Animal Models for the Study of Food Allergies
Sahar Kazemi, Sahar Kazemi, Ece Danisman, Ece Danisman, Michelle M. Epstein, Michelle M. Epstein
allergen elicitation
allergen sensitization
food allergy
genetically modified
humanized mouse models
IgE
mouse models
wild-type
Abstract
In the last decades there has been a parallel increase in the incidence of food allergies and the development of experimental mouse models. These models have improved our understanding of the disease but do have limitations. For instance, they do not entirely reproduce human pathophysiology; moreover, validated and predictive models are absent. Nevertheless, the models provide opportunities to further understand fundamental disease mechanisms. The selection of any of the many experimental models depends on the research aims. This overview focuses on IgE-mediated food allergy in wild-type, genetically modified, and humanized mouse models and presents a comprehensive overview of the currently used protocols, challenges, and limitations, as well as provides guidelines for model selection based on the three critical areas of research: 1) safety assessment, 2) evaluating treatment, and 3) elucidating pathophysiology. © 2023 The Authors. Current Protocols published by Wiley Periodicals LLC.
INTRODUCTION
List of Abbreviations
AHR | airway hyperresponsiveness |
BLG | β-lactoglobulin |
CT | cholera toxin |
DTH | delayed-type hypersensitivity |
FcR | Fc receptors |
GI | gastrointestinal |
GMOs | genetically modified organisms |
HSCs | hematopoietic stem cells |
i.d. | intradermal, route |
i.g. | intragastric, route |
i.n. | intranasal, route |
i.p. | intraperitoneal, route |
i.v. | intravenous |
ITH | immediate-type hypersensitivity |
KO | knockout |
LPS | lipopolysaccharide |
NOD | non-obese diabetic |
OVA | ovalbumin |
PBMCs | peripheral blood mononuclear cells |
p.o. | oral route |
s.c. | subcutaneous, route |
SCID | severe combined immunodeficient |
SEB | staphylococcal enterotoxin B |
SPF | specific pathogen-free |
tg | transgenic |
TNP | trinitrophenyl |
WASP | Wiskott-Aldrich syndrome protein |
FOOD ALLERGIES
Allergies to food proteins are abnormal immunologic responses following the ingestion of food that leads to adverse reactions in susceptible individuals (Yu, Freeland, & Nadeau, 2016b). It is predominantly a disease of industrialized regions, with over 220 million people worldwide suffering from food allergies (Berin & Sampson, 2013; Dunlop & Keet, 2018; Jones & Burks, 2017; Sicherer, 2011; Sicherer & Sampson, 2018). In the last 20 years, the prevalence increased in the USA by about 18% (Branum & Lukacs, 2009; Gupta et al., 2012). The current incidence is approximately 6 to 8% of young children and 3 to 4% of adults, and the estimated prevalence is 4 to 8% for children and 10% for adults (Branum & Lukacs, 2009; Gupta et al., 2019).
The main food allergens are peanuts, tree nuts, milk, egg, soy, wheat, fish, shellfish, and sesame (Smeekens & Kulis, 2021). They cause a range of mild to life-threatening symptoms, including GI symptoms (e.g., tingling and itching of the mouth and throat, nausea, abdominal pain, vomiting, and diarrhea), hives (urticaria), swelling (angioedema) and redness (erythema), difficulty breathing, bronchospasm, and anaphylaxis. Because trace amounts of allergens elicit an allergic response, patients must avoid accidental exposure, which in turn reduces their quality of life (Mali & Jambure, 2012; Yu, Freeland, & Nadeau, 2016a).
Generally, food proteins are either ignored by the immune system or induce immune tolerance, which prevents allergies in most people. However, in susceptible individuals, food allergens can induce IgE-mediated, mixed IgE- and non-IgE-mediated, non-IgE-mediated, or cell-mediated immune responses (Spergel, 2006; Waserman & Watson, 2011; Waserman, Begin, & Watson, 2018). Th2 cells, B cells, allergen-specific IgE, basophils, and mast cells mediate the response to most food allergens (Lee, 2016). De novo sensitization to food allergens is thought to occur in the GI tract, airways, and/or skin, but the exact conditions remain unclear (Satitsuksanoa, Daanje, Akdis, Boyd, & van de Veen, 2021). Mechanistically, antigen-presenting cells and lymphocytes, upon allergen encounter, generate allergen-specific IgE antibodies, which crosslinks the FcR on mast cells and basophils, leading to degranulation and the release of histamine, leukotrienes, prostaglandins, and other immune mediators (Anvari, Miller, Yeh, & Davis, 2019; Michelet, Balbino, Guilleminault, & Reber, 2021; Vickery, Chin, & Burks, 2011). Non-IgE-mediated/T cell-mediated food allergies are less common than IgE-mediated disease (Molkhou, 2016; Zhang, Sicherer, Berin, & Agyemang, 2021). The underlying mechanism for non-IgE-mediated allergy involves the generation of food protein-specific T cells that induce the release of inflammatory mediators, which cause GI tract and skin inflammation, e.g., dietary-protein-induced enterocolitis, celiac disease, and dermatitis herpetiformis.
The main experimental avenues for food allergy research include in silico , in vitro , and in vivo models aimed at the further understanding of the nature of allergens (Hayes, Rougé, Barre, Herouet-Guicheney, & Roggen, 2015), food protein permeability, stability, transport through the GI tract (Cubells-Baeza et al., 2015), barrier disruption of the epithelium (Gavrovic-Jankulovic, 2015), digestion (Dupont, 2015), sensitization, adaptive and innate immunity (Hussain, Epstein, & Noti, 2015; Smit, Noti, & O'Mahony, 2015), IgE and non-IgE mechanisms (Lozano-Ojalvo, Lezmi, Cortes-Perez, & Adel-Patient, 2015), the influence of the microbiome and diet (Barcik, Untersmayr, Pali-Schöll, O'Mahony, & Frei, 2015), and the potential allergenicity of novel foods including GMOs (Marsteller, Bøgh, Goodman, & Epstein, 2015).
The dawn of animal models for the study of food allergy was in 1911 when Wells and Osbourne followed up on the original finding by Besredka that feeding guinea pigs protein induced oral tolerance (Besredka, 1909; Wells, 1911; Wells & Osborne, 1911). Several decades later, oral tolerance was induced to bovine serum albumin (Thomas & Parrott, 1974) and red sheep blood cells (André, Heremans, Vaerman, & Cambiaso, 1975) in rats and mice, respectively. By 1977, an OVA model was developed in mice which showed feeding OVA caused oral tolerance (Vaz, Maia, Hanson, & Lynch, 1977). Soon after, Elson and Ealding found that CT could break oral tolerance in mice (Elson & Ealding, 1984), thus, establishing the basis for food allergy models.
In the last three decades, models of oral tolerance shifted towards modeling food allergy to elucidate the roles of the route of sensitization, consumption dose, e.g., timing and duration, adjuvants, maternal-fetal allergen transfer, cross-reactivity, e.g., pollen–food allergy syndrome (Chong, Ruiz-Garcia, Patel, Boyle, & Turner, 2020; Wang, Hu, Liu, Liu, & Li, 2020), co-morbidity, e.g., atopic dermatitis (Lack, 2012; Ohsaki et al., 2018; Sbihi et al., 2019) and inhalant allergies, the influence of the intestinal microbiome, and for evaluating novel therapeutics (Kanagaratham, Sallis, & Fiebiger, 2018; Smeekens & Kulis, 2021).
Mice are the preferred choice for investigators because of their closely related immune and allergic responses to humans. Rats, like the Brown Norway strain, are also favored for food allergy because of high IgE responses (Ballegaard, Madsen, & Bøgh, 2019) and ease of serial serum antibody monitoring (Pilegaard & Madsen, 2004). Compared to rats, mice have shorter breeding times, lower maintenance costs, larger numbers of wild-type, KO, tg strains, and immunological reagents, as well as requiring a lower amount of test materials (Bøgh et al., 2016). However, mouse models do have their limitations: 1) they do not entirely reproduce human pathology, 2) there are no validated and predictive models, 3) the models that require added adjuvants might not be useful for investigating sensitization or oral tolerance, because its role in human disease is unknown, 4) mice rarely develop anaphylaxis with low amounts of oral food protein, in contrast to patients (Arumugam et al., 2011; Burton et al., 2017; Capobianco et al., 2008; Lexmond et al., 2017; Li et al., 2000; Smeekens et al., 2020; Tordesillas et al., 2014), especially because ingested antigens must be systemically absorbed to induce anaphylaxis in mice (Strait et al., 2011), 5) except for polysensitized WASP-deficient mice, they do not develop spontaneous disease, and 6) both anaphylactic IgG1 and IgE mediate anaphylaxis in mice, but not in humans (Smeekens & Kulis, 2021). There are many protocols for inducing disease in mice, each with inherent strengths and weaknesses.
This comprehensive overview focuses on IgE-mediated food allergies in wild-type, GM, and humanized mouse models from 2000 to 2022 and includes a brief overview of rat models. We present protocols that are currently in use, the challenges and limitations of the models, and guidelines for model selection based on three critical areas of research: 1) safety assessment, 2) evaluating treatment, and 3) elucidating pathophysiology.
MOUSE MODELS
Although food allergy in mice is similar to human food allergy, it is not identical (Kanagaratham et al., 2018; Smeekens & Kulis, 2021). Nevertheless, in vivo food allergy models are a facsimile of disease and have improved our understanding of disease (Oyoshi, Oettgen, Chatila, Geha, & Bryce, 2014). Fifty-nine representative food allergy models, including 32 wild-type, 20 GM, and 7 humanized mice models, are listed in Tables 1, 4, and 5.
Allergen | * | Sensitization | Elicitation | Adjuvant | Strain | Reference |
---|---|---|---|---|---|---|
α−amylase inhibitor GM pea and bean, SSA-lupin, pinto bean seed meal suspensions; α−amylase inhibitor GM pea and Lupin SSA purified proteins | 2 | i.g. - seed meal suspension (GM- peas, beans, non-GM- beans, lupin, pinto beans) | s.c. - pea α−amylase inhibitor and Lupin SSA purified proteins | None | BALB/c | (Prescott et al., 2005) |
α−amylase inhibitor GM-pea and bean seed meal suspension α−amylase inhibitor GM-pea purified protein |
2 | i.g. - GM-peas and beans seed meal | i.n. - GM pea α−amylase inhibitor purified protein | None | BALB/c | (Lee et al., 2013) |
Bovine BLG | 1 | i.g. | i.g. | CT | C3H/HeN germ-free mice | (Feehley et al., 2019) |
Bovine BLG κ-casein (κ-CN) |
3 | i.p. | i.g. | i.p. - complete and incomplete Freund's adjuvant |
BALB/c/cmdb | (Zlotkowska et al., 2021) |
Bovine BLG Cow's milk |
1,3 | Cutaneous i.n. i.g. |
i.g. | CT | BALB/c | (Wavrin et al., 2015) |
Cow's milk whey protein BLG |
1 | i.g.-whey protein | i.g.- BLG | CT | C3H/HeN (germ-free and conventional) | (Rodriguez et al., 2012) |
Cow's milk whey protein | 2 | i.g. | i.d. | CT | C3H/HeOuJ | (Zhao et al., 2021) |
Gliadin from soy and wheat | 3 | i.p. | i.g. exercise |
Alum | B10.A | (Tanaka et al., 2011) |
Hazelnut/Filbert protein extract | 3 | Transdermal | i.g. | None | BALB/c | (Birmingham et al., 2007) |
OVA | 1,3 | Epicutaneous i.g. |
i.g. | CT | BALB/c | (Bartnikas et al., 2013) |
OVA Ovomucoid |
1 | i.g. - OVA or ovomucoid |
i.g.-OVA or ovomucoid i.p.-OVA after i.g. challenges |
CT | C3H/HeJ | (Leonard et al., 2012) |
OVA | 3 | s.c. | i.g. | Alum | 129SvEvBrd | (Arumugam et al., 2011) |
OVA | 3 | i.p. | i.g. | Alum | BALB/c C57BL/6 |
(Brandt et al., 2003) |
OVA | 3 | i.p. | i.g. | Alum | BALB/c | (Ando et al., 2017) |
OVA Egg white |
3 | i.p. - OVA | i.g. - egg white | Alum | BALB/c | (Burggraf et al., 2011) |
OVA | 3 | Epicutaneous | i.g. | Calcipotriol | C57BL/6J | (Hussain et al., 2018) |
Peach protein (Pru p 3) | 4 | i.n. | i.p. | LPS | BALB/c | (Rodriguez et al., 2017) |
Peanut (ground whole) Peanut crude extract |
2 | i.g. - ground whole peanut | i.p. - crude peanut extract | None | C3H/HeJ | (Proust et al., 2008) |
Peanut (ground whole) Peanut crude extract |
1 | i.g. - ground whole peanut | i.g. - crude peanut extract | CT | C3H/HeJ | (Li et al., 2000) |
Peanut (homogenized) CpG-coated poly (lactic-co-glycolic acid) nanoparticles containing peanut extract |
1 | i.g. - homogenized peanut |
i.g. - CpG-coated poly (lactic-co- glycolic acid) nanoparticles containing peanut extract | CT | C3H/HeJ | (Srivastava et al., 2016) |
Peanut crude extract | 2 | i.g. | i.p. | CT | C3H/HeJ | (Moutsoglou & Dreskin, 2016) |
Peanut crude extract Soy extract Green bean extract Cashew extract |
3,4 | Epicutaneous | i.g. i.p. |
CT or SEB | C3H/HeJ BALB/c |
(Tordesillas et al., 2014) |
Peanut extract | 2 | i.g. | i.p. | SEB | BALB/c | (Utsch et al., 2017) |
Peanut extract | 3 | Cutaneous | i.g. | CpG CT |
BALB/c | (Adel-Patient et al., 2007) |
Peanut extract (whole), Ara h 1, Ara h 2, or Ara h 3 | 2 | i.g. - whole peanut extract | i.p. - whole peanut extract, Ara h 1, Ara h 2, or Ara h 3 | CT | BALB/c | (Hardy et al., 2022) |
Peanut flour Peanut crude extract |
4 | i.n. - peanut flour | i.p. - crude peanut extract | None | BALB/c C57BL/6 |
(Dolence et al., 2018) |
Peanut | 4 | i.n. | i.p. | Indoor dust | C57BL/6J | (Smeekens et al., 2019) |
Peanut protein extract | 3,4 | Epicutaneous | i.g. i.d. |
None | BALB/c | (Strid et al., 2005) |
Shellfish protein extract | 3 | Transdermal | i.g. | None | BALB/c | (Parvataneni et al., 2015) |
Shrimp tropomyosin | 1 | i.g. | i.g. | CT | C3H/HeJ | (Capobianco et al., 2008) |
Shrimp tropomyosin rMet e 1 |
1 | i.g. | i.g. | CT | BALB/c | (Lam et al., 2015) |
- * 1) oral sensitization and challenge, 2) oral sensitization and non-oral challenge, 3) non-oral sensitization and oral challenge, and 4) non-oral sensitization and non-oral challenge with food proteins
Wild-Type Mouse Models
The majority of models use wild-type mice. Table 1 provides examples of wild-type mouse models and highlights the allergens, sensitizing, and eliciting routes of allergen administration, the use of adjuvants, and the mouse strain. Although BALB/c mice are most frequently used, other strains include B10.A (Tanaka et al., 2011), 129SvEvBrd (Arumugam et al., 2011), C57BL/6 (Brandt et al., 2003; Dolence et al., 2018), and C3H/HeJ (Li et al., 2000; Moutsoglou & Dreskin, 2016; Proust et al., 2008), and are primarily conventional mice, SPF and germ-free (Feehley et al., 2019) mice are also used. Wild-type mice do not appear to spontaneously develop food allergies like other animals, e.g., dogs and cats (Teuber et al., 2002). Therefore, it is necessary to sensitize them with protein. The proteins that have been commonly used are from food sources containing allergens, such as chicken egg white (e.g., OVA), cow's milk (e.g., BLG, whey), peanut (e.g., extract, Ara proteins), fruit (e.g., peach), legumes (e.g., lupin, peas, beans), and shellfish (e.g., shrimp tropomyosin). The allergenic proteins are administered as either whole food (most often as a suspension) (Burton et al., 2017; Lee et al., 2013; Smeekens & Kulis, 2021), purified proteins (Arumugam et al., 2011; Brandt et al., 2003; Burggraf et al., 2011; Capobianco et al., 2008; Hussain et al., 2018; Lam et al., 2015), or protein extracts (Dolence et al., 2018; Hardy et al., 2022; Li et al., 2000; Parvataneni, Gonipeta, Acharya, & Gangur, 2015; Smeekens & Kulis, 2021; Strid, Hourihane, Kimber, Callard, & Strobel, 2005; Tordesillas et al., 2014; Utsch, Logiantara, van Ree, & van Rijt, 2017).
There are two steps to induce allergy to food proteins or whole food: the first is to initiate an immune response to the protein in the animal, and the second is to elicit a secondary response. Sensitization induces allergic responses to one, or multiple, food proteins by administering proteins, extracts, or whole food by i.p., s.c., epicutaneous, transcutaneous, or i.g./p.o. routes. Once the animals are sensitized, the next step is the elicitation of an in vivo response, which is achieved by an allergen challenge administered by oral, dermal, systemic, or respiratory tract exposure. In some instances, sensitization and elicitation phases are induced with the same allergen, while in others, they may differ. For example, sensitizing with a purified protein and challenging with the whole food or vice versa (Burggraf et al., 2011; Lee et al., 2013; Li et al., 2000; Prescott et al., 2005; Proust et al., 2008).
A variety of adjuvants are used when administering purified proteins to reduce the risk of inducing oral tolerance (Mullins et al., 2022). Examples of adjuvants used in mouse models are CT, alum, and SEB (Ando et al., 2017; Arumugam et al., 2011; Brandt et al., 2003; Capobianco et al., 2008; Feehley et al., 2019; Lam et al., 2015; Moutsoglou & Dreskin, 2016; Rodriguez et al., 2012; Tanaka et al., 2011; Zhao et al., 2021; Zlotkowska et al., 2021), CpG oligonucleotide (Adel-Patient et al., 2007), LPS (Rodriguez et al., 2017), Freund's adjuvant (Zlotkowska et al., 2021), Calcipotriol (Hussain et al., 2018), and house dust mite extract or indoor dust (Dolence et al., 2018) but are usually unnecessary when immunizing with whole food.
The experimental in vivo endpoints for wild-type, GM, and humanized mouse models are similar (Castan et al., 2020) (Table 2). Briefly, in vivo readouts for food allergy include diarrhea, scratching, piloerection, and reduced activity. Clinical scores have been developed to assess clinical signs, e.g., (0) no symptoms; (1) scratching and rubbing around nose and head; (2) puffiness around eyes and mouth, diarrhea, pillar erect, reduced activity, and/or decreased activity with increased respiratory rate; (3) wheezing, labored respiration, and cyanosis around mouth and tail; (4) No activity after prodding, or tremor and convulsion; (5) death (Li et al., 2000).
Organ | Clinical signs | Test | Readout | Reference |
---|---|---|---|---|
Skin | Scratching | None | Observation | (Bøgh et al., 2009) |
Piloerection | None | Observation | (Strid et al., 2005) | |
Swelling, edema | ITH i.d. allergen |
Ear thickness | (Sun et al., 2007) | |
DTH s.c. ears or footpads |
Ear and footpad thickness | (Prescott et al., 2005; Strid et al., 2005) | ||
Edema (vascular leakage) | Passive cutaneous anaphylaxis | Blue color extremities and diameter of cutaneous reaction | (Dearman, Stone, Caddick, Basketter, & Kimber, 2003; Herouet-Guicheney et al., 2009) | |
GI tract | Diarrhea | Stool collection | Observation, clinical scores | (Brandt et al., 2003; Kinney et al., 2015; Kucuk et al., 2012) |
Systemic | Hypothermia | Rectal or s.c. | Temperature | (Ahrens et al., 2012;Branum & Lukacs, 2009; Hardy et al., 2022; Ito et al., 2021; Lexmond et al., 2016; Proust et al., 2008; Rodriguez et al., 2017; Smeekens & Kulis, 2021; Sun et al., 2007; Tanaka et al., 2011; Utsch et al., 2017; Yamani et al., 2021) |
Anaphylaxis | i.v. Evan's blue followed by an allergen challenge | Blue color in the extremities | (Balbino et al., 2017; Inagaki, Miura, Nagai, & Koda, 1992; Makabe-Kobayashi et al., 2002; Ogino, Sakazaki, Okuno, Arakawa, & Ueno, 2015; Park & Choi, 2016) | |
Neurological | Reduced activity | None | Observation, clinical scores | (de Theije et al., 2014) |
Lungs | Airway hypersensitivity | Airway hypersensitivity response following respiratory challenge | Airway resistance and compliance | (Wang et al., 2020) |
Anaphylaxis | Breathing rate | Breaths/minute count | (Proust et al., 2008) |
Anaphylaxis is assessed by a drop in core body temperature upon allergen challenge. Vascular leakage, an indicator of anaphylaxis, is evaluated following i.v. injection of Evan's blue immediately after an allergen challenge and visualized by blue color in the extremities (Evans, Killoran, & Mitre, 2014). Vascular leakage can also be measured by passive cutaneous anaphylaxis following an immediate allergen response, assessed by ear thickness and skin color after i.v. injection of Evans blue (Evans et al., 2014). Other in vivo readouts for allergen sensitization include i.d. allergen challenge in the ear, leading to an ITH readout as ear swelling (van Esch et al., 2010). Alternatively, allergen administered s.c. in the ears or footpads can be evaluated by measuring ear thickness, which is indicative of a DTH response (Prescott, Forbes, Foster, Matthaei, & Hogan, 2006). Lastly, AHR following an allergen challenge in the respiratory tract is an indicator of sensitization (Prescott et al., 2005).
Ex vivo endpoints for food allergy are similar to most immunological assessments for immunization with antigen, (Table 3) but focus on Th2 immune responses. The most common readouts are allergen-specific IgE and IgG1 titers (Birmingham et al., 2003). Although serum allergen-specific immunoglobulins are detected, a significant issue is that the IgE might be biologically inactive. The IgE functional activity can be tested with the in vitro rat basophil activation assay, which evaluates the ability of serum IgE to induce basophil degranulation (Bøgh et al., 2009). Other readouts include determining cell phenotype, activation and function of innate and adaptive immune cells from the GI tract, blood, and lymphoid organs, measuring cytokines, e.g., IL-4, IL-5, IL-13, IL-25, and IL-33, etc., and mediators, e.g., mast cell proteases, histamine, and tryptase. Evaluating inflammation and edema in the intestine with histological methods is also valuable (Hogan, Mishra, Brandt, Foster, & Rothenberg, 2000; Li et al., 2000; Mathias et al., 2011; Utsch et al., 2017; Walker et al., 2018; Yamani et al., 2018). Gene expression profiling, transcriptomics, proteomics, microbiota analysis, and epigenomics might provide valuable markers for sensitization and lead to novel mechanistic insights. Additional approaches include organ culture models (Tsilingiri et al., 2012), organoids and intestinal organ culture systems (Clevers, 2016), and gut-on-a-chip (Kim, Li, Collins, & Ingber, 2016).
Site | Tissue | Test | Readout | Reference |
---|---|---|---|---|
Blood | Sera | Serum allergen- specific IgE, IgG1 | Titers, gel electrophoresis, immunoblots, IgE binding epitope mapping | (Arumugam et al., 2011; Brandt et al., 2003; Burton et al., 2017; Hardy et al., 2022; Hogan et al., 2000; Lexmond et al., 2016; Lexmond et al., 2017; Li et al., 2000; Mathias et al., 2011; Moutsoglou & Dreskin, 2016; Pagovich et al., 2016; Smeekens et al., 2019; Srivastava et al., 2016; Strid et al., 2005; Sun et al., 2007; Tanaka et al., 2011; Utsch et al., 2017; Wang et al., 2020) |
Basophil activation test | Histamine levels | (Tordesillas et al., 2014) | ||
Plasma | Cytokines IL-4, IL-5, IL-13, IL-25, IL-33 | Cytokine expression | (Ito et al., 2021; Lexmond et al., 2016) | |
Mediators: mast cell proteases, histamine, tryptase, metabolites | Mediators and metabolites levels | (Arumugam et al., 2011; Brandt et al., 2003; Burggraf et al., 2011; Burton et al., 2017; Feehley et al., 2019; Ito et al., 2021; Lexmond et al., 2016; Moutsoglou & Dreskin, 2016; Utsch et al., 2017; Yamani et al., 2018) | ||
Transcriptomics | Transcriptome | (Burton et al., 2018) | ||
Cell flow cytometry | Phenotype, cellular composition, CD49b/IgE, CD200R, CD3, CD19, c-kit/IgE, CD107a, etc. | (Hogan et al., 2000; Leonard et al., 2012; Lexmond et al., 2016; Lexmond et al., 2017) | ||
GI | Feces | Fecal IgA, IgG | IgA or IgG titers | (Adel-Patient et al., 2007; Smeekens & Kulis, 2021) |
Intestine | Organ culture models, organoids, and intestinal organ culture systems | Mediators and metabolites levels | (Tsilingiri et al., 2012) (Clevers, 2016; Sato et al., 2009) |
|
Gut-on-a-chip | (Kim et al., 2016; Kim, Huh, Hamilton, & Ingber, 2012) | |||
Gene expression profiling | Tissue gene expression | (Arumugam et al., 2011; Brandt et al., 2003; Burton et al., 2013; Tomar et al., 2021) | ||
Microbiome | Microbiota | (Feehley et al., 2019; Hussain et al., 2018; Rodriguez et al., 2012; Smeekens & Kulis, 2021) | ||
Omics | Proteome, transcriptome, epigenome | (Brandt et al., 2003; Hussain et al., 2018; Lee et al., 2016; Lexmond et al., 2016; Platzer et al., 2015; Rodriguez et al., 2012; Tomar et al., 2021) | ||
Mast cell and eosinophil pathology i.g. treatment with FITC for evaluating intestinal permeability |
Cell counts, mast cell degranulation Concentration of fluorescein in fluorescence spectroscopy |
(Hogan et al., 2000; Li et al., 2000; Mathias et al., 2011; Utsch et al., 2017; Walker et al., 2018; Yamani et al., 2018) (Brandt et al., 2003; Hussain et al., 2019; Mathias et al., 2011) | ||
Endoscopy | Inflammation score | (Weigmann et al., 2012) | ||
Cell flow cytometry of small intestine lamina propria | Phenotype, cellular composition | (Burton et al., 2013; Hussain et al., 2019; Hussain et al., 2018) | ||
Peritoneal Lavage |
Cell flow cytometry | Mast cells, phenotype, cellular composition | (Arumugam et al., 2011) | |
Differential cell count | Inflammatory cell composition | (Sun et al., 2007) | ||
Lymphoid organs | Spleen, lymph node cells, bone marrow cells | IL-1a, IL-1β, IL-4, IL-5, IL-6, IL-13, IL-21, IL-25, IL-33, MCTP-1, IFNγ, TNFα, TLR4, NF- κB and TNFα | Immune cell secretion and quantities | (Burggraf et al., 2011; Feehley et al., 2019; Hogan et al., 2000; Mathias et al., 2011; Rodriguez et al., 2012; Smeekens et al., 2019; Srivastava et al., 2016; Strid et al., 2005; Sun et al., 2007; Tordesillas et al., 2014; Utsch et al., 2017; Walker et al., 2018) |
Cell proliferation, CFSE assay, apoptosis, MTT assay, suppression, degranulation | Cell proliferation, viability, mediator release | (Arumugam et al., 2011; Hogan et al., 2000; Li et al., 2000; Platzer et al., 2015; Rodriguez et al., 2017; Zlotkowska et al., 2021) | ||
Gene expression profiling, PCR | Tissue gene expression | (Mathias et al., 2011; Tordesillas et al., 2014) | ||
Omics | Proteome, transcriptome, epigenome | (Tordesillas et al., 2014) | ||
Protein expression of ERK1/2, Syk | Immunoblots for total or phosphorylated proteins | (Platzer et al., 2015) | ||
S100A8/A9, TLR4, and TNF-α-specific western blots | Protein levels | (Zhu, Wang, Ma, Sheng, & Li, 2021) | ||
Cell flow cytometry | Phenotype, cellular composition | (Dolence et al., 2018; Smeekens et al., 2020; Tordesillas et al., 2014) | ||
Chemotaxis assay with mast cell progenitors | Quantification of cell migration | (Platzer et al., 2015) | ||
Mast cell degranulation assay | Quantification of degranulating mast cells | (Arumugam et al., 2011; Lexmond et al., 2016; Lexmond et al., 2017) | ||
Lungs | Airways | Differential cell count | Inflammatory cell composition | (Dolence et al., 2018; Sun et al., 2007; Utsch et al., 2017) |
Lungs | Pathology | Inflammation score | (Sun et al., 2007; Utsch et al., 2017) | |
Omics | Transcriptome | (Platzer et al., 2015) | ||
Cytokines IL-4, IL-5, IL-13, IL-21, IL-1a, IL-1β, TSLP | Cytokine expression | (Dolence et al., 2018) | ||
Skin | Ears, footpads | Gene expression profiling, PCR | Cytokine expression | (Tordesillas et al., 2014) |
Genetically Modified Mouse Models
Transgenic and KO mice offer opportunities to derive novel mechanistic insights into the pathophysiology of food allergy. Table 4 presents examples of GM mouse models. Except for mice deficient in WASP that develop IgE-mediated reactions to food allergens (Lexmond et al., 2016), none of the previously used tg and KO mice spontaneously develop food allergies. The range of tg mice used in food allergy models include those expressing genes such as IL-4 (Hussain et al., 2018; Tomar et al., 2021), IL-4Rα (Burton et al., 2013; Ganesan, Sharma, Tomar, Schuler, & Hogan, 2022; Mathias et al., 2011; Tomar et al., 2021), IL-5 (Brandt et al., 2003), IL-9 (Ahrens et al., 2012; Ganesan et al., 2022; Yamani et al., 2021; Yamani et al., 2018), IL-13 (Tordesillas et al., 2014), IL-25 (Lee et al., 2016), GATA (Hussain et al., 2018), FcεRI (Ahrens et al., 2012; Brandt et al., 2003), human CD22 (Hardy et al., 2022), Baso-DTR (Noti et al., 2014), T cell receptor, e.g., DO11.10 (Tordesillas et al., 2014) and OT-II (Platzer et al., 2015), Abl1 (Yamani et al., 2018), TSLPR (Noti et al., 2014), filaggrin and Tmem79ma (Walker et al., 2018), CC027 (Smeekens & Kulis, 2021), WAS (Lexmond et al., 2016), Foxp3 (Lexmond et al., 2016), TGAX (Lexmond et al., 2016) combined with eGFP and assorted cell markers. Examples of KO mice used to study food allergy lack single or combinations of genes, including FcεRI (Brandt et al., 2003), FcRI-chain (Sun et al., 2007), IL-4 (Lexmond et al., 2017), IL-5 (Hogan et al., 2000), IL-17rb (Lee et al., 2016), eotaxin (Hogan et al., 2000), IgE, TSLP, and Igh7 (Noti et al., 2014), CD40L (Sun et al., 2007), Rag2 (Lexmond et al., 2016; Lexmond et al., 2017), and mice that lack B cells and mast cells (Sun et al., 2007). The food allergens used in the GM mouse models are similar to those used in wild-type models, e.g., related to milk, OVA, peanut, tree nuts, legumes, and fish, and used with and without adjuvants. The sensitizing and eliciting approaches are also similar to those used in wild-type mice, except for models using adoptive cell transfers with tg T cell receptor-expressing T cells from the DO11.10 and OT-II tg mice (Hussain et al., 2018; Platzer et al., 2015; Tordesillas et al., 2014).
Allergen | * | Sensitization | Elicitation | Adjuvant | Strain | Reference |
---|---|---|---|---|---|---|
OVA | 3 | s.c. | i.g. | Alum | IL4RαY709F IL9 tg | (Ganesan et al., 2022) |
OVA | 3 | i.p. | i.g. | Alum | FcεRI KO IL-5 tg IL-5/eotaxin-1 double KO |
(Brandt et al., 2003) |
OVA | 3 | i.p. | i.g. | Alum | OT-II TCR tg | (Platzer et al., 2015) |
OVA | 3 | i.p. | i.g. | Alum | Intestinal IL-9 tg (iIL-9Tg) | (Yamani et al., 2021) |
OVA | 3 | i.p. | i.g. | Alum | IL-5 KO Eotaxin KO |
(Hogan et al., 2000) |
OVA | 3 | i.p. | i.g. | Alum | IL-25 tg Il17rb (-/-) KO |
(Lee et al., 2016) |
OVA | 1 | i.g. | i.g. | CT SEB |
C.129.Il4ra F709/F709 F709/IgE −/− |
(Mathias et al., 2011) |
OVA | 1 | i.g. | i.g. | None | Il4rαF709 | (Burton et al., 2013) |
OVA | 1 | i.g. | i.g. | None | Was–/– KO | (Lexmond et al., 2017) |
OVA | 3 | Epicutaneous | i.g. | Calcipotriol | IL-4−IRES−eGFP (4Get) Il4raY709F IL-9-GFP (INFER) Batf-/- KO |
(Tomar et al., 2021) |
OVA | 3 | Epicutaneous | i.g. | Calcipotriol | C57BL/6 Il4-/- C57BL/6 4get C57BL/6 OT-II BALB/c ΔdblGATA C57BL/6 Il4-3′UTR |
(Hussain et al., 2018) |
OVA Anti-TNP-IgE |
3 | i.v.-anti-TNP-IgE i.p.- OVA |
i.g.-TNP-BSA i.g.- OVA |
None Alum |
FcεRI tg intestinal IL-9 transgenic (iIL9Tg) |
(Ahrens et al., 2012) |
OVA IL-4C, histamine anti-TNP-IgE OVA–2, 4, 6- TNP |
3 | i.p.-OVA i.v.-IL-4C,histamine,anti-TNP-IgE i.v.-anti- TNP-IgE |
i.g.-OVA-2, 4, 6-TNP | Alum | intestinal IL-9 tg Il4raY709F Cadherin-5Cre Abl1fl/fl |
(Yamani et al., 2018) |
OVA Peanut crude extract |
3 | Epicutaneous- OVA or peanut crude extract | i.g.- OVA or peanut crude extract | Calcipotriol | BALB/c Tslpr +/+ BALB/c Tslpr -/- C57BL/6 Baso-DTR BALB/c Igh-7 -/- (IgE-KO) |
(Noti et al., 2014) |
Peanut crude extract Soy extract Green bean extract Cashew extract |
3,4 | Epicutaneous | i.g. i.p. |
CT SEB |
4get DO11.10 IL-13eGFP |
(Tordesillas et al., 2014) |
Peanut Extract (whole) Peanut extract (whole), Ara h 1, Ara h 2, or Ara h 3 |
2 | i.g.- peanut extract | i.p. - whole peanut extract, Ara h 1, Ara h 2, or Ara h 3 i.v. cell transfer |
CT | human CD22 tg | (Hardy et al., 2022) |
Peanut protein extract OVA |
3 | Epicutaneous | i.g. | House dust mite extract Alternaria Alternata extract |
Flaky tail mice (heterozygous for the filaggrin (Flg)ft and Tmem79ma mutations) | (Walker et al., 2018) |
Peanut protein extract from peanut butter Peanut crude extract |
2 | i.g.- peanut protein extract | i.p. i.n. i.d. |
CT | B cell KO (B6.129S2-Igh-6tm1Cgn/J) CD40L KO (B6.129S2-CD40lgtm1Imx/J) Mast cell KO (WBB6F1/J-kitw/Kitw-v) FcRI -chain- KO (C.129S2-Fcer1atm1Knt/J) |
(Sun et al., 2007) |
Peanut Walnut Milk protein extract Egg protein extract |
1 | i.g. | i.g. | None | CC027/GeniUnc | (Smeekens & Kulis, 2021) |
Wheat, soybean meal, wheat middlings, yellow corn, fish meal (ground) | 1 | p.o.-wheat, soybean meal, wheat middlings, yellow corn, fish meal | i.g.- soy protein | None | Was–/– Was–/– Il4–/– Was–/– Rag2–/– floxed Was alleles Wasfl/fl Itgax-Cre Foxp3-Cre |
(Lexmond et al., 2016) |
- * 1) oral sensitization and challenge, 2) oral sensitization and non-oral challenge, 3) non-oral sensitization and oral challenge, and 4) non-oral sensitization and non-oral challenge with food proteins
Humanized Mouse Models
Humanized mouse models generated by engrafting human cells or tissues into immunodeficient mice (Walsh et al., 2017) are predominantly created by injecting human PBMCs or HSCs from healthy or allergic donors into SCID mice (Burton et al., 2018; Burton et al., 2017; Hardy et al., 2022; Parvataneni et al., 2015; Wang et al., 2022; Weigmann et al., 2012) (Table 5). The SCID mice lack B and T cells and, in some cases, have been crossed with tg and KO mice (Ito et al., 2021). The advantage of humanized mice is that they provide an opportunity to study an in vivo human immune system. However, there are limitations with these mice, including potential problems with the initial engraftment, graft-versus-host disease, and challenges related to distinct species-specific molecular, cellular, and organs that could alter the resulting pathophysiology. Additionally, humanized mice engrafted with an allergic donor cannot be used to evaluate sensitization because the T cells are already primed (Parvataneni et al., 2015; Wang et al., 2020; Weigmann et al., 2012). In contrast, elicitation can be assessed using various routes of allergen administration. It is also possible to elicit passive anaphylaxis with an injection of an i.v. antibody (Burton et al., 2018). In mice derived from non-allergic donors, sensitization has been done by i.p., i.g., and i.n. allergen administration with food proteins, including peanuts (Parvataneni et al., 2015; Wang et al., 2020), tree nuts (Weigmann et al., 2012), pollen (Weigmann et al., 2012), and house dust mite (Wang et al., 2020). Typically, adjuvants are not used in these models.
Allergen | * | Sensitization | Elicitation | Strain | Human cells | Reference |
---|---|---|---|---|---|---|
Birch pollen extract Grass pollen extract Hazelnut extract |
3,4 | i.p. | i.g.-rectal | NOD-scid-γc(-/-) | Allergic and healthy donor PBMCs | (Weigmann et al., 2012) |
BLG anti- BLG chimeric human IgE mAb |
3,4 | i.v. | p.o. i.v. |
NOG (NOD/Shi-SCID -IL2rgnull) NOG hIL- 3/hGM-CSF tg |
Healthy donor HSCs | (Ito et al., 2021) |
Peanut butter | 1 | i.g. | i.g. | NOD-scid-γc(-/-) | Healthy donor HSCs | (Burton et al., 2017) |
Peanut extract Passive anaphylaxis |
4 | i.v.-IgG-depleted serum from highly peanut allergic human donors | i.p-peanut extract | NOD-scid-γc(-/-) | Healthy donor HSCs | (Burton et al., 2018) |
Peanut crude extract House dust mite Peanut crude extract |
3 | i.p.- peanut crude extract i.n.- house dust mite |
i.g.- peanut crude extract | NOD-scid-γc(-/-) | Allergic donor PBMCs | (Wang et al., 2020) |
Peanut extract whole | 4 | i.v.- splenocytes of i.g. - sensitized mice |
i.p. | NOD-scid-γc(-/-) | Healthy donor PBMCs | (Hardy et al., 2022) |
Peanut protein extract | 3 | i.p. | i.g. | NOD-scid-γc(-/-) | Allergic & healthy donor PBMCs | (Pagovich et al., 2016) |
- * 1) oral sensitization and challenge, 2) oral sensitization and non-oral challenge, 3) non-oral sensitization and oral challenge, and 4) non-oral sensitization and non-oral challenge with food proteins
Rat Models
Food allergy is induced in the rat by epicutaneous, p.o., and i.g. administration of protein. Examples in Table 6 are in the Brown Norway rat with gluten and OVA allergens. Skin sensitization requires weekly abrasions before administering the protein samples without adjuvant (Ballegaard et al., 2019). The elicitation is done by i.p., i.g., i.d., or p.o. allergen exposure. The readouts are similar to those used in mouse models.
Allergen | * | Sensitization | Elicitation | Adjuvant | Reference |
---|---|---|---|---|---|
Gluten Acid-hydrolyzed gluten |
3,4 | Epicutaneous | i.p. i.g. |
None | (Ballegaard, Madsen, & Bøgh, 2019) |
OVA | 1,2 | p.o. | i.d. p.o. |
Carrageenan | (Atkinson, Johnson, Gee, Grigoriadou, & Miller, 1996) |
OVA | 2 | p.o. | None | None | (Pilegaard & Madsen, 2004) |
OVA | 1 | i.g. | i.g. | None | (Zhu et al., 2021) |
- * 1) oral sensitization and challenge, 2) oral sensitization and non-oral challenge, 3) non-oral sensitization and oral challenge, and 4) non-oral sensitization and non-oral challenge with food proteins
CHALLENGES AND LIMITATIONS
There are many challenges with food allergy models that could limit the experimental outcomes. These challenges can significantly influence model reproducibility as well as the translation to human disease. Designing a food allergy model is especially challenging because careful attention must be paid to the types of allergens and controls, the type and characteristics of the mice, e.g., age, gender, and strain, and the conditions for allergen exposure, including the use of adjuvants, environmental conditions, intestinal microbiome, and diet, which can influence the results.
Allergens
A significant challenge in food allergy model design is the selection of test allergens. Most investigators use known allergenic proteins and foods to ensure that the model reflects human allergy. Although there are few allergenic food proteins in humans (Bannon, 2004), most proteins, even non-allergenic ones, will induce an immune response in wild-type mice (Ladics et al., 2010). To date, there are no reports of non-allergenic food proteins in mice. However, a multi-laboratory study tested four wild-type mouse strains and clinically relevant allergenic proteins from peanut and cow's milk and non-allergenic proteins with a history of safe use in humans from spinach and soybean i.p., with or without adjuvant (Ladics et al., 2010; Thomas et al., 2005). They found that known allergens induced higher responses compared with the low immunogenic spinach rubisco and soy lipoxygenase (Ladics et al., 2010; Thomas et al., 2005). The lack of non-allergenic proteins in mice means that there are only naïve and vehicle-alone negative controls. Ideally, a clinically relevant reference set of proteins with known allergenic potency would be useful, but it is unclear whether establishing reference proteins is feasible. Another factor that might affect the test materials and the consequent experimental outcome is the source and preparation of the allergen, e.g., protein processing, isolation, and purification methods may differ. The selection of well-characterized allergen test materials is essential for model reproducibility.
Testing for Protein Allergenicity
While purified proteins and extracts can induce food allergy, it is ideal to establish food allergy models with whole food to fully understand sensitization to food proteins embedded within the food matrix. The proteins in whole food differ significantly from purified proteins because of possible changes in protein folding, exposure of hidden epitopes, protein content in the whole food, and other food components that could interact and alter allergenicity. For example, intrinsic adjuvants such as lipids, carbohydrates, and other proteins could augment the allergic response (del Moral & Martinez-Naves, 2017). The food matrix might also alter protein digestibility and bioavailability, or contain protease inhibitors and additives (Keshavarz, Jiang, Hsieh, & Rao, 2019; Schulten, Lauer, Scheurer, Thalhammer, & Bohle, 2011). The choice of whole food, and the processing used, is crucial because, for example, the proteins in cooked and raw foods also differ. The best approach is to use the food as it would be prepared for human consumption, i.e., baked, boiled, microwaved, etc. Industrial food processing methods like high heat, hydrolysis, defatting, and pH changes might also influence allergenicity (Bøgh et al., 2016). Purified proteins also have associated challenges compared to proteins in whole food, such as the possibility of different folding, post-translational modifications, and endotoxin contamination of recombinant proteins.
The type of allergen is important for inducing allergy, but the amount necessary for sensitization also varies. Some proteins at high oral doses might induce oral tolerance (Burggraf et al., 2011; Mathias et al., 2011; Strid et al., 2005; Walker et al., 2018). Allergen dose, frequency of administration, and exposure route might alter the responses (Wavrin, Bernard, Wal, & Adel-Patient, 2015). Therefore, when using a novel allergen for sensitization, it is essential to establish a dose-response curve.
Adjuvants
Often adjuvants are used to induce disease by breaking oral tolerance, though some adjuvant-free models have been reported (see Tables 1, 4, and 5). Adjuvants are mainly used when administering purified proteins and extracts compared to a whole food, which may have intrinsic adjuvants. A model without adjuvant is preferable because they tend to skew immune responses (Bøgh et al., 2016), but all models using OVA, for example, in Table 1 add adjuvant. Using adjuvants for sensitization depends upon the experimental aim and should be considered when the focus is not on sensitization.
Exposure
The sensitizing and eliciting exposure approaches are vastly different between models (see Tables 1, 4, and 5). Sensitization may occur by systemic and local immunization. I.p., i.d., and i.n. induction of a systemic immune response sensitizes the mice for GI tract challenges. It is also possible to generate disease with oral challenges, which tend to induce local GI tract and systemic responses. The challenges or elicitation of food allergy following sensitization depend on the desired readout and research aim. Oral challenges are preferred when inducing GI tract inflammation (Mathias et al., 2011). In contrast, i.p. challenges lead to anaphylactic reactions, mast cell responses, vascular permeability (Sun et al., 2007), and T cell proliferation (Rodriguez et al., 2017). Challenges in the skin for a DTH response (Prescott et al., 2005) or i.n. for a respiratory response (Sun et al., 2007; Wang et al., 2020) provide evidence of initial sensitization in another organ system.
Because oral challenges are probably the major sensitizing route in humans, oral challenges would be the most translatable route of exposure. The protocols in use have a wide range of doses and frequency of oral challenges that could be days to weeks (Fig. 1). Ideally, the quantity and frequency of oral test material exposure should be based on human consumption.
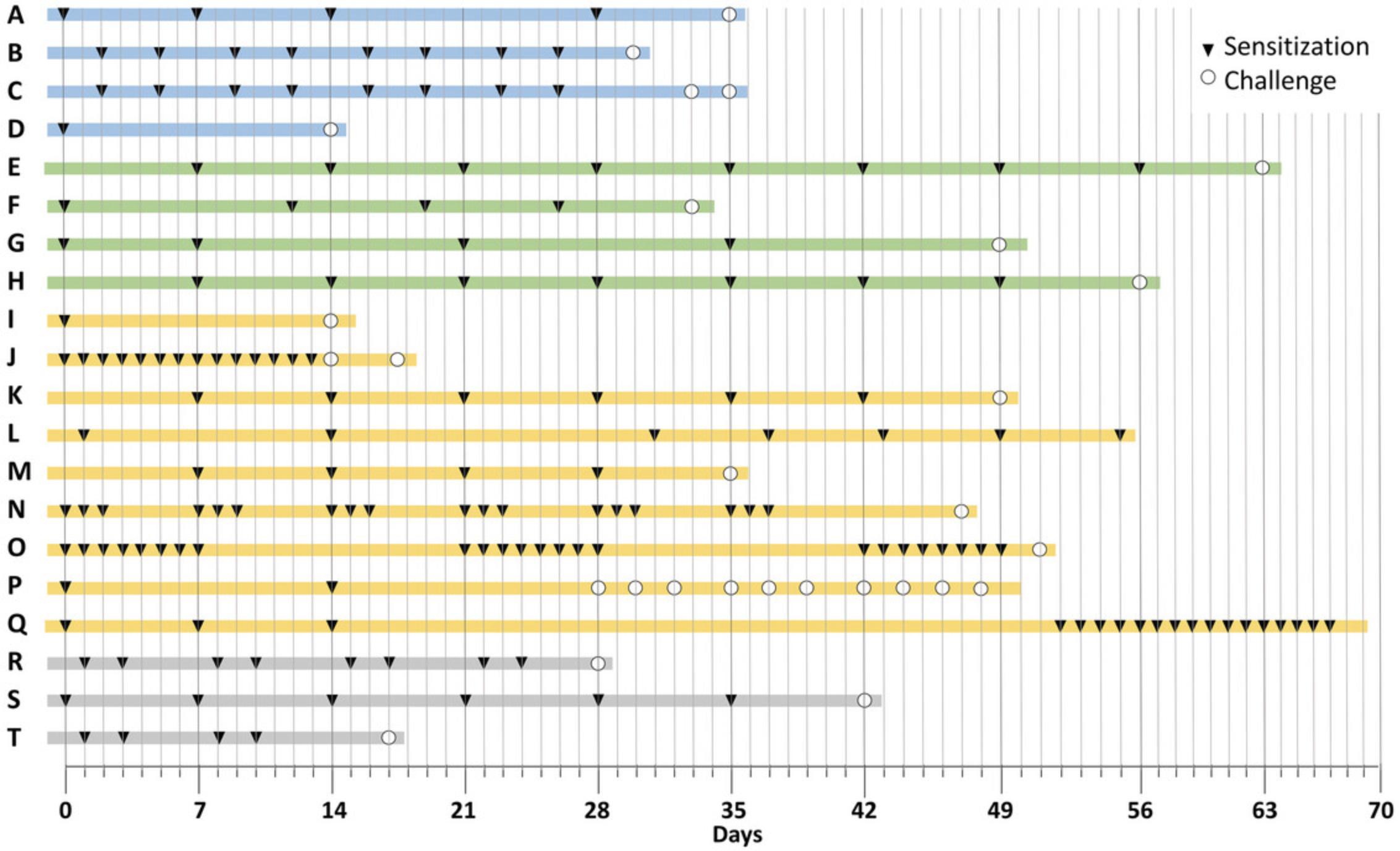
Environmental Factors
Many other factors are essential for establishing a reliable and reproducible model of food allergy, including environmental factors, e.g., housing conditions, light cycles, temperature, humidity, noise level, and gentle handling of the mice to reduce stress. The environment should be as consistent as possible. Even factors like changes in bedding and enrichments could potentially skew the results. Experienced personnel should conduct repeated allergen administrations to ensure consistency. Attention should also be given to the potential for contaminated test materials, e.g., mycotoxins and endotoxin, and to a consistent intestinal microbiome, e.g., the mice should be bred in-house or come from the same vendor and fed the same diet, etc.
Mouse Age, Gender, and Strain
The age and gender of the experimental mice should be consistent because responses in newborn mice differ significantly from older, e.g., 6–8-week-old mice, though there is some evidence that animals older than 8 weeks respond similarly (Akiyama et al., 2001; Pilegaard & Madsen, 2004). The gender should also be consistent because food allergy in female mice and rats appears more robust than in males (Parvataneni, Birmingham, Gonipeta, & Gangur, 2009; Pilegaard & Madsen, 2004). The mouse strain, i.e., genetic predisposition, can influence the type of immune response. Using mice from the same vendor or bred in the same facility reduces variability, like in other models used for studying immunity. The use of germ-free mice helps avoid issues relating to the intestinal microbiome but this is dependent upon the research aim.
Diet
The diet should be consistent for inter- and intra-experimental comparisons. The same feed should be used for the animals during an experiment and in parental generations before mating or weaning. The same food with the same nutritional ingredients should be used. A balanced diet is essential as deficiencies, like vitamin A or D deficiency, might influence the results (Matsui et al., 2018). Changes in fat consumption might alter the intestinal microbiome (Hussain et al., 2019), and cross-reactive proteins in the feed (Lee et al., 2013) could influence the development of food allergy and the reproducibility of the model. In some experiments, optimal allergen absorption is promoted by fasting the mice for a few hours before oral challenges (Brandt et al., 2003; Li et al., 2000; Pagovich et al., 2016; Wang et al., 2020).
Translation
Most food allergy models are isomorphic, which means that symptoms/clinical signs and treatments are shared. Ideally, food allergy models should meet the following criteria to be translational: 1) the mice should have similar biology and clinical signs as humans, 2) the mice should react similarly to non-allergenic and allergenic proteins (or a low to high range of protein allergenicity) as humans, and 3) the mice should have the same pathophysiological responses as humans. To date, none of the models exactly reproduce disease. Nevertheless, similarities exist between disease in mice and humans, creating promising prospects for further elucidation of the factors governing food allergy.
The biology underlying allergic disease in mice and humans is similar. In atopic dermatitis and allergic asthma, a similar array of genes is expressed in mice and humans (Ando et al., 2013; Yu et al., 2011), and a large number of additional pathophysiological similarities exist (Oyoshi et al., 2014).
Food proteins with a history of safe use in humans, i.e., non-allergenic, can be allergenic in mice (Ladics et al., 2010). Furthermore, they have similar biology, but the clinical signs might differ. For instance, after consuming peanuts, the most severe peanut allergy patients could have life-threatening anaphylaxis upon trace amounts of the allergen (Hourihane et al., 1997; Taylor et al., 2002). Inducing anaphylaxis with small amounts of allergen administered p.o. has not been reproduced in mice.
Although allergen sensitization in mice leads to allergen-specific IgG1 and IgE and other ex vivo readouts (Table 3), it might not mimic the initiation of food allergy in patients. The use of allergen co-administered with adjuvants to sensitize the mice differs from patients, though there are likely intrinsic adjuvants in food like endotoxins, etc. (Schwarzer et al., 2017). It is unlikely that patients would encounter purified proteins, except for in vaccines, and there may be an association between OVA-containing vaccines and an increased risk of egg allergy (Kiraly et al., 2016).
Many individuals have food protein-specific IgE without food allergies (Anvari et al., 2019; Hamilton & Oppenheimer, 2015). Thus, food protein-specific IgG1 and IgE titers indicate that sensitization might not lead to food allergy. The allergen needed to elicit clinical signs in sensitized mice might differ significantly in the amount, frequency, timing, and route of the allergen challenge compared with patients. The main challenge is our understanding of 1) when and how allergen-specific IgE sensitization occurs in patients and 2) what kind of challenge elicits the onset of clinical manifestations.
Wild-type mice likely do not spontaneously develop food allergies like humans or companion pets (Bryan & Frank, 2010; Gaschen & Merchant, 2011; Olivry & Mueller, 2017). Although spontaneous food allergy models have been defined as those induced without exogenous adjuvant (Kanagaratham et al., 2018), sensitization and elicitation might not be similar enough to patients, mainly because the exact conditions needed to establish disease in humans are unknown. However, the WASP-deficient mice might provide an opportunity to understand spontaneous sensitization further as they are the only mice that become polysensitized to food proteins (Lexmond et al., 2017).
MODEL PROTOCOLS
Although many models are listed in Tables 1, 4, and 5, their differences could be minimal. For example, the model might be the same, except that the timing of allergen administration differs. Table 7 highlights examples of protocols in use in wild-type mice as the most commonly used experimental animals and divides them into the following four categories based on allergen delivery: 1) oral sensitization and challenge, 2) oral sensitization and non-oral challenge, 3) non-oral sensitization and oral challenge, and 4) non-oral sensitization and non-oral challenge, with food proteins.
Sensitization | Allergen | Elicitation | Allergen | |||||||||||
---|---|---|---|---|---|---|---|---|---|---|---|---|---|---|
Skin | GI tract | Respiratory tract | Systemic | Whole food | Protein | Adjuvant | Skin | GI tract | Respiratory tract | Systemic | Whole food | Protein | Readout | Reference |
oral sensitization and challenge | ||||||||||||||
✓ | ✓ | ✓ | ✓ | ✓ | IgE, mMCP-1 levels, intestinal pathology | (Bartnikas et al., 2013) | ||||||||
✓ | ✓ | ✓ | ✓ | ✓ | IgE, T cell response, anaphylaxis | (Capobianco et al., 2008) | ||||||||
✓ | ✓ | ✓ | ✓ | ✓ | IgE, mast cell activation, intestine inflammation, blood & intestinal eosinophils, Th2 response, anaphylaxis | (Ganeshan et al., 2009) | ||||||||
✓ | ✓ | ✓ | ✓ | ✓ | IgE, intestinal mast cells, eosinophils and goblet cells, T cell response, anaphylaxis | (Lam et al., 2015) | ||||||||
✓ | ✓ | ✓ | ✓ | ✓ | IgE, mast cell activation, anaphylaxis | (Rodriguez et al., 2012) | ||||||||
✓ | ✓ | ✓ | ✓ | ✓ | IgE, plasma histamine, T cell response, anaphylaxis | (Srivastava et al., 2016) | ||||||||
oral sensitization and non-oral challenge | ||||||||||||||
✓ | ✓ | ✓ | ✓ | IgE, airway and lung inflammation | (Lee et al., 2013) | |||||||||
✓ | ✓ | ✓ | ✓ | IgE, DTH response | (Prescott et al., 2005) | |||||||||
✓ | ✓ | ✓ | ✓ | IgE, AHR, lung inflammation, T cell response | (Prescott et al., 2005) | |||||||||
✓ | ✓ | ✓ | ✓ | Anaphylaxis | (Proust et al., 2008) | |||||||||
✓ | ✓ | ✓ | ✓ | IgE, ear swelling, skin hypersensitivity reaction | (Proust et al., 2008) | |||||||||
✓ | ✓ | ✓ | ✓ | ✓ | ✓ | IgE, Acute skin response, anaphylaxis, mast cell activation, T cell response | (Zhao et al., 2021) | |||||||
non-oral sensitization and oral challenge | ||||||||||||||
✓ | ✓ | ✓ | ✓ | ✓ | IgE, diarrhea, intestinal pathology, mast cell | (Ando et al., 2017) | ||||||||
✓ | ✓ | ✓ | ✓ | ✓ | IgE, T cell response | (Adel-Patient et al., 2007) | ||||||||
✓ | ✓ | ✓ | ✓ | ✓ | IgE, hexosaminidase activity, mast cell, anaphylaxis | (Arumugam et al., 2011) | ||||||||
✓ | ✓ | ✓ | ✓ | IgE, mMCP-1, T cell response, intestinal pathology, anaphylaxis | (Bartnikas et al., 2013) | |||||||||
✓ | ✓ | ✓ | ✓ | IgE, T cell response, intestinal pathology | (Birmingham et al., 2007) | |||||||||
✓ | ✓ | ✓ | ✓ | ✓ | IgE, diarrhea, T cell response, anaphylaxis, intestinal pathology, mast cell | (Brandt et al., 2003) | ||||||||
✓ | ✓ | ✓ | ✓ | ✓ | IgE, GI signs, intestinal pathology, T cell response | (Burggraf et al., 2011) | ||||||||
✓ | ✓ | ✓ | ✓ | ✓ | IgE, intestinal mast cell, mast cell activation, skin inflammation, Th2 response | (Hussain et al., 2018) | ||||||||
✓ | ✓ | ✓ | ✓ | IgE, T cell response, anaphylaxis | (Parvataneni et al., 2015) | |||||||||
✓ | ✓ | ✓ | ✓ | ✓ | IgE, innate cytokines, T cell response | (Tordesillas et al., 2014) | ||||||||
✓ | ✓ | ✓ | ✓ | IgE, T cell response | (Wavrin et al., 2015) | |||||||||
✓ | ✓ | ✓ | ✓ | ✓ | ||||||||||
✓ | ✓ | ✓ | ✓ | ✓ | IgE, T cell response | (Zlotkowska et al., 2021) | ||||||||
non-oral sensitization and non-oral challenge | ||||||||||||||
✓ | ✓ | ✓ | ✓ | IgE, T cell response, airway and lung IL-1 cytokines | (Dolence et al., 2018) | |||||||||
✓ | ✓ | ✓ | ✓ | ✓ | IgE, T cell response, anaphylaxis | (Rodriguez et al., 2017) | ||||||||
✓ | ✓ | ✓ | ✓ | ✓ | IgE, cytokines, T cell response, anaphylaxis | (Smeekens et al., 2019) |
In category 1, six model protocols are shown that use p.o. administration routes for sensitization and elicitation. One protocol uses whole food for sensitization and elicitation, and others use protein and adjuvant to sensitize and protein with adjuvant, or protein alone, for challenges. These models aim to evaluate the immune responses, anaphylaxis, and GI tract effects.
In category 2, six models utilize oral sensitization followed by non-oral challenges via the respiratory tract, skin, or systemic (i.p.) to test for sensitization, i.e., to determine whether feeding whole food elicits immune responses. The readouts are in the skin, lungs, and in some cases, anaphylaxis.
Category 3 includes the most popular models. They are achieved by sensitizing the mice via the skin and followed by oral challenges. Most of these protocols use protein and adjuvant for sensitization, and protein for the oral challenge, though whole food is also used. These experiments aim to investigate the immune response to specific food allergens (including anaphylaxis in some) and their effect on the GI tract.
In category 4, three models sensitize via the lung with protein or whole food, and challenge systemically (i.p.). These models aim to study the allergenicity of food proteins.
Figure 1 provides an overview of twenty main types of protocols in a scheme that emphasizes the differences between the experimental designs. The protocols are clustered into the four categories above based on the route of allergen administration. The scheme illustrates the duration of the experiments runs from 2 to 10 weeks, and the number of times the allergen is administered, ranging from a total of 2 to 25 doses.
While it is tempting to argue that only protocols using oral sensitization and/or oral challenge are true food allergy models, this might be incorrect because the mechanisms underlying sensitization to food allergens remain elusive. For instance, there is growing evidence suggesting that food allergy may develop after non-oral sensitization routes, e.g., proteins found in topical agents, soaps, and cosmetics have been associated with food allergy (Codreanu, Morisset, Cordebar, Kanny, & Moneret-Vautrin, 2006; Fukutomi, Taniguchi, Nakamura, & Akiyama, 2014; Pootongkam & Nedorost, 2013) and the oral allergy syndrome is caused by cross-reactivity of food allergens with inhaled allergens like pollen (Popescu, 2015).
MODEL SELECTION GUIDELINES
There is a wide selection of models with distinct protocols (Table 7, Fig. 1) that makes model selection challenging. However, the choice of model(s) should be fit-for-purpose and address research aims and hypotheses for either 1) safety for an allergenicity risk assessment, 2) evaluation of novel therapeutic strategies, or 3) pathophysiology to acquire new insights into pathophysiology and new therapeutic targets.
Safety or Risk Assessment for Allergenicity
A safety or allergenicity risk assessment of food proteins is crucial for protecting the public from de novo sensitization to novel foods before entering the market. Several approaches are used for predicting the allergenicity of novel proteins, which are mainly based on the Codex Alimentarius (Codex Alimentarius Commission, 2003-2009) and (reviewed in (Mullins et al., 2022)). In vivo models for a safety assessment should ideally be robust, reliable, reproducible, and standardized. A safety allergenicity risk assessment has been used for predicting the allergenicity of the newly expressed proteins in GMOs (Marsteller et al., 2015) but has yet to be validated. For allergenicity testing, the models could be potentially done in wild-type and appropriate humanized mice. It is ideal to compare whole food and protein extracts to purified protein, even though the use of whole food is complex and difficult. Processed food might also be important for testing because of the potential for increasing or decreasing protein allergenicity. The dosing, timing, and frequency of the test materials should be close to what is expected for human consumption. Oral administration is better because GI tract digestion could play a key role in sensitization to food allergens within a whole food. Considerations about dosing are important because of the possibility of inducing oral tolerance. Establishing a dose-response curve would be essential for these models. Adjuvant-free models are preferable.
Using safety and risk assessment models could be practical for assessing potential cross-reactivity with other food proteins. Two examples illustrate the importance of using whole food, and one study showed that the bean alpha-amylase inhibitor protein used to produce GM peas cross-reacted with the intrinsic pea lectin protein in peas (Lee et al., 2013), and the other demonstrated cross-reactive proteins in soybean and cow's milk (Smaldini et al., 2012). An in vivo model has also been used for assessing whether newly expressed proteins in GMOs might be adjuvants (Andreassen et al., 2016; Guimaraes et al., 2008; Lee et al., 2013; Prescott et al., 2005; Reiner, Lee, Dekan, & Epstein, 2014; Tulinska et al., 2018; Vazquez-Padron, Moreno-Fierros, Neri-Bazan, de la Riva, & Lopez-Revilla, 1999), though none have convincingly shown an adjuvant effect.
Objective and reproducible readouts are essential for an allergenicity risk assessment. The currently used readouts seem appropriate and are mainly allergen-specific IgE and IgG1, basophil activation test, and clinical signs of anaphylaxis, e.g., reduced temperature and mobility, diarrhea, gut inflammation, and other possibilities might include intestinal permeability.
Treatment Efficacy
Wild-type and humanized mouse models could be used to evaluate the efficacy and effectiveness of treatment for food allergy (Leonard, Martos, Wang, Nowak-Wegrzyn, & Berin, 2012; Yamaki & Yoshino, 2012). The models can vary widely with the type of allergen, adjuvant, and routes of administration that reproducibly sensitizes the mice and can elicit an allergic response. If an allergen-specific therapy is intended, then the sensitizing allergen should be the same as the targeted treatment. While evaluating treatment effectiveness in the elicitation phase is important, prophylactic approaches can be tested before and during sensitization, e.g., inhibition of sensitization of oral tolerance. The treatment models should have objective and reproducible readouts, similar to the safety models above.
Pathophysiology and Disease Mechanisms
The selection of mouse models focused on pathophysiology is the most challenging because they are the most varied and depend on the type of research hypotheses and questions being addressed. The models could be established with wild-type, GM, and/or humanized mice, and any of the allergens, adjuvants, and routes of allergen administration. The readouts include the main disease parameters used for the other models, but also extend to cellular phenotype and function, gene expression profiling, transcriptomics, epigenetics, etc. Tg and KO mice provide opportunities for these experiments to elucidate the molecular and cellular mechanisms influencing sensitization and elicitation by addressing the existing challenges with the models and providing a system in which to directly interrogate the hypotheses.
Model Selection Criteria
Food allergy from any protocol can be achieved as long as the mice are sensitized and challenged using any of the indicated mouse strains, allergens, with or without adjuvants, routes of administration, and environmental conditions (Tables 1, 4, 5, 7, Fig. 1). On the one hand, having so many options allows for ease in the selection of a model, because there are so many to choose from. On the other hand, model selection can be challenging because it is difficult to choose when there are so many options. Importantly, selecting a model protocol and endpoints should be based upon the research aims.
The decision-making process should begin with the choice of a model that is fit for the intended purpose, based on whether the research is aimed at studying the 1) allergenicity of a protein, 2) treatment effectiveness, or 3) pathophysiology (Table 8).
Steps | Selection parameters | Options | Rationale and recommendations |
---|---|---|---|
1. Fit for purpose | Safety /allergenicity |
|
Research aims should guide the selection of experimental design and conditions: allergen, route, mouse strain, etc. |
Treatment effectiveness |
|
||
Pathophysiology |
|
||
2. Allergen sensitization | Purified protein | OVA (example) |
|
|
|||
|
|||
|
|||
|
|||
|
|||
|
|||
|
|||
|
|||
|
|||
Whole food (extracts and suspensions) | Peanut & seafood extracts (example) |
|
|
|
|||
|
|||
|
|||
|
|||
|
|||
|
|||
|
|||
|
|||
|
|||
3. Route of administration | Oral, skin, systemic, and respiratory tract |
|
|
|
|
||
|
|
||
|
|
||
4. Mice | Wild-type mice |
|
|
GM |
|
|
|
Humanized mice |
|
|
|
5. Costs | Model duration & dosing frequency |
|
|
|
|
||
|
|||
|
|||
Reagents and mice |
|
|
|
|
|
||
|
|
||
6. Investigator expertise | Investigator expertise and technology |
|
|
|
|
||
|
|
||
|
|
||
|
Based on the purpose of the model, the second step is choosing the allergen. Models using OVA are common because it is relatively inexpensive, has well-characterized immune responses and reagents, can cause oral tolerance and food allergy, is clinically relevant (egg allergy), requires adjuvant, and sensitization and elicitation can be done with purified protein as well as with whole food. Models with other relatively inexpensive allergens are whole food suspensions and extracts that are clinically relevant from peanuts and seafood. They can be done without adjuvants using a variety of allergen administration routes.
The third step is choosing the route of allergen administration and depends on whether the aim is to use whole food, which is best done by the p.o. route, or purified protein, which can be administered by any route and requires adjuvant. The choice between whole food and purified protein might be to mimic human consumption more closely, but it also depends on whether to focus on anaphylaxis or on GI tract inflammation, with or without anaphylaxis. Anaphylaxis is easier to elicit with purified protein.
The fourth step is choosing the mice. The most common strain is BALB/c, followed by C3H. There are strain differences, but not enough to warrant specifically using one over the other based on other criteria like type of allergen, route, etc. A tg or KO mouse strain might be ideal if the focus is on a particular gene or pathway. The choice to use humanized mice would also be possible depending on the aim being addressed.
The fifth step is selecting the models based on the research budget. For instance, the selection could be based on cost-effectiveness, e.g., a shorter model has lower mouse housing costs, requires less test material, and less labor. Other practical criteria might be the use of reagents available to the investigator, e.g., mouse strain, or GM mice, or a particular antigen.
The sixth step concerns investigator expertise and infrastructure. Establishing complex food allergy models would be facilitated by access to technology and facilities for creating tg, KO, and humanized mice and maintaining them in SPF and germ-free conditions. The selection of assays and readouts will depend upon the investigator's expertise and access to the needed instruments.
The wide variety of models provides a multitude of opportunities, but it is critical to base model selection on the hypothesis and research question. It is also possible that more than one model would be necessary. The selection of the best model(s) will become easier as our understanding of food allergy improves.
FUTURE DIRECTIONS
Translating basic lab results to patients with food allergies will require research leading to validated mouse models and a better understanding of the mechanisms underlying disease. A concerted effort integrating in silico , in vitro , and in vivo research findings will lead to discoveries and, ultimately, to clinical trials. Fundamental issues surrounding the disease in mice must address the development of translational models (Table 9).
Topic | Research need |
---|---|
Test materials: allergens, adjuvants, and controls | Defining non-allergenic test food proteins for negative controls |
Establishing a clinically relevant reference set of proteins with known allergenic potency | |
Establishing optimal approaches for characterizing allergen test materials | |
Determining how proteins in whole food differ from purified proteins | |
Establishing standardized protocols for administering food as it would be prepared and consumed for humans | |
Determining how quantity and frequency of test allergen exposure alters disease outcome | |
Determining how exogenous and endogenous adjuvants induce sensitization to food | |
Optimizing protocols for generating allergen dose-response curves | |
Establishing optimal approaches for characterizing allergen test materials | |
Route of administration | Understanding how administration routes for sensitizing and eliciting with distinct allergens influence disease outcomes |
Establishing standardized protocols for administering food as it would be prepared and consumed for humans | |
Disease mechanisms | Determining the mechanisms underlying factors that influence disease, e.g., environment, mouse age, gender, strain, diet, etc. |
Determining the steps necessary to progress from food protein-specific IgE without food allergies to symptomatic allergy | |
Identifying the methods for inducing anaphylaxis with small amounts of oral allergen | |
Establishing conditions for models of spontaneous disease |
CONCLUDING REMARKS
Although experimental mouse models have limitations, they have a potential role in understanding mechanisms underlying food allergy, establishing safety from unknown effects of novel foods, as well as evaluating novel therapeutics. The selection of the model should be fit-for-purpose. Notably, using more than one model might be necessary to address a hypothesis fully.
Implementing any model requires careful attention to the experimental design and the factors influencing disease, including mouse strains, allergens, the use of adjuvants, routes, frequency and timing of allergen administration, and environmental conditions.
There are numerous unanswered questions about the pathophysiology underlying food allergy. These knowledge gaps require complementary research with other areas of allergenicity research, including in silico and in vitro models, to generate more information on the nature and properties of allergens, and the role of the GI tract, e.g., digestion, transport, protein permeability, and barrier disruption. Further research is necessary to fully understand de novo sensitization mechanisms to develop optimal, standardized food allergy models.
AUTHOR CONTRIBUTIONS
Sahar Kazemi : Data curation and writing-original draft; Ece Danisman : Data curation and writing-original draft; Michelle M. Epstein : Conceptualization, data curation, supervision, and writing-original draft.
CONFLICT OF INTEREST
The authors do not have financial or personal relationships between themselves and others that might bias the work. The support source was not involved in the protocol design and in the collection, analysis, and interpretation of data.
Open Research
DATA AVAILABILITY STATEMENT
Data sharing is not applicable to this article as no datasets were generated or analyzed during the current study.
LITERATURE CITED
- Adel-Patient, K., Ah-Leung, S., Bernard, H., Durieux-Alexandrenne, C., Creminon, C., & Wal, J. M. (2007). Oral sensitization to peanut is highly enhanced by application of peanut extracts to intact skin, but is prevented when CpG and cholera toxin are added. International Archives of Allergy and Immunology , 143(1), 10–20. doi: 10.1159/000098221
- Ahrens, R., Osterfeld, H., Wu, D., Chen, C. Y., Arumugam, M., Groschwitz, K., … Hogan, S. P. (2012). Intestinal mast cell levels control severity of oral antigen-induced anaphylaxis in mice. American Journal of Pathology , 180(4), 1535–1546. doi: 10.1016/j.ajpath.2011.12.036
- Akiyama, H., Teshima, R., Sakushima, J. I., Okunuki, H., Goda, Y., Sawada, J. I., & Toyoda, M. (2001). Examination of oral sensitization with ovalbumin in Brown Norway rats and three strains of mice. Immunology Letters , 78(1), 1–5. doi: 10.1016/s0165-2478(01)00229-2
- Ando, T., Kashiwakura, J. I., Itoh-Nagato, N., Yamashita, H., Baba, M., Kawakami, Y., … Kawakami, T. (2017). Histamine-releasing factor enhances food allergy. Journal of Clinical Investigation , 127(12), 4541–4553. doi: 10.1172/JCI96525
- Ando, T., Matsumoto, K., Namiranian, S., Yamashita, H., Glatthorn, H., Kimura, M., … Kawakami, T. (2013). Mast cells are required for full expression of allergen/SEB-induced skin inflammation. Journal of Investigative Dermatology , 133(12), 2695–2705. doi: 10.1038/jid.2013.250
- André, C., Heremans, J. F., Vaerman, J. P., & Cambiaso, C. L. (1975). A mechanism for the induction of immunological tolerance by antigen feeding: Antigen-antibody complexes. Journal of Experimental Medicine , 142(6), 1509–1519. doi: 10.1084/jem.142.6.1509
- Andreassen, M., Bohn, T., Wikmark, O. G., Bodin, J., Traavik, T., Lovik, M., & Nygaard, U. C. (2016). Investigations of immunogenic, allergenic and adjuvant properties of Cry1Ab protein after intragastric exposure in a food allergy model in mice. BMC Immunology , 17(1), 10. doi: 10.1186/s12865-016-0148-x
- Anvari, S., Miller, J., Yeh, C. Y., & Davis, C. M. (2019). IgE-mediated food allergy. Clinical Reviews in Allergy & Immunology, 57(2), 244–260. doi: 10.1007/s12016-018-8710-3
- Arumugam, M., Ahrens, R., Osterfeld, H., Kottyan, L. C., Shang, X., Maclennan, J. A., … Hogan, S. P. (2011). Increased susceptibility of 129SvEvBrd mice to IgE-Mast cell mediated anaphylaxis. BMC Immunology , 12, 14. doi: 10.1186/1471-2172-12-14
- Atkinson, H. A., Johnson, I. T., Gee, J. M., Grigoriadou, F., & Miller, K. (1996). Brown Norway rat model of food allergy: Effect of plant components on the development of oral sensitization. Food and Chemical Toxicology , 34(1), 27–32. doi: 10.1016/0278-6915(95)00084-4
- Balbino, B., Sibilano, R., Starkl, P., Marichal, T., Gaudenzio, N., Karasuyama, H., … Galli, S. J. (2017). Pathways of immediate hypothermia and leukocyte infiltration in an adjuvant-free mouse model of anaphylaxis. Journal of Allergy and Clinical Immunology , 139(2), 584–596.e510. doi: 10.1016/j.jaci.2016.05.047
- Ballegaard, A. R., Madsen, C. B., & Bøgh, K. L. (2019). An animal model for wheat allergy skin sensitisation: A comparative study in naive versus tolerant brown Norway rats. International Archives of Allergy and Immunology , 178(2), 106–118. doi: 10.1159/000493802
- Bannon, G. A. (2004). What makes a food protein an allergen? Current Allergy and Asthma Reports , 4(1), 43–46. doi: 10.1007/s11882-004-0042-0
- Barcik, W., Untersmayr, E., Pali-Schöll, I., O'Mahony, L., & Frei, R. (2015). Influence of microbiome and diet on immune responses in food allergy models. Drug Discovery Today: Disease Models , 17-18, 71–80. doi: 10.1016/j.ddmod.2016.06.003
- Bartnikas, L. M., Gurish, M. F., Burton, O. T., Leisten, S., Janssen, E., Oettgen, H. C., … Oyoshi, M. K. (2013). Epicutaneous sensitization results in IgE-dependent intestinal mast cell expansion and food-induced anaphylaxis. Journal of Allergy and Clinical Immunology , 131(2), 451–460.e451–456. doi: 10.1016/j.jaci.2012.11.032
- Berin, M. C., & Sampson, H. A. (2013). Food allergy: An enigmatic epidemic. Trends in Immunology , 34(8), 390–397. doi: 10.1016/j.it.2013.04.003
- Besredka, A. (1909). De l'anaphylaxie lactique. Annales de l'Institut Pasteur , 23, 166–176.
- Birmingham, N., Payankaulam, S., Thanesvorakul, S., Stefura, B., HayGlass, K., & Gangur, V. (2003). An ELISA-based method for measurement of food-specific IgE antibody in mouse serum: An alternative to the passive cutaneous anaphylaxis assay. Journal of Immunological Methods , 275(1-2), 89–98. doi: 10.1016/s0022-1759(03)00008-5
- Birmingham, N. P., Parvataneni, S., Hassan, H. M., Harkema, J., Samineni, S., Navuluri, L., … Gangur, V. (2007). An adjuvant-free mouse model of tree nut allergy using hazelnut as a model tree nut. International Archives of Allergy and Immunology , 144(3), 203–210. doi: 10.1159/000103993
- Bøgh, K. L., Kroghsbo, S., Dahl, L., Rigby, N. M., Barkholt, V., Mills, E. N., & Madsen, C. B. (2009). Digested Ara h 1 has sensitizing capacity in brown Norway rats. Clinical and Experimental Allergy , 39(10), 1611–1621. doi: 10.1111/j.1365-2222.2009.03333.x
- Bøgh, K. L., van Bilsen, J., Glogowski, R., Lopez-Exposito, I., Bouchaud, G., Blanchard, C., … O'Mahony, L. (2016). Current challenges facing the assessment of the allergenic capacity of food allergens in animal models. Clinical and Translational Allergy , 6, 21. doi: 10.1186/s13601-016-0110-2
- Brandt, E. B., Strait, R. T., Hershko, D., Wang, Q., Muntel, E. E., Scribner, T. A., … Rothenberg, M. E. (2003). Mast cells are required for experimental oral allergen-induced diarrhea. Journal of Clinical Investigation , 112(11), 1666–1677. doi: 10.1172/JCI19785
- Branum, A. M., & Lukacs, S. L. (2009). Food allergy among children in the United States. Pediatrics , 124(6), 1549–1555. doi: 10.1542/peds.2009-1210
- Bryan, J., & Frank, L. A. (2010). Food allergy in the cat: A diagnosis by elimination. Journal of Feline Medicine and Surgery , 12(11), 861–866. doi: 10.1016/j.jfms.2010.09.005
- Burggraf, M., Nakajima-Adachi, H., Hachimura, S., Ilchmann, A., Pemberton, A. D., Kiyono, H., … Toda, M. (2011). Oral tolerance induction does not resolve gastrointestinal inflammation in a mouse model of food allergy. Molecular Nutrition & Food Research, 55(10), 1475–1483. doi: 10.1002/mnfr.201000634
- Burton, O. T., Darling, A. R., Zhou, J. S., Noval-Rivas, M., Jones, T. G., Gurish, M. F., … Oettgen, H. C. (2013). Direct effects of IL-4 on mast cells drive their intestinal expansion and increase susceptibility to anaphylaxis in a murine model of food allergy. Mucosal Immunology , 6(4), 740–750. doi: 10.1038/mi.2012.112
- Burton, O. T., Epp, A., Fanny, M. E., Miller, S. J., Stranks, A. J., Teague, J. E., … Oettgen, H. C. (2018). Tissue- specific expression of the low-affinity IgG receptor, FcgammaRIIb, on human mast cells. Frontiers in Immunology , 9, 1244. doi: 10.3389/fimmu.2018.01244
- Burton, O. T., Stranks, A. J., Tamayo, J. M., Koleoglou, K. J., Schwartz, L. B., & Oettgen, H. C. (2017). A humanized mouse model of anaphylactic peanut allergy. Journal of Allergy and Clinical Immunology , 139(1), 314–322. doi: 10.1016/j.jaci.2016.04.034
- Capobianco, F., Butteroni, C., Barletta, B., Corinti, S., Afferni, C., Tinghino, R., … Di Felice, G. (2008). Oral sensitization with shrimp tropomyosin induces in mice allergen-specific IgE, T cell response and systemic anaphylactic reactions. International Immunology , 20(8), 1077–1086. doi: 10.1093/intimm/dxn065
- Castan, L., Bøgh, K. L., Maryniak, N. Z., Epstein, M. M., Kazemi, S., O'Mahony, L., … Bastiaan-Net, S. (2020). Overview of in vivo and ex vivo endpoints in murine food allergy models: Suitable for evaluation of the sensitizing capacity of novel proteins? Allergy , 75(2), 289–301. doi: 10.1111/all.13943
- Chong, K. W., Ruiz-Garcia, M., Patel, N., Boyle, R. J., & Turner, P. J. (2020). Reaction phenotypes in IgE-mediated food allergy and anaphylaxis. Annals of Allergy, Asthma & Immunology, 124(5), 473–478. doi: 10.1016/j.anai.2019.12.023
- Clevers, H. (2016). Modeling development and disease with organoids. Cell , 165(7), 1586–1597. doi: 10.1016/j.cell.2016.05.082
- Codex Alimentarius Commission, J. F. W. F. S. P., Rome. (2003-2009). Codex Alimentarius, 2009. Foods Derived from Modern Biotechnology. Food & Agriculture Org.
- Codreanu, F., Morisset, M., Cordebar, V., Kanny, G., & Moneret-Vautrin, D. A. (2006). Risk of allergy to food proteins in topical medicinal agents and cosmetics. European Annals of Allergy and Clinical Immunology , 38(4), 126–130.
- Cubells-Baeza, N., Verhoeckx, K. C. M., Larre, C., Denery-Papini, S., Gavrovic-Jankulovic, M., & Diaz Perales, A. (2015). Applicability of epithelial models in protein permeability/transport studies and food allergy. Drug Discovery Today: Disease Models , 17-18, 13–21. doi: 10.1016/j.ddmod.2016.08.002
- de Theije, C. G., Wu, J., Koelink, P. J., Korte-Bouws, G. A., Borre, Y., Kas, M. J., & Kraneveld, A. D. (2014). Autistic-like behavioural and neurochemical changes in a mouse model of food allergy. Behavioural Brain Research , 261, 265–274. doi: 10.1016/j.bbr.2013.12.008
- Dearman, R. J., Stone, S., Caddick, H. T., Basketter, D. A., & Kimber, I. (2003). Evaluation of protein allergenic potential in mice: Dose-response analyses. Clinical and Experimental Allergy , 33(11), 1586–1594. doi: 10.1046/j.1365-2222.2003.01793.x
- del Moral, M. G., & Martinez-Naves, E. (2017). The role of lipids in development of allergic responses. Immune Network , 17(3), 133–143. doi: 10.4110/in.2017.17.3.133
- Dolence, J. J., Kobayashi, T., Iijima, K., Krempski, J., Drake, L. Y., Dent, A. L., & Kita, H. (2018). Airway exposure initiates peanut allergy by involving the IL-1 pathway and T follicular helper cells in mice. Journal of Allergy and Clinical Immunology , 142(4), 1144–1158.e1148. doi: 10.1016/j.jaci.2017.11.020
- Dunlop, J. H., & Keet, C. A. (2018). Epidemiology of food allergy. Immunology and Allergy Clinics of North America , 38(1), 13–25. doi: 10.1016/j.iac.2017.09.002
- Dupont, D., & Mackie, A. R. (2015). Static and dynamic in vitro digestion models to study protein stability in the gastrointestinal tract. Drug Discovery Today: Disease Models , 17-18, 23–27. doi: 10.1016/j.ddmod.2016.06.002
- Elson, C. O., & Ealding, W. (1984). Cholera toxin feeding did not induce oral tolerance in mice and abrogated oral tolerance to an unrelated protein antigen. Journal of Immunology , 133(6), 2892–2897.
- Evans, H., Killoran, K. E., & Mitre, E. (2014). Measuring local anaphylaxis in mice. Journal of Visualized Experiments: JoVE , (92), e52005. doi: 10.3791/52005
- Feehley, T., Plunkett, C. H., Bao, R., Choi Hong, S. M., Culleen, E., Belda-Ferre, P., & Nagler, C. R. (2019). Healthy infants harbor intestinal bacteria that protect against food allergy. Nature Medicine , 25(3), 448–453. doi: 10.1038/s41591-018-0324-z
- Fukutomi, Y., Taniguchi, M., Nakamura, H., & Akiyama, K. (2014). Epidemiological link between wheat allergy and exposure to hydrolyzed wheat protein in facial soap. Allergy , 69(10), 1405–1411. doi: 10.1111/all.12481
- Ganesan, V., Sharma, A., Tomar, S., Schuler, C. F. T., & Hogan, S. P. (2022). IL-4 receptor alpha signaling alters oral food challenge and immunotherapy outcomes in mice. Journal of Allergy and Clinical Immunology , 151(1), 182–191.e6. doi: 10.1016/j.jaci.2022.07.011
- Ganeshan, K., Neilsen, C. V., Hadsaitong, A., Schleimer, R. P., Luo, X., & Bryce, P. J. (2009). Impairing oral tolerance promotes allergy and anaphylaxis: A new murine food allergy model. Journal of Allergy and Clinical Immunology , 123(1), 231–238.e234. doi: 10.1016/j.jaci.2008.10.011
- Gaschen, F. P., & Merchant, S. R. (2011). Adverse food reactions in dogs and cats. The Veterinary Clinics of North America. Small Animal Practice , 41(2), 361–379. doi: 10.1016/j.cvsm.2011.02.005
- Gavrovic-Jankulovic, M., & Willemsen, L. E. M. (2015). Epithelial models to study food allergen-induced barrier disruption and immune activation. Drug Discovery Today: Disease Models , 17-18, 29–36. doi: 10.1016/j.ddmod.2016.09.002
- Guimaraes, V. D., Drumare, M. F., Ah-Leung, S., Lereclus, D., Bernard, H., Créminon, C., … Adel-Patient, K. (2008). Comparative study of the adjuvanticity of Bacillus thuringiensis Cry1Ab protein and cholera toxin on allergic sensitisation and elicitation to peanut. Food and Agricultural Immunology , 19(4), 325–337. doi: 10.1080/09540100802495651
- Gupta, R. S., Springston, E. E., Smith, B., Warrier, M. R., Pongracic, J., & Holl, J. L. (2012). Geographic variability of childhood food allergy in the United States. Clinical Pediatrics , 51(9), 856–861. doi: 10.1177/0009922812448526
- Gupta, R. S., Warren, C. M., Smith, B. M., Jiang, J., Blumenstock, J. A., Davis, M. M., … Nadeau, K. C. (2019). Prevalence and Severity of Food Allergies Among US Adults. JAMA Network Open , 2(1), e185630. doi: 10.1001/jamanetworkopen.2018.5630
- Hamilton, R. G., & Oppenheimer, J. (2015). Serological IgE analyses in the diagnostic algorithm for allergic disease. The Journal of Allergy and Clinical Immunology: In Practice , 3(6), 833–840. quiz 841-832. doi: 10.1016/j.jaip.2015.08.016
- Hardy, L. C., Smeekens, J. M., Raghuwanshi, D., Sarkar, S., Daskhan, G. C., Rogers, S., … Kulis, M. D. (2022). Targeting CD22 on memory B cells to induce tolerance to peanut allergens. Journal of Allergy and Clinical Immunology , 150(6), 1476–1485.e4. doi: 10.1016/j.jaci.2022.06.022
- Hayes, M., Rougé, P., Barre, A., Herouet-Guicheney, C., & Roggen, E. L. (2015). In silico tools for exploring potential human allergy to proteins. Drug Discovery Today: Disease Models , 17-18, 3–11. doi: 10.1016/j.ddmod.2016.06.001
- Herouet-Guicheney, C., Aldemir, H., Bars, R., de Barbeyrac, D., Kennel, P., Rouquie, D., … Dearman, R. J. (2009). Inter-laboratory comparisons of assessment of the allergenic potential of proteins in mice. Journal of Applied Toxicology , 29(2), 141–148. doi: 10.1002/jat.1391
- Hogan, S. P., Mishra, A., Brandt, E. B., Foster, P. S., & Rothenberg, M. E. (2000). A critical role for eotaxin in experimental oral antigen-induced eosinophilic gastrointestinal allergy. Proceedings of the National Academy of Sciences of the United States of America , 97(12), 6681–6686. doi: 10.1073/pnas.97.12.6681
- Hourihane, J. B., Kilburn, S. A., Nordlee, J. A., Hefle, S. L., Taylor, S. L., & Warner, J. O. (1997). An evaluation of the sensitivity of subjects with peanut allergy to very low doses of peanut protein: A randomized, double-blind, placebo-controlled food challenge study. Journal of Allergy and Clinical Immunology , 100(5), 596–600. doi: 10.1016/s0091-6749(97)70161-1
- Hussain, M., Bonilla-Rosso, G., Kwong Chung, C. K. C., Bariswyl, L., Rodriguez, M. P., Kim, B. S., … Noti, M. (2019). High dietary fat intake induces a microbiota signature that promotes food allergy. Journal of Allergy and Clinical Immunology , 144(1), 157–170.e158. doi: 10.1016/j.jaci.2019.01.043
- Hussain, M., Borcard, L., Walsh, K. P., Pena Rodriguez, M., Mueller, C., Kim, B. S., … Noti, M. (2018). Basophil-derived IL-4 promotes epicutaneous antigen sensitization concomitant with the development of food allergy. Journal of Allergy and Clinical Immunology , 141(1), 223–234.e225. doi: 10.1016/j.jaci.2017.02.035
- Hussain, M., Epstein, M. M., & Noti, M. (2015). Experimental food allergy models to study the role of innate immune cells as initiators of allergen-specific Th2 immune responses. Drug Discovery Today: Disease Models , 17-18, 55–62. doi: 10.1016/j.ddmod.2016.08.001
- Inagaki, N., Miura, T., Nagai, H., & Koda, A. (1992). Active cutaneous anaphylaxis (ACA) in the mouse ear. Japanese Journal of Pharmacology , 59(2), 201–208. doi: 10.1254/jjp.59.201
- Ito, R., Katano, I., Otsuka, I., Takahashi, T., Suemizu, H., Ito, M., & Simons, P. J. (2021). Bovine beta- lactoglobulin-induced passive systemic anaphylaxis model using humanized NOG hIL-3/hGM-CSF transgenic mice. International Immunology , 33(3), 183–189. doi: 10.1093/intimm/dxaa067
- Jones, S. M., & Burks, A. W. (2017). Food Allergy. New England Journal of Medicine , 377(12), 1168–1176. doi: 10.1056/NEJMcp1611971
- Kanagaratham, C., Sallis, B. F., & Fiebiger, E. (2018). Experimental models for studying food allergy. Cellular and Molecular Gastroenterology and Hepatology , 6(3), 356–369.e351. doi: 10.1016/j.jcmgh.2018.05.010
- Keshavarz, B., Jiang, X., Hsieh, Y. P., & Rao, Q. (2019). Matrix effect on food allergen detection-A case study of fish parvalbumin. Food Chemistry , 274, 526–534. doi: 10.1016/j.foodchem.2018.08.138
- Kim, H. J., Huh, D., Hamilton, G., & Ingber, D. E. (2012). Human gut-on-a-chip inhabited by microbial flora that experiences intestinal peristalsis-like motions and flow. Lab on a Chip , 12(12), 2165–2174. doi: 10.1039/c2lc40074j
- Kim, H. J., Li, H., Collins, J. J., & Ingber, D. E. (2016). Contributions of microbiome and mechanical deformation to intestinal bacterial overgrowth and inflammation in a human gut-on-a-chip. Proceedings of the National Academy of Sciences of the United States of America , 113(1), E7–15. doi: 10.1073/pnas.1522193112
- Kinney, S. R., Carlson, L., Ser-Dolansky, J., Thompson, C., Shah, S., Gambrah, A., … Mathias, C. B. (2015). Curcumin ingestion inhibits mastocytosis and suppresses intestinal anaphylaxis in a murine model of food allergy. Plos One , 10(7), e0132467. doi: 10.1371/journal.pone.0132467
- Kiraly, N., Koplin, J. J., Crawford, N. W., Bannister, S., Flanagan, K. L., Holt, P. G., … Allen, K. J. (2016). Timing of routine infant vaccinations and risk of food allergy and eczema at one year of age. Allergy , 71(4), 541–549. doi: 10.1111/all.12830
- Kucuk, Z. Y., Strait, R., Khodoun, M. V., Mahler, A., Hogan, S., & Finkelman, F. D. (2012). Induction and suppression of allergic diarrhea and systemic anaphylaxis in a murine model of food allergy. Journal of Allergy and Clinical Immunology , 129(5), 1343–1348. doi: 10.1016/j.jaci.2012.03.004
- Lack, G. (2012). Update on risk factors for food allergy. Journal of Allergy and Clinical Immunology , 129(5), 1187–1197. doi: 10.1016/j.jaci.2012.02.036
- Ladics, G. S., Knippels, L. M., Penninks, A. H., Bannon, G. A., Goodman, R. E., & Herouet-Guicheney, C. (2010). Review of animal models designed to predict the potential allergenicity of novel proteins in genetically modified crops. Regulatory Toxicology and Pharmacology: RTP , 56(2), 212–224. doi: 10.1016/j.yrtph.2009.09.018
- Lam, Y. F., Tong, K. K., Kwan, K. M., Tsuneyama, K., Shu, S. A., Leung, P. S., & Chu, K. H. (2015). Gastrointestinal immune response to the shrimp allergen tropomyosin: Histological and immunological analysis in an animal model of shrimp tropomyosin hypersensitivity. International Archives of Allergy and Immunology , 167(1), 29–40. doi: 10.1159/000431228
- Lee, J. B. (2016). Regulation of IgE-mediated food allergy by IL-9 producing mucosal mast cells and type 2 innate lymphoid cells. Immune Network , 16(4), 211–218. doi: 10.4110/in.2016.16.4.211
- Lee, J. B., Chen, C. Y., Liu, B., Mugge, L., Angkasekwinai, P., Facchinetti, V., … Wang, Y. H. (2016). IL-25 and CD4(+) TH2 cells enhance type 2 innate lymphoid cell-derived IL-13 production, which promotes IgE-mediated experimental food allergy. Journal of Allergy and Clinical Immunology , 137(4), 1216–1225.e1215. doi: 10.1016/j.jaci.2015.09.019
- Lee, R. Y., Reiner, D., Dekan, G., Moore, A. E., Higgins, T. J., & Epstein, M. M. (2013). Genetically modified alpha-amylase inhibitor peas are not specifically allergenic in mice. Plos One , 8(1), e52972. doi: 10.1371/journal.pone.0052972
- Leonard, S. A., Martos, G., Wang, W., Nowak-Wegrzyn, A., & Berin, M. C. (2012). Oral immunotherapy induces local protective mechanisms in the gastrointestinal mucosa. Journal of Allergy and Clinical Immunology , 129(6), 1579–1587.e1571. doi: 10.1016/j.jaci.2012.04.009
- Lexmond, W. S., Goettel, J. A., Lyons, J. J., Jacobse, J., Deken, M. M., Lawrence, M. G., … Fiebiger, E. (2016). FOXP3+ Tregs require WASP to restrain Th2-mediated food allergy. Journal of Clinical Investigation , 126(10), 4030–4044. doi: 10.1172/JCI85129
- Lexmond, W. S., Goettel, J. A., Sallis, B. F., McCann, K., Rings, E. H. H. M., Jensen-Jarolim, E., … Fiebiger, E. (2017). Spontaneous food allergy in Was(-/-) mice occurs independent of Fc epsilon RI- mediated mast cell activation. Allergy , 72(12), 1916–1924. doi: 10.1111/all.13219
- Li, X. M., Serebrisky, D., Lee, S. Y., Huang, C. K., Bardina, L., Schofield, B. H., … Sampson, H. A. (2000). A murine model of peanut anaphylaxis: T- and B-cell responses to a major peanut allergen mimic human responses. Journal of Allergy and Clinical Immunology , 106, 150–158. doi: 10.1067/mai.2000.107395
- Lozano-Ojalvo, D., Lezmi, G., Cortes-Perez, N., & Adel-Patient, K. (2015). Non-IgE mediated food allergy. Drug Discovery Today: Disease Models , 17-18, 45–53. doi: 10.1016/j.ddmod.2016.09.003
- Makabe-Kobayashi, Y., Hori, Y., Adachi, T., Ishigaki-Suzuki, S., Kikuchi, Y., Kagaya, Y., … Ohtsu, H. (2002). The control effect of histamine on body temperature and respiratory function in IgE-dependent systemic anaphylaxis. Journal of Allergy and Clinical Immunology , 110(2), 298–303. doi: 10.1067/mai.2002.125977
- Mali, S., & Jambure, R. (2012). Anaphyllaxis management: Current concepts. Anesthesia, Essays and Researches , 6(2), 115–123. doi: 10.4103/0259-1162.108284
- Marsteller, N., Bøgh, K. L., Goodman, R. E., & Epstein, M. M. (2015). A review of animal models used to evaluate potential allergenicity of genetically modified organisms (GMOs). Drug Discovery Today: Disease Models , 17-18, 81–88. doi: 10.1016/j.ddmod.2016.11.001
- Mathias, C. B., Hobson, S. A., Garcia-Lloret, M., Lawson, G., Poddighe, D., Freyschmidt, E. J., … Oettgen, H. C. (2011). IgE-mediated systemic anaphylaxis and impaired tolerance to food antigens in mice with enhanced IL-4 receptor signaling. Journal of Allergy and Clinical Immunology , 127(3), 795–805.e791–796. doi: 10.1016/j.jaci.2010.11.009
- Matsui, T., Yamashita, H., Saneyasu, K. I., Tanaka, H., Ito, K., & Inagaki, N. (2018). Vitamin D deficiency exacerbates sensitization and allergic diarrhea in a murine food allergy model. Allergology International , 67(2), 289–291. doi: 10.1016/j.alit.2017.08.010
- Michelet, M., Balbino, B., Guilleminault, L., & Reber, L. L. (2021). IgE in the pathophysiology and therapy of food allergy. European Journal of Immunology , 51(3), 531–543. doi: 10.1002/eji.202048833
- Molkhou, P. (2016). Non-IgE mediated food hypersensitivity. Revue Française d'Allergologie , 56(2), 76–83. doi: 10.1016/j.reval.2015.10.010
- Moutsoglou, D. M., & Dreskin, S. C. (2016). B cells establish, but do not maintain, long-lived murine anti-peanut IgE(a). Clinical and Experimental Allergy , 46(4), 640–653. doi: 10.1111/cea.12715
- Mullins, E., Bresson, J. L., Dalmay, T., Dewhurst, I. C., Epstein, M. M., George Firbank, L., … Moreno, F. J. (2022). Efsa panel on genetically modified organisms: Scientific opinion on development needs for the allergenicity and protein safety assessment of food and feed products derived from biotechnology. EFSA Journal , 20(1), e07044. doi: 10.2903/j.efsa.2022.7044
- Noti, M., Kim, B. S., Siracusa, M. C., Rak, G. D., Kubo, M., Moghaddam, A. E., … Artis, D. (2014). Exposure to food allergens through inflamed skin promotes intestinal food allergy through the thymic stromal lymphopoietin-basophil axis. Journal of Allergy and Clinical Immunology , 133(5), 1390–U1643. doi: 10.1016/j.jaci.2014.01.021
- Ogino, H., Sakazaki, F., Okuno, T., Arakawa, T., & Ueno, H. (2015). Oxidized dietary oils enhance immediate- and/or delayed-type allergic reactions in BALB/c mice. Allergology International , 64(1), 66–72. doi: 10.1016/j.alit.2014.07.004
- Ohsaki, A., Venturelli, N., Buccigrosso, T. M., Osganian, S. K., Lee, J., Blumberg, R. S., & Oyoshi, M. K. (2018). Maternal IgG immune complexes induce food allergen-specific tolerance in offspring. Journal of Experimental Medicine , 215(1), 91–113. doi: 10.1084/jem.20171163
- Olivry, T., & Mueller, R. S. (2017). Critically appraised topic on adverse food reactions of companion animals (3): Prevalence of cutaneous adverse food reactions in dogs and cats. BMC Veterinary Research , 13(1), 51. doi: 10.1186/s12917-017-0973-z
- Oyoshi, M. K., Oettgen, H. C., Chatila, T. A., Geha, R. S., & Bryce, P. J. (2014). Food allergy: Insights into etiology, prevention, and treatment provided by murine models. Journal of Allergy and Clinical Immunology , 133(2), 309–317. doi: 10.1016/j.jaci.2013.12.1045
- Pagovich, O. E., Wang, B., Chiuchiolo, M. J., Kaminsky, S. M., Sondhi, D., Jose, C. L., … Crystal, R. G. (2016). Anti-hIgE gene therapy of peanut-induced anaphylaxis in a humanized murine model of peanut allergy. Journal of Allergy and Clinical Immunology , 138(6), 1652–1662.e1657. doi: 10.1016/j.jaci.2016.03.053
- Park, J. H., & Choi, T. S. (2016). Splenocyte proliferation and anaphylaxis induced by BSA challenge in a D-galactose-induced aging mouse model. Central European Journal of Immunology , 41(3), 324–327. doi: 10.5114/ceji.2016.63134
- Parvataneni, S., Birmingham, N. P., Gonipeta, B., & Gangur, V. (2009). Dominant, non-MHC genetic control of food allergy in an adjuvant-free mouse model. International Journal of Immunogenetics , 36(5), 261–267. doi: 10.1111/j.1744-313X.2009.00860.x
- Parvataneni, S., Gonipeta, B., Acharya, H. G., & Gangur, V. (2015). An Adjuvant-Free Mouse Model of Transdermal Sensitization and Oral Elicitation of Anaphylaxis to Shellfish. International Archives of Allergy and Immunology , 168(4), 269–276. doi: 10.1159/000443736
- Pilegaard, K., & Madsen, C. (2004). An oral Brown Norway rat model for food allergy: Comparison of age, sex, dosing volume, and allergen preparation. Toxicology , 196(3), 247–257. doi: 10.1016/j.tox.2003.11.010
- Platzer, B., Baker, K., Vera, M. P., Singer, K., Panduro, M., Lexmond, W. S., … Fiebiger, E. (2015). Dendritic cell-bound IgE functions to restrain allergic inflammation at mucosal sites. Mucosal Immunology , 8(3), 516–532. doi: 10.1038/mi.2014.85
- Pootongkam, S., & Nedorost, S. (2013). Oat and wheat as contact allergens in personal care products. Dermatitis , 24(6), 291–295. doi: 10.1097/DER.0b013e3182a745f8
- Popescu, F. D. (2015). Cross-reactivity between aeroallergens and food allergens. World Journal of Methodology , 5(2), 31–50. doi: 10.5662/wjm.v5.i2.31
- Prescott, V. E., Campbell, P. M., Moore, A., Mattes, J., Rothenberg, M. E., Foster, P. S., … Hogan, S. P. (2005). Transgenic expression of bean alpha-amylase inhibitor in peas results in altered structure and immunogenicity. Journal of Agricultural and Food Chemistry , 53(23), 9023–9030. doi: 10.1021/jf050594v
- Prescott, V. E., Forbes, E., Foster, P. S., Matthaei, K., & Hogan, S. P. (2006). Mechanistic analysis of experimental food allergen-induced cutaneous reactions. Journal of Leukocyte Biology , 80(2), 258–266. doi: 10.1189/jlb.1105637
- Proust, B., Astier, C., Jacquenet, S., Ogier, V., Magueur, E., Roitel, O., … Kanny, G. (2008). A single oral sensitization to peanut without adjuvant leads to anaphylaxis in mice. International Archives of Allergy and Immunology , 146(3), 212–218. doi: 10.1159/000115889
- Reiner, D., Lee, R. Y., Dekan, G., & Epstein, M. M. (2014). No adjuvant effect of Bacillus thuringiensis-maize on allergic responses in mice. Plos One , 9(8), e103979. doi: 10.1371/journal.pone.0103979
- Rodriguez, B., Prioult, G., Hacini-Rachinel, F., Moine, D., Bruttin, A., Ngom-Bru, C., … Waligora-Dupriet, A. J. (2012). Infant gut microbiota is protective against cow's milk allergy in mice despite immature ileal T-cell response. FEMS Microbiology Ecology , 79(1), 192–202. doi: 10.1111/j.1574-6941.2011.01207.x
- Rodriguez, M. J., Aranda, A., Fernandez, T. D., Cubells-Baeza, N., Torres, M. J., Gomez, F., … Mayorga, C. (2017). LPS promotes Th2 dependent sensitisation leading to anaphylaxis in a Pru p 3 mouse model. Science Reports , 7, 40449. doi: 10.1038/srep40449
- Satitsuksanoa, P., Daanje, M., Akdis, M., Boyd, S. D., & van de Veen, W. (2021). Biology and dynamics of B cells in the context of IgE-mediated food allergy. Allergy , 76(6), 1707–1717. doi: 10.1111/all.14684
- Sato, T., Vries, R. G., Snippert, H. J., van de Wetering, M., Barker, N., Stange, D. E., … Clevers, H. (2009). Single Lgr5 stem cells build crypt-villus structures in vitro without a mesenchymal niche. Nature , 459(7244), 262–U147. doi: 10.1038/nature07935
- Sbihi, H., Boutin, R. C. T., Cutler, C., Suen, M., Finlay, B. B., & Turvey, S. E. (2019). Thinking bigger: How early-life environmental exposures shape the gut microbiome and influence the development of asthma and allergic disease. Allergy , 74(11), 2103–2115. doi: 10.1111/all.13812
- Schulten, V., Lauer, I., Scheurer, S., Thalhammer, T., & Bohle, B. (2011). A food matrix reduces digestion and absorption of food allergens in vivo. Molecular Nutrition & Food Research, 55(10), 1484–1491. doi: 10.1002/mnfr.201100234
- Schwarzer, M., Srutkova, D., Hermanova, P., Leulier, F., Kozakova, H., & Schabussova, I. (2017). Diet Matters: Endotoxin in the diet impacts the level of allergic sensitization in germ-free mice. Plos One , 12(1), e0167786. doi: 10.1371/journal.pone.0167786
- Sicherer, S. H. (2011). Epidemiology of food allergy. Journal of Allergy and Clinical Immunology , 127(3), 594–602. doi: 10.1016/j.jaci.2010.11.044
- Sicherer, S. H., & Sampson, H. A. (2018). Food allergy: A review and update on epidemiology, pathogenesis, diagnosis, prevention, and management. Journal of Allergy and Clinical Immunology , 141(1), 41–58. doi: 10.1016/j.jaci.2017.11.003
- Smaldini, P., Curciarello, R., Candreva, A., Rey, M. A., Fossati, C. A., Petruccelli, S., & Docena, G. H. (2012). In vivo evidence of cross-reactivity between cow's milk and soybean proteins in a mouse model of food allergy. International Archives of Allergy and Immunology , 158(4), 335–346. doi: 10.1159/000333562
- Smeekens, J. M., Immormino, R. M., Balogh, P. A., Randell, S. H., Kulis, M. D., & Moran, T. P. (2019). Indoor dust acts as an adjuvant to promote sensitization to peanut through the airway. Clinical and Experimental Allergy , 49(11), 1500–1511. doi: 10.1111/cea.13486
- Smeekens, J. M., Johnson-Weaver, B. T., Hinton, A. L., Azcarate-Peril, M. A., Moran, T. P., Immormino, R. M., … Kulis, M. D. (2020). Fecal IgA, antigen absorption, and gut microbiome composition are associated with food antigen sensitization in genetically susceptible mice. Frontiers in Immunology , 11, 599637. doi: 10.3389/fimmu.2020.599637
- Smeekens, J. M., & Kulis, M. D. (2021). Mouse models of food allergy in the pursuit of novel treatment modalities. Frontiers in Allergy , 2, 810067. doi: 10.3389/falgy.2021.810067
- Smit, J. J., Noti, M., & O'Mahony, L. (2015). The use of animal models to discover immunological mechanisms underpinning sensitization to food allergens. Drug Discovery Today: Disease Models , 17-18, 63–69. doi: 10.1016/j.ddmod.2016.09.001
- Spergel, J. M. (2006). Nonimmunoglobulin e-mediated immune reactions to foods. Allergy, Asthma & Clinical Immunology, 2(2), 78–85. doi: 10.1186/1710-1492-2-2-78
- Srivastava, K. D., Siefert, A., Fahmy, T. M., Caplan, M. J., Li, X. M., & Sampson, H. A. (2016). Investigation of peanut oral immunotherapy with CpG/peanut nanoparticles in a murine model of peanut allergy. Journal of Allergy and Clinical Immunology , 138(2), 536–543.e534. doi: 10.1016/j.jaci.2016.01.047
- Strait, R. T., Mahler, A., Hogan, S., Khodoun, M., Shibuya, A., & Finkelman, F. D. (2011). Ingested allergens must be absorbed systemically to induce systemic anaphylaxis. Journal of Allergy and Clinical Immunology , 127(4), 982–989. doi: 10.1016/j.jaci.2011.01.034
- Strid, J., Hourihane, J., Kimber, I., Callard, R., & Strobel, S. (2005). Epicutaneous exposure to peanut protein prevents oral tolerance and enhances allergic sensitization. Clinical and Experimental Allergy , 35(6), 757–766. doi: 10.1111/j.1365-2222.2005.02260.x
- Sun, J., Arias, K., Alvarez, D., Fattouh, R., Walker, T., Goncharova, S., … Jordana, M. (2007). Impact of CD40 ligand, B cells, and mast cells in peanut-induced anaphylactic responses. Journal of Immunology , 179(10), 6696–6703. doi: 10.4049/jimmunol.179.10.6696
- Tanaka, M., Nagano, T., Yano, H., Matsuda, T., Ikeda, T. M., Haruma, K., & Kato, Y. (2011). Impact of omega- 5 gliadin on wheat-dependent exercise-induced anaphylaxis in mice. Bioscience, Biotechnology, and Biochemistry , 75(2), 313–317. doi: 10.1271/bbb.100695
- Taylor, S. L., Hefle, S. L., Bindslev-Jensen, C., Bock, S. A., Burks, A. W. Jr., Christie, L., … Zeiger, R. S. (2002). Factors affecting the determination of threshold doses for allergenic foods: How much is too much? Journal of Allergy and Clinical Immunology , 109(1), 24–30. doi: 10.1067/mai.2002.120564
- Teuber, S. S., Del Val, G., Morigasaki, S., Jung, H. R., Eisele, P. H., Frick, O. L., & Buchanan, B. B. (2002). The atopic dog as a model of peanut and tree nut food allergy. Journal of Allergy and Clinical Immunology , 110(6), 921–927. doi: 10.1067/mai.2002.130056
- Thomas, H. C., & Parrott, M. V. (1974). The induction of tolerance to a soluble protein antigen by oral administration. Immunology , 27(4), 631–639.
- Thomas, K., Herouet, C., Bannon, G., Ladics, G., Macintosh, S., Privalle, L., & Woolhiser, M. (2005). Evaluation of IP mouse models for assessing the allergenic potential of proteins. Journal of Allergy and Clinical Immunology , 115(2), doi: 10.1016/j.jaci.2004.12.1007
- Tomar, S., Ganesan, V., Sharma, A., Zeng, C., Waggoner, L., Smith, A., … Hogan, S. P. (2021). IL-4-BATF signaling directly modulates IL-9 producing mucosal mast cell (MMC9) function in experimental food allergy. Journal of Allergy and Clinical Immunology , 147(1), 280–295. doi: 10.1016/j.jaci.2020.08.043
- Tordesillas, L., Goswami, R., Benede, S., Grishina, G., Dunkin, D., Jarvinen, K. M., … Berin, M. C. (2014). Skin exposure promotes a Th2-dependent sensitization to peanut allergens. Journal of Clinical Investigation , 124(11), 4965–4975. doi: 10.1172/JCI75660
- Tsilingiri, K., Barbosa, T., Penna, G., Caprioli, F., Sonzogni, A., Viale, G., & Rescigno, M. (2012). Probiotic and postbiotic activity in health and disease: Comparison on a novel polarised ex-vivo organ culture model. Gut , 61(7), 1007–1015. doi: 10.1136/gutjnl-2011-300971
- Tulinska, J., Adel-Patient, K., Bernard, H., Liskova, A., Kuricova, M., Ilavska, S., … Steinberg, P. (2018). Humoral and cellular immune response in Wistar Han RCC rats fed two genetically modified maize MON810 varieties for 90 days (EU 7th Framework Programme project GRACE). Archives of Toxicology , 92(7), 2385–2399. doi: 10.1007/s00204-018-2230-z
- Utsch, L., Logiantara, A., van Ree, R., & van Rijt, L. S. (2017). Experimental food allergy to peanut enhances the immune response to house dust mite in the airways of mice. Clinical and Experimental Allergy , 47(1), 121–128. doi: 10.1111/cea.12799
- van Esch, B. C., Schouten, B., Hofman, G. A., van Baalen, T., Nijkamp, F. P., Knippels, L. M., … Garssen, J. (2010). Acute allergic skin response as a new tool to evaluate the allergenicity of whey hydrolysates in a mouse model of orally induced cow's milk allergy. Pediatric Allergy and Immunology , 21(4 Pt 2), e780–786. doi: 10.1111/j.1399-3038.2009.00924.x
- Vaz, N. M., Maia, L. C. S., Hanson, D. G., & Lynch, J. M. (1977). Inhibition of homocytotropic antibody responses in adult inbred mice by previous feeding of the specific antigen. Journal of Allergy and Clinical Immunology , 60(2), 110–115. doi: 10.1016/0091-6749(77)90035-5
- Vazquez-Padron, R. I., Moreno-Fierros, L., Neri-Bazan, L., de la Riva, G. A., & Lopez-Revilla, R. (1999). Intragastric and intraperitoneal administration of Cry1Ac protoxin from Bacillus thuringiensis induces systemic and mucosal antibody responses in mice. Life Sciences , 64(21), 1897–1912. doi: 10.1016/s0024-3205(99)00136-8
- Vickery, B. P., Chin, S., & Burks, A. W. (2011). Pathophysiology of food allergy. Pediatric Clinics of North America , 58(2), 363–376. ix–x. doi: 10.1016/j.pcl.2011.02.012
- Walker, M. T., Green, J. E., Ferrie, R. P., Queener, A. M., Kaplan, M. H., & Cook-Mills, J. M. (2018). Mechanism for initiation of food allergy: Dependence on skin barrier mutations and environmental allergen costimulation. Journal of Allergy and Clinical Immunology , 141(5), 1711–1725.e1719. doi: 10.1016/j.jaci.2018.02.003
- Walsh, N. C., Kenney, L. L., Jangalwe, S., Aryee, K. E., Greiner, D. L., Brehm, M. A., & Shultz, L. D. (2017). Humanized mouse models of clinical disease. Annual Review of Pathology , 12, 187–215. doi: 10.1146/annurev-pathol-052016-100332
- Wang, B., Hu, J., Liu, Y., Liu, Q., & Li, D. (2020). Food allergy promotes a Th2/Th17 response that drives house dust mite-induced allergic airway inflammation in humanized mice. Clinical and Experimental Immunology , 202(3), 300–307. doi: 10.1111/cei.13504
- Wang, S., Zhang, R., Li, X., Gao, Y., Dai, N., Wei, Y., … Li, Z. (2022). Relationship between maternal-infant gut microbiota and infant food allergy. Frontiers in Microbiology , 13, 933152. doi: 10.3389/fmicb.2022.933152
- Waserman, S., Begin, P., & Watson, W. (2018). IgE-mediated food allergy. Allergy, Asthma & Clinical Immunology, 14(Suppl 2), 55. doi: 10.1186/s13223-018-0284-3
- Waserman, S., & Watson, W. (2011). Food allergy. Allergy, Asthma & Clinical Immunology, 7(Suppl 1), S7. doi: 10.1186/1710-1492-7-S1-S7
- Wavrin, S., Bernard, H., Wal, J. M., & Adel-Patient, K. (2015). Influence of the route of exposure and the matrix on the sensitisation potency of a major cows' milk allergen. Clinical and Translational Allergy , 5(1), 3. doi: 10.1186/s13601-015-0047-x
- Weigmann, B., Schughart, N., Wiebe, C., Sudowe, S., Lehr, H. A., Jonuleit, H., … Bellinghausen, I. (2012). Allergen-induced IgE-dependent gut inflammation in a human PBMC-engrafted murine model of allergy. Journal of Allergy and Clinical Immunology , 129(4), 1126–1135. doi: 10.1016/j.jaci.2011.11.036
- Wells, H. G. (1911). Studies on the chemistry of anaphylaxis (III). Experiments with isolated proteins, especially those of the hen's egg. The Journal of Infectious Diseases , 9(2), 147–171. doi: 10.1093/infdis/9.2.147
- Wells, H. G., & Osborne, T. B. (1911). The biological reactions of the vegetable proteins I. Anaphylaxis. The Journal of Infectious Diseases , 8(1), 66–124. doi: 10.1093/infdis/8.1.66
- Yamaki, K., & Yoshino, S. (2012). Tyrosine kinase inhibitor sunitinib relieves systemic and oral antigen- induced anaphylaxes in mice. Allergy , 67(1), 114–122. doi: 10.1111/j.1398-9995.2011.02717.x
- Yamani, A., Wu, D., Ahrens, R., Waggoner, L., Noah, T. K., Garcia-Hernandez, V., … Hogan, S. P. (2021). Dysregulation of intestinal epithelial CFTR-dependent Cl(-) ion transport and paracellular barrier function drives gastrointestinal symptoms of food-induced anaphylaxis in mice. Mucosal Immunology , 14(1), 135–143. doi: 10.1038/s41385-020-0306-6
- Yamani, A., Wu, D., Waggoner, L., Noah, T., Koleske, A. J., Finkelman, F., & Hogan, S. P. (2018). The vascular endothelial specific IL-4 receptor alpha-ABL1 kinase signaling axis regulates the severity of IgE- mediated anaphylactic reactions. Journal of Allergy and Clinical Immunology , 142(4), 1159–1172.e1155. doi: 10.1016/j.jaci.2017.08.046
- Yu, M., Eckart, M. R., Morgan, A. A., Mukai, K., Butte, A. J., Tsai, M., & Galli, S. J. (2011). Identification of an IFN-gamma/mast cell axis in a mouse model of chronic asthma. Journal of Clinical Investigation , 121(8), 3133–3143. doi: 10.1172/JCI43598
- Yu, W., Freeland, D. M. H., & Nadeau, K. C. (2016a). Food allergy: Immune mechanisms, diagnosis and immunotherapy. Nature Reviews Immunology , 16(12), 751–765. doi: 10.1038/nri.2016.111
- Yu, W., Freeland, D. M. H., & Nadeau, K. C. (2016b). Food allergy: Immune mechanisms, diagnosis and immunotherapy. Nature Reviews Immunology , 16(12), 751–765. doi: 10.1038/nri.2016.111
- Zhang, S., Sicherer, S., Berin, M. C., & Agyemang, A. (2021). Pathophysiology of non-IgE-mediated food allergy. ImmunoTargets and Therapy , 10, 431–446. doi: 10.2147/ITT.S284821
- Zhao, X., Thijssen, S., Chen, H., Garssen, J., Knippels, L. M. J., & Hogenkamp, A. (2021). Selenium modulates the allergic response to whey protein in a mouse model for cow's milk allergy. Nutrients , 13(8), 2479. doi: 10.3390/nu13082479
- Zhu, Q., Wang, J., Ma, J., Sheng, X., & Li, F. (2021). Changes in inflammatory factors in the Brown Norway rat model of food allergy. BMC Immunology [Electronic Resource] , 22(1), 8. doi: 10.1186/s12865-021-00398-9
- Zlotkowska, D., Stachurska, E., Fuc, E., Wroblewska, B., Mikolajczyk, A., & Wasilewska, E. (2021). Differences in regulatory mechanisms induced by beta-lactoglobulin and kappa-casein in cow's milk allergy mouse model-in vivo and ex vivo studies. Nutrients , 13(2), 349. doi: 10.3390/nu13020349
Citing Literature
Number of times cited according to CrossRef: 9
- Muyiwa Seyi Adegbaju, Titilayo Ajose, Ifeoluwa Elizabeth Adegbaju, Temitayo Omosebi, Shakirat Oloruntoyin Ajenifujah-Solebo, Olaitan Yetunde Falana, Olufunke Bolatito Shittu, Charles Oluwaseun Adetunji, Olalekan Akinbo, Genetic engineering and genome editing technologies as catalyst for Africa’s food security: the case of plant biotechnology in Nigeria, Frontiers in Genome Editing, 10.3389/fgeed.2024.1398813, 6 , (2024).
- A. Fernandez, E. Danisman, M. Taheri Boroujerdi, S. Kazemi, F. J. Moreno, M. M. Epstein, Research gaps and future needs for allergen prediction in food safety, Frontiers in Allergy, 10.3389/falgy.2024.1297547, 5 , (2024).
- Xing Zhang, Xiao Chen, Tianliang Bai, Xuanyi Meng, Yong Wu, Anshu Yang, Hongbing Chen, Xin Li, Egg White Diet Induces Severe Allergic Enteritis in an Animal Model Driven by Caspase-3 and Gasdermin C-Mediated Mucosal Alarmin Secretion, Journal of Agricultural and Food Chemistry, 10.1021/acs.jafc.4c06967, (2024).
- Katelin L. Davis, Estefania Claudio-Etienne, Pamela A. Frischmeyer-Guerrerio, Atopic dermatitis and food allergy: More than sensitization, Mucosal Immunology, 10.1016/j.mucimm.2024.06.005, 17 , 5, (1128-1140), (2024).
- Jiali Bao, Yushu Qiu, Xinyi Xu, Xiao Fu, Jingjing Song, Lei Wang, Lisu Huang, Weixi Zhang, Towards an optimized model of food allergy in zebrafish, Molecular Immunology, 10.1016/j.molimm.2024.07.014, 173 , (110-116), (2024).
- Lazar D. Nesovic, Pedro E. Gonzalez Cruz, Natalie Rychener, Logan R. Wilks, Harvinder S. Gill, Standardizing the skin tape stripping method for sensitization and using it to create a mouse model of peanut allergy, International Journal of Pharmaceutics, 10.1016/j.ijpharm.2024.124479, 662 , (124479), (2024).
- F. Javier Moreno, Marcello Laganaro, Antonio Fernandez-Dumont, Allergenicity risk assessment of GMOs and novel foods: An overview, Encyclopedia of Food Allergy, 10.1016/B978-0-323-96018-2.00148-6, (352-363), (2024).
- Gregory S. Ladics, Andre Silvanovich, Protein Allergy and Genetically Engineered Crops, Reference Module in Biomedical Sciences, 10.1016/B978-0-323-95488-4.00049-8, (2024).
- Shugui Zheng, Shuangyang Yin, Guixin Qin, Jiaqi Yao, Simiao Liu, Junfeng Han, Yang Zhou, Shuang Duan, Gastrointestinal digestion and absorption of soybean β-conglycinin in an early weaned piglet model: An initial step to the induction of soybean allergy, Food Chemistry, 10.1016/j.foodchem.2023.136640, 427 , (136640), (2023).