The Manufacture and Characterization of Biomimetic, Biomaterial-Based Scaffolds for Studying Physicochemical Interactions of Neural Cells in 3D Environments
Cian O'Connor, Cian O'Connor, Ian Woods, Ian Woods, Alan Hibbitts, Alan Hibbitts, Adrian Dervan, Adrian Dervan, Fergal J. O'Brien, Fergal J. O'Brien
Abstract
A particular challenge to the field of neuroscience involves translating findings from 2D in vitro systems to 3D in vivo environments. Standardized cell culture environments that adequately reflect the properties of the central nervous system (CNS) such as the stiffness, protein composition, and microarchitecture in which to study 3D cell–cell and cell–matrix interactions are generally lacking for in vitro culture systems. In particular, there remains an unmet need for reproducible, low-cost, high-throughput, and physiologically relevant environments comprised of tissue-native matrix proteins for the study of CNS microenvironments in 3D. Advances in the field of biofabrication over the past number of years have facilitated the production and characterization of biomaterial-based scaffolds. Typically developed for tissue engineering applications, they also provide sophisticated environments in which to study cell–cell and cell–matrix interactions and have been used for 3D modeling for a range of tissues. Here, we describe a simple and scalable protocol for the production of biomimetic, highly porous freeze-dried hyaluronic acid scaffolds with tunable microarchitecture, stiffness, and protein composition. Furthermore, we describe several different approaches that can be used to characterize a range of physicochemical properties and how to employ the scaffolds for the 3D culture of sensitive CNS cells in vitro. Finally, we detail several approaches for the study of key cell responses within the 3D scaffold environments. Overall, this protocol describes the manufacture and testing of a biomimetic and tunable macroporous scaffold system for neuronal cell culture applications. © 2023 The Authors. Current Protocols published by Wiley Periodicals LLC.
Basic Protocol 1 : Scaffold manufacture
Basic Protocol 2 : Scaffold characterization
Basic Protocol 3 : Cell culture and analysis of neurons in scaffolds
INTRODUCTION
The development of effective 3D in vitro models for the study of neural cells represents a bridge between simple but essential 2D culture and highly complex in vivo environments, improving the relevance of in vitro approaches while also reducing the use of animals in research (Hubrecht & Carter, 2019; Huch, Knoblich, Lutolf, & Martinez-Arias, 2017; Walczak, Perez-Esteban, Bassett, & Hill, 2021; Watson, Kavanagh, Allenby, & Vassey, 2017). Cellular interactions with the surrounding central nervous system (CNS) extracellular matrix (ECM) and neighboring cells are known to be essential for proper neuronal development, homeostasis, and signaling in both the healthy and diseased or injured CNS (Song & Dityatev, 2018), with altered cell–matrix interaction and composition known to underlie many pathologic states in the CNS (Guimarães, Gasperini, Marques, & Reis, 2020; Song & Dityatev, 2018; Walczak et al., 2021). It has become increasingly evident that biophysical cues and accompanying signaling pathways from the surrounding environment are strongly linked to the regulation of neural cell development and functionality (Barnes, Przybyla, & Weaver, 2017; Chighizola et al., 2019). Critically, in 3D environments mechanical properties and matrix composition drive several key changes such as growth and maturation in neuronal cells through a range of integrin-sensitive and mechanosignaling pathways, among others (Barnes et al., 2017; Chighizola et al., 2019). Mechanical cues from the surrounding macro- and microenvironment are known to impact cells and tissue in developing, healthy, and pathologic conditions throughout the body (Guimarães et al., 2020; Moeendarbary et al., 2017; Park et al., 2020; Sridharan, Cavanagh, Cameron, Kelly, & O'Brien, 2019). Notably, the same process can take different forms in 2D versus 3D environments. For example, axonal outgrowth switches from a standard cytoskeletal-pull mechanism in 2D to a more ameboid-like projection mechanism in 3D (Santos et al., 2020). Uniquely, the CNS is one of the softest tissues in the body, exhibiting a stiffness of <1.5 kPa. In contrast, the stiffness of hard, plastic, 2D environments used in many cell culture experiments are orders of magnitude higher, creating a physiologic mismatch in environmental properties (Barnes et al., 2017; Chighizola et al., 2019). In particular, cells make discrete contact in 3D environments with the surrounding ECM, whereas on 2D substrates only ∼50% of the cell surface is in contact with the ECM (Placone et al., 2015). Furthermore, ECM proteins can influence key neuronal processes such as neuronal outgrowth, proliferation, and compatibility (Hopkins et al., 2013; Leach, Brown, Jacot, Dimilla, & Wong, 2007; Lei et al., 2012). Generally, 2D culture models fail to match the complex microenvironments of human tissues including a complex network of ECM with defined physicochemical properties (Curtin et al., 2018; Gallagher, Murphy, O'Brien, & Piskareva, 2021; Karahuseyinoglu et al., 2021; Krencik et al., 2017).
The study of human neural cell interactions within more appropriate 3D environments is hampered in part due to the lack of tunable, reproducible in vitro 3D culture environments that attempt to replicate the native CNS environment. In order to improve the complex environments cells typically experience in vivo , complex sarcoma-derived ECM formulations are often used in vitro to support cell culture, adhesion, and differentiation in 2D environments. (Karahuseyinoglu et al., 2021; Placone et al., 2015; Polykandriotis, Arkudas, Horch, & Kneser, 2008). However, popular well-used and demonstrably trophic ECM formulations such as Matrigel (a commercial protein mixture produced from mouse sarcoma cells) consist of unknown compositions with high batch variability presenting challenges to designing reproducible cell culture systems (Aisenbrey & Murphy, 2020; Hughes, Postovit, & Lajoie, 2010; Polykandriotis et al., 2008). Although 2D culture systems are extremely useful for exploring individual cellular interactions, in recent years a stronger effort has been made to bridge the gap between 2D and in vivo systems through 3D model development (Hopkins, DeSimone, Chwalek, & Kaplan, 2015; Krencik et al., 2017; Placone et al., 2015). Organoid platforms have proven useful but still lack anatomical reproducibility and can become necrotic, collapsing without structural support (Huch et al., 2017). The field of organoid research has acknowledged that physicochemically defined scaffolds are required and are currently lacking for reproducible and controlled organoid assemblies (Aisenbrey & Murphy, 2020). Additionally, some models attempting to use materials such as polyacrylamide to create 3D environments have been advantageous for studying the effects of stiffness on neurons with a high degree of control over their physical properties (Norman & Aranda-Espinoza, 2010). However, cells are usually cultured on the surfaces of these polyacrylamide gels and therefore are not truly reflective of the native 3D CNS ECM environment (Norman & Aranda-Espinoza, 2010). Thus, there is a demand for tunable, high-resolution, easily manufactured, and reproducible 3D in vitro models of the human CNS to bridge the gap between 2D culture and the human in vivo condition that allows for the study of specific neuronal–environmental interactions (Hopkins et al., 2015).
The protocols presented here describe the development and use of freeze-dried scaffolds consisting of native CNS hyaluronic acid (HyA) of differing stiffness that can be functionalized with a desired selection of ECM proteins for 3D culture applications (Fig. 1). The developed scaffold systems can support several CNS cell types including neurons, glial cells (e.g., astrocytes), and peripheral nerve dorsal root ganglia in 3D culture (Woods et al., 2022). The application of the outlined freeze-drying fabrication approach allows the creation of highly porous, free-standing constructs that have a high degree of reproducibility in their mechanical properties, microarchitecture, and chemical composition (Woods et al., 2022). Additionally, anionic nonsulfated glycosaminoglycan HyA is the most abundant ECM constituent of the CNS (Gupta, Lall, Srivastava, & Sinha, 2019; Song & Dityatev, 2018) and is the ideal backbone for biomimetic CNS scaffold and model development (Seidlits et al., 2010). However, HyA lacks key integrin binding sites that facilitate cell–ECM interactions and primarily interacts with cells through CD44 binding sites (Kwon et al., 2019). Therefore, functionalization with additional ECM macromolecules is not only advantageous but also allows for the production of customizable 3D environments to study the desired neural cell–matrix interactions (Hibbitts et al., 2022). This set of protocols describes how to produce porous and customizable scaffolds using a straightforward freeze-dry fabrication process and thereafter provides detailed information on how to characterize different scaffold properties. Furthermore, we describe in detail the simple steps needed to produce 3D scaffold neuronal cultures with high fidelity.
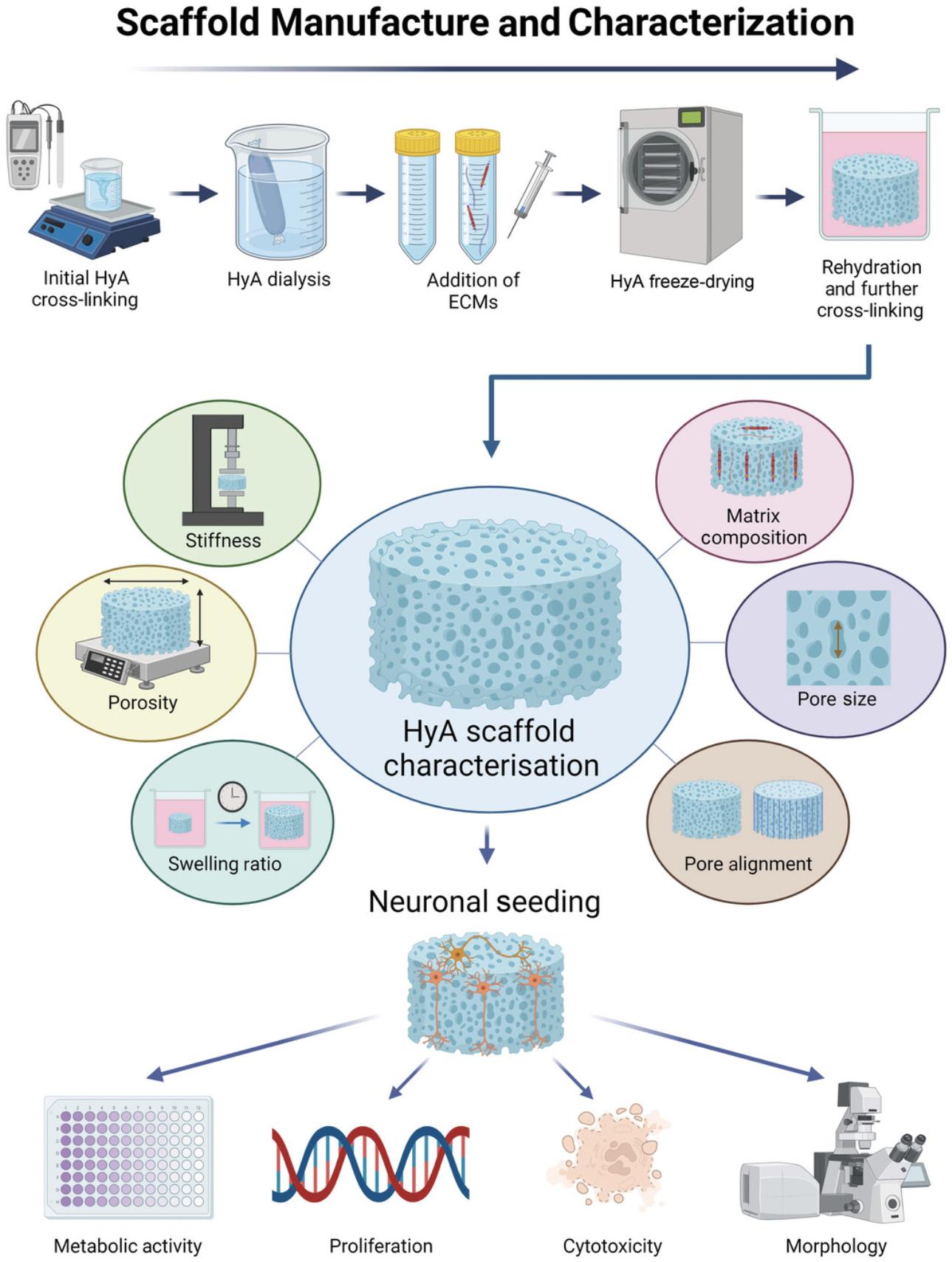
The outlined protocols describe the production of a flexible HyA-based platform for the study of neuronal cell behavior. The scaffolds can be tuned to exhibit a range of stiffnesses and pore architectures, and protocols are described for functionalization with native CNS matrix proteins (e.g., fibronectin, collagen IV). A simple set of protocols for characterizing the growth and development of neuronal cells within the scaffold is also provided. Specifically, a range of techniques is described to quantify the physiochemical properties of the scaffolds to ensure that the model system is defined and reproducible for the desired culture needs. In addition, we describe how to monitor the health of neurons in 3D and the proliferation of cells over time and how to characterize their morphological responses through immunostaining and imaging. From these data, the user can gather information on how their customized biomimetic scaffold properties can influence neuronal cell morphology, proliferation, and metabolic activity for up to 21 days in culture.
In summary, the described protocols outline the relatively simple manufacture and characterization of the scaffolds and subsequently how to seed, culture, and analyze neurons within the 3D environments.
Basic Protocol 1: SCAFFOLD MANUFACTURE
Porous freeze-dried 3D scaffolds have a high degree of tunability in which to accurately mimic native tissue environments in a simple, cost-effective, and reproducible manner (Curtin et al., 2018; Woods et al., 2022). Freeze-drying is a process through which a polymer solution is frozen and a vacuum is applied to bring the frozen solution to the critical point at which the solvent sublimates leaving behind an interconnected pore network between compressed polymer strands that become physically cross-linked during the process. Freeze-drying can produce a macroporous scaffold structure with a micrometer-scale porosity, which enables easy cell infiltration into the 3D material (O'Brien, Harley, Yannas, & Gibson, 2005). This protocol outlines the steps required to produce scaffolds of different stiffness, ECM composition, and topography. If conducted properly, the user will be able to customize and repeatedly produce biomimetic scaffolds with specific physicochemical properties for use in a wide range of CNS applications.
This protocol describes the manufacture of 3 mg/ml (∼900 Pa) and 10 mg/ml (∼3650 Pa) HyA solutions to manufacture scaffolds of different stiffness (Fig. 2). The procedure can be easily adapted to produce scaffolds of differing matrix composition and stiffness and allows the user to tailor specific extracellular conditions for their cell of study.
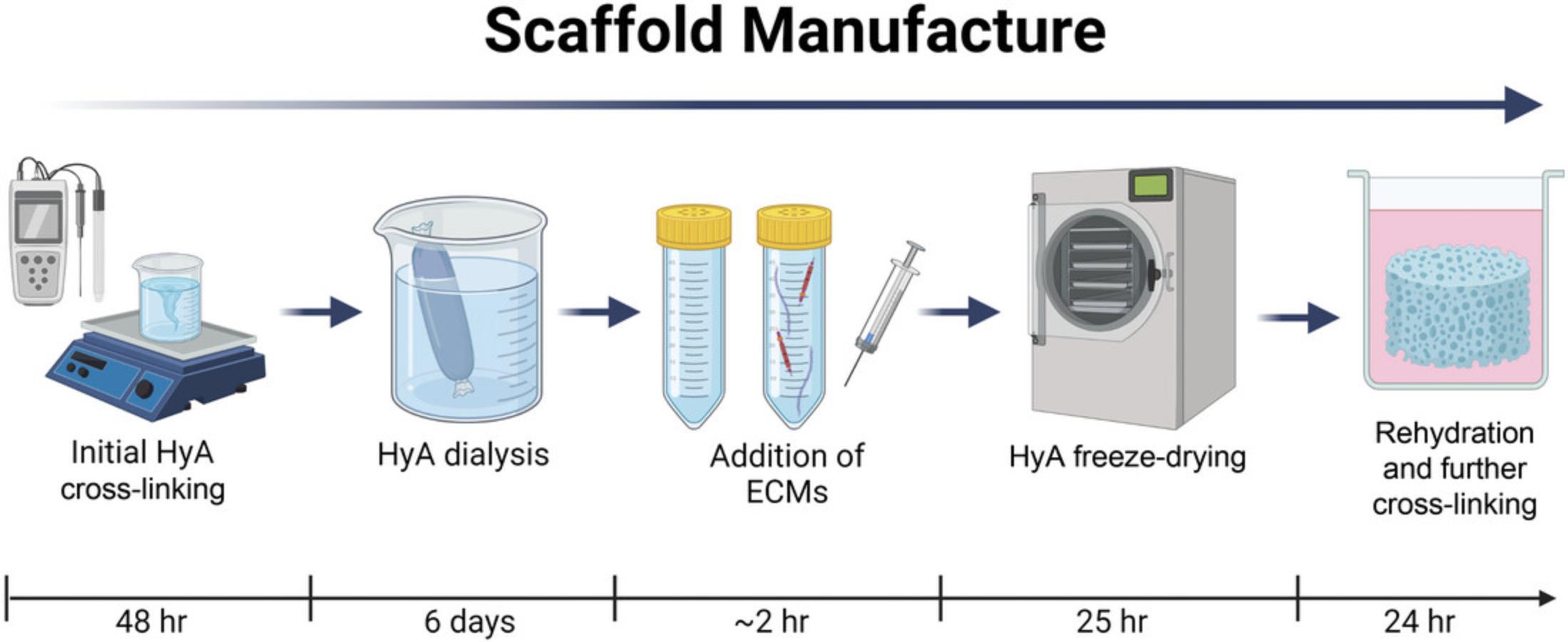
Materials
-
HyA sodium salt, 1.6 to 1.8 MDa (e.g., Sigma-Aldrich, cat. no. 53747-10G)
-
1-ethyl-3-(3-dimethylaminopropyl) carbodiimide (EDAC; e.g., Sigma-Aldrich, cat. no. E7750-5G)
-
Adipic acid dihydrazide (e.g., Sigma-Aldrich, cat. no. AO638-25G)
-
2 M HCl (e.g., Sigma-Aldrich, cat. no. 7647-01-0)
-
2 M NaOH (e.g., Sigma-Aldrich, cat. no. S5881)
-
NaCl (e.g., Fisher Scientific, cat. no. BP358-1)
-
100% ethanol (e.g., Sigma-Aldrich, cat. no. 10680993)
-
ECM solutions of interest (e.g., collagen IV, fibronectin)
-
Dulbecco's phosphate-buffered saline (PBS), sterile (e.g., Corning, cat. no. 21-040-CV)
-
Glass beakers, various sizes
-
Magnetic stir plate and stir bars (e.g., Fisher Scientific, cat. nos. 15298824 and 12661156)
-
Balance (e.g., Fisher Scientific, cat. no. 16314748)
-
pH meter (e.g., Mettler Toledo SevenExcellence pH meter S400-Bio-Kit )
-
Dialysis tubing (e.g., Sigma-Aldrich, cat. no. D9777-100FT)
-
Dialysis tubing closures (e.g., Sigma-Aldrich, cat. no. Z371092)
-
37°C incubator
-
Foil or cling film
-
Freeze dryer (e.g., SP VirTis AdVantage Pro Freeze Dryer)
-
10-ml syringe (e.g., Fisher Scientific, cat. no. 14955459)
-
18-G × 1.5-in needle (e.g., Terumo, cat. no. AN*1838R1)
-
1- to 2-mm thick stainless steel sheet (e.g., Radionics, cat. no. 770-2977)
-
6-mm thick polytetrafluoroethylene (PTFE) sheets (e.g., Radionics, cat. no. 197-0051)
-
Clamp, to hold down steel frame of prepared molds
-
Liquid N2 container
-
Fine forceps
-
24-well tissue culture plate
-
0.2-µm syringe filter (e.g., Fisher Scientific, cat. no. 15206869)
CAUTION : All steps involving chemical cross-linking and pH reagents must be performed in a suitable fume hood with efficient ventilation. Personal protective equipment (PPE), including safety glasses and reagent-impermeable protective gloves, should be worn and regularly exchanged when handling reagents.
Manufacture hyaluronic acid solutions
1.Remove stock HyA sodium salt from −20°C storage, and allow to equilibrate to room temperature (RT; ie, ∼22°C) to reduce buildup of moisture inside the container.
2.Calculate amount of HyA salt required to produce 3 and 10 mg/ml solutions in deionized, distilled water (dH2O) for the desired volume.
3.Pipet desired volume of dH2O into a clean glass beaker, and place on a stir plate with a magnetic stir bar.
4.Weigh out calculated amount of HyA sodium salt using a scale to two-decimal-point accuracy, and slowly add salt to the dH2O while stirring. Stir for 1 hr at RT, and check that HyA has dissolved into a uniform, viscous solution. If not fully dissolved, continue to stir, and check intermittently until there are no observable coagulations of HyA within the solution.
5.Once HyA solutions are fully mixed, weigh out cross-linker reagents (0.8 g EDAC per 100 ml solution and 9.16 g adipic acid multiplied by mg HyA). Starting with EDAC, sequentially and gradually add both cross-linkers to the continuously stirring HyA solution. Add both cross-linking reagents gradually to prevent coagulation, and leave to stir until fully dissolved (overnight, RT).
6.The next day (once cross-linking reagents are fully dissolved), prepare a fume hood with a magnetic stir plate and pH meter, and don appropriate PPE (gloves, goggles, laboratory coat). Transfer cross-linked HyA solution to fume hood and resume stirring.
7.Prepare 100 ml of 1 M HCl in dH2O, and ensure solution is uniformly mixed.
8.With calibrated pH probe inserted into the slurry, use a 1-ml pipette to slowly add 1 M HCl drop-wise to reduce pH of HyA solutions to 4 to initiate the cross-linkage reaction. Once achieved, place solution at 4°C for 18 hr overnight to allow further cross-linking to occur while ensuring slurry stability.
9.After the 18-hour incubation period, return slurry to the fume hood, and prepare 1 M NaOH in dH2O, ensuring a uniform solution.
10.With a calibrated pH meter and constant monitoring, use a 1-ml pipette to add 1 M NaOH drop-wise to slowly increase the pH of the stirring HyA solution to a final pH of 7.4 and in so doing halt the cross-linking reaction.
11.Prepare dialysis tubing to wash excess cross-linking reagents from the HyA solution. First, cut strips ∼30 cm in length, and incubate 5 min in stirring dH2O heated to 37°C to loosen and open the tubing. Fold one end over on itself, and clamp closed using a dialysis peg. Add each HyA solution to the open end of the dialysis tubing using a 5-ml pipette, and clamp tube opening closed with a second dialysis peg.
12.Prepare dialysis bath of 100 mM NaCl in a 5-L beaker with constant stirring on a magnetic stir plate. Ensure NaCl is fully dissolved and the final volume is large enough to cover the dialysis tubing before adding the loaded dialysis tubes. Leave dialysis bath to stir 24 hr at RT. Discard old bath, and change to a fresh bath of the same NaCl concentration. Incubate again 24 hr at RT.
13.Prepare sufficient 20% ethanol in dH2O from a 100% ethanol stock to cover the slurry-filled dialysis tubing. Discard NaCl solution from dialysis bath, and rinse briefly in dH2O to remove excess adipic acid. Incubate dialysis tubes in 20% ethanol 24 hr at RT.
14.Discard 20% ethanol, and replace with an equal volume dH2O. Leave to stir 24 hr at RT. Repeat step twice (two dH2O bath exchanges) to complete dialysis process.
15.Remove HyA-loaded dialysis tubing from dialysis bath, and prepare a 100-ml glass beaker for storage. Carefully, remove dialysis peg from one end, and transfer HyA solution from the tubing to the beaker. Cover in foil or cling film, and store at 4°C.
Manufacture porous hyaluronic acid scaffolds
To manufacture scaffolds with a unidirectionally aligned freeze-dried pore structure, set the shelf of a freeze drier to −40°C before preparing scaffolds to allow adequate time for the shelf to freeze. To manufacture scaffolds without unidirectional pore structure, keep the shelf at RT.
16.Remove dialyzed HyA slurry from storage, and allow to equilibrate to RT. Determine number of scaffolds required and volume of slurry needed. Add aqueous stock ECM solutions of interest alone or in combination directly into the aliquoted slurry to achieve the final desired concentration (0.1 mg/ml). Ensure pipetted ECM solution is fully purged from the pipette, and rinse pipette several times by pipetting up and down in the HyA solution.
17.To ensure complete mixing, use a 10-ml syringe with 18-G, 1.5-in. needle to take up HyA-ECM slurry, and thoroughly mix solution by passing through the needle several times (∼30 cycles to ensure complete dispersion of ECM proteins throughout the HyA slurry).
18.Use a freeze dryer to degas HyA solution (with and without additional ECM functionalization) by placing solution on freeze dryer shelf and applying pressure to <4000 mTorr until bubbling in the solution is no longer observed.
19.Assemble freeze-drying molds with a stainless-steel base and PTFE mold with cylindrical holes (6-mm height × 6-mm diameter), and clamp down with a steel frame so solutions cannot leak once loaded (Fig. 3).
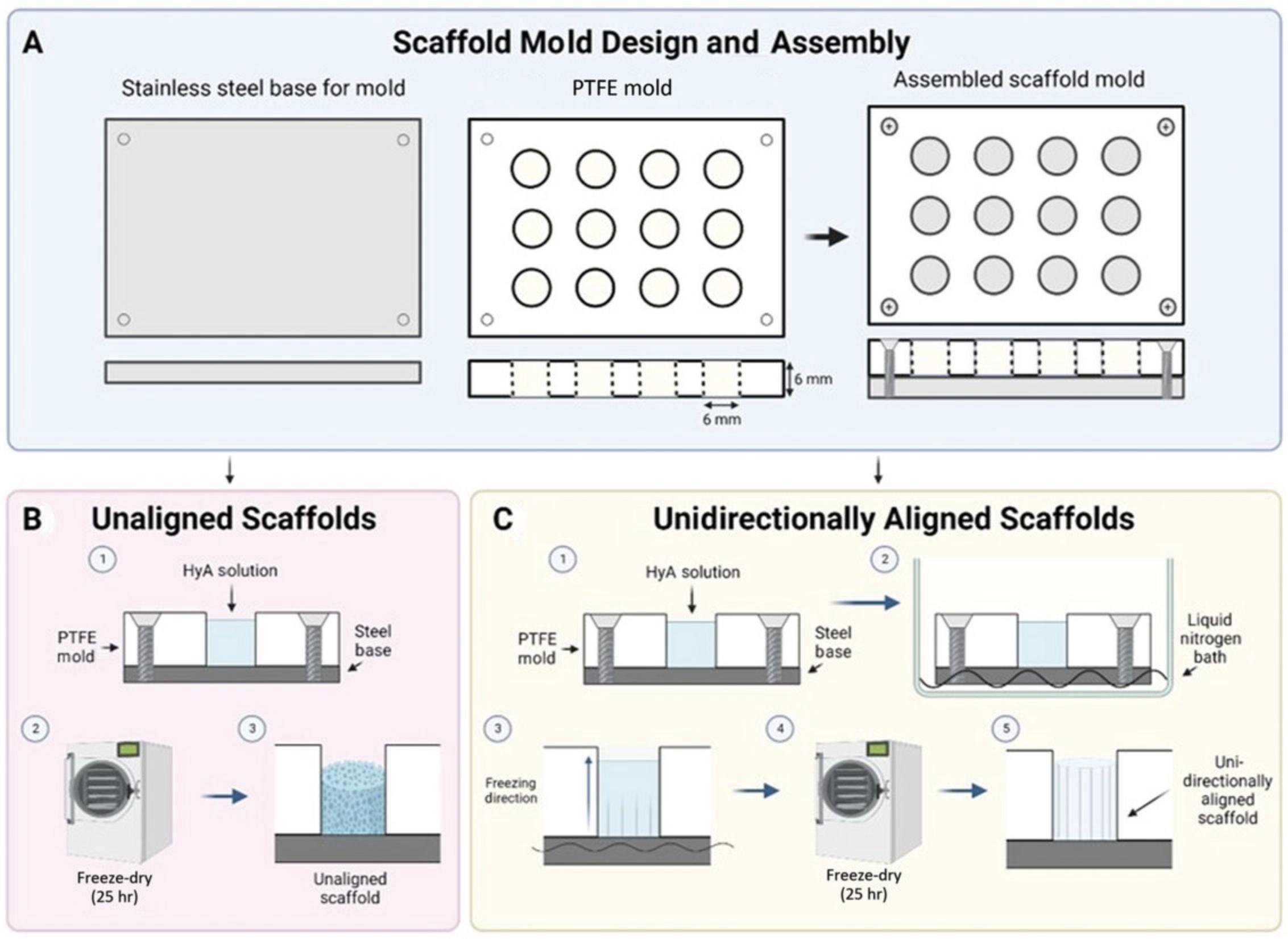
20.Calculate volume required per mold, and slowly pipette desired amount of HyA solution into each well by placing the pipette tip close to the bottom of each well and slowly withdrawing the pipette as the well fills to reduce bubble formation. Remove any bubbles inadvertently created during this process by using a fine needle and syringe (e.g., 18-G × 1.5-in. needle).
Nonaligned scaffold manufacture
21a. To manufacture scaffolds without a unidirectionally aligned architecture, load mold directly onto the freeze dryer shelf that has been kept at RT, and run program described in Figure 4 for 25 hr at 200 mTorr throughout. Proceed to step 24.
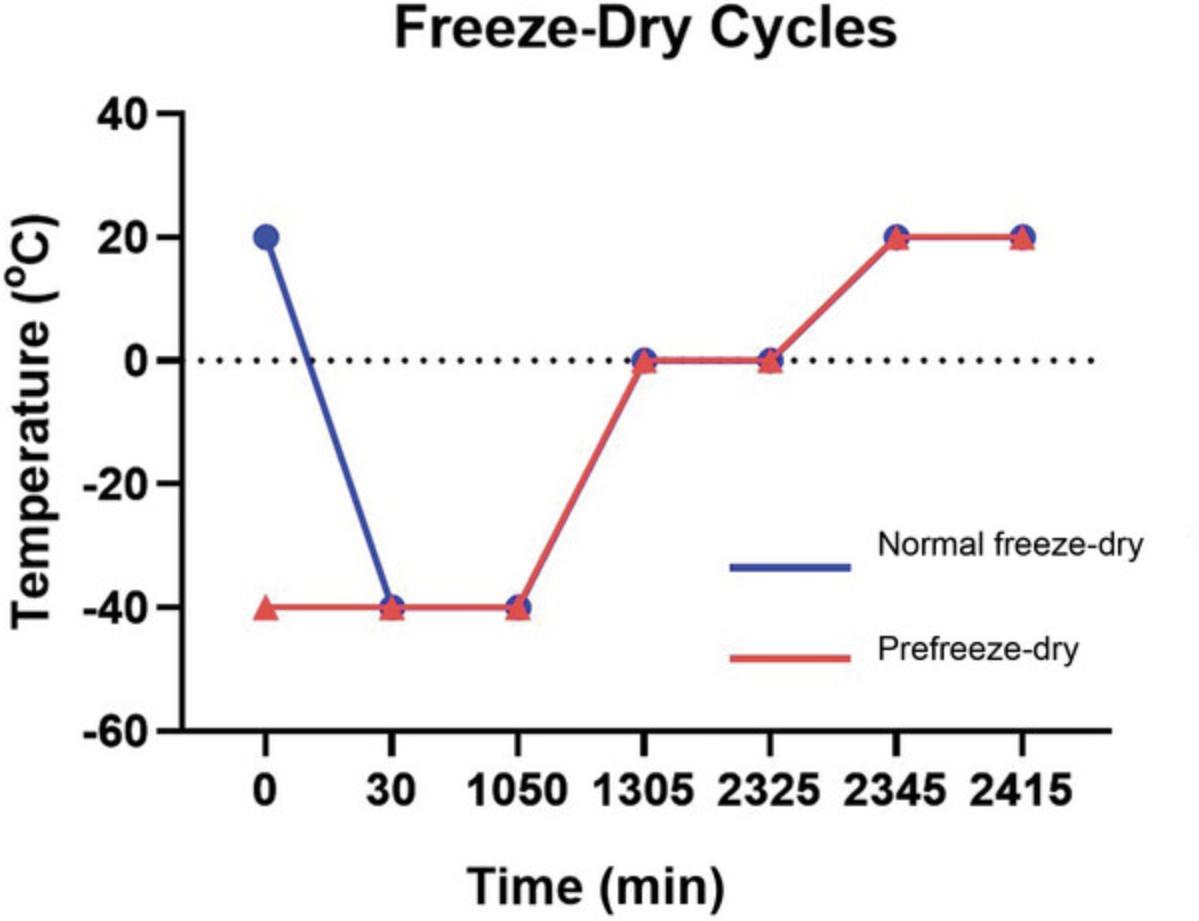
Unidirectionally aligned scaffold manufacture
21b. If a consistent porous longitudinally aligned internal scaffold architecture is required (e.g., to study process outgrowth), first prepare a well-insulated liquid N2 container with a flat bottom, and place a stainless steel sheet of similar size to the scaffold mold in the bottom of the container to indirectly snap freeze the scaffold mold.
22b. Using appropriate PPE, carefully fill container with liquid N2 so the conducting baseplate is fully immersed. Gently place filled mold apparatus on top of the baseplate to facilitate rapid metal-to-metal cooling and snap freezing of the ECM-filled wells (Fig. 3). Allow molds to incubate for 1 min.
23b. Immediately load snap-frozen HyA-loaded scaffold molds onto a prefrozen freeze dryer shelf set to −40°C, and run prefreeze program outlined in Figure 4 over 25 hr at 200 mTorr to complete scaffold formation. Proceed to step 24.
Processing of scaffolds post freeze-dryin
24.Once freeze-drying cycle is completed (regardless of aligned or unaligned manufacture approach used), remove mold from the freeze dryer. Uncouple baseplate from the scaffold-containing Teflon plate, and gently remove each formed scaffold from the mold using fine forceps.
25a. For dry scaffold characterization, immediately place scaffolds in a 24-well tissue culture plate at 4°C (Fig. 5).
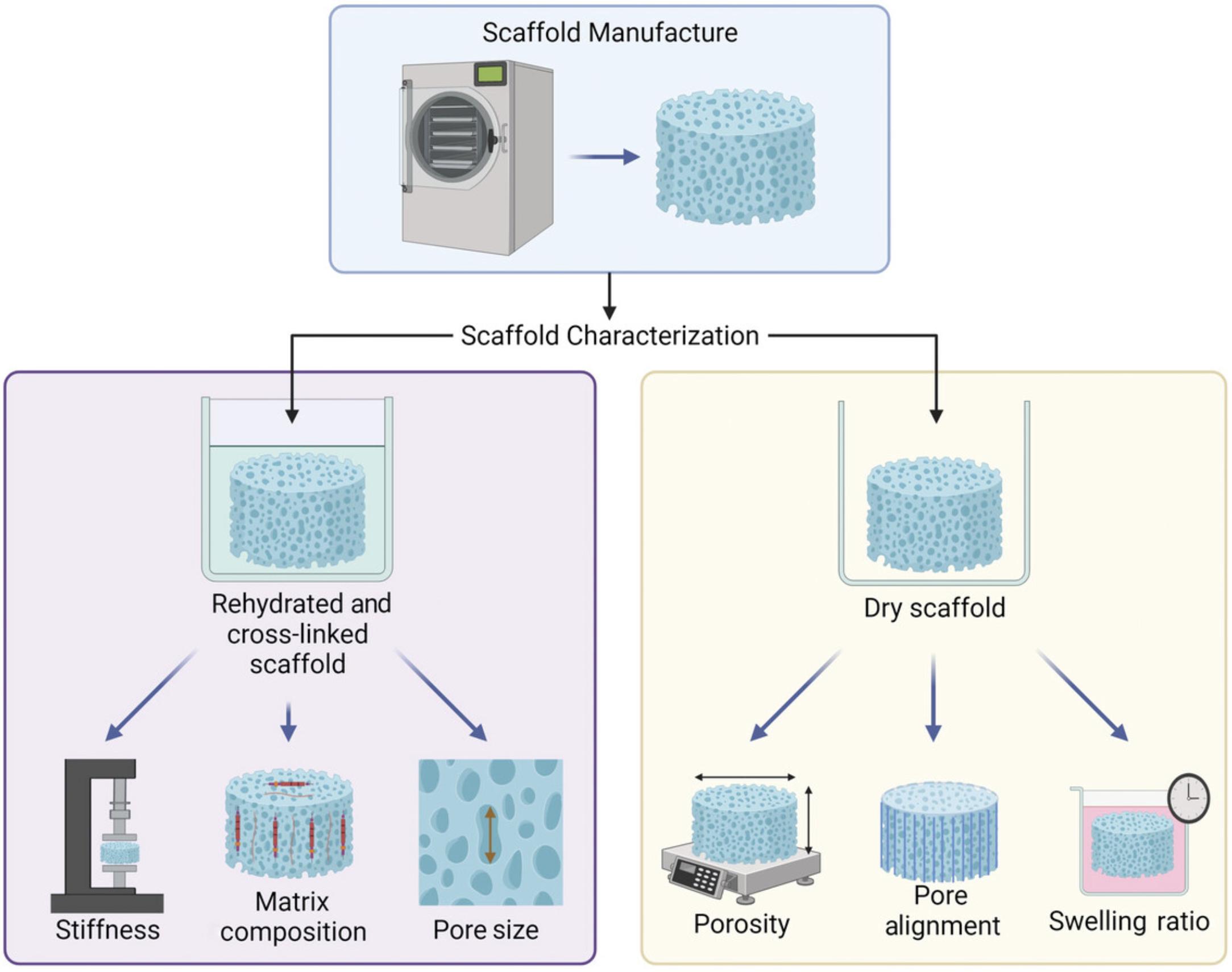
25b. For hydrated and cross-linked scaffold characterization or to prepare scaffolds for cell culture applications (Fig. 5), place each scaffold individually into a 24-well tissue culture plate, and immediately rehydrate scaffolds in 100% ethanol (1 hr, RT, 1 ml per scaffold), 90% ethanol (1 hr, RT, 1 ml per scaffold), and then 70% ethanol (overnight, 4°C).
26.In a sterile environment (e.g., laminar flow hood), prepare cross-linking solution containing 14 mM EDAC (2.68 mg/ml) and 5.5 mM NHS (0.63 mg/ml) in 70% ethanol, sequentially adding reagents and allowing each to dissolve. Filter sterilize using a 0.2-µm pore filter. Incubate each scaffold in 1 ml cross-linking solution for 2 hr at RT in a fresh culture plate in a sterile environment.
27.Remove scaffolds from cross-linking solutions using fine forceps, and wash scaffolds in sterile PBS three times before storing at 4°C. Dispose cross-linking reagents in appropriate chlorinated waste disposal facilities.
Basic Protocol 2: SCAFFOLD CHARACTERIZATION
A key advantage of freeze-dried scaffolds is the ability to easily tune physicochemical properties for specific applications (Woods et al., 2022). It is essential to fully characterize the produced scaffolds before embarking on a series of experiments to monitor any batch-to-batch variance and clearly understand the impact the surrounding physical environment may play on resultant cell behavior. Here we outline several procedures that can be used to characterize scaffold physicochemical properties such as porosity, pore size, mechanical properties, swelling ratio, microarchitecture, and ECM functionalization. These steps generate data for several key physicochemical properties that may affect cell behavior. Depending on the desired characterization approach, one scaffold sample can be used for multiple characterization techniques (e.g., serial sectioning to analyze both pore size and ECM functionalization). Batch-to-batch variability can be minimized by accurately determining the pH of the scaffolds during cross-linkage and consistently performing the cross-linking protocol in terms of materials used and timing.
The procedures required to characterize the physicochemical properties of produced porous freeze-dried HyA scaffolds are outlined in Figure 5.Previously reported characterization data can be found in Table 1 as reported by Woods et al. (2022).
Characteristic | 3 mg/ml HyA | 10 mg/ml HyA |
---|---|---|
Porosity, % | 98.63 | 96.33 |
Mean pore size, μm | 121.24 | 173.98 |
Swelling ratio, fold change in weight | 10.36 | 18.71 |
Mechanical properties, Pa | 896.5 | 3652.8 |
- a
Expected physicochemical characterization data of 3 and 10 mg/ml HyA freeze-dried scaffolds (based on data from Woods et al., 2022).
Materials
-
Scaffolds of interest (see Basic Protocol 1)
-
PBS (e.g., Corning, cat. no. 21-040-CV)
-
4% paraformaldehyde in PBS (e.g., Fisher Scientific, cat. no. 15670799)
-
Sucrose (e.g., Fisher Scientific, cat. no. 57-50-1)
-
OCT cryoembedding matrix (e.g., Epredia LAMB/OCT)
-
Toluidine blue (e.g., Sigma-Aldrich, cat. no. 89640)
-
DPX mounting medium (e.g., Sigma-Aldrich, cat. no. 06522)
-
3 M NaOH (e.g., Sigma-Aldrich, cat. no. S5881) in PBS
-
Bicinchoninic acid (BCA) protein assay kit (e.g., Thermo Fisher, cat. no. 23225)
-
Bovine serum albumin (BSA; e.g., Sigma-Aldrich, cat. no. A7030)
-
Primary antibodies for ECM proteins of interest (i.e., collagen IV monoclonal antibody; e.g., Invitrogen, cat. no. 14-9871-82)
-
Fluorescently labeled secondary antibodies appropriate for primary antibodies (i.e., Alexa Fluor 555 secondary antibody; e.g., Invitrogen, cat. no. A32727)
-
Flouromount-G mounting medium (e.g., Invitrogen, cat. no. 00-4958-02)
-
Fine-point forceps, 115 mm (e.g., S Murray, cat. no. SB44070)
-
Weight boat (e.g., VWR, cat. no. 611-1996P)
-
Vernier calipers (e.g., RS PRO)
-
Balance (e.g., Fisher Scientific, cat. no. 16314748)
-
24-well culture plate
-
Filter paper
-
Cryostat (e.g., Leica CM 1950)
-
Cryosection blades (e.g., Leica, cat. no. 3802105)
-
Superfrost microscope slides (e.g., Fisher Scientific, cat. no. 22-037-246)
-
Fine paint brush
-
Glass coverslips (e.g., Fisher Scientific, cat. no. 12343128)
-
Bright-field microscope with 10× objective
-
Computer running image analysis software (e.g., ImageJ)
-
Uniaxial mechanical tester (e.g., Zwick Roell Z005)
-
5 N Xforce P load cell (e.g., Zwick Roell)
-
1.5-ml microcentrifuge tubes (e.g., Fisher Scientific, cat. no. 11569914)
-
96-well flat-bottom assay plate, transparent (e.g., Greiner, cat. no. 655101)
-
Plate shaker
-
37°C incubator
-
Multimode plate reader (e.g., Tecan Infinite 200 PRO)
-
Hydrophobic barrier pen (e.g., Vector Laboratories, cat. no. H-4000)
-
Fluorescence microscope (e.g., Nikon 90i)
-
Razor blades or tissue chopper (e.g., McElwain 10180)
-
Scanning electron microscopy (SEM) double-sided carbon tape (e.g., Agar Scientific, cat. no. AGG3939)
-
Sputter coater (e.g., Polaron Emitech SC7640)
-
SEM mounting stubs (e.g., Oxford Instruments, cat. no. 51-1625-0259)
-
Scanning electron microscope (e.g., Tescan MIRA XMU)
CAUTION : All fixation and chemical reagent processing steps must be carried out in a suitable fume hood with efficient ventilation. Safety glasses and reagent-impermeable protective gloves should be worn when handling reagents. Care should be taken when handling any sharp objects.
NOTE : To save on materials, the scaffold porosity and swelling ratio can be calculated from the same scaffold samples by first performing the scaffold porosity measurements before hydrating the scaffolds for swelling ratio analysis.
Scaffold porosity
Porosity is a critical property in tissue engineering applications and provides a percentage metric of the matrix to free space in a scaffold. The greater the porosity, the easier it is for cells to infiltrate and for nutrient diffusion to occur (see Loh & Choong, 2013). Many scaffolds are designed to be porous, but freeze-dried tissue scaffolds in particular have high levels of porosity (typically >90%) while maintaining structural integrity, providing optimal diffusion both in vitro and in vivo (Curtin et al., 2018; O'Brien et al., 2005; Ryan et al., 2017). Steps 1 through 3 describe how to calculate the porosity of freeze-dried scaffolds.
1.Using fine forceps, place each individual dry scaffold in a clean weigh boat, and using digital Vernier calipers measure height and diameter of the scaffold, taking care not to crush the structure. Divide scaffold diameter by two to record the scaffold radius. Record values in millimeters for each respective scaffold, taking note of which values correspond to which scaffold.
2.Using fine forceps, transfer scaffolds to a balance and weigh, recording the mass of each respective scaffold in milligrams to three decimal places.
3.Using the following formulas, calculate porosity of the scaffolds:
where Pscaffold is the mass divided by the volume and Pmaterial is 1.8 ± 0.1 g/cm3 (i.e., density of HyA). To calculate the results as percent porosity, multiply the final values by 100.
Scaffold swelling ratio
The scaffold swelling ratio determines the ability of the material to absorb and retain water and affects the mechanical properties of scaffolds following rehydration (Raftery et al., 2016). Steps 4 through 7 describe how to characterize the swelling ratio of dry scaffolds in a simple and effective manner.
4.Following freeze-drying, immediately transfer dry scaffolds to individual wells of a 24-well culture plate, labeling each well before weighing each dry scaffold to record the respective scaffold's dry weight to three decimal places.
5.Immerse each scaffold in 1 ml PBS for 24 hr at RT. Ensure buffer fully immerses the scaffold.
6.Following incubation, using fine forceps gently blot individual scaffolds with filter paper to remove excess liquid before weighing again to record the respective wet weight of each scaffold.
7.Use the following formula to calculate swelling ratio:
Scaffold pore size
Scaffold pore size can significantly influence cell attachment, infiltration, and distribution within 3D scaffold environments. Characterization of pore size and distribution can offer insight into the structural layout and architecture in microscale detail.
8.Transfer individual hydrated and cross-linked scaffolds into a clean 24-well cell culture plate, and place plate in fume hood for fixation.
9.Fix each HyA scaffold in 1 ml of 4% (w/v) paraformaldehyde in PBS at RT for 20 min.
10.Gently remove 4% paraformaldehyde, and wash scaffolds with 1 ml PBS three times for 5 min per wash.
11.Prepare scaffolds for cryoembedding by immersing in successive and increasing concentrations of sucrose solutions: 1 ml of 10% (w/v) sucrose in PBS for 1 hr at RT; 1 ml of 20% (w/v) sucrose in PBS for 1 hr at RT; and then 30% (w/v) sucrose in PBS overnight at 4°C to ensure complete infiltration.
12.The next day, create a 50:50 solution of 30% (w/v) sucrose in PBS with OCT, and incubate each scaffold in 1 ml solution for 24 hr at 4°C.
13.To cut on the cryostat, set cryostat internal temperature to −20°C, and adjust slice thickness to 10 μm. Create an OCT platform by placing a small amount of OCT on the chuck and allowing it to freeze. Next, gently place each scaffold on the OCT platform, and allow scaffold to freeze in place. Ensure scaffold is oriented appropriately to produce the desired transverse or longitudinal cut sections. Cover scaffold completely in OCT, and allow it to freeze.
14.Collect cut sections from the mounted scaffold, and ensure uniform slices are produced. Immediately mount cut sections onto charged Superfrost slides, and allow sections to dry at RT or on a drying table.
15.Incubate dried slide-mounted sections in 10 mg/ml toluidine blue in dH2O for 5 min at RT. Then wash three times with dH2O (10 to 20 s per wash) before placing droplets of DPX mounting solution on each scaffold section and mounting with standard slide coverslips.
16.Image scaffold sections using a bright-field microscope at 10× magnification, taking at least three images of chosen representative regions of each section to acquire enough data to sufficiently represent the average scaffold pore size throughout the section.
17.Use ImageJ to measure pore size via manual tracing of the pore perimeters to analyze pore Feret diameter (the widest measure of pore diameter) and pore area, giving values for scaffold pore size.
Scaffold mechanical properties
The mechanical properties of the extracellular environment surrounding cells can directly influence neuronal and glial phenotypes, as well as the differentiation and maturation of neural cells (Moshayedi et al., 2014; Placone et al., 2015; Teixeira et al., 2009). To characterize scaffold mechanical properties, uniaxial mechanical testing (Zwick/Roell tensile testing machine) is the preferred approach and is performed with a 5-N load cell attachment for the machine.
18.Ensure appropriate safety guards are in place in the tensile testing machine before mounting the load cell. Once mounted, load software and prepare for operation.
19.Using a pair of fine forceps, transfer scaffold onto dry filter paper, and gently blot excess PBS. Using Vernier calipers, measure height and diameter of hydrated scaffold, and record for each scaffold.
20.Mount scaffold on the testing stage of the uniaxial mechanical testing machine so that the flat surface of the scaffold is in contact with the stage, and slowly immerse sample in PBS so that it is fully submerged.
21.Input scaffold dimensions into the mechanical testing software, and apply a strain rate until failure.
22.Use Young's modulus (Pa/N/m²) to calculate stiffness of each scaffold using the slope of the stress-strain curve from the values produced at two defined points that occur before failure (i.e., 1% to 10%) with the corresponding values in Newtons and strain in percentage. Calculate slope using the following formula to give stiffness values in Pascals for each scaffold:
Scaffold extracellular matrix functionalization
Here two approaches are described to characterize the addition of ECM proteins into the scaffolds: (1) BCA analysis and (2) immunofluorescence.
Nonspecific extracellular matrix proteins via bicinchoninic acid assay
23.Prepare 25 ml of 3 M NaOH in PBS.
24.Transfer dry and hydrated scaffolds that contain only HyA (control) or ECM functionalized scaffolds into separately labeled microcentrifuge tubes containing 500 μl of 3 M NaOH.
25.Incubate overnight at 4°C, allowing scaffold structure to fully dissolve in the solution.
26.Once fully dissolved, measure protein content of the scaffolds via a colorimetric BCA assay according to the manufacturer's instructions. First, calculate number of wells required to run the samples and the desired standard curve in duplicate ([number of scaffolds + number of wells required for desired standard curve] × 2).
27.Create working reagent (from BCA kit) by diluting reagent B in reagent A 1:50, ensuring the end volume supplies 200 μl/sample. Ensure working reagent is thoroughly mixed and yields a clear, green solution.
28.Prepare BSA standard curve solution, diluting across a range of 0 to 2000 μg/ml protein standards in 3 M NaOH, where the blank solution of 0 is 3 M NaOH.
29.Pipette 25 μl of each standard or sample in duplicate into each well of a clear 96-well assay plate. Ensure adequate mixing of the sample before pipetting to achieve uniform dispersion of the scaffold protein in the solution.
30.Add 200 μl working reagent to each sample or standard, and mix plate thoroughly on a plate shaker for 30 s. Cover plate and incubate at 37°C for 30 min.
31.Following incubation, measure absorbance at 562 nm on a plate reader.
32.To calculate results, subtract the average values of the blank standard replicates from the average values of the respective standards and unknown samples.
33.Prepare a standard curve by plotting the blank-corrected standard curve measurements vs the known concentration in μg/ml. Use the standard curve to determine the protein concentration of each unknown sample.
34.Compare values of the non-ECM and ECM-functionalized scaffolds to detect the difference in protein content, ensuring the added ECM is present.
Extracellular matrix functionalization via immunostaining
35.To characterize the presence and dispersion of specific ECM proteins throughout the scaffold structure via immunostaining, prepare scaffold cryosections of 10- to 50-μm thickness as per steps 8 to 17 (scaffold pore size analysis).
36.Using a hydrophobic pen, circle each scaffold section on the slide to help retain the solutions during incubations.
37.Prepare a working solution of 100 ml of 2.5% (w/v) BSA blocking solution (i.e., 2.5 g in 100 ml PBS), and apply to scaffold cryosections for 2 hr at RT to block nonspecific binding.
38.Prepare primary antibody solutions for the desired ECM to be detected by diluting in 1% (w/v) BSA in PBS according to the manufacturer's instructions.
39.Gently remove blocking solution by slowly dabbing the slide at a perpendicular angle on dry tissue. Perform a wash step by adding PBS for 5 min at RT to each sample before removing the solution by dabbing again. Perform this wash step three times.
40.Incubate cryosections in the desired volume of primary antibody solution, and incubate overnight at 4°C. Store slides flat in a humid container with water to prevent evaporation during incubation.
41.Prepare appropriate fluorescent secondary antibodies that match the incubated primary antibody species by diluting at a concentration recommended by the manufacturer in 1% (w/v) BSA in PBS.
42.Wash cryosection slides as described in step 39, and then apply the secondary antibody solution. Incubate at RT for 2 hr in a humidified chamber.
43.Perform a wash step as described in step 39.
44.Cover each scaffold cryosection slice in a 50-μl droplet of mounting medium. Immediately and gently place a coverslip across all samples on the slide. Store slides at 4°C in the dark, or image immediately.
45.To image stained ECM proteins, use a fluorescent microscope to detect immunostained ECM proteins within the scaffold cryosections.
Scaffold microarchitecture via scanning electron microscopy
SEM allows for a high-power, qualitative analysis of pore structure, matrix distribution, alignment, and fine morphological features of both the longitudinal and transverse features of the scaffolds (Hibbitts et al., 2022; Ryan et al., 2017).
46.Following freeze-drying, immediately transfer dry scaffolds to labeled containers. Do not rehydrate scaffolds as samples must be dry for SEM analysis (Fig. 5).
47.To prepare scaffolds for SEM imaging, transversely or longitudinally cut a scaffold using a fresh, single-backed blade in a single smooth movement or using a commercially available tissue chopper to produce a clean-cut surface. Alternatively, orient scaffold sample so that the tissue chopper cuts the scaffold longitudinally.
48.Prepare SEM stubs by placing double-sided carbon tape on the chuck.
49.Gently transfer cut scaffold sections using fine forceps onto the adhesive carbon tape stubs so that the exposed cut face of the scaffold points directly upward.
50.Carefully transfer the scaffold-loaded SEM stubs to a sputter coater, and run the recommended program for biological samples to evenly coat the samples with gold or palladium to enhance electron flow through the samples for SEM imaging.
51.Mount scaffolds in scanning electron microscope, and image at a range of desired magnifications to observe the transverse and longitudinal pore architecture of the different scaffold samples.
Basic Protocol 3: CELL CULTURE AND ANALYSIS OF NEURONAL RESPONSES IN 3D SCAFFOLDS
Once HyA scaffolds of desirable stiffness and matrix composition have been manufactured, cross-linked, and sterilized, they can then be directly seeded with cells. Neuronal lines (e.g., SH-SY5Y cells in this protocol) are advantageous for the optimization of seeding properties, migration, and colonization period and are useful to study the interactions of neurons within 3D environments owing to their relative homogeneity and ability to acquire high cell numbers (Dominguez-Alfaro et al., 2020).
The approaches outlined below can be used to study the metabolic, cytotoxic, proliferative, and morphologic responses of neurons to their surrounding microenvironment. Figure 6 demonstrates an experimental outline and timeline of when to perform each assay across a 21-day experimental period.
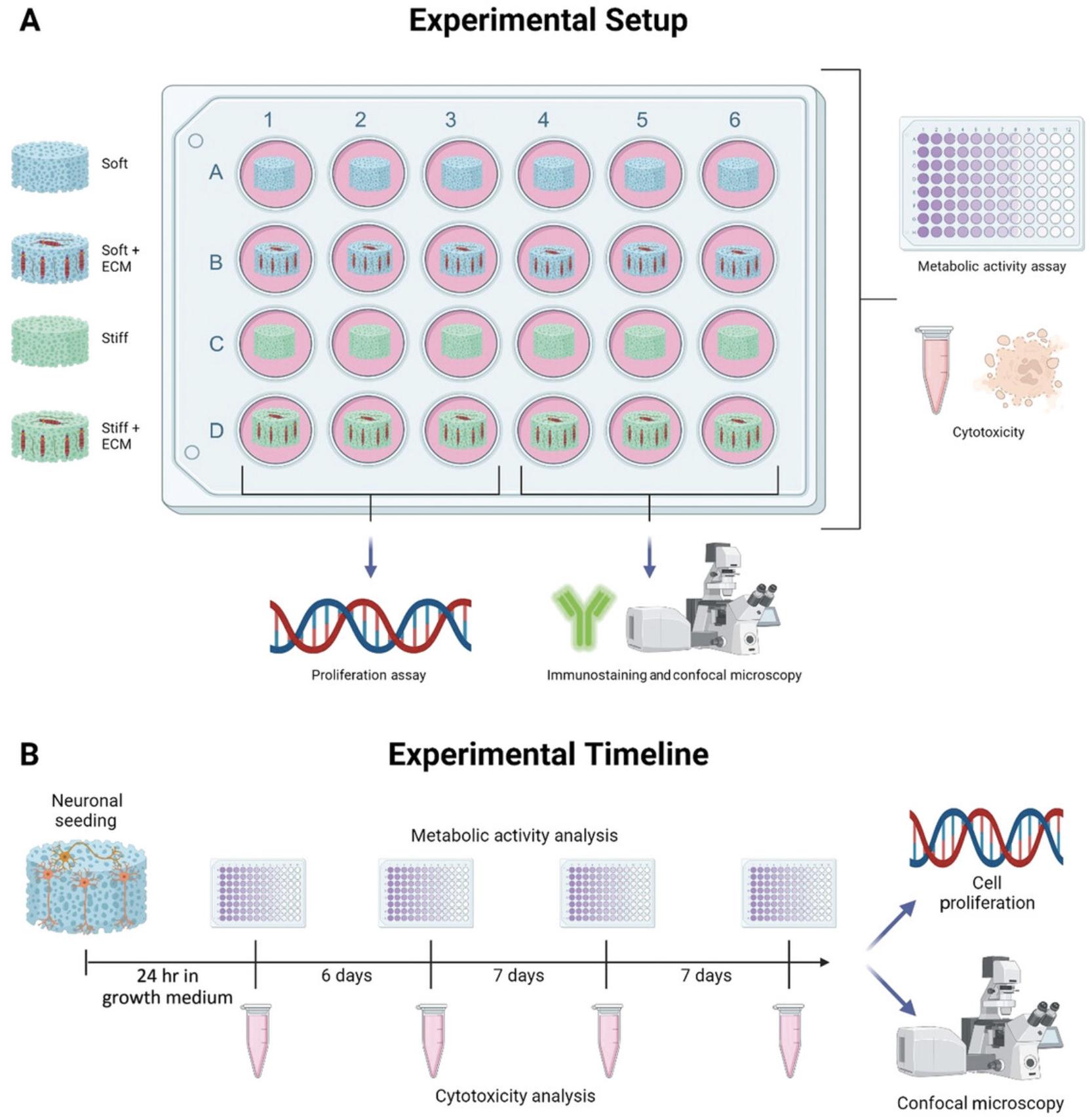
Materials
-
SH-SY5Y human-derived neuron-like cells (e.g., ATCC)
-
SH-SY5Y growth medium (see recipe)
-
Scaffolds of desirable properties (see Basic Protocols 1 and 2)
-
PBS (e.g., Corning, cat. no. 21-040-CV)
-
Trypsin-EDTA (e.g., Sigma-Aldrich, cat. no. T4049)
-
SH-SY5Y neuronal differentiation medium (see recipe)
-
4% (w/v) paraformaldehyde in PBS (e.g., Fisher Scientific, cat. no. 15670799)
-
Alamar Blue reagent (e.g., Invitrogen, cat. no. DAL1025)
-
Carbonate
-
Triton X-100 (e.g., Sigma-Aldrich, cat. no. T8787-100 ml)
-
Quant-iT™ PicoGreen™ dsDNA dsDNA Reagent and Kit (e.g., Invitrogen, cat. no. P7589) containing:
- Lambda DNA standard
- Quant-iT PicoGreen dsDNA reagent
- 20× TE buffer
-
Lactate dehydrogenase (LDH) CyQuant kit (e.g., Invitrogen, cat. no. C20301) containing:
- LDH positive control
- 5× assay buffer
- Substrate solution
- Stop solution
-
BSA (e.g., Sigma-Aldrich, cat. no. A7030)
-
Anti-β-tubulin III antibody, rabbit (e.g., Sigma-Aldrich, cat. no. T3952)
-
Goat anti-rabbit IgG secondary antibody, Alexa Fluor 488 (e.g., Invitrogen, cat. no. A-11008)
-
DAPI (e.g., Sigma-Aldrich, cat. no. D9542)
-
175-cm2 cell culture flasks (e.g., Greiner, cat. no. 660160)
-
Cell culture incubator (e.g., Panasonic)
-
Inverted microscope (e.g., Leica DM IL LED)
-
50-ml conical tubes (e.g., Greiner, cat. no. 227261)
-
Benchtop centrifuge (e.g., Eppendorf 5810R)
-
Hemocytometer (e.g., Hirschmann Neubauer)
-
24-well suspension culture plate (e.g., Greiner, cat. no. 662102)
-
Fine-point forceps, 115 mm (e.g., S Murray, cat. no. SB44070)
-
96-well flat-bottom assay plates, opaque and transparent (e.g., Greiner, cat. nos. 655076 and 655101)
-
Multimode plate reader (e.g., Tecan Infinite 200 PRO)
-
1.5-ml microcentrifuge tubes (e.g., Fisher Scientific, cat. no. 11569914)
-
Cryostat (e.g., Leica CM 1950)
-
Cryosection blades (e.g., Leica, cat. no. 3802105)
-
Glass microscope slides (e.g., Fisher Scientific, cat. no. 10219280)
-
Fluorescence microscope (e.g., Nikon 90i)
-
Confocal microscope (e.g., Zeiss Examiner.Z1)
-
Tissue chopper (e.g., McElwain 10180)
-
Glass rectangular coverslips (e.g., Fisher Scientific, cat. no. 12343128)
CAUTION : All cell culture work should be carried out under sterile conditions in a level 2 to 3 biosafety cabinet. All fixation steps must be run in a suitable fume hood with efficient ventilation. Safety glasses and reagent-impermeable protective gloves should be worn when handling reagents.
Seed and culture neurons in scaffolds
1.In 175-cm2 flasks, culture SH-SY5Y human neuroblastoma-derived cells to the desired population size for seeding into scaffolds via expansion in SH-SY5Y growth medium.
2.To prepare sterile scaffolds for cell seeding, remove excess PBS, and incubate in 1 ml SH-SY5Y growth medium per scaffold at 37°C for 60 min to completely infiltrate scaffolds with medium.
3.Discard excess medium from confluent SH-SY5Y flasks, and wash flask with 10 ml PBS. Immediately discard PBS to remove excess cellular debris.
4.Immediately incubate cells in 10 ml trypsin-EDTA for 5 min at 37°C to detach cells from the flask.
5.Following incubation, confirm most cells have dislodged from the flask using an inverted microscope, and collect cell suspension under sterile conditions into a 50-ml conical tube. Add 10 ml SH-SY5Y growth medium to neutralize trypsin. Mix gently and then centrifuge cells 5 min at 200 × g , RT, to form a pellet.
6.Once centrifuged, carefully discard cell supernatant without disturbing the cell pellet. Gently resuspend cells by adding 5 ml SH-SY5Y growth medium and pipetting up and down 8 to 12 times (until a uniform cell suspension is achieved). Load 20 µl concentrated cell solution into a hemocytometer according to the manufacturer's specifications, and count total number of cells.
7.When ready for seeding, transfer medium-soaked scaffolds into individual fresh wells of a 24-well suspension culture plate, which prevents cells from attaching to the plastic. Remove excess liquid from wells so that only the scaffold contains medium.
8.Dilute cell stock to 5 × 105 cells per 20 to 50 µl (i.e., roughly a droplet) to ensure most cell solution is absorbed into the scaffold. Carefully seed one side of the exposed scaffold with a cell droplet, and allow cells to adhere for 30 min at 37°C.
9.Gently flip each scaffold using sterile forceps so that the other side is exposed. Repeat cell seeding and incubating as described in step 8, and then flood each well with 1 ml SH-SY5Y growth medium. Incubate overnight at 37°C in a humidified incubator with 5% CO2.
10.Transfer each scaffold to a fresh well in a new 24-well suspension plate with 1 ml SH-SY5Y growth medium.
11.Discard all medium, and wash with PBS. Replace PBS with SH-SY5Y neuronal differentiation medium to promote differentiation of a neuronal phenotype. Replace 50% of the medium every 2 to 3 days for the desired culture period.
12.When the experiment has reached its end point, remove and store all medium at −20°C for further analysis. Wash scaffolds in 1 ml PBS.
13.Fix scaffolds with 1 ml of 4% (w/v) paraformaldehyde in PBS for 30 min at RT. Discard paraformaldehyde and then wash three times in 1 ml PBS, 5 min per wash, at RT. Store fixed scaffold samples at 4°C until ready for analysis.
Assess cell viability of neurons in scaffolds
Alamar blue assays offer a noninvasive and nondestructive assay for the measurement of cell metabolic activity. In the fluorometric dye, the active component resazurin is reduced to resorufin when in the presence of specific metabolic products when incubated with cells. The assay can be performed multiple times to study neuronal metabolic activity across an experimental timeframe with minimal disruption to the cells under study. Alamar blue reagent is highly light sensitive, so ensure all solutions are protected from light where possible (e.g., covering all reagent tubes with foil).
14.Calculate amount of Alamar blue reagent required:
15.Prepare desired working solution volume in sterile conditions by diluting Alamar blue reagent 1:10 in SH-SY5Y differentiation medium, ensuring the solution is evenly mixed and protected from light.
16.Discard medium from each well of the scaffold samples, and wash briefly with PBS. Incubate scaffolds with 1 ml Alamar blue working solution for 1 hr at 37°C in the dark. Additionally, incubate 1 ml Alamar blue working solution under the same conditions to serve as the blank (negative control).
17.Transfer 100 µl cell-incubated Alamar blue reagent and negative control in duplicate to each well of an opaque 96-well assay plate.
18.Cover plate and transfer to a plate reader to analyze at excitation/emission wavelengths of 545/585 nm.
19.Average duplicate readings before subtracting the blank values from all samples to obtain absolute values.
Determine proliferation rate of neurons in scaffolds
DNA content assays, such as those containing PicoGreen, can provide useful and accurate insight into neuronal proliferation within 3D scaffold environments. However, such assays require lysing of all cells on a scaffold to liberate neuronal DNA.
20.Prepare 100 ml lysis buffer comprising 0.2 M carbonate and 1% (v/v) Triton X-100 in dH2O.
21.Once the desired neuronal culture period has been reached, remove cell culture medium (retain for end of experiment assays by storing at −20°C), and rinse scaffold with 1 ml PBS. Transfer scaffold to a 1.5-ml microcentrifuge tube containing 1 ml lysis buffer.
22.Immediately transfer scaffold-containing microcentrifuge tube to −80°C, and store until samples are ready to be analyzed.
23.Before carrying out the assay, freeze-thaw (i.e., completely freeze at –80°C and then bring to RT) frozen, lysed samples to liberate DNA from the cultured neurons.
24.Using a PicoGreen assay kit, prepare a standard curve of the lambda DNA standard using concentrations ranging from 0 to 2000 pg/ml by diluting 1:2 in lysis buffer, where the 0 pg/ml is lysis buffer only (blank). Ensure adequate vortexing to obtain uniform mixing, and change pipette tips between each standard to avoid contamination.
25.Prepare 20 ml working reagent by adding 100 μl dsDNA PicoGreen reagent to 19.9 ml 1× TE buffer.
26.Pipette 100 µl of each sample and standard in duplicate into an opaque 96-well plate.
27.Using a multichannel pipette, load 100 µl PicoGreen working reagent to each sample and standard. Then immediately read fluorescence at an excitation/emission wavelength of 485/583 nm using a plate reader.
28.To analyze the results, subtract the average blank reading from all standards and samples. Plot the standard curve of the fluorescence values versus the known concentration of DNA in pg/ml to calculate the concentrations of DNA within the scaffold samples.
Analyze neuronal cytotoxicity in scaffolds
Environmental properties can influence cell survival and stress, which leads to the release of LDH owing to low-level damage to cell membranes (Aras, Hartnett, & Aizenman, 2008). LDH analysis can be performed on collected supernatant medium as a real-time noninvasive measurement of neuronal stress and cytotoxicity.
29.Warm stop solution from LDH assay kit, and thaw frozen conditioned medium to room temperature while preparing additional reagents.
30.Prepare 1 ml of 1× LDH positive control by adding 1.5 µl into 1 ml of 1% (w/v) BSA in PBS.
31.Prepare reaction mixture by diluting 300 μl assay buffer to 5.7 ml substrate solution. Mix thoroughly while protecting from light.
32.Transfer 50 µl of each sample's conditioned medium, prepared LDH positive control, and negative control (unconditioned neuronal medium) in triplicate into individual wells of a clear 96-well assay plate.
33.Transfer 50 µl reaction mixture to each sample and control well, and then mix gently by tapping the plate. Incubate for 30 min at RT in the dark.
34.Add 50 µl stop solution to each sample well, and gently mix by tapping the plate. After adding stop solution, read plate between 1 and 2 hr of incubation at RT. Verify positive control turned color, indicating the assay has been successfully run.
35.Measure absorbance at wavelengths of 490 and 680 nm using a plate reader.
36.Subtract 680-nm values from the respectively matched 480-nm values for each sample and control. Next, subtract negative control values from all sample values before calculating the average of the sample triplicates. Plot resulting absorbance values as relative absorbance units, or calculate as fold change compared with a desired control or time point.
Analyze neuronal morphology in scaffolds
Cell morphology such as neurite length can be used to assess neuronal vigor and behavior. It is known that both stiffness and matrix composition can influence neurite extension, branching, and complexity (Leach et al., 2007; Song & Dityatev, 2018; Sundararaghavan, Monteiro, Firestein, & Shreiber, 2009; Woods et al., 2022). Immunostaining combined with light and confocal microscopy can be used to assess not only individual neurites but also the 3D networks of neuronal processes within the scaffolds. Here we describe two approaches to preparing and imaging neuronal structures within scaffolds through: (1) cryosectioning and immunostaining to produce a high number of sections for staining various representative regions throughout the scaffold architecture and (2) scaffold hemisectioning, immunostaining, and imaging of regions of interest using confocal microscopy.
Scaffold cryosections
37.Prepare cryosections of 10- to 50-μM thick fixed neuronal scaffold as described in Basic Protocol 2, steps 8 to 17.
38.Apply blocking solutions and relevant primary antibodies (e.g., β-tubulin III) to scaffold cryosections, followed by appropriate fluorescently tagged secondary antibody as described in Basic Protocol 2, steps 35 to 45.
39.After secondary antibody incubation, wash sample slide three times for 5 min per wash with 1 ml PBS, dabbing excess solution off on dry tissue between individual washes.
40.Place DAPI (1:500 in PBS, protected from light) on the scaffold cryosections for 30 min at RT in the dark to stain cell nuclei.
41.Wash three times with PBS for 5 min per wash, dabbing excess fluid off before mounting as described in Basic Protocol 2, steps 35 to 45.Store mounted slides at 4°C until ready for imaging.
42.Using fluorescence/confocal microscopy, image scaffold samples at the desired magnification to analyze cellular infiltration, distribution, and neurite extension within the 3D scaffold.
Scaffold segments
43.Using a single-backed blade or fine forceps, transfer individual scaffolds to a tissue chopper, and cut scaffolds longitudinally and transversely as described in Basic Protocol 2, steps 46 to 51.
44.Once scaffolds have been cut into the desired number of segments (we recommend hemisections), incubate individual scaffold segments in 1 ml of 2.5% (w/v) BSA in PBS to block nonspecific binding.
45.Wash scaffolds three times in PBS for 5 min per wash.
46.Prepare primary antibodies using concentrations according to the manufacturer's recommendations in PBS containing 1% (w/v) BSA and 0.3% (v/v) Triton X-100. Incubate individual scaffold segments in 500 µl primary antibody solution overnight at 4°C.
47.Remove primary antibody solution, and wash scaffolds as described in step 45.
48.Prepare appropriate secondary antibodies at the manufacturer's recommended dilution in PBS containing 1% (w/v) BSA and 0.3% (v/v) Triton X-100. Incubate scaffold samples in 500 µl secondary antibody solution for at least 2 hr at RT in the dark.
49.Wash scaffold as described in step 45.
50.Incubate scaffolds in DAPI (1:500 in PBS, 500 µl/scaffold) for 30 min at RT in the dark.
51.Wash scaffolds as described in step 45.Store scaffolds in PBS at 4°C until ready for imaging via confocal microscopy.
52.To image via confocal microscopy, use forceps to carefully mount the scaffold on the underside of a coverslip, and gently invert so the scaffold hangs below the coverslip and is safely adhered owing to the surface tension of water.
53.Place coverslip onto the fluorescent/confocal microscope mounting stage, and add water or oil (depending on lens) to the top surface lens-facing side for optimal imaging.
54.Capture at least three images from different representative scaffold regions to study neurite outgrowth, network formation, and cellular distribution throughout the scaffold structures.
REAGENTS AND SOLUTIONS
SH-SY5Y growth medium
- DMEM, high glucose (e.g., Sigma-Aldrich, cat. no. D5671) supplemented with:
- 1% (v/v) L-glutamine (e.g., Sigma-Aldrich, cat. no. G7513)
- 1% (v/v) penicillin-streptomycin (e.g., Sigma-Aldrich, cat. no. P4333)
- 10% (v/v) fetal bovine serum (e.g., Sigma-Aldrich, cat. no. F7524)
- Store at 4°C for up to 2 weeks
SH-SY5Y differentiation medium
- Neurobasal medium (e.g., Gibco, cat. no. 21103049) supplemented with:
- 1% (v/v) B27 supplement (e.g., Gibco, cat. no. 17504044)
- 1% (v/v) GlutaMAX (e.g., Gibco, cat. no. 35050061)
- 1% (v/v) penicillin-streptomycin (e.g., Sigma-Aldrich, cat. no. P4333)
- 0.01 M trans -retinoic acid (e.g., Sigma-Aldrich, cat. no. 554720)
- Store at 4°C for up to 2 weeks
COMMENTARY
Background Information
Hyaluronic acid slurry production
HyA is a nonsulfated glycosaminoglycan and one of the main components of the human CNS, making it an ideal backbone material for CNS model applications (as opposed to less-native abundant collagens or foreign synthetic materials commonly used for CNS applications; Burnside & Bradbury, 2014; Khaing et al., 2011; Zuidema, Gilbert, & Gottipati, 2019). It is a natural polysaccharide and is made up of disaccharide monomers, which are present in the native CNS as high-molecular-weight polymer chains of D-glucuronic acid and N -acetyl-D-glucosamine (Seidlits et al., 2010). The described carbodiimide cross-linking process links together the carboxylate groups on the glucuronic acid monomer using a hydrazide cross-link, binding the polysaccharide chains together (Jeon et al., 2007; Woods et al., 2022). This forms a hydrogel where the viscosity is mainly dependent on the concentration of HyA added to the slurry, although the degree of cross-linking is also a contributory factor. The main advantage of this approach is the ease of production of a cross-linked HyA hydrogel whose mechanical properties (e.g., viscosity, stiffness) are easily tunable through different concentrations (Woods et al., 2022). The main drawback associated with HyA hydrogels is that, at concentrations >10 mg/ml, the slurries can be difficult to handle and process. It should also be noted that it is essential to faithfully use (and record) all volumes and concentrations as small changes can result in batch-to-batch variation in scaffolds, which may confound cell experiments.
Freeze-dry manufacture of macroporous scaffolds
Freeze-drying produces macroporous structures through the phase separation of polymers from aqueous solutions during freezing, during which ice crystals form within the solution, compressing the polymer phase into the spaces between the ice (Haugh, Murphy, & O'Brien, 2010). Compression of the polymer chains leads to a degree of physical cross-linking, and the ice can then be removed through sublimation by decreasing the pressure within the freeze dryer to the critical point at which water sublimates (Haugh et al., 2010). The sublimation of ice crystals directly from ice to vapor and the removal of vapor by the surrounding vacuum leaves behind an interconnected pore network. The morphology of this pore structure depends on the nature of the slurry used and the freezing parameters employed in the initial stages (Haugh et al., 2010). We describe a simple adaptation to the process in which a large thermal gradient is rapidly created through the use of liquid nitrogen and thermally insulated molds, which encourages the longitudinal growth of rapidly formed ice crystals from the ultracooled baseplate (Hibbitts et al., 2022; Ryan et al., 2017). Sublimation of these columnar crystals results in a uniaxially aligned pore structure. Previous studies have shown that this approach is an effective method of promoting the longitudinal growth of axons across macroporous scaffolds (Hibbitts et al., 2022; Ryan et al., 2017; Woods et al., 2022).
Addition of extracellular matrix proteins
One of the main mechanisms through which cell binding to HyA occurs is through the CD44 transmembrane receptor (Kim & Kumar, 2014; Kwon et al., 2019). Modification of HyA (e.g., through the addition of hydrazide cross-links) disrupts/replaces the native functional groups on HyA monomers leading to poorer CD44 binding. However, HyA itself is the source of attachment of several ECM proteins, and scaffold production can be modified with proteins that help provide a tunable environment for cell signaling and adhesion. For example, previously we incorporated the neurotrophic proteins collagen IV and fibronectin within the HyA scaffold at concentrations of 100 µg/ml before triturating into the slurry through repeated mixing through a syringe needle (Woods et al., 2022). This produced a homogenous slurry blend with the desired protein mix. Other water-soluble biomacromolecules can be added such as poly-L-lysine and laminins (Hibbitts et al., 2022; Ryan et al., 2017). Additionally, functionalization with more advanced macromolecules, synthetic materials, or conductive polymers within these 3D HyA scaffold structures may be possible depending on their solubility in water as demonstrated in other studies (Maughan et al., 2022), but functionalization remains untested for these HyA scaffold systems. The 100 µg/ml concentration of collagen IV and fibronectin was found to be effective for promoting axonal growth, but it is highly advisable that several concentrations be tested for novel protein combinations (Woods et al., 2022).
Porosity analysis
This article describes two methods of measuring porosity, which involve determining: (1) percent volume of material that is porous and (2) average size of the pores within the scaffold. The percent porosity is easily calculated using the specific density of the scaffold polymer, which along with the volume and weight quickly confirms the production of a porous structure; the porosity of macroporous scaffolds is generally >95% (Woods et al., 2022). Furthermore, increases in porosity promote increased diffusion of macromolecules and nutrients through the scaffold. The pore size measurement techniques described herein are a simple cross-sectional analysis of average pore diameters. Pore size plays an essential role in determining cellular infiltration and tissue development (O'Brien et al., 2005). For example, if pores are too small, cellular migration is limited (O'Brien, 2011). If pores are too large, cells cannot bridge across the pores, and the cells instead experience more of a curved 2D environment. For spinal cord applications, pore sizes of 100 to 250 µm are commonly used (Stokols & Tuszynski, 2004, 2006). It should be noted the serial-section approach to measuring average pore size and geometry provides an average relative comparison between similar scaffold types but cannot accurately recapitulate the precise dimensions of 3D pore networks with complex geometries.
Mechanical testing
The mechanical properties of the scaffold are key to understanding the physical force that cells can detect in 3D (Balgude, Yu, Szymanski, & Bellamkonda, 2001; Unal, Caliari, & Lampe, 2020; Willits & Skornia, 2004). Young's modulus is a measure of the innate capacity of a given material to elastically resist deformation under low-strain conditions (understood as stiffness or elasticity) and provides a useful measure of the mechanical properties of biological tissues (Guimarães et al., 2020). The advantage of this approach is that it is easily performed on a standard mechanical testing apparatus with little modification (requiring only the simple addition of PBS). The modulus of human CNS tissue is thought to range between 0.5 and 2 kPa, and uniaxial compression testing can provide a comparison of the stiffness of the polymer scaffold to native tissue (Guimarães et al., 2020). The key disadvantage of this approach is that it provides a simplified measurement of scaffold stiffness that does not account for the relative contribution of porosity within the overall material. More advanced methods such as nanoindentation using atomic force microscopy can give a more exact measurement of the actual “local” substrate stiffness that cells experience, and effective methodologies for this approach are described in other studies (Spedden & Staii, 2013).
Neuronal morphology
The analysis of neuronal cell growth throughout the scaffold structure can be analyzed effectively through immunohistochemical analysis of cryosections and hemisections of a fixed scaffold. Owing to the nature of the pore structure, several sections should be analyzed throughout the scaffold including the outer edges and core. Cell infiltration and colonization is usually denser toward the outer scaffold edge and less dense toward the center of the scaffold. Sections of 20 to 50 µm are generally sufficient to allow the capture of representative images of neuronal cell morphology. Longer neurites can form tortuous paths that make accurate analysis of neurite length difficult as the developing axons can pass in and out of the plane of the scaffold section. Staining and imaging of large scaffold hemisections can offer more information on the distribution and connection of neuronal clusters within the 3D scaffolds up to several hundred microns deep. However, standardization of imaging parameters and analysis between samples is more difficult in comparison to the imaging of cryosections because scaffold orientation, depth of imaging, and confocal laser intensities can all contribute to variability.
Critical Parameters and Troubleshooting
Hyaluronic acid slurry production
Adipic acid anhydride, EDAC, and HyA must be stored at −20°C to avoid degradation and loss of activity. HyA powder must always be slowly added when making up the base slurry and suitable time allowed for it to form an even solution. For higher-concentration slurries, this may take several hours. The pH has a significant effect on the activity of EDAC cross-linkage, and reducing the pH to 4 is essential for consistent and homogeneous cross-linkage. The degree of cross-linkage is also dependent on temperature (RT = 22°C) and time, and consistency in both of these conditions must be maintained. The cross-linked slurry can be stored at room temperature for up to 1 month. The slurry must be degassed before each freeze-drying run, otherwise any bubbles present will expand during the process leaving large defects and rendering the scaffolds unusable.
Freeze-dry manufacture of macroporous scaffolds
Once produced, the structural integrity of the scaffolds, particularly soft scaffolds, may become compromised if left in the dry state for too long once removed from the vacuum applied by the freeze dryer. Immediate removal from the Teflon holder and immersion in 100% ethanol helps prevent this effect. Some shrinkage can be visible, but scaffolds expand again following further rehydration. If soft scaffolds collapse following the freeze-drying process, a poorly cross-linked slurry may be responsible. Check the quality and expiration dates of the reagents used, and prepare the slurry again. Slurries of 3 mg/ml HyA are the lowest recommended concentration for unsupported scaffold production. Check that the freeze dryer is fully sealed and generates appropriate vacuums for each cycle during the freeze-dry run. Larger scaffolds may require longer drying stage times to fully dry. Cleaning of all mold elements following use is essential to enable the removal of scaffolds after freeze-drying. Periodic polishing of the molds is also recommended.
Functionalization with extracellular matrix proteins
The addition of ECM proteins before initial cross-linkage of the gel allows for the preparation of custom slurries and 3D tissue scaffolds for cell culture (Woods et al., 2022). More viscous slurry preparations may be difficult to triturate and may take more cycles of trituration to fully homogenize proteins throughout the slurry. Extended stirring, blending, or sonication can cause physical cross-linking of the HyA polymer or degradation of the HyA molecular weight and lead to inconsistencies in produced scaffolds. All cross-linked scaffolds must be well washed with PBS to remove toxic cross-linking residues, and larger scaffolds may require longer washing times for full removal. Failure to perform the second EDAC cross-linkage can lead to the rapid degradation of softer HyA scaffolds.
Porosity and mechanical characterization
Larger scaffolds apply more mass to scales and make it easier to calculate porosity. Similarly, although the stiffness of scaffolds of any size should remain consistent, the actual force detected by the load cell changes with the cross-sectional area of the scaffolds. As such, wider diameter scaffolds can be a better choice for mechanical testing of materials with lower-resolution load cells. Scaffolds should be fully swelled before mechanical testing (∼24 to 48 hr in PBS). Cryoembedding is preferable to paraffin embedding for analysis of porosity owing to the large changes in volume that occur during the dehydration steps typically needed for sectioning of paraffin-embedded materials.
Neuronal cell culture techniques and analysis
For a 6-mm diameter scaffold, the average number of cells seeded is ∼500,000 cells per scaffold, with 250,000 applied to each end of the scaffold. Incubating scaffolds in cell culture medium at 37°C following sterilization and PBS washes typically allows for enhanced cell adherence to the scaffold. For SH-SY5Y culture, it is advisable to leave seeded cells in the growth medium for ∼24 hr to encourage adherence and cell survival before switching to the neuron-inducing differentiation medium. Care should be taken to not overseed the scaffold with cells because excessive cell number and growth can prevent accurate assessment of individual cell morphologies. The gain applied to Alamar blue and PicoGreen sample solution fluorescence readings should be kept at the same level and should be comparable throughout all studies. This can be achieved by measuring the plate several times at different gain levels at each time point to identify the optimal gain parameters for the assay and the instrument. Furthermore, the amount of time the cells are allowed to incubate with Alamar blue reagent must also be kept consistent to ensure accurate comparisons at multiple time points.
Time Considerations
The protocols outlined within this article range in time commitment and are outlined via various timelines within the respective figures for each protocol. Of particular note, there are various points in the protocol where the process can be halted and the relevant reagents or samples can be stored until ready to be processed at a desired time point, allowing experimental flexibility. Additionally, steps for scaffold characterization outlined here are presented in a suggested order but can be performed step-wise or in parallel in any order at the discretion of the individual. Finally, although it is suggested to perform cell culture experiments for 21 days, culture timelines can be altered to better suit the desired experimental design (i.e., using alternative cell sources or cell types). It is estimated that all three protocols should take ∼35 days to complete if performed sequentially.
Acknowledgments
This research was funded by a joint funding initiative of the Irish Rugby Football Union Charitable Trust (IRFU-CT) and the Advanced Materials and Bioengineering Research (AMBER) Centre through Science Foundation Ireland (SFI/12/RC/2278), as well as by an Irish Research Council Postgraduate Fellowship (Government of Ireland, Grant Number: GOIPD/2021/262) and an Anatomical Society Student Fellowship.
Open access funding provided by IReL.
Author Contributions
Cian O'Connor : conceptualization, data curation, formal analysis, investigation, methodology, validation, visualization, writing–original draft, writing–review and editing; Ian Woods : conceptualization, data curation, formal analysis, investigation, methodology, validation, visualization, writing–original draft, writing–review and editing; Alan Hibbitts : conceptualization, methodology, validation, writing–review and editing; Adrian Dervan : conceptualization, project administration, supervision, validation, writing–review and editing; Fergal J. O'Brien : conceptualization, funding acquisition, project administration, resources, supervision, validation, visualization, writing–original draft, writing–review and editing.
Conflict of Interest
The authors declare no conflicts of interest.
Open Research
Data Availability Statement
The data that support the findings of this study have been published in Woods et al. (2022) and are available from the corresponding author upon reasonable request.
Literature Cited
- Aisenbrey, E. A., & Murphy, W. L. (2020). Synthetic alternatives to Matrigel. Nature Reviews Materials , 5, 539–551. doi: 10.1038/s41578-020-0199-8
- Aras, M. A., Hartnett, K. A., & Aizenman, E. (2008). Assessment of cell viability in primary neuronal cultures. Current Protocols in Neuroscience , 44, 7.18.1–7.18.15. doi: 10.1002/0471142301.ns0718s44
- Balgude, A. P., Yu, X., Szymanski, A., & Bellamkonda, R. V. (2001). Agarose gel stiffness determines rate of DRG neurite extension in 3D cultures. Biomaterials , 22(10), 1077–1084. doi: 10.1016/S0142-9612(00)00350-1
- Barnes, J. M., Przybyla, L., & Weaver, V. M. (2017). Tissue mechanics regulate brain development, homeostasis and disease. Journal of Cell Science , 130(1), 71–82. doi: 10.1242/jcs.191742
- Burnside, E. R., & Bradbury, E. J. (2014). Manipulating the extracellular matrix and its role in brain and spinal cord plasticity and repair. Neuropathology and Applied Neurobiology , 40(1), 26–59. doi: 10.1111/nan.12114
- Chighizola, M., Dini, T., Lenardi, C., Milani, P., Podestà, A., & Schulte, C. (2019). Mechanotransduction in neuronal cell development and functioning. Biophysical Reviews , 11(5), 701–720. doi: 10.1007/s12551-019-00587-2
- Curtin, C., Nolan, J. C., Conlon, R., Deneweth, L., Gallagher, C., Tan, Y. J., … Piskareva, O. (2018). A physiologically relevant 3D collagen-based scaffold–neuroblastoma cell system exhibits chemosensitivity similar to orthotopic xenograft models. Acta Biomaterialia , 70, 84–97. doi: 10.1016/j.actbio.2018.02.004
- Dominguez-Alfaro, A., Alegret, N., Arnaiz, B., Salsamendi, M., Mecerreyes, D., & Prato, M. (2020). Toward spontaneous neuronal differentiation of SH-SY5Y cells using novel three-dimensional electropolymerized conductive scaffolds. ACS Applied Materials and Interfaces , 12(51), 57330–57342. doi: 10.1021/acsami.0c16645
- Gallagher, C., Murphy, C., O'Brien, F. J., & Piskareva, O. (2021). Three-dimensional in vitro biomimetic model of neuroblastoma using collagen-based scaffolds. Journal of Visualized Experiments , 173, e62627. doi: 10.3791/62627-v
- Guimarães, C. F., Gasperini, L., Marques, A. P., & Reis, R. L. (2020). The stiffness of living tissues and its implications for tissue engineering. Nature Reviews Materials , 5(5), 351–370. doi: 10.1038/s41578-019-0169-1
- Gupta, R. C., Lall, R., Srivastava, A., & Sinha, A. (2019). Hyaluronic acid: Molecular mechanisms and therapeutic trajectory. Frontiers in Veterinary Science , 6, 192. doi: 10.3389/fvets.2019.00192
- Haugh, M. G., Murphy, C. M., & O'Brien, F. J. (2010). Novel freeze-drying methods to produce a range of collagen-glycosaminoglycan scaffolds with tailored mean pore sizes. Tissue Engineering. Part C, Methods , 16(5), 887–894. doi: 10.1089/ten.tec.2009.0422
- Hibbitts, A. J., Kočí, Z., Kneafsey, S., Matsiko, A., Žilić, L., Dervan, A., … O'Brien, F. J. (2022). Multi-factorial nerve guidance conduit engineering improves outcomes in inflammation, angiogenesis and large defect nerve repair. Matrix Biology , 106, 34–57. doi: 10.1016/j.matbio.2022.01.002
- Hopkins, A. M., De Laporte, L., Tortelli, F., Spedden, E., Staii, C., Atherton, T. J., … Kaplan, D. L. (2013). Silk hydrogels as soft substrates for neural tissue engineering. Advanced Functional Materials , 23(41), 5140–5149. doi: 10.1002/adfm.201300435
- Hopkins, A. M., DeSimone, E., Chwalek, K., & Kaplan, D. L. (2015). 3D in vitro modelling of the central nervous system. Progress in Neurobiology , 125, 1–25. doi: 10.1016/j.pneurobio.2014.11.003
- Hubrecht, R. C., & Carter, E. (2019). The 3Rs and humane experimental technique: Implementing change. Animals , 9(10), 754. doi: 10.3390/ani9100754
- Huch, M., Knoblich, J. A., Lutolf, M. P., & Martinez-Arias, A. (2017). The hope and the hype of organoid research. Development , 144(6), 938–941. doi: 10.1242/dev.150201
- Hughes, C. S., Postovit, L. M., & Lajoie, G. A. (2010). Matrigel: A complex protein mixture required for optimal growth of cell culture. Proteomics , 10(9), 1886–1890. doi: 10.1002/pmic.200900758
- Jeon, O., Song, S. J., Lee, K. J., Park, M. H., Lee, S. H., Hahn, S. K., … Kim, B. S. (2007). Mechanical properties and degradation behaviors of hyaluronic acid hydrogels cross-linked at various cross-linking densities. Carbohydrate Polymers , 70(3), 251–257. doi: 10.1016/j.carbpol.2007.04.002
- Karahuseyinoglu, S., Sekerdag, E., Aria, M. M., Cetin Tas, Y., Nizamoglu, S., Solaroglu, I., & Gürsoy-Özdemir, Y. (2021). Three-dimensional neuron–astrocyte construction on matrigel enhances establishment of functional voltage-gated sodium channels. Journal of Neurochemistry , 156(6), 848–866. doi: 10.1111/jnc.15185
- Khaing, Z. Z., Milman, B. D., Vanscoy, J. E., Seidlits, S. K., Grill, R. J., & Schmidt, C. E. (2011). High molecular weight hyaluronic acid limits astrocyte activation and scar formation after spinal cord injury. Journal of Neural Engineering , 8(4), 046033. doi: 10.1088/1741-2560/8/4/046033
- Kim, Y., & Kumar, S. (2014). CD44-mediated adhesion to hyaluronic acid contributes to mechanosensing and invasive motility. Molecular Cancer Research , 12(10), 1416–1429. doi: 10.1158/1541-7786.MCR-13-0629
- Krencik, R., Seo, K., van Asperen, J. V., Basu, N., Cvetkovic, C., Barlas, S., … Ullian, E. M. (2017). Systematic three-dimensional coculture rapidly recapitulates interactions between human neurons and astrocytes. Stem Cell Reports , 9(6), 1745–1753. doi: 10.1016/j.stemcr.2017.10.026
- Kwon, M. Y., Wang, C., Galarraga, J. H., Puré, E., Han, L., & Burdick, J. A. (2019). Influence of hyaluronic acid modification on CD44 binding towards the design of hydrogel biomaterials. Biomaterials , 222, 119451. doi: 10.1016/j.biomaterials.2019.119451
- Leach, J. B., Brown, X. Q., Jacot, J. G., Dimilla, P. A., & Wong, J. Y. (2007). Neurite outgrowth and branching of PC12 cells on very soft substrates sharply decreases below a threshold of substrate rigidity. Journal of Neural Engineering , 4(2), 26–34. doi: 10.1088/1741-2560/4/2/003
- Lei, W. L., Xing, S. G., Deng, C. Y., Ju, X. C., Jiang, X. Y., & Luo, Z. G. (2012). Laminin/β1 integrin signal triggers axon formation by promoting microtubule assembly and stabilization. Cell Research , 22(6), 954–972. doi: 10.1038/cr.2012.40
- Loh, Q. L., & Choong, C. (2013). Three-dimensional scaffolds for tissue engineering applications: Role of porosity and pore size. Tissue Engineering. Part B, Reviews , 19(6), 485–502. doi: 10.1089/ten.teb.2012.0437
- Luo, Y., Kirker, K. R., & Prestwich, G. D. (2000). Cross-linked hyaluronic acid hydrogel films: New biomaterials for drug delivery. Journal of Controlled Release , 69, 169–184. doi: 10.1016/s0168-3659(00)00300-x
- Maughan, J., Gouveia, P. J., Gutierrez, J., Leahy, L. M., Woods, I., Connor, C. O., … Brien, F. J. O. (2022). Collagen/pristine graphene as an electroconductive interface material for neuronal medical device applications. Applied Materials Today , 29, 101629. doi: 10.1016/j.apmt.2022.101629
- Moeendarbary, E., Weber, I. P., Sheridan, G. K., Koser, D. E., Soleman, S., Haenzi, B., … Franze, K. (2017). The soft mechanical signature of glial scars in the central nervous system. Nature Communications , 8, 14787. doi: 10.1038/ncomms14787
- Moshayedi, P., Ng, G., Kwok, J. C. F., Yeo, G. S. H., Bryant, C. E., Fawcett, J. W., … Guck, J. (2014). The relationship between glial cell mechanosensitivity and foreign body reactions in the central nervous system. Biomaterials , 35(13), 3919–3925. doi: 10.1016/j.biomaterials.2014.01.038
- Norman, L. L., & Aranda-Espinoza, H. (2010). Cortical neuron outgrowth is insensitive to substrate stiffness. Cellular and Molecular Bioengineering , 3(4), 398–414. doi: 10.1007/s12195-010-0137-8
- O'Brien, F. J. (2011). Biomaterials & scaffolds for tissue engineering. Materials Today , 14(3), 88–95. doi: 10.1016/S1369-7021(11)70058-X
- O'Brien, F. J., Harley, B. A., Yannas, I. V., & Gibson, L. J. (2005). The effect of pore size on cell adhesion in collagen-GAG scaffolds. Biomaterials , 26(4), 433–441. doi: 10.1016/j.biomaterials.2004.02.052
- Park, J. S., Burckhardt, C. J., Lazcano, R., Solis, L. M., Isogai, T., Li, L., … Danuser, G. (2020). Mechanical regulation of glycolysis via cytoskeleton architecture. Nature , 578(7796), 621–626. doi: 10.1038/s41586-020-1998-1
- Placone, A. L., McGuiggan, P. M., Bergles, D. E., Guerrero-Cazares, H., Quiñones-Hinojosa, A., & Searson, P. C. (2015). Human astrocytes develop physiological morphology and remain quiescent in a novel 3D matrix. Biomaterials , 42, 134–143. doi: 10.1016/j.biomaterials.2014.11.046
- Polykandriotis, E., Arkudas, A., Horch, R. E., & Kneser, U. (2008). To Matrigel or not to Matrigel. American Journal of Pathology , 172(5), 1441–1442. doi: 10.2353/ajpath.2008.071215
- Raftery, R. M., Woods, B., Marques, A. L. P., Moreira-Silva, J., Silva, T. H., Cryan, S. A., … O'Brien, F. J. (2016). Multifunctional biomaterials from the sea: Assessing the effects of chitosan incorporation into collagen scaffolds on mechanical and biological functionality. Acta Biomaterialia , 43, 160–169. doi: 10.1016/j.actbio.2016.07.009
- Ryan, A. J., Lackington, W. A., Hibbitts, A. J., Matheson, A., Alekseeva, T., Stejskalova, A., … O'Brien, F. J. (2017). A physicochemically optimized and neuroconductive biphasic nerve guidance conduit for peripheral nerve repair. Advanced Healthcare Materials , 6(24), 1700954. doi: 10.1002/adhm.201700954
- Santos, T. E., Schaffran, B., Broguière, N., Meyn, L., Zenobi-Wong, M., & Bradke, F. (2020). Axon growth of CNS neurons in three dimensions is amoeboid and independent of adhesions. Cell Reports , 32(3), 107907. doi: 10.1016/j.celrep.2020.107907
- Seidlits, S. K., Khaing, Z. Z., Petersen, R. R., Nickels, J. D., Vanscoy, J. E., Shear, J. B., & Schmidt, C. E. (2010). The effects of hyaluronic acid hydrogels with tunable mechanical properties on neural progenitor cell differentiation. Biomaterials , 31(14), 3930–3940. doi: 10.1016/j.biomaterials.2010.01.125
- Song, I., & Dityatev, A. (2018). Crosstalk between glia, extracellular matrix and neurons. Brain Research Bulletin , 136, 101–108. doi: 10.1016/j.brainresbull.2017.03.003
- Spedden, E., & Staii, C. (2013). Neuron biomechanics probed by atomic force microscopy. International Journal of Molecular Sciences , 14(8), 16124–16140. doi: 10.3390/ijms140816124
- Sridharan, R., Cavanagh, B., Cameron, A. R., Kelly, D. J., & O'Brien, F. J. (2019). Material stiffness influences the polarization state, function and migration mode of macrophages. Acta Biomaterialia , 89, 47–59. doi: 10.1016/j.actbio.2019.02.048
- Stokols, S., & Tuszynski, M. H. (2004). The fabrication and characterization of linearly oriented nerve guidance scaffolds for spinal cord injury. Biomaterials , 25(27), 5839–5846. doi: 10.1016/j.biomaterials.2004.01.041
- Stokols, S., & Tuszynski, M. H. (2006). Freeze-dried agarose scaffolds with uniaxial channels stimulate and guide linear axonal growth following spinal cord injury. Biomaterials , 27(3), 443–451. doi: 10.1016/j.biomaterials.2005.06.039
- Sundararaghavan, H. G., Monteiro, G. A., Firestein, B. L., & Shreiber, D. I. (2009). Neurite growth in 3D collagen gels with gradients of mechanical properties. Biotechnology and Bioengineering , 102(2), 632–643. doi: 10.1002/bit.22074
- Teixeira, A. I., Ilkhanizadeh, S., Wigenius, J. A., Duckworth, J. K., Inganäs, O., & Hermanson, O. (2009). The promotion of neuronal maturation on soft substrates. Biomaterials , 30(27), 4567–4572. doi: 10.1016/j.biomaterials.2009.05.013
- Unal, D., Caliari, S., & Lampe, K. (2020). 3D hyaluronic acid hydrogels for modeling oligodendrocyte progenitor cell behavior as a function of matrix stiffness. Biomacromolecules , 21(12), 4962–4971. doi: 10.1021/acs.biomac.0c01164
- Walczak, P. A., Perez-Esteban, P., Bassett, D. C., & Hill, E. J. (2021). Modelling the central nervous system: Tissue engineering of the cellular microenvironment. Emerging Topics in Life Sciences , 5(4), 507–517. doi: 10.1042/ETLS20210245
- Watson, P. M. D., Kavanagh, E., Allenby, G., & Vassey, M. (2017). Bioengineered 3D glial cell culture systems and applications for neurodegeneration and neuroinflammation. SLAS Discovery , 22(5), 583–601. doi: 10.1177/2472555217691450
- Willits, R. K., & Skornia, S. L. (2004). Effect of collagen gel stiffness on neurite extension. Journal of Biomaterials Science, Polymer Edition , 15(12), 1521–1531. doi: 10.1163/1568562042459698
- Woods, I., O'Connor, C., Frugoli, L., Kerr, S., Gonzalez, J. G., Stasiewicz, M., … O'Brien, F. J. (2022). Biomimetic scaffolds for spinal cord applications exhibit stiffness-dependent immunomodulatory and neurotrophic characteristics. Advanced Healthcare Materials , 11(3), 2101663. doi: 10.1002/adhm.202101663
- Zuidema, J. M., Gilbert, R. J., & Gottipati, M. K. (2019). Biomaterial approaches to modulate reactive astroglial response. Cells Tissues Organs , 205(5–6), 372–395. doi: 10.1159/000494667
Citing Literature
Number of times cited according to CrossRef: 2
- Pádraig Carroll, Éimear Smith, Adrian Dervan, Ciarán McCarthy, Ian Woods, Cliff Beirne, Geoff Harte, Dónal O'Flynn, John Quinlan, Fergal J. O'Brien, Michelle Flood, Frank Moriarty, The Development of Principles for Patient and Public Involvement (PPI) in Preclinical Spinal Cord Research: A Modified Delphi Study, Health Expectations, 10.1111/hex.14130, 27 , 4, (2024).
- Pádraig Carroll, Éimear Smith, Adrian Dervan, Ciarán McCarthy, Cliff Beirne, John Quinlan, Geoff Harte, Dónal O'Flynn, Fergal J. O'Brien, Frank Moriarty, Michelle Flood, Perspectives of researchers and clinicians on patient and public involvement (PPI) in preclinical spinal cord research: An interview study, Health Expectations, 10.1111/hex.13967, 27 , 1, (2024).