High-Resolution Multi-Isotope Imaging Mass Spectrometry (MIMS) Imaging Applications in Stem Cell Biology
G. McMahon, G. McMahon, C.P. Lechene, C.P. Lechene
Abstract
Multi-isotope imaging mass spectrometry (MIMS) allows the measurement of turnover of molecules within intracellular compartments with a spatial resolution down to 30 nm. We use molecules enriched in stable isotopes administered to animals by diet or injection, or to cells through the culture medium. The stable isotopes used are, in general, 15N, 13C, 18O, and 2H. For stem cell studies, we essentially use 15N-thymidine, 13C-thymidine, and 81Br from BrdU. This protocol describes the practical use of MIMS with specific reference to applications in stem cell research. This includes choice and administration of stable isotope label(s), sample preparation, best practice for high-resolution imaging, secondary ion mass spectrometry using the Cameca NanoSIMS 50L, and methods for robust statistical analysis of label incorporation in regions of interest (ROI). © 2021 The Authors. Current Protocols published by Wiley Periodicals LLC.
Basic Protocol 1 : Stable isotope labeling of DNA in cultured cells
Basic Protocol 2 : Stable isotope labeling of DNA in animals
Basic Protocol 3 : Preparation of Si chips, the general sample support for NanoSIMS analysis
Basic Protocol 4 : Stable isotope analysis of DNA replication in single nuclei in a population of cells with NanoSIMS
Basic Protocol 5 : Data reduction and processing
INTRODUCTION
Multi-isotope imaging mass spectrometry (MIMS) visualizes the incorporation of non-toxic stable isotopes into cells and tissues by combining a state-of-the-art secondary ion mass spectrometer, the use of stable isotope reporters, and intensive computation on samples which can be prepared by any laboratory experienced in electron microscopy sample preparation of cells and tissues. One may think of MIMS as a major leap over autoradiography, due to lateral imaging resolution similar to usual electron microscopy (EM).
This article provides a guide to studying stem cells by MIMS. We will discuss choice of DNA stable isotope precursor labels, methods for their administration in cells or live animals, and important parameters that differ from standard electron microscopy sample preparation protocols. Best practices for MIMS analysis using a Cameca nanoscale SIMS instrument (NanoSIMS 50L) are provided. Useful tips accumulated through our practical experience are also shared in the Commentary. The protocol may be easily adapted to other biological samples.
STRATEGIC PLANNING
MIMS uses stable isotopes to identify and quantify newly synthesized strands of DNA and can be used in experiments with cells or in live animal stem cell experiments. It allows the visualization and measurement of stable isotope−labeled thymidine incorporation into newly synthesized DNA. Thymidine labeled with different stable isotopes is commercially available (15N-thymidine, 13C-thymidine, 2H-thymidine) or can be custom made (18O-thymidine). Also, the thymidine analogs BrdU and IdU can be analyzed by MIMS by measuring the natural 81Br and 127I signals. Thus, within the same experiment, one can pulse serially using 15N-thymidine, 13C-thymidine, 2H-thymidine, and 18O-thymidine, and/or with BrdU or IdU. This allows the researcher to study DNA synthesis in cells or tissue from the same experiments at different times in the cell cycle or at different ages of animal. Stem cells can be tagged with several types of probes (Fu & Kraitchman, 2010), many of which contain elements not normally present in biological samples that can be directly identified with MIMS (e.g., CdSe, ZnSe quantum dots, fluorescent probes containing I). In this article, we assume that each laboratory will have their own general cell culture and animal tissue preparation procedures. We will only present steps that are relevant to MIMS analysis. Basic Protocol 1 addresses cultured cells and Basic Protocol 2 live animals. Basic Protocol 3 describes the preparation of Si chip substrates required in all MIMS experiments, and Basic Protocol 4 describes important parameters for high-precision NanoSIMS analysis. Basic Protocol 5 describes basic data processing and analysis.
Basic Protocol 1: STABLE ISOTOPE LABELING OF DNA IN CULTURED CELLS
We will describe the preparation of labeled thymidine solution and the stable isotopes that may be used. The choice of stable isotope label(s) should be carefully considered. Stable isotope distributions are studied by examining isotope ratio images. The sensitivity is the ability to measure a small excess of label over the natural abundance. A high sensitivity is easier to achieve with isotopes of low natural abundances. For example, 15N- or 18O-thymidine (natural abundances 0.36% and 0.20%, respectively) are preferable to 13C-thymidine (1.08% natural abundance).
Materials
-
Stable isotope−labeled thymidine and/or analogs (BrdU, IdU)
-
Cells or tissues of interest
-
Propylene oxide (Polysciences, Cat. No. 00236-1)
-
Epoxy, eg. Poly/Bed 812 (Polysciences, Cat. No. 08792-1)
-
35-mm culture dishes (see step 2, below)
-
Si chips (5 mm × 5 mm; see Basic Protocol 3)
-
Centrifuge
-
Vacuum oven
-
Embedding mold
-
Freeze-drying apparatus (see step 4, below)
1.Preparation of thymidine solution.
The solubility of thymidine in water is relatively low (50 mg/ml), giving a maximum concentration of 0.2 M. A useful trick is to prepare the thymidine stock solution directly in the bottle containing the powder received from the supplier.
Working concentrations of 15N-thymidine solution will vary depending upon the organ and duration of chase used in the study.
2.Cell culturing.
Normal cell culturing protocols may be followed. For MIMS analysis, more than enough cells are produced when seeded in 35-mm culture dishes.
Use of culture dishes commercially manufactured for optimal cell attachment must be avoided. The surface layer treatment acts as a “sponge” that can retain the stable isotope label after washing steps. If necessary, dishes can be prepared in the lab with attachment factors such as fibronectin. For similar reasons, ultra-low-attachment culture dishes that are treated with a hydrogel must be avoided.
For study of DNA replication, a molecule that is a precursor of DNA (in general thymidine) labeled with a stable isotope is added to the culture during the cell cycle, in the log phase of growth or in the lag phase. One can use serial labeling at different times in the cell cycle in a single experiment by using thymidine tagged with different stable isotopes (2H-thymidine, 13C-thymidine, 15N-thymidine, 18O-thymidine), or thymidine analogs such as BrdU and IdU. All of these are readily available except for 18O-thymidine. Radiolabels such as 3H-thymidine and 14C-thymidine can also be used. The labels incorporated into the replicating DNA will all be identified by the NanoSIMS on the same sample. Note that 2H-thymidine or 3H-thymidine should be used with caution, because even at room temperature substantial exchange with unlabeled hydrogen in cellular water can occur (Pinson, 1952).
Cells may be fixed, resin-embedded, and prepared as thin sections, or cultured directly on sterilized Si substrate carriers, freeze dried, and analyzed as whole cells in the NanoSIMS.
-
Thin-section preparation.
Cells can be prepared as thin sections (as in optical microscopy) but deposited on Si chip supports instead of glass slides. Thin-section preparations are also suitable for high-resolution SEM imaging. Excellent staining procedures for SEM imaging can be found in the literature (Bushong et al., 2015; Deerinck et al., 2010; Katchalski et al., 2018). In general, the heavy elements contained in the stain will not interfere with signals from stable isotopes incorporated in precursor molecules used for MIMS analysis.
- b.Whole mount preparation.
Cells can be cultured directly on the Si chips, freeze dried, and analyzed as whole mounts. The chips must be sterilized before use. As NanoSIMS analysis peels away (sputters) the sample in slices as thin as a few atomic monolayers, it produces successive isotopic image slices through the cell at the same x -y location. By analyzing whole mounts, we can reconstruct mass-resolved 3D images of, for example, DNA distribution within a single nucleus. It does, however, require long durations (days or weeks) of analysis at the NanoSIMS.
3.Fixation and embedding.
Cultured cells are fixed in situ while adhered to the culture dish and lifted off with propylene oxide to maintain cell structure (trypsinizing cells causes them to lose their original shape).
-
Add sufficient propylene oxide to cover the cells in the culture dish. Cells will detach and float. They should be removed, transferred to a centrifuge tube, and soaked in propylene oxide for 20-30 min. Centrifuge the cells to obtain a tight pellet. Resuspend in propylene oxide and repeat the 20-30 min soak followed by centrifugation to a tight pellet.
-
Infiltrate the cell pellet for several hours to overnight (covered) in equal parts epoxy (containing hardener and accelerator) and propylene oxide. The longer the better for embedding. If leaving overnight, place in the refrigerator to prevent the epoxy from becoming too sticky. The next day, let samples warm to room temperature in the hood before embedding.
-
To embed, warm an appropriate amount of 50/50 epoxy/propylene oxide blend (to make it less viscous) in a 60°C vacuum oven for 10-15 min. Pour the warmed epoxy into a suitable mold. Let the epoxy sit in the oven 5 min before embedding to let air bubbles rise. Immerse infiltrated cell pellet into the epoxy and let sit in a 60°C oven under vacuum until hardened (at least 8 hr).
Choice of embedding resin is important. For epoxy resins, one has to use a cross-linker for polymerization. These cross-linkers contain nitrogen. This fact has to be taken into account when one needs to measure small increments above the natural15N/14N ratio. Alternatively, one can use the acrylic resin LR White®, which is hardened without the use of a cross-linker, resulting in a resin with lower nitrogen content.
It is also important to avoid cross-linking agents when antibodies are used post fixation. The crosslinking agent may occupy the sites where the antibody would otherwise bind.
Note that the thickness of the sections should be around 500 nm. The usual 80- to 100-nm-thick histological sections may be analyzed if correlative TEM/MIMS is required, but they will be quickly sputtered in the NanoSIMS.
4.Whole cells prepared by freeze drying.
Cells cultured directly on Si chips and analyzed as whole mounts are prepared by freeze drying (Peteranderl & Lechene, 2004; Tang et al., 2014). Improper freeze drying can lead to growth of large ice crystals, which can cause morphological cell damage and redistribution of analyte. Thus, samples need to be quenched through the glass transition temperature (−138°C) of water to convert cellular water to vitreous ice. This may be achieved by quenching in solid nitrogen rather than liquid nitrogen, where nitrogen boiling considerably reduces thermal conductivity (solid nitrogen can be obtained by rapidly evaporating liquid nitrogen, for example by pumping liquid nitrogen in a cylindrical Dewar by a high-capacity mechanical pump). Store the samples in liquid nitrogen. At –80°C and above, the samples will be altered by growth of large crystals at the expense of smaller crystals because the sublimation pressure is higher with smaller radii of curvature. For the same reasons, freeze drying should not be performed with usual commercial equipment at –40°C, but at –80°C or below.
To suppress condensation from air water vapor, sample transfer should be done under a cloud of nitrogen. The cloud can be obtained by immersing paper laboratory wipes in liquid nitrogen and waving over the samples.
Basic Protocol 2: STABLE ISOTOPE LABELING OF DNA IN ANIMALS
Studies in stem cell research on live animals using MIMS introduce complexities that make defining a single protocol impossible. Each lab will have their own research project, to which each MIMS protocol will have to be tailored. Factors to consider are the length of time the stable isotope DNA precursor (thymidine) is administered, the length of chase period to be used, and the length of the cell cycle in the organ studied and its variation with age of animal. Surrogate parenting may also be useful in certain cases. Along with 15N-thymidine, additional DNA replication markers, 13C-thymidine, 2H-thymidine, 18O-thymidine, BrdU, and IdU, can be given at any time during the experiment.
In cell culture, cell cycles may differ in time scales on the order of days. In live animal experiments, the cell cycles in different organs can be different over a time scale of months to years. Cells in the small intestine will turn over every 2-4 days. Cardiomyocytes turn over at a rate of 1%/year in 25-year-old humans (Bergmann et al., 2009). This will affect how a MIMS label is best administered to the live animal. For those experiments where the cell cycle is short, the stable isotope–labeled DNA precursor can be administered over a few days by injection (intraperitoneal, subcutaneous, or via tail veins in rats and mice) and be observed in the stem and daughter cells. Note, however, that injection into muscle can result in inhomogenous temporal distribution of the nucleoside. For experiments where the cell cycle is much longer, delivery of the label using an osmotic pump is more convenient.
The length of chase period will dictate the dose of the labeled DNA precursor required. For example, if the study incorporates a long chase period and the organ of interest has a short cell cycle, higher doses will be required (while remaining below levels that will modify cell cycle) to ensure measurable label in the last generation of cell is achieved.
Examples of MIMS labeling protocols in mice for stem cell studies in mouse intestinal crypt (Steinhauser et al., 2012) and heart (Senyo et al., 2013) give specifics on labeled DNA precursor administration modes, durations, and doses.
The following examples are only rough guidelines for studying DNA replication in intestinal crypt stem cells. All parameters (primary and secondary DNA marker doses and time sequences, duration of chases) can be varied and adjusted to the organ studied.
In this section, we will present considerations relevant to MIMS analysis of samples prepared from animals that have been administered stable isotope precursor molecules (through diet or injections). Note that the use of stable isotopes may require the same administrative permissions as radiolabels.
Materials
-
Mice
-
Stable isotope−labeled thymidine and/or analogs (BrdU, IdU) or stable isotope−containing diet (always perform a bulk analysis of the food to determine its stable isotope concentration)
-
Osmotic minipump
-
Si chips (5 mm × 5 mm; see Basic Protocol 3)
-
Additional reagents and equipment for injection (see Current Protocols article: Donovan & Brown, 2006b) and euthanasia (see Current Protocols article: Donovan & Brown, 2006a) of mice
1.Examples of labeled nucleotide administration in mice.
We will give specifics of DNA labeling of intestinal cells, which have one of the fastest cell renewal rates in the body. The stable isotope−labeled thymidine concentrations given are at least one order of magnitude below doses used to modify the cell cycle. They are, however, sufficient for detection by NanoSIMS even if diluted by several rounds of DNA replication during chase. For organs such as the brain, heart, and lung—which practically do not renew or have much slower rates of renewal—parameters will have to be adjusted accordingly.
Specifics regarding thymidine doses, administration mode and duration, and chase duration are tailored to answer specific questions. The following examples are only rough guidelines for studying DNA replication in intestinal crypt stem cells.
2.Example of double labeling starting in utero.
-
Initiate labeling of the female before conception. Administer 500 µg/day of15N-labeled thymidine by subcutaneous injection (Donovan & Brown,2006b). Upon impregnation, administer 20 µg/hr by osmotic minipump for variable time during pregnancy.
-
Within 24 hr of birth, transfer pups to a surrogate mother that has not received any labeled thymidine.
-
After the desired duration of chase, one may administer 250 µg BrdU to the pups every 6 hr for 24 hr, then euthanize (Donovan & Brown,2006a).
3.Example of triple labeling starting in 4-day old pups.
-
Beginning on day 4 of life, administer15N-thymidine (50 µg/g) subcutaneously every 12 hr for as long as desired. Then, administer BrdU (50 µg/g) subcutaneously every 12 hr for up to more than 10 days.
-
Provide a chase period as desired.
-
Beginning 24 hr prior to euthanization, administer 250 µg of IdU every 6 hr.
4.Example of double labeling in adult mice.
-
Administer15N-thymidine at a dose rate of 20 µg/hr via osmotic minipump for 2 weeks.
-
Provide a 5-week chase period (can be shorter or longer, as desired).
-
Administer BrdU via a single 250-µg bolus injection, followed by 20 µg/hr delivered by osmotic minipump for 24 hr prior to sacrifice.
Basic Protocol 3: PREPARATION OF Si CHIPS, THE GENERAL SAMPLE SUPPORT FOR NanoSIMS ANALYSIS
Materials
-
Acetone (spectrophotometric grade ≥99.5%)
-
Methanol (spectrophotometric grade ≥99%)
-
Isopropanol (spectrophotometric grade ≥99.5%)
-
Ethanol (spectrophotometric grade ≥99.5%, 200-Proof)
-
Water, ultra-pure, spectrophotometric grade
-
Distilled water
-
Nitrogen gas
-
5 mm × 5−mm Si chips (Agar Scientific, Cat. No. AGG3390)
-
Wire mesh sink strainer
-
Magnetic stirrer and magnetic stir bars
-
600-ml clean beakers (at least six total)
-
Ultrasonic cell disruptor with ∼½-in. probe (e.g., Misonix, Sonicator 3000)
-
Powder- free gloves
-
Fine-tipped Teflon forceps
-
Sterile petri dishes
-
0.2-μm bottle top filter unit
1.Obtaining Si chips.
Samples to be analyzed by MIMS are supported by Si chips. The Si chips can be ordered or custom diced from a semiconductor-grade silicon wafer. We use wafers with <111> direction, with a resistivity of 0.005-0.020 Ω·cm.
The Si chips need to be extensively cleaned as described in step 2.
2.Cleaning Si chips
-
Place Si chips in a wire mesh sink strainer.
-
Place a magnetic stir bar in a clean, 600-ml glass beaker. Hang the strainer with the chips near the bottom of the beaker.
-
Add 200 ml spectrophotometric grade acetone to the beaker. Stir for ½ hr. Sonicate with an ultrasonic cell disruptor at a power of 20 W for 2 min. Pour off the acetone.
-
Add 200 ml spectrophotometric grade methanol to the beaker. Stir for ½ hr. Sonicate with an ultrasonic cell disruptor at a power of 20 W for 2 min. Pour off the methanol.
-
Add 200 ml spectrophotometric grade isopropanol to the beaker. Stir for ½ hr. Sonicate with an ultrasonic cell disruptor at a power of 20 W for 2 min. Pour off the isopropanol.
-
Gently agitate the chips within the strainer in a total of 2 L of filtered distilled water so that the chips are all well rinsed.
-
Place the strainer in 200 ml spectrophotometric grade water and stir moderately for ½ hr.
-
Place the strainer in 200 ml spectrophotometric grade ethanol and stir moderately for ½ hr .
-
Transfer the chips to a clean, sterile culture dish.
-
Cover the chips completely with filtered ethanol.
-
Individually dry the chips with a stream of dry, filtered nitrogen gas and transfer them to a culture dish, shiny side up.
Basic Protocol 4: STABLE ISOTOPE ANALYSIS OF DNA REPLICATION IN SINGLE NUCLEI IN A POPULATION OF CELLS WITH NanoSIMS
Note that Cameca Instruments has recently released (March 2021) a very detailed user manual to all NanoSIMS laboratories describing the use of the NanoSIMS. Here, we provide information gained by the practice of NanoSIMS analysis and MIMS studies of biological samples in general, and DNA replication in particular.
Materials
- Specimens
- NanoSIMS “Harvard” holder (Cameca Instruments, Cat. No. 45621567)
- Powder-free gloves
- Aluminum foil
- Fine-tipped Teflon forceps
- Screwdriver
- Vacuum oven
- Stereomicroscope with camera, or other means of acquiring low-magnification optical images
- Reflected light microscope with DIC capability, and camera
- Other types of microscopes (e.g., fluorescence, electron) are optional.
- NanoSIMS instrument (Cameca Instruments)
1.Mount the specimens in the NanoSIMS “Harvard” holder.
It is imperative to wear laboratory gloves when handling any part that will go into the NanoSIMS analysis chamber.
In this section, we describe the mounting of samples in the “Harvard” holder: it can accommodate up to 16, 5 mm × 5−mm Si chip sample supports. It is available from Cameca. Other holders (described in the Cameca manual, section 5.3.3.2.1 “Load”) that accommodate larger-sized Si sample supports, to a maximum of eight, are available from Cameca.
-
In a clean area, for example a laminar flow hood, place a sample holder front face down on clean aluminum foil.
-
Using the fine-tipped forceps, begin placing the Si chips supporting the samples within the insets of the holder windows. Ensure that all four corners of the chip are contacting the inner lip of the inset (Fig.1).
-
Map the location of the Si chips in the holder windows. A template as in Figure2is very useful.
-
Carefully align the holder component with the springs to the component with the samples. Make sure the two screw holes are aligned, and gently push down while tightening the two screws (Fig.3).
There should be no gaps between components when fully tightened.
-
Carefully examine the front face of the holder. It is imperative that no chips protrude from the holder, in order to protect the objective lens. If there are, disassemble the holder, adjust the positions of the offending chips, and reassemble.
-
Until analysis, place the sample holder in a heated vacuum oven.
Always include chip(s) with (i) an unlabeled “control” sample and (ii) dried microdroplets of all stable isotope−containing media (mother solution, growth medium). A patterned Si grid is also helpful to tune the instrument.
If one wants to perform MIMS analysis of TEM ultrathin sections, one can use the adapter for the Cameca “Biology” holder (Cameca manual, section 5.3.3.2.1 “Load”), or a resized version can be custom made to fit the “Harvard” holder.
Samples and sample holders must be perfectly dry in the NanoSIMS analysis chamber. They should be kept above ambient temperature until ready to be loaded (for example in an oven at 50°C) and in storage. They should never be breathed upon during transport to the NanoSIMS.
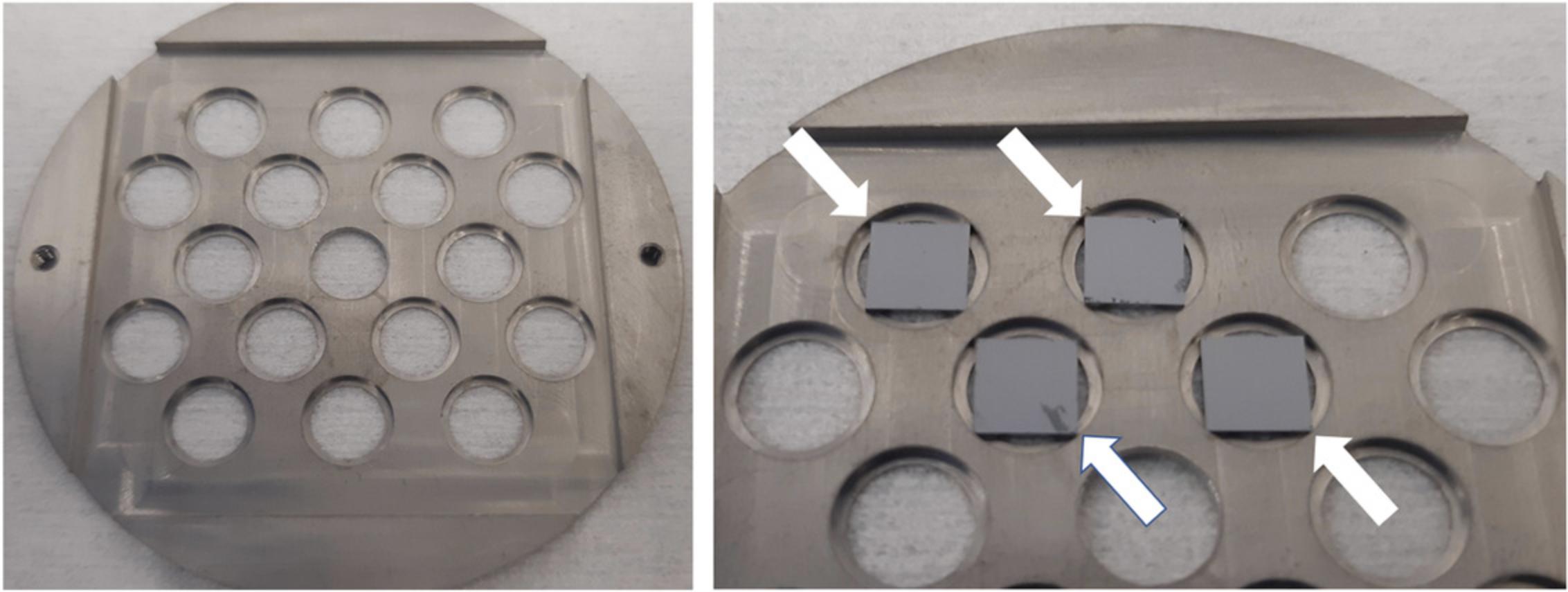
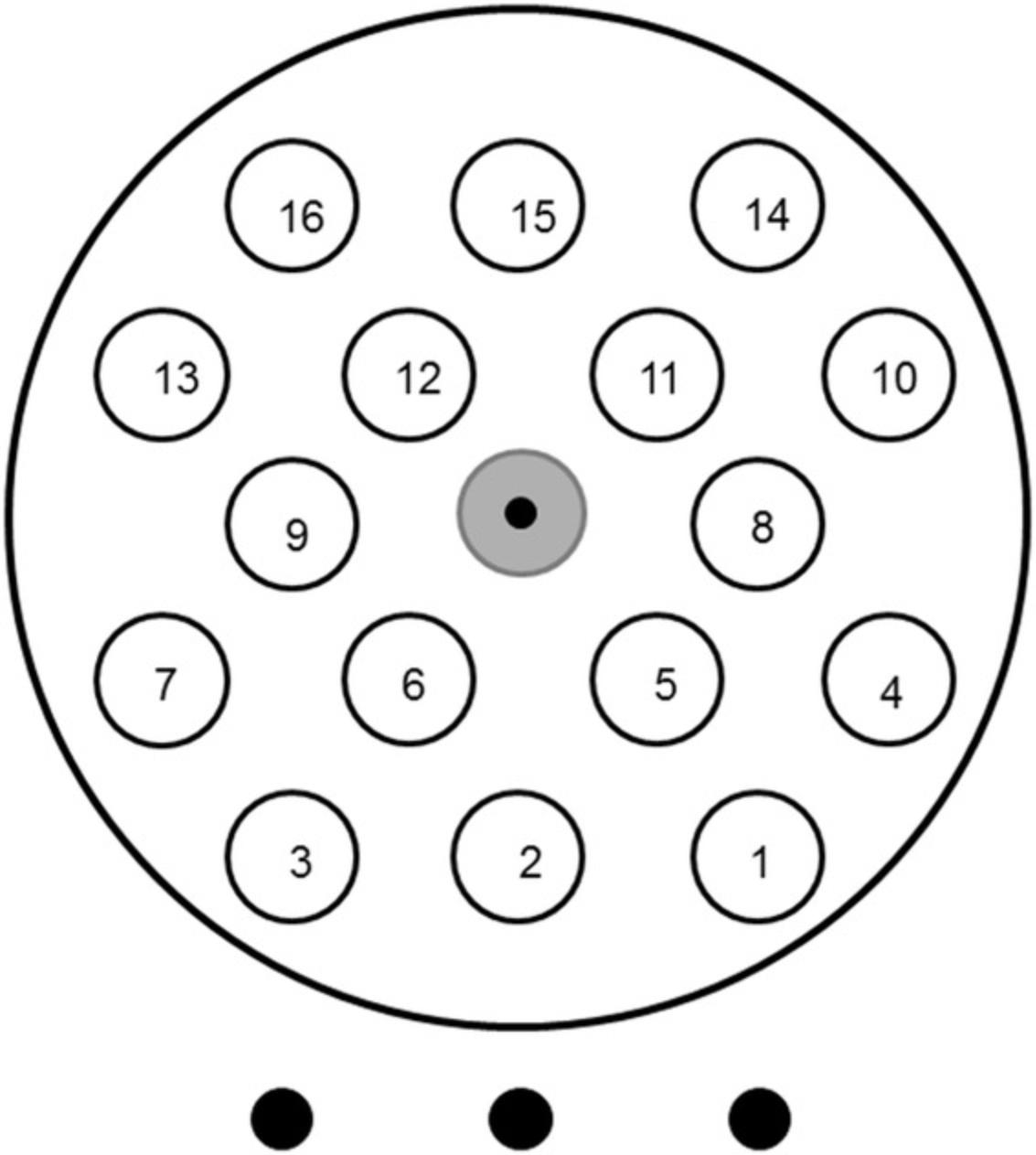
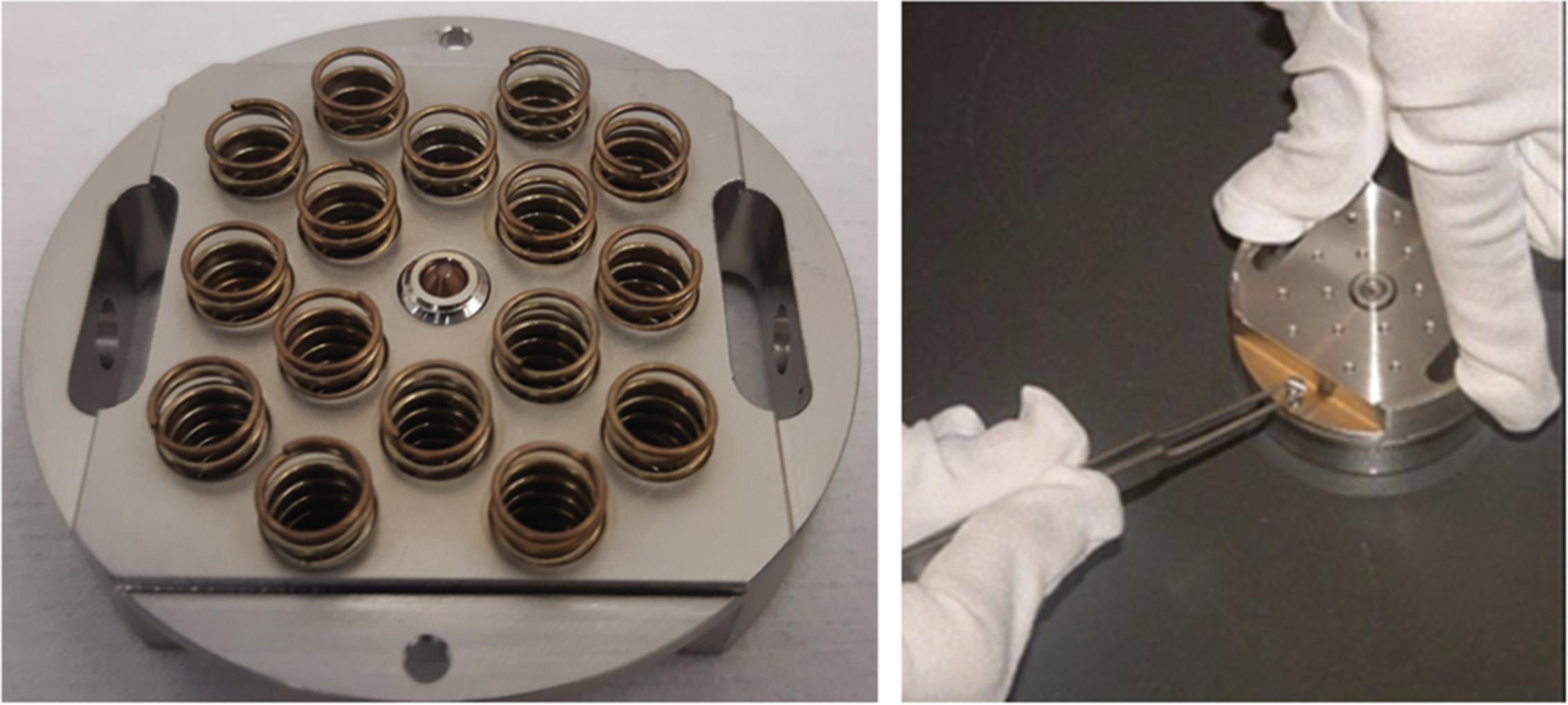
2.Acquire low-magnification optical image of the sample holder using a stereomicroscope or other means (tilting the holder slightly may provide more contrast) and differential interference contrast images of each section.
It is most useful to have low-magnification optical micrographs of the samples to aid navigation in the NanoSIMS. A low-magnification image using the NanoSIMS CCD camera is not possible, so navigation to the regions of interest in the sample can be time consuming and a waste of valuable instrument time.
-
Acquire an image of the entire sample holder (such as the one shown in Fig.4).
-
Using a reflected light microscope with an automated stage, acquire differential interference contrast (DIC) images. Samples are not always stained for SEM, so DIC can provide suitable contrast to identify specific sample locations. Record the stage positions.
This will allow location of samples in the NanoSIMS chamber by a coordinates transformation and reduce time spent navigating to the regions of interest, leaving more time for actual analysis. An example of a DIC image of a longitudinal section through mouse intestinal crypt is shown in Figure5.
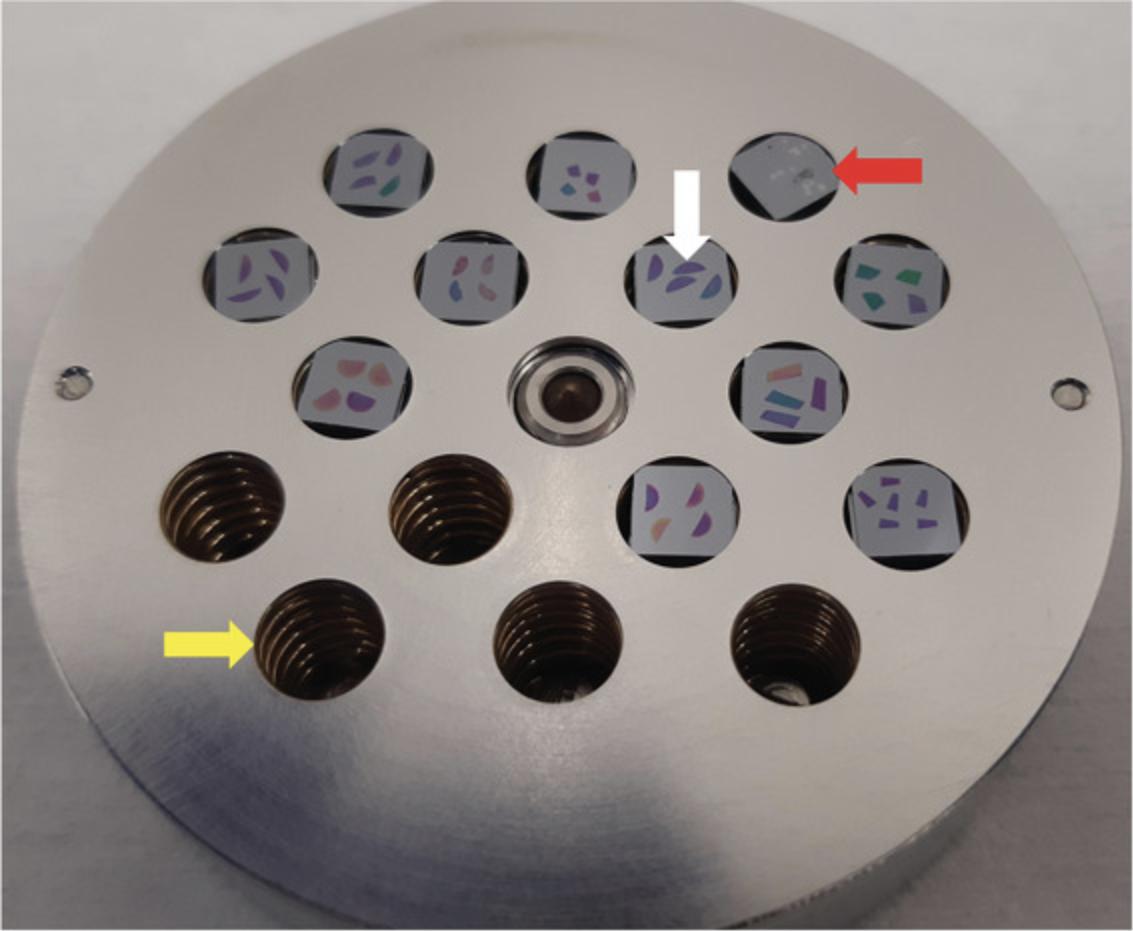
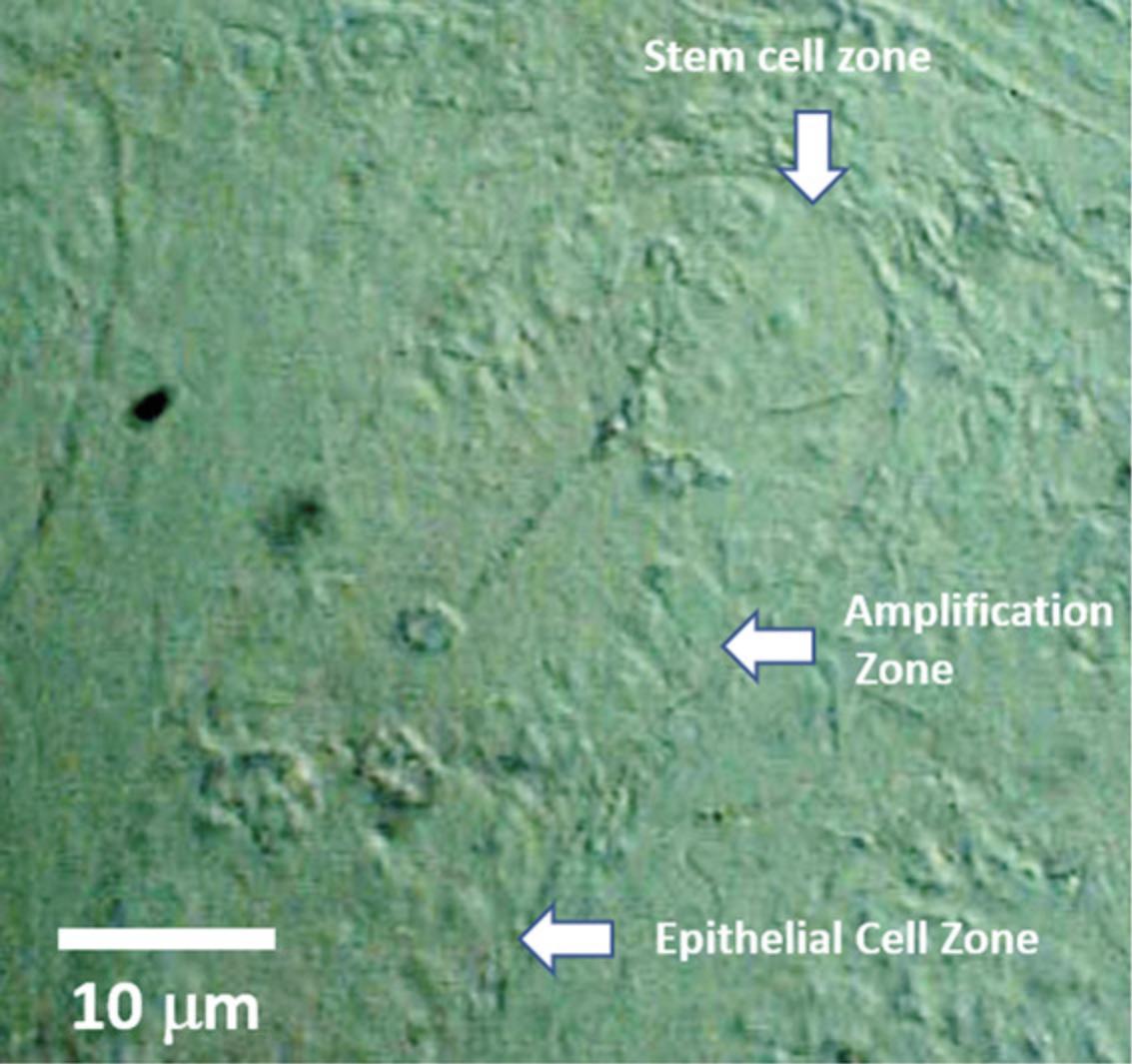
3.Start Cs source.
The procedure for starting the Cs+ source is described in the Cameca manual (section 9.1.2.1, “Cs+ primary ion source start”).
The source will be ready for use when a stable beam current is obtained, measured off-axis by a Faraday cup (Fcp) before all beam-focusing optics. The method for measuring the Fcp beam current is described in the Cameca manual (section 9.1.6, “Fcp and Fco beam current checking”). Target values for Cs+ ion currents vary from lab to lab, in general ranging from 20-40 nA. Higher currents will speed up analyses but necessitate more frequent source cleanings and replacement. A high Cs+ ion current (e.g., ∼ 80 nA) is also helpful when operating the NanoSIMS at high spatial resolution, allowing sufficient secondary ion currents to facilitate focusing the Cs+ beam on the sample
4.Stop Cs source.
The procedure for stopping the Cs source is described in the Cameca manual (section 9.1.3.1 “Cs+ source shutdown and restart”).
Note that when stopping the source, if the ionizer filament current is turned off first, the Cs will condense on the cold tungsten frit and effectively plug it—sometimes necessitating a change of source.
Note that we do not stop the source when performing several days of consecutive analyses, but leave it in “Standby” mode where the 8-kV extraction voltage and a reduced heating of the ionizer tip remain applied. This cleans Cs from the tip and speeds up the re-starting and stabilization of the source.
5.Load sample holder into NanoSIMS 50 airlock.
Attach holder to shuttle (see Cameca manual, section 9.1.1.1, “Sample mounting”). Load holder/shuttle assembly into the airlock (see Cameca manual section 9.1.1.2, “Sample loading in the airlock). Beware that an incorrectly attached sample may fall inside the NanoSIMS, resulting in at least 2 days recovery time.
Samples can be outgassed using the very hot halogen light source of the NanoSIMS airlock. To avoid burning, it is safer to degas biological samples in a vacuum oven around 50°C at least overnight before loading into the NanoSIMS.
6.Move sample holder into the NanoSIMS 50L vessel chamber.
The process of moving the sample holder to the vessel chamber is described in the Cameca user manual (section 9.1.1.3, “Sample transfer from airlock to vessel chamber). Always ensure that the sample holder is locked, first to the airlock stage, then to the transfer rod.
When the sample is docked into the vessel chamber carousel, retract the transfer rod slowly while observing through the vessel chamber viewport. Indeed, the sample holder may remain attached, but not locked, to the transfer rod and at risk of falling. If this is the case, push the holder forward so that it is fully back on the carousel, and try again to properly retract the transfer rod.
7.Move sample holder into the NanoSIMS 50L analysis chamber.
This process is described in the Cameca user manual (section 9.1.1.4, “Sample transfer from the vessel to the analysis chamber”).
Ensure that the sample holder is locked in the carousel by the pin on top of the vessel chamber. If the sample is not locked, the sample transfer rod will push the holder off the carousel into the NanoSIMS, causing significant downtime.
8.Set NanoSIMS 50L stage Z-position
The Z-position set for focusing the CCD camera observation is also the focal point for the secondary ion immersion lens (EOS). Adjusting the Z-position of the sample is therefore critical. The procedure is described in the Cameca manual [section 9.1.5.1, “Moving the sample under optical microscope (CCD)].
9.Measure primary ion beam current impacting the sample.
Measurement of Fcp (see step 3, above) provides a measure of the state of the Cs source. Far more important is the measurement of the primary beam current impinging on the sample (Fco). This value is necessary for ion dose and ion yield calculations. It is a function of several parameters: the value of Fcp, the diameter of the D1 apertures, and the L1 lens voltage. It is measured with a Faraday cup (Fco). The procedure is described in the Cameca manual (section 9.1.6, “Fcp and Fco beam current checking”).
Make note of Fco, because it is NOT recorded by the NanoSIMS software.
10.Record regions of interest (ROI) to be analyzed.
Use the NanoSIMS camera to locate the ROIs (Cameca manual section 5.3.1.3, “Getting Started”) or apply a coordinate transformation to ROI coordinates selected by other methods, e.g., optical microscopy, DIC, SEM (examples: scope2mims, Cameca manual section 6.2, “Point Logger”).
Any additional ROIs should be manually saved to the .prs file.
11.Implant Cs+ in region near analytical area for tuning the instrument.
The overall implantation procedure is described in the Cameca manual (section 9.1.10, “Pre-implantation”). Pay attention to detector damage prevention.
It is necessary to “implant” or deposit a sufficient amount of Cs onto the surface of the sample to elicit, maximize, and stabilize secondary ion emission. With biological samples, the CN ion count rate is useful for monitoring implantation. Initially, the count rate will increase very slowly with Cs implantation time, followed by a period of exponential growth, and finally reaching a constant rate, typically hundreds of thousands of counts when D1 Aperture 2 (Fco ∼2.5 pA) and Entrance Slit 3 are used. The dose of Cs+ ions required to reach a constant emission of ions is usually between 5 × 1016 and 5 × 1017 ions/cm2. This will be dependent on many parameters including embedding resin, and these dose values should only be used as a rough guide.
12.Set detector pulse height distributions (PHD).
Secondary ions are counted using electron multipliers (EMs). An immense advantage of EM detectors is that—when calibrated properly—they are noiseless and allow single ion counting. The design and principles of EM operation are described in Cameca manual section 2.1.4.3 (“Electron Multipliers”).
The entire procedure and an example of a proper PHD curve is described in the Cameca manual [section 9.1.12, “Manual EM pulse height distribution (PHD) adjustment”]. Check that the C4X deflector has been properly set (Cameca manual section 9.2.1.4, “Multi-collection”). The PHD should be measured on a sample that has been implanted until the count rate plateaus at a maximum value; this indicates that the sample has been fully implanted.
The distribution of voltage pulses should be centered around 220 mV, and is a function of the voltage gain applied to the EM. This is determined by recording the pulse height distribution (PHD) curve and adjusting the EM voltage gain accordingly. The high number of pulses at very low voltages is due to electronic noise and is filtered out by setting a threshold voltage below which all pulses are discarded from counting. This is usually around 50 mV.
To practically measure the PHD's on all detectors, position all movable detectors 2 amu apart from each other at around 450 mm magnet radius. Move to an Si chip and change the magnetic field so that 28Si is measured on detector 1.Measure the PHD and adjust voltage gain and threshold if necessary. Change the magnetic field so that 28Si is measured on detector 2, measure the PHD, and change gain and threshold. Repeat this process for detectors 3-6.The PHD on the fixed detector will need to be set separately. If the fixed detector (described below in step 13, paragraph 4) measures a significantly heavier ion than Si (for example measuring 197Au), the PHD is best measured from a standard sample containing the same ion species.
After calibrating the EMs, all conditions being the same, the count rate should be equivalent among detectors.
Detector PHDs should be regularly checked or after measuring samples with high count rate.
13.Gross positioning of the secondary ion detectors.
In stem cell studies with MIMS, one can identify replicating/replicated nuclei and can measure the number of divisions by using thymidine labeled with the stable isotopes 13C and/or 15N, and/or thymidine analogs containing 79Br or 81Br (BrdU) and 127I (IdU), which do not naturally occur in DNA.
Detectors can be positioned, for example, to count 12C and 13C to measure incorporation of 13C-thymidine, 12C14N and 12C15N to measure incorporation of 15N- thymidine, and 81Br, and/or 127I for the thymidine analogs. 31P is a useful nuclear marker. Measuring an isotope of Si is helpful to indicate when the sample has been consumed, or the location of any holes in the section. By using a variety of DNA markers, one can study DNA replication serially in the same experiment at time points extending from days to months.
Note that we analyze the nitrogen isotopes as the CN- ion. The secondary N- ion yield is very poor, and using the CN- ion improves ion yield by several orders of magnitude.
With six moveable detectors and one fixed detector, up to seven isotopes can be measured simultaneously in the NanoSIMS 50L. To measure a set of isotopes at the highest mass resolution, one should use the lowest magnetic field that allows the heaviest isotope to be measured on the fixed detector. The remaining isotopes are measured by moving the detectors to their appropriate positions along the magnetic radius.
The procedure for positioning the detectors is described below.
-
Ensure that multicollection is selected in both tuning and detection modes (Fig.6, red arrows).
-
In the Tuning/Counting window, enter the heaviest mass (either the value in amu or symbol) into the “Mass” location for detector 7 (Fig.6, black arrow). This will change the magnetic field to the approximate value required to measure these ions with detector 7 and change the mass values at the current locations of the movable detectors. Note it is not obligatory to use all seven detectors.
-
Turn the beam ON (Fig.6, green arrow).
-
If you do not see any counts appearing in the fixed detector (detector 7) window, change the magnetic field using the keyboard thumbwheel until counts appear.
-
Turn “NMR” on (Fig.6, brown arrow). This starts the high-precision magnetic field regulation. At this point, you should not change the magnetic field.
-
Enter the mass values of the other isotopes into the “Mass” location for the other detectors. This will move the detector to that position. Be careful to avoid possible detector collisions. It may be necessary to perform this step out of numerical detector order.
-
If no counts are observed on detectors 1 to 6 after movement, the fine adjustment arrows (Fig.6, orange arrows) may be used to move the detectors in small increments until signal is found. This is a trial-and-error process.
At this point, you should have counts on all seven detectors.
Note that the secondary ion column has not yet been aligned, which can greatly enhance count rates.
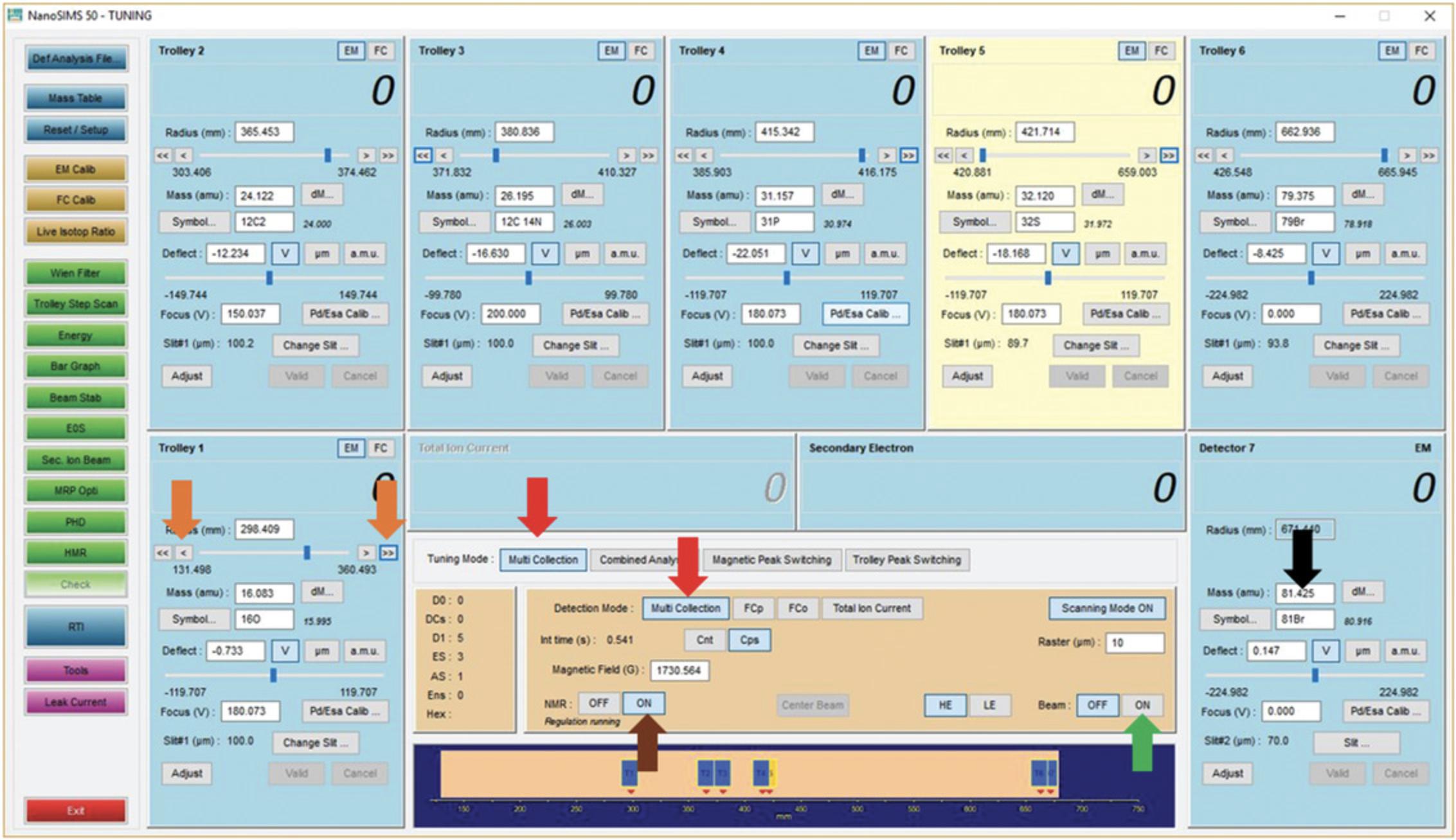
14.Align the secondary column.
The goal is to maximize the transmission of the secondary ions to the detectors. The procedure for aligning the secondary column is described in the Cameca manual (section 9.1.13, “Optimizing mass analyzer transmission”).
With biological samples, a faster method is to maximize the 12C14N- secondary ion current (count rate) using the thumbwheels by iteratively adjusting the triad [EOS-Cy-P2/P3] until no further increase in count rate is observed.
15.Precise positioning of secondary ion detectors.
The full procedure is described in the Cameca manual (section 9.1.14, “Optimizing mass resolving power”).
The HMR spectrum is acquired by scanning the focused secondary ion beam across the slit in front of each EM detector with a pair of voltage plates. This results in a plot of counts as a function of scanning voltage (Fig. 7). Because the resolving power of the instrument is not infinite, there may be a combination of elemental and molecular ions that are not separated. For example, the mass spectrum at mass 27 (Fig. 7) shows four plateaus, indicating the presence of three masses not completely separable by the mass resolving power of the instrument. From low to high mass, these are: 12C15N, 13C14N and 13C21H. Note that a greater separation between these mass lines could be achieved by using a narrower exit slit (see Cameca manual section 5.2.2, “EM/FC Detector Panel”), which however may create difficulty in peak stability.
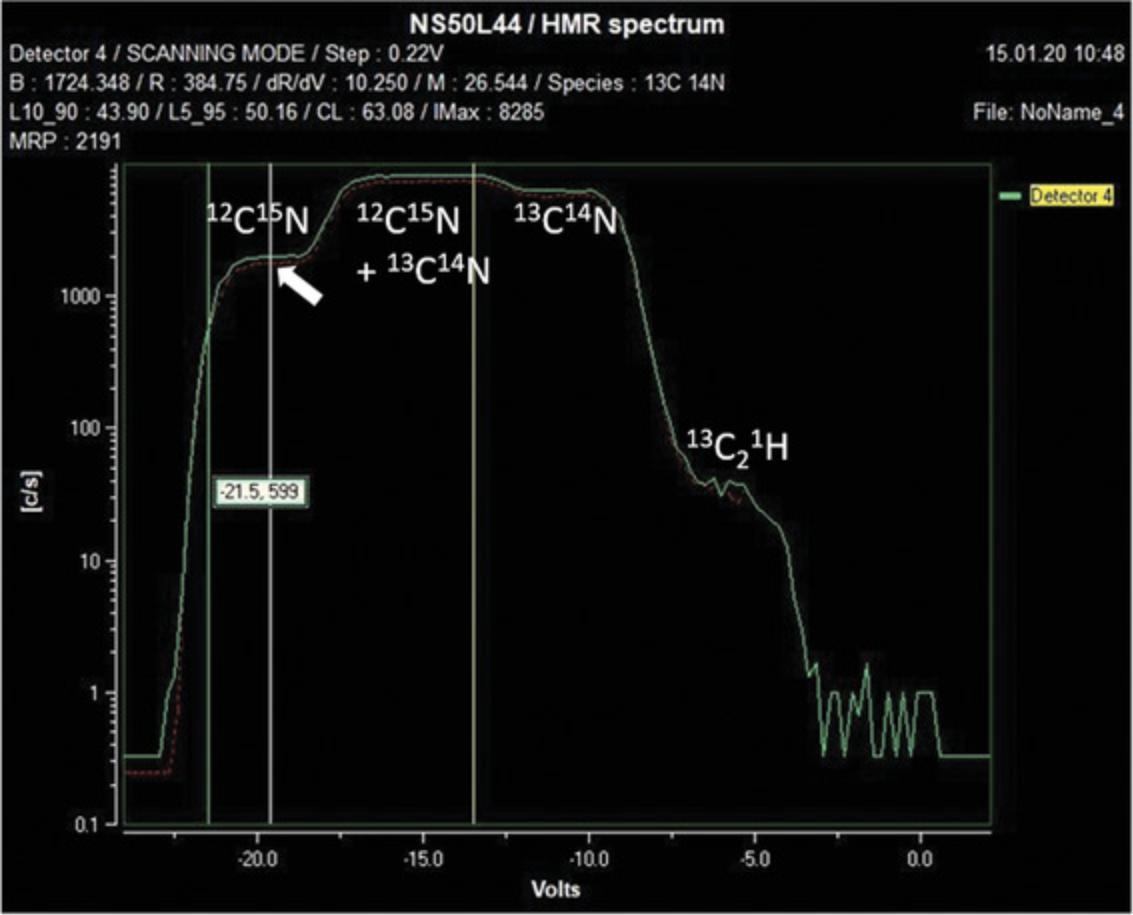
16.Optimizing mass resolving power MRP using the quadrupole lens (Q).
The procedure for optimizing the mass resolving power (MRP) with the quadrupole lens (Q) is described in the Cameca manual (section 9.1.14, “Optimizing mass resolving power”).
The optimum value for Q is dependent upon the magnetic radius. Thus, the best MRP value found by measuring the HMR on detector 7 will not be the same as that measured on detector 1. This discrepancy will be more pronounced the greater the difference in masses between detectors 1 and 7.Thus, Q should be optimized using the mass that requires the highest MRP, or one within a few amu (e.g., optimizing Q for best MRP for 12C15N can be done by optimizing Q using 12C14N). In some cases, a compromise value of Q may be required to span the entire mass range of the experiment.
The quadrupole lens has the largest effect on the MRP, but LF4 also has an effect and should be checked in a manner similar to Q. If the MRP still appears poor, the hexapole may also need to be checked. These procedures are described in the Cameca manual (section 9.3.1.3.2, “Secondary tuning in multicollection mode”). You should be able to get an MRP for 12C14N of at least 5000 using entrance slit 3 and aperture slit 1. If this cannot be achieved, it will be impossible to collect good data for mass 12C15N.
17.Prepare for image acquisition.
Basic image acquisition is described in the Cameca manual (section 9.1.15, “Basic image acquisition”).
Before launching the analysis, several acquisition parameters need to be selected. These are:
-
Pixel resolution (e.g., 256 × 256, 512 × 512 etc.).
-
Dwell time per pixel.
-
Single image plane or multiple image planes?
Single-scan images often provide all desired information, and for large fields of view this may be the preferred method. Long single-scan images will, however, be prone to drift effects and be more susceptible to sample charging. Multiple plane images can provide the data required for 3D information and imaging depth profiles, and a means for drift correction.
The pixel size of the final image should also be considered. One rule of thumb is that the spot size of the primary ion beam should be approximately twice the pixel size. This way, the tissue section or cell is neither undersampled nor heavily oversampled, which will consume the sample more rapidly with no added benefit.
Rather than randomly selecting a number of planes, the importance of counting statistics in MIMS should be considered. These play a large role in precision, which is given (in %) as 100/√N, where N is the number of counts (in a single pixel or ROI). Thus, in a measurement where the weakest-counting isotope yields 100 counts, one can expect no better than 10% precision in the ratio value. If only small increases in the isotopic ratio values are expected, then the length of the analysis should be sufficient for a precision based on counting statistics, to show that regions are indeed statistically significant in enrichment above the natural ratio value. The data can be processed during acquisition to allow a better judgement of how long it will require to achieve the desired counting statistics.
Basic Protocol 5: DATA REDUCTION AND PROCESSING
In a single plane analysis, the Cs primary beam dwells at each pixel address once and the intensities of the selected secondary ions measured by the detectors are stored in a single “.im” file. In multi-plane analysis, this procedure is repeated up to a thousand times, with data from successive planes also stored in the single .im file.
The data measured at each secondary ion mass can be displayed as a quantitative image and statistically analyzed.
We analyze data from the NanoSIMS using the OpenMIMS plug-in that we developed for ImageJ. A manual describing the general use of OpenMIMS is available at https://usermanual.wiki/Pdf/OpenMimsManual.682350371/help. Here we will provide additional information using examples from stem cells and DNA replication.
1.Processing raw NanoSIMS data files into quantitative MIMS images in a study of mouse intestinal stem cells.
In the example shown in Figure 8, the mouse received 15N-thymidine from day 4 until 8 weeks old [day 4 to 4 weeks, two daily subcutaneous injections (50 μg/g); 4 weeks to 8 weeks, osmotic pump at 20 μg/hr], followed by a chase period of 4 weeks. Prior to harvesting, BrdU was administered for 24 hr (1 mg bolus followed with 20 μg/hr by osmotic pump).
![Details are in the caption following the image Processing a .im file from a mouse intestine sample. Mouse was treated with <sup>15</sup>N-thymidine from day 4 until 8 weeks old [day 4 to 4 weeks: two daily subcutaneous injections (50 μg/g); 4 weeks to 8 weeks: osmotic pump at 20 μg/hr], followed by a chase period of four weeks. Prior to harvesting, BrdU was administered for 24 hr (1 mg as a bolus followed with 20 μg/hr by osmotic pump). Image field is 30 μm × 30 μm recorded at 512 × 512 pixels. Dwell time is 0.75 ms/pixel. Quantitative image data for seven masses is stored for each plane in an image stack containing 271 total planes. Bar: 5 µm. First image plane for each selected mass in the stack of a multiplane acquisition: (A) <sup>12</sup>C (B) <sup>13</sup>C (C) <sup>12</sup>C<sup>14</sup>N (D) <sup>12</sup>C<sup>15</sup>N (E) <sup>31</sup>P (F) <sup>32</sup>S, and (G) <sup>81</sup>Br. The image stack (containing 271 planes) is examined for bad images that should be discarded. (H) Example of a good <sup>12</sup>C<sup>14</sup>N image plane; (I) bad <sup>12</sup>C<sup>14</sup>N image plane showing non-uniform intensity distribution; (J) bad <sup>12</sup>C<sup>14</sup>N image plane where top third of image is absent. Inset shows a zoomed image of the Paneth cells, marked by red arrow. (H) Bad <sup>12</sup>C<sup>14</sup>N image with a zoomed image of the Paneth cells in (J) where the lower cell has clearly changed shape. All planes such as these should be removed from the stack of images. A total of 24 bad planes were found and discarded. The image planes for each mass are now summed, providing higher counts at each pixel and better statistical precision. If blurred sum images, such as those in (l) to (r) are observed, it indicates some spatial drift during the acquisition. The drift can be corrected by applying any necessary x-y translation to the images in the stack. The Autotrack algorithm in OpenMIMS computes and performs the x-y translations, using either the entire image or a selected smaller region of interest containing an object with high contrast (computationally faster). If the pixels in a single plane do not have a high enough count rate, it may be difficult to apply a drift correction and the “Compress” command may be helpful. Its function is to sum a few adjacent planes in the stack. No drift correction will be applied considering that adjacent images will have practically not drifted compared to the entire stack (acquired over several hours). Thus, for a stack of 1000 images and a compression of 10, the new stack will be of 100 images, with ∼10 times more counts per image, and it may then be possible to achieve a good drift correction on the compressed stack. After drift correction, much sharper sum images are observed: (s), <sup>12</sup>C sum image; (t), <sup>13</sup>C sum image; (u), <sup>12</sup>C<sup>14</sup>N sum image; (v), <sup>12</sup>C <sup>15</sup>N sum image; (w), <sup>31</sup>P sum image; (x), <sup>32</sup>S sum image; (y), <sup>81</sup>Br sum image.](https://static.yanyin.tech/literature_test/cpz1290-fig-0008-m.jpg)
2.Deriving isotope ratio images.
Deriving isotope ratios from the quantitative image data obtained by the NanoSIMS allows one to determine regions of the sample, if any, that have incorporated the stable isotopes. As the information content of a grayscale image is poor, we developed a method based on a hue saturation intensity (HSI) transformation of the ratio image, originally conceived by the Tsien lab (Poenie, Alderton, Steinhardt, & Tsien, 1986) for expression and fluorescence data. In an HSI image, the hue codes for the ratio value, and the intensity codes at each hue for the statistical precision. As we will show, HSI images provide a wealth of information at a glance.
Here, we had administered the DNA precursor thymidine enriched in 15N to mice for intestinal stem cell studies. The HSI images allow one to quickly observe which cells within the intestinal crypt have incorporated 15N into their DNA and how that DNA is distributed within the nuclei, and estimate the number of DNA replications. The process of producing an HSI image is described here and summarized in Figure 9.
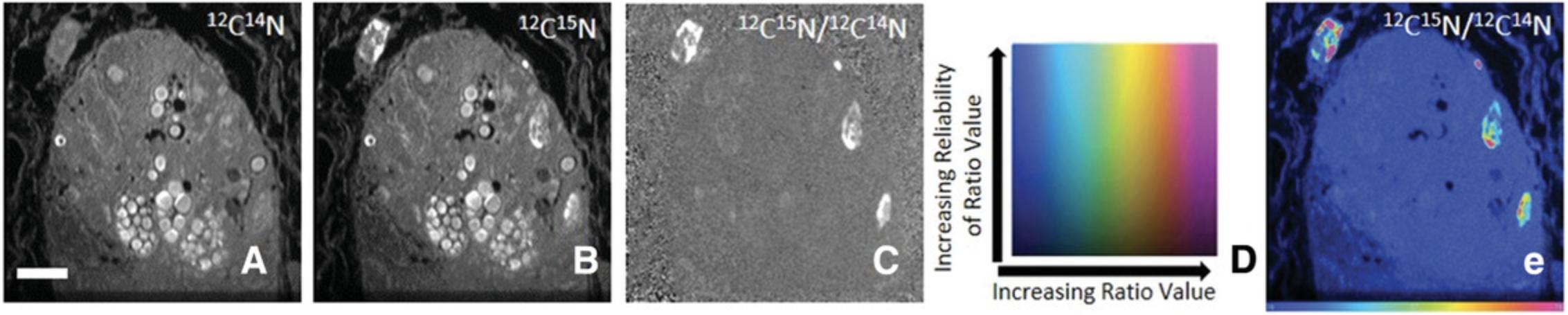
3.Region of interest (ROI) analysis.
Region of interest (ROI) analysis is performed to extract numerical values of the incorporation of the label into tables for statistical analysis. These regions can be individual cells in a population, subcellular structures in cells, and areas within the ratio image where one visually suspects label incorporation (brightness of un-normalized mass images of the label cannot be used because of matrix effects).
Regions of interest are recorded within OpenMIMS using the ROI manager (https://github.com/BWHCNI/OpenMIMS/wiki/Manual#roi-manager), and the numerical parameters are selected using the tomography window (https://github.com/BWHCNI/OpenMIMS/wiki/Manual#Tomography).
An example of ROI analysis is shown in Figures 10-12.Twelve regions of interest were drawn around chromosomes, guided by the 31P image (Fig. 10C). We selected the 12 ROIs shown in Figure 10 using the OpenMIMS ROI manager, and from each ROI we extracted the values for: (i) the 12C15N/12C14N ratio (mean and standard deviation), (ii) the Σ12C15N counts/Σ12C14N counts (N/D; Numerator/Denominator), and (iii) the Br counts (mean and standard deviation) using the OpenMIMS tomography window. If there is a discrepancy between the mean of the ratios and the ratio of the sums, it suggests insufficient counting statistics or highly inhomogenous labeling within the ROI. In Figure 11, we show the table of output from OpenMIMS. It can then be exported and analyzed using a statistical software like JMP (Fig. 12).
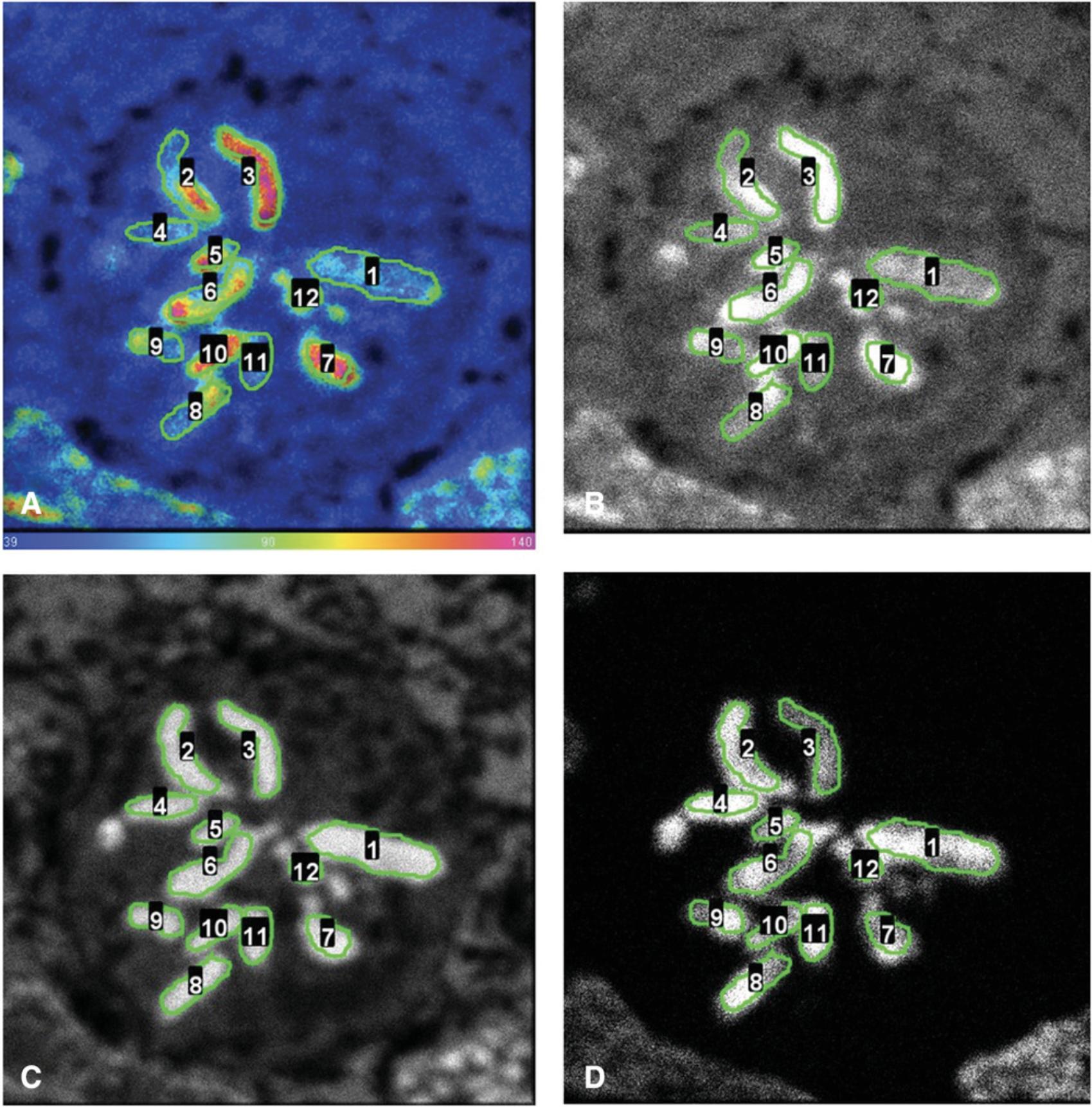
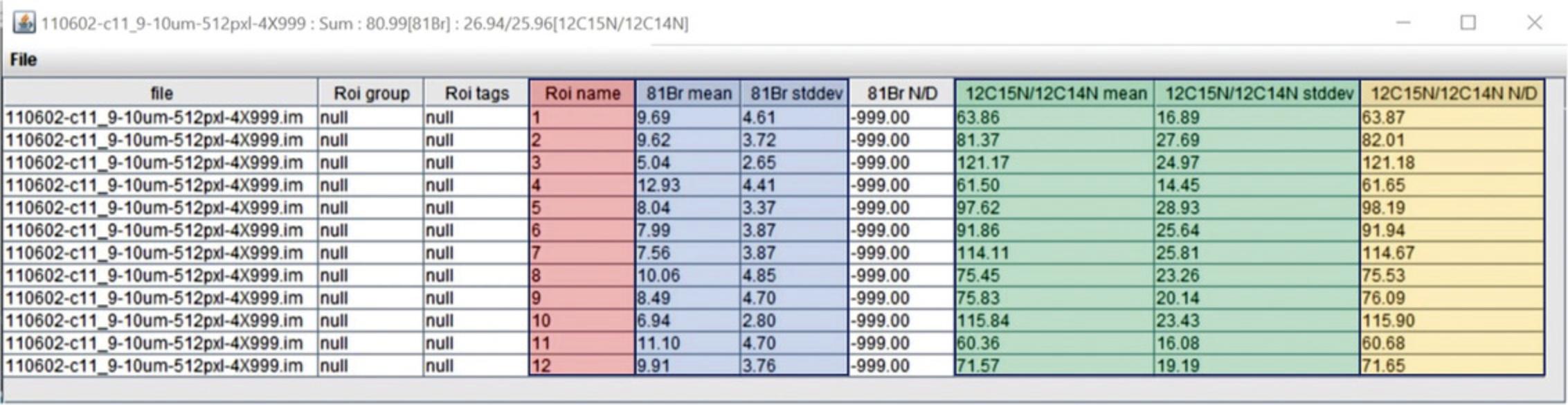
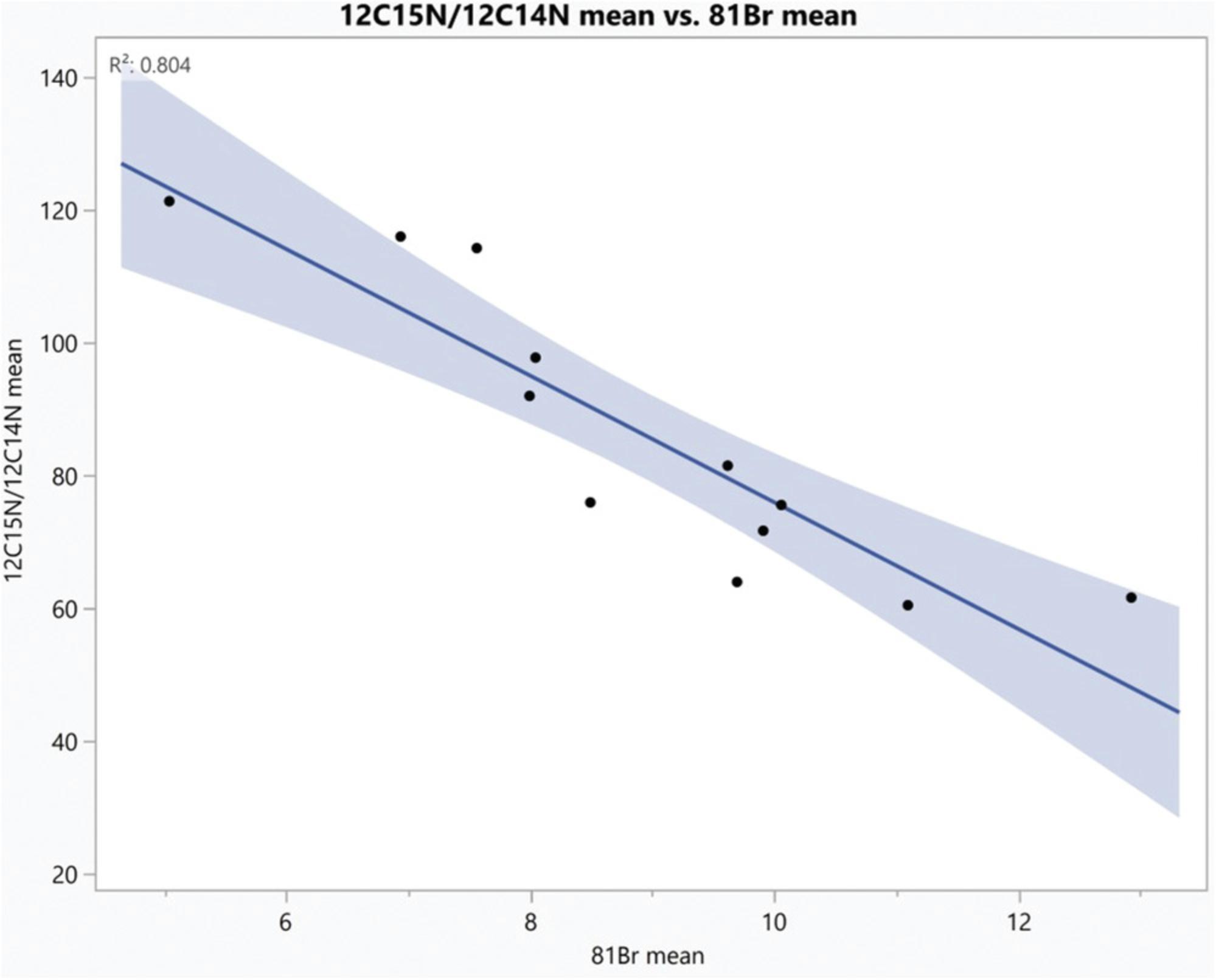
COMMENTARY
Background Information
Until the middle 1930s, it was believed that our constituents were permanent until death. Rudolf Schoenheimer left Germany in 1933 and joined the Department of Biochemistry of Columbia University. In 1934, he started collaborating with Harold C. Urey, who had isolated deuterium. Schoenheimer developed the method of isotope tagging of biomolecules. In “The Edward K. Dunham Lectures for the Promotion of the Medical Sciences 1941” at Harvard University, he mentioned that “Heavy nitrogen (N15), generously made available by Dr. Urey, has made possible a new technique for the investigation of protein metabolism”, and concluded that “all constituents of living matter, whether functional or structural, of simple or complex constitution, are in steady state of rapid flux.” (Schoenheimer, 1942).
These studies could not be pursued at the subcellular level because there was no radioactive isotope of nitrogen that could be used for autoradiography. Imaging of stable isotope tracers at the subcellular level only became possible with the development of secondary ion mass spectrometry (SIMS) quantitative imaging by Castaing and Slodzian (1962). Major advances in SIMS capability led by Slodzian and Hillion (Slodzian, Daigne, Girard, Boust, & Hillion, 1992) included a spatial resolution smaller than 50 nm and parallel collection of secondary ions, allowing one to measure isotope ratios at the submicron level. These developments provided the scientific community with an instrument having all the necessary capabilities to extend the pioneering work of Schoenheimer to the subcellular level (Lechene et al., 2006; McMahon, Glassner, & Lechene, 2006).
The development of MIMS provided a powerful new way of viewing and measuring, in all fields of biomedical research, important parameters that were previously impossible or difficult to see or measure. We have used MIMS to show for the first time how symbiotic bacteria convert atmospheric nitrogen to a form suitable for biosynthesis (Lechene, Luyten, McMahon, & Distel, 2007). MIMS allowed us to study protein renewal in sensory hair cell stereocilia (Zhang et al., 2012) and resolve “the immortal strand hypothesis” in the mouse intestinal crypt, where direct evidence against non-random template strand segregation in the small intestine was observed (Steinhauser et al., 2012). It has provided new insight into biological problems in a variety of domains including the source and generation of new heart cells in mammals (Senyo et al., 2013) and the antioxidant role of lipid droplets in stem cell niches (Bailey et al., 2015), and led to the proposition that age mosaicism across multiple scales is a fundamental principle of adult tissue, cell, and protein complex organization (Toyama et al., 2019). Other applications include studies in the fields of environmental microbiology and soil science (Eichorst et al., 2015; Finzi-Hart et al., 2009; Heister, Höschen, Pronk, Mueller, & Kögel-Knabner, 2012; Milucka et al., 2015; Pasulka et al., 2018; Wolfe-Simon et al., 2011) and numerous other biological and pharmaceutical-related interrogations (Cabin-Flaman et al., 2011; Guerquin-Kern, Wu, Quintana, & Croisy, 2005; Legin et al., 2014; Lovrić et al., 2017; Richter, Rizzoli, Jähne, Vogts, & Lovric, 2017; Vujic et al., 2018).
Critical Parameters
Before analysis
- 1.Choose an appropriate stable isotope label dose in the culture medium for cell preparations or food or injections for live animals. Factors to consider are both the absolute concentration of the label introduced into the cell culture medium or into the live animal, and the number of atoms in the molecule (e.g., thymidine) that have been replaced by the enriching isotopic label (e.g., 15N).
- 2.Be aware of isotopic effects (e.g., relative mass differences much greater for 2H and 1H compared to 13C and 12C, so biological activity may be affected when 2H is used as a label) and exchange effects between heavy isotope labels of H and O with those atoms in water molecules, which can lead to washing out of the label.
- 3.Ensure that drops of the labeled culture medium are placed on Si chips using micropipettes and loaded into the sample holder. This is particularly important for elements not commonly used as labels.
- 4.Always verify that natural ratios are measured after tuning of the NanoSIMS using an unlabeled control sample
- 5.Having optical microscopy and/or SEM images of regions of interest will be very useful when the sample is in the NanoSIMS.
Tuning of the NanoSIMS 50 before analysis
- 1.It is essential to ensure the pulse height distributions of the detectors are properly set (see Basic Protocol 4, step 12). It is essential to balance the count rates (see Basic Protocol 4, step 12) on the set of detectors used to measure isotope ratio or ratios.
- 2.Alignment of the Cs source can be done using the Si signal from any Si chip upon which the samples are deposited.
- 3.It is essential that the peaks in the HMR mass spectra contain flat tops and sharp shoulders (see Basic Protocol 4, step 16, and Fig. 7).
NanoSIMS analysis of samples
- 1.Do not implant the sample with a high Cs beam current (no diaphragm, D1-0). At least D1-1 must be used. Use of high beam currents will rapidly consume the sample.
- 2.Be aware that during implantation, and particularly if D1-0 is used, isotope label and cellular material may temporarily attach to the objective lens and possibly re-deposit on the sample during analysis, relocating isotope labels. This is known to occur in some dynamic SIMS instruments (sometimes referred to as the memory effect). We have not observed translocation of 15N-thymidine from the nucleus to the cytoplasm, which would be an indicator that this problem is occurring. When implanting samples, we never use the highest beam currents (D1-0), and this precaution seems to be sufficient to avoid this problem.
- 3.Ensure that the NMR regulation is on during analysis. If it has switched off, stop the analysis, switch the regulation back on, and re-check the HMR mass spectra before continuing analysis.
- 4.The analysis may be stopped when sufficient statistical data in the regions of interest have been acquired.
After analysis of samples
After processing the data from the first experiment in an analysis campaign, create a tracking document with the raw image data and ratio images. There are features in OpenMIMS to make this easy. Add to this document after each subsequent analysis. This will greatly facilitate future data analysis and manuscript writing.
Troubleshooting
Table 1 lists problems that may occur with the procedures in this article along with their possible causes and solutions.
Problem | Possible Cause | Solution(s) |
---|---|---|
No secondary ion signals |
Primary ion source not on. Primary ion beam badly out of alignment or gonio not in proper position. Problems with voltages on lenses or deflectors. Gate valve at Cs source or multicollector chamber is closed. No voltages applied to the detectors. Secondary ion beam grossly out of alignment. |
Ensure proper water circulation in cooling circuit. Verify beam currents both in primary column (Fcp) and at sample (Fco). Move to a bare Si area and attempt to detect 28Si counts. Remove all slits, if necessary. Use “Update Parameters” command to look for large differences in applied and measured voltages at various lenses/deflectors. Look for signal at total ion current detector to narrow down in which part of the instrument the problem may be occurring. |
Incorrect value of natural isotopic ratio from standards |
Detectors are not properly calibrated and PHDs have not been verified. Secondary ion beam is misaligned, such that the field is non-uniformly balanced with CN signal or C signal across the entire field of view. Control sample was contaminated or improperly labeled during sample mounting. C4x deflector not properly set at the plateau for all masses. Wrong peak selected in the HMR or mass resolution insufficient. |
Check PHDs on the electron multipliers and balance the count rate on the detectors. Check secondary alignments. Re-check HMRs to ensure proper peak has been selected and sufficient mass resolving power is attained. Check C4x scans. |
Higher than natural ratios are measured on control samples though all NanoSIMS 50L instrument parameters are found to be properly set | Cross contamination during preparation, possibly through pipette tip. | Casts suspicion on all samples for this culture experiment. Redo culturing and do not waste instrument time on dubious samples. |
Sample charging |
Sample has picked up moisture. Sample is intrinsically non-conductive. |
In our experience, we avoid and only exceptionally encounter sample charging by taking simple, yet extreme precautions to keep samples ultra-dry. They are stored in a vacuum oven at 50°C. When transferred from the oven to the analytical chamber of the NanoSIMS, they are never allowed to be at lower than room temperature. We take extreme precaution to avoid breathing upon them. In the rare case where we do encounter charging, we have found that implanting Cs from the Si chip to the periphery of the tissue section creates a conductive pathway that can help dissipate charge. Apply 5 nm conductive Au or Pt to sample. An evaporator is preferred over a sputter system, as there is a possibility that energetic Ar+ ions could sputter the sample and translocate material if the samples are placed too close to the plasma region. |
Statistical Analysis
MIMS provides results that are incredibly rich in statistical data, and the OpenMIMS plugin itself offers many statistical parameters to be analyzed and exported (see Basic Protocol 5, step 3). The data extracted from the OpenMIMS plugin can also be easily imported to other statistical analysis software packages.
Time Considerations
The time frame for completing a MIMS analysis can vary immensely. The sample labeling, especially in live models, can take just a few days to months. This would be followed by a period of several days for the actual preparation of the samples. MIMS image data could be acquired in as quickly as a few minutes or up to several days. There are many determining factors, including required level of spatial resolution in x , y , and z dimensions, as well as the statistical precision required and level of label incorporation. High-spatial-resolution acquisitions necessitate use of low primary ion beam currents, which reduces the secondary ion signals and sputter rate. The only recourse to retain high levels of statistical precision is to extend the acquisition time accordingly. Considering that the experiment will contain a standard control sample and perhaps different label concentrations and/or time points, it is not unreasonable for the entire MIMS analysis campaign to be of 10-14 days duration and perhaps longer. Finally, data processing and analysis can easily (and should) require 2-3 times the experimental time, but can be done off-line while other analyses are being acquired.
For correlative microscopy studies (and given the destructive nature of the MIMS technique), the NanoSIMS 50L analysis must be the last imaging modality used. Days to weeks may also be required for the acquisition of the correlative images (SEM, fluorescence, TEM).
Acknowledgments
The authors thank Max Sarfati, former CEO of Cameca, for donating the NanoSIMS prototype to Harvard Medical School/Brigham and Women's Hospital, and Georges Slodzian for continuously sharing with us his immense knowledge. The authors are deeply indebted to Francois Hillion for teaching us the finesse of the practical use of the prototype and NanoSIMS 50L. The authors thank Jeremy Brewer, Adam Cohen, Boris Epstein, Christelle Guillermier, Jamie Hill, Zeke Kaufman, Jason LeBeau, Colin Poczatek, Hugues-Francois St. Cyr, and Mei Wang for their invaluable contributions to the development of MIMS and Matt Steinhauser for development of stable isotope labeled thymidine pulsing techniques in live animals. The authors thank Louise Trakimas for superb assistance with sample preparation and Frederic Brochu for maintaining OpenMIMS. Heather Miniman helped in preparing the manuscript. C.P.L. acknowledges financial support from the National Institutes of Health (EB001974, AG034641), the Ellison Medical Foundation, and the Human Frontier Science Program. G.M. acknowledges funding from NPL's ISCF Medical Imaging Accelerator program financed by the U.K. Department for Business, Energy, and Industrial Strategy's Industrial Strategy Challenge Fund.
Author Contributions
G. McMahon : formal analysis, investigation, supervision, visualization, writing original draft; C.P. Lechene : conceptualization, data curation, formal analysis, funding acquisition, investigation, methodology, project administration, resources, software, supervision, visualization, writing review and editing.
Conflict of Interest
The authors declare no conflict of interest.
Open Research
Data Availability Statement
The data that support the findings of this study are available from the corresponding author upon reasonable request.
Literature Cited
- Bailey, A. P., Koster, G., Guillermier, C., Hirst, E. M. A., MacRae, J. I., Lechene, C. P. … Gould, A. P. (2015). Antioxidant role for lipid droplets in a stem cell niche of Drosophila. Cell , 163(2), 340–353. doi: 10.1016/j.cell.2015.09.020.
- Bergmann, O., Bhardwaj, R. D., Bernard, S., Zdunek, S., Barnabé-Heider, F., Walsh, S. … Frisén, J. (2009). Evidence for cardiomyocyte renewal in humans. Science , 324(5923), 98–102. doi: 10.1126/science.1164680.
- Bushong, E. A., Johnson, D. D., Kim, K.-Y., Terada, M., Hatori, M., Peltier, S. T. … Ellisman, M. H. (2015). X-ray microscopy as an approach to increasing accuracy and efficiency of serial block-face imaging for correlated light and electron microscopy of biological specimens. Microscopy and Microanalysis , 21(1), 231–238. doi: 10.1017/S1431927614013579.
- Cabin-Flaman, A., Monnier, A.-F., Coffinier, Y., Audinot, J.-N., Gibouin, D., Wirtz, T. … Norris, V. (2011). Combed single DNA molecules imaged by secondary ion mass spectrometry. Analytical Chemistry , 83(18), 6940–6947.doi: 10.1021/ac201685t.
- Castaing, R., & Slodzian, G. (1962). Microanalyse par emission ionique secondaire. Journal de Microscopie , 1, 395–410.
- Deerinck, T., Bushong, E., Lev-Ram, V., Shu, X., Tsien, R., & Ellisman, M. (2010). Enhancing serial block-face scanning electron microscopy to enable high resolution 3-D nanohistology of cells and tissues. Microscopy and Microanalysis , 16(S2), 1138–1139. doi: 10.1017/S1431927610055170.
- Donovan, J., & Brown, P. (2006b). Parenteral injections. Current Protocols in Immunology , 73, 1.6.1–1.6.10. doi: 10.1002/0471142735.im0106s73.
- Donovan, J., & Brown, P. (2006a). Euthanasia. Current Protocols in Immunology , 73, 1.8.1–1.8.4. doi: 10.1002/0471142735.im0108s73.
- Eichorst, S. A., Strasser, F., Woyke, T., Schintlmeister, A., Wagner, M., & Woebken, D. (2015). Advancements in the application of NanoSIMS and Raman microspectroscopy to investigate the activity of microbial cells in soils. FEMS Microbiology Ecology , 91(10), fiv106. doi: 10.1093/femsec/fiv106.
- Finzi-Hart, J. A., Pett-Ridge, J., Weber, P. K., Popa, R., Fallon, S. J., Gunderson, T. … Capone, D. G. (2009). Fixation and fate of C and N in the cyanobacterium Trichodesmium using nanometer-scale secondary ion mass spectrometry. Proceedings of the National Academy of Sciences , 106(15), 6345–6350. doi: 10.1073/pnas.0810547106.
- Fu, Y., & Kraitchman, D. L. (2010). Stem cell labeling for noninvasive delivery and tracking in cardiovascular regenerative therapy. Expert Review of Cardiovascular Therapy , 8(8), 1149–1160. doi: 10.1586/erc.10.106.
- Guerquin-Kern, J.-L., Wu, T.-D., Quintana, C., & Croisy, A. (2005). Progress in analytical imaging of the cell by dynamic secondary ion mass spectrometry (SIMS microscopy). Biochimica et Biophysica Acta , 1724(3), 228–238. doi: 10.1016/j.bbagen.2005.05.013.
- Heister, K., Höschen, C., Pronk, G. J., Mueller, C. W., & Kögel-Knabner, I. (2012). NanoSIMS as a tool for characterizing soil model compounds and organomineral associations in artificial soils. Journal of Soils and Sediments , 12(1), 35–47. doi: 10.1007/s11368-011-0386-8
- Katchalski, T., Case, T., Kim, K., Ramachandra, R., Bushong, E. A., Deerinck, T. J. … Ellisman, M. H. (2018). Iron-specific signal separation from within heavy metal stained biological samples using x-ray microtomography with polychromatic source and energy-integrating detectors. Scientific Reports , 8(1), 7553. doi: 10.1038/s41598-018-25099-z.
- Lechene, C., Hillion, F., McMahon, G., Benson, D., Kleinfeld, A. M., Kampf, J. P. … Slodzian, G. (2006). High-resolution quantitative imaging of mammalian and bacterial cells using stable isotope mass spectrometry. Journal of Biology , 5, 20. doi: 10.1186/jbiol42.
- Lechene, C. P., Luyten, Y., McMahon, G., & Distel, D. L. (2007). Quantitative imaging of nitrogen fixation by individual bacteria within animal cells. Science , 317(5844), 1563–1566. doi: 10.1126/science.1145557.
- Legin, A. A., Schintlmeister, A., Jakupec, M. A., Galanski, M., Lichtscheidl, I., Wagner, M., & Keppler, B. K. (2014). NanoSIMS combined with fluorescence microscopy as a tool for subcellular imaging of isotopically labeled platinum-based anticancer drugs. Chemical Science , 5(8), 3135. doi: 10.1039/c3sc53426j.
- Lovrić, J., Dunevall, J., Larsson, A., Ren, L., Andersson, S., Meibom, A. … Ewing, A. G. (2017). Nano secondary ion mass spectrometry imaging of dopamine distribution across nanometer vesicles. ACS Nano , 11(4), 3446–3455. doi: 10.1021/acsnano.6b07233.
- McMahon, G., Glassner, B. J., & Lechene, C. P. (2006). Quantitative imaging of cells with multi-isotope imaging mass spectrometry (MIMS)—Nanoautography with stable isotope tracers. Applied Surface Science , 252(19). doi: 10.1016/j.apsusc.2006.02.170.
- Milucka, J., Kirf, M., Lu, L., Krupke, A., Lam, P., Littmann, S. … Schubert, C. J. (2015). Methane oxidation coupled to oxygenic photosynthesis in anoxic waters. The ISME Journal , 9(9), 1991–2002. doi: 10.1038/ismej.2015.12.
- Pasulka, A. L., Thamatrakoln, K., Kopf, S. H., Guan, Y., Poulos, B., Moradian, A. … Orphan, V. J. (2018). Interrogating marine virus-host interactions and elemental transfer with BONCAT and nanoSIMS-based methods. Environmental Microbiology , 20(2), 671–692. doi: 10.1111/1462-2920.13996.
- Peteranderl, R., & Lechene, C. (2004). Measure of carbon and nitrogen stable isotope ratios in cultured cells. Journal of the American Society for Mass Spectrometry , 15(4), 478–485. doi: 10.1016/j.jasms.2003.11.019.
- Pinson, E. A. (1952). Water exchanges and barriers as studied by the use of hydrogen isotopes. Physiological Reviews , 32(2), 123–134. doi: 10.1152/physrev.1952.32.2.123.
- Poenie, M., Alderton, J., Steinhardt, R., & Tsien, R. (1986). Calcium rises abruptly and briefly throughout the cell at the onset of anaphase. Science , 233(4766), 886–889. doi: 10.1126/science.3755550.
- Richter, K. N., Rizzoli, S. O., Jähne, S., Vogts, A., & Lovric, J. (2017). Review of combined isotopic and optical nanoscopy. Neurophotonics , 4(2), 020901. doi: 10.1117/1.NPh.4.2.020901.
- Schoenheimer, R. (1942). The dynamic state of body constituents. Cambridge, MA: Harvard University Press..
- Senyo, S. E., Steinhauser, M. L., Pizzimenti, C. L., Yang, V. K., Cai, L., Wang, M. … Lee, R. T. (2013). Mammalian heart renewal by pre-existing cardiomyocytes. Nature , 493(7432), 433–436. doi: 10.1038/nature11682.
- Slodzian, G., Daigne, B., Girard, F., Boust, F., & Hillion, F. (1992). Scanning secondary ion analytical microscopy with parallel detection. Biology of the Cell , 74(1), 43–50. doi: 10.1016/0248-4900(92)90007-N.
- Steinhauser, M. L., Bailey, A. P., Senyo, S. E., Guillermier, C., Perlstein, T. S., Gould, A. P. … Lechene, C. P. (2012). Multi-isotope imaging mass spectrometry quantifies stem cell division and metabolism. Nature , 481(7382), 516–519. doi: 10.1038/nature10734.
- Tang, S. S., Guillermier, C., Wang, M., Poczatek, J. C., Suzuki, N., Loscalzo, J., & Lechene, C. (2014). Quantitative imaging of selenoprotein with multi-isotope imaging mass spectrometry (MIMS). Surface and Interface Analysis , 46(S1), 154–157. doi: 10.1002/sia.5625.
- Toyama, B. H., Arrojo e Drigo, R., Lev-Ram, V., Ramachandra, R., Deerinck, T. J., Lechene, C. … Hetzer, M. W. (2019). Visualization of long-lived proteins reveals age mosaicism within nuclei of postmitotic cells. Journal of Cell Biology , 218(2), 433–444. doi: 10.1083/jcb.201809123.
- Vujic, A., Lerchenmüller, C., Wu, T.-D., Guillermier, C., Rabolli, C. P., Gonzalez, E. … Rosenzweig, A. (2018). Exercise induces new cardiomyocyte generation in the adult mammalian heart. Nature Communications , 9(1), 1659. doi: 10.1038/s41467-018-04083-1.
- Wolfe-Simon, F., Blum, J. S., Kulp, T. R., Gordon, G. W., Hoeft, S. E., Pett-Ridge, J. … Oremland, R. S. (2011). A bacterium that can grow by using arsenic instead of phosphorus. Science , 332(6034), 1163–1166. doi: 10.1126/science.1197258.
- Zhang, D.-S., Piazza, V., Perrin, B. J., Rzadzinska, A. K., Poczatek, J. C., Wang, M. … Lechene, C. P. (2012). Multi-isotope imaging mass spectrometry reveals slow protein turnover in hair-cell stereocilia. Nature , 481(7382), 520–524. doi: 10.1038/nature10745.
Internet Resources
Fiji is an open-source image processing package based on ImageJ.
General description of OpenMIMS , an open-source data reduction and processing software.
The User Manual for OpenMIMS.
Download site for OpenMIMS plugin for Fiji.
JMP is a powerful statistical analysis platform. Import data extracted via OpenMIMS for further exploration and mining.
Citing Literature
Number of times cited according to CrossRef: 1
- Li-Xing Nie, Lie-Yan Huang, Xin-Ping Wang, Lin-Feng Lv, Xue-Xin Yang, Xiao-Fei Jia, Shuai Kang, Ling-Wen Yao, Zhong Dai, Shuang-Cheng Ma, Desorption Electrospray Ionization Mass Spectrometry Imaging Illustrates the Quality Characters of Isatidis Radix, Frontiers in Plant Science, 10.3389/fpls.2022.897528, 13 , (2022).