PROMIS: Global Analysis of PROtein-Metabolite Interactions
Aleksandra Skirycz, Aleksandra Skirycz, Ewelina M. Sokolowska, Ewelina M. Sokolowska, Dennis Schlossarek, Dennis Schlossarek, Marcin Luzarowski, Marcin Luzarowski
Abstract
Small molecules are not only intermediates of metabolism, but also play important roles in signaling and in controlling cellular metabolism, growth, and development. Although a few systematic studies have been conducted, the true extent of protein–small molecule interactions in biological systems remains unknown. PROtein–metabolite interactions using size separation (PROMIS) is a method for studying protein–small molecule interactions in a non-targeted, proteome- and metabolome-wide manner. This approach uses size-exclusion chromatography followed by proteomics and metabolomics liquid chromatography–mass spectrometry analysis of the collected fractions. Assuming that small molecules bound to proteins would co-fractionate together, we found numerous small molecules co-eluting with proteins, strongly suggesting the formation of stable complexes. Using PROMIS, we identified known small molecule–protein complexes, such as between enzymes and cofactors, and also found novel interactions. © 2019 The Authors.
Basic Protocol 1 : Preparation of native cell lysate from plant material
Support Protocol : Bradford assay to determine protein concentration
Basic Protocol 2 : Separation of molecular complexes using size-exclusion chromatography
Basic Protocol 3 : Simultaneous extraction of proteins and metabolites using single-step extraction protocol
Basic Protocol 4 : Metabolomics analysis
Basic Protocol 5 : Proteomics analysis
INTRODUCTION
PROtein–metabolite interactions using size separation (PROMIS) is a biochemical method for studying protein–small molecule interactions on a proteome- and metabolome-wide scale, based on the simple assumption that the small molecules or molecular complexes will exhibit similar chromatographic behavior (Veyel et al., 2017, 2018). Despite a few systematic studies, the true extent of protein–small molecule interactions in biological systems remains unknown (Diether, Nikolaev, Allain, & Sauer, 2019; Gallego et al., 2010; Li, Gianoulis, Yip, Gerstein, & Snyder, 2010; Piazza et al., 2018). Most currently available methods are limited by the availability of a recombinant protein, exploit compound libraries rather than complex metabolite extracts, or focus on a single, preselected small molecule (Luzarowski et al., 2017; Luzarowski & Skirycz, 2019; Maeda et al., 2013; Piazza et al., 2018; Savitski et al., 2014). PROMIS, however, takes advantage of the recent progress in mass spectrometry–based proteomics and metabolomics and enables analysis of the elution profiles of thousands of endogenous proteins and metabolites in a single experiment without the need to modify either proteins or metabolites. This makes PROMIS a powerful approach for identifying several hundred small molecules in a complex with proteins, including a set of their putative targets in the cell. Such analyses demonstrate the complexity of the protein-metabolite interactome and, similarly to recently published metabolome-wide approaches that report hundreds of novel protein-metabolite complexes, support the important regulatory role of metabolites in the cell.
The workflow for performing PROMIS experiments is divided into six parts, as shown in Figure 1: (i) the preparation of a native cell lysate from frozen plant material (Basic Protocol 1); (ii) fractionation of molecular complexes using size-exclusion chromatography (SEC; Basic Protocol 2); (iii) simultaneous extraction of proteins and metabolites from a single sample (Basic Protocol 3), liquid chromatography–mass spectrometry–based quantification of (iv) metabolites (Basic Protocol 4) and (v) proteins (Basic Protocol 5), and (vi) analysis of the obtained data and prediction of molecular complexes by calculating the Pearson correlation coefficient (PCC) between protein and metabolite elution profiles (Basic Protocol 6). A Support Protocol describes the Bradford assay used to determine protein concentrations in the native cell lysate and in samples obtained upon fractionation.
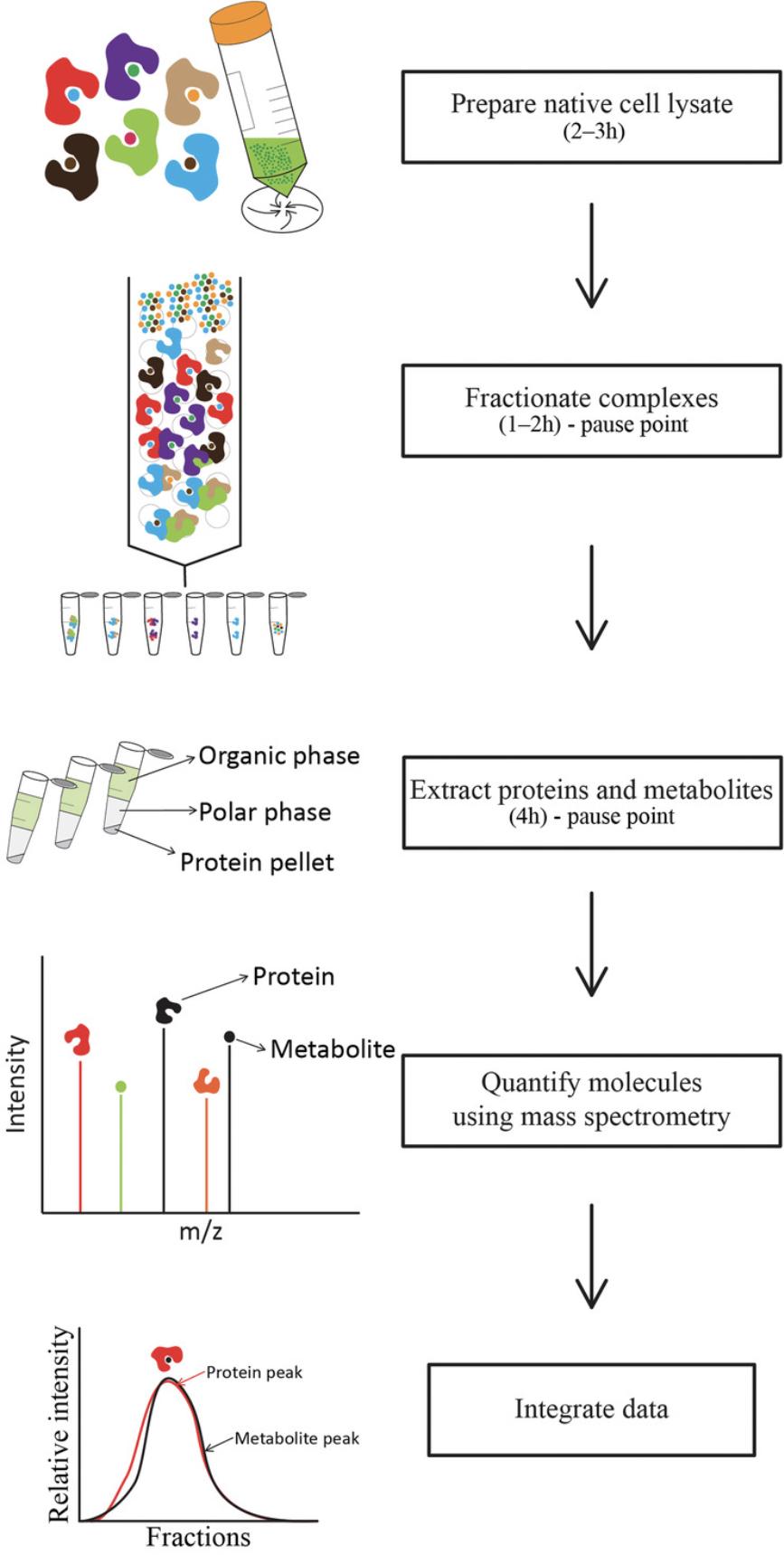
Basic Protocol 1: PREPARATION OF NATIVE CELL LYSATE FROM PLANT MATERIAL
Separation of intact protein-metabolite complexes requires an extraction procedure that does not interfere with their integrity. Here, we describe a protocol for extracting native, soluble protein-metabolite complexes from frozen plant material. Following mechanical grinding and addition of a lysis buffer containing protease and phosphatase inhibitors, the extract is subsequently centrifuged to pellet cellular debris, membranes, and larger aggregates.
Materials
-
Frozen plant material: to obtain sufficient protein amounts (40 mg) for size separation, 5 g of Arabidopsis rosette leaves or 3 g of Arabidopsis cell culture should be used.
-
Lysis buffer (see recipe)
-
Retsch mill (Retsch, cat. no. 207450001)
-
Stainless steel grinding balls (Retsch, cat. no. 05.368.0034)
-
Centrifuge tube, 50-ml (Sarstedt, cat. no. 62.559)
-
Vortex Shaker Genie® 2 2 Shaker (Scientific industries, cat. no. SI-T236)
-
Refrigerated centrifuge: e.g., Multifuge #1S-R with Sorvall rotor (Heraeus, cat. no. 75006449S)
-
Ultracentrifuge: e.g., Optima L-100K with swinging-bucket rotor SW 55 Ti (Beckman, cat. no. 342196)
-
Ultracentrifuge tubes: thick-wall polycarbonate tubes (Beckman, cat. no. 349622)
-
Amicon Ultra-15 centrifugal filter unit (10 kDa NMWL, Merck Millipore, cat. no. UFC901024)
-
5-ml tubes (Eppendorf)
-
Additional reagents and equipment for Bradford assay (Support Protocol)
1.Homogenize frozen plant material using a Retsch mill and 5-mm stainless steel grinding balls at 30 Hz for 1 min. Repeat this step four times in total. Place sample in liquid nitrogen between grinding steps.
2.Weigh 3 g (for Arabidopsis cell culture) of frozen plant material into a 50-ml tube.
3.Add 3 ml of lysis buffer and vortex vigorously until the material thaws.
4.Centrifuge total lysate 10 min at 4000 × g , 4°C.
5.Transfer the supernatant to 5-ml ultracentrifuge tubes and fill up the tube to the 5-ml mark with cold lysis buffer.
6.Ultracentrifuge for 1 hr at 165,052 × g (max.), 125,812 × g (avg.) at 4°C. Set acceleration and brake speed to maximum.
7.Transfer the supernatant (soluble fraction) to an Amicon Ultra-15 centrifugal filter unit and centrifuge 10 min at 4000 × g , 4°C, or until a volume of 2.5 ml is obtained.
8.Transfer supernatant to a 5-ml Eppendorf tube.
9.Determine protein concentration with Bradford assay (see Support Protocol).
10.Adjust the protein concentration using cold lysis buffer to reach a final concentration of 40 mg in 2 ml.
Support Protocol: BRADFORD ASSAY TO DETERMINE PROTEIN CONCENTRATION
Quantitation of proteins is a critical point in protein studies. In PROMIS, the Bradford assay is used to determine the protein concentration of soluble total extracts for SEC as well as to determine the protein concentration needed for protein trypsin digestion (Bradford, 1976).
Materials
- Bradford reagent (Sigma-Aldrich, cat. no. B6916)
- Bovine serum albumin (BSA; Sigma Aldrich, cat. no. 05470)
- Cell lysate to be assayed (Basic Protocol 1)
1.Pre-incubate Bradford reagent at room temperature.
2.Prepare a BSA standard in the range of 0.1 to 2 mg/ml in water.
3.Dilute the samples to 1:50 and 1:100 in water.
4.Prepare standard curve.
5.Add 200 µl of Bradford reagent to 5 µl of diluted sample, and measure absorbance at 595 nm.
Basic Protocol 2: SEPARATION OF MOLECULAR COMPLEXES USING SIZE-EXCLUSION CHROMATOGRAPHY
Here, we describe a workflow for separating native protein–small molecule complexes in cell lysates into fractions based on the sizes of the complexes, using SEC. As the sample passes through the column, smaller molecules enter the pores, which slow them down, while larger ones cannot enter any pores, thus passing through the column faster. Therefore, macromolecular complexes elute first, followed by unbound small molecules.
Materials
-
Separation buffer (see recipe)
-
Cell lysate
-
2-ml screw-cap tubes
-
ÄKTA Explorer 10 (GE Healthcare Life Science, cat. no. 18130000)
-
SEC column (SRT SEC-300 21.2 × 300 mm; Sepax, cat. no. 225300-21230)
-
Lyophilizer, Alpha 2-4 (Christ, cat. no. S/N:7911)
1.Equilibrate the SEC column with 2 column volumes of separation buffer.
2.Prepare 60 2-ml screw-cap tubes by piercing the caps with a needle or sharp forceps to make two holes.
3.For separation, set the flow rate of the ÄKTA Explorer chromatography apparatus to 7 ml/min, load 2 ml of cell lysate corresponding to 40 mg of protein by injecting the sample using a syringe, and clean the needle.
4.Collect 60 1-ml fractions from 61 ml to 121 ml elution volume in the previously prepared, open screw-cap tubes.
5.Close the tubes, place them in a cardboard rack inside a styrofoam box, and then snap-freeze the samples by gently pouring liquid nitrogen into the box.
6.Store samples at −80°C or lyophilize and store in a dry place at room temperature until further use.
Basic Protocol 3: SIMULTANEOUS EXTRACTION OF PROTEINS AND METABOLITES USING SINGLE-STEP EXTRACTION PROTOCOL
In the PROMIS experiment, we use a single-step extraction protocol for comprehensive and simultaneous analysis of lipids, polar metabolites, and proteins from a single fraction utilizing a complex extraction buffer containing solvents with different polarities (Giavalisco et al., 2011). Three independent fractions are separated: organic upper phase containing non-polar metabolites, such as lipids; lower polar phase containing polar primary and secondary metabolites; and protein/starch pellet. The obtained fractions are further analyzed using LC-MS.
Materials
-
Extraction buffer 1 (see recipe)
-
Extraction buffer 2 (see recipe)
-
Lyophilized sample (Basic Protocol 2)
-
1.5- and 2-ml tubes (Eppendorf, cat. no. 0030120086 and 0030120094)
-
Ultrasonication bath (Bandelin, cat. no. RK 31)
-
Benchtop centrifuge (Eppendorf, cat. no. 5417R)
-
Centrifugal evaporator: MaxiVac Alpha (Scanspeed, cat. no. 7.008.500.002)
1.Add 1 ml of pre-cooled (−20°C) extraction buffer 1 to the lyophilized sample and vortex vigorously.
2.Incubate 10 min at 4°C with shaking, then sonicate for 10 min by partially submerging the tubes in a sonication bath filled with water to ensure metabolite extraction from dry pellet.
3.Add 0.5 ml of extraction buffer 2 and mix well by vortexing.
4.Centrifuge samples 5 min at 20,817 × g , room temperature, in a benchtop centrifuge.
5.Remove the rest of the organic phase together with the interphase using a vacuum aspirator.
6.Transfer 500 µl of the lower (polar) phase to a fresh 1.5-ml tube and dry using a centrifugal evaporator. Continue with the polar metabolites analysis (Basic Protocol 4).
7.Remove remaining polar phase and air-dry the protein pellet. Freeze the pellets at −80°C or continue with digestion of proteins.
Basic Protocol 4: METABOLOMICS ANALYSIS
This protocol describes how metabolites are separated and quantified using reversed-phase HPLC and mass spectrometry, respectively. In addition, the protocol describes the conversion of acquired spectra and chromatograms to a matrix consisting of mass features, which are characterized by a unique mass-to-charge ratio (m /z), retention time (RT), and intensity. Quantified mass features are then assigned to actual metabolites by querying their m /z and RT against a library of commercially available standards. Here, a combination of SEC and HPLC-MS enables metabolome-wide identification of any metabolites remaining in the complexes with proteins.
Materials
-
Sample: aqueous phase from Basic Protocol 3
-
LC-MS mobile phase buffer A (see recipe)
-
LC-MS mobile phase buffer B (see recipe)
-
Ultrasonication bath (Bandelin, cat. no. RK 31)
-
Glass sample vials
-
UPLC (Waters, Acquity I Class System)
-
HSST3 C18 reversed-phase column (100 mm × 2.1 mm × 1.8 µm particles; Waters, cat. no. 186003539)
-
Mass spectrometer (Thermo Fisher Scientific, Exactive)
Sample preparation
1.Resuspend the dried aqueous phase from Basic Protocol 3, obtained after extraction, in 70 µl water and sonicate the sample by partially submerging the tubes in a sonication bath filled with water for 5 min.
2.Centrifuge the sample 10 min at 20,817 × g , room temperature, in a benchtop centrifuge, and then transfer the supernatant to a glass vial.
LC-MS measurement
Separate the polar metabolite extract using a UPLC equipped with an HSS T3 C18 reversed-phase column and acquire mass spectra using an Exactive mass spectrometer in positive and negative ionization modes.
3.Load 3 µl of the sample per injection onto the column for each ionization mode.
4.Separate metabolites at 400 µl/min using the following gradient: 1 min 1% LC-MS mobile phase buffer B, 11 min linear gradient from 1% to 40% buffer B, 13 min linear gradient from 40% to 70% buffer B, then 15 min linear gradient from 70% to 99% buffer B, and hold a 99% buffer B concentration until 16 min. Starting from 17 min, use a linear gradient from 99% to 1% buffer B. Re-equilibrate the column for 3 min with 1% buffer B before measuring the next sample.
5.Acquire mass spectra using the Exactive mass spectrometer covering a mass range from 100 to 1500 m /z. Set resolution to 25,000, with 10 scans/s, the AGC target set to 1e6, and the loading time restricted to 100 ms. Set the capillary voltage to 3 kV with sheath gas flow and auxiliary gas values of 60 and 20, respectively. Set the skimmer voltage to 25 V and capillary temperature to 250°C.
Metabolite data processing and annotation
Process the acquired chromatograms to extract the m /z , RT, and intensity of the associated peaks using, for example, Expressionist Refiner MS 12.0.3 (GeneData; http://www.genedata.com) or alternative software (Katajamaa & Orešič, 2007).
6.Open processing software by double-clicking the .exe file.
7.Create a new workflow and start chromatogram processing with the activity “Load from File.”
8.Open the activity settings and set the experiment's name. Select raw chromatograms to be processed.
9.Set “Profile Data Cutoff” to 0 intensity in the advanced settings tab.
10.Apply the “Data Sweep” activity. Open the activity settings.
11.In “General” settings, select “Centroid data” and “MS/MS Data.” Remove all MS/MS data by marking “Everything” in the selection panel.
12.Add the “Chromatogram Chemical Noise Subtraction” activity.
13.In the activity settings, mark “Chromatogram Smoothing” and set the number of scans to “3” and “Estimator” to “Moving average.” Set “RT Window” to 51 scans, “Quantile” to 50%, “Subtraction Method,” and “Threshold” to 750 Intensity.
14.In “Advanced” settings, mark “RT Structure Removal” and set “Minimum RT Length” to 5 scans and “Minimum m /z Length” to 3 points.
15.Add the activity “Chromatogram RT Alignment.” Open the activity settings.
16.In “General” settings, set “Alignment Scheme” to “Pairwise Alignment Base Tree” and “RT Search Interval” to 0.5 min.
17.Use default parameters for the “Advanced” settings.
18.Add the activity “Peak Detection” in the “Chromatogram” group of activities.
19.In the “General” settings of the activity, set “Summation Window” to 0.09 min, “Maximum Merge Distance” to 5 points, “Minimum Peak Size” to 0.03 min, and “Merge Strategy” to “Centers.” In the “Peak RT Splitting” box, set “Gap/Peak Ratio” to 50%.
20.In the “Advanced” settings, set “Refinement Threshold” to 80%, “Smoothing Window” to 5 points, and “Consistency Threshold” to 1.Set “Center Computation” to “Intensity-weighted” with “Intensity Threshold” set at 70%.
21.Add the activity “Isotope Clustering” in the “Chromatogram” group of activities.
22.In the “General” settings of the activity, set “RT tolerance” to 0.015 min and “ m /z Tolerance” to 5 ppm.
23.In the “Envelope fitting” settings, set “Method” to “No Shape Restriction” and “Ionization” to “Protonation” (for positive mode) and “Deprotonation” (for negative mode). Set “Minimum and Maximum charge” to 1 and 4, respectively.
24.Use default parameters for the “Advanced” settings.
25.Add the activity “Singleton Filter” and use the default settings.
26.Export the results by adding the activity “Analyst” in the “Export” group of activities.
27.In the “General” settings, set “Type” to “Clusters” and “Observable” to “Summed Intensity.” Finally, specify the directory to export files.
28.Use default parameters for the “Advanced” settings.
29.Annotate mass features using the in-house reference-compound database.
30.Analyze MSgrade reference compounds using UPLC-MS to acquire RT and m /z specifically for a certain compound. Use the same LC-MS method to analyze the reference compounds and mass features acquired in the PROMIS experiment.
31.Compare RT and m /z of the mass feature measured in the experiment and RT and m /z of the reference compound. Allow a deviation of 0.005 Da for m /z and 0.1 min for RT.
32.Use annotated metabolites for further analysis.
Basic Protocol 5: PROTEOMICS ANALYSIS
This protocol describes enzymatic digestion and desalting of the proteins obtained upon SEC fractionation (Promega), followed by the separation and quantification of C18-chromatographic peptides using a nanoAcquity high-performance liquid chromatograph coupled with a Q-Exactive HF high-sensitivity mass spectrometry. Acquired spectra and chromatograms are then converted using MaxQuant and matched using the integrated peptide search engine Andromeda (Cox & Mann, 2008; Cox et al., 2011).
Materials
-
Dried protein pellets from extraction (Basic Protocol 3)
-
Denaturation buffer (see recipe)
-
Reduction buffer: 100 mM dithiothreitol (DTT; Sigma-Aldrich, cat. no. D0632)
-
Alkylation buffer: 300 mM iodoacetamide (IAM; Sigma-Aldrich, cat. no. I1149)
-
LysC/trypsin mix (Promega, cat. no. V5071; also see Internet Resources)
-
50 mM ammonium bicarbonate (Sigma-Aldrich, cat. no. 9830), pH adjusted to 8 using acetic acid
-
Trifluoroacetic acid (TFA; Biosolve, cat. no. 202341)
-
Methanol (BioSolve UPLC grade, cat. no. 136806)
-
Solution A: 0.1% trifluoroacetic acid (TFA)
-
Solution B: 0.1% trifluoroacetic acid (TFA)/80% acetonitrile (ACN; BioSolve UPLC grade, cat. no. 12006)
-
Solution C: 0.1% trifluoroacetic acid (TFA)/60% acetonitrile (ACN; BioSolve UPLC grade, cat. no. 12006)
-
Resuspension buffer (see recipe)
-
Buffer A: 100% H2O (BioSolve UPLC grade, cat. no. 232106)/0.1% formic acid (FA; BioSolve UPLC grade, cat. no. 69141)
-
Buffer B: 100% acetonitrile (ACN; BioSolve UPLC grade, cat. no. 12006)/0.1% formic acid (FA; BioSolve UPLC grade, cat. no. 69141)
-
Ultrasonication bath (Bandelin, cat. no. RK 31)
-
pH indicator paper
-
Sep-Pak C18 96-well plate, 40 mg sorbent (Waters, cat. no. 186003966)96-well plates with 2-ml well volume for collection from Sep-Pak plate (washing and elution plates)
-
Refrigerated centrifuge: Multifuge #1S-R with Sorvall rotor #75006449S (Heraeus)
-
Centrifugal evaporator (e.g., SpeedVac)
-
UPLC (Waters, Acquity M Class System)
-
Glass sample vials
-
HSST3 C18 (100 mm × 2.1 mm × 1.8 µm particles; Waters, cat. no. 186003539)
-
Mass spectrometer (Thermo Fisher Scientific, Q-Exactive HF)
-
MaxQuant software (Version 1.6.0.16, MPI of Biochemistry, Germany)
-
Additional reagents and equipment for Bradford assay (Support Protocol)
In-solution protein digest
1.Dissolve the dried protein pellets from extraction by adding 50 µl denaturation buffer, and resuspend by partially submerging the tubes in a sonication bath filled with water for 15 min.
2.Determine protein concentration using a Bradford assay (Support Protocol).
3.Take a volume equivalent up to 50 µg protein, and adjust all your samples to an equal volume with denaturation buffer.
4.Add reduction buffer to a final DTT concentration of 5 mM, and incubate 30 min at room temperature.
5.Add alkylation buffer to a final IAM concentration of 15 mM, and incubate 30 min at room temperature in the dark.
6.Add LysC/trypsin mix to achieve a protein:protease ratio of 25:1 (w/w).
7.Dilute the urea concentration to 4 M with 50 mM ammonium bicarbonate buffer, pH 8, and incubate for 3 to 4 hr at 37°C.
8.Dilute the sample to 1 M urea with 50 mM ammonium bicarbonate buffer, pH 8, and continue incubation at 37°C overnight.
9.Acidify the samples with TFA to approximately 1% TFA final.
10.Check the pH with pH indicator paper; pH should be <3.
11.Store samples at −20°C, or continue with desalting.
Protein desalting
12.Place the Sep-Pak filter–column plate on top of a 96-well plate with a volume of 2 ml/well.
13.Wet the filter column with 1 ml 100% methanol, centrifuge for 1 min at 50 × g , room temperature, and discard the flowthrough.
14.Wet the filter column with 1 ml solution B, centrifuge for 1 min at 50 × g , room temperature, and discard the flowthrough.
15.Wash the filter column twice with 1 ml solution A, centrifuge for 1 min at 50 × g , room temperature, and discard the flowthrough.
16.Load the sample onto the filter column, centrifuge for 1 min at 50 × g , room temperature, and discard the flowthrough.
17.Wash the filter column twice with solution A, centrifuge for 1 min at 50 × g , room temperature, and discard the flowthrough.
18.Exchange washing plate for a fresh elution plate placed under the Sep-Pak plate.
19.Elute the peptides by adding 600 µl solution C per well of the Sep-Pak plate and collecting the flowthrough.
20.Transfer the flowthrough to 1.5-ml tubes and dry using a centrifugal evaporator to about 2 to 3 μl.
21.Store dried peptides at −20°C or continue with LC-MS.
LC-MS measurement
22.Resuspend dried peptides in 150 µl of resuspension buffer, and sonicate for 10 min.
23.Centrifuge the sample 1 min at 20,817 × g , room temperature, and then transfer 10 µl of a sample to a glass vial.
24.Measure samples with a Q-Exactive HF (Thermo Scientific) high-resolution mass spectrometer coupled to an ACQUITY UPLC M-Class System (Waters) using buffer A and buffer B as described in the following steps.
25.Load 3 µl sample per injection, and separate in 120-min gradients ramped from 3.2% buffer B (acetonitrile) to 7.2% buffer B over 20 min, then to 24.7% buffer B over 70 min and up to 35% buffer B over the next 30 min, followed by a 6-min washout with 75% buffer B. Use a flow rate of 300 nl/min and equilibrate the column with 10 µl of buffer A between samples.
26.Acquire mass spectra using the data-dependent MS/MS method with a full scan resolution of 120,000, an m /z ranging from 300 to 1600, an AGC target of 3 × 106 ions, and a maximum injection time of 50 ms.
27.Acquire MS2 scans using a maximum of 12 MS/MS scans per full scan (top 12) with a resolution of 15,000, an m /z ranging from 200 to 2000, an AGC target of 1 × 105, a maximum injection time of 100 ms, and an isolation window of 1.2 m /z. Set the collision energy to 27, the apex trigger from 4 to 9 s, and the dynamic exclusion to 30 s.
Proteomics data processing
28.Process the raw data using MaxQuant software.
29.Run MaxQuant analysis using the instrument settings for Orbitrap.
30.Set methionine oxidation and N -terminal acetylation as variable modifications.
31.Set cysteine carbamidomethylation as a fixed modification.
32.Select the following options for data analysis: “label-free quantification” and “match between runs.”
33.Set the minimum peptide length to at least seven amino acids, and allow a maximum of two missed cleavages.
34.Use the newest release of UniProt database (https://www.uniprot.org/) as a library. Also include the list of contaminants from The common Repository of Adventitious Proteins (cRAP, http://www.thegpm.org/crap).
35.In the further analysis, use only proteins with two or more unique peptides.
Basic Protocol 6: DATA INTEGRATION
Data integration is a crucial step in searching for captured co-eluting protein–metabolite pairs. The workflow described in Figure 2 can be performed using any table-calculating software, such as Microsoft Excel. However, it only represents the minimal requirements to match the obtained proteomics and metabolomics data. Additional steps can be included in the data analysis pipeline, such as data noise filtering, data smoothing, and peak deconvolution, and we highly recommend this (Gorka et al., 2019). Since data integration of large data sets can be time consuming, script-based tools for the automated analysis of such data sets might be expected considering the growing number of PROMIS users.
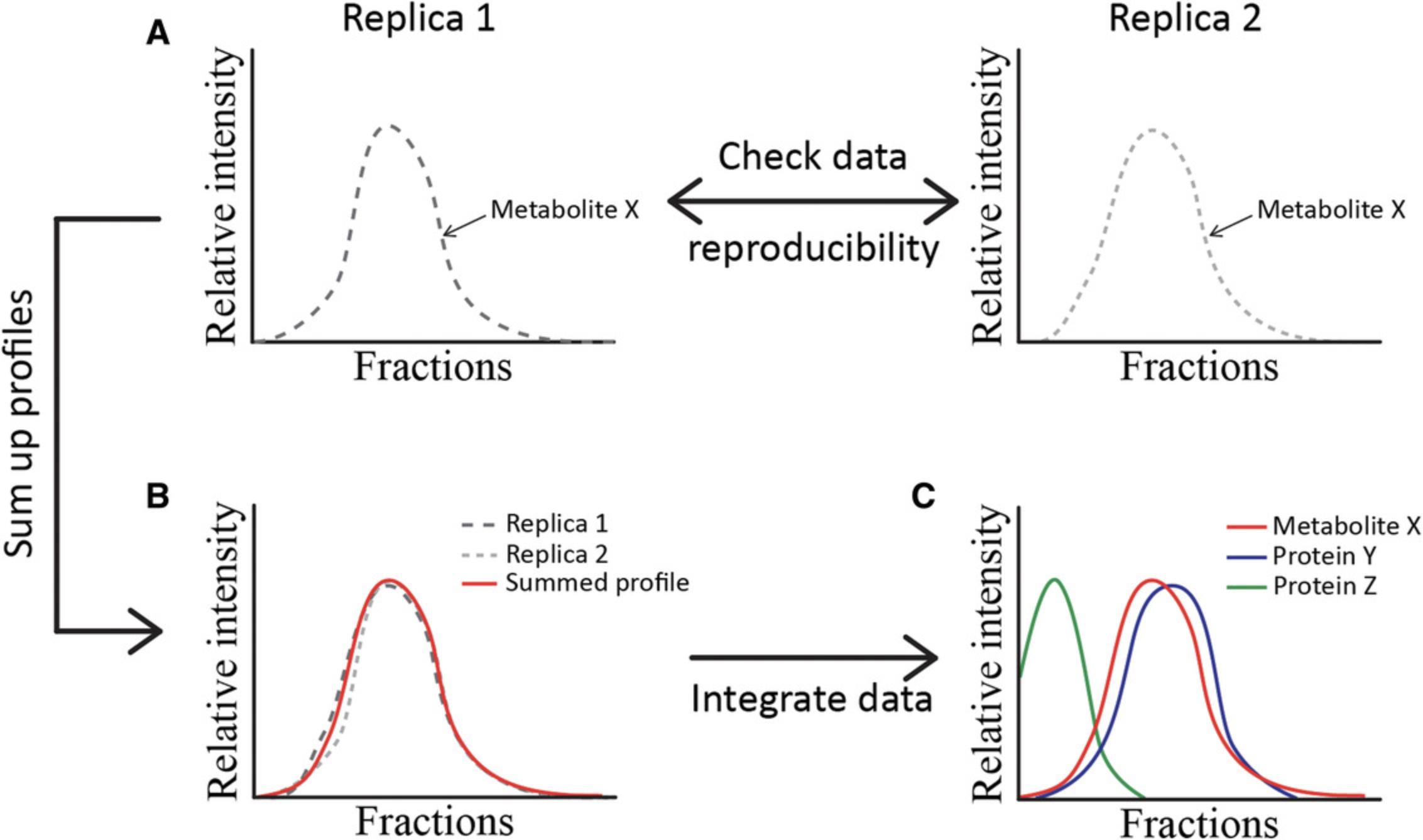
For data integration, data from different experimental replicates are combined. To do this, the elution profiles of replicates with high reproducibility, expressed as the Pearson correlation coefficient (PCC), are summed to one profile. Next, the resulting profile is normalized to its maximum intensity. Finally, the PCC between metabolite and protein profiles is calculated. A high PCC indicates co-eluting metabolite–protein pairs.
REAGENTS AND SOLUTIONS
Denaturation buffer
- 50 mM ammonium bicarbonate, pH adjusted to 8 using acetic acid (Sigma-Aldrich, cat. no. 9830)
- 2 M thiourea (Sigma-Aldrich, cat. no. T8656)
- 6 M urea (Sigma-Aldrich, cat. no. U5128)
- Prepare fresh
Extraction buffer 1
- 1 volume of methanol (BioSolve UPLC grade, cat. no. 136806)
- 3 volumes of methyl tert -butyl ether (MTBE; BioSolve UPLC grade, cat. no. 138906)
- 1 volume of water (BioSolve UPLC grade, cat. no. 232106)
- Store up to 1 month at 4°C
Extraction buffer 2
- 1 volume of methanol(BioSolve UPLC grade, cat. no. 136806)
- 3 volumes of water (BioSolve UPLC grade, cat. no. 232106)
- Store up to 1 month at 4°C
LC-MS mobile phase buffer A
- 100% H2O (BioSolve UPLC grade, cat. no. 232106)
- 0.1% formic acid (FA; BioSolve UPLC grade, cat. no. 69141)
- Store up to 2 weeks at 4°C
LC-MS mobile phase buffer B
- 100% acetonitrile (ACN; BioSolve UPLC grade, cat. no. 12006)
- 0.1% formic acid (FA; BioSolve UPLC grade, cat. no. 69141)
- Store up to 2 weeks at 4°C
Lysis buffer
- 50 mM ammonium bicarbonate, pH 7.5 (Sigma-Aldrich, cat. no. 9830)
- 150 mM NaCl (Sigma-Aldrich, cat. no. S7653)
- 1.5 mM MgCl2 (Carl Roth, cat. no. 2189.1)
- 5 mM dithiothreitol (DTT; Sigma-Aldrich, cat. no. D0632)
- 1 mM phenylmethylsulfonyl fluoride (PMSF; Sigma-Aldrich, cat. no. P7626)
- 1× protease inhibitor cocktail (Sigma Aldrich, cat. no. P9599)
- 0.1 mM sodium orthovanadate (Na3VO4; Sigma-Aldrich, cat. no. S6580)
- 1 mM sodium fluoride (NaF; Sigma-Aldrich, cat. no. S6776)
Resuspension buffer
- 3% acetonitrile (ACN; BioSolve UPLC grade, cat. no. 12006)
- 0.1% formic acid (FA; BioSolve UPLC grade, cat. no. 69141)
- Store up to 1 month at 4°C
Separation buffer
- 50 mM ammonium bicarbonate, pH 7.5 (Sigma-Aldrich, cat. no. 9830)
- 150 mM NaCl (Sigma-Aldrich, cat. no. S7653)
- 1.5 mM MgCl2 (Carl-Roth, cat. no. 2189.1)
COMMENTARY
Background Information
Small molecule signaling was classically studied using genetic-driven strategies, enabling identification of the small molecule protein receptors (Choi et al., 2014; Li & Chory, 1997). In forward genetic approaches, a mutant population is generated using a random mutagen and then screened for a phenotype of interest. Receptors are identified by mapping the causal mutations (Stockwell, 2000; Zheng, Chan, & Zhou, 2004; Zwiewka & Friml, 2012). These approaches, however, depend on the presence of a strong and easy-to-screen phenotype, and thus they are limited to narrow groups of signaling molecules. Moreover, forward genetics fails to identify the protein receptor if the functional protein is required for survival or if the protein is part of a redundant protein family. These limitations can be overcome by causing the changes in the phenotype by treatment with chemical compounds rather than by the mutagen, which may temporarily perturb the phenotype and affect a specific subset of the protein receptors (Rigal, Ma, & Robert, 2014; Stockwell, 2000; Tóth & Van der Hoorn, 2010).
Alternative strategies rely on biochemical methods and can be divided into three groups: (i) ligand-targeted, in which a metabolite is used as bait to determine the targets of the small molecule; (ii) protein-targeted, which aim to identify small molecules affecting the function of a signaling protein; and (iii) untargeted, which chart a map of the protein–metabolite interactome in a given organism (Luzarowski & Skirycz, 2019). Examples of ligand-targeted approaches include classical strategies like affinity purification, in which a chemical compound is immobilized to the matrix and used to capture interacting proteins from native cellular lysate, as well as the recent methods TPP/CETSA, SPROX, and LiP-SMAP, which monitor changes in the protein properties caused by ligand binding (Kosmacz et al., 2018; Ong et al., 2009; Piazza et al., 2018; Savitski et al., 2014; West, Tang, & Fitzgerald, 2008). These properties can be thermal stability of a protein, oxidation rate, or susceptibility of a protein to proteolysis. In comparison to ligand-targeted approaches, the development of protein-targeted strategies is lagging behind. Most of the existing protein-targeted methods rely on the availability of a recombinant protein and screen compound libraries for binding molecules. Examples include ligand-detected NMR, mass spectrometry integrated with equilibrium dialysis for the discovery of allostery systematically (MIDAS), the differential radial capillary action of ligand assay (DRACALA), or metabolite arrays (Cala, Guillière, & Krimm, 2014; Gallego et al., 2010; Nikolaev, Kochanowski, Link, Sauer, & Allain, 2016; Orr & Lee, 2017; Orsak et al., 2011; Current Protocols article: Seminara Turdiev, Turdiev, & Lee, 2018). One unique method that overcomes these limitations and allows for metabolome-wide analysis of protein-metabolite interactions in close to in vivo conditions is affinity purification using protein as bait. Affinity purification is a commonly used technique to study protein-protein interactions, but was recently adapted to study the interactions between proteins and small molecules, and many of the captured interactions have regulatory potential (Edwards & Dixon, 2018; Li et al., 2010; Luzarowski et al., 2017; Luzarowski, Wojciechowska, & Skirycz, 2018; Maeda et al., 2013; Sterlin et al., 2019).
All of the abovementioned techniques provide information about the interactome of a single, pre-selected metabolite or a protein. While this can be an advantage when searching for targets of drugs or ligands of proteins causing metabolic diseases, in situations when a signaling molecule is unknown, targeted approaches have no practical value. Examples include an unknown small-molecule component of the Sussex signal, putative regulatory protein–metabolite interactions involved in the response to biotic and abiotic stresses, and complexes important for development and growth (Kuhlemeier & Timmermans, 2016).
PROMIS is the only available non-targeted method that allows for metabolome- and proteome-wide analysis of protein–metabolite interactions at close to in vivo protein and metabolite concentrations (Veyel et al., 2017, 2018). It requires no modification of the protein or metabolite parts of the complex, as it relies on the assumption that components of the molecular complexes co-migrate through an SEC column. In case studies, we have tested the suitability of size separation–based methods (e.g., ultracentrifugal filters) to separate protein-metabolite complexes from free small molecules in complex cellular extracts (Veyel et al., 2017). Stable protein–metabolite complexes remained on the filter, whereas the pool of free small molecules was found in the flowthrough. Among the protein-bound metabolites, we found many cofactors (e.g., NAD, FMN, and pyridoxal-5′-phosphate), but more unexpectedly, we identified several dozen dipeptides and hundreds of unknown metabolites, which suggested an important regulatory role for metabolites in plant cells. Prompted by these observations, we decided to use SEC to fractionate protein-metabolite complexes and determine interaction partners using the chromatographic co-elution criterion. Similar attempts were proven to be successful for identifying protein-protein complexes in native cellular extracts and for monitoring interactions between proteins and drugs (Aryal, McBride, Chen, Xie, & Szymanski, 2017; Chan et al., 2012; Havugimana et al., 2012). In doing this, we developed protein-metabolite interactions using a size-separation method that we dubbed PROMIS. A single PROMIS experiment (i) detects hundreds of small molecules in protein complexes, many of which are unexpected and of regulatory nature; (ii) provides a list of putative protein-metabolite complexes based on the similarity of their elution profiles; and (iii) makes it possible to study targets of exogenous small molecules (drugs, inhibitors) in complex native cellular extracts and in the presence of endogenous metabolites. PROMIS, by charting a map of the interactome, creates a starting point for further identification of the molecular complexes formed in the cell, specific to the developmental stage or prevailing environmental conditions. In this article, we present a detailed PROMIS protocol to study protein–small molecule complexes in Arabidopsis thaliana.
Critical Parameters and Troubleshooting
Several parameters have to be considered before conducting the protocols, as they may drastically affect the outcome of the procedure.
- 1.Preparation of the native cell lysate : Throughout the whole procedure, protein degradation should be avoided by adding protease inhibitors to lysis buffer and by keeping the extract at a low temperature (4°C).
- 2.Amount of starting tissue that is required to perform separation : The developmental stage, efficiency of grinding, and volume of buffer used for cell lysis may all affect the yield of protein and metabolite recovery from the cell. Therefore, the amount of tissue needed for each PROMIS experiment will vary (e.g., younger, actively growing plants will exhibit substantially higher protein content compared to old, senescing plants). In general, the protein concentration of the lysate should be >20 mg/ml. If lower concentrations are obtained, proteins can be concentrated using an Amicon Ultra-15 centrifugal filter unit (see Basic Protocol 1).
- 3.The cell lysis procedure : Proteins must remain in their native conformation and the lysis procedure should not affect the composition of the molecular complexes. For example: (i) higher salt concentrations may favor hydrophobic interactions over ionic binding; (ii) sample overheating may cause protein denaturation and complex dissociation; and (iii) released components from crushed organelles may form false interactions the formation of which is not possible in vivo due to subcellular compartmentalization. Using isolated organelles instead of the whole cells as starting material may prevent the formation of false interactions caused by the disruption of intracellular structures. It is, however, laborious, time-consuming, and often impossible. Alternatively, we suggest filtering the predicted interactions using available subcellular localization data (Hooper et al., 2014; Krueger et al., 2011), and, based on that, verify if such interaction may happen in vivo.
- 4.Parameters of the size-exclusion chromatographic separation : Column properties (e.g., length, particle size, separation range), applied flow rate, volume of the loaded sample, composition of the mobile phase, and number of collected fractions will all have a detrimental effect on the resolution of the method (Jenkins, 2014; Striegel, Yau, Kirkland, & Bly, 2009). It is advisable to follow the recommendations of the SEC column manufacturer regarding the recommended flow rate and the maximum amount of loaded protein, and to keep the volume of the loaded sample below 2% of the total volume of the column. The column separation range should be adjusted to the size of the molecular complexes that will be separated. A separation range between 1 mDa and 10 kDa will be suitable for most uses, as the majority of the molecular complexes fall into this range.
- 5.Simultaneous extraction of metabolites and proteins : The extraction method used in the protocol allows analysis of a broad range of metabolites and proteins from a single sample. However, many of the molecules (e.g., ATP, sugar phosphates, polar amino acids, more polar triacylglycerols, and plant hormones) remain inaccessible, as their analysis requires specific extraction procedures and dedicated analytical instrumentation (Kim & Verpoorte, 2010; Müller & Munné-Bosch, 2011; Šimura et al., 2018; t'Kindt, De Veylder, Storme, Deforce, & Van Bocxlaer, 2008).
- 6.Processing of the raw chromatograms and data analysis : In the Basic Protocol 5, we use Expressionist Refiner MS and MaxQuant to process acquired metabolite and protein spectra. Several alternative software programs for extracting m /z , RT, and the intensities of associated peaks are available and can be used for this purpose (Katajamaa & Orešič, 2007; Matthiesen & Bunkenborg, 2013). The outcome table of the data-processing software should contain a list of proteins and metabolites with their absolute or relative intensities in fractions obtained upon fractionation. Changes in the relative abundance of the molecules are then used to determine the similarity between the chromatographic behavior of a specific protein and metabolite. The degree of similarity is estimated by calculating the PCC, which is the method of choice when searching for observations having the same overall profile regardless of their magnitudes. Deconvolution of elution profiles into separate peaks is critical for the method resolution and determining the number of captured interactions. Small molecules might have multiple protein targets, and therefore might be part of several molecular complexes, whereas monomer and homo/hetero-oligomers of a given protein may differ in their ability to bind small molecules.
Understanding Results
PROMIS should be seen as a discovery tool for identifying unknown signaling small molecules and their targets or for validating previously predicted binding events. On average, every metabolite co-elutes with several hundred proteins, which could be a source of spurious interactions. A successful PROMIS experiment separates small molecules, which co-elute with proteins that can be found in protein-containing fractions, from free small molecules. Although the majority of mass features (representing fragments and entire small molecules) are found outside the protein-separation range—approximately 80% of the total column volume—several hundred of them elute in protein-containing fractions, suggesting stable complexes. The elution profiles of proteins and small molecules are characterized by one or several separate peaks across the column separation range. In the case of proteins, the presence of a few discrete peaks might be explained by the differential migration of monomers and homo/hetero-oligomers of a given protein, whereas small molecules might have multiple protein targets, and are therefore part of several molecular complexes. In a case study, 4229 unique mass features eluted in protein-containing fractions, indicating that they create stable complexes with proteins and might be involved in regulation and signaling processes. Assignment of a metabolite to a potential protein target relies on the assumption that components of one molecular complex share similar chromatographic behavior. To narrow down potential candidates, we advise (i) combining the predicting power of PROMIS with biochemical knowledge regarding the proteins involved in the biosynthesis pathways of a given metabolite, and (ii) the use of binding evidence for experimentally confirmed protein-protein-metabolite interactions in other organisms, considering that molecular complexes are often evolutionarily conserved. This approach led us to the identification of putative feedback and feed-forward regulatory mechanisms in pantothenate and methylthioadenosine synthesis pathways, as we proved the interaction between pantothenate and ketopantoate hydroxymethyltransferase (KPHMT1) and between methylthioadenosine and methylthioribose-1-phosphate isomerase (MTI; Veyel et al., 2018).
When there is a lack of information about a given metabolite, the exact composition of a complex and regulatory mechanism can be characterized using orthogonal techniques such as affinity purification, thermal proteome profiling, or drug affinity responsive target stability. In such cases, PROMIS serves as a reference point and is useful for deciphering molecules forming stable complexes with proteins, and, therefore, in identifying novel regulatory small molecules.
Time Considerations
Preparation of native cell lysate from plant material followed by SEC takes about 5 hr, including waiting time during ultracentrifugation and SEC. Collected fractions are frozen with liquid nitrogen and stored at −80°C or lyophilized. Usually it takes 2 days to dry the samples completely. Lyophilized samples can be stored at room temperature in a dry place (protected from humidity).
Time necessary for simultaneous extraction of proteins and metabolites using the single-step extraction protocol highly depends on the number of samples to process. We recommend doing it in batches of 40 samples. In such cases, simultaneous extraction of proteins and metabolites in a single batch requires around 3 hr. After extraction, the samples are dried using a centrifugal evaporator (SpeedVac) for several hours or overnight. Dried samples can be stored at −20°C/−80°C for several weeks.
Literature Cited
- Aryal, U. K., McBride, Z., Chen, D., Xie, J., & Szymanski, D. B. (2017). Analysis of protein complexes in Arabidopsis leaves using size exclusion chromatography and label-free protein correlation profiling. Journal of Proteomics , 166, 8–18. doi: 10.1016/j.jprot.2017.06.004.
- Bradford, M. M. (1976). A rapid and sensitive method for the quantitation of microgram quantities of protein utilizing the principle of protein-dye binding. Analytical Biochemistry , 72(1–2), 248–254. doi: 10.1016/0003-2697(76)90527-3.
- Cala, O., Guillière, F., & Krimm, I. (2014). NMR-based analysis of protein–ligand interactions. Analytical and Bioanalytical Chemistry , 406(4), 943–956. doi: 10.1007/s00216-013-6931-0.
- Chan, J. N., Vuckovic, D., Sleno, L., Olsen, J. B., Pogoutse, O., Havugimana, P., … Musteata, M. F. (2012). Target identification by chromatographic co-elution: Monitoring of drug-protein interactions without immobilization or chemical derivatization. Molecular & Cellular Proteomics, 11(7), M111.016642. doi: 10.1074/mcp.M111.016642.
- Choi, J., Tanaka, K., Cao, Y., Qi, Y., Qiu, J., Liang, Y., … Stacey, G. (2014). Identification of a plant receptor for extracellular ATP. Science , 343(6168), 290–294. doi: 10.1126/science.343.6168.290.
- Cox, J., & Mann, M. (2008). MaxQuant enables high peptide identification rates, individualized ppb-range mass accuracies and proteome-wide protein quantification. Nature Biotechnology , 26(12), 1367–1372. doi: 10.1038/nbt.1511.
- Cox, J., Neuhauser, N., Michalski, A., Scheltema, R. A., Olsen, J. V., & Mann, M. (2011). Andromeda: A peptide search engine integrated into the MaxQuant environment. Journal of Proteome Research , 10(4), 1794–1805. doi: 10.1021/pr101065j.
- Diether, M., Nikolaev, Y., Allain, F. H., & Sauer, U. (2019). Systematic mapping of protein-metabolite interactions in central metabolism of Escherichia coli. Molecular Systems Biology , 15(8), e9008. doi: 10.15252/msb.20199008.
- Edwards, R., & Dixon, D. P. (2018). Protein-ligand fishing in planta for biologically active natural products using glutathione transferases. Frontiers in Plant Science , 9, 1659. doi: 10.3389/fpls.2018.01659.
- Gallego, O., Betts, M. J., Gvozdenovic-Jeremic, J., Maeda, K., Matetzki, C., Aguilar-Gurrieri, C., … Gavin, A. C. (2010). A systematic screen for protein–lipid interactions in Saccharomyces cerevisiae. Molecular Systems Biology , 6(1), 430. doi: 10.1038/msb.2010.87.
- Giavalisco, P., Li, Y., Matthes, A., Eckhardt, A., Hubberten, H. M., Hesse, H., … Willmitzer, L. (2011). Elemental formula annotation of polar and lipophilic metabolites using 13C, 15N and 34S isotope labelling, in combination with high-resolution mass spectrometry. The Plant Journal , 68(2), 364–376. doi: 10.1111/j.1365-313X.2011.04682.x.
- Gorka, M., Swart, C., Siemiatkowska, B., Martínez-Jaime, S., Skirycz, A., Streb, S.,…Graf, A. (2019). Protein complex identification and quantitative complexome by CN-PAGE. Scientific Reports , 9, 11523. doi:10.1038/s41598-019-47829-7.
- Havugimana, P. C., Hart, G. T., Nepusz, T., Yang, H., Turinsky, A. L., Li, Z., … Phanse, S. (2012). A census of human soluble protein complexes. Cell , 150(5), 1068–1081. doi: 10.1016/j.cell.2012.08.011.
- Hooper, C. M., Tanz, S. K., Castleden, I. R., Vacher, M. A., Small, I. D., & Millar, A. H. (2014). SUBAcon: A consensus algorithm for unifying the subcellular localization data of the Arabidopsis proteome. Bioinformatics , 30(23), 3356–3364. doi: 10.1093/bioinformatics/btu550.
- Jenkins, K. (2014). Beginner's guide to size-exclusion chromatography. Milford, CT: Waters Corporation.
- Katajamaa, M., & Orešič, M. (2007). Data processing for mass spectrometry-based metabolomics. Journal of Chromatography A , 1158(1–2), 318–328. doi: 10.1016/j.chroma.2007.04.021.
- Kim, H. K., & Verpoorte, R. (2010). Sample preparation for plant metabolomics. Phytochemical Analysis: An International Journal of Plant Chemical and Biochemical Techniques , 21(1), 4–13. doi: 10.1002/pca.1188.
- Kosmacz, M., Luzarowski, M., Kerber, O., Leniak, E., Gutierrez-Beltran, E., Beltran, J. C. M., … Graf, A. (2018). Interaction of 2′, 3′-cAMP with Rbp47b plays a role in stress granule formation. Plant Physiology , 177(1), 411–421. doi: 10.1104/pp.18.00285.
- Krueger, S., Giavalisco, P., Krall, L., Steinhauser, M.-C., Büssis, D., Usadel, B., … Steinhauser, D. (2011). A topological map of the compartmentalized Arabidopsis thaliana leaf metabolome. PLoS One , 6(3), e17806. doi: 10.1371/journal.pone.0017806.
- Kuhlemeier, C., & Timmermans, M. C. (2016). The Sussex signal: Insights into leaf dorsiventrality. Development , 143(18), 3230–3237. doi: 10.1242/dev.131888.
- Li, J., & Chory, J. (1997). A putative leucine-rich repeat receptor kinase involved in brassinosteroid signal transduction. Cell , 90(5), 929–938. doi: 10.1016/S0092-8674(00)80357-8.
- Li, X., Gianoulis, T. A., Yip, K. Y., Gerstein, M., & Snyder, M. (2010). Extensive in vivo metabolite-protein interactions revealed by large-scale systematic analyses. Cell , 143(4), 639–650. doi: 10.1016/j.cell.2010.09.048.
- Luzarowski, M., Kosmacz, M., Sokolowska, E., Jasińska, W., Willmitzer, L., Veyel, D., & Skirycz, A. (2017). Affinity purification with metabolomic and proteomic analysis unravels diverse roles of nucleoside diphosphate kinases. Journal of Experimental Botany , 68(13), 3487–3499. doi: 10.1093/jxb/erx183.
- Luzarowski, M., & Skirycz, A. (2019). Emerging strategies for the identification of protein-metabolite interactions. Journal of Experimental Botany , 70(18), 4605–4618. doi: 10.1093/jxb/erz228.
- Luzarowski, M., Wojciechowska, I., & Skirycz, A. (2018). 2 in 1: One-step affinity purification for the parallel analysis of protein-protein and protein-metabolite complexes. Journal of Visualized Experiments , 138. doi: 10.3791/57720.
- Maeda, K., Anand, K., Chiapparino, A., Kumar, A., Poletto, M., Kaksonen, M., & Gavin, A.-C. (2013). Interactome map uncovers phosphatidylserine transport by oxysterol-binding proteins. Nature , 501(7466), 257–261. doi: 10.1038/nature12430.
- Matthiesen, R., & Bunkenborg, J. (2013). Introduction to mass spectrometry-based proteomics. In R. Matthiesen (Ed.), Mass spectrometry data analysis in proteomics (Vol. 1007, pp. 1–45). Totowa, NJ: Humana Press.
- Müller, M., & Munné-Bosch, S. (2011). Rapid and sensitive hormonal profiling of complex plant samples by liquid chromatography coupled to electrospray ionization tandem mass spectrometry. Plant Methods , 7(1), 37. doi: 10.1186/1746-4811-7-37.
- Nikolaev, Y. V., Kochanowski, K., Link, H., Sauer, U., & Allain, F. H.-T. (2016). Systematic identification of protein–metabolite interactions in complex metabolite mixtures by ligand-detected nuclear magnetic resonance spectroscopy. Biochemistry , 55(18), 2590–2600. doi: 10.1021/acs.biochem.5b01291.
- Ong, S.-E., Schenone, M., Margolin, A. A., Li, X., Do, K., Doud, M. K., … Wood, J. L. (2009). Identifying the proteins to which small-molecule probes and drugs bind in cells. Proceedings of the National Academy of Sciences , 106(12), 4617–4622. doi: 10.1073/pnas.0900191106.
- Orr, M. W., & Lee, V. T. (2017). Differential radial capillary action of ligand assay (DRaCALA) for high-throughput detection of protein–metabolite interactions in bacteria. In P. Nordenfelt & M. Collin (Eds.), Bacterial pathogenesis (Vol. 1535, pp. 25–41). New York, NY: Humana Press.
- Orsak, T., Smith, T. L., Eckert, D., Lindsley, J. E., Borges, C. R., & Rutter, J. (2011). Revealing the allosterome: Systematic identification of metabolite–protein interactions. Biochemistry , 51(1), 225–232. doi: 10.1021/bi201313s.
- Piazza, I., Kochanowski, K., Cappelletti, V., Fuhrer, T., Noor, E., Sauer, U., & Picotti, P. (2018). A map of protein-metabolite interactions reveals principles of chemical communication. Cell , 172(1-2), 358–372. doi: 10.1016/j.cell.2017.12.006.
- Rigal, A., Ma, Q., & Robert, S. (2014). Unraveling plant hormone signaling through the use of small molecules. Frontiers in Plant Science , 5, 373. doi: 10.3389/fpls.2014.00373.
- Savitski, M. M., Reinhard, F. B., Franken, H., Werner, T., Savitski, M. F., Eberhard, D., … Klaeger, S. (2014). Tracking cancer drugs in living cells by thermal profiling of the proteome. Science , 346(6205), 1255784. doi: 10.1126/science.1255784.
- Seminara, A. B., Turdiev, A., Turdiev, H., & Lee, V. T. (2018). Differential radial capillary action of ligand assay (DRaCALA). Current Protocols in Molecular Biology , 126(1), e84. doi: 10.1002/cpmb.84 .
- Šimura, J., Antoniadi, I., Široká, J., Tarkowská, D., Strnad, M., Ljung, K., & Novák, O. (2018). Plant hormonomics: Multiple phytohormone profiling by targeted metabolomics. Plant Physiology , 177(2), 476–489. doi: 10.1104/pp.18.00293.
- Sterlin, Y., Pri-Tal, O., Zimran, G., Park, S. Y., Ben-Ari, J., Kourelis, J., … Mosquna, A. (2019). Optimized small-molecule pull-downs define MLBP 1 as an acyl-lipid-binding protein. The Plant Journal , 98(5), 928–941. doi: 10.1111/tpj.14272.
- Stockwell, B. R. (2000). Chemical genetics: Ligand-based discovery of gene function. Nature Reviews Genetics , 1(2), 116–125. doi: 10.1038/35038557.
- Striegel, A., Yau, W. W., Kirkland, J. J., & Bly, D. D. (2009). Modern size-exclusion liquid chromatography: Practice of gel permeation and gel filtration chromatography. Hoboken, NJ: John Wiley & Sons.
- t'Kindt, R., De Veylder, L., Storme, M., Deforce, D., & Van Bocxlaer, J. (2008). LC–MS metabolic profiling of Arabidopsis thaliana plant leaves and cell cultures: Optimization of pre-LC–MS procedure parameters. Journal of Chromatography B , 871(1), 37–43. doi: 10.1016/j.jchromb.2008.06.039.
- Tóth, R., & van der Hoorn, R. A. (2010). Emerging principles in plant chemical genetics. Trends in Plant Science , 15(2), 81–88. doi: 10.1016/j.tplants.2009.11.005.
- Veyel, D., Kierszniowska, S., Kosmacz, M., Sokolowska, E. M., Michaelis, A., Luzarowski, M., … Skirycz, A. (2017). System-wide detection of protein-small molecule complexes suggests extensive metabolite regulation in plants. Scientific Reports , 7, 42387. doi: 10.1038/srep42387.
- Veyel, D., Sokolowska, E. M., Moreno, J. C., Kierszniowska, S., Cichon, J., Wojciechowska, I., … Gorka, M. (2018). PROMIS, global analysis of PROtein-metabolite interactions using size separation in Arabidopsis thaliana. Journal of Biological Chemistry , 293(32), 12440–12453. doi: 10.1074/jbc.RA118.003351.
- West, G. M., Tang, L., & Fitzgerald, M. C. (2008). Thermodynamic analysis of protein stability and ligand binding using a chemical modification- and mass spectrometry-based strategy. Analytical Chemistry , 80(11), 4175–4185. doi: 10.1021/ac702610a.
- Zheng, X. S., Chan, T.-F., & Zhou, H. H. (2004). Genetic and genomic approaches to identify and study the targets of bioactive small molecules. Chemistry & Biology, 11(5), 609–618. doi: 10.1016/j.chembiol.2003.08.011.
- Zwiewka, M., & Friml, J. (2012). Fluorescence imaging-based forward genetic screens to identify trafficking regulators in plants. Frontiers in Plant Science , 3, 97. doi: 10.3389/fpls.2012.00097.
Internet Resources
Promega. Technical manual TM390, Trypsin/Lys-C Mix.
Citing Literature
Number of times cited according to CrossRef: 13
- Xi Zhou, Jing Yang, Yin Luo, Xiao Shen, HNCGAT: a method for predicting plant metabolite–protein interaction using heterogeneous neighbor contrastive graph attention network, Briefings in Bioinformatics, 10.1093/bib/bbae397, 25 , 5, (2024).
- Yogindra Kumari, Arshdeep Chopra, Rohit Bhatia, Recent Advancements and Applications of Size Exclusion Chromatography in Modern Analysis, Current Analytical Chemistry, 10.2174/1573411019666230526144816, 19 , 5, (374-390), (2023).
- Mei Luo, Bin Li, Georg Jander, Shaoqun Zhou, Non‐volatile metabolites mediate plant interactions with insect herbivores, The Plant Journal, 10.1111/tpj.16180, 114 , 5, (1164-1177), (2023).
- Dennis Schlossarek, Youjun Zhang, Ewelina M. Sokolowska, Alisdair R. Fernie, Marcin Luzarowski, Aleksandra Skirycz, Don't let go: co‐fractionation mass spectrometry for untargeted mapping of protein–metabolite interactomes, The Plant Journal, 10.1111/tpj.16084, 113 , 5, (904-914), (2023).
- Asaph Aharoni, Royston Goodacre, Alisdair R. Fernie, Plant and microbial sciences as key drivers in the development of metabolomics research, Proceedings of the National Academy of Sciences, 10.1073/pnas.2217383120, 120 , 12, (2023).
- Yan Zhang, Rui Chen, DuoDuo Zhang, Shuang Qi, Yan Liu, Metabolite interactions between host and microbiota during health and disease: Which feeds the other?, Biomedicine & Pharmacotherapy, 10.1016/j.biopha.2023.114295, 160 , (114295), (2023).
- Iman Tabatabaei, Saleh Alseekh, Mohammad Shahid, Ewa Leniak, Mateusz Wagner, Henda Mahmoudi, Sumitha Thushar, Alisdair R. Fernie, Kevin M. Murphy, Sandra M. Schmöckel, Mark Tester, Bernd Mueller-Roeber, Aleksandra Skirycz, Salma Balazadeh, The diversity of quinoa morphological traits and seed metabolic composition, Scientific Data, 10.1038/s41597-022-01399-y, 9 , 1, (2022).
- Qian Ma, Mingqin Chang, Georgia Drakakaki, Eugenia Russinova, Selective chemical probes can untangle the complexity of the plant cell endomembrane system, Current Opinion in Plant Biology, 10.1016/j.pbi.2022.102223, 68 , (102223), (2022).
- Maria Juliana Calderan-Rodrigues, Marcin Luzarowski, Carolina Cassano Monte-Bello, Romina I. Minen, Boris M. Zühlke, Zoran Nikoloski, Aleksandra Skirycz, Camila Caldana, Proteogenic Dipeptides Are Characterized by Diel Fluctuations and Target of Rapamycin Complex-Signaling Dependency in the Model Plant Arabidopsis thaliana, Frontiers in Plant Science, 10.3389/fpls.2021.758933, 12 , (2021).
- Marcin Luzarowski, Rubén Vicente, Andrei Kiselev, Mateusz Wagner, Dennis Schlossarek, Alexander Erban, Leonardo Perez de Souza, Dorothee Childs, Izabela Wojciechowska, Urszula Luzarowska, Michał Górka, Ewelina M. Sokołowska, Monika Kosmacz, Juan C. Moreno, Aleksandra Brzezińska, Bhavana Vegesna, Joachim Kopka, Alisdair R. Fernie, Lothar Willmitzer, Jennifer C. Ewald, Aleksandra Skirycz, Global mapping of protein–metabolite interactions in Saccharomyces cerevisiae reveals that Ser-Leu dipeptide regulates phosphoglycerate kinase activity, Communications Biology, 10.1038/s42003-021-01684-3, 4 , 1, (2021).
- Mohammad Amin Omidbakhshfard, Ewelina M. Sokolowska, Valerio Di Vittori, Leonardo Perez de Souza, Anastasiya Kuhalskaya, Yariv Brotman, Saleh Alseekh, Alisdair R. Fernie, Aleksandra Skirycz, Multi-omics analysis of early leaf development in Arabidopsis thaliana, Patterns, 10.1016/j.patter.2021.100235, 2 , 4, (100235), (2021).
- Dennis Schlossarek, Marcin Luzarowski, Ewelina Sokołowska, Michał Górka, Lothar Willmitzer, Aleksandra Skirycz, PROMISed: A novel web-based tool to facilitate analysis and visualization of the molecular interaction networks from co-fractionation mass spectrometry (CF-MS) experiments, Computational and Structural Biotechnology Journal, 10.1016/j.csbj.2021.08.042, 19 , (5117-5125), (2021).
- Taly Lapidot‐Cohen, Leah Rosental, Yariv Brotman, Liquid Chromatography–Mass Spectrometry (LC‐MS)‐Based Analysis for Lipophilic Compound Profiling in Plants, Current Protocols in Plant Biology, 10.1002/cppb.20109, 5 , 2, (2020).