High-Throughput Neurite Outgrowth Assay Using GFP-Labeled iPSC-Derived Neurons
Menghang Xia, Menghang Xia, Li Zhang, Li Zhang, Shuaizhang Li, Shuaizhang Li
Abstract
The potential neurotoxicity from an increasing number of drugs and untested environmental chemicals creates a need to develop reliable and efficient in vitro methods for identifying chemicals that may adversely affect the nervous system. An important process in neurodevelopment is neurite outgrowth, which can be affected by developmental neurotoxicity. Currently, neurite outgrowth assays rely mainly on staining, which requires multiple sample processing steps, particularly washing steps, that may introduce variation and limit throughput. Here, we describe a neurite outgrowth assay that uses induced pluripotent stem cell (iPSC)–derived human cortical glutamatergic neurons and/or spinal motor neurons labeled with green fluorescent protein (GFP) to test compounds in a high-content and high-throughput format. This method enables live and time-lapse imaging of GFP-labeled neurons using an assay plate that is continuously imaged at multiple times after chemical treatment. In this article, we describe how to thaw frozen GFP-labeled neurons, culture them, treat them with a compound of interest, and analyze neurite outgrowth using a high-content imaging platform. In this assay, GFP-labeled iPSC-derived human neurons represent a promising tool for identifying and prioritizing compounds with potential developmental neurotoxicity for further hazard characterization. © 2022 The Authors. Current Protocols published by Wiley Periodicals LLC. This article has been contributed to by U.S. Government employees and their work is in the public domain in the USA.
This article was corrected on 23 September 2022. See the end of the full text for details.
Basic Protocol 1 : Thawing and seeding of iPSC-derived neurons
Basic Protocol 2 : Compound plate preparation and treatment of neurons
Basic Protocol 3 : High-content imaging and analysis
INTRODUCTION
Many environmental chemicals, including heavy metals, pesticides, and food additives, exhibit neurotoxicity and developmental neurotoxicity (Alavanja, Hoppin, & Kamel, 2004; Aschner et al., 2017; Lau, McLean, Williams, & Howard, 2006; Wang et al., 2010), and are linked to the increasing incidence of neurological defects such as autism, attention deficit disorder, and hyperactivity disorder. With large numbers of chemicals being introduced into our environment, there is a great need to develop reliable and efficient high-throughput screening methods to identify compounds that could affect the nervous system.
Several in vitro assays have been developed to evaluate compounds for developmental neurotoxicity, and these are typically based on analysis of basic neuronal processes such as differentiation, proliferation, migration, and neurite outgrowth (Frimat et al., 2010; Harrill, Robinette, & Mundy, 2011; Krug et al., 2013; Radio & Mundy, 2008; Zimmer et al., 2012). Neurite outgrowth is characterized by axonal and dendritic processes with defined morphology. It can be characterized using a variety of metrics, including neurite length and area, which have been used extensively to evaluate developmental neurotoxicity and neurotoxicity (Radio & Mundy, 2008). High-content imaging (HCI) enables the assessment of multiple morphological parameters for neurite outgrowth and can differentiate specific effects on neurite outgrowth from nonspecific cytotoxicity (Harrill, Freudenrich, Machacek, Stice, & Mundy, 2010; Stiegler, Krug, Matt, & Leist, 2011). A high-throughput, HCI-based neurite outgrowth assay can be a promising tool for rapid profiling of a large number of chemicals for neurotoxicity and developmental neurotoxicity.
The effects of chemicals on neurite outgrowth have been studied in various in vitro models ranging from non-human primary cells to human induced pluripotent stem cell (iPSC)–derived neurons (Radio & Mundy, 2008; Schmidt et al., 2017). iPSC-derived neurons are more directly relevant to human biology and maintain the morphology and physiology of their in situ counterparts (Chai, Dage, & Citron, 2012; Haythornthwaite et al., 2012; Whitemarsh et al., 2012). These cells have been used successfully in high-content, high-throughput screening assays to evaluate compounds for developmental neurotoxicity and neurotoxicity (Ryan et al., 2016; Sirenko, Hesley, Rusyn, & Cromwell, 2014).
Neurite outgrowth assays commonly utilize βIII tubulin immunostaining or calcein AM staining (Krug et al., 2013; Radio, Breier, Shafer, & Mundy, 2008). While tubulin staining requires multiple washing steps, which is time-consuming and increases well-to-well variation, calcein AM can be used for live staining (no washing steps involved), but still cannot be used for time-lapse imaging due to cytotoxicity.
To address these issues, we developed and validated a robust, high-throughput, HCI-based neurite outgrowth assay for compound evaluation (Li et al., 2021). The assay uses commercially available human iPSC-derived cortical glutamatergic neurons and spinal motor neurons labeled with green fluorescent protein (GFP) to enable direct, live, and time-lapse imaging without wash steps. It can be performed in 96-, 384-, and 1536-well formats, greatly increasing throughput. Using this platform, neurons develop neurites rapidly and consistently, as quantified by automated HCI. Here, we provide a detailed neurite outgrowth assay protocol, including thawing and seeding of iPSC-derived neurons (see Basic Protocol 1), culturing of neurons and treating with test compounds (see Basic Protocol 2), and measurement and analysis of neurite outgrowth (see Basic Protocol 3). Figure 1 provides an outline of the entire workflow. Overall, this assay is reproducible and robust, and shows promise as a reliable high-throughput screening tool for identifying compounds with developmental neurotoxicity.
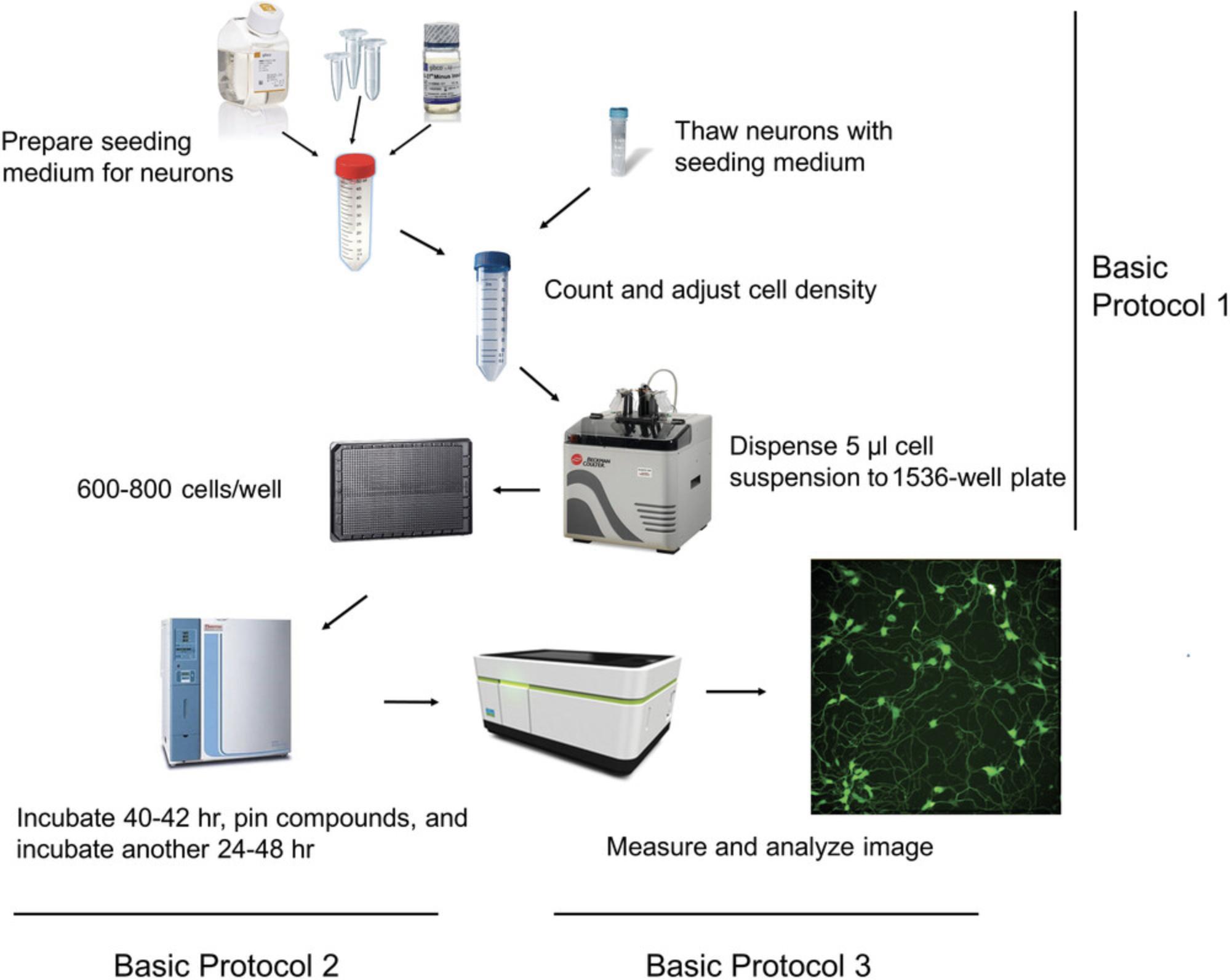
Basic Protocol 1: THAWING AND SEEDING OF iPSC-DERIVED NEURONS
This protocol explains how to prepare the seeding medium for neurons and thaw and seed neurons into a 1536-well plate. The iPSC-derived, GFP-labeled human neurons used here (cortical glutamatergic neurons and spinal motor neurons) are commercially available. Both cell types have high neuronal purity (>90%). Cortical glutamatergic neurons consist predominantly of excitatory neurons, whereas spinal motor neurons consist predominantly of motor neurons. We have successfully tested both neuron types in the neurite outgrowth assay described here. Users can choose the type that best suits their needs.
The seeding medium is prepared according to a recipe from BrainXell. It contains brain-derived neurotrophic factor (BDNF), which supports neuron proliferation and survival; glial-derived neurotrophic factor (GDNF, a disulfide-linked homodimer), which specifically promotes dopamine uptake, survival, and morphological differentiation of midbrain neurons; and transforming growth factor-β1 (TGF-β1), which regulates cell proliferation, growth, differentiation, motility, and the synthesis and deposition of extracellular matrix. BDNF, GDNF, and TGF-β1 are supplied as lyophilized powders and should be reconstituted according to manufacturer's instructions. For this assay, the recommended stock concentrations are 10 μg/ml for BDNF and GDNF and 1 μg/ml for TGF-β1.All stock solutions should be stored at −20°C in aliquots to avoid freeze-thaw cycles, which could compromise the integrity of the solutions.
Materials
- Common ingredients for seeding medium:
- DMEM/F12 medium, no phenol red (Thermo Fisher Scientific, cat. no. 11039021)
- Neurobasal medium, no phenol red (Thermo Fisher Scientific, cat. no. 12348017)
- B27 supplement (Thermo Fisher Scientific, cat. no. 17504-044)
- N2 supplement (Thermo Fisher Scientific, cat. no. 17502-048)
- Geltrex (Thermo Fisher Scientific, cat. no. A1413201)
- GlutaMAX (Thermo Fisher Scientific, cat. no. 35050-061)
- BDNF (Peprotech, cat. no. 450-02)
- GDNF (Peprotech, cat. no. 450-10)
- TGF-β1 (Peprotech, cat. no. 100-21C)
- Pen/strep (Thermo Fisher Scientific, cat. no. 15140-122)
- GFP-labeled human cortical glutamatergic neurons and supplement (BrainXell, cat. no. BX-0300)
- GFP-labeled human spinal motor neurons and supplement (BrainXell, cat. no. BX-0100)
- 70% (v/v) ethanol
- 0.4% trypan blue
- Laminar flow hood (Labconco Purifier BSC Class II or equivalent)
- 50-ml conical tubes
- 37°C water bath
- 10-ml serological pipette
- Sterile cell strainer (Corning, Fisher Scientific, cat. no. 07201430)
- Small plastic tubes (e.g., microcentrifuge tubes)
- Hemocytometer
- Tissue culture microscope (Nikon or equivalent)
- Automated reagent dispenser (e.g., BioRAPTR Flying Reagent Dispenser [FRD], Beckman Coulter, cat. no. BioRAPTR2 or Multidrop Combi Reagent Dispenser, Thermo Fisher Scientific, cat. no. 5840300)
- PDL-coated 1536-well black clear-bottom assay plates (Aurora Microplates, LoBase plates, cat. no. E8-1B1-210000-PDL)
- Stainless steel assay plate lid with holes (Wako Automation)
- 37°C humidified incubator with 5% CO2
Prepare seeding medium
1.Gather all components for the seeding medium in a cell culture hood (biological safety cabinet). Include the common ingredients as well as the cell-specific supplements provided with the cortical glutamatergic neurons and spinal motor neurons.
2.Thaw an aliquot of Geltrex (15 mg/ml) on ice and then dilute 1:10 using cold DMEM/F12 medium to give a working stock of 1.5 mg/ml.
3.Combine all ingredients in a sterile 50-ml conical tube, using separate tubes for cortical versus motor neuron seeding medium.
9.6 ml 1× DMEM/F12 medium (final 0.5×)
9.6 ml 1× Neurobasal medium (final 0.5×)
400 μl 50× B27 supplement (final 1×)
200 μl 100× N2 supplement (final 1×)
200 μl 1.5 mg/ml Geltrex (final 15 μg/ml)
50 μl 200 mM GlutaMAX (final 0.5 mM)
20 μl 10 μg/ml BDNF (final 10 ng/ml)
20 μl 10 μg/ml GDNF (final 10 ng/ml)
20 μl 1 μg/ml TGF-β1 (final 1 ng/ml)
20 μl 1000× cortical or motor neuron supplement (final 1×)
100 μl 200× penicillin/streptomycin (final 1×)
4.Allow medium to equilibrate to room temperature for 15 min. Do not warm in a 37°C water bath.
Thaw neurons and prepare suspension
5.Transfer a cryovial of neurons from liquid nitrogen to a 37°C water bath and gently move the vial within the water to increase the rate of thawing. To minimize contamination, be careful to avoid submerging the cap.
6.As soon as the last ice melts, remove the vial from the water bath, disinfect by spraying the vial with 70% ethanol, and transfer to the cell culture hood.
7.Slowly add 800 μl of the appropriate seeding medium to the vial at a rate of ∼1 drop/s using a 1-ml pipette tip.
8.Gently transfer the entire contents (1 ml total) to a new sterile 50-ml conical tube.
9.Gently add 1 ml of the appropriate seeding medium to the original vial to collect residual cells and transfer to the same 50-ml tube.
10.Slowly add an additional 3 ml seeding medium to the 50-ml tube using a 10-ml serological pipette, swirling the tube gently while adding the medium.
11.Mix suspension completely and filter through a cell strainer to a new 50-ml conical tube.
Determine neuron viability
12.Gently swirl the tube of cells, take a 10-μl aliquot, and mix with an equal volume of 0.4% trypan blue in a small plastic tube. Incubate ∼3 min at room temperature.
13.Apply a drop of trypan blue/cell mixture to a hemacytometer and count unstained (viable) and stained (nonviable) cells separately.
14.Determine the density of viable cells (D v in cells/ml) by multiplying the total number of viable cells by 2 (the dilution factor in trypan blue).
15.Determine the density of total cells (D t in cells/ml) by adding the number of viable and nonviable cells and multiplying by 2.
16.Calculate the percentage of viable cells as % viable cells = D v/D t × 100.
Seed neurons
17.Transfer the required number of cells to a new 50-ml conical tube and then bring the viable cell density to 1.2–1.6 × 105 cells/ml by adding the appropriate volume of seeding medium.
18.Dispense 5 μl cell suspension (600-800 cells) to each well of a PDL-coated 1536-well plate using a multi-drop Combi Dispenser or BioRAPTR.
19.Without moving the plate, cover it with a stainless steel lid and leave on the bench at room temperature for 15 min to allow the cells to attach.
20.After 15 min, observe the cells under a microscope to check cell density and distribution.
21.Gently transfer the assay plate to a 37°C humidified incubator with 5% CO2 and incubate 40-42 hr to allow neurite formation. Check daily under a microscope for neurite formation.
Basic Protocol 2: COMPOUND PLATE PREPARATION AND TREATMENT OF NEURONS
In addition to the seeded assay plate prepared in Basic Protocol 1, two additional 1536-well plates are needed: one for control compounds and one for test compounds. The control plate contains both a known positive control and a negative control, which are used to normalize the test compound data. Here, we use the neurotoxicant rotenone as a positive control for inhibition of neurite outgrowth. In the plate layout shown in Figure 2, rotenone is plated as a serial dilution (column 1) and as 16 replicates of three different concentrations (columns 2-3). The serial dilution can be used to calculate the IC50, which can be used to compare reproducibility for the assay plate. When we perform data analysis, we commonly use the maximum inhibition value of rotenone to normalize the data. By using three different concentrations in 16 replicates, we can pick the highest inhibition value to normalize the data. In addition to rotenone, we use tetraoctylammonium bromide as a positive control for cytotoxicity. As a negative control, we use DMSO (vehicle). For the test compound plate, compounds are diluted in DMSO and placed in columns 5-48.The final DMSO concentration in the wells is generally less than 0.5%, which has no toxic effect on the cells. Screening large numbers of test compounds may require multiple plates, but only a single control plate is needed. This is why control and test compounds are plated separately
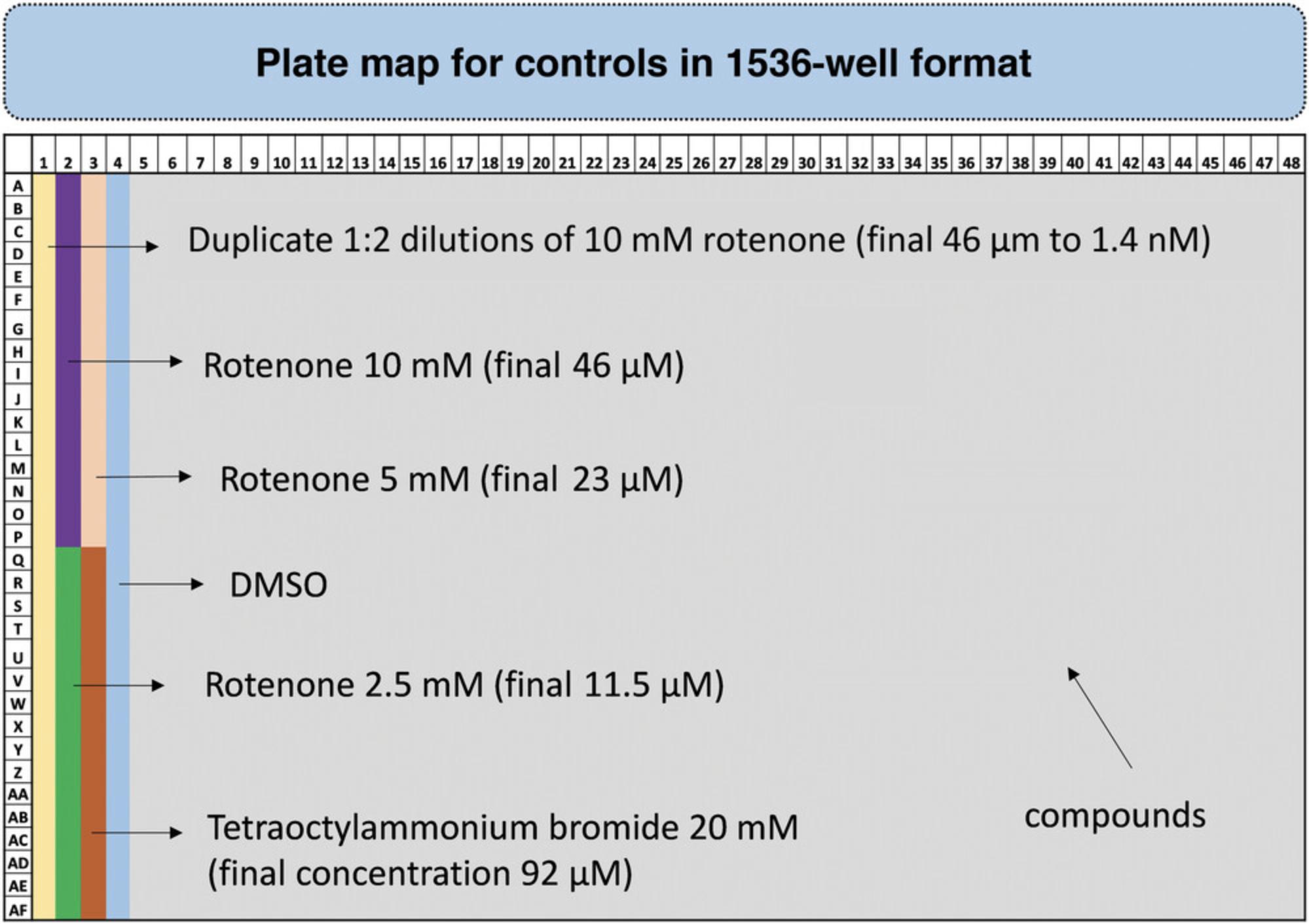
For compound treatment, 23 nl of each control and test compound is transferred to the assay plate using the PinTool workstation. This protocol describes how to prepare the control plate and transfer both control and test compounds to the assay plate containing cells. The control plate can be prepared as soon as the assay plate containing neurons is placed in the incubator (see Basic Protocol 1).
NOTE : This protocol can be adapted to treat neurons in a 96- or 384-well assay plate, but it is then critical to dilute compounds in medium or PBS instead of DMSO to avoid the effect of high DMSO concentrations on the neurons. The amount of compounds transferred to a 1536-well plate (23 nl) contains little enough DMSO to be ignored. For a 96- or 384-well format, the volume transferred is increased to 10 µl/well, significantly increasing the amount of DMSO.
Materials
- Rotenone (Sigma, cat. no. R-8875)
- Tetraoctylammonium bromide (Sigma, cat. no. 14866-33-2)
- Dimethyl sulfoxide (DMSO, Sigma, cat. no. D2650)
- Seeded assay plate (see Basic Protocol 1)
- 96-, 384-, and 1536-well clear round-bottom plates (Greiner, cat. nos. 650001 and 789270-C; Costar, cat. no. 3656)
- Multichannel pipetter (e.g., Capp C10-16 MultiChannel Pipettor) with tips
- Automated pipetting system (e.g., CyBi-Well Vario Multichannel Automated Pipetting System, Analytik Jena)
- Plate centrifuge
- Tissue culture microscope
- PinTool Compound Transfer Workstation (Wako Automation)
Prepare control and test compound plates
1.Dissolve positive control compounds (rotenone, tetraoctylammonium bromide) individually in DMSO (or the appropriate solvent) to make 10 mM stock solutions.
2.Prepare a 1:2 serial dilution of rotenone in the first two columns of a 96-well plate (16 wells), starting with 10 mM and ending at 305 nM (16 concentrations, 200 µl volume each). Use a multichannel pipetter to transfer the dilutions to the first column of a 384-well plate.
3.Add any other controls to the 384-well plate. In our example, this would be one column (16 wells) each for:
Three concentrations of rotenone (10, 5, and 2.5 mM)
Tetraoctylammonium bromide (20 mM)
DMSO
4.Use a multichannel pipettor or automated multichannel pipetting system to transfer 5 µl/well from the 384-well plate to rows 1-4 of a 1536-well plate as shown in Figure 2.
5.Prepare the test compound plate by adding 5 µl/well of the desired compounds and dilutions to rows 5-48 of a second 1536-well plate.
6.Centrifuge both 1536-well plates for 10 s at 1000 rpm, room temperature, and seal with film for later use.
Transfer compounds to assay plate
7.After the seeded assay plate has incubated 40-42 hr, check neurite formation under a microscope.
8.Remove the assay plate lid and place the plate inside the PinTool workstation along with the control and test compound plates.
9.Use the PinTool workstation to transfer 23 nl from each well of the compound plates to the assay plate.
10.Cover the assay plate with the stainless steel lid and return to the incubator for 24-48 hr.
Basic Protocol 3: HIGH-CONTENT IMAGING AND ANALYSIS
High-throughput imaging enables visualization and quantification by capturing many cellular features on a large scale using automated microscopy and image analysis platforms. High-content screening, or automated microscope-based screening, is one such approach that combines multiparametric microscopy with quantitation of a large dataset of morphological features. Measuring the length of neurite outgrowth can be done using an HCI platform to visually examine cells in a time-dependent manner (Li & Xia, 2019). Here, we describe how to measure neurite outgrowth and analyze the imaging data using the Operetta CLS High Content Analysis System. Images are taken from each well, and then the platform's image analysis software (here, Harmony 4.6) is used to convert the images into numerical data for comparison between treatment and controls. This protocol also describes how to analyze the data generated to extract the relevant information regarding the effect of test chemicals on neurite outgrowth.
Materials
- Treated assay plate (see Basic Protocol 2)
- Sterile breathable microporous sealing film (USA Scientific, cat. no. 2920-1010)
- Operetta CLS High Content Analysis System (PerkinElmer, cat. no. HH16000000) with Harmony 4.6 High Content Imaging and Analysis software package
Acquire images
1.Open Harmony software on the computer connected to the Operetta system and sign in.
2.Remove assay plate from the incubator and replace the lid with a sterilize breathable adhesive film.
3.Clean the bottom of the plate with fine paper towel to keep particles from interfering with imaging, and place the plate onto the plate holder. Click the “Load” button on the Harmony screen to load the plate into the system.
4.Under “Setup”, select the following parameters:
Plate type: | Aurora 1536-well low-base PDL-coated microplate |
Objective: | 20× water |
Mode: | Confocal |
Binning: | 2 (for a 1536-well plate) |
Channel: | EGFP (460-490 nm ex., 500-550 nm em.) |
5.Under “Navigation”, select one well and one field, and then adjust the exposure parameters (time, power, and height) to focus the image. To confirm the focus, test several other wells.
6.Under “Layout Selection”, select the outcome parameters (algorithmic outputs) for neurite outgrowth:
total neurite length per well
root number per well
neurite segments per well
maximum neurite length per well
7.Under “Navigation”, highlight all wells and fields to be imaged, provide a file name for images to be saved, and start measurements (run experiment).
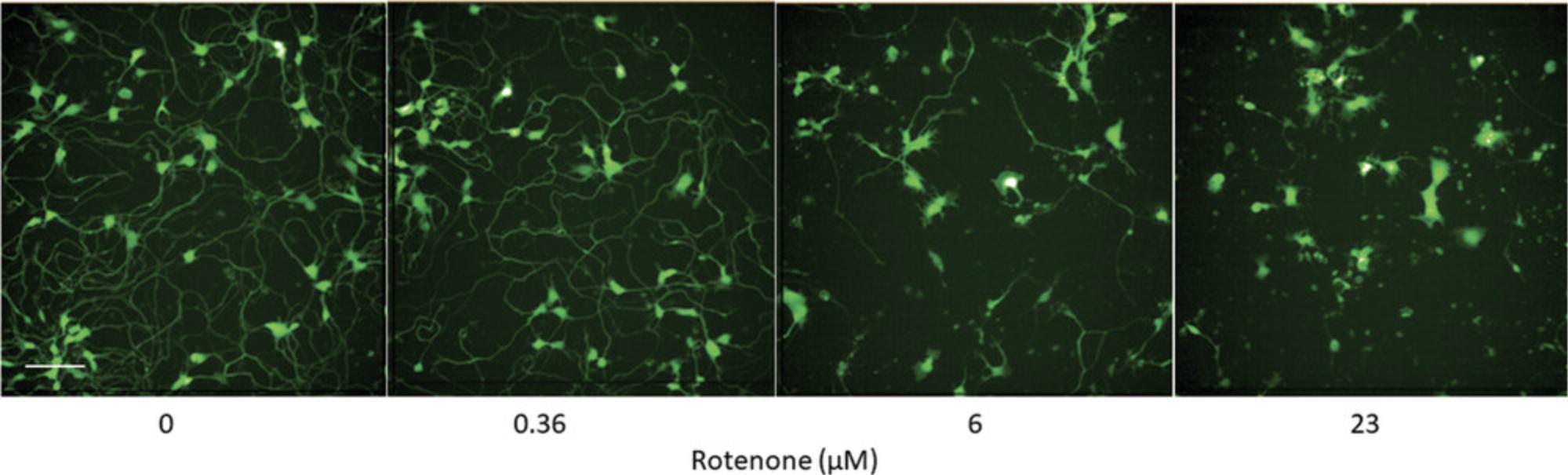
8.When measurement is complete, remove the plate. If it will be measured again (e.g., for a time course), replace the adhesive film with the lid and return the plate to the incubator until the next measurement.
Analyze images
9.Under the “Image Analysis” function in Harmony, select a measurement file and then select an image and field from one well.
10.When the image shows on the screen, find nuclei through the EGFP channel and name it as “All Cells” (number of objects) for data output.
11.Find neurites through the EGFP channel and use the “Population of All Cells”, “Region of Nucleus”, and “Method of CSIRO Neurite Analysis” tools.
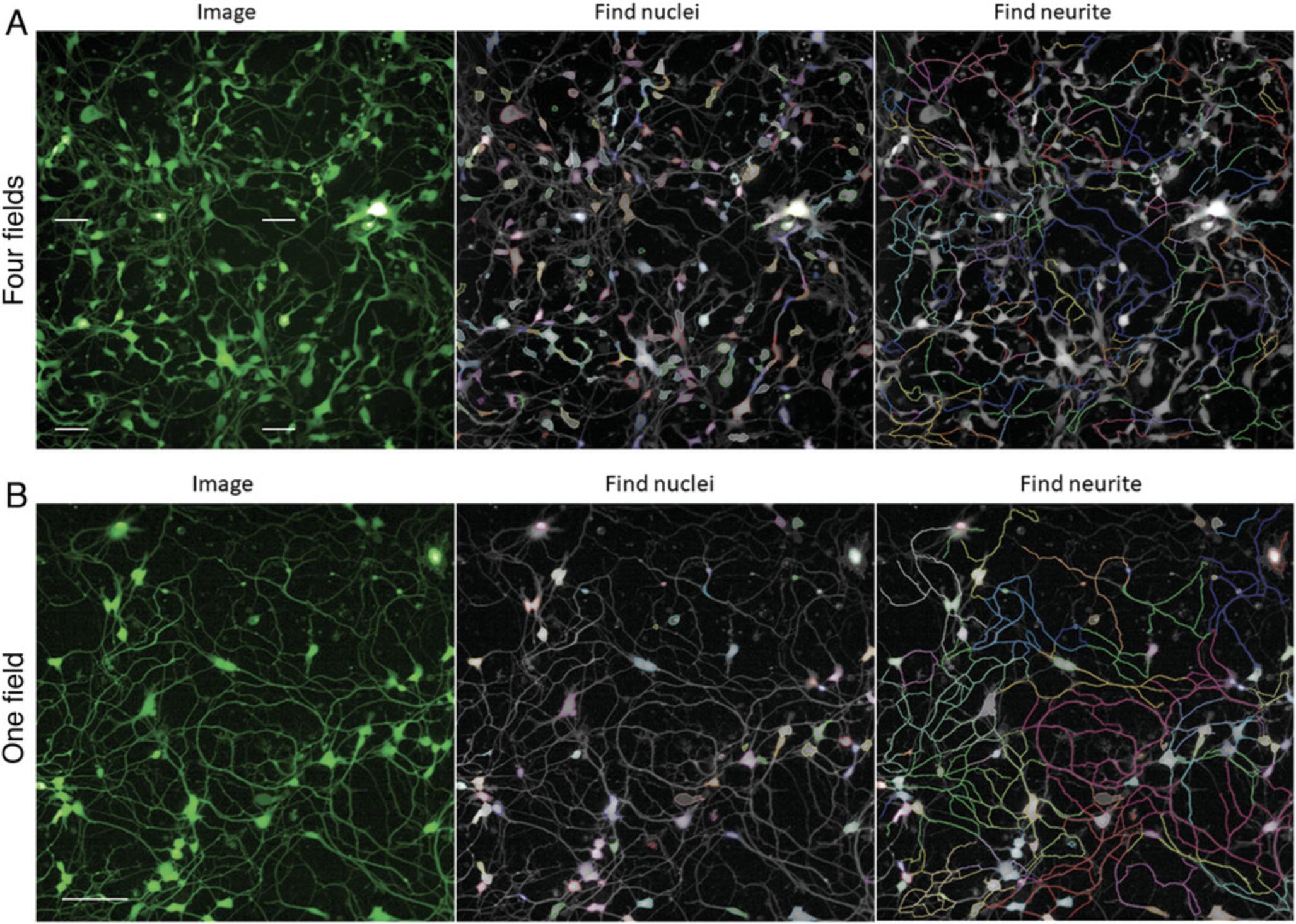
12.Select the outputs: neurite segments, total neurite length, root number, and maximum neurite length per well per cell.
13.Highlight fields in each well, select the images per well you wish to analyze, and start analyzing images by clicking “Evaluation”.
14.Select the table with numerical data and save as an Excel file for further analysis.
COMMENTARY
Background Information
Neurotoxicity and developmental neurotoxicity can alter the normal activity of the nervous system and is a major cause of neuro-related diseases. To evaluate environmental chemicals for their neurotoxicity potential, several in vitro neurotoxicity assays have been developed based on cell viability, neuronal differentiation, neurite outgrowth, and neuronal function (e.g., spontaneous electrical activity of neuronal networks) (Aschner et al., 2017). Each of these assays has limitations. Viability assays, for example, cannot assess phenotypic changes in neurons, and neuronal functional assays (e.g., multielectrode array assays) are not easy to use in a high-throughput screening platform.
Measurement of neurite outgrowth is considered a critical assay for evaluating nervous system development (Aschner et al., 2017). Indeed, neurite outgrowth assays in a high-throughput format and using a high-content platform have been reported for screening compounds for developmental neurotoxicity or neurotoxicity (Ryan et al., 2016; Sirenko et al., 2014). In general, immunostaining or dye staining is used to measure neurite outgrowth. For example, Radio et al. (2008) used βIII tubulin immunostaining to measure neurite outgrowth in PC-12 rat neuronal cells. However, tubulin staining requires multiple washing steps, which is time-consuming and prone to well-to-well variation. Another type of neurite outgrowth assay using calcein AM to stain live cell structures has been developed for HCI (Krug et al., 2013). However, the calcein AM method still requires time-consuming staining steps. Furthermore, calcein AM causes cytotoxicity when extended staining times are used, so this method is not amenable to time-lapse readouts.
The assay described here can measure neurite outgrowth in real time because it uses GFP-labeled human neurons (Li et al., 2021). This robust method does not require washing steps, which greatly improves screening throughput and reduces well-to-well variation (Li et al., 2021), as well as reducing cell disturbance and assay time. More importantly, this assay enables live and time-lapse imaging of neurite outgrowth, allowing for observation of chemical effects over time. We have obtained neurite outgrowth read-outs 24 and 48 hr after compound treatment within one continuous experimental assay.
Details about the development, optimization, and validation of this assay have been reported previously (Li et al., 2021) and demonstrate the efficiency and reliability of the protocol. Using this assay, we have shown that the well-known neurotoxic compound rotenone displays concentration-dependent inhibition of neurite outgrowth in both cortical and motor neurons. Its relative activity was compared to the vehicle (DMSO) using multiple endpoints (total neurite outgrowth, number of neurite segments, maximum neurite length, and number of objects) in 1536-well plates. Our data demonstrated that human iPSC-derived neurons are amenable to high-throughput assays of neurite outgrowth using HCI, supporting the use of this platform for testing the effect of test compounds for neurotoxicity potential.
When performing the neurite outgrowth assay, the number of objects can be measured in the same well and used to identify and count the number of cells in order to evaluate neuronal cell viability (cytotoxicity) due to compound treatment. Additionally, at the end of imaging, cytotoxicity can be evaluated using Calcein Red AM (AAT Bioquest; Li et al., 2021).
Critical Parameters
Preparation
It is important to have all materials and reagents ready before the experiment. Users should pay special attention to the specific requirements of the neurons, medium, plate types, plate dispenser, compound transfer system (e.g., PinTool workstation), and high-content imaging system.
All solutions used in this assay, including the seeding medium, should be freshly and carefully prepared before the experiment. Geltrex, which is used in the seeding medium to improve cell attachment, should be thawed on ice and diluted 1:10 before being added to the medium. If desired, it can be aliquoted either straight or as a 1:10 dilution and stored at −20°C for up to one month. DMEM/F12 medium should be used straight out of 4°C for maximum activity. To protect cells from contamination, pen/strep is added to the seeding medium at half the concentration used in cell culture.
It is especially important that plates are coated with PDL so that neurons attach firmly to the plate. We previously tested eight different coating conditions for optimization: PDL, laminin, polyethyleneimine (PEI), collagen I, fibronectin, laminin, Cultrex Basement Membrane Extract (BME), PDL-BME, and uncoated. In our hands, PDL provided the highest imaging quality as a coating agent. The use of low-base PDL-coated plates (which have a shorter distance between the cell surface and the microscope lens) improved image quality even further.
Thawing and seeding neurons
To obtain high-quality images and reproducible results, proper thawing and seeding of neurons is critical. Neurons should be stored in liquid nitrogen or at −150°C, and thawing should be performed gently and slowly, using freshly prepared medium. Thawed neurons should be filtered through a cell strainer to obtain a single-neuron suspension and remove clumps before counting.
Viable neurons should be counted with a hemocytometer to determine their density. The culture density should be adjusted to 1.2–1.6 × 105 viable cells/ml to obtain 600-800 cells/well in 1536-well plates. However, the number of cells per well should be optimized before a formal experiment. One vial of neurons (∼5 × 106 cells) is typically enough for three 1536-well plates. For high-quality results, cell viability should be more than 80%.
Two automated dispensing systems can be used. The BioRAPTR FRD is a liquid handling system that can transfer 0.2-10 µl of up to four different reagents or cell suspensions simultaneously into a 1536-well plate. The Multidrop Combi Reagent Dispenser is a high-speed dispenser capable of dispensing one reagent or cell suspension at a time using an eight-channel detachable dispensing cassette. When using any automated dispenser, it is especially important to start with a single-neuron suspension to minimize clogging of the tubing.
The stainless steel plate lids should have small, evenly spaced holes to allow for air exchange, which is necessary in cellular assays. This recommended assay lid also has a rubber gasket that sits around the top outer edge of the plate. The weight of the lid allows the gasket to form a strong barrier around the plate, virtually eliminating edge effects. The lid should be disinfected with 70% (v/v) ethanol before use. The assay plate should be kept in a 37°C incubator with 5% CO2 and 95% humidity.
Plates should be checked under a microscope every day to evaluate neuron and neurite conditions.
Handling controls and test compounds
A CyBi-Well Vario Pipettor (96-, 384-, and 1536-channel) is the most suitable piece of equipment for preparing the control plate in a 1536-well format. It requires the use of disposable tips.
A PinTool workstation is needed to transfer 23 nl of test and control compounds from the 1536-well compound and control plate to the 1536-well assay plate with neurons.
We commonly use a 10 mM stock compound concentration because most compounds are soluble at this concentration. When making multiple concentrations, we usually use a 1:2 or 1:3 serial dilution in DMSO.
Imaging
During imaging, it is critical to select the right plate type at “Setup” and to use confocal mode and a 20× water-immersion objective for a 1536-well format. Due to the small size of these wells, focusing is important for image quality. Exposure parameters such as time, power, and height should be optimized to make the image clear. Using low-base 1536-well plates can also increase image quality.
An HCI instrument with proper analysis software is required to capture images and convert image data to numerical data. We use the Operetta CLS High Content Analysis System (PerkinElmer, HH16000000) with Harmony software v. 4.6.
Troubleshooting
This high-throughput, HCI neurite outgrowth assay is robust, reliable, and reproducible. It is not difficult to perform when done carefully. Nonetheless, great attention must be given to each step, especially when thawing and seeding neurons. Common problems encountered with the protocol are listed in Table 1 along with possible causes and solutions.
Problem | Possible cause | Solution |
---|---|---|
Neurons dying or not growing | Inappropriate seeding medium | Use correct medium according to BrainXell protocol |
DMSO toxicity | Test DMSO concentrations on neurons during assay optimization | |
Neurites did not form | Inappropriate plate | Use coated plate |
Inappropriate thawing and seeding | Check seeding medium; thaw neurons slowly and gently | |
Inappropriate culture conditions | Check incubator temperature, humidity, and CO2 | |
No GFP signal | Wrong channel used for imaging | Select correct channel (e.g., 460-490 nm ex., 500-550 nm em.) |
Inappropriate plate | Use plate with clean, clear bottom | |
Image out of focus | Adjust setup parameters to focus the bottom of the plate | |
Images are crowded | Too many cells seeded per well | Adjust cell density (for a 1536- well plate, 600-800 neurons/well is recommended) |
Images have neuron clumps | Neurons clumped during thawing | Filter suspension through a cell strainer to ensure a single-cell suspension |
Images have spotty background | Contamination | Add pen/strep to seeding medium |
Images not clear | Out of focus | Check plate type in setup; readjust exposure parameters (time, power, height) |
Large well-to-well variation | Different numbers of neurons in wells | Use a cell dispenser (e.g., BioRAPTR) to plate cells |
Inappropriate algorithmic outputs | Optimize algorithmic outputs (total neurite length, root number, neurite segments, and maximum neurite length); select the best output to measure neurite outgrowth |
Understanding Results
Neurite outgrowth is analyzed using four parameters: total neurite length, number of neurite segments, maximum neurite length, and number of objects. The raw data are saved in Microsoft Excel and then normalized using the positive and DMSO controls. The normalized data can be transferred to any plotting software (e.g., GraphPad) to calculate the IC50 (concentration of half-maximal inhibition) and efficacy (% inhibition) of each compound. Figure 5 shows total neurite length per cell per well measured at different times from the same plate. It demonstrates that a neurite grows faster in the first three days of culture and then slows down. We selected the treatment time and duration (i.e., 40-42 hr initial culture; 24-48 hr treatment) based on this observation. Figure 6 shows the inhibitory effect of the positive control rotenone on neurite outgrowth.
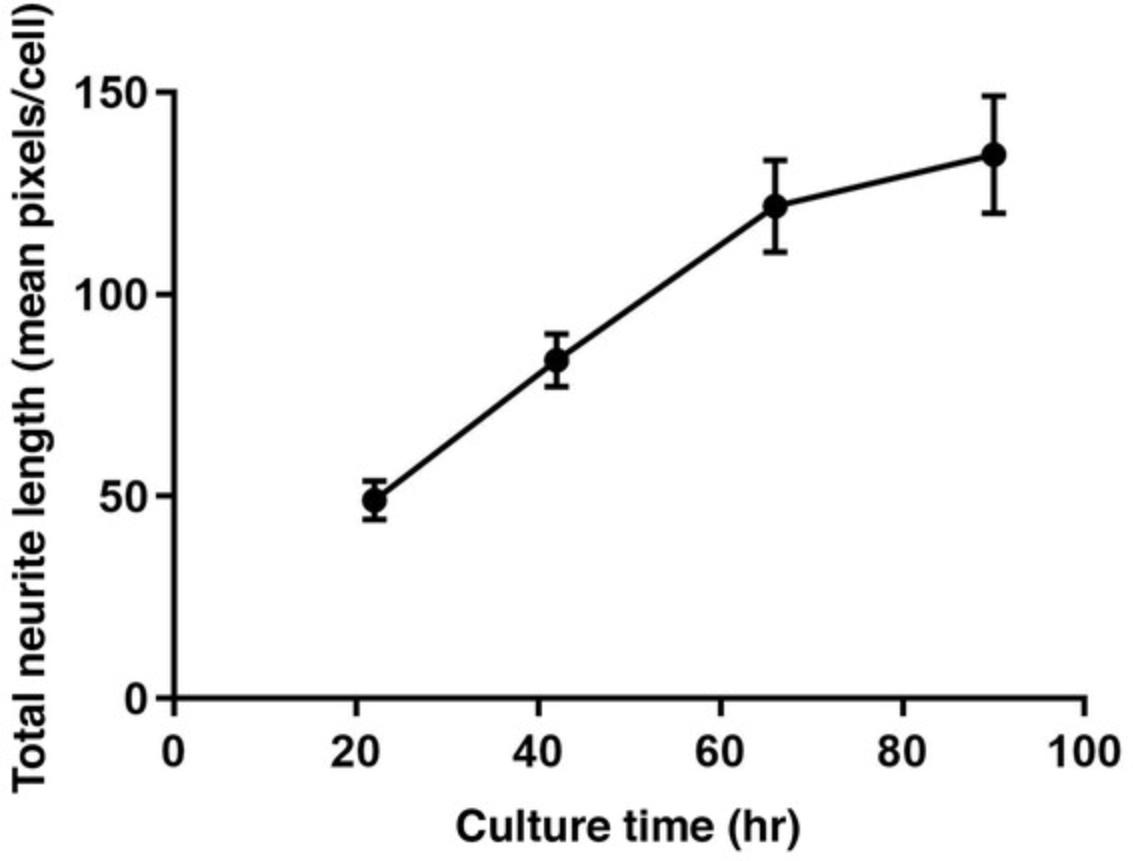
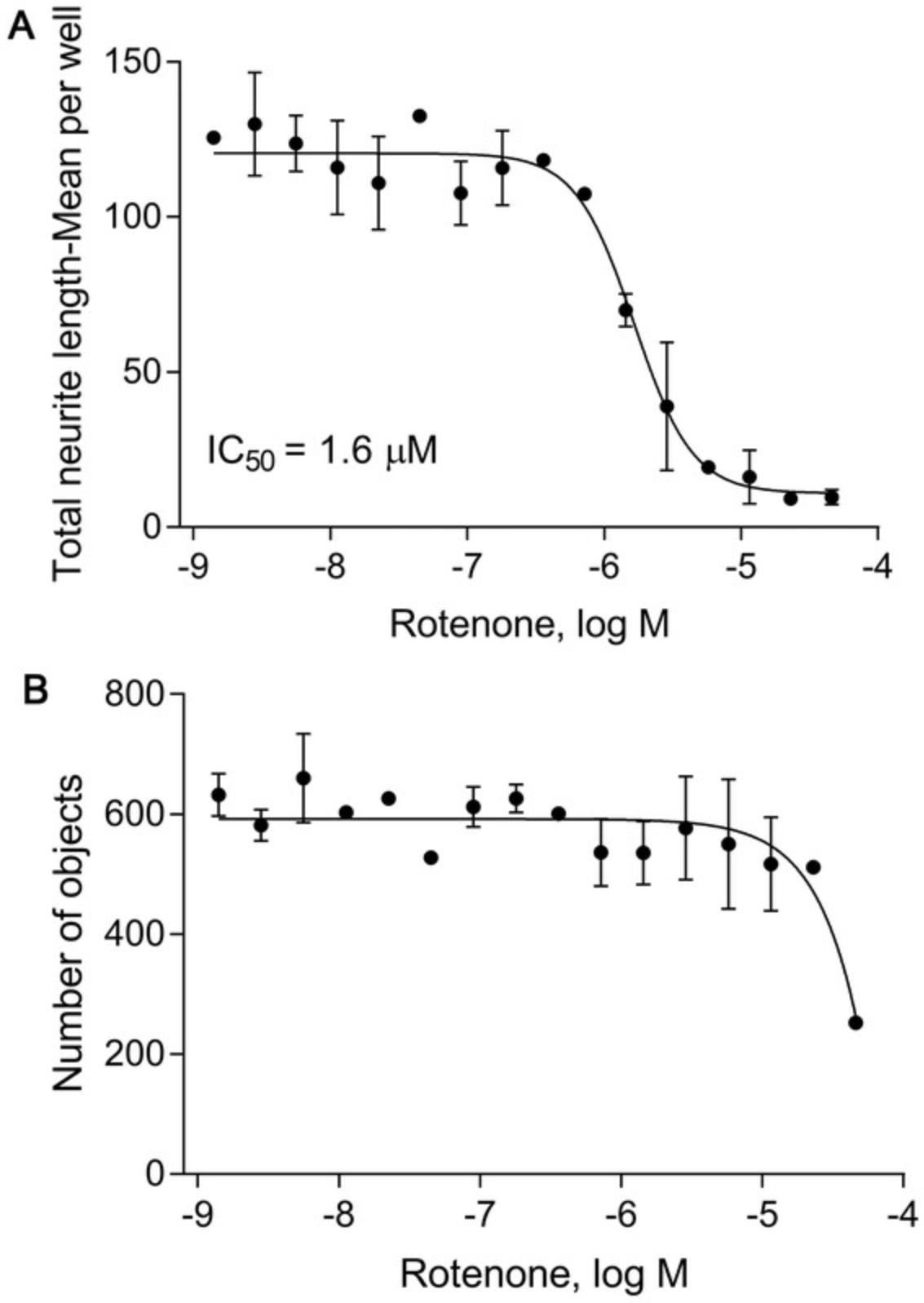
For comparison, the number of objects can be used when evaluating cell viability in the presence of each compound. For instance, Figure 6 shows the inhibitory effect of rotenone on neurite outgrowth (Fig. 6A) as well as the cytotoxicity generated from the assay treatment (Fig. 6B). Rotenone inhibits neurite outgrowth with an IC50 of 1.6 μM, but only affects the number of neurons at higher concentrations (46 μM).
Neurite outgrowth can also be examined qualitatively by direct visualization of the images. Figure 3 shows images of neurite outgrowth following rotenone treatment at three concentrations at a single time point (i.e., 48 hr). This demonstrates that rotenone inhibits neurite outgrowth in a concentration-dependent manner.
Time Considerations
It takes 4-5 days to complete the assay, including incubation for cell attachment and neurite formation. We suggest thawing neurons on Monday afternoon (day 1), treating with compounds on Wednesday morning (day 3, after 40-42 hr incubation), and taking images on Thursday morning (day 4, after 24 hr treatment) or Friday morning (day 5, after 48 hr treatment).
It takes 90 min to acquire an image of four fields in each well of a 1536-well plate using a 20× water objective and confocal mode. It takes 10-15 min to then evaluate the images. For quick image measurement, one field can be considered, cutting the time down to 24 min per plate.
Acknowledgments
We thank Dr. Caitlin Lynch for editing this manuscript. This work was supported in part by the Intramural Research Program of the National Center for Advancing Translational Sciences (NCATS), National Institutes of Health (NIH).
Author Contributions
Li Zhang : Data curation, formal analysis, methodology, visualization, writing (original draft); Shuaizhang Li : Methodology, writing (review and editing); Menghang Xia : Conceptualization, data curation, supervision, validation, visualization, writing (review and editing).
Conflict of Interest
The authors declare no conflicts of interest. All authors read and approved the final manuscript.
Open Research
Data Availability Statement
Data sharing is not applicable to this article as no new data were created or analyzed in this study.
Literature Cited
- Alavanja, M. C. R., Hoppin, J. A., & Kamel, F. (2004). Health effects of chronic pesticide exposure: Cancer and neurotoxicity. Annual Review of Public Health , 25, 155–197. doi: 10.1146/annurev.publhealth.25.101802.123020
- Aschner, M., Ceccatelli, S., Daneshian, M., Fritsche, E., Hasiwa, N., Hartung, T., … Lein, P. J. (2017). Reference compounds for alternative test methods to indicate developmental neurotoxicity (DNT) potential of chemicals: Example lists and criteria for their selection and use. ALTEX , 34(1), 49–74. doi: 10.14573/altex.1604201
- Chai, X., Dage, J. L., & Citron, M. (2012). Constitutive secretion of tau protein by an unconventional mechanism. Neurobiology of Disease , 48(3), 356–366. doi: 10.1016/j.nbd.2012.05.021
- Frimat, J. P., Sisnaiske, J., Subbiah, S., Menne, H., Godoy, P., Lampen, P., … West, J. (2010). The network formation assay: A spatially standardized neurite outgrowth analytical display for neurotoxicity screening. Lab on a Chip , 10(6), 701–709. doi: 10.1039/b922193j
- Harrill, J. A., Freudenrich, T. M., Machacek, D. W., Stice, S. L., & Mundy, W. R. (2010). Quantitative assessment of neurite outgrowth in human embryonic stem cell-derived hN2 cells using automated high-content image analysis. Neurotoxicology , 31(3), 277–290. doi: 10.1016/j.neuro.2010.02.003
- Harrill, J. A., Robinette, B. L., & Mundy, W. R. (2011). Use of high content image analysis to detect chemical-induced changes in synaptogenesis in vitro. Toxicology in Vitro , 25(1), 368–387. doi: 10.1016/j.tiv.2010.10.011
- Haythornthwaite, A., Stoelzle, S., Hasler, A., Kiss, A., Mosbacher, J., George, M., … Fertig, N. (2012). Characterizing human ion channels in induced pluripotent stem cell-derived neurons. Journal of Biomolecular Screening , 17(9), 1264–1272. doi: 10.1177/1087057112457821
- Krug, A. K., Balmer, N. V., Matt, F., Schonenberger, F., Merhof, D., & Leist, M. (2013). Evaluation of a human neurite growth assay as specific screen for developmental neurotoxicants. Archives of Toxicology , 87(12), 2215–2231. doi: 10.1007/s00204-013-1072-y
- Lau, K., McLean, W. G., Williams, D. P., & Howard, C. V. (2006). Synergistic interactions between commonly used food additives in a developmental neurotoxicity test. Toxicological Sciences , 90(1), 178–187. doi: 10.1093/toxsci/kfj073
- Li, S., & Xia, M. (2019). Review of high-content screening applications in toxicology. Archives of Toxicology , 93(12), 3387–3396. doi: 10.1007/s00204-019-02593-5
- Li, S., Zhang, L., Huang, R., Xu, T., Parham, F., Behl, M., & Xia, M. (2021). Evaluation of chemical compounds that inhibit neurite outgrowth using GFP-labeled iPSC-derived human neurons. Neurotoxicology , 83, 137–145. doi: 10.1016/j.neuro.2021.01.003
- Radio, N. M., Breier, J. M., Shafer, T. J., & Mundy, W. R. (2008). Assessment of chemical effects on neurite outgrowth in PC12 cells using high content screening. Toxicological Sciences , 105(1), 106–118. doi: 10.1093/toxsci/kfn114
- Radio, N. M., & Mundy, W. R. (2008). Developmental neurotoxicity testing in vitro : Models for assessing chemical effects on neurite outgrowth. Neurotoxicology , 29(3), 361–376. doi: 10.1016/j.neuro.2008.02.011
- Ryan, K. R., Sirenko, O., Parham, F., Hsieh, J. H., Cromwell, E. F., Tice, R. R., & Behl, M. (2016). Neurite outgrowth in human induced pluripotent stem cell-derived neurons as a high-throughput screen for developmental neurotoxicity or neurotoxicity. Neurotoxicology , 53, 271–281. doi: 10.1016/j.neuro.2016.02.003
- Schmidt, B. Z., Lehmann, M., Gutbier, S., Nembo, E., Noel, S., Smirnova, L., … Dinnyes, A. (2017). In vitro acute and developmental neurotoxicity screening: An overview of cellular platforms and high-throughput technical possibilities. Archives of Toxicology , 91(1), 1–33. doi: 10.1007/s00204-016-1805-9
- Sirenko, O., Hesley, J., Rusyn, I., & Cromwell, E. F. (2014). High-content high-throughput assays for characterizing the viability and morphology of human iPSC-derived neuronal cultures. Assay and Drug Development Technologies , 12(9-10), 536–547. doi: 10.1089/adt.2014.592
- Stiegler, N. V., Krug, A. K., Matt, F., & Leist, M. (2011). Assessment of chemical-induced impairment of human neurite outgrowth by multiparametric live cell imaging in high-density cultures. Toxicological Sciences , 121(1), 73–87. doi: 10.1093/toxsci/kfr034
- Wang, X., Meng, D., Chang, Q., Pan, J., Zhang, Z., Chen, G., … Shi, X. (2010). Arsenic inhibits neurite outgrowth by inhibiting the LKB1-AMPK signaling pathway. Environmental Health Perspectives , 118(5), 627–634. doi: 10.1289/ehp.0901510
- Whitemarsh, R. C., Strathman, M. J., Chase, L. G., Stankewicz, C., Tepp, W. H., Johnson, E. A., & Pellett, S. (2012). Novel application of human neurons derived from induced pluripotent stem cells for highly sensitive botulinum neurotoxin detection. Toxicological Sciences , 126(2), 426–435. doi: 10.1093/toxsci/kfr354
- Zimmer, B., Lee, G., Balmer, N. V., Meganathan, K., Sachinidis, A., Studer, L., & Leist, M. (2012). Evaluation of developmental toxicants and signaling pathways in a functional test based on the migration of human neural crest cells. Environmental Health Perspectives , 120(8), 1116–1122. doi: 10.1289/ehp.1104489
Key Reference
- Li et al. (2021). See above.
- Detailed description of the use of GFP-labeled iPSC-derived human neurons for neurite outgrowth assay.
Corrections
In this publication, the following corrections have been made.
Article Introduction, 4th paragraph (p.2): The sentence:
“While tubulin staining requires multiple washing steps, which is time-consuming and increases well-to-well variation, calcein AM staining also requires washing steps and in addition cannot be used for time-lapse imaging due to cytotoxicity.”
has been edited to read:
“While tubulin staining requires multiple washing steps, which is time-consuming and increases well-to-well variation, calcein AM can be used for live staining (no washing steps involved), but still cannot be used for time-lapse imaging due to cytotoxicity.”
Commentary, Background Information, 2nd paragraph (p. 10): The sentence:
“However, calcein AM staining also requires time-consuming washing steps.”
has been edited to read:
“However, the calcein AM method still requires time-consuming staining steps.”
The current version online now includes this information and may be considered the authoritative version of record.