Lightsheet Microscopy
Laura Dyer, Laura Dyer, Andrew Parker, Andrew Parker, Keanu Paphiti, Keanu Paphiti, Jeremy Sanderson, Jeremy Sanderson
airy beam
Bessel beam
cilia imaging
clearing
cochlear imaging
fluorescence microscopy
Gaussian beam
lattice lightsheet
lightsheet microscopy
selective plane illumination microscopy
spheroid imaging
Abstract
In this paper, we review lightsheet (selective plane illumination) microscopy for mouse developmental biologists. There are different means of forming the illumination sheet, and we discuss these. We explain how we introduced the lightsheet microscope economically into our core facility and present our results on fixed and living samples. We also describe methods of clearing fixed samples for three-dimensional imaging and discuss the various means of preparing samples with particular reference to mouse cilia, adipose spheroids, and cochleae. © 2022 The Authors. Current Protocols published by Wiley Periodicals LLC.
INTRODUCTION
Developments in microscopy never stop. Fritz Zernicke, who devised phase contrast and was awarded the Nobel Prize for this work, was once famously told by a well-established microscope company that “if this had any practical value, we would ourselves have invented it long ago.” The development of the lightsheet is a remarkable story of how a 100-year-old technique was reused in the 21st century to allow both fast and gentle imaging (Scherf & Huisken, 2015). The stories of the development of phase contrast and specifically that of lightsheet microscopy are a powerful argument for funding and promoting pure “blue-sky thinking” research without the funding constraints that are currently fashionable.
The mouse remains the model organism for studying mammalian, especially human, pathology (Clark, Dinsmore, & Soriano, 2020; Phifer-Rixey & Nachman, 2015). Moreover, in vivo research is central to the functional dissection and understanding of multiorgan systems and whole organism physiology (e.g., Kikkawa et al., 2012; Li, Chen, et al., 2018). Researchers are increasingly turning to “mesoscopy” (Cardin, Crair, & Higley, 2020; Gualda et al., 2013; Perkel, 2019) to image large samples at high resolution. One option particularly suited to mouse embryos is the Mesolens (Chhetri & Keller, 2016; McConnell et al., 2016), but here, we review our implementation of the lightsheet microscope at MRC Harwell Institute.
The lightsheet microscope was devised to study developing cells (Mayr et al., 2019; Scherf & Huisken, 2015; Stock & Pauli, 2021; Zinner, Lukonin, & Liberali, 2020), mammalian embryos (de Medeiros, Balázs, & Hufnagel, 2016; Eisenstein, 2015; Follain, Mercier, Osmani, Harlepp, & Goetz, 2017), and organs including the cochlea (Brody, Hampson, Cho, Johnson, & O'Leary, 2020; Hutson, Pulver, Ariel, Naso, Fitzpatrick, 2021; Moatti et al., 2020; Voie, Burns, & Spelman, 1993). Along with technological advances in genomics and the Deep Genome Project (Lloyd et al., 2020), live-cell microscopy and in vivo research (Huang et al., 2021; Wang & Fan, 2021; Waylen, Nim, Martelotto, & Ramialison, 2020) remain central to mouse genetics, irrespective of the short-term decisions of funding bodies and our political masters.
In a conventional fluorescence widefield or confocal microscope, the objective acts as its own condenser with both the illuminating light and emitted signal travelling on-axis through the objective. The lightsheet microscope also creates optical sections, as does the confocal microscope, but with an objective forming a sheet of light set orthogonally side-on to a separate objective used for creating the image (Fig. 1; also see Box 2, page 334 in Pantazis & Supatto, 2014). The sample is passed through the lightsheet at each z-plane. It is also possible to rotate the sample in the lightsheet; this is generally done with thick samples to avoid unevenly illuminated datasets and striping artifacts.
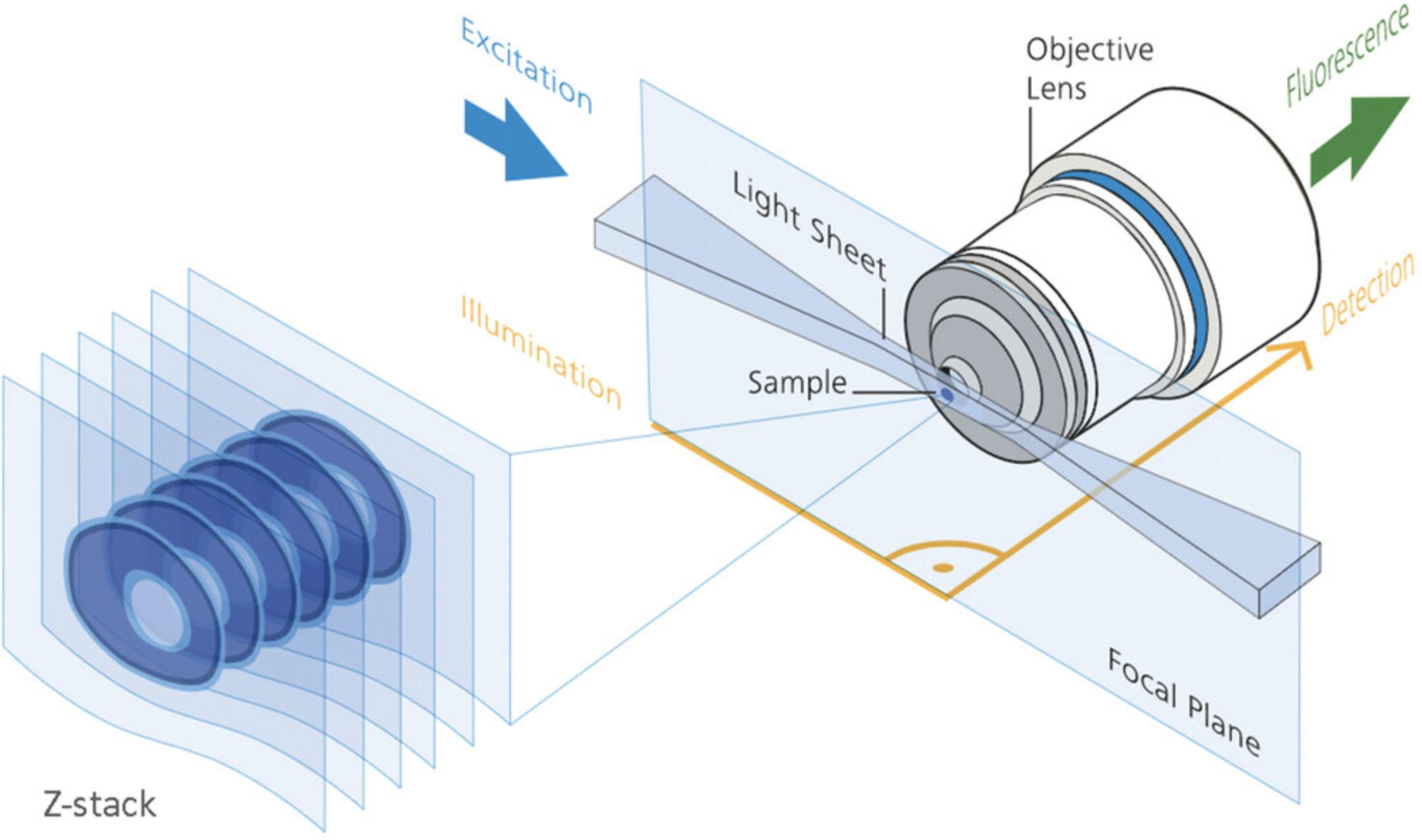
By rotating the sample, 3D tomographic datasets (Huang et al., 2021; Rawson, Maksimcuka, Withers, & Cartmell, 2020) of cells, tissues, embryos, and entire small organisms can be produced. Other forms of tomographic imaging (Fig. 1 in Follain et al., 2017; Norris et al., 2013; Singh et al., 2016; Tsien, 2003; Wang, Larina, & Larin, 2020), which include MRI (Mandino et al., 2020; Zhang, Wu, & Turnbull, 2018), micro-CT (Cole et al., 2018; Holdsworth & Thornton, 2002; Rawson et al., 2020), OCT (Burwood, Fridberger, Wang, & Nuttall, 2019; Drexler et al., 2014; Lopez, Wang, & Larina, 2020; Wu et al., 2017), OPT (Bassi, Schmid, & Huisken, 2015; Gualda, Moreno, Tomancak, & Martins, 2014; Liu et al., 2019; Sharpe, 2004; Watson et al., 2017; Wong, Dazai, Walls, Gale, & Henkelman, 2013), and SLOT (Tinne et al., 2017), were developed to image macroscopic specimens, but a large volume capability comes at the expense of limited resolving power and limited depth of tissue penetration.
Huisken and Stainier (2009) and Hutson et al. (2021) provide comparative reviews of these tomographic methods with lightsheet microscopy, but Hutson et al. conclude that confocal microscopy is still dominant for studying cochlea (e.g., MacDonald & Rubel, 2008). Nevertheless, lightsheet microscopy allows functional imaging of fluorescently labeled cells and tissues at high resolution with a relatively large field of view and minimal phototoxicity at very high frame rates (Jemielita, Taormina, Delaurier, Kimmel, & Parthasarathy, 2013; Scherf & Huisken, 2015; Stelzer, 2015). It shows huge potential for future imaging in biomedical and life science research, higher than what even confocal microscopy showed over three decades ago. As such, a decade after its introduction, light sheet fluorescence microscopy was named “Method of the Year” by Nature Methods for 2014. Eisenstein (2015) provides an excellent overview that can be read in 20 min.
BACKGROUND INFORMATION
The confocal microscope is the workhorse of fluorescence imaging (Sanderson, 2022), optically sectioning the sample to create blur-free images. Since its introduction in the mid-1980s (Amos & White, 2003), this microscope has had a profound impact on life science research, and its effectiveness is not in dispute. However, confocal imaging comes at a price; the specimen is illuminated both above and below the focal plane with the same light flux, leading to fluorophore photobleaching and sample phototoxicity (Laissue, Alghamdi, Tomancak, Reynaud, & Shroff, 2017). Nonlinear two-photon microscopy (Gopal, Kazarine, Dubach, & Wiseman, 2021; Helmchen & Denk, 2005; Lecoq, Orlova, & Grewe, 2019; Parodi et al., 2020) offers a partial advantage; the intensity of the focal spot falls off quadratically because excitation is dependent upon the square of the laser intensity. Although signal scattering is improved with longer wavelength excitation and long-term embryonic development has been achieved with two-photon microscopy (Huang et al., 2020; Karreman, Hyenne, Schwab, & Goetz, 2016), the problems of photobleaching and potential sample phototoxicity remain, as does the time-consuming method of illuminating the sample point by point.
Life is a four-dimensional (x,y,z, time) fast-paced event. Although the confocal optical sectioning microscope is perfectly good for many applications, it is not ideal for imaging delicate living samples which are, more often than not, thick entities (Li & Kilian, 2015) rather than being thin adherent cells on a coverslip, and it is too slow to cover most physiological processes. It is possible to reduce image acquisition speeds using multipoint or tandem scanners, and swept-field or line scanners, but these suffer from compromises in optical sectioning, either due to crosstalk or because the optical section capability of the line scanner is fixed. An alternative solution is to use a spatial light modulator in a programmable array microscope (PAM; Wang, Grooms, Civale, & Chung, 2021) to create computer-controlled masks for optical sectioning.
The advantage of the lightsheet microscope lies in disengaging the illuminating and imaging pathways, thus optimizing the efficiency of photon collection. The sheet illumination creates optical sectioning capability and minimizes photobleaching (Scherf & Huisken, 2015). The lightsheet was developed in 2004 by Ernst Stelzer's group at EMBL (Huisken, Swoger, Del Bene, Wittbrodt, & Stelzer, 2004) to use fluorophores efficiently and to detect as much in-focus signal as possible. A useful illustrative timeline of the development of the lightsheet is shown in Figure 1 (page 659) of Wan, McDole, and Keller (2019).
The definitive historical account is given by Mappes et al. (2012), who recreated the early orthogonal illumination colloidal microscope. Zsigmondy wanted to determine the sizes of the suspended colloidal gold particles (Siedentopf & Zsigmondy, 1903). To do so, he developed a dark-field illumination method jointly with Siedentopf, who was an employee of Carl Zeiss in Jena at the time. To reduce the scattering of light, they separated the illumination and detection light paths. This design was later refined as a slit ultramicroscope. The short working distance of high-numerical-aperture objectives meant it proved impossible to orientate one objective orthogonally to the other (still a problem at times today unless a careful selection is made); therefore, the front lenses and mounts of both objectives were cut away at a 45° angle and polished flat.
In the early 1990s, the Spelman group (Voie et al., 1993) used orthogonal-plane fluorescence optical sectioning (OPFOS; Buytaert, Descamps, Adriaens, & Dirckx, 2012; Hofman, Segenhout, & Wit, 2009) to quantitatively assess hair cell structure and cochlear function. For an account of this work and the introduction of OPFOS, see Santi (2011). However, lightsheet microscopy became widely appreciated as a new technology only a decade later, when the work of Stelzer's group was published in Science. Stelzer recalls (Abbott, 2009; Greger, Swoger, & Stelzer, 2007) being concerned about the 2D nature of the classic microscope slide preparation to study 3D tissues (e.g., Jensen & Teng, 2020; Sakalem, De Sibio, da Costa, & de Oliveira, 2021) and the inevitable photodamage associated with fluorescence microscopy. He had already experimented with confocal theta microscopy (Stelzer & Lindek, 1994; Stelzer et al., 1995) to improve the overall resolving power using two objectives to simultaneously collect a greater number of rays diffracted from the specimen. This configuration was extremely tricky to align and use, but it also improved the signal-to-noise ratio by reducing background fluorescence above and below the focal plane. The selective plane illumination microscope (SPIM) is the widefield equivalent (Greger et al., 2007) of the confocal theta microscope. As lightsheet technology has evolved and developed, several confusing acronyms have been used, as Reynaud observes (Sanderson, 2019: Table 19.1; Lemon & McDole, 2020: Abstract) for selective plane illumination microscopy. We have chosen to use “lightsheet” as the simplest generic term.
Besides studies on the cochlea and inner ear, the lightsheet microscope has also been used to record entire embryonic development processes (Andilla et al., 2017; Bardot & Hadjantonakis, 2020; Keller, 2013; Nowotschin, Garg, Piliszek, & Hadjantonakis, 2019; Sutherland, 2016) and both preimplanation (Reichmann, Eguren, Lin, Schneider, & Ellenberg, 2018; Strnad et al., 2016) and postimplantation (McDole et al., 2018; Udan, Piazza, Hsu, Hadjantonakis, & Dickinson, 2014; Wan et al., 2019) studies of mouse embryo development for which knock-in reporters are now becoming available (Gu, 2020; Li, Chen, et al., 2018). Ichikawa et al. (2014) and Candeo et al. (2016) have respectively published protocols for studying gastrulation and the mouse intestine with the lightsheet microscope. Nevertheless, the eventual size of the mouse embryo and the fact that it cannot be immobilized for lengthy periods (Xavier da Silveira Dos Santos & Liberali, 2019) is a challenge peculiar to mouse developmental biology, particularly when imaging adult mice or complete organs (McClelland & Bowles, 2016). Phillipp Keller at the Janelia Research Campus has had some success (McDole et al., 2018; Strack, 2018) in doing this by using a custom sample chamber for longitudinal embryo culturing and automated software to keep the growing embryo in focus.
Light sheet microscopy only illuminates a single Z-layer of a sample at a time. This is achieved by uncoupling the illumination and detection axes, illuminating a single plane from a 90o angle relative to the detection system. Essentially, the lightsheet microscope consists of five basic units (Greger et al., 2007):
- illuminating laser(s)
- the unit for illumination beam shaping
- an objective, filter sets, and CCD/sCMOS camera detection unit
- (stage) movement/sample mounting unit
- computer/software and controller to synchronize the operation of the above four units.
Using high-quality cameras for image acquisition and imaging the whole illuminated plane at once allow for much larger fields of view and faster imaging. Because the illumination and detection pathways are decoupled and entirely separate, the lightsheet can be formed in several ways without being constrained by the detection objective and signal collection optics. Although the theoretical lateral resolving power of the lightsheet microscope is given by the well-known formula for widefield fluorescence microscopy (0.61.λ/NA; Sanderson, 2020) and is in practice limited by the resolution of the camera chip (0.5-2.0 μm), the axial resolving power is determined by the numerical aperture (NA) of the detection objective and the thickness of the lightsheet (2-6 μm), which also sets the optical section thickness of the Z-stack.
LIGHTSHEET DESIGN
In its simplest configuration, the lightsheet is generated as a Gaussian beam (Saghafi, Becker, Hahn, & Dodt, 2014; Sheppard & Saghafi, 1996) with cylindrical optics. Extra lenses, slits, and mirrors are used to shape and steer the beam. The profile of the Gaussian beam profile has minimum thickness at its focal point, called the beam waist, ω0 (Fig. 2), where ω0 = 1.4 f λ/ 2 Dlens and ω0 ≈ λ/πNA.
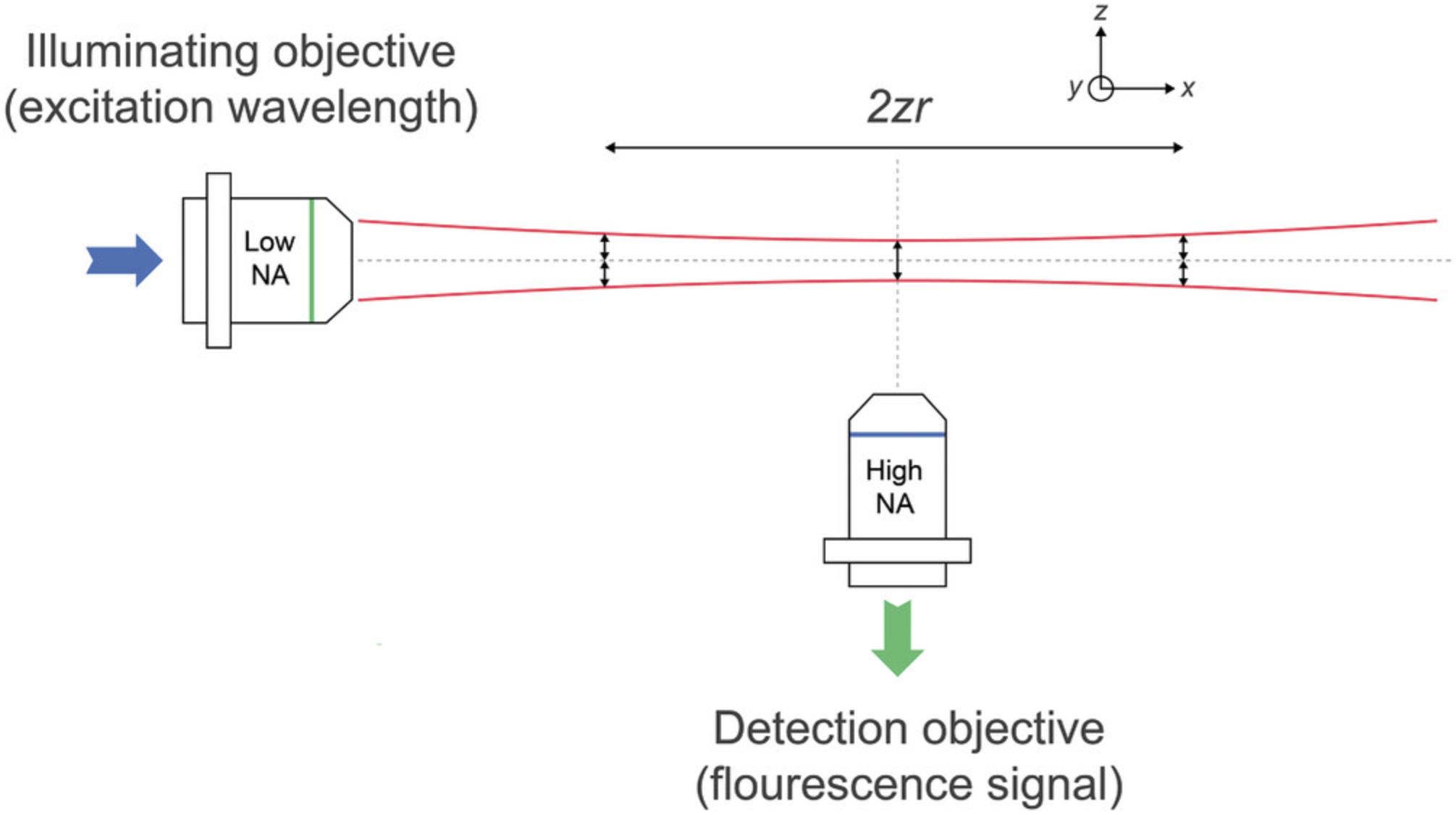
The Rayleigh length (Rayleigh range), ZR, is the distance along the propagation direction of the Gaussian lightsheet from the waist to where the area of the cross section is doubled. The confocal parameter is twice the Rayleigh length. These quantities can be expressed in terms of the numerical aperture of the illuminating objective as ZR = (λ.n) / (π. NAill2) (Daniels, 2019; Power & Huisken, 2017).
This distance where the thickness remains reasonably constant is called the “confocal parameter,” which determines the axial resolution and optical sectioning ability. The thicker the illumination sheet, the further laterally in the x-y plane will the confocal parameter be maintained. For example, a Gaussian beam with a 1-μm-thick beam waist radius has a depth of field of nearly 13 μm and a confocal parameter of 0.8 mm at a 488 nm wavelength.
The axial resolution in the final image is 2nλ/π.NAill, and it is largely determined by the thickness of the lightsheet. Therefore, for low-magnification lightsheet microscopes, the axial resolving power is superior to equivalent fluorescence microscopes, and the 3D isotropy of the PSF is much improved.
The lateral resolution is determined by the detection objective, which is 0.61.λem/NAdet, where NAdet is the NA of the detection or imaging objective. The effective resolution is also partially influenced by the spatial and temporal resolution of the camera, that is, its pixel density and frame rate.
For lightsheets created with Gaussian beams, the beam thickness and depth of field are proportional to each other and thus only extend for small fields of view. Hence, a Gaussian lightsheet with a confocal parameter of several micrometers requires a relatively thick beam waist which may, in turn, compromise axial resolution and expose the sample to unnecessary irradiation and cause light scattering (Dodt et al., 2015). Moreover, Gaussian beams are diffractive, which generates artifacts and reduces image contrast. Therefore, a Gaussian beam lightsheet is not necessarily ideal for intracellular studies that require high contrast and high axial resolution over an extended field of view spanning tens of micrometers. This is why the lightsheet has not taken over completely from the OPT for low-resolution studies of large samples (Liu et al., 2019; Nguyen et al., 2017). OPT tends to give better images of very large samples that scatter light and permits 3D fluorescence imaging to be combined with transmitted-light microscopy.
Significantly thinner lightsheets—for isotropic resolution in both x-y and z directions and improved resolving power—can be generated by two-photon microscopy (Lavagnino et al., 2016; Mahou, Vermot, Beaurepaire, & Supatto, 2014; Truong, Supatto, Koos, Choi, & Fraser, 2011), by employing a Bessel beam (Durnin, Miceli, & Eberly, 1987; Gao, Shao, Chen, & Betzig, 2014; Khonina, Kazanskiy, Karpeev, & Butt, 2020), using an Airy beam (Piksarv et al., 2017; Vettenburg et al., 2014), or using interference methods to generate a lattice sheet (Chang, Dean, & Fiolka, 2020; Planchon et al., 2011) from either of these nondiffractive beams.
Because Bessel and Airy beams are nondiffractive, the lightsheet remains at a constant thickness over a much larger distance (a field of view three to five times larger) than an equivalent Gaussian beam (Durnin, Miceli, & Eberly, 1988). Bessel beams are also “self-healing,” meaning that if the illumination beam is partially obstructed by the sample, then the beam will reform further along the lightsheet axis (Fahrbach & Rohrbach, 2012; Fahrbach, Simon, & Rohrbach, 2010). This property helps reduce light scattering and aberrations induced by imaging deep into tissues. The Airy beam is also self-healing and is both thinner and longer than equivalent Gaussian or Bessel lightsheets, but its curved beam profile with a number of areas of peak intensity and the ease of implementing Bessel beams means most lightsheets are formed from Bessel beams.
Further improvement occurred with digital scanning (Kafian, Lalenejad, Moradi-Mehr, Birgani, & Abdollahpour, 2020; Keller & Stelzer, 2008; Keller et al., 2010) using galvanomic mirrors to scan the orthogonal focal plane. Consequently, dynamic lightsheets are more homogenous than static ones. As no beam-shaping units are required, illumination efficiency is very high (>95%) and laser powers can be reduced. Although slower than SPIM, digital scanning lightsheet microscopy (DSLM) is still faster by two orders of magnitude than confocal laser scanning. In addition, because the illumination is incoherent, it is less affected by scatter than static light sheets, and imaging artifacts due to obstacles and denser regions in the sample are reduced. Bessel beams also reduce the occurrence of striping artifacts (Chen, Glaser, & Liu, 2017). However, the “side lobes” of the Bessel beam contribute to background fluorescence by reducing contrast, and these must be suppressed, possibly by forming a lattice lightsheet.
In lattice lightsheet microscopy (Chen et al., 2014), a fast-switching spatial light modulator generates the Bessel beam before it enters the illuminating objective. Scanners, similar to those used in a confocal microscope, dither the lattice structure to create a smooth lightsheet. Betzig's group (Gao et al., 2012, 2014) found that a Bessel beam combined with super-resolution SIM gives the best isotropic images, reducing the Bessel beam side lobes and improving resolution. Although the static Gaussian beam is economical, is better suited to very fast acquisition times, and is less powerful and thus less phototoxic, the lattice lightsheet method of forming an artifact-free illumination beam is likely to be the future design of choice.
Striping pattern artifacts in the image (Ding et al., 2018; Rohrbach, 2009) are caused by absorption of light at the sample surface along the lightsheet propagation path, particularly in large samples. Clearing samples will reduce—but not eliminate—shadowing and striping artifacts, and clearing cannot be used on living specimens. Several destriping algorithms have been developed (e.g., Chang, Fang, Yan, & Liu, 2013; Liang et al., 2016; Liu, Lauderdale, & Kner, 2019; Salili, Harrington, & Durian, 2018), but most algorithms are designed for lightsheets coming only from one side and are not suited for more complex noise patterns; Liang et al. (2016) developed their algorithm specifically for multidirectional lightsheet microscope configurations. These destriping methods either use digital filtering in the Fourier domain (which can potentially also remove some structural information having the same frequency), or they involve histogram matching or moment matching of pixel intensities with respect to a reference image.
The need to collect high SNR images of opaque specimens while retaining high temporal resolution and low phototoxicity has also led to the development of dual-excitation LSFM configurations. Light will always become attenuated the further it travels through a sample. As light usually penetrates the sample from one side, obstacles lying in the path of the lightsheet can disturb its quality by scattering and/or absorption. A simple solution is to rotate the sample, which is sequentially illuminated from alternate sides, and fuse the image into a single dataset (Verveer et al., 2007); however, this slows image acquisition and can potentially overexpose the center of the sample. Another solution is to add a third objective lens so that the sample can be illuminated from two sides (mSPIM; Glaser et al., 2018; Hu, Bolus, & Brown, 2017; Huisken & Stainier 2007; Wu et al., 2011) by rapidly tilting or pivoting the beam (Huisken & Stainier, 2007). This is useful when the sample cannot be rotated, and it offers isotropic resolution. For faster image acquisition, a fourth objective is used for detection (Krzic, Gunther, Saunders, Streichan, & Hufnagel, 2012; Tomer, Khairy, Amat, & Keller, 2012) to acquire images simultaneously from two opposing views. Our lightsheet design splits the laser illumination into two lightsheets orthogonal to one another at 90° in a single illumination sheet.
More recently, various lightsheet microscope configurations have been developed that either use the same objective for illumination and detection or illuminate the sample and detect the image from the same side (Fig. 3). This frees researchers from the restriction of imaging relatively small samples within a confined space, and it potentially allows the investigation of much larger specimens. These so-called “open-top” configurations are not discussed here at length because we chose to build our lightsheet around a zoom macroscope, which still allows us sufficient space to image mouse organs and embryos. For those wishing to investigate open-top lightsheet microscopes, we recommend the following references as starting points: Bishop, Glaser, and Liu (2020); Glaser et al. (2019); McGorty et al. (2015); Yordanov et al. (2021); and Strack (2021) for an overview.
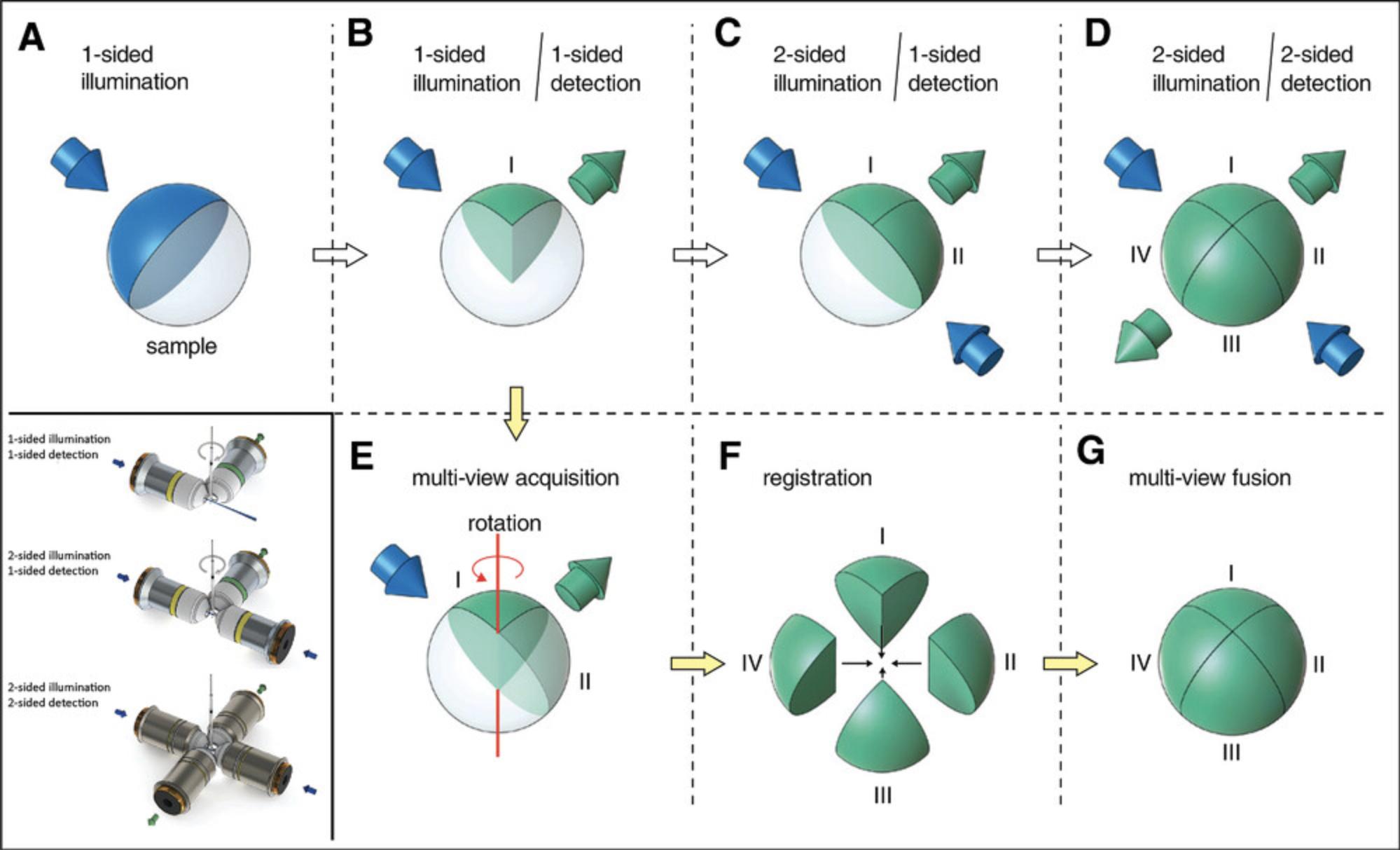
Key Points
- Lightsheet microscopy only illuminates a single Z-layer of a sample at a time. This is achieved by uncoupling the illumination and detection axes.
- Imaging the entire illuminated plane at once allows for much larger fields of view and faster imaging.
- The lateral resolution is determined by the numerical aperture of the detection objective.
- The axial resolving power is determined by the numerical aperture of the detection objective and the thickness of the lightsheet, which also sets the optical section thickness of the Z-stack.
- In its simplest form, the lightsheet is generated as a Gaussian beam with cylindrical optics.
- The distance where the thickness of the lightsheet remains reasonably constant is called the “confocal parameter,” which determines the axial resolution and optical sectioning ability. This is determined by the numerical aperture of the illuminating objective.
- Significantly thinner lightsheets can be generated using a Bessel beam or an Airy beam or generating a lattice sheet from either of these nondiffractive beams.
- Dynamic lightsheets formed by digital scanning are more homogenous than static ones.
- Striping pattern artifacts in the image are caused by absorption of light at the sample surface, along the lightsheet propagation path. Destriping algorithms exist.
- Open-top lightsheet microscope configurations allow sufficient space to image mouse organs and embryos.
SELF-BUILDING
As with any other emerging technology, the lightsheet microscope was first home-built in a few reference laboratories. As a technology matures, becomes widely adopted, and establishes itself, more people tend to buy rather than building themselves. The same trend was seen with the development of laser-scanning and multiphoton microscopy. Nowadays, approximately 1% of laser-scanning microscopes, 15% of spinning-disc microscopes, and 30% of multiphoton microscopes are home-built. In major research groups, these are home-built either because of economic constraints or the requirement for unique and radically different architectures for bespoke applications.
Zeiss Z1, the first commercially available turnkey system introduced in 2012, took six years to develop, and the price inevitably reflects development costs. As technology matures, additional sophistication and building under license means an entry-level turnkey system can cost $600,000 and above. Many research groups are left with no financially accessible method of benefitting from lightsheet microscopy. Fortunately, academics and institutions behind the development and introduction of lightsheet and SPIM have long promoted open access for all. Thus, OpenSPIM (Marx, 2016; Pitrone et al., 2013) exists to create an open-source and community-driven group for the development and improvement of open-source lightsheet microscopy.
A sophisticated wealth of material (Garbellotto & Taylor, 2018; Girstmair et al., 2016; Gualda et al., 2013; Keomanee-Dizon, Fraser, & Truong, 2020; Voigt et al., 2019), including a full parts list, costings, and construction guide is available with step-by-step instructions for building a microscope. The final cost is very much dependent upon the quality of the camera, but it is in the range of
The advantages of home-building lie in the adaptability and control of the design, which may need to be upgraded in the future. It is even possible to build a very economical miniSPIM either by 3D printing (Diederich et al., 2020; Wincott et al., 2021) or employing a smartphone as the recording device (Hedde, 2021). There is also a lively and helpful online self-build community to save you from wasting time in re-inventing the wheel. The disadvantage is that a self-build project will take considerable time; you will not have access to commercial microscope software with which you are familiar; you will have to learn your way around open-source software, such as Micro-Manager and ImageJ/Fiji. The quality of this open-source system is limited mainly by the quality of the components used and the accuracy of your construction, which may result in a lower-quality microscope than a commercial alternative. Additionally, the costs saved in terms of the materials may well be outweighed by the time taken to build not only the microscope but also to learn how to operate and manage both the hardware and associated software. We had funds for a lightsheet microscope, but only $90,000 was available over two years. Therefore, given our financial constraints, we chose to partner with Cairn Research to adapt existing microscope stands within our institution. We built the essential components onto an upright Zeiss Axioskop 2 in the first year and added extra laser lines and transferred the Gaussian lightsheet system onto a macroscopic stand (Fig. 4) in the second year. The microscope is operated by Micro-Manager (Biehlmaier, Hehl, & Csucs, 2011; Edelstein, Amodaj, Hoover, Vale, & Stuurman, 2010; Saska, Pichler, Qian, Buckley, & Lagnado, 2021) through the Zeiss Axio-Zoom V.16 macroscope interface.
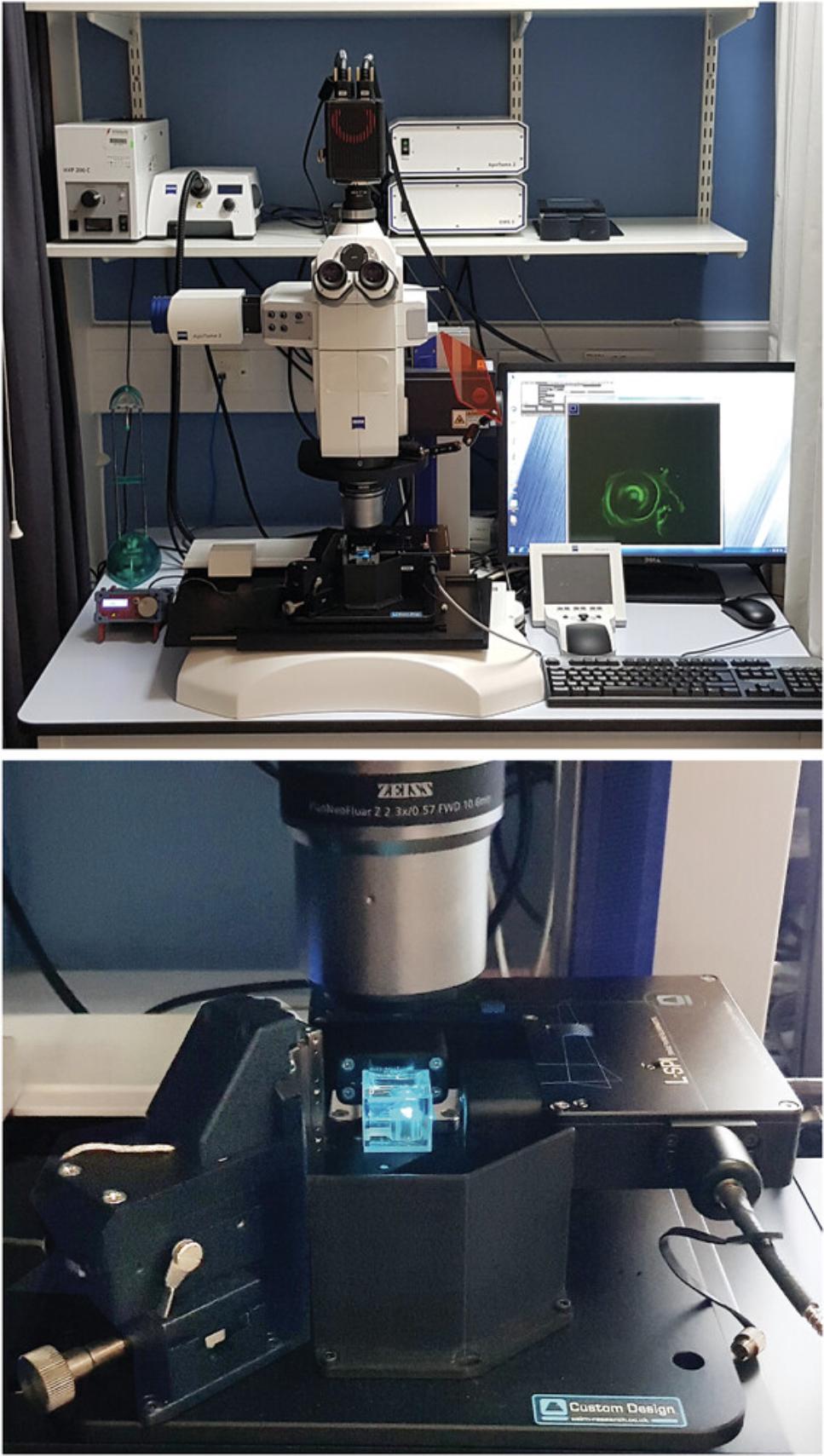
Within our budget, we have introduced a cost-effective three-color lightsheet system. It is possible to image cochleae (Moatti et al., 2020) with a custom-built microscope of a specific architecture incorporating adaptive pitch and yaw control of the illumination (Royer, Lemon, Chhetri, & Keller, 2018; Royer et al., 2016), but we chose a different configuration. This was partly because we wanted to achieve a narrower beam waist (2 μm diameter instead of ≈ 8 μm) for economic reasons and for imaging other samples.
Key Points
- Research groups home-build for economic reasons or for developing unique architectures for bespoke applications.
- OpenSPIM exists to create an open-source and community-driven group for the development and improvement of open-source lightsheet microscopy.
ARRANGEMENTS FOR HOLDING THE SAMPLE
One of the advantages of using lightsheet microscopy is the possibility of imaging whole embryos and organs, rather than preparing thin samples or growing cells mounted on a coverslip. A range of mounting methods are possible. These are not shown here, but they can easily be found in the Zeiss Lightsheet Z1 White Paper on Sample Preparation [(URL in the References) and Figure 2(c) in Reynaud, Peychl, Huisken, and Tomancak, 2015]. The classic method is to hold the sample within a molded agarose chamber (Fig. 3, Keller, Pampaloni, & Stelzer, 2006) or extrude a low-melting-point agarose cylinder into the sample chamber from a glass capillary tube (Fig. 5; also Fig. 8 in Pampaloni, 2013), or formed within Teflon™ FEP tubing (Kaufmann, Mickoleit, Weber, & Huisken, 2012) with a refractive index (RI) similar to water and extruded in the same way. Although the purpose of using the lightsheet microscope was to eliminate the ubiquitous coverslip, it is sometimes advantageous to image thin diverse samples such as cell monolayers or filamentous fungi on coverslips. These can be attached to a rod acting as a specimen holder (as shown in Fig. 2 and Fig. 3 in Pampaloni, Knuppertz, Hamann, Osiewacz, & Stelzer, 2017).
![Details are in the caption following the image Mounting samples in agarose. Agarose is commonly used to mount specimens for lightsheet microscopy. We identified the position of the sample [e.g., spheroid ball derived from stromal vascular fraction (SVF) cells of mouse white adipose tissue] under a dissection stereomicroscope, cut down the extruded agarose cylinder and mounted it onto a metal SEM stub with cyanoacrylate glue. The stub and sample can then be placed in clearing media within the glass cuvette. An image of the dual-stained adipose spheroid derived from SVF cells of mouse white adipose tissue (Hoechst 33342: nuclei; BODIPY™ 493/503: lipid droplets; cleared in 4M urea with 10% glycerol) is shown. Scale bar = 200 μm. Mounting samples in agarose – own figure.](https://static.yanyin.tech/literature_test/cpz1448-fig-0005-m.jpg)
The short working distances of both the illuminating and detection objectives mean that there is, in practice, very little space to insert or mount a sample between two regular microscope objectives. Indeed, some objectives with a high NA and short working distance may require their front faces to be ground away to make room. Despite all its proven advantages, sample mounting is the Achilles heel of the lightsheet microscope and can often be cumbersome. This is the primary reason we moved away from an upright microscope configuration, with high-NA water-dipping objectives on the Axioskop 2 stand, to the Axio-zoom V.16 macroscope with its high-quality air objectives having very long working distances. We had tried fitting a dipping cap onto the front of the air objectives using coverslips and custom-made caps from Perspex (in a similar manner to that described in Dodt et al., 2015), but this proved unsuccessful on the upright Axioskop 2 stand. The long working distance of the dedicated macroscope objectives also allows us to image larger tissues, organ slices, organoids (Kim, Koo, & Knoblich, 2020; Susaki & Takasato, 2021), and samples than what would otherwise be the case on a conventional upright microscope adapted for lightsheet microscopy.
A critical consideration when embedding samples is to ensure that the embedding matrix has the same RI as the fluid used for immersing your sample (for two overview papers on cell RI, see Gul, Ashraf, Khan, Nisar, & Ahmad, 2021 and Khan, Gul, Khan, Nisar, & Ahmad, 2021). This will prevent detrimental interference with the lightsheet when light enters the material, and the importance of this cannot be overemphasized. Classically, materials such as agarose dissolved in water are often used for specimen support. Agarose can easily be melted to a liquid and then solidified by cooling to room temperature. This approach is favored for samples such as whole organs or whole organisms (McClelland & Bowles, 2016; McClelland, Ng, & Bowles, 2016; Swoger, Pampaloni, & Stelzer, 2014). The embedding material should not be excessively heated to the point where it will damage a living specimen. Furthermore, if the concentration of agarose is too low, the supporting cylinder can fall apart when extruded from a syringe or the widely used FEP tubing. If the concentration is too high, the agarose block can either inhibit specimen growth and/or scatter both the illumination and signal beams. Although supporting samples in agarose is effective for our studies of cilia and spheroids and widely adopted for Drosophila, zebrafish and smaller early postimplantation embryos (Fig. 5; Figs. 1 and 2 in Udan et al., 2014), it is not suited for larger mouse embryos. Therefore, we used a large-walled glass cuvette, approximately 34 mm3 with 2-mm-thick glass walls (No. Z805866, Hellma OG Müllheim).
McDole et al. (2018) developed a static culture method using hollow glass capillaries filled with Matrigel. For imaging, the embryos are transferred to chambers or ultrathin Teflon™ FEP tubing (25 mm wall thickness), which helps minimize changes in the RI along the illumination light path. Laroche, Burri, Dubey, and Seitz (2019) adapted a sliding rod holder specifically for the Zeiss Lightsheet Z1, but this can easily be adapted for other lightsheet microscope designs. Two other groups (Desmaison, Lorenzo, Rouquette, Ducommun, & Lobjois, 2013; Jeandupeux, Lobjois, & Ducommun, 2015) have reported success in 3D printing of sample holders for lightsheet microscopy. Inverted lightsheet microscopes offer more freedom to mount samples. Russell and Rees (2020) present 3D CAD files to print a versatile chamber that can hold coverslips as well as small embryos and tissues. A relevant concern is the potential for cell-toxic materials to be present in 3D printing substrates; this issue has been investigated by Tepperman et al. (2020).
Key Points
- Typically, the sample is held within an extruded agarose cylinder in the sample chamber.
- There is usually very little space to insert or mount a sample between two regular microscope objectives.
- The embedding agarose must have the same RI as the fluid used for immersing your sample to prevent aberration of the lightsheet.
- For mouse embryos, we used a large-walled glass cuvette to hold the sample.
CLEARING FIXED SAMPLES
Biological tissues are, for the most part, opaque and therefore require some form of chemical clearing to visualize components several millimeters deep within the tissue itself without resorting to classical microtomy. Zebrafish are widely used as model organisms because of their transparent larval stages, but even they require clearing (Antinucci & Hindges, 2016) to view highly pigmented tissues, such as the eye. Because the lightsheet microscope enables extremely fast imaging of whole tissues and organ explants in three dimensions with minimal phototoxicity, the field of tissue clearing has grown significantly alongside the development of lightsheet/SPIM microscopy. For example, searching PubMed with the term “tissue clearing” throws up 71,000 references between 1975 and 2021, with the majority published in the 21st century.
Clearing tissues is not a new phenomenon, and it is a routine procedure in histology. The anatomist Werner Spalteholz developed the benzyl alcohol and methyl salicylate clearing solution (RI = 1.54) to render heart tissue transparent to study the cause of myocardial infarctions (Spalteholz, 1914). Together with BABB (benzyl alcohol and benzyl benzoate), methyl salicylate clears tissues and organs very effectively (Dodt et al., 2007). An intermediate ethanol-based dehydration step is required because organic solvents such as benzyl benzoate are immiscible with watery tissues. If preserving cell morphology is important, 2,2’-thiodiethanol (TDE; Staudt, Lang, Medda, Engelhardt, & Hell, 2007) may be a better choice.
Clearing methods for preserving tissue morphology are principally divided into three categories:
- solvent-based methods (for a review, see Zhan, Wu, Liu, Lin, & Zhang, 2021)
- aqueous-based methods (Tainaka et al., 2018)
- hydrogel-based methods (Choi, Guan, & Chung, 2021; Flood, Page, & Reynaud, 2016; Gradinaru, Treweek, Overton, & Deisseroth, 2018)
Some reviews (e.g., Para-Damas & Saura, 2020; Zhao, Lai, Qi, He, & Sun, 2021) include a fourth category, tissue expansion, but here (Table 1), we use the more commonly recognized solvent-based, hydrophilic aqueous-based, and hydrogel-based methods.
Solvent-based | Clearing media | Active ingredient(s) | Detergent | Lipid removal | Tissue size change | Fluorescence/antibody signal | Timescale | References |
---|---|---|---|---|---|---|---|---|
BABB | benzyl alcohol and benzyl benzoate | No | Yes | Yes, reduces | No |
hours days |
Becker et al. (2012) Foster et al. (2019) |
|
FluoClear BABB | 1-propanol or tert-butanol (dehydration) | No | Yes | Yes, reduces |
partial fluorescence immuno = no |
hours |
Schwarz et al. (2015) Stefaniuk et al. (2016) |
|
3DISCO | dibenzyl ether (DBE) | No | Yes | Yes, reduces |
partial fluorescence immuno = partial |
hours | Ertürk et al. (2012) Molbay et al. (2021) | |
iDISCO plus | Dichloromethane and DBE | Yes | Yes | Yes, reduces |
Yes; partial, poor for FPs immuno = good |
days/weeks |
Renier et al. (2016) Li, Xu, Zhu, Yu, and Zhu (2020) |
|
FDISCO plus | tetrahydrofuran and dichloromethane | No | Yes | Yes, reduces | Yes, good | days |
Wan et al. (2021) Qi et al. (2019) |
|
FOCM | DMSO, urea, and glycerol | No | No | Yes, increases | Yes, good | hours | Zhu et al. (2019) | |
Aqueous-based | ||||||||
TDE | 2,2′-thiodiethanol | No | No | None |
quenches eGFP immuno = yes |
days |
Staudt et al. (2007) Aoyagi et al. (2015) |
|
ClearT-2 | formamide (FM) and poly ethylene glycol (PEG) | No | No | None | Yes, good | days/weeks |
Kuwajima et al. (2013) Costa, Moreira, de Melo-Diogo, and Correia (2018) |
|
RTF | triethanolamineand FM | No | No | None | Yes, excellent | hours/days | Yu et al. (2018) | |
SeeDB-2 | iohexol | Yes, saponin | No | None | Yes | weeks | Ke et al. (2016) | |
FRUIT (SeeBD derived) | fructose, thioglycerol, and urea | No | No | Yes, slight increase |
partial fluorescence immuno = poor |
days | Hou et al. (2015) Hildebrand et al. (2020) | |
CUBIC | ethylenediamine, urea, and sucrose | Yes, triton X-100 | Yes | Yes, slight increase |
partial fluorescence immuno = partial |
days | Susaki et al. (2015) | |
Scale/S | urea and sorbitol | Yes, triton X-100 | Yes | None | Yes, excellent | weeks + | Hama et al. (2015) | |
FLASH | urea, boric acid, and Zwittergent 3-10 | Yes, Na dodecyl sulphate (SDS) | Yes | Yes, reduces | Yes, excellent | hours/days | Messal et al. (2021) | |
Hydrogel-based | ||||||||
SWITCH | lithium hydroxide and boric acid | Yes, SDS | Yes | Yes, slight increase |
partial fluorescence immuno = yes |
hours/days | Murray et al. (2015) | |
CLARITY | acrylamide and glycerol | Yes, SDS | Yes | Yes, slight increase | Yes, good | hours/days | Chung et al. (2013) Du, Hou, Wang, Wang, and Li (2019) | |
ACT-Presto | as for CLARITY and CUBIC | Yes, SDS | yes | Yes, slight increase | Yes, good | days | Lee et al. (2016) |
The solvent-based methods are the first clearing techniques to be widely used; these involve dehydration, lipid solvation, and matching the RI to that of the remaining tissue and can achieve high level of tissue transparency relatively quickly within several days, but they introduce the additional problems of substantial tissue shrinkage and quenching of endogenous fluorescence signals (Wang et al., 2020) due to the dehydration step. To try and preserve fluorescent markers in tissue, either the alcohol dehydration step can be replaced with tetrahydrofuran (THF) as in 3DISCO (Ertürk et al., 2012; Molbay, Kolabas, Todorov, Ohn, & Ertürk, 2021) or iDISCO (Molbay et al., 2021; Renier et al., 2014) with a rehydration step to permit immunolabeling against the fluorescent (protein) label. An alternative is to use tert-butanol (FluoClear BABB; Schwarz et al., 2015; Stefaniuk et al., 2016) instead of THF to preserve fluorescent labeling. Shrinkage may be advantageous to image whole mounts and large tissue volumes, and uDISCO (Pan et al., 2016) and modified a-uDISCO (Li, Xu, Wan, Yu, & Zhu, 2018) for better preserved fluorescent signals are useful; however, uDISCO can take a long time to clear tissues, and hence FDISCO (Qi et al., 2019) and FDISCO+ (Wan et al., 2021) are developed for superior fluorescence-preserving abilities. Becker, Jährling, Saghafi, Weiler, and Dodt (2012) found that a mixture of THF and dibenzyl ether cleared tissues well and preserved GFP labeling, whereas adjusting the pH to about 8.2 may help preserve endogenous fluorescence. Scott et al. (2018) report an ultrastable GFP for use with the clearing agent Clarity (see below), and Cai et al. (2019) used nanobodies in vDISCO to preserve fluorescence signals.
Aqueous clearing methods were developed to preserve fluorescence and prevent tissue shrinkage. Most soft tissues contain 10% lipids, so clearing is achieved by one of the following methods:
- passive immersion in a matching RI solution
- lipid removal followed by hyperhydration to lower the RI of the tissue
- lipid removal followed by immersion in a matching RI solution
An RI greater than 1.45 is generally required to achieve clearing of hydrated lipid-containing samples. As the RI of most aqueous solutions cannot be increased above 1.48, an alternative stratagem is to reduce the RI of the tissue through a process known as hyperhydration, either with detergents (Scale, Hama et al., 2011, 2015; CUBIC, Susaki et al., 2015) or without them (ClearT, Kuwajima et al., 2013; FRUIT, Hou et al., 2015). Detergents will denature and remove proteins from tissues, and urea-containing clearing solutions can denature epitopes. Permeabilization is essential for promoting the diffusion of high-RI media and antibodies into a 3D tissue. The commonly used permeabilizing reagents include solvents, hyperhydration reagents, and delipidation reagents (Richardson & Litchman, 2017). Some high-RI matching clearing solutions (See DB, Ke, & Imai, 2014) use high concentrations of sugars, but these are viscous and take a long time to penetrate tissues. A low viscosity alternative, RIMS, has been proposed by Yang et al. (2014), but TDE (Aoyagi, Kawakami, Osanai, Hibi, & Nemoto, 2015; Staudt et al., 2007) and the FRUIT clearing media (Hou et al., 2015) are better alternatives. TDE is useful in that its concentration can be adjusted to match the RI of tissues and minimize spherical aberration.
To preserve immunostaining of tissues, proteins can be crosslinked in hydrogels to prevent their extraction by detergents and subsequent loss. CLARITY was introduced as a clearing method specifically for lipid extraction, both with electrophoresis (Chung & Deisseroth, 2013; Tomer, Ye, Hsueh, & Deisseroth, 2014; Zach et al., 2019) and without it (Poguzhelskaya, Artamonov, Bolshakova, Vlasova, & Bezprozvanny, 2014; Yang et al., 2014) with minimal tissue swelling. The hydrogel (Flood et al., 2016) is used to fix tissue proteins in their correct morphological location, and subsequent lipid removal may be accelerated by electrophoresis. Some investigators (Orlich & Kiefer, 2018), including us, have found the electrophoresis step to be inconsistent.
Therefore, CUBIC (Matsumoto et al., 2019; Susaki et al., 2015)—a modification of Scale—was developed to overcome the technical challenge of using electrophoresis devices for CLARITY, and as a passive hyperhydrating clearing solution to allow for scaling up the clearing of multiple tissues (PACT; Yang et al., 2014). This clearing agent has been improved from the original as CUBIC-L, CUBIC-R+, and CUBIC-X (Tainaka et al., 2018). We have chosen to use CUBIC and FluoClear BABB as the clearing agents in this study, preferring FluoClear BABB to CUBIC because of the nontoxicity and much reduced viscosity of the former clearing agent. The high triton concentration in CUBIC may reduce epitopes and weaken immunostaining, and this needs to be monitored.
Mixtures of iDISCO and CUBIC have successfully been used (McKey, Cameron, Lewis, Batchvarov, & Capel, 2020) to clear and improve antibody penetration in opaque immunostained ovary tissues that generally present challenges when clearing owing to the medullary connective tissue. Yu et al. (2018) combined ClearT and CUBIC to develop RTF (a derivation of ClearT2) to give fast clearing that preserved fluorescent signals, particularly in lipophilic dyes.
Most biological tissues have an intrinsic milky appearance; this translucence is caused by the scattering of light. The extent to which tissues scatter light is dependent upon several factors, the principal one being the heterogeneity and inhomogeneity in the amount of scattering between different regions of the tissues and their components. The second major factor is the RI mismatch between tissue components and the extracellular mounting media. In essence, cytoplasm has low RI and organelles have high RI. Although a little dated, it is recommended you read the excellent review by Richardson and Lichtman (2015) to understand how tissues scatter light. For example, a mounting medium may either be watery (RI = 1.33) or a solvent-based medium with an RI close to that of glass (RI range = 1.5-1.7); however, the RI of nuclei is 1.39, and that of cytoplasmic organelles is between 1.35 and 1.37. Some high-density protein and lipid components, which effectively scatter light, may have RIs between 1.39 and 1.47, much higher than the RI of water, which occupies 70%-80% of the tissue volume.
The effect of RI mismatch becomes apparent when trying to image through thick tissues, even when they have been cleared (e.g., Dodt et al., 2015). At a depth of just 1.5 mm, the lateral resolving power deteriorates twofold and the axial resolving power by fourfold when using a water-immersion objective to view samples cleared with a solution of RI = 1.49 (Hell, Reiner, Cremer, & Stelzer, 1993; Marx, 2014). Other factors that determine the scattering of light by tissues are collagen disassociation, delipidation, decalcification, dehydration, and autofluorescence arising from inherently fluorescent molecules present in the tissue, or introduced during processing, giving rise to autofluorescence that can mask the fluorescent label of interest. The key to acquiring good images on the lightsheet microscope is effective clearing.
The choice of the clearing method depends upon a number of factors, based on the tissue: tissue thickness and required imaging depth, labeling, whether immuno-staining is employed, antibody or fluorescent protein robustness, and the resources available. An earlier paper (Muntifering et al., 2018) gives protocols for using CUBIC, CLARITY, 3DISCO, and SeeDB. Dense, heavily pigmented tissues such as the liver are particularly challenging to clear, but Hough et al. (2021) report success with a modified urea-amino sugar (UbasM; Chen, Li, et al., 2017) protocol. Various clearing methods either cause expansion (CLARITY, CUBIC, ScaleS) or shrinkage (See DB). An ultrafast clearing method is FOCM (Zhu et al., 2019), a DMSO/urea-based reagent, which only causes 2% expansion without the tissue fragility and risk of fragmentation caused by clearing with urea-based Scale. A very promising technique, FLASH (fast light-microscopic analysis of antibody-stained whole organs) for successfully immunostaining and clearing whole organs quickly has been published by Messal et al. (2021). FLASH is tolerant of a wide range of fixatives and antibodies and does not use hydrogel crosslinking. The complete protocol from tissue collection to imaging can take as little as 4-7 days, compared to ScaleS and iDISCO, which can take between two and four times as long.
As with methods of fixation, different tissues are suited to different means of clearing. Likewise, some tissue moieties are better labeled with antibodies and others by endogenous fluorescent reporters. Several reviews of clearing methods have been published. Richardson and Lichtman (2015) is cited above; a recent mechanistic overview is given by Yu, Zhu, Li, and Zhu (2021). A very recent summary that should be read in conjunction with Richardson and Lichtman is Eisenstein (2021). Seo et al. (2016); Matryba, Kaezmarek, and Golab (2019); Ueda, Dodt, et al. (2020); Gomez-Gaviro et al. (2020); and Xu, Ma, Yu, and Zhu (2019) are also recommended as the other overviews of clearing techniques, whereas Ueda, Ertürk, et al. (2020) and Para-Damas, and Saura (2020) are reviews for neuroscientists. A very good recent practical review tutorial has been published by Weiss, Voigt, Shepherd, and Huisken (2021) and contains good summary tables of protocols and considerations for using organic solvents, aqueous clearing solutions, or hydrogel-embedding protocols. We recommend you read this prior to planning an experiment using lightsheet microscopy.
Key Points
- Biological tissues are opaque and thus require some form of chemical clearing to see and resolve internal cellular details.
- Clearing methods are either solvent-based, aqueous-based, or hydrogel-based ones.
- The solvent-based methods are rapid and can achieve a high level of tissue transparency, but they may shrink tissues and quench fluorescence signals.
- Aqueous clearing methods preserve fluorescence and prevent tissue shrinkage. Either lipids are removed, or tissues are immersed in a matching RI solution.
- Permeabilization is essential for promoting the diffusion of high-RI media and antibodies into a 3D tissue. The detergent concentration needs to be carefully balanced to preserve immunoreactivity.
- Proteins can be crosslinked in hydrogels to prevent their extraction and loss of immunoreactivity. Different clearing media can be combined to exploit the advantages of each.
- RI mismatch limits imaging through thick tissues, even when cleared.
DATA HANDLING
Lightsheet microscopy, generating 4D datasets over large time frames, creates a huge amount of data, up to 10 TB per hour (Chhetri et al., 2015). These large datasets require significant storage space, which attracts further costs for the user. A dedicated server infrastructure may be necessary if data output is sufficiently high.
Moreover, to be useful, the dataset must be processed once imaging is completed. Even a high-quality computer will struggle to handle the colossal datasets, so virtual servers may be necessary. Additional problems involve the bandwidth of data that can be transmitted from the device to the storage system. If not high enough, a bottleneck could form during data transfer, which could abruptly end an imaging run early. The simplest answer is to avoid acquiring new data until the data at hand have been processed. Deleting data once used may also be a solution, although it may be a legal requirement to keep raw data for several years. Compression algorithms allow 3D projections and Z-stacks to be transformed into 2D cartographic projections, and specialist companies, such as Acquifer, help life scientists manage their data (Fig. 6, Video 1, and Fig. 7).
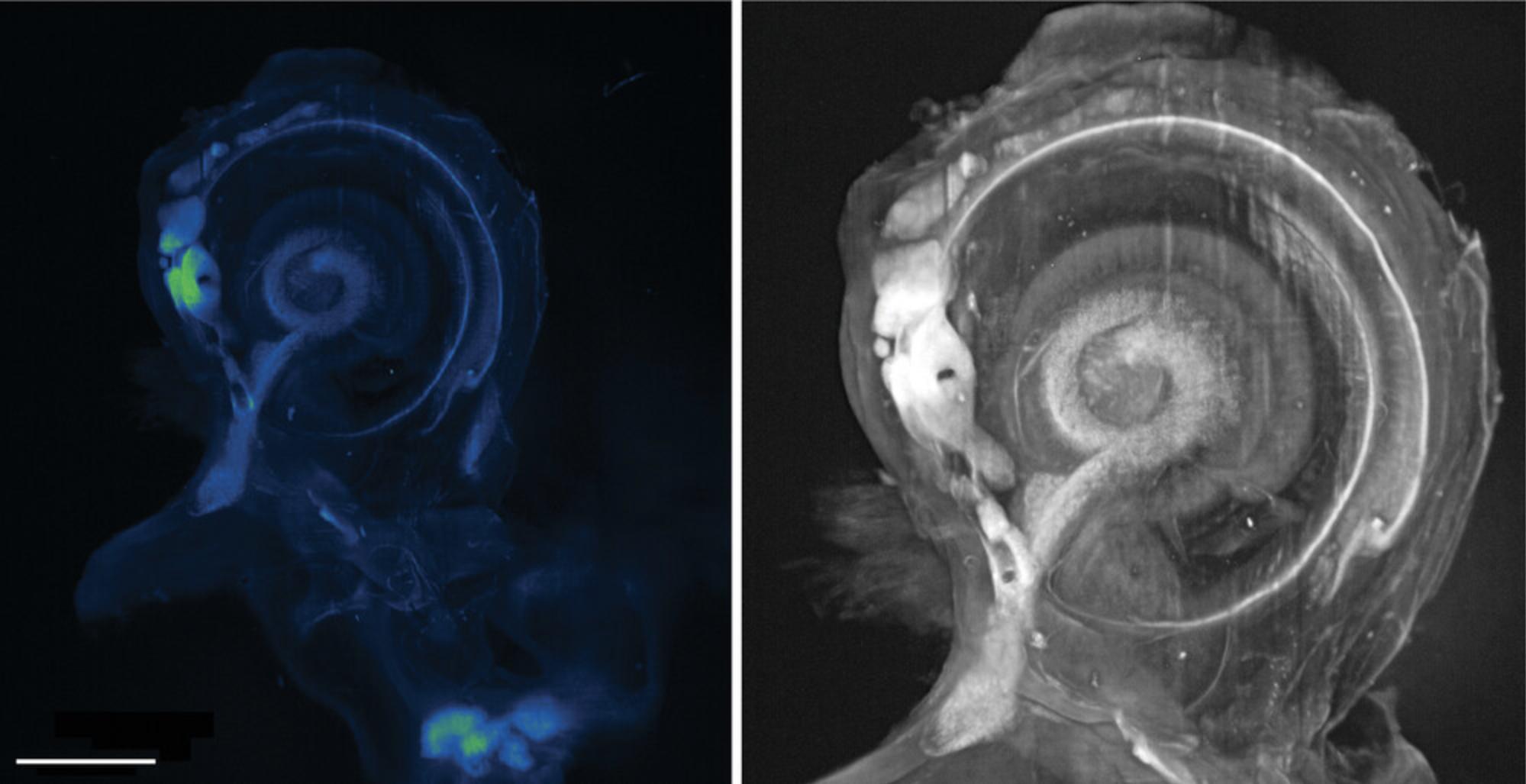
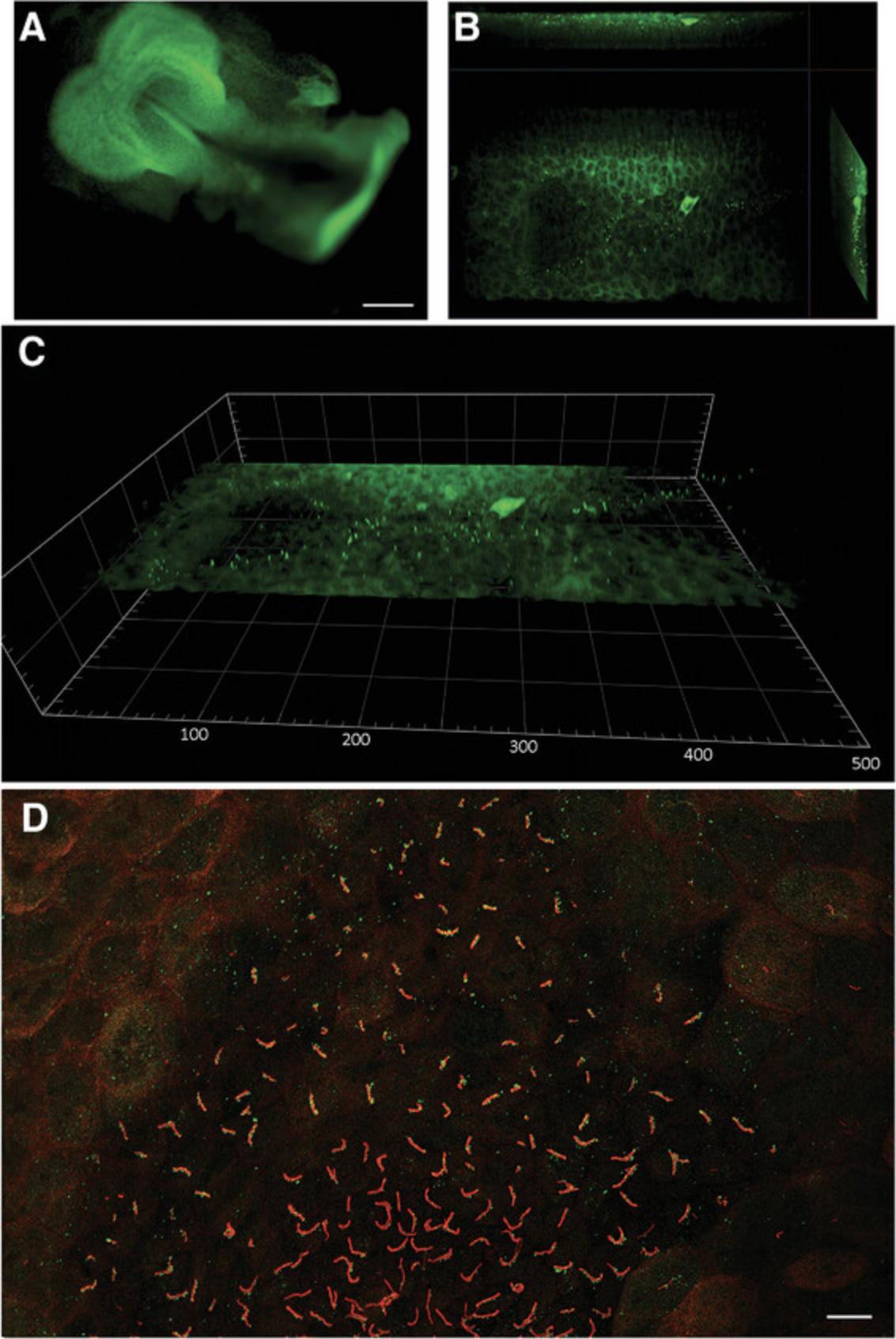
Open Research
DATA AVAILABILITY STATEMENT
The authors choose to not share data.
LITERATURE CITED
- Abbott, A. (2009). Microscopic marvels: Seeing the system. Nature , 459(7247), 630–631. doi: 10.1038/459630a
- Amos, W. B., & White, J. G. (2003). How the confocal laser scanning microscope entered biological research. Biology of the Cell , 95, 335–342. doi: 10.1016/s0248-4900(03)00078-9
- Andilla, J., Jorand, R., Olarte, O. E., Dufour, A. C., Cazales, M., Montagner, Y. L., … Lorenzo, C. (2017). Imaging tissue-mimic with light sheet microscopy: A comparative guideline. Science Reports , 7, 44939. doi: 10.1038/srep44939
- Antinucci, P., & Hindges, R. (2016). A crystal-clear zebrafish for in vivo imaging. Science Reports , 6, 29490. doi: 10.1038/srep29490
- Aoyagi, Y., Kawakami, R., Osanai, H., Hibi, T., & Nemoto, T. (2015). A rapid optical clearing protocol using 2,2'-thiodiethanol for microscopic observation of fixed mouse brain. PLoS ONE , 10(1), e0116280. doi: 10.1371/journal.pone.0116280
- Bardot, E. S., & Hadjantonakis, A. K. (2020). Mouse gastrulation: Coordination of tissue patterning, specification and diversification of cell fate. Mechanisms of Development , 163, 103617. doi: 10.1016/j.mod.2020.103617
- Bassi, A., Schmid, B., & Huisken, J. (2015). Optical tomography complements light sheet microscopy for in toto imaging of zebrafish development. Development , 142(5), 1016–1020. doi: 10.1242/dev.116970
- Becker, K., Jährling, N., Saghafi, S., Weiler, R., & Dodt, H. U. (2012). Chemical clearing and dehydration of GFP expressing mouse brains. PLoS ONE , 7(3), e33916. doi: 10.1371/journal.pone.0033916
- Biehlmaier, O., Hehl, J., & Csucs, G. (2011). Acquisition speed comparison of microscope software programs. Microscopy Research and Technique , 74(6), 539–545. doi: 10.1002/jemt.20944
- Bishop, K. W., Glaser, A. K., & Liu, J. T. C. (2020). Performance tradeoffs for single- and dual-objective open-top light-sheet microscope designs: A simulation-based analysis. Biomedical Optics Express , 11(8), 4627–4650. doi: 10.1364/BOE.397052
- Brody, K. M., Hampson, A. J., Cho, H. J., Johnson, P., & O'Leary, S. J. (2020). A new method for three-dimensional immunofluorescence study of the cochlea. Hearing Research , 392, 107956. doi: 10.1016/j.heares.2020.107956
- Burwood, G. W. S., Fridberger, A., Wang, R. K., & Nuttall, A. L. (2019). Revealing the morphology and function of the cochlea and middle ear with optical coherence tomography. Quantitative Imaging in Medicine and Surgery , 9(5), 858–881. doi: 10.21037/qims.2019.05.10
- Buytaert, J. A., Descamps, E., Adriaens, D., & Dirckx, J. J. (2012). The OPFOS microscopy family: High-resolution optical sectioning of biomedical specimens. Anatomy Research International , 2012, 206238. doi: 10.1155/2012/206238
- Cai, R., Pan, C., Ghasemigharagoz, A., Todorov, M. I., Förstera, B., Zhao, S., … Ertürk, A. (2019). Panoptic imaging of transparent mice reveals whole-body neuronal projections and skull-meninges connections. Nature Neuroscience , 22(2), 317–327. doi: 10.1038/s41593-018-0301-3
- Candeo, A., Sana, I., Ferrari, E., Maiuri, L., D'Andrea, C., Valentini, G., & Bassi, A. (2016). Virtual unfolding of light sheet fluorescence microscopy dataset for quantitative analysis of the mouse intestine. Journal of Biomedial Optics , 21(5), 56001. doi: 10.1117/1.JBO.21.5.056001
- Cardin, J. A., Crair, M. C., & Higley, M. Y. (2020). Mesocopic imaging: Shining a wide light on large-scale neural dynamics. Neuron , 108(1), 33–43. doi: 10.1016/j.neuron.2020.09.031
- Chang, B-J., Dean, K. M., & Fiolka, R. (2020). Systematic and quantitative comparison of lattice and Gaussian light-sheets. Optics Express , 28(18), 27052–27077. doi: 10.1364/OE.400164
- Chang, Y., Fang, H., Yan, L., & Liu, H. (2013). Robust destriping method with unidirectional total variation and framelet regularization. Optics Express , 21(20), 23307–23323. doi: 10.1364/OE.21.023307
- Chen, B. C., Legant, W. R., Wang, K., Shao, L., Milkie, D. E., Davidson, M. W., … Betzig, E. (2014). Lattice light-sheet microscopy: Imaging molecules to embryos at high spatiotemporal resolution. Science , 346(6208), 1257998. doi: 10.1126/science.1257998
- Chen, L., Li, G., Li, Y., Li, Y., Zhu, H., Tang, L., … Ruan, S. (2017). UbasM: An effective balanced optical clearing method for intact biomedical imaging. Science Reports , 7(1), 12218. doi: 10.1038/s41598-017-12484-3
- Chen, Y., Glaser, A., & Liu, J. T. (2017). Bessel-beam illumination in dual-axis confocal microscopy mitigates resolution degradation caused by refractive heterogeneities. Journal of Biophotonics , 10(1), 68–74. doi: 10.1002/jbio.201600196
- Chhetri, R. K., Amat, F., Wan, Y., Höckendorf, B., Lemon, W. C., & Keller, P. J. (2015). Whole-animal functional and developmental imaging with isotropic spatial resolution. Nature Methods , 12(12), 1171–1178. doi: 10.1038/nmeth.3632
- Chhetri, R. K., & Keller, P. J. (2016). Imaging far and wide. Elife , 5, e21072. doi: 10.7554/eLife.21072
- Choi, S. W., Guan, W., & Chung, K. (2021). Basic principles of hydrogel-based tissue transformation technologies and their applications. Cell , 184(16), 4115–4136. doi: 10.1016/j.cell.2021.07.009
- Chung, K., & Deisseroth, K. (2013). CLARITY for mapping the nervous system. Nature Methods , 10(6), 508–513. doi: 10.1038/nmeth.2481. Erratum in: Nature Methods , 2013 Oct,10(10):1035.
- Clark, J. F., Dinsmore, C. J., & Soriano, P. (2020). A most formidable arsenal: Genetic technologies for building a better mouse. Genes & Development, 34(19-20), 1256–1286. doi: 10.1101/gad.342089.120
- Cole, J. M., Symes, D. R., Lopes, N. C., Wood, J. C., Poder, K., Alatabi, S., … Najmudin, Z. (2018). High-resolution μCT of a mouse embryo using a compact laser-driven x-ray betatron source. Proceedings of the National Academy of Sciences of the United States of America , 115(25), 6335–6340. doi: 10.1073/pnas.1802314115
- Costa, E. C., Moreira, A. F., de Melo-Diogo, D., & Correia, I. J. (2018). Polyethylene glycol molecular weight influences the ClearT2 optical clearing method for spheroids imaging by confocal laser scanning microscopy. Journal of Biomedial Optics , 23(5), 1–11. doi: 10.1117/1.JBO.23.5.055003
- de Medeiros, G., Balázs, B., & Hufnagel, L. (2016). Light-sheet imaging of mammalian development. Seminars in Cell & Developmental Biology, 55, 148–155. doi: 10.1016/j.semcdb.2015.11.001
- Desmaison, A., Lorenzo, C., Rouquette, J., Ducommun, B., & Lobjois, V. (2013). A versatile sample holder for single plane illumination microscopy. Journal of Microscopy , 251(2), 128–132. doi: 10.1111/jmi.12051
- Diederich, B., Lachmann, R., Carlstedt, S., Marsikova, B., Wang, H., Uwurukundo, X., … Heintzmann, R. (2020). A versatile and customizable low-cost 3D-printed open standard for microscopic imaging. Nature Communication , 11(1), 5979. doi: 10.1038/s41467-020-19447-9
- Ding, Y., Bailey, Z., Messerschmidt, V., Nie, J., Bryant, R., Rugonyi, S., … Hsiai, T. K. (2018). Light-sheet fluorescence microscopy for the study of the murine heart. Journal of Visualized Experiments: JoVE , 139, 57769. doi: 10.3791/57769
- Dodt, H. U., Leischner, U., Schierloh, A., Jährling, N., Mauch, C. P., Deininger, K., … Becker, K. (2007). Ultramicroscopy: Three-dimensional visualization of neuronal networks in the whole mouse brain. Nature Methods , 4(4), 331–336. doi: 10.1038/nmeth1036
- Dodt, H. U., Saghafi, S., Becker, K., Jährling, N., Hahn, C., Pende, M., … Niendorf, A. (2015). Ultramicroscopy: Development and outlook. Neurophotonics , 2(4), 041407. doi: 10.1117/1.NPh.2.4.041407
- Drexler, W., Liu, M., Kumar, A., Kamali, T., Unterhuber, A., & Leitgeb, R. A. (2014). Optical coherence tomography today: Speed, contrast, and multimodality. Journal of Biomedical Optics , 19(7), 071412. doi: 10.1117/1.JBO.19.7.071412
- Du, H., Hou, P., Wang, L., Wang, Z., & Li, Q. (2019). Modified CLARITY achieving faster and better intact mouse brain clearing and immunostaining. Scientific Reports , 9(1), 10571. doi: 10.1038/s41598-019-46814-4
- Durnin, J., Miceli, J. Jr, & Eberly, J. H. (1987). Diffraction-free beams. Physical Review Letters , 58(15), 1499–1501. doi: 10.1103/PhysRevLett.58
- Durnin, J., Miceli, J. J., & Eberly, J. H. (1988). Comparison of Bessel and Gaussian beams. Optics Letters , 13(2), 79–80. doi: 10.1364/ol.13.000079
- Edelstein, A., Amodaj, N., Hoover, K., Vale, R., & Stuurman, N. (2010). Computer control of microscopes using μManager. Current Protocols in Molecular Biology , 92, 14.20.1–14.20.17. doi: 10.1002/0471142727.mb1420s92
- Eisenstein, M. (2015). Pump up the volume. Nature Methods , 12(1), 19–22. doi: 10.1038/nmeth.3220
- Eisenstein, M. (2021). Clarifying a crowded field of techniques. Lab Animal , 50(7), 159–161. doi: 10.1038/s41684-021-00796-x
- Ertürk, A., Becker, K., Jährling, N., Mauch, C. P., Hojer, C. D., Egen, J. G., … Dodt, H. U. (2012). Three-dimensional imaging of solvent-cleared organs using 3DISCO. Nature Protocols , 7(11), 1983–1995. doi: 10.1038/nprot.2012.119
- Fahrbach, F. O., & Rohrbach, A. (2012). Propagation stability of self-reconstructing Bessel beams enables contrast-enhanced imaging in thick media. Nature Communication , 3, 632. doi: 10.1038/ncomms1646
- Fahrbach, F. O., Simon, P., & Rohrbach, A. (2010). Microscopy with self-reconstructing beams. Nature Photon , 4, 780–785. doi: 10.1038/nphoton.2010.204
- Flood, P., Page, H., & Reynaud, E. G. (2016). Using hydrogels in microscopy: A tutorial. Micron , 84, 7–16. doi: 10.1016/j.micron.2016.02.002
- Follain, G., Mercier, L., Osmani, N., Harlepp, S., & Goetz, J. G. (2017). Seeing is believing - multi-scale spatio-temporal imaging towards in vivo cell biology. Journal of Cell Science , 130(1), 23–38. doi: 10.1242/jcs.189001
- Foster, D. S., Nguyen, A. T., Chinta, M., Salhotra, A., Jones, R. E., Mascharak, S., … Longaker, M. T. (2019). A clearing technique to enhance endogenous fluorophores in skin and soft tissue. Science Reports , 9(1), 15791. doi: 10.1038/s41598-019-50359-x
- Gao, L., Shao, L., Chen, B. C., & Betzig, E. (2014). 3D live fluorescence imaging of cellular dynamics using Bessel beam plane illumination microscopy. Nature Protocols , 9(5), 1083–1101. doi: 10.1038/nprot.2014.087
- Gao, L., Shao, L., Higgins, C. D., Poulton, J. S., Peifer, M., Davidson, M. W., … Betzig, E. (2012). Noninvasive imaging beyond the diffraction limit of 3D dynamics in thickly fluorescent specimens. Cell , 151(6), 1370–1385. doi: 10.1016/j.cell.2012.10.008
- Garbellotto, C., & Taylor, J. M. (2018). Multi-purpose SLM-light-sheet microscope. Biomedical Optics Express , 9(11), 5419–5436. doi: 10.1364/BOE.9.005419
- Girstmair, J., Zakrzewski, A., Lapraz, F., Handberg-Thorsager, M., Tomancak, P., Pitrone, P. G., … Telford, M. J. (2016). Light-sheet microscopy for everyone? Experience of building an OpenSPIM to study flatworm development. BMC Developmental Biology , 16(1), 22. doi: 10.1186/s12861-016-0122-0
- Glaser, A. K., Chen, Y., Yin, C., Wei, L., Barner, L. A., Reder, N. P., & Liu, J. T. C. (2018). Multidirectional digital scanned light-sheet microscopy enables uniform fluorescence excitation and contrast-enhanced imaging. Scientific Reports , 8(1), 13878. doi: 10.1038/s41598-018-32367-5
- Glaser, A. K., Reder, N. P., Chen, Y., Yin, C., Wei, L., Kang, S., … Liu, J. T. C. (2019). Multi-immersion open-top light-sheet microscope for high-throughput imaging of cleared tissues. Nature Communication , 10(1), 2781. doi: 10.1038/s41467-019-10534-0
- Gómez-Gaviro, M. V., Sanderson, D., Ripoll, J., & Desco, M. (2020). Biomedical applications of tissue clearing and three-dimensional imaging in health and disease. iScience , 23(8):101432.
- Gopal, A. A., Kazarine, A., Dubach, J. M., & Wiseman, P. W. (2021). Recent advances in nonlinear microscopy: Deep insights and polarized revelations. International Journal of Biochemistry & Cell Biology, 130(1), 105896. doi: 10.1016/j.biocel.2020.105896
- Gradinaru, V., Treweek, J., Overton, K., & Deisseroth, K. (2018). Hydrogel-tissue chemistry: Principles and applications. Annual Review of Biophysics , 47, 355–376. doi: 10.1146/annurev-biophys-070317-032905
- Greger, K., Swoger, J., & Stelzer, E. H. (2007). Basic building units and properties of a fluorescence single plane illumination microscope. Review of Scientific Instruments , 78(2), 023705. doi: 10.1063/1.2428277
- Gu, B. (2020). Light up the embryos: Knock-in reporter generation for mouse developmental biology. Animal Reproduction , 17(3), e20200055. doi: 10.1590/1984-3143-AR2020-0055. Erratum in: Animal Reproduction , 2020 Sep 17,17(3):e20200078.
- Gualda, E., Moreno, N., Tomancak, P., & Martins, G. G. (2014). Going "open" with mesoscopy: A new dimension on multi-view imaging. Protoplasma , 251(2), 363–372. doi: 10.1007/s00709-013-0599-3
- Gualda, E. J., Vale, T., Almada, P., Feijó, J. A., Martins, G. G., & Moreno, N. (2013). OpenSpin Microscopy: An open-source integrated microscopy platform. Nature Methods , 10(7), 599–600. doi: 10.1038/nmeth.2508
- Gul, B., Ashraf, S., Khan, S., Nisar, H., & Ahmad, I. (2021). Cell refractive index: Models, insights, applications and future perspectives. Photodiagnosis and Photodynamic Therapy , 33, 102096. doi: 10.1016/j.pdpdt.2020.102096
- Hama, H., Hioki, H., Namiki, K., Hoshida, T., Kurokawa, H., Ishidate, F., … Miyawaki, A. (2015). ScaleS: An optical clearing palette for biological imaging. Nature Neuroscience , 18(10), 1518–1529. doi: 10.1038/nn.4107
- Hama, H., Kurokawa, H., Kawano, H., Ando, R., Shimogori, T., Noda, H., … Miyawaki, A. (2011). Scale: A chemical approach for fluorescence imaging and reconstruction of transparent mouse brain. Nature Neuroscience , 14(11), 1481–1488. doi: 10.1038/nn.2928
- Hedde, P. N. (2021). miniSPIM - A miniaturized light-sheet microscope. ACS Sensors , 6(7), 2654–2663. doi: 10.1021/acssensors.1c00607
- Hell, S., Reiner, G., Cremer, C., & Stelzer, E. H. K. (1993). Aberrations in confocal fluorescence microscopy induced by mismatches in refractive index. Journal of Microscopy , 169(3), 391–405. doi: 10.1111/j.1365-2818.1993.tb03315.x
- Helmchen, F., & Denk, W. (2005). Deep tissue two-photon microscopy. Nature Methods , 2(12), 932–940. doi: 10.1038/nmeth818. Erratum in: Nature Methods , 2006 Mar,3(3):235.
- Hildebrand, S., Schueth, A., Wangenheim, K. V., Mattheyer, C., Pampaloni, F., Bratzke, H., … Galuske, R. A. W. (2020). hFRUIT: An optimized agent for optical clearing of DiI-stained adult human brain tissue. Scientific Reports , 10(1), 9950. doi: 10.1038/s41598-020-66999-3
- Hofman, R., Segenhout, J. M., & Wit, H. P. (2009). Three-dimensional reconstruction of the guinea pig inner ear, comparison of OPFOS and light microscopy, applications of 3D reconstruction. Journal of Microscopy , 233(2), 251–257. doi: 10.1111/j.1365-2818.2009.03115.x
- Holdsworth, D. W., & Thornton, M. M. (2002). Micro-CT in small animal and specimen imaging. Trends Biotech , 20/8, S34–S39. doi: 10.1016/S0167-7799(02)02004-8
- Hörl, D., Rojas Rusak, F., Preusser, F., Tillberg, P., Randel, N., Chhetri, R. K., … Preibisch, S. (2019). BigStitcher: Reconstructing high-resolution image datasets of cleared and expanded samples. Nature Methods , 16(9), 870–874. doi: 10.1038/s41592-019-0501-0
- Hou, B., Zhang, D., Zhao, S., Wei, M., Yang, Z., Wang, S., … Jiang, T. (2015). Scalable and DiI-compatible optical clearance of the mammalian brain. Frontiers in Neuroanatomy , 9, 19. doi: 10.3389/fnana.2015.00019
- Hough, M., Fenlon, M., Glazier, A., Short, C., Fernandez, G. E., Xu, J., … Wang, K. S. (2021). Urea-based amino sugar agent clears murine liver and preserves protein fluorescence and lipophilic dyes. Biotechniques , 70(2), 72–80. doi: 10.2144/btn-2020-0063
- Hu, B., Bolus, D., & Brown, J. Q. (2017). Improved contrast in inverted selective plane illumination microscopy of thick tissues using confocal detection and structured illumination. Biomedical Optics Express , 8(12), 5546–5559. doi: 10.1364/BOE.8.005546
- Huang, Q., Cohen, M. A., Alsina, F. C., Devlin, G., Garrett, A., McKey, J., … Shen, X. (2020). Intravital imaging of mouse embryos. Science , 368(6487), 181–186. doi: 10.1126/science.aba0210
- Huang, Q., Garrett, A., Bose, S., Blocker, S., Rios, A. C., Clevers, H., & Shen, X. (2021). The frontier of live tissue imaging across space and time. Cell Stem Cell , 28(4), 603–622. doi: 10.1016/j.stem.2021.02.010
- Huisken, J., & Stainier, D. Y. (2007). Even fluorescence excitation by multidirectional selective plane illumination microscopy (mSPIM). Optics Letters , 32(17), 2608–2610.doi: 10.1364/ol.32.002608
- Huisken, J., & Stainier, D. Y. (2009). Selective plane illumination microscopy techniques in developmental biology. Development , 136(12), 1963–1975. doi: 10.1242/dev.022426
- Huisken, J., Swoger, J., Del Bene, F., Wittbrodt, J., & Stelzer, E. H. K. (2004). Optical sectioning deep inside live embryos by selective plane illumination microscopy. Science , 305, 1007–1009. doi: 10.1126/science.1100035
- Hutson, K. A., Pulver, S. H., Ariel, P., Naso, C., & Fitzpatrick, D. C. (2021). Light sheet microscopy of the gerbil cochlea. Journal of Comparative Neurology , 529(4), 757–785. doi: 10.1002/cne.24977
- Ichikawa, T., Nakazato, K., Keller, P. J., Kajiura-Kobayashi, H., Stelzer, E. H., Mochizuki, A., & Nonaka, S. (2014). Live imaging and quantitative analysis of gastrulation in mouse embryos using light-sheet microscopy and 3D tracking tools. Nature Protocols , 9(3), 575–585. doi: 10.1038/nprot.2014.035
- Jeandupeux, E., Lobjois, V., & Ducommun, B. (2015). 3D print customized sample holders for live light sheet microscopy. Biochemical and Biophysical Research Communications , 463(4), 1141–1143. doi: 10.1016/j.bbrc.2015.06.072
- Jemielita, M., Taormina, M. J., Delaurier, A., Kimmel, C. B., & Parthasarathy, R. (2013). Comparing phototoxicity during the development of a zebrafish craniofacial bone using confocal and light sheet fluorescence microscopy techniques. Journal of Biophotonics , 6(11-12), 920–928. doi: 10.1002/jbio.201200144
- Jensen, C., & Teng, Y. (2020). Is it time to start transitioning from 2D to 3D cell culture? Frontiers in Molecular Biosciences , 7, 33. doi: 10.3389/fmolb.2020.00033
- Kafian, H., Lalenejad, M., Moradi-Mehr, S., Birgani, S. A., & Abdollahpour, D. (2020). Light-sheet fluorescence microscopy with scanning non-diffracting beams. Scientific Reports , 10(1), 8501. doi: 10.1038/s41598-020-63847-2
- Karreman, M. A., Hyenne, V., Schwab, Y., & Goetz, J. G. (2016). Intravital correlative microscopy: Imaging life at the nanoscale. Trends in Cell Biology , 26(11), 848–863. doi: 10.1016/j.tcb.2016.07.003
- Kaufmann, A., Mickoleit, M., Weber, M., & Huisken, J. (2012). Multilayer mounting enables long-term imaging of zebrafish development in a light sheet microscope. Development , 139(17), 3242–3247. doi: 10.1242/dev.082586
- Ke, M. T., & Imai, T. (2014). Optical clearing of fixed brain samples using SeeDB. Current Protocols in Neuroscience , 66, 2.22.1–2.22.19. doi: 10.1002/0471142301.ns0222s66
- Ke, M. T., Nakai, Y., Fujimoto, S., Takayama, R., Yoshida, S., Kitajima, T. S., … Imai, T. (2016). Super-resolution mapping of neuronal circuitry with an index-optimized clearing agent. Cell Reports , 14(11), 2718–2732. doi: 10.1016/j.celrep.2016.02.057
- Keomanee-Dizon, K., Fraser, S. E., & Truong, T. V. (2020). A versatile, multi-laser twin-microscope system for light-sheet imaging. Review of Scientific Instruments , 91(5), 053703. doi: 10.1063/1.5144487
- Keller, P. J. (2013). In vivo imaging of zebrafish embryogenesis. Methods , 62(3), 268–278. doi: 10.1016/j.biocel.2016.12.009
- Keller, P. J., Pampaloni, F., & Stelzer, E. H. (2006). Life sciences require the third dimension. Current Opinion in Cell Biology , 18(1), 117–124. doi: 10.1016/j.ceb.2005.12.012
- Keller, P. J., Schmidt, A. D., Santella, A., Khairy, K., Bao, Z., Wittbrodt, J., & Stelzer, E. H. (2010). Fast, high-contrast imaging of animal development with scanned light sheet-based structured-illumination microscopy. Nature Methods , 7(8), 637–642. doi: 10.1038/nmeth.1476
- Keller, P. J., & Stelzer, E. H. (2008). Quantitative in vivo imaging of entire embryos with digital scanned laser light sheet fluorescence microscopy. Current Opinion in Neurobiology , 18(6), 624–632. doi: 10.1016/j.conb.2009.03.008
- Khan, R., Gul, B., Khan, S., Nisar, H., & Ahmad, I. (2021). Refractive index of biological tissues: Review, measurement techniques, and applications. Photodiagnosis and Photodynamic Therapy , 33, 102192. doi: 10.1016/j.pdpdt.2021.102192
- Khonina, S. N., Kazanskiy, N. L., Karpeev, S. V., & Butt, M. A. (2020). Bessel beam: significance and applications—A progressive review. Micromachines , 11(11), 997. doi: 10.3390/mi11110997
- Kikkawa, Y., Seki, Y., Okumura, Ohshiba, Y., Miyasaka, Y., Suzuki, S., … Yonekawa, H. (2012). Advantages of a mouse model for human hearing impairment. Experimental Animals , 61(2), 85–98. doi: 10.1538/expanim.61.85
- Kim, J., Koo, B. K., & Knoblich, J. A. (2020). Human organoids: Model systems for human biology and medicine. Nature Reviews Molecular Cell Biology , 21(10), 571–584. doi: 10.1038/s41580-020-0259-3
- Krzic, U., Gunther, S., Saunders, T. E., Streichan, S. J., & Hufnagel, L. (2012). Multiview light-sheet microscope for rapid in toto imaging. Nature Methods , 9(7), 730–733. doi: 10.1038/nmeth.2064
- Kuwajima, T., Sitko, A. A., Bhansali, P., Jurgens, C., Guido, W., & Mason, C. (2013). ClearT: A detergent- and solvent-free clearing method for neuronal and non-neuronal tissue. Development , 140(6), 1364–1368. doi: 10.1242/dev.091844
- Laissue, P. P., Alghamdi, R. A., Tomancak, P., Reynaud, E. G., & Shroff, H. (2017). Assessing phototoxicity in live fluorescence imaging. Nature Methods , 14(7), 657–661. doi: 10.1038/nmeth.4344
- Laroche, T., Burri, O., Dubey, L. K., & Seitz, A. (2019). Development of sample-adaptable holders for lightsheet microscopy. Frontiers in Neuroanatomy , 13, 26. doi: 10.3389/fnana.2019.00026
- Lavagnino, Z., Sancataldo, G., d'Amora, M., Follert, P., DePietri Tonelli, D., Diaspro, A., & Cella Zanacchi, F. (2016). 4D (x-y-z-t) imaging of thick biological samples by means of two-photon inverted selective plane illumination microscopy (2PE-iSPIM). Scientific Reports , 6, 23923. doi: 10.1038/srep23923
- Lecoq, J., Orlova, N., & Grewe, B. F. (2019). Wide. fast. deep: Recent advances in multiphoton microscopy of in vivo neuronal activity. Journal of Neuroscience , 39(46), 9042–9052. doi: 10.1523/JNEUROSCI.1527-18.2019
- Lee, E., Choi, J., Jo, Y., Kim, J. Y., Jang, Y. J., Lee, H. M., … Sun, W. (2016). ACT-PRESTO: Rapid and consistent tissue clearing and labeling method for 3-dimensional (3D) imaging. Scientific Reports , 6, 18631. doi: 10.1038/srep18631
- Lemon, W. C., & McDole, K. (2020). Live-cell imaging in the era of too many microscopes. Current Opinion in Cell Biology , 66, 34–42. doi: 10.1016/j.ceb.2020.04.008
- Li, S., Chen, L. X., Peng, X. H., Wang, C., Qin, B. Y., Tan, D., … Zhou, X. H. (2018). Overview of the reporter genes and reporter mouse models. Animal Models and Experimental Medicine , 1(1), 29–35. doi: 10.1002/ame2.12008
- Li, Y., & Kilian, K. A. (2015). Bridging the gap: From 2D cell culture to 3D microengineered extracellular matrices. Advanced Healthcare Materials , 4(18), 2780–2796. doi: 10.1002/adhm.201500427
- Li, Y., Xu, J., Wan, P., Yu, T., & Zhu, D. (2018). Optimization of GFP fluorescence preservation by a modified uDISCO clearing protocol. Frontiers in Neuroanatomy , 12, 67. doi: 10.3389/fnana.2018.00067
- Li, Y., Xu, J., Zhu, J., Yu, T., & Zhu, D. (2020). Three-dimensional visualization of intramuscular innervation in intact adult skeletal muscle by a modified iDISCO method. Neurophotonics , 7(1), 015003. doi: 10.1117/1.NPh.7.1.015003
- Liang, X., Zang, Y., Dong, D., Zhang, L., Fang, M., Yang, X., … Tian, J. (2016). Stripe artifact elimination based on nonsubsampled contourlet transform for light sheet fluorescence microscopy. Journal of Biomedcial Optics , 21(10), 106005. doi: 10.1117/1.JBO.21.10.106005
- Liu, A., Xiao, W., Li, R., Liu, L., & Chen, L. (2019). Comparison of optical projection tomography and light-sheet fluorescence microscopy. Journal of Microscopy , 275(1), 3–10. doi: 10.1111/jmi.12796
- Liu, Y., Lauderdale, J. D., & Kner, P. (2019). Stripe artifact reduction for digital scanned structured illumination light sheet microscopy. Optics Letters , 44(10), 2510–2513. doi: 10.1364/OL.44.002510
- Lloyd, K. C. K., Adams, D. J., Baynam, G., Beaudet, A. L., Bosch, F., Boycott, K. M., … Brown, S. D. M. (2020). The deep genome project. Genome Biology , 21(1), 18. doi: 10.1186/s13059-020-1931-9
- Lopez, A. L. 3rd, Wang, S., & Larina, I. V. (2020). Embryonic mouse cardiodynamic OCT imaging. Journal of Cardiovascular Development and Disease , 7(4), 42. doi: 10.3390/jcdd7040042
- MacDonald, G. H., & Rubel, E. W. (2008). Three-dimensional imaging of the intact mouse cochlea by fluorescent laser scanning confocal microscopy. Hearing Research , 243(1-2), 1–10. doi: 10.1016/j.heares.2008.05.009
- Mahou, P., Vermot, J., Beaurepaire, E., & Supatto, W. (2014). Multicolor two-photon light-sheet microscopy. Nature Methods , 11(6), 600–601. doi: 10.1038/nmeth.2963
- Mandino, F., Cerri, D. H., Garin, C. M., Straathof, M., van Tilborg, G. A. F., Chakravarty, M. M., … Grandjean, J. (2020). Animal functional magnetic resonance imaging: Trends and path toward standardization. Frontiers in Neuroinformatics , 13, 78. doi: 10.3389/fninf.2019.00078
- Mappes, T., Jahr, N., Csaki, A., Vogler, N., Popp, J., & Fritzsche, W. (2012). The invention of immersion ultramicroscopy in 1912—the birth of nanotechnology? Angewandte Chemie (International ed in English) , 51(45), 11208–11212. doi: 10.1002/anie.201204688
- Marx, V. (2014). Microscopy: Seeing through tissue. Nature Methods , 11(12), 1209–1214. doi: 10.1038/nmeth.3181
- Marx, V. (2016). Microscopy: OpenSPIM 2.0. Nature Methods , 13(12), 979–982. doi: 10.1038/nmeth.4070
- Matryba, P., Kaczmarek, L., & Golab, J. (2019). Advances in ex situ tissue optical clearing. Laser & Photonics Reviews, 13(8), 1800292. doi: 10.1002/lpor.201800292
- Matsumoto, K., Mitani, T. T., Horiguchi, S. A., Kaneshiro, J., Murakami, T. C., Mano, T., … Ueda, H. R. (2019). Advanced CUBIC tissue clearing for whole-organ cell profiling. Nature Protocols , 14(12), 3506–3537. doi: 10.1038/s41596-019-0240-9
- Mayr, R., Serra, D., & Liberali, P. (2019). Exploring single cells in space and time during tissue development, homeostasis and regeneration. Development , 146(12), dev176727. doi: 10.1242/dev.176727.
- McClelland, K. S., & Bowles, J. (2016). Culturing murine embryonic organs: Pros, cons, tips and tricks. Differentiation , 91(4-5), 50–56. doi: 10.1016/j.diff.2016.01.008
- McClelland, K. S., Ng, E. T., & Bowles, J. (2016). Agarose/gelatin immobilisation of tissues or embryo segments for orientated paraffin embedding and sectioning. Differentiation , 91(4-5), 68–71. doi: 10.1016/j.diff.2015.12.001
- McConnell, G., Trägårdh, J., Amor, R., Dempster, J., Reid, E., & Amos, W. B. (2016). A novel optical microscope for imaging large embryos and tissue volumes with sub-cellular resolution throughout. Elife , 5, e18659. doi: 10.7554/eLife.18659
- McDole, K., Guignard, L., Amat, F., Berger, A., Malandain, G., Royer, L. A., … Keller, P. J. (2018). In toto imaging and reconstruction of post-implantation mouse development at the single-cell level. Cell , 175(3), 859–876.e33. doi: 10.1016/j.cell.2018.09.031
- McGorty, R., Liu, H., Kamiyama, D., Dong, Z., Guo, S., & Huang, B. (2015). Open-top selective plane illumination microscope for conventionally mounted specimens. Optics Express , 23(12), 16142–16153. doi: 10.1364/OE.23.016142
- McKey, J., Cameron, L. A., Lewis, D., Batchvarov, I. S., & Capel, B. (2020). Combined iDISCO and CUBIC tissue clearing and lightsheet microscopy for in toto analysis of the adult mouse ovary. Biology of Reproduction , 102(5), 1080–1089. doi: 10.1093/biolre/ioaa012
- Messal, H. A., Almagro, J., Zaw Thin, M., Tedeschi, A., Ciccarelli, A., Blackie, L., … Behrens, A. (2021). Antigen retrieval and clearing for whole-organ immunofluorescence by FLASH. Nature Protocols , 16(1), 239–262. doi: 10.1038/s41596-020-00414-z
- Moatti, A., Cai, Y., Li, C., Sattler, T., Edwards, L., Piedrahita, J., … Greenbaum, A. (2020). Three-dimensional imaging of intact porcine cochlea using tissue clearing and custom-built light-sheet microscopy. Biomedical Optics Express , 11(11), 6181–6196. doi: 10.1364/BOE.402991
- Molbay, M., Kolabas, Z. I., Todorov, M. I., Ohn, T. L., & Ertürk, A. (2021). A guidebook for DISCO tissue clearing. Molecular Systems Biology , 17(3), e9807. doi: 10.15252/msb.20209807
- Muntifering, M., Castranova, D., Gibson, G. A., Meyer, E., Kofron, M., & Watson, A. M. (2018). Clearing for deep tissue imaging. Current Protocols in Cytometry , 86(1), e38. doi: 10.1002/cpcy.38
- Murray, E., Cho, J. H., Goodwin, D., Ku, T., Swaney, J., Kim, S. Y., … Chung, K. (2015). Simple, scalable proteomic imaging for high-dimensional profiling of intact systems. Cell , 163(6), 1500–1514. doi: 10.1016/j.cell.2015.11.025
- Nguyen, D., Marchand, P. J., Planchette, A. L., Nilsson, J., Sison, M., Extermann, J., … Lasser, T. (2017). Optical projection tomography for rapid whole mouse brain imaging. Biomedical Optics Express , 8(12), 5637–5650. doi: 10.1364/BOE.8.005637
- Norris, F. C., Wong, M. D., Greene, N. D., Scambler, P. J., Weaver, T., Weninger, W. J., … Lythgoe, M. F. (2013). A coming of age: Advanced imaging technologies for characterising the developing mouse. Trends in Genetics , 29(12), 700–711. doi: 10.1016/j.tig.2013.08.004
- Nowotschin, S., Garg, V., Piliszek, A., & Hadjantonakis, A. K. (2019). Ex utero culture and imaging of mouse embryos. Methods in Molecular Biology , 1920, 163–182. doi: 10.1007/978-1-4939-9009-2_11
- Orlich, M., & Kiefer, F. (2018). A qualitative comparison of ten tissue clearing techniques. Histology and Histopathology , 33(2), 181–199. doi: 10.14670/HH-11-903
- Pampaloni, F. (2013). Spheroids with light-sheet-based fluorescence microscopy. Cell and Tissue Research , 352(1), 161–177. doi: 10.1007/s00441-013-1589-7
- Pampaloni, F., Knuppertz, L., Hamann, A., Osiewacz, H. D., & Stelzer, E. H. K. (2017). Three-dimensional live imaging of filamentous fungi with light sheet-based fluorescence microscopy (LSFM). Methods in Molecular Biology , 1563, 19–31. doi: 10.1007/978-1-4939-6810-7_2
- Pan, C., Cai, R., Quacquarelli, F. P., Ghasemigharagoz, A., Lourbopoulos, A., Matryba, P., … Ertürk, A. (2016). Shrinkage-mediated imaging of entire organs and organisms using uDISCO. Nature Methods , 13(10), 859–867. doi: 10.1038/nmeth.3964
- Pantazis, P., & Supatto, W. (2014). Advances in whole-embryo imaging: A quantitative transition is underway. Nature Reviews Molecular Cell Biology , 15(5), 327–339. doi: 10.1038/nrm3786
- Para-Damas, A., & Saura, C. A. (2020). Tissue clearing and expansion methods for imaging brain pathology in neurodegeneration: From circuits to synapses and beyond. Frontiers in Neuroscience , 14, 914. doi: 10.3389/fnins.2020.00914
- Parodi, V., Jacchetti, E., Osellame, R., Cerullo, G., Polli, D., & Raimondi, M. T. (2020). Nonlinear optical microscopy: From fundamentals to applications in live bioimaging. Frontiers in Bioengineering and Biotechnology , 8, 585363. doi: 10.3389/fbioe.2020.585363
- Perkel, J. M. (2019). The microscope makers putting ever-larger biological samples under the spotlight. Nature , 575(7784), 715–717. doi: 10.1038/d41586-019-03632-y
- Phifer-Rixey, M., & Nachman, M. W. (2015). Insights into mammalian biology from the wild house mouse Mus musculus. Elife , 4, e05959. doi: 10.7554/eLife.05959
- Pietzsch, T., Saalfeld, S., Preibisch, S., & Tomancak, P. (2015). BigDataViewer: Visualization and processing for large image data sets. Nature Methods , 12(6), 481–483. doi: 10.1038/nmeth.3392
- Piksarv, P., Marti, D., Le, T., Unterhuber, A., Forbes, L. H., Andrews, M. R., … Dholakia, K. (2017). Integrated single- and two-photon light sheet microscopy using accelerating beams. Scientific Reports , 7(1), 1435. doi: 10.1038/s41598-017-01543-4
- Pitrone, P. G., Schindelin, J., Stuyvenberg, L., Preibisch, S., Weber, M., Eliceiri, K. W., … Tomancak, P. (2013). OpenSPIM: An open-access light-sheet microscopy platform. Nature Methods , 10(7), 598–599. doi: 10.1038/nmeth.2507
- Planchon, T. A., Gao, L., Milkie, D. E., Davidson, M. W., Galbraith, J. A., Galbraith, C. G., & Betzig, E. (2011). Rapid three-dimensional isotropic imaging of living cells using Bessel beam plane illumination. Nature Methods , 8(5), 417–423. doi: 10.1038/nmeth.1586
- Poguzhelskaya, E., Artamonov, D., Bolshakova, A., Vlasova, O., & Bezprozvanny, I. (2014). Simplified method to perform CLARITY imaging. Molecular Neurodegeneration , 9, 19. doi: 10.1186/1750-1326-9-19
- Power, R. M., & Huisken, J. (2017). A guide to light-sheet fluorescence microscopy for multiscale imaging. Nature Methods , 14(4), 360–373. doi: 10.1038/nmeth.4224
- Preibisch, S., Saalfeld, S., Schindelin, J., & Tomancak, P. (2010). Software for bead-based registration of selective plane illumination microscopy data. Nature Methods , 7(6), 418–419. doi: 10.1038/nmeth0610-418
- Qi, Y., Yu, T., Xu, J., Wan, P., Ma, Y., Zhu, J., … Zhu, D. (2019). FDISCO: Advanced solvent-based clearing method for imaging whole organs. Science Advances , 5(1), eaau8355. doi: 10.1126/sciadv.aau8355
- Rawson, S. D., Maksimcuka, J., Withers, P. J., & Cartmell, S. H. (2020). X-ray computed tomography in life sciences. BMC Biology , 18(1), 21. doi: 10.1186/s12915-020-0753-2
- Reichmann, J., Eguren, M., Lin, Y., Schneider, I., & Ellenberg, J. (2018). Live imaging of cell division in preimplantation mouse embryos using inverted light-sheet microscopy. Methods in Cell Biology , 145, 279–292. doi: 10.1016/bs.mcb.2018.03.030
- Renier, N., Adams, E. L., Kirst, C., Wu, Z., Azevedo, R., Kohl, J., … Tessier-Lavigne, M. (2016). Mapping of brain activity by automated volume analysis of immediate early genes. Cell , 165(7), 1789–1802. doi: 10.1016/j.cell.2016.05.007
- Renier, N., Wu, Z., Simon, D. J., Yang, J., Ariel, P., & Tessier-Lavigne, M. (2014). iDISCO: A simple, rapid method to immunolabel large tissue samples for volume imaging. Cell , 159(4), 896–910. doi: 10.1016/j.cell.2014.10.010
- Reynaud, E. G., Peychl, J., Huisken, J., & Tomancak, P. (2015). Guide to light-sheet microscopy for adventurous biologists. Nature Methods , 12(1), 30–34. doi: 10.1038/nmeth.3222
- Richardson, D. S., & Lichtman, J. W. (2015). Clarifying tissue clearing. Cell , 162(2), 246–257. doi: 10.1016/j.cell.2015.06.067
- Richardson, D. S., & Lichtman, J. W. (2017). SnapShot: Tissue clearing. Cell , 171(2), 496–496.e1. doi: 10.1016/j.cell.2017.09.025
- Rohrbach, A. (2009). Artifacts resulting from imaging in scattering media: A theoretical prediction. Optics Letters , 34(19), 3041–3043. doi: 10.1364/OL.34.003041
- Royer, L. A., Lemon, W. C., Chhetri, R. K., & Keller, P. J. (2018). A practical guide to adaptive light-sheet microscopy. Nature Protocols , 13(11), 2462–2500. doi: 10.1038/s41596-018-0043-4
- Royer, L. A., Lemon, W. C., Chhetri, R. K., Wan, Y., Coleman, M., Myers, E. W., & Keller, P. J. (2016). Adaptive light-sheet microscopy for long-term, high-resolution imaging in living organisms. Nature Biotechnology , 34(12), 1267–1278. doi: 10.1038/nbt.3708
- Royer, L. A., Weigert, M., Günther, U., Maghelli, N., Jug, F., Sbalzarini, I. F., & Myers, E. W. (2015). ClearVolume: Open-source live 3D visualization for light-sheet microscopy. Nature Methods , 12(6), 480–481. doi: 10.1038/nmeth.3372
- Russell, C. T., & Rees, E. J. (2020). An open-hardware sample mounting solution for inverted light-sheet microscopes with large detection objective lenses. Journal of Microscopy , 280(1), 63–68. doi: 10.1111/jmi.12940
- Saghafi, S., Becker, K., Hahn, C., & Dodt, H. U. (2014). 3D-ultramicroscopy utilizing aspheric optics. Journal of Biophotonics , 7, 117–125. doi: 10.1002/jbio.201300048
- Sakalem, M. E., De Sibio, M. T., da Costa, F. A. D. S., & de Oliveira, M. (2021). Historical evolution of spheroids and organoids, and possibilities of use in life sciences and medicine. Biotechnology Journal , 16(5), e2000463. doi: 10.1002/biot.202000463
- Salili, S. M., Harrington, M., & Durian, D. J. (2018). Note: Eliminating stripe artifacts in light-sheet fluorescence imaging. Review of Scientific Instruments , 89(3), 036107. doi: 10.1063/1.5016546
- Sanderson, J. B. (2019). Understanding light microscopy. Chichester: John Wiley & Sons.
- Sanderson, J. (2020). Fundamentals of microscopy. Current Protocols in Mouse Biology , 10(2), e76. doi: 10.1002/cpmo.76
- Sanderson, J. B. (2022) (in press). Confocal microscopy. In Principles of light microscopy: From basic to advanced. Switzerland: Springer Nature AG.
- Santi, P. A. (2011). Light sheet fluorescence microscopy: A review. Journal of Histochemistry and Cytochemistry , 59(2), 129–138. doi: 10.1369/0022155410394857
- Saska, D., Pichler, P., Qian, C., Buckley, C. L., & Lagnado, L. (2021). μSPIM Toolset: A software platform for selective plane illumination microscopy. Journal of Neuroscience Methods , 347, 108952. doi: 10.1016/j.jneumeth.2020.108952
- Scherf, N., & Huisken, J. (2015). The smart and gentle microscope. Nature Biotechnology , 33(8), 815–818. doi: 10.1038/nbt.3310
- Schwarz, M. K., Scherbarth, A., Sprengel, R., Engelhardt, J., Theer, P., & Giese, G. (2015). Fluorescent-protein stabilization and high-resolution imaging of cleared, intact mouse brains. PLoS ONE , 10(5), e0124650. doi: 10.1371/journal.pone.0124650
- Scott, D. J., Gunn, N. J., Yong, K. J., Wimmer, V. C., Veldhuis, N. A., Challis, L. M., … Griffin, M. D. W. (2018). A novel ultra-stable, monomeric green fluorescent protein for direct volumetric imaging of whole organs using CLARITY. Scientific Reports , 8(1), 667. doi: 10.1038/s41598-017-18045-y
- Seo, J., Choe, M., & Kim, S.-Y. (2016). Clearing and labeling techniques for large-scale biological tissues. Molecular Cell , 39(6), 439–446. doi: 10.14348/molcells.2016.0088
- Sharpe, J. (2004). Optical projection tomography. Annual Review of Biomedical Engineering , 6, 209–228. doi: 10.1146/annurev.bioeng.6.040803.140210
- Sheppard, C. J. R., & Saghafi, S. (1996). Flattened light beams. Optics Communications , 132(1–2), 144–152. doi: 10.1016/0030-4018(96)00310-0
- Siedentopf, H., & Zsigmondy, R. (1903). Über Sichbarmachung und Größenbestimmung ultramikroskopischer Teilchen, mit besonderer Anwendung auf Goldrubingläser. Annalen Der Physik , 315, 1–39. doi: 10.1002/andp.19023150102
- Singh, M., Raghunathan, R., Piazza, V., Davis-Loiacono, A. M., Cable, A., Vedakkan, T. J., … Larin, K. V. (2016). Applicability, usability, and limitations of murine embryonic imaging with optical coherence tomography and optical projection tomography. Biomedical Optics Express , 7(6), 2295–2310. doi: 10.1364/BOE.7.002295
- Spalteholz, W. (1914). Über das Durchsichtigmachen von menschlichen und tierischen Präparaten und seine theoretischen Bedingungen [On making human and animal specimens transparent and its theoretical conditions]. Leipzig: S.Hirzel.
- Staudt, T., Lang, M. C., Medda, R., Engelhardt, J., & Hell, S. W. (2007). 2,2'-thiodiethanol: A new water soluble mounting medium for high resolution optical microscopy. Microscopy Research and Technique , 70(1), 1–9. doi: 10.1002/jemt.20396
- Stefaniuk, M., Gualda, E. J., Pawlowska, M., Legutko, D., Matryba, P., Koza, P., … Kaczmarek, L. (2016). Light-sheet microscopy imaging of a whole cleared rat brain with Thy1-GFP transgene. Scientific Reports , 6, 28209. doi: 10.1038/srep28209
- Stelzer, E. H. (2015). Light-sheet fluorescence microscopy for quantitative biology. Nature Methods , 12(1), 23–26. doi: 10.1038/nmeth.3219
- Stelzer, E. H. K., Lindek, S., Albrecht, S., Pick, R., Ritter, G., Salmon, N. J., & Stricker, R. (1995). A new tool for the observation of embryos and other large specimens: Confocal theta microscopy. Journal of Microscopy , 179(1), 1–10. doi: 10.1111/j.1365-2818.1995.tb03608.x
- Stelzer, E. H. K., & Lindek, S. (1994). Fundamental reduction of the observation volume in far-field light microscopy by detection orthogonal to the illumination axis: Confocal theta microscopy. Optics Communication , 111, 536–547. doi: 10.1016/0030-4018(94)90533-9
- Stock, J., & Pauli, A. (2021). Self-organized cell migration across scales–from single cell movement to tissue formation. Development , 148(7), dev191767. doi: 10.1242/dev.191767
- Strack, R. (2018). Mapping mouse development. Nature Methods , 15(12), 998. doi: 10.1038/s41592-018-0241-6
- Strack, R. (2021). Single-objective light sheet microscopy. Nature Methods , 18(1), 28. doi: 10.1038/s41592-020-01027-w
- Strnad, P., Gunther, S., Reichmann, J., Krzic, U., Balazs, B., de Medeiros, G., … Ellenberg, J. (2016). Inverted light-sheet microscope for imaging mouse pre-implantation development. Nature Methods , 13(2), 139–142. doi: 10.1038/nmeth.3690
- Susaki, E. A., & Takasato, M. (2021). Perspective: Extending the utility of three-dimensional organoids by tissue clearing technologies. Frontiers in Cell and Developmental Biology , 9, 679226. doi: 10.3389/fcell.2021.679226
- Susaki, E. A., Tainaka, K., Perrin, D., Yukinaga, H., Kuno, A., & Ueda, H. R. (2015). Advanced CUBIC protocols for whole-brain and whole-body clearing and imaging. Nature Protocols , 10(11), 1709–1727. doi: 10.1038/nprot.2015.085
- Sutherland, A. E. (2016). Tissue morphodynamics shaping the early mouse embryo. Seminars in Cell & Developmental Biology, 55, 89–98. doi: 10.1016/j.semcdb.2016.01.033
- Swoger, J., Pampaloni, F., & Stelzer, E. H. (2014). Imaging MDCK cysts with a single (selective) plane illumination microscope. Cold Spring Harbor Protocols , 2014(1), 114–118. doi: 10.1101/pdb.prot080184
- Tainaka, K., Murakami, T. C., Susaki, E. A., Shimizu, C., Saito, R., Takahashi, K., … Ueda, H. R. (2018). Chemical landscape for tissue clearing based on hydrophilic reagents. Cell Reports , 24(8), 2196–2210.e9. doi: 10.1016/j.celrep.2018.07.056
- Tepperman, A., Zheng, D. J., Taka, M. A., Vrieze, A., Le Lam, A., & Heit, B. (2020). Customizable live-cell imaging chambers for multimodal and multiplex fluorescence microscopy. Biochemistry and Cell Biology , 98(5), 612–623. doi: 10.1139/bcb-2020-0064
- Tinne, N., Antonopoulos, G. C., Mohebbi, S., Andrade, J., Nolte, L., Meyer, H., … Ripken, T. (2017). Three-dimensional hard and soft tissue imaging of the human cochlea by scanning laser optical tomography (SLOT). PLoS ONE , 12(9), e0184069. doi: 10.1371/journal.pone.0184069
- Tomer, R., Khairy, K., Amat, F., & Keller, P. J. (2012). Quantitative high-speed imaging of entire developing embryos with simultaneous multiview light-sheet microscopy. Nature Methods , 9(7), 755–763. doi: 10.1038/nmeth.2062
- Tomer, R., Ye, L., Hsueh, B., & Deisseroth, K. (2014). Advanced CLARITY for rapid and high-resolution imaging of intact tissues. Nature Protocols , 9(7), 1682–1697. doi: 10.1038/nprot.2014.123
- Tosi, S., Bardia, L., Barallobre, M. J., Muñoz-Barrutia, A., Soto-Montenegro, M. L., & Colombelli, J. (2020). MosaicExplorerJ: Interactive stitching of terabyte-size tiled datasets from lightsheet microscopy. F1000Research , 9, 1308. doi: 10.12688/f1000research.27112.2
- Truong, T. V., Supatto, W., Koos, D. S., Choi, J. M., & Fraser, S. E. (2011). Deep and fast live imaging with two-photon scanned light-sheet microscopy. Nature Methods , 8(9), 757–760. doi: 10.1038/nmeth.1652
- Tsien, R. Y. (2003). Imagining imaging's future. Nature Reviews Molecular Cell Biology , Sep, Suppl SS16–21. doi: 10.1038/nrm1196
- Udan, R. S., Piazza, V. G., Hsu, C. W., Hadjantonakis, A. K., & Dickinson, M. E. (2014). Quantitative imaging of cell dynamics in mouse embryos using light-sheet microscopy. Development , 141(22), 4406–4414. doi: 10.1242/dev.111021
- Ueda, H. R., Dodt, H. U., Osten, P., Economo, M. N., Chandrashekar, J., & Keller, P. J. (2020). Whole-brain profiling of cells and circuits in mammals by tissue clearing and light-sheet microscopy. Neuron , 106(3), 369–387. doi: 10.1016/j.neuron.2020.03.004
- Ueda, H. R., Ertürk, A., Chung, K., Gradinaru, V., Chédotal, A., Tomancak, P., & Keller, P. J. (2020). Tissue clearing and its applications in neuroscience. Nature Reviews Neuroscience , 21(2), 61–79. doi: 10.1038/s41583-019-0250-1. Erratum in: Nature Reviews Neuroscience , 2020 May,21(5):298.
- Verveer, P. J., Swoger, J., Pampaloni, F., Greger, K., Marcello, M., & Stelzer, E. H. (2007). High-resolution three-dimensional imaging of large specimens with light sheet-based microscopy. Nature Methods , 4(4), 311–313. doi: 10.1038/nmeth1017
- Vettenburg, T., Dalgarno, H. I., Nylk, J., Coll-Lladó, C., Ferrier, D. E., Čižmár, T., … Dholakia, K. (2014). Light-sheet microscopy using an Airy beam. Nature Methods , 11(5), 541–544. doi: 10.1038/nmeth.2922
- Voie, A. H., Burns, D. H., & Spelman, F. A. (1993). Orthogonal-plane fluorescence optical sectioning: Three-dimensional imaging of macroscopic biological specimens. Journal of Microscopy , 170(3), 229–236. doi: 10.1111/j.1365-2818.1993.tb03346.x
- Voigt, F. F., Kirschenbaum, D., Platonova, E., Pagès, S., Campbell, R. A. A., Kastli, R., … Helmchen, F. (2019). The mesoSPIM initiative: Open-source light-sheet microscopes for imaging cleared tissue. Nature Methods , 16(11), 1105–1108. doi: 10.1038/s41592-019-0554-0
- Wan, P., Li, Y., Zhu, J., Xu, J., Liu, X., Yu, T., & Zhu, D. (2021). FDISCO+: A clearing method for robust fluorescence preservation of cleared samples. Neurophotonics , 8(3), 035007. doi: 10.1117/1.NPh.8.3.035007
- Wan, Y., McDole, K., & Keller, P. J. (2019). Light-sheet microscopy and its potential for understanding developmental processes. Annual Review of Cell and Developmental Biology , 35, 655–681. doi: 10.1146/annurev-cellbio-100818-125311
- Wang, P., Zhang, D., Bai, S., Tao, B., Li, S., Wang, T., & Shang, A. (2020). Feasibility of commonly used fluorescent dyes and viral tracers in aqueous and solvent-based tissue clearing. Neuroscience Letters , 737, 135301. doi: 10.1016/j.neulet.2020.135301
- Wang, S., Larina, I. V., & Larin, K. V. (2020). Label-free optical imaging in developmental biology. Biomedical Optics Express , 11(4), 2017–2040. doi: 10.1364/BOE.381359
- Wang, X., & Fan, J. (2021). Spatiotemporal molecular medicine: A new era of clinical and translational medicine. Clinical and Translational Medicine , 11(1), e294. doi: 10.1002/ctm2.294
- Wang, Y. L., Grooms, N. W. F., Civale, S. C., & Chung, S. H. (2021). Confocal imaging capacity on a widefield microscope using a spatial light modulator. PLoS ONE , 16(2), e0244034. doi: 10.1371/journal.pone.0244034
- Watson, T., Andrews, N., Davis, S., Bugeon, L., Dallman, M. D., & McGinty, J. (2017). OPTiM: Optical projection tomography integrated microscope using open-source hardware and software. PLoS ONE , 12(7), e0180309. doi: 10.1371/journal.pone.0180309
- Waylen, L. N., Nim, H. T., Martelotto, L. G., & Ramialison, M. (2020). From whole-mount to single-cell spatial assessment of gene expression in 3D. Communications Biology , 3(1), 602. doi: 10.1038/s42003-020-01341-1
- Weber, M., & Huisken, J. (2011). Light sheet microscopy for real-time developmental biology. Current Opinion in Genetics & Development, 21(5), 566–572. doi: 10.1016/j.gde.2011.09.009
- Weiss, K. R., Voigt, F. F., Shepherd, D. P., & Huisken, J. (2021). Tutorial: Practical considerations for tissue clearing and imaging. Nature Protocols , 16(6), 2732–2748. doi: 10.1038/s41596-021-00502-8
- Wincott, M., Jefferson, A., Dobbie, I. M., Booth, M. J., Davis, I., & Parton, R. M. (2021). Democratising "Microscopi": A 3D printed automated XYZT fluorescence imaging system for teaching, outreach and fieldwork. Wellcome Open Research , 6, 63. doi: 10.12688/wellcomeopenres.16536.1
- Wolff, C., Tinevez, J. Y., Pietzsch, T., Stamataki, E., Harich, B., Guignard, L., … Pavlopoulos, A. (2018). Multi-view light-sheet imaging and tracking with the MaMuT software reveals the cell lineage of a direct developing arthropod limb. Elife , 7, e34410. doi: 10.7554/eLife.34410
- Wong, M. D., Dazai, J., Walls, J. R., Gale, N. W., & Henkelman, R. M. (2013). Design and implementation of a custom built optical projection tomography system. PLoS ONE , 8(9), e73491. doi: 10.1371/journal.pone.0073491
- Wu, C., Le, H., Ran, S., Singh, M., Larina, I. V., Mayerich, D., … Larin, K. V. (2017). Comparison and combination of rotational imaging optical coherence tomography and selective plane illumination microscopy for embryonic study. Biomedical Optics Express , 8(10), 4629–4639. doi: 10.1364/BOE.8.004629
- Wu, Y., Ghitani, A., Christensen, R., Santella, A., Du, Z., Rondeau, … Shroff, H. (2011). Inverted selective plane illumination microscopy (iSPIM) enables coupled cell identity lineaging and neurodevelopmental imaging in Caenorhabditis elegans. Proceedings National Academy of Science USA , 108(43), 17708–17713. doi: 10.1073/pnas.1108494108
- Xavier da Silveira Dos Santos, A., & Liberali, P. (2019). From single cells to tissue self-organization. FEBS Journal , 286(8), 1495–1513. doi: 10.1111/febs.14694
- Xu, J., Ma, Y., Yu, T., & Zhu, D. (2019). Quantitative assessment of optical clearing methods in various intact mouse organs. Journal of Biophotonics , 12(2), e201800134. doi: 10.1002/jbio.201800134
- Yang, B., Treweek, J. B., Kulkarni, R. P., Deverman, B. E., Chen, C. K., Lubeck, E., … Gradinaru, V. (2014). Single-cell phenotyping within transparent intact tissue through whole-body clearing. Cell , 158(4), 945–958. doi: 10.1016/j.cell.2014.07.017
- Yordanov, S., Neuhaus, K., Hartmann, R., Díaz-Pascual, F., Vidakovic, L., Singh, P. K., & Drescher, K. (2021). Single-objective high-resolution confocal light sheet fluorescence microscopy for standard biological sample geometries. Biomedical Optics Express , 12(6), 3372–3391. doi: 10.1364/BOE.420788
- Yu, T., Zhu, J., Li, D., & Zhu, D. (2021). Physical and chemical mechanisms of tissue optical clearing. iScience , 24(3), 102178. doi: 10.1016/j.isci.2021.102178
- Yu, T., Zhu, J., Li, Y., Ma, Y., Wang, J., Cheng, X., … Zhu, D. (2018). RTF: A rapid and versatile tissue optical clearing method. Science Reports , 8(1), 1964. doi: 10.1038/s41598-018-20306-3
- Zach, P., Mrzílková, J., Pala, J., Uttl, L., Kútna, V., Musil, V., … Tůma, P. (2019). New design of the electrophoretic part of CLARITY technology for confocal light microscopy of rat and human brains. Brain Sciences , 9(9), 218. doi: 10.3390/brainsci9090218
- Zhan, Y., Wu, H., Liu, L., Lin, J., & Zhang, S. (2021). Organic solvent-based tissue clearing techniques and their applications. Journal of Biophotonics , 14(6), e202000413. doi: 10.1002/jbio.202000413
- Zhang, J., Wu, D., & Turnbull, D. H. (2018). In utero MRI of mouse embryos. Methods in Molecular Biology , 1718, 285–296. doi: 10.1007/978-1-4939-7531-0_17
- Zhao, J., Lai, H. M., Qi, Y., He, D., & Sun, H. (2021). Current status of tissue clearing and the path forward in neuroscience. ACS Chemical Neuroscience , 12(1), 5–29. doi: 10.1021/acschemneuro.0c00563
- Zhu, X., Huang, L., Zheng, Y., Song, Y., Xu, Q., Wang, J., … Gong, W. (2019). Ultrafast optical clearing method for three-dimensional imaging with cellular resolution. Proceedings National Academy of Science USA , 116(23), 11480–11489. doi: 10.1073/pnas.1819583116
- Zinner, M., Lukonin, I., & Liberali, P. (2020). Design principles of tissue organisation: How single cells coordinate across scales. Current Opinion in Cell Biology , 67, 37–45. doi: 10.1016/j.ceb.2020.07.004
INTERNET RESOURCES
Applied Scientific Instrumentation blog 9 Mar 2019: Does “n” belong? (or, getting light sheet equations correct.
BioStudies
Image Data Resource (IDR)
Micro-Manager
Sample preparation
iDISCO protocols
Citing Literature
Number of times cited according to CrossRef: 7
- Yuta Kaneko, Tsukasa Masuda, Kimiharu Takamatsu, Shuji Mikami, Kohei Nakamura, Hiroshi Nishihara, Ryuichi Mizuno, Nobuyuki Tanaka, Mototsugu Oya, Volumetric imaging of the tumor microvasculature reflects outcomes and genomic states of clear cell renal cell carcinoma, The Journal of Pathology: Clinical Research, 10.1002/2056-4538.12388, 10 , 4, (2024).
- Oscar Leong, Angela F. Gao, He Sun, Katherine L. Bouman, Discovering Structure From Corruption for Unsupervised Image Reconstruction, IEEE Transactions on Computational Imaging, 10.1109/TCI.2023.3325752, 9 , (992-1005), (2023).
- Elise Delage, Thomas Guilbert, Frank Yates, Successful 3D imaging of cleared biological samples with light sheet fluorescence microscopy, Journal of Cell Biology, 10.1083/jcb.202307143, 222 , 11, (2023).
- Biswajoy Ghosh, Krishna Agarwal, Viewing life without labels under optical microscopes, Communications Biology, 10.1038/s42003-023-04934-8, 6 , 1, (2023).
- Dmitry S. Ushakov, Stefan Finke, Tissue optical clearing and 3D imaging of virus infections, Imaging in Virus Research, 10.1016/bs.aivir.2023.06.003, (89-121), (2023).
- Jeremy Sanderson, Multi‐Photon Microscopy, Current Protocols, 10.1002/cpz1.634, 3 , 1, (2023).
- Clara Gomez-Cruz, Sonia Laguna, Ariadna Bachiller-Pulido, Cristina Quilez, Marina Cañadas-Ortega, Ignacio Albert-Smet, Jorge Ripoll, Arrate Muñoz-Barrutia, Single Plane Illumination Microscopy for Microfluidic Device Imaging, Biosensors, 10.3390/bios12121110, 12 , 12, (1110), (2022).