Human iPSC-Derived Blood-Brain Barrier Models: Valuable Tools for Preclinical Drug Discovery and Development?
Antje Appelt-Menzel, Antje Appelt-Menzel, Sabrina Oerter, Sabrina Oerter, Sanjana Mathew, Sanjana Mathew, Undine Haferkamp, Undine Haferkamp, Carla Hartmann, Carla Hartmann, Matthias Jung, Matthias Jung, Winfried Neuhaus, Winfried Neuhaus, Ole Pless, Ole Pless
blood-brain barrier (BBB) in vitro model
CNS disease
drug permeability screening
human-induced pluripotent stem cells (hiPSC)
preclinical drug discovery
Abstract
Translating basic biological knowledge into applications remains a key issue for effectively tackling neurodegenerative, neuroinflammatory, or neuroendocrine disorders. Efficient delivery of therapeutics across the neuroprotective blood-brain barrier (BBB) still poses a demanding challenge for drug development targeting central nervous system diseases. Validated in vitro models of the BBB could facilitate effective testing of drug candidates targeting the brain early in the drug discovery process during lead generation. We here review the potential of mono- or (isogenic) co-culture BBB models based on brain capillary endothelial cells (BCECs) derived from human-induced pluripotent stem cells (hiPSCs), and compare them to several available BBB in vitro models from primary human or non-human cells and to rodent in vivo models, as well as to classical and widely used barrier models [Caco-2, parallel artificial membrane permeability assay (PAMPA)]. In particular, we are discussing the features and predictivity of these models and how hiPSC-derived BBB models could impact future discovery and development of novel CNS-targeting therapeutics. © 2020 The Authors.
INTRODUCTION
Effectively targeting diseases of the central nervous system (CNS) remains an unmet clinical need. Efficient delivery of therapeutics across the blood-brain barrier (BBB) poses a demanding challenge for CNS drug development and translation of basic biological findings towards application. 98% of small-molecule and nearly 100% of large-molecule drugs are not able to cross the BBB (Pardridge, 2005). Furthermore, clinical studies reported by the largest companies involved in drug discovery and development indicate a continued low translation between first-in-man studies and approval of novel therapeutics, particularly in CNS-linked diseases, where the overall success rate is low, even though the clinical candidates have previously been tested in cellular in vitro or in vivo models (Dowden & Munro, 2019; Kola & Landis, 2004; Waring et al., 2015). This indicates the urgent need for alternative methods and implementation of appropriate testing strategies. One of the key determinants for the below-average success in targeting CNS disease is the non-standardized and often contradictory use of in vitro and in vivo test systems for characterizing the effects of potential therapeutic agents (small molecule compounds and biologicals, in particular antibodies). The reasons behind this phenomenon are complex and specific to the respective drug development program but include on- and off-target related toxicities, insufficient efficacy, inadequate validation of the disease-target linkage (poor target validation), and insufficient availability of the agent at the intended site of action in the brain. Accurate determination of efficacy of a test substance is a key readout parameter in early drug discovery projects. In addition, the establishment and validation of disease-relevant in vitro and in vivo models is essential to program success. If the intended compound cannot enter the brain, then efficacy cannot be achieved, regardless of the degree of target validation and failure is guaranteed. Therefore, data on the permeability of substances across the BBB are ultimately indispensable for estimating the availability and effectiveness of CNS drugs. However, some aspects have to be considered for a reliable prediction of the BBB permeability, e.g., species differences between humans and non-human primates/rodent models, as well as the substitution of brain capillary endothelial cells (BCECs) with peripheral tissue-specific endothelial cell (EC) sources. Cell sources with divergent functional characteristics, for example epithelial cell lines (e.g., Caco-2 or MDCK) or ECs of non-cerebral origin (e.g., HUVEC), provide another reason why these transport models are inadequate for the task at hand. Compounding this problem are the lack of regulatory guidelines to assist in the validation of new models for BBB permeation. Functionally relevant and validated human BBB models accurately predicting the transport of substances into the brain together with other methods such as serum binding, brain slice uptake, or brain homogenate binding assays will greatly reduce the requirement for in vivo testing of compound distribution and action in the brain and is highly relevant for all CNS-related toxicity, pharmacokinetic, pharmacodynamics and efficacy studies. A physiological and disease-relevant BBB model would act as a pre-screening platform to evaluate the capability of chemical or biological agents to penetrate or actively overcome the BBB. Only substances with the potential to cross the BBB would be taken into account to determine direct and indirect neurotoxic effects. Preclinical safety and efficacy studies for newly developed drug candidates could be a main field of application of human BBB models. Especially in the early phase of lead optimization, it is expected to induce an improved selection of the drug candidates but also an improved predictivity in later developmental stages. In 2012, the first BBB models derived from human-induced pluripotent stem cells (hiPSCs) were invented and are now reaching a level of validation that might make them suitable for utilization in preclinical drug development programs in the pharmaceutical industry. In addition, these models could provide insights into mechanisms of CNS diseases, which are often associated with general or specific pathophysiological alterations at the BBB.
In this review we discuss how hiPSC-derived BBB models compare to widely applied barrier test systems, such as Caco-2 and parallel artificial membrane permeability assay (PAMPA), as well as to BBB models from primary human or non-human BCECs and rodent in vivo models. In particular, we are focusing on the predictivity of the model types, the current technological status to generate hiPSC-derived BBB models and future trends in pharmaceutical development.
STRUCTURE AND FUNCTION OF THE BBB IN HEALTH AND DISEASE
The average adult human brain consists of ∼100 billion neurons and weighs around 1.5 kg with a volume of around 1.2 L. Although it constitutes only 2% of the total body weight, around 20% of the basal metabolic energy is consumed. Proper functioning of the vasculature supplying the brain with nutrients is required to maintain this high energy demand. The capillaries of the brain parenchyma have a length of around 600 km with a diameter of 7 μm (Keller, 2013; Wong et al., 2013). The circumference of these brain capillaries are lined with specialized ECs, representing the main component of the BBB and interacting with pericytes, astrocytes, neurons, microglial cells, and extracellular matrix components (ECM). The complex and dynamic association and communication of the elements led to the definition of the term neurovascular unit (NVU). The BCECs show specialized characteristics due to lack of fenestrations, higher mitochondria numbers, minimal pinocytotic activities, low rate of transcytosis, low expression of leucocyte adhesion molecules, higher pericyte coverage, special astrocyte end feet coverage, and the expression of dense tight junctions (TJs), as well as an increased expression of solute carriers and efflux transporters when compared to peripheral vasculature (Hawkins & Davis, 2005; Keller, 2013). This complex interaction of the cell types and functionalities make up the BBB. The main functions of the BBB can be divided into three subgroups, the physical-, metabolic-, and transport-barrier (Neuhaus & Noe, 2010). The BBB regulates and modulates biochemical and activated cellular traffic at the NVU thereby maintaining the homeostasis of the brain microenvironment (Abbott & Friedman, 2012).
Tight Junctions at the BBB
Entry of hydrophilic molecules and immune cells to the CNS is restricted by the presence of junctional protein complexes. These complexes are responsible for sealing the paracellular space and the degree of BBB tightness is determined via interaction of junctional proteins on neighboring BCECs. TJs limit the paracellular transport of substances to the brain and are also crucial for the polarization of the BCECs. The main transmembrane proteins involved in the formation of TJs are claudins, occludins, and junction adhesion molecules (JAMs). Adherence junctions (AJs) represent an additional type cellular junctions of BCECs and are a prerequisite for proper TJ formation. Especially claudin-5 is highly expressed in rodent BMECs. KO mice lacking claudin-5 are characterized by a size-selective leakage of the BBB. Occludin is highly enriched in CNS BCECs compared to other tissues. JAMs are known to regulate leucocyte extravasations, particularly JAM4 has been identified in the BBB of mice. The tight junction complexes are linked to the cytoskeleton via a series of adaptors and cytoplasmic accessory proteins such as zonula occludens (ZO)-1 and -2, cingulin, jacop, membrane-associated guanylate kinases, afadin, and 7H6 antigen. Additionally, the TJs interact with AJs. Main proteins of AJs are vascular endothelial cadherin (VE-cadh) and platelet EC adhesion molecule (PECAM-1), which are further linked to the cytoskeleton via catenins (Bauer, Krizbai, Bauer, & Traweger, 2014; Daneman & Prat, 2015; Liu, Wang, Zhang, Wei, & Li, 2012; Sweeney, Zhao, Montagne, Nelson, & Zlokovic, 2019; Weiss, Miller, Cazaubon, & Couraud, 2009). These TJs are responsible for barrier integrity which can for example be measured by the transendothelial electrical resistance (TEER). In frogs, average TEER values of around 1900 Ωcm2 (Crone & Olesen, 1982) and in rats of around 1500 Ωcm2 (Butt, Jones, & Abbott, 1990) were determined. Until now, no human in vivo reference TEER data is available.
Transporters at the BBB
The high energy demand of neurons is met by the selective entry of various monosaccharides, amino acids, and ions. Glucose is actively transported via glucose transporters-1 (GLUT-1) and GLUT-3. Small hydrophobic molecules, gases, and uncharged polar molecules can pass the membrane by simple diffusion. Short chain monocarboxylic acids, such as L-lactate, acetate, pyruvates, thyroid hormones, aromatic amino acids, and ketone bodies are transported via monocarboxylic acid transporters (MCTs) (Vijay & Morris, 2014). Proteins, such as insulin-like growth factors, vasopressin and transferrin, are transported into the brain via receptor-mediated transcytosis mechanisms (RMT). RMT mechanisms are specially investigated in the delivery of drugs to the brain, among the most importantly studied ones are the transferrin receptors (Tfr), low density lipoprotein receptors (LDLR) and insulin receptors (INSR) (Pulgar, 2018). Multispecific transporters of the ATP-binding cassette transporter families (ABCs) and solute carrier families (SLC) play crucial roles in regulating the entry of blood-delivered molecules, such as drugs into the brain. They recognize active endogenous compounds such as prostaglandins, leukotrienes, and steroid hormones and prevent the brain from potential over-accumulation of these compounds. ABC transporters are responsible for the efflux of lipophilic and amphiphilic toxic compounds including several anti-inflammatory, anti-infectious, anti-depressant, and psychotropic agents (Strazielle & Ghersi-Egea, 2015). These transporters include P-glycoprotein (P-gp/ABCB1), breast cancer resistance protein (BCRP/ABCG2), and several multidrug-resistance associated proteins (MRPs/ABCCs). P-gp actively effluxes various xenobiotic compounds. P-gp, BCRP and MRPs show overlaps in substrate specificities thereby preventing the therapeutic entry of pharmaceuticals into the brain (Wevers & de Vries, 2016). BCRPs exclude therapeutics such as cytostatics and MRPs are known to efflux neutral organic drugs. Almost all transporters not belonging to the ABC transporter family belong to the SLC group, which consists of three main sub families. These carriers can be either unidirectional or bidirectional. The SLC22 subfamily includes charged organic transporters called organic anionic transporters (OATs) and organic cationic transporters (OCTs). The SLC21/SLCO forms a family of organic anion transport polypeptides (OATPs). SLCO members accept a broad range of substrates like environmental pollutants, nucleosidic antiviral drugs and non-steroidal anti-inflammatory agents. Members of the SLC15 family are required to transport endogenous di- and tri-peptides, peptidomimetic drugs (β-lactam) and antibiotics (Strazielle & Ghersi-Egea, 2015; Sweeney et al., 2019).
Disease- and Age-Associated Changes at the BBB
Altered BBB functions are shown in numerous brain disorders such as stroke, epilepsy, brain trauma, multiple sclerosis, Huntington´s disease, amyotrophic lateral sclerosis, schizophrenia (SCZ), Parkinson´s disease (PD), and Alzheimer´s disease (AD) (Greene et al., 2018; Neuwelt et al., 2011). Munji and co-workers recently analyzed BBB endothelial cells in mice suffering from neurological diseases including stroke, multiple sclerosis, traumatic brain injury, and seizures (Munji et al., 2019). BBB endothelial cells were analyzed using transcriptomics, and obtained gene expression profiles exhibited comparable gene expression changes in the context of different diseases. These data suggest that different diseases share common disease mechanisms affecting BBB functionality. One major result is that these changes induce loss of BBB characteristics and support a peripheral identity. Mice with seizures showed changes likely due to the increased metabolic activity of neurons highlighting that BCECs dynamically alter their properties in response to neural activity. Viruses such as poliovirus, adenovirus, Epstein-Barr virus, and West Nile virus can directly infect the BCECs while targeting JAMs or GLUTs. These infections can downregulate TJ proteins promoting chemokine production while host immune responses can attenuate BBB damage (Sweeney et al., 2019). In addition, the Zika virus infects and replicates in BCECs strain-independent, but changes in BBB permeability occurred strain-dependent (Leda et al., 2019). With the recent outbreak of the Coronavirus disease 2019 (COVID-19), researchers debate the need for neurological tissue models to understand the biology of SARS-CoV-2 infections. The brain has been reported to express angiotensin converting 2 enzyme (ACE-2) receptors, responsible for virus uptake, and brain autopsies of patients suffering from severe acute respiratory syndrome (SARS) presented with virus-infected brain tissue (Puelles et al., 2020). Furthermore, recent studies have proposed these manifestations in hospitalized patients affirming the neurotropic potential of the virus (Mao et al., 2020). Nevertheless, current data do not allow to conclude that SARS-CoV-2 can permeate across the BBB. It is known that the thickness of the basement membrane of the BBB increases during postnatal development and continually increases in aged animals. Other changes include gliofibrillar proliferations, loss of BCECs, decreased mitochondria/mitochondrial dysfunction, and region-specific alterations in cross-sections of capillary walls and lumens (Erdo, Denes, & de Lange, 2017; Goodall et al., 2018). Notably, interspecies differences in these phenotypes exist: Recent studies in mice revealed ultrastructural changes of the BBB of mice with increasing age, showing an enhanced thickness and lipid accumulations compared to the human BBB (Ceafalan et al., 2019). Some studies using advanced magnetic resonance imaging were able to quantify regional BBB permeability in living human brains, showing an age-dependent BBB breakdown in the hippocampus (Montagne et al., 2015). In this article we will focus on disease-associated alterations at the BBB in AD. AD is the most common cause of dementia with a steep increase of 5.8 million Americans diagnosed with AD with around 122,019 deaths in 2018 compared to previous years (“2020 Alzheimer's disease facts and figures,” 2020). AD is characterized by impaired cognitive functions. The two main pathologies in AD affected brains are the formation of amyloid β (Aβ) plaques and tau tangles. A “two hit hypothesis” was suggested to explain the etiology of AD: Initially, damage occurs at the blood vessels resulting in BBB dysfunctions which in turn leads to Aβ accumulation in the brain and neurodegeneration. Studies from AD post mortem brains applying immunohistochemistry and immunoblotting show reduced TJ protein expression and capillary leakages of blood derived proteins, in particular albumins, immunoglobulins, thrombins, iron-containing proteins, and fibrinogens in brain areas with increased plaque depositions. Imaging studies show leaky BBBs in AD patients suggesting this phenotype to be an early biomarker for AD (Neuwelt et al., 2011; Sweeney, Sagare, & Zlokovic, 2018). In addition to non-specific leakages, non-functional BBB transport can drive AD pathology. Molecular changes such as low levels of GLUT-1 expression in BCECs were shown to result in diminished glucose transport (Erickson & Banks, 2019). The efflux transporters P-gp and the receptor LRP-1 are major regulators of Aβ CNS levels. Patients with AD show decreased protein levels of LRP-1 and P-gp. This led to the hypothesis that inefficient efflux at the BBB can lead to progression of AD via reduced clearance of Aβ from brain parenchyma. LRP-1 surface receptors on BCECs are responsible for Aβ clearance. They were shown to be diminished in AD brain microvessels leading to reduced clearance of Aβ promoting intracerebral accumulations. AD mouse models with an EC-specific knockout of LRP-1 show increased levels of soluble brain Aβ and severe learning and memory deficits while P-gp deficiency decreases Aβ clearance rates (Banks, 2016; Desai, Monahan, Carvey, & Hendey, 2007). In addition, BBB breakdown also leads to the increased uptake of inflammatory mediators like cytokines, chemokines, peripheral leukocytes, and CD4+T cells into the brain parenchyma thereby accelerating disease progression. Red blood cell extravasation, as well as infiltration by peripheral macrophages and neutrophils reported in AD post mortem studies further suggest innate immune system activation in the brain contributing to the pathophysiological changes (Engelhardt, Vajkoczy, & Weller, 2017; Nishihara et al., 2020; Sweeney et al., 2019). ABCA7, which shares a significant sequence homology to ABCA1, mainly promotes cholesterol and phospholipid transport across membranes, but involvement in phagocytic activity and Aβ clearance pathway was also shown in mouse models (Jehle et al., 2006; Kaminski et al., 2000; Kim et al., 2013; Sakae et al., 2016). ABCA7 levels were found to be increased in AD brains due to a compensatory regulation as suggested by the authors (Karch et al., 2012; Vasquez, Fardo, & Estus, 2013). Further, ABCA7 was identified by GWAS as a susceptibility locus contributing to the late-onset form of AD and loss-of-function variants of ABCA7 were reported for AD patients (Almeida, dos Santos, Trancozo, & de Paula, 2018; Hollingworth et al., 2011; Lambert et al., 2013; Steinberg et al., 2015; Vasquez et al., 2013). Recently, it was shown that an ABCA7 polymorphism (rs3764650) within apolipoprotein E carriers of a homozygous risk haplotype (APOE-ε4) results in memory impairment and functional network connectivity in AD patients (Chang et al., 2019). Many mechanisms underlying the onset, progression, and severity of CNS diseases are not completely understood, in particular with regard to BBB functionality (Profaci, Munji, Pulido, & Daneman, 2020). Furthermore, it is not yet understood if the disease-specific BBB degradation is cause or effect of neurological diseases.
APPLICATION OF BBB MODELS IN PRECLINICAL DRUG DISCOVERY
Value of BBB Models for Drug Discovery and Development
The increasing age of the world's population is significantly associated with a growing number of CNS-related diseases, including AD, PD, brain cancer, or stroke, resulting in an urgent need to develop cost-effective packages of medical and social care. A major problem in the CNS drug discovery process is the BBB penetration of effective therapeutic agents in sufficient amounts (Ghose, Herbertz, Hudkins, Dorsey, & Mallamo, 2012). This is one of the reasons for low success rates in CNS drug discovery, resulting in halted drug development programs in pharmaceutical companies for these indications. The increasing number of patients, however, is reflecting the dramatic need of effective drugs: Only for dementia it is predicted that the number of patients in the United Nations population will double every 20 years from 36 million people in 2010 to 115 million in 2050 (Prince et al., 2013). In order to increase the market release of CNS drugs, two major issues need to be overcome in preclinical development: First, the understanding of physicochemical and structural drug characteristics, which correlate with good penetrability needs to be improved; second, better translational models have to be provided to evaluate BBB penetration capability and efficacy of CNS therapeutics. BBB in vitro models are useful tools to study BBB permeability and to predict pharmacological availability of drugs, such as small molecules, antibodies, proteins, and peptides. The pharmaceutical industry seeks for standardized and predictive human BBB in vitro models in order to differentiate between CNS active and non-active compounds early on in the drug development process.
Currently Applied BBB Models in Early Stage Drug Discovery
Due to the high demand for test systems in basic and preclinical research of drug development and transport studies, a range of different BBB models have been implemented. Besides the in silico , acellular in vitro and in vivo models, numerous cell-based BBB models have been developed. However, standardized models based on immortalized human cell lines show only moderate TJ expression and possess low barrier integrity, which is detected through TEER values below 150 Ωcm2 (Deli, Ábrahám, Kataoka, & Niwa, 2005). In comparison, the TEER values in animal experiments reached average values of more than 1500 Ωcm2 at the BBB (Butt et al., 1990; Crone & Olesen, 1982). These values are valuable benchmarks from in vivo experiments for the in vitro model validation, but it should be highlighted that also significantly lower and higher values have been measured in these reports. The availability of human primary BBB cells and healthy human brain tissue is very limited and ethically troublesome. Isolated primary cells change their characteristics rapidly during in vitro cultivation. Furthermore, the limited possibility to subculture or cryo-preserve primary cells make them unsuitable for a standardized industrial application. Most widely used primary BBB models in the pharmaceutical industry are based on bovine and porcine brain microvascular endothelial cells (BMECs), but the isolation and cultivation processes are very labor intensive and lead to variable results (Reichel, 2006). In this regard, it should be mentioned that the isolation of pure BCECs is quite difficult. Most procedures yield BMECs and not BCECs, since many protocols end up with a mixture of BCECs with other cells from the microvasculature such as brain arteriole or venule endothelial cells. Furthermore, due to the increasing knowledge of BBB-specific species differences, reproducible human in vitro models become more and more important. Most prominent differences are known in the expression of the transporters P-gp versus MDR as well as claudin subtypes in rodents and humans (Aday, Cecchelli, Hallier-Vanuxeem, Dehouck, & Ferreira, 2016). By aid of quantitative targeted absolute proteomics (QTAP) of human brain transporters and receptors, it was shown that P-gp expression in humans is 2.33-fold less compared to mdr1a expression in mice. More than 2-fold differences in protein expression were also detected for multidrug resistance-associated protein 4 (ABCC4/MRP4), monocarboxylate transporter 1 (MCT1/SLC16A1), l-type amino acid transporter (LAT1/SLC7A5), and organic anion transporter 3 (OAT3/SLC22A8) in comparative studies of human and rat BBBs. The amounts of ABCG2/BCRP, SLC2A1/GLUT-1, and INSR were similar in both species. These data together with others highlighted that the expression of ABCG2/BCRP was higher than the one of ABCB1/P-gp in humans (Shawahna et al., 2011). Moreover, the specific transporter MRP could not be detected in humans but is reported to be expressed in the rodent BBB. In addition, the expression level of LAT1/SLC7A5 was decreased 5-fold compared to mice (Hoshi et al., 2013; Uchida et al., 2011). Thus, intraspecies differences have an important impact on reproducibility and translation of drug transport efficacy studies (Aday et al., 2016; Bhalerao et al., 2020). Beside the expression of transporters, differences in the presence and quantity of tight junction proteins as well as receptors are reported between humans and rodents. The expression of claudin-3, -5, and -12 was reported to be most relevant in mouse models (Krause et al., 2008; Pfeiffer et al., 2011) until recently the role of claudin-3 and -12 for the barrier function of the BBB in mice was questioned (Castro Dias, Coisne, Baden et al., 2019; Castro Dias, Coisne, Lazarevic et al., 2019). On the contrary, the expression of claudin-1 was only evident at the human BBB (Berndt et al., 2019; Liebner et al., 2000). Claudin-5 expression dominates the BBB in vitro , but this does not reflect the in vivo situation. The expression profile of mouse and human TJ proteins in vivo is much more complex, but their complexity is largely lost under in vitro conditions (Berndt et al., 2019). In general, the properties of BCECs in vivo are better comparable to primary human BBB models than to immortalized cell lines (Bagchi et al., 2019). Due to the simple and cost-efficient usage, cell-free PAMPA assays and non-cerebral cell lines, such as Caco-2 or kidney epithelial cells (MDCK), are widely utilized in BBB assays. The MDCK cell line for example is characterized by a tighter barrier and has therefore been used to rank order passive permeation characteristics. Despite its routine use, permeability data obtained by the intestinal cell line Caco-2 cannot be used to evaluate CNS penetration, due to lacking tissue-specificity and, therefore, significance (Reichel, 2006). PAMPA assays are based on transwell systems containing a lipid artificial membrane in order to predict BBB permeation. PAMPA represents a predictive surrogate test for transcellular passive diffusion. Using this assays, compounds can be classified into high, low or uncertain BBB permeation categories. In general, PAMPA assays are considered a high throughput, low cost, and reproducible method. Nevertheless, active transport processes cannot be examined using PAMPA assays, resulting in an overestimation of in vivo penetration of tested substances (Bicker, Alves, Fortuna, & Falcao, 2014). In summary, no currently applied in vitro test system is able to mimic the in vivo complexity of the BBB and its properties (Bicker et al., 2014). As long as the ideal model, representing all critical aspects of BBB penetration characteristics at the same time, is not available, industry-driven drug development programs rely on the combination of several models to separately investigate the passive permeability and specific transport processes of compounds (Reichel, 2006). An alternative to avoid the aforementioned problems and to provide standardized human BBB models by the use of reproducible conditions, could be the application of hiPSC-derived test systems.
CURRENT TECHNOLOGICAL STATUS IN HIPSC-DERIVED BBB MODELING
Comparison of Differentiation Methods and Cellular Characterization
Within the last eight years a variety of different hiPSC-derived BBB models have been established, following alternative differentiation strategies and test system complexities. As summarized in Table 1, numerous methods of successful in vitro hiPSC differentiation, under reproducible conditions, were already developed. The BCECs were examined for the presence and the functionality of endothelial-specific markers, as well as specific transporters using transcriptional and proteomic methods. The most extensively used protocol for the derivation of BCECs is the co-differentiation protocol developed by Lippmann and colleagues (Lippmann et al., 2012). The protocol comprises of the following main steps: Firstly, the hiPSCs are differentiated to neural cells and ECs, followed by selective maturation of ECs and finally the elimination of neural subtypes via sub-culture onto collagen IV and fibronectin ECM. Furthermore, retinoic acid (RA) was identified as a suitable component in the enhancement of BBB phenotypes (Lippmann, Al-Ahmad, Azarin, Palecek, & Shusta, 2014; Stebbins et al., 2016; Wilson, Canfield, Hjortness, Palecek, & Shusta, 2015). The protocol has been reproduced by us and various other groups (Appelt-Menzel et al., 2017; Appelt-Menzel, Cubukova, & Metzger, 2018; Katt, Xu, Gerecht, & Searson, 2016; Yamashita, Aoki, Hashita, Iwao, & Matsunaga, 2020). Updates have been made to the original method in accelerating the differentiation process, use of serum-free media or efforts to eliminate additional purification steps (Hollmann et al., 2017; Neal et al., 2019; Ribecco-Lutkiewicz et al., 2018). Inhibition of TGFβ pathway induced the expression of cellular markers and cell-specific characteristics, as well as reduced damages induced by freezing/thawing processes (Yamashita et al., 2020). The use of embryoid bodies (EBs) and co-culture with rat glioma cells or conditioned medium has been explored (Minami et al., 2015). A shift from the co-differentiation protocol to direct BCECs generation was achieved by using chemically defined factors (Grifno et al., 2019; Praca et al., 2019; Qian et al., 2017). Recent advancements have been focused on the overexpression of transcription factors in upregulating BBB phenotypes (Roudnicky et al., 2020). Here, Roudnicky et al. identified 17 transcription factors, including among others TAL1, SOX7, SOX18, ETS1, and LEF1, to improve the direct differentiation of vascular endothelial cells and analyzed them using gain-of-function assays (Patsch et al., 2015; Roudnicky et al., 2020).
Strategy | Co-differentiation | Directed differentiation | Transcription factor overexpression | EBs + Co-culture | ||||||
---|---|---|---|---|---|---|---|---|---|---|
Original reference | Lippmann et al. (2012) | Lippmann et al.,(2014) | Hollmann et al.,(2017) | Ribecco-Lutkiewicz et al. (2018) | Neal et al. (2019) | Yamashita et al. (2020) | Qian et al. (2017) | Praca et al. (2019) | Roudnicky et al. (2020) | Minami et al. (2015) |
Days required | ∼13 | ∼13 | ∼8 | ∼21 | ∼8 | ∼13 | ∼10 | ∼15-20 | ∼6 | ∼14 |
Purification step | + | + | + | - | + | + | - | + | - | + |
TEER (Ω*cm2) monoculture | ∼200 | ∼3000 | ∼1000-4500 | ∼300-800 | ∼2000-8000 | ∼1500-4000 | ∼3000 | ∼50-60 | ∼20-25 | ∼22-28 |
TEER (Ω*cm2) co-culture (cells/conditioned medium) | ∼1500 | ∼5000 | ∼6500 | ∼1000-1500 | ∼9000-10,500 | N/A | ∼4000 | ∼50-60 | N/A | ∼55 |
Tube formation in vitro | + | N/A | N/A | N/A | N/A | + | + | N/A | N/A | + |
Marker expression (immuno-fluorescence) | Claudin−5, GLUT−1, occludin, PECAM−1, P−gp, VE−cadh, von Willebrand Factor (vWF), ZO−1 | BCRP, claudin−5, GLUT−1, MRP−1, occludin, PECAM−1, P−gp, VE−cadh | Claudin−5, GLUT−1, occludin, PECAM−1, VE−cadh | Claudin-5, GLUT−1, occludin, P−gp, PECAM−1,vWF, ZO−1 | Claudin−5, GLUT−1, occludin, PECAM−1, VE−cadh | BCRP, claudin−5, GLUT−1, occludin, P−gp, VE−cadh, ZO−1 | BCRP, claudin−5, GLUT−1, occludin, MRP−1, PECAM−1, P-gp, VE−cadh, vWF, ZO−1 | Claudin−5, GLUT−1, occludin, PECAM−1, P−gp, Tie−2, VE−cadh, vWF, ZO−1 | Claudin−5, occludin, VE−cadh, ZO−1 | PECAM−1, vWF |
Types of hiPSC-Derived In Vitro BBB Models
Static transwell models in mono- and co-culture
Transwell-based models mainly consist of BCECs cultured on a semipermeable cell culture membrane, providing a separated apical and basolateral culture compartment. These models are easy to use; the main advantage is the suitability for unsophisticated co-culture, TEER measurement, and permeability studies. This model is static and the plastic insert membrane acts as an extrinsic barrier, which can be considered as a drawback (Gastfriend, Palecek, & Shusta, 2018). Depending on the protocol used for differentiation, BBB models using these mono-cultures led to varying TEER values (brief summary provided in Table 1) (Delsing et al., 2018; Hollmann et al., 2017; Katt, Linville, Mayo, Xu, & Searson, 2018; Lippmann et al., 2014; Lippmann et al., 2012; Lippmann, Al-Ahmad, Palecek, & Shusta, 2013; Neal et al., 2019; Qian et al., 2017; Ribecco-Lutkiewicz et al., 2018; Stebbins et al., 2016). Recent comparisons of these models have been made to porcine-based systems, resulting in similar drug permeability data for a set of 23 CNS targeting compounds with a correlation coefficient of R2 = 0.8. In addition, activity differences of transporters were highlighted for efflux transporters, as well as GLUT-1 and LAT-1 (Di Marco et al., 2020). Co-culture with pericytes or hiPSC-derived ACs and neurons increased TEER values in transwell models by 30%, while monocultures had values around 3000 Ωcm2 (Qian et al., 2017). HiPSC-derived BCECs and PCs co-cultures enhanced BBB phenotypes with reduced levels of transcytosis (Stebbins et al., 2019). Previously, we have analyzed a set of ten different BBB culture models using hiPSC-derived BCECs, multipotent (fetal) neural stem cells, ACs, and PCs. The most complex culture models were able to investigate the distinct upregulation of typical BBB genes, as well as significant increase of TEER compared to the mono-cultures (Appelt-Menzel et al., 2017; Appelt-Menzel et al., 2018). Studies like ours confirmed that the combination of cell types of the NVU enhances barrier properties compared to mono-cultures of hiPSC-derived BCECs. Ribecco and colleagues developed the first syngeneic stem cell model to study receptor-mediated transcytosis and its application in evaluation of antibody-based BBB carriers. The mono-cultures yielded TEER between 300 and 800 Ωcm2, while with AC conditioned medium the TEER is elevated to ∼1000 Ω*cm2 (Ribecco-Lutkiewicz et al., 2018). Transwell models based on hiPSC-derived BCECs can generally also be used to mimic aspects of neurological diseases, as already shown for example for cerebral ischemia (Kokubu, Yamaguchi, & Kawabata, 2017; Page, Raut, & Al-Ahmad, 2019), Huntington's disease (Lim et al., 2017), MCT8 deficiency (Vatine et al., 2017) or cerebral amyloid angiopathy (CAA) and AD (Blanchard et al., 2020). An overview of hiPSC-derived transwell models is provided in Figure 1.
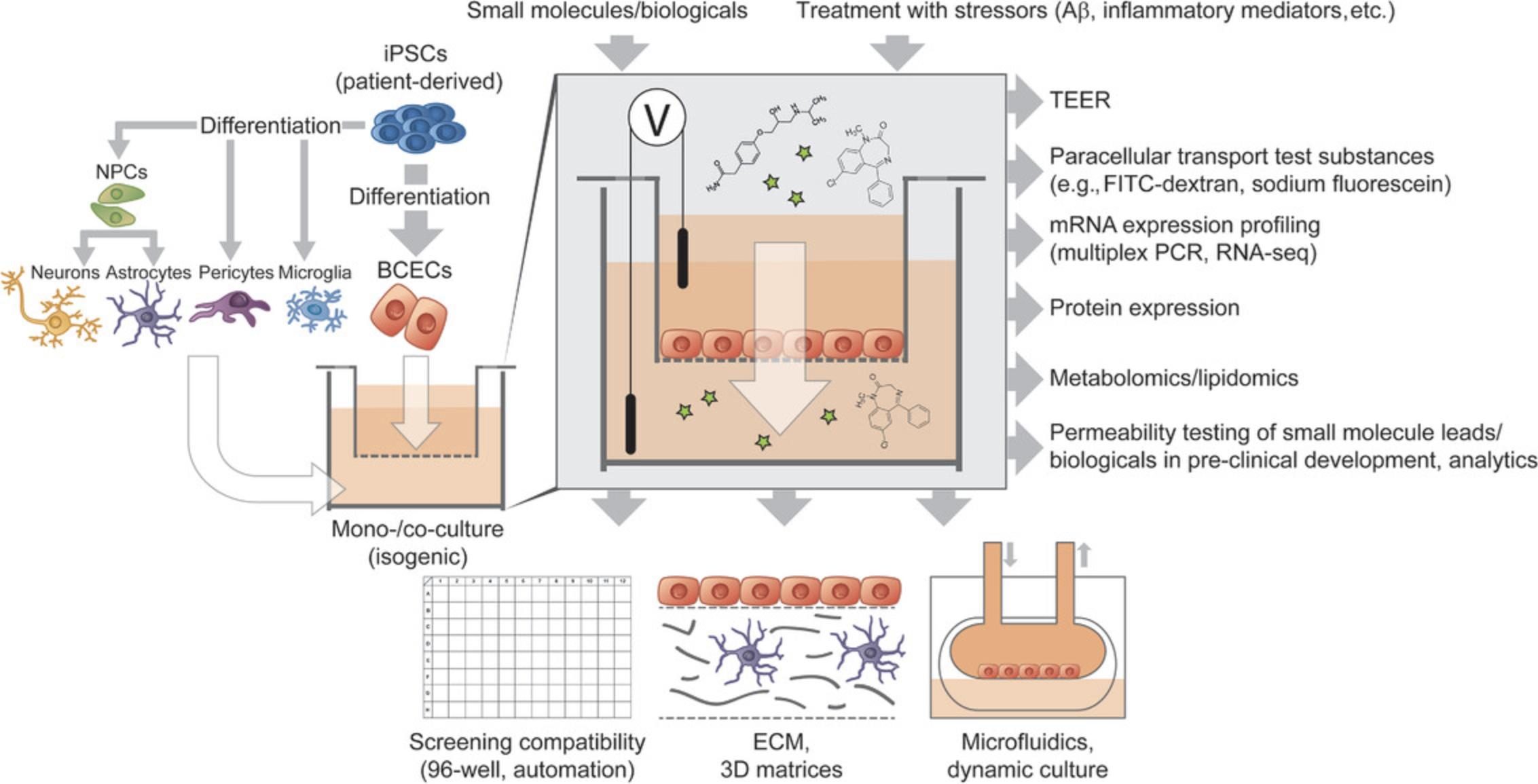
Planning and performing co-culture experiments face challenges like choosing the ideal media composition for every cell type of the NVU to ensure e.g., viability of co-cultured cells and the maintenance of cell type-specific markers. Considering the effect of cell maturity to signaling pathways, another aspect is finding the optimal timing of bringing together several cell types or various differentiation protocols to one timepoint.
Microfluidic models
Compared to transwell models the microfluidic test systems offer benefits like imitation of fluid flow and shear stress conditions found in physiological situation; however, the scalability and requirement of special expertise is a drawback when using these models for drug discovery applications (Gastfriend et al., 2018). Perfused hydrogels show that hiPSC-derived BCECs form confluent 3D monolayers with barrier integrity up to 3 weeks in culture. Perfusion of the cell-lined channels in low stress conditions stabilizes the barrier integrity over non-perfused controls, hence, indicating that perfusion with shear stress enables long-term barrier functions due to mechanical cues and continuous medium circulations. As a downside, angiogenesis was reported in the perfused channels, presumably due to lack of other NVU cell types and their secreted factor or cell-cell contacts (Faley et al., 2019). DeStefano and colleagues have shown that shear stress does not significantly affect the proliferation, rate of apoptosis, elongation, alignment, and gene expressions in hiPSC-derived BCECs (DeStefano, Jamieson, Linville, & Searson, 2018). Recently, hiPSC-derived BCECs co-cultured with ACs in perfused microfluidic channels were reported to maintain in vivo - like TEER values for 12 days, whereby the authors concluded that shear forces were not essential for the establishment of strong intercellular junctions but required to stabilize barrier integrity over time (Wang, Abaci, & Shuler, 2017). HiSC-derived BCECs co-cultured with rat primary ACs in a pumpless microfluidic platform for 10 days showed maintained TEER of ∼2000 Ω*cm2. This system was used as a drug candidate screening platform (Wang et al., 2017). A first-of-a-kind human hypoxic BBB chip model permitting analysis of BBB penetrating peptides and TfRc shuttling mechanisms has been described by Park and colleagues (Park et al., 2019). Channels with cylindrical geometry mimicking the three-dimensional architecture of the BBB have been introduced in proof-of-concept studies (Grifno et al., 2019; Katt et al., 2018; Linville et al., 2019).
Isogenic models
Canfield and colleagues reported for the first time that an NVU transwell model with hiPS-derived neurons, ACs, and BCECs in physiological cell ratios facilitated the investigation of the BBB. The endothelial stimulation by neurons, as well as ACs to the co-culture models induced barrier tightening, tight junction continuity and elevated TEER values, while P-gp efflux transport activity remained unchanged. The main limitation in this study was the absence of isogenic PCs (Canfield et al., 2016; Xiang, Andjelkovic, Wang, & Keep, 2017). In order to overcome the limitation of the previous study, the authors further included the effects of isogenic PCs in a new study, which led to the reduction of the rate of non-specific transcytosis. In this model, the authors noticed no differences in efflux activity compared to mono-cultures, although the co-cultures demonstrated barrier tightening and significant increases in expression of junctional proteins, especially occludin (Canfield et al., 2019). Patel and colleagues investigated the variability on phenotype and cell yield after differentiation in isogenic BBB models using asymptomatic patient samples. Differences in differentiation efficiencies of hiPSC-derived BCECs were noticed for PECAM-1, GLUT-1, and P-gp expression and drug efflux pump activities. The observation of such differences suggest interindividual polymorphisms or sexual dimorphisms; therefore, these observations must be taken into consideration for isogenic disease model development (Patel, Page, & Al-Ahmad, 2017). Recently, novel isogenic NVU chip models are developed supporting co-culture of hiPSC-derived BCECs and ACs. These chip models support media flow, which can be beneficial in recapitulating physiological conditions (Motallebnejad, Thomas, Swisher, & Azarin, 2019).
Preclinical Permeability Prediction and Potential Application of hiPSC-derived BBB Models
Robust and standardized hiPSC-derived BBB in vitro models could be valuable tools for preclinical drug-discovery because they fulfill two fundamental important criteria: They demonstrate physiological BBB relevant in vivo -like characteristics and simultaneously are compatible with the high-throughput demands of the pharmaceutical industry. Upscaling technologies using stirred tank bioreactors, spinner or suspension flasks are already routinely used for standardized hiPSC maintenance, as well as differentiation (Ackermann et al., 2018; Halloin et al., 2019; Kropp et al., 2016; Schwedhelm et al., 2019; Silva et al., 2015). On this basis, a high-throughput drug-compatible BBB assay should be feasible. For every developed CNS targeting compound, but also for substances acting in the periphery in order to exclude CNS-mediated side effects, it is mandatory to determine the brain targeting efficacy, as well as the toxicity. BBB in vitro models can be applied to perform transport studies, visualize transport routes, analyze drug transporter functionalities, perform drug interaction, or targeting studies, ensure pharmacological safety, examine disease-relevant BBB functions and conduct drug discovery studies (Prieto et al., 2004). The process of developing novel therapeutic agents follows a strategy that is based on the same principle across the entire pharmaceutical industry. Usually, the process of drug discovery is divided into different phases sequentially including target identification, hit identification, lead identification, and lead optimization. There are, however, differences between companies, in particular concerning the detailed organization of the various phases of the drug discovery and development processes. For compounds destined to act within the CNS, the penetrability of the BBB is an important additional property, which is typically investigated during lead generation (Reichel, 2006). A typical high-throughput screen (HTS) results in up to 1% hits of the initial screening library, which after hit validation (comprising of confirmation of single dose hits from the primary screen in replicates, assessment of compound identity and purity) and hit qualification (comprising of in vitro potency and selectivity towards related targets, early structure-activity relationships, physiochemistry, pharmacokinetics, and toxicology) narrow down to a handful of lead series (ideally providing a chemically diverse lead-like compound series with sufficient potential for chemical optimization). The characterization of lead compounds and lead series involves in silico tools, in vitro models and in vivo studies to examine pharmacological, pharmacokinetic and early toxicological properties. In programs aiming for the development of CNS therapeutics, the pharmacokinetic studies involve tests for CNS penetration, ranging from in silico classification systems, in vivo permeability assays, and for selected compounds the determination of brain-to-plasma or CSF-to-plasma ratios in vivo. Leads will serve as starting points for iterative steps of chemical optimization with the goal to increase in vitro potency and selectivity and to improve in vivo pharmacokinetic and pharmacodynamics properties by rational medicinal chemistry efforts. Ultimately, compounds need to be efficacious in relevant animal disease models in order to be progressed. The most promising compounds of these lead series which show in vivo efficacy are thoroughly characterized in order to identify the most critical properties and hence, the determining characteristics for the direction of chemical optimization in the subsequent drug development phase. The transition from discovery to development represents a major decision point, as all further activities on the candidate drug will demand a substantial commitment in terms of manpower, resources and budget. Drug development begins with a preclinical phase, which investigates in great detail the metabolism and pharmacokinetics, as well as the toxicology and safety profile of the compound in several (rodent and non-rodent) animal species in order to prepare the testing of the compound into human studies. Current strategies aim at combining elements of drug discovery and development phases earlier in the process to optimize safety and efficacy parameters. Clinical studies establish the safety and pharmacokinetics of the compound in humans (phase I study), from which the dosing scheme is derived for the subsequent phase II and phase III studies to demonstrate clinical efficacy in multi-center trials. To prevent misinterpretations and incorrect predictions resulting from species differences with regard to the expression of transporters, receptors and TJ proteins which influence BBB permeability, predictive human in vitro BBB models should also to be taken into account in drug discovery studies to complement the results (Aday et al., 2016). Obviously, the importance of the BBB and their integrity should be considered to estimate the penetration capacity of newly developed drugs and their metabolites, but on the other hand should also not be ignored in neurotoxicity screenings. The combination of predictive and robust BBB models with downstream located neurological systems can be a future testing strategy of choice. To increase standardization and simplicity, as well as to reduce workload and cell culture costs, cryopreserved hiPSC-derived BCECs can potentially be utilized for customer-specific applications (Wilson, Faubion, Hjortness, Palecek, & Shusta, 2016). The cryopreservation and banking of hiPSC-derived BCECs or respective progenitors will be a future trend for a standardized application of hiPSC-derived BBB models in drug discovery. Several pan-European, US, and Japanese strategies led to the establishment of sophisticated cell banks to address this need over the last decade and could serve as a source for high-quality reference lines used for hiPSC-derived BCEC progenitor generation (De Sousa et al., 2017; McKernan & Watt, 2013; O'Shea, Steeg, Chapman, Mackintosh, & Stacey, 2020).
In Vitro -In Vivo Correlation of Permeability Data Obtained from hiPSC-derived BBB Models
Especially in vitro models derived from human cell lines and stem cells have been demonstrated as useful tools for preclinical drug discovery (Aday et al., 2016). During the last years, different hiPSC-derived BBB in vitro models were evaluated for their applicability to predict drug permeability of novel treatment modalities, providing promising future concepts for CNS drug development. After differentiation of hiPSCs into BCECs and model characterization, the group of Lippmann et al. correlated in 2012 for the first time permeability values with in vivo rodent brain uptake measured by in situ brain perfusion, confirming high correlative values for the transport prediction of small molecules with a correlation coefficient of R2 = 0.98 (Lippmann et al., 2012). Le Roux and colleagues differentiated hiPSCs in accordance to protocols described by Lippmann et al. and included an additional puromycin-based selection process for EC purification. The morphology of the differentiated cells was comparable to primary human BMECs and expression of ZO-1, as well as claudin-5 was confirmed on protein level. Except for PECAM-1 and ABCB1, the mRNA expression level of transporters and receptors including ABCC1, ABCG2, TfRc, and INSR was similar to primary cell cultures. Furthermore, they compared BBB permeability of eight clinical positron emission tomography (PET) radioligands in comparison to the human BBB in vivo , showing a highly significant correlation (R2 = 0.83, P = 0.008) (Roux et al., 2019). With another panel of 18 compounds, the group aimed to further evaluate the ability of the hiPSC-derived BBB in vitro test systems to distinguish between CNS and non-CNS drugs. Indeed, the permeability values between both groups significantly differed, showing high values of CNS active drugs (mean Papp = 30.1 ± 4 × 10−6 cm/s) and only low transport rates of non-CNS drugs (mean Papp = 2.1 ± 0.6 × 10−6 cm/s), but a relationship between permeability and physicochemical properties of the compounds could not be established. In this context, Papp describes the apparent permeability coefficient and is often used to describe transport velocities of drugs. In intestinal models this value correlates with the absorption. Moreover, species-related transport differences could be confirmed comparing the transport data to an in vitro BBB model based on primary rat cells. Prediction of in vivo human BBB permeability was also investigated by Ohshima and colleagues using hiPSC-derived mono- and co-culture models (Ohshima et al., 2019). Transporter expression profiles of the hiPSC-derived BCECs was similar to that of human primary BMECs, barrier integrity with TEER values >1000 Ω*cm2 and expression of relevant TJ markers, such as claudin-5, ZO-1, and occludin was confirmed. In detail, the expression of transporters and other tissue relevant markers was not affected by co-cultures with pericytes, astrocytes, or neurons. Especially the expression level of P-gp/ABCB1 was similar between the different BBB set-ups, but significantly lower than in the analyzed Caco-2 models. Drug permeability assays yielded a better correlation between hiPSC-derived BBB models and data obtained from in vivo assays than rat BBB models or Caco-2 assays. Antibody-triggered RMT was studied by Ribecco-Lutkiewicz and colleagues (Ribecco-Lutkiewicz et al., 2018). The applied hiPSC-derived BBB model expressed RMT-associated receptors, as LDLR, TfR, INSR, or TMEM30A (receptor for BBB-crossing antibody FC5) and allowed the discrimination of species-selective antibody-mediated transcytosis mechanisms. Correlation of Papp values derived from specific antibody transport and the apparent CNS exposure in rat [simultaneous pharmacokinetic measurements of antibodies in cerebrospinal fluid (CSF) and in the serum] was significantly high with R2 = 0.96.
REGULATORY REQUIREMENTS FOR PRECLINICAL DRUG TESTING AT THE BBB
Regulatory Status Quo
A related key problem within the domain of drug permeability testing at the BBB is that until now no regulatory guidelines for the validation of in vitro models are available. An exception are Caco-2 models mimicking the gut epithelium (Volpe, 2011; Volpe et al., 2007). Already in 1993, the European Union Reference Laboratory for alternatives to animal testing (EURL ECVAM) defined the prevalidation and validation of non-animal methods for predicting the penetration of chemicals through the BBB as a current priority (https://cordis.europa.eu/article/id/10815-alternative-methods-in-biosciences, accessed on 21.04.2020). Furthermore, within the report of the ECVAM Workshop 49, the need for in vitro models to determine BBB transport was again underlined. As there are large quantitative and qualitative differences in BBB systems, EURL ECVAM defined the presence of a restrictive paracellular permeability, the presence of a physiologically plausible cell architecture/morphology, the expression of in vivo relevant transporter mechanisms, as well as the simplicity of cell culture as minimal requirements for useful BBB models (Prieto et al., 2004). To sum up the recommendations for the evaluation of the performance of promising in vitro models, it was suggested to use an appropriate and defined set of selected compounds to better characterize and standardize the models. To define acceptance criteria of in vitro models, the expression of BBB relevant (active) transporters has to be compared to the in vivo situation. To ensure the comparability and model standardization, all applied methods have to be defined in standard operation procedures. Moreover, a more precise definition of the abovementioned minimal requirements for model characterization is required, stressing in particular the importance on studying cellular polarity and the restriction of paracellular transport. Model performance has been evaluated by analyzing influx and efflux rates, as well as barrier integrity. All obtained permeability and CNS toxicity data should be provided for setting up an EURL ECVAM database. Endpoints for model validation prior to substance testing have to be defined for valid BBB in vitro models. This includes for example the specification of permeability values describing the paracellular tightness of the barrier as well as the transport velocities of BBB relevant reference drugs. Furthermore, the definition of relevant substances, as well as optimal testing concentrations for the (pre-)validation of BBB models are missing, thereby providing an adequate model quality control. Moreover, this topic remains challenging due to the insufficient availability of human in vivo data. Nevertheless a wide acceptance of a validated human BBB in vitro model is prognosed, urging the supply of a guidance provided by regulatory authorities.
Requirements in Preclinical Drug Discovery
To develop more effective neuropharmacological drugs with the capability to cross the BBB, standardized, robust and highly predictive humanized BBB models should be applied to determine the penetration capacity, as well as to understand the related expression and functionality of transporters at the BBB. The results obtained by use of adequate human BBB test systems should valorize the toxicological results generated in animals, in order to improve the outputs of toxicity tests and to enhance the evaluation of risk and safety in comparison with clinical data in humans (Cecchelli et al., 2007). A challenge to be fulfilled for permeability screenings is the application of in vitro models that recapitulate essential aspects of the in vivo physiology of the BBB and are compatible with the high-throughput requirements of the pharmaceutical industry (Bicker et al., 2014). In order to evaluate efficiently the large numbers of compounds generated by the pharmaceutical and chemical industry, assays have to be compatible to 96- or 384-well formats, allowing at least a partial automatization of the experimental workflow and resulting data analysis. Furthermore, a simplified handling of the test systems should be feasible, including an easy assay procedure with well-defined ready-to-use components/reagents (“mix-and-ready”) and robust readout parameters using state-of-the-art detection technologies. An appropriate turnaround time ensures the milestone-based compound progression. Beside time efficiency, the assay costs have to be justifiable. A successful usage of hiPSC-derived BBB models in preclinical testing requires the availability of robust in vitro test systems, characterized by the presence of a physiologically relevant morphology and cellular polarity, a reproducible permeability of reference compounds, an adequate screening capacity, and the expression of complex tight junction proteins and transporters, as well as their functionality (Cecchelli et al., 2007). To avoid misinterpretations of preclinical toxicity and bioavailability data and to efficiently translate this towards the clinic, the following criteria should be considered: According to the EURL ECVAM principle on test validation, robust protocols to reproducibly generate hiPSC-derived BCECs and resultant BBB models should be provided, describing the purpose of the test method, especially specifying the test system, the readout of the method, defined endpoints, the derivation and expression of results, acceptance criteria, the interpretation of the results and the implementation of adequate controls. Secondly, within-laboratory variability of the assay over time as well as the transferability to another laboratory have to be examined. In addition, the between-laboratory reproducibility should be addressed using a group of at least three qualified laboratories/test facilities at different sites performing a blinded study (Hartung et al., 2004). Advices in good cell culture practice for human primary and stem cell-derived models should be complied to increase the reproducibility of the assay and to ensure high-quality control standards (Pamies et al., 2018). By comparing the obtained results with a reference method, as for example the expression profile of cell-specific markers and transporters to human brain tissue biopsies or freshly isolated BMECs, the predictiveness of the test system can be evaluated. Furthermore, the transport results have to be compared to established in vivo and in vitro assays (e.g., PAMPA, Caco-2, in vivo studies of analyze brain penetration) to determine the robustness of the developed in vitro assay. For validation of transport studies a panel of well-defined reference substances, including BBB relevant drugs, small molecules, and biopharmaceuticals of different substance classes, covering the whole spectrum of permeability rates from slow to fast permeating substances, also including substrates of active transporters, should be used. If the predictive power of the hiPSC-derived BBB models should be compared to different species, it is advisable to investigate similar transport targets and processes, but also to include in vivo as well as in vitro models of the other species in order to exclude in vitro model set-up dependent effects. In order to increase the successful development of neurotherapeutics, particular attention has to be focused on the identification of disease-relevant transport targets and mechanisms, taking into account the relevant cellular populations based on hiPSC-derived disease models and variations in gene expression depending on age, sex, or disease stage. To advance the exchange of improved knowledge and to make data available, for example by installation of a online database, for academia as well as for industry, exchange of permeability data from in vitro and in vivo studies, protocols of standardized test procedures and drug reference sets might lead to an improved data quality for proper comparison of the results obtained by different researchers. This could enable faster validation and approval processes, as well as an increased predictive power of transport studies performed by means of in vitro models including an increased correlation to in vivo data.
FUTURE TRENDS IN DRUG DEVELOPMENT APPLYING hiPSC-DERIVED BBB MODELS
Monolayer models of the human BBB using transwells as a basis are most frequently used to study signaling pathways, transporter kinetics, binding affinities, or to characterize leads. To fully simulate the BBB integrity and complexity, including cell-cell communications and cell-matrix interactions more complex models are required (Bagchi et al., 2019). The interaction between different brain cell types, directly or indirectly via secreted soluble factors, as well as dynamic shear stress increase for example the expression of EC transporters, as well as TJs and promote cellular polarity. Implementing these factors might be decisive in the future development of e.g. personalized and disease-relevant BBB models.
Precision Medicine
Precision medicine (PM), also known as personalized medicine, is a novel approach of medical treatment taking into account the individual characteristics of each patient. The approach relies on tailored therapeutic strategies based on identifying and understanding a person's unique molecular and genetic profile and vulnerability to certain diseases. Compared to the traditional approach (“one size fits all”), PM takes into account a patient's genetic and epigenetic risk factors or other biomarkers in addition to clinical information and can increase the chance to predict the most effective and safe medical treatment. Individual BBB properties recently became more and more relevant in this context (Vatine et al., 2019).
Patients suffering from AD and PD show altered BBB functionality at the beginning of the disease and a BBB breakdown in later stages, which is accompanied with neuronal dysfunction, loss of neuronal connectivity, and neurodegeneration (Bowman et al., 2007; Gray & Woulfe, 2015). PM taking into account patient-specific BBB properties could improve therapeutic success for patients suffering from neurodegenerative disorders like AD or PD because alterations of the BBB accompany disease progression (Sweeney et al., 2018). However, modifying BBB functions for treating AD still remains challenging for different treatment strategies including antibody-based approaches, increasing brain clearance of Aβ or antagonize aggregation (Panza, Lozupone, Logroscino, & Imbimbo, 2019). PM focuses on the analysis of genetic mutations of patients to identify suitable drug targets for therapy or risk assessment including the prediction of negative side-effects and the exclusion of non-responders. In AD, this may include considering the genotypes of APOE and ACE (both expressed in BCECs) as recently reported for the treatment with ACE inhibitors (de Oliveira, Chen, Smith, & Bertolucci, 2018). Moreover, modulation of calcineurin-nuclear factor of activated T cell (NFAT) signaling could be another therapeutic option in APOE4-mediated CAA and AD (Blanchard et al., 2020).
HiPSC-based therapies for treating age-related macular degeneration have been successfully conducted (Zarbin, Sugino, & Townes-Anderson, 2019) and cell replacement therapies for human brain cells are currently being tested in clinical trials. A fascinating approach in PM is the transplantation of autografts into the CNS aiming for a curative approach of neurological disease (Barker, Parmar, Studer, & Takahashi, 2017), in particular in PD. The increasing number of clinical trials involving therapies based on hiPSC-derived cells shows the general applicability of autologous clinical-grade stem cells for cell replacement therapies. Additionally, gene therapy is discussed as a promising technology to restore functionality in diseased tissue. Recently, primary rodent BCECs were successfully transduced targeting the lysosomal cholesterol storage disease Niemann Pick type C2 (Hede et al., 2019). To target AD- and PD-related BBB symptoms, there is a need to first understand the consequence of disease-associated genetic variants. Then, patient-specific hiPSCs could be genetically modified to produce healthy BBB tissue for autologous transplantation, although current technologies and associated costs are still prohibitive.
Disease Models Mimicking BBB Alterations
The major promises of disease models are to improve our understanding of pathomechanisms and associated biomarkers. This could be achieved by hiPSCs since one key limitation in drug development for disorders of the CNS is the inaccessibility of (intact) brain tissue. Accordingly, there is a lack of adequate models with high predictive value.
The application of disease models with patient- and disease-specific hiPSC-derived cell types opens up the possibility (i) to develop more effective personalized therapies, (ii) to characterize novel drugs, (iii) to characterize disease-associated mutations and resulting malformations that occur in embryogenesis, childhood, adulthood or old age, (iv) to develop disease- and/or tissue-specific disease models in vitro , and (v) to mimic disease-associated mutations or to introduce artificial mutations (e.g. gene knockout) by gene editing (e.g. CRISPR/cas technology).
Diseases of the CNS are often multifactorial including a variety of environmental and genetic risk factors. Genetic risk factors are DNA variations including copy number variations (CNVs), single-nucleotide polymorphisms (SNPs), and functional homozygous mutations. DNA variations influence onset, progression, and treatment of neurodevelopmental and neurodegenerative diseases as well. In this context, DNA variations in genes regulating BBB functionality may not only have an impact on disease onset and progression, but may also play a role for disease treatment. Accordingly, the APOE status is detected as a biomarker in many preclinical studies. Disease models provide a powerful tool to interpret findings from genetic studies including the validation of AD risk variants such as loss-of-function mutation found in ABCA7 representing a transporter expressed on BCECs (Sims et al., 2017). To investigate BBB dysfunction depending on individual genetic differences, individual cellular models need to be established according to patient-specific genetic profiles. HiPSC-derived models are ideal candidates for disease recapitulation enabling the analysis of molecular and cellular pathways in order to nominate suitable molecular targets addressing a dysfunctional BBB. Patient-derived hiPSCs can be used to investigate the underlying genetically driven pathomechanisms and pathways in BBB dysfunction, since they most likely maintain patient-specific genetic mutations also after transduction and differentiation. Genetic studies have already been conducted for over 20 years and the first familial AD-correlated mutation on chromosome 21 was already suggested in 1987 (St George-Hyslop et al., 1987). Later on, highly multiplexed genotyping sped up the identification of genetic causes of neurological disorders in patients (Sims et al., 2017). Following results from these genome-wide association studies, numerous projects aimed for the identification of disease-specific mutations and the resulting dysregulation or functional impairment of proteins in neurodegenerative disorders. The number of genetic risk factors associated with BBB dysfunction in neurodegenerative disorders is constantly growing due to the growing number of cohort studies and the enlargement of existing cohorts (Savage et al., 2018). The technological advancement in multi-omics technologies will further accelerate and enlarge such studies. Meta-analysis and epidemiological studies will support these developments. These data will help to fill gaps in our knowledge regarding of the contribution of altered BBB functionality in AD, PD, and other diseases. However, identifying the causal relationship between specific mutations and pathophysiological changes of the BBB and especially BCECs has only been addressed scarcely.
Cellular two-dimensional and organotypic three-dimensional hiPSC-derived in vitro models hold a great promise for the functional characterization of DNA variations necessary to further advance the field of translational medicine aiming at the transfer of insight from neuroscience research to clinical application. Patient-specific hiPSC-derived cells can also mimic aspects of BBB associated glial cell types including microglia which are key modulators of CNS disease (Ormel et al., 2018). Complex BBB models are suggested to be more predictive for disease modeling and toxicity screenings (Qian et al., 2018). Advanced co-culture CNS models composed of patient-specific hiPSC-derived cell types allow for the analysis of cell-cell communication and provide deeper insights into complex brain physiology (Raja et al., 2016; Smits et al., 2019). Thus, the impact of disease-associated mutations on BBB integrity in AD and PD patients can be studied in different cell types of the NVU. It is important to mention that mutations associated with AD occur in genes that are expressed by a variety of different cell types including BCECs, PCs, ACs, and neurons (Anttila et al., 2013; Blanchard et al., 2020; Challen et al., 2011). Therefore, in complex diseases such as AD and PD, however, multifactorial etiologies have to be considered, thus just cultivating AD hiPSC-derived BCECs might be not sufficient to mimic the disease phenotype and factors such as additional cell types of the NVU, inflammatory cytokines, growth medium adaptations or further disease-relevant stimuli have to be considered for in vitro model optimization.
Personalized in vitro cell models of the BBB, generated from patient-derived hiPSCs, could be used to validate drug candidates or to repurpose launched drugs for different indications. In preclinical testing, these models might have the potential to improve the success rate in clinical trials of CNS disorders affecting the BBB including AD and PD. Over 5000 clinical trials analyzing a variety of interventions for the treatment of neurodevelopmental diseases are currently listed in the United States National Institute of Health database ClinicalTrials.gov. Only 37 of them are related to the BBB. Application of preclinical hiPSC-derived BBB models to (i) improve the prediction of drug transport across the BBB to the target location, (ii) optimize efficient drug delivery, (iii) avoid potential risks, and (iv) eliminate unsuitable drug candidates early in the discovery/development phase could streamline this process and increase awareness for BBB-related aspects for CNS drugs.
Innovative Matrices and Three-Dimensional BBB In Vitro Models
In recent years, there has been a shift towards the development of more complex BBB models to better mimic brain functionality and physiology. To this end, the cellular composition and extracellular milieu of the in vitro models was further improved. The ECM has a significant role in influencing cellular behavior, like differentiation, proliferation and cell attachment. As of now, single proteins are typically in use to increase specific cell behavior; however, this does not represent the complex in vivo extracellular microenvironment. Recent advances in biomaterial engineering has enabled the development from standard two-dimensional monolayer cell culture to three-dimensional approaches of CNS models. Cell-loaded hydrogels are in focus as an ECM for three-dimensional brain models, as it possesses many aspects of the natural ECM including stiffness, enzymatic degradability, and binding ligands for cell adhesion (Katt et al., 2018; Tibbitt & Anseth, 2009). Especially for neural stem cell engineering, the understanding and the role of ECM is of growing importance (Lam et al., 2019; Murphy, Haynes, Laslett, Cameron, & O'Brien, 2020; Yang et al., 2012), which is related to the increasing interest in the development of neural transplants for therapy of neurodegenerative diseases and stroke. Neuronal progenitor cells (NPCs) and glia cells can be differentiated from hiPSCs; however, there is a lack of efficient in vitro models that generate functional neuronal cells. Novel hydrogels seem to be able to provide promising ECMs for neural differentiation to matured and functional cells for e.g. stroke implants (Lam, Lowry, Carmichael, & Segura, 2014; Moshayedi et al., 2016), and are therefore a current focus for biomolecular engineered stem cell transplants (Nih et al., 2017). Tissue engineered scaffolds like decellularized porcine brain tissue may also be utilized to build up a three-dimensional model of the CNS, since it consists of brain-specific matrix components, like glycosaminoglycans, collagen I, collagen III, collagen IV, collagen V, collagen VI, perlecan, as well as laminin (DeQuach, Yuan, Goldstein, & Christman, 2011). This porcine brain matrix can be used as a platform for hiPSC-derived cells of the NVU to build up a three-dimensional brain model and to support vascularization. Alternatively, it can be applied as coating reagent to enhance cell adhesion or to generate artificial hydrogel-based nanofibrous scaffolds (DeQuach et al., 2011). Despite of the great potential of such natural brain-specific tissue scaffolds, they are still from animal origin, which might pose a problem of species specificity. In this regard, it was shown that the usage of human-specific laminin led to better astroglia differentiation compared to the murine protein (Delsing, Kallur, Zetterberg, Hicks, & Synnergren, 2019), also the integrity of hiPSC-derived BCECs was even improved by human laminin compared to BCECs differentiated on (mouse-derived) Matrigel (Aoki, Yamashita, Hashita, Iwao, & Matsunaga, 2020). Cho et al. established a promising electrospun nanofibrous scaffold based on human brain ECM derived from decellularized human brain tissue to enhance the differentiation of hiPSCs into functional, myelin-expressing oligodendrocytes (Cho et al., 2019). Similar approaches might work also for the establishment of improved BBB models. To avoid possible ethical issues due to the usage of human tissue, an increasing number of studies focus on advanced self-assembling cell types to simulate in vivo three-dimensional tissue architecture, such as BBB spheroids, neuronal organoids and mini-brains. These approaches avoid the application of additional ECM constituents (Campisi et al., 2018; Lancaster et al., 2013; Pasca et al., 2015; Urich et al., 2013). The major advantage of these three-dimensional models over two-dimensional co-cultures is the direct cell-cell contact, which is crucial for the activity of key signaling pathways. Furthermore, hiPSC-derived three-dimensional in vitro BBB models allow to replicate in vivo architecture and offer an exciting possibility to study species-specific processes in health and disease in order to develop novel assays suitable for drug discovery (Cho et al., 2017; Nzou et al., 2018).
Dynamic Flow and Microfluidic Culture Systems
BCECs, lining the lumen of capillaries and microvessels, are under constant shear stress generated by blood flow. Although it is known that the application of shear stress affects BCEC characteristics, the majority of the BBB models are represented as static models, (Cucullo, Hossain, Puvenna, Marchi, & Janigro, 2011; DeStefano, Xu, Williams, Yimam, & Searson, 2017; Faley et al., 2019; Garcia-Polite et al., 2017; Wang et al., 2017). Vascular ECs under laminar flow show elongation, polarization, and alignment in the direction of the flow (Ballermann, Dardik, Eng, & Liu, 1998; Tkachenko et al., 2013). The effects of shear stress on BCECs seem to be dependent on the BCECs origin and their tightness status. Especially, hiPSC-derived BCECs showed increased resistance to shear stress mediated elongation in comparison to immortalized BCEC lines with lower basic tightness (DeStefano et al., 2017; Reinitz, DeStefano, Ye, Wong, & Searson, 2015; Ye et al., 2014). Despite the contradicting data on the role of shear stress and continuous supply of fresh media in different models, the influence of flow is not negligible for BCECs, but it is currently unclear how decisive this factor is for the generation of functional barriers. Different kinds of dynamic BBB models including medium flow are in use to mimic the in vivo situation more closer. One example is the application of spinning bioreactors to adapt and maintain mini-brains or spheroids in order to ensure an optimal nutrient distribution within the culture vessel (Qian et al., 2018; Qian et al., 2016; Yan, Song, Madinya, Ma, & Li, 2018). Thereby, the generation of large scale hiPSC-derived BBB spheroid models seems to be feasible and could be used to provide a platform for modeling human brain development and disease (Miranda et al., 2015; Qian et al., 2018; Qian et al., 2016). Engineered microvessels and chip models are another example to apply physiological sheer stress on BCECs (de Graaf et al., 2019). Hydrogel casts with a few hundred-micron range thickness allow the observation of cellular behavior in a more biomimetic environment including fabricated channels supporting fluid flow within the gel (Bertassoni et al., 2014; Lee et al., 2020; Miller et al., 2012). In engineered microfluidic models, such as three-dimensional gelatin/hydrogel channels or so-called brains-on-a-chip, hiPSC-derived BCECs represented a much more stable barrier function and a significant barrier tightness compared to static controls (Faley et al., 2019; Katt et al., 2018; Wang et al., 2017). Moreover, the addition of shear stress on hiPSC-derived BBB models increased the longevity and highlighted their potential application for studies lasting up to three weeks (Faley et al., 2019). The future developments in advanced microfluidics and their application will show (if they are able to hold their promise to recapitulate in vivo BBB physiology). To make them ready for industrial use, additional distinct efforts are necessary.
CONCLUSION
The decisive criterion for BBB model selection is the purpose of the study to be conducted (Bagchi et al., 2019). One could follow the credo: As easy as possible, as complex as necessary. A comparison of the currently available drug permeability datasets obtained from BBB in vitro models is difficult due to the diversity of the model setups applied, the characterization of variable sets of compounds targeting a variety of transport mechanisms, and the differences of the applied BCECs (Stanimirovic, Bani-Yaghoub, Perkins, & Haqqani, 2015). Moreover, complex dynamic in vivo processes, such as drug pharmacokinetics, brain exposure, distribution, elimination, target engagement, and also efficacy, cannot be fully replaced by in vitro approaches (Stanimirovic et al., 2015). We see human BBB models as an initial drug screening platform to reduce overall costs in drug development (Aday et al., 2016). Specific questions like the capacity of paracellular diffusion, directional transport across the BBB, rates of transcellular transport, efflux of substances, carrier-mediated transport, receptor-mediated transcytosis, drug metabolism/degradation by BCECs, immune cell interactions with the BBB, screening for potential BBB Trojan horses, species differences/selectivity, as well as cell-based toxicity can be answered by this technology (Stanimirovic et al., 2015). Therefore, the development, validation, and standardization of BBB models is key. HiPSC-derived BBB in vitro models showed high correlation to the human in vivo situation, but much effort has to be invested on validating these in vitro models in order to achieve their broad acceptance, also in industry. Additional aspects requiring critical evaluation are hiPSC-specific model variations, the long-term stability of these BBB models (Aday et al., 2016), and the integration of age-related phenotypes, since BBB models derived from pluripotent stem cells mostly represent young individuals (Lauschke, Frederiksen, & Hall, 2017). Recently, a variety of improved and complex hiPSC-derived BBB models was developed, considering relevant factors such as direct cell-cell contacts, modulation of barrier properties by specified ECM components or flow-induced shear stress, and three-dimensional structures. Nevertheless, due to cost efficiency, handling simplicity, options for automatization, time efficiency for reaching steady-state and comparative reasons, transwell-based BBB models currently seem to be the models with the most potential to be integrated into industrial testing regimes. Monolayer- or isogenic co-culture in vitro models of the NVU are considered the gold standard in the field of BBB research and should be incorporated into CNS drug discovery and development programs after evaluation and optimization of the desired features. The combination of standardized in vitro and in vivo approaches will improve the translation of data and hopefully clinical success of drug development targeting CNS disease by designing safer and more efficient drug delivery systems (Bicker et al., 2014; Stanimirovic et al., 2015).
LITERATURE CITED
- 2020 Alzheimer's disease facts and figures. (2020). Alzheimers Dement. doi: 10.1002/alz.12068.
- Abbott, N. J., & Friedman, A. (2012). Overview and introduction: The blood-brain barrier in health and disease. Epilepsia , 53(Suppl 6), 1–6. doi: 10.1111/j.1528-1167.2012.03696.x.
- Ackermann, M., Kempf, H., Hetzel, M., Hesse, C., Hashtchin, A. R., Brinkert, K., … Lachmann, N. (2018). Bioreactor-based mass production of human iPSC-derived macrophages enables immunotherapies against bacterial airway infections. Nature Communications , 9(1), 5088. doi: 10.1038/s41467-018-07570-7.
- Aday, S., Cecchelli, R., Hallier-Vanuxeem, D., Dehouck, M. P., & Ferreira, L. (2016). Stem cell-based human blood-brain barrier models for drug discovery and delivery. Trends in Biotechnology , 34(5), 382–393. doi: 10.1016/j.tibtech.2016.01.001.
- Almeida, J. F. F., dos Santos, L. R., Trancozo, M., & de Paula, F. (2018). Updated meta-analysis of BIN1, CR1, MS4A6A, CLU, and ABCA7 variants in Alzheimer's disease. Journal of Molecular Neuroscience , 64(3), 471–477. doi: 10.1007/s12031-018-1045-y.
- Anttila, V., Winsvold, B. S., Gormley, P., Kurth, T., Bettella, F., McMahon, G., … Consortium, I. H. G. (2013). Genome-wide meta-analysis identifies new susceptibility loci for migraine. Nature Genetics , 45(8), 912–U255. doi: 10.1038/ng.2676.
- Aoki, H., Yamashita, M., Hashita, T., Iwao, T., & Matsunaga, T. (2020). Laminin 221 fragment is suitable for the differentiation of human induced pluripotent stem cells into brain microvascular endothelial-like cells with robust barrier integrity. Fluids and Barriers of the CNS , 17(1), 25. doi: 10.1186/s12987-020-00186-4.
- Appelt-Menzel, A., Cubukova, A., Gunther, K., Edenhofer, F., Piontek, J., Krause, G., … Metzger, M. (2017). Establishment of a human blood-brain barrier co-culture model mimicking the neurovascular unit using induced pluri- and multipotent stem cells. Stem Cell Reports , 8(4), 894–906. doi: 10.1016/j.stemcr.2017.02.021.
- Appelt-Menzel, A., Cubukova, A., & Metzger, M. (2018). Establishment of a human blood-brain barrier co-culture model mimicking the neurovascular unit using induced pluripotent stem cells. Current Protocols in Stem Cell Biology , 47(1), e62. doi: 10.1002/cpsc.62.
- Bagchi, S., Chhibber, T., Lahooti, B., Verma, A., Borse, V., & Jayant, R. D. (2019). In-vitro blood-brain barrier models for drug screening and permeation studies: An overview. Drug Design, Development and Therapy , 13, 3591–3605. doi: 10.2147/DDDT.S218708.
- Ballermann, B. J., Dardik, A., Eng, E., & Liu, A. (1998). Shear stress and the endothelium. Kidney International. Supplement , 67, S100–108. doi: 10.1046/j.1523-1755.1998.06720.x.
- Banks, W. A. (2016). From blood-brain barrier to blood-brain interface: New opportunities for CNS drug delivery. Nature Reviews Drug Discovery , 15(4), 275–292. doi: 10.1038/nrd.2015.21.
- Barker, R. A., Parmar, M., Studer, L., & Takahashi, J. (2017). Human trials of stem cell-derived dopamine neurons for Parkinson's disease: Dawn of a new era. Cell Stem Cell , 21(5), 569–573. doi: 10.1016/j.stem.2017.09.014.
- Bauer, H. C., Krizbai, I. A., Bauer, H., & Traweger, A. (2014). “You Shall Not Pass”-tight junctions of the blood brain barrier. Frontiers in Neuroscience , 8, 392. doi: 10.3389/fnins.2014.00392.
- Berndt, P., Winkler, L., Cording, J., Breitkreuz-Korff, O., Rex, A., Dithmer, S., … Haseloff, R. F. (2019). Tight junction proteins at the blood-brain barrier: Far more than claudin-5. Cellular and Molecular Life Sciences , 76(10), 1987–2002. doi: 10.1007/s00018-019-03030-7.
- Bertassoni, L. E., Cecconi, M., Manoharan, V., Nikkhah, M., Hjortnaes, J., Cristino, A. L., … Khademhosseini, A. (2014). Hydrogel bioprinted microchannel networks for vascularization of tissue engineering constructs. Lab on A Chip , 14(13), 2202–2211. doi: 10.1039/c4lc00030g.
- Bhalerao, A., Sivandzade, F., Archie, S. R., Chowdhury, E. A., Noorani, B., & Cucullo, L. (2020). in vitro modeling of the neurovascular unit: Advances in the field. Fluids and Barriers of the CNS , 17(1), 22. doi: 10.1186/s12987-020-00183-7.
- Bicker, J., Alves, G., Fortuna, A., & Falcao, A. (2014). Blood-brain barrier models and their relevance for a successful development of CNS drug delivery systems: A review. European Journal of Pharmaceutics and Biopharmaceutics , 87(3), 409–432. doi: 10.1016/j.ejpb.2014.03.012.
- Blanchard, J. W., Bula, M., Davila-Velderrain, J., Akay, L. A., Zhu, L., Frank, A., … Tsai, L. H. (2020). Reconstruction of the human blood-brain barrier in vitro reveals a pathogenic mechanism of APOE4 in pericytes. Nature Medicine , 26(6), 952–963. doi: 10.1038/s41591-020-0886-4.
- Bowman, G. L., Kaye, J. A., Moore, M., Waichunas, D., Carlson, N. E., & Quinn, J. F. (2007). Blood-brain barrier impairment in Alzheimer disease: Stability and functional significance. Neurology , 68(21), 1809–1814. doi: 10.1212/01.wnl.0000262031.18018.1a.
- Butt, A. M., Jones, H. C., & Abbott, N. J. (1990). Electrical resistance across the blood-brain barrier in anaesthetized rats: A developmental study. Journal of Physiology , 429, 47–62. doi: 10.1113/jphysiol.1990.sp018243.
- Campisi, M., Shin, Y., Osaki, T., Hajal, C., Chiono, V., & Kamm, R. D. (2018). 3D self-organized microvascular model of the human blood-brain barrier with endothelial cells, pericytes and astrocytes. Biomaterials , 180, 117–129. doi: 10.1016/j.biomaterials.2018.07.014.
- Canfield, S. G., Stebbins, M. J., Faubion, M. G., Gastfriend, B. D., Palecek, S. P., & Shusta, E. V. (2019). An isogenic neurovascular unit model comprised of human induced pluripotent stem cell-derived brain microvascular endothelial cells, pericytes, astrocytes, and neurons. Fluids and Barriers of the CNS , 16(1), 25. doi: 10.1186/s12987-019-0145-6.
- Canfield, S. G., Stebbins, M. J., Morales, B. S., Asai, S. W., Vatine, G. D., Svendsen, C. N., … Shusta, E. V. (2016). An isogenic blood-brain barrier model comprising brain endothelial cells, astrocytes and neurons derived from human induced pluripotent stem cells. Journal of Neurochemistry , doi: 10.1111/jnc.13923.
- Castro Dias, M., Coisne, C., Baden, P., Enzmann, G., Garrett, L., Becker, L., … Engelhardt, B. (2019). Claudin-12 is not required for blood-brain barrier tight junction function. Fluids and Barriers of the CNS , 16(1), 30. doi: 10.1186/s12987-019-0150-9.
- Castro Dias, M., Coisne, C., Lazarevic, I., Baden, P., Hata, M., Iwamoto, N., … Engelhardt, B. (2019). Claudin-3-deficient C57BL/6J mice display intact brain barriers. Scientific Reports , 9(1), 203. doi: 10.1038/s41598-018-36731-3.
- Ceafalan, L. C., Fertig, T. E., Gheorghe, T. C., Hinescu, M. E., Popescu, B. O., Pahnke, J., & Gherghiceanu, M. (2019). Age-related ultrastructural changes of the basement membrane in the mouse blood-brain barrier. Journal of Cellular and Molecular Medicine , 23(2), 819–827. doi: 10.1111/jcmm.13980.
- Cecchelli, R., Berezowski, V., Lundquist, S., Culot, M., Renftel, M., Dehouck, M. P., & Fenart, L. (2007). Modelling of the blood-brain barrier in drug discovery and development. Nature Reviews Drug Discovery , 6(8), 650–661. doi: 10.1038/nrd2368.
- Challen, G. A., Sun, D., Jeong, M., Luo, M., Jelinek, J., Vasanthakumar, A., … Goodell, M. A. (2011). Dnmt3a is essential for hematopoietic stem cell differentiation. Blood , 118(21), 178–178. doi: 10.1182/blood.V118.21.386.386.
- Chang, Y. T., Hsu, S. W., Huang, S. H., Huang, C. W., Chang, W. N., Lien, C. Y., … Chang, C. C. (2019). ABCA7 polymorphisms correlate with memory impairment and default mode network in patients with APOEepsilon4-associated Alzheimer's disease. Alzheimer's Research and Therapy , 11(1), 103. doi: 10.1186/s13195-019-0563-3.
- Cho, A. N., Jin, Y., Kim, S., Kumar, S., Shin, H., Kang, H. C., & Cho, S. W. (2019). Aligned brain extracellular matrix promotes differentiation and myelination of human-induced pluripotent stem cell-derived oligodendrocytes. ACS Applied Materials and Interfaces , 11(17), 15344–15353. doi: 10.1021/acsami.9b03242.
- Cho, C. F., Wolfe, J. M., Fadzen, C. M., Calligaris, D., Hornburg, K., Chiocca, E. A., … Lawler, S. E. (2017). Blood-brain-barrier spheroids as an in vitro screening platform for brain-penetrating agents. Nature Communications , 8, 15623. doi: 10.1038/ncomms15623.
- Crone, C., & Olesen, S. P. (1982). Electrical resistance of brain microvascular endothelium. Brain Research , 241(1), 49–55. doi: 10.1016/0006-8993(82)91227-6.
- Cucullo, L., Hossain, M., Puvenna, V., Marchi, N., & Janigro, D. (2011). The role of shear stress in Blood-Brain Barrier endothelial physiology. BMC Neuroscience , 12, 40. doi: 10.1186/1471-2202-12-40.
- Daneman, R., & Prat, A. (2015). The blood-brain barrier. Cold Spring Harbor Perspectives in Biology , 7(1), a020412. doi: 10.1101/cshperspect.a020412.
- de Graaf, M. N. S., Cochrane, A., van den Hil, F. E., Buijsman, W., van der Meer, A. D., van den Berg, A., … Orlova, V. V. (2019). Scalable microphysiological system to model three-dimensional blood vessels. APL Bioengineering , 3(2), 026105. doi: 10.1063/1.5090986.
- de Oliveira, F. F., Chen, E. S., Smith, M. C., & Bertolucci, P. H. F. (2018). Pharmacogenetics of angiotensin-converting enzyme inhibitors in patients with Alzheimer's disease dementia. Current Alzheimer Research , 15(4), 386–398. doi: 10.2174/1567205014666171016101816.
- De Sousa, P. A., Steeg, R., Wachter, E., Bruce, K., King, J., Hoeve, M., … Allsopp, T. E. (2017). Rapid establishment of the European Bank for induced Pluripotent Stem Cells (EBiSC) - the hot start experience. Stem Cell Research , 20, 105–114. doi: 10.1016/j.scr.2017.03.002.
- Deli, M. A., Ábrahám, C. S., Kataoka, Y., & Niwa, M. (2005). Permeability studies on in vitro blood-brain barrier models: Physiology, pathology, and pharmacology. Cellular and Molecular Neurobiology , 25(1), 59–127. doi: 10.1007/s10571-004-1377-8.
- Delsing, L., Donnes, P., Sanchez, J., Clausen, M., Voulgaris, D., Falk, A., … Synnergren, J. (2018). Barrier properties and transcriptome expression in human iPSC-derived models of the blood-brain barrier. Stem Cells , 36(12), 1816–1827. doi: 10.1002/stem.2908.
- Delsing, L., Kallur, T., Zetterberg, H., Hicks, R., & Synnergren, J. (2019). Enhanced xeno-free differentiation of hiPSC-derived astroglia applied in a blood-brain barrier model. Fluids and Barriers of the CNS , 16(1), 27. doi: 10.1186/s12987-019-0147-4.
- DeQuach, J. A., Yuan, S. H., Goldstein, L. S., & Christman, K. L. (2011). Decellularized porcine brain matrix for cell culture and tissue engineering scaffolds. Tissue Engineering Part A , 17(21-22), 2583–2592. doi: 10.1089/ten.TEA.2010.0724.
- Desai, B. S., Monahan, A. J., Carvey, P. M., & Hendey, B. (2007). Blood-brain barrier pathology in Alzheimer's and Parkinson's disease: Implications for drug therapy. Cell Transplantation , 16(3), 285–299. doi: 10.3727/000000007783464731.
- DeStefano, J. G., Jamieson, J. J., Linville, R. M., & Searson, P. C. (2018). Benchmarking in vitro tissue-engineered blood-brain barrier models. Fluids and Barriers of the CNS , 15(1), 32. doi: 10.1186/s12987-018-0117-2.
- DeStefano, J. G., Xu, Z. S., Williams, A. J., Yimam, N., & Searson, P. C. (2017). Effect of shear stress on iPSC-derived human brain microvascular endothelial cells (dhBMECs). Fluids and Barriers of the CNS , 14(1), 20. doi: 10.1186/s12987-017-0068-z.
- Di Marco, A., Vignone, D., Gonzalez Paz, O., Fini, I., Battista, M. R., Cellucci, A., … Munoz-Sanjuan, I. (2020). Establishment of an in vitro human blood-brain barrier model derived from induced pluripotent stem cells and comparison to a porcine cell-based system. Cells , 9(4), 994. doi: 10.3390/cells9040994.
- Dowden, H., & Munro, J. (2019). Trends in clinical success rates and therapeutic focus. Nature Reviews Drug Discovery , 18(7), 495–496. doi: 10.1038/d41573-019-00074-z.
- Engelhardt, B., Vajkoczy, P., & Weller, R. O. (2017). The movers and shapers in immune privilege of the CNS. Nature Immunology , 18(2), 123–131. doi: 10.1038/ni.3666.
- Erdo, F., Denes, L., & de Lange, E. (2017). Age-associated physiological and pathological changes at the blood-brain barrier: A review. Journal of Cerebral Blood Flow and Metabolism , 37(1), 4–24. doi: 10.1177/0271678×16679420.
- Erickson, M. A., & Banks, W. A. (2019). Age-associated changes in the immune system and blood-brain barrier functions. International Journal of Molecular Sciences , 20(7), 1632. doi: 10.3390/ijms20071632.
- Faley, S. L., Neal, E. H., Wang, J. X., Bosworth, A. M., Weber, C. M., Balotin, K. M., … Bellan, L. M. (2019). iPSC-derived brain endothelium exhibits stable, long-term barrier function in perfused hydrogel scaffolds. Stem Cell Reports , 12(3), 474–487. doi: 10.1016/j.stemcr.2019.01.009.
- Garcia-Polite, F., Martorell, J., Del Rey-Puech, P., Melgar-Lesmes, P., O'Brien, C. C., Roquer, J., … Balcells, M. (2017). Pulsatility and high shear stress deteriorate barrier phenotype in brain microvascular endothelium. Journal of Cerebral Blood Flow and Metabolism , 37(7), 2614–2625. doi: 10.1177/0271678×16672482.
- Gastfriend, B. D., Palecek, S. P., & Shusta, E. V. (2018). Modeling the blood-brain barrier: Beyond the endothelial cells. Current Opinion in Biomedical Engineering , 5, 6–12. doi: 10.1016/j.cobme.2017.11.002.
- Ghose, A. K., Herbertz, T., Hudkins, R. L., Dorsey, B. D., & Mallamo, J. P. (2012). Knowledge-based, central nervous system (CNS) lead selection and lead optimization for CNS drug discovery. ACS Chemical Neuroscience , 3(1), 50–68. doi: 10.1021/cn200100h.
- Goodall, E. F., Wang, C., Simpson, J. E., Baker, D. J., Drew, D. R., Heath, P. R., … Wharton, S. B. (2018). Age-associated changes in the blood-brain barrier: Comparative studies in human and mouse. Neuropathology and Applied Neurobiology , 44(3), 328–340. doi: 10.1111/nan.12408.
- Gray, M. T., & Woulfe, J. M. (2015). Striatal blood-brain barrier permeability in Parkinson's disease. Journal of Cerebral Blood Flow and Metabolism , 35(5), 747–750. doi: 10.1038/jcbfm.2015.32.
- Greene, C., Kealy, J., Humphries, M. M., Gong, Y., Hou, J., Hudson, N., … Campbell, M. (2018). Dose-dependent expression of claudin-5 is a modifying factor in schizophrenia. Molecular Psychiatry , 23(11), 2156–2166. doi: 10.1038/mp.2017.156.
- Grifno, G. N., Farrell, A. M., Linville, R. M., Arevalo, D., Kim, J. H., Gu, L., & Searson, P. C. (2019). Tissue-engineered blood-brain barrier models via directed differentiation of human induced pluripotent stem cells. Scientific Reports , 9(1), 13957. doi: 10.1038/s41598-019-50193-1.
- Halloin, C., Schwanke, K., Lobel, W., Franke, A., Szepes, M., Biswanath, S., … Zweigerdt, R. (2019). Continuous WNT control enables advanced hPSC cardiac processing and prognostic surface marker identification in chemically defined suspension culture. Stem Cell Reports , 13(2), 366–379. doi: 10.1016/j.stemcr.2019.06.004.
- Hartung, T., Bremer, S., Casati, S., Coecke, S., Corvi, R., Fortaner, S., … Zuang, V. (2004). A modular approach to the ECVAM principles on test validity. Alternatives to Laboratory Animals , 32(5), 467–472. doi: 10.1177/026119290403200503.
- Hawkins, B. T., & Davis, T. P. (2005). The blood-brain barrier/neurovascular unit in health and disease. Pharmacological Reviews , 57(2), 173–185. doi: 10.1124/pr.57.2.4.
- Hede, E., Heegaard, C., Korbelin, J., Schwanninger, M., Moos, T., & Burkhart, A. (2019). Gene therapy to the blood-brain barrier with resulting protein secretion as a strategy for treatment of NPC2 disease. Human Gene Therapy , 30(11), A100–A101.
- Hollingworth, P., Harold, D., Sims, R., Gerrish, A., Lambert, J. C., Carrasquillo, M. M., … Consortium, E. (2011). Common variants at ABCA7, MS4A6A/MS4A4E, EPHA1, CD33 and CD2AP are associated with Alzheimer's disease. Nature Genetics , 43(5), 429–+. doi: 10.1038/ng.803.
- Hollmann, E. K., Bailey, A. K., Potharazu, A. V., Neely, M. D., Bowman, A. B., & Lippmann, E. S. (2017). Accelerated differentiation of human induced pluripotent stem cells to blood-brain barrier endothelial cells. Fluids and Barriers of the CNS , 14(1), 9. doi: 10.1186/s12987-017-0059-0.
- Hoshi, Y., Uchida, Y., Tachikawa, M., Inoue, T., Ohtsuki, S., & Terasaki, T. (2013). Quantitative atlas of blood-brain barrier transporters, receptors, and tight junction proteins in rats and common marmoset. Journal of Pharmaceutical Sciences , 102(9), 3343–3355. doi: 10.1002/jps.23575.
- Jehle, A. W., Gardai, S. J., Li, S. Z., Linsel-Nitschke, P., Morimoto, K., Janssen, W. J., … Tall, A. R. (2006). ATP-binding cassette transporter A7 enhances phagocytosis of apoptotic cells and associated ERK signaling in macrophages. Journal of Cell Biology , 174(4), 547–556. doi: 10.1083/jcb.200601030.
- Kaminski, W. E., Orso, E., Diederich, W., Klucken, J., Drobnik, W., & Schmitz, G. (2000). Identification of a novel human sterol-sensitive ATP-binding cassette transporter (ABCA7). Biochemical and Biophysical Research Communications , 273(2), 532–538. doi: 10.1006/bbrc.2000.2954.
- Karch, C. M., Jeng, A. T., Nowotny, P., Cady, J., Cruchaga, C., & Goate, A. M. (2012). Expression of novel Alzheimer's disease risk genes in control and Alzheimer's disease brains. Plos One , 7(11), e50976. doi: 10.1371/journal.pone.0050976.
- Katt, M. E., Linville, R. M., Mayo, L. N., Xu, Z. S., & Searson, P. C. (2018). Functional brain-specific microvessels from iPSC-derived human brain microvascular endothelial cells: The role of matrix composition on monolayer formation. Fluids and Barriers of the CNS , 15(1), 7. doi: 10.1186/s12987-018-0092-7.
- Katt, M. E., Xu, Z. S., Gerecht, S., & Searson, P. C. (2016). Human brain microvascular endothelial cells derived from the BC1 iPS cell line exhibit a blood-brain barrier phenotype. Plos One , 11(4), e0152105. doi: 10.1371/journal.pone.0152105.
- Keller, A. (2013). Breaking and building the wall: The biology of the blood-brain barrier in health and disease. Swiss Medical Weekly , 143, w13892. doi: 10.4414/smw.2013.13892.
- Kim, W. S., Li, H., Ruberu, K., Chan, S., Elliott, D. A., Low, J. K., … Garner, B. (2013). Deletion of Abca7 increases cerebral amyloid-beta accumulation in the J20 mouse model of Alzheimer's disease. Journal of Neuroscience , 33(10), 4387–4394. doi: 10.1523/JNEUROSCI.4165-12.2013.
- Kokubu, Y., Yamaguchi, T., & Kawabata, K. (2017). in vitro model of cerebral ischemia by using brain microvascular endothelial cells derived from human induced pluripotent stem cells. Biochemical and Biophysical Research Communications , 486(2), 577–583. doi: 10.1016/j.bbrc.2017.03.092.
- Kola, I., & Landis, J. (2004). Can the pharmaceutical industry reduce attrition rates? Nature Reviews Drug Discovery , 3(8), 711–715. doi: 10.1038/nrd1470.
- Krause, G., Winkler, L., Mueller, S. L., Haseloff, R. F., Piontek, J., & Blasig, I. E. (2008). Structure and function of claudins. Biochimica Et Biophysica Acta , 1778(3), 631–645. doi: 10.1016/j.bbamem.2007.10.018.
- Kropp, C., Kempf, H., Halloin, C., Robles-Diaz, D., Franke, A., Scheper, T., … Olmer, R. (2016). Impact of feeding strategies on the scalable expansion of human pluripotent stem cells in single-use stirred tank bioreactors. Stem Cells Translational Medicine , 5(10), 1289–1301. doi: 10.5966/sctm.2015-0253.
- Lam, D., Enright, H. A., Cadena, J., Peters, S. K. G., Sales, A. P., Osburn, J. J., … Fischer, N. O. (2019). Tissue-specific extracellular matrix accelerates the formation of neural networks and communities in a neuron-glia co-culture on a multi-electrode array. Scientific Reports , 9(1), 4159. doi: 10.1038/s41598-019-40128-1.
- Lam, J., Lowry, W. E., Carmichael, S. T., & Segura, T. (2014). Delivery of iPS-NPCs to the stroke cavity within a hyaluronic acid matrix promotes the differentiation of transplanted cells. Advanced Functional Materials , 24(44), 7053–7062. doi: 10.1002/adfm.201401483.
- Lambert, J. C., Ibrahim-Verbaas, C. A., Harold, D., Naj, A. C., Sims, R., Bellenguez, C., … Amouyel, P. (2013). Meta-analysis of 74,046 individuals identifies 11 new susceptibility loci for Alzheimer's disease. Nature Genetics , 45(12), 1452–1458. doi: 10.1038/ng.2802.
- Lancaster, M. A., Renner, M., Martin, C. A., Wenzel, D., Bicknell, L. S., Hurles, M. E., … Knoblich, J. A. (2013). Cerebral organoids model human brain development and microcephaly. Nature , 501(7467), 373–379. doi: 10.1038/nature12517.
- Lauschke, K., Frederiksen, L., & Hall, V. J. (2017). Paving the way toward complex blood-brain barrier models using pluripotent stem cells. Stem Cells and Development , 26(12), 857–874. doi: 10.1089/scd.2017.0003.
- Leda, A. R., Bertrand, L., Andras, I. E., El-Hage, N., Nair, M., & Toborek, M. (2019). Selective disruption of the blood-brain barrier by zika virus. Frontiers in Microbiology , 10, 2158. doi: 10.3389/fmicb.2019.02158.
- Lee, U. N., Day, J. H., Haack, A. J., Bretherton, R. C., Lu, W., DeForest, C. A., … Berthier, E. (2020). Layer-by-layer fabrication of 3D hydrogel structures using open microfluidics. Lab on A Chip , 20(3), 525–536. doi: 10.1039/c9lc00621d.
- Liebner, S., Fischmann, A., Rascher, G., Duffner, F., Grote, E. H., Kalbacher, H., & Wolburg, H. (2000). Claudin-1 and claudin-5 expression and tight junction morphology are altered in blood vessels of human glioblastoma multiforme. Acta Neuropathologica , 100(3), 323–331. doi: 10.1007/s004010000180.
- Lim, R. G., Quan, C., Reyes-Ortiz, A. M., Lutz, S. E., Kedaigle, A. J., Gipson, T. A., … Thompson, L. M. (2017). Huntington's disease iPSC-derived brain microvascular endothelial cells reveal WNT-mediated angiogenic and blood-brain barrier deficits. Cell Reports , 19(7), 1365–1377. doi: 10.1016/j.celrep.2017.04.021.
- Linville, R. M., DeStefano, J. G., Sklar, M. B., Xu, Z., Farrell, A. M., Bogorad, M. I., … Searson, P. C. (2019). Human iPSC-derived blood-brain barrier microvessels: Validation of barrier function and endothelial cell behavior. Biomaterials , 190-191, 24–37. doi: 10.1016/j.biomaterials.2018.10.023.
- Lippmann, E. S., Al-Ahmad, A., Azarin, S. M., Palecek, S. P., & Shusta, E. V. (2014). A retinoic acid-enhanced, multicellular human blood-brain barrier model derived from stem cell sources. Scientific Reports , 4, 4160. doi: 10.1038/srep04160.
- Lippmann, E. S., Al-Ahmad, A., Palecek, S. P., & Shusta, E. V. (2013). Modeling the blood-brain barrier using stem cell sources. Fluids and Barriers of the CNS , 10(1), 2. doi: 10.1186/2045-8118-10-2.
- Lippmann, E. S., Azarin, S. M., Kay, J. E., Nessler, R. A., Wilson, H. K., Al-Ahmad, A., … Shusta, E. V. (2012). Derivation of blood-brain barrier endothelial cells from human pluripotent stem cells. Nature Biotechnology , 30(8), 783–791. doi: 10.1038/nbt.2247.
- Liu, W. Y., Wang, Z. B., Zhang, L. C., Wei, X., & Li, L. (2012). Tight junction in blood-brain barrier: An overview of structure, regulation, and regulator substances. CNS Neuroscience and Therapeutics , 18(8), 609–615. doi: 10.1111/j.1755-5949.2012.00340.x.
- Mao, L., Jin, H., Wang, M., Hu, Y., Chen, S., He, Q., … Hu, B. (2020). Neurologic manifestations of hospitalized patients with coronavirus disease 2019 in Wuhan, China. JAMA Neurology , doi: 10.1001/jamaneurol.2020.1127.
- McKernan, R., & Watt, F. M. (2013). What is the point of large-scale collections of human induced pluripotent stem cells? Nature Biotechnology , 31(10), 875–877. doi: 10.1038/nbt.2710.
- Miller, J. S., Stevens, K. R., Yang, M. T., Baker, B. M., Nguyen, D. H., Cohen, D. M., … Chen, C. S. (2012). Rapid casting of patterned vascular networks for perfusable engineered three-dimensional tissues. Nature Materials , 11(9), 768–774. doi: 10.1038/nmat3357.
- Minami, H., Tashiro, K., Okada, A., Hirata, N., Yamaguchi, T., Takayama, K., … Kawabata, K. (2015). Generation of brain microvascular endothelial-like cells from human induced pluripotent stem cells by co-culture with C6 glioma cells. Plos One , 10(6), e0128890. doi: 10.1371/journal.pone.0128890.
- Miranda, C. C., Fernandes, T. G., Pascoal, J. F., Haupt, S., Brustle, O., Cabral, J. M., & Diogo, M. M. (2015). Spatial and temporal control of cell aggregation efficiently directs human pluripotent stem cells towards neural commitment. Biotechnology Journal , 10(10), 1612–1624. doi: 10.1002/biot.201400846.
- Montagne, A., Barnes, S. R., Sweeney, M. D., Halliday, M. R., Sagare, A. P., Zhao, Z., … Zlokovic, B. V. (2015). Blood-brain barrier breakdown in the aging human hippocampus. Neuron , 85(2), 296–302. doi: 10.1016/j.neuron.2014.12.032.
- Moshayedi, P., Nih, L. R., Llorente, I. L., Berg, A. R., Cinkornpumin, J., Lowry, W. E., … Carmichael, S. T. (2016). Systematic optimization of an engineered hydrogel allows for selective control of human neural stem cell survival and differentiation after transplantation in the stroke brain. Biomaterials , 105, 145–155. doi: 10.1016/j.biomaterials.2016.07.028.
- Motallebnejad, P., Thomas, A., Swisher, S. L., & Azarin, S. M. (2019). An isogenic hiPSC-derived BBB-on-a-chip. Biomicrofluidics , 13(6), 064119. doi: 10.1063/1.5123476.
- Munji, R. N., Soung, A. L., Weiner, G. A., Sohet, F., Semple, B. D., Trivedi, A., … Daneman, R. (2019). Profiling the mouse brain endothelial transcriptome in health and disease models reveals a core blood-brain barrier dysfunction module. Nature Neuroscience , 22(11), 1892–+. doi: 10.1038/s41593-019-0497-x.
- Murphy, A. R., Haynes, J. M., Laslett, A. L., Cameron, N. R., & O'Brien, C. M. (2020). Three-dimensional differentiation of human pluripotent stem cell-derived neural precursor cells using tailored porous polymer scaffolds. Acta Biomaterialia , 101, 102–116. doi: 10.1016/j.actbio.2019.10.017.
- Neal, E. H., Marinelli, N. A., Shi, Y., McClatchey, P. M., Balotin, K. M., Gullett, D. R., … Lippmann, E. S. (2019). A simplified, fully defined differentiation scheme for producing blood-brain barrier endothelial cells from human iPSCs. Stem Cell Reports , 12(6), 1380–1388. doi: 10.1016/j.stemcr.2019.05.008.
- Neuhaus, W., & Noe, C. R. (2010). Transport at the blood-brain barrier. Wiley.
- Neuwelt, E. A., Bauer, B., Fahlke, C., Fricker, G., Iadecola, C., Janigro, D., … Drewes, L. R. (2011). Engaging neuroscience to advance translational research in brain barrier biology. Nature Reviews Neuroscience , 12(3), 169–182. doi: 10.1038/nrn2995.
- Nih, L. R., Moshayedi, P., Llorente, I. L., Berg, A. R., Cinkornpumin, J., Lowry, W. E., … Carmichael, S. T. (2017). Engineered HA hydrogel for stem cell transplantation in the brain: Biocompatibility data using a design of experiment approach. Data in Brief , 10, 202–209. doi: 10.1016/j.dib.2016.11.069.
- Nishihara, H., Soldati, S., Mossu, A., Rosito, M., Rudolph, H., Muller, W. A., … Engelhardt, B. (2020). Human CD4(+) T cell subsets differ in their abilities to cross endothelial and epithelial brain barriers in vitro. Fluids and Barriers of the CNS , 17(1), 3. doi: 10.1186/s12987-019-0165-2.
- Nzou, G., Wicks, R. T., Wicks, E. E., Seale, S. A., Sane, C. H., Chen, A., … Atala, A. J. (2018). Human cortex spheroid with a functional blood brain barrier for high-throughput neurotoxicity screening and disease modeling. Scientific Reports , 8(1), 7413. doi: 10.1038/s41598-018-25603-5.
- O'Shea, O., Steeg, R., Chapman, C., Mackintosh, P., & Stacey, G. N. (2020). Development and implementation of large-scale quality control for the European bank for induced Pluripotent Stem Cells. Stem Cell Research , 45, 101773. doi: 10.1016/j.scr.2020.101773.
- Ohshima, M., Kamei, S., Fushimi, H., Mima, S., Yamada, T., & Yamamoto, T. (2019). Prediction of drug permeability using in vitro blood-brain barrier models with human induced pluripotent stem cell-derived brain microvascular endothelial cells. BioResearch Open Access , 8(1), 200–209. doi: 10.1089/biores.2019.0026.
- Ormel, P. R., de Sa, R. V., van Bodegraven, E. J., Karst, H., Harschnitz, O., Sneeboer, M. A. M., … Pasterkamp, R. J. (2018). Microglia innately develop within cerebral organoids. Nature Communications , 9, 4167. doi: 10.1038/s41467-018-06684-2.
- Page, S., Raut, S., & Al-Ahmad, A. (2019). Oxygen-glucose deprivation/reoxygenation-induced barrier disruption at the human blood-brain barrier is partially mediated through the HIF-1 pathway. NeuroMolecular Medicine , 21(4), 414–431. doi: 10.1007/s12017-019-08531-z.
- Pamies, D., Bal-Price, A., Chesne, C., Coecke, S., Dinnyes, A., Eskes, C., … Daneshian, M. (2018). Advanced Good Cell Culture Practice for human primary, stem cell-derived and organoid models as well as microphysiological systems. Altex , 35(3), 353–378. doi: 10.14573/altex.1710081.
- Panza, F., Lozupone, M., Logroscino, G., & Imbimbo, B. P. (2019). A critical appraisal of amyloid-beta-targeting therapies for Alzheimer disease. Nature Reviews Neurology , 15(2), 73–88. doi: 10.1038/s41582-018-0116-6.
- Pardridge, W. M. (2005). The blood-brain barrier: Bottleneck in brain drug development. NeuroRx , 2(1), 3–14. doi: 10.1602/neurorx.2.1.3.
- Park, T. E., Mustafaoglu, N., Herland, A., Hasselkus, R., Mannix, R., FitzGerald, E. A., … Ingber, D. E. (2019). Hypoxia-enhanced Blood-Brain Barrier Chip recapitulates human barrier function and shuttling of drugs and antibodies. Nature Communications , 10(1), 2621. doi: 10.1038/s41467-019-10588-0.
- Pasca, A. M., Sloan, S. A., Clarke, L. E., Tian, Y., Makinson, C. D., Huber, N., … Pasca, S. P. (2015). Functional cortical neurons and astrocytes from human pluripotent stem cells in 3D culture. Nature Methods , 12(7), 671–678. doi: 10.1038/nmeth.3415.
- Patel, R., Page, S., & Al-Ahmad, A. J. (2017). Isogenic blood-brain barrier models based on patient-derived stem cells display inter-individual differences in cell maturation and functionality. Journal of Neurochemistry , 142(1), 74–88. doi: 10.1111/jnc.14040.
- Patsch, C., Challet-Meylan, L., Thoma, E. C., Urich, E., Heckel, T., O'Sullivan, J. F., … Cowan, C. A. (2015). Generation of vascular endothelial and smooth muscle cells from human pluripotent stem cells. Nature Cell Biology , 17(8), 994–1003. doi: 10.1038/ncb3205.
- Pfeiffer, F., Schafer, J., Lyck, R., Makrides, V., Brunner, S., Schaeren-Wiemers, N., … Engelhardt, B. (2011). Claudin-1 induced sealing of blood-brain barrier tight junctions ameliorates chronic experimental autoimmune encephalomyelitis. Acta Neuropathologica , 122(5), 601–614. doi: 10.1007/s00401-011-0883-2.
- Praca, C., Rosa, S. C., Sevin, E., Cecchelli, R., Dehouck, M. P., & Ferreira, L. S. (2019). Derivation of brain capillary-like endothelial cells from human pluripotent stem cell-derived endothelial progenitor cells. Stem Cell Reports , 13(4), 599–611. doi: 10.1016/j.stemcr.2019.08.002.
- Prieto, P., Blaauboer, B. J., de Boer, A. G., Boveri, M., Cecchelli, R., Clemedson, C., … Tahti, H. (2004). Blood-brain barrier in vitro models and their application in toxicology. The report and recommendations of ECVAM Workshop 49. Alternatives to Laboratory Animals , 32(1), 37–50. doi: 10.1177/026119290403200107.
- Prince, M., Bryce, R., Albanese, E., Wimo, A., Ribeiro, W., & Ferri, C. P. (2013). The global prevalence of dementia: A systematic review and metaanalysis. Alzheimer's and Dementia , 9(1), 63–75.e62. doi: 10.1016/j.jalz.2012.11.007.
- Profaci, C. P., Munji, R. N., Pulido, R. S., & Daneman, R. (2020). The blood-brain barrier in health and disease: Important unanswered questions. Journal of Experimental Medicine , 217(4), e20190062. doi: 10.1084/jem.20190062.
- Puelles, V. G., Lutgehetmann, M., Lindenmeyer, M. T., Sperhake, J. P., Wong, M. N., Allweiss, L., … Huber, T. B. (2020). Multiorgan and renal tropism of SARS-CoV-2. New England Journal of Medicine , doi: 10.1056/NEJMc2011400.
- Pulgar, V. M. (2018). Transcytosis to cross the blood brain barrier, new advancements and challenges. Frontiers in Neuroscience , 12, 1019. doi: 10.3389/fnins.2018.01019.
- Qian, T., Maguire, S. E., Canfield, S. G., Bao, X., Olson, W. R., Shusta, E. V., & Palecek, S. P. (2017). Directed differentiation of human pluripotent stem cells to blood-brain barrier endothelial cells. Science Advances , 3(11), e1701679. doi: 10.1126/sciadv.1701679.
- Qian, X., Jacob, F., Song, M. M., Nguyen, H. N., Song, H., & Ming, G. L. (2018). Generation of human brain region-specific organoids using a miniaturized spinning bioreactor. Nature Protocols , 13(3), 565–580. doi: 10.1038/nprot.2017.152.
- Qian, X., Nguyen, H. N., Song, M. M., Hadiono, C., Ogden, S. C., Hammack, C., … Ming, G. L. (2016). Brain-region-specific organoids using mini-bioreactors for modeling ZIKV exposure. Cell , 165(5), 1238–1254. doi: 10.1016/j.cell.2016.04.032.
- Raja, W. K., Mungenast, A. E., Lin, Y. T., Ko, T., Abdurrob, F., Seo, J., & Tsai, L. H. (2016). Self-Organizing 3D human neural tissue derived from induced pluripotent stem cells recapitulate Alzheimer's disease phenotypes. Plos One , 11(9), e0161969. doi: 10.1371/journal.pone.0161969.
- Reichel, A. (2006). The role of blood-brain barrier studies in the pharmaceutical industry. Current Drug Metabolism , 7(2), 183–203. doi: 10.2174/138920006775541525.
- Reinitz, A., DeStefano, J., Ye, M., Wong, A. D., & Searson, P. C. (2015). Human brain microvascular endothelial cells resist elongation due to shear stress. Microvascular Research , 99, 8–18. doi: 10.1016/j.mvr.2015.02.008.
- Ribecco-Lutkiewicz, M., Sodja, C., Haukenfrers, J., Haqqani, A. S., Ly, D., Zachar, P., … Bani-Yaghoub, M. (2018). A novel human induced pluripotent stem cell blood-brain barrier model: Applicability to study antibody-triggered receptor-mediated transcytosis. Scientific Reports , 8(1), 1873. doi: 10.1038/s41598-018-19522-8.
- Roudnicky, F., Kim, B. K., Lan, Y., Schmucki, R., Kuppers, V., Christensen, K., … Cowan, C. A. (2020). Identification of a combination of transcription factors that synergistically increases endothelial cell barrier resistance. Scientific Reports , 10(1), 3886. doi: 10.1038/s41598-020-60688-x.
- Roux, G. L., Jarray, R., Guyot, A. C., Pavoni, S., Costa, N., Theodoro, F., … Mabondzo, A. (2019). Proof-of-concept study of drug brain permeability between in vivo human brain and an in vitro iPSCs-human blood-brain barrier model. Scientific Reports , 9(1), 16310. doi: 10.1038/s41598-019-52213-6.
- Sakae, N., Liu, C. C., Shinohara, M., Frisch-Daiello, J., Ma, L., Yamazaki, Y., … Kanekiyo, T. (2016). ABCA7 Deficiency accelerates amyloid-beta generation and Alzheimer's neuronal pathology. Journal of Neuroscience , 36(13), 3848–3859. doi: 10.1523/JNEUROSCI.3757-15.2016.
- Savage, J. E., Jansen, P. R., Stringer, S., Watanabe, K., Bryois, J., de Leeuw, C. A., … Posthuma, D. (2018). Genome-wide association meta-analysis in 269,867 individuals identifies new genetic and functional links to intelligence. Nature Genetics , 50(7), 912–+. doi: 10.1038/s41588-018-0152-6.
- Schwedhelm, I., Zdzieblo, D., Appelt-Menzel, A., Berger, C., Schmitz, T., Schuldt, B., … Hansmann, J. (2019). Automated real-time monitoring of human pluripotent stem cell aggregation in stirred tank reactors. Scientific Reports , 9(1), 12297. doi: 10.1038/s41598-019-48814-w.
- Shawahna, R., Uchida, Y., Decleves, X., Ohtsuki, S., Yousif, S., Dauchy, S., … Scherrmann, J. M. (2011). Transcriptomic and quantitative proteomic analysis of transporters and drug metabolizing enzymes in freshly isolated human brain microvessels. Molecular Pharmaceutics , 8(4), 1332–1341. doi: 10.1021/mp200129p.
- Silva, M. M., Rodrigues, A. F., Correia, C., Sousa, M. F., Brito, C., Coroadinha, A. S., … Alves, P. M. (2015). Robust expansion of human pluripotent stem cells: Integration of bioprocess design with transcriptomic and metabolomic characterization. Stem Cells Translational Medicine , 4(7), 731–742. doi: 10.5966/sctm.2014-0270.
- Sims, R., van der Lee, S. J., Naj, A. C., Bellenguez, C., Badarinarayan, N., Jakobsdottir, J., … Schellenberg, G. D. (2017). Rare coding variants in PLCG2, ABI3, and TREM2 implicate microglial-mediated innate immunity in Alzheimer's disease. Nature Genetics , 49(9), 1373–1384. doi: 10.1038/ng.3916.
- Smits, L. M., Reinhardt, L., Reinhardt, P., Glatza, M., Monzel, A. S., Stanslowsky, N., … Schwamborn, J. C. (2019). Modeling Parkinson's disease in midbrain-like organoids. NPJ Parkinson's Disease , 5, 5. doi: 10.1038/s41531-019-0078-4.
- St George-Hyslop, P. H., Tanzi, R. E., Polinsky, R. J., Haines, J. L., Nee, L., Watkins, P. C., … et al. (1987). The genetic defect causing familial Alzheimer's disease maps on chromosome 21. Science , 235(4791), 885–890. doi: 10.1126/science.2880399.
- Stanimirovic, D. B., Bani-Yaghoub, M., Perkins, M., & Haqqani, A. S. (2015). Blood-brain barrier models: in vitro to in vivo translation in preclinical development of CNS-targeting biotherapeutics. Expert Opinion on Drug Discovery , 10(2), 141–155. doi: 10.1517/17460441.2015.974545.
- Stebbins, M. J., Gastfriend, B. D., Canfield, S. G., Lee, M. S., Richards, D., Faubion, M. G., … Shusta, E. V. (2019). Human pluripotent stem cell-derived brain pericyte-like cells induce blood-brain barrier properties. Science Advances , 5(3), eaau7375. doi: 10.1126/sciadv.aau7375.
- Stebbins, M. J., Wilson, H. K., Canfield, S. G., Qian, T., Palecek, S. P., & Shusta, E. V. (2016). Differentiation and characterization of human pluripotent stem cell-derived brain microvascular endothelial cells. Methods , 101, 93–102. doi: 10.1016/j.ymeth.2015.10.016.
- Steinberg, S., Stefansson, H., Jonsson, T., Johannsdottir, H., Ingason, A., Helgason, H., … DemGene (2015). Loss-of-function variants in ABCA7 confer risk of Alzheimer's disease. Nature Genetics , 47(5), 445–U424. doi: 10.1038/ng.3246.
- Strazielle, N., & Ghersi-Egea, J. F. (2015). Efflux transporters in blood-brain interfaces of the developing brain. Frontiers in Neuroscience , 9, 21. doi: 10.3389/fnins.2015.00021.
- Sweeney, M. D., Sagare, A. P., & Zlokovic, B. V. (2018). Blood-brain barrier breakdown in Alzheimer disease and other neurodegenerative disorders. Nature Reviews Neurology , 14(3), 133–150. doi: 10.1038/nrneurol.2017.188.
- Sweeney, M. D., Zhao, Z., Montagne, A., Nelson, A. R., & Zlokovic, B. V. (2019). Blood-brain barrier: From physiology to disease and back. Physiological Reviews , 99(1), 21–78. doi: 10.1152/physrev.00050.2017.
- Tibbitt, M. W., & Anseth, K. S. (2009). Hydrogels as extracellular matrix mimics for 3D cell culture. Biotechnology and Bioengineering , 103(4), 655–663. doi: 10.1002/bit.22361.
- Tkachenko, E., Gutierrez, E., Saikin, S. K., Fogelstrand, P., Kim, C., Groisman, A., & Ginsberg, M. H. (2013). The nucleus of endothelial cell as a sensor of blood flow direction. Biology Open , 2(10), 1007–1012. doi: 10.1242/bio.20134622.
- Uchida, Y., Ohtsuki, S., Katsukura, Y., Ikeda, C., Suzuki, T., Kamiie, J., & Terasaki, T. (2011). Quantitative targeted absolute proteomics of human blood-brain barrier transporters and receptors. Journal of Neurochemistry , 117(2), 333–345. doi: 10.1111/j.1471-4159.2011.07208.x.
- Urich, E., Patsch, C., Aigner, S., Graf, M., Iacone, R., & Freskgard, P. O. (2013). Multicellular self-assembled spheroidal model of the blood brain barrier. Scientific Reports , 3, 1500. doi: 10.1038/srep01500.
- Vasquez, J. B., Fardo, D. W., & Estus, S. (2013). ABCA7 expression is associated with Alzheimer's disease polymorphism and disease status. Neuroscience Letters , 556, 58–62. doi: 10.1016/j.neulet.2013.09.058.
- Vatine, G. D., Al-Ahmad, A., Barriga, B. K., Svendsen, S., Salim, A., Garcia, L., … Svendsen, C. N. (2017). Modeling psychomotor retardation using iPSCs from MCT8-deficient patients indicates a prominent role for the blood-brain barrier. Cell Stem Cell , 20(6), 831–843. doi: 10.1016/j.stem.2017.04.002.
- Vatine, G. D., Barrile, R., Workman, M. J., Sances, S., Barriga, B. K., Rahnama, M., … Svendsen, C. N. (2019). Human iPSC-derived blood-brain barrier chips enable disease modeling and personalized medicine applications. Cell Stem Cell , 24(6), 995–1005.e1006. doi: 10.1016/j.stem.2019.05.011.
- Vijay, N., & Morris, M. E. (2014). Role of monocarboxylate transporters in drug delivery to the brain. Current Pharmaceutical Design , 20(10), 1487–1498. doi: 10.2174/13816128113199990462.
- Volpe, D. A. (2011). Drug-permeability and transporter assays in Caco-2 and MDCK cell lines. Future Medicinal Chemistry , 3(16), 2063–2077. doi: 10.4155/fmc.11.149.
- Volpe, D. A., Faustino, P. J., Ciavarella, A. B., Asafu-Adjaye, E. B., Ellison, C. D., Yu, L. X., & Hussain, A. S. (2007). Classification of drug permeability with a Caco-2 cell monolayer assay. Clinical Research and Regulatory Affairs , 24(1), 39–47. doi: 10.1080/10601330701273669.
- Wang, Y. I., Abaci, H. E., & Shuler, M. L. (2017). Microfluidic blood-brain barrier model provides in vivo -like barrier properties for drug permeability screening. Biotechnology and Bioengineering , 114(1), 184–194. doi: 10.1002/bit.26045.
- Waring, M. J., Arrowsmith, J., Leach, A. R., Leeson, P. D., Mandrell, S., Owen, R. M., … Weir, A. (2015). An analysis of the attrition of drug candidates from four major pharmaceutical companies. Nature Reviews Drug Discovery , 14(7), 475–486. doi: 10.1038/nrd4609.
- Weiss, N., Miller, F., Cazaubon, S., & Couraud, P. O. (2009). The blood-brain barrier in brain homeostasis and neurological diseases. Biochimica Et Biophysica Acta , 1788(4), 842–857. doi: 10.1016/j.bbamem.2008.10.022.
- Wevers, N. R., & de Vries, H. E. (2016). Morphogens and blood-brain barrier function in health and disease. Tissue Barriers , 4(1), e1090524. doi: 10.1080/21688370.2015.1090524.
- Wilson, H. K., Canfield, S. G., Hjortness, M. K., Palecek, S. P., & Shusta, E. V. (2015). Exploring the effects of cell seeding density on the differentiation of human pluripotent stem cells to brain microvascular endothelial cells. Fluids and Barriers of the CNS , 12, 13. doi: 10.1186/s12987-015-0007-9.
- Wilson, H. K., Faubion, M. G., Hjortness, M. K., Palecek, S. P., & Shusta, E. V. (2016). Cryopreservation of brain endothelial cells derived from human induced pluripotent stem cells is enhanced by rho-associated coiled coil-containing kinase inhibition. Tissue Engineering Part C: Methods , 22(12), 1085–1094. doi: 10.1089/ten.TEC.2016.0345.
- Wong, A. D., Ye, M., Levy, A. F., Rothstein, J. D., Bergles, D. E., & Searson, P. C. (2013). The blood-brain barrier: An engineering perspective. Frontiers in Neuroengineering , 6, 7. doi: 10.3389/fneng.2013.00007.
- Xiang, J., Andjelkovic, A. V., Wang, M. M., & Keep, R. F. (2017). Blood-brain barrier models derived from individual patients: A new frontier: An Editorial Highlight on ‘An isogenic blood-brain barrier model comprising brain endothelial cells, astrocytes, and neurons derived from human induced pluripotent stem cells’. Journal of Neurochemistry , 140(6), 843–844. doi: 10.1111/jnc.13961.
- Yamashita, M., Aoki, H., Hashita, T., Iwao, T., & Matsunaga, T. (2020). Inhibition of transforming growth factor beta signaling pathway promotes differentiation of human induced pluripotent stem cell-derived brain microvascular endothelial-like cells. Fluids and Barriers of the CNS , 17(1), 36. doi: 10.1186/s12987-020-00197-1.
- Yan, Y., Song, L., Madinya, J., Ma, T., & Li, Y. (2018). Derivation of cortical spheroids from human induced pluripotent stem cells in a suspension bioreactor. Tissue Engineering Part A , 24(5-6), 418–431. doi: 10.1089/ten.TEA.2016.0400.
- Yang, K., Lee, J. S., Kim, J., Lee, Y. B., Shin, H., Um, S. H., … Cho, S. W. (2012). Polydopamine-mediated surface modification of scaffold materials for human neural stem cell engineering. Biomaterials , 33(29), 6952–6964. doi: 10.1016/j.biomaterials.2012.06.067.
- Ye, M., Sanchez, H. M., Hultz, M., Yang, Z., Bogorad, M., Wong, A. D., & Searson, P. C. (2014). Brain microvascular endothelial cells resist elongation due to curvature and shear stress. Scientific Reports , 4, 4681. doi: 10.1038/srep04681.
- Zarbin, M., Sugino, I., & Townes-Anderson, E. (2019). Concise review: Update on retinal pigment epithelium transplantation for age-related macular degeneration. Stem Cells Translational Medicine , 8(5), 466–477. doi: 10.1002/sctm.18-0282.
KEY REFERENCES
- Abbott & Friedman, 2012. See above.
Gave a fundamental overview and introduction to understand the BBB in health and disease.
- Lippmann et al., 2012. See above.
Published for the first time a differentiation method to generate purified BCECs from hiPSCs. These hiPSC-derived BCECs are characterized by in vivo-like properties. This work pioneered the application of patient-specific hiPSC-derived BBB models in research.
- Urich et al., 2013. See above.
Investigated a three-dimensional multicellular spheroid model of the BBB, presenting for the first time a spontaneous and complex self-organization of multiple BBB cell types. Most importantly, this process was not dependent on artificial scaffolds, supporting the hypothesis that the formation and cellular architecture of the BBB is intrinsically programmed within the specific cell types.
- Patel, Page, & Al-Ahmad, 2017. See above.
Established an isogenic complex BBB model derived from patient-specific hiPSCs and investigated the impact of inter-individual genetic variations on the yield and phenotype of isogenic BBB models. Differences in differentiation capacity, maturation, and barrier properties between the models derived from different hiPSC lines supported the impact of individual polymorphisms in stem cell research and for precision medicine.
- Prieto et al., 2004. See above.
Within the report of the forty-ninth workshop organized by the EURL ECVAM this key reference gave fundamental recommendations on the application and validation of BBB in vitro models in toxicology screening strategies.
- Reichel, 2006. See above.
Reviewed the role of BBB studies for the pharmaceutical industry in preclinical drug discovery and development. Furthermore, the specific requirements of the pharmaceutical industry for the development of effective and safe new CNS medicines were summarized.
Citing Literature
Number of times cited according to CrossRef: 28
- Gabriela Xavier, Alexander Navarrete Santos, Carla Hartmann, Marcos L. Santoro, Nicole Flegel, Jessica Reinsch, Annika Majer, Toni Ehrhardt, Jenny Pfeifer, Andreas Simm, Thomas Hollemann, Sintia I. Belangero, Dan Rujescu, Matthias Jung, Comparison of Extracellular Vesicles from Induced Pluripotent Stem Cell-Derived Brain Cells, International Journal of Molecular Sciences, 10.3390/ijms25073575, 25 , 7, (3575), (2024).
- Ramyar Rahimi Darehbagh, Seyedeh Asrin Seyedoshohadaei, Rojin Ramezani, Nima Rezaei, Stem cell therapies for neurological disorders: current progress, challenges, and future perspectives, European Journal of Medical Research, 10.1186/s40001-024-01987-1, 29 , 1, (2024).
- Zimeng Cao, Fanshu Kong, Jiaqi Ding, Chunxia Chen, Fumei He, Wenbin Deng, Promoting Alzheimer’s disease research and therapy with stem cell technology, Stem Cell Research & Therapy, 10.1186/s13287-024-03737-w, 15 , 1, (2024).
- Shifaa M Abdin, Friederike Mansel, Anna Rafiei Hashtchin, Mania Ackermann, Gesine Hansen, Björn Becker, Benjamin Kick, Nhi Pham, Hendrik Dietz, Christoph Schaniel, Ulrich Martin, Ingo Spreitzer, Nico Lachmann, Sensor macrophages derived from human induced pluripotent stem cells to assess pyrogenic contaminations in parenteral drugs, Biofabrication, 10.1088/1758-5090/ad4744, 16 , 3, (035017), (2024).
- Asmaa Khalil, Alexandre Barras, Rabah Boukherroub, Ching-Li Tseng, David Devos, Thierry Burnouf, Winfried Neuhaus, Sabine Szunerits, Enhancing paracellular and transcellular permeability using nanotechnological approaches for the treatment of brain and retinal diseases, Nanoscale Horizons, 10.1039/D3NH00306J, 9 , 1, (14-43), (2024).
- Joanna M. Wasielewska, Kathryn Szostak, Lachlan E. McInnes, Hazel Quek, Juliana C. S. Chaves, Jeffrey R. Liddell, Jari Koistinaho, Lotta E. Oikari, Paul S. Donnelly, Anthony R. White, Patient-Derived Blood-Brain Barrier Model for Screening Copper Bis(thiosemicarbazone) Complexes as Potential Therapeutics in Alzheimer’s Disease, ACS Chemical Neuroscience, 10.1021/acschemneuro.3c00743, 15 , 7, (1432-1455), (2024).
- Ronny Vargas, Catalina Lizano-Barrantes, Miquel Romero, Kevin Valencia-Clua, David A. Narváez-Narváez, Josep Ma Suñé-Negre, Pilar Pérez-Lozano, Encarna García-Montoya, Noelia Martinez-Martinez, Cristina Hernández-Munain, Carlos Suñé, Marc Suñé-Pou, The piper at the gates of brain: A systematic review of surface modification strategies on lipid nanoparticles to overcome the Blood-Brain-Barrier, International Journal of Pharmaceutics, 10.1016/j.ijpharm.2024.124686, 665 , (124686), (2024).
- Sanjana Mathew-Schmitt, Matthias Peindl, Philipp Neundorf, Gudrun Dandekar, Marco Metzger, Vera Nickl, Antje Appelt-Menzel, Blood-tumor barrier in focus - investigation of glioblastoma-induced effects on the blood-brain barrier, Journal of Neuro-Oncology, 10.1007/s11060-024-04760-w, 170 , 1, (67-77), (2024).
- Arnaud Martino Capuzzo, Riccardo Ossanna, Lindsey Alejandra Quintero Sierra, Federica Virla, Alessandro Negri, Anita Conti, Andrea Sbarbati, Sheila Veronese, From the Classification of Stem Cells to the Release of Potential in Cell Therapies: Limits, Considerations and Future Aspects in Regenerative Medicine, Possibilities and Limitations in Current Translational Stem Cell Research, 10.5772/intechopen.110572, (2023).
- Vanessa Hall, Katja Maria Sahlgren Bendtsen, Getting closer to modeling the gut-brain axis using induced pluripotent stem cells, Frontiers in Cell and Developmental Biology, 10.3389/fcell.2023.1146062, 11 , (2023).
- Burak Ozgür, Elena Puris, Andreas Brachner, Antje Appelt-Menzel, Sabrina Oerter, Viktor Balzer, Mikkel Roland Holst, Rasmus Folmann Christiansen, Kathrine Hyldig, Stephen T. Buckley, Mie Kristensen, Seppo Auriola, Allan Jensen, Gert Fricker, Morten Schallburg Nielsen, Winfried Neuhaus, Birger Brodin, Characterization of an iPSC-based barrier model for blood-brain barrier investigations using the SBAD0201 stem cell line, Fluids and Barriers of the CNS, 10.1186/s12987-023-00501-9, 20 , 1, (2023).
- Undine Haferkamp, Carla Hartmann, Chaudhry Luqman Abid, Andreas Brachner, Alevtina Höchner, Anna Gerhartl, Bernadette Harwardt, Selin Leckzik, Jennifer Leu, Marco Metzger, Marina Nastainczyk-Wulf, Winfried Neuhaus, Sabrina Oerter, Ole Pless, Dan Rujescu, Matthias Jung, Antje Appelt-Menzel, Human isogenic cells of the neurovascular unit exert transcriptomic cell type-specific effects on a blood-brain barrier in vitro model of late-onset Alzheimer disease, Fluids and Barriers of the CNS, 10.1186/s12987-023-00471-y, 20 , 1, (2023).
- Alessandra Granata, Functional genomics in stroke: current and future applications of iPSCs and gene editing to dissect the function of risk variants, BMC Cardiovascular Disorders, 10.1186/s12872-023-03227-6, 23 , 1, (2023).
- Stéphane D. Girard, Ingrid Julien‐Gau, Yves Molino, Benjamin F. Combes, Louise Greetham, Michel Khrestchatisky, Emmanuel Nivet, High and low permeability of human pluripotent stem cell–derived blood–brain barrier models depend on epithelial or endothelial features, The FASEB Journal, 10.1096/fj.202201422R, 37 , 2, (2023).
- Kawthar K. Abla, Mohammed M. Mehanna, The battle of lipid-based nanocarriers against blood-brain barrier: a critical review, Journal of Drug Targeting, 10.1080/1061186X.2023.2247583, 31 , 8, (832-857), (2023).
- Ann-Kristin Reinhold, Beate Hartmannsberger, Malgorzata Burek, Heike L. Rittner, Stabilizing the neural barrier – A novel approach in pain therapy, Pharmacology & Therapeutics, 10.1016/j.pharmthera.2023.108484, 249 , (108484), (2023).
- Matthias Jung, Juliane-Susanne Jung, Jenny Pfeifer, Carla Hartmann, Toni Ehrhardt, Chaudhry Luqman Abid, Jenny Kintzel, Anne Puls, Anne Navarrete Santos, Thomas Hollemann, Dagmar Riemann, Dan Rujescu, Neuronal Stem Cells from Late-Onset Alzheimer Patients Show Altered Regulation of Sirtuin 1 Depending on Apolipoprotein E Indicating Disturbed Stem Cell Plasticity, Molecular Neurobiology, 10.1007/s12035-023-03633-z, 61 , 3, (1562-1579), (2023).
- Jihyoung Choi, Sanjana Mathew, Sabrina Oerter, Antje Appelt-Menzel, Jan Hansmann, Tobias Schmitz, Online Measurement System for Dynamic Flow Bioreactors to Study Barrier Integrity of hiPSC-Based Blood–Brain Barrier In Vitro Models, Bioengineering, 10.3390/bioengineering9010039, 9 , 1, (39), (2022).
- Ekta Jagtiani, Mihika Yeolekar, Shivraj Naik, Vandana Patravale, In vitro blood brain barrier models: An overview, Journal of Controlled Release, 10.1016/j.jconrel.2022.01.011, 343 , (13-30), (2022).
- Jann Harberts, Malte Siegmund, Carina Hedrich, Wonjong Kim, Anna Fontcuberta i Morral, Robert Zierold, Robert H. Blick, Generation of Human iPSC‐Derived Neurons on Nanowire Arrays Featuring Varying Lengths, Pitches, and Diameters, Advanced Materials Interfaces, 10.1002/admi.202200806, 9 , 24, (2022).
- Mark J. Lynch, Oliviero L. Gobbo, Advances in Non-Animal Testing Approaches towards Accelerated Clinical Translation of Novel Nanotheranostic Therapeutics for Central Nervous System Disorders, Nanomaterials, 10.3390/nano11102632, 11 , 10, (2632), (2021).
- Alberto Williams-Medina, Michael Deblock, Damir Janigro, In vitro Models of the Blood–Brain Barrier: Tools in Translational Medicine, Frontiers in Medical Technology, 10.3389/fmedt.2020.623950, 2 , (2021).
- Dariush Skowronek, Robin A. Pilz, Konrad Schwefel, Christiane D. Much, Ute Felbor, Matthias Rath, Bringing CCM into a dish: cell culture models for cerebral cavernous malformations, Medizinische Genetik, 10.1515/medgen-2021-2091, 33 , 3, (251-259), (2021).
- Jann Harberts, Katja Bours, Malte Siegmund, Carina Hedrich, Michael Glatza, Hans R. Schöler, Undine Haferkamp, Ole Pless, Robert Zierold, Robert H. Blick, Culturing human iPSC-derived neural progenitor cells on nanowire arrays: mapping the impact of nanowire length and array pitch on proliferation, viability, and membrane deformation, Nanoscale, 10.1039/D1NR04352H, 13 , 47, (20052-20066), (2021).
- Jann Harberts, Malte Siegmund, Matteo Schnelle, Ting Zhang, Yakui Lei, Linwei Yu, Robert Zierold, Robert H. Blick, Robust neuronal differentiation of human iPSC-derived neural progenitor cells cultured on densely-spaced spiky silicon nanowire arrays, Scientific Reports, 10.1038/s41598-021-97820-4, 11 , 1, (2021).
- Sara Wellens, Lucie Dehouck, Vidya Chandrasekaran, Pranika Singh, Rodrigo Azevedo Loiola, Emmanuel Sevin, Thomas Exner, Paul Jennings, Fabien Gosselet, Maxime Culot, Evaluation of a human iPSC-derived BBB model for repeated dose toxicity testing with cyclosporine A as model compound, Toxicology in Vitro, 10.1016/j.tiv.2021.105112, 73 , (105112), (2021).
- Zameel Cader, Human Blood-Brain-Barrier In Vitro Models: Overview and Applications, Physiology, Pharmacology and Pathology of the Blood-Brain Barrier, 10.1007/164_2021_562, (205-222), (2021).
- Daniel Besser, Advancing Stem Cell Technologies and Applications: A Special Collection from the PluriCore Network in the German Stem Cell Network (GSCN), Current Protocols in Stem Cell Biology, 10.1002/cpsc.129, 55 , 1, (2020).