Repli-seq Sample Preparation using Cell Sorting with Cell-Permeant Dyes
Silvia Meyer-Nava, Silvia Meyer-Nava, Anala V. Shetty, Anala V. Shetty, Juan Carlos Rivera-Mulia, Juan Carlos Rivera-Mulia
Abstract
Replication timing is significantly correlated with gene expression and chromatin organization, changes dynamically during cell differentiation, and is altered in diseased states. Genome-wide analysis of replication timing is performed in actively replicating cells by Repli-seq. Current methods for Repli-seq require cells to be fixed in large numbers. This is a barrier for sample types that are sensitive to fixation or are in very limited numbers. In this article, we outline optimized methods to process live cells and intact nuclei for Repli-seq. Our protocol enables the processing of a smaller number of cells per sample and reduces processing time and sample loss while obtaining high-quality data. Further, for samples that tend to form clumps and are difficult to dissociate into a single-cell suspension, we also outline methods for isolation, staining, and processing of nuclei for Repli-seq. The Repli-seq data obtained from live cells and intact nuclei are comparable to those obtained from the standard protocols. © 2023 The Authors. Current Protocols published by Wiley Periodicals LLC.
Basic Protocol : Live cell isolation and staining
Alternate Protocol : Nuclei isolation and staining
INTRODUCTION
All eukaryotic organisms duplicate their DNA in a specific temporal order; this program is known as DNA replication timing (RT) (Rivera-Mulia & Gilbert, 2016a, 2016b). RT is a robust cell type-specific chromatin feature (Rivera-Mulia et al., 2015) and is highly conserved across species (Massey & Koren, 2022; Solovei et al., 2016; Yang et al., 2018). Moreover, RT correlates with important genomic features, such as transcription (Liu et al., 2021; Rivera-Mulia & Gilbert, 2016; Rivera-Mulia et al., 2019; Sarni et al., 2020;), chromatin states (Klein et al., 2021; Pope et al., 2014; Poulet et al., 2019; Ryba et al., 2010), genome evolution (Bracci et al., 2023), and is misregulated in cancer and disease (Rivera-Mulia et al., 2017; Rivera-Mulia et al., 2019; Rivera-Mulia et al., 2022; Sasaki et al., 2017; Zhang et al., 2020), and during replication stress (Kodali et al., 2022). Thus, RT constitutes a measurable functional readout of large-scale chromatin organization that can be exploited to detect disease-related alterations in genomic function (Rivera-Mulia et al., 2017).
The development of several RT profiling techniques, such as Repli-chip and Repli-seq that map nascent DNA molecules and DNA copy number-based strategies, enabled genome-wide evaluation of the temporal replication program (Farkash-Amar et al., 2008; Hiratani et al., 2008; Hiratani et al., 2010; Hulke et al., 2020; MacAlpine et al., 2004; Marchal et al., 2018; Rivera-Mulia et al., 2022; Ryba et al., 2010; Ryba et al., 2011; Schübeler et al., 2002; Zhao et al., 2020). Repli-seq is the current standard method to assess RT genome-wide by next-generation sequencing (Marchal et al., 2018; Rivera-Mulia et al., 2022). In this method nucleotide analogs, such as BrdU, are introduced into cell media and are used to mark nascent DNA during replication. Subsequently, early and late S-phase cell populations are separated by flow cytometry and the nascent replicated DNA is purified and sequenced (Hansen et al., 2010; Marchal et al., 2018; Rivera-Mulia et al., 2022). This technique is reliable and reproducible, but one of the major drawbacks is the need for high numbers of growing cells (Marchal et al., 2018; Rivera-Mulia et al., 2022; Zhao et al., 2020). Moreover, it can be difficult to process uncommon, limited samples, such as those derived from diseased patients, from which typically only a small percentage of cells are proliferating (Rivera-Mulia et al., 2018, 2019, 2022). In addition, the standard sample preparation protocol requires cell fixation in ethanol, which leads to a significant sample loss. Thus, improved sample preparation methods are necessary to facilitate the analysis of the temporal order of DNA replication as well as its links to gene regulation.
Recently, we optimized distinct steps of the Repli-seq method to enable the analysis of samples with limited amounts of cells (Rivera-Mulia et al., 2022). These improvements include distinct changes aimed at avoiding sample loss during cell lysis, DNA purification, and library preparation for next-generation sequencing, by sorting the sample directly into lysis buffer and performing DNA fragmentation and library preparation in a single tube. However, the standard sample preparation continues to be a limiting step, as typically 25% to 60% of the cells are lost after the fixation step. Here, we have developed an improved sample preparation protocol for Repli-seq, including processing and collecting cells from distinct cell cycle phases by flow cytometry based on cell-permeant dyes. These improvements enable RT analyses of samples sensitive to fixation and reduce cell loss, achieving retention of ≥90% of the sample. In this protocol, we provide a step-by-step method for cell sample preparation for the Repli-seq. Our live cell isolation and staining method (Basic Protocol), overcomes the sample loss caused by the standard fixing process, enabling higher yields of cells for Repli-seq. Moreover, for samples that are challenging to dissociate and for which single-cell suspensions cannot be obtained, we developed an alternative method (Alternate Protocol) of intact nuclei isolation and staining for Repli-seq (Fig. 1). The Alternate Protocol improves sample preparation and cell cycle analysis of samples, such as human embryonic stem cells (hESC), human fibroblasts, and mouse embryonic fibroblasts (MEFs), which are difficult to dissociate and tend to form aggregates after dissociation.
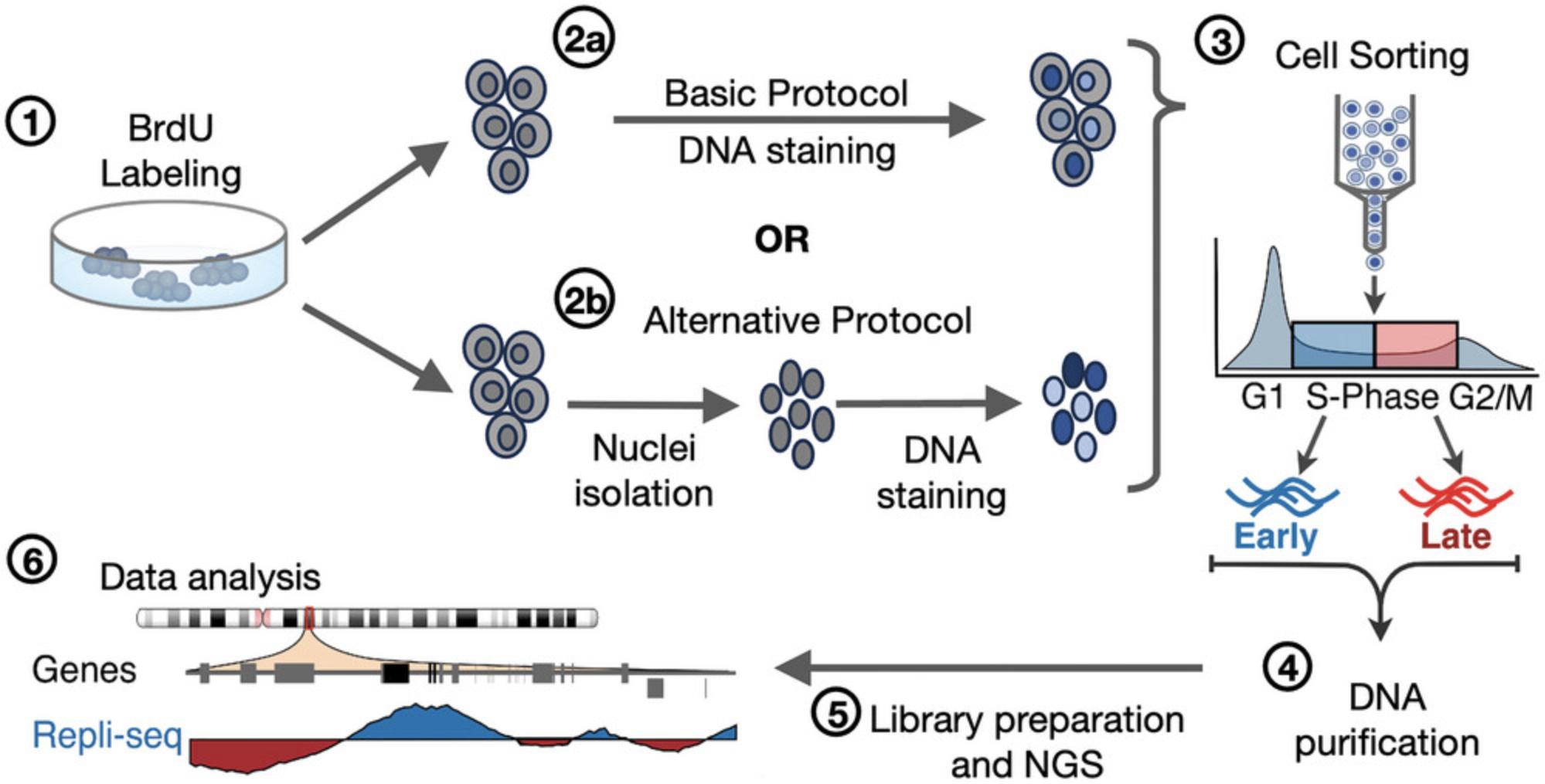
Basic Protocol: LIVE CELL ISOLATION AND STAINING
This protocol can be applied to any cell type actively proliferating. We have successfully used this protocol to profile RT of different cell lines, such as human fibroblast (IMR90), human lymphocytes (Jurkat), human hepatic cells (HepG2), osteosarcoma cells (U2OS), human embryonic stem cells (hESCs), and mouse embryonic fibroblasts (MEFs). With this protocol intact, live cells can be prepared to facilitate analyses of the RT program by Repli-seq (Rivera-Mulia et al., 2022).
Materials
-
5–10 × 106 cells of interest (see step 1 for details on starting cell numbers)
-
Medium for the cell type of interest
-
BrdU (Sigma, cat. no. B5002, or equivalent)
-
1× PBS (Corning, cat. no. 21-040-CV)
-
Dissociation agents, e.g., trypsin-EDTA (Life Technologies, cat. no. A011105-01)
-
FBS (Corning, cat. no. 35011CV)
-
Trypan blue solution, 0.4% (Sigma, T8154-100ml)
-
Cell-permeable DNA staining dye, e.g., Vybrant DyeCycle Violet (Thermo Fisher, cat. no. V35003)
-
Quick-DNA Microprep Kit (Zymo, cat. no. D3020)
-
DNA/RNA shield (Zymo, cat. no. R1100), optional
-
Plates/flasks to grow the cells of interest, e.g., 100-mm × 20-mm style dish (Corning cat. no. 430167)
-
37°C incubator
-
15-ml round-bottom tubes (Falcon, cat. no. 2059)
-
Automated cell counter (e.g., CountessII-FL, or equivalent)
-
5-ml, 12-mm × 75-mm round polypropylene bottom culture tube (VWR, cat. no. 60818-500)
-
Benchtop centrifuge
-
36-μm nylon mesh (Fisher Scientific, cat no. NC05156770
-
1.5-ml collection tubes, low binding (Thermo Scientific cat. no. 90410)
-
Sony SH800 cell sorter, or equivalent
-
Vortex
-
Fluorescence microscope, EVOS M5000 imaging system (Invitrogen, cat. no. AMF5000, or equivalent)
CAUTION : All procedures involving BrdU should be protected from light since BrdU is sensitive to light.
1.Start with healthy growing cells at ≤65% confluency.
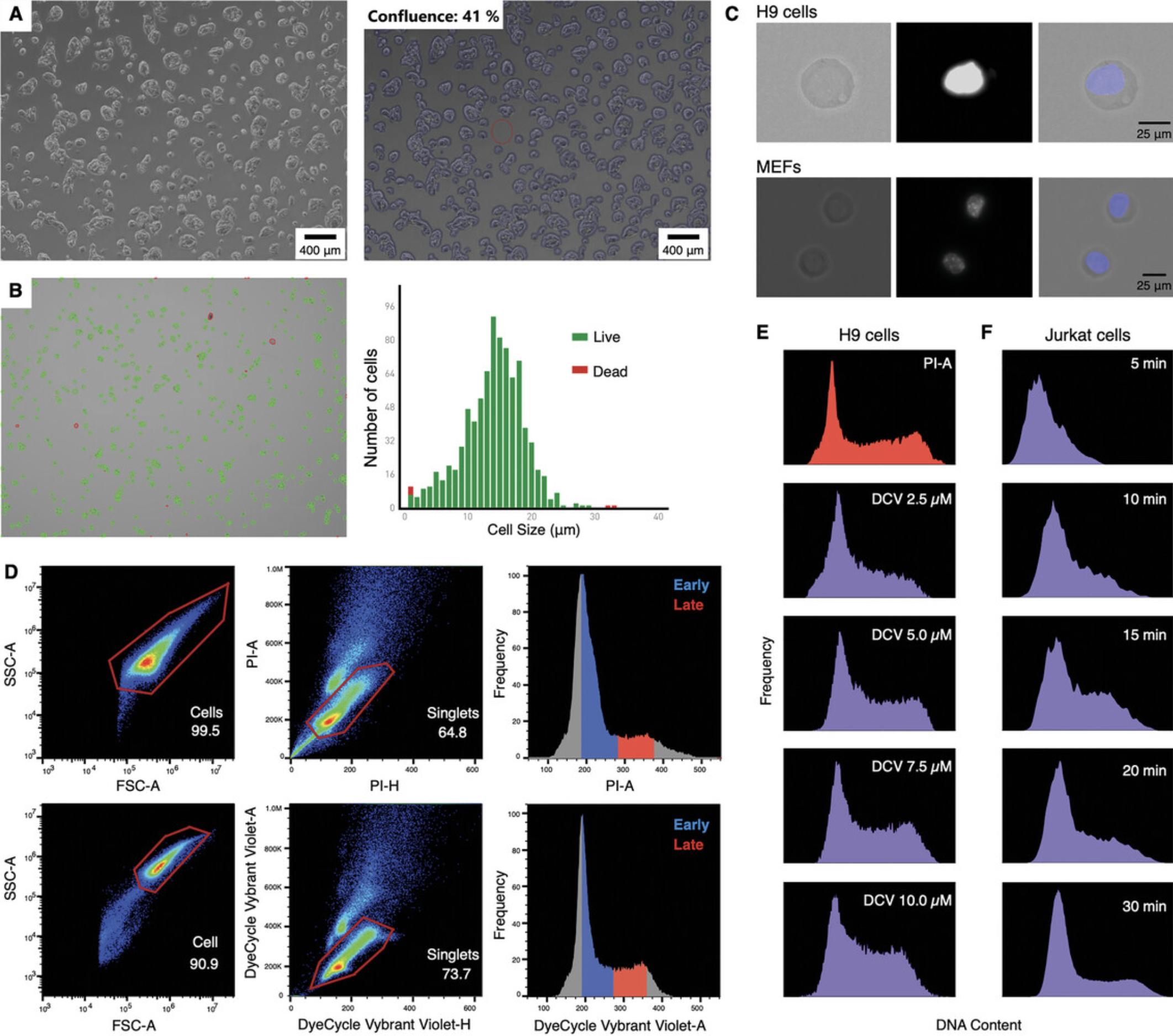
2.Add BrdU into the cell culture medium at a final concentration of 100 μM (46 µl of 10 mg/ml BrdU for 15 ml). Incubate cells for 2 hr at 37˚C to allow BrdU incorporation into newly synthesized DNA.
3.Rinse the cells in culture gently with PBS twice using an add, swirl and aspirate procedure.
4.Dissociate the cells from the culture plates using its specific/validated dissociation agents for the specific cell type of interest (trypsin-EDTA, accutase, or TrypLE, etc.).
5.Inactivate the dissociation agent by adding 3 ml of medium supplemented with FBS per every ml of trypsin. Pipette gently but thoroughly to obtain a single-cell suspension. Transfer cell suspension to a 15-ml round bottom tube.
6.Assess cell viability using trypan blue staining. A viability of >90% is desirable. Count the cells and prepare aliquot 1 × 106 cells per replicate in 5-ml round-bottom culture tubes (Fig. 2B).
7.Centrifuge 5 min at ∼200 × g , room temperature, and carefully discard the supernatant by pipetting (do not aspirate to avoid sample loss).
8.Resuspend the cells in 1 ml of medium and filter using 36-μm nylon mesh filters to remove aggregates to ensure good staining.
9.Per 1 × 106 cells, add 1 µl of the cell-permeable DNA dye DyeCycle Vybrant Violet Stain (other colors are also available, see Table 1 and Fig. 2C).
Dye | Excitation (nm) | Emission (nm) |
---|---|---|
Hoechst 33342 | 350 | 461 |
DyeCycle Vybrant Violet | 405 | 440 |
DyeCycle Green | 488 | 520 |
DyeCycle Orange | 532 | 570 |
DyeCycle Ruby | 633 | 670 |
DRAQ5 | 647 | 681/697 |
Whole cells | Nuclei | ||||
---|---|---|---|---|---|
Cell line | Vybrant DyeCycle (μl) | Incubation time (min) | Lysis time (min) | Vybrant DyeCycle (μl) | Incubation time (min) |
HepG2 | 2 | 40-45 | 3-5 | 1.5 | 15 |
IMR90 | 1 | 30 | ND | ND | ND |
U2OS | 1 | 30 | ND | ND | ND |
hESCs | 1 | 40-50 | 5 | 1.5 | 25 |
MEFs | 1 | 40-50 | 5 | 1.5 | 15 |
Jurkat | 1 | 20-30 | ND | ND | ND |
-
aAmount of Vybrant DyeCycle is per 1 × 106 cells/nuclei.
10.Incubate the cells at 37°C for 30 to 90 min, protected from light, and invert the tube gently every 10 min. Keep cells at 37˚C until ready for sorting.
11.Prepare at least six collection tubes per sample (6 × 1.5-ml tubes, for three replicates of early S phase and three replicates of late S phase fractions). Add 400 μl of Zymo lysis buffer or DNA/RNA Shield per collection tube.
12.After staining with Vybrant DyeCycle Violet proceed immediately to FACS cell sorting. Sort cells directly into collection tubes containing 400 μl of Zymo lysis buffer or DNA/RNA Shield from step 11.
13.For DyeCycle Vybrant Violet sort samples use the ∼405 nm excitation and ∼440 nm emission wavelength settings. For other colors, use corresponding excitation and detection settings. See other dye examples in Table 1.
14.Perform sorting calibration before each experiment to ensure accurate cell cycle analysis.
15.Adjust cell sorter parameters to use low FSC thresholds to detect all particles in the sample (on the SONY SH800 we use 1.0% FSC). Minimize gain settings to enable accurate measurement of DNA staining (FSC of 2, and BSC in 25% to 30% and FL1 Brilliant Violet 40%).
16.Cell sorter gating strategy:
-
Using the log scale, select most events by plotting the BSC area on the y-axis and the FSC area on the x-axis.
-
Gate singlets with Vybrant Violet area on the y-axis and Vybrant Violet height on the x-axis.
-
Gating for cell collection is based on the cell cycle stages based on DNA content, i.e., by plotting events on the y-axis and the Vybrant Violet area on the x-axis (Fig.2D).
-
For a typical sample with normal cell cycle, discrimination of cells in particular phases of the cell cycle based on differences in their DNA content is made by selecting the higher peak as G1, later a plateau S phase, and finally a second peak, not as big as the first one, G2 phase (cells under treatments that affect the cell cycle would have distinct profiles and untreated controls would be needed).
Set a low flow rate (<500 events/s) for optimal resolution.
17.Sort between 20,000 to 40,000 cells from each S-phase fraction directly into collection tubes from step 11 containing Zymo lysis buffer or DNA/RNA Shield and mix well by vortexing.
18.Purify DNA following the Zymo Quick DNA Microprep kit instructions.
19.Purified DNA samples can be stored in the dark indefinitely at −20°C or can be processed by library preparation immediately as described in Rivera-Mulia et al. (2022). Briefly, continue Repli-seq processing by performing DNA fragmentation, DNA clean-up, end-repair, adapter ligation, and indexing as described in Rivera-Mulia et al. (2022) (see Understanding Results).
Alternate Protocol: NUCLEI ISOLATION AND STAINING
A starting cell number of 2 to 2.5 million cells per replicate is necessary for nuclei isolation, staining and sorting. We recommend using a starting cell number of 5 to 10 million cells. This should be enough to obtain sufficient replicates from samples with at least 5% of S-phase cells.
Additional Materials (also see Basic Protocol)
- Nuclei isolation lysis buffer (see recipe)
- Nuclei wash and resuspension buffer (see recipe)
1.Follow steps 1 to 5 from Basic Protocol 1 (Fig. 3A).
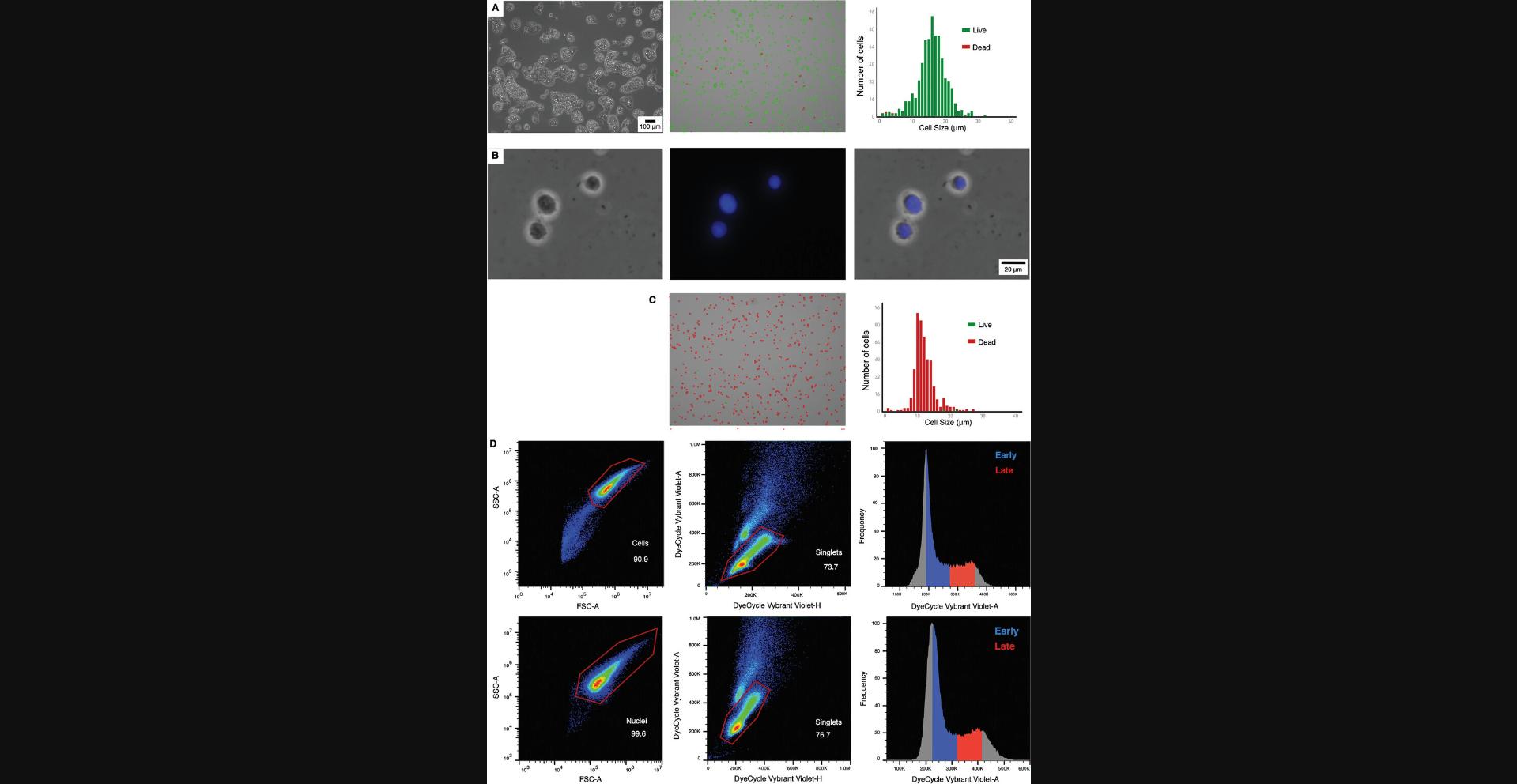
2.Assess cell viability with trypan blue staining (>90% is desirable), count the cells, and aliquot 2 million cells per replicate in 1.5-ml tubes.
3.Prepare fresh nuclei isolation lysis buffer. Store the lysis buffer on ice until use.
4.Prepare 50 ml of the nuclei wash and resuspension buffer. Once prepared, this can be stored at –20˚C and used for up to 6 months. Always store on ice when used.
5.Centrifuge the cells 5 min at ∼200 × g , room temperature.
6.Carefully remove the supernatant without disturbing the cell pellet.
7.Resuspend the cells in 200 μl of lysis buffer and pipette up and down 5 times or until the cells have fully resuspended in the lysis buffer, avoiding the formation of air bubbles.
8.Transfer the cells to ice and allow lysis for 1 to 5 min (this time needs to be optimized based on the type of cells being used).
9.Add 800 μl of nuclei wash and resuspension buffer and pipette up and down 5 times to quench lysis.
10.Centrifuge the nuclei 10 min at 500 × g , 4°C.
11.Carefully remove the supernatant.
12.Add 1 ml of nuclei wash and resuspension buffer.
13.Centrifuge the nuclei 10 min at 500 × g , 4°C.
14.Carefully remove the supernatant.
15.Repeat steps 12 to 14 twice.
16.Resuspend the nuclei in 1 ml of nuclei wash buffer and resuspension buffer and pipette gently 10 times or until the nuclei are fully resuspended.
17.Count the final number of nuclei using standard trypan blue dye staining.
18.Stain the nuclei with DNA Vybrant DyeCycle Violet. Add 1.5 μl of Vybrant DyeCycle Violet per 1 × 106 nuclei per ml for each replicate and then incubate in the dark at 37˚C for 15 min before sorting.
19.After staining, pipette 5 times, and filter the stained nuclei into FACS tubes using a 36-μm nylon mesh filter into 1.5-ml FACs tubes to get rid of any clumps or debris.
20.Observe the nuclei under the microscope using a bright field and DAPI filter to ensure they are intact and well stained (see Fig. 3B).
21.Prepare at least six collection tubes per experiment (6 × 1.5 ml tubes, three replicates for early S phase and three for late S phase fractions). Add 400 μl of Zymo lysis buffer or DNA/RNA Shield per collection tube.
22.Sort samples on the cell sorter using the ∼405 nm excitation and ∼440 nm emission wavelength settings on the cell sorter.
23.Adjust cell sorter parameters:
-
Use low FSC thresholds to detect all particles in the sample (on the SONY SH800 we use 1.0% FSC).
-
Minimize gain settings to enable accurate measurement of DNA staining (FSC of 2, and BSC in 25% to 30%).
-
Cell sorter gating strategy:Using the linear scale, select most events putting the BSC area on the y-axis and the FSC area on the x-axis.Gate singlets with Vybrant Violet area on the y-axis and Vybrant Violet Height on the x-axis.Exclude doublets by creating a combination of same-channel bivariate plots utilizing area versus height.Then, the gate is based on the cell cycle by plotting events on the y-axis and the Vybrant Violet area on the x-axis (Fig.3D).
24.Sort 20,000 to 40,000 intact nuclei from each S-phase fraction directly into collection tubes (step 21) containing Zymo lyis buffer or DNA/RNA Shield and mix well by vortexing.
25.Purify DNA following the Zymo Quick DNA Microprep kit instructions.
26.The samples can be stored in the dark indefinitely at −20°C or proceed with library preparation for Repli-seq using purified DNA as described in Rivera-Mulia et al. (2022) (see Understanding Results).
REAGENTS AND SOLUTIONS
Lysis reagent, 25%
- 250 μl of 100% IGEPAL CO-630 lysis reagent (Millipore Sigma cat. no. 542334)
- 750 μl 1× PBS (Thermo Fisher, cat. no. 20012027)
- Store up to 6 months at room temperature
Nuclei isolation lysis buffer
- 100 μl of 1 M Tris·HCl, pH 7.4 (Current Protocols, 2006)
- 20 μl of 5 M NaCl (Current Protocols, 2006)
- 30 μl of 1 M MgCl2 (Sigma, cat. no. M1028-100ML)
- 10 μl of 25% lysis reagent (see recipe)
- 9.84 ml of 1× PBS (Thermo Fisher, cat. no. 20012027)
- Prepare fresh with each use
Nuclei wash and resuspension buffer
50 ml 1× PBS (Thermo Fisher, cat. no. 20012027) supplemented with 1% (v/v) FBS. Store up to 6 months at 4˚C.
COMMENTARY
Background Information
DNA replication occurs in a precise spatiotemporal pattern known as replication time (RT). The first evidence of a RT program was obtained by cytological techniques incorporating nucleotide analogs into replicating DNA (Taylor, 1960). Assays that quantify RT over the entire genome have been available in the last 10 years (Hansen et al., 2010; Hiratani et al., 2008; Ryba et al., 2010). These assays consist of briefly labeling the nascent DNA with a nucleotide analog, such as BrdU, collecting cells at specific S-phase stages according to their total DNA content by flow cytometry, and measuring the labeled DNA. Using antibodies against BrdU, DNA is immunoprecipitated, and is either hybridized into microarrays (Repli-chip) (Pope et al., 2014) or processed by next-generation sequencing (Repli-seq) (Marchal et al., 2018; Rivera-Mulia et al., 2022).
In this protocol, we developed methods for live cell preparation and intact nuclei isolation for Repli-seq without the need for sample fixation. Both live cell and intact nuclei preparation have several advantages over the standard use of fixed sample protocols. With live cells and intact nuclei preparation, there is no need for fixation of the cells, which saves time and reduces sample loss. While choosing between live cell and nuclei sorting, several factors need to be considered. The advantages of sorting live cells are that live cells do not require nuclei isolation and sample loss can be minimized (intact nuclei isolation typically leads to loss of 20% of starting cell number). Staining live cells for DNA also allows simultaneous staining with cell surface markers and vital dyes, which allows for sorting specific subpopulations via FACS and the removal of dead cells. Conversely, the advantage of using intact nuclei is that it allows for the processing of cells that tend to form clumps and/or are difficult to dissociate. This also prevents problems, such as cell breakage and the formation of clumps that can lead to nozzle clogging during cell sorting. Cell cycle analysis is also facilitated using nuclei compared to live/fixed cells as DNA staining is more efficient.
A limitation of this protocol is the process of samples with <100,000 cells, i.e., mammalian embryos in early development or rare populations of patient-derived samples. This limitation is due to the minimum number of cells necessary for cell sorting (100,000 cells for the SONY SH800 cell sorter). In these cases, single-cell approaches could be used to assess the RT program (Dileep & Gilbert, 2018; Miura et al., 2020).
Critical Parameters
Staining of live cells and nuclei
The practical application of fluorochromes in flow cytometry involves several significant aspects of their interaction with DNA. Firstly, these interactions are reversible rather than covalent, involving processes like intercalation between base pairs in the double helix or binding to the minor groove. For live cells and intact nuclei, distinct cell-permeable DNA-specific dyes can be used (such as DAPI, Hoechst, Acridine Orange, Calcein, etc). Here, we described the optimized method using Vybrant DyeCycle Violet Stain (Fig. 1). Other DyeCycle colors can also be used, such as DyeCycle Green, DyeCycle Orange, and DyeCycle Ruby. The incubation period for staining the DNA must be optimized for different cell types while performing live cell staining. An incubation period of 10 to 15 min for nuclei staining is sufficient for all cell types tested. When the dye concentration is low and not all potential binding sites are saturated, the number of sites interacting with the dye follows the law of mass action. As a result, the intensity of DNA staining varies based on the number of binding sites available (cell number per sample). To maintain stable fluorescence levels, an equilibrium is required, ensuring a substantial excess of dye molecules compared to binding sites. This excess of dye helps minimize the impact of minor variations in cell number on the concentration of unbound free dye, thereby maintaining consistent DNA stainability. However, it is essential to note that even when the cell number is the same, the number of binding sites can vary.
Lysis time for nuclei isolation
The nuclei isolation protocol involves timed lysis of single cells in solution to digest through the cell membrane and expose the nuclei. This is followed by three washing steps using a wash solution of PBS supplemented with 1% (v/v) FBS to eliminate cell debris and enrich nuclei in the solution. The exposure time to the lysis buffer needs to be optimized for each sample (Fig. 3C) to obtain complete lysis and efficient nuclei isolation. A shorter lysis time will not allow complete lysis of all the cells in the culture. In contrast, a longer than necessary lysis time will lead to the degradation of the nuclei and reduce the quality of the starting sample. From the starting cell number of 2 to 2.5 million cells, ∼1.5 to 2 million nuclei can be obtained after any cell/nuclei loss during the lysis and wash steps.
FACs parameters for sorting live cells and nuclei
After staining, live cells or intact nuclei are resuspended in PBS with 1% (v/v) FBS and filtered with a 36-µm nylon mesh into 1.5-ml tubes. A 100-µm (nuclei) or 130-µm sorting chip was used. FACS sorting of specific cell numbers was performed in our laboratory SONY SH800 cell sorter, set up and adjusted a flow cytometer for the 405 nm laser with FSC 2 and BSC at 26%. Still, the parameters must be adjusted for different cell types.
Troubleshooting
Several issues can be present during the sample preparation. Common problems with the protocols, their causes, and potential solutions are listed in Table 3.
Problem | Possible cause | Solution |
---|---|---|
Not seeing a clear pattern in the cell cycle profile | Cells are not incorporating the stain correctly | Try to adjust the time, see step 10 in Basic Protocol 1 |
Cell clumping leading to pausing the sorting machine | Some cells are prone to clump | Pause sorting, pipette ∼5 times and filter the solution again |
Not a clear difference between singlets or nuclei gating selection | A lot of debris during nuclei extraction; poor incorporation of the dye | Do another extra step in the selection gated: (1) proceed to select all events putting the BSC area on the y-axis and the FSC area on the x-axis; (2) select only nuclei with Vybrant Violet area on the y-axis and Vybrant Violet height on the x-axis; (3) open a new selection parameter using FSC height in the y-axis and FSC area in the x-axis, only select the events close to the diagonal; (4) finally select the base in the cell cycle having events in the y-axis and Vybrant Violet area in the x-axis |
Sample loss after nuclei isolation | The nuclei were broken during the process of extraction | Decrease the time in the lysis buffer or sort with whole cells |
High debris in intact nuclei solution | Broken nuclei | Optimize lysis buffer incubation time; increase PBS/FBS washes; filter nuclei sample again |
Incomplete rupture of the cell to release the nuclei | The cells need more time in the lysis buffer | Increase the lysis buffer incubation time by 1 min; check the sample again in the microscope, if the nuclei are still not released completely, add another minute |
Nuclei broken | Excess incubation time in lysis buffer or excess force of pipetting | Try to pipette more gently and decrease the amount of incubation time in the lysis buffer |
Understanding Results
The results of cellular DNA content measurements are generally presented as frequency histograms (see Figs. 2D and 3D). The protocol presented here can be used to process any cell line and the dye is selected according to the content of DNA. In Figure 2, with the FACS profiles, consider that each cell line will vary in the number of events present in each phase of the cell cycle. For normal diploid cells, two peaks are expected, the first corresponding to the more prominent G1 and the second from G2/M (Figs. 2D and 3D).
Collecting a minimum of 20,000 cells or nuclei in each fraction is recommended for samples with a good incorporation of BrdU. Alternatively, up to 40,000 in each fraction can be collected for samples with low BrdU incorporation. Total expected amount of DNA from 20,000 cells is ∼60 ng.
After genomic DNA isolation, follow the steps from the optimized Repli-seq protocol (Rivera-Mulia et al., 2022). Representative RT profiles are shown in Figure 4. Comparable RT profiles were produced by both methods (sorting ethanol-fixed cells stained with propidium iodide (PI) or intact nuclei stained with Vybrant DyeCycle Violet, Fig. 4A). Moreover, genome-wide correlation of RT programs confirms comparable results from standard sample preparation and cells processed with this protocol (Fig. 4B). In fact, the correlation value (r = 0.944) is as comparable to those obtained between Repli-seq technical replicates (ENCODE Project Consortium et al., 2020; Rivera-Mulia et al., 2015; Rivera-Mulia et al., 2022; Sarni et al., 2020).
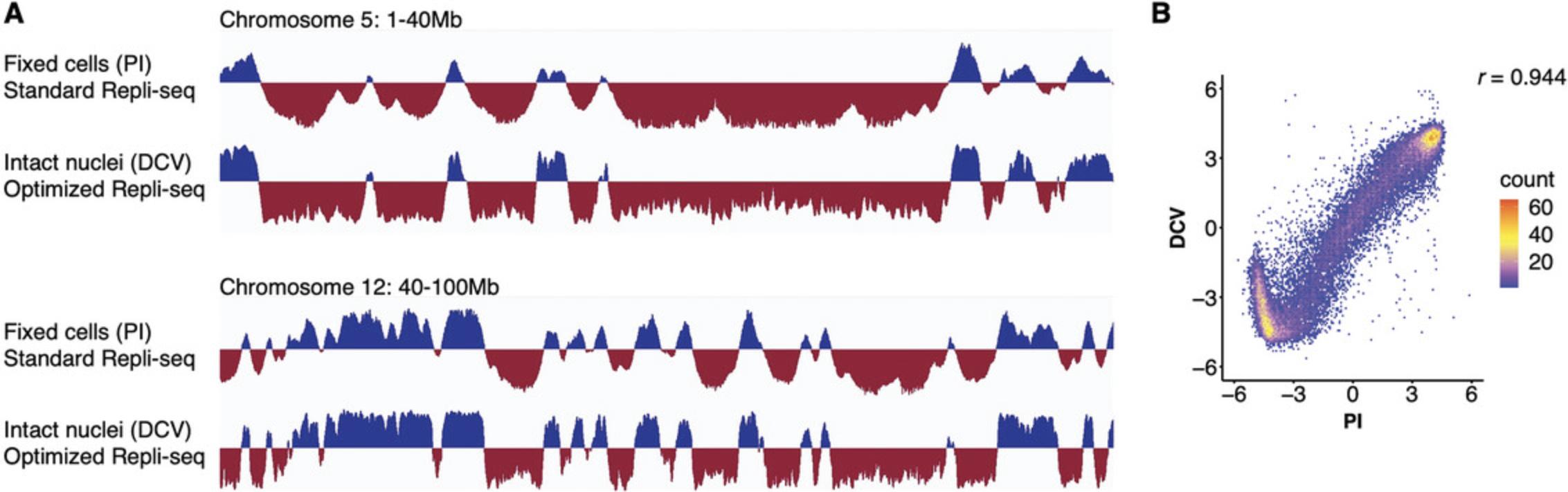
Time Considerations
The complete process for live cell isolation, staining, and sorting takes ∼5 hr. For nuclei, an additional hour is required for the nuclei isolation steps involving the lysis buffer incubation and the subsequent washes. The nuclei isolation steps should be performed quickly on ice to minimize sample loss. After obtaining the DNA from each fraction, it can be stored for several months at –20°C.
Acknowledgments
This work was supported by NIH R35GM137950 and Regenerative Medicine Minnesota RMM-091621-DS-006 to JCRM.
Author Contributions
Silvia Meyer-Nava : Data curation; formal analysis; writing original draft; writing review and editing. Anala V. Shetty : Formal analysis; validation; writing original draft. Juan Carlos Rivera-Mulia : Conceptualization; formal analysis; funding acquisition; validation; visualization; writing original draft; writing review and editing.
Conflict of Interest
The authors declare no conflict of interest.
Open Research
Data Availability Statement
The data that support the protocol are openly available in the Gene Expression Omnibus at (http://www.ncbi.nlm.nih.gov/geo/), reference number GSE196749.
Literature Cited
- Boquest, A. C., Day, B. N., & Prather, R. S. (1999). Flow cytometric cell cycle analysis of cultured porcine fetal fibroblast cells. Biology of Reproduction , 60(4), 1013–1019. https://doi.org/10.1095/biolreprod60.4.1013
- Bracci, A. N., Dallmann, A., Ding, Q., Hubisz, M. J., Caballero, M., & Koren, A. (2023). The evolution of the human DNA replication timing program. Proceedings of the National Academy of Sciences of the United States of America , 120(10), e2213896120. https://doi.org/10.1073/pnas.2213896120
- Current Protocols. (2006). Commonly Used Reagents. Current Protocols in Microbiology , 00, A.2A.1–A.2A.15. https://doi.org/10.1002/9780471729259.mca02as00
- Dileep, V., & Gilbert, D. M. (2018). Single-cell replication profiling to measure stochastic variation in mammalian replication timing. Nature Communications , 9(1), 427. https://doi.org/10.1038/s41467-017-02800-w
- ENCODE Project Consortium, Moore, J. E., Purcaro, M. J., Pratt, H. E., Epstein, C. B., Shoresh, N., Adrian, J., Kawli, T., Davis, C. A., Dobin, A., Kaul, R., Halow, J., van Nostrand, E. L., Freese, P., Gorkin, D. U., Shen, Y., He, Y., Mackiewicz, M., Pauli-Behn, F., … Weng, Z. (2020). Expanded encyclopaedias of DNA elements in the human and mouse genomes. Nature , 583, 699–710. https://doi.org/10.1038/s41586-020-2493-4
- Farkash-Amar, S., Lipson, D., Polten, A., Goren, A., Helmstetter, C., Yakhini, Z., & Simon, I. (2008). Global organization of replication time zones of the mouse genome. Genome Research , 18, 1562–1570. https://doi.org/10.1101/gr.079566.108
- Hansen, R. S., Thomas, S., Sandstrom, R., Canfield, T. K., Thurman, R. E., Weaver, M., Dorschner, M. O., Gartler, S. M., & Stamatoyannopoulos, J. A. (2010). Sequencing newly replicated DNA reveals widespread plasticity in human replication timing. Proceedings of the National Academy of Sciences of the United States of America , 107(1), 139–144. https://doi.org/10.1073/pnas.0912402107
- Hiratani, I., Ryba, T., Itoh, M., Yokochi, T., Schwaiger, M., Chang, C. W., Lyou, Y., Townes, T. M., Schübeler, D., & Gilbert, D. M. (2008). Global reorganization of replication domains during embryonic stem cell differentiation. PLoS Biology , 6(10), 2220–2236. https://doi.org/10.1371/journal.pbio.0060245
- Hiratani, I., Ryba, T., Itoh, M., Rathjen, J., Kulik, M., Papp, B., Fussner, E., Bazett-Jones, D. P., Plath, K., Dalton, S., Rathjen, P. D., & Gilbert, D. M. (2010). Genome-wide dynamics of replication timing revealed by in vitro models of mouse embryogenesis. Genome Research , 20, 155–169. https://doi.org/10.1101/gr.099796.109
- Hulke, M. L., Massey, D. J., & Koren, A. (2020). Genomic methods for measuring DNA replication dynamics. Chromosome Research , 9, 1–19. https://doi.org/10.1007/s10577-019-09624-y
- Klein, K. N., Zhao, P. A., Lyu, X., Sasaki, T., Bartlett, D. A., Singh, A. M., Tasan, I., Zhang, M., Watts, L. P., Hiraga, S. I., Natsume, T., Zhou, X., Baslan, T., Leung, D., Kanemaki, M. T., Donaldson, A. D., Zhao, H., Dalton, S., Corces, V. G., & Gilbert, D. M. (2021). Replication timing maintains the global epigenetic state in human cells. Science , 372(6540), 371–378. https://doi.org/10.1126/science.aba5545
- Kodali, S., Meyer-Nava, S., Landry, S., Chakraborty, A., Rivera-Mulia, J. C., & Feng, W. (2022). Epigenomic signatures associated with spontaneous and replication stress-induced DNA double strand breaks. Frontiers in Genetics , 13, 907547. https://doi.org/10.3389/fgene.2022.907547
- Liu, Y., Ai, C., Gan, T., Wu, J., Jiang, Y., Liu, X., Lu, R., Gao, N., Li, Q., Ji, X., & Hu, J. (2021). Transcription shapes DNA replication initiation to preserve genome integrity. Genome Biology , 22, 176. https://doi.org/10.1186/s13059-021-02390-3
- MacAlpine, D. M., Rodríguez, H. K., & Bell, S. P. (2004). Coordination of replication and transcription along a Drosophila chromosome. Genes & Development, 18, 3094–3105. https://doi.org/10.1101/gad.1246404
- Marchal, C., Sasaki, T., Vera, D., Wilson, K., Sima, J., Rivera-Mulia, J. C., Trevilla-García, C., Nogues, C., Nafie, E., & Gilbert, D. M. (2018). Genome-wide analysis of replication timing by next-generation sequencing with E/L Repli-seq. Nature Protocols , 13(5), 819–839. https://doi.org/10.1038/nprot.2017.148
- Massey, D. J., & Koren, A. (2022). High-throughput analysis of single human cells reveals the complex nature of DNA replication timing control. Nature Communications , 13, 2402. https://doi.org/10.1038/s41467-022-30212-y
- Miura, H., Takahashi, S., Shibata, T., Nagao, K., Obuse, C., Okumura, K., Ogata, M., Hiratani, I., & Takebayashi, S.-I. (2020). Mapping replication timing domains genome wide in single mammalian cells with single-cell DNA replication sequencing. Nature Protocols , 15, 4058–4100. https://doi.org/10.1038/s41596-020-0378-5
- Pope, B. D., Ryba, T., Dileep, V., Yue, F., Wu, W., Denas, O., Vera, D. L., Wang, Y., Hansen, R. S., Canfield, T. K., Thurman, R. E., Cheng, Y., Gülsoy, G., Dennis, J. H., Snyder, M. P., Stamatoyannopoulos, J. A., Taylor, J., Hardison, R. C., Kahveci, T., & Gilbert, D. M. (2014). Topologically associating domains are stable units of replication-timing regulation. Nature , 515(7527), 402–405. https://doi.org/10.1038/nature13986
- Poulet, A., Li, B., Dubos, T., Rivera-Mulia, J. C., Gilbert, D. M., & Qin, Z. S. (2019). RT States: Systematic annotation of the human genome using cell type-specific replication timing programs. Bioinformatics , 35(13), 2167–2176. https://doi.org/10.1093/bioinformatics/bty957
- Rivera-Mulia, J. C., Buckley, Q., Sasaki, T., Zimmerman, J., Didier, R., Nazor, K., Loring, J. F., Lian, Z., Weissman, S., Robins, A. J., Schulz, T. C., Menendez, L., Kulik, M. J., Dalton, S., Gabr, H., Kahveci, T., & Gilbert, D. M. (2015). Dynamic changes in replication timing and gene expression during lineage specification of human pluripotent stem cells. Genome Research , 25(8), 1091–1103. http://www.genome.org/cgi/doi/10.1101/gr.187989.114
- Rivera-Mulia, J. C., & Gilbert, D. M. (2016a). Replication timing and transcriptional control: Beyond cause and effect-part III. Current Opinion in Cell Biology , 40, 168–178. https://doi.org/10.1016/j.ceb.2016.03.022
- Rivera-Mulia, J. C., & Gilbert, D. M. (2016b). Replicating large genomes: Divide and conquer. Molecular Cell , 62(5), 756–765. https://doi.org/10.1016/j.molcel.2016.05.007
- Rivera-Mulia, J. C., Sasaki, T., Trevilla-Garcia, C., Nakamichi, N., Knapp, D. J. H. F., Hammond, C. A., Chang, B. H., Tyner, J. W., Devidas, M., Zimmerman, J., Klein, K. N., Somasundaram, V., Druker, B. J., Gruber, T. A., Koren, A., Eaves, C. J., & Gilbert, D. M. (2019). Replication timing alterations in leukemia affect clinically relevant chromosome domains. Blood Advances , 3(21), 3201–3213. https://doi.org/10.1182/bloodadvances.2019000641
- Rivera-Mulia, J. C., Schwerer, H., Besnard, E., Desprat, R., Trevilla-Garcia, C., Sima, J., Bensadoun, P., Zouaoui, A., Gilbert, D. M., & Lemaitre, J. M. (2018). Cellular senescence induces replication stress with almost no affect on DNA replication timing. Cell Cycle , 17(13), 1667–1681. https://doi.org/10.1080/15384101.2018.1491235
- Rivera-Mulia, J. C., Desprat, R., Trevilla-Garcia, C., Cornacchia, D., Schwerer, H., Sasaki, T., Sima, J., Fells, T., Studer, L., Lemaitre, J. M., & Gilbert, D. M. (2017). DNA replication timing alterations identify common markers between distinct progeroid diseases. Proceedings of the National Academy of Sciences of the United States of America , 114(51), E10972–E10980. https://doi.org/10.1073/pnas.1711613114
- Rivera-Mulia, J. C., Trevilla-Garcia, C., & Martinez-Cifuentes, S. (2022). Optimized Repli-seq: Improved DNA replication timing analysis by next-generation sequencing. Chromosome Research , 30(4), 401–414. https://doi.org/10.1007/s10577-022-09703-7
- Ryba, T., Hiratani, I., Lu, J., Itoh, M., Kulik, M., Zhang, J., Schulz, T. C., Robins, A. J., Dalton, S., & Gilbert, D. M. (2010). Evolutionarily conserved replication timing profiles predict long-range chromatin interactions and distinguish closely related cell types. Genome Research , 20(6), 761–770. https://doi.org/10.1101/gr.099655.109
- Ryba, T., Battaglia, D., Pope, B. D., Hiratani, I., & Gilbert, D. M. (2011). Genome-scale analysis of replication timing: From bench to bioinformatics. Nature Protocols , 6(6), 870–895. https://doi.org/10.1038/nprot.2011.328
- Sarni, D., Sasaki, T., Irony Tur-Sinai, M., Miron, K., Rivera-Mulia, J. C., Magnuson, B., Ljungman, M., Gilbert, D. M., & Kerem, B. (2020). 3D genome organization contributes to genome instability at fragile sites. Nature Communications , 11(1), 3613. https://doi.org/10.1038/s41467-020-17448-2
- Sasaki, T., Rivera-Mulia, J. C., Vera, D., Zimmerman, J., Das, S., Padget, M., Nakamichi, N., Chang, B. H., Tyner, J., Druker, B. J., Weng, A. P., Civin, C. I., Eaves, C. J., & Gilbert, D. M. (2017). Stability of patient-specific features of altered DNA replication timing in xenografts of primary human acute lymphoblastic leukemia. Experimental Hematology , 71–82.e3. https://doi.org/10.1016/j.exphem.2017.04.004
- Schübeler, D., Scalzo, D., Kooperberg, C., van Steensel, B., Delrow, J., & Groudine, M. (2002). Genomewide DNA replication profile for Drosophila melanogaster: A link between transcription and replication timing. Nature Genetics , 32, 438–442. https://doi.org/10.1038/ng1005
- Solovei, I., Thanisch, K., & Feodorova, Y. (2016). How to rule the nucleus: Divide et impera. Current Opinion in Cell Biology , 40, 47–59. https://doi.org/10.1016/j.ceb.2016.02.014
- Taylor, J. H. (1960). Asynchronous duplication of chromosomes in cultured cells of Chinese hamster. The Journal of Biophysical and Biochemical Cytology , 7(3), 455–464. https://doi.org/10.1083/jcb.7.3.455
- Topman, G., Sharabani-Yosef, O., & Gefen, A. (2011). A method for quick, low-cost automated confluency measurements. Microscopy and Microanalysis , 17(6), 915–922. https://doi.org/10.1017/S1431927611012153
- Yang, Y., Gu, Q., Zhang, Y., Sasaki, T., Crivello, J., O'Neill, R. J., Gilbert, D. M., & Ma, J. (2018). Continuous-trait probabilistic model for comparing multi-species functional genomic data. Cell Systems , 7(2), 208–218. https://doi.org/10.1016/j.cels.2018.05.022
- Zhao, P. A., Sasaki, T., & Gilbert, D. M. (2020). High-resolution Repli-Seq defines the temporal choreography of initiation, elongation and termination of replication in mammalian cells. Genome Biology , 21(1), 76. https://doi.org/10.1186/s13059-020-01983-8
- Zhang, J., Bellani, M. A., James, R. C., Pokharel, D., Zhang, Y., Reynolds, J. J., McNee, G. S., Jackson, A. P., Stewart, G. S., & Seidman, M. M. (2020). DONSON and FANCM associate with different replisomes distinguished by replication timing and chromatin domain. Nature Communications , 11(1), 3951. https://doi.org/10.1038/s41467-020-17449-1