Chromatin Immunoprecipitation and Sequencing (ChIP-seq) Optimized for Application in Caenorhabditis elegans
Ilke Sen, Ilke Sen, Alan Kavšek, Alan Kavšek, Christian G. Riedel, Christian G. Riedel
Caenorhabditis elegans
ChIP-seq
chromatin immunoprecipitation
protein-DNA interaction
sequencing library construction
Abstract
Chromatin immunoprecipitation followed by next-generation sequencing (ChIP-seq) has become one of the most popular methods to study protein-DNA interactions and can be used, for instance, to identify the binding sites of transcription factors or to determine the distributions of histones with specific post-translational modifications throughout the genome. Although standard ChIP-seq protocols work well in most experimental systems, there are exceptions, and one of these is the popular model organism Caenorhabditis elegans. Even though this system is very amenable to genetic and cytological methods, biochemical approaches are challenging. This is due to both the animals’ cuticle, which impairs lysis as well as penetration by cross-linkers, and the rather low protein and chromatin content per body weight. These issues have rendered standard ChIP-seq protocols inefficient in C. elegans and raised a need for their improvement. Here, we describe improved protocols, with the most important advances being the efficient breakage of the C. elegans cuticle by freeze-grinding and the use of a very sensitive sequencing library construction procedure, optimized for the relatively low DNA content per body weight of C. elegans. The protocols should therefore improve the reproducibility, sensitivity, and uniformity across tissues of ChIP-seq in this organism. © 2021 The Authors. Current Protocols published by Wiley Periodicals LLC.
Basic Protocol 1 : Growth and harvesting of synchronized Caenorhabditis elegans
Basic Protocol 2 : Chromatin immunoprecipitation (ChIP)
Basic Protocol 3 : Library construction for Illumina sequencing
INTRODUCTION
The nematode Caenorhabditis elegans is a very powerful model organism, particularly due to its good genetic amenability, fully mapped cellular lineage, and excellent suitability for in vivo imaging. As a result, C. elegans is widely used in diverse research areas, such as developmental biology, small RNA biology, epigenetics, neurobiology, metabolism, stress responses, and aging, just to name a few (Corsi, Wightman, & Chalfie, 2015; Fischer, 2010; Greenwald, 2016; Kenyon, 2005; see Current Protocols article; Meneely, Dahlberg, & Rose, 2019; Mullaney & Ashrafi, 2009; Sulston & Horvitz, 1977; Wenzel, Palladino, & Jedrusik-Bode, 2011; White, 2013). However, despite being a powerful system for genetic and cytological approaches, addressing the majority of biological questions also requires biochemical methods, and these have remained uncommonly used and challenging in C. elegans (Corsi, 2006). The main reasons for this are 1) the animals’ cuticle, which hinders their lysis and can impair penetration by chemicals needed for certain biochemical methods (Bhaskaran et al., 2011; Kumar & Mukhopadhyay, 2018), and 2) a rather low content of intracellular protein, RNA, and DNA per body weight. To illustrate the second point, we can compare C. elegans with human HeLa cells. Considering their estimated weights and genome sizes and the fact that C. elegans contains 959 somatic cells (Andrássy, 1995; Saier, 2008; Park et al., 2008), we estimate the chromatin content of HeLa cells to be about 100-fold higher. To overcome these challenges, many common biochemical methods need to be specifically adapted for C. elegans.
In the protocols described here, we focus on the study of protein-DNA interactions using chromatin immunoprecipitation (ChIP) followed by next-generation sequencing (ChIP-seq). This method has become the current standard to study distributions of proteins of interest across the genome and identify the sites, genomic features, or sequence motifs with which they associate. Basic ChIP-seq protocols, e.g., those used in mammalian cells (Lee, Johnstone, & Young, 2006; see Current Protocols article; Lloyd & Bao, 2019; see Current Protocols article; Raha, Hong, & Snyder, 2010), are inefficient in C. elegans due to three main shortcomings. First, chemicals, including cross-linkers, are unable to rapidly penetrate the animals’ cuticle (Burns et al., 2010), leading to insufficient or variable cross-linking of the protein of interest to DNA. Second, chromatin release and its shearing by sonication work poorly in C. elegans , again due to obstruction by the cuticle (Bhaskaran et al., 2011; Kumar & Mukhopadhyay, 2018). This ultimately leads to a very low yield of DNA of the appropriate length to be immunoprecipitated and identified by sequencing. Finally, even if these previous issues did not exist, the yield of immunoprecipitated chromatin still remains very low due to the animal's low intracellular protein and DNA content.
We present a series of three comprehensive and in-depth protocols that together address these issues. In Basic Protocol 1, we describe how to grow and harvest synchronized C. elegans. In Basic Protocol 2, we describe the ChIP procedure. In contrast to simple protocols typically used for mammalian cells (Lee et al., 2006) or protocols previously reported for C. elegans (Mukhopadhyay, Deplancke, Walhout, & Tissenbaum, 2008; Zhong et al., 2010), we partially break the animals by freeze-grinding prior to cross-linking. This facilitates penetration by the cross-linker, ensures more homogenous cross-linking results, leads to more homogenous shearing of the chromatin into fragments of appropriate length by sonication, and ultimately increases the yield of immunoprecipitated chromatin. In addition, we improve reproducibility of the protocol by cleaning and concentrating the final DNA samples using a commercially available spin column–based DNA cleanup kit, thereby replacing typically used—but more error-prone—phenol-chloroform-based extraction methods. In Basic Protocol 3, we describe the construction of sequencing libraries from the input and immunoprecipitated DNA samples obtained in Basic Protocol 2. Given the low DNA amounts available when conducting ChIP for C. elegans , we employ a very sensitive picogram-scale library construction procedure adapted from work in other systems (Bowman et al., 2013). We further provide examples of data that can be obtained by following these protocols, namely the genome-wide distributions of RNA polymerase II (RNA Pol II) and the transcription factor HLH-30 in long-lived daf-2 mutant animals (Lin et al., 2018; Sen et al., 2020).
Basic Protocol 1: GROWTH AND HARVESTING OF SYNCHRONIZED Caenorhabditis elegans
In this protocol, we describe a typical procedure for growing C. elegans to obtain 4 to 5 ml of freeze-ground animals, which is the required starting material for our ChIP protocol (Basic Protocol 2). We outline the steps to synchronize, harvest, and resuspend the worms in the appropriate buffer for subsequent use. Our overall workflow has been optimized to obtain ChIP-seq data for transcription factors or for RNA Pol II. Given that these proteins only bind to specific parts of the genome, a substantial amount of starting material is needed. In some cases, for instance, when immunoprecipitating highly expressed and broadly distributed chromatin-associated proteins like histones in certain modification states, a lesser amount of starting material might be sufficient, but we encourage users to start at the described scale when trying the protocol for the first time.
Materials
-
Stationary-phase OP50-1 Escherichia coli culture, 2-, 10-, or 50-fold concentrated and resuspended in M9 buffer (see recipe)
-
Worm strain(s) of interest [created in own lab, obtained from other labs, or ordered from the Caenorhabditis Genetics Center (CGC)]
-
Sterile M9 buffer (see recipe), room temperature and 4°C
-
Hypochlorite solution (see recipe; make fresh)
-
M9 buffer (see recipe) supplemented with 1 mM MgSO4 (from 1 M MgSO4 stock solution)
-
Phosphate-buffered saline (PBS; see recipe), 4°C
-
PBS supplemented with protease inhibitors (see recipe), 4°C
-
Liquid nitrogen
-
Temperature-controlled incubators, one at temperature used to grow C. elegans strain of interest (usually 20°C), one at 15°C, and one at 37°C
-
Worm pick
-
Sterilized metal spatula
-
Standard benchtop centrifuge for 15- and 50-ml centrifuge tubes (e.g., Thermo Fisher Scientific Megafuge 8)
-
15- and 50-ml centrifuge tubes
-
Vortex mixer
-
Stereomicroscope (e.g., Olympus SZX7)
-
Tube rotator (e.g., Thermo Scientific Tube Revolver)
-
Refrigerated benchtop centrifuge for 15- and 50-ml centrifuge tubes (e.g., Thermo Fisher Scientific Megafuge 40R), 4°C
-
500-ml Dewar beaker
-
Additional reagents and equipment for preparing NGM plates (6-, 10-, and 15-cm diameter; see recipe)
NOTE : All buffers used from step 25 onward for harvesting and washing the animals should be ice-cold to slow down physiological changes.
Growth of worms to scale and egg preparation
1.Prepare several NGM plates (6-, 10-, and 15-cm diameter). Seed these plates with stationary-phase OP50-1 E. coli culture.
2.Thaw desired worm strain(s) of interest and transfer worms to one of the bacteria-seeded 6-cm NGM plates. Place plate inside a temperature-controlled incubator.
3.The next day, transfer any live worms to a fresh bacteria-seeded 6-cm NGM plate by using a worm pick. Place plate inside a temperature-controlled incubator and let it grow at desired temperature until the plate is crowded and the food runs low.
4.Cut agar of the crowded plate into four pieces with a sterilized metal spatula and transfer all four of them with their worms onto a single bacteria-seeded 10-cm NGM plate. Grow until this NGM plate is crowded with unsynchronized animals and the food runs low.
5.Cut agar of the crowded plate into 10 pieces, each carrying a similar number of worms, with a sterilized metal spatula. Transfer each agar piece to a separate bacteria-seeded 10-cm NGM plate and grow them at desired temperature until the plates are crowded and the food is running low.
6.Wash worms off the plates using a pipet containing 15 ml sterile M9 buffer. Collect all worm suspension into a single 50-ml centrifuge tube.
7.Distribute worm suspension across 10 bacteria-seeded 15-cm NGM plates and grow them until mostly gravid adults are present.
8.Wash animals off the plates using a pipet and 25 ml sterile M9 buffer per plate. Collect worm suspensions in multiple 15-ml centrifuge tubes. Centrifuge 1 min at 1500 × g at room temperature to pellet animals.
9.For each tube, remove supernatant and resuspend animals in 10 ml sterile M9 buffer. Then, centrifuge 1 min at 1500 × g at room temperature.
10.Repeat step 9 until all OP50-1 bacteria have been removed and supernatant is clear.
11.For each tube, remove supernatant and resuspend worms in 7.5 ml sterile M9 buffer.
12.For each tube, add 2.5 ml fresh hypochlorite solution and mix by vortexing.
13.Every 30 s, briefly examine tubes under a stereomicroscope to determine whether the worm corpses have disappeared and released eggs can be seen. Continue vortexing until ≤5% of the worm corpses remain.
14.Centrifuge tubes for 1 min at 1500 × g at room temperature to pellet the released eggs.
15.Carefully discard supernatant and resuspend eggs in 10 ml sterile M9 buffer.
16.Repeat steps 14 and 15 at least three more times. If the sample is split across several tubes, combine it into a single tube.
17.Centrifuge 1 min at 1500 × g at room temperature and discard supernatant.
18.Resuspend eggs in 10 ml M9 buffer supplemented with 1 mM MgSO4.
19.Rotate tube containing the eggs at 10 to 12 rpm on a tube rotator for 1 day at room temperature or for 2 days at 15°C in an incubator.
Synchronized growth and harvest
20.Prepare thirty 15-cm NGM plates and seed them with stationary-phase OP50-1 E. coli culture.
21.Incubate these plates overnight at 37°C.
22.The next day, prepare 1 ml of a 1:10 dilution of the worm suspension from step 19 in sterile M9 buffer. Pipet 10 µl of the dilution onto a 6-cm NGM plate. Count worms under the stereomicroscope. Repeat this step two more times and calculate average worm number per milliliter.
23.Seed each of the 30 bacteria-seeded NGM plates with 20,000 to 25,000 of these worms.
24.Grow animals at the desired temperature until the desired stage.
25.Wash animals off the plates using ice-cold sterile M9 buffer and collect them in 50-ml centrifuge tubes on ice.
26.Centrifuge tubes for 2 min at 650 × g , 4°C. Discard supernatant.
27.Resuspend animals in 40 ml ice-cold sterile M9 buffer. If the sample is split across several tubes, combine it into a single tube, so that the pellet volume is between 5 and 10 ml.
28.Centrifuge 2 min at 650 × g , 4°C. Discard supernatant.
29.Repeat steps 27 and 28 two more times.
30.Resuspend pellet in 40 ml ice-cold PBS.
31.Centrifuge 2 min at 650 × g , 4°C. Discard supernatant.
32.Add 0.5 pellet-volumes of ice-cold PBS supplemented with protease inhibitors.
33.Resuspend using a 10-ml pipet and then dispense sample, drop by drop, into a 500-ml Dewar beaker filled with liquid nitrogen.
34.Chill a 50-ml centrifuge tube using liquid nitrogen and transfer worm beads into it.
35.Store beads at –80°C.
Basic Protocol 2: CHROMATIN IMMUNOPRECIPITATION (ChIP)
Here, we describe how to cross-link chromatin in C. elegans , which locks not only its protein-DNA interactions but also its protein-protein interactions in place. We then describe how to lyse the worms and isolate the cross-linked chromatin and how to best shear the chromatin into fragments of appropriate size for immunoprecipitation. These steps have been specifically developed or optimized for use in C. elegans and may differ significantly from what you find in protocols used for other organisms or for cultured cells. Most notably, the C. elegans cuticle is difficult to penetrate with chemicals like formaldehyde (Burns et al., 2010), which makes it impossible to achieve efficient and uniform cross-linking of chromatin across tissues. We circumvent this problem, first by flash-freezing the worms in liquid nitrogen (see Basic Protocol 1, step 33), then by gently breaking them into several fragments by grinding in liquid nitrogen, and finally by thawing these animals in the presence of formaldehyde. This approach leads to much better penetration by the cross-linker and, hence, improved ChIP results. Upon cross-linking, the samples are further homogenized by bead-beating, and then we use sonication to fully lyse the animals and shear the chromatin into fragments of appropriate size. Lastly, we describe the immunoprecipitation of these fragments via protein pull-down using an antibody against the protein of interest bound to Protein A or G Dynabeads.
Materials
-
Protein A Dynabeads (Invitrogen, 10002D) or Protein G Dynabeads (Invitrogen, 10004D)
-
B-ChIP-BL (see recipe; make fresh)
-
Purified antibody or antiserum (antibody of choice for immunoprecipitation; e.g., BioLegend, 920102)
-
Liquid nitrogen
-
Frozen worm beads (see Basic Protocol 1)
-
Phosphate-buffered saline (PBS; see recipe)
-
16% (w/v) formaldehyde, methanol-free (Thermo Fisher Scientific, 28908)
-
100 mM phenylmethylsulfonyl fluoride (PMSF) solution (Sigma-Aldrich, 93482)
-
2.5 M glycine
-
PBS supplemented with protease inhibitors (see recipe), 4°C
-
B-ChIP-L0 (see recipe), 4°C
-
Acid-washed glass beads (Sigma-Aldrich, G8772)
-
10% (v/v) Triton X-100, diluted in water
-
B-ChIP-Lys (see recipe), 4°C
-
B-ChIP-Lys containing 200 mM NaCl (see recipe), 4°C
-
B-ChIP-W (see recipe), 4°C
-
Containing 50 mM NaCl, 4°C
-
B-ChIP-EL (see recipe)
-
10 mg/ml RNase A (Roche, 10109142001), diluted in water
-
10 mg/ml proteinase K (Roche, 3115836001), diluted in water
-
ChIP DNA cleanup kit, including elution buffer (Zymo Research, D5205)
-
1 M Tris·HCl, pH 8.8 (optional)
-
10% (w/v) sodium dodecyl sulfate (SDS; optional)
-
1 M dithiothreitol (DTT; optional)
-
Vortex mixer
-
1.5- and 2.0-ml Safe-Lock tubes (Eppendorf, 0030120086 and 022363352)
-
Magnetic rack for Dynabeads (e.g., Invitrogen DynaMag-2)
-
Tube rotator (e.g., Thermo Scientific Tube Revolver)
-
Biopulverizer and hammer (Biospec Products, 59014N)
-
Mortar and pestle (Biospec Products, 206)
-
Metal spatula
-
15- and 50-ml centrifuge tubes
-
Glass slides
-
Stereomicroscope (e.g., Olympus SZX7)
-
42°C water bath
-
Refrigerated benchtop centrifuge for 15- and 50-ml centrifuge tubes (e.g., Thermo Fisher Scientific Megafuge 40R), 4°C
-
15-ml polymethylpentene centrifuge tubes (Diagenode, C30010009)
-
Bioruptor Plus sonicator (Diagenode, B01020001) with actively cooled water bath set to 4°C
-
Refrigerated benchtop centrifuge for 1.5- and 2-ml tubes (e.g., Eppendorf 5424R), 4°C
-
Thermomixer (ThermoMixer C with thermoblock, Eppendorf, 5382000015 and 5360000038)
-
95°C (optional) and 100°C heat block
-
Additional reagents and equipment for agarose gel electrophoresis (optional; see Current Protocols article; Voytas, 2000), SDS-PAGE (optional; see Current Protocols article; Gallagher, 2012), and western blotting (optional; see Current Protocols article; Gallagher, Winston, Fuller, & Hurrell, 2011)
Preparation of affinity resin
1.Vortex the stock of protein A Dynabeads or protein G Dynabeads for 20 s and then immediately transfer 100 µl bead suspension into a 2.0-ml Safe-Lock tube.
2.Add 1.5 ml fresh B-ChIP-BL and mix by pipetting up and down with a 1-ml pipet.
3.Settle beads by placing the tube in a magnetic rack for Dynabeads and waiting for 1 min.
4.With the tube in the rack, remove supernatant. Then, remove tube from the rack.
5.Repeat steps 2 to 4 two more times.
6.Resuspend beads in 250 µl fresh B-ChIP-BL and add 10 µg purified antibody or 4 µl antiserum.
7.Incubate on a tube rotator at 10 rpm for 6 to 16 hr at 4°C.
8.Settle beads by placing the tube in a magnetic rack and waiting for 1 min.
9.Remove supernatant.
10.Remove tube from the rack, add 1.5 ml B-ChIP-BL, and mix by pipetting up and down with a 1-ml pipet.
11.Settle beads by placing the tube back in the magnetic rack and waiting for 1 min.
12.Remove supernatant.
13.Repeat steps 10 to 12 two more times.
14.Resuspend beads in 100 µl fresh B-ChIP-BL and store them at 4°C.
Freeze-grinding and cross-linking
15.Pre-chill a Biopulverizer and hammer, a mortar and pestle, a metal spatula, and a few 15-ml centrifuge tubes by submerging them in liquid nitrogen.
16.Transfer ≤5 ml frozen worm beads from Basic Protocol 1 into the pre-cooled Biopulverizer and break them by three rounds of five blows with the included hammer. After each round of five blows, use the pre-cooled spatula to collect the powder into the middle of the Biopulverizer again.
17.Re-cool same spatula in liquid nitrogen and use it to transfer the powder to the pre-cooled mortar. Using the pestle, grind for ∼5 min.
18.Transfer a small amount of powder to a glass slide, let it thaw, and check under a stereomicroscope that all worms have broken into at least 4 to 5 pieces.
19.Using the re-cooled spatula, transfer powder to the pre-cooled 15-ml centrifuge tubes from step 15.
20.Store these tubes at –80°C.
21.Prepare 40 ml PBS in a 50-ml centrifuge tube and heat tube to 42°C in a water bath for ≥30 min.
22.Just before use, add 16% formaldehyde and 100 mM PMSF solution to final concentrations of 1.1% and 1 mM, respectively.
23.Get tubes from step 20 out of the freezer and transfer the 4 to 5 ml frozen ground worm powder into the PBS/formaldehyde/PMSF-containing 50-ml tube.
24.Use 2 to 4 ml suspension from step 23 to wash remaining powder out of the 15-ml tube then transfer into the 50-ml tube.
25.Incubate on a tube rotator for 7 min at room temperature.
26.Stop cross-linking by adding 2.3 ml of 2.5 M glycine to achieve a final glycine concentration of 125 mM.
27.Incubate on a tube rotator for 5 min at room temperature.
28.Centrifuge 3 min at 4000 × g , 4°C, remove supernatant, and resuspend pellet in 10 ml ice-cold PBS supplemented with protease inhibitors.
29.Transfer suspension to a 15-ml centrifuge tube.
30.Centrifuge 3 min at 4000 × g , 4°C, and then discard supernatant.
31.Resuspend pellet in 10 ml ice-cold PBS supplemented with protease inhibitors.
32.Repeat steps 30 and 31.
33.Centrifuge 3 min at 4000 × g , 4°C, and then discard supernatant.
34.Resuspend pellet in ice-cold B-ChIP-L0 (use 650 µl B-ChIP-L0 per 100 µl pellet volume).
Worm lysis
35.Take 1.7 ml suspension from step 34 and transfer it to a 2.0-ml Safe-Lock tube.
36.Add 200 µl acid-washed glass beads.
37.Vortex for 30 s (preferably in a cold room) and then place on ice for 60 s.
38.Repeat step 37 two more times.
39.Transfer 1.4 ml sample to a 15-ml polymethylpentene centrifuge tube.
Shearing of the chromatin
40.Position sonicator probe in the tube and place it inside a Bioruptor Plus sonicator with an actively cooled water bath set to 4°C.
41.Set sonicator to full power and set its sonication cycle to 30 s ON, 90 s OFF.
42.Run sonicator for eight cycles.
43.Take tube out of the water bath and vortex it gently to homogenize sample.
44.Repeat steps 42 and 43 two more times, leading to 12 min total sonication time.
45.Transfer sample to a 2.0-ml Safe-Lock tube, add 10% Triton X-100 to a final concentration of 1%, and rotate on a tube rotator for 10 min at 4°C.
46.Centrifuge sample for 5 min at 20,000 × g , 4°C, and transfer supernatant to a 1.5-ml Safe-Lock tube.
47.Centrifuge sample for 15 min at 20,000 × g , 4°C, and transfer supernatant to a fresh 1.5-ml Safe-Lock tube.
Immunoprecipitation
48.Store 50 µl of the cleared sample at –20°C.
49.Store another 50 µl of the cleared sample in a cold room on ice.
50.Add remaining cleared sample to the tube with Dynabeads from step 14, resuspend by pipetting, and then incubate on a tube rotator overnight at 4°C.
51.Write down total volume of the sample used in step 50.
52.Settle beads by placing the tube in a magnetic rack and waiting for 1 min. Store 50 µl of the supernatant at –20°C and discard remaining supernatant.
53.Remove tube from the magnetic rack and add 1.5 ml ice-cold B-ChIP-Lys to the beads. Resuspend by pipetting.
54.Rotate tube for 5 min at 4°C.
55.Settle beads by placing the tube in a magnetic rack and waiting for 1 min. Discard supernatant.
56.Repeat steps 53 to 55 one more time.
57.Remove tube from the magnetic rack and add 1.5 ml ice-cold B-ChIP-Lys containing 200 mM NaCl. Resuspend by pipetting and then transfer sample to a new 2.0-ml Safe-Lock tube.
58.Rotate tube for 5 min at 4°C.
59.Settle beads by placing the tube in a magnetic rack and waiting for 1 min. Discard supernatant.
60.Remove tube from the magnetic rack and add 1.5 ml ice-cold B-ChIP-W. Resuspend by pipetting and then transfer sample to a new 2.0-ml Safe-Lock tube.
61.Rotate tube for 5 min at 4°C.
62.Settle beads by placing the tube in a magnetic rack and waiting for 1 min. Discard supernatant.
63.Repeat steps 60 to 62 without changing Safe-Lock tube.
64.Add 1.2 ml ice-cold TE (pH 8.0) containing 50 mM NaCl, resuspend by pipetting, and then transfer sample to a new 1.5-ml Safe-Lock tube.
65.Rotate tube for 5 min at 4°C.
66.Settle beads by placing the tube in a magnetic rack and waiting for 1 min. Discard supernatant.
67.Repeat steps 64 to 66 without changing Safe-Lock tube.
68.Add 280 µl B-ChIP-EL and resuspend by pipetting.
69.Incubate for 15 min at 65°C and 800 rpm in a Thermomixer.
70.Denature required amount of 10 mg/ml RNase A by incubating it in a heat block for 10 min at 100°C and afterward letting it cool again to room temperature.
71.Settle beads from step 69 by placing the tube in a magnetic rack and waiting for 1 min.
72.Transfer supernatant to a new 1.5-ml Safe-Lock tube.
73.Spin tube for 20 s at 20,000 × g to remove any contaminating Dynabeads and transfer 250 µl supernatant to a new 1.5-ml Safe-Lock tube. Set aside 10 µl and store it at –20°C.
DNA purification
74.Add 200 µl B-ChIP-EL to the stored D-IN samples from step 49.
75.Add 10 µl of 10 mg/ml RNase A from step 70 to each D-IN and D-EL tube and incubate for 1 hr at 37°C and 800 rpm in a Thermomixer.
76.Add 10 µl of 10 mg/ml proteinase K to each of those tubes and incubate for 2 hr at 55°C and 800 rpm in a Thermomixer.
77.Shake tubes overnight (ideally for 16 to 18 hr) at 65°C and 800 rpm in a Thermomixer to reverse any cross-links.
78.Clean and concentrate each D-IN and D-EL sample by using a ChIP DNA cleanup kit according to the manufacturer's instructions. Use 50 µl elution buffer for final elution of the samples.
79.Dilute 10 µl of each D-IN sample with TE (pH 8.0) to 500 µl. Then, take 10 µl of each of these dilutions and dilute them further with TE to 100 µl to reach a final dilution of 1:500.
Checking the extent of shearing (optional)
80.Run 25 µl undiluted D-IN sample on a 1.5% agarose gel (see Current Protocols article; Voytas, 2000). Use a gel without dye and only stain after the run.
Checking the efficiency of the protein pull-down (optional)
81.Add 1 M Tris·HCl (pH 8.8) to a final concentration of 200 mM, 10% SDS to a final concentration of 1%, and 1 M DTT to a final concentration of 50 mM to all P-IN, P-FT, and P-EL samples.
82.Incubate at 95°C in a heat block for 25 min.
83.Run all samples on an SDS-PAGE gel (e.g., a 4% to 20% gradient gel) (see Current Protocols article; Gallagher, 2012) and detect the protein of interest by western blotting (for details about western blotting, see Current Protocols article; Gallagher, Winston, Fuller, & Hurrell, 2011).
Basic Protocol 3: LIBRARY CONSTRUCTION FOR ILLUMINA SEQUENCING
Here, we describe how to create a library from the input (D-IN) and eluted (D-EL) DNA samples from Basic Protocol 2 that can be sequenced on any Illumina platform sequencer, e.g., a HiSeq 2500 or HiSeq 3000. This part is challenging, as one obtains very little DNA in the eluted samples, commonly in the range of only 10 to 100 pg. The herein described protocol, derived from previous work by the laboratory of Robert Kingston (Bowman et al., 2013), has been chosen accordingly.
For most C. elegans ChIP-seq libraries, it is sufficient to sequence them at a depth of 10 to 20 million reads. Given that modern Illumina sequencers easily generate more than 200 million reads per sample, C. elegans ChIP-seq libraries should be multiplexed. For this purpose, this protocol introduces barcodes into each library that allow for their pooled sequencing and subsequent demultiplexing.
The protocol entails four major steps, which are end repair, A-tailing, ligation of the adapters, and library amplification. DNA shearing by sonication results in non-homogenous DNA fragments. The DNA end-repair step ensures that each fragment is blunt ended and contains 5’-phosphate and 3’-hydroxyl groups. During A-tailing, an A-overhang is created at the 3’ ends of each DNA strand to convert the fragments’ blunt ends into sticky ends that facilitate ligation. Resulting fragments are then ligated to adapters with complementary T-overhangs, which allow for the PCR-based amplification of the fragments using defined primers. Finally, that PCR-based amplification is conducted to introduce barcodes for demultiplexing and increase the amount of the library material to a level that is sufficient for sequencing.
Materials
- AMPure XP magnetic bead suspension (Beckman Coulter, A63881)
- D-EL or diluted D-IN samples from Basic Protocol 2
- Nuclease-free water (e.g., Thermo Fisher, AM9930)
- 10 mM dNTPs (e.g., New England Biolabs, N0447L)
- 10× T4 DNA ligase reaction buffer (New England Biolabs, B0202)
- 3 U/µl T4 DNA polymerase (New England Biolabs, M0203)
- 5 U/µl DNA polymerase I, large (Klenow) fragment (New England Biolabs, M0210)
- 10 U/µl T4 polynucleotide kinase (New England Biolabs, M0201)
- 80% (v/v) ethanol, diluted in water (make fresh)
- NEB buffer 2 (New England Biolabs, B7002)
- 1 mM dATP (New England Biolabs, N0440)
- 5 U/µl Klenow fragment (3'→5' exo-) (New England Biolabs, M0212)
- TE, pH 8.0 (see recipe), containing 50 mM NaCl
- HPLC-purified oligonucleotides (see Table 1)
- 2× rapid ligation buffer (Enzymatics, B1010)
- 600 U/µl T4 DNA ligase (rapid; Enzymatics, L6030-HC-L)
- Phusion High-Fidelity (HF) PCR master mix (with HF buffer; New England Biolabs, M0531)
- SYBR Green nucleic acid stain (Thermo Fisher Scientific, S7563)
- High-sensitivity DNA kit for Bioanalyzer (Agilent, 5067-4626)
PCR.1 | CAAGCAGAAGACGGCATACGAGATCGTGATGTGACTGGAGTTCAGACGTGTGCTCTTCCGATC*T |
PCR.2 | CAAGCAGAAGACGGCATACGAGATACATCGGTGACTGGAGTTCAGACGTGTGCTCTTCCGATC*T |
PCR.3 | CAAGCAGAAGACGGCATACGAGATGCCTAAGTGACTGGAGTTCAGACGTGTGCTCTTCCGATC*T |
PCR.4 | CAAGCAGAAGACGGCATACGAGATTGGTCAGTGACTGGAGTTCAGACGTGTGCTCTTCCGATC*T |
PCR.5 | CAAGCAGAAGACGGCATACGAGATCACTGTGTGACTGGAGTTCAGACGTGTGCTCTTCCGATC*T |
PCR.6 | CAAGCAGAAGACGGCATACGAGATATTGGCGTGACTGGAGTTCAGACGTGTGCTCTTCCGATC*T |
PCR.7 | CAAGCAGAAGACGGCATACGAGATGATCTGGTGACTGGAGTTCAGACGTGTGCTCTTCCGATC*T |
PCR.8 | CAAGCAGAAGACGGCATACGAGATtCAAGTGTGACTGGAGTTCAGACGTGTGCTCTTCCGATC*T |
PCR.9 | CAAGCAGAAGACGGCATACGAGATCTGATCGTGACTGGAGTTCAGACGTGTGCTCTTCCGATC*T |
PCR.10 | CAAGCAGAAGACGGCATACGAGATAAGCTAGTGACTGGAGTTCAGACGTGTGCTCTTCCGATC*T |
Universal primer | AATGATACGGCGACCACCGAGATCTACACTCTTTCCCTACACGACGCTCTTCCGATC*T |
Universal adapter oligo 1 | ACACTCTTTCCCTACACGACGCTCTTCCGATC*T |
Universal adapter oligo 2 | 5’Phos-GATCGGAAGAGCACACGTCTGAACTCCAGTCAC |
- 96-well real-time PCR plates (e.g., Bio-Rad, HSP9601)
- Thermal cycler with non-heated lid (e.g., Bio-Rad C1000 Touch)
- Vortex mixer
- Magnetic rack for 96-well plates (e.g., Thermo Fisher, AM10027)
- Real-time PCR machine (e.g., Bio-Rad CFX96 Touch)
- PCR tubes (optional)
- Bioanalyzer (e.g., Agilent 2100 Bioanalyzer Instrument)
Repairing the ends of the DNA fragments
1.Place an aliquot of AMPure XP magnetic bead suspension at room temperature for ≥30 min.
2.For each sample, prepare the following mixture (50 µl total) in a 96-well real-time PCR plate:
15 µl | DNA sample (D-EL or diluted D-IN samples from Basic Protocol 2) |
22.5 µl | Nuclease-free water |
2 µl | 10 mM dNTPs |
5 µl | 10× T4 DNA ligase reaction buffer |
2.5 µl | 3 U/µl T4 DNA polymerase |
0.5 µl | 5 U/µl DNA polymerase I, large (Klenow) fragment |
2.5 µl | 10 U/µl T4 polynucleotide kinase |
3.Incubate mixture for 30 min at 20°C in a thermal cycler with a non-heated lid.
4.To isolate the end-repaired DNA fragments, mix the AMPure XP magnetic bead suspension from step 1 well by vortexing and then add 1.8 volumes of magnetic bead suspension to each well (90 µl bead suspension for 50 µl sample).
5.Mix immediately by pipetting up and down 10 times.
6.Incubate for 5 min at room temperature.
7.Pellet beads by placing the 96-well plate on a magnetic rack for 96-well plates for 8 min. Then, remove supernatant.
8.Add 200 µl freshly made 80% ethanol without disturbing pellet and wait 30 s.
9.Remove supernatant.
10.Repeat steps 8 and 9.
11.Dry pellet for 5 min at room temperature.
12.Remove plate from the magnetic rack and add 16.5 µl nuclease-free water.
13.Pipet up and down 10 times to resuspend pellet.
14.Incubate for 1 min at room temperature.
15.Pellet beads by placing the 96-well plate on a magnetic rack for 1 min and then transfer 16 µl supernatant to a well in a new 96-well plate and keep this new plate at room temperature.
A-tailing the DNA fragments
16.To each sample, add the following mixture, resulting in a total volume of 25 µl:
2.5 µl | NEB buffer 2 |
5 µl | 1 mM dATP |
1.5 µl | 5 U/µl Klenow fragment (3'→5' exo-) |
17.Incubate for 30 min at 37°C in a thermal cycler with a non-heated lid.
18.To isolate the A-tailed DNA fragments, mix the AMPure XP magnetic bead suspension from step 1 well by vortexing and then add 1.8 volumes of magnetic bead suspension (45 µl bead suspension for 25 µl sample).
19.Repeat steps 5 to 11.
20.Remove plate from the magnetic rack and add 9.5 µl nuclease-free water.
21.Pipet up and down 10 times to resuspend pellet.
22.Incubate for 1 min at room temperature.
23.Pellet beads by placing the 96-well plate on a magnetic rack for 1 min and then transfer 9 µl supernatant to a well of a new 96-well plate and keep this new plate at room temperature.
Ligating the adapters
24.To anneal universal adapter oligos (see HPLC-purified oligonucleotides in Table 1), prepare the mixture (100 µl total) below. Next, using a real-time PCR machine, heat this mixture to 95°C for 1 min, then decrease the temperature at a rate of 0.1°C/s for 800 s, and finally bring it to 14°C.
50 µl | TE (pH 8.0) containing 50 mM NaCl |
25 µl | 100 µM universal adapter oligo 1 (see Table 1) |
25 µl | 100 µM universal adapter oligo 2 (see Table 1) |
25.To each sample, add the following reagents, resulting in a total volume of 25 µl:
12.5 µl | 2× rapid ligation buffer |
1 µl | 0.25 µM universal adapter (annealed universal adapter oligos 1 and 2 from step 24) |
2.5 µl | 600 U/µl T4 DNA ligase (rapid) |
26.Incubate for 15 min at room temperature.
27.To isolate the ligation products, mix the AMPure XP magnetic bead suspension from step 1 well by vortexing and then add 1.6 volumes of magnetic bead suspension (40 µl bead suspension for 25 µl sample).
28.Repeat steps 5 to 11.
29.Remove plate from the magnetic rack and add 10.5 µl nuclease-free water.
30.Pipet up and down 10 times to resuspend pellet.
31.Incubate for 1 min at room temperature.
32.Pellet beads by placing the 96-well plate on a magnetic rack for 1 min and then transfer 10 µl supernatant to a well in a new 96-well plate or a new PCR tube. Keep new plate or tube at room temperature.
Amplification of the library
33.For each sample, prepare the following PCR mixture in a well of a real-time PCR plate (50 µl total):
25 µl | Phusion HF PCR master mix |
1 µl | 10 µM universal primer (see Table 1) |
1 µl | 10 µM barcoded primer (any out of PCR.1 to PCR.10; see Table 1) |
1 µl | SYBR Green nucleic acid stain (1:2000 dilution) |
6 µl | Adapter-ligated DNA (from step 32) |
16 µl | Nuclease-free water |
34.Place the plate in a real-time qPCR machine and run the following program:
Initial step: | 30 s | 98°C |
40 cycles: | 10 s | 98°C |
20 s | 64°C | |
45 s | 72°C. |
35.Monitor qPCR run closely as it is ongoing and immediately stop it at the end of the corresponding extension step (the last step of the qPCR program) when the exponential DNA amplification phase comes to an end.
36.To isolate the ligation products, mix the AMPure XP magnetic bead suspension from step 1 well by vortexing and then add 1.6 volumes of magnetic bead suspension (80 µl bead suspension for 50 µl sample).
37.Repeat steps 5 to 11.
38.Remove plate from the magnetic rack and add 10.5 µl nuclease-free water.
39.Pipet up and down 10 times to resuspend pellet.
40.Incubate for 1 min at room temperature.
41.Pellet beads by placing the PCR plate on a magnetic rack for 1 min and then transfer 10 µl supernatant to a well in a new 96-well plate or a new PCR tube. Keep the new plate or tubes at room temperature.
Quality control of the sequencing libraries (recommended)
42.Take 1 μl of each DNA library and run it on a Bioanalyzer using a high-sensitivity DNA kit for the Bioanalyzer according to the manufacturer's instructions.
43.Determine concentration of the libraries from the Bioanalyzer software following the manufacturer's instructions.
REAGENTS AND SOLUTIONS
B-ChIP-BL
Dissolve 0.5% (w/v) bovine serum albumin (BSA) in PBS. Prepare fresh immediately before use and keep on ice.
B-ChIP-EL
- 50 mM Tris·HCl, pH 8
- 10 mM EDTA, pH 8
- 1% (w/v) SDS
- Sterile MilliQ water
- Store ≤2 years at room temperature
Always keep this buffer at room temperature and not in the cold to prevent the precipitation of SDS.
B-ChIP-L0
Prepare as for B-ChIP-Lys (see recipe) but without Triton X-100. Store ≤2 years at 4°C. Add the protease inhibitors just prior to use.
B-ChIP-Lys
- 50 mM HEPES-KOH, pH 7.5
- 300 mM NaCl
- 1 mM EDTA, pH 8
- 1% (v/v) Triton X-100 (from aqueous stock solution)
- 0.1% (w/v) sodium deoxycholate (from aqueous stock solution)
- 0.5% (v/v) N-lauroylsarcosine (from aqueous stock solution)
- Sterile MilliQ water
- Store ≤2 years at 4°C
- Prior to use, add PMSF (from 100 mM stock solution; Sigma-Aldrich, 93482) to 1 mM and cOmplete (EDTA-free) Protease Inhibitor Cocktail (Sigma-Aldrich, 11873580001) to 1× and keep the resulting buffer on ice
B-ChIP-Lys containing 200 mM NaCl
Prepare as for B-ChIP-Lys (see recipe) but additionally add NaCl (from 5 M stock solution) to 200 mM. Store ≤2 years at 4°C. Add the protease inhibitors just prior to use.
B-ChIP-W
- 10 mM Tris·HCl, pH 8
- 250 mM LiCl
- 1 mM EDTA
- 0.5% (w/v) sodium deoxycholate (from aqueous stock solution)
- 0.5% (v/v) NP-40 (from aqueous stock solution; Merck, 492016)
- Sterile MilliQ water
- Store ≤2 years at 4°C
- Prior to use, add PMSF (from 100 mM stock solution; Sigma-Aldrich, 93482) to 1 mM and cOmplete (EDTA-free) Protease Inhibitor Cocktail (Sigma-Aldrich, 11873580001) to 1× and keep the resulting buffer on ice
Hypochlorite solution
- 1 volume of 10 M NaOH
- 4 volumes of 10% to 15% (w/v) sodium hypochlorite solution in water
- 5 volumes of sterile MilliQ water
- Prepare fresh immediately before use
M9 buffer
- 6 g KH2PO4
- 12 g Na2HPO4
- 10 g NaCl
- Sterile MilliQ water to 2 L
- Sterilize by autoclaving
- Store ≤3 years, with some at room temperature and some at 4°C
Nematode growth medium (NGM) plates
- 3 g NaCl
- 17 g agar
- 7.5 g Bacto Peptone
- Sterile MilliQ water to 975 ml
- Autoclave
- After cooling, add the following ingredients:
- 1 ml 5 mg/ml cholesterol in ethanol
- 1 ml 1 M CaCl2, sterile
- 1 ml 1 M MgSO4, sterile
- 25 ml potassium phosphate buffer, pH 6, sterile
- 1.25 ml 4400 U/ml nystatin suspension, sterile
- 2 ml 100 mg/ml streptomycin, sterile
- Pour into appropriately sized Petri dishes while maintaining sterility
- Store ≤3 months at 4°C
PBS supplemented with protease inhibitors
- 8 g NaCl
- 0.2 g KCl
- 1.44 g Na2HPO4
- 0.24 g KH2PO4
- Sterile MilliQ water to 1 L
- Adjust pH to 7.4 using NaOH or HCl
- Store ≤2 years at 4°C
- Prior to use, add PMSF (from 100 mM stock solution; Sigma-Aldrich, 93482) to 2 mM and cOmplete (EDTA-free) Protease Inhibitor Cocktail (Sigma-Aldrich, 11873580001) to 2× and keep the resulting buffer on ice
Phosphate-buffered saline (PBS)
- 8 g NaCl
- 0.2 g KCl
- 1.44 g Na2HPO4
- 0.24 g KH2PO4
- Sterile MilliQ water to 1 L
- Adjust pH to 7.4 with HCl
- Store ≤2 years, with some at room temperature and some at 4°C
Tris-EDTA (TE), pH 8.0
- 10 mM Tris·HCl, pH 8
- 1 mM EDTA, pH 8
- Sterile MilliQ water
- Store ≤2 years at room temperature
COMMENTARY
Background Information
The method of ChIP originated in the 1980s, most notably when Gilmour and Lis cross-linked DNA and its associated proteins, then immunoprecipitated DNA polymerases, and eventually determined regions bound by these polymerases by probe hybridization methods (Gilmour & Lis, 1984, 1985). Since then, the method has seen continuous improvements, first in the laboratory of Alexander Varshavsky (Solomon, Larsen, & Varshavsky, 1988) and later in many others (Orlando, 2000). A big step forward was taken during the early 2000s, when it became feasible to analyze the immunoprecipitated chromatin by genome-wide methods, initially by the hybridization to whole-genome DNA microarrays (a method called ChIP-chip; Buck & Lieb, 2004) and later by next-generation sequencing (ChIP-seq, the method described here; Johnson, Mortazavi, Myers, & Wold, 2007). Thanks to these developments, it has now become feasible and affordable for the average researcher to determine the genome-wide distribution of DNA-associated proteins of interest at good resolution (normally ∼50 bp).
As mentioned in the introduction, existing ChIP-seq methods still have a few limitations. One of these is addressed here, namely that basic ChIP protocols, which work well, for example, with cultured mammalian cells (e.g., Lee et al., 2006), still perform poorly in a subset of tissues or organisms, particularly if the intracellular material is protected by a rigid extracellular matrix or cell wall. For many such scenarios, solutions have been found and ChIP-seq protocols have been adjusted accordingly, with typical examples being today's protocols used for yeast (Lelandais, Blugeon, & Merhej, 2016) or plants (Cortijo, Charoensawan, Roudier, & Wigge, 2018). ChIP-seq has also been challenging in the popular model organism C. elegans , largely because C. elegans is surrounded by a cuticle that hinders its penetration by chemicals (Burns et al., 2010), including formaldehyde, the most commonly used cross-linker in ChIP experiments. The cuticle also impairs lysis and efficient DNA shearing by sonication (Bhaskaran et al., 2011; Kumar & Mukhopadhyay, 2018). In addition, ChIP experiments in C. elegans suffer from the animals’ rather low chromatin content per body weight, which can be up to 100-fold below that of mammalian cells.
Because of these challenges, few studies have actually conducted ChIP-seq experiments in C. elegans , and even though their authors have tried to adjust basic ChIP protocols to meet the challenges of this model organism (e.g., Mukhopadhyay et al., 2008; Zhong et al., 2010), we have found these adjustments not to be ideal, mostly because they lacked good enough strategies to permeate the animals for the cross-linker. Additionally, most prior ChIP studies in this animal have used quantitative PCR rather than sequencing as a method to analyze the precipitated DNA.
A typical ChIP-seq protocol can be divided into the following steps: cross-linking, cell lysis, shearing of the chromatin, immunoprecipitation, DNA cleanup, and library construction for sequencing. In order to obtain efficient and uniform cross-linking across tissues, we find it important to properly permeate the cuticle by partial breakage of the animals. Previous studies have already tried to achieve similar results by Dounce homogenization (Kumar & Mukhopadhyay, 2018; Mukhopadhyay et al., 2008), but we found Dounce homogenization to result in varying degrees of breakage depending on the developmental stage of the animals, demanding extensive optimization of the homogenization conditions for different experiments. Furthermore, the stage bias of this method would be unsuitable for mixed-stage C. elegans populations. Finally, partial breakage by Dounce homogenization occurs at 4°C, and it takes many minutes before animals are fully permeated, during which protein-DNA and also protein-protein interactions may get disturbed before they become fixed by cross-linking. In our experience, freeze-grinding (Basic Protocol 2) provides a better alternative. It ensures more uniform partial breakage of the animals and is less affected by developmental stage. In addition, it better preserves protein-DNA and protein-protein interactions because the partial breakage occurs at temperatures at or below –80°C. When we then thaw these samples to 4°C in the presence of formaldehyde, the animals are already permeated, and the chromatin should become exposed to the cross-linker within seconds.
Next, we focused on improving the lysis and sonication of these rigid animals by introducing a bead-beating homogenization step (Basic Protocol 2). Even though this step may strain the sample, e.g., by causing increased aeration, this risk should be negligible, because, at this stage of the protocol, the chromatin has already been cross-linked and relevant protein-DNA and protein-protein interactions are unlikely to be lost. On the plus side, this bead-beating step leads to more uniform lysis and therefore more uniform shearing of the chromatin during sonication. For sonication, most sonicators should be suitable, but we recommend using a Bioruptor (Diagenode), which can process multiple samples in parallel, leading to improved reproducibility of lysis and shearing throughout the experiment.
The subsequent immunoprecipitation steps in Basic Protocol 2 are similar to those of most other ChIP protocols. One noteworthy change is that for the eventual cleanup and concentration of the input and eluate DNA samples, we avoid the most commonly used phenol-chloroform extraction methods. Instead, we use ion-exchange chromatography in the form of a commercially available spin-column kit (see Basic Protocol 2). We find the use of this kit to improve the handling and reproducibility of the DNA cleanup and concentration.
The last important improvement in our protocol has been the choice of library construction protocol (Basic Protocol 3). As already mentioned, the intracellular protein and DNA content of C. elegans is quite low compared to its overall weight, ultimately leading to very small amounts of DNA being obtained after chromatin immunoprecipitation. For example, ChIP of a transcription factor may yield only 10 to 100 pg DNA. To ensure generation of high-quality and Illumina-compatible sequencing libraries from such small amounts of DNA, we adapted a protocol that was previously used in other systems in scenarios with similarly low amounts of input DNA (Bowman et al., 2013). Even though this protocol is not the only option and alternative commercial offerings and kits exist that can generate sequencing libraries from such low amounts of DNA, the chosen protocol excels due to its simplicity, its fairly low cost, and the relatively short time that it takes to complete.
Critical Parameters and Troubleshooting
The protein of interest
When considering ChIP-seq in C. elegans , one needs to be aware that although this method can produce very good and informative data for many proteins of interest, it is still unsuitable for some. An ideal and easy-to-work-with protein of interest is well expressed in most tissues, has a direct and tight interaction with DNA, and has a specific antibody available (ideally, already validated by other ChIP studies) that can be used for its precipitation. However, if your protein of interest is expressed at very low levels or expressed in only a few cells in the C. elegans body, you might have difficulties precipitating enough chromatin for generation of a sequencing library. To determine if this is the case for your protein of interest, you can, for example, look at published whole-animal proteomic or mRNA sequencing data and compare your protein's expression level to that of proteins for which the tissue distribution and abundance are known and that have been successfully studied by ChIP-seq before. Alternatively, you can estimate the expression level and tissue distribution of your protein of interest by imaging a GFP fusion of this protein. Similarly, if your protein of interest has a very weak interaction with DNA or binds to it indirectly (e.g., via other proteins that are in direct contact with the DNA), not enough DNA may be co-precipitated. Absence of a conserved DNA-binding domain in your protein of interest could indicate that you will face such problem. Another concern is proteins that interact with chromatin in a non-sequence-specific manner (e.g., histones). For such proteins, it needs to be kept in mind that the resulting ChIP-seq signal may look more diffuse than for proteins with sequence specificity, making it sometimes harder to distinguish such signal from background. Finally, if you only have an antibody with poor affinity or specificity for your protein of interest, you may suffer from low signal or from cross-reactivity of the antibody with other proteins and thus nonspecific background signal.
In summary, if you want to try ChIP-seq for the first time, we recommend that you choose a protein that others have done ChIP-seq with before. It should be a) a protein expressed in all tissues and at least at a level comparable to that of broadly expressed transcription factors; b) a protein that has a well-defined distribution on DNA, e.g., due to a sequence-specific DNA-binding motif; and c) a protein that has a good antibody available. Starting with such a protein will maximize your chances of a successful first ChIP-seq experience, allow you to compare your results to prior results, and enable you to set a benchmark of what a good ChIP-seq experiment should look like. If you are ultimately interested in more challenging proteins, you can try those next.
Growth, synchrony, and reproducibility of C. elegans cultures
By following Basic Protocol 1, users should achieve good growth, synchrony, and reproducibility of C. elegans cultures to ultimately harvest animal populations of appropriate size and developmental stage within a reasonable amount of time. Many parameters can influence the success of Basic Protocol 1. First, the C. elegans strain matters, e.g., plenty of mutant or transgenic lines grow slower, produce less offspring, or grow less synchronously than wild-type animals. Second, growth conditions matter. Always make sure that sufficient amounts of OP50-1 bacteria are supplied, that plates are not overpopulated, and that the animals do not burrow in the agar. Also consider the temperature that you use to grow your animals. In particular, temperatures <20°C will slow down growth and expansion of your animal population. Finally, you should be careful when conducting the egg preparation. Excessive bleaching of your animals may kill not only gravid adults but also their eggs and thereby stall your experiment. Similarly, make sure that you remove all bleach from the egg suspension during the washes. Bleach that trails through into the overnight hedging phase may kill the larvae or cause delays or asynchrony in their development.
Harvesting worms
All buffers used for harvesting and washing of the animals in Basic Protocol 1 should be ice-cold to slow physiological changes that might otherwise occur during the harvesting process. We only recommend differently when using temperature-sensitive strains. With these, you may want to use buffers that maintain the temperature that the animals were grown at up until step 32 of Basic Protocol 1. Finally, when freezing the worm suspension by dripping it in liquid nitrogen, make sure to drip it slowly enough that the worm beads do not merge into larger clumps; those would be difficult to break later.
Freeze-grinding
For successful freeze-grinding in Basic Protocol 2, it is very important to keep all instruments that come in contact with your sample very cold. The Bioruptor, mortar, pestle, and spatula should all be pre-chilled in liquid nitrogen and be re-chilled whenever possible during use. Otherwise, the sample may deteriorate due to partial thawing and the powder generated by the grinding will clump, which in turn impairs the grinding process.
Cross-linking
Optimal cross-linking conditions (Basic Protocol 2) will differ depending on your protein of interest. For example, for proteins that bind tightly to DNA, less cross-linking tends to be sufficient and leads to less noise in the data, whereas proteins that are only loosely or indirectly associated with DNA may require more cross-linking to capture their interaction. The intensity of cross-linking can be changed by altering the concentration of formaldehyde used or by altering the duration of the cross-linking step. We recommend starting with the standard conditions that we describe in Basic Protocol 2. Should you obtain unsatisfactory results, you can consider changing your cross-linking conditions as one of the ways to optimize your ChIP.
It also is important to know that the cross-linking efficiency can differ substantially between various brands and quality grades of formaldehyde. We recommend 16% (w/v) formaldehyde that is provided methanol free in single-use glass ampules (Thermo Fisher Scientific, 28908) to improve the reproducibility of your results.
Homogenization of the samples using glass beads
While vortexing the samples in Basic Protocol 2, make sure that the glass beads swirl in the tube and do not just sit at the bottom. Otherwise, homogenization will not occur.
Sonication
When following Basic Protocol 2, you must test and optimize the sonication conditions empirically, even if you use the same sonicator as recommended here. Every sonicator performs differently. If you use a Bioruptor, use the sonication conditions that we describe in our protocol as a starting point. Begin with these settings and then test a range of shorter, similar, and longer sonication times. If you use a different sonicator, obtain reference settings from other researchers who have successfully used this sonicator for ChIP experiments and once again test a range of sonication times. In general, sonication of C. elegans chromatin takes longer than, for example, the sonication of chromatin from human cultured cells. You want to shear your chromatin so that you maximize the yield of fragments in the range of 150 to 300 bp. If you shear less and have longer fragments, this will lower the resolution of your ChIP-seq. If you shear too much, you will have fewer fragments of suitable size and thus will lose signal. Figure 1A shows an example of a properly sonicated sample.
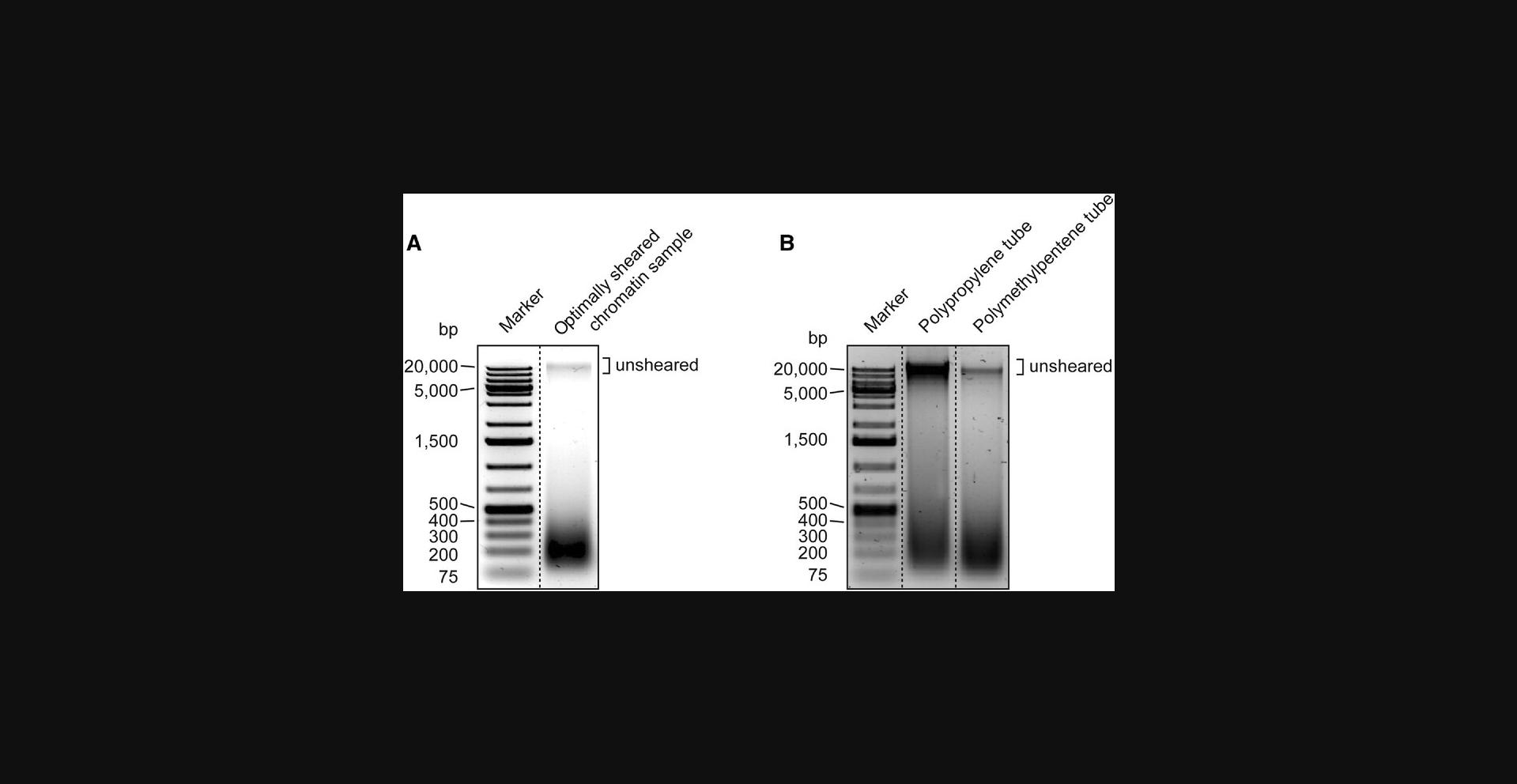
Further, when using a water bath–based sonicator like a Bioruptor, it is crucial to use the recommended tubes. Figure 1B shows how sonication efficiency can decline when choosing tubes of the wrong material, e.g., polypropylene.
Finally, make sure to keep your samples cold during sonication. Conduct sonication in a cold room, chill the water bath of your Bioruptor, or, if you use a conventional probe sonicator, make sure to sonicate on ice. Also make sure to include sufficiently long OFF phases in your sonication protocol to allow for cooling between ON phases. Your sonication tubes should never feel warmer than room temperature. Excessive heat during sonication may cause denaturation of your samples and the partial reversal of cross-links.
Antibody and immunoprecipitation
The choice of antibody for your ChIP experiment (Basic Protocol 2) is very important. Not every antibody that works well for western blotting or conventional protein immunoprecipitation experiments will yield efficient chromatin immunoprecipitation or show a good resolution and signal-to-noise ratio in the final ChIP-seq results. Here, it is helpful if you can use antibodies that others have already found to be suitable for ChIP. If such antibodies are not available for your protein of interest, we recommend evaluating and comparing up to three different antibodies empirically by conducting either full ChIP-seq experiments according to our protocol or by conducting ChIP-qPCR assays, evaluating regions that are thought to be bound by the immunoprecipitated protein. Alternatively, you can work with a strain that expresses an epitope-tagged version of your protein of interest. In this case, you only need to identify a good antibody for the tag once and then can use this tag to conduct ChIP of other proteins in the future. Figure 2 provides an example in which we compared two different anti-GFP antibodies for their suitability to immunoprecipitate a GFP-tagged transgene of the transcription factor DAF-16 in the context of a ChIP experiment. The readout used here is qPCR at two promoter regions known to be bound by DAF-16 compared to qPCR at an unbound control region.
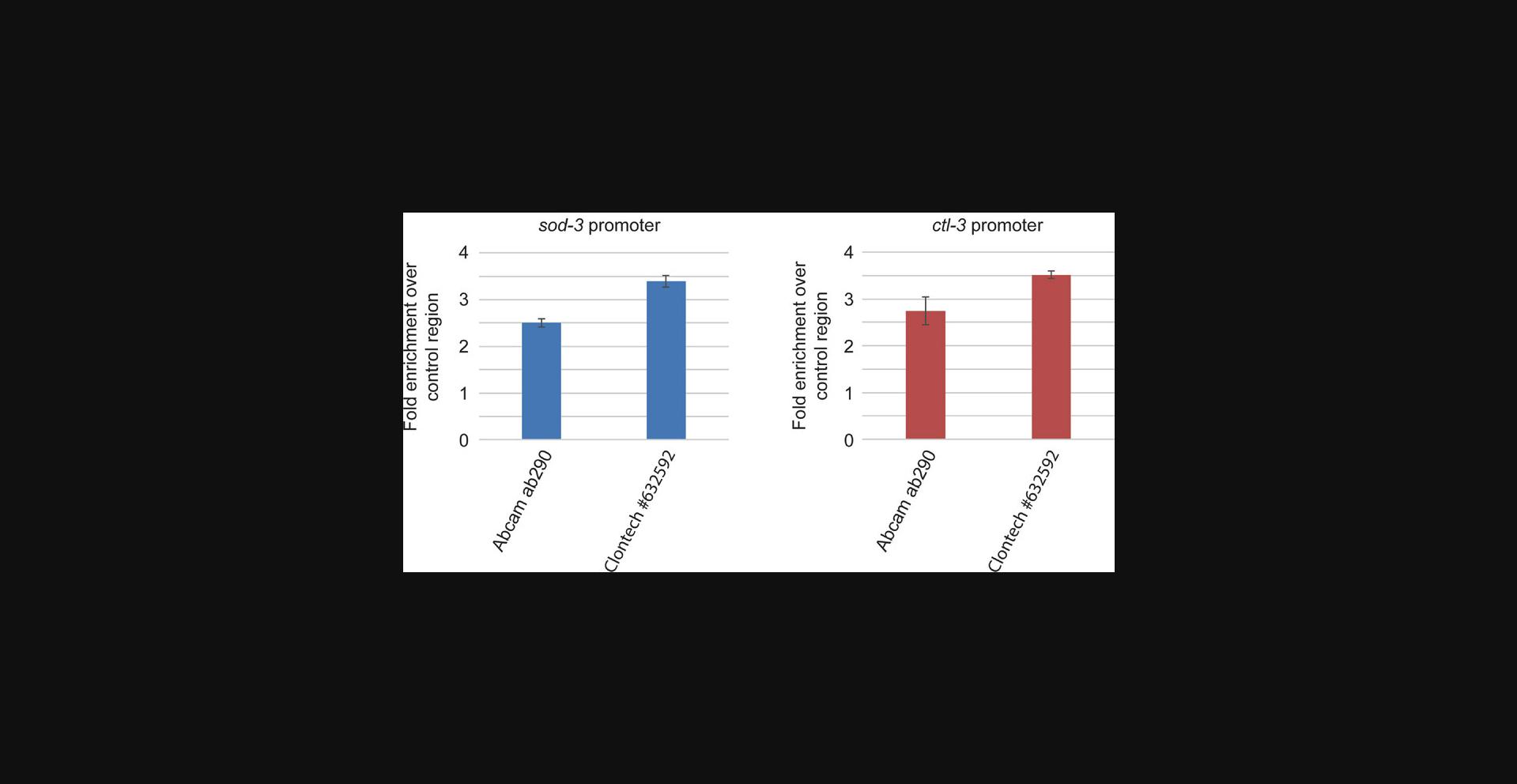
If, at the end of Basic Protocol 2, you realize that your protein of interest was not efficiently precipitated, try alternative antibodies, or you can also try to increase the amount of antibody and Dynabeads.
Working with magnetic beads
Magnetic beads (including Dynabeads and AMPure XP beads; Basic Protocols 2 and 3) may clump together, even if this might not be clearly visible by eye. Thus, make sure to always resuspend them well, e.g., by pipetting up and down 10 times, using an appropriate pipet than can hold the entire sample volume.
Construction of sequencing libraries
Be very meticulous and careful while going through Basic Protocol 3. Many of the pipetting steps handle very small volumes. Take your time and make sure that all pipetting steps go well. Consider creating master mixes wherever appropriate. Additionally, be careful about the use of multichannel pipets, as they may have faulty individual channels, among other issues.
The first step in which you can evaluate the success of the workflow is the real-time PCR step (step 34) in Basic Protocol 3, when you amplify your library material. As stated in the protocol, we usually reach the end of the exponential amplification phase after 12 to 15 cycles. You should expect all of your D-IN and D-EL samples to amplify similarly and reach the end of the exponential amplification phase within a few cycles of each other. Excessively high cycle numbers would suggest that too little DNA was present when starting library construction or that there was some sample loss during library construction. Excessively low cycle numbers can indicate that your antibody lacks specificity or that there was insufficient stringency during the immunoprecipitation in Basic Protocol 2.
Quality control of the final libraries
As already stated at the end of Basic Protocol 3, we recommend checking the quality of the constructed libraries with a Bioanalyzer. This device determines the size distribution and quantity of the DNA fragments that constitute your library. Users should aim for most fragments to be in the range of 200 to 500 bp. Larger fragments (>1.5 kb) should mostly be absent. If you have them, this suggests insufficient shearing of your DNA. Similarly, the library should mostly be devoid of adapter dimers. These can arise during Basic Protocol 3, especially if the input amount of DNA into the protocol is low. Figure 3 shows some examples of Bioanalyzer traces from a good library as well as libraries with issues that need to be addressed.
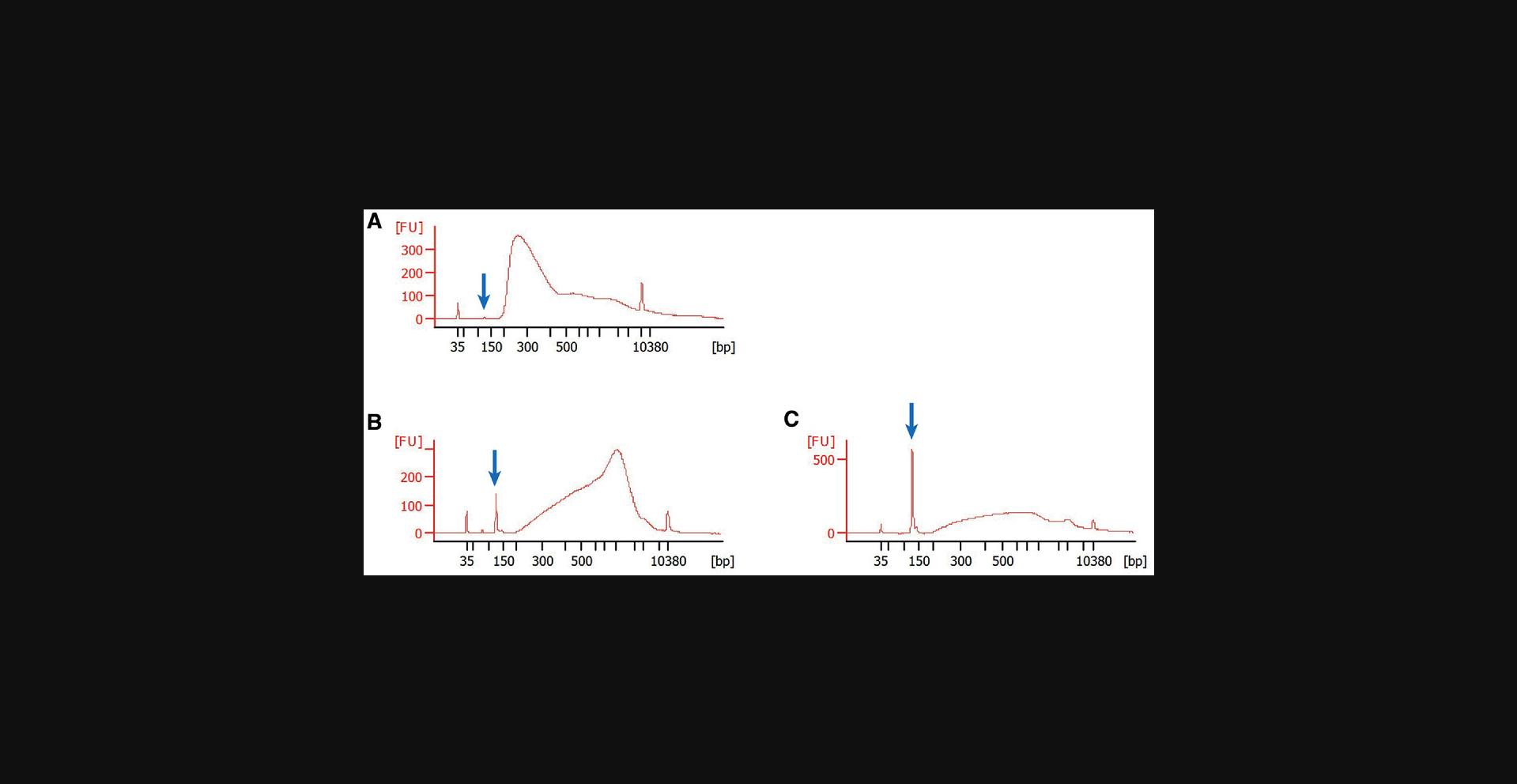
Quite often you will find that you have a good library with a decent concentration (10 to 50 nM) of fragments in the 200- to 500-bp range but that you also have contaminating DNA of smaller or larger size that you would like to remove. This can be done quite easily. A conventional strategy would be to run your library on an agarose gel and to then excise the size range of interest. However, we recommend size selection using AMPure XP beads. If you would like to remove smaller fragments, e.g., adapter dimers, you can take your library, bring it to 50 µl by adding nuclease-free water, and then repeat steps 36 to 41 of Basic Protocol 3, with the difference that you would not use 1.6× volumes of AMPure XP beads but a lower amount, e.g., 1.4× or 1.2×. You may have to determine the optimal amount empirically. If you want to remove larger fragments, you can again take your library, bring it to 50 µl by adding nuclease-free water, and now add an even lower amount of AMPure XP beads, e.g., 0.7× volumes. Next, you can follow steps 5 to 6 of Basic Protocol 3 and then pellet the beads by placing your sample plate on a magnetic rack for 8 min, transfer the supernatant to a new plate well, and finally re-concentrate it, as shown in steps 36 to 41 of Basic Protocol 3.
For all the gel-based or AMPure XP bead–based size selection methods described here, keep in mind that they will lead not only to size selection but also to overall sample loss. Thus, you can only size-select once or twice before the concentration of your library becomes insufficient for sequencing.
If you find that your D-IN library at the end of Basic Protocol 3 contains hardly any DNA, e.g., below a concentration of 5 nM, this suggests an experimental error while purifying the input DNA or while conducting Basic Protocol 3. In the case of a D-EL library, this could also suggest an experimental error, but more likely, it means that too little DNA was obtained from the ChIP. In this latter case, if your protein immunoprecipitated efficiently, this argues for your protein not being DNA associated or for the association not being strong enough. Increasing the duration of cross-linking or increasing the formaldehyde concentration may help you to capture weaker interactions.
Understanding Results
We have described here three basic protocols that should enable researchers to conduct successful and reproducible ChIP-seq experiments in C. elegans. Our protocols describe the growth and harvest of C. elegans samples (Basic Protocol 1), the actual chromatin immunoprecipitation (Basic Protocol 2), and the construction of DNA libraries from input and immunoprecipitated materials that can be sequenced on an Illumina sequencer (Basic Protocol 3). Throughout the previous sections, we have already discussed the expected outcomes from these protocols, we have highlighted the steps that need special attention, and we have tried our best to give troubleshooting advice for any problems that may arise.
In this section, we briefly illustrate what information can be obtained when taking the constructed libraries further, through sequencing and the bioinformatic analysis of the resulting data. For this, we turn to previous work from our lab, in which we have studied transcriptional events that promote stress resistance and longevity under conditions of reduced insulin/IGF-like signaling (IIS) (Riedel et al., 2013; Sen et al., 2020). Specifically, we describe here ChIP-seq results for a transcription factor called HLH-30 that is required for this stress resistance and longevity to occur and ChIP-seq results for the transcription machinery that is ultimately influenced by this transcription factor. To introduce reduced IIS conditions, we have conducted this work at non-permissive temperature in animals carrying a temperature-sensitive mutation of the insulin/IGF receptor gene [the mutant allele is called daf-2(e1370)]. For ChIP-seq of HLH-30, we further introduced a transgene expressing a GFP fusion of HLH-30 under the control of the hlh-30 promoter and used an anti-GFP antibody (Takara, 632592) to immunoprecipitate it. ChIP-seq of the transcription machinery was conducted using an antibody against the large subunit of RNA Pol II, AMA-1 (BioLegend, 920102).
Animals were grown to scale, shifted to non-permissive temperature to reduce IIS, harvested, and subjected to ChIP-seq according to the basic protocols described above. We recommend conducting the ChIP-seq in duplicate or triplicate to obtain dependable results. The DNA fragments in the resulting libraries were sequenced for 50 bp from one end using an Illumina HiSeq 3000. Note that the exact sequencer should not impinge on the results. Additionally, read length should not matter, as long as it is longer than ∼30 bp. Single-end sequencing is sufficient, but paired-end sequencing can sometimes facilitate read mapping or improve resolution of the ChIP-seq results. We analyzed the obtained sequencing data as described before (Lin et al., 2018; Sen et al., 2020). In brief, the quality of the sequencing reads was verified using FastQC (Simon, 2021). Then, the reads were mapped to the C. elegans genome using Bowtie 2 (Langmead & Salzberg, 2012). Such result can be visualized, e.g., by loading the mapped reads as a BigWig file into a genome browser like IGV (Robinson et al., 2011). For transcription factors like HLH-30 or for RNA Pol II, one expects them to localize to specific sites or regions, i.e., promoter regions (HLH-30 and RNA Pol II) or gene bodies (RNA Pol II). To determine these sites or regions (often also referred to as “peaks”), we used MACS 2 (Zhang et al., 2008). It identifies genomic regions that are overrepresented in the D-EL samples when compared to D-IN and thus have specifically co-precipitated with your ChIP bait protein. In our data, a total of 4932 such peaks were found for HLH-30. Many of these peaks were located in promoter regions, suggesting that HLH-30 binds to these promoters to regulate the expression of immediately downstream genes. In previous work, we have further explored this by performing mRNA-seq under the same conditions (Lin et al., 2018). This indeed identified many genes whose promoters are directly bound by HLH-30 and whose expression is affected by the presence of HLH-30. They are important to promote stress resistance and longevity, which seems to explain the importance of HLH-30 for conferring these phenotypes. For RNA Pol II, MACS 2 identified 17033 peaks, most of which were located in promoters, gene bodies, and 3’-UTR regions.
Figure 4 shows three examples of genes bound by HLH-30 and RNA Pol II. Peaks, as determined by MACS 2, are indicated in green. As expected for a transcription factor, HLH-30 localizes to the promoter region of all three genes, just upstream of the transcriptional start site. In contrast, RNA Pol II is found across the promoter (where it is initially recruited), the gene body (where it transcribes the gene), and the 3’-UTR region (where transcription terminates). The amount and distribution of RNA Pol II, though, differ in these examples: atg-18 represents a gene that is bound by a comparably large amount of RNA Pol II throughout the locus, which would be consistent with it being strongly transcribed; daf-15 is bound by a comparably small amount of RNA Pol II, which would be consistent with less transcription; and hsp-3 resembles a special case where the gene is transcribed but also poised for even further induction because some of the RNA Pol II is pausing just downstream of the transcriptional start site after transcriptional initiation, presumably awaiting additional signals that promote transcriptional elongation (Adelman & Lis, 2012).
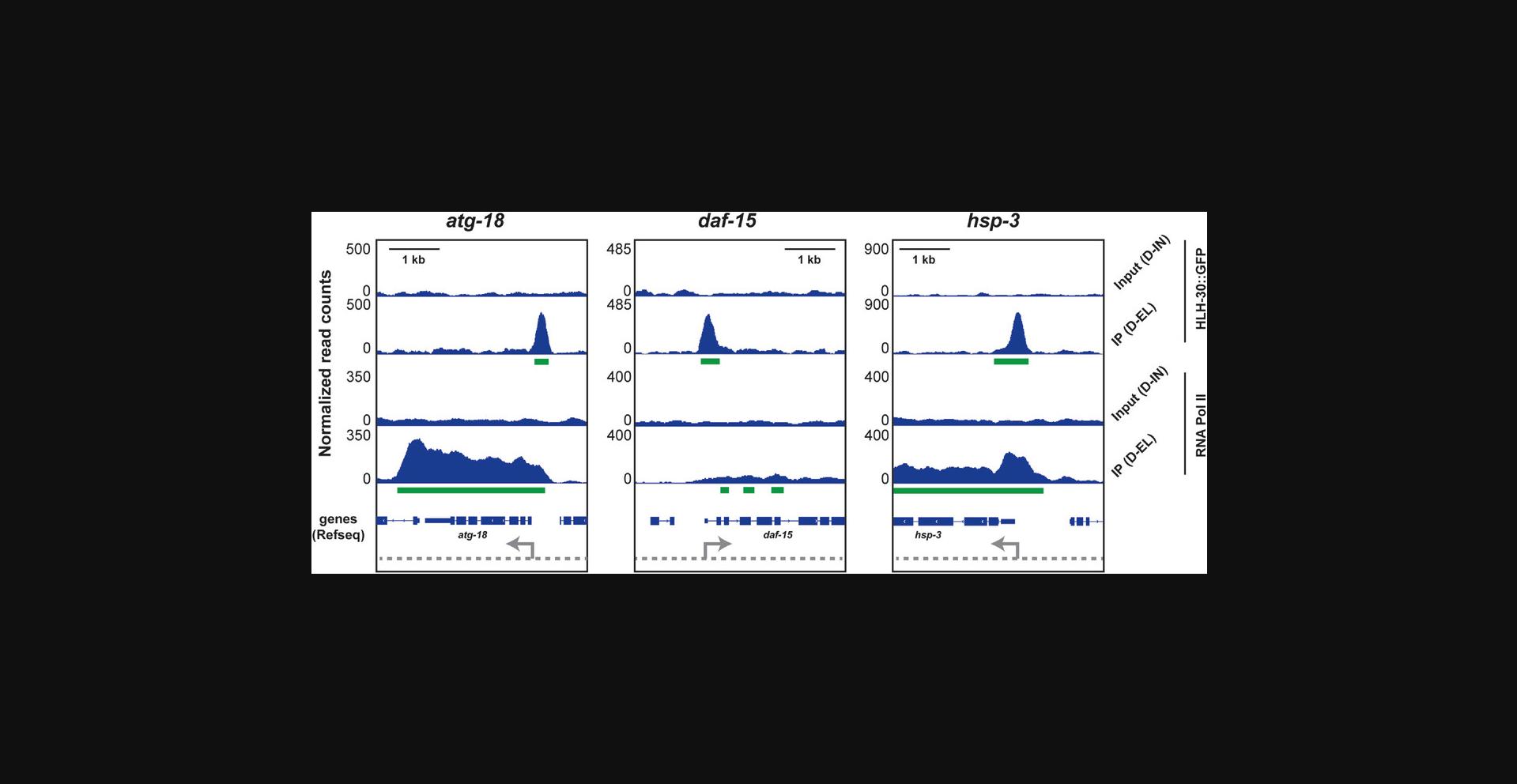
Time Considerations
Because we describe three consecutive protocols with various optional stopping points, it is difficult to estimate their overall duration. The time that it takes to grow C. elegans (Basic Protocol 1) depends on various parameters, i.e., the strain used (some mutant strains grow slower than the wild-type strain), environmental conditions (i.e., temperature), and the developmental stage that the animals should grow to before harvest. To provide a rough estimate, it may take 2 to 3 weeks to complete Basic Protocol 1, starting from a single crowded 6-cm NGM plate in step 3. Additional time is required if C. elegans strains first need to be thawed and grown to this scale. The ChIP procedure (Basic Protocol 2) can be performed within 2 or 3 days, depending on the number of samples. Library preparation (Basic Protocol 3) takes 1 day, but additional time may be required to confirm the quality of the resulting libraries and their optimization (i.e., size selection) for sequencing.
Acknowledgments
We would like to thank Sevinc Ercan (New York University, New York, USA) for advice on C. elegans cross-linking and lysis. We would like to thank Sarah Bowman and Robert Kingston (Massachusetts General Hospital, Boston, USA) for help with optimizing the library construction protocol (Basic Protocol 3). Development of the protocol and analysis of the presented data were supported by the Swedish Research Council (VR) grants 2015-03740, 2017-06088, and 2019-04868; the Swedish Cancer Society (Cancerfonden) grant 20 1034 Pj; the COST grant BM1408 (GENiE); and an ICMC project grant to C.G.R.
Author Contributions
Ilke Sen : Formal analysis, Methodology, Writing-review and editing. Alan Kavšek : Methodology, Writing-original draft, Writing-review and editing. Christian G. Riedel : Conceptualization, Data curation, Formal analysis, Funding acquisition, Methodology, Resources, Supervision, Visualization, Writing-review and editing.
Conflict of Interest
The authors declare no conflict of interest.
Open Research
Data Availability Statement
Data sharing is not applicable to this article as no new datasets were generated for the current study.
Literature Cited
- Adelman, K., & Lis, J. T. (2012). Promoter-proximal pausing of RNA polymerase II: Emerging roles in metazoans. Nature Reviews Genetics , 13, 720. doi: 10.1038/nrg3293.
- Andrássy, I. (1995). Free-living nematodes of Hungary (Nematoda errantia), Vol. 1. Budapest, Hungary: Hungarian Natural History Museum.
- Bhaskaran, S., Butler, J. A., Becerra, S., Fassio, V., Girotti, M., & Rea, S. L. (2011). Breaking Caenorhabditis elegans the easy way using the Balch homogenizer: An old tool for a new application. Analytical Biochemistry , 413, 123–132. doi: 10.1016/j.ab.2011.02.029.
- Bowman, S. K., Simon, M. D., Deaton, A. M., Tolstorukov, M., Borowsky, M. L., & Kingston, R. E. (2013). Multiplexed Illumina sequencing libraries from picogram quantities of DNA. BMC Genomics , 14, 1–7. doi: 10.1186/1471-2164-14-466.
- Buck, M. J., & Lieb, J. D. (2004). ChIP-chip: Considerations for the design, analysis, and application of genome-wide chromatin immunoprecipitation experiments. Genomics , 83, 349–360. doi: 10.1016/j.ygeno.2003.11.004.
- Burns, A. R., Wallace, I. M., Wildenhain, J., Tyers, M., Giaever, G., Bader, G. D., … Roy, P. J. (2010). A predictive model for drug bioaccumulation and bioactivity in Caenorhabditis elegans. Nature Chemical Biology , 6, 549–557. doi: 10.1038/nchembio.380.
- Corsi, A. K. (2006). A biochemist's guide to Caenorhabditis elegans. Analytical Biochemistry , 359, 1–17. doi: 10.1016/j.ab.2006.07.033.
- Corsi, A. K., Wightman, B., & Chalfie, M. (2015). A transparent window into biology: A primer on Caenorhabditis elegans. Genetics , 200, 387–407. doi: 10.1534/genetics.115.176099.
- Cortijo, S., Charoensawan, V., Roudier, F., & Wigge, P. A. (2018). Root development, methods and protocols. Methods in Molecular Biology , 1761, 231–248. doi: 10.1007/978-1-4939-7747-5_18.
- Fischer, S. E. J. (2010). Small RNA-mediated gene silencing pathways in C. elegans. The International Journal of Biochemistry and Cell Biology , 42, 1306–1315. doi: 10.1016/j.biocel.2010.03.006.
- Gallagher, S. R. (2012). One-dimensional SDS gel electrophoresis of proteins. Current Protocols in Molecular Biology , 97, 10.2A.1–10.2A.44. doi: 10.1002/0471142727.mb1002as97.
- Gallagher, S., Winston, S. E., Fuller, S. A., & Hurrell, J. G. R. (2011). Immunoblotting and immunodetection. Current Protocols in Molecular Biology , 52, 10.8.1–10.8.28. doi: 10.1002/0471143030.cb0602s52.
- Gilmour, D. S., & Lis, J. T. (1984). Detecting protein-DNA interactions in vivo: Distribution of RNA polymerase on specific bacterial genes. Proceedings of the National Academy of Sciences , 81, 4275–4279. doi: 10.1073/pnas.81.14.4275.
- Gilmour, D. S., & Lis, J. T. (1985). In vivo interactions of RNA polymerase II with genes of Drosophila melanogaster. Molecular and Cellular Biology , 5, 2009–2018. doi: 10.1128/MCB.5.8.2009.
- Greenwald, I. (2016). WormBook: WormBiology for the 21st Century. Genetics , 202, 883–884. doi: 10.1534/genetics.116.187575.
- Johnson, D. S., Mortazavi, A., Myers, R. M., & Wold, B. (2007). Genome-wide mapping of in vivo protein-DNA interactions. Science , 316, 1497–1502. doi: 10.1126/science.1141319.
- Kenyon, C. (2005). The plasticity of aging: Insights from long-lived mutants. Cell , 120, 449–460. doi: 10.1016/j.cell.2005.02.002.
- Kumar, N., & Mukhopadhyay, A. (2018). FOXO transcription factors, methods and protocols. Methods in Molecular Biology , 1890, 115–130. doi: 10.1007/978-1-4939-8900-3_10.
- Langmead, B., & Salzberg, S. L. (2012). Fast gapped-read alignment with Bowtie 2. Nature Methods , 9, 357–359. doi: 10.1038/nmeth.1923.
- Lee, T. I., Johnstone, S. E., & Young, R. A. (2006). Chromatin immunoprecipitation and microarray-based analysis of protein location. Nature Protocols , 1, 729–748. doi: 10.1038/nprot.2006.98.
- Lelandais, G., Blugeon, C., & Merhej, J. (2016). Yeast functional genomics, methods and protocols. Methods in Molecular Biology , 1361, 185–202. doi: 10.1007/978-1-4939-3079-1_11.
- Lin, X.-X., Sen, I., Janssens, G. E., Zhou, X., Fonslow, B. R., Edgar, D., … Riedel, C. G. (2018). DAF-16/FOXO and HLH-30/TFEB function as combinatorial transcription factors to promote stress resistance and longevity. Nature Communications , 9, 4400. doi: 10.1038/s41467-018-06624-0.
- Lloyd, S. M., & Bao, X. (2019). Pinpointing the genomic localizations of chromatin-associated proteins: The yesterday, today, and tomorrow of ChIP-seq. Current Protocols in Cell Biology , 84, e89. doi: 10.1002/cpcb.89.
- Meneely, P. M., Dahlberg, C. L., & Rose, J. K. (2019). Working with worms: Caenorhabditis elegans as a model organism. Current Protocols Essential Laboratory Techniques , 19, e35. doi: 10.1002/cpet.35.
- Mukhopadhyay, A., Deplancke, B., Walhout, A. J. M., & Tissenbaum, H. A. (2008). Chromatin immunoprecipitation (ChIP) coupled to detection by quantitative real-time PCR to study transcription factor binding to DNA in Caenorhabditis elegans. Nature Protocols , 3, 698–709. doi: 10.1038/nprot.2008.38.
- Mullaney, B. C., & Ashrafi, K. (2009). C. elegans fat storage and metabolic regulation. Biochimica et Biophysica Acta (BBA) - Molecular and Cell Biology of Lipids , 1791, 474–478. doi: 10.1016/j.bbalip.2008.12.013.
- Orlando, V. (2000). Mapping chromosomal proteins in vivo by formaldehyde-crosslinked-chromatin immunoprecipitation. Trends in Biochemical Sciences , 25, 99–104. doi: 10.1016/S0968-0004(99)01535-2.
- Park, K., Jang, J., Irimia, D., Sturgis, J., Lee, J., Robinson, J. P., … Bashir, R. (2008). ‘Living cantilever arrays’ for characterization of mass of single live cells in fluids. Lab on a Chip , 8, 1034–1041. doi: 10.1039/b803601b.
- Raha, D., Hong, M., & Snyder, M. (2010). ChIP-Seq: A method for global identification of regulatory elements in the genome. Current Protocols in Molecular Biology , 91, 21.19.1–21.19.14. doi: 10.1002/0471142727.mb2119s91.
- Riedel, C. G., Dowen, R. H., Lourenco, G. F., Kirienko, N. V., Heimbucher, T., West, J. A., … Ruvkun, G. (2013). DAF-16 employs the chromatin remodeller SWI/SNF to promote stress resistance and longevity. Nature Cell Biology , 15, 491–501. doi: 10.1038/ncb2720.
- Robinson, J. T., Thorvaldsdóttir, H., Winckler, W., Guttman, M., Lander, E. S., Getz, G., & Mesirov, J. P. (2011). Integrative genomics viewer. Nature Biotechnology , 29, 24–26. doi: 10.1038/nbt.1754.
- Saier, M. H. Jr. (2008). The bacterial chromosome. Critical Reviews in Biochemistry and Molecular Biology , 43, 89–134. doi: 10.1080/10409230801921262.
- Sen, I., Zhou, X., Chernobrovkin, A., Puerta-Cavanzo, N., Kanno, T., Salignon, J., … Riedel, C. G. (2020). DAF-16/FOXO requires Protein Phosphatase 4 to initiate transcription of stress resistance and longevity promoting genes. Nature Communications , 11, 138. doi: 10.1038/s41467-019-13931-7.
- Simon (2021). FastQC: A Quality Control Tool for High Throughput Sequence Data. Retrieved from https://www.bioinformatics.babraham.ac.uk/projects/fastqc/.
- Solomon, M. J., Larsen, P. L., & Varshavsky, A. (1988). Mapping proteinDNA interactions in vivo with formaldehyde: Evidence that histone H4 is retained on a highly transcribed gene. Cell , 53, 937–947. doi: 10.1016/S0092-8674(88)90469-2.
- Sulston, J. E., & Horvitz, H. R. (1977). Post-embryonic cell lineages of the nematode, Caenorhabditis elegans. Developmental Biology , 56, 110–156. doi: 10.1016/0012-1606(77)90158-0.
- Voytas, D. (2000). Agarose gel electrophoresis. Current Protocols in Molecular Biology , 51, 2.5A.1–2.5A.9. doi: 10.1002/0471142727.mb0205as51.
- Wenzel, D., Palladino, F., & Jedrusik-Bode, M. (2011). Epigenetics in C. elegans: Facts and challenges. Genesis , 49, 647–661. doi: 10.1002/dvg.20762.
- White, J. G. (2013). Getting into the mind of a worm—a personal view. WormBook , 1–10. doi: 10.1895/wormbook.1.158.1.
- Zhang, Y., Liu, T., Meyer, C. A., Eeckhoute, J., Johnson, D. S., Bernstein, B. E., … Liu, X. S. (2008). Model-based analysis of ChIP-Seq (MACS). Genome Biology , 9, R137. doi: 10.1186/gb-2008-9-9-r137.
- Zhong, M., Niu, W., Lu, Z. J., Sarov, M., Murray, J. I., Janette, J., … Snyder, M. (2010). Genome-wide identification of binding sites defines distinct functions for Caenorhabditis elegans PHA-4/FOXA in development and environmental response. PLOS Genetics , 6, e1000848. doi: 10.1371/journal.pgen.1000848.
Citing Literature
Number of times cited according to CrossRef: 2
- Duo Chen, Huan Li, Jing Chen, Yuying Han, Xuehai Zheng, Yixin Xiao, Xupeng Chen, Tao Chen, Jiannan Chen, Youqiang Chen, Ting Xue, Combined analysis of chromatin accessibility and gene expression profiles provide insight into Fucoxanthin biosynthesis in Isochrysis galbana under green light, Frontiers in Microbiology, 10.3389/fmicb.2023.1101681, 14 , (2023).
- Felicity J. Emerson, Siu Sylvia Lee, CUT&RUN for Chromatin Profiling in Caenorhabditis elegans, Current Protocols, 10.1002/cpz1.445, 2 , 6, (2022).