Rfoot-seq: Transcriptomic RNase Footprinting for Mapping Stable RNA-Protein Complexes and Rapid Ribosome Profiling
Qianru Li, Qianru Li, Emily K. Stroup, Emily K. Stroup, Zhe Ji, Zhe Ji
Abstract
Ribosome profiling isolates ribosome-protected fragments for sequencing and is a valuable method for studying different aspects of RNA translation. However, conventional protocols require millions of input cells and time-consuming steps to isolate translating ribosome complexes using ultracentrifugation or immunoprecipitation. These limitations have prevented their application to rare physiological samples. To address these technical barriers, we developed an RNase footprinting approach named Rfoot-seq to map stable transcriptomic RNA-protein complexes that allows rapid ribosome profiling using low-input samples (Li, Yang, Stroup, Wang, & Ji, 2022). In this assay, we treat a cell lysate with concentrated RNase without complex crosslinking and retained only RNA footprints associated with stable complexes for sequencing. The footprints in coding regions represent ribosome-protected fragments and can be used to study cytosolic and mitochondrial translation simultaneously. Rfoot-seq achieves comparable results to conventional ribosome profiling to quantify ribosome occupancy and works robustly for various cultured cells and primary tissue samples. Moreover, Rfoot-seq maps RNA fragments associated with stable non-ribosomal RNA-protein complexes in noncoding domains of small noncoding RNAs and some long noncoding RNAs. Taken together, Rfoot-seq opens an avenue to quantify transcriptomic translation and characterize functional noncoding RNA domains using low-input samples. © 2023 The Authors. Current Protocols published by Wiley Periodicals LLC.
Basic Protocol 1 : Harvesting and lysing adherent cells
Alternate Protocol 1 : Harvesting and lysing suspension cells
Alternate Protocol 2 : Harvesting and lysing primary tissue samples
Basic Protocol 2 : RNase treatment and footprint purification for low-input samples
Alternate Protocol 3 : RNase treatment and footprint purification for ultra-low-input samples
Basic Protocol 3 : Library preparation for high-throughput sequencing
Support Protocol : Preparation of dsDNA markers for library size selection
Basic Protocol 4 : Data analysis and quality control after sequencing
INTRODUCTION
Ribosome profiling represents a state-of-the-art technology to study active translation with single-nucleotide resolution in vivo. It uses RNase to digest RNA fragments not protected by ribosomes, isolates the translating ribosome complexes, and converts the protected footprints to a DNA library for deep sequencing (Ingolia et al., 2009; Ingolia, 2014). It has been widely used to study different aspects of protein synthesis, such as regulation of translation efficiency (Brar et al., 2012; Guo et al., 2010), codon usage (Radhakrishnan et al., 2016), ribosome pausing (Liu et al., 2013; Wu et al., 2019), alternative translation initiation (Ingolia et al., 2011; Lee et al., 2012), and identifying translated noncanonical open reading frames (ORFs; Calviello et al., 2016; Chen et al., 2020; Ji et al., 2015).
The major limitation of conventional ribosome profiling protocols is that they require complicated experimental procedures to isolate 80S cytosolic ribosomes, including ultracentrifugation through a sucrose cushion/gradient (Ingolia et al., 2009; McGlincy & Ingolia, 2017), size-exclusion chromatography (Khajuria et al., 2018; Van Heesch et al., 2019), or ribosome immunoprecipitation (Clamer et al., 2018). To map mitochondrial ribosome footprints, 55S mitoribosomes are purified using sucrose gradient centrifugation (Couvillion et al., 2016; Morscher et al., 2018; Rooijers et al., 2013). Due to these complex isolation steps, the protocols typically recommend 1.5 to 10 million input cells, creating a technical barrier that has prevented the application of ribosome profiling to rare cell types, especially primary tissue samples, which typically show low global translation activity.
To address this technical challenge, we recently established a low-input RNase footprinting assay called Rfoot-seq to map transcriptomic stable RNA-protein complexes. Our approach allows rapid simultaneous quantification of cytosolic and mitochondrial ribosome footprints (Li et al., 2022). The assay is based on the stronger protection affinity that translating ribosomes (i.e., 80S cytosolic ribosomes and 55S mitoribosomes) demonstrate for RNA fragments compared to other RNA-binding proteins (RBPs) in coding regions (e.g., splicing factors and 40S scanning ribosomes). Unlike CLIP-seq or RIP-seq, we did not perform RNA-protein crosslinking using formaldehyde or UV (Archer et al., 2016; Darnell, 2010; Van Nostrand et al., 2017), and we treated cell lysates with concentrated RNase. Rfoot-seq selectively mapped footprints from stable RNA-protein complexes, including cytosolic and mitochondrial translating ribosomes as well as non-ribosomal complexes from small noncoding RNAs (sncRNAs) and some long noncoding RNAs (lncRNAs). The footprints from protein-coding regions of RNAs were comparable to those obtained from conventional ribosome profiling protocols and can be applied to study different aspects of translational regulation.
In this article, we describe the step-by-step experimental procedures of Rfoot-seq, which is divided into four protocols (Fig. 1). Basic Protocol 1 introduces the steps of preparing and lysing samples. Adherent cultured cells, suspension cells, and snap-frozen tissues are described separately in Basic Protocol 1 and Alternate Protocols 1 and 2. Basic Protocol 2 describes the RNase treatment and footprint purification steps. RNase digestion is a critical step for generating a high-quality Rfoot-seq library. Regardless of their original biological types, we grouped samples into low-input and ultra-low-input based on the total amount of RNA. The steps for determining RNase treatment conditions and recovering RNase footprints of each group are described in Basic Protocol 2 and Alternate Protocol 3. Basic Protocol 3 details the preparation of the high-throughput sequencing library and Basic Protocol 4 introduces the data analysis and library quality control steps.
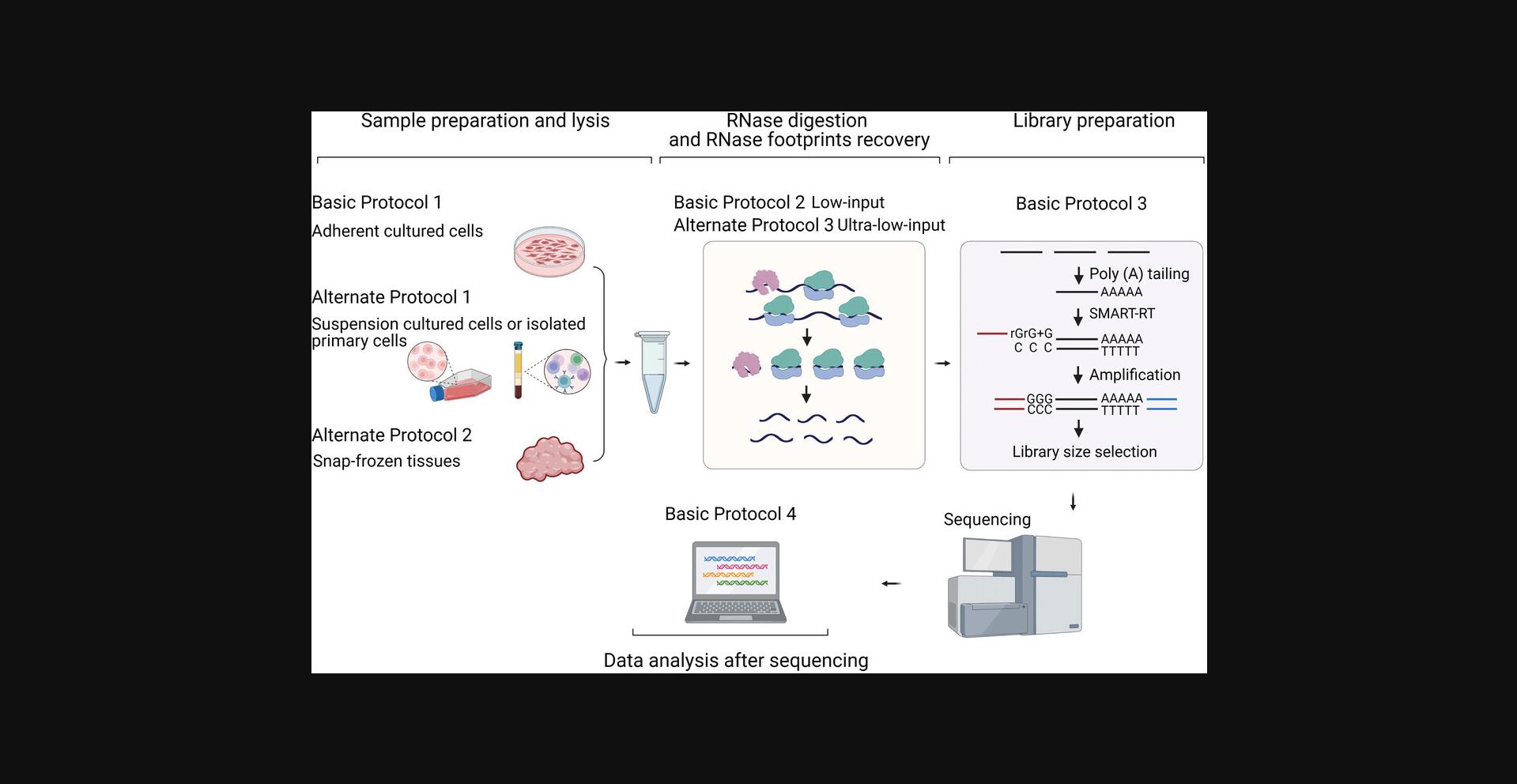
STRATEGIC PLANNING
Rfoot-seq works robustly for various mammal-derived biological samples. Previous studies showed that ribosomes from other model species can display different stability upon ribonuclease treatment (Gerashchenko & Gladyshev, 2017). The RNase digestion conditions may need to be optimized for specific cell types from these species. The total RNA input for Rfoot-seq ranges from 20 ng to 1.2 µg. Users can plan experiments following suitable protocols for their cell types and the total amount of RNA obtained from the cells. If 200 ng to 1.2 µg total RNA can be obtained, the sample should be processed as low-input. If 20-200 ng total RNA is expected, the sample should be considered ultra-low-input. For example, we generally obtain 200-1000 ng total RNA from 15,000-50,000 cultured cells (e.g., HEK293T and K562 cells) and ∼1 µg total RNA from 1 mg snap-frozen tissues, and we considered these to be low-input samples. Primary immune cells show >20-fold lower translation activity than cultured cells. We generally obtain <100 ng total RNA from 100,000 human peripheral blood mononuclear cells (PBMCs) and considered this to be an ultra-low-input sample.
Most of the procedures described in Basic Protocol 1, Alternate Protocol 1, and Basic Protocol 3 are identical for both low- and ultra-low-input samples. However, several experimental conditions have been further optimized for ultra-low-input samples in order to generate a high-quality Rfoot-seq library. In Basic Protocol 1 and Alternate Protocol 1, we lyse ultra-low-input samples with a smaller volume of lysis buffer. In Basic Protocol 3, we extend the polyadenylation time and increase PCR1 and PCR2 cycle numbers for ultra-low-input samples. For these steps, conditions for low- and ultra-low-input samples are described individually and the user can follow the appropriate one.
Rfoot-seq does not require large equipment. It uses only standard lab instruments and is affordable for most labs.
NOTE : Users should ensure they have obtained all the necessary permissions and ethical approvals for work with mammalian tissue samples.
Basic Protocol 1: HARVESTING AND LYSING ADHERENT CELLS
This protocol describes how to prepare a cell lysate for Rfoot-seq using HEK293T cells and can be adapted to other adherent cultured mammalian cells. Most conditions are identical for low-input and ultra-low-input samples; exceptions are described in the appropriate steps. We recommend plating cells in an appropriate multiwell plate and culturing them in an incubator overnight to allow attachment to the plate. Typical cell confluency before harvesting is 70%-80%.
Materials
-
HEK293T cells (ATCC, cat. no. CRL-3216)
-
Complete DMEM: DMEM (Gibco, cat. no. 11965092) with 10% FBS (Gibco, cat. no. 26140079)
-
DPBS, no calcium, no magnesium (Gibco, cat. no. 14190094)
-
Cell lysis buffer (see recipe)
-
48- or 96-well plate (Corning, cat. nos. 3548 and 3595)
-
CO2 incubator (NuAire or equivalent)
-
1.5-ml low-retention microcentrifuge tubes (Thermo Scientific, cat. no. 3451)
1.The day before cell harvest, seed HEK293T cells in complete DMEM in a 48-well plate at 4–5 × 104 cells per well. Grow overnight in a CO2 incubator to allow cells to adhere.
2.Remove and discard medium from the wells. Quickly rinse cells twice with 200 µl cold DPBS and aspirate DPBS thoroughly.
3.Add 80-90 µl cold cell lysis buffer to each well place the plate on ice.
4.Transfer the entire cell lysate to a pre-cooled 1.5-ml microcentrifuge tube, pipette up and down 10 times, and place on ice for 10 min.
5.Proceed directly to RNase digestion (see Basic Protocol 2 or Alternate Protocol 3).
Alternate Protocol 1: HARVESTING AND LYSING SUSPENSION CELLS
This protocol describes how to prepare a cell lysate for Rfoot-seq using suspension cells. We use cultured K562 cells and isolated primary human PBMCs as examples, but the procedure can be adapted to other suspension cells or isolated primary cells. Most conditions are identical for low-input and ultra-low-input samples; exceptions are described in the appropriate steps.
Additional Materials (also see Basic Protocol 1)
-
K562 cells (ATCC, cat. no. CCL-243) or primary human PBMCs
-
Complete IMDM: IMDM (Gibco, cat. no. 12440061) with 10% FBS
-
Complete RPMI: RPMI 1640 (Gibco, cat. no. A1049101) with 10% FBS
-
0.4% trypan blue (Gibco, cat. no. 15250061)
-
Culture flask (Greiner, cat. no. 658195)
-
Automated cell counter (e.g., Countess 3, Invitrogen, cat. no. AMQAX2000)
-
Cell counting chamber slides (e.g., Countess, Invitrogen, cat. no. C10312)
1.For K562 cells, grow a fresh culture in complete IMDM to a density of ∼500,000 cells/ml in a culture flask. For PBMCs, isolate according to manufacturer's instructions and suspend in complete RPMI.
2.Aspirate 10 µl cell suspension to a 1.5-ml microcentrifuge tube and mix well with 10 µl 0.4% trypan blue.
3.Transfer 10 µl to a chamber slide and measure the cell density using a cell counter.
4.Transfer medium containing 50,000 K562 cells or 100,000 PBMCs to a 1.5-ml microcentrifuge tube and centrifuge 3 min at 100 × g.
5.Quickly rinse cells by resuspending with 200 µl cold DPBS, centrifuging 3 min at 100 × g , and aspirating off the DPBS.
6.Repeat rinse and remove all residual DPBS with a pipette.
7.Add 80-90 µl cold lysis buffer to the pellet, pipette up and down 10 times, and incubate on ice for 10 min.
8.Proceed directly to RNase digestion (see Basic Protocol 2 or Alternate Protocol 3).
Alternate Protocol 2: HARVESTING AND LYSING PRIMARY TISSUE SAMPLES
This protocol describes how to prepare a cell lysate for Rfoot-seq using snap-frozen tissues. We use snap-frozen spleen tissue from a mouse as an example, but the procedure can be adapted to other snap-frozen tissues.
Additional Materials (also see Basic Protocol 1)
- Snap-frozen spleen tissue from a mouse
- Liquid nitrogen
- Scalpel
- Mortar and pestle (BioSpec, cat. no. 206; Bel-Art, cat. no. H37260-0100; or equivalent)
- 26-G needle (BD, cat. no. 305111)
- 1-ml syringe (BD, cat. no. 309659)
1.Weigh 1-2 mg snap-frozen spleen tissue using an analytical balance. Place in a pre-cooled mortar and powder the tissue using a pestle while continuously adding liquid nitrogen.
2.Transfer powdered tissue to a pre-cooled 1.5-ml microcentrifuge tube.
3.Add 100 µl cold lysis buffer, triturate through a 26-G needle ten times, and incubate on ice for 10 min.
4.Centrifuge lysate 5 min at 1000 × g , 4°C.
5.Transfer supernatant (∼80-90 µl lysate) to a new pre-cooled 1.5-ml microcentrifuge tube.
6.Place on ice and proceed directly to RNase digestion (see Basic Protocol 2 or Alternate Protocol 3).
Basic Protocol 2: RNase TREATMENT AND FOOTPRINT PURIFICATION FOR LOW-INPUT SAMPLES
RNase treatment is a key step of Rfoot-seq as library quality is highly dependent on RNase digestion. This protocol describes how to determine the conditions for RNase digestion and recover RNase footprints after digestion for low-input samples. This protocol is used when 200 ng to 1.2 µg total RNA can be obtained from your sample (e.g., 50,000 HEK293T cells or K562 cells or 1 mg tissue).
Materials
-
Qubit RNA HS Assay (Invitrogen, cat. no. Q32852)
-
Qubit 4 fluorometer and assay tubes (Invitrogen, cat. no. Q33238 and Q32856)
-
Polysome buffer (see recipe; optional)
-
10 U/μl RNase I (Lucigen, LGC Biosearch Technologies, cat. no. N6901K)
-
TRIzol Reagent (Invitrogen, cat. no. 15596018)
-
Chloroform (molecular biology grade, Sigma-Aldrich, cat. no. C2432)
-
15 mg/ml GlycoBlue (Invitrogen, cat. no. AM9515)
-
3 M sodium acetate (NaOAc), pH 5.5 (RNase-free, Invitrogen, cat. no. AM9740)
-
Isopropanol (molecular biology grade, Fisher Scientific, cat. no. BP2618)
-
80% (v/v) ethanol (Fisher Scientific, cat. no. BP2818) in nuclease-free water, freshly prepared
-
Size markers for analysis of RNA footprints (optional):
- 26- and 34-nt RNAs: 5′-ACGAACAUCUUGAUAUGGUCCACUGC-3′ and 5′-AGUAAUAACACCAGAAACGUGACCAGCAAUAAGC-3′ (synthesized by Integrated DNA Technologies)
- Low-range ssRNA ladder (New England Biolabs, cat. no. N0364S)
-
Parafilm (Bemis, cat. no. PM996)
-
Tube nutator (Fisher Scientific, cat. no. NC0597936)
-
1.5-ml low-retention microcentrifuge tube (Thermo Scientific, cat. no. 3451)
Perform digestion
1.Measure the RNA concentration in the cell lysate using the Qubit RNA high-sensitivity assay kit according to the manufacturer's protocol.
2.Calculate the total amount of RNA in the lysate using the equation:
- Total RNA (µg) = RNA concentration × volume cell lysate (µl)
3.Calculate the required amount of RNase using the equation:
- RNase 1 (U) = 50 U/µg × total RNA amount (µg)
4.Calculate the resulting concentration of RNase 1 in the reaction using the equation:
- RNase 1 (U/µl) = RNase 1 (U) / volume cell lysate (µl)
If it is between 0.5 and 1 U/µl, proceed to the next step. If it is <0.5 U/µl, add RNase 1 to reach a minimum of 0.5 U/µl (see Table 1 for examples).
Lysate volume (µl) | RNA amount (µg) | Calculated amount of RNase (U) | Reaction volume (µl)a | Calculated [RNase] (U/µl) | Is [RNase] within 0.5-1 U/µl? | Adjust RNasea | Calculated [RNase] (U/µl) | Is [RNase] within 0.5-1 U/µl? |
---|---|---|---|---|---|---|---|---|
80 | 1.2 | 60 | 86 | 0.698 | Yes | — | — | — |
90 | 1.2 | 60 | 96 | 0.625 | Yes | — | — | — |
80 | 1 | 50 | 85 | 0.588 | Yes | — | — | — |
90 | 1 | 50 | 95 | 0.526 | Yes | — | — | — |
80 | 0.8 | 40 | 84 | 0.476 | No | +5 U | 0.533 | Yes |
90 | 0.8 | 40 | 94 | 0.426 | No | +10 U | 0.526 | Yes |
- a
Concentrations are calculated based on RNase I from Lucigen, which is provided at 10 U/μl. If another source provides the enzyme at a different concentration, the volume change must be taken into account.
5.Add the calculated volume of RNase 1 to the cell lysate, mix gently by pipetting, and seal the tube with Parafilm. Digest for 1.5 hr at room temperature (25°C) with gentle agitation (e.g., on a tube nutator).
6.Add 400 µl TRIzol to stop the digestion, vortex to mix thoroughly, and place the tube on ice.
Extract and precipitate RNA
7.Add 100 µl chloroform, vortex thoroughly, and centrifuge 15 min at 12,000 × g , 4°C.
8.Transfer the upper (aqueous) layer to a new microcentrifuge tube.
9.Precipitate RNA fragments by adding 20 µg GlycoBlue, 0.1 vol. of 3 M NaOAc, pH 5.5, and 1.5 vol. isopropanol.
10.Incubate overnight (at least 6 hr) at −20°C.
11.Pellet RNA by centrifuging 30 min at 15,000 × g , 4°C.
12.Remove and discard the supernatant, being careful not to disturb the RNA pellet.
13.Add 500 µl of 80% ethanol to the pellet and incubate on ice for 1 min.
14.Centrifuge 5 min at 15,000 × g , 4°C.
15.Remove all liquid from the tube and allow the RNA pellet to air-dry for 8 min.
16.Place the RNA pellet on ice and proceed directly to library preparation (see Basic Protocol 3).
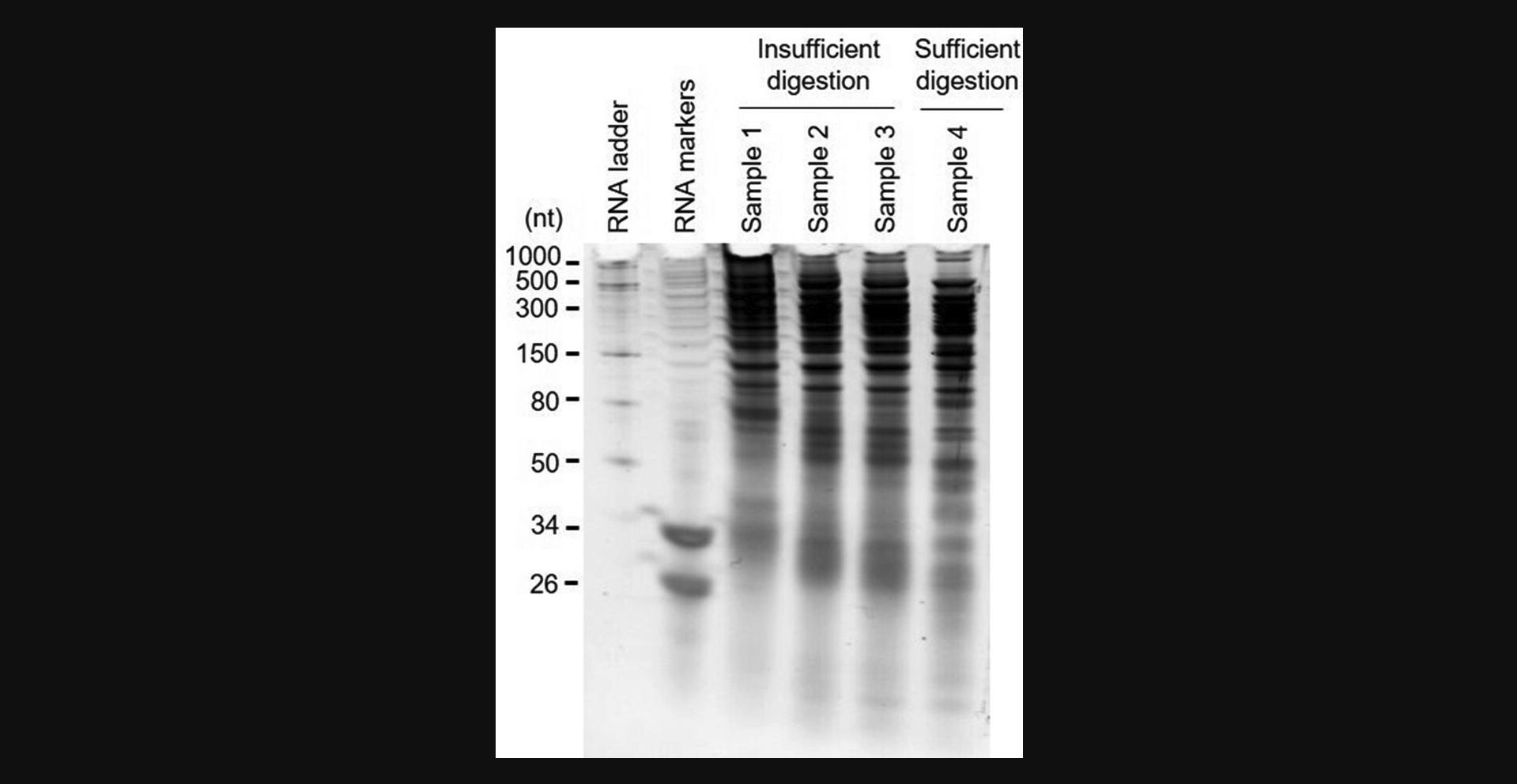
Alternate Protocol 3: RNase TREATMENT AND FOOTPRINT PURIFICATION FOR ULTRA-LOW-INPUT SAMPLES
The protocol describes how to determine the condition of RNase digestion and recover footprints after the digestion for ultra-low-input samples. We use a different equation to calculate the RNase amount and extend the RNase treatment time. If 20 ng to 200 ng total RNA can be obtained from your sample (e.g., 1000 cultured K562 cells or 100,000 PBMCs), follow the procedures described in this section.
For materials, see Basic Protocol 2.
1.Determine the RNA concentration and total amount of RNA in the cell lysate as described (see Basic Protocol 2, steps 1-2).
2.Calculate the required amount of RNase using the equation:
- RNase 1 (U) = 1 U/µl × volume cell lysate (µl)
3.Add the calculated amount of RNase 1 to the lysate and mix gently by pipetting.
4.Seal the microcentrifuge tube with Parafilm and digest for 2 hr at room temperature (25°C) with gentle agitation.
5.Proceed with the rest of the protocol as described (see Basic Protocol 2, steps 6-16), but increase the amount of GlycoBlue in step 9 from 20 μg to 25 μg.
Basic Protocol 3: LIBRARY PREPARATION FOR HIGH-THROUGHPUT SEQUENCING
In this protocol, we describe how to generate a DNA library for sequencing using the total RNA fragments isolated from Basic Protocol 2.We outline the procedures for end-healing of RNase footprints, polyA-tailing of end-repaired RNase footprints, SMART-RT, removal of RNA fragments, and PCR1 and PCR2. Size selection is conducted on dsDNA after PCR amplification but not on RNA fragments. Most of the experimental conditions are identical for low- and ultra-low-input samples; any differences are noted in the appropriate steps.
Additional Materials (also see Basic Protocol 2)
- RNase footprints (see Basic Protocol 2 or Alternate Protocol 3)
- T4 polynucleotide kinase (PNK) and 10× reaction buffer (New England Biolabs, cat. nos. M0201L and B0201S)
- SUPERase⋅In RNase inhibitor (Invitrogen, cat. no. AM2694)
- Nuclease-free water
- 5× SuperScript II first-strand buffer (Invitrogen, cat. no. 18064014)
- E. coli polyA polymerase (New England Biolabs, cat. no. M0276L)
- Adenosine-5′-triphosphate (ATP, New England Biolabs, cat. no. B0756A)
- 10 µM SMART-RT primer (synthesized by IDT; see Table 2 for sequence)
- SuperScript II reverse transcriptase (Invitrogen, cat. no. 18064014)
- 10 mM dNTP mix (Invitrogen, cat. no. 18427-013)
- 100 mM DTT (Invitrogen, cat. no. 18064014)
- 5 mM betaine (Sigma, cat. no. B0300)
- 1 M MgCl2 (RNase free, Invitrogen, cat. no. AM9530G)
- 100 µM SMARTer oligo (synthesized by QIAGEN; see Table 2 for sequence)
- 5000 U/ml RNase H (New England Biolabs, cat. no. M0297L)
- 2× Ultra II Q5 Master Mix (New England Biolabs, cat. no. M0544L)
- PCR1 and PCR2 forward and reverse primers (synthesized by IDT; see Table 2 for sequences)
- DNA Clean & Concentrator-5 (Zymo Research, cat. no. D4014)
- 6× Gel Loading Dye (New England Biolabs, cat. no. B7024S)
- 4% UltraPure agarose gel (precast, see recipe)
- 174- and 194-bp dsDNA markers (see Support Protocol)
- 100-bp DNA ladder (New England Biolabs, cat. no. N3231L)
- 10× TBE (molecular biology grade, Promega, cat. no. V4251)
- Zymoclean gel DNA recovery kit (Zymo Research, cat. no. D4008)
- Qubit 1× dsDNA high sensitivity (HS) kit (Invitrogen, cat. no. Q33231)
Primer | Sequence |
---|---|
SMART-RT primer | 5′-ACGTGTGCTCTTCCGATCTNNNNNNNNNNNNNNNTTTTTTTTTTTTTTTVN-3′ |
SMARTer oligo | 5′-CTCTTTCCCTACACGACGCTCTTCCGATCTNNNNrGrG+G-3′ |
PCR1 forward primer | 5′-GATCTACACTCTTTCCCTACACGACGC-3′ |
PCR1 reverse primer | 5′-GTGACTGGAGTTCAGACGTGTGCTCTTCCGATCT-3′ |
PCR2 forward primer | 5′-AATGATACGGCGACCACCGAGATCTACACTCTTTCCCTACAC-3′ |
PCR2 reverse primer | 5′-CAAGCAGAAGACGGCATACGAGATNNNNNNGTGACTGGAGTTCAGACGTGTG-3′ |
-
0.2-ml PCR tubes, DNA/DNase/RNase free
-
Thermal cycler (e.g., Applied Biosystems, cat. no. 4375786)
-
Blue-light transilluminator (e.g., Safe Imager 2.0, Invitrogen, cat. no. G6600)
-
Agilent High Sensitivity D1000 ScreenTape (run on the Agilent 2200 TapeStation)
-
Additional reagents and equipment for agarose gel electrophoresis
End-heal RNase footprints
1.Prepare the T4 PNK reaction mixture on ice as follows and mix well by pipetting:
- 0.5 µl 10× PNK buffer
- 1 µl T4 PNK
- 0.5 µl SUPERase⋅In RNase inhibitor
- 3 µl nuclease-free water
2.Suspend RNase footprints in 5 µl T4 PNK reaction mixture.
3.Run in a thermal cycler with the following program:
- 37°C for 45 min
- 50°C for 90 s
- 37°C for 15 min
- 65°C for 5 min
- Hold at 4°C
Perform polyA tailing
4.Prepare the polyadenylation mix as follows and keep on ice:
- 2 µl SuperScript II first-strand buffer
- 2 µl E. coli polyA polymerase
- 0.25 µl 10 mM ATP
- 0.5 µl SUPERase⋅In RNase inhibitor
- 0.25 µl nuclease-free water
5.Add 5 µl polyadenylation mix to end-repaired RNase footprints and mix well.
6.Incubate in a thermal cycler at 37°C for 2-3 hr for low-input samples or 4 hr for ultra-low input samples.
Perform SMART-RT
7.Add 2.5 µl of 10 µM SMART-RT primer to the polyA-tailed samples and mix well by pipetting.
8.Anneal primer by running the following program in a thermal cycler:
- 72°C for 5 min
- Hold at 4°C
9.Prepare the SMART-RT reaction mix as follows and keep on ice:
- 4 µl SuperScript II first-strand buffer
- 2 µl SuperScript II reverse transcriptase
- 0.5 µl SUPERase⋅In RNase Inhibitor
- 2.5 µl 10 mM dNTP mix
- 1.5 µl 100 mM DTT
- 6 µl 5 mM betaine
- 0.12 µl 1 M MgCl2
- 0.5 µl 100 µM SMARTer oligo
- 0.5 µl nuclease-free water
10.Add the SMART-RT mix (17.12 μl) to the reaction and mix well.
11.Run the following RT program in a thermal cycler:
- 1 cycle:
- 1 hr at 42°C
- 10 cycles:
- 2 min at 50°C
- 2 min at 42°C
- 1 cycle:
- 15 min at 70°C
- Hold at 4°C
Remove RNA fragments
12.Add 1 µl of 5000 U/ml RNase H and incubate in a thermal cycler as follows:
- 15 min at 37°C
- 15 min at 65°C (to inactivate RNase H)
Amplify cDNA by SMART-RT (PCR1)
13.Prepare the PCR1 mix and mix well:
- 50 µl 2× Ultra II Q5 Master Mix
- 5 µl 10 µM PCR1 forward primer
- 5 µl 10 µM PCR1 reverse primer
- 30 µl cDNA (from step 12)
- 10 µl nuclease-free water
14.Run the PCR1 program in a thermal cycler:
- 1 cycle:
- 3 min at 98°C
- 1 cycle:
- 20 s at 98°C
- 30 s at 65°C
- 90 s at 72°C
- 6-8 cycles:
- 20 s at 98°C
- 20 s at 67°C
- 60 s at 72°C
- 1 cycle:
- 3 min at 72°C
- Hold at 4°C
15.Purify the PCR1 product using a DNA Clean & Concentrator-5 column, eluting the product in 20 µl nuclease-free water.
Amplify PCR1 product with extended primers (PCR2)
16.Prepare the following PCR2 mix and mix well:
- 25 µl 2× Ultra II Q5 Master Mix
- 2.5 µl 10 µM PCR2 forward primer
- 2.5 µl 10 µM PCR2 reverse primer
- 20 µl purified PCR1 eluent
17.Run the PCR2 program in a thermal cycler:
- 1 cycle:
- 3 min at 98°C
- 4-6 cycles:
- 20 s at 98°C
- 20 s at 67°C
- 30 s at 72°C
- 1 cycle:
- 3 min at 72°C
- Hold at 4°C
18.Purify the PCR2 product using a DNA Clean & Concentrator-5 column, eluting in 20 µl nuclease-free water.
Perform size selection
19.Add 4 µl of 6× Gel Loading Dye to each sample and load on a precast 4% agarose gel.
20.Prepare dsDNA markers for one lane by mixing:
- 100 ng 174-bp library marker (equivalent to a 15-bp insert)
- 100 ng 194-bp library marker (equivalent to a 35-bp insert)
- 6× loading buffer
- Nuclease-free water to bring loading buffer concentration to 1×
21.Load dsDNA library markers and 10 µl of 100-bp DNA ladder in separate lanes of the gel.
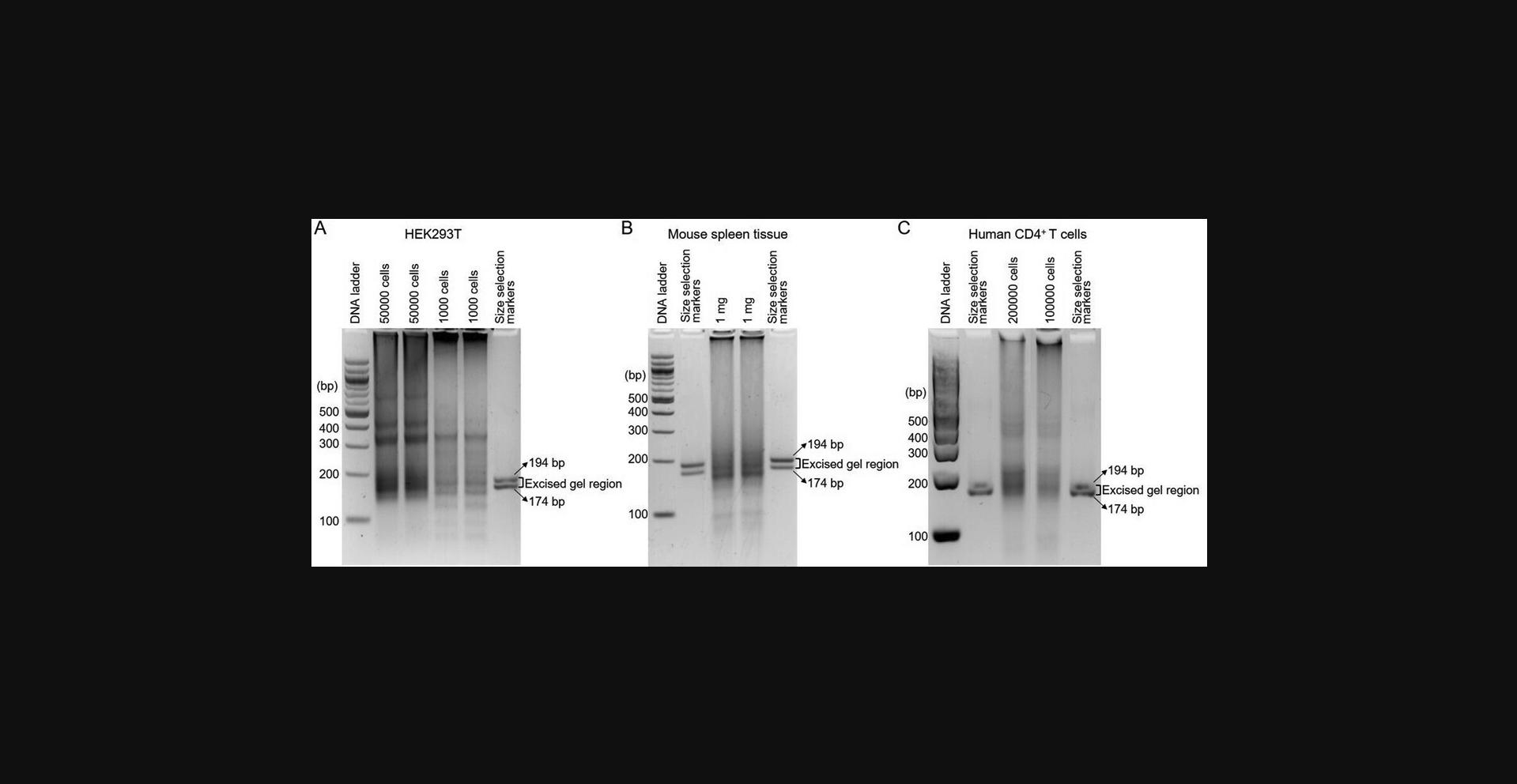
22.Run gel in 1× TBE buffer for 60 min at 150 V.
23.Visualize the gel on a blue-light transilluminator and excise the DNA product with 15-35 bp insert.
24.Use a DNA gel recovery kit to recover the size-selected DNA library.
25.Quantify dsDNA in the library using the Qubit dsDNA high-sensitivity kit.
Sequence dsDNA library
26.Check the DNA size distribution of the Rfoot-seq library with a high-sensitivity D1000 ScreenTape assay according to manufacturer's instructions.
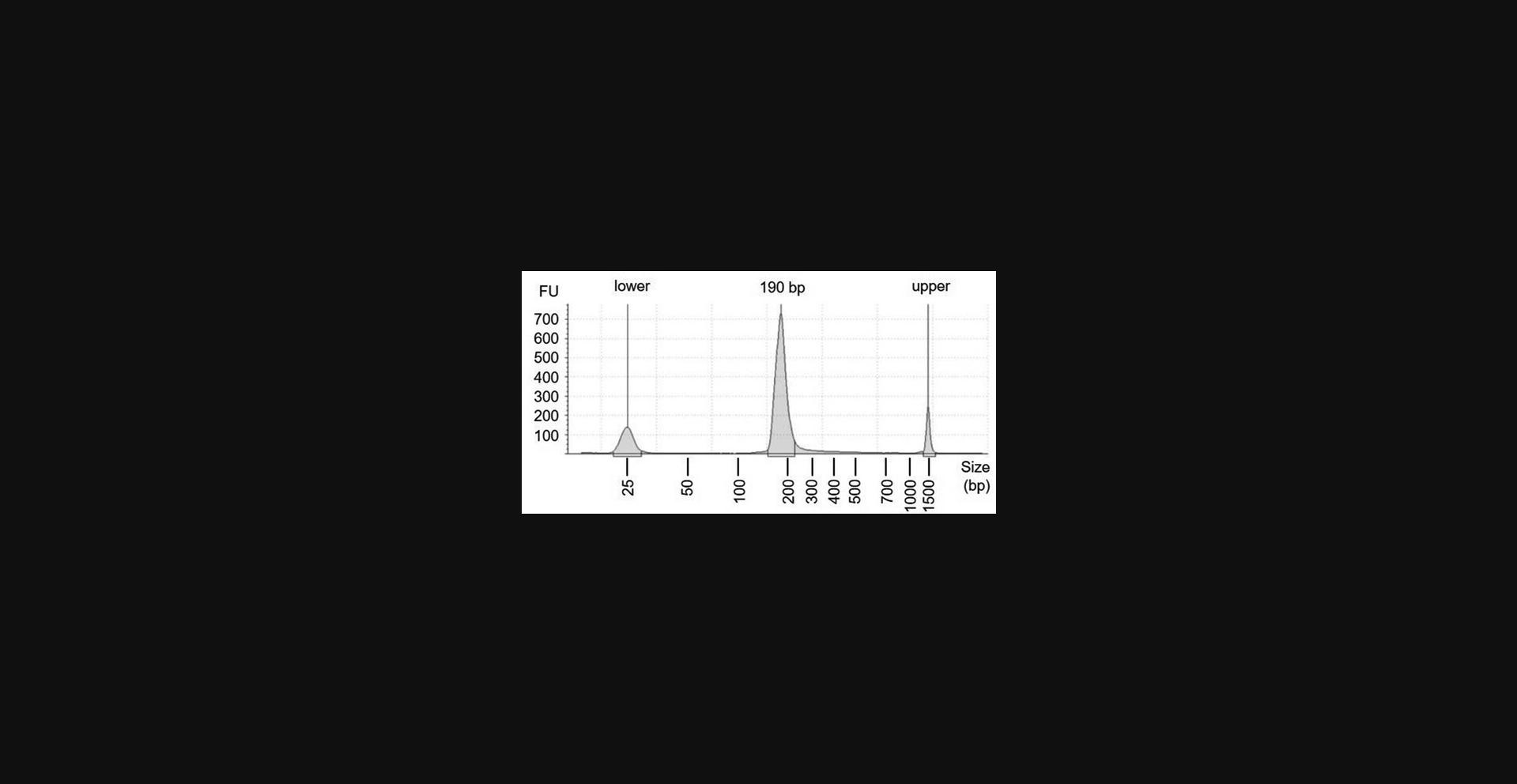
27.Sequence Rfoot-seq libraries with an Illumina sequencer (HiSeq or similar) to obtain at least 50 million reads per sequencing library.
Support Protocol: PREPARATION OF dsDNA MARKERS FOR LIBRARY SIZE SELECTION
Two dsDNA fragments (174 and 194 bp) are used as markers to guide library size selection. Both markers are generated by PCR amplification from the same synthesized 174-nt ssDNA template using separate sets of primers.
Materials
- ssDNA template and forward and reverse primers (see Table 3)
- 2× Ultra II Q5 Master Mix (New England Biolabs, cat. no. M0544L)
- DNA Clean & Concentrator-5 (Zymo Research, cat. no. D4014)
- Qubit 1× dsDNA high sensitivity (HS) kit (Invitrogen, cat. no. Q33231)
- Thermal cycler (e.g., Applied Biosystems, cat. no. 4375786)
Oligonucleotide | Sequence |
---|---|
ssDNA library marker template | 5′-AATGATACGGCGACCACCGAGATCTACACTCTTTCCCTACACGACGCTCTTCCGATCTATCGGGGATCTGCACTAGCCATTTCAACCAAAGTGTTTTCATTCAAATACTGAGATCGGAAGAGCACACGTCTGAACTCCAGTCACATCGCTATCTCGTATGCCGTCTTCTGCTTG-3′ |
Forward primer for 194-bp marker | 5′-AGCAGGGTAGAATGATACGGCGACCACCGA-3′ |
Reverse primer for 194-bp marker | 5′-AGTTGGTGCTCAAGCAGAAGACGGCATACG-3′ |
Forward primer for 174-bp marker | 5′-AATGATACGGCGACCACCGA-3′ |
Reverse primer for 174-bp marker | 5′-CAAGCAGAAGACGGCATACG-3′ |
1.Prepare 10 µM stock solutions of all oligos listed in Table 3.
2.Prepare two reactions, one using the appropriate primers for the 174-bp marker and the other using the primers for the 194-bp marker (see Table 3):
- 50 µl 2× Ultra II Q5 Master Mix
- 5 µl 10 µM forward primer
- 5 µl 10 µM reverse primer
- 0.1 µl 10 µM marker ssDNA template
- 40 µl nuclease-free water
3.Amplify in a thermal cycler with the following program:
- 1 cycle:
- 3 min at 98°C
- 20 cycles:
- 20 s at 98°C
- 20 s at 67°C
- 20 s at 72°C
- 1 cycle:
- 3 min at 72°C
- Hold at 4°C
4.Clean amplified DNA fragments using DNA Clean & Concentrator-5 columns.
5.Quantify DNA concentration using the Qubit dsDNA High Sensitivity (HS) kit.
6.Store dsDNA library markers indefinitely at −20°C.
Basic Protocol 4: DATA ANALYSIS AND QUALITY CONTROL AFTER SEQUENCING
This protocol describes the computational data analysis of the Rfoot-seq data. When Rfoot-seq is applied to a new cell type of interest, it is generally necessary to optimize the RNase treatment and library preparation procedures. Here, we introduce the library data quality analysis steps and describe the procedures to calculate ribosome occupancy in protein-coding regions. As this assay also maps RNA fragments from stable non-ribosomal complexes in noncoding regions (e.g., sncRNAs and some lncRNAs), we describe the analysis needed to distinguish ribosomal from non-ribosome footprints based on read distribution features.
As an example, we use Rfoot-seq data derived from HeLa cells, a human epithelial cell line derived from cervical cancer. The example command lines are available on GitHub (https://github.com/zhejilab/RNaseFootprinting). This procedure can be adapted to analyze data from other cell types or model species.
NOTE : Where appropriate, we have specified the use of eight threads to speed up alignment and coverage track creation steps. This number should be adjusted based on the computational resources available.
Materials
- Python/Anaconda
- R
- Bowtie 2 (Langmead & Salzberg, 2012)
- TopHat (Kim et al., 2013)
- deepTools (Ramírez et al., 2016)
- SAMtools (Danecek et al., 2021)
- HTSeq (Anders et al., 2015)
- SRA Toolkit (https://github.com/ncbi/sra-tools)
- Cutadapt (https://github.com/marcelm/cutadapt)
- RibORF 2.0 (https://github.com/zhejilab/RibORF)
- Optional : Rfoot (https://github.com/zhejilab/Rfoot)
Read mapping
1.Download reference files from the UCSC Genome Browser and GENCODE (Frankish et al., 2019) databases. The “refFlat.txt.gz” file is processed into genePred format to meet the RibORF input requirements. All reference files are moved to the “annotation” directory for organizational purposes.
-
-
mkdir ./annotation
-
gunzip GRCh38.primary_assembly.genome.fa.gz
-
mv GRCh38.primary_assembly.genome.fa ./annotation/genome.GRCh38.fa
-
wget "https://ftp.ebi.ac.uk/pub/databases/gencode/Gencode_human/release_42/gencode.v42.annotation.gtf.gz"
-
gunzip gencode.v42.annotation.gtf.gz
-
mv gencode.v42.annotation.gtf ./annotation/gencode.v42.gtf
-
wget "https://hgdownload.cse.ucsc.edu/goldenpath/hg38/database/refFlat.txt.gz"
-
gunzip refFlat.txt.gz
-
cut refFlat.txt -f2-11 > ./annotation/refseq.genePred
-
rm refFlat.txt
-
2.Make alignment indices for Bowtie 2 and TopHat.
-
-
mkdir ./annotation/rRNA
-
bowtie2-build -f ./annotation/rRNA.fa ./annotation/rRNA/rRNA
-
mv ./annotation/rRNA.fa ./annotation/rRNA/rRNA.fa
-
mkdir ./annotation/genome
-
bowtie2-build -f ./annotation/genome.GRCh38.fa ./annotation/genome/genome
-
mv ./annotation/genome.GRCh38.fa ./annotation/genome/genome.fa
-
mkdir ./annotation/transcriptome
-
tophat -G ./annotation/gencode.v42.gtf --transcriptome-index=./annotation/transcriptome/known ./annotation/genome/genome
-
mv ./annotation/gencode.v42.gtf ./annotation/transcriptome/known.gtf
-
3.Optional : Download the FASTQ file of interest from NIH Gene Expression Omnibus (GEO) repository. The data for “SRR11950060” are analyzed here as an example.
-
- fasterq-dump --outdir ./data/SRR11950060
- gzip ./data/SRR11950060_1.fastq
- gzip ./data/SRR11950060_2.fastq
-
4.Trim read adapters for higher accuracy of downstream read alignment. As the RNase footprints are generally short, the R1 sequencing reads can cover the whole footprint and are used for downstream analyses. The first seven nucleotides are from the SMARTer oligo (rGrG+G + 4 random nucleotides) and are trimmed from the beginning of each read (“-u 7”). The polyA adapter is removed from the end of reads (“-a AAAAAAAA”), allowing for one mismatch within the adapter sequence (“-e 0.2”, where “-e” sets the maximum allowed error rate in the adapter sequence). Reads with a length between 18 and 35 nt are retained for downstream analysis to facilitate quick and accurate alignment (“-m 18 -M 35”, where “-m” and “-M” are the minimum and maximum read lengths).
-
- cutadapt -a AAAAAAAA -e 0.2 -u 7 -m 18 -M 35 \
- -o ./data/SRR11950060_1.trimmed.fastq.gz \
- ./data/SRR11950060_1.fastq.gz > ./logs/SRR11950060_cutadapt.log
-
5.Align trimmed reads to rRNA. Trimmed input reads are aligned to rRNA reference sequences. The reads unmapped to rRNA sequences are used for further analyses. About 80% of Rfoot-seq reads were mapped to rRNAs. These reads cannot be aligned to a reference transcriptome to study RNA translation.
-
- mkdir ./align_rRNA
- bowtie2 -p 8 \
- -x ./annotation/rRNA/rRNA \
- -U ./data/SRR11950060_1.trimmed.fastq.gz \
- --un ./align_rRNA/unmapped.fastq \
- -S ./align_rRNA/accepted_hits.sam 2> ./logs/SRR11950060_align_rRNA.log
-
6.Align rRNA-unmapped reads to the transcriptome and then genome. To prevent an error during alignment, the output directory needs to be specified with a trailing forward slash.
-
- mkdir ./align_transcriptome
- tophat -p 8 \
- --transcriptome-index=./annotation/transcriptome/known \
- -o ./align_transcriptome/ \
- ./annotation/genome/genome \
- ./align_rRNA/unmapped.fastq &> ./logs/SRR11950060_align_transcriptome.log
-
7.Extract uniquely mapped reads and quality check results. Coverage tracks (BigWig format) for the forward and reverse strands can be viewed in the Integrative Genomics Viewer (IGV).
-
- samtools view -H ./align_transcriptome/accepted_hits.bam > ./align_transcriptome/unique_hits.sam
- samtools view ./align_transcriptome/accepted_hits.bam | grep -w "NH:i:1" >> ./align_transcriptome/unique_hits.sam
- samtools view -S -b ./align_transcriptome/unique_hits.sam | samtools sort -o ./align_transcriptome/unique_hits.bam
- samtools index ./align_transcriptome/unique_hits.bam
- bamCoverage -p 8 -b ./align_transcriptome/unique_hits.bam -o ./align_transcriptome/unique_hits.str1.bw --filterRNAstrand reverse --normalizeUsing CPM --binSize 1
- bamCoverage -p 8 -b ./align_transcriptome/unique_hits.bam -o ./align_transcriptome/unique_hits.str2.bw --filterRNAstrand forward --normalizeUsing CPM --binSize 1
-
Data quality control using RibORF
RibORF takes the read alignment from the step above and examines the read fragment sizes and their distribution around the start and stop codons of mRNAs.
8.Examine the read fragment sizes and plot the distribution of read 5′ end locations surrounding the start and stop codons grouped by read length. High-quality data should show strong read enrichment in coding regions compared to UTRs. The coding region reads should be enriched for lengths of ∼21 and ∼29 nt with 3-nt periodicity (Fig. 5A,B). If this pattern is not observed, see Table 4 for troubleshooting of experimental conditions.
-
- mkdir -p ./riborf/readdist
- perl ./software/RibORF.2.0/readDist.pl \
- -f ./align_transcriptome/unique_hits.sam \
- -g ./annotation/gencode.v38.genePred \
- -o ./riborf/readdist \
- -d 18,19,20,21,22,23,24,25,26,27,28,29,30,31,32,33,34,35,
-
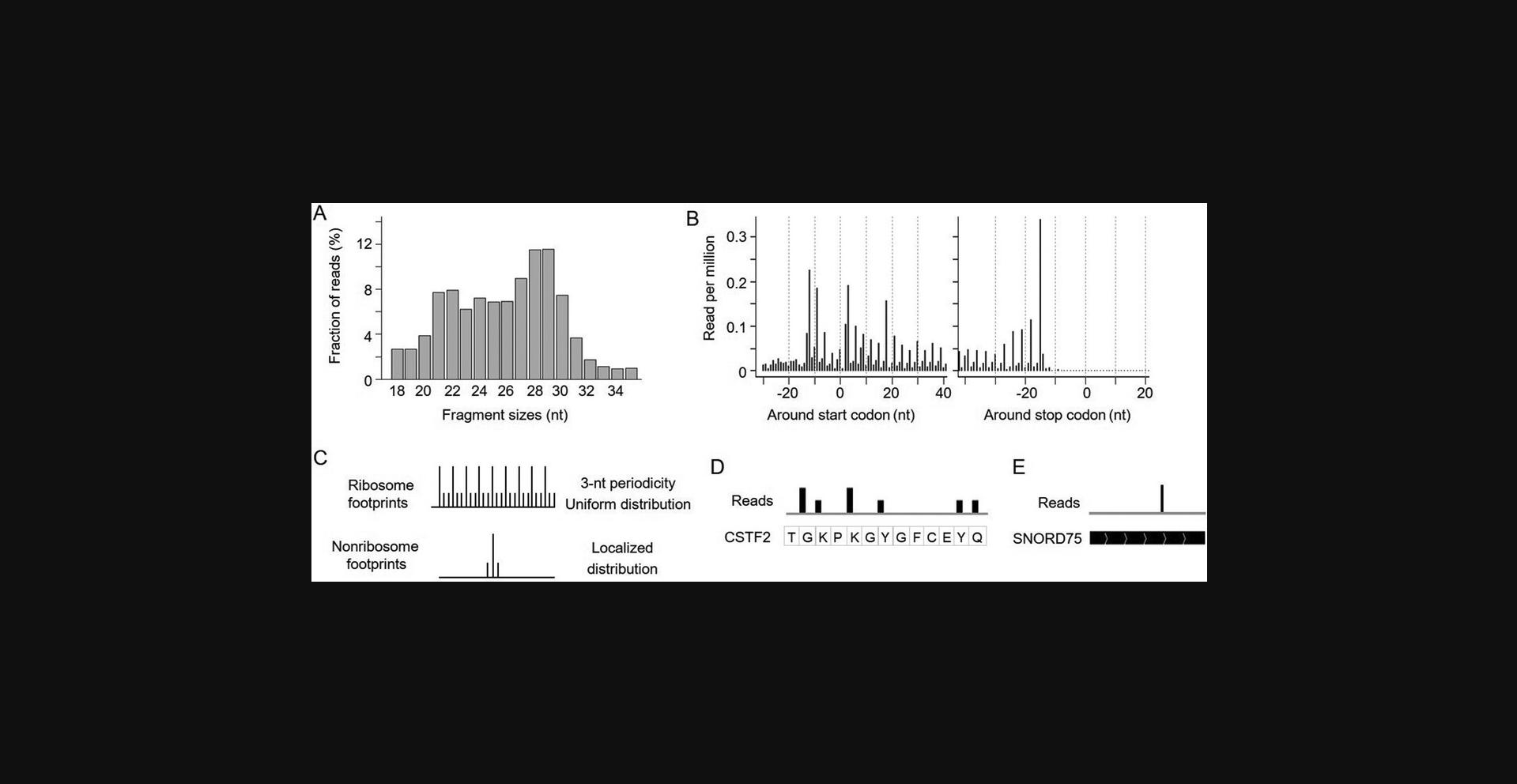
Problem | Possible Cause | Solution |
---|---|---|
Read size peak >29 nt | Insufficient RNase digestion | Follow Basic Protocol 2 or Alternate Protocol 3 to optimize digestion conditions |
Confirm performance of RNase 1, especially its unit definition | ||
Increase RNase 1 in the reaction so it is close to 1 U/µl | ||
Read size peak <28 nt | Overdigestion by RNase | Follow Basic Protocol 2 or Alternate Protocol 3 to optimize digestion conditions |
Decrease RNase 1 in the reaction so it is close to 0.5 U/µl | ||
Lack of 3 nt and read size peak is ∼29 nt | Insufficient RNase digestion or cell-specific ribosome conformation | Confirm performance of RNase1, especially its unit definition |
Increase RNase 1 in the reaction so it is close to 1 U/µl | ||
Try on another cell or tissue to see if it is cell/tissue-specific | ||
Low yield | RNA degradation | Clean bench and pipetters with RNase decontamination solution before library preparation |
Unoptimized library construction conditions | Increase incubation time for polyA tailing | |
Increase cycle numbers for PCR and PCR2 | ||
Reads show strong bias across coding regions covering few codons | Too much starting material | Use no more than 1200 ng starting material |
Unoptimized SMART-RT conditions | Follow Basic Protocol 3 to optimize SmartRT conditions | |
Low sequencing depth | Obtain more sequencing reads | |
Low mappable reads | Inaccurate dsDNA size selection | Use 4% agarose gel |
Run gel longer for better DNA separation |
Quantifying ribosome occupancy in protein-coding regions
9.Quantify ribosome occupancy in coding regions using HTSeq. We quantify only reads that overlap CDS regions according to the annotation file (“--type CDS”). Reads that overlap a portion of a gene but are not fully contained within that gene are also counted (“--mode union”). Reads that overlap multiple transcript isoforms are counted toward the gene expression (“--nonunique all”). The data is processed in a strand-specific manner (“--stranded yes”).
-
- htseq-count \
- --format="bam" \
- --stranded="yes" \
- --type="CDS" \
- --mode="union" \
- --nonunique="all" \
- ./align_transcriptome/unique_hits.bam \
- ./annotation/transcriptome/known.gff > ./align_transcriptome/unique_hits.counts.txt
-
Read distribution features distinguishing ribosome from non-ribosome footprints
10.Correct the location of reads based on their read length and the provided offset parameters, which will need to be manually curated before this step. This will assign the read mapping locations to ribosomal A-sites, correcting for the offset distance between the 5′ end of reads and ribosomal A-sites. These correction parameters are inferred from the RibORF readDist plots. Here, we only include fragment sizes showing >60% of reads assigned to the first nucleotides of codons, including 21, 27, 28, and 29 nt. Details are described in a previously published protocol (Ji, 2018b). Users can load corrected reads (BigWig files) to IGV. The translated ORFs show 3-nt periodicity and the non-ribosome footprints show a localized distribution without periodicity (Fig. 5C-E).
-
-
mkdir -p ./riborf/corrected
-
perl ./software/RibORF.2.0/offsetCorrect.pl \
-
-r ./align_transcriptome/unique_hits.sam \
-
-p ./riborf/offset.correction.parameters.txt \
-
-o ./riborf/corrected/corrected_hits.sam
-
perl ./software/RibORF.2.0/readDist.pl \
-
-f ./riborf/corrected/corrected_hits.sam \
-
-g ./annotation/refseq.genePred \
-
-o ./riborf/corrected \
-
-d 1
-
Rscript --vanilla ./software/RibORF.2.0/combine_plots.R ./riborf/corrected 1
-
samtools view -S -b ./riborf/corrected/corrected_hits.sam | samtools sort -o ./riborf/corrected/corrected_hits.bam
-
samtools index ./riborf/corrected/corrected_hits.bam
-
bamCoverage -p 8 -b ./riborf/corrected/corrected_hits.bam -o ./riborf/corrected/corrected_hits.str1.bw --filterRNAstrand reverse --normalizeUsing CPM --binSize 1
-
bamCoverage -p 8 -b ./riborf/corrected/corrected_hits.bam -o ./riborf/corrected/corrected_hits.str2.bw --filterRNAstrand forward --normalizeUsing CPM --binSize 1
-
11.Optional : Users can further apply RibORF to identify genome-wide translated ORFs and Rfoot to identify non-ribosome footprints using the Rfoot-seq data. The detailed analysis steps have been described in previous Current Protocols articles (Ji, 2018a,b).
REAGENTS AND SOLUTIONS
Agarose gel for library size selection
Dilute 100 ml of 10× TBE electrophoresis buffer to 1× in distilled water. Dissolve 2 g UltraPure agarose-1000 (Invitrogen, cat. no. 16550-100) completely in 50 ml of 1× TBE buffer. Add SYBR Safe dye (Invitrogen, cat. no. S33102) and mix well. Typically, the agarose gel is prepared immediately before use but it can be stored for a few days at 4°C.
Cell lysis buffer
- 10 ml polysome buffer (see recipe)
- 1% (v/v) Triton X-100 (molecular biology grade, Calbiochem, cat. no. 648466)
- 10 µl 1 M dithiothreitol (DTT, BioUltra, Sigma-Aldrich, cat. no. 43816)
- 10 µl 100 mg/ml cycloheximide (Sigma-Aldrich, cat. no. C4859)
Lysis buffer without DTT and cycloheximide can be stored up to 1 month at 4°C. Add DTT and cycloheximide immediately before use and then keep on ice.
Polysome buffer
- 1 ml 1 M Tris, pH 7.5 (Invitrogen, cat. no. 15567027)
- 1.5 ml 5 M NaCl (molecular biology grade, Invitrogen, cat. no. AM9760G)
- 250 µl 1 M MgCl2 (molecular biology grade, Invitrogen, cat. no. AM9530G)
- 47.25 ml RNase-free water (Invitrogen, cat. no. AM9930)
- Store up to 6 months at 4°C
COMMENTARY
Background Information
A rate-limiting step of conventional ribosome profiling is the experimental procedure to isolate translating ribosome complexes. The original protocol used a sucrose gradient to isolate 80S cytosolic ribosome complexes (Ingolia et al., 2009). To simplify the procedure, a sucrose cushion or size-exclusion chromatography was used to enrich protein complexes with higher molecular weights (McGlincy & Ingolia, 2017). These complex ribosome isolation methods are time-consuming and require a large amount of starting material. Here, we leveraged the stronger protection of RNA fragments by translating ribosomes (80S ribosomes and 55S mitoribosomes) compared to other RNA-binding proteins in coding regions (e.g., splicing factors). We optimized the digestion conditions using concentrated RNase and effectively retained ribosome-protected fragments while digesting other RNA fragments. We significantly simplified the procedure to select translating ribosome–protected footprints without ultracentrifugation or immunoprecipitation steps. By also preparing the sequencing library using A-tailing and SMARTer oligo–based template switching, Rfoot-seq can work robustly using as few as 1000 cultured cells, which is about 1000-fold less starting material than is required in other published protocols (Li et al., 2022).
The development of this assay was inspired by our previous analyses of conventional ribosome profiling data (Ji et al., 2015, 2016). We found that ultracentrifugation through a sucrose cushion is not specific for 80S ribosomes. By calculating the ultracentrifugation parameters, we found that protein complexes with much smaller molecular weights were also isolated during this step. Due to the nature of transcriptomic RNase footprinting, ribosome profiling reveals both ribosome and non-ribosome footprints, which can be computationally distinguished based on read distribution features (Ingolia et al., 2014; Ji et al., 2016). These findings motivated us to omit the ultracentrifugation step.
Compared with conventional ribosome profiling, Rfoot-seq is simple, rapid, and inexpensive. It requires no specialized equipment and the total hands-on working time is about 1.5 days. The assay can quantitatively measure different aspects of protein synthesis. The calculated gene-level ribosome occupancy, codon usage level, and ORF translation probability are well correlated with conventional ribosome profiling datasets (Li et al., 2022). The assay is widely applicable to rare cell types for both basic scientific research and clinical prognosis.
Despite its many advantages, there are several limitations to the Rfoot-seq assay. First, the template switch (SMART-RT) used for library preparation tends to amplify footprints with a 5′ G nucleotide (Li et al., 2022). This bias needs to be considered when interpreting ribosome occupancy in individual genomic locations, for example, when studying ribosome pausing. However, comparative analyses with ribosome profiling data show that this bias does not affect the quantification of overall ribosome occupancy across coding regions. Second, similar to conventional ribosome profiling, ∼80% of the reads were mapped to rRNAs and ∼1% of reads were aligned to non-ribosome footprints in sncRNAs and some lncRNAs. Future effort is needed to deplete these rRNA-mapping reads to increase the fraction that can be used to study RNA translation.
Critical Parameters
Cell lysis
To capture ribosome footprints that more accurately reflect translation activity in vivo , a cardinal rule is to harvest and lyse samples quickly. We routinely use lysis buffer with cycloheximide to stabilize translating ribosomes, but pretreatment of cultured cells with cycloheximide is optional and we have generated high-quality libraries with and without this pretreatment. Pretreatment with other translation inhibitors such as harringtonine or lactimidomycin is compatible with our assay if the user is interested in a specific translation step.
Although cell lysates can be snap-frozen in liquid nitrogen and stored at −80°C, we find that an uninterrupted RNase digestion yields better results, especially for cells with low translation activity, such as primary immune cells. If a stopping point is required on day 1, a safer stopping point is after TRIzol has been added to the RNase-digested lysate. At that point, the lysate can be stored for several days at −80°C.
RNase treatment
RNase treatment is the critical step of Rfoot-seq, as library quality is highly dependent on digestion. There are multiple commercially available RNase 1 enzymes, but their activity varies more than 10-fold due to different unit definitions. Our assay was developed using RNase 1 produced by Lucigen (LGC Biosearch Technologies), which defines one unit as that which degrades 100 ng E. coli ribosomal RNA per second into acid-soluble nucleotides at 37 °C.
We have described how to determine whether RNase digestion is sufficient using an agarose gel. Ultra-low-input samples may not have enough RNA material to do this, but a pilot experiment can be performed using 50,000 cultured cells (e.g., HEK293T cells) by following Basic Protocol 2. If your RNase 1 works well on low-input samples, high-quality libraries of ultra-low-input samples can be generated by following Alternate Protocol 3.
RNase footprint recovery
After RNase digestion, RNA fragments are extracted using TRIzol and chloroform and then precipitated using isopropanol. Although some column-based RNA preparation kits can be used to recover and purify RNA fragments, isopropanol-based precipitation does a better job of retaining small RNase footprints and gives a better yield, especially for ultra-low-input samples.
Library preparation
We have standardized most of the experimental conditions of library preparation to work for both low-input and ultra-low-input samples. Some experimental conditions were further optimized for ultra-low input samples, including extending the polyA tailing time to 4 hr and increasing the PCR1 and PCR2 cycle numbers.
Size selection
Accurate size selection will save more desired reads that can be mapped during computational analysis. We have described a gel-based size selection here, but a method with higher sensitivity could also be used.
Troubleshooting
See Table 4 for common problems encountered when performing these protocols and suggested solutions.
Understanding Results
We typically obtain 0.8-1.2 µg total RNA from the lysate of 50,000 cultured mammalian cells or 1 mg snap-frozen mammalian tissues, and 20-200 ng total RNA from the lysate of 1000 cultured mammalian cells or 100,000 primary immune cells. The agarose gel quality check of RNase digestion in Basic Protocol 2 is an optional step that new users can perform using one additional replicate to get a preliminary idea of how RNase 1 works for their input sample. Sample electrophoresis results of RNA fragments with insufficient and sufficient RNase digestion are shown in Figure 2.
Evaluation of the library using Qubit after PCR1 and PCR2 amplification is the opportunity to determine if an Rfoot-seq assay was likely successful. If the library preparation worked, more than 1 µg dsDNA product could be achieved after PCR1 and a higher yield should be detected after PCR2. Agarose gel electrophoresis results of DNA libraries generated using 1000 and 50,000 cultured HEK293T cells, 1 mg snap-frozen mouse spleen tissue, and 100,000 primary human CD4+ T cells are shown in Figure 3. Two dsDNA markers are used to guide the DNA size selection (Fig. 3). Tapestation High Sensitivity D1000 analysis results of a multiplexed Rfoot-seq library are shown in Figure 4. The size distribution should be from 174 to 205 bp, peaking at ∼190 bp.
After the quality control computational analyses, we can observe fragment size peaks at ∼29 and ∼21 nt (minor in some samples) for high-quality libraries. The reads are highly enriched in coding regions and are depleted in 3′ UTRs. The fragments with peak sizes show 3-nt periodicity across translated ORFs and can be used to identify noncanonical translated ORFs. For some datasets, the 3-nt periodicity of the read distribution may not be very clear. If the reads show the right size distribution and are highly enriched in coding regions, they can be used to quantify ribosome occupancy but are less useful to identify noncanonical ORFs.
Rfoot-seq maps footprints associated with stable protein complexes across annotated noncoding RNAs. For sncRNAs, the footprints represent non-ribosomal RNA-protein complexes. For lncRNAs, there can be a mixture of ribosomal and non-ribosome footprints. Emerging studies have shown that some annotated lncRNAs encode functional microproteins (Ji, 2018b). Characterizing non-ribosome footprints can also lead to novel insights into the RNA domain function (Ji, 2018a).
Time Considerations
Rfoot-seq library preparation was designed to take one and a half days. On day 1, the workflow involves sample lysis (10-30 min), RNase digestion (1.5-2 hr), RNA extraction (20 min), and precipitation (overnight). On day 2, it involves RNase footprint suspension (40 min), DNA library preparation (6.5-8.5 hr), and size selection and gel DNA recovery (1-1.5 hr)
On day 1, a break can be taken after RNase digestion has been stopped by addition of TRIzol. TRIzol-stabilized samples can be stored for several days at −80°C. On day 2, there are several points when a break can be taken safely: after reverse transcription, after PCR1, and after PCR2. cDNA and dsDNA can be stored long term at −20°C or short term at 4°C.
Library quality control by bioanalysis and library sequencing will take 3-4 days. Data analysis will take 1-2 days depending on computational resources available.
Acknowledgments
This work was supported by grants to Z.J. from the National Institutes of Health (R35GM138192, R01HL161389, and R00CA207865) and the Lynn Sage Scholar Fund from the Lynn Sage Breast Cancer Research Foundation.
Author Contributions
Qianru Li : Conceptualization, methodology, visualization, writing (original draft, review and editing); Emily K. Stroup : Formal analysis, software, writing (original draft, review and editing); Zhe Ji : Conceptualization, data curation, formal analysis, funding acquisition, investigation, methodology, project administration, resources, software, supervision, validation, visualization, writing (original draft, review and editing).
Conflict of Interest
Northwestern University has filed patent applications related to the work described here. The title of the patent application is “Methods for mapping personalized translatome”. The U.S. Provisional Application was filed on December 4, 2020 (application number 63/121,680).
Open Research
Data Availability Statement
Data supporting the findings of this study are openly available in the Gene Expression Omnibus (GEO) at https://www.ncbi.nlm.nih.gov/geo/query/acc.cgi?acc=GSE151989 (reference number GSE151989).
Literature Cited
- Anders, S., Pyl, P. T., & Huber, W. (2015). HTSeq—a Python framework to work with high-throughput sequencing data. Bioinformatics , 31(2), 166–169. https://doi.org/10.1093/bioinformatics/btu638
- Archer, S. K., Shirokikh, N. E., Beilharz, T. H., & Preiss, T. (2016). Dynamics of ribosome scanning and recycling revealed by translation complex profiling. Nature , 535(7613), 570–574. https://doi.org/10.1038/nature18647
- Brar, G. A., Yassour, M., Friedman, N., Regev, A., Ingolia, N. T., & Weissman, J. S. (2012). High-resolution view of the yeast meiotic program revealed by ribosome profiling. Science , 335(6068), 552–557. https://doi.org/10.1126/science.1215110
- Calviello, L., Mukherjee, N., Wyler, E., Zauber, H., Hirsekorn, A., Selbach, M., Landthaler, M., Obermayer, B., & Ohler, U. (2016). Detecting actively translated open reading frames in ribosome profiling data. Nature Methods , 13(2), 165–170. https://doi.org/10.1038/nmeth.3688
- Chen, J., Brunner, A.-D., Cogan, J. Z., Nuñez, J. K., Fields, A. P., Adamson, B., Itzhak, D. N., Li, J. Y., Mann, M., Leonetti, M. D., & Weissman, J. S. (2020). Pervasive functional translation of noncanonical human open reading frames. Science , 367(6482), 1140–1146. https://doi.org/10.1126/science.aay0262
- Clamer, M., Tebaldi, T., Lauria, F., Bernabò, P., Gómez-Biagi, R. F., Marchioretto, M., Kandala, D. T., Minati, L., Perenthaler, E., Gubert, D., Pasquardini, L., Guella, G., Groen, E. J. N., Gillingwater, T. H., Quattrone, A., & Viero, G. (2018). Active ribosome profiling with RiboLace. Cell Reports , 25(4), 1097–1108.e1095. https://doi.org/10.1016/j.celrep.2018.09.084
- Couvillion, M. T., Soto, I. C., Shipkovenska, G., & Churchman, L. S. (2016). Synchronized mitochondrial and cytosolic translation programs. Nature , 533(7604), 499–503. https://doi.org/10.1038/nature18015
- Danecek, P., Bonfield, J. K., Liddle, J., Marshall, J., Ohan, V., Pollard, M. O., Whitwham, A., Keane, T., Mccarthy, S. A., Davies, R. M., & Li, H. (2021). Twelve years of SAMtools and BCFtools. Gigascience , 10(2), giab008. https://doi.org/10.1093/gigascience/giab008
- Darnell, R. B. (2010). HITS-CLIP: Panoramic views of protein-RNA regulation in living cells. Wiley Interdisciplinary Reviews: RNA , 1(2), 266–286. https://doi.org/10.1002/wrna.31
- Frankish, A., Diekhans, M., Ferreira, A.-M., Johnson, R., Jungreis, I., Loveland, J., Mudge, J. M., Sisu, C., Wright, J., Armstrong, J., Barnes, I., Berry, A., Bignell, A., Carbonell Sala, S., Chrast, J., Cunningham, F., Di Domenico, T., Donaldson, S., Fiddes, I. T., … Flicek, P. (2019). GENCODE reference annotation for the human and mouse genomes. Nucleic Acids Research , 47(D1), D766–D773. https://doi.org/10.1093/nar/gky955
- Gerashchenko, M. V., & Gladyshev, V. N. (2017). Ribonuclease selection for ribosome profiling. Nucleic Acids Research , 45(2), e6. https://doi.org/10.1093/nar/gkw822
- Guo, H., Ingolia, N. T., Weissman, J. S., & Bartel, D. P. (2010). Mammalian microRNAs predominantly act to decrease target mRNA levels. Nature , 466(7308), 835–840. https://doi.org/10.1038/nature09267
- Ingolia, N. T. (2014). Ribosome profiling: New views of translation, from single codons to genome scale. Nature Reviews Genetics , 15(3), 205–213. https://doi.org/10.1038/nrg3645
- Ingolia, N. T., Brar, G. A., Stern-Ginossar, N., Harris, M. S., Talhouarne, G. J. S., Jackson, S. E., Wills, M. R., & Weissman, J. S. (2014). Ribosome profiling reveals pervasive translation outside of annotated protein-coding genes. Cell Reports , 8(5), 1365–1379. https://doi.org/10.1016/j.celrep.2014.07.045
- Ingolia, N. T., Ghaemmaghami, S., Newman, J. R., & Weissman, J. S. (2009). Genome-wide analysis in vivo of translation with nucleotide resolution using ribosome profiling. Science , 324(5924), 218–223. https://doi.org/10.1126/science.1168978
- Ingolia, N. T., Lareau, L. F., & Weissman, J. S. (2011). Ribosome profiling of mouse embryonic stem cells reveals the complexity and dynamics of mammalian proteomes. Cell , 147(4), 789–802. https://doi.org/10.1016/j.cell.2011.10.002
- Ji, Z. (2018a). Rfoot: Transcriptome-scale identification of RNA-protein complexes from ribosome profiling data. Current Protocols in Molecular Biology , 124(1), e66. https://doi.org/10.1002/cpmb.66
- Ji, Z. (2018b). RibORF: Identifying genome-wide translated open reading frames using ribosome profiling. Current Protocols in Molecular Biology , 124(1), e67. https://doi.org/10.1002/cpmb.67
- Ji, Z., Song, R., Huang, H., Regev, A., & Struhl, K. (2016). Transcriptome-scale RNase-footprinting of RNA-protein complexes. Nature Biotechnology , 34(4), 410–413. https://doi.org/10.1038/nbt.3441
- Ji, Z., Song, R., Regev, A., & Struhl, K. (2015). Many lncRNAs, 5′UTRs, and pseudogenes are translated and some are likely to express functional proteins. eLife , 4, e08890. https://doi.org/10.7554/eLife.08890
- Khajuria, R. K., Munschauer, M., Ulirsch, J. C., Fiorini, C., Ludwig, L. S., Mcfarland, S. K., Abdulhay, N. J., Specht, H., Keshishian, H., Mani, D. R., Jovanovic, M., Ellis, S. R., Fulco, C. P., Engreitz, J. M., Schütz, S., Lian, J., Gripp, K. W., Weinberg, O. K., Pinkus, G. S., … Sankaran, V. G. (2018). Ribosome levels selectively regulate translation and lineage commitment in human hematopoiesis. Cell , 173(1), 90–103.e119. https://doi.org/10.1016/j.cell.2018.02.036
- Kim, D., Pertea, G., Trapnell, C., Pimentel, H., Kelley, R., & Salzberg, S. L. (2013). TopHat2: Accurate alignment of transcriptomes in the presence of insertions, deletions and gene fusions. Genome Biology , 14(4), R36. https://doi.org/10.1186/gb-2013-14-4-r36
- Langmead, B., & Salzberg, S. L. (2012). Fast gapped-read alignment with Bowtie 2. Nature Methods , 9(4), 357–359. https://doi.org/10.1038/nmeth.1923
- Lee, S., Liu, B., Lee, S., Huang, S. X., Shen, B., & Qian, S. B. (2012). Global mapping of translation initiation sites in mammalian cells at single-nucleotide resolution. Proceedings of the National Academy of Sciences of the United States of America , 109(37), E2424–2432. https://doi.org/10.1073/pnas.1207846109
- Li, Q., Yang, H., Stroup, E. K., Wang, H., & Ji, Z. (2022). Low-input RNase footprinting for simultaneous quantification of cytosolic and mitochondrial translation. Genome Research , 32(3), 545–557. https://doi.org/10.1101/gr.276139.121
- Liu, B., Han, Y., & Qian, S. B. (2013). Cotranslational response to proteotoxic stress by elongation pausing of ribosomes. Molecular Cell , 49(3), 453–463. https://doi.org/10.1016/j.molcel.2012.12.001
- McGlincy, N. J., & Ingolia, N. T. (2017). Transcriptome-wide measurement of translation by ribosome profiling. Methods , 126, 112–129. https://doi.org/10.1016/j.ymeth.2017.05.028
- Morscher, R. J., Ducker, G. S., Li, S. H., Mayer, J. A., Gitai, Z., Sperl, W., & Rabinowitz, J. D. (2018). Mitochondrial translation requires folate-dependent tRNA methylation. Nature , 554(7690), 128–132. https://doi.org/10.1038/nature25460
- Radhakrishnan, A., Chen, Y. H., Martin, S., Alhusaini, N., Green, R., & Coller, J. (2016). The DEAD-box protein Dhh1p couples mRNA decay and translation by monitoring codon optimality. Cell , 167(1), 122–132.e129. https://doi.org/10.1016/j.cell.2016.08.053
- Ramírez, F., Ryan, D. P., Grüning, B., Bhardwaj, V., Kilpert, F., Richter, A. S., Heyne, S., Dündar, F., & Manke, T. (2016). deepTools2: A next generation web server for deep-sequencing data analysis. Nucleic Acids Research , 44(W1), W160–165. https://doi.org/10.1093/nar/gkw257
- Rooijers, K., Loayza-Puch, F., Nijtmans, L. G., & Agami, R. (2013). Ribosome profiling reveals features of normal and disease-associated mitochondrial translation. Nature Communications , 4, 2886. https://doi.org/10.1038/ncomms3886
- Van Heesch, S., Witte, F., Schneider-Lunitz, V., Schulz, J. F., Adami, E., Faber, A. B., Kirchner, M., Maatz, H., Blachut, S., Sandmann, C.-L., Kanda, M., Worth, C. L., Schafer, S., Calviello, L., Merriott, R., Patone, G., Hummel, O., Wyler, E., Obermayer, B., … Hubner, N. (2019). The translational landscape of the human heart. Cell , 178(1), 242–260.e229. https://doi.org/10.1016/j.cell.2019.05.010
- Van Nostrand, E. L., Nguyen, T. B., Gelboin-Burkhart, C., Wang, R., Blue, S. M., Pratt, G. A., Louie, A. L., & Yeo, G. W. (2017). Robust, cost-effective profiling of RNA binding protein targets with single-end enhanced crosslinking and immunoprecipitation (seCLIP). Methods in Molecular Biology , 1648, 177–200. https://doi.org/10.1007/978-1-4939-7204-3_14
- Wu, C. C., Zinshteyn, B., Wehner, K. A., & Green, R. (2019). High-resolution ribosome profiling defines discrete ribosome elongation states and translational regulation during cellular stress. Molecular Cell , 73(5), 959–970.e955. https://doi.org/10.1016/j.molcel.2018.12.009
Key Reference
- Li et al. (2022). See above.
Internet Resource
Site for example computational analysis command lines on GitHub.