Single Genomic Loci Labeling and Manipulation Using SIMBA System
Yanwei Chen, Yanwei Chen, Ziliang Huang, Ziliang Huang, Qin Peng, Qin Peng, Yingxiao Wang, Yingxiao Wang
Abstract
The SIMBA (Simultaneous Imaging and Manipulation of genomic loci by Biomolecular Assemblies) system is an innovative CRISPR-based imaging technique that leverages dCas9-SunTag and FRB-mCherry-HP1α, with scFv-FKBP acting as a bridge. This powerful system enables simultaneous visualization and manipulation of genomic loci. The dCas9-SunTag fusion protein allows for precise targeting of specific genomic sites, and the FRB-mCherry-HP1α fusion protein facilitates the condensation of chromatin at the targeted loci. The scFv-FKBP bridge protein links dCas9-SunTag and FRB-mCherry-HP1α, ensuring efficient and specific recruitment of HP1α to the desired genomic loci. This integrated approach allows us to visualize and manipulate genomic regions of interest, opening up new avenues for studying genome organization, gene expression regulation, and chromatin dynamics in living cells. © 2023 The Authors. Current Protocols published by Wiley Periodicals LLC.
Basic Protocol 1 : Cloning of genetic constructs
Basic Protocol 2 : Transient transfection in mammalian cells and live-cell imaging
Basic Protocol 3 : Generation of SIMBA-expressing stable cell lines
Basic Protocol 4 : Manipulation of genomic loci using SIMBA
INTRODUCTION
In recent years, significant advancements have been made in the development of various microscopic techniques aimed at visualizing and accurately mapping the localization of chromatin compartments. One widely used approach is the application of DNA fluorescent in situ hybridization (FISH) techniques (Manuelidis, 1985; Price, 1993). By leveraging the power of fluorescently labeled DNA probes, these methods enable researchers to precisely target and visualize specific genomic regions within the nucleus. The traditional FISH design was combined with super-resolution imaging techniques, leading to the development of high-resolution intranuclear imaging methods (Jerkovic & Cavalli, 2021; Wang et al., 2017). These innovative approaches push the boundaries of optical resolution, allowing for the visualization of chromatin compartments at an unprecedented level of detail.
Although DNA FISH has proven to be a valuable tool in chromatin research, it does have certain limitations. One such limitation is its restriction to fixed cell samples, which do not provide temporal information or allow capture of dynamic processes occurring within the cell. This lack of temporal resolution poses challenges when studying time-dependent phenomena such as chromatin remodeling, gene expression dynamics, or the spatial reorganization of chromatin during cellular processes.
The emerging usage of the CRISPR/Cas9 system provides us with more opportunity in tracking genome dynamics at the live-cell level (Liu et al., 2017; Wang et al., 2019; Wu et al., 2016). For example, the live-FISH system uses nuclease-defective Cas9 (dCas9) and fluorescent-labeled guide RNA (gRNA) complex (Wang et al., 2019). dCas9/gRNA complex recognizes the target gene sequence, which carries its chromophore to the loci to be visualized by a confocal microscope. Various catalytically inactive CRISPR/Cas9 (dCas9)-based labeling systems have been developed to visualize genomic loci in living cells, aiming to overcome challenges such as low signal-to-noise ratio (SNR) at specific genomic loci, particularly those with non-repetitive sequences (Chen et al., 2013; Cheng et al., 2016; Hong et al., 2018; Ma et al., 2016; Qin et al., 2017; Ye et al., 2017). Strategies to enhance the SNR and amplify signals for locus labeling include bimolecular fluorescence complementation (BiFC) (Chen et al., 2013; Ma et al., 2018), tandem repeats of MS2 stem-loop (Gu et al., 2018; Ye et al., 2017), and multiple repeats of SunTag (Chen et al., 2013; Hong et al., 2018; Ye et al., 2017), typically integrated with the dCas9-single guide RNA (sgRNA) system. However, for non-repetitive loci, where there is only one anchoring site for a dCas9-sgRNA complex, the efficiency of locus-specific labeling using a single sgRNA is generally low. Currently, multiple sgRNAs targeting neighboring regions of the target locus are still required for efficient labeling of non-repetitive loci (Cheng et al., 2016; Gilbert et al., 2013; Mao et al., 2019; Shao et al., 2018; Ye et al., 2017). However, this approach introduces increased complexity, reduced efficiency, and a higher risk of off-target labeling.
Oligomers have demonstrated the capacity to form membrane-less organelles, including condensed biomolecular assemblies (BAs) within the nucleus. These assemblies have the ability to interact with chromatin, thereby influencing genome architecture and gene expression (Brangwynne et al., 2009; Cheutin et al., 2003; Frey et al., 2006; Qi & Zhang, 2021). One key player in this context is heterochromatin protein 1α, often abbreviated as HP1α, which serves as a significant component of constitutive heterochromatin. HP1α plays pivotal roles in shaping chromatin structure, upholding genome stability, and governing gene expression (Larson et al., 2017; Wang et al., 2016). HP1α exhibits the capability to condense both in vitro and in vivo , driven by weak hydrophobic interactions primarily facilitated by an intrinsically disordered region (IDR) situated in the N-terminal extension and hinge domain (Keenen et al., 2021; Strickfaden et al., 2020; Strom et al., 2017). This increased local concentration of HP1α encourages the formation of BAs at genomic loci, involving intricate interactions, both homotypic and heterotypic, with proteins, RNAs, and DNAs (Wang et al., 2020). Furthermore, specific buffer conditions and environmental co-factors present at these genomic regions can further promote the formation of condensed chromatin structures (Strickfaden et al., 2020).
The structural makeup of HP1α encompasses distinct domains, including an N-terminal chromodomain (CD) responsible for binding to K9-trimethylated histone H3 (H3K9me3), a middle positively charged hinge domain (HD) that facilitates interactions with DNA and RNA, and a C-terminal chromo shadow domain (CSD) crucial for self-association (Strom et al., 2017). HP1α exhibits direct interactions with histone methyltransferases, such as SUV39H1/2 and SETDB1, thereby enabling self-propagation and sequential methylation of adjacent nucleosomes and the recruitment of additional HP1α molecules within the local genomic region (Chaudhary et al., 2020; Krouwels et al., 2005; Schotta et al., 2002; Schultz et al., 2002). These unique characteristics of HP1α make it a promising candidate for triggering BA formation for genomic locus labeling. Additionally, the transcription suppression capability of HP1α may allow for localized gene silencing at the labeled loci in living cells. Thus, HP1α is emerging as a promising candidate for initiating the formation of BAs at genomic loci for the purpose of labeling. Additionally, its ability to suppress transcription suggests the potential for localized gene silencing at labeled loci in living cells.
Here, we introduce basic protocols for the CRISPR-based SIMBA (Simultaneous Imaging and Manipulation of genomic loci by Biomolecular Assemblies) system (Peng et al., 2022). In this design, dCas9 recognizes specific gene loci, recruiting fluorescent protein–fused HP1α in a rapamycin-dependent manner, which facilitates chromatin condensation through HP1α and SUV39H1-mediated heterochromatin formation. The system could be easily turned on by rapamycin induction, and the condensed chromatin at specific genomic loci (repetitive and non-repetitive) not only enables precise imaging with an enhanced SNR but also alters the local H3K9me3 level, leading to gene silencing.
Basic Protocol 1: CLONING OF GENETIC CONSTRUCTS
The SIMBA system involves the utilization of three key components: dCas9-sgRNA complex carrying multiple (24×, 8×, 4×, 2×, 1×) SunTag, rapamycin-inducible dimerization system–based scFv-FKBP, and FRB-mCherry-HP1α as the reporter unit. The cloning procedures can be summarized as follows: (1) generation of the dCas9-SunTag/scFv-FKBP/FRB-mCherry fragments, (2) integration of components by ligating coding fragments and inserting them into linearized vector by Gibson Assembly or Golden Gate Assembly, and (3) validation of the construct by restriction enzyme digestion and/or Sanger sequencing (Fig. 1).
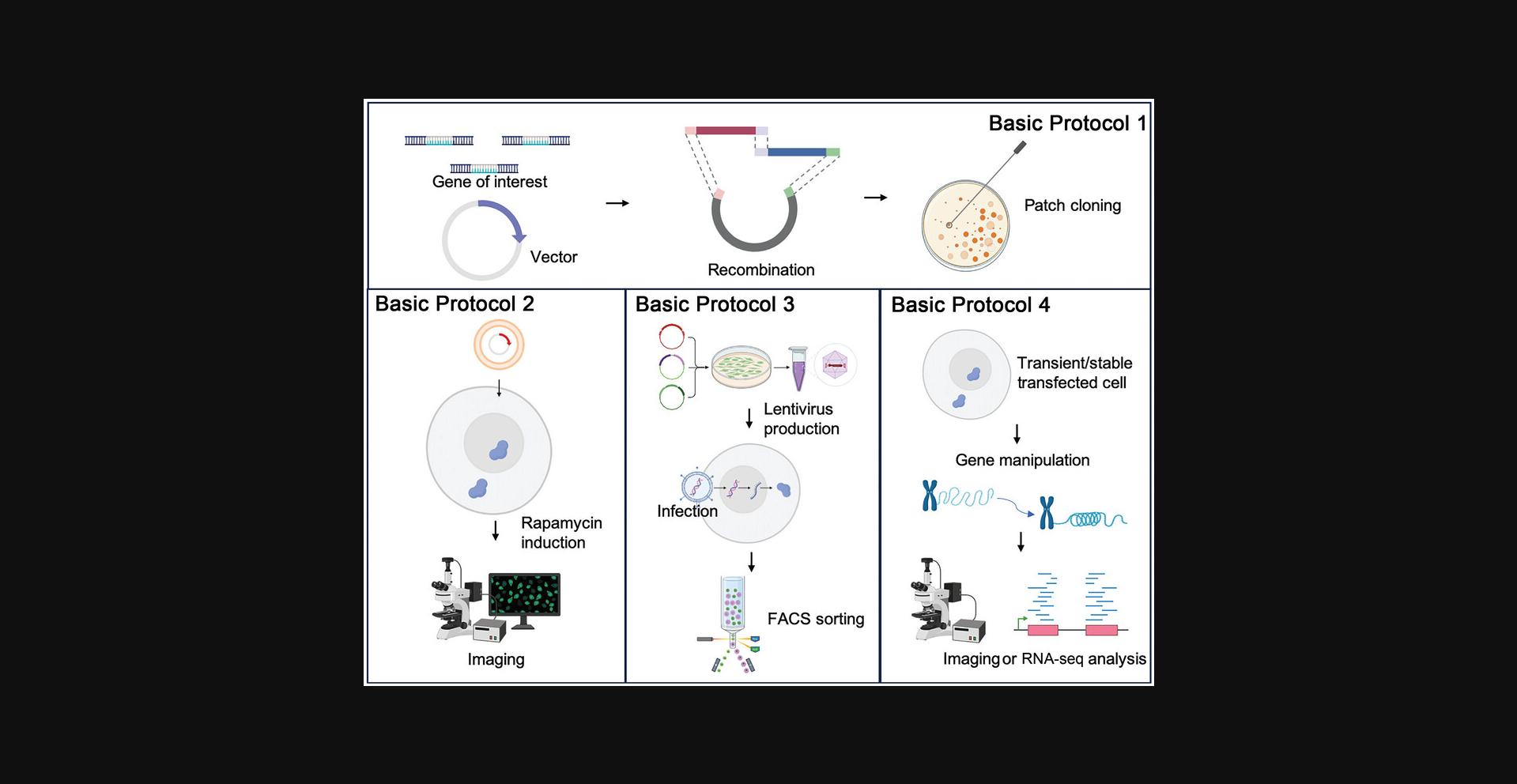
Materials
- Addgene plasmids (Table 1)
- 1× ampicillin LB agar plates (see recipe)
- Stbl3 competent cells (Invitrogen, C737303)
- S.O.C. medium (Invitrogen, 15544034)
- 1× ampicillin LB culture medium (see recipe)
- 50% (w/v) glycerol, sterile (filter-sterilize with 0.22-μm filter)
- GenElute HP Plasmid DNA Miniprep Kit (Sigma, NA0150-1KT; for plasmid extraction from bacterial culture)
- Molecular biology–grade water (Fisher, BP2819-1)
- Appropriate primers for cassette cloning (Table 2)
- Q5 High-Fidelity DNA Polymerase (New England Biolabs, M0491L)
- 10× rCutSmart Buffer (New England Biolabs, B6004; for restriction enzyme reactions)
- Restriction enzymes (New England Biolabs):
- EcoRI (EcoRI-HF, R3101S), NotI (NotI-HF, R3189S), BamHI (BamHI-HF, R3136S), and XhoI(R0146S) for Gibson Assembly subcloning and plasmid digestion fingerprint identification
- BbsI (R3539S), for Golden Gate Assembly
Usage | Plasmid | Source |
---|---|---|
dCas9-SunTag fragment cloning | pHRdSV40-NLS-dCas9-24×GCN4_v4-NLS-P2A-BFP-dWPRE | Addgene, #60910 |
scFv cloning | pHR-scFv-GCN4-sfGFP-GB1-NLS-dWPRE | Addgene, #60906 |
pHR vector backbone, for restriction enzyme digestion | pHR-PGK-antiCD19-synNotch-Gal4VP64 | Addgene, #79125 |
For BiFC component cloning | pBiFC-bJunVN155(I152L) | Addgene, #27098 |
pBiFC-bFosVC155 | Addgene, #22013 | |
pHAGE-EFS-MCP-3XBFP-NLS | Addgene, #75384 | |
pHAGE-EFS-PCP-3XGFP-NLS | Addgene, #75385 | |
pLH-sgRNA1-MS2-PP7 | Addgene, #75392 | |
pHR-FUSN-mCh-Cry2WT | Addgene, #101223 | |
All-in-one construct building | PiggyBac vector PB-iMYOD1-P2A-GFP-Puro | Addgene, #168804 |
Forward primer (5′-3′) | Reverse primer (5′-3′) | Template | |
---|---|---|---|
NFAT1 | TAAAGCGGCCGCGACTCTAG | CATGGTGGCGACCGGTGGATC | |
8× SunTag | GATCCACCGGTCGCCACCATGGACGGCATTGGTAGTGGG | TCTAGAGTCGCGGCCGCTTTAACCTTGCGGCCGCTGACC | Template from full-length 24×SunTag sequence |
FUSN | agcggcgggaccatggcctcaaacgattatacccaacaag | cacgcatgttgcaggtgggagttgcaccttgcgcttcttcttgggactagttcctccacctccacggtcctgctgtc | pHR-FUSN-mCh-Cry2WT (Addgene, #101223) |
P2A | GCTACTAACTTCAGCCTGCTGAAGCAGGCTGGAGACGTGGAGGAGAACCCTGGACCT | ||
T2A | GAGGGCAGAGGAAGTCTGCTAACATGCGGTGACGTCGAGGAGAATCCTGGCCCA | ||
PBVector | TAAACCGGTTAGTAATGAGTTTAAAC | CATGGTGGCTAGCTCTAG | PB-iMYOD1-P2A-GFP-Puro (Addgene, #168804) |
NFAT1-8×SunTag | GTCTAGAGCTAGCCACCATGATCTTTTACCCATACGATGTTCCTGACTATGCG | TCCACGTCTCCAGCCTGCTTCAGCAGGCTGAAGTTAGTAGCACCTTGCGGCCGCTGACC | Gibson Assembly product: NFAT-SunTag |
ScFv-FKBP | TGCTGAAGCAGGCTGGAGACGTGGAGGAGAACCCTGGACCTATGGGCCCCGACATCGTG | CCTCGACGTCACCGCATGTTAGCAGACTTCCTCTGCCCTCCACCTTGCGCTTCTTCTTGG | Gibson assembly product: scFv-FKBP |
FRB-mCh-HP1α | CTGCTAACATGCGGTGACGTCGAGGAGAATCCTGGCCCAATGGAGATGTGGCACGAG | ACTCATTACTAACCGGTTTATTACACCTTGCGCTTCTTC | Gibson Assembly product: FRB-mCh-HP1a |
-
Bacteria-spreading glass beads
-
42°C water bath (for competent cell transformation)
-
37°C bacterial incubator
-
Disposable 14-ml incubation tubes (Falcon, 352059)
-
37°C incubator shaker
-
1.5-ml Eppendorf tubes or cryovials
-
Standard tabletop centrifuge
-
Spectrophotometer (NanoDrop One 2000, Thermo Fisher)
-
Thermocycler
-
0.2-ml PCR tubes
-
Additional reagents and equipment for preparing Addgene plasmids, for Sanger sequencing and/or restriction enzyme digestion, for PCR using high-fidelity DNA polymerase (see manufacturer's protocol), for agarose gel electrophoresis, for oligo synthesis (see Table 3 for oligos in sgRNA vector), and for subcloning (see manufacturer's protocol)
gRNA targeting locus/gene | Sequence (5′->3′) | Reference |
---|---|---|
Telomere | 5′-tagggttagggttagggtta-3′ | (Chen et al., 2013) |
MUC4-E3 | 5′-gtggcgtgacctgtggatgctg-3′ | |
non-repetitive MUC4 (MUC4.1) | 5′-gtaaagtagaaaaggcataaa-3′ | (Qin et al., 2017) |
IL-1B | 5′-tgagataattctctggttca-3′ | (Konermann et al., 2015) |
NOTE : Experiments involving PCR require extremely careful technique to prevent contamination.
Addgene plasmid preparation and expansion
1.Prepare the basic Addgene plasmids (see Table 1) containing the components for SIMBA and the related systems. Order any necessary constructs with building cassettes from Addgene (https://www.addgene.org) and check if any plasmid or bacterial stock already exists in the lab.
2.Amplify the plasmid components to obtain a sufficient number of copies. For those in bacterial glycerol stock, streak on 1× ampicillin LB agar plates with screening antibiotic using bacteria-spreading glass beads to obtain single colonies. For simple plasmid amplification, transform Stbl3 competent cells in S.O.C. medium with 42°C heat shock in a water bath following the manufacturer's protocol. Then, streak transformed bacteria on LB agar plates to obtain single colonies. Incubate at 37°C overnight (16 to 18 hr) in a bacterial incubator.
3.Pick a single colony on the LB agar plate for each plasmid and inoculate into 5 ml of 1× ampicillin LB culture medium with selective antibiotics in a disposable 14-ml incubation tube. Incubate at 37°C and 250 rpm overnight (16 to 18 hr) in an incubator shaker with sufficient aeration.
4.Take a small aliquot (100 to 200 μl bacterial culture) and mix with 1 volume of 50% (v/v) glycerol in a 1.5-ml Eppendorf tube or cryovial to prepare glycerol stock. Store stock at −80°C for long-term storage. Spin down the remaining culture for 5 min at 5000 × g. Extract plasmid DNA using GenElute HP Plasmid DNA Miniprep Kit following the manufacturer's protocol.
5.Elute plasmid DNA with 30 to 60 μl molecular biology–grade water. Measure the DNA concentration using a spectrophotometer.
6.Verify the amplified plasmid by Sanger sequencing or restriction enzyme digestion.
Preparation of basic coding cassettes and vector backbone for recombination
7.Design and obtain the appropriate primers for cassette cloning (containing 20- to 25-bp annealing region) with a homologous arm region (16 to 25 bp with Tm ≥ 48°C) for Gibson Assembly.
8.For plasmid construct for the expression of basic components of SIMBA system, first acquire the following coding sequences by PCR using Q5 High-Fidelity DNA Polymerase following the manufacturer's protocol: dCas9-24×SunTag from pHRdSV40-NLS-dCas9-24xGCN4_v4-NLS-P2A-BFP-dWPRE (Addgene, #60910), scFv from pHR-scFv-GCN4-sfGFP-GB1-NLS-dWPRE (Addgene, #60906), FKBP, FRB, mCherry (from template pHR-FUSN-mCh-Cry2WT, Addgene, #101223), and HP1α from human cDNA (sequences are provided in Supporting Information).
9.Prepare the pHR vector backbone from pHR_PGK_antiCD19_synNotch_Gal4VP64 by double digestion with EcoRI and NotI using the following digestion reaction: 400 ng plasmid, 2 μl of 10× rCutSmart Buffer, 0.5 μl EcoRI, 0.5 μl NotI, and molecular biology–grade water to 20 μl.
10.Recover the linearized vector fragment by agarose gel electrophoresis and gel recovery using the Zymoclean Gel DNA Recovery Kit.
11.Build expression vector for each of the components by Gibson Assembly, including dCas9-24×SunTag, scFv-FKBP, and FRB-mCherry-HP1α, preparing the reaction mix using Gibson Assembly Master Mix following the manufacturer's protocol. Mix by pipetting, perform a quick spin, and then incubate at 50°C for 15 min in a thermocycler.
12.Amplify and screen for successful clones by transformation of reaction product into Stbl3 competent cells and streak on an ampicillin-selective LB plate.
13.Verify the construct by Sanger sequencing or restriction enzyme digestion.
sgRNA expression vector subcloning
14.Design the 20-nt targeting sequence sgRNA sequence for specific labeling loci or look up from previous publications.
15.Synthesize sgRNA oligos carrying specific targeting sequences with 5′-annealing sequence as indicated below:
- Forward: 5′-ACCG-(+) targeting sequence-3′
- Reverse: 5′-AAAC-(-) targeting sequence-3′
16.Anneal the sgRNA oligo pair in the following reaction system in a 0.2-ml PCR tube: 40 μl annealing buffer (100 mM Tris·HCl, pH 8.0, 50 mM NaCl, and 1 mM EDTA), 5 μl of 100 μM Forward oligo, 5 μl of 100 μM Reverse oligo (total volume: 50 μl).
17.Incubate the PCR tube containing reaction system in a thermocycler at 95°C for 3 min and then slowly cool to 25°C with a ramp rate of 0.5°C/s. Then, dilute 5 μl annealed oligos with 245 μl water to a final concentration of 200 nM, which is ∼3 ng/μl and ready for use.
18.Subclone the annealed sgRNA to the pLH vector by Golden Gate Assembly with the following reaction mix: 100 ng pLH-sgRNA1-MS2-PP7, 0.3 μl T7 DNA ligase, 1 μl of 10× rCutSmart Buffer, 0.5 μl BbsI, 1 μl of 10 mM ATP, 1 μl of 200 nM annealed oligos, and water to 10 μl.
19.Incubate the reaction at 37°C for 15 min in a thermocycler or incubator and then transform 2 μl reaction mix into 25 μl Stbl3 competent cells for further amplification to obtain single clones.
20.Examine the single clones by Sanger sequencing or restriction enzyme digestion.
SIMBA constructs
21.For SIMBA imaging and further lentivirus packaging, subclone the sgRNA to the pHR vector by Gibson Assembly following the manufacturer's protocol.
22.For orthogonal fluorescent labeling of dCas9-sgRNA complex using BiFC, visualize the transcribed sgRNA-MS2-PP7 by the complementary Venus fluorescent protein. To this end, perform PCR on the N- and C-split Venus components from pBiFC-bJunVN155(I152L) and pBiFC-bFosVC155, respectively. Then, insert N-Venus into pHAGE-EFS-MCP-3×BFP-NLS and C-Venus into pHAGE-EFS-PCP-3×GFP-NLS in between the BamHI and XhoI sites, to replace 3×BFP or 3×GFP, by Gibson Assembly or ligation, respectively.
23.Amplify the corresponding plasmids for either experimental usage or storage.
Basic Protocol 2: TRANSIENT TRANSFECTION IN MAMMALIAN CELLS AND LIVE-CELL IMAGING
Basic Protocol 1 yields the essential constructs needed for delivery and expression in mammalian cells. To assess the functionality of the SIMBA system, transient transfection is performed in HEK 293T cells (ATCC®, CRL-3216). Upon successful transfection, rapamycin treatment is administered to the transfected cells, initiating the dimerization of FKBP-FRB. Subsequently, images of BAs in the transfected cells are captured following rapamycin treatment, facilitating the visualization of genomic loci (refer to Fig. 2). Specific SIMBA-labeled puncta undergo analysis based on SNR, employing established methods for SNR assessment as detailed in prior studies (Mao et al., 2019). This experimental trajectory serves to validate the potential of the SIMBA system at the live-cell level.
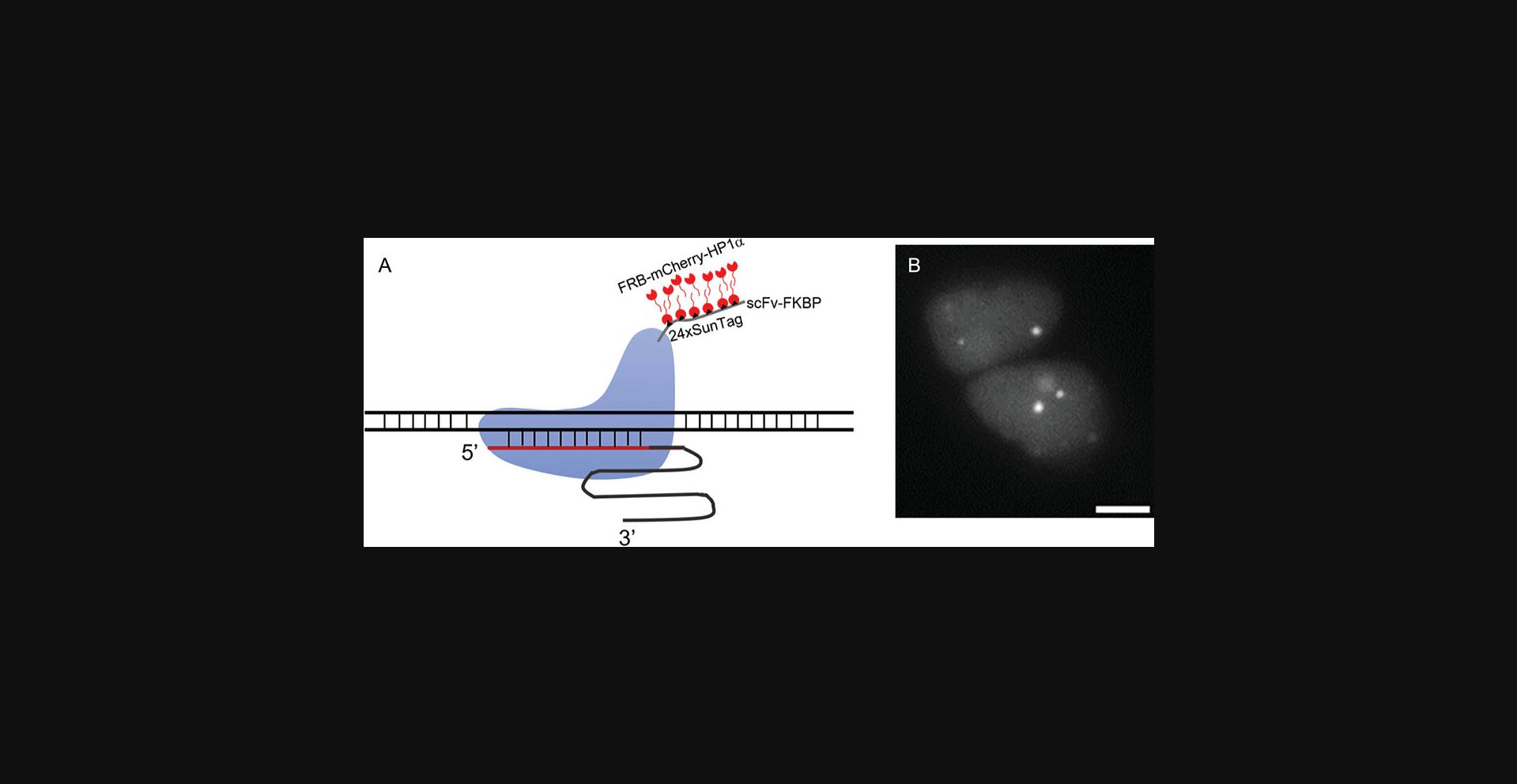
Materials
-
HEK 293T cells (ATCC®, CRL-3216)
-
Complete DMEM (see recipe)
-
Lipofectamine 3000 transfection reagent, with P3000 reagent (Invitrogen, L3000075)
-
Opti-MEM (Opti-MEM I Reduced Serum Medium, Gibco, 31985062)
-
Glass-bottom dishes (Cell E&G, GBD00001-200) coated with fibronectin (20 µg/ml; fibronectin bovine plasma, Sigma-Aldrich, F1141)
-
100 μM rapamycin stock (see recipe)
-
Dimethyl sulfoxide (DMSO)
-
Live-cell imaging solution without phenol red (Thermo Fisher, A1896701)
-
12-well plates (GenClone, 25-106)
-
1.5-ml Eppendorf tubes
-
Confocal microscope
-
MetaFluor software (ver. 7.10.4.450; Molecular Devices) and/or ImageJ (ver. 2.3.0; NIH)
-
Additional reagents and equipment for preparing plasmids of SIMBA imaging components (see Basic Protocol 1) for gene locus visualization (see Table 4)
SIMBA only | SIMBA-BiFC orthogonal imaging |
---|---|
dCas9-24×SunTag | dCas9-24×SunTag-P2A-BFP |
scFv-FKBP | sgRNA-MS2-PP7 |
FRB-mCherry-HP1α | scFv-FKBP |
sgRNA | FRB-mCherry-HP1α |
MCP-N-Venus | |
PCP-C-Venus |
NOTE : All solutions and equipment coming into contact with cells must be sterile, and proper sterile technique should be used accordingly.
NOTE : All culture incubations are performed in a humidified 37°C, 5% CO2 incubator unless otherwise specified.
1.Prepare the plasmids of SIMBA imaging components for gene locus visualization for transfection (see Table 4): dCas9-24×SunTag, sgRNA-encoding plasmid targeting the locus of interest, the binding unit scFv-FKBP, and the reporter unit FRB-mCherry-HP1α.
2.One day before transfection, seed 0.2 × 106 HEK 293T cells per well onto 12-well plates to obtain 70% to 90% confluency by the time of transfection (no longer than 24 hr after cell seeding). Culture cells in complete DMEM in a humidified incubator at 37°C supplemented with 5% CO2.
3.Incubate overnight to reach 70% to 90% confluency for optimal transfection performance. Exchange medium with fresh medium before transfection.
4.Transfect the corresponding plasmids using Lipofectamine 3000 transfection reagent following the manufacturer's protocol. For transfection of one well of cells, prepare two 1.5-ml Eppendorf tubes separately: (a) 50 μl Opti-MEM medium with 1.5 μl Lipofectamine 3000 reagent and (b) 50 μl Opti-MEM with plasmid to transfect and P3000 reagent at a ratio of 1:2 (w/v), i.e., 2 μl P3000 reagent per 1 µg plasmid. Mix contents of tube (a) with contents of tube (b) and incubate for 10 min at room temperature. Then, add the mixture to the culture well.
5.Seed the transfected cells onto glass-bottom dishes (one well per dish in complete DMEM) coated with fibronectin at 24 hr after transfection.
6.Add 100 μM rapamycin stock to medium to a final concentration of 100 nM at 2 hr before imaging. Add the same amount of DMSO to the cells of control group. At 36 to 48 hr after transfection, switch medium to live-cell imaging solution without phenol red for best imaging quality and image the cells using a confocal microscope.
7.Use MetaFluor software and/or ImageJ for imaging data analysis. Spot SIMBA puncta manually to determine their average intensity. Quantify background signals from regions within the nucleus outside of the puncta area and the nucleolus, allowing measurement of the average background intensity and standard deviation.
8.Determine the SNRs by dividing the corrected SIMBA puncta signal (average SIMBA puncta intensity subtracted mean background intensity) by the standard deviation of the background signal. Utilize the designated SNR value of 10 as a threshold for identifying specific SIMBA puncta in response to rapamycin induction.
Basic Protocol 3: GENERATION OF SIMBA-EXPRESSING STABLE CELL LINES
Generating a stable cell line expressing the desired components can provide stable expression and induction levels for further imaging and analysis. In this protocol, we demonstrate that human U2OS cells can be transduced by lentiviral vectors encoding the SIMBA components to generate stable cell lines, which can be verified by fluorescence microscopy imaging and/or flow cytometry.
Additional Materials (also see Basic Protocol 2)
-
Plasmids:
- SIMBA system component constructs: 1) pHR-dCas9-8×SunTag-P2A-EGFP, 2) pHR-scFv-FKBP-T2A-FRB-mCherry-HP1α, and 3) pLH-sgRNA construct (refer to Basic Protocol 1 for detailed construct information)
- pCMV-VSV-G (Addgene, #8454)
- pCMV ΔR8.2 (Addgene, #12263)
-
ProFection® Mammalian Transfection System (Promega, E1200)
-
Clontech Lenti-X Concentrator (Takara, 631232)
-
U2OS cells (ATCC®, HTB-96)
-
Complete McCoy's 5A (Modified) Medium (see recipe)
-
10 mg/ml polybrene (Biosharp, BL628A)
-
10-cm cell culture dishes
-
0.45-μm low-binding filter
-
FACS sorter (SONY SH800S)
-
Additional reagents and equipment for cell passaging (see step 6, annotation)
Lentivirus production
1.Day 0: Seed 5 × 106 HEK 293T cells in 10 ml complete DMEM in each 10-cm cell culture dish 1 day before transfection. Culture cells in a humidified incubator at 37°C supplemented with 5% CO2.
2.Day 1: Ensure that cells cultured overnight have reached ∼80% confluency for optimal transfection performance. Exchange medium with fresh culture medium at 3 hr before transfection. Then, transfect the cells with the following plasmids: 5 μg pCMV-VSV-G, 5 μg pCMV ΔR8.2, and 10 μg of the SIMBA lentiviral plasmids (dCas9-8×SunTag-P2A-EGFP, scFv-FKBP-T2A-FRB-mCherry-HP1α, sgRNA). Perform transfection using ProFection® Mammalian Transfection System following the manufacturer's protocol.
3.Day 3: Prepare for lentivirus collection at 48 hr after transfection by collecting supernatant containing lentivirus and filtering through a 0.45-μm low-binding filter to remove any residue cell debris. Concentrate the virus using Clontech Lenti-X Concentrator following the manufacturer's protocol. Immediately store the concentrated virus at −80°C in single-use aliquots in 1.5-ml Eppendorf tubes (∼10 μl/aliquot).
Cell line generation
4.Seed U2OS cells in complete McCoy's 5A (Modified) Medium into 10-cm culture dishes (2 × 106 cells in 10 ml medium per dish) or 12-well plates (0.2 × 106 cells in 1 ml medium per well) and culture in humidified incubator at 37°C with 5% CO2 to an optimal confluence (about 50% to 70%) for infection. Exchange medium with fresh medium before virus infection.
5.For lentivirus infection, pre-mix virus with 4 µg/ml polybrene (from 10 mg/ml stock). Then, dilute 10 μl SIMBA virus from step 3 10-fold in complete DMEM and add to cells. Infect the cells for 24 hr.
6.Sort the SIMBA-transduced cells (mCherry-positive) using a FACS sorter 3 days post-infection. Passage the stable cell lines at least six times after sorting before rapamycin induction for experiments (see step 7).
Imaging using SIMBA stable cell lines
7.Seed the stable cell line in 1 ml medium in a glass-bottom dish coated with fibronectin and prepare for imaging with 100 nM rapamycin induction 6 hr in advance. Add the same amount of DMSO to the cells of control group.
8.Image cells using a confocal microscope and analyze as in Basic Protocol 2, steps 7 and 8.
Basic Protocol 4: MANIPULATION OF GENOMIC LOCI USING SIMBA
The SIMBA system allows genomic loci labeling and manipulation via the BAs. At its core, the system comprises two fundamental units: the dCas9-sgRNA complex as the targeting unit and HP1α as the functional unit. By custom-designing for specific genomic loci, the system allows for straightforward substitution of sgRNA, thereby achieving precise labeling for different gene loci. Further characterization of target gene transcription, often carried out through quantitative PCR (qPCR), unveils the transcriptional status of the labeled gene within its genomic context.
For the simultaneous labeling of multiple genomic loci, the SIMBA system offers strategies such as parallel labeling at multiple sites by mixing sgRNA that targets different loci. Moreover, the system is useful to directly manipulate transcription at specific sites, leveraging downstream genes affected by transcription factors, which facilitates comprehensive analysis of transcription factor impact on gene regulation across the entire genome, offering profound insights into the intricacies of gene regulatory networks.
Another key feature of the system is the capability to replace HP1α with other IDR sequences, thereby achieving phase separation effects without necessitating chromatin condensation. This could enhance our understanding of gene distribution and interactions within three-dimensional chromatin space. Therefore, the SIMBA system stands as a versatile tool empowering researchers in gene tracing and manipulation studies.
Additional Materials (also see Basic Protocols 1 and 2)
-
Plasmids:
- All-in-one construct of dCas9-8×SunTag-P2A-scFv-FRB-T2A-FKBP-mCherry-HP1α
- Target sgRNA expressing vector
- NFAT1-8×SunTag-P2A-scFv-FRB-T2A-FKBP-mCherry-HP1α
-
RNeasy Mini Kit (Qiagen, 74104)
-
SuperScript IV Reverse Transcriptase (Invitrogen, 18090050)
-
SYBR Green Mix (Bio-Rad, 1725125)
-
24-well plates
-
Additional reagents and equipment for qPCR and RNA-seq
Part 1: Gene manipulation at specific loci on chromosome
1.Seed HEK 293T cells with good viability in 12-well plates at 0.2 × 106 cells in 1 ml medium per well. Incubate until about 70% to 80% confluent and then exchange medium with fresh medium for transfection preparation.
2.Transfect cells with 100 ng dCas9-8×SunTag-P2A-scFv-FKBP-T2A-FRB-mCherry-HP1α and 250 ng corresponding sgRNAs with Lipofectamine 3000 reagent following the manufacturer's protocol.
3.Incubate the cells in humidified cell culture incubator at 37°C supplemented with 5% CO2 for 36 to 48 hr.
4.Collect the cell sample, sort out mCherry-positive cells by FACS, and re-seed them in 500 μl culture medium in 24-well plates.
5.Incubate the mCherry-positive cells to optical confluence and then treat with 100 nM rapamycin to induce BA formation.
6.Six hours after rapamycin induction, extract total RNA from cell sample using RNeasy Mini Kit following the manufacturer's protocol.
7.Once fresh total RNA sample is acquired, perform reverse transcription PCR using SuperScript IV Reverse Transcriptase following the manufacturer's protocol.
8.Design and obtain qPCR primers for the gene targets based on previous publications or database. Perform qPCR on cDNA samples using SYBR Green Mix. Use beta-actin (ACTB) as an internal control for qPCR analysis.
9.Analyze qPCR results and evaluate the manipulation efficiency of SIMBA system.
Part 2: Gene manipulation by using NFAT1 transcription factor as targeting unit for puncta formation
10.Transfect cells with 100 ng all-in-one construct of NFAT1-8×SunTag-P2A-scFv-FKBP-T2A-FRB-mCherry-HP1α or NFAT1-8×SunTag-P2A-scFv-FKBP-T2A-FRB-mCherry with Lipofectamine 3000 reagent following the manufacturer's protocol.
11.Incubate the cells in humidified culture incubator at 37°C with 5% CO2 for 36 to 48 hr.
12.Collect the cell sample and sort out mCherry-positive cells with FACS sorter.
13.Re-seed the sorted cells into a 24-well plate with 500 μl growth medium.
14.Incubate the mCherry-positive cells to optimal confluency and treat cells with 100 nM (final concentration) rapamycin to induce BA formation.
15.Harvest the cells at 6 hr after rapamycin induction. Extract total RNA from cell sample using RNeasy Mini Kit by following the manufacturer's protocol.
16.Send collected RNA samples for RNA-seq (including library preparation and next-generation sequencing).
17.Analyze RNA-seq data for gene expression level change resulting from SIMBA puncta formation (Fig. 3).
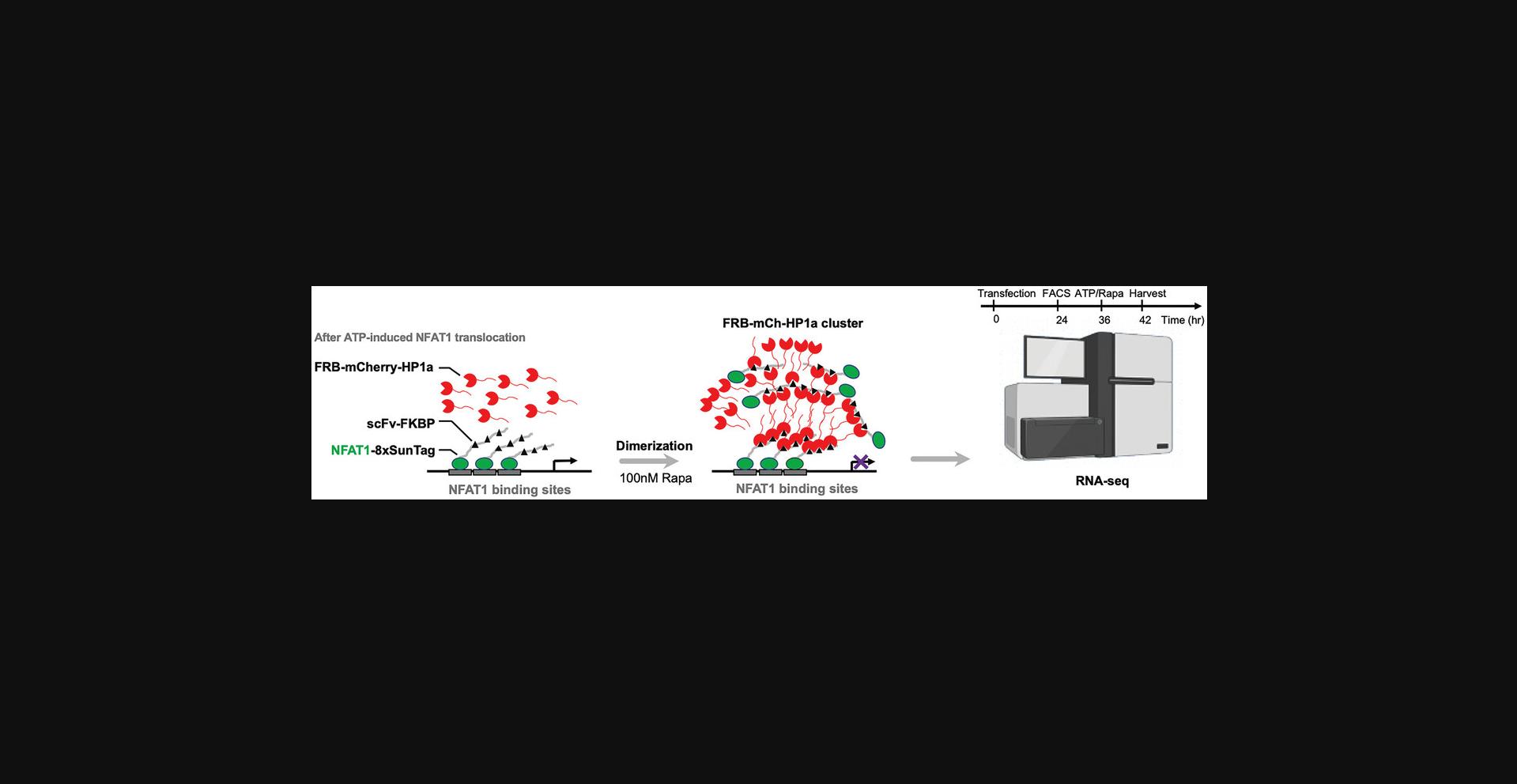
REAGENTS AND SOLUTIONS
1× ampicillin LB agar plates
- 5 g yeast extract
- 10 g tryptone
- 10 g sodium chloride (NaCl)
- 15 g agar
- Dissolve in deionized H2O with heating
- Adjust pH to 7.0 using NaOH
- Deionized H2O to 1 L
- Autoclave to sterilize
- When cooled to <60°C, add 1000× ampicillin stock (see recipe) to 1×
- Pour into culture plates and allow to solidify
- Store ≤1 month at 4°C
1× ampicillin LB culture medium
- 5 g yeast extract
- 10 g tryptone
- 10 g sodium chloride (NaCl)
- Dissolve in deionized H2O
- Adjust pH to 7.0 using NaOH
- Deionized H2O to 1 L
- Autoclave to sterilize
- When cooled to <60°C, add 1000× ampicillin stock (see recipe) to 1×
- Store short-term at room temperature and store ≤2 months at 4°C
Ampicillin stock, 1000×
- 1 g ampicillin sulfate powder
- Milli-Q H2O to 10 ml
- Shake until ampicillin completely dissolves
- Sterilize with 0.22-μm filters
- Store ≤6 months at −20°C
Complete DMEM
- Dulbecco's Modified Eagle's Medium (DMEM Gibco, 11995065)
- 10% (v/v) heat-inactivated FBS (Gibco, 10099141C)
- 100 U/ml penicillin/streptomycin (10,000 U/ml stock; Gibco, 15140122)
- Store ≤1 month at 4°C
Complete McCoy's 5A (Modified) Medium
- Complete McCoy's 5A (Modified) Medium (Gibco, 16600082)
- 10% (v/v) heat-inactivated FBS (Gibco, 10099141C)
- 2 mM L-glutamine
- 100 U/ml penicillin/streptomycin (10,000 U/ml stock; Gibco, 15140122)
- Store ≤1 month at 4°C
Rapamycin stock, 100 μM
Dissolve rapamycin powder in DMSO to a concentration of 100 μM (1000× stock). Dispense in aliquots and store ≤3 months at −20°C. Avoid repeated freeze-thaw cycles.
COMMENTARY
Background Information
Emerging CRISPR-Cas9 systems
The advent of CRISPR-Cas9 technology has sparked a revolution in gene editing, providing researchers with a powerful tool for precise genetic modifications (Doudna & Charpentier, 2014). CRISPR-Cas9, initially harnessed from the bacterial immune system (Jinek et al., 2012), has been ingeniously repurposed to facilitate targeted DNA cleavage and subsequent repair processes (Cofsky et al., 2022; Jinek et al., 2014; O'Connell et al., 2014). This revolutionary technique enables the manipulation of specific genetic sequences, holding immense promise for therapeutic applications, disease modeling, and fundamental biological research.
In parallel, the emergence of the catalytically inactive Cas9 mutant, known as dCas9, has expanded the toolkit of genetic manipulation strategies (Gilbert et al., 2013; Lebar et al., 2020; Zalatan et al., 2015). Unlike its active counterpart, dCas9 lacks endonuclease activity, rendering it incapable of inducing DNA double-strand breaks (Gilbert et al., 2013). However, this deficiency has proven to be its strength, as dCas9 can be tailored for locus-specific targeting without altering the DNA sequence. By utilizing dCas9 fused with effector domains or reporter molecules, the system allows for precise localization and dynamic monitoring of genomic loci (Lebar et al., 2020; Zalatan et al., 2015). The synergy of dCas9 with these functional moieties has laid the foundation for the SIMBA system, which combines precise targeting with real-time visualization, opening up new avenues for exploring gene function within living cells.
Phase separation and biomolecule assembly in cells and their functions
Recent advancements in cell biology have unveiled the phenomenon of phase separation and BA within cells (Lafontaine et al., 2021; Rippe, 2022). Intracellular condensates, characterized by the dynamic aggregation of biomolecules, play pivotal roles in orchestrating various cellular processes (Lu et al., 2018; Strom et al., 2017). These liquid-liquid phase-separated compartments enable the spatial organization of cellular components, offering a dynamic platform for biochemical reactions and signaling cascades.
Phase separation is driven by multivalent interactions between intrinsically disordered protein domains, RNA molecules, and other cellular components (Lu et al., 2018; Sanulli et al., 2019; Schilling et al., 2021). These interactions generate liquid-like compartments, facilitating the concentration and sequestration of molecules, thereby enhancing reaction efficiency and specificity. Examples of phase-separated compartments include stress granules, nucleoli, and P bodies, each fulfilling distinct cellular functions (Riback et al., 2020; Yang et al., 2020). Importantly, the recognition of phase separation's role in cellular processes has expanded our understanding of gene regulation, protein homeostasis, and the stress response.
SIMBA system
The SIMBA (Simultaneous Imaging and Manipulation with Biomolecular Assemblies) system represents a remarkable integration of CRISPR-Cas9 technology, phase separation principles, and BA dynamics (Peng et al., 2022). At its core, the SIMBA system leverages the dCas9-SunTag fusion to achieve precise locus-specific targeting. The FKBP-mCherry-HP1α reporting unit enables the visualization and manipulation of targeted genomic loci, whereas the scFv-FRB serves as a bridging component to allow the assembly of the system in response to rapamycin, triggering dynamic gene manipulation events.
To establish the SIMBA system, several key steps are involved. First, the dCas9-SunTag construct is generated and validated through cloning and functional assays. The FRB-mCherry-HP1α fusion is then introduced, enabling fluorescence-based readouts of gene-targeting events, as well as HP1α-mediated transcriptional manipulation. The scFv-FKBP bridge is incorporated, ensuring the inducible assembly of biomolecules upon rapamycin treatment. The culmination of these components creates a synergistic platform that merges live-cell imaging with precise gene manipulation.
One of the remarkable features that distinguish the SIMBA system is its inherent modular design, offering the flexibility to tailor both the targeting and the sensing units to address specific experimental requirements. This modular architecture empowers researchers to customize the system according to their investigational needs, thereby expanding its applicability and versatility. For instance, the targeting unit within the SIMBA system can be seamlessly substituted with different molecular entities to achieve locus-specific gene manipulation. A prime illustration of this flexibility is the integration of transcription factors like NFAT1.Leveraging NFAT1's inherent binding specificity, it becomes possible to precisely target downstream genomic loci and exert a controlled influence on gene expression patterns. This ability to introduce different targeting modules not only demonstrates the versatility of the SIMBA system but also opens avenues for probing intricate gene regulatory networks within living cells.
Moreover, the underlying strength that magnifies the signal of low-repeat or single-copy loci within the SIMBA system is the orchestrated assembly of HP1α, a component facilitated by the phenomenon of phase separation. This remarkable effect enhances the localization and concentration of HP1α, leading to an amplified readout at specific genomic sites. By harnessing phase separation, the SIMBA system transcends the limitations of low-repeat or single-repeat regions, enabling precise investigations even in challenging genetic contexts. Furthermore, the potential utilization of IDRs, such as the commonly used FUS protein, introduces an additional layer of adaptability to the SIMBA system. As demonstrated in our experiments, the incorporation of FUSN as part of the system showcases its compatibility without inducing disruptive chromatin remodeling.
In essence, the SIMBA system represents a paradigm shift in live-cell imaging and gene manipulation. Its unique fusion of theoretical underpinnings and practical implementation unlocks a new dimension of cellular exploration, as well as promising transformative insights into the complexity of living systems.
Critical Parameters
The SIMBA system approach includes the following basic requirements for DNA cloning, lentivirus packaging, cell transfection, and puncta induction for imaging and manipulation.
For basic cloning of the constructs for SIMBA system delivery, the following need to be considered:
- 1.DNA quality and storage: High-quality template DNA or fragment of cassettes and vector backbone is crucial for effective construct assembly. Store DNA from clones of varying insert sizes at −20°C to preserve its integrity.
- 2.Clone verification: Selecting 2 to 3 colonies from each recombineering reaction enhances the identification of correct clones. Validate using restriction enzyme DNA fingerprinting for robust confirmation.
- 3.Enzyme selection: Opt for efficient and cost-effective restriction enzymes for confirming recombineering outcomes. Compare with unmodified clones and perform parallel digestions for accurate verification.
- 4.Gel electrophoresis and uncut DNA: Employ agarose gel electrophoresis with uncut DNA samples alongside plasmid digestions.
Beyond the realm of DNA recombination, achieving efficient delivery of recombinant DNA into target cells stands as another pivotal facet of the experimental process. This critical step encompasses both transient transfections facilitated by Lipofectamine 3000 and stable transfection via lentiviral vectors, each requiring distinct considerations to optimize successful gene transfer.
In the case of transient transfection utilizing Lipofectamine 3000, several key parameters profoundly impact the process. The choice of transfection reagent concentration, DNA-to-reagent ratio, and cell density during transfection significantly influences transfection efficiency. Optimization of these factors ensures optimal DNA uptake and expression. Moreover, the duration of transfection plays a crucial role; a balance must be struck to achieve sufficient gene expression without inducing cellular stress. Co-transfection with reporter genes aids in monitoring transfection efficiency and facilitating downstream analyses.
Alternatively, stable transfection through lentiviral vectors offers a more enduring genetic modification. Critical parameters here include titration of lentiviral particles to determine the optimal multiplicity of infection (MOI) for target cells, ensuring effective gene delivery without inducing cytotoxic effects. The selection of appropriate selection markers, such as antibiotic resistance or fluorescence, is vital for isolating and maintaining cells with stable transgene expression. Proper timing of selection pressure application and proper maintenance duration guarantee robust establishment of stable transfectants.
In both transient and stable transfection approaches, the choice of target cell type is paramount. Considerations encompassing cell proliferation rate, susceptibility to transfection, and compatibility with downstream applications dictate the most suitable cellular context for the experiment. Maintenance of consistent cell culture conditions, including medium composition, temperature, and CO2 levels, further ensures reproducibility and reliability across experiments.
The key for best imaging and gene manipulation after successful delivery of the SIMBA system is the induction of particle assembly by rapamycin. Thorough gene expression monitoring, transfection validation, and downstream assays validate the relevance of introduced DNA. Effective delivery of recombinant DNA involves optimizing parameters like transfection reagents, ratios, cell density, duration, and selection markers. Precise target cell selection and post-transfection analysis ensure successful SIMBA puncta formation in response to rapamycin, aligning with the experiment's goal.
Troubleshooting
For a list of common problems with different aspects of the protocols, their causes, and potential solutions, please see Table 5.
Problem | Possible cause | Solution |
---|---|---|
Category: molecule cloning | ||
Low cloning efficiency | Nonspecific binding or low binding of primer sequence | Optimize primer design, including GC content and melting temperature (Tm) value, to the length of annealing sequence |
Inappropriate system setup | Change the program setup of thermocycler and consider alternative cloning methods if efficiency remains low | |
Category: transient transfection | ||
Low transfection efficiency | Reagent/plasmid ratio is not optimal | Optimize transfection reagents and conditions |
Low cell viability | Change the cell line and optimize cell culture conditions | |
Category: stable cell line generation | ||
Insufficient lentivirus packaging | Low viability of HEK 293T cell line | Check cell lineage and optimize cell culture conditions |
Inconsistent construct expression | Insufficient selection pressure | Increase selection pressure |
Low lentivirus titer | Check lentivirus packaging and the titer of lentivirus | |
Category: imaging and genomic locus manipulation | ||
Insufficient puncta formation | Insufficient response to rapamycin induction | Optimize the induction time and concentration of rapamycin |
Low labeling efficiency | Insufficient transfection | Check transfection efficiency |
Off-target effect of dCas9 complex | Check the sgRNA sequence |
Understanding Results
Our experimental design hinges on the foundational process of construct cloning (Basic Protocol 1), which serves as the linchpin of the SIMBA system's implementation. Cloning stands as the essential framework upon which the entire endeavor is built. Careful attention is paid to the nuances of each cloning step, employing high-fidelity enzymes to ensure accurate replication of genetic components. Rigorous validation methods, such as PCR and enzymatic fingerprinting, bolster the reliability of each clone, ultimately confirmed by Sanger DNA sequencing to ensure precision. The pivotal factor underpinning the effective utilization of the SIMBA system, as guided by Basic Protocols 2 to 4, revolves around the precise targeting of repetitive or non-repetitive sequences through the sensing unit of the dCas9-sgRNA complex or transcription factors such as NFAT1 aimed at its downstream genes. Additionally, the assembly of fluorescence molecules is triggered by the induction of rapamycin. The initial step involves the validation of dot formation using established methods to ensure robust SNR representation (Fig. 4).
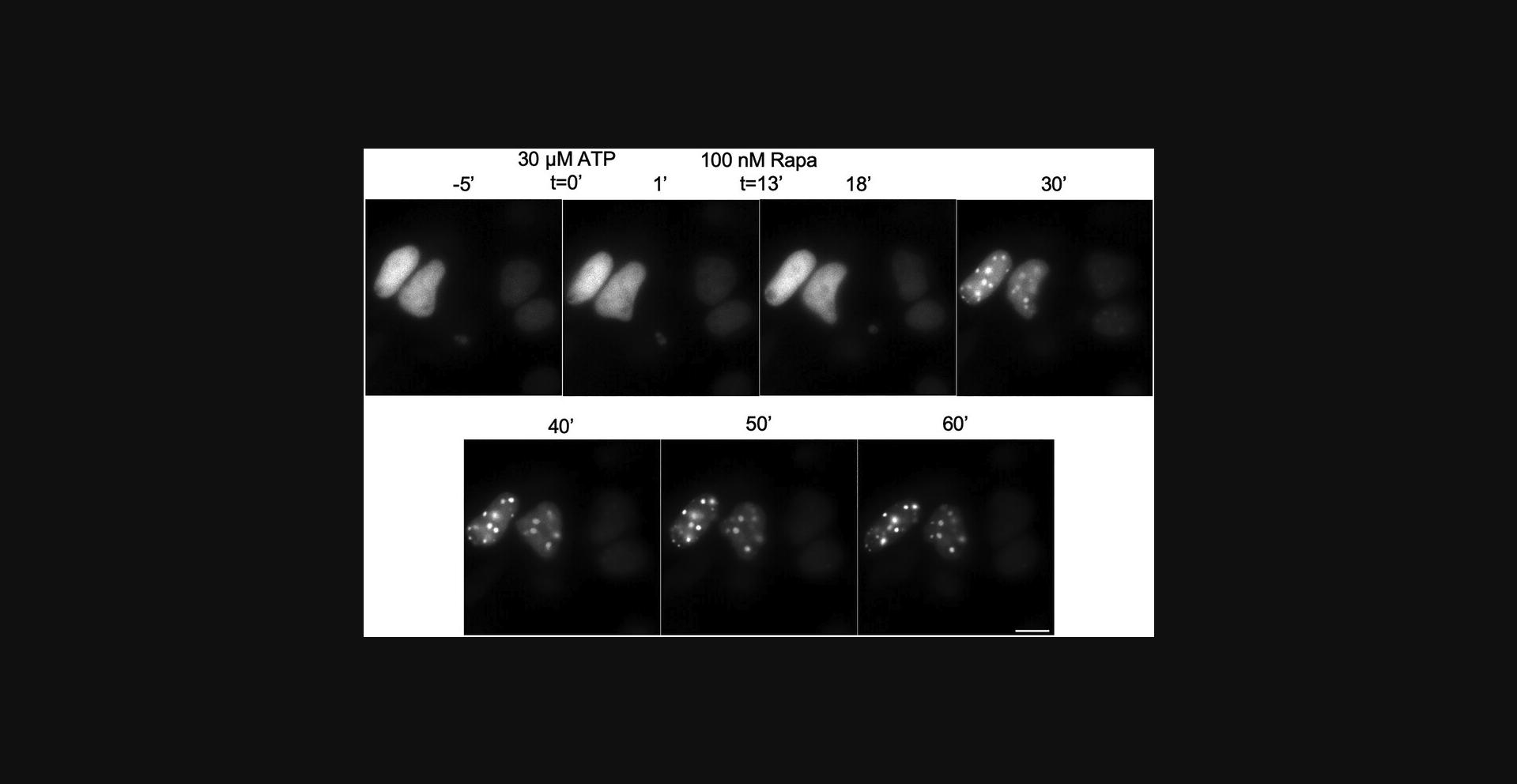
The formation of SIMBA puncta is readily observable following a brief, 30-min rapamycin induction. These puncta become visually evident under the lens of an epi-fluorescence microscope, providing a direct and tangible visualization of the underlying cellular processes. Once these distinctive dots materialize, their dynamic progression can be conveniently tracked (Fig. 5). This enables the real-time monitoring of their behavior, shedding light on the intricate dynamics of chromatin within the cellular milieu.
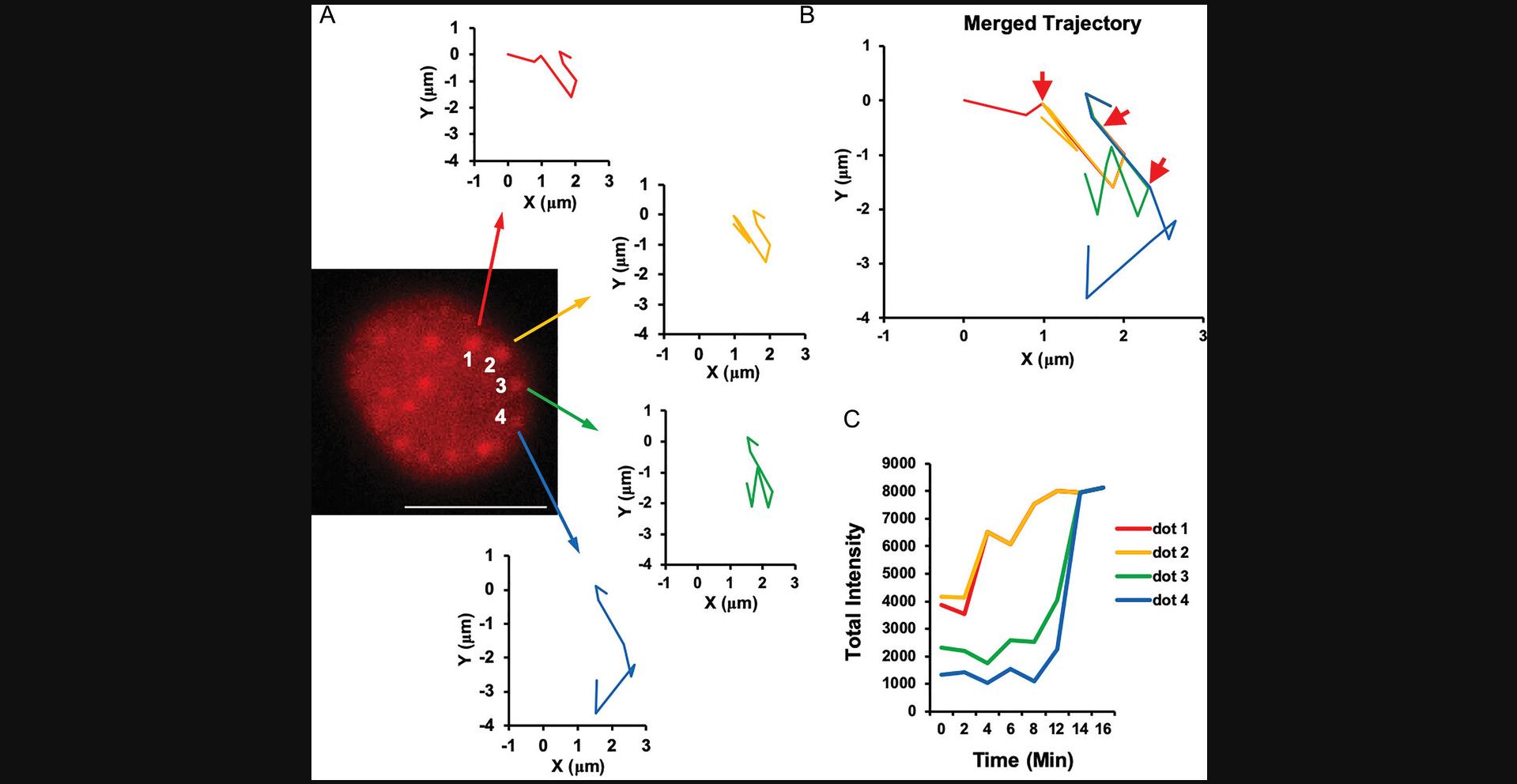
Following imaging validation, the potential for gene manipulation within the SIMBA system becomes evident. Utilizing conventional transformation and induction techniques, we can assess variations in transcription levels induced by different SIMBA strategies. Whether through HP1a-mediated chromatin rearrangement or the NFAT1 transcription factor's downstream gene network regulation, both single-gene and network-level modifications of cellular expression can be achieved. This underscores the versatility of the SIMBA system, enabling not only visualization but also controlled manipulation of cellular processes at both individual gene and network levels.
Time Considerations
Construct cloning
Upon receipt of the required primers and plasmids, the preparation of recombineering templates for construct cloning in Basic Protocol 1 can be accomplished within an estimated time frame of ∼5 days. This process unfolds as follows:
Day 1: PCR amplification is executed to generate recombineering cassettes for SIMBA puncta formation. Anneal the sgRNA oligos for Golden Gate Assembly.
Day 2: Either Gibson Assembly or Golden Gate Assembly is employed for the assembly of PCR-amplified recombineering cassettes. This involves chemical transformation of the assembly reaction and plating onto LB agar plates to generate individual colonies.
Day 3: The colonies obtained from the previous day are established on plates, and cultures are initiated to facilitate the subsequent isolation of plasmids encoding the recombineering templates.
Day 4: Plasmid isolation is carried out, followed by the verification of these plasmids through restriction enzyme DNA fingerprinting. Subsequently, the verified plasmids are submitted for Sanger DNA sequencing to ensure their accuracy and fidelity.
Day 5: Analysis of the Sanger DNA sequencing results is conducted, and recombineering templates are linearized to prepare them for subsequent usage.
Transient transfection
Upon acquisition of the requisite cell lines and transfection reagents, the process of transient cell transfection in Basic Protocol 2 unfolds over an estimated 3-day period:
Day 1: Cells are seeded in appropriate culture vessels to achieve optimal confluency for transfection. Transfection complexes are prepared by combining plasmids with transfection reagents and are subsequently added to the cells.
Day 2: The transfection process is allowed to proceed, enabling the introduction of various SIMBA components into the cells. This period also facilitates the establishment of gene expression and the necessary cellular responses.
Day 3: Post-transfection analysis is conducted, encompassing the assessment of gene expression, transfection efficiency, and other relevant parameters. This step confirms the successful delivery of recombinant DNA and sets the stage for subsequent experimentation.
Stable cell line generation
The creation of stable cell lines in Basic Protocol 3 spans an approximate duration of 3 to 4 weeks, depending on cell growth and selection dynamics:
Week 1: Cells are cultured and maintained, ensuring optimal growth and confluency. Transduction with lentiviral vectors containing the SIMBA system components is performed, initiating the integration of these elements into the host cell genome.
Week 2: Following transduction, cells undergo a selection process using appropriate markers or antibiotics. This step facilitates the enrichment of cells harboring the integrated SIMBA system components.
Weeks 3 to 4: Selected cells are expanded and characterized for stable integration and expression of the SIMBA system. Verification may involve gene expression analysis, fluorescence imaging, or other relevant assays. Successful stable cell line generation establishes a robust foundation for subsequent experimentation.
Evaluating gene manipulation effects with SIMBA system
Gene manipulation with the SIMBA system followed by RNA-seq or qPCR usually takes 1 week's time:
Days 1 to 2: Cells are seeded in culture vessels to achieve optimal confluency for subsequent transfection. Then, they are prepared for transfection.
Day 3: Post-transfection analysis is conducted, including the assessment of gene expression, transfection efficiency, and other relevant parameters.
This step confirms the successful delivery of recombinant DNA and sets the stage for subsequent experimentation.
Day 4: Positively expressing cells are sorted based on the established parameters from the post-transfection analysis. The sorting process ensures the isolation of cells expressing SIMBA system components.
Day 5 onward: RNA sample is extracted from cells and qPCR or RNA-seq analysis is performed. qPCR is performed to quantify gene expression levels, providing insights into the success of SIMBA system expression. If utilizing RNA-seq, library preparation is conducted. RNA-seq analysis is performed to comprehensively explore gene expression changes induced by the SIMBA system.
Acknowledgments
This work was financially supported by the National Natural Science Foundation of China (32100450 to Q. P.) and Guangdong Pearl River Talent Program (2021QN02Y781 to Q. P.).
Author Contributions
Yanwei Chen : Writing—original draft; writing—review and editing. Ziliang Huang : Methodology; validation; writing—review and editing. Qin Peng : Conceptualization; funding acquisition; methodology; project administration; supervision; writing—original draft; writing—review and editing. Yingxiao Wang : Conceptualization; funding acquisition; project administration; supervision; writing—review and editing.
Conflict of Interest
Y. W. is a scientific co-founder of Cell E&G Inc. and Acoustic Cell Therapy LLC. However, these financial interests did not affect the content of this article.
Open Research
Data Availability Statement
Data sharing is not applicable to this article as no new data were created or analyzed in this study.
Supporting Information
Filename | Description |
---|---|
cpz1947-sup-0001-SuppMat.docx15.7 KB | Annotation. |
Please note: The publisher is not responsible for the content or functionality of any supporting information supplied by the authors. Any queries (other than missing content) should be directed to the corresponding author for the article.
Literature Cited
- Brangwynne, C. P., Eckmann, C. R., Courson, D. S., Rybarska, A., Hoege, C., Gharakhani, J., Jülicher, F., & Hyman, A. A. (2009). Germline P granules are liquid droplets that localize by controlled dissolution/condensation. Science , 324(5935), 1729–1732. https://doi.org/10.1126/science.1172046
- Chaudhary, N., Nho, S. H., Cho, H., Gantumur, N., Ra, J. S., Myung, K., & Kim, H. (2020). Background-suppressed live visualization of genomic loci with an improved CRISPR system based on a split fluorophore. Genome Research , 30(9), 1306–1316. https://doi.org/10.1101/gr.260018.119
- Chen, B., Gilbert, L. A., Cimini, B. A., Schnitzbauer, J., Zhang, W., Li, G. W., Park, J., Blackburn, E. H., Weissman, J. S., Qi, L. S., & Huang, B. (2013). Dynamic imaging of genomic loci in living human cells by an optimized CRISPR/Cas system. Cell , 155(7), 1479–1491. https://doi.org/10.1016/j.cell.2013.12.001
- Cheng, A. W., Jillette, N., Lee, P., Plaskon, D., Fujiwara, Y., Wang, W., Taghbalout, A., & Wang, H. (2016). Casilio: A versatile CRISPR-Cas9-Pumilio hybrid for gene regulation and genomic labeling. Cell Research , 26(2), 254–257. https://doi.org/10.1038/cr.2016.3
- Cheutin, T., McNairn, A. J., Jenuwein, T., Gilbert, D. M., Singh, P. B., & Misteli, T. (2003). Maintenance of stable heterochromatin domains by dynamic HP1 binding. Science , 299(5607), 721–725. https://doi.org/10.1126/science.1078572
- Cofsky, J. C., Soczek, K. M., Knott, G. J., Nogales, E., & Doudna, J. A. (2022). CRISPR-Cas9 bends and twists DNA to read its sequence. Nature Structural & Molecular Biology, 29(4), 395–402. https://doi.org/10.1038/s41594-022-00756-0
- Doudna, J. A., & Charpentier, E. (2014). Genome editing. The new frontier of genome engineering with CRISPR-Cas9. Science , 346(6213), 1258096. https://doi.org/10.1126/science.1258096
- Frey, S., Richter, R. P., & Gorlich, D. (2006). FG-rich repeats of nuclear pore proteins form a three-dimensional meshwork with hydrogel-like properties. Science , 314(5800), 815–817. https://doi.org/10.1126/science.1132516
- Gilbert, L. A., Larson, M. H., Morsut, L., Liu, Z., Brar, G. A., Torres, S. E., Stern-Ginossar, N., Brandman, O., Whitehead, E. H., Doudna, J. A., Lim, W. A., Weissman, J. S., & Qi, L. S., & Qi, L. S. (2013). CRISPR-mediated modular RNA-guided regulation of transcription in eukaryotes. Cell , 154(2), 442–451. https://doi.org/10.1016/j.cell.2013.06.044
- Gu, B., Swigut, T., Spencley, A., Bauer, M. R., Chung, M., Meyer, T., & Wysocka, J. (2018). Transcription-coupled changes in nuclear mobility of mammalian cis-regulatory elements. Science , 359(6379), 1050–1055. https://doi.org/10.1126/science.aao3136
- Hong, Y., Lu, G., Duan, J., Liu, W., & Zhang, Y. (2018). Comparison and optimization of CRISPR/dCas9/gRNA genome-labeling systems for live cell imaging. Genome Biology , 19(1), 39. https://doi.org/10.1186/s13059-018-1413-5
- Jerkovic, I., & Cavalli, G. (2021). Understanding 3D genome organization by multidisciplinary methods. Nature Reviews Molecular Cell Biology , 22(8), 511–528. https://doi.org/10.1038/s41580-021-00362-w
- Jinek, M., Chylinski, K., Fonfara, I., Hauer, M., Doudna, J. A., & Charpentier, E. (2012). A programmable dual-RNA-guided DNA endonuclease in adaptive bacterial immunity. Science , 337(6096), 816–821. https://doi.org/10.1126/science.1225829
- Jinek, M., Jiang, F., Taylor, D. W., Sternberg, S. H., Kaya, E., Ma, E., Anders, C., Hauer, M., Zhou, K., Lin, S., Kaplan, M., Iavarone, A. T., Charpentier, E., Nogales, E., & Doudna, J. A. (2014). Structures of Cas9 endonucleases reveal RNA-mediated conformational activation. Science , 343(6176), 1247997. https://doi.org/10.1126/science.1247997
- Keenen, M. M., Brown, D., Brennan, L. D., Renger, R., Khoo, H., Carlson, C. R., Huang, B., Grill, S. W., Narlikar, G. J., & Redding, S. (2021). HP1 proteins compact DNA into mechanically and positionally stable phase separated domains. eLife , 10, e64563. https://doi.org/10.7554/eLife.64563
- Konermann, S., Brigham, M. D., Trevino, A. E., Joung, J., Abudayyeh, O. O., Barcena, C., Hsu, P. D., Habib, N., Gootenberg, J. S., Nishimasu, H., Nureki, O., & Zhang, F. (2015). Genome-scale transcriptional activation by an engineered CRISPR-Cas9 complex. Nature , 517(7536), 583–588. https://doi.org/10.1038/nature14136
- Krouwels, I. M., Wiesmeijer, K., Abraham, T. E., Molenaar, C., Verwoerd, N. P., Tanke, H. J., & Dirks, R. W. (2005). A glue for heterochromatin maintenance: Stable SUV39H1 binding to heterochromatin is reinforced by the SET domain. Journal of Cell Biology , 170(4), 537–549. https://doi.org/10.1083/jcb.200502154
- Lafontaine, D. L. J., Riback, J. A., Bascetin, R., & Brangwynne, C. P. (2021). The nucleolus as a multiphase liquid condensate. Nature Reviews Molecular Cell Biology , 22(3), 165–182. https://doi.org/10.1038/s41580-020-0272-6
- Larson, A. G., Elnatan, D., Keenen, M. M., Trnka, M. J., Johnston, J. B., Burlingame, A. L., Agard, D. A., Redding, S., & Narlikar, G. J. (2017). Liquid droplet formation by HP1alpha suggests a role for phase separation in heterochromatin. Nature , 547(7662), 236–240. https://doi.org/10.1038/nature22822
- Lebar, T., Lainscek, D., Merljak, E., Aupic, J., & Jerala, R. (2020). A tunable orthogonal coiled-coil interaction toolbox for engineering mammalian cells. Nature Chemical Biology , 16(5), 513–519. https://doi.org/10.1038/s41589-019-0443-y
- Liu, X., Zhang, Y., Chen, Y., Li, M., Zhou, F., Li, K., Cao, H., Ni, M., Liu, Y., Gu, Z., Dickerson, K. E., Xie, S., Hon, G. C., Xuan, Z., Zhang, M. Q., Shao, Z., & Xu, J. (2017). In situ capture of chromatin interactions by biotinylated dCas9. Cell , 170(5), 1028–1043.e1019. https://doi.org/10.1016/j.cell.2017.08.003
- Lu, H., Yu, D., Hansen, A. S., Ganguly, S., Liu, R., Heckert, A., Darzacq, X., & Zhou, Q. (2018). Phase-separation mechanism for C-terminal hyperphosphorylation of RNA polymerase II. Nature , 558(7709), 318–323. https://doi.org/10.1038/s41586-018-0174-3
- Ma, H., Tu, L. C., Naseri, A., Chung, Y. C., Grunwald, D., Zhang, S., & Pederson, T. (2018). CRISPR-Sirius: RNA scaffolds for signal amplification in genome imaging. Nature Methods , 15(11), 928–931. https://doi.org/10.1038/s41592-018-0174-0
- Ma, H., Tu, L. C., Naseri, A., Huisman, M., Zhang, S., Grunwald, D., & Pederson, T. (2016). Multiplexed labeling of genomic loci with dCas9 and engineered sgRNAs using CRISPRainbow. Nature Biotechnology , 34(5), 528–530. https://doi.org/10.1038/nbt.3526
- Manuelidis, L. (1985). Individual interphase chromosome domains revealed by in situ hybridization. Human Genetics , 71(4), 288–293. https://doi.org/10.1007/BF00388453
- Mao, S., Ying, Y., Wu, X., Krueger, C. J., & Chen, A. K. (2019). CRISPR/dual-FRET molecular beacon for sensitive live-cell imaging of non-repetitive genomic loci. Nucleic Acids Research , 47(20), e131. https://doi.org/10.1093/nar/gkz752
- O'Connell, M. R., Oakes, B. L., Sternberg, S. H., East-Seletsky, A., Kaplan, M., & Doudna, J. A. (2014). Programmable RNA recognition and cleavage by CRISPR/Cas9. Nature , 516(7530), 263–266. https://doi.org/10.1038/nature13769
- Peng, Q., Huang, Z., Sun, K., Liu, Y., Yoon, C. W., Harrison, R. E. S., Schmitt, D. L., Zhu, L., Wu, Y., Tasan, I., Zhao, H., Zhang, J., Zhong, S., Chien, S., & Wang, Y. (2022). Engineering inducible biomolecular assemblies for genome imaging and manipulation in living cells. Nature Communications , 13(1), 7933. https://doi.org/10.1038/s41467-022-35504-x
- Price, C. M. (1993). Fluorescence in situ hybridization. Blood Reviews , 7(2), 127–134. https://doi.org/10.1016/s0268-960x(05)80023-2
- Qi, Y., & Zhang, B. (2021). Chromatin network retards nucleoli coalescence. Nature Communications , 12(1), 6824. https://doi.org/10.1038/s41467-021-27123-9
- Qin, P., Parlak, M., Kuscu, C., Bandaria, J., Mir, M., Szlachta, K., Singh, R., Darzacq, X., Yildiz, A., & Adli, M. (2017). Live cell imaging of low- and non-repetitive chromosome loci using CRISPR-Cas9. Nature Communications , 8, 14725. https://doi.org/10.1038/ncomms14725
- Riback, J. A., Zhu, L., Ferrolino, M. C., Tolbert, M., Mitrea, D. M., Sanders, D. W., Wei, M. T., Kriwacki, R. W., & Brangwynne, C. P. (2020). Composition-dependent thermodynamics of intracellular phase separation. Nature , 581(7807), 209–214. https://doi.org/10.1038/s41586-020-2256-2
- Rippe, K. (2022). Liquid-liquid phase separation in chromatin. Cold Spring Harbor Perspectives in Biology , 14(2), a040683. https://doi.org/10.1101/cshperspect.a040683
- Sanulli, S., Trnka, M. J., Dharmarajan, V., Tibble, R. W., Pascal, B. D., Burlingame, A. L., Griffin, P. R., Gross, J. D., & Narlikar, G. J. (2019). HP1 reshapes nucleosome core to promote phase separation of heterochromatin. Nature , 575(7782), 390–394. https://doi.org/10.1038/s41586-019-1669-2
- Schilling, M., Prusty, A. B., Boysen, B., Oppermann, F. S., Riedel, Y. L., Husedzinovic, A., Rasouli, H., König, A., Ramanathan, P., Reymann, J., Erfle, H., Daub, H., Fischer, U., & Gruss, O. J. (2021). TOR signaling regulates liquid phase separation of the SMN complex governing snRNP biogenesis. Cell Reports , 35(12), 109277. https://doi.org/10.1016/j.celrep.2021.109277
- Schotta, G., Ebert, A., Krauss, V., Fischer, A., Hoffmann, J., Rea, S., Jenuwein, T., Dorn, R., & Reuter, G. (2002). Central role of Drosophila SU(VAR)3-9 in histone H3-K9 methylation and heterochromatic gene silencing. EMBO Journal , 21(5), 1121–1131. https://doi.org/10.1093/emboj/21.5.1121
- Schultz, D. C., Ayyanathan, K., Negorev, D., Maul, G. G., & Rauscher, F. J. 3rd. (2002). SETDB1: A novel KAP-1-associated histone H3, lysine 9-specific methyltransferase that contributes to HP1-mediated silencing of euchromatic genes by KRAB zinc-finger proteins. Genes & Development, 16(8), 919–932. https://doi.org/10.1101/gad.973302
- Shao, S., Chang, L., Sun, Y., Hou, Y., Fan, X., & Sun, Y. (2018). Multiplexed sgRNA expression allows versatile single nonrepetitive DNA labeling and endogenous gene regulation. ACS Synthetic Biology , 7(1), 176–186. https://doi.org/10.1021/acssynbio.7b00268
- Strickfaden, H., Tolsma, T. O., Sharma, A., Underhill, D. A., Hansen, J. C., & Hendzel, M. J. (2020). Condensed chromatin behaves like a solid on the mesoscale in vitro and in living cells. Cell , 183(7), 1772–1784.e1713. https://doi.org/10.1016/j.cell.2020.11.027
- Strom, A. R., Emelyanov, A. V., Mir, M., Fyodorov, D. V., Darzacq, X., & Karpen, G. H. (2017). Phase separation drives heterochromatin domain formation. Nature , 547(7662), 241–245. https://doi.org/10.1038/nature22989
- Wang, H., Nakamura, M., Abbott, T. R., Zhao, D., Luo, K., Yu, C., Nguyen, C. M., Lo, A., Daley, T. P., La Russa, M., Liu, Y., & Qi, L. S. (2019). CRISPR-mediated live imaging of genome editing and transcription. Science , 365(6459), 1301–1305. https://doi.org/10.1126/science.aax7852
- Wang, J., Jia, S. T., & Jia, S. (2016). New insights into the regulation of heterochromatin. Trends in Genetics , 32(5), 284–294. https://doi.org/10.1016/j.tig.2016.02.005
- Wang, L., Hu, M., Zuo, M. Q., Zhao, J., Wu, D., Huang, L., Wen, Y., Li, Y., Chen, P., Bao, X., Dong, M. Q., Li, G., & Li, P. (2020). Rett syndrome-causing mutations compromise MeCP2-mediated liquid-liquid phase separation of chromatin. Cell Research , 30(5), 393–407. https://doi.org/10.1038/s41422-020-0288-7
- Wang, Y., Ratna, P., & Shivashankar, G. V. (2017). Superresolution imaging of nanoscale chromosome contacts. Scientific Reports , 7, 42422. https://doi.org/10.1038/srep42422
- Wu, J., Huang, B., Chen, H., Yin, Q., Liu, Y., Xiang, Y., Zhang, B., Liu, B., Wang, Q., Xia, W., Li, W., Li, Y., Ma, J., Peng, X., Zheng, H., Ming, J., Zhang, W., Zhang, J., Tian, G., … Xie, W. (2016). The landscape of accessible chromatin in mammalian preimplantation embryos. Nature , 534(7609), 652–657. https://doi.org/10.1038/nature18606
- Yang, P., Mathieu, C., Kolaitis, R. M., Zhang, P., Messing, J., Yurtsever, U., Yang, Z., Wu, J., Li, Y., Pan, Q., Yu, J., Martin, E. W., Mittag, T., Kim, H. J., & Taylor, J. P. (2020). G3BP1 Is a tunable switch that triggers phase separation to assemble stress granules. Cell , 181(2), 325–345.e328. https://doi.org/10.1016/j.cell.2020.03.046
- Ye, H., Rong, Z., & Lin, Y. (2017). Live cell imaging of genomic loci using dCas9-SunTag system and a bright fluorescent protein. Protein Cell , 8(11), 853–855. https://doi.org/10.1007/s13238-017-0460-0
- Zalatan, J. G., Lee, M. E., Almeida, R., Gilbert, L. A., Whitehead, E. H., La Russa, M., Tsai, J. C., Weissman, J. S., Dueber, J. E., Qi, L. S., & Lim, W. A. (2015). Engineering complex synthetic transcriptional programs with CRISPR RNA scaffolds. Cell , 160(1–2), 339–350. https://doi.org/10.1016/j.cell.2014.11.052