Automated Quantification of Subcellular Particles in Myogenic Progenitors
Chloë van Oostende-Triplet, Chloë van Oostende-Triplet, Romina L. Filippelli, Romina L. Filippelli, Natasha C. Chang, Natasha C. Chang, Amr Omer, Amr Omer, Shulei Li, Shulei Li, Imed E. Gallouzi, Imed E. Gallouzi
automated quantification
autophagosome
fluorescence microscopy
messenger ribonucleoprotein granule
myoblast isolation
Abstract
Fluorescence microscopy is a powerful tool enabling the visualization of protein localization within cells. In this article, we outline an automated and non-biased way to detect and quantify subcellular particles using immunocytochemistry, fluorescence microscopy, and the program CellProfiler. We discuss the examination of two types of subcellular particles: messenger ribonucleoprotein (mRNP) granules, namely processing bodies and stress granules, and autophagosomes. Fluorescent microscopy Z-stacks are acquired and deconvolved, and maximum intensity images are generated. The number of subcellular particles per cell is then quantified using the described CellProfiler pipeline. We also explain how to isolate primary myoblast progenitor cells from mice, which were used to obtain the presented results. Last, we discuss the critical parameters to be considered for each of these techniques. Both mRNP granules and autophagosomes play important roles in sequestering intracellular cargo, such as messenger RNAs and RNA-binding proteins for mRNP granules and cytoplasmic waste for autophagosomes. The methods outlined in this article are widely applicable for studies relating to subcellular particle formation, localization, and flux during homeostasis, following stimuli, and during disease. © 2021 The Authors. Current Protocols published by Wiley Periodicals LLC.
Basic Protocol 1 : Immunofluorescence microscopy of messenger ribonucleoprotein granules in primary myoblasts
Alternate Protocol : Immunofluorescence microscopy of autophagosomes in primary myoblasts
Support Protocol : Isolation of primary myoblasts from mice
Basic Protocol 2 : Automated quantification of subcellular particles
INTRODUCTION
Sequestration of cellular constituents allows for the compartmentalization of macromolecules, as well as the timely and integrated regulation of cellular responses to stress and stimuli. mRNA regulation is one crucial mechanism in cell biology for controlling gene expression and enabling rapid and local control over the biological functions of specific mRNA transcripts (Buchan, 2014). mRNA biosynthesis, translation, stability, and localization directly influence mRNA regulation and thus the expression of a given protein (Buchan, 2014; Khong & Parker, 2020). Messenger ribonucleoprotein (mRNP) granules, such as stress granules and processing bodies (P-bodies), self-assemble into visible cytoplasmic structures lacking a limiting membrane (Buchan, 2014). Another example of cellular sequestration is macroautophagy (hereafter referred to as autophagy), a catabolic and intracellular degradative process involving the encapsulation of cellular waste contents into double-membrane vesicles named autophagosomes (Boya, Reggiori, & Codogno, 2013). Both mRNP granules and autophagosomes represent types of subcellular particles that are present during cellular homeostasis and that can be induced by specific stimuli.
Adult stem cells are critically dependent on cellular mechanisms that maintain cellular integrity and homeostasis in order to preserve the regenerative capacity of the stem cell pool throughout life. Muscle stem cells, also known as satellite cells, serve as a useful model for studying stem cell biology. Indeed, mRNA regulation and autophagy are two mechanisms that are critical for satellite cell quiescence and activation. Importantly, dysregulation of the organization and homeostasis of these pathways has been implicated in skeletal myopathies (Chen & Shyu, 2014; Crist, Montarras, & Buckingham, 2012; Margeta, 2020; Mensch & Zierz, 2020). Visualization of these structures by immunofluorescent staining of proteins found within mRNP granules and autophagosomes in fixed cells provides insight into the homeostatic status of these entities as well as into perturbations to their homeostasis in response to stress or disease conditions.
In this article, we outline how to visualize and quantify mRNP granules and autophagosomes in satellite cell–derived myogenic progenitor cells, which are also known as myoblasts (Fig. 1). First, in Basic Protocol 1 and the Alternate Protocol, we describe immunofluorescence protocols to stain against proteins that can be used to identify mRNP granules and autophagosomes, respectively. In the Support Protocol, we outline how to isolate primary myoblasts from the hind limb muscle tissue of mice for use in these experiments. Last, in Basic Protocol 2, we detail an analysis pipeline using CellProfiler for automated quantification of subcellular particles (Carpenter et al., 2006).
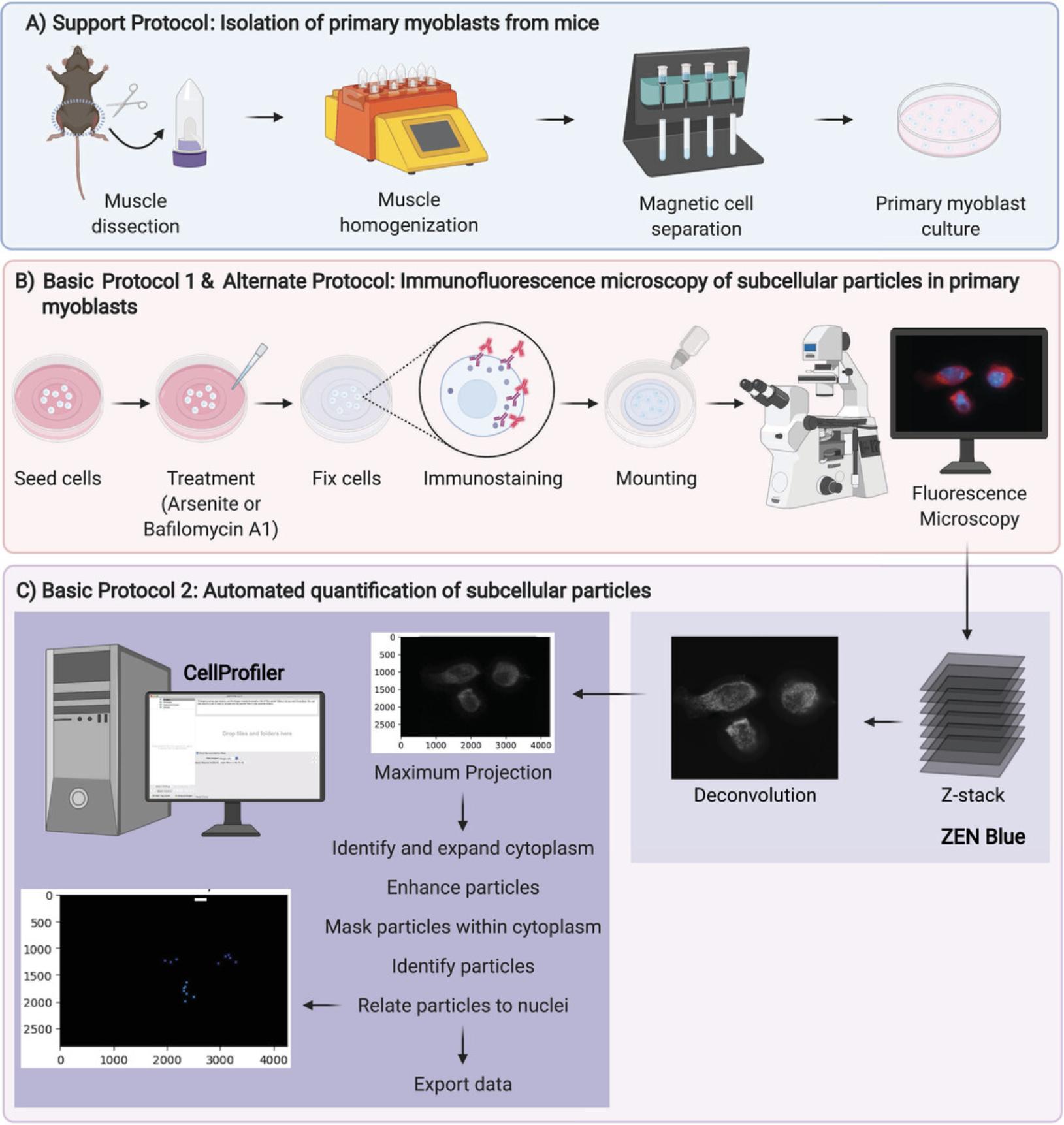
NOTE : All solutions that come into contact with living cells should be sterile, and proper sterile technique should be used when manipulating live cells. We recommend that all solutions be prepared fresh immediately before performing the protocols.
NOTE : Basic Protocol 1 and the Alternate Protocol describe the visualization of mRNP granules and autophagosomes in myogenic progenitor cells. These protocols can be modified and adapted to other stem/progenitor cell types. Additionally, other subcellular entities that can be identified by immunofluorescence are compatible with these protocols.
Basic Protocol 1: IMMUNOFLUORESCENCE MICROSCOPY OF MESSENGER RIBONUCLEOPROTEIN GRANULES IN PRIMARY MYOBLASTS
Immunofluorescent staining of proteins found within mRNP granules allows for the visualization of subcellular mRNP granule homeostasis. Cells are grown on microscopy-optimized plates and treated with sodium arsenite, which induces stress granule formation in mammalian cells in an eIF2α phosphorylation–dependent manner (Bernstam & Nriagu, 2000). Following treatment, the cells are fixed and permeabilized. Primary antibodies are used to bind to the protein of interest, that is, an mRNP granule marker. Subsequently, a secondary antibody, raised against the species of the primary antibody and conjugated to a specific fluorophore, binds to the primary antibody. The localization and quantity of subcellular particles present within a given cell provide insight into a number of aspects related to mRNP granule biology, such as mRNA stability and mRNP granule homeostasis and dynamics.
Materials
-
Collagen solution (see recipe)
-
Primary myoblasts (see Support Protocol)
-
Complete myoblast growth medium (see recipe), 37°C
-
Sterile phosphate-buffered saline (PBS; see recipe)
-
0.125 mM sodium arsenite (Sigma, cat. no. S7400) or vehicle (sterile MilliQ water) diluted in complete myoblast growth medium (see recipe) (optional)
-
Formaldehyde solution (see recipe)
-
IF permeabilization solution (see recipe; make fresh)
-
IF washing solution (see recipe; make fresh), 4°C
-
IF blocking solution (see recipe; make fresh), 4°C
-
Mounting medium with DAPI (ProLong™ Gold Antifade Mountant with DAPI, Thermo Fisher, cat. no. P36935)
-
35-mm imaging plates (35-mm µ-Dish ibiTreat plates, Ibidi, cat. no. 81156)
-
37°C, 5% CO2 humidified incubator
-
Aluminum foil
-
P200 micropipet
-
Tweezers
-
18-mm-diameter circular coverslips (Electron Microscopy Sciences, cat. no. 72222)
-
Inverted microscope with motorized stage allowing Z-stack capability [e.g., Zeiss Axio Observer 7 (Zeiss, cat. no. 491917-0001-000) fitted with Colibri 5 Type RGB-UV Light Source (Zeiss, cat. no. 423052-9640-000) and Axiocam 512 Mono Camera, 12 Megapixel, 14 Bit (Zeiss, cat. no. 426560-9020-000)]
-
Microscope software (Zeiss ZEN Blue, Version 3.2.0.0000)
-
Additional reagents and equipment for preparing cold IF primary antibody solution (see recipe and Table 1) and cold IF secondary antibody solution (see recipe and Table 2)
Antigen | Vendor | Cat. no. | Source | Dilution | Target |
---|---|---|---|---|---|
G3BP1 | Abcam | ab181150 | Rabbit | 1:500 | Stress granules |
DCP1A | Santa Cruz | sc-100706 | Mouse | 1:200 | P-bodies |
RCK | Santa Cruz | sc-376433 | Mouse | 1:100 | Stress granules and P-bodies |
WIPI2 | Bio-Rad | MCA5780GA | Mouse | 1:200 | Autophagosomes |
Antigen | Vendor | Cat. no. | Dilution | Fluorophore |
---|---|---|---|---|
Donkey anti-mouse IgG (H+L) | Invitrogen | A21202 | 1:1000 | Alexa Fluor 488 |
Donkey anti-mouse IgG (H+L) | Invitrogen | A31571 | 1:1000 | Alexa Fluor 647 |
Donkey anti-rabbit IgG (H+L) | Invitrogen | A31573 | 1:1000 | Alexa Fluor 647 |
Cell culture
1.To coat plates, pipet 400 µl collagen solution onto coverslip (inner circular area) of a 35-mm imaging plate, covering its surface area. After 30 min, remove collagen solution and leave plate to dry for ≥2 hr.
2.Seed primary myoblasts in 2 ml pre-warmed complete myoblast growth medium onto collagen-coated plate. Culture cells in a 37°C, 5% CO2 humidified incubator until they reach a confluency of ∼70% (2 to 3 days).
3.Optional: Aspirate medium from the plate and wash cells once with sterile PBS. Add 2 ml of 0.125 mM sodium arsenite or vehicle (sterile MilliQ water) diluted in complete myoblast growth medium (treatment medium) to a total volume of 2 ml and incubate cells for 1 hr in the incubator.
Fixation, permeabilization, and antibody staining
4.Aspirate complete myoblast growth medium from step 2 or treatment medium from step 3 and wash cells once with PBS. Add 400 µl formaldehyde solution to cells on the coverslip in the plate and incubate samples for 20 min at room temperature.
5.Aspirate formaldehyde solution and wash plate three times with PBS for 5 min each.
6.Aspirate PBS and incubate cells on the coverslip with 400 µl IF permeabilization solution for 10 min at room temperature.
7.Aspirate IF permeabilization solution and wash with cold IF washing solution for 10 min at room temperature. Repeat this step for a total of three times.
8.Aspirate IF washing solution and incubate cells on the coverslip with 400 µl cold IF blocking solution for ≥1 hr at room temperature.
9.Using primary antibodies that stain against mRNPs, such as G3BP1, DCP1A, and RCK, prepare cold IF primary antibody solution as indicated in Table 1.Aspirate IF blocking solution from the plate and add 250 µl IF primary antibody solution to cells on the coverslip. Incubate overnight at 4°C.
10.Aspirate IF primary antibody solution from the plate. Wash cells with cold IF washing solution for 10 min at room temperature. Repeat this wash step for a total of three times.
11.Prepare cold IF secondary antibody solution as indicated in Table 2.Aspirate IF washing solution from the plate and add 250 µl to cells on the coverslip. From this point, cover plate in aluminum foil to protect the samples from light. Incubate for 1 hr at room temperature.
12.Aspirate IF secondary antibody solution from the plate. Wash cells with cold IF washing solution for 10 min at room temperature. Repeat this wash step for a total of three times.
Sample mounting and imaging
13.Aspirate IF washing solution and let plate air-dry on an angle for 5 min. Collect any remaining washing solution at bottom of the angled plate with a P200 micropipet. Add one drop of mounting medium with DAPI to center of the coverslip in the plate. With tweezers, place one 18-mm-diameter circular coverslip above the mounting medium and drop at an angle. If air bubbles are trapped under the 18-mm coverslip, tap coverslip gently with tweezers or a P200 micropipet tip to remove them, as air bubbles may interfere with imaging. Dry coverslip overnight at room temperature in the dark. The next day, store samples at 4°C until imaging.
14.Using an inverted microscope with a motorized stage allowing Z-stack capability, capture Z-stacks of samples in the microscope software using the optimal spacing for Z-stacks.
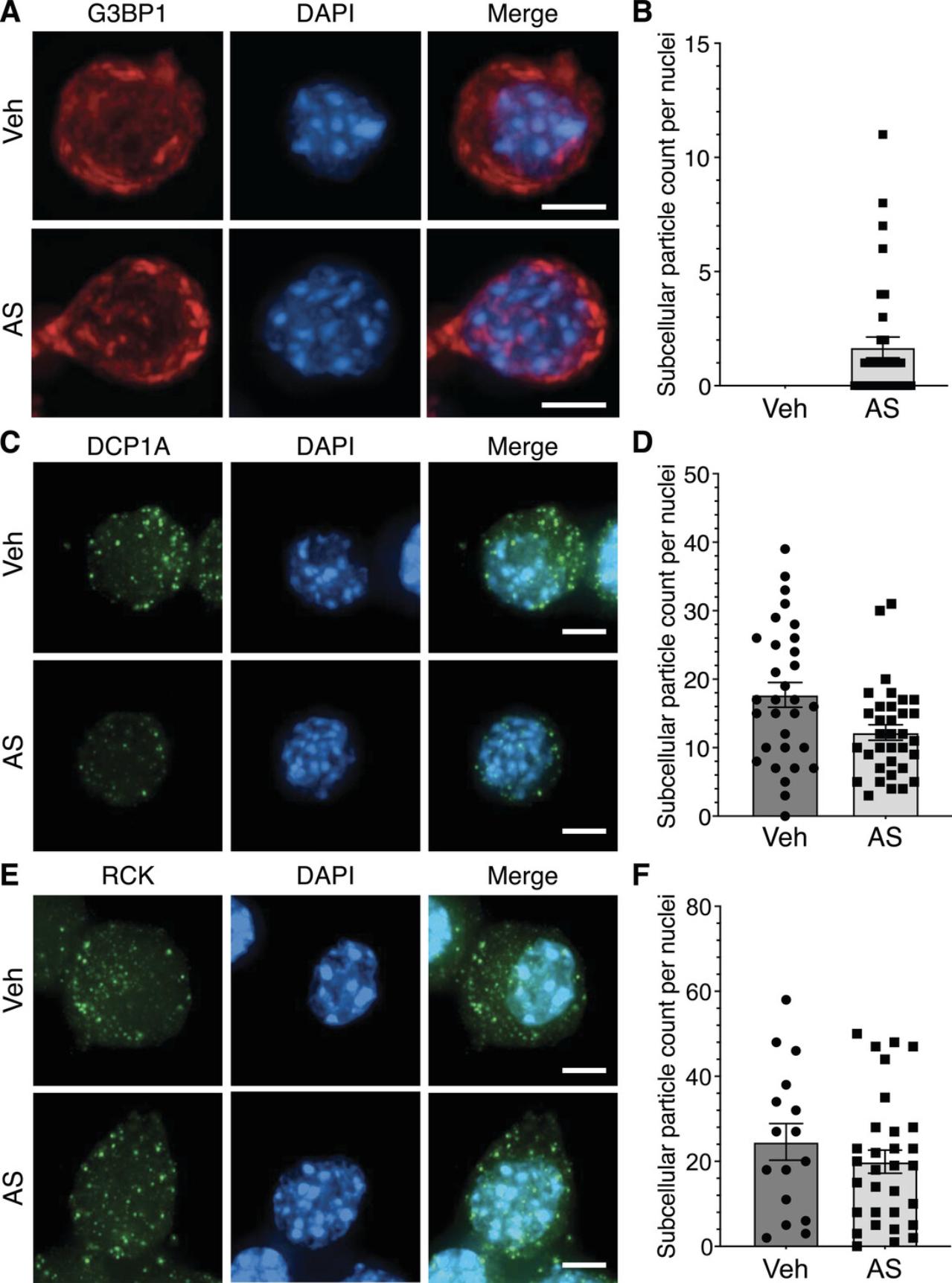
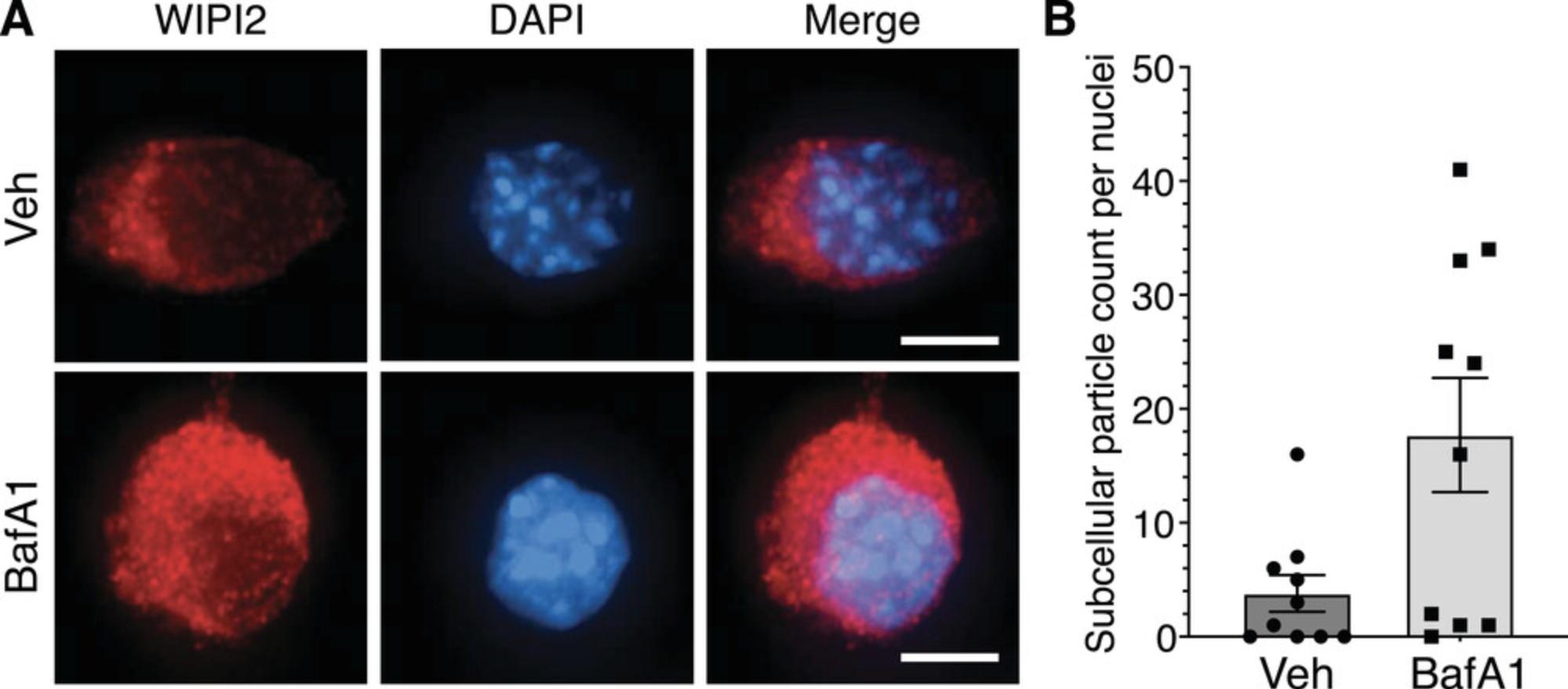
Alternate Protocol: IMMUNOFLUORESCENCE MICROSCOPY OF AUTOPHAGOSOMES IN PRIMARY MYOBLASTS
Autophagy is a catabolic and self-destructive process involving the degradation of intracellular aggregates and damaged organelles (Glick, Barth, & Macleod, 2010). In the case of autophagy, cargo is sequestered within a double-membrane entity known as the autophagosome (Glick et al., 2010). Here, we outline an automated method to count the number of autophagosomes per cell to gain insight into the autophagic state of primary myoblasts. We alter the protocol presented in Basic Protocol 1 to include the inhibition of lysosomal proteases using bafilomycin A1 (BafA1), an inhibitor of the vacuolar-type H+-ATPase, as well as the use of anti-WIPI2 antibody, which identifies autophagosomes, for immunodetection.
Additional Materials (also see Basic Protocol 1)
- 100 nM BafA1 (Sigma, cat. no. 196000, CAS: 88899-55-2) or vehicle (sterile 100% dimethyl sulfoxide; DMSO; BioShop, cat. no. DMS666, CAS: 67-68-5) diluted in complete myoblast growth medium
Cell culture
1.Perform steps 1 and 2 of Basic Protocol 1.
2.Aspirate complete myoblast growth medium from the plate and wash once with sterile PBS. Add appropriate volume of 100 nM BafA1 or vehicle (sterile 100% DMSO) diluted in complete myoblast growth medium (treatment medium) to the plate.
Antibody staining, sample mounting, and imaging
3.Continue with Basic Protocol 1, steps 4 to 8.
4.Using primary antibodies that stain against proteins present in autophagosomes, such as WIPI2, prepare IF primary antibody solution as indicated in Table 1.Aspirate IF blocking solution from the plate and add IF primary antibody solution. Incubate overnight at 4°C.
5.Continue with Basic Protocol 1, steps 10 to 14.
Support Protocol: ISOLATION OF PRIMARY MYOBLASTS FROM MICE
Magnetic-activated cell sorting (MACS) is an efficient and gentle approach for isolating primary cells from tissue. Here, we describe the isolation of primary myoblasts from skeletal muscle tissue obtained from the hind limbs of mice. Dissected muscles are enzymatically digested and homogenized using a gentleMACS Octo Dissociator with Heaters instrument. Muscle homogenates are subjected to filtration and centrifugation, followed by negative cell selection and subsequently positive cell selection using magnetic cell separators. This protocol yields a pure population of primary myoblasts that can be passaged and cultured on collagen-coated tissue culture dishes (Basic Protocol 1 and Alternate Protocol).
Materials
-
PBS (see recipe), sterile, 4°C
-
Collagenase/dispase solution (see recipe)
-
Fetal bovine serum (FBS; Gibco, cat. no. 12483020)
-
MACS buffer (see recipe), 4°C
-
Satellite Cell Isolation Kit, mouse, including satellite cell isolation cocktail (Miltenyi Biotec, cat. no. 130-104-268)
-
Anti-integrin α-7 MicroBeads, mouse (Miltenyi Biotec, cat. no. 130-104-261)
-
Complete myoblast growth medium (see recipe), 37°C
-
Sharp dissection scissors (Fine Science Tools, cat. no. 14060-11 and 14184-09)
-
Non-coated and collagen-coated (see Basic Protocol 1, step 1) 6-cm tissue culture dishes (Fisher, cat. no. 08772B)
-
gentleMACS C tubes (Miltenyi Biotec, cat. no. 130-093-237)
-
gentleMACS Octo Dissociator with Heaters (Miltenyi Biotec, cat. no. 130-096-427)
-
70-μm cell strainer (Falcon, cat. no. 352350)
-
15- and 50-ml conical tubes, sterile (FroggaBio, cat. no. TB15-500 and TB50-500)
-
Tabletop centrifuge
-
LD separation column (Miltenyi Biotec, cat. no. 130-042-901)
-
MidiMACS or QuadroMACS separator (Miltenyi Biotec, cat. no. 130-042-302, 130-090-976)
-
MACS MultiStand (Miltenyi Biotec, cat. no. 130-042-303)
-
MS separation column (Miltenyi Biotec, cat. no. 130-042-201)
-
MiniMACS or OctoMACS separator (Miltenyi Biotec, cat. no. 130-042-102 and 130-042-109)
-
37°C, 5% CO2 humidified incubator
-
Additional reagents and equipment for mouse sacrifice (see Current Protocols article; Donovan & Brown, 2006)
Muscle tissue preparation
1.Sacrifice mice according to institutional animal care guidelines. Using dissection scissors, remove fur and skin of the hind limbs to expose the hind limb muscles.
2.Dissect out hind limb muscles and place muscles in a non-coated 6-cm tissue culture dish filled with cold sterile PBS kept on ice. Remove any visible fur, fat, and bones and avoid contaminating muscle preparation with blood. Transfer muscles to a new non-coated 6-cm tissue culture dish containing PBS to further clean the muscles.
3.Transfer muscles to an empty non-coated 6-cm tissue culture dish and remove as much PBS as possible. Then, transfer muscles to a gentleMACS C tube. Mince muscles directly in the tube with sharp dissection scissors. When the tissue chunks reach ∼2 mm3 in size, add 5 ml collagenase/dispase solution. Continue mincing tissue and rinse scissors in the solution.
4.Secure cap onto the C tube and invert tube, ensuring that all muscle pieces come down into the solution. Insert C tube into the gentleMACS Octo Dissociator with Heaters and place heater element on top of the C tube. Perform a 2-min “Slice and Dice” using the following program parameters: 1.temp ON; 2.loop 10×; 3.spin 360 rpm, 5”; 4.spin –360 rpm, 5”; 5.end loop; 6.end. Then, perform a 25-min “Muscle Digestion” program: 1. temp ON; 2. spin 60 rpm, 3’ 0”; 3. spin –30 rpm, 9’ 0”; 4. loop 10×; 5. spin 360 rpm, 5”; 6. spin –360 rpm, 5”; 7.end loop; 8.spin –30 rpm, 12’ 0”; 9.end. At the end of the program, remove heater element and C tube from the instrument.
Homogenate preparation
5.To quench the digestion, open C tube and add 5 ml FBS. Mix well by pipetting up and down.
6.Place a 70-μm cell strainer on top of a 50-ml conical tube and pre-wet strainer with 2 ml FBS. Add muscle cell suspension gradually to the strainer and collect flow-through. Rinse empty C tube with 8 ml PBS by closing the cap and shaking vigorously to maximize cell yield and pass PBS through the cell strainer. Rinse C tube again with another 8 ml PBS and pass solution through the cell strainer.
7.Transfer filtered cell suspension into two 15-ml conical tubes and centrifuge 10 min at 600 × g. Aspirate fatty floating layer sitting on top of the supernatant and then aspirate supernatant, leaving ∼100 µl of supernatant volume above the pellet. Resuspend each pellet in 100 µl cold MACS buffer and combine the two pellets into one 15-ml conical tube.
Cell labeling and separation
8.For negative cell labeling, add 20 µl satellite cell isolation cocktail (from Satellite Cell Isolation Kit). Mix by gently agitating tube and incubate at 4°C for 15 min. Then, add 300 µl cold MACS buffer to adjust volume of the cell lysate to ∼500 µl.
9.Place an LD separation column in a MidiMACS or QuadroMACS separator placed onto a MACS MultiStand. Add 2 ml cold MACS buffer to column to pre-wet the column.
10.For negative selection, place a clean 15-ml conical tube under column and add 500-µl cell suspension to the column. Collect the unlabeled cells that pass through the column. When there is no more flow-through coming out of the column, wash column with 1 ml cold MACS buffer. Perform a second wash with an additional 1 ml cold MACS buffer. Collect all flow-through in the same tube.
11.Centrifuge cells for 5 min at 600 × g. Aspirate supernatant, leaving ∼100 µl of supernatant volume above pellet. Resuspend cell pellet in 100 µl cold MACS buffer.
12.For positive cell labeling, add 20 µl anti-integrin α-7 MicroBeads. Mix by gently agitating tube and incubate at 4°C for 15 min. Then, add 400 µl cold MACS buffer to adjust volume of the cell lysate to ∼500 µl.
13.Place an MS separation column in a MiniMACS or OctoMACS separator placed onto a MACS MultiStand. Add 500 µl cold MACS buffer to column to pre-wet the column.
14.For positive selection, add 500-µl cell suspension to the column. When there is no more flow-through coming out of the column, wash column three times with 500 µl cold MACS buffer. Discard flow-through, which contains the unlabeled cell fraction.
15.Remove column from the MACS MultiStand and place column into a clean 15-ml conical tube. Add 1 ml cold MACS buffer to column and immediately flush out cells by pushing the provided plunger through the column. Repeat this step with another 1 ml cold MACS buffer and combine the two flow-throughs. Centrifuge cells for 5 min at 600 × g.
16.Remove supernatant, making sure to leave 100 µl of supernatant volume above pellet (which should be barely visible). Resuspend cell pellet in 5 ml pre-warmed complete myoblast growth medium and transfer to a collagen-coated 6-cm tissue culture dish. Incubate cells in a 37°C, 5% CO2 humidified incubator for 2 days.
Basic Protocol 2: AUTOMATED QUANTIFICATION OF SUBCELLULAR PARTICLES
For an unbiased and automated approach to quantifying stress granules, P-bodies, and autophagosomes (referred to as subcellular particles), we use CellProfiler (Carpenter et al., 2006). This software allows for high-throughput analysis of a large number of images to relate subcellular particle count to specific cells following immunofluorescence imaging (Basic Protocol 1 and Alternate Protocol). Deconvolution of Z-stacked images, where each slice from each fluorescent channel is exported as a TIFF, is recommended to allow for particle counts across many focal planes within a cell. Maximum intensity projections of DAPI images and subcellular particle images are generated using slices from the appropriate fluorescent channel in CellProfiler. DAPI staining is used to identify individual nuclei of cells, and subcellular particle layer(s) are also independently split into gray 8-bit images for analysis. The nuclei, using DAPI images, and cytoplasms, using particle images, of individual cells are then identified after smoothing using global Otsu thresholding (Otsu, 1979). Cytoplasms are also expanded to ensure complete encapsulation of each cell's cytoplasm. Once appropriately identified, the 8-bit gray-scaled particle images are enhanced using provided modules within CellProfiler to increase the intensity of particles within each image. The enhanced particles are then overlayed within the expanded cytoplasm to generate a mask to restrict particle counting within each cell. Following this, individual particles are identified based on fluorescence using adaptive Otsu thresholding (used in this case to adjust for non-uniform cytoplasmic staining) (Otsu, 1979). Finally, particle counts are directly related to independent cells using previously identified nuclei, allowing for counting of “particles” per cell, exported into .csv format. Importantly, prior to high-throughput/batch analysis of large numbers of images, 20% of images are randomly selected to calibrate and validate particle counts prior to quantification for each experiment.
Materials
- Zeiss ZEN Blue software (Version 3.2.0.0000)
- Computer
- CellProfiler (download for free at https://cellprofiler.org/releases)
1.To deconvolve Z-stacks, open Zeiss ZEN Blue software on a computer:
-
Open Z-stacks using ZEN image processing within the software.
-
Under the “Processing” header, open “Method” tab. Select “Deconvolution (defaults)” in “Method” tab.
-
Under the “Method Parameters” section of “Processing,” open “Parameters” tab. Select deconvolution method best suited to the given sample.
There are three different deconvolution settings offered: “Simple, very fast (Nearest Neighbor),” “Better, fast (Regularized Inverse Filter),” and “Good, medium speed (Fast Iterative).” Deconvoluted Z-stacks in this paper were generated using “Simple, very fast (Nearest Neighbor)” for presentation purposes and qualitative analysis. However, the “Nearest Neighbor” algorithm is not quantitative so should not be used to quantify fluorescence intensity. To obtain quantitative deconvoluted Z-stacks, we suggest using the “Fast Iterative” algorithm. To learn more about quantitative deconvolution, we refer the reader to the referenced chapter (Goodwin,2014). Deconvolution can also be performed using third-party software, such as the DeconvolutionLab2 plugin for FIJI.
Deconvolution uses model-based methods to increase the sharpness of images by subtracting the out-of-focus fluorescence due to the diffracted light, which causes the typical blur of wide-field images (see Current Protocols article; Biggs,2010; Goodwin,2014). This increases the signal-to-background ratio, which allows for clearer presentation of puncta and thus subcellular particles.
-
Under the “Image Parameters” section of “Processing,” open “Input” tab. Drag desired Z-stack into the “Input” tab.
-
Under the “Image Parameters” section of “Processing,” open “Output” tab. Specify name of the output Z-stack by typing it into the “Custom Output Name” box.
-
Click “Apply” button at the top of the “Processing” display.
-
Save deconvoluted Z-stack in .czi file format. Save each slice of each fluorescent channel within Z-stack as a TIFF.
For faster high-throughput analysis, it is recommended to perform the deconvolution processing in batch format. In the “Processing” tab, select the “Batch” function. Click “+ Add” to select all your images to deconvolve. Under the “Batch method” section, choose “Deconvolution,” which will be applied only to the first image in the table. Select this first image and then select “Copy Parameters.” Select all the other images in the table and select “Paste parameters,” followed by “Run Selected.”
2.To generate maximum intensity images, using CellProfiler (Carpenter et al., 2006), create a maximum intensity image from slices within each Z-stack:
-
In the “Metadata” module, enable metadata extraction. To allow for grouping of multiple files, in “Groups” module, select the appropriate subfolder name to group by metadata category.
-
Create and name maximum projection images using “MakeProjection” module, selecting “Maximum” as the type of projection.
-
Save maximum projection images using “SaveImages” module.
Maximum projection images must be generated prior to feeding these images into the subcellular particle counting pipeline, described in step 3, as the “MakeProjection” module does not become available in subsequent modules until completion of all the image processing cycles.
3.To create the analysis pipeline for subcellular particle counting, using CellProfiler (Carpenter et al., 2006), generate a pipeline for automated image analysis through addition of individual modules made available in CellProfiler using the following steps:
- Identify nuclei by addition of “IdentifyPrimaryObjects” module using the grayscale maximum intensity DAPI image as the input image. Name identified primary objects (here, we use “Nuclei” as an example). Within the “IdentifyPrimaryObjects” module, select “Global Otsu Thresholding” using two-class thresholding.
Smoothing can optionally be used to simplify nucleus identification. Clumped objects can optionally be distinguished by shape.
- Identify cytoplasms by addition of “IdentifySecondaryObjects” module using the grayscale maximum projection image as the input image and using the identified nuclei (see step 3a) as the input objects. Name identified secondary objects (here, we use “Cytoplasm” as an example). Within the “IdentifySecondaryObjects” module, select “Global Otsu Thesholding” using two-class thresholding.
In our work, cytoplasms were identified using the “Watershed” option within the module (Shafarenko, Petrou, & Kittler,1997). Smoothing can optionally be used to simplify cytoplasm identification.
- To ensure complete encapsulation of each cytoplasm, expand size of each cytoplasm by 2 to 5 pixels (depending on the magnification of the image) using the “ExpandOrShrinkObjects” module and using the identified “Cytoplasm” as the input object.
We term the input object “ExpandedCytoplasm.”
- To enhance particles within images, use “EnhanceOrSuppressFeatures” module to apply filtering and opt to enhance the intensity of particle features specifically based on their size.
We name the output image “EnhancedParticles.”
- Overlay “EnhancedParticles” images within the “ExpandedCytoplasm” using the “MaskImage” module to generate a mask to restrict particle counting within each cell.
This image is named “MaskParticles.”
- To identify the individual particles within each image, use “IdentifyPrimaryObjects” module with the “MaskParticles” image as the input image. Name identified primary objects (here, we use “Particles” as an example). Ensure measurement of average diameter and intensity of particles to limit identification. Within the “IdentifyPrimaryObjects” module, select “Adaptive Otsu Thresholding” using two-class thresholding.
We recommend to avoid using smoothing or any distinguishing of clumped objects.
- After particles found within “MaskImage” have been identified, use “RelateObjects” module to directly relate particle counts to independent cells.
More specifically, we use “Nuclei” objects as the parent objects and “Particles” objects as the child objects within the module. Ultimately, this allows for the counting of “Particles” per cell.
- Export counts of particles per cell to .csv format using the “ExportToSpreadsheet” module.
Specifically, the “Nuclei” data should be exported to ensure that the particle-to-cell relationship is being studied. Counts will be separate based on image and the cell within each image.
-
Save pipeline.
4.To run the analysis pipeline for subcellular particle counting, organize deconvoluted Z-stack slices as TIFFs within subfolders, where the subfolder name and file name distinguish the channel (for example, “SubfolderC1” subfolder name and file name prefix “C1_” for Alexa Fluor 488 slices in TIFF format). Drag parent folder into Cell Profiler. Click “Analyze Images” button.
REAGENTS AND SOLUTIONS
Collagenase/dispase solution
- 2.5 g collagenase B from Clostridium histolyticum (Sigma, cat. no. 11088831001)
- 1 g dispase II (neutral protease, grade II; Sigma, cat. no. 04942078001)
- 250 ml Ham's F-10 Nutrient Mix (Gibco, cat. no. 12390035)
- Mix well using magnetic stir bar and stirring plate for ∼20 min
- Filter-sterilize using 0.22-μm filter
- Aliquot (we recommend 10-ml aliquots)
- Store ≤6 months at –20°C
Collagen solution
- 998 ml MilliQ H2O
- 2 ml glacial acetic acid
- Filter-sterilize using 0.2-μm filter
- Add 1 bottle of 100 mg Collagen Type I, Rat Tail (Corning, cat. no. 354236)
- Mix well by inversion
- Aliquot (we recommend 100-ml aliquots)
- Store ≤6 months at 4°C
CAUTION: The acetic acid should be added in the fume hood.
The solution should be filter-sterilized before adding the collagen. The collagen solution can be reused up to five times.
Complete myoblast growth medium
- 400 ml Ham's F-10 Nutrient Mix (Gibco, cat. no. 12390035)
- 100 ml FBS (Gibco, cat. no. 12483020; 20% final)
- 5 ml penicillin-streptomycin solution (Wisent, cat. no. 450-201-EL; 1% final)
- 25 μl 50 ng/μl fibroblast growth factor-basic (bFGF; Gibco, cat. no. PHG0261)
- Store ≤1 month at 4°C
The solution should be made in the tissue culture hood to keep it sterile.
The 50 ng/μl bFGF is made by dissolving 100 μg bFGF powder in 2 ml Ham's F-10 Nutrient Mix. Filter-sterilize the solution with a 0.2-μm filter. Aliquot in 25-μl aliquots and store ≤6 months at –20°C (or at –80°C for long-term storage).
Formaldehyde solution
- One 10-ml vial of 8% (w/v) formaldehyde aqueous solution (Electron Microscopy Sciences, cat. no. 157-8)
- 2 ml 10× PBS
- 8 ml MilliQ H2O
- Store ≤1 month at 4°C
The final solution is 4% (w/v) formaldehyde in 1× PBS.
IF blocking solution
- 45 µl donkey serum (Gibco, cat. no. 16050122)
- 0.18 mg BSA (BioShop, cat. no. ALB001, CAS: 9048-46-8)
- 9 µl 10% Triton X-100 (Bio Basic, cat. no. TB0198)
- 4.5 µl 10% polyoxyethylene-20 (Tween 20; Bio Basic, cat. no. TB0560)
- 1× PBS (see recipe) to 900 µl
- Prepare fresh immediately before use and store at 4°C for duration of experiment
Volumes presented are sufficient for one 35-mm imaging plate (e.g., 35-mm µ-Dish ibiTreat plate). The total volume is sufficient for blocking, primary antibody solution application, and secondary antibody solution application. Volumes should be scaled up depending on the total number of samples. The final solution is 5% (v/v) donkey serum, 2% (w/v) BSA, 0.1% (v/v) Triton X-100, and 0.05% (v/v) Tween 20 in 1× PBS.
IF permeabilization solution
- 4 µl 10% Triton-X-100 (Bio Basic, cat. no. TB0198)
- 40 µl 1 M glycine (Bio Basic, cat. no. GB0235; CAS: 56-40-6)
- 356 µl 1× PBS (see recipe)
- Prepare fresh immediately before use and store at room temperature for duration of experiment
Volumes presented are sufficient for one 35-mm imaging plate (e.g., 35-mm µ-Dish ibiTreat plate). Volumes should be scaled up depending on the total number of samples. The final solution is 0.1% (v/v) Triton X-100 and 0.1 M glycine in 1× PBS.
IF primary antibody solution
- 250 µl IF blocking solution (see recipe)
- Primary antibody (see Table 1)
- Prepare fresh immediately before use and store at 4°C for duration of experiment
The volume presented is sufficient for one 35-mm imaging plate (e.g., 35-mm µ-Dish ibiTreat plate). The volume should be scaled up depending on the total number of samples.
IF secondary antibody solution
- 250 µl IF blocking solution (see recipe)
- Secondary antibody (see Table 2)
- Prepare fresh immediately before use and store at 4°C for duration of experiment
The volume presented is sufficient for one 35-mm imaging plate (e.g., 35-mm µ-Dish ibiTreat plate). The volume should be scaled up depending on the total number of samples.
IF washing solution
- 36 µl donkey serum (Gibco, cat. no. 16050122)
- 3564 µl 1× PBS (see recipe)
- Prepare fresh immediately before use and store at 4°C for duration of experiment
The volume presented is sufficient for one 35-mm imaging plate (e.g., 35-mm µ-Dish ibiTreat plate). The volume should be scaled up depending on the total number of samples. The final solution is 1% (v/v) donkey serum in 1× PBS.
MACS buffer
- 500 ml 1× PBS (see recipe)
- 2 ml 0.5 M EDTA solution (Bio Basic, cat. no. SD8135; 2 mM final)
- 2.5 g BSA (BioShop, cat. no. ALB001; 5% final)
- Filter-sterilize with 0.2 μm filter
- Store ≤1 month at 4°C
Phosphate-buffered saline (PBS)
- 8 g NaCl (137 mM final)
- 0.2 g KCl (2.7 mM final)
- 1.44 g Na2HPO4 (10 mM final)
- 0.24 g KH2PO4 (1.8 mM final)
- Dissolve in 800 ml MilliQ H2O
- Adjust pH to 7.4 with HCl
- MilliQ H2O to 1 L
- Sterilize by autoclaving
- Store ≤1 year at room temperature
This can also be made as a 10× stock and diluted to 1× as needed.
COMMENTARY
Background Information
Examining mechanisms of mRNA regulation through visualization of mRNP granules
mRNA regulation enables rapid changes in protein synthesis at local points of mRNA translation (Buchan, 2014). Furthermore, the regulation of translation and, consequentially, mRNA localization is critical for ensuring mRNA vitality during periods of stress or mRNA decay (Buchan, 2014). In eukaryotic cells, one way that this is attained is through the gathering of non-translating mRNAs bound with RNA proteins, termed mRNPs, into subcellular particles that lack a limiting membrane, known as mRNP granules (Buchan, 2014). Although all mRNP granules share many features, including the ability to direct mRNA harbored within a granule back toward the translation machinery, they can be further classified by their function and their composition of specific RNA-binding proteins (Buchan & Parker, 2009).
P-bodies are cytoplasmic RNA granules that contain the 5’-3’ mRNA decay machinery, proteins related to the nonsense-mediated decay pathway, and the RNA-induced silencing complex (RISC) (Anderson & Kedersha, 2006). P-bodies are conserved in eukaryotes (Luo, Na, & Slavoff, 2018). The discovery and characterization of P-bodies stemmed from the visualization of the mammalian 5’-3’ exonuclease, XRN1, into distinct subcellular foci (Anderson & Kedersha, 2006; Parker & Sheth, 2007). P-bodies are uniform in shape, taking on a spheroid conformation (Anderson & Kedersha, 2006). Along with XRN1, P-bodies also contain enzymes functional within each stage of the mRNA decay pathway, such as the deadenylase complex Ccr4-Not, Lsm1-7, DCP1/2, and RCK decapping enzymes (Anderson & Kedersha, 2006). Mammalian P-bodies additionally function as sites of miRNA-mediated translational silencing, which has not yet been observed in yeast (Buchan, 2014). P-bodies are not the only mechanism mediating mRNA decay, as the decay of transcripts has been observed in yeast strains lacking genes functional for P-body formation (edc3 and lsm4) (Luo et al., 2018). Thus, their function is most likely in serving as a storage unit for translationally repressed mRNAs as well as in sequestration of inactivated mRNA decay enzymes, though this remains a somewhat unanswered question, as mRNA degradation is difficult to observe using direct visualization (Luo et al., 2018).
Stress granules were first discovered in 1989 in heat-shocked tomato cells (Buchan, 2014). In mammalian cells, their formation can be triggered by various sources of environmental stress, including heat, UV irradiation, and hypoxia (Buchan, 2014). Their formation, which occurs minutes after exposure to stress stimuli via liquid-liquid phase separation, is a survival mechanism to repair damage, adapt to changes in growth conditions, and perform quality control (Wheeler et al., 2016). Stress granules are heterogeneous in both size and shape (Anderson & Kedersha, 2006). At their core, stress granules contain the stalled 48S initiation complex; thus, they hold small but not large ribosomal subunits (Buchan, 2014). Stress granules can therefore be described as temporary storage for untranslated mRNAs as a means of translation triage (Buchan, 2014; Sheinberger & Shav-Tal, 2017). Stress granules are in dynamic equilibrium, demonstrated by their inhibition or stimulation of assembly using translation-inhibiting drugs that promote polysome stabilization (such as cycloheximide) or destabilization (such as puromycin) (Buchan, 2014). In addition to stalled preinitiation complexes and mRNAs, stress granules harbor numerous ribosome-binding proteins (RBPs), including poly(A)-binding protein (PABP), fragile X mental retardation protein (FMRP), Au-rich element-binding protein (HuR), and eIF4E (Anderson & Kedersha, 2006; Gallouzi et al., 2000; Kedersha et al., 2002; Kedersha, Gupta, Li, Miller, & Anderson, 1999).
Dysregulation of mRNA stability can be pathological, as mRNAs and the proteins that result from their translation can become aberrantly over-accumulated, as is the case in Alzheimer's disease (Guhaniyogi & Brewer, 2001). Indeed, dysregulation of mRNP granule homeostasis is the underlying cause of numerous degenerative diseases, such as Pagets disease, amyotropic lateral sclerosis (ALS), and multi-system proteinopathy (MSP) (Buchan, 2014). Recent evidence demonstrates that mRNP granules are also implicated in muscle stem cell differentiation (known as myogenesis) and myopathies. The mRNP granule proteins FMRP and DCP1A both play important yet different roles in maintaining muscle stem cell quiescence (Roy et al., 2021). Whereas FMRP-positive subcellular particles are abundant during muscle stem cell quiescence, DCP1A-positive subcellular particles only appear during activation and proliferation of muscle stem cells (Roy et al., 2021). Further, DCP1A knockdown increases cell proliferation and cyclin expression (Roy et al., 2021). Conversely, FMRP knockdown causes decreased proliferation and cyclin expression (Roy et al., 2021).
mRNP granules also play an active role in priming muscle satellite stem cells for myogenesis by influencing the localization of the myogenic regulatory factor transcript Myf5 (Crist et al., 2012). Translation of Myf5 mRNA is prevented in quiescent muscle satellite cells through its sequestration with microRNA-31 (miR-31) within mRNP granules (Crist et al., 2012). Upon satellite cell activation, these mRNP granules dissociate, miR-31 levels are repressed, and Myf5 is translated (Crist et al., 2012). Given the important role that mRNP granules play in myogenesis, it is essential to have tools to enable the visualization of mRNP granule homeostasis in different stem cell contexts. In this article, we outline an automated and unbiased technique for quantifying the number of mRNP granules in myoblasts isolated from mice (Basic Protocols 1 and 2). Isolation of mRNP granules, such as through differential centrifugation and pull-down techniques, is technically challenging. Thus, performing immunofluorescence microscopy is a simple technique to gain insight into the mRNP granule status within a cell. Here, we outline an automated method for quantifying the number of P-bodies and stress granules per cell in a rapid and unbiased fashion (Basic Protocols 1 and 2).
Assessing autophagy through visualization of autophagosomes
Autophagy is an evolutionarily conserved, catabolic, and degradative process named after the Greek word for “self-eating,” through which the cell turns over its own constituents, thereby releasing the building blocks necessary for cellular metabolism (Boya et al., 2013). Autophagy is critical for the maintenance of homeostasis, both on a cellular level and an organism level (Boya et al., 2013). During basal autophagy or after the induction of autophagy, ATG proteins assemble at a location termed the isolation membrane assembly site and coordinate the formation of an isolation membrane, also known as a phagophore (Boya et al., 2013; Mizushima, Yoshimori, & Ohsumi, 2011). The isolation membrane expands with the help of ATG proteins, SNAP receptors (SNAREs), and other tethering factors to encapsulate intracellular cargo (Boya et al., 2013). This results in the formation of the autophagosome, a unique organelle responsible for mediating autophagy (Boya et al., 2013; Mizushima et al., 2011). Cytosolic LC3/ATG8 (LC3-I) is then conjugated to phosphatidylethanolamine (LC3-II) and is recruited to the autophagosome membrane (Tanida, Ueno, & Kominami, 2008). In eukaryotic cells, autophagosomes subsequently fuse with the lysosome, where the cargo sequestered by the autophagosome is degraded by lysosomal acid proteases. This is followed by the return of amino acids and other byproducts to the cytoplasm (Boya et al., 2013).
Microscopy has served as a vital tool in understanding autophagy, as the vacuole in the yeast Saccharomyces cerevisiae , equivalent to the lysosome in mammals, was identified by phase-contrast microscopy (Ohsumi, 2014). LC3 fused with green fluorescent protein (GFP) was the first ATG protein visualized by a fluorescent microscope and provided evidence of the pre-autophagosomal structure (Ohsumi, 2014; Suzuki et al., 2001). Use of fluorescence microscopy to monitor autophagosome formation was revolutionary and empowered the autophagy field with the ability to understand autophagosome formation and localization and therefore autophagy-related protein movement in vivo (Legakis & Klionsky, 2008; Mizushima, Yamamoto, Matsui, Yoshimori, & Ohsumi, 2004; Ohsumi, 2014). Proteins used to identify autophagosomes by fluorescence microscopy include LC3 and WIPI2, a WD-repeat phosphoinositide-interacting protein associated with ATG proteins and required for LC3 conjugation (Dooley et al., 2014; Mizushima & Yoshimori, 2007).
Of note, basal autophagy is active at a constitutive level in all cells and is a dynamic process. Thus, the amount of LC3-II present at a given time is not reflective of autophagic flux (Mizushima & Yoshimori, 2007). If cells are subjected to short periods of starvation, LC3-I levels decrease, whereas LC3-II levels increase (Mizushima & Yoshimori, 2007). If cells are exposed to long periods of starvation, both LC3-I and LC3-II levels are reduced (Mizushima & Yoshimori, 2007). A more accurate representation of autophagic flux is the difference in LC3-II levels between samples in the presence or absence of an inhibitor of autophagic flux (Mizushima & Yoshimori, 2007). BafA1 is a widely used macrolide that inhibits autophagosome-lysosome fusion (Furuchi, Aikawa, Arai, & Inoue, 1993). BafA1 targets the vacuolar H+-ATPase of the lysosome, thereby inhibiting the activation of lysosomal proteases by preventing the passage of protons into the lysosomal lumen and subsequently lysosomal acidification (Furuchi, Aikawa, Arai, & Inoue, 1993; Mauvezin & Neufeld, 2015). Use of BafA1 prevents autophagic degradation of LC3-II, but not LC3-I, and therefore can be used to assess autophagic flux (Mizushima & Yoshimori, 2007).
Autophagy is well implicated in ensuring the proper function and health of skeletal muscle tissue. Skeletal muscles exhibit a high demand for energy production (Sandri, 2010). Autophagy is an important mechanism used to clear cellular waste in skeletal muscle, as the accumulation of dysfunctional organelles and waste byproducts results in muscle atrophy (Masiero et al., 2009). Additionally, during exercise, autophagy is activated alongside tissue remodeling, where autophagy plays a role in supporting muscle plasticity (Lira et al., 2013; Sanchez, Bernardi, Py, & Candau, 2014). Autophagy has also been shown to regulate satellite cell homeostasis during quiescence and is induced during satellite cell activation as a means to provide additional energy (Garcia-Prat et al., 2016; Tang & Rando, 2014). Given autophagy's important role in skeletal muscle, defects in autophagy lead to the impairment of muscle homeostasis, and thus, dysfunctional autophagy is linked to various myopathies (Margeta, 2020). In this article, we outline an automated method for quantifying the number of autophagosomes per cell in the presence of an autophagic flux inhibitor (Alternate Protocol and Basic Protocol 2).
Critical Parameters
Various critical parameters throughout these protocols may impact data quality and reproducibility. Firstly, it is of utmost importance to culture primary myoblasts (Basic Protocol 1, Alternate Protocol, and Support Protocol) under optimal conditions. If primary myoblasts are passaged prematurely (i.e., before reaching 60% to 70% confluency), the cells will show slow growth. Conversely, if primary myoblasts are overgrown and reach a high confluency (above 80% to 90%), the cells may show signs of differentiation, evidenced by an elongated spindle morphology. Moreover, the number of cell passages may influence experimental data. The passage number should be optimized for the specific cell type being studied.
For immunofluorescence experiments (Basic Protocol 1 and Alternate Protocol), we recommend fixing cells when they have reached an adequate confluency (∼70%). If cellular confluency is too low, this impacts the number of cellular events that can be visualized and imaged, resulting in low cell numbers, which may impact the statistical robustness of the results. If samples are fixed at too high of a confluency, the cells will have grown too close to each other to be able to properly discriminate between individual cytoplasms. This will undoubtedly affect the subcellular particle count per cell. After fixation, it is important to keep IF solutions on ice (except for the permeabilization solution, which can be stored at room temperature). We recommend that all solutions be made fresh before performing the protocols.
While imaging, it is vital to maintain the same exposure and LED intensity in a given fluorescent channel across all samples to ensure that intensity and fluorescence are comparable. Moreover, samples should ideally be imaged within the first 2 weeks after staining to prevent fading of the secondary antibody signal. It is also important to image samples that were grouped together (e.g., vehicle versus treated condition) on the same day, as extended time delays between staining and imaging may impact fluorescence intensity.
Once Z-stacks have been obtained at the microscope, we recommend performing deconvolution (Basic Protocol 2). Deconvolved Z-stacks should be saved with a file name that is different from the non-deconvolved counterpart to ensure the maintenance of original data files. Lastly, when using CellProfiler, users of this protocol should manually validate their pipeline with 20% of their images. This allows the pipeline to be tested and adjusted if needed. Moreover, each image should be examined for cells that may need to be rejected prior to CellProfiler analysis. The subcellular particle count of these cells should be removed after running the pipeline to remove outliers.
Troubleshooting
Please see Table 3 for a troubleshooting guide.
Problem | Possible cause | Solution |
---|---|---|
Poor cellular growth on 35-mm imaging plates | Cells may not be exposed to optimal growth conditions | Optimize the coating of the coverslip surface to promote cell growth. Alternatively, cells can be grown directly on coated #1.5 microscopy glass coverslips. Following immunofluorescence, mount the coverslips to a standard microscopy slide. |
Over-saturated speckles present in microscopy image | Primary antibody is too concentrated | Due to lot-to-lot variation, antibody dilution factors may need optimization. Dilute the antibody in IF blocking buffer and test a lower concentration. |
Overlap in signal is observed between fluorescent channels | Wavelengths of secondary antibodies bleed through | It is highly recommended to choose fluorophores that have well-separated spectra. If four channels are necessary, we recommend DAPI, Alexa Fluor 488, Alexa Fluor 568, and Alexa Fluor 647. |
Nuclear DAPI signal is bleeding through in the Alexa Fluor 488 fluorescent channel | Excitation wavelengths of Alexa Fluor 488 and DAPI overlap | Perform a secondary antibody test by staining a sample with DAPI and the Alexa Fluor 488 secondary antibody, but no primary antibody (Test 1). If, after Test 1, there is still a nuclear signal, perform another secondary antibody test by staining a sample with only the Alexa Fluor 488 secondary antibody, and no primary antibody or DAPI (Test 2). If, after Test 2, there is still a nuclear signal, image your sample with a different Alexa Fluor, as either the antibody is exhibiting nonspecific binding or the cells exhibit autofluorescence (which can be tested by imaging a completely unstained sample). |
While imaging, fluorescence of the samples is fading | Samples are being photobleached during imaging process | Decrease the LED intensity while increasing the exposure time to minimize bleaching |
CellProfiler does not recognize subcellular particles and/or nuclei, despite their being visible by eye | Range of nuclei and/or particle size is too narrow | Because the size of nuclei and particles fluctuates across cell lines, their size range may need to be adjusted. Using 20% of the images, adjust this range until an accurate number of particles and/or nuclei is recognized by CellProfiler. |
Signal is too faint or signal-to-background ratio is too small | Ensure that the intensity of the signal emitted by the sample covers ≥30% of the dynamic range of the camera (refer to the histogram) |
Understanding Results
Basic Protocol 1 and the Alternate Protocol use primary myoblasts isolated from mice (Support Protocol) to perform indirect immunofluorescence against protein markers of subcellular particles, which are subsequently quantified using CellProfiler (Basic Protocol 2).
Sodium arsenite treatment (Basic Protocol 1) induces stress granule formation through the creation of oxidative stress, which leads to the phosphorylation of eIF2α and stalled translation (Bernstam & Nriagu, 2000). Stress granules, marked by G3BP1, will be visible under the fluorescent microscope as bright foci in the arsenite-treated condition (Fig. 2A). CellProfiler analysis (Basic Protocol 2) of G3BP1-stained images shows stress granules in the treated condition (Fig. 2B). Stress granule counts for the vehicle condition were not quantified, as stress granules are not present under homeostatic conditions in the absence of stress. P-bodies, visualized by staining against DCP1A, are present under both basal and stress conditions (Luo et al., 2018). Therefore, DCP1A-containing granules will be detected as bright puncta under the microscope, as shown in Figure 2C. CellProfiler quantification of the number of P-bodies per cell shows P-bodies present in both the vehicle- and the arsenite-treated conditions (Fig. 2D). RCK, a dual marker of P-bodies and stress granules, shows bright puncta under the microscope (Fig. 2E). CellProfiler data also show detectable subcellular particles in both the vehicle- and the arsenite-treated conditions (Fig. 2F).
Autophagy occurs at basal levels, so autophagosomes can be detected as fluorescent puncta when stained with anti-WIPI2 antibody in the absence of any treatment (Boya et al., 2013) (Fig. 3A, vehicle condition). BafA1 is an inhibitor of the vacuolar H+-ATPase that is used to prevent autophagosome degradation and visualize autophagic flux (Mizushima & Yoshimori, 2007). Thus, following BafA1 treatment (Alternate Protocol), autophagosomes accumulate in comparison to the vehicle condition and can be visualized under the microscope (Fig. 3A, BafA1 condition). Similarly, CellProfiler quantification (Basic Protocol 2) of both vehicle- and BafA1-treated samples reveals increased cellular autophagosome content following treatment with BafA1 (Fig. 3B).
Time Considerations
Please see Table 4 for time considerations.
Step | Time |
---|---|
Basic Protocol Basic Protocol 1/ Alternate Protocol: Immunofluorescence microscopy of messenger ribonucleoprotein granules or autophagosomes in primary myoblasts | |
Coating of plates | 2.5 hr |
Seeding of cells | 15 min |
Cell treatment | 1 hr |
Formaldehyde fixation | 20 min |
Quenching of formaldehyde | 15 min |
Permeabilization | 10 min |
Washing | 30 min |
Blocking | 1-2 hr |
Primary antibody incubation | Overnight |
Secondary antibody incubation | 1 hr |
Washing | 30 min |
Mounting | Overnight |
Microscopy imaging | 15-20 min per sample |
Support Protocol: Isolation of primary myoblasts from mice | |
Dissection | 5 min per mouse |
Muscle digestion and homogenization | 30 min |
Homogenate filtration and centrifugation | 15 min |
Negative cell labeling | 15 min |
Negative selection and collection | 15 min |
Positive cell labeling | 15 min |
Positive selection and collection | 15 min |
Cell culturing | 2+ days (until cells reach desired confluency) |
Basic Protocol 2: Automated quantification of subcellular particles | |
Deconvolution of Z-stacks | 5 min per Z-stack; batch processing will depend on the number of Z-stacks |
Generation of maximum intensity images | Depends on the number of files |
Creation of the analysis pipeline for subcellular particle counting | 1 hr |
Running of the analysis pipeline for subcellular particle counting | Depends on the number of files |
Acknowledgments
Research in the Chang laboratory is supported by research grants from the Stem Cell Network, Jesse's Journey, Fonds de recherche du Québec - Santé, and the Canadian Institutes of Health Research. RLF is supported by a Maysie MacSporran Studentship from McGill University and a Canada Graduate Scholarships Master's award from the Canadian Institutes of Health Research. NCC is supported by a Chercheurs-boursiers - Junior 1 award from the Fonds de recherche Québec - Santé. Figure 1 was created with BioRender.com.
Author Contributions
Romina L. Filippelli : Conceptualization, data curation, formal analysis, investigation, methodology, writing-original draft, writing-review and editing; Amr Omer : Data curation, formal analysis, investigation, methodology, writing-original draft, writing-review and editing; Shulei Li : Investigation; Chloë van Oostende-Triplet : Methodology, writing-review and editing; Imed E. Gallouzi : Conceptualization, resources, supervision; Natasha C. Chang : Conceptualization, funding acquisition, methodology, resources, supervision, writing-original draft, writing-review and editing.
Conflict of Interest
The authors declare no conflict of interest.
Open Research
Data Availability Statement
The data that support the findings of this study are available from the corresponding author upon reasonable request.
Literature Cited
- Anderson, P., & Kedersha, N. (2006). RNA granules. Journal of Cell Biology , 172(6), 803–808. doi: 10.1083/jcb.200512082.
- Bernstam, L., & Nriagu, J. (2000). Molecular aspects of arsenic stress. Journal of Toxicology and Environmental Health, Part B Critical Review , 3(4), 293–322. doi: 10.1080/109374000436355.
- Biggs, D. S. (2010). 3D deconvolution microscopy. Current Protocols in Cytometry , 52, 12.19.11–12.19.20. doi: 10.1002/0471142956.cy1219s52.
- Boya, P., Reggiori, F., & Codogno, P. (2013). Emerging regulation and functions of autophagy. Nature Cell Biology , 15(7), 713–720. doi: 10.1038/ncb2788.
- Buchan, J. R. (2014). mRNP granules. Assembly, function, and connections with disease. RNA Biology , 11(8), 1019–1030. doi: 10.4161/15476286.2014.972208.
- Buchan, J. R., & Parker, R. (2009). Eukaryotic stress granules: The ins and outs of translation. Molecular Cell , 36(6), 932–941. doi: 10.1016/j.molcel.2009.11.020.
- Buchwalow, I., Samoilova, V., Boecker, W., & Tiemann, M. (2018). Multiple immunolabeling with antibodies from the same host species in combination with tyramide signal amplification. Acta Histochemica , 120(5), 405–411. doi: 10.1016/j.acthis.2018.05.002.
- Carpenter, A. E., Jones, T. R., Lamprecht, M. R., Clarke, C., Kang, I. H., Friman, O. … Sabatini, D. M. (2006). CellProfiler: Image analysis software for identifying and quantifying cell phenotypes. Genome Biology , 7(10), R100. doi: 10.1186/gb-2006-7-10-r100.
- Chen, C. Y., & Shyu, A. B. (2014). Emerging mechanisms of mRNP remodeling regulation. Wiley Interdisciplinary Reviews: RNA , 5(5), 713–722. doi: 10.1002/wrna.1241.
- Crist, C. G., Montarras, D., & Buckingham, M. (2012). Muscle satellite cells are primed for myogenesis but maintain quiescence with sequestration of Myf5 mRNA targeted by microRNA-31 in mRNP granules. Cell Stem Cell , 11(1), 118–126. doi: 10.1016/j.stem.2012.03.011.
- Dooley, H. C., Razi, M., Polson, H. E., Girardin, S. E., Wilson, M. I., & Tooze, S. A. (2014). WIPI2 links LC3 conjugation with PI3P, autophagosome formation, and pathogen clearance by recruiting Atg12-5-16L1. Molecular Cell , 55(2), 238–252. doi: 10.1016/j.molcel.2014.05.021.
- Donovan, J., & Brown, P. (2006). Euthanasia. Current Protocols in Immunology , 13, 1.8.1–1.8.4. doi: 10.1002/0471142735.im0108s73.
- Furuchi, T., Aikawa, K., Arai, H., & Inoue, K. (1993). Bafilomycin A1, a specific inhibitor of vacuolar-type H(+)-ATPase, blocks lysosomal cholesterol trafficking in macrophages. The Journal of Biological Chemistry , 268(36), 27345–27348 doi: 10.1016/S0021-9258(19)74255-0.
- Gallouzi, I. E., Brennan, C. M., Stenberg, M. G., Swanson, M. S., Eversole, A., Maizels, N., & Steitz, J. A. (2000). HuR binding to cytoplasmic mRNA is perturbed by heat shock. Proceedings of the National Academy of Sciences of the United States of America , 97(7), 3073–3078. doi: 10.1073/pnas.97.7.3073.
- Garcia-Prat, L., Martínez-Vicente, M., Perdiguero, E., Ortet, L., Rodríguez-Ubreva, J., Rebollo, E., Ruiz-Bonilla, V. … Muñoz-Cánoves, P. (2016). Autophagy maintains stemness by preventing senescence. Nature , 529(7584), 37–42. doi: 10.1038/nature16187.
- Glick, D., Barth, S., & Macleod, K. F. (2010). Autophagy: Cellular and molecular mechanisms. Journal of Pathology , 221(1), 3–12. doi: 10.1002/path.2697 doi: 10.1002/path.2697.
- Goodwin, P. C. (2014). Quantitative deconvolution microscopy. Methods in Cell Biology , 123, 177–192. doi: 10.1016/B978-0-12-420138-5.00010-0.
- Guhaniyogi, J., & Brewer, G. (2001). Regulation of mRNA stability in mammalian cells. Gene , 265(1-2), 11–23. doi: 10.1016/s0378-1119(01)00350-x.
- Kedersha, N., Chen, S., Gilks, N., Li, W., Miller, I. J., Stahl, J., & Anderson, P. (2002). Evidence that ternary complex (eIF2-GTP-tRNA(i)(Met))-deficient preinitiation complexes are core constituents of mammalian stress granules. Molecular Biology of the Cell , 13(1), 195–210. doi: 10.1091/mbc.01-05-0221.
- Kedersha, N. L., Gupta, M., Li, W., Miller, I., & Anderson, P. (1999). RNA-binding proteins TIA-1 and TIAR link the phosphorylation of eIF-2 alpha to the assembly of mammalian stress granules. Journal of Cell Biology , 147(7), 1431–1442. doi: 10.1083/jcb.147.7.1431.
- Khong, A., & Parker, R. (2020). The landscape of eukaryotic mRNPs. RNA , 26(3), 229–239. doi: 10.1261/rna.073601.119.
- Legakis, J. E., & Klionsky, D. J. (2008). Analysis of autophagosome membrane cycling by fluorescence microscopy. Methods in Molecular Biology , 445, 135–145. doi: 10.1007/978-1-59745-157-4_9.
- Lira, V. A., Okutsu, M., Zhang, M., Greene, N. P., Laker, R. C., Breen, D. S. … Yan, Z. (2013). Autophagy is required for exercise training-induced skeletal muscle adaptation and improvement of physical performance. FASEB Journal , 27(10), 4184–4193. doi: 10.1096/fj.13-228486.
- Luo, Y., Na, Z., & Slavoff, S. A. (2018). P-bodies: Composition, properties, and functions. Biochemistry , 57(17), 2424–2431. doi: 10.1021/acs.biochem.7b01162.
- Margeta, M. (2020). Autophagy defects in skeletal myopathies. Annual Review of Pathology , 15, 261–285. doi: 10.1146/annurev-pathmechdis-012419-032618.
- Masiero, E., Agatea, L., Mammucari, C., Blaauw, B., Loro, E., Komatsu, M. … Sandri, M. (2009). Autophagy is required to maintain muscle mass. Cell Metabolism , 10(6), 507–515. doi: 10.1016/j.cmet.2009.10.008.
- Mauvezin, C., & Neufeld, T. P. (2015). Bafilomycin A1 disrupts autophagic flux by inhibiting both V-ATPase-dependent acidification and Ca-P60A/SERCA-dependent autophagosome-lysosome fusion. Autophagy , 11(8), 1437–1438. doi: 10.1080/15548627.2015.1066957.
- Mensch, A., & Zierz, S. (2020). Cellular stress in the pathogenesis of muscular disorders-from cause to consequence. International Journal of Molecular Sciences , 21(16), 5830. doi: 10.3390/ijms21165830.
- Mizushima, N., Yamamoto, A., Matsui, M., Yoshimori, T., & Ohsumi, Y. (2004). In vivo analysis of autophagy in response to nutrient starvation using transgenic mice expressing a fluorescent autophagosome marker. Molecular Biology of the Cell , 15(3), 1101–1111. doi: 10.1091/mbc.e03-09-0704.
- Mizushima, N., & Yoshimori, T. (2007). How to interpret LC3 immunoblotting. Autophagy , 3(6), 542–545. doi: 10.4161/auto.4600.
- Mizushima, N., Yoshimori, T., & Ohsumi, Y. (2011). The role of Atg proteins in autophagosome formation. Annual Review of Cell and Developmental Biology , 27, 107–132. doi: 10.1146/annurev-cellbio-092910-154005.
- North, A. J. (2006). Seeing is believing? A beginners’ guide to practical pitfalls in image acquisition. Journal of Cell Biology , 172(1), 9–18. doi: 10.1083/jcb.200507103.
- Ohsumi, Y. (2014). Historical landmarks of autophagy research. Cell Research , 24(1), 9–23. doi: 10.1038/cr.2013.169.
- Otsu, N. (1979). A threshold selection method from gray-level histograms. IEEE Transactions on Systems, Man, and Cybernetics , 9(1), 62–66. doi: 10.1109/TSMC.1979.4310076.
- Parker, R., & Sheth, U. (2007). P bodies and the control of mRNA translation and degradation. Molecular Cell , 25(5), 635–646. doi: 10.1016/j.molcel.2007.02.011.
- Roy, N., Sundar, S., Pillai, M., Patell-Socha, F., Ganesh, S., Aloysius, A., Rumman, M. … Dhawan, J. (2021). mRNP granule proteins Fmrp and Dcp1a differentially regulate mRNP complexes to contribute to control of muscle stem cell quiescence and activation. Skeletal Muscle , 11(1), 18. doi: 10.1186/s13395-021-00270-9.
- Sanchez, A. M., Bernardi, H., Py, G., & Candau, R. B. (2014). Autophagy is essential to support skeletal muscle plasticity in response to endurance exercise. American Journal of Physiology Regulatory, Integrative and Comparative Physiology , 307(8), R956–969. doi: 10.1152/ajpregu.00187.2014.
- Sandri, M. (2010). Autophagy in skeletal muscle. FEBS Letters , 584(7), 1411–1416. doi: 10.1016/j.febslet.2010.01.056.
- Shafarenko, L., Petrou, M., & Kittler, J. (1997). Automatic watershed segmentation of randomly textured color images. IEEE Trans Image Process , 6(11), 1530–1544. doi: 10.1109/83.641413.
- Sheinberger, J., & Shav-Tal, Y. (2017). mRNPs meet stress granules. FEBS Letters , 591(17), 2534–2542. doi: 10.1002/1873-3468.12765.
- Suzuki, K., Kirisako, T., Kamada, Y., Mizushima, N., Noda, T., & Ohsumi, Y. (2001). The pre-autophagosomal structure organized by concerted functions of APG genes is essential for autophagosome formation. EMBO Journal , 20(21), 5971–5981. doi: 10.1093/emboj/20.21.5971.
- Tang, A. H., & Rando, T. A. (2014). Induction of autophagy supports the bioenergetic demands of quiescent muscle stem cell activation. EMBO Journal , 33(23), 2782–2797. doi: 10.15252/embj.201488278.
- Tanida, I., Ueno, T., & Kominami, E. (2008). LC3 and autophagy. Methods in Molecular Biology , 445, 77–88. doi: 10.1007/978-1-59745-157-4_4.
- Wheeler, J. R., Matheny, T., Jain, S., Abrisch, R., & Parker, R. (2016) Distinct stages in stress granule assembly and disassembly. elife , 5, e18413. doi: 10.7554/eLife.18413.
Citing Literature
Number of times cited according to CrossRef: 1
- Cordell A. VanGenderen, Jules A. Granet, Romina L. Filippelli, Yiyang Liu, Natasha C. Chang, Modulating Myogenesis: An Optimized In Vitro Assay to Pharmacologically Influence Primary Myoblast Differentiation, Current Protocols, 10.1002/cpz1.565, 2 , 9, (2022).