Detecting RNA–Protein Interactions With EGFP-Cy3 FRET by Acceptor Photobleaching
Shagufta Rehman Alam, Shagufta Rehman Alam, Mani S. Mahadevan, Mani S. Mahadevan, Ammasi Periasamy, Ammasi Periasamy
Abstract
Förster Resonance Energy Transfer (FRET) is a great tool for cell biologists to investigate molecular interactions in live specimens. FRET is a distance-dependent phenomenon which can detect molecular interactions at distances between 1-10 nm. Several FRET approaches are reported in the literature for live and fixed cells to study protein-protein interactions; this protocol provides details of acceptor photobleaching as a FRET method to study RNA-Protein interactions. Cy3-labeled RNA foci (FRET acceptors) are photobleached at the intra-cellular site of interest (the nuclei) and the intensity of the EGFP-tagged proteins (FRET donors) at that same site are measured pre- and post- photobleaching. In principle, FRET is detected if the intensity of EGFP increases after photobleaching of Cy3. This protocol describes necessary steps and appropriate controls to conduct FRET measurements by the acceptor photobleaching method. Successful applications of this protocol will provide data to support the conclusion that EGFP-labeled proteins directly interact with Cy3-labeled RNA at the site of photobleaching. © 2023 The Authors. Current Protocols published by Wiley Periodicals LLC.
Basic Protocol : FRET in fixed cells
Alternate Protocol : FRET in live cells
INTRODUCTION
This paper describes a protocol for detecting molecular interactions between RNA and proteins at a specific location within a cell. We previously developed this FRET assay using acceptor photobleaching for identifying deleterious mutant RNA-protein interactions in cells derived from patients of myotonic dystrophy type 1 (DM1) (Rehman, Gladman, Periasamy, Sun, & Mahadevan, 2014).
Key physiological and cellular processes require molecular interactions which may be stable or transient in nature. Currently, in vitro methods like UV crosslinking assays and immunoprecipitation (IP), which require cell disruption and homogenization under denaturing conditions, are used to identify the binding target molecules, which disrupts the spatial and temporal resolution of the interacting partners, otherwise identified by FRET.
Disease pathogenesis due to RNA toxicity is increasingly being recognized in other disorders with repeat expansion such as myotonic dystrophy. DM1 is caused by the (CTG)n repeat expansion in the 3′ UTR of the DMPK gene which results in the nuclear retention of DMPK transcripts in RNA foci. The toxic gain-of-function of the mutant RNA modifies the functions of RNA binding proteins like MBNL1. Using acceptor photobleaching FRET we were able to identify a direct interaction between mutant RNA foci and MBNL1 in DM1 patient cells (Rehman et al., 2014). FRET interactions were identified in intact cells without cell disruption or homogenization.
Like any other experimental technique, a positive and a negative control is required for FRET measurements. For most FRET imaging experiments, three sets of specimens (slides) are required for both positive and negative control samples: double labeled (DA), donor alone (D), and acceptor alone (A). To perform this assay, stable or transiently transfected DM1 cells (fibroblasts or myoblasts) expressing the following is required: (1) EGFP-protein (MBNL1) and mutant RNA forming RNA foci FISH labeled with Cy3, used as a DA positive control ; (2) cells expressing both unfused EGFP and FISH-labeled Cy3-RNA should be used as a DA negative control ; (3) cells expressing EGFP-protein or unfused EGFP (D); and (4) cells expressing only the FISH-labeled Cy3-RNA (A) should be used for optimization of the photobleaching cycle. Inclusion of unlabeled, untransfected cells as a dark control for background noise is highly encouraged. After performing the Basic Protocol (see below), the slides were imaged on a Leica TCS SP5 X confocal microscope with a white light laser (WLL); any other comparable confocal microscope would be suitable, with appropriate excitation and emission filters to detect EGFP and Cy3 FRET pairs, respectively. In this protocol, a WLL at 488 nm for EGFP (donor) and at 552 nm for Cy3 (acceptor) were used on the Leica TCS SP5 X. Acceptor-Cy3-labeled RNA foci were photobleached at a specific location (using the ROI function) within cells with a 552 nm setting of the WLL at 100% power level for 8 iterations. Donor-EGFP tagged protein fluorescence intensity was quantified pre- and post-photobleaching by the acceptor (Cy3). FRET is detected if the fluorescence intensity of the donor (MBNL1-EGFP, positive control) increases significantly above the fluorescence intensity of the negative control and the donor alone specimens, at the same intracellular site after photobleaching the acceptor. This indicates molecular interactions between the protein (MBNL1) and RNA (mutant RNA foci) at the location of the photobleach. Differences in FRET signals expressed as energy transfer efficiency (E%) in the positive control represent differences in proximities between the protein and RNA within different ribonuclear foci (as can be seen in Fig. 1). The Basic Protocol can be modified for live cells (see Alternate Protocol) by other in vivo RNA labeling methods described elsewhere (Bao, Won, & Tsourkas, 2009).
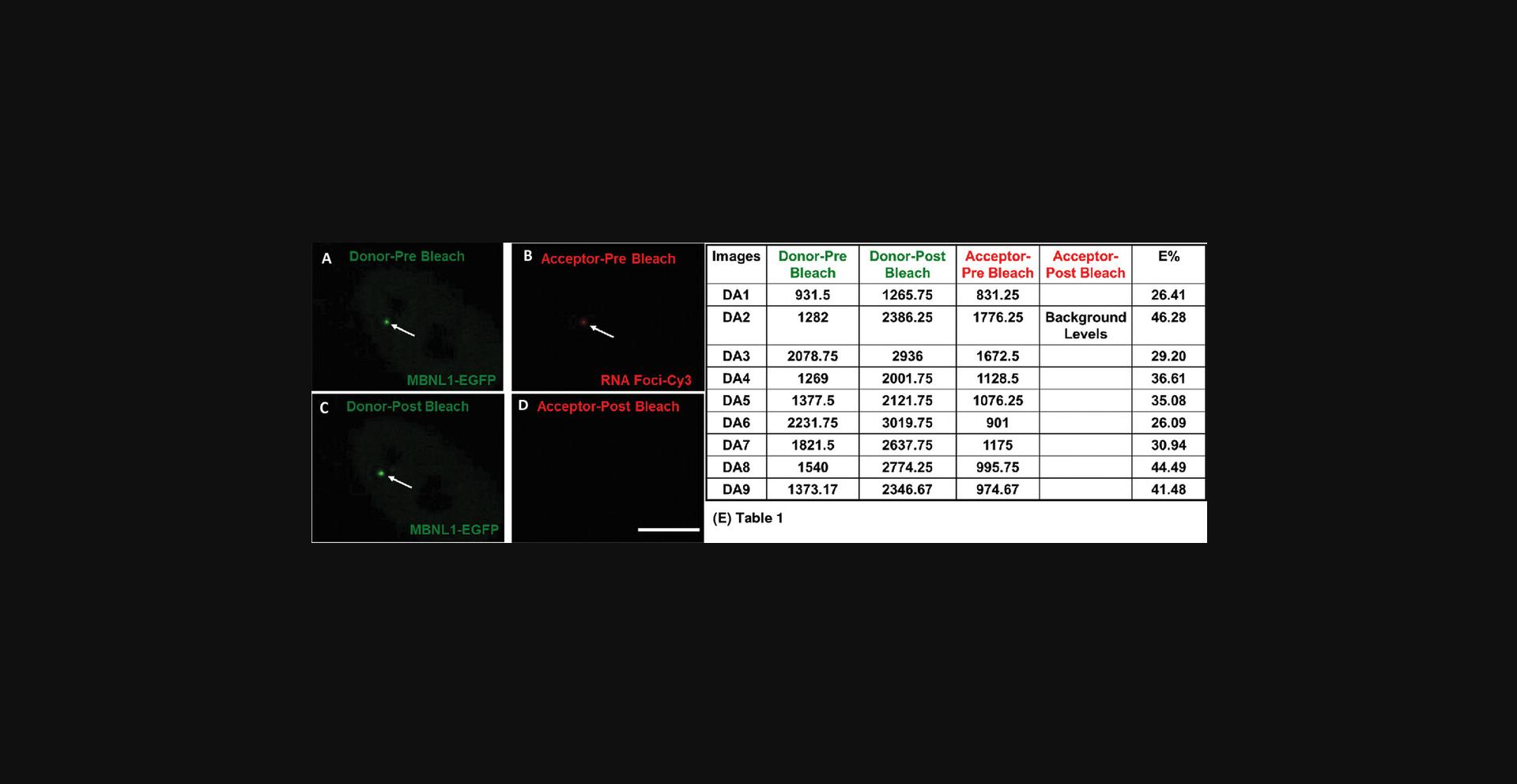
Basic Protocol: FRET IN FIXED CELLS
This protocol outlines a method we previously developed to identify deleterious mutant RNA interactions in DM1 (Rehman et al., 2014) and is therefore highly recommended. EGFP and Cy3 were used as FRET pairs to identify molecular interaction between the mutant RNA foci and the MBNL1 protein.
Materials
-
Plasmids: EGFP-Protein (MBNL1) and EGFP empty vector (see “Prepare plasmids, cell lines, transfection, slides for FRET”)
-
Cell line of interest expressing the RNA foci (mutant RNA) endogenously (Genomics Institute of the Novartis Research Foundation, CA)
-
Transfection reagent (lipofectamine, Invitrogen, cat. no. L3000001 or Nucleofector, Lonza, cat. no. VPD-1001)
-
Reagents for RNA-FISH:
- Phosphate-buffered saline (PBS) (Gibco, cat. no. 10010023)
- 4% (v/v) paraformaldehyde (Sigma-Aldrich, cat. no. 158127) in PBS
- 2% (v/v) cold acetone (Sigma-Aldrich, cat. no. 179124) in PBS
- 30% (v/v) formamide (Sigma-Aldrich, cat. no. F9037) in 2× SSC buffer (Fisher Scientific, cat. no. BP132520)
- Cy3-labeled (CAG)10 anti-sense probe (Integrated DNA Technologies)
- Sequence: 5′-/5Cy3/CAG CAG CAG CAG CAG CAG CAG CAG CAG CAG -3′
- Hybridization buffer (see recipe)
-
Mounting medium without antifade (Vectashield, Vector Laboratories, cat. no. H-1000-10)
-
No. 1.5 coverslips (Fisher Scientific, cat. no. NC1272770)
-
6-well tissue-culture plate (Greiner Bio-One, CellStar, cat. no. 657160)
-
Laser scanning confocal microscope e.g., Leica TCS SP5 X or a similar system with:
-
63× oil immersion objective, numerical aperture, 1.4
-
Excitation wavelengths: 488 nm for EGFP, 552 nm for Cy3
-
500-550 nm emission filter for EGFP
-
565-700 nm emission filter for Cy3
-
Additional reagents for transfection (Seidman, Struhl, Sheen, & Jessen, 2001) or nucleofection (Lonza), for mammalian cell culture, and RNA-FISH (Rehman et al., 2014) can be found in the cited references.
Prepare plasmids, cell lines, transfection, slides for FRET
1.To test FRET between RNA (mutant RNA) endogenously expressed in DM1 cells and protein (MBNL1), construct or obtain the expression plasmids (Fardaei, 2001; Rehman et al., 2014).
2.Perform experiments to assess the functionality of the fusion protein and the mutant RNA.
3.Construct or obtain plasmids to be used as negative FRET controls. In this case, unconjugated EGFP or an empty vector may be obtained (Clontech).
4.Perform transfections (Seidman et al., 2001) using standard transfection procedure or by nucleofection with the plasmids obtained in steps 1-3 to obtain five different coverslips of cells containing the following:
-
Protein-EGFP (MBNL1-EGFP) and RNA foci (mutant RNA); DA Positive Control.
-
Protein-EGFP (MBNL1-EGFP); Donor Alone.
-
RNA foci (mutant RNA); Acceptor Alone.
-
Unconjugated EGFP and RNA foci (mutant RNA); DA Negative Control.
-
Unconjugated EGFP; Donor Alone.
NOTE: For FRET studies, transfection with 1 μg of plasmid DNA was sufficient in our hands.
5.Allow the transfected cells to grow on no. 1.5 glass coverslips in a 6-well plate until the full expression of the plasmids are attained (24-48 hr).
Details on mammalian cell culture used in this protocol can be found elsewhere (Rehman et al., 2014).
6.Fix the transfected DM1 cells with 4% paraformaldehyde and proceed with RNA-FISH using a Cy3-labeled anti-sense oligonucleotide probe for the mutant RNA as described in detail by us (Rehman et al., 2014) and mentioned here:
-
Following fixation of DM1 cells, permeabilize the cells in cold 2% acetone in PBS for 5 min at room temperature.
-
Wash the cells with PBS three times.
-
Incubate the cells with 30% formamide in 2× SSC buffer at 37°C for 10 min.
-
Proceed with hybridization with Cy3 labeled (CAG)10 probe at 0.1 ng/µl for 2 hr at 37°C in the hybridization buffer (seerecipe).
-
After the hybridization, wash the cells in 30% formamide in 2× SSC at 45°C for 30 min.
7.After RNA-FISH, wash the DM1 cells with PBS (three times, 10 min each) and mount the coverslips on a glass slide with a mounting medium such as Vectashield without anti-fade. Seal the glass coverslip with a transparent nail paint, air dry and proceed with FRET imaging by the acceptor photobleaching method. Alternatively, Fluoromount G (Thermo Fisher Scientific, cat. no. 00-4958-02) may be used as a mounting medium. It forms a semi-permanent seal and does not require the use of nail paint for sealing.
Configure the confocal microscope for EGFP and Cy3 imaging
8.Prepare the Leica TCS SP5 X confocal microscope used here with WLL or with a comparable laser system for excitation with 488 nm and 552 nm laser lines.
9.Select the magnification objective and zoom settings.
10.Make sure to have the correct filters and dichroic in the optical path for EGFP and Cy3 imaging. For the Leica TCS SP5 X, adjust the tunable Acousto-Optical Tunable Filters (AOBS) to 500-550 nm for EGFP and 565-700 nm for Cy3.
11.Select PMT2 for EGFP and PMT3 for Cy3.Set the PMT gain to 650-750V.
12.Select 12-bit image on the acquisition-scan control window.
13.Select sequential scan by frame for fixed cells (select line scan for live cells).
14.Use the Leica AP wizard or a comparable workflow on a different system for acceptor photobleaching FRET.
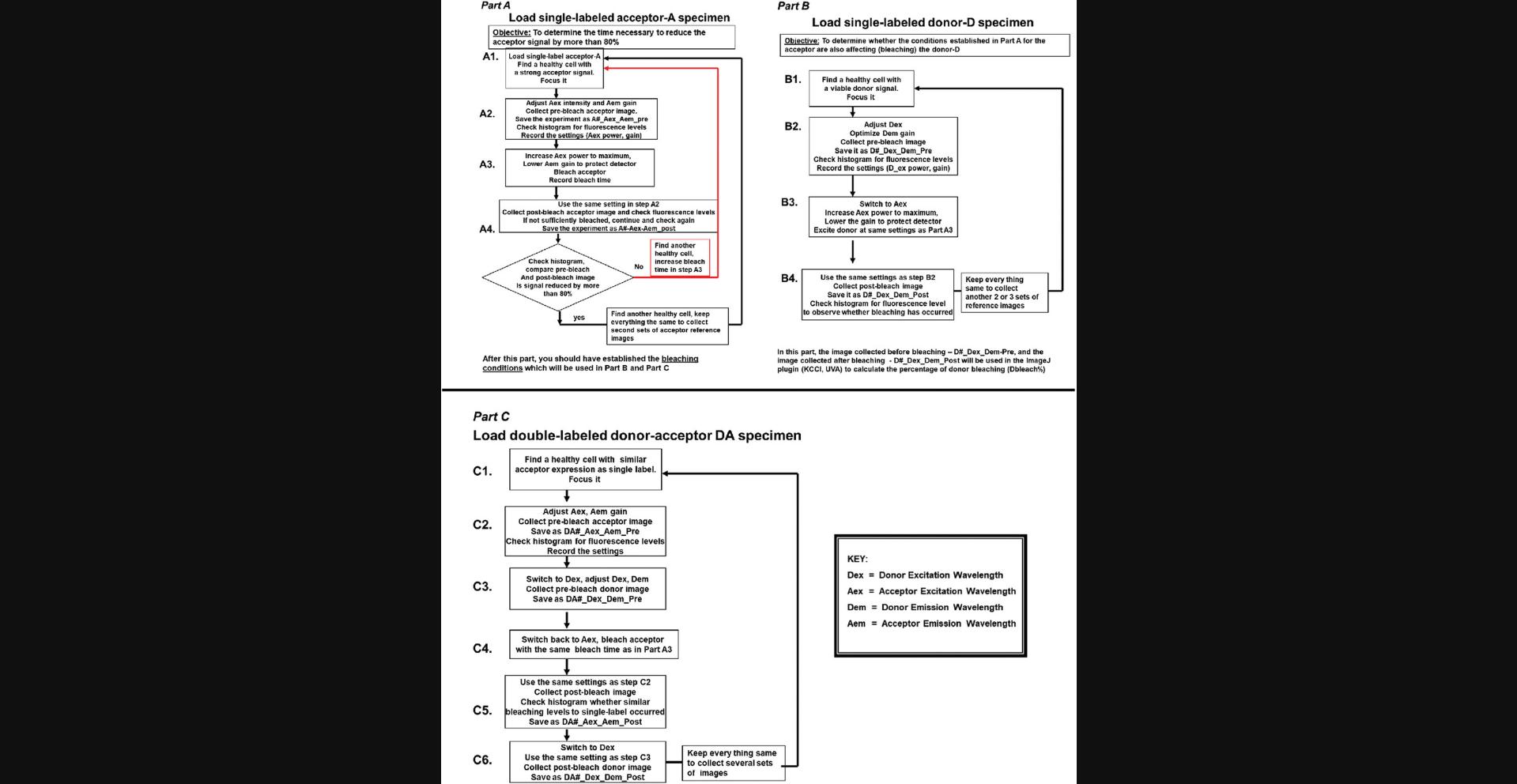
Optimization of the imaging parameters for dual confocal imaging of EGFP and Cy3
Perform steps 15-20 on the slides with protein-EGFP and then Cy3 alone, and then again with the protein-EGFP slide. Optimization of imaging parameters (laser excitation, PMT gain, offset, and emission filters) are necessary to make sure that there is no bleed-through or crosstalk between the 2 channels, and that there is no photobleaching of the fluorophores during normal image acquisition. While selecting the field of view of cells for the experiment, check the morphology of the cells using the bright field or phase contrast function of the microscope. These steps are again repeated for the negative control unconjugated EGFP slide.
15.Load the protein-EGFP (or EGFP) slide first on the microscope stage. Use the microscope ocular to find representative cells using a mercury vapor arc (or LED) lamp and the corresponding filter cube to EGFP or Cy3. Focus the cells to the center of the Field of View (FoV).
16.Switch to the confocal acquisition mode. Use the settings for the EGFP channel as described above. To keep the thickness of the optical section for the EGFP channel identical to the Cy3 channel, first select the pinhole for EGFP to 1 airy unit and then do the same for the Cy3 channel. Turn on the 488 nm laser to 5%-20% acousto optical tunable filter (AOTF) depending on the intensity of the protein-EGFP (or unconjugated EGFP).
17.Both EGFP and Cy3 detectors can be kept ON to check for a bleed-through signal. Set the detector or PMT gain between 650-750v such that the gray level intensity does not exceed more than ∼2000 for EGFP and ∼3000 intensity units for Cy3 on a 12-bit image depth.
18.If the gray level intensity of EGFP is lower, try increasing the PMT gain by an additional 50v and/or the 488 nm laser excitation by 1%-2%.
19.Check the average background signal using the dark control, the untransfected cells, and select the offset if needed to reduce the background noise.
20.Load the Cy3 slide and repeat steps 15-19 using the 552 nm laser to 10%-12% AOTF.
21.Save the optimized imaging parameters (steps 15-20) and do not change for subsequent imaging.
Perform the acceptor photobleaching
Steps 22-25 are to be performed on slides a, b, c, d, & e prepared in step 4.First, to optimize the bleaching cycle proceed with “c” for the RNA foci-Cy3 acceptor alone (A) slide.
22.Place the slide “c” with the RNA foci-Cy3 acceptor alone (A) specimen on the microscope stage. Using the microscope ocular, focus the cells to the center of the stage as described in step 15.
23.Select the region of interest (ROI) over the RNA foci. Set up the bleaching cycle with the 552 nm laser with 100% AOTF. The bleaching cycle can be defined by activating the FRET AB wizard on the Leica TCS SP5 X or a similar workflow macro on a standard commercial confocal microscope as outlined in Figure 2.A time series module in lieu of a wizard/macro could also be used to set up the bleaching cycle.
24.Once the acceptor bleaching cycle is optimized, donor alone slides with specimen “b” (protein-EGFP) and “e” (unconjugated EGFP) can be tested. Donor intensity prebleach and with the bleaching cycle can be quantified over time in targeted bleached and non-bleached ROIs using a time series module.
25.If donor photobleaching is observed, reduce the 552 nm laser power and iterations as described in the section “critical considerations in acceptor photobleaching and donor image acquisition.” Also see Figure 2.
FRET data collection and calculation of efficiency of energy transfer (E%)
26.Proceed with FRET data collection with the double labeled DA and control slides using the specimens a, b, d, and e from step 4, and the dark control with untransfected cells (for measuring the background noise) with the optimized settings described in steps 15-19 and 22-25.One can use the Leica TCS SP5 X AB wizard or a similar workflow as described below (Fig. 2). Image at least 30-40 cells for independent ROIs.
27.Using the Leica TCS SP5 X AB wizard or a similar software for image quantification, draw a 2 × 2 pixel ROI over the RNA foci and quantify pre- and post-bleach intensities of donor and acceptor (as shown in Fig. 1) for the slides a, b, d, and e from step 4.
28.Calculate FRET efficiency (E%) in the targeted bleached ROIs using the formula
29.Calculate FRET efficiency (E%) in the non-targeted, non-bleached control (Ctrl) ROIs to get Ctrl-E%.
30.Calculate Expt-E% and Ctrl-E% in all the 4 slides a, b, d, and e to assess whether or not FRET occurred between the target mutant RNA foci and MBNL1 protein as described under “Understanding Results.”
31.A more generalized approach for carrying out FRET by acceptor photobleaching using any FRET pair is outlined below in Figure 2.
Alternate Protocol: FRET IN LIVE CELLS
The basic protocol is suitable for fixed cell applications because of the requirement of the RNA-FISH labeling step for visualization of the endogenous mutant RNA foci in DM1 cells. However, the basic protocol can be applied to live cells if the visualization of the endogenous RNA in living cells can be modified and achieved by other in vivo RNA labeling techniques, for example by the use of targeted oligonucleotide probes or molecular beacons as described elsewhere (Bao et al., 2009).
The basic protocol can be applied to live cells if the targets (protein and RNA) do not exchange or diffuse rapidly. This can be checked by photobleaching protein and RNA separately in live cells and collecting time lapse images to see if there is recovery of fluorescence after photobleaching (Day & Davidson, 2012). If there is recovery, follow the photobleaching step as described below.
Additional materials (also see Basic Protocol)
-
DMEM fluorobrite (Invitrogen-Gibco, cat. no. A1896701)
-
Collagen-coated glass bottom dishes (MatTek, cat. No. P35GCOL-1.5-14-C) for seeding cells for transfections, labeling, and live-cell imaging of protein and RNA
-
Live-cell imaging chamber or heated stage inserts with humidified gas flow for maintaining live-cell imaging conditions
-
If the modified alternate protocol is used for the visualization of the protein-EGFP and RNA of interest in live cells, skip the steps of fixation and RNA-FISH (steps 6-7) and proceed to imaging in basic cell culture media without phenol red or other suitable imaging media like DMEM fluorobrite. Imaging in phenol red-free media is required to keep the background noise low.
-
When performing photobleaching of the acceptor, use the whole or half of the cell to photobleach, such that all or most of the acceptor fluorescence signal will be destroyed after photobleaching.
-
This is required to prevent the recovery of acceptor molecules from the vicinity into the targeted ROIs for FRET analysis which will otherwise confound the effect of acceptor photobleaching on the donor fluorescence and evaluation of E%. Another caveat for FRET by acceptor photobleaching in live cells could be the contribution of the EGFP donor “mobile fraction” from the vicinity which could lead to false positivity of FRET signal.
Prepare plasmids, cell lines, transfection, and slides for FRET
Prepare plasmids, cell lines, transfection, and slides for FRET as described in the Basic Protocol (steps 1-5) and as mentioned above in the section “FRET in live cells” for labeling the endogenous RNA for live-cell imaging.
1.For the alternate protocol skip the steps of fixation and RNA-FISH (steps 6-7) and proceed with live-cell imaging in phenol red-free or DMEM fluorobrite.
2.Wash the cells (expressing the protein-EGFP and the labeled RNA) growing on the collagen-coated glass bottom dishes with PBS (three washes, 10 min each).
3.Mount the culture dish with the cells in DMEM fluorobrite onto the microscope stage with the live-cell imaging chamber or heated stage inserts under humidified gas flow.
4.Proceed with steps 8-30 as described in the Basic Protocol.
REAGENTS AND SOLUTIONS
Hybridization buffer (freshly prepared)
- Prepare 0.1% diethyl pyrocarbonate (DEPC) (Sigma-Aldrich, cat. no. D5758) using autoclaved water and add the following to the required volume
- 2× SCC (Fisher Scientific, cat. no. BP132520)
- 30% formamide (Sigma-Aldrich, cat. no. F9037)
- 0.02% BSA (Sigma-Aldrich, cat. no. A3858)
- 66 µg/ml yeast tRNA (Thermo Fisher Scientific, cat. no. AM7119)
- 2 mM vanadyl complex (Sigma-Aldrich, cat. no. R3380)
- Store at 4°C up to 2 weeks
COMMENTARY
Background Information
Basics of FRET
All physiological cellular processes are dependent on molecular interactions. Some of these interactions form stable complexes like large macro-molecular complexes like the ribonuclear foci (used here as an example); while others are transient in nature depending on the role of that protein. Most of these interactions are still identified by in vitro biochemical assays like immunoprecipitation (IP) for protein-protein, RNA-IP for RNA-protein, and for DNA-protein by UV cross linking assays. Homogenization of the tissues and cells in these assays disrupts identification of spatial or temporal resolution of the molecular interaction in their native environment. Also, the nature of the homogenization and extraction protocols could lead to artifacts due to non-specific binding of proteins.
With fluorescence microscopy one could identify if there is co-localization between the 2 target molecules labeled with two different (spectrally apart) color fluorophores within the cells and tissues without the need for homogenization. However, light microscopy approaches are limited by the constraints of diffraction-limit of light (Abbe, 1873). Co-localization, though a pre-requisite to FRET studies, is not synonymous to interaction. If the two target molecules are observed to co-localize together by fluorescence microscopy, this simply means that they reside within the focal volume (voxel), and not necessarily directly interact with each other. For FRET to occur, the molecular interactions take place at distances between 1-10 nm. The advent of super resolution microscopy allows visualization of target proteins beyond the diffraction-limit (Betzig, 1995; Hell & Wichmann, 1994; Moerner & Kador, 1989) depending on the type of the super resolution modality used, which will have its own technical requirements and challenges (Betzig et al., 2006; Klar, Jakobs, Dyba, Egner, & Hell, 2000).
Förster Resonance Energy Transfer (FRET) is an advanced light microscopy technique widely used to identify molecular interactions. FRET is a distant-dependent phenomenon in which the energy transfer takes place from donor fluorophore in the excited state to an acceptor fluorophore in the ground state, via long range dipole-dipole coupling (Förster, 1948, 2012). FRET is described as the non-radiative transfer of energy which occurs when the emission spectrum of the donor fluorophore overlaps with the excitation spectrum of the acceptor fluorophore in proximity. FRET can occur only when the three conditions of FRET are satisfied, as explained below (Periasamy & Day, 2005; Sun & Periasamy, 2015).
There are three main criteria for FRET to occur: (a) spectral overlap integral, meaning there should be a spectral overlap of at least 30% or more between emission spectrum of the donor and the absorption spectrum of the acceptor fluorophores; (b) molecular distance, the distance separating the donor and acceptor fluorophores should be within 1-10 nm; and (c) matching dipole orientation (K2) between donor and acceptor fluorophores, meaning that the emission dipole of the donor and the absorption dipole of the acceptor must be oriented to each other and not perpendicular to each other (Lakowicz, 2006). Due to FRET, there is quenching of the donor fluorescence and sensitized emission from the acceptor fluorophore. The amount of FRET, or its efficiency E , is given by the equation E = R 06/(R 06 + r 6). E is inversely proportional to the sixth power of the distance (r) separating the donor and acceptor molecule subject to the Förster distance (R 0). R 0 is the distance between the donor and acceptor fluorophores at which the energy transfer efficiency is 50%. Most of the FRET pairs have R 0 values between 2-6 nm (Karpova & McNally, 2006). R 0 between EGFP and Cy3 is 6 nm (Snapp & Hegde, 2006). Similarly, R 0 values of suitable FRET pairs with high quantum yields can be found elsewhere (Bajar et al., 2016), or in molecular probes handbooks and the 2006 Leica Application notes, and chosen based on the experimental strategy. In principle, E decreases dramatically if r is more than their R 0 (Periasamy & Day, 2005).
Since, the efficiency of the energy transfer varies with the sixth power of the distance separating the donor and acceptor molecules, the molecular distance at which FRET can occur is limited to 1-10 nm. Therefore, FRET acts as a spectroscopic ruler and provides a non-invasive method to visualize protein-protein interactions (Day, Periasamy, & Schaufele, 2001; Periasamy & Day, 2005), DNA-protein (Parkhurst, Parkhurst, Powell, Wu, & Williams, 2002) and RNA–protein (Rehman et al., 2014) interactions in intact cells.
FRET applications
The discovery of GFP and its application to research has revolutionized in vivo fluorescence imaging (Chalfie, Tu, Euskirchen, Ward, & Prasher, 1994; Prasher, Eckenrode, Ward, Prendergast, & Cormier, 1992). Visible fluorescent proteins (VFP) now covering almost the whole range of the visible spectrum (Day & Davidson, 2012) has led to tremendous increase in FRET applications. Application of GFP variants (Day & Davidson, 2012; Heim, Prasher, & Tsien, 1994) has enabled detection of spatial-temporal resolution of the dynamics of interactions between different interacting target molecules. Use of FRET to identify protein-protein interactions from the cell surface receptors (Maurel et al., 2008) to cytoplasmic organelle (Rehman, Brown-Steinke, Palmer, & Periasamy, 2015) and to the nuclei (Day et al., 2001; Rehman et al., 2014) has spanned over the years. Earlier FRET studies were limited to either direct or indirect labeling of target molecules. Direct labeling methods of protein were tedious and cumbersome, involving first in vitro labeling, purification, and then introduction into the cells for imaging. Indirect methods like immunofluorescence using an antibody are still prevalent but steric hindrance is an important concern in FRET detection. No detection of FRET does not necessarily mean that the two proteins do not interact, but possibly that a particular method of FRET was not able to detect an interaction due to several reasons ranging from steric hinderance to FRET conditions not being satisfied. One needs to try different approaches available for FRET detection to see which approach fits better with the experimental need. Like any experiment, FRET is “no stand alone” technique and it is important to have supporting evidence from other biochemical assays like Co-IP or RNA-IP. Also, before conducting FRET studies it is important to first identify if the two target molecules in question co-localize intracellularly with each other, meaning if they are within the resolution limit of a microscope or not. If the two targets do not co-localize, there is no point in doing FRET. Also, to identify true FRET interactions, perturbation like a drug treatment or generating deletion mutants of the binding domains in the protein is required, which should result in the decrease or loss of FRET signal (Rehman et al., 2014).
Techniques of FRET
Further details of basics on FRET can be found in the following references (Clegg, 2006; Förster, 1948, 2012; Lakowicz, 2006; Periasamy, 2014; Periasamy & Clegg, 2009; Periasamy & Day, 2005; Periasamy, Wallrabe, Chen, & Barroso, 2008; Sun, Rombola, Jyothikumar, & Periasamy, 2013; Wallrabe & Periasamy, 2005). There are different methods of detecting FRET such as intensity and lifetime-based methods. Intensity based methods include Confocal FRET (Elangovan et al., 2003; Rehman et al., 2015), Spectral FRET (Chen, Mauldin, Day, & Periasamy, 2007), and acceptor photobleaching (Kenworthy, 2001; Kenworthy, Petranova, & Edidin, 2000), whereas lifetime-based methods involves FLIM-FRET (Sun & Periasamy, 2015; Wallrabe & Periasamy, 2005).
In intensity-based FRET methods, sensitized emission from the acceptor follows after excitation of the donor. Due to FRET, there is quenched donor signal and sensitized emission of the acceptor after excitation of the donor, known as uFRET (uncorrected FRET). Because of the spectral overlap of the FRET pairs, intensity-based FRET methods are not direct measurements and require algorithms to remove bleed-through signal from the channel (PMT) used for collecting acceptor emission to identify true FRET signal. The bleed-through signal includes: (1) donor emission into the acceptor channel, and (2) acceptor emission due to cross-excitation by donor excitation. Imaging of donor and acceptor alone specimens at the identical FRET imaging settings allow for these corrections to be applied through different algorithms (Chen, Elangovan, & Periasamy, 2005; Elangovan et al., 2003; Gordon, Berry, Liang, Levine, & Herman, 1998; van Rheenen, Langeslag, & Jalink, 2004; Xia & Liu, 2001; Youvan et al., 1997). FRET software on commercial systems like Leica can also perform bleed-through corrections. Intensity based methods for FRET detection can be performed on wide-field and confocal microscopes, and on live and fixed specimens.
In photobleaching methods, either the donor (Arndt-Jovin & Jovin, 1989) or acceptor (Kenworthy, 2001) is photobleached. Photobleaching the acceptor is a popular and direct method of detecting FRET (Kenworthy, 2001; Kenworthy et al., 2000). FRET detection by the acceptor photobleaching method is the most straight forward method for detecting molecular interactions. In FRET as mentioned above, there is quenching of the donor fluorescence and sensitized emission from the acceptor fluorophore because of the non-radiative energy transfer from the donor to the acceptor molecules. In acceptor photobleaching FRET, since the acceptor is photobleached, the donor is no longer able to transfer its energy which results in de-quenched (increased) donor signal post acceptor photobleaching.
Since donor signal pre- and post-bleach is quantified after complete photobleaching of the acceptor, in principle it does not require bleed-through corrections. E% is calculated by the equation
FRET by the acceptor photobleaching method presented here can be performed on any confocal laser scanning microscope or wide-field fluorescence microscope with appropriate excitation laser lines. The in-built workflow or wizard facilitates faster (a few seconds) photobleaching by the laser via targeted ROIs versus photobleaching performed by mercury or xenon vapor arc lamps which could take longer time (a few minutes) in which there could be recovery after photobleaching. Also, the FoV or the cells could drift out of focus, making measurements inaccurate. Using a confocal system also allows making multiple ROIs for photobleaching in a single cell and comparing with the non-targeted, non-bleached intra-cellular compartments or cells in the same FoV as controls.
In lifetime-based FRET measurements, FRET events are identified if there is reduction in the donor lifetimes, because of quenching of fluorescence in the presence of the acceptor. The efficiency of energy transfer or E% is calculated from the following equation
Critical Parameters and Troubleshooting
Setting up for FRET imaging
FRET measurements require a dual imaging setup for the FRET pairs. Because of the spectral overlap integral in the FRET pairs, correct emission filters, dichroic with optimized settings of laser excitation (steps 8-11), AOTF, detector gain, and offsets are required to minimize the bleed-through (steps 15-20).
Once all the imaging parameters are set, they should not be changed in the middle of the experiment. If changed midway, one must repeat all the imaging from the beginning with new settings. To avoid problems like this, it is advised first to examine all the specimens in slides (a-e from step 4) before starting with FRET imaging to get a sense of the level of expression and intensity of the fluorophores. Ideally, cells showing an optimal level of expression representative in all the slides should be imaged and it would be better to avoid over- or under-expressed cells. Imaging cells expressing in common intensity ranges in all the specimens avoids the problem of changing laser excitation AOTF or PMT gain in the middle of the experiment.
Critical considerations in acceptor photobleaching and donor image acquisition
As the donor intensity is quantified pre- and post-bleach of the acceptor, it is important to check that photobleaching of the donor does not occur either during the acceptor photobleaching cycles or during normal image acquisition. If donor bleaching occurs, then correction should be implemented as explained in Figure 2. Laser power used for imaging, photobleaching and the AOTF settings should be carefully optimized using the negative controls like the EGFP and Cy3 alone slides.
First, the Cy3-acceptor (A) alone slide should be used to optimize the photobleaching cycles. The percentage of laser power and number of iterations are critical factors. For example, if photobleaching of the acceptor can be achieved with 75% laser power with 5-6 iterations there is no point in using a 100% laser power or more iterations, because the goal is to achieve the photobleaching of the acceptor without photobleaching the donor.
Once the acceptor photobleaching is optimized, the EGFP-donor (D) alone slides should be tested out by completing the FRET imaging by acceptor photobleaching workflow (see Fig. 2). Donor intensities in non-bleach control ROIs and in the targeted bleached ROIs over time can be plotted and compared. If no significant drop in donor intensities is observed, the photobleaching cycle is optimized.
It is also important to make sure that the 488 nm laser used for the EGFP-donor image acquisition does not itself photobleach. Again, donor intensities in non-bleach control ROIs over time can be plotted and compared. There should be some changes or fluctuations in donor intensity with time but if it is insignificant or less than 10%-12%, the laser power should be okay for donor image acquisition.
Pseudo FRET
In acceptor photobleaching FRET measurements, sometimes pseudo-FRET can be observed, which is described as the increase in donor fluorescence intensity in non-bleach control regions of the double labeled specimens or in the donor alone negative controls after photobleaching the acceptor (Karpova & McNally, 2006). However, the possible causes of this phenomenon are not clearly identified. Heating of the specimen during FRET imaging due to high excitation power to photobleach, fixation process, and the ingredients of the mounting medium could possibly account for it (Karpova & McNally, 2006). If pseudo-FRET occurs commonly, it is advised to try changing an alternative fixation protocol or mounting medium. The use of aqueous buffers in the imaging chamber could also help dissipate local heating (Karpova & McNally, 2006). In the author's experience, some pseudo-FRET in donor alone slides were observed, which were significantly less than the calculated FRET efficiency (E%).
No FRET
If the efficiency of energy transfer (E%) is not significantly more than the controls (negative controls and control ROIs in the non-bleached region of the experimental double labeled slide), no FRET was detected between the RNA and protein using this method. As mentioned above, FRET is not a ‘stand-alone’ technique and if there is biochemical supportive evidence of interaction, FRET detection can be optimized by incorporating different FRET pairs or an alternative method including confocal, spectral or FLIM-FRET to identify molecular interactions.
In acceptor photobleaching FRET, the donor emission is measured pre- and post- bleach; if the intensity of the donor fluorophore is weaker, it can be improved (a) by increasing the amount of plasmid DNA in transfection, and (b) by selecting brighter and more photostable fluorophore alternatives to get a good donor signal. Several new generation FRET pairs are available (Day & Davidson, 2012). Similarly, Alexa Fluor dyes instead of Cy3 could also be better alternatives. Incomplete photobleaching of the acceptor will also result in a lack of evidence of a FRET interaction. Sometimes, it is harder to photobleach the acceptor, especially when they are in dense aggregates. Activating two laser lines to simultaneously photobleach the acceptor to background levels may help.
Since FRET is a distance-dependent phenomenon, the lack of evidence of a FRET interaction may also mean that the FRET pairs used could be more spatially apart, even though the target molecules interact with one another. In such scenarios, generating a N′- to C′ protein fusion or alternating the fluorescent tag to the oligonucleotide for RNA may help improving the efficiency of energy transfer.
Understanding Results
The authors suggest the following steps to evaluate the occurrence of FRET between RNA and protein in this particular example using the slides described in the section “Prepare plasmids, cell lines, transfection, slides for FRET.”
The first step is to examine the results from the DA positive control slide or Expt with protein-EGFP and RNA foci-Cy3 (slide a), and calculate E% from the targeted bleached ROIs (Expt-E%). For visualization and a better understanding of how the acceptor photobleaching technique works, the images and results from some of the data points from this experiment are shown in Figure 1. Compare Expt-E% with the Ctrl-E% for non-targeted, non-bleached control regions on the same slide. Expt-E% should be more than Ctrl-E%.
If Expt-E% < Ctrl-E%, there is potential photobleaching of the EGFP reporter taking place with the 552 nm laser. If Expt-E% is equal to Ctrl-E%, there could be a problem with the FRET imaging setup. If the microscope is configured properly for FRET imaging by acceptor photobleaching, one should see Expt-E% > Ctrl-E% and this should be statistically different.
The second step is to do the same evaluation in the negative control-Neg slide (d) with unconjugated EGFP and RNA foci-Cy3. Here, the expected result is that Neg-E% would be like Ctrl-E%. Few data points showing higher Neg-E% is possible because of random interactions of unconjugated EGFP with Cy3, coming in close proximity, which could result in occasional FRET. However, the overall Neg-E% should be < Expt-E%. The Expt-E% should be higher and statistically different than Neg-E% (Rehman et al., 2014).
The third step is to repeat the same evaluation in the protein-EGFP (b) slide and the unconjugated EGFP, donor alone (e) slide. Here, the expected result is that Donor alone-E% ≤ Ctrl-E%. Some donor photobleaching is expected while photobleaching the acceptor. However, the Donor alone-E% should be < Expt-E%. The Expt-E% should be much higher and statistically different than the Donor alone-E%.
If all the FRET conditions are met and the FRET imaging setup is correctly done on the microscope, one would see FRET interaction between the targets to conclude that the there is indeed evidence of interaction between the RNA-protein in question.
Time Considerations
FRET by acceptor photobleaching can be performed within 4-5 hr with optimization and data acquisition for at least 25-30 cells, giving approximately 1 hr to each specimen. For reproducibility of the results, one should perform 2-3 independent experiments, and plan for a 4-5 hr session on each day.
Acknowledgments
This research was supported by funding from NIH RR025616 & AG06704 (AP) and Muscular Dystrophy Association (MM). The authors thank Dr. David Brook (University of Nottingham, UK) and Dr. Scott Vande Pol (University of Virginia, VA) for plasmids and empty vector; and Dr. I. H. Engels (Genomics Institute of the Novartis Research Foundation, CA) for immortalized DM1 fibroblasts/myoblasts used in developing this protocol. Author AP acknowledges support from the Ministry of Education, New Delhi (Sanction No. 11/9/2019-U.3(A)), and the Centre of Excellence Biosensing, Indian Institute of Technology – Madras, India.
Author Contributions
Shagufta Rehman Alam : Conceptualization, data curation, formal analysis, investigation, methodology, validation, writing - original draft, writing - review & editing; Mani S. Mahadevan : Conceptualization, funding acquisition, project administration, supervision; Ammasi Periasamy : Conceptualization, funding acquisition, investigation, methodology, project administration, supervision, writing - review & editing.
Conflict of Interest
The authors declare that they have no conflict of interest.
Open Research
Data Availability Statement
The data that support the findings of this study are available from the corresponding author upon reasonable request.
Literature Cited
- Abbe, E. (1873). Beiträge zur Theorie des Mikroskops und der mikroskopischen Wahrnehmung. Archiv für Mikroskopische Anatomie , 9, 413–468. doi: 10.1007/BF02956173
- Arndt-Jovin, D. J., & Jovin, T. M. (1989). Fluorescence labeling and microscopy of DNA. Methods in cell biology , 30, 417–448. doi: 10.1016/s0091-679x(08)60989-9
- Bajar, B. T., Wang, E. S., Zhang, S., Lin, M. Z., & Chu, J. (2016). A guide to fluorescent protein FRET pairs. Sensors (Switzerland) , 16, 1488. doi: 10.3390/s16091488
- Bao, G., Won, J. R., & Tsourkas, A. (2009). Fluorescent probes for live-cell RNA detection. Annual Review of Biomedical Engineering , 11, 25–47. doi: 10.1146/annurev-bioeng-061008-124920
- Betzig, E. (1995). Proposed method for molecular optical imaging. Optics Letters , 20, 237. doi: 10.1364/OL.20.000237
- Betzig, E., Patterson, G. H., Sougrat, R., Lindwasser, O. W., Olenych, S., Bonifacino, J. S., … Hess, H. F. (2006). Imaging intracellular fluorescent proteins at nanometer resolution. Science (New York, N.Y.) , 313(5793), 1642–1645. doi: 10.1126/science.1127344
- Chalfie, M., Tu, Y., Euskirchen, G., Ward, W. W., & Prasher, D. C. (1994). Green Fluorescent Protein as a Marker for Gene Expression. Science , 263, 802–805. doi: 10.1126/science.8303295
- Chen, Y. E., Elangovan, M., & Periasamy, A. (2005). FRET data analysis: The algorithm. In A. Periasamy & R.N. Day (Eds.), Molecular imaging: FRET microscopy and spectroscopy (pp. 126–145). New York, USA, New York: Oxford University Press.
- Chen, Y., Mauldin, J. P., Day, R. N., & Periasamy, A. (2007). Characterization of spectral FRET imaging microscopy for monitoring nuclear protein interactions. Journal of Microscopy , 228, 139–152. doi: 10.1111/j.1365-2818.2007.01838.x
- Clegg, R. M. (2006). The history of FRET: From conception through the labors of birth. Reviews in Fluorescence , 2006, 1–45. doi: 10.1007/0-387-33016-X_1
- Day, R. N., & Davidson, M. W. (2012). Fluorescent proteins for FRET microscopy: Monitoring protein interactions in living cells. BioEssays , 34, 341–350. doi: 10.1002/bies.201100098
- Day, R. N., Periasamy, A., & Schaufele, F. (2001). Fluorescence resonance energy transfer microscopy of localized protein interactions in the living cell nucleus. Methods , 25, 4–18. doi: 10.1006/meth.2001.1211
- Elangovan, M., Wallrabe, H., Chen, Y., Day, R. N., Barroso, M., & Periasamy, A. (2003). Characterization of one- and two-photon excitation fluorescence resonance energy transfer microscopy. Methods , 29, 58–73. doi: 10.1016/S1046-2023(02)00283-9
- Fardaei, M. (2001). In vivo co-localisation of MBNL protein with DMPK expanded-repeat transcripts. Nucleic Acids Research , 29, 2766–2771. doi: 10.1093/nar/29.13.2766
- Fessenden, J. D. (2009). Förster resonance energy transfer measurements of ryanodine receptor type 1 structure using a novel site-specific labeling method. PLoS ONE , 4, e7338. doi: 10.1371/journal.pone.0007338
- Förster, T. (1948). Intermolecular energy migration and fluorescence. Energetics , 148, 55–75.
- Förster, T. (2012). Energy migration and fluorescence. Journal of Biomedical Optics , 17, 110021–1100210. doi: 10.1117/1.JBO.17.1.011002
- Gordon, G. W., Berry, G., Liang, X. H., Levine, B., & Herman, B. (1998). Quantitative fluorescence resonance energy transfer measurements using fluorescence microscopy. Biophysical Journal , 74, 2702–2713. doi: 10.1016/S0006-3495(98)77976-7
- Haj, F. G., Verveer, P. J., Squire, A., Neel, B. G., & Bastiaens, P. I. H. (2002). Imaging sites of receptor dephosphorylation by PTP1B on the surface of the endoplasmic reticulum. Science , 295, 1708–1711. doi: 10.1126/science.1067566
- Heim, R., Prasher, D. C., & Tsien, R. Y. (1994). Wavelength mutations and posttranslational autoxidation of green fluorescent protein. Proceedings of the National Academy of Sciences , 91, 12501–12504. doi: 10.1073/pnas.91.26.12501
- Hell, S. W., & Wichmann, J. (1994). Breaking the diffraction resolution limit by stimulated emission: Stimulated-emission-depletion fluorescence microscopy. Optics Letters , 19, 780. doi: 10.1364/OL.19.000780
- Karpova, T., & McNally, J. G. (2006). Detecting protein–protein interactions with CFP-YFP FRET by acceptor photobleaching. Current Protocols in Cytometry , Unit 12.7 , 12.7.1–12.7.11. doi: 10.1002/0471142956.cy1207s35
- Kenworthy, A. K. (2001). Imaging protein-protein interactions using fluorescence resonance energy transfer microscopy. Methods , 24, 289–296. doi: 10.1006/meth.2001.1189
- Kenworthy, A. K., Petranova, N., & Edidin, M. (2000). High-resolution FRET microscopy of cholera toxin B-subunit and GPI-anchored proteins in cell plasma membranes. Molecular Biology of the Cell , 11, 1645–1655. doi: 10.1091/mbc.11.5.1645
- Klar, T. A., Jakobs, S., Dyba, M., Egner, A., & Hell, S. W. (2000). Fluorescence microscopy with diffraction resolution barrier broken by stimulated emission. Proceedings of the National Academy of Sciences , 97, 8206–8210. doi: 10.1073/pnas.97.15.8206
- J. R. Lakowicz (Ed). (2006). Principles of fluorescence spectroscopy ( 3rd ed.). Springer, USA.
- Maurel, D., Maurel, D., Comps-Agrar, L., Brock, C., Rives, M. L., Bourrier, E., … Pin, J. P. (2008). Cell-surface protein-protein interaction analysis with time-resolved FRET and snap-tag technologies: application to GPCR oligomerization. Nature Methods , 5(6), 561–567. doi: 10.1038/nmeth.1213
- Moerner, W. E., & Kador, L. (1989). Optical detection and spectroscopy of single molecules in a solid. Physical Review Letters , 62, 2535–2538. doi: 10.1103/PhysRevLett.62.2535
- Parkhurst, L. J., Parkhurst, K. M., Powell, R., Wu, J., & Williams, S. (2002). Time-resolved fluorescence resonance energy transfer studies of DNA bending in double-stranded oligonucleotides and in DNA-protein complexes. Biopolymers , 61, 180–200. doi: 10.1002/bip.10138
- Periasamy, A. (2014). Advanced light microscopy. Methods (San Diego, Calif.) , 66, 121–123. doi: 10.1016/j.ymeth.2014.03.011
- Periasamy, A., & Clegg, R. M. (2009). FLIM microscopy in biology and medicine. Chapman and Hall/CRC.
- Periasamy, A., & Day, R. N. (2005). Molecular imaging: FRET microscopy and spectroscopy. New York: Oxford University Press.
- Periasamy, A., Wallrabe, H., Chen, Y., & Barroso, M. (2008). Chapter 22: Quantitation of protein-protein interactions: confocal FRET microscopy. Methods in cell biology , 89, 569–598. doi: 10.1016/S0091-679X(08)00622-5
- Prasher, D. C., Eckenrode, V. K., Ward, W. W., Prendergast, F. G., & Cormier, M. J. (1992). Primary structure of the Aequorea victoria green-fluorescent protein. Gene , 111, 229–233. doi: 10.1016/0378-1119(92)90691-H
- Rehman, S., Brown-Steinke, K., Palmer, L., & Periasamy, A. (2015). Development of confocal immunofluorescence FRET microscopy to Investigate eNOS and GSNOR localization and interaction in pulmonary endothelial cells. In A. Periasamy, P. T. C. So, & K. König (Eds.), Proceedings of the SPIE (Vol. 9329), id. 93290G 6 pp.
- Rehman, S., Gladman, J. T., Periasamy, A., Sun, Y., & Mahadevan, M. S. (2014). Development of an AP-FRET based analysis for characterizing RNA-protein interactions in myotonic dystrophy (DM1). PLoS ONE , 9(4), e95957. doi: 10.1371/journal.pone.0095957
- Sambrook, J., & Russell, D. W. (2001). Molecular cloning: A laboratory manual. Cold Spring Harbor Laboratory Press, N.Y.: Cold Spring Harbor.
- Seidman, C. E., Struhl, K., Sheen, J., & Jessen, T. (2001). Introduction of plasmid DNA into cells. Current Protocols in Molecular Biology , Unit 1.8 , 1.8.1–1.8.10. doi: 10.1002/0471142727.mb0108s37
- Snapp, E. L., & Hegde, R. S. (2006). Rational design and evaluation of FRET experiments to measure protein proximities in cells. Current Protocols in Cell Biology , Unit 17.9 , 17.9.1–17.9.20. doi: 10.1002/0471143030.cb1709s32
- Sun, Y., & Periasamy, A. (2015). Localizing protein-protein interactions in living cells using fluorescence lifetime imaging microscopy. Methods in Molecular Biology (Clifton, N.J.) , 1251, 83–107. doi: 10.1007/978-1-4939-2080-8_6
- Sun, Y., Rombola, C., Jyothikumar, V., & Periasamy, A. (2013). Förster resonance energy transfer microscopy and spectroscopy for localizing protein-protein interactions in living cells. Cytometry. Part A : The journal of the International Society for Analytical Cytology , 83, 780–793. doi: 10.1002/cyto.a.22321
- van Rheenen, J., Langeslag, M., & Jalink, K. (2004). Correcting confocal acquisition to optimize imaging of fluorescence resonance energy transfer by sensitized emission. Biophysical Journal , 86, 2517–2529. doi: 10.1016/S0006-3495(04)74307-6
- Wallrabe, H., & Periasamy, A. (2005). Imaging protein molecules using FRET and FLIM microscopy. Current Opinion in Biotechnology , 16, 19–27. doi: 10.1016/j.copbio.2004.12.002
- Wouters, F. S., & Bastiaens, P. I. H. (1999). Fluorescence lifetime imaging of receptor tyrosine kinase activity in cells. Current Biology , 9, 1127–1130.
- Xia, Z., & Liu, Y. (2001). Reliable and global measurement of fluorescence resonance energy transfer using fluorescence microscopes. Biophysical Journal , 81, 2395–2402. doi: 10.1016/S0006-3495(01)75886-9
- Youvan, D. C., Silva, C. M., Bylina, E. J., Coleman, W. J., Dilworth, M. R., & Yang, M. (1997). Calibration of fluorescence resonance energy transfer in microscopy using genetically engineered GFP derivatives on nickel chelating beads. Biotechnology et Alia , 3, 1–18.