CRISPR-Cas9 Based Genome Editing in Wheat
Mark A. Smedley, Mark A. Smedley, Sadiye Hayta, Sadiye Hayta, Martha Clarke, Martha Clarke, Wendy A. Harwood, Wendy A. Harwood
Abstract
The development and application of high precision genome editing tools such as programmable nucleases are set to revolutionize crop breeding and are already having a major impact on fundamental science. Clustered regularly interspaced short palindromic repeats (CRISPR), and its CRISPR-associated protein (Cas), is a programmable RNA-guided nuclease enabling targeted site-specific double stranded breaks in DNA which, when incorrectly repaired, result in gene knockout. The two most widely cultivated wheat types are the tetraploid durum wheat (Triticum turgidum ssp. durum L.) and the hexaploid bread wheat (Triticum aestivum L.). Both species have large genomes, as a consequence of ancient hybridization events between ancestral progenitors. The highly conserved gene sequence and structure of homoeologs among subgenomes in wheat often permits their simultaneous targeting using CRISPR-Cas9 with single or paired single guide RNA (sgRNA). Since its first successful deployment in wheat, CRISPR-Cas9 technology has been applied to a wide array of gene targets of agronomical and scientific importance. The following protocols describe an experimentally derived strategy for implementing CRISRP-Cas9 genome editing, including sgRNA design, Golden Gate construct assembly, and screening analysis for genome edits. © 2021 The Authors.
This article was corrected on 26 July 2022. See the end of the full text for details.
Basic Protocol 1 : Selection of sgRNA target sequence for CRISPR-Cas9
Basic Protocol 2 : Construct assembly using Golden Gate (MoClo) assembly
Basic Protocol 3 : Screening for CRISPR-Cas9 genome edits
Alternate Protocol : BigDye Terminator reactions for screening of CRISPR-Cas9 genome edits
INTRODUCTION
The development of high precision genome editing tools like targeted nucleases has accelerated advances in fundamental plant science and crop breeding (Zhang, Massel, Godwin, & Gao, 2018). Clustered regularly interspaced short palindromic repeats (CRISPR), and its CRISPR-associated protein (Cas), is an RNA-guided nuclease developed from the microbial adaptive immune system by repurposing for genome editing (Barrangou & Marraffini, 2014). CRISPR-Cas technologies can facilitate efficient genome engineering within eukaryotic cells (Komor, Badran, & Liu, 2017). Precise targeting of the Cas9 protein is achieved by specifying a 20-nucleotide (nt) targeting sequence within its single guide RNA (sgRNA; Ran et al., 2013). The application of genome editing tools such as CRISPR-Cas9 in genetic studies and for engineering crops with desirable traits has become a major focus of plant scientists and precision plant breeders alike (Borrill, 2020). The induction of targeted site-specific double stranded breaks in DNA and the subsequent repair through direct ligation sometimes results in errors such as insertions or deletions (indels) leading to a loss of gene function (knockouts; Rodgers & McVey, 2016).
Modern domesticated wheats are derivatives from ancient hybridization events between ancestral progenitor species. The two most extensively cultivated wheats are the tetraploid durum or pasta wheat (Triticum turgidum ssp . durum L.) and hexaploid bread wheat (Triticum aestivum L.) (Matsuoka, 2011). Both species have large genomes—durum, ∼12 and hexaploidy, ∼16 Gbp—consisting mostly of repetitive elements. Within these polyploid species each gene usually exists as two copies in the tetraploid durum wheat or as three in hexaploid bread wheat. Homoeologous gene copies are usually highly conserved in gene structure and sequence among the subgenomes (>95%; Adamski et al., 2020).
The first reported use of CRISPR-Cas9 to produce a stably genome edited wheat plant was the targeted knockout of the Mildew Locus O (Mlo), conferring resistance to the powdery mildew pathogen Blumeria graminis f.sp. tritici (Bgt), this was achieved in combination with an earlier gene editing technology, transcription activator-like effector nucleases (TALEN; Wang et al., 2014). Since this first report, wheat genes of agronomical and fundamental scientific interest have been targeted using CRISPR-Cas9 technology, such as α-gliadin genes to lower gluten grain content (Sánchez-León et al., 2018), TaGW2 to increase grain weight (Zhang, Li, et al., 2018), TaZIP4-B2 to understand meiotic homologous crossover (Rey et al., 2018), TaQsd1 to reduce preharvest sprouting (Abe et al., 2019), TaMTL and CENH3 for haploid plant induction (Liu et al., 2019; Lv et al., 2020).
Here, we describe experimentally derived protocols for the selection of sgRNA targets, construct assembly, and screening analysis for genome editing in hexaploid wheat.
STRATEGIC PLANNING
Cas9 is an RNA-guided nuclease for genome editing derived from Streptococcus pyogenes. The specificity of Cas9 nuclease is determined by the 20-nt guide sequence within the sgRNA. When using the S. pyogenes Cas9, the target sequence (e.g., 5′- GAATATTGCTCGTGTCAGGG-3′) must be directly followed by a 3′-NGG protospacer adjacent motif (PAM). Cas9 cleavage occurs at ∼3 base pairs upstream (5′) of the PAM (Ran et al., 2013). It should be noted that the PAM sequence does not form part of the sgRNA guide sequence.
In hexaploid wheat, genome editing using the CRISPR-Cas9 system usually requires the knocking out or modifying of all three homoeologous copies of a gene target within each subgenome (A, B, and D). In order to assess the homology between target gene copies it is advisable to align the target sequences using an alignment tool. For knockouts, if possible, guides should be designed close to the ATG start codon to cause a frameshift early on in the coding sequences (CDS). The two main considerations when selecting the 20-nt guide sequence are the presence of the 3′-NGG PAM and the minimizing of off-target activity. There are many online tools and freeware that can scan the sequence and identify potential guide target sequences; however, few are designed specifically for wheat. The WheatCRISPR tool publicly available at https://crispr.bioinfo.nrc.ca/WheatCrispr/ selects effective sgRNAs based on their predicted high on-target and low off-target activity scores (Cram et al., 2019), allowing researchers to browse all possible sgRNAs within a target gene or sequence of interest. The WheatCRISPR tool is unique in that it takes into consideration hexaploidy in bread wheat allowing the researcher to target either a single gene copy or all three homoeologs by selecting a tick box option. It should be noted that, presently, wheat guide design tools are directed towards the Chinese Spring (CS) sequence within databases and therefore there may be differences between the CS sequence and the cultivar being transformed and edited. Presently, the most transformable wheat cultivar is the hexaploid spring wheat cultivar “Fielder”; therefore, it is suggested, that if using this cultivar your target sequence be checked by BLASTn at this publicly available portal: https://grassroots.tools/public/service/blast-blastn
Another consideration when designing sgRNAs is the type of polymerase III promoter used to express the guide. For instance, the preferred first base of transcription of the wheat polymerase III promoters TaU3 or TaU6 differ, either adenine (A) or guanine (G), respectively. The example in this protocol is for the polymerase III promoter TaU6. Where the 20-nt guide sequence does not begin with G, an extra G is added at the 5′ end of the sgRNA (21 nt) for transcription start but also to enable cloning.
Once sgRNAs have been identified, PCR primers should be designed spanning the target area and tested using genomic DNA extracted from the transformable wheat cultivar “Fielder.” In this protocol we assume that Fielder is the cultivar used. The primers should be designed as described below.
Screening
There are two approaches for Sanger sequence screening in wheat; the first uses a set of target generic primers that amplify all target gene copies within the plant. This first PCR is then used as an enriched DNA template for amplification of subgenome specific (SgS) target regions, using SgS nested primers. This nested approach is useful for amplicons that are particularly difficult to amplify.
The second approach, for screening wheat, uses SgS primers that amplify the individual target regions directly from “Fielder” genomic DNA. The SgS target area amplicons are then directly sequenced using the forward SgS primer. This direct approach is less time consuming and uses fewer reagents than the nested primer approach, and is therefore preferred.
In both the above approaches, SgS screening primers should be designed spanning the target area, at least 120 to 150 bp from the first and last sgRNA target sequence, therefore, producing an ∼400-bp sized PCR amplicon, which facilitates easy identification of large (∼50 to 100 bp) deletions in edited plants using gel electrophoresis and produces a good-sized amplicon for direct sequencing. An initial test PCR should produce a clean single band on a gel and be of good quality capable of being directly sequenced. Direct sequencing is usually performed using the relevant forward SgS primer.
Primer design starts by aligning the target gene sequences, ensuring that there is sufficient sequence data flanking the target area (∼200 bp up and downstream). For SgS primer design, look for polymorphisms between the different subgenome target genes ∼120 to 150 bp from the first and last sgRNA target sequence. Often, a single nucleotide polymorphism (SNP), specific to one subgenome, incorporated at the 3′ end of a primer is enough to make it subgenome specific. Primers can be designed by eye, and the annealing temperatures checked with online tools or can be designed using online tools such as Primer3 (Untergasser et al., 2012).
Basic Protocol 1: SELECTION OF sgRNA TARGET SEQUENCE FOR CRISPR-Cas9
This protocol describes designing sgRNAs for your chosen target sequence (see Strategic Planning for detailed background information). It is recommended that the design should include four sgRNAs for each gene target, two guides per construct, and two constructs per target gene. Ideally, paired guides are designed 50 to 150 bp apart, which will give an easily recognizable deletion if the guides work simultaneously.
Materials
Computer with alignment software and Internet access to the following websites:
- http://plants.ensembl.org/Triticum_aestivum
- https://crispr.bioinfo.nrc.ca/WheatCrispr/
- https://grassroots.tools/public/service/blast-blastn
1.The International Wheat Genome Sequencing Consortium (IWGSC) genomic information for wheat cultivar “Chinese Spring” is available at http://plants.ensembl.org/Triticum_aestivum. Collate information for your target gene of interest. Ensure that you can identify exonic and intronic sequences and the start of translation (ATG).
2.Align the three homoeologs of your gene of interest from the three subgenomes. Look for areas of homology where guides can be designed to target all homoeologs.
3.Either paste the sequence into WheatCrispr or Enter an IWGSC v1 gene name such as: TraesCS6B02G093900homologous sequence. Select to target promoter or coding region. Tick the “Target Homoeologues?” box if all three subgenome homoeologs are to be targeted. Run the program to identify 20-nt sgRNA guide sequences within the target gene sequence. When using SpCas9, the target site of the sgRNA will be the 20-bp preceding a PAM (5′-NGG-3′). The WheatCrispr website will display an overall score consisting of a weighted average of the on-target and off-target scores (https://crispr.bioinfo.nrc.ca/WheatCrispr/).
4.Ensure that the chosen sgRNA guides will target the desired gene target in the wheat cultivar “Fielder” by carrying out BLASTn analysis. Add the target sequence to https://grassroots.tools/public/service/blast-blastn and check that the sgRNA sequence does not differ between CP and “Fielder.”
5.Design sets of primers for amplification of the target area of each subgenome individually, to be able to directly Sanger sequence the amplicons (see Strategic Planning, Screening section).
6.Order complementary oligonucleotides with the sgRNA sequence with the addition of 4 nt at the 5′ end for construct assembly (Fig. 1A).
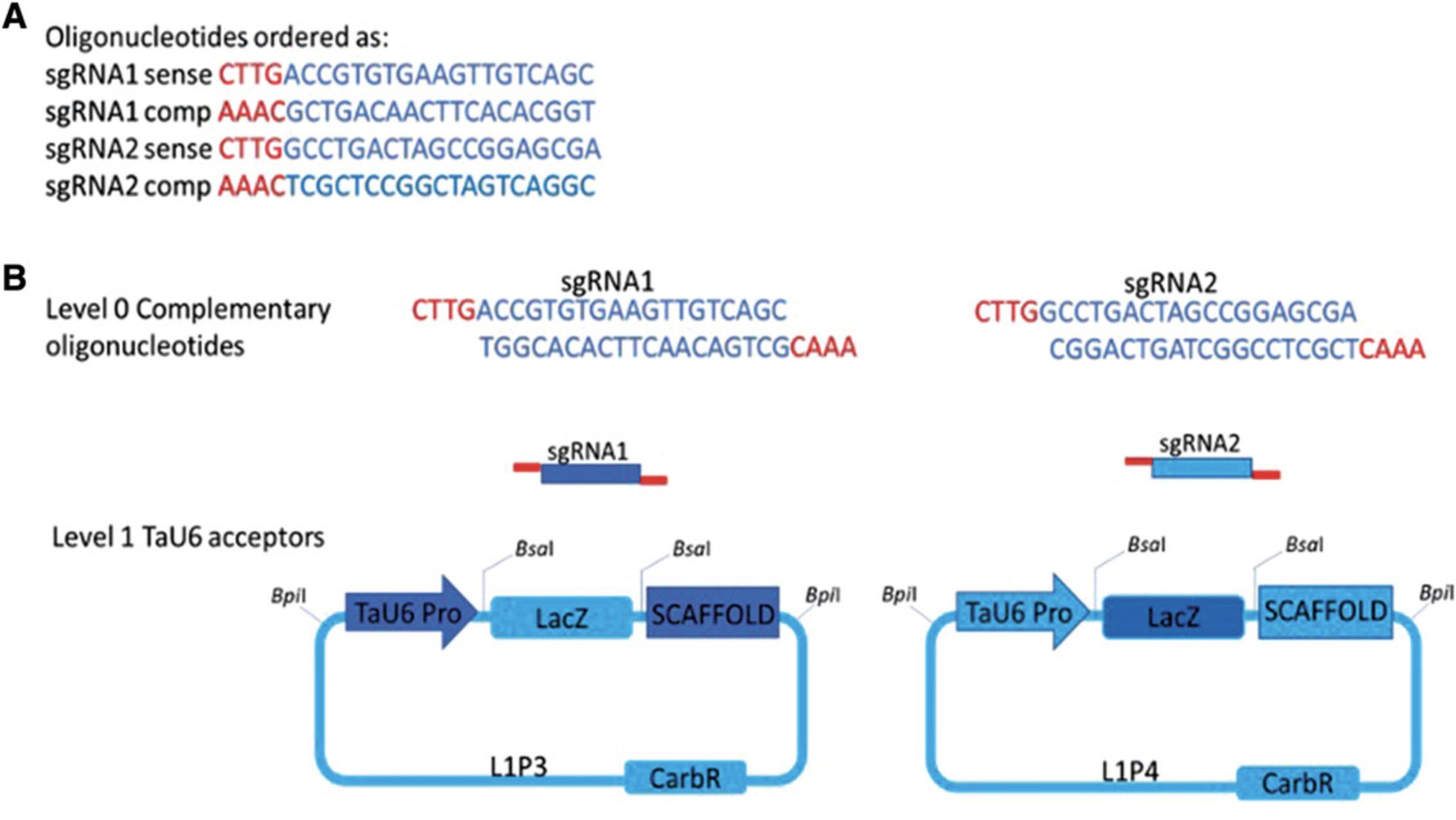
Basic Protocol 2: CONSTRUCT ASSEMBLY USING GOLDEN GATE (MoClo) ASSEMBLY
This basic protocol describes how to assemble plasmid vectors for CRISPR-Cas9 induced targeted gene knockout using the Type IIS restriction enzyme mediated Golden Gate (GG) modular cloning (MoClo) assembly (Weber, Engler, Gruetzner, Werner, & Marillonnet, 2011). The GG based MoClo system is a hierarchical cloning system that uses standardized DNA parts and/or overhangs; e.g., promoters, coding sequences, or terminators (Level 0), which are assembled into expression cassettes (Level 1) and these in turn can be assembled into multigene binary vector constructs (Level 2). The assembly is performed in a “one-pot” digestion-ligation reaction, where multiple DNA fragments are combined in a defined linear order into a recipient vector. The defined linear order of the level 1 expression cassettes within the multigene level 2 binary vector run from the left border (LB in Fig. 2) repeat denoted as Position 1 (P1) to Position 7 (P7) at the right border (RB) repeat. The finished binary vector containing two guides will have the plant selection resistance gene hpt at P1, Cas9 P2, and TaU6-sgRNAs at Positions P3 and P4 (Fig. 2).
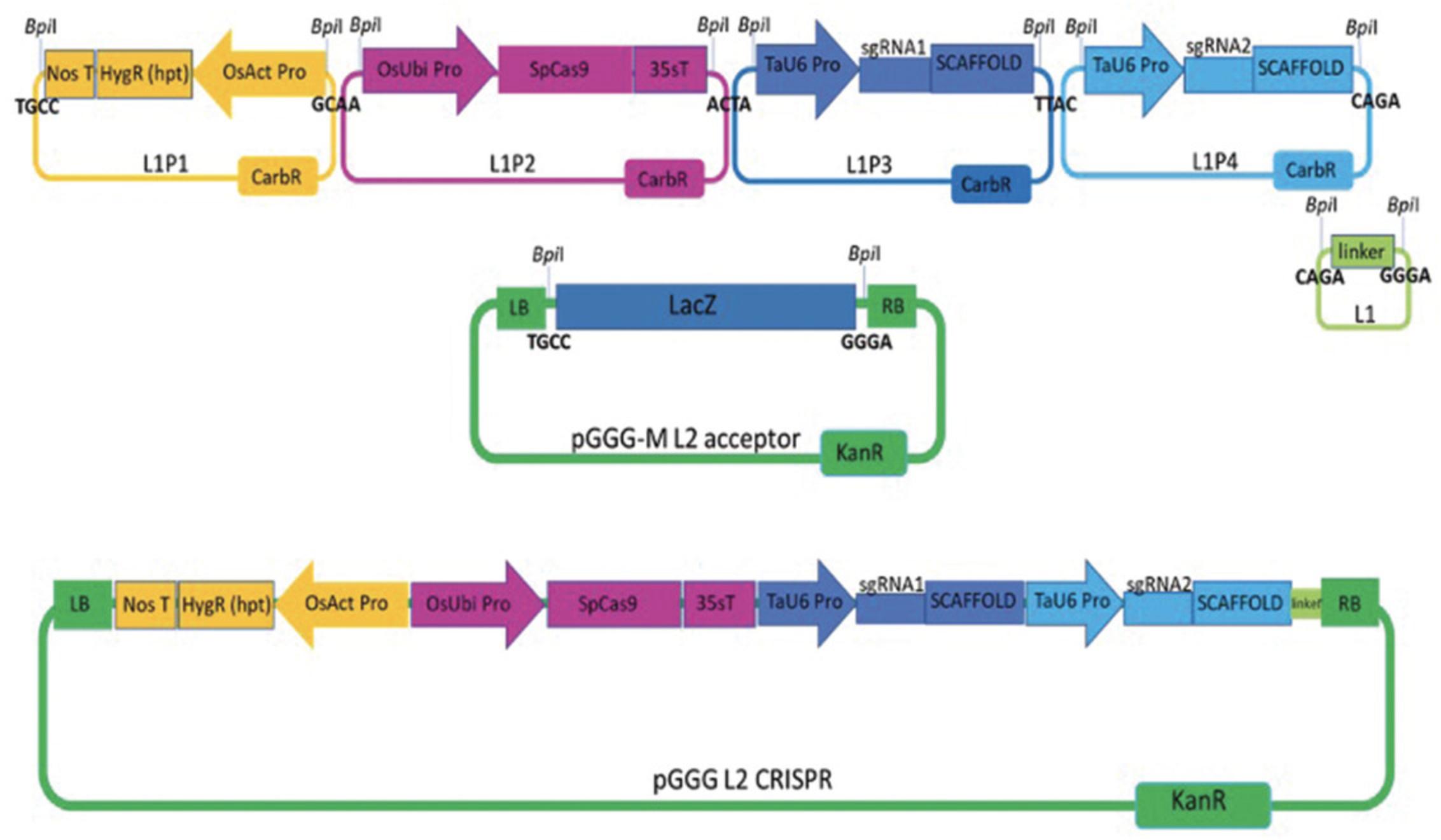
The first procedure is to introduce your chosen target sequence into the sgRNA invariant scaffold downstream of the TaU6 promoter; this is a simple and rapid single cloning step. The complementary oligonucleotide pair (level 0 component) encoding the 20-nt guide sequences with the addition of four base overhangs at the 5′ ends are annealed and ligated into a level 1 acceptor plasmid directly between the TaU6 promoter and the sgRNA scaffolding site (Fig. 1B). Once the level 1 plasmids containing your sgRNAs are prepared, these can then be incorporated into your final level 2 binary vector along with the selection cassette and Cas9.
Materials
-
sgRNA oligonucleotides, sense and complement, resuspended at 100 µM
-
Level 1 P1 OsActinP:hpt -int:35sT plasmid at ∼80 ng µl-1 (∼50-100 ng µl-1; Addgene, #165423)
-
Level 1 P2 OsUbiP:Cas9:NosT plasmid at ∼80 ng µl-1 (∼50-100 ng µl-1; Addgene, #165424)
-
Level 1 P3 TaU6 acceptor plasmid at ∼80 ng µl-1 (∼50-100 ng µl-1; Addgene, #165599)
-
Level 1 P4 TaU6 acceptor plasmid at ∼80 ng µl-1 (∼50-100 ng µl-1; Addgene, #165600)
-
Level 1 end-linker 4 plasmid pELE-4 at ∼80 ng µl-1 (∼50-100 ng µl-1; Addgene, #48019)
-
Level 2 acceptor plasmid pGGG-M at ∼80 ng µl-1 (∼50-100 ng µl-1; Addgene, #165422)
-
Restriction enzyme Eco 31I (Bsa I), 10 U µl-1 (Thermo Fisher Scientific, cat. no. ER0291)
-
Restriction enzyme Bpi I (Bbs I), 10 U µl-1 (Thermo Fisher Scientific, cat. no. ER1011)
-
Restriction enzyme Bgl II, 10 U µl-1 (Invitrogen, Thermo Fisher Scientific, cat. no. IVGN0196)
-
T4 DNA ligase and its accompanying 10× buffer (New England Biolabs, cat. no. M0202S)
-
Escherichia coli DH5α competent cells (includes SOC medium, Hanahan, 1983; Invitrogen, Thermo Fisher Scientific, cat. no. 18263-012)
-
LB solid plates, X-Gal/IPTG/carbenicillin, 50 µg ml-1
-
LB solid plates, X-Gal/IPTG/kanamycin, 50 µg ml-1
-
LB liquid medium, 10 ml aliquots
-
Analytical grade sterile water
-
TaU6 Forward sequencing primer 5′-TAGGAGGGAATCGAACTAGGAATATTG-3′
-
LB Forward 5′-GTGGTGTAAACAAATTGACGC-3′
-
Actin P Forward 5′-CGTATCAAAGTACCGACAAAAACA-3′
-
Cas9-3′end Forward 5′-CTTCAAGTACTTCGACACCACCATAGA-3′
-
RB-R1 Reverse 5′-CCCGCCAATATATCCTGTCA-3′
-
QIAprep Spin Miniprep Kit (50; Qiagen, cat. no. 27104)
-
Thermocycler, incubator set at 37°C, shaker-incubator, heat block or water bath set at 42°C
-
Additional reagents and equipment for gel electrophoresis (Current Protocols article: Gallagher, 2012)
Level 1 construct assembly
Maintain reagents and reactions on ice.
1.To an Eppendorf tube, add 5 µl of both the sense and antisense oligos (100 µM) and mix. Add 1 µl of this oligo mix to 49 µl analytical grade sterile water to make a 1:50 dilution (2 µM final concentration).
2.In a 0.2-ml thin-walled Eppendorf tube, setup diglig reaction as outlined below:
1.1 µl TaU6 acceptor plasmid (P3 or P4) at ∼80 ng µl-1 (∼50-100 ng µl-1) 2.1 µl oligo mix (2 µM) 3.1 µl ligase buffer (10×) 4.0.5 µl Eco 31I (BsaI), 10 U µl-1 5.0.5 µl T4 DNA ligase 6.6 µl analytical grade sterile water
Mix and briefly centrifuge to bring contents to the bottom of the tube.
3.Place tube in a thermocycler and run the following diglig program with heated lid:
-
37°C for 5 min;
-
16°C for 5 min;
-
Cycle step a. nine times;
-
37°C for 7 min;
-
80°C for 10 min;
-
4°C hold.
This program will run for ∼2 hr, 20 min.
4.Thaw E. coli DH5α competent cells on ice. To a prechilled 1.5-ml Eppendorf tube, gently transfer 50 µl competent cells and add 2 µl diglig reaction. Gently mix and place on ice, incubating for 20 min.
5.Heat shock cells at 42°C for 35 s in a preheated hot block or water bath.
6.Immediately place back on ice for 2 min.
7.Add 500 µl room temperature SOC medium and incubate tube of cells horizontally at 37°C; shake at 180 rpm for 1-1.5 hr.
8.Spread 20 µl and 40 µl of the E. coli transformation onto LB solid plates, X-Gal/IPTG/carbenicillin (50 µg ml-1) medium and incubate upside down at 37°C overnight (∼16 hr).
9.Select white colonies (two for each construct) of each level 1 TaU6 sgRNA you have assembled and inoculate 10 ml LB liquid medium supplemented with carbenicillin at 50 µg ml-1. Incubate at 37°C, shaking at 220 rpm overnight (∼16 hr).
Level 2 binary vector assembly
Once the level 1 TaU6P:sgRNA integrity has been confirmed by sequencing, assembly of the final level 2 binary vector can commence. Maintain reagents and reactions on ice.
10.Add the following to a 0.2-ml thin-walled Eppendorf tube to perform the level 2 diglig reaction:
- 1 µl Level 1 P1 OsActinP:hpt -int:35sT plasmid at ∼80 ng µl-1 (∼50-100 ng µl-1)
- 1 µl Level 1 P2 OsUbiP:Cas9:NosT plasmid at ∼80 ng µl-1 (∼50-100 ng µl-1)
- 1 µl Level 1 P3 TaU6P:sgRNA plasmid ∼80 ng µl-1 (∼50-100 ng µl-1)
- 1 µl Level 1 P4 TaU6P:sgRNA plasmid ∼80 ng µl-1 (∼50-100 ng µl-1)
- 1 µl Level 1 end-linker 4 plasmid pELE-4 at ∼80 ng µl-1 (∼50-100 ng µl-1)
- 1 µl Level 2 acceptor plasmid pGGG-M at ∼80 ng µl-1 (∼50-100 ng µl-1) 7.1.5 µl ligase buffer (10×) 8.0.5 µl Bpi I (Bbs I), 10 U µl-1 9.0.5 µl T4 DNA ligase 10.6.5 µl analytical grade sterile water.
Mix and briefly centrifuge to bring contents to the bottom of the tube.
11.Place tube in a thermocycler and run diglig program as described above under level 1 assembly, step 3.
12.Transform 50 µl E. coli DH5α competent cells with 3.5 µl of the level 2 diglig reaction by heat shock as previously described in steps 4-6 of level 1 construct assembly above.
13.Spread 40 µl and 80 µl of the E. coli transformation onto LB solid plates, X-Gal/IPTG/kanamycin (50 µg ml-1) medium and incubate upside down at 37°C overnight (∼16 hr).
14.Select two white colonies for each level 2 construct assembled and inoculate 10 ml LB liquid medium supplemented with kanamycin at 50 µg ml-1. Incubate at 37°C, shaking at 220 rpm overnight, ∼16 hr.
15.Perform a diagnostic digest using Bgl II restriction enzyme. Each digest consists of the following in a 1.5-ml Eppendorf tube (volume in µl):
- 2 µl mini-prepped Level 2 plasmid DNA (∼200 ng µl-1)
- 1 µl Bgl II restriction enzyme (10 U µl-1)
- 1 µl digestion buffer red (10×)
- 6 µl analytical grade sterile water.
Incubate at 37°C in a water bath for ∼1 hr.
16.Perform gel electrophoresis of the pGGG level 2 Bgl II restriction enzyme digests on a 1% agarose gel containing ethidium bromide at 0.1 µg ml-1. A 1-kb size marker should be included on the gel.
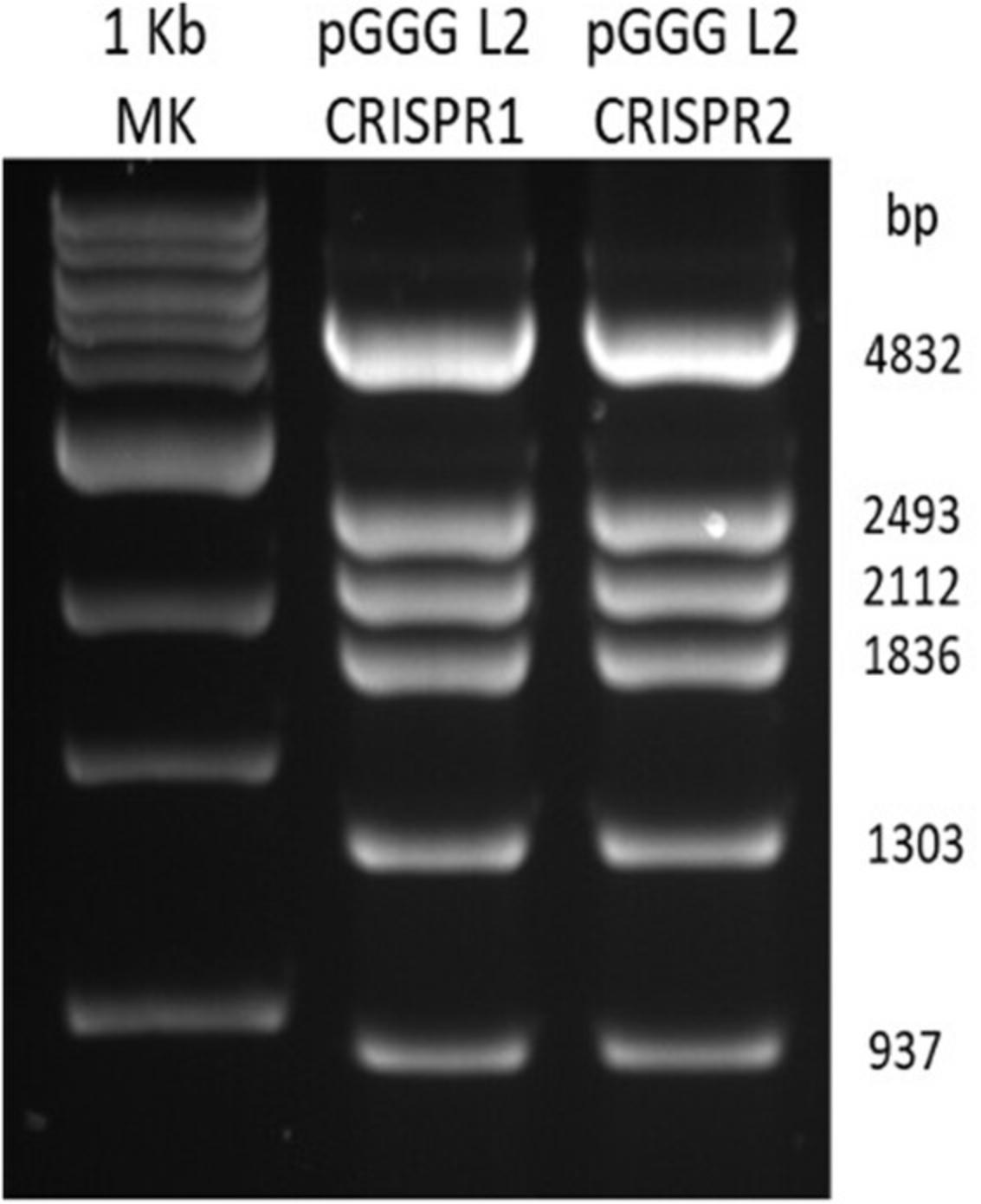
Sequencing primers | |
---|---|
LB forward | 5′-GTGGTGTAAACAAATTGACGC-3′ |
Actin P forward | 5′-CGTATCAAAGTACCGACAAAAACA-3′ |
Cas9, 3′ end forward | 5′-CTTCAAGTACTTCGACACCACCATAGA-3′ |
RB-R1 reverse | 5′-CCCGCCAATATATCCTGTCA-3′ |
Basic Protocol 3: SCREENING FOR CRISPR-Cas9 GENOME EDITS
This protocol describes the screening of primary transgenic plants for genome editing events using direct Sanger sequencing of PCR amplicons (see Strategic Planning for detailed background information). For screening hexaploid wheat using Sanger sequencing, PCR amplicons need to be identified that span the gene target (Fig. 4) within each subgenome (A, B, and D). When screening primary transgenics for genome editing events using direct Sanger sequencing of amplicons, due to the usual mosaic nature of editing, expect to see mixtures of unedited and edited DNA within samples. Identification of editing is done by looking at the sequence chromatogram traces. The chromatogram trace should be of good quality with well-defined peaks; active editing is identified by seeing a dramatic drop of signal and double peaks in the trace at an sgRNA target site (Fig. 5). This drop/double peaks will continue to the end of the trace. Screening this way in the primary transgenics allows the identification of wheat lines with active editing. These “active” lines can be taken through to the T1 generation where the edits and transfer DNA (T-DNA) are segregated. For more in-depth analysis of the primary transgenics, the PCR amplicon can be inserted into a cloning vector such as pGEM-T, transformed into E. coli , and a number (around ten, for example) of individual colonies selected, prepped, and sequenced. For a large number of wheat lines this would be a highly impractical way of screening. Next generation sequencing (NGS) can provide more detailed and quantitative information about editing events. NGS generates large amounts of data and requires competence in bioinformatics to handle and analyze such data (Bahassi & Stambrook, 2014).
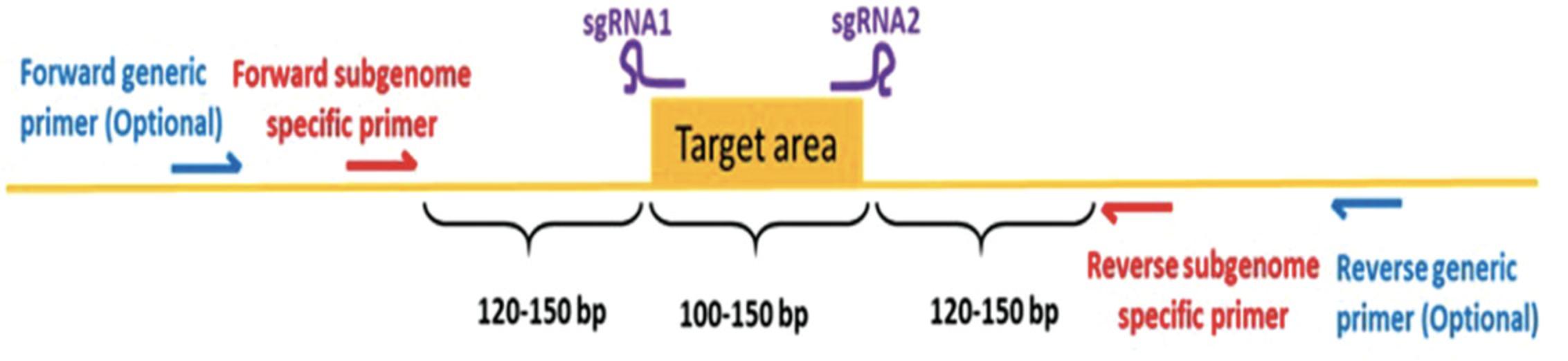
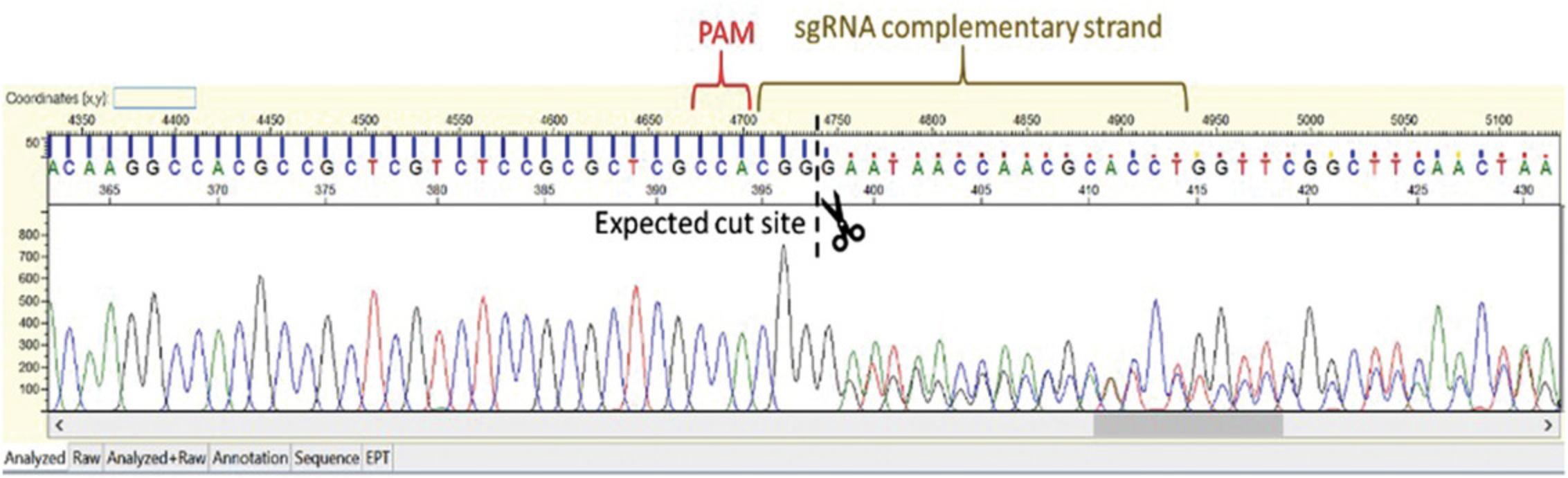
Materials
-
Predesigned and tested screening primer pairs (see Basic Protocols 1 and 2)
-
ReadyMix™ Taq PCR Reaction Mix with MgCl2 (MilliporeSigma, cat. no. P4600-100RXN)
-
Template DNA (extracted according to manufacturer's instructions from leaf material using Qiagen DNeasy Plant Mini Kit, cat. no. 69106)
-
1-kb DNA Ladder (New England Biolabs, cat. no. N3232S)
-
Gel Loading Dye, Purple (6×; New England Biolabs, cat. no. B7025)
-
Analytical grade sterile water
-
Thermocycler
-
AB Sequence Scanner Software v2.0 (Applied Biosystems) or equivalent freeware (e.g., https://benchling.com)
-
Additional reagents and equipment for gel electrophoresis (Current Protocols article: Gallagher, 2012)
Screening by amplicon Sanger sequencing
1.Set up and run initial PCR, either with the SgS primer sets or with generic primers. Each 0.2-ml thin-walled Eppendorf tube will contain the following:
1.10 µl ReadyMix™ Taq PCR Reaction Mix (2×) 2.4 µl template DNA 3.1 µl forward primer (10 µM) 4.1 µl reverse primer (10 µM) 5.4 µl analytical grade sterile water.
If the first PCR is to be used as an enriched template for the second SgS PCR, use 1 µl as template and increase the water to 7 µl in the second PCR reaction. An untransformed wheat DNA wild-type (wt) control and a water negative control should be included in all PCR runs.
2.Resolve 5 µl of the SgS PCR product on a 1% agarose gel containing ethidium bromide at 0.1 μg ml−1. Add 1 µl loading dye (6×) to each sample. A 1-kb DNA ladder should be included on the gel. Alternatively, users can perform BigDye Terminator reactions (see Alternate Protocol).
3.Send samples to be directly sequenced using the forward SgS primer.
Analysis of sequence data
On receiving your sequence data from the sequencing company, check the quality and length of reads; this information is usually provided by the company. Do not dismiss short good quality reads as these may indicate a larger deletion.
4.Open the Applied Biosystems Inc Format (ABIF) files signified by the file extension “ab1” using suitable software such as AB Sequence Scanner Software v2.0 or freeware such as https://benchling.com in order to view the sequence chromatogram traces.
5.Paste into the sequence search tool the first 12-13 bases of the sgRNA target sequence (or if the sgRNA is on the complementary strand, ∼15 bp upstream of the target sequence) and scroll through the trace file until the target sequence.
6.If no editing has been identified, repeat process with the second sgRNA target within the sequence.
Alternate Protocol: BigDye TERMINATOR REACTIONS FOR SCREENING OF CRISPR-Cas9 GENOME EDITS
Perform BigDye Terminator reactions as per the manufacturer's instructions before sequencing as a ready-to-run reaction. For BigDye Terminator reactions, make 1/10 dilutions of the SgS PCR reaction and use 1 µl of this dilution as sequencing template.
Additional Materials (also see Basic Protocols 1 and 2)
-
BigDye Terminator v3.1 Cycle Sequencing Kit (Applied Biosystems, cat. no. 4337454)
-
Predesigned and tested screening primer pairs
-
Template DNA (extracted according to the manufacturer's instructions from leaf material using Qiagen DNeasy Plant Mini Kit, cat. no. 69106)
-
Analytical grade sterile water
-
Thermocycler
1.Complete steps 1-2 of Basic Protocol 3.
2.Each 0.2-ml thin-walled Eppendorf tube will contain the following:
1.1 µl template 1:10 diluted PCR reaction (DNA ∼20-40 ng µl−1) 2.1 µl BigDye Terminator v3.1 3.1 µl forward primer (5 µM) 4. 1.2 µl BigDye buffer (10×) 5. 7.8 µl analytical grade sterile water.
3.Place tubes in a thermocycler and run the following BigDye Terminator program.
-
95°C for 1 min;
-
95°C for 30 s;
-
56°C for 30 s;
-
60°C for 1 min;
-
Cycle step a. 24 times;
-
60°C for 5 min;
-
4°C hold.
Once the program has completed, the samples are now ready for Sanger sequencing.
REAGENTS AND SOLUTIONS
Carbenicillin, 1,000× stock solution
- 50 mg ml-1 suspended in water.
- Store as 1-ml aliquots at −20°C for up to 6 months.
Isopropyl β-D-1-thiogalactopyranoside (IPTG), 1,000× stock solution
- 250 mg ml-1 suspended in ethanol.
- Store as 1-ml aliquots at −20°C for up to 6 months.
Kanamycin, 1,000× stock solution
- 50 mg ml-1 suspended in water.
- Store as 1-ml aliquots at −20°C for up to 6 months.
LB liquid medium
- 5 g L-1 yeast extract (Duchefa Y1333)
- 10 g L-1 NaCl
- 10 g L-1 tryptone
- After autoclaving, medium can be stored under sterile conditions at room temperature for ∼3 months.
Reference: Bertani, 1951.
LB solid medium
- 5 g L-1 yeast extract (Duchefa Y1333)
- 10 g L-1 NaCl
- 10 g L-1 tryptone
- 1 g L-1 Bacto agar (Difco)
- After autoclaving, medium can be stored under sterile conditions at room temperature for ∼3 months.
LB solid plates, X-Gal/IPTG/carbenicillin, 50 µg ml-1
- Add 1 ml each 1,000× carbenicillin, X-gal, and IPGT stocks (see recipes) to 1 L of LB solid medium (see recipe).
LB solid plates, X-Gal/IPTG/kanamycin, 50 µg ml-1
- Add 1 ml each 1,000× kanamycin, X-gal, and IPGT stocks (see recipes) to 1 L of LB solid medium (see recipe).
X-gal, 1,000× stock solution
- 5-Bromo-4-chloro-3-indolyl β-D-galactopyranoside (X-gal), 80 mg ml-1 suspended in dimethyl sulfoxide (DMSO).
- Store as 1-ml aliquots at −20°C for up to 6 months.
COMMENTARY
Background Information
The first genome edited wheat plant obtained through the use of CRISPR-Cas9 was reported by Wang et al. (2014). This was achieved in combination with TALEN genome editing technology to knockout all three subgenomes of the Mlo gene to confer powdery mildew resistance in wheat. CRISPR-Cas9 technology has since been used for targeted knockout of agronomically and scientifically important genes. Traits have been modified using CRISPR-Cas9 which include: lower gluten grain content (Sánchez-León et al., 2018), increased grain weight (Zhang, Li, et al., 2018), meiotic crossover (Rey et al., 2018), reduced preharvest sprouting (Abe et al., 2019), and for haploid plant induction (Liu et al., 2019; Lv et al., 2020).
The CRISPR-Cas9 technology is advancing at a tremendous rate; new discoveries and new applications are reported frequently (Ansari et al., 2020). Multiplexing genome editing in hexaploid wheat was reported by Wang et al. (2018). Three wheat genes, TaGW2 , TaLpx-1 , and TaMLO were successfully targeted using three gRNAs combined in a tRNA array polycistronic cassette controlled transcriptionally by a single TaU3 promoter (Wang et al., 2018). The Cas9 protein itself has undergone developments and new forms with flexible PAMs have been generated, two such derivatives are xCas9 and Cas9-NG (Hu et al., 2018; Nishimasu et al., 2018). Proteins have been fused to Cas9 in order to change single bases, known as base editors (Komor, Kim, Packer, Zuris, & Liu, 2016) or for rewriting short stretches of genetic code deemed prime editing (Anzalone et al., 2019). Base editing in wheat has been performed using a Cas9-cytidine deaminase fusion (Zong et al., 2017) and a Cas9-adenosine deaminase fusion (Li et al., 2018). Primer editing in wheat was recently described by Lin et al. (2020).
Critical Parameters and Troubleshooting
Problems occurring with cloning are generally due to enzymes not being fresh or DNA preparations being of poor quality. In general, if the plates contain mainly blue colonies this would suggest a problem with the restriction enzyme being used. A lack of colonies on the plates would suggest an assembly problem and the first thing to check and/or change would be the ligase. Note, ligase buffer contains the ligase cofactor ATP which is sensitive to thaw and freeze cycles. Contemplate making small aliquots of ligase buffer. If purchasing fresh enzyme stocks does not resolve the problem, consider preparing new DNA stocks. DNA diluted with pure water from a concentrated stock prepared using a midi kit works well.
Time Considerations
Allow ∼4 weeks for construct preparation:
- Week 1: bioinformatics and experimental design;
- Weeks 2 and 3: construct assembly;
- Week 4: sequencing and QA checks.
The wheat transformation takes ∼12 weeks from embryo isolation to the transfer of primary transgenic plants to soil. Allow a further 1 week for plants to establish in soil. Allow a further 3 weeks for screening, consisting of PCRs, sequencing, and data analysis.
Acknowledgments
The authors acknowledge and thank Macarena Forner for excellent technical support. The authors acknowledge and thank Tom Lawrenson of the John Innes Centre for supplying the TaU6 acceptors and Mark Youles of TSL SynBio for supplying L0 Golden Gate components. The authors acknowledge funding support from the Biotechnology and Biological Sciences Research Council (BBSRC) Institute Strategic Programme GEN [BB/P013511/1] to the John Innes Centre.
Author Contributions
Mark A. Smedley : Conceptualization; Investigation; Methodology; Supervision; writing-original draft. Sadiye Hayta : Supervision; writing-review & editing. Martha Clarke : Methodology. Wendy A. Harwood : Funding acquisition; Resources; writing-review & editing.
Conflict of Interest
The authors declare no conflict of interest.
Open Research
Data Availability Statement
Data sharing not applicable–no new data generated.
Literature Cited
- Abe, F., Haque, E., Hisano, H., Tanaka, T., Kamiya, Y., Mikami, M., … Sato, K. (2019). Genome-edited triple-recessive mutation alters seed dormancy in wheat. Cell Reports , 28(5), 1362–1369.e1364. doi: 10.1016/j.celrep.2019.06.090.
- Adamski, N. M., Borrill, P., Brinton, J., Harrington, S. A., Marchal, C., Bentley, A. R., … Uauy, C. (2020). A roadmap for gene functional characterisation in crops with large genomes: Lessons from polyploid wheat. eLife , 9, e55646. doi: 10.7554/eLife.55646.
- Ansari, W. A., Chandanshive, S. U., Bhatt, V., Nadaf, A. B., Vats, S., Katara, J. L., … Deshmukh, R. (2020). Genome editing in cereals: Approaches, applications and challenges. International Journal of Molecular Sciences , 21(11), 4040. doi: 10.3390/ijms21114040.
- Anzalone, A. V., Randolph, P. B., Davis, J. R., Sousa, A. A., Koblan, L. W., Levy, J. M., … Liu, D. R. (2019). Search-and-replace genome editing without double-strand breaks or donor DNA. Nature , 576(7785), 149–157. doi: 10.1038/s41586-019-1711-4.
- Bahassi, E. M., & Stambrook, P. J. (2014). Next-generation sequencing technologies: Breaking the sound barrier of human genetics. Mutagenesis , 29(5), 303–310. doi: 10.1093/mutage/geu031.
- Barrangou, R., & Marraffini, L. A. (2014). CRISPR-Cas systems: Prokaryotes upgrade to adaptive immunity. Molecular Cell , 54(2), 234–244. doi: 10.1016/j.molcel.2014.03.011.
- Bertani, G. (1951). Studies on lysogenesis. I. The mode of phage liberation by lysogenic Escherichia coli. Journal of Bacteriology , 62(3), 293–300. doi: 10.1128/jb.62.3.293-300.1951.
- Borrill, P. (2020). Blurring the boundaries between cereal crops and model plants. New Phytologist , 228, 1721–1727. doi: 10.1111/nph.16229.
- Cram, D., Kulkarni, M., Buchwaldt, M., Rajagopalan, N., Bhowmik, P., Rozwadowski, K., … Kagale, S. (2019). WheatCRISPR: A web-based guide RNA design tool for CRISPR/Cas9-mediated genome editing in wheat. BMC Plant Biology , 19(1), 474. doi: 10.1186/s12870-019-2097-z.
- Gallagher, S. R. (2012). One-dimensional SDS gel electrophoresis of proteins. Current Protocols in Molecular Biology , 97, 10.2A.1-10.2A.44. doi: 10.1002/0471142727.mb1002as97.
- Hanahan, D. (1983). Studies on transformation of Escherichia coli with plasmids. Journal of Molecular Biology , 166(4), 557–580. doi: 10.1016/s0022-2836(83)80284-8.
- Hayta, S., Smedley, M. A., Clark, M., Forner, M., & Harwood, W. A. (2021). An efficient Agrobacterium -mediated transformation protocol for hexaploid and tetraploid wheat varieties. Current Protocols , e58. doi: 10.1002/cpz1.58.
- Hu, J. H., Miller, S. M., Geurts, M. H., Tang, W., Chen, L., Sun, N., … Liu, D. R. (2018). Evolved Cas9 variants with broad PAM compatibility and high DNA specificity. Nature , 556(7699), 57–63. doi: 10.1038/nature26155.
- Komor, A. C., Badran, A. H., & Liu, D. R. (2017). CRISPR-Based technologies for the manipulation of eukaryotic genomes. Cell , 168(1-2), 20–36. doi: 10.1016/j.cell.2016.10.044.
- Komor, A. C., Kim, Y. B., Packer, M. S., Zuris, J. A., & Liu, D. R. (2016). Programmable editing of a target base in genomic DNA without double-stranded DNA cleavage. Nature , 533(7603), 420–424. doi: 10.1038/nature17946.
- Li, C., Zong, Y., Wang, Y., Jin, S., Zhang, D., Song, Q., … Gao, C. (2018). Expanded base editing in rice and wheat using a Cas9-adenosine deaminase fusion. Genome Biology , 19(1), 59. doi: 10.1186/s13059-018-1443-z.
- Lin, Q., Zong, Y., Xue, C., Wang, S., Jin, S., Zhu, Z., … Gao, C. (2020). Prime genome editing in rice and wheat. Nature Biotechnology , 38(5), 582–585. doi: 10.1038/s41587-020-0455-x.
- Liu, H., Wang, K., Jia, Z., Gong, Q., Lin, Z., Du, L., … Ye, X. (2019). Efficient induction of haploid plants in wheat by editing of TaMTL using an optimized Agrobacterium -mediated CRISPR system. Journal of Experimental Botany , 71(4), 1337–1349. doi: 10.1093/jxb/erz529.
- Lv, J., Yu, K., Wei, J., Gui, H., Liu, C., Liang, D., … Kelliher, T. (2020). Generation of paternal haploids in wheat by genome editing of the centromeric histone CENH3. Nature Biotechnology , 38, 1397–1401. doi: 10.1038/s41587-020-0728-4.
- Matsuoka, Y. (2011). Evolution of polyploid Triticum wheats under cultivation: The role of domestication, natural hybridization and allopolyploid speciation in their diversification. Plant and Cell Physiology , 52(5), 750–764. doi: 10.1093/pcp/pcr018.
- Nishimasu, H., Shi, X., Ishiguro, S., Gao, L., Hirano, S., Okazaki, S., … Nureki, O. (2018). Engineered CRISPR-Cas9 nuclease with expanded targeting space. Science , 361(6408), 1259–1262. doi: 10.1126/science.aas9129.
- Ran, F. A., Hsu, P. D., Wright, J., Agarwala, V., Scott, D. A., & Zhang, F. (2013). Genome engineering using the CRISPR-Cas9 system. Nature Protocols , 8(11), 2281–2308. doi: 10.1038/nprot.2013.143.
- Rey, M.-D., Martín, A. C., Smedley, M., Hayta, S., Harwood, W., Shaw, P., & Moore, G. (2018). Magnesium increases homoeologous crossover frequency during meiosis in ZIP4 (Ph1 gene) mutant wheat-wild relative hybrids. Frontiers in Plant Science , 9, 509–509. doi: 10.3389/fpls.2018.00509.
- Rodgers, K., & McVey, M. (2016). Error-prone repair of DNA double-strand breaks. Journal of Cellular Physiology , 231(1), 15–24. doi: 10.1002/jcp.25053.
- Sánchez-León, S., Gil-Humanes, J., Ozuna, C. V., Giménez, M. J., Sousa, C., Voytas, D. F., & Barro, F. (2018). Low-gluten, nontransgenic wheat engineered with CRISPR/Cas9. Plant Biotechnology Journal , 16(4), 902–910. doi: 10.1111/pbi.12837.
- Untergasser, A., Cutcutache, I., Koressaar, T., Ye, J., Faircloth, B. C., Remm, M., & Rozen, S. G. (2012). Primer3—new capabilities and interfaces. Nucleic Acids Research , 40(15), e115–e115. doi: 10.1093/nar/gks596.
- Wang, W., Pan, Q., Fei, H., Akhunova, A., Chao, S., Trick, H., & Akhunov, E. (2018). Transgenerational CRISPR-Cas9 activity facilitates multiplex gene editing in allopolyploid wheat. The CRISPR Journal , 1(1), 65–74. doi: 10.1089/crispr.2017.0010.
- Wang, Y., Cheng, X., Shan, Q., Zhang, Y., Liu, J., Gao, C., & Qiu, J. L. (2014). Simultaneous editing of three homoeoalleles in hexaploid bread wheat confers heritable resistance to powdery mildew. Nature Biotechnology , 32(9), 947–951. doi: 10.1038/nbt.2969.
- Weber, E., Engler, C., Gruetzner, R., Werner, S., & Marillonnet, S. (2011). A modular cloning system for standardized assembly of multigene constructs. PLoS One , 6(2), e16765. doi: 10.1371/journal.pone.0016765.
- Zhang, Y., Li, D., Zhang, D., Zhao, X., Cao, X., Dong, L., … Wang, D. (2018). Analysis of the functions of TaGW2 homoeologs in wheat grain weight and protein content traits. The Plant Journal , 94(5), 857–866. doi: 10.1111/tpj.13903.
- Zhang, Y., Massel, K., Godwin, I. D., & Gao, C. (2018). Applications and potential of genome editing in crop improvement. Genome Biology , 19(1), 210. doi: 10.1186/s13059-018-1586-y.
- Zong, Y., Wang, Y., Li, C., Zhang, R., Chen, K., Ran, Y., … Gao, C. (2017). Precise base editing in rice, wheat and maize with a Cas9-cytidine deaminase fusion. Nature Biotechnology , 35(5), 438–440. doi: 10.1038/nbt.3811.
Corrections
In this publication, author's conflict of interest and data availability statement have been added.
The current version online now includes this information and may be considered the authoritative version of record.
Citing Literature
Number of times cited according to CrossRef: 22
- Vijay K. Tiwari, Gautam Saripalli, Parva K. Sharma, Jesse Poland, Wheat genomics: genomes, pangenomes, and beyond, Trends in Genetics, 10.1016/j.tig.2024.07.004, (2024).
- Adam Gauley, Marianna Pasquariello, Guilherme V. Yoshikawa, Abdul Kader Alabdullah, Sadiye Hayta, Mark A. Smedley, Laura E. Dixon, Scott A. Boden, Photoperiod-1 regulates the wheat inflorescence transcriptome to influence spikelet architecture and flowering time, Current Biology, 10.1016/j.cub.2024.04.029, 34 , 11, (2330-2343.e4), (2024).
- Hirendra Kumar Das, Megha Kaushik, Pranab Kumar Mandal, Inadequate lysine content of wheat endosperm proteins - possibility of correcting it by CRISPR-Cas system of genome editing, Journal of Plant Biochemistry and Biotechnology, 10.1007/s13562-024-00881-5, 33 , 2, (103-107), (2024).
- Alpana Joshi, Seo-Yeon Yang, Hyung-Geun Song, Jiho Min, Ji-Hoon Lee, Genetic Databases and Gene Editing Tools for Enhancing Crop Resistance against Abiotic Stress, Biology, 10.3390/biology12111400, 12 , 11, (1400), (2023).
- Tracie N. Draeger, María-Dolores Rey, Sadiye Hayta, Mark Smedley, Azahara C. Martin, Graham Moore, DMC1 stabilizes crossovers at high and low temperatures during wheat meiosis, Frontiers in Plant Science, 10.3389/fpls.2023.1208285, 14 , (2023).
- Tracie N. Draeger, María-Dolores Rey, Sadiye Hayta, Mark Smedley, Abdul Kader Alabdullah, Graham Moore, Azahara C. Martín, ZIP4 is required for normal progression of synapsis and for over 95% of crossovers in wheat meiosis, Frontiers in Plant Science, 10.3389/fpls.2023.1189998, 14 , (2023).
- Yaqi Jia, Huimin Zhao, Yani Niu, Yucheng Wang, Long noncoding RNA from Betula platyphylla, BplncSIR1, confers salt tolerance by regulating BpNAC2 to mediate reactive oxygen species scavenging and stomatal movement, Plant Biotechnology Journal, 10.1111/pbi.14164, 22 , 1, (48-65), (2023).
- Lauren Crossland-Marr, Alexandru Giurca, Maya Tsingos, Matthew A. Schnurr, Adrian Ely, Dominic Glover, Glenn Davis Stone, Klara Fischer, Siloed discourses: a year-long study of twitter engagement on the use of CRISPR in food and agriculture, New Genetics and Society, 10.1080/14636778.2023.2248363, 42 , 1, (2023).
- María Cecilia Pérez-Pizá, Francisco José Sautua, Agnieszka Szparaga, Andrea Bohata, Sławomir Kocira, Marcelo Aníbal Carmona, New Tools for the Management of Fungal Pathogens in Extensive Cropping Systems for Friendly Environments, Critical Reviews in Plant Sciences, 10.1080/07352689.2023.2268921, 43 , 2, (63-93), (2023).
- Marinus J. M. Smulders, Luud J. W. J. Gilissen, Martina Juranić, Jan G. Schaart, Clemens C. M. van de Wiel, Gene Editing of Wheat to Reduce Coeliac Disease Epitopes in Gluten, A Roadmap for Plant Genome Editing, 10.1007/978-3-031-46150-7_13, (203-222), (2023).
- Domenica Nigro, Mark A. Smedley, Francesco Camerlengo, Sadiye Hayta, Using Gene Editing Strategies for Wheat Improvement, A Roadmap for Plant Genome Editing, 10.1007/978-3-031-46150-7_12, (183-201), (2023).
- Tanushri Kaul, Rachana Verma, Sonia Khan Sony, Jyotsna Bharti, Khaled Fathy Abdel Motelb, Arul Prakash Thangaraj, Rashmi Kaul, Mamta Nehra, Murugesh Eswaran, CRISPR/Cas Enables the Remodeling of Crops for Sustainable Climate‐Smart Agriculture and Nutritional Security, Global Climate Change and Plant Stress Management, 10.1002/9781119858553.ch9, (71-111), (2023).
- Eun Ji Park, Jae-Ryeong Sim, Yu-Jeong Yang, Saet Buyl Lee, Beom-Gi Kim, Sewon Kim, Jong-Yeol Lee, Agrobacterium -Mediated Transformation of Wheat , Korean Journal of Breeding Science, 10.9787/KJBS.2022.54.4.358, 54 , 4, (358-368), (2022).
- Habtamu Kefale, Sewnet Getahun, Gene Editing Improves the Agronomic Important Traits of Wheat – CRISPR-Cas9 and Cas12/Cpf1, Wheat, 10.5772/intechopen.103867, (2022).
- Joseph Oddy, Sarah Raffan, Mark D. Wilkinson, J. Stephen Elmore, Nigel G. Halford, Understanding the Relationships between Free Asparagine in Grain and Other Traits to Breed Low-Asparagine Wheat, Plants, 10.3390/plants11050669, 11 , 5, (669), (2022).
- Leena Tripathi, Kanwarpal S. Dhugga, Valentine O. Ntui, Steven Runo, Easter D. Syombua, Samwel Muiruri, Zhengyu Wen, Jaindra N. Tripathi, Genome Editing for Sustainable Agriculture in Africa, Frontiers in Genome Editing, 10.3389/fgeed.2022.876697, 4 , (2022).
- Jilin Chen, Shaoya Li, Yubing He, Jingying Li, Lanqin Xia, An update on precision genome editing by homology-directed repair in plants, Plant Physiology, 10.1093/plphys/kiac037, 188 , 4, (1780-1794), (2022).
- Ajjamada C. Kushalappa, Niranjan G. Hegde, Kalenahalli N. Yogendra, Metabolic pathway genes for editing to enhance multiple disease resistance in plants, Journal of Plant Research, 10.1007/s10265-022-01409-5, 135 , 6, (705-722), (2022).
- Ramesh Katam, Fatemeh Hasanvand, Vinson Teniyah, Jessi Noel, Virginia Gottschalk, Biosafety Issue Related to Genome Editing in Plants Using CRISPR-Cas9, Genome Editing, 10.1007/978-3-031-08072-2_16, (289-317), (2022).
- Shubham Kumar Sinha, Koppolu Raja Rajesh Kumar, Heat Stress in Wheat: Impact and Management Strategies Towards Climate Resilience, Plant Stress: Challenges and Management in the New Decade, 10.1007/978-3-030-95365-2_13, (199-214), (2022).
- Shaoya Li, Chen Zhang, Jingying Li, Lei Yan, Ning Wang, Lanqin Xia, Present and future prospects for wheat improvement through genome editing and advanced technologies, Plant Communications, 10.1016/j.xplc.2021.100211, 2 , 4, (100211), (2021).
- Sadiye Hayta, Mark A. Smedley, Martha Clarke, Macarena Forner, Wendy A. Harwood, An Efficient Agrobacterium‐Mediated Transformation Protocol for Hexaploid and Tetraploid Wheat, Current Protocols, 10.1002/cpz1.58, 1 , 3, (2021).