Mouse Models of Sepsis
Shravan Kumar Kannan, Shravan Kumar Kannan, Caleb Y. Kim, Caleb Y. Kim, Mohammad Heidarian, Mohammad Heidarian, Roger R. Berton, Roger R. Berton, Isaac J. Jensen, Isaac J. Jensen, Thomas S. Griffith, Thomas S. Griffith, Vladimir P. Badovinac, Vladimir P. Badovinac
Abstract
Human sepsis is a complex disease that manifests with a diverse range of phenotypes and inherent variability among individuals, making it hard to develop a comprehensive animal model. Despite this difficulty, numerous models have been developed that capture many key aspects of human sepsis. The robustness of these models is vital for conducting pre-clinical studies to test and develop potential therapeutics. In this article, we describe four different models of murine sepsis that can be used to address different scientific questions relevant to the pathology and immune response during and after a septic event. Basic Protocol 1 details a non-synchronous cecal ligation and puncture (CLP) model of sepsis, where mice are subjected to polymicrobial exposure through surgery at different time points within 2 weeks. This variation in sepsis onset establishes each mouse at a different state of inflammation and cytokine levels that mimics the variability observed in humans when they present in the clinic. This model is ideal for studying the long-term impact of sepsis on the host. Basic Protocol 2 is also a type of polymicrobial sepsis, where injection of a specific amount of cecal slurry from a donor mouse into the peritoneum of recipient mice establishes immediate inflammation and sepsis without any need for surgery. Basic Protocol 3 describes infecting mice with a defined gram-positive or -negative bacterial strain to model a subset of sepsis observed in humans infected with a single pathogen. Basic Protocol 4 describes administering LPS to induce sterile endotoxemia. This form of sepsis is observed in humans exposed to bacterial toxins from the environment. © 2024 The Authors. Current Protocols published by Wiley Periodicals LLC.
This article was corrected on 08 July 2024. See the end of the full text for details.
Basic Protocol 1 : Non-synchronous cecal ligation and puncture
Basic Protocol 2 : Cecal slurry model of murine sepsis
Basic Protocol 3 : Monomicrobial model of murine sepsis
Basic Protocol 4 : LPS model of murine sepsis
INTRODUCTION
Sepsis in humans is defined by an overwhelming response to an infection or toxin that causes hyper-inflammation and immune cell death, which can eventually lead to multi-organ failure and death (Singer et al., 2016). While some patients develop sepsis before being admitted to the hospital, others develop sepsis days after hospitalization for other medical reasons. Consequently, treatments need to be tailored to each sepsis patient depending on the severity of the symptoms. These features make it important to develop robust mouse models that capture the multitude of phenotypic and developmental aspects of human sepsis to empower scientists to translate their research from bench to bedside. The oldest published record of mouse septicemia in PubMed dates to 1892 (Moore, 1892). Monomicrobial sepsis models using bacteria, e.g., Escherichia coli or Pseudomonas aeruginosa , in mice garnered early interest (Fig. 1D and E). In the attempt to find therapies from bacterial and fungal components, scientists isolated endotoxins like lipopolysaccharide (LPS). Once we learned the significance of these endotoxins in establishing inflammation, the LPS endotoxemia model came into existence (Parant et al., 1977). The ease of execution and reproducibility of the LPS model have made it the most published model of sepsis (Fig. 1A and C). The mouse models have also evolved to complement the scientific unraveling of complex sepsis phenotypes in humans. Cecal ligation and puncture (CLP) is one such model that was first established in mice in 1983 (Baker et al., 1983). The CLP model captures some of the most important hallmarks of human sepsis, specifically the inflammation, lymphopenia, and long-term immunosuppression, making it the most comprehensive model used today. The cecal slurry model of sepsis is relatively new compared to the other models and has been slowly gaining traction since 2007 (Fig. 1F; Wynn et al., 2007). Publication data from PubMed (as of August 2023) show the number of publications using the LPS or CLP models has increased 2-fold in the last decade (Fig. 1B and C), while papers using a monomicrobial sepsis model appear to be reaching saturation (Fig. 1D and E). It is important to emphasize that all these sepsis models are still relevant for preclinical use depending on the scientific question(s) being investigated.
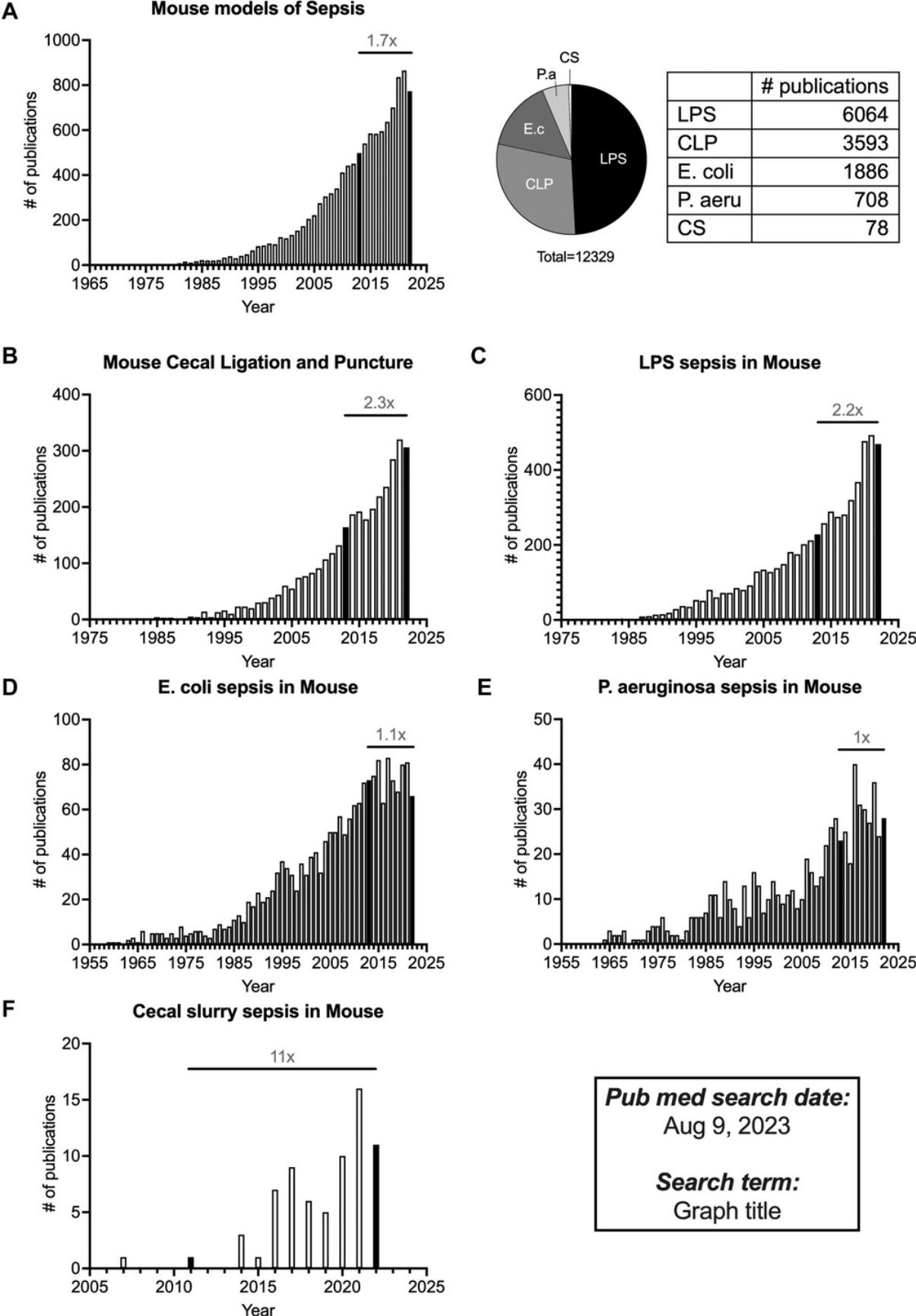
In 2020, we published a protocol detailing the CLP model of sepsis in Current Protocols in Immunology (Sjaastad et al., 2020). In this update, we will be describing the intricacies and strengths/weaknesses of other sepsis mouse models used routinely to address different scientific questions. Basic Protocol 1 outlines a non-synchronous model of polymicrobial sepsis, where CLP surgery is performed on different days but all analyses of that cohort of mice is performed on the same day. This variation of the CLP model will capture the variability observed in humans in terms of their state of inflammation, lymphopenia, and disease kinetics at the time of clinical presentation, and thus serves as a better pre-clinical model to assess the efficacy of immunotherapies to treat sepsis. Basic Protocol 2 describes the methodology for the cecal slurry model, where a defined amount of cecal slurry is directly injected into the peritoneum of recipient mice to induce sepsis. This model can also be used to study cytokine kinetics and lymphopenia (especially at early time points) without the complications of surgery. Basic Protocol 3 describes the steps in a monomicrobial model of sepsis, where mice are infected with a predetermined dose of gram-positive or -negative bacteria to induce sepsis. This model is useful to measure pathogen-specific sepsis phenotypes along with immune responses. Basic Protocol 4 describes an LPS endotoxemia model, where a septic response is induced in mice without any infection. This model is useful for assessing short-term inflammatory cytokine responses and testing therapies that target the cytokines (or other circulating molecules) without the added complications of a cellular response to infection.
NOTE : Protocols involving live animals must be reviewed and approved by the investigator's Institutional Animal Care and Use Committee (IACUC). Appropriate personal protective equipment (e.g., gloves, surgical mask, hair net, lab coat, protective sleeves) should be worn based on institutional guidance and regulations. In addition, the use of any controlled substances (e.g., ketamine) requires appropriate licensure and storage. Bacterial pathogens, e.g., E. coli and P. aeruginosa , are Biosafety Level 2 (BSL-2) pathogens. Therefore, follow all appropriate guidelines and regulations for the use and handling of pathogenic microorganisms. All bacterial usage requires the preparation of sterile media, plates, and glassware.
Basic Protocol 1: NON-SYNCHRONOUS CECAL LIGATION AND PUNCTURE
This protocol describes the method for inducing polymicrobial murine sepsis by non-synchronous cecal ligation and puncture (non-sync CLP). CLP is the most used model of polymicrobial sepsis, which has received outstanding attention over the past decade with >3500 publications in PubMed (Fig. 1). Here, we propose a non-synchronous variation to the CLP model, where instead of performing all CLP surgeries at one sitting, mice are staggered into different groups where they undergo surgeries at different days/hours (Fig. 2D). While all the technical aspects for each CLP surgery remain identical to the previously-published protocol (Sjaastad et al., 2020), inclusion of mice on different time courses after the septic insult attempts to imitate the real-world situation where sepsis patients are admitted during various stages of sepsis, with little-to-no information about the exact onset, and allows one to account for such heterogeneity that is often neglected during conventional CLP.
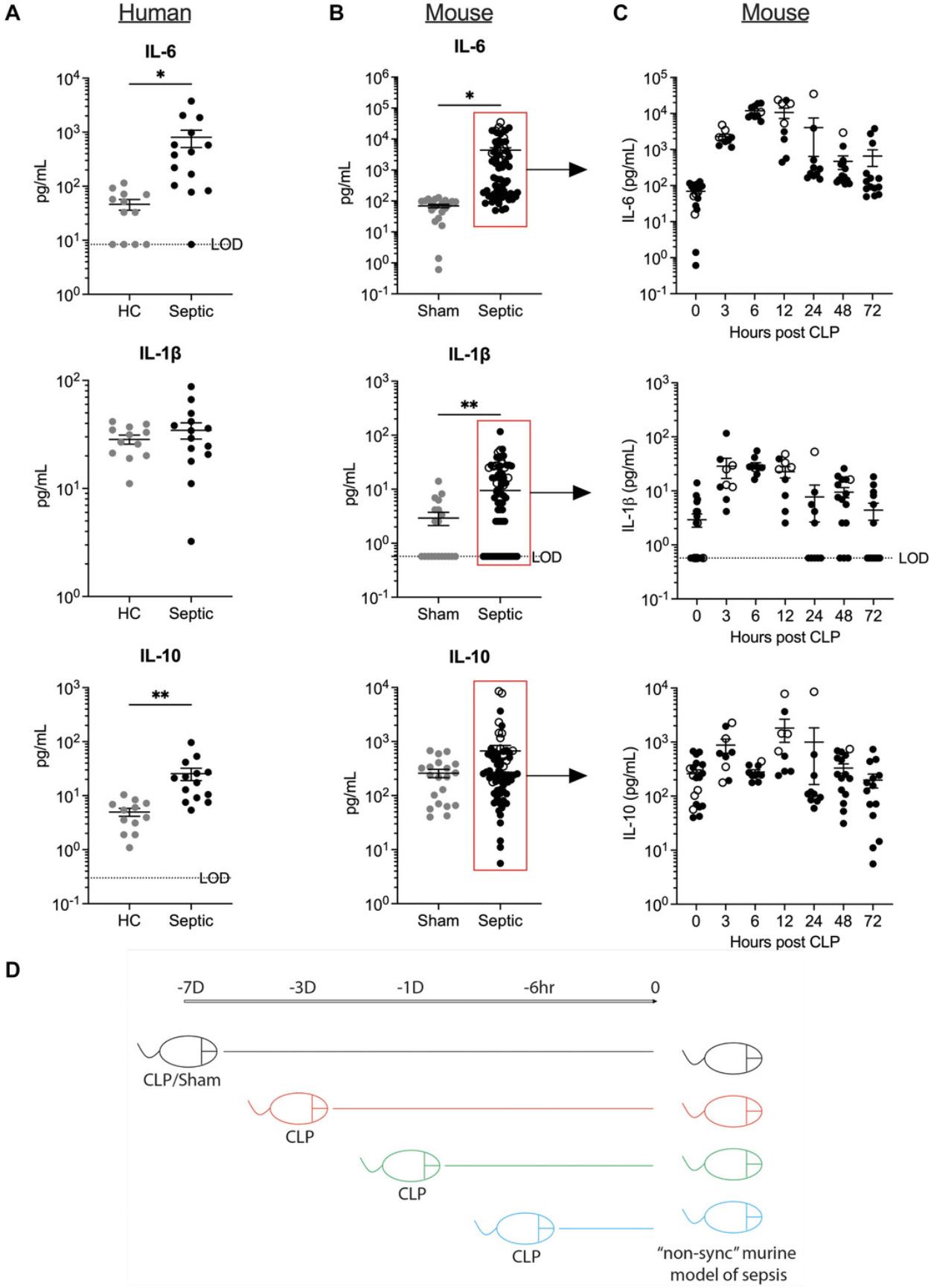
Materials
- See Sjaastad et al. (2020)
Induction of non-synchronous CLP in the mouse
Please refer to Sjaastad et al. (2020) for the annotated protocol steps to induce polymicrobial sepsis via CLP surgery. Specifically, the details can be found about how to “ Prepare for surgery,” “Anesthetize and prepare first mouse,” “Perform CLP,” “Perform post-operative care,” and “Perform additional CLPs.” To adjust the previous CLP protocol to a non-sync CLP method, one needs to perform CLP surgeries at different days/times, and we recommend scattering mice so that after all the surgeries are performed, there are mice that received CLP at 7 days, 3 days, 1 day, and 6 hr before using the CLP mice for the experiment. Mice in the control group underwent sham surgery 7 days before the mice were ready for the experiment. Refer to the previous article (Sjaastad et al., 2020) for the post-operative care and monitoring procedures. For the non-synchronous CLP protocol, make sure that the mice undergoing CLP at 7 days, 3 days, 1 day, and 6 hr should be given the corresponding post-operative care and monitoring separately.
Basic Protocol 2: CECAL SLURRY MODEL OF MURINE SEPSIS
This protocol describes the method for preparing a cecal slurry (CS) for inducing polymicrobial sepsis after intraperitoneal injection into recipient mice. The protocol is designed for use in adult mice but can be modified for use in neonatal mice. Any strain, sex, or age of mouse can in theory be used in the protocol, but donor and recipient mice should be matched.
Materials
-
Donor mice, age- and strain-matched mice to recipient mice, e.g., 12-week-old female C57BL/6 mice (The Jackson Laboratory)
-
15% glycerol (Sigma, cat. no. G2025) in phosphate-buffered saline (PBS) (see recipe), autoclave sterilized
-
Primaxin I.V., imipenem 500 mg stabilized in cilastatin (Merck, NDC 006-3516-59)
-
Saline solution (NDC 57319-555-06)
-
Phosphate-buffered saline (PBS) (see recipe)
-
60-mm × 10-mm sterile culture dish plate (Corning)
-
Ice
-
50-ml conical tubes (Corning Falcon)
-
Balance
-
Cell screen mesh, 860-, 190-, and 94-µm (Bellco Glass, cat. nos. 1985-00020, 1985-00080, and 1985-00150, respectively)
-
Plunger from 5 ml Luer-Lok syringe (BD, cat. no. 309646)
-
50-ml glass beaker
-
Magnetic stir bar
-
Cryovials
-
Therma waterproof type T high precision thermacouple meter with a mini rectal probe, type T, 0.1°C (ThermoWorks, cat. nos. THS-232-107 and RET-3, respectively)
-
23-gauge needle
Slurry stock preparation
1.Euthanize the donor mouse and open the peritoneum to access the cecum.
2.Cut the cecum where it meets the intestine. It will visibly have a three-way intersection. Transfer cecum in an empty 60-mm × 10-mm culture dish over ice.
3.Remove the cecal contents by squeezing from the closed end to the opening into a previously weighed 50-ml conical tube. Weigh the total mass of cecal contents.
4.Resuspend the cecal content in 1 ml of 15% glycerol in PBS solution for every 100 mg (wet weight) cecal content.
5.Pass the cecal contents through a sterile 860-µm mesh strainers over another 50-ml conical tube. Using the back end of a plunger from a 5 ml syringe, scrape back and forth across the mesh until only the large contents remain.
6.Repeat the process with 190- and 94-µm mesh strainers, respectively, into fresh 50-ml conical tubes.
7.Transfer the slurry contents into a 50-ml glass beaker, along with a magnetic stir bar.
8.Under continuous stirring, dispense the slurry in 1 ml aliquots into cryovials and store at –80°C.
Preparation of antibiotics
9.Weigh desired amount of Primaxin powder and reconstitute in sterile saline at 5 mg/ml. Aliquot and store frozen up to 1 week at –20°C (Starr et al., 2014; Steele et al., 2017).
Inducing polymicrobial sepsis
10.Weigh mice and calculate the volume of slurry contents to be injected (1.3 mg cecal slurry per gram body weight).
11.Take frozen CS stock and thaw immediately before injection.
12.Mix thoroughly with a 23-gauge needle and inject intraperitoneally (i.p.).
13.Begin resuscitation interventions 10 hr after cecal slurry injection by administering 300 µl of 5 mg/ml Primaxin in saline (1.5 mg/mouse) i.p. and 1 ml pre-warmed sterile saline subcutaneously.
14.Administer resuscitation interventions every 12 hr until mice body temperature recovered to at least 35°C.
15.Within 24 to 48 hr of CS administration, perform peripheral bleeds (50 to 100 µl) for serum collection (to assess the magnitude of the cytokine storm) and/or lymphopenia evaluation.
16.21 days post-CS injection, mice are ready for post-sepsis studies. Mice that did not develop severe hypothermia (<30°C at 10 hr) should be excluded from the study.
Mice should still be monitored on a regular basis (daily for first 5 to 7 days after CS injection, and then every 2 to 3 days until use in post-sepsis studies. Body temperatures and weights can be measured as long as desired, but they should return to pre-CS values within 7 days.
We routinely evaluate mice for clinical signs of sepsis for scoring disease severity in both the CLP and CS models. Clinical scores can be assessed using an ascending morbidity scale as previously described (Berton et al., 2022; Shrum et al., 2014; Silva, Moioffer et al., 2023):
1.Grooming: 0, (normal); 1, fur that has lost shine/become matte (dusty); 2, fur becomes erect or bristling (ruffled). 2.Mobility: 0, mobile without stimulation (normal); 1, mice are less responsive/mobile to stimuli (reduced); 2, mice are unresponsive to stimuli (immobile). 3.Body position: 0, body is fully extended (normal); 1, back is arched/curved (hunched); 2, laying on side at rest (on side). 4.Weight loss: 0, due to minimal weight loss that occurs in sham control mice, weight loss has been adjusted to allow for surgery-specific weight loss to be mitigated (<10%); 1, moderate weight loss (10% to 15%); 2, severe weight loss (>15%).
After giving one score for each category, the sum of all categories indicates disease score. Importantly, dead mice are given highest score (8) on the day of death, and thereafter removed from scoring. Healthy scores range from 0 to 2; moderate disease scores range from 3 to 5; and severe disease scores range from 6 to 8.
Using the doses of cecal slurry described here, mice will show signs of sepsis (e.g., increased serum cytokines, increased pathogen burden in the blood, decrease in immune cells in the blood and lymphoid organs, decrease in body temperature) within 48 hr of infection. However, the dose can be increased/decreased to modulate disease severity (survival, serum levels, weight loss, lymphopenia, and body temperature).
Basic Protocol 3: MONOMICROBIAL MODEL OF MURINE SEPSIS
Experimental monomicrobial sepsis can be induced in mice by direct injection of a single viable pathogen. This model mimics what would be a systemic infection derived from a primary infection (e.g., severe urinary tract infection/pyelonephritis or bacterial pneumonia). Bacterial infections are the primary causes of pathogenic sepsis, but systemic fungal and viral infections can also induce sepsis (especially in immunocompromised individuals). Because of the breadth of microbes that can induce sepsis and the extensive number of monomicrobial models that could be used, we will describe a model where mice experience a systemic uropathogenic E. coli infection by intravenous inoculation.
Materials
-
Kanamycin-resistant uropathogenic E. coli strain UTI89-kan-RFP (Mora-Bau et al., 2015)
-
Miller's LB agar (see recipe)
-
Miller's LB broth (see recipe)
-
Kanamycin
-
PBS (see recipe)
-
C57BL/6 mice (The Jackson Laboratory)
-
Other strains or genetically modified mice can be used depending on the experimental question being asked.
-
Spectrophotometer (Spectronic 20D+, Thermo Scientific, cat. no. 333183000)
-
Microcentrifuge tube
-
Microcentrifuge
-
Vortex
-
Insulin syringes with needle (29G × 0.5-in.) (McKesson, cat no. 102-SM05C2905P)
Preparation of bacteria prior to infection
1.Pick a single colony of uropathogenic E. coli strain UTI89-kan-RFP bacteria grown on LB agar plates containing kanamycin (50 μg/ml) using a sterile pipette tip or inoculating loop and add it to 10 ml LB broth containing kanamycin (50 μg/ml; Mora-Bau et al., 2015). Grow bacteria statically (i.e., no shaking) overnight at room temperature.
2.The following morning, measure the optical density of the culture using a spectrophotometer set to a wavelength of 600 nm (OD600).
3.Determine the number of bacteria to prepare based on the infectious dose needed to induce the desired magnitude of sepsis and the number of mice to infect.
4.Transfer the necessary volume of bacterial culture to a microcentrifuge tube (or multiple tubes if required). Centrifuge the tube(s) 1 min at 13,000 × g , room temperature, to pellet the bacteria. Aspirate the culture medium from the tube, being careful not to disturb the bacterial pellet. Resuspend the bacteria in the predetermined volume (1000 µl in the above example) in sterile PBS. The bacteria are now ready for injection.
Administration of bacteria to mice
5.Anesthetize mice using approved methodology (e.g., isoflurane or ketamine/xylazine).
6.Briefly vortex bacteria prior to drawing up into a 29G × 0.5-in. insulin syringe with needle.
7.Deliver 50 μl of the bacterial suspension intravenously (via the retro-orbital plexus) per mouse for an infectious dose of 2 × 108 CFU/mouse.
8.Within 24 to 48 hr of bacterial injection, perform peripheral bleeds (50 to 100 µl) for serum collection (to assess magnitude of cytokine storm) and/or lymphopenia evaluation.
9.Mice are returned to caging and housed under BSL-2 conditions for the duration of the experiment. Mice should be monitored daily (at least) for signs of morbidity using the ascending score system previous described.
Using the doses of uropathogenic E. coli described here, mice will show signs of sepsis (e.g., increased serum cytokines, increased pathogen burden in the blood, decrease in immune cells in the blood and lymphoid organs, decrease in body temperature) within 48 hr of infection. However, the infectious dose can be increased/decreased to modulate disease severity (survival, serum levels, weight loss, lymphopenia, and body temperature).
Basic Protocol 4: LPS MODEL OF MURINE SEPSIS
Lipopolysaccharide (LPS) injection is used extensively to mimic the septic shock observed in humans. The model uses purified LPS (usually from E. coli) injected intraperitoneally (i.p.) or intravenously (i.v.) into mice to induce endotoxemia. LPS is a pathogen-associated molecule pattern (PAMP) that signals through Toll-like receptor-4 (TLR4) on innate immune cells. Dose-dependent administration can generate varying severity of sepsis phenotype.
Materials
-
C57BL/6 mice (The Jackson Laboratory)
-
Purified LPS from E. coli O111:B4 (InvivoGen, cat. no. tlrl-eblps)
-
Balance
-
Syringe with 25G needle
Administration of LPS to mice
1.Weigh mice to be injected with LPS.
2.Prepare purified LPS in endotoxin-free water supplied with LPS to a working concentration of 10 (for severe inflammation), 5 (for moderate inflammation), or 1 (for mild inflammation) mg/kg body weight.
3.Inject 200 µl LPS solution into the mouse in a single dose intra-peritoneally (i.p.).
4.Within 24 to 48 hr of bacterial injection, perform peripheral bleeds (50 to 100 µl) for serum collection (to assess magnitude of cytokine storm) and/or circulating immune cell evaluation.
5.Mice are returned to caging and housed under BSL-1 conditions for the duration of the experiment. Mice should be monitored daily (at least) for signs of morbidity using the ascending score system previous described (see Basic Protocol 2, text after step 16).
REAGENTS AND SOLUTIONS
Miller's LB agar
Dissolve 40 g Miller's LB agar (Research Products International, cat. no. L24020-500.0) in 1 L distilled water. Adjust the pH to 7.2 using NaOH or HCl. Autoclave for 15 min. Cool to 50°C, add appropriate antibiotic, and mix. Pour 23 ml per sterile 100-mm × 15-mm petri dish. Cool and store up to 1 month at 4°C.
Miller's LB broth
Dissolve 25 g Miller's LB broth (Research Products International, cat. no. L24040-500.0) in 1 L distilled water. Adjust the pH to 7.2 using NaOH or HCl. Sterilize by autoclaving for 15 min. Store up to 1 month at room temperature.
Phosphate-buffered saline (PBS)
- 2.3 g NaH2PO4 (anhydrous sodium phosphate monobasic) (Fisher BioReagents, cat. no. BP329-1)
- 11.5 g Na2HPO4 (anhydrous sodium phosphate dibasic) (Fisher BioReagents, cat. no. BP332)
- 90 g NaCl (Fisher BioReagents, cat. no. BP358)
- 9.75 L dH2O
- Adjust pH to 7.2 to 7.4 using NaOH or HCl
- Sterilize by autoclaving
- Store up to 6 months at room temperature
COMMENTARY
Background Information
The first international consensus definition for sepsis and septic shock was detailed in 1991 (SEPSIS-1: Bone et al., 1992) to help clinicians and scientists consolidate the veritable clinical manifestations and variability observed among different patients. Despite this consorted effort in defining the characteristics of sepsis, there was a need to revise this definition twice: first in 2001 (SEPSIS-2: Levy et al., 2003), and more recently in 2016 (SEPSIS-3: Shankar-Hari et al., 2016). The need for such revisions is attributed to the constant uncovering of new sepsis pathophysiology, recent availability of quality data through electronic health records, and improvements in epidemiological statistics that help delineate true variation among populations (Shankar-Hari et al., 2016). While the SEPSIS-3 definition (along with its earlier generations) has been immensely helpful in regard to making life saving decisions in ICUs, it has become more challenging for the existing animal models to accommodate newly defined attributes.
CLP murine model of sepsis is currently considered the ‘gold standard’ owing to its ability to capture diverse aspects of human sepsis (Table 1). Firstly, prolonged release of gut bacteria into the peritoneal cavity leads to the release of an array of pro- and anti-inflammatory cytokines (Silva, Skon-Hegg et al., 2023; Tamayo et al., 2011). Secondly, tissue necrosis resulting from the cecal ligation leads to organ-specific abnormalities (Abraham et al., 2020) shortly after the surgery. Thirdly, CLP mice also exhibit transient lymphopenia followed by a long-lasting state of immune suppression (Berton et al., 2023; Heidarian et al., 2023; Martin et al., 2020), a phenomenon commonly observed in human sepsis survivors (Donnelly et al., 2015; Drewry et al., 2014). This robustness of the CLP model has enabled sepsis researchers to investigate both short- and long-term impacts of sepsis. Despite these strengths, all animal models of sepsis, specifically CLP, are criticized for their inability to predict the outcome of therapeutic interventions in sepsis patients (>200 failed randomized clinical trials; Marshall, 2014). While this could be inherent to the model itself, we should remember that the CLP model does not replicate the variability in sepsis pathology observed among patients at the time of enrollment to the study. For example, upon admission to the hospital, a sepsis patient could be experiencing the early pro-inflammatory stage of sepsis, the peak of the anti-inflammatory response, or the resolution of the cytokine storm where they suffer from lymphopenia. Therefore, the primary goal of the non-synchronous CLP protocol described herein is to achieve the variation in sepsis states among mice within the study groups.
Murine model of sepsis | Description | Manipulable factors to modulate severity | Aspect of human sepsis captured by the model | Pros | Cons | References |
---|---|---|---|---|---|---|
Conventional CLP sepsis | CLP performed at the same time for all mice in the study | Length of the cecum ligated; needle size; number of punctures; amount of feces extruded | Pro- and anti- inflammatory cytokine response; lymphopenia and organ dysfunction; long-term immune paralysis | Captures most immunological and physiological aspects of human sepsis; ideal for studies requiring long-term sepsis phenotype | Surgical training and practice are required to achieve consistency; time to perform the surgery makes it harder to include higher n numbers | Silva, Moioffer et al., 2023; Heidarian et al., 2023; Iskander et al., 2016; Wang et al., 2022; Zingarelli et al., 2019 |
Non-synchronous CLP sepsis | CLP performed at different times for mice in each experimental group | Everything listed for conventional CLP; days chosen to perform CLP to achieve non-sync | Captures the variability in immune phenotypes as seen in humans; similar organ dysfunction to humans | Captures most immunological and physiological aspects of human sepsis; ideal for studies requiring long-term sepsis phenotype; better model to test therapeutics | Surgical training and practice are required to achieve consistency; high variability within groups, thus requiring a higher n number | Cai et al., 2023; Berton et al., 2023; Donnelly et al., 2015; Drewry et al., 2014; Martin et al., 2020 |
Cecal slurry | Intraperitoneal administration of cecal slurry at a fixed concentration | Concentration of cecal slurry; microbiome composition | Achieve comparable cytokine response kinetics | Achieves hyperinflammation within 24 hr without surgery; ease of induction makes it ideal for studies that require data within a few hours of induction; includes the use of antibiotics for source control, which mimics the clinical setting | Short term inflammation and organ dysfunction; source and batch of cecal slurry can affect repeatability | Saito et al., 2021; Fujinami et al., 2021; Fallon et al., 2021; Ashina et al., 2020; Fujioka et al., 2018 |
Monomicrobial | Infection with a gram-positive or -negative bacteria (or fungi) | Gram nature of the microbe; CFUs administered; route of infection (i.v. or i.p.) | Recaps a subset of human sepsis caused by monomicrobial infection; model's host immune response against the pathogen during sepsis | Easy to set up the model and reproduce | Lacks the immunological and physiological complexities of polymicrobial sepsis; requires empirical determination of the optimal infectious dose for the desired outcome; immune cells involved in the response to infection may differ depending on the microbe used | Martin et al., 2023; Herout et al., 2023; LeBlanc & Lefort, 2021; Dimitrijevic et al., 2021; Merino et al., 2020; Martinez-Jéhanne et al., 2012 |
LPS | Administration of a fixed dose of LPS that acts as a PAMP, and mediate inflammatory signals in cells expressing PRRs | Number of LPS doses; concentration of LPS; route of administration; source of LPS (bacterial or fungal) | Replicates the endotoxemia mediated sepsis models of humans; models human innate immune inflammation mediated sepsis | Easy to set up the model and reproduce; consistent phenotype with less variation due to the lack of complexities involved with microbial composition | Lacks the key human sepsis phenotype of immunosuppression following hyperinflammation; LPS lot and manufacturer source can influence the outcome | Gabarin et al., 2021; Huggins et al., 2019; Warren et al., 2010; Rittirsch et al., 2007; Copeland et al., 2005 |
The CS model of sepsis is not used as frequently as other models (e.g., CLP or monomicrobial infection), but there are some unique advantages to the CS model (Table 1). Sepsis lethality is disproportionally high in humans at the extremes of age (i.e., neonates and older adults). While CLP can be performed on adult mice, it is technically not feasible with neonatal mice. Consequently, the CS model is the preferred method for inducing murine neonatal sepsis. Additional similarities/differences between the CLP and CS models include the technical complexities of inducing polymicrobial sepsis. The severity of sepsis can be modulated by the amount of cecal contents extruded from the ligated cecum or injected as a slurry. The technical difficulties of the CLP surgery open the possibility for some inherent operator subtleties that may be difficult to fully replicate. In contrast, intraperitoneal injection of CS is a much easier procedure to perform and does not require anesthesia.
Compared to polymicrobial sepsis, monomicrobial sepsis is more common in humans. There are many protocols available using different bacterial species to induce sepsis (He et al., 2021; LeBlanc & Lefort, 2021; Spencer et al., 2021), while fungal models of sepsis are also prevalent (Carreras et al., 2019; Fajardo et al., 2021). In each of the models, evaluating systemic inflammation, lymphopenia, temperature changes, and weight loss will be guides to determine a sepsis-causing pathogen dose used. Furthermore, the intravenous or intraperitoneal administration of the microbe bypasses the initial site of infection (e.g., lungs, bladder, kidney, or gastrointestinal tract). This may be an oversight and weakness of the model if there are unknown mechanisms about tissue specific roles of infection in sepsis. However, there are advantages to using this model as many standard protocols are available to prepare microbes, and injections are easy to administer.
LPS endotoxemia is yet another model of sepsis that shares the same ease of induction as the monomicrobial sepsis. It is critical to note that sensitivity to LPS differs between humans and SPF mice (Rittirsch et al., 2007). Mice require 250- to 500-fold higher LPS doses to produce effects (e.g., cytokine response) comparable to that seen in humans (Copeland et al., 2005; Warren et al., 2010). The exact mechanism for this difference remains undefined, but TLR4 sensitivity can be increased in mice with microbial exposure (Huggins et al., 2019). These microbially-experienced mice have a higher frequency of cells expressing TLR4 in the circulation compared to mice housed in specific pathogen-free conditions, which lead to a more robust cytokine storm and increased mortality after LPS challenge. Table 1 elaborates some of the pros and cons of each protocol described here, and the aspect of human sepsis that they capture.
Critical Parameters and Troubleshooting
Non-synchronous CLP model of murine sepsis
All the critical parameters and troubleshooting options described in our previous article for CLP (Sjaastad et al., 2020) are very much applicable to this protocol as well. As for the critical parameters specific to non-synchronous CLP, careful attention to the quality and consistency of the surgeries (e.g., being performed by the same person) becomes even more critical because they are performed on different days for each experimental group of mice. The time of starting the surgery on each day should be the same so that the kinetics can be tracked accurately at later time points. The time needed to complete the surgery per mouse varies from person to person. Therefore, calculating the time to complete the surgery for all the mice on the last day is crucial, so that the time at the middle of the surgery can be set as the starting point to calculate the time to start the treatment. For instance, in Figure 2D if the surgery for mice in the blue group (−6 hr) takes 3 hr (i.e., 9 AM to 12 PM), then 4:30 PM (i.e., 6 hr starting from 10:30 AM) is set as the starting point of treatment or analysis. Also, mice that die before day 0 (D0) can be excluded, which means it may be necessary to perform CLP surgeries on a higher number of mice to achieve the desired number of testable mice at D0. It is important to note that the mice that die from −6 hr and −24 hr group could be an artefact of poor aseptic technique. So, one should be careful while excluding mice after D0 and/or interpreting results, especially from mice that underwent CLP up to 24 hr before D0. Finally, note that we used SPF mice in this model, and by doing that we are excluding the contributions of genetic heterogeneity and infection history in humans that can influence sepsis outcome. However, studies have shown that in-bred, out-bred, and dirty mice with specific pathogen experience are less susceptible to CLP sepsis compared to SPF mice (Berton et al., 2022). Therefore, it is crucial to understand that this model only accounts for the heterogeneity in the immune system states, but not the heterogeneity inherent to the host.
CS model of murine sepsis
Mice tend to eat more during the night and will have more contents in their cecum in the morning for harvesting. One full mouse cecum can yield enough fecal content to produce ∼3 ml of slurry. Collecting cecal contents from multiple donor mice can generate a large bank of CS samples that can be used in multiple experiments, providing continuity, as well as increased flexibility for planning experiments. Fresh CS can also be produced on the day of each experiment, if preferred. The microbial composition of the cecal material collected and used in the slurry can vary depending on the housing conditions of the donor mice. Thus, investigators need to consider the potential impact of microbiome differences when comparing the response of recipient mice receiving CS from different donors. Like the CLP model, the use and timing of antibiotics and fluids influences the severity of the cytokine storm and recovery of the mice. Changing the timing and dosage of antibiotic treatment will influence the readouts of sepsis.
Monomicrobial model of murine sepsis
We will broadly speak about critical parameters using any monomicrobial model of sepsis. It is important to use a clinically relevant microbe where observations of the pathogen induce hallmarks of sepsis, beyond normal infection. Examples of clinical readouts, e.g., survival, cytokine production, and lymphopenia, are considerations when developing a monomicrobial model of murine sepsis. Weight loss and rectal temperatures are not demonstrated in this model, but these are other potential readouts to evaluate the severity of the response to the systemic infection. The uropathogenic E. coli described herein has a high mortality rate (80%) by day 3 using the infectious dose listed, but it gives the investigator the ability to evaluate acute innate immune responses to a systemic infection by a gram-negative bacterium. When evaluating this model of sepsis for long term readouts, titer the infectious dose to induce a less severe sepsis and be sure to administer antibiotics and saline fluids to boost recovery.
LPS endotoxemia model
LPS injection is an easily repeatable procedure where the dosage can be modulated. It is a simple and straightforward procedure that can easily induce sterile endotoxemia and inflammation. LPS purity can slightly differ depending on the source, microbe, and vendor. While LPS model is a one-time injection that generates high concentrations of inflammatory cytokines, it is cleared rapidly as it is one component of a complex gram-negative pathogen. The rapid clearance does not correlate with humans as microbes replicate causing a prolonged inflammatory response. Furthermore, LPS does not induce the long-term immunosuppression phenotype seen in the CLP model of sepsis, which are not useful for studying long-term effects of sepsis.
Understanding Results
Non-synchronous CLP model of murine sepsis
By performing non-synchronous CLP in mice (Fig. 2D), we compared the serum cytokine profile of all the mice to that of human samples (Fig. 2). Analysis of serum cytokine profile of septic patients shortly after their admission to hospital showed an expected increase in the levels of both pro- and anti-inflammatory cytokines. However, closer examination of the data, points to a larger spread in the cytokine levels, highlighting significant diversity with respect to the inflammatory status and sepsis progression of human patients at the time of hospitalization (Fig. 2A). This variability is only replicated in CLP mice when the serum cytokine levels of mice are pooled and measured at different time intervals within 72 hr after CLP surgery (Fig. 2B). Within the first 24 hr after induction of CLP, the levels of pro-inflammatory cytokines IL-6 and IL-1β quickly rise followed by an increase in anti-inflammatory cytokine IL-10. The mice that survived (closed circles) the CLP-induced heightened inflammation demonstrated gradual return to the homeostatic cytokine levels by 72 hr post-CLP (Fig. 2C). Hence, the use of non-synchronous groups of CLP mice that exhibit different inflammatory status is advantageous to better mimic the heterogeneity of immune system state of sepsis patients. One can envision that this model might provide a better platform to test efficacy of the treatments to ameliorate sepsis-induced symptoms. It can even be done in a blinded fashion, so that the person performing the surgery can be different form the investigator using those mice to ask relevant questions. Once the experiment is completed, whether successful or not, it would be possible to identify if, for instance, the timing of treatment post sepsis (i.e., early vs late after sepsis induction) matters.
CS model of murine sepsis
As described in Basic Protocol 2, one should expect a decline in body temperature within 10 to 12 hr of sepsis induction, followed by recovery within 48 hr (Fig. 3A). Depending on the CS dose used, reduction in body temperature can be seen as early as 4 hr. After inducing CS sepsis, it is important to monitor mouse body temperature until it reaches 35°C. Peripheral blood collected 46 hr post CS sepsis can be used to verify loss of white blood cells (WBCs; Fig. 3B), which is also an important phenotype observed in human sepsis. Compared to CLP, CS sepsis leads to inflammation early after induction. The levels of different cytokines in the serum of vehicle and 1.3 mg/g CS treated mice was determined before and 10 hr after CS injection (Fig. 3C). Cytokine levels were below the limit of detection at baseline (before vehicle or CS injection), and importantly remained low even after 10 hr in the mice given the vehicle. As for the CS injected mice, the levels of multiple pro-inflammatory cytokines IL-1β, IL-2, IL-6, IL-18, IFNβ, IFNγ, TNFα, and anti-inflammatory cytokine IL-10 were increased at the 10-hr time point. Together, these parameters suggest this CS dose is capable of inducing sepsis in mice.
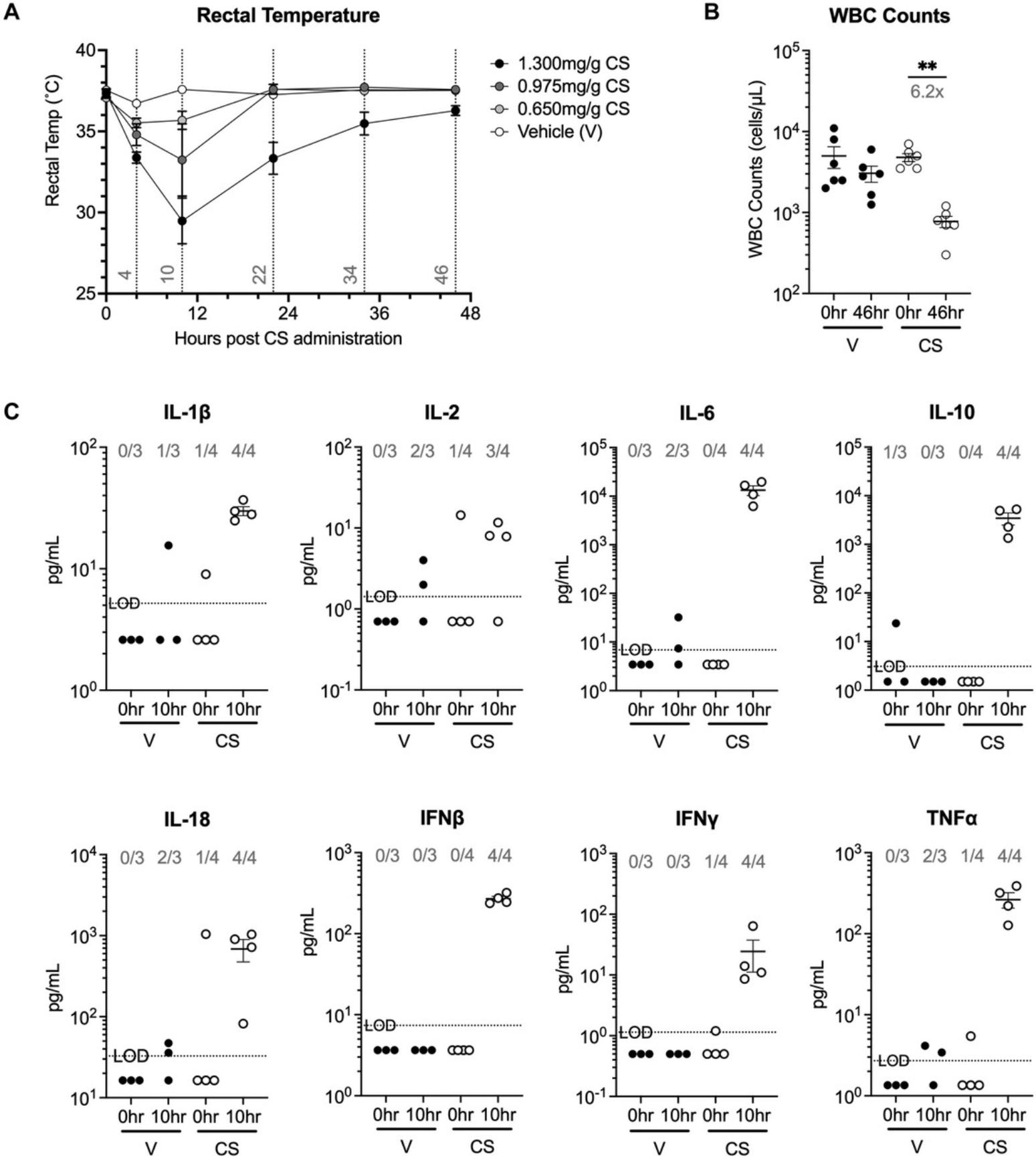
Monomicrobial model of murine sepsis
Using the information provided in protocol 3, infection of B6 mice with 1 × 108 CFU uropathogenic E. coli induces the rapid production of multiple cytokines and chemokines, including IL-1β, IL-6, TNFα, and IL-10/CXCL10, which can be detected in the serum 3 hr post-infection (Fig. 4A). By 24 hr post-infection, cytokine/chemokine levels decrease but are elevated compared to pre-infection values. A numerical reduction in T cells, B cells, and NK cells in blood can also be seen at the 24-hr post-infection time point by flow cytometry (Fig. 4B).
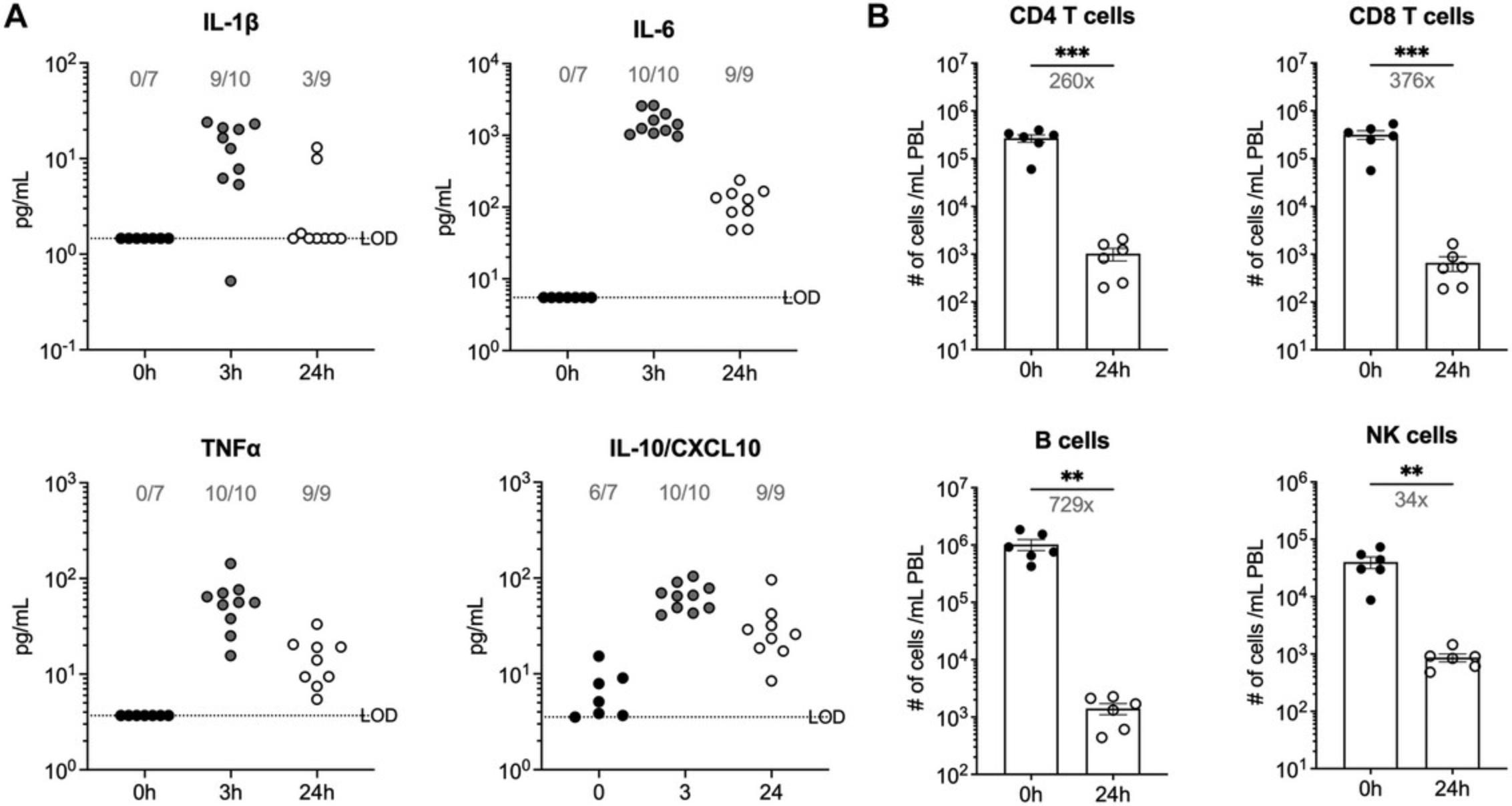
Time Considerations
Preparing the surgery station for CLP, performing the surgery, and waiting for the mice to recover from anesthesia (especially when ketamine/xylazine is used) can take some time. With practice, one can perform CLP surgeries at the rate of 10 to 15 min per mouse. However, it should be kept in mind that with a bigger group of mice, it takes longer to perform the surgery. Non-synchronous CLP models thus have a smaller number of mice undergoing surgery each day, while still having enough mice at D0 making it reactively easy compared to conventional CLP.
As for the CS, monomicrobial, and LPS models, preparation of the materials also takes some time, but the sepsis induction by itself can be performed considerably faster compared to conventional and non-synchronous CLP sepsis since the injections can be done without putting the mice under anesthesia.
Acknowledgments
This study was supported by National Institutes of Health grants GM134880 (to V.P.B.), GM140881 (to T.S.G.), and a Veterans Administration Merit Review Award (BX001324; to T.S.G.). V.P.B. is a University of Iowa Distinguished Scholar. T.S.G. is the recipient of a Research Career Scientist award (IK6BX006192) from the Department of Veterans Affairs. We also wish to extend our gratitude to Dr. Patricia De Assis (University of Michigan) for providing essential information for executing the described cecal slurry model of sepsis.
Author Contributions
Shravan-Kumar Kannan : Conceptualization; data curation; formal analysis; investigation; methodology; visualization; writing original draft. Caleb Y. Kim : Conceptualization; data curation; formal analysis; investigation; methodology; visualization; writing original draft. Mohammad Heidarian : Conceptualization; formal analysis; investigation; writing original draft. Roger R. Berton : Data curation. Isaac J. Jensen : Data curation; formal analysis. Thomas S. Griffith : Conceptualization; funding acquisition; project administration; resources; supervision; visualization; writing review and editing. Vladimir P. Badovinac : Conceptualization; funding acquisition; methodology; project administration; resources; supervision; visualization; writing review and editing.
Conflict of Interest
The authors declare no competing interests.
Open Research
Data Availability Statement
Data available on request from the authors.
Literature Cited
- Abraham, M. N., Kelly, A. P., Brandwein, A. B., Fernandes, T. D., Leisman, D. E., Taylor, M. D., Brewer, M. R., Capone, C. A., & Deutschman, C. S. (2020). Use of organ dysfunction as a primary outcome variable following cecal ligation and puncture: Recommendations for future studies. Shock (Augusta, Ga.) , 54(2), 168–182. https://doi.org/10.1097/SHK.0000000000001485
- Ashina, M., Fujioka, K., Nishida, K., Okubo, S., Ikuta, T., Shinohara, M., & Iijima, K. (2020). Recombinant human thrombomodulin attenuated sepsis severity in a non-surgical preterm mouse model. Scientific Reports , 10(1), 333. https://doi.org/10.1038/s41598-019-57265-2
- Baker, C. C., Chaudry, I. H., Gaines, H. O., & Baue, A. E. (1983). Evaluation of factors affecting mortality rate after sepsis in a murine cecal ligation and puncture model. Surgery , 94(2), 331–335.
- Berton, R. R., Jensen, I. J., Harty, J. T., Griffith, T. S., & Badovinac, V. P. (2022). Inflammation controls susceptibility of immune-experienced mice to sepsis. ImmunoHorizons , 6(7), 528–542. https://doi.org/10.4049/immunohorizons.2200050
- Berton, R. R., McGonagil, P. W., Jensen, I. J., Ybarra, T. K., Bishop, G. A., Harty, J. T., Griffith, T. S., & Badovinac, V. P. (2023). Sepsis leads to lasting changes in phenotype and function of naïve CD8 T cells. PLoS Pathogens , 19(10), e1011720. https://doi.org/10.1371/journal.ppat.1011720
- Bone, R. C., Balk, R. A., Cerra, F. B., Dellinger, R. P., Fein, A. M., Knaus, W. A., Schein, R. M., & Sibbald, W. J. (1992). Definitions for sepsis and organ failure and guidelines for the use of innovative therapies in sepsis. The ACCP/SCCM Consensus Conference Committee. American College of Chest Physicians/Society of Critical Care Medicine. Chest , 101(6), 1644–1655. https://doi.org/10.1378/chest.101.6.1644
- Cai, L., Rodgers, E., Schoenmann, N., & Raju, R. P. (2023). Advances in rodent experimental models of sepsis. International Journal of Molecular Sciences , 24(11), 9578. https://doi.org/10.3390/ijms24119578
- Carreras, E., Velasco de Andrés, M., Orta-Mascaró, M., Simões, I. T., Català, C., Zaragoza, O., & Lozano, F. (2019). Discordant susceptibility of inbred C57BL/6 versus outbred CD1 mice to experimental fungal sepsis. Cellular Microbiology , 21(5), e12995. https://doi.org/10.1111/cmi.12995
- Copeland, S., Warren, H. S., Lowry, S. F., Calvano, S. E., Remick, D., & Inflammation and the Host Response to Injury Investigators. (2005). Acute inflammatory response to endotoxin in mice and humans. Clinical and Diagnostic Laboratory Immunology , 12(1), 60–67. https://doi.org/10.1128/CDLI.12.1.60-67.2005
- Dimitrijevic, Z., Paunovic, G., Tasic, D., Mitic, B., & Basic, D. (2021). Risk factors for urosepsis in chronic kidney disease patients with urinary tract infections. Scientific Reports , 11(1), 14414. https://doi.org/10.1038/s41598-021-93912-3
- Donnelly, J. P., Hohmann, S. F., & Wang, H. E. (2015). Unplanned readmissions after hospitalization for severe sepsis at academic medical center-affiliated hospitals. Critical Care Medicine , 43(9), 1916–1927. https://doi.org/10.1097/CCM.0000000000001147
- Drewry, A. M., Samra, N., Skrupky, L. P., Fuller, B. M., Compton, S. M., & Hotchkiss, R. S. (2014). Persistent lymphopenia after diagnosis of sepsis predicts mortality. Shock (Augusta, Ga.) , 42(5), 383–391. https://doi.org/10.1097/SHK.0000000000000234
- Fajardo, P., Cuenda, A., & Sanz-Ezquerro, J. J. (2021). A mouse model of candidiasis. Methods in Molecular Biology (Clifton, N.J.) , 2321, 63–74. https://doi.org/10.1007/978-1-0716-1488-4_7
- Fallon, E. A., Chung, C. S., Heffernan, D. S., Chen, Y., De Paepe, M. E., & Ayala, A. (2021). Survival and pulmonary injury after neonatal sepsis: PD1/PDL1's contributions to mouse and human immunopathology. Frontiers in Immunology , 12, 634529. https://doi.org/10.3389/fimmu.2021.634529
- Fujinami, Y., Inoue, S., Ono, Y., Miyazaki, Y., Fujioka, K., Yamashita, K., & Kotani, J. (2021). Sepsis induces physical and mental impairments in a mouse model of post-intensive care syndrome. Journal of Clinical Medicine , 10(8), 1593. https://doi.org/10.3390/jcm10081593
- Fujioka, K., Kalish, F., Zhao, H., Wong, R. J., & Stevenson, D. K. (2018). Heme oxygenase-1 deficiency promotes severity of sepsis in a non-surgical preterm mouse model. Pediatric Research , 84(1), 139–145. https://doi.org/10.1038/s41390-018-0028-6
- Gabarin, R. S., Li, M., Zimmel, P. A., Marshall, J. C., Li, Y., & Zhang, H. (2021). Intracellular and extracellular lipopolysaccharide signaling in sepsis: Avenues for novel therapeutic strategies. Journal of Innate Immunity , 13(6), 323–332. https://doi.org/10.1159/000515740
- He, X. H., Ouyang, D. Y., & Xu, L. H. (2021). Injection of Escherichia coli to induce sepsis. Methods in Molecular Biology (Clifton, N.J.) , 2321, 43–51. https://doi.org/10.1007/978-1-0716-1488-4_5
- Heidarian, M., Griffith, T. S., & Badovinac, V. P. (2023). Sepsis-induced changes in differentiation, maintenance, and function of memory CD8 T cell subsets. Frontiers in Immunology , 14, 1130009. https://doi.org/10.3389/fimmu.2023.1130009
- Herout, R., Vappala, S., Hanstock, S., Moskalev, I., Chew, B. H., Kizhakkedathu, J. N., & Lange, D. (2023). Development of a high-throughput urosepsis mouse model. Pathogens (Basel, Switzerland) , 12(4), 604. https://doi.org/10.3390/pathogens12040604
- Huggins, M. A., Sjaastad, F. V., Pierson, M., Kucaba, T. A., Swanson, W., Staley, C., Weingarden, A. R., Jensen, I. J., Danahy, D. B., Badovinac, V. P., Jameson, S. C., Vezys, V., Masopust, D., Khoruts, A., Griffith, T. S., & Hamilton, S. E. (2019). Microbial exposure enhances immunity to pathogens recognized by TLR2 but increases susceptibility to cytokine storm through TLR4 sensitization. Cell Reports , 28(7), 1729–1743.e5. https://doi.org/10.1016/j.celrep.2019.07.028
- Iskander, K. N., Vaickus, M., Duffy, E. R., & Remick, D. G. (2016). Shorter duration of post-operative antibiotics for cecal ligation and puncture does not increase inflammation or mortality. PloS One , 11(9), e0163005. https://doi.org/10.1371/journal.pone.0163005
- LeBlanc, B. W., & Lefort, C. T. (2021). A mouse model of Pseudomonas aeruginosa pneumonia. Methods in Molecular Biology (Clifton, N.J.) , 2321, 53–61. https://doi.org/10.1007/978-1-0716-1488-4_6
- Levy, M. M., Fink, M. P., Marshall, J. C., Abraham, E., Angus, D., Cook, D., Cohen, J., Opal, S. M., Vincent, J. L., Ramsay, G., & International Sepsis Definitions Conference. (2003). 2001 SCCM/ESICM/ACCP/ATS/SIS international sepsis definitions conference. Intensive Care Medicine , 29(4), 530–538. https://doi.org/10.1007/s00134-003-1662-x
- Marshall, J. C. (2014). Why have clinical trials in sepsis failed? Trends in Molecular Medicine , 20(4), 195–203. https://doi.org/10.1016/j.molmed.2014.01.007
- Martin, M. D., Badovinac, V. P., & Griffith, T. S. (2020). CD4 T cell responses and the sepsis-induced immunoparalysis state. Frontiers in Immunology , 11, 1364. https://doi.org/10.3389/fimmu.2020.01364
- Martin, M. D., Skon-Hegg, C., Kim, C. Y., Xu, J., Kucaba, T. A., Swanson, W., Pierson, M. J., Williams, J. W., Badovinac, V. P., Shen, S. S., Ingersoll, M. A., & Griffith, T. S. (2023). CD115+ monocytes protect microbially experienced mice against E. coli -induced sepsis. Cell Reports , 42(11), 113345. https://doi.org/10.1016/j.celrep.2023.113345
- Martinez-Jéhanne, V., Pichon, C., du Merle, L., Poupel, O., Cayet, N., Bouchier, C., & Le Bouguénec, C. (2012). Role of the vpe carbohydrate permease in Escherichia coli urovirulence and fitness in vivo. Infection and Immunity , 80(8), 2655–2666. https://doi.org/10.1128/IAI.00457-12
- Merino, I., Porter, S. B., Johnston, B., Clabots, C., Thuras, P., Ruiz-Garbajosa, P., Cantón, R., & Johnson, J. R. (2020). Molecularly defined extraintestinal pathogenic Escherichia coli status predicts virulence in a murine sepsis model better than does virotype, individual virulence genes, or clonal subset among E. coli ST131 isolates. Virulence , 11(1), 327–336. https://doi.org/10.1080/21505594.2020.1747799
- Moore, V. A. (1892). Mouse Septicæmia Bacilli in a pig's spleen. The Journal of Comparative Medicine and Veterinary Archives , 13(6), 333–341.
- Mora-Bau, G., Platt, A. M., van Rooijen, N., Randolph, G. J., Albert, M. L., & Ingersoll, M. A. (2015). Macrophages subvert adaptive immunity to urinary tract infection. PLoS Pathogens , 11(7), e1005044. https://doi.org/10.1371/journal.ppat.1005044
- Parant, M., Parant, F., & Chedid, L. (1977). Inheritance of lipopolysaccharide-enhanced nonspecific resistance to infection and of susceptibility to endotoxic shock in lipopolysaccharide low-responder mice. Infection and Immunity , 16(2), 432–438. https://doi.org/10.1128/iai.16.2.432-438.1977
- Rittirsch, D., Hoesel, L. M., & Ward, P. A. (2007). The disconnect between animal models of sepsis and human sepsis. Journal of Leukocyte Biology , 81(1), 137–143. https://doi.org/10.1189/jlb.0806542
- Saito, M., Fujinami, Y., Ono, Y., Ohyama, S., Fujioka, K., Yamashita, K., Inoue, S., & Kotani, J. (2021). Infiltrated regulatory T cells and Th2 cells in the brain contribute to attenuation of sepsis-associated encephalopathy and alleviation of mental impairments in mice with polymicrobial sepsis. Brain, Behavior, and Immunity , 92, 25–38. https://doi.org/10.1016/j.bbi.2020.11.010
- Shrum, B., Anantha, R. V., Xu, S. X., Donnelly, M., Haeryfar, S. M., McCormick, J. K., & Mele, T. (2014). A robust scoring system to evaluate sepsis severity in an animal model. BMC Research Notes , 7, 233. https://doi.org/10.1186/1756-0500-7-233
- Silva, E. E., Moioffer, S. J., Hassert, M., Berton, R. R., Smith, M. G., van de Wall, S., Meyerholz, D. K., Griffith, T. S., Harty, J. T., & Badovinac, V. P. (2023). Defining parameters that modulate susceptibility and protection to respiratory murine coronavirus MHV1 infection. Journal of Immunology (Baltimore, Md.: 1950) , ji2300434. Advance online publication. https://doi.org/10.4049/jimmunol.2300434
- Silva, E. E., Skon-Hegg, C., Badovinac, V. P., & Griffith, T. S. (2023). The calm after the storm: Implications of sepsis immunoparalysis on host immunity. Journal of Immunology (Baltimore, Md. : 1950) , 211(5), 711–719. https://doi.org/10.4049/jimmunol.2300171
- Singer, M., Deutschman, C. S., Seymour, C. W., Shankar-Hari, M., Annane, D., Bauer, M., Bellomo, R., Bernard, G. R., Chiche, J. D., Coopersmith, C. M., Hotchkiss, R. S., Levy, M. M., Marshall, J. C., Martin, G. S., Opal, S. M., Rubenfeld, G. D., van der Poll, T., Vincent, J. L., & Angus, D. C. (2016). The third international consensus definitions for sepsis and septic shock (sepsis-3). JAMA , 315(8), 801–810. https://doi.org/10.1001/jama.2016.0287
- Sjaastad, F. V., Jensen, I. J., Berton, R. R., Badovinac, V. P., & Griffith, T. S. (2020). Inducing experimental polymicrobial sepsis by cecal ligation and puncture. Current Protocols in Immunology , 131(1), e110. https://doi.org/10.1002/cpim.110
- Spencer, C. T., Ramos Muniz, M. G., Setzu, N. R., & Sanchez Guillen, M. A. (2021). Francisella tularensis infection of mice as a model of sepsis. Methods in Molecular Biology (Clifton, N.J.) , 2321, 75–100. https://doi.org/10.1007/978-1-0716-1488-4_8
- Starr, M. E., Steele, A. M., Saito, M., Hacker, B. J., Evers, B. M., & Saito, H. (2014). A new cecal slurry preparation protocol with improved long-term reproducibility for animal models of sepsis. PloS One , 9(12), e115705. https://doi.org/10.1371/journal.pone.0115705
- Steele, A. M., Starr, M. E., & Saito, H. (2017). Late Therapeutic intervention with antibiotics and fluid resuscitation allows for a prolonged disease course with high survival in a severe murine model of sepsis. Shock (Augusta, Ga.) , 47(6), 726–734. https://doi.org/10.1097/SHK.0000000000000799
- Tamayo, E., Fernández, A., Almansa, R., Carrasco, E., Heredia, M., Lajo, C., Goncalves, L., Gómez-Herreras, J. I., de Lejarazu, R. O., & Bermejo-Martin, J. F. (2011). Pro- and anti-inflammatory responses are regulated simultaneously from the first moments of septic shock. European Cytokine Network , 22(2), 82–87. https://doi.org/10.1684/ecn.2011.0281
- Wang, N., Lu, Y., Zheng, J., & Liu, X. (2022). Of mice and men: Laboratory murine models for recapitulating the immunosuppression of human sepsis. Frontiers in Immunology , 13, 956448. https://doi.org/10.3389/fimmu.2022.956448
- Warren, H. S., Fitting, C., Hoff, E., Adib-Conquy, M., Beasley-Topliffe, L., Tesini, B., Liang, X., Valentine, C., Hellman, J., Hayden, D., & Cavaillon, J. M. (2010). Resilience to bacterial infection: Difference between species could be due to proteins in serum. The Journal of Infectious Diseases , 201(2), 223–232. https://doi.org/10.1086/649557
- Wynn, J. L., Scumpia, P. O., Delano, M. J., O'Malley, K. A., Ungaro, R., Abouhamze, A., & Moldawer, L. L. (2007). Increased mortality and altered immunity in neonatal sepsis produced by generalized peritonitis. Shock (Augusta, Ga.) , 28(6), 675–683. https://doi.org/10.1097/SHK.0b013e3180556d09
- Zingarelli, B., Coopersmith, C. M., Drechsler, S., Efron, P., Marshall, J. C., Moldawer, L., Wiersinga, W. J., Xiao, X., Osuchowski, M. F., & Thiemermann, C. (2019). Part I: Minimum quality threshold in preclinical sepsis studies (MQTiPSS) for study design and humane modeling endpoints. Shock (Augusta, Ga.) , 51(1), 10–22. https://doi.org/10.1097/SHK.0000000000001243
Corrections
In this publication, the following correction has been made:
A sentence has been added to the Acknowledgements to thank Dr. Patricia De Assis for her contribution to this article.
The current version online now includes this correction and may be considered the authoritative version of record.
Citing Literature
Number of times cited according to CrossRef: 2
- Tiago R. Velho, Helena Raquel, Nuno Figueiredo, Ana Neves-Costa, Dora Pedroso, Isa Santos, Katharina Willmann, Luís F. Moita, Immunomodulatory Effects and Protection in Sepsis by the Antibiotic Moxifloxacin, Antibiotics, 10.3390/antibiotics13080742, 13 , 8, (742), (2024).
- V. I. Lyakhovskyi, V. I. Shepitko, O. S. Osipov, V. S. Drabovskiy, S. M. Suprunenko, S. M. Bilash, G. A. Yeroshenko, ACTUAL METHODS OF EXPERIMENTAL MODELING OF PERITONITIS: OVERVIEW AND PERSPECTIVES, Bulletin of Problems Biology and Medicine, 10.29254/2077-4214-2024-3-174-24-35, 1 , 3, (24), (2024).