greenCUT&RUN: Efficient Genomic Profiling of GFP-Tagged Transcription Factors and Chromatin Regulators
Stefanie Koidl, Stefanie Koidl, H. T. Marc Timmers, H. T. Marc Timmers
CUT&RUN
genome localization
GFP-fusion proteins
micrococcal nuclease mapping
transcription factor binding
genomic profiling
greenCUT&RUN
Abstract
Genome-wide mapping of transcription factors and chromatin regulators is important to distinguish their direct from indirect effects on gene transcription or chromatin function. Novel approaches for studying their genomic localization under native conditions, such us cleavage under target and release using nuclease (CUT&RUN), offer higher resolution and lower sequencing costs than classical chromatin immunoprecipitation (ChIP) assays, and require fewer cells but they still depend on the availability of high-quality antibodies. Here, we describe detailed and robust protocols for greenCUT&RUN, which is a generic CUT&RUN-based approach for mapping the genome-wide localization of green fluorescent protein (GFP)-tagged factors in intact mammalian cells. The greenCUT&RUN method makes use of a micrococcal nuclease (MNase) coupled to a high affinity nanobody against GFP, which exploits the accessibility of multiple surfaces of the GFP tag, thus eliminating issues of antibody variability and availability. We also provide efficient protocols for the expression and purification of two different GFP nanobodies, which recognize non-overlapping GFP epitopes and can be combined for a further gain in sensitivity and accuracy. Compared to traditional CUT&RUN, genomic localization by greenCUT&RUN reduces handling time and experimental variability. GreenCUT&RUN is a versatile, robust, and universal procedure for surveying the genome-wide localization of GFP-tagged versions of proteins that drive key transcriptional programs and regulate chromatin function. © 2021 The Authors. Current Protocols published by Wiley Periodicals LLC.
Basic Protocol : Standard greenCUT&RUN for GFP-tagged proteins in mammalian cells
Alternate Protocol : High-Ca++/low-salt greenCUT&RUN for GFP-tagged histone proteins in mammalian cells
Support Protocol : Expression and purification of GFP nanobody-MNase fusion proteins for greenCUT&RUN
INTRODUCTION
Chromatin function and structure is intertwined with the regulation of mRNA biosynthesis, which depends on extensive sets of proteins and multi-protein complexes controlling gene transcription and mRNA processing. To distinguish direct from indirect functions of transcription factors and chromatin regulators, several experimental strategies have been developed over the years, including genome-wide localization techniques like chromatin immunoprecipitation (ChIP) coupled to high-throughput sequencing, or ChIPseq (Johnson, Mortazavi, Myers, & Wold, 2007). ChIP involves formaldehyde crosslinking, which fixes DNA-protein contacts, followed by sonification of crosslinked chromatin and immunoprecipitation with specific antibodies. While powerful, important drawbacks of ChIP relate to issues with formaldehyde fixation, sonication procedures, and data normalization (Meyer & Liu, 2014; Skene & Henikoff, 2017). To tackle some of these issues, Steven Henikoff and coworkers developed the cleavage under target and release using nuclease (CUT&RUN) method for the genome-wide detection of native protein-DNA interactions (Meers, Bryson, Henikoff, & Henikoff, 2019; Skene & Henikoff, 2017; Skene, Henikoff, & Henikoff, 2018). CUT&RUN involves two consecutive incubation steps, consisting of a first step with the primary antibody, followed by a second incubation with an immunoglobulin-binder like protein A or protein G fused to micrococcal nuclease (pA/G-MNase in Fig. 1, left). Targeted chromatin complexes are released from bulk chromatin by in situ MNase cleavage of permeabilized cells or nuclei. Compared to ChIPseq, CUT&RUN typically exhibit lower background signals, which allows reduced sequencing depth (and hence, costs) and the use of fewer cells. The Henikoff laboratory also developed cleavage under targets and tagmentation (CUT&Tag), in which the MNase protein is replaced by a hyperactive Tn5 transposase, which generates chromatin fragments ready for PCR amplification and DNA sequencing (Kaya-Okur et al., 2019; Kaya-Okur, Janssens, Henikoff, Ahmad, & Henikoff, 2020).
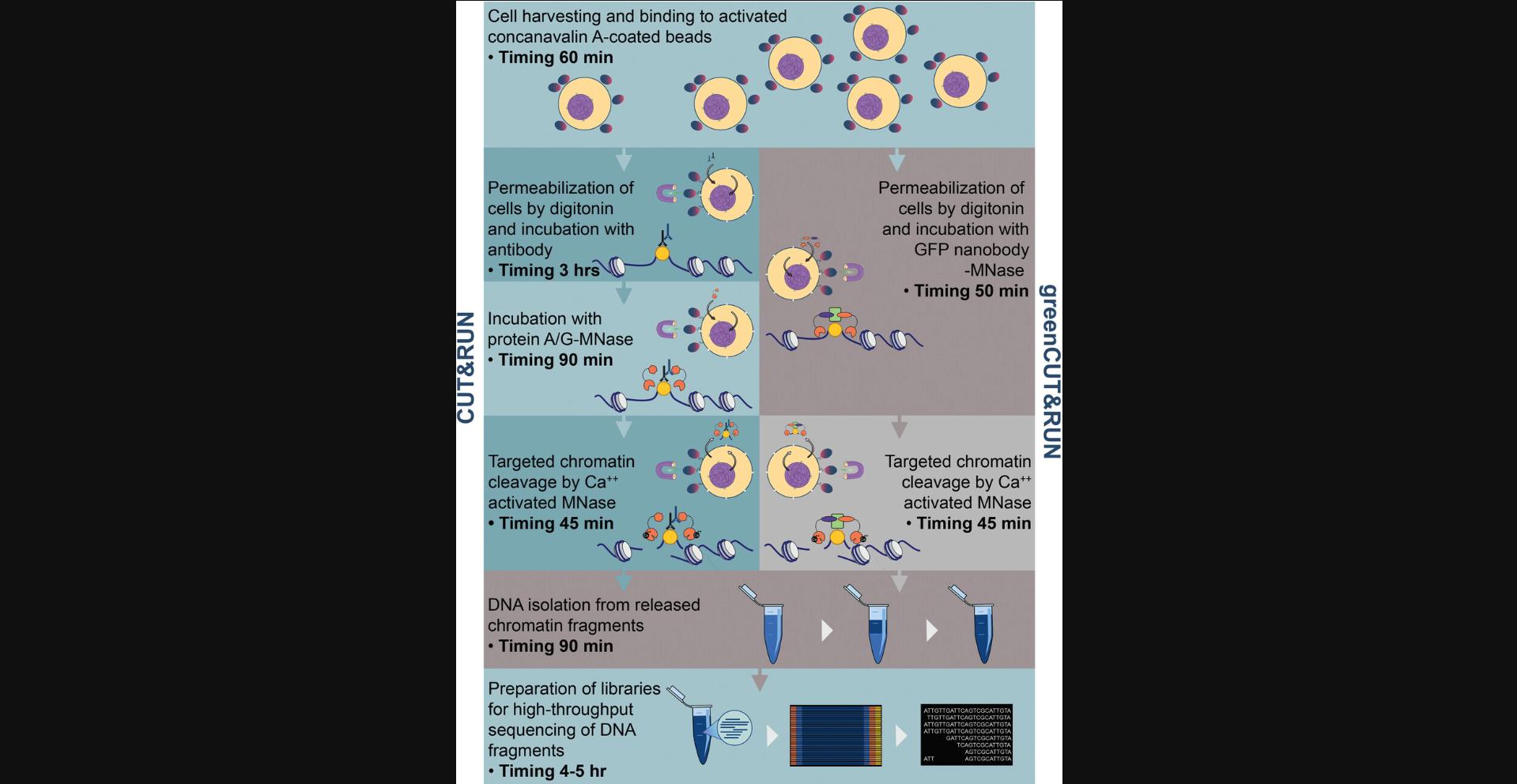
Like ChIP-based methods, CUT&RUN and CUT&Tag depend on the availability of high-quality antibodies and, importantly, of epitope accessibility in the chromatin-bound state. To circumvent such antibody issues, we developed the greenCUT&RUN approach for GFP-tagged proteins that are expressed upon doxycycline treatment of cells (Nizamuddin et al., 2021). In greenCUT&RUN, the primary antibody and protein A/G-MNase steps are combined through the use of a GFP nanobody-MNase fusion protein (Fig. 1, right). The sub-nanomolar affinity of these nanobodies for the GFP moiety (Kubala, Kovtun, Alexandrov, & Collins, 2010) allows incubation times of the GFP nanobody-MNase fusion of only 20 min to 2 hr at 4°C, which reduces handling time from ∼4.5 hr to 50 min (Fig. 1) and may help in preserving protein-DNA interactions. GFP nanobody-MNase fusions display low non-specific binding to chromatin and are efficiently removed by washing. Recombinant GFP nanobody-MNase proteins can be expressed and purified in large amounts and are stable upon long-term storage. Genome localization by greenCUT&RUN is characterized by high sensitivity, specificity, and reproducibility of the mapping results, and by high spatial genomic resolution (Nizamuddin et al., 2021).
Here, we provide a comprehensive set of protocols for performing greenCUT&RUN mapping using permeabilized mammalian cells expressing GFP fusions. Basic Protocol describes the steps of greenCUT&RUN for mapping the genome-wide localization of GFP-tagged transcription factors in human tissue culture cells. Alternate Protocol details greenCUT&RUN cleavage under low-salt and high-Ca++ concentrations, which can be advantageous for the detection of variant or mutant nucleosomes by suppression of secondary cleavages (Meers et al., 2019). In addition, we provide Support Protocol, which describes the expression, purification, and activity assays for two different GFP nanobody-MNase fusions (Support Protocol). These GFP nanobodies recognize non-overlapping GFP epitopes and can be combined to further increase the sensitivity of greenCUT&RUN. The provided protocols, however, do not cover the downstream bioinformatic analyses for the sequencing results, for which we refer the reader to previous publications (Hainer, Boskovic, McCannell, Rando, & Fazzio, 2019; Meers, Tenenbaum, & Henikoff, 2019; Nizamuddin et al., 2021; Zhu, Liu, Orkin, & Yuan, 2019). It is worth mentioning that these protocols for mammalian tissues can form the starting point for greenCUT&RUN of cells and tissues from various organisms, including plants.
STRATEGIC PLANNING
The greenCUT&RUN procedure requires users to have purified GFP nanobody-MNase at their disposal and to have actively growing mammalian cells expressing the GFP-tagged target protein.
GFP nanobody-MNase proteins are expressed in bacteria as glutathione-S-transferase (GST)-fusion proteins and are purified by glutathione affinity purification, followed by thrombin cleavage of the GST-moiety and ion chromatography, to yield a large amount of highly purified GFP nanobody-MNase proteins (Support Protocol).
For target cells, we mostly use derivatives of HeLa Flp-In/T-REx cells, which express the GFP fusion in a doxycycline-dependent manner from a single FRT-marked chromosomal locus (van Nuland et al., 2013). The Flp-In/T-REx derivative of the human embryonic kidney 293 cell line is commercially available from Thermo Fisher Scientific (cat. no. R78007). We expect, however, that stable cell lines with continuous GFP-fusion expression created using a variety of expression vectors can also be used. Flp-In variants of baby hamster kidney, mouse 3T3, African green monkey CV-1, Chinese hamster ovary, or human Jurkat cells for continuous expression of GFP-fusions from a single chromosomal locus are available from Thermo Fisher Scientific (cat. no. R76007, R76107, R75207, R75807, and R76207, respectively). We recommend users avoid transient expression of GFP-tagged proteins for greenCUT&RUN, as this results in heterogenous expression levels and in experimental variability, although we do not exclude that transient expression may work for selected cases. We have found that greenCUT&RUN mapping works well with both N-terminal and C-terminal GFP tags, and with a variety of human cell lines (HeLa, COLO205, U-2 OS, and HEK293).
The effectiveness of cell permeabilization by digitonin varies between cell lines and between digitonin batches. Full permeability can be easily tested by titrating in the range of 0% to 0.1% (w/v) digitonin and using a standard trypan blue exclusion test. We strongly recommend determining the conditions for effective permeabilization by digitonin before starting the experiment. The digitonin test can be done by mixing 5 μl of trypan blue solution with 5 μl of cell suspensions and incubating for 10 min in wash buffers with varying digitonin concentrations. Then, simply apply ∼5 μl of this mix to a microscopy glass slide, place a coverslip, and observe under the light microscope for the next 10 min. For permeabilization, choose the lowest digitonin concentration that results in blue staining of all or nearly all cells. We typically use 0.05% (w/v) digitonin for greenCUT&RUN of HeLa cells. For K562 cells, Henikoff and coworkers reported no variation in CUT&RUN results with 0.02% to 0.1% (w/v) digitonin but they also reported lot-to-lot variation between digitonin batches (Skene et al., 2018).
As a negative control in the greenCUT&RUN protocol, we typically use a parallel incubation, which is not treated with CaCl2 (the “no Ca++ control” in Basic Protocol, step 29 or Alternate Protocol, step 5). Other controls, like isogenic cells lacking the GFP-target protein, perform equally well (Nizamuddin et al., 2021). Isogenic cell lines expressing GFP alone or GFP fused to a nuclear localization signal can also be used, but due to their small size, these GFP control proteins are washed out from permeabilized cells during steps 17 to 26 of the Basic Protocol. We note that in the case of doxycycline-induced expression of the GFP fusion, a “no dox-control” will still give specific signals, which is due to the high sensitivity of greenCUT&RUN.
To allow normalization of greenCUT&RUN signals, we include spike-in DNA from mono-nucleosomes isolated from Drosophila melanogaster, as this gives a nice size distribution and provides sufficient genomic complexity (Nizamuddin et al., 2021). Others use bacterial DNA contaminating the recombinant MNase fusion preparations for normalization (Meers et al., 2019). Heterologous genomic DNA of 200 to 300 bp from other sources for spike-in normalization can be prepared by sonication (Sambrook & Russell, 2006) and Epicypher offers prepared spike-in DNA from Escherichia coli (cat. no. SKU: 18-1401). As such, users should prepare spike-in DNA or chromatin from their source of choice before starting the greenCUT&RUN protocol.
In the Support Protocol, cool the Fiberlite F14-6 × 250y rotor (or equivalent) and Sorvall Lynx 400 medium-speed centrifuge (or equivalent) to 4°C prior to harvesting induced bacteria in step 12. Similarly, cool the SW 28 rotor and Beckmann ultracentrifuge to 4°C before use.
For bioinformatic analyses of CUT&RUN data, we refer the experimenter to previous publications (Hainer et al., 2019; Meers et al., 2019; Nizamuddin et al., 2021; Zhu et al., 2019).
Basic Protocol: STANDARD GREENCUT&RUN FOR GFP-TAGGED PROTEINS IN MAMMALIAN CELLS
This protocol describes greenCUT&RUN in human HeLa cell derivatives expressing the GFP-tagged protein of interest. This protocol requires only 250,000 intact HeLa cells per sample. The cells are first coated with magnetic concanavalin A beads to allow rapid subsequent washing steps. Next, the coated cells are permeabilized with digitonin-containing buffers to allow diffusion of the GFP nanobody-MNase proteins into the nucleus. In a separate step, the enzymatic activity of MNase is activated by millimolar concentrations of Ca++ ions, to allow chromatin cleavage and release of GFP-tagged complexes. Lastly, the DNA is purified from these chromatin complexes via phenol/chloroform/iso-amyl alcohol extraction, followed by ethanol precipitation. This is done because in greenCUT&RUN assays for transcription factors, only minute amounts of DNA are typically released and commercial DNA extraction kits do not perform well with such low amounts of small DNA fragments.
Materials
-
Mammalian cells expressing the tagged protein of interest (see Strategic Planning)
-
PBS (Thermo Fisher Scientific, cat. no. 14190-094)
-
Mammalian cell growth medium: DMEM with 4.5 g/L glucose (Thermo Fisher Scientific, cat. no. 41966-029), supplemented with 10% FBS (Thermo Fisher Scientific, cat. no. 10270-106) and 1% penicillin-streptomycin solution (Merck, cat. no. P0781-100ML)
-
Concanavalin A-coated magnetic beads (Bangs Laboratories, cat. no. PB531)
-
Binding buffer (see recipe)
-
Wash buffer (see recipe)
-
Digitonin buffer (see recipe)
-
Permeabilization buffer (see recipe)
-
Purified recombinant GFP nanobody-MNase (1 mg/ml) expressed from Addgene plasmid (cat. no. 166035 and/or cat. no. 170978), and purified as described in Support Protocol
-
Trypan blue solution (e.g., Merck, cat. no. 93595-50ML)
-
Digitonin stock solution (see recipe)
-
100 mM CaCl2 (see recipe)
-
STOP buffer (see recipe)
-
Proteinase K (20 mg/ml; Merck, cat. no. 3115801001)
-
Glycogen (20 mg/ml; Merck, cat. no. 10901393001) or equivalent
-
RNase A (10 mg/ml; Merck, cat. no. R6513-50MG)
-
10% (v/v) SDS (see recipe)
-
Tris-buffered phenol/chloroform/iso-amyl alcohol mixture (e.g., Carl Roth GmbH, cat. no. A156.2)
-
Chloroform (e.g., Carl Roth GmbH, cat. no. 3313.1)
-
99.8% ethanol (e.g., Carl Roth GmbH, cat. no. 9065.4)
-
GlycoBlue, 15 mg/ml (Invitrogen, cat. no. AM9515)
-
TE buffer (see recipe)
-
NEBNext Ultra II library prep kit for Illumina (New England Biolabs, cat. no. E7645S or E7645L)
-
NEBNext Multiplex Oligos for Illumina (New England Biolabs, cat. no. E7335S, E7335L, E7500S, E7500L, E7710L, E7710S, E7730S, or E7730L)
-
Microscopy glass slides (e.g., Thermo Fisher Scientific, cat. no. AA00000112E01MNZ10)
-
Microscopy cover slips (e.g., Paul Marienfeld GmbH, cat. no. 0101222)
-
Automated Casey cell counter (OLS OMNI Life Science GmbH, cat. no. CASY TT45) or equivalent
-
Centrifuge for 15-ml conical tubes (e.g., 5810 R, Eppendorf, cat. no. 5811000015) or equivalent
-
Magnetic stand for 1.5-ml microcentrifuge tubes (e.g., Bio-Rad, cat. no. 1614916) or equivalent
-
Standard light microscope with phase contrast (e.g., Zeiss Axiovert 25) or equivalent
-
Bio-Rad ZOE fluorescent cell imager (Bio-Rad, cat. no. 1450031) or equivalent
-
Refrigerated microcentrifuge (e.g., 5427 R, Eppendorf, cat. no. 5409000010) or equivalent
-
Metal block (e.g., Merck, cat. no. Z743497-1EA) or equivalent
-
Thermomixer (e.g., Mixer HC, Starlab GmbH, cat. no. S8012-0000) or equivalent
-
Vortex mixer (e.g., Scientific Industries, cat. no. SI-0236) or equivalent
-
Rotator for microcentrifuge tubes (e.g., IKA, cat. no. 0004016000) or equivalent
-
Qubit 4 fluorometer (e.g., Thermo Fisher Scientific, cat. no. Q33238) or equivalent
-
1.5-ml safe-lock microcentrifuge tubes (e.g., Eppendorf, cat. no. 0030120.086) or equivalent
-
1.5-ml low-binding microcentrifuge tubes (e.g., Sarstedt, cat. no. 72.706.700) or equivalent
-
2-ml microcentrifuge tubes (e.g., Eppendorf, cat. no. 0030120.094) or equivalent
-
15-ml conical polypropylene tubes (e.g., Greiner bio-one, cat. no. 188271) or equivalent
-
Additional reagents and equipment for cell culture and cell counting using a hemocytometer and trypan blue (Current Protocols article: Phelan, 2006)
NOTE : Make sure all buffers and materials are at room temperature, except the 100 mM CaCl2 solution and digitonin buffer, which should be chilled to 0°C on wet ice before use.
Preparation of concanavalin A-coated magnetic beads (time: 10 min)
Prior to cell harvesting, concanavalin A-coated magnetic beads need to be activated by a short incubation with divalent cations. While we routinely perform this step just prior to cell harvesting, activated beads can be kept for 1 day on ice without loss in binding activity.
1.Resuspend the 50% slurry of concanavalin A-coated magnetic beads by gentle vortexing and/or by inversion.
2.Per sample, withdraw 20 μl of the concanavalin A-coated magnetic bead slurry (∼10 μl of packed beads) and transfer it to a 2-ml microcentrifuge tube.
3.Add 1.5 ml binding buffer to the 2-ml tube and mix by inversion or gentle pipetting. Spin down the liquid from the cap and sides of the tube with a quick pulse on a microcentrifuge (<100 × g for ∼1 s at room temperature) and remove. Place tube on the magnetic stand until the solution is clear (1-2 min).
4.Aspirate and discard the clear liquid and remove tube from the magnetic stand. Add 1.5 ml binding buffer, mix by inversion or gentle pipetting, and spin down the liquid from the cap and sides of the tube with a quick pulse on a microcentrifuge (<100 × g for ∼1 s at room temperature). Remove liquid. Place tube back on the magnetic stand until the solution is clear (1-2 min).
5.Aspirate and discard the clear liquid and resuspend the beads in a volume of binding buffer equal to the initial volume of bead suspension (20 μl per sample). The concanavalin A-coated beads are now activated. Keep beads on wet ice until the cells are ready for binding.
Cell harvesting and binding to activated concanavalin A-coated beads (time: 60 min)
To minimize genomic DNA breakage throughout the protocol, we recommend avoiding rigorous resuspension and/or vigorous vortexing to prevent cavitation. Per GFP-tagged target and/or condition, we prepare two samples: The test sample and the no Ca++ control.
6.Grow cells to 60%-80% confluency in a 10-cm tissue culture dish or T175 flask and harvest by trypsinization. Add mammalian cell growth medium to reach a 12-ml volume and transfer cells to a 15-ml centrifuge tube.
7.Draw a 50-µl sample of the cell suspension and determine cell density using an automated Casy cell counter.
8.In the meantime, centrifuge the 15-ml centrifuge tube with the cell suspension (from step 6) at 600 × g for 3 min at room temperature and remove supernatant. Resuspend cell pellet in wash buffer to reach 1,000,000 cells per ml.
9.Label two 1.5-ml microcentrifuge tubes, one for the test sample and one for the no Ca++ control sample. Dispense 250 µl (equivalent to 250,000 cells) of the cell suspension to each tube. Add 1.25 ml wash buffer at room temperature and mix by inversion or gentle pipetting.
10.Centrifuge tubes for 3 min at 600 × g at room temperature and aspirate supernatant.
11.Add 1.5 ml wash buffer at room temperature and mix by inversion or gentle pipetting.
12.Centrifuge tubes for 3 min at 600 × g at room temperature and aspirate supernatant.
13.Resuspend pelleted cells in 1 ml wash buffer at room temperature by gently pipetting.
14.Set vortex mixer at its lowest speed setting. While gently vortexing tubes at room temperature, add the activated concanavalin A-coated magnetic beads prepared in step 1-5 (20 µl of the 50% slurry in binding buffer).
15.Rotate tubes 5-10 min at room temperature.
Permeabilization of cells by digitonin and incubation with the GFP nanobody-MNase (time: 50 min)
16.Place tubes with bead-bound cells (from step 15) on the magnetic stand until the solution turns clear (2 min) and then aspirate the liquid.
17.Remove tubes from the magnetic stand and add 1 ml permeabilization buffer per tube to wash the bead-bound cells. Resuspend carefully with a 1-ml pipet tip. Incubate 3 min at room temperature. Place tubes with the bead-coated cells back onto the magnetic stand for 2 min and remove liquid by aspiration.
18.Prepare a 4 µg/ml GFP nanobody-MNase solution in cold digitonin buffer (100 μl is needed per incubation) and chill on wet ice until use.
19.Place tubes with bead-bound cells for 3 min on wet ice to chill. Set vortex at low speed (∼1,100 rpm) and place each tube on it at a low angle. Add 100 μl cold GFP nanobody-MNase solution along the side of the tube, while gently vortexing to allow the solution to dislodge most or all of the bead-bound cells. Tap to dislodge remaining beads.
20.Incubate 20 min at room temperature and flick the tube every 5 min.
21.Spin down the liquid from the cap and sides of the tubes with a quick pulse (<100 × g for ∼1 s at room temperature) on a microcentrifuge, if necessary.
22.Place tubes on the magnetic stand until the solution turns clear (∼2 min) and remove all liquid by aspiration.
23.Add 1 ml cold digitonin buffer. Mix by flicking and inverting the tubes or by gentle pipetting using a 1-ml tip, if clumps persist. Spin down the liquid from the cap and the sides of the tubes with a quick pulse on a microcentrifuge (<100 × g for ∼1 s at room temperature).
24.Place tubes on the magnetic stand until the solution turns clear (∼2 min) and remove all liquid.
25.Add 1 ml cold digitonin buffer. Mix by flicking and inverting the tubes or by gentle pipetting using a 1-ml tip, if clumps persist. Spin down the liquid from the cap and the sides of the tube with a quick pulse on a microcentrifuge (<100 × g for ∼1 s at room temperature).
26.Place tubes on the magnetic stand until the solution turns clear (∼2 min) and remove all liquid.
27.Set the vortex mixer at a low speed (1,100 rpm). Place each tube at a low angle on it and add 150 μl cold digitonin buffer per sample along the side of the tube while gently vortexing to allow the solution to dislodge most or all of the beads. Tap to dislodge the remaining beads.
Targeted chromatin cleavage by Ca++-activated MNase (time: 90 min)
At this point in the protocol, the GFP nanobody-MNase fusion protein has bound to the chromatin-associated GFP-tagged target protein but it is catalytically inactive due to the absence of Ca++ ions. It is crucial to chill the tubes to 0°C prior to addition of the CaCl2 solution.
28.Place a metal block in wet ice. Insert tubes into the wells of the metal to chill the tubes to 0°C; this will take ∼5 min.
29.Add 3 μl ice-cold 100 mM CaCl2 to the test samples and 3 µl H2O to the no Ca++ controls. Mix by flicking or gentle vortexing of the tubes, and immediately place tubes back in the chilled metal block in wet ice.
30.Incubate tubes at 0°C for 30 min in the metal block in wet ice.
31.Add 100 μl ice-cold STOP buffer and mix by gentle vortexing.
32.Incubate tubes for 30 min at 37°C to release the cleaved chromatin fragments from the insoluble chromatin.
33.Centrifuge tubes at 16,000 × g for 5 min at 4°C. Place tubes on a magnetic stand for 2 min stand and proceed to the DNA isolation step.
DNA isolation from released chromatin fragments (time: 1.5 hr)
For a quantitative recovery of DNA fragments of all sizes, we prefer DNA isolation from the chromatin fragments using proteinase K digestion and phenol/chloroform/iso-amyl alcohol extraction followed by overnight precipitation by ethanol. This retains the small DNA fragments of ≤80 bp, which provide the highest spatial resolution on transcription factor binding.
34.Transfer supernatant (∼250 µl) from step 33 into a 1.5-ml safe-lock microcentrifuge tube. Be careful not to touch or transfer any bead-bound cells.
35.To each sample, add 2.5 μl of 10% (v/v) SDS and 1.5 μl proteinase K (20 mg/ml). Mix by inversion and incubate 10 min at 65°C.
36.Add 250 μl of phenol/chloroform/iso-amyl alcohol and mix by full-speed vortexing for ∼5 s. Centrifuge tubes for 5 min at 16,000 × g and room temperature.
37.Carefully transfer the upper aqueous phase to a 1.5-ml safe-lock microcentrifuge tube without disturbing the interphase. A small volume of the aqueous phase can be left behind.
38.Add 250 μl chloroform to the aqueous phase, vortex 5 s, and centrifuge 5 min at 16,000 × g and room temperature.
39.Add 2 μl of a 2 mg/ml GlycoBlue solution (freshly diluted in H2O from the 15 mg/ml stock solution) to a new 1.5-ml safe-lock microcentrifuge tube. Then transfer the upper phase into this safe-lock microcentrifuge tube.
40.Add 500 μl of 99.8% ethanol and mix by vigorous vortexing for 5 s.
41.Precipitate the DNA by placing the tubes at −20°C overnight (14-20 hr).
42.On the next day, centrifuge tubes in a pre-cooled microcentrifuge at 16,000 × g and 4°C for 20 min.
43.Decant supernatant carefully and be careful not to dislodge the pellet, which is hardly visible at this stage.
44.Rinse pellet carefully by adding 500 µl of 99.8% ethanol and centrifuge again at 16,000 × g and 4°C for 5 min.
45.Decant supernatant carefully and be careful not to dislodge the pellet. Air dry pellet for at least 5 min in order to remove the last traces of ethanol.
46.Dissolve DNA pellet in 20 µl TE buffer by gently pipetting up and down.
47.Measure DNA concentrations using a Qubit instrument. Proceed to library preparation. Alternatively, store samples at −20°C for library preparations at a later date (within 1 week).
48.Prepare sequencing libraries using 0.5-10 ng of DNA and the NEBNext Ultra II library prep kit for Illumina from New England Biolabs following the manufacturer's instructions. In order to increase recovery of small fragments, however, implement the following modifications:
-
omit the size selection step to prevent the loss of small DNA fragments, which are the major contributor to high-spatial resolutions by CUT&RUN mapping methods;
-
reduce the temperature and increase the incubation time of enzyme inactivation of the End Prep step of the NEBNext Ultra II protocol from 30 min at 65°C to 60 min at 50°C. This reduces the denaturation of small DNA fragments, which increases the efficiency of adaptor ligation to small DNA fragments;
-
reduce the annealing/extension time of the PCR amplification step from 75 s at 65°C to 10 s at 65°C.
Alternate Protocol: HIGH-Ca++/LOW-SALT GREENCUT&RUN FOR GFP-TAGGED HISTONE PROTEINS IN MAMMALIAN CELLS
Ca++ activation of the targeted MNase (Basic Protocol, step 29) cleaves GFP targeted chromatin on both sides and this released complex could now digest freely accessible DNA (Skene & Henikoff, 2017). In order to minimize such secondary cleavages, it is crucial to perform the digestion at 0°C and to limit digestion time. Suppression of secondary cuts is particularly important for abundant chromatin complexes like nucleosomes. Steven Henikoff and coworkers developed an alternate CUT&RUN protocol for (modified) histones, which exploits the inherent instability of nucleosome core particles in high-divalent/low-salt conditions (Meers et al., 2019). This high-calcium/low-salt protocol reduces the risk of over digestion in standard CUT&RUN assays for abundant histone modifications like H3K27me3 (Meers et al., 2019). MNase activation under these conditions results in cleaved chromatin, which is retained in the cells and requires 150 mM NaCl, 20 mM ethylene glycol-bis(2-aminoethylether)-N,N,N′,N′-tetraacetic acid (EGTA), and 25 µg/ml RNase A to be released into the supernatant. Here, we provide the high-divalent/low-salt digestion procedure for greenCUT&RUN, which is useful for the genome profiling of GFP-tagged (mutant) histones. We note, however, that we have also performed greenCUT&RUN analyses of transcription factors like TATA-binding protein (TBP) and the A subunit of nuclear factor Y (NF-YA) using both Basic Protocol and Alternate Protocol, and found that both work equally well.
Additional Materials (also see Basic Protocol)
- Low-salt rinse buffer (see recipe)
- High-calcium/low-salt buffer (see recipe)
- No-calcium/low-salt buffer (see recipe)
- EGTA-STOP buffer (see recipe)
Targeted chromatin cleavage under low salt conditions by Ca++-activated MNase (time: 90 min)
1.Perform steps 1 to 27 of Basic Protocol to bind the cells expressing the GFP-tagged target to concanavalin A-coated beads to permeabilize cells with digitonin under physiological salt conditions and to bind the GFP nanobody-MNase fusion protein to its nuclear targets.
2.Place a metal block in wet ice and then insert tubes into the metal block to chill down to 0° C (∼5 min).
3.Add 1 ml cold low-salt rinse buffer. Mix by flicking and inverting tubes or by gently pipetting using a 1-ml tip, if clumps persist. Spin down the liquid from the cap and the sides of the tube with a quick pulse on a microcentrifuge (<100 × g for ∼1 s at room temperature).
4.Place tubes on the magnetic stand until the solution turns clear (∼2 min) and remove all liquid by aspiration. Place tubes back in the metal block on wet ice.
5.Set vortex mixer to low speed (∼1,100 rpm). Place each tube at a low angle on the vortex mixer and add 150 μl high-calcium/low-salt buffer per test sample or 150 μl no-calcium/low-salt buffer per control sample along the side of the tube, while gently vortexing, to allow the solution to dislodge most or all of the beads. Tap to dislodge the remaining beads. Place tubes immediately back in the metal block in wet ice as cleavage starts immediately.
6.Incubate tubes at 0°C for 30 min in the metal block on ice.
7.Place tube on the magnetic stand until the solution turns clear and remove all liquid. Remove tube from the stand, add 250 μl cold EGTA-STOP buffer, and mix by gentle vortexing.
8.Incubate tubes for 30 min at 37°C to release the cleaved chromatin fragments from the insoluble chromatin.
9.Centrifuge tubes at 16,000 × g for 5 min and at 4°C. Place tubes on a magnetic stand.
10.Proceed with steps 34-48 of Basic Protocol for the isolation of DNA from released chromatin fragments and preparation of sequencing libraries.
Support Protocol: EXPRESSION AND PURIFICATION OF GFP NANOBODY-MNASE FUSION PROTEINS FOR GREENCUT&RUN
Genome-wide profiling by greenCUT&RUN requires purified GFP nanobody-MNase fusion proteins, which are not yet commercially available. We use two different nanobodies, recognizing non-overlapping GFP epitopes, and provide the protocols to express these proteins as a fusion to GST. The Support Protocol results in high yields of GFP nanobody-MNase fusion proteins, which are free of contaminating DNase activities. The protocols can be performed in any molecular biology lab equipped with minimal protein purification equipment. The time investment for expression, purification, and activity assays per GFP nanobody-MNase is 6 to 8 days. The protocol can be scaled down easily but this does not result in a large gain in time.
The protocol starts with a fresh colony of BL21(DE3) bacteria transformed with the pRPN-enhancer-MNase or pRPN-LaG16-MNase plasmid, which express the GFP nanobody-MNase as a GST fusion protein. A 1-L culture of bacteria containing the GST-GFP nanobody-MNase expression plasmids is induced at the proper density. The induced bacteria are then lysed by sonification and a clear lysate is obtained by centrifugation. The GST moiety of the expressed proteins allows efficient purification using glutathione affinity matrices and can be cleaved off by thrombin to release the GFP nanobody-MNase protein. The LaG16-MNase fusion requires solid-phase thrombin cleavage, while for the enhancer-MNase, both solid-phase and in-solution thrombin digestion work well (Nizamuddin et al., 2021). The in-solution cleavage procedure allows better control of the optimal amount of thrombin but the procedure is more time and labor intensive. The glutathione affinity chromatography step combined with in-column thrombin cleavage already results in highly purified GFP nanobody-MNase preparations of reasonable concentrations. We prefer to clean up these preparations using a strong cation exchanger, which will remove low-abundant contaminants, including the inactivated thrombin. The enhancer-MNase (predicted pI 9.0) elutes at ∼330 mM KCl in the phosphate buffer of pH 7.0, while the LaG16-MNase (predicted pI 8.3) elutes at ∼225 mM KCl from the Mono S or HiTrap S HP matrices. Additional advantages of this cation exchange step are the increase in concentration and in specific activity of the GFP nanobody-MNase. After determination of nuclease purity and of GFP nanobody-MNase concentrations, glycerol is added to 55% and the preparation is aliquoted for long-term storage at −80°C. The expected yields from a 1-L bacterial culture are ∼15 mg for the enhancer-MNase and ∼5 mg for the LaG16-MNase protein. The obtained GFP nanobody-MNase preparations will be sufficient for many greenCUT&RUN assays as a single incubation only needs 400 ng GFP nanobody-MNase.
Our purification protocol employs a low-pressure ÅKTAprime plus/ÅKTAstart or medium-pressure ÅKTApure chromatography system (Cytiva), but the protocol can be adapted easily to other chromatography systems, or even loading and elution by syringe. We include a simple assay for the GFP nanobody-MNase preparations to assess their specific activities. We do not provide a detailed protocol for protein analysis by SDS-PAGE and Coomassie staining as we expect this to be a standard technique for a molecular biology lab. Interested readers are referred to Current Protocols article: Gallagher, 2012 for details.
Materials
-
pRPN-enhancer-MNase plasmid (Addgene, cat. no. 166035)
-
pRPN-LaG16-MNase plasmid (Addgene, cat. no. 170978)
-
Escherichia coli BL21(DE3) strain (New England Biolabs, cat. no. C2527H or Thermo Fisher Scientific, cat. no. EC0114)
-
Luria-Bertani (LB) medium (see recipe)
-
10× MNase buffer (see recipe)
-
LB agar plates with ampicillin (see recipe)
-
Ampicillin stock (see recipe)
-
1 M IPTG (see recipe)
-
Bacterial lysis buffer (see recipe)
-
Chicken lysozyme (25 mg/ml; see recipe)
-
Sample buffer (see recipe)
-
Glutathione Sepharose 4 Fast Flow resin (Cytiva, cat. no. 17513202 for 100 ml resin) or glutathione chromatography cartridges (5 ml; Thermo Fisher Scientific, cat. no. 16110 or Cytiva, cat. no. 17513001)
-
Glutathione wash buffer I (see recipe)
-
Glutathione wash buffer II (see recipe)
-
Glutathione elution buffer (see recipe)
-
Thrombin (Merck, cat. no. T6634 or Serva, cat. no. 3640202)
-
Thrombin buffer (see recipe)
-
200 mM phenylmethylsulfonylfluoride (PMSF; see recipe)
-
Benzamidine Sepharose 4 Fast Flow (Cytiva, cat. no. 17512310 for 25 ml resin)
-
Buffer A (see recipe)
-
Buffer B (see recipe)
-
Micrococcal nuclease or MNase (New England Biolabs, cat. no. M0247S)
-
Glycerol p.a. quality (e.g., Carl Roth, cat. no. 3783.1) or equivalent
-
Bio-Rad Bradford protein assay (Bio-Rad, cat. no. 5000001)
-
Genomic DNA of high molecular weight DNA (50-250 kb) prepared via commercial kits (e.g., Qiagen, cat. no. 10223 or New England Biolabs, cat. no. T3060)
-
Incubator shaker with temperature control for bacterial cultures (Multitron Standard from Infors, cat. no. 29530) or equivalent
-
Sterile 100-ml Erlenmeyer flasks (e.g., Fisherbrand, cat. no. FB33131) or equivalent
-
Sterile 3-L Erlenmeyer flasks (e.g., Fisherbrand, cat. no. FB33136) or equivalent
-
Plastic 1-ml cuvettes (e.g., BRAND, cat. no. 759015) or equivalent
-
DeNovix spectrophotometer (e.g., DeNovix, cat. no. DS-11 FX+) or equivalent
-
Microcentrifuge (e.g., Eppendorf 5427R, cat. no. 5409000010) or equivalent
-
Sorvall Lynx 400 medium-speed centrifuge (Thermo Fisher Scientific, cat. no. 75006581) or equivalent
-
Fiberlite F14-6 × 250y fixed-angle rotor (Thermo Fisher Scientific, cat. no. 096-062075) or equivalent
-
Nalgene 250-ml buckets for the Fiberlite rotor (Thermo Fisher Scientific, cat. no. 3120-0250PK) or equivalent
-
Vortex mixer (e.g., Scientific Industries, cat. no. SI-0236) or equivalent
-
Water bath (e.g., GFL, cat. no. 1083) or equivalent
-
Branson Sonifier W-450D sonicator (e.g., Marshall Scientific, cat. no. B450) or equivalent
-
SW 28 Ti swing-out rotor (e.g., Beckman Coulter, cat. no. 342207) or equivalent
-
SW 28 polypropylene tubes (e.g., Beckman Coulter, cat. no. 326823) or equivalent
-
Optima XE-90 ultracentrifuge (e.g., Beckman Coulter, cat. no. A94471) or equivalent
-
Chromatography column with inner diameter of 1.5-2 cm (e.g., XK16/20 with one adaptor; Cytiva, cat. no. 28988937 and 28989876) or equivalent
-
Chromatography system (e.g., ӒKTApure 25 M, Cytiva, cat. no. 29018226 or ӒKTAstart, Cytiva, cat. no. 29022094) or equivalent
-
Mono S 5/50 GL column (Cytiva, cat. no. 17516801) or HiTrap S HP column (Cytiva, cat. no. 29051324 or 17115101) or equivalent
-
1.5-ml microcentrifuge tubes (e.g., Eppendorf, cat. no. 0030120.086) or equivalent
-
15-ml conical polypropylene centrifuge tubes (e.g., Greiner, cat. no. 188271) or equivalent
-
50-ml conical polypropylene centrifuge tubes (e.g., Greiner, cat. no. 227261) or equivalent
-
Rotator for 1.5-ml microcentrifuge and 15-ml centrifuge tubes (e.g., IKA Loopster digital, cat. no. 0004016000) or equivalent
-
Centrifuge for 15-ml conical tubes (e.g., Eppendorf, cat. no. 5702) or equivalent
-
Additional reagents and equipment for polyacrylamide/SDS gel electrophoresis and Coomassie staining of protein gels (Current Protocols article: Gallagher, 2012), and for agarose gel electrophoresis and ethidium bromide staining (Current Protocols article: Voytas, 2001)
NOTE : Make sure all buffers for bacterial lysis and protein purification are at 4°C.
Expression of GST-nanobody-MNase and lysate preparation (time: 2-3 days)
Remember to pre-cool the relevant rotor and centrifuges for use in steps 12 and 19 (see Strategic Planning).
1.Inoculate a single colony of BL21(DE3) harboring the pRPN-enhancer-MNase or pRPN-LaG16-MNase plasmid from a fresh LB-ampicillin plate into a 100-ml Erlenmeyer flask containing 15 ml LB with 100 µg/ml ampicillin.
2.Grow overnight at 37°C with shaking at 200 rpm.
3.The next day, prepare 1 L LB medium supplemented with 50 µg/ml ampicillin and distribute it over two sterile 3-L Erlenmeyer flasks (500 ml each). Take a 1-ml sample to be used as a reference (blank) for OD600 measurements to follow the growth of the culture.
4.Add 5 ml of the overnight culture (final dilution 1:100) into each flask.
5.Incubate at 37°C with shaking at 200 rpm.
6.At 1-hr intervals, draw a 1-ml sample to measure OD600 of the cultures against the reference (blank) sample.
7.When the cultures reach an OD600 of 0.5-0.6, remove 1.5 ml from the culture and transfer it to a 1.5-ml microcentrifuge tube. Centrifuge at 6,000 × g for 2 min at room temperature in a microcentrifuge to pellet the bacteria. Aspirate supernatant and resuspend pellet in 300 µl sample buffer. Keep this sample as the non-induced control at −20°C for analysis of protein induction by Coomassie staining of protein gels (step 21).
8.Add 500 µl of 1 M IPTG to each 3-L Erlenmeyer flask to induce expression of the GST-GFP nanobody-MNase protein.
9.For the GST-enhancer-MNase, continue incubation in the incubator at 200 rpm for 3 hr at 37°C. For the GST-LaG16-MNase, cool the shaking incubator to 18°C and continue incubation overnight (16-20 hr).
10.After incubation (for GST-LaG16-MNase, this will be the next morning) measure OD600. Take a 200-µl sample of the culture and dilute to 1 ml in LB medium before measuring.
11.For later analysis by Coomassie staining of protein gels, take a 500 µl sample and transfer to a 1.5-ml microcentrifuge tube. Centrifuge tube at 6,000 × g for 2 min at room temperature to pellet the bacteria. Aspirate supernatant and resuspend pellet in 300 µl sample buffer. Keep at −20°C for analysis of protein induction by Coomassie staining of protein gels (step 21).
12.Transfer the bacterial cultures to 250-ml buckets and harvest bacteria by centrifugation at 2,000 × g (3,600 rpm in a Fiberlite F14-6 × 250y rotor) for 12 min at 4°C.
13.Carefully decant supernatant and vortex pellet for 10-15 s to facilitate resuspension. Add 12.5 ml cold bacterial lysis buffer to each 250-ml bucket. Vortex pelleted bacteria until all clumps are resuspended.
14.Combine bacterial suspension into one of the 250-ml buckets and divide suspension over four 50-ml conical centrifuge tubes. Keep tubes on wet ice from now on.
15.Add 250 µl lysozyme (25 mg/ml) to each tube and mix by vortexing.
16.Subject each 50-ml tube to three freeze-thaw cycles, which alternate between freezing in a dry ice/ethanol bath and thawing in a 16°C water bath. The bacterial suspension should become very viscous, which can be checked by pipetting. If the solution is not viscous and lysis is not apparent, repeat the freeze-thaw cycles. End with a thawed bacterial suspension.
17.Sonicate lysate in each 50-ml tube three times for 20 s using a 7-mm tip at the maximum power setting. Keep tubes on ice during sonication and between cycles to prevent heating of the samples. The lysates should lose their viscosity. If this is not the case, repeat sonication for one to three cycles. Take a 50-µl aliquot of the lysate into a 1.5-ml microcentrifuge tube. Keep at −20°C for analysis of protein induction by Coomassie staining of protein gels (step 21).
18.In order to remove insoluble proteins, bacterial debris, and non-lysed bacteria by ultracentrifugation, transfer lysates to SW 28 ultracentrifuge tubes. Combine lysates from two 50-ml tubes into one ultracentrifuge tube, which would result in two SW 28 tubes, with 25-30 ml of lysate each. Balance tubes carefully to a weight difference of <100 mg.
19.Centrifuge tubes at 110,000 × g (25,000 rpm) and 4°C for 90 min in a precooled SW 28 rotor and ultracentrifuge.
20.Transfer each clear supernatant to a 50-ml tube. Take a 50-µl aliquot of each lysate into a 1.5-ml microcentrifuge tube. Keep at −20°C for analysis of protein induction by Coomassie staining of protein gels (step 21). Also, quick-freeze the clarified lysates in a dry ice/ethanol bath and store at −80°C until further purification.
21.Analyze the expression of the GST-nanobody-MNase fusion proteins by loading:
-
20 µl of the total lysates (obtained in steps 7 and 11),
-
5 µl of the sonicated lysate before centrifugation (step 17), and
-
5 µl of the soluble lysate (step 20) on a 12% polyacrylamide/SDS gel.
Add 15 µl sample buffer to the sonicated and soluble lysates (step 17 and 20). Incubate all samples for 5 min at 95°C before gel loading. Perform gel electrophoresis and Coomassie staining as detailed in Current Protocols article: Gallagher, 2012.
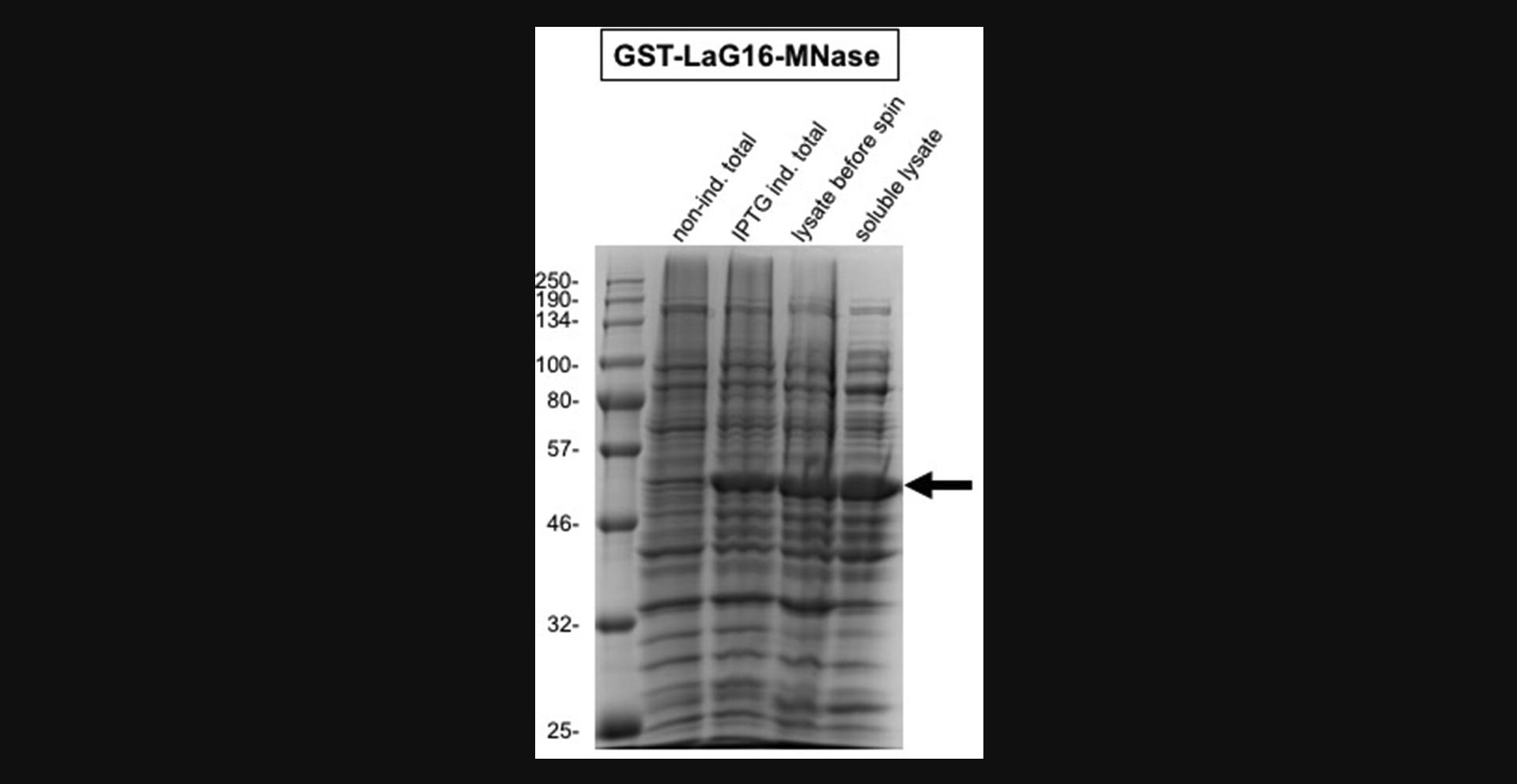
Purification over glutathione resin and in-column digestion with thrombin (time: 2-3 days)
In this part, the GST-nanobody-MNase fusion is purified using glutathione-affinity chromatography. We prefer applying the bacterial lysate to a prepared glutathione column in a chromatography system rather than performing a batch-binding procedure. We find that batch binding results in partial capture of the GST fusion, dilution of the eluted proteins, and increased concentrations of bacterial contaminants. Prior to step 23, cool the SW 28 rotor and Beckmann ultracentrifuge to 4°C.
22.Prepare an ∼5-ml glutathione Sepharose FF matrix in a chromatography column (XK16/20 or equivalent). Alternatively, a 5-ml glutathione cartridge can be used as the chromatography column. Connect the glutathione column to the chromatography system, wash out the preservatives with 0.22-µm filtered pure water, and equilibrate column with ∼50 ml of bacterial lysis buffer.
23.Thaw the clarified lysate from step 20 (which is at −80°C) and transfer to two SW 28-tubes, adding 25-30 ml lysate to each. In order to remove any precipitates formed by the freeze-thaw cycle, centrifuge lysates in a pre-cooled SW 28 rotor and ultracentrifuge at 110,000 × g (25,000 rpm) and 4°C for 30 min.
24.Load the lysate with a flow rate of 0.5 ml/min onto the 5-ml glutathione column. As the volume of the lysate is ∼50 ml, this loading step will take ∼100 min. Collect the flow-through of the column in 50-ml polypropylene centrifuge tubes.
25.Transfer ∼100 µl lysate and ∼100 µl flow-through to 1.5-ml microcentrifuge tubes. Store both tubes at −20°C for analysis by Coomassie staining of SDS-PAGE gels in step 45.
26.Freeze the 50-ml tubes with the flow-through and store at −80°C.
27.Change the loading tube of the chromatography system to glutathione wash buffer and wash the column with 30 ml of glutathione wash buffer I at a flow rate of 1 ml/min. Collect the wash I in a 50-ml polypropylene centrifuge tube and keep on wet ice. This step will take ∼ 30 min.
28.Transfer ∼100 µl of wash I to a 1.5-ml microcentrifuge tube. Store this tube at −20°C for analysis by Coomassie staining of SDS-PAGE gels in step 45.Freeze the 50-ml tube with wash I and store at −80°C.
29.Wash the column with 30 ml glutathione wash buffer II at 1 ml/min. Collect this wash II in 50-ml polypropylene centrifuge tubes. This step will take ∼30 min.
30.Transfer ∼100 µl wash II to a 1.5-ml microcentrifuge tube. Store this tube at −20°C for analysis by Coomassie staining of SDS-PAGE gels at step 45.Freeze the 50-ml tube with wash II and store at −80°C.
31.Prepare thrombin digestion solution by adding 350 µl of thrombin (500 U/ml) to 7 ml thrombin buffer. Take up this solution in a 10-ml syringe with a Luer lock. Make sure to remove any air bubbles from the solution in the syringe.
32.Disconnect the glutathione column with the GST-fusion protein from the purification system. Make sure that no air gets trapped in the tubing or connectors. Attach the 10-ml syringe with the thrombin solution to the column outlet. Slowly inject the complete thrombin solution into the column and push out ∼2 ml of this buffer from the column inlet.
33.Close the column inlet with a cap and keep the syringe connected to the column outlet. Keep the column overnight at room temperature to allow in-column digestion by thrombin.
34.The next morning, fill a 10-ml syringe with a Luer lock with 10 ml fresh thrombin buffer (without the addition of thrombin enzyme). Remove cap from the inlet and connect this 10-ml syringe to the inlet.
35.Slowly push the fresh thrombin buffer into the column. This will elute the released GFP nanobody-MNase from the column, which will be transferred into the syringe at the outlet. Continue until all fresh buffer passes through the column into the outlet syringe.
36.Transfer the elution with the GFP nanobody-MNase from the 10-ml syringe into a 15-ml conical centrifuge tube. Add 50 µl of 200 mM PMSF to the elution to inactivate the thrombin. Mix by inverting and place on wet ice.
37.Using a cut-off 200-µl pipet tip, transfer ∼100 µl benzamidine Sepharose matrix to a 1.5-ml microcentrifuge tube. Add 1.2 ml pure water, invert three times, and spin at 600 × g for 1 min in a microcentrifuge. Remove supernatant without disturbing the benzamidine Sepharose matrix. Repeat this step.
38.Add 1.2 ml thrombin buffer (without thrombin enzyme) to the matrix, invert three times, and spin at 600 × g for 1 min in a microcentrifuge. Remove supernatant without disturbing the benzamidine Sepharose matrix. Repeat this step.
39.Resuspend the benzamidine Sepharose matrix in ∼300 µl thrombin buffer. Using a cut-off 1,000-µl pipet tip, transfer the resuspended matrix to the elution fraction containing the GFP nanobody-MNase in the 15-ml conical tube.
40.Place tube on a rotator and rotate at 12 rpm and 4°C for 2 hr.
41.Spin tube for 5 min at 600 × g and 4°C in a pre-cooled centrifuge. Transfer supernatant to a 50-ml centrifuge tube on wet ice.
42.Take up this GFP nanobody-MNase solution into a 15-ml syringe. Place a 0.22-µm filter on the syringe and slowly filter the solution into a 15-ml centrifuge tube on wet ice.
43.Take a 100-µl aliquot into a 1.5-ml microcentrifuge tube. Store tubes at −20°C for determination of protein concentration in step 44 and analysis by Coomassie staining of SDS-PAGE gels in step 45. Store the remainder in the 15-ml centrifuge tube at −80°C until further cation exchange purification.
44.Determine protein concentration of the elution fraction with the GFP nanobody-MNase with the Bradford assay from Bio-Rad.
45.Analyze the aliquots of the glutathione load (step 25), flow-through (step 25), wash I (step 28), wash II (step 30), and thrombin elution (step 43) by SDS-PAGE. Take 5 µl of the load, flow-through, and elution, and 10 µl of wash I and wash II, and add sample buffer to 20 µl. Incubate all samples for 5 min at 95°C before gel loading. Perform gel electrophoresis and Coomassie staining as detailed in Current Protocols article: Gallagher, 2012.
Purification over cation exchange column (time: 1 day)
46.Take the GFP nanobody-MNase solution obtained in step 43 from the −80°C freezer and thaw on wet ice.
47.Filter the solution using a 0.22-µm filter to remove any aggregates that may have formed by freeze-thawing. Keep the filtered GFP nanobody-MNase solution on wet ice.
48.Equilibrate the 1-ml Mono S HR5/5 or HiTrap S HP column with 10 ml of 8% buffer B.
49.Load the GFP nanobody-MNase solution onto the column with a flow rate of 0.25 ml/min. Take a 50-µl aliquot of the load into a 1.5-ml microcentrifuge tube. Store tube at −20°C for analysis by Coomassie staining of SDS-PAGE gels in step 55.
50.Collect the flow-through fraction in a 50-ml centrifuge tube and take a 100-µl aliquot into a 1.5-ml microcentrifuge tube. Store tube at −20°C for analysis by Coomassie staining of SDS-PAGE gels in step 55.Freeze the 50-ml tube with flow-through and store at −80°C.
51.After loading of the GFP nanobody-MNase solution, wash column with 10 ml of 8% buffer B at a flow rate of 0.5 ml/min. Collect wash fraction in a 50-ml centrifuge tube and take up a 200-µl aliquot into a 1.5-ml microcentrifuge tube. Store tube at −20°C for analysis by Coomassie staining of SDS-PAGE gels in step 55. Freeze the 50-ml tube with wash and store at −80°C.
52.Elute the bound proteins in a 10-ml linear gradient from 8% buffer B to 60% buffer B, in buffer A, at a flow rate of 0.5 ml/min. Collect 300 µl fractions of the 10-ml gradient (∼34 fractions in total) and store on ice.
53.Wash column with 3 ml of 100% buffer B, collect this high-salt wash in a single fraction, and store on ice.
54.Determine protein concentration of the elution fractions with the Bradford assay.
55.Analyze aliquots of the load, flow-through, wash, and elution fractions by SDS-PAGE. Take 5 µl of the load (step 49) and flow-through (step 50), 10 µl of the wash fraction (step 51), and 1 µl of the peak elution fractions (step 52) and add sample buffer to 20 µl. Incubate all samples for 5 min at 95°C before gel loading. Perform gel electrophoresis and Coomassie staining as detailed in Current Protocols article: Gallagher, 2012.
56.Place a 15-ml centrifuge tube on wet ice to pre-cool. Combine peak fractions with the lowest relative amount of contaminating proteins into the pre-cooled 15-ml centrifuge tube. Dilute to 2 mg/ml with cold buffer A. Determine the exact volume and add an equivalent volume of 100% glycerol to reach a final concentration of 55% (v/v) glycerol and 1 mg/ml of purified GFP nanobody-MNase. Mix well by inverting until the solution is homogenous.
57.Divide the 1 mg/ml GFP nanobody-MNase solution over pre-cooled 1.5-ml microcentrifuge tubes in 300 µl aliquots. Store at −80°C.
MNase activity assay (time: 2 hr)
The specific activity of the purified GFP nanobody-MNase protein is determined by comparison with MNase from a commercial source with a defined specific activity (e.g., New England Biolabs, cat. no. M0247S). This step consists of a short incubation with genomic DNA under digestion conditions. As MNase substrate, we use purified genomic DNA of high molecular weight from HeLa cells but DNA from other cell lines or tissues should also work. Parallel incubations lacking Ca++ ions are included to detect any contaminating DNase activities. We do not provide a detailed protocol for DNA analysis by agarose gel electrophoresis as this is a standard technique for a molecular biology lab. Interested readers can find details in Current Protocols article: Voytas, 2001.
58.Use one aliquot of the purified GFP nanobody-MNase proteins to freshly prepare a five-point 1:3 dilution series in 1× MNase buffer. Select a range from 30 to 0.3 ng/µl (i.e., 30, 10, 3, 1, 0.3 ng/µl).
59.Freshly prepare a five-point dilution series of commercial MNase in 1× MNase buffer in a range from 20 units/µl to 0.2 units/µl (i.e., 20, 6, 2, 0.6, 0.2 units/µl).
60.Prepare a dilution of high MW DNA with a final concentration of 150 ng/µl in 1× MNase buffer. Add 8 µl of this solution to ten new tubes.
61.Add 1 µl of 50 mM CaCl2 (freshly diluted from the 100 mM CaCl2 stock) to each of the ten tubes from step 60.
62.Add 1 µl of each MNase dilution series sample (steps 58 and 59) per tube and incubate at 37°C for 15 min.
63.Stop MNase digestion by adding 2 µl 0.5 M EDTA to each tube.
64.Analyze 5 µl of each DNA digestion by a 1.2% agarose/TBE gel electrophoresis followed by staining with ethidium bromide.
65.Compare the DNA digestion patterns of the purified GFP nanobody-MNase preparation with those of the commercial MNase (to determine the specific activity of the GFP nanobody-MNase preparation in comparison to the commercial MNase).
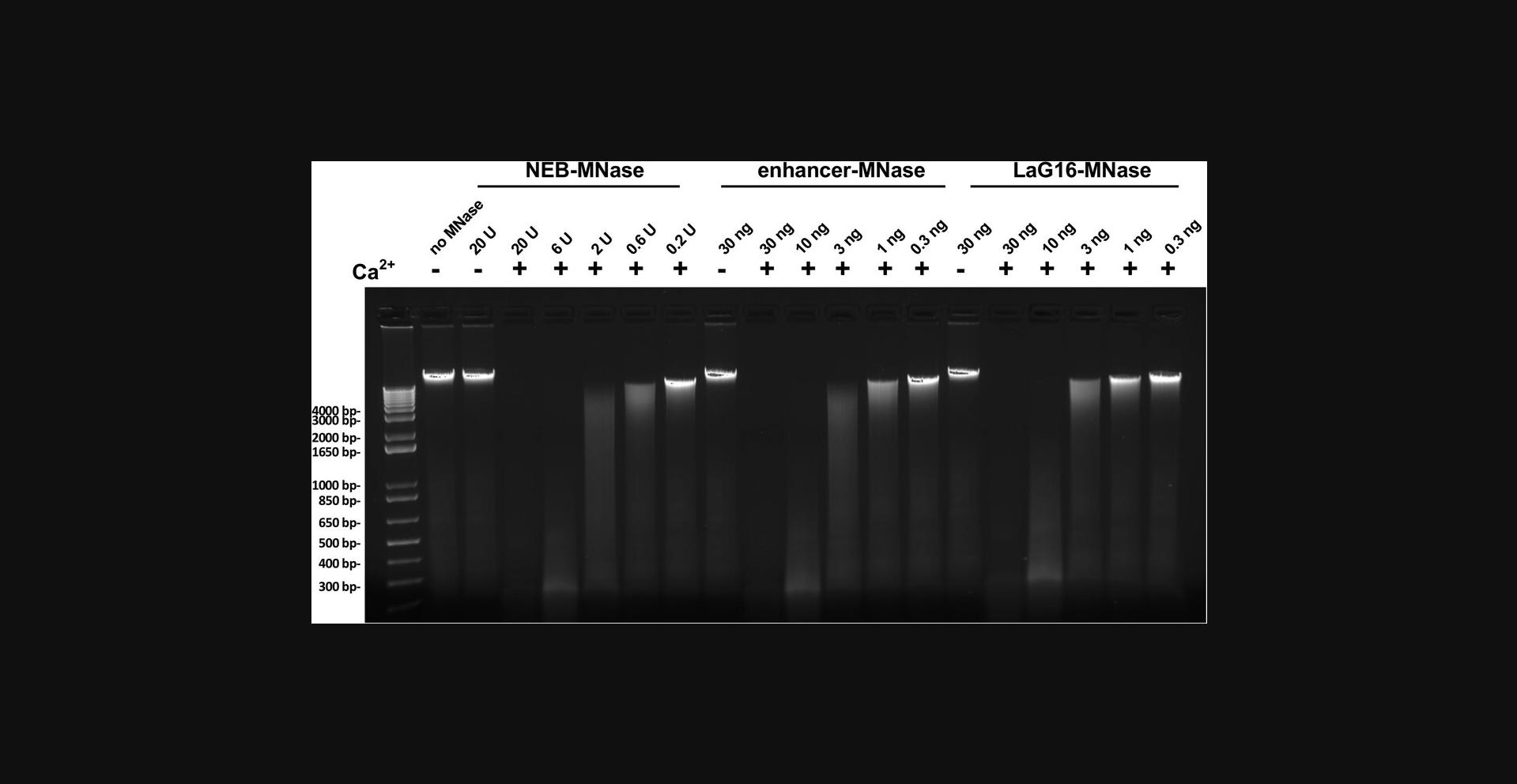
REAGENTS AND SOLUTIONS
Except for the 100 mM CaCl2 solution, digitonin buffer, and the STOP buffer, all other greenCUT &RUN buffers are used at room temperature. Store and use the buffers for preparation of bacterial lysates and chromatography of the GFP nanobody-MNase proteins at 4°C.
Ampicillin stock, 100 mg/ml
Dissolve 2 g of ampicillin (Merck, cat. no. A9393-5G) in 20 ml of pure water. Sterilize by filtration using a 0.22-µm filter unit. Divide into 1.5-ml microcentrifuge tubes in aliquots of 1.0 ml and store at −20°C for up to 2 years.
Bacterial lysis buffer
Mix 50 ml of 1 M Tris·HCl, pH 8.0 (Current Protocols article: Moore, 2001), 200 g of sucrose, 300 ml of 1 M KCl (Current Protocols article: Moore, 2001), 4 ml of 0.5 M EDTA, pH 8.0 (Current Protocols article: Moore, 2001), and 10 ml of 10% Triton X (Applichem, cat. no. A4975.0500) in a 1-L graduated cylinder. Add pure water to 1 L. Mix well to dissolve the sucrose and store at 4°C for up to 2 months. Prior to use, add one Complete Protease Inhibitor tablet (Roche, cat. no. 11836145001) per 50 ml of buffer. After dissolving the tablet, add 50 µl of 1 M DTT to this buffer.
Binding buffer
Mix 400 μl of 1 M HEPES-NaOH, pH 7.9 (see recipe), 200 μl of 1 M KCl (Current Protocols article: Moore, 2001), 20 μl of 1 M CaCl2 (Current Protocols article: Moore, 2001), and 20 μl of 1 M MnCl2 (see recipe). Bring the final volume to 20 ml with pure water.
Store at 4°C for up to 6 months.
Buffer A
Mix 100 ml of 0.1 M K2HPO4, pH 7.0 (Current Protocols article: Moore, 2001), 50 ml of 100% glycerol, 0.5 ml of 0.5 M EDTA, pH 8.0 (Current Protocols article: Moore, 2001), and 0.5 ml of 200 mM PMSF (see recipe) in a 500-ml graduated cylinder. Add pure water to 500 ml, mix, and leave for 10 min at room temperature. Add 0.5 ml of 1 M DTT and mix again. Sterilize by filtration using a 0.22-µm filter unit.
Store at 4°C for up to 3 months.
Buffer B
Mix 100 ml of 0.1 M K2HPO4, pH 7.0 (Current Protocols article: Moore, 2001), 50 ml of 100% glycerol, 0.5 ml of 0.5 M EDTA, pH 8.0 (Current Protocols article: Moore, 2001), and of 0.5 ml 200 mM PMSF (see recipe) in a 500-ml graduated cylinder. Add pure water to 500 ml. Add 37.3 g of solid KCl, dissolve by mixing, and leave for 10 min at room temperature. Add 0.5 ml of 1 M DTT and mix again. Sterilize by filtration using a 0.22-µm filter unit.
Store at 4°C for up to 3 months.
CaCl2, 100 mM
Dilute 1 ml of 1 M CaCl2 (Current Protocols article: Moore, 2001) with pure water to 10 ml and chill on ice before use.
Store at room temperature for up to 2 years.
Chicken lysozyme, 25 mg/ml
Dissolve 250 mg of lysozyme from hen egg white (Merck, cat. no. 10837059001) into 10 ml of pure water. Remove aggregates using a 0.22-µm filter and divide into 1-ml aliquots in 1.5-ml microcentrifuge tubes.
Store at −20°C for up to 2 years.
Digitonin buffer, 0.05%
Mix 1 ml of 1 M HEPES-NaOH, pH 7.5 (see recipe), 1.5 ml of 5 M NaCl (Current Protocols article: Moore, 2001), 250 μl of 10% digitonin (see recipe), and 25 μl of 1 M spermidine (see recipe). Bring the final volume to 50 ml with pure water and add one Complete Protease Inhibitor (EDTA-free) tablet (Roche, cat. no. 11873580001) to dissolve.
Store at 4°C for up to 1 week.
Digitonin stock solution, 10% (w/v)
Add 10 ml of DMSO (e.g., Genaxxon, cat. no. M6324.0500) to the vial containing 1 g of digitonin (Merck, cat. no. 300410-1GM). Mix well. Divide into 1-ml aliquots in 1.5-ml microcentrifuge tubes.
Store at −20°C for up to 1 year.
EGTA-STOP buffer
Mix 300 μl of 5 M NaCl (Current Protocols article: Moore, 2001), 200 μl of 0.5 M EGTA (see recipe), 100 μl of 1 M HEPES-NaOH, pH 7.5 (see recipe), 25 μl of 10% digitonin (see recipe), 12.5 μl of 10 mg/ml RNase A (Merck, cat. no. R6513-50MG), and 12.5 µl of 20 mg/ml glycogen (Merck, cat. no. 10901393001). Bring the final volume to 5 ml with 4.35 ml pure water.
Store at 4°C for up to 1 week.
Add spike-in mono-nucleosomes from Drosophila or fragmented genomic DNA from other heterologous sources directly before use to the EGTA-STOP buffer at 10 pg per sample. For GPF-tagged histones, use 100-1,000 pg per sample.
Ethylene glycol-bis(2-aminoethylether)-N,N,N′,N′-tetraacetic acid (EGTA), 0.5 M
While stirring, dissolve 19 g of EGTA powder (MilliporeSigma, cat. no. E4378-250G) in 90 ml of pure water by adding NaOH pellets (Carl Roth, cat. no. 6771.1) until pH 7.9 is reached. Bring the final volume to 100 ml with pure water. Sterilize by filtration using a 0.22-µm filter unit and store at room temperature. Sterile stock solutions are stable for at least 1 year.
Glutathione wash buffer I
Mix 50 ml of 1 M Tris·HCl, pH 8.0 (Current Protocols article: Moore, 2001), 200 g of sucrose, 300 ml of 1 M KCl (Current Protocols article: Moore, 2001), and 4 ml of 0.5 M EDTA, pH 8.0 (Current Protocols article: Moore, 2001) in a 1-L graduated cylinder. Add pure water to 1 L. Mix well to dissolve the sucrose and store at 4°C for up to 2 months.
Prior to use, add one Complete Protease Inhibitor tablet (Roche, cat. no. 11836145001) per 50 ml of buffer. After dissolving the tablet, add 50 µl of 1 M DTT to this buffer.
Glutathione wash buffer II
Mix 50 ml of 1 M K2HPO4, pH 7.0 (Current Protocols article: Moore, 2001), 100 ml of 1 M KCl (Current Protocols article: Moore, 2001), 2 ml of 0.5 M EDTA, pH 8.0 (Current Protocols article: Moore, 2001), and 1 ml of 1 M DTT in a 1-L graduated cylinder. Add pure water to 1 L. Mix well.
Store at 4°C for up to 2 months.
HEPES-NaOH, 1 M, pH 7.5
Dissolve 23.8 g of HEPES powder (MilliporeSigma, cat. no. H3375-1KG) in 50 ml of pure water, add NaOH pellets (Carl Roth, cat. no. 6771.1) with stirring until pH 7.5 is reached, and bring the volume to 100 ml with pure water. Sterilize by filtration using a 0.22-µm filter unit and store at room temperature. Sterile stock solutions are stable for at least 1 year.
HEPES-NaOH, 1 M, pH 7.9
Dissolve 23.8 g HEPES powder (MilliporeSigma, cat. no. H3375-1KG) in 50 ml of pure water, add NaOH pellets (Carl Roth, cat. no. 6771.1) with stirring until pH 7.9 is reached, and bring the volume to 100 ml with pure water. Sterilize by filtration using a 0.22-µm filter unit and store at room temperature. Sterile stock solutions are stable for at least 1 year.
High-calcium/low-salt buffer
Mix 7 μl of 1 M HEPES-NaOH, pH 7.5 (see recipe), 10 μl of 10% digitonin (see recipe), and 20 μl of 1 M CaCl2 (Current Protocols article: Moore, 2001). Bring the final volume to 2 ml with pure water.
In a separate tube, dissolve a Complete Protease Inhibitor (EDTA-free) tablet (Roche, cat. no. 11873580001) in 1 ml pure water, and then add 40 μl of this solution to the buffer.
Store at 4°C for up to 1 week.
IPTG, 1 M
Dissolve 2.38 g of IPTG (dioxane free; Thermo Fisher Scientific, cat. no. R0392) in 10 ml of pure water. Sterilize by filtration using a 0.22-µm filter unit. Divide into 1.5-ml microcentrifuge tubes in aliquots of 1.0 ml and store at −20°C for up to 2 years.
Low-salt rinse buffer
Mix 200 μl of 1 M HEPES-NaOH, pH 7.5 (see recipe), 50 μl of 10% digitonin (see recipe), and 5 μl of 1 M spermidine (see recipe). Bring the final volume to 9.8 ml with pure water.
In a separate tube, dissolve a Complete Protease Inhibitor (EDTA-free) tablet (Roche, cat. no. 11873580001) in 1 ml of pure water. Add 200 μl of this solution to the mix for a final buffer volume of 10 ml.
Store at 4°C for up to 1 week.
Luria-Bertani (LB) medium
Place 20 LB capsules (Fisher BioReagents, cat. no. BP9733-500) into a 1-L bottle. Add 1,000 ml of pure water and sterilize by autoclaving for 20 min at 121°C.
Store at room temperature for up to 6 months.
MNase buffer, 10×
Prepare 10 ml of 500 mM Tris·HCl, pH 7.9 (Current Protocols article: Moore, 2001). Add 10 mg of BSA (MilliporeSigma, cat. no. A9418-100G) and dissolve. Sterilize by filtration using a 0.22-µm filter unit and use this as a 10× buffer. Aliquot in 1.5-ml microcentrifuge tubes and store at −20°C.
Store at −20°C for up to 2 years.
MnCl2, 1 M
Dissolve 1.98 g of MnCl2 (Carl Roth, cat. no. 4320.1) in 10 ml filtered pure water. Stir at room temperature until dissolved and sterilize by filtration using a 0.22-µm filter unit.
Store at room temperature for up to 1 year in a 15-ml conical tube covered with foil.
No-calcium/low-salt buffer
Mix 7 μl 1 M HEPES-NaOH, pH 7.5 (see recipe) and 10 μl 10% digitonin (see recipe). Bring the final volume to 2 ml with pure water. In a separate tube, dissolve a Complete Protease Inhibitor (EDTA-free) tablet (Roche, cat. no. 11873580001) in 1 ml pure water and then add 40 μl of this solution to the buffer.
Store at 4°C for up to 1 week.
Permeabilization buffer
Mix 40 μl of 0.5 M EDTA (Current Protocols article: Moore, 2001) with 10 ml of digitonin buffer (see recipe) and place on ice.
Store at 4°C for up to 1 week.
Phenylmethylsulfonylfluoride (PMSF), 200 mM
Dissolve 0.348 g of PMSF (Merck, cat. no. 10837091001) in 10 ml of iso-propanol. Sterilize using a 0.22-µm filter and divide over 1.5-ml microcentrifuge tubes in aliquots of 0.5 ml.
Store at −20°C for up to 1 year.
Before use, warm the PMSF solution to 37°C in order to re-dissolve PMSF crystals.
Sample buffer
Add 2.5 ml of 1 M Tris·HCl, pH 6.8 (Current Protocols article: Moore, 2001), 10 ml of 10% SDS (see recipe), 10 ml of 50% glycerol, 2 ml of 0.5 M EDTA (Current Protocols article: Moore, 2001), and 500 µl of β-mercaptoethanol (MilliporeSigma, cat. no. 8057400250) in a 50-ml centrifuge tube. Add pure water to 50 ml and 0.02% bromophenol (Serva, cat. no. 15375).
Store at room temperature for up to 6 months.
Spermidine, 1 M
Add 2.9 g of spermidine (MilliporeSigma, cat. no. S-2501) to 20 ml pure water. Stir at room temperature until dissolved. Sterilize by filtration using a 0.22-µm filter unit. Divide into 1-ml aliquots in 1.5-ml microcentrifuge tubes and store at −20°C for up to 2 years.
STOP buffer
Mix 340 μl of 5 M NaCl (Current Protocols article: Moore, 2001), 200 μl 0.5 M EDTA (Current Protocols article: Moore, 2001), 100 μl of 0.5 M EGTA (see recipe), 10 μl of 10% digitonin (see recipe), 25 μl of 10 mg/ml RNase A (Merck, cat. no. R6513-50MG), and 12.5 μl of 20 mg/ml glycogen (Merck, cat. no. 10901393001). Bring to a final volume of 5 ml with 4.2 ml pure water.
Store at 4°C for up to 1 week.
Add spike-in mono-nucleosomes from Drosophila S2 cells (Nizamuddin et al., 2021) or sonicated genomic DNA from other sources (e.g., Drosophila melanogaster, Saccharomyces cerevisiae or E. coli ; for preparation of sonicated DNA see Sambrook and Russell, 2006) at 10 pg per sample to the STOP buffer just before use.
SDS, 10%
Add 10 g of SDS to 80 ml filtered pure water. Stir at room temperature until completely dissolved. Bring to final volume of 100 ml.
Store at room temperature for up to 1 year.
TE buffer
Mix 1 ml of 1 M Tris·HCl, pH 8.0 (Current Protocols article: Moore, 2001) with 20 µl of 0.5 M EDTA (Current Protocols article: Moore, 2001) and add pure water to 100 ml. Sterilize using a 0.22-µm filter.
Store at room temperature for up to 6 months.
Thrombin buffer
Mix the following in a 50-ml centrifuge tube: 2.5 ml of 0.1 M K2HPO4, pH 7.0 (Current Protocols article: Moore, 2001), 5 ml of 100% glycerol, 4 ml of 1 M KCl (Current Protocols article: Moore, 2001), and 50 µl of 1 M DTT. Add pure water to 50 ml. Filter through a 0.22-µm filter to remove small particles.
Store at 4°C and use within 1 week.
Wash buffer
Mix 1 ml of 1 M HEPES-NaOH, pH 7.5 (see recipe), 1.5 ml of 5 M NaCl (Current Protocols article: Moore, 2001), and 25 μl of 1 M spermidine (see recipe). Bring the final volume to 50 ml with pure water and add one Complete Protease Inhibitor (EDTA-free) tablet (Roche, cat. no. 11873580001) to dissolve.
Store at 4°C for up to 1 week.
COMMENTARY
Background Information
Sixty years ago, Jacob and Monod used bacterial genetics to define regulation of adaptive gene expression into cis- and trans -acting genetic elements (Jacob & Monod, 1961). This marked the beginning of the interest in identifying the trans -acting DNA-binding factors and their cis -acting operator sequences in bacterial operons. The paradigms developed in bacteria then guided the studies of eukaryotic gene transcription and chromatin functions, which similarly required the identification of cis -acting elements for a given transcription or chromatin factor in human cells.
Binding-site identification through in vivo DNA footprinting methods using UV light, chemical compounds (dimethyl sulfate), or enzymatic probes (DNase I) for a single genomic locus were first developed in the 1980s (Becker & Wang, 1984; Ephrussi, Church, Tonegawa, & Gilbert, 1985; Zinn & Maniatis, 1986). These methods were characterized by high spatial resolution (up to single base pairs). However, the identity of the protein responsible for the observed footprint could not be ascertained. Alternatively, Drosophila polytene chromosome immunostaining methods focused on specific transcription factors (TFs), but the spatial resolution was limited to chromosome bands (Plagens, Greenleaf, & Bautz, 1976).
Formaldehyde treatment of cells enables fixation of the dynamic contacts of chromatin-bound factors with genomic DNA. Combined with antibody-mediated isolation of crosslinked chromatin complexes after chromatin fragmentation by sonication, formaldehyde crosslinking formed the foundation of chromatin immunoprecipitation (ChIP) assays. The advantage of formaldehyde fixation lies in its reversibility, allowing efficient recovery of the bound DNA (Solomon & Varshavsky, 1985), which can then be detected by DNA hybridization with selected probes (Solomon, Larsen, & Varshavsky, 1988) or, alternatively, with DNA microarrays to provide (increased) genome coverage and resolution (Ren et al., 2000). Advances in DNA sequencing technologies then led to ChIP sequencing (ChIPseq; Johnson et al., 2007; Current Protocols article: Raha, Hong, & Snyder, 2010), which enabled the sequencing of all immunoprecipitated DNA. ChIPseq combined, in a genome-wide manner, the spatial resolution of in vivo DNA footprinting with knowledge of the identity of the chromatin-bound protein in cells or tissues from any species with a well-annotated genome sequence.
While ChIPseq provided a breakthrough for the field of gene transcription and chromatin function, important drawbacks of the technique became apparent later, which are related to issues with formaldehyde fixation, sonication procedures, and data normalization (Meyer & Liu, 2014; Skene & Henikoff, 2017). In addition, ChIP depends on the availability of high-quality antibodies with a strong affinity and selectivity for crosslinked protein-DNA complexes. These drawbacks motivated the development of assays to detect native chromatin complexes bound by regulatory factors or marked by specific histone modifications. Of these, cleavage under target and release using nuclease (CUT&RUN), developed by Steven Henikoff and coworker in 2017 (Skene & Henikoff, 2017), and its derivative, cleavage under target and tagmentation (CUT&Tag), also developed by the Henikoff laboratory 2 years later (Kaya-Okur et al., 2019), do not rely on formaldehyde-mediated crosslinking of protein-DNA complexes. Instead, permeabilized intact mammalian cells or isolated nuclei are coated with magnetic beads and incubated with an antibody to the nuclear target of interest. In a subsequent incubation step, an immunoglobulin binder like protein A or protein A/G fused to micrococcal nuclease (for CUT&RUN) or to the Tn5 transposase loaded with adapters (for CUT&Tag) is added to bind to the chromatin-associated antibody-targeted chromatin complex. CUT&RUN requires subsequent activation of the micrococcal nuclease (MNase) by the addition of micromolar amounts of Ca++ ions to release the targeted chromatin complexes from bulk chromatin into the supernatant. Both CUT&RUN and CUT&Tag provide high spatial resolution and can yield improved signal-to-noise ratios as compared to ChIP, which significantly lowers sequencing costs and the required number of cells. CUT&RUN and CUT&Tag have been applied successfully to single cells and individual pre-implantation embryos (Hainer et al., 2019; Kaya-Okur et al., 2019; Skene et al., 2018). In general, the number of reads required for saturation mapping of binding sites by CUT&RUN are up to ten fold lower compared to ChIPseq (Skene et al., 2018), which could range from 3 million for a DNA sequence-specific binder like CTCF to 30 million reads for an abundant histone modification. CUT&RUN has quickly become a popular method also due the generosity of the Henikoff laboratory, which kindly provided aliquots of protein A-MNase (pA-MNase) to laboratories interested in setting up CUT&RUN (including our laboratory). The pA-MNase, pG-MNase, and pA/G-MNase enzymes and other reagents for CUT&RUN (including validated antibodies) can now be purchased directly from Epicypher, Cell Signaling, or Merck.
The success of both CUT&RUN and CUT&Tag, however, still depends on the availability of high-quality antibodies, with their epitopes accessible in the chromatin-bound state. We have sought to circumvent this through the use of (1) high-affinity single-chain camelid antibodies (so-called nanobodies) directed towards the green fluorescent protein (GFP) from the jellyfish Aequorea victoria and (2) mammalian cell lines expressing a GFP-tagged version of the transcription factors or chromatin regulators of interest under the control of doxycycline (Nizamuddin et al., 2021). We fused GFP nanobodies directly to the catalytic domain of MNase for expression in bacteria. Optimization of the biochemical purification yielded milligram amounts of the active GFP nanobody-MNase, which is devoid of contaminating nucleases and has a specific activity similar to that of commercial MNase preparations. We coined the name greenCUT&RUN for this genome profiling approach, and showed that it is superior to or equally good as CUT&RUN for transcription factors for which high-quality antibodies are available (Nizamuddin et al., 2021). As greenCUT&RUN requires only a single 20-min incubation step with the GFP nanobody-MNase compared to the longer antibody incubation (2 hr to overnight) followed by the protA/G-MNase incubation typical of CUT&RUN (Fig. 1), greenCUT&RUN reduces handling time and experimental variation. The GFP nanobody-MNase fusions employed display low non-specific binding to chromatin and are efficiently removed by washing. In addition, GFP nanobodies binding to non-overlapping epitopes can be combined to improve the signal of low abundant GFP-tagged proteins. Similar to CUT&RUN, greenCUT&RUN data are characterized by high accuracy, sensitivity, resolution, reproducibility, and specificity (Nizamuddin et al., 2021). GreenCUT&RUN, however, depends on the exogenous expression of GFP-tagged proteins and it is not suited for the profiling of histone modifications or other post-translationally modified proteins
The provided greenCUT&RUN protocols use 250,000 intact HeLa cells and we have obtained similar results with higher cell numbers (1,000,000). We have not titrated cell numbers down for greenCUT&RUN but published CUT&RUN protocols provide guidelines for this (Current Protocols article: Hainer & Fazzio, 2019; Skene et al., 2018). In contrast to the CUT&RUN protocol for isolated nuclei of Hainer and Fazzio (Current Protocols article: Hainer & Fazzio, 2019), we use intact cells for greenCUT&RUN but it should be straightforward to adapt our protocols to isolated nuclei. Using human tissue culture cells, we have successfully applied greenCUT&RUN to a variety of gene-specific transcription factors (NF-Y, JUND, FOS, SMAD4), components of the basal transcription machinery (TBP, TAF1, TAF4, TAF9, BTAF1, and RPB1), and chromatin regulators (Menin, KDM6A/UTX), and this list is growing. For some applications, the conditional (mild) overexpression of GFP-tagged proteins can be a drawback (see discussion below), which can be circumvented by using cells with GFP knock-in alleles or by expressing the GFP allele from single-copy bacterial artificial chromosomes (Poser et al., 2008). On the other hand, conditional expression allows structure-function analyses of (basal) transcription factors, chromatin regulators, or histones by expressing GFP-tagged mutant proteins that would not support cell viability.
A unique advantage of greenCUT&RUN is that it can be integrated with GFP-based quantitative mass spectrometry to detect and to quantify “piggy-back” DNA binding events in which a transcription factor binds DNA through another sequence-specific transcription factor (Nizamuddin et al., 2021). The principle of greenCUT&RUN to fuse the catalytic domains of MNase directly to a camelid nanobody can also be exploited for epitopes other than GFP and exchanging the MNase moiety with the Tn5 transposase would enable greenCUT&Tag approaches. By analogy to this principle, similar strategies using any high-affinity nanobody directed against (modified) endogenous proteins or other tags used for cell imaging and affinity purifications can be fused to MNase to produce an antigen-specific CUT&RUN reagent of stable quality and of unlimited supply.
Critical Parameters
Suppression of secondary MNase cleavages
For all CUT&RUN-based approaches, it is critical to minimize secondary MNase cleavages by the targeted protein/DNA complexes released from their primary site. Therefore, the reaction tubes should be chilled at 0°C on wet ice before addition of cold CaCl2 to activate the cleavage activity of the targeted GFP nanobody-MNase (step 29 of Basic Protocol or step 5 of Alternate Protocol). Throughout the cleavage reaction, the tube should be kept at 0°C on wet ice. While we found that 30 min on wet ice is optimal for the detection of primary cleavages with GFP-tagged NF-YA or TBP, shorter times may be better for abundant proteins, like GFP-tagged histones. We found that incubations of 90 min lead to an unacceptable level of secondary cleavages, which are revealed by longer fragment sizes in the sequencing libraries (S. Nizamuddin, S. Koidl, and H. T. M. Timmers, unpublished observations). Paired-end sequencing allows the identification of such long fragments, which can be removed from subsequent bioinformatic analyses. For NF-YA, we found that fragments >220 bp lack the cognate DNA binding sequence and, therefore, are most likely the result of secondary cleavages (S. Nizamuddin, S. Koidl, and, H. T. M. Timmers, unpublished observations). These long reads typically constitute <10% of the total number of reads.
Background controls for greenCUT&RUN can either be the no calcium control or a cell line lacking any GFP protein. As greenCUT&RUN does not exhibit any preference for open chromatin, both controls are equally fine (Nizamuddin et al., 2021). The improved signal-to-noise ratios that can be obtained with CUT&RUN approaches compared to ChIPseq makes the inclusion of heterologous spike-in DNA for normalization purposes essential. We typically aim for spike-in controls to constitute 1% to 10% of the total reads. To obtain a similar number of spike-in reads, control libraries require only 25% to 35% of the reads from the test sample libraries. In general, we find that two to ten million reads are sufficient to determine all genomic binding sites for human DNA sequence-specific transcription factors like NF-YA, FOS, JUND, SMAD4, or TBP, while chromatin regulatory factors displaying broader binding, such as KDM6A/UTX or Menin, require ten to fifteen million reads to reach saturation. We do not remove (apparent) duplicate reads from the sequencing results as they mostly likely do not result from PCR duplicates but are genuine experimental duplicates.
Dealing with tiny amounts of released chromatin
The strength of CUT&RUN-based methods is that only the targeted chromatin complexes are released and that the vast majority of genomic DNA remains behind. This means that for the majority of gene-specific transcription factors, chromatin regulators, and basal transcription factors, only tiny amounts of small DNA fragments are isolated. In general, commercial DNA purification columns do not perform well with such tiny amounts of small fragments. To prevent the loss of these DNA fragments, we prefer to use phenol/chloroform/iso-amyl alcohol extraction and a prolonged ethanol precipitation step over the use of columns for the cleanup of released DNA (steps 34 to 45 of the Basic Protocol). However, the DNA precipitate obtained after ethanol is barely visible and special care is required to prevent loss of this pellet. Saving the supernatants from step 43 and 45 of Basic Protocol may be prudent for the first greenCUT&RUN experiments.
Bioinformatic analyses of greenCUT&RUN data
The high signal-to-noise ratio of CUT&RUN libraries complicates peak calling based on local backgrounds, which work well for noisy ChIPseq data. To address this, Henikoff and colleagues developed the SEACR software (Meers et al., 2019), but we and others found that HOMER software performs well for peak calling of greenCUT&RUN data (Hainer et al., 2019; Nizamuddin et al., 2021). Interpretation of greenCUT&RUN results can be aided by knowledge of the DNA binding motifs or cognate gene targets, which require dedicated bioinformatic analyses. While a comprehensive understanding of greenCUT&RUN data requires such analyses, a visual inspection of genome browser tracks of specific genomic regions can already provide a rapid assessment of the data quality (see Understanding Results).
Troubleshooting
Expression levels of GFP-tagged proteins
The expression level of the GFP-tagged protein of interest is important for the quality of greenCUT&RUN data. In general, we aim for (sub-)endogenous levels of the tagged protein, which can be assessed by immunoblotting using antibodies directed against the protein of interest to compare endogenous and exogenous levels. When the expression levels of the GFP-tagged protein greatly exceed endogenous levels, this can result in spurious chromatin binding events. We found this to be the case for GFP-tagged versions of JUN and MYC. The greenCUT&RUN data of these TFs showed many spurious binding events revealed by the lack of cognate DNA binding sites (S. Nizamuddin, S. Koidl and H. T. M. Timmers, unpublished observations). In case of undesired high expression of the GFP-tagged protein, expression levels can be limited by reducing doxycycline levels or the length of induction. Overexpression of the GFP-tagged protein is less of an issue when analyzing proteins which bind DNA as part of larger heteromeric complexes, like NF-YA, FOS, TBP, TAF1, SMAD4, Menin, or KDM6A/UTX. In these cases spurious chromatin binding of the GFP-tagged protein is suppressed because this would also require larger amounts of the other subunits of their cognate complex. On the other hand, expression levels of the GFP-tagged factor can also be too low to detect chromatin binding events. We found this to be the case for a TBP regulator, BTAF1, and for the largest subunit of pol II, RPB1 (Fig. 4). Both proteins are large in size (>150 kDa), which limits their expression levels in HeLa Flp-In/T-REx cells. Inclusion of intron II of the human β-globin gene in the expression construct enhances expression levels of BTAF1 and of RPB1 three to five fold (S. Koidl and H. T. M. Timmers, unpublished observations). This enhanced expression allowed detection of proper chromatin binding events, as shown in Figure 5. Alternatives to the Flp-In/T-REx expression system for GFP-tagged proteins could be a different (rtTA3-dependent) induction system, expression from bacterial artificial chromosomes (Poser et al., 2008), or the use of GFP knock-in alleles to achieve endogenous expression levels.
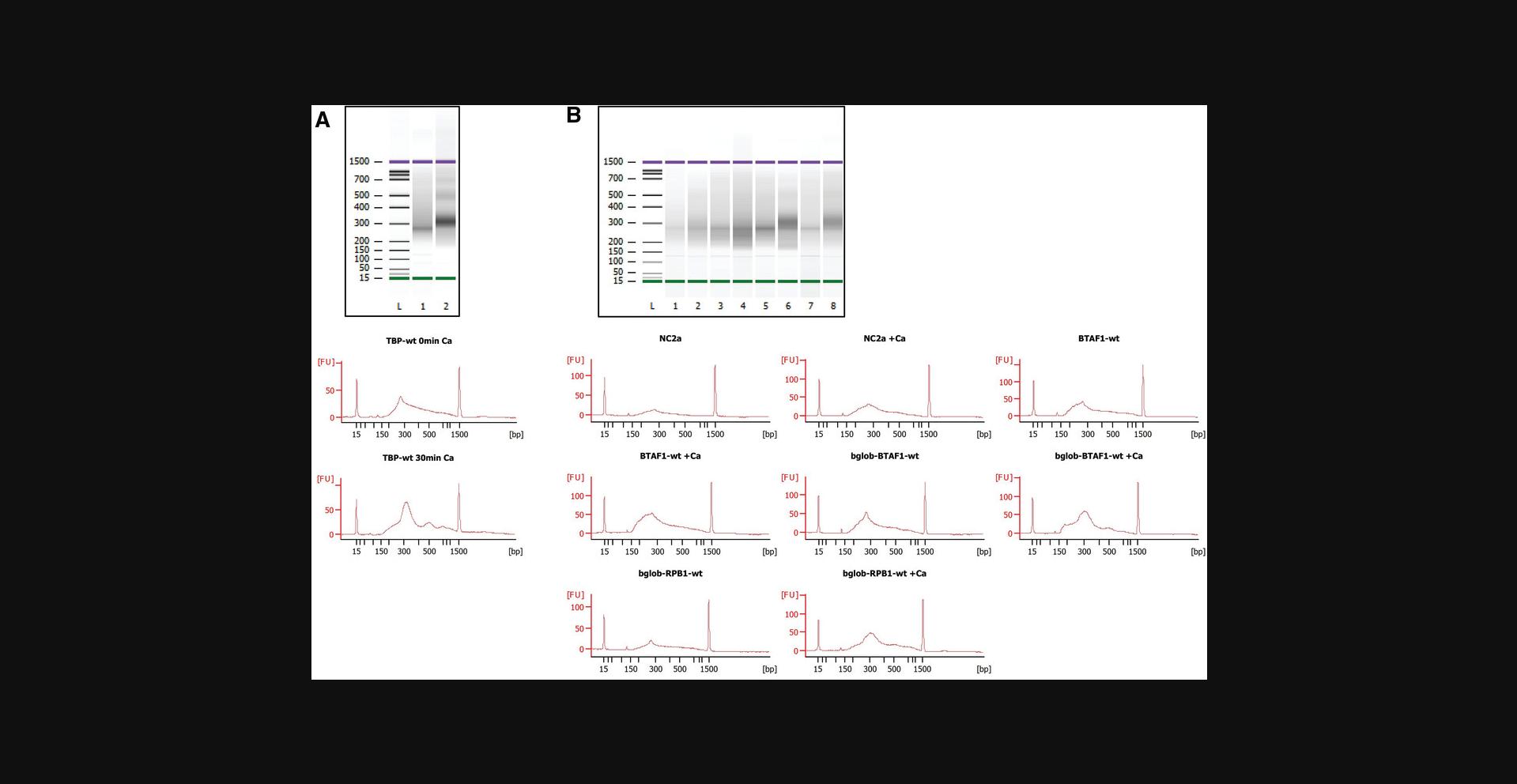
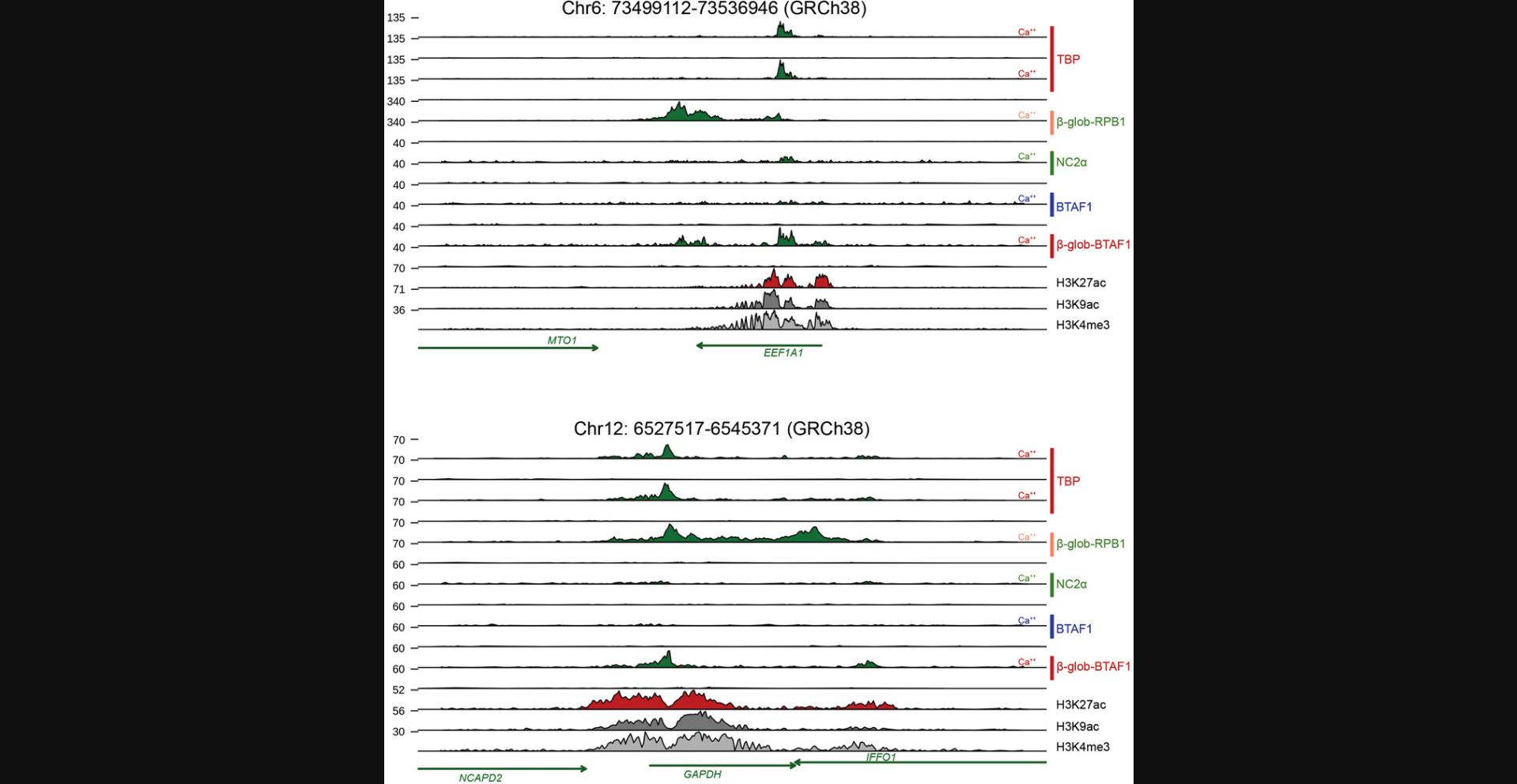
Position of the GFP tag
Cleavage of chromatin complexes in greenCUT&RUN depends on the accessibility of the targeted MNase to the surrounding DNA. As mentioned, we found that the combination of two different GFP nanobody-MNases can augment the signal for greenCUT&RUN for certain proteins, like TAF1, while it had no effect on greenCUT&RUN with TBP or NF-YA (S. Nizamuddin, S. Koidl and H. T. M. Timmers, unpublished observations). DNA cleavage by the targeted MNase also depends on the vicinity of the GFP-tagged protein to genomic DNA. In principle, chromatin complexes targeted in greenCUT&RUN contain at least two flexible hinges: One flexible linker between GFP and the target protein and the other between the GFP nanobody and MNase. This flexibility even allows the MNase to access the DNA site on the opposite surface of the transcription factor (Nizamuddin et al., 2021). However, it is theoretically possible that the GFP moiety of the fused transcription or chromatin factor is too far removed from the surrounding DNA and/or that steric hindrance by other proteins or adjacent nucleosomes prevents efficient DNA cleavage around the target protein-binding site. This would be revealed during the bioinformatic analyses by the lack of expected binding events and/or binding peaks. We believe that this is the case for the α-subunit of the NC2 complex, which restricts TBP activity in supporting transcription initiation by pol II. While an N-terminal GFP fusion of NC2α is well expressed, only very weak promoter peaks were detected by greenCUT&RUN (Fig. 5). It is possible that the N-terminal GFP tag affects NC2α binding to NC2β and the formation of the functional heterodimeric NC2 complex. The possibility to determine the interactome of the GFP-tagged protein by quantitative mass spectrometry (Smits, Jansen, Poser, Hyman, & Vermeulen, 2013; van Nuland et al., 2013) provides a direct quality check to determine functional interference by the GFP moiety but we have not yet investigated this for GFP-NC2α. A potential solution to the problem of MNase accessibility would be to fuse the GFP part to the other end of the target protein. It is good advice, in any case, to avoid fusing GFP to functional domains located at the very beginning or very end of the target protein.
Coating of cells with concanavalin beads
In rare cases, we noted that cells coated by the magnetic concanavalin A (ConA) beads were not attracted by the magnet. While we do not yet have a clear explanation for this phenomenon, it is possible that this is related to a low beads-to-cells ratio. Increasing the ratio of ConA beads to cells may solve this problem, which can be monitored by attraction of the colored beads to the magnetic stand in the first steps in the protocol (steps 16 and 17 of Basic Protocol).
Expression and solubility of GFP nanobody-MNase proteins
Camelid nanobodies differ in their solubility and proper folding when expressed in the cytoplasm of bacteria (de Marco, 2020). While the enhancer nanobody fusion is well expressed as a soluble protein, the LaG16 variant requires overnight induction at 18°C as described in Support Protocol. These conditions should result in expression levels of soluble GST-LaG16-MNase as presented in Figure 2. When this is not the case, we recommend optimizing soluble expression before moving on to the protein purification steps. Soluble expression of GST-LaG16-MNase can be improved, for instance, by chilling the culture to 18°C before IPTG addition in step 8 of Support Protocol, reducing the IPTG concentration from 1 mM to 0.1 mM at step 8, or changing bacterial strains for expression, from BL21(DE3) to Origami2(DE3).
Understanding Results
The success of a greenCUT&RUN experiment is only revealed after bioinformatic data analysis but some hints can be obtained prior to this. In general, the recovery of DNA from released chromatin is low but differences in recovered DNA from test and control samples can be observed in step 47 of Basic Protocol for gene-specific TFs, like NF-YA and FOS, which both produce high-quality greenCUT&RUN data (Nizamuddin et al., 2021) and for GFP-tagged histone proteins. Such differences are less obvious for proteins like TBP. For most transcription factors or chromatin regulators, differences in DNA yield may not be apparent in step 47 of Basic Protocol. However, for several (but not all) proteins, clear differences between test and control samples can be observed in the Bioanalyzer profiles of test and control greenCUT&RUN libraries. To illustrate this, we compared Bioanalyzer results with sequencing data of greenCUT&RUN libraries prepared for components of the basal pol II transcription machinery. As shown in Figure 4A, the GFP-TBP library (lane 2) displays stronger peaks at 300 and 500 bp compared to the control library (lane 1). Figure 4B shows similar signal increases with β-globin expression constructs for BTAF1 (compare lanes 5 and 6) and for RPB1 (lanes 7 and 8). Such increases are not observed with GFP-NC2α (lanes 1 and 2) or the standard GFP-BTAF1 expression construct (lanes 3 and 4). Subsequent bioinformatic analyses of the sequencing results of these libraries (Fig. 5) confirmed that GFP-β-RPB1 yields signals over the transcribed regions of two (highly) expressed genes (EEF1A1 and GAPDH), while GFP-TBP and GFP-β-BTAF1 peaks were detected over the promoter regions of these genes. GFP-NC2α and GFP-BTAF1 only yielded weak promoter signals. For comparison, genome tracks for the activating histone marks H3K4m3, H3K9ac, and H3K27ac are included.
Time Considerations
The full procedure for greenCUT&RUN can be completed in 1.5 days by an experienced researcher. On day 1, the harvesting of the cells, coating with ConA beads, permeabilization, GFP nanobody-MNase incubation, MNase activation, and purification of released DNA takes an afternoon. These steps cannot be interrupted, except at the GFP nanobody-MNase incubation step, which could also be performed overnight. However, we do not recommend this, as it may result in lower signals. On day 2, DNA recovery after ethanol precipitation and subsequent library preparation takes a full day. At the end of day 2, the libraries can be loaded on the flow cell for high-throughput DNA sequencing. Purification of each GFP nanobody-MNase protein takes a full week. This represents a significant time investment but it is only needed once as it yields sufficient recombinant protein for 10,000 to 30,000 greenCUT&RUN reactions. In all described protocols, we indicated flexible stopping points.
Acknowledgments
The authors thank all members of the Timmers lab for their constructive comments and discussions during the development of greenCUT&RUN. In particular, the authors thank Laura Pulido Cortés and Simona Capponi, who worked hard in trying to establish chromatin immunocleavage sequencing (ChICseq) in HeLa cells, and Sheikh Nizamuddin, who performed the bioinformatic analyses guiding optimization of greenCUT&RUN protocols. The authors also thank Sheik Nizamuddin and Timothy En Haw Chan for the critical reading of this manuscript. In addition, the authors are grateful to Tamara Werner and Silke Lassmann of the Department of Pathology of the Medical Center-University of Freiburg and to Chiara Bella and Thomas Manke from the Deep Sequencing facility of the Max Planck Institute of Immunology and Epigenetics in Freiburg, who performed high-throughput sequencing during the initial phase of greenCUT&RUN. This work was financially supported by grants from the Deutsche Forschungsgemeinschaft (DFG, German Research Foundation) with the project-IDs 192904750-SFB 992, 431984000-SFB 1453, and TI688/1-1. Open access funding enabled and organized by Projekt DEAL.
Author Contributions
Stefanie Koidl : Conceptualization, formal analysis, methodology, visualization, writing review and editing; Marc Timmers : Conceptualization, funding acquisition, investigation, project administration, resources, supervision, writing original draft, writing review and editing.
Conflict of Interest
The authors declare no conflict of interest.
Open Research
Data Availability Statement
The data that support the findings of this study are available from the corresponding author upon reasonable request.
Literature Cited
- Becker, M. M., & Wang, J. C. (1984). Use of light for footprinting DNA in vivo. Nature , 309(5970), 682–687. doi: 10.1038/309682a0.
- de Marco, A. (2020). Recombinant expression of nanobodies and nanobody-derived immunoreagents. Protein Expression and Purification , 172, 105645. doi: 10.1016/j.pep.2020.105645.
- Ephrussi, A., Church, G. M., Tonegawa, S., & Gilbert, W. (1985). B lineage–specific interactions of an immunoglobulin enhancer with cellular factors in vivo. Science , 227(4683), 134–140. doi: 10.1126/science.3917574.
- Gallagher, S. R. (2012). One-dimensional SDS gel electrophoresis of proteins. Current Protocols in Molecular Biology , 97, 10.2A.1-10.2A.44. doi: 10.1002/0471142727.mb1002as97.
- Hainer, S. J., Boskovic, A., McCannell, K. N., Rando, O. J., & Fazzio, T. G. (2019). Profiling of pluripotency factors in single cells and early embryos. Cell , 177(5), 1319–1329 e1311. doi: 10.1016/j.cell.2019.03.014.
- Hainer, S. J., & Fazzio, T. G. (2019). High-resolution chromatin profiling using CUT&RUN. Current Protocols in Molecular Biology , 126(1), e85. doi: 10.1002/cpmb.85.
- Jacob, F., & Monod, J. (1961). Genetic regulatory mechanisms in the synthesis of proteins. Journal of Molecular Biology , 3, 318–356. doi: 10.1016/s0022-2836(61)80072-7.
- Johnson, D. S., Mortazavi, A., Myers, R. M., & Wold, B. (2007). Genome-wide mapping of in vivo protein-DNA interactions. Science , 316(5830), 1497–1502. doi: 10.1126/science.1141319.
- Kaya-Okur, H. S., Janssens, D. H., Henikoff, J. G., Ahmad, K., & Henikoff, S. (2020). Efficient low-cost chromatin profiling with CUT&Tag. Nature Protocol , 15(10), 3264–3283. doi: 10.1038/s41596-020-0373-x.
- Kaya-Okur, H. S., Wu, S. J., Codomo, C. A., Pledger, E. S., Bryson, T. D., Henikoff, J. G., … Henikoff, S. (2019). CUT&Tag for efficient epigenomic profiling of small samples and single cells. Nature Communication , 10(1), 1930. doi: 10.1038/s41467-019-09982-5.
- Kubala, M. H., Kovtun, O., Alexandrov, K., & Collins, B. M. (2010). Structural and thermodynamic analysis of the GFP:GFP-nanobody complex. Protein Science , 19(12), 2389–2401. doi: 10.1002/pro.519.
- Meers, M. P., Bryson, T. D., Henikoff, J. G., & Henikoff, S. (2019). Improved CUT&RUN chromatin profiling tools. Elife , 8. doi: 10.7554/eLife.46314.
- Meers, M. P., Tenenbaum, D., & Henikoff, S. (2019). Peak calling by sparse enrichment analysis for CUT&RUN chromatin profiling. Epigenetics & Chromatin, 12(1), 42. doi: 10.1186/s13072-019-0287-4.
- Meyer, C. A., & Liu, X. S. (2014). Identifying and mitigating bias in next-generation sequencing methods for chromatin biology. Nature Reviews Genetics , 15(11), 709–721. doi: 10.1038/nrg3788.
- Moore, D. D. (2001). Commonly used reagents and equipment. Current Protocols in Molecular Biology , Appendix 235(1). doi: 10.1002/0471142727.mba02s35.
- Nizamuddin, S., Koidl, S., Bhuiyan, T., Werner, T. V., Biniossek, M. L., Bonvin, A., … Timmers, H. T. (2021). Integrating quantitative proteomics with accurate genome profiling of transcription factors by greenCUT&RUN. Nucleic Acids Research , 49, e49. doi: 10.1093/nar/gkab038.
- Phelan, M. C. (2006). Techniques for mammalian cell tissue culture. Current Protocols in Molecular Biology , Appendix 3F , 74(1). doi: 10.1002/0471142727.mba03fs74.
- Plagens, U., Greenleaf, A. L., & Bautz, E. K. (1976). Distribution of RNA polymerase on Drosophila polytene chromosomes as studied by indirect immunofluorescence. Chromosoma , 59(2), 157–165. doi: 10.1007/BF00328484.
- Poser, I., Sarov, M., Hutchins, J. R., Heriche, J. K., Toyoda, Y., Pozniakovsky, A., … Hyman, A. A. (2008). BAC TransgeneOmics: A high-throughput method for exploration of protein function in mammals. Nature Methods , 5(5), 409–415. doi: 10.1038/nmeth.1199.
- Raha, D., Hong, M., & Snyder, M. (2010). ChIP-Seq: A method for global identification of regulatory elements in the genome. Current Protocols in Molecular Biology , 91, 21.19.1-21.19.14. doi: 10.1002/0471142727.mb2119s91.
- Ren, B., Robert, F., Wyrick, J. J., Aparicio, O., Jennings, E. G., Simon, I., … Young, R. A. (2000). Genome-wide location and function of DNA binding proteins. Science , 290(5500), 2306–2309. doi: 10.1126/science.290.5500.2306.
- Sambrook, J., & Russell, D. W. (2006). Fragmentation of DNA by sonication. CSH Protocols , 2006(4). doi: 10.1101/pdb.prot4538.
- Skene, P. J., Henikoff, J. G., & Henikoff, S. (2018). Targeted in situ genome-wide profiling with high efficiency for low cell numbers. Nature Protocol , 13(5), 1006–1019. doi: 10.1038/nprot.2018.015.
- Skene, P. J., & Henikoff, S. (2017). An efficient targeted nuclease strategy for high-resolution mapping of DNA binding sites. Elife , 6. doi: 10.7554/eLife.21856.
- Smits, A. H., Jansen, P. W., Poser, I., Hyman, A. A., & Vermeulen, M. (2013). Stoichiometry of chromatin-associated protein complexes revealed by label-free quantitative mass spectrometry-based proteomics. Nucleic Acids Research , 41(1), e28. doi: 10.1093/nar/gks941.
- Solomon, M. J., Larsen, P. L., & Varshavsky, A. (1988). Mapping protein-DNA interactions in vivo with formaldehyde: Evidence that histone H4 is retained on a highly transcribed gene. Cell , 53(6), 937–947. doi: 10.1016/s0092-8674(88)90469-2.
- Solomon, M. J., & Varshavsky, A. (1985). Formaldehyde-mediated DNA-protein crosslinking: A probe for in vivo chromatin structures. Proceedings of the National Academy of Sciences USA , 82(19), 6470–6474. doi: 10.1073/pnas.82.19.6470.
- van Nuland, R., Smits, A. H., Pallaki, P., Jansen, P. W., Vermeulen, M., & Timmers, H. T. (2013). Quantitative dissection and stoichiometry determination of the human SET1/MLL histone methyltransferase complexes. Molecular and Cellular Biology , 33(10), 2067–2077. doi: 10.1128/MCB.01742-12.
- Voytas, D. (2001). Agarose gel electrophoresis. Current Protocols in Molecular Biology , 51(1), 2.5A.1-23.3.6. doi: 10.1002/0471142727.mb0205as51.
- Zhu, Q., Liu, N., Orkin, S. H., & Yuan, G. C. (2019). CUT&RUNTools: A flexible pipeline for CUT&RUN processing and footprint analysis. Genome Biology , 20(1), 192. doi: 10.1186/s13059-019-1802-4.
- Zinn, K., & Maniatis, T. (1986). Detection of factors that interact with the human beta-interferon regulatory region in vivo by DNAase I footprinting. Cell , 45(4), 611–618. doi: 10.1016/0092-8674(86)90293-x.
Key References
- Skene and Henikoff, 2017. See above.
First description of CUT&RUN using transcription factors (Abf1 and Reb1), SNF2 family members (Sth1 and Mot1), and histones (Cse4 and H2A) in yeast cells, and CTCF and H3K27me3 in human cells.
- Skene et al., 2018. See above.
Describes a detailed protocol for CUT&RUN for human cells using CTCF and H3K27me3 to determine the lowest cell numbers required.
- Hainer and Fazzio, 2019. See above.
Describes a detailed protocol for CUT&RUN using low numbers of isolated nuclei.
- Nizamuddin et al., 2021. See above.
Describes the greenCUT&RUN method with the transcription factors NF-YA, FOS, and TBP as examples.
Internet Resources
Website from the Henikoff lab with information on CUT&RUN and CUT&Tag protocols and bioinformatic analyses, and a useful frequently asked questions section.
Commercial information on CUT&RUN protocols and reagents by Epicypher.
Commercial information on CUT&RUN protocols and reagents by Cell Signaling.
Frequently asked questions section for CUT&RUN by Cell Signaling.
Citing Literature
Number of times cited according to CrossRef: 5
- Liucong Ling, Bettina Mühling, Rita Jaenichen, Nicolas Gompel, Increased chromatin accessibility promotes the evolution of a transcriptional silencer in Drosophila , Science Advances, 10.1126/sciadv.ade6529, 9 , 7, (2023).
- Tao Chen, Xiaolu Wei, Cécile Courret, Min Cui, Lin Cheng, Jing Wu, Kami Ahmad, Amanda M. Larracuente, Yikang S. Rong, The nanoCUT&RUN technique visualizes telomeric chromatin in Drosophila, PLOS Genetics, 10.1371/journal.pgen.1010351, 18 , 9, (e1010351), (2022).
- Koen M. A. Dreijerink, Ezgi Ozyerli-Goknar, Stefanie Koidl, Ewoud J. van der Lelij, Priscilla van den Heuvel, Jeffrey J. Kooijman, Martin L. Biniossek, Kees W. Rodenburg, Sheikh Nizamuddin, H. T. Marc Timmers, Multi-omics analyses of MEN1 missense mutations identify disruption of menin–MLL and menin–JunD interactions as critical requirements for molecular pathogenicity, Epigenetics & Chromatin, 10.1186/s13072-022-00461-8, 15 , 1, (2022).
- Steven Henikoff, Kami Ahmad, In situ tools for chromatin structural epigenomics, Protein Science, 10.1002/pro.4458, 31 , 11, (2022).
- Ray Scheid, James A. Dowell, Dean Sanders, Jianjun Jiang, John M. Denu, Xuehua Zhong, Histone Acid Extraction and High Throughput Mass Spectrometry to Profile Histone Modifications in Arabidopsis thaliana, Current Protocols, 10.1002/cpz1.527, 2 , 8, (2022).