Activation of Dendritic Cells Isolated from the Blood of Patients with Prostate Cancer by Ex Vivo Fluid Shear Stress Stimulation
Jenna A. Dombroski, Jenna A. Dombroski, Monika Antunovic, Monika Antunovic, Kerry R. Schaffer, Kerry R. Schaffer, Paula J. Hurley, Paula J. Hurley, Michael R. King, Michael R. King
Abstract
Prostate cancer is one of the most common cancers among men in the United States and a leading cause of cancer-related death in men. Treatment options for patients with advanced prostate cancer include hormone therapies, chemotherapies, radioligand therapies, and immunotherapies. Provenge (sipuleucel-T) is an autologous cancer-vaccine-based immunotherapy approved for men with asymptomatic or minimally symptomatic metastatic castration-resistant prostate cancer (mCRPC). Administration of sipuleucel-T involves leukapheresis of patient blood to isolate antigen-presenting cells (APCs), including dendritic cells (DCs), and subsequent incubation of isolated APCs with both an antigen, prostatic acid phosphatase (PAP), and granulocyte macrophage-colony stimulating factor (GM-CSF) before their infusion back into the patient. Although sipuleucel-T has been shown to improve overall survival, other meaningful outcomes, such as prostate-specific antigen (PSA) levels and radiographic response, are inconsistent. This lack of robust response may be due to limited ex vivo activation of DCs using current protocols. Earlier studies have shown that many cell types can be activated ex vivo by external forces such as fluid shear stress (FSS). We hypothesize that novel fluid shear stress technologies and methods can be used to improve ex vivo efficacy of prostate cancer DC activation in prostate cancer. Herein, we report a new protocol for activating DCs from patients with prostate cancer using ex vivo fluid shear stress. Ultimately, the goal of these studies is to improve DC activation to expand the efficacy of therapies such as sipuleucel-T. © 2023 The Authors. Current Protocols published by Wiley Periodicals LLC.
Basic Protocol 1 : Sample collection and DC isolation
Basic Protocol 2 : Determination and application of fluid shear stress
Basic Protocol 3 : Flow cytometry analysis of DCs after FSS stimulation
INTRODUCTION
One in eight men in the United States will be diagnosed with prostate cancer in his lifetime (Siegel et al., 2023). As with other cancers, 5-year survival rates vary based on stage, dropping from over 99% for localized disease to 32% for advanced prostate cancer (National Cancer Institute, 2022). Mortality was heavily reduced after 1993 by early detection through prostate-specific antigen (PSA) screening, but this decline has severely slowed more recently due to a reduction in screening for PSA (Siegel et al., 2023). Current therapies for advanced prostate cancer include hormone therapies, chemotherapies, radioligand therapies, and immunotherapies (Chen & Zhao, 2013; Litwin & Tan, 2017; Rodrigues et al., 2014; Sumanasuriya & De Bono, 2018). Androgen-deprivation therapies, which commonly improve disease prognosis and reduce reported cancer symptoms, are successful for an average of 18-36 months before castration resistance emerges (Sumanasuriya & De Bono, 2018). Despite advances in prostate cancer therapies, improving the effectiveness of current treatments remains an ongoing need in the field, particularly for patients who have metastatic prostate cancer.
In mCRPC, following castration, chemotherapy is often the first line of treatment, but the duration of treatment is often limited either by toxicities or by the development of chemotherapy-refractory cancer (Hashemi et al., 2023; Posdzich et al., 2023). Chemotherapies have shown better results when used in combination with immunotherapy and radiotherapy (Posdzich et al., 2023). Radiation therapies have demonstrated effectiveness in clinical trials, but problems remain, such as patient resistance and potential for toxicity (Gafita et al., 2022; Hennrich & Eder, 2022; Wang et al., 2022). Recently, strides have been made in advanced prostate cancer therapeutics with the development of Pluvicto (177Lu-PSMA-617; Parent & Kase, 2022), which was approved by the FDA as the first radioligand therapy for prostate cancer (Hennrich & Eder, 2022). Pluvicto is a beta-emitter theranostic involving PSMA-617, a PSMA-specific peptidomimetic, which directly targets PSMA+ prostate cancer cells and allows the therapeutic to be endocytosed, after which the radionuclide, lutetium1-77, causes DNA damage within the cell and surrounding cells (Hennrich & Eder, 2022). Although many patients benefit from Pluvicto, a proportion of those on Pluvicto therapy demonstrate de novo or acquired resistance (Gafita et al., 2022; Wang et al., 2022). Because of the limited effectiveness and known cumulative toxicities of these currently approved therapies, there is now interest in combining chemotherapies and radioligand therapies with immunotherapy, given the potential to generate neoantigens with both chemotherapy and radiation therapy, and also acknowledging the durable potential of immunotherapy. Patients with either chemotherapy- or radioligand-therapy-resistant mCRPC may be ideal candidates for novel immunotherapeutic approaches.
Provenge (sipuleucel-T), the first cancer immunotherapy authorized by the U.S. Food and Drug Administration (FDA), was approved in 2010 for the treatment of men who have metastatic castration-resistant prostate cancer (mCRPC) with no or few symptoms (Anassi & Ndefo, 2011). Sipuleucel-T is an autologous antigen-presenting cell (APC) cancer vaccine that works by activating a patient's immune cells after patient APCs are isolated, cultured with GM-CSF and the antigen prostatic acid phosphatase (PAP) ex vivo , and then reintroduced back into the patient for enhanced lymphoid homing and T cell priming (Anassi & Ndefo, 2011; Wei et al., 2015). Although sipuleucel-T increases overall survival in patients with mCRPC, its effectiveness remains limited because of several factors, including marrow suppression due to disease infiltration and systemic treatments causing limited marrow reserve and potential for DC activation (Hafron et al., 2022; He et al., 2023; Kantoff et al., 2010; Wang et al., 2022). DC-based immunotherapies hold enormous potential as therapeutics because they enhance T cell priming and homing to lymphoid organs, allowing more effective cancer cell targeting and killing (Gu et al., 2020). In recent work, we assessed the ability of a novel fluid shear stress protocol to activate DCs from patients with mCRPC who are receiving one of two approved, robust systemic therapies: chemotherapy and radioligand therapy. A number of immunotherapies and other systemic therapies for advanced prostate cancer are currently under development. As these move forward, it will be critical to better understand the previous lack of robust impact from immunotherapy on prostate cancer and to identify potential mechanisms by which to enhance immune activation in patients.
Notably, immunotherapeutic strategies may have increased efficacy in more advanced mCRPC with improved DC ex vivo activation. These pitfalls motivate the development of a more effective protocol for the ex vivo activation of a cancer patient's immune cells, which could circumvent many of the difficulties with radiotherapies. Studies have shown that cells can be activated by forces, and our laboratory has demonstrated that immune cells such as T cells respond to fluid shear stress (FSS; Hagihara et al., 2004; Hope et al., 2022; Yamamoto et al., 2000). Cells sense and respond to forces via the opening of mechanosensitive ion channels (MSCs), which allow an influx of ions and thereby enable a host of responses (Haswell et al., 2011; Dombrowski et al., 2023). In our study, we aimed to determine whether DCs isolated from cancer patients could be activated ex vivo within a cone-and-plate flow device, and we analyzed how specific subpopulations of DCs respond to FSS.
Provided below are three sequential protocols, developed as part of that study, for the collection, isolation, and FSS stimulation of human-derived DCs followed by the analysis of their state of activation. In these protocols, whole blood is collected from a cohort of patients, peripheral blood mononuclear cells (PBMCs) are isolated from the collected whole blood, DCs are isolated from that population according to manufacturer's instructions, and those cells are stimulated with FSS for subsequent flow cytometry assays. Our results demonstrated the activation of specific subsets of DCs due to this FSS stimulation.
Strategic Planning
Cell culture
Dendritic cells (DCs) are suspended and/or grown in culture medium consisting of RPMI-1640 (Gibco) supplemented with 10% fetal bovine serum (FBS) and penicillin-streptomycin (P/S). Cells are maintained at 37°C with 5% CO2.
Whole blood collection
In our study applying these protocols, deidentified information on prostate cancer patients was collected in the Department of Medicine at Vanderbilt University Medical Center, Nashville, Tennessee, from September 2021 to January 2023. The information collected includes age of diagnosis, age at time of sample collection, self-reported race and ethnicity, treatment course, Gleason score at diagnosis (this refers to the grading system used for the patients with prostate cancer and is correlated with prognosis), and site(s) of metastatic disease (Tagai et al., 2019). The cohort consisted of eight patients with heavily treated prostate adenocarcinoma (see Table 1), including four patients receiving radioligand therapy.
Patient | Race | Ethnicity | Age at diagnosis | Age at sample collection | Gleason | Disease site(s) | Prior treatments (in order, following castration)a | Summary of treatment history | PSA at time of collection |
---|---|---|---|---|---|---|---|---|---|
K1 | CA | NR | 58 | 73 | 4 + 4 = 8 | Bone, liver | Xtandi Abiraterone Docetaxel | Discontinued chemotherapy, on ADT monotherapy at time of collection | NR |
K2 | CA | NH | 58 | 64 | 4 + 3 = 7 | Bone, LN | Darolutamide Docetaxel and carboplatin | On chemotherapy at time of collection | NR |
K3 | CA | NH | 67 | 79 | 4 + 3 = 7 | Bone, LN | Apalutamide Abiraterone Docetaxel | On chemotherapy at time of collection | NR |
K5 | CA | NR | 76 | 78 | 5 + 4 = 9 | Bone, liver | Xtandi Radium-223 Docetaxel | On chemotherapy at time of collection | NR |
K14 | CA | NH | NR | 69 | 4 + 5 = 9 | LN | Zytiga with PARPi Sipuleucel T Xtandi Docetaxel | On 177Lu at time of collection | NR |
K15 | CA | NH | 74 | 84 | 3 + 4 = 7 | Bone, LN | Sipuleucel T Xtandi Abiraterone Docetaxel 177Lu | On 177Lu at time of collection | NR |
K17 | CA | NH | 60 | 78 | 3 + 4 = 7 | Bone, LN, liver | Zytiga Xtandi Docetaxel Cabazitaxel Sipuleucel T | On 177Lu at time of collection | 6,667 |
K18 | CA | NH | 76 | 81 | 5 + 4 = 9 | Bone | Sipuleucel T Zytiga Xtandi Radium-223 Docetaxel 177Lu | On 177Lu at time of collection | 26,000 |
- a All patients were castrated via orchiectomy or androgen-deprivation therapy.
- CA, Caucasian; NH, non-Hispanic; NR, not reported; LN, lymph node; PARPi, poly(ADP-ribose) polymerase inhibition; PSA, prostate-specific antigen; 177Lu, lutetium (177Lu) vipivotide tetraxetan.
Human dendritic cell isolation
All experimental protocols involving humans or human tissue samples were approved by Vanderbilt University Institutional Review Board (IRB), Protocol #210748. All patients provided written informed consent before enrollment in the study and sample collection. All methods were carried out in accordance with relevant guidelines and regulations, and patient samples remained deidentified throughout the entire study. The cohort consisted of prostate cancer patients undergoing laboratory testing at the Vanderbilt-Ingram Cancer Center (VICC) in Nashville, Tennessee.
NOTE : All experimental protocols involving humans or human tissue samples should be approved by the appropriate IRB(s) for your institution and location, and informed consent must be obtained from the individuals involved.
Basic Protocol 1: SAMPLE COLLECTION AND DC ISOLATION
In this protocol, we collect samples of whole blood from a cohort of patients with prostate cancer. Peripheral blood mononuclear cells (PBMCs) are isolated from this whole blood via differential centrifugation, and DCs are subsequently isolated from that population using a magnetic bead isolation kit.
Materials
-
CAS 9005-64-5 (P1379) Ficoll-Paque PLUS (GE17-1440-03; Sigma-Aldrich)
-
Blood Dendritic Cell Isolation Kit II, human (130-091-379; Miltenyi Biotec)
-
HBSS (Gibco)
-
RPMI medium: RPMI-1640 (Invitrogen)/10% fetal bovine serum (FBS) with penicillin/streptomycin
-
Vacutainer blood collection tubes (366643; BD)
-
15-ml conical tubes
-
Serological pipettor
-
Serological pipets
-
Centrifuge: e.g., Thermo Sorvall ST16
-
Pipettor
-
Pipet tips
1.Draw ∼8 ml of whole blood per patient into BD Vacutainer collection tubes containing 3.2% sodium citrate (BD 366643).
2.Isolate PBMCs via gradient centrifugation using Ficoll-Paque. Pipet 4 ml Ficoll-Paque into each of two separate 15-ml conical tubes.
-
Centrifuge 20 min at 2000 ×g(at 6 acceleration and 2 deceleration in Thermo Sorvall ST16 centrifuge), room temperature.
-
Carefully pipet the buffy coat (cloudy layer) out of the tube and into a new tube.
-
Add HBSS to the buffy coat and centrifuge 5 min at 300 ×g, room temperature, with standard acceleration and deceleration.
-
Remove the supernatant and proceed to step 3 with the cell pellet.
3.Separate the DCs from the PBMCs isolated in the previous step using the manufacturer's directions for magnetic bead isolation (Miltenyi Biotec's Blood Dendritic Cell Isolation Kit II).
-
Count cells and resuspend the pellet based on the desired concentration of cells.
-
Perform first isolation, negatively selecting for non-DC populations.
Count cells after this step.
- Perform second isolation, positively selecting for DCs.
Count cells after this step.
- Resuspend DCs in RPMI medium at 1 × 105cells/ml.
Cells are immediately processed.
Basic Protocol 2: APPLICATION OF FLUID SHEAR STRESS TO CELL SAMPLES
In this protocol, we stimulate previously isolated human DCs with fluid shear stress (FSS). The protocol includes appropriate controls to account for any activation that could potentially be a result of the instrument setup as opposed to the applied FSS. This protocol is straightforward, with no anticipated difficulties.
Materials
-
70% (v/v) ethanol
-
5.0% (w/v) bovine serum albumin (BSA; Sigma-Aldrich)
-
DC suspension in RPMI (Basic Protocol 1, step 3d)
-
RPMI medium: RPMI-1640 (Invitrogen) containing 10% (v/v) fetal bovine serum (FBS; Invitrogen) and penicillin/streptomycin
-
Cone-and-Plate Viscometers (Brookfield)
-
Cone spindles (CPA-41Z; Brookfield)
-
15-ml conical tubes
-
Serological pipettor
-
Serological pipets
-
Pipettor
-
Pipet tips
-
12-well plates (optional)
Activation of DCs using shear stress
1.Clean cone-and-plate flow devices and spindles using 70% ethanol. Use two cone-and-plate devices per patient (one will be turned on for stimulation and one will remain stationary for an unstimulated control condition).
2.Calibrate the cone-and-plate flow device according to the manufacturer's directions using CPA-41Z cone spindles.
3.Block all cone-and-plate flow devices with 2 ml of 5.0% BSA for 1 hr in order to prevent nonspecific binding.
4.Pipet 2 ml of DC suspension in RPMI into the plate of each corresponding cone-and-plate flow device. Secure the cone and plate.
5.Stimulate cells with 5 dyn/cm2 FSS by turning on the flow device at 100 rpm.
6.Cells may now be immediately processed by transferring them using a pipet to correspondingly labeled 1.5-µl microcentrifuge tubes (see Basic Protocol 3 for following steps). For experiments being performed after 4 or more hours, cells should be plated into a 12-well plate and incubated at 37°C (see Basic Protocol 3 for following steps).
7.For representative data to confirm the correct execution of these procedures, see the final step of Basic Protocol 3, below.
Basic Protocol 3: FLOW CYTOMETRY ANALYSIS OF DCs AFTER FSS STIMULATION
In this protocol, DCs stimulated immediately after being collected from the cone-and-plate flow device, or after being lifted and washed following an overnight incubation, are analyzed (along with unstimulated control DCs) via flow cytometry. This procedure is used to assess their expression of costimultory molecules required for effective DC priming of T cells for attack of cancer cells. This protocol is performed following Basic Protocol 2, step 7. For cells plated for 24 h, first follow steps 1a-c to lift the cells. To immediately begin working with the cells, proceed to step 2a or 2b.
Materials
-
FSS-simulated cells (from Basic Protocol 2) in 1.5-µl microcentrifuge tubes (from any preferred plastics supplier; preferably easy-open tubes to facilitate working with many tubes at a time)
-
RPMI medium: RPMI-1640 (Invitrogen) with fetal bovine serum (FBS; Invitrogen) and penicillin/streptomycin
-
0.25% trypsin (Gibco)
-
Hank's balanced salt solution (HBSS) without calcium and magnesium (Gibco)
-
32% paraformaldehyde aqueous solution (PFA; Electron Microscopy Sciences)
-
100% methanol, 4°C
-
Bovine serum albumin (BSA; Sigma-Aldrich)
-
PE-phospho-NF-κB p65 (Ser529; Thermo Fisher) or PE-phospho-c-Fos (AP-1; Ser32; cFosS32-BA9; Thermo Fisher)
-
Anti-human PE-CD40 (5C3; Biolegend), anti-human PE-CD80 (305207; Biolegend), or PE-anti-CD86 human monoclonal (305438; BioLegend)
-
Appropriate sample tubes
-
96-well U-bottom plate
-
Flow cytometer
1.Prepare the FSS-simulated cells for staining by one of two methods: if you are evaluating an intracellular target, proceed to step 2a; if evaluating an extracellular target, proceed to step 2b. This step is performed either immediately after FSS application or after 24 hr. In the latter case, to analyze cell response after 24 hr, perform the following actions before proceeding to step 2a or 2b.
-
Pipet cell suspensions into correspondingly labeled tubes, add 200 µl of 0.25% trypsin per tube to lift the cells, and let sit for 5 min. Neutralize the reaction with 200 µl RPMI and then pipet the cells into labeled tubes.
-
Centrifuge the cells for 5 min at 300 ×g, room temperature.
-
Wash cells in 1 ml HBSS and centrifuge for 5 min at 300 ×g, room temperature.
2a. For intracellular staining of proteins such as phospho-NF-κB and phospho-c-Fos, use the following protocol:
-
Fix cells for 10 min with 4% PFA in HBSS.
-
Centrifuge 5 min at 300 ×g, room temperature.
-
Wash cells with 1 ml HBSS.
-
Centrifuge 5 min at 300 ×g, room temperature.
-
Permeabilize cells with 1 ml of 100% methanol (4°C).
-
Wash cells with 1 ml HBSS.
-
Prepare the following antibody cocktail (per sample): 3:100 phospho-NF-κBor2:100 phospho-c-Fos in 100 µl of 1% BSA. Add to the cells and allow cells to stain for 15 min in the absence of light.
-
Wash cells with 1 ml HBSS.
-
Resuspend pellet in 250 µl HBSS. Pipet into a 96-well U-bottom plate for flow cytometry analysis.
2b. For extracellular staining (after 24 hr) of proteins such as CD40, CD80, or CD86, use the following:
-
Wash cells with 1 ml HBSS.
-
Prepare the following antibody cocktail: 1:100 CD40, CD80,orCD86 in 100 µl of 1% BSA. Add to cells and allow cells to stain for 15 min in the absence of light.
-
Wash cells with 1 ml HBSS.
-
Resuspend pellet in 250 µl HBSS. Pipet into a 96-well U-bottom plate for flow cytometry analysis.
3.Load 96-well plate with 250 µl cell suspensions into the flow cytometer, detecting at 575-nm wavelength corresponding to each of the PE-conjugated antibodies. Collect cells at 10,000 events and gate for DCs.
4.After loading cells into the flow cytometer, gate in forward scatter and side scatter, and then gate for specific populations such as NF-κB or specific DC populations of interest. An example of results obtained using this gating strategy for a typical data set derived from healthy donors can be seen in Figure 1.
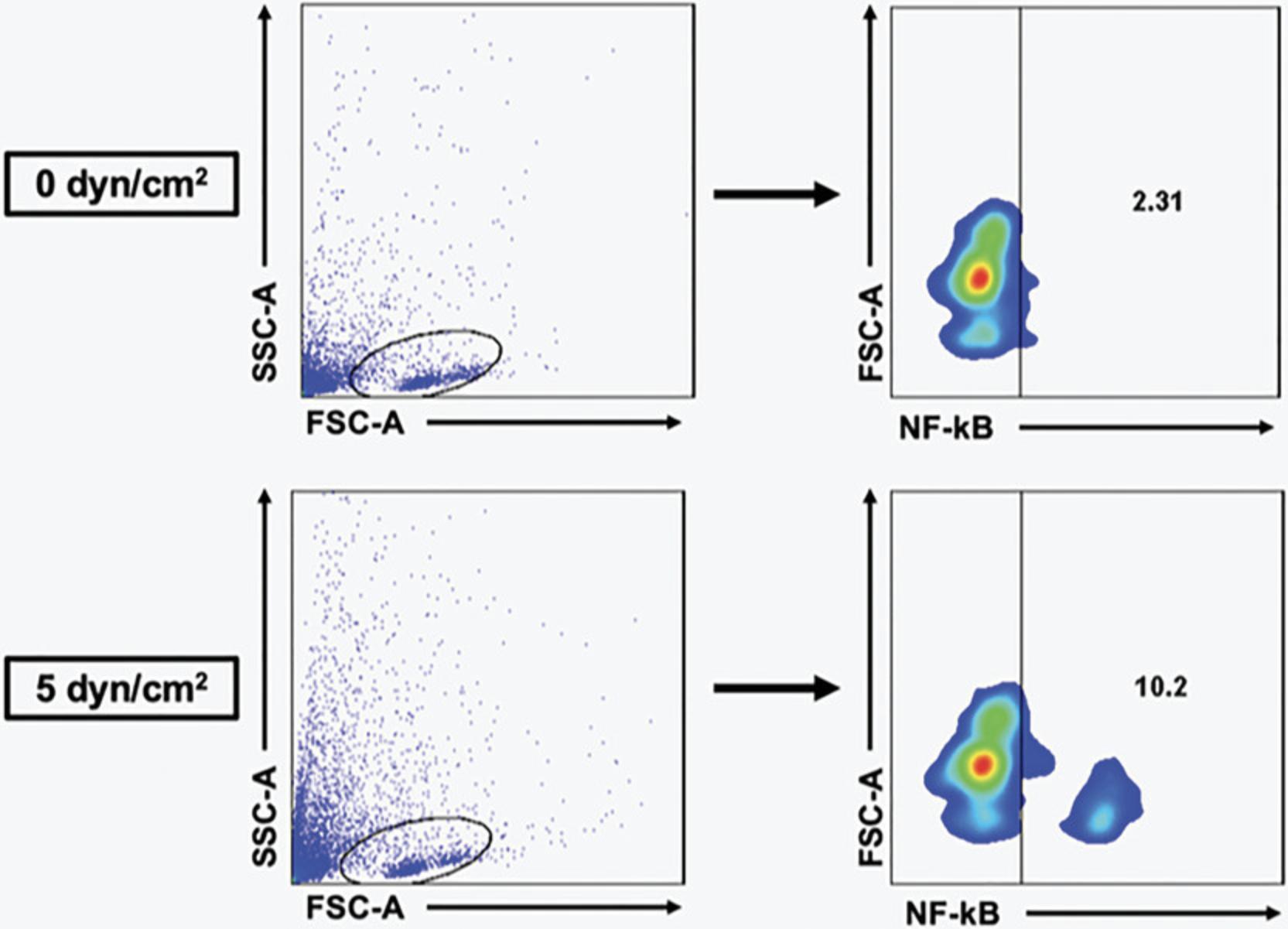
COMMENTARY
Background Information
FSS is applied to DCs via an array of Brookfield Cone-and-Plate Viscometers, which consist of a stationary plate and rotating cone in close contact. The protocol for applying FSS to cells has been previously described (Hope et al., 2022; Hope et al., 2019; Mitchell & King, 2013; Wayne et al., 2016). In our work, we collected whole blood from a cohort of eight patients with prostate cancer undergoing lab blood draw at VICC following informed consent (Table 1). PBMCs are isolated by combining blood with Ficoll-Paque and carrying out gradient centrifugation. Using a Blood Dendritic Cell Isolation Kit II from Miltenyi Biotec, PBMCs are passed through columns and DCs isolated according to the manufacturer's instructions. Following isolation, DCs are resuspended in complete RPMI medium at 1 × 105 cells/ml and exposed to 0 dyn/cm2 (“static”) or 5 dyn/cm2 of FSS (“shear”). Cells are analyzed immediately afterward or plated overnight (Fig. 2).
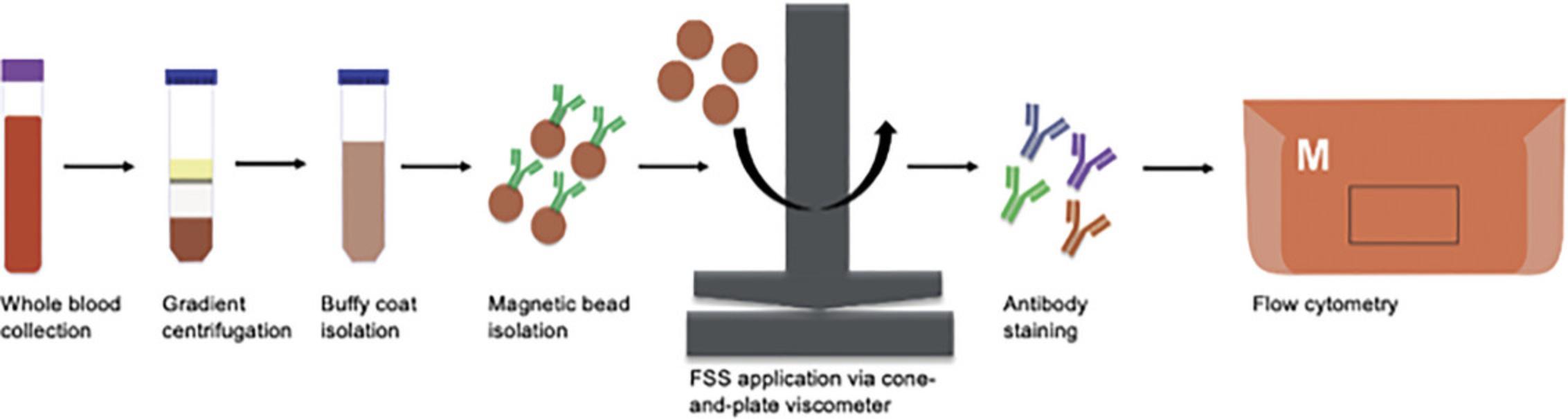
It is important to note that the cells in the flow device receive the same shear rate at all locations within the fluid, the fluid is assumed to be Newtonian, and flow is assumed to be laminar. As such, the equation used to determine the local shear rate is
Once the shear rate is set, the shear stress is determined by
Critical Parameters and Troubleshooting
It is essential that the double elution in Basic Protocol 1 result in the greatest number of cells as possible; if the cell count is too low, that will create difficulties for the success of, and subsequent analysis of, the applied FSS in Basic Protocol 2.It is therefore recommended that the steps of Basic Protocol 1 and the isolation kit manufacturer's instructions be followed very carefully, especially those pertaining to elution and passing the buffer through the column, to ensure maximal efficiency.
In the event of this protocol not producing sufficient DCs for analysis, some troubleshooting steps include the following.
1.Negative selection : Counting cells during the process that have already passed through the magnetic field to see if enough have gone through; passing additional buffer through the column (Basic Protocol 1, step 3b).
2.Positive selection : passing additional buffer through the column, passing the elution back through the column to account for any cells that have been missed (Basic Protocol 1, step 3c).
Statistical Analysis
All data are reported as mean and standard error of the mean. Statistical differences were tested using a paired t test unless otherwise indicated. GraphPad Prism software was used to perform statistical comparisons and produce figures for this article.
Understanding Results
Identification of DC subtypes
PBMCs consist of multiple cell types, including different populations of DCs. Plasmacytoid dendritic cells (pDCs) are a subset of DCs that secrete large quantities of type I interferons (IFN-I) in response to antigen exposure, particularly via toll-like receptors (TLRs) -7 and -9 (Li et al., 2017; Ye et al., 2020). Another type of DC are conventional dendritic cells (cDCs), which are professional antigen-presenting cells (APCs) and are further divided into subtypes 1 and 2, where cDC1s perform cross-presentation and cDC2s activate innate lymphoid cells (Saito et al., 2022; Sichien et al., 2017).
In our work, we analyzed DCs under static conditions for the presence of the following markers: CD303 for pDCs, CD1c for cDC2, and CD141 for cDC1 (Fig. 3A and B). These subpopulations were identified via flow cytometry, and gating and analyses were performed with FlowJo software. The total number of DCs from the subpopulations was determined for static conditions for each patient and compared to the whole population of DCs for a comparative study (Fig. 3C and D).
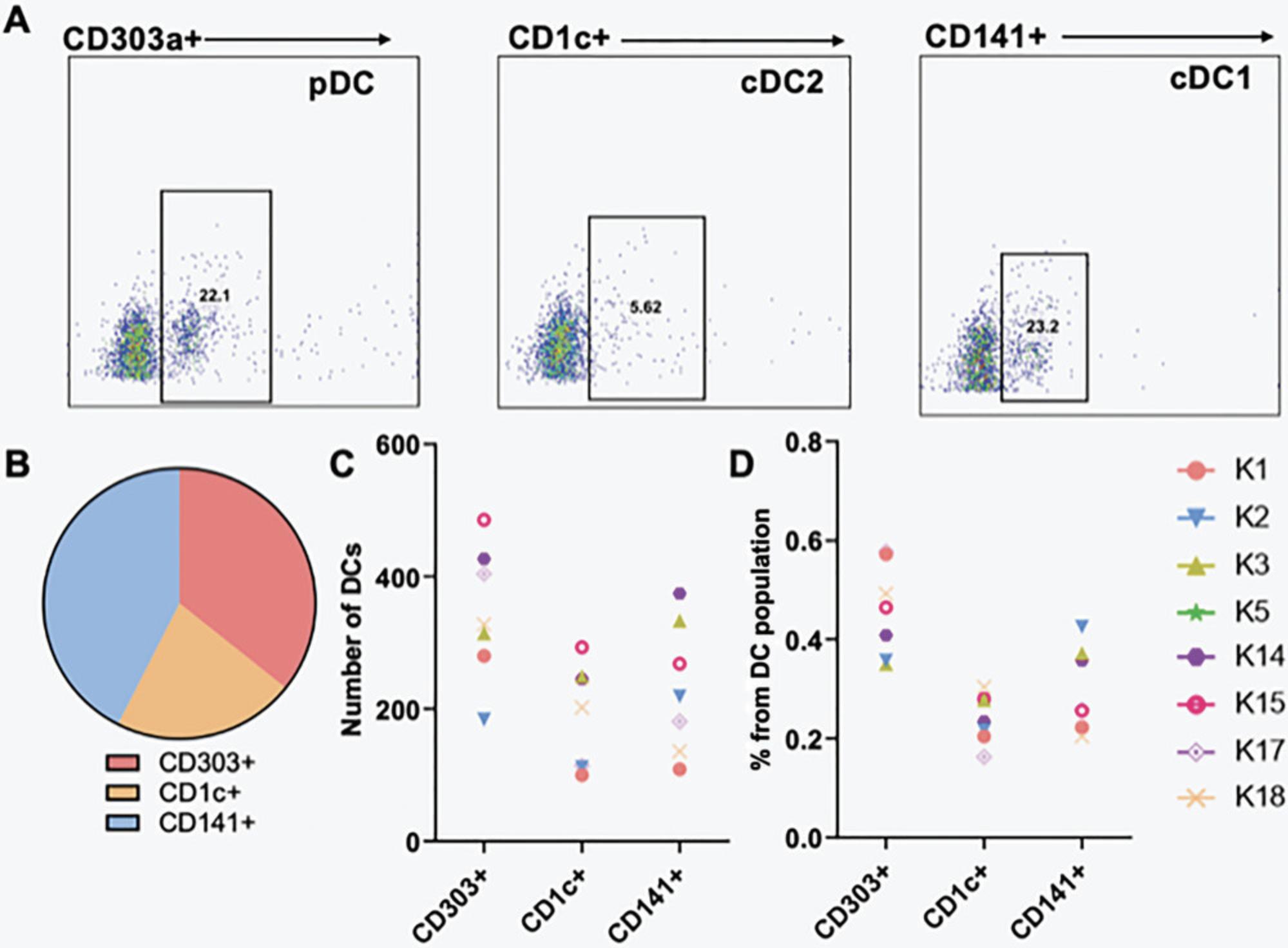
Assessment of changes in activation markers following FSS
The transcription factor NF-κB is regulated by an influx of calcium ions, and its phosphorylation ultimately leads to enhanced cytokine release as well as DC maturation and cell cycle progression (Ade et al., 2007; Ouaaz et al., 2002; Rescigno et al., 1998; Takenaka et al., 2016; Vuong et al., 2015). We used flow cytometry to analyze NF-κB phosphorylation (phospho-NF-κB) of DCs following FSS stimulation. DCs from patients with prostate cancer showed an overall increase in phospho-NF-κB following FSS stimulation compared to static condition, and this trend was observed in most patients (Fig. 4A) and in the different DC subtypes tested—pDC, cDC1, and cDC2 (Fig. 4B-D).
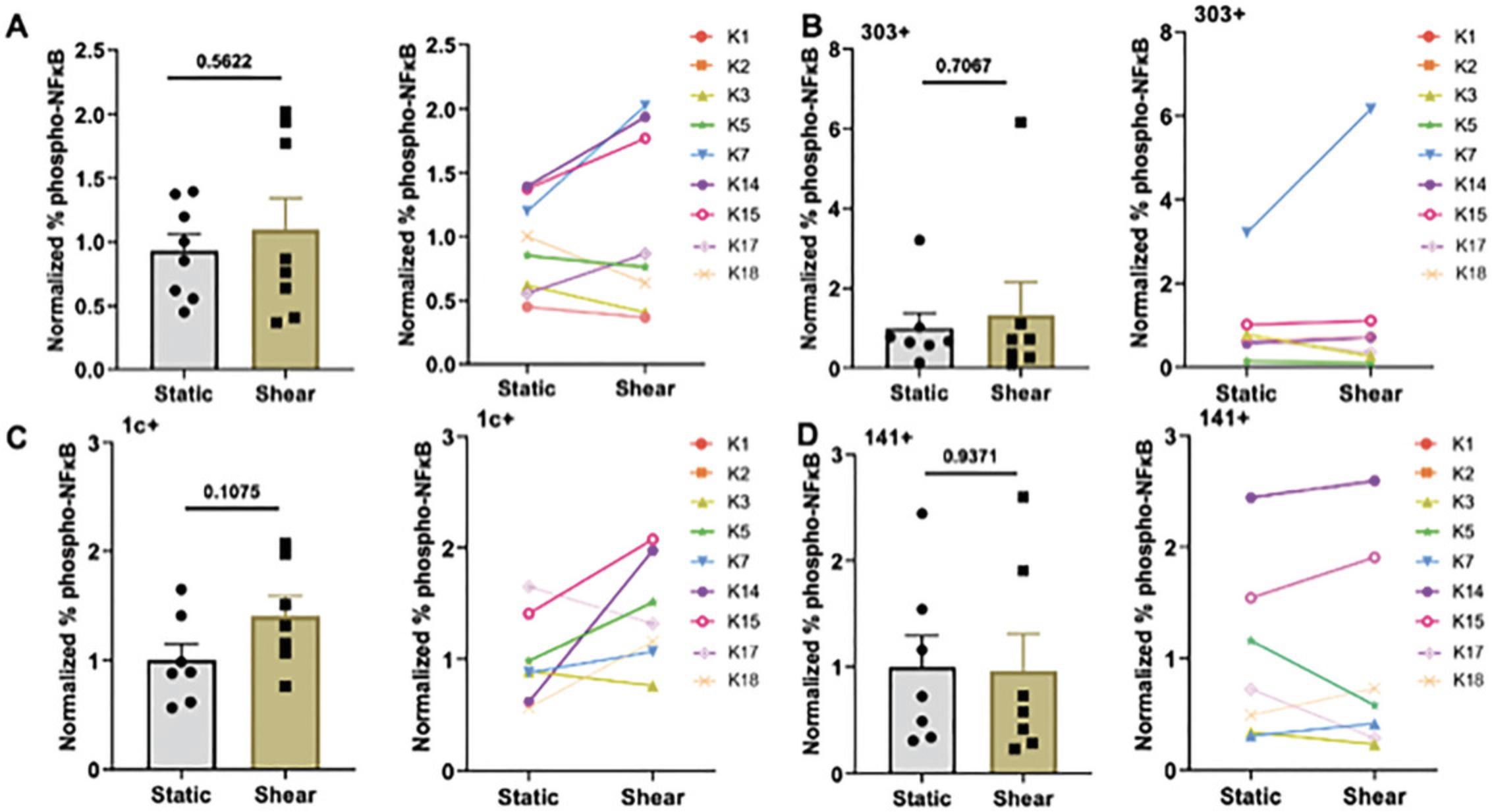
Similar to NF-κB, the transcription factor AP-1 is regulated by calcium influx and controls cytokine release and costimulatory molecule expression and proliferation in DCs. We used an antibody against phospho-c-Fos to analyze its activation in the patient DCs. Interestingly, AP-1 and NF-κB are simultaneously activated in DCs, and the balance of this activation is important to DC function (Kriehuber et al., 2005; Remoli et al., 2007; Takenaka et al., 2016). Following FSS stimulation, we analyzed the patient DCs for phosphorylation of c-Fos (phospho-c-Fos). No clear trend was observed in phospho-c-Fos (Fig. 5A). However, when categorized by pDC, cDC1, and cDC2 subtypes, clearer trends were observed with an increase in phosphorylation (Fig. 5B-D).
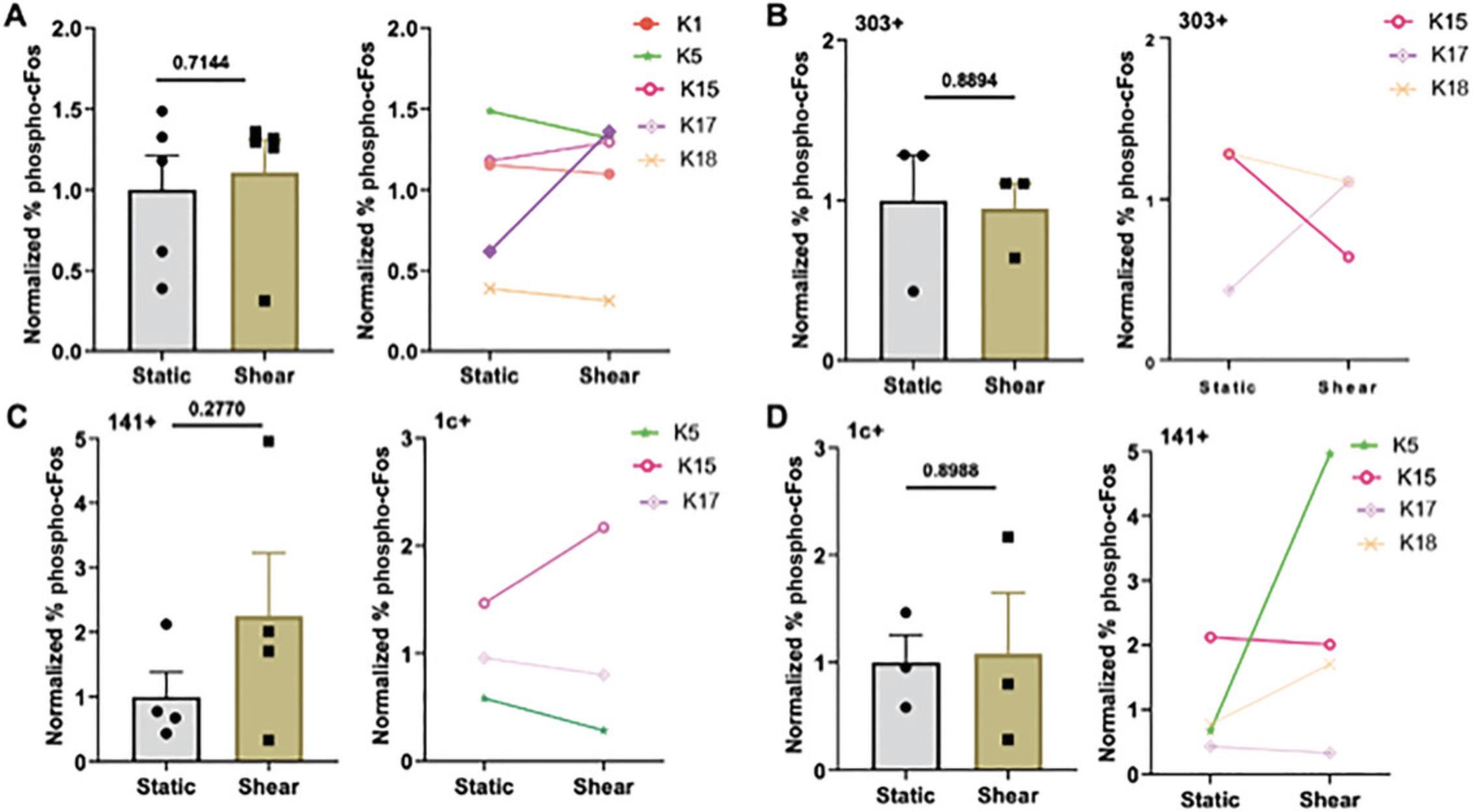
Assessment of changes in costimulatory molecule expression following FSS
Costimulatory molecules such as CD40, CD80, and CD86 are necessary for DCs to effectively form an immune synapse with T cells and initiate a response (Benvenuti, 2016; Leithner et al., 2021; Sallusto & Lanzavecchia, 2002). Following FSS stimulation, we plated DCs overnight and then analyzed them for extracellular markers via flow cytometry, comparing DCs subjected to static and shear conditions (Fig. 6A-C). There was a wide range of responses between patients, indicating that if enhanced costimulatory molecule expression was desired for the study outcomes, an additional stimulating factor would likely be necessary.
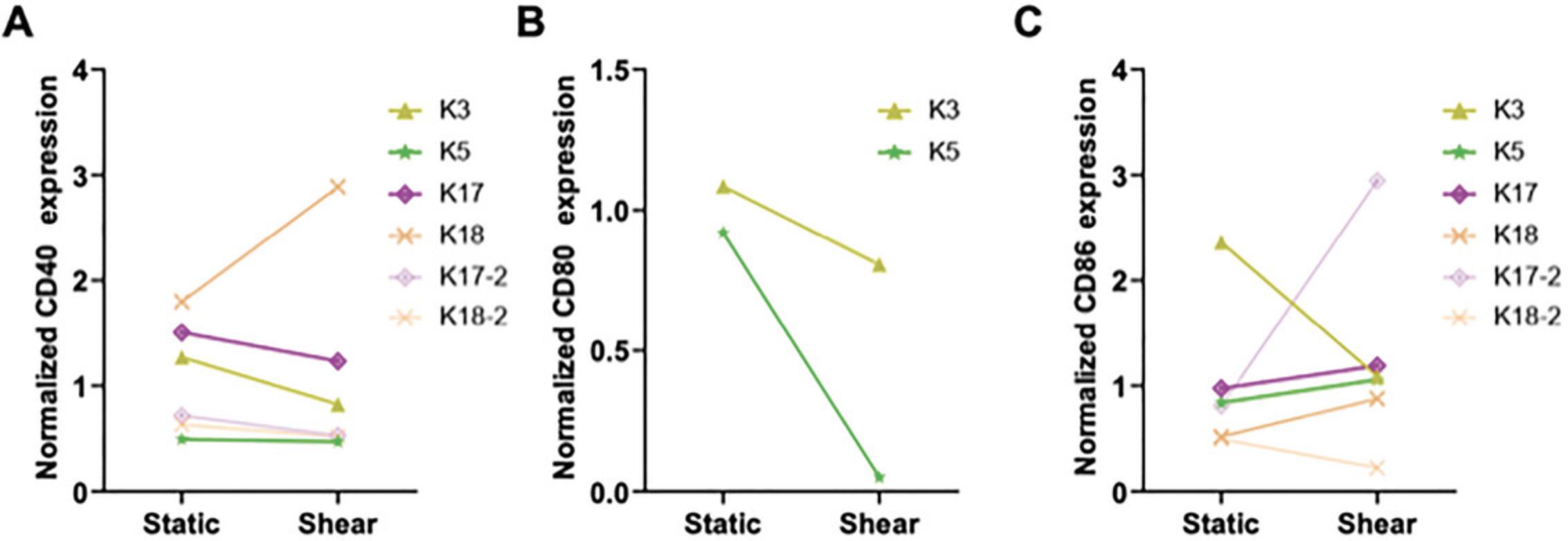
To the best of our knowledge, this was the first study of its kind, i.e. assessing DCs from patients with prostate cancer for population subtypes and then stimulating them with FSS. Our goal in developing this procedure was to learn more about DCs from patients with cancer and to study the potential for immune cell activation within these populations, motivated by the idea of applying this to combination therapy to improve the response rate and durability of systemic treatments. This patient cohort consisted of heavily treated patients with advanced mCRPC. We were able to gain insight into the different DC populations in whole blood from these patients, which comprised plasmacytoid and conventional DCs of similar counts across patients.
We were also able to determine that we could successfully activate cells ex vivo using FSS. With just 1 hr of stimulation, we saw increases in phospho-NF-κB and phospho-c-Fos, observable on a patient-by-patient basis as well as for distinct DC subsets. CDCs from patients responded most substantially to ex vivo FSS stimulation. Overall, patients receiving Pluvicto treatment responded most positively to fluid forces, indicating the potential of our methodology as a combination therapy with radioligands. The variance observed in costimulatory molecule expression following FSS indicates that an additional stimulating factor may be necessary for initiating robust T cell priming if this methodology is to be used in prostate cancer therapy. Further studies should be conducted to better understand why DC costimulatory molecule expression was heightened in specific patients after FSS stimulation.
This study is unique in that it included a relatively wide age range of patients, from 64 to 84 years old at the time of sample collection. This broader age spectrum reduces potential age-related bias that could occur due to differing immune function across age groups. This is one of the first studies to evaluate immune function in patients receiving Pluvicto and compare it to that of individuals with recent or ongoing chemotherapy. A limitation of this study was the lack of racial diversity in this small cohort. Larger studies to explore these intriguing findings further will help to identify the optimal conditions to improve prostate cancer immunotherapies using FSS cell activation.
Acknowledgments
This study was funded by U.S. National Institutes of Health (NIH) grant no. CA256054 to M.R.K. and by the U.S. National Science Foundation (NSF). This material is based on work supported by a NSF Graduate Research Fellowship Program award to J.A.D (award no. 1937963).
Author Contributions
Jenna A. Dombroski : Conceptualization; investigation; methodology; writing—original draft; writing—review and editing. Monika Antunovic : Data curation; investigation; project administration. Kerry R. Schaffer : Conceptualization; project administration; resources; supervision; writing—review and editing. Paula J. Hurley : Conceptualization; project administration; supervision; writing—review and editing. Michael R. King : Conceptualization; funding acquisition; supervision; writing—review and editing.
Conflict of Interest
The authors have no conflicts of interest to disclose.
Open Research
Data Availability Statement
The data, tools, and material (or their source) that support the protocol are available from the corresponding author upon reasonable request.
Literature Cited
- Ade, N., Antonios, D., Kerdine-Romer, S., Boisleve, F., Rousset, F., & Pallardy, M. (2007). NF-κB plays a major role in the maturation of human dendritic cells induced by NiSO4 but not by DNCB. Toxicological Sciences , 99(2), 488–501. https://doi.org/10.1093/toxsci/kfm178
- Anassi, E., & Ndefo, U. A (2011). Sipuleucel-T (Provenge) injection: The first immunotherapy agent (vaccine) for hormone-refractory prostate cancer. P & T: A Peer-Reviewed Journal for Formulary Management, 36(4), 197–202.
- Benvenuti, F. (2016). The dendritic cell synapse: A life dedicated to T cell activation. Frontiers in Immunology , 7, 70. https://doi.org/10.3389/fimmu.2016.00070
- Chen, F.-Z., & Zhao, X.-K (2013). Prostate cancer: Current treatment and prevention strategies. Iranian Red Crescent Medical Journal , 15(4), 279–284. https://doi.org/10.5812/ircmj.6499
- Dombroski, J. A., Rowland, S. J., Fabiano, A. R., Knoblauch, S. V., Hope, J. M., & King, M. R. (2023). Fluid shear stress enhances dendritic cell activation. Immunobiology , 228(6), 152744.
- Gafita, A., Marcus, C., Kostos, L., Schuster, D. M., Calais, J., & Hofman, M. S. (2022). Predictors and real-world use of prostate-specific radioligand therapy: PSMA and beyond. American Society of Clinical Oncology Educational Book , 42, 366–382. https://doi.org/10.1200/EDBK_350946
- Gu, Y.-Z., Zhao, X., & Song, X.-R. (2020). Ex vivo pulsed dendritic cell vaccination against cancer. Acta Pharmacologica Sinica , 41(7), 959–969. https://doi.org/10.1038/s41401-020-0415-5
- Hafron, J. M., Wilfehrt, H. M., Ferro, C., Harmon, M., Flanders, S. C., & McKay, R. R (2022). Real-world effectiveness of Sipuleucel-T on overall survival in men with advanced prostate cancer treated with androgen receptor-targeting agents. Advances in Therapy , 39(6), 2515–2532. https://doi.org/10.1007/s12325-022-02085-6
- Hagihara, M., Higuchi, A., Tamura, N., Ueda, Y., Hirabayashi, K., Ikeda, Y., Kato, S., Sakamoto, S., Hotta, T., Handa, S., & Goto, S. (2004). Platelets, after exposure to a high shear stress, induce IL-10-producing, mature dendritic cells in vitro. Journal of Immunology , 172(9), 5297–5303. https://doi.org/10.4049/jimmunol.172.9.5297
- Hashemi, M., Zandieh, M. A., Talebi, Y., Rahmanian, P., Shafiee, S. S., Nejad, M. M., Babaei, R., Sadi, F. H., Rajabi, R., Abkenar, Z. O., Rezaei, S., Ren, J., Nabavi, N., Khorrami, R., Rashidi, M., Hushmandi, K., Entezari, M., & Taheriazam, A. (2023). Paclitaxel and docetaxel resistance in prostate cancer: Molecular mechanisms and possible therapeutic strategies. Biomedicine & Pharmacotherapy, 160, 114392. https://doi.org/10.1016/j.biopha.2023.114392
- Haswell, E. S., Phillips, R., & Rees, D. C. (2011). Mechanosensitive channels: What can they do and how do they do it? Structure (London, England) , 19(10), 1356–1369. https://doi.org/10.1016/j.str.2011.09.005
- He, M., Roussak, K., Ma, F., Borcherding, N., Garin, V., White, M., Schutt, C., Jensen, T. I., Zhao, Y., Iberg, C. A., Shah, K., Bhatia, H., Korenfeld, D., Dinkel, S., Gray, J., Ulezko Antonova, A., Ferris, S., Donermeyer, D., Lindestam Arlehamn, C., … Klechevsky, E. (2023). CD5 expression by dendritic cells directs T cell immunity and sustains immunotherapy responses. Science , 379(6633), eabg2752. https://doi.org/10.1126/science.abg2752
- Hennrich, U., & Eder, M. (2022). [177Lu]Lu-PSMA-617 (PluvictoTM): The first FDA-approved radiotherapeutical for treatment of prostate cancer. Pharmaceuticals , 15(10), 1292. https://doi.org/10.3390/ph15101292
- Hope, J. M., Dombroski, J. A., Pereles, R. S., Lopez-Cavestany, M., Greenlee, J. D., Schwager, S. C., Reinhart-King, C. A., & King, M. R. (2022). Fluid shear stress enhances T cell activation through Piezo1. BMC Biology , 20(1), 61. https://doi.org/10.1186/s12915-022-01266-7
- Hope, J. M., Lopez-Cavestany, M., Wang, W., Reinhart-King, C. A., & King, M. R. (2019). Activation of Piezo1 sensitizes cells to TRAIL-mediated apoptosis through mitochondrial outer membrane permeability. Cell Death & Disease, 10(11), 837. https://doi.org/10.1038/s41419-019-2063-6
- Kantoff, P. W., Higano, C. S., Shore, N. D., Berger, E. R., Small, E. J., Penson, D. F., Redfern, C. H., Ferrari, A. C., Dreicer, R., Sims, R. B., Xu, Y., Frohlich, M. W., Schellhammer, P. F, & IMPACT Study Investigators. (2010). Sipuleucel-T immunotherapy for castration-resistant prostate cancer. New England Journal of Medicine , 363(5), 411–422. https://doi.org/10.1056/NEJMoa1001294
- Kriehuber, E., Bauer, W., Charbonnier, A. S., Winter, D., Amatschek, S., Tamandl, D., Schweifer, N., Stingl, G., & Maurer, D. (2005). Balance between NF-κB and JNK/AP-1 activity controls dendritic cell life and death. Blood , 106(1), 175–183. https://doi.org/10.1182/blood-2004-08-3072
- Leithner, A., Altenburger, L. M., Hauschild, R., Assen, F. P., Rottner, K., Stradal, T. E. B., Diz-Muñoz, A., Stein, J. V., & Sixt, M. (2021). Dendritic cell actin dynamics control contact duration and priming efficiency at the immunological synapse. Journal of Cell Biology , 220(4), e202006081. https://doi.org/10.1083/jcb.202006081
- Li, S., Wu, J., Zhu, S., Liu, Y. J., & Chen, J. (2017). Disease-associated plasmacytoid dendritic cells. Frontiers in Immunology , 8, 1268. https://doi.org/10.3389/fimmu.2017.01268
- Litwin, M. S., & Tan, H. J. (2017). The diagnosis and treatment of prostate cancer: A review. JAMA , 317(24), 2532. https://doi.org/10.1001/jama.2017.7248
- Mitchell, M. J., & King, M. R. (2013). Fluid shear stress sensitizes cancer cells to receptor-mediated apoptosis via trimeric death receptors. New Journal of Physics , 15(1), 015008. https://doi.org/10.1088/1367-2630/15/1/015008
- National Cancer Institute. (2022). Cancer Stat Facts: Prostate Cancer. National Cancer Institute Surveillance Epidemiology End Results Program. https://seer.cancer.gov/statfacts/html/prost.html
- Ouaaz, F., Arron, J., Zheng, Y., Choi, Y., & Beg, A. A. (2002). Dendritic cell development and survival require distinct NF-κB subunits. Immunity , 16(2), 257–270. https://doi.org/10.1016/S1074-7613(02)00272-8
- Parent, E. E., & Kase, A. M. (2022). A treatment paradigm shift: Targeted radionuclide therapies for metastatic castrate resistant prostate cancer. Cancers , 14(17), 4276. https://doi.org/10.3390/cancers14174276
- Posdzich, P., Darr, C., Hilser, T., Wahl, M., Herrmann, K., Hadaschik, B., & Grünwald, V. (2023). Metastatic prostate cancer—A review of current treatment options and promising new approaches. Cancers , 15(2), 461. https://doi.org/10.3390/cancers15020461
- Remoli, M. E., Ragimbeau, J., Giacomini, E., Gafa, V., Severa, M., Lande, R., Pellegrini, S., & Coccia, E. M. (2007). NF-κB is required for STAT-4 expression during dendritic cell maturation. Journal of Leukocyte Biology , 81(1), 355–363. https://doi.org/10.1189/jlb.0506319
- Rescigno, M., Martino, M., Sutherland, C. L., Gold, M. R., & Ricciardi-Castagnoli, P. (1998). Dendritic cell survival and maturation are regulated by different signaling pathways. Journal of Experimental Medicine , 188(11), 2175–2180. https://doi.org/10.1084/jem.188.11.2175
- Rodrigues, D. N., Butler, L. M., Estelles, D. L., & de Bono, J. S. (2014). Molecular pathology and prostate cancer therapeutics: From biology to bedside: Molecular pathology and prostate cancer therapeutics. Journal of Pathology , 232(2), 178–184. https://doi.org/10.1002/path.4272
- Saito, Y., Komori, S., Kotani, T., Murata, Y., & Matozaki, T. (2022). The role of type-2 conventional dendritic cells in the regulation of tumor immunity. Cancers , 14(8), 1976. https://doi.org/10.3390/cancers14081976
- Sallusto, F., & Lanzavecchia, A. (2002). The instructive role of dendritic cells on T-cell responses. Arthritis Research , 4(Suppl 3), S127. https://doi.org/10.1186/ar567
- Sichien, D., Lambrecht, B. N., Guilliams, M., & Scott, C. L. (2017). Development of conventional dendritic cells: From common bone marrow progenitors to multiple subsets in peripheral tissues. Mucosal Immunology , 10(4), 831–844. https://doi.org/10.1038/mi.2017.8
- Siegel, R. L., Miller, K. D., Wagle, N. S., & Jemal, A. (2023). Cancer statistics, 2023. CA: A Cancer Journal for Clinicians , 73(1), 17–48. https://doi.org/10.3322/caac.21763
- Sumanasuriya, S., & de Bono, J. (2018). Treatment of advanced prostate cancer—A review of current therapies and future promise. Cold Spring Harbor Perspectives in Medicine , 8(6), a030635. https://doi.org/10.1101/cshperspect.a030635
- Tagai, E. K., Miller, S. M., Kutikov, A., Diefenbach, M. A., Gor, R. A., Al-Saleem, T., Chen, D. Y. T., Fleszar, S., & Roy, G. (2019). Prostate cancer patients’ understanding of the Gleason scoring system: Implications for shared decision-making. Journal of Cancer Education , 34(3), 441–445. https://doi.org/10.1007/s13187-018-1320-1
- Takenaka, M. C., Araujo, L. P., Maricato, J. T., Nascimento, V. M., Guereschi, M. G., Rezende, R. M., Quintana, F. J., & Basso, A. S. (2016). Norepinephrine controls effector T cell differentiation through β2-adrenergic receptor–mediated inhibition of NF-κB and AP-1 in dendritic cells. Journal of Immunology , 196(2), 637–644. https://doi.org/10.4049/jimmunol.1501206
- Vuong, B., Hogan-Cann, A. D. J., Alano, C. C., Stevenson, M., Chan, W. Y., Anderson, C. M., Swanson, R. A., & Kauppinen, T. M (2015). NF-κB transcriptional activation by TNFα requires phospholipase C, extracellular signal-regulated kinase 2 and poly(ADP-ribose) polymerase-1. Journal of Neuroinflammation , 12(1), 229. https://doi.org/10.1186/s12974-015-0448-8
- Wang, I., Song, L., Wang, B. Y., Rezazadeh Kalebasty, A., Uchio, E., & Zi, X. (2022). Prostate cancer immunotherapy: A review of recent advancements with novel treatment methods and efficacy. American Journal of Clinical and Experimental Urology , 10(4), 210–233.
- Wayne, E. C., Chandrasekaran, S., Mitchell, M. J., Chan, M. F., Lee, R. E., Schaffer, C. B., & King, M. R (2016). TRAIL-coated leukocytes that prevent the bloodborne metastasis of prostate cancer. Journal of Controlled Release , 223, 215–223. https://doi.org/10.1016/j.jconrel.2015.12.048
- Wei, X. X., Fong, L., & Small, E. J. (2015). Prostate cancer immunotherapy with Sipuleucel-T: Current standards and future directions. Expert Review of Vaccines , 14(12), 1529–1541. https://doi.org/10.1586/14760584.2015.1099437
- Yamamoto, K., Korenaga, R., Kamiya, A., & Ando, J. (2000). Fluid shear stress activates Ca2+ influx into human endothelial cells via P2X4 purinoceptors. Circulation Research , 87(5), 385–391. https://doi.org/10.1161/01.RES.87.5.385
- Ye, Y., Gaugler, B., Mohty, M., & Malard, F. (2020). Plasmacytoid dendritic cell biology and its role in immune-mediated diseases. Clinical & Translational Immunology, 9(5), e1139. https://doi.org/10.1002/cti2.1139