Cloning and Overexpressing Membrane Proteins Using Pichia pastoris (Komagataella phaffii)
Magali Schwob, Magali Schwob, Valérie Kugler, Valérie Kugler, Renaud Wagner, Renaud Wagner
G protein-coupled receptors
immunodetection
integral membrane proteins
Komagataella phaffii
ligand binding
membrane preparation
Pichia pastoris
recombinant expression
Abstract
Understanding the structure and function of key proteins located within biological membranes is essential for fundamental knowledge and therapeutic applications. Robust cell systems allowing their actual overexpression are required, among which stands the methylotrophic yeast Pichia pastoris. This system proves highly efficient in producing many eukaryotic membrane proteins of various functions and structures at levels and quality compatible with their subsequent isolation and molecular investigation. This article describes a set of basic guidelines and directions to clone and select recombinant P. pastoris clones overexpressing eukaryotic membrane proteins. Illustrative results obtained for a panel of mammalian membrane proteins are presented, and hints are given on a series of experimental parameters that may substantially improve the amount and/or the functionality of the expressed proteins. © 2023 The Authors. Current Protocols published by Wiley Periodicals LLC.
Basic Protocol 1 : Designing and cloning a P. pastoris expression vector
Basic Protocol 2 : Integrative transformation of P. pastoris and selection of recombinant clones
Basic Protocol 3 : Culturing transformed P. pastoris for membrane protein expression
Basic Protocol 4 : Yeast cell lysis and membrane preparation
Basic Protocol 5 : Immunodetection of expressed membrane proteins: western blot
Alternate Protocol 1 : Immunodetection of expressed membrane proteins: dot blot
Alternate Protocol 2 : Immunodetection of expressed membrane proteins: yeastern blot
Basic Protocol 6 : Activity assay: ligand-binding analysis of an expressed GPCR
INTRODUCTION
Integral membrane proteins (IMPs) represent about one third of the proteome of every living organism (Wallin & von Heijne, 1998) where they carry out diverse, critical, and finely tuned cellular functions, including selective transport of ions and solutes, intra- and intercellular contacts and communication, enzymatic activities, and energy metabolism. In humans, they are associated with numerous disorders and diseases, making them the principal target for about two third of marketed drugs (Santos et al., 2017). Accessing the molecular details of their structure, dynamics, and interplay with their environment is thus highly desired to better understand how they function and to develop efficient and tailored therapeutics. However, the experimental conditions that are needed for producing, extracting, and purifying IMPs at levels compatible with their biochemical and biophysical characterizations are complicated by their intrinsic nature that requires an adapted hydrophobic environment for their proper folding and function (Guyot et al., 2020; Kesidis et al., 2020).
The methylotrophic yeast Komagataella phaffii , best known as Pichia pastoris in applied sciences for historical reasons (Bernauer et al., 2021), stands as one of the most efficient recombinant hosts for producing these difficult-to-handle proteins. Hundreds of IMPs, essentially of eukaryotic origin, have indeed been successfully expressed with and studied from this cell system, in quality and quantity levels that notably led to 3D-structure resolution for 62 of them (see Internet Resources). Most remarkably, these IMP structures are representative of a wide diversity of protein topologies, supramolecular assemblies, and membrane functions, making P. pastoris one of the most versatile hosts for this endeavor.
The main advantages of P. pastoris as a potent system for the overexpression of IMPs are the handling simplicity of a unicellular microbe and the cellular sophistication of a eukaryotic organism. While the former aspect has a positive and direct impact on the experimental timing and on cost and scale-up issues, the latter endows this host with most of the cellular machineries needed to achieve the synthesis, maturation, and trafficking of complex proteins, including membrane proteins from mammals. In addition, P. pastoris is one of the few types of yeast that have developed a singular methanol utilization pathway relying on some of the strongest and most tightly regulated known promoters (Ata et al., 2021) that can be used for very high-level expression of recombinant genes.
This article is intended to give general guidelines and instructions to operate the P. pastoris system for (over)expressing IMPs. Included are details on DNA sequence design and assembly, transformation and selection of recombinant yeast clones, conditions for expression, and preparation and analyses of membrane samples containing the proteins of interest. The initial and crucial step is the choice of the expression vector and selected fusion sequences that impact not only the yields and activity of the recombinant IMPs, but also the subsequent detection and purification strategies. The main objective of Basic Protocol 1 is to provide the reader with some guidance on the important sequence elements required to assemble IMP-adapted expression vectors. As an example, the design of one vector developed for the expression of G Protein-Coupled Receptors (GPCRs) (André et al., 2006) is described, together with an illustrative standard protocol for introducing one target gene in this vector.
Basic Protocol 2 details the integrative transformation of P. pastoris and the subsequent selection of recombinant clones. This section first gives a global overview on the existing strains and transforming strategies and then focuses on the most frequently used methods for generating and selecting recombinant clones based on phenotypic criteria.
Once these clones have been obtained, the operator is then ready to proceed to the expression of the foreign IMP, which is usually achieved in an inducible mode. Basic Protocol 3 outlines the methods to culture the yeast cells, both at the analytical scale to evaluate several clones in parallel, and at the preparative scale to generate larger amounts of cells for further analyses.
In contrast with cytosoluble recombinant proteins that can be easily secreted outside the cells and purified from the culture medium, preparing IMPs for analyses requires a cell disruption phase followed by the recovery of the membrane fractions. Efficient methods to lyse this yeast are described in Basic Protocol 4, together with a standard protocol for preparing the total membrane fractions containing the IMPs of interest before analysis.
A panel of approaches that assess both the yields and functionality of the expressed proteins are then illustrated with different human IMPs. Whereas Basic Protocol 5 and Alternate Protocols 1 and 2 present different immunodetection methods to evaluate the overall amounts of the produced IMPs, Basic Protocol 6 describes an example of a radiometric assay fitted to a model GPCR to evaluate its activity. Finally, hints are given in the Commentary section on the major parameters that can be modulated to potentiate the expression level of IMPs.
Basic Protocol 1: DESIGNING AND CLONING A P. PASTORIS EXPRESSION VECTOR
P. pastoris expression vectors are built on a classical E. coli /yeast shuttle model with components required for E. coli amplification (classically one origin of replication and one antibiotic selection marker) and specific elements for heterologous gene expression in P. pastoris. These typically include a selectable auxotrophy marker and/or an antibiotic resistance bacterial gene, as well as a promoter and a terminator sequence that surround a cloning cassette and optional fusion sequences added to improve the yields and proper folding of the expressed proteins and to facilitate their detection and their purification. Most of these vectors are built on, or derived from, the pPIC series of plasmids and are commercially available (e.g., Invitrogen). Table 1 summarizes the vectors most frequently employed for the expression of IMPs.
Name | Selection markers | Phenotype of transformants | Promoter | Secretion sequence | Added tags |
---|---|---|---|---|---|
pAO815 | HIS4 | His+ | PAOX1 | none | none |
pPIC3.5K | HIS4, Kan | His+, G418R | PAOX1 | none | none |
pPIC9K | HIS4, Kan | His+, G418R | PAOX1 | α Factor | none |
pPICZ A, B, C | Ble | ZeoR | PAOX1 | none | c-Myc/His6 |
pPICZα A, B, C | Ble | ZeoR | PAOX1 | α Factor | c-Myc/His6 |
pPink-HC | ADE2 | Ade+ | PAOX1 | none | none |
pPinkα-HC | ADE2 | Ade+ | PAOX1 | α Factor | none |
- a
HIS4 gene from P. pastoris, encodes a histidinol dehydrogenase and restores histidine prototrophy (His+); Kan gene, confers resistance to G418 (G418R); Ble gene from Streptoalloteichus hindustanus, confers resistance to Zeocin (ZeoR); ADE2 gene from P. pastoris, encodes a phosphoribosylaminoimidazole carboxylase, restore adenine prototrophy; PAOX1, promoter sequence of the AOX1 gene from P. pastoris encoding an alcohol oxidase; α Factor, encodes the native S. cerevisiae α factor secretion signal; c-Myc, C-terminal myc epitope, EQKLISEEDL; His6, hexahistidine tag.
Designing the expression vector
The first decision in setting up a P. pastoris expression system is the choice of the expression vector(s) that will be best adapted to a specific IMP. Usually there are two scenarios: no information is available in the literature, or similar proteins have already been successfully produced in this system and the published data can serve as a useful starting point. In the latter case, it is advisable to consult the few authoritative surveys that have been conducted on various IMPs expressed in P. pastoris (Alkhalfioui et al., 2011; Bill & Hedfalk, 2021; Byrne, 2015; Hausjell et al., 2020; Popova et al., 2023). When no published information is available, a combination of vectors that comprise the following sequence elements must be considered. First, plasmids bearing the HIS4 /Kan or the ble selection markers are generally preferred. Similarly, the inducible P AOX1 promoter from the alcohol oxidase AOX1 gene of P. pastoris is also favored. Among the panel of constitutive and inducible promoters that are available in expression vectors, P AOX1 is one of the most tightly repressed by sugar-based carbon sources and strongly induced by methanol (Ata et al., 2021; Hartner & Glieder, 2006), allowing a finely controlled and efficient overexpression of IMPs that may be toxic to the host. Additional methanol-regulated and potent promoters have been recently investigated (Vogl et al., 2016), but not yet used for the recombinant expression of IMPs.
The sequences that can be fused to the IMP gene are usually very dependent on the particular protein, with various impacts. For instance, adding a secretion sequence, such as the alpha-factor secretion signal from S. cerevisiae , may be highly beneficial for some IMPs, such as the seven transmembrane (7TM) GPCRs (Grunewald et al., 2004; Weiss et al., 1995; Weiss et al., 1998). Alternatively, for several 6TM aquaporins where the N and C termini are both located intracellularly, protein expression led to high yields of high-quality protein suitable for structural studies, whether a secretion signal was present or not (Fisher et al., 2009; Tornroth-Horsefield et al., 2006).
Similarly, various tag sequences can be inserted to improve the downstream detection and purification steps. While it is difficult to decide on the best sequence and position to use, an ideal tag should have (1) no effect on the tertiary structure and the biological activity of the protein; (2) suitability with the shortest, affinity-based purification strategy; and (3) easy and specific removal to recover the native protein for further use. A large panel of such tags has been used with IMPs, ranging from hexa- or decahistidine (the most widely employed tags), c-Myc, FLAG, HA and StrepII epitopes, to larger peptidic domains such as a biotinylation domain (bio) and the green fluorescent protein GFP. It is noteworthy that C-terminal bio fusions have been shown to enhance the functional expression of GPCRs (Grunewald et al., 2004; Weiss et al., 1998). In addition, protease cleavage sites, such as the factor-Xa or TEV sequences inserted in some constructs, are sometimes included in the constructs for eliminating the fused tags after or during the purification step.
Cloning into the expression vector
Once the expression vector(s) has been chosen, cloning is the next step. Basically, this is achieved by directly introducing the gene of interest into either a standard ready-made expression vector (see Table 1), or after modifying one of these vectors to incorporate any chosen additional or alternate fusion sequence(s). In both cases, the final constructs can be obtained by common cloning strategies, such as subcloning DNA fragments, insertion of PCR fragments, or recombinational cloning.
As an illustration of how to handle such a task, the following sections present the information and protocols needed for the cloning of a member of the human GPCR family into an engineered pPIC9K plasmid. This vector was modified to incorporate a FLAG-tag, a TEV protease cleavage sequence and a decahistidine-tag (His10) upstream the Bam HI and Spe I cloning sites for insertion of the target gene, as well as a second TEV site and a bio-tag downstream (André et al., 2006). This combination was selected on the basis of previous studies showing dramatically enhanced expression levels of other GPCRs when fused to the α-MF signal sequence and the bio-tag (Grunewald et al., 2004; Weiss et al., 1998). The FLAG and His10 tags were inserted for detection and purification purposes; the TEV cleavage sites were added to allow cleavage of the N- and C-terminally fused sequences following purification. The protocols below illustrate a standard way to generate the desired P. pastoris expression plasmid, as well as its preparation prior to yeast transformation. The human alpha-1A adrenergic receptor is employed as an example.
Materials
-
cDNA template containing the full-length ADRA1A_HUMAN encoding gene (cDNA Resource Center, cat. no. AR0A1A0001)
-
ADRA1A-specific forward primer bearing an additional 5′ adapter specifically designed to introduce a Bam HI restriction site (5'-GGATCCATGGTGTTTCTCTCGGGAAATGCTTCCGAC-3'), and a similar reverse primer, bearing a 5′ adapter introducing Spe I (5'-ACTAGTGACTTCCTCCCCGTTCTCACTGAGGGAGAT-3')
-
High-fidelity DNA polymerase, e.g., Phusion (Thermo Fisher, cat. no. F530S), and its specific buffer
-
dNTP mix, 2.5 mM each (Thermo Fisher, cat. no. AM8228G)
-
H2O, sterile
-
Loading dye, e.g., 6× DNA loading dye (Thermo Fisher, cat. no. R0621)
-
1% (w/v) agarose gels supplemented with 0.5 µg/ml ethidium bromide (Euromedex, cat. no. EU0070)
-
Nucleic acid extraction and purification kit, e.g., NucleoSpin kit (Macherey-Nagel, cat. no. 740609.250)
-
Restriction enzymes and their related buffers, e.g., FastDigest Bam HI and Spe I (Thermo Fisher, cat. nos. FD0054 and FD1254, respectively)
-
T4 DNA ligase, e.g., DNA ligation kit (Thermo Fisher, cat. no. EL001)
-
E. coli competent cells, e.g., Stellar chemically competent cells (Takara, cat. no. 636763)
-
SOC medium (see recipe)
-
Liquid and agar plates of LB medium (see recipe) supplemented with 50 μg/ml kanamycin (Euromedex, cat. no. EU0420-A)
-
80% (v/v) glycerol, autoclave-sterilized
-
Thermal cycler
-
1.5- and 2-ml microtubes
-
37°C incubator
-
37°C shaking incubator
-
Cryotubes
-
–20°C and –80°C freezers
-
Vortex
-
Additional reagents and equipment for polymerase chain reaction (Kramer & Coen, 2002), DNA separation and visualization, agarose gel electrophoresis (Voytas, 2001), UV spectrophotometry, and E. coli culturing
Perform PCR amplification and prepare the ADRA1A cDNA fragment
1.Prepare the PCR reaction mix on ice. Typically:
- 1 to 10 ng cDNA template
- 2.5 μl each of the 10 μM stock solution of the forward and reverse primers
- 10 μl 5× Phusion GC buffer
- 14 μl dNTP mixture (2.5 mM each)
- 1.5 μl 50 mM MgCl2
- 1.5 μl 100% DMSO
- 1 U high-fidelity polymerase
- Sterile water to a final volume of 50 μl
2.Run the PCR reaction in a thermal cycler with a standard 30-cycle protocol alternating 15 s at 98°C, 15 s at 55°C, and 1 min at 72°C.
3.Mix 25 μl of the PCR reaction with 5 μl of 6× loading dye and load the mixture onto a 1% (w/v) agarose gel to analyze the amplified product after migration.
4.Extract the desired DNA fragment using the protocol detailed in the NucleoSpin kit.
Ligate the pPIC9K plasmid with the insert DNA
5.In 1.5-ml microtubes, digest in parallel the amplified insert fragment coding for the gene of interest and the pPIC9K vector with the Bam HI and Spe I enzymes (according to the manufacturer's instructions).
6.Load the digestion products on a 1% (w/v) agarose gel, migrate the DNA fragments, and extract them separately using a NucleoSpin kit.
7.Prepare the ligation reaction with a 3:1 molar ratio of insert over plasmid. Typically, 50 to 100 ng of linearized plasmid is used. Add 1 U of T4 DNA ligase together with the ligase buffer and adjust to 20 μl with sterile water. Incubate the mixture for at least 1 hr at 22°C.
Transform E. coli cells and analyze recombinant clones
8.Mix ∼5 μl of the ligation mixture with 50 μl chemically of competent E. coli cells. Incubate on ice for 5 to 30 min.
9.Heat-shock the cells for 45 s at 42°C and immediately transfer the tubes to wet ice.
10.Add 250 μl SOC medium and let the cells regenerate for 1 hr at 37°C.
11.Spread 100 to 200 μl of the transformation mixture on pre-warmed LB agar plates supplemented with 50 μg/ml kanamycin and incubate overnight at 37°C.
12.The following day, pick six to twelve colonies and use each of them to inoculate 2 ml LB supplemented with 50 μg/ml kanamycin. Grow the cultures overnight at 37°C in a shaking incubator.
13.Purify the plasmid DNA of each clone using a plasmid purification kit (e.g., NucleoSpin kit from Macherey Nagel) following the manufacturer's instructions.
14.Perform restriction digest of the plasmids using the Bam HI and Spe I enzymes. After electrophoresis on a 1% agarose gel, verify the presence of two bands of sizes corresponding to the pPIC9K plasmid and the insert DNA used for the ligation.
15.Confirm the integrity of the insert by DNA sequencing.
16.Store the plasmid containing the correct sequence up to 6 months at –20°C. In addition, prepare a glycerol stock by adding 800 μl of the LB culture of the corresponding bacterial clone to 200 μl of 80% (v/v) sterile glycerol in cryotubes. Thoroughly mix by vortexing and store up to several years at –80°C.
Basic Protocol 2: INTEGRATIVE TRANSFORMATION OF P. PASTORIS AND SELECTION OF RECOMBINANT CLONES
As for the majority of the available expression vectors, those used for IMP expression are designed to be integrated in the genome of P. pastoris. Following yeast transformation, recombination events between linearized sequences borne by the plasmids (typically HIS4 or P AOX1) and their homologous sequence counterparts in the genome result in targeted insertion of the expression vectors into the P. pastoris genome. Moreover, such plasmid insertions frequently occur in tandem in yeasts and thus lead to multiple integration of the gene of interest with an associated impact on subsequent expression levels.
In an alternative strategy, integration can be obtained by replacing the P. pastoris AOX1 gene by the construct of interest, leading to clones exhibiting a reduced growth speed on methanol-containing media (methanol utilization slow –MutS phenotype). Such a gene replacement strategy is, however, not recommended for IMP expression. For a complete overview of the different existing integration possibilities and methods, see Cregg et al. (2010).
The strains usually employed for heterologous expression of IMPs (see Table 2) are all histidine auxotrophs due to a deficiency in the histidinol dehydrogenase gene (his4). The prototrophy for histidine can be restored upon transformation with pPIC vectors bearing the HIS4 gene, thereby serving as a positive selection marker. Strains from the SMD series bear additional deficiencies in endogeneous proteases and are often preferred for this convenient phenotype. Additional engineered strains have been described, notably for their capacity in performing “human-like” N-glycosylation (Hamilton & Gerngross, 2007), but none of them have been reported yet in IMP expression studies.
Strain | Genotype | Phenotype | Reference |
---|---|---|---|
GS115 | his4 | Mut+, His- | Cregg & Madden (1987) |
KM71 | his4, arg4, aox1::ARG4 | MutS, His-, Arg+ | Cregg & Madden (1987) |
SMD1163 | his4, pep4, prb1 | Mut+, His-, Prot- (A-, B-, CarbY-) | White et al. (1995) |
SMD1165 | his4, prb1 | Mut+, His-, Prot- (B-) | White et al. (1995) |
SMD1168 | his4, ura3, pep4::URA3 | Mut+, His-, Prot- (A-, BS, CarbY-) | White et al. (1995) |
- a
his4: deficient histidinol dehydrogenase encoding gene (responsible for the histidine auxotrophy, His-, phenotype); Mut+: methanol utilization plus; ARG4/arg4: wild-type / deficient arginosuccinate lyase encoding genes; aox1: deficient alcohol oxydase encoding gene (responsible for the methanol utilization slow, MutS, phenotype); pep4: deficient aspartyl protease encoding gene (responsible for the proteinase A minus, ProtA-, and carboxypeptidase minus, CarbY-, phenotype); prb1: deficient proteinase B encoding gene (responsible for the proteinase B minus, ProtB-, and carboxypeptidase minus, CarbY-, phenotype); URA3/ura3: wild-type / deficient orotidine 5'-phosphate decarboxylase encoding gene.
Several robust methods are available for transforming P. pastoris , either based on chemically competent or electrocompetent cells. While several reliable procedures can be found in other publications (Cregg et al., 2010), described here is a protocol for the electroporation of yeast cells, which is the method of choice for most researchers. It is convenient to carry out, it yields transformants at high frequencies, and it is compatible with multicopy integration events. In the present example, the previously cloned pPIC9K vector bearing the ADRA1A GPCR gene is used to transform a SMD1163 strain (his4, pep4, prb1).
Additional Materials (also see Basic Protocol 1)
-
Purified expression vector (from Basic Protocol 1)
-
Restriction enzyme Pme I and its specific buffer (e.g., Thermo Fisher, cat. no. ER1342)
-
24:24:1 (v/v/v) phenol:chloroform:isoamyl alcohol
-
Chloroform
-
100% (v/v) ethanol, ice-cold
-
3 M sodium acetate, pH 4.8
-
70% (v/v) ethanol, ice-cold
-
YPD medium (see recipe)
-
A fresh SMD1163 colony streaked on a YPD agar plate (see recipe for YPD plates)
-
1 M HEPES, pH 8 (Euromedex, cat. no. 10-110)
-
1 M dithiothreitol (DTT) (Euromedex, cat. no. EU0006-D)
-
Ice-cold sterile H2O
-
1 M sorbitol, ice-cold (Sigma, cat. no. S6021)
-
MD agar plates (see recipe)
-
YPD plates supplemented with 0.1 and 0.25 mg/ml geneticin sulfate (G418 sulfate) (Gibco, cat. no. 11811-064)
-
Centrifuge
-
30°C shaking incubator
-
Spectrophotometer
-
0.2-cm electroporation cuvettes, sterile (Dutscher, cat. no. 4905021)
-
Electroporation instrument, e.g., Gene Pulser system from BioRad
-
Sterile cell scraper
NOTE : All materials and solutions must be sterile.
Prepare the expression vector prior to transformation
1.Prepare a restriction digest solution by adding 5 to 7 μg of purified expression vector to 25 U of Pme I, 20 μl of 10× corresponding buffer, and sterile water to a final volume of 200 μl. Incubate the reaction for 2 hr at 37°C.
2.Add 400 μl of 24:24:1 (v/v/v) chloroform:phenol:isoamyl alcohol to the 200 μl digestion mixture.
3.Centrifuge 5 min at 18,000 × g , room temperature, and transfer the upper aqueous phase into a new 1.5-ml microtube.
4.Add 400 μl chloroform and vortex thoroughly for ∼20 s.
5.Centrifuge 5 min at 18,000 × g , room temperature, and transfer the upper aqueous phase into a new 1.5-ml microtube.
6.Add 1 ml of 100% ethanol, 50 μl of 3 M sodium acetate and incubate for at least 1 hr at –20°C to precipitate the DNA.
7.Centrifuge 30 min at 18,000 × g , 4°C. Discard the supernatant.
8.Wash the pellet with 100 μl of ice-cold 70% (v/v) ethanol and centrifuge for 5 min at at 18,000 × g , 4°C. Discard the supernatant.
9.Air dry the pellet for 15 min and then resuspend in 15 μl sterile H2O.
10.Check the DNA linearization by loading 1 μl of the solution on a 1% (w/v) agarose gel.
Prepare P. pastoris electrocompetent cells
11.Inoculate 100 ml YPD medium with a fresh SMD1163 colony and incubate the preculture overnight at 30°C in a shaking incubator.
12.Measure the OD600 of the culture with a spectrophotometer, dilute it in 400 ml of fresh YPD to obtain an OD600 of 0.25, and incubate at 30°C.
13.When the culture reaches an OD600 of 1 (after ∼4 hr), harvest the cells by centrifuging in sterile tubes for 5 min at 4000 × g , 4°C.
14.Resuspend the cells in 100 ml YPD, 20 ml of 1 M HEPES, pH 8, and 2.5 ml of 1 M DTT. Mix gently until the pellet is resuspended.
15.Incubate for 15 min at 30°C.
16.Transfer onto ice and add ice-cold sterile H2O to a final volume of 500 ml.
17.Pellet the cells by centrifuging for 5 min at 4000 × g , 4°C.
18.Decant the supernatant and wash the cell pellet with 250 ml of ice-cold sterile H2O.
19.Pellet the cells by centrifuging for 5 min at 4000 × g , 4°C.
20.Decant the supernatant and resuspend the pellet in 20 ml of ice-cold 1 M sorbitol by gently mixing.
21.Pellet the cells by centrifuging for 5 min at 4000 × g , 4°C.
22.Decant the supernatant and resuspend the pellet in 500 μl of ice-cold 1 M sorbitol by gently mixing.
Electrotransform P. pastoris cells
23.Place an electroporation cuvette on ice at least 10 to 15 min before performing the transformation.
24.Mix 40 μl of competent cells (from step 22) with 7.5 μl of the linearized DNA (see step 10) in the cuvettes, mix gently with the pipet, and incubate for 5 min on ice.
25.Adjust the electroporation settings as follows: 1 500 V, 25 μF, 600 Ω.
26.Place the cuvette in the electroporator chamber and apply the electric pulse.
27.Immediately resuspend the electroporated mixture in 1 ml of ice-cold 1 M sorbitol and transfer into a sterile 1.5-ml microtube.
28.Allow the cells to recover for ∼1 hr at 30°C then pellet the cells by centrifuging 5 min at 4000 × g , room temperature.
29.Discard the supernatant and resuspend the pellet in 500 μl of 1 M sorbitol. Proceed to step 30.
Select the recombinant clones
In the present protocol involving a pPIC9K-derived expression vector bearing both the HIS4 and KanR markers, the selection of the recombinant clones is managed in two steps. The first one corresponds to the selection of His+ transformants growing on minimal medium (MD) agar plates, and the second is dedicated to the identification of multicopy transformants resistant to increased concentrations of the G418 sulfate antibiotic contained in YPD plates. In the present case, as the SMD1163 strain is more sensitive to G418 sulfate because of the prb1 and pep4 mutation it bears, a concentration range of antibiotic comprised between 0.05 and no more than 0.25 mg/ml is usually applied. In addition, whatever the strain used, the direct selection of transformants on G418 sulfate is not recommended, mainly because the level of resistance is dependent on the cell density and false positive clones may be isolated.
30.Spread separately 50 μl and 450 µl of electro-transformed cells on each of two MD plates and incubate for 2 to 3 days at 30°C.
31.Harvest the His+ transformants by adding 1 ml YPD medium onto the plates and scrape off all the clones using a sterile scraper.
32.Perform 10× and 100× dilutions in YPD and measure the OD600 for each dilution.
33.Spread an equivalent of 105 cells/plate (OD600 of 1 is equivalent to ∼5 × 107 cells/ml) on YPD plates supplemented with 0.1 or 0.25 mg/ml G418 sulfate.
34.Incubate for 2 to 3 days at 30°C.
35.Pick six to twelve representative colonies from the different G418 sulfate concentration plates, streak them onto a fresh YPD plates and let them grow for 1 to 2 days at 30°C. Directly use these colonies for further expression tests (see Basic Protocol 3) and/or resuspend them in 1 ml sterile YPD supplemented with 20% (v/v) glycerol and store them up to several years at –80°C.
Basic Protocol 3: CULTURING TRANSFORMED P. PASTORIS FOR MEMBRANE PROTEIN EXPRESSION
Once transformants have been obtained, the next step consists of screening for the clones exhibiting the best expression levels of the recombinant IMPs. As mentioned previously, tandem multicopy integration is indeed relatively frequent in the transforming conditions used, leading to recombinant clones with a variable number of copies of the expression vector. However, a high number of integrations does not necessarily correlate with higher expression levels, so several representative clones typically need to be assayed. For expression based on P AOX1 -dependent vectors, this is practically achieved by growing the clones in repressive medium to an appropriate cell density and growth phase before starting the expression phase by transferring the cells to a methanol-containing induction medium. For clones and expression condition screening, small-scale culturing procedures most often rely on shaken baffled-flasks or tubes of smaller volumes, provided an appropriate aeration is maintained. Such a standard procedure is described below.
When upscaling the production of IMPs to larger amounts (in the mg range), shake-flask culturing is often still the method of choice. Alternatively, when expression levels are low, when large volumes of culture are required (> 10 L), or when experimental parameters need to be finely monitored (oxygen transfer, pH, substrate feeding), bioreactors can be employed. Accordingly, a number of robust fermentation processes including fed-batch techniques or continuous culturing procedures have been developed. Detailed descriptions of these methods with their benefits and limitations can be found in several excellent and comprehensive publications (Cos et al., 2006; Li et al., 2007; Looser et al., 2015), including for IMP production (Singh et al., 2012).
Materials
-
BMGY medium (see recipe)
-
Recombinant clones (from Basic Protocol 2) freshly streaked on a YPD agar plate
-
BMMY medium (see recipe)
-
Phosphate-buffered saline (PBS) (e.g., Euromedex, cat. no. ET330)
-
500-ml baffled-flasks
-
50-ml sterile conical disposable tubes
-
30°C shaking incubator
1.Inoculate 50 ml of freshly prepared BMGY medium in a 500-ml baffled-flask with a fresh recombinant colony isolated on a YPD agar plate. Incubate overnight on a shaker at 250 rpm, 30°C.
2.On the next day, measure OD600 of the culture. Dilute the cells into 50 ml fresh BMGY medium in a 500-ml flask to achieve an OD600 of 1 (∼5.107 cells/ml) and incubate on a shaker at 250 rpm, 30°C.
3.When the culture reaches ∼5 OD600 (∼4 to 5 hr later), pellet the cells by centrifuging in sterile tubes for 5 min at 4000 × g , room temperature.
4.Decant the supernatant and resuspend the cell pellet with 50 ml fresh BMMY medium. Incubate 18 to 24 hr in a shaker at 30°C, 250 rpm.
5.After induction, harvest the cells by centrifuging for 5 min at 4000 × g , 4°C.
6.Decant the supernatant and wash the cell pellet with 50 ml PBS, pH 7.Centrifuge the suspension for 5 min at 4000 × g , 4°C.
7.Discard the supernatant and weigh the cell pellet.
Basic Protocol 4: YEAST CELL LYSIS AND MEMBRANE PREPARATION
The first critical step when preparing P. pastoris membranes is a robust cell lysis method that effectively disrupts the thick protective cell wall. Shearing-based methods involving microbeads are preferred since they are very efficient, compatible with a broad range of sample volumes, and directly accessible to most laboratories. In the simplest and widest-used mode, cells in cold buffers are violently shaken with 500-μm diameter beads using a basic vortex apparatus in 4 to 8 cycles of alternating shaking (30 s) and ice-cooling (30 s) phases. To achieve the most reliable and reproducible results, programmable equipment is recommended, such as the Tissue Lyser from Qiagen or the FastPrep 24 from MP Biomedicals that accommodate various sample volumes and formats (up to 50 ml), or the more sophisticated and expensive grinder series from Dyno Mills that can operate large volumes both in batch or continuous modes. In addition, pressure-based instruments have also proven efficient for the lysis of P. pastoris cells, and besides the well-known French pressure cell press, a panel of cell disruptors is available from Constant Systems that can handle from 0.5 to 40 ml of batch samples, and up to 24 L/hr with the continuous flow models. Here again, programmable equipment is recommended for reproducible results. Alternative methods can involve glucanase enzymes (e.g., Helicase from snail digestive juice, Zymolyase or Lyticase from microbial sources) to remove the cell wall and yield protoplast preparations that can be easily burst (Hartmann et al., 2017).
Of importance, keep in mind that the longer the induction phase, the thicker the cell wall and the less efficient the lysis step. This issue must be considered when optimizing the induction time in order to strike a balance between expression level and cell lysis efficiency.
Following cell disruption in the presence of protease inhibitors (typically PMSF or other commercially available cocktails), the unbroken cells and large debris are separated from the lysate with a low-speed centrifugation step (typically 10 min at 2000 × g). The whole membrane fraction in the supernatant is then pelleted by ultracentrifugation before being resuspended in an appropriate buffer for further analysis. Optionally, cycles of ultracentrifugation and stringent washes may be applied to eliminate unwanted membrane-associated proteins, resulting in samples enriched with the IMP of interest.
Described below is a protocol optimized for the preparation of membranes of P. pastoris using a bead-based disruption method and standard centrifugation procedures.
Materials
-
Yeast cell pellet (from Basic Protocol 3)
-
TNGE buffer (see recipe)
-
Acid-washed glass beads (425- to 600-µm diameter, Sigma-Aldrich, cat. no. G8772)
-
TNG buffer (see recipe)
-
High-speed benchtop homogenizer (e.g., FastPrep 24 from MP Biomedicals)
-
26-ml polycarbonate bottles for ultracentrifuge
-
Ultracentrifuge equipped with an appropriate fixed-angle rotor
-
3-ml Potter homogenizer
-
Additional reagents and equipment recommended for the determination of protein concentration (Olson & Markwell, 2007), e.g., BCA assay
1.Resuspend 1 g of the yeast pellet obtained in Basic Protocol 3 with 10 ml of ice-cold TNGE buffer.
2.Add 5 ml of acid-washed glass beads.
3.Place the tubes on the cell breaker device and proceed with cell lysis by alternating shaking and cooling steps on ice (3 cycles of 40 s each at 6.5 m/s on a FastPrep 24).
4.Centrifuge the samples 5 min at 4000 × g , 4°C and collect the supernatant in a bottle stored at 4°C.
5.Dissolve the remaining pellet in 10 ml of ice-cold TNGE buffer and repeat steps 3 to 5 three additional times (or until the supernatant is clear).
6.Centrifuge the collected supernatants for an additional 5 min at 4000 × g , 4°C.
7.Transfer the supernatant from step 3 into ultracentrifugation bottles and centrifuge them at 30 min at 100,000 × g , 4°C.
8.Discard the supernatant, transfer the membrane pellet in a Potter homogenizer and carefully resuspend it in 3 ml ice-cold TNG buffer until a homogeneous suspension is obtained.
9.Determine the protein concentration following the BCA assay procedure described in Olson & Marwell (2007).
Basic Protocol 5: IMMUNODETECTION OF EXPRESSED MEMBRANE PROTEINS: WESTERN BLOT
Expression levels of IMPs are usually assessed through specific immunodetection tests and activity assays. Immunodetection methods, such as western blot, dot blot, or ELISA are rather straightforward for evaluating the overall amounts of produced IMP. This is facilitated by the presence of a tag epitope fused to the protein of interest (see Basic Protocol 1), particularly when no specific antibody is commercially available, which is a recurrent issue with IMPs.
This protocol presents a western blot protocol where the expression level analysis of an IMP is illustrated with the His-tagged human ABHD12 monoacylglycerol lipase after a denaturing SDS-PAGE.
Materials
-
Membrane preparation samples (from Basic Protocol 4)
-
Blocking buffer (see recipe)
-
Monoclonal anti-His antibody from mouse (e.g., Qiagen Penta-His antibody, cat. no. 34660)
-
Blotting buffer (see recipe)
-
IRDye800-conjugated anti-mouse IgG antibody (e.g., Euromedex, cat. no. A90-337D8)
-
Phosphate-buffered saline (PBS) (e.g., Euromedex, cat. no. ET330)
-
Nitrocellulose membrane
-
Orbital shaker
-
Fluorescent imager, e.g., the Odyssey Clx Imager from Licor
-
Additional reagents and equipment for SDS-PAGE (Gallagher, 2012) and electrotransfer of proteins (Goldman et al., 2015)
1.Prepare a 10% SDS-polyacrylamide gel and load 10 μg of membrane preparation pre-incubated in a standard Laemmli buffer.
2.After electrophoresis and protein separation, transfer the proteins from the gel to a nitrocellulose membrane by electroblotting in a tank unit for ∼90 min at 100 V.
3.Transfer the membrane in a new tank and incubate in 50 ml blocking buffer for 1 hr at room temperature on an orbital shaker. Alternatively, incubate the membrane overnight at 4°C.
4.Remove the blocking buffer and incubate the membrane with the monoclonal anti-His antibody diluted in the blocking buffer (1:2000, v/v) for 1 hr at room temperature on an orbital shaker.
5.Wash the membrane three times, each time with 50 ml blotting buffer on an orbital shaker for 10 min at room temperature.
6.Remove the blotting buffer and incubate the membrane with the IRDye800 anti-mouse antibody diluted in the blocking buffer (1:5000, v/v) in the dark for 1 hr at room temperature on an orbital shaker.
7.Wash the membrane three times, each time in the dark with 50 ml blotting buffer on an orbital shaker for 10 min at room temperature.
8.Replace the blotting buffer with 50 ml PBS and keep the membrane in the dark until its analysis in the fluorescent imager.
9.Proceed to membrane revelation according to the fluorescent imager instructions.
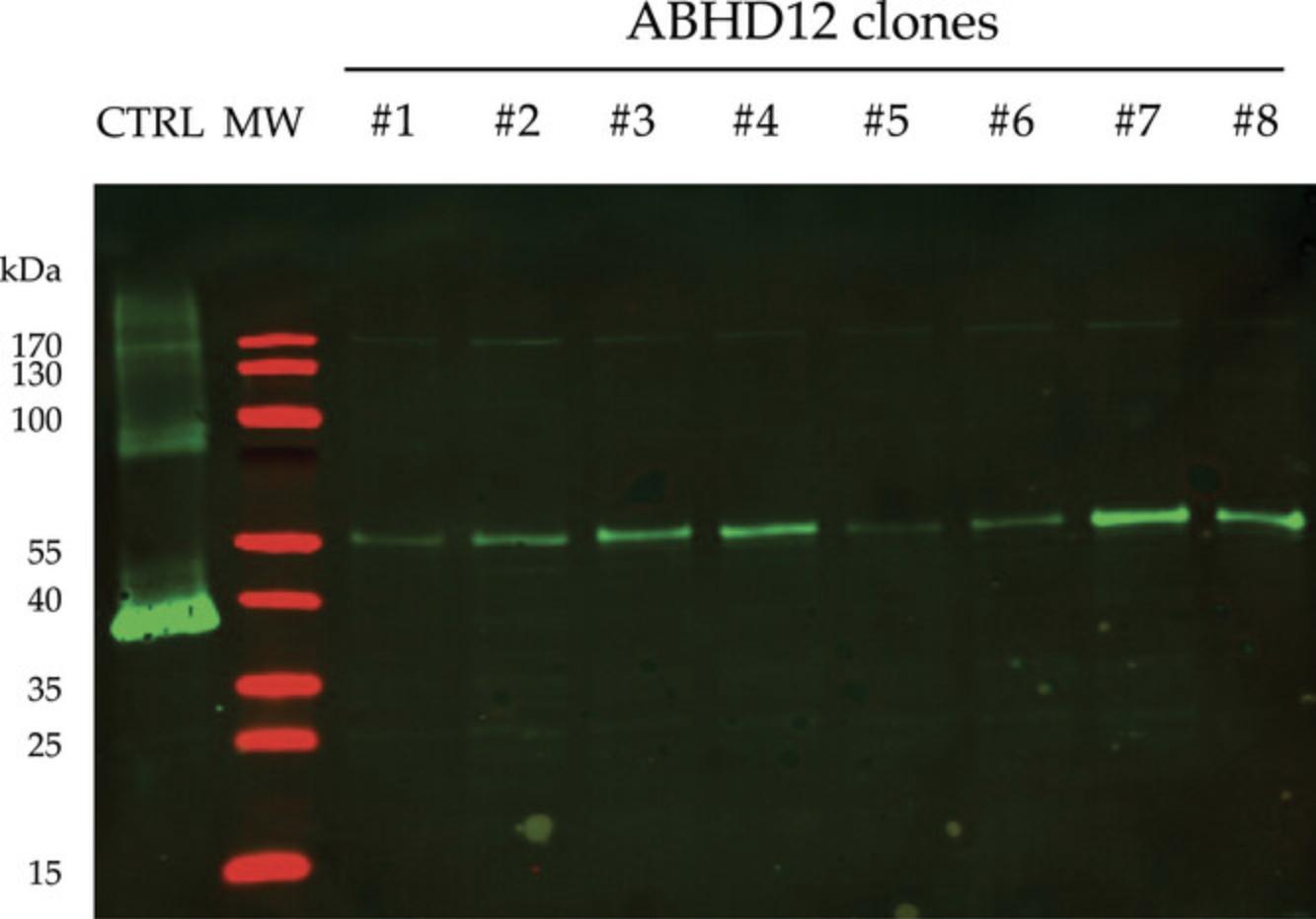
Alternate Protocol 1: IMMUNODETECTION OF EXPRESSED MEMBRANE PROTEINS: DOT BLOT
While western blot analysis (Basic Protocol 5) yields useful information on both the expression levels and the size and integrity of the immunodetected protein of interest, the throughput is generally low. Actually, SDS-PAGE separation and protein transfer onto western blotting membranes require a number of handling steps that are not adapted for the comparison of dozens of samples obtained from a large panel of recombinant IMP-expressing clones. In such a situation, screening for the best expressing clones can be more readily achieved by a dot blot approach, as it has been validated for GPCR expressing clones (Zeder-Lutz et al., 2006). Such a method is described below.
Additional Materials (also see Basic Protocol 5)
-
Membrane preparation samples (from Basic Protocol 4)
-
Microfiltration blotting device (e.g., Bio-Dot apparatus from Bio-Rad)
1.Soak a piece of nitrocellulose membrane with PBS and fix it tightly into the microfiltration blotting device (following the manufacturer's instructions).
2.Pipet 100 μl PBS into each well of the blotting device and let it flow through the nitrocellulose membrane by applying the vacuum.
3.Turn the vacuum off and pipet 10 μg of membrane protein sample into the appropriate wells. Incubate 5 min before applying the vacuum.
4.Turn the vacuum on and wash the wells 3 times, each time with 100 μl PBS.
5.Remove the nitrocellulose membrane from the blotting device and proceed to the blocking and immunodetection reactions as described in Basic Protocol 5, steps 3 to 9.
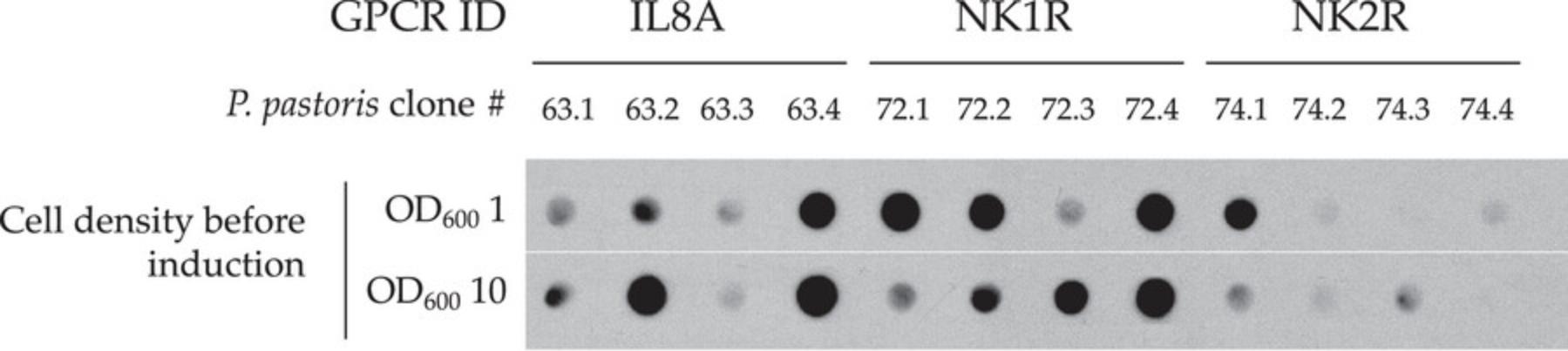
Alternate Protocol 2: IMMUNODETECTION OF EXPRESSED MEMBRANE PROTEINS: YEASTERN BLOT
As exemplified above, dot blot immunodetection is well-suited for screening purposes but is limited by the low throughput of all the manipulations required for sample preparation, from clone culturing and induction in liquid formats, to cell harvesting, cell lysis, and whole membranes isolation steps. The yeastern blot protocol presented below was developed for overcoming this issue (Hartmann et al., 2016). Recombinant clones isolated on selective medium are directly spotted on a nitrocellulose membrane that is successively exposed to solid repressive BMGY medium and inducible BMMY medium on agar plates. The membrane is further processed for in situ yeast cells lysis, and the resulting cell fractions adsorbed on the nitrocellulose are directly analyzed via a standard anti-IMP immunodetection procedure.
This protocol is particularly well suited for the evaluation of 96 individual clones at once, which therefore allows skipping the second selection step on G418 containing plates (see Basic Protocol 2), thus further limiting the handling steps post-transformation.
Additional Materials (also see Basic Protocol 5)
-
YPD medium (see recipe)
-
G418 sulfate
-
Recombinant clones on MD plate (from Basic Protocol 2)
-
BMGY agar (see recipe)
-
BMMY agar (see recipe)
-
Yeastern lysis buffer (see recipe)
-
Sterile 96-well microplate and lid (e.g., Falcon, cat. no. 353072)
-
Sterile toothpicks
-
96-well microplate shaker
-
Multichannel pipet
-
Large petri dishes, 14 cm diameter (e.g., Greiner Bio-one, cat. no. 639160)
1.Sterilely fill each well of a 96-well microplate with 100 µl YPD supplemented with 0.05 mg/ml G418 sulfate.
2.Using sterile toothpicks, individually inoculate each well with a His+ colony from an MD plate.
3.Incubate overnight on a plate shaker at 250 rpm, 30°C.
4.The day after, sterilely place a nitrocellulose membrane of a microplate dimensions onto a BMGY-agar large plate without trapping air bubbles.
5.Spot 5 µl of each well from the YPD + G418 preculture onto the nitrocellulose membrane with a multichannel pipet and incubate at 30°C.
6.After ∼12 hr incubation, transfer the membrane onto a BMMY-agar large plate avoiding air bubbles.
7.Incubate for ∼20 hr at 30°C.
8.In a large petri dish, place 12 sheets of Whatman paper and soak them with lysis buffer without recovering the last upper sheet.
9.Transfer the nitrocellulose membrane on top of the Whatman papers avoiding air bubbles.
10.Incubate for 4 hr at 65°C.
11.Extensively wash the membrane with water until no remnants of yeast colonies are visible.
12.Proceed to the blocking and immunodetection reactions as described in Basic Protocol 5, steps 3 to 9.
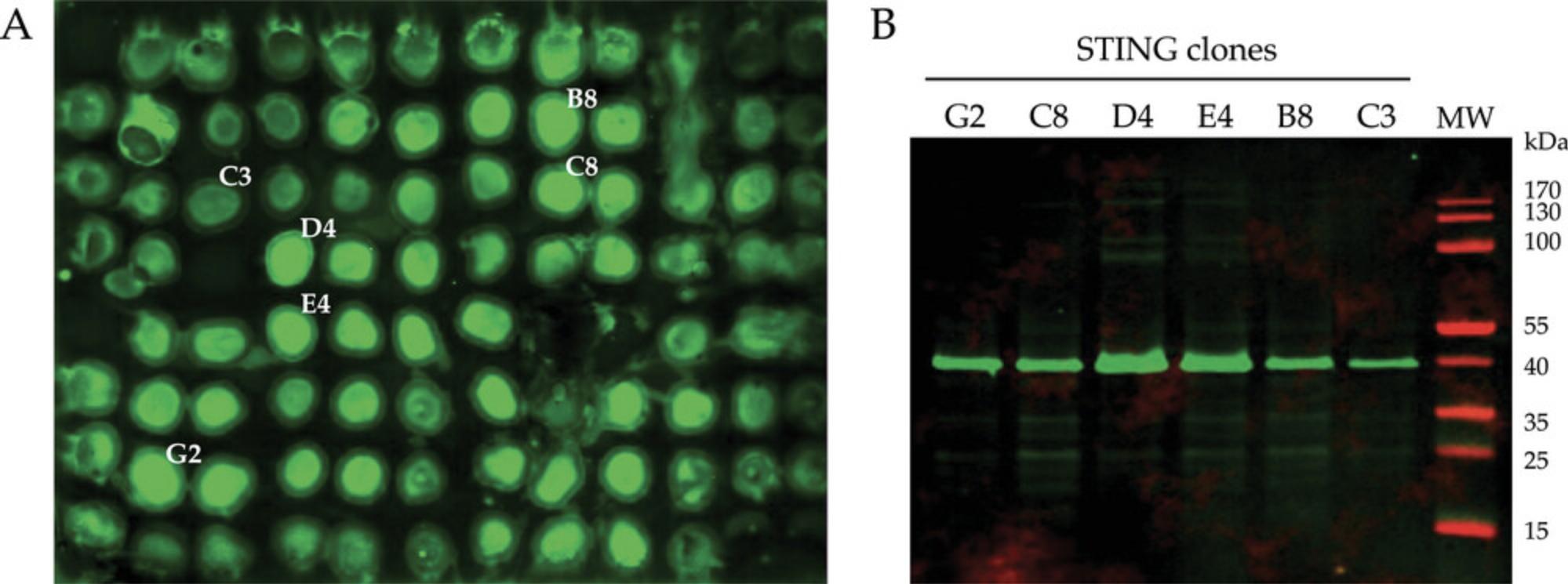
Basic Protocol 6: ACTIVITY ASSAY: LIGAND-BINDING ANALYSIS OF AN EXPRESSED GPCR
Immunodetection analysis is an essential means to evaluate the actual synthesis and integrity of a recombinant protein. However, these methods do not inform on the proper folding and functionality of the protein, which is often an issue with IMPs that need to be properly translocated in biological membranes during their production to fulfill their function. As far as possible, activity assays are thus required to evaluate this aspect and to properly validate expression conditions. However, such assays are often not accessible for IMPs, in particular for transporters, channels or enzymes that require two separate compartments to exert their function, which is usually not applicable to membrane preparation samples. In the case of GPCRs and other liganded-IMPs, ligand-binding assays are valuable methods to determine the amounts of active receptors (Bmax) and their affinity (Kd) for specific ligands. This protocol assesses the ligand-binding parameters of the ADRA1A receptor toward a specific radiolabeled antagonist. In brief, membrane preparations are incubated in triplicate with a given concentration of radioactive ligands until equilibrium is reached. For nonspecific binding determination, similar incubations are performed in parallel in the presence of an excess of unlabeled specific ligand. Bound and free ligands are separated by rapid filtration on glass-fiber filters and the retained radioactivity is measured by liquid-scintillation counting. Saturation curves are obtained with increasing concentrations of radioligand, and data are analyzed by nonlinear regression with appropriate software (Prism from GraphPad Software or KaleidaGraph from Synergy Software). Detailed description of the theoretical aspects, benefits, and limitations of ligand binding assays can be found in more specific reviews (for instance, see Bylund & Toews, 2011).
Materials
-
Membrane preparation samples (from Basic Protocol 4)
-
Ice-cold binding buffer (see recipe)
-
Ice-cold wash buffer (see recipe)
-
Filter buffer (see recipe)
-
[3H]-Prasozin (Perkin Elmer, cat. no. NET823250UC)
-
Prasozin hydrochloride (Biotechne, cat. no. 0623)
-
Scintillation cocktail (e.g., Microscint-O, Perkin Elmer, cat. no. 6013611)
-
96-well plate, low protein binding (e.g., Treff, cat. no. 96.08996.9.01)
-
96-well microplate shaker
-
GF/C-grade glass-fiber filters (e.g., Unifilters GF/C 96-microplate, Perkin Elmer, cat. no. 6055690)
-
Manifold vacuum filtration apparatus, e.g., Unifilter-96 Cell harvester (Perkin Elmer).
-
Scintillation counter, e.g., TopCount NXT (Perkin Elmer)
-
Analysis software (e.g., Prism7, GraphPad)
Incubate membranes and ADRA1A-specific ligands
1.Thaw and homogenize membrane preparations on ice.
2.For each concentration of [3H]-Prasozin assayed (e.g., 1, 2, 5, 10 and 20 nM), label three wells for total (T) binding and three additional wells for non-specific (NS) binding.
3.Add 160 µl binding buffer containing 10 µg of membrane protein to each T- and NS-labeled well.
4.Add 20 µl binding buffer to the T wells and 20 µl of 100 µM nonradioactive prazosin (final concentration 10 µM) to the NS wells so that the total volume in each well is 180 µl.
5.Finally, add to each well 20 µl of 10-fold concentrated radioactive [3H]-Prasozin to reach 1, 2, 5, 10 and 20 nM in a final volume of 200 µl.
6.Incubate at room temperature on a microplate shaker for 1 hr to achieve ligand-binding equilibrium.
7.During the incubation or at least 15 min prior to filtration, presoak the GF/C filters in filter buffer.
Separate free from bound ligands and analyze
8.Terminate the reaction by a rapid filtration of the samples through the presoaked GF/C filters using a vacuum manifold (following the manufacturer's instructions).
9.Wash the filters three times, each time with 5 ml of ice-cold wash buffer.
10.Allow the filters to air dry for at least 1 hr at room temperature.
11.Incubate the filters with an adapted volume of scintillation cocktail, e.g., 40 µl per well for Unifilter GF/C 96-microplate.
12.Incubate 2 hr in the dark at room temperature before proceeding to counting using a scintillation counter following standard program for tritiated radionucleid.
13.Subtract the NS from the T values to determine specific binding values. Analyze the data by nonlinear regression using appropriate software.
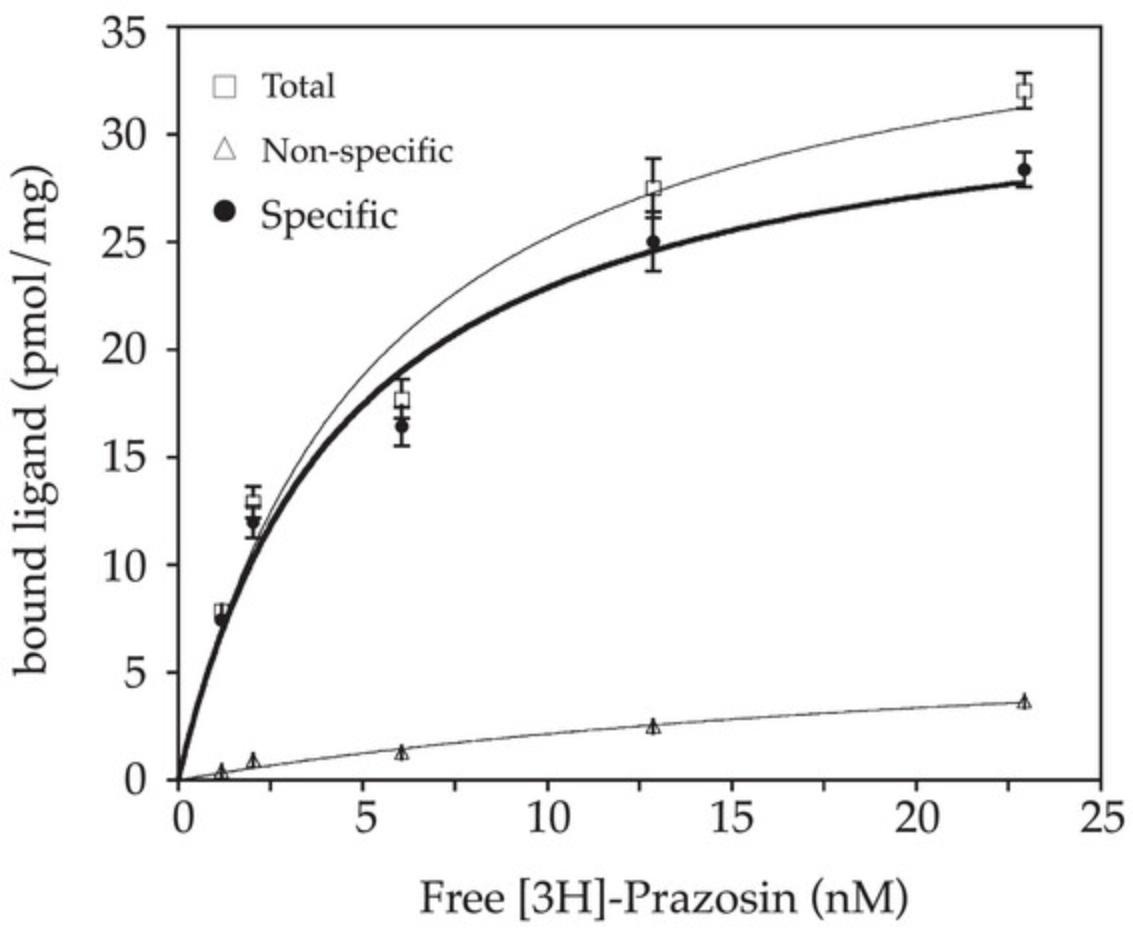
REAGENTS AND SOLUTIONS
Binding buffer
- 30 mM HEPES, pH 7.5 (Euromedex, cat. no. 10-110)
- 150 mM NaCl (Euromedex, cat. no. 1112-A)
- 1 mg/ml BSA (Euromedex, cat. no. 04-100-812-E)
- Prepare fresh
Blocking buffer
- Blotting buffer (see recipe) with 5% (w/v) nonfat dry milk
- Prepare fresh
Blotting buffer
- 25 mM Tris·Cl, pH 7.4 (Euromedex, cat. no. 26-128-30-94-B)
- 0.15 M NaCl (Current Protocols, 2006)
- 0.1% (v/v) Tween 20 (Sigma-Aldrich, cat. no. P1754)
- Prepare fresh
BMGY agar
- 700 ml YEP agar (see recipe, still liquid and warm)
- 100 ml 13.4% (w/v) yeast nitrogen base w/o amino acids (10× stock solution, filter-sterilized; Formedium, cat.no. CYN0410)
- 100 ml 10% (v/v) glycerol (10× stock solution, filter-sterilized; Euromedex, cat. no. EU3550)
- 100 ml 1 M phosphate buffer, pH 6 (10× solution, filter-sterilized; see recipe)
- Pour the solution in large, 14 cm diameter, Petri dishes
- Store up to 1 month at 4°C
BMGY medium
- For 1 L of BMGY solution, prepare 700 ml YEP [10 g yeast extract (e.g., Formedium, cat. no. YEA03), 20 g peptone (e.g., Formedium, cat. no. PEP03) and complete with deionized water] and sterilize by autoclaving.
- Just before use, add the following volumes of filter-sterilized stock solutions:
- 100 ml of 13.4% (w/v) yeast nitrogen base w/o amino acids (10× stock solution)
- 100 ml of 10% (v/v) glycerol (10× stock solution)
- 100 ml of 1 M phosphate buffer, pH 6 (10× stock solution; see recipe)
YEP and 10× stock solutions can be stored up to 2 months at 4°C.
BMMY agar
Prepare as for BMGY agar (see recipe) but replace glycerol with 100 ml of 5% methanol (10× stock solution, filter-sterilized; Sigma-Aldrich, cat. no. 179957).
BMMY medium
Prepare as for BMGY medium (see recipe) but replace glycerol with 100 ml of 5% methanol (10× stock solution).
Filter buffer
- 50 mM Tris·Cl, pH 7.4 (Current Protocols, 2006)
- 0.3% (v/v) polyethylenimine (Sigma-Aldrich, cat. no. P3143)
- Prepare fresh
LB agar
- 35 g LB agar Lennox (e.g., Formedium, cat. no. LBX0202)
- Bring to a final volume of 1 L with water
- Sterilize by autoclaving
- Store up to 6 months at 4°C
LB medium
- 20 g LB broth Lennox (e.g., Formedium, cat. no. LBX0102)
- Bring to a final volume of 1 L with water
- Sterilize by autoclaving
- Store up to 6 months at 4°C
MD agar
- 1.34 g yeast nitrogen base w/o amino acids (e.g., Euromedex, cat. no. CYN0410)
- 2 g dextrose (e.g., Euromedex, cat. no. UG3050)
- 2 g bacto agar (e.g., Euromedex, cat. no. 1330-D)
- Bring to a final volume of 100 ml with water
- Heat sterilize in an autoclave
- Let the solution cool to ∼50°C and sterilely pour into petri dishes
- Store up to 1 month at 4°C
Phosphate buffer
- 3.1% (w/v) dipotassium hydrogen phosphate trihydrate (K2HPO4·3H2O; Sigma-Aldrich, cat. no. P5504)
- 11.81% (w/v) potassium dihydrogen phosphate (KH2PO4; Sigma-Aldrich, cat. no. P0662)
- Filter-sterilize
- Store up to 2 months at 4°C
SOC medium
- 31.5 g SOC broth (e.g., Formedium, cat. no. SOC0201)
- Bring to a final volume of 1 L with water
- Heat sterilize in an autoclave
- Divide into 5- to 10-ml sterile aliquots
- Store up to 6 months at –20°C
TNG buffer
- 50 mM Tris·Cl, pH 7.4 (Current Protocols, 2006)
- 0.5 M NaCl (Current Protocols, 2006)
- 1 mM PMSF
- 10% (v/v) glycerol
- Prepare fresh
TNGE buffer
Prepare as for TNG buffer (see recipe) adding 1 mM EDTA (e.g., Euromedex, cat. no. EU0007).
Wash buffer
- 50 mM Tris·Cl, pH 7.4 (Current Protocols, 2006)
- Prepare fresh from a 1 M Tris·Cl, pH 7.4 stock solution
Yeastern lysis buffer
- 100 mM Tris·Cl pH 7.4 (Current Protocols, 2006)
- 150 mM β-mercaptoethanol (e.g., Sigma-Aldrich, cat. no. M3148)
- 20 mM EDTA
- 2% (v/v) SDS (e.g., Euromedex, cat. no. EU0660-B)
- 9 M urea (e.g., Euromedex, cat. no. EU0014-B)
- Dissolve the components at 65°C
- Prepare fresh
YEP agar
- 10 g yeast extract (e.g., Formedium, cat. no. YEM03)
- 20 g peptone (e.g., Formedium, cat. no. PEP03)
- 20 g bacto agar (e.g., Euromedex, cat. no. 1330-D)
- Dissolve in 700 ml water
- Heat-sterilize in autoclave
- Prepare fresh
YPD agar
Dissolve 70 g YPD agar (e.g., Formedium, cat. no. CCM0110) in water to a final volume of 1 L. Sterilize by autoclaving. Allow solution to cool to ∼50°C and sterilely pour into Petri dishes. Store up to 1 month at 4°C.
YPD medium
Dissolve 50 g YPD broth (e.g., Formedium, cat. no. CCM0210) in water to a final volume of 1 L. Sterilize by autoclaving. Store up to 1 month at 4°C.
COMMENTARY
Background Information
The use of P. pastoris as a robust micro-factory has expanded over the years, delivering thousands of proteins for both academic research and industrial applications, including products for the chemical and food industries, and an increasing number of biopharmaceuticals (Barone et al., 2023; Love et al., 2018). This popularity and versatility are largely attributable to a series of advantages related to both its yeast nature and its methylotrophic metabolism. This microorganism indeed holds a short generation time (∼2 hr on rich medium) and is easy to grow on very simple and inexpensive media, potentially reaching very high cell densities [up to 130 g/l dry cell weight (Cereghino & Cregg, 2000)]. In addition, it is amenable to various culturing formats and schemes in bioreactors, including at large-scale levels (Cereghino et al., 2002; Cos et al., 2006; Nieto-Taype et al., 2020). Comprehensive panels of plasmids and strategies are available for the expression of recombinant genes, and genetic manipulation is relatively straightforward. This issue has notably benefited recently from the validation of dedicated genome editing CRISPR/Cas9 approaches (Gassler et al., 2019; Weninger et al., 2018), as well as the development of novel and specific engineering methodologies and tools (Fischer & Glieder, 2019). As a methylotroph, P. pastoris possesses a peculiar methanol utilization pathway relying on some of the strongest and most tightly regulated known promoters that can be used for very high-level expression of recombinant genes (Ata et al., 2021).
Contrary to bacteria, P. pastoris is a eukaryote and is thus capable of complex posttranslational modifications, including disulfide isomerization, sulfation, phosphorylation, amino-terminal acetylation, carboxy-terminal methylation, myristoylation, farnesylation and glycosylation (reviewed in Eckart & Bussineau, 1996), which are often very essential for the proper targeting, the biological activity, and the stability of the expressed recombinant proteins. Concerning glycosylation modifications, even if the oligosaccharide chains attached to proteins are much shorter and authentic than in S. cerevisiae (Li et al., 2007), P. pastoris is not able to graft the complex carbohydrate motifs achieved in mammalian cells, which may be critical for the functionality of certain proteins. In addition, non-homogeneous N-glycosylation of recombinant proteins is frequently observed, leading to some degrees of heterogeneity (Shukla et al., 2007; Tate et al., 2003; Weiss et al., 1998) that can be detrimental in various applications including structural studies. Several strains have been engineered to generate more complex (human-like) and more homogeneous N-glycosylations (Hamilton & Gerngross, 2007); none of them yet reported for their use with IMP overexpression.
Thanks to its special physiology and low operating cost, P. pastoris is also one of the very few organisms adapted to the production of isotopically labeled recombinant proteins for their fine structural and dynamic characterizations by NMR spectroscopies (Wood & Komives, 1999; Whittaker & Whittaker, 2005). Several of these production strategies have been recently transposed and adapted to the production of IMPs, either uniformly or selectively labeled (Barret et al., 2022; Clark et al., 2018; Munro et al., 2020).
As for every highly potent technological system, P. pastoris also displays some limitations. First, a common characteristic for eukaryotic systems that are efficiently overexpressing recombinant genes is that an overload of the translocation and folding machineries often creates a stress that triggers the activation of sorting and degradation processes, resulting in lowered expression levels and heterogeneity of recombinant proteins. This is often observed with IMP overexpression in P. pastoris , an issue that may be efficiently improved after some optimization (see the Critical Parameters and Troubleshooting section below).
Another characteristic that can have a direct impact on the expression of mammalian IMPs is related to the lipidic composition of yeast membranes, which varies significantly from that of higher eukaryotes (Opekarova & Tanner, 2003). As they require specific lipids for their proper functions or for their correct folding and stability, these differences may influence both the expression level, functionality, and stability of recombinant IMPs (Haviv et al., 2007; Lifshitz et al., 2007). In particular, the main sterol in yeast membranes, ergosterol, is different from the cholesterol counterpart found in mammalian membranes, which may be detrimental to the activity and stability of certain IMPs as it was shown in several studies (Talmont et al., 2020; Wriessnegger & Pichler, 2013). To overcome this issue, Pichler and his colleagues developed a cholesterol-producing P. pastoris strain that significantly improved the functional expression and stability of a human Na,K-ATPase (Hirz et al., 2013).
Critical Parameters and Troubleshooting
As is true for every other expression system, its performance depends not only on the characteristics of the IMPs to be expressed, but also on the experimental conditions applied. Therefore, optimization of the expression conditions is often very helpful for the recovery of higher amounts of functional recombinant IMPs. From the seminal work of Helmut Reilander and his colleagues (Weiss et al., 1995), more than a hundred different GPCRs have been expressed in P. pastoris thus far, with several of them evaluated via activity assays, representing an ideal source of data to exemplify the different directions that can be undertaken to enhance the expression levels of functional IMPs.
As mentioned in Basic Protocol 1, adjustments of the coding sequence, the fused tags, and the gene dosage often reveal beneficial for improving the expression levels of functional GPCRs (Grunewald et al., 2004; Sarramegna et al., 2002; Weiss et al,, 1998). Importantly, it was very often observed that clones presenting the highest amounts of immunodetected GPCRs rarely exhibited the highest ligand binding activities (André et al., 2006). This strongly suggests that bottlenecks for producing functional receptors lie not only in the transcription and translation steps but also in folding and/or posttranslational processing.
Accordingly, further improvements can be implemented by appropriately adjusting some of the experimental parameters that influence the host cell physiology, hence, its performance for heterologous gene expression, correct protein folding, and proper trafficking.
As a first step, evaluating the production time-course of an IMP often reveals very useful information, as the outcome may importantly vary from one receptor to another. For instance, 10 hr was determined as the optimal induction time for different constructs expressing a mu opioid receptor (Sarramegna et al., 2002), whereas the highest expression levels were obtained in the range of 18 to 24 hr post-induction for a majority of other receptors, and up to 60 hr for an engineered ACM2 muscarinic receptor (Yurugi-Kobayashi et al., 2009). Similarly, cell density before the induction phase in methanol-containing medium is another important parameter that may differently affect the expression level of GPCRs, as it was observed for the different receptors presented in Figure 2 when comparing cell densities of 1 OD600/ml and 10 OD600/ml.
Formulation of the induction medium is also an important issue. Adjustments in the composition of buffered medium, pH values, and methanol concentration usually did not bring major benefits in GPCR expression, and a typical induction is generally performed at pH 5 to 7 in buffered complex-medium containing 0.5% (v/v) methanol. For these IMPs, substantial improvements, however, can be gained by supplementing these media with some small compounds that are believed to facilitate the folding and processing of the recombinant proteins. Among these molecules, dimethyl sulfoxide (DMSO) added in the induction medium remarkably increased the production yield of 16 out of 20 tested receptors up to 6-fold relative to standard conditions (André et al., 2006). DMSO possibly influences the translocation of membrane proteins and is also believed to act as a stabilizer of folding intermediates, therefore being qualified a chemical chaperone (Bernier et al., 2004). In a comparable fashion, adding ligands specific to a given GPCR proved highly beneficial for a large majority of tested receptors (André et al., 2006). Such small molecules are considered pharmacological chaperones as they have been shown to selectivity promote the proper folding and trafficking of the targeted GPCR (Bernier et al., 2004). In addition, it was shown in several studies that lowering temperature during expression to a typical range of 18°C to 24°C was optimal for various receptors, as measured by ligand binding (Singh et al., 2012). Possible explanations for the temperature effect include slowing of protein production and a reduced overload of the translocation machinery, protein processing, or intracellular trafficking. Lowering temperature has also been shown to reduce proteolytic activities and upregulate cold-shock proteins, such as chaperones.
Overall, adjusting these different culturing parameters very often allows enhancing the expression levels of active GPCRs (André et al., 2006). Importantly, when the Bmax is significantly increased upon optimization, it is usually observed that the total amount of receptors remains unchanged. This recurrent observation with GPCRs and other membrane proteins strongly suggests that IMPs are expressed in P. pastoris under a functional/non-functional equilibrium that can be modulated with the expression conditions that are used.
Accordingly, and aside from the improvements that could be applied at the genetic level (optimization of the coding sequence, fused tags, and gene dosage), a systematic approach we recommend for the successful expression of a given IMP in P. pastoris is to perform a primary screen for a set of separate expression conditions by varying time, temperature, and additives (DMSO, ligand, etc.). Further improvement could then be gained in combining and in refining every positive parameter as a subsequent screen.
Understanding Results
The different protocols described in this article provide a convenient starting point for expressing any IMP in P. pastoris. As mentioned, hundreds of IMPs of various origins, topologies, and functions have been successfully overexpressed with this system, and the corresponding reports can serve as good starting references. Using such methodologies along with the optimizations mentioned above, the authors have contributed to the successful expression of dozens of various IMPs for different applications and characterizations (André et al., 2006; Barret et al., 2022; Bornert et al., 2013; Chaptal et al., 2017; Damian et al., 2018; Fernandes et al., 2022; Guyot et al., 2020; Hansen et al., 2016; Lecas et al., 2020; Logez et al., 2014; Lunstrom et al., 2006; Singh et al., 2008; Vasseur et al., 2019).
Time Considerations
When employing P. pastoris for the recombinant expression of IMPs, the required time between DNA cloning and obtaining a validated expressing clone is somewhat longer than with bacterial systems, but much more straightforward compared to other stable eukaryotic expression systems. Practically, once the expression vector has been designed, it typically takes less than one month to perform all the steps for the identification of an expressing clone, with a time schedule that can be summarized as follows:
- Yeast transformation : Starting from a freshly streaked colony, 1 day is needed for the preparation of yeast competent cells and their subsequent electro-transformation, and 2 to 4 days are required for the growth of recombinant clones on an agar plate. The second step of phenotypic selection for multicopy transformants then requires 2 to 3 additional days;
- Expression step : Starting from a recombinant colony freshly isolated on a YPD agar plate, cultures of small to large volumes of cells (10 ml to 10 L) need ∼3 days, including 2 days for pre-culturing in BMGY medium plus another 1 day for methanol induction in BMMY medium;
- Sample preparation and analysis : Yeast cell lysis and membrane preparation can be performed in one day, and analyses by immunoblotting and ligand binding activity assays (in the case of GPCR expression) generally takes 2 to 3 more days.
Accordingly, a clone evaluated in standard expression conditions can be obtained in 2 to 3 weeks. From there, the number of conditions that can be subsequently screened for optimizing the yield and functionality of the expressed IMP will determine the additional time needed.
Acknowledgments
The authors are supported by the CNRS and the University of Strasbourg, and by grants from French research agencies (ANR-21-CE29-0012 and CIFRE 2021/1599).
Author Contributions
Magali Schwob : Investigation; methodology; writing original draft; writing review and editing. Valérie Kugler : Investigation; methodology; writing review and editing. Renaud Wagner : Conceptualization; funding acquisition; investigation; methodology; project administration; supervision; writing original draft; writing review and editing.
Conflict of Interest
The authors declare no conflict of interest.
Open Research
Data Availability Statement
The data that support the findings of this study are available from the corresponding author upon reasonable request.
Literature Cited
- Alkhalfioui, F., Logez, C., Bornert, O., & Wagner, R. (2011). Expression systems: Pichia pastoris. In A. S. Robinson (Ed.), Production of membrane proteins—Strategies for expression and isolation (pp. 75–108). Wiley-VCH.
- André, N., Cherouati, N., Prual, C., Steffan, T., Zeder-Lutz, G., Magnin, T., Pattus, F., Michel, H., Wagner, R., & Reinhart, C. (2006). Enhancing functional production of G protein-coupled receptors in Pichia pastoris to levels required for structural studies via a single expression screen. Protein Science , 15, 1115–1126. https://doi.org/10.1110/ps.062098206
- Ata, Ö., Ergün, B. G., Fickers, P., Heistinger, L., Mattanovich, D., Rebnegger, C., & Gasser, B. (2021). What makes Komagataella phaffii non-conventional? FEMS Yeast Research , 21, foab059. https://doi.org/10.1093/femsyr/foab059
- Barone, G. D., Emmerstorfer-Augustin, A., Biundo, A., Pisano, I., Coccetti, P., Mapelli, V., & Camattari, A. (2023). Industrial production of proteins with Pichia pastoris-Komagataella phaffii. Biomolecules , 13, 441. https://doi.org/10.3390/biom13030441
- Barret, L., Schubeis, T., Kugler, V., Guyot, L., Pintacuda, G., & Wagner, R. (2022). Production and preparation of isotopically labeled human membrane proteins in Pichia pastoris for Fast-MAS-NMR analyses. Methods in Molecular Biology , 2507, 201–221. https://doi.org/10.1007/978-1-0716-2368-8_11
- Bernauer, L., Radkohl, A., Lehmayer, L. G. K., & Emmerstorfer-Augustin, A. (2021). Komagataella phaffii as emerging model organism in fundamental research. Frontiers in Microbiology , 11, 607028. https://doi.org/10.3389/fmicb.2020.607028
- Bernier, V., Lagacé, M., Bichet, D. G., & Bouvier, M. (2004). Pharmacological chaperones: Potential treatment for conformational diseases. Trends in Endocrinology and Metabolism , 15, 222–228. https://doi.org/10.1016/j.tem.2004.05.003
- Bill, R. M., & Hedfalk, K. (2021). Aquaporins - Expression, purification and characterization. Biochimica et Biophysica Acta Biomembranes , 1863, 183650. https://doi.org/10.1016/j.bbamem.2021.183650
- Bornert, O., Møller, T. C., Boeuf, J., Candusso, M. P., Wagner, R., Martinez, K. L., & Simonin, F. (2013). Identification of a novel protein-protein interaction motif mediating interaction of GPCR-associated sorting proteins with G protein-coupled receptors. PLoS One , 8, e56336. https://doi.org/10.1371/journal.pone.0056336
- Bylund, D. B. (2007). Characterization of Adrenoceptors. Current Protocols in Pharmacology , 36(1), 1.5.1–1.5.18. https://doi.org/10.1002/0471141755.ph0105s36
- Bylund, D. B., & Toews, M. L. (2011). Radioligand binding methods for membrane preparations and intact cells. Methods in Molecular Biology , 746, 135–164. https://doi.org/10.1007/978-1-61779-126-0_8
- Byrne, B. (2015). Pichia pastoris as an expression host for membrane protein structural biology. Current Opinion in Structural Biology , 32, 9–17. https://doi.org/10.1016/j.sbi.2015.01.005
- Cereghino, J. L., & Cregg, J. M. (2000). Heterologous protein expression in the methylotrophic yeast Pichia pastoris. FEMS Microbiology Reviews , 24, 45–66. https://doi.org/10.1111/j.1574-6976.2000.tb00532.x
- Cereghino, G. P. L., Cereghino, J. L., Ilgen, C., & Cregg, J. M. (2002). Production of recombinant proteins in fermenter cultures of the yeast Pichia pastoris. Current Opinion in Biotechnology , 13, 329–332. https://doi.org/10.1016/s0958-1669(02)00330-0
- Chaptal, V., Delolme, F., Kilburg, A., Magnard, S., Montigny, C., Picard, M., Prier, C., Monticelli, L., Bornert, O., Agez, M., Ravaud, S., Orelle, C., Wagner, R., Jawhari, A., Broutin, I., Pebay-Peyroula, E., Jault, J. M., Kaback, H. R., le Maire, M., & Falson, P. (2017). Quantification of detergents complexed with membrane proteins. Scientific Reports , 7, 41751. https://doi.org/10.1038/srep41751
- Clark, L., Dikiy, I., Rosenbaum, D. M., & Gardner, K. H. (2018). On the use of Pichia pastoris for isotopic labeling of human GPCRs for NMR studies. Journal of Biomolecular NMR , 71, 203–211. https://doi.org/10.1007/s10858-018-0204-3
- Cos, O., Ramón, R., Montesinos, J. L., & Valero, F. (2006). Operational strategies, monitoring and control of heterologous protein production in the methylotrophic yeast Pichia pastoris under different promoters: A review. Microbial Cell Factories , 5, 17. https://doi.org/10.1186/1475-2859-5-17
- Cregg, J. M., & Madden, K. R. (1987). Development of yeast transformation systems and construction of methanol-utilization-defective mutants of Pichia pastoris gene disruption. Biological Research on Yeasts , II, 1–18.
- Cregg, J. M., Tolstorukov, I., Kusari, A., Sunga, A. J., Madden, K., & Chappell, T. (2010). Expression of recombinant genes in the yeast Pichia pastoris. Current Protocols Essential Laboratory Techniques , 4, 13.2.1–13.2.14. https://doi.org/10.1002/9780470089941.et1302s04
- Current Protocols. (2006). Commonly Used Reagents. Current Protocols in Microbiology , 00, A.2A.1–A.2A.15. https://doi.org/10.1002/9780471729259.mca02as00
- Damian, M., Pons, V., Renault, P., M'Kadmi, C., Delort, B., Hartmann, L., Kaya, A. I., Louet, M., Gagne, D., Ben Haj Salah, K., Denoyelle, S., Ferry, G., Boutin, J. A., Wagner, R., Fehrentz, J. A., Martinez, J., Marie, J., Floquet, N., Galès, C., … Banères, J. L. (2018). GHSR-D2R heteromerization modulates dopamine signaling through an effect on G protein conformation. Proceedings of the National Academy of Sciences of the United States of America , 115, 4501–4506. https://doi.org/10.1073/pnas.1712725115
- Eckart, M. R., & Bussineau, C. M. (1996). Quality and authenticity of heterologous proteins synthesized in yeast. Current Opinion in Biotechnology , 7, 525–530. https://doi.org/10.1016/s0958-1669(96)80056-5
- Fernandes, C. A. H., Zuniga, D., Fagnen, C., Kugler, V., Scala, R., Péhau-Arnaudet, G., Wagner, R., Perahia, D., Bendahhou, S., & Vénien-Bryan, C. (2022). Cryo-electron microscopy unveils unique structural features of the human Kir2.1 channel. Science Advances , 8, eabq8489. https://doi.org/10.1126/sciadv.abq8489
- Fischer, G., Kosinska-Eriksson, U., Aponte-Santamaria, C., Palmgren, M., Geijer, C., Hedfalk, K., Hohmann, S., de Groot, B. L., Neutze, R., & Lindkvist-Petersson, K. (2009). Crystal structure of a yeast aquaporin at 1.15 Å reveals a novel gating mechanism. PLoS Biology , 7, e1000130. https://doi.org/10.1371/journal.pbio.1000130
- Fischer, J. E., & Glieder, A. (2019). Current advances in engineering tools for Pichia pastoris. Current Opinion in Biotechnology , 59, 175–181. https://doi.org/10.1016/j.copbio.2019.06.002
- Gallagher, S. R. (2012). SDS-Polyacrylamide Gel Electrophoresis (SDS-PAGE). Current Protocols Essential Laboratory Techniques , 6(1), 7.3.1–7.3.28. https://doi.org/10.1002/9780470089941.et0703s06
- Gassler, T., Heistinger, L., Mattanovich, D., Gasser, B., & Prielhofer, R. (2019). CRISPR/Cas9-Mediated homology-directed genome editing in Pichia pastoris. Methods in Molecular Biology , 1923, 211–225. https://doi.org/10.1007/978-1-4939-9024-5_9
- Goldman, A., Ursitti, J. A., Mozdzanowski, J., & Speicher, D. W. (2015). Electroblotting from polyacrylamide gels. Current Protocols in Protein Science , 82, 10.7.1–10.7.16. https://doi.org/10.1002/0471140864.ps1007s82
- Grunewald, S., Haase, W., Molsberger, E., Michel, H., & Reilander, H. (2004). Production of the human D2S receptor in the methylotrophic yeast P. pastoris. Receptors and Channels , 10, 37–50. https://doi.org/10.3109/10606820490279466
- Guyot, L., Hartmann, L., Mohammed-Bouteben, S., Caro, L., & Wagner, R. (2020). Preparation of recombinant membrane proteins from Pichia pastoris for molecular investigations. Current Protocols in Protein Science , 100, e104. https://doi.org/10.1002/cpps.104
- Hamilton, S. R., & Gerngross, T. U. (2007). Glycosylation engineering in yeast: The advent of fully humanized yeast. Current Opinion in Biotechnology , 18, 387–392. https://doi.org/10.1016/j.copbio.2007.09.001
- Hansen, R. W., Wang, X., Golab, A., Bornert, O., Oswald, C., Wagner, R., & Martinez, K. L. (2016). Functional stability of the human kappa opioid receptor reconstituted in nanodiscs revealed by a time-resolved scintillation proximity assay. PLoS One , 11, e0150658. https://doi.org/10.1371/journal.pone.0150658
- Hartmann, L., Kugler, V., & Wagner, R. (2016). Expression of eukaryotic membrane proteins in Pichia pastoris. Methods in Molecular Biology , 1432, 143–162. https://doi.org/10.1007/978-1-4939-3637-3_10
- Hartmann, L., Metzger, E., Ottelard, N., & Wagner, R. (2017). Direct extraction and purification of recombinant membrane proteins from Pichia pastoris protoplasts. Methods in Molecular Biology , 1635, 45–56. https://doi.org/10.1007/978-1-4939-7151-0_3
- Hartner, F. S., & Glieder, A. (2006). Regulation of methanol utilisation pathway genes in yeasts. Microbial Cell Factories , 5, 39. https://doi.org/10.1186/1475-2859-5-39
- Haviv, H., Cohen, E., Lifshitz, Y., Tal, D. M., Goldshleger, R., & Karlish, S. J. D. (2007). Stabilization of Na+,K+-ATPase purified from Pichia pastoris membranes by specific interactions with lipids. Biochemistry , 46, 12855–12867. https://doi.org/10.1021/bi701248y
- Hausjell, J., Schendl, D., Weissensteiner, J., Molitor, C., Halbwirth, H., & Spadiut, O. (2020). Recombinant production of a hard-to-express membrane-bound cytochrome P450 in different yeasts-Comparison of physiology and productivity. Yeast , 37, 217–226. https://doi.org/10.1002/yea.3441
- Hirz, M., Richter, G., Leitner, E., Wriessnegger, T., & Pichler, H. (2013). A novel cholesterol-producing Pichia pastoris strain is an ideal host for functional expression of human Na,K-ATPase α3β1 isoform. Applied Microbiology and Biotechnology , 97, 9465–9478. https://doi.org/10.1007/s00253-013-5156-7
- Kesidis, A., Depping, P., Lodé, A., Vaitsopoulou, A., Bill, R. M., Goddard, A. D., & Rothnie, A. J. (2020). Expression of eukaryotic membrane proteins in eukaryotic and prokaryotic hosts. Methods , 180, 3–18. https://doi.org/10.1016/j.ymeth.2020.06.006
- Kramer, M. F., & Coen, D. M. (2002). The polymerase chain reaction. Current Protocols in Protein Science , Appendix 4 , A.4J.1–A.4J.8. https://doi.org/10.1002/0471140864.psa04js29
- Lecas, L., Hartmann, L., Caro, L., Mohamed-Bouteben, S., Raingeval, C., Krimm, I., Wagner, R., Dugas, V., & Demesmay, C. (2020). Miniaturized weak affinity chromatography for ligand identification of nanodiscs-embedded G-protein coupled receptors. Analytica et Chimica Acta , 1113, 26–35. https://doi.org/10.1016/j.aca.2020.03.062
- Li, P., Anumanthan, A., Gao, X. G., Ilangovan, K., Suzara, V. V., Düzgünes, N., & Renugopalakrishnan, V. (2007). Expression of recombinant proteins in Pichia pastoris. Applied Biochemistry and Biotechnology , 142, 105–124. https://doi.org/10.1007/s12010-007-0003-x
- Lifshitz, Y., Petrovich, E., Haviv, H., Goldshleger, R., Tal, D. M., Garty, H., & Karlish, S. J. D. (2007). Purification of the human α2 isoform of Na,K-ATPase expressed in Pichia pastoris. Stabilization by lipids and FXYD1. Biochemistry , 46, 14937–14950.
- Logez, C., Berger, S., Legros, C., Banères, J. L., Cohen, W., Delagrange, P., Nosjean, O., Boutin, J. A., Ferry, G., Simonin, F., & Wagner, R. (2014). Recombinant human melatonin receptor MT1 isolated in mixed detergents shows pharmacology similar to that in mammalian cell membranes. PLoS One , 9, e100616. https://doi.org/10.1371/journal.pone.0100616
- Looser, V., Bruhlmann, B., Bumbak, F., Stenger, C., Costa, M., Camattari, A., Fotiadis, D., & Kovar, K. (2015). Cultivation strategies to enhance productivity of Pichia pastoris : A review. Biotechnology Advances , 33, 1177–1193. https://doi.org/10.1016/j.biotechadv.2015.05.008
- Love, K. R., Dalvie, N. C., & Love, J. C. (2018). The yeast stands alone: The future of protein biologic production. Current Opinion in Biotechnology , 53, 50–58. https://doi.org/10.1016/j.copbio.2017.12.010
- Lundstrom, K., Wagner, R., Reinhart, C., Desmyter, A., Cherouati, N., Magnin, T., Zeder-Lutz, G., Courtot, M., Prual, C., André, N., Hassaine, G., Michel, H., Cambillau, C., & Pattus, F. (2006). Structural genomics on membrane proteins: Comparison of more than 100 GPCRs in 3 expression systems. Journal of Structural and Functional Genomics , 7, 77–91. https://doi.org/10.1007/s10969-006-9011-2
- Munro, R., de Vlugt, J., Ladizhansky, V., & Brown, L. S. (2020). Improved protocol for the production of the low-expression eukaryotic membrane protein human Aquaporin 2 in Pichia pastoris for solid-state NMR. Biomolecules , 10, 434. https://doi.org/10.3390/biom10030434
- Nieto-Taype, M. A., Garcia-Ortega, X., Albiol, J., Montesinos-Seguí, J. L., & Valero, F. (2020). Continuous cultivation as a tool toward the rational bioprocess development with Pichia pastoris cell factory. Frontiers in Bioengineering and Biotechnology , 8, 632. https://doi.org/10.3389/fbioe.2020.00632
- Olson, B. J., & Markwell, J. (2007). Assays for determination of protein concentration. Current Protocols in Protein Science , 48, 3.4.1–3.4.29. https://doi.org/10.1002/0471140864.ps0304s48
- Opekarova, M., & Tanner, W. (2003). Specific lipid requirements of membrane proteins–a putative bottleneck in heterologous expression. Biochimica et Biophysica Acta , 1610, 11–22. https://doi.org/10.1016/s0005-2736(02)00708-3
- Popova, L. G., Khramov, D. E., Nedelyaeva, O. I., & Volkov, V. S. (2023). Yeast heterologous expression systems for the study of plant membrane proteins. International Journal of Molecular Sciences , 24, 10768. https://doi.org/10.3390/ijms241310768
- Santos, R., Ursu, O., Gaulton, A., Bento, A. P., Donadi, R. S., Bologa, C. G., Karlsson, A., Al-Lazikani, B., Hersey, A., Oprea, T. I., & Overington, J. P. (2017). A comprehensive map of molecular drug targets. Nature Reviews Drug Discovery , 16, 19–34. https://doi.org/10.1038/nrd.2016.230
- Sarramegna, V., Demange, P., Milon, A., & Talmont, F. (2002). Optimizing functional versus total expression of the human mu-opioid receptor in Pichia pastoris. Protein Expression and Purification , 24, 212–220. https://doi.org/10.1006/prep.2001.1564
- Shukla, A. K., Haase, W., Reinhart, C., & Michel, H. (2007). Heterologous expression and comparative characterization of the human neuromedin U subtype II receptor using the methylotrophic yeast Pichia pastoris and mammalian cells. The International Journal of Biochemistry and Cell Biology , 39, 931–942. https://doi.org/10.1016/j.biocel.2007.01.016
- Singh, S., Gras, A., Fiez-Vandal, C., Ruprecht, J., Rana, R., Martinez, M., Strange, P. G., Wagner, R., & Byrne, B. (2008). Large-scale functional expression of WT and truncated human adenosine A2A receptor in Pichia pastoris bioreactor cultures. Microbial Cell Factories , 7, 28. https://doi.org/10.1186/1475-2859-7-28
- Singh, S., Gras, A., Fiez-Vandal, C., Martinez, M., Wagner, R., & Byrne, B. (2012). Large-scale production of membrane proteins in Pichia pastoris : The production of G protein-coupled receptors as a case study. Methods in Molecular Biology , 866, 197–207. https://doi.org/10.1007/978-1-61779-770-5_17
- Talmont, F., Lebrun, C., & Zajac, J. M. (2020). Agonist binding of human mu opioid receptors expressed in the yeast Pichia pastoris : Effect of cholesterol complementation. Neurochemistry International , 132, 104588. https://doi.org/10.1016/j.neuint.2019.104588
- Tate, C. G., Haase, J., Baker, C., Boorsma, M., Magnani, F., Vallis, Y., & Williams, D. C. (2003). Comparison of seven different heterologous protein expression systems for the production of the serotonin transporter. Biochimica et Biophysica Acta , 1610, 141–153. https://doi.org/10.1016/s0005-2736(02)00719-8
- Tornroth-Horsefield, S., Wang, Y., Hedfalk, K., Johanson, U., Karlsson, M., Tajkhorshid, E., Neutze, R., & Kjellbom, P. (2006). Structural mechanism of plant aquaporin gating. Nature , 439, 688–694. https://doi.org/10.1038/nature04316
- Vasseur, L., Cens, T., Wagner, R., Saint, N., Kugler, V., Chavanieu, A., Ouvry, C., Dupré, C., Ferry, G., & Boutin, J. A. (2019). Importance of the choice of a recombinant system to produce large amounts of functional membrane protein hERG. International Journal of Molecular Science , 20, 3181. https://doi.org/10.3390/ijms20133181
- Vogl, T., Sturmberger, L., Kickenweiz, T., Wasmayer, R., Schmid, C., Hatzl, A. M., Gerstmann, M. A., Pitzer, J., Wagner, M., Thallinger, G. G., Geier, M., & Glieder, A. (2016). A toolbox of diverse promoters related to methanol utilization: Functionally verified parts for heterologous pathway expression in Pichia pastoris. ACS Synthetic Biology , 5, 172–186. https://doi.org/10.1021/acssynbio.5b00199
- Voytas, D. (2001). Agarose gel electrophoresis. Current Protocols in Protein Science , Appendix 4 , https://doi.org/10.1002/0471140864.psa04fs13
- Wallin, E., & von Heijne, G. (1998). Genome-wide analysis of integral membrane proteins from eubacterial, archaean, and eukaryotic organisms. Protein Science , 7, 1029–1038. https://doi.org/10.1002/pro.5560070420
- Weninger, A., Fischer, J. E., Raschmanová, H., Kniely, C., Vogl, T., & Glieder, A. (2018). Expanding the CRISPR/Cas9 toolkit for Pichia pastoris with efficient donor integration and alternative resistance markers. Journal of Cellular Biochemistry , 119, 3183–3198. https://doi.org/10.1002/jcb.26474
- Weiss, H. M., Haase, W., Michel, H., & Reilander, H. (1995). Expression of functional mouse 5-HT5A serotonin receptor in the methylotrophic yeast Pichia pastoris : Pharmacological characterization and localization. FEBS Letter , 377, 451–456. https://doi.org/10.1016/0014-5793(95)01389-x
- Weiss, H. M., Haase, W., Michel, H., & Reilander, H. (1998). Comparative biochemical and pharmacological characterization of the mouse 5HT5A 5-hydroxytryptamine receptor and the human ß2-adrenergic receptor produced in the methylotrophic yeast Pichia pastoris. Biochemical Journal , 330, 1137–1147. https://doi.org/10.1042/bj3301137
- White, C. E., Hunter, M. J., Meininger, D. P., White, L. R., & Komives, E. A. (1995). Large-scale expression, purification and characterization of small fragments of thrombomodulin: the roles of the sixth domain and of methionine 388. Protein Engineering , 8(11), 1177–1187. https://doi.org/10.1093/protein/8.11.1177
- Whittaker, M. M., & Whittaker, J. W. (2005). Construction and characterization of Pichia pastoris strains for labeling aromatic amino acids in recombinant proteins. Protein Expression and Purification , 41, 266–274. https://doi.org/10.1016/j.pep.2004.11.008
- Wood, M. J., & Komives, E. A. (1999). Production of large quantities of isotopically labeled protein in Pichia pastoris by fermentation. Journal of Biomolecular NMR , 13, 149–159. https://doi.org/10.1023/a:1008398313350
- Wriessnegger, T., & Pichler, H. (2013). Yeast metabolic engineering–targeting sterol metabolism and terpenoid formation. Progress in Lipid Research , 52, 277–293. https://doi.org/10.1016/j.plipres.2013.03.001
- Yurugi-Kobayashi, T., Asada, H., Shiroishi, M., Shimamura, T., Funamoto, S., Katsuta, N., Ito, K., Sugawara, T., Tokuda, N., Tsujimoto, H., Murata, T., Nomura, N., Haga, K., Haga, T., Iwata, S., & Kobayashi, T. (2009). Comparison of functional non-glycosylated GPCRs expression in Pichia pastoris. Biochemical and Biophysical Research Communications , 380, 271–276. https://doi.org/10.1016/j.bbrc.2009.01.053
- Zeder-Lutz, G., Cherouati, N., Reinhart, C., Pattus, F., & Wagner, R. (2006). Dot-blot immunodetection as a versatile and high-throughput assay to evaluate recombinant GPCRs produced in the yeast Pichia pastoris. Protein Expression and Purification , 50, 118–127. https://doi.org/10.1016/j.pep.2006.05.017
Internet Resources
List of proteins that were produced with this system and led to 3D-structure resolution (62 as of September 2023).