Nanopore-Enabled Microbiome Analysis: Investigating Environmental and Host-Associated Samples in Rainbow Trout Aquaculture
Giulia Zarantonello, Giulia Zarantonello, Argelia Cuenca, Argelia Cuenca
Abstract
Microbiome sequencing is at the forefront of health management development, and as such, it is becoming of great interest to monitor the microbiome in the aquaculture industry as well. Oxford Nanopore Technologies (ONT) platforms are gaining popularity to study microbial communities, enabling faster sequencing, extended read length, and therefore, improved taxonomic resolution. Despite this, there is a lack of clear guidelines to perform a metabarcoding study, especially when dealing with samples from non-mammalian species, such as aquaculture-related samples. In this article, we provide general guidelines for sampling, nucleic acid extraction, and ONT-based library preparation for both environmental (water, sediment) and host-associated (gill or skin mucus, skin, gut content, or gut mucosa) microbiome analysis. Our procedures focus specifically on rainbow trout (Oncorhynchus mykiss) reared in experimental facilities. However, these protocols can also be transferred to alternative types of samples, such as environmental DNA (eDNA) monitoring from alternative water sources, or to different fish species. The additional challenge posed by the low biomass and limited bacterial diversity inherent in fish-associated microbiomes is addressed through the implementation of troubleshooting solutions. Furthermore, we describe a bioinformatic pipeline starting from raw reads and leading to taxonomic abundance tables using currently available tools and software. Finally, we provide a set of specific guidelines and considerations related to the strategic planning of a microbiome study within the context of aquaculture. © 2024 The Authors. Current Protocols published by Wiley Periodicals LLC.
Basic Protocol 1 : Environmental sample collection
Basic Protocol 2 : Host-associated sample collection
Alternate Protocol : Host-associated sample collection: Alternative sample types
Basic Protocol 3 : Sample pre-treatment and nucleic acid extraction
Basic Protocol 4 : Quality control and preparation for 16S rRNA gene sequencing
Support Protocol 1 : Assessment of inhibition by quantitative PCR
Support Protocol 2 : Bioinformatic analysis from raw files to taxonomic abundance tables
INTRODUCTION
Increasing evidence is pointing to the host and environmental microbiomes as key components involved in the general health status of an organism. This is also the case in the aquaculture field, where, supporting this hypothesis, several recent studies found that microbial dysbiosis, i.e., an imbalance in the microbiota composition, was correlated to distress or disease conditions in the host (Infante-Villamil et al., 2021). Several methods are available to investigate microbiomes. The most common culture-independent high throughput sequencing (HTS)-based microbiome studies start with sample collection, followed by nucleic acid extraction, and subsequently either PCR amplification and sequencing of a marker gene (metabarcoding) or untargeted (whole metagenome) sequencing. With metabarcoding, the chosen marker gene—e.g., 16S rRNA gene (Caporaso et al., 2012)—is used to estimate the relative abundance of the bacterial taxa present in the sample. To date, such studies were predominantly based on short-read sequencing (Illumina), but more recently there has been a shift towards long reads, using platforms such as Oxford Nanopore Technologies (ONT) or PacBio. ONT in particular offers the added advantages of a shorter sequencing time, portability, the possibility of real-time analysis, and feasibility for small-scale projects. Importantly, in the context of metabarcoding studies, longer reads translate to enhanced taxonomic resolution (Johnson et al., 2019).
Microbiome studies based on HTS have revolutionized the way microbial communities are studied thanks to the amount of information that can be gathered at once from a single sample. However, the field faces a substantial challenge in terms of reproducibility. Even with extensively studied sample types, finding a consensus on robust protocols remains elusive, hindering the ability to conduct meaningful meta-analyses (Thompson et al., 2017; Tourlousse et al., 2021). In the field of aquaculture, microbiome studies are still relatively new, and the field is lacking comprehensive protocols and guidelines for appropriate and effective sampling techniques, nucleic acid extraction, and preparation for sequencing. Additionally, from the few shotgun metagenomics studies in salmonids (Collins et al., 2021; Rasmussen et al., 2021; Riiser et al., 2019), it is now known that both the biomass and biodiversity of microbial communities in these host species are extremely low in comparison to those in mammals (Limborg et al., 2023). This observation introduces further challenges during library preparation, requiring specific considerations that will be addressed in this article.
Here we describe the methods we optimized in our laboratory to study microbiomes through 16S rRNA gene metabarcoding. We will mainly address methodologies appropriate to being coupled with Oxford Nanopore Technology (ONT) sequencing, but also easily adaptable to short-read Illumina sequencing. We particularly describe procedures related to environmental (water, sediment) and host-associated microbiomes, which were optimized by focusing on one of the most farmed fish species worldwide, the rainbow trout (Oncorhynchus mykiss). Specifically, we first provide step-by-step guidance for the collection of microbiomes residing within the biological barriers interfacing the host and its environment, including the skin, skin mucus, gill mucus, gut content and mucosa (Basic Protocols 1 and 2). After collection, a pre-processing and extraction method that proved to be effective for all kinds of samples mentioned is presented (Basic Protocol 3). Finally, quality controls and appropriate tests to perform prior library preparation with ONT kits are described (Basic Protocol 4). Resulting from these protocols, full-length (hypervariable regions V1 to V9) 16S rRNA gene amplicons are generated in a sufficient amount to undergo sequencing. While we focus on metabarcoding, the following protocols could potentially be adapted for metagenomics or metatranscriptomics studies with suitable modifications, such as omitting the PCR amplification and treating the sample with specific nucleases. The protocols are supplemented by best practices and guidance concerning the design of a microbiome study in the field of aquaculture, applicable to experimental facilities and field investigations. Among others, we provide practical considerations for laboratory procedures, instructions on the choice of relevant controls, and finally a bioinformatics workflow to obtain taxonomic abundance tables.
NOTE : All protocols involving animals must be reviewed and approved by the appropriate Animal Care and Use Committee and must follow regulations for the care and use of laboratory animals.
Basic Protocol 1: ENVIRONMENTAL SAMPLE COLLECTION
Here we describe the optimized methods to collect environmental microbial samples in our experimental facilities. We will focus on two environmental sample types, namely water and sediment.
Generally, the collection of water is a straightforward procedure in experimental tank facilities, as it can usually be sampled from the surface or, if present, from the outlet of recirculating pumps. Since conditions and equipment availability can vary considerably when performing on-site sampling (e.g., in a fish farm), this step can be subject to modifications and can be easily modified to suit field sampling (see “Adaptations for field sampling” in the Critical Parameters section).
On the other hand, with most experimental tank designs, it is impractical or even not at all feasible to collect the sedimented organic matter from the tank's bottom compartment repeatedly and reproducibly over the course of an experimental trial. Thus, when interested in collecting and analyzing sediment from experimental fish tanks, it is necessary to place the suitable sampling equipment in the tank prior the start of the experiment. In our laboratory setup, we use a system based on the communicating vessels principle: a flexible rubber conduit is positioned to connect the tank's base and the exterior. The terminal end of the tube external to the tank is securely plugged, while the lower aperture remains unobstructed. Upon de-capping the upper end, the water pressure facilitates the ingress of sediment through the rubber conduit, resulting in a continuous outward efflux (Fig. 1). This method ensures that the samples are consistently collected from the same site, and additionally, neither the water nor the fish are disturbed during collection.
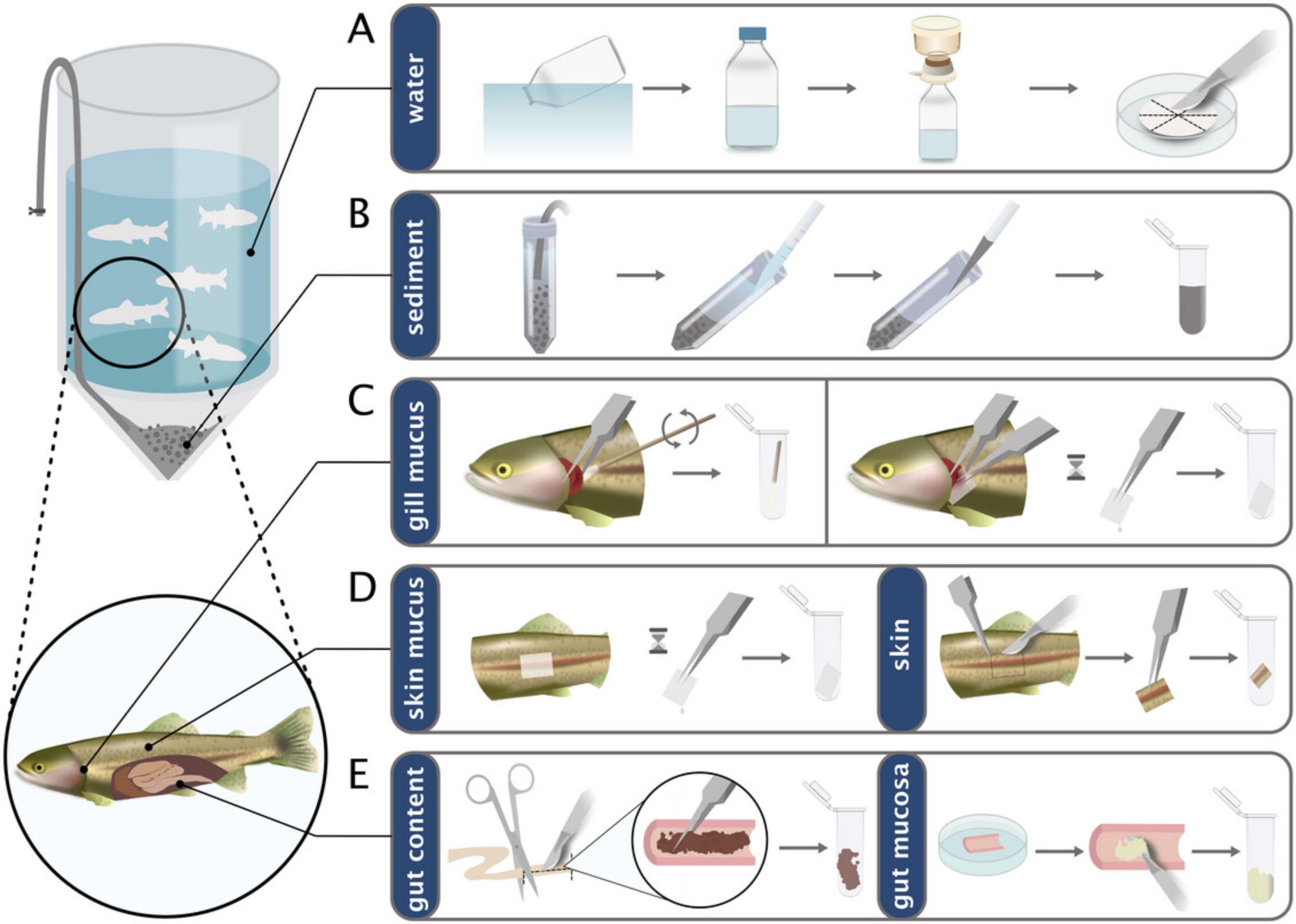
Materials
-
70% (v/v) ethanol
-
Personal protective equipment (PPE), including lab coat and gloves
-
Autoclaved 1- to 2-L glass bottles (DURAN graduated laboratory bottles, or equivalent)
-
Laminar flow hood
-
Autoclaved water filtration apparatus (Nalgene Reusable Bottle Top Filters, cat. no. DS0320, or equivalent)
-
0.22-µm PVDF filters, 47-mm diameter (EMD Millipore Durapore, or equivalent)
-
Sterile surgical tweezers and scalpels
-
Laboratory vacuum pump (ScanVac VacSafe 15, or equivalent)
-
Sterile 60-mm Petri dishes (Falcon, cat. no. 35007, or equivalent)
-
Sterile 2-ml tubes (Eppendorf, cat. no. 022363352, or equivalent)
-
50-ml sterile conical tubes
-
Laboratory rack for 50-ml conical tubes
-
20-ml serological pipettes
-
Pipettor
-
Vortex
-
Laboratory spoon
-
Laboratory balance
-
Dry ice
Water collection and filtration
1.Before starting, wear PPE and wipe the exterior part of the glass bottle with 70% (v/v) ethanol.
2.Collect 1 L water in a sterile glass bottle. Take notes of physical parameters of the water, at least temperature and depth at which the water was collected (e.g., surface level; 50 cm depth; tank bottom; etc.).
3.Set up the vacuum filtration apparatus in a laminar flow hood to avoid capturing dust and air particles during filtration.
4.Place a 0.22-µm PVDF sterile filter on the membrane support plate using sterile surgical tweezers, then place and tighten the upper chamber.
5.Pour the water in the upper chamber and start the vacuum pump. Continue adding and filtering water until the desired sample volume is reached (e.g., 500 ml) or until the filter membrane clogs.
6.Recover the filter using sterile tweezers and place it in a sterile Petri dish.
7.Using a sterile scalpel, section the filter as shown in Figure 1A. Place the sectioned filter in a 2-ml sterile tube.
8.Store immediately at −80°C until extraction.
Sediment collection
9.Uncap the rubber tube that was set up prior the start of the experimental trial for the purpose of sediment collection (see Basic Protocol 1, Introduction), and let the sediment flow out for a few seconds.
10.Using a 50-ml sterile conical tube, collect ∼50 ml of sediment (Fig. 1B).
11.By this method, a mix of water and sediment is collected. Place the 50-ml tube in a rack in a vertical position to let the sediment accumulate on the bottom of the tube.
12.Discard the water on the top of the tube using a serological pipette and pipettor.
13.Vortex mix the sediment.
14.With either a laboratory spoon or a pipette with a cropped 1-ml tip, transfer a fixed amount of sediment (at least 300 mg) into a sterile 2-ml tube.
15.Snap freeze on dry ice and store immediately at −80°C until extraction.
Basic Protocol 2: HOST-ASSOCIATED SAMPLE COLLECTION
For the collection of host-associated samples, we will mainly focus on the microbiomes that, together with the fish immune system, constitute the interface between the animal and the environment. In an experimental study that involves living animals, it is important to consider non-lethal sampling options to reduce the number of individuals that will be included (see “Non-lethal and non-invasive sampling options” in the Critical Parameters section). For this reason, here we will first describe two non-lethal sampling methods to collect gill mucus and skin mucus. Nevertheless, these non-lethal sample types will not always be suitable for the kind of study that is being conducted. In the Alternate Protocol, we describe other host-associated sample collection methods, which detail how to collect skin tissue, gut content, and gut mucosal samples. All these sample types were tested and optimized on rainbow trout but can also be transferred to other fish species.
Materials
-
Live fish specimen (e.g., rainbow trout)
-
Anesthetic (benzocaine, Sigma-Aldrich, cat. no. E1501, or equivalent)
-
Personal protective equipment (PPE), including lab coat and gloves
-
Flame sterilizer
-
Toothed dissecting forceps (Kruuse, cat. no. 130667, or similar) and tweezers
-
Medical wipe sections, 1 to 2 cm2, or sterile swab
-
Sterile 2-ml tubes (Eppendorf, cat. no. 022363352, or equivalent)
-
Dry ice
Gill mucus collection
1.Before starting, wear PPE and clean lab surfaces and equipment.
Anesthetize or euthanize or the fish. For anesthesia, benzocaine concentration should be 75 to 100 mg/L in oxygenated water, and bath immersion should last for 2 to 5 min, until achievement of stage 4 to 5 anesthesia. In this case, after the procedure, the fish should be placed and monitored in a separate water basin until complete recovery, before being returned to the experimental tank. For euthanasia, bath immersion must be performed in water containing an overdose of benzocaine (>250 mg/L) for a minimum of 10 min.
2.Lay the fish down on a flame-sterilized surface.
3.With sterile, toothed dissecting forceps open the operculum to reveal the gill arches. With tweezers, place a piece of medical wipe (Ivanova et al., 2021) on the gill arches and filaments to absorb the gills mucus (Fig. 1C). Repeat on both left and right gills of the fish using the same section of medical wipe.
4.Place the medical wipe (or swab) in a sterile 2-ml tube.
5.Place the tube on dry ice to snap freeze it. Store immediately at −80°C until extraction.
Skin mucus collection
6.With sterile tweezers, quickly lay a section of sterile medical wipe on the surface of the skin (Ivanova et al., 2021), being careful not to damage the skin.
7.After absorption, pick up the section of medical wipe with sterile tweezers and place it in a new 2-ml tube (Fig. 1D).
8.Place the tube on dry ice to snap freeze it. Store immediately at −80°C until extraction.
Alternate Protocol: HOST-ASSOCIATED SAMPLE COLLECTION: ALTERNATIVE SAMPLE TYPES
The following procedure describes the collection of skin tissue and subsequently gut content and gut mucosal samples. In this protocol, we use the generic term “skin” to refer to the cutis, i.e., the combination of the epidermal and dermal layers.
Additional Materials (also see Basic Protocol 2)
-
1× phosphate-buffered saline (PBS) (see recipe)
-
Sterile pointed scalpel
-
Scissors
-
Sterile 60-mm Petri dishes (Falcon, cat. no. 35007, or equivalent)
Skin samples collection
1.Before starting, wear PPE and clean lab surfaces and equipment.
2.Euthanize the fish. For euthanasia, bath immersion must be performed in water containing an overdose of benzocaine (> 250 mg/L) for a minimum of 10 min.
3.Lay the fish on a flame-sterilized surface.
4.Select and identify the fish side (right or left), skin site (e.g., mid-lateral line), and surface measurement (cm2) of the skin portion that will be sampled.
5.The sampled skin area should be selected according to the purpose of the study. As for the skin mucus sampling, the sampled region must be chosen on a precise anatomical area that is easily identifiable. This is crucial to ensure consistency in the sampling site across specimens in the same experiment. With a sterile, pointed scalpel, perform a ∼2 mm deep incision in the shape of a square (Fig. 1D) to excise the dermal and epidermal layer (Schmidt et al., 2021).
6.With toothed dissecting forceps, firmly grab one corner of the incised skin square and pull tightly to lift the skin. The epidermal layer should easily detach from the muscular layer beneath.
7.Place the skin section in a sterile 2-ml tube.
8.Place the tube on dry ice to snap freeze it. Store immediately at −80°C until extraction.
Gut content and mucosa collection
9.With sterile toothed dissecting forceps, firmly grab on the operculum and perform a long incision along the lateral line using surgical scissors. The incision should then curve down towards the vent. With tissue forceps, move the tissue to reveal the abdominal cavity. With new tweezers and scalpel or surgical scissors, perform an incision caudally to the pyloric caeca and at the vent to dissect the mid or distal intestine (MI and DI).
10.Place the intestine on a sterile surface and use sterile scissors to isolate the portion of interest of the intestinal tube. The collected section (e.g., PC, AI, MI, DI) must be consistent for all samples.
11.With a sterile scalpel, perform a longitudinal incision on the intestinal tube section (Fig. 1E).
12.Using tweezers, collect the luminal content (digesta) and place the sample in a sterile 2-ml tube.
13.If necessary, quickly rinse the open intestinal tube section in a sterile 60-mm Petri dish containing sterile PBS, then place it on a sterile surface with the luminal side facing up.
14.With a cell scraper or the blunt side of a new scalpel, gently scrape the mucosa and collect it in a new 2 ml tube.
15.Place both samples on dry ice to snap freeze them. Store immediately at −80°C until extraction.
Basic Protocol 3: SAMPLE PRE-TREATMENT AND NUCLEIC ACID EXTRACTION
One of the main challenges in microbiome research is the lack of a global standard in processing and analyzing samples, which in turn often impairs comparison and meta-analyses between studies (Thompson et al., 2017; Tourlousse et al., 2021). Furthermore, it is advised to use the same extraction kit among sample types included in the same study (Salter et al., 2014).
For this reason, here we propose a pre-treatment and extraction protocol combination that works for a broad range of aquaculture-derived samples. Most protocols for total microbial nucleic acid extraction include either mechanical cell disruption or enzymatic lysis to ensure that no bias is present against difficult-to-lyse bacteria. In our case, we use a combination of mechanical and enzymatic lysis that is suitable for all our sample types. The lysozyme incubation step is particularly useful when dealing with difficult-to-lyse gram-positive bacteria. However, it is optional and can be omitted if preservation of RNA integrity in the sample is a priority. The extraction kit of choice is the MagMAX Microbiome Ultra nucleic acid isolation kit, which effectively purified all the sample types mentioned above with slight modifications. Other kits can also be used, taking into account the considerations described in the Critical Parameters subsection “Choice of extraction kit”.
Materials
-
Microbial community standard (e.g., ZymoBIOMICS Microbial Community Standard, cat. no. D6300)
-
Environmental (filter membranes, sediment) or host-associated (skin, skin mucus, gill mucus, gut content or mucosa) samples from Basic Protocols 1, 2 and/or Alternate Protocol
-
Nucleic acid purification kit (MagMAX Microbiome Ultra nucleic acid isolation kit, Applied Biosystems, cat. no. A42358, or equivalent)
-
1× PBS (see recipe), optional
-
80 mg/ml lysozyme solution (see recipe)
-
Personal protective equipment (PPE), including lab coat and gloves
-
Laminar flow cabinet (Labogene, ScanLaf Mars B2)
-
Laboratory balance
-
Sterile 2-ml tubes (Eppendorf, cat. no. 022363352, or equivalent)
-
5-mm beads, sterile stainless steel (Qiagen, cat. no. 69989 or equivalent)
-
100-µm zirconia beads (part of the MagMAX Microbiome Ultra nucleic acid isolation kit, but also separately available from Applied Biosystems, cat. no. A42351, or equivalent)
-
Pipettes, P10 and P1000
-
10- and 1000-µl sterile pipette tips
-
Vortex mixer
-
Mechanical disruptor (e.g., Qiagen, TissueLyser II)
-
Microcentrifuge (Eppendorf, MiniSpin Plus)
-
Shaking thermal block (Eppendorf, ThermoMixer C)
-
96 deep–well plates for automated sample extraction (Thermo Fisher Scientific, cat. no. 95040460), optional
-
Purification system for automated sample extraction (e.g., Thermo Fisher Scientific, KingFisher Flex), optional
-
Sterile tubes for nucleic acid storage (e.g., Labcon PurePlus 0.2-ml 8-well PCR tube strips, Labcon, cat. no. 392-755-000-9, or equivalent)
NOTE : Remember to include negative control samples (see “Control samples” in the Critical Parameters section) and an aliquot of mock community standards (ZymoBIOMICS Microbial Community Standard) among the samples to extract.
Mechanical lysis
1.Before starting, wear PPE and clean lab surfaces and equipment. Perform all steps in a laminar flow cabinet.
2.Weigh each sample (sediment, skin tissue, gut content or mucosa) up to 300 mg and move it to a sterile 2-ml tube.
3.Add a 5-mm sterile stainless-steel bead and 1 g of 100-µm zirconia beads directly in the 2-ml tube containing the sample.
4.Add 800 µl lysis buffer (from the MagMAX Microbiome Ultra nucleic acid isolation kit) to the sample tube.
5.Vortex to mix the sample and beads.
6.Lyse in a mechanical disruptor at 25 Hz for 2 min or until completely homogenized.
Lysozyme treatment and extraction
7.Centrifuge 5 min at 14,000 × g , room temperature, in a microcentrifuge.
8.Add 9.2 µl of 80 mg/ml lysozyme solution.
9.Vortex the sample and spin down.
10.Incubate at 37°C for 1 hr on a shaking thermal block.
11.Centrifuge 5 min at 14,000 × g , room temperature, in a microcentrifuge.
12.Save 500 µl of supernatant for extraction, and load in a 96 deep-well plate.
13.Extract nucleic acids from the homogenate supernatant using the chosen nucleic acid extraction kit (e.g., MagMAX Microbiome Ultra nucleic acid isolation kit) according to the manufacturer's instructions (see “Remarks on choice of extraction kit” in the Critical Parameters section). The program MagMAX_Microbiome_Soil_Flex is used on a KingFisher Flex automatic extraction system with the following modifications:
-
Elution volume is modified to 100 µl.
-
Elution time is prolonged (“Elution” was set to 5; “Elution time collection” was set to 10 s).
14.After elution, unload the elution plate and transfer the extracted DNA samples into sterile tubes.
15.Store the DNA at −80°C until further processing.
Basic Protocol 4: QUALITY CONTROL AND PREPARATION FOR 16S rRNA GENE SEQUENCING
Standard DNA quality control (QC) steps are followed before library preparation. The subsequent 16S rRNA gene V1 to V9 regions PCR amplification tests are aligned with and serve as a precursor to the library preparation protocol designed for the ONT 16S barcoding kit (SQK-16S024). The test endpoint PCR amplification should be performed for all the sample types in analysis (and controls). This step is particularly crucial when a relatively low bacterial biomass is expected, e.g., tissue-rich samples where it is inevitable to obtain a high amount of host DNA. Specifically for these samples, conducting a preliminary amplification test will enable the optimization of PCR conditions prior to library preparation. This is useful to determine the required ng of initial template, number of optimal PCR cycles, as well as the possible need of replicate PCR reactions to pool. Test the same DNA template amount, cycling conditions, reaction volume, and primers that will be later used for library preparation.
Materials
-
Extracted genomic DNA from Basic Protocol 3
-
Fluorometric assay for dsDNA (e.g., Thermo Fisher Scientific, Qubit dsDNA quantification assay, cat. no. Q33230)
-
H2O, nuclease-free
-
27F primer, 5´-AGAGTTTGATCMTGGCTCAG-3´ (from IDT or equivalent)
-
1492R primer, 5´-CGGTTACCTTGTTACGACTT-3´ (from IDT or equivalent)
-
LongAmp Hot Start Taq 2× master mix (New England BioLabs, cat. no. M0533S)
-
Personal protective equipment (PPE), including lab coat and gloves
-
Microvolume spectrophotometer (e.g., Thermo Fisher Scientific, Nanodrop)
-
Fluorometer (Thermo Fisher Scientific, Qubit, or equivalent)
-
P10, P200, and P1000 pipettes
-
10-, 200-, and 1000-µl sterile pipette tips
-
2-ml tubes, sterile (Eppendorf, cat. no. 022363352, or equivalent)
-
0.2-ml PCR tubes (e.g., Labcon PurePlus 0.2-ml 8-well PCR tube strips, Labcon, cat. no. 392- 755- 000- 9)
-
PCR thermal cycler (Applied Biosystems, MiniAmp Plus Thermal Cycler)
-
Additional reagents and equipment for gel electrophoresis (Voytas, 2000)
DNA purity, integrity, and concentration assessment
1.Before starting, wear PPE and clean lab surfaces and equipment.
2.To assess purity, measure the absorbance of the purified DNA by loading 1 µl of the eluted sample on a microvolume spectrophotometer.
3.Perform agarose gel electrophoresis to evaluate the integrity of the nucleic acids—see Voytas (2000) for detailed instructions.
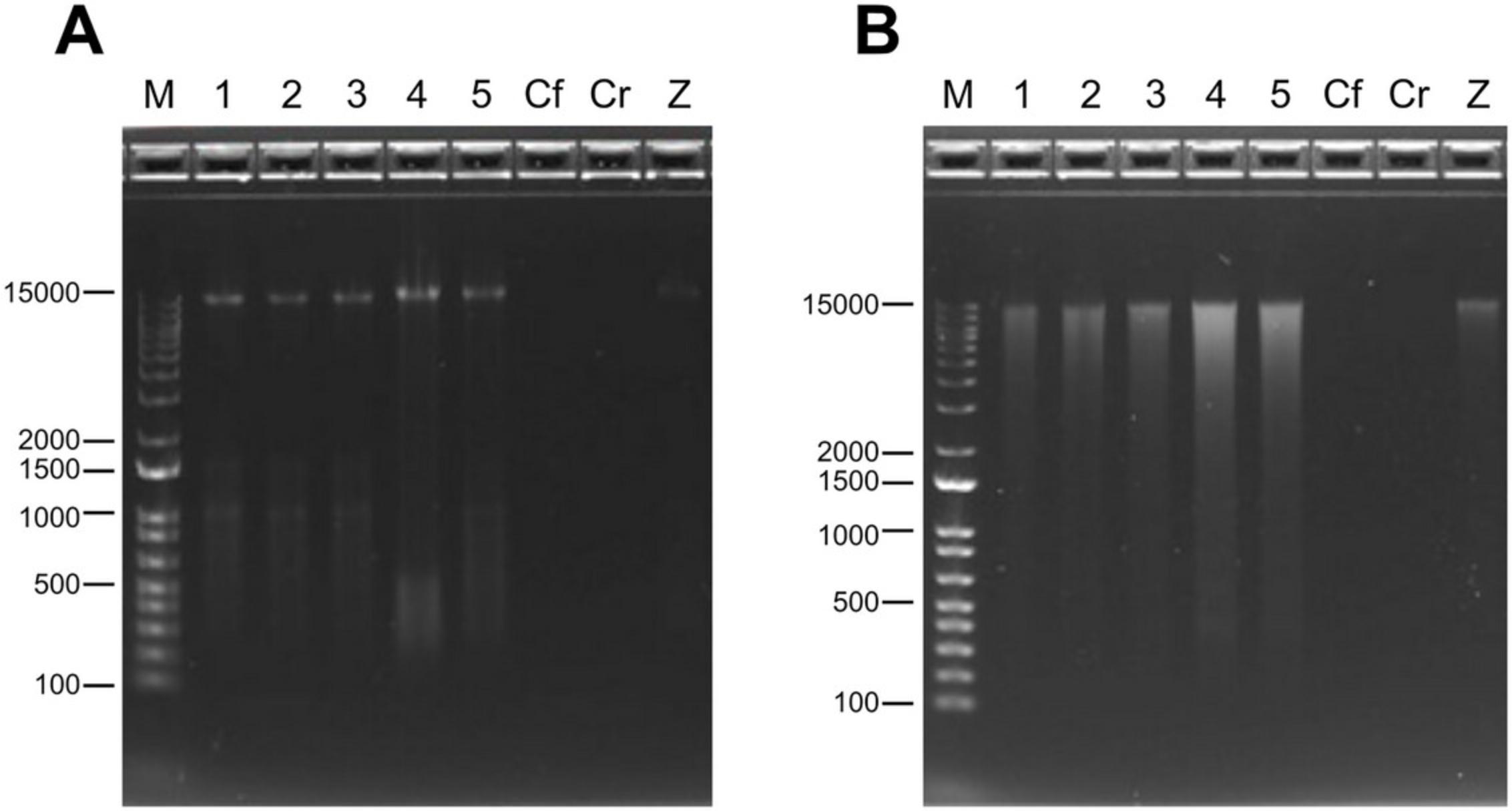
4.Before moving on to library preparation, accurately measure the concentration of DNA in the samples with a fluorometric assay of choice, e.g., the Qubit dsDNA quantification assay.
Test PCR amplification with selected 16S rRNA gene primers
5.Thaw the DNA samples to test. Among samples to test, include positive control (Microbial Community Standard) and negative controls from the extraction step. Additionally, prepare at least one blank PCR reaction, where nuclease-free water is added instead of DNA template.
6.Dilute both primer oligonucleotides to a final concentration of 10 μM in nuclease-free water. Use the same primers sequences as for library preparation (for the purpose of testing, they can be deprived of 5’ tags/barcodes/spacers). The most used couple of primers for full-length 16S rRNA gene sequencing are the following V1 to V9 primers, which are also the ones provided in the ONT 16S barcoding kit (SQK-16S024):
- 27F (5´-AGAGTTTGATCMTGGCTCAG-3´)
- 1492R (5´-CGGTTACCTTGTTACGACTT-3´)
7.In a sterile 2-mL tube, prepare the master mix for the PCR reactions as described in Table 1.Do not add the DNA template yet.
Component | Quantity for a 50 µl reaction | Final concentration |
---|---|---|
LongAmp Hot Start Taq 2× master mix | 25 µl | 1× |
27F (10 μM) | 1 µl | 0.2 μM |
1492R (10 μM) | 1 µl | 0.2 μM |
H2O, nuclease-free | To 50 µl | |
Template DNA | Variable (10 ng recommended by ONT) | <1000 ng total |
8.Aliquot the master mix in 0.2-ml PCR tubes.
9.Add the DNA template for each sample (or an equal volume of nuclease-free water, in the control blank reaction) to the 0.2-ml tubes where the master mix has been aliquoted.
Sample type | Average template DNA starting quantity (ng) | Average amplification cycles | Pooled PCR reactions |
---|---|---|---|
Water | 10 | 28 | 1 |
Sediment | 10 | 28 | 1 |
Gill mucus (swab) | 50 | 35 | 1 |
Skin | 50 | 40 | 3 |
Skin mucus | 50 | 30 | 1 |
Gut content | 750 | 30 | 1 |
Gut mucosa | 750 | 30 | 3 |
Gut (entire section) | 750 | 30 | 1 |
- a
Information is provided for each sample type in the protocol, as previously obtained in our laboratory, based on at least 5 replicates per sample type.
10.Set up the PCR reaction in a thermal cycler using the thermocycling conditions detailed in Table 3.
Step | Temperature (°C) | Time | Cycles |
---|---|---|---|
Initial denaturation | 94 | 1 min | 1 |
Denaturation | 94 | 20 s | 25 to 40 |
Annealing | 55 | 30 s | 25 to 40 |
Extension | 65 | 2 min | 25 to 40 |
Final extension | 65 | 5 min | 1 |
Hold | 4-10 | ∞ | 1 |
11.Use an aliquot of each sample to perform an agarose gel electrophoresis to visualize the PCR products. This step will assess possible failed reactions and specificity of amplification.
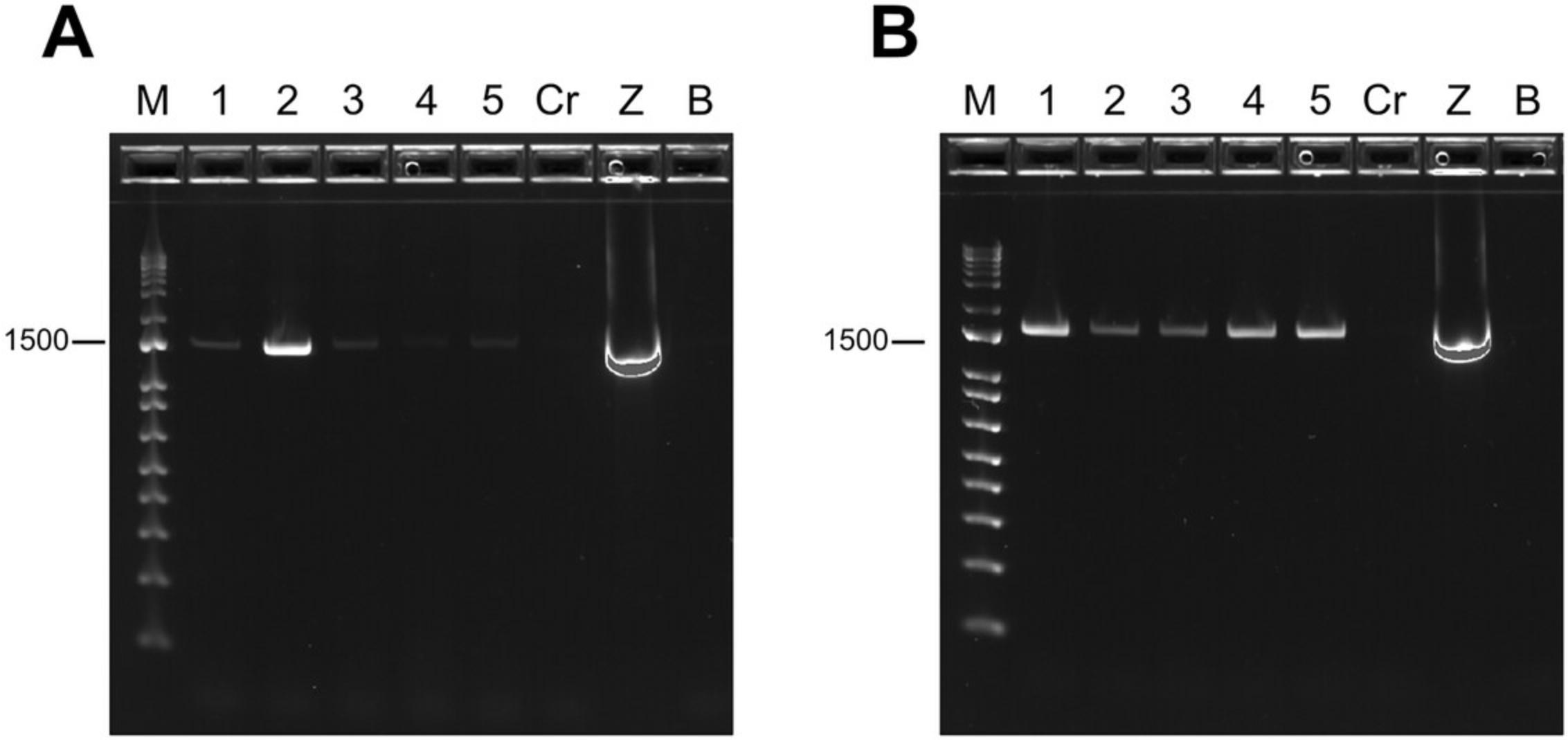
12.If DNA bands are bright and visible at the correct size (1.5 kbp), with no unexpected bands, the sample can be subject to library preparation with the ONT 16S metabarcoding kit (SQK-16S024 kit or chosen kit) without further optimization (Fig. 3B).
Support Protocol 1: ASSESSMENT OF INHIBITION BY QUANTITATIVE PCR
If the last step of Basic Protocol 4 (test PCR amplification) failed, testing different DNA dilutions through a qPCR assay can help assess if failure is due to PCR inhibition. This protocol can help determine optimal DNA template dilution and number of PCR cycles needed for library preparation. Importantly, it must be taken into consideration that DNA polymerase and primers, as well as target region and amplicon size, are not the same that will be used during library preparation for ONT long-read sequencing. For this reason, this test will only give an indication on the optimal range of cycles or amount of PCR inhibition that may be occurring during amplification.
This Support Protocol can also be used to estimate the bacterial DNA proportion relative to the host DNA, allowing for the efficacy assessment of alternative sampling methods or host DNA exclusion protocols. To perform this additional estimation, an assay targeting the host DNA (e.g., rainbow trout) should be included.
Additional Materials (also see Basic Protocol 4)
- Bacterial universal primer oligos, 10 µM each, e.g., primers in Table 4
- SYBR assay master mix, e.g., 2× Brilliant II SYBR Green qPCR MasterMix (Agilent Technologies, cat. no. 600843)
Primer names | Primer sequences |
---|---|
515F-Y (Parada et al., 2016) | 5'-GTGYCAGCMGCCGCGGTAA-3' |
806R (Apprill et al., 2015) | 5'-GGACTACNVGGGTWTCTAAT-3' |
- a
These primers were used in Support Protocol 1 to assess the possible presence of inhibition and to estimate the number of cycles needed for sufficient amplification.
- 0.2-ml optical plate with caps (e.g., MicroAmp Optical 96-well reaction plate, Applied Biosystems, cat. no. N8010560, with MicroAmp Optical 8- cap strips, Life Technologies, cat. no. 4323032)
- Real-time PCR thermal cycler (BioRad, CFX Opus 96)
1.Before starting, wear PPE and clean lab surfaces and equipment.
2.Dilute the DNA samples in nuclease-free water to decreasing 10-fold concentrations (e.g., 1:1, 1:10, 1:100, 1:1000).
3.Choose universal 16S rRNA gene primers that can work for a qPCR assay. To perform this test, we recommend the 16S rRNA gene universal primers from the Earth Microbiome Project (EMP) (Thompson et al., 2017), in Table 4.
4.Prepare the master mix by mixing the chosen primers and the SYBR assay master mix following the manufacturer's instructions. In a 0.2-ml optical plate, aliquot the prepared master mix and add the diluted DNA samples. Seal the plate with optical caps and perform qPCR using the real-time PCR thermal cycler.
5.After running the qPCR, import the data to the qPCR analysis software of choice (e.g., CFX Maestro, BioRad), and observe the amplification curves plotted in the logarithmic scale for each sample.
6.Assess the possible presence of PCR inhibition by calculating the amplification efficiency and by observing the ΔCq values between 10-fold dilutions. Choose the optimal DNA sample dilution accordingly.
7.Determine the optimal number of cycles, which lies in the range where the amplification curve is in the exponential phase and the PCR amplification efficiency is constant (Ruiz-Villalba et al., 2021).
Support Protocol 2: BIOINFORMATIC ANALYSIS FROM RAW FILES TO TAXONOMIC ABUNDANCE TABLES
Most bioinformatic tools are intended for processing of short read sequences, as Illumina sequencing platform has dominated the field until recent years. As such, tools to process Nanopore-derived long reads are being continuously developed and optimized, both by ONT and by third-party developers. For scientists with no command-line experience, a graphical user interface application from ONT exists, the EPI2ME data-analysis platform (https://epi2me.nanoporetech.com), which takes FASTQ files and can run a multitude of pre-assembled data analysis pipelines, called workflows. Among them, the “Fastq 16S” workflow was designed specifically to accept FASTQ files as input, and to produce taxonomical tables featuring relative abundances. Alternatively, when more flexibility is needed to finely adjust the analysis parameters, custom pipelines can be constructed. Here we describe the pipeline in use in our laboratory, starting from raw reads to obtain taxonomic relative abundance tables. The pipeline we propose is based on Linux command-line scripts and it only requires a minimal experience with bash scripting. The pre-processing pipeline, from FAST5 files to polished and demultiplexed FASTQ files (steps 2 to 9), is also available on GitHub (https://github.com/zrngiulia/16S_ONT_preprocessreads).
Necessary Resources
Hardware
- Computer equipped with a Linux-based operating system
- NVIDIA Graphic Processing Unit (GPU)
Software
- CUDA toolkit, NVIDIA (https://developer.nvidia.com/cuda-downloads?target_os=Linux)
- Guppy basecalling software v6.5.7 (https://cdn.oxfordnanoportal.com/software/analysis/ont-guppy_6.5.7_linux64.tar.gz)
- Fastqc software (https://www.bioinformatics.babraham.ac.uk/projects/fastqc/)
- MultiQC software (https://github.com/MultiQC/MultiQC)
- Emu software (https://github.com/treangenlab/emu)
Files
- FAST5 files generated by the MinKNOW software (https://community.nanoporetech.com/nanopore_learning/lessons/minknow-installation) in the ONT sequencing device
NOTE : All steps are annotated with related pseudocode. The examples are based on a run performed with a SpotON Flow Cell (e.g., FLO-MIN106) using the sequencing kit SQK-16S024.
1.Retrieve the raw reads (FAST5) from the Nanopore sequencing device, e.g., MinION.
2.Use Guppy Basecalling Software (ONT) to perform GPU-accelerated High-Accuracy (HAC) basecalling. If reads were split into ‘pass’ and ‘fail’ folders during the sequencing run, use the parent folder to process all reads. By default, the HAC-basecalling model also quality filters reads with a qscore threshold of 9.
3.Concatenate all ‘pass’ FASTQ files.
4.Perform a preliminary quality control step using fastqc.
5.Perform demultiplexing and trim barcodes, primers, and adapter sequences from the resulting FASTQ files using Guppy. Importantly, adding the ‘--detect_mid_strand_barcodes’ flag will detect and filter chimeric sequences, i.e., a PCR-associated artifact that is significantly affected by the number of cycles.
6.For each barcode, concatenate the fastq files.
7.Filter reads by length. Common thresholds to length-filter V1 to V9 16S rRNA gene reads, which are on average 1500 bp long, are 1000 bp as a minimum and 2000 bp as a maximum length. To achieve this, here we use awk, a Linux program for text files manipulation.
8.Perform a second quality control on the pre-processed, concatenated reads using fastqc.
9.If interested in an overview of how the pre-processing steps affected the quality of the original reads, place all fastqc reports in the same folder and run multiqc (Ewels et al., 2016) afterwards.
10.Perform taxonomical classification of the reads to the species level using Emu (Curry et al., 2022). As a database, either the default Emu database [based on rrnDB (Stoddard et al., 2015) and NCBI taxonomy (O'Leary et al., 2016)] or alternatives [e.g., SILVA (Quast et al., 2013)] can be used, which are already pre-built for use with Emu. Perform this step for each barcode.
11.Taxonomic classification can be collapsed to the desired taxonomic rank with an additional Emu command using ‘collapse-taxonomy’.
12.The taxonomy tables can then be imported to R statistical software (R Core Team, 2018) for subsequent data analysis and visualization.
REAGENTS AND SOLUTIONS
Preparation should be conducted in a laminar flow hood to avoid microbial contamination of the stock solution.
Lysozyme solution, 80 mg/ml
Mix 2.2 ml nuclease-free water (Qiagen, cat. no. 129115) with 2.4 ml Triton X-100 solution (Sigma, cat. no. 93443) and 400 µl of 100× Tris-EDTA buffer solution (Sigma, cat. no. T9285). Dissolve 400 mg lysozyme from chicken egg white (Sigma, cat. no. 62970-1G-F) for a final concentration of 80 mg/ml. Aliquots can be stored up to 1 year at −20°C.
Phosphate-buffered saline (PBS), 1×
Dilute 50 ml of 10× PBS (Thermo Fisher, cat. no. 10204733) with 450 ml nuclease-free water (Qiagen, cat. no. 129115). Aliquots can be stored up to 1 year at 4°C.
COMMENTARY
Background Information
Since the advent of high-throughput sequencing (HTS), the scientific community's interest in investigating complex microbial networks has been rising, starting from human-colonizing microbiomes, and in more recent years extending to a multitude of fields, such as animal, plant, and environmental microbiome studies. In particular, the opportunity to monitor a large number of microbes at the same time is naturally appealing in agriculture and the food production chain, and as expected, the fish farming industry has recently joined in the collective interest towards microbiome investigation as well (Llewellyn et al., 2014). In fact, shifts in the host and/or surrounding environment microbial communities’ compositions have been related to exposure of fish to antibiotics (Almeida et al., 2021) or experimental phage therapy (Donati et al., 2022), pollutants (Bellec et al., 2022; Hano et al., 2021), diet variations (Serra et al., 2021) and prebiotics administration (Menanteau-Ledouble et al., 2022), hypoxia conditions (Gatesoupe et al., 2016), early-life stress (Uren Webster et al., 2021), and to deteriorating health status (Bozzi et al., 2021; Brown et al., 2021; Tongsri et al., 2023; Vasemägi et al., 2017), with compelling possibilities for disease prevention, management, and informed decision making in the fish farm industries. The vast majority of the current literature on the subject was generated based on short reads (Illumina sequencing platform) up to 600 bp. Thus, with this platform, it was only possible to sequence fragments of the 16S rRNA gene encompassing a maximum of three variable regions (e.g., V1 to V3). These were subsequently being used to infer the identity of bacteria and their relative abundance in the community, although with different taxonomic assignment performance outcomes depending on the chosen regions (Ghyselinck et al., 2013). Third generation sequencing platforms, e.g., Oxford Nanopore Technology (ONT), allow long-read sequencing, virtually up to the length of the generated nucleic acid fragments. Consequently, full 16S rRNA gene sequencing (hypervariable regions V1 to V9) becomes possible, which in turn can provide a higher taxonomical resolution than sequencing of shorter variable regions (Johnson et al., 2019). When studying teleosts’ microbiomes, additional considerations must be considered based on the unique nature of their associated communities. The total biomass and diversity of bacterial communities associated with salmonid species are lower compared to those of most well studied warm-blooded mammals (Limborg et al., 2023). This results in a low proportion of microbial DNA (related to the sample total nucleic acids content), and thus poses an additional challenge during library preparation. For this reason, in our sample collection protocols, we focused on the enrichment of microbial biomass by minimizing the collection of host tissue. Moreover, considering that a study might comprise two or more of the presented sample types, we tested and optimized an extraction method that proved to be effective for all sample types in this protocol albeit with slight modifications. Finally, we provide guidance for all the key steps leading to library preparation. That involves quality control of the extracted metagenomes, test amplification of 16S rRNA gene amplicons, and further troubleshooting tests related to the low microbial biomass issues referenced above. All tests are aimed to use the ONT 16S metabarcoding kit, which relies on the 27F and 1942R primer couple. However, they can easily be adapted to alternative primer pairs that can be coupled with ONT commercialized sequencing kits that are currently available.
Critical Parameters
Sample collection considerations
When designing an experiment, especially a longitudinal study, it is crucial to consider additional variables when establishing sampling days and times. First, unless it is the variable of interest, the time of the day for the sampling will generally need to be consistent throughout the experiment. For instance, previous studies have shown that the skin microbiota composition of rainbow trout exhibits circadian fluctuations and is profoundly affected by photoperiods (Ellison et al., 2021). Importantly, routine interventions, such as feeding, are going to impact the microbial quality of the entire system; therefore, sampling must always take place in a similar timeframe in relation to, e.g., feeding times. Similarly, the time elapsed from the last tank cleaning, or any other maintenance intervention, needs to be consistent among sampling points. Such measures must be taken to avoid external confounders that can add an unwanted source of variability among microbiome samples.
Non-lethal and non-invasive sampling options
Considerations about welfare and ethics are a prerequisite of any study involving living animals. All experimentations must comply with the laboratory animals care and welfare regulations standards (Directive 2010/63/EU, 2010). While we refer to the European Legislation, it is crucial for each scientist to verify and adhere to their local regulations, as they may differ based on jurisdiction. According to these regulations, individuals sacrificed for sample collection should be euthanized using humane methods. For fish, these generally involve an anesthetic overdose by immersion drugs, such as benzocaine hydrochloride or MS-222 (Tricaine Methane Sulfonate) solutions (Schroeder et al., 2021). However, when possible, the experimental design should consider the principle of the three R's (replacement, reduction, and refinement) to minimize the distress on the animals involved and the number of animals involved and sacrificed in the study (Tannenbaum & Bennett, 2015). In this framework, the interest to monitor animal's health by obtaining samples with non-lethal methods is growing. Among the sample types in this article, alternatives to lethal samples are presented (e.g., gill mucus; skin mucus). Choosing to focus on non-lethal sample types not only minimizes the number of animals sacrificed in one experiment, but also, when coupled to individual tagging, it opens the possibility of collecting samples from the same individuals over time along the same study. This is particularly important when time-dependent processes, such as disease dynamics or exposure to treatments or stressors, are investigated. To collect such samples, the animal must be anesthetized or at the very least sedated. As any procedure can introduce unwanted sources of variation, it is advisable to be consistent with the chosen methods for anesthesia or euthanasia in the same study. Finally, environmental DNA (eDNA)-based studies are paving the way for future molecular investigations that further minimize the sacrifice of animals, both in research and in commercial settings. Sampling water or sediment represents one of the non-invasive sample collection methods with the potential to enable monitoring of the health status of an entire community, from microbial surveillance of human populations (Ko et al., 2022) to farmed animals (Peters et al., 2018).
Adaptation to field sampling
Although the protocols described here were optimized in experimental facilities, they can be transferred to field sampling. The first concern when transferring the protocol to the field is the potentially limited ability to snap-freeze and immediately preserve or process the samples. For this reason, when dry ice and −80°C preservation are not an option, cooling and transportation on ice or at 4°C can be implemented until long term storage at −80°C or downstream processing. The elapsed time between sampling and storage at −80°C must be consistent among all samples, as any variation may contribute to biases in the detection of microbial composition. For water samples specifically, given the potential challenges associated with filtration in the field, a widely accepted approach is to transport them on ice or at 4°C before processing for up to 24 hr (Perrin et al., 2019; van Rossum et al., 2015). Regarding other samples, which need to be transported in tubes, preservation solutions can be considered. For skin and mucus samples, when choosing a preservation solution, we recommend using a solution where the sample can be homogenized, and that can be carried over to extraction (e.g., DNA/RNA Shield, Zymo Research). This is because, in the case of preserving agents that need to be removed at the time of extraction, the act of separating and rinsing the sample from the solution could result in a variable dispersion and subsequent loss of bacteria within the solution. Concerning gut content and mucosal scrapes from rainbow trout specifically, previous reports showed that 96% ethanol is a suitable preservation method (Hildonen et al., 2019).
An additional concern about transferring this protocol to field samples arises from the expectation that a fish farm industry may exhibit higher overall microbial biomasses and a different degree of microbiome complexity. As such, adjustments might be required not only in the experimental design, but also in some of the sampling methods. For instance, water is expected to be more turbid and richer in waste particles, which might prematurely obstruct the membranes and therefore impair the vacuum filtration step. Sequential filtrations using membranes of reducing pore size could be necessary in that case to separate the suspended particles fractions, as recommended in protocols focusing on the analysis of microbiomes from rivers (Reddington et al., 2019).
Furthermore, the metadata that need to be collected will also vary from experimental facilities to an aquaculture farm. For instance, information on eventual water treatments (antibiotics, formalin), disease outbreaks, and mortality of the farmed biomass, will need to be collected at the time of sampling.
Choice of extraction kit
Several commercially accessible kits are available for extraction of total nucleic acid content from complex microbial communities. The choice between column-based and magnetic bead-based, or manual and automatic extraction methods depends on both the available equipment in the laboratory and the number of samples to process. Although some of the most used extraction kits for microbiome samples are column-based (e.g., PowerSoil DNA Isolation kit, MO BIO), when starting from environmental samples (water and sediment) and gut contents, magnetic bead-based extraction is commonly favored over column-based methods. This preference is due to the selective retention of nucleic acids on magnetic beads throughout the various steps, facilitating a more effective removal of contaminants and PCR inhibitors, which are commonly found in such samples (Schrader et al., 2012). The current recommendations from the EMP regarding DNA extraction also involve a magnetic bead-based process on an automated system (L. Marotz et al., 2018). Ideally, all samples should be processed at the same time to avoid batch effects as a confounder. Consequently, especially when dealing with a considerable number of samples, it is preferrable to use an automated extraction system over manual extraction.
Different extraction kits lead to significantly different yields and different biases in terms of bacterial taxa over- or under-representation. Therefore, when planning an investigation that aims to compare different sample types, it is recommended to use an extraction kit that works for all kinds of samples included in the study (Salter et al., 2014). In this protocol, we describe the application and adaptation of a magnetic bead-based extraction using an automatic purification system. The kit of choice in our case is the MagMAX Microbiome Ultra nucleic acid isolation kit, which proved to work effectively for extraction from all the previously mentioned sample types.
As a side note, in studies including also metatranscriptomics, it is advised to use a kit that preserves both DNA and RNA, followed by either DNase or RNase treatment, so that the same eluted sample can be used for both applications.
Control samples
As every processing phase is likely to introduce bias or contamination, controls must be included in every step. It is crucial to include both positive and negative controls that are appropriate for the system and methods of interest.
As a positive control, at least one aliquot of a known bacterial mock community must be used. This is commonly included to assess possible bias in the DNA isolation, in the marker gene amplification step, as well as in the final sequencing. The ideal extraction method should prove the same performance on bacteria with different cell wall compositions. Similarly, since non-proportional amplification can affect the final representation of the sample composition, the use of a known bacterial community will allow the optimization of the PCR step. An increasing number of commercial options are available to suit several types of samples and studies. As microbiome research is still mainly focusing on the human biomedical field, most of the mock bacterial communities that have been developed and are commercially available will be composed of human-associated strains (Highlander, 2013). However, the existing mock bacterial communities may not be relevant for research fields other than biomedicine. As aquaculture is a relatively new system for microbiome studies, future studies should aim to develop a custom mock community that includes the most relevant, prevalent, or challenging bacterial taxa in the sample type of interest (Colovas et al., 2022).
Most importantly, negative controls must be included to assess the presence of contamination during the pre-processing and extraction steps. Such controls are constituted of all the extraction reagents and must undergo the same treatments at the same time as the experimental samples. Negative controls also must be included in the sequencing runs, as they will constitute a baseline to identify putative contaminant OTUs during the bioinformatic analysis.
As a final note, the microbiome field has seen an increased interest in absolute quantification of copy numbers as opposed to the mere calculation of relative microbial abundance. This can be achieved by using a spike-in synthetic community standard, which is added into the samples in a defined quantity prior to extraction, processing, and sequencing. Synthetic spike-ins have been developed for both 16S rRNA gene metabarcoding (Tourlousse et al., 2017) and for shotgun metagenomics (Hardwick et al., 2018) applications. The read number of spiked-in standards can subsequently be used as a reference to normalize read counts, or to calculate absolute microbial abundances.
Replicate samples
In the analysis of host-associated microbiomes, it is important to account for individual variation and expected complexity in the study design and the sample size estimation. For instance, wild populations are expected to display a much higher metagenomic complexity with respect to production animals (Alberdi et al., 2022). With increasing degree of expected complexity, a higher number of individuals must be included in the study. On the other hand, when dealing with the study of a particular environment microbiome, it can be challenging to use “biological” replicates. For instance, a specific aquaculture facility, or river, might be the study subject. In that case, replicate sampling of the same environment, such as replicate filtration of different aliquots of water from the same source, can be performed. This strategy has been previously used in the study of river microbiomes (Urban et al., 2021).
Technical replicates can also be used in the amplification step. The Earth Microbiome Project (EMP) recommendation for 16S rRNA gene metabarcoding is to perform triplicate amplification reactions for each sample, and to later pool them before amplicon electrophoresis, quantification, and purification (Thompson et al., 2017). While such a protocol is appropriate for sequencing projects based on the Illumina short-read platforms, most studies using the ONT sequencing kit (SQK-16S024) perform one amplification reaction for each sample, since primers in the kit are already barcoded, and pooling is only possible if the same barcoded primers are used for each replicate. However, the kit provides more than one primer set; therefore, such an approach is a feasible option. Another common approach to technical replicates of amplification (mostly for short-read Illumina sequencing) is to prepare replicate PCR reactions and, instead of pooling them, sequence them separately (Alberdi et al., 2018). After separately sequencing PCR replicates, two analysis methods are possible, depending on the purpose of the study. An additive method, which will add all the detected taxa in the replicates, will maximize the detection of microbial diversity. A more restrictive (and less common) method of analysis will instead only keep the OTUs that appear in all sequenced replicates, therefore minimizing the false positives and experimental artifacts originating during library preparation. However, replicate PCR reactions of the same samples were often reported to yield different results in terms of detected taxa and richness, especially when the initial sample has a low bacterial biomass and a low amount of DNA template is used (Kennedy et al., 2014). Therefore, in cases where the template DNA amount is exceptionally low, the PCR stochasticity will be higher, and each PCR replicate will not represent the diversity of the original sample. The restrictive approach is thus not advised in such circumstances, as it could result in the loss of real diversity (Alberdi et al., 2018).
Troubleshooting
16S rRNA gene PCR troubleshooting
One of the most common issues with PCR-based library preparation, especially starting from host-associated samples, is an unsuccessful or suboptimal 16S rRNA gene amplification. There are distinct reasons why the PCR can fail, but in this section, we will mainly deal with problems related to the sampling methods described above, i.e., mixed host-microbial DNA.
First, the overall microbial biomass in the sample might be too low. Therefore, the DNA template quantity may be raised to increase the starting material for amplification, or the number of PCR cycles can be increased. Second, and intimately related with low bacterial biomass, is a sample where the host to bacteria nucleic acid ratio is too high. In some kinds of samples (e.g., entire fish gut sections), the host DNA can easily exceed 90% of the extracted material. In this case, adding the advised starting quantity of DNA template could still result in a failed amplification, because the majority of starting DNA derives from the host. Also in this case, increasing the starting material or raising the number of amplification cycles can be beneficial. On the other hand, a failed PCR could be caused by inhibition, and diluting the sample could solve the issue (Wang et al., 2017). A qPCR using universal 16S rRNA gene primers can be performed prior to library preparation and subsequently used as a starting point to identify the optimal template DNA dilution and to optimize the number of PCR cycles needed.
Previous studies have highlighted the challenges related to cross-kingdom amplification of host DNA when using universal primers targeting the bacterial 16S rRNA gene (Galkiewicz & Kellogg, 2008; Walker et al., 2020). Such lack of specificity can outcompete the amplification of target bacterial sequences and result in unexpected DNA bands among the products of test PCR reactions. Possible solutions to this issue include purification of the expected amplicon after size-separation on agarose gel (McDevitt-Irwin et al., 2019), changing the universal primers used in the study, or by using peptide nucleic acid (PNA) clamps to impair off-target amplification (Reigel et al., 2020).
Another issue with library preparation might be related to a subsequent step, i.e., the amplicon purification step. Most library preparation kits rely on magnetic-bead purification (e.g., AMPure XP beads, Beckman Coulter), which needs to yield an appropriate concentration to undergo sequencing. For instance, according to the ONT 16S barcoding kit, the final concentration of pooled barcoded libraries needs to be in the range of 50 to 100 ng in a volume of 10 µl for optimal performance. Thus, according to the desired degree of multiplexing, ranging from 1 to 24 samples for such ONT kit, the concentration of purified amplicons for each sample must exceed a minimum threshold (from 5 down to 0.2 ng/µl). However, it is likely that the purification step will result in the loss of a certain amount of amplicon, to the point that the final concentration will not be sufficient for sequencing. Strategies to solve this issue include preparing replicate PCR reactions to pool prior purification or testing alternative PCR purification kits to achieve a higher amplicon recovery rate.
In Table 2, we provide examples of average initial template DNA quantity, number of amplification cycles and replicate PCR reactions to pool that previously worked for our samples, producing sufficient amplicon to undergo sequencing.
Alternatively, adjustments to the sampling method could be required to try to minimize the collection of host tissue. However, there are also cases in which the tissue is important per se, e.g., when interested in bacterial infiltration in tissues (e.g., skin diseases) or in intracellular bacterial taxa. In these situations, the exclusion of host tissue is not possible, and thus additional protocols intended for exclusion of host DNA (Heravi et al., 2020; C. A. Marotz et al., 2018) can be taken in consideration.
Table 5 summarizes all possible troubleshooting solutions for library preparation that we mentioned in this section.
Problem | Possible cause | Solution |
---|---|---|
No amplicon band is visible | The amount of starting bacterial DNA template is too low, causing the amplification to produce little to no amplicon band; PCR inhibition could possibly be impairing the amplification | Assess band presence by an alternative method (e.g., Bioanalyzer); increase starting quantity of template DNA and/or number of amplification cycles; test dilutions by qPCR to assess presence of possible inhibitors |
Faint amplicon band | The initial template DNA may be too low | Increase starting quantity of template DNA and/or number of amplification cycles; prepare replicate PCR reactions to pool prior amplicon purification |
Test qPCR on sample dilutions shows >110% efficiency | PCR inhibitors might be present in the sample | Dilute sample; test additives to inactivate inhibitors (e.g., BSA); test alternative DNA extraction protocols |
Unexpected amplicon bands are present | Unexpected bands are usually due to non-specific cross-amplification | Purification of the expected band (1.5 kbp) upon agarose gel size-separation; PNA clamps to reduce off-target amplification; use of alternative universal 16S rRNA gene primers |
The concentration of purified amplicon is too low | The amplicon quantity resulting from the PCR may be too low, or amplicon may have been lost during the purification step | Prepare replicate PCR reactions to pool prior purification; test alternative PCR purification kits |
It is not possible to add enough bacterial template DNA without over-saturating PCR reaction | When dealing with samples that show a low bacterial biomass and high host DNA contamination, it might be necessary to add more initial DNA template, but the proportion of host DNA might be so high that it is not possible to do so without over-saturating the PCR reaction | Consider alternative sampling methods (e.g., sampling methods that exclude as much host tissue as possible); consider host DNA exclusion protocols or bacterial DNA enrichment protocols |
Understanding Results
The quality of the extraction step is assessed prior to library preparation. As described in Basic Protocol 4, this is usually done in three phases. First, purity of the nucleic acids is evaluated by reading the absorbance values on a spectrophotometer. The ratio between nucleic acids and protein is generally assessed by 260/280 absorbance ratio. The purity over proteins is deemed satisfactory when the value is between 1.7 and 2.0. The 260/230 absorbance ratio can be used to estimate the purity of nucleic acids over salts or contaminants, and it is usually acceptable above 1.8. DNA integrity is assessed by agarose gel electrophoresis. A clear band of high molecular weight DNA should be visible in the agarose gel (Fig. 2A). If the genomic DNA appears as a long “smear” (Fig. 2B), this could indicate that DNA shearing occurred either during the extraction step or during a suboptimal preservation of the sample. This could impact the ability to amplify long amplicons (1.5 kbp) in subsequent steps, and as such, it might be a source of potential bias in the results. Accurately measuring the DNA concentration in the eluted sample using a fluorometric assay will be useful to evaluate the DNA yield for each sample. This is particularly important for low-biomass samples, as fluorometric assays are usually more sensitive and accurate to low DNA concentrations than measurements on a spectrophotometric device. Positive controls are important to assess the efficacy of the extraction method (see “Control samples” in the Critical Parameters section).
Next, a test PCR amplification is performed with universal bacterial primers targeting the V1 to V9 hypervariable regions of the 16S rRNA gene. The resulting PCR products are evaluated through gel electrophoresis. Under optimal conditions, a single bright, clear DNA band is expected to appear at 1.5 kbp size (Fig. 3B). A faint DNA band (Fig. 3A) may indicate that the PCR amplification step requires optimization (see Troubleshooting). If no bands are visible, or if increasing template and/or PCR cycles number does not solve the issue, PCR inhibitors, e.g., specific dissolved organic compounds commonly found in stool or environmental samples (Schrader et al., 2012) may be present in the sample. An additional qPCR test can be performed to assess this (see Support Protocol 1).
The results of such qPCR assay can be interpreted using a qPCR analysis software of choice. Calculation of the amplification efficiency (E= −1+10(−1/slope)) for each group of sample dilutions is usually performed automatically by the analysis software. The optimal range is between values of 90% to 110%; if it exceeds 110%, this could indicate that the amplification is affected by inhibition. If the efficiency of the PCR is in the optimal range, each 10-fold dilution should amplify with a difference of ∼3.3 Cq values from one dilution to the next. If a moderate degree of inhibition is present, the undiluted samples will show a delay in their exponential amplification phase, while more diluted samples will display a better efficiency of amplification and excluding the highest concentration samples will improve the efficiency value. If that is the case, lower amounts of DNA template for library preparation are recommended, and a new endpoint PCR test (see Basic Protocol 4, “Test PCR amplification with selected 16S rRNA gene primers”) can be performed with optimized conditions. If the efficiency of amplification is overall suboptimal, or no amplification is at all possible, application of additives to overcome inhibition (Schrader et al., 2012) can be considered.
After optimization of the PCR step, it is still possible that the amplicon quantity might be too low to undergo sequencing. A successful library preparation will yield an appropriate concentration of amplicon. As detailed in the Troubleshooting section, for the ONT 16S barcoding kit this means that, for each sample, the minimum concentration after amplicon purification should be from 5 ng/ µl down to 0.2 ng/ µl (depending on the samples to be multiplexed, 1 to 24). A test purification from the test PCR step can determine if the amplicon amount will be sufficient, or if multiple reactions will need to be prepared for each sample to be pooled prior purification and sequencing.
With the optimized conditions, the samples can finally undergo library preparation and sequencing by following the manufacturer's instructions from the kit of choice (e.g., SQK-16S024). Starting from a sample type of unknown complexity, it is important to perform a test sequencing run and data analysis to determine the minimum sequencing depth for the sample type and study purpose. For instance, the approximate number of reads that are needed to reach saturation (plateau) of the read rarefaction curves can be calculated and used as a target sequencing depth. Such curves can be simply plotted as number of features (taxa) over number of reads—e.g., using vegan (Dixon, 2003) in R. This knowledge will also help determining how many samples can be multiplexed together in the same run. For instance, if samples exhibit a relatively low complexity and rarefaction curves are saturated around 50,000 reads, it will be possible to run multiple libraries in the same run (e.g., all 24 barcodes in an SQK-16S024 kit). After sequencing, raw FAST5 files will be produced. Support Protocol 2 shows an example of a bioinformatics pipeline that can be used to retrieve and process the raw reads to produce clean and filtered FASTQ reads. The Emu taxonomic assignment algorithm is finally used to generate taxonomic abundance tables, which can be then imported to R Statistical Software for further analysis. A plethora of R packages were developed for microbiome datasets analysis, such as vegan (Dixon, 2003) and phyloseq (McMurdie & Holmes, 2013). When selecting the analysis tools, it is imperative to consider that, during statistical analysis, tests should match the compositional nature of microbiome datasets (Gloor et al., 2017).
Time Considerations
Sampling procedures
Water sampling and filtration: up to 1 hr/sample (highly dependent on water turbidity and sampling volume).
Sediment sampling: 10 min/sample.
Gill/skin mucus; skin sampling: 5 min/sample.
Gut sampling: 10 min/sample.
Nucleic acid extraction
Sample homogenization and pre-treatment: 1 hr 30 min (dependent on number of samples to process).
DNA extraction: 1 hr for up to 96 samples (time relative to DNA extraction using an automated extraction system, e.g., KingFisher Flex).
Quality control and test amplification
DNA purity assessment: 10 s/sample.
DNA electrophoresis: 30 min.
Fluorometric quantification: 2 min/sample.
Test 16S rRNA gene amplification: 3 hr (dependent on the number of PCR cycles).
Inhibition assessment by qPCR: 2 hr.
Acknowledgments
The authors thank Jacob Schmidt for advice on rainbow trout skin samples collection, Niccolò Vendramin and experimental facilities technician Henrik Andersen for designing the sediment collection method in the animal facilities, and Juliane Sørensen for initial guidance on the operation of automated extraction systems.
Funding
This project has received funding from the European Union's Horizon 2020 research and innovation programme under grant agreement No. 871108 (AQUAEXCEL3.0). This output reflects only the author's view and the European Commission cannot be held responsible for any use that may be made of the information contained therein.
Author Contributions
Giulia Zarantonello : Conceptualization; data curation; methodology; software; validation; visualization; writing—original draft; writing—review and editing. Argelia Cuenca : Conceptualization; funding acquisition; methodology; project administration; resources; supervision; writing—original draft; writing— review and editing.
Conflict of Interest
The authors declare no conflict of interest.
Open Research
Data Availability Statement
Data sharing not applicable as no new data were generated.
Literature Cited
- Alberdi, A., Aizpurua, O., Gilbert, M. T. P., & Bohmann, K. (2018). Scrutinizing key steps for reliable metabarcoding of environmental samples. Methods in Ecology and Evolution , 9(1), 134–147. https://doi.org/10.1111/2041-210X.12849
- Alberdi, A., Andersen, S. B., Limborg, M. T., Dunn, R. R., & Gilbert, M. T. P. (2022). Disentangling host–microbiota complexity through hologenomics. Nature Reviews Genetics , 23(5), 5. https://doi.org/10.1038/s41576-021-00421-0
- Almeida, A. R., Domingues, I., & Henriques, I. (2021). Zebrafish and water microbiome recovery after oxytetracycline exposure. Environmental Pollution (Barking, Essex : 1987) , 272, 116371. https://doi.org/10.1016/J.ENVPOL.2020.116371
- Apprill, A., McNally, S., Parsons, R., & Weber, L. (2015). Minor revision to V4 region SSU rRNA 806R gene primer greatly increases detection of SAR11 bacterioplankton. Aquatic Microbial Ecology , 75(2), 129–137. https://doi.org/10.3354/ame01753
- Bellec, L., Le Du-Carrée, J., Almeras, F., Durand, L., Cambon-Bonavita, M.-A., Danion, M., & Morin, T. (2022). Glyphosate-based herbicide exposure: Effects on gill microbiota of rainbow trout (Oncorhynchus mykiss) and the aquatic bacterial ecosystem. FEMS Microbiology Ecology , 98, fiac076. https://doi.org/10.1093/femsec/fiac076
- Bozzi, D., Rasmussen, J. A., Carøe, C., Sveier, H., Nordøy, K., Gilbert, M. T. P., & Limborg, M. T. (2021). Salmon gut microbiota correlates with disease infection status: Potential for monitoring health in farmed animals. Animal Microbiome , 3(1), 30. https://doi.org/10.1186/s42523-021-00096-2
- Brown, R., Moore, L., Mani, A., Patel, S., & Salinas, I. (2021). Effects of ploidy and salmonid alphavirus infection on the skin and gill microbiome of Atlantic salmon (Salmo salar). PloS One , 16(2), e0243684. https://doi.org/10.1371/journal.pone.0243684
- Caporaso, J. G., Lauber, C. L., Walters, W. A., Berg-Lyons, D., Huntley, J., Fierer, N., Owens, S. M., Betley, J., Fraser, L., Bauer, M., Gormley, N., Gilbert, J. A., Smith, G., & Knight, R. (2012). Ultra-high-throughput microbial community analysis on the Illumina HiSeq and MiSeq platforms. The ISME Journal , 6(8), 1621–1624. https://doi.org/10.1038/ismej.2012.8
- Collins, F. W. J., Walsh, C. J., Gomez-Sala, B., Guijarro-García, E., Stokes, D., Jakobsdóttir, K. B., Kristjánsson, K., Burns, F., Cotter, P. D., Rea, M. C., Hill, C., & Ross, R. P. (2021). The microbiome of deep-sea fish reveals new microbial species and a sparsity of antibiotic resistance genes. Gut Microbes , 13(1), 1–13. https://doi.org/10.1080/19490976.2021.1921924
- Colovas, J., Bintarti, A. F., Mechan Llontop, M. E., Grady, K. L., & Shade, A. (2022). Do-it-yourself mock community standard for multi-step assessment of microbiome protocols. Current Protocols , 2(9), e533. https://doi.org/10.1002/cpz1.533
- Curry, K. D., Wang, Q., Nute, M. G., Tyshaieva, A., Reeves, E., Soriano, S., Wu, Q., Graeber, E., Finzer, P., Mendling, W., Savidge, T., Villapol, S., Dilthey, A., & Treangen, T. J. (2022). Emu: Species-level microbial community profiling of full-length 16S rRNA Oxford Nanopore sequencing data. Nature Methods , 19(7), 7. https://doi.org/10.1038/s41592-022-01520-4
- Directive 2010/63/EU, L276 § Official Journal of the European Union. (2010). http://data.europa.eu/eli/dir/2010/63/2019-06-26/eng
- Dixon, P. (2003). VEGAN, a package of R functions for community ecology. Journal of Vegetation Science , 14(6), 927–930. https://doi.org/10.1111/j.1654-1103.2003.tb02228.x
- Donati, V. L., Madsen, L., Middelboe, M., Strube, M. L., & Dalsgaard, I. (2022). The gut microbiota of healthy and flavobacterium psychrophilum-infected rainbow trout fry is shaped by antibiotics and phage therapies. Frontiers in Microbiology , 13, 771296. https://doi.org/10.3389/fmicb.2022.771296
- Ellison, A. R., Wilcockson, D., & Cable, J. (2021). Circadian dynamics of the teleost skin immune-microbiome interface. Microbiome , 9(1), 222. https://doi.org/10.1186/s40168-021-01160-4
- Ewels, P., Magnusson, M., Lundin, S., & Käller, M. (2016). MultiQC: Summarize analysis results for multiple tools and samples in a single report. Bioinformatics , 32(19), 3047–3048. https://doi.org/10.1093/bioinformatics/btw354
- Galkiewicz, J. P., & Kellogg, C. A. (2008). Cross-kingdom amplification using bacteria-specific primers: Complications for studies of coral microbial ecology. Applied and Environmental Microbiology , 74(24), 7828–7831. https://doi.org/10.1128/AEM.01303-08
- Gatesoupe, F. J., Huelvan, C., Le Bayon, N., Le Delliou, H., Madec, L., Mouchel, O., Quazuguel, P., Mazurais, D., & Zambonino-Infante, J. L. (2016). The highly variable microbiota associated to intestinal mucosa correlates with growth and hypoxia resistance of sea bass, Dicentrarchus labrax, submitted to different nutritional histories. BMC Microbiology , 16(1), 1–13. https://doi.org/10.1186/S12866-016-0885-2
- Ghyselinck, J., Pfeiffer, S., Heylen, K., Sessitsch, A., & De Vos, P. (2013). The effect of primer choice and short read sequences on the outcome of 16S rRNA gene based diversity studies. PLoS ONE , 8(8), e71360. https://doi.org/10.1371/journal.pone.0071360
- Gloor, G. B., Macklaim, J. M., Pawlowsky-Glahn, V., & Egozcue, J. J. (2017). Microbiome datasets are compositional: And this is not optional. Frontiers in Microbiology , 8, 2224. https://doi.org/10.3389/fmicb.2017.02224
- Hano, T., Ito, M., Ito, K., & Uchida, M. (2021). Alterations of stool metabolome, phenome, and microbiome of the marine fish, red sea bream, Pagrus major, following exposure to phenanthrene: A non-invasive approach for exposure assessment. Science of the Total Environment , 752, 141796. https://doi.org/10.1016/j.scitotenv.2020.141796
- Hardwick, S. A., Chen, W. Y., Wong, T., Kanakamedala, B. S., Deveson, I. W., Ongley, S. E., Santini, N. S., Marcellin, E., Smith, M. A., Nielsen, L. K., Lovelock, C. E., Neilan, B. A., & Mercer, T. R. (2018). Synthetic microbe communities provide internal reference standards for metagenome sequencing and analysis. Nature Communications , 9(1), 3096. https://doi.org/10.1038/s41467-018-05555-0
- Heravi, F. S., Zakrzewski, M., Vickery, K., & Hu, H. (2020). Host DNA depletion efficiency of microbiome DNA enrichment methods in infected tissue samples. Journal of Microbiological Methods , 170, 105856. https://doi.org/10.1016/j.mimet.2020.105856
- Highlander, S. (2013). Mock community analysis. In K. E. Nelson (Ed.), Encyclopedia of Metagenomics (pp. 1–7). Springer. https://doi.org/10.1007/978-1-4614-6418-1_54-1
- Hildonen, M., Kodama, M., Puetz, L. C., Gilbert, M. T. P., & Limborg, M. T. (2019). A comparison of storage methods for gut microbiome studies in teleosts: Insights from rainbow trout (Oncorhynchus mykiss). Journal of Microbiological Methods , 160, 42–48. https://doi.org/10.1016/j.mimet.2019.03.010
- Infante-Villamil, S., Huerlimann, R., & Jerry, D. R. (2021). Microbiome diversity and dysbiosis in aquaculture. Reviews in Aquaculture , 13(2), 1077–1096. https://doi.org/10.1111/raq.12513
- Ivanova, L., Rangel-Huerta, O. D., Tartor, H., Gjessing, M. C., Dahle, M. K., & Uhlig, S. (2021). Fish skin and gill mucus: A source of metabolites for non-invasive health monitoring and research. Metabolites , 12(1), 28. https://doi.org/10.3390/metabo12010028
- Johnson, J. S., Spakowicz, D. J., Hong, B. Y., Petersen, L. M., Demkowicz, P., Chen, L., Leopold, S. R., Hanson, B. M., Agresta, H. O., Gerstein, M., Sodergren, E., & Weinstock, G. M. (2019). Evaluation of 16S rRNA gene sequencing for species and strain-level microbiome analysis. Nature Communications , 10(1), 5029. https://doi.org/10.1038/s41467-019-13036-1
- Kennedy, K., Hall, M. W., Lynch, M. D. J., Moreno-Hagelsieb, G., & Neufeld, J. D. (2014). Evaluating bias of illumina-based bacterial 16S rRNA gene profiles. Applied and Environmental Microbiology , 80(18), 5717–5722. https://doi.org/10.1128/AEM.01451-14
- Ko, K. K. K., Chng, K. R., & Nagarajan, N. (2022). Metagenomics-enabled microbial surveillance. Nature Microbiology , 7(4), 4. https://doi.org/10.1038/s41564-022-01089-w
- Limborg, M. T., Chua, P. Y. S., & Rasmussen, J. A. (2023). Unexpected fishy microbiomes. Nature Reviews Microbiology , 21(6), 6. https://doi.org/10.1038/s41579-023-00879-1
- Llewellyn, M. S., Boutin, S., Hoseinifar, S. H., & Derome, N. (2014). Teleost microbiomes: The state of the art in their characterization, manipulation and importance in aquaculture and fisheries. Frontiers in Microbiology , 5, 207. https://doi.org/10.3389/fmicb.2014.00207
- Marotz, C. A., Sanders, J. G., Zuniga, C., Zaramela, L. S., Knight, R., & Zengler, K. (2018). Improving saliva shotgun metagenomics by chemical host DNA depletion. Microbiome , 6(1), 1–9. https://doi.org/10.1186/s40168-018-0426-3
- Marotz, L., Schwartz, T., Thompson, L., Humphrey, G., Gogul, G., Gaffney, J., Amir, A., & Knight, R. (2018). Earth Microbiome Project (EMP) high throughput (HTP) DNA extraction protocol. Protocols.Io , https://doi.org/10.17504/protocols.io.pdmdi46
- McDevitt-Irwin, J. M., Garren, M., McMinds, R., Vega Thurber, R., & Baum, J. K. (2019). Variable interaction outcomes of local disturbance and El Niño-induced heat stress on coral microbiome alpha and beta diversity. Coral Reefs , 38(2), 331–345. https://doi.org/10.1007/s00338-019-01779-8
- McMurdie, P. J., & Holmes, S. (2013). phyloseq: An R package for reproducible interactive analysis and graphics of microbiome census data. PLOS ONE , 8(4), e61217. https://doi.org/10.1371/journal.pone.0061217
- Menanteau-Ledouble, S., Skov, J., Lukassen, M. B., Rolle-Kampczyk, U., Haange, S.-B., Dalsgaard, I., von Bergen, M., & Nielsen, J. L. (2022). Modulation of gut microbiota, blood metabolites, and disease resistance by dietary β-glucan in rainbow trout (Oncorhynchus mykiss). Animal Microbiome , 4(1), 58. https://doi.org/10.1186/s42523-022-00209-5
- Nguyen, C. D. H., Amoroso, G., Ventura, T., & Elizur, A. (2020). Assessing the pyloric caeca and distal gut microbiota correlation with flesh color in atlantic salmon (Salmo salar L., 1758). Microorganisms , 8(8), 1244. https://doi.org/10.3390/microorganisms8081244
- O'Leary, N. A., Wright, M. W., Brister, J. R., Ciufo, S., Haddad, D., McVeigh, R., Rajput, B., Robbertse, B., Smith-White, B., Ako-Adjei, D., Astashyn, A., Badretdin, A., Bao, Y., Blinkova, O., Brover, V., Chetvernin, V., Choi, J., Cox, E., Ermolaeva, O., … Pruitt, K. D. (2016). Reference sequence (RefSeq) database at NCBI: Current status, taxonomic expansion, and functional annotation. Nucleic Acids Research , 44(D1), D733–745. https://doi.org/10.1093/nar/gkv1189
- Parada, A. E., Needham, D. M., & Fuhrman, J. A. (2016). Every base matters: Assessing small subunit rRNA primers for marine microbiomes with mock communities, time series and global field samples. Environmental Microbiology , 18(5), 1403–1414. https://doi.org/10.1111/1462-2920.13023
- Perrin, Y., Bouchon, D., Delafont, V., Moulin, L., & Héchard, Y. (2019). Microbiome of drinking water: A full-scale spatio-temporal study to monitor water quality in the Paris distribution system. Water Research , 149, 375–385. https://doi.org/10.1016/j.watres.2018.11.013
- Peters, L., Spatharis, S., Dario, M. A., Dwyer, T., Roca, I. J. T., Kintner, A., Kanstad-Hanssen, Ø., Llewellyn, M. S., & Praebel, K. (2018). Environmental DNA: A new low-cost monitoring tool for pathogens in salmonid aquaculture. Frontiers in Microbiology , 9, 3009. https://doi.org/10.3389/fmicb.2018.03009
- Quast, C., Pruesse, E., Yilmaz, P., Gerken, J., Schweer, T., Yarza, P., Peplies, J., & Glöckner, F. O. (2013). The SILVA ribosomal RNA gene database project: Improved data processing and web-based tools. Nucleic Acids Research , 41(Database issue), D590–596. https://doi.org/10.1093/nar/gks1219
- R Core Team. (2018). R: A language and environment for statistical computing. [Computer software]. R Foundation for Statistical Computing. https://www.r-project.org/
- Rasmussen, J. A., Villumsen, K. R., Duchêne, D. A., Puetz, L. C., Delmont, T. O., Sveier, H., Jørgensen, L. von G., Præbel, K., Martin, M. D., Bojesen, A. M., Gilbert, M. T. P., Kristiansen, K., & Limborg, M. T. (2021). Genome-resolved metagenomics suggests a mutualistic relationship between Mycoplasma and salmonid hosts. Communications Biology , 4(1), 1. https://doi.org/10.1038/s42003-021-02105-1
- Reddington, K., Eccles, D., O'Grady, J., Drown, D. M., Hansen, L. H., Nielsen, T. K., Ducluzeau, A.-L., Leggett, R. M., Heavens, D., Peel, N., Snutch, T. P., Bayega, A., Oikonomopoulos, S., Ragoussis, J., Barry, T., van der Helm, E., Jolic, D., Richardson, H., Jansen, H., … Brown, B. L. (2019). DNA extraction and purification for MARC phase 3 global river water sequencing. Protocols.Io , https://doi.org/10.17504/protocols.io.qtgdwjw
- Reigel, A. M., Owens, S. M., & Hellberg, M. E. (2020). Reducing host DNA contamination in 16S rRNA gene surveys of anthozoan microbiomes using PNA clamps. Coral Reefs , 39(6), 1817–1827. https://doi.org/10.1007/s00338-020-02006-5
- Riiser, E. S., Haverkamp, T. H. A., Varadharajan, S., Borgan, Ø., Jakobsen, K. S., Jentoft, S., & Star, B. (2019). Switching on the light: Using metagenomic shotgun sequencing to characterize the intestinal microbiome of Atlantic cod. Environmental Microbiology , 21(7), 2576–2594. https://doi.org/10.1111/1462-2920.14652
- Ruiz-Villalba, A., Ruijter, J. M., & van den Hoff, M. J. B. (2021). Use and misuse of Cq in qPCR data analysis and reporting. Life , 11(6), 496. https://doi.org/10.3390/life11060496
- Salter, S. J., Cox, M. J., Turek, E. M., Calus, S. T., Cookson, W. O., Moffatt, M. F., Turner, P., Parkhill, J., Loman, N. J., & Walker, A. W. (2014). Reagent and laboratory contamination can critically impact sequence-based microbiome analyses. BMC Biology , 12, 87. https://doi.org/10.1186/s12915-014-0087-z
- Schmidt, J. G., Henriksen, N. H., & Olesen, N. J. (2021). Antibiotic treatment alleviates red mark syndrome symptoms in rainbow trout (Oncorhynchus mykiss) and reduces load of Midichloria-like organism. Aquaculture , 532, 736008. https://doi.org/10.1016/j.aquaculture.2020.736008
- Schrader, C., Schielke, A., Ellerbroek, L., & Johne, R. (2012). PCR inhibitors—Occurrence, properties and removal. Journal of Applied Microbiology , 113(5), 1014–1026. https://doi.org/10.1111/j.1365-2672.2012.05384.x
- Schroeder, P., Lloyd, R., McKimm, R., Metselaar, M., Navarro, J., O'Farrell, M., Readman, G. D., Speilberg, L., & Mocho, J.-P. (2021). Anaesthesia of laboratory, aquaculture and ornamental fish: Proceedings of the first LASA-FVS symposium. Laboratory Animals , 55(4), 317–328. https://doi.org/10.1177/0023677221998403
- Serra, C. R., Oliva-Teles, A., Enes, P., & Tavares, F. (2021). Gut microbiota dynamics in carnivorous European seabass (Dicentrarchus labrax) fed plant-based diets. Scientific Reports , 11(1), 1–13. https://doi.org/10.1038/s41598-020-80138-y
- Stoddard, S. F., Smith, B. J., Hein, R., Roller, B. R. K., & Schmidt, T. M. (2015). rrnDB: Improved tools for interpreting rRNA gene abundance in bacteria and archaea and a new foundation for future development. Nucleic Acids Research , 43(Database issue), D593–598. https://doi.org/10.1093/nar/gku1201
- Tannenbaum, J., & Bennett, B. T. (2015). Russell and Burch's 3Rs then and now: The need for clarity in definition and purpose. Journal of the American Association for Laboratory Animal Science: JAALAS , 54(2), 120–132.
- Thompson, L. R., Sanders, J. G., McDonald, D., Amir, A., Ladau, J., Locey, K. J., Prill, R. J., Tripathi, A., Gibbons, S. M., Ackermann, G., Navas-Molina, J. A., Janssen, S., Kopylova, E., Vázquez-Baeza, Y., González, A., Morton, J. T., Mirarab, S., Zech Xu, Z., Jiang, L., … Earth Microbiome Project Consortium. (2017). A communal catalogue reveals Earth's multiscale microbial diversity. Nature , 551(7681), 457–463. https://doi.org/10.1038/nature24621
- Tongsri, P., Cheng, G., Huang, Z., Wang, Z., Dong, F., Wu, Z., Kong, W., Yu, Y., & Xu, Z. (2023). Mucosal immunity and microbiota change in the rainbow trout (Oncorhynchus mykiss) gills after being challenged with infectious hematopoietic necrosis virus. Fish & Shellfish Immunology, 142, 109166. https://doi.org/10.1016/j.fsi.2023.109166
- Tourlousse, D. M., Narita, K., Miura, T., Sakamoto, M., Ohashi, A., Shiina, K., Matsuda, M., Miura, D., Shimamura, M., Ohyama, Y., Yamazoe, A., Uchino, Y., Kameyama, K., Arioka, S., Kataoka, J., Hisada, T., Fujii, K., Takahashi, S., Kuroiwa, M., … Terauchi, J. (2021). Validation and standardization of DNA extraction and library construction methods for metagenomics-based human fecal microbiome measurements. Microbiome , 9(1), 95. https://doi.org/10.1186/s40168-021-01048-3
- Tourlousse, D. M., Yoshiike, S., Ohashi, A., Matsukura, S., Noda, N., & Sekiguchi, Y. (2017). Synthetic spike-in standards for high-throughput 16S rRNA gene amplicon sequencing. Nucleic Acids Research , 45(4), e23. https://doi.org/10.1093/nar/gkw984
- Toxqui-Rodríguez, S., Naya-Català, F., Sitjà-Bobadilla, A., Piazzon, M. C., & Pérez-Sánchez, J. (2023). Fish microbiomics: Strengths and limitations of MinION sequencing of gilthead sea bream (Sparus aurata) intestinal microbiota. Aquaculture , 569, 739388. https://doi.org/10.1016/j.aquaculture.2023.739388
- Urban, L., Holzer, A., Baronas, J. J., Hall, M. B., Braeuninger-Weimer, P., Scherm, M. J., Kunz, D. J., Perera, S. N., Martin-Herranz, D. E., Tipper, E. T., Salter, S. J., & Stammnitz, M. R. (2021). Freshwater monitoring by nanopore sequencing. eLife , 10, 1–27. https://doi.org/10.7554/eLife.61504
- Uren Webster, T. M., Consuegra, S., & Garcia de Leaniz, C. (2021). Early life stress causes persistent impacts on the microbiome of Atlantic salmon. Comparative Biochemistry and Physiology. Part D, Genomics & Proteomics, 40, 100888. https://doi.org/10.1016/j.cbd.2021.100888
- van Rossum, T., Peabody, M. A., Uyaguari-Diaz, M. I., Cronin, K. I., Chan, M., Slobodan, J. R., Nesbitt, M. J., Suttle, C. A., Hsiao, W. W. L., Tang, P. K. C., Prystajecky, N. A., & Brinkman, F. S. L. (2015). Year-long metagenomic study of river microbiomes across land use and water quality. Frontiers in Microbiology , 6, 1405. https://doi.org/10.3389/fmicb.2015.01405
- Vasemägi, A., Visse, M., & Kisand, V. (2017). Effect of environmental factors and an emerging parasitic disease on gut microbiome of wild salmonid fish. mSphere , 2(6), 418–435. https://doi.org/10.1128/MSPHERE.00418-17
- Voytas, D. (2000). Agarose gel electrophoresis. Current Protocols in Molecular Biology , 51(1), 2.5A.1–2.5A.9. https://doi.org/10.1002/0471142727.mb0205as51
- Walker, S. P., Barrett, M., Hogan, G., Flores Bueso, Y., Claesson, M. J., & Tangney, M. (2020). Non-specific amplification of human DNA is a major challenge for 16S rRNA gene sequence analysis. Scientific Reports , 10(1), 16356. https://doi.org/10.1038/s41598-020-73403-7
- Wang, H., Qi, J., Xiao, D., Wang, Z., & Tian, K. (2017). A re-evaluation of dilution for eliminating PCR inhibition in soil DNA samples. Soil Biology and Biochemistry , 106, 109–118. https://doi.org/10.1016/j.soilbio.2016.12.011
Internet Resources
Earth Microbiome Project's website contains useful resources on sample preparation and protocol standardization for metabarcoding studies. While it focuses on Illumina sequencing, these protocols (up to the library preparation step) can be applied for any kind of sequencing study.
- https://az.research.umich.edu/animalcare/guidelines/guidelines-fish-anesthesia-analgesia-and-surgery
Guidelines on fish anesthesia, analgesia, and surgery from the Unit for Laboratory Animal Medicine of the University of Michigan. General guidance on anesthesia and post-anesthesia care procedures for fish can be found on the webpage. Please be aware of your local regulations and recommendations regarding laboratory animal procedures and approval of laboratory activities.
Oxford Nanopore Technologies website Resource Centre containing articles, workflows, and the latest news regarding Nanopore sequencing applications.
Oxford Nanopore Technologies YouTube Channel, with video collections encompassing practical workflows, masterclasses, and bioinformatic resources to start working with the sequencing platforms, with examples of different study applications.
EPI2ME website, with software and resources related to the EPI2ME workflows, from Oxford Nanopore Technologies.
Introduction to FAST5 files from EPI2ME labs (Oxford Nanopore Technologies).
“Introduction to Long-Read Data Analysis”, a useful collection of tutorials for long reads analysis tools, focusing on Oxford Nanopore data. Basecalling, quality control, read filtering, trimming and adapter removal tutorials can be useful for 16S rRNA gene metabarcoding reads pre-processing.