DNA Fiber Assay for the Analysis of DNA Replication Progression in Human Pluripotent Stem Cells
Jason A. Halliwell, Jason A. Halliwell, Polly Gravells, Polly Gravells, Helen E. Bryant, Helen E. Bryant
Abstract
Human pluripotent stem cells (PSC) acquire recurrent chromosomal instabilities during prolonged in vitro culture that threaten to preclude their use in cell-based regenerative medicine. The rapid proliferation of pluripotent cells leads to constitutive replication stress, hindering the progression of DNA replication forks and in some cases leading to replication-fork collapse. Failure to overcome replication stress can result in incomplete genome duplication, which, if left to persist into the subsequent mitosis, can result in structural and numerical chromosomal instability.
We have recently applied the DNA fiber assay to the study of replication stress in human PSC and found that, in comparison to somatic cells states, these cells display features of DNA replication stress that include slower replication fork speeds, evidence of stalled forks, and replication initiation from dormant replication origins. These findings have expanded on previous work demonstrating that extensive DNA damage in human PSC is replication associated. In this capacity, the DNA fiber assay has enabled the development of an advanced nucleoside-enriched culture medium that increases replication fork progression and decreases DNA damage and mitotic errors in human PSC cultures.
The DNA fiber assay allows for the study of replication fork dynamics at single-molecule resolution. The assay relies on cells incorporating nucleotide analogs into nascent DNA during replication, which are then measured to monitor several replication parameters. Here we provide an optimized protocol for the fiber assay intended for use with human PSC, and describe the methods employed to analyze replication fork parameters. © 2020 Wiley Periodicals LLC.
Basic Protocol 1 : DNA fiber labeling
Basic Protocol 2 : DNA fiber spreading
Basic Protocol 3 : Immunostaining
Support Protocol 1 : Microscopy/data acquisition
Support Protocol 2 : Data analysis
INTRODUCTION
Human pluripotent stem cells (PSC) possess the ability to endlessly renew and differentiate into any cell type of the body, making them a promising resource for cell-based regenerative medicine (Takahashi et al., 2007, Thomson et al., 1998). To capitalize on this potential, researchers may need to expand PSC for long periods of time in a genetically stable and undifferentiated state. However, recurrent genetic changes have been reported to arise during prolonged in vitro culture (Draper et al., 2004, Olariu et al., 2010). These changes occur through mutation and subsequent selection of variant cells in which the genetic change provide a growth advantage (Avery et al., 2013, Blum, Bar-Nur, Golan-Lev, & Benvenisty, 2009). Although extensive resources have been applied to understanding the mechanism of selection, comparatively little is known about the mechanism of mutation in these cells.
DNA damage and genome stress, including replication stress, can lead to genetic instability like that often observed in cancer (Burrell et al., 2013). Previous studies have highlighted that human PSC are prone to replication stress and DNA damage (Halliwell et al., 2020, Simara et al., 2017, Vallabhaneni et al., 2018), which may also drive the high frequency of mitotic errors that has been reported elsewhere (Lamm et al., 2016, Zhang et al., 2019). Yet, despite this, the underlying mutation rate in these cells is low (Thompson et al., 2020). This apparent contradiction can be reconciled by observations that cell cycle checkpoints such as CHK1, responsible for relay signaling in response to replication stress, are relaxed in human PSC, causing these cells to die in response to genomic damage (Desmarais et al., 2012, Desmarais, Unger, Damjanov, Meuth, & Andrews, 2016). These characteristics may reflect the requirements of early embryogenesis, where a relentless need to proliferate while maintaining genetic stability is critical to ensure successful development. Nevertheless, this system is not perfect and mutations still arise both in vivo and in vitro.
To better understand the origins of genome instability in human PSC, the appropriate assays must be optimized for use in these systems. The DNA fiber assay has become the gold standard in directly monitoring DNA replication fork dynamics, and is thus an important tool for the understanding of DNA replication stress. However, its application has been primarily focused on understanding replication in cancer cell lines (Nieminuszczy, Schwab, & Niedzwiedz, 2016). This article describes the basic protocols required to perform the DNA fiber assay in human PSC: the sequential pulse labeling of actively replicating DNA (Basic Protocol 1), spreading of labeled DNA fibers onto glass slides (Basic Protocol 2), immunolabeling of nascent DNA fibers (Basic Protocol 3) for visualization by confocal microscopy (Support Protocol 1), and data analysis and replication event characterization (Support Protocol 3) will be described (Fig. 1). Using our protocol, DNA fiber length, replication fork speed, inter-replication origin distance, and fork symmetry can be measured, and replication events including the detection of stalled/terminated forks, new origins, bidirectional forks, and fork terminations can be quantified.
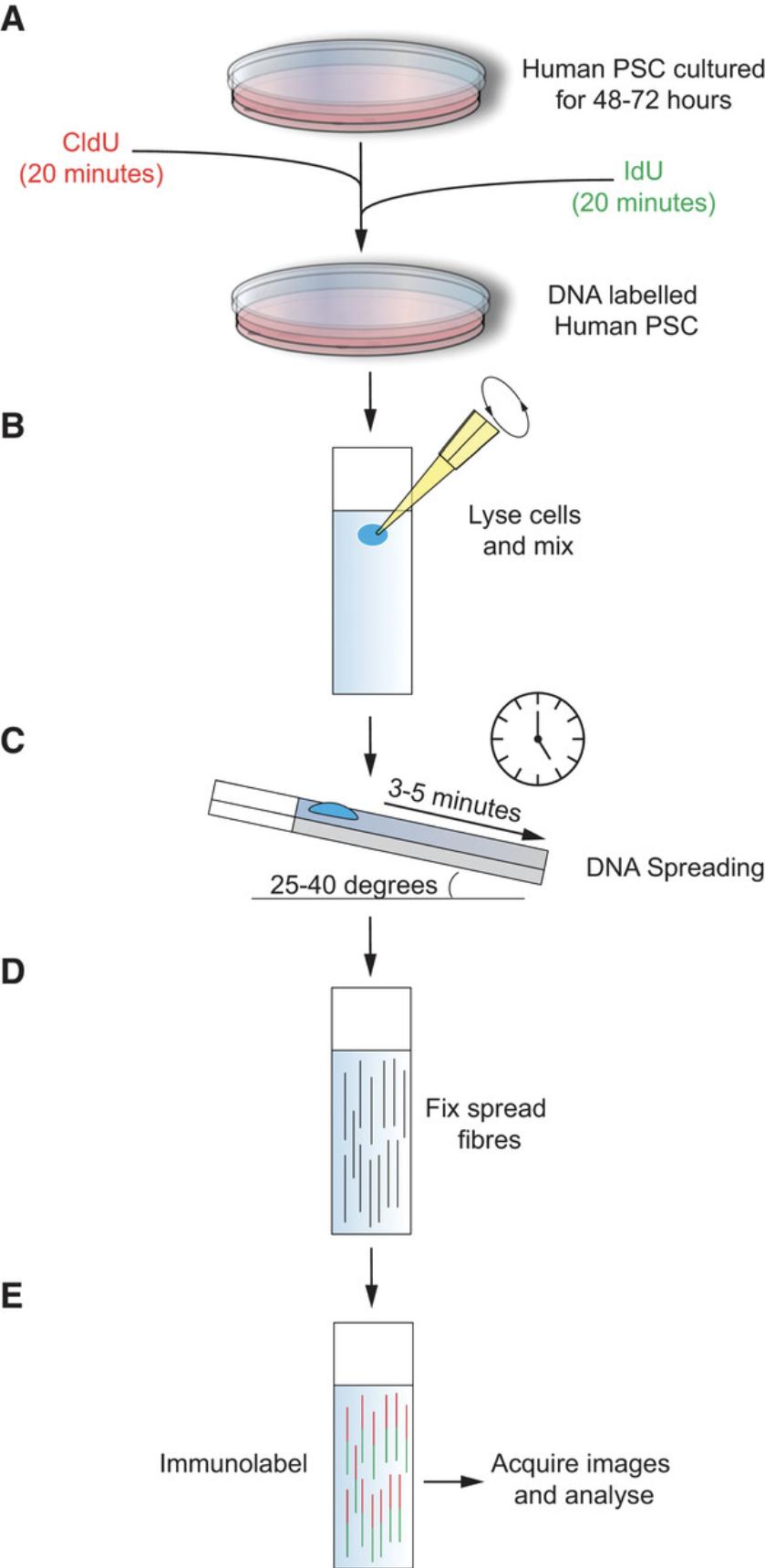
The protocols in this article have been successfully used to monitor replication dynamics in several human iPSC and ES cell lines cultured in feeder-free conditions using mTeSR, Nutristem, or E8 on a matrix of recombinant vitronectin−coated plates (Halliwell et al., 2020).
Basic Protocol 1: DNA FIBER LABELING
This protocol describes the pulse labeling of DNA and harvesting of the labeled human PSC intended for use in the DNA fiber assay. Human PSC should be seeded at least 48 hr before starting the experiment. Pulse labeling is performed sequentially with two thymidine analogs—chlorodeoxyuridine (CldU) and iododeoxyuridine (IdU)—for 20 min each (Fig. 1A). DNA labeling exploits the ability of in vitro−cultured cells to incorporate nucleotide analogs into newly replicating DNA strands. To ensure consistent and robust labeling, the cells should be at ∼50% confluency at the start of the experiment: this will ensure the cells are still in the growth phase and replication is not suppressed. Further, the labeling time should be strictly monitored. A deviation in timing will introduce inaccuracy and confound experimental results. Once labeled, the cells should be dissociated into single cells using TrypLE, to minimize stress and allow for accurate cell counting. Dissociated cells should be kept on ice to inhibit further enzymatic activity before spreading (Basic Protocol 2).
This protocol should produce a homogenous single-cell suspension of pulse-labeled cells in ice-cold PBS for DNA spreading (Basic Protocol 2).
Materials
-
1× PBS (see recipe)
-
Human PSC culture medium: mTeSR 1 (STEMCELL Technologies, 85850), E8 (in house; Chen et al., 2011), or Nutristem XF (Biological Industries, 05-100-1A)
-
Rho-associated, coiled-coil containing protein kinase 1 inhibitor (Y-27632; see recipe)
-
One T-12.5 flask of human embryonic or induced pluripotent stem cells
-
TrypLE Express single-cell dissociation solution (Life Technologies, 12604-021)
-
2.5 mM 5-chloro-2′-deoxyuridine (CldU) stock solution (see recipe)
-
2.5 mM 5-iodo-2′-deoxyuridine (IdU stock solution (see recipe)
-
Ice cold 1× Dulbecco's phosphate buffered saline (PBS; see recipe)
-
Vitronectin-coated 6-well plate (see recipe)
-
15-ml conical centrifuge tubes (Falcon or equivalent)
-
Cell counter
-
Centrifuge
Seed human PSC for DNA labeling
1.Add 2 ml of human PSC culture medium, supplemented with Y-27632 (1:1000), to each well of a vitronectin-coated 6-well plate. Pre-warm the plate for 30 min at 37°C in a 5% CO2 incubator to allow the vitronectin to set.
2.Aspirate the cell culture medium from the T-12.5 flask of human PSC.
3.Add 1 ml TrypLE to the T-12.5 flask and incubate for 3 min at 37°C in a 5% CO2 incubator.
4.Add 4 ml of human PSC culture medium to the T-12.5 flask to detach the cells. Transfer the cell suspension into a 15-ml tube.
5.Count the total cell number and centrifuge the cells 3 min at 300 × g , room temperature, then resuspend the pellet in 1 ml human PSC culture medium.
6.Seed 80,000 cells per well of the 6-well plate prepared in step 1.Culture for 72 hr (minimum of 48 hr, although cell numbers will need to be adjusted accordingly) with daily batch feeding, removing the Y-27632 after 24 hr.
DNA pulse labeling
7.Prewarm CldU and IdU stock solutions to 37°C.
8.Prewarm TrypLE solution to 37°C.
9.Add CldU stock solution to the seeded cells (from step 1-6) for a final concentration of 25 μM (Fig. 1A).
10.Incubate for precisely 20 min at 37°C in a 5% CO2 incubator.
11.Add IdU stock solution to the seeded cells for a final concentration of 250 μM (Fig. 1A).
12.Incubate for precisely 20 min at 37°C in a 5% CO2 incubator.
13.Aspirate the medium.
14.Wash twice, each time with 2 ml ice-cold PBS, aspirating medium after each wash.
15.Add 1 ml pre-warmed TrypLE solution to each well. Coat the wells thoroughly and aspirate off the excess solution.
16.Incubate for 3 min.
17.Aspirate off the excess TrypLE and add 0.5 ml ice-cold PBS to release the cells from the 6-well plate. Transfer the cell suspension to a 15-ml conical tube.
18.Count the total cell number and dilute with ice-cold PBS to 400,000 cells per ml.
19.Keep on ice and spread (Basic Protocol 2) within 30 min.
Basic Protocol 2: DNA SPREADING
This protocol aims to describe the technique of DNA spreading (Parra & Windle, 1993). Following DNA labeling (Basic Protocol 1), a small droplet of cell suspension is lysed on a glass slide (Fig. 1B) and then tilted to allow spreading. The droplet of cell lysate solution generates cohesive tension with the slide as it runs its length, stretching the DNA into fibers that can be later stained and visualized by immunofluorescence (Basic Protocol 3 and Support Protocol 1).
The major factor requiring optimization in this protocol is the type of glass slide used. Before initiating this protocol, different brands and batches of slides should be tested to ensure the droplet runs slowly down the length of the glass slide when tilted at 25°-40°. If this protocol is conducted properly, a droplet of cell lysate solution should run the length of a glass slide in 3 to 5 min. Once dried, the path of the droplet should leave behind a cloudy precipitate. Depending on the temperature and humidity, it may be necessary to alter the volume of the spreading buffer or the angle at which the slide is tilted to ensure that the droplet runs at a slow, constant rate.
Materials
-
Cell suspension (Basic Protocol 1)
-
Spreading buffer (see recipe)
-
Methanol/acetic acid fixative (see recipe)
-
Super premium microscope slides (BDH Laboratory Supplies)
- The lid of a multi-well plate (or something similar) to hold the tilted slides in place
Cell lysis
1.Working with one sample at a time, pipette 2 μl of ice-cold cell suspension at the top of each of 3-5 microscope slides, depending on the number of replicates to be performed (Fig. 1B).
2.Allow to dry for 5-7 min.
3.Add ∼7 μl of spreading buffer and stir with a pipette tip (Fig. 1B).
4.Incubate for 2 min.
Spreading
5.Tilt each slide at an angle of 25° to 40° and hold in place on the lid of a multi-well plate.
6.Let the droplet run down the slide slowly and at a constant speed. The droplet should reach the bottom edge after 3 to 5 min (Fig. 1C).
7.Lay the slides flat and allow to air dry completely (approximately 15 min).
8.Go back to step 1 and prepare the next sample.
Fixation
9.Using a 1-ml pipette, gently add 1 ml methanol/acetic acid fixative to the bottom right-hand corner of the slide and allow it to spread over the entirety of the slide (Fig. 1D).
10.Incubate for 10 min.
11.Tilt the slide to allow the excess fixative to run off, and allow to air dry completely.
Basic Protocol 3: IMMUNOSTAINING
This protocol describes the immunolabeling of DNA fibers spread onto glass slides. The protocol begins with an acid treatment to denature the DNA fibers before blocking for unspecific binding and labeling the CldU and IdU labeled fibers with rat and mouse anti-BrdU primary antibodies, respectively. Detection of the labeled DNA fibers is possible by immunostaining with fluorescently labeled secondary antibodies (Fig. 1E).
Following this immunostaining protocol, it will be possible to detect the labeled DNA fibers by confocal microscopy. The labeled DNA should be detectable as bi-labeled fibers in the 555 and 488 Alexa Fluor visible spectrum. Further details and expected results from this protocol can be found in the “Understanding Results” section.
Materials
-
Slides with spread DNA fibers (Basic Protocol 2)
-
2.5 M hydrochloric acid (see recipe)
-
1× PBS (see recipe)
-
Blocking solution (see recipe), pre-chilled
-
Rat monoclonal anti-BrdU antibody [BU1/75 (ICR1)] (Abcam, ab6326)
-
Mouse monoclonal anti-BrdU antibody (clone B44) (Becton Dickson, 347580 (7580))
-
4% paraformaldehyde (PFA; see recipe)
-
Alexa Fluor 555 goat anti−rat IgG (Thermo Fisher Scientific, A21434)
-
Alexa Fluor 488 F (ab′)2 goat anti−mouse IgG (Thermo Fisher Scientific, A-11017)
-
Fluoroshield mounting medium (Sigma, F6182)
-
Glass staining troughs with slide racks (e.g., Sigma, BR472200)
-
Coplin staining jar (e.g., Sigma, S5766)
-
Coverslips, 60 × 22 mm (ThermoFisher Scientific, BB02200600A113MNT0)
Wash, denature, and block
1.Wash slides twice with double-distilled H2O in a staining trough.
2.Rinse the slides once in 2.5 M hydrochloric acid in a staining trough.
3.Denature in 2.5 M hydrochloric acid in a staining trough for 1 hr.
4.Rinse the slides twice with 1× PBS.
5.Wash the slides twice with blocking solution in a Coplin jar.
6.Incubate the slides in fresh blocking solution in the Coplin jar for 1 hr.
Primary antibody immunolabeling
7.Make a fresh solution of primary antibodies by mixing rat monoclonal anti-BrdU antibody (1:500) and mouse monoclonal anti-BrdU antibody (1:500) in blocking solution.
8.Using a 1-ml pipette, gently add 750 μl of the antibody solution to the bottom right-hand corner of the slide and allow it to spread over the entirety of the slide.
9.Incubate for 1 hr.
10.Rinse three times with 1× PBS in a staining trough. Remove the slides and stand them upright on a paper towel to remove any excess PBS.
11.Using a 1-ml pipette, gently add 1 ml of 4% PFA to the bottom right-hand corner of the slide and allow it to spread over the entirety of the slide.
12.Incubate for 10 min.
13.Rinse three times with 1× PBS in a staining trough.
14.Wash three times with blocking solution in a Coplin jar.
Secondary antibody immunolabeling
All steps should be performed in the dark to reduce photobleaching of the fluorophore labels. Use a dark staining trough and Coplin jar or wrap in aluminum foil to block the light.
15.Make a fresh solution of secondary antibodies by mixing Alexa Fluor 555 goat anti-rat IgG (1:500) and Alexa Fluor 488 F (ab′)2 goat anti−mouse IgG (1:500) in blocking solution.
16.Using a 1-ml pipette, gently add 750 μl of the secondary antibody mix to the bottom right-hand corner of the slide, and allow it to spread over the entirety of the slide (Fig. 1E).
17.Incubate for 2 hr in the dark at room temperature
18.Rinse twice with 1× PBS in a staining trough.
19.Wash three times with blocking solution in a Coplin jar.
20.Rinse twice with 1× PBS in a staining trough.
21.Using Fluoroshield, mount a coverslip onto the glass slide and allow to dry for 30 min in the dark.
Support Protocol 1: MICROSCOPY/DATA ACQUSITION
In support of the basic protocols above, we provide microscopy parameters and data-acquisition instructions to facilitate accurate data analysis (Support Protocol 2). It is important to take pictures of fibers from across the entire slide where fibers do not overlap, in order to ensure that robust measurements will be obtained. In total, 150-200 fibers should be acquired per experimental condition to enable reliable estimation of replication parameters. Three or more independent repeats should be performed.
Microscopy parameters
The parameters in Table 1 have provided robust measurements when using an Olympus FV1000 confocal microscope.
Parameter | Setting | Description/notes |
---|---|---|
Objective | ×63 | Oil-immersion lens |
Channel (CldU) | 568 | Detection of CldU-labeled Alexa Fluor 555 label |
Channel (IdU) | 488 | Detection of IdU-labeled Alexa Fluor 488 label |
Zoom | 1 | Increasing the zoom will limit the number of fibers per field and could confound measurements (Support Protocol 2) |
Scan format | 1080 × 1080 | Number of pixels in the field |
Scan speed | ∼8 μs | This can be optimized to produce the best image quality while minimizing photobleaching |
File export | TIFF | Contains metadata of scale |
Support Protocol 2: DATA ANALYSIS
This protocol will describe the data analysis and replication-event characterization that can be achieved from DNA fiber assays as described above. In particular, we will describe how to measure DNA fiber length, replication fork speed, fork symmetry, and inter-replication origin distance, and how to detect and quantify different replication events.
In each case, a total of 150-200 fibers should be analyzed per condition for a reliable estimation of fiber length, and to account for the range of fiber lengths that can be attributed to the varied dynamics of replication forks. Only clear fibers that do not overlap should be included (Fig. 2). It is also important to image fibers from across the entire slide to ensure robust measurement of replication fork dynamics. Data should be plotted as scatter dot plots, or in box-and-whiskers plots. Statistical significance between two groups is normally assessed using the Mann-Whitney U test (unpaired and nonparametric).
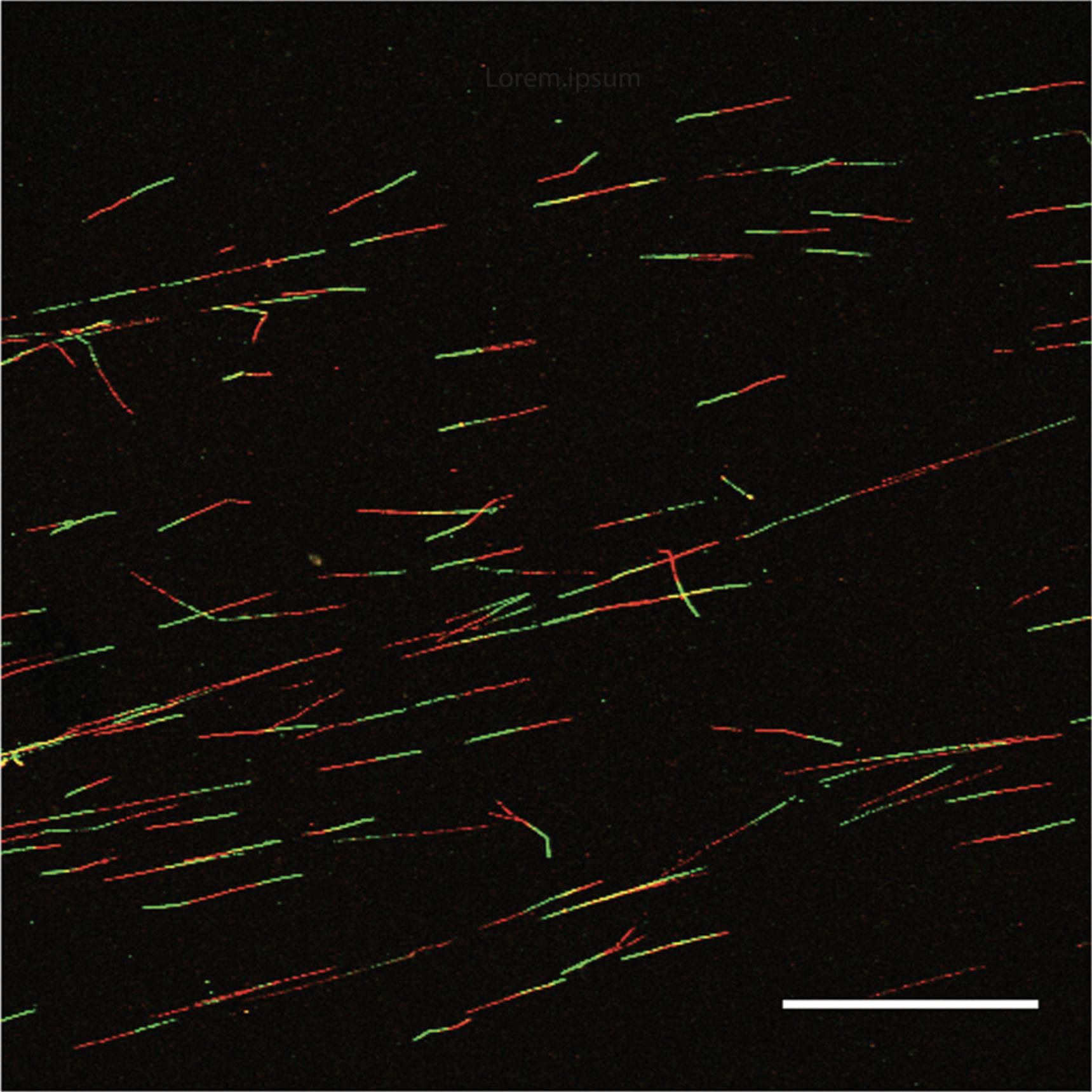
Materials
- TIFF images from Support Protocol 1
- ImageJ software (Schneider, Rasband, & Eliceiri, 2012)
Determining DNA fiber length (μm)
The following steps are for determining total length covered by the replication fork within the 40-min pulse labelings.
1.Open each TIFF image from Support Protocol 1 in ImageJ.
2.In the Analyze menu, select measure.
3.For each fiber, measure the length of the CldU (red) followed by the IdU (green).
4.Find the sum of the red and green lengths (Fig. 3A).
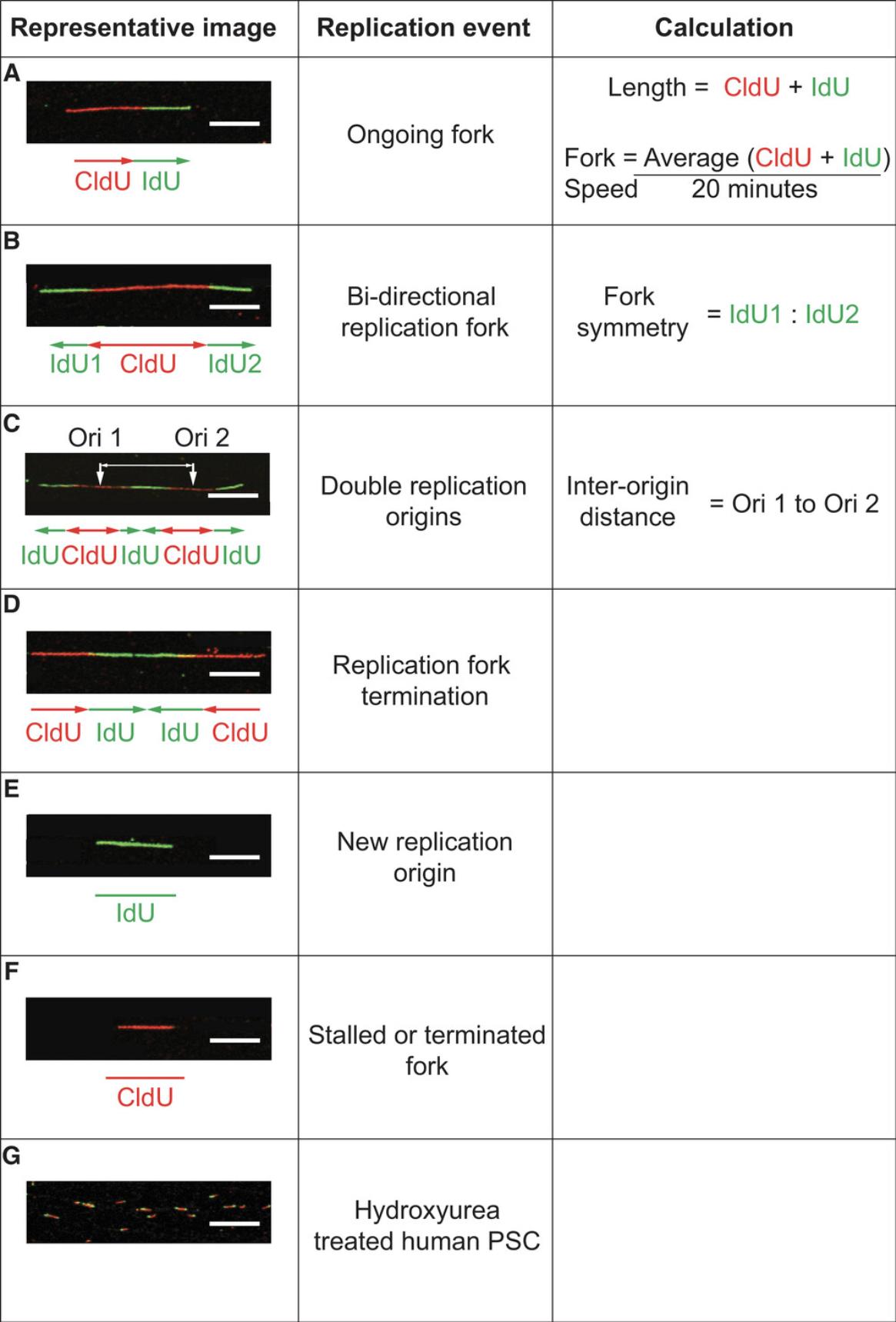
Determining replication fork speed of a progressing fork
The following steps are for determining the average speed of the replication fork over the two sequential 20-min pulse labelings.
5.Perform steps 1-3.
6.Find the average length of each of CldU and IdU.
7.Divide by the pulse length (20 min).
8.To convert from μm/min to kb/min, a commonly used conversion factor of 2.59 kb per μm (Jackson & Pombo, 1998; Fig. 3A) can be applied.
Fork symmetry
The following steps are for analysis of the synchronized progression of sister forks emanating from a single origin. Fork asymmetry suggests replication-fork stalling events. This analysis is performed on fibers that have contiguous IdU-CldU-IdU (green-red-green) signals.
9.Perform steps 1-2.
10.Measure the length of the two IdU (green) tracts emanating from a single CldU (red) origin (Fig. 3B).
11.Calculate the ratio between the two green tracts.
Inter-origin distance
The following steps are for determining distance between origins in two consecutive DNA tracts. Care should be taken when measuring the inter-origin distance using the DNA spreading technique, selecting only consecutive DNA tracts that are certain to be on the same DNA fiber. Ideally, DNA should be counterstained with a fluorescent dye, such as YOYO-1.
12.Perform step 1-2.
13.Measure the distance between two CldU (red) tracts of two consecutive and contiguous IdU-CldU-IdU (green-red-green) fibers (Fig. 3C).
Replication events
The frequency of replication events, detailed in Figure 3, can be quantified and normalized to the total number of fibers present. These measurements can provide insight into replication response to loss of protein or replication stress.
REAGENTS AND SOLUTIONS
Blocking solution
Thoroughly dissolve 5 g BSA and 0.5 ml of Tween 20 in 500 ml of 1× Dulbecco's PBS (see recipe). Make fresh and chill to 4°C prior to use.
CldU stock solution, 25 mM
Dissolve 6.6 mg CldU (Sigma, C6891) in 10 ml DMEM/F12 medium (Sigma, D6421). Vortex until fully dissolved. Divide into 0.25-ml aliquots and store at −20°C for up to 1 year.
Dulbecco's PBS, 1×
Dilute 10× concentrate stock, without calcium and magnesium (Sigma, D1408) 1:10 with double-distilled H2O. Autoclave the 1× solution before use. Store up to 1 year at room temperature.
Hydrochloric acid, 2.5 M
Slowly add 83.2 ml hydrochloric acid (Sigma, 320331) to 316.8 ml double-distilled H2O. Prepare fresh for each use.
IdU stock solution, 2.5 mM
Dissolve 8.9 mg IdU (Sigma, I7125) in 10 ml DMEM/F12 medium (Sigma, D6421). Heat to 60°C and vortex for 5 min to dissolve. Make 0.25-ml aliquots and store at −20°C for up to 1 year.
Methanol/acetic acid fixative
Mix methanol (Sigma, 179337) and acetic acid (Sigma, 695092) at a 3:1 ratio. For example, to make 50 ml of fixative, mix 37.5 ml of methanol with 12.5 ml acetic acid. Store at room temperature in an air-tight container for up to 1 year.
Paraformaldehyde solution, 4%
Dilute 8% paraformaldehyde solution (see recipe) in 1× Dulbecco's PBS (see recipe) at a 1:1 ratio. Store up to 2 weeks at 4°C.
Paraformaldehyde solution, 8%
Add 40 g of paraformaldehyde (Sigma, P6148) to 400 ml double-distilled H2O. Heat the solution to 60°C and stir at a medium speed under a fume hood. Add ∼10 drops of 1 N NaOH to dissolve the PFA granules. Continue to mix for 2 hr. Allow the solution to cool, and add double-distilled H2O for a total volume of 500 ml. Aliquot and store at −20°C for up to 1 year.
Rho-associated, coiled-coil containing protein kinase 1 inhibitor (Y-27632)
Dissolve Y-27632 dihydrochloride (TOCRIS, 1254) in DMSO (Sigma, 472301) for a 10 mM stock solution. Store for up to 1 year at −20°C.
Spreading buffer
Solution 1: Dissolve 3.152 g of Tris hydrochloride in 40 ml of double-distilled H2O and adjust to pH 7.4.
Solution 2: Dissolve 1.46 g of disodium EDTA in 40 ml of double-distilled H2O.
Combine solution 1 and 2 and add 0.5 g SDS. Make the solution up to 100 ml with double-distilled H2O and vortex to dissolve. Store at room temperature for up to 1 year.
Vitronectin-coated 6-well plate
Thaw 120 μl vitronectin recombinant protein (ThermoFisher Scientific, A14700) at room temperature and combine with 11.88 ml of 1× Dulbecco's PBS (see recipe). Mix well and add 2 ml to each well of a 6-well cell culture plate. Incubate at room temperature for 1 hr. For longer-term storage, keep at 4°C and use within 2 weeks.
COMMENTARY
Background Information
Human PSC hold great promise in the field of regenerative medicine. Yet, in order to reach the full potential of these cells, we must first capitalize on their ability to rapidly and endlessly renew to generate large numbers of genetically stable and undifferentiated cells. However, it has become apparent that human PSC acquire genetic changes during long-term culture, which raise concerns over the safety of stem cell−derived products that are destined for the clinic (Draper et al., 2004, Olariu et al., 2010). The recurrent nature of certain karyotypic changes, such as amplifications to chromosomes 1q, 12p, 17q, and 20q, have highlighted that certain mutations provide a growth advantage to the variant cell, which becomes selected for in a culture over time (Amps et al., 2011, Baker et al., 2016, Olariu et al., 2010). Despite the mechanism of selection now being well defined, relatively little is known about the underlying mutational mechanisms, although the observations of replication stress and genomic damage in human PSC are similar to the oncogene-induced model of genetic instability in cancer development and progression (Halazonetis, Gorgoulis, & Bartek, 2008). The self-renewal of human PSC is characterized by an abbreviated G1 phase that bypasses the Rb/E2F checkpoint and is driven by high expression of cyclin D2 and constitutive expression of cyclin E (Becker et al., 2006, Filipczyk, Laslett, Mummery, & Pera, 2007). Maintaining this rapid proliferation over extensive culture periods may expose these cells to replication stress, characteristics of which, such as reduced replication rates, have been defined in human PSC using the DNA fiber assay (Halliwell et al., 2020). Furthermore, we have recently identified regions of microhomology at the breakpoint of chromosome 20 tandem amplification, which implicates that template-switching mechanisms at stalled or collapsed forks are responsible for these mutations (J.A. Halliwell, D. Baker, P.W. Andrews, I. Barbaric, unpub. observ.). Collectively, these studies have determined that genetic stability in human PSC is overtly linked to DNA replication. This article provides experimental details and protocols required to perform the DNA fiber assay, which have been optimized for studying replication stress in human PSC.
The DNA fiber assay has become the gold-standard assay in direct monitoring of DNA replication-fork dynamics. The protocols described here are based on the previously described DNA fiber assay (Merrick, Jackson, & Diffley, 2004), which itself was modified from the original DNA fiber labeling (DIRVISH) technique (Jackson & Pombo, 1998). Two distinguishable modified nucleotides, CldU and IdU, are added sequentially to the cell culture medium to pulse label nascent DNA strands. The labeled nascent DNA is then removed from the cells by lysis and stretched onto glass slides. By tilting the glass slide, the droplet of DNA solution runs down its length under the force of gravity, stretching the fibers as it runs (Parra & Windle, 1993). Stretching the DNA by spreading is fast, and requires little material or preparation. It is therefore affordable and accessible to most research laboratories. However, the DNA fiber assay described here has relatively low resolution and does not detect ssDNA discontinuities, which means that analysis of the results must be done carefully to ensure that broken fibers and crossed fibers are not included. The addition of replication-stalling and DNA-damaging agents prior to and during the DNA-labeling procedure can facilitate the study of fork progression following stalling, and when encountering DNA lesions. Human PSC are particularly sensitive to genotoxic agents, radiation, or agents used to induce replication block, even at doses that have little effect on cancer or somatic cells (Desmarais et al., 2012; Desmarais et al., 2016; Hyka-Nouspikel et al., 2012; Luo et al., 2012; Simara et al., 2017). However, these experiments have been reviewed extensively elsewhere (Quinet, Carvajal-Maldonado, Lemacon, & Vindigni, 2017).
The procedure described in this article has been optimized for use with human PSC, and has been successfully applied to several human iPSC and human ESC cell lines (Halliwell et al., 2020). We have utilized this assay to improve culture conditions: supplementing cultures with nucleosides alleviates replication stress and decreases the frequency of mitotic errors, highlighting that these events are linked in human PSC (Halliwell et al., 2020). The DNA fiber assay has revealed approaches that can be used to reduce the appearance of genetic instability in human PSC, which is necessary for the safe application of human PSC in regenerative medicine.
Critical Parameters
We have observed little difference in fiber assay results whether human PSC are cultured in mTeSR, E8, or Nutristem cell culture medium. Currently, feeder layer−dependent cell culture practices have not been tested. The whole assay can be done in a single day, although we have included a stop point following the fixation step in Basic Protocol 2, which allows the protocol to be run over a period of 2 days.
We advise allowing the human PSC to recover for at least 48 hr following plating. This will permit 24 hr in culture without Rho-associated, coiled-coil containing protein kinase 1 inhibitor (Y-27632). It will also allow recovery from stressful re-plating, and for the cells to re-enter logarithmic growth phase. It is critical, where the results of experiments are to be compared, that the density of cells initially seeded and the confluency at the point of starting the experiment be consistent. Higher confluency may cause cell proliferation and DNA replication to slow.
With regard to labeling of DNA, it is possible to increase or decrease the pulse labeling times, but this will result in longer and shorter DNA fibers, respectively. Again, the labeling time must be constant across comparable experiments. It is also important that the labeling time be accurately measured. Deviations one of 1 min will increase labeling by 5%, and will confound the results of the experiment. To ensure timely labeling, it is advised that the reagents be prepared well ahead of starting the experiment. Also, when adding the second nucleotide analog (IdU), the plate should be removed from the incubator 1 min before the end of the first incubation period, to give a time buffer when preparing the second label.
Once harvested, the cells should be suspended in ice-cold PBS and kept on ice. DNA spreading should then be performed within 30 min, to minimize cell death.
When spreading DNA fibers, the user should select slides where the droplet spreads down the length of the slide in 3 to 5 min at a constant rate. In our experience, batches of slides can differ from one another, and each batch should be optimized.
Troubleshooting
Table 2 describes problems that can arise with various steps in the assay, along with their possible causes and Solutions.
Step | Problem | Possible reason | Solution |
---|---|---|---|
Basic Protocol 1 (step 18) | Too many overlapping DNA fibers | Density of labeled cells for spreading is too high | Dilute the harvested labeled cell solution |
Basic Protocol 2 (step 6) | Fibers spreading occur too slow or fast | Room temperature | Increase or decrease the volume of spreading buffer |
Humidity | Increase or decrease the slide tilt angle | ||
Microscope slide | Optimize spreading conditions for each brand and batch of microscope slides prior to use | ||
Support Protocol 2 | Inconsistent results between biological and technical replicates | Length of pulse labeling | Ensure CldU or IdU is added for 20 min exactly |
Time between cell harvesting and spreading | Perform spreading sooner after cell harvesting. Reduce the number of samples to become more manageable. |
Statistical Analysis
It is important to take images of fields with minimum fiber cross overs across the whole slide to ensure a robust measurement of the progressing replication forks. A minimum of 150-200 individual fibers must be measured to account for the heterogeneity between progressing forks. The conversion factor for stretched DNA fibers is 2.59 kb/μm (Jackson & Pombo, 1998).
Consideration should be given to the presentation of data. Histograms and scatter plots are appropriate for capturing normal differences in replication fork progression. Statistical significance can be calculated using an unpaired Mann-Whitney U- test.
Understanding Results
The DNA fibers produced can be variable but, in our experience, under normal growth conditions, they should contain equal lengths of CldU and IdU staining. When measured, we find the replication fork speed to vary between 0.5 and 0.75 kb/min. A reduced fiber length or fork speed can indicate replication stress.
Monitoring the frequency of replication events can provide valuable information regarding the replication processes going on during the culture of human PSC (Fig. 3). A strict control of replication origin density is required to maintain chromosomal stability (Prioleau & MacAlpine, 2016). Excessive replication-origin firing can deplete necessary protein and metabolites required for efficient DNA replication (Sørensen & Syljuåsen, 2012). A decrease in inter-origin distance or a higher density of IdU-only labeled fibers is a measure of increased origin density, suggesting greater numbers of simultaneously firing origins of replication. Fork stalling is a prerequisite for DNA breakage, which can become a substrate for genetic instability (Toledo, Neelsen, & Lukas, 2017). Measuring the frequency of CldU-only fibers or the ratio of the IdU labels on a bi-directional fork can be used to measure fork stalling. A shorter IdU fiber on one side of a bi-directional fork implies a fork-stalling event, and will result in a greater ratio between the IdU tracts.
Time Considerations
Basic Protocol 1
Cells should be grown for a minimum of 48 hr following seeding; we have found 72 hr to be optimal. Steps 1 to 6: ∼40 min will be needed for cell seeding. ∼20 min per day will be needed to refresh the medium.
Steps 7 to 18: ∼1 hr will be needed to pulse label and harvest the cells.
Basic Protocol 2
Step 1 to 4: preparation of cell lysate for DNA spreading will require ∼15 min.
Step 5 to 7: DNA spreading will require ∼20 min for spreading one set of three slides simultaneously.
Step 8 to 10: fixation of DNA fibers will require ∼15 min.
Basic Protocol 3
Step 1 to 18: DNA immunolabeling steps will require ∼6.5 hr.
Support Protocol 1 and 2
Several hours will be required for image acquisition and data analysis, but this will be variable depending on the quality of the DNA fibers and the biological question being asked.
Acknowledgments
This project has received funding from the European Union's Horizon 2020 research and innovation program under grant agreement No. 668724.
This work was partly funded by the European Union's Horizon 2020 research and innovation program under grant agreement No. 668724 and partly by the UK Regenerative Medicine Platform, MRC reference MR/R015724/1.
Literature Cited
- Amps, K., Andrews, P. W., Anyfantis, G., Armstrong, L., Avery, S., Baharvand, H., … Zhou, Q. (2011). Screening ethnically diverse human embryonic stem cells identifies a chromosome 20 minimal amplicon conferring growth advantage. Nature Biotechnology , 29, 1132–1144.
- Avery, S., Hirst, A. J., Baker, D., Lim, C. Y., Alagaratnam, S., Skotheim, R. I., … Knowles, B. B. (2013). BCL-XL mediates the strong selective advantage of a 20q11.21 amplification commonly found in human embryonic stem cell cultures. Stem Cell Reports , 1, 379–386. doi: 10.1016/j.stemcr.2013.10.005.
- Baker, D., Hirst, A. J., Gokhale, P. J., Juarez, M. A., Williams, S., Wheeler, M., … Barbaric, I. (2016). Detecting genetic mosaicism in cultures of human pluripotent stem cells. Stem Cell Reports , 7, 998–1012. doi: 10.1016/j.stemcr.2016.10.003.
- Becker, K. A., Ghule, P. N., Therrien, J. A., Lian, J. B., Stein, J. L., Van Wijnen, A. J., & Stein, G. S. (2006). Self-renewal of human embryonic stem cells is supported by a shortened G1 cell cycle phase. Journal of Cellular Physiology , 209, 883–893. doi: 10.1002/jcp.20776.
- Blum, B., Bar-Nur, O., Golan-Lev, T., & Benvenisty, N. (2009). The anti-apoptotic gene survivin contributes to teratoma formation by human embryonic stem cells. Nature Biotechnology , 27, 281–287. doi: 10.1038/nbt.1527.
- Burrell, R. A., Mcclelland, S. E., Endesfelder, D., Groth, P., Weller, M. C., Shaikh, N., … Swanton, C. (2013). Replication stress links structural and numerical cancer chromosomal instability. Nature , 494, 492–496. doi: 10.1038/nature11935.
- Chen, G., Gulbranson, D. R., Hou, Z., Bolin, J. M., Ruotti, V., Probasco, M. D., … Thomson, J. A. (2011). Chemically defined conditions for human iPSC derivation and culture. Nature Methods , 8, 424–429. doi: 10.1038/nmeth.1593.
- Desmarais, J. A., Hoffmann, M. J., Bingham, G., Gagou, M. E., Meuth, M., & Andrews, P. W. (2012). Human embryonic stem cells fail to activate CHK1 and commit to apoptosis in response to DNA replication stress. Stem Cells , 30, 1385–1393. doi: 10.1002/stem.1117.
- Desmarais, J. A., Unger, C., Damjanov, I., Meuth, M., & Andrews, P. (2016). Apoptosis and failure of checkpoint kinase 1 activation in human induced pluripotent stem cells under replication stress. Stem Cell Research & Therapy, 7, 17.
- Draper, J. S., Smith, K., Gokhale, P., Moore, H. D., Maltby, E., Johnson, J., … Andrews, P. W. (2004). Recurrent gain of chromosomes 17q and 12 in cultured human embryonic stem cells. Nature Biotechnology , 22, 53–54. doi: 10.1038/nbt922.
- Filipczyk, A. A., Laslett, A. L., Mummery, C., & Pera, M. F. (2007). Differentiation is coupled to changes in the cell cycle regulatory apparatus of human embryonic stem cells. Stem Cell Research , 1, 45–60. doi: 10.1016/j.scr.2007.09.002.
- Halazonetis, T. D., Gorgoulis, V. G., & Bartek, J. (2008). An oncogene-induced DNA damage model for cancer development. Science , 319, 1352–1355. doi: 10.1126/science.1140735.
- Halliwell, J. A., Frith, T. J. R., Laing, O., Price, C. J., Bower, O. J., Stavish, D., … Andrews, P. W. (2020). Nucleosides rescue replication-mediated genome instability of human pluripotent stem cells. Stem Cell Reports , 14, P1009–1017. doi: 10.1016/j.stemcr.2020.04.004.
- Hyka-Nouspikel, N., Desmarais, J., Gokhale, P. J., Jones, M., Meuth, M., Andrews, P. W., & Nouspikel, T. (2012). Deficient DNA damage response and cell cycle checkpoints lead to accumulation of point mutations in human embryonic stem cells. Stem Cells , 30, 1901–1910. doi: 10.1002/stem.1177.
- Jackson, D. A., & Pombo, A. (1998). Replicon clusters are stable units of chromosome structure: Evidence that nuclear organization contributes to the efficient activation and propagation of S phase in human cells. Journal of Cell Biology , 140, 1285–1295. doi: 10.1083/jcb.140.6.1285.
- Lamm, N., Ben-David, U., Golan-Lev, T., Storchová, Z., Benvenisty, N., & Kerem, B. (2016). Genomic instability in human pluripotent stem cells arises from replicative stress and chromosome condensation defects. Cell Stem Cell , 18, 253–261. doi: 10.1016/j.stem.2015.11.003.
- Luo, L. Z., Gopalakrishna-Pillai, S., Nay, S. L., Park, S. W., Bates, S. E., Zeng, X., … O'connor, T. R. (2012). DNA repair in human pluripotent stem cells is distinct from that in non-pluripotent human cells. PloS One , 7, e30541. doi: 10.1371/journal.pone.0030541.
- Merrick, C. J., Jackson, D., & Diffley, J. F. (2004). Visualization of altered replication dynamics after DNA damage in human cells. Journal of Biological Chemistry , 279, 20067–20075. doi: 10.1074/jbc.M400022200.
- Nieminuszczy, J., Schwab, R. A., & Niedzwiedz, W. (2016). The DNA fibre technique—Tracking helicases at work. Methods , 108, 92–98. doi: 10.1016/j.ymeth.2016.04.019.
- Olariu, V., Harrison, N. J., Coca, D., Gokhale, P. J., Baker, D., Billings, S., … Andrews, P. W. (2010). Modeling the evolution of culture-adapted human embryonic stem cells. Stem Cell Research , 4, 50–56. doi: 10.1016/j.scr.2009.09.001.
- Parra, I., & Windle, B. (1993). High resolution visual mapping of stretched DNA by fluorescent hybridization. Nature Genetics , 5, 17–21. doi: 10.1038/ng0993-17.
- Prioleau, M. N., & Macalpine, D. M. (2016). DNA replication origins—Where do we begin? Genes & Development, 30, 1683–1697.
- Quinet, A., Carvajal-Maldonado, D., Lemacon, D., & Vindigni, A. (2017). DNA fiber analysis: Mind the gap! Methods in Enzymology , 591, 55–82. doi: 10.1016/bs.mie.2017.03.019.
- Schneider, C. A., Rasband, W. S., & Eliceiri, K. W. (2012). NIH Image to ImageJ: 25 years of image analysis. Nature Methods , 9, 671–675. doi: 10.1038/nmeth.2089.
- Simara, P., Tesarova, L., Rehakova, D., Matula, P., Stejskal, S., Hampl, A., & Koutna, I. (2017). DNA double-strand breaks in human induced pluripotent stem cell reprogramming and long-term in vitro culturing. Stem Cell Research & Therapy, 8, 73.
- Sørensen, C. S., & Syljuåsen, R. G. (2012). Safeguarding genome integrity: The checkpoint kinases ATR, CHK1 and WEE1 restrain CDK activity during normal DNA replication. Nucleic Acids Research , 40, 477–486. doi: 10.1093/nar/gkr697.
- Takahashi, K., Tanabe, K., Ohnuki, M., Narita, M., Ichisaka, T., Tomoda, K., & Yamanaka, S. (2007). Induction of pluripotent stem cells from adult human fibroblasts by defined factors. Cell , 131, 861–872. doi: 10.1016/j.cell.2007.11.019.
- Thompson, O., Von Meyenn, F., Hewitt, Z., Alexander, J., Wood, A., Weightman, R., … Andrews, P. W. (2020). Low rates of mutation in clinical grade human pluripotent stem cells under different culture conditions. Nature Communications , 11, 1528. doi: 10.1038/s41467-020-15271-3.
- Thomson, J. A., Itskovitz-Eldor, J., Shapiro, S. S., Waknitz, M. A., Swiergiel, J. J., Marshall, V. S., & Jones, J. M. (1998). Embryonic stem cell lines derived from human blastocysts. Science , 282, 1145–1147. doi: 10.1126/science.282.5391.1145.
- Toledo, L., Neelsen, K. J., & Lukas, J. (2017). Replication catastrophe: When a checkpoint fails because of exhaustion. Molecular Cell , 66, 735–749. doi: 10.1016/j.molcel.2017.05.001.
- Vallabhaneni, H., Lynch, P. J., Chen, G., Park, K., Liu, Y., Goehe, R., … Hursh, D. A. (2018). High basal levels of γH2AX in human induced pluripotent stem cells are linked to replication-associated DNA damage and repair. Stem Cells , 36, 1501–1513. doi: 10.1002/stem.2861.
- Zhang, J., Hirst, A. J., Duan, F., Qiu, H., Huang, R., Ji, Y., … Na, J. (2019). Anti-apoptotic mutations desensitize human pluripotent stem cells to mitotic stress and enable aneuploid cell survival. Stem Cell Reports , 12, 557–571. doi: 10.1016/j.stemcr.2019.01.013.
Citing Literature
Number of times cited according to CrossRef: 12
- Jing Qian, Yanxia Ma, William M. Tahaney, Cassandra L. Moyer, Amanda Lanier, Jamal Hill, Darian Coleman, Negar Koupaei, Susan G. Hilsenbeck, Michelle I. Savage, Brent D. G. Page, Abhijit Mazumdar, Powel H. Brown, The novel phosphatase NUDT5 is a critical regulator of triple-negative breast cancer growth, Breast Cancer Research, 10.1186/s13058-024-01778-w, 26 , 1, (2024).
- Jia Feng, You Heng Chuah, Yajing Liang, Nadia Omega Cipta, Yingying Zeng, Tushar Warrier, Gamal Ahmed Rashed Elsayed Elfar, Jeehyun Yoon, Oleg V Grinchuk, Emmy Xue Yun Tay, Ker-Zhing Lok, Zong-Qing Zheng, Zi Jian Khong, Zheng-Shan Chong, Jackie Teo, Emma May Sanford, Cheryl Jia Yi Neo, Hsin Yao Chiu, Jia Yu Leung, Loo Chien Wang, Yan Ting Lim, Tianyun Zhao, Radoslaw M Sobota, Karen Carmelina Crasta, Vinay Tergaonkar, Reshma Taneja, Shi-Yan Ng, Chit Fang Cheok, Shuo-Chien Ling, Yuin-Han Loh, Derrick Sek Tong Ong, PHF2 regulates genome topology and DNA replication in neural stem cells via cohesin, Nucleic Acids Research, 10.1093/nar/gkae457, 52 , 12, (7063-7080), (2024).
- Mónika Mórocz, Erda Qorri, Emese Pekker, Gabriella Tick, Lajos Haracska, Exploring RAD18-dependent replication of damaged DNA and discontinuities: A collection of advanced tools, Journal of Biotechnology, 10.1016/j.jbiotec.2023.12.001, 380 , (1-19), (2024).
- Xiaqing Zhang, Jing Guo, Xin Shi, Xin Zhou, Qiang Chen, LUC7L3 is a downstream factor of SRSF1 and prevents genomic instability, Cell Insight, 10.1016/j.cellin.2024.100170, 3 , 3, (100170), (2024).
- MegAnn K. Haubold, Jessica N. Pita Aquino, Sarah R. Rubin, Isabella K. Jones, Clairine I. S. Larsen, Edward Pham, Kinjal Majumder, Genomes of the autonomous parvovirus minute virus of mice induce replication stress through RPA exhaustion, PLOS Pathogens, 10.1371/journal.ppat.1011203, 19 , 5, (e1011203), (2023).
- Michèle Beniey, Audrey Hubert, Takrima Haque, Alexia Karen Cotte, Nelly Béchir, Xiaomeng Zhang, Danh Tran-Thanh, Saima Hassan, Sequential targeting of PARP with carboplatin inhibits primary tumour growth and distant metastasis in triple-negative breast cancer, British Journal of Cancer, 10.1038/s41416-023-02226-w, 128 , 10, (1964-1975), (2023).
- Huan Zhang, Huanyao Gao, Yayun Gu, August John, Lixuan Wei, Minhong Huang, Jia Yu, Adeyemi A. Adeosun, Richard M. Weinshilboum, Liewei Wang, 3D CRISPR screen in prostate cancer cells reveals PARP inhibitor sensitization through TBL1XR1-SMC3 interaction, Frontiers in Oncology, 10.3389/fonc.2022.999302, 12 , (2022).
- Qiu Tu, Xiuyun Liu, Xiaoqing Yao, Ruixue Li, Gaojing Liu, Honglv Jiang, Kaiqin Li, Qiongfang Chen, Xiaoyan Huang, Qing Chang, Guoqiang Xu, Hong Zhu, Peng Shi, Bo Zhao, RETSAT associates with DDX39B to promote fork restarting and resistance to gemcitabine based chemotherapy in pancreatic ductal adenocarcinoma, Journal of Experimental & Clinical Cancer Research, 10.1186/s13046-022-02490-3, 41 , 1, (2022).
- Longjie Li, Arun Mouli Kolinjivadi, Kok Haur Ong, David M Young, Gabriel Pik Liang Marini, Sock Hoai Chan, Siao Ting Chong, Ee Ling Chew, Haoda Lu, Laurent Gole, Weimiao Yu, Joanne Ngeow, Automatic DNA replication tract measurement to assess replication and repair dynamics at the single-molecule level, Bioinformatics, 10.1093/bioinformatics/btac533, 38 , 18, (4395-4402), (2022).
- Madhura Deshpande, Theodore Paniza, Nahed Jalloul, Gouri Nanjangud, Jerzy Twarowski, Amnon Koren, Nikica Zaninovic, Qiansheng Zhan, Kalyani Chadalavada, Anna Malkova, Hossein Khiabanian, Advaitha Madireddy, Zev Rosenwaks, Jeannine Gerhardt, Error-prone repair of stalled replication forks drives mutagenesis and loss of heterozygosity in haploinsufficient BRCA1 cells, Molecular Cell, 10.1016/j.molcel.2022.08.017, 82 , 20, (3781-3793.e7), (2022).
- Marcelina W. Musiałek, Dorota Rybaczek, Hydroxyurea—The Good, the Bad and the Ugly, Genes, 10.3390/genes12071096, 12 , 7, (1096), (2021).
- Yilin Fan, Marielle S. Köberlin, Nalin Ratnayeke, Chad Liu, Madhura Deshpande, Jeannine Gerhardt, Tobias Meyer, LRR1-mediated replisome disassembly promotes DNA replication by recycling replisome components, Journal of Cell Biology, 10.1083/jcb.202009147, 220 , 8, (2021).