Production of Structured RNA Fragments by In Vitro Transcription and HPLC Purification
Lorenzo Baronti, Lorenzo Baronti, Hampus Karlsson, Hampus Karlsson, Katja Petzold, Katja Petzold, Hannes Feyrer, Hannes Feyrer
Abstract
The understanding of the functional importance of RNA has increased enormously in the last decades. This has required research on the RNA molecules themselves, with the concomitant need for obtaining purified RNA samples, such as for structural studies by NMR or other methods. The main method to create labeled and unlabeled RNA, T7 in vitro transcription, suffers from sequence-dependent yield and often low homogeneity for short constructs (<100 nt) and requires laborious purification. Additionally, the design of structured RNA fragments mimicking the structure of a larger biological RNA is often not straightforward. Secondary structure simulations can be used to make reliable predictions about the folding of a particular RNA fragment. In this article, we describe how to design an RNA construct of interest from a larger sequence, and we combine several previously published improvements of the in vitro transcription method, such as the use of 2′-methoxy modifications and dimethyl sulfoxide or the use of tandem repeats, to increase yield and purity of in vitro–transcribed RNA. Together with a high-performance liquid chromatography (HPLC) purification procedure using both reversed-phase ion-pairing and ion-exchange HPLC, we provide a robust protocol to obtain highly pure RNA of short to intermediate length in large quantities. The protocol optimizes yield, especially for RNA starting with nucleotides other than G. At the same time, it is simplified, and the required time is reduced. The protocols described here constitute a versatile pipeline for the production of purified RNA samples and are suitable for users with little experience in liquid chromatography. © 2021 The Authors.
Basic Protocol 1 : RNA construct design
Basic Protocol 2 : DNA template production and in vitro transcription
Alternate Protocol : Tandem transcription and RNase H cleavage
Basic Protocol 3 : Reversed-phase ion-pairing HPLC purification
Basic Protocol 4 : Ion-exchange HPLC purification
INTRODUCTION
RNAs are becoming increasingly important as regulators (Dethoff, Petzold, Chugh, Casiano-Negroni, & Al-Hashimi, 2012), drug targets (Dethoff et al., 2012; Wacker et al., 2020), or even as vaccines (Mulligan et al., 2020) and drugs (Schlagnitweit et al., 2019). Experiments performed to elucidate these RNA functions and the correlation to their structures rely heavily on samples prepared in vitro, mostly through transcription with the bacteriophage enzyme T7 RNA polymerase (T7 in vitro transcription [IVT]; Milligan & Uhlenbeck, 1989; Milligan, Groebe, Witherell, & Uhlenbeck, 1987). This method has been used extensively to study structured RNA fragments from a larger RNA molecule with methods like NMR spectroscopy (Baisden, Boyer, Zhao, Hammond, & Zhang, 2021; Baronti et al., 2020; Dethoff et al., 2012; Pabis et al., 2019; Petzold et al., 2007; Wang et al., 2014), which impose size limitations. Such RNA fragments are typically between 20 and 100 nucleotides (nt) long. Despite its usefulness, IVT has several drawbacks: the starting sequence is limited to 5′-GG for high yield; a large number of side products need to be removed for high purity; and purification through polyacrylamide gel electrophoresis (PAGE) uses toxic acrylamide and can be cumbersome. The RNA sample preparation pipeline consists of the design of the RNA fragment from the full-length biological context, production of the RNA with T7 IVT, and final purification of the target-length RNA constructs. This requires production of an RNA fragment that contains the structured region of interest, while omitting the surrounding RNA, the so called “divide and conquer” approach. In such workflow, double-stranded structures are usually stabilized by generation as a hairpin construct containing a UUCG tetraloop. This approach first requires a sample design step that has, so far, not been addressed in detail in the literature.
The main limitation in the T7 IVT sample preparation procedure is heterogeneous transcription, which complicates purification of the target-length transcript from transcripts of similar length. The T7 polymerase sample production methodology has been used since the 1980s (Milligan & Uhlenbeck, 1989; Milligan et al., 1987) and has been improved over time. Issues with length heterogeneity when producing the sample using T7 IVT have been addressed by the use of cleaving enzymes (e.g., hammerhead ribozymes; Price, Ito, Oubridge, Avis, & Nagai, 1995; Shields, Mollova, Marie, Hansen, & Pardi, 1999) or the use of 5′ end 2′-methoxy-modified DNA templates (Helmling et al., 2015; Kao, Zheng, & RüDisser, 1999) together with dimethyl sulfoxide (DMSO; Chen & Zhang, 2005). These methods somewhat address the 5′ and sometimes 3′ inhomogeneity, but a comprehensive protocol has been missing. Another limitation of T7 IVT is the requirement for 5′-GG in the starting sequence to gain high-yield transcription initiation.
Basic Protocol 1 describes how to design an RNA construct of interest from a larger sequence and obtain a corresponding RNA fold using the secondary structure simulation tool MC-Fold (Parisien & Major, 2008). For this purpose, 5′-GG/CC-3′ and central UUCG tetraloop sequences are typically added to the target sequence to stabilize the thermodynamic equilibrium of the hairpin if termini or central loop are not predefined by sequence restraints. The 5′-GG sequence serves to enhance transcription yield and to stabilize potential fraying ends of the stem-loop structure.
Basic Protocol 2 instructs on how to generate a modified single-stranded DNA (ssDNA) template from the RNA sequence designed in Basic Protocol 1 and how to perform the IVT. As IVT efficiency is strongly sequence dependent, a screen of reaction conditions is recommended, ensuring higher yields and initial purity. As an alternative to using a ssDNA template, we provide an Alternate Protocol, which describes how to use a plasmid template to produce a tandem transcript of the target RNA. This tandem transcript is then processed to single units of the target RNA by the use of the site-specific enzyme RNase H, guided by a chimeric oligo. This method has proven particularly useful when dealing with sequences that do not start with a 5′-GG sequence and suffer from low yield and length heterogeneity. After synthesis, purifying the RNA sample is absolutely required, as side products cannot be completely eliminated, just reduced. The most common purification technique has historically been denaturing PAGE excision, which can be tedious and prone to sample loss and contamination. Recent developments have led to the use of preparative high-performance liquid chromatography (HPLC) as a more modern approach to RNA purification. Basic Protocol 3 describes the use of reversed-phase ion-pairing (RP-IP) HPLC for initial purification based on hydrophobicity of the RNA complexed with a lipophilic counterion. This step is optional as it does produce pure RNA free of reaction contaminants, but RNA products of similar length can still be present. Finally, in Basic Protocol 4 we describe the separation of the target RNA sample from RNA products of similar length to yield a pure sample suitable for structural studies. Figure 1 shows the entire workflow and offers a decision tree for the user.
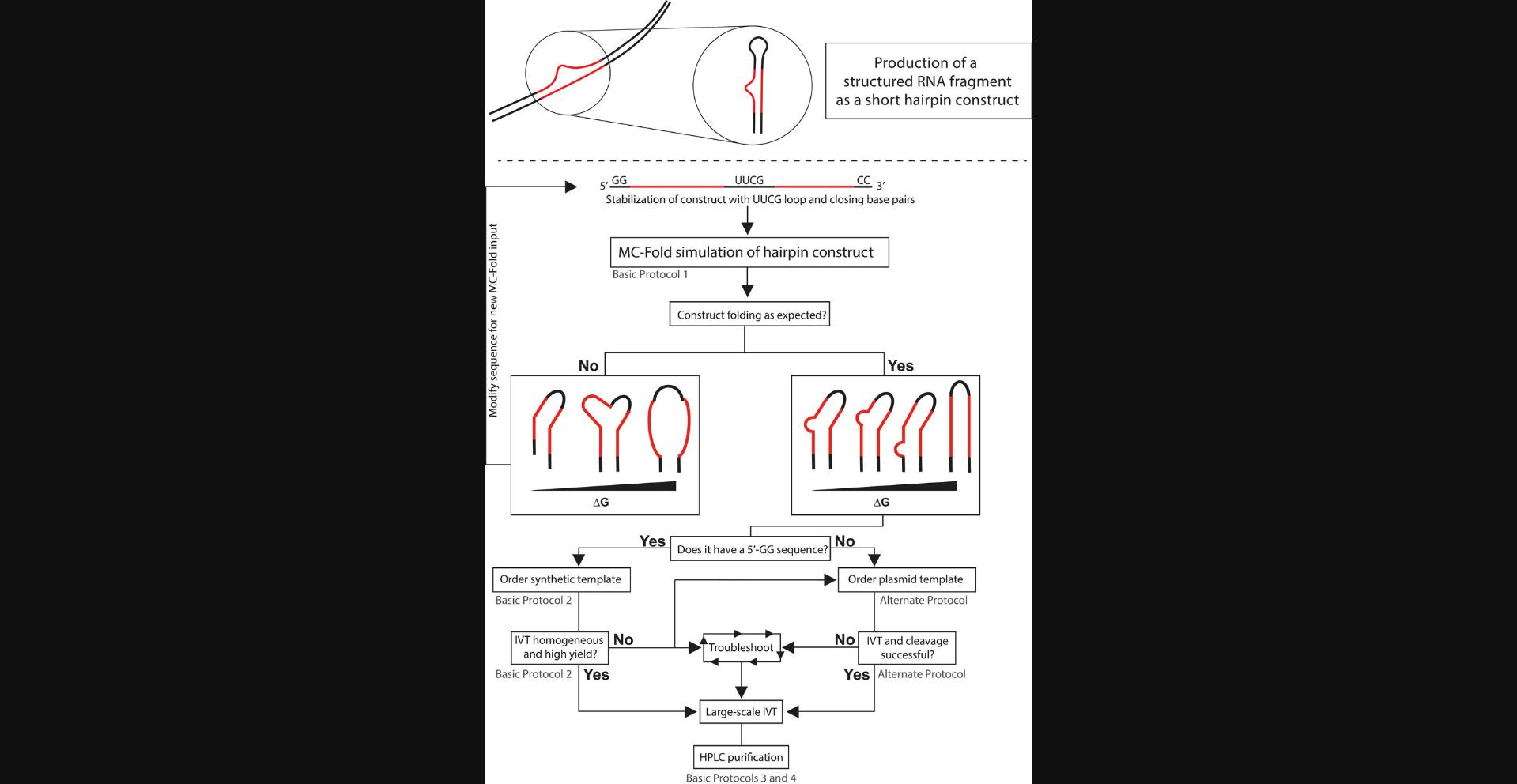
Basic Protocol 1: RNA CONSTRUCT DESIGN
In this protocol, the user will design the target RNA as a hairpin construct. The user will perform secondary structure prediction to confirm that the target RNA retains the structural features of the native (wild-type) sequence. A stable scaffold is crucial when studying small structures like bulges, base mismatches, or base pair switches. Therefore, features like 5′-GG/CC-3′ and UUCG tetraloop are introduced to stabilize the structural region in the RNA stem (see Figs. 1 and 2). The protocol describes an iterative procedure in which the user modifies the RNA sequence, performs a secondary structure prediction, and compares the fold of the generated target RNA with the native sequence. In addition to the minimum-free-energy structure, higher-energy structures are also analyzed to account for potential variability in the conformational ensemble. After Basic Protocol 1, the user will obtain an RNA sequence that will be used to design a DNA template, which will be then used to synthesize and purify the RNA target. Typically, a 5′-GG sequence is introduced to stabilize the stem and to provide higher yields in the IVT reaction, as described in Basic Protocol 2.If this is not possible (e.g., due to destabilization of the predicted structure), the user should consider the Alternate Protocol, as it is not dependent on the initiation sequence of the RNA.
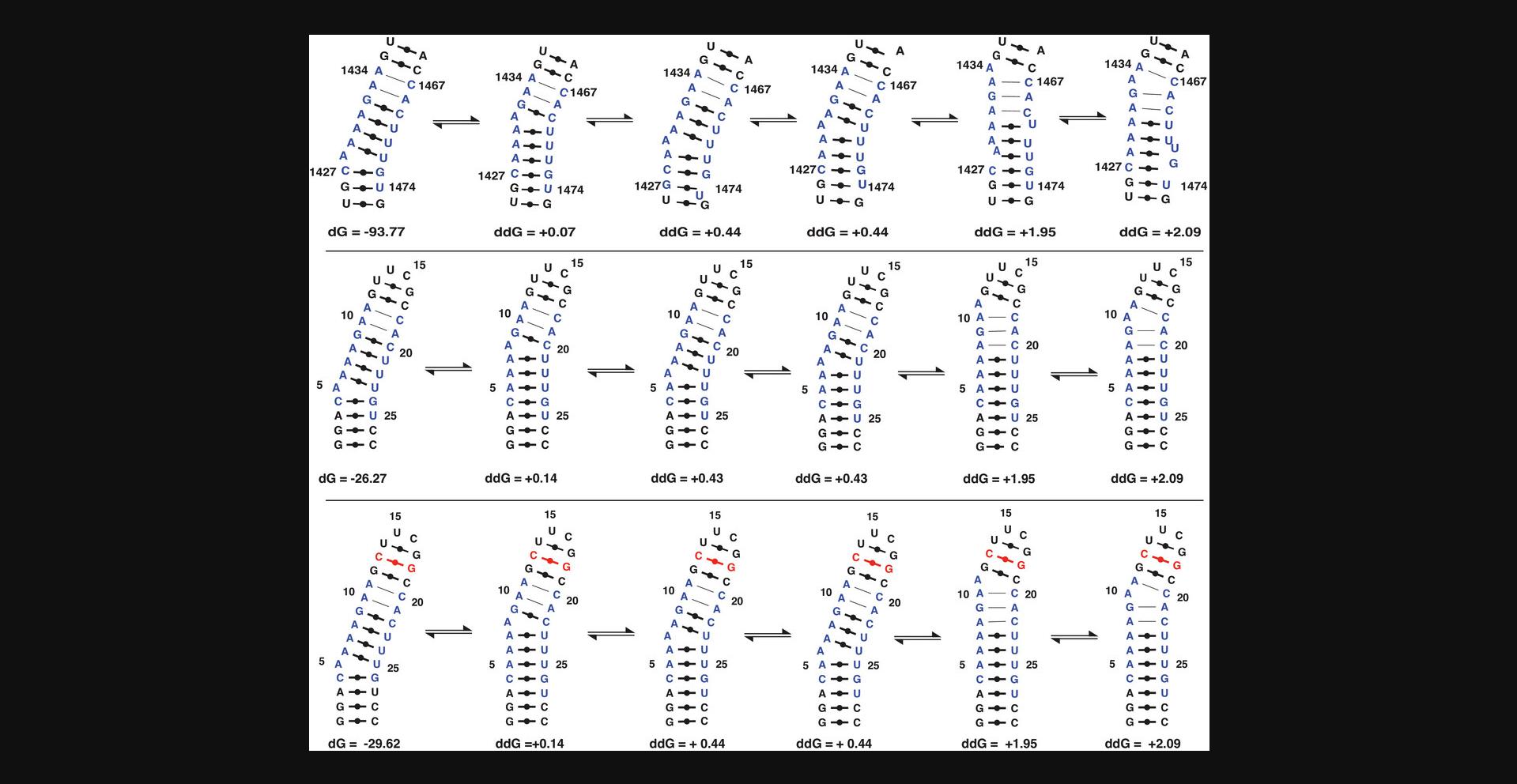
Materials
- Computer running MC-Fold 2.3.2 or higher (https://major.iric.ca/Web/mctools)
1.Input target RNA sequence 5′ to 3′ as one string of letters (A/U/G/C) into MC-Fold.
2.Consider whether addition of 5′-GG/CC-3′ or a central UUCG loop can be incorporated into the sequence or if the sequences would perturb the biological function. If the sequences would not perturb function, add them to the MC-Fold input.
3.Inspect output and folding properties of the RNA sequence with and without the added 5′-GG/CC-3′ ends, considering:
- Does the molecule fold as expected? That is, does the smaller RNA fragment show a similar base-pairing pattern or secondary structure compared with a known secondary structure or the predicted structure of the sequence within the larger RNA molecule (Figs.1and2)?
A known secondary structure could, for instance, come from existing structural data.
-
Are the wanted secondary structure properties stable over a large energy range? In other words, does the change in base paring pattern agree between the smaller RNA fragment and the secondary structure pattern of the large RNA when looking at higher energy structures?
-
How would deletion or addition (±1) products affect the folding? For instance, would deletion products be more prone to fold differently or show unfolded structures? Would ±1 products lead to more excessive base pair openings within the same free energy interval as the full-length product?
Note that deletion and addition products are unwanted common byproducts of IVT, and while the protocols described here will aid in reducing them, they might still be present.
If adding 5′-GG/3′-CC or ±1 products leads to unwanted folding, consider using theAlternate Protocolto create a DNA template for tandem transcription instead.
- Is it feasible to add GG to the 5′ end and CC to the 3′ ea end of the RNA to improve transcription?
If the intention is to create a size-reduced hairpin construct that contains a part of a larger biological molecule, addition of 5′-GG and 3′-CC is feasible and advisable. An example where this might not be feasible is if a biologically active RNA imposes restraints on the sequence at the 5′ or 3′ ends, such as a microRNA or part of a messenger RNA.
4.Repeat steps 1 through 3, modifying your RNA sequence, and study how sequence modifications are predicted to impact the overall fold.
Basic Protocol 2: DNA TEMPLATE PRODUCTION AND IN VITRO TRANSCRIPTION
This protocol describes how to take the RNA sequence designed in Basic Protocol 1 and generate a ssDNA template for it. The user will add a T7 promoter to the target sequence. As the T7 RNA polymerase can transcribe from both ssDNA or double-stranded DNA (dsDNA) templates but requires a 17-base-pair dsDNA promoter region, the user will then anneal a T7 promoter DNA oligo to the target sequence. Despite the selected sequence having a 5′-GG, yield and length heterogeneity are sequence dependent, and thus the transcription reaction should be optimized. Initial conditions for such an optimization screen, in a small-scale volume (50 µl), are provided (Table 1). The results of the screen are then analyzed via denaturing PAGE (Fig. 3) to select the optimal transcription conditions. The best conditions (i.e., those producing the highest yield) are then used for a large-scale IVT reaction (>10 ml), which is then purified (see Basic Protocols 3 and 4). The protocol can be modified for the production of 13C-/15N-labeled RNA samples by using the corresponding labeled nucleotides only in the large-scale IVT step.
Optimization reaction no. | Water | 0.5 M Tris | 0.25 M MgCl2 | 2.5 M DTT | 0.25 M spermidine | DMSO | PEG | 0.1 M XMP | 0.1 M NT(M)Psc | RNaseOUT | iPPase | 25 μM DNA | T7 polymerase |
---|---|---|---|---|---|---|---|---|---|---|---|---|---|
1 | 24.8 | 5 | 6 | 6 | 0.4 | 0 | 0 | 0 | 1.5 | 0 | 0 | 0.8 | 1 |
2 | 19.8 | 10 | 6 | 6 | 0.4 | 0 | 0 | 0 | 1.5 | 0 | 0 | 0.8 | 1 |
3 | 14.8 | 15 | 6 | 6 | 0.4 | 0 | 0 | 0 | 1.5 | 0 | 0 | 0.8 | 1 |
4 | 21.8 | 10 | 4 | 6 | 0.4 | 0 | 0 | 0 | 1.5 | 0 | 0 | 0.8 | 1 |
5 | 17.8 | 10 | 8 | 6 | 0.4 | 0 | 0 | 0 | 1.5 | 0 | 0 | 0.8 | 1 |
7 | 13.8 | 10 | 12 | 6 | 0.4 | 0 | 0 | 0 | 1.5 | 0 | 0 | 0.8 | 1 |
8 | 20.2 | 10 | 6 | 6 | 0.4 | 0 | 0 | 0 | 1.5 | 0 | 0 | 0.4 | 1 |
9 | 18.3 | 10 | 6 | 6 | 0.4 | 0 | 0 | 1.5 | 1.5 | 0 | 0 | 0.8 | 1 |
10 | ‒ | 10 | 6 | 6 | 0.4 | 0 | 0 | 0 | 1.5 | Xd | 0 | 0.8 | 1 |
11 | ‒ | 10 | 6 | 6 | 0.4 | 0 | 0 | 0 | 1.5 | 0 | Xd | 0.8 | 1 |
12 | 14.8 | 10 | 6 | 6 | 0.4 | 5 | 0 | 0 | 1.5 | 0 | 0 | 0.8 | 1 |
13 | 9.8 | 10 | 6 | 6 | 0.4 | 10 | 0 | 0 | 1.5 | 0 | 0 | 0.8 | 1 |
14 | 4.8 | 10 | 6 | 6 | 0.4 | 15 | 0 | 0 | 1.5 | 0 | 0 | 0.8 | 1 |
-
DMSO, dimethyl sulfoxide; DTT, dithiothreitol; iPPase, inorganic pyrophosphatase; NT(M)P, nucleoside tri(mono)phosphate; PEG, poly(ethylene glycol); XMP, NMP of starting nucleotide.
- a
As this is the first optimization round, it is recommended to further optimize conditions (e.g., smaller increments of concentrations that were found to work best in the first optimization round).
- b
Volumes listed in μl. Volumes in bold indicate deviations from the default volume of the reagent.
- c
Unlabeled NTPs are used for optimization; labeled NTPs can be used for large-scale reactions if the user wants to prepare labeled RNA.
- d
Use according to manufacturer's specification.
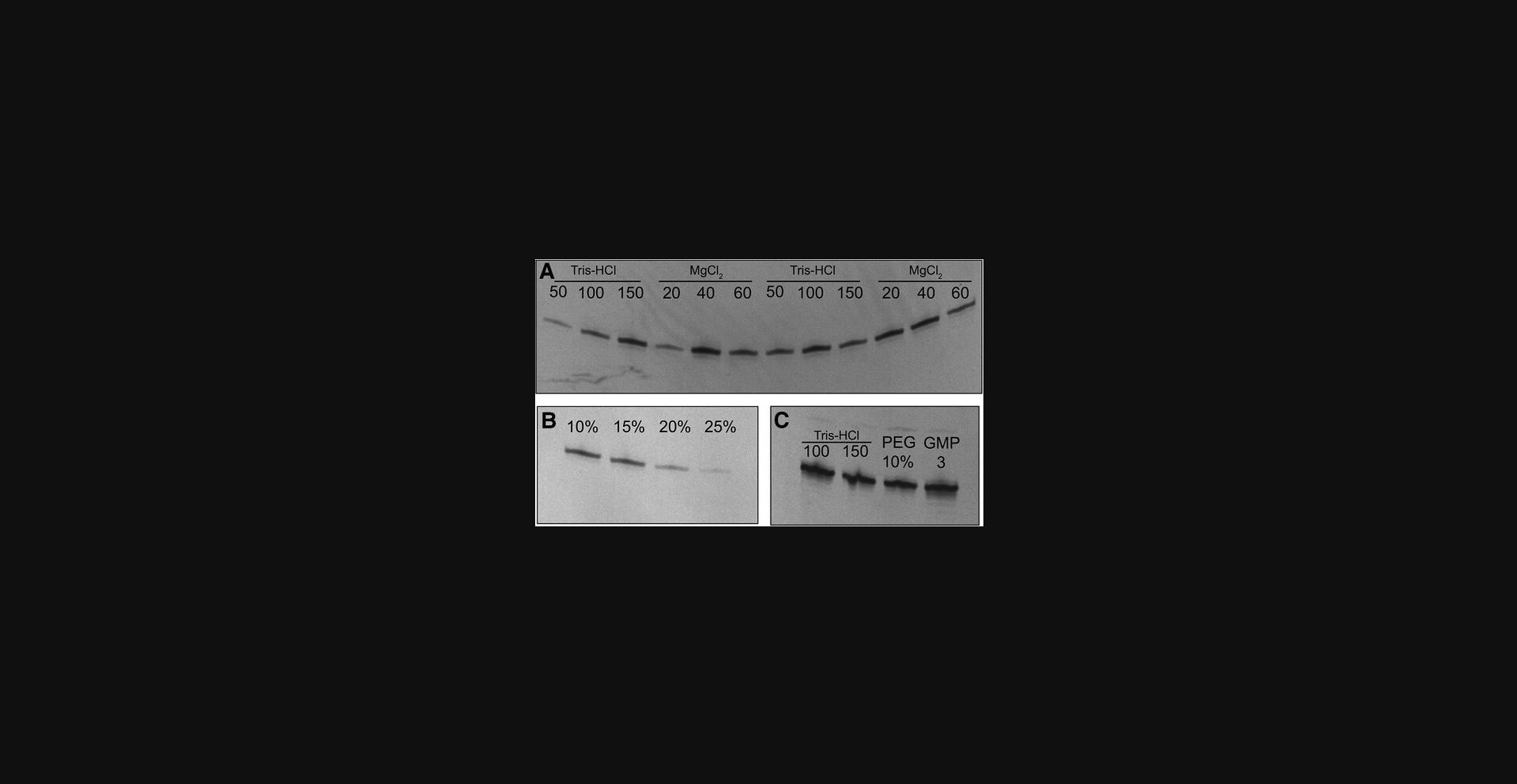
Materials
-
RNA sequence output (see Basic Protocol 1)
-
100 μM coding DNA template
-
100 μM T7 promotor complementary DNA oligo
-
0.25 M MgCl2 (e.g., Sigma-Aldrich, cat. no. 63068) in ultrapure water, sterile
-
Tris·HCl (see recipe)
-
0.25 M dithiothreitol (DTT; e.g., Sigma-Aldrich, cat. no. 43815) in ultrapure water, sterile
-
0.25 M spermidine trihydrochloride (e.g., Sigma-Aldrich, cat. no. 85578) in ultrapure water, sterile
-
DMSO (e.g., Sigma-Aldrich, cat. no. D8418)
-
Poly(ethylene glycol) (PEG)
-
NMP/NTP stock solutions (see recipe)
-
Inorganic pyrophosphatase (iPPase; e.g., Sigma-Aldrich, cat. no. I1643-100UN; UniProt ref. P00817)
-
RNaseOUTTM (e.g., Thermo Fisher, cat. no. 10777019)
-
T7 RNA polymerase (e.g., Sigma-Aldrich, cat. no. 10881767001; UniProt ref. P00573)
-
PAGE solution (see recipe)
-
10% (v/v) ammonium persulfate (APS; see recipe)
-
TEMED (e.g., Sigma-Aldrich, cat. no. T9281)
-
10× Tris-borate-EDTA buffer (TBE; e.g., Sigma-Aldrich, cat. no. T4415)
-
Formamide-based gel loading solution (see recipe)
-
Molecular weight marker (e.g., New England BioLabs, cat. nos. N0364S or N2102S)
-
Ethidium bromide (e.g., Sigma-Aldrich, cat. no. 1.11608.0030) or SYBR™ Gold (e.g., Thermo Fisher, cat. no. S11494)
-
Computer running IDT oligo analyzer tool (https://eu.idtdna.com/calc/analyzer) or similar
-
1.5-ml microcentrifuge tube (e.g., Sarstedt, cat. no. 72.690.001)
-
95°C heating block (e.g., VWR, cat. no. 75838-268)
-
Ice/NaCl bath
-
37°C water bath (e.g., VWR, cat. no. 461-3117)
-
Bio-Rad PROTEAN® II electrophoresis gel casting equipment or similar
-
Bio-Rad Mini-PROTEAN® gel electrophoresis system or similar
-
Syringe
-
UV gel imaging system (e.g., VWR, cat. no.730-1470)
Generate single-stranded DNA template
1.Analyze RNA sequence from Basic Protocol 1 with an IDT oligo analyzer tool to obtain the complementary DNA sequence.
2.Add T7 promoter sequence, 5′-TATAGTGAGTCGTATTAA-3′, to the 3′ end of the complementary DNA sequence.
3.Order resulting DNA template from IDT or other commercial provider. Specify that the 2 nt closest to the 5′ end should carry a C2′-methoxy group by adding “m” prior to their base identity.
4.Order complementary DNA strand for the T7 promoter (5′-TTAATACGACTCACTATA-3′).
Anneal DNA template
5.Create a double-stranded T7 promoter region by mixing equimolar amounts of coding DNA template and T7 promoter complementary DNA oligo (25 μM final) in the presence of 3 mM Mg2+ in a 1.5-ml microcentrifuge tube (30 μl total):
- 7.5 μl of 100 μM DNA template
- 7.5 μl of 100 μM T7 promoter complementary DNA strand
- 9 μl of 10 mM MgCl2
- 6 μl ddH2O.
6.Heat 1.5-ml microcentrifuge tubes with the annealing mixtures in a heating block at 95°C for 5 min.
7.Cool immediately on ice mixed with a small amount of NaCl to increase cooling effect, and continue cooling for 30 min.
Optimize in vitro transcription
8.Optimize transcription reaction conditions by performing test reactions on a total volume of 50 μl with different amounts of reactants. Mix all reactants in a 1.5-ml microcentrifuge tube (do not add T7 polymerase yet):
- 50 to 150 mM Tris·HCl
- 20 to 60 mM MgCl2
- 30 mM DTT
- 2 mM spermidine
- 10% to 30% (v/v) DMSO
- 10% (v/v) PEG
- 3 mM NMP starting nucleotide
- iPPase (according to manufacturer's specifications)
- RNaseOUT (according to manufacturer's specifications)
- 4 × 10–7 M DNA template with annealed promoter region.
9.Once all reactants—except T7 polymerase—have been mixed in the microcentrifuge tubes, preheat tubes in a water bath at 37°C for 5 min.
10.Add T7 polymerase to each test tube according to the manufacturer's specification.
11.Incubate reaction tubes in a water bath at 37°C for 2 hr.
Perform analytical gel electrophoresis
12.Set up Protean II gel casting system, or equivalent available system, according to the manufacturer's specifications.
13.Mix 50 ml PAGE solution with 300 μl of 10% (w/v) APS and 30 μl TEMED, and pour into gel casting system. Let gel polymerize for 15 min.
14.Mount polymerized gel in the electrophoresis system, and fill apparatus with 1× TBE. Preheat gel at 12 W power (or according to manufacturer's instructions for other systems) for ∼15 min.
15.Mix 1 μl of each individual transcription reaction with 9 μl formamide-based gel loading solution in a new, labeled 1.5-ml microcentrifuge tube.
16.Heat tubes at 95°C for 1 to 2 min.
17.Clean wells of the gel from excess urea with a syringe.
18.Load 9.5 μl mixtures from step 15 onto the gel. Add ladder or reference with the correct expected size. Run gel at 12 to 14 W for 2 hr to achieve good resolution (until the first dye is at the end of the gel).
19.Stain gel for 10 min in ethidium bromide prepared by adding 20 μl of 1% (v/v) ethidium bromide solution to 1 L water (alternative: SYBR™ Gold).
20.Destain gel in water for 10 min to obtain an even stain.
21.Inspect how the transcription reactions performed by analyzing gel using a UV board and camera (Fig. 3).
Perform large-scale transcription reaction
22.Based on the outcome of the analytical gel electrophoresis, band intensities, and distribution of deletion products, choose the best-performing reaction conditions.
23.Scale up optimum reaction condition, commonly at a 10- to 15-ml reaction scale, depending on required yield (3% to 7% typical yield of input-limiting NMP). Prepare reaction in the same way as the small-scale reactions, but incubate at 37°C overnight.
Alternate Protocol: TANDEM TRANSCRIPTION AND RNase H CLEAVAGE
This protocol can be used instead of Basic Protocol 2 in order to produce the target RNA designed in Basic Protocol 1.We have found this protocol to be particularly useful when addition of a 5′-GG sequence to the target RNA is not feasible or when the approach described in Basic Protocol 1 yields little and/or impure RNA. The procedure is based on transcribing repeated units (in tandem) from a plasmid template, followed by RNase H processing, which releases the target RNA units for further HPLC purification in a sequence-specific manner (Feyrer, Munteanu, Baronti, & Petzold, 2020). The use of the tandem template and further cleavage of target units allows the use of a high-yield initiation site. The high sequence specificity of RNase H is provided by a chimeric cleavage oligo (12 nt), which results in high-purity target RNA at the end of the protocol (see Fig. 4). Since a plasmid template is used, initial cost and effort are increased, as the plasmid needs to be amplified in bacteria first. Design and preparation of both the plasmid template and the cleavage guide oligo are described at the beginning of the protocol. In vitro transcription and RNase H cleavage are tested first in a small scale, similar to Basic Protocol 2, and are thereafter scaled up for RNA production. Escherichia coli RNase H is fully active in T7 IVT buffers, and the transcription and cleavage reactions can therefore be performed in the same tube. Many constructs do not need an annealing procedure for the RNase H cleavage guide, and the cleavage reaction can be performed simultaneously to the transcription reaction. After this protocol, the user will obtain an RNA sample ready for purification with Basic Protocol 4 (ion-exchange [IE] HPLC). The RNA produced following this protocol contains fewer abortion products, which facilitates purification. Therefore, Basic Protocol 3 (RP-IP HPLC) is generally skipped when following the Alternate Protocol.
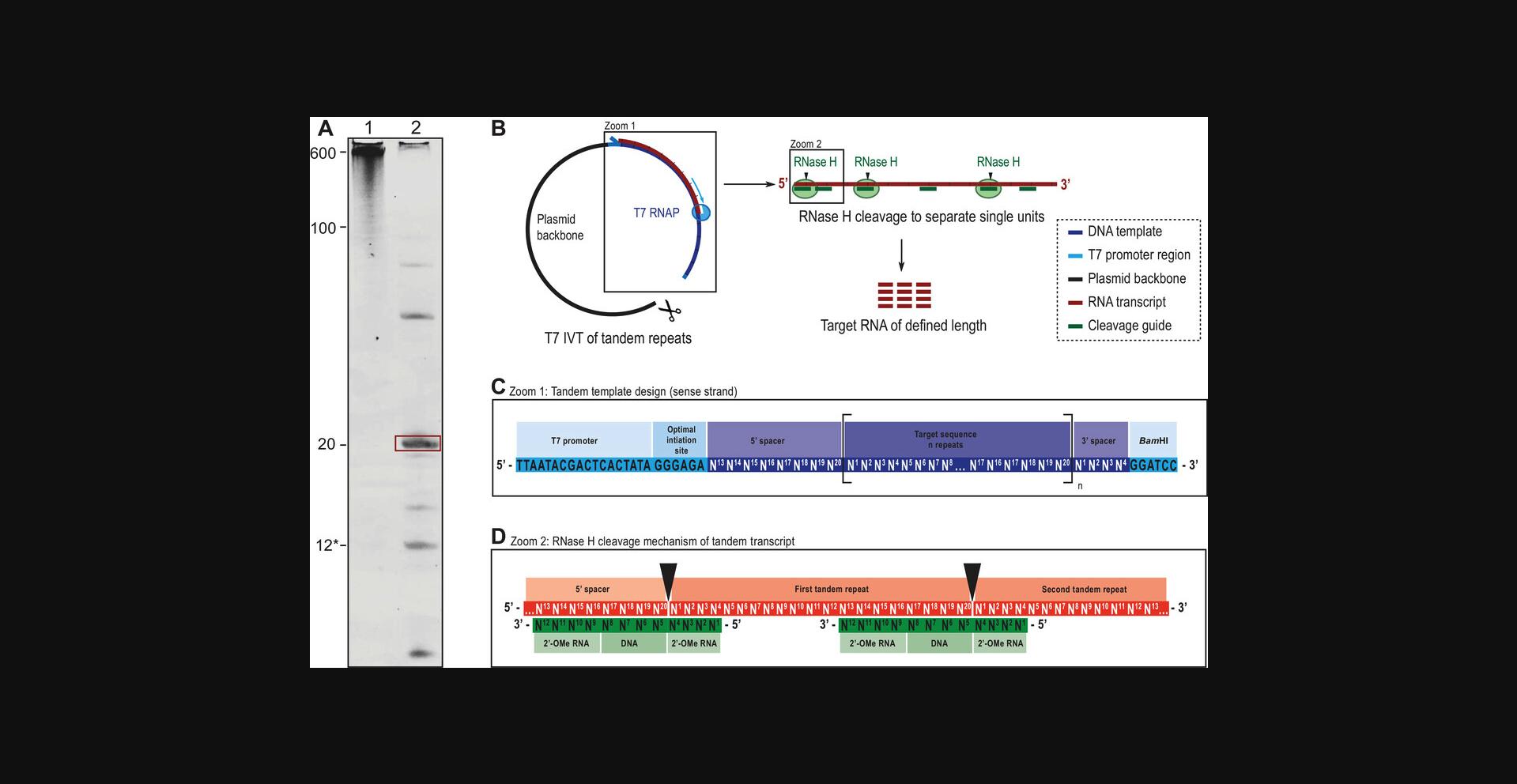
Additional Materials (also see Basic Protocol 2)
-
High-copy transcription plasmid (e.g., pUC19) containing insert designed in Basic Protocol 1
-
Plasmid purification kit (e.g., QIAGEN Plasmid Maxi, Machery-Nagel NucleoBond Xtra Maxi)
-
Bam HI restriction enzyme and reaction buffer (e.g., New England BioLabs, cat. no. R0136L)
-
Agarose (e.g., Sigma-Aldrich, cat, no. A9414)
-
GelRed (e.g., VWR, cat. no. 41003)
-
DNA ladder (e.g., GeneRuler 1kbp Plus; Fisher Scientific, cat. no. SM1333)
-
iPPase (e.g., Sigma-Aldrich, cat. no. I1643-100UN; UniProt ref. P00817)
-
E. coli RNase H (e.g., New England BioLabs, cat. no. M0297L; UniProt ref. P0A7Y4)
-
EDTA (e.g., Sigma-Aldrich, cat. no. E6758)
-
0.22-μm syringe filter (e.g., VWR, cat. no. 514-0068)
-
Amicon Ultra centrifugal filter unit (e.g., Sigma-Aldrich, cat. no. UFC900324)
-
37°C incubator
-
Conventional microwave oven
-
Horizontal gel electrophoresis system (e.g., VWR, cat no. 700-0056)
-
50-ml centrifuge tube
-
Vortex mixer
Design plasmid
In brief, the goal is to design and order a plasmid that contains a common T7 promoter sequence, a 5′ spacer sequence, a number of repeats of the target sequence, a 3′ spacer sequence, and a restriction site for linearization (see Fig. 4C). 5′ and 3′ spacers act as cleavage guide binding sites to separate the initiation sequence and plasmid overhangs from the first and last repeat sequence, respectively. In the example described here, the target sequence is 20 nt long. All sequences are written as “sense” strands, meaning the target RNA sequence can simply be converted to a DNA sequence by changing U to T. Gene synthesis will automatically produce the reverse complement template.
1.Open cloning tool of choice (e.g., SnapGene or SerialCloner) to design the sense strand of the template.
2.Start with the T7 promoter sequence as a sense strand, and add the high-yield initiation sequence (standard T7 promotor: 5′-TAA TAC GAC TCA CTA TA^G GGA GA-3′).
3.Add the last 8 nt of the target RNA sequence as a 5′ spacer (using T instead of U; Fig. 4C).
4.Add tandem repeats of the RNA target sequence (using T instead of U) until nucleotide limit of the chosen manufacturer is reached (e.g., 600 nt limit from GenScript).
5.Add the first 4 nt of the target RNA sequence at the end as a 3′ spacer (using T instead of U).
6.Add a Bam HI restriction sequence (or other unique restriction site; Fig. 4C).
7.Order high-copy plasmid (e.g., pUC19) with above designed insert.
Design cleavage guide oligo
The 12 nt cleavage guide oligo is comprised of four central DNA nucleotides, which are crucial for the activity of E. coli RNase H. The enzyme cleaves the RNA strand opposite the DNA 5′ end. This sequence is flanked by 2′-OMe modified nucleotides, which enhance specificity and cleavage efficiency but do not change the cleavage site. In brief, the cleavage guide oligo is designed to overlap with 8 nt of the first repeat and with 4 nt of the second repeat and cleaves so that the DNA 5′ end aligns with the separation of first and last repeats (Inoue, Hayase, Iwai, & Ohtsuka, 1987; see Fig. 4D).
8.Take the last 8 nt of the RNA sequence (5′–3′), and add the first 4 nt on the 3′ end.
9.Create the complimentary DNA strand of this sequence (e.g., with IDT oligo analyzer: https://eu.idtdna.com/calc/analyzer).
10.Convert the first four and the last 4 nt to 2′-OMe-modified versions by adding “m” before the base identity (U stays mU for synthesis reasons). Order the oligo from IDT or other commercial supplier.
Prepare plasmid
11.Amplify plasmid (ordered in step 7) in E. coli using a commercial maxiprep kit following the manufacturer's instructions.
12.Linearize plasmid using the Bam HI restriction enzyme:
- a.Mix reagents:
- 1 µg amplified plasmid (in water or TE buffer)
- 5 µl of 10× reaction buffer
- Nuclease-free water to 49 µl
- 1 µl Bam HI.
- b.Incubate for 1 hr at 37°C.
- c.
Store reaction in freezer until further use.
The reaction can be scaled up to 1 ml at full restriction yield. The plasmid can be stored at –20°C for several months and thawed right before use. A cleanup of the reaction is not necessary but is recommended (e.g., using Monarch DNA/PCR cleanup kit; New England BioLabs, cat. no. T1030S).
13.Confirm linearization on a 1% (w/v) agarose gel:
-
Dissolve 1 g agarose in 100 ml of 0.5× TBE by heating in a microwave.
-
Add 10 µl GelRed and mix well.
-
Pour hot agarose solution into electrophoresis chamber, and let cool for ∼30 min. Insert comb.
-
Once solidified, insert chamber into the running tank, and fill with 0.5× TBE until the gel is covered.
-
Prepare samples at a total amount of 50 to 100 ng plasmid, and add DNA loading dye (shipped with restriction enzyme).
-
Remove comb and load samples by carefully pipetting into wells. Add DNA ladder.
-
Run gel at 150 V for 30 min.
-
Image gel using a UV illuminator.
Successful linearization is shown by a single band that is slightly shifted upward compared with the band representing the nonlinearized plasmid. Note that the circular plasmid (run as a negative control) often shows multiple bands from multiple supercoiling states.
Perform small-scale in vitro transcription
14.Prepare transcription reaction in a volume of 50 µl:
- 10 μl of 0.5 M Tris·HCl (100 mM final)
- 2 μl of 0.25 M MgCl2 (10 mM final)
- 2 μl of 0.25 M DTT (10 mM final)
- 5 μl of 0.25 M spermidine (25 mM final)
- 2.5 μl of 0.1 M GMP (5 mM final)
- 1.5 μl of 0.1 M NTPs each (3 mM final)
- 5 µl of 20 ng/µl digested plasmid from step 12 (100 ng final)
- 10 µl of 100 µM cleavage guide (20 μM final)
- 0.1 mg/ml iPPase
- 0.2 µg/ml E.coli RNase H
- 0.3 mg/ml T7 RNA polymerase.
15.Incubate at 37°C for 1 hr.
16.Analyze transcription and cleavage on a 20% denaturing polyacrylamide gel as described in Basic Protocol 2 steps 12 to 21.
Perform large-scale in vitro transcription reaction
17.Prepare 10-ml reaction with the concentrations described in step 14.
18.Run reaction overnight at 37°C.
19.Analyze transcription and cleavage on a 20% denaturing polyacrylamide gel. If only the target band and cleavage guide are visible, move on to IE HPLC purification (Basic Protocol 4), and skip RP-IP HPLC (Basic Protocol 3).
20.If higher molecular compounds are still present, perform an annealing step and another RNase H cleavage reaction:
- Heat reaction mixture (from step 15) in a conventional microwave for 10 to 15 s at 450 W in a closed 50-ml centrifuge tube.
The solution might reach the boiling point.
- Let solution cool for 30 min at 37°C and then for 30 min at room temperature.
A precipitate may form.
-
Add the same amounts of iPPase and RNase H as in step 14.
-
Incubate at 37°C for 3 hr, and analyze cleavage success on a denaturing gel.
-
If cleavage is still incomplete, repeat step 20.
Heating large amounts of RNA solution can take a long time, which allows for Mg2+-catalyzed RNA hydrolysis. Use of a microwave oven significantly reduces this time and avoids the need for aliquoting the sample. So far, we have not seen adverse effects of microwave irradiation.
21.Quench reaction by adding EDTA to 50 mM and vortex thoroughly.
22.Filter solution through a 0.22-µm syringe filter, and concentrate using an Amicon Ultra concentrator for injection into HPLC, depending on injection loop size (e.g., 5 ml).
Basic Protocol 3: REVERSED-PHASE ION-PAIRING HPLC PURIFICATION
This protocol describes the purification of the in vitro‒transcribed RNA material from Basic Protocol 2, first with a crude purification step using RP-IP HPLC. During the preparation phase, precipitated magnesium pyrophosphates (iPP) are dissolved with an EDTA solution (Fig. 2; Karlsson, Baronti, & Petzold, 2020) and proteins precipitated by heat denaturation. After this preparation, initial purification with RP-IP HPLC removes many of the shorter IVT products, reaction components, and possible residual, microscopic iPP particles. Therefore, performing the RP-IP purification step reduces the load on the IE column in the second HPLC purification step described in Basic Protocol 4.Basic Protocol 3 yields semi-purified material that might be considered sufficiently pure for some purposes (e.g., NMR spectroscopy, UV melting studies, other biophysical characterization techniques), but most likely the user will want to proceed to Basic Protocol 4 and further purify the material in the IE purification step.
Materials
-
IVT reaction (see Basic Protocol 2)
-
40 mM EDTA (see recipe)
-
RP-IP buffer A (see recipe)
-
RP-IP buffer B (see recipe)
-
Refrigerated centrifuge (e.g., Eppendorf 5804R) with swinging-bucket rotor
-
1.5-ml microcentrifuge tube (e.g., Sarstedt, cat. no. 72.690.001)
-
Heating block (e.g., VWR, no. 75838-268)
-
Ice
-
Microcentrifuge (e.g., VWR, cat. no. 521-2161P)
-
Syringe filter (e.g., VWR, cat. no. 514-0068)
-
Amicon Ultra-15 Centrifugal Filter Unit (e.g., Sigma-Aldrich, cat. no. UFC900324)
-
HPLC system (e.g., Dionex Ultimate 3000 UHPLC) and associated equipment:
- LPG-3400RS pump (e.g., Thermo Scientific, cat. no. 5040.0036)
- TCC-3000RS column thermostat (e.g., Thermo Scientific, cat. no. 5730.0000)
- VWD-3100 detector (e.g., Thermo Scientific, cat. no. 5074.0005)
- AFC-3000 HPLC fraction collector (e.g., Thermo Scientific, cat. no. 5702.1000)
- Hypersil Gold 250 × 10 reversed-phase semi prep column (e.g., Thermo Scientific, cat. no. 25005-259070)
- Hypersil Gold 10 × 10 guard column (e.g., Thermo Scientific, cat. no. 25005-019023)
- HPLC injection syringe (e.g., Sigma-Aldrich)
Prepare in vitro transcription for reversed-phase ion-pairing HPLC
1.Remove large-scale transcription reaction from the water bath (Basic Protocol 2 step 23), and centrifuge potential iPP pellet 5 min at 2000 × g , 4°C.
2.Distribute supernatant between several microcentrifuge tubes (∼1 ml each), taking care not to disturb the pellet.
3.Dissolve iPP pellet using 40 mM EDTA. Aliquot this material into new 1.5-ml microcentrifuge tubes (∼1 ml each).
4.Heat all microcentrifuge tubes (ones containing supernatants and ones containing dissolved iPP pellet) in a heating block for ∼3 min to denature proteins. Cool tubes on ice, forming a precipitate.
5.Once tubes are cold, briefly centrifuge using a table-top microcentrifuge.
6.Using a syringe and syringe filter, filter supernatants into an Amicon Ultra-15 centrifugal filter unit.
7.Concentrate filtered material to a volume suitable for the injection loop of the HPLC system. Keep RNA material on ice.
Equilibrate column
8.Equilibrate RP system (10 × 250 Hypersil Gold Reversed-Phase Column) using the following suggested gradient:
0 min | 2.5 ml/min | 0% buffer B |
5 min | 2.5 ml/min | 100% buffer B |
15 min | 2.5 ml/min | 100% buffer B |
20 min | 2.5 ml/min | 7% buffer B |
45 min | 2.5 ml/min | 7% buffer B. |
9.Place microcentrifuge tubes into fraction collector.
Purify by reversed-phase ion-pairing HPLC
10.Prepare HPLC system for purification of RNA using the following suggested gradient:
0 min | 3 ml/min | 7% buffer B |
15 min | 3 ml/min | 7% buffer B |
17 min | 3 ml/min | 25% buffer B |
47 min | 3 ml/min | 35% buffer B |
52 min | 3 ml/min | 100% buffer B |
62 min | 3 ml/min | 100% buffer B |
67 min | 3 ml/min | 7% buffer B |
80 min | 3 ml/min | 7% Buffer B. |
11.Inject RNA material onto the RP column using the gradient from step 10.Once the HPLC run is completed, label fraction tubes, and store in the freezer.
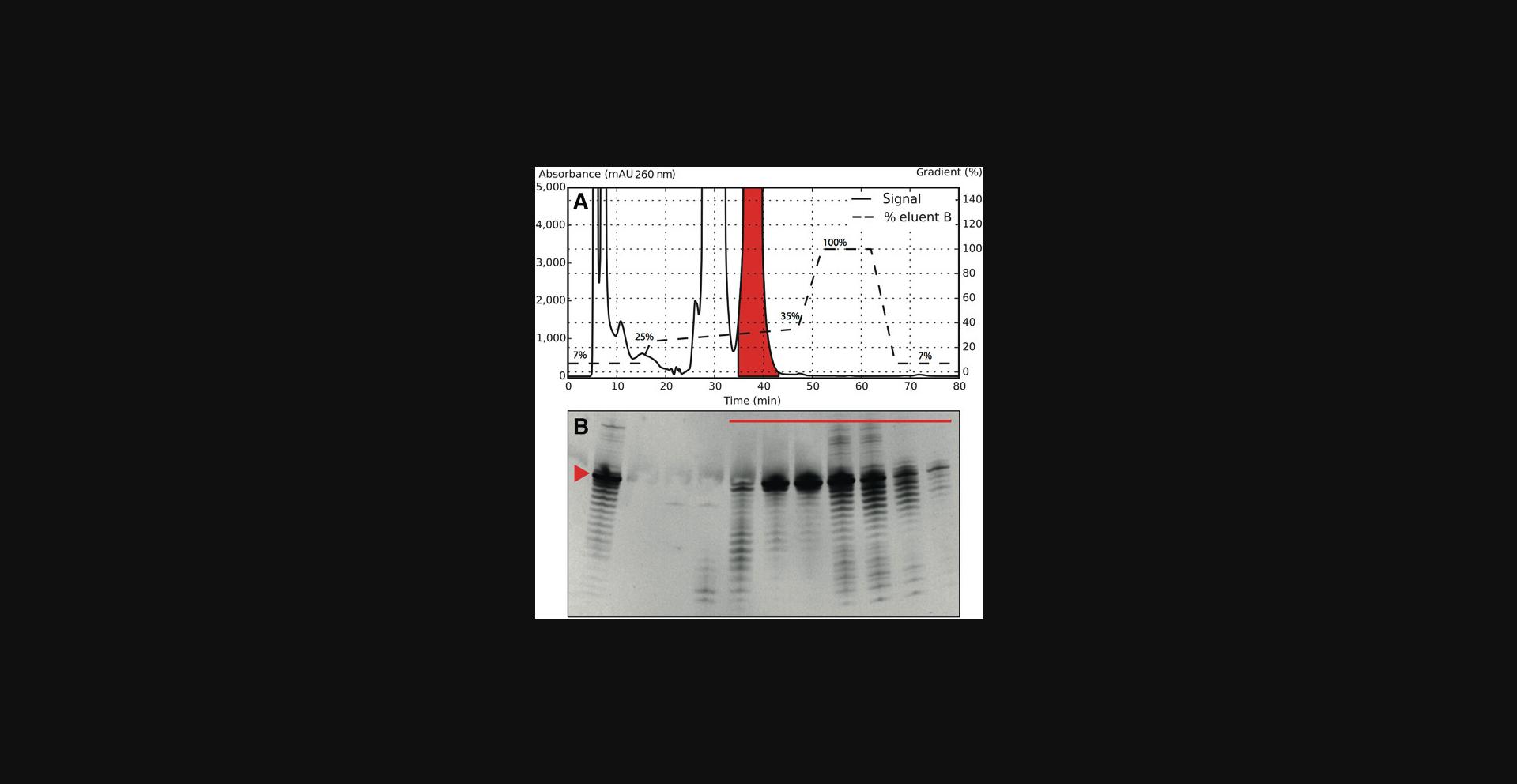
12.Repeat injections on the RP system according to steps 10 and 11 until all material has been purified by RP-IP HPLC.
13.Prepare PAGE according to Basic Protocol 2 steps 12 to 21.
14.Prepare HPLC fractions that are expected to contain the desired target. Load 1 to 5 μl on a gel, and analyze according to Basic Protocol 2 steps 12 to 21.
15.Based on PAGE results, pool all HPLC fractions in which the transcript of interest is dominating (i.e., band intensity of the wanted transcription product is higher than of unwanted products; Fig. 5).
16.Concentrate pooled fractions using an Amicon Ultra concentrator. Store concentrate at –20°C if the IE step is not performed immediately.
Basic Protocol 4: ION-EXCHANGE HPLC PURIFICATION
This protocol describes the final purification step of the workflow, in which RNA is purified with IE HPLC. Here, the user purifies the RNA material by distributing it between several injections onto a heated IE column and separates it using a perchlorate-based buffer system. The source RNA material has either been prepurified by RP-IP HPLC in Basic Protocol 3 or comes directly from an IVT reaction as described in the Alternate Protocol. This protocol purifies the material further and yields HPLC fractions of high purity that are then once again analyzed with PAGE, after which the purest fractions are chosen, pooled, concentrated, and buffer exchanged to yield the final RNA sample.
Additional Materials (also see Basic Protocol 3)
-
IE buffer A (see recipe)
-
IE buffer B (see recipe)
-
RNA sample (see Basic Protocol 3 or Alternate Protocol)
-
NMR buffer (optional; see recipe)
-
DNAPac PA200 22 × 250 semi-prep column (e.g., Thermo Scientific, cat. no. SP6734)
-
DNAPac PA200 22 × 50 guard column (e.g., Thermo Scientific, cat. no. SP6731)
Equilibrate column
1.Equilibrate IE column at 75°C using the following suggested gradient:
0 min | 4 ml/min | 0% buffer B |
10 min | 4 ml/min | 0% buffer B |
11 min | 4 ml/min | 20% buffer B |
25 min | 4 ml/min | 30% buffer B |
27 min | 4 ml/min | 100% buffer B |
33 min | 4 ml/min | 100% buffer B |
35 min | 4 ml/min | 0% buffer B |
45 min | 4 ml/min | 0% buffer B. |
2.While the column is equilibrating (45 min), prepare 1.5-ml microcentrifuge tubes, and put in the fraction collector for fraction collection.
Purify by ion-exchange HPLC
3.After column equilibration, inject RNA material that has been prepurified using RP-IP HPLC in Basic Protocol 3 or that comes directly from IVT in the Alternate Protocol using the following suggested starting gradient:
0 min | 4.5 ml/min | 0% buffer B |
10 min | 4.5 ml/min | 0% buffer B |
11 min | 4.5 ml/min | 17% buffer B |
45 min | 4.5 ml/min | 42% buffer B |
46 min | 4.5 ml/min | 100% buffer B |
59 min | 4.5 ml/min | 100% buffer B |
60 min | 4.5 ml/min | 0% buffer B |
75 min | 4.5 ml/min | 0% buffer B. |
4.Once the HPLC run is completed, label fraction tubes, and store at –20°C. Repeat injections on the IE system according to step 3 until all material has been purified by IE HPLC.
5.Perform PAGE according to Basic Protocol 2 steps 12 to 21.
6.Prepare HPLC fractions that are expected to contain the desired target. Load fractions on a gel, and analyze according to Basic Protocol 2 steps 12 to 21 (see Fig. 6).
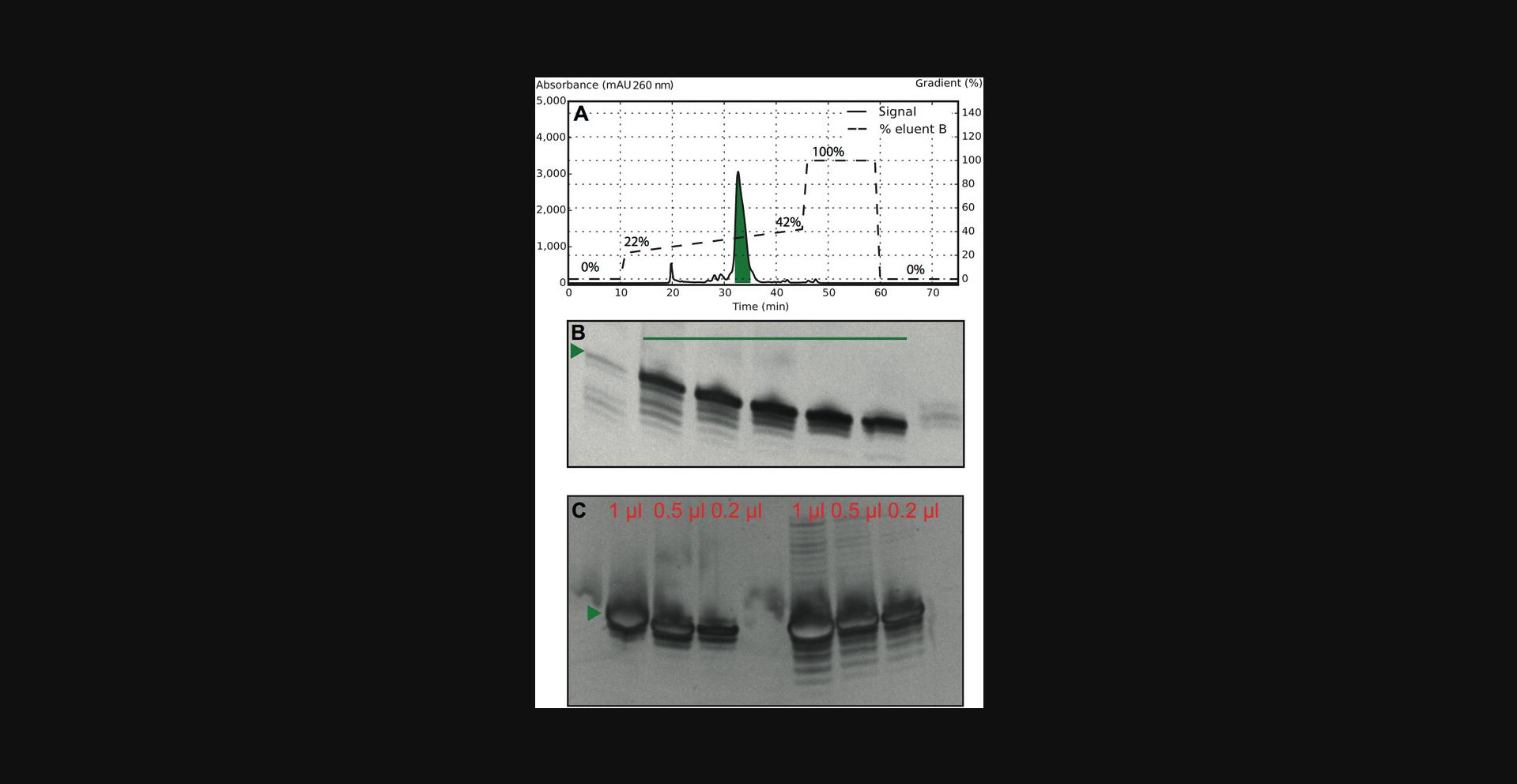
7.Pool fractions considered pure in an Amicon Ultra-15 centrifugal filter unit, and start concentrating material in a centrifuge at 2000 × g , 4°C.
8.Once the material has been concentrated to <1.5 ml, exchange buffer to one suitable for subsequent experiments of interest (e.g., NMR buffer) by performing a 10-fold dilution and reconcentrating at least three times.
9.Fold RNA by distributing the sample (∼10 ml) into several microcentrifuge tubes, heating to 95°C for 3 min, and cooling rapidly on ice.
10.Once the material has cooled after the folding step, continue with the final concentration down to the final volume.
REAGENTS AND SOLUTIONS
APS, 10%
- 1 g APS (e.g., Sigma-Aldrich, cat. no. A3678)
- 10 ml ultrapure type 1 water, 18.2 MΩ**·** cm at 25°C
- Store aliquots at –20°C for up to 12 months
EDTA, 40 mM
- 40 mM EDTA (e.g., Sigma-Aldrich, cat. no. 1.11608.0030)
- Ultrapure type 1 water, 18.2 MΩ**·** cm at 25°C
- Adjust pH from 6.5 to 8.0 slowly by titrating with 1 M NaOH (e.g., Sigma-Aldrich, cat. no. 72068)
- Sterilize by filtering through a Sterflip filter unit (e.g., Sigma-Aldrich, cat. no. SCNY00100)
- Store at room temperature for up to 3 months
Formamide-based gel loading solution
- 50 ml formamide, >99.5% (e.g., Sigma-Aldrich, cat. no. 47671)
- 50 mM EDTA (e.g., Sigma-Aldrich, cat. no. E6758)
- 13 mg bromophenol blue (e.g., Sigma-Aldrich, cat. no. 1081220005)
- Store 1-ml aliquots at room temperature for up to 2 weeks
Store larger amounts in the fridge (2°C to 8°C).
IE buffer A
- 20 mM sodium acetate (e.g., Sigma-Aldrich, cat. no. S2889)
- 20 mM NaClO4 (e.g., Sigma-Aldrich, cat. no. 71853)
- Ultrapure type 1 water, 18.2 MΩ**·** cm at 25°C
- Adjust pH to 6.5 using acetic acid (e.g., Sigma-Aldrich, cat. no. 49199)
- Sterilize and degas by filtering through a bottle-top filter (e.g., VWR, cat. no. 514-0340)
- Store at room temperature for up to 3 months
IE buffer B
- 20 mM sodium acetate (.g., Sigma-Aldrich, cat. no. S2889)
- 600 mM NaClO4 (.g., Sigma-Aldrich, cat. no. 71853)
- Ultrapure type 1 water, 18.2 MΩ.cm at 25°C
- Adjust pH to 6.5 using acetic acid (e.g., Sigma-Aldrich, cat. no. 49199)
- Sterilize and degas by filtering through a bottle-top filter (e.g., VWR, cat. no. 514-0340)
- Store at room temperature for up to 3 months
NMP/NTPs
- Prepare individual solutions containing 100 mM each of:
- GMP (e.g., Sigma-Aldrich, cat. no. G8377)
- UMP (e.g., Sigma-Aldrich, cat. no. U6375)
- ATP (e.g., Sigma-Aldrich, cat. no. A2383)
- UTP (e.g., Sigma-Aldrich, cat. no. U6625)
- GTP (e.g., Sigma-Aldrich, cat. no. G8877)
- CTP (e.g., Sigma-Aldrich, cat. no. C1506)
- Ultrapure type 1 water, 18.2 MΩ**·** cm at 25°C
- Sterilize by filtering through a Sterflip filter unit (e.g., Sigma-Aldrich, cat. no. SCNY00100)
- Store at –20°C for up to 12 months
As an alternative 13C-/15N-labeled NTPs can instead be used: GMP-13C10,15N5 (e.g., Sigma-Aldrich, cat. no. 650684), UMP-13C9,15N2 (e.g., Sigma-Aldrich, cat. no. 651370), ATP-13C10,15N5 (e.g., Sigma-Aldrich, cat. no. 645702), UTP-13C10,15N5 (e.g., Sigma-Aldrich, cat. no. 645672), GTP-13C10,15N5 (e.g., Sigma-Aldrich, cat. no. 645680), and CTP-13C10,15N5 (e.g., Sigma-Aldrich, cat. no. 645699).
NMR buffer
- 1.Prepare stock solution A containing 15 mM NaH2PO4 monobasic (e.g., Sigma-Aldrich, cat no. S3139), 25 mM NaCl (e.g., Sigma-Aldrich, cat. no. S3014), and 0.1 mM EDTA (e.g., Sigma-Aldrich, cat. no. E6758) in ultrapure type 1 water, 18.2 MΩ**·** cm at 25°C.
- 2.Prepare stock solution B containing 15 mM NaHPO4 dibasic (e.g., Sigma-Aldrich, cat. no. S3264), 25 mM NaCl (e.g., Sigma-Aldrich, cat. no. S3014), and 0.1 mM EDTA (e.g., Sigma-Aldrich, cat. no. E6758) in ultrapure type 1 water, 18.2 MΩ**·** cm at 25°C.
- 3.Mix stock solutions A and B at a 2:1 ratio (A in excess). Slowly add more solution A while measuring pH until the final pH is 6.5.
- 4.Sterilize by filtering using a bottle-top filter (e.g., VWR, cat. no. 514-1019).
- 5.Store at room temperature for up to 12 months.
PAGE solution
- 500 ml of 40% acrylamide/bis solution, 19:1 (e.g., Bio-Rad, cat. no. 161-0144)
- 480 g of 8 M urea (e.g., Sigma-Aldrich, cat. no. U5378)
- 100 ml of 10× TBE (e.g., Sigma-Aldrich, cat. no. T4415)
- Bring to 1 L with ultrapure type 1 water, 18.2 MΩ**·** cm at 25°C
- Sterilize by filtering using a bottle-top filter (e.g., VWR, cat. no. 514-1019)
- Store at room temperature for up to 3 months
RP-IP buffer A
- 100 mM ammonium acetate (e.g., Fisher Scientific, cat. no. 10598410)
- 2 mM tetrabutylammonium hydrogen sulfate (e.g., Fisher Scientific, cat. no. 10095650)
- Ultrapure type 1 water, 18.2 MΩ**·** cm at 25°C
- Adjust pH to 6.5 using NaOH (e.g., Sigma-Aldrich, cat. no. 72068)
- Degas using a bottle-top filter (e.g., VWR, cat. no. 514-0340)
- Store at room temperature for up to 3 months
RP-IP buffer B
- 4 mM ammonium acetate (e.g., Fisher Scientific, cat. no. 10598410)
- 0.4 mM tetrabutylammonium hydrogen sulfate (from 100× stock solution; e.g., Fisher Scientific, cat. no. 10095650)
- Ultrapure type 1 water, 18**·** 2 MΩ.cm at 25°C
- Adjust pH to 6.5 using NaOH (e.g., Sigma-Aldrich, cat. no. 72068)
- Add acetonitrile (e.g., Sigma-Aldrich, cat. no. 34851) to 80% (v/v)
- Degas using an ultrasound bath
- Store at room temperature for up to 3 months
Tris·HCl
- 0.5 M Tris base (e.g., Fisher Scientific, cat. no. 10103203)
- Ultrapure type 1 water, 18.2 MΩ**·** cm at 25°C
- Adjust pH to pH 8.0 using HCl (e.g., Sigma-Aldrich, cat. no. H1758)
- Sterilize by filtering through a Sterflip filter unit (e.g., Sigma-Aldrich, cat. no. SCNY00100)
- Store at –20°C for up to 12 months
COMMENTARY
Background Information
The protocols presented in this article are based on sample production methods published previously by our group (Feyrer et al., 2020; Karlsson et al., 2020). They are a consolidation of previous advancements of RNA production with T7 IVT and are put together in one coherent and robust protocol. We additionally describe how to design an RNA fragment, which should preserve the structure of a larger biological RNA for structural studies (Fig. 1). This approach compares the simulated structure of the larger native RNA to that of the fragment of interest to be produced. The simulations are performed with the software MC-Fold, as it takes non-canonical base pairs into account. An inference about thermodynamic stability and the conformational ensemble of both RNA constructs can be made by analyzing the simulated structures over a large energy range (Fig. 2). Using this approach, the likelihood of correct folding of the RNA fragment produced can be increased before starting the production of the sample.
Since the development of the T7 RNA polymerase as a tool in molecular biology (Milligan et al., 1987; Milligan & Uhlenbeck, 1989), several aspects of the process have been advanced to overcome limitations in the in vitro production of RNA. For synthetic DNA templates, the last 2 nt of the template have been modified with 2′-OMe groups (Helmling et al., 2015; Kao et al., 1999), which yield RNA of higher 3′ homogeneity (Gholamalipour, Karunanayake Mudiyanselage, & Martin, 2018; Gholamalipour, Johnson, & Martin, 2019) in the presence of DMSO. DMSO has also been shown to increase the yield of RNA in IVT reactions (Chen & Zhang, 2005). As transcription products of similar length to the target (±3) can never be fully avoided, these similar-sized RNA molecules need to be purified from the RNA of interest. Our pipeline uses HPLC purification instead of fast protein liquid chromatography (FPLC) or PAGE excision. The RP step employs an IP agent to allow separation by size in native conditions. As a counterion in IE HPLC, perchlorate has shown high separation power for nucleic acids. Both of these purification steps overcome issues with low separation seen in FPLC and with residual contaminants seen in PAGE excision. By using RP-IP HPLC prepurification, the injection load on the IE column is significantly reduced, and fewer abortive RNA products and transcription contaminants are injected. Distribution of the RNA material between several different injections also reduces resolution issues and allows the user to obtain purer HPLC fractions. Finally, the first transcribed nucleotides can influence the yield of the IVT. The incorporation of a 5′-GG starting sequence can partly overcome this limitation, although this is not an approach suitable for all RNA target sequences. For RNA sequences that require a non-GG starting sequence, we developed the Alternate Protocol, which overcomes all aforementioned problems by using a high-yield starting sequence and cleaving tandem units with high precision using E. coli RNase H. This exact pipeline has been used to produce a variety of samples in our laboratory, some of which have been previously published (Baronti et al., 2020; Schlagnitweit, Steiner, Karlsson, & Petzold, 2018; Steiner, Schlagnitweit, Lundström, & Petzold, 2016). Even though we usually follow the protocols described here to produce samples for studies with NMR spectroscopy, the RNA preparations are not limited to this particular method. In principle, any application requiring highly pure RNA fragments can benefit from this pipeline (e.g., small angle X-ray scattering, electrophoretic shift assays, X-ray crystallography).
Despite the many advantages of the described approach, the method has a number of limitations. Prediction of secondary structure cannot guarantee exact folding, leaving some uncertainty and the need for further structure probing. In addition, even with the introduction of 5′-GG sequences or using the tandem transcript approach, sequence dependency cannot be eliminated entirely, giving rise to a certain variation in yield between different RNA constructs. Further, the RP-IP HPLC step does reduce wear and tear on the IE column but adds more time to the protocol and can even lead to product loss. Throughout the protocols, we have consistently suggested the use of polyacrylamide gels stained with ethidium bromide. Despite the well-known health hazards of these chemicals and the fact that more modern alternatives such as the SYBR® series of nucleic acid stains are now available, we have chosen ethidium bromide for staining owing to its robustness and ease of use. A detailed discussion on the use of ethidium bromide has been done (Karlsson et al., 2020). However, staining protocols using different stains provide similar sensitivity and do not limit the applicability of the protocol.
Critical Parameters
Construct and sequence design
The performance of the T7 transcription reaction will depend on the sequence of the RNA to be transcribed. As mentioned in Basic Protocol 1, a sequence starting with a 5′-GG will transcribe better (Price et al., 1995). As emphasized in Basic Protocol 1, it is important to consider the folding behavior of possible deletion and addition products. Even if the protocols described here will result in final fractions of high purity, deletion and addition products might be present to some extent.
Cleavage guide design
The tandem transcription excels over conventional IVT because RNase H produces more precise cleavage sites than T7 polymerase produces defined 5′ and 3′ ends. Therefore, off-target cleavage due to unspecific binding of the cleavage guide is detrimental to the method. Screen the sequence for complementary matches of the central DNA fragment and in the flanking regions. We have not experienced this issue; however, Duss, Maris, von Schroetter, & Allain (2010) mentioned that extending the flanks can increase specificity in case of off-target or incomplete cleavage.
In vitro transcription optimization
The goal of the IVT optimization steps (Basic Protocol 2 steps 8 to 11) is to maximize the RNA transcript yield in relation to amount/cost of the reactants. In the protocols described here, when analytical gel electrophoresis with ethidium bromide staining and UV illumination is used for evaluation of the reaction, it is important to not overinterpret the results of the gel. That is, it should be kept in mind that quite large concentration variations can give rise to bands of similar appearance on a stained gel, depending on loading accuracy, staining efficiency, and side products. Once a condition allows for clear transcription and results in intense bands on the gel, choose that condition, especially if it requires smaller amounts of expensive reagents.
RNase H cleavage
In the case of a successful tandem IVT, all of the transcript can be cleaved to full-length target RNA, leaving only the 5′ and 3′ spacer regions, containing the initiation sequence and restriction site residue, respectively. No higher molecular weight species should be visible on an analytical PAGE.
HPLC system
For a successful outcome of the protocols, it is important that the HPLC system is equilibrated properly. If the HPLC columns are correctly equilibrated, the reproducibility between injections is usually high, and the regions of the chromatograms in which the main transcript elutes are almost identical between injections if equal amounts are injected.
RNase-free working routines
Throughout all of the steps of the protocol, it is important to ensure that the HPLC system and laboratory equipment for the IVT, buffer exchange steps, and other steps are free from RNases. The best way to ensure this is, in addition to ordinary sterile technique routines (e.g., ethanol, gloves), to clean workspaces and equipment with >95% ethanol and RNase-removing products such as RNaseZap.
Analytical gel electrophoresis (PAGE)
The HPLC fractions in Basic Protocols 3 and 4 are analyzed with analytical gel electrophoresis. What is important to consider during gel staining of HPLC fractions are the dilution effects from HPLC use and that contaminant transcripts might be poorly visible on a gel from a HPLC fraction, but once pooled and concentrated down into a final sample, they might appear more clearly. It is important to keep this in mind when choosing fractions to pool for further processing.
Troubleshooting
Table 2 outlines some common problems that users may encounter when following the protocols, their likely causes, and potential solutions.
Problem | Possible reason | Solution |
---|---|---|
Poor or no transcription (standard IVT) | Erroneous transcription reaction stock solutions; RNA degradation; RNase contamination; unsuitable reaction conditions; failed DNA template annealing | Prepare new stock solutions of reactants; change reaction conditions; test enzyme of other supplier; check DNA template on gel for correct size and purity; run IVT reaction that has worked before as a positive control; consider tandem IVT |
Poor or no transcription (plasmid IVT) | As above for standard IVT; unsuccessful linearization | Test linearization on agarose gel |
Incomplete RNase H cleavage | Cleavage guide or RNase H concentration too low or time too short | Heat to 95°C for 2 min and cool slowly; add more iPPase and RNase H for 1 hr |
Small peak in chromatogram; loss of material during concentration steps | Leakage through concentrator membrane during concentration steps | Check A260 of concentrator flow-through; use concentrator filter unit for shorter time; ensure acetonitrile content is below limit specified by manufacturer |
Gel contains unexpected bands when loading concentrated material | HPLC purifications dilute the RNA material, so when loading a fraction contaminant bands might be poorly visible on gel | Consider possible dilution effects when analyzing HPLC fractions and choosing fractions to pool together as sample |
Nonhomogeneous migration of bands on gel | Temperature differences within gel during running; high salt content | Fill gel apparatus with water to even out temperature within gel; clean gel wells quickly before loading |
RNA does not bind to HPLC column | Column is poorly equilibrated; HPLC buffer system is erroneous | Re-equilibrate column; expose column to larger amount of eluting buffers; check HPLC buffers |
Retention time shift; RNA elutes in wrong part of gradient | Column is poorly equilibrated; HPLC buffer system is erroneous | Re-equilibrate column; expose column to larger amount of eluting buffers; check HPLC buffers; clean HPLC system |
- A260, absorbance at 260 nm; HPLC, high-performance liquid chromatography; iPPase, inorganic pyrophosphatase; IVT, in vitro transcription.
Understanding Results
The ultimate goal of the protocols described here is to obtain an RNA sample of high enough purity for the user's needs. One starts with creating a suitable sample that contains the region of interest (Basic Protocol 1) as shown in Figure 1. An example of how to evaluate the selection of the correct construct is shown in Figure 2. This is a flexible protocol, with options to use different protocols for RNA transcription (Basic Protocol 2 and Alternate Protocol). Use of Basic Protocol 4 or a combination of Basic Protocols 3 and 4 offers additional flexibility and the possibility to produce even poorly transcribing RNA with high yield and purity. The examples provided in Figures 3, 5, and 6 show gels and chromatograms from the optimization and purification of a 29mer RNA, which ultimately had a yield of 5.6%, corresponding to 1.8 mg of RNA material. Figure 3 demonstrates the impact of reactant concentration on transcription performance (e.g., increasing DMSO suppresses transcription, resulting in reduced band intensity). This figure therefore indicates the need for optimization of reaction conditions in Basic Protocol 2 and shows how to elucidate and interpret the results thereof. The Alternate Protocol, on the other hand, does not require such an optimization as the template size allows for a sequence-independent IVT.
Figure 5 illustrates the purity distribution of HPLC fractions from the RP-IP purification procedure described in Basic Protocol 3. The red line in Figure 5B indicates fractions under the target peak in the chromatogram in Figure 5A. The main band, representing the target, is enriched compared with the complete IVT mixture; however, shorter and longer products are still present. As these fractions will have reduced concentrations of side products and IVT reactants, a more efficient preparation can be achieved in Basic Protocol 4. Finally, Figure 6B shows pure HPLC fractions the user can obtain by following Basic Protocols 3 and 4 together. Figure 6C shows both the final pure and less pure RNA fractions that have been combined and analyzed at high concentration by denaturing PAGE to visualize how abundant side products in the final material are. The 29mer sample—exemplified in Figures 3, 5, and 6—was transcribed with RNA with a 5′-GG, which increased the overall yield. It is also possible to purify longer RNAs with this method (Karlsson et al., 2020).
Time Considerations
We estimate that sequence design and IVT optimization (Basic Protocols 1 and 2) can be done in 5 working days, not considering delivery time of DNA templates (oligos or plasmids). Workup and RP-IP purification (Basic Protocol 3) of a large-scale (∼10-ml) transcription reaction could be done in 2 to 3 working days, and a similar amount of time could be estimated for Basic Protocol 4. An estimate of the total time from large-scale transcription reaction to final sample is thus around 1 week. Bacterial amplification of plasmids requires ∼3 days. Once enough plasmid has been purified, the Alternate Protocol and Basic Protocol 4 together can be done in 4 days from the linearization reaction to obtaining pure and clean RNA product.
Acknowledgments
The authors thank the Swedish Foundation for Strategic Research (project no. ICA14-0023 and FFL15-0178), the Swedish Research Council (2014-04303 and 2018-00250), the Ragnar Söderberg Foundation (M91/14), the Karolinska Institutet (KI FoAss and KID 2-3707/2013), and the Department of Medical Biochemistry and Biophysics (for support for the purchase of a 600-MHz Bruker NMR spectrometer). The authors thank the protein science facility (PSF) at the Karolinska Institutet for expression and purification of T7 RNA polymerase and E. coli RNase H and Martin Hällberg for the generous gift of the inorganic phosphatase. The authors also thank Andrea Coulthard Sørensen for contributing during the IVT optimization of the 29mer RNA exemplified in Figure 3.
Author Contributions
Hampus Karlsson : conceptualization, data curation, investigation, methodology, writing–original draft, writing–review and editing. Hannes Feyrer : conceptualization, data curation, investigation, methodology, writing–original draft, writing–review and editing. Lorenzo Baronti : conceptualization, data curation, investigation. Katja Petzold : conceptualization, investigation, methodology, project administration, resources, supervision, writing–review and editing.
Conflict of Interest
The authors declare no conflict of interest.
Open Research
Data Availability Statement
The data that support the findings of this study are available from the corresponding author upon reasonable request.
Literature Cited
- Baisden, J. T., Boyer, J. A., Zhao, B., Hammond, S. M., & Zhang, Q. (2021). Visualizing a protonated RNA state that modulates microRNA-21 maturation. Nature Chemical Biology , 17(1), 80–88. doi: 10.1038/s41589-020-00667-5.
- Baronti, L., Guzzetti, I., Ebrahimi, P., Friebe Sandoz, S., Steiner, E., Schlagnitweit, J., … Petzold, K. (2020). Base-pair conformational switch modulates miR-34a targeting of Sirt1 mRNA. Nature , 583(7814), 139–144. doi: 10.1038/s41586-020-2336-3.
- Chen, Z., & Zhang, Y. (2005). Dimethyl sulfoxide targets phage RNA polymerases to promote transcription. Biochemical and Biophysical Research Communications , 333(3), 664–670. doi: 10.1016/j.bbrc.2005.05.166.
- Dethoff, E. A., Petzold, K., Chugh, J., Casiano-Negroni, A., & Al-Hashimi, H. M. (2012). Visualizing transient low-populated structures of RNA. Nature , 491(7426), 724–728. doi: 10.1038/nature11498.
- Duss, O., Maris, C., von Schroetter, C., & Allain, F. H.-T. (2010). A fast, efficient and sequence-independent method for flexible multiple segmental isotope labeling of RNA using ribozyme and RNase H cleavage. Nucleic Acids Research , 38(20), e188. doi: 10.1093/nar/gkq756.
- Feyrer, H., Munteanu, R., Baronti, L., & Petzold, K. (2020). One-pot production of RNA in high yield and purity through cleaving tandem transcripts. Molecules , 25(5), 1142. doi: 10.3390/molecules25051142.
- Gholamalipour, Y., Johnson, W. C., & Martin, C. T. (2019). Efficient inhibition of RNA self-primed extension by addition of competing 3′-capture DNA improved RNA synthesis by T7 RNA polymerase. Nucleic Acids Research , 47(19), e118. doi: 10.1093/nar/gkz700.
- Gholamalipour, Y., Karunanayake Mudiyanselage, A., & Martin, C. T. (2018). 3′ end additions by T7 RNA polymerase are RNA self-templated, distributive and diverse in character—RNA-Seq analyses. Nucleic Acids Research , 46(18), 9253–9263. doi: 10.1093/nar/gky796.
- Helmling, C., Keyhani, S., Sochor, F., Fürtig, B., Hengesbach, M., & Schwalbe, H. (2015). Rapid NMR screening of RNA secondary structure and binding. Journal of Biomolecular NMR , 63(1), 67–76. doi: 10.1007/s10858-015-9967-y.
- Inoue, H., Hayase, Y., Iwai, S., & Ohtsuka, E. (1987). Sequence-dependent hydrolysis of RNA using modified oligonucleotide splints and RNase H. FEBS Letters , 215(2), 327–330. doi: 10.1016/0014-5793(87)80171-0.
- Kao, C., Zheng, M., & RüDisser, S. (1999). A simple and efficient method to reduce nontemplated nucleotide addition at the 3′ terminus of RNAs transcribed by T7 RNA polymerase. RNA , 5(9), 1268–1272. doi: 10.1017/S1355838299991033.
- Karlsson, H., Baronti, L., & Petzold, K. (2020). A robust and versatile method for production and purification of large-scale RNA samples for structural biology. RNA , 26(8), 1023–1037. doi: 10.1261/rna.075697.120.
- Milligan, J. F., Groebe, D. R., Witherell, G. W., & Uhlenbeck, O. C. (1987). Oligoribonucleotide synthesis using T7 RNA polymerase and synthetic DNA templates. Nucleic Acids Research , 15(21), 8783–8798. doi: 10.1093/nar/15.21.8783.
- Milligan, J. F., & Uhlenbeck, O. C. (1989). [5]Synthesis of small RNAs using T7 RNA polymerase. In J. E. Dahlberg, & J. N. Abelson (Eds) Methods in Enzymology (vol. 180, pp. 51–62). Academic Press. doi: 10.1016/0076-6879(89)80091-6.
- Moazed, D., Stern, S., & Noller, H. F. (1986). Rapid chemical probing of conformation in 16 S ribosomal RNA and 30 S ribosomal subunits using primer extension. Journal of Molecular Biology , 187(3), 399–416. doi: 10.1016/0022-2836(86)90441-9.
- Mulligan, M. J., Lyke, K. E., Kitchin, N., Absalon, J., Gurtman, A., Lockhart, S., … Jansen, K. U. (2020). Phase I/II study of COVID-19 RNA vaccine BNT162b1 in adults. Nature , 586(7830), 589–593. doi: 10.1038/s41586-020-2639-4.
- Pabis, M., Popowicz, G. M., Stehle, R., Asami, S., Warner, L., Martınez-Chantar, L., … Sattler, M. (2019). HuR biological function involves RRM3-mediated dimerization and RNA binding by all three RRMs. Nucleic Acids Research , 47(2), 1011–1029. doi: 10.1093/nar/gky1138.
- Parisien, M., & Major, F. (2008). The MC-Fold and MC-Sym pipeline infers RNA structure from sequence data. Nature , 452(7183), 51–55. doi: 10.1038/nature06684.
- Petzold, K., Duchardt, E., Flodell, S., Larsson, G., Kidd-Ljunggren, K., Wijmenga, S., & Schleucher, J. (2007). Conserved nucleotides in an RNA essential for hepatitis B virus replication show distinct mobility patterns. Nucleic Acids Research , 35(20), 6854–6861. doi: 10.1093/nar/gkm774.
- Price, S. R., Ito, N., Oubridge, C., Avis, J. M., & Nagai, K. (1995). Crystallization of RNA-protein complexes I. Methods for the large-scale preparation of RNA suitable for crystallographic studies. Journal of Molecular Biology , 249(2), 398–408. doi: 10.1006/jmbi.1995.0305.
- Schlagnitweit, J., Friebe Sandoz, S., Jaworski, A., Guzzetti, I., Aussenac, F., Carbajo, R. J., … Petzold, K. (2019). Observing an antisense drug complex in intact human cells by in-cell NMR. ChemBioChem , 20(19), 2474–2478. doi: 10.1002/cbic.201900297.
- Schlagnitweit, J., Steiner, E., Karlsson, H., & Petzold, K. (2018). Efficient detection of structure and dynamics in unlabeled RNAs: The SELOPE approach. Chemistry A European Journal , 24(23), 6067–6070. doi: 10.1002/chem.201800992.
- Shields, T. P., Mollova, E., Marie, L. Ste., Hansen, M. R., & Pardi, A. (1999). High-performance liquid chromatography purification of homogenous-length RNA produced by trans cleavage with a hammerhead ribozyme. RNA , 5(9), 1259–1267. doi: 10.1017/S1355838299990945.
- Steiner, E., Schlagnitweit, J., Lundström, P., & Petzold, K. (2016). Capturing excited states in the fast-intermediate exchange limit in biological systems using 1H NMR spectroscopy. Angewandte Chemie (International Edition in English) , 128, 16101–16104. doi: 10.1002/anie.201609102.
- Wacker, A., Weigand, J. E., Akabayov, S. R., Altincekic, N., Bains, J. K., Banijamali, E., … Zetzsche, H. (2020). Secondary structure determination of conserved SARS-CoV-2 RNA elements by NMR spectroscopy. Nucleic Acids Research , 48(22), 12415–12435. doi: 10.1093/nar/gkaa1013.
- Wang, I., Hennig, J., Jagtap, P. K. A., & Sonntag, M., Valcarcel, J., & Sattler, M. (2014). Structure, dynamics and RNA binding of the multi-domain splicing factor TIA-1. Nucleic Acids Research , 42(9), 5949–5966. doi: 10.1093/nar/gku193.
Key References
- Feyrer et al. (2020). See above.
Original source for the tandem IVT method as presented in the Alternate Protocol.
- Karlsson et al. (2020). See above.
Primary research article describing RNA sample preparation, Basic Protocols 1 and 2, and the HPLC methods underlying Basic Protocols 3 and 4.
Internet Resources
Provides access to MC-Fold 2.32.
Gives complementary DNA sequence for RNA, as well as biophysical data.
Citing Literature
Number of times cited according to CrossRef: 2
- Hannes Feyrer, Cenk Onur Gurdap, Maja Marušič, Judith Schlagnitweit, Katja Petzold, Enzymatic incorporation of an isotope-labeled adenine into RNA for the study of conformational dynamics by NMR, PLOS ONE, 10.1371/journal.pone.0264662, 17 , 7, (e0264662), (2022).
- Ge Han, Yi Xue, Rational design of hairpin RNA excited states reveals multi-step transitions, Nature Communications, 10.1038/s41467-022-29194-8, 13 , 1, (2022).