Cryogel-based Injectable 3D Microcarrier Co-culture for Support of Hematopoietic Progenitor Niches
Daniel Naveed Tavakol, Daniel Naveed Tavakol, Fabien Bonini, Fabien Bonini, Josefine Tratwal, Josefine Tratwal, Martina Genta, Martina Genta, Joé Brefie-Guth, Joé Brefie-Guth, Thomas Braschler, Thomas Braschler, Olaia Naveiras, Olaia Naveiras
biomaterial
bone marrow
cryogel
hematopoietic
injectable
scaffold
stem cell niche
tissue engineering
Abstract
Although hematopoietic stem cell (HSC) transplantation can restore functional hematopoiesis upon immune or chemotherapy-induced bone marrow failure, complications often arise during recovery, leading to up to 25% transplant-related mortality in treated patients. In hematopoietic homeostasis and regeneration, HSCs in the bone marrow give rise to the entirety of cellular blood components. One of the challenges in studying hematopoiesis is the ability to successfully mimic the relationship between the stroma and hematopoietic stem and progenitor cells (HSPCs). This study and the described protocols propose an advantageous method for culturing and assessing stromal hematopoietic support in three dimensions, representing a simplified in vitro model of the bone marrow niche that can be transplanted in vivo by injection. By co-culturing OP9 bone marrow–derived stromal cells (BMSCs) and cKit+ Sca-1+ Lin– (KLS+) HSPCs on collagen-coated carboxymethylcellulose scaffolds for 2 weeks in the absence of cytokines, we established a methodology for in vivo subcutaneous transplantation. With this model we were able to detect early signs of extramedullary hematopoiesis. This work can be useful for studying various stromal cell populations in co-culture, as well as simple transfer by injection of these scaffolds in vivo for heterotopic regeneration of the marrow microenvironment. © 2021 The Authors. Current Protocols published by Wiley Periodicals LLC.
[Correction added on May 17, 2022, after first online publication: CSAL funding statement has been added.]
Basic Protocol 1 : Isolation of HSPCs from mice
Basic Protocol 2 : Co-seeding of HSPCs and BMSCs on collagen-coated CCMs
Basic Protocol 3 : Maintenance, real-time imaging, and analysis of co-seeded scaffolds
Basic Protocol 4 : End-point analysis of co-seeded scaffolds using flow cytometry and CFU assays
Basic Protocol 5 : Transplantation of scaffolds by subcutaneous injection
Support Protocol : Preparation of custom scaffold drying device
INTRODUCTION
Bone marrow (BM) failure, whether secondary to chemotherapy in cancer patients or to inherited or immune-mediated bone marrow failure syndromes, is accompanied by massive remodeling of the BM microenvironment, which typically entails a reversible adipocytic conversion of the marrow (Tratwal et al., 2020). Although hematopoietic stem cell (HSC) transplantation can restore functional hematopoiesis, complications often arise during the first few weeks of recovery, leading to up to 25% mortality in treated patients (Gooley et al., 2010; Jenq & van den Brink, 2010). Identifying putative supportive stromal cells and engineering artificial and functional HSC niches that allow expansion of repopulating HSCs is thus of intense interest for HSC regenerative therapies, as outlined by the American Society of Hematology (ASH) in its most recent research agenda (Bianco, Riminucci, Gronthos, & Robey, 2001; Morrison & Scadden, 2014; Mullighan, 2018).
HSCs are the primary population of cells that give rise to the entirety of cellular blood components through hematopoiesis. They reside in the BM and give rise to myeloid, erythrocytic, megakaryocytic, and lymphoid progenitors. In vivo , the HSC niche within the BM modulates both the self-renewal and differentiation capacity of HSCs (Pinho & Frenette, 2019).
In vitro engineered models aim to recapitulate the physiological and pathophysiological functions of organ systems in the body, while balancing throughput and complexity (Tavakol, Fleischer, & Vunjak-Novakovic, 2021). Previous models of BM, including organ-on-a-chip microfluidic platforms and ossicle-based scaffolds, have demonstrated multicellular complexity, but have limited ease of use (Bourgine et al., 2018; Chou et al., 2020; Ferreira et al., 2012; Raic, Rödling, Kalbacher, & Lee-Thedieck, 2014; Torisawa et al., 2014). Many of these existing models are xenogeneic, or require complex hematopoietic cytokines and even daily intraperitoneal injections for extended periods of time to enhance ossification (Reinisch et al., 2016). Moreover, transplantable systems often require non-standardized extracellular matrix components (e.g., Matrigel).
We have developed a co-culture system to study interactions between murine HSPCs and murine OP9 bone marrow–derived stromal cells (BMSCs) in vitro (Fig. 1). Using collagen-coated carboxymethylcellulose cryogel microscaffolds (CCMs) with the capacity to covalently interlock, we co-cultured HSPCs and BMSCs for up to 2 weeks. We then performed in vivo subcutaneous injection in mice through a process that triggers particle interlocking. Through this work, we have developed methodologies that support hematopoietic cells in 3D cultures without exogenous cytokines, allow imaging of 3D co-seeded scaffolds over time, and generate cellularized scaffolds that can be injected subcutaneously. We show maintenance of hematopoiesis after 12 days of in vitro co-culture and robust cellularization of implants with evidence of in situ hematopoiesis 12 weeks post-implantation.
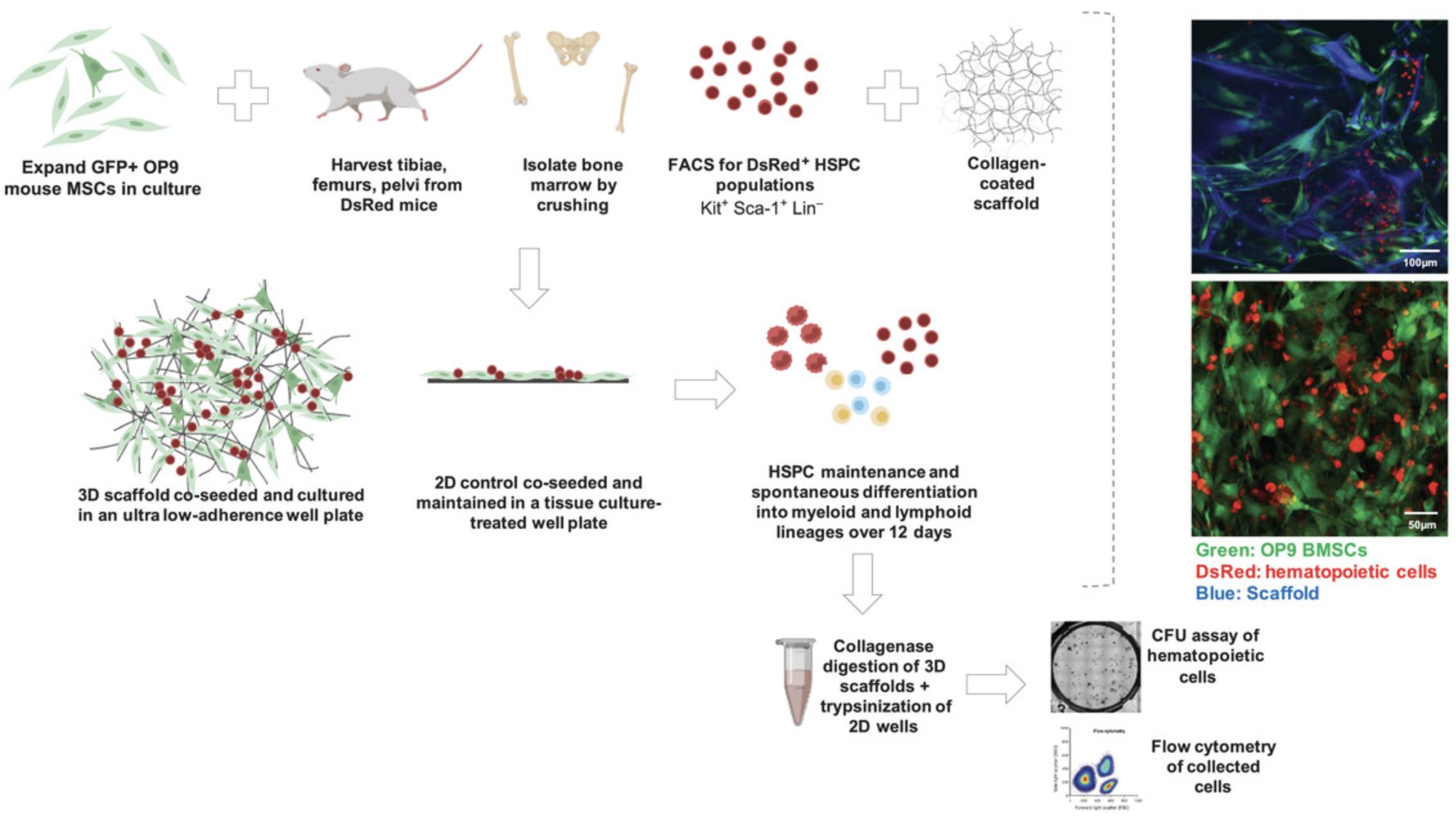
In this article, we expand upon our recent work in Tavakol et al. (2020). We present in detailed steps for the isolation of murine HSPCs (see Basic Protocol 1), seeding of HSPCs and BMSCs in vitro (see Basic Protocol 2), maintenance and imaging of the co-cultured scaffolds (see Basic Protocol 3), end-point analysis of the 3D culture system (see Basic Protocol 4), preparation of cellularized 3D scaffolds to fit injection parameters (see Basic Protocol 5), and in vivo subcutaneous transplantation in immunodeficient mice (see Basic Protocol 6).
STRATEGIC PLANNING
Many of the methodologies and figures described here are an elaboration of the work in Tavakol et al. (2020). Here, we describe the use of two specific cell populations from murine origin: GFP OP9 BMSCs and DsRed HSPCs. The BMSCs were provided by a collaborating laboratory and have been carefully maintained at subconfluency in our laboratory since 2013. The HSPCs were harvested from endogenously labeled C57Bl/6 mice (specifically, B6.Cg-Tg(CAG-DsRed*MST)Nagy/J), also maintained in-house since 2013. These DsRed mice are commercially available (Jackson Laboratory, strain 006051). Additional experiments made use of tdTomato+ KLS+ cells from mTmG reporter mice (Jackson Laboratory, strain 007676). The methodologies described here are not limited to these cell types; any BMSCs and HSPCs may be tested in these protocols, as the fluorescent labeling was only essential in characterization and imaging of the scaffolds over time. There is also potential to use these methodologies to study human hematopoiesis.
Fabrication of the CCM scaffolds is described in Tavakol et al. (2020). The underlying collagen adsorption and immobilization to carboxymethylcellulose scaffolds has been studied in depth (Serex et al., 2018), whereas the rheological and in vivo tissue reconstruction properties are part of a manuscript currently available as a preprint (Béduer, Bonini, Verheyen, Burch, & Braschler, 2020).
NOTE : All protocols involving live animals must be reviewed and approved by an Institutional Animal Care and Use Committee (IACUC) and must conform to government regulations for the care and use of laboratory animals.
NOTE : All solutions and equipment coming into contact with cells must be sterile, and proper sterile technique should be used accordingly.
Basic Protocol 1: ISOLATION OF HSPCs FROM MICE
To isolate hematopoietic stem and progenitor cells from mice, the long bones are extracted and thoroughly cleaned of all soft tissue. The bones are crushed with a mortar and pestle to release the hematopoietic cells, and the cell suspension is filtered to remove debris. Red blood cells (RBCs) are lysed, and the remaining cells are stained with antibodies for HSPC isolation based on fluorescence-activated cell sorting (FACS). The cells are sorted into medium based on surface marker expression and stored on ice. Typically, one mouse will yield 60,000 cKit+ Sca-1+ Lin– (i.e., KLS+) HSPCs.
Materials
-
Endogenously labeled mice (see Strategic Planning)
-
Wild-type control mouse
-
Phosphate-buffered saline (PBS)
-
FACS buffer (see recipe)
-
1× RBC lysis buffer (BioLegend, 420301), ice cold
-
Lineage depletion kit (BD Pharmingen, 558451), including lineage cocktail and magnetic bead suspension
-
Blocking solution (see recipe)
-
Anti-streptavidin-PO (Invitrogen, S32365)
-
Anti-cKit PE-Cy7 (BioLegend, 105814)
-
Anti-Sca-1 APC (BioLegend, 108112)
-
DAPI (Applichem, A4099.005)
-
Hematopoietic culture medium (HCM; see recipe)
-
Mortar and pestle
-
10-ml pipette
-
70-µm cell strainers (Corning, cat. no. CLS431751)
-
15- and 50-ml Falcon tubes
-
0.2-µm strainer
-
Cell counter or hemocytometer
-
AutoMACS magnetic cell separator (Miltenyi Biotec)
-
1.5-ml microcentrifuge tubes
-
0.85-μm mesh strainers
-
FACS machine (e.g., Aria Fusion)
-
Additional reagents and equipment for euthanasia and bone removal
NOTE : If an AutoMACS instrument is not available, depletion can be performed using manual midi LD columns (Miltenyi Biotec) according to manufacturer's recommendations.
Prepare hematopoietic cell suspension
1.Isolate all tibiae, femurs, and pelvic bones from two to three endogenously labeled (DsRed) mice and remove soft tissue using sterile lab tissues.
2.Place bones from all mice in a single tube containing PBS on ice.
3.Shake tube to rinse the bones and further remove any soft tissue. Repeat.
4.Crush bones with a mortar and pestle in a small amount of FACS buffer (enough to cover the bones).
5.Using a 10-ml pipette, filter the suspension through a 70-µm cell strainer into a 50-ml Falcon tube.
6.Rinse the bone pieces with FACS buffer (2 ml) and continue to crush them and filter the suspension, repeating until no large bone chunks are visible and the crushed bone debris is white.
7.Centrifuge the suspension 10 min at 300 × g , 4°C.
8.Carefully discard the supernatant and gently resuspend the pellet in 5 ml ice-cold RBC lysis buffer per mouse for 30 s.
9.Stop lysis by adding 20 ml FACS buffer and centrifuging 5 min at 300 × g , 4°C.
10.Discard supernatant and resuspend in 1 ml FACS buffer.
11.Count cells for yield calculations in case of low output.
Stain and sort cells
12.Add 50 µl lineage antibody cocktail per mouse and incubate for 20 min on ice.
13.Wash by adding 20 ml FACS buffer and centrifuging 5 min at 300 × g , 4°C.
14.Carefully discard the supernatant, resuspend the pellet in 1 ml FACS buffer, and keep on ice.
15.Take a 50 µl WT sample for a Lin+ single-color control (SCC), add 50 µl FACS buffer, and keep on ice.
16.Add 50 µl magnetic bead suspension per mouse to the WT (control) and DsRed (labeled cells of interest) samples. Incubate 15 min on ice.
17.Start AutoMACS instrument and run the Rinse program.
18.Wash each suspension with 20 ml FACS buffer and centrifuge 5 min at 300 × g , 4°C.
19.Discard supernatant and resuspend suspension in 3 ml FACS buffer.
20.Pass through a 70-µm cell strainer into a 15-ml Falcon tube and bring to the AutoMACS along with collection tubes (i.e., 15-ml Falcon tubes) for positive and negative fractions.
21.Run Deplete + QRinse on the AutoMACS.
22.Take the Lin– fractions and centrifuge 5 min at 300 × g , 4°C. Count cells.
23.Discard supernatant and resuspend the Lin– fraction as the purified DsRed sample in 100 µl blocking solution and the WT sample in 400 µl blocking solution. Also add 100 µl blocking solution to Lin+ SCC. Incubate for 15 min on ice.
24.Divide the WT sample into four 1.5-ml microcentrifuge tubes on ice (100 µl suspension each). Add 100 µl FACS buffer to each tube. Keep two on ice for SCC samples, one unstained and one DAPI-stained.
25.Stain the DsRed tube with KLS antibody cocktail using the following final concentrations, and stain the WT tubes using each single-color control. Incubate these solutions 1 hr on ice.
- 1:200 streptavidin-PO
- 1:200 cKit PE-Cy7
- 1:100 Sca-1 APC
26.Wash each microcentrifuge tube with 1 ml FACS buffer and centrifuge 5 min at 300 × g , 4°C.
27.Resuspend the DsRed Lin– samples in 1.5 ml FACS buffer. For optimal sorting, count cells and dilute, if necessary, to a maximum of 1–2 × 107 cells/ml.
28.Resuspend the DAPI-SCC sample in 200 µl DAPI (1:5000) and the WT SCC samples in 200 µl FACS buffer.
29.Filter all samples through 85-µm mesh strainers into 15-ml Falcon tubes containing 2 ml HCM. Invert tubes prior to sorting to ensure that walls are wet with HCM.
30.Once the sort is completed, perform a purity control by mixing the sorting tube three times by inversion, then mixing 10 μl of the final solution with 90 μl HCM and reanalyzing the total volume of the suspension in the sorter.
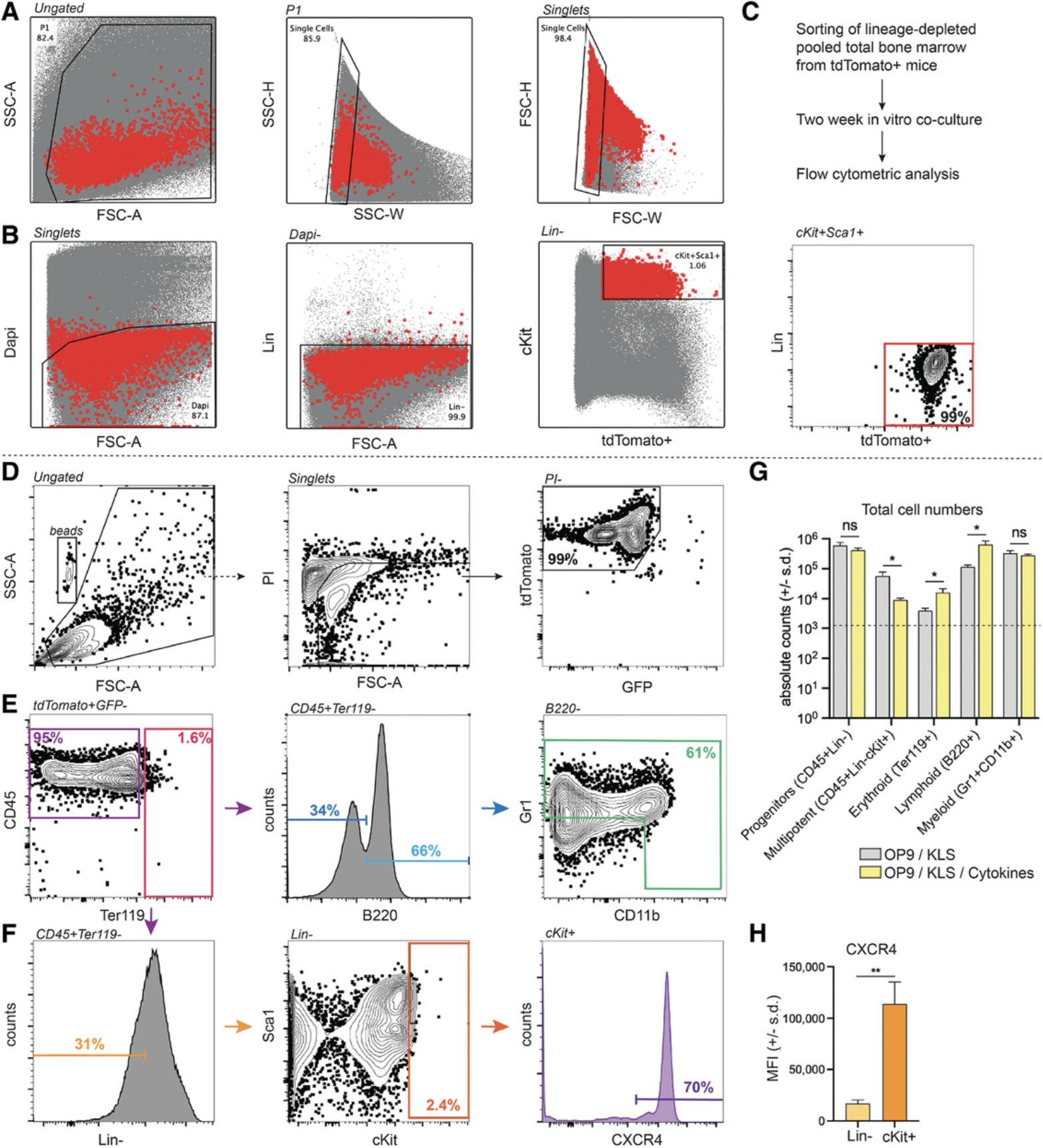
Basic Protocol 2: CO-SEEDING OF HSPCs AND BMSCs ON COLLAGEN-COATED CARBOXYMETHYLCELLULOSE MICROPARTICLES
Freshly sorted HSPCs and trypsinized OP9 cells are counted and the appropriate ratio of cells is mixed. The CCM scaffolds are dried as a small clump in a 70-µm cell strainer on a SteriCup 500-ml media filtration device, and then carefully placed in an ultra-low-adhesion 6-well plate. The cell suspension is then seeded onto the CCM scaffolds, which are rehydrated through this process. The plate is placed in the incubator for 1 hr, after which additional medium is added and the plate is returned to the incubator.
OP9 BMSCs were donated by the Daley laboratory (McKinney-Freeman, Naveiras, & Daley, 2008), who received them from the Nakano laboratory (Nakano, Kodama, & Honjo, 1994) and lentivirally transduced them for GFP expression as described in Tavakol et al. (2020). Both the parental and GFP+ lines were authenticated by the ATCC on June 24, 2021, by short tandem repeat (STR) analysis, indicating an exact match of the ATCC reference OP9 cell line (CRL-2749). OP9 cells are available from the ATCC (CRL-2749), but this source has not been tested for hematopoietic support in our laboratory.
For preparation of stock 13.5 mg/ml collagen-coated CCM scaffolds, see Tavakol et al. (2020). This procedure is described for 370 µl (5 mg) of CCM scaffolds.
Materials
-
OP9 BMSCs (see protocol introduction)
-
BMSC medium (see recipe)
-
Phosphate-buffered saline (PBS)
-
0.05% (w/v) trypsin-EDTA (Life Technologies, 25300054)
-
Trypan blue
-
13.5 mg/ml collagen-coated CCM scaffolds in PBS (Tavakol et al., 2020), stored at 4°C
-
Sorted KLS+ HSPCs (see Basic Protocol 1)
-
Co-culture medium (see recipe)
-
Incubator at 37°C, 5% CO2
-
T150 cell culture flasks (Corning, CLS430825)
-
10-ml serological pipettes
-
15-ml Falcon tubes
-
Cell counter slide
-
70-µm cell strainers (Corning, CLS431751)
-
SteriCup filtration systems (Millipore, C3240)
-
6-well ultra-low-adhesion plate (Corning, CLS347)
Prepare OP9 BMSCs
1.Expand OP9 BMSCs in BMSC medium in T150 flasks at 70%-80% confluency, typically using 1:3 passages every 3-4 days for 1-2 weeks.
2.Identify a flask of 70%-80% confluent BMSCs for co-seeding. Remove BMSC medium and wash once with PBS.
3.Remove PBS and trypsinize with 3 ml of 0.05% trypsin-EDTA for 2-3 min at 37°C, 5% CO2.
4.Tap the flask firmly in the tissue culture hood and stop trypsinization by adding 4 ml BMSC medium.
5.Gently wash the bottom of the flask with BMSC medium using a 10-ml pipette to remove loosely attached cells. Transfer suspension to a 15-ml Falcon tube.
6.Centrifuge 5 min at 300 × g , 4°C.
7.Carefully discard supernatant, resuspend pellet in 500 µl BMSC medium, and count viable cells using trypan blue and a cell counter.
8.Adjust concentration to 5 × 106 cells/ml.
Prepare and seed CCM scaffolds
9.Dry 370 µl collagen-coated CCM scaffolds in a 70-µm cell strainer in the top chamber of a SteriCup filtration system.
10.With scaffolds still in the strainer, wash sequentially with 1 ml PBS and then 1 ml medium. Dry scaffolds carefully each time.
11.Using a 2-ml serological pipette or a cell scraper, scratch the CCM off the strainer and place in a single well of a 6-well ultra-low-adhesion plate, forming a viscous cluster or “bubble” in the center of the well. Repeat for the desired number of wells.
12.Confirm cell counts for BMSCs and sorted KLS+ HSPCs. Prepare mixed suspensions at 1:10 and 1:100 ratios of HSPCs to BMSCs. For optimal seeding, prepare mixtures in a total volume of ∼100 µl in co-culture medium.
13.Add 100 μl mixed cell suspension to each dried CCM scaffold bubble in the 6-well plate and incubate for 1 hr at 37°C, 5% CO2.
14.Add 3 ml co-culture medium and incubate overnight at 37°C, 5% CO2.
Basic Protocol 3: MAINTENANCE, REAL-TIME IMAGING, AND ANALYSIS OF CO-SEEDED SCAFFOLDS
As many CCMs are in each well, it is easy to collect a small sample (e.g., a few scaffolds) for serial imaging over time. Hoechst stain, traditionally a live nuclear stain, also stains the scaffolds. Combined with the endogenous cell labels (GFP for BMSCs and DsRed for HSPCs), this makes imaging useful for looking at proliferation of cells over time. End-point imaging with additional markers via fluorescent antibody staining was not performed, though it could be performed if the cell populations are not endogenously labeled prior to the start of the experiment.
Materials
-
Silicone glue
-
Co-seeded scaffolds (see Basic Protocol 2)
-
Co-culture medium (see recipe)
-
0.1 μg/ml Hoechst 33258
-
FACS buffer (see recipe)
-
2.5-mm-thick polypropylene sheet
-
Laser cutter
-
Coverslips
-
Incubator at 37°C, 5% CO2
-
200-μl pipette tips with tip cut off
-
Zeiss LSM 700 Inverted Confocal Microscope
-
Fiji/ImageJ software (NIH)
Fabricate custom observation chambers
1.Cut pieces of 2.5-mm-thick polypropylene sheet with the following dimensions using a laser cutter:
- 75 × 25 mm for the slide
- 40 × 14 mm for the central chamber
2.Adhere a coverslip to one side using silicone glue to form the bottom of the chamber (Fig. 3).
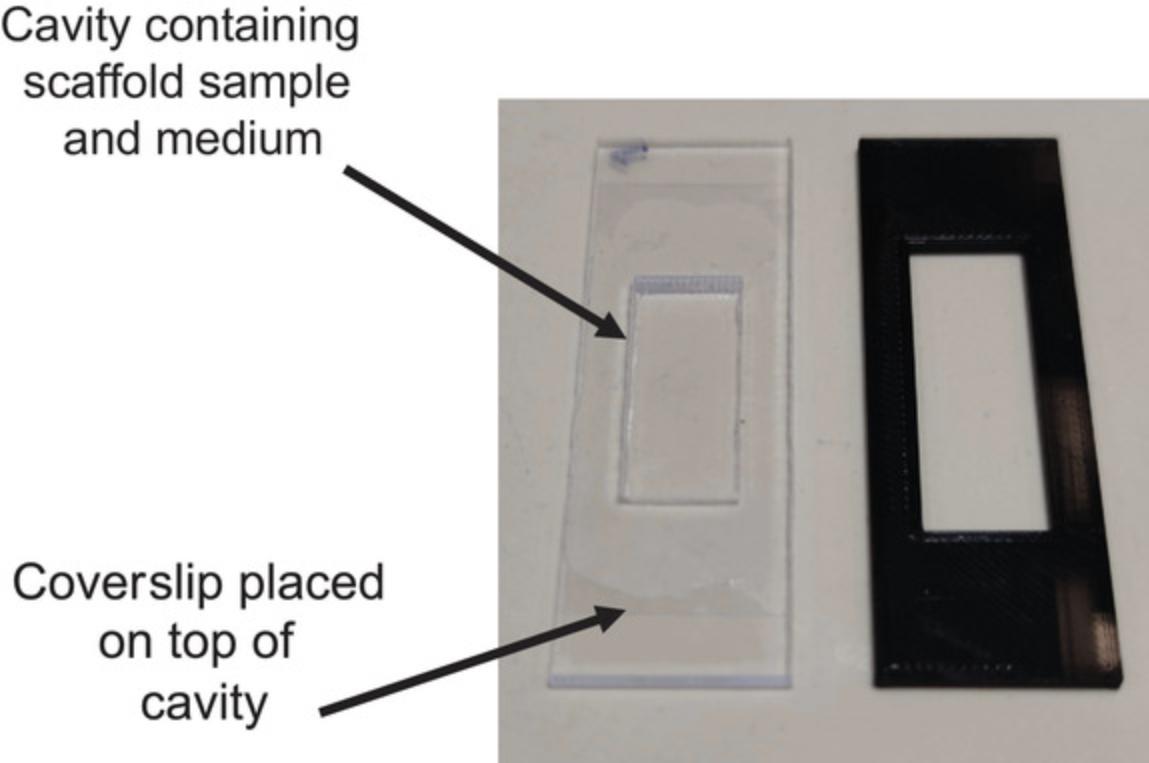
Maintain and image co-cultures
3.Maintain co-seeded scaffolds in co-culture medium for 2 weeks at 37°C, 5% CO2. Add 3 ml medium at 1 week post-seeding.
4.On days 1, 4, 7, and 11, take a small volume of suspended CCMs (∼100 µl) and transfer to an observation chamber.
5.Supplement this volume with 100 µl of 1:1000 Hoechst 33258 (if staining the scaffold) or 100 µl FACS buffer. For Hoechst staining, incubate 15-20 min and wash with PBS just before imaging.
6.Image co-seeded scaffolds within 1 hr of transferring to the chamber. When ready to image, apply a coverslip on top of the sample, being careful to avoid air bubbles.
Analyze images
7.Using Fiji/ImageJ analysis tools, for each image, separate each fluorescent channel and create a compiled volume-rendered image for each cell type (HSPCs and BMSCs).
8.Threshold each channel using the Li algorithm (“image > threshold > Li algorithm”). If necessary, convert the image to 8-bit.
9.Analyze the fluorescent area corresponding to each channel using “Analyze > Analyze Particle area” and the following parameters:
- Size range: 4 to infinity (to remove nonspecific fluorescence background)
- Circularity: 0-1 (default)
- Display result: checked
- Exclude on edges checked
If necessary, use the option “Includes holes” for scaffold area quantification to increase the accuracy of the measurements. Scaffold areas can also be quantified manually using the “Freehand selections” tool.
10.Calculate the fluorescent “total area” for each label (i.e., GFP and DsRed for HSPCs and BMSCs) as well as the “total scaffold area” for each image by summing all the “Area” values in Excel.
11.Calculate the percentage of the total particle fluorescent area for each fluorescent label (HSPCs or BMSCs):
- % total particle fluorescent area = (HSPC or BMSC “total area”)/(“total scaffold area”) × 100
12.Calculate this value for each label and create a ratio for HSPC to BMSC relative fluorescent areas for each image over time.
Basic Protocol 4: END-POINT ANALYSIS OF CO-SEEDED SCAFFOLDS USING FLOW CYTOMETRY AND CFU ASSAYS
To assess hematopoietic expansion, cells are isolated from the co-culture and prepared for flow cytometric analysis as a single-cell suspension. In parallel, to assess hematopoietic progenitor function, cells are seeded for hematopoietic colony-forming unit (CFU) assays in semi-solid methylcellulose-based medium. After 1 week, CFUs are counted and categorized according to size and complexity. Colony assays to specifically assay long-term hematopoietic stem cell activity (LT-HSC) are described elsewhere (Kerenyi, 2014).
The protocol below uses MethoCult methylcellulose-based medium from Stemcell Technologies and their STEMvision colony-counting instrument. Alternatively, counting can be performed using a brightfield microscope equipped with 4× and 10× objectives and manual scoring according to manufacturer's instructions (https://static.yanyin.tech/literature/current_protocol/10.1002/cpz1.275/attachments/MA28405-Mouse_Colony_Forming_Unit_Assays_Using_MethoCult.pdf).
Materials
- Co-seeded scaffolds (see Basic Protocol 2)
- Serum-free medium: HCM (see recipe) without FBS
- 0.04% (w/v) collagenase I (Thermo Fisher Scientific, 17100-017) in Hanks' balanced salt solution (HBSS)
- Co-culture medium (see recipe)
- FACS buffer (see recipe)
- Blocking solution (see recipe)
- Lineage cocktail from lineage depletion kit (BD Pharmingen, 558451)
- Flow cytometry antibody cocktail (see Table 1)
- BrightCount beads (1000 beads/µl, Invitrogen, C36950)
- DAPI (Applichem, A4099.005)
- MethoCult medium (Stemcell Technologies, M3434)
- Penicillin/Streptavidin (Thermo Fisher Scientific, 15140122)
Panel | Antibody | Clone | Isotype | Conjugation | Dilution | Supplier, cat. no. |
---|---|---|---|---|---|---|
HSPC sorting | cKit (CD117) | 2B8 | Rat IgG2b | PE-Cy7 | 1:100 | BioLegend, 105814 |
Sca-1 (Ly-6A/E) | E13-161.7 | APC | 1:50 | BioLegend, 122508 | ||
Lineage cocktail | Biotin | 1:20 | BD Biosciences, 51-9000794 | |||
Streptavidin | Pacific Orange | 1:100 | Life Technologies, S32365 | |||
DAPI | UV | 1:5000 | Axon Lab, A4099.0005 | |||
HSPC panel | cKit (CD117) | 2B8 | Rat IgG2b | PE-Cy7 | 1:100 | BioLegend, 105814 |
Sca-1 (Ly-6A/E) | E13-161.7 | APC | 1:50 | BioLegend, 122508 | ||
Lineage cocktail | Biotin | 1:20 | BD Biosciences, 51-9000794 | |||
Streptavidin | Pacific Orange | 1:100 | Life Technologies, S32365 | |||
CD184 (CXCR4) | 2B11 | PerCP-eFluor710 | 1:25 | Invitrogen, 46-9991-82 | ||
PI | 1:5000 | |||||
Mature lineage panel | pan-CD45 | 30-F11 | Rat IgG2b | Alexa Fluor 700 | 1:50 | BioLegend, 1013128 |
Ter119 | TER-119 | Rat | BUV395 | 1:200 | BD Biosciences, 563827 | |
B220 (CD45R) | RA3-6B2 | PE-Cy5 | 1:25 | BioLegend, 103210 | ||
Gr1 (Ly-6G/Ly-6C) | RB6-8C5 | APC | 1:250 | BioLegend, 108412 | ||
CD11b | M1/70 | APCeFluor 780 | 1:500 | Invitrogen, 47-0112-82 | ||
PI | UV | 1:5000 |
- a
Exploration of murine lineage commitment can be determined with alternative combinations of surface markers including CD45 pan-hematopoietic marker for better separation from stromal components (e.g., granulocytic: Gr1; monocytic: CD11b; erythroid: Ter119/CD71; lymphoid: B220/CD3).
- 2- or 5-ml serological pipettes
- 70-µm cell strainer (Corning, CLS431751)
- 50-ml Falcon tubes
- 1.5-ml microcentrifuge tubes
- 85-μm mesh filter
- Flow cytometer (e.g., BD Biosciences LSR II SORP or Beckman Coulter CytoFLEX LX)
- 16-G blunt-end needles and 3-cc syringes (Stemcell Technologies, 28110 and 28240)
- 6-well meniscus-free plates (e.g., Stemcell Technologies SmartDish, 27371)
- Humidifying chamber
- Incubator at 37°C, 5% CO2
- STEMvision colony counter (Stemcell Technologies)
Digest scaffolds
1.Carefully collect most of the medium from the co-culture wells and set aside on ice as the non-adherent fraction.
2.Gently wash CCM particles twice with serum-free medium.
3.Remove excess medium and add 2 ml of 0.04% collagenase I per well of the 6-well plate. Incubate 25 min at 37°C, 5% CO2.
4.Inactivate collagenase by adding 4 ml co-culture medium using a 2- or 5-ml serological pipette and pipetting up and down for 1-2 min.
5.Pass through a 70-µm cell strainer into a 50-ml Falcon to isolate cells from particles.
6.Repeat steps 4-5 twice more, then discard strainer and residual CCM particles.
7.Pipette sample up and down to obtain a single-cell suspension (the collagenase-digested adherent fraction).
8.Centrifuge non-adherent and adherent fractions for 10 min at 300 × g , 4°C.
9.Resuspend pellets for a total of 500 µl cell suspension in FACS buffer.
10.Split each fraction to two microcentrifuge tubes for CFU and flow cytometry analysis.
Stain for flow cytometry
11.Centrifuge cytometry aliquots (50 μl at a 1:10 allocation) at 300 × g and resuspend pellets (∼20 µl) with 75 µl blocking solution. Transfer to fresh microcentrifuge tubes and incubate 10 min at room temperature.
12.Add 5 µl lineage cocktail and incubate 20 min on ice.
13.Wash with 1 ml FACS buffer and pass through a 85-µm mesh filter.
14.Centrifuge 10 min at 300 × g , 4°C, and aspirate supernatant.
15.Incubate with the antibody cocktail in Table 1 for 45 min on ice. To count cell numbers, include 10 µl BrightCount beads.
16.Add 200 µl DAPI and incubate ∼10 min before acquiring using a flow cytometer.
Perform CFU assay
17.Thaw a 100-ml bottle of MethoCult medium the day prior to use and add 1% penicillin/streptomycin directly to the bottle. Shake vigorously, let sit to allow bubbles to settle, and dispense 2.3-ml aliquots in 15-ml Falcon tubes.
18.To each MethoCult aliquot, add the appropriate volume of cell suspension to give ∼100 KLS+ cells per 6-well plate.
19.Mix cells and MethoCult using a 16-G blunt-end needle and let sit for 5 min to allow bubbles to rise to the surface.
20.Slowly collect cell/methylcellulose suspension from below the bubbles and carefully add 1.1 ml to each well of a meniscus-free 6-well plate.
21.Place each plate in a humidifying chamber and leave in an incubator for 7 and 14 days without manipulation.
22.Image colonies using the STEMvision instrument (or score live using a brightfield microscope) according to manufacturer's protocols for imaging and analysis.
Basic Protocol 5: TRANSPLANTATION OF SCAFFOLDS BY SUBCUTANEOUS INJECTION
To perform in vivo experiments, co-culture scaffolds are injected subcutaneously in anesthetized mice. Prior to injection, the scaffolds must be gently packed (concentrated). To achieve this, a controlled-pressure, cell-protecting drying device was developed (Fig. 4). The scaffold suspension is loaded into the column of the drying device and controlled capillary action is used to gently pack the scaffolds. The steps below describe drying of the scaffolds and injection into mice. For preparation of the drying device, see the Support Protocol that follows. Video 1 demonstrates the process of loading scaffolds on the column for delicate drying, loading the syringe with scaffolds in the transfer tip, and performing the subcutaneous injection. In our experiments, injected scaffolds were maintained for up to 12 weeks in vivo.
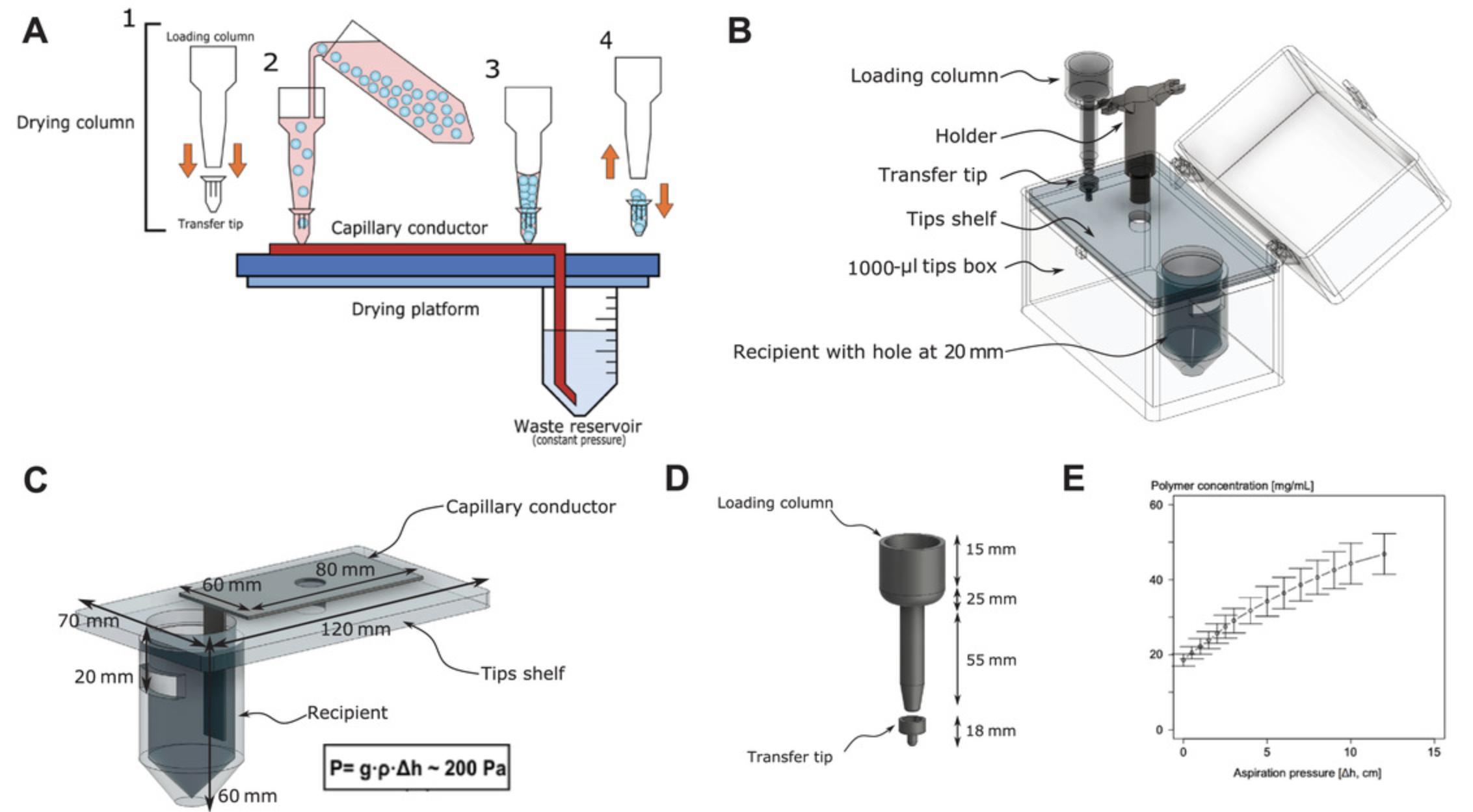
Support Protocol: PREPARATION OF CUSTOM SCAFFOLD DRYING DEVICE
Instructions are provided here for constructing the device from common laboratory materials. The assembled device can be seen in Figure 4 and Video 1.Alternatively, the device can be assembled from 3D-printed parts made using the 3D printing maps provided (see Supplementary Information).
All plastic consumables used should be made from polypropylene, as this allows assembly using a standard crafting hot glue gun and soldering iron. Additionally, it makes the device and columns suitable for sterilization by autoclaving.
Materials
- 15- and 50-ml Falcon tubes
- Metal saw or sharp knife
- 1000-µl pipette tip (Axygen, 7.9 cm)
- 20-µl barrier pipette tip (MultiGuard, Sorenson BioScience)
- 5-ml syringe (BD Biosciences)
- 1000-µl pipette tip box (Axygen Scientific)
- Soldering iron
- Hot glue gun (traditionally with ethylene vinyl acetate polymer glue)
- Commercial or craft felt material, autoclavable (e.g., 82% viscose, 18% polypropylene, Coop, Switzerland)
Fabricate loading column
1.Cut a 15-ml Falcon tube in three parts by cutting at the 3-ml and 12-ml lines using a saw or a sharp knife. Discard the middle section.
2.Assemble the bottom and top pieces by melting the rim of both pieces with a hot glue gun and rapidly pressing them together.
3.Cut off the very bottom conical part of the tube so that the diameter of the hole is the same as the top of a 1000-μl pipette tip (typically, 1.9 cm o.d.).
4.Attach a 1000-μl tip to the column by melting the rim of both pieces with the hot glue gun and pressing together tightly.
5.Cut off the bottom of the tip to remove 1.2-1.5 cm (0.4 cm o.d.).
Fabricate transfer tip
6.Cut the top section of a 20-µl barrier pipette tip at 1.2 cm from the top (larger opening).
7.Cut off the bottom section (the narrowest part) at 2.3 cm from the tip, below the filter. Attach the two pieces using the hot glue gun to make the transfer tip.
Fabricate column holder
8.Cut a 5-ml syringe at 2 cm from the top and keep the top section (the part with handles).
9.Attach one handle of the syringe to the top part of a 1000-µl tip.
10.Repeat steps 8 and 9 up to four times on the same tip to allow multiple dried scaffolds to be prepared at once.
Fabricate drying box
11.Cut a 50-ml Falcon tube at 6 cm from the tip and keep the bottom piece to serve as the waste reservoir (recipient; Fig. 4).
12.In the top shelf of an empty 1000-µl pipette tip box, make a hole with same diameter as the 50-ml Falcon tube.
13.Insert the recipient in the hole and attach by melting with a soldering iron.
14.Make a hole in the Falcon tube 2 cm from the top (Fig. 4B,C).
15.Cut a cleaning cloth or felt material into an 8 × 7–cm rectangle with a protruding tail of at least 6 cm on one side to serve as the capillary conductor (Fig. 4A,C).
16.Assemble pieces as shown in Figure 4 and test for leakage.
17.Autoclave and use under the hood as described (see Basic Protocol 5).
REAGENTS AND SOLUTIONS
Antibiotic drinking solution
- 225 ml drinking water
- 25 ml co-amoxi mepha (final 10% v/v)
- 300 μl of 10% Baytril
- Two bags (250 mg each) Dafalgan/paracetamol
- Prepare fresh each week in a 250-ml red/photoprotective water bottles
Blocking solution
- FACS buffer (see recipe)
- 5 μg/ml hIgG (I4506, Sigma-Aldrich)
- Prepare fresh and store on ice
BMSC medium
- Alpha-minimum essential medium (a-MEM) + Glutamax (Thermo Fisher Scientific, 32561)
- 10% (v/v) fetal bovine serum (FBS, Gibco, 10270-106)
- 1% penicillin/streptavidin (Thermo Fisher Scientific, 15140122)
- Store up to 1 month at 4°C
Co-culture medium
First, prepare conditioned HCM by culturing confluent BMSCs in HCM (see recipe) for 48 hr. Collect conditioned medium, filter with a 0.2-µm filter, and store up to 2 months at −20°C.
Prepare co-culture medium by mixing conditioned medium with an equal volume of fresh HCM. Store up to 1 month at 4°C protected from light.
FACS buffer
- Phosphate-buffered saline (PBS)
- 1 mM EDTA (Thermo Fisher Scientific, 15575020)
- 2% (v/v) fetal bovine serum
- Store up to 1 month at 4°C
Hematopoietic culture medium (HCM)
- IMDM + Glutamax (25 mM HEPES)
- 10% (v/v) fetal bovine serum (FBS, Gibco, 10270-106)
- 1% penicillin/streptomycin (Thermo Fisher Scientific, 15140122)
- Store up to 1 month at 4°C
COMMENTARY
Background Information
Since the first in vitro description of mixed bone marrow stromal cultures with hematopoietic output in 2D conditions (Dexter, Wright, Krizsa, & Lajtha, 1977), more refined methods have demonstrated the capacity for limited hematopoietic expansion in vitro in highly controlled conditions, often stroma-free, and with potential use for HSPC expansion in clinical transplantation (Bujko, Kucia, Ratajczak, & Ratajczak, 2019; Derakhshani et al., 2019). These methods, however, do not allow the study of HSPC interactions with the niche that regulates their behavior in homeostatic conditions. This microenvironment-mediated regulation is thought to be critical in early stages of hematological neoplastic transformation, such as in myelodysplastic syndromes (reviewed in Le, Andreeff, & Battula, 2018). Moreover, it is thought that microenvironment-directed pharmacological modulation could constitute a novel approach for treatment of hematological diseases (Behrmann, Wellbrock, & Fiedler, 2020). For these reasons, the need for “engineering artificial and functional hematopoietic stem cell niches” has been identified as one of the top priorities for hematological research by the 2018 roadmap of the American Society of Hematology (Mullighan, 2018). Methods for heterotopic implantation of bone marrow ossicles to model hematopoietic niches and associated hematological malignancies have been extensively reviewed elsewhere in recent years (Abarrategi et al., 2018; Dupard, Grigoryan, Farhat, Coutu, & Bourgine, 2020), and novel approaches are rapidly emerging (Shah et al., 2019; Tavakol et al., 2020). Here, we present in detail the method for a scalable approach to maintain a minimal hematopoietic niche in vitro whose intact cellular interactions can be transferred in vivo for creation of highly vascularized subcutaneous nodules with persistence of in situ hematopoiesis in the absence of ossification. Advantages of our method as compared to current standards are ease of use, scalability, remarkable vascularization upon in vivo transfer, and the potential for clinical-grade manufacturing of the proposed scaffold.
Critical Parameters and Troubleshooting
Critical parameters that influence this protocol are the source of HSPCs and BMSCs, as well as the choice of recipients for in vivo transplantation. In particular, the minimalistic culture protocol in absence of exogenous cytokines presented here depends on the utilization of a highly supportive marrow stromal line. Complementation with exogenous cytokines is definitely possible, but requires optimization depending on the purpose of the experimental approach. Readouts should be also adapted to the particular application. In our example, progenitor readouts were used (CFU assays and CD45+ cKit+ Lin– quantification by flow cytometry), as our interest was in short-term hematopoietic outputs. Readouts of stem cell function will require sequential CFU assays in vitro (e.g., serial CFU-IC or LT-HSC CFU assays) and long-term multi-lineage repopulation assays in vivo. Additionally, lineage-specific panels and appropriate histological readouts need to be tailored to the specific experimental question being addressed.
For troubleshooting guidelines, see Table 2.
Procedure | Problem | Possible reason | Solution |
---|---|---|---|
Isolation of HSPCs | Low yield of KLS+ cells | Young mice were used; not enough marrow cavities were harvested | Sacrifice multiple mice in anticipation of low yields |
Co-seeding on scaffolds | Cells do not fully adhere to scaffolds | Seeding volume too high; cells disperse and do not attach | Decrease seeding volume to just cover scaffold particles |
Incubate cells in suspension on scaffolds for >1 hr | |||
Make sure to hydrate the scaffold bubble with extra medium to prevent drying | |||
CFU assay | Colony density is too high to count | Too many HSPCs seeded in MethoCult at start of assay | Count KLS+ cell fraction prior to plating and optimize ratios prior to experiment |
Plate 5-10× serial dilutions if ratio is unknown | |||
Save a fraction of HSPCs on ice while analyzing flow cytometry data and only plate once the expected progenitor enrichment is known by FACS | |||
Implantation | Co-seeded scaffolds stick in syringe during injection | Over-drying of scaffolds prior to injection | Take up some PBS in the syringe to moisturize it prior to injection (this should not affect the injection site other than to ensure full delivery of scaffolds) |
Understanding Results
In the protocols described here, the culture of HSPCs can be assessed through co-cultures with marrow stromal cells in 3D, mimicking some of the basic interactions between these cells in mammalian BM. To that end, these culture methodologies can be applied towards facile transplantation of co-cultured scaffolds in vivo for the purposes of BM regeneration post-radiation. Here, we outline the use of murine cell sources for 3D culture, including primary bone marrow–derived KLS+ HSPCs and the OP9 BMSC cell line.
In these protocols, we outline the appropriate metrics for culturing HSPCs in basal cytokine-free conditions. In these methods, the only exogenous cytokines provided to the co-culture system were from OP9 BMSC conditioned medium, which was only employed to increase the stromal cell supportive mechanisms of BMSCs towards the KLS+ cells during in vitro culture. Therefore, the results described here are only a subset of the envisioned results that may be generated from 3D co-culture systems. By using exogenous cytokines, HSPCs in co-culture can be directed towards myeloid and lymphoid differentiation lineages. For example, adding macrophage colony stimulating factor (M-CSF) would push cells into a myeloid/monocytic lineage in vitro. To that end, we show brief characterization of how low levels of cytokines may increase the total cell yield and progenitor/downstream cell population types (Fig. 2G). If using human hematopoietic cells, the context of a starting CD34+ progenitor population would alter the results described here; however, we believe these methodologies can be applied towards human CD34+ cells with the use of proper (equivalent) controls as compared to the KLS+ cells derived in this work. Many in vitro 3D co-culture models using human cord blood–derived CD34+ cells use exogenous cytokines such as FMS-like tyrosine kinase 3 ligand (FLT-3L), thrombopoietin (TPO), and stem cell factor (SCF) to increase the yield of HSPCs in culture. Our results demonstrate that a large percentage of progenitors were also found in suspension, against the hypothesis that the larger proportion of immature cells are attached to the BMSCs and scaffold. This finding may vary from group to group, as different 3D systems and in vitro models may affect HSPC attachment to the surrounding stroma. In future work, additional stromal cell types (adipocytes, endothelial cells, etc.) may be incorporated into the model, which will further influence the ability of such in vitro systems to support hematopoiesis, though advancements in effectively vascularizing tissues and in vitro models remain a challenge in the field (Fleischer, Tavakol, & Vunjak-Novakovic, 2020).
In our in vitro culture techniques, we provide appropriate characterization of structural and functional metrics to assess the efficiency of co-culture with BMSCs and HSPCs. Through serial imaging, we are able to understand the relative location of HSPCs to BMSCs using endogenously labeled cells, which is important in mimicking the role of BMSCs in providing the appropriate stromal support needed to maintain hematopoiesis. To functionally characterize the co-culture system, we looked at fold expansion and percentage of immature to mature hematopoietic cells post-culture using flow cytometry. Similarly, flow cytometric analysis of 2-week 3D co-cultures revealed the presence of the main hematopoietic lineages with CD45+ as a pan-hematopoietic marker (Fig. 2D-G).
To corroborate findings from flow cytometry, CFU assays provide an indication of the number of colonies that can be formed from a single HSPC. Further identification of colonies and their categorization can give insight into their multipotent potential, including CFU-GEMM (granulocyte, erythroid, monocyte, megakaryocyte), CFU-GM (granulocyte, monocyte), and BFU-E (erythroid) colonies.
For transplantation applications, our methodologies demonstrate ease of use and feasibility of collecting, drying, and injecting 3D scaffolds in vivo. Subcutaneous transplantation applications for hematopoietic transplant are of significant interest to clinicians, as an easier route of stem cell delivery may help optimize delivery of gene-edited HSCs or prevent adverse effects in HSC transplant patients. Scaffolds seeded with OP9 cells show little to no vascularization versus the readily vascularized (CD31+ staining) of OP9/KLS co-seeded implants, which also show a significant presence of tdTomato+ donor-derived cells as previously reported (Tavakol et al., 2020). Twelve weeks of implantation was originally chosen as the end point due to this classical time point for ossicle formation, but vascularization associated to hematopoietic cellularity can already be observed at 1.5 weeks post-implantation (Fig. 5). Our techniques can help give insight into both injection of 3D scaffolds and recovery of transplanted tissue after many months in vivo. We hope this work can inform future researchers to design multi-faceted tissue-engineered tools to model hematopoiesis in vitro (Basic Protocols 1-4) and regenerate bone marrow in vivo (Basic Protocol 5).
Time Considerations
Isolation of HSPCs
Approximately 1-2 hr are needed to euthanize animals, collect bones, and crush them for marrow isolation. Staining for MACS sorting requires ∼1 hr, and MACS sorting requires ∼15-30 min. Final staining and FACS requires another ∼2-3 hr.
Co-seeding HSPCs and BMSCs on CCMs
Expansion of BMSCs requires over 1-2 weeks. Once they are ready, ∼30 min are required to prepare BMSCs in suspension, ∼30 min are required to prepare scaffolds for cell seeding, and ∼1.5 hr are needed to incubate the cell suspensions and scaffolds. After additional medium is added, co-cultures are maintained for 2 weeks prior to evaluation and use for transplantation.
Analysis of co-seeded scaffolds by flow cytometry and CFU assays
To prepare the adherent fraction, ∼1.5 hr is required for collagenase digestion, collection of the cell suspension, and washing. This may vary depending on number of samples. For flow cytometry, ∼3 hr are required for blocking, staining, and running the samples. For CFU assays, ∼2 hr are required to calculate the optimal cell seeding density, aliquot cell suspensions, and plate aliquots on 6-well plates. Two weeks after plating, manual or automatic scoring and then visual verification of colony assignation requires ∼15 min per well of a 6-well plate. Serial imaging will require ∼1-3 hours each day.
Implantation
It takes ∼2 hrs to design the drying device. All materials should be autoclaved ∼1 day prior to the experiment. On the day of implantation, ∼15-20 min is needed to prepare each mouse, ∼5-10 min are needed for scaffold drying and syringe preparation, and ∼10-15 min are needed per mouse for injection. After the desired time in vivo (in our case, 12 weeks), ∼15-20 min are needed per mouse for euthanasia and harvesting scaffolds.
Acknowledgments
We would like acknowledge funding sources from the Whitaker International Program (D.N.T), the Anna Fuller Fund (J.T.), and the Swiss National Science Foundation (PP00P3_183725, PP00P3_176990, and CRSII5_186271 to O.N.; PP00P2_163684 and PZ00P2_161347 to T.B.; PP00P2_194813 to J.B.-G.). We would also like to acknowledge the experimental help of Aurelien Oggier, Dr. Vasco Campos, Dr. Sylke Hoehnel, Dr. Shanti Rojas-Sutterlin, Dr. Philippe Renaud (EPFL); Patrick Burch and Amélie Béduer (Volumina Medical); and Dr. Marco Alessandrini (University of Geneva).
Open access funding provided by Universite de Lausanne.
Author Contributions
D. N. Tavakol, F. Bonini, J. Tratwal, T. Braschler, and O. Naveiras contributed to the design of the study. All authors contributed to the writing and proofing of the manuscript. D. N. Tavakol, F. Bonini, J. Tratwal, and J. Brefie-Guth performed and optimized the in vitro study. J. Brefie-Guth and F. Bonini prepared the biomaterial and optimized the collagen modification. F. Bonini designed the 3D maps. F. Bonini and T. Braschler designed the first version of the dehydration device, and D. N. Tavakol, J. Tratwal, M. Genta, J. Brefie-Guth helped with device optimization. J. Tratwal performed FACS and analyzed the results in Figure 2 together with O. Naveiras. J. Tratwal, D.N. Tavakol, M. Genta, and J. Brefie-Guth carried out the in vivo studies. J. Tratwal and F. Bonini analyzed the in vivo data.
Conflict of Interest
The authors declare no conflict of interest.
Open Research
Data Availability Statement
Data sharing is not applicable to this article as no new data were created or analyzed in this study.
Supporting Information
Filename | Description |
---|---|
fwmissing3dprintingmapsforcurrentprotocols.zip766.2 KB | Supporting Information |
Please note: The publisher is not responsible for the content or functionality of any supporting information supplied by the authors. Any queries (other than missing content) should be directed to the corresponding author for the article.
Literature Cited
- Abarrategi, A., Mian, S. A., Passaro, D., Rouault-Pierre, K., Grey, W., & Bonnet, D. (2018). Modeling the human bone marrow niche in mice: From host bone marrow engraftment to bioengineering approaches. Journal of Experimental Medicine , 215(3), 729–743. doi: 10.1084/jem.20172139.
- Béduer, A., Bonini, F., Verheyen, C. A., Genta, M., Martins, M., Brefie-Guth, J., … Braschler, T. (2021). An injectable meta-biomaterial: From design and simulation to in vivo shaping and tissue induction. Advanced Materials , 33, 2102350. doi: 10.1002/adma.202102350.
- Behrmann, L., Wellbrock, J., & Fiedler, W. (2020). The bone marrow stromal niche: A therapeutic target of hematological myeloid malignancies. Expert Opinion on Therapeutic Targets , 24(5), 451–462. doi: 10.1080/14728222.2020.1744850.
- Bianco, P., Riminucci, M., Gronthos, S., & Robey, P. G. (2001). Bone marrow stromal stem cells: Nature, biology, and potential applications. Stem Cells , 19(3), 180–192. doi: 10.1634/stemcells.19-3-180.
- Bourgine, P. E., Klein, T., Paczulla, A. M., Shimizu, T., Kunz, L., Kokkaliaris, K. D., … Martin, I. (2018). In vitro biomimetic engineering of a human hematopoietic niche with functional properties. Proceedings of the National Academy of Sciences of the United States of America , 115(25), e5688–e5695. doi: 10.1073/pnas.1805440115.
- Bujko, K., Kucia, M., Ratajczak, J., & Ratajczak, M. Z. (2019). Hematopoietic stem and progenitor cells (HSPCs). Advances in Experimental Medicine and Biology , 1201, 49–77. doi: 10.1007/978-3-030-31206-0_3.
- Chou, D. B., Frismantas, V., Milton, Y., David, R., Pop-Damkov, P., Ferguson, D., … Ingber, D. E. (2020). On-chip recapitulation of clinical bone marrow toxicities and patient-specific pathophysiology. Nature Biomedical Engineering , 4(4), 394–406. doi: 10.1038/s41551-019-0495-z.
- Derakhshani, M., Abbaszadeh, H., Movassaghpour, A. A., Mehdizadeh, A., Ebrahimi-Warkiani, M., & Yousefi, M. (2019). Strategies for elevating hematopoietic stem cells expansion and engraftment capacity. Life Sciences , 232, 116598. doi: 10.1016/j.lfs.2019.116598.
- Dexter, T. M., Wright, E. G., Krizsa, F., & Lajtha, L. G. (1977). Regulation of haemopoietic stem cell proliferation in long term bone marrow cultures. Biomedicine , 27(9-10), 344–349.
- Dupard, S. J., Grigoryan, A., Farhat, S., Coutu, D. L., & Bourgine, P. E. (2020). Development of humanized ossicles: Bridging the hematopoietic gap. Trends in Molecular Medicine , 26(6), 552–569. doi: 10.1016/j.molmed.2020.01.016.
- Ferreira, M. S., Jahnen-Dechent, W., Labude, N., Bovi, M., Hieronymus, T., Zenke, M., … Neuss, S. (2012). Cord blood-hematopoietic stem cell expansion in 3D fibrin scaffolds with stromal support. Biomaterials , 33(29), 6987–6997. doi: 10.1016/j.biomaterials.2012.06.029.
- Fleischer, S., Tavakol, D. N., & Vunjak-Novakovic, G. (2020). From arteries to capillaries: Approaches to engineering human vasculature. Advanced Functional Materials , 30(37), 1910811. doi: 10.1002/adfm.201910811.
- Gooley, T. A., Chien, J. W., Pergam, S. A., Hingorani, S., Sorror, M. L., Boeckh, M., … McDonald, G. B. (2010). Reduced mortality after allogeneic hematopoietic-cell transplantation. New England Journal of Medicine , 363(22), 2091–2101. doi: 10.1056/NEJMoa1004383.
- Jenq, R. R., & van den Brink, M. R. (2010). Allogeneic haematopoietic stem cell transplantation: Individualized stem cell and immune therapy of cancer. Nature Reviews Cancer , 10(3), 213–221. doi: 10.1038/nrc2804.
- Kerenyi, M. A. (2014). LT-HSC methylcellulose assay. Bio-protocol , 4(5), e1067. doi: 10.21769/bioprotoc.1067.
- Le, P. M., Andreeff, M., & Battula, V. L. (2018). Osteogenic niche in the regulation of normal hematopoiesis and leukemogenesis. Haematologica , 103(12), 1945–1955. doi: 10.3324/haematol.2018.197004.
- McKinney-Freeman, S. L., Naveiras, O., & Daley, G. Q. (2008). Isolation of hematopoietic stem cells from mouse embryonic stem cells. Current Protocols in Stem Cell Biology , 4(1), 1F.3.1–1F.3.10. doi: 10.1002/9780470151808.sc01f03s4.
- Morrison, S. J., & Scadden, D. T. (2014). The bone marrow niche for haematopoietic stem cells. Nature , 505(7483), 327–334. doi: 10.1038/nature12984.
- Mullighan, C. G. (2018). The ASH agenda for hematology research: A roadmap for advancing scientific discovery and cures for hematologic diseases. Blood Advances , 2(19), 2430–2432. doi: 10.1182/bloodadvances.2018025403.
- Nakano, T., Kodama, H., & Honjo, T. (1994). Generation of lymphohematopoietic cells from embryonic stem cells in culture. Science , 265(5175), 1098–1101. doi: 10.1126/science.8066449.
- Pinho, S., & Frenette, P. S. (2019). Haematopoietic stem cell activity and interactions with the niche. Nature Reviews Molecular Cell Biology , 20(5), 303–320. doi: 10.1038/s41580-019-0103-9.
- Raic, A., Rödling, L., Kalbacher, H., & Lee-Thedieck, C. (2014). Biomimetic macroporous PEG hydrogels as 3D scaffolds for the multiplication of human hematopoietic stem and progenitor cells. Biomaterials , 35(3), 929–940. doi: 10.1016/j.biomaterials.2013.10.038.
- Reinisch, A., Thomas, D., Corces, M. R., Zhang, X., Gratzinger, D., Hong, W. J., … Majeti, R. (2016). A humanized bone marrow ossicle xenotransplantation model enables improved engraftment of healthy and leukemic human hematopoietic cells. Nature Medicine , 22(7), 812–821. doi: 10.1038/nm.4103.
- Serex, L., Braschler, T., Filippova, A., Rochat, A., Béduer, A., Bertsch, A., & Renaud, P. (2018). Pore size manipulation in 3D printed cryogels enables selective cell seeding. Advanced Materials Technologies , 3(4), 1700340. doi: 10.1002/admt.201700340.
- Shah, N. J., Mao, A. S., Shih, T. Y., Kerr, M. D., Sharda, A., Raimondo, T. M., … Scadden, D. T. (2019). An injectable bone marrow-like scaffold enhances T cell immunity after hematopoietic stem cell transplantation. Nature Biotechnology , 37(3), 293–302. doi: 10.1038/s41587-019-0017-2. Erratum: Nat. Biotechnol. 2021 Oct 18.
- Tavakol, D. N., Tratwal, J., Bonini, F., Genta, M., Campos, V., Burch, P., … Braschler, T. (2020). Injectable, scalable 3D tissue-engineered model of marrow hematopoiesis. Biomaterials , 232, 119665. Epub: 2019 Dec 11. doi: 10.1016/j.biomaterials.2019.119665.
- Tavakol, D. N., Fleischer, S., & Vunjak-Novakovic, G. (2021). Harnessing organs-on-a-chip to model tissue regeneration. Cell Stem Cell , 28(6), 993–1015. doi: 10.1016/j.stem.2021.05.008.
- Torisawa, Y.-S., Spina, C. S., Mammoto, T., Mammoto, A., Weaver, J. C., Tat, T., … Ingber, D. E. (2014). Bone marrow-on-a-chip replicates hematopoietic niche physiology in vitro. Nature Methods , 11(6), 663–669. doi: 10.1038/nmeth.2938.
- Tratwal, J., Bekri, D., Boussema, C., Sarkis, R., Kunz, N., Koliqi, T., … Naveiras, O. (2020). MarrowQuant across aging and aplasia: A digital pathology workflow for quantification of bone marrow compartments in histological sections. Frontiers in Endocrinology , 11, 480. doi: 10.3389/fendo.2020.00480.
- Wilson, A., Laurenti, E., Oser, G., van der Wath, R. C., Blanco-Bose, W., Jaworski, M., … Trumpp, A. (2008). Hematopoietic stem cells reversibly switch from dormancy to self-renewal during homeostasis and repair. Cell , 135(6), 1118–1129. doi: 10.1016/j.cell.2008.10.048.
Citing Literature
Number of times cited according to CrossRef: 4
- Yuxuan Pang, Lin Guan, Yanlin Zhu, Ruijuan Niu, Song Zhu, Quan Lin, Gallic acid-grafted chitosan antibacterial hydrogel incorporated with polydopamine-modified hydroxyapatite for enhancing bone healing, Frontiers in Bioengineering and Biotechnology, 10.3389/fbioe.2023.1162202, 11 , (2023).
- Bangheng Liu, Min Jin, Dong-An Wang, In vitro expansion of hematopoietic stem cells in a porous hydrogel-based 3D culture system, Acta Biomaterialia, 10.1016/j.actbio.2023.01.057, 161 , (67-79), (2023).
- Oleg Kandarakov, Alexander Belyavsky, Ekaterina Semenova, Bone Marrow Niches of Hematopoietic Stem and Progenitor Cells, International Journal of Molecular Sciences, 10.3390/ijms23084462, 23 , 8, (4462), (2022).
- Ryan Sarkar, Francesco Pampaloni, In Vitro Models of Bone Marrow Remodelling and Immune Dysfunction in Space: Present State and Future Directions, Biomedicines, 10.3390/biomedicines10040766, 10 , 4, (766), (2022).