CRISPR/Cas9-enhanced Targetron Insertion for Delivery of Heterologous Sequences into the Genome of Gram-Negative Bacteria
Elena Velázquez, Elena Velázquez, Yamal Al-Ramahi, Yamal Al-Ramahi, Víctor de Lorenzo, Víctor de Lorenzo
Abstract
Targetron technology, a gene-editing approach based on the use of mobile group II introns, is particularly useful for bacterial strains deficient in homologous recombination. Specifically, the Ll.LtrB intron from Lactococcus lactis can be used in a wide range of species and can be easily retargeted, that is, modified for integration into any locus of interest. Targetron technology is thus a powerful tool for generating genomic insertions in a broad range of genetic backgrounds, mainly when no other techniques can be efficiently employed. Notably, the approach can be coupled to CRISPR/Cas9 counterselection of wildtype DNA sequences to decrease the population of unmodified cells and ultimately improve Ll.LtrB insertion efficiency. Here, we describe a step-by-step protocol for delivering exogenous sequences into the genome of Gram-negative bacteria by means of targetron technology and CRISPR/Cas9 counterselection using Pseudomonas putida as a model. We describe the retargeting of the Ll.LtrB intron to the locus selected for insertion, the design of specific spacers for eliminating unmutated cells through CRISPR/Cas9 counterselection, and the cloning of exogenous sequences into Ll.LtrB. We also provide a protocol for delivering a specific cargo to the locus of choice once all necessary components of the system are ready. Lastly, we describe a general protocol for curing the engineered strain of all plasmids. CRISPR/Cas9-enhanced Ll.LtrB insertion can be an efficient alternative for overcoming low recombination-based editing efficiency and can be used in numerous bacterial species. © 2022 The Authors. Current Protocols published by Wiley Periodicals LLC.
Basic Protocol 1 : Retargeting the Ll.LtrB intron to the target locus
Support Protocol 1 : Preparation of competent E. coli
Basic Protocol 2 : Design and cloning of CRISPR spacers to counterselect Ll.LtrB insertions
Support Protocol 2 : Interference assay to check efficiency of selected spacers
Basic Protocol 3 : Cloning cargos into Ll.LtrB
Basic Protocol 4 : Ll.LtrB/CRISPR/Cas9-mediated insertion
Basic Protocol 5 : Curing the engineered strain of plasmids
INTRODUCTION
Genetic editing of bacterial genomes has become easier thanks to the large number of techniques that have been developed in recent years. Nonetheless, insertion of large sequences at specific loci is a time-consuming procedure and most tools rely on homologous recombination (Martinez-Garcia & de Lorenzo, 2011). These methods often cause unforeseen genome rearrangements and, importantly, cannot be employed in recA -deficient strains (Jiang, Bikard, Cox, Zhang, & Marraffini, 2013). To overcome these issues, various methods have been developed that are independent of homologous recombination, such as using CRISPR-associated transposases (Klompe, Vo, Halpin-Healy, & Sternberg, 2019; Strecker et al., 2019) or recombineering (Ellis, Yu, DiTizio, & Court, 2001). Despite these advances, there is still a dearth of genetic tools that can be used in recA – strains.
Group II introns have the ability to splice and split from an mRNA and insert into specific DNA sequences in a process called retrohoming. This mechanism is aided by an intron-encoded protein (IEP), which is normally encoded within each mobile group II intron. The IEP also assists in recognition of the target molecule, although this is mostly driven by complementarity between the target locus and specific regions within the intron. The intronic sites are called exon-binding sites 1 and 2 (EBS1 and 2) and ∂, and their complementary counterparts in the target locus are called intron-binding sites (IBS1 and 2) and ∂′. After recognition, a reverse splicing event takes place that links the intronic RNA to one of the DNA strands of the target molecule. Later, the IEP retrotranscribes the group II intron into DNA, and then different mechanisms are used to completely insert the intron sequence into its target region. After insertion, the intron is flanked by IBS1, IBS2, and ∂′, and can be expressed and spliced again to invade non-inserted target sites.
The targetron platform, which was developed as a nucleic acid delivery system, is based on the Ll.LtrB group II intron from Lactococcus lactis and its EBS1, EBS2, and ∂ components (Perutka, Wang, Goerlitz, & Lambowitz, 2004). These introns can be modified to carry heterologous cargo and can stably integrate into the genomes of a wide range of species (Frazier, San Filippo, Lambowitz, & Mills, 2003; Plante & Cousineau, 2006; Rawsthorne, Turner, & Mills, 2006). Furthermore, they have high sequence recognition specificity (Xiang, Qin, Michels, Freeland, & Pyle, 1998) that can be modified at will and, importantly, work in a recA- independent fashion (Cousineau et al., 1998). Unfortunately, the use of these retroelements for bearing and delivering DNA cargo has been limited thus far by their low efficiency. The addition of selectable markers to these group II introns was tested as a mean to improve the system (Zhong, Karberg, & Lambowitz, 2003), but this approach hinders their capability to transport exogenous sequences, as their efficiency drops with long fragments, with cargos longer than 1 kb typically being difficult to handle (Plante & Cousineau, 2006).
By using CRISPR/Cas9 as a tool to counterselect against wildtype genotypes, we have recently shown that cargos of different sizes can be effectively incorporated into the genome of various Gram-negative bacteria using the targetron system (Velazquez, Al-Ramahi, Tellechea-Luzardo, Krasnogor, & de Lorenzo, 2021; Velazquez, Lorenzo, & Al-Ramahi, 2019). Briefly, we adopted the CRISPR/Cas9 system from Streptococcus pyogenes , the components of which include a DNA spacer that is expressed to produce a CRISPR RNA (crRNA), a trans -activating RNA (tracrRNA), and an endonuclease, in this case S. pyogenes Cas9 (Spy Cas9). The crRNA base pairs with the tracrRNA to form a molecule that mediates the interaction of the crRNA with Spy Cas9. The crRNA then directs Spy Cas9 activity to a specific region in the genome via complementarity. If there is a protospacer-adjacent motif (PAM) in the immediate vicinity of the recognition site, the Spy Cas9 is activated and generates a double-strand break (DSB) at this genomic location. On this basis, designing a CRISPR spacer that recognizes the wildtype locus for Ll.LtrB insertion generates a crRNA-Cas9 complex that identifies this sequence and generates a DSB that, in most cases, cannot be repaired. Suitably, the insertion of Ll.LtrB alters the recognition mediated by crRNA-Cas9 so that only mutated cells survive counterselection. In this way, we reduced the population of unmutated bacteria that did not incorporate the group II intron at the correct site, thereby making the process of identifying mutated clones significantly easier and more efficient. As the parts included in the insertion/counterselection events are virtually orthogonal with respect to the host, the described strategy can be adapted to many types of organisms, both prokaryotic and eukaryotic. Moreover, we have introduced the coding sequence of Ll.LtrB and its IEP (LtrA) into standardized SEVA plasmid vectors (Silva-Rocha et al., 2013), facilitating the application of the procedure to many types of Gram-negative bacteria.
In this article, we explain the steps for delivering cargos of less than 600 bp to the genome of the soil bacterium and metabolic engineering platform P. putida using Ll.LtrB and CRISPR/Cas9 counterselection. This methodology is divided into five Basic Protocols (outlined in Fig. 1) and two Support Protocols. In Basic Protocol 1, we describe the programming of Ll.LtrB for targeting new loci, including the use of the Perutka algorithm (Perutka et al., 2004), which predicts the best target loci within a given sequence and is freely accessible at the ClosTron website (http://clostron.com/). We also explain how to perform a splicing by overlap extension or splicing by overhang extension protocol (SOEing PCR; Horton, Hunt, Ho, Pullen, & Pease, 1989) to retarget Ll.LtrB into the selected locus. In this variant of PCR, more than two primers are used to introduce specific changes into a DNA sequence. Finally, we describe cloning of the generated SOEing PCR fragment into pSEVA6511-GIIi via Gibson assembly (Gibson et al., 2009, 2010). In Basic Protocol 2, we describe the design of specific spacers to counterselect Ll.LtrB insertions and their cloning into pSEVA231-CRISPR. In Basic Protocol 3, we detail the cloning of the cargo of interest with the Ll.LtrB intron in the retargeted pSEVA6511-GIIi vector. In Basic Protocol 4, we describe the steps for insertion of the Ll.LtrB::cargo in a P. putida strain with the help of CRISPR/Cas9 counterselection. Finally, we provide Basic Protocol 5 as an optional protocol for curing an engineered strain of all plasmids. In Support Protocol 1, we describe a general procedure for preparing chemically competent E. coli cells. In Support Protocol 2, we describe an interference assay for testing the efficiency of the spacers cloned into pSEVA231-CRISPR. Throughout the article, we illustrate the approach using the insertion of a 300-bp cargo sequence from the luxC gene retrieved from pSEVA256 (Silva-Rocha et al., 2013) into the pyrF gene of P. putida.
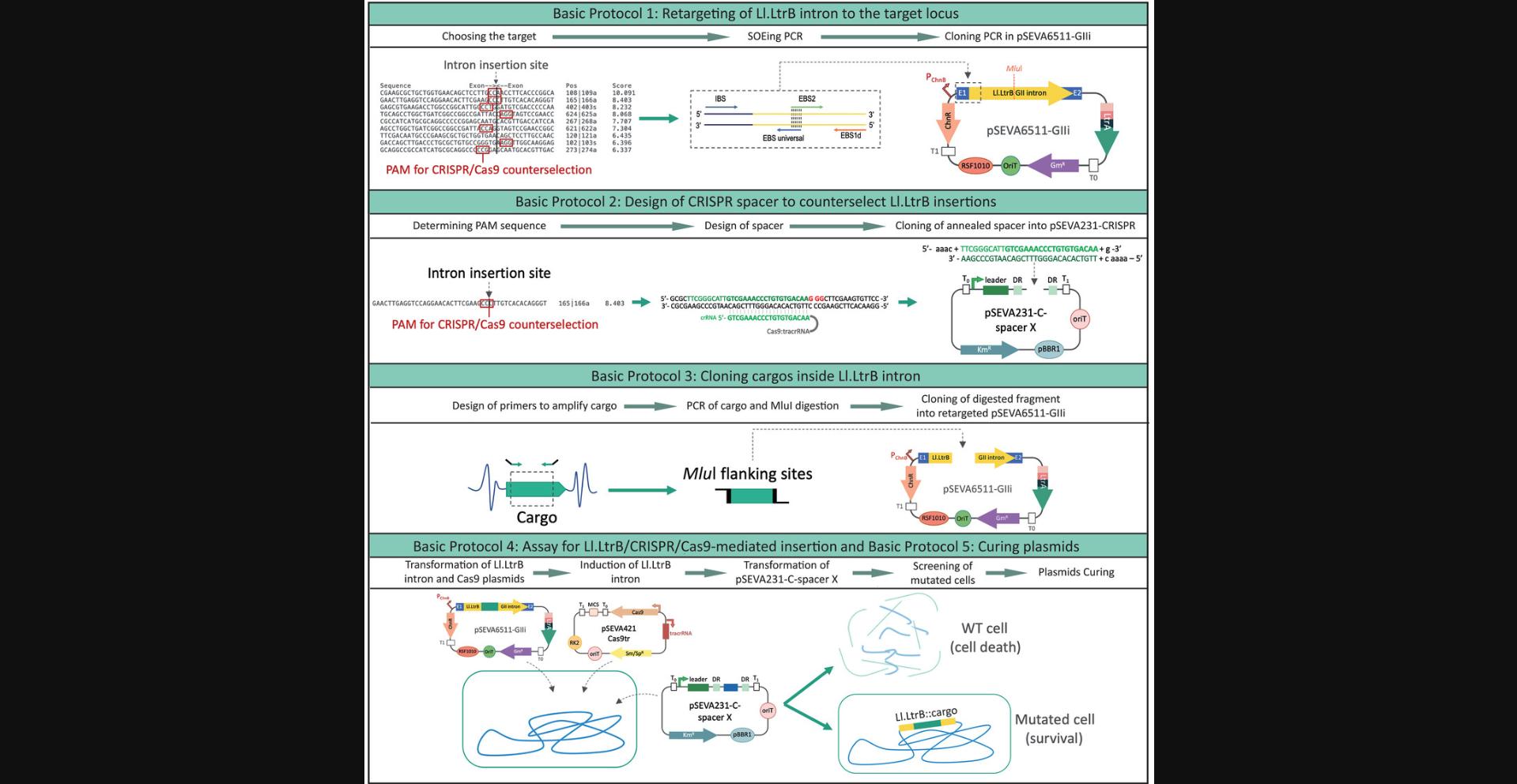
STRATEGIC PLANNING
Plasmid pSEVA6511-GIIi bears an Ll.LtrB group II intron that has been retargeted to insert into the 1063a locus of the E. coli lacZ gene. Specifically, Ll.LtrB was designed to insert between nucleotides 1063 and 1064 in the antisense orientation of the lacZ ORF. The modified intron includes an additional Hpa I restriction site that is necessary for retargeting Ll.LtrB to new genomic locations (Fig. 2). For this reason, the order for retargeting the intron (Basic Protocol 1) and introducing the desired cargo (Basic Protocol 3) will depend on the final goal of the experiment. If a particular cargo is to be delivered to different loci, it will save time to clone the cargo first and then retarget the intron. On the other hand, if different cargos are to be delivered to the same locus to generate different strains of the same species, it is best to retarget the intron and then introduce different cargos. Despite the apparent complexity, Gibson assembly can also be adapted to complete cloning of the necessary plasmids in just one attempt by combining Basic Protocols 1 and 3 into a single cloning step (for further details, see Background Information).
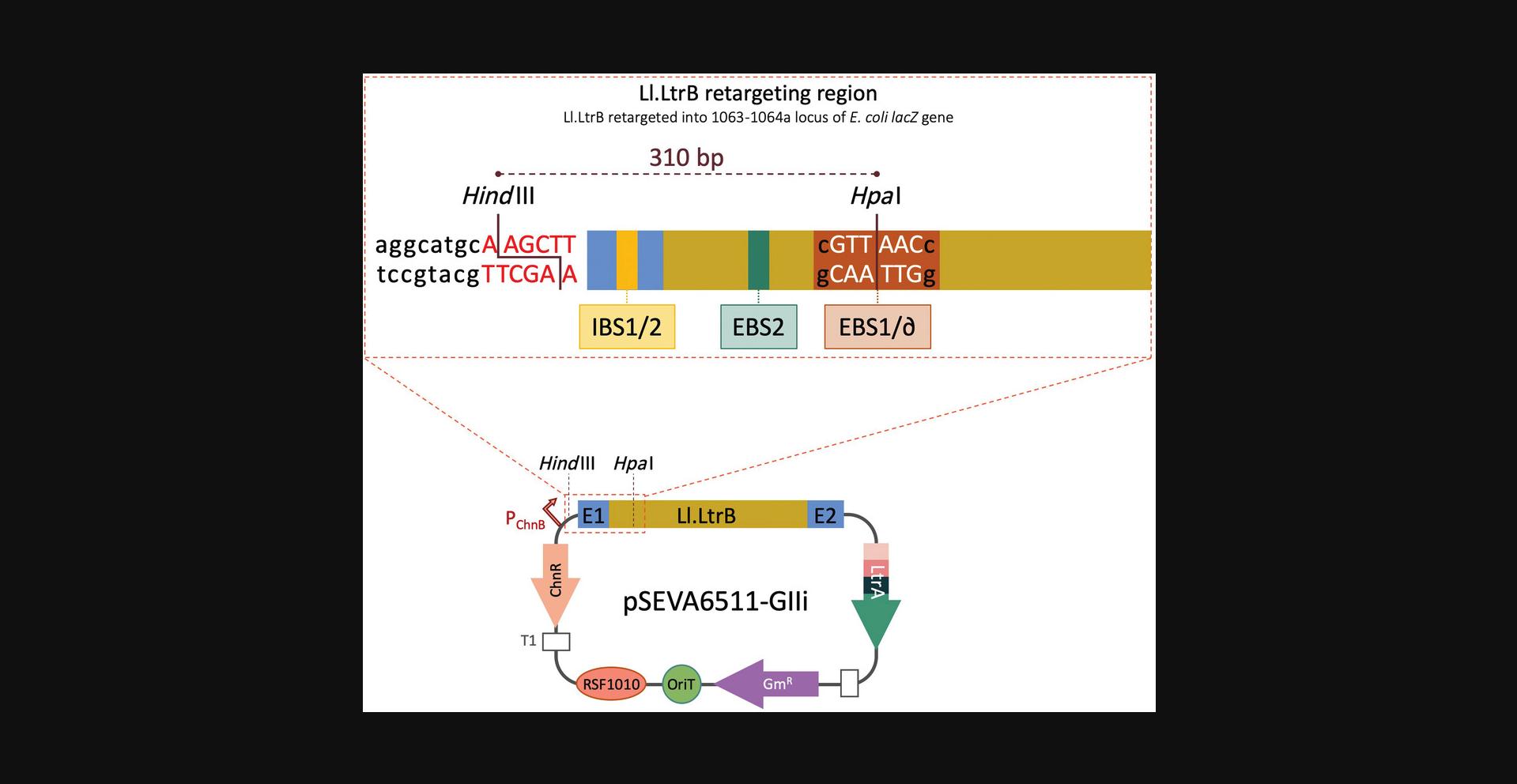
When selecting an insertion locus, we recommend selecting more than one, as spacer efficiency for directing Cas9 activity may vary greatly. This allows one to identify and use the spacer with the best efficiency, making selection of insertion mutants easier.
In each protocol we provide suppliers and catalog numbers for reagents and equipment we normally use. These can be substituted for similar items per user preference.
Basic Protocol 1: RETARGETING THE Ll.LtrB INTRON TO THE TARGET LOCUS
The native Ll.LtrB intron inserts into the ltrB gene of L. lactis (Mills, McKay, & Dunny, 1996; Shearman, Godon, & Gasson, 1996). In pSEVA6511-GIIi, this intron has been modified to recognize a different target, and this region can be further modified to use this intron to integrate into other loci of interest. Three regions within Ll.LtrB interact with the target sequence and start the retrohoming process: EBS1, EBS2, and ∂. To alter Ll.LtrB target recognition, these three components can be modified along with the corresponding IBS1 and IBS2 sites, which are homologous to the target site and are present next to the 5′ intron sequence to help with intron folding and splicing (Karberg et al., 2001; Mohr, Smith, Belfort, & Lambowitz, 2000). For retargeting, EBS1 and ∂ can be considered a single region because they are next to each other, so they are normally called EBS1d. Accordingly, IBS1 and IBS2 are usually referred to as the IBS region and are complementary to EBS1d and EBS2.Since three different regions need to be modified (EBS1d, EBS2, and IBS), three primers bearing the desired changes are included in the PCR reaction, along with a fourth primer that carries a short overlap region at 5′ (the EBS universal primer). After two smaller PCR products are generated, this overlap allows the coupling and amplification of both fragments in a single amplicon. This is called splicing by overlap extension or splicing by overhang extension PCR (SOEing PCR; Horton et al., 1989) and is used here to yield a single PCR fragment with retargeted EBS and IBS sequences that will later be cloned into Ll.LtrB (Fig. 2).
In this protocol, the user will alter EBS1d, EBS2, and IBS by cloning the new targeting sequence generated by SOEing PCR into pSEVA6511-GIIi via Gibson assembly (Gibson et al., 2009, 2010). As an example, we implement the steps to generate an Ll.LtrB intron that targets a region within the pyrF gene of P. putida (Galvao & de Lorenzo, 2005). First, the ClosTron website (http://clostron.com/; Heap, Pennington, Cartman, Carter, & Minton, 2007) is used to design primers for generating a PCR product with the retargeted region via SOEing PCR (Horton et al., 1989). Then, this fragment is reamplified for introducing the homology regions needed to assemble the generated PCR amplicon into the linearized pSEVA6511-GIIi via Gibson cloning. The resulting plasmid will carry an intron that is able to insert into the selected region of pyrF , allowing either the generation of disruption mutants or insertion of specific sequences in this locus.
Materials
- QIAprep Spin Miniprep Kit (Qiagen, cat. no. 27106), including columns and buffers P1 and P2
- LB liquid medium (Nelson et al., 2002; see recipe)
- 10 mg/ml gentamycin (Gm; Sigma-Aldrich, cat. no. G1264)
- E. coli strain CC118 carrying pSEVA6511-GIIi (Manoil & Beckwith, 1985)
- Milli-Q H2O
- 10× Cutsmart buffer (New England Biolabs, cat. no. B7204S)
- Hpa I (New England Biolabs, cat. no. R0105S)
- Hin dIII (New England Biolabs, cat. no. R0104S)
- Agarose D1 medium (Condalab, cat. no. 8019)
- 50× TAE buffer (see recipe)
- SYBR safe DNA gel stain (Invitrogen/Thermo Fisher Scientific, cat. no. S33102)
- 5× Nucleic Acid Sample Loading Buffer (Bio-Rad, cat. no. 161-0767)
- Molecular standard (EZ Load 500-bp Molecular Ruler, Bio-Rad, cat. no. 1708354)
- NucleoSpin Gel and PCR Clean-up Kit (Macherey-Nagel, cat. no. 740609.250)
- Retargeting primers (Table 1; Merck or similar):
- Universal primer pEBSuniv
- Specific primers (in our example, pyrF_165a-EBS2, pyrF_165a-EBS1d, and pyrF_165a-IBS)
- Primers for adding homology regions: pRetarget-rev and pRetarget-fwd
Use | Name | Sequence (5′→3′) | Tm (°C) |
---|---|---|---|
Retargeting of Ll.LtrB group II intron to insert into the selected locus inside pyrF gene (Between nucleotides 165 and 166 in the antisense orientation) | pyrF_165a-EBS1d | CAG ATT GTA CAA ATG TGG TGA TAA CAG ATA AGT CGA AGC CCT TAA CTT ACC TTT CTT TGT | 80.3 |
pyrF_165a-EBS2 | TGA ACG CAA GTT TCT AAT TTC GGT TAA GTG TCG ATA GAG GAA AGT GTC T | 79.6 | |
pyrF_165a-IBS | AAA GCT TAT AAT TAT CCT TAC ACT TCG AAG CCG TGC GCC CAG ATA GGG TG | 82.9 | |
pEBS universal | CGA AAT TAG AAA CTT GCG TTC AGT AAA C | 66.2 | |
Primers to add homology regions to the retargeting PCR fragment in order to clone it through Gibson assembly | pRetarget-rev | GTT CTC CTA CAG ATT GTA CAA ATG TGG TGA TAA CAG ATA | 73.0 |
pRetarget-fwd | AGA GTC GAC CTG CAG GCA TGC AAG CTT ATA ATT ATC CTT A | 77.6 | |
Sequencing primer to verify correct retargeting of Ll.LtrB | R24 | AGC GGA TAA CAA TTT CAC ACA GGA | 67.3 |
Primers to build the specific spacer through annealing for its cloning into pSEVA231-CRISPR | Spacer_S | AAA CTT CGG GCA TTG TCG AAA CCC TGT GTG ACA AG | |
Spacer_AS | AAA ACT TGT CAC ACA GGG TTT CGA CAA TGC CCG AA | ||
Sequencing of pSEVA backbone from Terminator T1 | pS1 | AGG GCG GCG GAT TTG TCC | 71.7 |
Sequencing of pSEVA backbone from Terminator T0 | pS2 | GCG GCA ACC GAG CGT TC | 70.6 |
Amplification of Lux2 cargo | pLux_fwd | TTA TTA TAC GCG TAT GAC TAA AAA AAT TTC ATT CAT TAT TAA CGG | 71.7 |
pLux2_rev | TTA TTA TAC GCG TCT CTA GCT TAG CCA TTT CTT CTG | 71.5 | |
Primers to check positive clones after cloning cargo sequences into the Mlu site inside Ll.LtrB | pgII_cargo_fwd | TAG TAG TCT GAG AAG GGT AAC G | 52.8 |
pGIIi_cargo_rev | GTA TAC GGC TCT GTT ATT GTT C | 51.0 | |
Primer to check Ll.LtrB insertion into pyrF gene in combination with primer pgII_cargo_fwd | pyrF_F | ACT TGC CAA GAG ACC CTG | 60.5 |
-
100-ml Erlenmeyer glass flask
-
2-ml microcentrifuge tubes (e.g., Eppendorf Safe-Lock, Merck, cat. no. 0030 120.094)
-
37°C orbital shaker incubator
-
SpeedVac concentrator (DNA120, ThermoSavant, Thermo Fisher Scientific)
-
Spectrophotometer (NanoVue Plus, GE Healthcare)
-
Sterile surgical blade (B. Braun, cat. no. BB520)
-
ClosTron website (http://clostron.com/)
-
0.2-ml 8-tube PCR strips and 8-cap strips (Bio-medical Laboratory Supplies, cat. nos. K77101 and B79501)
-
Thermocycler (Bio-Rad)
-
Heating block at 42° and 50°C
-
1.5-ml microcentrifuge tubes (Sarstedt, cat. no. 72.690.001)
-
4-mm sterile glass plating beads or Drigalski spatula
-
1.8-ml cryogenic tubes (Thermo Fisher Scientific, cat. no. 375418)
-
Additional reagents and equipment for agarose gel electrophoresis
Prepare plasmid DNA
1.Inoculate a 100-ml flask containing 20 ml LB plus 20 µg/ml Gm with E. coli strain CC118 from a frozen stock. Grow aerobically (shaking at 170 rpm or 11 × g) overnight at 37°C.
2.Split culture into four 2-ml microcentrifuge tubes, spin in a benchtop centrifuge for 1 min at ∼15,900 × g , and discard the supernatant. Repeat for a total of three spins to concentrate the cells.
3.Add 250 µl buffer P1 from the QIAprep plasmid extraction kit to each tube and pipette up and down to resuspend the pellets.
4.Add 250 µl buffer P2 and proceed by following manufacturer's instructions. Elute plasmid DNA using 100 µl Milli-Q H2O for each extraction column.
5.Concentrate DNA in a SpeedVac for 30 min.
6.Combine DNA from the four tubes and quantify spectrophotometrically.
Linearize pSEVA6511-GIIi
7.Set up the following double digestion with Hin dIII and Hpa I (Fig. 2):
- 5 µl 10× CutSmart buffer
- 1 µg pSEVA6511-GIIi DNA
- 1 µl HpaI
- 1 µl HindIII
- 42 µl Milli-Q H2O
Alternatively, linearized pSEVA6511-GIIi can be prepared by PCR using primers bearing homology regions with the retargeting fragment.
8.Incubate 2 hr at 37°C.
9.Prepare a 0.8% (w/v) agarose gel in 1× TAE buffer with SYBR safe DNA gel stain.
10.Add 10 µl of 5× loading buffer to digestion and mix well. Split the 60 µl across separate wells in the gel, and add 5 µl molecular standard in a separate well. Run at 100 V until the molecular ladder is sufficiently separated.
11.Visualize DNA fragments on a transilluminator.
12.Excise the 9.95-kb fragment from the gel using a razor blade and then purify the DNA using a gel purification kit.
13.Quantify the gel-extracted linearized vector and concentrate if the yield is >20 ng/µl.
Select the Ll.LtrB insertion site
14.Select the genomic region that Ll.LtrB should recognize and to which it should retrohome (>200 bp is recommended).
15.Go to http://clostron.com/. Select the first “ClosTron” tab and then “Go to intron design tool”.
16.Accept the terms and conditions, select “Perutka algorithm implemented at ClosTron.com”, and click “Start design tool”.
17.Enter a query name (e.g., pyrF) and click “OK” (Fig. 3A).
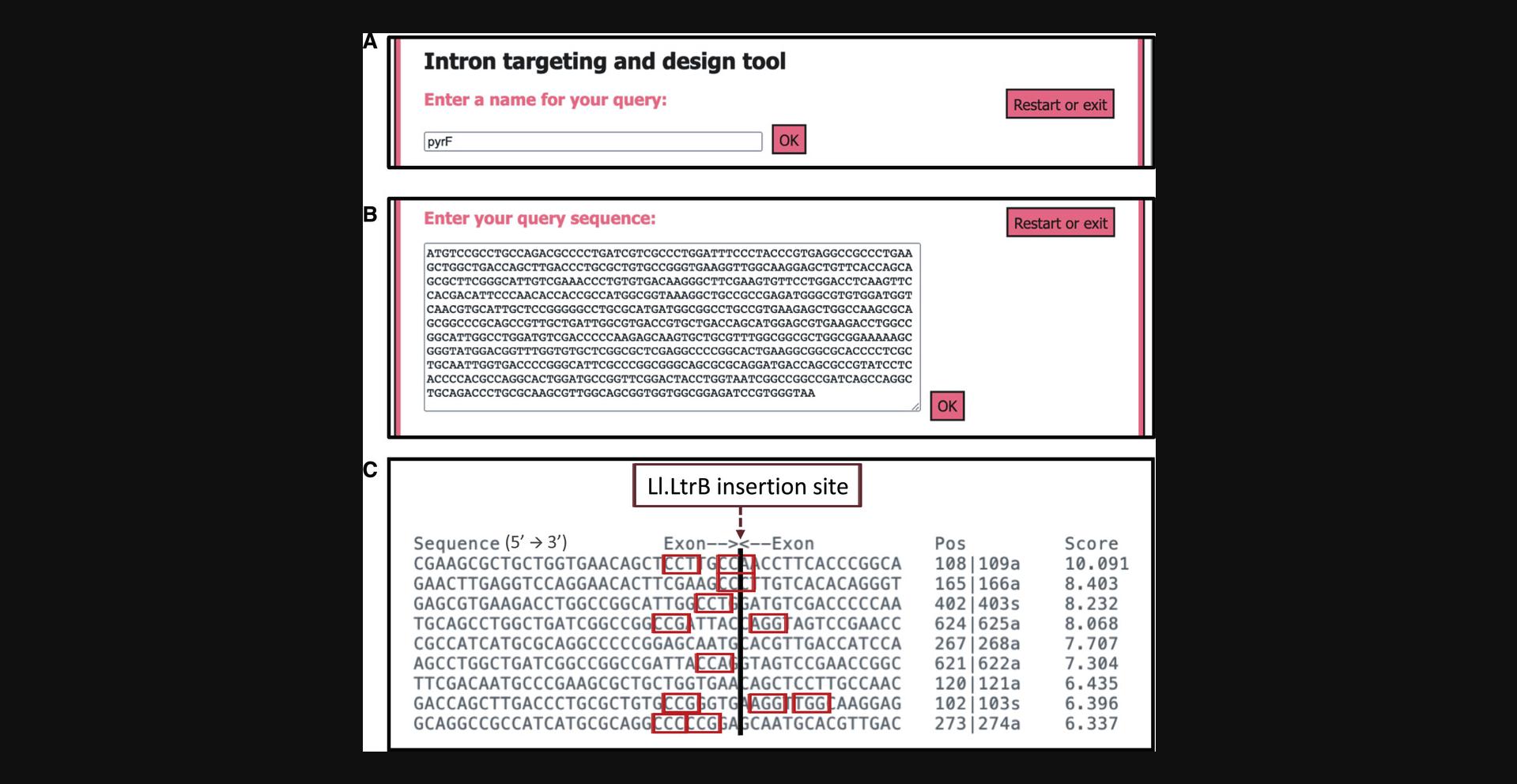
18.Enter the sequence of the selected genomic region (e.g., the 702-nt sequence of pyrF) and click “OK” (Fig. 3B).
19.Select the insertion locus that has the highest score and is close to a Spy Cas9 CRISPR PAM sequence (5′-NGG-3′).
20.Introduce a name for the selected locus (e.g., pyrF165a) and click “OK”.
21.Introduce the sequence of the selected locus and click “OK”.
22.Download the generated text file with the four primer sequences and the rest of the information (sequence used as query, whole list of target sites with scores, selected locus, and the fragment that will be generated after SOEing PCR).
Perform first-round PCR
23.Order the four primers (standard desalting purification, 0.025-µmol scale) and resuspend in Milli-Q H2O to the following concentrations:
- 100 μM pyrF_165a-IBS
- 100 μM pyrF_165a-EBS1d
- 20 μM pyrF_165a-EBS2
- 20 μM pEBSuniv
pEBSuniv is a universal EBS primer common for all retargeting sequences, and thus only needs to be ordered once.
24.Prepare a primer mix by adding 2 µl of each diluted primer to 12 µl Milli-Q H2O in a 0.2-ml PCR tube (total 20 μl).
25.Prepare the following SOEing PCR mix in another 0.2-ml PCR tube:
- 1.25 µl primer mix
- 1 ng undigested pSEVA6511-GIIi DNA
- 5 µl 5× Q5 Reaction Buffer
- 0.5 µl 10 mM dNTPs
- 0.25 µl Q5 HighFidelity DNA Polymerase
- Milli-Q H2O to 25 µl
One PCR reaction should be enough to obtain the necessary amount of DNA for the next step, but this can be scaled up as needed.
26.Run in a thermocycler with following program:
- Denaturation: 30 s at 98°C
30 cycles:
- 10 s at 98°C (denaturation)
- 10 s at 60°C (annealing)
- 15 s at 72°C (extension)
- Final extension: 2 min at 72°C
Reactions can be kept at 10°C in the thermocycler until the next step.
27.Run product on a 0.8% agarose gel, purify the 350-bp band using a DNA extraction kit (Fig. 4, first gel), and quantify the purified fragment spectrophotometrically.
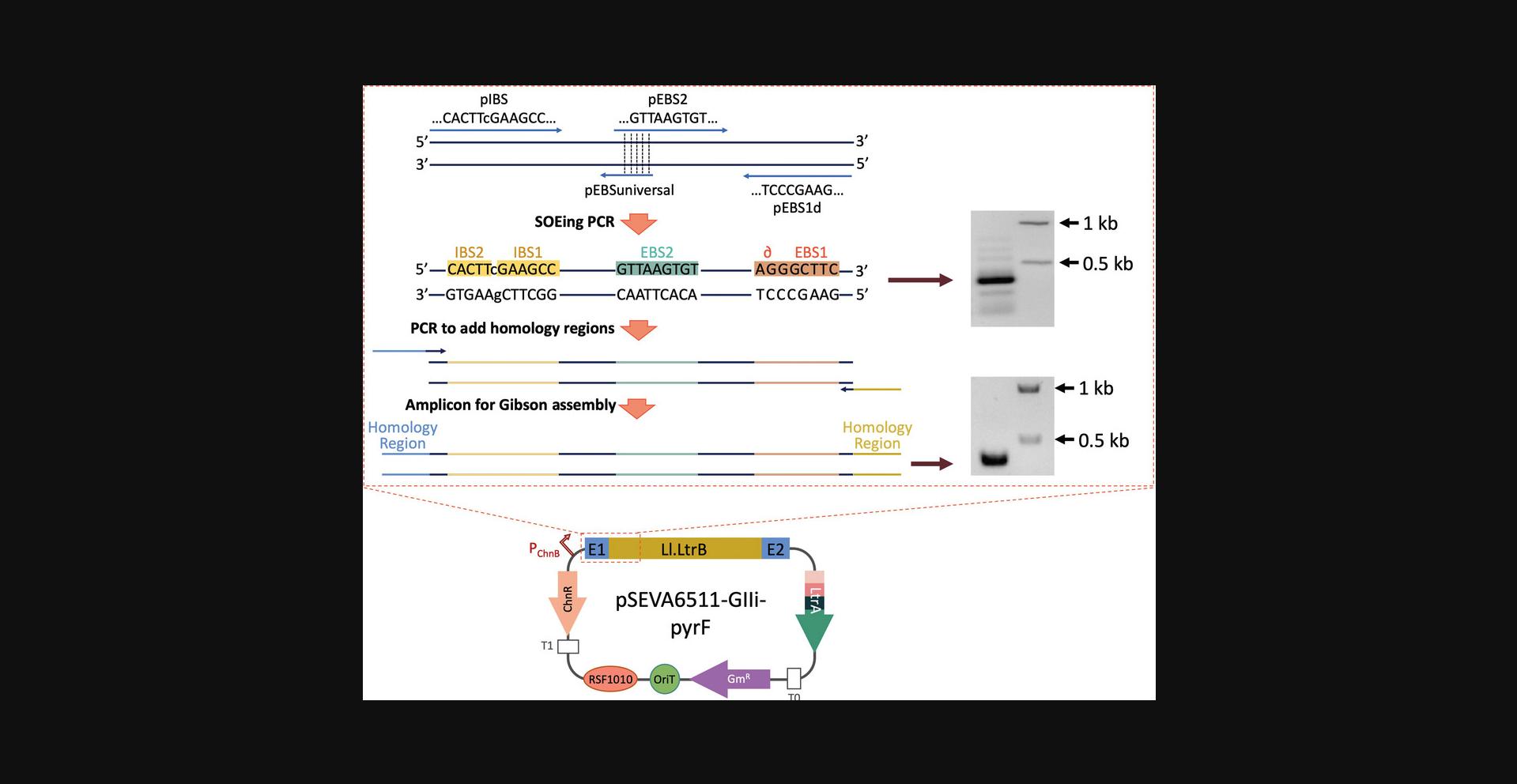
Perform second-round PCR
The goal of the second PCR is to add homology regions that flank the SOEing PCR fragment for cloning into the linearized vector. An alternative is to add homology regions to the IBS (5′-AGAGTCGACCTGCAGGCATGC-3′) and IBS1d (5′-GTTCTCCTACAGAT-3′) primers generated by the ClosTron website after the Hin dIII site (AAGCTT) in the case of the IBS primer or after the Bsr GI site (TGTACA) in the case of IBS1d.
28.Prepare the second PCR mix as in step 25, but replace the primer mix and vector template with the following:
- 1.25 µl 5 μM pRetarget-rev primer (final 0.25 μM)
- 1.25 µl 5 μM pRetarget-fwd primer (final 0.25 μM)
- 0.5-1 ng purified amplicon from step 27
29.Run with the following program:
- Denaturation: 30 s at 98°C
30 cycles:
- 10 s at 98°C (denaturation)
- 10 s at 70°C (annealing)
- 10 s at 72°C (extension)
- Final extension: 2 min at 72°C
Again, reactions can be kept at 10°C in the thermocycler until the next step.
30.Purify product using a PCR clean-up kit (e.g., NucleoSpin) and quantify spectrophotometrically.
Perform Gibson assembly
31.Mix the following reagents in a 0.2-ml tube:
- 7.5 µl 2× Gibson Assembly Master Mix
- 100 ng digested pSEVA6511-GIIi (step 13)
- 10 ng PCR fragment (step 30)
- Milli-Q H2O to 15 µl
32.Incubate 1 hr at 50°C in a heating block.
Transform bacteria, isolate plasmid DNA, and verify clone
33.Mix 7 µl of the reaction with 70 µl competent E. coli CC118 cells in a 1.5-ml microcentrifuge tube.
34.Incubate 30 min on ice (4°C), then 1 min at 42°C in a heating block, and finally 2 min on ice (4°C).
35.Add 1 ml fresh LB and incubate 1 hr aerobically in a 37°C shaker incubator at 170 rpm.
36.Centrifuge 1 min at ∼15,900 × g to pellet cells.
37.Discard most of the supernatant (∼900 µl) and plate the rest on LB-Gm plates (or other appropriate antibiotic) using glass plating beads or a Drigalski spatula. Incubate overnight at 37°C.
38.Select several colonies, extract plasmid DNA (see steps 1-6), and send for sequencing to ensure that Ll.LtrB was modified as desired.
39.Name the pSEVA6511-GIIi derivative plasmid(s).
Prepare glycerol stock
40.Re-streak a colony of a sequence-confirmed clone onto a fresh LB-Gm plate (or appropriate antibiotic) and incubate overnight at 37°C in the case of E. coli (P. putida should be grown at 30°C).
41.Add 3 ml LB with 20% (v/v) glycerol to the plate and scrape all cells using a bent 20-µl pipette tip or Drigalski spatula.
42.Transfer supernatant (∼1.5 ml) to a 1.8-ml cryogenic tube and store long-term at −80°C.
Support Protocol 1: PREPARATION OF COMPETENT E. coli CELLS
Competent E. coli cells are needed for transformation of Gibson assembly and ligation reactions in Basic Protocols 1–3.Below is the protocol we routinely use to prepare chemical competent E. coli CC118 cells with enough transformation efficiency to carry out the cloning procedures in each protocol. Any other protocol or commercial E. coli cloning strain can be used (e.g., DH5α) provided the resulting cells have high transformation efficiency.
Additional Materials (also see Basic Protocol 1)
-
E. coli CC118 cells in frozen LB-glycerol stock (Manoil & Beckwith, 1985)
-
LB agar plates (see recipe)
-
Solution 1 (see recipe)
-
Solution 2 (see recipe)
-
Spectrophotometer
-
500-ml Erlenmeyer flask
-
50-ml Falcon tubes (VWR, cat. no. 525-1099)
1.Scrape E. coli CC118 cells from a frozen stock and streak onto an LB plate to isolate single colonies. Incubate overnight at 37°C.
2.Scrape a single colony and use to inoculate 7 ml LB. Incubate overnight at 37°C with shaking (170 rpm or 11 × g).
3.Measure the OD600 of the overnight culture.
4.Inoculate 100 ml LB in a 500-ml Erlenmeyer flask with enough overnight culture to give a final OD600 of 0.05.
5.Grow at 37°C with shaking to an OD600 of 0.4 to 0.5.
6.Place the flask on ice for 10 min.
7.Transfer culture to two 50-ml Falcon tubes and centrifuge 5 min at ∼3200 × g , 4°C.
8.Discard supernatant and gently resuspend each pellet in 15 ml cold solution 1.
9.Centrifuge again for 5 min at ∼3200 × g.
10.Discard supernatant and gently resuspend each pellet in 2 ml cold solution 2.
11.Dispense 150-μl aliquots into 1.5-ml microcentrifuge tubes on ice.
12.Close tubes and store long-term at −80°C.
Basic Protocol 2: DESIGN AND CLONING OF CRISPR SPACERS TO COUNTERSELECT Ll.LtrB INSERTIONS
This protocol explains how to design and clone the specific spacer that recognizes the chosen Ll.LtrB insertion site to mediate CRISPR/Cas9-mediated counterselection. It is based on two different plasmids, pSEVA231-CRISPR and pSEVA421-Cas9tr. pSEVA231-CRISPR is the backbone plasmid for cloning the designed spacer and for expressing the corresponding crRNA. The spacer is cloned between two direct repeats (DRs) that contain sequences required for processing of the pre-crRNA and for interaction with the tracrRNA (Deltcheva et al., 2011). pSEVA421-Cas9tr expresses both the Spy Cas9 and tracrRNA.
After transforming both plasmids into the desired strain, the crRNA::Spy Cas9 complex is formed and the designed crRNA will direct Spy Cas9 nuclease activity to the selected insertion locus of Ll.LtrB in the bacterial genome. If the Spy Cas9 PAM (5′-NGG-3′) is adjacent to the recognized sequence, the endonuclease introduces a DSB that is lethal if not repaired (Cui & Bikard, 2016; Jiang et al., 2013). However, if the Ll.LtrB intron is inserted in its target sequence, the crRNA will no longer recognize this region and mutated cells will survive. Therefore, the first step to design a spacer is always to identify a PAM sequence in the immediate vicinity of the insertion locus. The further away the PAM is located, the lower the efficiency of the counterselection system, since there is a possibility that the crRNA could still bind to a part of its target and enable Spy Cas9 to induce the DSB. To avoid this, a distance no greater than 6 nt is recommended (Aparicio, de Lorenzo, & Martinez-Garcia, 2018). After finding the PAM sequence, the next step is to select the corresponding nucleotides that will compose the spacer and to design the correct sense (S) and antisense (AS) oligonucleotides that will be annealed and cloned directly into Bsa I-digested pSEVA231-CRISPR. In Support Protocol 2, we explain how to perform an interference assay to test the efficiency of the designed spacer to kill the bacterial population, since it has been reported that this varies depending on the spacers (Aparicio et al., 2018).
In the example described here, the resulting plasmid, pSEVA231-C-pyrF1, will bear a spacer that recognizes the vicinity of the insertion locus in pyrF. This way, if Ll.LtrB retrohomes and inserts itself into its selected locus, the crRNA will no longer recognize its target and Spy Cas9 will not cleave the genomic DNA. Otherwise, the cells that did not incorporate the group II intron at the expected location will have their genomes cleaved by Spy Cas9.
This protocol was adapted from (Aparicio et al., 2018).
Additional Materials (also see Basic Protocol 1)
- pSEVA231-CRISPR plasmid DNA (Addgene, plasmid no. 138712)
- Bsa I (New England Biolabs, , cat. no. R0535S)
- 1.0 M NaCl
- Quick Ligation Kit (New England Biolabs, cat. no. M2200L), including Quick Ligase and 2× buffer
- LB agar plates (see recipe) with kanamycin (Km, 50 μg/ml) (Panreac Química S.L.U., cat. no. A4789.0010)
- pS1 and/or pS2 sequencing primers (100 µM; Table 1; Merck)
Design spacer sequences
1.Scan the possible insertion locus and manually identify PAM sequences (Fig. 3C, red boxes).
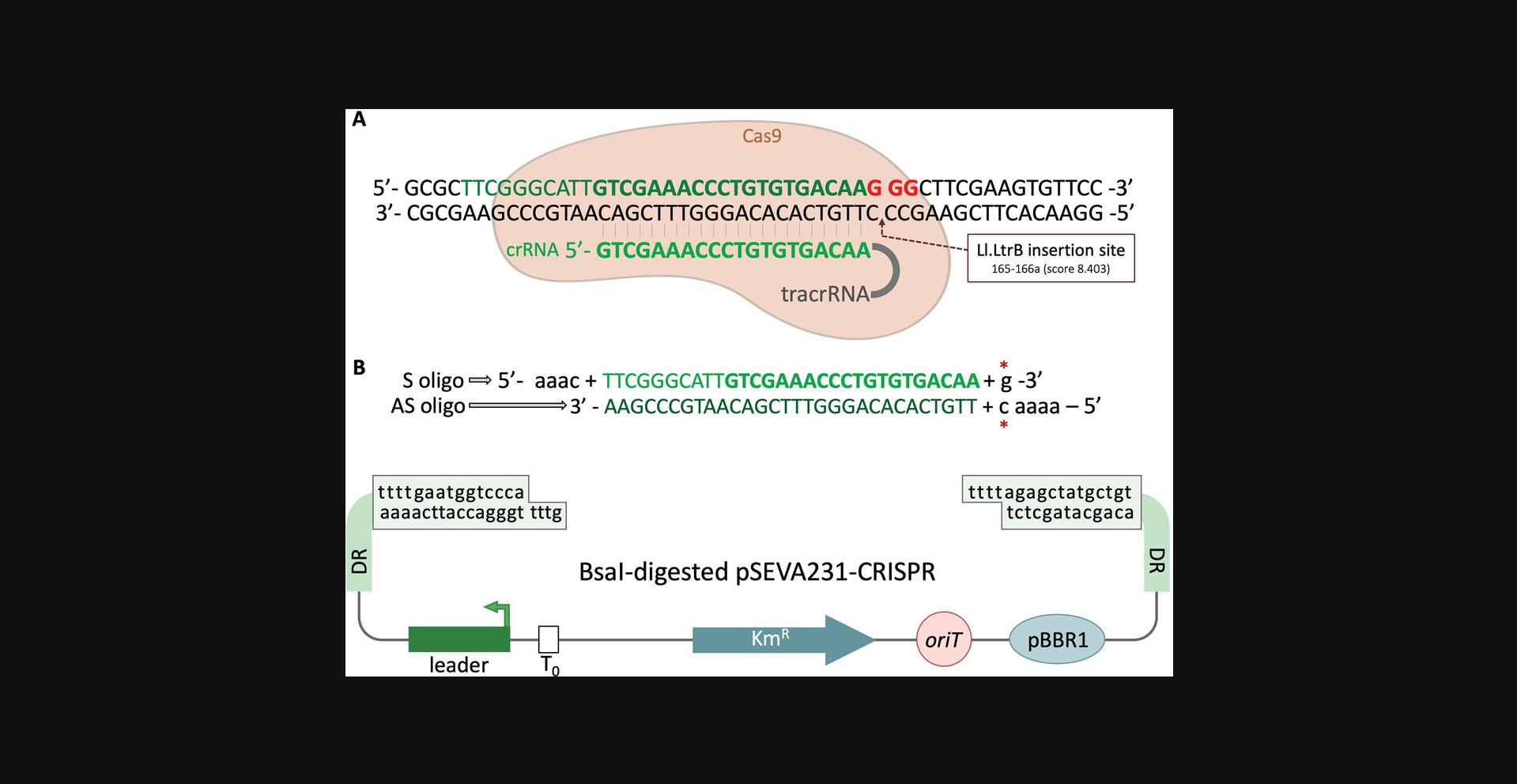
2.For the spacer, select the 30 nucleotides adjacent to the PAM sequence in the 5′ direction, i.e., 5′-N30-NGG-3′, where N is any of the four nucleotides.
3.Add the sequence AAAC to the 5′ end and a G to the 3′ end of the S oligo (Fig. 5B). Add AAAAC to the 5′ end of the AS oligo.
4.Order oligos S and AS (standard desalting, 0.025-µmol scale).
Linearize pSEVA231-CRISPR
5.Set up the following Bsa I digestion:
- 1 µg pSEVA231-CRISPR
- 5 µl 10× CutSmart buffer
- 1 µl Bsa I
- Milli-Q H2O to 50 µl
6.Incubate for 1 hr at 37°C
7.Purify the linearized fragment on a 0.8% (w/v) agarose gel as described (see Basic Protocol 1, steps 9-13).
Anneal spacer S and AS oligonucleotides
8.Add Milli-Q H2O to each spacer oligo (S and AS) to make a 100 µM stock.
9.Prepare the following annealing mix in a 0.2-ml PCR tube:
- 45.5 µl Milli-Q H2O
- 1 µl each 100 μM oligo (S and AS)
- 2.5 µl 1.0 M NaCl
10.Place in a thermocycler and run the following annealing program:
Initial denaturation:
- 5 min at 95°C
Ramp down:
- 1 min at 95°C
- decrease 1°C per cycle (1 min each) for 70 cycles
- Hold at 12°C.
11.Make a 1:10 dilution by adding 5 µl annealed S:AS oligos to 45 µl Milli-Q H2O.
Ligate annealed oligos and pSEVA231-CRISPR
12.Set up the following ligation reaction:
- 50 ng Bsa I-digested and purified pSEVA231-CRISPR (step 7)
- 6 µl Milli-Q H2O
- 1 µl diluted S:AS oligos (step 11)
- 10 µl 2× Quick Ligation Buffer
- 1 µl Quick Ligase
Any ligase and protocol can be used.
13.Incubate 5 min at room temperature (25°C).
Transform cells
14.Transform 7 µl ligation reaction directly into 70 µl competent E. coli CC118 cells as described (see Basic Protocol, steps 33-37), but plate cells on LB-Km plates.
15.Re-streak several colonies on LB-Km plates to isolate individual colonies.
16.Do a plasmid miniprep from isolated clones (see Basic Protocol 1, steps 1-6) and send for sequencing of the cloned spacer using primer pS1 and/or pS2 (Table 1).
17.Select a correct clone, prepare a frozen LB-glycerol stock, and store at −80°C (see Basic Protocol 1, steps 40-42).
Support Protocol 2: INTERFERENCE ASSAY TO CHECK EFFICIENCY OF SELECTED SPACERS
Spacers exhibit different efficiencies at guiding the Spy Cas9 endonuclease to the target sequence for reasons that are not well understood (Aparicio, de Lorenzo, & Martinez-Garcia, 2019). This protocol describes how to test the efficiency of the selected spacers (Basic Protocol 2) to guide Spy Cas9 and kill the population of unmutated WT cells, allowing users to choose the best spacer before proceeding to Basic Protocol 4.If a spacer is very efficient at guiding Spy Cas9, the nuclease will kill a larger fraction of WT bacteria, making it easier to identify mutated clones with Ll.LtrB integrated in the right genomic locus. To this end, the P. putida strain bearing pSEVA421-Cas9tr is transformed with pSEVA231-CRISPR (control plasmid) or its derivative plasmid(s) bearing the designed spacer(s). pSEVA231-CRISPR has a spacer that is not operative in the P. putida genome, so its product will produce a crRNA that is not effective at guiding Cas9.On the other hand, derivative plasmids will produce crRNAs that are able to direct Cas9 to a target region in the P. putida genome, causing Cas9 to induce DSBs in the bacterial genomic DNA and cause cell death. The interference protocol is also adapted from Aparicio et al. (2018).
Additional Materials (also see Basic Protocol 1)
-
P. putida or desired strain previously transformed with pSEVA421-Cas9tr (Addgene, plasmid no. 138709)
-
50 mg/ml streptomycin (Sm, Sigma-Aldrich, cat. no. S6501)
-
300 mM sucrose (Merck, cat. no. 1.07687.1000)
-
100 ng pSEVA231-CRISPR and derivative plasmid(s) (see Basic Protocol 2)
-
LB agar plates (see recipe) with Sm (100 μg/ml) and with Sm (100 μg/ml) plus Km (50 μg/ml)
-
30°C orbital shaker incubator
-
50-ml Falcon tubes (VWR, , cat. no. 525-1099)
-
Electroporator (Bio-Rad, , cat. no. 1652100)
-
2-mm gap electroporation cuvettes (Bio-Rad, cat. no. 1652086)
-
10-ml culture tubes (Deltalab, cat. no. 300904)
Prepare electrocompetent cells
1.Inoculate 20 ml LB with 100 µg/ml Sm in a 100-ml flask with the P. putida strain bearing pSEVA421-Cas9tr. Grow aerobically (170 rpm or 11 × g) overnight at 30°C.
2.Transfer culture to a 50-ml Falcon tube and centrifuge 10 min at 3200 × g , room temperature.
3.Gently discard supernatant and resuspend pellet in 1 ml of 300 mM sucrose by pipetting up and down.
4.Transfer culture to a 1.5-ml microcentrifuge tube and spin cells 1 min at ∼15,900 × g in a benchtop centrifuge.
5.Repeat steps 3-4 for a total of at least five washes. Resuspend the final pellet in 400 µl of 300 mM sucrose.
6.Divide competent cells into 100-µl aliquots in 1.5-ml microcentrifuge tubes.
Perform transformation
7.Add 100 ng of the desired plasmids (pSEVA231-CRISPR control or derivatives) to separate aliquots of electrocompetent cells and mix gently.
8.Transfer each mixture to a separate 2-mm-gap electroporation cuvette.
9.Electroporate at 2.5 kV and immediately add 1 ml fresh LB with 100 µg/ml Sm. Mix well and transfer to separate 10-ml culture tubes.
10.Allow electroporated cells to recover 2 hr at 30°C with shaking at 170 rpm.
11.Plate appropriate dilutions of each electroporation reaction on two plates: LB-Sm plates to assess viability after electroporation and LB-Sm-Km plates to count transformant clones.
12.Incubate plates at 30°C until colonies appear (16-32 hr) and count CFU.
Evaluate transformation efficiency
13.Calculate transformation efficiency:
-
Divide CFU on LB-Sm-Km (transformant clones) by CFU on LB-Sm (viable cells).
-
Normalize to 109viable cells to be able to compare the two conditions.
Any other type of normalization is fine.
14.Divide the transformation efficiency of pSEVA231-CRISPR by the transformation efficiency of the derivative(s) to obtain the ratio of efficiency.
Basic Protocol 3: CLONING CARGO INTO Ll.LtrB
This protocol describes the basic steps for inserting the desired cargo sequence (in this example, a 300-bp fragment) into Ll.LtrB. The best region for this within Ll.LtrB is domain IVb (Plante & Cousineau, 2006). In pSEVA6511-GIIi, Ll.LtrB has been engineered to contain an Mlu I restriction site at this location. By performing a simple PCR step to add Mlu I sites to the cargo of interest, users can clone heterologous sequences into Ll.LtrB that will be delivered to the bacterial genome of choice.
Additional Materials (also see Basic Protocol 1)
- Plasmid DNAs:
- pSEVA256 (available upon request from the SEVA collection, http://seva-plasmids.com/)
- Plasmid DNA of retargeted pSEVA6511-GIIi (see Basic Protocol 1)
- Plasmid DNA of original pSEVA6511-GIIi (if this protocol is performed before retargeting; see Strategic Planning)
- Mlu I (New England Biolabs, cat. no. R01985S)
- NEBuffer 3.1 (New England Biolabs, cat. no. B7203S)
- Quick Ligation Kit (New England Biolabs, cat. no. M2200L), including Quick Ligase and 2× buffer
- Primers pgII_cargo_fwd and pGIIi_cargo_rev (100 µM; Table 1; Merck)
- 2× AmpliTools Green Master Mix (Biotools, cat. no. 10.432-4750)
Prepare cargo with MluI sites
1.Select the sequence of the fragment to be delivered.
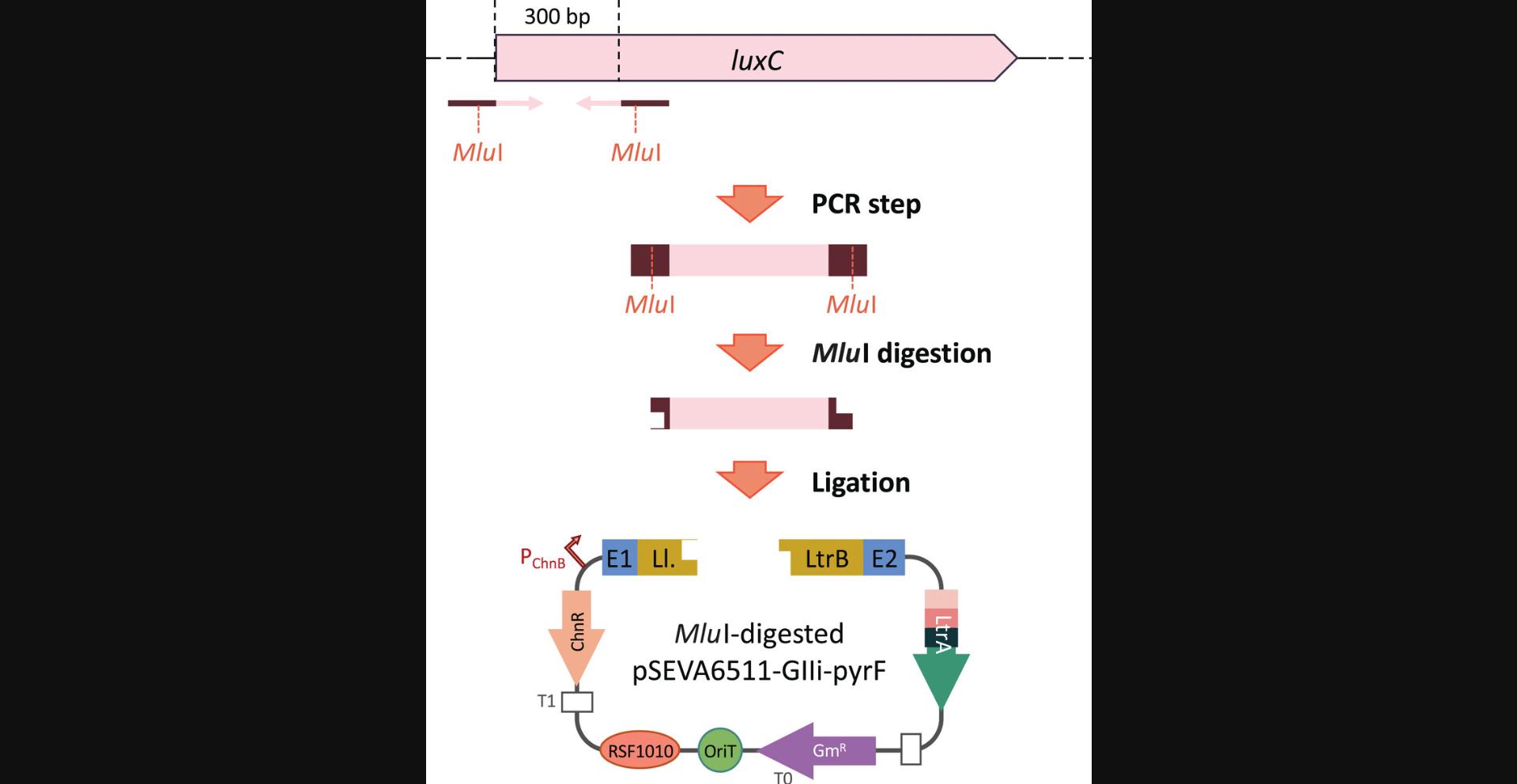
2.Design forward and reverse primers for amplification of the cargo and add Mlu I sites at the 5′ end of both primers.
3.Order primers (standard desalting purification, 0.025-µmol scale) and resuspend in Milli-Q H2O to a concentration of 100 μM. Then prepare a separate stock of each primer by diluting an aliquot in Milli-Q H2O to a final concentration of 5 µM.
4.Set up the following PCR reaction:
- 1 ng template DNA (in our case, pSEVA256 plasmid DNA)
- 10 µl forward primer (final 1 µM) (in our case, pLux_fwd)
- 10 µl reverse primer (final 1 µM) (in our case, pLux2_rev)
- 0.5 µl Q5 polymerase
- 10 µl 5× Q5 reaction buffer
- Milli-Q H2O to 50 µl
5.Run in a thermocycler with the following program:
- Denaturation: 30 s at 98°C
30 cycles:
- 10 s at 98°C
- 10 s at the annealing temperature (61°C in our case)
- 10 s at 72°C for extension
- Final extension: 2 min at 72°C
The annealing temperature was chosen using the NEB Tm calculator tool (https://tmcalculator.neb.com/#!/main). Reactions can be kept at 10°C in the thermocycler until the next steps are performed.
6.Purify PCR fragment on a 0.8% (w/v) agarose gel and quantify the DNA spectrophotometrically (see Basic Protocol 1, steps 9-13).
Ligate cargo and vector
7.Set up two separate digestions, one with retargeted pSEVA6511-GIIi (in our case, pSEVA6511-GIIi-pyrF) and one with purified cargo PCR fragment (step 5):
- 5 µl 10× NEBuffer 3.1
- 1 µg pSEVA6511-GIIi derivative or cargo
- 1 µl Mlu I
- Milli-Q H2O to 50 µl
8.Incubate 2 hr at 37°C.
9.Purify both DNAs and quantify spectrophotometrically.
- a. For Mlu I-digested pSEVA6511-GIIi derivatives, gel purify on a 0.8% (w/v) agarose gel (see Basic Protocol 1, steps 9-13) to ensure high purity of the linearized vector.
- b. For Mlu I-digested cargo, use a PCR clean-up kit (e.g., NucleoSpin).
10.Set up the following ligation reaction (1:3 molar ratio of vector to insert):
- 50 ng Mlu I-digested pSEVA6511-GIIi
- 4.5 ng Mlu I-digested cargo
- 10 µl 2× Quick Ligation Buffer
- 1 µl Quick Ligase
- Milli-Q H2O to 20 µl
Any other ligation protocol and kit can be used.
11.Incubate 5 min at room temperature (25°C).
Perform transformation and select desired transformants
12.Transform 7 µl ligation reaction into chemically competent E. coli CC118 cells (or other E. coli cloning strain) as described (see Basic Protocol 1, steps 33-37), plating transformed cells on LB-Gm plates.
13.Set up colony PCR reactions to identify positive clones using the following volumes per reaction:
- 4.8 µl Milli-Q H2O
- 0.6 µl 5 μM pgII_cargo_fwd (final 0.25 µM)
- 0.6 µl 5 μM pGIIi_cargo_rev (final 0.25 µM)
- 6 µl 2× DNA AmpliTools Green Master Mix
14.Use a 20-μl pipette tip to scrape out a single colony from an LB-Gm plate and make a small patch on a fresh, labeled LB-Gm plate. Next, introduce the tip to a correspondingly labeled tube with the reaction mix and swirl or pipette up and down to release the cells. Repeat with all colonies to be checked.
15.Place PCR tubes in a thermocycler and run the following program:
- Denaturation: 5 min at 95°C
30 cycles:
- 30 s at 95°C
- 30 s at annealing temperature (50°C in our case)
- 60 s per kb at 72°C for extension (40 s in our case)
- Final extension: 5 min at 72°C
The time needed for extension depends on the length of the insert. Primers pgII_cargo_fwd and pGIIi_cargo_rev anneal in vector pSEVA6511-GIIi flanking the MluI site. If the 300-bp insert is not cloned, this colony PCR will yield a 351-bp fragment. If it is cloned, the final fragment will be 651 bp. For this reason, the amplification time is 40 s.
The annealing temperature was chosen using the NEB Tm calculator tool (https://tmcalculator.neb.com/#!/main). Reactions can be kept in the thermocycler at 12°C until use.
16.Run 6 µl of each reaction on a 0.8% (w/v) agarose gel to check for clones with the right amplicon size.
17.Select positive clones and start overnight cultures in 6-ml LB with 20 µg/ml Gm.
18.Prepare a miniprep of plasmid DNA and send for sequencing with either the pgII_cargo_fwd or pGIIi_cargo_rev primer.
19.Identify clones with no mutations and with the cargo inserted in the desired orientation.
20.Prepare stocks in LB-glycerol and store at −80°C (see Basic Protocol 1, steps 40-42).
Basic Protocol 4: Ll.LtrB/CRISPR/Cas9-MEDIATED INSERTION
This protocol describes how to perform an Ll.LtrB insertion assay in combination with CRISPR/Cas9-mediated counterselection employing the plasmids generated in Basic Protocols 1–3.After completing those protocols, users will have the following two plasmids: (1) a pSEVA6511-GIIi derivative plasmid (in our case, pSEVA6511-GIIi(Lux2)-pyrF) that has an Ll.LtrB intron retargeted to the desired insertion locus (in our case, the 165a locus of pyrF) and a cargo fragment (in our case, a 300-bp fragment from luxC gene) cloned into the Mlu I site within the Ll.LtrB sequence, and (2) a pSEVA231-CRISPR derivative with a specific spacer to counterselect the WT insertion locus (in our case, pSEVA231-C-pyrF1) (Aparicio et al., 2018). Once these two vectors are ready, the insertion experiment can be carried out as described here (Fig. 7). It should be noted that the induction conditions can be adapted based on users’ needs and constraints and are not necessarily optimal.
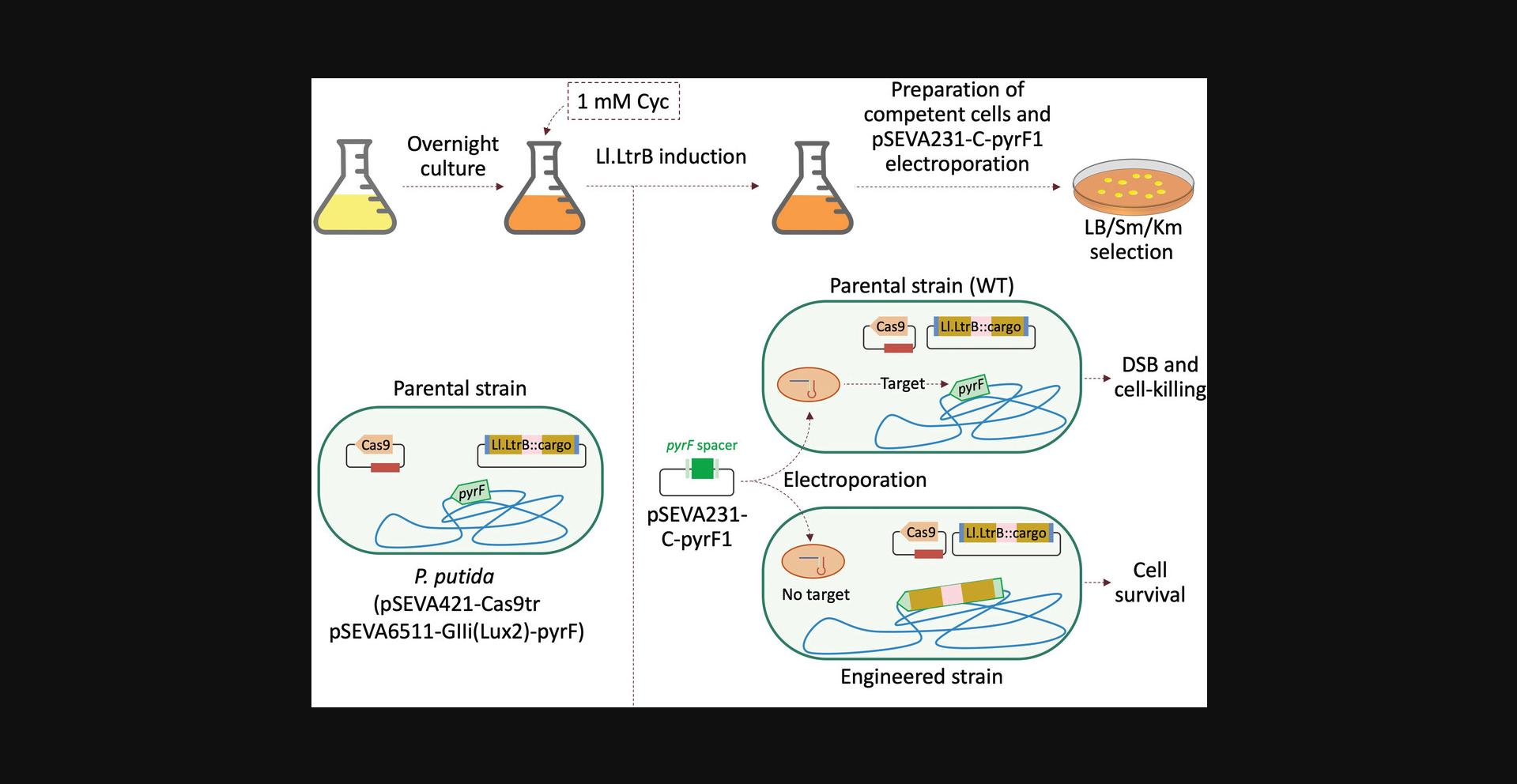
Additional Materials (also see Basic Protocol 1 and Support Protocol 2)
- Plasmid DNAs:
- pSEVA6511-GIIi derivative with cargo (see Basic Protocol 3; in our case, pSEVA6511-Gii(Lux2)-pyrF)
- pSEVA421-Cas9tr (Aparicio et al., 2018)
- pSEVA231-CRISPR derivative (see Basic Protocol 2; in our case, pSEVA231-C-pyrF1)
- Selection antibiotics:
- 10 mg/ml gentamycin (Gm, Sigma-Aldrich, cat. no. G1264)
- 50 mg/ml streptomycin (Sm, Sigma-Aldrich, cat. no. S6501)
- 50 mg/ml kanamycin (Km, Panreac Química S.L.U., cat. no. A4789.0010)
- 9.6 M cyclohexanone (Sigma-Aldrich, cat. no. 29140)
- Primers to detect Ll.LtrB insertion (in our case, pgII_cargo_fwd and pyrF_F; Table 1; Merck)
- 30°C orbital shaker incubator
- 50-ml Falcon tubes (VWR, cat. no. 525-1099)
Transform cells with plasmids
1.Prepare electrocompetent P. putida cells and sequentially transform with pSEVA6511-GIIi derivative (e.g., pSEVA6511-Gii(Lux2)-pyrF) and pSEVA421-Cas9tr (see Support Protocol 2, steps 1-12), selecting transformants on LB plates with the corresponding antibiotic (100 µg/ml Sm and/or 30 µg/ml Gm).
2.Check the integrity of both plasmids by performing a miniprep and enzyme digestion.
3.Prepare glycerol stocks of the generated strain containing both plasmids and store at −80°C (see Basic Protocol 1, steps 40-42).
Perform insertion of Ll.LtrB
4.Inoculate a 100-ml flask containing 20 ml LB with Gm (30 µg/ml) and Sm (100 µg/ml) with the generated strain (Fig. 7, top).
5.Incubate aerobically (170 rpm or 11 × g) overnight at 30°C.
6.Induce Ll.LtrB expression by adding cyclohexanone at a final concentration of 1 mM and incubating 4 hr in a 30°C shaker incubator (170 rpm).
7.Transfer cells to a 50-ml Falcon tube and centrifuge 10 min at 3200 × g , room temperature.
8.Prepare electrocompetent cells (see Support Protocol 2, steps 3-6).
9.Add 100 ng pSEVA231-CRISPR derivative (in our case, pSEVA231-C-pyrF1) to 100 µl electrocompetent cells in a 1.5-ml tube.
10.Transfer mixture to a 2-mm-gap electroporation cuvette and electroporate at 2.5 kV.
11.Immediately add 1 ml LB with Sm (100 µg/ml), transfer to a 10-ml culture tube, and incubate aerobically (170 rpm) for 2 hr at 30°C
12.Plate 100 µl of appropriate dilutions (we recommend undiluted and a 1/10 dilution) on LB-Sm-Km plates using glass plating beads or a Drigalski spatula. Grow overnight to 2 days at 30°C.
Identify correct insertion by colony PCR
13.Test Sm/Km-resistant colonies by colony PCR as described (see Basic Protocol 3, steps 13-16), but use the following primers in step 13 and an amplification time of 1 min:
- 0.6 µl 5 µM pgII_cargo_fwd (final 0.25 µM)
- 0.6 µl 5 µM primer pyrF_F (final 0.25 µM)
To detect insertion in the chromosome, one primer should be in the vicinity of the selected target locus. Because our target is in the pyrF gene, we designed primer pyrF_F (Table 1). The second primer should anneal inside the cargo sequence or Ll.LtrB intron (in our case, primer pgII_cargo_fwd).
In our example, and with the suggested primers, the amplification product will be a 928-bp band if there is indeed an insertion. No band will be seen from negative insertion clones (Fig. 8, bottom).
Because the efficiency of each insertion may differ, there is no clear number of clones that should always be tested. We advise checking at least 16 different colonies, and increasing this number with the size of the insert (i.e., the bigger the insert, the more clones should be screened).
Alternatively, a PCR reaction using both flanking primers can be performed and purified to send for sequencing to corroborate the correct insertion of the Ll.LtrB and cargo sequences.
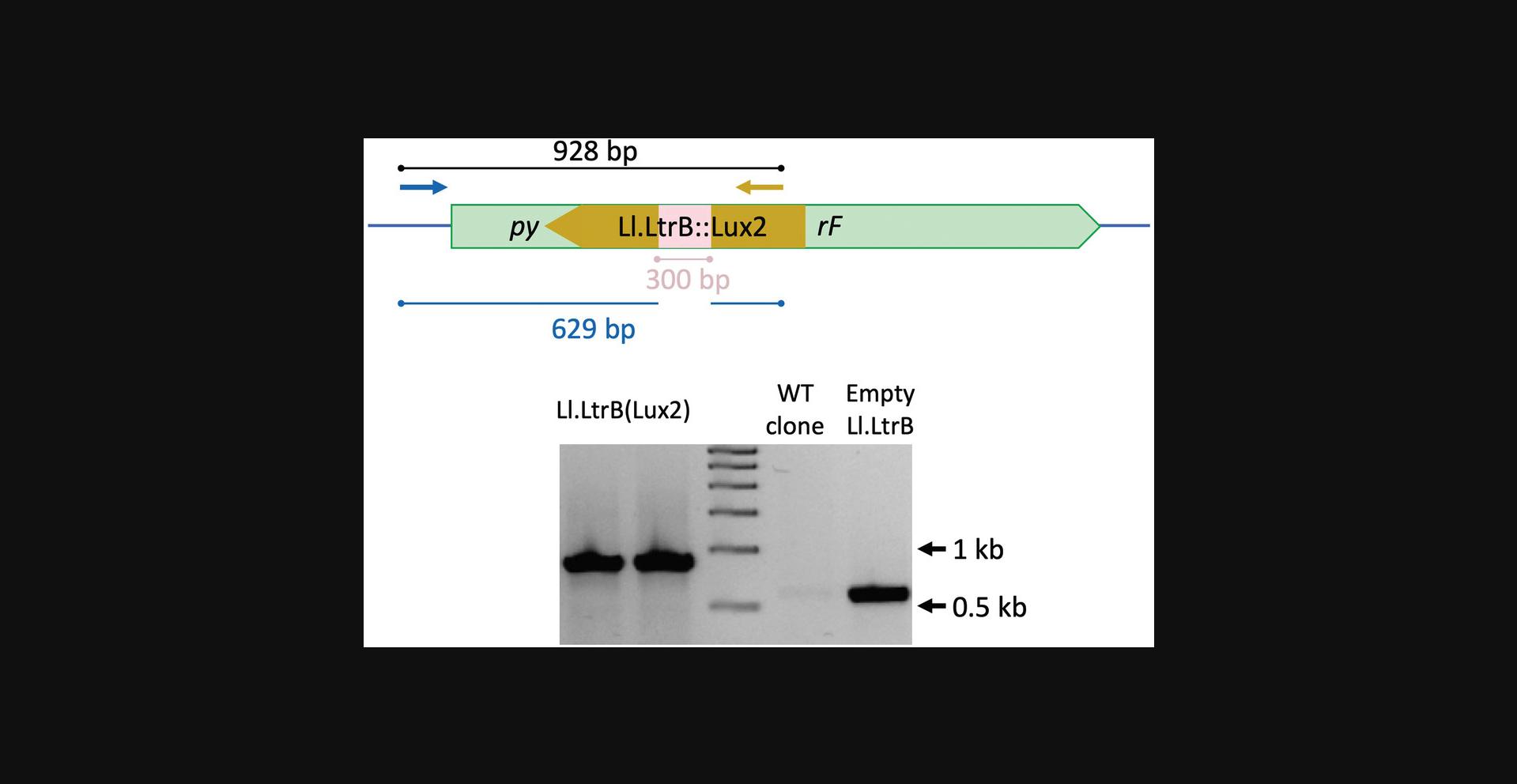
14.Prepare an LB-glycerol stock of the final strain and store at −80°C (Basic Protocol 1, steps 40-42).
Basic Protocol 5: CURING THE ENGINEERED STRAIN OF PLASMIDS
At the end of the insertion protocol, a resulting bacterial strain harbors three plasmids that should be eliminated to avoid interference in future applications, e.g., if new plasmids are to be introduced for different purposes. This protocol explains the main steps to remove the plasmids from bacterial cells and can be adapted to species other than P. putida. The general idea is to carry out several passages without selection pressure (i.e., without antibiotics) until a cell can be found that is sensitive to all the antibiotic markers in the plasmids.
Materials
- Sequence-confirmed insertion strain (see Basic Protocol 4), plate or frozen stock
- LB medium (see recipe)
- LB agar plates (see recipe) with Km (50 µg/ml), Gm (30 µg/ml), or Sm (100 µg/ml)
- Primers to detect Ll.LtrB insertion (in our case, pgII_cargo_fwd and pyrF_F; Table 1)
- 10-ml culture tubes
- 30°C orbital shaker incubator
1.Inoculate 3 ml LB in a 10-ml culture tube with the desired strain and incubate overnight at 30°C with shaking (170 rpm or 11 × g).
2.Dilute overnight 1/1000 in fresh LB and then inoculate 3 ml fresh LB in a second 10-ml tube with 3 µl diluted culture. Incubate 6 hr at 30°C with shaking.
3.Inoculate 3 ml fresh LB in a third 10-ml tube with 3 µl culture and grow overnight at 30°C.
4.Repeat steps 2-3 at least ten times.
5.Streak final culture on an LB plate to obtain individual clones.
6.Streak individual clones on separate LB-Gm, LB-Km, and LB-Sm plates.
7.Select one colony that is sensitive to all antibiotics and confirm by PCR that the desired modification is present (see Basic Protocol 4, step 13).
8.Prepare a glycerol stock and store at −80°C (see Basic Protocol 1, steps 40-42).
REAGENTS AND SOLUTIONS
Lysogeny broth (LB), 1×
- 10 g tryptone (Condalab, cat. no. 1612)
- 5 g yeast extract (Condalab, cat. no. 1702)
- 5 g sodium chloride (CARLO ERBA Reagents, cat. no. CE-91669)
- Bring to 1 L with distilled water
- Autoclave
- Store up to 6 months at room temperature or 1 year at 4°C
LB agar plates
- 1 L LB (see recipe)
- 15 g bacteriological agar (Condalab, cat. no. 1800.00)
- Autoclave
- Optional : After medium has cooled to ∼55°-60°C, add antibiotics at the recommended concentration
- Pour 20 ml into 100-mm plates
- Store plates up to 1 month at 4°C
LB with 20% (v/v) glycerol
- 200 ml LB (see recipe)
- 51 ml of 98% glycerol (CARLO ERBA Reagents, cat. no. 453752)
- Sterilize by filtration
- Store at 4°C for up to 6 months
We use Nalgene Rapid-Flow sterile single-use vacuum filter units (Thermo Fisher Scientific, cat. no. 567-0020).
Solution 1
- 0.41 g sodium acetate (final 10 mM)
- 3.1 g MnCl2 (final 50 mM)
- 0.146 g NaCl (final 5 mM)
- Bring to 0.5 L with distilled water
- Filter sterilize
- Store up to 6 months at 4°C
The final pH should be ∼5.6-6. The MnCl2 may precipitate, but the solution can be filtered again to remove the precipitate.
Solution 2
- 0.41 g sodium acetate (final 10 mM)
- 3.88 g CaCl2 (final 70 mM)
- 0.31 g MnCl2 (final 5 mM)
- 25.5 ml 98% (v/v) glycerol (final 5%)
- Bring to 0.5 L with distilled water
- Filter sterilize
- Store up to 6 months at 4°C
The final pH should be ∼5.6-6.
Tris-acetate-EDTA (TAE) buffer, 50×
- 242 g Tris base (Panreac Química S.L.U., cat. no. A1086)
- 100 ml 0.5 M EDTA disodium salt dihydrate (Fluka/Sigma-Aldrich, cat. no. 03679)
- 57.1 ml glacial acetic acid (Panreac Química S.L.U., cat. no. 141008)
- Dissolve Tris in distilled water (∼700 ml), then add the remaining components, and bring to 1 L with distilled water. Store up to 6 months at room temperature.
The pH should be ∼8.5.
COMMENTARY
Background Information
Although different are techniques available for generating stable insertions in bacterial genomes, such as recombineering (Ellis et al., 2001) or homologous recombination–based methodologies (Martinez-Garcia & de Lorenzo, 2011), these tools exhibit limitations regarding host-range applicability and insertion size. For this reason, newer genetic tools are under development that offer the ability to engineer all kinds of biological entities in a desired way. Group II introns are retroelements that were first identified in the genomes of chloroplasts and other eukaryotic organelles (Michel, Umesono, & Ozeki, 1989; Zimmerly, Hausner, & Wu, 2001). Later, microbial group II introns were described that have the capability of inserting into specific DNA regions, a process termed retrohoming. Ll.LtrB from L. lactis is one of the best characterized group II introns, and its retrohoming process has been well studied. First, the intron is transcribed into RNA along with the exons of the gene it is interrupting. Mobile group II introns contain an ORF in domain IV that encodes an essential protein for their splicing and mobility. This protein is generally referred as intron-encoded protein (IEP); in the case of Ll.LtrB, it is called LtrA (Matsuura et al., 1997). After transcription, the ltrA mRNA is translated and aids in splicing of Ll.LtrB from the exons. After splicing, LtrA remains attached to Ll.LtrB to form a ribonucleoprotein complex that starts scanning DNA molecules for a target site to reverse-splice and re-insert into the genome.
Recognition of the target site is driven mostly by complementarity between specific regions in the intron sequence that are generally called exon-binding sites 1 and 2 (EBS1 and 2) and ∂. Perutka et al. (2004) were able to modify these three regions to obtain a retargeted version that can insert into genomic loci different than its native one. This achievement opened the possibility of using Ll.LtrB as a new gene disruption technology, which was named targetron. The main advantage of this technique is its independence from the cell's homologous recombination system, because Ll.LtrB retrohoming can take place even in ∆ recA strains (Cousineau et al., 1998). This is a useful feature, because some bacterial strains have low recombination efficiency and because homologous recombination is usually associated with unwanted genome rearrangements (Jiang et al., 2013). Furthermore, Ll.LtrB has been proven to work in many different bacterial strains and even eukaryotic cells, corroborating its broad host-range functionality (Akhtar & Khan, 2012; Guo et al., 2000; Heap et al., 2007; Mastroianni et al., 2008; Perutka et al., 2004; Yao & Lambowitz, 2007; Yao et al., 2006).
Additional studies have revealed that LtrA can be expressed in trans to increase the efficiency of Ll.LtrB insertion (Guo et al., 2000). This allows the implementation of Ll.LtrB as a delivery system, as cargo fragments can be introduced in place of the ltrA gene to be mobilized with the intron. However, the efficiency of splicing and mobilization is diminished with increasing cargo size (Plante & Cousineau, 2006). In this context, the application of a counterselection method to reduce the population of WT cells (in which the intron did not insert) became important. The addition of CRISPR/Cas9-based counterselection increased the efficiency of identifying bacterial cells with a retrohomed intron at the correct locus, as shown in both E. coli (Velazquez et al., 2019) and P. putida (Velazquez et al., 2021).
To implement a combined CRISPR/Cas9 and targetron technology and ensure a broad host range, the Ll.LtrB expression system and other characteristics of the commercialized TargeTron plasmid had to be changed. Since the original TargeTron plasmid (pACD4K-C) cannot replicate in P. putida , targetron components (Ll.LtrB intron and ltrA sequences) were transferred to a pSEVA plasmid to increase the host range to any Gram-negative bacteria. As the implemented CRISPR/Cas9 platform was already based on two derived SEVA plasmids (pSEVA231-CRISPR and pSEVA421-Cas9tr), we selected pSEVA651 as the backbone because it has a different origin of replication and antibiotic resistance gene, which make it compatible with the CRISPR/Cas9 vectors. Further, a ChnR/PChnb -based induction system was introduced to control expression of both Ll.LtrB and ltrA by addition of cyclohexanone (Velazquez et al., 2021). The resulting plasmid, pSEVA6511-GIIi, is compatible with the CRISPR/Cas9 SEVA plasmids, which are characterized elsewhere (Aparicio et al., 2018). The Ll.LtrB intron in this plasmid was chosen for its additional Hpa I restriction site, which is used for linearization and retargeting and is lost after this process. Alternatively, a PCR reaction to amplify the whole backbone plasmid with the correct primers can be performed. However, pSEVA6511-GIIi is 11 kb long, which can make amplification difficult, and users run the risk of introducing mutations during the PCR step. For this reason, we usually perform a double-digest of pSEVA6511-GIIi with Hin dIII and Hpa I. Furthermore, the CRISPR/Cas9 counterselection system chosen for this protocol was previously characterized, but different plasmids can be used, as well as single plasmids containing both Cas9 ORF and CRISPR expression cassettes.
The rest of the reagents are recommendations but can be substituted with similar reagents from different manufacturers, and general cloning protocols can be adapted to the user's needs. Conversely, Support Protocols 1 and 2 can also be used in other procedures, as they are general methodologies for preparing chemically competent cells and identifying efficient spacers for CRISPR/Cas9 experiments. The same is true of Basic Protocol 2 (design of CRISPR spacers) and Basic Protocol 5 (plasmid curing).
The system described here is not limited to use in gene delivery, but can also be used to generate disruption mutants when no other selection methods for Ll.LtrB insertion are available. Depending on the desired application, the Ll.LtrB/CRISPR/Cas9 system can be of great help as performing the insertion is very straightforward once the system is ready. The system can be employed to produce the same modification in different strains of the same species or to insert different cargo genes in one particular genomic site to generate diverse strains for testing. One interesting application could be the introduction of small synthetic pieces of DNA (i.e., genetic barcodes) for molecular labeling of different bacterial strains (Tellechea-Luzardo et al., 2020, 2022). Since the main limitation of group II introns is the size of the insert that can be delivered (>600-bp cargos can be difficult depending on the species), the insertion of small barcodes (∼150 bp) is a good example of the applicability of this system. Another potential limitation, particularly in bacteria with low GC content, is the identification of a PAM close to the Ll.LtrB insertion site. Different Cas nuclease effectors have been studied and developed with this limitation in mind and can be incorporated into the system. A final limitation involves identification of appropriate insertion sites, as it is possible that the ClosTron algorithm will yield only low-scored sites in the genomic region of interest. With the help of the CRISPR/Cas9 counterselection system, we have successfully obtained insertions with such a locus (score of 4.4) (Velazquez et al., 2021).
Critical Parameters
A high-scoring retargeting region and an efficient spacer are the key components of this procedure. The first parameter involves the efficiency of Ll.LtrB to splice and retrohome into the given target site. Because the algorithm used has a probabilistic nature, low-scoring target sites can still result in insertions. In this context, the presence of a very specific and efficient spacer can allow the identification of mutants even when the insertion frequency is very low. As stated in Support Protocol 2, it is important to conduct a preliminary test of the efficiency of each designed spacer for inducing cell death.
Regarding the cargo sequences to be delivered, it has already been stated that fragments longer than 600 bp in P. putida or 1 kb in L. lactis usually generate introns with deficient mobility. This also depends on the selected insertion site and the bacterial species used, so longer fragments should be tested first before discarding this methodology.
Another aspect to consider is the distance of the insertion site from the PAM sequence. It is essential that the insertion site be very close to the PAM sequence. The first eight to ten nucleotides adjacent to the PAM sequence are crucial in nucleating the binding of the crRNA and the target sequence, and mutations in this region can abolish crRNA/Cas9-induced cleavage (Jinek et al., 2012; Semenova et al., 2011; Wiedenheft et al., 2011). Since Ll.LtrB mutants have to escape the scanning of Cas9 complexes to survive, the insertion site must be inside this seed region. If there are no other options, insertion targets that are further away from PAMs (>10 nt) can be assayed, but it needs to be kept in mind that the specificity of the selection is reduced, and that Cas9 complexes have the potential to recognize mutated regions as well as wildtype sequences.
It is crucial that the first 5′ nucleotide of the Ll.LtrB sequence be a guanosine (G) and that the last 3′ one be cytosine (C) (Plante & Cousineau, 2006). This means that the insertion locus cannot be between the two Gs of the PAM sequence of Spy Cas9 (5′-NGG-3′). In this insertion site, retrohoming of the intron would restore the wildtype PAM sequence (i.e., with no Ll.LtrB) and this would abolish the counterselection.
The specific type of bacterial strain to be engineered plays an important role, as we previously detected differences in the efficiency of this technology between P. putida KT2440 recA + and recA – derivatives. This means the induction protocol and insertion site should be optimized for each strain to improve the efficiency of the insertion assay if initial results are not as expected.
In summary, the key elements in this protocol are the efficiency of the spacer and its specificity to differentiate between wildtype regions and Ll.LtrB-containing sequences. For this, the Ll.LtrB insertion site should be contained in the first ten nucleotides adjacent to the PAM. In addition, insertion sites with high scores are preferred, as they are expected to result in an Ll.LtrB with higher capacity to splice and retrohome correctly.
Troubleshooting
Retargeting the Ll.LtrB intron to the target locus
See Table 2 for a list of common problems with the protocol for retargeting the Ll.LtrB intron to the target locus, their causes, and potential solutions.
Protocol | Problem | Possible cause | Solution |
---|---|---|---|
Basic Protocol 1 | No target sites found on ClosTron | DNA sequence too short | Try increasing the size of the region used in the algorithm. DNA sequences <100 bp have a low probability of having highly scored insertion sites. |
Unsuccessful restriction digestion of backbone | Incubation time too short | Increase digestion time to improve plasmid linearization | |
Too much DNA | Reduce amount of DNA. We suggest adding no more than 1 µg DNA per 1 µl restriction enzyme. | ||
Unsuccessful SOEing PCR amplification | Wrong annealing temperature | Use a gradient of temperatures for annealing of primers as well as a longer extension time (e.g., 30 s instead of 15 s) | |
No or few colonies after transformation of Gibson reactions into E. coli | Unsuccessful Gibson reaction | Scale up reaction volume (final volume of 20 µl) | |
Add more insert to reaction (i.e., increase insert:vector ratio) | |||
Use new Gibson reagents or check that they are functional | |||
Increase reaction volume used for transformation and, consequently, increase volume of competent cells. | |||
Incubate Gibson reactions for 1 hr at 50°C if the time was shortened in previous attempts | |||
Unsuccessful transformation | Check transformation ability of competent cells | ||
Try to recover cells for a longer time after transformation | |||
Basic Protocol 3 | Unsuccessful restriction digestion of backbone | Incubation time too short | Increase incubation time to improve plasmid linearization |
Too much DNA | Reduce amount of DNA. We suggest adding no more than 1 µg DNA per 1 µl restriction enzyme. | ||
Unsuccessful ligation reaction | Inactive ligase | Use a new ligase and buffer | |
Use a different ligase and increase ligation time | |||
Try Gibson assembly as an alternative | |||
Increase the molar ratio of insert:vector from 3:1 to 7:1 | |||
Unsuccessful transformation | Competent cells | Check competence of cells | |
Allow cells to recover longer | |||
Unsuccessful identification of clones with cargo in correct orientation | Insufficient number screened clones | Screen more clones (20 or more, depending on initial amount tested) | |
Use one primer annealing inside the cargo and one in the corresponding region of the intron to directly identify the orientation of the cargo cloned into Ll.LtrB through a single PCR step | |||
Bias to one orientation of cargo | Increase the molar ratio of insert:vector from 3:1 to 7:1 | ||
Increase number of clones screened | |||
Use one primer annealing inside the cargo and one in the corresponding region of the intron to directly identify the orientation of the cargo cloned into Ll.LtrB through a single PCR step | |||
Basic Protocol 4 | No transformants | Low transformation efficiency | Prepare new competent cells and electroporate a larger amount of plasmid DNA |
Try mating to introduce plasmids into the desired strain; pSEVA backbones are endowed with an oriT so they can be transferred by conjugation | |||
No or few colonies after transformation of pSEVA231-CRISPR derivative and no positive clones | Low transformation efficiency | Prepare new competent cells and electroporate a larger amount of plasmid DNA | |
Low efficiency of intron insertion | Change the induction protocol: Use longer inductions or induce the culture at exponential phase | ||
Electroporate more than one aliquot of cells with pSEVA231-CRISPR derivative | |||
Choose a different insertion site when possible | |||
Check for correct design of spacer and insertion region once the intron is retrohomed to make sure that spacer recognition is disrupted after Ll.LtrB insertion | |||
Perform the same experiment with no cargo to see if the problem is the size of the fragment | |||
Many colonies after transformation but no positive clones | Low counterselection efficiency | Change the insertion site when possible to test a different spacer | |
Check a larger number of clones. For example, pool PCR reactions where several colonies are tested per reaction. | |||
Lawn of cells after transformation of pSEVA231-CRISPR derivative | Counterselection does not work | Check the integrity of plasmids by miniprep and digestion. As pSEVA plasmids share common sequences, plasmid rearrangement can occur. | |
Check pSEVA231-CRISPR derivative |
Designing CRISPR spacers
If no PAM is found close to the insertion site, a different Cas effector with alternative PAM sequence recognition can be employed instead of Spy Cas9.
When no transformants are obtained after carrying out Basic Protocol 2, we suggest trying 5′-phosphorylated oligonucleotides for the annealing step. Phosphorylation is not normally needed because the Bsa I-digested pSEVA231-CRISPR backbone provides the 5′-phosphate needed for ligation with the annealed oligonucleotides, even though just one strand of the annealed oligos will be ligated to the plasmid. This nicked plasmid will still transform efficiently into cloning cells and will be fixed after transformation. It has been shown that phosphorylated primers work slightly better, but they are more expensive and generally take longer to be delivered (Aparicio et al., 2018).
Cloning of cargos into Ll.LtrB
See Table 2 for a list of common problems with the cloning of cargos into Ll.LtrB.
Insertion assay
See Table 2 for an extensive troubleshooting guide with the most common problems encountered with the Ll.LtrB/CRISPR/Cas9-mediated insertion assay.
Understanding Results
An engineered strain with Ll.LtrB inserted in the correct genomic location along with the desired cargo can be generated by following the approach described in these protocols. For a fragment of 300 bp and using the P. putida KT2440 ∆ recA strain, we identified mutated cells with a ∼40% efficiency (Velazquez et al., 2021). The efficiency of the method depends greatly on the selected Ll.LtrB insertion site as well as the interference efficiency of the designed spacer. The bacterial strain used also plays a key role, as differences in the performance of this technology have been reported between the recA + and recA – derivatives of P. putida KT2440 (Velazquez et al., 2021), as discussed above. Nonetheless, the optimization of the induction protocol as well as the insertion site should improve the efficiency of the insertion assay in any strain to be tested. In this way, we have succeeded in delivering small fragments (150 bp) into insertion loci even with low expected efficiency (low score) and a low-efficiency spacer (90% cleavage efficiency) (Tellechea-Luzardo et al., 2022; Velazquez et al., 2021).
For the retargeting step, even though more bands are generated during the SOEing PCR reaction (Fig. 4, top gel), note that the 350-bp band should be visually more intense than the rest. On the contrary, reamplification of the fragment for the Gibson assembly reaction should only give a single, clear, and intense band (Fig. 4, bottom gel).
Time Considerations
Generation of a retargeted pSEVA6511-GIIi (Basic Protocol 1) and a pSEVA231-CRISPR derivative with the desired spacer (Basic Protocol 2) can be performed in parallel and should take no more than 6 days if no unexpected problems arise with cloning. The design, ordering, and shipping of primers can take 3-4 days, but digestion of the backbone plasmids can be performed during this time, so that linearized vector is ready once the primers arrive. The retargeting PCR and annealing of spacer oligos can be carried out in a single day, as well as the ligation and transformation into E. coli. After this, preparing cultures for miniprep and sequencing takes another 6 days.
Generation of a cargo-containing Ll.LtrB (Basic Protocol 3) requires having previously retargeted the intron, so cloning of this cargo fragment cannot start until Basic Protocol 1 has been completed. Nevertheless, the design of primers and amplification of the chosen cargo can be performed in parallel with Basic Protocols 1–2. Once the retargeted pSEVA6511-GIIi has been generated, the digestion, ligation, and transformation of the cargo fragment can be performed in a single day. Again, preparing cultures for miniprep and sequencing will take another 3 days. This means that it takes an average of 9 days to obtain the two plasmids necessary for the insertion protocol.
Regarding the insertion of Ll.LtrB and CRISPR/Cas9 counterselection (Basic Protocol 4), sequentially transforming pSEVA421-Cas9tr and the derivative pSEVA6511-GIIi can take 6 days in total. Some users transform both plasmids at the same time, but we recommend sequential transformations to avoid DNA rearrangement or other mutations. pSEVA421-Cas9tr is available from the authors (Aparicio et al., 2018; Velazquez et al., 2021; Velazquez et al., 2019) and from Addgene (plasmid no. 138709), and can be transformed into the desired bacterial strain in parallel with the execution of Basic Protocols 1–3. Once a bacterial species is transformed with pSEVA421-Cas9tr, the resulting strain can be used as the starting material to transform a different pSEVA6511-GIIi if different types of Ll.LtrB insertions are desired in the same strain. This will save one transformation step and 3 days of work. Once the bacterial cells bear both plasmids, the insertion protocol can be completed in a total of 3 days, although the induction time can be modified depending on the users’ needs.
The preparation of competent E. coli (Support Protocol 1) takes 3 days of work but can be carried out before the other protocols. Preparing and storing batches of competent cells can save time and allows them to be used for other cloning procedures. Alternatively, users can purchase commercial competent cells. Support Protocol 2 is performed once the corresponding pSEVA231-CRISPR derivatives are ready (Basic Protocol 2) and after transforming pSEVA421-Cas9tr into the desired strain (Basic Protocol 4). Therefore, it can be carried out at the same time as Basic Protocol 3 if you have previously transformed pSEVA421-Cas9tr into the strain. Since this transformation has to be done for Basic Protocol 4 and can be done independently of the rest of the protocols, it should take less than 4 days to carry out Support Protocol 2. Therefore, the Support Protocols do not necessarily increase the total time needed to complete the entire protocol.
Taking all of this into consideration, engineered bacteria can be generated in a total of 15 days. Curing the strain of plasmids (Basic Protocol 5) can take longer, and this will depend on the bacterial species used. Given the repeated overnight incubations, one should expect this process to take 1 week or more.
Acknowledgments
This work was funded by the SETH ( RTI2018-095584-B-C42 of MCIN/ AEI /10.13039/501100011033/ FEDER) and SYCOLIM (ERA-COBIOTECH 2018 - PCI2019-111859-2 of MCIN/AEI /10.13039/501100011033/EU) Projects of the Spanish Ministry of Science and Innovation; MADONNA (H2O20-FET-OPEN-RIA-2017-1-766975), SYNBIO4FLAV (H2O20-NMBP-TR-IND/H2O20-NMBP-BIO-2018-814650), and MIX-UP (MIX-UP H2O20-BIO-CN-2019-870294) Contracts of the European Union; InGEMICS-CM (S2017/BMD-3691) and BIOSINT-CM (Y2020/TCS- 6555) Projects of the Comunidad de Madrid - European Structural and Investment Funds (FSE, FECER). E.V. was the recipient of a Fellowship from the Education Ministry, Madrid, Spanish Government (FPU15/04315).
Author Contributions
Elena Velázquez : Conceptualization, formal analysis, investigation, methodology, writing (original draft); Yamal Al-Ramahi : Conceptualization, methodology, validation; Víctor de Lorenzo : Conceptualization, formal analysis, funding acquisition, supervision, writing (review and editing).
Conflict of Interest
The authors declare no conflict of interest.
Open Research
Data Availability Statement
All relevant data regarding this protocol can be found in the article. Plasmids pSEVA231-CRISPR and pSEVA421-Cas9tr can be purchased from Addgene (plasmid nos. 138712 and 138709, respectively). These plasmids and pSEVA6511-GIIi can also be requested from the authors. Plasmid pSEVA256, used as template for the Lux2 fragment, is available at the SEVA webpage (http://seva-plasmids.com/find-your-plasmid/).
Literature Cited
- Akhtar, P., & Khan, S. A. (2012). Two independent replicons can support replication of the anthrax toxin-encoding plasmid pXO1 of Bacillus anthracis. Plasmid , 67(2), 111–117. doi: 10.1016/j.plasmid.2011.12.012
- Aparicio, T., de Lorenzo, V., & Martinez-Garcia, E. (2018). CRISPR/Cas9-based counterselection boosts recombineering efficiency in Pseudomonas putida. Biotechnology Journal , 13(5), e1700161. doi: 10.1002/biot.201700161
- Aparicio, T., de Lorenzo, V., & Martinez-Garcia, E. (2019). CRISPR/Cas9-enhanced ssDNA recombineering for Pseudomonas putida. Microbial Biotechnology , 12(5), 1076–1089. doi: 10.1111/1751-7915.13453
- Cousineau, B., Smith, D., Lawrence-Cavanagh, S., Mueller, J. E., Yang, J., Mills, D., … Belfort, M. (1998). Retrohoming of a bacterial group II intron: Mobility via complete reverse splicing, independent of homologous DNA recombination. Cell , 94(4), 451–462. doi: 10.1016/s0092-8674(00)81586-x
- Cui, L., & Bikard, D. (2016). Consequences of Cas9 cleavage in the chromosome of Escherichia coli. Nucleic Acids Research , 44(9), 4243–4251. doi: 10.1093/nar/gkw223
- Deltcheva, E., Chylinski, K., Sharma, C. M., Gonzales, K., Chao, Y., Pirzada, Z. A., … Charpentier, E. (2011). CRISPR RNA maturation by trans-encoded small RNA and host factor RNase III. Nature , 471(7340), 602–607. doi: 10.1038/nature09886
- Ellis, H. M., Yu, D., DiTizio, T., & Court, D. L. (2001). High efficiency mutagenesis, repair, and engineering of chromosomal DNA using single-stranded oligonucleotides. Proceedings of the National Academy of Sciences of the United States of America , 98(12), 6742–6746. doi: 10.1073/pnas.121164898
- Frazier, C. L., San Filippo, J., Lambowitz, A. M., & Mills, D. A. (2003). Genetic manipulation of Lactococcus lactis by using targeted group II introns: Generation of stable insertions without selection. Applied and Environmental Microbiology , 69(2), 1121–1128. doi: 10.1128/AEM.69.2.1121-1128.2003
- Galvao, T. C., & de Lorenzo, V. (2005). Adaptation of the yeast URA3 selection system to Gram-negative bacteria and generation of a ΔbetCDE Pseudomonas putida strain. Applied and Environmental Microbiology , 71(2), 883–892. doi: 10.1128/AEM.71.2.883-892.2005
- Gibson, D. G., Glass, J. I., Lartigue, C., Noskov, V. N., Chuang, R. Y., Algire, M. A., … Venter, J. C. (2010). Creation of a bacterial cell controlled by a chemically synthesized genome. Science , 329(5987), 52–56. doi: 10.1126/science.1190719
- Gibson, D. G., Young, L., Chuang, R. Y., Venter, J. C., Hutchison, C. A. 3rd, & Smith, H. O. (2009). Enzymatic assembly of DNA molecules up to several hundred kilobases. Nature Methods , 6(5), 343–345. doi: 10.1038/nmeth.1318
- Guo, H., Karberg, M., Long, M., Jones, J. P. 3rd, Sullenger, B., & Lambowitz, A. M. (2000). Group II introns designed to insert into therapeutically relevant DNA target sites in human cells. Science , 289(5478), 452–457. doi: 10.1126/science.289.5478.452
- Heap, J. T., Pennington, O. J., Cartman, S. T., Carter, G. P., & Minton, N. P. (2007). The ClosTron: A universal gene knock-out system for the genus Clostridium. Journal of Microbiological Methods , 70(3), 452–464. doi: 10.1016/j.mimet.2007.05.021
- Horton, R. M., Hunt, H. D., Ho, S. N., Pullen, J. K., & Pease, L. R. (1989). Engineering hybrid genes without the use of restriction enzymes: Gene splicing by overlap extension. Gene , 77(1), 61–68. doi: 10.1016/0378-1119(89)90359-4
- Jiang, W., Bikard, D., Cox, D., Zhang, F., & Marraffini, L. A. (2013). RNA-guided editing of bacterial genomes using CRISPR-Cas systems. Nature Biotechnology , 31(3), 233–239. doi: 10.1038/nbt.2508
- Jinek, M., Chylinski, K., Fonfara, I., Hauer, M., Doudna, J. A., & Charpentier, E. (2012). A programmable dual-RNA-guided DNA endonuclease in adaptive bacterial immunity. Science , 337(6096), 816–821. doi: 10.1126/science.1225829
- Karberg, M., Guo, H., Zhong, J., Coon, R., Perutka, J., & Lambowitz, A. M. (2001). Group II introns as controllable gene targeting vectors for genetic manipulation of bacteria. Nature Biotechnology , 19(12), 1162–1167. doi: 10.1038/nbt1201-1162
- Klompe, S. E., Vo, P. L. H., Halpin-Healy, T. S., & Sternberg, S. H. (2019). Transposon-encoded CRISPR-Cas systems direct RNA-guided DNA integration. Nature , 571(7764), 219–225. doi: 10.1038/s41586-019-1323-z
- Manoil, C., & Beckwith, J. (1985). TnphoA: A transposon probe for protein export signals. Proceedings of the National Academy of Sciences of the United States of America , 82(23), 8129–8133. doi: 10.1073/pnas.82.23.8129
- Martinez-Garcia, E., & de Lorenzo, V. (2011). Engineering multiple genomic deletions in Gram-negative bacteria: Analysis of the multi-resistant antibiotic profile of Pseudomonas putida KT2440. Environmental Microbiology , 13(10), 2702–2716. doi: 10.1111/j.1462-2920.2011.02538.x
- Mastroianni, M., Watanabe, K., White, T. B., Zhuang, F., Vernon, J., Matsuura, M., … Lambowitz, A. M. (2008). Group II intron-based gene targeting reactions in eukaryotes. PLoS One , 3(9), e3121. doi: 10.1371/journal.pone.0003121
- Matsuura, M., Saldanha, R., Ma, H., Wank, H., Yang, J., Mohr, G., … Lambowitz, A. M. (1997). A bacterial group II intron encoding reverse transcriptase, maturase, and DNA endonuclease activities: Biochemical demonstration of maturase activity and insertion of new genetic information within the intron. Genes & Development, 11(21), 2910–2924. doi: 10.1101/gad.11.21.2910
- Michel, F., Umesono, K., & Ozeki, H. (1989). Comparative and functional anatomy of group II catalytic introns — a review. Gene , 82(1), 5–30. doi: 10.1016/0378-1119(89)90026-7
- Mills, D. A., McKay, L. L., & Dunny, G. M. (1996). Splicing of a group II intron involved in the conjugative transfer of pRS01 in Lactococci. Journal of Bacteriology , 178(12), 3531–3538. doi: 10.1128/jb.178.12.3531-3538.1996
- Mohr, G., Smith, D., Belfort, M., & Lambowitz, A. M. (2000). Rules for DNA target-site recognition by a lactococcal group II intron enable retargeting of the intron to specific DNA sequences. Genes & Development, 14(5), 559–573.
- Nelson, K. E., Weinel, C., Paulsen, I. T., Dodson, R. J., Hilbert, H., Martins dos Santos, V. A., … Fraser, C. M. (2002). Complete genome sequence and comparative analysis of the metabolically versatile Pseudomonas putida KT2440. Environmental Microbiology , 4(12), 799–808. doi: 10.1046/j.1462-2920.2002.00366.x
- Perutka, J., Wang, W., Goerlitz, D., & Lambowitz, A. M. (2004). Use of computer-designed group II introns to disrupt Escherichia coli DExH/D-box protein and DNA helicase genes. Journal of Molecular Biology , 336(2), 421–439. doi: 10.1016/j.jmb.2003.12.009
- Plante, I., & Cousineau, B. (2006). Restriction for gene insertion within the Lactococcus lactis Ll.LtrB group II intron. RNA , 12(11), 1980–1992. doi: 10.1261/rna.193306
- Rawsthorne, H., Turner, K. N., & Mills, D. A. (2006). Multicopy integration of heterologous genes, using the lactococcal group II intron targeted to bacterial insertion sequences. Applied and Environmental Microbiology , 72(9), 6088–6093. doi: 10.1128/AEM.02992-05
- Semenova, E., Jore, M. M., Datsenko, K. A., Semenova, A., Westra, E. R., Wanner, B., … Severinov, K. (2011). Interference by clustered regularly interspaced short palindromic repeat (CRISPR) RNA is governed by a seed sequence. Proceedings of the National Academy of Sciences of the United States of America , 108(25), 10098–10103. doi: 10.1073/pnas.1104144108
- Shearman, C., Godon, J. J., & Gasson, M. (1996). Splicing of a group II intron in a functional transfer gene of Lactococcus lactis. Molecular Microbiology , 21(1), 45–53. doi: 10.1046/j.1365-2958.1996.00610.x
- Silva-Rocha, R., Martinez-Garcia, E., Calles, B., Chavarria, M., Arce-Rodriguez, A., de Las Heras, A., … de Lorenzo, V. (2013). The Standard European Vector Architecture (SEVA): A coherent platform for the analysis and deployment of complex prokaryotic phenotypes. Nucleic Acids Research , 41, D666–675. doi: 10.1093/nar/gks1119
- Strecker, J., Ladha, A., Gardner, Z., Schmid-Burgk, J. L., Makarova, K. S., Koonin, E. V., & Zhang, F. (2019). RNA-guided DNA insertion with CRISPR-associated transposases. Science , 365(6448), 48–53. doi: 10.1126/science.aax9181
- Tellechea-Luzardo, J., Hobbs, L., Velazquez, E., Pelechova, L., Woods, S., de Lorenzo, V., & Krasnogor, N. (2022). Versioning biological cells for trustworthy cell engineering. Nature Communication , 13(1), 765. doi: 10.1038/s41467-022-28350-4
- Tellechea-Luzardo, J., Winterhalter, C., Widera, P., Kozyra, J., de Lorenzo, V., & Krasnogor, N. (2020). Linking engineered cells to their digital twins: A version control system for strain engineering. ACS Synthetic Biology , 9(3), 536–545. doi: 10.1021/acssynbio.9b00400
- Velazquez, E., Al-Ramahi, Y., Tellechea-Luzardo, J., Krasnogor, N., & de Lorenzo, V. (2021). Targetron-assisted delivery of exogenous DNA sequences into Pseudomonas putida through CRISPR-aided counterselection. ACS Synthetic Biology , 10(10), 2552–2565. doi: 10.1021/acssynbio.1c00199
- Velazquez, E., Lorenzo, V., & Al-Ramahi, Y. (2019). Recombination-independent genome editing through CRISPR/Cas9-enhanced TargeTron delivery. ACS Synthetic Biology , 8(9), 2186–2193. doi: 10.1021/acssynbio.9b00293
- Wiedenheft, B., van Duijn, E., Bultema, J. B., Waghmare, S. P., Zhou, K., Barendregt, A., … Doudna, J. A. (2011). RNA-guided complex from a bacterial immune system enhances target recognition through seed sequence interactions. Proceedings of the National Academy of Sciences of the United States of America , 108(25), 10092–10097. doi: 10.1073/pnas.1102716108
- Xiang, Q., Qin, P. Z., Michels, W. J., Freeland, K., & Pyle, A. M. (1998). Sequence specificity of a group II intron ribozyme: Multiple mechanisms for promoting unusually high discrimination against mismatched targets. Biochemistry , 37(11), 3839–3849. doi: 10.1021/bi972661n
- Yao, J., & Lambowitz, A. M. (2007). Gene targeting in Gram-negative bacteria by use of a mobile group II intron (“targetron”) expressed from a broad-host-range vector. Applied and Environmental Microbiology , 73(8), 2735–2743. doi: 10.1128/AEM.02829-06
- Yao, J., Zhong, J., Fang, Y., Geisinger, E., Novick, R. P., & Lambowitz, A. M. (2006). Use of targetrons to disrupt essential and nonessential genes in Staphylococcus aureus reveals temperature sensitivity of Ll.LtrB group II intron splicing. RNA , 12(7), 1271–1281. doi: 10.1261/rna.68706
- Zhong, J., Karberg, M., & Lambowitz, A. M. (2003). Targeted and random bacterial gene disruption using a group II intron (targetron) vector containing a retrotransposition-activated selectable marker. Nucleic Acids Research , 31(6), 1656–1664. doi: 10.1093/nar/gkg248
- Zimmerly, S., Hausner, G., & Wu, X. (2001). Phylogenetic relationships among group II intron ORFs. Nucleic Acids Research , 29(5), 1238–1250. doi: 10.1093/nar/29.5.1238
Internet Resources
Website for retargeting of Ll.LtrB to a region of interest.
Tm calculator tool from NEB website was used to calculate the specific annealing temperature for each primer pair used in a PCR reaction.
Source for pSEVA231-CRISPR and pSEVA421-Cas9tr plasmids.
Source for any pSEVA backbone such as pSEVA256.