Rapid Synthesis of Nucleoside Triphosphates and Analogues
Alexander Ripp, Alexander Ripp, Jyoti Singh, Jyoti Singh, Henning J. Jessen, Henning J. Jessen
cyclotriphosphate
non-hydrolyzable nucleotide analogues
nucleoside 5′-triphosphate
phosphoramidite
phosphorylation
Abstract
Nucleoside triphosphates (NTPs) are essential biomolecules involved in almost all biological processes, and their study is therefore critical to understanding cellular biology. Here, we describe a chemical synthesis suitable for obtaining both natural and highly modified NTPs, which can, for example, be used as surrogates to probe biological processes. The approach includes the preparation of a reagent that enables the facile introduction and modification of three phosphate units: cyclic pyrophosphoryl P-amidite (c-PyPA), derived from pyrophosphate (PV) and a reactive phosphoramidite (PIII). By using non-hydrolyzable analogues of pyrophosphate, the reagent can be readily modified to obtain a family of non-hydrolyzable analogues containing CH2, CF2, CCl2, and NH that are stable in solution for several weeks if stored appropriately. They enable the synthesis of NTPs by reaction with nucleosides to give deoxycyclotriphosphate esters that are then oxidized to cyclotriphosphate (cyclo-TP) esters. The use of different oxidizing agents provides an opportunity for modification at P-α. Furthermore, terminal modifications at P-γ can be introduced by linearization of the cyclo-TP ester with various nucleophiles. © 2020 The Authors.
Basic Protocol 1 : Synthesis of cyclic pyrophosphoryl P-amidite (c-PyPA) and derivatives (c-PyNHPA, c-PyCH2PA, c-PyCCl2PA, c-PyCF2PA)
Basic Protocol 2 : Synthesis of 3′-azidothymidine 5′-γ-P - propargylamido triphosphates and analogues
Basic Protocol 3 : Synthesis of 2′-deoxythymidine 5′-γ-P-propargylamido triphosphate (15)
Basic Protocol 4 : Synthesis of adenosine 5′-γ-P-amido triphosphate (19) and adenosine 5′-γ-P-propargylamido triphosphate (20)
Basic Protocol 5 : Synthesis of d4T 5′-γ-propargylamido β,γ-(difluoromethylene)triphosphate
Support Protocol : Synthesis of diisopropylphosphoramidous dichloride
INTRODUCTION
(Deoxy)nucleoside triphosphates are building blocks for essential biomacromolecules (DNA and RNA), and furthermore have fundamental importance in signaling processes and metabolic pathways (Hollenstein, 2012; Roy, Depaix, Périgaud, & Peyrottes, 2016). Chemical syntheses of natural and unnatural/modified NTPs provide many significant opportunities for the study of their versatile functions (Dutta, Captain, & Jessen, 2017; Ermert, Marx, & Hacker, 2017; Hocek, 2014). Yet, despite impressive progress over the years (Liao, Bala, Ngor, Yik, & Chaput, 2019; Shepard & Cummins, 2019; Shepard, Windsor, Raines, & Cummins, 2019; Singh, Ripp et al., 2019), novel, easy-to-implement synthetic methods are still very much needed (Liao et al., 2019). In this context, we recently reported a family of reagents, the so-called cyclic pyrophosphoryl P-amidites (c-PyPA) (Singh, Steck et al., 2019), that allow an easy and straightforward synthesis of α,β- and γ-modified NTPs in a one-flask operation (Singh, Ripp et al., 2019). Due to the selectivity of the initial phosphitylation reaction, nucleosides can be customized without protecting groups, although placing protecting groups on the nucleosides will increase yield and facilitate purification. Figure 1 illustrates the syntheses of NTPs by this approach, highlighting the possible modifications that can be introduced.
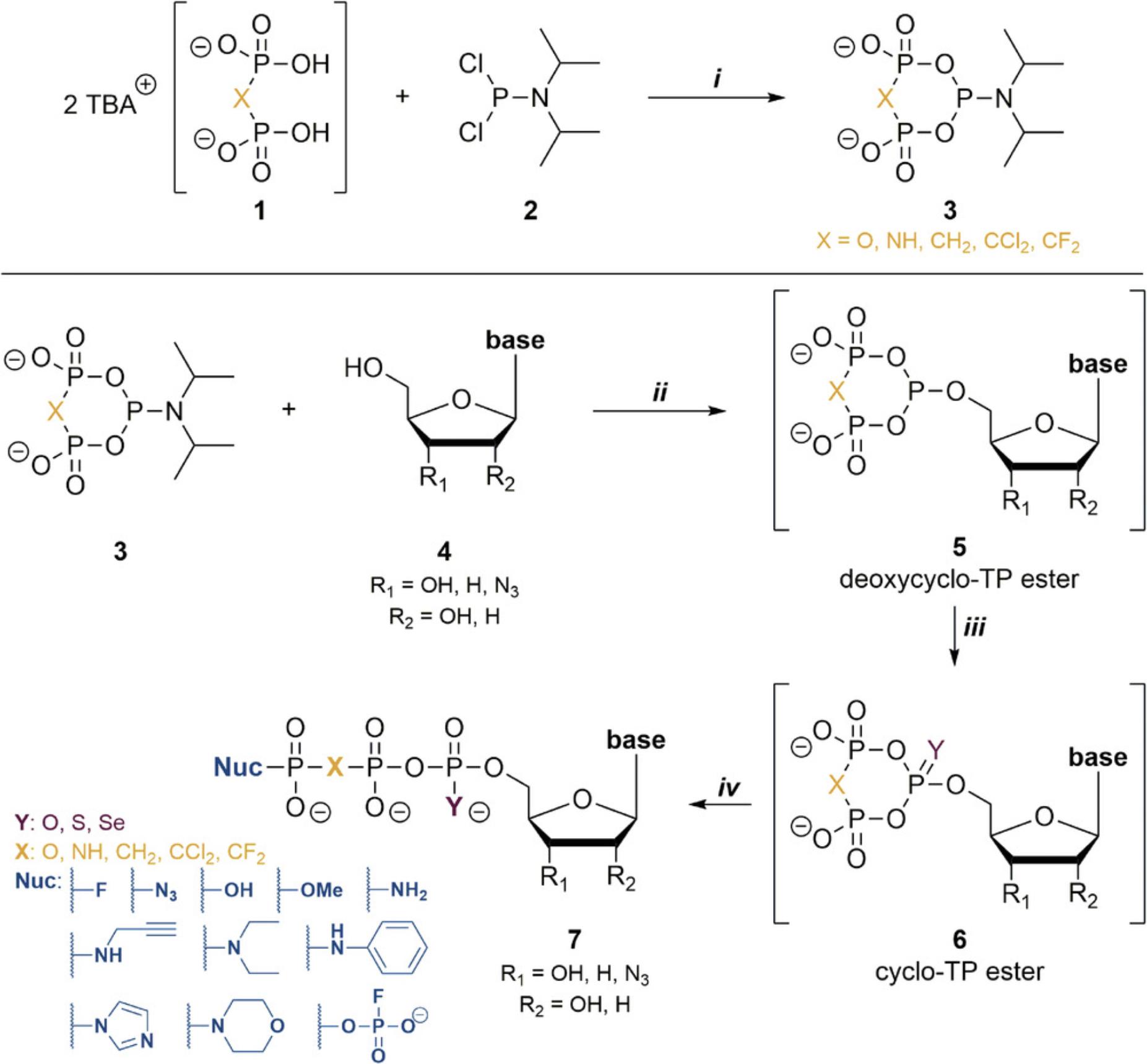
c-PyPA can be prepared before NTP synthesis and stored, thereby enabling the synthesis of several analogues in multiple reactions. The reactions of different pyrophosphate derivatives (such as pyrophosphate, imidodiphosphate, methylene diphosphonate, dichloromethylene diphosphonate, and difluoromethylenediphosphonate; (1)) with diisopropylphosphoramidous dichloride (2) allow access to a variety of c-PyPA-type reagents (3). The resulting c-PyPA and its analogues (c-PyNHPA, c-PyCH2PA, c-PyCCl2PA, c-PyCF2PA) are stable for at least 4 weeks under an argon atmosphere at –20°C, except for the imidodiphosphate analogue.
The c-PyPA reagents can be used directly in solution without purification for NTP synthesis and, in the presence of an acidic activator, will react preferentially with the sterically least hindered alcohol of the nucleoside. Thus, there is a pronounced preference for primary over secondary alcohols. Due to this selectivity, nucleosides can be used without protecting groups provided they have some solubility in polar organic solvents (such as DMF, DMSO, MeCN, or ionic liquids). After the coupling step, the deoxycyclo-TP ester (5) is oxidized and then linearized through a nucleophilic attack on the cyclo-TP intermediate (6), which generally results in linearization and places the incoming nucleophile in the γ position. This pathway was validated both experimentally and by computation (Singh, Ripp et al., 2019). Variations of the oxidizing reagent and the nucleophile for linearization provide diverse options for modification and can be mutually combined. For instance, using imidazole or morpholine as nucleophiles enables the synthesis of well-known activated NTPs (γ-morpholidates, γ-imidazolides; Wanat et al., 2015; Warminski, Sikorski, Kowalska, & Jemielity, 2017), which can be used to generate long oligophosphate chains. As another example, the use of propargylamine as nucleophile yields precursors for azide-alkyne cycloadditions ("click chemistry"; Azevedo et al., 2018; Rostovtsev, Green, Fokin, & Sharpless, 2002; Serdjukow, Kink, Steigenberger, Tomás-Gamasa, & Carell, 2014; Wanat et al., 2015).
This protocol is designed to guide users in applying c-PyPA reagents and benefiting from their versatility. We describe potential pitfalls and how to avoid them, so that high yields and easy purifications can be achieved in every laboratory that wants to include these novel reactions in their NTP synthesis portfolio.
Basic Protocol 1 presents a systematic procedure for the synthesis of c-PyPA deriving from pyrophosphate. This protocol can also be applied to the rest of this reagent family (c-PyNHPA, c-PyCH2PA, c-PyCCl2PA, c-PyCF2PA) by using the corresponding pyrophosphate analogues. However, for the synthesis of c-PyNHPA, c-PyCH2PA, c-PyCCl2PA, and c-PyCF2PA, some minor deviations, as compared to the constitutive c-PyPA protocol detailed here, need to be considered. These deviations are indicated directly after the respective steps. In Basic Protocol 2, the use of c-PyPA for the synthesis of 3′-azidothymidine (AZT) triphosphate (AZTTP) and its modification on P-α using different oxidizing agents is discussed. Basic Protocol 3 provides further insights into the triphosphorylation of unprotected 2′-deoxynucleosides, with discussion of selectivity issues and separation of regioisomers. The triphosphorylation of adenosine as a prototype ribonucleoside is highlighted in Basic Protocol 4. Finally, in Basic Protocol 5 we discuss the application of c-PyPA analogues to generate non-hydrolyzable NTPs with modifications between the β-γ phosphates. Finally, a Support Protocol describes the synthesis of diisopropylphosphoramidous dichloride, a necessary reagent for the preparation of cyclic pyrophosphoryl P-amidite (c-PyPA) and its analogues in Basic Protocol 1.
IMPORTANT NOTE : Several steps of the following procedures require anhydrous conditions. These steps should be carried out under a dry argon atmosphere in oven-dried glassware and anhydrous solvents. The anhydrous solvents can be purchased from commercial suppliers unless otherwise noted.
IMPORTANT NOTE : To guarantee a strictly dry, inert atmosphere during reactions, use the Schlenk technique. In addition, dry the argon gas by passing it through a column containing phosphorous pentoxide–coated boiling stones. Transfer the liquid chemical reagents by using a syringe and needles under a flow of dry argon. An argon-filled balloon can provide a dry argon atmosphere in a septum-sealed flask.
IMPORTANT NOTE : Both syringes and needles should be purged with argon three times before filling with chemicals.
IMPORTANT NOTE : Be careful not to contaminate chemical bottles while transferring liquid reagents. Be aware that chemical containers are not under pressure; therefore, slight positive pressure is required.
Basic Protocol 1: SYNTHESIS OF CYCLIC PYROPHOSPHORYL P-AMIDITE (c-PyPA) AND DERIVATIVES (c-PyNHPA, c-PyCH2PA, c-PyCCl2PA, c-PyCF2PA)
This protocol describes the synthesis of cyclic pyrophosphoryl P-amidite (c-PyPA) and its analogues (c-PyNHPA, c-PyCH2PA, c-PyCCl2PA, and c-PyCF2PA) (3a-e) deriving from the reaction of diisopropylphosphoramidous dichloride (2) with the corresponding pyrophosphate analogues (1 ; Fig. 2). Pyrophosphate and the other analogues, which are commercially available as sodium salts (8), must first be converted into the corresponding tetrabutylammonium (TBA) salts. The resulting bis-TBA pyrophosphate, imidodiphosphate, methylene diphosphonate, dichloromethylene diphosphonate, and difluoromethylenediphosphonate, now soluble in polar organic solvents, then react with 2 in the presence of a tertiary amine base (trimethylamine or diisopropylethylamine) under strictly anhydrous conditions. After quantitative conversion into the desired reagents (3a-e), monitored by 31P-NMR (diagnostic triplet at 130-125 ppm for PIII atom), the reaction mixture can be directly used for the triphosphorylation of nucleosides.
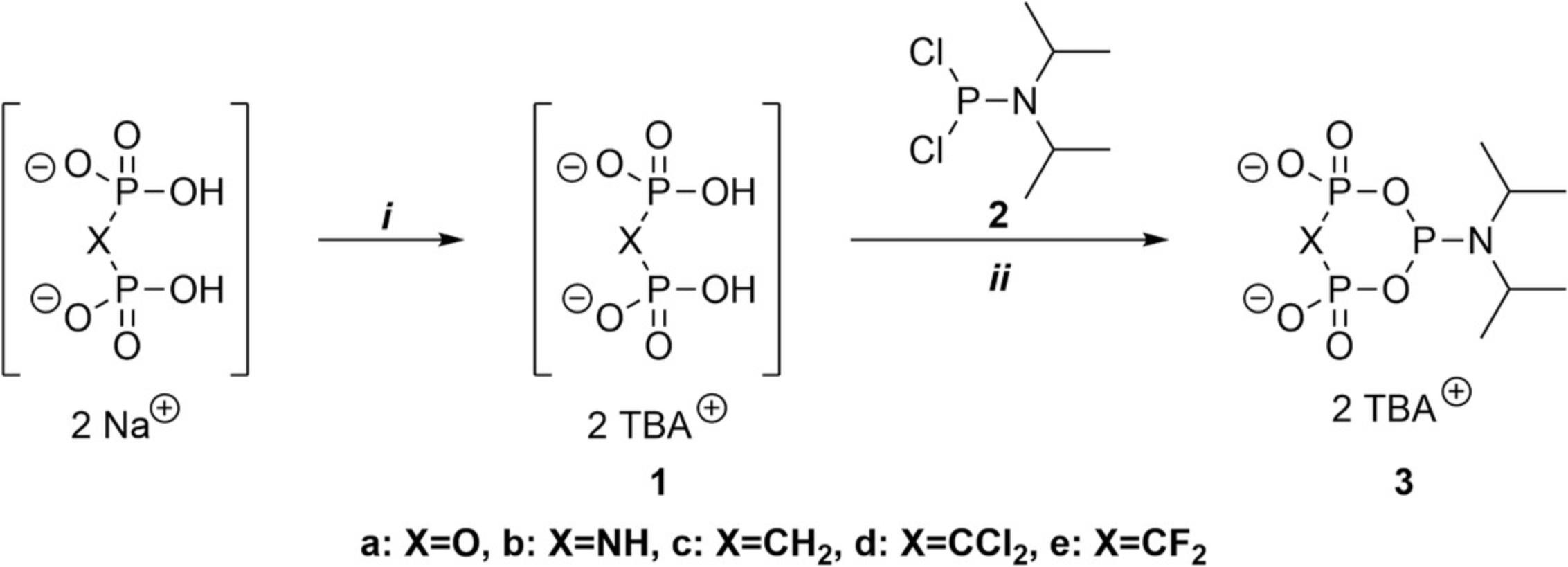
The synthesis of c-PyPA (3a), c-PyCH2PA (3c), c-PyCCl2PA (3d), and c-PyCF2PA (3e) starting from pyrophosphate or phosphonate analogues is readily implemented, whereas c-PyNHPA (3b) is much more difficult to handle and proved to be unstable in the reaction mixture. This is further complicated by the fact that the commercially available salt is impure. Therefore, 3b must be used directly for the coupling reactions, as it cannot be stored, and yields are typically lower. Because c-PyPA (3a), c-PyCH2PA (3c), c-PyCCl2PA (3d), and c-PyCF2PA (3e) are stable over extended periods of time if stored appropriately (argon, anhydrous conditions), the preparation of more significant amounts of these compounds is recommended, as this facilitates the correct weighing of reagents.
The reagents (3a-d) can be prepared less concentrated (0.075 M) in anhydrous MeCN or more concentrated (0.5 M) in anhydrous DMF, depending on the solubility of the nucleoside substrate.
Materials
-
Starting reagent, i.e., one of the following:
- Sodium dihydrogen diphosphate, +99% (ChemPUR, cat. no. 007023-500)
- Imidodiphosphate sodium salt, >97% (Sigma-Aldrich, cat. no. I0631)
- Methylene pyrophosphate acid (Acros)
- Dichloromethylene diphosphonic acid disodium salt (Sigma-Aldrich)
- Difluoromethylene bisphosphonic acid (prepared according to Crans et al., 2007)
-
Deionized water
-
Tetrabutylammonium hydroxide 30-hydrate (TBAOH; Acros) or ∼40% (∼1.5 M) TBAOH in water (Sigma-Aldrich, cat. no. 86854)
-
Acetone, P.A. grade
-
Tetramethyl phosphonium bromide (Acros or Sigma-Aldrich)
-
Anhydrous acetonitrile (MeCN; Acros cat. no. 10353732)
-
Anhydrous triethylamine (TEA; Sigma-Aldrich, cat. no. 387649) or anhydrous diisopropylethylamine (DIPEA; Sigma-Aldrich, cat. no. 471283)
-
Diisopropylphosphoramidous dichloride ((i Pr)2N-PCl2; see Support Protocol)
-
Chloroform-D (CDCl3; Acros)
-
10-ml beaker
-
Dowex 50WX8 (H+) column (Sigma-Aldrich; for reuse, see step 2)
-
100- and 500-ml round-bottom flasks
-
Universal pH paper
-
Dry ice
-
Rotary evaporator
-
Lyophilizer (Alpha 1-4 LD plus Freeze Dryer, Christ)
-
Dry argon gas
-
3-Å molecular sieves (Carl Roth, cat. no. 8487.2; see recipe)
-
Rubber septum
-
Magnetic stirring bars (triangular or oval-shaped) and stirring plate
-
Schlenk line
-
Vacuum source (membrane pump: ∼1 mbar; oil pump for Schlenk line: ∼1.0 × 10–3 mbar)
Synthesis of tetrabutylammonium pyrophosphate salt
IMPORTANT NOTE : Confirm the quality of the pyrophosphate and derivates by 31P-NMR before use, as high quality of the material is essential for reliable results (>99%). Pure sodium dihydrogen diphosphate resonates in D2O as a singlet at –10 ppm. Monophosphate, a common impurity, generates a singlet at 0 ppm in D2O.
The procedure is optimized for pyrophosphate and its diphosphonate analogues. Because imidodiphosphate sodium salt is already unstable during cation exchange on the Dowex column and also later in the reaction mixture, the procedure will yield less reliable results for this reagent.
1.Weigh 3.0 g sodium dihydrogen diphosphate (13.5 mmol, 1.0 eq.) into a 10-ml beaker and dissolve in 20 ml deionized water.
2.Apply the solution to a protonated Dowex 50WX8 (H+) column to exchange the sodium cations with protons.
3.Elute the target compound with ∼300-350 ml deionized water, collecting it as one fraction in a 500-ml flask. Monitor the elution of the pyrophosphoric acid by checking the pH value using universal indicator paper.
4.The elution of the target compound into the 500-ml flask will be complete as soon as the pH value of the eluate is neutral again.
5.Add 21.6 g TBAOH trihydrate (27.0 mmol, 2.0 eq.) in one portion to the flask.
6.Attach the flask to a rotary evaporator and replace the heating bath with a dewar filled with acetone and dry ice (−78°C). Rotate slowly to freeze the water inside the flask.
7.Remove water by lyophilization on a freeze dryer overnight.
8.Calculate the exact ratio of TBA cation in the pyrophosphate salt. To do this, perform a 1H- and 31P-NMR measurements of the product (∼9 mg) with ∼6 mg tetramethylphosphonium bromide (PMe4Br; 35 µmol) as an internal standard in ∼0.75 ml D2O.
9.Dissolve the solidified product in 30 ml anhydrous MeCN and transfer the liquid into a precisely weighed 100-ml single-neck round-bottom flask. Wash the 500-ml flask with 20 ml anhydrous MeCN to complete the transfer into the 100 ml flask.
10.Evaporate the solvent under reduced pressure by using a rotary evaporator.
11.Repeat step 10 twice with 10 ml anhydrous MeCN and dry the obtained solid (solidified oil) further under high vacuum overnight.
12.Weigh out the flask and calculate the exact yield (in millimoles) of the respective TBA pyrophosphate salt in consideration of the already determined number of equivalents of TBA cation.
13.Store the TBA pyrophosphate salt under an argon atmosphere.
Synthesis of cyclic pyrophosphoryl P-amidite
14.Place 2 g activated 3-Å molecular sieves and a stirring bar into a 100-ml flask containing 2.09 g tetrabutylammonium pyrophosphate salt (TBA PPi, 3.02 mmol, 1.0 eq.).
15.Seal the flask with a rubber septum.
16.Exchange the atmosphere three times with dry argon, and dissolve TBA PPi in 40 ml anhydrous MeCN (13.3 ml per mmol TBA PPi).
17.Let the solution stand for at least 4 h to enable efficient removal of residual water.
18.Cool the reaction mixture to −10°C and add 1.50 ml anhydrous TEA (10.5 mmol, 3.5 eq.) to the flask.
19.Add 520 μl (i Pr)2N-PCl2 (3.02 mmol, 1.0 eq.) dropwise within 1 min into the reaction mixture and stir 5-10 min.
20.Monitor progress of the reaction by 31P-NMR: Mix 250 µl of the reaction mixture and 250 µl CDCl3 in an NMR tube under argon atmosphere. Completion of the reaction is revealed by the disappearance of a singlet at +170 ppm (resonance of (i Pr)2N-PCl2) and the appearance of a triplet at +130 ppm (resonance of PIII of the c-PyPA).
21.Seal the reaction flask with parafilm and store it at −20°C.
22.Use the clear solution obtained (approximate molarity 0.075 M) directly for the coupling reactions without further manipulations (calculate the molarity of the solution by dividing the initial amount of TBA PPi by the added amount of anhydrous MeCN, assuming quantitative conversion.)
23.Using an aliquot of the reaction mixture, characterize the product, 3a-e , by 31P-NMR and HR-MS.
Basic Protocol 2: SYNTHESIS OF 3′-AZIDOTHYMIDINE 5′-γ-P-PROPARGYLAMIDO TRIPHOSPHATES AND ANALOGUES
In the following, the synthesis of 3′-azidothymidine (AZT) 5′-γ-P - propargylamido triphosphate and P-α analogues is described in detail (Fig. 3). Under dry conditions and in the presence of an acidic activator, c-PyPA reacts with AZT (8), forming the deoxycyclo-TP ester (9). Three options are described for the subsequent oxidization of the ester: Oxidation of 9 with m CPBA transfers an oxygen atom, while oxidation with Beaucage's reagent or KSeCN transfer S or Se, respectively. The obtained cyclo-TP esters (10a-c) are then linearized by a nucleophile. By using propargylamine as the nucleophile, 3′-azidothymidine 5′-γ-P-propargylamido triphosphate and P-α derivatives (S or Se) (11a-c) are obtained. In most cases, facile isolation by precipitation as sodium salts is sufficient to obtain the product in high purity.
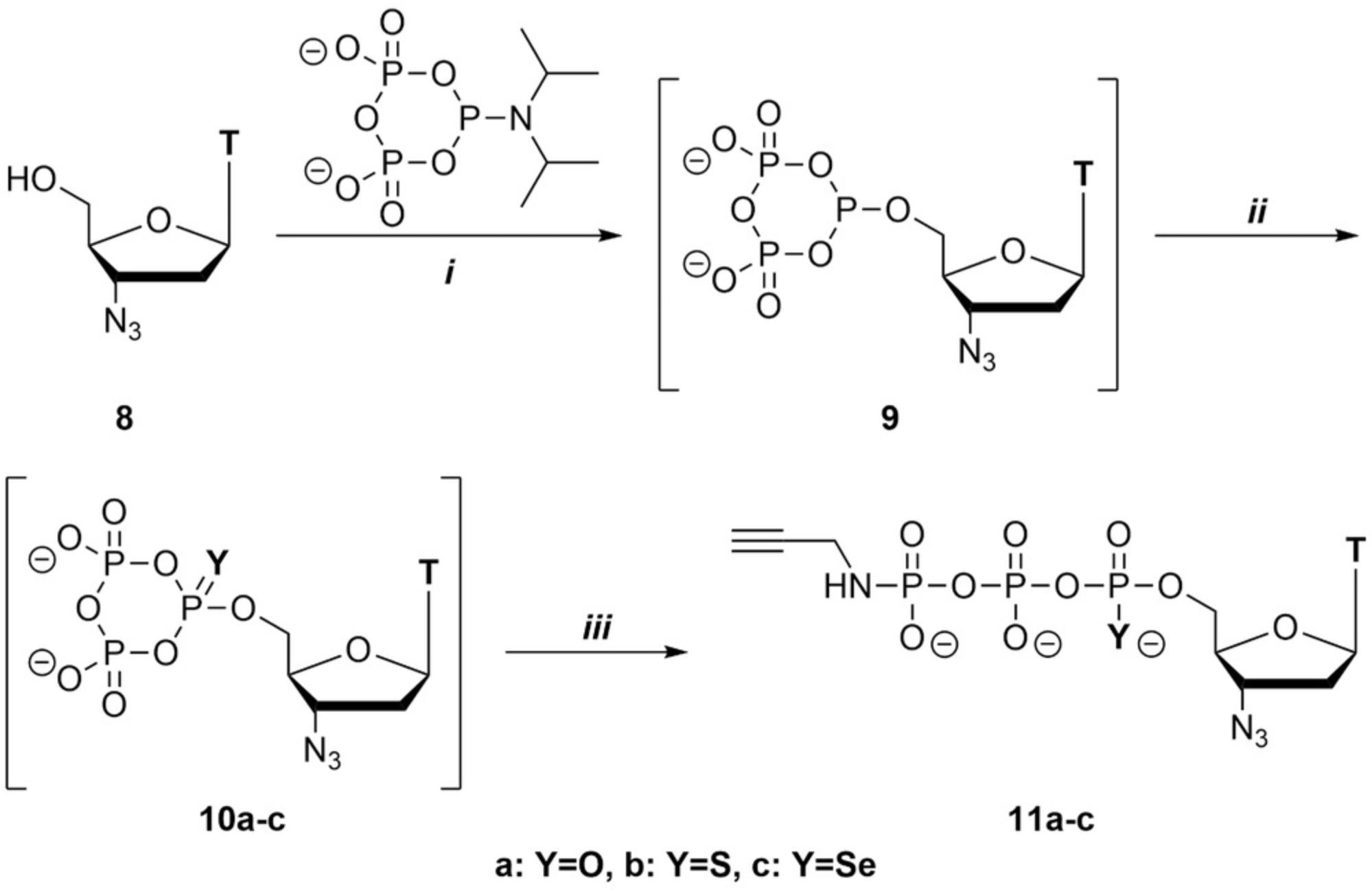
Materials
-
3′-Azidothymidine (AZT)
-
5-(Ethylthio)-1 H -tetrazole (ETT; ChemPUR)
-
Anhydrous acetonitrile (MeCN; Acros, cat. no. 10353732)
-
0.075 M cyclic pyrophosphoryl-P-amidite (c-PyPA) in in MeCN (Basic Protocol 1), −20°C
-
≤77% meta- chloroperbenzoic acid (m CPBA; Sigma-Aldrich)
-
Propargylamine (Acros)
-
0.5 M NaClO4 in acetone (see recipe)
-
3 H -1,2-Benzodithiol-3-one 1,1-dioxide (Beaucage's reagent; Acros)
-
Potassium selenocyanate (KSeCN; Acros)
-
Deuterium oxide (D2O; Acros)
-
Chloroform-D (CDCl3; Acros)
-
10-, 25-, 50-, and 100-ml round-bottom flasks and pear-shaped flask, oven dried
-
Magnetic stirring bars (triangular shape) and stirring plate
-
Rubber septa
-
Heat gun
-
Water bath, 50-60°C
-
Cooling baths: mixtures of ice and sodium chloride (NaCl, technical grade) in correct proportion to set the desired temperatures of −4°C and −20°C
-
Schlenk line
-
Vacuum source (membrane pump: ∼1 mbar; oil pump for Schlenk line: ∼1.0 × 10–3 mbar)
-
Dry argon gas
-
10- or 50-ml Falcon tubes
-
Strong anion-exchange chromatography (SAX) system (e.g., ÄktaPURE)
-
Centrifuge
Part 1: Preparation of deoxycyclo-TP ester (9)
1.Perform the reaction in an oven-dried 25-ml pear-shaped single-neck flask equipped with a triangular-shaped magnetic stirring bar.
2.Seal the flask with a rubber septum; heat it with a heat gun under high vacuum.
3.Weigh 344 mg AZT (8 , 1.28 mmol, 1.0 eq.) and 540 mg ETT (4.15 mmol, 3.2 eq.) into this flask.
4.Co-evaporate the solids with 8 ml MeCN as follows: Add 4 ml anhydrous MeCN to the flask. Place a 50-60°C water bath under the flask, and remove the solvent under vigorous stirring using high vacuum over a Schlenk line. Repeat this process at least twice and then remove the water bath.
5.Purge three times with argon.
6.Remove the solution of 0.075 M c-PyPA (3a) in MeCN from the −20°C freezer, and immediately add 17.0 ml (1.27 mmol, 1.0 eq.) of solution to the solids (do not wait until the solution reaches room temperature). Stir the mixture 5-10 min at room temperature.
7.Monitor the formation of the deoxycyclo-TP ester by 31P-NMR. A shift of the PIII atom from +130 ppm toward +100 ppm will be observed.
Part 2a: Oxidation of deoxycyclo-TP ester (9) with mCPBA (α = O)
8a. Cool the solution from part 1 to –4°C and add 439 mg m CPBA (2.55 mmol, 2.0 eq.) in one portion.
9a. Stir the reaction mixture for 5-10 min and monitor complete oxidation to the cyclo-TP ester 10a via 31P-NMR: a triplet at around –25 ppm should be observed.
Part 3a: Linearization of cyclo-TP ester (10a) to obtain 3′-azidothymidine 5′-γ-P-propargylamido triphosphate (11a) as its sodium salt
10a. Remove the reaction flask from the cooling bath.
11a. Add 520 μl propargylamine (8.28 mmol, 6.5 eq.) and stir the mixture for 15 min at room temperature.
12a. Fill two 50-ml centrifuge tubes (Falcon tubes) with 30 ml 0.5 M NaClO4 in acetone, at –20°C, and place them into a –20°C cooling bath.
13a. Distribute the reaction mixture in equal parts into the two centrifuge tubes and shake them. The sodium salt of the triphosphate (11a) will precipitate.
14a. After the tubes have spent 5-10 min at –20°C, collect the precipitate by centrifugation. Centrifuge 5-7 min at 7700 relative centrifugal force (rcf; × g), 0°C.
15a. Dispose of the organic layer in the beaker.
16a. Resuspend the precipitate in 30 ml acetone, centrifuge again, and dispose of the supernatant. Repeat this washing step once.
17a. Dry the precipitate in high vacuum and determine the yield of 3′-azidothymidine 5′-γ-P-propargylamido triphosphate 11a.
Part 2b: Oxidation of deoxycyclo-TP ester (9) with Beaucage's reagent (α = S)
IMPORTANT NOTE : In part 1, deoxycyclo-TP ester (9) was prepared on a smaller scale (0.11 mmol). The described oxidation was optimized for this scale, but scaling up should be straightforward.
8b. Cool the solution from part 1 (being careful with quantities, as the scale of the experiment is different) to 0°C and add 4.5 mg Beaucage's reagent (0.22 mol, 2.0 eq.) in one portion.
9b. Stir the reaction mixture for 5 min and control the formation of α-(S)-cyclo-TP ester (10b) via 31P-NMR. A characteristic signal at ∼43 ppm should appear.
Part 3b: Linearization of cyclo-TP ester (10b) with propargylamine to obtain 3′-azidothymidine 5′-γ-P-propargylamido α-(S)-triphosphate (11b) as its sodium salt
10b. Perform ring opening and precipitation by following steps 10a-16a from part 3a of this protocol.
11b. Dry the obtained solid under high vacuum and determine the yield of AZT 5′-γ propargylamido triphosphate 11b.
Part 2c: Oxidation of deoxycyclo-TP ester (9) with KSeCN (α = Se)
IMPORTANT NOTE : Part 1, the preparation of the deoxy cyclo-TP intermediate (9), was performed on a smaller scale (0.36 mmol).
8c. Cool the solution from part 1 (being careful with quantities, as the scale of the experiment is different) to 0°C and carefully add 650 mg KSeCN (4.51 mmol, 12.5 eq.) in one portion.
9c. Stir the reaction mixture for 15 min. The α-(Se)-cyclo-TP ester (10c) will precipitate.
10c. To achieve complete precipitation, add 30 ml acetone to the flask, collect the precipitate by centrifugation (5-7 min at 7700 rcf), and analyze an aliquot of the precipitate by 31P-NMR.
Part 3c: Linearization of cyclo-TP ester 10c with propargylamine to obtain 3′-azidothymidine 5′-γ-P-propargylamido α-(Se)-triphosphate (11c) as its sodium salt
11c. Dissolve the precipitate in 1 ml D2O and add 1 ml propargylamine. Stir the mixture for 15 min at room temperature.
12c. Perform precipitation of the product by following steps 10a-16a of this protocol, adjusting the volume of the solution of 0.5 M NaClO4 in acetone to your reaction scale.
13c. Load this sample on a strong anion-exchange chromatography system (e.g., ÄktaPURE).
14c. Elute the product at (300-500 mM) of 1 M NH4HCO3 buffer.
15c. Freeze-dry the product-containing fractions, as judged from the 31P-NMR, to obtain product 11c as a mixture of diastereoisomers in the eluate range of 300-500 mM.
Basic Protocol 3: SYNTHESIS OF 2′-DEOXYTHYMIDINE 5′-γ-P-PROPARGYLAMIDO TRIPHOSPHATE (15)
The following procedure focuses on the regioselective synthesis of 2′-deoxythymidine 5′-γ-P-propargylamido triphosphate (Fig. 4). 2′-Deoxythymidine (dT), as a representative of the 2′-deoxynucleosides, contains a 5′-primary alcohol moiety and a 3′-secondary alcohol moiety. A reaction with c-PyPA in the presence of acidic activator results in a preferential formation of the 5′-deoxycyclo-TP ester (13). This intermediate is then oxidized with m CPBA (alternative oxidizing reagent are possible; see Basic Protocol 2) and linearized with propargylamine. The minor 3′-phosphorylated product can be separated from the major 5′-phosphorylated product (ratio 0.15:1.0 according to 31P-NMR), also on a preparative scale, by using an MPLC gradient-separation protocol.
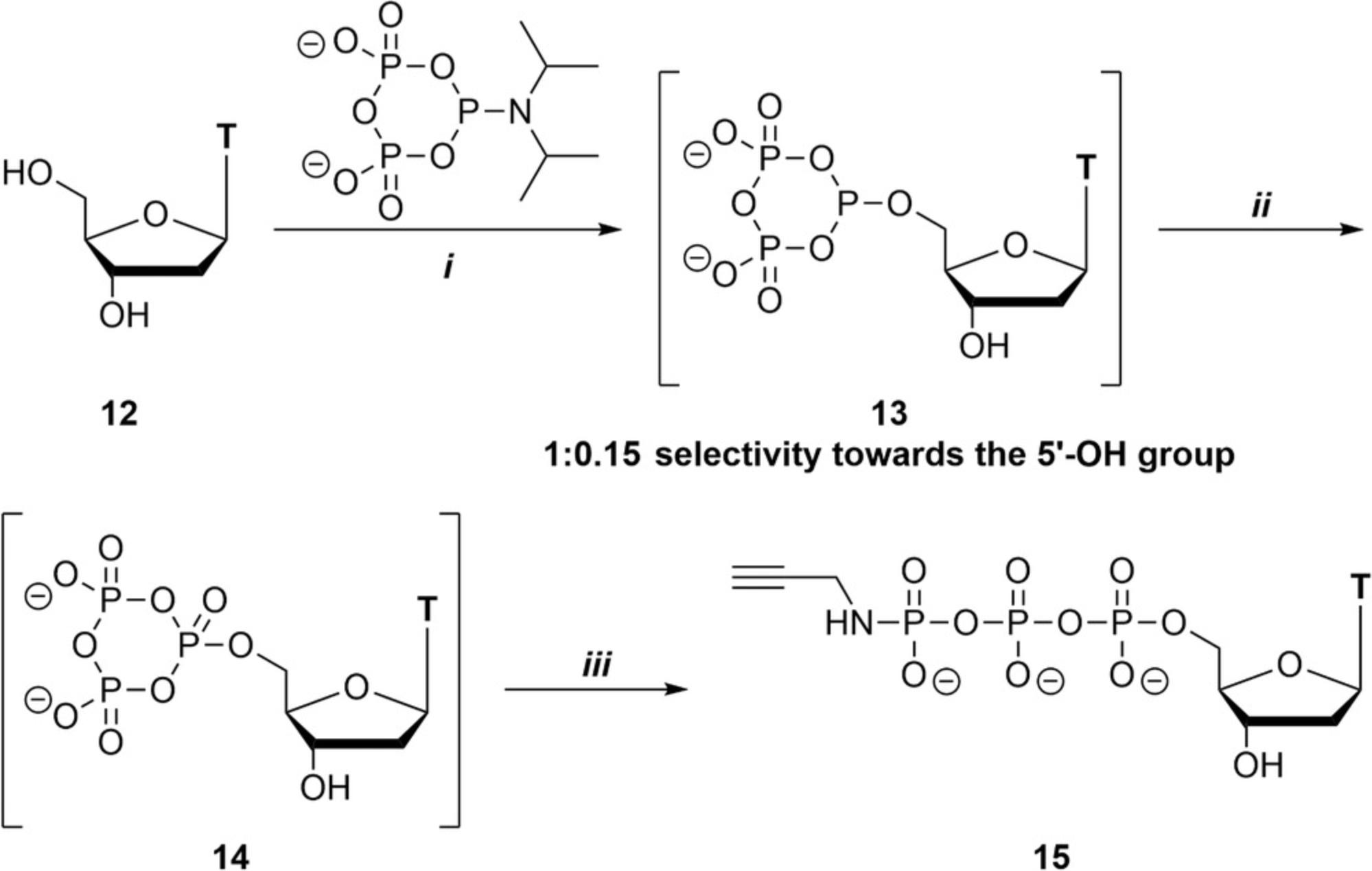
Materials
-
2′-Deoxythymidine (dT)
-
Anhydrous N,N -dimethylformamide (DMF; Sigma-Aldrich cat. no. 227056-100ML)
-
4,5-Dicyanoimidazole (DCI; Acros or ChemPUR)
-
Dry argon gas
-
0.075 M cyclic pyrophosphoryl-P-amidite (c-PyPA) in MeCN (Basic Protocol 1), −20°C
-
≤77% meta- chloroperbenzoic acid (m CPBA; Sigma-Aldrich)
-
Propargylamine (Acros)
-
0.5 M NaClO4 in acetone (see recipe)
-
Deuterium oxide (D2O; Acros)
-
Chloroform-D (CDCl3; Acros)
-
Triethyl ammonium acetate buffer (TEAAc; pH 8.5)
-
10-, 25-, 50-, and 100-ml round-bottom flasks and pear-shaped flask, oven dried
-
Rubber septa
-
Magnetic stirring bars (triangular shape) and stirring plate
-
Heat gun
-
Vacuum source (membrane pump: ∼1 mbar; oil pump for Schlenk line: ∼1.0 × 10–3 mbar)
-
Cooling baths: mixtures of ice and sodium chloride (NaCl, technical grade) in correct proportion to set the desired temperatures of −4°C and −20°C
-
Schlenk line
-
Reverse-phase analytical HPLC system
-
10- or 50-ml Falcon tubes
-
Centrifuge
-
Reverse-phase HPLC system with 3 × 150-mm Hypersil GOLD aQ column
-
Medium-pressure liquid chromatography (MPLC) system (e.g., PuriFlash430®, Interchim®)
-
Lyophilizer (Alpha 1-4 LD plus Freeze Dryer, Christ)
Part 1: Preparation of deoxycyclo-TP esters (13)
1.Perform the reaction in a 50-ml oven-dried pear-shaped single-neck flask equipped with a triangular-shaped magnetic stirring bar.
2.Seal the flask with a rubber septum; heat it with a heat gun under high vacuum.
3.Weigh 470 mg dT (1.95 mmol, 1.3 eq.) and 700 mg DCI (6.00 mmol, 4.0 eq.) into this flask.
4.Co-evaporate the solids with 10 ml MeCN as described in Basic Protocol 2, step 4.
5.Dry the solids for at least 30 min under high vacuum and purge three times with argon.
6.Dissolve the dried solids in 5 ml anhydrous DMF and add 20 ml 0.075 M c-PyPA (3a) in MeCN (1.5 mmol, 1.0 eq.), at −20°C, to the reaction mixture. Stir the mixture for 10 min at room temperature.
7.Monitor the formation of the deoxycyclo-TP ester (13) by 31P-NMR. A shift of the PIII resonance from +130 ppm toward +100 ppm should be observed.
Part 2: Oxidation of deoxycyclo-TP ester (13) with mCPBA (α = O)
8.Cool the solution from part 1 to −4°C and add 460 mg m CPBA (2.70 mmol, 1.8 eq.) in one portion.
9.Stir the reaction mixture for 5-10 min and monitor complete oxidation to the cyclo-TP ester (14) via 31P-NMR (triplet at around –22 ppm).
Part 3: Linearization of cyclo-TP ester (14) to obtain 2′-deoxythymidine 5′-γ-P-propargylamido triphosphate as its sodium salt
10.Add 1.52 ml propargylamine (24.0 mmol, 16.0 eq.) to the reaction flask. Remove the sample from the cooling bath and stir the mixture for 15 min.
11.Isolate the sodium salt of the product triphosphate by NaClO4 precipitation as described in Basic Protocol 2, steps 12a-16a.
12.Dry the obtained solid under high vacuum.
13.Analyze an aliquot on a reverse-phase analytical HPLC to identify conditions for the separation of the 5′- and 3′-regioisomer on a preparative scale (an exemplary chromatogram is shown in Fig. 5). Use the following HPLC conditions:
14.For large-scale separation of the regioisomers, use an MPLC system. Dissolve half of the sample (to avoid column overload) in 1 ml deionized water and load it onto a C18 column. Use the following separation conditions:
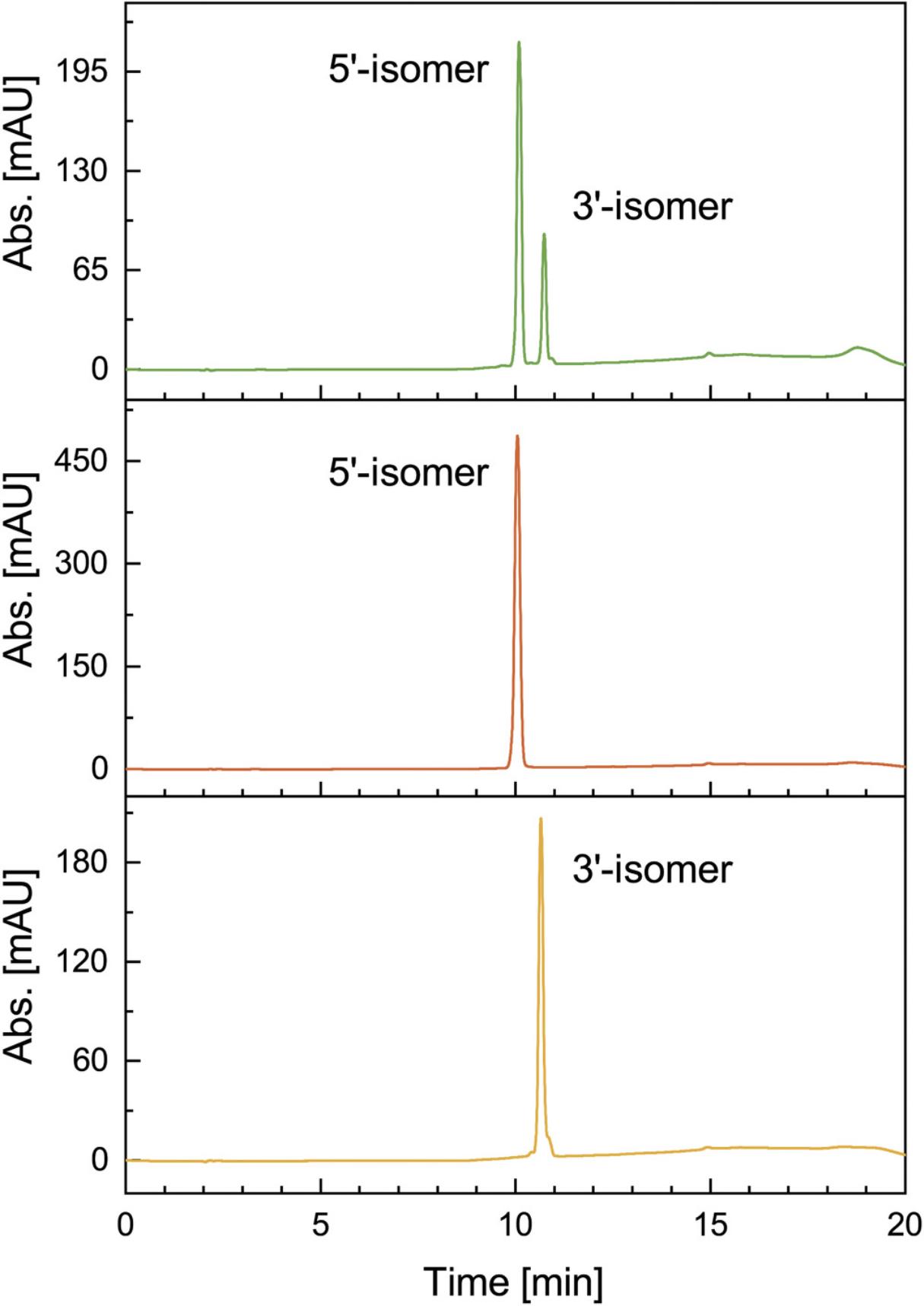
15.Evaporate the collected fractions of the 5′- and 3′-regioisomers under reduced pressure and isolate the regioisomers by NaClO4 precipitation (Basic Protocol 1, step 3a, 10-16).
16.Obtain the separated regioisomers as colorless solids after drying them under high vacuum.
Basic Protocol 4: SYNTHESIS OF ADENOSINE 5′-γ-P-AMIDO TRIPHOSPHATE (19) AND ADENOSINE 5′-γ-P-PROPARGYLAMIDO TRIPHOSPHATE (20)
The synthesis of P-γ modified 5′-adenosine triphosphates (19 , 20) is described in the following procedure (Fig. 6). Adenosine (18), as a representative of the ribonucleosides, has one primary alcohol moiety at the 5′ position and two secondary alcohol moieties in the 2′ and 3′ positions. A triphosphorylation with c-PyPA reagent leads to the desired 5′-phosphorylated product 19 or 20 , depending on the nucleophile used for linearization, as a major component and 2′,3′-cyclic AMP (2′,3′-c AMP) as a byproduct. 2′,3′-c AMP, which generates a characteristic singlet at ∼20 ppm in the 31P-NMR, can be easily removed by ion-exchange chromatography given its significant difference in overall charge. To prevent precipitation during the coupling step with c-PyPA, since adenosine is only soluble in DMF, a concentrated (0.5 M) c-PyPA mixture in DMF is preferred to a less concentrated (0.075 M) c-PyPA mixture in MeCN.
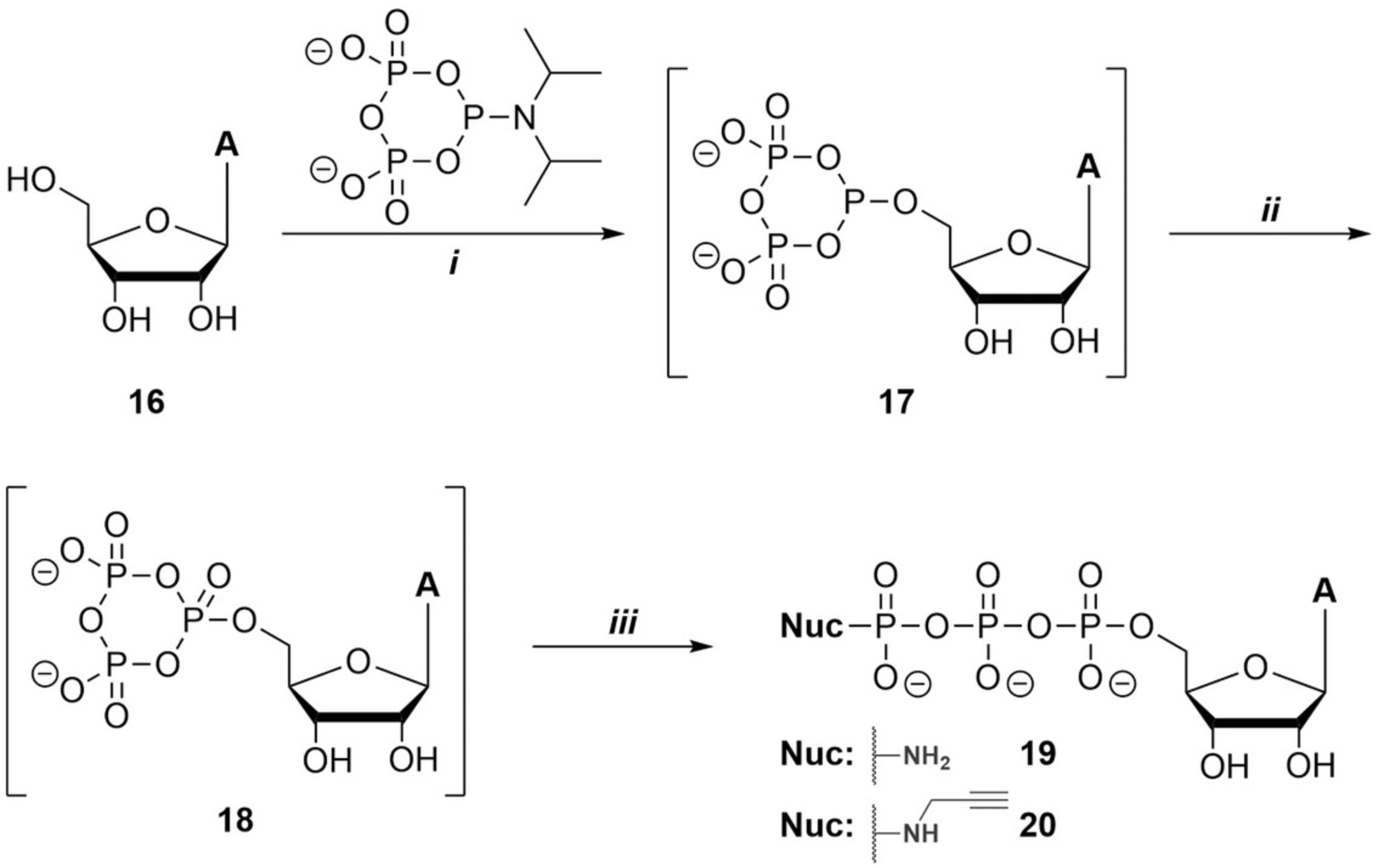
Materials
-
Adenosine (A; Carl Roth)
-
4,5-Dicyanoimidazole (DCI; Acros or ChemPUR)
-
Dry argon gas
-
Anhydrous acetonitrile (MeCN; Acros, cat. no. 10353732)
-
Anhydrous N,N -dimethylformamide (DMF; Sigma-Aldrich, cat. no. 227056-100ML)
-
0.5 M cyclic pyrophosphoryl-P-amidite (c-PyPA) in DMF (Basic Protocol 1), −20°C
-
≤77% meta- chloroperbenzoic acid (m CPBA; Sigma-Aldrich)
-
25% (w/w) aqueous ammonia solution (conc. aq. NH3; VWR)
-
Propargylamine (Acros)
-
Sodium perchlorate monohydrate (NaClO4, Sigma-Aldrich)
-
Acetone, P.A. grade
-
1 M ammonium bicarbonate (NH4HCO3) buffer
-
Deuterium oxide (D2O; Acros)
-
Chloroform-D (CDCl3; Acros)
-
10-, 25-, 50-, and 100-ml round-bottom flasks and pear-shaped flask, oven dried
-
Rubber septa
-
Magnetic stirring bars (triangular shape) and stirring plate
-
Rotary evaporator
-
Cooling baths: mixtures of ice and sodium chloride (NaCl, technical grade) in correct proportion to set the desired temperatures of −4°C and −20°C
-
Schlenk line
-
Vacuum source (membrane pump: ∼1 mbar; oil pump for Schlenk line: ∼1.0 × 10–3 mbar)
-
0.1-ml NMR tube
-
20- or 50-ml Falcon tubes
-
Centrifuge
-
Strong anion-exchange chromatography (SAX) system (e.g., ÄKTApureTM system and HiTrapTM column)
-
Lyophilizer (Alpha 1-4 LD plus Freeze Dryer from Christ)
Part 1: Preparation of deoxycyclo-TP ester (17)
1.Perform the reaction in a 25-ml oven-dried, pear-shaped, single-neck flask equipped with a triangular-shaped magnetic stirring bar.
2.Seal the flask with a rubber septum; heat it with a heat gun under high vacuum.
3.Weigh 120 mg adenosine (0.45 mmol, 1.5 eq.) and 94 mg DCI (0.80 mmol, 2.7 eq.) into this flask.
4.Co-evaporate the solids with 10 ml MeCN as described in Basic Protocol 2, step 4.
5.Purge three times with argon.
6.Dissolve the dried solids in 5.5 ml anhydrous DMF and add 0.6 ml 0.5 M c-PyPA (3a) in DMF (0.3 mmol, 1.0 eq.), at −20°C, to the reaction mixture. Stir 10 min at room temperature.
7.Monitor the formation of the deoxycyclo-TP ester (17) by 31P-NMR. A shift of the PIII atom from +130 ppm toward +104 ppm should be observed.
Part 2: Oxidation of deoxycyclo-TP ester (17) with mCPBA (α = O)
8.Cool the solution from part 1 to −4°C and add 104 mg m CPBA (0.60 mmol, 2.0 eq.) in one portion.
9.Stir the reaction mixture for 5-10 min and monitor complete oxidation to the cyclo-TP ester (18) via 31P-NMR. A triplet at around -22 ppm should be observed.
Part 3: Linearization of cyclo-TP ester (18) to obtain adenosine 5′-γ-modified triphosphates (19, 20) as their ammonium salts
IMPORTANT NOTE : The process of ring opening with different nucleophiles is performed by combining the reaction mixture in an NMR tube also containing the nucleophile and D2O.
10.Add 0.5 ml (∼25 µmol) of the reaction mixture, with a calculated maximal concentration of 0.049 M cyclo-TP ester, to a 0.1-ml NMR tube containing D2O and 0.1 ml of either conc. aq. NH3, to give 19 , or propargylamine, to give 20.
11.Isolate the sodium triphosphate salt by NaClO4 precipitation as described in Basic Protocol 2, steps 12a-16a.
12.Dry the obtained solid under high vacuum.
13.Dissolve the crude product in deionized water.
14.Load this sample on an ion-exchange chromatography system (e.g., ÄktaPURE) and elute the product with 1 M NH4HCO3 buffer.
15.Combine the fractions according to the chromatogram (product fractions: 6.4-9.2% of 1 M NH4HCO3).
16.Remove water and excess buffer by lyophilization on a freeze dryer overnight.
17.Redissolve the product in deionized water and freeze-dry it again. Product 19 and 20 are obtained as ammonium salts.
Basic Protocol 5: SYNTHESIS OF d4T 5′-γ-PROPARGYLAMIDO β,γ-(DIFLUOROMETHYLENE)TRIPHOSPHATE
This procedure highlights the synthesis of β,γ non-hydrolyzable nucleotides by utilizing the family of c-PyPA reagents, e.g., c-PyCH2-PA and c-PyCF2-PA. The protocol details the synthesis of d4T 5′-γ-propargylamido β,γ-(difluoromethylene)triphosphate as an example. This protocol can be easily adopted to generate a range of other compounds, including nucleosides, and by combination with the other protocols in regard to, for example, the oxidation or linearization steps. Several examples are discussed in the primary publications (Singh, Ripp et al., 2019). Figure 7 summarizes the possibilities of this approach.
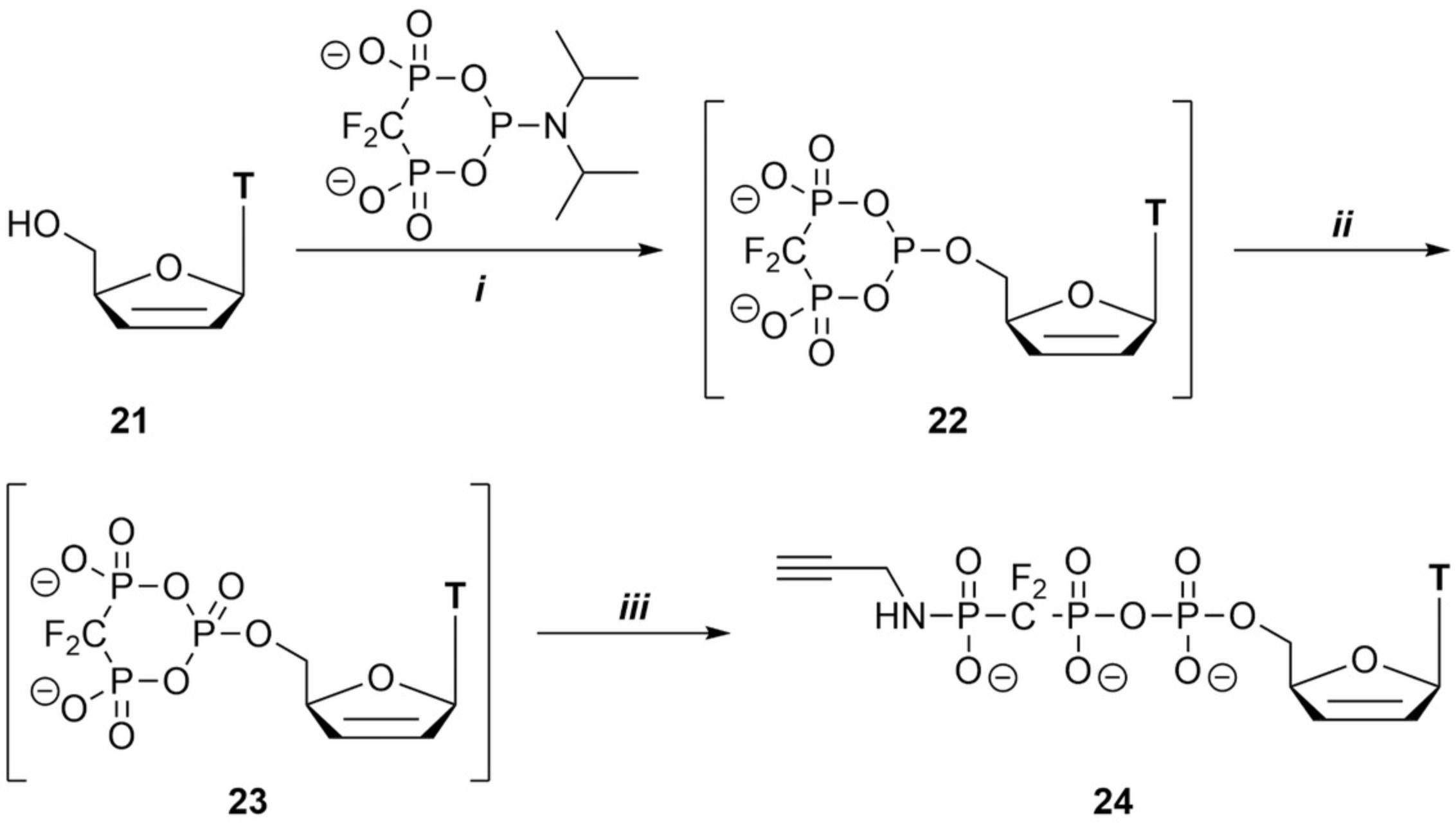
Materials
-
Stavudine (d4T)
-
5-(Ethylthio)-1 H -tetrazole (ETT; ChemPUR)
-
Anhydrous acetonitrile (MeCN; Acros, cat. no. 10353732)
-
Dry argon gas
-
0.073 M cyclic pyrophosphoryl-P-amidite (c-PyCF2PA) in MeCN (Basic Protocol 1), −20°C
-
meta- Chloroperbenzoic acid (m CPBA; Sigma-Aldrich)
-
Propargylamine (Acros)
-
Sodium perchlorate monohydrate (NaClO4; Sigma-Aldrich)
-
Acetone, P.A. grade
-
Deuterium oxide (D2O; Acros)
-
Chloroform-D (CDCl3; Acros)
-
10-, 25-, 50-, and 100-ml round-bottom flasks and pear-shaped flask, oven dried
-
Magnetic stirring bars (triangular shape) and stirring plate
-
Cooling bath: mixture of ice and sodium chloride (NaCl, technical grade) in correct proportion to set the desired temperature of −4°C
-
Schlenk line
-
Vacuum source (membrane pump: ∼1 mbar; oil pump for Schlenk line: ∼1.0 × 10–3 mbar)
-
10- or 50-ml Falcon tubes
-
Centrifuge
Part 1: Preparation of deoxycyclo-TP ester (22)
1.Perform the reaction in a 25 ml oven-dried, pear-shaped single-neck flask equipped with a triangular-shaped magnetic stirring bar.
2.Seal the flask with a rubber septum; heat it with a heat-gun under high vacuum.
3.Weigh 40 mg d4T (0.17 mmol, 1.2 eq.) and 67 mg ETT (0.5 mmol, 3.5 eq.) into this flask.
4.Co-evaporate the solids with 8 ml MeCN as described in Basic Protocol 2.
5.Purge three times with Ar.
6.Add 1 ml anhydrous DMF to dissolve the solids.
7.Remove 0.073 M c-PyCF2PA (3e) solution in MeCN from the −20°C freezer, add 2.0 ml (0.15 mmol, 1.0 eq.) to the reaction mixture, and stir at room temperature for 5-10 min.
8.The reaction results in the formation of a deoxycyclo-TP ester (22), which can be monitored by 31P-NMR. A shift of the PIII atom from +130 ppm toward +108 ppm can be observed.
Part 2: Oxidation of deoxycyclo-TP ester (22) with mCPBA (α = O)
9.Cool the solution from part 1 to –4°C and add 439 mg m CPBA (2.55 mmol, 2.0 eq.) in one portion.
10.Stir the reaction mixture for 3 min and monitor complete oxidation to the cyclo-TP ester (23) via 31P-NMR. A triplet will appear at around –25 ppm.
Part 3: Linearization of cyclo-TP ester (23) to obtain d4T 5′-γ-propargylamido β,γ-(difluoromethylene)triphosphate (24) as its sodium salt
IMPORTANT NOTE : The ring opening is performed similarly to that in Basic Protocol 4: The reaction mixture is added to an NMR tube containing the nucleophile and D2O.
11.Add 0.25 ml reaction mixture of step 2a, with a calculated concentration of 0.049 M β,γ-modified cyclo-TP (23) (12.2 µmol), into an NMR-tube containing D2O (0.1 ml) and propargylamine (0.25 ml).
12.Isolation the sodium triphosphate salt by the NaClO4 precipitation method as described in Basic Protocol 2 steps 12-16.
13.Dry the obtained solid under high vacuum.
Support Protocol: SYNTHESIS OF DIISOPROPYLPHOSPHORAMIDOUS DICHLORIDE
This protocol describes the synthesis of diisopropylphosphoramidous dichloride (2), necessary as a reagent for the preparation of the cyclic pyrophosphoryl P-amidite (c-PyPA) and its analogues (c-PyNHPA, c-PyCH2PA, c-PyCCl2PA, c-PyCF2PA) (3a-e) (Basic Protocol 1). Although diisopropylphosphoramidous dichloride (2) is commercially available, we recommend performing an in-laboratory synthesis when it is used on a larger scale. The preparation we describe here is based on published synthesis from 1985 using anhydrous diethyl ether as solvent (King & Sadanani, 1985). We also found another synthetic approach for this compound, reported by Amigues et al., to be very suitable (Amigues, Hardacre, Keane, & Migaud, 2008). By using an ionic liquid as a solvent, the pure product could be easily obtained by distillation directly out the reaction mixture, and recycling of the ionic liquid is possible.
Materials
-
Phosphorus trichloride (PCl3, Sigma Aldrich), freshly distilled
-
Diisopropylamine
-
Anhydrous diethyl ether (Et2O)
-
Chloroform-D (CDCl3, Acros)
-
25, 100-, and 500-ml round-bottom flasks and pear-shaped flask, oven dried
-
Magnetic stirring bars (oval and triangular shaped) and stirring plate
-
Mechanical KPG stirrer
-
Heat gun
-
Vacuum source (membrane pump: ∼1 mbar; oil pump for Schlenk line: ∼1.0 × 10–3 mbar)
-
Dry argon gas
-
Schlenk frit
-
Rotary evaporator
-
Oil bath, 100°C
-
Schlenk line
1.Perform the reaction in a 500-ml oven-dried round-bottom three-necked flask, containing an oval-shaped magnetic stirrer, attached to the central neck of the flask by a mechanical KPG stirrer.
2.Use a heat gun to dry the flask under vacuum and purge with argon.
3.Add 30 ml freshly distilled PCl3 (0.34 mol, 1.0 eq.) and dissolve in 180 ml anhydrous diethyl ether.
4.Cool the mixture to 0°C, and then add a solution of 95 ml freshly distilled diisopropylamine (0.68 mol, 2.0 eq.) in 150 ml anhydrous ethanol dropwise over 1 h.
5.Stir the mixture vigorously overnight at room temperature.
6.Carefully filter the reaction mixture by the use of Schlenk frit under an inert atmosphere.
7.Remove the solvent under reduced pressure on a rotary evaporator.
8.Transfer the obtained yellow oil into a 100-ml pear-shaped single-neck flask, equipped with a triangular-shaped magnetic stirring bar.
9.Purify the obtained yellow oil by vacuum distillation, and use oil bath at 100°C (5 mbar; boiling point of the product 75°C) to give the target compound as a colorless oil, which crystallizes at 4°C.
10.Seal the flask with parafilm under argon atmosphere and store the compound in a −20°C freezer.
REAGENTS AND SOLUTIONS
Activated molecular sieves
Activate molecular sieves are prepared by heating commercially available 3-Å molecular sieves under high vacuum in a heating furnace. First, attach high vacuum and slowly heat to 450°C in 1 hr intervals. Afterward, keep the temperature at 450°C overnight. Let it cool down slowly, and store the activated molecular sieve in a sealed flask under argon atmosphere for up to 4 months.
NaClO4 in acetone, 0.5 M
Dissolve 70 g sodium perchlorate monohydrate (NaClO4; Sigma-Aldrich) in 1 liter P.A.-grade acetone and stir for 2-5 min. Store for up to several weeks at –20°C.
COMMENTARY
Background Information
Native nucleoside triphosphates (NTPs) and unnatural analogues are heavily studied in various different fields of research, as they have versatile functions in living organisms. NTPs are building blocks of nucleic acids (DNA and RNA), interfere in signaling processes, and provide energy for metabolic pathways (Hollenstein, 2012; Roy et al., 2016). The energy is structurally conserved in the phosphoanhydride bonds of the triphosphate ester (Kamerlin, Sharma, Prasad, & Warshel, 2013; Surya Prakash et al., 2010; Westheimer, 1987). This charged ionic moiety, in combination with the more lipophilic nucleoside residue, complicates both chemical synthesis and the purification (Burgess & Cook, 2000). In most cases, the triphosphorylation is the most challenging step of NTP synthesis. Several approaches for synthesizing NTPs have previously been reported and are summarized in various reviews and book chapters (Burgess & Cook, 2000; Duro, Mustafa, Kashemirov, & McKenna, 2019; Hollenstein, 2012; Kore, & Srinivasan, 2013; Monir-Vaghefi, 2005; Roy et al., 2016; Sherstyuk & Abramova, 2015; Weinschenk & Meier, 2013). Despite their enormous range of biological functions, a universal synthesis procedure for all NTPs remains elusive (Liao et al., 2019).
In this article, we described a regioselective, highly modifiable NTP synthesis based on phosphoramidite chemistry. The so-called cyclic pyrophosphoryl P-amidite (c-PyPA), derived from pyrophosphate (PV) and a reactive phosphoramidite (PIII), is used for the triphosphorylation (Singh, Ripp et al., 2019; Singh, Steck et al., 2019). By using non-hydrolyzable analogues of the pyrophosphate, a family of c-PyPA analogues (CH2, CF2, CCl2, NH) can be obtained, providing facile access to the corresponding non-hydrolyzable NTPs (β,γ-modified; Dutta et al., 2017). A largely regioselective reaction on the sterically least hindered alcohol on the nucleoside initiates this one-pot triphosphorylation. A modification in P-α can be easily introduced by using different oxidizing agents (Caton-Williams, Lin, Smith, & Huang, 2011; Caton-Williams, Smith, Carrasco, & Huang, 2011). The resulting cyclo-triphosphate ester can then be opened by various nucleophiles, allowing the synthesis of P-γ modified NTPs.
Critical Parameters and Troubleshooting
It is crucial to exclude moisture during the synthesis of c-PyPA and its analogues to avoid the formation of unwanted hydrolysis products (Singh, Steck et al., 2019). Therefore, rigorously dry all glassware and chemical reagents used during the synthesis. Dry the argon flow with a phosphorus pentoxide (P2O5) column integrated into the Schlenk line. Use only anhydrous or freshly distilled tertiary amine bases during the c-PyPA synthesis. The precise amounts of reagents used in assembling the c-PyPA reagent affects its quality and conversions. Hence, determine the exact number of TBA counterions and weigh only the dry TBA pyrophosphate salts to prevent miscalculation of the equivalents.
Excluding moisture is also critical for the first step of the triphosphorylation reaction. Use dry glassware, anhydrous chemical reagents, and dry argon flow. We found that pear-shaped flasks enable more efficient co-evaporation of the reagents, and hence a smoother reaction, compared to round-bottom flasks. While performing the precipitation with cold NaClO4 solution, let the slurry stand for a few minutes at −20°C to complete the precipitation. Monitor the formation of the different phosphorus species during this one-pot process by analyzing aliquots of the reaction mixture via 31P-NMR. Use oven-dried NMR tubes, anhydrous deuterated solvents (stored over molecular sieves), and an argon atmosphere to avoid hydrolysis within the NMR tube and consequent misinterpretations.
Time Considerations
Although the reaction time for the synthesis of c-PyPA and its analogues is quite short, the drying step of the TBA salt requires a significant time investment and dedication, as it is critical for success. Therefore, allot 2 days for this synthesis and prepare larger stocks to allow some to be used for future reactions. Once c-PyPA (and analogues) are obtained as a stock solution, the NTP synthesis that follows is very rapid. The triphosphorylated target compound can also be obtained rapidly. If the linearization with different nucleophiles is performed in NMR tubes (with NaClO4 precipitation), you can obtain various γ-modified NTPs within a single day in good quality.
Acknowledgments
Funded by the Deutsche Forschungsgemeinschaft (DFG, German Research Foundation) under Germany's Excellence Strategy – EXC-2193/1 – 390951807. H. J. J. also acknowledges support from the Human Frontier Science Program HFSP (RGP0025/2016).
Literature Cited
- Amigues, E. J., Hardacre, C., Keane, G., & Migaud, M. E. (2008). Solvent-modulated reactivity of PCl3 with amines. Green Chemistry , 10, 660–669. doi: 10.1039/b718849h.
- Azevedo, C., Singh, J., Steck, N., Hofer, A., Ruiz, F. A., Singh, T., … Saiardi, A. (2018). Screening a protein array with synthetic biotinylated inorganic polyphosphate to define the human PolyP-ome. ACS Chemical Biology , 13, 1958–1963. doi: 10.1021/acschembio.8b00357.
- Burgess, K., & Cook, D. (2000). Syntheses of nucleoside triphosphates. Chemical Reviews , 100, 2047–2060. doi: 10.1021/cr990045m.
- Caton-Williams, J., Lin, L., Smith, M., & Huang, Z. (2011). Convenient synthesis of nucleoside 5′-triphosphates for RNA transcription. Chemical Communications (Cambridge, UK) , 47, 8142–8144. doi: 10.1039/c1cc12201k.
- Caton-Williams, J., Smith, M., Carrasco, N., & Huang, Z. (2011). Protection-free one-pot synthesis of 2′-deoxynucleoside 5′-triphosphates and DNA polymerization. Organic Letters , 13, 4156–4159. doi: 10.1021/ol201073e.
- Crans, D. C., Holder, A. A., Saha, T. K., Prakash, G. K. S., Yousufuddin, M., Kultyshev, R., … Florián, J. (2007). Chelation of vanadium (V) by difluoromethylene bisphosphonate, a structural analogue of pyrophosphate. Inorganic Chemistry , 46, 6723–6732. doi: 10.1021/ic062484r.
- Duro, M. V. V., Mustafa, D., Kashemirov, B. A., & McKenna, C. E. (2019). Phosphorus in chemical biology and medicinal chemistry. In V. Iaroshenko (Ed.), Organophosphorus chemistry: From molecules to applications (Vol. 406, pp. 499–544). Weinheim: Wiley-VCH.
- Dutta, A. K., Captain, I., & Jessen, H. J. (2017). New synthetic methods for phosphate labeling. Topics in Current Chemistry , 375, 51. doi: 10.1007/s41061-017-0135-6.
- Ermert, S., Marx, A., & Hacker, S. M. (2017). Phosphate-modified nucleotides for monitoring enzyme activity. Topics in Current Chemistry , 375, 28. doi: 10.1007/s41061-017-0117-8.
- Hocek, M. (2014). Synthesis of base-modified 2′-deoxyribonucleoside triphosphates and their use in enzymatic synthesis of modified DNA for applications in bioanalysis and chemical biology. Journal of Organic Chemistry , 79, 9914–9921. doi: 10.1021/jo5020799.
- Hollenstein, M. (2012). Nucleoside triphosphates—building blocks for the modification of nucleic acids. Molecules (Basel, Switzerland) , 17, 13569–13591. 10.3390/molecules171113569.
- Kamerlin, S. C. L., Sharma, P. K., Prasad, R. B., & Warshel, A. (2013). Why nature really chose phosphate. Quarterly Reviews of Biophysics , 46, 1–132. doi: 10.1017/S0033583512000157.
- King, R. B., & Sadanani, N. D. (1985). Dialkylaminodichlorophosphines. Synthesis and Reactivity in Inorganic and Metal-Organic Chemistry , 15, 149–153. doi: 10.1080/00945718508059375.
- Kore, A. R., & Srinivasan, B. (2013). Recent advances in the syntheses of nucleoside triphosphates. Current Organic Synthesis , 10, 903–934. 10.2174/15701794113109990001.
- Liao, J.-Y., Bala, S., Ngor, A. K., Yik, E. J., & Chaput, J. C. (2019). P(V) reagents for the scalable synthesis of natural and modified nucleoside triphosphates. Journal of the American Chemical Society , 141, 13286–13289. doi: 10.1021/jacs.9b04728.
- Monir-Vaghefi, S.-M. (2005). Nucleoside triphosphates and their analogs: Chemistry, biotechnology, and biological applications. Boca Raton: Taylor & Francis.
- Rostovtsev, V. V., Green, L. G., Fokin, V. V., & Sharpless, K. B. (2002). A stepwise Huisgen cycloaddition process: Copper(I)-catalyzed regioselective “ligation” of azides and terminal alkynes. Angewandte Chemie International Edition , 41, 2596–2599. doi: 10.1002/1521-3773(20020715)41:14<2596::AID-ANIE2596>3.0.CO;2-4.
- Roy, B., Depaix, A., Périgaud, C., & Peyrottes, S. (2016). Recent trends in nucleotide synthesis. Chemical Reviews , 116, 7854–7897. doi: 10.1021/acs.chemrev.6b00174.
- Serdjukow, S., Kink, F., Steigenberger, B., Tomás-Gamasa, M., & Carell, T. (2014). Synthesis of γ-labeled nucleoside 5′-triphosphates using click chemistry. Chemical Communications (Cambridge, UK) , 50, 1861–1863. doi: 10.1039/C3CC48937J.
- Shepard, S. M., & Cummins, C. C. (2019). Functionalization of intact trimetaphosphate: A triphosphorylating reagent for C, N, and O nucleophiles. Journal of the American Chemical Society , 141, 1852–1856. doi: 10.1021/jacs.8b12204.
- Shepard, S. M., Windsor, I. W., Raines, R. T., & Cummins, C. C. (2019). Nucleoside tetra- and pentaphosphates prepared using a tetraphosphorylation reagent are potent inhibitors of ribonuclease A. Journal of the American Chemical Society , 141, 18400–18404. doi: 10.1021/jacs.9b09760.
- Sherstyuk, Y. V., & Abramova, T. V. (2015). How to form a phosphate anhydride linkage in nucleotide derivatives. Chembiochem , 16, 2562–2570. doi: 10.1002/cbic.201500406.
- Singh, J., Ripp, A., Haas, T. M., Qiu, D., Keller, M., Wender, P. A., … Jessen, H. J. (2019). Synthesis of modified nucleoside oligophosphates simplified: Fast, pure, and protecting group free. Journal of the American Chemical Society , 141, 15013–15017. doi: 10.1021/jacs.9b08273.
- Singh, J., Steck, N., De, D., Hofer, A., Ripp, A., Captain, I., … Jessen, H. J. (2019). A phosphoramidite analogue of cyclotriphosphate enables iterative polyphosphorylations. Angewandte Chemie International Edition , 58, 3928–3933. doi: 10.1002/anie.201814366.
- Surya Prakash, G. K., Zibinsky, M., Upton, T. G., Kashemirov, B. A., McKenna, C. E., Oertell, K., … Olah, G. A. (2010). Synthesis and biological evaluation of fluorinated deoxynucleotide analogs based on bis-(difluoromethylene)triphosphoric acid. Proceedings of the National Academy of Sciences of the United States of America , 107, 15693–15698. doi: 10.1073/pnas.1007430107.
- Wanat, P., Walczak, S., Wojtczak, B. A., Nowakowska, M., Jemielity, J., & Kowalska, J. (2015). Ethynyl, 2-propynyl, and 3-butynyl C-phosphonate analogues of nucleoside di- and triphosphates: Synthesis and reactivity in CuAAC. Organic Letters , 17, 3062–3065. doi: 10.1021/acs.orglett.5b01346.
- Warminski, M., Sikorski, P. J., Kowalska, J., & Jemielity, J. (2017). Applications of phosphate modification and labeling to study (m)RNA caps. Topics in Current Chemistry , 375, 16. doi: 10.1007/s41061-017-0106-y.
- Weinschenk, L., & Meier, C. (2013). Chemical syntheses of nucleoside triphosphates. In P. Merino (Ed.), Chemical synthesis of nucleoside analogues ( 1st ed., Vol. 100, pp. 209–227). Hoboken: Wiley.
- Westheimer, F. (1987). Why nature chose phosphates. Science , 235, 1173–1178. doi: 10.1126/science.2434996.
Citing Literature
Number of times cited according to CrossRef: 2
- Alina I. Novgorodtseva, Alexander A. Lomzov, Svetlana V. Vasilyeva, Synthesis and Properties of α-Phosphate-Modified Nucleoside Triphosphates, Molecules, 10.3390/molecules29174121, 29 , 17, (4121), (2024).
- Roza Pawlowska, Ewa Radzikowska-Cieciura, Sepideh Jafari, Julia Fastyn, Eliza Korkus, Edyta Gendaszewska-Darmach, Gangyin Zhao, Ewa Snaar-Jagalska, Arkadiusz Chworos, Double-modified, thio and methylene ATP analogue facilitates wound healing in vitro and in vivo, Scientific Reports, 10.1038/s41598-024-63759-5, 14 , 1, (2024).