Human Umbilical Cord Mesenchymal Stem Cell–Based in vitro Model for Neurotoxicity Testing
Teresa Coccini, Teresa Coccini, Arsenio Spinillo, Arsenio Spinillo, Marianna Roccio, Marianna Roccio, Elisa Lenta, Elisa Lenta, Chiara Valsecchi, Chiara Valsecchi, Uliana De Simone, Uliana De Simone
Abstract
Neurotoxicity (NT) testing for regulatory purposes is based on in vivo animal testing. There is general consensus, however, about the need for the development of alternative methodologies to allow researchers to more rapidly and cost effectively screen large numbers of chemicals for their potential to cause NT, or to investigate their mode of action. In vitro assays are considered an important source of information for making regulatory decisions, and human cell–based systems are recommended as one of the most relevant models in toxicity testing, to reduce uncertainty in the extrapolation of results from animal-based models. Human neuronal models range from various neuroblastoma cell lines to stem cell–derived systems, including those derived from mesenchymal stem/stromal cells (hMSC). hMSCs exhibit numerous advantages, including the fact that they can be obtained in high yield from healthy human adult tissues, can be cultured with a minimal laboratory setup and without genetic manipulations, are able of continuous and repeated self-renewal, are nontumorigenic, and can form large populations of stably differentiated cells representative of different tissues, including neuronal cells. hMSCs derived from human umbilical cord (hUC) in particular possess several prominent advantages, including a painless, non-invasive, and ethically acceptable collection procedure, simple and convenient preparation, and high proliferation capacity. In addition, hMSCs can be efficiently differentiated into neuron-like cells (hNLCs), which can then be used for the assessment of neuronal toxicity of potential neurotoxic compounds in humans. Here, we describe a step-by-step procedure to use hMSCs from the umbilical cord for in vitro neurotoxicity testing. First, we describe how to isolate, amplify, and store hMSCs derived from the umbilical cord. We then outline the steps to transdifferentiate these cells into hNLCs, and then use the hNLCs for neurotoxicity testing by employing multiple common cytotoxicity assays after treatment with test compounds. The approach follows the most updated guidance on using human cell–based systems. These protocols will allow investigators to implement an alternative system for obtaining primary NLCs of human origin, and support advancement in neurotoxicity research. © 2022 The Authors. Current Protocols published by Wiley Periodicals LLC.
Basic Protocol 1 : Isolation and maintenance of human mesenchymal stem/stromal cells (hMSCs) obtained from the umbilical cord lining membrane
Basic Protocol 2 : Transdifferentiation of hMSCs into neuron-like cells (hNLCs) and basic neurotoxicity assessment
INTRODUCTION
The gold standard for neurotoxicity (NT) testing for regulatory purposes is based on in vivo animal tests, following Test Guidelines (TGs) defined by the Organization for Economic Co-operation and Development (OECD). For example, Neurotoxicity Study in Rodents , TG 424, involves daily oral dosing of rats for acute, subchronic, or chronic assessments (28 days, 90 days, or 1 year or longer; OECD TG 424, OECD, 1997). Primary endpoints include behavioral assessments and evaluation of nervous system histopathology. Developmental neurotoxicity (DNT) evaluation is also performed on animal models (Bal-Price et al., 2015; Makris et al., 2009) using standardized guidelines such as OECD TG 426 (OECD, 2007; US-EPA, 1998) and the OECD TG 443 (OECD, 2011). While informative, these in vivo tests are complex and often too laborious and expensive; they are not suitable for high-throughput toxicity screening, and translatability of findings to human is often unclear due to species-specific differences (Lee & Lee, 2020; Leist et al., 2014). U.S. government agencies as well as several institutions (i.e., ICCVAM, CAAT, EPA) and the EU Parliament (i.e., REACH legislation) emphasize the use of high-throughput, human-relevant alternative methods, and encourage keeping the number of animals for testing to a minimum (Bal-Price & Pistollato, 2019; Daneshian, Busquet, Hartung, & Leist, 2015; European Commission, 2013; Hartung, 2010; http://ec.europa.eu/growth/sectors/cosmetics/animal-testing_en; https://www.science.org/content/article/us-epa-eliminate-all-mammal-testing-2035; Krewski et al., 2020; also see Directive 2010/63/EU). However, international validation authorities such as OECD, EURL ECVAM, and ICCVAM have not yet declared a validated non-animal method or alternative testing strategy to assess neurotoxicity.
Major considerations for progress in replacing animal use in neurotoxicity testing include (i) the functional mechanistic endpoints of NT that need to be represented in a test battery that capture the complexity of the nervous system and the processes involved in NT; (ii) development of in vitro test methods to elucidate toxicity mechanisms and to identify the target cells of NT; and (iii) validation of individual in vitro methods and a test battery as part of a tiered testing strategy. The first testing tier would distinguish neurotoxicant from cytotoxic chemicals, and the second would consist of mechanism-specific tests. A minimum battery can consist of methods for assessing basal cytotoxicity and energy metabolism (Harry et al., 1998; Prieto et al., 2005; Worth & Balls, 2002).
In recent years, the development of alternative methods and approaches to conventional animal testing that can be used as tools for NT and DNT testing has intensified (Costa, Pellacani, & Guizzetti, 2017). This goal has been pursued not only because of ethical concerns or the increasing time and cost burden of in vivo studies, but also because of the requirement to investigate mode and/or mechanism of action of chemicals, particularly related to early, upstream events in the neurotoxic process, and to screen chemicals of unknown toxicity to flag compounds for further in vitro and in vivo neurotoxicity studies (Costa et al., 2017).
In vitro cellular models are among the different alternative methods considered for neurotoxicology studies and testing. These include (i) rodent primary cells: neurons and glia (microglia, oligodendrocyte, astrocyte) from different brain regions; (ii) human and rodent cell lines—neuroblastoma, astrocytoma, glioma, pheochromocytoma; and (iii) neural stem/progenitor populations—multipotent cells which are able to self-renew and proliferate without limit (Breier et al., 2009; Coecke et al., 2007; Harry & Tiffany-Castiglioni, 2005; Prieto et al., 2005; Worth & Balls, 2002). Primary cell cultures require the use of animals for obtaining the cells. Continuous cell lines, on the other hand, originally derived from human or animal brain tissues, can typically be propagated, frozen, and thawed, and therefore maintained for research and testing purposes for many years. Human primary cells can actually be isolated from almost any tissue (Ramos, Mathew, Thompson, & Ehrhardt, 2014), but the one organ from which it is difficult to isolate primary cells is the human brain. With the recent rapid development of stem cell technology, many studies are seeking to differentiate human stem cells (SCs) into specific cells of interest, and this approach is also pursued in the area of NT and DNT testing (Kim, Che, & Yun, 2019; Masjosthusmann et al., 2018; Singh et al., 2016). SCs have the advantage over primary and immortalized cells of being capable of continuous, repeated self-renewal, and of forming large populations of stably differentiated cells representative of different tissues of human origin, including cerebral cells (e.g., neuronal and glial cells; Kim et al., 2019; Singh & Kashyap, 2016; Suma & Mohanan, 2015; Zakrzewski, Dobrzyński, Szymonowicz, & Rybak, 2019). There are many different types of SCs derived from various tissues or that are formed at different times in our lives, and they include pluripotent stem cells (embryonic)—whose use is ethically and politically controversial—and induced pluripotent stem cells (iPSCs)—which have to be engineered in the lab by converting tissue-specific cells into cells that behave like embryonic stem cells. iPSCs share many of the same characteristics as embryonic stem cells, including the ability to give rise to all the cell types in the body; however, they are not exactly the same and this reprogramming could generate tumors (Patel & Yang, 2010). Another type of stem cell includes multipotent stem cells, which comprise the “mesenchymal stem cells” (MSCs), i.e., cells isolated from the stroma, the connective tissue that surrounds other tissues and organs. These cells, more accurately called “stromal cells” (Viswanathan et al., 2019; Zakrzewski et al., 2019), can be obtained from different human healthy adult tissues (e.g., bone marrow, adipose tissue, and umbilical cord; Heo, Choi, Kim, & Kim, 2016; Lim & Phan, 2014).
MSCs derived from the human umbilical cord (hUC) possess several prominent advantages, including a painless, non-invasive, and ethically acceptable collection procedure, fast self-renewal (Jeschke, Gauglitz, Phan, Herndon, & Kita, 2011; Nagamura-Inoue & He, 2014; Saleh & Reza, 2017; Stubbendorff et al., 2013), and simple and convenient preparation. In addition, they exhibit primitive properties (i.e., fetal in nature) and a high proliferation capacity. Furthermore, the number of human MSCs that can be obtained from the UC exceeds that from umbilical blood, bone marrow, and adipose tissue. These MSCs can also be efficiently differentiated in vitro into cells of nonmesodermal origin, including neuron-like cells (hNLCs; Cortés-Medina et al., 2019; Czarnecka, Porowińska, Bajek, Hołysz, & Roszek, 2017; Hernández et al., 2020; Kil, Choi, & Park, 2016; Shahbazi et al., 2016; Shi et al., 2018; Singh & Kashyap, 2016), which can be used as a powerful tool for chemical neurotoxicity/developmental neurotoxicity assessment in humans (Buzanska et al., 2009; Kashyap et al., 2015; Singh & Kashyap, 2016; Singh et al., 2016; Zychowicz et al., 2014). Indeed, many neurotoxic mechanisms can be studied using hNLCs, with functional and morphological endpoints that have the potential for medium- to high-throughput testing.
In this respect, we have set up a new 2D cell-based model, namely, primary neuron-like cells generated from human MSCs derived from umbilical cord lining membranes (Coccini, Pignatti, Spinillo, & De Simone, 2020; De Simone, Spinillo, Caloni, Gribaldo, & Coccini, 2020). These hNLCs, serving as a source of “healthy” cells (that are not immortalized or cancer-derived cell lines), can be used with a testing battery that allows evaluation of a range of parameters, such as mitochondrial function and membrane integrity, and enable protein expression profiling of the neuronal differentiation process. hNLCs can be utilized as an in vitro cell-based model for reproducing, studying, and monitoring various stages of neuronal maturation and function over time.
Here, we describe two easy, step-by-step protocols to establish this in vitro system. First, we describe how to isolate, amplify, and store human MSCs derived from the outer lining membrane of the hUC obtained from full-term pregnant women during elective caesarean section (Basic Protocol 1). Then, we provide the steps to transdifferentiate these cells into neuron-like cells (Basic Protocol 2). Some examples of the application of the hNLCs for neurotoxicity testing are also presented, using standard tests. In particular, we comment on tests to use for the assessment of the cytotoxicity effects of two different compounds (MAM 2201, a new psychoactive drug, and Fe3O4NPs, a magnetite nanoparticle), via evaluation of cell viability (by MTT assay and trypan blue exclusion test), mitochondrial function (using ATP content), and expression of relevant neuronal markers. An overview of the two protocols is shown in Figure 1.
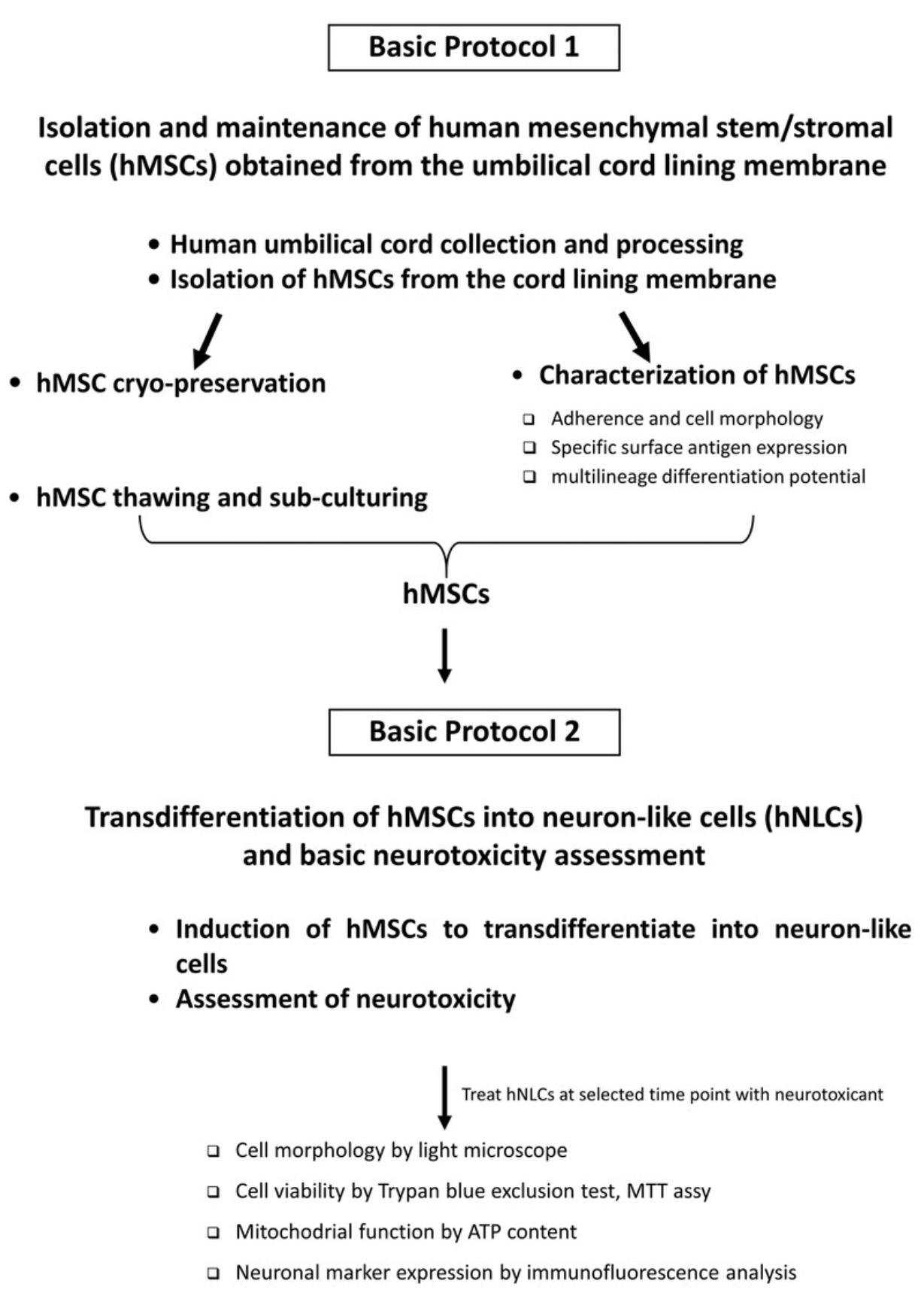
Together, these protocols will allow the establishment of human primary neuron-like cells as a tool for not only speeding up chemicals/xenobiotics screening, but also to provide some mechanistic data related to brain alterations in support of hazard assessment to prioritize chemicals/xenobiotics for further in-depth testing. This approach could be used as a first step, permitting the screening of a large number of chemicals/drugs/xenobiotics/emerging contaminants (including nanomaterials) over a wide range of concentrations in a time and cost-efficient manner.
STRATEGIC PLANNING
The use of human-derived material needs to satisfy all the requirements of the local applicable statutes and regulations. Informed consent from the donor is an essential prerequisite, as well as ethical approval obtained from the relevant Institutional Review Board/independent ethics committee. Further, consent for scientific publication must be gained from the mothers, preserving their privacy, confidentiality, and anonymity.
The experimental protocols described here, allowing the isolation of human MSCs and the generation of human primary neuron-like cells, respect guidelines and regulations on Good Cell Culture Practice (Bal-Price & Coecke, 2011; Coecke et al., 2005), laboratory safety regulations, and Internal Operating Procedures for safe handling and storage of chemicals.
When working with live materials (cord/placenta samples or cells), particular caution and sterile technique should be adopted to avoid contamination of the samples/cells. Use a laminar flow cabinet (Biosafety Cabinet, Class II), sterile labware, and aseptic procedures.
Before starting, and to minimize laboratory personnel contamination and biological risk, wear the Personal Protective Equipment (PPE) required by your laboratory's safety regulations (protective clothing and gloves).
Prior to use, the laminar hood should be cleaned with 70% (v/v) ethanol in water and kept aseptic during the whole procedure.
Basic Protocol 1: ISOLATION AND MAINTENANCE OF HUMAN MESENCHYMAL STEM/STROMAL CELLS (hMSCs) OBTAINED FROM THE UMBILICAL CORD LINING MEMBRANE
This protocol describes how to isolate a large number of high-quality MSCs from a human umbilical cord (about 65 million MSCs from 3 cm of umbilical cord at Passage 1). We first describe the steps for the collection and processing of the umbilical cord, and isolation of hMSCs from the cord lining membrane. We then outline the procedure for hMSC characterization (see below), cryo-preservation, thawing, and sub-culturing. The entire procedure is shown in Figure 2.
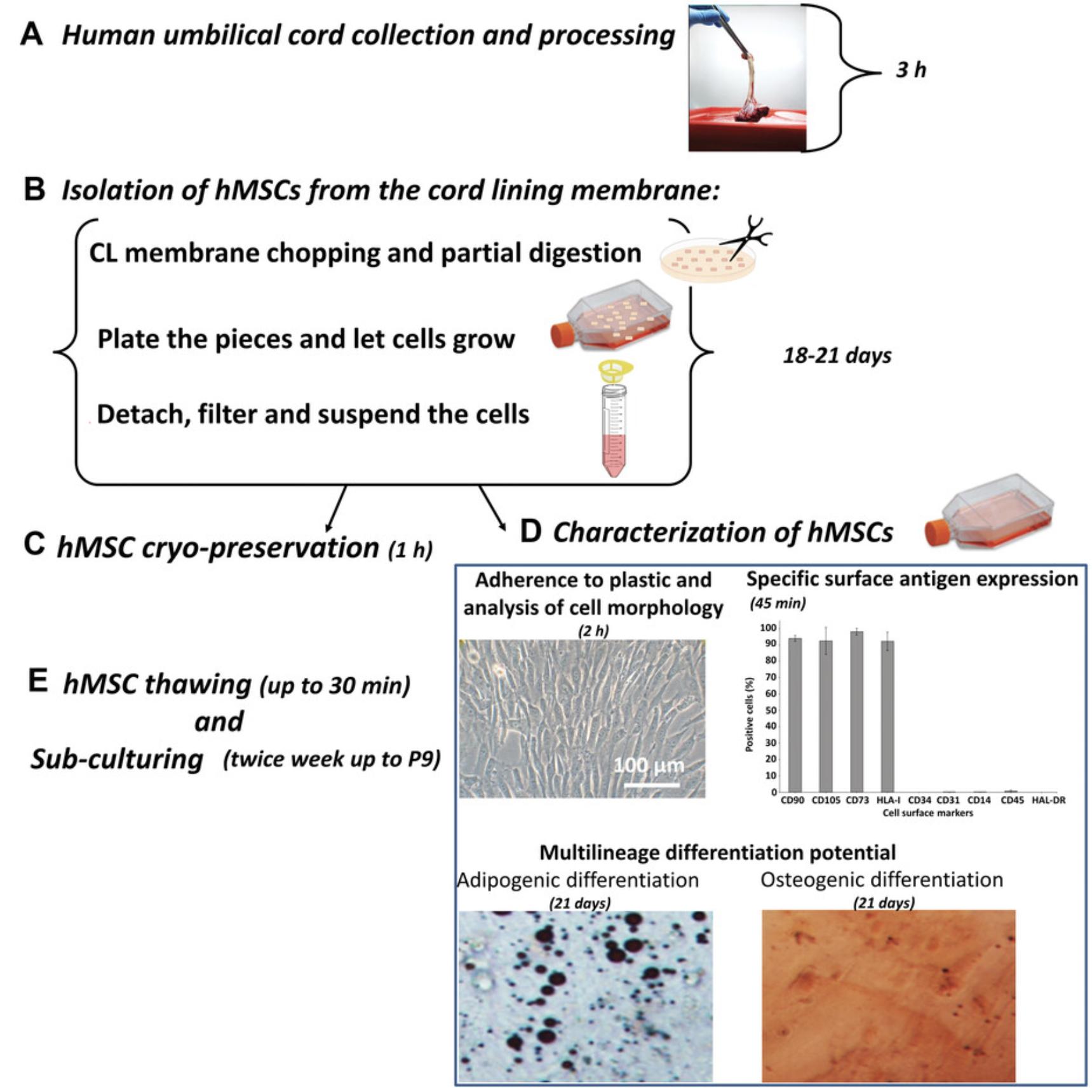
After the cells are isolated, it is imperative to proceed to MSC characterization to determine whether the isolated cells exhibit the typical characteristics of MSCs. This is done by evaluating whether the cells meet the set of criteria proposed by the Mesenchymal and Tissue Stem Cell Committee of the ISCT (International Society for Cellular Therapy) (Bosch et al., 2012; Dominici et al., 2006), and we describe the steps for this here. First, the isolated cells, during culture, should exhibit a fibroblast-like morphology. In addition, the cells must satisfy the following criteria:
- (i)Adherence to plastic : MSCs must be plastic-adherent when maintained in standard culture conditions using tissue culture flasks or similar;
- (ii)Specific surface antigen (Ag) expression : 90%-95% of the MSC population must express CD105, CD73 CD90, and HLA class I, and they must lack expression (max 5%-10% positive) of CD45, CD34, CD31, CD14 or CD11b, and HLA class II;
- (iii)Multipotent differentiation potential : cells must be able to differentiate into osteoblasts, adipocytes, and/or chondroblasts under standard in vitro differentiating conditions.
Cells that meet all these criteria can be designated as MSCs and then can be used for a specific project.
Materials
-
Human umbilical cord/placenta
-
Physiological saline solution (0.9% NaCl; PanReac AppliChem, cat. no. APLIA1671.0500)
-
Phosphate-buffered saline (1× PBS), without calcium, without magnesium (Carlo Erba Reagents, cat. no. LJ67802AP)
-
Trypsin solution (Sigma-Aldrich, cat. no. T4549)
-
Complete DMEM medium (see recipe)
-
Complete mesenchymal stem cell growth medium 2 (MSC medium; see recipe)
-
Accutase solution (DUTSCHER, cat. no. L0950)
-
Trypan blue solution, 0.4% (Corning, cat. no. 25-900-CI)
-
Human serum (HS), pooled (Sigma Aldrich, cat. no. H-5667)
-
Primary antibodies:
- Anti-human CD90 BV421 (Becton Dickinson, cat. no. 562556)
- Anti-human CD73 FITC (Becton Dickinson, cat. no. 561254)
- Anti-human CD105 (Becton Dickinson, cat. no. 562408)
- HLA DR-APC-H7 (Becton Dickinson, cat. no. 641411)
- HLA I (Becton Dickinson, cat. no. 567581)
- Anti-human CD34 PE (Becton Dickinson, cat. no. 345802)
- Anti-human CD31 (Becton Dickinson, cat. no. 563651)
- Anti-human CD45 (Becton Dickinson, cat. no. 655873)
- Anti-human CD14 (Becton Dickinson, cat. no. 560180)
-
7-Amino-actinomycin (D7-AAD; Becton Dickinson, cat no. 559925)
-
Adipogenic differentiation medium (see recipe)
-
Human Mesenchymal Stem Cells (optional; PromoCell, cat. no. C-12971)
-
Oil red-O (ready to use; Bio-Optica, cat. no. 30-30112)
-
60% isopropanol (see recipe)
-
Osteogenic differentiation medium (see recipe)
-
Alkaline phosphatase substrate solution (see recipe)
-
Alizarin red S solution (see recipe)
-
Freezing medium: Cryo-SFM (PromoCell, cat. no. C29912)
-
Liquid nitrogen
-
70% v/v ethanol (Carlo Erba Reagents, cat. no. 41605)
-
Laminar flow hood (Gelaire, BSB 4 - S, Class II)
-
Sterile 150-mm plastic Petri dishes (Nunc, cat. no. 168381)
-
Sterile Pasteur pipettes (glass; WVR, 230 mm, cat. no. 612-1702)
-
Sterile scissors (straight blade; 145 mm)
-
Sterile 100-mm plastic Petri dishes (BD FalconTM, cat. no. 353003)
-
Sterile forceps (1 × 2 teeth; 145 mm)
-
Sterile scraper (Corning, cat. no. 3008)
-
CO2 incubator (Thermo Scientific, Hera Cell 150i)
-
Inverted microscope (Zeiss Axiovert 25 microscope equipped with a 10× objective)
-
Sterile polypropylene tubes (Corning, 15 ml: cat. no. 430052; 50 ml: cat. no. 430290)
-
Vacuum aspiration pump (Vacuum Technology s.r.l., DVP)
-
Sterile forceps (toothed oval jaw; 255 mm)
-
T-75 (75-cm2) cell culture flasks, vented cap (Corning, cat. no. CC431464U)
-
100-µm sterile cell strainer (ClearLine, cat. no. 141380C)
-
Single-use plastic pipettes (Life Sciences, 5 ml: cat. no. GSP010005, 10 ml: cat. no. GSP211010)
-
Micropipettes and tips (Diamond D200V, 2-200 µl: cat no. F161930, or similar)
-
Microcentrifuge tubes (VWR, 1.5 ml: cat. no. 20170-038, or similar)
-
Burker Chamber (BLAUBRAND®, cat. no. 718920)
-
Polystyrene round-bottom tubes (Falcon®, 5 ml: cat. no. 352052)
-
Microcentrifuge (Thermo Scientific, PICO 21)
-
Flow cytometer FACSCanto II (Becton Dickinson) combined with FACS Diva Software (Becton Dickinson)
-
24-well plates (Corning, cat. no. CC3524)
-
Cryogenic vials (Nalgene®, cat. no. 5000-0020)
-
Cryo freezing container (Nalgene®, Mr. Frosty™, cat. no. 5000-0001)
-
Liquid nitrogen container (Taylor Wharton, RS SERIES)
-
Styrofoam box
-
Sterile centrifuge tubes (ClearLine, 15 ml: cat. no. CL482; 50 ml: cat. no. CL474)
-
37°C water bath (ASAL S.R.L., 720 D)
-
Additional reagents and equipment for counting cells with a hemocytometer (e.g., Burker chamber; see Current Protocols article Strober, 2015)
Human umbilical cord collection and processing
In this section, we describe the collection and processing of UC tissue from full-term pregnant women (sourced during elective cesarean section) in order to obtain the cord lining membrane for further isolating the MSCs.
In hospital
1.Aseptically collect the sample of cord/placenta from full-term pregnant woman during elective cesarean section, after informed consent.
2.Immediately place the sample in a collection cup containing cold physiological saline solution (0.9%NaCl) and store at 4°C until arrival at the lab. Continue to next steps within 2 hr.
In laboratory
3.Transfer the cord/placenta sample to a Petri dish (150 mm) containing PBS (about 20 ml) placed on ice under laminar flow hood.
4.Rinse the sample multiple times with ice-cold PBS using a sterile glass Pasteur pipette in order to remove blood clots.
5.Separate the umbilical cord (UC) from the placenta using a pair of sterile scissors.
6.Collect the waste (placenta and cord residues) in a cup and follow your institution's biohazard disposal waste regulations.
7.Transfer UC (approximately 3 cm in length) to a Petri dish (100 mm) placed on ice.
8.Cut the UC longitudinally using sterile scissors and forceps (1 × 2 teeth) (Fig. 3).
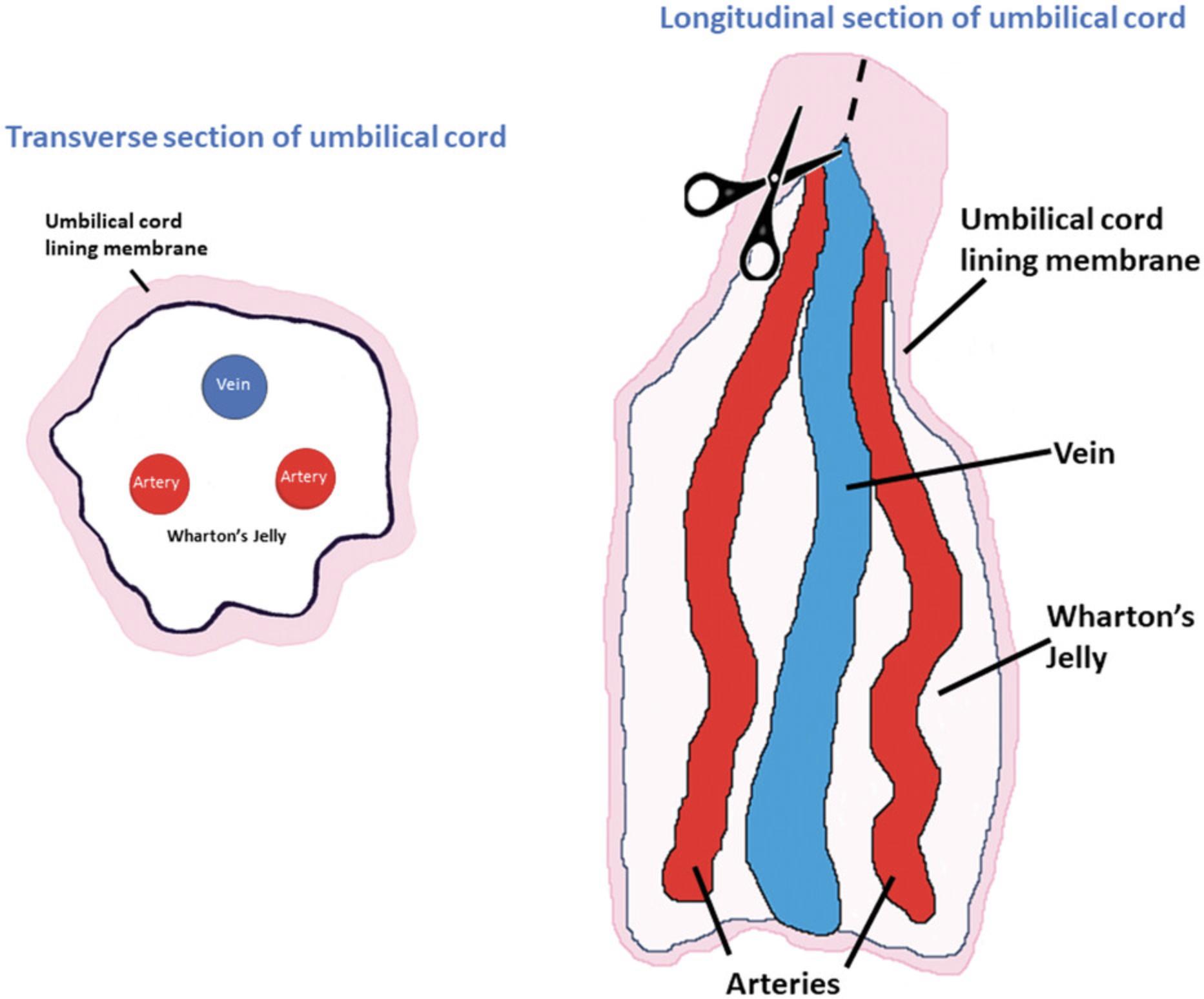
9.Expose the blood vessels (vein and arteries) and the surrounding Wharton's jelly (WJ) without damaging the epithelium.
10.Scrape the WJ away from the blood vessels and inner epithelium of the subamnion region using a scraper.
11.Cut/remove blood vessels and discard them as indicated in step 6.
12.Collect the outer lining membrane, the cord lining (CL), in a separate Petri dish (100 mm) placed on ice, containing PBS (5 ml). Proceed to isolate the MSCs as described below.
Isolation of hMSCs from the cord lining membrane
13.Discard PBS from the Petri dish containing the CL membrane using a vacuum aspiration system and add 5 ml of 1× trypsin solution.
14.Cut CL membrane into 1- to 2-mm pieces using a pair of sterile scissors.
15.Incubate CL pieces at 37°C for 30 min in a 5% CO2 incubator for partial digestion of samples.
16.Monitor the partial digestion after 30 min using an inverted microscope in bright-field mode (the released cells from pieces should be visualized in the Petri dish).
17.At the end of the enzymatic incubation, add an equal volume (5 ml) of complete DMEM medium in order to inactivate the trypsin.
18.Transfer the suspension with pieces into a 50-ml centrifuge tube and let the partially digested tissue pieces settle for 5 min.
19.Carefully aspirate the supernatant using a vacuum pump.
20.Select CL pieces (1-2 mm only) and align them with the help of sterile forceps (toothed oval jaw): place 20 partially digested tissue pieces per 75-cm2 tissue culture flask.
21.Gently add 10 ml of MSC medium to each flask.
22.Incubate at 37°C in a 5% CO2 incubator and do not disturb the culture flasks for 2-3 days, to allow for the adherence of tissue pieces.
23.Change the MSC medium every 3-4 days and monitor for the appearance of outgrowth of cells from the explant pieces using an inverted microscope in bright-field mode.
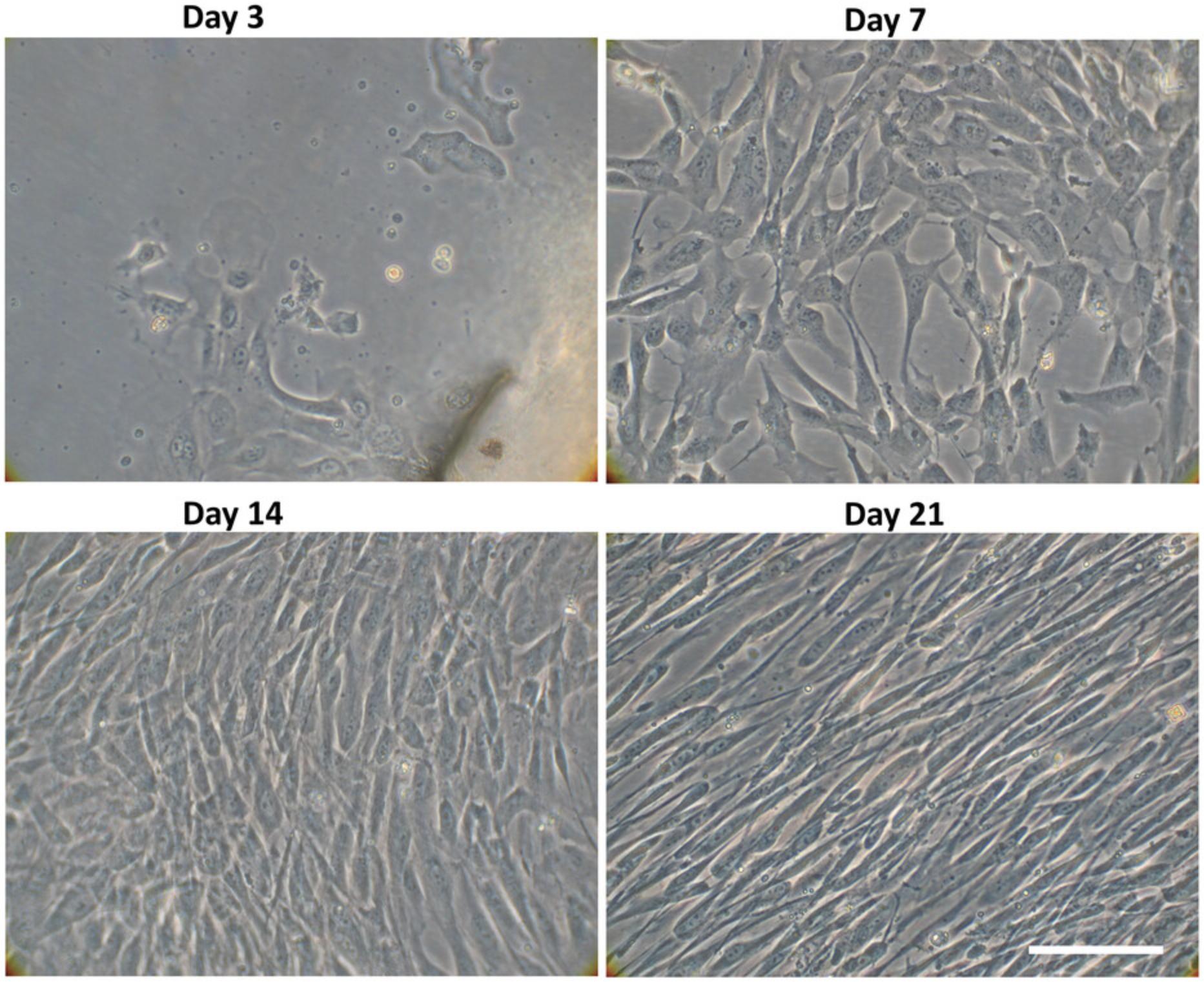
24.Let cells grow from the tissue explants until 70%-80% cell confluence is reached (about 3 weeks). At this point, the cells are considered at passage 0.
25.Gently remove the medium from the 75-cm2 culture flask using a vacuum aspiration system, and wash the cells once with 5 ml of PBS.
26.Discard the PBS and add 2 ml of Accutase solution per 75-cm2 flask in order to detach the pieces and the cells. Incubate for 5 min at room temperature.
27.Stop the reaction by adding 5 ml of MSC medium to each 75-cm2 flask.
28.Pool the contents (cells + pieces + medium) of each 75-cm2 flask culture (total = 28 ml) and filter with a 100-µm sterile cell strainer to separate cells from tissue fragments into a 50-ml centrifuge tube, carefully pipetting them up and down in order to obtain a single cell suspension (without cell agglomeration) (cells at Passage 1).
29.Take a 90-µl aliquot of the cell suspension and mix with 10 µl of 0.4% trypan blue solution in a microcentrifuge tube.
30.Transfer 10 µl of the mix to a Burker chamber and count viable cells.
31.Based on the number of cells obtained at this point (about 65 million cells at Passage 1), transfer 3 × 106 isolated cells to a 50-ml tube: these cells will be used for the characterization of the MSCs. Immediately cryopreserve the remaining cells (preparing cryovials at 1-1.5 × 106 cells/tube; see “Cryopreservation” below).
Characterization of hMSCs
Adherence to plastic and analysis of cell morphology
32.Seed the isolated cells (cells at Passage 1) in a 75-cm2 culture flask at a density of 4000 cells/cm2 in 12 ml of MSC medium.
33.After 3 days, when 70%-80% cell confluence is reached, observe the cells to detect whether they are attached.
34.Monitor morphology. Cells should change towards a fibroblast-like shape/phenotype, appearing as a homogeneous monolayer of long spindle-like cells with a whirlpool like-array.
35.If cells meet this first criterion (i.e., adherence of fibroblast-like cells to plastic), use them and proceed to the evaluation of the other two criteria below.
Specific surface antigen expression
36.Use the isolated cells in the flask mentioned above (step 33).
37.Discard the MSC medium using a vacuum aspiration system.
38.Wash the cells once with PBS (5 ml PBS per 75-cm2 culture flask).
39.Discard the PBS and add 2 ml of Accutase solution (incubate for 5 min at room temperature) to each flask in order to detach the cells.
40.Stop the reaction by adding 5 ml of MSC medium.
41.Combine a 90-µl aliquot of the cell suspension with 10 µl of 0.4% trypan blue solution in a microcentrifuge tube (1.5 ml) and mix.
42.Transfer 10 µl of the mix to a Burker chamber and count viable cells.
43.Resuspend 1 × 106 cells in 1 ml of MSC medium and transfer to a microcentrifuge tube.
44.Centrifuge the suspension 10 min at 100 × g (1000 rpm), room temperature.
45.Remove the supernatant.
46.Add 100 µl of cell suspension buffer (PBS containing 5% pooled human serum; HS) to the pellet
47.Split cell suspension into two acquisition tubes marked as "Tube 1" (50 µl) and "Tube 2" (50 µl).
48.Add 10 µl, from stock (as reported on product data sheet), of anti-human monoclonal antibodies specific for CD90-BV421, CD105-APC, CD73-FITC, HLA I-PE, CD34-PE, CD31-PECy7, CD14-APC-H7, CD45-V500, and HLA-DR-APC-H7 to Tube 2 (containing 50 µl of cell suspension).
49.Incubate the cells for 30 min at 4°C.
50.Add 1 ml of cell suspension buffer (PBS containing 5% pooled human serum; HS) to both tubes.
51.Centrifuge the suspension 10 min at 100 × g , room temperature.
52.Remove the supernatant.
53.Resuspend the pellet in 400 µl of physiological saline (0.9% NaCl).
54.Before acquisition in flow cytometer, add 5 µl of 7-AAD to both tubes.
55.Acquire 20,000 live cells in flow cytometer.
56.Analyze the cells by flow cytometry.
Multilineage differentiation potential: Adipogenic differentiation
57.Use the isolated cells (at the cell confluence, described in steps 32-33 of the “adherence to plastic and analysis of cell morphology”).
58.Discard the MSC medium using a vacuum aspiration system.
59.Wash the cells once with PBS (5 ml PBS/75-cm2 culture flask).
60.Discard the PBS and add 2 ml of Accutase solution (incubate for 5 min at room temperature) in order to detach the cells.
61.Stop the reaction by adding 5 ml of MSC medium.
62.Combine a 90-µl aliquot of the cell suspension with 10 µl of 0.4% trypan blue solution in a microcentrifuge tube and mix.
63.Transfer 10 µl of the mix to a Burker chamber and count viable cells.
64.Place 17 × 103/cm2 cells in 1 ml/well of adipogenic differentiation medium in a 24-well plate (this corresponds to ∼30,000 cells/well). Incubate at 37°C in a 5% CO2 incubator.
65.Also use commercially available hMSCs or hMSCs that have already been tested, as a positive control for the ability to differentiate into adipocytes.
66.Change medium twice a week.
67.Remove medium after 3 weeks and wash twice with 500 µl/well of PBS.
68.Add 400 µl/well of Oil red O solution.
69.Incubate at room temperature for 20 min.
70.Remove Oil red O solution.
71.Add 400 µl/well of 60% isopropanol and incubate at room temperature for 10 s.
72.Remove and wash twice with 500 µl/well of PBS.
73.Visualize the red-stained lipid droplets, indicative of adipogenic cell lineage, using an inverted microscope.
Multilineage differentiation potential: Osteogenic differentiation
74.Follow steps 57-63 in “Adipogenic differentiation.”
75.Place 17 × 103 cells/cm2 in 1 ml/well of osteogenic differentiation medium in a 24-well plate (this corresponds to ∼30,000 cells/well). Incubate (at 37°C in a 5% CO2 incubator)
76.Use also commercially available hMSCs or hMSCs already tested, as a positive control for the ability to differentiate into osteoblasts.
77.Change medium twice a week.
78.Remove medium after 3 weeks and wash with 500 µl/well of PBS.
79.Add 400 µl/well of alkaline phosphatase substrate solution and incubate at room temperature for 10 min.
80.Remove solution and wash twice with PBS (500 µl/well).
81.Add 400 µl/well of Alizarin red S solution and incubate for 20 min at room temperature.
82.Remove and wash twice with 500 µl/well of distilled water.
83.Visualize the red staining of the calcium deposits, and violet staining for the presence of alkaline phosphatase, using an inverted microscope.
hMSC cryo-preservation
Once the cells have been verified, use the remaining part of the cells/MSCs (about 60 × 106) obtained at Passage 1 and proceed to their cryopreservation using a defined, animal-component free, and protein-free cryo-SFM preservation medium, in order to store hMSCs long-term in house.
84.Take the cell suspension from step 31 in the 50-ml tube, at Passage 1, and centrifuge 4 min at 100 × g , room temperature.
85.Aspirate the supernatant (without disturbing the cells) from the pellet.
86.Resuspend the cells in freezing medium at a density of 1-1.5 × 106 viable cells/ml in cryogenic vials (2 ml).
87.Place the cryovials, containing cells and freezing medium, in a Cryo freezing container with isopropanol (Mr. Frosty). Store Mr. Frosty at –80°C overnight.
88.The next day, transfer the cryovials to a liquid nitrogen container and store the cells until use.
hMSCs thawing and sub-culturing
89.Use one or more cryogenic vials (at P1) (depending on the number of planned experiments) for thawing and sub-culturing.
90.Rapidly transfer a cryogenic vial containing cryopreserved hMSCs (1 ml) from the liquid nitrogen container to a 37°C water bath.
91.Thaw cells by gentle agitation until most, but not all, of the contents are thawed.
92.Remove the cryogenic vial from the water bath, rinse the cryovial externally with 70% ethanol solution, and then transfer it into laminar flow hood. Let the remaining cells thaw.
93.Transfer the thawed hMSCs suspension (1 ml) into a sterile 15-ml tube and slowly add 4 ml of MSC medium (pre-warmed at 37°C).
94.Gently pipette solution up and down to ensure homogenous cell distribution.
95.Combine a 90-µl aliquot of the cell suspension with 10 µl of 0.4% trypan blue solution in a microcentrifuge tube and mix.
96.Transfer 10 µl of the mix to a Burker chamber and count viable cells.
97.Prepare the appropriate number of flasks for your experiment with the corresponding amount of pre-warmed MSC medium (12 ml of medium/75 cm2 flask) and seed cells at a density of 4000 cells/cm2.
98.Incubate the flask with hMSCs in a CO2 incubator at 37°C.
99.The next day, replace MSC medium with 12 ml of fresh MSC medium (pre-warmed to 37°C).
100.When 70%-80% cell confluence is reached (about 3-4 days after the thawing), prewarm the solutions, namely PBS and MSC medium, to 37°C for at least 30 min before starting the procedure; let Accutase solution warm at room temperature.
101.Remove the medium using a vacuum aspiration system, and wash the cells once with 5 ml of PBS (prewarmed).
102.Discard the PBS using a vacuum aspiration system.
103.Add 2 ml of Accutase solution and incubate for 5 min for cell detachment.
104.Stop the reaction by adding 3 ml of MSC medium (prewarmed).
105.Transfer the hMSCs with Accutase solution + medium to a 15-ml centrifuge tube and carefully pipette up and down to obtain a single-cell suspension (i.e., without the cell aggregation).
106.Combine a 90-µl aliquot of the cell suspension with 10 µl of 0.4% trypan blue solution in a microcentrifuge tube and mix.
107.Transfer 10 µl of the mix to Burker chamber and count viable MSCs.
108.At this point, freeze a portion of the hMSCs to prepare a cell bank before initiating neuronal transdifferentiation (Basic Protocol 2). For the remaining cells (about 5-7 × 106), follow the next steps to subculture them.
109.For subculturing (e.g., P2), plate the cells at a density of 4000 cells/cm2 cells in a 75-cm2 flask with 12 ml of MSC medium.
110.Incubate at 37°C in atmosphere of 5% CO2 and split the cells when 70%-80% confluence is reached (after 3-4 days).
111.Follow steps 37-42 in “Specific surface antigen expression.”
112.Use part of these cells (P3) for neuronal transdifferentiation (Basic Protocol 2).
Basic Protocol 2: TRANSDIFFERENTIATION OF hMSCs INTO NEURON-LIKE CELLS (hNLCs) AND BASIC NEUROTOXICITY ASSESSMENT
This protocol describes how to differentiate the hMSCs generated in Basic Protocol 1 into neuron-like cells (hNLCs), to establish a human primary cell in vitro model for neurotoxicity testing.
The transdifferentiation process into hNLCs, from undifferentiated (1 hr after induction) to the fully differentiated (8 days) stage, can be monitored by the accompanying striking changes in cell morphology, with the formation of neural dendrites and axons, and by analyzing the expression of some neuron-specific markers such as β-Tubulin III (β-Tub III), microtubule-associated protein 2 (MAP-2), and enolase (NSE), although other endpoints can be evaluated.
The effects caused by chemicals/drugs/xenobiotics/emerging contaminants (including nanomaterials) can be assessed at various stages of the neuronal differentiation process of hMSCs, such as at the early- (at 2-3 days after induction) or mid-differentiation (at 4-5 days after induction) stages, and at fully differentiated neuron-like stages (at 8 days after differentiation).
A general approach to use hNLCs for assessing developmental neurotoxicity is also described here, and is based on studying different endpoints, such as evaluating changes in neuronal morphology, cell viability, and mitochondrial function (Buzanska et al., 2009; Radio & Mundy, 2008; Shi et al., 2018; Singh et al., 2016). Morphological changes of the hMSCs versus a neuronal-like phenotype can be observed during different stages of the neuronal differentiation process using phase-contrast microscopy; cell viability can be evaluated through the assessment of the membrane integrity using the trypan blue exclusion test or mitochondrial dehydrogenase activity by MTT assay; mitochondrial function can be evaluated by assessing cytosolic ATP content. In addition, expression of various neuronal markers can be evaluated by immunofluorescence staining. For this, some commonly studied markers include nestin (an intermediate filament protein that is specifically found in the precursors to neuron), glial fibrillary acidic protein (GFAP, an astrocyte marker), β-Tub III (a microtubule element of the tubulin family, structural marker predominantly in neurons), MAP-2 (a mature neuron marker), NSE (cytoplasmic protein expressed by mature neurons), and SOX-2, a key transcriptional factor for pluripotent stem cell regulation and a marker for undifferentiated stem cells.
An outline of the workflow of this protocol is shown in Fig. 5.
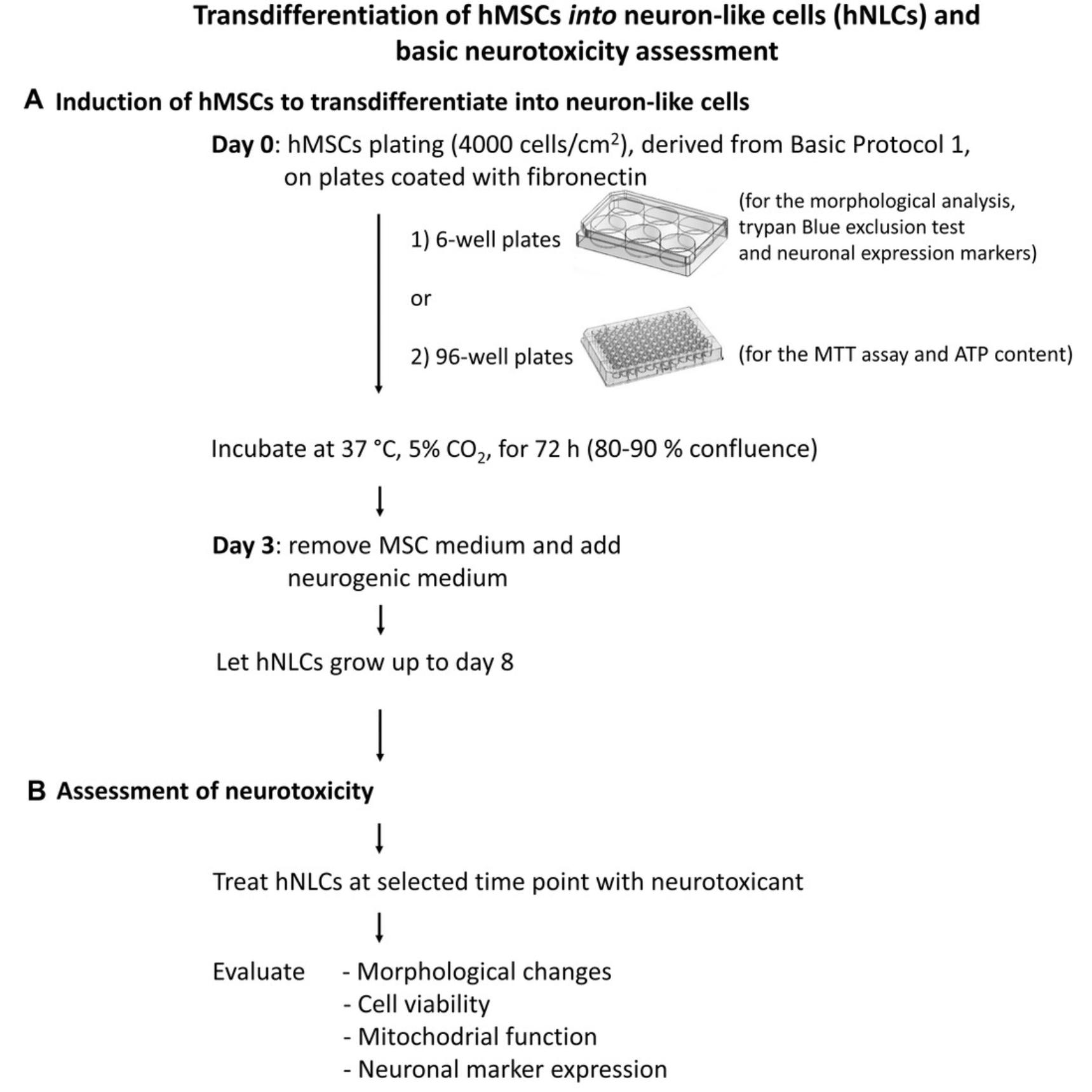
Materials
-
1 mg/ml human fibronectin solution (PromoCell, cat. no. C43060)
-
hMSCs (from Basic Protocol 1)
-
Mesenchymal stem cell growth medium 2, ready-to-use (MSC medium; see recipe)
-
Complete mesenchymal stem cell neurogenic differentiation medium (Ready-to-use) (neurogenic medium; see recipe)
-
Test drug/chemical of interest
-
Trypan blue solution 0.4% (Corning, cat. no. 25-900-CI)
-
MTT ((3-(4,5-dimethylthiazol-2-yl)-2,5-diphenyltetrazolium bromide) (Sigma-Aldrich, cat. no. M-2128)
-
ATP reagent (CellTiter-Glo® 3D reagent, Promega, cat. no. G9681)
-
Phosphate-buffered saline (1× PBS), without calcium, without magnesium (Carlo Erba Reagents, cat. no. LJ67802AP)
-
4% paraformaldehyde (PF; see recipe)
-
Triton X-100 (Sigma-Aldrich, cat no. X100)
-
Dry milk (BioRad, cat. no. 170-6404)
-
Primary antibodies:
- Anti-human NSE conjugated to Alexa-Fluor® 594 (Santa Cruz Biotechnology, cat. no. sc-271384-AF594)
- Anti-human β-Tub III conjugated to Alexa-Fluor® 488 (Merck, cat. no. AB15708A4)
- Anti-human MAP-2 conjugated to Alexa-Fluor® 488 (Merck, cat. no. MAB3418X)
- Anti-human nestin conjugated to Cy3 (Merck, cat. no. MAB5326C3)
- Anti-human SOX2 conjugated to Alexa-Fluor® 488 (BioLegend, cat. no. 656110)
- Anti-human GFAP (Santa Cruz Biotechnology, cat. no sc-33673)
-
Secondary antibody:
- Goat anti-Mouse IgG2b conjugated to Alexa-Fluor® 488 (Invitrogen, cat. no. A-21141)
-
Hoechst 33258 (Invitrogen, cat. no. H3569)
-
Fluoroshield (Sigma-Aldrich, cat. no. F6182)
-
Laminar flow hood (Gelaire, BSB 4 - S, Class II)
-
6-well plates (SPL, cat. no. 30006)
-
96-well plates (SPL, cat. no. 330035)
-
CO2 incubator (Thermo Scientific, Hera Cell 150i)
-
Vacuum aspiration pump (Vacuum Technology s.r.l., DVP)
-
Inverted microscope (Zeiss Axiovert 25 microscope equipped with 10× objective and 32× phase contrast objective)
-
Digital camera
-
96-well plates: black well/clear bottom plate (ThermoFisher Scientific, cat. no. 165305)
-
Adhesive white light-reflecting film (VWR, cat. no. 89087-696)
-
Aluminum foil
-
Humidity chamber: e.g., plastic box with moistened paper towels
-
Fluoroskan™ FL Microplate Fluorometer and Luminometer (Fluoroskan Ascent FL, Thermo Scientific)
-
Water bath 37°C (ASAL S.R.L., 720 D)
-
Orbital shaker (TWISTER TW 3)
-
Fluorescence microscope (Olympus, CX41)
-
Microscope coverglass (VWR, 22 × 22 mm, cat. no. ECN631-1570)
-
Additional reagents and equipment for MTT assay (Aras, Hartnett, & Aizenman, 2008) or trypan blue exclusion (TB) test (see Current Protocols article: Strober, 2015)
Induction of hMSCs for transdifferentiaton into neuron-like cells
1.Coat 96- or 6-well plates with a 10 µg/ml solution of human fibronectin for 1 hr at room temperature, under laminar flow hood.
2.After 1 hr of incubation, remove the coating solution and let dry under laminar flow hood for 20-30 min before plating hMSCs.
3.Thaw hMSCs from one cryopreserved vial (1 ml) (higher than Passage 1, from Basic Protocol 1). To do this, follow the procedure described in steps 90-107 in Basic Protocol 1.
4.Seed the hMSCs at a density of 4000 cells/cm2 using MSC medium: 2 ml of medium/well for coated 6-well plates or 100 µl of medium/well for coated 96-well plates.
5.Incubate at 37°C in an atmosphere of 5% CO2 and let the hMSCs adhere until they reach 80% confluence (after 72 hr).
6.Change the medium every 48 hr.
7.When the cells reach 80% confluency, aspirate the MSC medium from each well using a vacuum aspiration system.
8.Proceed to the neuronal transdifferentiation of hMSCs by adding 2 ml/well to the 6-well plates or 100 µl/well to the 96-well plates of neurogenic medium (prewarmed at 37°C). Incubate the cells at 37°C in an atmosphere of 5% CO2 for at least 3 days and then up to 8 days.
9.Change the neurogenic medium every 48 hr up to 8 days (fully differentiated neuronal stage) and use the cells for testing at different time points, from day 2 (early differentiated neuronal stage) to day 8 (fully differentiated neuronal stage), depending on your experimental goals/design.
10.Proceed with the treatment of hNLCs with the putative testing compound at different concentrations using one of these two approaches, depending on your experimental goals/design:
-
Treat the hMSCs at the beginning of the neuronal induction and proceed to test at selected time points of the neuronal differentiation process: e.g., at day 2 (early differentiated neuronal stage), at day 4 (mid differentiated neuronal stage), or at day 8 (fully differentiated neuron-like cells).
or
- b.Treat hNLCs when fully differentiated (at day 8) and proceed to test after the scheduled exposure time (e.g., after 24 and 48 hr) defined in your project.
11.Follow the next steps to evaluate the impact of toxicant exposure on the hNLCs using your endpoint of interest.
Assessment of neurotoxicity
Cell morphology
12.After exposure to a compound (with any of the approaches described in step 10), observe the cells by phase-contrast microscopy and verify whether the treatment affects the neuron-like phenotype, including the retraction of the cytoplasm towards the nucleus, cytoplasmic extensions, and loss of interaction between cells, compared to controls.
13.Capture microscopic fields, randomly selected, using a digital camera, and store them, in order to record any morphological change.
14.Count the number of cells exhibiting neurites and report it as the percentage compared to untreated hNLCs.
Cell viability
15.After treatment of the hNLCs with the test compound, evaluate cell viability using the MTT assay (Aras, Hartnett, & Aizenman, 2008) or trypan blue exclusion (TB) test (see Current Protocols article: Strober, 2015) according to standard procedures for each assay.
Mitochondrial function
16.After exposure to a compound, evaluate mitochondrial function using a commercial kit (CellTiter-Glo® 3D reagent) and perform the assay according to the manufacturer's specifications.
Neuronal expression markers
17.After exposure to a compound, aspirate the neurogenic medium from each well of the 6-well plates containing hNLCs and wash once with PBS (2 ml/well).
18.Discard PBS and proceed to fix the hNLCs with the addition of 4% PF (2 ml/well) for 30 min at room temperature.
19.Remove PF and permeabilize for 5 min using 0.1% Triton X-100 in PBS.
20.Rinse three times with PBS (2 ml/well).
21.Block using 2% dry milk in PBS (2 ml/well) for 30 min at room temperature.
22.Remove the blocking solution, then:
- For direct immunofluorescence: Incubate cells with the Alexa-Fluor®488 or 594 or Cy3 conjugated primary antibodies against: β-Tub III (1:100), MAP-2 (1:100), NSE (1:100), nestin (1:100), and SOX-2 (2 µg/ml) (all diluted in blocking solution) for 60 min in the dark (using aluminum foil to cover the plate) on an orbital shaker at room temperature.
If you use antibodies with different emission wavelengths, you can use different ones in the same well: for example, human β-Tub III antibody conjugated to Alexa-Fluor®488 and nestin conjugated to Cy3 can be added in the same well, since the emission wavelength of each antibody is different (the emission for β-Tub III is green, while that for nestin is red). In this case, it is possible to differentiate the two protein expression profiles. On the contrary, since human antibodies β-Tub III conjugated to Alexa-Fluor®488 and SOX2 conjugated to Alexa-Fluor®488 have the same emission wavelength, each of them should be added to cells in different wells.
-
For indirect immunofluorescence: Incubate cells with a primary antibody against GFAP (1:100) overnight at 4°C (in a humidity chamber) and then wash three times with PBS (2 ml/well), for 5 min each time. Stain with secondary antibody (Alexa-Fluor®488-labeled; dilution 1:100) for 60 min in the dark (using aluminum foil to cover the plate) on an orbital shaker at room temperature.
23.After incubation with conjugated primary or secondary antibodies, wash three times (5 min each washing) with PBS (2 ml/well).
24.Remove PBS and then stain the nuclei using 1 ml/well of Hoechst 33258 5 µM solution for 10 min at room temperature.
25.Wash twice with PBS (2 ml/well) and remove it.
26.For each well, mount with Fluoroshield (two drops/well) and then cover well with a coverglass and let dry.
27.Visualize immunostaining using a fluorescent microscope equipped with oil-immersion objective (100×) lens using appropriate filters.
28.Capture, using a digital camera, randomly selected microscopic fields, store them, and analyze the fluorescence signal using imaging software. A decrease in fluorescence signal, compared to that evidenced in control, indicates the degree of toxicity caused by the test compound.
REAGENTS AND SOLUTIONS
Alkaline phosphatase substrate solution
- 1 Tablet of Sigma Fast (Sigma-Aldrich, cat. no. B5655)
- 10 ml distilled H2O
Alizarin red S solution
- 2 g Alizarin red S (Sigma-Aldrich, cat. no. A5533)
- 100 ml distilled H2O
- Adjust to pH 5.3-5.5 with 0.5% NH4OH (Sigma-Aldrich, cat. no. 221228)
- Adjust volume to 100 ml
- Filter with 0.2-µm flow filter unit (Thermo Scientific, cat. no. 565-0020)
Adipogenic differentiation medium (for 20 ml)
- 20 ml α-MEM 10% Mesencult (Stem Cell Technology, cat. no. 05402)
- 20 µl dexamethasone stock solution (see recipe)
- 200 µl sodium l-ascorbate stock solution (see recipe)
- 20 µl insulin stock solution (see recipe)
- 2 µl IBMX stock solution (see recipe)
- 4 µl indomethacin stock solution (see recipe)
- All solutions should be prepared fresh
β-Glycerophosphate stock solution
- 2.16 g β-glycerophosphate (Sigma-Aldrich, cat. no. G9891)
- 10 ml 1× PBS, without calcium, without magnesium (Carlo Erba Reagents, cat. no. LJ67802AP)
- Store at –80°C in working aliquots (500 µl)
Complete DMEM medium (for 500 ml)
- 500 ml Dulbecco's Modified Eagle's Medium, high glucose (DMEM; Sigma-Aldrich, cat. No. D5671)
- 50 ml sterile fetal bovine serum (FBS; USA origin Sigma-Aldrich, cat. no. F2442)
- 5 ml 200 mM l-glutamine solution (BioXtra, cat. no. G7513)
- 2.5 ml penicillin/streptomycin with 10,000 U penicillin and 10 mg streptomycin per ml (Sigma-Aldrich, cat. no. P0781)
Medium can be stored up to 2 months at 4°C. Avoid heating to reduce degradation of glutamine and growth factors.
Complete mesenchymal stem cell growth medium 2 (for 500 ml)
- Ready-to-use Kit (PromoCell, cat. no. C28009) including:
- 450 ml Basal Medium
- 50 ml Supplement Mix
Medium can be stored up to 6 weeks at 4°C in the dark. Avoid heating, to reduce degradation of glutamine and growth factors.
For use, prewarm only an aliquot of the complete medium and keep the remaining refrigerate at 4°C.
Complete mesenchymal stem cell neurogenic differentiation medium (for 100 ml)
- Ready-to-use Kit (PromoCell, cat. no. C28015) including:
- 90 ml Basal Medium
- 10 ml Supplement Mix
Dexamethasone stock solution, 10-4 M
- 1 mg dexamethasone (Sigma-Aldrich, cat. no. D1756)
- 1 ml ethanol (Sigma-Aldrich, cat. no. 443611)
- 49 ml α-MEM (Gibco, cat. no. 32561-029)
- Store at –80°C in working aliquots (500 µl)
Indomethacin stock solution
- 1 g indomethacin (Sigma-Aldrich, cat. no. I7378)
- 11.5 ml DMSO (Alchimia, cat. no. CRN001-00)
- Store at –80°C in working aliquots (500 µl)
Insulin stock solution
- 350 mg insulin (Sigma-Aldrich, cat. no. I4011)
- 35 ml 1× PBS, without calcium, without magnesium (Carlo Erba Reagents, cat. no. LJ67802AP)
- 0.12 ml 5 M HCl (Sigma-Aldrich, cat. no. 320331)
- Store at –80°C in working aliquots (500 µl)
3-Isobutyl-1-methylxanthine stock solution (IBMX)
- 100 mg IBMX (Sigma-Aldrich, cat. no. I5879)
- 9 ml absolute ethanol (Sigma-Aldrich, cat. no. 443611)
- Store at –80°C in working aliquots (500 µl)
Isopropanol, 60%
- 30 ml 99.5% isopropanol (Sigma Aldrich, cat. no. I9516)
- 20 ml distilled H2O
Osteogenic differentiation medium (for 20 ml)
- 20 ml α-MEM 10% Mesencult (Stem cell Technology, cat. no. 05402)
- 20 µl dexamethasone stock solution (see recipe)
- 200 µl sodium l-ascorbate stock solution (see recipe)
- 100 µl β-glycerophosphate stock solution (see recipe)
- All solutions should be prepared fresh
Paraformaldehyde (PF) solution (for 100 ml), 4%
- 4 g paraformaldehyde (Sigma-Aldrich, cat. no. P6148)
- 100 ml 1× PBS without calcium, without magnesium (Carlo Erba Reagents, cat. no. LJ67802AP)
Store the 4% PF at 4°C and use it up to 1 week, or store at –20°C for up to 12 months in order to preserve reactivity of aldehydes. Do not re-freeze aliquots once thawed.
CAUTION: PF is classified as harmful if inhaled and swallowed, irritating to the eyes and the skin, sensitizing after contact with the skin, and possibly carcinogenic. Therefore, it is compulsory to work under a chemical hood and wear all the appropriate personal protective equipment, which are, at least: gloves, lab clothes, glasses, and mask with protection filter type P3. Keep available the Material Safety Data Sheet of the paraformaldehyde. It is strongly recommended to perform all procedures under the chemical hood.
Sodium l-ascorbate stock solution
- 200 mg sodium l-ascorbate (Sigma-Aldrich, cat. no. A7631)
- 40 ml 1× PBS, without calcium, without magnesium (Carlo Erba Reagents, cat. no. LJ67802AP)
- Store at –80°C in working aliquots (500 µl)
COMMENTARY
Background Information
The methods described in this article outline a procedure to obtain human primary neuron-like cells from human UC lining membrane to establish an in vitro model for the assessment of neuronal toxicity of potential neurotoxic compounds in humans.
Current test methods for the evaluation of the neurotoxicity potential of chemicals use complex in vivo tests with animal models, which are often too laborious and expensive, and might also not properly reflect the human situation because of inter-species variation (Leist et al., 2014). Hence, alternative methods are being pursued, including in vitro and in silico methods. These are particularly relevant given the general interest in reducing the number of animals used in testing to a minimum. It is now recognized that the future of xenobiotic safety assessment must move away from animal tests towards a combination of complementary approaches that address functional mechanistic endpoints tied to adverse outcomes of regulatory concern (Bal-Price et al., 2015; Bal-Price & Pistollato, 2019; Daneshian et al., 2015; European Commission, 2013; Hartung, 2010; http://ec.europa.eu/growth/sectors/cosmetics/animal-testing_en; https://www.science.org/content/article/us-epa-eliminate-all-mammal-testing-2035; Krewski et al., 2020; Makris et al., 2009).
Primary and human stem cell models have gained increased interest in toxicology research (Zakrzewski et al., 2019; Wobus & Löser, 2011). Various stem cell types can potentially be used, including (i) embryonic stem cells (ESC), isolated from the inner cell mass of blastocysts; (ii) stem cells isolated from adult tissues, like the mesenchymal stem cells (MSC); and (iii) induced pluripotent stem cells (iPSC), which are adult somatic cells reprogrammed to pluripotency (Patel & Yang, 2010). iPS cells are the most promising among those classified as pluripotent because of their high plasticity, similar to ESC, but without the ethical issues associated with their isolation. iPS cells can be derived directly from adult cells through gene reprogramming. The level of expertise required for iPSC culture, however, limits their application as a screening tool in general cell culture laboratories (Zomer, Vidane, Gonçalves, & Ambrósio, 2015).
Human MSCs, on the other hand, can be isolated from discarded tissues post surgery, and cultured by explant/enzymatic methods with minimal laboratory set-up and without genetic manipulations (Patil, Kharat, Kulkarni, Kheur, & Bhonde, 2018). They can be obtained in large quantities and through noninvasive methods from adult tissues. Moreover, they are nontumorigenic and have been widely studied. MSCs were first described by Friedenstein and colleagues in 1966 as a population of adherent, colony-forming, fibroblast-like cells able to undergo osteogenic differentiation (Friedenstein, Piatetzky-Shapiro, & Petrakova, 1966). Since then, MSCs have been isolated from numerous human tissues, including bone marrow, adipose tissue, muscles, peripheral blood, umbilical cord, placenta, fetal and amniotic fluid, and dental pulp, and have been cultured in vitro (Taran et al., 2014; Zomer et al., 2015). MSCs were originally isolated from the bone marrow (BM) (Friedenstein, Petrakova, Kurolesova, & Frolova, 1968), and this population is still considered the gold standard for MSC applications. Nevertheless, the BM has several limitations as a source of MSCs, including low frequency of MSCs in this compartment, a painful isolation procedure, and the decline in MSC characteristics with donor's age. Compared to BM stem cells, hUC-MSCs have a painless collection procedure and faster self-renewal properties. Importantly, the number of MSCs that can be obtained from one UC greatly exceeds the mesenchymal stem cells that can be derived from bone marrow, cord blood, and adipose tissue (Lim & Phan, 2014).
In particular, the hMSCs derived from the UC have multiple advantages over other tissues, including:
- (i) Simple and convenient preparation—MSCs from the UC or umbilical blood (UB) have the same advantage of being a source of young donor cells, but crucially the UC-hMSCs are easier and less expensive to harvest and grow compared to UB cells (Raileanu et al., 2019).
- (ii) Feasible source—UC-hMSCs can be easily obtained from healthy donors following a non-invasive and ethically acceptable collection procedure.
- (iii) More “primitive” properties (i.e., fetal in nature)—UC-hMSCs show a gene expression profile similar to that of pluripotent SCs, such as embryonic SCs (Jin et al., 2013; Nagamura-Inoue & He, 2014). Moreover, UC-hMSCs have a unique combination of prenatal and postnatal SC properties, and they exhibit genetic stability for several in vitro passages (Arutyunyan, Elchanino, Makaro, & Fatkhudino, 2016);
- (iv) Higher proliferation capacity—UC-hMSCs possess rather fast self-renewal. For example, doubling time is about 20 hr for UC vs. 70 hr for bone marrow-derived ones (Coccini et al., 2019).
Furthermore, the number of human MSCs that can be obtained from UC (about 65 million MSCs from 3 cm of UC at Passage 1) exceeds that from UB, bone marrow (about 1500-3000 MSCs/ml BM), and adipose tissue (2-6 million/300 ml AT) (Coccini et al., 2020; Goulart et al., 2018; Lim & Phan 2014; Pittenger et al., 1999).
The procedure to isolate the MSCs, described in the present protocol, is based on an approach applied by other groups (Amari et al., 2015; Beeravolu et al., 2017; Chen et al., 2016; Mennan et al., 2013; Salehinejad et al., 2015; Wang et al., 2004), with some modifications, and consists in manually removing, from the UC segment, the umbilical cord vessels, scraping off WJ, and then cutting and treating the tissue pieces enzymatically. Others, instead, directly cut down the whole UC into smaller segments, strip the vessels, and then proceed with the enzymatic digestion, followed by filtering or centrifugation to remove the tissue fragments (Karahuseyinoglu et al., 2007; Kikuchi-Taura et al., 2012; Kita, Gauglitz, Phan, Herndon, & Jeschke, 2010; La Rocca et al., 2009; Majore, Moretti, Stahl, Hass, & Kasper, 2011; Mennan et al., 2016; Mitchell et al., 2003; Pereira, Khushnooma, Madkaikar, & Ghosh, 2008). Other authors have also reported subjecting the UC segment to a single longitudinal cut, without dissection or enzyme digestion, with the disadvantage that some residual endothelial contamination is present in the first passages (De Bruyn et al., 2011; La Rocca et al., 2009). Indeed, the optimal method for an efficient isolation of a homogeneous and well-defined population of UC-MSCs has not been defined thus far. In this context, our method could be considered as a robust alternative, since it relies on two important advancements: first, the simplicity of MSC isolation, leading to well-characterized and highly proliferating human MSCs; and secondly, the achievement of a large number of hMSCs to use for differentiation into human primary neuron-like cells.
There are two crucial components in our procedure. First, we use a commercial medium, namely, mesenchymal growth medium 2, a medium with an optimized low-serum formulation designed for the in vitro expansion and routine culture of human MSCs (patented formulation by PromoCell). This specific medium has been used by other labs to maintain in culture hMSCs derived from bone marrow and adipose tissue (Padiolleau et al., 2020; Takahashi et al., 2017). Second, we use an Accutase solution, developed for very gentle and effective detachment and dissociation of anchorage-dependent cells from flask surfaces. Moreover, the Accutase solution, containing a well-balanced combination of proteolytic and collagenolytic enzymes, ensures that surface proteins and epitopes stay intact.
The ability of MSCs to proliferate and differentiate into multiple lineages makes them ideal for testing the impact of test compounds on different cell types (Ankrum, Ong, & Karp, 2014; Viswanathan et al., 2019). In particular, MSCs can differentiate into adipocytes, chondrocytes, and osteoblasts, and into endodermal lineages, e.g., cardiomyocytes and hepatocytes, as well as into ectodermal lineages, especially neuronal-lineage cells consisting of neurons and glia (George, Hamblin, & Abrahamse, 2019). Notably, the derived neuron-like cells may represent a human cell–based model for neurotoxicity testing, as discussed in this article. One of the major considerations for progress in replacing animals in neurotoxicity testing embraces the development of in vitro assays needed as part of a test battery that encompasses relevant endpoints of neurotoxicity and identification of the mechanisms of neurotoxicity (Worth & Balls, 2002; Prieto et al., 2005).
The procedure detailed here allows the use of UC-MSCs for obtaining hNLCs as a promising source of human primary cells. The procedure is easy and based on optimized serum-free medium, namely, mesenchymal stem cell neurogenic differentiation medium, consisting of a basal medium and a mix of growth factors and supplements (patented and sold by PromoCell), which has been subjected to comprehensive quality control tests for maintenance of differentiation capacity of hMSCs. In addition, it is free from animal-derived culture supplements that can induce a xenogeneic response. Several studies have used a protocol similar to ours, including the same neurogenic medium, for the transdifferentiation of hMSCs from different sources (e.g., bone marrow, UC, dental pulp) (Czarnecka et al., 2017; Díez, Bauman, Gajardo, & Jorquera, 2015; Koaykul, Kim, Kawahara, Yuge, & Kino-Oka, 2019; Seonwoo et al., 2018). A drawback of this medium, however, is its high price. Other protocols thus use neural induction media that are lab-made. These are generally serum-free and are composed of a cocktail of growth factors (e.g., BDNF, bFGF, NGF, etc.) and/or small molecules (e.g., retinoic acid, forskolin, etc.) added in different concentrations and combinations for driving hMSCs towards neuronal lineage (Cortés-Medina et al., 2019; Jang, Cho, Cho, Park, & Jeong, 2010; Kil et al., 2016; Nan et al., 2016; Shi et al., 2018; Yang et al., 2013). These protocols, however, can take several weeks.
In contrast, hMSCs transdifferentiation into hNLCs using the protocol described here is an easy procedure that takes a maximum of 8 days. The high cost of neurogenic medium is certainly a limitation; however, it is ready-to-use and does not require special attention from the user. Again, using this commercially standardized formulation medium can help overcome the problem of extensive lot-to-lot variability associated with the use of lab-made medium.
Human primary NLCs derived as described in this article have been used in our lab to evaluate the cytotoxicity induced by different compounds, such as a new psychoactive drug, namely MAM 2201 (Coccini et al., 2021), and magnetite nanoparticles (Fe3O4NPs) (Coccini et al., 2020; De Simone et al., 2020). The cytotoxic effects were assessed at various stages of neuronal maturity, such as in early (at 2-3 days after induction) or mid-differentiation (at 4-5 days after induction) cells, and on fully differentiated neuron-like cells (at 8 days after differentiation). When the fully differentiated cells (at day 8) were treated with increasing MAM 2201 concentrations (1-30 µM; 4-48 hr), the cytotoxic effects included increased cell mortality and apoptosis, changes in morphology, mitochondrial dysfunction, and decrease of neuronal marker expression (i.e., MAP-2 and NSE) already at 5 μM, appearing early (3 hr) and persisting after 24 and 48 hr. Comparatively, differently from hNLCs, human SH-SY5Y-neurons, a cancer-derived cell line, were not susceptible to MAM 2201 tested under the same conditions (Coccini et al., 2021). So far, the in vitro neurotoxicity of MAM 2201 has been predominantly evaluated on rodent brain cultures (Costain et al., 2016; Irie et al., 2015; Tomiyama & Funada, 2011, 2014; Tauskela et al., 2016). By comparing in vitro animal data with that obtained from hNLCs after MAM 2201 treatment, higher susceptibility was observed in the human NLCs, suggesting that human cells may be more susceptible than animal models (Coccini et al., 2021).
hNLCs (at day 8) have also been used to evaluate the cytotoxicity effects of Fe3O4NPs (10-100 µg/ml, 24-48 hr). The study evidenced that hNLCs were susceptible to NP treatment, and exhibited a concentration- and time-dependent reduction of cell viability, ATP intracellular content, cell density, and apoptotic effects (De Simone et al., 2020). When the effects of these nanoparticles on hNLCs were compared with those in the SH-SY5Y cell line, an extensively used in vitro model for CNS toxicity, the hNLCs were more susceptible than the tumor cell line (Coccini, Caloni, Cando, , & De Simone, 2017).
Our recent study also demonstrated that Fe3O4NPs affected, in a concentration-dependent manner, the neuronal differentiation process of primary human NLCs, with effects starting at an early stage (day 2) of the neuronal process and persisting up to day 8, in terms of cell viability and ATP content decrease, a decrease in the expression of neuron-specific markers (i.e., β-Tub III, MAP-2 and NSE) and the neuronal precursor marker nestin, and apoptotic effects (Coccini et al., 2020). Other studies, using murine neuronal stem cells, demonstrated effects of iron oxide NPs on the actin cytoskeleton and microtubule network architectures, although the changes were evident only at higher concentrations compared to those found in our human neuronal model (hNLCs) (Soenen, Nuytten, De Meyer, De Smedt, & De Cuyper, 2010). The possibility that NPs could interfere with neuronal differentiation has been observed in previous investigations using different types of NPs tested on human cell lines (i.e., SH-SY5Y) and murine neuronal or embryonic stem cells. The studies evidenced interference on cytoskeleton and microtubule network architectures, and a reduction of neuron specific β-Tub III expression and reduction of neurite generation (Ducray et al., 2017; Gliga et al., 2017; Rostami, Kouchesfahani, Kiani, & Fakheri, 2015). Comparatively, these findings showed, once again, less susceptibility of these cells (i.e., animal models and tumor cell lines) compared to human primary NLCs.
The proposed in vitro cell-based model (hNLCs) described here, together with an array of in vitro assays covering different modes of action, has the potential to be used as a powerful tool for predicting human-specific neurotoxicity induced by emerging toxic compounds.
Critical Parameters
Obtaining large quantities of high-quality hMSCs from cord lining membrane is critical for the success of the procedure outlined in Basic Protocol 1. Umbilical cord samples must be neither contaminated nor degraded during transport or sample processing. In addition, rapid and refrigerated transport from the hospital to the lab has to be ensured: the sample must be harvested in a collection cup containing physiological solution, stored at 4°C, and immediately transported, on ice, to the lab, and processed within 2 hr from collection, under strict aseptic conditions. In our experience, the number of hMSCs at P0, obtained at the end of Base Protocol 1, depends on different critical factors, such as user expertise and the number and size of seeded CL pieces. The collection of the CL tissue is the most critical aspect in terms of manipulation from the user. It is important to develop the skills to properly identify the umbilical cord parts (CL membrane, Wharton's jelly, and arteries and vein) and for removing all Wharton's jelly and vessels (vein and arteries) from CL membranes, since (i) their partial elimination could result in contamination of the CL-hMSCs with hMSCs derived from the other components, and (ii) a small or damaged CL sample can be obtained, with the consequent isolation of few cells.
Another critical point for the robustness of the results is to obtain perfect adherence of the partially digested CL pieces to the bottom of the culture flask. Following the attachment of the pieces, cell release is promoted in the plane of the solid substrate. Specifically, users must pay close attention during medium addition, since this procedure can cause detachment of some CL pieces, resulting in lower cell production. We recommend adding a small amount of medium to the culture flasks, only covering the tissue pieces, for the first 2 hr of culture, and then carefully adding the remaining medium and incubating the flasks for 3-4 days. Even for the next 10 days, improper handling of the culture flasks can cause the pieces to detach, so it is recommended to pay close attention when moving the culture flasks from the incubator to the hood and during microscope observation. If there is detachment during the first 3 days, users can transfer the floating pieces and rescue them into new culture flasks for re-attachment and culturing. If this procedure does not lead to re-attachment of these CL pieces, it is advisable to discard the flasks; cell yield will be low without the necessary number of CL pieces. The choice of the correct CL piece size is also a determining factor for obtaining a high number of isolated cells. Contrary to what many users might expect, culturing large explanted CL pieces does not improve cell outgrowth efficiency, and instead users should carefully select very small and partially digested tissue pieces, to increase cellular release. In addition, the number of explanted pieces should be no more than 20 per 75-cm2 tissue culture flask, since too little space between the pieces or too many pieces per flask are inhibitory for cell outgrowth.
Another crucial element is the culture medium used. In our protocols, optimized media (ready to use) are utilized for both isolation of human MSCs and transdifferentiation of hMSCs into hNLCs: the media are subjected, as stated by the company, to comprehensive quality control tests for maintenance of multipotency, growth promoting activity, adherence rate, and typical morphology of the MSCs, as well as differentiation capacity. In addition, using standardized media can avoid xenogeneic responses. These include uncontrolled influences on MSC differentiation caused by animal-derived culture supplements that are not well characterized. The correct management of the medium is another crucial requirement, since the medium-handling conditions have to be strictly followed to obtain high-quality hMSCs (e.g., avoid repeated warming of complete medium; opt, instead, to aliquot the volume needed for each use). The hMSCs should be characterized as described in Basic Protocol 1 before proceeding to Basic Protocol 2. In addition, we suggest using hMSCs at early passages (<P5) for neuronal transdifferentiation, since long-term in vitro culture of the hMSCs may lead to senescence, which results in cell growth arrest, morphological changes (from spindle shape to a flattened square shape), and reduction of differentiation capacity.
Troubleshooting
Common problems encountered during the isolation of hMSC from CL and their transdifferentiation into hNLCs, and their potential solutions, are summarized in Table 1.
Problem | Possible Cause | Solution |
---|---|---|
Contamination during MSC medium changes and during monitoring of cell growth from the explant pieces | Microbiological contaminants (e.g., bacteria and fungi) | Work in laminar flow hood and under strict aseptic conditions. |
Low or no expression of specific surface markers in hMSCs | Loss of fluorescence staining quality caused by antibodies stored or handled inappropriately (e.g., protect from light) | Repeat the assay making sure to use the antibodies per the manufacturer's instructions. If the issue persists, discard the cells and start again with a new human umbilical cord for isolating hMSCs. |
Isolated cells are not hMSCs | It is recommended to repeat the entire isolation procedure using a new human umbilical cord. | |
Isolated cells fail to undergo adipogenic or osteogenic differentiation | The isolated cells were passaged several times before initiating the multilineage differentiation | Use isolated cells at early passages. |
Culture conditions are not correct:
|
Prepare the specific differentiated medium (e.g., osteogenic differentiation medium or adipogenic differentiation medium) again and repeat the procedure. If the issue persists in the isolated cells only but the positive control works correctly, proceed to isolate hMSCs from a new human umbilical cord. |
|
Low cell survival rate of MSCs after thawing and sub-culturing | hMSCs stressed or damaged during cryopreservation |
Ensure that hMCSs are neither under- nor over-confluent prior to cryopreservation. Use cells at 70%-80% confluency for harvesting. Ensure Mr. Frosty Freezing Container contains the appropriate amount of 100% isopropyl alcohol. Refresh the container with fresh isopropyl alcohol every 5 freeze cycles. Ensure dropwise addition of the cryomedium to the hMSCs to avoid osmotic shock. Do not disturb the Mr. Frosty freezing container for up to 4 hr after placement in the –80°C freezer. |
hMSCs stressed or damage during recovery from cryopreservation |
Ensure that cells are not left at 37°C for periods >2 min. Thaw hMSCs until only a small ice crystal remains before adding the medium. Ensure dropwise addition of growth medium to the hMSCs to avoid osmotic shock to the cells. |
|
Errors in home-made stock preparation, leading to a drop in viable cells |
Freeze cells at a density of 1-1.5 × 106 viable cells/ml. Use low-passage cells to make your own stocks. Be careful to freeze slowly and thaw fast. |
|
Cells not handled gently | Treat your hMSCs gently; do not vortex, bang the flasks to detach the cells, or centrifuge the cells at high speeds. | |
MSCs and NLCs exhibit morphological alterations or are damaged (e.g., round shape, cell debris, cell shrinkage) | Any force can represent an exogenous source of cellular damage | Handle gently. Carefully pipet up and down the cell suspension when needed. |
Long enzymatic digestion can cause membrane damage during detachment from plates/flasks | Pay close attention to the incubation time with Accutase solution. | |
Low neuronal differentiation efficiency | Incorrect cell density for seeding | Use the recommended cell density of 4000 hMSCs per cm2. |
Presence of residual mesenchymal medium together with neurogenic medium | Ensure that the mesenchymal stem cell growth medium 2 is completely removed before adding mesenchymal stem cell neurogenic differentiation medium. | |
Culture medium not reconstituted correctly | Use new bottles to reconstitute the neurogenic medium and proceed again to differentiation. | |
hMSCs fail to differentiate into hNLCs | hMSCs have been passaged too many times | Use healthy hMSCs, under passage 4-5. |
hMSCs detachment during neural induction | Your culture plate is not coated |
Be sure to use coated plate with human fibronectin. Check quality of human fibronectin (e.g., storage conditions, contamination). |
Understanding Results
Basic Protocol 1
Well-characterized and highly proliferating human MSCs from the umbilical cord lining membrane can be obtained following the procedure described in Basic Protocol 1 (Fig. 2). In our hands, hMSC properties are preserved even after thawing, and persist until cellular senescence, with >90%-95% cell viability. Normally, around 65 million hMSCs are generated at passage 1 from 3 centimeters of umbilical cord. Figure 4 shows the cell outgrowth from the CL piece explants over time from day 3 after explant to day 21 (when the cells can be detached). Specifically, cells proliferate rapidly and, after 3 days, they adhere completely to plastic at the flask bottom. The typical spindle-shaped fibroblast-like cells appear at day 7 of the cell culture, and a homogeneous monolayer can be observed between days 10 and 17; confluence (80%-90%) is reached approximately after 21 days. The hMSC cell cultures require splitting approximately twice a week from P1 to P9, and as the number of passages increases, the hMSCs become senescent, i.e., their proliferation rate decreases, and their morphology changes from a spindle shape to a flattened square shape. It has been noticed that the hMSCs derived from CL exhibit more proliferation capacity than human MSC derived from bone marrow (Coccini et al., 2019). In previous experiments, hMSCs displayed a fast population doubling time, which was about 21-25 hr at P1-P3 and remained almost constant from P4 to P9 (30-38 hr) (Coccini et al., 2019).
Basic Protocol 2
We have demonstrated that hMSCs can easily be differentiated into neuron-like cells (hNLCs) (Fig. 6) by using commercially available neurogenic medium, which allows for efficient transdifferentiation, which can be observed as phenotypic changes and expression of different markers involved in the neuronal differentiation process. Specifically, the hMSCs exhibit morphological changes over time (up to 8 days) when they are cultured in the neurogenic medium. After 8 days, hMSCs, from human CL, adopt a neural-like morphology, switching from spindle-shaped to spherical, characterized by light-refractive and transparent cell bodies with long branching processes (elongated protrusions from cytoplasm–axon- and dendrite-like cellular structures). This stepwise morphological differentiation is perfectly visible from days 3 to 8 under light microscopy (Fig. 6).
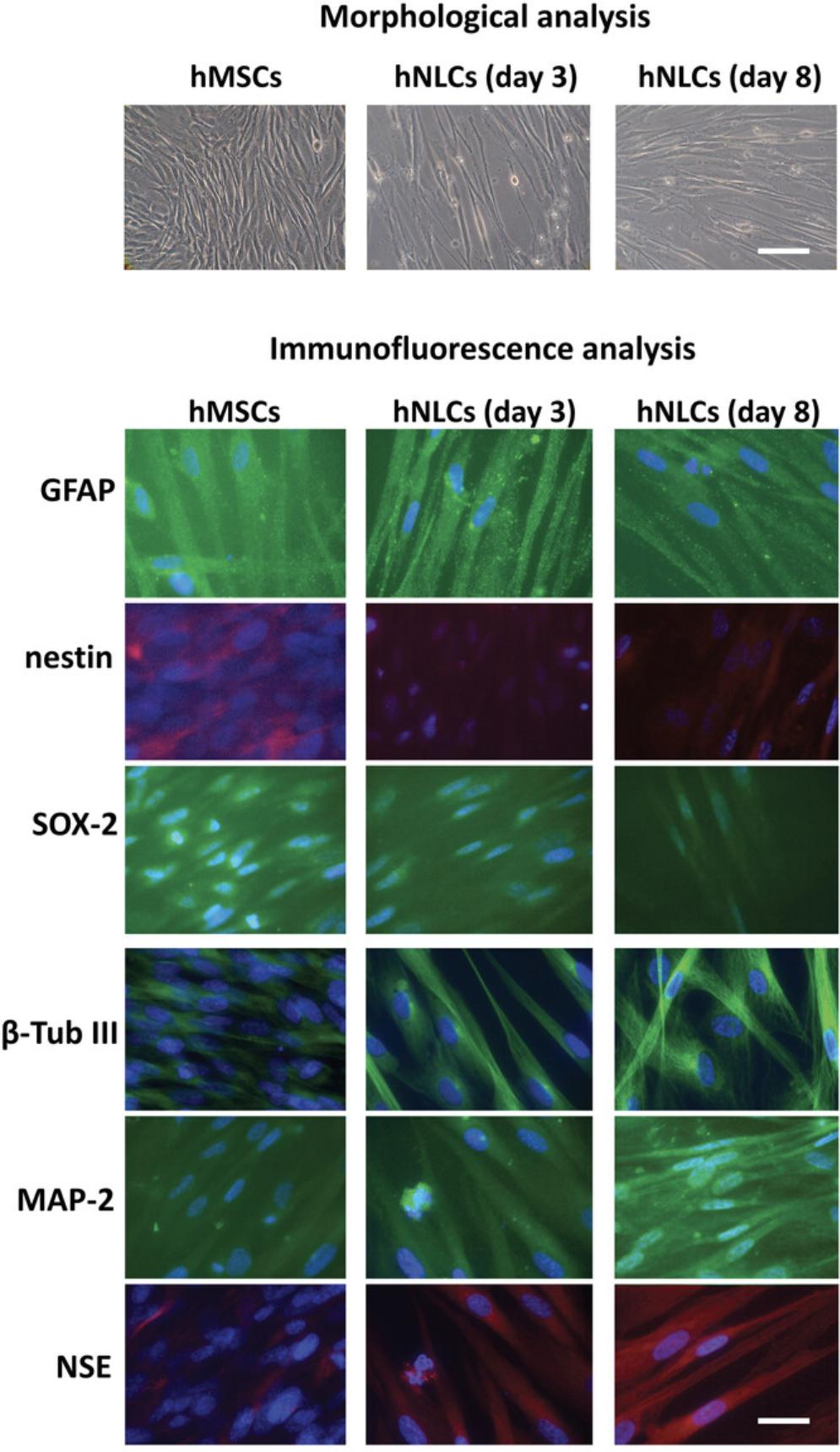
Regarding expression of neuronal markers during the hMSC neuronal differentiation process into hNCLs (up to 8 days), it is possible to observe an early increase followed by a later downregulation of immature marker proteins, namely nestin and SOX-2 (Fig. 6), and a gradual increase up to day 8 (fully differentiated hNLCs) of mature neuron marker expression, namely β-Tub III, MAP-2, and NSE (Fig. 6).
Increased GFAP expression at undifferentiated and early stages of the neuronal transdifferentiation process indicates that hMSCs have the potential capacity to also differentiate into astrocytes. However, as expected, in fully differentiated neuronal-like cells, the fluorescence intensity signal of GFAP is low (Fig. 6).
hNLCs can be easily obtained at different stages of neuronal maturity, namely as early (at 3 days after induction) and fully differentiated neuron-like cells (at 8 days after differentiation), which can be utilized for neurotoxicity testing by applying classical in vitro cytotoxicity assays to evaluate different endpoints such as cell morphology (e.g., changes in size, shape; axons and dendrites in live cells by microscopy), cell viability, mitochondrial function, and analysis of neuronal marker expression (Fig. 7).
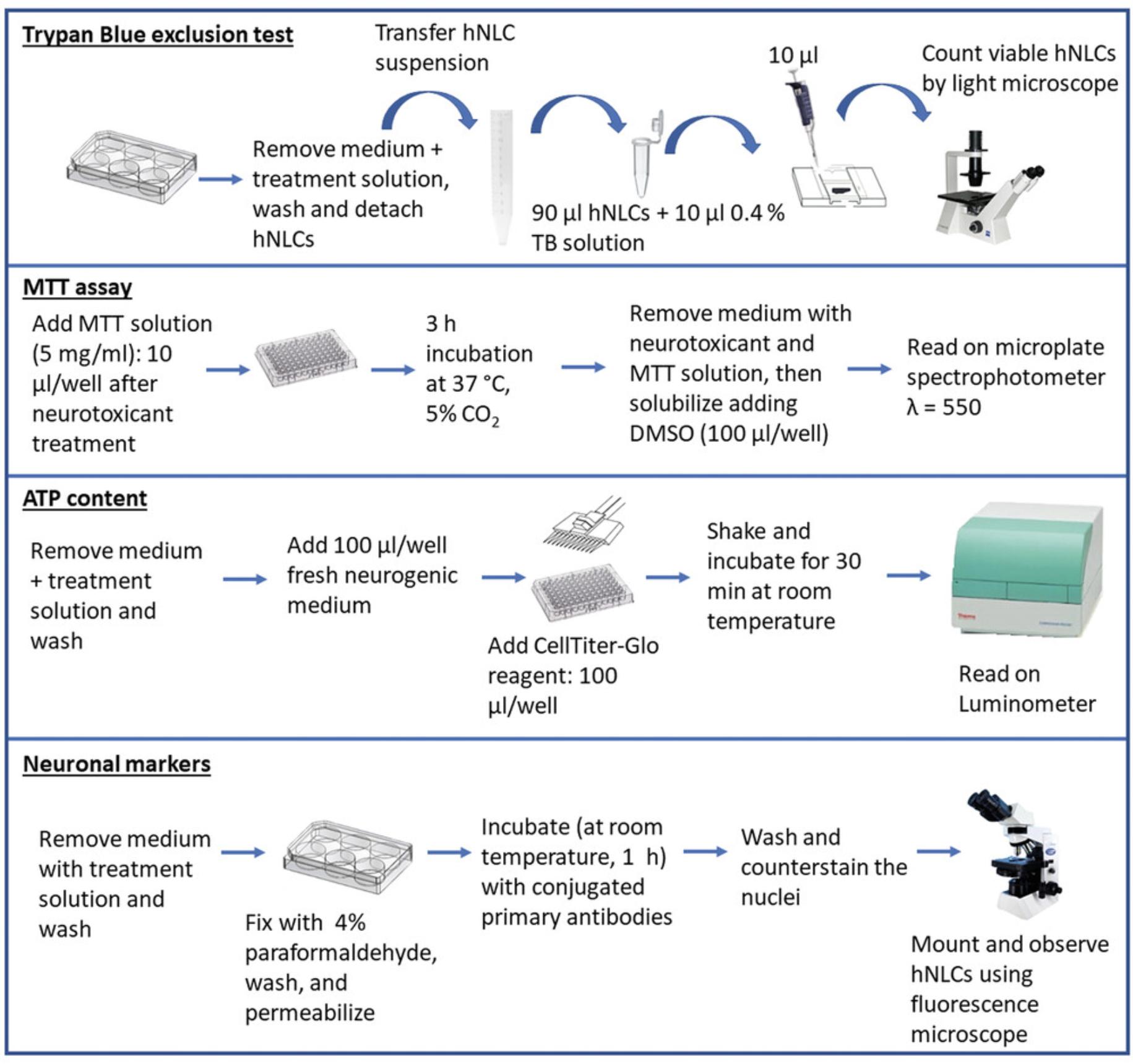
As discussed above, these hNLCs have been utilized to test different compounds, such as a new psychoactive drug i.e., MAM 2201 and magnetite nanoparticles (Fe3O4NPs). When the fully differentiated cells (at day 8) were treated with MAM 2201, using the conventional MTT cell viability test, we found hNLCs to be susceptible to this new psychoactive drug (Fig. 8B) (Coccini et al., 2021). Similarly, when hNLCs (at day 8) were treated with Fe3O4NPs, decreased cell viability was observed by the TB exclusion test, a standard method to estimate cell viability by evaluating cell membrane integrity (Fig. 8A). A decrease in ATP content, another assay widely used to evaluate mitochondrial function, was also detected upon Fe3O4NP treatment of hNLCs (Fig. 8C) (De Simone et al., 2020). Moreover, hNLCs were shown to be susceptible to NP exposure, evidenced by a decrease in the expression of the specific neuronal markers β-Tub III, MAP-2, and NSE, by using immunofluorescence staining (Fig. 9) (Coccini et al., 2020).
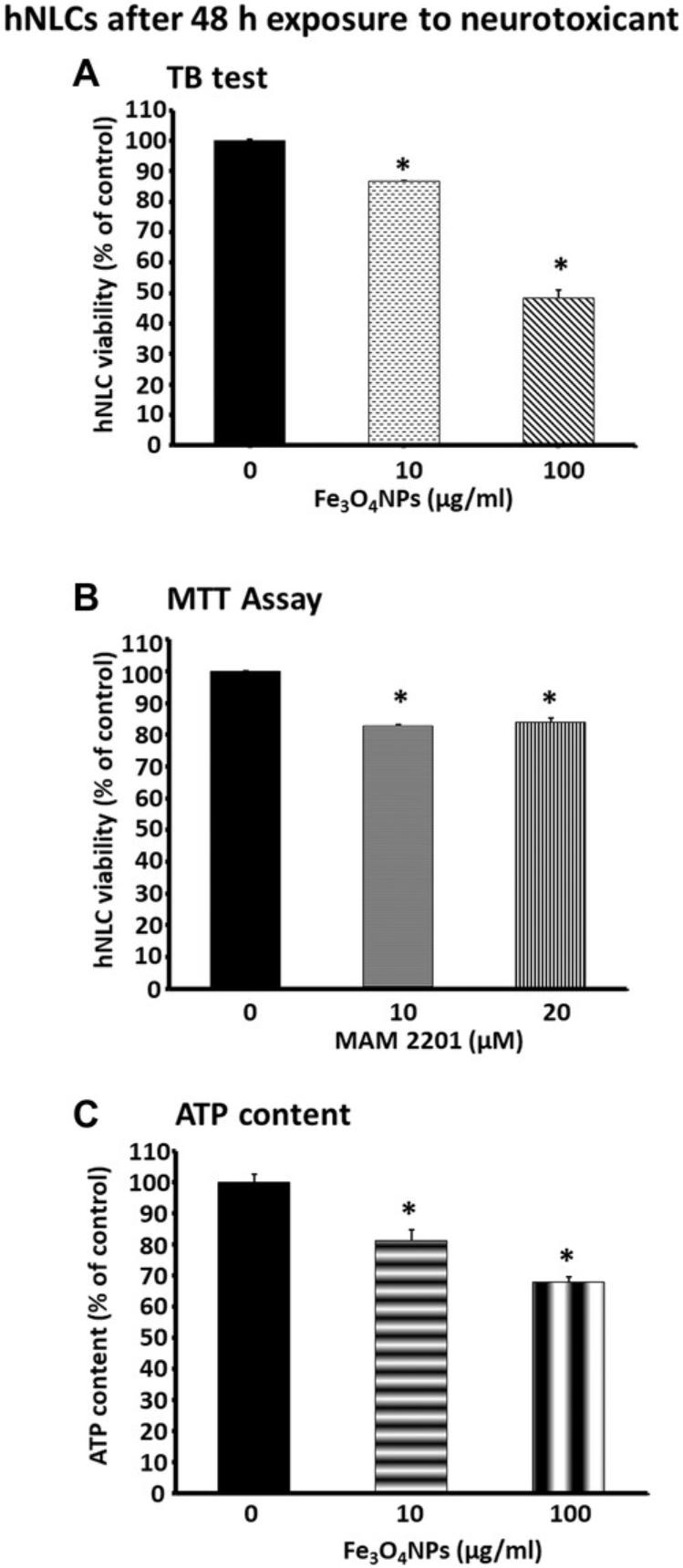
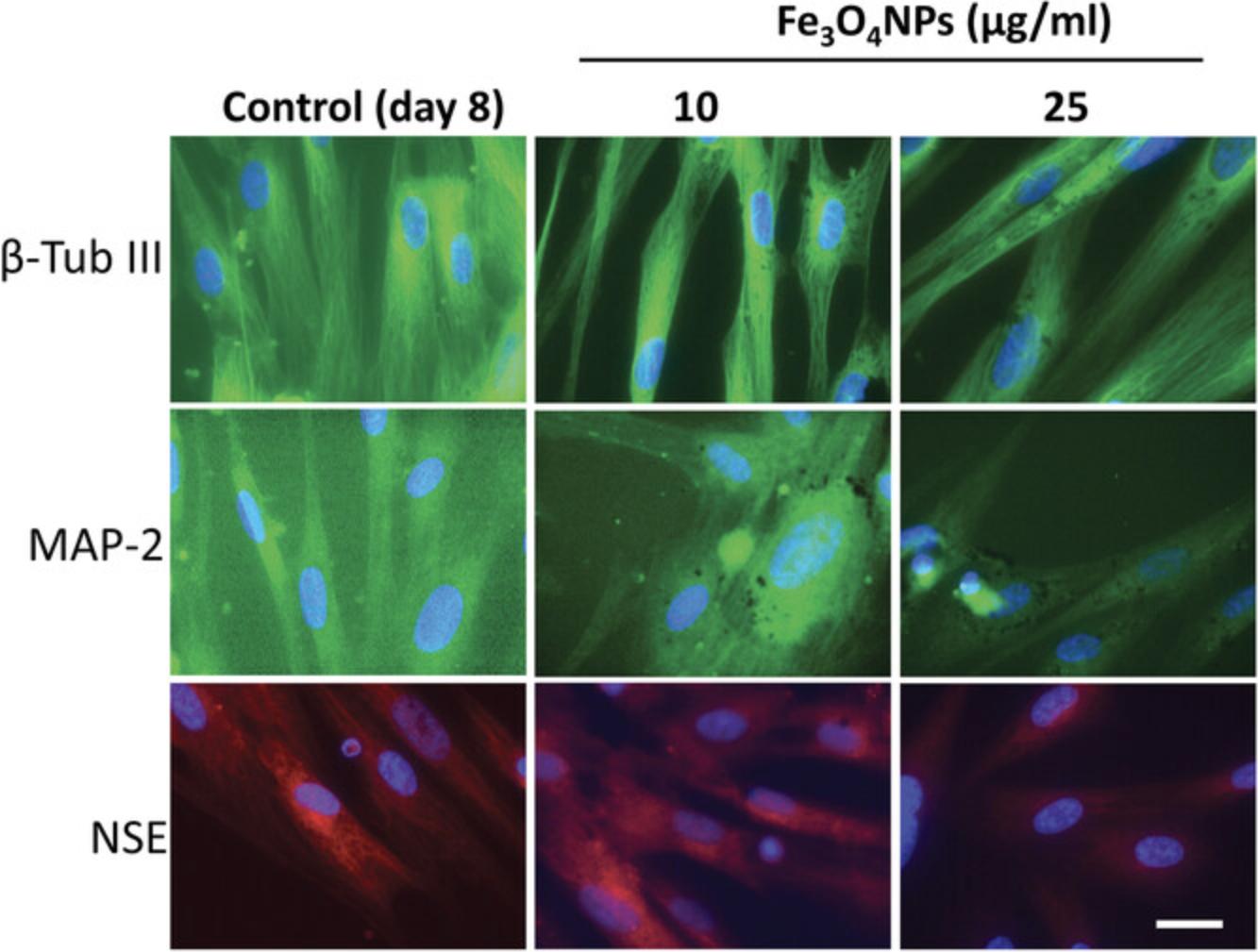
Time Considerations
Figures 2 and 5 show the relative time for each procedure during the implementation of the protocols herein described. In Basic Protocol 1, human umbilical cord processing to obtain the cord lining membrane (CL) takes no longer than 3 hr, while the isolation and culturing of the cells from the cord lining membrane takes about 18-21 days. Cells can then be harvested and fractionated to be frozen at passage 1 or to be characterized. Within Basic Protocol 1, cell characterization is the most time-consuming substep, since several types of analyses are needed: (i) monitoring (by inverted microscope) cell adherence and cell morphology takes about 2 hr, and this check has to be repeated every 3-4 days, for up to 21 days; (ii) detection of specific surface antigen expression (by flow cytometer) takes about 45 min; and (iii) multilineage differentiation potential into adipogenic and osteogenic cells requires 21 days. Cell cryo-preservation takes about 24 hr:1 hr for preparing cell aliquots and an overnight incubation at –80°C before the cells are stored in liquid nitrogen. The hMSC thawing and sub-culturing entail 2 min for thawing the cryogenic vial containing hMSCs, and about 30 min to seed the cells on a flask for culturing. The hMSCs should then be passaged twice a week up to P3-P5, when they are used for the neuronal induction.
hMSC differentiation into hNLCs (Basic Protocol 2) takes 8 days maximum to obtain fully differentiated neurons. Generally, the hMSCs can be seeded in MSC medium on Friday (requiring about 1 hr), and this medium is then replaced with neurogenic medium after 72 hr (requiring about 30 min). After that, medium changes with fresh neurogenic should be done every 48 hr up to day 8.
The various cytotoxicity tests require different amounts of time: cell morphology evaluation takes no longer than 2 hr, as does the trypan blue exclusion test; the MTT and ATP content assays require 3 hr and 30 min, respectively. Analysis of neuronal marker expression, evaluated by direct immunofluorescence, takes about 2 hr and, if evaluated by indirect immunofluorescence, takes 1 day. Fluorescence signal observation by fluorescence microscopy takes about 2 hr.
Acknowledgments
This work was supported in part by the Ricerca Corrente funding scheme of the Ministry of Health, Italy.
Author Contribution
Teresa Coccini : conceptualization, data curation, investigation, methodology, resources, supervision, visualization, writing original draft, writing review and editing; Arsenio Spinillo : resources, writing review and editing; Marianna Roccio : resources, writing review and editing; Elisa Lenta : methodology, resources; Chiara Valsecchi : methodology, resources; Uliana De Simone : data curation, investigation, methodology, resources, visualization, writing original draft, writing review and editing.
Conflict of Interest
The authors declare no conflict of interest.
Open Research
Data Availability Statement
The data that support the findings of this study are available from the corresponding author upon reasonable request.
Literature Cited
- Amari, A., Ebtekar, M., Moazzeni, S. M., Soleimani, M., Amirabad, L. M., Tahoori, M. T., & Massumi, M. (2015). Investigation of immunomodulatory properties of human Wharton's Jelly-derived mesenchymal stem cells after lentiviral transduction. Cellular Immunology , 293(2), 59–66. doi: 10.1016/j.cellimm.2014.12.003.
- Ankrum, J. A., Ong, J. F., & Karp, J. M. (2014). Mesenchymal stem cells: Immune evasive, not immune privileged. Nature Biotechnology , 32(3), 252–260. doi: 10.1038/nbt.2816.
- Aras, M. A., Hartnett, K. A., & Aizenman, E. (2008). Assessment of cell viability in primary neuronal cultures. Current Protocols in Neuroscience , 44, 7.18.1–7.18.10. doi: 10.1002/0471142301.ns0718s44.
- Arutyunyan, I., Elchanino, A., Makaro, A., & Fatkhudino, T. (2016). Umbilical cord as prospective source for mesenchymal stem cell-based therapy. Stem Cells International , 2016, 1–17. doi: 10.1155/2016/6901286.
- Bal-Price, A., & Coecke, S. (2011). Guidance on Good Cell Culture Practice (GCCP). In M. Aschner, C. Suñol, & A. Bal-Price (Eds). Cell culture technique: Neuromethods (Vol. 56, pp, 1–25). New York: Springer.
- Bal-Price, A., Crofton, K. M., Leist, M., Allen, S., Arand, M., Buetler, T., … Fritsche, E. (2015). International STakeholder NETwork (ISTNET): Creating a developmental neurotoxicity (DNT) testing road map for regulatory purposes. Archives of Toxicology , 89(2), 269–287. doi: 10.1007/s00204-015-1464-2.
- Bal-Price, A. K., & Pistollato, F. (2019). Application of non-animal methods to more effective neurotoxicity testing for regulatory purposes. In M. Aschner & L. Costa (Eds.), Cell culture techniques, neuromethods (Vol. 145). New York: Springer. doi: 10.1007/978-1-4939-9228-7_15.
- Beeravolu, N., McKee, C., Alamri, A., Mikhael, S., Brown, C., Perez-Cruet, M., & Chaudhry, G. R. (2017). Isolation and characterization of mesenchymal stromal cells from human umbilical cord and fetal placenta. Journal of Visualized Experiments , 122, e55224, 1–13. doi: 10.3791/55224.
- Bosch, J., Houben, A. P., Radke, T. F., Stapelkamp, D., Bünemann, E., Balan, P., … Kögler, G. (2012). Distinct differentiation potential of “MSC” derived from cord blood and umbilical cord: Are cord-derived cells true mesenchymal stromal cells? Stem Cells and Development , 21, 1977–1988. doi: 10.1089/scd.2011.0414.
- Breier, J. M., Gassmann, K., Kayser, R., Stegeman, H., De Groot, D., Fritsche, E., & Shafer, T. J. (2009). Neural progenitor cells as models for high-throughput screens of developmental neurotoxicity: State of the science. Neurotoxicology and Teratology , 32(1), 4–15. doi: 10.1016/j.ntt.2009.06.005.
- Buzanska, L., Sypecka, J., Nerini-Molteni, S., Compagnoni, A., Hogberg, H. T., del Torchio, R., … Coecke, S. (2009). A human stem cell-based model for identifying adverse effects of organic and inorganic chemicals on the developing nervous system. Stem Cells , 27, 2591–2601. doi: 10.1002/stem.179.
- Chen, S., Zhang, W., Wang, J. M., Duan, H. T., Kong, J. H., Wang, Y. X., … Song, J. (2016). Differentiation of isolated human umbilical cord mesenchymal stem cells into neural stem cells. International Journal of Ophthalmology , 9(1), 41–47. doi: 10.18240/ijo.2016.01.07.
- Coccini, T., Caloni, F., Cando, R. L. J., & De Simone, U. (2017). Cytotoxicity and proliferative capacity impairment induced on human brain cell cultures after short- and long-term exposure to magnetite nanoparticles. Journal of Applied Toxicology , 37(3), 361–373. doi: 10.1002/jat.3367.
- Coccini, T., De Simone, U., Roccio, M., Croce, S., Lenta, E., Zecca, M., … Avanzini, M. A. (2019). In vitro toxicity screening of magnetite nanoparticles by applying mesenchymal stem cells derived from human umbilical cord lining. Journal of Applied Toxicology , 39, 1320–1336. doi: 10.1002/jat.3819.
- Coccini, T., Pignatti, P., Spinillo, A., & De Simone, U. (2020). Developmental neurotoxicity screening for nanoparticles using neuron-like cells of human umbilical cord mesenchymal stem cells: Example with magnetite nanoparticles. Nanomaterials , 10, 1–28. doi: 10.3390/nano10081607.
- Coccini, T., De Simone, U., Lonati, D., Scaravaggi, G., Marti, M., & Locatelli, C. A. (2021). MAM-2201, one of the most potent-naphthoyl Indole derivative-synthetic cannabinoids, exerts toxic effects on human cell-based models of neurons and astrocytes. Neurotoxicity Research , 39(4), 1251–1273. doi: 10.1007/s12640-021-00369-3.
- Coecke, S., Balls, M., Bowe, G., Davis, J., Gstraunthaler, G., Hartung, T., … Stokes, W. (2005). Guidance on good cell culture practice. A Report of the Second ECVAM Task force on good cell culture practice. Alternatives to Laboratory Animals , 33, 261–287. doi: 10.1177/026119290503300313.
- Coecke, S., Goldberg, A. M., Allen, S., Buzanska, L., Calamandrei, G., Crofton, K., … Bal-Price, A. (2007). Workgroup report: Incorporating in vitro alternative methods for developmental neurotoxicity into international hazard and risk assessment strategies. Environmental Health Perspectives , 115(6), 924–931. doi: 10.1289/ehp.9427.
- Cortés-Medina, L. V., Pasantes-Morales, H., Aguilera-Castrejon, A., Picones, A., Lara-Figueroa, C. O., Luis, E., … Ramos-Mandujano, G. (2019). Neuronal transdifferentiation potential of human mesenchymal stem cells from neonatal and adult sources by a small molecule cocktail. Stem Cells International , 2019, 7627148. doi: 10.1155/2019/7627148.
- Costa, L. G., Pellacani, C., & Guizzetti, M. (2017). In vitro and alternative approaches to developmental neurotoxicity. In R. C. Gupta (Ed.), Reproductive and developmental toxicology ( 2nd ed., pp. 241–253). San Diego: Academic Press. doi: 10.1016/B978-0-12-804239-7.00014-7.
- Costain, W. J., Tauskela, J. S., Rasquinha, I., Comas, T., Hewitt, M., Marleau, V., & Soo, E. C. (2016). Pharmacological characterization of emerging synthetic cannabinoids in HEK293T cells and hippocampal neurons. European Journal of Pharmacology , 786, 234–245. doi: 10.1016/j.ejphar.2016.05.040.
- Czarnecka, J., Porowińska, D., Bajek, A., Hołysz, M., & Roszek, K. (2017). Neurogenic differentiation of mesenchymal stem cells induces alterations in extracellular nucleotides metabolism. Journal of Cellular Biochemistry , 118(3), 478–486. doi: 10.1002/jcb.25664.
- Daneshian, M., Busquet, F., Hartung, T., & Leist, M. (2015). Animal use for science in Europe. ALTEX , 32(4), 261–274. doi: 10.14573/altex.1509081.
- De Bruyn, C., Najar, M., Raicevic, G., Meuleman, N., Pieters, K., Stamatopoulos, B., … Lagneaux, L. (2011). A rapid, simple, and reproducible method for the isolation of mesenchymal stromal cells from Wharton's jelly without enzymatic treatment. Stem Cells and Development , 20(3), 547–557. doi: 10.1089/scd.2010.0260.
- De Simone, U., Spinillo, A., Caloni, F., Gribaldo, L., & Coccini, T. (2020). Neuron-like cells generated from human umbilical cord lining-derived mesenchymal stem cells as a new in vitro model for neuronal toxicity screening: Using magnetite nanoparticles as an example. International Journal of Molecular Sciences , 21(1), 271. doi: 10.3390/ijms21010271.
- Díez, J. M., Bauman, E., Gajardo, R., & Jorquera, J. I. (2015). Culture of human mesenchymal stem cells using a candidate pharmaceutical grade xeno-free cell culture supplement derived from industrial human plasma pools. Stem Cell Research & Therapy, 6(1), 28. doi: 10.1186/s13287-015-0016-2.
- Dominici, M., Le Blanc, K., Mueller, I., Slaper-Cortenbach, I., Marini, F., Krause, D., … Horwitz, E. (2006). Minimal criteria for defining multipotent mesenchymal stromal cells. The International Society for Cellular Therapy position statement. Cytotherapy , 8(4), 315–317. doi: 10.1080/14653240600855905.
- Ducray, A. D., Stojiljkovic, A., Möller, A., Stoffel, M. H., Widmer, H. R., Frenz, M., & Mevissen, M. (2017). Uptake of silica nanoparticles in the brain and effects on neuronal differentiation using different in vitro models. Nanomedicine , 13, 1195–1204. doi: 10.1016/j.nano.2016.11.001.
- European Commission. (2013). Seventh Report on the Statistics on the Number of Animals used for Experimental and other Scientific Purposes in the Member States of the European Union. Available at http://ec.europa.eu/environment/chemicals/labanimals/reportsen.htm.
- Friedenstein, A. J., Piatetzky-Shapiro, I. I., & Petrakova, K. V. (1966). Osteogenesis in transplants of bone marrow cells. Journal of Embryology and Experimental Morphology , 16(3), 381–390.
- Friedenstein, A. J., Petrakova, K. V., Kurolesova, A. I., & Frolova, G. P. (1968). Heterotopic of bone marrow. Analysis of precursor cells for osteogenic and hematopoietic tissues. Transplantation , 6, 230–247. doi: 10.1097/00007890-196803000-00009.
- George, S., Hamblin, M. R., & Abrahamse, H. (2019). Differentiation of mesenchymal stem cells to neuroglia: In the context of cell signalling. Stem Cell Reviews and Reports , 15(6), 814–826. doi: 10.1007/s12015-019-09917-z.
- Gliga, A. R., Edoff, K., Caputo, F., Källman, T., Blom, H., Karlsson, H. L., … Fadeel, B. (2017). Cerium oxide nanoparticles inhibit differentiation of neural stem cells. Scientific Reports , 71, 9284. doi: 10.1038/s41598-017-09430-8.
- Goulart, V. A., Braga Ferreira, L., Angélico Duarte, C., Lemos de Lima, I., Ferreira, E. R., Candido de Oliveira, B., … de Souza Castro-Filice, L. (2018). Mesenchymal stem cells from human adipose tissue and bone repair: A literature review. Biotechnology Research and Innovation , 2(1), 74–80, doi: 10.1016/j.biori.2017.10.005.
- Harry, G. J., Billingsley, M., Bruinink, A., Campbell, I. L., Classen, W., Dorman, D. C., … Tilson, H. A. (1998). In vitro techniques for the assessment of neurotoxicity. Environmental Health Perspectives , 106(Suppl 1), 131–158. doi: 10.1289/ehp.98106s1131.
- Harry, G. J., & Tiffany-Castiglioni, E. (2005). Evaluation of neurotoxic potential by use of in vitro systems. Expert Opinion on Drug Metabolism & Toxicology, 1(4), 701–713. doi: 10.1517/17425255.1.4.701.
- Hartung, T. (2010). Lessons learned from alternative methods and their validation for a new toxicology in the 21st century. Journal of Toxicology and Environmental Health, Part B, Critical Reviews , 13(2-4), 277–290. doi: 10.1080/10937404.2010.483945.
- Heo, J. S., Choi, Y., Kim, H. S., & Kim, H. O. (2016). Comparison of molecular profiles of human mesenchymal stem cells derived from bone marrow, umbilical cord blood, placenta and adipose tissue. International Journal of Molecular Medicine , 37(1), 115–125. doi: 10.3892/ijmm.2015.2413.
- Hernández, R., Jiménez-Luna, C., Perales-Adán, J., Perazzoli, G., Melguizo, C., & Prados, J. (2020). Differentiation of human mesenchymal stem cells towards neuronal lineage: Clinical trials in nervous system disorders. Biomolecules & Therapeutics, 28(1), 34–44. doi: 10.4062/biomolther.2019.065.
- Irie, T., Kikura-Hanajiri, R., Usami, M., Uchiyama, N., Goda, Y., & Sekino, Y. (2015). MAM-2201, a synthetic cannabinoid drug of abuse, suppresses the synaptic input to cerebellar Purkinje cells via activation of presynaptic CB1 receptors. Neuropharmacology , 95, 479–491. doi: 10.1016/j.neuropharm.2015.02.025.
- Jang, S., Cho, H. H., Cho, Y. B., Park, J. S., & Jeong, H. S. (2010). Functional neural differentiation of human adipose tissue-derived stem cells using bFGF and forskolin. BMC Cell Biology , 11, 25. doi: 10.1186/1471-2121-11-25.
- Jeschke, M. G., Gauglitz, G. G., Phan, T. T., Herndon, D. N., & Kita, K. (2011). Umbilical cord lining membrane and wharton's jelly-derived mesenchymal stem cells: The similarities and differences. The Open Tissue Engineering and Regenerative Medicine Journal , 4, 21–27. doi: 10.2174/1875043501104010021.
- Jin, J. H., Bae, K. Y., Kim, M., Kwon, S. J., Jeon, H. B., Choi, S. J., … Chang, J. W. (2013). Comparative analysis of human mesenchymal stem cells from bone marrow, adipose tissue, and umbilical cord blood as sources of cell therapy. International Journal of Molecular Sciences , 14, 17986–18001. doi: 10.3390/ijms140917986.
- Karahuseyinoglu, S., Cinar, O., Kilic, E., Kara, F., Akay, G. G., Demiralp, D. O., … Can, A. (2007). Biology of stem cells in human umbilical cord stroma: In situ and in vitro surveys. Stem Cells , 25, 319–331. doi: 10.1634/stemcells.2006-0286.
- Kashyap, M. P., Kumar, V., Singh, A. K., Tripathi, V. K., Jahan, S., Pandey, A., … Pant, A. B. (2015). Differentiating neurons derived from human umbilical cord blood stem cells work as a test system for developmental neurotoxicity. Molecular Neurobiology , 51, 791–807. doi: 10.1007/s12035-014-8716-7.
- Kikuchi-Taura, A., Taguchi, A., Kanda, T., Inoue, T., Kasahara, Y., Hirose, H., … Soma, T. (2012). Human umbilical cord provides a significant source of unexpanded mesenchymal stromal cells. Cytotherapy , 14, 441–450. doi: 10.3109/14653249.2012.658911.
- Kil, K., Choi, M. Y., & Park, K. H. (2016). In vitro differentiation of human Wharton's jelly-derived mesenchymal stem cells into auditory hair cells and neurons. Journal of International Advanced Otology , 12, 37–42. doi: 10.5152/iao.2016.1190.
- Kim, T. W., Che, J. H., & Yun, J. W. (2019). Use of stem cells as alternative methods to animal experimentation in predictive toxicology. Regulatory Toxicology and Pharmacology , 105, 15–29. doi: 10.1016/j.yrtph.2019.03.016.
- Kita, K., Gauglitz, G. G., Phan, T. T., Herndon, D. N., & Jeschke, M. G. (2010). Isolation and characterization of mesenchymal stem cells from the sub-amniotic human umbilical cord lining membrane. Stem Cells and Development , 19(4), 491–502. doi: 10.1089/scd.2009.0192.
- Koaykul, C., Kim, M. H., Kawahara, Y., Yuge, L., & Kino-Oka, M. (2019). Maintenance of neurogenic differentiation potential in passaged bone marrow-derived human mesenchymal stem cells under simulated microgravity conditions. Stem Cells and Development , 28(23), 1552–1561. doi: 10.1089/scd.2019.0146.
- Krewski, D., Andersen, M. E., Tyshenko, M. G., Krishnan, K., Hartung, T., Boekelheide, K., … Cote, I. (2020). Toxicity testing in the 21st century: Progress in the past decade and future perspectives. Archives of Toxicology , 94(1), 1–58. doi: 10.1007/s00204-019-02613-4.
- La Rocca, G., Anzalone, R., Corrao, S., Magno, F., Loria, T., Lo Iacono, M., … Farina, F. (2009). Isolation and characterization of Oct-4+/HLA-G+ mesenchymal stem cells from human umbilical cord matrix: Differentiation potential and detection of new markers. Histochemistry and Cell Biology , 131(2), 267–282. doi: 10.1007/s00418-008-0519-3.
- Lee, S. J., & Lee, H. A. (2020). Trends in the development of human stem cell-based non-animal drug testing models. The Korean Journal of Physiology & Pharmacology, 24(6), 441–452. doi: 10.4196/kjpp.2020.24.6.441.
- Leist, M., Hasiwa, N., Rovida, C., Daneshian, M., Basketter, D., Kimber, I., … Hartung, T. (2014). Consensus report on the future of animal-free systemic toxicity testing. ALTEX , 31(3), 341–356. doi: 10.14573/altex.1406091.
- Lim, I. J., & Phan, T. T. (2014). Epithelial and mesenchymal stem cells from the umbilical cord lining membrane. Cell Transplantation , 23, 497–503. doi: 10.3727/096368914x678346.
- Majore, I., Moretti, P., Stahl, F., Hass, R., & Kasper, C. (2011). Growth and differentiation properties of mesenchymal stromal cell populations derived from whole human umbilical cord. Stem Cell Reviews and Reports , 7, 17–31. doi: 10.1007/s12015-010-9165-y.
- Makris, S. L., Raffaele, K., Allen, S., Bowers, W. J., Hass, U., Alleva, E., … Crofton, K. M. (2009). A retrospective performance assessment of the developmental neurotoxicity study in support of OECD test guideline 426. Environmental Health Perspectives , 117, 17–25. doi: 10.1289/ehp.11447.
- Masjosthusmann, S., Barenys, M., El-Gamal, M., Geerts, L., Gerosa, L., Gorreja, A., … Fritsche, E. (2018). Literature review and appraisal on alternative neurotoxicity testing methods. EFSA, External Scientific Report , 15(4), 1–125. doi: 10.2903/sp.efsa.2018.EN-1410.
- Mennan, C., Wright, K., Bhattacharjee, A., Balain, B., Richardson, J., & Roberts, S. (2013). Isolation and characterisation of mesenchymal stem cells from different regions of the human umbilical cord. BioMed Research International , 2013, 916136. doi: 10.1155/2013/916136.
- Mennan, C., Brown, S., McCarthy, H., Mavrogonatou, E., Kletsas, D., Garcia, J., … Roberts, S. (2016). Mesenchymal stromal cells derived from whole human umbilical cord exhibit similar properties to those derived from Wharton's jelly and bone marrow. FEBS Open Bio , 6(11), 1054–1066. doi: 10.1002/2211-5463.12104.
- Mitchell, K. E., Weiss, M. L., Mitchell, B. M., Martin, P., Davis, D., Morales, L., … Medicetty, S. (2003). Matrix cells from Wharton's jelly form neurons and glia. Stem Cells , 21, 50–60. doi: 10.1634/stemcells.21-1-50.
- Nagamura-Inoue, T., & He, T. (2014). Umbilical cord-derived mesenchymal stem cells: Their advantages and potential clinical utility. World Journal of Stem Cells , 6(2), 195–202. doi: 10.4252/wjsc.v6.i2.195.
- Nan, C., Guo, L., Zhao, Z., Ma, S., Liu, J., Yan, D., … Liu, H. (2016). Tetramethylpyrazine induces differentiation of human umbilical cord-derived mesenchymal stem cells into neuron-like cells in vitro. International Journal of Oncology , 48(6), 2287–2294. doi: 10.3892/ijo.2016.3449.
- OECD. (1997). Test Guideline 424. Neurotoxicity Study in Rodents.
- OECD. (2007). Test guideline 426. OECD guideline for testing of chemicals. Developmental Neurotoxicity Study.
- OECD. (2011). Test Guideline 443. Extended One-Generation Reproductive Toxicity Study.
- Padiolleau, L., Chanseau, C., Durrieu, S., Ayela, C., Laroche, G., & Durrieu, M. C. (2020). Directing hMSCs fate through geometrical cues and mimetics peptides. Journal of Biomedical Materials Research Part A , 108(2), 201–211. doi: 10.1002/jbm.a.36804.
- Patel, M., & Yang, S. (2010). Advances in reprogramming somatic cells to induced pluripotent stem cells. Stem Cell Reviews , 6, 367–380. doi: 10.1007/s12015-010-9123-8.
- Patil, V. R., Kharat, A. H., Kulkarni, D. G., Kheur, S. M., & Bhonde, R. R. (2018). Long term explant culture for harvesting homogeneous population of human dental pulp stem cells. Cell Biology International , 42(12), 1602–1610. doi: 10.1002/cbin.11065.
- Pereira, W. C., Khushnooma, I., Madkaikar, M., & Ghosh, K. (2008). Reproducible methodology for the isolation of mesenchymal stem cells from human umbilical cord and its potential for cardiomyocyte generation. Journal of Tissue Engineering and Regenerative Medicine , 2, 394–399. doi: 10.1002/term.107.
- Prieto, P., Clemedson, C., Meneguz, A., Pfaller, W., Sauer, U. G., & Westmoreland, C. (2005). Subacute and subchronic toxicity. Alternatives to Laboratory Animals , 33(Suppl 1), 109–116. doi: 10.1177/026119290503301s12.
- Pittenger, M. F., Mackay, A. M., Beck, S. C., Jaiswal, R. K., Douglas, R., Mosca, J. D., … Marshak, D. R. (1999). Multilineage potential of adult human mesenchymal stem cells. Science , 284, 143–147. doi: 10.1126/science.284.5411.143.
- Radio, N. M., & Mundy, W. R. (2008). Developmental neurotoxicity testing in vitro: Models for assessing chemical effects on neurite outgrowth. Neurotoxicology , 29(3), 361–376. doi: 10.1016/j.neuro.2008.02.011.
- Raileanu, V. N., Whiteley, J., Chow, T., Kollara, A., Mohamed, A., Keating, A., & Rogers, I. M. (2019). Banking mesenchymal stromal cells from umbilical cord tissue: Large sample size analysis reveals consistency between donors. Stem Cells Translational Medicine , 8(10), 1041–1054. doi: 10.1002/sctm.19-0022.
- Ramos, T. V., Mathew, A. J., Thompson, M. L., & Ehrhardt, R. O. (2014). Standardized cryopreservation of human primary cells. Current Protocols in Cell Biology , 64, A.3I., 1–8. doi: 10.1002/0471143030.cba03is64.
- Rostami, A. A., Kouchesfahani, H. M., Kiani, S., & Fakheri, R. (2015). Iron oxide nanoparticles reduced retinoic acid induced-neuronal differentiation of mouse embryonic stem cells by ROS generation. Archives of Iranian Medicine , 18(9), 586–590.
- Saleh, R., & Reza, H. M. (2017). Short review on human umbilical cord lining epithelial cells and their potential clinical applications. Stem Cell Research & Therapy, 8(1), 222. doi: 10.1186/s13287-017-0679-y.
- Salehinejad, P., Alitheen, N. B., Ali, A. M., Omar, A. R., Moshrefi, M., Motamedi, B., & Nematollahi-Mahani, S. N. (2015). Neural differentiation of human umbilical cord matrix-derived mesenchymal cells under special culture conditions. Cytotechnology , 67(3), 449–460. doi: 10.1007/s10616-014-9703-6.
- Seonwoo, H., Jang, K. J., Lee, D., Park, S., Lee, M., Park, S., … Chung, J. H. (2018). Neurogenic differentiation of human dental pulp stem cells on graphene-polycaprolactone hybrid nanofibers. Nanomaterials , 8(7), 554. doi: 10.3390/nano8070554.
- Shahbazi, A., Safa, M., Alikarami, F., Kargozar, S., Asadi, M. H., Joghataei, M. T., & Soleimani, M. (2016). Rapid induction of neural differentiation in human umbilical cord matrix mesenchymal stem cells by camp-elevating agents. International Journal of Molecular and Cellular Medicine , 5(3), 167–177.
- Shi, Y., Nan, C., Yan, Z., Liu, L., Zhou, J., Zhao, Z., & Li, D. (2018). Synaptic plasticity of human umbilical cord mesenchymal stem cell differentiating into neuron-like cells in vitro induced by edaravone. Stem Cells International , 2018, 5304279. doi: 10.1155/2018/5304279.
- Singh, S., Srivastava, A., Kumar, V., Pandey, A., Kumar, D., Rajpurohit, C. S., … Pant, A. B. (2016). Stem cells in neurotoxicology/developmental neurotoxicology: Current scenario and future prospects. Molecular Neurobiology , 53, 6938–6949. doi: 10.1007/s12035-015-9615-2.
- Singh, A. K., & Kashyap, M. P. (2016). An overview on human umbilical cord blood stem cell-based alternative in vitro models for developmental neurotoxicity assessment. Molecular Neurobiology , 53, 3216–3226. doi: 10.1007/s12035-015-9202-6.
- Soenen, S. J. H., Nuytten, N., De Meyer, S. F., De Smedt, S. C., & De Cuyper, M. (2010). High intracellular iron oxide nanoparticle concentrations affect cellular cytoskeleton and focal adhesion kinase-mediated signalling. Small , 6(7), 832–842. doi: 10.1002/smll.200902084.
- Strober, W. (2015). Trypan blue exclusion test of cell viability. Current Protocols in Immunology , 111, A.3B.1–A.3B.4.21. doi: 10.1002/0471142735.ima03bs21.
- Stubbendorff, M., Deuse, T., Hua, X., Phan, T. T., Bieback, K., Atkinson, K., … Schrepfer, S. (2013). Immunological properties of extraembryonic human mesenchymal stromal cells derived from gestational tissue. Stem Cells and Development , 22(19), 2619–2629. doi: 10.1089/scd.2013.0043.
- Suma, R. N., & Mohanan, P. V. (2015). Stem cells, a new generation model for predictive nanotoxicological assessment. Current Drug Metabolism , 16, 932–939. doi: 10.2174/1389200216666151015113720.
- Takahashi, D., Mori, T., Sohara, E., Tanaka, M., Chiga, M., Inoue, Y., … Uchida, S. (2017). WNK4 is an adipogenic factor and its deletion reduces diet-induced obesity in mice. EBioMedicine , 18, 118–127. doi: 10.1016/j.ebiom.2017.03.011.
- Taran, R., Mamidi, M. K., Singh, G., Dutta, S., Parhar, I. S., John, J. P., … Das, A. K. (2014). In vitro and in vivo neurogenic potential of mesenchymal stem cells isolated from different sources. Journal of Biosciences , 39(1), 157e69. doi: 10.1007/s12038-013-9409-5.
- Tauskela, J. S., Comas, T., Hewitt, M., Aylsworth, A., Zhao, X., Martina, M., & Costain, W. J. (2016). Effect of synthetic cannabinoids on spontaneous neuronal activity: Evaluation using Ca2+ spiking and multi-electrode arrays. European Journal of Pharmacology , 786, 148–160. doi: 10.1016/j.ejphar.2016.05.038.
- Tomiyama, K., & Funada, M. (2011). Cytotoxicity of synthetic cannabinoids found in “Spice” products: The role of cannabinoid receptors and the caspase cascade in the NG 108-15 cell line. Toxicology Letters , 207, 12–17. doi: 10.1016/j.toxlet.2011.08.021.
- Tomiyama, K., & Funada, M. (2014). Cytotoxicity of synthetic cannabinoids on primary neuronal cells of the forebrain: The involvement of cannabinoid CB1 receptors and apoptotic cell death. Toxicology and Applied Pharmacology , 274, 17–23. doi: 10.1016/j.taap.2013.10.028.
- US-EPA. (1998). Health Effects Test Guidelines OPPTS 870.6300 Developmental Neurotoxicity Study. (EPA 712-C-98-239).
- Viswanathan, S., Shi, Y., Galipeau, J., Krampera, M., Leblanc, K., Martin, I., … Sensebe, L. (2019). Mesenchymal stem versus stromal cells: International Society for Cell & Gene Therapy (ISCT) Mesenchymal Stromal Cell committee position statement on nomenclature. Cytotherapy , 21, 1019–1024. doi: 10.1016/j.jcyt.2019.08.002.
- Wang, H. S., Hung, S. C., Peng, S. T., Huang, C. C., Wei, H. M., Guo, Y. J., … Chen, C. C. (2004). Mesenchymal stem cells in the Wharton's jelly of the human umbilical cord. Stem Cells , 22, 1330–1337. doi: 10.1634/stemcells.2004-0013.
- Wobus, A. M., & Löser, P. (2011). Present state and future perspectives of using pluripotent stem cells in toxicology research. Archives of Toxicology , 85(2), 79–117. doi: 10.1007/s00204-010-0641-6.
- Worth, A. P., & Balls, M. (2002). Alternative (non-animal) methods for chemical testing: Current status and future prospects. ATLA , 30(Suppl 1), 1–125.
- Yang, H., Xie, Z. H., Wei, L. F., Yang, H. N., Yang, S. N., Zhu, Z. Y., … Bi, J. Z. (2013). Human umbilical cord mesenchymal stem cell-derived neuron-like cells rescue memory deficits and reduce amyloid-beta deposition in an AβPP/PS1 transgenic mouse model. Stem Cell Research & Therapy, 4(4), 76. doi: 10.1186/scrt227.
- Zakrzewski, W., Dobrzyński, M., Szymonowicz, M., & Rybak, Z. (2019). Stem cells: Past, present, and future. Stem Cell Research & Therapy, 10(1), 68. doi: 10.1186/s13287-019-1165-5.
- Zomer, H. D., Vidane, A. S., Gonçalves, N. N., & Ambrósio, C. E. (2015). Mesenchymal and induced pluripotent stem cells: General insights and clinical perspectives. Stem Cells Cloning , 8, 125–134. doi: 10.2147/SCCAA.S88036.
- Zychowicz, M., Dziedzicka, D., Mehn, D., Kozlowska, H., Kinsner-Ovaskainen, A., Stepien, P. P., … Buzanska, L. (2014). Developmental stage dependent neural stem cells sensitivity to methylmercury chloride on different biofunctional surfaces. Toxicology in Vitro , 28, 76–87. doi: 10.1016/j.tiv.2013.06.023.
Citing Literature
Number of times cited according to CrossRef: 3
- Teresa Coccini, Azzurra Schicchi, Carlo Alessandro Locatelli, Francesca Caloni, Sara Negri, Elena Grignani, Uliana De Simone, Methylglyoxal‐induced neurotoxic effects in primary neuronal‐like cells transdifferentiated from human mesenchymal stem cells: Impact of low concentrations, Journal of Applied Toxicology, 10.1002/jat.4515, 43 , 12, (1819-1839), (2023).
- Tian Xue, Xiaolin Wang, Jing Ru, Lixing Zhang, Huancai Yin, The inhibitory effect of human umbilical cord mesenchymal stem cells expressing anti-HAAH scFv-sTRAIL fusion protein on glioma, Frontiers in Bioengineering and Biotechnology, 10.3389/fbioe.2022.997799, 10 , (2022).
- Yizhuo Wang, Shuyun Liu, Lan Li, Ling Li, Xueli Zhou, Meihua Wan, Peng Lou, Meng Zhao, Ke Lv, Yujia Yuan, Younan Chen, Yanrong Lu, Jingqiu Cheng, Jingping Liu, Peritoneal M2 macrophage-derived extracellular vesicles as natural multitarget nanotherapeutics to attenuate cytokine storms after severe infections, Journal of Controlled Release, 10.1016/j.jconrel.2022.06.063, 349 , (118-132), (2022).