic disturbance model may represent aspects of increased joint loading caused by obesity and local/systemic inflammation associated with the metabolic OA phenotype. The aging OA phenotype may be studied best through genetically modified and spontaneous preclinical models. Finally, preclinical models of OA pain may help understand mechanisms of OA pain in humans and may allow for testing of interventions aimed at pain reduction. Understanding the specific etiologies of OA phenotypes may help in making treatment decisions, such as exercise interventions, drug interventions, or hormone therapies.
In our view, continued effort to develop, validate, and assess the translational potential of OA animal models is required to support progression of preclinical discoveries to the clinical setting. In addition, the procedures and methods used in these models should be better described.
Although translational animal models are complex and costly and their use comes with ethical challenges, a responsible path needs to be established to better address the significant needs of OA patients and society. The following paper in this series of articles will deal with larger animal models that can be defined as translational models of OA.
AUTHOR CONTRIBUTIONS
Jaqueline Lourdes Rios : conceptualization, writing original draft, writing review & editing; Dora Sapède : writing original draft; Farida Djouad : writing original draft; Anna E. Rapp : writing original draft, writing review & editing; Annemarie Lang : writing original draft, writing review & editing; Jonathan Larkin : funding acquisition, resources, writing original draft, writing review & editing; Christoph Ladel : conceptualization, funding acquisition, resources, supervision, writing original draft, writing review & editing; Ali Mobasheri : conceptualization, funding acquisition, resources, supervision, writing original draft, writing review & editing.
CONFLICT OF INTEREST
The authors do not have any financial and personal relationships that might bias this work. This work did not receive external funding, and there were no sponsors involved in the collection, analysis, and interpretation of published data.
DISCLOSURES
JLR is a part-time employee of Percuros BV. JL is the president and founder of SynOA Therapeutics. CL is an independent consultant. AM is the Immediate Past President of the Osteoarthritis Research Society International (OARSI) and serves on the scientific advisory boards of Kolon TissueGene Inc., Aptissen S.A., Research Square, and the European Society for Clinical and Economic Aspects of Osteoporosis, Osteoarthritis and Musculoskeletal Diseases (ESCEO).
Open Research
DATA AVAILABILITY STATEMENT
Not applicable
LITERATURE CITED
- Adães, S., Mendonça, M., Santos, T. N., Castro-Lopes, J. M., Ferreira-Gomes, J., & Neto, F. L. (2014). Intra-articular injection of collagenase in the knee of rats as an alternative model to study nociception associated with osteoarthritis. Arthritis Research & Therapy, 16, R10. doi: 10.1186/ar4436
- Adams, M. E. (1983). Target tissue models: Cartilage changes in experimental osteoarthritis in the dog. The Journal of Rheumatology. Supplement , 11, 111–113.
- Andriacchi, T. P., & Mündermann, A. (2006). The role of ambulatory mechanics in the initiation and progression of knee osteoarthritis. Current Opinion in Rheumatology , 18, 514–518. doi: 10.1097/01.bor.0000240365.16842.4e
- Apschner, A., Schulte-Merker, S., & Witten, P. E. (2011). Not all bones are created equal–using zebrafish and other teleost species in osteogenesis research. Methods in Cell Biology , 105, 239–255. doi: 10.1016/B978-0-12-381320-6.00010-2
- Arzi, B., Wisner, E. R., Huey, D. J., Kass, P. H., Hu, J., & Athanasiou, K. A. (2011). A proposed model of naturally occurring osteoarthritis in the domestic rabbit. Lab Animal , 41, 20–25. doi: 10.1038/laban0112-20
- Askary, A., Smeeton, J., Paul, S., Schindler, S., Braasch, I., Ellis, N. A., … Crump, J. G. (2016). Ancient origin of lubricated joints in bony vertebrates. eLife , 5, e16415. doi: 10.7554/eLife.16415
- Baker-LePain, J. C., & Lane, N. E. (2010). Relationship between joint shape and the development of osteoarthritis. Current Opinion in Rheumatology , 22, 538–543. doi: 10.1097/BOR.0b013e32833d20ae
- Bendele, A. M. (2001). Animal models of osteoarthritis. Journal of Musculoskeletal & Neuronal Interactions, 1, 363–376.
- Bergen, D. J. M., Kague, E., & Hammond, C. L. (2019). Zebrafish as an emerging model for osteoporosis: A primary testing platform for screening new osteo-active compounds. Frontiers in Endocrinology , 10, 6. doi: 10.3389/fendo.2019.00006
- Bijlsma, J. W. J., Berenbaum, F., & Lafeber, F. P. J. G. (2011). Osteoarthritis: An update with relevance for clinical practice. The Lancet , 377, 2115–2126. doi: 10.1016/S0140-6736(11)60243-2
- Billiau, A., & Matthys, P. (2011). Collagen-induced arthritis and related animal models: How much of their pathogenesis is auto-immune, how much is auto-inflammatory? Cytokine & Growth Factor Reviews, 22, 339–344. doi: 10.1016/j.cytogfr.2011.11.003
- Blaker, C. L., Clarke, E. C., & Little, C. B. (2017). Using mouse models to investigate the pathophysiology, treatment, and prevention of post-traumatic osteoarthritis. Journal of Orthopaedic Research , 35, 424–439. doi: 10.1002/jor.23343
- Blalock, D., Miller, A., Tilley, M., & Wang, J. (2015). Joint instability and osteoarthritis. Clinical Medicine Insights. Arthritis and Musculoskeletal Disorders , 8, 15–23. doi: 10.4137/CMAMD.S22147
- Bliddal, H., Leeds, A. R., & Christensen, R. (2014). Osteoarthritis, obesity and weight loss: Evidence, hypotheses and horizons - a scoping review. Obesity Reviews , 15, 578–586. doi: 10.1111/obr.12173
- Brun, P., Castagliuolo, I., Di Leo, V., Buda, A., Pinzani, M., Palù, G., & Martines, D. (2007). Increased intestinal permeability in obese mice: New evidence in the pathogenesis of nonalcoholic steatohepatitis. American Journal of Physiology. Gastrointestinal and Liver Physiology , 292, G518–G525. doi: 10.1152/ajpgi.00024.2006
- Brunner, A. M., Henn, C. M., Drewniak, E. I., Lesieur-Brooks, A., Machan, J., Crisco, J. J., & Ehrlich, M. G. (2012). High dietary fat and the development of osteoarthritis in a rabbit model. Osteoarthritis and Cartilage , 20, 584–592. doi: 10.1016/j.joca.2012.02.007
- Brunt, L. H., Roddy, K. A., Rayfield, E. J., & Hammond, C. L. (2016). Building finite element models to investigate zebrafish jaw biomechanics. Journal of Visualized Experiments , 118, e54811. doi: 10.3791/54811
- Buckwalter, J. A., & Brown, T. D. (2004). Joint injury, repair, and remodeling: Roles in post-traumatic osteoarthritis. Clinical Orthopaedics and Related Research , 423, 7–16. doi: 10.1097/01.blo.0000131638.81519.de
- Cani, P. D., Amar, J., Iglesias, M. A., Poggi, M., Knauf, C., Bastelica, D., … Burcelin, R. (2007). Metabolic endotoxemia initiates obesity and insulin resistance. Diabetes , 56, 1761–1772. doi: 10.2337/db06-1491
- Chakraborty, C., Hsu, C. H., Wen, Z. H., Lin, C. S., & Agoramoorthy, G. (2009). Zebrafish: A complete animal model for in vivo drug discovery and development. Current Drug Metabolism , 10, 116–124. doi: 10.2174/138920009787522197
- Chan, K. W., Ngai, H. Y., Ip, K. K., Lam, K. H., & Lai, W. W. (2009). Co-morbidities of patients with knee osteoarthritis. Hong Kong Medical Journal , 15, 168–172.
- Choi, W.-S., Yang, J.-I., Kim, W., Kim, H.-E., Kim, S.-K., Won, Y., … Chun, J.-S. (2019). Critical role for arginase II in osteoarthritis pathogenesis. Annals of the Rheumatic Diseases , 78, 421–428. doi: 10.1136/annrheumdis-2018-214282
- Cluny, N. L., Eller, L. K., Keenan, C. M., Reimer, R. A., & Sharkey, K. A. (2015). Interactive effects of oligofructose and obesity predisposition on gut hormones and microbiota in diet-induced obese rats. Obesity , 23, 769–778. doi: 10.1002/oby.21017
- Collins, K. H., Hart, D. A., Reimer, R. A., Seerattan, R. A., & Herzog, W. (2016). Response to diet-induced obesity produces time-dependent induction and progression of metabolic osteoarthritis in rat knees. Journal of Orthopaedic Research , 34, 1010–1018. doi: 10.1002/jor.23103
- Collins, K. H., Paul, H. A., Reimer, R. A., Seerattan, R. A., Hart, D. A., & Herzog, W. (2015a). Relationship between inflammation, the gut microbiota, and metabolic osteoarthritis development: Studies in a rat model. Osteoarthritis and Cartilage , 23, 1989–1998. doi: 10.1016/j.joca.2015.03.014
- Collins, K. H., Reimer, R. A., Seerattan, R. A., Leonard, T. R., & Herzog, W. (2015b). Using diet-induced obesity to understand a metabolic subtype of osteoarthritis in rats. Osteoarthritis and Cartilage , 23, 957–965. doi: 10.1016/j.joca.2015.01.015
- Conaghan, P. G., Porcheret, M., Kingsbury, S. R., Gammon, A., Soni, A., Hurley, M., … Birrell, F. (2015). Impact and therapy of osteoarthritis: The Arthritis Care OA Nation 2012 survey. Clinical Rheumatology , 34, 1581–1588. doi: 10.1007/s10067-014-2692-1
- Courties, A., Berenbaum, F., & Sellam, J. (2019). The phenotypic approach to osteoarthritis: A look at metabolic syndrome-associated osteoarthritis. Joint, Bone, Spine: Revue du Rhumatisme , 86, 725–730. doi: 10.1016/j.jbspin.2018.12.005
- Courties, A., Sellam, J., & Berenbaum, F. (2017). Metabolic syndrome-associated osteoarthritis. Current Opinion in Rheumatology , 29, 214–222. doi: 10.1097/BOR.0000000000000373
- Creely, S. J., McTernan, P. G., Kusminski, C. M., Fisher, f. f. M., Da Silva, N. F., … Kumar, S. (2007). Lipopolysaccharide activates an innate immune system response in human adipose tissue in obesity and type 2 diabetes. American Journal of Physiology. Endocrinology and Metabolism , 292, E740–E747. doi: 10.1152/ajpendo.00302.2006
- Cross, M., Smith, E., Hoy, D., Nolte, S., Ackerman, I., Fransen, M., … March, L. (2014). The global burden of hip and knee osteoarthritis: Estimates from the global burden of disease 2010 study. Annals of the Rheumatic Diseases , 73, 1323–1330. doi: 10.1136/annrheumdis-2013-204763
- Culley, K. L., Dragomir, C. L., Chang, J., Wondimu, E. B., Coico, J., Plumb, D. A., … Goldring, M. B. (2015). Mouse models of osteoarthritis: Surgical model of posttraumatic osteoarthritis induced by destabilization of the medial meniscus. Methods in Molecular Biology , 1226, 143–173. doi: 10.1007/978-1-4939-1619-1_12
- Culley, K. L., Singh, P., Lessard, S., Wang, M., Rourke, B., Goldring, M. B., & Otero, M. (2021). Mouse models of osteoarthritis: Surgical model of post-traumatic osteoarthritis induced by destabilization of the medial meniscus. Methods in Molecular Biology , 2221, 223–260. doi: 10.1007/978-1-0716-0989-7_14
- Das, B. C., McCormick, L., Thapa, P., Karki, R., & Evans, T. (2013). Use of zebrafish in chemical biology and drug discovery. Future Medicinal Chemistry , 5, 2103–2116. doi: 10.4155/fmc.13.170
- De Ceuninck, F., Fradin, A., & Pastoureau, P. (2014). Bearing arms against osteoarthritis and sarcopenia: When cartilage and skeletal muscle find common interest in talking together. Drug Discovery Today , 19, 305–311. doi: 10.1016/j.drudis.2013.08.004
- Driever, W., Stemple, D., Schier, A., & Solnica-Krezel, L. (1994). Zebrafish: Genetic tools for studying vertebrate development. Trends in Genetics , 10, 152–159. doi: 10.1016/0168-9525(94)90091-4
- Eckstein, F., Hochberg, M. C., Guehring, H., Moreau, F., Ona, V., Bihlet, A. R., … Conaghan, P. G. (2021). Long-term structural and symptomatic effects of intra-articular sprifermin in patients with knee osteoarthritis: 5-year results from the FORWARD study. Annals of the Rheumatic Diseases , 80(8), 1062–1069. doi: 10.1136/annrheumdis-2020-219181
- Edd, S. N., Giori, N. J., & Andriacchi, T. P. (2015). The role of inflammation in the initiation of osteoarthritis after meniscal damage. Journal of Biomechanics , 48, 1420–1426. doi: 10.1016/j.jbiomech.2015.02.035
- Englund, M. (2009). The role of the meniscus in osteoarthritis genesis. Medical Clinics of North America , 93, 37–43. doi: 10.1016/j.mcna.2008.08.005
- Fang, H., & Beier, F. (2014). Mouse models of osteoarthritis: Modelling risk factors and assessing outcomes. Nature Reviews. Rheumatology , 10, 413–421. doi: 10.1038/nrrheum.2014.46
- Felson, D. T., Anderson, J. J., Naimark, A., Walker, A. M., & Meenan, R. F. (1988). Obesity and knee osteoarthritis. The Framingham Study. Annals of Internal Medicine , 109, 18–24. doi: 10.7326/0003-4819-109-1-18
- Felson, D. T., & Chaisson, C. E. (1997). Understanding the relationship between body weight and osteoarthritis. Bailliere's Clinical Rheumatology , 11, 671–681. doi: 10.1016/S0950-3579(97)80003-9
- Felson, D. T. (2004). Risk factors for osteoarthritis: Understanding joint vulnerability. Clinical Orthopaedics and Related Research , 427, S16–S21. doi: 10.1097/01.blo.0000144971.12731.a2
- Frisbie, D. D., Cross, M. W., & McIlwraith, C. W. (2006). A comparative study of articular cartilage thickness in the stifle of animal species used in human pre-clinical studies compared to articular cartilage thickness in the human knee. Veterinary and Comparative Orthopaedics and Traumatology , 19, 142–146. doi: 10.1055/s-0038-1632990
- Gerwin, N., Bendele, A. M., Glasson, S., & Carlson, C. S. (2010). The OARSI histopathology initiative–recommendations for histological assessments of osteoarthritis in the rat. Osteoarthritis and Cartilage , 18, S24–S34. doi: 10.1016/j.joca.2010.05.030
- Gibert, Y., Trengove, M. C., & Ward, A. C. (2013). Zebrafish as a genetic model in pre-clinical drug testing and screening. Current Medicinal Chemistry , 20, 2458–2466. doi: 10.2174/0929867311320190005
- Glasson, S. S., Chambers, M. G., Van Den Berg, W. B., & Little, C. B. (2010). The OARSI histopathology initiative–recommendations for histological assessments of osteoarthritis in the mouse. Osteoarthritis and Cartilage , 18, S17–S23. doi: 10.1016/j.joca.2010.05.025
- Glyn-Jones, S., Palmer, A. J. R., Agricola, R., Price, A. J., Vincent, T. L., Weinans, H., & Carr, A. J. (2015). Osteoarthritis. The Lancet , 386, 376–387. doi: 10.1016/S0140-6736(14)60802-3
- Goetz, J. E., Fredericks, D., Petersen, E., Rudert, M. J., Baer, T., Swanson, E., … Tochigi, Y. (2015). A clinically realistic large animal model of intra-articular fracture that progresses to post-traumatic osteoarthritis. Osteoarthritis and Cartilage , 23, 1797–1805. doi: 10.1016/j.joca.2015.05.022
- Gregory, M. H., Capito, N., Kuroki, K., Stoker, A. M., Cook, J. L., & Sherman, S. L. (2012). A review of translational animal models for knee osteoarthritis. Arthritis , 2012, 764621. doi: 10.1155/2012/764621
- Grenier, S., Bhargava, M. M., & Torzilli, P. A. (2014). An in vitro model for the pathological degradation of articular cartilage in osteoarthritis. Journal of Biomechanics , 47, 645–652. doi: 10.1016/j.jbiomech.2013.11.050
- Griffin, T. M., Fermor, B., Huebner, J. L., Kraus, V. B., Rodriguiz, R. M., Wetsel, W. C., … Guilak, F. (2010). Diet-induced obesity differentially regulates behavioral, biomechanical, and molecular risk factors for osteoarthritis in mice. Arthritis Research & Therapy, 12, R130. doi: 10.1186/ar3068
- Griffin, T. M., Huebner, J. L., Kraus, V. B., Yan, Z., & Guilak, F. (2012). Induction of osteoarthritis and metabolic inflammation by a very high-fat diet in mice: Effects of short-term exercise. Arthritis and Rheumatism , 64, 443–453. doi: 10.1002/art.33332
- Harte, A. L., da Silva, N. F., Creely, S. J., McGee, K. C., Billyard, T., Youssef-Elabd, E. M., … McTernan, P. G. (2010). Elevated endotoxin levels in non-alcoholic fatty liver disease. Journal of inflammation , 7, 15. doi: 10.1186/1476-9255-7-15
- Hart, D. J., & Spector, T. D. (1993). The relationship of obesity, fat distribution and osteoarthritis in women in the general population: The Chingford Study. The Journal of Rheumatology , 20, 331–335.
- Hawker, G. A. (2019). Osteoarthritis is a serious disease. Clinical and Experimental Rheumatology , 37, S3–S6.
- Howe, K., Clark, M. D., Torroja, C. F., Torrance, J., Berthelot, C., Muffato, M., … Stemple, D. L. (2013). The zebrafish reference genome sequence and its relationship to the human genome. Nature , 496, 498–503. doi: 10.1038/nature12111
- Huebner, J. L., & Kraus, V. B. (2006). Assessment of the utility of biomarkers of osteoarthritis in the guinea pig. Osteoarthritis and Cartilage , 14, 923–930. doi: 10.1016/j.joca.2006.03.007
- Hügle, T., Geurts, J., Nüesch, C., Müller-Gerbl, M., & Valderrabano, V. (2012). Aging and osteoarthritis: An inevitable encounter? Journal of Aging Research , 2012, 950192. doi: 10.1155/2012/950192
- Hunter, D. J., March, L., & Chew, M. (2020). Osteoarthritis in 2020 and beyond: A Lancet Commission. The Lancet , 396, 1711–1712. doi: 10.1016/S0140-6736(20)32230-3
- Hu, K., Xu, L., Cao, L., Flahiff, C. M., Brussiau, J., Ho, K., … Li, Y. (2006). Pathogenesis of osteoarthritis-like changes in the joints of mice deficient in type IX collagen. Arthritis and Rheumatism , 54, 2891–2900. doi: 10.1002/art.22040
- Iannone, F., & Lapadula, G. (2003). The pathophysiology of osteoarthritis. Aging Clinical and Experimental Research , 15, 364–372. doi: 10.1007/BF03327357
- Jimenez, P. A., Glasson, S. S., Trubetskoy, O. V., & Haimes, H. B. (1997). Spontaneous osteoarthritis in Dunkin Hartley guinea pigs: Histologic, radiologic, and biochemical changes. Laboratory Animal Science , 47, 598–601.
- Johnson, R. G. (1986). Transection of the canine anterior cruciate ligament: A concise review of experience with this model of degenerative joint disease. Experimental Pathology , 30, 209–213. doi: 10.1016/S0232-1513(86)80079-2
- Karsdal, M. A., Christiansen, C., Ladel, C., Henriksen, K., Kraus, V. B., & Bay-Jensen, A. C. (2014). Osteoarthritis–a case for personalized health care? Osteoarthritis and Cartilage , 22, 7–16. doi: 10.1016/j.joca.2013.10.018
- Kikuchi, T., Sakuta, T., & Yamaguchi, T. (1998). Intra-articular injection of collagenase induces experimental osteoarthritis in mature rabbits. Osteoarthritis and Cartilage , 6, 177–186. doi: 10.1053/joca.1998.0110
- King, K. B., & Rosenthal, A. K. (2015). The adverse effects of diabetes on osteoarthritis: Update on clinical evidence and molecular mechanisms. Osteoarthritis and Cartilage , 23, 841–850. doi: 10.1016/j.joca.2015.03.031
- Kollias, G., Papadaki, P., Apparailly, F., Vervoordeldonk, M. J., Holmdahl, R., Baumans, V., … Gay, S. (2011). Animal models for arthritis: Innovative tools for prevention and treatment. Annals of the Rheumatic Diseases , 70, 1357–1362. doi: 10.1136/ard.2010.148551
- Kraus, V. B., Burnett, B., Coindreau, J., Cottrell, S., Eyre, D., Gendreau, M., … OARSI FDA Osteoarthritis Biomarkers Working Group. (2011). Application of biomarkers in the development of drugs intended for the treatment of osteoarthritis. Osteoarthritis and Cartilage , 19, 515–542. doi: 10.1016/j.joca.2010.08.019
- Kraus, V. B., Huebner, J. L., DeGroot, J., & Bendele, A. (2010). The OARSI histopathology initiative–recommendations for histological assessments of osteoarthritis in the guinea pig. Osteoarthritis and Cartilage , 18, S35–S52. doi: 10.1016/j.joca.2010.04.015
- Kraus, V. B., & Karsdal, M. A. (2022). Foundations of osteoarthritis section 3: Clinical monitoring in OA chapter 15: Biomarkers. Osteoarthritis and Cartilage , 30, 1159–1173. doi: 10.1016/j.joca.2021.04.019
- Kuyinu, E. L., Narayanan, G., Nair, L. S., & Laurencin, C. T. (2016). Animal models of osteoarthritis: Classification, update, and measurement of outcomes. Journal of Orthopaedic Surgery and Research , 11, 19. doi: 10.1186/s13018-016-0346-5
- Lampropoulou-Adamidou, K., Lelovas, P., Karadimas, E. V., Liakou, C., Triantafillopoulos, I. K., Dontas, I., & Papaioannou, N. A. (2014). Useful animal models for the research of osteoarthritis. European Journal of Orthopaedic Surgery & Traumatology : Orthopedie Traumatologie, 24, 263–271. doi: 10.1007/s00590-013-1205-2
- Lapveteläinen, T., Hyttinen, M., Lindblom, J., Långsjö, T. K., Sironen, R., Li, S. W., … Helminen, H. J. (2001). More knee joint osteoarthritis (OA) in mice after inactivation of one allele of type II procollagen gene but less OA after lifelong voluntary wheel running exercise. Osteoarthritis and Cartilage , 9, 152–160. doi: 10.1053/joca.2000.0370
- Latourte, A., Kloppenburg, M., & Richette, P. (2020). Emerging pharmaceutical therapies for osteoarthritis. Nature Reviews. Rheumatology , 16, 673–688. doi: 10.1038/s41584-020-00518-6
- Lawrence, E. A., Aggleton, J., van Loon, J., Godivier, J., Harniman, R., Pei, J., … Hammond, C. (2021). Exposure to hypergravity during zebrafish development alters cartilage material properties and strain distribution. Bone & Joint Research, 10, 137–148. doi: 10.1302/2046-3758.102.BJR-2020-0239.R1
- Lawrence, E. A., Kague, E., Aggleton, J. A., Harniman, R. L., Roddy, K. A., & Hammond, C. L. (2018). The mechanical impact of col11a2 loss on joints; col11a2 mutant zebrafish show changes to joint development and function, which leads to early-onset osteoarthritis. Philosophical Transactions of the Royal Society of London. Series B, Biological Sciences , 373, 20170335. doi: 10.1098/rstb.2017.0335
- Lawson, N. D., & Wolfe, S. A. (2011). Forward and reverse genetic approaches for the analysis of vertebrate development in the zebrafish. Developmental Cell , 21, 48–64. doi: 10.1016/j.devcel.2011.06.007
- Lessman, C. A. (2011). The developing zebrafish (Danio rerio): A vertebrate model for high-throughput screening of chemical libraries. Birth Defects Research. Part C, Embryo Today: Reviews , 93, 268–280. doi: 10.1002/bdrc.20212
- Ley, R. E., Bäckhed, F., Turnbaugh, P., Lozupone, C. A., Knight, R. D., & Gordon, J. I. (2005). Obesity alters gut microbial ecology. Proceedings of the National Academy of Sciences of the United States of America , 102, 11070–11075. doi: 10.1073/pnas.0504978102
- Lieschke, G. J., & Currie, P. D. (2007). Animal models of human disease: Zebrafish swim into view. Nature Reviews. Genetics , 8, 353–367. doi: 10.1038/nrg2091
- Little, C. B., & Hunter, D. J. (2013). Post-traumatic osteoarthritis: From mouse models to clinical trials. Nature Reviews. Rheumatology , 9, 485–497. doi: 10.1038/nrrheum.2013.72
- Little, C. B., Smith, M. M., Cake, M. A., Read, R. A., Murphy, M. J., & Barry, F. P. (2010). The OARSI histopathology initiative – recommendations for histological assessments of osteoarthritis in sheep and goats. Osteoarthritis and Cartilage , 18, S80–S92. doi: 10.1016/j.joca.2010.04.016
- Little, C. B., & Zaki, S. (2012). What constitutes an “animal model of osteoarthritis”–the need for consensus? Osteoarthritis and Cartilage , 20, 261–267. doi: 10.1016/j.joca.2012.01.017
- Liu, K., Petree, C., Requena, T., Varshney, P., & Varshney, G. K. (2019). Expanding the CRISPR toolbox in zebrafish for studying development and disease. Frontiers in Cell and Developmental Biology , 7, 13. doi: 10.3389/fcell.2019.00013
- Li, H., George, D. M., Jaarsma, R. L., & Mao, X. (2016). Metabolic syndrome and components exacerbate osteoarthritis symptoms of pain, depression and reduced knee function. Annals of Translational Medicine , 4, 133. doi: 10.21037/atm.2016.03.48
- Li, N., Felber, K., Elks, P., Croucher, P., & Roehl, H. H. (2009). Tracking gene expression during zebrafish osteoblast differentiation. Developmental Dynamics , 238, 459–466. doi: 10.1002/dvdy.21838
- Lorenz, J., & Grässel, S. (2014). Experimental osteoarthritis models in mice. Methods in Molecular Biology , 1194, 401–419. doi: 10.1007/978-1-4939-1215-5_23
- Luong, M.-L. N., Cleveland, R. J., Nyrop, K. A., & Callahan, L. F. (2012). Social determinants and osteoarthritis outcomes. Aging Health , 8, 413–437. doi: 10.2217/ahe.12.43
- Macfadyen, M. A., Daniel, Z., Kelly, S., Parr, T., Brameld, J. M., Murton, A. J., & Jones, S. W. (2019). The commercial pig as a model of spontaneously-occurring osteoarthritis. BMC Musculoskeletal Disorders , 20, 70. doi: 10.1186/s12891-019-2452-0
- Malfait, A-M., & Little, C. B. (2015). On the predictive utility of animal models of osteoarthritis. Arthritis Research & Therapy, 17, 225. doi: 10.1186/s13075-015-0747-6
- Malfait, A-M., & Tortorella, M. D. (2019). The “elusive DMOAD”: Aggrecanase inhibition from laboratory to clinic. Clinical and Experimental Rheumatology , 37, S130–S134.
- Manninen, P., Riihimaki, H., Heliövaara, M., & Suomalainen, O. (2004). Weight changes and the risk of knee osteoarthritis requiring arthroplasty. Annals of the Rheumatic Diseases , 63, 1434–1437. doi: 10.1136/ard.2003.011833
- Martel-Pelletier, J., Barr, A. J., Cicuttini, F. M., Conaghan, P. G., Cooper, C., Goldring, M. B., … Pelletier, J.-P. (2016). Osteoarthritis. Nature Reviews. Disease Primers , 2, 16072. doi: 10.1038/nrdp.2016.72
- Matthews, G. L. (2013). Disease modification: Promising targets and impediments to success. Rheumatic Diseases Clinics of North America , 39, 177–187. doi: 10.1016/j.rdc.2012.10.006
- McCoy, A. M. (2015). Animal models of osteoarthritis: Comparisons and key considerations. Veterinary Pathology , 52, 803–818. doi: 10.1177/0300985815588611
- McIlwraith, C. W., Frisbie, D. D., & Kawcak, C. E. (2012). The horse as a model of naturally occurring osteoarthritis. Bone & Joint Research, 1, 297–309. doi: 10.1302/2046-3758.111.2000132
- McNulty, M. A., Loeser, R. F., Davey, C., Callahan, M. F., Ferguson, C. M., & Carlson, C. S. (2011). A comprehensive histological assessment of osteoarthritis lesions in mice. Cartilage , 2, 354–363. doi: 10.1177/1947603511402665
- Meeson, R. L., Todhunter, R. J., Blunn, G., Nuki, G., & Pitsillides, A. A. (2019). Spontaneous dog osteoarthritis – a One Medicine vision. Nature Reviews. Rheumatology , 15, 273–287. doi: 10.1038/s41584-019-0202-1
- Metcalfe, D., Harte, A. L., Aletrari, M. O., Al Daghri, N. M., Al Disi, D., Tripathi, G., & McTernan, P. G. (2012). Does endotoxaemia contribute to osteoarthritis in obese patients? Clinical Science , 123, 627–634. doi: 10.1042/CS20120073
- Mitchell, R. E., Huitema, L. F. A., Skinner, R. E. H., Brunt, L. H., Severn, C., Schulte-Merker, S., & Hammond, C. L. (2013). New tools for studying osteoarthritis genetics in zebrafish. Osteoarthritis and Cartilage , 21, 269–278. doi: 10.1016/j.joca.2012.11.004
- Mobasheri, A., Rayman, M. P., Gualillo, O., Sellam, J., van der Kraan, P., & Fearon, U. (2017). The role of metabolism in the pathogenesis of osteoarthritis. Nature Reviews. Rheumatology , 13, 302–311. doi: 10.1038/nrrheum.2017.50
- Modeling osteoarthritis genetics in zebrafish. (2013). BoneKEy Reports , 2 , 349. doi: 10.1038/bonekey.2013.83
- Moriyama, H., Kanemura, N., Brouns, I., Pintelon, I., Adriaensen, D., Timmermans, J.-P., … Deie, M. (2012). Effects of aging and exercise training on the histological and mechanical properties of articular structures in knee joints of male rat. Biogerontology , 13, 369–381. doi: 10.1007/s10522-012-9381-8
- Myers, S. L., Brandt, K. D., O'Connor, B. L., Visco, D. M., & Albrecht, M. E. (1990). Synovitis and osteoarthritic changes in canine articular cartilage after anterior cruciate ligament transection. Effect of surgical hemostasis. Arthritis and Rheumatism , 33, 1406–1415. doi: 10.1002/art.1780330913
- Nasevicius, A., & Ekker, S. C. (2000). Effective targeted gene “knockdown” in zebrafish. Nature Genetics , 26, 216–220. doi: 10.1038/79951
- O'Connor, M. I. (2006). Osteoarthritis of the hip and knee: Sex and gender differences. The Orthopedic Clinics of North America , 37, 559–568. doi: 10.1016/j.ocl.2006.09.004
- O'Neill, T. W., McCabe, P. S., & McBeth, J. (2018). Update on the epidemiology, risk factors and disease outcomes of osteoarthritis. Best Practice & Research. Clinical Rheumatology, 32, 312–326. doi: 10.1016/j.berh.2018.10.007
- Oo, W. M., Little, C., Duong, V., & Hunter, D. J. (2021). The development of disease-modifying therapies for osteoarthritis (DMOADs): The evidence to date. Drug Design , Development and Therapy , 15, 2921–2945. doi: 10.2147/DDDT.S295224
- Oo, W. M., Yu, S. P.-C., Daniel, M. S., & Hunter, D. J. (2018). Disease-modifying drugs in osteoarthritis: Current understanding and future therapeutics. Expert Opinion on Emerging Drugs , 23, 331–347. doi: 10.1080/14728214.2018.1547706
- Pataki, A., Fries, R., Ochsner, K., & Witzemann, E. (1987). Qualitative radiographic diagnosis of osteo-arthritis of the knee joint in the C57BL mouse. Agents and Actions , 22, 123–130. doi: 10.1007/BF01968827
- Patton, E. E., Zon, L. I., & Langenau, D. M. (2021). Zebrafish disease models in drug discovery: From preclinical modelling to clinical trials. Nature Reviews. Drug Discovery , 20, 611–628. doi: 10.1038/s41573-021-00210-8
- Peterson, N. C., Nunamaker, E. A., & Turner, P. V. (2017). To treat or not to treat: The effects of pain on experimental parameters. Comparative Medicine , 67, 469–482.
- Poole, R., Blake, S., Buschmann, M., Goldring, S., Laverty, S., Lockwood, S., … Yaksh, T. (2010). Recommendations for the use of preclinical models in the study and treatment of osteoarthritis. Osteoarthritis and Cartilage , 18, S10–S16. doi: 10.1016/j.joca.2010.05.027
- Pregizer, S. K., Kiapour, A. M., Young, M., Chen, H., Schoor, M., Liu, Z., … Capellini, T. D. (2018). Impact of broad regulatory regions on Gdf5 expression and function in knee development and susceptibility to osteoarthritis. Annals of the Rheumatic Diseases , 77, 450. doi: 10.1136/annrheumdis-2017-212475
- Proffen, B. L., McElfresh, M., Fleming, B. C., & Murray, M. M. (2012). A comparative anatomical study of the human knee and six animal species. The Knee , 19, 493–499. doi: 10.1016/j.knee.2011.07.005
- Quinn, R. (2005). Comparing rat's to human's age: How old is my rat in people years? Nutrition , 21, 775–777. doi: 10.1016/j.nut.2005.04.002
- Reynard, L. N., & Barter, M. J. (2020). Osteoarthritis year in review 2019: Genetics, genomics and epigenetics. Osteoarthritis and Cartilage , 28, 275–284. doi: 10.1016/j.joca.2019.11.010
- Rios, J. L., Bomhof, M. R., Reimer, R. A., Hart, D. A., Collins, K. H., & Herzog, W. (2019). Protective effect of prebiotic and exercise intervention on knee health in a rat model of diet-induced obesity. Scientific Reports , 9, 3893. doi: 10.1038/s41598-019-40601-x
- Sabha, M., Siaton, B. C., & Hochberg, M. C. (2020). Lorecivivint, an intra-articular potential disease-modifying osteoarthritis drug. Expert Opinion on Investigational Drugs , 29, 1339–1346. doi: 10.1080/13543784.2020.1842357
- Safiri, S., Kolahi, A.-A., Smith, E., Hill, C., Bettampadi, D., Mansournia, M. A., … Cross, M. (2020). Global, regional and national burden of osteoarthritis 1990-2017: A systematic analysis of the Global Burden of Disease Study 2017. Annals of the Rheumatic Diseases , 79, 819–828. doi: 10.1136/annrheumdis-2019-216515
- Schott, E. M., Farnsworth, C. W., Grier, A., Lillis, J. A., Soniwala, S., Dadourian, G. H., … Zuscik, M. J. (2018). Targeting the gut microbiome to treat the osteoarthritis of obesity. Journal of Clinical Investigation Insight , 3, e95997. doi: 10.1172/jci.insight.95997
- Sellam, J., & Berenbaum, F. (2013). Is osteoarthritis a metabolic disease? Joint, Bone, Spine: Revue du Rhumatisme , 80, 568–573. doi: 10.1016/j.jbspin.2013.09.007
- Stoop, R., van der Kraan, P. M., Buma, P., Hollander, A. P., Billinghurst, R. C., Poole, A. R., & van den Berg, W. B. (1999). Type II collagen degradation in spontaneous osteoarthritis in C57Bl/6 and BALB/c mice. Arthritis and Rheumatism , 42, 2381–2389. doi: 10.1002/1529-0131(199911)42:11%3c2381::AID-ANR17%3e3.0.CO;2-E
- Talbot, J. C., & Amacher, S. L. (2014). A streamlined CRISPR pipeline to reliably generate zebrafish frameshifting alleles. Zebrafish , 11, 583–585. doi: 10.1089/zeb.2014.1047
- Tonelli, F., Bek, J. W., Besio, R., De Clercq, A., Leoni, L., Salmon, P., … Forlino, A. (2020). Zebrafish: A resourceful vertebrate model to investigate skeletal disorders. Frontiers in Endocrinology , 11, 489. doi: 10.3389/fendo.2020.00489
- Tonge, D. P., Pearson, M. J., & Jones, S. W. (2014). The hallmarks of osteoarthritis and the potential to develop personalised disease-modifying pharmacological therapeutics. Osteoarthritis and Cartilage , 22, 609–621. doi: 10.1016/j.joca.2014.03.004
- Valdes, A. M., & Spector, T. D. (2011). Genetic epidemiology of hip and knee osteoarthritis. Nature Reviews. Rheumatology , 7, 23–32. doi: 10.1038/nrrheum.2010.191
- van der Kraan, P. M., Stoop, R., Meijers, T. H., Poole, A. R., & van den Berg, W. B. (2001). Expression of type X collagen in young and old C57Bl/6 and Balb/c mice. Relation with articular cartilage degeneration. Osteoarthritis and Cartilage , 9, 92–100. doi: 10.1053/joca.2000.0364
- van der Weyden, L., Wei, L., Luo, J., Yang, X., Birk, D. E., Adams, D. J., … Chen, Q. (2006). Functional knockout of the matrilin-3 gene causes premature chondrocyte maturation to hypertrophy and increases bone mineral density and osteoarthritis. The American Journal of Pathology , 169, 515–527. doi: 10.2353/ajpath.2006.050981
- Van Spil, W. E., Kubassova, O., Boesen, M., Bay-Jensen, A.-C., & Mobasheri, A. (2019). Osteoarthritis phenotypes and novel therapeutic targets. Biochemical Pharmacology , 165, 41–48. doi: 10.1016/j.bcp.2019.02.037
- Vos, T., Flaxman, A. D., Naghavi, M., Lozano, R., Michaud, C., Ezzati, M., … Memish, Z. A. (2012). Years lived with disability (YLDs) for 1160 sequelae of 289 diseases and injuries 1990-2010: A systematic analysis for the Global Burden of Disease Study 2010. The Lancet , 380, 2163–2196. doi: 10.1016/S0140-6736(12)61729-2
- Walton, M. (1977a). Degenerative joint disease in the mouse knee; radiological and morphological observations. The Journal of Pathology , 123, 97–107. doi: 10.1002/path.1711230206
- Walton, M. (1979). Patella displacement and osteoarthrosis of the knee joint in mice. The Journal of Pathology , 127, 165–172. doi: 10.1002/path.1711270402
- Walton, M. (1977b). Studies of degenerative joint disease in the mouse knee joint; scanning electron microscopy. The Journal of Pathology , 123, 211–217. doi: 10.1002/path.1711230403
- Yan, J.-Y., Tian, F.-M., Wang, W.-Y., Cheng, Y., Xu, H.-F., Song, H.-P., … Zhang, L. (2014). Age dependent changes in cartilage matrix, subchondral bone mass, and estradiol levels in blood serum, in naturally occurring osteoarthritis in Guinea pigs. International Journal of Molecular Sciences , 15, 13578–13595. doi: 10.3390/ijms150813578
- Zheng, L., Zhang, Z., Sheng, P., & Mobasheri, A. (2020). The role of metabolism in chondrocyte dysfunction and the progression of osteoarthritis. Ageing Research Reviews , 66, 101249. doi: 10.1016/j.arr.2020.101249
- Zhou, S., Lu, W., Chen, L., Ge, Q., Chen, D., Xu, Z., … Jiang, Q. (2017). AMPK deficiency in chondrocytes accelerated the progression of instability-induced and ageing-associated osteoarthritis in adult mice. Scientific Reports , 7, 43245. doi: 10.1038/srep43245
- Zhuo, Q., Yang, W., Chen, J., & Wang, Y. (2012). Metabolic syndrome meets osteoarthritis. Nature Reviews. Rheumatology , 8, 729–737. doi: 10.1038/nrrheum.2012.135