Preparation of a Convertible Spacer Containing a Disulfide Group for Versatile Functionalization of Oligonucleotides
Alexander Pontarelli, Alexander Pontarelli, Jiang Tian Liu, Jiang Tian Liu, Jung Kwon Oh, Jung Kwon Oh, Christopher J. Wilds, Christopher J. Wilds
oligonucleotide functionalization
oligonucleotide conjugation
disulfide linker
redox-responsive release
Abstract
The protocols described in this article provide details regarding the synthesis and characterization of a disulfide containing linker phosphoramidite for terminal functionalization of synthetic oligonucleotides. The linker is first synthesized from 6-mercaptohexanol in two steps and is incorporated at the 5′ end of short DNA oligonucleotides using automated solid-phase synthesis. The linker contains a terminal tosylate group which is post-synthetically displaced by altering the deprotection conditions to yield a variety of functional handles (N3, NH2, OMe, SH) or alternatively, the tosylate can be displaced directly with primary amines such as tert -butylamine. The linker system is also compatible with RNA oligonucleotides enabling the introduction of various functional handles (N3, NH2). The protocol outlined in this procedure provides access to a versatile linker for the terminal functionalization of oligonucleotides containing a disulfide bond which may serve useful in the synthesis of reduction-responsive oligonucleotide conjugates. As a proof of concept, in this protocol the linker is used to modify a dT10 oligonucleotide and then conjugated by copper(I)-mediated azide-alkyne cycloaddition (CuAAC) to an alkyne-modified poly(ethylene glycol) which shows concentration dependent release of the oligonucleotide upon treatment with 1,4-dithiothreitol, a reducing agent. © 2023 The Authors. Current Protocols published by Wiley Periodicals LLC.
Basic Protocol 1 : Preparation of disulfide linker phosphoramidite 3
Basic Protocol 2 : Synthesis, functionalization, and characterization of DNA oligonucleotides containing disulfide linker phosphoramidite 3
Basic Protocol 3 : Displacement of terminal tosylate functionalized DNA with primary aliphatic amines
Basic Protocol 4 : Synthesis of oligonucleotide-PEG conjugate
Support Protocol : Preparation of PEG-alkyne
INTRODUCTION
Chemical modification of oligonucleotides is a significant component in the development of enhanced nucleic acid-based drugs capable of modulating genetic expression via the antisense or small interfering RNA mechanisms. While incorporation of potent base, sugar, and backbone modifications into therapeutic oligonucleotides has afforded several clinical advances in this field, numerous issues regarding cellular uptake and targeted delivery still pose significant challenges to their universal clinical application (Crooke, Baker, Crooke, & Liang, 2021; Khvorova & Watts, 2017). In that regard, a novel generation of oligonucleotide therapeutics has emerged which aims to conjugate oligonucleotides to ligands with the goal of enhancing the pharmacokinetic properties of the underlying drug (Juliano, Ming, & Nakagawa, 2012). Oligonucleotides conjugated to peptides (Patutina et al., 2018), polymers (Bakardzhiev et al., 2020), lipids (Wang, Allen, Prakash, Liang, & Crooke, 2019), and antibodies (Arnold et al., 2018)—among copious other ligands—have been reported, and their clinical success has been exemplified with the approval of Givosiran (Givlaari™), which contains a covalently bonded, trivalent N -acetylgalactosamine (GalNAc) ligand for targeted delivery (Scott, 2020). Several methods for the preparation of oligonucleotide conjugates have been reported which to date rely on key chemical reactions such as Michael additions, amidations with amino-modified oligonucleotides, copper-catalyzed azide-alkyne cycloadditions (CuAACs), and thiol-disulfide exchanges (Singh, Murat, & Defrancq, 2010). The latter approach has garnered particular interest as the resulting disulfide tether offers a means for reductive-responsive release of the therapeutic upon entry into the mildly reductive extracellular space (Le, Kosbar, & Veedu, 2020; Welch, Swanekamp, King, Dean, & Nilsson, 2016). Conjugates formed by the thiol-disulfide exchange reaction however poses certain synthetic limitations such as the selective incorporation of activated pyridyl disulfide groups and reactive thiols, necessitating at times lengthy and difficult synthetic approaches (Danial & Postma, 2017; Stasińska, Putaj, & Chmielewski, 2019). Moreover, conjugation using this method poses additional issues regarding the location of the disulfide tether when various thiol functionalities are present.
With the goal of improving the efficiency as well as the simplicity of oligonucleotide conjugates bearing a disulfide bond, herein we describe the synthesis of a novel disulfide containing linker phosphoramidite (3 , Fig. 1, Scheme A). The described linker is incorporated efficiently using automated solid-phase synthesis (SPS) and allows for post-synthetic incorporation of common terminal functionalities (azide, amine, thiol, or methoxy) or for direct displacement with primary aliphatic amines via displacement of a terminal tosylate group (Fig. 1, Scheme B). Modified oligonucleotides are deprotected with minor modification to standard procedures and can be purified by common purification methods such as ion-exchange high-performance liquid chromatography (IEX-HPLC). Moreover, the methodology described is focused primarily on DNA sequences, but the linker demonstrates compatibility with RNA oligonucleotides depending on the desired functional handle. Lastly, the potential of such a system for the synthesis of oligonucleotide conjugates is highlighted by the conjugation of an alkyne-terminated poly(ethylene glycol) (Mn 5000, PEG-alkyne) via CuAAC and reduction mediated release of the oligonucleotide using 1,4-dithiothreitol (DTT) as monitored by polyacrylamide gel-electrophoresis (Fig. 1, Scheme B).
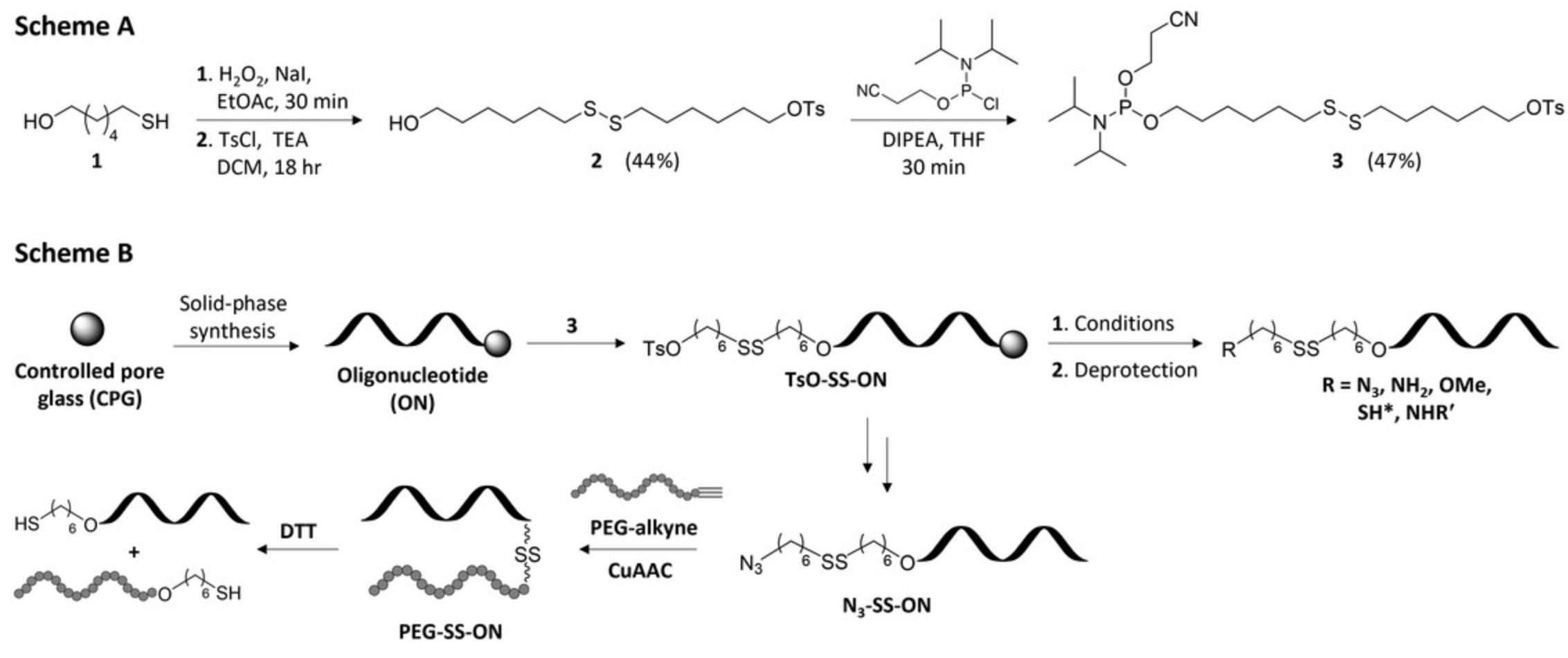
The protocols given below for the synthesis of linker 3 (Pontarelli et al., 2022) describe the chemical synthesis, purification, and characterization of the disulfide linker phosphoramidite (Basic Protocol 1) starting from commercially available 6-mercaptohexanol. Basic Protocol 2 provides details regarding the incorporation of the linker phosphoramidite into short chemically synthesized DNA or RNA oligonucleotides using SPS and their post-synthetic functionalization during deprotection. Moreover, purification using a suitable technique such as IEX-HPLC and characterization by electro-spray ionization mass spectrometry (ESI-MS) is described. In Basic Protocol 3, post-synthetic displacement of the tosylate group of functionalized DNA oligonucleotides with some primary aliphatic amines in addition to their purification and characterization is provided. Finally, conjugation of an azide functionalized DNA oligonucleotide to a PEG-alkyne (Supporting Protocol) and its DTT-mediated release is provided in Basic Protocol 4.
CAUTION : All reactions must be performed in a suitable fume hood with efficient ventilation. Some of the chemicals used in this protocol are toxic and/or flammable. Appropriate protective gear (goggles, lab coat, and gloves) should be worn at all times.
NOTE : Glassware and needles used for reactions involving anhydrous solvents should be oven-dried before use. Anhydrous solvents were purchased and used as is and no additional drying techniques were employed.
NOTE : Distilled water was used for all organic transformations (aqueous work ups), however for all manipulations involving oligonucleotides, autoclaved HPLC quality water was used and the use of gloves is highly recommended.
Basic Protocol 1: PREPARATION OF DISULFIDE LINKER PHOSPHORAMIDITE 3
Synthesis of the disulfide containing phosphoramidite linker 3 is performed in two steps starting from commercially available 6-mercapto-1-hexanol (1 , Fig. A). Oxidation of 1 with hydrogen peroxide provides the symmetric disulfide quantitatively, which is subsequently tosylated yielding 2 in a 44% yield over two steps. Phosphitylation of the remaining hydroxyl group yields the corresponding linker phosphoramidite 3 , ready for use in SPS. Caution must be taken during the preparation of 3 as the presence of impurities in the final product can significantly hinder the yields of the functionalized oligonucleotide.
Materials
-
6-Mercapto-1-hexanol (1 , MilliporeSigma, cat. no. 451088)
-
Ethyl acetate (EtOAc, ACS reagent, MilliporeSigma, CAS no. 141-78-6)
-
Sodium iodide (MilliporeSigma, CAS no. 7681-82-5)
-
Hydrogen peroxide (30%, w/w, Thermo Fisher Scientific, cat. no. H325-500)
-
Sodium thiosulfate (MilliporeSigma, CAS no. 7772-98-7)
-
Sodium bicarbonate (NaHCO3, MilliporeSigma, CAS no. 144-55-8)
-
Anhydrous sodium sulfate (MilliporeSigma, CAS no. 7757-82-6)
-
Dichloromethane (DCM, ACS reagent, MilliporeSigma, CAS no. 75-09-2)
-
Triethylamine (TEA, ≥99%, MilliporeSigma, CAS no. 121-44-8)
-
4-Toluenesulfonyl chloride, ≥98% (MilliporeSigma, CAS no. 98-59-9)
-
Argon gas (Ar, ≥99.999%, Air Liquide Canada)
-
Silica gel (60 Å pore size, 230-400 mesh, 40-63 μm particle size; ZEO prep, Zeochem)
-
Anhydrous tetrahydrofuran, ≥99.9% (MilliporeSigma, CAS no. 109-99-9)
-
Anhydrous N,N -diisopropylethylamine, ≥99.5% (MilliporeSigma, CAS no. 7087-68-5)
-
N,N -Diisopropylamino cyanoethyl phosphonamidic chloride (ChemGenes, cat. no. RN1505)
-
Sodium chloride, ≥99% (MilliporeSigma, CAS no. 7647-14-5)
-
Hexanes (mixture of isomers, ACS reagent, MilliporeSigma, CAS no. 107-83-5)
-
Distilled water
-
Hot plate with stirrer
-
Magnetic stir bar
-
Round-bottom flask (100 and 500 ml)
-
Erlenmeyer flask (250 and 500 ml)
-
Rubber stopper
-
Glass stopper
-
Addition funnel (25 ml)
-
Plastic Syringes (1, 3, 5, and 10 ml, BD Plastipak® or equivalent)
-
Needles (304 stainless steel, 18 gauge, MilliporeSigma)
-
Disposable Pasteur pipets (5¾ in. and 9 in. borosilicate glass)
-
250-ml separatory funnel
-
Graduated cylinder (10 ml, 100 ml, and 500 ml)
-
Glass chromatography column (30-mm column width)
-
Thin-layer chromatography plates (silica gel, 60 Å pore size, aluminum backing, F254, Merck, cat. no. 1.05554)
-
Hand-held UV lamp (254 nm)
-
Rotary evaporator with water bath and pump
-
High-vacuum pump
Perform oxidation
1.Add 1.00 g 6-mercapto-1-hexanol (1 , 7.45 mmol) to a tared 100-ml round-bottom flask containing a stir bar using a glass Pasteur pipet and cap with a rubber stopper.
2.Add 12 ml EtOAc and 12 mg sodium iodide (0.08 mmol) with stirring.
3.Add 836 μl hydrogen peroxide using a 1-ml syringe and stir 30 min.
4.Prepare 30 ml of 10% (w/v) sodium thiosulfate in distilled water and place in a 250-ml separatory funnel.
5.Pour contents of the reaction into the separatory funnel, rinsing the round-bottom with EtOAc (3 × 15 ml).
6.Mix and collect the organic layer in a clean 250-ml Erlenmeyer flask, repeating this step with an additional 50 ml EtOAc. Discard aqueous layer.
7.Repeat steps 5-6, washing with 50 ml 3% (w/v) aqueous NaHCO3.
8.Add 2 g anhydrous sodium sulfate to the collected organic layer and wait 20 min.
9.Decant organic solvent into a clean 500-ml round-bottom flask, rinsing the Erlenmeyer with EtOAc (3 × 10 ml).
10.Concentrate contents of the round-bottom flask using a rotary evaporator.
11.Transfer the resulting oil to a clean, dried 100-ml round-bottom flask, rinsing with EtOAc (2 × 5 ml).
12.Concentrate using a rotary evaporator and place flask under vacuum using a high-vacuum pump overnight.
Perform tosylation
13.Close the high-vacuum pump and introduce argon into the stoppered round-bottom flask.
14.Add a stir bar to the flask and place in an ice-water bath on a hot plate.
15.Add 10 ml anhydrous DCM using a 10-ml syringe and dry needle, and begin stirring.
16.Add 1.05 ml anhydrous triethylamine (7.45 mmol) using a 3-ml syringe and dry needle.
17.Transfer 1.06 g 4-toluenesulfonyl chloride (5.59 mmol) to a dry 25-ml addition funnel.
18.Add 10 ml anhydrous DCM to the addition funnel and cap with a rubber septum with an argon filled balloon.
19.Attach the addition funnel to the round-bottom flask and begin adding the tosyl chloride solution dropwise to the reaction flask with stirring (five to ten drops per minute).
20.Remove addition funnel and cap round-bottom flask with a rubber septum.
21.Stir an additional 30 min on the ice-water bath.
22.Remove flask from the ice-water bath and stir at room temperature (21°C) for 18 hr.
23.Add 50 ml 3% (w/v) aqueous NaHCO3 to a 250-ml separatory funnel.
24.Pour the contents of the reaction flask into the separatory funnel, rinsing the flask with DCM (3 × 15 ml).
25.Mix by inverting the separatory funnel, collect organic layer, and repeat this step with an additional 50 ml DCM. Discard aqueous layer.
26.Wash combined organic layers twice with 50 ml 3% (w/v) aqueous NaHCO3.
27.Collect organic layer in a 250-ml Erlenmeyer flask and add 2 g anhydrous sodium sulfate.
28.Wait 20 min and decant solution into a 500-ml round-bottom flask, rinsing with DCM (3 × 10 ml).
29.Concentrate contents of the round-bottom flask using a rotary evaporator.
30.Purify the resulting yellow oil with flash-column chromatography using silica gel (40 g) with EtOAc/hexanes (2:8, v/v) as eluent at a flow rate of 2 in. (∼5 cm) per minute.
31.Dry purified 2 on the high vacuum for a minimum of 2 hr.
32.Characterize 2 using 1H NMR, 13C NMR, TLC, and MS.
Perform phosphitylation
33.Transfer 0.25 g 2 (0.59 mmol) to an argon filled 25-ml round-bottom flask containing a stir bar on a hot plate.
34.Add 8 ml anhydrous tetrahydrofuran using a 10-ml syringe and dry needle to the flask, and begin stirring.
35.Add 206 μl anhydrous N,N -diisopropylethylamine (1.2 mmol) using a 1-ml syringe and dry needle.
36.Add 164 μl N,N -diisopropylamino cyanoethyl phosphonamidic chloride (0.94 mmol) using a 1-ml syringe and dry needle dropwise over 1 min.
37.Stir reaction at room temperature (21°C) for 1 hr.
38.Evaporate solvent under reduced pressure using a rotary evaporator.
39.Add 50 ml 3% (w/v) aqueous NaHCO3 to a 250-ml separatory funnel.
40.Prepare 100 ml of EtOAc/triethylamine solution (99.5:0.5, v/v).
41.Transfer the contents of the reaction flask to the separatory funnel using the EtOAc/TEA solution (70 ml).
42.Discard aqueous phase and wash organic phase with 70 ml saturated aqueous sodium chloride.
43.Collect organic layer into a 250-ml Erlenmeyer flask and add 2 g anhydrous sodium sulfate.
44.Wait 20 min and decant organic liquid into a 500-ml round-bottom flask, rinsing with the remaining EtOAc/TEA solution (3 × 10 ml).
45.Evaporate solvent under reduced pressure using a rotary evaporator.
46.Purify crude phosphoramidite 3 with flash-column chromatography using silica gel (35 g) with EtOAc/hexanes/TEA (2:79:1, v/v) as eluent at a flow rate of 2 in. (∼5 cm) per minute.
47.Characterize 3 using 1H NMR, 13C NMR, 31P NMR, TLC, and MS.
Basic Protocol 2: SYNTHESIS, FUNCTIONALIZATION, AND CHARACTERIZATION OF DNA OLIGONUCLEOTIDES CONTAINING DISULFIDE LINKER PHOSPHORAMIDITE 3
Oligonucleotides are prepared using SPS on an Applied Biosystems Model 3400 DNA synthesizer, but the protocol outlined here may be adapted to other automated DNA synthesizers. Solid-phase synthesis is carried out on long-chain alkylamine controlled pore glass (LCAA-CPG, 500 Å) containing the appropriate 5′-O-dimethoxytritylated (DMTr) nucleoside tethered via a succinyl linker at the 3′-hydroxyl functionality and using standard β-cyanoethylphosphoramidite chemistry. Synthesis cycles begin with detritylation of the support-bound nucleoside with 3% (w/v) trichloroacetic acid in dichloromethane to liberate the free 5′-hydroxyl group. DNA phosphoramidites are dissolved in anhydrous acetonitrile at a concentration of 0.1 M and coupling is achieved using 5-(ethylthio)-1H-tetrazole (0.25 M) in anhydrous acetonitrile for 120 s. Capping of oligonucleotides is carried out using 1:1:8 acetic anhydride/pyridine/tetrahydrofuran (v/v/v; solution A) and 4:21 1-methyl-imidazole/tetrahydrofuran (w/v; solution B), while oxidation is performed using 0.02 M iodine solution in 7:2:1 tetrahydrofuran/pyridine/water (v/v/v). RNA synthesis is achieved using the same auxiliary reagents with 2′-O-tert -butyldimethylsilyl protected phosphoramidites dissolved at a concentration of 0.15 M and coupled for 450 s. Phosphoramidite linker 3 is dissolved at a concentration of 0.1 M and coupled at the final position of the desired oligonucleotide for 180 s followed by standard capping and oxidation procedures. The final detritylation is not necessary for oligonucleotide synthesis. Support-bound DNA oligonucleotides containing linker 3 are functionalized with a series of different reagents to produce a variety of functional handles (N3, SH, NH2, OMe) depending on the needs of the user. RNA oligonucleotides are treated in an analogous manner but amongst the functional groups investigated, are only compatible with N3 and NH2 functionalization. Crude oligonucleotides are purified by IEX-HPLC on a Dionex DNAPAC PA-100 column (0.4 cm × 25 cm) with UV detection at 260 nm. Purified oligonucleotides are desalted using C18 SEP-PAK™ cartridges and are characterized by ESI-MS on an Agilent 1100 LC system coupled to a Thermo LTQ Orbitrap Velos mass spectrometer equipped with a heated electrospray ion source at negative mode. The protocol described here produces purified oligonucleotides containing a variety of functional handles which may be used for conjugation to a variety of ligands producing oligonucleotide conjugates with a reduction-responsive release mechanism.
Materials
-
Disulfide linker phosphoramidite 3 (obtained from Basic Protocol 1)
-
3% (w/v) trichloroacetic acid in dichloromethane (Glen Research, cat. no. 40-4140-57)
-
Activator: 5-ethylthio-1H-tetrazole in acetonitrile, 0.25 M (Glen Research, cat. no. 30-3140-57)
-
Cap Mix A: 80:10:10 tetrahydrofuran/pyridine/acetic anhydride (v/v/v; Glen Research, cat. no 40-4110-57)
-
Cap Mix B: 16% 1-methylimidazole in tetrahydrofuran (v/v; Glen Research, cat. no. 40-4220-57)
-
Oxidizing Solution: 0.02 M iodine in 7:2:1 tetrahydrofuran/pyridine/water (v/v/v; Glen Research cat. no. 40-4330-57)
-
Acetonitrile (ACN; HPLC grade, MilliporeSigma, CAS no. 75-05-8)
-
Dichloromethane (DCM, ACS reagent, MilliporeSigma, CAS no. 75-09-2)
-
Argon gas (Ar), ≥99.999% (Air Liquide Canada)
-
Controlled pore glass with succinyl linked protected nucleobase (CPG, Glen Research)
-
DNA phosphoramidites:
- Bz-dA-CE phosphoramidite (Glen Research, cat. no. 10-1000)
- iBu-dG-CE phosphoramidite (Glen Research, cat. no. 10-1020)
- Ac-dC-CE phosphoramidite (Glen Research, cat. no. 10-1015)
- dT-CE phosphoramidite (Glen Research, cat. no. 10-1030)
-
RNA phosphoramidites:
- Bz-rA-CE phosphoramidite (Glen Research, cat. no. 10-3003)
- iBu-rG-CE phosphoramidite (Glen Research, cat. no. 10-1020)
- Ac-rC-CE phosphoramidite (Glen Research, cat. no.10-3015)
- U-CE phosphoramidite (Glen Research, cat. no.10-3034)
-
Sodium azide, ≥99.5% (MilliporeSigma, CAS no. 26628-22-8)
-
Anhydrous dimethylformamide, ≥99.9% (MilliporeSigma, CAS no. 67-68-5)
-
Dithiothreitol (DTT, >99.0, BioShop, CAS no. 3483-12-3)
-
Autoclaved water (HPLC grade, MilliporeSigma, CAS no. 7732-18-5)
-
Ethanol, 95.0%, ACS reagent (MilliporeSigma, CAS no. 64-17-5)
-
Ammonium hydroxide solution, 28.0%-30.0%, ACS reagent (MilliporeSigma, CAS no. 1336-21-6)
-
Sodium hydroxide (pellets, certified ACS, Thermo Fisher Scientific, CAS no. 1310-73-2)
-
Methanol, ≥99.9%, HPLC grade (MilliporeSigma, CAS no. 67-56-1)
-
Triethylamine trihydrofluoride (TEA·3HF), ≥98.0% (MilliporeSigma, CAS no. 73602-61-6)
-
Anhydrous ethanol, ≥99.5% (MilliporeSigma, CAS no. 64-17-5)
-
IEX-HPLC buffer A (see recipe)
-
IEX-HPLC buffer B (see recipe)
-
C18 SEP-PAK™ elution buffer (see recipe)
-
DNA synthesizer (Applied Biosystems Model 3400 or equivalent)
-
Amber glass bottles (for attachment to synthesizer) with caps
-
Solid-phase synthesis column (Glen Research, cat. no. 20-0030-00)
-
Plastic Syringes (1-, 3-, 5-, and 10-ml, BD Plastipak® or equivalent)
-
Vortex mixer
-
Disposable Pasteur pipets (5¾ in. and 9 in. borosilicate glass)
-
Dry glass vials with cap (2 ml)
-
2-ml screw-top tubes with O-ring cap
-
1.5-ml microcentrifuge tubes
-
Mini centrifuge
-
Automatic pipet (P20, P200, and P1000) and autoclaved tips
-
Digital water bath
-
SpeedVac concentrator (with pump and cold trap)
-
Refrigerated centrifuge
-
Freezer
-
Spectrophotometer
-
Quartz cuvette (1 cm path length)
-
High-performance liquid chromatography instrument (equipped with column heater and spectrophotometer)
-
Dionex DNAPAC™ PA-100 column (0.4 cm × 25 cm)
-
Centrifuge tubes (5- and 15-ml)
-
C18 SEP-PAK™ cartridges (Waters, cat. no. WAT051910)
-
Luer 3-way stopcock
-
Electrospray ionization mass spectrometry (ESI-MS) system
Perform automated oligonucleotide solid-phase synthesis
1.Weigh out appropriate quantities of nucleoside phosphoramidites into amber bottles.
2.Dissolve phosphoramidites in anhydrous acetonitrile and place on synthesizer.
3.Add desired CPG (1 μmol) to synthesis column and place on synthesizer.
4.Equip synthesizer with all appropriate auxiliary reagents and perform SPS of desired oligonucleotide in DMT-ON mode with 3 as the final base.
5.Remove synthesis column and dry with a gentle stream of argon.
Functionalization and deprotection of linker modified oligonucleotide
6.Transfer oligonucleotide bound CPG to a clean 2-ml screw-top tube with an O-ring cap.
7.Add functionalizing reagent depending on the desired functional handle:
- Terminal azide (N3): 1 ml saturated sodium azide in DMF.
- Terminal amine (NH2): 1 ml ammonium hydroxide solution.
- Terminal thio (SH): 1 ml ammonium hydroxide solution and 7.7 mg DTT.
- Terminal methoxy (OMe): 1 ml 0.4 M NaOH in 4:1 methanol/water (v/v).
The use of saturated sodium azide solution at elevated temperatures in plastic screw-top tubes has been reported to produce impurities which could hinder the overall yield of isolated oligonucleotides. It is recommended that the azide treatment is performed in a clean 2-ml glass vial as no significant reduction in conversion/yield is observed when compared to functionalization in plastic screw-top tubes. Note, only terminal azide functionalization and amine functionalization were found to be compatible for RNA oligonucleotides.
8.Cap tube and let react at room temperature or at elevated temperature in a digital water bath.
- For terminal azide (N3): 30 min at 60°C.
- For terminal amine (NH2): 2 hr at room temperature (21°C) then 4 hr at 55°C.
- For terminal thiol (SH): 16 hr at 55°C.
- For terminal methoxy (OMe): 16 hr at room temperature (21°C).
Treatment of the CPG with sodium azide may be done at room temperature for 16 hr if oligonucleotides are sensitive to elevated temperatures, followed by deprotection at room temperature (see below) with no loss in conversion or isolated yield. All conditions are sufficiently basic enough to result in complete cleavage of the succinyl linker and removal of the protecting groups on the heterocyclic bases except for treatment with sodium azide which did not show any cleavage from the solid support at 60°C for 4 hr.
Only perform steps 9-15 if performing azide functionalization.
9.Vortex and centrifuge screw-top tube at 2000 × g for 10 s.
10.Carefully remove supernatant using an automatic pipet so as not to disturb the CPG.
11.Wash the CPG with 1 ml water, 1 ml ethanol, and finally 1 ml acetonitrile by adding the solvent, vortexing then centrifuging at 2000 × g for 10 s, discarding the washes at each step.
12.Dry the CPG in a SpeedVac concentrator for ∼10 min or until no solvent remains.
13.Add 1 ml ammonium hydroxide solution, vortex, and place in digital water bath for 4 hr at 55°C.
14.Remove sample from water bath and allow to cool for 5 min.
15.Centrifuge screw-top tube at 2000 × g for 10 s and carefully transfer supernatant to a new screw-top tube using an automatic pipet, rinsing the CPG with 200 μl acetonitrile and 200 μl water.
16.Dry the transferred oligonucleotide in a SpeedVac concentrator.
17.Add 200 μl TEA·3HF to the dried RNA oligonucleotide and briefly vortex the screw-top tube.
18.Place screw-top tube in a digital water bath at 65°C for 2 hr.
19.Remove screw-top tube and allow to cool for 5 min.
20.Add 800 μl cold methanol, vortex, and place in -20°C freezer for 30 min.
21.Isolate precipitated RNA using centrifugation at 14,000 × g for 15 min (4°C).
22.Wash pellet with 200 μl cold methanol, discard washing, and dry pellet in a SpeedVac concentrator.
Purify modified oligonucleotide
23.Add 1 ml water to the dried crude oligonucleotide and vortex to dissolve.
24.Quantitate crude oligonucleotide using a spectrophotometer at λ = 260 nm.
25.Analyze ∼0.1 OD260 of crude oligonucleotide by IEX-HPLC equipped with a Dionex DNAPAC™ PA-100 column (0.4 cm × 25 cm), degasser, column heater, and UV detector at 260 nm.
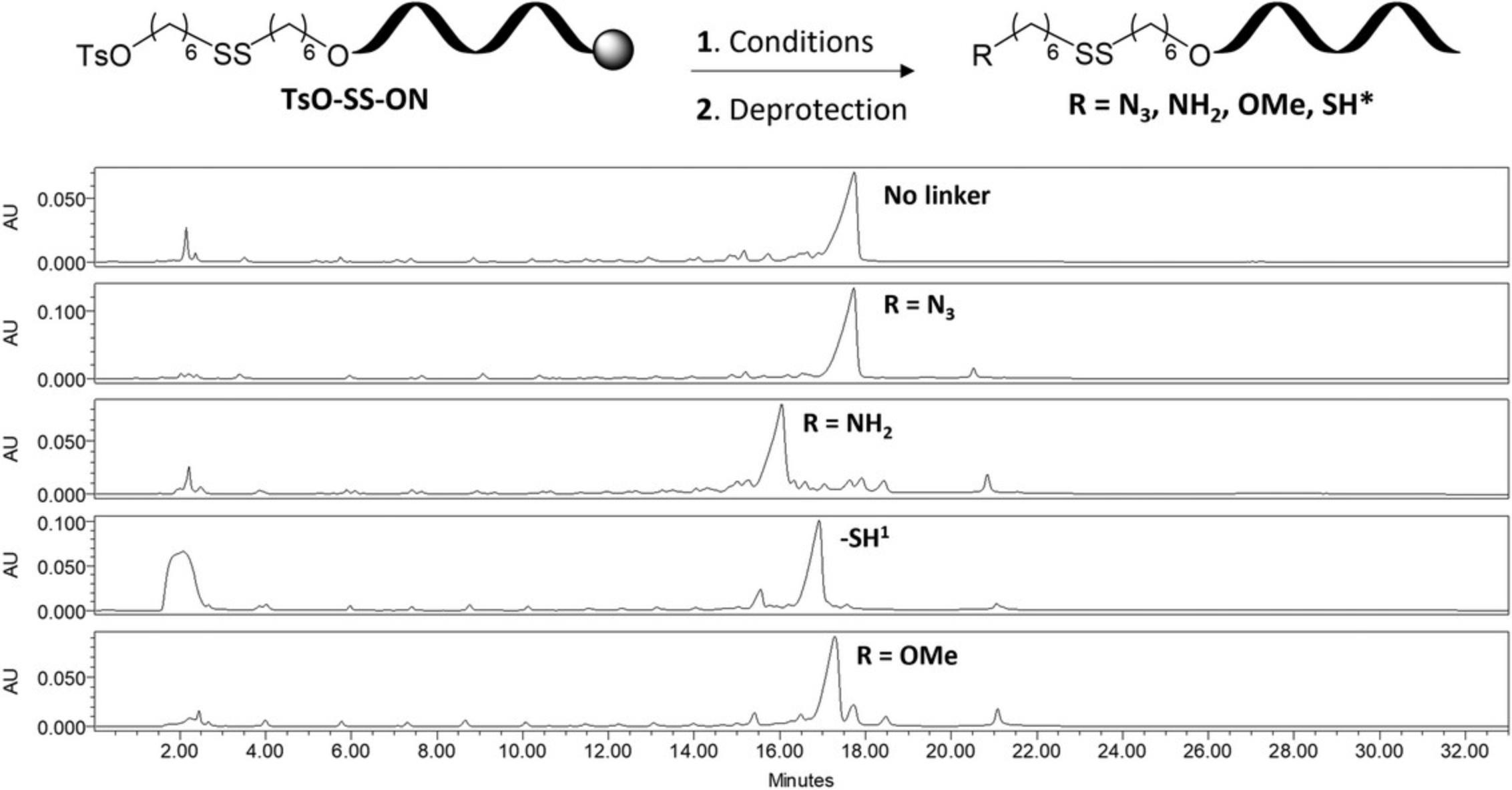
26.Purify ∼15 OD260 of modified oligonucleotide by IEX-HPLC using the optimal gradient at 260 nm, collecting the desired product in a 15-ml centrifuge tube.
27.Dilute collected desired product to 10 ml with water.
Desalt purified oligonucleotide using C18 SEP-PAK™ column
28.Attach C18 SEP-PAK™ cartridge to a Luer 3-way stopcock attached to a 10-ml plastic syringe.
29.Equilibrate column by washing with 5 ml ACN, 5 ml 50% water/ACN (v/v) and 5 ml water, consecutively.
30.Load diluted purified oligonucleotide and slowly pass through the column (one drop per s), collecting the eluent back into the same 15-ml centrifuge tube.
31.Vortex the contents of the tube, repeat the loading process two times, and measure the A 260 of the collected flow-through to ensure the oligonucleotide was retained. Repeat as needed.
32.Wash column twice with 10 ml water and collect in a waste beaker.
33.Elute oligonucleotide with 3-4 ml C18 SEP-PAK™ elution buffer into a 5-ml centrifuge tube.
34.Measure the A 260 of the eluted mixture to ensure complete retrieval of the oligonucleotide.
35.Dry transferred oligonucleotide in a SpeedVac concentrator.
Analyze purified oligonucleotide
36.Dissolve dried oligonucleotide in 1 ml water and measure A 260. Calculate the concentration.
37.Analyze ∼0.1 OD260 of purified oligonucleotide by IEX-HPLC using the same method for the crude analysis to estimate the purity of the oligonucleotide.
38.Analyze purified oligonucleotide by ESI-MS.
Basic Protocol 3: DISPLACEMENT OF TERMINAL TOSYLATE FUNCTIONALIZED DNA WITH PRIMARY ALIPHATIC AMINES
Support-bound oligonucleotide containing linker 3 is used for post-synthetic incorporation of ligands by direct substitution of the terminal tosylate with primary aliphatic amines. Modifications are introduced by immersing the bound oligonucleotide in an aqueous solution of the amine (25%, v/v) for 16 hr at 40°C in a water bath followed by removal of the protecting groups with concentrated aqueous ammonia. The crude oligonucleotides are purified by IEX-HPLC and characterized by ESI-MS to assess the identity and purity of the modified oligonucleotides (see Fig. 3 for IEX-HPLC chromatogram). As proof of example, the protocol outlined here utilizes tert -butylamine and n -butylamine for substitution, on a 14mer mixed-base oligonucleotide modified with linker 3 (Basic Protocol 2, Ts-SS-ON). Isolated oligonucleotides are obtained in moderate yields depending on the amine used, however conditions could be optimized for each individual amine if higher yields are desired. Moreover, while the protocol outlined here utilizes select amines, this approach could easily be adapted to various amine containing ligand molecules as an alternative method to generate oligonucleotide conjugates containing a reductive-responsive disulfide tether.
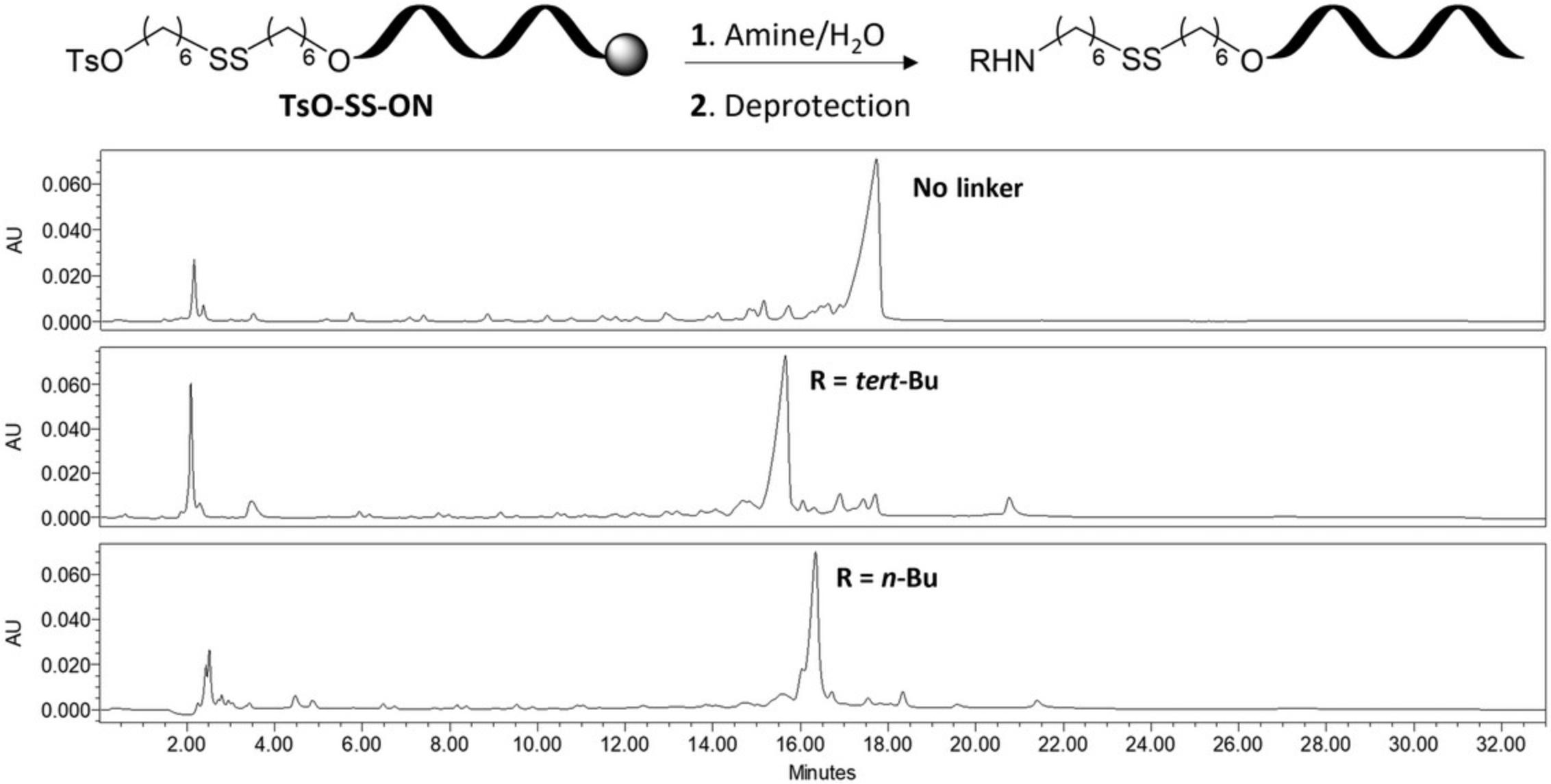
Materials
-
Tosylate modified ON (Ts-SS-ON) on CPG (Basic Protocol 2)
-
Acetonitrile (HPLC grade, MilliporeSigma, CAS no. 75-05-8)
-
Autoclaved water (HPLC grade, MilliporeSigma, CAS no. 7732-18-5)
-
Ammonium hydroxide solution, 28.0%-30.0%, ACS reagent (MilliporeSigma, CAS no. 1336-21-6)
-
tert -Butylamine, ≥99.5% (MilliporeSigma, CAS no. 75-64-9)
-
n -Butylamine, ≥99.5% (MilliporeSigma, CAS no. 109-73-9)
-
Benzylamine, ≥99.0% (MilliporeSigma, CAS no. 100-46-9)
-
6-Aminohexanol, ≥97.0% (MilliporeSigma, CAS no. 4048-33-3)
-
Anhydrous ethanol, ≥99.5% (MilliporeSigma, CAS no. 64-17-5)
-
IEX-HPLC buffer A (see recipe)
-
IEX-HPLC buffer B (see recipe)
-
C18 SEP-PAK™ elution buffer (see recipe)
-
Plastic Syringes (10-ml, BD Plastipak® or equivalent)
-
Vortex mixer
-
2-ml screw-top tubes with O-ring cap
-
1.5-ml microcentrifuge tubes
-
Mini centrifuge
-
Automatic pipet (P20, P200, and P1000) and autoclaved tips
-
Digital water bath
-
SpeedVac concentrator (with pump and cold trap)
-
Spectrophotometer
-
Quartz cuvette (1 cm path length)
-
High-performance liquid chromatography instrument (equipped with column heater and spectrophotometer)
-
Dionex DNAPAC™ PA-100 column (0.4 cm × 25 cm)
-
Centrifuge tubes (5- and 15-ml)
-
C18 SEP-PAK™ cartridges
-
Luer 3-way stopcock
-
Electrospray ionization mass spectrometry (ESI-MS) system
Perform substitution with amine and deprotection
1.Transfer dry support-bound Ts-SS-ON to a clean 2-ml screw-top tube.
2.Add 375 μl water and 125 μl amine to the tube, vortex, and place in a digital water bath at 40°C for ∼16 hr.
3.Remove sample from the water bath and centrifuge at 2000 × g for 10 s.
4.Transfer solution to a clean 2-ml screw-top tube, carefully avoiding any CPG and remove the solvent using a SpeedVac concentrator.
5.Add 1 ml concentrated aqueous ammonia solution, vortex, and place in a water bath at 55°C for 4 hr.
6.Remove sample from the bath and allow to cool to room temperature. Remove solution in vacuo using a SpeedVac concentrator.
Purify crude oligonucleotide
7.Dissolve dried oligonucleotide in 1 ml water and measure A 260. Calculate the concentration.
8.Analyze ∼0.1 OD260 of crude oligonucleotide by IEX-HPLC equipped with a Dionex DNAPAC™ PA-100 column (0.4 cm × 25 cm), degasser, column heater, and UV detector at 260 nm.
9.Purify ∼15 OD260 of modified oligonucleotide by IEX-HPLC using the optimal gradient at 260 nm, collecting fractions in a 15-ml centrifuge tube.
10.Merge collected fractions in a 15-ml centrifuge tube and dilute to 10 ml with water.
Desalt purified oligonucleotide using C18 SEP-PAK™ column
11.Attach C18 SEP-PAK™ cartridge to a Luer 3-way stopcock attached to a 10-ml plastic syringe.
12.Equilibrate column by washing with 5 ml ACN, 5 ml 50% water/ACN (v/v) and 5 ml water, consecutively.
13.Load the diluted purified oligonucleotide and slowly pass through the column (one drop per s), collecting the eluent back into the same 15-ml tube.
14.Vortex contents of the tube, repeat the loading process two times, and measure the A 260 of the collected flow-through to ensure the oligonucleotide was retained. Repeat as needed.
15.Wash column twice with 10 ml water and collect in a waste beaker.
16.Elute oligonucleotide with 3-4 ml of C18 SEP-PAK™ elution buffer into a 5-ml centrifuge tube.
17.Measure the A 260 of the eluted mixture to ensure complete retrieval of the oligonucleotide.
18.Dry transferred oligonucleotide in a SpeedVac concentrator.
Analyze purified oligonucleotide
19.Dissolve dried oligonucleotide in 1 ml water and measure the A 260. Calculate the concentration.
20.Analyze ∼0.1 OD260 of purified oligonucleotide by IEX-HPLC using the same method described in step 8 for the crude analysis to estimate the purity of the oligonucleotide.
21.Analyze the purified oligonucleotide by ESI-MS.
Basic Protocol 4: SYNTHESIS OF OLIGONUCLEOTIDE-PEG CONJUGATE
Conjugation of an azide functionalized dT10 oligonucleotide prepared using disulfide linker 3 (Basic Protocol 2) with an alkyne functionalized PEG (Mn = 5000) via CuAAC is described (Fig. 4). The conjugation reaction is performed in aqueous sodium chloride solution and is complete in 16 hr at room temperature using copper(I) iodide and N,N,N′,N′′,N′′ -pentamethyldiethylenetriamine (PMDTA) in dimethyl sulfoxide. The conjugate is isolated by desalting using a C18 SEP-PAK™ cartridge and analyzed using 20% denaturing PAGE as evident by a band with significantly reduced mobility (see Fig. 4). The prepared conjugate is treated with varying concentrations of DTT at room temperature for 4 hr to cleave the disulfide tether which is monitored by PAGE.
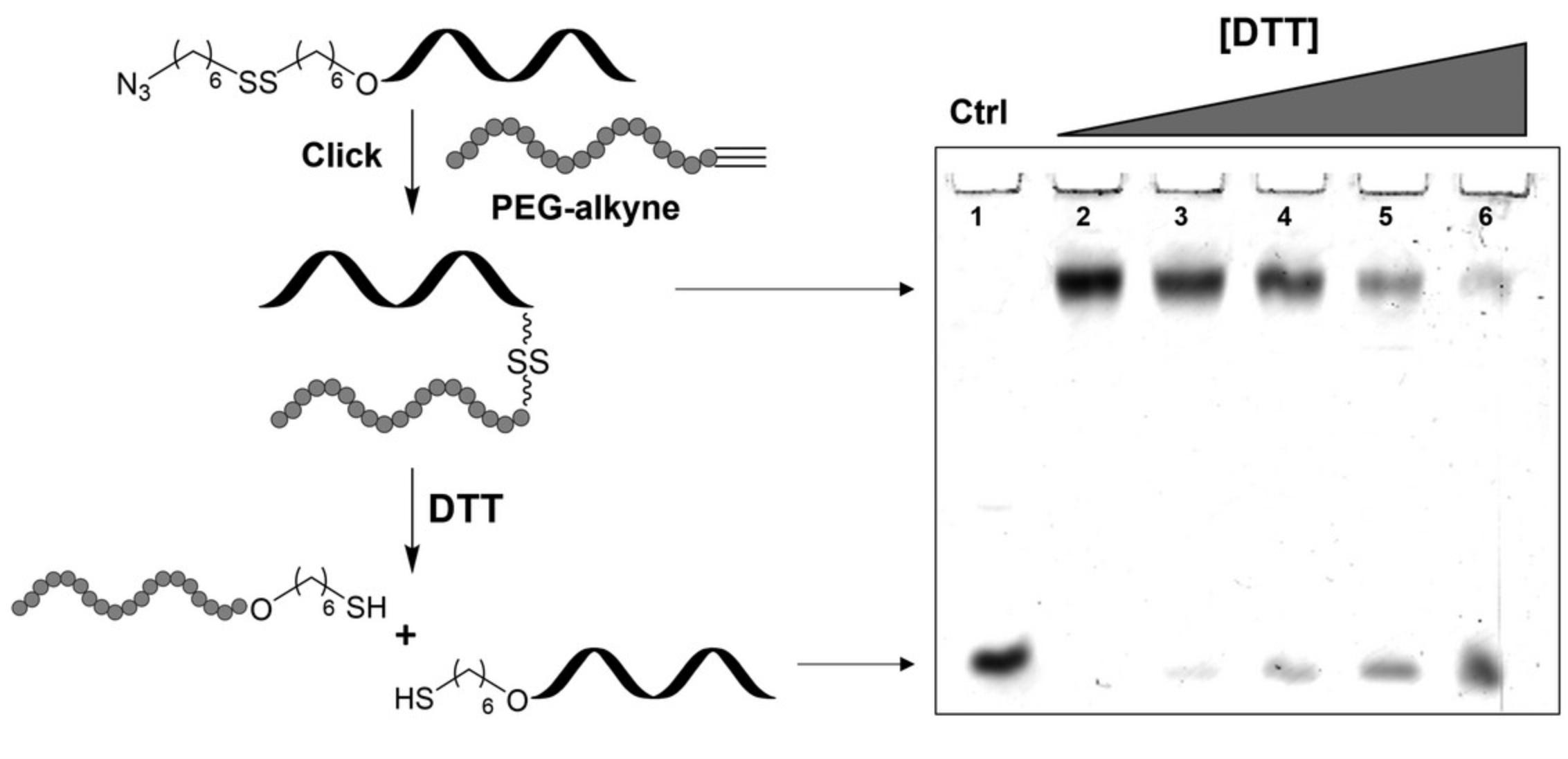
Materials
-
Azide modified dT10 (N3-SS-dT10) using phosphoramidite 3 (Basic Protocol 2)
-
PEG-alkyne (Support Protocol)
-
Aqueous sodium chloride (0.2 M)
-
Copper(I) iodide, ≥99.5% (MilliporeSigma, CAS no. 7681-65-4)
-
N,N,N′,N′′,N′′ -Pentamethyldiethylenetriamine, ≥99.0% (MilliporeSigma, CAS no. 3030-47-5)
-
Dimethylsulfoxide, ≥99.9% (MilliporeSigma, CAS no. 67-68-5)
-
Acetonitrile (HPLC grade, MilliporeSigma, CAS no. 75-05-8)
-
Water (HPLC grade, MilliporeSigma, CAS no. 7732-18-5)
-
C18 SEP-PAK™ elution buffer (see recipe)
-
2% (v/v) aqueous EDTA, ≥99.0% (MilliporeSigma, CAS no. 60-00-4)
-
Dithiothreitol (DTT, >99.0, BioShop, CAS no. 3483-12-3)
-
Ethyl acetate (EtOAc), ≥99.8% (MilliporeSigma, CAS no. 141-78-6)
-
Formamide, ≥99.5% (BioShop, CAS no. 75-12-7)
-
20% ammonium persulfate (APS) solution (see recipe)
-
Tetramethylethylenediamine (TEMED), ≥99.0% (BioShop, CAS no. 110-18-9)
-
20% denaturing PAGE solution (see recipe)
-
10× TBE solution (Biotech grade, BioBasic, cat. no. A0026)
-
Invitrogen SYBR™ Safe DNA Gel Stain (Thermo Fisher Scientific) or other similar DNA stain
-
C18 SEP-PAK™ cartridges (Waters, cat. no. WAT051910)
-
1.5-ml microcentrifuge tubes
-
Nutator mixer
-
Centrifuge tubes (5- and 15-ml)
-
Plastic Syringes (10-ml, BD Plastipak® or equivalent)
-
Vortex mixer
-
Mini centrifuge
-
SpeedVac concentrator (with pump and cold trap)
-
Automatic pipet (P20, P200, and P1000) and autoclaved tips
-
Spectrophotometer
-
Quartz cuvette (1 cm path length)
-
C18 SEP-PAK™ cartridges
-
Luer 3-way stopcock
-
0.75-mm analytical gel plates (10.1 × 7.3 cm)
-
0.75-mm analytical spacer gel plates (10.1 × 8.2 cm)
-
0.75-mm 10-well comb
-
Gel electrophoresis setup (casting tray, chamber, and power supply)
-
Plastic container (Rubbermaid, Square, 669 ml, 6.2 cm × 6.2 cm × 2.1 cm, W × L × H)
-
Amersham™ Typhoon™ 5 Biomolecular Imager (Cytiva)
-
Electrospray ionization mass spectrometry (ESI-MS) system
Perform CuAAC
1.Transfer 60 nmol of N3-SS-dT10 to a 1.5-ml microcentrifuge tube and dry in a SpeedVac concentrator.
2.Add 6 mg PEG-alkyne (Support Protocol, 1200 nmol).
3.Add 950 μl 0.2 M aqueous sodium chloride and vortex to dissolve.
4.Add 2.3 mg copper(I) iodide (12 μmol) in a separate 1.5-ml microcentrifuge tube and dissolve in 100 μl DMSO.
5.Add 2.0 μl PMDTA (12 μmol) to the copper solution and vortex to mix.
6.Degas both tubes by placing them in a SpeedVac concentrator for 2 min with the caps slightly loosened.
7.Transfer 50 μl of the copper mixture to the oligonucleotide-PEG mixture, and gently mix on a nutator mixer for ∼16 hr at room temperature in the dark.
8.Transfer contents of the reaction tube to a 15-ml centrifuge tube, rinsing twice with 1 ml water and dilute to 10 ml with water.
9.Attach a C18 SEP-PAK™ cartridge to a Luer 3-way stopcock attached to a 10-ml plastic syringe.
10.Equilibrate column by washing with 5 ml ACN, 5 ml 50% water/ACN (v/v), and 5 ml water, consecutively.
11.Load the diluted purified oligonucleotide and slowly pass through the column (one drop per s), collecting the eluent back into the same 15-ml tube.
12.Vortex contents of the tube and repeat loading process two times.
13.Wash column with 2 ml aqueous EDTA and collect in a waste beaker.
14.Wash column twice with 10 ml water and collect in a waste beaker.
15.Elute the oligonucleotide with 4 ml C18 SEP-PAK™ elution buffer into a 5-ml centrifuge tube.
16.Measure the A 260 of the eluted mixture to ensure complete retrieval of the oligonucleotide.
17.Dry oligonucleotide in a SpeedVac concentrator.
Reduce conjugate with DTT
18.Dissolve dried conjugate in 1 ml water and measure the A 260. Calculate the concentration.
19.Transfer 50 pmol of conjugate to five labeled 1.5-ml microcentrifuge tubes and dry in a SpeedVac concentrator.
20.Prepare solutions of 0.01, 0.1, 0.5, and 1.0 M DTT in 500 μl water.
21.Add the DTT solutions to four separate microcentrifuge tubes containing the conjugate and gently mix all samples on a nutator mixer 4 hr at room temperature.
22.Add 500 μl EtOAc to the microcentrifuge tubes, vortex, and allow to separate.
23.Remove the upper layer of the solution with an automatic pipet, being careful not to remove the aqueous layer.
24.Repeat steps 22-23 three times to remove any excess DTT and discard the washings.
25.Dry remaining aqueous layer in a SpeedVac concentrator.
Visualize using denaturing PAGE
26.Dissolve dried samples in 10 μl formamide, vortex to dissolve, and centrifuge at 2000 × g for 10 s.
27.Prepare a 20% denaturing gel using 5 ml of 20% denaturing PAGE solution, 20% APS solution, and 10 μl TEMED on 0.75-mm analytical gel plates containing a 10-well comb.
28.Wash wells of the gel with running buffer (1× TBE) and place gel cassette in the appropriate electrophoresis chamber. Fill with fresh 1× running buffer.
29.Load samples in individual lanes, connect to the power supply, and run gel at 200 V for 1.5 hr.
30.Add 20 ml water to a clean plastic container and add 2 μl SYBR™ Safe DNA Gel Stain in the dark.
31.Remove gel from the electrophoresis assembly carefully and place in the plastic container using clean gloves.
32.Gently mix the contents of the plastic container in the dark for 20 min.
33.Decant diluted stain and rinse three times with distilled water.
34.Image the gel using Amersham™ Typhoon™ 5 Biomolecular Imager.
Support Protocol: PREPARATION OF PEG-ALKYNE
Preparation of alkyne functionalized PEG-5k is performed in one step using potassium hydroxide and propargyl bromide. The reaction yields PEG molecules containing terminal alkyne groups so that they may be conjugated to linker functionalized oligonucleotides using the copper(I) catalyzed azide alkyne cycloaddition reaction.
Materials
-
Poly(ethylene glycol) methyl ether (Mn 5000, MilliporeSigma, cat. no. 81323)
-
Anhydrous toluene, 99.8% (MilliporeSigma, CAS no. 108-88-3)
-
Potassium hydroxide (ACS reagent, MilliporeSigma, CAS no. 1310-58-3)
-
80% propargyl bromide in toluene, v/v (MilliporeSigma, cat. no. 81831)
-
Acetone, ≥99.5% (MilliporeSigma, CAS no. 67-64-1)
-
Diethyl ether, ≥99.0% (MilliporeSigma, CAS no. 60-29-7)
-
Celite (Alfa Aesar, cat. no. L14552)
-
Hot plate with stirrer
-
Magnetic stir bar
-
Round-bottom flask (250 ml)
-
Rubber septum
-
Addition funnel (10 ml)
-
Plastic Syringes (5 ml, BD Plastipak® or equivalent)
-
Dry Luer needles
-
Silicon oil bath
-
Glass Buchner funnel with filter
-
Rotary evaporator
-
Scintillation vial (20 ml)
-
High Vacuum pump
Perform propargylation
1.Add 5.0 g poly(ethylene glycol) methyl ether (Mn 5000, 1 mmol) to a dry 250-ml round-bottom flask containing a stir bar and stopper the flask.
2.Add 40 ml anhydrous toluene to the reaction flask and begin stirring.
3.Add 0.70 g potassium hydroxide (7.1 mmol) to the reaction flask.
4.Place the reaction flask in a heated oil bath at 60°C on a hot plate and stir for 30 min.
5.Add 3.4 ml propargyl bromide (36 mmol) to a 10-ml addition funnel and stopper with rubber septum.
6.Install addition funnel on top of the reaction flask and begin adding propargyl bromide dropwise over a period of 5 min, with stirring at 60°C.
7.Remove addition funnel, cap reaction flask with a rubber septum, and continue stirring at 60°C overnight.
8.Filter the hot mixture over a Celite plug in a glass Buchner funnel into a clean round-bottom flask.
9.Wash Celite with acetone (3 × 20 ml) and concentrate the combined filtrate in vacuo using a rotary evaporator.
10.Precipitate the concentrated mixture with diethyl ether.
11.Filter precipitate and wash with cold diethyl ether.
12.Transfer precipitate to a tared scintillation vial and dry on the high vacuum overnight.
13.Characterize PEG-alkyne using 1H NMR and FT-IR.
REAGENTS AND SOLUTIONS
Ammonium persulfate (APS) solution, 20%
- 0.2 g ammonium persulfate (Thermo Fisher Scientific, cat. no. 17874)
- 1 ml distilled water
- Store at 4°C for up to 1 month
C18 SEP-PAK™ elution buffer
- 10 ml acetonitrile (for HPLC, gradient grade, MilliporeSigma, cat. no. 34851)
- 20 ml methanol, ≥99.9%, HPLC grade (MilliporeSigma, CAS no. 67-56-1)
- 10 ml water
- Store at room temperature for up to 12 months
Final concentrations: acetonitrile: 25% (v/v); methanol: 50% (v/v); water: 25% (v/v).
Denaturing PAGE solution, 20%
- 76 g acrylamide (electrophoresis grade, BioShop, cat. no. ACR002)
- 4 g bis-acrylamide (Bio UltraPure grade, BioShop, cat. no. BIS001)
- 168 g urea (Bio UltraPure grade, BioShop, cat. no. URE001)
- 40 ml 10× TBE solution (Biotech grade, BioBasic, cat. no. A0026)
- Dilute to 400 ml with water
- Store in an amber bottle at 4°C for up to 4 months
- Preparation should be conducted in a fume hood and solution should be covered and stirred with a magnetic stir bar on a hotplate until all reagents are dissolved.
- Acrylamide, bis-acrylamide, and urea are toxic. Caution should be taken when preparing this solution and an appropriate mask (N95) should be worn when weighing out these materials.
IEX-HPLC buffer A
- 100 ml acetonitrile (for HPLC, gradient grade, MilliporeSigma, cat. no. 34851)
- 100 ml Trizma® hydrochloride solution, pH 7.4 (BioPerformance Certified, MilliporeSigma, cat. no. T2194)
- 800 ml water (HPLC grade, MilliporeSigma, CAS no. 7732-18-5)
- Store for up to 6 months at 4°C
- Acetonitrile should be added in a fume hood.
Final concentrations: acetonitrile: 10% (v/v); Trizma® hydrochloride solution: 0.1 M; water: 80% (v/v).
IEX-HPLC buffer B
- 100 ml acetonitrile (for HPLC, gradient grade, MilliporeSigma, cat. no. 34851)
- 100 ml Trizma® hydrochloride solution, pH 7.4 (BioPerformance Certified, MilliporeSigma, cat. no. T2194)
- 250 ml 4 M aqueous sodium chloride solution
- 450 ml water (HPLC grade, MilliporeSigma, CAS no. 7732-18-5)
- Store for up to 6 months at 4°C
- Acetonitrile should be added in a fume hood.
Final concentrations: acetonitrile: 10% (v/v); Trizma® hydrochloride solution: 0.1 M; sodium chloride solution: 1 M; water: 80% (v/v).
COMMENTARY
Background Information
Harnessing the favorable properties of various ligands through covalent conjugation to oligonucleotides is a promising approach to enhance the therapeutic, diagnostic, and biophysical properties of nucleic acids. Moreover, the nature of the covalent tether has been of interest as the length, chemical composition, and stability of the linkage is strongly correlated with the performance of the oligonucleotide conjugate (Dragovich et al., 2021a, 2021b; Wada et al., 2016). To date, various linkages have been developed via common reactions such as amide bond formation, click reactions, and thiol-disulfide exchanges through incorporation of functional chemical handles on oligonucleotides post-synthetically or using automated SPS with custom phosphoramidites (Singh et al., 2010). Of particular significance, synthesis of oligonucleotide conjugates via thiol-disulfide exchange is of particular interest given the nature of the resulting reduction-responsive disulfide tether which could be harnessed to trigger release of oligonucleotides (Saito, Swanson, & Lee, 2003).
The most common approach for the preparation of oligonucleotide conjugates containing disulfide linkages is via the thiol-disulfide exchange reaction between a thiol and pyridyl disulfide installed on either coupling partner (Winkler, 2013). This approach consequently requires installation of both functional groups which at times can be synthetically challenging and requires protection of the reactive thiol group prior to conjugation (Danial & Postma, 2017). Moreover, the presence of multiple thiol groups poses additional issues with site-specific conjugation (Stasińska et al., 2019). With these issues in mind, we sought to synthesize a new linker that may be introduced into the oligonucleotide scaffold using automated SPS which would allow for the synthesis of conjugates bearing a disulfide using alternative chemical approaches. In addition, given the tremendous success of the CuAAC (Kolb, Finn, & Sharpless, 2001; Rostovtsev, Green, Fokin, & Sharpless, 2002; Tornøe, Christensen, & Meldal, 2002), we sought to combine the two approaches which would allow for disulfide linked conjugates to be formed using click chemistry. With these goals, we developed a disulfide phosphoramidite linker containing a tosylate group which may be introduced at the terminal position of synthetic oligonucleotides using automated SPS (Fig. 1, Scheme B). Displacement of the terminal tosylate group with NaN3 followed by standard deprotection yielded azide functionalized oligonucleotides bearing a disulfide oligonucleotide which we demonstrate can be used for the synthesis of a reduction-responsive oligonucleotide-PEG conjugate via CuAAC. Pleasantly, treatment of the tosylate group with ammonia during deprotection rather than NaN3 results in displacement of the tosylate to yield a terminal amino functionality which could be used for further functionalization. This led us to briefly explore a variety of other functional handles that could be introduced using the described linker as well as the possibility for direct substitution of the tosylate with primary aliphatic amines. This protocol describes the synthesis, incorporation, functionalization, and characterization of the disulfide linker, and its potential in oligonucleotide conjugation is highlighted.
Critical Parameters
The protocols explained here depend primarily on the quality of the synthesized phosphoramidite linker outlined in Basic Protocol 1. The chemical reactions described are not of advanced difficulty however the success of the synthesized linker is strongly affected by the skill of the organic chemist. In that regard, this protocol should be performed by a chemist familiar with basic organic chemistry operations including but not limited to thin-layer chromatography, column chromatography, and organic extractions. Moreover, training with the various laboratory instruments described in all protocols is necessary to achieve the results outlined in this procedure. With respect to all organic transformations under anhydrous conditions (tosylation and phosphitylation), it is imperative that care to exclude moisture is taken to ensure the quality of the final product produced. One key parameter in the synthesis of the phosphoramidite is the presence of other impurities which can severely impact the yield of the final oligonucleotide; therefore, it is imperative that the linker phosphoramidite is purified and analyzed correctly by means of NMR, to ensure the highest quality is obtained and if not, additional purification be performed.
Incorporation of the linker phosphoramidite is completely compatible with DNA SPS using the reagents described here. Most users will likely not be equipped with the exact synthesizer and/or reagents in this protocol, but we envision no potential issue adapting this protocol to other systems given certain key elements are retained. Notably, the choice of oxidizing agent can have an impact on the disulfide bond and therefore it is suggested that dilute I2 (0.02 M) be used to eliminate any potential oxidative cleavage of the linker during SPS. Moreover, the phosphoramidite should be mixed well with ACN (up to 10 min) prior to synthesis as it can at times be difficult to dissolve given its viscous nature. In our case, we have used the same phosphoramidite bottle several times to functionalize oligonucleotides over the course of a 6-month period by removing excess solvent in vacuo and keeping the reagent at -20°C with only a slight decrease in overall yield. However, for optimal results we suggest preparing the linker and portioning single-injection quantities into individual bottles at -20°C for use within 3 months.
Upon successful incorporation, the linker can be modified quite robustly using the protocols described here depending on the functional handle desired. Nonetheless, all protocols described here should be performed by someone with at least basic training with synthetic oligonucleotides including knowledge of the laboratory techniques described (e.g., pipetting, HPLC analysis, UV analysis). Moreover, the quality of the oligonucleotide is largely dependent on the HPLC parameters and skills of the user with oligonucleotide purification. Therefore, we highly encourage all users have access to all proper instrumentation and experience to achieve appropriate results.
Troubleshooting
In our experience, any issues regarding functionalization of oligonucleotides using 3 is directly related to the quality of the synthesized phosphoramidite. These issues include but are not limited to poor coupling of 3 during solid-phase synthesis, poor yield of functionalized oligonucleotide, and the presence of several full length impurities. If at any time these issues arise, we recommend repurifying the linker and analyzing as described in the protocol (1H and 31P NMR). In addition, poor yields can also arise from the use of a strong oxidizing solution during solid-phase synthesis (>0.02 M) and thus use of more dilute oxidant should resolve the issue. In addition, some users may encounter difficulties with purification of the functionalized oligonucleotides by IEX-HPLC. In this case, we suggest performing several analytical analyses to obtain the ideal gradient and temperature to facilitate purification. It should also be noted that for longer oligonucleotides or those capable of forming stable secondary structures, IEX-HPLC may not be a suitable purification option and alternative methods such as denaturing polyacrylamide gel electrophoresis may be required.
Further, during our initial investigation with conjugation of the azide functionalized oligonucleotide using click-chemistry we discovered it is imperative to use a ligand to avoid copper-mediated degradation of the oligonucleotide. In our case, N,N,N′,N′′,N′′ -pentamethyldiethylenetriamine (PMDTA) gave optimal results however, it is possible depending on the system that alternative ligands may provide better results. In addition, the success of the click reaction is highly dependent upon many variables including but not limited to the oligonucleotide sequence, the ligand, the copper source, length of the reaction, and solubility of the alkyne-functionalized polymer. Therefore, if in other cases the conjugation reaction does not produce the intended conjugate, optimization of any of these variables may be required.
Understanding Results
The phosphoramidite linker 3 described in this protocol is obtained in moderate yields and easily characterized by 1H NMR and mass spectrometry. Synthesis of the linker beginning with 1.0 g of 6-mercapto-1-hexanol yields 0.17 g of disulfide phosphoramidite which may be used for nearly seven incorporations using the protocols outlined here. The coupling efficiency of the linker is difficult to obtain as no dimethoxytrityl group is present on the linker itself and as a result could only be estimated by HPLC analysis (see Figs. 2 and 3 for examples of crude HPLC chromatograms). Similarly, efficiency for the displacement of the tosylate groups may be estimated by integrating the corresponding peaks on the HPLC chromatogram. In all cases, the major species was purified and determined to be the intended product, however mass spectrometry is crucial in determining the identity of the isolated species. The synthesis of oligonucleotide conjugates using this approach has, to date, been limited to click chemistry using CuAAC with an alkyne functionalized PEG polymer. The reaction is robust and the linker is stable to the conditions used for this ligation. The oligonucleotide conjugate is visualized using denaturing PAGE in which the larger conjugate has a significantly reduced mobility compared to the oligonucleotide control (Fig. 4). Subjecting the synthesized conjugate to reducing agents such as DTT will result in cleavage of the disulfide bond and release of the oligonucleotide which can be monitored by denaturing PAGE.
Time Considerations
The synthesis of the phosphoramidite linker described in Basic Protocol 1 can be accomplished within 2 to 3 days depending if additional purification is required for the phosphoramidite linker and the instruments available (NMR and MS). Incorporation of the linker phosphoramidite (Basic Protocol 2) into oligonucleotides occurs quickly (normally 10 min for complete cycle) if the desired CPG-bound oligonucleotide is prepared previously. Depending on the functional handle required, displacement of the tosylate and deprotection of the oligonucleotide can be achieved within 4.5 to 17 hr. Displacement of the tosylate with primary amines requires 1 day followed by standard deprotection times. Purification of oligonucleotides depends on the difficulty of purification, however in most cases, desalted pure oligonucleotides can be obtained within 2 days. In the example described here, the linker phosphoramidite is synthesized, incorporated into a dT10 oligonucleotide, functionalized with a terminal azide (dT10-SS-N3), and purified in 5 days. Conjugation of this oligonucleotide to an alkyne-modified PEG and DTT mediated cleavage of the conjugate (Basic Protocol 4) is performed in 2 days.
Acknowledgments
The authors would like to thank Hourieh Movasat and Sarah Ménard for preliminary work with prior systems which led to the conception of this work in addition to Dr. Anne Noronha and Gabrielle Juneau for help with SPS (Concordia University). This research was supported by the Fonds de recherche du Québec – Nature et technologies (FRQNT) Projet de recherche en équipe program (to J.K.O. and C.J.W., 2019-PR-255255), the Natural Science and Engineering Research Council (NSERC) of Canada as Discovery Grants to J.K.O. (RGPIN-2016-04989 and RGPIN-2021-03473) and C.J.W. (RGPIN-2017-06683), and a Canada Research Chair (CRC) Award to J.K.O. (Tier II CRC in Nanobioscience, renewed in 2016, 950−231058). A.P. was supported with postgraduate fellowships from NSERC, Concordia University, and the NSERC Collaborative Research and Training Experience Program in Programmed Molecules for Therapeutics, Sensing, and Diagnostics (PROMOTE).
Author Contributions
Alexander Pontarelli : Conceptualization, data curation, formal analysis, investigation, methodology, writing original draft; Jiang Tian Liu : Investigation, methodology; Jung Kwon Oh : Conceptualization, funding acquisition, project administration; Christopher J. Wilds : Funding acquisition, investigation, methodology, project administration, supervision, writing review and editing.
Conflict of Interest
The authors declare no conflict of interest.
Open Research
Data Availability Statement
The data that support the findings of this study are available from the corresponding author upon reasonable request.
Literature Cited
- Arnold, A. E., Malek-Adamian, E., Le, P. U., Meng, A., Martínez-Montero, S., Petrecca, K., … Shoichet, M. S. (2018). Antibody-antisense oligonucleotide conjugate downregulates a key gene in glioblastoma stem cells. Molecular Therapy - Nucleic Acids , 11, 518–527. doi: 10.1016/j.omtn.2018.04.004
- Bakardzhiev, P., Toncheva-Moncheva, N., Mladenova, K., Petrova, S., Videv, P., Moskova-Doumanova, V., … Rangelov, S. (2020). Assembly of amphiphilic nucleic acid-polymer conjugates into complex superaggregates: Preparation, properties, and in vitro performance. European Polymer Journal , 131, 109692. doi: 10.1016/j.eurpolymj.2020.109692
- Crooke, S. T., Baker, B. F., Crooke, R. M., & Liang, X. (2021). Antisense technology: An overview and prospectus. Nature Reviews Drug Discovery , 20, 427–453. doi: 10.1038/s41573-021-00162-z
- Danial, M., & Postma, A. (2017). Disulfide conjugation chemistry: A mixed blessing for therapeutic drug delivery? Therapeutic Delivery , 8, 359–362. doi: 10.4155/tde-2017-0003
- Dragovich, P. S., Pillow, T. H., Blake, R. A., Sadowsky, J. D., Adaligil, E., Adhikari, P., … Zhu, X. (2021a). Antibody-mediated delivery of chimeric BRD4 degraders. Part 1: Exploration of antibody linker, payload loading, and payload molecular properties. Journal of Medicinal Chemistry , 64, 2534–2575. doi: 10.1021/acs.jmedchem.0c01845
- Dragovich, P. S., Pillow, T. H., Blake, R. A., Sadowsky, J. D., Adaligil, E., Adhikari, P., … Zhu, X. (2021b). Antibody-mediated delivery of chimeric BRD4 degraders. Part 2: Improvement of in vitro antiproliferation activity and in vivo antitumor efficacy. Journal of Medicinal Chemistry , 64, 2576–2607. doi: 10.1021/acs.jmedchem.0c01846
- Juliano, R. L., Ming, X., & Nakagawa, O. (2012). The chemistry and biology of oligonucleotide conjugates. Accounts of Chemical Research , 45, 1067–1076. doi: 10.1021/ar2002123
- Khvorova, A., & Watts, J. K. (2017). The chemical evolution of oligonucleotide therapies of clinical utility. Nature Biotechnology , 35, 238–248. doi: 10.1038/nbt.3765
- Kolb, H. C., Finn, M. G., & Sharpless, K. B. (2001). Click chemistry: Diverse chemical function from a few good reactions. Angewandte Chemie International Edition , 40, 2004–2021. doi: 10.1002/1521-3773(20010601)40:11<2004::AID-ANIE2004>3.0.CO;2-5
- Le, B. T., Kosbar, T. R., & Veedu, R. N. (2020). Novel disulfide-bridged bioresponsive antisense oligonucleotide induces efficient splice modulation in muscle myotubes in vitro. ACS Omega , 5, 18035–18039. doi: 10.1021/acsomega.0c01463
- Patutina, O. A., Bazhenov, M. A., Miroshnichenko, S. K., Mironova, N. L., Pyshnyi, D. V., Vlassov, V. V., & Zenkova, M. A. (2018). Peptide-oligonucleotide conjugates exhibiting pyrimidine-X cleavage specificity efficiently silence miRNA target acting synergistically with RNase H. Scientific Reports , 8, 14990. doi: 10.1038/s41598-018-33331-z
- Pontarelli, A., Liu, J. T., Movasat, H., Ménard, S., Oh, J. K., & Wilds, C. J. (2022). Synthesis of a convertible linker containing a disulfide group for oligonucleotide functionalization. Organic Letters , 24, 5579–5583. doi: 10.1021/acs.orglett.2c02149
- Rostovtsev, V. V., Green, L. G., Fokin, V. V., & Sharpless, K. B. (2002). A stepwise Huisgen cycloaddition process: Copper(I)-catalyzed regioselective “ligation” of azides and terminal alkynes. Angewandte Chemie International Edition , 41, 2596–2599. doi: 10.1002/1521-3773(20020715)41:14<2596::AID-ANIE2596>3.0.CO;2-4
- Saito, G., Swanson, J. A., & Lee, K.-D. (2003). Drug delivery strategy utilizing conjugation via reversible disulfide linkages: Role and site of cellular reducing activities. Advanced Drug Delivery Reviews , 55, 199–215. doi: 10.1016/S0169-409X(02)00179-5
- Scott, L. J. (2020). Givosiran: First approval. Drugs , 80, 335–339. doi: 10.1007/s40265-020-01269-0
- Singh, Y., Murat, P., & Defrancq, E. (2010). Recent developments in oligonucleotide conjugation. Chemical Society Reviews , 39, 2054–2070. doi: 10.1039/b911431a
- Stasińska, A. R., Putaj, P., & Chmielewski, M. K. (2019). Disulfide bridge as a linker in nucleic acids’ bioconjugation. Part I: An overview of synthetic strategies. Bioorganic Chemistry , 92, 103223. doi: 10.1016/j.bioorg.2019.103223
- Tornøe, C. W., Christensen, C., & Meldal, M. (2002). Peptidotriazoles on solid phase: [1,2,3]-triazoles by regiospecific copper(I)-catalyzed 1,3-dipolar cycloadditions of terminal alkynes to azides. The Journal of Organic Chemistry , 67, 3057–3064. doi: 10.1021/jo011148j
- Wada, S., Yasuhara, H., Wada, F., Sawamura, M., Waki, R., Yamamoto, T., … Obika, S. (2016). Evaluation of the effects of chemically different linkers on hepatic accumulations, cell tropism and gene silencing ability of cholesterol-conjugated antisense oligonucleotides. Journal of Controlled Release , 226, 57–65. doi: 10.1016/j.jconrel.2016.02.007
- Wang, S., Allen, N., Prakash, T. P., Liang, X.-H., & Crooke, S. T. (2019). Lipid conjugates enhance endosomal release of antisense oligonucleotides into cells. Nucleic Acid Therapeutics , 29, 245–255. doi: 10.1089/nat.2019.0794
- Welch, J. J., Swanekamp, R. J., King, C., Dean, D. A., & Nilsson, B. L. (2016). Functional delivery of siRNA by disulfide-constrained cyclic amphipathic peptides. ACS Medicinal Chemistry Letters , 7, 584–589. doi: 10.1021/acsmedchemlett.6b00031
- Winkler, J. (2013). Oligonucleotide conjugates for therapeutic applications. Therapeutic Delivery , 4, 791–809. doi: 10.4155/tde.13.47