Protocol for the Growth and Maturation of hiPSC-Derived Kidney Organoids using Mechanically Defined Hydrogels
Ivan Krupa, Ivan Krupa, Niall J. Treacy, Niall J. Treacy, Shane Clerkin, Shane Clerkin, Jessica L. Davis, Jessica L. Davis, Aline F. Miller, Aline F. Miller, Alberto Saiani, Alberto Saiani, Jacek K. Wychowaniec, Jacek K. Wychowaniec, Emmanuel G. Reynaud, Emmanuel G. Reynaud, Dermot F. Brougham, Dermot F. Brougham, John Crean, John Crean
gelatin methacryloyl
GelMA
hiPSC-derived kidney organoids
human induced pluripotent stem cells
hydrogels
self-assembling peptide hydrogels
stiffness
Abstract
With recent advances in the reprogramming of somatic cells into induced Pluripotent Stem Cells (iPSCs), gene editing technologies, and protocols for the directed differentiation of stem cells into heterogeneous tissues, iPSC-derived kidney organoids have emerged as a useful means to study processes of renal development and disease. Considerable advances guided by knowledge of fundamental renal developmental signaling pathways have been made with the use of exogenous morphogens to generate more robust kidney-like tissues in vitro. However, both biochemical and biophysical microenvironmental cues are major influences on tissue development and self-organization. In the context of engineering the biophysical aspects of the microenvironment, the use of hydrogel extracellular scaffolds for organoid studies has been gaining interest. Two families of hydrogels have recently been the subject of significant attention: self-assembling peptide hydrogels (SAPHs), which are fully synthetic and chemically defined, and gelatin methacryloyl (GelMA) hydrogels, which are semi-synthetic. Both can be used as support matrices for growing kidney organoids. Based on our recently published work, we highlight methods describing the generation of human iPSC (hiPSC)-derived kidney organoids and their maturation within SAPHs and GelMA hydrogels. We also detail protocols required for the characterization of such organoids using immunofluorescence imaging. Together, these protocols should enable the user to grow hiPSC-derived kidney organoids within hydrogels of this kind and evaluate the effects that the biophysical microenvironment provided by the hydrogels has on kidney organoid maturation. © 2024 The Authors. Current Protocols published by Wiley Periodicals LLC.
Basic Protocol 1 : Directed differentiation of human induced pluripotent stem cells (hiPSCs) into kidney organoids and maturation within mechanically tunable self-assembling peptide hydrogels (SAPHs)
Alternate Protocol : Encapsulation of day 9 nephron progenitor aggregates in gelatin methacryloyl (GelMA) hydrogels.
Support Protocol 1 : Human induced pluripotent stem cell (hiPSC) culture.
Support Protocol 2 : Organoid fixation with paraformaldehyde (PFA)
Basic Protocol 2 : Whole-mount immunofluorescence imaging of kidney organoids.
Basic Protocol 3 : Immunofluorescence of organoid cryosections
INTRODUCTION
Human induced pluripotent stem cell (hiPSC)-derived kidney organoids are three-dimensional (3D) cellularly heterogeneous aggregates that recapitulate key structural features of the human kidney. As such, kidney organoids hold great potential as next-generation human tissue models for drug discovery and disease modeling applications. In recent years, kidney organoids have been utilized to model diseases such as polycystic kidney disease (Shimizu et al., 2020), renal fibrosis (Davis et al., 2022), and the effect of hyperglycemia on SARS-COV2 infection of the kidney (Garreta et al., 2022). The past decade of work in the field has seen the establishment of protocols that derive kidney organoids from human pluripotent stem cells (hPSCs) through the temporal addition of exogenous morphogens to drive developmental pathways analogous to fetal kidney organogenesis (Freedman et al., 2015; Garreta et al., 2019; Morizane et al., 2015; Takasato et al., 2015). These methods of generating kidney organoids typically first differentiate hPSCs towards intermediate mesoderm, generating the two kidney progenitor populations, the metanephric mesenchyme and the ureteric bud, which are then used to give rise to kidney organoids with segmented nephron and collecting duct-like structures. Recent modifications to these protocols, such as the introduction of flow by culturing organoids on a millifluidic chip (Homan et al., 2019), as well as separate induction of ureteric bud and metanephric mesenchyme progenitors (Taguchi & Nishinakamura, 2017), have yielded considerable improvements in kidney organoid maturation. In addition, the timing and concentration of certain factors used in kidney organoid differentiation have also been shown to affect the proportion of different renal cell types generated in the resulting kidney organoids (Low et al., 2019; Taguchi & Nishinakamura, 2017; Takasato et al., 2015). While precise biochemical control is crucial for guiding organoid differentiation, the role of extracellular matrix (ECM) stiffness in the development of kidney organoids has also been studied in recent years with considerable progress made (Garreta et al., 2019; Geuens et al., 2021; Ruiter et al., 2022; Treacy et al., 2023).
Tissue stiffness has been shown to influence a wide array of developmental processes and has also been implicated in disease states. For instance, pre-implantation mouse embryos cultured on soft (G’ = 1 kPa) Collagen I hydrogels had an improved frequency of differentiation to blastocyst and hatching blastocyst stages of development compared to control embryos cultured on polystyrene (Kolahi et al., 2012). Early differentiation events of embryonic stem cells (ESCs) have also been shown to be affected by stiffness (Evans et al., 2009). In the context of kidney fibrosis, it has been found that renal fibroblasts respond more to profibrotic TGFβ signaling when cultured on stiff substrates (Szeto et al., 2016). This association with matrix stiffness and renal fibrosis is supported by shear wave elastography (SWE) stiffness measurements of the renal cortex in diabetic kidney disease (DKD), which have shown that the stiffness of the renal cortex in patients with DKD can reach values of E = 30 kPa in comparison to healthy human kidney being in the range of E = 6–11 kPa (Hassan et al., 2016). Regional differences in kidney stiffness have also been identified by magnetic resonance elastography (MRE) measurements, with the renal sinus being stiffer (G’ ∼6.8 kPa) than the medullary region (G’ ∼5.5 kPa), which was found to be stiffer than the renal cortex (G’ ∼4.3 kPa) (Bensamoun et al., 2011). This highlights a spatial gradient of stiffness in the kidney from the cortex to the sinus. Given its influence on cell fate, correlation with disease states, and spatial regions of the kidney, it is likely that stiffness and surrounding viscoelasticity of the tissue play a major role in kidney development and, consequently, kidney organoid formation from hiPSCs.
Mechanically tunable hydrogels can be used to control the stiffness of the microenvironment kidney organoids differentiate within. Hydrogels are 3D structures composed of physically- or chemically crosslinked polymeric chains that can absorb and retain large amounts of water. One particularly interesting class of physically crosslinked hydrogel we have recently utilized as a mechanically tunable support matrix for kidney organoid growth and maturation is β-sheet forming self-assembling peptide hydrogels, or SAPHs (Treacy et al., 2023). SAPHs are physically crosslinked hydrogels composed of short amino acid sequences that undergo self-assembly into fibrillar-like β-sheet-rich structures above a critical gelation concentration to form a high-water content hydrogel network (Gao et al., 2017; Wychowaniec et al., 2020). One popular SAPH design is based on peptides with alternating hydrophobic and hydrophilic residues that allow for the self-assembly process to take place via intermolecular hydrogen bonding and hydrophobic interactions (Gao et al., 2017; Yokoi et al., 2005). Their mechanical properties can be tuned by altering peptide concentration, amino acid sequence, and solvent electrolyte ion composition (Gao et al., 2017). The SAPHs we have used to grow kidney organoids, Alpha5 and Alpha4 PeptiGels, were both shown to have similar in vivo-like fibrillar ECM microstructure via TEM whilst being mechanically distinct as shown through rheological measurements, thereby providing a means to compare the effects of soft (Alpha4) and stiff (Alpha5) microenvironments on cell fate determination in the context of hiPSC-derived kidney organoid maturation (Treacy et al., 2023).
Here, we describe a protocol for the encapsulation of hiPSC-derived nephron progenitor aggregates and their maturation into kidney organoids within hydrogels. The protocol primarily describes organoid encapsulation and maturation within the above-mentioned SAPHs. We also describe a protocol that utilizes gelatin methacryloyl (GelMA) hydrogels for organoid encapsulation, which has been the focus of more recent work in our group. The GelMA hydrogel formulations, comprised of 5% (w/v) GelMA and 15% (w/v) GelMA, with 0.5% (w/v) Irgacure-2959, roughly mimic the mechanical properties of Alpha4 and Alpha5 SAPHs, respectively. GelMA hydrogel formation relies on UV crosslinking to form crosslinked polymeric hydrogels.
In Basic Protocol 1, we present a method for the encapsulation of hiPSC-derived nephron progenitor aggregates within SAPHs and their subsequent maturation to form kidney organoids. The Alternate Protocol describes the same procedure but with (GelMA) hydrogels. In Basic Protocols 2 and 3, we detail the immunofluorescence imaging and sample preparation techniques required for the characterization of kidney organoids generated using either the SAPH or GelMA protocol. Support Protocol 1 describes the culture of hiPSCs, which are required to produce kidney organoids. Support Protocol 2 describes the fixation of kidney organoids in paraformaldehyde (PFA) and is a prerequisite step for either Basic Protocol 2 or Basic Protocol 3.
CAUTION : hiPSCs are a Biosafety Level 2 (BSL-2) hazard. Follow all appropriate guidelines and regulations for the use and handling of hiPSCs.
NOTE : All work related to hiPSC culture or the generation and maturation of hiPSC-derived kidney organoids must be performed with strict adherence to aseptic technique. All related materials used must also be sterile.
STRATEGIC PLANNING
Before beginning this protocol, the user should be competent in the technique of culturing human induced pluripotent stem cells (hiPSCs). Support Protocol 1 describes in detail the feeder-free culture of hiPSCs. It is essential that high-quality hiPSCs are used for the completion of this protocol to ensure satisfactory and reproducible results (see Fig. 1). hiPSCs should be checked regularly for their level of pluripotency, genetic abnormalities, and contamination. We regularly use the StemCell Technologies PCR-based hPSC Genetic Analysis Kit (StemCell Technologies, cat. no. 07550) to test for genetic abnormalities. However, it is important to note that this kit only tests for the most commonly observed genetic abnormalities in PSCs. Karyotyping can also be employed to test for genetic abnormalities (Taapken et al., 2011). Maintenance of pluripotency throughout routine hiPSC culture can be assessed in various ways, including visual assessment of hiPSC morphology (see Fig. 1), qPCR analysis of pluripotency markers such as OCT4, NANOG, and SOX2, and by trilineage differentiation assay using a trilineage differentiation kit. (e.g., StemCell Technologies, cat. no. 05230). hiPSCs should also be tested regularly for mycoplasma contamination. hiPSCs should be cultured for a minimum of 2–3 passages after thawing from liquid nitrogen storage before being used for differentiation experiments (Uhrig et al., 2022).
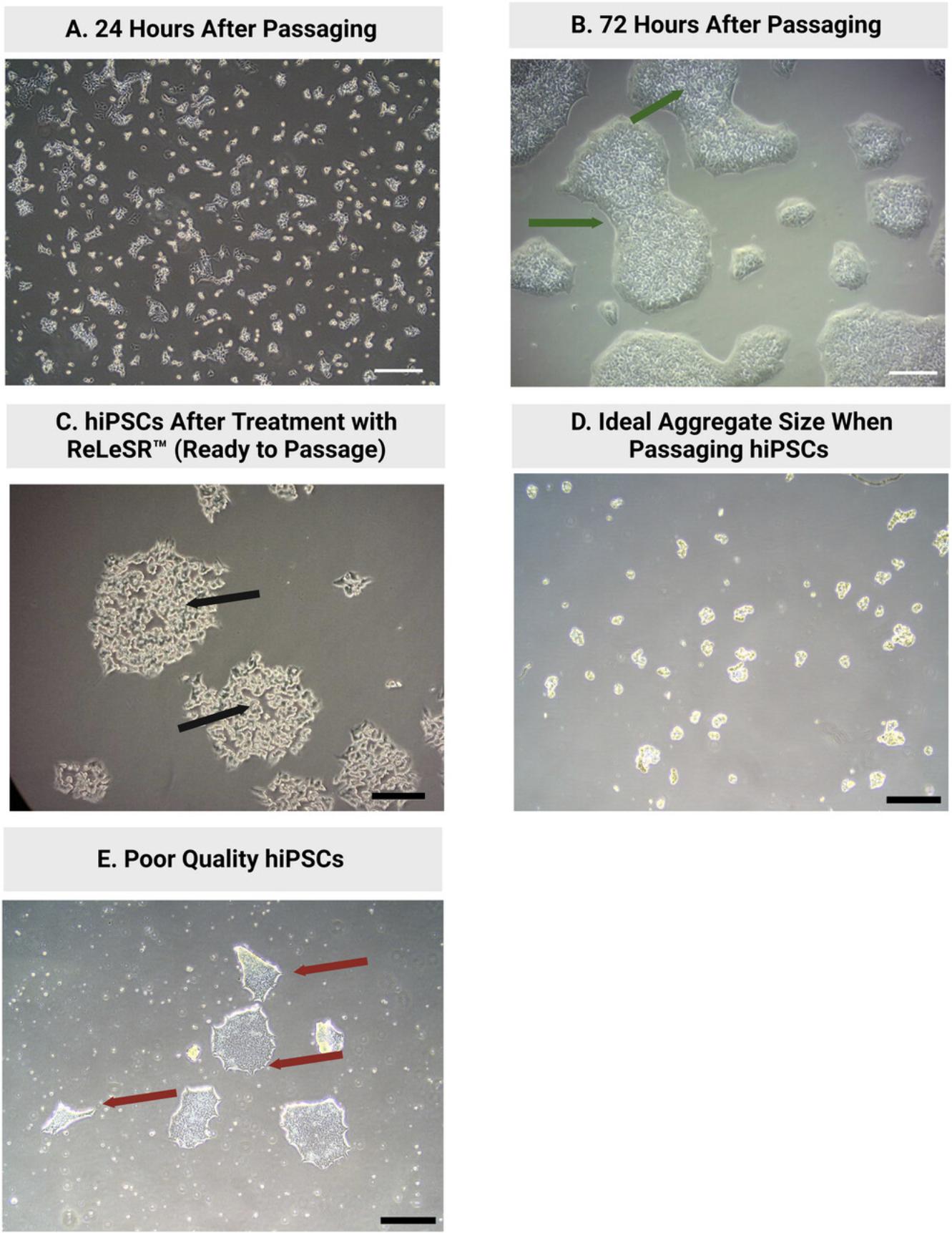
As a core component of this kidney organoid differentiation protocol, the user should possess some SAPHs for the encapsulation of nephron progenitor aggregates on day 9 of the protocol. SAPHs with similar biophysical properties to Alpha4 and Alpha5 (Treacy et al., 2023) can be purchased from Cell Guidance Systems (https://www.cellgs.com/).
The biophysical and mechanical properties of the hydrogel(s) employed by the user when attempting this protocol should be comprehensively characterized. Properties such as mechanical strength, porosity, and swelling behavior can all influence kidney organoid differentiation and maturation. Techniques used to measure peptide hydrogel microstructure, such as nanofiber width, typically involve electron microscopy techniques, such as transmission electron microscopy (TEM). In our work, TEM micrographs revealed a nanofiber width in the 3–4 nm range for both the soft (Alpha4) and stiff (Alpha5) SAPHs used for growing kidney organoids (Treacy et al., 2023), which closely resembles in vivo fibrillar ECM components (Prince & Kumacheva, 2019). The storage modulus (G’) of a hydrogel is typically measured using rheology or atomic force microscopy (AFM) (Norman et al., 2021; Zuidema et al., 2014). Hydrogel mechanical parameters such as stiffness and swelling should be monitored throughout organoid growth. Characterization methods for hydrogels have been reviewed more comprehensively elsewhere (Raghuwanshi & Garnier, 2019).
Lastly, the biocompatibility of the hydrogels for organoid culture should also be considered and tested. This can be done relatively easily with LIVE/DEAD staining kits (e.g., Invitrogen, cat. no. L3224). For further information on how to conduct a LIVE/DEAD assay on hydrogel-encapsulated organoids, please refer to Treacy et al., 2023.
These pre-requisite considerations required for planning a kidney organoid hydrogel maturation experiment are summarized in Figure 2A.
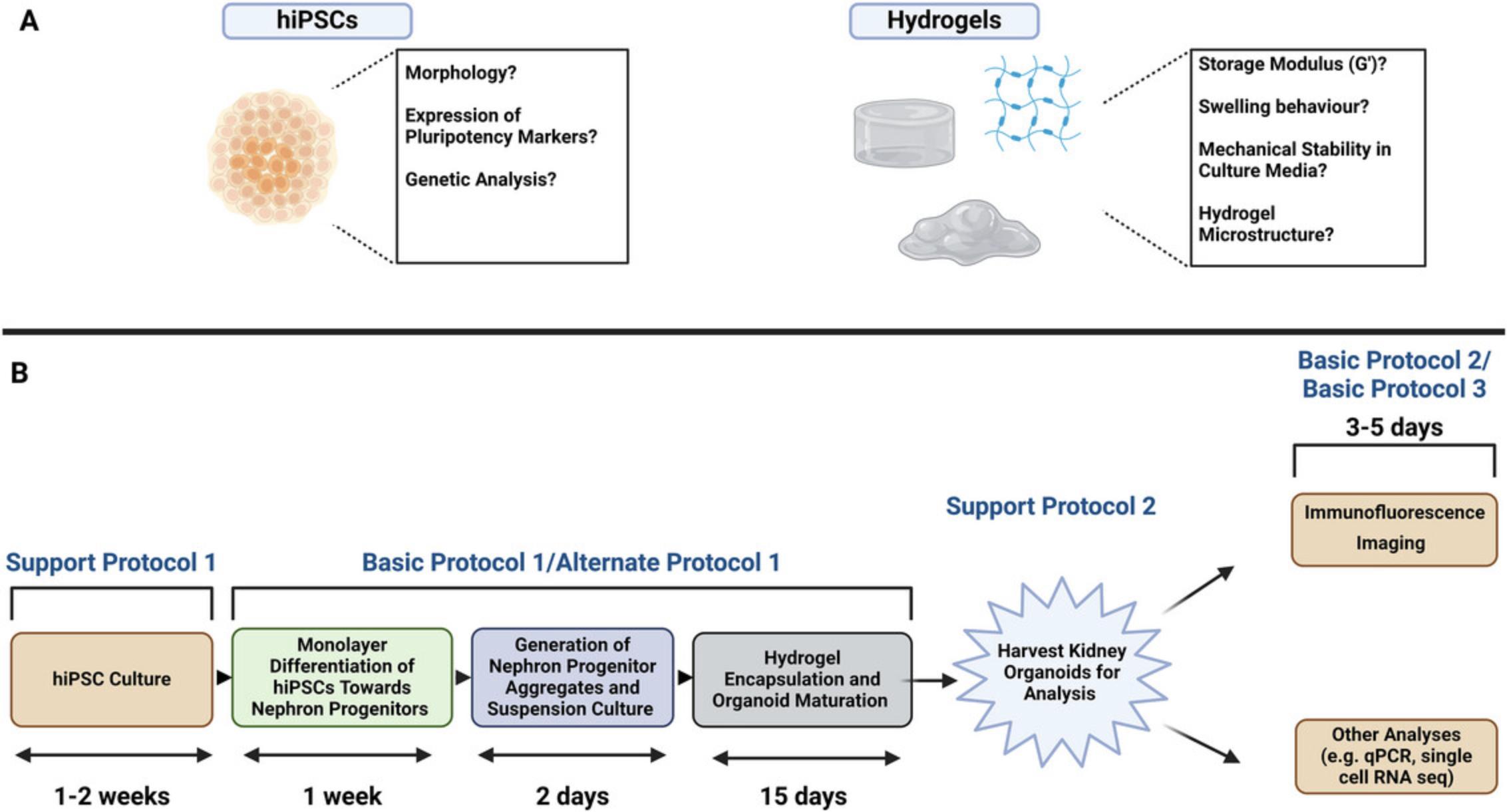
Basic Protocol 1: DIRECTED DIFFERENTIATION OF HUMAN INDUCED PLURIPOTENT STEM CELLS (hiPSCs) INTO KIDNEY ORGANOIDS AND MATURATION WITHIN MECHANICALLY TUNABLE SELF-ASSEMBLING PEPTIDE HYDROGELS (SAPHs)
In this protocol, we describe the method for generating kidney organoids from hiPSCs and their subsequent growth and maturation in SAPHs of defined storage modulus. The first 7 days of the protocol are adapted from Takasato et al. (2015) and involve the directed differentiation of hiPSCs into a mixed kidney progenitor population by day 7 (Fig. 3). To do this, CHIR99021, a GSK-3 inhibitor, is used to indirectly promote WNT signaling and induce differentiation to posterior primitive streak (PPS) by day 3.Subsequent addition of recombinant human fibroblast growth factor 9 (FGF9), with heparin as a cofactor, is used to induce intermediate mesoderm (IM) and generate nephron progenitor populations. These progenitor cells are used to form nephron progenitor aggregates on day 7 of the protocol. Following this, a 48 hr suspension culture is used to facilitate compaction of nephron progenitor aggregates before encapsulation within hydrogels on day 9 of the protocol (see Fig. 3). If performed correctly, using high-quality hiPSCs, kidney organoids containing glomerular structures with podocytes, tubular epithelia and supporting stromal cells can be generated using this protocol (Fig. 4). At the end of the protocol, the organoids can be fixed or harvested for other analyses by the user.
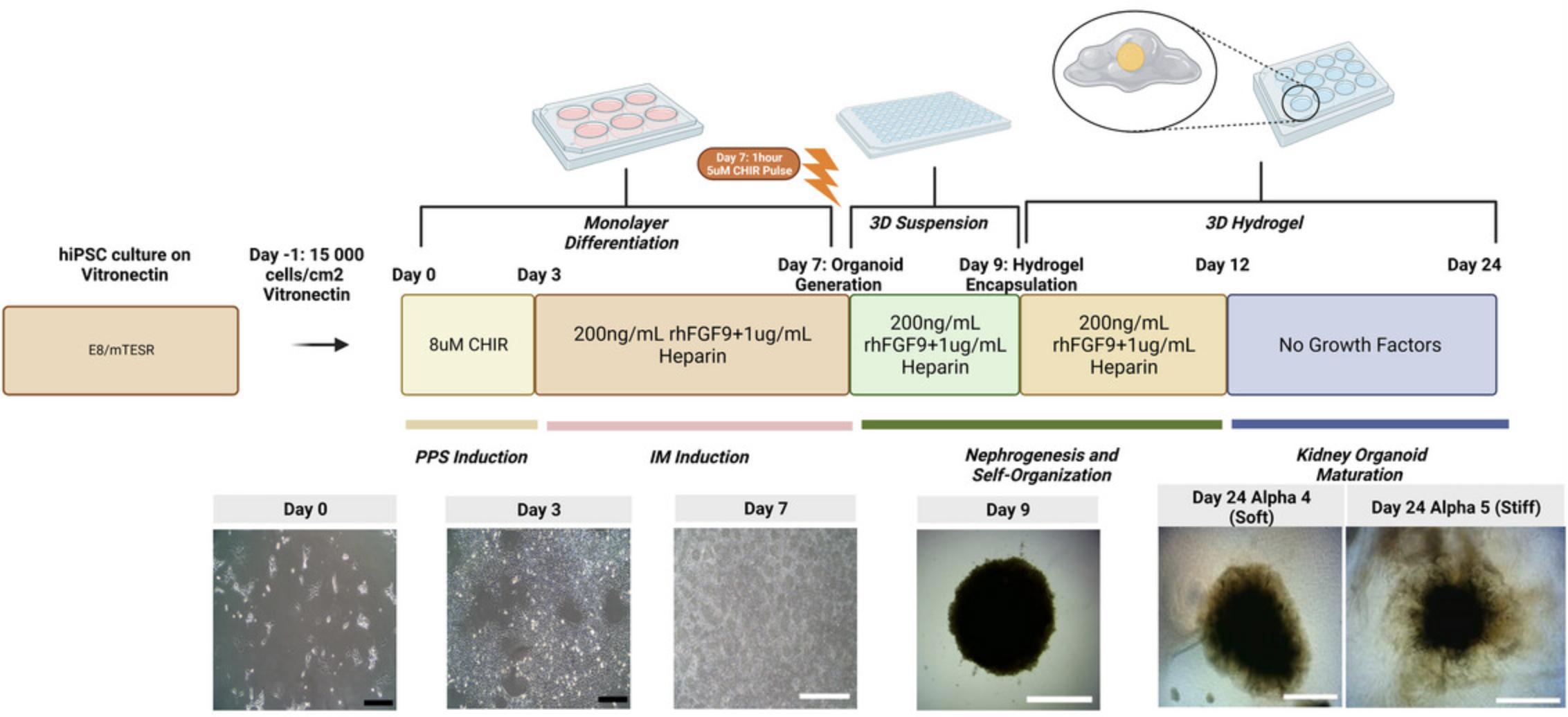
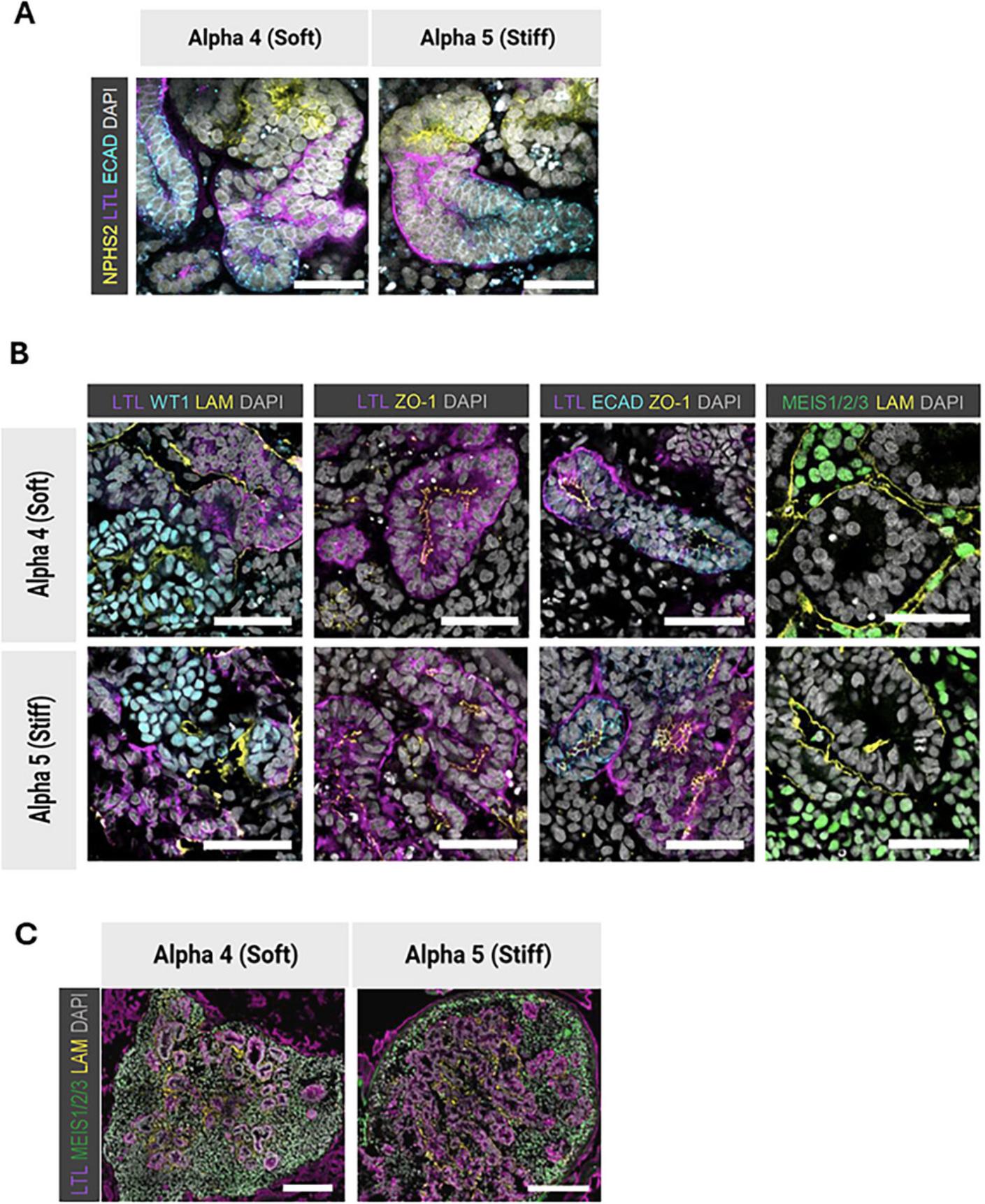
Materials
-
Human induced pluripotent stem cells (hiPSCs, ideally HPSI1213i-babk2 line ECACC, cat. no. 66540098; Wellcome Trust Sanger Institute, Cambridge, UK)
-
Vitronectin XF (StemCell Technologies, cat. no. 07180)
-
10 mM ROCK inhibitor Y-27632 (see recipe)
-
ReLeSR™ (StemCell Technologies, cat. no. 05872)
-
Alternative: TrypLE™ Express-with Phenol Red (Thermo Fisher Scientific cat. no. 12605010)
-
mTeSR™ Plus (‘mTESR’) (StemCell Technologies, cat. no. 100-0276)
-
0.05% Trypsin-EDTA (Gibco, cat. no. 25300062) (see recipe)
-
Alternative: TrypLE™ Express-with phenol red (Thermo Fisher Scientific cat. no. 12605010)
-
DPBS, no calcium, no magnesium (Thermo Fisher Scientific, cat. no. 14190144)
-
Basal APEL medium (STEMdiff™ APEL™2 Medium with 5% PFHM, 1% Antibiotic/Antimycotic 100×)
-
0.4% Trypan blue stain (Thermo Fisher Scientific, cat. no. 15250061)
-
8 µM CHIR99021 in basal APEL medium (see recipe)
-
5 µM CHIR99021 pulse medium (see recipe)
-
200 ng/ml rhFGF9, 1 µg/ml sterile filtered heparin in basal APEL medium (see recipe)
-
Low-glucose DMEM (DMEM, low glucose, GlutaMAX™ supplement, pyruvate supplemented with 10% (v/v) fetal bovine serum and 100 U/ml penicillin, 100 µg/ml streptomycin) (see recipe)
-
Self-assembling peptide hydrogels (SAPHs)
-
SAPHs similar to the hydrogels used by our group (Alpha4 and Alpha5) to grow kidney organoids can be purchased at https://www.cellgs.com/items/defined-surfaces-and-ecms/PeptiGels.html
-
Corning® Costar® non-tissue culture treated 6-well plates (Corning, cat. no. 3736)
-
96-well clear round-bottom ultra-low attachment (ULA) microplates (Corning, cat. no. 7007)
-
12-well tissue culture plates (e.g., Greiner CELLSTAR® 12 well culture plates, Sigma-Aldrich, cat. no. M8687)
-
6-well tissue culture plates (e.g., Greiner CELLSTAR® 6 well culture plates, Sigma-Aldrich, cat. no. M8562)
-
Micropipettes (P2/P10/P200/P1000) (e.g., Gilson, cat. no. FA10006M, FA10005P FA10003P, FA10001P)
-
P200 wide bore tips (e.g., Thermo Fisher Scientific, cat. no. 2069G)
-
Serological 10-ml or 5-ml pipettes (e.g., Greiner Bio-One, cat. no. 607180)
-
Positive displacement micropipette (e.g., Gilson, cat. no. FD10005)
-
Cell culture incubator, set to 37°C, 5% CO2 (e.g., Thermo Fisher Scientific, cat. no. 51023121)
-
15-ml conical tubes, sterile (e.g., Corning, cat. no. 05-538-53F)
-
Sterile or autoclavable 1.5-ml microcentrifuge tubes (e.g., Greiner Bio-One, cat. no. 616201)
Plating hiPSCs for differentiation (Day −1)
1.Coat the required number of wells of a 6-well plate with 10 µg/ml Vitronectin XF 1 hr prior to beginning the differentiation experiment (as described in Support Protocol 1).
2.Detach hiPSCs using ReLeSR, as described in Support Protocol 1.Collect the resulting cell suspension in a 15-ml conical tube.
3.Alternative : More recently, we have adapted the protocol below for the detachment of hiPSCs with TrypLE™ Express at this stage of the procedure. First, aspirate medium from hiPSCs and wash once with DPBS.
4.Incubate colonies with 300 µl TrypLE™ Express for 3 min in a 37°C/5%CO2 incubator.
5.Neutralize the TrypLE™ Express with 2 ml mTESR and transfer suspension to a 15-ml conical tube.
6.Centrifuge the cell suspension at 120 × g for 4 min at room temperature.
7.Resuspend cells in 2 ml mTESR. Pipette gently (2–3 times) when resuspending, and do not over-pipette.
8.Count viable cells using a hemocytometer. Take a representative 50 µl aliquot of cell suspension and mix with 50 µl Trypan blue dye in a sterile 1.5-ml microcentrifuge tube. Then, pipette the suspension up and down 5–10 times to generate small cell clusters. Pipette 10 µl of the cell suspension into the hemocytometer and manually count the viable cells.
9.Calculate the volume of the cell suspension needed to seed 15,000 cells/cm2 per well.
10.Wash the Vitronectin XF-coated 6-well plates with DPBS, as described in Support Protocol 1.
11.Add the cell suspension containing 144,000 cells per well of a 6-well plate and bring to a final volume of 2 ml with mTESR.
12.Add 2 µl of 10 mM ROCK inhibitor Y-27632 to each well containing the medium added in Step 5 (final concentration: 10 µM).
13.Gently move the plate side to side and towards and away from you 2–3 times to ensure an even distribution of hiPSCs aggregates on the plate surface.
14.Culture the iPSCs in a 37°C/5%CO2 incubator for 24 hr prior to commencing differentiation.
Monolayer differentiation of hiPSCs into nephron progenitor cells (days 0 to 7)
Once iPSC colonies have reached 10% to 20% confluency (see day 0 image, Fig. 3), directed differentiation towards kidney organoids can commence.
15.On day 0, make up the required amount of 8 µM CHIR99021 basal APEL medium.
16.Aspirate mTESR from iPSC colonies and wash once in DPBS to remove any residual mTESR and ROCK inhibitor.
17.Commence differentiation by adding 2 ml basal APEL medium containing 8 µM CHIR99021 per well.
18.On day 2, exchange the spent medium with freshly made 8 µM CHIR99021 basal APEL medium.
19.On day 3, remove spent medium and increase the volume to 4 ml basal APEL medium containing 200 ng/ml rhFGF9 and 1 µg/ml heparin.
20.On day 5, exchange the spent medium with 4 ml fresh 200 ng/ml rhFGF9, 1 µg/ml heparin basal APEL medium.
Day 6: Preparation for generating nephron progenitor aggregates
Due to the labor-intensive nature of generating nephron progenitor aggregates on day 7 and aggregate encapsulation with hydrogels on day 9, we recommend preparing some materials in advance:
21.Prepare stock of basal APEL medium for organoid generation.
22.Aliquot the required amount of low glucose DMEM (see recipe) into a 50-ml conical tube and store at 4°C.
23.Thaw a tube of aliquoted 0.05% Trypsin-EDTA and store at 4°C.
24.If not using pre-bought P200 wide bore tips for aggregate transfer steps on days 7 and 9, it is possible to prepare these from standard tips. To do so, cut P200 tips roughly 1–2 cm from the tip end and pass them through a lighter or Bunsen burner flame. Sterilize the prepared wide-bore tips by autoclaving.
Aggregation of nephron progenitors and 3D suspension culture (days 7 to 9)
It is important to work quickly on these days. Progressing too slowly can negatively impact cell viability and subsequent self-organization and maturation of the organoids.
25.On day 7, remove the spent medium and incubate 1 hr at 37°C/5% CO2 in basal APEL medium containing 5 µM CHIR99021 (5 µM CHIR99021 pulse medium; see recipe) to induce nephrogenesis.
26.Meanwhile, prepare the required number of sterile 1.5-ml microcentrifuge tubes for organoid generation by filling each tube with 250 µl basal APEL medium containing 200 ng/ml rhFGF9 and 1 µg/ml heparin.
27.Following the 1-hr incubation, wash cells once with DPBS and add 300 µl of 0.05% Trypsin-EDTA per well.
28.Incubate the cells with trypsin in a 37°C/5%CO2 incubator for 2 min.
29.Following incubation, neutralize trypsin with 3 ml low-glucose DMEM supplemented with 10% (v/v) FBS, 100 U/ml penicillin, and 100 µg/ml streptomycin.
30.Pipette the cell suspension 3–4 times while washing down any cells that may still be adhered in the process.
31.Collect the cell suspension in a 15-ml conical tube and centrifuge at 400 × g and room temperature for 3 min.
32.Resuspend cells in 3 ml (per well of a six-well plate) basal APEL medium containing 200 ng/ml rhFGF9 and 1 µg/ml heparin. Pipette gently while doing so, approximately 4–6 times.
33.Perform a cell count as described in Step 8 of this protocol and add enough cell suspension to achieve 500,000 cells per microcentrifuge tube containing the 250 µl basal APEL medium with 200 ng/ml rhFGF9 and 1 µg/ml heparin media (Step 26).
34.Centrifuge the tubes four times at 400 × g and room temperature for 2 mins, performing 180° horizontal rotations between each centrifugation.
35.Following centrifugation, use a wide-bore P200 tip to individually transfer organoids from the microcentrifuge tubes to the 96-well ULA round-bottom plate.
36.Fill each well of the 96-well ULA round-bottom plate with 150 µl of basal APEL medium containing 200 ng/ml rhFGF9 and 1 µg/ml heparin.
37.Once all aggregates have been transferred to the 96-well ULA round-bottom plate, place in a 37°C/5%CO2 incubator. Leave undisturbed for 48 hr.
Encapsulation of Day 9 nephron progenitor aggregates into SAPHs (days 9 to 12) (see Fig. 5 schematic)
Following 48-hr suspension culture, Day 9 nephron progenitor aggregates are ready for encapsulation within SAPHs of variable mechanical strength.
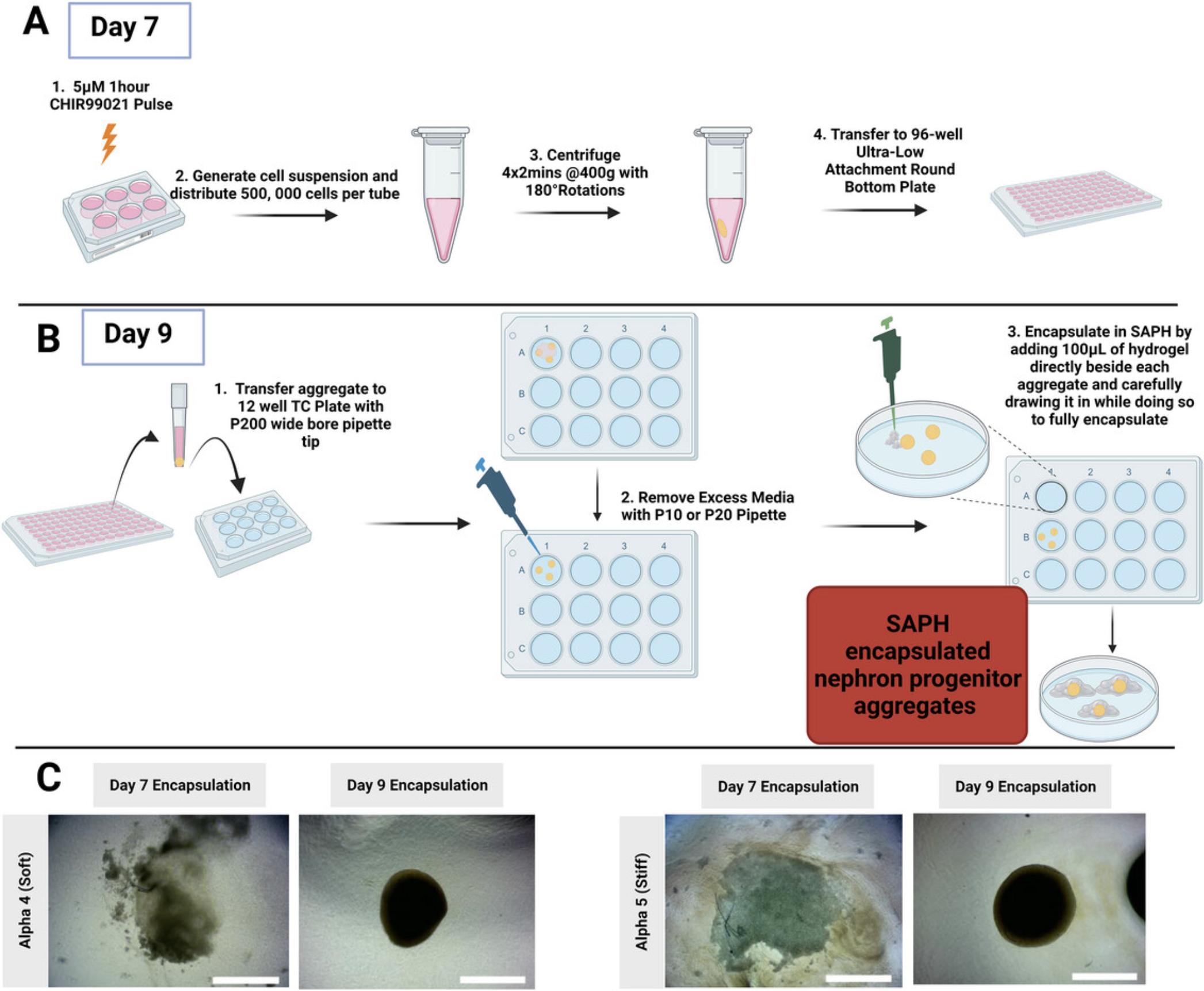
38.Transfer three organoids, one at a time, from the 96-well ULA plate into a 12-well plate with minimal medium carryover:
Using a wide-bore P200 tip, take up a small volume of the medium before aspirating the aggregate into the wide-bore tip. Once you can confirm the aggregate is in the tip by eye (it should appear like a small white pellet/dot), let it fall by gravity to the bottom of the tip by pointing the tip straight down. Then, release onto the surface of a 12-well plate without carrying over any additional medium.
39.Remove as much medium as possible around the aggregates with a P10 or P20 pipette. Point the tip away from the aggregate to avoid sucking it up inside the tip.
40.Using a positive displacement pipette, dispense 100 µl of the desired PeptiGel or other SAPH directly beside each aggregate while carefully drawing it in such that the aggregate is taken up into the hydrogel.
41.Once all aggregates are encapsulated, add 1 ml freshly prepared 200 ng/ml rhFGF9, 1 µg/ml heparin basal APEL medium per well of a 12-well plate and culture in a 37°C/5%CO2 incubator.
42.On day 10, exchange the spent medium with the same volume of freshly prepared 200 ng/ml rhFGF9, 1 µg/ml heparin basal APEL medium.
Maturation of kidney organoids (days 12 to 24)
From day 12, encapsulated organoids self-organize and mature in basal APEL medium in the absence of growth factors or small molecules.
43.On day 12, replace the spent medium with basal APEL medium without growth factors.
44.Exchange the spent medium with fresh basal APEL medium every 2–3 days until day 24–26.
Alternate Protocol: ENCAPSULATION OF DAY 9 NEPHRON PROGENITOR AGGREGATES IN GELATIN METHACRYLOYL (GelMA) HYDROGELS
As part of our ongoing work, we recently evaluated the potential utility of chemically crosslinked matrices such as gelatin methacryloyl (GelMA) as matrix supports for the growth of kidney organoids. We synthesize GelMA monomers via a chemical process involving a reaction of porcine gelatin type-A with methacrylic anhydride. This results in the replacement of lysine and hydroxyl lysine amino acid residues within gelatin with methacryloyl groups. Details on the synthesis of GelMA can be found elsewhere (Yue et al., 2015). For our kidney organoid studies, we have consistently used lyophilized GelMA monomer with a degree of methacrylate functionalization (DoF) of 59% to 63%. GelMA monomer with a similar DoF can also be purchased from commercial vendors.
GelMA monomers must be crosslinked via photopolymerization to form a hydrogel support matrix for the developing organoid. We use Irgacure-2959 for this purpose at a final concentration of 0.5% (w/v). The Irgacure-2959 is dissolved in 100% ethanol at a concentration of 25% (w/v) before being added to the GelMA hydrogel solution. The 5% (w/v) GelMA hydrogels have a storage modulus of 400–1000 Pa, while 15% (w/v) GelMA hydrogels have a storage modulus of 6000–8000 Pa. We have used both GelMA hydrogel formulations to grow kidney organoids.
Materials
-
Day 9 hiPSC-derived nephron progenitor aggregates in 96-well clear round-bottom ultra-low attachment (ULA) microplates generated using Basic Protocol 1 Steps 1–37.
-
GelMA with a DoF of 59% to 63% (e.g., Sigma-Aldrich; cat. no. 900622-1G)
-
Irgacure-2959 (Sigma-Aldrich, cat. no. 410896)
-
DPBS, no calcium, no magnesium (Thermo Fisher Scientific, cat. no. 14190144)
-
Basal APEL medium (STEMdiff™ APEL™2 Medium with 5% PFHM, 1% Antibiotic/Antimycotic 100×) (see recipe)
-
200 ng/ml rhFGF9, 1 µg/ml sterile filtered heparin in basal APEL medium (see recipe)
-
UV light source capable of a radiation power of 16 mW/cm2 and a wavelength of 365 nm (Fig. 6)
-
12-well tissue culture plates (e.g., Greiner CELLSTAR® 12 well culture plates, Sigma-Aldrich, cat. no. M8687)
-
6-well tissue culture plates (e.g., Greiner CELLSTAR® 6 well culture plates, Sigma-Aldrich, cat. no. M8562)
-
Micropipettes (P2/P10/P200/P1000) (e.g., Gilson, cat. no. FA10006M, FA10005P FA10003P, FA10001P)
-
P200 wide-bore tips (e.g., Thermo Fisher Scientific, cat. no. 2069G)
-
Serological 10- or 5-ml pipettes (e.g., Greiner Bio-One, cat. no. 607180)
-
37°C, 5% CO2 cell culture incubator (e.g., Thermo Fisher Scientific, cat. no. 51023121)
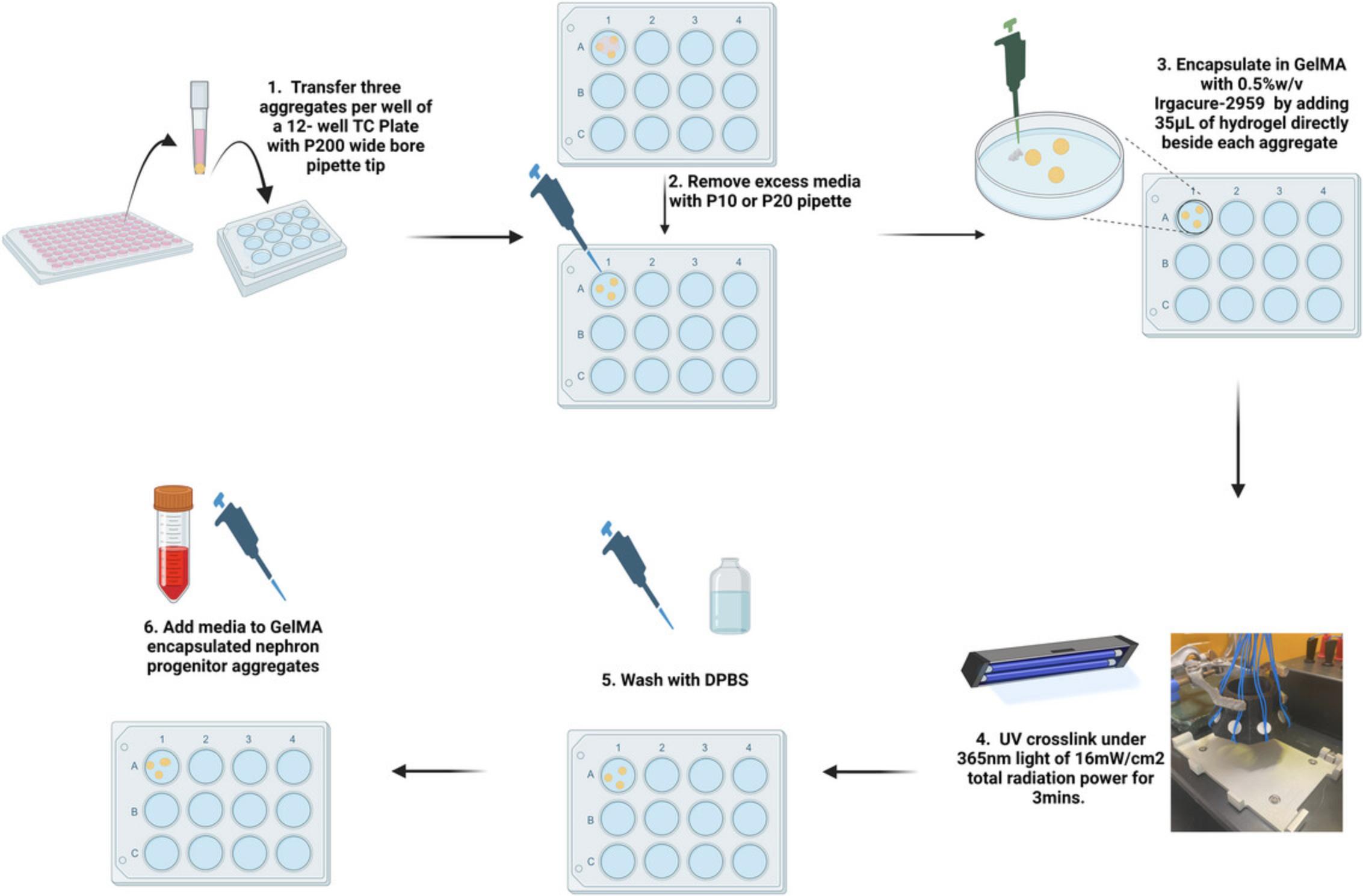
Procedure (see Fig. 6 schematic)
1.Pre-warm GelMA monomer solutions to 50°C for 1 hr prior to proceeding
2.Follow the same procedure (Steps 38 and 39) as with encapsulation in SAPHs up to the point of removing excess medium from around the organoids (see Fig. 5)
3.Using a P100 pipette set to 35 µl, slowly add the warm hydrogel solution beside an aggregate so that it is drawn into the hydrogel drop.
4.Ensure each aggregate is centered within its droplet of GelMA hydrogel solution. If aggregates are on the edge of a droplet, center them by taking up a small volume of GelMA hydrogel solution with a P2 tip and ‘nudging’ the aggregate gently towards the center.
5.Follow Steps 2 and 3 above until all 2–3 aggregates in the well are encapsulated within GelMA hydrogel droplets.
6.Photo-crosslink the hydrogels encapsulating the aggregates by placing them under a 365-nm UV light source capable of producing UV light with a radiation power of 16 mW/cm2 and a wavelength of 365 nm.
7.Turn on the UV light to crosslink the hydrogel for exactly 180 s.
8.After crosslinking, perform three 5-min washes in DPBS, with the last wash taking place in a 37°C/5%CO2 incubator.
9.Following the last wash, add 1 ml of 200 ng/ml rhFGF9, 1 µg/ml heparin basal APEL medium per well of a 12-well plate or 2 ml if using a 6-well plate.
10.Replace medium on the following day (day 10) and follow the same procedure as with SAPHs from this point on (Steps 43 and 44).
Support Protocol 1: HUMAN INDUCED PLURIPOTENT STEM CELL (hiPSC) CULTURE
In this protocol, we describe the cell culture methods required to maintain hiPSCs. hiPSCs are created by direct cellular reprogramming of human somatic cells. These cells can give rise to multiple cell types, including all three embryonic lineages. hiPSCs are derived from adult somatic cells through the ectopic expression of a combination of the pluripotency factors KLF4, OCT3/4, SOX2, C-MYC, and NANOG. Methods for hiPSC generation have been described elsewhere (Bayart et al., 2013). hiPSCs are morphologically akin to embryonic stem cells (ESCs) with round shapes, large nucleoli, and scant cytoplasm. Additionally, iPSCs form sharp-edged, flat, and tightly packed colonies (see Fig. 1). They are also mitotically active, actively self-renewing, and proliferate and divide at a rate equal to that of ESCs. hiPSCs express human embryonic stem cell (hESC) specific markers such as TRA-1-60, Nanog, TRA1-81, Oct3/4, Sox2, and REX1 (Reviewed in Nordin et al., 2011)
This procedure for hiPSC maintenance has been optimized to the HPSI1213i-babk_2 line derived from human dermal fibroblasts obtained from the Wellcome Trust Sanger Institute in Cambridge, UK. This maintenance protocol describes a feeder-free culture system for hiPSCs on a vitronectin-coated substrate using mTeSRTM Plus medium (mTESR).
Materials
-
Human induced pluripotent stem cells (hiPSCs, HPSI1213i-babk2 line ECACC, cat. no. 66540098; Wellcome Trust Sanger Institute, Cambridge, UK)
-
Vitronectin XF (StemCell Technologies, cat. no. 07180)
-
10 mM ROCK inhibitor Y-27632 (see recipe)
-
ReLeSR™ (StemCell Technologies, cat. no. 05872)
-
mTeSR™ Plus (StemCell Technologies, cat. no. 100-0276)
-
DPBS, no calcium, no magnesium (Thermo Fisher Scientific, cat. no. 14190144)
-
DMSO (Dimethyl sulfoxide) (Sigma-Aldrich, cat. no. D2650)
-
hESC grade fetal bovine serum (FBS) (Gibco, cat. no. 10270-106)
-
Corning® Costar® non-treated 6-well plates (Corning, cat. no. 3736)
-
Micropipettes (P2/P10/P200/P1000) (e.g., Gilson, cat. no. FA10006M, FA10005P FA10003P, FA10001P)
-
Serological 10- or 5-ml pipettes (e.g., Greiner Bio-One, cat. no. 607180)
-
37°C, 5% CO2 incubator (e.g., Thermo Fisher Scientific, cat. no. 51023121)
-
15-ml conical tubes, sterile (e.g., Corning, cat. no. 05-538-53F)
-
Mr. Frosty (Thermo Fisher Scientific, cat. no. 5100-0001)
-
Cryoprotective vials (e.g., Greiner Bio-One, cat. no. 126278)
Thawing frozen hiPSCs from liquid nitrogen storage
1.One hour before thawing out iPSCs, coat two wells of an untreated 6-well plate with vitronectin. Add 80 µl freshly thawed vitronectin XF per 2 ml DPBS (no calcium, no magnesium) in a 15-ml tube to a final concentration of 10 µg/ml. Roll the 15-ml tube gently to mix the contents (avoid bubble formation and do not vortex) and add 1 ml diluted vitronectin per well of the 6-well plate. Add another 1 ml DPBS on top of the well to ensure complete coating. Gently rock the plate to evenly coat the wells and incubate at room temperature for 60 min.
2.Prepare a 12.5-ml stock of mTESR containing 10 µM ROCK inhibitor.
3.Partially thaw a frozen vial of iPSCs using a water bath heated to 37°C until a small ice crystal remains.
4.Add 1 ml mTESR containing 10 µM ROCK inhibitor dropwise to the cryovial, then gently collect and transfer the entire contents to a 15-ml conical tube.
5.Add 7 ml mTESR with 10 µM ROCK inhibitor to cell suspension.
6.Centrifuge at 120 × g and room temperature for 3 min.
7.Meanwhile, gently tilt the plate now coated with vitronectin XF to allow the excess to collect at the edge. Remove the excess solution using a pipette or by aspiration. Ensure the coated surface is not scratched.
8.Wash the coated plate three times with DPBS, employing the same technique as in Step 7.
9.Add 1 ml of 10 µM ROCK inhibitor mTESR into each of the two vitronectin XF-coated wells.
10.Gently resuspend the cell pellet in 2 ml of 10 µM ROCK inhibitor mTESR.
11.Check cells under the microscope to ensure the plated iPSC aggregates are adequately sized (Fig. 1D)
12.Incubate the plate in a 37°C/5% CO2 incubator for 24 hr before replenishing with mTESR without ROCK inhibitor.
Passaging hiPSCs
Once colonies are at 60% to 80% confluency, have been on the same plate for ∼4 days, or have moderate levels of differentiated cells, hiPSCs should be passaged (Fig. 1B). The protocol below describes iPSC passaging using the EDTA-based dissociation reagent ReLeSr™. This is the protocol used in our lab and has been optimized for the HPSI1213i-babk_2 line. The passaging method and protocol may differ between hiPSC lines.
13.Prior to passaging, warm mTESR and DPBS to room temperature (15°C to 25°C).
14.60 min prior to passaging, coat the desired number of wells of a 6-well plate with vitronectin XF.
15.Remove spent medium from the now confluent well of iPSCs and wash the cells with 1 ml DPBS.
16.Using a P1000, remove DPBS and add 400 µl ReLeSR. Gently rock the plate, and once the entire surface is covered with ReLeSR, immediately aspirate to expose colonies to a thin film of liquid.
17.Incubate cells at 37°C for 6–8 min. Monitor cell morphology under a phase contrast microscope. A gradual separation between cells within hiPSC colonies should be observed over the period of ReLeSR™ incubation.
18.Meanwhile, aspirate vitronectin from the coated plate, ensuring that the coated surface is not scratched.
19.Wash the coated plate three times with DPBS.
20.Remove DPBS and add an appropriate amount of mTESR to cover the coated surface (e.g., 1 ml on a 6-well plate) to ensure the vitronectin does not dry out.
21.Add 2 µl of 10 mM ROCK inhibitor Y-27632 to the prepared well. This will ensure the final concentration of ROCK inhibitor in the medium is 10 µM once hiPSCs are transferred following passaging and the medium is brought to a 2-ml volume.
22.Following incubation with ReLeSR, and once colonies have detached from one another (Fig. 1C), hold the plate with one hand and use the other hand to firmly tap the sides of the plate 15–20 times to aid in lifting/separating the cells in colonies.
23.Once appropriate separation is obtained within colonies, add 1 ml mTESR. Again, hold the plate with one hand and use the other hand to firmly tap the sides of the plate for approximately 15–30 s to aid in detaching the colonies.
24.Using a 5-ml pipette, take up the medium with the detached cells and wash the well gently three times using this cell suspension. View detached cells under a microscope. Cell aggregates should be appropriately sized for plating (mean aggregate size of approximately 50–200 µm at 10× magnification). Aggregates should range in size from 4–12 cells (Fig. 1D).
25.If detached cell aggregates are mainly in large clumps (>50–200 µm at 10× magnification), then the cell suspension should be pipetted gently up and down and monitored using the phase contrast microscope.
26.Split cells according to Table 1.
Confluency before passage | mTeSR plus split ratio |
---|---|
<50% | 1:6 |
50%–60% | 1:8-1:10 |
70%–80% | 1:12-1:14 |
Gently pipette the required amount of the aggregate suspension mixture (depending on the split ratio) up and down and transfer (dropwise) to the vitronectin-coated plate containing mTESR.
27.Bring to required volume (2 ml per well of a 6-well plate).
28.Place the plate in a 37°C/5%CO2 incubator. Move the plate in several quick, short, back-and-forth and side-to-side motions to evenly distribute the cell aggregates. Do not disturb for 24 hr.
29.Visually assess cultures to monitor growth until the next passage. Feed every 48 hours. If feeding over the weekend, a double feed of 4 ml medium can be done.
Cryopreservation of hiPSCs
30.Prepare a freezing medium consisting of 10% DMSO, 40% hESC-grade FBS, and 50% mTESR.
31.Passage hiPSCs as previously described. After washing the well in mTESR, transfer the suspension to a 15-ml tube and centrifuge at 150 × g and room temperature for 3 min.
32.Resuspend the pellet in 1 ml, 2 ml, or 3 ml freezing medium.
33.Dispense 1 ml of the cell suspension generated in Step 2 into pre-chilled, sterile cryo-protective vials and place at −80°C in a pre-chilled Mr. Frosty overnight.
34.The next day, place frozen hiPSC vials into liquid nitrogen storage for an indefinite period.
Support Protocol 2: ORGANOID FIXATION WITH PARAFORMALDEHYDE (PFA)
Proper fixation of kidney organoid samples following a differentiation experiment is crucial for the preservation of organoid structure and the antigenicity of proteins of interest, both of which are necessary for reliable results when conducting immunofluorescence imaging characterization of kidney organoids. A 1-hr fixation with 2% PFA at 4°C has been found to be a suitable fixation protocol for kidney organoids generated using the methods described here. The PFA fixation protocol presented below applies as a prerequisite step for both Basic Protocol 2 (whole-mount immunofluorescence) and Basic Protocol 3 (immunofluorescence of cryosections).
CAUTION : PFA is highly toxic. Any work related to PFA fixation or handling of PFA waste must be done while wearing appropriate PPE and in a laminar flow hood.
Materials
-
hiPSC-derived kidney organoids
-
2% paraformaldehyde (PFA; see recipe)
-
DPBS, no calcium, no magnesium (Thermo Fisher Scientific, cat. no. 14190144)
-
Stereomicroscope (e.g., Bresser cat. no. 5803850)
1.Remove medium from hydrogel-encapsulated organoids.
2.Wash with 1–2 ml DPBS per well (assuming 12-well plate format) on a rocker at low speed for 5 min at room temperature.
3.Fix organoids in 2% (w/v) PFA at 4°C for 1 hr on a rocker set to low speed.
4.Wash organoids three times for 5 min each in ice-cold DPBS.
5.Using a scalpel and a fine spatula, remove as much of the excess hydrogel surrounding the organoids as possible.
Basic Protocol 2: WHOLE-MOUNT IMMUNOFLUORESCENCE OF KIDNEY ORGANOIDS
Whole-mount immunofluorescence imaging allows for 3D visualization of organoid microstructure without the need for sectioning. This technique requires optical clearing to avoid refractive index mismatches that can occur between different cellular components in 3D. The whole-mount immunofluorescence protocol used by our lab is based on a fructose-glycerol tissue-clearing method described by Dekkers et al. (2019). This protocol should allow the user to image fixed and cleared hiPSC-derived kidney organoids grown within hydrogels in three dimensions without the need for sectioning.
Materials
- Fixed hiPSC-derived kidney organoids in DPBS
- DPBS, no calcium, no magnesium (Thermo Fisher Scientific, cat. no. 14190144)
- Blocking buffer (see recipe)
- Streptavidin and Biotin Blocking Kit (Vector Laboratories, cat. no. SP-2002)
- Primary and secondary antibodies (Table 2, Table 3)
- Hoescht33342 trihydrochloride (Invitrogen, cat. no. H3570)
- Triton X-100 (Sigma Aldrich, cat. no. T8787)
- Fructose-glycerol clearing solution (see recipe)
Antibody | Source | Supplier | cat. no. | Primary antibody dilution (in blocking buffer) | Secondary Antibody Dilution (in 5% Goat serum) |
---|---|---|---|---|---|
E-Cadherin (ECAD) | Mouse | BD Biosciences, | 610181 | 1:300 | 1:500 |
Laminin | Rabbit | Sigma-Aldrich | L9393 | 1:300 | 1:500 |
LTL | Biotinylated | Vector Laboratories | B-1325 | 1:200 | 1:400a |
MEIS1/2/3 | Mouse | Santa Cruz | sc-101850 | 1:100 | 1:200 |
NPHS2 | Rabbit | Sigma-Aldrich | P0372 | 1:300 | 1:500 |
WT1 | Mouse | Santa Cruz | cs-7385 | 1:100 | 1:200 |
Z0-1 | Rabbit | Invitrogen | 61-7300 | 1:300 | 1:500 |
- a
LTL secondary antibody (DyLight® 649 Streptavidin) is diluted in DPBS and is not included in the overnight incubation with other secondary antibodies.
2° Antibody or Stain | Supplier | Cat no. |
---|---|---|
Alexa Fluor 488 goat anti-rabbit IgG (H+L) | Invitrogen | A-11034 |
Alexa Fluor 568 goat anti-mouse IgG (H+L) | Invitrogen | A-11004 |
Alexa Fluor 555 donkey anti-goat IgG (H+L) | Invitrogen | A-21432 |
Texas Red goat anti-rabbit IgG (H+L) | Invitrogen | T-2767 |
DyLight® 649 Streptavidin | Vector Laboratories | SA-5649 |
Hoechst 33342 Trihydrochloride | Invitrogen | H3570 |
- Oven/hotplate (e.g., Sigma Aldrich cat. no. Z761249)
- Fine spatula (e.g., Thermo Fisher Scientific, cat. no. 21-401-10)
- µ-Slide 8-well chamber slide (Ibidi, cat. no. 80806)
- 48-well plates (e.g., Greiner Bio-one, cat. no. 677180)
- Confocal microscope for immunofluorescence imaging (e.g., Leica SP8 confocal microscope)
1.Transfer organoids using a fine spatula to 48-well plates for further processing.
2.Aspirate DPBS from fixed organoid samples.
3.Replace DBPS with 300 µl blocking buffer per well of the 48-well plate. Incubate at room temperature for a minimum of 2 hr on a rocker set to low speed.
4.While blocking, prepare primary antibodies in blocking buffer (see Table 2). Prepare 150 µl of each antibody solution per well at 2× the concentration shown in Table 2.
5.If staining with LTL primary antibody, an additional blocking step with the Streptavidin/Biotin Blocking Kit (Vector Laboratories, cat. no. SP-2002) is necessary to block endogenous biotin. First, remove 150 µl blocking solution per well.
DPBS washes
6.Add 500 µl DPBS (per well). Wait 2 min, then remove 500 µl.
7.Add 500 µl DPBS (per well). Wait 2 min, then remove 500 µl.
Streptavidin block
8.Add 150 µl streptavidin blocking solution (provided in the kit) per well.
9.Incubate on a rocker set to low speed at room temperature for a minimum of 20 min.
10.Remove 150 µl streptavidin blocking solution per well.
DPBS washes
11.Add 500 µl DPBS (per well). Wait 2 min, then remove 500 µl.
12.Add 500 µl DPBS (per well). Wait 2 min, then remove 500 µl.
13.Add 500 µl DPBS per well and incubate on a rocker set to low speed at room temperature for 5 min.
14.Remove 500 µl from each well.
15.Add 500 µl DPBS (per well). Wait 2 min, then remove 500 µl.
16.Add 500 µl DPBS (per well). Wait 2 min, then remove 500 µl.
Biotin block
17.Add 150 µl biotin blocking solution per well.
18.Incubate on a rocker set to low speed at room temperature for a minimum of 20 min.
19.Remove 150 µl biotin blocking solution per well.
DPBS washes
20.Add 500 µl DPBS (per well). Wait 2 min, then remove.
21.Add 500 µl DPBS (per well). Wait 2 min, then remove 500 µl.
22.Add 500 µl DPBS per well and incubate on a rocker set to low speed at room temperature for 5 min.
23.Remove 500 µl from each well.
24.Add 500 µl DPBS (per well). Wait 2 min, then remove 500 µl.
25.Add 500 µl DPBS (per well). Wait 2 min, then remove 500 µl.
Primary antibody incubation
26.Remove 150 µl blocking buffer and add 150 µl primary antibody solution at 2× the concentration given in Table 2 per well of the 48-well plate.
27.Incubate overnight at 4°C on a rocker set to low speed.
Three 1 hr washes in 0.1%Triton X-100
28.Remove 150 µl primary antibody solution and add 500 µl of 0.1%Triton X-100 in DPBS per well.
29.Wait 2 min, then remove 500 µl from each well.
30.Add 500 µl of 0.1% Triton X-100 per well and incubate at room temperature for 1 hr on a rocker set to low speed to wash the organoids.
31.Remove 500 µl from each well.
32.Add 500 µl of 0.1%Triton X-100 to each well, wait 2 min, then remove 500 µl from each well.
33.Add 500 µl of 0.1% Triton X-100 per well and incubate at room temperature for 1 hr on a rocker set to low speed to wash the organoids.
34.Remove 500 µl from each well.
35.Add 500 µl of 0.1%Triton X-100 to each well, wait 2 min, then remove 500 µl from each well.
36.Add 500 µl of 0.1% Triton X-100 per well and incubate at room temperature for 1 hr on a rocker set to low speed to wash the organoids.
Secondary antibody incubation
37.During this final wash step, prepare secondary antibodies at 2× the concentration in Table 3 in 5% goat serum containing 1:750 Hoescht33342 trihydrochloride.
38.Remove 500 µl from each well. Each well should now contain 150 µl.
39.Add 150 µl secondary antibody (prepared in Step 17) per well.
40.Fully cover the plate with tin foil to protect it from light and incubate overnight at 4°C on a rocker set to low speed.
LTL secondary antibody incubation and three 1-hr washes in 0.1%Triton X-100
If not staining for LTL, repeat Steps 28-36 to complete three 1 hr washes in 0.1%Triton X-100, then move on to Step 48
41.Prepare DyLight® 649 Streptavidin secondary antibody in 0.1% Triton X-100 at 2× concentration (see Table 3)
42.Remove 150 µl primary antibody solution and add 500 µl 0.1% Triton X-100 in DPBS per well.
43.Wait 2 min, then remove 500 µl from each well.
44.Add 150 µl DyLight® 649 Streptavidin secondary antibody in 0.1% Triton X-100 at 2× concentration per well. Incubate on a rocker set to low speed at room temperature for 1 hr.
45.Remove 150 µl from each well. There should now be 150 µl remaining in each well.
46.Add 500 µl 0.1%Triton X-100. Wait 2 min, then remove 500 µl.
47.Repeat Steps 32–36 for an additional two 1-hr washes in 0.1% Triton X-100 in DPBS.
DPBS washes
48.Remove 500 µl from each well.
49.Add 500 µl DPBS (per well). Wait 2 min, then remove 500 µl.
50.Add 500 µl DPBS (per well). Wait 2 min, then remove 500 µl.
Fructose-glycerol clearing
51.Transfer organoids to µ-Slide 8-well chamber slide using P1000 or P200 wide-bore tips.
52.Add fructose-glycerol clearing reagent (200 µl per well of the µ-Slide 8-well dish) to the organoids. Use a positive displacement pipette to avoid bubbles.
53.Incubate at room temperature with clearing reagent for a minimum of 20 min before imaging on a confocal microscope.
Basic Protocol 3: IMMUNOFLUORESCENCE OF ORGANOID CRYOSECTIONS
Immunofluorescence imaging using organoid cryosections can also be used in place of or in conjunction with the whole-mount immunofluorescence techniques described above for the characterization of hiPSC-derived kidney organoids. This mode of imaging allows comparatively deeper organoid access compared to the whole-mount staining protocol described, as it obviates the issues of antibody and imaging penetration depth, which are known limitations of whole-mount immunofluorescence imaging. However, preparing cryosections may be more technically challenging for the user. The cryosection immunofluorescence protocol involves first cryopreserving fixed organoid tissue in a sucrose gradient followed by cryoembedding in a gelatin-sucrose mixture. Gelatin is used to improve section quality by providing homogeneity and mechanical support. The frozen organoid tissue blocks generated with this protocol are then cut into sections for immunofluorescence staining and imaging using a cryotome, such as the Leica CM1950 Rotary Microtome.
Materials
-
Fixed hiPSC-derived kidney organoids in DPBS
-
Sucrose (Sigma-Aldrich, cat. no. S0389)
-
DPBS, no calcium, no magnesium (Thermo Fisher Scientific, cat. no. 14190144)
-
Blocking buffer (see recipe)
-
Streptavidin and Biotin Blocking Kit (Vector Laboratories, cat. no. SP-2002)
-
Primary and secondary antibodies (Table 2 and Table 3)
-
Hoescht33342 Trihydrochloride (Invitrogen, cat. no. H3570)
-
Ibidi Mounting medium (Ibidi, cat. no. 50001)
-
Gelatin (Sigma - Aldrich, cat. no. G1890)
-
OCT (Tissue-Tek, Cat. no. 4583)
-
Isopropanol (2-propanol) (Thermo Fisher Scientific, cat. no. 383910025)
-
Dry ice (e.g., Polar Ice Ltd. Dry Ice Pellets, 9 mm)
-
Small square weighing boats (e.g., VWR, cat. no. 10803-138)
-
Scalpel (e.g., VWR, cat. no. 233-0034)
-
Oven/Hotplate (e.g., Sigma Aldrich, cat. no. Z761249)
-
Tin foil (e.g., Millbrook Foods Market Premier Catering Foil 90 m)
-
Plastic forceps (e.g., Sigma Aldrich, cat. no. Z708356)
-
Fine spatula (e.g., Thermo Fisher Scientific, cat. no. 21-401-10)
-
1- or 2- graduated glass beaker (e.g., Sigma Aldrich, cat. no. CLS10032L)
-
Micropipettes (P2/P10/P200/P1000) (e.g., Gilson, cat. no. FA10006M, FA10005P FA10003P, FA10001P)
-
Cryotome for sectioning of frozen organoid tissue (e.g., Leica CM1950 rotary microtome for sectioning frozen tissues)
-
SuperFrost Plus™ Adhesion slides (EprediaTM, Thermo Fisher Scientific, cat. no. 10149870)
-
Glass coverslips suitable for confocal immunofluorescent microscopy (e.g., Eisco Labs, cat. no. BI0094GBR)
-
Hydrophobic pen/PAP pen (e.g., Vector Laboratories, cat. no. H-4000)
-
Fast-drying clear nail polish (e.g., Catrice Quick Dry Top Coat)
-
Autoclave tape (e.g., VWR, cat. no. 489-1311)
-
Permanent marker/lab marker (e.g., Staedtler Lumocolour Pen Permanent Fine Black)
-
Slide storage boxes (e.g., Sigma Aldrich, cat. no. Z374385)
-
Freezer capable of −80°C. (e.g., Haier Biomedical, cat. no. SLS1092)
-
Confocal microscope for immunofluorescent imaging (e.g., Leica SP8 confocal microscope)
Cryoprotecting and cryoembedding
1.Prepare 10% (w/v), 15% (w/v), and 30% (w/v) sucrose in DPBS (see recipe).
2.Remove DPBS from organoids (now in a 48-well plate following PFA fixation) and incubate in 10% (w/v) sucrose solution for 2 hr at 4°C.
3.Remove 10% sucrose and incubate in 15% sucrose for 3 hr at 4°C.
4.Remove 15% sucrose and incubate in 30% sucrose overnight at 4°C.
5.The next day, using a serological pipette, add 1 ml of 10% sucrose/7.5% gelatin into a small weigh boat, ensuring that the entire bottom is covered.
6.Solidify in a fridge (2°C to 8°C) for 5 to 10 min.
7.Replace the 30% sucrose solution on organoids with 10% sucrose/7.5% gelatin and allow it to equilibrate for 15 min at 37°C.
8.Meanwhile, prepare an ice-cold bath using isopropanol and dry ice. Isopropanol should be placed in a container surrounded by dry ice or dry ice pellets. Cool isopropanol for a minimum of 20 min before use.
9.Carefully transfer the organoids to the solidified gelatin/sucrose mixture in the weigh boat. Avoid bubbles.
10.Fill weigh boats containing organoids and solidified gelatin/sucrose with more 10% sucrose/7.5% gelatin so that the organoid is fully covered. Set for 15 min at 4°C.
11.Meanwhile, test the freezing time of gelatin/sucrose blocks in the isopropanol bath that was prepared. Do this by dropping a block of polymerized gelatin/sucrose without an organoid into the isopropanol bath for 2 min. Remove, cut in half, and see if the middle is frozen. Repeat if necessary and increase the time until an appropriate freezing time is found.
12.Cut out the gelatin/sucrose blocks containing the organoids (now covered with solidified 10% sucrose/7.5% gelatin) using a sharp scalpel, ensuring that the block is straight.
13.Drop the gelatin/sucrose blocks containing the organoid samples into the isopropanol bath and allow them to freeze for the allocated time (tested in Step 11 above), then remove the frozen block to a piece of tissue paper using plastic forceps.
14.Quickly dry off isopropanol, then wrap samples in appropriately labeled tinfoil.
15.Store at −80°C until sectioning.
Cryosectioning
16.Equilibrate the organoid tissue gelatin/sucrose block to the temperature within the cryostat for 10 min. Do this by placing it inside the cryostat.
17.Mount frozen block on metal disk/chuck using OCT.
18.Cut sections at anywhere from 8 to 20 µm and avoid rolling by using an anti-roll plate and a fine brush to unroll and position sections for collection.
19.Collect sections on Superfrost Plus slides
20.Allow sections to dry on the slide, then store at −80°C.
Immunofluorescence of cryosections
When adding or removing solutions during the following incubations and wash steps, always pipette slowly and gently while pointing the pipette tip away from the organoid on the slide. This will prevent the sample from getting damaged or lifting off.
Preparation of slides
21.Allow the slides to equilibrate to room temperature overnight. Do this by leaving them with the sample side facing up on a piece of tissue paper covered by a box or other suitable object to prevent dust or moisture from getting on them.
22.Draw around sections with a hydrophobic marker.
23.Rehydrate the slides for 15 min in DPBS. Slowly add 50–100 µl DPBS to each hydrophobic marker-traced section. Incubate at room temperature for 15 min.
Blocking and antibody incubations
24.Block slides for 1 hr at room temperature in 50–200 µl blocking buffer per slide.
25.Remove blocking solution.
26.Wash sections twice with DPBS
27.Add 10–30 µl (depending on the size of the section) streptavidin blocking solution (provided in the streptavidin/biotin blocking kit) per organoid section. Incubate at room temperature for 15 min.
28.Remove streptavidin blocking solution and wash twice with DPBS.
29.Remove DPBS and add 10–30 µl biotin blocking solution (provided in the streptavidin/biotin blocking kit) per organoid section. Incubate in a humid chamber at room temperature for 15 min.
30.Wash sections twice with DPBS.
31.Add 50–200 µl primary antibody at the appropriate dilution in blocking buffer (see Table 2) per slide overnight at 4°C in a humid chamber.
32.Wash slides three times for 5 min in DPBS.
33.Add secondary antibodies (see Table 3) and incubate overnight at 4°C in a humid chamber.
34.Wash slides three times for 5 min in DPBS.
35.Prepare DyLight® 649 Streptavidin secondary antibody in DBPS (see Table 3).
36.Add 50–200 µl secondary antibody per slide.
37.Incubate 15 min at room temperature.
38.Wash slides three times for 5 min in DPBS.
39.Meanwhile, prepare coverslips by first cleaning with 100% ethanol, then air-dry.
Mount with Ibidi mounting media and seal with nail polish
40.Pipette 10–30 µl of the mounting medium directly onto each section.
41.Carefully lower the coverslip at an angle before dropping it down when it is almost touching the slide.
42.Seal the corners and edges with small dabs of fast-drying nail polish.
43.Leave the slides at room temperature, protected from light for a minimum of 2 hr to ensure appropriate sealing.
44.Store slides in a dedicated slide box or proceed to imaging on a confocal microscope (suggested: Leica SP8 confocal microscope).
Imaging on the Leica SP8 confocal microscope
A Leica SP8 confocal microscope was used for the images presented in this protocol article, which are adapted from Treacy et al. (2023). Z-stacks of organoid sections or whole-mount fructose-glycerol cleared organoids were acquired with system optimized z step size. Raw files were exported from the microscope software, and Fiji/ImageJ software was used to process the images. Brightness and contrast settings were adjusted, and maximum intensity projections of z-stacks were used for visualization. Immunofluorescence imaging of hiPSC-derived kidney organoids generated using the described protocols should confirm the expression and nephron-like structural organization of key renal markers, including WT1, NPHS2 (podocytes), Laminin (basement membrane), LTL (proximal tubules), ECAD (distal tubules), and Meis1/2/3 (stromal cells) (see Fig. 4).
REAGENTS AND SOLUTIONS
Basal APEL medium
Thaw freshly purchased STEMdiff™ APEL™2 Medium at 2°C to 8°C overnight.
- To make 10 ml basal APEL medium (STEMdiff™ APEL™2 Medium supplemented with 5% PFHM, 1% Antibiotic/Antimycotic 100×):
- 9.4 ml STEMdiff™ APEL 2 medium (StemCell Technologies, cat. no. 05275)
- 100 µl Antibiotic-Antimycotic 100× (Gibco, cat. no. 15240062)
- 500 µl PFHM (Protein-Free Hybridoma Media) (Gibco, cat. no. 12040077)
Basal APEL medium can be stored at 2°C to 8°C for up to 2 weeks. Warm to room temperature before use. Never warm in a 37°C water bath.
Blocking buffer (0.06% Triton X, 5% goat serum in DPBS)
- 38 ml DPBS, no calcium, no magnesium (Gibco, cat. no. 5814190094)
- 2 ml goat serum (Sigma-Aldrich, cat. no. G9023)
- 24 µl Triton X-100 (Sigma-Aldrich, cat. no. T8787)
Aliquot and store indefinitely at −20°C. Thaw at room temperature before use.
CHIR99021, 8 mM stock solution
- 2.5 mg CHIR99021 (Sigma-Aldrich, cat. no. SML1046)
- 672 µl DMSO (Sigma-Aldrich, cat. no. D2650)
Dissolve CHIR99021 in DMSO and mix by pipetting to ensure homogeneity. Aliquot in 20–50 µl aliquots and store for up to 6 months at −20°C.
CHIR99021 APEL medium, 8 µM
- 10 ml basal APEL medium
- 10 µl of 8 mM CHIR99021 stock solution
Warm to room temperature before use. Make up fresh each time. Any leftover medium should be stored at 2°C to 8°C and used within 3 days.
CHIR99021 pulse medium, 5 µM
- 10 ml basal APEL medium
- 6.25 µl of 8 mM CHIR99021 stock solution
Warm to room temperature before use. Make up fresh each time. Any leftover medium should be stored at 2°C to 8°C and used within 3 days.
Cryoembedding solution (10% Sucrose/7.5% Gelatin)
- 500 ml DPBS, no calcium, no magnesium (Gibco, cat. no. 5814190094)
- 50 g sucrose (Sigma-Aldrich, cat. no. S0389)
- 37.5 g type-A gelatin (Sigma-Aldrich, cat. no. G2500)
Dissolve using a stirrer bar and a heated stirring plate set to 37°C while gradually adding sucrose powder. Once the 10% sucrose solution is dissolved (solution is homogenously clear), slowly add the gelatin powder to the heated stirring plate set to 37°C until homogenously dissolved. Aliquot 45 ml into 50-ml tubes and freeze at −20°C. Store no longer than one year.
Fructose-glycerol clearing solution (2.5 M fructose, 60%v/v glycerol)
- Slowly dissolve 45 g fructose (Sigma-Aldrich, cat. no. F0127) in 40 ml dH2O in a graduated glass beaker on a hotplate set to 40°C to 50°C, stirring with a magnetic stirrer.
- Slowly add 60 ml of 100% glycerol (Sigma-Aldrich, cat. no. 104093) to the stirred and heated solution.
- Stir on a hotplate set to 40°C to 50°C until the solution appears clear and homogenous.
- Bring to 100 ml with dH2O to account for evaporation.
Store at 2°C to 8°C for up to 2 weeks. Bring to room temperature (19°C to 23°C) before use.
CAUTION: Solutions are very viscous. Pipette slowly and carefully to avoid introducing bubbles.
GelMA hydrogel monomer
- Dissolve 0.5 to 1.5 g GelMA monomer (e.g., Sigma-Aldrich; cat. no. 900622-1G) in 9.5 ml deionized water at 37°C overnight to achieve a concentration of 5% to 15% (w/v) GelMA.
- Add Irgacure-2959 (Sigma-Aldrich, cat. no. 410896) dissolved in 100% ethanol to the GelMA solution to achieve a concentration of 0.5% (w/v).
- Warm at 50°C for 1 hr.
- Mix thoroughly by gently pipetting solutions up and down, avoiding bubble formation, before passing through a 0.22-µm PES filter (e.g., Merck, cat. no. GPWP02500) to sterilize.
- Store at 2°C to 8°C protected from light for up to 3 months.
Goat serum, 5%
- 47.5 ml DPBS, no calcium, no magnesium (Gibco, cat. no. 5814190094)
- 2.5 ml goat serum (Sigma-Aldrich, cat. no. G9023)
Aliquot and store indefinitely at −20°C. Thaw at room temperature before use.
Heparin, 1 mg/ml
- 50 mg heparin (Sigma-Aldrich, cat. no. H4784)
- 50 ml dH2O
Dissolve heparin in water and mix well to ensure homogeneity before passing through a 0.22-µm PES filter (e.g., Merck, cat. no. GPWP02500) to sterilize. Store at 2°C to 8°C for up to a year.
Low-glucose DMEM (DMEM, low glucose, GlutaMAX™ Supplement, pyruvate supplemented with 10% (v/v) fetal bovine serum and 100 U/ml penicillin, 100 µg/ml streptomycin)
- 450 ml DMEM, low glucose, GlutaMAX™ Supplement, pyruvate (Gibco, cat. no. 10567014)
- 50 ml fetal bovine serum (Gibco cat. no. 10270106)
- 5 ml of 10 000 U/ml penicillin, 10,000 µg/ml streptomycin (Gibco cat. no. 15140122)
Heat in a 37°C water bath until all are liquid. Combine and invert the container to mix components together thoroughly. Store at 2°C to 8°C up to the date of expiry. Heat to 37°C before use.
mTESR1 complete medium (‘mTESR’)
Thaw the mTeSR™1 Complete Kit (StemCell Technologies, cat. no. 85850) at 2°C to 8°C overnight before adding the entire contents of the 5× supplement to the basal medium. Mix thoroughly by inverting. Aliquot and store at 2°C to 8°C for up to 2 weeks or at −20°C until expiry. Frozen complete mTeSRTM Plus may be thawed once—do NOT repeatedly freeze-thaw. Bring to room temperature before use.
Paraformaldehyde, 2% (PFA)
- 70 ml DPBS, no calcium, no magnesium (Gibco, cat. no. 5814190094).
- 10 ml of 16% PFA (Electron Microscopy Sciences, cat. no. 15710)
CAUTION: PFA is highly toxic. Wear sufficient PPE and perform all work with PFA in a laminar flow hood. Store at room temperature or at 2°C to 8°C indefinitely.
rhFGF9, 100 µg/ml
- X1 50 µg vial Recombinant Human Fibroblast Growth Factor 9 (rhFGF9) (StemCell Technologies, cat. no. 78161.1)
- 500 µl of 0.1% w/v sterile bovine serum albumin (BSA) (Sigma-Aldrich, cat. no. A1595) in DPBS
Thaw rhFGF9 at room temperature and reconstitute in 500 µl of 0.1% BSA. Using a P1000 pipette, pipette the solution up and down several times to fully dissolve rhFGF9 and homogenize the solution. Aliquot in sterile microcentrifuge tubes and store for up to 6 months at −80°C.
rhFGF9, 200 ng/ml; sterile-filtered heparin APEL medium, 1 µg/ml
- 10 ml basal APEL medium
- 20 µl of 100 µg/ml rhFGF9 stock solution
- 10 µl of 1 mg/ml sterile filtered heparin stock solution
Warm to room temperature before use. Make up fresh each time. Any leftover medium should be stored at 2°C to 8°C and used within 3 days.
ROCK inhibitor Y-27632, 10 mM
- 10 mg lyophilized ROCK inhibitor Y-27632 (Tocris, cat. no. 1254)
- 3.12 ml sterile dH2O
Mix thoroughly, incubate for 3 min at room temperature, aliquot into sterile microcentrifuge tubes, and store for up to 6 months at −20°C. Avoid repeated freeze-thawing. Y-27632 is light-sensitive, so special care should be taken to avoid and minimize exposure to light.
Sucrose in DPBS, 30% (w/v)
- 500 ml DPBS, no calcium, no magnesium (Gibco, cat. no. 5814190094)
- 150 g sucrose (Sigma-Aldrich, cat. no. S0389)
Dissolve using a stirrer bar and a heated stirring plate set to 37°C while gradually adding sucrose powder. Store up to 2 weeks at 2°C to 8°C prior to use. Use this to make up 10% and 15% (w/v) sucrose solutions during cryoprotection of organoids.
Trypsin-EDTA, 0.05% (Gibco, cat. no. 25300062)
- Thaw frozen bottle overnight at 2°C to 8°C. Aliquot and store at −20°C until date of expiry.
COMMENTARY
Background Information
The generation of kidney organoids entirely from hPSCs was first described in 2015 by Takasato et al. and Morizane et al. The design of these (and future) kidney organoid generation protocols was guided by a knowledge of in vivo renal development processes and pathways (reviewed in Krause et al., 2015). Thus, to generate kidney organoids from hPSCs (human pluripotent stem cells), both groups (Morizane et al., 2015; Takasato et al., 2015) used comparable strategies, first differentiating hPSCs towards a mesodermal lineage, which ultimately produced a population of multipotent nephron progenitors in monolayer 2D culture. These progenitors were then aggregated into a three-dimensional mass of cells, which was then further matured in 3D “organotypic” culture conditions, self-organizing to form a kidney organoid over the course of roughly 2 weeks. The 3D environment for organoid maturation was provided either through suspension culture (Morizane et al., 2015) or Transwell air-liquid interface insert culture (Takasato et al., 2015). The kidney organoids generated in these landmark studies were shown to consist of a cellularly heterogeneous mixture of renal cell types, including renal interstitial cells, endothelial cells, and proximal and distal tubule epithelia, all organized in a manner resembling the segmented structure of the human nephron.
However, transcriptional analysis of kidney organoids generated using these methods revealed they were the most similar to first-trimester fetal kidneys (Wu et al., 2018). Moreover, attempts to culture kidney organoids using these protocols for longer periods of time to drive further maturation proved unsuccessful, with no evidence of further tubular or glomerular maturation with stromal expansion and a fibrotic phenotype being observed upon prolonged in vitro culture (Geuens et al., 2021; van den Berg et al., 2018). A single-cell transcriptomic study conducted by Wu et al. in 2018 also showed that such kidney organoids had immature transcriptional profiles compared to fetal and adult kidneys and consisted of up to 20% ‘off-target’ non-renal cell types such as neurons and muscle-like cells.
The above-mentioned barriers to translational utility prompted researchers in the field to investigate various strategies for improving the generation of hPSC-derived kidney organoids with the aim of advancing structural, transcriptional, and functional maturity. As mentioned previously, various biochemical-based approaches yielded some considerable improvements in this regard, including improved higher-order structure (Taguchi & Nishinakamura, 2017) and vascularization (Low et al., 2019). However, the knowledge that both the biochemical and the biophysical properties of the extracellular microenvironment influence cell fate determination and tissue morphogenesis (reviewed in Vining et al., 2017; Muncie & Weaver, 2018; Bissell et al., 1982) soon prompted many to investigate how the mechanical properties of the extracellular microenvironment may influence the maturation of kidney organoids and how biomaterials can be used to control these properties. Many noteworthy results have come out of such endeavors in recent years. For instance, through the use of mechanically tunable thiol-ene crosslinked alginate hydrogels, Geuens et al. (2021) were able to show that encapsulation of kidney organoids initially cultured on an air-liquid interface in a soft (G’ 100–200 Pa) hydrogel improved tubular polarity and lumen morphology. This soft hydrogel encapsulation also resulted in a downregulation of genes linked to the fibrotic-like ECM accumulation and stromal expansion observed during extended in vitro kidney organoid culture, whereas these effects were not observed with encapsulation in a stiff hydrogel (G’ 20 kPa) or air-liquid interface culture (Geuens et al., 2021; Ruiter et al., 2022).
In our investigations with Alpha4 and Alpha5 SAPHs, we were able to show that the maturation of nephron progenitor aggregates into kidney organoids over the course of 15 days (day 9 to day 24) within the stiffer Alpha5 matrix resulted in an increased proportion of renal cell types with podocytes displaying a more mature transcriptional profile compared to the soft matrix (Alpha4), matrigel, or air-liquid interface culture. Moreover, kidney organoids grown in SAPHs had a decreased proportion of non-renal ‘off-target’ cell types compared to air-liquid interface culture (Treacy et al., 2023). It is important to note that in the thiol-ene crosslinked alginate (Geuens et al., 2021; Ruiter et al., 2022) and the SAPH (Treacy et al., 2023) encapsulation experiments organoids were encapsulated in the respective hydrogels at different timepoints, for different durations of time and in a different 3D environment (air-liquid interface transwells were used by Geuens and Ruiter) and therefore direct comparison should be made with care. Hydrogels have also been leveraged to gain improvements in kidney organoid generation in a 2D context where kidney organoids derived from hPSCs initially differentiated on soft (G’ 1 kPa) 2D hydrogels displayed a more mature gene expression signature and a larger quantity of proximal tubular and glomerular structures compared to hPSCs initially differentiated on a stiffer (G’ 60 kPa) hydrogel (Garreta et al., 2019).
In the Alternate Protocol, we describe a protocol for encapsulation of nephron progenitor aggregates in GelMA hydrogels. There are a few key differences in their properties when compared to SAPHs that should be taken into consideration. Firstly, SAPHs fall into the category of physically crosslinked hydrogels, undergoing spontaneous self-assembly into a hydrogel network through non-covalent interactions, while GelMA hydrogels are crosslinked chemically in a reaction catalyzed by UV light and Irgacure-2959 resulting in covalent bonds between GelMA monomers. This gives SAPHs an advantage as they do not require any potentially cytotoxic (Irgacure-2959 is cytotoxic) chemical crosslinking agents or UV light. Nevertheless, we have also been able to generate kidney organoids of comparable quality and structural maturity within GelMA hydrogels of similar mechanical profiles to the SAPHs employed by Treacy et al. (2023).
Bulk shear rheological analysis of hydrogels throughout organoid growth is possible with physically crosslinked SAPHs due to the relative ease with which organoids can be removed without damage being done to the hydrogels. This is not possible with the chemically crosslinked GelMA hydrogels mentioned in Alternate Protocol 1, as organoid removal causes the hydrogel to crack, preventing reliable rheological readouts.
Recent work in our lab has shown that the storage modulus of GelMA hydrogels remains stable in the presence of organoid culture medium (no organoids) over the 15-day period that is required for the maturation of nephron progenitor aggregates into kidney organoids using the protocols described here. On the other hand, SAPHs are known to be sensitive to the composition of cell culture medium, particularly its ionic strength and other components present in the media or released by the cells. In our group's experiments, the SAPHs exhibited an increase in stiffness in the presence of the organoid culture medium used over the 15-day timespan (Treacy et al., 2023). Nonetheless, similar effects on renal cell fate specification and maturation were observed both in our GelMA and SAPH studies, most notably the improved maturation of podocytes in organoids cultured within stiffer hydrogels (Alpha5 or 15%w/v GelMA), suggesting that these effects were due to hydrogel mechanical properties. It should also be pointed out that the mechanical properties of 5%w/v GelMA and 15%w/v GelMA hydrogels were roughly equivalent to the Alpha4 and Alpha5 SAPHs, respectively, at day 0 of encapsulation (corresponding to day 9 of the kidney organoid differentiation protocol described here).
As previously mentioned, both types of hydrogels outlined in the discussed protocols are available for purchase from commercial providers (examples earlier in this text). While SAPHs can be purchased formulated and ready to use, GelMA, on the other hand, is typically purchased as a powder or kit (including crosslinker) and requires user intervention to generate the basic solutions for the crosslinking reaction (see reagents section for details). The two hydrogel variants differ in their origin, methods of synthesis, and classification. GelMA is made from an animal-derived material, gelatin, which is methacrylated via a chemical process (see Yue et al., 2015) and thus classed as semi-synthetic, while SAPHs are fully synthetic, being formulated from β-sheet forming peptides. SAPHs therefore represent a fully synthetic, animal product-free option providing the intrinsic benefit of a lesser ethical burden along with improved reproducibility due to the fully controlled and synthetic nature of their origin. GelMA, however, relies on the use of an animal-derived material whose exact composition may vary from batch to batch but can be obtained at a lower cost. In summary, while both biomaterials can be utilized similarly for the growth of kidney organoids, as depicted in the protocols outlined herein, the decision on which to employ is influenced by the above-mentioned factors, which users may wish to consider depending on the intended application.
Despite showing that we can achieve a physiological resemblance to the in vivo nephron using the kidney organoid differentiation protocols and hydrogels described here, there are some key limitations that the user may want to bear in mind. Differentiation of hiPSCs towards organoids is a highly sensitive process that is known to result in off-target cell types with varying results depending on the hiPSC line used and poor higher-order structural organization compared to the organ being mimicked (Bhaduri et al., 2020; Fowler et al., 2020; Subramanian et al., 2019). We also have not been able to establish extensive vascularization of kidney organoids using the methods described here, and hence, oxygen diffusion and transport of molecules throughout the organoid remain limited. Hydrogel biomaterials can also present biological and practical difficulties. For instance, GelMA hydrogel encapsulation relies on the use of UV light and potentially cytotoxic chemical crosslinkers such as Irgacure-2959, which may impede some of the long-term functionality or desired processing. In the case of SAPHs, their pre-formulated state and, therefore, viscosity can make it difficult to handle the materials.
Critical Parameters
hiPSC culture
When passaging hiPSCs, it is crucial that aggregates are of the correct size and that they are evenly distributed following passaging. Passaging aggregates that are not of the correct size may result in excessive cell death and/or increased spontaneous differentiation of hiPSCs, negatively impacting downstream differentiation experiments. The incubation times with ReleSR should ideally be optimized by the user also as too long incubation times can result in poor cell viability 24 to 48 hr after passaging, while an incubation time that is too short will result in poor detachment of hiPSCs when passaging. It is also important to use a gentle pipetting technique while passaging and feeding hiPSCs so that cell viability is not compromised. As already mentioned, we also recommend that hiPSCs are cultured for at least 2 to 3 passages before commencing with differentiation experiments.
2D monolayer differentiation
The confluence of seeded hiPSCs on day 0 is critically important for the successful differentiation of hiPSCs towards nephron progenitors. If cells are not at 10% to 20% confluence 24 hr after seeding (see day 0 image in Fig. 3), do not commence differentiation. Doubling the volume of the medium on day 3 is necessary for efficient mesodermal differentiation and cell growth.
Aggregate generation and hydrogel encapsulation
On day 7, it is necessary to work quickly so that the viability and subsequent self-organization of kidney organoids are not compromised. For experiments where a larger number of organoids need to be generated, it is advisable to perform the procedure for generating kidney organoids in batches (e.g., 2 wells of day 7 nephron progenitors at a time). During steps where cells are to be resuspended in media or detached from their plate, it is important not to over-pipette, as this can also negatively affect downstream results.
We employed 48 hr suspension culture so that sufficient compaction of the aggregates occurs and organoids maintain structural integrity within the hydrogel support matrix as they grow and mature.
On day 9, it is important that aggregates have little to no residual media surrounding them just before the hydrogel solution is applied to encapsulate them. This is because components of the media can interfere with hydrogel crosslinking (this applies both in the case of SAPHs and for GelMA). Good pipetting technique is also crucial, as introducing bubbles into the hydrogels encapsulating the aggregate can negatively impact the structural integrity of the hydrogel. For this reason, we decided to use a positive displacement pipette for casting hydrogels during encapsulation.
In the case of GelMA encapsulation (Alternate Protocol 1), the stated number of washes in DPBS after encapsulation is necessary, as any uncrosslinked monomer and residual Irgacure-2959 can be cytotoxic to the organoid if not washed away adequately. Do not shorten the wash times.
Immunofluorescence staining and imaging
For whole-mount immunofluorescence, 0.1% Triton X-100 must be used as the wash buffer to minimize non-specific binding. Do not shorten the wash times. For best clearing results, we also recommend preparing fresh fructose-glycerol solution each time.
During cryoembedding, it is essential to avoid introducing bubbles as this negatively affects subsequent sectioning on the cryostat. It is also necessary to remove as much hydrogel surrounding the organoid as possible, as too much hydrogel can negatively impact sectioning. While staining organoid sections, it is necessary to use a humid chamber during incubations as otherwise, the antibody solutions, as well as the sections themselves, may dry out. Another crucial point in the protocol is bringing the slides—stored at −80°C—to room temperature before blocking. Drying allows proper adherence of the section to the slide, and sections should not lift off during subsequent staining steps.
Troubleshooting
See Table 4 for a troubleshooting guide regarding organoid generation and downstream immunofluorescence characterization.
Problem | Possible cause | Possible solution(s) |
---|---|---|
Larger aggregates are obtained when passaging hiPSCs (mean aggregate size >200 µm) |
|
|
Smaller aggregates are obtained when passaging hiPSCs (mean aggregate size <50 µm) |
|
|
Excessive cell death after passaging hiPSCs |
|
|
Excessive cell death after adding CHIR99021 to commence differentiation |
|
|
Loss of structural integrity of aggregates following hydrogel encapsulation |
|
|
Absence of certain renal cell types or structures seen upon immunofluorescent imaging |
|
|
Understanding Results
By following the protocols described herein, users should be able to generate kidney organoids that contain structures resembling the human nephron upon immunofluorescence imaging for the relevant markers (Fig. 4). WT1+ve podocytes should be seen to lead into LTL+ve proximal tubular cells, adjacent to ECAD+ve distal tubular epithelia, both containing Z0-1+ve tight junctions (Fig. 4B). MEIS1/2/3+ve stromal cells and a Laminin+ve basement membrane should also be observed within organoids (Fig. 4B). If the user wishes to compare maturation of kidney organoids within soft and stiff hydrogels, some qualitative differences may be noted when imaging.
Aside from imaging, the user may also wish to investigate transcriptional differences between kidney organoids grown within different mechanical environments. For single-cell RNA sequencing, we used the 10× Genomics Chromium platform following the Single-Cell 3′ v3.1 protocol. This allowed us to comprehensively investigate the impact of stiffness on kidney organoid maturation. Through single-cell transcriptomics, we were able to show that the stiffer matrix, Alpha5, generated organoids with more mature podocyte transcriptional signatures in comparison to the softer matrix, Alpha4, air-liquid interface, or Matrigel. We also found that organoids generated within 3D matrices (SAPHs or Matrigel) had comparatively reduced off-target cell types compared to organoids grown on transwells. For full details on these single-cell RNAseq results, refer to Treacy et al. (2023). Once techniques for growing kidney organoids within SAPHs or GelMA hydrogels have been established, the user may also wish to conduct toxicological or model disease state studies through the addition of small drug molecules, morphogens, or other treatments (reviewed in Bejoy et al., 2022, Miyoshi et al., 2020, Przepiorski et al., 2020). We have shown that day 22 kidney organoids matured within SAPHs contain LTL+ve proximal tubules possessing endocytic functionality, as shown by the uptake of fluorescently labeled dextran (Treacy et al., 2023). Hence, we would recommend that such studies commence on organoids aged between day 22 and day 24. As mentioned above, similar organoids were obtained with GelMA, and therefore, a similar recommendation applies to organoids grown in GelMA hydrogels.
Time Considerations
The times required to complete each aspect of the protocols described here can be seen graphically in Figure 2B.
Acknowledgments
Certain components of figures were generated using BioRender.com. This work has been conducted with the financial support of Science Foundation Ireland (SFI) and is co-funded under the European Regional Development Fund 13/RC/2073_P2. The authors acknowledge financial support from SFI 16/IA/4584 and 19/FFP/6833 and the SFI-funded Centre for Research in Medical Devices, CURAM. J.K.W. would also like to acknowledge the Royal Society of Chemistry grant M19-6613. I.K would also like to acknowledge the Irish Research Council grant GOIPG/2023/3604. Immunofluorescence images used in this manuscript were acquired using facilities at the Nano Research Facility, Dublin City University, funded under the Programme for Research in Third Level Institutions (PRTLI) Cycle 5. The PRTLI is co-funded through the European Regional Development Fund (ERDF), part of the European Union Structural Funds Programme 2011–2015.
Open access funding provided by IReL.
Author Contributions
Ivan Krupa : Methodology; writing—original draft. Niall J. Treacy : Conceptualization; data curation; formal analysis; investigation; methodology; writing—review and editing. Shane Clerkin : Conceptualization; data curation; formal analysis; investigation; methodology; writing—review and editing. Jessica L. Davis : Methodology; writing—review and editing. Aline F. Miller : Conceptualization; resources. Alberto Saiani : Conceptualization; resources; writing—review and editing. Jacek K. Wychowaniec : Conceptualization; methodology. Emmanuel. G. Reynaud : Conceptualization; methodology. Dermot F. Brougham : Conceptualization; funding acquisition; resources; supervision. John Crean : Conceptualization; funding acquisition; resources; supervision; writing—review and editing.
Conflict of Interest
The work to which the protocols described here refer (Treacy et al., 2023) was supported, in part, by a contribution from Manchester BIOGEL. AS was a founder and consultant for Manchester BIOGEL (a now-defunct company) and is currently a consultant for Cell Guidance Systems.
Open Research
Data Availability Statement
Data available on request from the authors
Literature Cited
- Bayart, E., & Cohen-Haguenauer, O. (2013). Technological overview of iPS induction from human adult somatic cells. Current Gene Therapy , 13(2), 73–92. https://doi.org/10.2174/1566523211313020002
- Bejoy, J., Qian, E. S., & Woodard, L. E. (2022). Tissue culture models of AKI: From tubule cells to human kidney organoids. Journal of the American Society of Nephrology , 33(3), 487–501. https://doi.org/10.1681/ASN.2021050693
- Bensamoun, S. F., Robert, L., Leclerc, G. E., Debernard, L., & Charleux, F. (2011). Stiffness imaging of the kidney and adjacent abdominal tissues measured simultaneously using magnetic resonance elastography. Clinical Imaging , 35(4), 284–287. https://doi.org/10.1016/j.clinimag.2010.07.009
- Bhaduri, A., Andrews, M. G., Mancia Leon, W., Jung, D., Shin, D., Allen, D., Jung, D., Schmunk, G., Haeussler, M., Salma, J., Pollen, A. A., Nowakowski, T. J., & Kriegstein, A. R. (2020). Cell stress in cortical organoids impairs molecular subtype specification. Nature , 578(7793), 142–148. https://doi.org/10.1038/s41586-020-1962-0
- Bissell, M. J., Hall, H. G., & Parry, G. (1982). How does the extracellular matrix direct gene expression? Journal of Theoretical Biology , 99(1), 31–68. https://doi.org/10.1016/0022-5193(82)90388-5
- Davis, J. L., Kennedy, C., Clerkin, S., Treacy, N. J., Dodd, T., Moss, C., Murphy, A., Brazil, D. P., Cagney, G., Brougham, D. F., Murad, R., Finlay, D., Vuori, K., & Crean, J. (2022). Single-cell multiomics reveals the complexity of TGFβ signalling to chromatin in iPSC-derived kidney organoids. Communications Biology , 5(1), 1301. https://doi.org/10.1038/s42003-022-04264-1
- Dekkers, J. F., Alieva, M., Wellens, L. M., Ariese, H. C. R., Jamieson, P. R., Vonk, A. M., Amatngalim, G. D., Hu, H., Oost, K. C., Snippert, H. J. G., Beekman, J. M., Wehrens, E. J., Visvader, J. E., Clevers, H., & Rios, A. C. (2019). High-resolution 3D imaging of fixed and cleared organoids. Nature Protocols , 14(6), 1756–1771. https://doi.org/10.1038/s41596-019-0160-8
- Evans, N. D., Minelli, C., Gentleman, E., LaPointe, V., Patankar, S. N., Kallivretaki, M., Chen, X., Roberts, C. J., & Stevens, M. M. (2009). Substrate stiffness affects early differentiation events in embryonic stem cells. European Cells & Materials, 18(1), e13.
- Fowler, J. L., Ang, L. T., & Loh, K. M. (2020). A critical look: Challenges in differentiating human pluripotent stem cells into desired cell types and organoids. Wiley Interdisciplinary Reviews: Developmental Biology , 9(3), e368. https://doi.org/10.1002/wdev.368
- Freedman, B. S., Brooks, C. R., Lam, A. Q., Fu, H., Morizane, R., Agrawal, V., Saad, A. F., Li, M. K., Hughes, M. R., Werff, R. V., Peters, D. T., Lu, J., Baccei, A., Siedlecki, A. M., Valerius, M. T., Musunuru, K., McNagny, K. M., Steinman, T. I., Zhou, J., … Bonventre, J. V. (2015). Modelling kidney disease with CRISPR-mutant kidney organoids derived from human pluripotent epiblast spheroids. Nature Communications , 6(1), 8715. https://doi.org/10.1038/ncomms9715
- Gao, J., Tang, C., Elsawy, M. A., Smith, A. M., Miller, A. F., & Saiani, A. (2017). Controlling self-assembling peptide hydrogel properties through network topology. Biomacromolecules , 18(3), 826–834. https://doi.org/10.1021/acs.biomac.6b01693
- Garreta, E., Prado, P., Tarantino, C., Oria, R., Fanlo, L., Martí, E., Zalvidea, D., Trepat, X., Roca-Cusachs, P., Gavaldà-Navarro, A., Cozzuto, L., Campistol, J. M., Izpisúa Belmonte, J. C., Hurtado Del Pozo, C., & Montserrat, N. (2019). Fine tuning the extracellular environment accelerates the derivation of kidney organoids from human pluripotent stem cells. Nature Materials , 18(4), 397–405. https://doi.org/10.1038/s41563-019-0287-6
- Garreta, E., Prado, P., Stanifer, M. L., Monteil, V., Marco, A., Ullate-Agote, A., Moya-Rull, D., Vilas-Zornoza, A., Tarantino, C., Romero, J. P., Jonsson, G., Oria, R., Leopoldi, A., Hagelkruys, A., Gallo, M., González, F., Domingo-Pedrol, P., Gavaldà, A., Del Pozo, C. H., … Montserrat, N. (2022). A diabetic milieu increases ACE2 expression and cellular susceptibility to SARS-CoV-2 infections in human kidney organoids and patient cells. Cell Metabolism , 34(6), 857–873. https://doi.org/10.1016/j.cmet.2022.04.009
- Geuens, T., Ruiter, F. A. A., Schumacher, A., Morgan, F. L. C., Rademakers, T., Wiersma, L. E., van den Berg, C. W., Rabelink, T. J., Baker, M. B., & LaPointe, V. L. S. (2021). Thiol-ene cross-linked alginate hydrogel encapsulation modulates the extracellular matrix of kidney organoids by reducing abnormal type 1a1 collagen deposition. Biomaterials , 275, 120976. https://doi.org/10.1016/j.biomaterials.2021.120976
- Hassan, K., Loberant, N., Abbas, N., Fadi, H., Shadia, H., & Khazim, K. (2016). Shear wave elastography imaging for assessing the chronic pathologic changes in advanced diabetic kidney disease. Therapeutics and Clinical Risk Management , 1615–1622. https://doi.org/10.2147/TCRM.S118465
- Homan, K. A., Gupta, N., Kroll, K. T., Kolesky, D. B., Skylar-Scott, M., Miyoshi, T., Mau, D., Valerius, M. T., Ferrante, T., Bonventre, J. V., Lewis, J. A., & Morizane, R. (2019). Flow-enhanced vascularization and maturation of kidney organoids in vitro. Nature Methods , 16(3), 255–262. https://doi.org/10.1038/s41592-019-0325-y
- Krause, M., Rak-Raszewska, A., Pietilä, I., Quaggin, S. E., & Vainio, S. (2015). Signaling during kidney development. Cells , 4(2), 112–132. https://doi.org/10.3390/cells4020112
- Kolahi, K. S., Donjacour, A., Liu, X., Lin, W., Simbulan, R. K., Bloise, E., Maltepe, E., & Rinaudo, P. (2012). Effect of substrate stiffness on early mouse embryo development. PloS One , 7(7), e41717. https://doi.org/10.1371/journal.pone.0041717
- Low, J. H., Li, P., Chew, E. G. Y., Zhou, B., Suzuki, K., Zhang, T., Lian, M. M., Liu, M., Aizawa, E., Rodriguez Esteban, C., Yong, K. S. M., Chen, Q., Campistol, J. M., Fang, M., Khor, C. C., Foo, J. N., Izpisua Belmonte, J. C., & Xia, Y. (2019). Generation of human PSC-derived kidney organoids with patterned nephron segments and a de novo vascular network. Cell Stem Cell , 25(3), 373–387. https://doi.org/10.1016/j.stem.2019.06.009
- Miyoshi, T., Hiratsuka, K., Saiz, E. G., & Morizane, R. (2020). Kidney organoids in translational medicine: Disease modeling and regenerative medicine. Developmental Dynamics , 249(1), 34–45. https://doi.org/10.1002/dvdy.22
- Morizane, R., Lam, A. Q., Freedman, B. S., Kishi, S., Valerius, M. T., & Bonventre, J. V. (2015). Nephron organoids derived from human pluripotent stem cells model kidney development and injury. Nature Biotechnology , 33(11), 1193–1200. https://doi.org/10.1038/nbt.3392
- Muncie, J. M., & Weaver, V. M. (2018). The physical and biochemical properties of the extracellular matrix regulate cell fate. Current Topics in Developmental Biology , 130 , 1–37.
- Nordin, N., Lai, M. I., Veerakumarasivam, A., Ramasamy, R., Abdullah, S., Wendy-Yeo, W. Y., & Rosli, R. (2011). Induced pluripotent stem cells: History, properties and potential applications. Medical Journal of Malaysia , 66(1), 4–9.
- Norman, M. D., Ferreira, S. A., Jowett, G. M., Bozec, L., & Gentleman, E. (2021). Measuring the elastic modulus of soft culture surfaces and three-dimensional hydrogels using atomic force microscopy. Nature Protocols , 16(5), 2418–2449. https://doi.org/10.1038/s41596-021-00495-4
- Prince, E., & Kumacheva, E. (2019). Design and applications of man-made biomimetic fibrillar hydrogels. Nature Reviews Materials , 4(2), 99–115. https://doi.org/10.1038/s41578-018-0077-9
- Przepiorski, A., Crunk, A. E., Espiritu, E. B., Hukriede, N. A., & Davidson, A. J. (2020). The utility of human kidney organoids in modeling kidney disease. In Seminars in nephrology (Vol. 40, No. 2, pp. 188–198). WB Saunders.
- Raghuwanshi, V. S., & Garnier, G. (2019). Characterisation of hydrogels: Linking the nano to the microscale. Advances in Colloid and Interface Science , 274, 102044. https://doi.org/10.1016/j.cis.2019.102044
- Ruiter, F. A. A., Morgan, F. L. C., Roumans, N., Schumacher, A., Slaats, G. G., Moroni, L., LaPointe, V. L. S., & Baker, M. B. (2022). Soft, dynamic hydrogel confinement improves kidney organoid lumen morphology and reduces epithelial–mesenchymal transition in culture. Advanced Science , 9(20), 2200543. https://doi.org/10.1002/advs.202200543
- Shimizu, T., Mae, S. I., Araoka, T., Okita, K., Hotta, A., Yamagata, K., & Osafune, K. (2020). A novel ADPKD model using kidney organoids derived from disease-specific human iPSCs. Biochemical and Biophysical Research Communications , 529(4), 1186–1194. https://doi.org/10.1016/j.bbrc.2020.06.141
- Subramanian, A., Sidhom, E. H., Emani, M., Vernon, K., Sahakian, N., Zhou, Y., Kost-Alimova, M., Slyper, M., Waldman, J., Dionne, D., Nguyen, L. T., Weins, A., Marshall, J. L., Rosenblatt-Rosen, O., Regev, A., & Greka, A. (2019). Single cell census of human kidney organoids shows reproducibility and diminished off-target cells after transplantation. Nature Communications , 10(1), 5462. https://doi.org/10.1038/s41467-019-13382-0
- Szeto, S. G., Narimatsu, M., Lu, M., He, X., Sidiqi, A. M., Tolosa, M. F., Chan, L., De Freitas, K., Bialik, J. F., Majumder, S., Boo, S., Hinz, B., Dan, Q., Advani, A., John, R., Wrana, J. L., Kapus, A., & Yuen, D. A. (2016). YAP/TAZ are mechanoregulators of TGF-β-Smad signaling and renal fibrogenesis. Journal of the American Society of Nephrology , 27(10), 3117–3128. https://doi.org/10.1681/ASN.2015050499
- Taguchi, A., & Nishinakamura, R. (2017). Higher-order kidney organogenesis from pluripotent stem cells. Cell Stem Cell , 21(6), 730–746. https://doi.org/10.1016/j.stem.2017.10.011
- Taapken, S. M., Nisler, B. S., Newton, M. A., Sampsell-Barron, T. L., Leonhard, K. A., McIntire, E. M., & Montgomery, K. D. (2011). Karyotypic abnormalities in human induced pluripotent stem cells and embryonic stem cells. Nature Biotechnology , 29(4), 313–314. https://doi.org/10.1038/nbt.1835
- Takasato, M., Er, P. X., Chiu, H. S., Maier, B., Baillie, G. J., Ferguson, C., Parton, R. G., Wolvetang, E. J., Roost, M. S., Chuva de Sousa Lopes, S. M., & Little, M. H. (2015). Kidney organoids from human iPS cells contain multiple lineages and model human nephrogenesis. Nature , 526(7574), 564–568. https://doi.org/10.1038/nature15695
- Treacy, N. J., Clerkin, S., Davis, J. L., Kennedy, C., Miller, A. F., Saiani, A., Wychowaniec, J. K., Brougham, D. F., & Crean, J. (2023). Growth and differentiation of human induced pluripotent stem cell (hiPSC)-derived kidney organoids using fully synthetic peptide hydrogels. Bioactive Materials , 21, 142–156. https://doi.org/10.1016/j.bioactmat.2022.08.003
- Uhrig, M., Ezquer, F., & Ezquer, M. (2022). Improving cell recovery: Freezing and thawing optimization of induced pluripotent stem cells. Cells , 11(5), 799. https://doi.org/10.3390/cells11050799
- van den Berg, C. W., Ritsma, L., Avramut, M. C., Wiersma, L. E., van den Berg, B. M., Leuning, D. G., Lievers, E., Koning, M., Vanslambrouck, J. M., Koster, A. J., Howden, S. E., Takasato, M., Little, M. H., & Rabelink, T. J. (2018). Renal subcapsular transplantation of PSC-derived kidney organoids induces neo-vasculogenesis and significant glomerular and tubular maturation in vivo. Stem Cell Reports , 10(3), 751–765. https://doi.org/10.1016/j.stemcr.2018.01.041
- Vining, K. H., & Mooney, D. J. (2017). Mechanical forces direct stem cell behaviour in development and regeneration. Nature reviews Molecular cell biology , 18(12), 728–742. https://doi.org/10.1038/nrm.2017.108
- Wychowaniec, J. K., Smith, A. M., Ligorio, C., Mykhaylyk, O. O., Miller, A. F., & Saiani, A. (2020). Role of sheet-edge interactions in β-sheet self-assembling peptide hydrogels. Biomacromolecules , 21(6), 2285–2297. https://doi.org/10.1021/acs.biomac.0c00229
- Wu, H., Uchimura, K., Donnelly, E. L., Kirita, Y., Morris, S. A., & Humphreys, B. D. (2018). Comparative analysis and refinement of human PSC-derived kidney organoid differentiation with single-cell transcriptomics. Cell Stem Cell , 23(6), 869–881. https://doi.org/10.1016/j.stem.2018.10.010
- Yokoi, H., Kinoshita, T., & Zhang, S. (2005). Dynamic reassembly of peptide RADA16 nanofiber scaffold. Proceedings of the National Academy of Sciences , 102(24), 8414–8419. https://doi.org/10.1073/pnas.0407843102
- Yue, K., Trujillo-de Santiago, G., Alvarez, M. M., Tamayol, A., Annabi, N., & Khademhosseini, A. (2015). Synthesis, properties, and biomedical applications of gelatin methacryloyl (GelMA) hydrogels. Biomaterials , 73, 254–271. https://doi.org/10.1016/j.biomaterials.2015.08.045
- Zuidema, J. M., Rivet, C. J., Gilbert, R. J., & Morrison, F. A. (2014). A protocol for rheological characterization of hydrogels for tissue engineering strategies. Journal of Biomedical Materials Research Part B: Applied Biomaterials , 102(5), 1063–1073. https://doi.org/10.1002/jbm.b.33088