Murine Models of Salmonella Infection
Gregory T. Walker, Gregory T. Walker, Romana R. Gerner, Romana R. Gerner, Sean-Paul Nuccio, Sean-Paul Nuccio, Manuela Raffatellu, Manuela Raffatellu
Abstract
The pathogen Salmonella enterica encompasses a range of bacterial serovars that cause intestinal inflammation and systemic infections in humans. Mice are a widely used infection model due to their relative simplicity and versatility. Here, we provide standardized protocols for culturing the prolific zoonotic pathogen S. enterica serovar Typhimurium for intragastric inoculation of mice to model colitis or systemic dissemination, along with techniques for direct extraintestinal infection. Furthermore, we present procedures for quantifying pathogen burden and for characterizing the immune response by analyzing tissue pathology, inflammatory markers, and immune cells from intestinal tissues. © 2023 The Authors. Current Protocols published by Wiley Periodicals LLC.
Basic Protocol 1 : Murine colitis model utilizing oral streptomycin pretreatment and oral S. Typhimurium administration
Basic Protocol 2 : Intraperitoneal injection of S. Typhimurium for modeling extraintestinal infection
Support Protocol 1 : Preparation of S. Typhimurium inoculum
Support Protocol 2 : Preparation of mixed S. Typhimurium inoculum for competitive infection
Basic Protocol 3 : Assessment of S. Typhimurium burden
Support Protocol 3 : Preservation and pathological assessment of S. Typhimurium–infected tissues
Support Protocol 4 : Measurement of inflammatory marker expression in intestinal tissues by qPCR
Support Protocol 5 : Preparation of intestinal content for inflammatory marker quantification by ELISA
Support Protocol 6 : Immune cell isolation from Salmonella -infected intestinal tissues
INTRODUCTION
The genus Salmonella covers an array of Gram-negative facultative anaerobic bacteria that in general colonize a broad range of hosts. Salmonella enterica is divided into six subspecies that represent over 2500 serovars (Grimont & Weill, 2007). Infection of humans and livestock is associated most commonly with serovars of the subspecies enterica (S. enterica subsp. enterica). Salmonella serovars are often termed typhoidal or non-typhoidal based on disease presentation in humans. Non-typhoidal Salmonella (NTS) serovars (e.g., S. enterica subsp. enterica serovar Typhimurium; hereafter, S. Typhimurium) are zoonotic generalists that cause inflammatory colitis during infection of otherwise healthy humans and represent the majority of serovars. In contrast, typhoidal serovars (e.g., S. Typhi) have evolved into human-restricted pathogens that typically present as a more severe extraintestinal disease known as typhoid or enteric fever (Gal-Mor et al., 2014; Hiyoshi et al., 2018).
Responsible for over 100 million human infections per year worldwide, S. enterica is a major concern for public health (Majowicz et al., 2010). The emergence of multidrug resistance and invasive disease among NTS serovars is an increasingly significant problem (Ao et al., 2015; CDC, 2019). Due to its well-characterized virulence systems (Bao et al., 2020; Larock et al., 2015; Sanchez-Garrido et al., 2021), relative ease of genetic manipulation, and availability of mutant strains and libraries (Geng et al., 2016; Porwollik et al., 2014), S. enterica has been widely studied as a model intestinal, extraintestinal, and intracellular bacterial pathogen, particularly using strains of the NTS serovar S. Typhimurium.
In humans, infections with NTS such as S. Typhimurium typically remain localized to the gut, where the pathogen triggers inflammatory diarrhea, a disease characterized by the recruitment of neutrophils (Acheson & Hohmann, 2001). Systemic NTS infection generally occurs in highly susceptible individuals (e.g., children and the elderly) or during coinfection with malaria or human immunodeficiency virus (Acheson & Hohmann, 2001). In contrast, in commonly employed laboratory mouse strain backgrounds (e.g., C57BL/6 and BALB/c), S. Typhimurium causes a systemic infection with a limited intestinal inflammatory response followed by rapid mortality (typically 3-10 days, depending on infectious dose, mouse strain, and mouse microbiota). Thus, S. Typhimurium infection of mice has historically been used to model aspects of the extraintestinal Salmonella disease caused by typhoidal serovars in humans, and alternative mammalian models (e.g., cow, monkey, pig, rabbit) have been used to investigate mechanisms of NTS-associated colitis in vivo (Everest et al., 1999; Rout et al., 1974; Santos et al., 2001; Tsolis et al., 2011).
More recently, researchers have developed models to investigate NTS colitis in mice. Some models use genetically resistant mouse lines that are more capable of controlling the extraintestinal infection of S. Typhimurium (Jacobson et al., 2018; Rivera-Chávez et al., 2016). Others require administration of antibiotics (e.g., streptomycin) to perturb the commensal microbiota and prime the exudative inflammatory responses that follow S. Typhimurium gut colonization (Kaiser et al., 2012; Palmer & Slauch, 2017; Stecher et al., 2007; Tsolis et al., 2011). These advances have enabled researchers to employ “genetics-squared” approaches, wherein the host and pathogen can each be genetically altered in order to probe the combined interactions of host immunity and pathogen virulence and metabolism on the outcome of infection (Byndloss, Olsan, et al., 2017; Persson & Vance, 2007).
This article provides detailed protocols as examples of commonly used mouse infection models for studying Salmonella disease. The primary focus is on S. Typhimurium as a model pathogen, but the protocols can be adapted to other S. enterica serovars. Basic Protocol 1 describes steps for the streptomycin-pretreatment Salmonella colitis model in mice, including the preparation and administration of streptomycin and the intragastric infection of mice with S. Typhimurium on the next day. Basic Protocol 2 provides procedures for a direct extraintestinal infection model with S. Typhimurium by intraperitoneal injection. Support Protocol 1 describes preparation of the inoculum for these models. As an alternative, Support Protocol 2 outlines the preparation of a mixed S. Typhimurium inoculum to study the competitive fitness of the pathogen. Basic Protocol 3 describes procedures for quantifying intestinal and tissue pathogen burden. Support Protocol 3 outlines the assessment of infected tissue histopathology. Support Protocol 4 describes extraction of RNA from intestinal tissues and provides validated qPCR primers for markers and mediators of colitis. Support Protocol 5 describes the collection and preparation of intestinal content to measure markers of inflammation by ELISA, including myeloperoxidase and lipocalin-2. Finally, Support Protocol 6 provides methods for isolating immune cells (including lymphocytes, infiltrating granulocytes, monocytes, and innate lymphoid cells) from infected intestinal tissues for immunophenotyping or ex vivo activity assays.
CAUTION: S. enterica strains are commonly regarded as Biosafety Level 2 (BSL-2) pathogens. All appropriate institutional and governmental guidelines and regulations for the use and handling of pathogenic microorganisms should be followed. Infected mice should be housed in a BSL-2 facility. Appropriate personal protective equipment (PPE) including laboratory coat, gloves, and eye protection should be used when handling Salmonella cultures or infected animals/tissues.
NOTE : All protocols involving animals must be reviewed and approved by the appropriate Animal Care and Use Committee (IACUC) and must follow regulations for the care and use of laboratory animals.
NOTE : General cleanliness, surface decontamination, materials sterilization, and aseptic technique are all critical to the preparation, performance, and conclusion of every experimental design and workflow. These help to ensure the safety of laboratory personnel and the surrounding community (by keeping infectious material contained), the integrity and reproducibility of results (by avoiding contamination), and respect for the privilege of utilizing animal subjects (by minimizing experimental repeats).
Basic Protocol 1: MURINE COLITIS MODEL UTILIZING ORAL STREPTOMYCIN PRETREATMENT AND ORAL S. TYPHIMURIUM ADMINISTRATION
Intragastric inoculation of streptomycin-treated mice with S. Typhimurium should induce severe inflammation of the cecum and colon beginning 24 hr post-infection (Barthel et al., 2003). In susceptible mice (e.g., C57BL/6 mice; Simon et al., 2011), significant S. Typhimurium dissemination from the gut to systemic sites can be observed during infection over time, resulting in mortality after 4-5 days (Barthel et al., 2003), depending on the S. Typhimurium strain, the vivarium, and the gut microbiota. In the absence of streptomycin pretreatment, susceptible mice will usually develop few intestinal signs of gastroenteritis and are considered a model for typhoid disease, given the substantial dissemination of Salmonella from the gut to systemic tissues including blood, liver, and spleen. However, in some mouse lines that display greater immunological control of systemic S. Typhimurium infection (“resistant” lines; e.g., CBA/J; Simon et al., 2011), S. Typhimurium infection without antibiotic pretreatment can generate significant intestinal inflammation by around 7-10 days of infection (Faber et al., 2017; Rivera-Chávez et al., 2016). For a more detailed classification of mouse lines displaying resistance and susceptibility to S. Typhimurium, refer to Simon et al. (2011).
Pretreatment of mice with streptomycin inhibits microbiota-associated intestinal colonization resistance against S. Typhimurium, prompting a neutrophilic inflammatory response to the pathogen in the cecum and colon (the murine colitis model) (Barthel et al., 2003; Bohnhoff & Miller, 1962). In this model, antibiotic administration is performed by gavage 24 hr prior to S. Typhimurium inoculation to allow for both reduced colonization resistance and clearance of most of the antibiotic from the gastrointestinal tract prior to infection. Uninfected mice treated with streptomycin restore the microbiota by day 4 post-infection (Barthel et al., 2003; Stecher et al., 2007). Inoculation of mice is also performed by gavage.
Materials
-
Mice, typically 8-12 weeks old (sex-matched) and acclimated to their housing for ≥1 week
-
Streptomycin sulfate (EMD Millipore Corp)
-
Sterile water
-
70% (v/v) ethanol
-
Sterile PBS (see recipe; optional)
-
Sterile 1.5-ml tube
-
Sterile 1-ml syringes
-
0.22-µm syringe filter (Acrodisc; Pall Corporation)
-
Reusable gavage needle/feeding tube (22-gauge, 1.5 inches), stored in 70% ethanol
-
Scale (accurate in the 10-50 g range)
-
Additional reagents and equipment for preparing the S. Typhimurium inoculum (see Support Protocol 1 or 2)
Administer streptomycin (24 hr prior to inoculation)
1.Prepare a fresh 200 mg/ml solution of streptomycin sulfate in sterile water.
2.Filter sterilize into a sterile 1.5-ml tube (e.g., autoclaved microcentrifuge tube) and keep on ice until ready to administer.
3.Immediately before administration, flush a gavage needle with 70% ethanol and then enough sterile water to remove any residual ethanol.
4.Change the syringe on the gavage needle, agitate the streptomycin solution to mix evenly, and draw up an adequate volume for treatment.
5.With the gavage needle pointing upwards, tap the syringe and depress the plunger to remove any air bubbles.
6.Wipe the outside of the gavage needle with 70% ethanol and a Kimwipe to remove any external droplets.
7.Scruff the first mouse firmly, hold it in an upright position, and gently insert the gavage needle down the esophagus into the stomach (i.e., intragastrically).
8.Dispense 0.1 ml streptomycin solution and gently withdraw the needle.
9.Return mouse to its cage and monitor for signs of respiratory distress or abnormal behavior.
10.Wipe the gavage needle with 70% ethanol and a Kimwipe before repeating with the remaining mice.
11.After the last mouse, clean and disinfect the gavage needle and work surfaces with 70% ethanol.
Perform infection
12.Optional : Remove mouse chow from the cages 3-4 hr before inoculation.
13.Record the weight of each mouse and identify using marker lines on the tail or ear tags.
14.Prepare the inoculum (see Support Protocol 1 or 2) at a concentration of 2.5 × 108 to 1 × 1010 CFU/ml.
15.Perform gavage as in steps 3-11.
16.If animals were fasted prior to infection, return food to their cages.
17.Monitor and weigh animals at least once or twice daily to assess the course of infection.
Basic Protocol 2: INTRAPERITONEAL INJECTION OF S. TYPHIMURIUM FOR MODELING EXTRAINTESTINAL INFECTION
For some research questions, it is desirable to determine whether an observed phenotype depends on the intestinal or extraintestinal stage of infection (e.g., whether higher systemic levels of Salmonella are due to increased extraintestinal growth/survival or are downstream of gut-associated factors). A simple model to investigate this question is performing an infection by intraperitoneal (i.p.) injection, allowing Salmonella to colonize systemic tissues while bypassing the selective pressures present in the gut. If the phenotype is absent following i.p. infection, it can be concluded that the mechanism driving the phenotype involves the intestinal stage of infection.
Additional Materials (also see Basic Protocol 1)
- Mice, typically 6-8 weeks old (sex-matched) and acclimated to their housing for ≥1 week
- 26-G PrecisionGlide needles (Becton Dickinson)
1.Weigh mice prior to inoculation.
2.Prepare the inoculum (see Support Protocol 1 or 2) at a concentration of 1 × 103 to 1 × 105 CFU/ml.
3.Agitate the inoculum to mix evenly, then draw it up into a 1-ml syringe (at least 0.1 ml per mouse) and attach the needle.
4.With the needle pointing upwards, tap the syringe and depress the plunger to remove any air bubbles.
5.Wipe the outside of the needle with 70% ethanol and a Kimwipe to remove any external inoculum droplets.
6.Scruff the mouse firmly and maneuver it underside-up, angling it away from you, with the head pointed downward at an ∼30° angle.
7.Insert the needle ∼0.5 cm deep at an angle almost parallel to the body into the peritoneal cavity, aiming for either side between the nipples.
8.Inject the inoculum (0.1-0.2 ml) into the peritoneal cavity and then gently withdraw the needle. Confirm that the inoculum is not expelled out of the hole left by the needle, which would indicate it was not properly inserted into the peritoneal cavity.
9.Repeat for additional mice.
10.Clean and disinfect the area with 70% ethanol and dispose of the syringe and needle in a biohazardous sharps disposal container.
11.Monitor and weigh the animals at least daily to assess the course of infection.
Support Protocol 1: PREPARATION OF S. TYPHIMURIUM INOCULUM
This protocol provides directions for preparing a S. Typhimurium inoculum from a frozen glycerol stock. This protocol requires 3 days.
Materials
-
70% (v/v) ethanol, 10% bleach, or other disinfectant
-
Glycerol stock (see recipe) of wild-type S. Typhimurium (e.g., strain ATCC 14028s, SL1344, or antibiotic-resistant derivative such as IR715)
-
LB or MacConkey lactose agar plates (see recipes) with appropriate selective antibiotic (50 µg/ml nalidixic acid for IR715; 100 µg/ml streptomycin for SL1344)
-
LB broth (see recipe)
-
Sterile PBS (see recipe)
-
Insulated culture transport vessel containing dry ice or chilled to and capable of maintaining approximately −80°C
-
Sterile inoculation loops (disposable plastic or reusable metal)
-
37°C incubator with shaker or tube roller
-
15-ml sterile test tubes with loose-fitting caps
-
Spectrophotometer
Plate cells from frozen stock
1.Clean work surfaces with 70% ethanol, fresh 10% bleach, or another approved/compatible disinfectant.
2.To minimize contamination, light an active flame and perform all steps within 2 feet of the flame to maintain a sterile field and limit the chance of contaminating cultures with environmental microbes.
3.Remove a glycerol stock of S. Typhimurium from the −80°C freezer and place in an insulated cold transport vessel.
4.Gently scrape the top layer of the stock with a sterile inoculation loop and run it across the top of a room-temperature LB agar plate containing selective antibiotics using the quadrant or T-streak technique to generate isolated colonies.
5.Incubate plate upside-down in a 37°C incubator overnight (14-18 hr).
Prepare overnight culture
6.Use a sterile inoculation loop to collect a few colonies or confluent growth from the plate and transfer to a 15-ml sterile test tube containing ∼5 ml fresh LB broth. Gently rub the loop against the side of the tube in the broth, then remove the loop and cap the tube loosely.
7.Prepare a second tube with 5-ml LB broth alone as a control for contamination of the broth stock and supplies used.
8.Incubate 12-16 hr at 37°C with aeration (shaking at ∼200 rpm or rotating).
9.Optional : Dilute the overnight culture 1:50 (v/v) in fresh LB broth (5-50 ml total volume, depending on the amount of inoculum required) and incubate an additional 3-4 hr at 37°C with shaking.
10.Measure the OD600 and calculate the required volume needed for the desired inoculum concentration (see Critical Parameters for more information on typical S. Typhimurium quantities used for inocula).
11.Harvest bacteria by centrifuging at least 5 min at 3200 × g , 4°C, to pellet the cells. Discard the supernatant.
12.Wash cells at least once by resuspending (e.g., vortex) in 5-10 ml sterile PBS. Pellet again by centrifugation and discard the supernatant.
13.Resuspend pellet in the volume of PBS calculated to produce an inoculum at the desired concentration and use immediately (i.e., within 10 min) for infection (see Basic Protocol 1).
14.Plate serial dilutions of the inoculum (typically 10–6, 10–7, 10–8, and 10–9 for an inoculum of 1 × 1010 CFU/ml) and incubate plates upside-down in a 37°C incubator overnight (14-18 hr). Count CFUs to confirm that the actual dose was close to the concentration estimated by OD600 approximation.
Support Protocol 2: PREPARATION OF MIXED S. TYPHIMURIUM INOCULUM FOR COMPETITIVE INFECTION
This protocol is used to create an inoculum consisting of two different S. Typhimurium strains (typically, a wild-type and an isogenic mutant strain) that can be used for competitive fitness infection assays in vivo or in vitro. In a competitive infection experiment, the input ratio of the inoculum (i.e., strain A CFU/strain B CFU) is compared to the output ratio at the end of the infection to determine the competitive index (CI), a measure of the relative fitness advantage of one strain over another in a given environment (Beuzón & Holden, 2001). The average CI will be equal to 1 if there is no difference in fitness between strains, >1 if strain A is more fit than strain B, and <1 if strain B is more fit than strain A.
This technique requires that the two strains of interest be discernible when plated, typically by using different encoded antibiotic resistances. Below, two examples are provided. Example 1 uses one strain that is nalidixic acid–resistant and streptomycin sensitive (NalR StrepS) and one that is the opposite (NalS StrepR). Example 2 uses two strains that are NalR, but one is KanS and the other is KanR. Example 2 is a common setup for comparison of wild-type and mutant strains.
IMPORTANT : Be wary when using plasmids to mark strains with an antibiotic resistance for the purpose of quantification ex vivo from a host. Many commonly employed plasmids are not stably maintained within a population of cells without constant selective pressure for a bacterium to possess the plasmid (e.g., growth in an antibiotic-containing medium). As a result, the plasmid can begin to titrate out of the bacterial population (i.e., following cell division, daughter cells that do not receive a copy of an antibiotic resistance plasmid will still be able to replicate in the environment). Thus, plating on antibiotic-containing agar media selecting for the plasmid's presence would undercount the strain of interest, as some percentage of the population will have lost the plasmid. As such, an observed infection phenotype could simply be measuring loss of a marker plasmid rather than the impact of a mutation of interest, and this will be further magnified the longer the experiment runs.
Additional Materials (also see Support Protocol 1)
- Glycerol stocks (see recipe) of two S. Typhimurium strains:
- Example 1: IR715 (NalR StrepS) and SL1344 (NalS StrepR)
- Example 2: IR715 Δ iroN ::KSAC (NalR KanR) and IR715 (NalR KanS)
1.Prepare separate inocula of both strains as described (see Support Protocol 1, steps 1-13). Resuspend the strains to the same concentration.
2.Mix the inocula 1:1 (v/v) and use immediately (i.e., within 10 min) for infection (see Basic Protocol 1).
3.To more accurately quantify the concentration of each strain in the inoculum (the input ratio), serially dilute and plate a sample of the mixed inoculum on separate LB agar plates supplemented with the appropriate selective antibiotics.
-
Example 1: If strain A is NalRand strain B is StrepR, plate the mixed inoculum on both LB/Nal and LB/Strep plates. The abundance of each strain is directly quantifiable from the colonies counted on the respective plates.
-
Example 2: If strain A is NalRKanRand strain B is NalRKanS, plate the mixed inoculum on both LB/Nal and LB/Kan plates. Strain A is directly quantifiable on LB/Kan. Strain B is quantified by subtracting the LB/Kan count from the LB/Nal count.
The approach for example 2 is valid only in the following scenarios: (1) strain A (a mutant of strain B) is less abundant than strain B; (2) strains A and B are roughly equal in abundance; (3) strain A is at most a few-fold more abundant than strain B (i.e., one loses the ability to easily quantify strain B amidst strain A as strain A becomes relatively more abundant). In scenarios where strain A is more abundant than strain B, one may “pick and patch” several hundred colonies from each LB/Nal plate and transfer them to LB/Kan (test condition) and LB alone (positive control) in order to quantify strain B. Genetics permitting, in such a scenario, one should consider reconstructing the strains for easier quantification.
As the relationship between OD600absorbance units and actual live bacterial cells can vary between strains and growth state, quantifying inoculum concentration by CFU levels of each strain provides a more accurate input ratio to assess the competitive index.
4.Measure infection outcomes. Use the methods for quantifying S. Typhimurium burden in intestinal content and tissues (see Basic Protocol 3), while plating each sample's dilutions on both selective agars to assess the output ratio in that sample. Finally, calculate CI by dividing the output ratio by the input ratio.
Basic Protocol 3: ASSESSMENT OF S. TYPHIMURIUM BURDEN
This protocol describes methods for assessing the course of S. Typhimurium infection of mice, including the intestinal and systemic arms of infection. After orogastric infection, S. Typhimurium rapidly colonizes the mouse gut (terminal ileum, cecum, and colon). The increased levels of S. Typhimurium in the distal gut (especially in colitis models) are most readily measured over time in live mice by plating fecal samples. Feces are collected, processed and then spread on selective agar plates to assess the number of S. Typhimurium CFUs. Colonization levels are more variable in mice that are not treated with antibiotics and more consistent when mice are pretreated with streptomycin. Part 1 of this protocol provides instructions for collecting and preparing murine fecal samples and quantifying Salmonella abundance by counting CFUs. For tracking S. Typhimurium intestinal burden in live mice, fecal pellets can be collected daily.
At the end of infection, various terminal analyses can be performed at necropsy. Intestinal tissue from different sections (terminal ileum, colon, cecum) may be collected and homogenized to quantify S. Typhimurium levels in the gut. S. Typhimurium also colonizes Peyer's patches, intestinal lymphoid tissues that are found mostly throughout the small intestine (Fig. 1). Because wild-type mice are incapable of stopping S. Typhimurium from spreading beyond the intestine, measuring the S. Typhimurium burden in extraintestinal sites can help assess the relative severity of the infection in a mouse. Commonly collected tissues that display substantial S. Typhimurium extraintestinal colonization following infection by oral gavage include the mesenteric lymph nodes, spleen, liver, and blood. For intraperitoneal infection, blood, spleen, and liver are typically collected. Part 2 describes each of these sample collection methods.
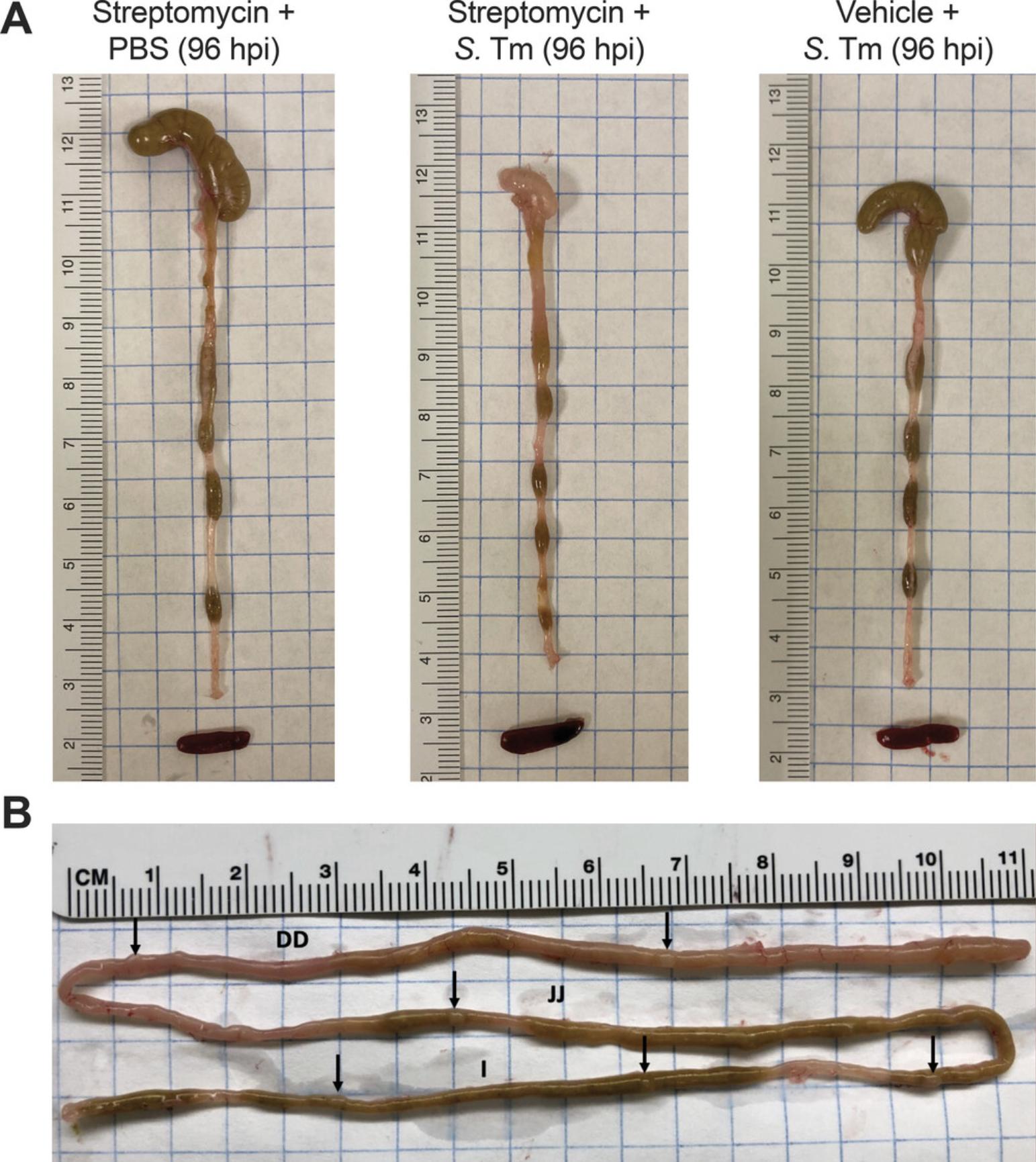
Materials
-
S. Typhimurium–infected mice (see Basic Protocol 1 or 2)
-
70% (v/v) ethanol
-
Sterile PBS (see recipe)
-
LB or MacConkey agar plates (see recipes) with appropriate antibiotics
-
0.5 M EDTA, sterile
-
Sterile 1.5- or 2-ml microcentrifuge tubes
-
Milligram scale
-
Clean paper towels
-
500-ml clear glass beaker with a pouring lip (or similar), disinfected with 70% ethanol
-
Forceps, disinfected with 70% ethanol
-
Vortex mixer with multi-tube attachment (e.g., Horizontal-(24) Microtube Holder, Scientific Industries)
-
Sterile wide-bore and standard 200-µl micropipette tips
-
37°C incubator
-
Sterile 26-G PrecisionGlide needles (Becton Dickinson)
-
Sterile 1-ml syringes (Becton Dickinson)
-
Sterile 15- or 50-ml conical tubes (Corning)
-
Sterile dissection tools (forceps, scissors)
-
Tissue homogenizer/disperser (e.g., IKA T25 digital ULTRA-TURRAX), fixed on a lab stand support, inside a biosafety cabinet
-
Additional reagents and equipment for euthanasia
Part 1: Quantification of fecal S. Typhimurium levels
IMPORTANT : To limit the potential for Salmonella cross-contamination between animals or samples, use a fresh, clean paper towel for each mouse and wipe down beakers and forceps with 70% ethanol between mice. Allow to dry by evaporation before use.
1.Prepare a labeled microcentrifuge tube for each infected mouse. Weigh each tube on a milligram scale and record the weight.
2.Place a mouse on a clean paper towel and cover it with an inverted sterile glass beaker.
3.Use sterile forceps to collect one to three pellets from each mouse (∼25-100 mg fecal content) into the empty tubes. Close firmly and place immediately on ice.
4.Weigh feces-containing tubes and record the weight. Determine the weight of each sample by subtracting the empty tube weight.
5.Add sterile PBS to each sample at a 9:1 ratio (e.g., 900 µl PBS to 100 mg feces).
6.Homogenize samples into a fecal slurry by vortexing at top speed for 5-10 min.
7.Perform 10-fold serial dilutions of the fecal slurry in PBS (e.g., 100 µl slurry in 900 µl PBS), vortexing each dilution before proceeding to the next.
8.Plate the dilutions on LB agar with selective antibiotics, using wide-bore micropipette tips for the first two dilutions. Incubate the plates inverted overnight (14-16 hr) at 37°C.
9.Record the number of colonies (CFUs) that form from each dilution that exhibits separate, distinct, countable colonies.
10.Calculate the fecal load of S. Typhimurium in each sample as:
Fecal load (CFU/mg) = # CFUs on least-diluted countable sample / (concentration of fecal slurry × dilution × volume plated)
Part 2: Quantification of S. Typhimurium loads at necropsy
IMPORTANT : Use disinfected scissors and forceps to open the mouse and collect relevant tissues in preweighed/labeled sterile tubes. Store tissue-containing tubes on ice until they can be processed. Disinfect tools with 70% ethanol between collecting different tissues to minimize the potential of cross-contamination between samples.
11.Prepare tubes needed for sample collection.
-
For blood: Prepare a 26-G needle and 1-ml syringe for each mouse by drawing up and expelling 1 ml of sterile 0.5 M EDTA, leaving behind a residual amount as an anticoagulant. Also prepare one 1.5-ml sterile microcentrifuge tube for each mouse by adding 50 µl of 0.5 M EDTA and then weighing.
-
For intestinal content from small intestine, cecum, and colon: Label and weigh three microcentrifuge tubes for each mouse.
-
For intestinal and extraintestinal tissues: Label and weigh a sterile 15- or 50-ml polypropylene tube containing 1-5 ml sterile PBS for each tissue from each mouse.
12.Humanely euthanize the mouse by CO2 asphyxiation followed by cervical dislocation (or similar method approved in your IACUC protocol).
13.Disinfect the fur and outer skin by wetting with 70% ethanol. Open the mouse using disinfected scissors and forceps.
14.Collect tissues:
-
Blood: Locate the heart in the thoracic cavity and perform a cardiac puncture in either ventricle with the EDTA-treated needle. Pull the plunger back slowly to draw up blood and gently relocate the needle (without withdrawing it from the heart) to relieve pressure. Once an adequate volume has been collected (200-600 µl), remove the needle and expel the blood into the preweighed 1.5-ml tube containing EDTA.
-
Spleen: Excise the spleen by blunt dissection from the left side of the body cavity. Transfer part or all of the spleen in a prepared tube.
-
Mesenteric lymph nodes: Locate the mesenteric lymph nodes, which look like a white translucent tube in the fatty mass adjacent to the intestines (they will often be larger/more prominent in infected animals). Dissect out the nodes and collect them in a prepared tube.
-
Intestinal content/tissues: Gently move the intestines to locate the distal end of the colon. Cut the colon as distally as possible and use forceps to pull and straighten out the intestines onto a clean paper towel, being careful not to tear the organs. Separate the colon, cecum, and small intestine by cutting where they join at the cecum. Make sure to orient the tissue before cutting it, as the colon and small intestine can be hard to distinguish once cut. If the mice are pretreated with streptomycin before infection with wild-typeS. Typhimurium, observe that the cecum is small, thick, whitish in color, and devoid of content (Fig.1A). If luminal content is present, squeeze it out from each section and collect in prepared tubes. Collect samples of the terminal ileum, cecum, and colon tissue into separate tubes prepared with PBS.
The cecum can be sectioned with scissors during necropsy for different downstream analyses (e.g., CFU counts, RNA analysis, histopathology). If performing histopathological analysis of the cecum, be sure to handle it only minimally while collecting the cecal tip (distal third to half of the cecum) for this analysis.
- Peyer's patches: The Peyer's patches can be located at various points along the small intestine, appearing as white, raised, oval spots (they will often be larger/more prominent in infected animals) (Fig.1B). They can be identified more easily if the intestinal content is present. Peyer's patches can be cut off the small intestine most readily using fine-tip curved dissecting scissors. For CFU assessment, the three to four most-distal Peyer's patches in the ileum from each animal may be pooled in a single prepared tube.
Due to their small size, it is often easier to collect a consistent number of Peyer's patches (e.g., three) from each subject and then normalize CFU counts as an average per Peyer's patch rather than per milligram tissue.
- Liver: Mice have four liver lobes, which may be found immediately below the lungs, attached to the diaphragm. Cut a section of liver tissue (e.g., third to half of a lobe) and collect it in a prepared tube.
Avoid rupturing the gallbladder (the small, yellow-to-black sack in the middle of the median liver lobe), which may contain high loads of Salmonella and can contaminate liver tissue samples if damaged.
15.Dispose of infected mouse carcasses as biohazardous waste.
16.Process samples:
-
Blood: Weigh tubes and determine the total volume of blood collected by (a) subtracting the weight of the empty tube and (b) using a blood density of ∼1 g/ml.
-
Intestinal content: Weigh tubes and determine the weight of the collected material by subtracting the weight of the empty tubes. Homogenize intestinal content by vortexing at top speed for 5-10 min (similar to processing of fecal pellets in step 6).
-
Intestinal and extraintestinal tissues: Weigh tubes and determine the tissue weight by subtracting the weight of the empty tubes. Use a homogenizer to thoroughly disrupt the tissues in their tubes with the PBS. This typically takes 3-10 s per sample tube.
Clean and disinfect the homogenizer's dispersing element between samples by running it through two washes of 70% ethanol (two separate 50-ml conical tubes containing enough ethanol to sufficiently cover the disperser) and one wash of sterile PBS. Use tweezers (disinfected with 70% ethanol) to remove any residual tissue that remains stuck in the homogenizer. Process one type of tissue at a time and change the washing media between tissues and additionally as needed.
17.For all processed samples, perform 10-fold serial dilutions in sterile PBS and plate on appropriate selective agar as described for quantifying S. Typhimurium CFUs in feces.
Support Protocol 3: PRESERVATION AND PATHOLOGICAL ASSESSMENT OF S. TYPHIMURIUM–INFECTED TISSUES
Non-typhoidal Salmonella colitis is characterized by the influx of inflammatory monocytes and neutrophils into the lamina propria and lumen of the gut, primarily in the cecum and colon (Barthel et al., 2003; Faber et al., 2017; Rivera-Chávez et al., 2016). In the absence of streptomycin treatment, a slowly developing mononuclear infiltrate can be observed, primarily in the small intestine (Santos et al., 2001). Here, we describe procedures for preservation of S. Typhimurium–infected intestinal tissues for histopathology and provide a sample rubric for quantifying inflammation in the intestine based on scoring tissues stained with hematoxylin & eosin (H&E). This scoring is most effectively performed by a trained professional (medical or veterinary pathologist), and samples should be blinded to limit potential bias in the analysis.
Materials
-
S. Typhimurium–infected mice (see Basic Protocol 1 or 2)
-
70% (v/v) ethanol
-
10% (v/v) neutral-buffered formalin (NBF; Fisher Scientific)
-
Sterile PBS (see recipe)
-
Tissue-processing histology cassettes (Sakura Finetek)
-
Histology pencil (VWR International or lead pencil)
-
Sterile dissecting instruments (scissors, forceps)
-
Dissection cutting board
-
Sterile Petri dishes
-
20-ml syringe and 21-G needle (Becton Dickinson)
-
Biopsy foam pad (Simport)
-
Additional reagents and equipment for euthanasia, paraffin embedding, sectioning, and H&E staining
1.Assign each sample a unique ID and label histology cassettes using a histology pencil.
2.At critical time points after infection or upon reaching a preset criterion to terminate the experiment (e.g., weight loss or morbidity), humanely euthanize mice using an approved method such as CO2 asphyxiation followed by cervical dislocation.
3.Thoroughly wet the fur with 70% ethanol and open the abdomen to dissect out the entire large intestine (cecum and colon). Separate the cecum from the colon using scissors and place immediately in a Petri dish containing 10% NBF.
4.If parts of the cecum are needed for RNA or protein analysis, the cecal tip is usually sufficient for histological scoring. If the entire organ is available for histological preservation, cut open the cecum and gently flush the tissue with PBS using a syringe to remove fecal content/pus, and then carefully pat dry on a clean paper towel. Place the cecum lumen-side up onto a biopsy foam pad and transfer to a labeled histology cassette placed in the Petri dish with NBF.
5.Cut 1-cm portions of the proximal, middle, and distal part of the colon and gently push out the luminal content using forceps. Transfer tissue pieces to a single histology cassette or to separate ones, depending the experimental design.
6.Fix samples in 10% NBF for at least 24-48 hr to allow for proper morphological preservation.
7.After fixation, transfer cassettes to a container with 70% ethanol. Collect used fixative in a waste container for appropriate disposal.
8.Perform paraffin-embedding, sectioning, and H&E staining according to standard histological procedures or submit to a histology lab.
9.Analyze H&E-stained sections on a light microscope or digital pathology scanner (e.g., Nanozoomer by Hamamatsu) to determine the extent of intestinal inflammation according to the semi-quantitative scoring scheme described in Table 1.
Score | Description | Area affected (extent)a | Max total score |
---|---|---|---|
Mononuclear inflammation | |||
0 1 2 3 |
Absent (normal sparse lymphocytic infiltrate) Mild (diffuse increase in LP) Moderate (LP with increased basal immune aggregates displacing crypts) Severe (LP with submucosal infiltrate) |
0-4 | 12 |
Neutrophilic inflammation | |||
0 1 2 3 |
None Mild (LP only) Moderate (LP and cryptitis/crypt abscesses) Sheet-like or submucosal infiltration |
0-4 | 12 |
Submucosal edema/width | |||
0 1 2 3 |
No pathological changes Mild (<50% of the diameter of the intestinal wall) Moderate (SM accounts for 50-80% of the intestinal wall diameter) Severe (SM accounts for >80% of the intestinal wall diameter) |
0-4 | 12 |
Epithelial injury | |||
0 1 2 3 |
None Mild (crypt dropout, epithelial desquamation) Moderate (focal ulceration) Severe (multifocal or extensive ulceration) |
0-4 | 12 |
Total | 48 |
- a
Each sample is investigated for the described parameters and multiplied by the factor of the respective extent. Extent is scored on a five-point scale based on the % of the section affected: 0 = none; 1 = <10% of section; 2 = <10%-25% of section; 3 = <25%-50% of section; 4 = >50% of section. The total score is a sum score of each parameter multiplied by extent. Abbreviations: LP, lamina propria; SM, submucosa.
Support Protocol 4: MEASUREMENT OF INFLAMMATORY MARKER EXPRESSION IN INTESTINAL TISSUES BY qPCR
This protocol provides recommended methods for collection and preparation of cecal tissue mRNA to measure S. Typhimurium–associated changes in gene expression, and provides a sample set of validated qPCR primers of known inflammatory markers relevant to S. Typhimurium infection. Increased expression of these factors in infected tissues is a hallmark of the host response to S. Typhimurium infection and can be measured by qPCR of the tissue mRNA.
Materials
- S. Typhimurium–inoculated mice (see Basic Protocol 1 or 2)
- Liquid nitrogen
- 70% (v/v) ethanol
- RNaseZAP (Sigma)
- TRI Reagent (Sigma-Aldrich)
- Chloroform or 1-bromo-3-chloropropane (BCP)
- Isopropanol
- 75% (v/v) ethanol made with ultrapure, DEPC-treated water
- Ultrapure, RNase-free water
- Reverse transcription kit (e.g., SuperScript VILO cDNA Synthesis Kit, Invitrogen)
- SYBR Green Master Mix (Applied BioSystems)
- mRNA-targeted primers (Table 2)
Mouse target gene | Primer sequences | Source |
---|---|---|
Il1b (IL-1β) | Forward: 5′-CTCTCCAGCCCAAGCTTCCTTGTGC-3′ | Perez-Lopez et al. (2019) |
Reverse: 5′-GCTCTCATCAGGACAGCCCAGGT-3′ | ||
Il12a (IL-12) | Forward: 5′-GGAAGCACGGCAGCAGAATA-3′ | Perez-Lopez et al. (2019) |
Reverse: 5′-AACTTGAGGGGAGAATGAGGAATGG-3′ | ||
Il17a (IL-17A) | Forward: 5′-GCTCCAGAAGGCCCTCAGA-3′ | Godinez et al. (2009); Perez-Lopez et al. (2019) |
Reverse: 5′-AGCTTTCCCTCCGCATTGA-3′ | ||
Il22 (IL-22) | Forward: 5′-GGCCAGCCTTGCAGATAACA-3′ | Godinez et al. (2009); Perez-Lopez et al. (2019) |
Reverse: 5′-GCTGATGTGACAGGAGCTGA-3′ | ||
Ifng (IFN-γ) | Forward: 5′-TCAAGTGGCATAGATGTGGAAGAA-3′ | Deriu et al. (2013); Godinez et al. (2009) |
Reverse: 5′-TGGCTCTGCAGGATTTTCATG-3′ | ||
Tnfa (TNF-α) | Forward: 5′-CATCTTCTCAAAATTCGAGTGACAA-3′ | Deriu et al. (2013) |
Reverse: 5′-TGGGAGTAGACAAGGTACAACCC-3′ | ||
Cxcl1 (CXCL1) | Forward: 5′-TGCACCCAAACCGAAGTCAT-3′ | Godinez et al. (2009); Perez-Lopez et al. (2019) |
Reverse: 5′-TTGTCAGAAGCCAGCGTTCAC-3′ | ||
Lcn2 (LCN2) | Forward: 5′-ACATTTGTTCCAAGCTCCAGGGC-3′ | Deriu et al. (2013) |
Reverse: 5′-CATGGCGAACTGGTTGTAGTCCG-3′ | ||
Actb (β-actin, housekeeping) | Forward: 5′-GGCTGTATTCCCCTCCATCG-3′ | Perez-Lopez et al. (2019) |
Reverse: 5′-CCAGTTGGTAACAATGCCATGT-3′ |
-
Cryovials (2.0 ml, Thermo Fisher) and appropriate transport vessel
-
Sterile 50-ml conical tubes (Corning)
-
Mortars and pestles, cleaned and treated with RNaseZAP
-
1.5-ml RNase-free microcentrifuge tubes
-
NanoDrop spectrophotometer or Qubit fluorometer and kit
-
Additional reagents and equipment for dissection (see Basic Protocol 3)
Extract tissue RNA
1.At necropsy (see Basic Protocol 3), carefully remove cecal content, collect a portion of cecal tissue (∼1 cm2) into an empty cryotube, and immediately snap-freeze the tissue in liquid nitrogen.
2.Prepare the benchtop by disinfecting with 70% ethanol and then treating with RNaseZAP to eliminate environmental RNase contamination.
3.In a fume hood, aliquot 1 ml TRI Reagent into one 50-ml conical tube for each sample.
4.Clean a mortar and pestle set for each sample with RNaseZAP and dry thoroughly with a Kimwipe. Cool each mortar and pestle set with liquid nitrogen until the liquid no longer evaporates.
5.Add tissue sample to the mortar and make a very fine powder using the pestle, adding liquid nitrogen as needed to keep everything as cold as possible.
6.Condense the powdered tissue by adding liquid nitrogen and tilting the mortar as it evaporates. Gently transfer the powder to a 50-ml conical tube containing TRI Reagent (step 3) and vortex to mix.
7.Leave homogenates for 5 min at room temperature and transfer to RNase-free 1.5-ml tubes in the fume hood.
8.Add 200 µl chloroform (or 100 µl BCP) to each tube, vortex to mix thoroughly, and let stand at room temperature for 5-10 min.
9.Centrifuge 10 min at 12,000 × g , 4°C, to separate the upper clear aqueous phase (RNA) from the middle interphase (DNA) and red organic phase (protein).
10.Carefully remove the aqueous phase (∼500 µl) to a clean, RNase-free microcentrifuge tube.
11.Add an equal volume (500 µl) of isopropanol and keep at −20°C for 30 min or overnight to precipitate nucleic acids.
12.Centrifuge 8 min at 12,000 × g , 4°C, and discard the supernatant.
13.Wash RNA pellet by adding 1 ml of 75% ethanol and invert to mix.
14.Centrifuge again, decant the ethanol, and air-dry the pellet with the tubes inverted on a clean surface for at least 3-5 min.
15.Resuspend pellet in ultrapure RNase-free water (30-100 µl, depending on pellet size), recording how much water was used for each sample. Incubate 10 min at 50°-55°C to dissolve the RNA thoroughly.
16.Quantify RNA concentration by NanoDrop or Qubit.
17.Use a reverse transcription kit to prepare cDNA from 1 µg total RNA for each sample.
Quantify expression by qPCR
18.Set up 11-μl qPCR reactions for each gene of interest and a housekeeping gene such as Actb (β-actin) for normalization using SYBR Green Master Mix, ∼35 ng cDNA, and 500 nM mRNA-targeted primers. See Table 2 for validated sets of mouse transcript-targeted primers (preferably intron-spanning) for a sample panel of genes expected to display increased expression during S. Typhimurium colitis.
Support Protocol 5: PREPARATION OF INTESTINAL CONTENT FOR INFLAMMATORY MARKER QUANTIFICATION BY ELISA
Many inflammatory mediators and host-derived byproducts of the response to Salmonella in the gut are soluble and can be directly detected by ELISA on supernatant from intestinal content or homogenized tissue samples. This protocol provides directions for acquiring intestinal content samples suitable for quantification of inflammatory markers by ELISA, such as the antimicrobial peptide lipocalin-2 and myeloperoxidase (a product of neutrophil activity).
Materials
-
S. Typhimurium–inoculated mice (see Basic Protocol 1 or 2)
-
Sterile PBS (see recipe)
-
Protease inhibitor cocktail (e.g., cOmplete Protease Inhibitor Cocktail Tablets, Roche)
-
ELISA kit(s) of interest, e.g., Mouse Myeloperoxidase Duoset ELISA and/or Mouse Lipocalin-2/NGAL Duoset ELISA (R&D Systems)
-
Sterile 1.5-ml microcentrifuge tubes
-
Milligram scale
-
96-well flat-bottom NUNC MaxiSorp Plates (Thermo Fisher)
-
Plate reader with tunable monochromator or absorbance filters at 450 nm and 540 or 570 nm
-
Additional reagents and equipment for collection of intestinal content (see Basic Protocol 3)
1.Label and weigh sterile microcentrifuge tubes containing 1 ml PBS or appropriate reagent diluent (usually 1% BSA in PBS) supplemented with protease inhibitor cocktail.
2.Collect cecal or other intestinal content into labeled tubes (see Basic Protocol 3, step 14d). Weigh tubes and determine the intestinal content weight. Keep on ice until processing.
3.Homogenize intestinal content by vortexing (see Basic Protocol 3, step 16b).
4.Centrifuge 5-10 min at 10,000 × g and transfer supernatant (containing soluble inflammatory markers) to new labeled microcentrifuge tubes.
5.Perform ELISA according to manufacturer's instructions, diluting samples as necessary to be in the linear range of the provided standard.
6.Correct concentration measurements to the weight of content collected for comparisons between samples.
Support Protocol 6: IMMUNE CELL ISOLATION FROM SALMONELLA-INFECTED INTESTINAL TISSUES
S. Typhimurium colitis is associated with the influx and activation of several cell types at the intestinal mucosa, including lymphocytes, macrophages, neutrophils, and innate lymphoid cells (ILCs). This protocol provides directions for isolating intraepithelial lymphocytes (IELs) and lamina propria lymphocytes (LPLs) along with associated myeloid cells and ILCs from Salmonella- infected intestinal tissues for downstream analyses including immunophenotyping by flow cytometry and ex vivo activation assays. All steps should be completed on the same day the tissues are collected.
Materials
-
S. Typhimurium–inoculated mice (see Basic Protocol 1 or 2)
-
Sterile PBS (see recipe)
-
Shaking medium (see recipe)
-
1 M DTT
-
0.5 M EDTA
-
Harvest medium (see recipe)
-
Digest medium (see recipe)
-
Iscove's modified Dulbecco's medium (IMDM, Gibco, cat. no. 21980)
-
Trypan blue
-
Sterile Petri dishes
-
Wide-bore needles (18-G or larger)
-
3- or 10-ml syringes (Becton Dickinson)
-
Sterile 50-ml conical tubes (Corning)
-
Tabletop shaker
-
Sterile 5-, 10-, and 25-ml serological pipettes
-
40-, 70-, and 100-µm strainers (Corning)
-
37°C incubator
-
Hemocytometer or automated cell counter
-
Additional reagents and equipment for collecting intestinal tissue (see Basic Protocol 3, step 14d)
Collect intestinal tissue
1.Collect desired intestinal tissue (small intestine, cecum, and/or colon) and place in ice-cold sterile PBS in a sterile Petri dish.
2.Using a wide-bore needle, flush the luminal content out of the intestine with ice-cold sterile PBS.
3.Carefully remove fatty tissue adherent to the intestine.
4.Optional : Remove Peyer's patches from small intestine.
5.Use scissors to open the intestine longitudinally, then dice it into fragments ∼5 mm in length. Transfer to a 50-ml conical tube containing 10 ml shaking medium at room temperature.
Isolate immune cells
6.Add 5 µl of 1 M DTT and 40 µl of 0.5 M EDTA to each tube and shake vertically on a tabletop shaker (250-300 rpm) for 10 min at room temperature.
7.Add another 5 µl of 1 M DTT and shake for another 10 min.
8.Vortex 4 min at maximum speed (e.g., setting 10 on Vortex Genie II) to dislodge intraepithelial cells, then allow tissue fragments to settle.
9.Use a serological pipette to collect the supernatant containing intraepithelial cells (including IELs) and dispense it through a 100-µm cell strainer into a new 50-ml tube (“IEL fraction”). Keep on ice.
10.To increase the yield of intraepithelial cells, add 10 ml fresh harvest medium to the tube containing the remaining tissue fragments and vortex for 2 min to release additional cells. Collect supernatant and strain through the 100-µm cell strainer into the same IEL fraction tube.
11.To isolate lamina propria cells (including LPLs, neutrophils, and other immune cells of potential interest), add 5 ml prewarmed (37°C) digest medium to the tissue fragments and shake for 60 min in a 37°C incubator.
12.Decant the digest and strain sequentially through 100-, 70-, and 40-µm cell strainers. At each filtration step, mash the digested tissue fragments on the filter with the plunger of a 1-ml syringe (changing the plunger between samples) and flush the strainers with 5 ml IMDM.
13.Centrifuge filtered cells 5 min at 350 × g , 4°C. Decant supernatant.
14.Resuspend cell pellet in sterile PBS or harvest medium. Dilute an aliquot of cells 1:1 with trypan blue and count viable cells using a hemocytometer or automated cell counter.
15.Centrifuge cells 5 min at 350 × g , 4°C. Decant supernatant.
16.Resuspend cell pellet in sterile PBS or desired medium at an appropriate concentration (cells/ml) for analysis. Store on ice and use as soon as possible (within a few hours).
REAGENTS AND SOLUTIONS
Digest medium
- 89 ml Iscove's modified Dulbecco's medium (IMDM, Gibco, cat. no. 21980)
- 10 ml fetal bovine serum (FBS, 20% final)
- 1 ml 100× antibiotic/antimycotic (Gibco)
- Store up to 1 month at 4°C
- Immediately before use, add:
- 0.33 mg/ml collagenase VIII (Sigma, cat. no. C2139)
- 17.5 µg/ml DNase I (Sigma, cat. no. DN25)
For better enzyme activity, prepare collagenase and DNase as fresh 100× stocks the day of isolation and add 50 µl per 5 ml medium.
Harvest medium
- 395 ml IMDM (Gibco, cat. no. 21980)
- 100 ml FBS (20% final)
- 5 ml 100× antibiotic/antimycotic (Gibco)
- Store up to 1 month at 4°C
LB agar plates (Miller)
- 10 g/L tryptone
- 5 g/L yeast extract
- 10 g/L NaCl
- 15 g/L agar
- Selective antibiotics (see recipe)
Disperse in ultrapure water with a stir bar and autoclave. Cool to ∼55°C (until the flask base can be handled without autoclave gloves) while stirring at low speed (to disperse agar but avoid bubbles). Add antibiotic at appropriate concentration for selection and stir for 1 min. Aliquot into labeled, sterile, disposable Petri dishes (∼25-30 ml per plate) and let solidify overnight at room temperature. The next day, invert plate stacks and let dry an additional 2-3 days (depending laboratory humidity) at room temperature. Store plate stacks inverted in closed plastic sleeves at 4°C.
LB broth (Miller)
- 10 g/L tryptone
- 5 g/L yeast extract
- 10 g/L NaCl
- Dissolve in ultrapure water and autoclave
- Store autoclaved broth up to up to 6-12 months at room temperature
MacConkey lactose agar plates
- 40 g/L MacConkey agar base
- 10 g/L lactose
Disperse in ultrapure water with a stir bar and autoclave. Let cool and then poor plates as described for LB agar plates. Store inverted plates in closed plastic sleeves for up to 4-8 months at 4°C.
Phosphate-buffered saline (PBS)
- 900 ml ultrapure water
- 8 g NaCl
- 0.2 g KCl
- 1.44 g Na2HPO4 (anhydrous)
- 0.25 g KH2PO4 (anhydrous)
- Adjust pH to 7.4 with HCl
- Bring to 1 L with ultrapure water
- Aliquot and autoclave to sterilize
- Store autoclaved PBS up to 2 years at room temperature
Salmonella glycerol stocks
Prepare 60% (w/v) glycerol in ultrapure H2O (glycerol density = 1.26 g/ml). Filter-sterilize (0.22 µm) or autoclave. Prepare an overnight culture of S. Typhimurium in LB broth (OD600 ∼1-4). Combine 500 µl 60% glycerol and 500 µl overnight culture in 1.5- or 2.0-ml screw-cap cryovials (final 30% glycerol). Close caps tightly and vortex to mix. Store at −80°C.
Selective antibiotics
1000 stock solutions :
- Carbenicillin: 100 mg/ml in sterile ultrapure water; store in 1-ml aliquots at −20°C
- Chloramphenicol: 30 mg/ml in 100% ethanol; store in 50-ml aliquots at −20°C
- Kanamycin: 100 mg/ml in sterile ultrapure water; store in 50-ml aliquots at 4°C
- Nalidixic acid: 50 mg/ml in sterile 1 N NaOH; store in 50-ml aliquots at 4°C
- Streptomycin: 100 mg/ml in sterile ultrapure water; store in 1-ml aliquots at −20°C
Filter-sterilize antibiotics prepared in water or 1 N NaOH using a 0.22-µm filter. The stocks indicated for storage at 4°C are generally stable for 6 months; those stored at −20°C for at least 12 months. Older stocks should be performance-tested before use. Stocks that remain liquid in storage (e.g., nalidixic acid) should be removed from storage for only as long as necessary to take aliquots and promptly returned to storage. Once a frozen aliquot is thawed, any remainder should be discarded and not refrozen for future use.
Working solutions :
- Dilute stocks in medium at 1 concentration (i.e., 1 ml stock in 1 liter medium)
Shaking medium
- 445 ml Hank's Buffered Saline Solution (HBSS; Gibco)
- 50 ml FBS (10% final)
- 5 ml 100× antibiotic/antimycotic (Gibco)
- Store up to 1 month at 4°C
COMMENTARY
Background Information
To infect a host and cause illness, both non-typhoidal and typhoidal Salmonella rely on a core set of virulence factors that includes two distinct Type-3 secretion systems (T3SS-1 and T3SS-2) and their related effector proteins. These virulence factors enable Salmonella to interact directly with mammalian host cells and shape a host response that benefits pathogen growth and transmission (Byndloss, Rivera-Chávez, et al., 2017). Studies using secretion system mutants have shown that T3SS-1, encoded by Salmonella pathogenicity island 1 (SPI-1), is necessary for invasion of the intestinal epithelium (Larock et al., 2015). T3SS-2, encoded by SPI-2, promotes Salmonella intracellular survival inside host cells, particularly phagocytes (Jennings et al., 2017).
Infection with NTS triggers an inflammatory response characterized by the secretion of proinflammatory cytokines and chemokines that attract immune cells, particularly neutrophils, to the gut. This process helps restrict the pathogen to the gut and limit its dissemination to systemic sites (Perez-Lopez et al., 2016). In contrast, typhoidal serovars such as S. Typhi have acquired virulence mechanisms to avoid detection by the innate immune system and persist in the host for weeks to years, with some hosts becoming chronic carriers of the pathogen. The capacity to evade immunity has been linked to functional stealth adaptations of S. Typhi, including the loss of fepE -regulated very-long O-antigen chains and the acquisition of a polysaccharide capsule (the Vi antigen) encoded by viaB , which helps to mask LPS and limits complement deposition and phagocytosis of the pathogen (Crawford et al., 2013; Wangdi et al., 2014; Wilson et al., 2008, 2011). Further adaptations by S. Typhi include the altered structure and decreased expression of pathogen-associated molecules such as flagellin that otherwise allow for rapid immune recognition of non-typhoidal serovars via Toll-like receptors (Atif et al., 2014; Winter et al., 2014).
The generation of relevant and appropriate animal models to study the pathogenesis of different Salmonella serovars involved in human disease has been a long journey that is nicely summarized by Tsolis et al. (2011). Typhoidal Salmonella serovars are human-adapted and neither colonize nor cause disease in conventional mice. Thus, experimental research with typhoidal Salmonella serovars such as S. Typhi has been primarily limited to in vitro assays. More recently, humanized mice have been used to investigate S. Typhi virulence mechanisms in vivo (Libby et al., 2010; Song et al., 2010), but these models present challenges and are not widely available. Of note, although a Tlr11 –/– mouse model of S. Typhi infection was proposed (Mathur et al., 2012), it was subsequently disproven by multiple leaders in the field (Song et al., 2016). Meanwhile, oral infection of commonly used mouse lines such as C57BL/6 and BALB/c with non-typhoidal serovars such as S. Typhimurium yields a typhoid-like disease with minimal colitis and significant extraintestinal pathogen spread resulting in rapid mortality (Santos et al., 2001). S. Typhimurium infection of susceptible mice has been used to model human typhoid disease and contributed to identifying virulence factors important for pathogenesis, including those mentioned above. Nevertheless, an important limitation of this model is that NTS serovars, including S. Typhimurium, lack many important features of S. Typhi's genome (Nuccio & Bäumler, 2014) and do not cause enteric fever in humans, instead causing inflammatory diarrhea.
To interrogate the virulence and immunological bases of NTS colitis, much in vivo research prior to the 2000s employed bovine and rabbit intestinal loop infection models, which display substantial inflammation and neutrophil influx to the infected intestine compared to conventional mice (Everest et al., 1999; Santos et al., 2001). In 2003, Barthel and colleagues revived an infection model originally described in a series of studies by Marjorie Bonhoff and C. Philip Miller 40 years prior (Bohnhoff & Miller, 1962; Bohnhoff et al., 1954), utilizing mice pretreated with streptomycin to transiently disrupt colonization resistance prior to S. Typhimurium oral infection (Barthel et al., 2003). Compared to S. Typhimurium oral infection without pretreatment, streptomycin pretreatment resulted in efficient colonization of the pathogen to high levels in the intestinal lumen (>109 CFU/g in the cecum and colon) and pronounced cecal inflammation, including edema, epithelial degradation, and neutrophil infiltration (Barthel et al., 2003). Similar to the calf model (Santos et al., 2001), intestinal inflammation was found to depend on T3SS virulence factors, and the model has been adopted as the gold standard for studying the host-microbe molecular interactions involved in Salmonella colitis (Hapfelmeier et al., 2005; Kaiser et al., 2012). This model is independent of the wild-type strain and mouse lineage, so it can be adapted to interrogate the interplay between Salmonella virulence factors and mammalian genetics on colitis.
In streptomycin-pretreated mice, S. Typhimurium colitis is accompanied by a neutrophil-dominated inflammatory response akin to that observed in humans (Acheson & Hohmann, 2001). This inflammatory response results from T3SS-dependent and PAMP/TLR-mediated transcriptional activation of proinflammatory cytokines produced by macrophages (TNF-α, IL-1β) and of Type-1 (IFN-γ, IL-12, IL-18) and Type-17 (IL-23, IL-17, IL-22) cytokines, which collectively promote antibacterial functions to limit the infection. IL-23 produced by dendritic and mononuclear cells in the gut in response to S. Typhimurium infection triggers IL-17 and IL-22 production by TH17 cells, γδ T cells, and innate lymphoid cells. IL-17 and IL-22 stimulate intestinal epithelial cells to rapidly amplify the production of antimicrobial proteins (REG3γ, lipocalin-2, and calprotectin) and chemokines. Upregulated chemokines include CXCL1, CXCL2, and CXCL5, which recruit neutrophils to the gut (Perez-Lopez et al., 2016). Even though aspects of these inflammatory responses benefit the host and promote control of S. Typhimurium infection, numerous studies have demonstrated that the pathogen exploits host immunity to colonize the host and thrive (Behnsen et al., 2015).
Recent research has focused on how intestinal inflammation benefits NTS and related pathogens/pathobionts such as E. coli by creating new nutrient and respiratory metabolic niches in the gut that promote the transient expansion of Enterobacteriaceae (Winter and Bäumler 2014). Other models, like the high-fat diet model and a model with a low-complexity microbiota, have been shown to promote intestinal inflammation and enhance S. Typhimurium colonization (Stecher et al., 2005, 2010; Wotzka et al., 2019). One major drawback of the streptomycin model is the ablation of microbiota-mediated colonization resistance, which is an important factor in understanding NTS infection dynamics (Rogers et al., 2021). Using fecal microbiota transfer to germ-free mice, comparative studies of the microbiota found in mice from different vendors have begun elucidating critical factors in microbiota-derived colonization resistance (Stecher, 2021; Velazquez et al., 2019). Additionally, genetically resistant CBA/J mice infected with S. Typhimurium have been found to eventually develop cecal inflammation without antibiotic treatment (Faber et al., 2017; Rivera-Chávez et al., 2016), even though the inflammation levels are typically lower and more variable. These mice have been used, for example, to determine whether phenotypes observed after streptomycin pretreatment are also present in mice with intact microbiota. Systemic infection still occurs in such resistant mice, but the host controls S. Typhimurium replication at systemic sites, and infection studies can be continued for two weeks or more. Long-term infection models using mice from the resistant (Nramp +/+) 129 Sv-lineage have also been developed to study S. Typhimurium dynamics at extraintestinal sites such as the mesenteric lymph nodes for up to a year (Monack et al., 2004). These mice allow for the study of the underlying genetics and mechanisms by which Salmonella promotes granuloma formation during infection and how the microbiota impacts transmission by chronic carriers, both aspects observed in human typhoid fever (Gopinath et al., 2013; Lawley et al., 2006, 2008; Monack et al., 2004; Nagy et al., 2013).
There has been an interest in using mouse Salmonella infections to model and probe the potential immune deficiencies associated with NTS bacteremia, which is responsible for most of the “all-cause” Salmonella -associated mortality (Ao et al., 2015). Based on epidemiological evidence, underlying risk factors promoting NTS bacteremia include compromised immunity resulting from HIV infection in adults, nutritional deficiencies, and co-infection with malaria in very young children (Ao et al., 2015; Bronzan et al., 2007; Feasey et al., 2012). The latter risk factors have been modeled in diet studies and parasite co-infection models in mice, which may help explain potential mechanisms boosting extraintestinal Salmonella (Mottram et al., 2016; Roux et al., 2010; Stull-Lane et al., 2020). For a detailed protocol on murine malaria models, which may be combined with the S. Typhimurium infection models presented here to study co-infection, please refer to Huang et al. (2015).
Critical Parameters
When planning S. Typhimurium infection experiments, choosing the most appropriate model for the relevant research question is important. All animal experiments require Institutional Animal Care and Use Committee review and approval to ensure that animal welfare is always given consideration.
Mouse considerations and sources of model variability
As with any mouse infection model, mice should be age, strain, and sex-matched between experimental groups to ensure robust and replicable data. Typically, young adult mice 8-12 weeks of age are used for infections, though for intraperitoneal infection models, younger mice (6-8 weeks old) may be used to obtain more consistent, reproducible results with fewer animals. Genetically susceptible mice will typically succumb to infection with S. Typhimurium in 3-5 days with streptomycin pretreatment or 5-10 days without pretreatment. Resistant mouse lines can usually control S. Typhimurium for longer (10 days or more) and may even clear the infection. Mouse resistance and susceptibility to S. Typhimurium are multifactorial, but in general are largely impacted by allelic variation (point mutation) in the phagosomal transition metal transporter gene Slc11a1 (also known as Nramp1) of susceptible lines such as C57BL/6 and BALB/c (Monack et al., 2004; Vidal et al., 1996). Resistant mice (i.e., encode functional SLC11A1; particularly mouse strain 129SvJ) have been used as models of chronic Salmonella carriage, a phenotype observed in human S. Typhi cases (Gunn et al., 2014; Ruby et al., 2012; Stecher et al., 2006). Resistant mice (particularly CBA/J) have also proved to be useful for studying gut microbiota-associated phenotypes during colitis that can be hard to track following administration of streptomycin or other antibiotics (Faber et al., 2017; Rivera-Chávez et al., 2016). One caveat of using 129SvJ or CBA/J mice is the general lack of readily available transgenic and knockout strains, which are mostly available in more commonly used susceptible backgrounds (e.g., C57BL/6). Because many knockout mouse lines were generated as a chimera of 129S2 and C57BL/6, knockout mice should be genotyped for the Slc11a1 allele. Moreover, knockouts of loci in relatively close proximity to the Slc11a1 gene (e.g., Il17a , Cxcr2) should also be genotyped for Slc11a1 status before use, as wild-type and knockout littermates may harbor different alleles.
The same strain of mice from different vendors can harbor vastly different microbiota compositions, which may influence the colonization and competition of S. Typhimurium in the gut. For example, specific-pathogen-free C57BL/6J mice from The Jackson Laboratory typically lack common commensal Enterobacteriaceae (such as E. coli), which are present in many other vendors’ mice and can compete with Salmonella in the gut for nutrients (Sassone-Corsi et al., 2016; Velazquez et al., 2019). While co-housing different mouse strains (e.g., wild-type and knockout) prior to infection experiments can help normalize their microbiota, this is not always effective. Thus, breeding littermate controls is the preferred option for maintaining consistent microbiota between groups. Characterizing microbiota-associated infection findings will often require fecal microbiota transfer to germ-free animals. Diet composition also influences the microbiota, inflammation, and Salmonella pathogenesis (Daniel et al., 2014; Desai et al., 2016; Kreuzer & Hardt, 2020; Nickerson et al., 2014). For example, a high-fat diet stimulates bile acid production, which affects the gut microbiota but not Salmonella (Wotzka et al., 2019). It is best to work with a veterinary dietitian to select an appropriate control diet when testing the impact of food components.
The timing of infection can also be important due to circadian fluctuations in feeding behaviors and inflammatory mediators, so infection times should remain consistent between experiments (Bellet et al., 2013).
Salmonella considerations
For each experiment, following the same procedure to generate the inoculum is necessary for reproducibility of results. Usually, a dose of 5 × 107 to 1 × 109 CFU of S. Typhimurium per mouse is used for oral infection. Lower concentrations are necessary for intraperitoneal infection, typically ∼1 × 103 CFU per mouse. While some protocols may call for direct inoculation of liquid cultures from a glycerol stock, even small amounts of glycerol can alter Salmonella gene expression in the inoculum, which may impact experimental outcomes. Instead, inoculating cultures from colonies or confluent growth on a plate, as this protocol directs, promotes both active Salmonella growth and more consistently reproducible infections with the resulting inoculum (Kragh et al., 2018). We have also found that growing overnight cultures in LB with selective antibiotics can influence stress responses in the bacteria, potentially altering disease phenotypes and competitive fitness. Thus, antibiotics should in general be added to cultures used for inocula only when necessary to maintain selection for an unstable genetic locus or plasmid. In both intragastric and intraperitoneal infection models, uninfected control mice should be mock-infected with the appropriate vehicle (LB or PBS) to control for the impact of the inoculum and for stress responses induced by the procedure.
It is unfortunately common in some research fields to utilize a Salmonella infection model without being aware of the specific serovar or substrain used for experiments, despite that being critical to understanding the relevance of the findings. In particular, many immunological studies utilize an S. Typhimurium aroA mutant, which is a metabolically deficient strain often used as a live-attenuated vaccine vector in mice (Felgner et al., 2016; Hoiseth & Stocker, 1981). Infection studies with this strain are not equivalent to fully virulent wild-type strains such as SL1344 and ATCC 14028s (including its IR715 derivative) (Everest et al., 1999), and findings should always be conveyed in that context. Even between wild-type strains, genomic variations such as the presence or absence of a single gene (e.g., the phage-encoded effector gene sopE ; present in SL1344 but absent in ATCC 14028s) have been shown to impact colitis severity (Hapfelmeier et al., 2004; Lopez et al., 2012), which can impede the comparison of infection phenotypes between model strains. Of note, although initial experiments in the streptomycin pretreatment model were performed using a strain with relatively high resistance to streptomycin (SL1344), strains more sensitive to streptomycin (such as ATCC 14028s and its derivative IR715) have also been shown to colonize at high levels and induce colitis in the model. Nevertheless, when the streptomycin pretreatment model is used for competitive infections, the two competing strains must have similar sensitivity/resistance to the antibiotic in order to limit the potential for the antibiotic itself to directly influence the competitive index. One option to normalize resistance between strains with dissimilar resistances is to transform them with a plasmid conferring streptomycin resistance (e.g., pHP45Ω).
The intragastric gavage technique described here is the most commonly employed method for delivering an S. Typhimurium inoculum to the gut. However, some alternate oral inoculation techniques have been reported to generate equivalent colonization results in mice; for example, foodborne inoculation by providing mice small amounts of feed coated with a defined quantity of S. Typhimurium was shown to successfully implant the infection (Nilsson et al., 2019) and better mimic the typical food-associated route of infection. Non-invasive techniques like this also help limit animal distress and reduce the odds of inoculum mis-delivery during inoculation, but the inoculum dose delivered can be less precise and less consistent from animal to animal.
Understanding Results
The overall outcomes of the S. Typhimurium infection models will be influenced by many variables, including but not limited to mouse strain genetics, gut microbiota composition, S. Typhimurium strain(s) used, preparation of the inoculum, the infection route, and the timing of infection. Maintaining consistency in these factors helps generate reproducible results between experiments. Wild-type S. Typhimurium colonization tends to follow log-normal distributions in mouse infection studies, so raw CFU/g values should be logarithmically transformed prior to comparing S. Typhimurium loads between infected mouse groups by parametric statistical tests. Similarly, competitive indices should usually be log-transformed for comparisons to properly weight ratios less than 1 (i.e., more mutant strain recovered than wildtype). For an intragastric infection of 1 × 109 CFU S. Typhimurium 14028s in Salmonella -susceptible C57BL/6J mice without streptomycin pretreatment, one would expect to detect colonization of 103 to 106 CFU/g feces 1 day after infection, and anywhere from 105 to 109 CFU/g feces or cecal content by 4 days post-infection (Velazquez et al., 2019). In Salmonella -resistant CBA/J mice without streptomycin pretreatment, one would expect 103 to 106 CFU/g feces early in the infection (1-3 days) with a transient increase up to 106 to 1010 CFU/g after 5-7 days (Rivera-Chávez et al., 2016). The same intragastric infection in streptomycin-pretreated susceptible mice should result in substantially higher fecal S. Typhimurium loads at 1 day post-infection (≥107 CFU/g), while inflammation-associated phenotypes will usually develop within 3-4 days. S. Typhimurium levels in systemic tissues such as the liver and spleen can vary throughout the infection and are dependent on mouse lines, but are generally held to <109 CFU/g tissue in susceptible mice. Resistant mice exhibit low-level S. Typhimurium colonization in the spleen, as they can control the pathogen's replication at this site. Planning to continue an S. Typhimurium infection past 4 days in susceptible mice is risky, as it will result in significant mortality and a consequent loss of important experimental samples.
On a gross pathology level, inflamed colons are generally shorter than healthy colons and can appear hyperemic, and the cecum typically shrinks during infection (Fig. 1). Exemplary H&E-stained sections of streptomycin-pretreated uninfected and S. Typhimurium-infected cecal tissues are provided in Figure 2. Histological inflammation can be quite severe, as shown in Figure 2, whereas no signs of inflammation are observed in preserved tissue from uninfected animals.
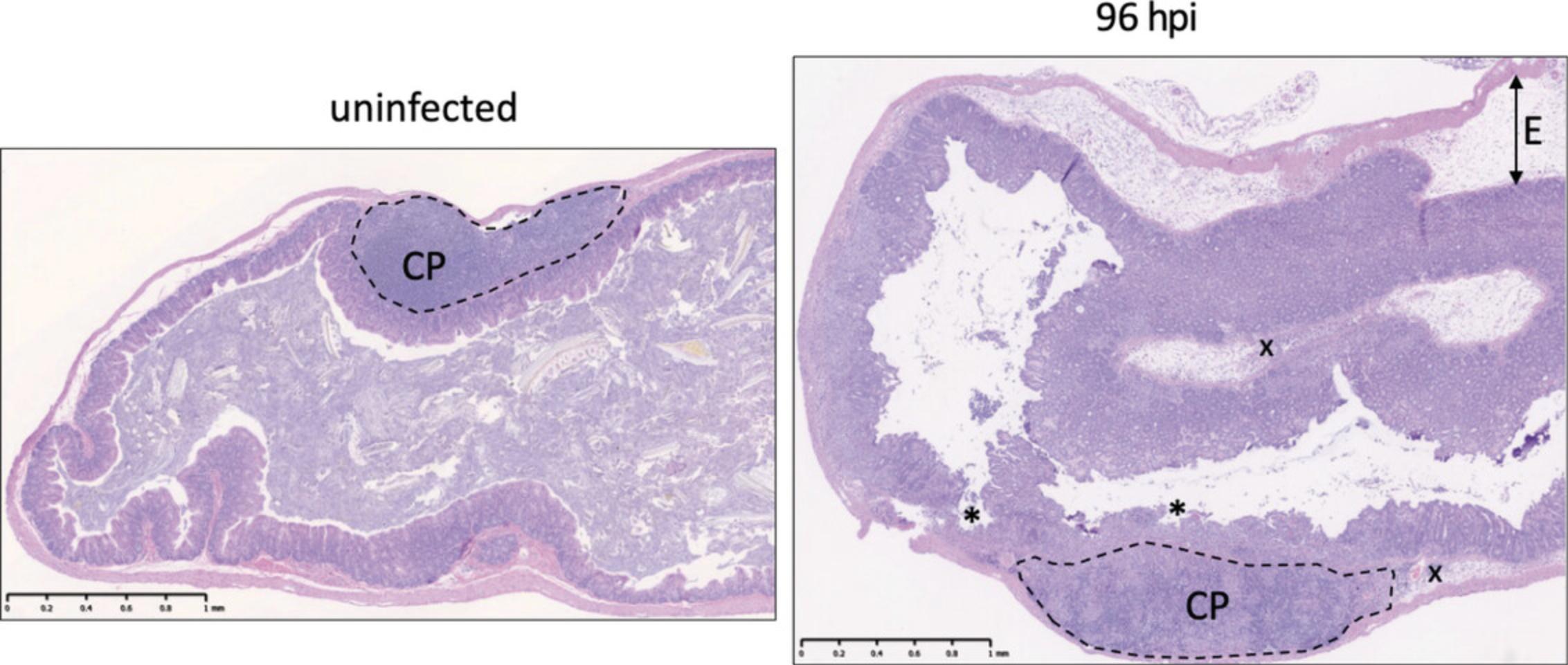
In colitis models, S. Typhimurium can induce 10-fold to 1000-fold changes in the expression of colitis-associated genes (see Table 2) relative to uninfected controls by day 3-4 of infection (Godinez et al., 2008). Changes in gene expression can be measured by qPCR of cecal RNA (ΔΔCt method, normalized to mouse Actb levels) (Deriu et al., 2013; Godinez et al., 2008; Perez-Lopez et al., 2019). In intestinal content and tissue of streptomycin-pretreated mice, protein levels of inflammatory markers (e.g., myeloperoxidase, lipocalin-2, calprotectin) will typically be higher (2- to 1000-fold) in samples from S. Typhimurium–infected mice relative to healthy control mice at the peak of infection. A general increase of T cell numbers and T cell cytokine production (particularly IL-22, IL-17, IFNγ) can be observed in the gut beginning at 24 to 48 hr after S. Typhimurium infection (Godinez et al., 2008, 2009; Perez-Lopez et al., 2019; Raffatellu et al., 2008). These cells amplify the inflammatory response during S. Typhimurium colitis, including the recruitment of neutrophils. Together with lymphocyte and neutrophil isolation, analysis of changes in gene expression and histopathology provide a comprehensive understanding of the immune response during NTS colitis.
Time Considerations
Mice should be allowed to acclimate to a new room at least 1 week before infection. Preparation of S. Typhimurium cultures for inoculation will take 3 days in total, although the active portions involving streaking of glycerol stocks onto agar plates and inoculating of LB broth cultures should only take minutes. Streptomycin preparation will take 10-20 min and should be done as soon as possible before use. Scruffing restraint and gavage or intraperitoneal infection of mice can take 1-5 min per mouse, depending on the experience of the operator. After inoculation, S. Typhimurium infection will typically result in mortality within 1 week in susceptible mice. Animals should be monitored and weighed at least daily during that time.
Collection of fecal samples from live infected mice typically takes 5-15 min per mouse, although it may take 30 min or longer when colitis is more severe. Humane euthanasia of animals takes ∼5 min, and dissection and tissue removal take 10-20 min per mouse. Blood collection should be performed as soon as possible after euthanasia to limit coagulation in the mouse. Weighing sample tubes, homogenization, preparing serial dilutions and plating of fecal or tissue slurries can take 20 min per mouse, but processing multiple samples in parallel will greatly speed the per-mouse time (i.e., processing samples from ten mice takes ∼1 hr total). Plated dilutions should be incubated at 37°C for 14-18 hr to generate CFUs of appropriate size for enumeration. Counting CFUs can take 1-2 min per plate.
Organs recovered for histopathological assessment during necropsy should be placed in formalin expeditiously to limit degradation of tissue structures. Histological cassettes should be fixed in formalin for 24-48 hr, then moved into 70% ethanol for long-term storage. Analysis and scoring of H&E-stained tissue sections can take 5-10 min per section.
Tissues sampled for RNA extraction should be snap-frozen in liquid nitrogen immediately at necropsy, then stored at −80°C for later processing. Mortar-and-pestle grinding of tissues can take 5 min per sample. Phenol-chloroform RNA extraction from tissue homogenates takes ∼2 hr for four to ten samples. Synthesizing cDNA takes 1-2 hr, depending on the number of RNA samples that need to be diluted. Preparing SYBR Green reaction mixes and samples in a 96- or 384-well plate for qPCR takes 1-2 hr and the qPCR reaction takes 1.5-2 hr.
ELISAs that do not come with pre-coated plates will need to be set up to incubate with capture antibodies the evening before the assay. The assays typically take 5-7 hr including incubation steps.
Media for immune cell isolation from intestinal tissues can be made prior to necropsy. For good-quality cell preparations, the isolation steps should be performed as soon as possible (within a few hours) following tissue collection in harvest medium. The isolation takes 3-4 hr overall for tissues from six to ten mice. Cells should be used for immunophenotyping or other ex vivo stimulation assays the same day as collection.
Acknowledgments
G.T.W. was funded by the National Institutes of Health (NIH, grant T32AI007036). R.R.G. was funded by a fellowship from the Crohn's & Colitis Foundation (award number 649744). M.R. was funded by the NIH (grants AI126277, AI145325, AI154644, AI114625), by the Chiba University-UCSD Center for Mucosal Immunology, Allergy, and Vaccines, and by the UCSD Department of Pediatrics. M.R. also holds an Investigator in the Pathogenesis of Infectious Disease Award from the Burroughs Wellcome Fund.
Author Contributions
Gregory T. Walker : Investigation, methodology, writing (original draft, review and editing); Romana R. Gerner : Methodology; writing (original draft, review and editing); Sean-Paul Nuccio : Writing (review and editing); Manuela Raffatellu : Writing (review and editing).
Conflict of Interest
The authors declare no conflict of interest.
Open Research
Data Availability Statement
There are no data associated with this manuscript.
Literature Cited
- Acheson, D., & Hohmann, E. L. (2001). Nontyphoidal salmonellosis. Clinical Infectious Diseases , 32(2), 263–269. https://doi.org/10.1086/318457
- Ao, T. T., Feasey, N. A., Gordon, M. A., Keddy, K. H., Angulo, F. J., & Crump, J. A. (2015). Global burden of invasive nontyphoidal Salmonella disease, 2010. Emerging Infectious Diseases , 21(6), 941–949. https://doi.org/10.3201/eid2106.140999
- Atif, S. M., Winter, S. E., Winter, M. G., McSorley, S. J., & Bäumler, A. J. (2014). Salmonella enterica serovar Typhi impairs CD4 T cell responses by reducing antigen availability. Infection and Immunity , 82(6), 2247–2254. https://doi.org/10.1128/IAI.00020-14
- Bao, H., Wang, S., Zhao, J. H., & Liu, S. L. (2020). Salmonella secretion systems: Differential roles in pathogen-host interactions. Microbiological Research , 241(December), 126591. https://doi.org/10.1016/j.micres.2020.126591
- Barthel, M., Hapfelmeier, S., Quintanilla-Martínez, L., Kremer, M., Rohde, M., Hogardt, M., Pfeffer, K., Rüssmann, H., & Hardt, W. D. (2003). Pretreatment of mice with streptomycin provides a Salmonella enterica serovar Typhimurium colitis model that allows analysis of both pathogen and host. Infection and Immunity , 71(5), 2839–2858. https://doi.org/10.1128/IAI.71.5.2839-2858.2003
- Behnsen, J., Perez-Lopez, A., Nuccio, S. P., & Raffatellu, M. (2015). Exploiting host immunity: The Salmonella paradigm. Trends in Immunology , 36(2), 112–120. https://doi.org/10.1016/j.it.2014.12.003
- Bellet, M. M., Deriu, E., Liu, J. Z., Grimaldi, B., Blaschitz, C., Zeller, M., Edwards, R. A., Sahar, S., Dandekar, S., Baldi, P., George, M. D., Raffatellu, M., & Sassone-Corsi, P. (2013). Circadian clock regulates the host response to Salmonella. Proceedings of the National Academy of Sciences of the United States of America , 110(24), 9897–9902. https://doi.org/10.1073/pnas.1120636110
- Beuzón, C. R., & Holden, D. W. (2001). Use of mixed infections with Salmonella strains to study virulence genes and their interactions in vivo. Microbes and Infection , 3(14-15), 1345–1352. https://doi.org/10.1016/S1286-4579(01)01496-4
- Bohnhoff, M., Drake, B. L., & Miller, C. P. (1954). Effect of streptomycin on susceptibility of intestinal tract to experimental Salmonella infection. Experimental Biology and Medicine , 86(1), 132–137. https://doi.org/10.3181/00379727-86-21030
- Bohnhoff, M., & Miller, C. P. (1962). Enhanced susceptibility to Salmonella infection in streptomycin-treated mice. The Journal of Infectious Diseases , 111(Sept.), 117–127. https://doi.org/10.1093/infdis/111.2.117
- Bouladoux, N., Harrison, O. J., & Belkaid, Y. (2017). The mouse model of infection with Citrobacter rodentium. Current Protocols in Immunology , 119, 19.15.1–19.15.25. https://doi.org/10.1002/cpim.34
- Bronzan, R. N., Taylor, T. E., Mwenechanya, J., Tembo, M., Kayira, K., Bwanaisa, L., Njobvu, A., Kondowe, W., Chalira, C., Walsh, A. L., Phiri, A., Wilson, L. K., Molyneux, M. E., & Graham, S. M. (2007). Bacteremia in Malawian children with severe malaria: Prevalence, etiology, HIV coinfection, and outcome. The Journal of Infectious Diseases , 195(6), 895–904. https://doi.org/10.1086/511437
- Byndloss, M. X., Olsan, E. E., Rivera-Chávez, F., Tiffany, C. R., Cevallos, S. A., Lokken, K. L., Torres, T. P., Byndloss, A. J., Faber, F., Gao, Y., Litvak, Y., Lopez, C. A., Xu, G., Napoli, E., Giulivi, C., Tsolis, R. M., Revzin, A., Lebrilla, C. B., & Bäumler, A. J. (2017). Microbiota-activated PPAR-γ signaling inhibits dysbiotic Enterobacteriaceae expansion. Science , 357(6351), 570–575. https://doi.org/10.1126/science.aam9949
- Byndloss, M. X., Rivera-Chávez, F., Tsolis, R. M., & Bäumler, A. J. (2017). How bacterial pathogens use type III and type IV secretion systems to facilitate their transmission. Current Opinion in Microbiology , 35(Feb.), 1–7. https://doi.org/10.1016/j.mib.2016.08.007
- Centers for Disease Control and Prevention (CDC). (2019). Antibiotic Resistance Threats in the United States, 2019. U.S. Department of Health and Human Services, CDC.
- Crawford, R. W., Wangdi, T., Spees, A. M., Xavier, M. N., Tsolis, R. M., & Bäumler, A. J. (2013). Loss of very-long O-antigen chains optimizes capsule-mediated immune evasion by Salmonella enterica serovar Typhi. mBio , 4(4), e00232–13. https://doi.org/10.1128/mBio.00232-13
- Daniel, H., Gholami, A. M., Berry, D., Desmarchelier, C., Hahne, H., Loh, G., Mondot, S., Lepage, P., Rothballer, M., Walker, A., Böhm, C., Wenning, M., Wagner, M., Blaut, M., Schmitt-Kopplin, P., Kuster, B., Haller, D., & Clavel, T. (2014). High-fat diet alters gut microbiota physiology in mice. The ISME Journal , 8(2), 295–308. https://doi.org/10.1038/ismej.2013.155
- Deriu, E., Liu, J. Z., Pezeshki, M., Edwards, R. A., Ochoa, R. J., Contreras, H., Libby, S. J., Fang, F. C., & Raffatellu, M. (2013). Probiotic bacteria reduce salmonella typhimurium intestinal colonization by competing for iron. Cell Host & Microbe, 14(1), 26–37. https://doi.org/10.1016/j.chom.2013.06.007
- Desai, M. S., Seekatz, A. M., Koropatkin, N. M., Kamada, N., Hickey, C. A., Wolter, M., Pudlo, N. A., Kitamoto, S., Terrapon, N., Muller, A., Young, V. B., Henrissat, B., Wilmes, P., Stappenbeck, T. S., Núñez, G., & Martens, E. C. (2016). A dietary fiber-deprived gut microbiota degrades the colonic mucus barrier and enhances pathogen susceptibility. Cell , 167(5), 1339–1353.e21. https://doi.org/10.1016/j.cell.2016.10.043
- Everest, P., Ketley, J., Hardy, S., Douce, G., Khan, S., Shea, J., Holden, D., Maskell, D., & Dougan, G. (1999). Evaluation of Salmonella typhimurium mutants in a model of experimental gastroenteritis. Infection and Immunity , 67(6), 2815–2821. https://doi.org/10.1128/IAI.67.6.2815-2821.1999
- Faber, F., Thiennimitr, P., Spiga, L., Byndloss, M. X., Litvak, Y., Lawhon, S., Andrews-Polymenis, H. L., Winter, S. E., & Bäumler, A. J. (2017). Respiration of microbiota-derived 1,2-propanediol drives Salmonella expansion during colitis. PLoS Pathogens , 13(1), e1006129. https://doi.org/10.1371/journal.ppat.1006129
- Feasey, N. A., Dougan, G., Kingsley, R. A., Heyderman, R. S., & Gordon, M. A. (2012). Invasive non-typhoidal Salmonella disease: An emerging and neglected tropical disease in Africa. The Lancet , 379(9835), 2489–2499. https://doi.org/10.1016/S0140-6736(11)61752-2
- Felgner, S., Frahm, M., Kocijancic, D., Rohde, M., Eckweiler, D., Bielecka, A., Bueno, E., Cava, F., Abraham, W. R., Curtiss, R., Häussler, S., Erhardt, M., & Weiss, S. (2016). aroA -Deficient Salmonella enterica serovar Typhimurium is more than a metabolically attenuated mutant. mBio , 7(5), e01220–16. https://doi.org/10.1128/mBio.01220-16
- Gal-Mor, O., Boyle, E. C., & Grassl, G. A. (2014). Same species, different diseases: How and why typhoidal and non-typhoidal Salmonella enterica serovars differ. Frontiers in Microbiology , 5(August), 391. https://doi.org/10.3389/fmicb.2014.00391
- Geng, S., Tian, Q., An, S., Pan, Z., Chen, X., & Jiao, X. (2016). High-efficiency, two-step scarless-markerless genome genetic modification in Salmonella enterica. Current Microbiology , 72(6), 700–706. https://doi.org/10.1007/s00284-016-1002-3
- Godinez, I., Haneda, T., Raffatellu, M., George, M. D., Paixão, T. A., Rolán, H. G., Santos, R. L., Dandekar, S., Tsolis, R. M., & Bäumler, A. J. (2008). T cells help to amplify inflammatory responses induced by Salmonella enterica serotype Typhimurium in the intestinal mucosa. Infection and Immunity , 76(5), 2008–2017. https://doi.org/10.1128/IAI.01691-07
- Godinez, I., Raffatellu, M., Chu, H., Paixão, T. A., Haneda, T., Santos, R. L., Bevins, C. L., Tsolis, R. M., & Bäumler, A. J. (2009). Interleukin-23 orchestrates mucosal responses to Salmonella enterica serotype Typhimurium in the intestine. Infection and Immunity , 77(1), 387–398. https://doi.org/10.1128/IAI.00933-08
- Gopinath, S., Hotson, A., Johns, J., Nolan, G., & Monack, D. (2013). The systemic immune state of super-shedder mice is characterized by a unique neutrophil-dependent blunting of TH1 responses. PLoS Pathogens , 9(6), e1003408. https://doi.org/10.1371/journal.ppat.1003408
- Grimont, P. A. D., & Weill, F.-X. (2007). Antigenic formulae of the Salmonella serovars ( 9th ed.). World Health Organization, WHO Collaborating Centre for Reference and Research on Salmonella.
- Gunn, J. S., Marshall, J. M., Baker, S., Dongol, S., Charles, R. C., & Ryan, E. T. (2014). Salmonella chronic carriage: Epidemiology, diagnosis, and gallbladder persistence. Trends in Microbiology , 22(11), 648–655. https://doi.org/10.1016/j.tim.2014.06.007
- Hapfelmeier, S., Ehrbar, K., Stecher, B., Barthel, M., Kremer, M., & Hardt, W. D. (2004). Role of the Salmonella pathogenicity island 1 effector proteins SipA, SopB, SopE, and SopE2 in Salmonella enterica subspecies 1 serovar Typhimurium colitis in streptomycin-pretreated mice. Infection and Immunity , 72(2), 795–809. https://doi.org/10.1128/IAI.72.2.795-809.2004
- Hapfelmeier, S., Stecher, B., Barthel, M., Kremer, M., Müller, A. J., Heikenwalder, M., Stallmach, T., Hensel, M., Pfeffer, K., Akira, S., & Hardt, W. D. (2005). The Salmonella pathogenicity island (SPI)-2 and SPI-1 type III secretion systems allow Salmonella serovar Typhimurium to trigger colitis via MyD88-dependent and MyD88-independent mechanisms. Journal of Immunology , 174(3), 1675–1685. https://doi.org/10.4049/jimmunol.174.3.1675
- Hiyoshi, H., Tiffany, C. R., Bronner, D. N., & Bäumler, A. J. (2018). Typhoidal Salmonella serovars: Ecological opportunity and the evolution of a new pathovar. FEMS Microbiology Reviews , 42(4), 527–541. https://doi.org/10.1093/femsre/fuy024
- Hoiseth, S. K., & Stocker, B. A. D. (1981). Aromatic-dependent Salmonella Typhimurium are non-virulent and effective as live vaccines. Nature , 291(5812), 238–239. https://doi.org/10.1038/291238a0
- Huang, B., Pearman, E., & Kim, C. (2015). Mouse models of uncomplicated and fatal malaria. Bio-Protocol , 5(13), e1514. https://doi.org/10.21769/bioprotoc.1514
- Ibarra, J. A., Knodler, L. A., Sturdevant, D. E., Virtaneva, K., Carmody, A. B., Fischer, E. R., Porcella, S. F., & Steele-Mortimer, O. (2010). Induction of Salmonella pathogenicity island 1 under different growth conditions can affect Salmonella -host cell interactions in vitro. Microbiology , 156(Pt 4), 1120–1133. https://doi.org/10.1099/mic.0.032896-0
- Jacobson, A., Lam, L., Rajendram, M., Tamburini, F., Honeycutt, J., Pham, T., Van Treuren, W., Pruss, K., Stabler, S. R., Lugo, K., Bouley, D. M., Vilches-Moure, J. G., Smith, M., Sonnenburg, J. L., Bhatt, A. S., Huang, K. C., & Monack, D. (2018). A gut commensal-produced metabolite mediates colonization resistance to Salmonella infection. Cell Host & Microbe, 24(2), 296–307.e7. https://doi.org/10.1016/j.chom.2018.07.002
- Jennings, E., Thurston, T. L. M., & Holden, D. W. (2017). Salmonella SPI-2 type III secretion system effectors: Molecular mechanisms and physiological consequences. Cell Host & Microbe, 22(2), 217–231. https://doi.org/10.1016/j.chom.2017.07.009
- Kaiser, P., Diard, M., Stecher, B., & Hardt, W. D. (2012). The streptomycin mouse model for Salmonella diarrhea: Functional analysis of the microbiota, the pathogen's virulence factors, and the host's mucosal immune response. Immunological Reviews , 245(1), 56–83. https://doi.org/10.1111/j.1600-065X.2011.01070.x
- Kragh, K. N., Alhede, M., Rybtke, M., Stavnsberg, C., Jensen, P. Ø., Tolker-Nielsen, T., Whiteley, M., & Bjarnsholt, T. (2018). The inoculation method could impact the outcome of microbiological experiments. Applied and Environmental Microbiology , 84(5), https://doi.org/10.1128/AEM.02264-17
- Kreuzer, M., & Hardt, W. D. (2020). How food affects colonization resistance against enteropathogenic bacteria. Annual Review of Microbiology , 74(September), 787–813. https://doi.org/10.1146/annurev-micro-020420-013457
- Larock, D. L., Chaudhary, A., & Miller, S. I. (2015). Salmonellae interactions with host processes. Nature Reviews. Microbiology , 13(4), 191–205. https://doi.org/10.1038/nrmicro3420
- Lawley, T. D., Bouley, D. M., Hoy, Y. E., Gerke, C., Relman, D. A., & Monack, D. M. (2008). Host transmission of Salmonella enterica serovar Typhimurium is controlled by virulence factors and indigenous intestinal microbiota. Infection and Immunity , 76(1), 403–416. https://doi.org/10.1128/IAI.01189-07
- Lawley, T. D., Chan, K., Thompson, L. J., Kim, C. C., Govoni, G. R., & Monack, D. M. (2006). Genome-wide screen for Salmonella genes required for long-term systemic infection of the mouse. PLoS Pathogens , 2(2), e11. https://doi.org/10.1371/journal.ppat.0020011
- Lee, C. A., & Falkow, S. (1990). The ability of Salmonella to enter mammalian cells is affected by bacterial growth state. Proceedings of the National Academy of Sciences of the United States of America , 87(11), 4304–4308. https://doi.org/10.1073/pnas.87.11.4304
- Libby, S. J., Brehm, M. A., Greiner, D. L., Shultz, L. D., McClelland, M., Smith, K. D., Cookson, B. T., Karlinsey, J. E., Kinkel, T. L., Porwollik, S., Canals, R., Cummings, L. A., & Fang, F. C. (2010). Humanized nonobese diabetic-scid IL2rγnull mice are susceptible to lethal Salmonella Typhi infection. Proceedings of the National Academy of Sciences of the United States of America , 107(35), 15589–15594. https://doi.org/10.1073/pnas.1005566107
- Lopez, C. A., Winter, S. E., Rivera-Chávez, F., Xavier, M. N., Poon, V., Nuccio, S. P., Tsolis, R. M., & Bäumler, A. J. (2012). Phage-mediated acquisition of a type III secreted effector protein boosts growth of Salmonella by nitrate respiration. mBio , 3(3), e00143–12. https://doi.org/10.1128/mBio.00143-12
- Majowicz, S. E., Musto, J., Scallan, E., Angulo, F. J., Kirk, M., O'Brien, S. J., Jones, T. F., Fazil, A., & Hoekstra, R. M. (2010). The global burden of nontyphoidal Salmonella gastroenteritis. Clinical Infectious Diseases , 50(6), 882–889. https://doi.org/10.1086/650733
- Mathur, R., Oh, H., Zhang, D., Park, S. G., Seo, J., Koblansky, A., Hayden, M. S., & Ghosh, S. (2012). A mouse model of Salmonella Typhi infection. Cell , 151(3), 590–602. https://doi.org/10.1016/j.cell.2012.08.042
- Monack, D. M., Bouley, D. M., & Falkow, S. (2004). Salmonella Typhimurium persists within macrophages in the mesenteric lymph nodes of chronically infected Nramp1+/+ mice and can be reactivated by IFNγ neutralization. The Journal of Experimental Medicine , 199(2), 231–241. https://doi.org/10.1084/jem.20031319
- Mottram, L., Speak, A. O., Selek, R. M., Cambridge, E. L., McIntyre, Z., Kane, L., Mukhopadhyay, S., Grove, C., Colin, A., Brandt, C., Duque-Correa, M. A., Forbester, J., Nguyen, T. A. P., Hale, C., Vasilliou, G. S., Arends, M. J., Wren, B. W., Dougan, G., & Clare, S. (2016). Infection susceptibility in gastric intrinsic factor (Vitamin B12)-defective mice is subject to maternal influences. mBio , 7(3), e00830–16. https://doi.org/10.1128/mBio.00830-16
- Nagy, T. A., Moreland, S. M., Andrews-Polymenis, H., & Detweiler, C. S. (2013). The ferric enterobactin transporter Fep is required for persistent Salmonella enterica serovar Typhimurium infection. Infection and Immunity , 81(11), 4063–4070. https://doi.org/10.1128/IAI.00412-13
- Nickerson, K. P., Homer, C. R., Kessler, S. P., Dixon, L. J., Kabi, A., Gordon, I. O., Johnson, E. E., De La Motte, C. A., & McDonald, C. (2014). The dietary polysaccharide maltodextrin promotes Salmonella survival and mucosal colonization in mice. PLoS One , 9(7), e101789. https://doi.org/10.1371/journal.pone.0101789
- Nilsson, O. R., Kari, L., & Steele-Mortimer, O. (2019). Foodborne infection of mice with Salmonella Typhimurium. PLoS One , 14(8), e0215190. https://doi.org/10.1371/journal.pone.0215190
- Nuccio, S. P., & Bäumler, A. J. (2014). Comparative analysis of Salmonella genomes identifies a metabolic network for escalating growth in the inflamed gut. mBio , 5(2), e00929–14. https://doi.org/10.1128/mBio.00929-14
- Palmer, A. D., & Slauch, J. M. (2017). Mechanisms of Salmonella pathogenesis in animal models. Human and Ecological Risk Assessment , 23(8), 1877–1892. https://doi.org/10.1080/10807039.2017.1353903
- Perez-Lopez, A., Behnsen, J., Nuccio, S. P., & Raffatellu, M. (2016). Mucosal immunity to pathogenic intestinal bacteria. Nature Reviews. Immunology , 16(3), 135–148. https://doi.org/10.1038/nri.2015.17
- Perez-Lopez, A., Nuccio, S. P., Ushach, I., Edwards, R. A., Pahu, R., Silva, S., Zlotnik, A., & Raffatellu, M. (2019). CRTAM shapes the gut microbiota and enhances the severity of infection. Journal of Immunology , 203(2), 532–543. https://doi.org/10.4049/jimmunol.1800890
- Persson, J., & Vance, R. E. (2007). Genetics-squared: Combining host and pathogen genetics in the analysis of innate immunity and bacterial virulence. Immunogenetics , 59(10), 761–778. https://doi.org/10.1007/s00251-007-0248-0
- Porwollik, S., Santiviago, C. A., Cheng, P., Long, F., Desai, P., Fredlund, J., Srikumar, S., Silva, C. A., Chu, W., Chen, X., Canals, R., Reynolds, M. M., Bogomolnaya, L., Shields, C., Cui, P., Guo, J., Zheng, Y., Endicott-Yazdani, T., Yang, H. J., … Mcclelland, M. (2014). Defined single-gene and multi-gene deletion mutant collections in Salmonella enterica sv Typhimurium. PLoS One , 9(7), e99820. https://doi.org/10.1371/journal.pone.0099820
- Raffatellu, M., Santos, R. L., Verhoeven, D. E., George, M. D., Wilson, R. P., Winter, S. E., Godinez, I., Sankaran, S., Paixao, T. A., Gordon, M. A., Kolls, J. K., Dandekar, S., & Bäumler, A. J. (2008). Simian immunodeficiency virus-induced mucosal interleukin-17 deficiency promotes Salmonella dissemination from the gut. Nature Medicine , 14(4), 421–428. https://doi.org/10.1038/nm1743
- Rivera-Chávez, F., Zhang, L. F., Faber, F., Lopez, C. A., Byndloss, M. X., Olsan, E. E., Xu, G., Velazquez, E. M., Lebrilla, C. B., Winter, S. E., & Bäumler, A. J. (2016). Depletion of butyrate-producing Clostridia from the gut microbiota drives an aerobic luminal expansion of Salmonella. Cell Host & Microbe, 19(4), 443–454. https://doi.org/10.1016/j.chom.2016.03.004
- Rogers, A. W. L., Tsolis, R. M., & Bäumler, A. J. (2021). Salmonella versus the microbiome. Microbiology and Molecular Biology Reviews , 85(1), e00027–19. https://doi.org/10.1128/MMBR.00027-19
- Rout, W. R., Formal, S. B., Dammin, G. J., & Giannella, R. A. (1974). Pathophysiology of Salmonella diarrhea in the Rhesus monkey: Intestinal transport, morphological and bacteriological studies. Gastroenterology , 67(1), 59–70. https://doi.org/10.1016/S0016-5085(19)32926-9
- Roux, C. M., Butler, B. P., Chau, J. Y., Paixao, T. A., Cheung, K. W., Santos, R. L., Luckhart, S., & Tsolis, R. M. (2010). Both hemolytic anemia and malaria parasite-specific factors increase susceptibility to nontyphoidal Salmonella enterica serovar Typhimurium infection in mice. Infection and Immunity , 78(4), 1520–1527. https://doi.org/10.1128/IAI.00887-09
- Ruby, T., Mclaughlin, L., Gopinath, S., & Monack, D. (2012). Salmonella ’s long-term relationship with its host. FEMS Microbiology Reviews , 36(3), 600–615. https://doi.org/10.1111/j.1574-6976.2012.00332.x
- Sanchez-Garrido, J., Ruano-Gallego, D., Choudhary, J. S., & Frankel, G. (2021). The type III secretion system effector network hypothesis. Trends in Microbiology , 30(6), 524–533. https://doi.org/10.1016/j.tim.2021.10.007
- Santos, R. L., Zhang, S., Tsolis, R. M., Kingsley, R. A., Garry Adams, L., & Bäumler, A. J. (2001). Animal models of Salmonella infections: Enteritis versus typhoid fever. Microbes and Infection , 3(14-15), 1335–1344. https://doi.org/10.1016/S1286-4579(01)01495-2
- Sassone-Corsi, M., Nuccio, S. P., Liu, H., Hernandez, D., Vu, C. T., Takahashi, A. A., Edwards, R. A., & Raffatellu, M. (2016). Microcins mediate competition among Enterobacteriaceae in the inflamed gut. Nature , 540(7632), 280–283. https://doi.org/10.1038/nature20557
- Simon, R., Tennant, S. M., Galen, J. E., & Levine, M. M. (2011). Mouse models to assess the efficacy of non-typhoidal Salmonella vaccines: Revisiting the role of host innate susceptibility and routes of challenge. Vaccine , 29(32), 5094–5106. https://doi.org/10.1016/j.vaccine.2011.05.022
- Song, J., Wilhelm, C. L., Wangdi, T., Maira-Litran, T., Lee, S. J., Raetz, M., Sturge, C. R., Mirpuri, J., Pei, J., Grishin, N. V., Mcsorley, S. J., Gewirtz, A. T., Bäumler, A. J., Pier, G. B., Galán, J. E., & Yarovinsky, F. (2016). Absence of TLR11 in mice does not confer susceptibility to Salmonella Typhi. Cell , 164(5), 827–828. https://doi.org/10.1016/j.cell.2016.02.015
- Song, J., Willinger, T., Rongvaux, A., Eynon, E. E., Stevens, S., Manz, M. G., Flavell, R. A., & Galán, J. E. (2010). A mouse model for the human pathogen Salmonella Typhi. Cell Host & Microbe, 8(4), 369–376. https://doi.org/10.1016/j.chom.2010.09.003
- Stecher, B. (2021). Establishing causality in Salmonella -microbiota-host interaction: The use of gnotobiotic mouse models and synthetic microbial communities. International Journal of Medical Microbiology , 311(3), 151484. https://doi.org/10.1016/j.ijmm.2021.151484
- Stecher, B., Chaffron, S., Käppeli, R., Hapfelmeier, S., Freedrich, S., Weber, T. C., Kirundi, J., Suar, M., Mccoy, K. D., Von Mering, C., Macpherson, A. J., & Hardt, W. D. (2010). Like will to like: Abundances of closely related species can predict susceptibility to intestinal colonization by pathogenic and commensal bacteria. PLoS Pathogens , 6(1), e1000711. https://doi.org/10.1371/journal.ppat.1000711
- Stecher, B., MacPherson, A. J., Hapfelmeier, S., Kremer, M., Stallmach, T., & Hardt, W. D. (2005). Comparison of Salmonella enterica serovar Typhimurium colitis in germfree mice and mice pretreated with streptomycin. Infection and Immunity , 73(6), 3228–3241. https://doi.org/10.1128/IAI.73.6.3228-3241.2005
- Stecher, B., Paesold, G., Barthel, M., Kremer, M., Jantsch, J., Stallmach, T., Heikenwalder, M., & Hardt, W. D. (2006). Chronic Salmonella enterica serovar Typhimurium-induced colitis and cholangitis in streptomycin-pretreated Nramp1+/+ mice. Infection and Immunity , 74(9), 5047–5057. https://doi.org/10.1128/IAI.00072-06
- Stecher, B., Robbiani, R., Walker, A. W., Westendorf, A. M., Barthel, M., Kremer, M., Chaffron, S., Macpherson, A. J., Buer, J., Parkhill, J., Dougan, G., Von Mering, C., & Hardt, W. D. (2007). Salmonella enterica serovar Typhimurium exploits inflammation to compete with the intestinal microbiota. PLoS Biology , 5(10), 2177–2189. https://doi.org/10.1371/journal.pbio.0050244
- Stull-Lane, A. R., Lokken-Toyli, K. L., Diaz-Ochoa, V. E., Walker, G. T., Cevallos, S. A., Winter, A. L. N., Muñoz, A. D. H., Yang, G. G., Velazquez, E. M., Wu, C. Y., & Tsolis, R. M. (2020). Vitamin A supplementation boosts control of antibiotic-resistant Salmonella infection in malnourished mice. PLoS Neglected Tropical Diseases , 14(10), e0008737. https://doi.org/10.1371/journal.pntd.0008737
- Tsolis, R. M., Xavier, M. N., Santos, R. L., & Bäumler, A. J. (2011). How to become a top model: Impact of animal experimentation on human Salmonella disease research. Infection and Immunity , 79(5), 1806–1814. https://doi.org/10.1128/IAI.01369-10
- Velazquez, E. M., Nguyen, H., Heasley, K. T., Saechao, C. H., Gil, L. M., Rogers, A. W. L., Miller, B. M., Rolston, M. R., Lopez, C. A., Litvak, Y., Liou, M. J., Faber, F., Bronner, D. N., Tiffany, C. R., Byndloss, M. X., Byndloss, A. J., & Bäumler, A. J. (2019). Endogenous Enterobacteriaceae underlie variation in susceptibility to Salmonella infection. Nature Microbiology , 4(6), 1057–1064. https://doi.org/10.1038/s41564-019-0407-8
- Vidal, S. M., Pinner, E., Lepage, P., Gauthier, S., & Gros, P. (1996). Natural resistance to intracellular infections: Nramp1 encodes a membrane phosphoglycoprotein absent in macrophages from susceptible (Nramp1 D169) mouse strains. Journal of Immunology , 157(8), 3559–3568. https://doi.org/10.4049/jimmunol.157.8.3559
- Wangdi, T., Lee, C. Y., Spees, A. M., Yu, C., Kingsbury, D. D., Winter, S. E., Hastey, C. J., Wilson, R. P., Heinrich, V., & Bäumler, A. J. (2014). The Vi capsular polysaccharide enables Salmonella enterica serovar Typhi to evade microbe-guided neutrophil chemotaxis. PLoS Pathogens , 10(8), e1004306. https://doi.org/10.1371/journal.ppat.1004306
- Wilson, R. P., Raffatellu, M., Chessa, D., Winter, S. E., Tükel, Ç., & Bäumler, A. J. (2008). The Vi-capsule prevents Toll-like receptor 4 recognition of Salmonella. Cellular Microbiology , 10(4), 876–890. https://doi.org/10.1111/j.1462-5822.2007.01090.x
- Wilson, R. P., Winter, S. E., Spees, A. M., Winter, M. G., Nishimori, J. H., Sanchez, J. F., Nuccio, S. P., Crawford, R. W., Tükel, Ç., & Bäumler, A. J. (2011). The Vi capsular polysaccharide prevents complement receptor 3-mediated clearance of Salmonella enterica serotype Typhi. Infection and Immunity , 79(2), 830–837. https://doi.org/10.1128/IAI.00961-10
- Winter, S. E., & Bäumler, A. J. (2014). Dysbiosis in the inflamed intestine: Chance favors the prepared microbe. Gut Microbes , 5(1), 71–73. https://doi.org/10.4161/gmic.27129
- Winter, S. E., Winter, M. G., Poon, V., Keestra, A. M., Sterzenbach, T., Faber, F., Costa, L. F., Cassou, F., Costa, E. A., Alves, G. E. S., Paixão, T. A., Santos, R. L., & Bäumler, A. J. (2014). Salmonella enterica serovar Typhi conceals the invasion-associated type three secretion system from the innate immune system by gene regulation. PLoS Pathogens , 10(7), e1004207. https://doi.org/10.1371/journal.ppat.1004207
- Wotzka, S. Y., Kreuzer, M., Maier, L., Arnoldini, M., Nguyen, B. D., Brachmann, A. O., Berthold, D. L., Zünd, M., Hausmann, A., Bakkeren, E., Hoces, D., Gül, E., Beutler, M., Dolowschiak, T., Zimmermann, M., Fuhrer, T., Moor, K., Sauer, U., Typas, A., … Hardt, W. D. (2019). Escherichia coli limits Salmonella Typhimurium infections after diet shifts and fat-mediated microbiota perturbation in mice. Nature Microbiology , 4(12), 2164–2174. https://doi.org/10.1038/s41564-019-0568-5