Culture of Rat Primary Cortical Cells for Microelectrode Array (MEA) Recordings to Screen for Acute and Developmental Neurotoxicity
Lora-Sophie Gerber, Lora-Sophie Gerber, Lennart V.J. van Melis, Lennart V.J. van Melis, Regina G.D.M. van Kleef, Regina G.D.M. van Kleef, Aart de Groot, Aart de Groot, Remco H.S. Westerink, Remco H.S. Westerink
acute
subchronic and chronic exposure
microelectrode array (MEA)
recordings
neuronal activity analysis
neurotoxicity screening
rat primary cortical cultures
Abstract
Neurotoxicity testing of chemicals, drug candidates, and environmental pollutants still relies on extensive in vivo studies that are very costly, time-consuming, and ethically debated due to the large number of animals typically used. Currently, rat primary cortical cultures are widely used for in vitro neurotoxicity studies, as they closely resemble the in vitro brain with respect to the diversity of cell types, their physiological functions, and the pathological processes that they undergo. Common in vitro assays for neurotoxicity screening often focus on very target-specific endpoints such as morphological, biochemical, or electrophysiological changes, and such narrow focus can hamper translation and interpretation. Microelectrode array (MEA) recordings provide a non-invasive platform for extracellular recording of electrical activity of cultured neuronal cells, thereby enabling the evaluation of changes in neuronal (network) function as a sensitive and integrated endpoint for neurotoxicity screening. Here, we describe an in vitro approach for assessing changes in neuronal network function as a measure for neurotoxicity, using rat primary cortical cultures grown on MEAs. We provide a detailed protocol for the culture of rat primary cortical cells, and describe several experimental procedures to address acute, subchronic, and chronic exposure scenarios. We additionally describe the steps for processing and analyzing MEA and cell viability data. © 2021 The Authors. Current Protocols published by Wiley Periodicals LLC.
Basic Protocol 1 : Isolation and culture of rat primary cortical cells on 48-well MEA plates
Support Protocol 1 : Pretreatment and washing of 48-well MEA plates before first use or for re-use
Support Protocol 2 : Coating of 48-well MEA plates with 0.1% PEI solution
Basic Protocol 2 : MEA measurements during acute exposure
Alternate Protocol 1 : MEA measurements during subchronic exposure
Alternate Protocol 2 : MEA measurements during chronic exposure
Support Protocol 3 : Determination of cell viability after MEA experiments
Basic Protocol 3 : MEA data processing
Basic Protocol 4 : Analyzing MEA experiments after acute and subchronic exposure
Alternate Protocol 3 : Analyzing MEA experiments after chronic exposure
INTRODUCTION
A major challenge faced by in vitro neurotoxicity testing approaches is that the cell model used should closely reflect the complexity of the in vivo nervous system, especially with respect to the diversity of cell types, their physiological functions, and the pathophysiological processes that affect them (Westerink, 2013). Currently, general neurotoxicity testing of chemicals, drug candidates, and environmental pollutants still mostly relies on extensive and costly in vivo studies that require a large number of animals and specific expertise (Accardi et al., 2016; Bal-Price et al., 2008). Consequently, there is a clear need for the implementation of alternative in vitro neurotoxicity testing strategies that are robust and economically feasible, provide high throughput, and have an adequate predictive capacity (Bal-Price et al., 2008; Bal-Price, Hogberg, Buzanska, & Coecke, 2010a).
Many approaches for in vitro neurotoxicity screening focus on specific biochemical, morphological, or electrophysiological endpoints. However, given the wide potential diversity of toxicity mechanisms and modes of action, it is virtually impossible to cover all relevant ones with target-specific assays. Assessing neuronal network function, on the other hand, provides an efficient and sensitive way to cover multiple neurotoxicity endpoints within the same experimental setup (McConnell, McClain, Ross, LeFew, & Shafer, 2012; Robinette, Harrill, Mundy, & Shafer, 2011; Zwartsen, Hondebrink, & Westerink, 2018).
Microelectrode arrays (MEAs) consist of a cell culture surface with an integrated array of microelectrodes constituting a non-invasive platform to record the electrophysiological activity of excitable cells in vitro (Johnstone et al., 2010). Extracellular field potentials generated by neuronal activity (spikes) are detected simultaneously at several locations on the electrode array. Comparing neuronal activity patterns before and after exposure to test compounds allows for the investigation of the potential impact of these compounds on neuronal (network) function (Johnstone et al., 2010). MEA recordings thereby provide a functional endpoint for neurotoxicity testing that covers multiple intrinsic, physiologically relevant mechanisms important for neuronal function, including acute disturbance of calcium homeostasis, receptor and ion channel functionality, and synaptic transmission (Johnstone et al., 2010; Vassallo et al., 2017).
For many test compounds, a (sub)chronic exposure better resembles a real-life scenario (Dingemans et al., 2016; Zwartsen, Hondebrink, & Westerink, 2019). As MEA recordings are non-invasive, measurements can be performed at several time points over days or even weeks, providing an efficient way of collecting large amounts of in-depth electrophysiology data (for a review, see Johnstone et al., 2010; also see Spira & Hai, 2013). (Sub)chronic exposure experimental designs allow researchers to study not only changes in neuronal activity due to acute disturbance of calcium homeostasis, receptor and ion channel functionality, as well as synaptic transmission, but also changes in network integrity, expression of ion channels and neurotransmitter receptors, and cell viability occurring after prolonged exposure. Further, subchronic-exposure MEA experiments are also suitable to study the reversibility of functional neurotoxicity (Gopal, Miller, & Gross, 2007) or, with some minor adaptations to the experimental protocol, the ability of the neuronal network to recover from the insult (Zwartsen et al., 2019).
Various cell types can be cultured on MEAs, including primary cultures. Rat primary cortical cultures consist of excitatory and inhibitory neurons, and supportive cells such as astrocytes, thereby largely representing the in vivo cellular diversity (for details see Hondebrink et al., 2016; Tukker et al., 2020a). The cortical cultures form networks and develop spontaneous and synchronized neuronal activity that reflects many functions of neurons in vivo (Charlesworth, Cotterill, Morton, Grant, & Eglen, 2015; Chiappalone, Vato, Berdondini, Koudelka-Hep, & Martinoia, 2007). The neuronal activity pattern displayed by rat primary cortical cultures grown on MEAs includes spiking, bursting, and network bursting (Chiappalone et al., 2007; Cotterill et al., 2016; Robinette et al., 2011). Spikes basically represent extracellular field recordings of action potentials, whereas bursting is defined as a series of spikes with a short interval recorded by a single electrode. Network bursts are more complex, and represent the simultaneous occurrence of bursting at several electrodes throughout the array of a single well (Johnstone et al., 2010). Importantly, the activity of rat primary cortical cultures can be modulated by different physiological, toxicological, and pharmacological compounds, indicating the functional relevance of diverse intra- and intercellular signaling pathways (de Groot, Westerink, & Dingemans, 2013; Hondebrink et al., 2016; McConnell et al., 2012; Nicolas et al., 2014; Puia, Gullo, Dossi, Lecchi, & Wanke, 2012; Strickland, Martin, Richard, Houck, & Shafer, 2018; Valdivia et al., 2014; Vassallo et al., 2017). While the use of human cells can avoid the need for interspecies translation, the high costs and long culture times involved currently hamper the use of human iPSC-derived neurons for in vitro neurotoxicity testing. Consequently, the availability of MEA data derived from human cells is still scarce. The sensitivity between cells from different species can differ, with rat cortical cultures being more sensitive to methoxetamine (Hondebrink et al., 2017), and, on the other hand, hiPSC-derived neurons being more sensitive to several seizureogenic compounds (Tukker, Wijnolts, de Groot, & Westerink, 2020b). Notably, however, differences in sensitivity between cells from different species are generally small, i.e., less than a factor of 10 (Tukker et al., 2020b), or negligible (Kasteel & Westerink, 2017). As a result of the often small differences observed with human-derived cells, and also considering the relatively low costs and short culture duration, rat cortical cultures are still the standard for (in vitro) neurotoxicity testing (Authier et al., 2016). Notably, it has been demonstrated in several studies that rat primary cortical cultures grown on 48-well MEA plates are well suited for in vitro neurotoxicity screening, exhibiting considerable throughput, inter-laboratory reproducibility, and high sensitivity and specificity (McConnell et al., 2012; Nicolas et al., 2014; Novellino et al., 2011; Valdivia et al., 2014; Vassallo et al., 2017). Therefore, rat primary cortical cultures grown on MEAs are increasingly used as a robust, high-throughput screening tool to investigate acute, subchronic, and developmental neurotoxicity of chemicals, environmental pollutants, and endogenous molecules (Hogberg et al., 2011; Hondebrink et al., 2016; Johnstone et al., 2010; McConnell et al., 2012; Tukker et al., 2020b; Valdivia et al., 2014).
In this article, we describe the isolation and culture of rat primary cortical cells and their applicability for neurotoxicity screening by MEA recordings in several experimental exposure scenarios, including acute, subchronic, and chronic (developmental) exposure. Figure 1 shows an overview of the experimental procedure for the different exposure scenarios. Basic Protocol 1 describes in detail the dissection of cortices from Wistar rats on postnatal day 0 to 1, and the isolation, seeding, and culture of the primary cortical cells on 48-well MEA plates. Support Protocols 1 and 2 describe the required treatment of 48-well MEA plates prior to culture of primary rat cortical cells, and the washing procedure to reuse 48-well MEA plates, respectively. In Basic Protocol 2, we describe how to perform an acute exposure experiment. Furthermore, we describe the required software settings for recording spontaneous neuronal activity of rat primary cortical cells using the Maestro (Axion BioSystems) multi-well microelectrode array platform. Alternate Protocols 1 and 2 outline the experimental procedure for repeated MEA recordings during subchronic (up to 48 hr) and chronic (21 days) exposure, respectively. Support Protocol 3 describes the option to combine MEA recordings with cell viability assessment, which is recommended at the end of MEA experiments. Basic Protocol 3 explains the general spike train data processing, which applies to all obtained MEA data. Basic Protocol 4 and Alternate Protocol 3 describe the procedure we have implemented to assess changes in neuronal activity by chemically induced acute/subchronic and chronic exposure, respectively. Altogether, these protocols will allow investigators to use MEA experiments to study the potential impact of test compound(s) on neuronal functionality and synchronicity after different exposure durations.
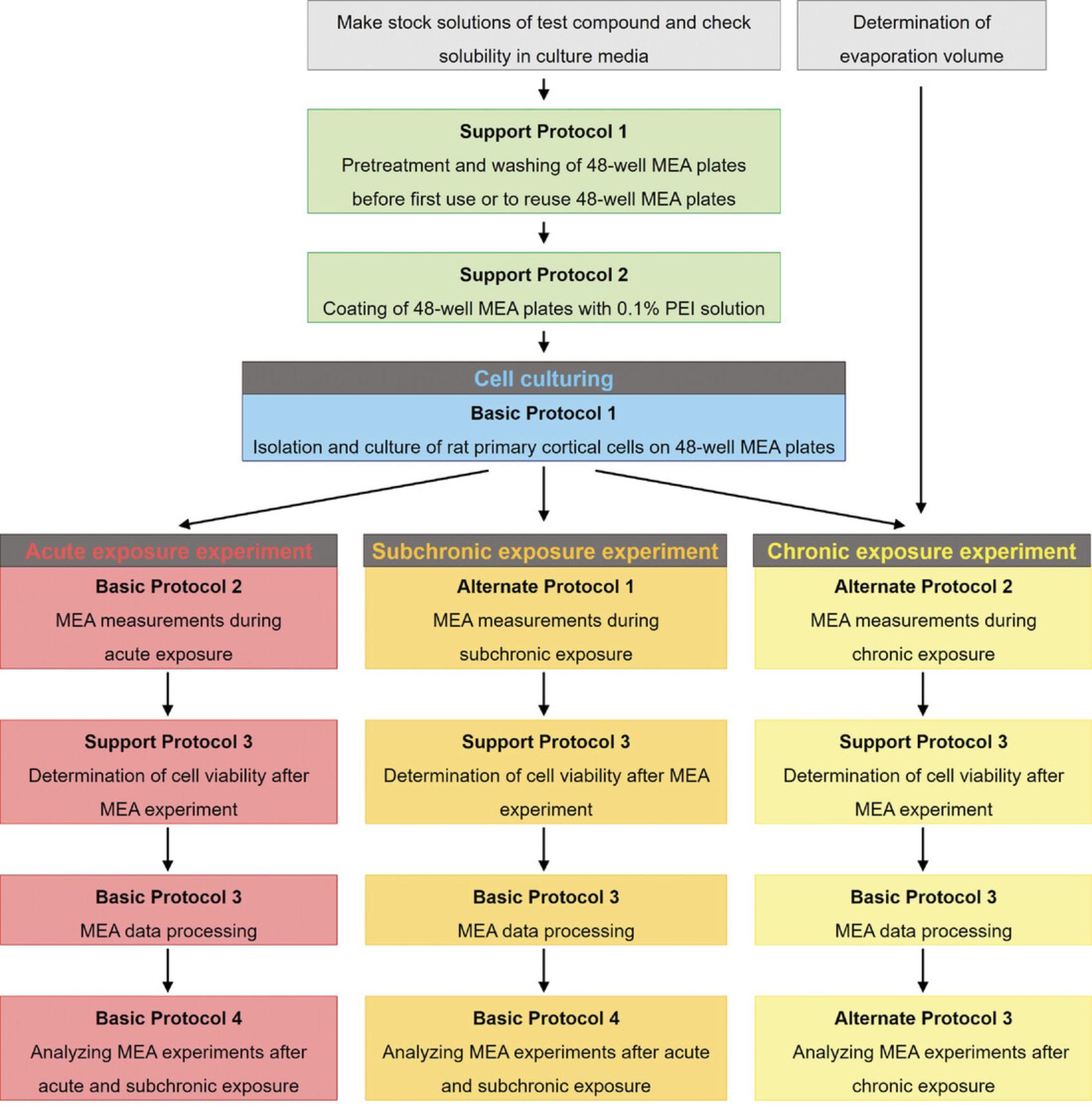
Strategic Planning
In this article, we describe several experimental setups for neurotoxicity screening using rat primary cortical cultures grown on MEA. Please note that these are long experiments (4–7 weeks) and, therefore, planning is essential. For a detailed overview of the required time, see Time Considerations.
First, ordering the rat pups needs to be done well in advance. To avoid medium changes or experiments on the weekends, we recommend using timed pregnant rats that give birth on Sundays.
Importantly, when planning a chronic exposure experiment, the user should first check how much volume evaporates from a 48-well MEA plate after 3-4 days in culture. The evaporation volume differs between different types of plates and laboratories. Please note that the evaporation volume is usually larger in the outer wells than in the inner wells. To check for the different evaporation volumes in the outer and inner wells of a 48-well MEA plate, fill up each well with 500 µl of sterile laboratory water and put the 48-well MEA plate in a humidified incubator (37°C, 5% CO2 and 95% air atmosphere). After 3-4 days of incubation, weigh the plate without the lid and note the weight (weight 1). Then, remove the entire volume of all outer wells, weigh the 48-well MEA plate again, and note the weight (weight 2). Also remove the entire volume from the inner wells, weigh the 48-well MEA plate again, and note the weight (weight 3). The evaporated volumes can now be calculated. First, subtract weight 2 from weight 1, and divide the result by 24 (because there are 24 outer wells). This value is the volume that remains in each outer well after 3-4 days in the incubator. Subtract this number from 500 µl (the starting volume in each well) to calculate how much water evaporated during 3-4 days incubation at 37°C, 5% CO2, and 95% air atmosphere. To calculate the evaporation volume for the inner wells, subtract weight 3 from weight 2 and continue as described above for the outer wells.
Further preparatory steps include the pretreatment, washing, and coating of 48-well MEA plates (see Support Protocols 1 and 2), which takes about 2 weeks in total. At least 1 day before the experiment starts (i.e., 1 day before Basic Protocol 1), the user should have coated the 48-well MEA plate with a 0.1% polyethyleneimine (PEI) solution (see Support Protocol 2), since the MEA plate needs to be completely air-dried at room temperature before plating the cells.
In our laboratory, the isolation of rat primary cortical cells (see Basic Protocol 1) takes place on Mondays (which here represents day in vitro (DIV) 0), and the medium is replaced on DIV 4 (Friday). In the second week, neuronal baseline activity can be recorded on DIV 7 (for chronic exposure experiment) or on DIV 9-11 (for acute and subchronic exposure experiments), and the cells are subsequently exposed to test compound(s). Before the exposure, the user needs to decide which compounds to test and at which concentrations. When this has been done, the user needs to prepare the test concentrations in a predetermined solvent, which can be water, ethanol, or DMSO, depending on the test compound. The solubility of the compounds needs to be tested beforehand to see whether each concentration of the test compound dissolves in the appropriate medium. If this is not the case, the user can decide to test an alternative solvent or omit the highest test concentration(s), as these are most likely to give solubility problems. This issue should be addressed before the (first) exposure day.
Basic Protocol 1: ISOLATION AND CULTURE OF RAT PRIMARY CORTICAL CELLS ON 48-WELL MEA PLATES
Primary cortical cultures closely resemble the complexity and diversity of cell types in the in vivo brain. Over time, cortical cultures form networks that exhibit spontaneous electrical activity, which makes for a robust screening model for (developmental) neurotoxicity testing. Here, we describe the isolation and culture of rat primary cortical cells, a procedure that takes at least 1 week and requires careful planning (see Strategic Planning). 48-well MEA plates need to be pretreated (see Support Protocol 1) and precoated with 0.1% polyethyleneimine (PEI, see Support Protocol 2) before the cell isolation takes place. On the day of isolation, cortices from postnatal day 0 to 1 Wistar rat pups are dissected, and cortical cells are isolated and seeded in glutamate-supplemented dissection medium on the 0.1% PEI−precoated 48-well MEA plate. To culture cells on the microelectrode array, the cell suspension is seeded in a droplet immediately on the electrode array and cells are allowed to attach to the array for 2 hr before the remaining medium is added. On DIV 4, glutamate-supplemented dissection medium is replaced with culture medium without glutamate. Within the first week of culture, the neuronal network develops spontaneous neuronal activity, reaching a plateau at DIV 9-11, after which the activity gradually decreases (Dingemans et al., 2016). For long-term maintenance, 50% of the medium is exchanged every 3–4 days after volume adjustment with sterile water. Rat primary cortical cultures can be maintained at least up to DIV 28 without loss of neuronal activity (also see Understanding Results, which shows the development of neuronal activity of a rat primary cortical culture under control conditions).
In some countries, the preparation and use of primary cultures may be restricted to a Biological Safety Level 1 (BSL-1)–approved laboratory environment. Unless otherwise indicated, all experiments should be performed under sterile conditions in a laminar flow hood. All reagents and biologicals must be sterilized by autoclaving or filter sterilization (0.2 μm filter) prior to use in cell culture, and must be maintained under sterile conditions during the duration of the experiment. All culture incubations are performed in a humidified 37°C/5% CO2 incubator.
Materials
-
Phosphate-buffered saline (PBS; ThermoScientific GibcoTM, cat. no. 10010-023)
-
Dissection medium (see recipe)
-
Wistar rat pups on postnatal day 0 to 1 (Envigo, Horst, the Netherlands)
-
0.4% trypan blue solution (Sigma-Aldrich, cat. no. T8154)
-
Culture medium (see recipe)
-
Polystyrene box (30 × 25 × 10 cm; length × width × height) to contain ice
-
Petri dishes (10 cm diameter)
-
Sterile dissection tools (scissor, microdissection scissor, curved forceps, forceps with fine tips, spatula, scalpel)
-
Water bath at 37°C
-
50-ml conical polypropylene centrifuge tubes
-
EASYstrainerTM (100 µm cell strainer; Greiner bio-one, cat. no. 542 000)
-
Syringe (10 ml)
-
Dissection microscope
-
Biological safety hood
-
Serological pipettes (5, 10, and 25 ml)
-
Tabletop centrifuge
-
Hemocytometer (Bürker-Türk counting chamber) and light microscope, or automatic cell counter
-
6- or 8-channel electronic pipettor (Integra Bioscience, Voyager)
-
Sterile sample container (125 ml; VWR, cat. no. 216-1823)
-
Pretreated and 0.1% PEI precoated 48-well MEA plates (Axion BioSystems, cat. no. M768-KAP-48; for pretreatment and precoating, see Support Protocols 1 and 2, respectively)
-
Additional reagents and equipment for cell culture techniques including counting cells (see Current Protocols article: Phelan & May, 2015)
Preparing for dissection and cell isolation
Primary cells are very vulnerable. It is, therefore, recommended to dissect the cortices and to isolate cells as quickly as possible to ensure good quality of the final primary culture. To reduce procedural time, this section describes several preparation steps (see Fig. 2A-C) that should be done before starting the dissection and cell isolation.
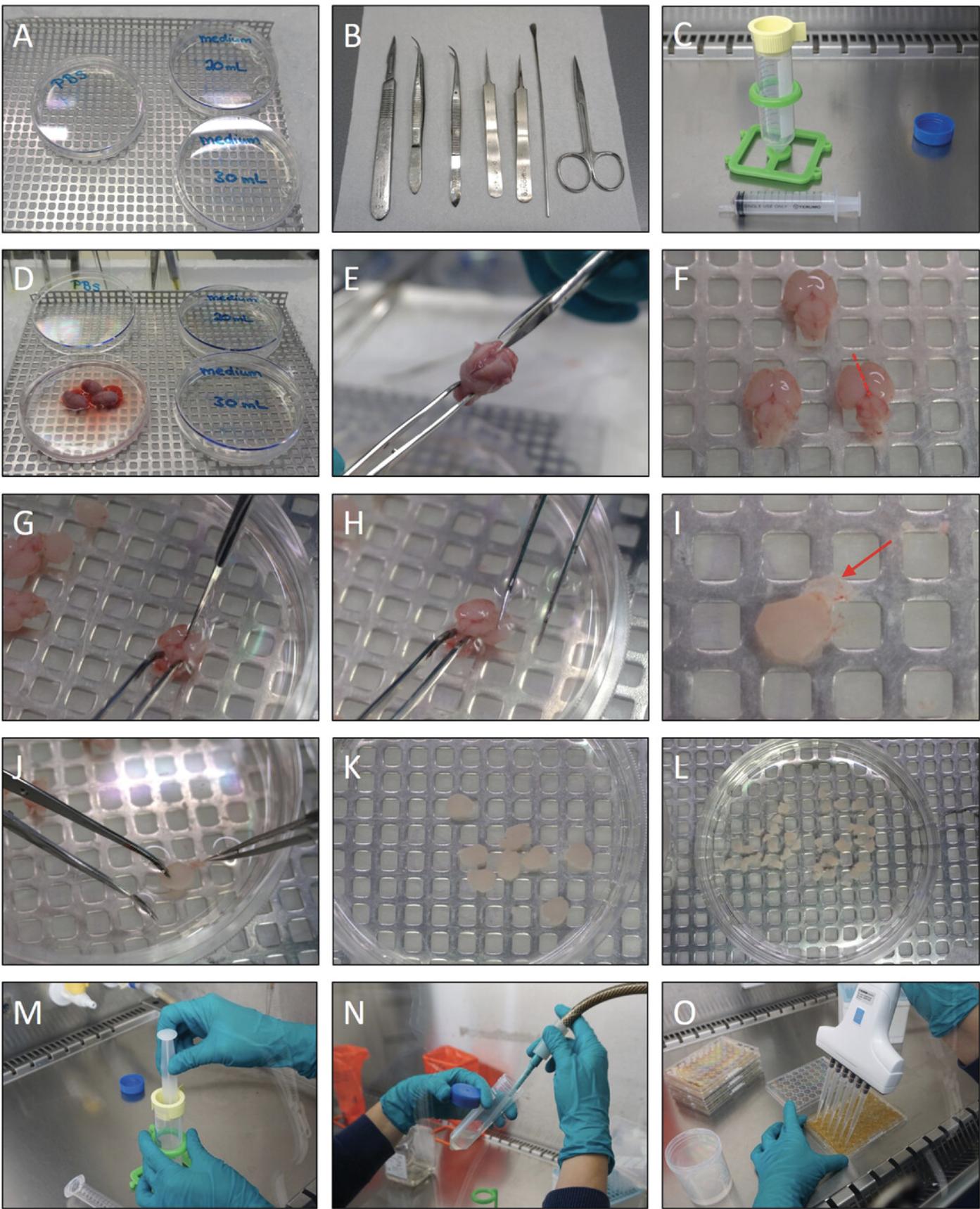
Prepare the dissection area (Fig. 2A)
1.Fill the polystyrene box with ice.
2.Add 30 ml of 4°C cold PBS to one 10-cm petri dish, and 20 and 30 ml of dissection medium to two other 10-cm petri dishes, respectively. Keep the petri dishes on ice in the polystyrene box.
3.Place the sterile dissection tools next to the polystyrene box (Fig. 2B).
4.Prewarm the remaining dissection medium to 37°C in a water bath.
Prepare for cell isolation in the biological safety hood (Fig. 2C)
5.Put the 100-µm sterile cell strainer on a 50-ml centrifuge tube.
6.Place a 10-ml syringe next to the centrifuge tube.
Dissection of rat cortices
The pups are sacrificed and the cortices are dissected from the cranium, under sterile conditions (Fig. 2D-L). For large litters (more than 10 pups), we recommend splitting the litter and performing the cortex dissection, cell isolation, and cell counting in two separate runs. After the desired cell density of 2 × 106 cells/ml is achieved, the cell suspensions can be combined and used for seeding.
7.Sacrifice the pups by holding them above the petri dish containing PBS (on ice; see step 2) and decapitating them using scissors in such a way that the cranium drops into the PBS (Fig. 2D).
8.Hold the nose portion of the head with forceps. Use the microdissection scissors to gently cut the skin along the midline advancing toward the nose, plus a lateral cut on each side. Afterwards, cut the skull in the same manner (Fig. 2E).
9.Remove the brain using the spatula and transfer the brain to the petri dish containing 30 ml of dissection medium prepared in step 2 (Fig. 2F).
10.With the brain dorsal side up, separate the cortices from the midbrain by cutting the tissue in a medial to lateral fashion (Fig. 2G; the red dotted line in Fig. 2F indicates where to cut).
11.Hold the brain with curved forceps (or stick forceps in the midbrain) and remove the cortex by peeling with curved forceps (Fig. 2H).
12.Remove residual parts of the midbrain and hippocampus area from the cortices using curved forceps (Fig. 2I; the red arrow in Fig. 2I points to the meninges, which need to be removed in step 13).
13.Remove any residual meninges from the cortices using forceps with thin tips. Use a dissection microscope to better visualize the meninges (Fig. 2J). Collect the cortices from all brains in the petri dish containing 20 ml of dissection medium prepared in step 2 (Fig. 2K).
14.Cut the cortices into smaller pieces (∼2 mm each) using a scalpel (Fig. 2L), and transfer the petri dish to the biological safety hood.
Isolation of primary cells from rat cortices
Work under sterile conditions in a biological safety hood when isolating the primary cortical cells.
15.Transfer the cortex pieces from the petri dish to the cell strainer on top of the 50-ml sterile polypropylene centrifuge tube from step 5 using a 10-ml serological pipette.
16.Mince the cortices in the strainer with the plunger from a 10-ml syringe (Fig. 2M).
17.Repeat steps 15-16 as often as necessary to mince all the cortex pieces.
18.Rinse the strainer with the remaining medium from the petri dish (from step 15).
19.Centrifuge the cell suspension for 5 min at 100 × g in a tabletop centrifuge at room temperature.
20.Remove the supernatant carefully, without disrupting the cell pellet (Fig. 2N).
21.Add 1 ml of prewarmed (37°C) dissection medium per brain (0.5 ml per cortex) to the cell pellet, and resuspend the pellet.
22.Measure and note the total volume of the cell suspension.
23.Determine the number of viable cells per ml in the suspension by mixing 90 µl of 0.4% trypan blue and 10 µl of the cell suspension in a 0.5-ml microcentrifuge tube, and using either a hemocytometer chamber (see Current Protocols article: Phelan & May, 2015) and a light microscope, or an automatic cell counter to count the cells.
24.Adjust the cell density of the remaining suspension to 2 × 106 cells/ml by adding prewarmed (37°C) dissection medium.
Seeding and culturing of primary rat cortical cells on 48-well MEA plates
Work under sterile conditions in a biological safety hood when seeding the primary cortical cells. Refer to Current Protocols article Phelan & May (2015) for basic cell culture techniques.
25.Gently but thoroughly resuspend the cell suspension to ensure equal cell distribution. To use the 6- or 8-channel electronic pipettor for seeding, transfer the cell suspension to a 125-ml sterile sample container.
26.Seed the cells by adding a 50-µl drop of the cell suspension to the center of the bottom of each well of a PEI-coated 48-well MEA plate (Fig. 2O; see Support Protocol 1 for the coating procedure). In each well, the final number of cells will be approximately 1 × 105, which is the optimal cell density for MEA recordings.
27.Keep the cells for 2 hr in a humidified incubator at 37°C with 5% CO2, to allow them to attach to the array surface. Then, add 450 µl of prewarmed (37°C) dissection medium to each well.
28.Culture the cells until DIV 4 in a humidified incubator at 37°C with 5% CO2.
29.On DIV 4, replace 450 µl of the dissection medium with culture medium (dissection medium without l-glutamate).
30.Keep the cells in a humidified incubator at 37°C with 5% CO2 until the start of the MEA experiment on DIV 7 (for developmental/chronic exposure, Alternate Protocol 2) or DIV 9-11 (for acute or subchronic exposure, Basic Protocol 2 or Alternate Protocol 1, respectively).
Support Protocol 1: PRETREATMENT AND WASHING OF 48-WELL MEA PLATES BEFORE FIRST USE OR FOR RE-USE
In this protocol, the user will pretreat and wash 48-well MEA plates, which are required steps before the first use or reuse of a 48-well MEA plate. In our experience, cell survival and neuronal activity are improved when MEA plates are incubated in protein-containing medium before first use. While MEA plates are designed for single use, it can be cost-effective to reuse them. Note, however, that reusing plates can result in leakage of medium and serious damage to the hardware. Also, reusing MEA plates can cause damage to the electrode array, resulting in a decreased signal-to-noise ratio. In practice, MEA plates can be reused up to four more times.
After the pretreatment, or to reuse MEA plates, the plates need to undergo a specific washing procedure, described in the steps below. In the case of reuse, MEA plates are first rinsed several times with demineralized water to remove the test compound(s). Detached cells or their debris are removed by incubating the plate with 0.5% trypsin/EDTA and further rinsing with demineralized water. Plates are sterilized by rinsing and incubating with 70% ethanol, then drying the plates at 55°C overnight. Afterwards, the labeling is removed from the plate lids and the plates are ready to be reused.
Materials
-
Protein-containing culture medium
- Any type of medium containing proteins (e.g., fetal bovine serum, B27 Plus supplement, etc., is suitable; see step 1).
-
Sterile laboratory-grade demineralized water
-
0.5% trypsin-EDTA (10×; ThermoScientific GibcoTM, cat. no. 15400-054)
-
70% ethanol
-
6- or 8-channel electronic pipettor (Integra Bioscience, Voyager)
-
New or used 48-well MEA plates (Axion BioSystems, cat. no. M768-KAP-48)
-
55°C oven
Pretreatment of 48-well MEA plates before first use
1.Under sterile conditions, add 300 µl of protein-containing culture medium to each well and incubate the plate for at least 24 hr.
Washing of 48-well MEA plates (after pretreatment or for reuse of MEA plates)
All washing steps should be performed under sterile conditions to avoid bacterial, fungal, or other contamination.
2.Rinse each well of the 48-well MEA plate with 300 µl of demineralized water three times.
3.Fill the whole 48-well MEA plate (wells and inter-well space) with demineralized water.
4.Rinse the plates three times with 300 µl of demineralized water.
5.Add 100 µl of 0.5% trypsin-EDTA (10×) to each well.
6.Incubate the plates overnight at 37°C, with 5% CO2 and 95% air atmosphere.
7.Rinse the plates three times with 300 µl of demineralized water.
8.Rinse the plates three times with 300 µl of 70% ethanol.
9.Fill up the whole 48-well MEA plate (wells and inter-well space) with 70% ethanol.
10.Incubate the plates overnight at room temperature in a fume hood.
11.Rinse the plates two times with 300 µl of 70% ethanol.
12.Discard the 70% ethanol and put the plates upside down on paper towels.
13.Dry the plates for at least 16 hr (overnight) at 55°C in an oven.
14.Clean the lids of the 48-well MEA plates with 70% ethanol.
Support Protocol 2: COATING OF 48-WELL MEA PLATES WITH 0.1% PEI SOLUTION
Primary cells require a protein matrix to attach to, which is why plastic culture plates need to be coated before seeding the cells. For MEA plates, a coating with a 0.1% PEI solution is used to ensure adequate adhesion of the cortical cultures to the electrode array. The MEA plates need to be coated at least 1 day prior to the generation of the primary culture (Basic Protocol 1). MEA plates coated with 0.1% PEI can be stored for up to 2 weeks at room temperature. Use a biological safety hood for the coating procedure to avoid bacterial, fungal, or other contamination.
Materials
-
0.1% polyethyleneimine solution (0.1% PEI; see recipe)
-
Sterile laboratory-grade water
-
48-well MEA plate (Axion BioSystems, cat. no. M768-KAP-48), pretreated as in Support Protocol 1
-
6- or 8-channel electronic pipettor (Integra Bioscience, Voyager)
1.Add a droplet of 0.1% PEI solution (50 μl/well) on the bottom of every well.
2.Incubate the 48-well MEA plate for 1 hr at room temperature.
3.Aspirate the 0.1% PEI solution and rinse each well four times with 300 μl of sterile laboratory-grade water.
4.Air-dry the 0.1% PEI-coated 48-well MEA plate overnight at room temperature.
Basic Protocol 2: MEA MEASUREMENTS DURING ACUTE EXPOSURE
In this protocol, the user will perform MEA measurements during acute exposure of rat cortical cells seeded on MEA plates (Basic Protocol 1) to a (set of) test compound(s). These acute exposure experiments can be used to investigate if short-term exposure to a test compound affects spontaneous neuronal activity. Acute exposure experiments will be performed on DIV 9-11, since at this developmental stage, the neuronal networks already exhibit a high degree of activity (Dingemans et al., 2016), including (network) burst behavior (Brown et al., 2016). Users will first perform a 30-min baseline MEA measurement, followed by another 35-min MEA measurement in which the exposure to the test compound(s) takes place. Since the cells will not be cultured further after acute exposure experiments, the exposure does not have to be performed under sterile conditions. To ensure the robustness and reproducibility of MEA data, we recommend having a sample size of at least 20 wells per condition, spread out over three MEA plates. At the end of this protocol, the user will have MEA data output (.RAW files), which can be further analyzed using Basic Protocol 3: MEA data processing.
Materials
-
48-well MEA plate (Axion BioSystems, cat. no. M768-KAP-48) with rat cortical cells cultured until DIV 9-11 (from Basic Protocol 1)
-
Stock concentrations of test compound(s) in solvent (see Strategic Planning)
-
Culture medium (see recipe)
-
Maestro Middleman (Axion BioSystems)
-
Computer
-
Axion Integrated Studio (AxIS) software (Axion BioSystems; version 2.5.2)
MEA settings and recording
1.Turn on the computer and the Maestro Middleman, and start the AxIS software on the computer.
2.In the Environment Control module, turn ‘heater control’ on and set the temperature to 37°C.
3.Select the type of plate that will be measured at ‘Active Plate’ (top left of the screen).
4.In the ‘Experiment Setup Properties’ panel, select the appropriate settings for the Maestro. Right-click Maestro to change the settings. In the pop-up screen (see Fig. 3A), sampling frequency should be at 12.5 kHz (this is fixed); set Analog Settings to ’Neural: Spikes,’ which has a sample gain of 1200× and a band-pass filter of 200-5000 Hz. Low-pass filter is disabled when using ‘Neural: Spikes’ in Analog Settings. Referencing should be set to ‘median’ to reduce noise common to the electrode groups and improve detection of low amplitude signals.
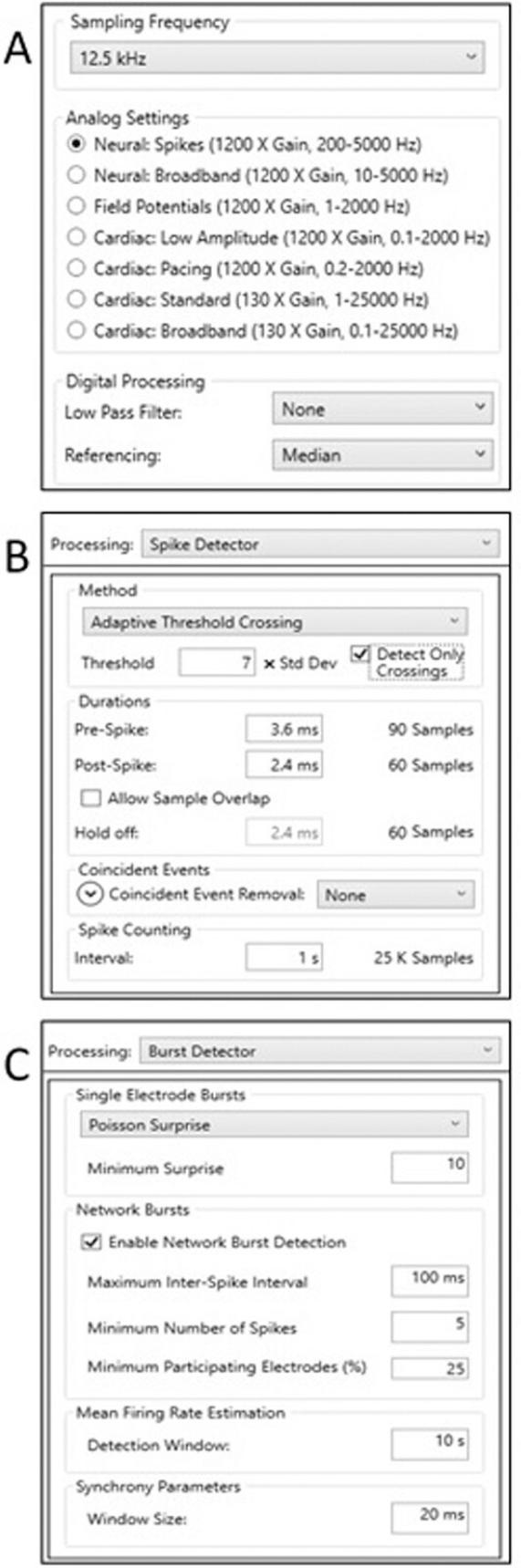
5.To visually inspect the activity of each plate before and during each recording, add the data processors ‘Spike Detector’ (to enable visualization of spikes) and ’Burst Detector’ (to enable visualization of (network) bursts). The output of the spike and burst detector should not be saved.
6.Select the folder in which you want to save the experiment by clicking ‘Browse’ in the Experiment Folder field and name the experiment at ‘Continuous Streams.’ Provide additional information in the experiment description section if needed.
7.In the Scheduled Recording Setup module, set the desired recording time and desired recording start time. For a single recording, select ‘Execute: Once.’
8.When the set temperature for the MEA Maestro has been reached, place the 48-well MEA plate containing the primary cortical cells from Basic Protocol 1, step 30, in the device by lifting the Maestro handle, placing the MEA plate into the docking bay, and gently lowering the handle to engage it to the system. Start live play by clicking the play button in the AxIS software.
9.Allow the plate to equilibrate for ∼5 min.
10.Start a 30-min baseline recording by clicking ‘Start schedule’ in the Scheduled Recording Setup panel.
11.During baseline recording, prepare exposure solutions of the test compound(s) in culture medium. Prewarm the exposure solutions to 37°C. It is advisable to test a maximum of five conditions per plate, since this allows for eight replicates per condition.
12.After ∼20 min of baseline recording, count the number of active electrodes per well at the Spike Plots module. Each field here represents a single electrode. If the number of active electrodes is less than four, do not expose the cells in this well to the test compound(s), since such a low number of electrodes will hinder proper detection of network bursts.
13.Make an exposure schedule (for an example, see Fig. 4). Since each plate is normalized to the average of its own controls, make sure to include enough control wells, in which the cell culture is exposed to a solvent (diluted just as the test compounds), water, or medium control. If you will be using different compounds diluted in different solvents, include sufficient control wells for each particular solvent. In our experience, the number of control wells should preferably be eight, but six at a minimum.
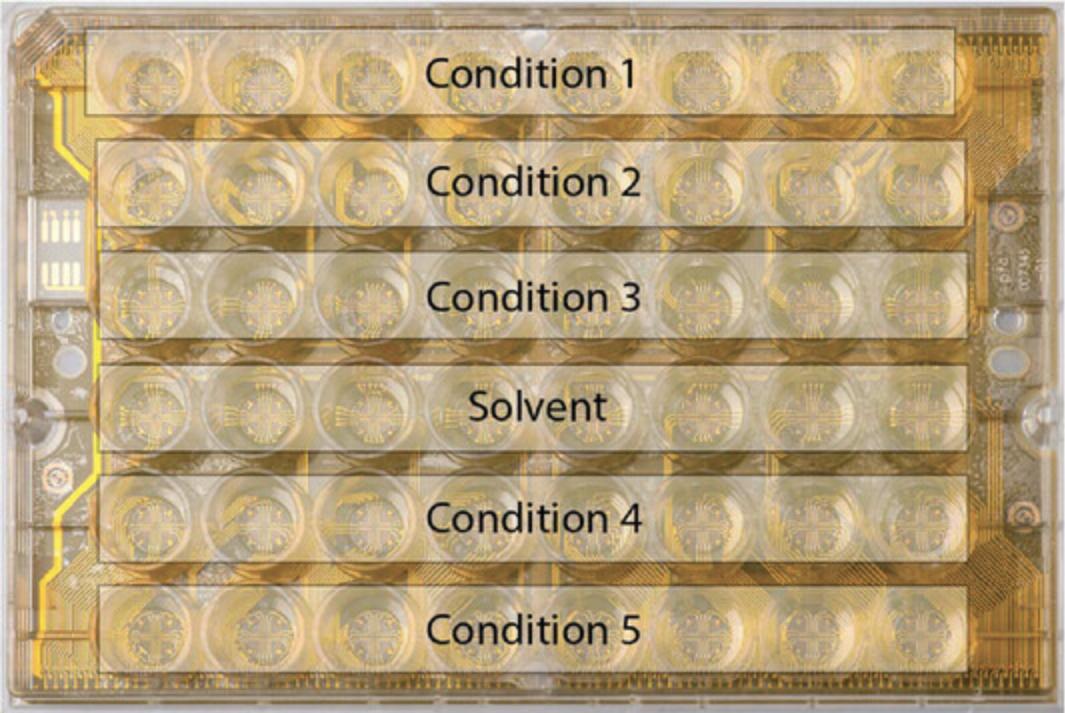
14.Start a 35-min exposure recording. Expose the culture by adding the appropriate exposure solution to each well during the first 5 min of the exposure recording.
15.After the MEA measurement, close the AxIS software, and turn off the Maestro MiddleMan and computer.
16.Determine cell viability using the Alamar Blue assay (see Support Protocol 3).
17.After the cell viability assay, clean the MEA plate as described in Support Protocol 1 and proceed to MEA data processing (Basic Protocol 3).
Alternate Protocol 1: MEA MEASUREMENTS DURING SUBCHRONIC EXPOSURE
In this protocol, the user will perform MEA measurements during subchronic exposure of rat cortical cells seeded on MEA plates (Basic Protocol 1) to a (set of) test compound(s). These subchronic exposure experiments will show the impact of exposure to a test compound(s) on neuronal function by recording spontaneous neuronal (network) activity at various time points during subchronic exposure: after 30 min (to reveal any acute effects), and after 24 and 48 hr exposure (to assess whether or not effects develop or aggravate over time).
Subchronic exposure measurements will be performed on DIV 9-11, since at this developmental stage the neuronal networks exhibit a high and stable degree of activity (Dingemans et al., 2016), including (network) burst behavior (Brown et al., 2016; Zwartsen et al., 2018). On DIV 9, users will perform a 30-min baseline MEA measurement, followed by exposure to the test compound(s) under sterile conditions in a biological safety hood to enable further culturing. Immediately after this exposure, users will perform another 30-min MEA measurement to determine acute effects. On DIV 10 (after 24 hr exposure) and DIV 11 (after 48 hr exposure), users will again perform 30-min MEA measurements. After the MEA measurement on DIV 11, users will determine cell viability (Support Protocol 3: “Determination of cell viability after MEA experiments”). To ensure the robustness and reproducibility of MEA data, we recommend having a sample size of at least 20 wells per condition, spread out over three MEA plates. At the end of this protocol, the user will have MEA data output (.RAW files), which can be further analyzed following Basic Protocol 3.
For materials, see Basic Protocol 2.
1.Record baseline spontaneous neuronal activity as described in Basic Protocol 2, steps 1-10.
2.Prepare exposure solutions during baseline recording (see Basic Protocol 2, step 11). Prewarm the exposure solutions to 37°C. It is advisable to test a maximum of five conditions per plate, since this allows for eight replicates per condition.
3.After ∼20 min of baseline recording, count the number of active electrodes per well at the Spike Plots module. Each field here represents a single electrode. If the number of active electrodes is less than four, do not expose the cells in this well to the test compound(s), since such a low number of electrodes will hinder proper detection of network bursts.
4.Make an exposure schedule (see Fig. 4; Basic Protocol 2, step 13). Since each plate is normalized to the average of its own controls, make sure to include enough control wells in which the culture is exposed to a solvent, water, or medium control, as appropriate. In our experience, the number of control wells should be at least six, but preferably eight.
5.Expose the culture by adding 55.5 µl of exposure solution to each well under sterile conditions.
6.Wait 30 min and record the spontaneous neuronal activity again (see Basic Protocol 2, steps 1-10). This is timepoint ‘0.5 hr.’
7.After completing the MEA recording, close the AxIS software, turn off the Maestro MiddleMan and computer, and incubate the exposed culture for 23 hr at 37°C, with 5% CO2 and 95% air atmosphere.
8.Record the spontaneous neuronal activity for 30 min (see Basic Protocol 2, steps 1-10). This is timepoint ‘24 hr.’ After the run, close the AxIS software, turn off the Maestro MiddleMan and computer, and incubate the exposed culture for another 23.5 hr at 37°C, with 5% CO2 and 95% air atmosphere.
9.Record the spontaneous neuronal activity for 30 min (see Basic Protocol 2, steps 1-10). This is timepoint ‘48 hr.’
10.After the MEA measurement, close the AxIS software, and turn off the Maestro MiddleMan and computer.
11.Determine cell viability using the Alamar Blue assay (see Support Protocol 3).
12.After the cell viability assay, clean the MEA plate as described in Support Protocol 1 and proceed to MEA data processing (Basic Protocol 3).
Alternate Protocol 2: MEA MEASUREMENTS DURING CHRONIC EXPOSURE
In this protocol, the user will perform MEA measurements during chronic exposure of rat cortical cells seeded on MEA plates (Basic Protocol 1) to a (set of) test compound(s). These chronic exposure experiments investigate the effect of a prolonged exposure on the development of spontaneous neuronal activity over time, i.e., developmental neurotoxicity. These chronic exposure experiments could, in principle, start at DIV 0, but in our laboratory, we start measuring at DIV 7, since at this time, neuronal networks have started developing and there is already spontaneous (network) activity (Dingemans et al., 2016). On DIV 7, users will perform a 30-min baseline MEA measurement, followed by exposure to the test compound(s). To ensure that the exposure concentration remains constant over time, and to minimize disturbance to the cells, half medium changes will be done after each MEA measurement. In these half medium changes, half of the medium is removed from each well and replaced by fresh medium with the desired exposure concentration. Since the cells can be cultured and maintained for at least 28 days, exposures and half medium changes are performed under sterile conditions in a biological safety hood. On DIV 10, 14, 17, 21, and 24, users will again perform 30-min MEA measurements followed by a half medium changes. On DIV 28, users will perform the last 30-min MEA measurement, followed by a cell viability check (Support Protocol 3). To ensure the robustness and reproducibility of MEA data, we recommend having a sample size of at least 20 wells per condition, spread out over three MEA plates. At the end of this protocol, the user will have MEA data output (.RAW files), which can be further analyzed using Basic Protocol 3.
For materials, see Basic Protocol 2.
1.Record the baseline spontaneous neuronal activity as described in Basic Protocol 2, steps 1-10.
2.Prepare 2× exposure solutions during the baseline recording. Prewarm the exposure solutions to 37°C.
3.After ∼20 min of baseline recording, count the number of active electrodes per well at the Spike Plots module. Each field here represents a single electrode. If the number of active electrodes is less than four, do not expose the cells in this well to the test compound(s), since such a low number of electrodes will hinder proper detection of network bursts.
4.Make an exposure schedule (see Fig. 4; Basic Protocol 2, step 13). Since each plate is normalized to the average of its own controls, make sure to include enough control wells in which the culture is exposed to a solvent, water, or medium control. In our experience, the number of control wells should be eight at minimum for chronic MEA experiments, since some of the control wells may lose activity during the 28 days culture.
5.After the baseline recording, remove the plate from the MEA Maestro.
6.Perform the half medium change. First, correct the volume in each well, since this changes over time due to evaporation.
7.Carefully resuspend and remove 250 µl of the medium from each well.
8.Gently add 250 µl of the 2× exposure solution at the concentrations of interest to the appropriate wells.
9.Place the 48-well MEA plate in a humidified incubator at 37° with 5% CO2. Wait for 3 days, until DIV 10.
10.Start another 30-min recording as described in Basic Protocol 2, steps 1-10.After the MEA measurement, close the AxIS software, and turn off the Maestro MiddleMan and computer.
11.Prepare exposure solutions in culture medium. In this step, contrary to step 2, the concentration of the exposure solution should be equal to the desired exposure concentration (i.e., 1×). Warm the exposure solutions to 37°C and keep at this temperature until used.
12.Repeat steps 6-9.
13.At DIV 14, 17, 21, and 24, repeat steps 10-12.
14.At DIV 28, start another 30-min recording. After the MEA measurement, close the AxIS software, and turn off the Maestro MiddleMan and computer.
15.Determine cell viability using the Alamar Blue assay (see Support Protocol 3).
16.After the cell viability assay, clean the MEA plate as described in Support Protocol 1 and proceed to MEA data processing (Basic Protocol 3).
Support Protocol 3: DETERMINATION OF CELL VIABILITY AFTER MEA EXPERIMENTS
Combining acute (Basic Protocol 2), subchronic (Alternate Protocol 1), or chronic MEA (Alternate Protocol 2) experiments with a fluorescence or colorimetric-based cell viability assay (e.g., Alamar Blue assay, WST-1 assay, MTT assay) enables distinguishing chemical-induced direct and functional neurotoxic effects from changes in neuronal activity that result from cytotoxicity (Wallace, Strickland, Valdivia, Mundy, & Shafer, 2015). The following protocol describes the evaluation of cell viability using the Alamar Blue assay, which is based on the reduction of the non-fluorescent, cell-permeable resazurin to highly fluorescent resorufin. After the (final) MEA recording is completed, the culture medium is replaced with prewarmed (37°C) Alamar Blue cell viability working solution, and cells are incubated for 1.5 hr. Afterwards, the Alamar Blue cell viability reagent is transferred to a transparent 96-well culture plate, and changes in cell viability can be assessed by measuring fluorescence. We further describe how to analyze the resulting data. The results will be expressed as mitochondrial activity (% of control), which is an indirect measure of viability.
Materials
-
48-well MEA plate from acute-, subchronic-, or chronic-exposure MEA experiment (Basic Protocol 2, step 17, Alternate Protocol 1, step 10, or Alternate Protocol 2, step 15, respectively)
-
25 µM Alamar Blue cell viability working solution (see recipe)
-
6- or 8-channel electronic pipettor (Integra Bioscience, Voyager)
-
Transparent 96-well plate suitable for the microplate reader
-
Microplate reader
Measuring metabolic activity
1.At the end of the MEA measurement, replace culture medium with 300 µl of Alamar Blue cell viability reagent (25 µM).
2.Incubate the plate for 1.5 hr at 37°C with 5% CO2 and 95% air atmosphere.
3.Transfer 200 µl of the Alamar Blue cell viability working solution from each well of the 48-well MEA plate to a well on a transparent 96-well culture plate. Include a minimum of four wells for the blank control (which consists of Alamar Blue cell viability working solution that has not been added to the 48-well MEA plate) per 96-well plate.
4.Measure fluorescence using a microplate reader (excitation 540 nm and emission 590 nm).
Data analysis
5.Determine the mean blank value and subtract this value from all other fluorescence values.
6.Determine the mean and SD for the control values and exclude values exceeding mean ± 2×SD, since these can be considered outliers.
7.Normalize the fluorescence values for all wells to the mean fluorescence value of the control by using the following equation:
FluorescencevalueexposedwellMeanfluorescencevaluecontrol∗100=mitochondrialactivity(%ofcontrol)
8.If applicable, combine data from different plates.
9.For each condition, exclude values where the treatment ratio > mean ± 2×SD (for their respective condition), since these can be considered outliers. Do not do this again for control values as this has been performed already in step 6.The data is now ready for statistical analysis.
Basic Protocol 3: MEA DATA PROCESSING
After the MEA recordings are completed (following Basic Protocol 2, Alternate Protocol 1, or Alternate Protocol 2), the Axion Integrated Studio (AxIS) software is used to generate AxIS Spike files (.spk files) from the .RAW files for further data analysis. In this step, a specific threshold is used to differentiate between spikes and background noise. The .spk files can be loaded into the NeuralMetrics Tool. In this software, further criteria are specified to identify bursts and network bursts in the recorded data. The NeuralMetrics Tool generates .CSV files, which contain the raw values for each parameter for each recording and will be used for analysis in Basic Protocol 4 (for acute and subchronic exposure experiments) or Alternate Protocol 3 (for chronic exposure experiments).
Materials
- Computer
- Axion Integrated Studio (AxIS) software (Axion BioSystems; version 2.5.2)
- Data from MEA experiments (.RAW files from Basic Protocol 2, Alternate Protocol 1, or Alternate Protocol 2)
- NeuralMetric Tool (Axion BioSystems; version 3.1.7)
Obtaining .spk files
1.Open the Axion software on the computer. Select the MEA output .RAW files that need to be pre-processed by clicking File → New batch process. In the pop-up screen, click Add → Select the files → OK.
2.Add a spike detection method. Right-click on the batch process, click on ‘Add processing,’ and select ‘Spike Detector.’
3.In the pop-up screen (see Fig. 3B), use the method ‘Adaptive Threshold Crossing’ and set the threshold to the appropriate setting. Check the ‘Detect Only Crossings’ box. Set Coincident Event Removal to ‘none’ and ‘Spike counting interval’ to 1 s. Set the output file to ‘AxIS Spike’ and start the batch process.
Spike train analysis with NeuralMetrics Tool
The .spk files generated can be analyzed by loading them into NeuralMetrics Tool (version 3.1.7, Axion Biosystems). The settings typically used in the NeuralMetrics Tool can be seen in Figure 5, but will also be explained in detail below.
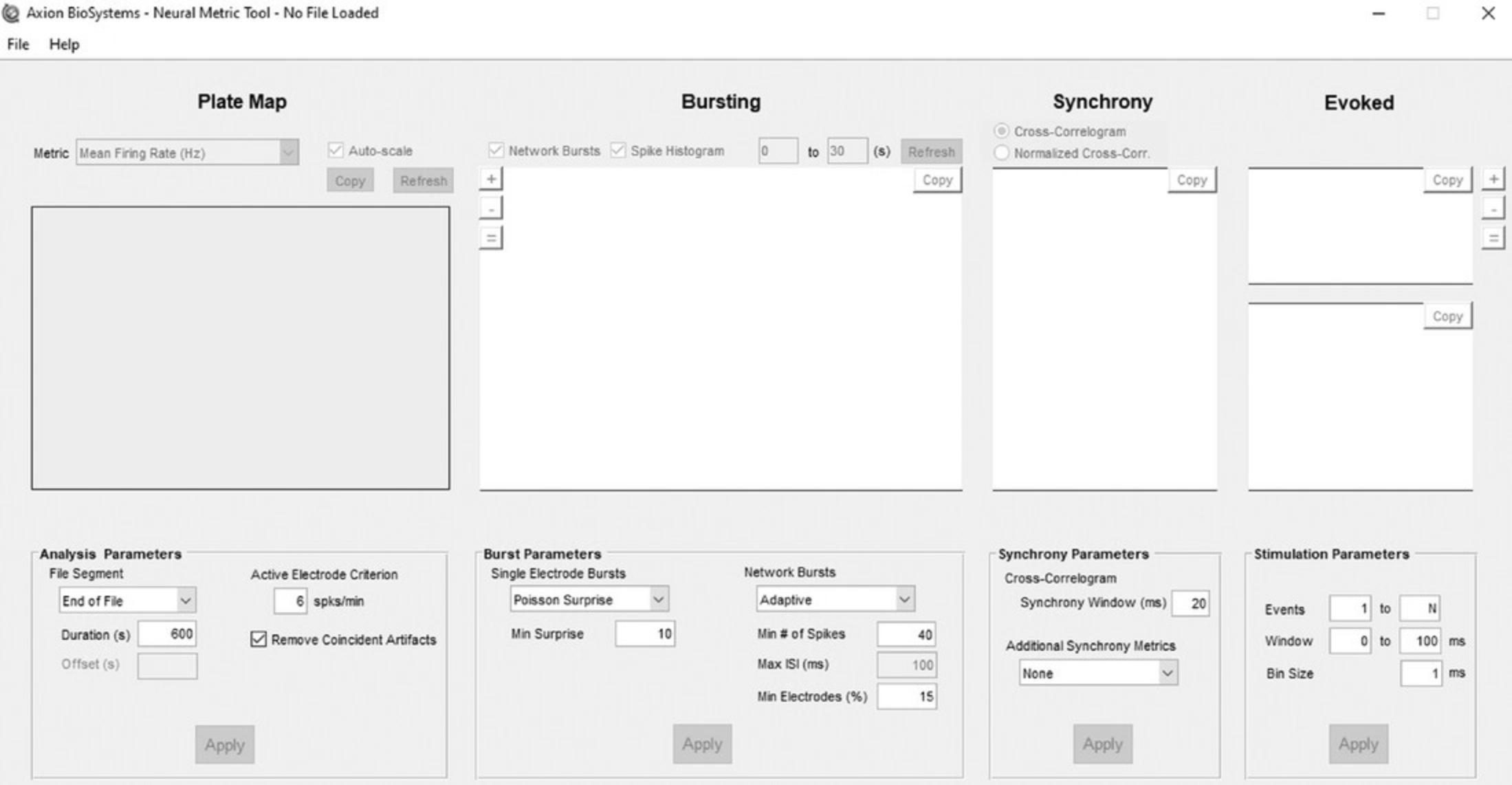
4.Open the NeuralMetrics Tool on the computer.
5.Set the file segment to 600 s (acute experiment) or 1200 s (chronic experiment) from the end of each measurement by selecting ‘End of File’ and setting the duration to 600 or 1200 s, respectively.
6.Set the Active Electrode Criterion to 6 spikes/min and check the box ‘Remove coincident Artifacts,’ which are artificial spikes that are detected on multiple electrodes at the exact same time and may occur due to environmental interference.
7.For burst parameters, select the same burst analysis settings as used during the MEA recording.
8.Select ‘Adaptive’ under Network Burst. This separates the spikes by a maximum interval set automatically on a well-by-well basis based on the mean spike rate for each well. Set the minimum number of spikes (Min # of Spikes) to 40, and the minimum of electrodes per well (Min Electrodes (%)) to 15%.
9.For Synchrony Parameters, set the synchrony window at 20 ms and additional synchrony metrics to ‘none.’
10.Since there is no evoked stimulation, do not change any settings for stimulation parameters.
11.Select the files that need to be loaded into NeuralMetrics Tool by clicking File → Batch process multiple files. In the pop-up screen, click OK after making sure the analysis and parameters settings are correct. In the next pop-up screen, click ‘Add Files’ to select the files. Make sure to set the timeframe in the pop-up screen to 600 s (for acute experiments) or 1200 s (for chronic experiments) from the end of each measurement. From the export options, select ‘Export Recommended Metrics to CSV.’
12.Start the NeuralMetrics Tool process by clicking ‘Run Batch.’
Basic Protocol 4: ANALYZING MEA EXPERIMENTS AFTER ACUTE AND SUBCHRONIC EXPOSURE
For acute and subchronic MEA experiments, the .CSV files are combined into burst macro files using, for instance, a custom-made Excel macro. In this burst macro, the raw values for baseline and exposure recordings are grouped per condition. This step and the final pre-processing steps before statistical analysis, including normalization to control, combining experiments, and outlier analysis, are shown in Figure 6 and will be explained in detail below. At the end of this protocol, the user will have treatment ratios (defined as % change to control) for each specific parameter measured, which will show the effect of the exposure to the test compound(s) on neuronal activity. These treatment ratios can be used in statistical analyses and, for example, for concentration-response fitting.
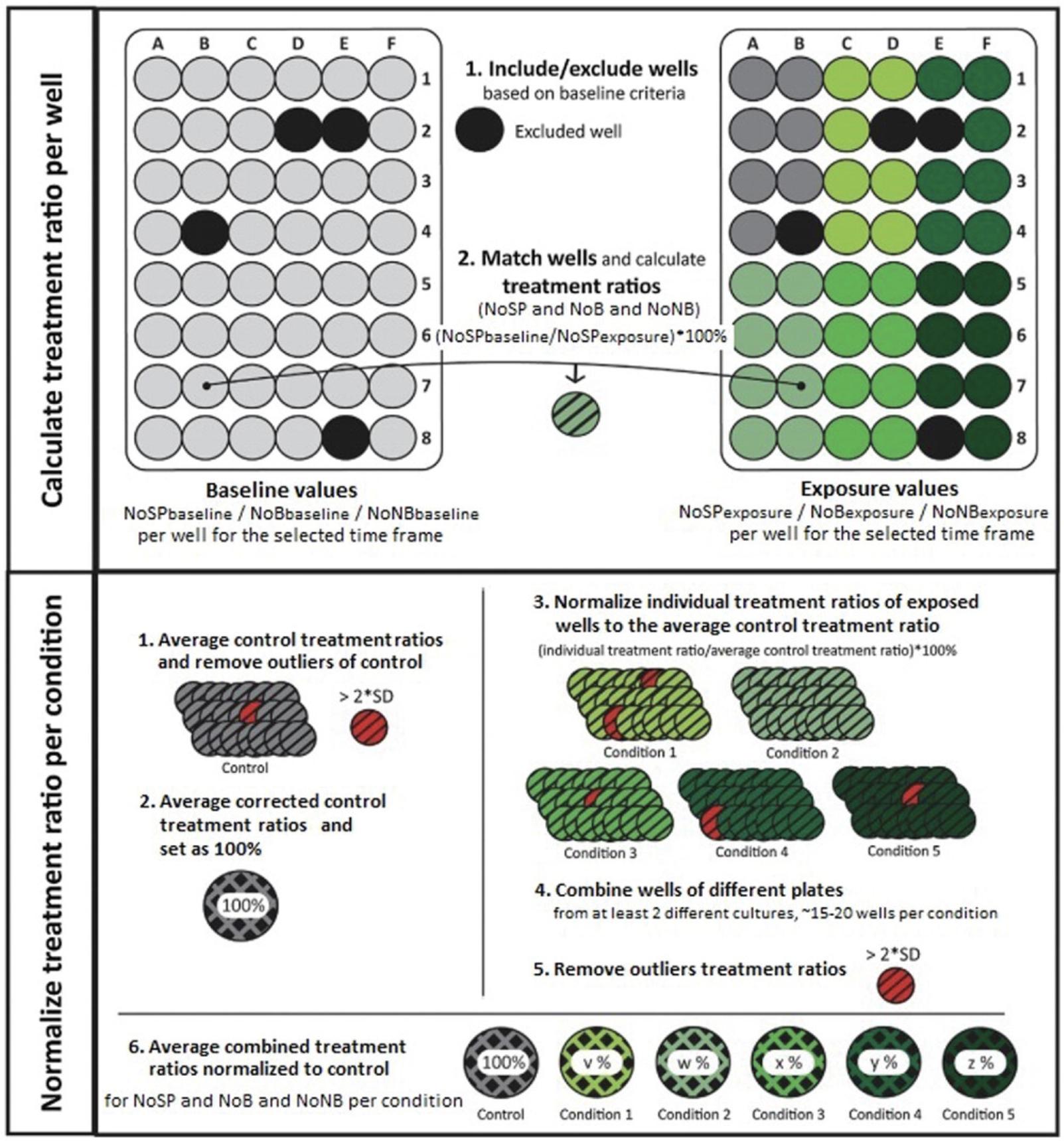
Materials
- Output from NeuralMetric Tools (.CSV files, from Basic Protocol 3)
1.The NeuralMetric Tool calculates the parameters of interest (see Table 1 for more information per parameter) per well prior to exposure (baseline activity) and after exposure. These values can be found in the .CSV files generated in Basic Protocol 3.Use these values to calculate a paired-comparison for each well. For each parameter of interest and each well, take the value at parameterexposure, divide this by the value at parameterbaseline, and multiply by 100. This expresses the parameterexposure as a percentage change compared to the parameterbaseline, and is the treatment ratio per well. In our lab, we do this paired-comparison with a custom-made Excel macro.
Category | Metrics parameters | Description |
---|---|---|
Spike parameters |
Number of spikes ISI coefficient of variation |
Total number of spikes over the duration of the analysis Standard deviation ISI (time between spikes) divided by the mean ISI. Measure for spike regularity: 0 indicates perfect spike distribution, >1 indicates bursting |
Burst parameters | Number of bursts | Total number of bursts over the duration of the analysis |
Burst duration | Average time from the first spike in a burst till the last spike (s). Longer bursts indicate more excitation, as it takes longer to shut down a burst. | |
Number of spikes per burst | Average number of spikes occurring in a burst | |
Mean inter-spike interval (ISI) within burst | Mean inter-spike interval within a burst (s). Smaller values indicate more intense bursts. | |
Inter-burst interval (IBI) | Time between the last spike of a burst and the first spike of a subsequent burst (s) | |
IBI coefficient of variation | Standard deviation of IBI divided by the mean IBI. Measure for burst regularity. | |
Burst percentage | Percentage of total number of spikes occurring in a burst | |
Network burst parameters | Number of network bursts | Total number of network bursts over the duration of the analysis |
Network burst duration | Average time from the first spike till the last spike in a network burst (s). Longer bursts indicate more excitation as it takes longer to shut down a burst. | |
Number of spikes per network burst | Average number of spikes occurring in a network burst | |
Mean ISI within network burst | Average of the mean ISIs within a network burst (s) | |
Network burst percentage | Percentage of total spikes occurring in a network burst | |
Network IBI coefficient of variation | Standard deviation of network IBI divided by the mean network IBI. Measure of network burst rhythmicity: value is small when bursts occur at a regular interval and increases when bursts occur more sporadically | |
Synchronicity parameters | Area under normalized cross-correlation | Area under inter-electrode cross-correlation normalized to the auto-correlations. The higher the value, the greater the synchronicity of the network. |
Full width at half height of normalized cross-correlation | Width at half height of the normalized cross-correlogram. Measure for network synchrony: the higher the value, the less synchronized the network. |
2.Exclude wells with less than four bursting electrodes in the baseline recording.
3.Sort all treatment ratios per concentration per test compound.
4.Remove outliers in the control values (value > mean ± 2×SD) and set the average control treatment ratio to 100% (which constitutes no change compared to baseline).
5.Normalize the treatment ratios of individual exposed wells to the average treatment ratio of the control wells.
Alternate Protocol 3: ANALYZING MEA EXPERIMENTS AFTER CHRONIC EXPOSURE
For chronic MEA experiments that last up to DIV 28 (Alternate Protocol 2), the NeuralMetrics Tool process described in Basic Protocol 3 produces .CSV files for seven different measurements (time points). The user will need to combine the output files from these seven measurements, after which the final pre-processing steps before statistical analysis, including normalization to time-matched control, combining experiments, and outlier analysis, can be done. At the end of this protocol, the user will obtain treatment ratios (defined as % change to time-matched control) for each specific parameter measured, which will show the effect of the exposure to the test compound(s) on neuronal activity. These treatment ratios can be used in statistical analysis and, for example, for concentration-response fitting.
Materials
- Output from NeuralMetric Tools (.CSV files from Basic Protocol 3)
1.The NeuralMetric Tool calculates the parameters of interest per well for each timepoint measured. These values can be found in the .CSV files generated in Basic Protocol 3.Each .CSV file contains the raw values for all parameters measured at a specific timepoint. Combine the values from all seven .CSV files to obtain a file that contains the raw values per parameter from all timepoints, grouped by condition. In our lab, we do this with a custom-made Excel macro, but any way of combining the values is appropriate.
2.Exclude wells with less than four bursting electrodes in the baseline recording.
3.Determine the mean and SD for the raw values of the control per parameter measured during the MEA recordings per time point and exclude values > mean ± 2×SD (of their respective timepoint), since these can be considered outliers.
4.For each parameter, normalize the raw values per time point to their time-matched average control value to obtain treatment ratios.
5.Set the time-matched average control treatment ratios to 100% (which constitutes no change compared to baseline).
6.For each parameter, exclude exposure values in which the treatment ratio is greater than the mean ± 2×SD (of their respective timepoint), since these can be considered outliers. Do not do this again for control values, as this has been performed already in step 3. The data is now fit for statistical analysis.
REAGENTS AND SOLUTIONS
Alamar Blue cell viability reagent (5 mM)
Add 1 g of resazurin sodium salt (Sigma-Aldrich, cat. no. 199303) to 40 ml of phosphate-buffered saline (PBS; GibcoTM, cat. no. 10010-023), resulting in a stock solution of 100 mM. To make the 5 mM Alamar Blue cell viability reagent, further dilute the stock solution 1:20 by adding 10 ml of the stock solution to 190 ml PBS. Prepare 5-ml aliquots of the Alamar Blue cell viability reagent and store protected from light at 4°C.
Alamar Blue cell viability working solution (25 µM)
Prepare a 1× dilution of 10× Hanks’ Balanced Salt Solution (GibcoTM, cat. no. 14065-049) with sterile laboratory-grade water. Dilute the 5 mM Alamar blue cell viability reagent (see recipe) 1:200 with 1× Hanks’ Balanced Salt solution to make an Alamar Blue Cell Viability working solution of 25 µM.
Borate buffer (24 mM sodium borate/50 mM boric acid; pH 8.4)
Dissolve 3.1 g boric acid (Sigma-Aldrich, cat. no. B6768) and 4.75 g sodium tetraborate (Sigma-Aldrich, cat. no. 221732) in 1 L sterile laboratory-grade water by stirring for 3 hr at 60°C using a magnetic stirrer. Adjust pH to 8.4 with hydrochloric acid.
Culture medium
Reagent | Supplier | Volume required | Final concentration |
Neurobasal-A medium (phenol red-free) | GibcoTM, cat. no. 12349-015 | 450 ml | |
Sucrose (28 g/100 ml; see recipe) | Sigma-Aldrich, cat. no. S8501 | 50 ml | 14 g sucrose/500 ml |
l-Glutamine (200 mM) | GibcoTM, cat. no. 25030-024 | 1.25 ml | 0.5 mM |
Penicillin-streptomycin (5000 U/ml) | GibcoTM, cat. no. 15070-063 | 5 ml | 50 U/ml |
B27 Plus Serum-Free Supplement (50×) | GibcoTM, cat. no. A35828-01 | 10 ml | 1× |
After preparing, sterilize the solution by filtration using a 250-ml vacuum filter system (Corning, cat. no. 431096) |
Dissection medium
Reagents | Supplier | Volume required | Final concentration |
Neurobasal-A medium (phenol red-free) | GibcoTM, cat. no. 12349-015 | 450 ml | |
Sucrose (28 g/100 ml; see recipe) | Sigma-Aldrich, cat. no. S8501 | 50 ml | 14 g sucrose/500 ml |
l-Glutamine (200 mM) | GibcoTM, cat. no. 25030-024 | 1.25 ml | 0.5 mM |
Penicillin-streptomycin (5000 U/ml) | GibcoTM, cat. no. 15070-063 | 5 ml | 50 U/ml |
B27 Plus Serum-Free Supplement (50×) | GibcoTM, cat. no. A35828-01 | 10 ml | 1× |
Glutamate solution (3.5 mM; see recipe) | Sigma-Aldrich, cat. no. 49621 | 5 ml | ∼35 µM |
After preparing, sterilize the solution by filtration using a 250-ml vacuum filter system (Corning, cat. no. 431096). |
Glutamate solution (3.5 mM)
Dissolve 131 mg l-glutamic acid monosodium salt monohydrate (Sigma-Aldrich, cat. no. 49621) in 200 ml sterile water. After preparing, sterilize the solution by filtration using a 250-ml vacuum filter system (Corning, cat. no. 431096). Glutamate solution can be stored for 6 months at 4°C.
Polyethyleneimine solution, 0.1%
Dissolve 500 µg of 50% (w/v) polyethyleneimine (PEI; Fluka, cat. no. P3143) in 250 ml borate buffer (see recipe) and filter sterilize using a 250-ml vacuum filter system (Corning, cat. no. 431096).
Sucrose (28 g/100 ml)
Dissolve 28 g sucrose (Sigma-Aldrich, cat. no. S8501) in 100 ml Neurobasal-A medium (GibcoTM, cat. no. 12349-015), minus phenol red. After preparing, sterilize the solution by filtration using a 250-ml vacuum filter system (Corning, cat. no. 431096). Sucrose solution can be stored for 2 months at 4°C.
COMMENTARY
Background Information
Neurotoxicity refers to adverse changes in the structure or function of the nervous system due to exposure to chemicals, drugs, environmental pollutants, or endogenous molecules, and is of significant public concern (Costa et al., 2014; U.S. Environmental Protection Administration, 1998; Grandjean & Landrigan, 2006). Current testing guidelines from, for example, the Organisation for Economic Co-operation and Development (OECD) or U.S. Environmental Protection Agency (EPA), for hazard characterization of adult and developmental neurotoxicity still largely rely on in vivo animal experiments evaluating neurobehavioral changes in motor, sensory, and cognitive function, as well as neuropathology (Bal-Price et al., 2008; for an overview, see Moser, 2011). Besides ongoing ethical debate about the high number of animals needed for such assays, the high costs and time requirements (up to 2 years to complete an in vivo neurodevelopment study) of these studies makes it untenable to use them for the screening of large numbers of chemicals (Bal-Price et al., 2008; Crofton, Mundy, & Shafer, 2012), which has promoted the widespread use of in vitro assays. Common in vitro assays to assess neurotoxicity, however, are often very target-specific and/or based on biochemical or morphological endpoints. For instance, in vitro screening assays for important processes often cover endpoints like gene expression (Hogberg, Kinsner-Ovaskainen, Hartung, Coecke, & Bal-Price, 2009), neurite outgrowth (Radio & Mundy, 2008; Radio, Breier, Shafer, & Mundy, 2008), synaptogenesis (Harrill, Robinette, & Mundy, 2011), and ion channel function (Accardi et al., 2016). While undoubtedly relevant, such endpoint-specific measurements often lack important functional aspects of the nervous system as a whole and fail to detect, for example, disturbances in network connectivity and synchronicity (see Current Protocols article: Bradley & Strock, 2019; also see Robinette et al., 2011). Although these assays can have a complementary role in studies on hazard characterization (Bal-Price 2010), integrated approaches, such as MEA recordings that assess activity, functionality, and synchronicity of neuronal networks, are desirable.
In addition to MEA recordings, common techniques to detect changes in neuronal function include Ca2+ imaging, intracellular recording by the patch clamp technique, or extracellular recording in hippocampal brain slices (Authier et al., 2016; Bradley, Luithardt, Metea, & Strock, 2018; Easter, Sharp, Valentin, & Pollard, 2007). Although well accepted, these techniques are often also too endpoint-specific, require extensive training and expertise, are chemically or mechanically invasive (thereby precluding long-term measurements), and/or lack the required throughput to be applicable as screening methods for hazard identification and characterization (Tukker et al., 2020a).
Assessment of neuronal (network) function using MEA recordings can bypass many of the limitations mentioned above. MEA recordings cover the entire neuronal network-specific functionality, for example, electrical activity, receptor and ion channel activation, synaptogenesis, neurotransmission, excitotoxicity, enzyme activity, and network formation and function (Bal-Price et al., 2010b; Johnstone et al., 2010). Further, MEA recordings permit simultaneous, non-invasive recordings of extracellular field potentials over a long period (up to 4-5 weeks) (Johnstone et al., 2010; Potter & DeMarse, 2001). Comparison of baseline to post-exposure neuronal activity patterns enables assessing changes induced by test compounds on several levels of neuronal function and at multiple time points after exposure, thereby allowing the study of the effects of acute, subchronic, and chronic exposures. Further, the development of multiwell microelectrode arrays makes it possible to test multiple compounds or concentrations, including several replicates and an appropriate number of controls in one experiment.
In this article, we describe a medium-throughput in vitro method to screen compounds for hazard identification and characterization in the context of neurotoxicology research. This approach uses rat primary cortical cultures, which resemble the in vivo brain as closely as possible (Authier et al., 2016; Charlesworth et al., 2015; Chiappalone et al., 2007; Tukker et al., 2020b). Compared to previously published protocols, our model includes cortical neurons and supportive cells that cover functional perturbations resulting from test compound−induced direct or indirect molecular effects. Furthermore, we describe in detail how to apply this approach to study distinct acute, subchronic, and chronic (developmental) exposure scenarios.
Critical Parameters
PEI coating
PEI coating creates a matrix on the surface of the electrode array ensuring cell adhesion and robust neurite outgrowth, which is critical for the development of a healthy neural network. The 48-well MEA plates must be allowed to dry completely (overnight) after the PEI coating and rinse steps, to enable seeding the cells in a droplet. Ideally, PEI coating of MEA plates should take place the week before cell isolation, to ensure that the wells are completely dry. In our laboratory, 0.1% PEI−coated 48-well MEA plates are air-dried and stored in a regular cupboard. To avoid bacterial, fungal, or other contamination, make sure the 0.1% PEI−coated 48-well MEA plate is covered with the lid at all times. Plates can be stored at room temperature for a maximum of 2 weeks before use. Longer storage impairs the PEI coating, which may cause cells to agglomerate rather than form a uniform monolayer over the electrode array.
Dissection and isolation of rat primary cortical cells
The dissection and subsequent cell isolation should be performed rapidly and uninterruptedly to avoid bacterial, fungal, or other contamination, or increased cell mortality. Therefore, preparing the working area before starting the dissection is essential. As soon as the cells are in suspension, they can be kept for a short period (10-20 min) in the water bath at 37°C to prepare the seeding step. Resuspend the cells gently and avoid intense pipetting or introduction of bubbles, since these will damage the cells.
Medium change
During medium change, particularly on DIV 4, carefully remove the dissection medium, taking care not to remove the cells as well. Add prewarmed culture medium using a low dispense speed, to avoid disturbing the neuronal network.
Medium evaporation
As the medium is refreshed using half medium changes, consider that maintaining the cells for chronic experimental setups requires adjustment of the evaporated volume. A higher volume evaporates from the outer—compared to the inner—wells. The exact evaporation volume should be determined beforehand and for the specific types of plates and incubators used, since there may be inter-laboratory differences (see Strategic Planning). Not adjusting for the evaporation volume will result in a lower volume of the culture medium over prolonged culture, which subsequently increases the concentration of supplements as well as of any test compound(s).
Non-test compound−induced responses
Neuronal activity is sensitive to electrolyte changes in the medium. Therefore, it is recommended to keep the percentage of solvents (DMSO, PBS, sterile laboratory-grade water) in the final volume at or below 0.1% (v/v) after the stock concentration has been dissolved in medium.
Exposure to test compound(s) can induce a mechanical response in the first minutes following application. Therefore, for acute-exposure MEA experiments, the first 5 min of the recording should not be used for data analysis. Further, it is important to check for the optimal effect window using the NeuralMetrics Tool. In case a test compound induces a transient response within the 30 min after exposure, due, for example, to rapid receptor desensitization, the time window for the data processing needs to be adjusted accordingly.
Sample size
To ensure the robustness and reproducibility of MEA data, we recommend having a sample size of at least 20 wells per condition, spread out over three MEA plates. Most preferably, these experiments should be done using three different cortical cultures to avoid “batch effects” such as differences in seeding or less viable cell cultures. For prioritization purposes, a smaller sample size can be sufficient, although it is most likely less reliable.
Troubleshooting
Table 2 lists some common problem that users may face when culturing rat primary cortical cultures and performing subsequent MEA experiments, and potential solutions (see Table 2).
Problem | Possible cause | Solution |
---|---|---|
Insufficient active electrodes per well | Unsatisfactory coupling between electrodes and cells | When handling the 48-well MEA plates, make sure that the electrodes within the wells are not touched |
With use, the electrodes wear out | Reuse 48-well MEA plates max. 2-3 times | |
Low neuronal (network) activity, possibly due to an unsatisfactory coupling between electrodes and cells | Deficient PEI coating, rinsing, or drying | Ensure that PEI drop is on the electrode array during coating, that each well is rinsed at least 4 times with sterile water, and that 48-well MEA plates are completely dry when seeding the cells |
Insufficient cell density per 48-well MEA plate | Check cell counting | |
When replacing the entire medium on DIV 4, take care not to aspirate cells or to destroy the neuronal network | ||
Increased cell mortality | Dissect the cortices and isolate the cells as quickly as possible, as extended processing times increase cell mortality rate | |
Unequal distribution of neuronal activity throughout the 48-well MEA plate | Unequal seeding of primary cortical cells | During seeding, keep remaining cells in suspension by carefully—but constantly—shaking the tube. |
When replacing the entire medium on DIV 4, take care not to aspirate cells or to destroy the neuronal network | ||
Low reproducibility across wells causing high SDs | Poor cell culture, differences in seeding between wells, or bad plates | Perform the experiment on multiple plates derived from multiple cell cultures to increase sample size and avoid batch and plate effects |
Parameter goes to zero after treatment, resulting in abnormal SDs | Treatment causes complete inhibition | Some parameters (such as number of spikes and bursts) are measured directly on the MEA and can still be used. Other parameters (such as inter-spike and inter-burst-interval) are, however, dependent on these parameters and are technically undefined when the parameter they are derived from goes to zero. Exclude these parameters if this problem arises. For example, the parameter ‘number of spikes’ can go to zero after treatment, but ‘inter-spike-interval,’ which is derived from the timestamps at which spikes are found, is undefined when there are no spikes. |
Difficulties with detecting spikes and bursts | High signal-to-noise ratio | Compare different spike detection thresholds and NeuralMetrics Tool settings to see which settings work best |
No detection of immediate acute effects | Effect occurs outside the chosen analysis time window | Compare different analysis time windows to see which time window can detect the desired effects |
Data not normally distributed | Using treatment ratios normalized to control | Choose your statistical analysis method with care and consider using non-parametric tests |
Understanding Results
After performing the steps in Basic Protocol 4 (for acute and subchronic experiments), Alternate Protocol 3 (for chronic experiments), and Support Protocol 3 (for cell viability experiments), the data is ready for statistical analysis. Carefully choose a statistical analysis method that fits the data, since MEA data are often not normally distributed and, thus, do not pass the criteria for parametric tests. Moreover, the analysis method may differ depending on the conditions that were tested (e.g., a one-way ANOVA when only testing a concentration-effect for a single test compound, or a repeated-measures ANOVA when performing a chronic experiment). Some example data are shown below. For clarity and simplicity, we show only the number of spikes, bursts, and network bursts for the MEA data. The replicates shown in each data example are the number of wells per concentration, derived from multiple MEA plates.
Acute MEA experiments
Plotting the treatment ratios for each different concentration of a test compound for a given parameter results in a concentration-response curve. Figure 7 shows concentration-response curves for the number of spikes (left), bursts (middle), and network bursts (right) during acute exposure to different concentrations (0.01-100 µM) of the neurotoxic insecticide alpha-cypermethrin. Alpha-cypermethrin shows a concentration-dependent inhibition for all parameters shown here, in line with previous results (Dingemans et al., 2016).
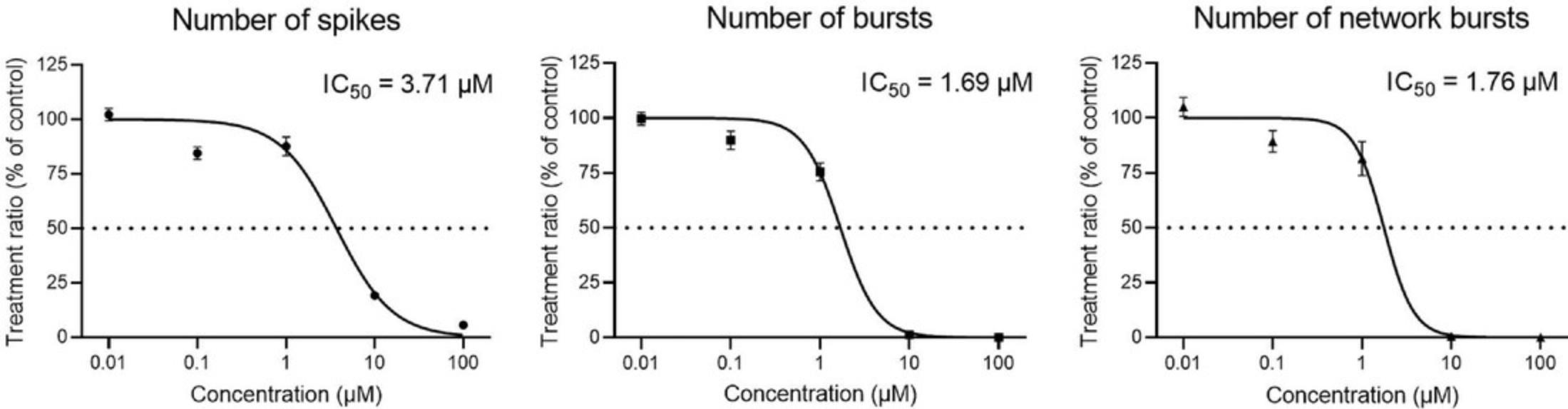
Such concentration-response curves, obtained for different compounds could be used for calculations of, for example, IC50 values, to determine the rank order potency of a set of test compounds.
Chronic MEA experiments
Figure 8 shows the mean count per well for the number of spikes (left), bursts (middle), and network bursts (right) over time after exposure to solvent (DMSO), to illustrate the development of neuronal activity under control conditions. Neuronal activity increases until DIV 10, after which activity remains fairly constant or increases only gradually over time.
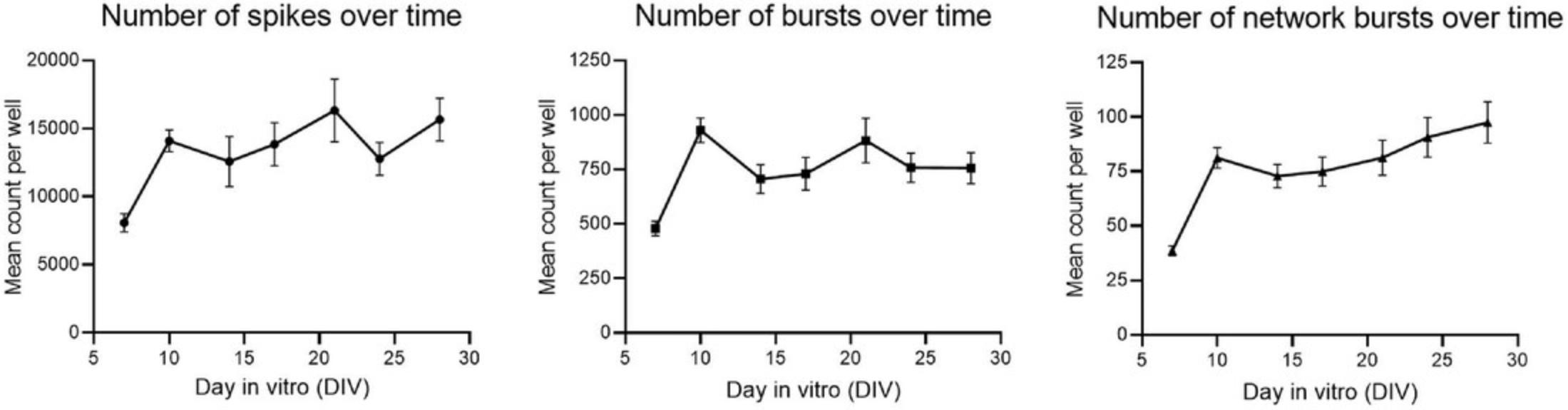
Figure 9 shows the treatment ratios for the number of spikes (left), bursts (middle), and network bursts (right) after chronic exposure from DIV 7-28 to different concentrations (0.01-100 µM) of the environmental pollutant bisphenol-A (BPA). The treatment ratios shown here for chronic MEA experiments are normalized to their time-matched controls. This means that a treatment effect does not necessarily reflect a change in neuronal activity over time, but a change in neuronal activity compared to control development at that time point. For 0.01 µM BPA, treatment ratios remain close to 100% of control, indicating that chronic exposure has little effect. At higher concentrations (0.1-10 µM), BPA increases neuronal activity in a time- and concentration-dependent manner. Interestingly, exposure to the highest concentration of BPA (100 µM) induces a sharp decrease in neuronal activity, which slowly recovers over time and is most pronounced for the number of spikes and bursts.
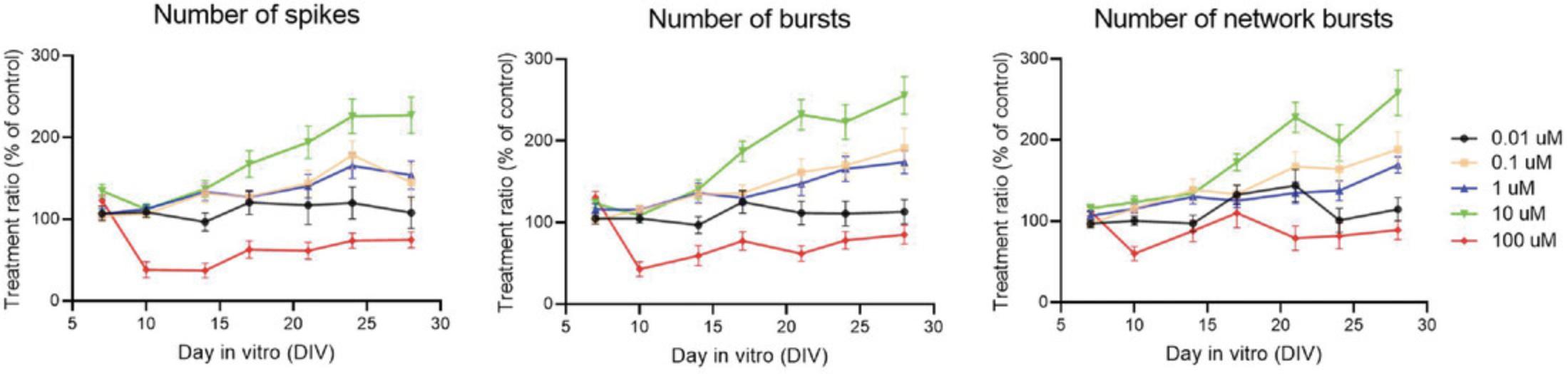
Cell viability experiments
Figure 10 shows the effect of chronic exposure from DIV 7-28 to different concentrations (0.01-100 µM) of the environmental pollutant bisphenol-A (BPA) on cell viability at DIV 28. Cell viability can be indirectly assessed by measuring the effects of BPA on mitochondrial activity, as described in Support Protocol 3. Exposure to 0.01-10 µM BPA did not affect mitochondrial activity, while exposure to 100 µM BPA resulted in a small decrease in mitochondrial activity, thus indicating a decrease in cell viability.
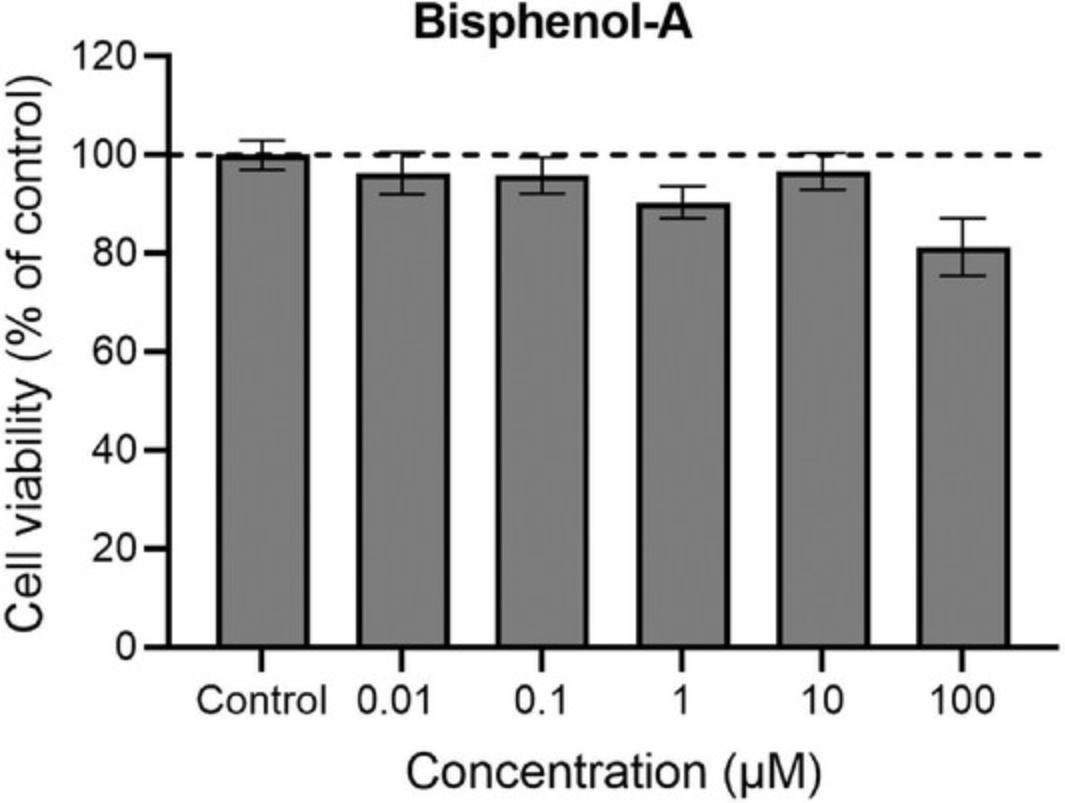
Time Considerations
The time it takes to perform an entire experiment, including preparatory work, culturing the cells, and the MEA measurements, ranges from 4 weeks for acute and subchronic exposure experiments to 7 weeks for chronic exposure experiments. Table 3 describes relative time considerations for the generation and maintenance of rat primary cortical cultures on MEA plates, performing MEA experiments for acute, subchronic, and chronic exposure scenarios and of the cell viability assay, and data processing.
Stage | Objective | Active time | Total time |
---|---|---|---|
Preparation of 48-well MEA plates | Pretreatment | 30 min | 3-5 day, with overnight incubation |
Washing procedure | 2 hr | 3 day, with overnight incubation | |
PEI coating | 2 hr | 1 day, with overnight drying | |
Cell culture | Generation and seeding of primary cortical culture | 1.5 hr | 4 hr, including incubation until cells attach and addition of medium |
Medium change on DIV 4 | 1 hr | 1.5 hr, with preparation and prewarming of medium | |
MEA experiments | Acute exposure | 2 hr | 2 hr |
Subchronic exposure | 3 hr | 2 days, with MEA recordings after 24 hr and 48 hr exposure | |
Chronic exposure | 10 hr | 21 days, with MEA recording on DIV 7, 10, 14, 17, 21, 24, and 28, and seven half-medium changes | |
Cell viability assay | Alamar Blue assay | 30 min | 2 hr, with incubation for 1.5 hr |
Data analysis | Acute MEA experiment | 1 hr | 3 hr, with obtaining .spk files and generation of NeuralMetric files |
Subchronic MEA experiment | 2 hr | 5 hr, with obtaining .spk files and generation of NeuralMetric files | |
Chronic MEA experiment | 1 hr | 5 hr, with obtaining .spk files and generation of NeuralMetric files | |
Cell viability assay | 30 min |
Acknowledgments
The authors acknowledge funding support from ENDpoiNTs (this project has received funding from the European Union's Horizon 2020 research and innovation programme under grant agreement No 825759), TUBE (this project has received funding from the European Union's Horizon 2020 research and innovation programme under grant agreement No 814978), and the Faculty of Veterinary Medicine (Utrecht University).
Author Contributions
Lora-Sophie Gerber : conceptualization, formal analysis, investigation, methodology, visualization, writing original draft; Lennart van Melis : conceptualization, formal analysis, investigation, methodology, visualization, writing original draft; Regina G.D.M. van Kleef : conceptualization, methodology; Aart de Groot : conceptualization, methodology, software; Remco Westerink : conceptualization, funding acquisition, methodology, supervision, writing review and editing
Conflict of Interest
The authors declare no conflicts of interest.
Open Research
Data Availability Statement
The data that support the findings of this study are available from the corresponding authors upon reasonable request.
Literature Cited
- Accardi, M. V., Pugsley, M. K., Forster, R., Troncy, E., Huang, H., & Authier, S. (2016). The emerging role of in vitro electrophysiological methods in CNS safety pharmacology. Journal of Pharmacological and Toxicological Methods , 81, 47–59. doi: 10.1016/j.vascn.2016.03.008.
- Authier, S., Arezzo, J., Delatte, M. S., Kallman, M. J., Markgraf, C., Paquette, D., … Curtis, M. J. (2016). Safety pharmacology investigations on the nervous system: An industry survey. Journal of Pharmacological and Toxicological Methods , 81, 37–46. doi: 10.1016/j.vascn.2016.06.001.
- Bal-Price, A. K., Hogberg, H. T., Buzanska, L., & Coecke, S. (2010a). Relevance of in vitro neurotoxicity testing for regulatory requirements: Challenges to be considered. Neurotoxicology and Teratology , 32, 36–41. doi: 10.1016/j.ntt.2008.12.003.
- Bal-Price, A. K., Hogberg, H. T., Buzanska, L., Lenas, P., van Vliet, E., & Hartung, T. (2010b). In vitro developmental neurotoxicity (DNT) testing: Relevant models and endpoints. NeuroToxicology , 31, 545–554. doi: 10.1016/j.neuro.2009.11.006.
- Bal-Price, A. K., Suñol, C., Weiss, D. G., van Vliet, E., Westerink, R. H. S., & Costa, L. G. (2008). Application of in vitro neurotoxicity testing for regulatory purposes: Symposium III summary and research needs. NeuroToxicology , 29, 520–531. doi: 10.1016/j.neuro.2008.02.008.
- Bradley, J. A., Luithardt, H. H., Metea, M. R., & Strock, C. J. (2018). In vitro screening for seizure liability using microelectrode array technology. Toxicological Sciences , 163, 240–253. doi: 10.1093/toxsci/kfy029.
- Bradley, J. A., & Strock, C. J. (2019). Screening for neurotoxicity with microelectrode array. Current Protocols in Toxicology , 79, e67, doi: 10.1002/cptx.67.
- Brown, J. P., Hall, D., Frank, C. L., Wallace, K., Mundy, W. R., & Shafer, T. J. (2016). Editor's highlight: Evaluation of a microelectrode array-based assay for neural network ontogeny using training set chemicals. Toxicological Sciences , 154, 126–139. doi: 10.1093/toxsci/kfw147.
- Charlesworth, P., Cotterill, E., Morton, A., Grant, S. G. N., & Eglen, S. J. (2015). Quantitative differences in developmental profiles of spontaneous activity in cortical and hippocampal cultures. Neural Development , 10, 1, doi: 10.1186/s13064-014-0028-0.
- Chiappalone, M., Vato, A., Berdondini, L., Koudelka-Hep, M., & Martinoia, S. (2007). Network dynamics and synchronous activity in cultured cortical neurons. International Journal of Neural Systems , 17, 87–103. doi: 10.1142/S0129065707000968.
- Costa, L. G., Cole, T. B., Coburn, J., Chang, Y. C., Dao, K., & Roque, P. (2014). Neurotoxicants are in the air: Convergence of human, animal, and in vitro studies on the effects of air pollution on the brain. BioMed Research International , 2014, 8. doi: 10.1155/2014/736385.
- Cotterill, E., Hall, D., Wallace, K., Mundy, W. R., Eglen, S. J., & Shafer, T. J. (2016). Characterization of early cortical neural network development in multiwell microelectrode array plates. Journal of Biomolecular Screening , 21, 510–519. doi: 10.1177/1087057116640520.
- Crofton, K. M., Mundy, W. R., & Shafer, T. J. (2012). Developmental neurotoxicity testing: A path forward. Congenital Anomalies , 52, 140–146. doi: 10.1111/j.1741-4520.2012.00377.x.
- Dingemans, M. M. L., Schütte, M. G., Wiersma, D. M. M., de Groot, A., van Kleef, R. G. D. M., Wijnolts, F. M. J., & Westerink, R. H. S. (2016). Chronic 14-day exposure to insecticides or methylmercury modulates neuronal activity in primary rat cortical cultures. NeuroToxicology , 57, 194–202. doi: 10.1016/j.neuro.2016.10.002.
- Easter, A., Sharp, T. H., Valentin, J.-P., & Pollard, C. E. (2007). Pharmacological validation of a semi-automated in vitro hippocampal brain slice assay for assessment of seizure liability. Journal of Pharmacological and Toxicological Methods , 56, 223–233. doi: 10.1016/j.vascn.2007.04.008.
- Frank, C. L., Brown, J. P., Wallace, K., Mundy, W. R., & Shafer, T. J. (2017). Developmental neurotoxicants disrupt activity in cortical networks on microelectrode arrays: Results of screening 86 compounds during neural network formation. Toxicological Sciences , 160, 121–135. doi: 10.1093/toxsci/kfx169.
- Gopal, K. V., Miller, B. R., & Gross, G. W. (2007). Acute and sub-chronic functional neurotoxicity of methylphenidate on neural networks in vitro. Journal of Neural Transmission , 114, 1365–1375. doi: 10.1007/s00702-007-0759-8.
- Grandjean, P., & Landrigan, P. (2006). Developmental neurotoxicity of industrial chemicals. Lancet , 368, 2167–2178. doi: 10.1016/S0140-6736(06)69665-7.
- de Groot, M. W. G. D. M., Westerink, R. H. S., & Dingemans, M. M. L. (2013). Don't judge a neuron only by its cover: Neuronal function in in vitro developmental neurotoxicity testing. Toxicological Sciences , 132, 1–7. doi: 10.1093/toxsci/kfs269.
- Harrill, J. A., Robinette, B. L., & Mundy, W. R. (2011). Use of high content image analysis to detect chemical-induced changes in synaptogenesis in vitro. Toxicology in Vitro , 25, 368–387. doi: 10.1016/j.tiv.2010.10.011.
- Hogberg, H. T., Kinsner-Ovaskainen, A., Hartung, T., Coecke, S., & Bal-Price, A. K. (2009). Gene expression as a sensitive endpoint to evaluate cell differentiation and maturation of the developing central nervous system in primary cultures of rat cerebellar granule cells (CGCs) exposed to pesticides. Toxicology and Applied Pharmacology , 235, 268–286. doi: 10.1016/j.taap.2008.12.014.
- Hogberg, H. T., Sobanski, T., Novellino, A., Whelan, M., Weiss, D. G., & Bal-Price, A. K. (2011). Application of micro-electrode arrays (MEAs) as an emerging technology for developmental neurotoxicity: Evaluation of domoic acid-induced effects in primary cultures of rat cortical neurons. NeuroToxicology , 32, 158–168. doi: 10.1016/j.neuro.2010.10.007.
- Hondebrink, L., Kasteel, E. E. J., Tukker, A. M., Wijnolts, F. M. J., Verboven, A. H. A., & Westerink, R. H. S. (2017). Neuropharmacological characterization of the new psychoactive substance methoxetamine. Neuropharmacology , 123, 1–9. doi: 10.1016/j.neuropharm.2017.04.035.
- Hondebrink, L., Verboven, A. H. A., Drega, W. S., Schmeink, S., de Groot, M. W. G. D. M., van Kleef, R. G. D. M., … Westerink, R. H. S. (2016). Neurotoxicity screening of (illicit) drugs using novel methods for analysis of microelectrode array (MEA) recordings. NeuroToxicology , 55, 1–9. doi: 10.1016/j.neuro.2016.04.020.
- Johnstone, A. F. M., Gross, G. W., Weiss, D. G., Schroeder, O. H. U., Gramowski, A., & Shafer, T. J. (2010). Microelectrode arrays: A physiologically based neurotoxicity testing platform for the 21st century. NeuroToxicology , 31, 331–350. doi: 10.1016/j.neuro.2010.04.001.
- Kasteel, E. E. J., & Westerink, R. H. S. (2017). Comparison of the acute inhibitory effects of Tetrodotoxin (TTX) in rat and human neuronal networks for risk assessment purposes. Toxicology Letters , 270, 12–16. doi: 10.1016/j.toxlet.2017.02.014.
- Kosnik, M. B., Strickland, J. D., Marvel, S. W., Wallis, D. J., Wallace, K., Richard, A. M., … Shafer, T. J. (2020). Concentration–response evaluation of ToxCast compounds for multivariate activity patterns of neural network function. Archives of Toxicology , 94, 469–484. doi: 10.1007/s00204-019-02636-x.
- Legendy, C. R., & Salcman, M. (1985). Bursts and recurrences of bursts in the spike trains of spontaneously active striate cortex neurons. Journal of Neurophysiology , 53, 926–939. doi: 10.1152/jn.1985.53.4.926.
- Mack, C. M., Lin, B. J., Turner, J. D., Johnstone, A. F. M., Burgoon, L. D., & Shafer, T. J. (2014). Burst and principal components analyses of MEA data for 16 chemicals describe at least three effects classes. NeuroToxicology , 40, 75–58. doi: 10.1016/j.neuro.2013.11.008.
- McConnell, E. R., McClain, M. A., Ross, J., LeFew, W. R., & Shafer, T. J. (2012). Evaluation of multi-well microelectrode arrays for neurotoxicity screening using a chemical training set. NeuroToxicology , 33, 1048–1057. doi: 10.1016/j.neuro.2012.05.001.
- Moser, V. C. (2011). Functional assays for neurotoxicity testing. Toxicologic Pathology , 39, 36–45. doi: 10.1177/0192623310385255.
- Nicolas, J., Hendriksen, P. J. M., van Kleef, R. G. D. M., de Groot, A., Bovee, T. F. H., Rietjens, I. M. C. M., & Westerink, R. H. S. (2014). Detection of marine neurotoxins in food safety testing using a multielectrode array. Molecular Nutrition and Food Research , 58, 2369–2378. doi: 10.1002/mnfr.201400479.
- Novellino, A., Scelfo, B., Palosaari, T., Price, A., Sobanski, T., Shafer, T. J. … Whelan M. (2011). Development of micro-electrode array based tests for neurotoxicity: Assessment of interlaboratory reproducibility with neuroactive chemicals. Frontiers in Neuroengineering , 4, 1–14. doi: 10.3389/fneng.2011.00004.
- Phelan, K. and May, K.M. (2015). Basic techniques in mammalian cell tissue culture. Current Protocols in Cell Biology , 66, 1.1.1–1.1.22. doi: 10.1002/0471143030.cb0101s66.
- Potter, S. M., & DeMarse, T. B. (2001). A new approach to neural cell culture for long-term studies. Journal of Neuroscience Methods , 110, 17–24. doi: 10.1016/S0165-0270(01)00412-5.
- Puia, G., Gullo, F., Dossi, E., Lecchi, M., & Wanke, E. (2012). Novel modulatory effects of neurosteroids and benzodiazepines on excitatory and inhibitory neurons excitability: A multi-electrode array recording study. Frontiers in Neural Circuits , 6, 94. doi: 10.3389/fncir.2012.00094.
- Radio, N. M., Breier, J. M., Shafer, T. J., & Mundy, W. R. (2008). Assessment of chemical effects on neurite outgrowth in PC12 cells using high content screening. Toxicological Sciences , 105, 106–118. doi: 10.1093/toxsci/kfn114.
- Radio, N. M., & Mundy, W. R. (2008). Developmental neurotoxicity testing in vitro: Models for assessing chemical effects on neurite outgrowth. NeuroToxicology , 29, 361–376. doi: 10.1016/j.neuro.2008.02.011.
- Robinette, B. L., Harrill, J. A., Mundy, W. R., & Shafer, T. J. (2011). In vitro assessment of developmental neurotoxicity: Use of microelectrode arrays to measure functional changes in neuronal network ontogeny. Frontiers in Neuroengineering , 4, 1–9. doi: 10.3389/fneng.2011.00001.
- Spira, M. E., & Hai, A. (2013). Multi-electrode array technologies for neuroscience and cardiology. Nature Nanotechnology , 8, 83–94. doi: 10.1038/nnano.2012.265.
- Strickland, J. D., Martin, M. T., Richard, A. M., Houck, K. A., & Shafer, T. J. (2018). Screening the ToxCast phase II libraries for alterations in network function using cortical neurons grown on multi-well microelectrode array (mwMEA) plates. Archives of Toxicology , 92, 487–500. doi: 10.1007/s00204-017-2035-5.
- Tukker, A. M., van Kleef, R. G. D. M., Wijnolts, F. M. J., de Groot, A., & Westerink, R. H. S. (2020a). Towards animal-free neurotoxicity screening: Applicability of hiPSC-derived neuronal models for in vitro seizure liability assessment. Altex , 37, 121–135. doi: 10.14573/altex.1907121.
- Tukker, A. M., Wijnolts, F. M. J., de Groot, A., & Westerink, R. H. S. (2020b). Applicability of hiPSC-derived neuronal cocultures and rodent primary cortical cultures for in vitro seizure liability assessment. Toxicological Sciences , 178, 71–87. doi: 10.1093/toxsci/kfaa136.
- U.S. Environmental Protection Administration (1998). Guidelines for Neurotoxicity Risk Assessment. Federal Register , 63, 26926–26954.
- Valdivia, P., Martin, M., LeFew, W. R., Ross, J., Houck, K. A., & Shafer, T. J. (2014). Multi-well microelectrode array recordings detect neuroactivity of ToxCast compounds. NeuroToxicology , 44, 204–217. doi: 10.1016/j.neuro.2014.06.012.
- Vassallo, A., Chiappalone, M., De Camargos Lopes, R., Scelfo, B., Novellino, A., Defranchi, E. … Shafer, T. J. (2017). A multi-laboratory evaluation of microelectrode array-based measurements of neural network activity for acute neurotoxicity testing. NeuroToxicology , 60, 280–292. doi: 10.1016/j.neuro.2016.03.019.
- Wallace, K., Strickland, J. D., Valdivia, P., Mundy, W. R., & Shafer, T. J. (2015). A multiplexed assay for determination of neurotoxicant effects on spontaneous network activity and viability from microelectrode arrays. NeuroToxicology , 49, 79–85. doi: 10.1016/j.neuro.2015.05.007.
- Westerink, R. H. S. (2013). Do we really want to REACH out to in vitro? NeuroToxicology , 39, 169–172. doi: 10.1016/j.neuro.2013.10.001.
- Zwartsen, A., Hondebrink, L., & Westerink, R. H. (2019). Changes in neuronal activity in rat primary cortical cultures induced by illicit drugs and new psychoactive substances (NPS) following prolonged exposure and washout to mimic human exposure scenarios. NeuroToxicology , 74, 28–39. doi: 10.1016/j.neuro.2019.05.004.
- Zwartsen, A., Hondebrink, L., & Westerink, R. H. (2018). Neurotoxicity screening of new psychoactive substances (NPS): Effects on neuronal activity in rat cortical cultures using microelectrode arrays (MEA). NeuroToxicology , 66, 87–97. doi: 10.1016/j.neuro.2018.03.007.
Citing Literature
Number of times cited according to CrossRef: 6
- Katrien C. K. Poelaert, Regina G. D. M. van Kleef, Mengying Liu, Arno van Vliet, Heyrhyoung Lyoo, Lora-Sophie Gerber, Yoshiki Narimatsu, Christian Büll, Henrik Clausen, Erik de Vries, Remco H. S. Westerink, Frank J. M. van Kuppeveld, Enterovirus D-68 Infection of Primary Rat Cortical Neurons: Entry, Replication, and Functional Consequences, mBio, 10.1128/mbio.00245-23, 14 , 2, (2023).
- Luise Schlotterose, Megane Beldjilali-Labro, Gaya Schneider, Ofir Vardi, Kirsten Hattermann, Uzi Even, Esther Shohami, Herman D. Haustein, Yael Leichtmann-Bardoogo, Ben M. Maoz, Traumatic Brain Injury in a Well: A Modular Three-Dimensional Printed Tool for Inducing Traumatic Brain Injury In vitro , Neurotrauma Reports, 10.1089/neur.2022.0072, 4 , 1, (255-266), (2023).
- Anke M. Tukker, Misha F. Vrolijk, Regina G.D.M. van Kleef, Dick T.H.M. Sijm, Remco H.S. Westerink, Mixture effects of tetrodotoxin (TTX) and drugs targeting voltage-gated sodium channels on spontaneous neuronal activity in vitro, Toxicology Letters, 10.1016/j.toxlet.2022.11.005, 373 , (53-61), (2023).
- Lora-Sophie Gerber, Regina G.D.M. van Kleef, Paul Fokkens, Flemming R. Cassee, Remco HS Westerink, In vitro neurotoxicity screening of engine oil- and hydraulic fluid-derived aircraft cabin bleed-air contamination, NeuroToxicology, 10.1016/j.neuro.2023.04.010, 96 , (184-196), (2023).
- Lora-Sophie Gerber, Harm J. Heusinkveld, Celine Langendoen, Burkhard Stahlmecke, Roel PF Schins, Remco HS Westerink, Acute, sub-chronic and chronic exposures to TiO2 and Ag nanoparticles differentially affects neuronal function in vitro, NeuroToxicology, 10.1016/j.neuro.2022.10.010, 93 , (311-323), (2022).
- Marta Nowakowska, Marie Jakešová, Tony Schmidt, Aleksandar Opančar, Mathias Polz, Robert Reimer, Julia Fuchs, Silke Patz, Daniel Ziesel, Susanne Scheruebel, Karin Kornmueller, Theresa Rienmüller, Vedran Đerek, Eric D. Głowacki, Rainer Schindl, Muammer Üçal, Light‐Controlled Electric Stimulation with Organic Electrolytic Photocapacitors Achieves Complex Neuronal Network Activation: Semi‐Chronic Study in Cortical Cell Culture and Rat Model, Advanced Healthcare Materials, 10.1002/adhm.202401303.